- Division of Infectious Diseases, Center for Inflammation and Tolerance, Cincinnati Children’s Hospital Medical Center, Department of Pediatrics, University of Cincinnati College of Medicine, Cincinnati, OH, United States
Bidirectional exchange of cells between mother and fetus occurs during pregnancy, and persistence of these genetically foreign cells establishes long-term microchimerism in both individuals after parturition. Since women can have multiple pregnancies, and all mothers were once daughters themselves, the microchimeric milieu in each woman could theoretically contain cells from a variety of origins, including from their own mothers as well as their babies from each pregnancy. Interestingly and in sharp contrast to this prediction, we recently showed preexisting populations of microchimeric cells are lost following pregnancy and associated with seeding of new fetal microchimeric cells. Complete loss of preexisting microchimeric cells in this context draws parallels to immunological rejection with synchronized elimination of cells and tissues that express defined discordant antigens. This perspective evaluates this provocative hypothesis regarding pregnancy induced rejection of microchimeric cells, including new experimental data comparing microchimerism levels in mice simultaneously lacking B and T cells before pregnancy, and after parturition with primary and secondary pregnancies.
Introduction
Presence of genetically discordant cells in individuals is called chimerism, and persistence of these cells at very low frequency (<1 in 105 cells) is called microchimerism (1–6). Although chimerism is intentionally induced after solid-organ and stem cell transplantation, microchimerism primarily stems from the bidirectional exchange of cells between mother and fetus during pregnancy, with persistence of these cells in both individuals after parturition. Persistence of fetal cells in mothers is called fetal microchimerism, and includes classical examples of Y chromosome expressing cells in the peripheral blood of mothers decades after giving birth to sons (7). Reciprocally, persistence of maternal cells in offspring after delivery is called maternal microchimerism, classically demonstrated by cells expressing two X chromosomes in male offspring (8).
Directly related to the presence of maternal microchimeric cells is expanded immune tolerance in offspring that extends to non-inherited maternal antigen (NIMA) that these cells express (9, 10). Classical examples include resistance to Rhesus (Rh) sensitization in Rh negative women born to Rh positive mothers (11), selective B cell hypo-responsiveness to noninherited maternal HLA haplotypes in transfusion dependent individuals (12), and prolonged renal allograft survival in sibling donor-recipient pairings when matched for NIMA compared with noninherited paternal MHC haplotypes (13). To further dissect the immunological basis responsible for NIMA-specific tolerance, we previously investigated the “chicken and egg” relationship between expanded tolerance in this context and persistence of NIMA-expressing maternal microchimeric cells. We showed experimental depletion of maternal microchimeric cells overturned NIMA-specific tolerance, thereby solidifying their necessity for sustained tolerance in offspring (14). In particular, allogeneic pregnancies sired by males expressing NIMA-matched MHC haplotypes associated with expanded accumulation of immune-suppressive FOXP3+ regulatory T cells (Tregs) with NIMA-specificity were highly resilient to fetal wastage induced by perturbations in fetal tolerance (14). These phenotypes, including protection against fetal wastage and NIMA-specific Treg accumulation, were each overturned in mice depleted of maternal microchimeric cells (14). Given the dominant role reproductive fitness plays in trait selection, cross-generational reproductive fitness driven by maternal microchimeric cells in this context highlights one aspect of nature’s intent with what seems like added immunological complexity associated with individuals being constitutively chimeric (1).
Building upon this line of reasoning that maternal microchimeric cells promote reproductive fitness by enforcing fetal tolerance in the future pregnancies of their daughters, we reasoned partner specific protection conferred by prior pregnancy against complications in future pregnancy might similarly require fetal microchimeric cells that persist in mothers after parturition (15–17). Considering all mothers were each at one time daughters themselves, this reasoning further led to the expectation of an active “microchiome” comprised by an assortment of antigenically diverse genetically foreign cells purposefully retained in reproductive age females to promote reproductive fitness (18). Besides for reproductive benefits, microchimeric cells may at the same time confer other physiological benefits related to tissue regeneration and repair (19–22), but also with potential offsetting negative proinflammatory consequences related to autoimmune disorders such as type 1 diabetes and scleroderma (23–25). Together, these considerations opened up new questions regarding how the assortment of microchimeric cells from unique genetic origin interact with each other in mothers, and whether the niche for microchimeric cells is fixed or capable of expansion in perpetuity.
These questions were recently addressed by evaluating how pregnancy with seeding of new fetal microchimeric cells impacts levels of maternal microchimeric cells; and how secondary pregnancy impacts levels of fetal microchimeric cells retained in mothers from prior pregnancy. In each of these contexts, pregnancy with seeding of new fetal microchimeric cells was shown to displace preexisting microchimeric cells in maternal tissues (26). Preexisting maternal microchimeric cells retained in female mice were eliminated to near completion and replaced by fetal microchimeric cells retained in parous microchimeric cells after primary pregnancy. Likewise, fetal microchimeric cells retained in parous female mice after primary pregnancy were eliminated to near completion and replaced by fetal microchimeric cells from the second pregnancy. Loss of preexisting maternal and fetal microchimerism was surprising given how rare maternal and fetal microchimerism exists in maternal tissues each on the order of 1 in 105 to 107 cells (26). Why can't tissues contain another set of cells of such rarity – similar to how the compartment of memory T cells grows in size with immunological experience (27)?
