- 1Department of Neurosurgery, West China Hospital, Sichuan University, Chengdu, Sichuan, China
- 2Department of Neurosurgery, Jiujiang No. 1 People’s Hospital, Jiujiang, China
Glioma is one of the common tumors in the central nervous system, and its treatment methods (surgery, radiotherapy, and chemotherapy) lack specificity and have a poor prognosis. With the development of immunology, cell biology, and genomics, tumor immunotherapy has ushered in a new era of tumor therapy, achieving significant results in other invasive cancers such as advanced melanoma and advanced non-small cell lung cancer. Currently, the clinical trials of immunotherapy in glioma are also progressing rapidly. Here, this review summarizes promising immunotherapy methods in recent years, reviews the current status of clinical trials, and discusses the challenges and prospects of glioma immunotherapy.
Introduction
Glioma refers to a tumor originating from glial cells and is the most common primary tumor in the central nervous system (1). Glioma, with an incidence of 6.6/100,000 of which about half were glioblastomas (1), accounts for almost 90% of all malignant brain and other central nervous system tumors (2). The incidence of glioma increases with age (3), and the incidence of glioblastoma was highest in males, persons aged more than 65 years, and non-Hispanic White (2). The symptoms of glioma depend on their location, size, type, and growth rate. Glioma treatment usually begins with surgery and is followed by radiation therapy, chemotherapy, and targeted therapy. However, most patients are not sensitive to traditional treatment and have a poor prognosis. Available treatment options include second-line surgery, radiotherapy, alkylating agent chemotherapy, and bevacizumab therapy. Unfortunately, once progression or recurrence occurs, the median overall survival (OS) is only 6 to 9 months. Therefore, there is an urgent need for new therapeutic strategies to treat recurrent GBM (4). With the advancement of immunology, cell biology, and molecular biology, tumor immunotherapy has ushered in a new era of cancer treatment (5). Immunotherapy as a new treatment method may be beneficial for delaying glioma recurrence and improving the therapeutic effect of glioma. Recently, immunotherapy has achieved some exciting and encouraging results even though there are still many challenges in practical clinical applications. A deeper understanding of the biology and immune microenvironment, along with the development of new therapeutic combinations, may potentially change the current challenges faced by immunotherapy in GBM. We provide a review of the current status and new developments in immunotherapy, including tumor vaccines, immune checkpoint inhibitors, chimeric antigen receptor T cells (CAR-T), and oncolytic virotherapy, for glioma (Figure 1).
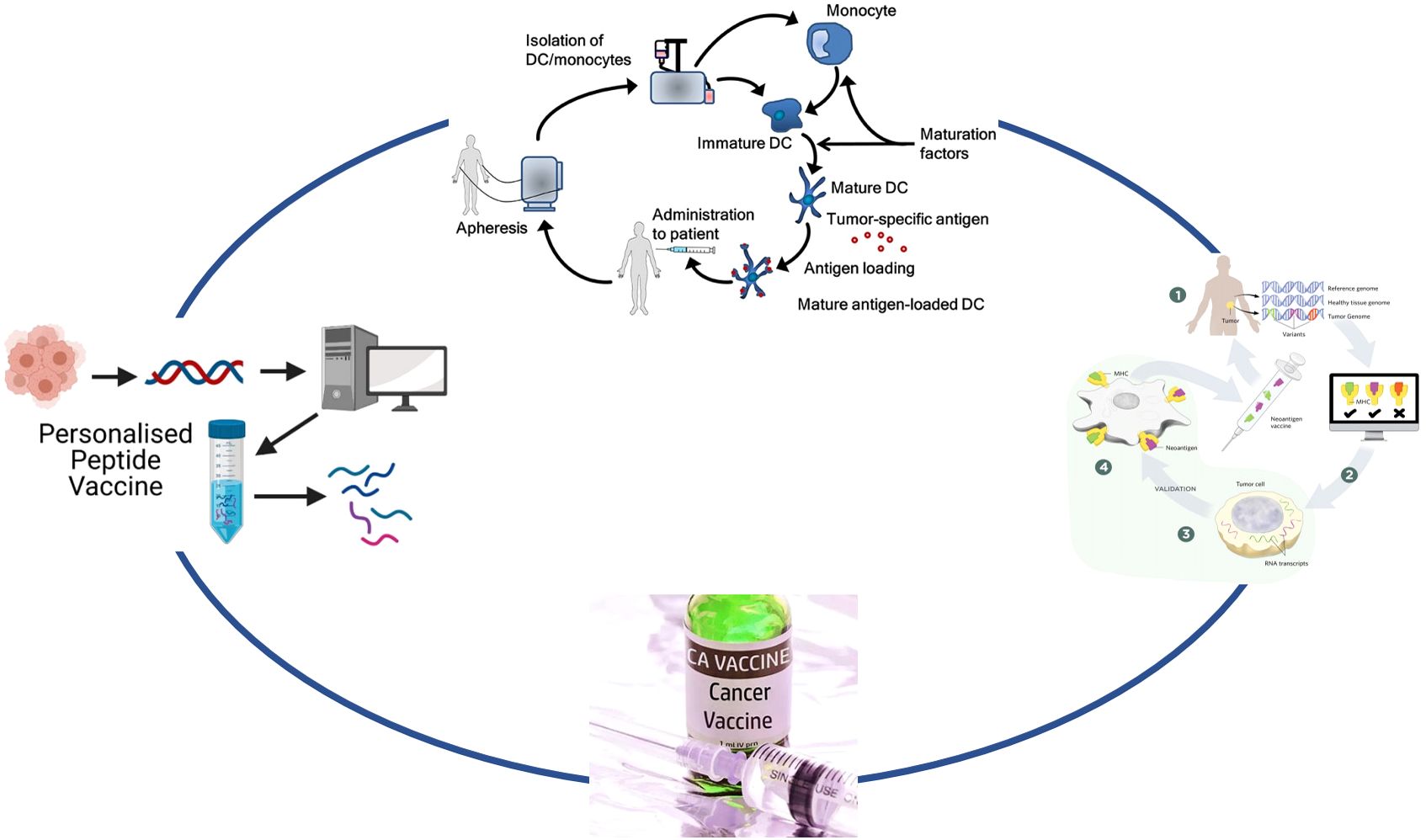
Figure 1. Strategies to overcome gliomas. The diagram illustrates various immunotherapy strategies for targeting and eliminating cancer cells. On the left, cancer vaccines stimulate an immune response, while immune checkpoint inhibitors like Ipilimumab, Nivolumab, Pembrolizumab, and Cemiplimab block the PD-1 pathway to activate T-cells. PD-L1 inhibitors such as Atezolizumab, Durvalumab, and Avelumab further promote the killing of cancer cells by preventing cancer cells from evading immune detection. CAR-T cell therapy is depicted, showing how T-cells are engineered with a chimeric antigen receptor (CAR) to directly attack tumor cells. Oncolytic viruses are also represented, illustrating their role in selectively infecting and destroying cancer cells.
Tumor vaccines
The use of vaccines to treat confirmed malignant tumors can be traced back to 1910s and 1950s (6). Tumor vaccines can utilize the adaptive immune system to produce tumor-specific antibodies and thereby exert anti-tumor effects (7), mainly divided into peptide vaccine, dendritic cell vaccine, and tumor neoantigen vaccine (8) (Figure 2).
