- Department of Veterinary Medicine, University of Cambridge, Cambridge, United Kingdom
More affordable and effective vaccines against bacterial meningitis caused by Neisseria meningitidis serogroup B are still required for global prevention. We have previously shown that modified outer membrane vesicles (mOMVs) from commensal Neisseria cinerea can be used as a platform to induce immune responses against meningococcal antigens. The aim of the present study was to use a combination of two genetically engineered mOMVs to express multiple antigens from N. meningitidis known to be involved in protective immunity to meningococcal meningitis (different variants of factor H binding protein (fHbp), Neisseria Heparin Binding Antigen (NHBA) and Neisseria Adhesin A (NadA)). Antigen expression in the mOMVs was confirmed by Western blotting; detoxification of the lipooligosaccharide (LOS) was confirmed by measuring human Toll-like receptor 4 (hTLR4) activation using in vitro cell assays. Mice immunised with a combination of two mOMVs expressing fHbp, NHBA and NadA produced antibodies to all the antigens. Furthermore, serum bactericidal activity (SBA) was induced by the immunisation, with mOMVs expressing NadA displaying high SBA titres against a nadA+ MenB strain. The work highlights the potential of mOMVs from N. cinerea to induce functional immune responses against multiple antigens involved in the protective immune response to meningococcal disease.
Introduction
Neisseria meningitidis is a Gram-negative diplococcus that asymptomatically colonises the nasopharyngeal mucosa of healthy carriers. On some occasions, the bacterium traverses the mucosal epithelium and invades the bloodstream, leading to invasive meningococcal disease (IMD), a potentially life-threatening disease and a major cause of bacterial meningitis and septicaemia worldwide (1). There are 13 serogroups of N. meningitidis based on the composition of polysaccharide capsule, with most IMD cases primarily caused by serogroups A, B, C, W, Y and X (2).
Although conjugated polysaccharide vaccines are used for immunization against N. meningitidis serogroups A, C, Y, and W (2), this has not been the case for N. meningitidis serogroup B (MenB). A polysaccharide-based vaccine for MenB was disregarded for two major reasons: its theoretical risk for autoimmunity due to the similarity of the chemical composition to sialic acid found on many human cell types and its low immunogenicity (3, 4). Therefore, several non-capsular outer membrane vesicle (OMV) vaccines were used during epidemics of MenB (5), and vaccines based on recombinant proteins identified by proteomic and reverse vaccinology approaches are currently available (6, 7).
Two protein-based vaccines against MenB currently in use are the four-component 4CMenB vaccine (Bexsero®, GlaxoSmithKline) and the bivalent fHbp2086 vaccine (Trumenba®, Pfizer). These vaccines use subcapsular proteins that are widely present not only in serogroup B strains, but also across different meningococcus serogroups (8, 9). The factor H binding protein (fHbp) vaccine (formally known as LP2086) consists of two lipidated fHbp (8). The 4CMenB vaccine contains three components that were identified by reverse vaccinology based on the complete genome sequence of a pathogenic reference MenB strain (strain MC58): the fHbp variant 1.1 fused to the genome-derived Neisseria antigen (GNA) 2091, the Neisseria adhesin A variant 2/3 peptide 8 (NadA-8), and the Neisseria Heparin binding antigen peptide 2 (NHBA-2) fused to GNA1030 (9). Besides these three recombinant surface-exposed protein antigens, the vaccine contains detergent-extracted OMVs from the New Zealand strain NZ98/254 containing porin A (PorA) P1.4 (10). While these vaccines have successfully decreased the incidence of group B meningitis in both infants and adults, the challenge of high production costs persists (11).
FHbp is a surface-exposed protein expressed in almost all N. meningitidis strains and divided in two subfamilies, A and B (12). Due to the variations in amino acid sequences, each fHbp protein can be identified by an ID number (12). FHbp binds to human factor H, a negative regulator of the alternative pathway of complement activation and is an immune-evasion factor in Neisseria (13, 14). NHBA is a surface protein that binds to heparin, which can confer a level of serum resistance as well as binding to heparin sulphate proteoglycans to aid in bacterial adherence to epithelium (15, 16). An additional role of NHBA is to promote biofilm development through the binding with extracellular DNA involved in the initial stages of cell-to-cell attachment (17). NHBA is present in all N. meningitidis, N. gonorrhoeae and some commensal Neisseria species (18). NadA is an outer membrane protein (OMP) that promotes adhesion to and invasion of host cells during bacterial infection and colonisation of the human respiratory tract (18). There are four variants of NadA, with variants 1 and 2/3 found in invasive meningococci (19). Unlike fHbp and NHBA, the nadA gene is only found in approximately 30% of N. meningitidis isolates, but importantly it is present in approximately 75% of hypervirulent MenB strains (18, 20, 21).
In vitro measurement of functional antibodies has been considered for decades as a correlate of protection against many bacterial species (22). Serum bactericidal activity (SBA) assays are commonly used as correlates of protection for vaccines against N. meningitidis (23–26), with SBA titres having been used to prioritise antigens for incorporation into the Bexsero® vaccine.
OMVs are an attractive vaccine platform for their excellent adjuvanticity (27, 28), the possibility of modifying them to include surface-expressed heterologous antigens (29–32), and the simplicity of their production and purification process (33). Indeed, OMV-based vaccines against bacterial pathogens have already reached the market (34, 35), while others are in advanced clinical phases (36, 37). Vaccines developed against MenB based on OMVs include VA-MENGOC-BC [Finlay Institute] used in Cuba, MenBvac [Norwegian Institute of Public Health] in Norway, and MeNZB [Novartis] in New Zealand. However, as they were designed for specific strains, this results in poor coverage across the diverse range of MenB strains.
Our group has previously shown the immunogenicity of genetically modified OMVs (mOMVs) from the commensal BSL-1 Neisseria cinerea with key antigens such as NHBA and fHbp being expressed (32). OMVs purified from the commensal biosafety level 1 (BSL-1) Neisseria lactamica have also been studied as a potential vaccine against N. meningitidis (38–40). The use of a non-pathogenic Neisseria strain has enormous benefits in terms of ease and safety of manipulation in the laboratory, ease of production, and low production costs. OMVs from commensal Neisseria spp. that are phylogenetically placed near N. meningitidis also have the potential to induce immune responses against antigens with a high level of homology (and therefore potential immunological cross-reactivity) between Neisseria species (32, 39).
In the present study, we capitalised on our previous proof-of-principle work (32) on the use of N. cinerea OMV. In the current study we have leapt towards the delivery of a combination of several Neisseria antigens known to be involved in the protective response against meningococcal meningitis. We have used the commensal strain N. cinerea ATCC® 14685™ that naturally expresses fHbpID100 from subfamily B, with 97% aa identity to the fHbp present in Bexsero® (32, 41). Along with fHbpID45 from subfamily A and NHBA peptide 2 (NHBA-2, included in Bexsero®), we have also incorporated NadA variant 2/3 peptide 8 (NadA-8, included in Bexsero®) into the mOMVs to provide a broader coverage of MenB strains. Antigen combinations of fHbp, NHBA and NadA are known to play a key role in the protective activity of the currently licensed vaccines against MenB (9). We immunised mice with two different vesicles incorporating the antigens listed above and tested antibody responses by ELISA and Western blotting (WB), as well as measuring SBA as a correlate of protection.