Another consideration relates to the efficiency whereby preexisting microchimeric cells are eliminated after pregnancy. Efficient loss of preexisting microchimeric cells in this context draws parallels to immunological rejection with synchronized elimination of cells which express defined discordant antigens. These considerations led us to refocus our established platform for measuring maternal and fetal microchimerism to investigate whether similar dynamics and replacement occurs in mothers simultaneously lacking B and T cells, and to evaluate whether these adaptive immune components driving classical rejection phenotypes are essential for pregnancy induced displacement of preexisting maternal and fetal microchimeric cells.
Maternal B and T cells non-essential for fetal microchimeric cell displacement
For investigating the necessity of maternal B and T cells, Rag1-/- female mice deficient in these adaptive immune components on the H-2b C57BL/6 background were used for breeding (28). Similar to our recently published breeding scheme, primary allogeneic pregnancy were sired by transgenic male mice ubiquitously expressing a recombinant cell surface protein containing ovalbumin (OVA) in all cells on the H-2d Balb/c background so that fetal microchimeric cells retained after primary pregnancy can be enumerated by quantifying OVA+ DNA levels (Figure 1A) (14, 26). Thereafter for a subset of mice, non-transgenic (non-OVA expressing) H-2k+ third party male mice on the CBA background were used to sire secondary pregnancy so that H-2k+ fetal microchimeric cells seeded in secondary pregnancy can be distinguished from OVA+ fetal microchimeric cells seeded after primary pregnancy (Figure 1A).
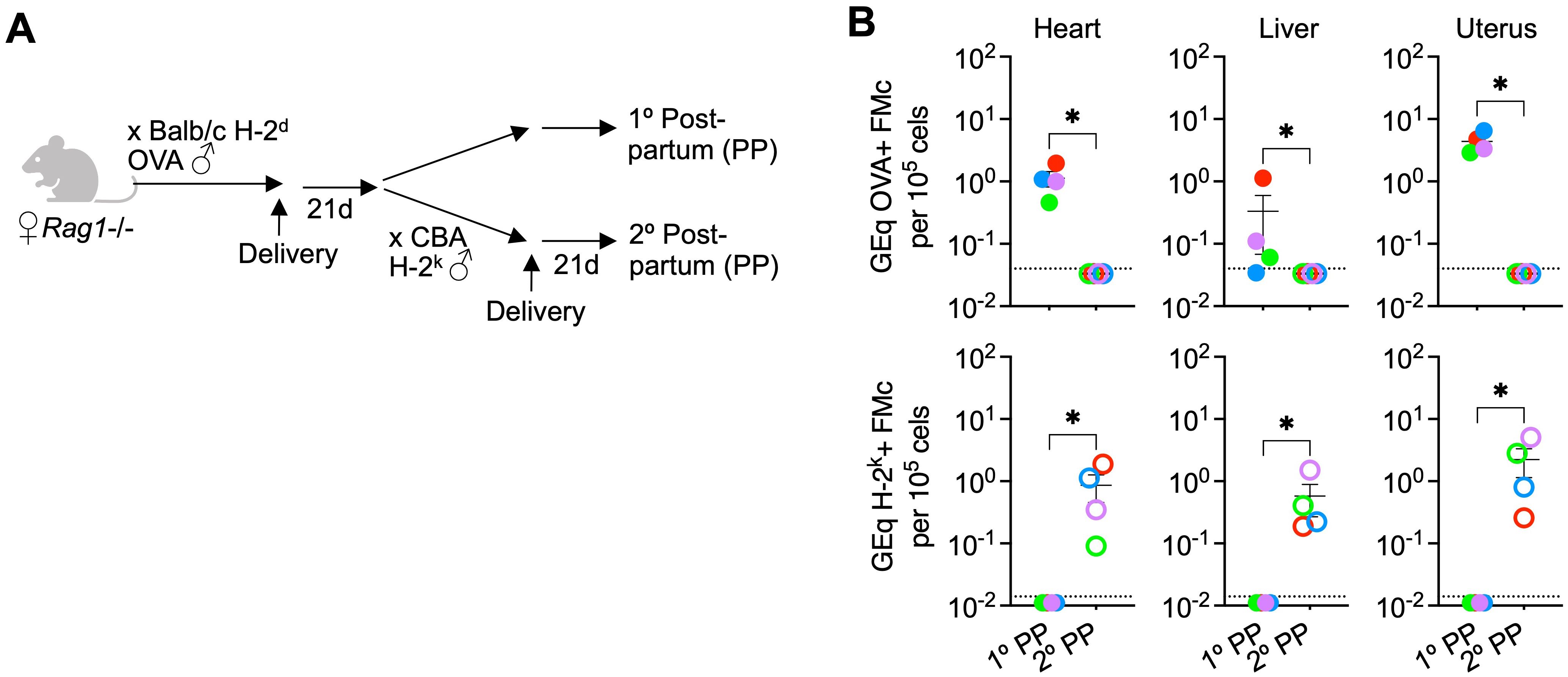
Figure 1. Fetal microchimeric cells displaced after pregnancy despite lack of maternal B and T cells. (A) Mating scheme for Rag1-/- (Jackson laboratory, strain #002216) sired by OVA+ transgenic males allowing identification of microchimeric cells by quantifying OVA+ DNA (primary postpartum) or after secondary pregnancy sired by non-transgenic CBA H-2k males allowing identification of microchimeric cells by quantifying H-2k+ DNA (secondary postpartum). (B) Levels of OVA+ DNA specific to OVA+ fetal microchimeric cells or H-2k+ DNA specific to H-2k+ fetal microchimeric cells in each tissue among Rag1-/- mice 21 days postpartum after primary allogeneic pregnancy sired by OVA+ H-2d Balb/c males (filled), or secondary pregnancy sired by H-2k+ CBA males (open) quantified using DNA extraction and quantitative PCR methods described in reference (26). Each point represents the data from an individual mouse, with colors used to depict data from tissues of the same mouse, and representative of at least two independent experiments each with similar results. Bar, mean ± standard error. *P < 0.05. Mouse figures were prepared using Biorender; graphs and statistics analyzed using Graphpad Prism (Version 10.1).