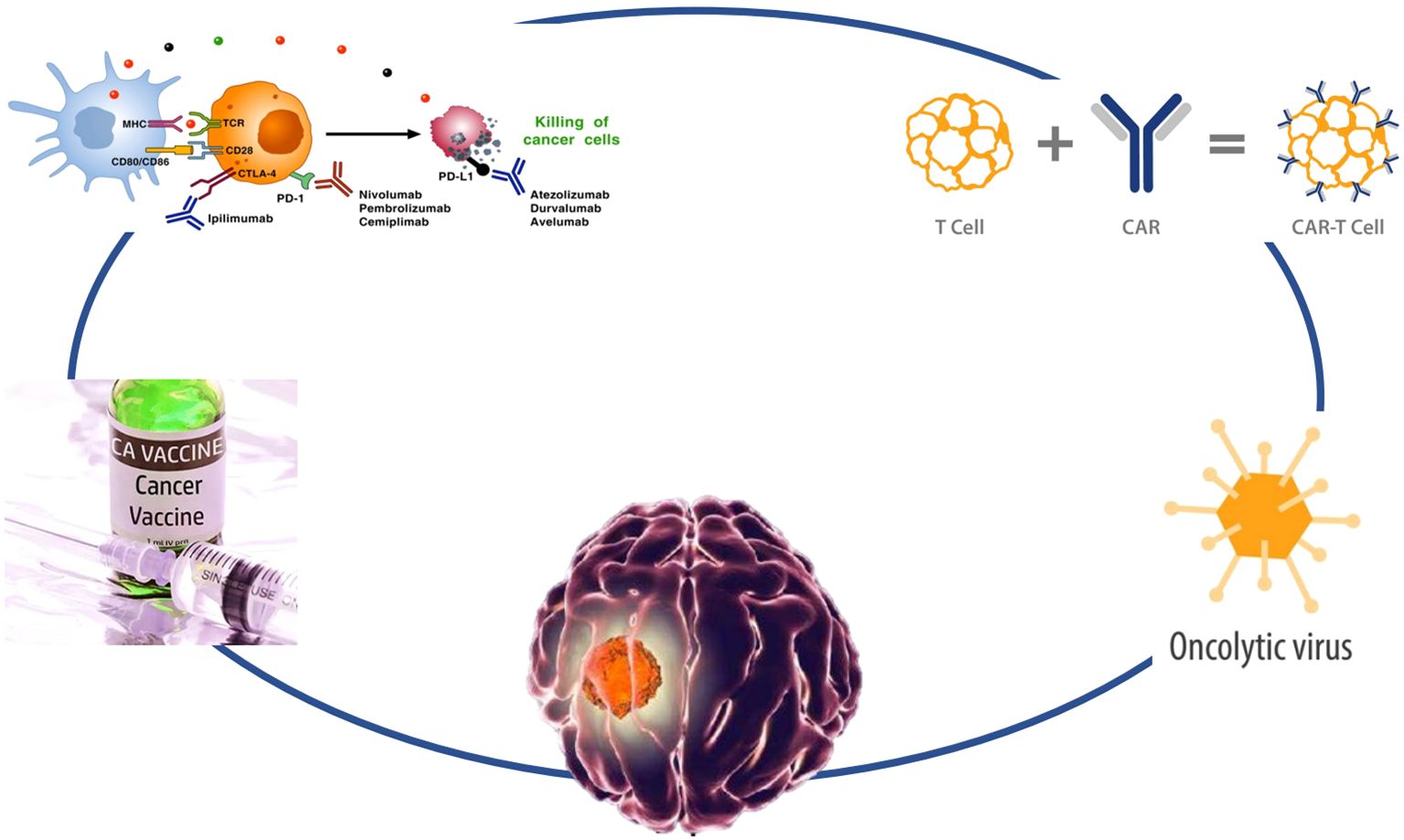
Figure 2. Classification of tumor vaccines. This diagram illustrates two personalized immunotherapy approaches for cancer: peptide vaccines and dendritic cell (DC) therapy. On the left, the process of creating a personalized peptide vaccine begins with the identification of tumor-specific antigens from the patient’s tumor cells, followed by the generation of a peptide vaccine tailored to the patient. On the right, dendritic cell therapy involves isolating monocytes from the patient, differentiating them into immature DCs, and loading them with tumor-specific antigens. These mature antigen-loaded DCs are then reintroduced into the patient to stimulate an immune response.
Peptide vaccine
Peptide vaccine is a vaccine made by artificially synthesizing protective short peptides in the amino acid sequence of natural proteins, connecting them with carriers, and adding adjuvants. A phase I clinical study conducted in Japan indicated that WT1 peptide vaccine induced WT1-specific CD8+ T cells and CD4+ T cells and was tolerable in patients with WT1+ malignant glioma (9), and the phase II clinical trial further showed WT1 peptide vaccine was safe and produced a clinical response in patients with WT1/HLA-A*2402+ recurrent glioblastoma multiforme (10). During the administration of the WT1 peptide vaccine, the maintenance of WT1 expression in tumor cells is significantly associated with a longer progression free and overall survival (11). ACT IV, a randomized, double-blind, phase 3 study done conducted in 22 countries, was terminated prematurely due to the significant adverse events and ineffectiveness on overall survival for rindopepimut (a vaccine targeting EGFRvIII) in newly diagnosed EGFRvIII+ glioblastoma patients who underwent maximal surgical resection and completed standard radiotherapy with concomitant temozolomide (12). However, another phase II, multicenter, prospective trial completed in American indicated that the toxicity of PEPvIII-KLH (another vaccine targeting EGFRvIII) was generally minimal in adults with newly diagnosed EGFRvIII+ glioblastoma patients who underwent maximal surgical resection and completed standard radiotherapy with concomitant temozolomide and the overall survival of patients with EGFRvIII specific antibody response was longer than that of patients without, and there was still a statistical difference after adjusting for factors such as age, Karnofsky performance status, and methylguanine methyltransferase methylation (13). ReACT, a double-blind, randomized, phase II study done in American, further confirmed the potential of rindopepimut for targeted immunotherapy among patients with recurrent EGFRvIII+ glioblastoma (14). Neurooncology Working Group of the German Cancer Society trial 16 (NOA-16), a non-controlled, open-label, single-arm, multicenter, first-in-humans phase I trial conducted in Germany, demonstrated that IDH1(R132H)-specific peptide vaccine (IDH1-vac) prolonged the pseudoprogression and survival time of patients with IDH1(R132H)+, non-1p/19q co-deleted, ATRX− World Health Organization (WHO) grade 3 and 4 gliomas and the vaccine-related adverse events were restricted to grade 1 (15). Moreover, the new antigen IDH1 (R132H) is immunogenic across multiple HLA alleles and effectively induced an IDH1-specific immune response (15). MultIceNTER Phase I Peptide VaCcine Trial for the Treatment of H3-Mutated Gliomas (INTERCEPT-H3), a non-controlled, open-label, single arm, multicenter phase I trial done in Germany, showed that H3K27M-specific long peptide vaccine (H3K27M-vac) induced neoepitope-specific CD4+ T cell-dominated colocalization immune responses with HLA class II-DR in patients with newly diagnosed H3K27M diffuse midline gliomas and these patients with immune responses showed radiographic improvement (16).
Dendritic cell vaccine
Dendritic cell vaccine is a vaccine that utilizes the patient’s own dendritic cells to activate the immune system and enhance its ability to attack tumor cells. The main function of dendritic cells is to initiate and activate the recognition and response of initial T cells to protein antigens, present viral and tumor antigens. It is the only dedicated antigen presenting cell that can directly activate initial T cells. Several phase I clinical trials found that three biweekly intradermal administration of autologous tumor lysate-pulsed dendritic cell vaccine among malignant glioma patients elicited antigen-specific systemic and intracranial cytotoxic and memory T-cell infiltration (17–19). A later phase I/II clinical study done in Japan showed the safety and clinical response of autologous tumor lysate-pulsed dendritic cell therapy for patients with malignant glioma (20, 21). A phase 3 randomized, double-blinded, placebo-controlled clinical trial conducted in the American, Canada, Germany, and the United Kingdom showed that addition of autologous tumor lysate-pulsed dendritic cell vaccine (DCVax®-L) to standard therapy was feasible and safe in newly diagnosed or recurrent glioblastoma patients and extended progression free survival and overall survival (22, 23). A randomized, double-blind, multicenter phase II placebo-controlled study found autologous tumor lysate-pulsed dendritic cell vaccine (ICT-107) improved progression free survival and maintained quality of life in recurrent glioma patients (24). A single-arm phase 2 clinical trial completed in American indicated that the Aivita glioblastoma vaccine (AV-GBM-1) was well-tolerated and extended the median progression free survival with numerous treatment-emergent central nervous system adverse events (25). A single-center, randomized, open-label multi-arm phase II clinical trial conducted in American indicated that combining autologous tumor lysate-pulsed dendritic cell vaccine with poly-ICLC, a Toll-like receptor agonist, induced a polarized interferon activation in circulating monocytes and CD8+ T cells, which may represent an important blood biomarker for immunotherapy in patients with newly-diagnosed or recurrent WHO Grade III-IV malignant gliomas (26). There are different variants for dendritic cell vaccine. The cytomegalovirus-specific dendritic cell vaccine patients improved long-term progression free survival and overall survival in patients with newly diagnosed glioblastoma (27) and pre-conditioning the cytomegalovirus-specific dendritic cell vaccine site with a potent recall antigen significantly improves the lymph node homing and efficacy of vaccine (28), later sequential clinical trials utilizing cytomegalovirus-specific dendritic cell vaccine associated with extended overall survival for newly diagnosed glioblastoma (29). The other one is autologous dendritic cell vaccine pulsed with lysate derived from an allogeneic stem-like cell line, which was safe and well tolerated in newly diagnosed and recurrent glioblastoma (30).
Tumor neoantigen vaccine
Neoantigen originates from tumor specific protein coding mutations and is not affected by central tolerance. It can generate strong immune responses and act as an unquestionable antigen to promote tumor rejection (31) (Table 1, Supplementary Figure 1). Single-cell T cell receptor analysis of phase I/Ib study involving personalized neoantigen-targeting vaccines for patients with newly diagnosed glioblastoma demonstrated neoantigen-specific T cells from the peripheral blood can migrate into intracranial glioblastoma (32). NeoVax, a personalized neoantigen-targeting vaccine, significantly increased neoantigen-specific effector T cells in newly diagnosed glioblastoma patients (33). These encouraging results contribute to the development of future clinical trials for glioma patients based on neoantigen vaccines.