Materials and methods
Bacterial strains and growth conditions
All commensal Neisseria strains (Supplementary Table S1) were grown on gonococcal (GC) base medium agar plates (Oxoid) with Kellogg’s supplements (42) or in GC liquid broth (Oxoid) with Kellogg’s supplements and 0.042% (w/v) sodium bicarbonate at 37C, 5% CO2. N. meningitidis MC58 was grown on horse blood agar plates at 37C, 5% CO2. Erythromycin (10 μg/mL), gentamicin (10 μg/mL), kanamycin (40 μg/mL) and chloramphenicol (2.5 μg/mL) were added to GC agar or GC broth as required for selective growth conditions.
Generation of genetically modified N. cinerea ATCC® 14685™ strains
Briefly, all the insertions and deletions of genes into the chromosome of N. cinerea ATCC® 14685™ were performed using constructs synthesised by GenScript. The constructs were inserted into pUC18 vector (Supplementary Table S2), linearised with the restriction enzyme HindIII (NEB) and purified using the Monarch PCR and DNA Cleanup Kit (NEB). The fragments were amplified with Q5 Hot Start High Fidelity 2X Master Mix (NEB) by PCR with the appropriate primer pairs (Supplementary Table S3). The resulting fragments were purified using the Monarch PCR and DNA Cleanup Kit (NEB), confirmed by Sanger Sequencing (Source Biosciences) and transformed into the appropriate N. cinerea ATCC® 14685™ strains (Supplementary Table S1).
mOMV production
For production of mOMVs, bacteria were streaked onto GC agar plates containing the appropriate antibiotics and incubated at 37°C, 5% CO2 for 16-48 hours. Colonies were picked and used to inoculate 5 mL of GC broth to obtain an optical density (OD) at 600 nm (OD600) of ~0.1, and then incubated at 37°C, 5% CO2, shaking at 150 rpm. Once the culture reached OD600 of ~1.0, 5 mL of the broth was used to inoculate 50 mL of fresh, pre-warmed GC broth (1:10 dilution) and incubated at 37°C, 5% CO2, shaking at 150 rpm. When the culture reached OD600 of ~1.0 (typically 3-4 hours later) and the OD600 value remained constant or started to decrease after a further hour, the culture was removed from incubation and the bacteria were separated from the media by centrifuging at 2,200 x g, 4°C for 40 minutes. The supernatant was then filtered with a 0.22 μm polyethersulfone membrane (Millipore). To check for absence of bacterial growth, 10 μL of supernatant was streaked on a GC agar plate and incubated overnight at 37°C, 5% CO2.
To isolate the mOMVs that were blebbed during culturing, the supernatant was ultracentrifuged at 45,000 rpm at 4°C for 2 hours using a Beckman Coulter L-80 Ultracentrifuge (Type 50.2 Ti rotor), before the pellet was resuspended in 1X PBS. Afterwards, the suspension was spun again, and the pellet was allowed to resuspend in 100-200 μL of fresh 1X PBS overnight, before finally being spun through 0.22 μm spin filters (Corning). The resulting OMV sample was then stored at -20°C until further analysis.
Recombinant proteins, monoclonal and polyclonal antibodies
Recombinant proteins fHbpID100 (rID100), fHbpID45 (rID45), NHBA-2 (rNHBA-2) and NadA-8 (rNadA-8) were custom-made by GenScript. Briefly, genes expressing fHbpID100, fHbpID45, NHBA-2 and NadA-8 were cloned into the pET30a vector using the NdeI/HindIII cloning sites. A C-terminal His-tag was used for fHbp genes, while an N-terminal His-tag was used for nhba-2 and nadA-8 genes. The signal peptide sequence was removed from the N-terminal end of NHBA-2 and NadA-8. rID100 was purified by nickel chromatography and size exclusion chromatography while rID45, rNHBA-2 and rNadA-8 were purified by nickel chromatography. For plasmid generation, competent Escherichia coli TOP10 was used, while for protein expression E. coli BL21 StarTM (DE3) was used.
The commercially available monoclonal antibodies JAR5 and JAR13 were purchased from NIBSC and Merck, respectively. JAR5 is specific for fHbp subfamily B and JAR13 is specific for fHbp subfamily A (43). To generate polyclonal antibodies against NHBA-2 and NadA-8, the purified recombinant proteins were used to immunise rabbits in three doses (carried out by Genscript, see Supplementary Table S4).
Confirmation of mOMV antigenic profile
The Pierce™ bicinchoninic acid (BCA) Protein Assay Kit (ThermoFisher) was used to determine the total protein concentration of purified mOMVs in relation to a standard curve obtained with commercially available bovine serum albumin (BSA) and followed the manufacturer’s instruction.
To determine expression of antigens in OMV preparations, SDS-PAGE was used to separate the individual proteins by molecular weight. For each preparation, 1.5 μg of OMVs was mixed with 2X sample buffer (NuPage Sample Reducing Agent (10X) and NuPAGE LDS Sample Buffer (4X) in MilliQ water) and heated at 95°C for 20 minutes. Recombinant proteins (rID100, rID45, rNHBA2 and rNadA) were also prepared as a positive control to 0.1 μg in 1X sample buffer and heated at 95°C for 20 minutes. Controls and samples were then loaded on either NuPAGE Novex 12% Bis-Tris Protein Gels (Thermo Scientific), using NuPAGE MOPS SDS Running Buffer (Thermo Scientific) to check fHbp expression or NuPAGE 7% Tris-Acetate Protein Gels (Thermo Scientific) using NuPAGE Tris-Acetate Running Buffer (ThermoFisher) to check NHBA and NadA expression. The gels were also loaded with 10-250 kDa Colour Prestained Protein Standard Ladder (NEB). NuPAGE Novex 12% Bis-Tris Protein Gels were then ran at 100 V for 25 minutes and then 200 V for 40 minutes, whilst NuPAGE 7% Tris-Acetate Protein Gels were ran at 80 V for 25 minutes followed by 120 V for 40 minutes.
Subsequently, gels were used for WB. The Bio-Rad Turbo Transfer System was used to transfer the separated proteins from the gels to a nitrocellulose membrane using a ‘Mixed Molecular Weight’ program (25 V for 7 minutes). All membranes were then blocked with 10% (w/v) milk in 1X PBS for 2 hours at room temperature on a rocking platform, before being washed in wash buffer (0.05% Tween-20 in 1X PBS) six times. For incubation with a primary antibody solution, the relevant membranes were incubated overnight with the specific antibodies prepared as indicated in Supplementary Table S4 in buffer solution (10% (w/v) milk, 0.05% Tween- 20 in 1X PBS). After another wash step, membranes were incubated for a further 2 hours with the relevant secondary antibody solution (See Supplementary Table S4). After a final wash, the membranes were developed using 1 mL BCIP®/NBT-Purple Liquid Substrate System (Sigma) and the reaction was then stopped by washing with MilliQ water before images were taken.
Dynamic light scattering
Particle size distribution of mOMVs was evaluated by dynamic light scattering (DLS), with a Malvern Zetasizer Nano S containing a HE-Ne laser of 633 nm wavelength. mOMV samples were diluted to a 0.1 ug/uL dilution in PBS, then read at 25°C. Each sample was read in triplicates to measure distribution of OMVs, and the resulting readouts included average diameter and polydispersity index (PdI), using the Zetasizer 7.11 software (Malvern).