These experiments showed consistent recovery of OVA+ fetal microchimeric cells after primary pregnancy in the tissues of Rag1-/- female mice (Figure 1B), and to levels similar to that previously described for B and T cell sufficient WT mice (26). Remarkably, and in contrast to the prediction that rejection by maternal adaptive immune cells drives displacement of these fetal microchimeric cells, OVA+ fetal microchimeric cells declined to below the limits of detection (1 in 3.3 x 106 cells) in the heart, liver and uterus of Rag1-/- female mice after secondary pregnancy sired by H-2k+ CBA males (Figure 1B). For evaluating potential microchimeric cell displacement, we focused particularly on the heart, liver and uterus because these tissues consistently contain the highest levels of microchimeric cells (14, 26). Loss of OVA+ fetal microchimeric cells seeded in primary pregnancy was associated with the accumulation of H-2k+ microchimeric cells after secondary pregnancy compared with H-2k+ cells consistently below the limits of detection (1 in 107 cells) in the tissues of Rag1-/- control mice after primary pregnancy but without secondary pregnancy (Figure 1B). Our interpretation of these results is that maternal B and T cells are simultaneously non-essential for pregnancy induced displacement of fetal microchimeric cells, given similar levels of OVA+ fetal microchimerism after primary pregnancy, and replacement by H-2k+ fetal microchimeric cells after secondary pregnancy in Rag1-/- female mice.
Maternal B and T cells non-essential for maternal microchimeric cell displacement
We next evaluated the necessity for maternal B and T cells for pregnancy induced displacement of maternal microchimerism by comparing levels of maternal microchimeric cells in Rag1-/- female mice after primary pregnancy compared with Rag1-/- virgin control mice. Using our validated breeding scheme (14, 26), non-transgenic female Rag1-/- offspring born to Rag1+/- mothers heterozygous for the aforementioned transgene encoding constitutive expression of a recombinant cell surface protein containing OVA were utilized, allowing maternal microchimeric cells retained in offspring to be enumerated by quantifying OVA+ DNA levels (Figure 2A). Thereafter for investigating how fetal microchimeric cells seeded in pregnancy impacts levels of these maternal microchimeric cells, non-transgenic H-2k+ third party male mice on the CBA background were used to sire primary pregnancy so that H-2k+ fetal microchimeric cells can be distinguished from preexisting OVA+ maternal microchimeric cells (Figure 2A).
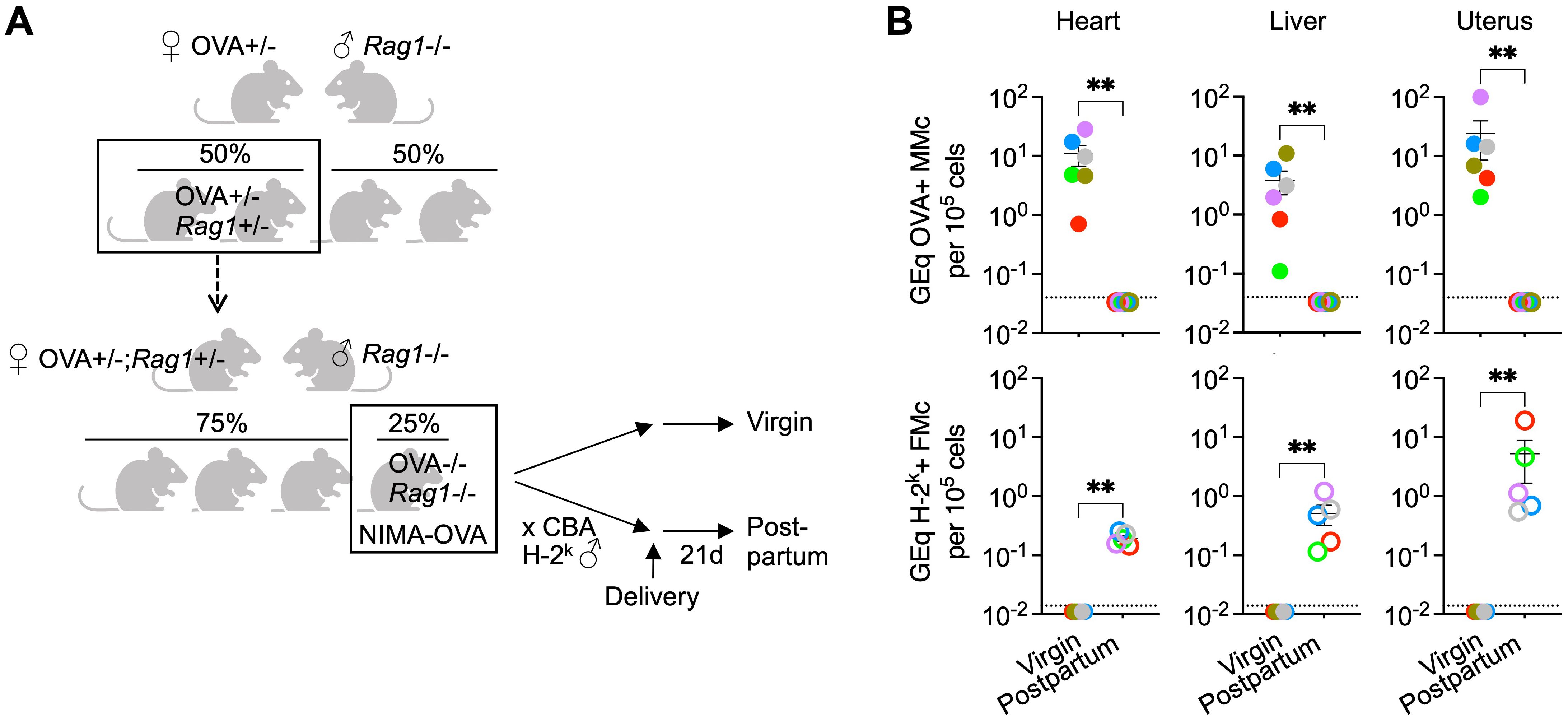