Immune checkpoint inhibitors
Immune checkpoint inhibitors, including programmed cell death 1 (PD-1)/programmed cell death ligand 1 (PD-L1) and cytotoxic T lymphocyte-associated antigen 4 (CTLA-4), are important immunosuppressive targets for tumor escape (34)(Table 2, Supplementary Figure 2). The PD-1/PD-L1 axis promotes glioma tumor growth and invasion (35). CheckMate 143 confirmed the safety and tolerability of nivolumab in patients with newly diagnosed glioblastoma, median overall survival with nivolumab was 33.38 (16.2 to not estimable) and 16.49 (12.94-22.08) months in patients with methylated and unmethylated MGMT promoter, respectively (36). Later CheckMate 143 demonstrated median overall survival with nivolumab and bevacizumab was 9.8 (8.2-11.8) and 10.0 (9.0-11.8) months, respectively, in recurrent glioblastoma population, the objective response rate was 7.8% (4.1%-13.3%) and 23.1% (16.7%-30.5%) with nivolumab and with bevacizumab, respectively (37). Given the potential benefits of nivolumab for methylated patients, CheckMate 548 has emerged. However, CheckMate 548 did not achieve the primary endpoints with the median overall survival with nivolumab and placebo was 28.9 (24.4-31.6) and 32.1 (29.4-33.8) months, respectively, and the median progression free survival with nivolumab and placebo was 10.6 (8.9-11.8) and 10.3 (9.7-12.5) months, respectively (38). The above studies are all aimed at the basic treatment of simultaneous radiotherapy and temozolomide chemotherapy, while CheckMate 498 compared nivolumab with temozolomide chemotherapy on the basis of radiotherapy. The median overall survival with nivolumab and temozolomide chemotherapy was 13.4 (12.6-14.3) and 14.9 (13.3-16.1) months, respectively (hazard ratio =1.31 (1.09-1.58), P = 0.0037), and the median progression free survival with nivolumab and temozolomide chemotherapy was 6.0 (5.7-6.2) and 6.2 (5.9-6.7) months, respectively, in patients with newly diagnosed glioblastoma with unmethylated MGMT promoter (39). Changes in the immune status of gliomas may affect the patient’s responsiveness to anti-PD-1 immunotherapy (nivolumab or pembrolizumab) (40). Resectable glioblastoma tumor tissue pre- and post-nivolumab dosing resulted in higher immune cell infiltration, enhanced chemokine transcripts, and augmented T cell receptor clonal diversity, supporting a local immunomodulatory effect of treatment (41). Recurrent glioblastoma patients receiving neoadjuvant pembrolizumab had significantly extended overall survival, pembrolizumab was associated with upregulated T cell- and interferon-γ-related gene expression as well as downregulated cell-cycle-related gene expression, which enhancing both the intratumoral and systemic immune responses (42). The median survival of responders was longer than non-responders to neoadjuvant nivolumab or pembrolizumab in a retrospective analysis, MAPK pathway alterations were enriched in responders while PTEN mutations associated with immunosuppressive signature from CD44 + tumor cells were enriched in non-responders, and immune infiltration that reflect the tumor’s clonal evolution during treatment (43). Following 10 mg nivolumab intravenously administration, 27 recurrent glioblastoma patients underwent a maximal safe resection, followed by 10 mg ipilimumab or 5mg ipilimumab plus 10 mg nivolumab injection. The overall survival was better compared with historical cohorts (Belgian and GliAvAx trials) (44). CheckMate 908 investigated nivolumab and nivolumab plus ipilimumab in pediatric patients with high-grade central nervous system malignancies. The median overall survival with nivolumab and nivolumab plus ipilimumab was 1.7 (10.3-16.5) and 10.8 (9.1-15.8) months, respectively, in newly diagnosed diffuse intrinsic pontine glioma and the median progression free survival with nivolumab and nivolumab plus ipilimumab was 1.7 (1.4-2.7) and 1.3 (1.2-1.5) months, respectively, in high-grade glioma; 1.4 (1.2-1.4) and 2.8 (1.5-4.5) months, respectively, in medulloblastoma; 1.4 (1.4-2.6) and 4.6 (1.4-5.4) months in ependymoma; 1.2 (1.1-1.3) and 1.6 (1.3-3.5) months, respectively, in other recurrent/progressive central nervous system tumors (45). Bevacizumab could enhance the tolerability and efficacy of pembrolizumab in patients with recurrent high-grade gliomas receiving hypofractionated stereotactic irradiation. The median overall survival of bevacizumab-naïve and bevacizumab-resistant patients was 13.45 (9.46-18.46) and 9.3 (8.97-18.86) months, respectively, and the progression free survival was 7.92 (6.31-12.45) and 6.54 (5.95-18.86) months, respectively (46). Isatuximab plus atezolizumab had acceptable safety and tolerability and reduced CD38+ immune cells in the glioblastoma microenvironment (47).
CAR-T therapy
CAR-T can specifically recognize tumor cell surface antigens, independent of MHC activation, and produce stronger anti-tumor immune responses (48) (Table 3, Supplementary Figure 3). There are also many practices in gliomas. Among them, GD2, EGFR-vIII, HER2, and IL13Ra2 are the three most common targets and have been tested in early phase trial.
GD2-targeted CAR-T seemed safe in recurrent or refractory advanced-stage neuroblastoma and was associated with tumor regression or necrosis, thereby resulting in longer survival (49). GD2-targeted CAR-T was tolerable in H3K27M-mutated diffuse midline gliomas and improved the clinical and radiographic outcomes through the pro-inflammatory cytokine levels in the plasma and cerebrospinal fluid (50). Recent trial assessed the administration methods of GD2-targeted CAR-T and found that intravenous alone or intravenous combined with intracavitary administration of GD2-targeted CAR-T were safe and well tolerated in glioblastoma and GD2-targeted CAR-T mediated antigen loss and activated immune responses in the glioblastoma microenvironment (51). NCT02209376 was the first-in-human study of EGFRvIII-targeted CAR-T in recurrent glioblastoma. Intravenous infusion of EGFRvIII-targeted CAR-T was feasible and safe, increased inhibitory molecules expression and regulatory T cells infiltration (52). The dose-escalating phase I study indicated persistence of CAR-T correlated with EGFRvIII-targeted CAR-T dose, but there were no objective responses (53). Infusion of autologous HER2-targeted CAR-T was tolerated and was associated with clinical benefit for patients with progressive glioblastoma (54). NCT00730613 was the first-in-human pilot study to evaluate the safety and feasibility of IL13Rα2-targeted CAR-T in recurrent glioblastoma. Intracranial delivery of the IL13Rα2-targeted CAR-T was well-tolerated, induced transient anti-glioma responses, and increased tumor necrotic volume (55). A case report indicated intracranial infusion of IL13Rα2-targeted CAR-T was associated with no toxic effects of grade 3 or higher. After IL13Rα2-targeted CAR-T treatment, all intracranial and spinal tumors were regressed along with increased levels of cytokines and immune cells in the cerebrospinal fluid (56). Brown and his colleagues generated an off-the-shelf, steroid-resistant, IL13Rα2-targeted CAR-T and found it was safety and induced transient tumor reduction and/or tumor necrosis in patients with glioblastoma (57).
In addition to the targets reviewed above, CD7 (58) and EphA2 (59, 60) have conducted relevant research. Some research have also emerged. Local intracranial CAR-T elicits superior anti-tumor efficacy as compared to intravenous CAR-T, with intraventricular administration exhibiting possible benefits over intracranial administration in a multifocal disease model (61). Cyclophosphamide and fludarabine but not PD-1 inhibitor enhanced the expansion or persistence of GD2-targeted CAR-T (62). Trivalent CAR-T (HER2, IL13Rα2, and EphA2-targeted CAR-T) mediated immunoreaction forming polarized microtubule organizing centers, exhibited improved cytotoxicity and cytokine release, and overcame antigenic heterogeneity in glioblastoma thereby improving treatment outcomes (63). Moreover, HER2-targeted CAR-NK cells (64) and ErbB2-targeted CAR-NK cells (65) might be feasible and safe in recurrent glioblastoma. In a first-in-human trial published in 2024, three patients with recurrent glioblastoma received CARv3-TEAM-E T cell therapy (66). CARv3-TEAM-E T cells are chimeric antigen receptor (CAR) T cells that target both the epidermal growth factor receptor variant III (EGFRvIII), a tumor-specific antigen, and wild-type EGFR protein, attacking via secreted T cell-engaging antibody molecules (TEAM). The treatment did not cause any grade 3 or higher adverse events or dose-limiting toxicities. Radiological imaging revealed a rapid and significant reduction in tumor size, with responses occurring within days after a single intraventricular injection. However, two of the patients experienced only short-lived responses.
Oncolytic virotherapy
Oncolytic viruses are a type of tumor-killing virus with replication ability. They not only proliferate infinitely within tumor cells, leading to their death, but also directly or indirectly activate the anti-tumor immune system, specifically killing tumor cells (67) (Table 4, Supplementary Figure 4). Due to its ability to recognize tumor cells specifically through corresponding receptors and its replication relying on tumor-specific promoters, oncolytic viruses do not affect normal brain tissue cells.