In vitro toxicity assays with mOMVs, using HEK-blue cell lines
To determine the toxicity of mOMVs, in vitro human Toll-like receptor (hTLR4) stimulation assay was carried out using the commercially available HEK-Blue hTLR4 and HEK-Blue Null2 cells (InvivoGen). Cells were seeded and maintained as per manufacturer’s instruction (InvivoGen). In a 96-well flat bottom microplate, 20 μL of OMV sample were aliquoted from 1,000 ng/μL and serially diluted to 0.1 ng/μL. As a positive control, 20 μL of ultrapure lipopolysaccharide from Escherichia coli K12 (LPS-EK, InvivoGen) were aliquoted from 1,000 ng/μL and serially diluted to 0.1 ng/μL. OMV sample from N. cinerea ATCC® 14685™ WT (OMV WT) was also used as a control to compare with mutant mOMVs. 80μL of either HEK-Blue cell suspension was added to the relevant wells and the plates were then incubated at 37°C in 5% CO2 for 24 hours. Subsequently, 100 μL of each supernatant was transferred to a new 96-well microplate and read using a microplate reader (Byonoy), at 620 nm (OD620). The ratio of SEAP induced colour change was then calculated:
Transmission electron microscopy
mOMVs were imaged by transmission electron microscopy (TEM), using a Tecnai G20 transmission electron microscope (FEI/ThermoFisher Scientific). Approximately 1 μg of each OMV preparation, diluted in 1X PBS, were place under a glow-discharged 400 mesh copper/carbon film grids (EM Resolutions) and left to be adsorbed for two minutes. The grids were then washed twice by passing over deionised water drops to remove buffer salts, before being passed on 2% (w/v) aqueous uranyl acetate to improve negative staining. After excess uranyl acetate was removed, the grids were then left to dry at room temperature. Images were then made at an accelerating voltage of 200 kV using a 20 μm objective aperture to improve contrast, using an ORCA HR CCD camera (Advanced Microscopy Techniques Corp, USA) for capture.
Mouse immunogenicity studies
The study was performed at the University of Cambridge, under the appropriate Home Office licences and Ethical Review Committee approval. The dose formulations were prepared on the day of dosing, where the required concentration of relevant mOMV was added with Alhydrogel adjuvant (14 mg of Alhydrogel per 1 mg of total mOMVs) in 1X PBS and allowed to mix for one hour by rotation at room temperature. In each study groups of 6-8 weeks old female C57BL/6 mice were immunised intraperitoneally (IP) in three doses with 10 μg or 20 μg mOMVs (whole protein content), each ~21-28 days apart with control animals receiving Alhydrogel only (see Table 1).
Blood samples were collected ~21 days after the third dose, by cardiac puncture under terminal anaesthesia and sera were stored at -20C until further use.
Enzyme linked immunosorbent assay on mice sera
For the purpose of testing mice sera with indirect ELISAs, 96-well, flat bottomed, clear immunoassay plates (Thermo Scientific) were coated with 2 μg/mL of mOMVs or 0.25-2 μg/mL recombinant proteins and left at 4°C overnight. The plates were then washed six times in wash buffer (PBS-Tween-20 (v/v 0.05%) (PBS-T)), dried, and incubated at room temperature for 1 hour in 100 μL of blocking buffer (PBS-milk (w/v 5%)). Then, the plates were washed in wash buffer six more times, dried, before 2 hours of incubation with 50 μL of mouse sera diluted in PBS-T with 5% (w/v) milk in triplicate wells. For mOMV-coated plates, sera were diluted 1:27,000, then serially diluted, 1:3, three more times. For rID100, rID45 and rNHBA-2 coated plates, sera were diluted 1:20, then serially diluted, 1:2, three more times. For rNadA-8 coated plates, sera were diluted 1:2,000, then serially diluted, 1:2, three more times. Following a further washing step, wells were either incubated for 1 hour with 50 μL of anti-mouse IgG in PBS-T or anti-rabbit IgG in PBS-T (see Supplementary Table S4).
For development, plates were washed six times, and 100 μL of development solution was added (5x diethanolamine buffer (ThermoFisher) diluted in deionised water along with 1mg/mL 4-nitrophenylphosphate) and incubated at room temperature. The OD was read after 20 minutes of development time, using a microplate reader (Byonoy) at 405 nm (OD405), then again, every 5 minutes for a further 35 minutes. The OD at 405 nm (OD405) in each well was measured using a microplate reader (Byonoy) after 35 minutes for mOMV-coated plates or 45 minutes for mOMV-coated plates, ensuring readings represent the linear part of the curve. The average OD405 value was calculated between the technical triplicates, and the average ‘Blank’ OD405 reading was subtracted to yield the final titre value.
For data representation, the OD405 at 1:27,000 serum dilution was used for mOMV-coated plates, 1:40 serum dilution was used for plates coated with rID45 and rNHBA-2, 1:80 serum dilution was used for plates coated with rID100 and 1:4,000 serum dilution was used for plates coated with rNadA, as these dilutions are within the linear part of the curve.
JAR5, JAR13, and the rabbit polyclonal antibodies -NHBA-2, -NadA-8 were used as positive controls to ascertain reproducibility between different ELISA plates, at dilutions that fall in the linear part of predetermined OD405 vs. dilution curves (see Supplementary Table S4 for concentration). Using these controls, the differences in OD405 between plates were <20%.
Serum bactericidal activity assay
SBA assays were carried out by UK Health Security Agency, Vaccine Evaluation Unit (Manchester, UK), as described by Lucidarme et al. (44). N. meningitidis serogroup B strains 5/99 (Mar07) and M17 240102 were used for the assay. Pooled sera were heated before testing to deactivate the innate complement. For the assay, complement-preserved human serum was used. It should be noted that the lower limit of quantification for the SBA due to the specified stating dilution is 8. If any samples have SBA titres below 8, this will be reported as <8.
Statistical analyses
Quantile-quantile plots have revealed that the ELISA data were not normally distributed and therefore distribution of ELISA data was analysed using the Kruskal-Wallis test with Dunn’s correction for multiple comparisons. hTLR4 stimulation assay was analysed using two-way ANOVA with Dunnett’s multiple comparisons test. All analyses were performed on GraphPad Prism Version 9.4.1.
Results
Expression of fHbpID100 and NadA-8 in N. cinerea ATCC® 14685™ mOMVs
While it has been observed from genome sequencing that some N. cinerea strains contain intact homologues of the nadA gene (45), N. cinerea ATCC® 14685™ contains remnants of nadA variant 4/5 (see Supplementary Results and Supplementary Figure S1-S3). Therefore, to express a functional copy of nadA, a construct containing the nadA-2/3.8 gene (variant 2/3, peptide 8) was transformed into N. cinerea ATCC14685 lpxL1 (see Supplementary Table S1), which naturally expresses fHbpID100. The nadA construct contains the gene under the control of nadA-porA fusion promoter (46) and an upstream erythromycin resistance gene, flanked by regions homologous to the nadA-4/5 remnant region. mOMVs generated from N. cinerea ATCC® 14685™ lpxL1 nadA-4/5::nadA-2/3.8 containing functional copies of the fHbpID100 and nadA genes, are referred as OMV-ID100 NadA-8. Antigen expression of fHbpID100 and NadA-8 on the surface of the bacteria were confirmed by flow cytometry (data not shown).
Following mOMV purification, the expression of fHbpID100 and NadA-8 was determined by WB. As controls for the WB, OMVs were also extracted from N. cinerea ATCC® 14685™ WT, N. cinerea ATCC® 14685™ ΔlpxL1 and N. cinerea ATCC® 14685™ ΔfHbp ΔlpxL1, which are referred as OMV WT, OMV ID100 and OMV ΔfHbp, respectively. As shown in Figure 1A, the presence of fHbpID100 from OMV-ID100 and OMV-ID100 NadA-8 can be detected by WB. FHbp bands are at the expected molecular weight (MW, ~30 kDa), with the recombinant protein having a higher MW than their counterparts in the mOMV due to the presence of the His-tag.