Figure 2. Maternal microchimeric cells displaced after pregnancy despite lack of maternal B and T cells. (A) Mating scheme for generating Rag1-/- born to OVA+/- mothers transforming OVA into a surrogate NIMA and tracking maternal microchimeric cells after quantifying OVA+ DNA in virgin control or after pregnancy sired by non-transgenic CBA H-2k males (postpartum). (B) Levels of OVA+ DNA specific to OVA+ maternal microchimeric cells or H-2k+ DNA specific to H-2k+ fetal microchimeric cells in each tissue among Rag1-/- NIMA-OVA mice 21 days postpartum after allogeneic pregnancy sired by H-2k+ CBA males (open) or age-matched virgin Rag1-/- NIMA-OVA mice (filled) quantified using DNA extraction and quantitative PCR methods described in reference (26). Each point represents the data from an individual mouse, with colors used to depict data from tissues of the same mouse, and representative of at least two independent experiments each with similar results. Bar, mean ± standard error. **P < 0.01. Mouse figures were prepared using Biorender; graphs and statistics analyzed using Graphpad Prism (Version 10.1).
These experiments showed the expected recovery of OVA+ maternal microchimeric cells in the heart, liver and uterus of Rag1-/- virgin female offspring (Figure 2B), and to levels similar to that previously described for B and T cell sufficient WT mice (26). Similar to non-essential roles for maternal B and T cells for displacement of fetal microchimerism, OVA+ maternal microchimeric cells consistently declined to below the limits of detection (1 in 3.3 x 106 cells) in maternal tissues of Rag1-/- female mice after primary pregnancy sired by H-2k+ CBA males (Figure 2B). Loss of OVA+ maternal microchimerism was associated with the accumulation of H-2k+ fetal microchimeric cells after primary pregnancy, compared with H-2k+ cells consistently below the limits of detection (1 in 107 cells) in the tissues of virgin control Rag1-/- mice (Figure 2B). Our interpretation of these results is that maternal B and T cells are simultaneously non-essential for pregnancy induced displacement of maternal microchimeric cells given similar OVA+ maternal microchimerism levels in Rag1-/- virgin mice, and their efficient replacement by H-2k+ fetal microchimeric cells after primary pregnancy in Rag1-/- female mice.
Fetal B and T cells non-essential for microchimeric cell displacement
Although using non-leaky Rag1-/- mice conclusively demonstrates non-essential roles for maternal B and T cells, we recognize that microchimerism may also extend to these adaptive immune cells. For example, preconceptually primed maternal pathogen-specific T cells have been described to be vertically transferred and protect neonatal mice against infection (29), and microchimerism in fetal or maternal antibody producing cells are associated with accumulation of arthritis-specific autoantibodies (30). Thus to further investigate the necessity of newly seeded fetal microchimeric B and T cells for displacement of preexisting microchimeric cells, Rag1-/- males on the C57BL/6 background were used instead of CBA H-2k males in the aforementioned platforms for evaluating microchimeric cell displacement.