A phase I dose-escalating clinical study showed that 24 patients with recurrent malignant gliomas received intracerebral injections of ONYX-015, an E1B-attenuated adenovirus. None of the patients had any adverse events related to ONYX-015 injection, confirming the relative safety of ONYX-015 in the treatment of malignant gliomas (68). DNX-2401, an E1A-attenuated adenovirus, resulted in more than 3 years of progression free survival in 12% of patients with recurrent malignant gliomas that were probably due to direct oncolytic effects of DNX-2401 followed by elicitation of an immune-mediated response (69, 70). DNX-2401 promoted a pro-inflammatory microenvironment and M1 characteristics of tumoral macrophages in glioblastoma (71), a later phase I clinical trial also indicated that DNX-2401 locally delivered by convection-enhanced delivery in tumor and surrounding brain induced local inflammatory reaction in patients with recurrent glioblastoma (72). DNX-2401 is also used in conjunction with other treatment methods. Intertumoral infusion of DNX-2401 followed by radiotherapy in pediatric patients with newly diagnosed diffuse intrinsic pontine glioma resulted in changes in T-cell activity and a reduction in or stabilization of tumor size in some patients but was associated with adverse events (73). The first-in-human investigation of combined DNX-2401 with pembrolizumab for recurrent glioblastoma confirmed the safety with notable survival benefits in select patients and response to treatment informed by the balance between immune infiltration and expression of immune checkpoint inhibitors (74). In addition, intratumoral infusion of PVSRIPO, recombinant nonpathogenic polio-rhinovirus chimera, in patients with recurrent malignant glioma confirmed the absence of neurovirulent potential and improved survival (75). A first-in-human, open-label, phase 1, dose-escalation trial indicated neural stem cell delivery of an oncolytic adenovirus (NSC-CRAd-S-pk7) in newly diagnosed malignant gliomas was feasible and safe (76). Ling AL, Solomon IH, Landivar AM, et al. report a phase I clinical trial linking oncolytic virus-mediated immune activation to survival in glioblastoma patients. In this study, 41 patients with recurrent glioblastoma were treated with CAN-3110, an oncolytic herpes virus designed for preferential tumor replication. No dose-limiting toxicities were observed. Improved survival was notably associated with HSV1 seropositivity, which also correlated with enhanced T cell response and immune activation signatures in the tumor microenvironment. These findings provide evidence supporting the therapeutic potential of oncolytic viruses in immunosuppressive tumors like glioblastoma (77).
Discussion
Glioma is one of the most concerning fields in neurological tumors, and its treatment is a clinical challenge. Although the standard post-surgical treatment for newly diagnosed glioblastoma, involving concurrent radiotherapy and the alkylating chemotherapeutic agent temozolomide (TMZ) followed by adjuvant TMZ, has been established for over a decade, glioblastoma inevitably recurs and develops resistance to further chemotherapy. One of the earliest mechanisms of resistance to TMZ is the upregulation of DNA methyltransferase (MGMT), which removes methyl adducts from DNA, enabling mismatch repair and allowing tumor DNA replication to continue (78). Immunotherapy is a research hotspot, and many immunotherapy studies are targeting glioma. Here, we reviewed the current concepts of immunotherapy in glioma (Tables 1–4), the current clinical research results may be encouraging, but there is still much room for improvement.
Tumor vaccines activate T cells in patients by introducing tumor antigens, thereby inducing an immune response to kill tumor cells. Compared with traditional radiotherapy and chemotherapy, tumor vaccines have become highly promising immunotherapy due to their convenient operation process, strong specificity, good safety, and ability to establish long-term immune memory. The core of tumor vaccine development lies in accurately screening suitable tumor antigens and determining effective antigen delivery methods. To overcome these challenges, researchers have proposed various strategies. Firstly, the combination of tumor vaccines and immune checkpoint inhibitors is used to enhance immune response, thereby enhancing treatment efficacy. NEO-PV-01, a personalized neoantigen vaccine, in combination with pembrolizumab, supports the safety and immunogenicity in patients with advanced non-squamous non-small cell lung cancer (79). Secondly, the personalized vaccine strategy based on neoantigens improves the targeting and efficacy of tumor vaccines by accurately identifying tumor-specific antigens. The future research focus will be on combining clinical efficacy and artificial intelligence technology to improve the accuracy of predicting neoantigens, thereby optimizing the therapeutic effect and application scope of vaccines. Finally, utilizing systems biology methods could optimize vaccine design and improve vaccine delivery systems. By utilizing advanced biomaterials such as nanoparticles to optimize drug delivery systems could improve the vaccine efficacy and reduce side effects (80). KK2DP7, a simple dendrimer polypeptide nanoparticle, enhances antitumor immunity of a neoantigen-based vaccine (81). CRISPR-Cas9 technology enhances the immune response by precisely modifying the genetic information of immune cells or tumor cells. The engineered therapeutic tumor cells, repurposed from interferon-β sensitive to resistant using CRISPR-Cas9 by knocking out the interferon-β-specific receptor, eliminated established glioblastoma tumors in mice (82).
The research progress on immune checkpoint inhibitors is rapid, especially monoclonal antibodies targeting PD-1/PD-L1 and CTLA-4, which have been approved by the FDA for first-line treatment of melanoma and/or lung cancer. At the same time, research is also underway, including targeting LAG3, TIM3, and other checkpoint inhibitors. The combination immunotherapy method of PD-1 blockade was successfully used for advanced glioma. However, intratumoral heterogeneity (83, 84), low PD-L1 expression (85), low mutation burden (86), and chemotherapy-induced mutation properties (87, 88) in diffuse midline glioma might explain why no survival benefits have been observed with immune checkpoint inhibitors monotherapy (16, 89). Therefore, the FDA has not yet approved malignant glioma as a treatment indication for immune checkpoint inhibitors. In the future, further research is needed on neuroimmunology and the possibility of combining immune checkpoint inhibitors with other therapies to treat glioma.
Due to the heterogeneity of glioma and the immune suppression of CAR-T by the tumor microenvironment, increasing the number of CAR-T coverage antigens and combination therapy is key to improving the efficacy of CAR-T. Combination therapy with Lp2-targeted CAR-T and oncolytic virus G47Δ further inhibited the glioblastoma growth and improved survival (90). Although CAR-T treatment for glioma is still in its early stages, the emergence and application of advanced biotechnology will accelerate the search for new strategies for glioma. The method of genome-scale screening using CRISPR-Cas9 can significantly shorten the time for discovering key genes that can be interfered with to improve the therapeutic effect of CAR-T, a three-dimensional model of glioblastoma organoids that summarizes the cellular heterogeneity, structure, and function of primary tissues can be used for better preclinical studies of CAR-T therapy, and single-cell sequencing technology can accurately reveal the intratumoral heterogeneity of glioma cells and other cellular components of tumor microenvironment to provide rich information for monitoring immune response and predict therapeutic efficacy during the treatment process.
The progress of basic research and the iteration of technology have made oncolytic viruses more specific, effective, and safe in the treatment of glioma. More and more oncolytic viruses are entering phase I, II, and even III clinical trials. However, there are still many challenges in the treatment of glioma with oncolytic viruses. Firstly, in terms of safety, although existing clinical trial results have not reported significant safety events, there is still a risk of off-target treatment. In addition, although genetically modified viruses enhance their ability to recognize tumor cells, elderly people and immunocompromised patients may still be infected with the virus and cause serious consequences. Secondly, in terms of drug delivery routes, most oncolytic viruses are currently administered locally through direct intratumoral injection in the treatment of gliomas, reducing the risk of virus replication in non-target cells (91). However, there are problems such as bleeding, infection, and difficulty in drug delivery in deep lesions, and continuous improvement of targeted guidance techniques and the technical standards for intratumoral drug delivery should be emphasized. Compared with direct intratumoral injection, intravenous injection is undoubtedly more convenient and safer (92). However, due to the dilution of systemic blood, rapid neutralization of antibodies, and isolation of non-target organs and the blood-brain barrier, the application of intravenous medication is greatly limited. Increasing the dose of oncolytic viruses used for intravenous injection, improving oncolytic virus vectors, and using new ultrasound to open the blood-brain barrier (93) may improve the effectiveness of intravenous administration. Finally, how to improve the therapeutic effect of oncolytic viruses is also a difficult problem. Attention should be paid to the application of combining oncolytic virotherapy with other treatments such as chemotherapy, radiotherapy, and immune checkpoint inhibitors in the treatment of glioma to increase anti-tumor synergistic effects. Because there are currently no biomarkers available to predict the dose of relevant oncolytic viruses and their potential for in vivo replication (94), in addition to central imaging review, molecular pathology, and immune monitoring, in-depth research on virus replication and clinical anti-tumor response should be conducted in preclinical models and clinical trials.