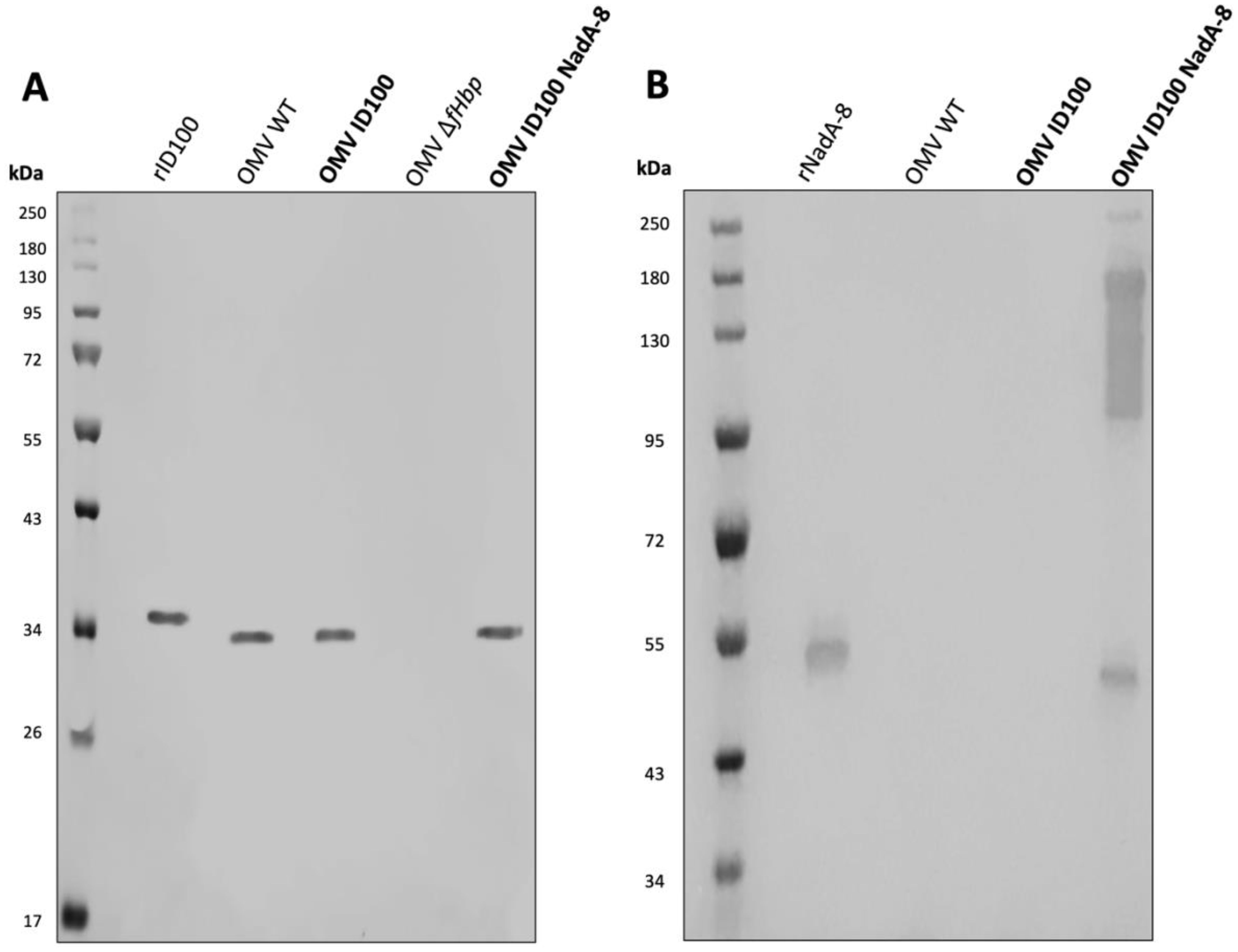
Figure 1. Analysis of the expression of fHbp ID100 and NadA-8 in mOMV. The presence of fHbpID100 in the mOMV from N. cinerea strains were analysed by WB with (A) JAR5 and the presence of NadA-8 were analysed by WB with (B) a polyclonal α-NadA-8. Each lane was loaded with either 2.5 μg of OMVs or 0.1 μg of recombinant proteins. Recombinant fHbpID100 and NadA-8 were used as positive controls.
The expression of NadA-8 from the mOMV was also detected by WB (Figure 1B), with multiple bands representing NadA-8 found in OMV ID100 NadA, while the bands were absent in OMV WT and OMV ID100. While the lower MW band represents the monomeric form of NadA-8 (~50 kDa), the higher-MW represents the oligomeric form of the antigen. It has been previously reported that NadA can form oligomers (18).
Expression of fHbpID45 and NHBA-2 in N. cinerea ATCC® 14685™ mOMVs
To express NHBA-2, a construct containing the nhba-2 gene and an upstream kanamycin resistance gene, flanked by regions homologous to the lpxL1 gene was transformed into N. cinerea ATCC® 14685™ ΔfHbpID100::fHbpID45 (see Supplementary Table S1). mOMVs generated from N. cinerea ATCC® 14685™ ΔfHbpID100::fHbpID45 ΔlpxL1::nhba-2 containing functional copies of fHbpID45 and nhba-2 genes, are referred as OMV-ID45 NHBA-2. Antigen expression of fHbpID45 and NHBA-2 on the surface of the bacteria was confirmed by flow cytometry (data not shown).
Following mOMV purification, the expression of fHbpID45 and NHBA-2 was determined by WB. As controls for the WB, OMVs were also extracted from N. cinerea ATCC® 14685™ WT and N. cinerea ATCC® 14685™ ΔfHbpID100::fHbpID45 ΔlpxL1, which are referred as OMV WT and OMV ID45, respectively. As shown in Figure 2A, the presence of fHbpID45 from OMV-ID45 and OMV-ID45 NHBA-2 can be detected by WB, while it is absent in OMV WT. FHbp bands are at the expected molecular weight (MW, ~30 kDa), with the recombinant protein having a higher MW than their counterparts in the mOMV due to the presence of the His-tag. The expression of NHBA-2 from the mOMV was also detected by WB (Figure 2B) while it is absent in OMV WT, with the bands representing NHBA-2 found at the expected MW of ~80 kDa.