We reasoned use of Rag1-/- male to sire secondary pregnancy in Rag1-/- females with primary pregnancy sired by OVA expressing H-2d Balb/c allows the importance of fetal microchimeric B and T cells in displacing OVA+ fetal microchimeric cells to be evaluated (Figure 1A). Likewise, use of Rag1-/- male to sire primary pregnancy in non-transgenic female Rag1-/- offspring born to Rag1+/- mothers heterozygous for the OVA transgene allows evaluating the necessity for fetal microchimeric B and T cells in displacing OVA+ maternal microchimeric cells (Figure 2A). Together these experiments showed non-essential roles for fetal microchimeric B and T cells in displacement of preexisting microchimeric cells. OVA+ DNA was reduced to below the limits of detection (1 in 3.3 X 106 cells) in the heart, liver and uterus for Rag1-/- females with primary pregnancy sired by OVA expressing H-2d Balb/c mice after secondary pregnancy with Rag1-/- males (6 of 6 tissues from 2 postpartum mice). In turn, OVA+ DNA was similarly reduced to below the limits of detection in the heart, liver and uterus for Rag1-/- virgin females with OVA+ maternal microchimeric cells after primary pregnancy sired by Rag1-/- males (12 of 12 tissues from 4 postpartum mice). Along with demonstrating non-essential roles for fetal microchimeric B and T cells in loss of preexisting microchimeric cells, use of Rag1-/- males on the C57BL/6 H-2b background for establishing syngeneic pregnancy also show displacement in this context is not limited only allogeneic pregnancy.
Discussion
One of the most fascinating and perplexing immunological considerations pertaining to microchimeric cells is how these cells persist and escape rejection, despite being genetically foreign. Persistence in this context appears to be life-long, given recovery of fetal microchimeric cells in women decades after giving birth and recovery of maternal microchimeric cells in adult individuals (1, 2, 5, 7). An interesting new twist to this conundrum is how pregnancy appears to so efficiently reset the microchimeric cell niche, with near complete replacement of preexisting microchimeric cells with new fetal microchimeric cells. Herein we describe our rationale and experience investigating the hypothesis that loss of preexisting microchimeric cells reflects rejection by evaluating this process using Rag1-deficient mice simultaneously lacking B and T cells. Remarkably, we find absence of these adaptive immune components known to drive rejection of genetically foreign cells and tissues in transplantation does not override pregnancy induced displacement of either preexisting maternal microchimeric cells in mice after primary pregnancy, or preexisting fetal microchimeric cells seeded by primary pregnancy in mice after secondary pregnancy. We recognize that adaptive features associated with rejection have been described for more traditional innate immune cell types including natural killer cells, innate lymphoid cells and monocyte-macrophage cells remaining in Rag1-/- mice. For example, natural killer cells are capable of context-specific self versus non-self discrimination, and with transplant induced activation licensed to kill allogeneic target cells (31). Phenotypically distinct proinflammatory compared with protective innate lymphoid cells are increasing associated with allograft rejection versus acceptance (32, 33), and macrophage cells with trained activation may reduce the threshold of stimulation T cell mediated allograft rejection (34). Thus evaluating the contribution of these more classical innate immune cells in pregnancy induced microchimerism dynamics and displacement, including earlier time points after parturition, are important areas for future investigation.
Perhaps a broader consideration is whether viewing loss and replacement of microchimeric cells through the lens of traditional rejection and loss of tolerance to non-self antigens expressed by microchimeric cells is too simplistic. For example, second pregnancy induced loss of fetal microchimeric cells seeded in primary pregnancy does not functionally erase tolerance to fetal antigens encountered in first pregnancy. We recently showed enforced tolerance is sustained after encounter with these same antigens in tertiary pregnancy through expanded accumulation of FOXP3+ Tregs derived from a pool of cells that lose FOXP3-expression called exTregs (26). Thus, if rejection drives the loss of preexisting fetal microchimeric cells allowing seeding of new fetal microchimeric cells in subsequent pregnancy, it is difficult to imagine why tolerance is not overturned, and instead reinforced. This example also illustrates an interesting distinction between how fetal microchimeric cells and maternal microchimeric cells prime tolerance. Tolerance to NIMA appears to be completely erased after depletion or displacement of maternal microchimerism, whereas tolerance to fetal antigens encountered even in past pregnancies seem to be retained in mothers despite intervening pregnancy sired by genetically discordant male partners (26, 35). Thus, outstanding questions remain regarding whether NIMA-specific tolerance is transformed to NIMA-specific sensitization in women after pregnancy, and especially with male partners without overlapping NIMAs. Here a tantalizing consideration is the biphasic effects on renal allograft survival where NIMA-matching leads to a higher incidence of early rejection in some individuals despite overall improved long-term allograft survival (13). If rejection drives loss of maternal microchimeric cells, we would hypothesize parous women would be highly enriched among individuals with early rejection, as a complementary explanation to “split tolerance” for the biphasic response to NIMA-matched tissues (36).