The challenge of immunotherapy for glioma is still limited by objective factors. From an anatomical perspective, it is difficult to obtain central nervous system tumor section specimens, and due to the presence of the blood-brain barrier, some drugs are difficult to accurately reach the tumor periphery. To address anatomical difficulties, it is possible to simultaneously obtain tumor pathological tissue specimens on the basis of maximizing tumor tissue resection, and analyze individual tumor phenotype characteristics using multiple immunohistochemistry, proteomics, spatial transcriptomics techniques, etc. It is also possible to collect tumor-derived circulating DNA from cerebrospinal fluid before surgical resection, supplemented by tumor genome analysis, to obtain immunotherapy target information for a certain individual. Local administration (intratumoral delivery) may be feasible for addressing the challenges of the blood-brain barrier. Meanwhile, with the development of nanotechnology, it can cross the blood-brain barrier and target tumor sites, bringing new ways for immunotherapy of glioma. From the perspective of peritumoral characteristics, the immunosuppressive microenvironment is dynamic, which poses challenges to micro level research. Enhanced monitoring of individual patients may help capture immune dynamics, and non-invasive methods may be urgently needed due to increased frequency. With the widespread application of second-generation sequencing technology, the current research direction is mainly to search for new tumor mutations and antigens; in addition, research on immune related pathways and immunosuppressive mechanisms is also a new direction in tumor immunotherapy. Gliomas also exhibit a tendency to recur, further limiting the effectiveness of immunotherapy. It is possible to enhance the function of memory lymphocytes, search for specific receptors on the surface of memory lymphocytes, promote the function of activating receptors, and block the function of inhibitory receptors.
In addition, clinical research design also needs improvement. In clinical trials, the number of glioma patients is relatively small compared to more common solid tumors, which requires new therapies, including immunotherapy, to be tested in small, underpowered, and non-randomized designs. Nearly half of the published clinical studies are single-arm studies with a sample size of no more than 50 patients. These limitations make it difficult to demonstrate the efficacy of new therapies in glioma. Low randomization rate, insufficient use of the controls, and overestimation of benefits/effects, especially in early trials, may limit the widespread applicability of the results. The suboptimal design may be driven by accrual challenges, emphasizing the need for more collaborative efforts and creating incentives in these areas to enable larger-scale experiments, ideally using synchronous controls, but in appropriate cases, inequal randomization can be considered. The use of historical data can lead to bias in patient selection. Therefore, it is recommended to conduct randomized controlled clinical studies. Another area that needs improvement is the outcome indicators. Response rate is not an ideal endpoint for immunotherapy. Similarly, progression free survival is often used, but progression free survival also has the same issue in terms of response rate dependent on MRI evaluation, which may be inaccurate as pseudoprogression and pseudoresponse are common in glioblastoma. The Response Assessment in Neuro-Oncology criteria may provide some assistance. The most powerful clinical endpoint is overall survival. However, overall survival need longer follow-up time. Finally, the regulatory and technical authorities for trials should strengthen their supervision of the design and implementation of trials, and if necessary, terminate trials promptly to ensure that trials are designed and conducted in a safe, effective, and feasible manner for glioma patients.
Overall, despite the uneven quality and mixed results of available clinical trials, we still see the dawn of immunotherapy for glioma. Molecular biomarkers are of great significance in providing assistance and evaluating prognosis (95). With the advancement of cutting-edge technologies (artificial intelligence, CRISPR-Cas9, and single-cell sequencing), not only new evaluation methods can be provided for the immunotherapy of glioma, but more importantly, it is conducive to the rapid development of new immunotherapy strategies. The field of clinical trials of immunotherapy may contribute to individualized and personalized treatment and ultimately be applied in clinical practice, achieving the ultimate goal of improving the prognosis of patients with glioma (96).
Author contributions
JL: Conceptualization, Data curation, Formal analysis, Funding acquisition, Investigation, Methodology, Project administration, Resources, Software, Supervision, Validation, Visualization, Writing – original draft, Writing – review & editing. JP: Formal analysis, Funding acquisition, Investigation, Methodology, Project administration, Resources, Software, Supervision, Writing – original draft. JJ: Data curation, Formal analysis, Funding acquisition, Investigation, Project administration, Resources, Software, Supervision, Validation, Writing – review & editing. YL: Data curation, Formal analysis, Funding acquisition, Investigation, Methodology, Project administration, Resources, Software, Supervision, Validation, Writing – original draft.
Funding
The author(s) declare that no financial support was received for the research, authorship, and/or publication of this article.
Conflict of interest
The authors declare that the research was conducted in the absence of any commercial or financial relationships that could be construed as a potential conflict of interest.
Publisher’s note
All claims expressed in this article are solely those of the authors and do not necessarily represent those of their affiliated organizations, or those of the publisher, the editors and the reviewers. Any product that may be evaluated in this article, or claim that may be made by its manufacturer, is not guaranteed or endorsed by the publisher.
Supplementary material
The Supplementary Material for this article can be found online at: https://www.frontiersin.org/articles/10.3389/fimmu.2024.1476436/full#supplementary-material
References
1. Weller M, Wick W, Aldape K, Brada M, Berger M, Pfister SM, et al. Glioma. Nat Rev Dis Primers. (2015) 1:15017. doi: 10.1038/nrdp.2015.17
2. Forjaz G, Barnholtz-Sloan JS, Kruchko C, Siegel R, Negoita S, Ostrom QT, et al. An updated histology recode for the analysis of primary Malignant and nonmalignant brain and other central nervous system tumors in the Surveillance, Epidemiology, and End Results Program. Neurooncol Adv. (2021) 3:vdaa175. doi: 10.1093/noajnl/vdaa175
3. Ostrom QT, Gittleman H, Liao P, Rouse C, Chen Y, Dowling J, et al. CBTRUS statistical report: primary brain and central nervous system tumors diagnosed in the United States in 2007-2011. Neuro Oncol. (2014) 16 Suppl 4:iv1–63. doi: 10.1093/neuonc/nou223
4. Weller M, Cloughesy T, Perry JR, Wick W. Standards of care for treatment of recurrent glioblastoma–are we there yet? Neuro-oncology. (2013) 15:4–27. doi: 10.1093/neuonc/nos273
5. Huang Y, Wang Y, Huang Z. A specific peptide vaccine against IDH1(R132H) glioma. Neurosci Bull. (2022) 38:223–5. doi: 10.1007/s12264-021-00791-9
6. Old LJ, Clarke DA, Benacerraf B. Effect of Bacillus Calmette-Guerin infection on transplanted tumours in the mouse. Nature. (1959) 184:291–2. doi: 10.1038/184291a0
7. Lin MJ, Svensson-Arvelund J, Lubitz GS, Marabelle A, Melero I, Brown BD, et al. Cancer vaccines: the next immunotherapy frontier. Nat Cancer. (2022) 3:911–26. doi: 10.1038/s43018-022-00418-6
8. Saxena M, van der Burg SH, Melief CJM, Bhardwaj N. Therapeutic cancer vaccines. Nat Rev Cancer. (2021) 21:360–78. doi: 10.1038/s41568-021-00346-0
9. Tsuboi A, Hashimoto N, Fujiki F, Morimoto S, Kagawa N, Nakajima H, et al. A phase I clinical study of a cocktail vaccine of Wilms' tumor 1 (WT1) HLA class I and II peptides for recurrent Malignant glioma. Cancer Immunol Immunother. (2019) 68:331–40. doi: 10.1007/s00262-018-2274-1
10. Izumoto S, Tsuboi A, Oka Y, Suzuki T, Hashiba T, Kagawa N, et al. Phase II clinical trial of Wilms tumor 1 peptide vaccination for patients with recurrent glioblastoma multiforme. J Neurosurg. (2008) 108:963–71. doi: 10.3171/JNS/2008/108/5/0963
11. Yokota C, Kagawa N, Takano K, Chiba Y, Kinoshita M, Kijima N, et al. Maintenance of WT1 expression in tumor cells is associated with a good prognosis in Malignant glioma patients treated with WT1 peptide vaccine immunotherapy. Cancer Immunology Immunotherapy. (2022) 71:189–201. doi: 10.1007/s00262-021-02954-z
12. Weller M, Butowski N, Tran DD, Recht LD, Lim M, Hirte H, et al. Rindopepimut with temozolomide for patients with newly diagnosed, EGFRvIII-expressing glioblastoma (ACT IV): a randomised, double-blind, international phase 3 trial. Lancet Oncol. (2017) 18:1373–85. doi: 10.1016/S1470-2045(17)30517-X
13. Sampson JH, Heimberger AB, Archer GE, Aldape KD, Friedman AH, Friedman HS, et al. Immunologic escape after prolonged progression-free survival with epidermal growth factor receptor variant III peptide vaccination in patients with newly diagnosed glioblastoma. J Clin Oncol. (2010) 28:4722–9. doi: 10.1200/JCO.2010.28.6963
14. Reardon DA, Desjardins A, Vredenburgh JJ, O'Rourke DM, Tran DD, Fink KL, et al. Rindopepimut with bevacizumab for patients with relapsed EGFRvIII-expressing glioblastoma (ReACT): results of a double-blind randomized phase II trial. Clin Cancer Res. (2020) 26:1586–94. doi: 10.1158/1078-0432.CCR-18-1140
15. Platten M, Bunse L, Wick A, Bunse T, Cornet LL, Harting I, et al. A vaccine targeting mutant IDH1 in newly diagnosed glioma. Nature. (2021) 592:463–8. doi: 10.1038/s41586-021-03363-z
16. Grassl N, Poschke I, Lindner K, Bunse L, Mildenberger I, Boschert T, et al. A H3K27M-targeted vaccine in adults with diffuse midline glioma. Nat Med. (2023) 29:2586–92. doi: 10.1038/s41591-023-02555-6
17. Liau LM, Prins RM, Kiertscher SM, Odesa SK, Kremen TJ, Giovannone AJ, et al. Dendritic cell vaccination in glioblastoma patients induces systemic and intracranial T-cell responses modulated by the local central nervous system tumor microenvironment. Clin Cancer Res. (2005) 11:5515–25. doi: 10.1158/1078-0432.CCR-05-0464
18. Yu JS, Wheeler CJ, Zeltzer PM, Ying H, Finger DN, Lee PK, et al. Vaccination of Malignant glioma patients with peptide-pulsed dendritic cells elicits systemic cytotoxicity and intracranial T-cell infiltration. Cancer Res. (2001) 61:842–7.