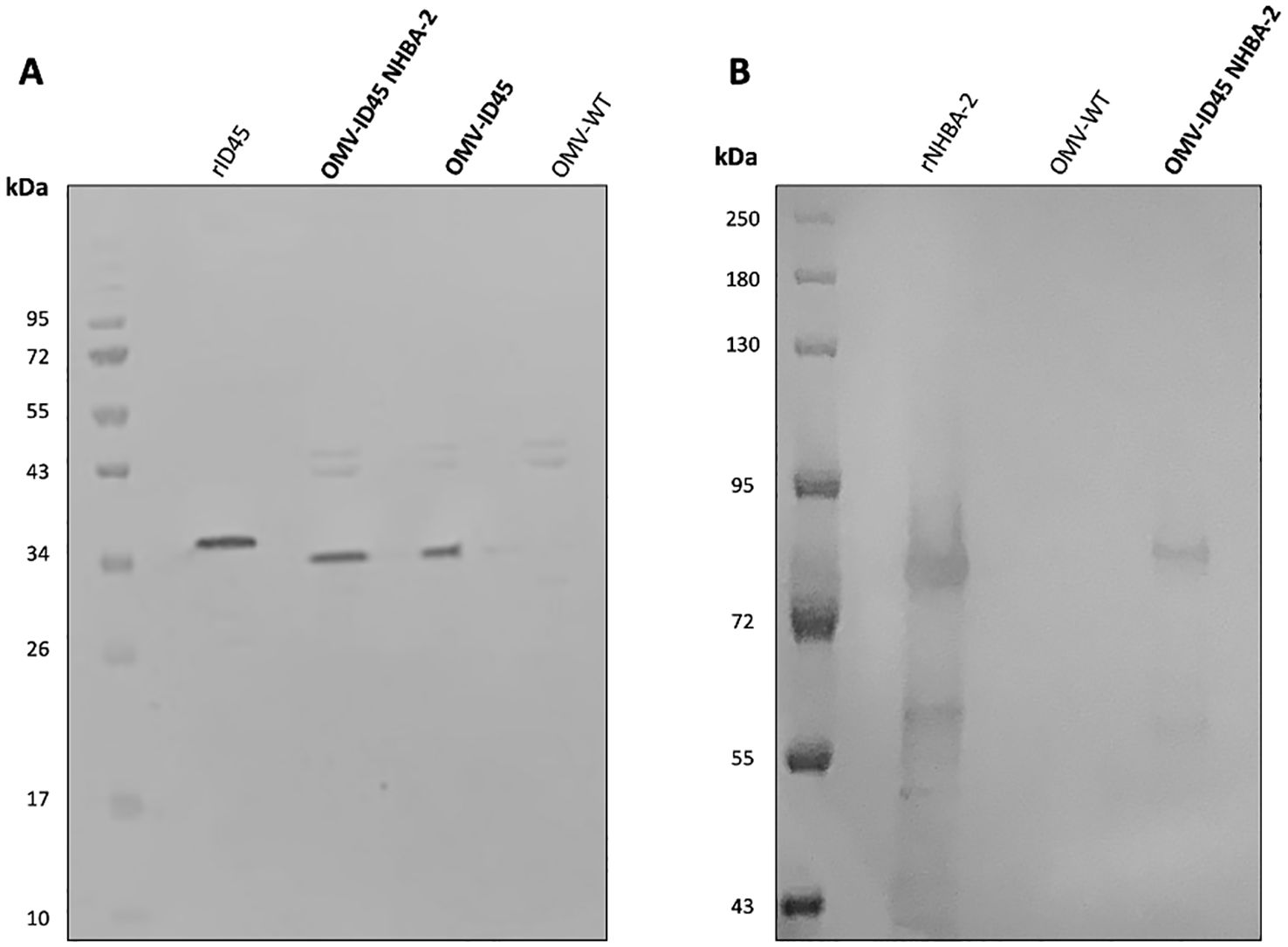
Figure 2. Analysis of the expression of fHbp ID45 and NHBA-2 in mOMV. The presence of fHbpID45 was analysed by WB with (A) JAR13 and the presence of NHBA-2 in the mOMV from N. cinerea strains was analysed by WB with (B) a monoclonal α-NHBA 10C3. Each lane was loaded with either 2.5 μg of OMVs or 0.1 μg of recombinant proteins. Recombinant fHbpID45 and NHBA-2 were used as positive controls.
Characterisation of vesicles by DLS and transmission electron microscopy
To assess the vesicle size, mOMV generated in this study were analysed by DLS. The DLS profiles (Supplementary Figure S4) indicated a uniform distribution of nanoparticles within the reads consisting of three individual measurements. The nanoparticles were ranging between 81.09 to 98.22 nm (Table 2), which falls within the 20-250 nm known range, confirming that the samples contained the expected size of vesicles (47, 48). The average diameter and the polydispersity index (PdI) of each set of mOMV is shown in Table 2. All samples displayed low PdI, suggesting near-homogenous mOMVs were extracted.
mOMVs from N. cinerea ATCC® 14685™ strains carrying different mutations and heterologous antigens were also visualised using TEM, with the OMVs appearing intact and sizes of vesicles being between 100-200 nm (Figure 3).
Confirmation of reduced reactogenicity of mOMVs in vitro
To ensure that the lpxL1 deletion in the mutant strains results in the expected reduction of reactogenicity (49, 50), hTLR4 activation in HEK-Blue hTLR4 cells was studied after stimulation with mOMVs from ΔlpxL1 and parental N. cinerea ATCC® 14685™ strains, to confirm the reduced engagement of TLR4 by LOS. As shown in Figure 4, OMVs from N. cinerea ATCC® 14685™ WT induced hTLR4 activation in a dose-dependent manner. In comparison, all mOMV preparations from N. cinerea ATCC® 14685™ containing the ΔlpxL1 deletion showed a greatly reduced or no stimulation of hTLR4 (p<0.05 with 10 ng/μL or higher concentrations of mOMVs). Thus, mOMV produced from N. cinerea ATCC® 14685™ containing the ΔlpxL1 deletion showed a reduced hTLR4 activation in vitro, irrespective of the different heterologous antigens expressed.
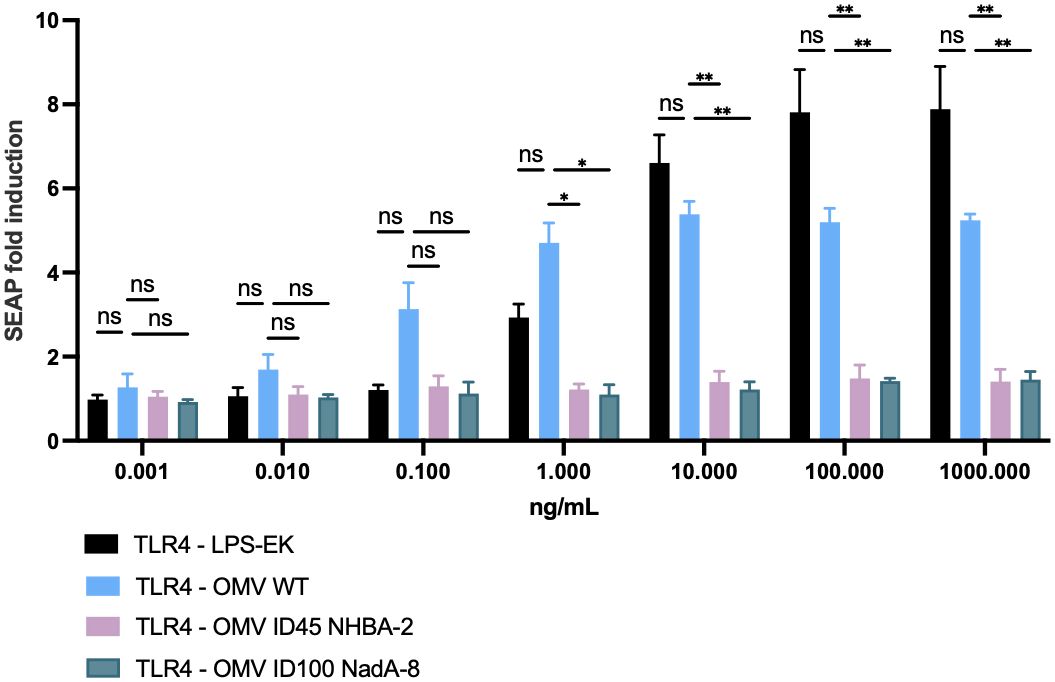
Figure 4. Cellular in vitro assay to assess TLR4 activation after stimulation with mOMVs. 2.5 x 104 cells/well of HEK-293 hTLR4, MD2, CD14 were stimulated for 22 hours with 10-fold serial dilutions of mOMV preparations. hTLR4 activation correlates with SEAP induction, measured as per manufacturer’s protocol. Data shown are from three biological replicates, with each replicates containing technical triplicates; data are shown as mean with standard deviation (vertical error bars). LPS-EK: ultrapure lipopolysaccharide from E. coli K12; it served as positive control for the assay. HEK-Null cells (which contained the gene reporter system, but lacking hTLR4, MD2 and CD14) were used as a negative control for the assay (data not shown) and showed SEAP fold induction of less than 1.5 when stimulated with OMV or purified LPS-EK throughout the dose range used for the assays. Statistical analysis was performed using Two-Way ANOVA with Dunnett’s multiple comparisons test, where * denotes p-value of <0.05, ** denotes p-value of <0.01, and ns denotes not significant.