Regardless of whether classical rejection mechanisms are applicable for microchimeric cell replacement, another fascinating consideration is the surprisingly fixed niche of these exceptionally rare cells that appears to allow the presence of cells from only a single source at a time. Data we present here further show non-essential roles for B and T cells in restricting this remarkably fixed niche given efficient replacement of maternal microchimeric cells by fetal microchimeric cells in Rag1-/- after primary pregnancy, and similarly efficient replacement of preexisting fetal microchimeric cells in Rag1-/- mothers by new fetal microchimeric cells in subsequent pregnancy. How this physiological niche is controlled, and apparently reset by pregnancy remains uncertain. Given the wide heterogeneity in maternal microchimeric and fetal microchimeric cell levels within each tissue and across multiple tissues in individual mice (14, 26), this niche is unlikely to be simply numerical where individual tissues can only accommodate a fixed number of rare microchimeric cells. If not numerical and not immunological, how does it work?
One intriguing hypothesis for why microchimerism may be restricted to cells from only a single source at a time is to minimize immunological complexity. By extension, inefficient or incomplete microchimeric cell replacement allowing for multiple sets of genetically discordant cells to be present in women at the same time leading to more immunological complexity may increase the risk of autoimmune or autoinflammatory disorders. Thus, incomplete microchimeric cells replacement may offer an additional explanation for increased incidence of autoimmune disorders in women compared with men (37–39). Potential negative consequences of microchimerism in this context is consistent with the average age of onset after puberty for most autoimmune disorders that disproportionately impact women including systemic lupus erythematosus, systemic sclerosis, and rheumatoid arthritis (40). Comparatively, since males with maternal microchimeric cells do not become pregnant, displacement by fetal microchimeric cells should never occur, precluding expanded immunological complexity caused by inefficient or incomplete microchimeric cell replacement. Thus, additional important areas for future investigation include evaluating levels of microchimeric cells from a variety of likely sources (maternal microchimerism; fetal microchimerism from each pregnancy) in the circulation and diseased tissues of parous women with autoimmunity.
In the larger scientific context, while pregnancy has been and remains arguably the most physiological model of immune tolerance, it remains underutilized for investigating how immune cells work. Systemic seeding of fetal cells into the tissues of women during pregnancy may represent only a small part of the larger puzzle for why mothers do not reject their babies during pregnancy, why babies do not reject their mothers, and pregnancy induced shifts in severity of systemic and organ-specific autoimmune disorders. Within this reproductive framework and behind the scenes, there also appears to be dynamic forces allowing for near complete displacement of preexisting microchimeric cells and replacement by new fetal microchimeric cells in maternal tissues during pregnancy. We are just beginning to scratch the surface in unraveling how our bodies work and reproduce, including the importance of these exceptionally rare but intriguing cells that defy classical immunological rules related to tolerance and rejection. These points are further highlighted in this brief perspective suggesting similar non-classical means for the displacement and replacement of these cells which occurs with remarkable efficiency. In turn understanding what microchimeric cells do and how they work, including synchronized disappearance to make space for a new set of cells during pregnancy, holds exceptional promise for innovative new ways for investigating how to promote acceptance or rejection of genetically foreign cells in other physiological contexts including transplantation and cancer.
Data availability statement
The original contributions presented in the study are included in the article/supplementary material. Further inquiries can be directed to the corresponding author.
Ethics statement
The animal study was approved by Cincinnati Children’s Hospital Research Foundation IACUC. The study was conducted in accordance with the local legislation and institutional requirements.
Author contributions
GP: Writing – review & editing, Visualization, Investigation, Data curation. TS: Writing – review & editing, Visualization. JK: Conceptualization, Writing – review & editing. YP: Methodology, Writing – review & editing. LT: Writing – review & editing, Conceptualization. SW: Resources, Writing – review & editing, Writing – original draft, Visualization, Validation, Supervision, Project administration, Investigation, Funding acquisition, Formal analysis, Data curation, Conceptualization, Methodology.
Funding
The author(s) declare financial support was received for the research, authorship, and/or publication of this article. This work was supported in part by NIH-NIAID through grants R01-AI175431 and R01-AI184537, the Burroughs Wellcome Fund Investigator in the Pathogenesis of Infectious Disease Award (grant #1011031) and March of Dimes Ohio Prematurity Research Collaborative. LT was supported by the NIAID through Vaccinology Training Program NIH-NIAID T32-AI165396.
Conflict of interest
The authors declare that the research was conducted in the absence of any commercial or financial relationships that could be construed as a potential conflict of interest.
Publisher’s note
All claims expressed in this article are solely those of the authors and do not necessarily represent those of their affiliated organizations, or those of the publisher, the editors and the reviewers. Any product that may be evaluated in this article, or claim that may be made by its manufacturer, is not guaranteed or endorsed by the publisher.