19. Yu JS, Liu G, Ying H, Yong WH, Black KL, Wheeler CJ. Vaccination with tumor lysate-pulsed dendritic cells elicits antigen-specific, cytotoxic T-cells in patients with Malignant glioma. Cancer Res. (2004) 64:4973–9. doi: 10.1158/0008-5472.CAN-03-3505
20. Yamanaka R, Homma J, Yajima N, Tsuchiya N, Sano M, Kobayashi T, et al. Clinical evaluation of dendritic cell vaccination for patients with recurrent glioma: results of a clinical phase I/II trial. Clin Cancer Res. (2005) 11:4160–7. doi: 10.1158/1078-0432.CCR-05-0120
21. Yamanaka R, Abe T, Yajima N, Tsuchiya N, Homma J, Kobayashi T, et al. Vaccination of recurrent glioma patients with tumour lysate-pulsed dendritic cells elicits immune responses: results of a clinical phase I/II trial. Br J Cancer. (2003) 89:1172–9. doi: 10.1038/sj.bjc.6601268
22. Liau LM, Ashkan K, Tran DD, Campian JL, Trusheim JE, Cobbs CS, et al. First results on survival from a large Phase 3 clinical trial of an autologous dendritic cell vaccine in newly diagnosed glioblastoma. J Transl Med. (2018) 16:142. doi: 10.1186/s12967-018-1507-6
23. Liau LM, Ashkan K, Brem S, Campian JL, Trusheim JE, Iwamoto FM, et al. Association of autologous tumor lysate-loaded dendritic cell vaccination with extension of survival among patients with newly diagnosed and recurrent glioblastoma: A phase 3 prospective externally controlled cohort trial. JAMA Oncol. (2023) 9:112–21. doi: 10.1001/jamaoncol.2022.5370
24. Wen PY, Reardon DA, Armstrong TS, Phuphanich S, Aiken RD, Landolfi JC, et al. A randomized double-blind placebo-controlled phase II trial of dendritic cell vaccine ICT-107 in newly diagnosed patients with glioblastoma. Clin Cancer Res. (2019) 25:5799–807. doi: 10.1158/1078-0432.CCR-19-0261
25. Bota DA, Taylor TH, Piccioni DE, Duma CM, LaRocca RV, Kesari S, et al. Phase 2 study of AV-GBM-1 (a tumor-initiating cell targeted dendritic cell vaccine) in newly diagnosed Glioblastoma patients: safety and efficacy assessment. J Exp Clin Cancer Res. (2022) 41:344. doi: 10.1186/s13046-022-02552-6
26. Everson RG, Hugo W, Sun L, Antonios J, Lee A, Ding L, et al. TLR agonists polarize interferon responses in conjunction with dendritic cell vaccination in Malignant glioma: a randomized phase II Trial. Nat Commun. (2024) 15:3882. doi: 10.1038/s41467-024-48073-y
27. Batich KA, Reap EA, Archer GE, Sanchez-Perez L, Nair SK, Schmittling RJ, et al. Long-term survival in glioblastoma with cytomegalovirus pp65-targeted vaccination. Clin Cancer Res. (2017) 23:1898–909. doi: 10.1158/1078-0432.CCR-16-2057
28. Mitchell DA, Batich KA, Gunn MD, Huang MN, Sanchez-Perez L, Nair SK, et al. Tetanus toxoid and CCL3 improve dendritic cell vaccines in mice and glioblastoma patients. Nature. (2015) 519:366–9. doi: 10.1038/nature14320
29. Batich KA, Mitchell DA, Healy P, Herndon JE II, Sampson JH. Once, twice, three times a finding: reproducibility of dendritic cell vaccine trials targeting cytomegalovirus in glioblastoma. Clin Cancer Res. (2020) 26:5297–303. doi: 10.1158/1078-0432.CCR-20-1082
30. Hu JL, Omofoye OA, Rudnick JD, Kim S, Tighiouart M, Phuphanich S, et al. A phase I study of autologous dendritic cell vaccine pulsed with allogeneic stem-like cell line lysate in patients with newly diagnosed or recurrent glioblastoma. Clin Cancer Res. (2022) 28:689–96. doi: 10.1158/1078-0432.CCR-21-2867
31. Peng M, Mo Y, Wang Y, Wu P, Zhang Y, Xiong F, et al. Neoantigen vaccine: an emerging tumor immunotherapy. Mol Cancer. (2019) 18:128. doi: 10.1186/s12943-019-1055-6
32. Keskin DB, Anandappa AJ, Sun J, Tirosh I, Mathewson ND, Li S, et al. Neoantigen vaccine generates intratumoral T cell responses in phase Ib glioblastoma trial. Nature. (2019) 565:234–9. doi: 10.1038/s41586-018-0792-9
33. Johanns TM, Garfinkle EAR, Miller KE, Livingstone AJ, Roberts KF, Venkata LPR, et al. Integrating multisector molecular characterization into personalized peptide vaccine design for patients with newly diagnosed glioblastoma. Clin Cancer Res. (2024) 30(13):2729–42. doi: 10.1158/1078-0432.27034444.v1
34. Zhou JG, Liang B, Liu JG, Jin SH, He SS, Frey 2B, et al. Identification of 15 lncRNAs signature for predicting survival benefit of advanced melanoma patients treated with anti-PD-1 monotherapy. Cells. (2021) 10(5):977. doi: 10.3390/cells10050977
35. Wang XP, Guo W, Chen YF, Hong C, Ji J, Zhang XY, et al. PD-1/PD-L1 axis is involved in the interaction between microglial polarization and glioma. Int Immunopharmacol. (2024) 133:112074. doi: 10.1016/j.intimp.2024.112074
36. Omuro A, Reardon DA, Sampson JH, Baehring J, Sahebjam S, Cloughesy TF, et al. Nivolumab plus radiotherapy with or without temozolomide in newly diagnosed glioblastoma: Results from exploratory phase I cohorts of CheckMate 143. Neurooncol Adv. (2022) 4:vdac025. doi: 10.1093/noajnl/vdac025
37. Reardon DA, Brandes AA, Omuro A, Mulholland P, Lim M, Wick A, et al. Effect of nivolumab vs bevacizumab in patients with recurrent glioblastoma: the checkMate 143 phase 3 randomized clinical trial. JAMA Oncol. (2020) 6:1003–10. doi: 10.1001/jamaoncol.2020.1024
38. Lim M, Weller M, Idbaih A, Steinbach J, Finocchiaro G, Raval RR, et al. Phase III trial of chemoradiotherapy with temozolomide plus nivolumab or placebo for newly diagnosed glioblastoma with methylated MGMT promoter. Neuro-Oncology. (2022) 24:1935–49. doi: 10.1093/neuonc/noac116
39. Omuro A, Brandes AA, Carpentier AF, Idbaih A, Reardon DA, Cloughesy T, et al. Radiotherapy combined with nivolumab or temozolomide for newly diagnosed glioblastoma with unmethylated MGMT promoter: An international randomized phase III trial. Neuro-Oncology. (2022) 25:123–34. doi: 10.1093/neuonc/noac099
40. Ito H, Nakashima H, Chiocca EA. Molecular responses to immune checkpoint blockade in glioblastoma. Nat Med. (2019) 25:359–61. doi: 10.1038/s41591-019-0385-7
41. Schalper KA, Rodriguez-Ruiz ME, Diez-Valle R, López-Janeiro A, Porciuncula A, Idoate MA, et al. Neoadjuvant nivolumab modifies the tumor immune microenvironment in resectable glioblastoma. Nat Med. (2019) 25:470–6. doi: 10.1038/s41591-018-0339-5
42. Cloughesy TF, Mochizuki AY, Orpilla JR, Hugo W, Lee AH, Davidson TB, et al. Neoadjuvant anti-PD-1 immunotherapy promotes a survival benefit with intratumoral and systemic immune responses in recurrent glioblastoma. Nat Med. (2019) 25:477–86. doi: 10.1038/s41591-018-0337-7
43. Zhao J, Chen AX, Gartrell RD, Silverman AM, Aparicio L, Chu T, et al. Immune and genomic correlates of response to anti-PD-1 immunotherapy in glioblastoma. Nat Med. (2019) 25:462–9. doi: 10.1038/s41591-019-0349-y
44. Duerinck J, Schwarze JK, Awada G, Tijtgat J, Vaeyens F, Bertels C, et al. Intracerebral administration of CTLA-4 and PD-1 immune checkpoint blocking monoclonal antibodies in patients with recurrent glioblastoma: a phase I clinical trial. J Immunother Cancer. (2021) 9:e002296. doi: 10.1136/jitc-2020-002296