In vivo immunogenicity study of mOMVs expressing fHbp, NadA and NHBA antigens
mOMVs expressing meningococcal antigens were tested in a preclinical murine model to determine whether the N. cinerea ATCC® 14685™ mOMVs would induce immune responses against antigens present within the mOMVs. Six groups of 10-15 C57BL/6 mice were immunised with different doses of OMV ID100 NadA-8, OMV ID45 NHBA-2, or with a combination of the two mOMVs or with Alhydrogel adjuvant only (Table 1). The dose concentrations used were 10 μg and 20 μg. Mice received three IP injections of each preparation on days 0, 21 and 49. To assess immunogenicity of the mOMVs, serum samples were tested by ELISAs against the same type of mOMVs used to immunise the mice or against recombinant proteins.
Immunisation with the various mOMV preparations at different dose concentrations induced a high level of IgG (high OD405 values at a 1:27,000 dilution) against their respective mOMVs (corresponding to the same mOMVs that were used to dose each group) (Figures 5A, B). The mean OD405 ranged from 2.34-2.39 on plates coated with OMV ID100 NadA-8, and 2.62-2.66 for plates coated with OMV ID45 NHBA-2. Immunisation with Alhydrogel only yielded low OD405 values of <0.05 (Figures 5A, B).
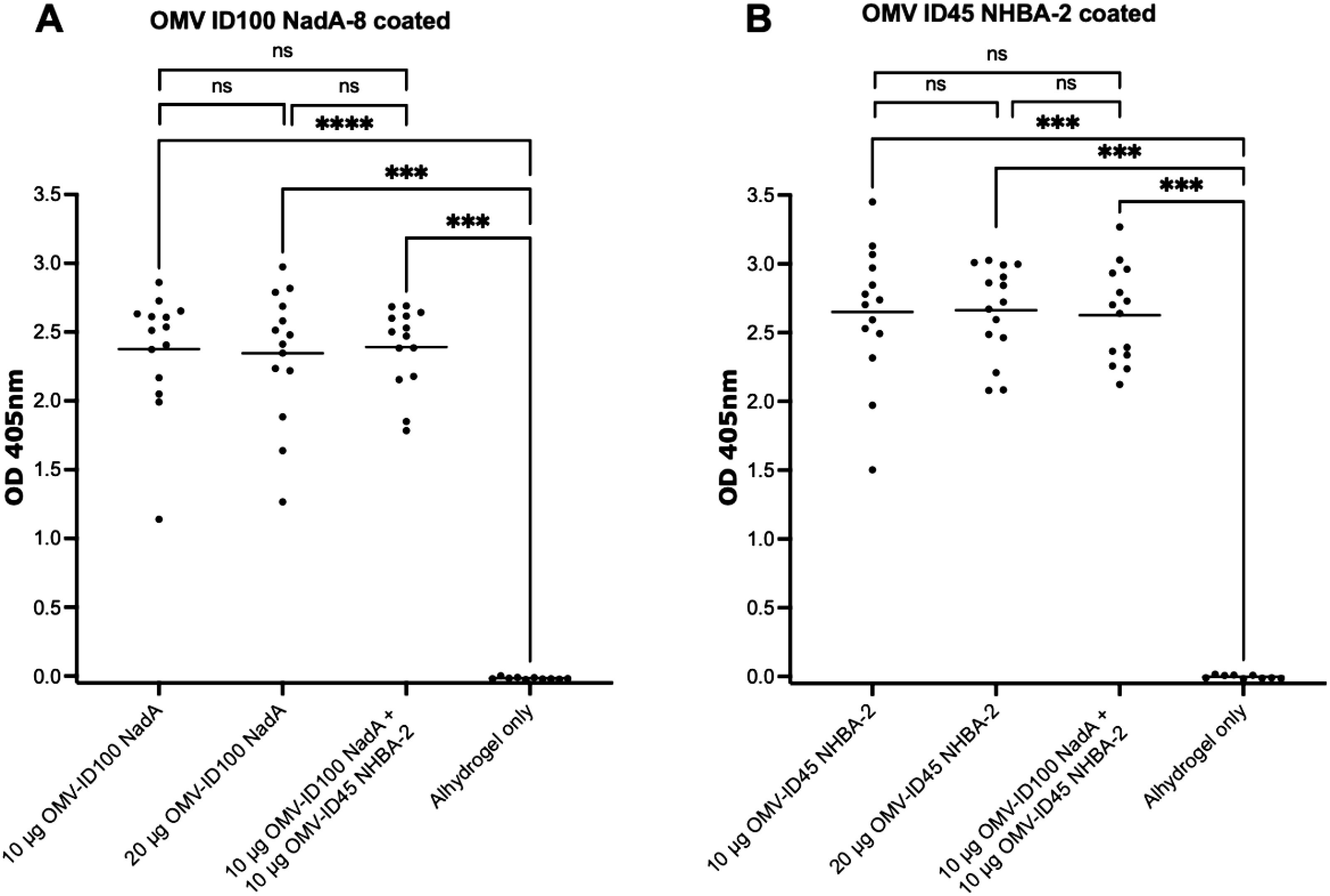
Figure 5. Sera responses of mice immunized with OMV-ID100 NadA-8 and/or OMV-ID45 NHBA-2, when plates were coated with their respective mOMVs. IgG antibody levels measured in sera diluted at 1:27,000 when plates were coated with A) OMV-ID100 NadA and B) OMV-ID45 NHBA-2. Statistical analysis was performed using Kruskal-Wallis test with Dunn’s multiple comparison post-test, where ns denotes not significant, *** denotes p-value of <0.001 and **** denotes p-value of <0.0001.
For ELISA plates coated with recombinant proteins, the OD405 at 1:40 serum dilution is shown for plates coated with rID45 and rNHBA-2, 1:80 serum dilution is shown for plates coated with rID100 and 1:4,000 serum dilution is shown for plates coated with rNadA, as these dilutions are within the linear part of the OD405 curve. Sera from mice immunised with OMV-ID100 NadA-8 yielded significantly higher OD405 readings towards rID100 compared to sera from mice injected with Alhydrogel only (Figure 6A). The mean OD405 is statistically higher at 20 μg compared to 10 μg of mOMVs. (Figure 6A). Immunisation with OMV ID100 NadA-8 induced a significantly higher levels of antibodies against rNadA-8 compared to mice immunised with Alhydrogel only (Figure 6B) with no statistical difference observed between the dose of 10 μg and 20 μg (Figure 6B).