References
1. Nelson JL. The otherness of self: microchimerism in health and disease. Trends Immunol. (2012) 33:421–7. doi: 10.1016/j.it.2012.03.002
2. Gammill H, Nelson J. Naturally acquired microchimerism. Int J Dev Biol. (2010) 54:531–43. doi: 10.1387/ijdb.082767hg
3. Bianchi DW, Khosrotehrani K, Way SS, MacKenzie TC, Bajema I, O’Donoghue K. Forever connected: the lifelong biological consequences of fetomaternal and maternofetal microchimerism. Clin Chem. (2021) 67:351–62. doi: 10.1093/clinchem/hvaa304
4. Boddy AM, Fortunato A, Wilson Sayres M, Aktipis A. Fetal microchimerism and maternal health: a review and evolutionary analysis of cooperation and conflict beyond the womb. Bioessays. (2015) 37:1106–18. doi: 10.1002/bies.201500059
5. Stevens AM. Maternal microchimerism in health and disease. Best Pract Res Clin Obstet Gynaecol. (2016) 31:121–30. doi: 10.1016/j.bpobgyn.2015.08.005
6. O’Donoghue K. Fetal microchimerism and maternal health during and after pregnancy. Obstet Med. (2008) 1:56–64. doi: 10.1258/om.2008.080008
7. Bianchi D, Zickwolf G, Weil G, Sylvester S, DeMaria M. Male fetal progenitor cells persist in maternal blood for as long as 27 years postpartum. PNAS. (1996) 93:705–8. doi: 10.1073/pnas.93.2.705
8. Stevens AM, Hermes HM, Rutledge JC, Buyon JP, Nelson JL. Myocardial-tissue-specific phenotype of maternal microchimerism in neonatal lupus congenital heart block. Lancet. (2003) 362:1617–23. doi: 10.1016/S0140-6736(03)14795-2
9. Dutta P, Molitor-Dart M, Bobadilla JL, Roenneburg DA, Yan Z, Torrealba JR, et al. Microchimerism is strongly correlated with tolerance to noninherited maternal antigens in mice. Blood. (2009) 114:3578–87. doi: 10.1182/blood-2009-03-213561
10. Mold JE, Michaelsson J, Burt TD, Muench MO, Beckerman KP, Busch MP, et al. Maternal alloantigens promote the development of tolerogenic fetal regulatory T cells in utero. Science. (2008) 322:1562–5. doi: 10.1126/science.1164511
11. Owen RD, Wood HR, Foord AG, Sturgeon P, Baldwin LG. Evidence for actively acquired tolerance to Rh antigens. Proc Natl Acad Sci USA. (1954) 40:420–4. doi: 10.1073/pnas.40.6.420
12. Claas FH, Gijbels Y, van der Velden-de Munck J, van Rood JJ. Induction of B cell unresponsiveness to noninherited maternal HLA antigens during fetal life. Science. (1988) 241:1815–7. doi: 10.1126/science.3051377
13. Burlingham WJ, Grailer AP, Heisey DM, Claas FH, Norman D, Mohanakumar T, et al. The effect of tolerance to noninherited maternal HLA antigens on the survival of renal transplants from sibling donors. N Engl J Med. (1998) 339:1657–64. doi: 10.1056/NEJM199812033392302
14. Kinder JM, Jiang TT, Ertelt JM, Xin L, Strong BS, Shaaban AF, et al. Cross-generational reproductive fitness enforced by microchimeric maternal cells. Cell. (2015) 162:505–15. doi: 10.1016/j.cell.2015.07.006
15. Rowe JH, Ertelt JM, Xin L, Way SS. Pregnancy imprints regulatory memory that sustains anergy to fetal antigen. Nature. (2012) 490:102–6. doi: 10.1038/nature11462
16. Li DK, Wi S. Changing paternity and the risk of preeclampsia/eclampsia in the subsequent pregnancy. Am J Epidemiol. (2000) 151:57–62. doi: 10.1093/oxfordjournals.aje.a010122
17. Zhang J, Patel G. Partner change and perinatal outcomes: a systematic review. Paediatr Perinat Epidemiol. (2007) 21:46–57. doi: 10.1111/j.1365-3016.2007.00837.x
18. Kinder JM, Stelzer IA, Arck PC, Way SS. Immunological implications of pregnancy-induced microchimerism. Nat Rev Immunol. (2017) 17:483–94. doi: 10.1038/nri.2017.38
19. Bou-Gharios G, Amin F, Hill P, Nakamura H, Maxwell P, Fisk NM. Microchimeric fetal cells are recruited to maternal kidney following injury and activate collagen type I transcription. Cells Tissues Organs. (2011) 193:379–92. doi: 10.1159/000321172
20. Mahmood U, O’Donoghue K. Microchimeric fetal cells play a role in maternal wound healing after pregnancy. Chimerism. (2014) 5:40–52. doi: 10.4161/chim.28746
21. Nassar D, Droitcourt C, Mathieu-d’Argent E, Kim MJ, Khosrotehrani K, Aractingi S. Fetal progenitor cells naturally transferred through pregnancy participate in inflammation and angiogenesis during wound healing. FASEB J. (2012) 26:149–57. doi: 10.1096/fj.11-180695
22. O’Donoghue K, Sultan HA, Al-Allaf FA, Anderson JR, Wyatt-Ashmead J, Fisk NM. Microchimeric fetal cells cluster at sites of tissue injury in lung decades after pregnancy. Reprod BioMed Online. (2008) 16:382–90. doi: 10.1016/S1472-6483(10)60600-1