45. Dunkel IJ, Doz F, Foreman NK, Hargrave D, Lassaletta A, André N, et al. Nivolumab with or without ipilimumab in pediatric patients with high-grade CNS Malignancies: Safety, efficacy, biomarker, and pharmacokinetics-CheckMate 908. Neuro Oncol. (2023) 25:1530–45. doi: 10.1093/neuonc/noad031
46. Sahebjam S, Forsyth PA, Tran ND, Arrington JA, Macaulay R, Etame AB, et al. Hypofractionated stereotactic re-irradiation with pembrolizumab and bevacizumab in patients with recurrent high-grade gliomas: results from a phase I study. Neuro Oncol. (2021) 23:677–86. doi: 10.1093/neuonc/noaa260
47. Simonelli M, Garralda E, Eskens F, Gil-Martin M, Yen CJ, Obermannova R, et al. Isatuximab plus atezolizumab in patients with advanced solid tumors: results from a phase I/II, open-label, multicenter study. ESMO Open. (2022) 7:100562. doi: 10.1016/j.esmoop.2022.100562
48. Brown MP, Ebert LM, Gargett T. Clinical chimeric antigen receptor-T cell therapy: a new and promising treatment modality for glioblastoma. Clin Transl Immunol. (2019) 8:e1050. doi: 10.1002/cti2.2019.8.issue-5
49. Pule MA, Savoldo B, Myers GD, Rossig C, Russell HV, Dotti G, et al. Virus-specific T cells engineered to coexpress tumor-specific receptors: persistence and antitumor activity in individuals with neuroblastoma. Nat Med. (2008) 14:1264–70. doi: 10.1038/nm.1882
50. Majzner RG, Ramakrishna S, Yeom KW, Patel S, Chinnasamy H, Schultz LM, et al. GD2-CAR T cell therapy for H3K27M-mutated diffuse midline gliomas. Nature. (2022) 603:934–41. doi: 10.1038/s41586-022-04489-4
51. Liu Z, Zhou J, Yang X, Liu Y, Zou C, Lv W, et al. Safety and antitumor activity of GD2-Specific 4SCAR-T cells in patients with glioblastoma. Mol Cancer. (2023) 22:3. doi: 10.1186/s12943-022-01711-9
52. O’Rourke DM, Nasrallah MP, Desai A, Melenhorst JJ, Mansfield K, Morrissette JJD, et al. A single dose of peripherally infused EGFRvIII-directed CAR T cells mediates antigen loss and induces adaptive resistance in patients with recurrent glioblastoma. Sci Trans Med. (2017) 9:eaaa0984. doi: 10.1126/scitranslmed.aaa0984
53. Goff SL, Morgan RA, Yang JC, Sherry RM, Robbins PF, Restifo NP, et al. Pilot trial of adoptive transfer of chimeric antigen receptor-transduced T cells targeting EGFRvIII in patients with glioblastoma. J Immunother. (2019) 42:126–35. doi: 10.1097/CJI.0000000000000260
54. Ahmed N, Brawley V, Hegde M, Bielamowicz K, Kalra M, Landi D, et al. HER2-specific chimeric antigen receptor–modified virus-specific T cells for progressive glioblastoma: A phase 1 dose-escalation trial. JAMA Oncol. (2017) 3:1094–101. doi: 10.1001/jamaoncol.2017.0184
55. Brown CE, Badie B, Barish ME, Weng L, Ostberg JR, Chang WC, et al. Bioactivity and safety of IL13Rα2-redirected chimeric antigen receptor CD8+ T cells in patients with recurrent glioblastoma. Clin Cancer Res. (2015) 21:4062–72. doi: 10.1158/1078-0432.CCR-15-0428
56. Brown Christine E, Alizadeh D, Starr R, Weng L, Wagner JR, Naranjo A, et al. Regression of glioblastoma after chimeric antigen receptor T-cell therapy. New Engl J Med. (2016) 375:2561–9. doi: 10.1056/NEJMoa1610497
57. Brown CE, Rodriguez A, Palmer J, Ostberg JR, Naranjo A, Wagner JR, et al. Off-the-shelf, steroid-resistant, IL13Rα2-specific CAR T cells for treatment of glioblastoma. Neuro-Oncology. (2022) 24:1318–30. doi: 10.1093/neuonc/noac024
58. Jin L, Ge H, Long Y, Yang C, Chang YE, Mu L, et al. CD70, a novel target of CAR T-cell therapy for gliomas. Neuro Oncol. (2018) 20:55–65. doi: 10.1093/neuonc/nox116
59. Chow KK, Naik S, Kakarla S, Brawley VS, Shaffer DR, Yi Z, et al. T cells redirected to EphA2 for the immunotherapy of glioblastoma. Mol Ther. (2013) 21:629–37. doi: 10.1038/mt.2012.210
60. Lin Q, Ba T, Ho J, Chen D, Cheng Y, Wang L, et al. First-in-human trial of ephA2-redirected CAR T-cells in patients with recurrent glioblastoma: A preliminary report of three cases at the starting dose. Front Oncol. (2021) 11:694941. doi: 10.3389/fonc.2021.694941
61. Brown CE, Aguilar B, Starr R, Yang X, Chang WC, Weng L, et al. Optimization of IL13Rα2-targeted chimeric antigen receptor T cells for improved anti-tumor efficacy against glioblastoma. Mol Ther. (2018) 26:31–44. doi: 10.1016/j.ymthe.2017.10.002
62. Heczey A, Louis CU, Savoldo B, Dakhova O, Durett A, Grilley B, et al. CAR T cells administered in combination with lymphodepletion and PD-1 inhibition to patients with neuroblastoma. Mol Ther. (2017) 25:2214–24. doi: 10.1016/j.ymthe.2017.05.012
63. Bielamowicz K, Fousek K, Byrd TT, Samaha H, Mukherjee M, Aware N, et al. Trivalent CAR T cells overcome interpatient antigenic variability in glioblastoma. Neuro Oncol. (2018) 20:506–18. doi: 10.1093/neuonc/nox182
64. Burger MC, Forster MT, Romanski A, Straßheimer F, Macas J, Zeiner PS, et al. Intracranial injection of natural killer cells engineered with a HER2-targeted chimeric antigen receptor in patients with recurrent glioblastoma. Neuro Oncol. (2023) 25:2058–71. doi: 10.1093/neuonc/noad087
65. Zhang C, Burger MC, Jennewein L, Genßler S, Schönfeld K, Zeiner P, et al. ErbB2/HER2-specific NK cells for targeted therapy of glioblastoma. J Natl Cancer Inst. (2016) 108(5). doi: 10.1093/jnci/djv375
66. Choi BD, Gerstner ER, Frigault MJ, Leick MB, Mount CW, Balaj L, et al. Intraventricular CARv3-TEAM-E T cells in recurrent glioblastoma. New Engl J Med. (2024) 390:1290–8. doi: 10.1056/NEJMoa2314390
67. Wang JL, Scheitler KM, Wenger NM, Elder JB. Viral therapies for glioblastoma and high-grade gliomas in adults: a systematic review. Neurosurg Focus. (2021) 50:E2. doi: 10.3171/2020.11.FOCUS20854
68. Chiocca EA, Abbed KM, Tatter S, Louis DN, Hochberg FH, Barker F, et al. A phase I open-label, dose-escalation, multi-institutional trial of injection with an E1B-attenuated adenovirus, ONYX-015, into the peritumoral region of recurrent Malignant gliomas, in the adjuvant setting. Mol Ther. (2004) 10:958–66. doi: 10.1016/j.ymthe.2004.07.021
69. Lang FF, Conrad C, Gomez-Manzano C, Yung WKA, Sawaya R, Weinberg JS, et al. Phase I study of DNX-2401 (Delta-24-RGD) oncolytic adenovirus: replication and immunotherapeutic effects in recurrent Malignant glioma. J Clin Oncol. (2018) 36:1419–27. doi: 10.1200/JCO.2017.75.8219
70. Martínez-Vélez N, Garcia-Moure M, Marigil M, González-Huarriz M, Puigdelloses M, Pérez-Larraya JG, et al. The oncolytic virus Delta-24-RGD elicits an antitumor effect in pediatric glioma and DIPG mouse models. Nat Commun. (2019) 10:2235. doi: 10.1038/s41467-019-10043-0
71. van den Bossche WBL, Kleijn A, Teunissen CE, Voerman JSA, Teodosio C, Noske DP, et al. Oncolytic virotherapy in glioblastoma patients induces a tumor macrophage phenotypic shift leading to an altered glioblastoma microenvironment. Neuro Oncol. (2018) 20:1494–504. doi: 10.1093/neuonc/noy082