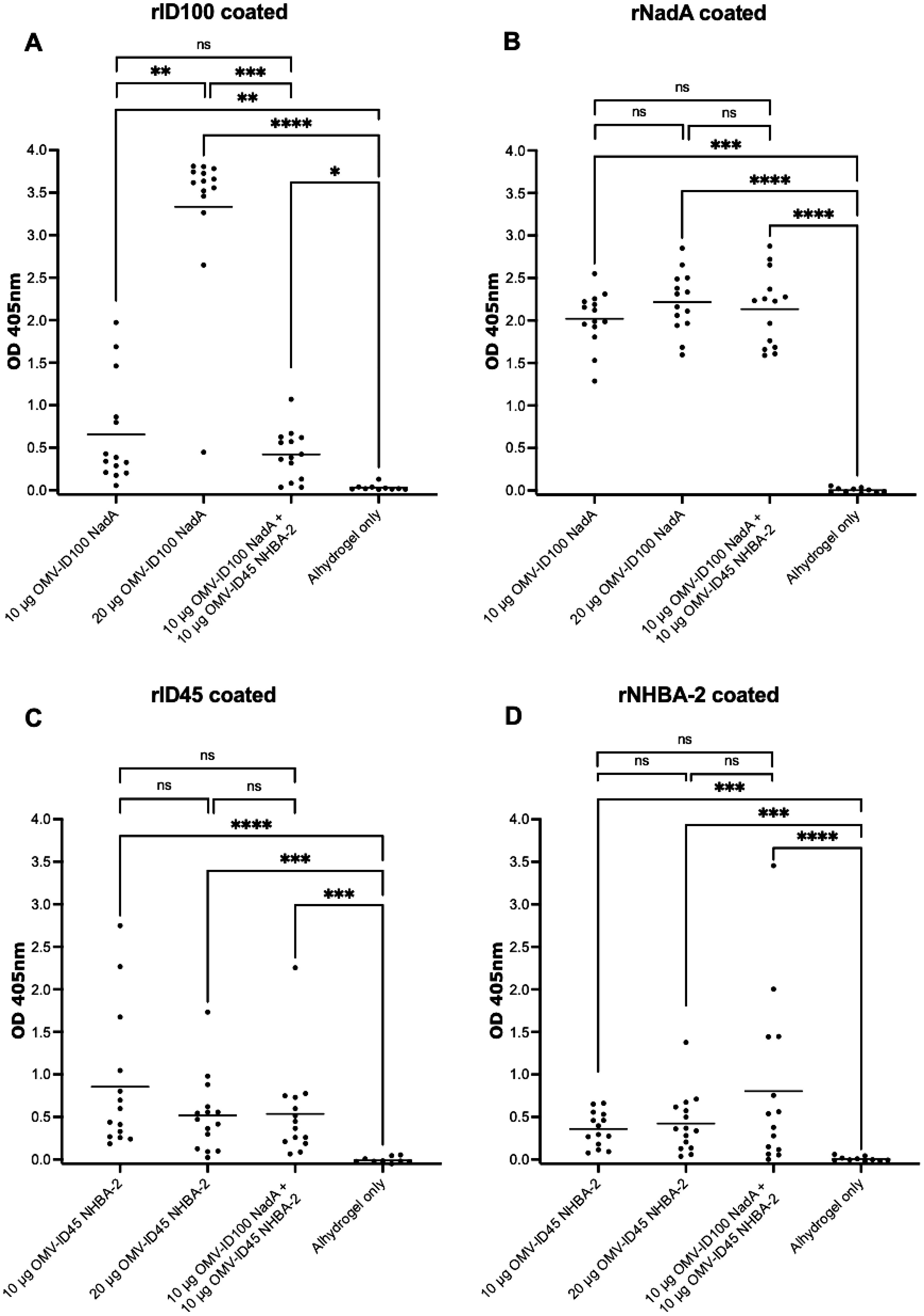
Figure 6. Sera responses of mice immunized with OMV-ID100 NadA-8 and/or OMV-ID45 NHBA-2, when plates were coated with recombinant proteins. (A) IgG antibody levels measured in sera diluted at 1:80 when plates were coated with rID100 protein. (B) IgG antibody levels measured in sera diluted at 1:4,000 when plates were coated with rNadA-8 protein. (C) IgG antibody levels measured in sera diluted at 1:40 when plates were coated with rID45 protein. (D) IgG antibody levels measured in sera diluted at 1:40 when plates were coated with rNHBA-2 protein. Line denotes the mean of IgG antibody levels. Statistical analysis was performed using Kruskal-Wallis test with Dunn’s multiple comparison post-test, where ns denotes not significant, * denotes p-value of <0.05, ** denotes p-value of <0.01, *** denotes p-value of <0.001 and **** denotes p-value of <0.0001.
Sera from animals immunised with OMV-ID45 NHBA-2 yielded significantly higher OD405 values against rID45 and rNHBA-2 compared to sera from animals injected with Alhydrogel only (Figures 6C, D). No significant difference was observed between the dose of 10 μg compared to 20 μg (Figures 6C, D).
Serum antibodies cross-react with proteins from representative MenB and commensal Neisseria strains
To examine the cross-reactivity of mOMV-induced serum antibodies against MenB, WB were performed against whole cell lysates of MenB MC58. Whole cell lysates of commensal strains N. cinerea ATCC® 14685™, Neisseria sicca ATCC® 29256™ and N. sicca ATCC® 29259™ were also used, as these strains are phylogenetically placed near N. meningitidis (45, 51, 52).
Pooled sera from mice immunized with mOMVs (OMV ID100 NadA-8 and/or OMV ID45 NHBA-2) recognised prominent bands in N. meningitidis MenB MC58 of apparent MW of ~55 kDa, 34 kDa and 20 kDa (Figure 7). The sera were also cross-reacting against commensal Neisseria lysates, including N. cinerea ATCC® 14685™, from which the mOMVs were extracted from. In comparison, pooled sera from mice immunised with Alhydrogel only did not display any reactivity to any Neisseria strains (Figure 7).