23. Nelson JL, Gillespie KM, Lambert NC, Stevens AM, Loubiere LS, Rutledge JC, et al. Maternal microchimerism in peripheral blood in type 1 diabetes and pancreatic islet beta cell microchimerism. Proc Natl Acad Sci U.S.A. (2007) 104:1637–42. doi: 10.1073/pnas.0606169104
24. Artlett CM, Smith JB, Jimenez SA. Identification of fetal DNA and cells in skin lesions from women with systemic sclerosis. N Engl J Med. (1998) 338:1186–91. doi: 10.1056/NEJM199804233381704
25. Nelson JL, Furst DE, Maloney S, Gooley T, Evans PC, Smith A, et al. Microchimerism and HLA-compatible relationships of pregnancy in scleroderma. Lancet. (1998) 351:559–62. doi: 10.1016/S0140-6736(97)08357-8
26. Shao TY, Kinder JM, Harper G, Pham G, Peng Y, Liu J, et al. Reproductive outcomes after pregnancy-induced displacement of preexisting microchimeric cells. Science. (2023) 381:1324–30. doi: 10.1126/science.adf9325
27. Vezys V, Yates A, Casey KA, Lanier G, Ahmed R, Antia R, et al. Memory CD8 T-cell compartment grows in size with immunological experience. Nature. (2009) 457:196–9. doi: 10.1038/nature07486
28. Mombaerts P, Iacomini J, Johnson RS, Herrup K, Tonegawa S, Papaioannou VE. RAG-1-deficient mice have no mature B and T lymphocytes. Cell. (1992) 68:869–77. doi: 10.1016/0092-8674(92)90030-G
29. Yuzen D, Urbschat C, Schepanski S, Thiele K, Arck PC, Mittrucker HW. Pregnancy-induced transfer of pathogen-specific T cells from mother to fetus in mice. EMBO Rep. (2023) 24:e56829. doi: 10.15252/embr.202356829
30. Hemon M, Giassi M, Ghaffar Y, Martin M, Roudier J, Auger I, et al. Microchimeric cells promote production of rheumatoid arthritis-specific autoantibodies. J Autoimmun. (2024) 146:103238. doi: 10.1016/j.jaut.2024.103238
31. Benichou G, Yamada Y, Aoyama A, Madsen JC. Natural killer cells in rejection and tolerance of solid organ allografts. Curr Opin Organ Transplant. (2011) 16:47–53. doi: 10.1097/MOT.0b013e32834254cf
32. Kang J, Loh K, Belyayev L, Cha P, Sadat M, Khan K, et al. Type 3 innate lymphoid cells are associated with a successful intestinal transplant. Am J Transplant. (2021) 21:787–97. doi: 10.1111/ajt.16163
33. Mak ML, Reid KT, Crome SQ. Protective and pathogenic functions of innate lymphoid cells in transplantation. Clin Exp Immunol. (2023) 213:23–39. doi: 10.1093/cei/uxad050
34. Ochando J, Fayad ZA, Madsen JC, Netea MG, Mulder WJM. Trained immunity in organ transplantation. Am J Transplant. (2020) 20:10–8. doi: 10.1111/ajt.15620
35. Porrett PM. How mothers tolerate their children. Science. (2023) 381:1286. doi: 10.1126/science.adk1218
36. Bracamonte-Baran W, Florentin J, Zhou Y, Jankowska-Gan E, Haynes WJ, Zhong W, et al. Modification of host dendritic cells by microchimerism-derived extracellular vesicles generates split tolerance. Proc Natl Acad Sci U.S.A. (2017) 114:1099–104. doi: 10.1073/pnas.1618364114
37. Kronzer VL, Bridges SL Jr., Davis JM. 3rd, Why women have more autoimmune diseases than men: An evolutionary perspective. Evol Appl. (2021) 14:629–33. doi: 10.1111/eva.13167
38. Ngo ST, Steyn FJ, McCombe PA. Gender differences in autoimmune disease. Front Neuroendocrinol. (2014) 35:347–69. doi: 10.1016/j.yfrne.2014.04.004
39. Whitacre CC. Sex differences in autoimmune disease. Nat Immunol. (2001) 2:777–80. doi: 10.1038/ni0901-777
Keywords: microchimerism, RAG1, T cell, B cell, non-inherited maternal antigen, microchiome, immune tolerance, reproductive fitness
Citation: Pham G, Shao T-Y, Kinder JM, Peng Y, Turner LH and Way SS (2024) Pregnancy induced displacement of preexisting microchimeric cells in the absence of maternal B and T cells. Front. Immunol. 15:1478465. doi: 10.3389/fimmu.2024.1478465
Received: 12 August 2024; Accepted: 11 October 2024;
Published: 30 October 2024.
Edited by:
Fadi G. Lakkis, University of Pittsburgh, United StatesReviewed by:
Anita S. Chong, The University of Chicago, United StatesCopyright © 2024 Pham, Shao, Kinder, Peng, Turner and Way. This is an open-access article distributed under the terms of the Creative Commons Attribution License (CC BY). The use, distribution or reproduction in other forums is permitted, provided the original author(s) and the copyright owner(s) are credited and that the original publication in this journal is cited, in accordance with accepted academic practice. No use, distribution or reproduction is permitted which does not comply with these terms.
*Correspondence: Sing Sing Way, c2luZ3Npbmcud2F5QGNjaG1jLm9yZw==
†Present addresses: Tzu-Yu Shao, Amgen, South San Francisco, CA, United States
Jeremy M. Kinder, Sana Biotechnology Inc., Seattle, WA, United States