72. van Putten EHP, Kleijn A, van Beusechem VW, Noske D, Lamers CHJ, Goede ALD, et al. Convection enhanced delivery of the oncolytic adenovirus delta24-RGD in patients with recurrent GBM: A phase I clinical trial including correlative studies. Clin Cancer Res. (2022) 28:1572–85. doi: 10.1158/1078-0432.CCR-21-3324
73. Gállego Pérez-Larraya J, Garcia-Moure M, Labiano S, Patiño-García A, Dobbs J, Gonzalez-Huarriz M, et al. Oncolytic DNX-2401 virus for pediatric diffuse intrinsic pontine glioma. N Engl J Med. (2022) 386:2471–81. doi: 10.1056/NEJMoa2202028
74. Nassiri F, Patil V, Yefet LS, Singh O, Liu J, Dang RMA, et al. Oncolytic DNX-2401 virotherapy plus pembrolizumab in recurrent glioblastoma: a phase 1/2 trial. Nat Med. (2023) 29:1370–8. doi: 10.1038/s41591-023-02347-y
75. Desjardins A, Gromeier M, Herndon JE 2nd, Beaubier N, Bolognesi DP, Friedman AH, et al. Recurrent glioblastoma treated with recombinant poliovirus. N Engl J Med. (2018) 379:150–61. doi: 10.1056/NEJMoa1716435
76. Fares J, Ahmed AU, Ulasov IV, Sonabend AM, Miska J, Lee-Chang C, et al. Neural stem cell delivery of an oncolytic adenovirus in newly diagnosed Malignant glioma: a first-in-human, phase 1, dose-escalation trial. Lancet Oncol. (2021) 22:1103–14. doi: 10.1016/S1470-2045(21)00245-X
77. Ling AL, Solomon IH, Landivar AM, Nakashima H, Woods JK, Santos A, et al. Clinical trial links oncolytic immunoactivation to survival in glioblastoma. Nature. (2023) 623:157–66. doi: 10.1038/s41586-023-06623-2
78. Ou A, Yung WKA, Majd N. Molecular mechanisms of treatment resistance in glioblastoma. Int J Mol Sci. (2020) 22(1):351. doi: 10.3390/ijms22010351
79. Awad MM, Govindan R, Balogh KN, Spigel DR, Garon EB, Bushway ME, et al. Personalized neoantigen vaccine NEO-PV-01 with chemotherapy and anti-PD-1 as first-line treatment for non-squamous non-small cell lung cancer. Cancer Cell. (2022) 40:1010–26.e11. doi: 10.1016/j.ccell.2022.08.003
80. Liu Z, Shi M, Ren Y, Xu H, Weng S, Ning W, et al. Recent advances and applications of CRISPR-Cas9 in cancer immunotherapy. Mol Cancer. (2023) 22:35. doi: 10.1186/s12943-023-01738-6
81. Zhang R, Tang L, Wang Y, Tian Y, Wu S, Zhou B, et al. A dendrimer peptide (KK2DP7) delivery system with dual functions of lymph node targeting and immune adjuvants as a general strategy for cancer immunotherapy. Adv Sci (Weinh). (2023) 10:e2300116. doi: 10.1002/advs.202300116
82. Chen KS, Reinshagen C, Van Schaik TA, Rossignoli F, Borges P, Mendonca NC, et al. Bifunctional cancer cell-based vaccine concomitantly drives direct tumor killing and antitumor immunity. Sci Transl Med. (2023) 15:eabo4778. doi: 10.1126/scitranslmed.abo4778
83. Johnson KC, Anderson KJ, Courtois ET, Gujar AD, Barthel FP, Varn FS, et al. Single-cell multimodal glioma analyses identify epigenetic regulators of cellular plasticity and environmental stress response. Nat Genet. (2021) 53:1456–68. doi: 10.1038/s41588-021-00926-8
84. Vinci M, Burford A, Molinari V, Popov S, Clarke M, Taylor KR, et al. Functional diversity and cooperativity between subclonal populations of pediatric glioblastoma and diffuse intrinsic pontine glioma cells. Nat Med. (2018) 24:1204–15. doi: 10.1038/s41591-018-0086-7
85. Jha P, Manjunath N, Singh J, Mani K, Garg A, Kaur K, et al. Analysis of PD-L1 expression and T cell infiltration in different molecular subgroups of diffuse midline gliomas. Neuropathology. (2019) 39:413–24. doi: 10.1111/neup.12594
86. Alexandrov LB, Nik-Zainal S, Wedge DC, Aparicio SAJR, Behjati S, Biankin AV, et al. Signatures of mutational processes in human cancer. Nature. (2013) 500:415–21. doi: 10.1038/nature12477
87. Touat M, Li YY, Boynton AN, Spurr LF, Iorgulescu JB, Bohrson CL, et al. Mechanisms and therapeutic implications of hypermutation in gliomas. Nature. (2020) 580:517–23. doi: 10.1038/s41586-020-2209-9
88. McGranahan N, Furness AJ, Rosenthal R, Ramskov S, Lyngaa R, Saini SK, et al. Clonal neoantigens elicit T cell immunoreactivity and sensitivity to immune checkpoint blockade. Science. (2016) 351:1463–9. doi: 10.1126/science.aaf1490
89. Cacciotti C, Choi J, Alexandrescu S, Zimmerman MA, Cooney TM, Chordas C, et al. Immune checkpoint inhibition for pediatric patients with recurrent/refractory CNS tumors: a single institution experience. J Neurooncol. (2020) 149:113–22. doi: 10.1007/s11060-020-03578-6
90. Chalise L, Kato A, Ohno M, Maeda ZS, Yamamichi A, Kuramitsu S, et al. Efficacy of cancer-specific anti-podoplanin CAR-T cells and oncolytic herpes virus G47Δ combination therapy against glioblastoma. Mol Ther Oncolytics. (2022) 26:265–74. doi: 10.1016/j.omto.2022.07.006
91. Harrington K, Freeman DJ, Kelly B, Harper J, Soria JC. Optimizing oncolytic virotherapy in cancer treatment. Nat Rev Drug Discovery. (2019) 18:689–706. doi: 10.1038/s41573-019-0029-0
92. LaRocca CJ, Warner SG. Oncolytic viruses and checkpoint inhibitors: combination therapy in clinical trials. Clin Transl Med. (2018) 7:35. doi: 10.1186/s40169-018-0214-5
93. Sonabend AM, Gould A, Amidei C, Ward R, Schmidt KA, Zhang DY, et al. Repeated blood-brain barrier opening with an implantable ultrasound device for delivery of albumin-bound paclitaxel in patients with recurrent glioblastoma: a phase 1 trial. Lancet Oncol. (2023) 24:509–22. doi: 10.1016/S1470-2045(23)00112-2
94. Kaufman HL, Kohlhapp FJ, Zloza A. Oncolytic viruses: a new class of immunotherapy drugs. Nat Rev Drug Discovery. (2015) 14:642–62. doi: 10.1038/nrd4663
95. Louis DN, Perry A, Wesseling P, Brat DJ, Cree IA, Figarella-Branger D, et al. The 2021 WHO classification of tumors of the central nervous system: a summary. Neuro-oncology. (2021) 23:1231–51. doi: 10.1093/neuonc/noab106
Keywords: immunotherapy, gliomas, tumor vaccines, immune checkpoint inhibitors, CAR-T, oncolytic virotherapy
Citation: Liu J, Peng J, Jiang J and Liu Y (2024) Clinical immunotherapy in glioma: current concepts, challenges, and future perspectives. Front. Immunol. 15:1476436. doi: 10.3389/fimmu.2024.1476436
Received: 05 August 2024; Accepted: 16 October 2024;
Published: 01 November 2024.
Edited by:
Jiaheng Xie, Central South University, ChinaReviewed by:
Yuxuan Song, Peking University People’s Hospital, ChinaKok-Siong Chen, Brigham and Women’s Hospital and Harvard Medical School, United States
Qihang Yuan, Dalian Medical University, China
Copyright © 2024 Liu, Peng, Jiang and Liu. This is an open-access article distributed under the terms of the Creative Commons Attribution License (CC BY). The use, distribution or reproduction in other forums is permitted, provided the original author(s) and the copyright owner(s) are credited and that the original publication in this journal is cited, in accordance with accepted academic practice. No use, distribution or reproduction is permitted which does not comply with these terms.
*Correspondence: Yanhui Liu, bGl1eWhAc2N1LmVkdS5jbg==