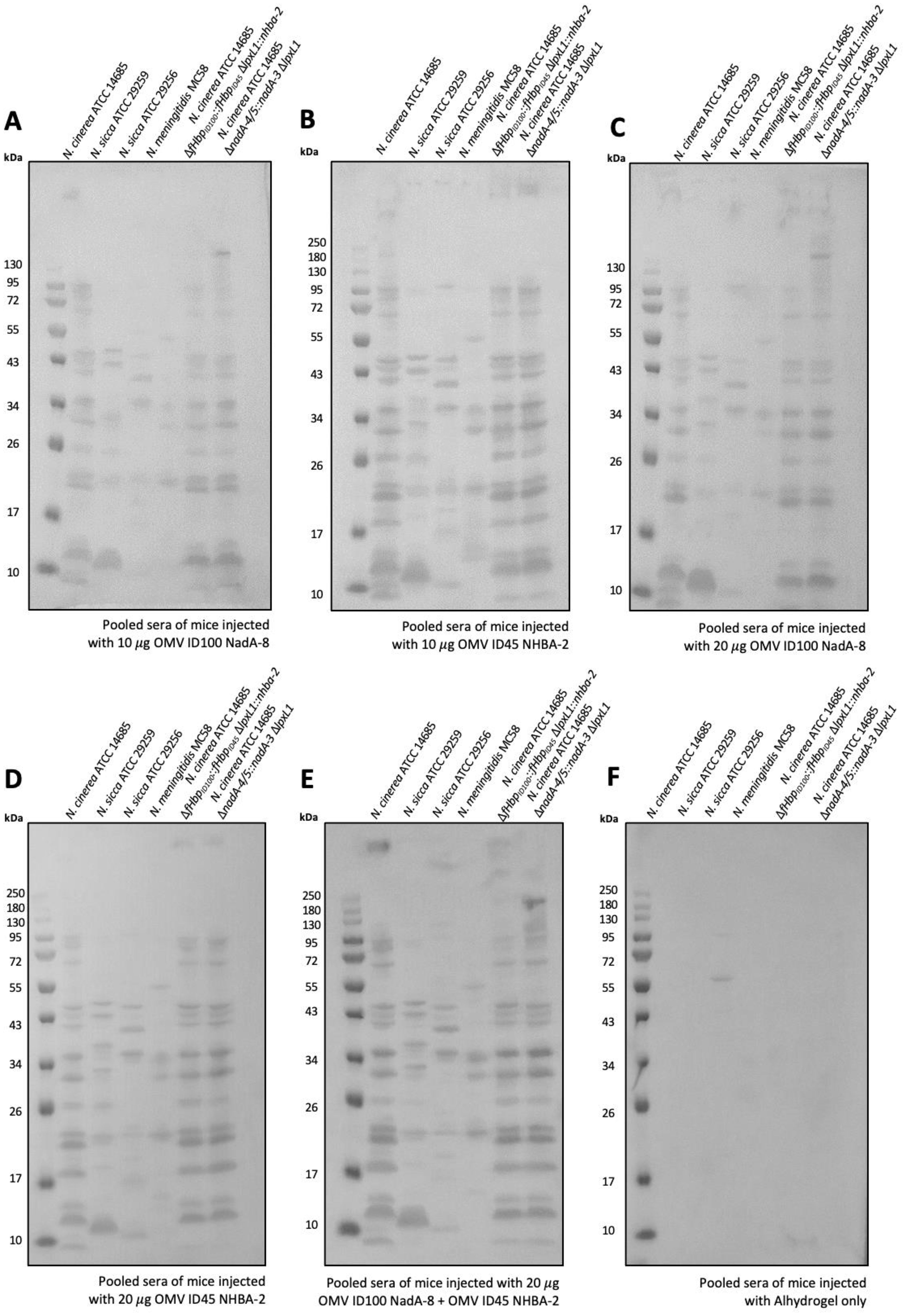
Figure 7. Sera antibodies induced by immunization with mOMVs expressing meningococcal antigens cross-react with MenB. Sera from mice immunized with (A) 10 μg OMV-ID100 NadA-8 (B) 10 μg OMV-ID45 NHBA-2, with (C) 20 μg OMV-ID100 NadA-NadA-8 (D) 20 g OMV-ID45 NHBA-2 (E) 10 μg OMV-ID100 NadA-8 + 10 μg OMV-ID45 NHBA-2 and (F) Alhydrogel only were used to probe whole-cell lysates of MenB MC58, as well as N. cinerea ATCC® 14685TM and N. sicca ATCC® 29259TM.
mOMV induced strong serum bactericidal activity against a NadA-expressing MenB strain
The functional activity of the mouse sera was investigated by serum bactericidal activity (SBA) assays. With studies identifying that antibodies to NHBA alone lack bactericidal activity (53–55), we have selected MenB strains that express either NadA and/or variants of fHbp. SBA was performed against N. meningitidis serogroup B strains 5/99 (Mar 07) and M17 240102, with strain 5/99 (Mar 07) carrying fHbp from subfamily A and NadA from variant 3, and strain M17 240102 carrying fHbp from subfamily B.
Pooled sera from mice immunised with OMV ID100 NadA elicited bactericidal activity against MenB strain 5/99 (Mar07) with a titre of 32,768; pooled sera from mice immunised with Alhydrogel only had SBA titre of <8 (Table 3). No difference in SBA titres was observed between the sera of mice immunised with 10 μg and 20 μg of mOMVs. In addition, sera from mice dosed with 10 μg of OMV ID45 NHBA-2 displayed a titre of 64, while sera from mice dosed with 20 g of OMV ID45 NHBA-2 displayed a titre of <8 (Table 3). A combination of OMV ID100 NadA and OMV ID45 NHBA-2 at a total protein concentration of 20 μg for each dose also elicited bactericidal activity, with a titre of 16,384 (Table 3).
Against strain M17 240102, which expresses fHbp from subfamily B and does not contain a functional nadA gene, pooled sera from all the groups did not display any bactericidal activity (Table 3). This suggests that although OMV ID100 NadA expresses fHbp from subfamily B, pooled sera from mice immunised with OMV ID100 NadA could not elicit bactericidal activity against N. meningitidis expressing fHbp subfamily B.
Discussion
To address the need for an affordable MenB vaccine, we conducted a study to evaluate the design and immunogenicity of two mOMVs from commensal N. cinerea using a mouse model. This study aimed to investigate the possibility to deliver multiple Neisseria antigens to the immune system in a two vesicle mOMV preparation. We demonstrated that the use of mOMVs (OMV ID100 NadA and OMV ID45 NHBA-2) from commensal N. cinerea induces immune responses in vivo against total OMV preparations and against individual bacterial antigens that are expressed in the mOMV after recombinant cloning of their genetic sequences.
In this study, we have expressed variants of fHbp and NHBA-2 in N. cinerea mOMVs, which are antigens that are present in all MenB strains (12, 18). We have additionally inserted the nadA-8 gene in N. cinerea ATCC® 14685™ ΔlpxL1. Although nadA is absent in some virulent strains and most non-virulent strains of N. meningitidis, the gene is generally associated with hypervirulent strains and found in approximately 75% of hypervirulent MenB strains (18, 20, 21). NadA-8 has previously been highlighted to be potently immunogenic (56), which led to its incorporation in the multicomponent Bexsero vaccine (57).
N. cinerea provides an attractive parental species to produce a vaccine candidate due to being a BSL-1 bacteria (58). All mOMVs were proven to possess the correct antigen profiles by WB. Toxicity studies were conducted to measure the in vitro response by hTLR4 to the presence of Neisseria mOMVs. It was demonstrated that mutants with the lpxL1 gene deletion could produce mOMVs that lowered stimulatory potential of hTLR4. This consistently shows that this modification detoxifies the LOS compared to OMV WT and prevent activation of downstream innate proinflammatory pathways such as transcription factor NF-κB activation.
Parenteral administration of mOMVs from N. cinerea ATCC® 14685™ expressing at least two meningococcal antigens induced immune responses in mice against total mOMV preparations and against individual bacterial antigens present in the mOMVs, in a three-dose regimen. The potency of immune responses varied between the different mOMVs used in the mice experiment. In particular, while NadA and fHbpID100 induced high levels of serum antibody responses, fHbpID45 and NHBA-2 induced low levels of serum antibody responses, as shown by the OD values in ELISAs. The reason for this could be due to expression levels of the antigens on the outer membrane. Future work will include measuring the levels of antigen expression and optimizing the expression of the antigens by investigating different promoter sequences as well as exploring the effect of the deletion of immunodominant OMPs such as PorB. Deletion of PorA, PorB and/or RmpM has been shown to be achievable in N. meningitidis and the mOMVs enhanced the cross-protective effect of OMV vaccine (59).
Sera from mice dosed with mOMVs were able to recognise multiple proteins on all the bacterial lysates tested, ranging in sizes from 10 kDa to 130 kDa. As expected, these groups of sera recognised proteins from the parental strain (N. cinerea wild-type) but also several proteins from the meningococcal strain MC58, showing a level of cross-reactivity with the pathogenic strain used as the antigenic basis of fHbp and NHBA variants in the 4CMenB vaccine.
The levels of antibody recognition in ELISAs were later paralleled by the potency of sera in SBA. SBAs tested against N. meningitidis 5/99 (Mar07), which expresses fHbp subfamily A and NadA, demonstrated very high bactericidal activity by antibodies from mice dosed with OMV ID100 NadA with a titre of 32,768, while a titre of 64 or below was observed with OMV ID45 NHBA depending on the concentration. A combination of OMV ID100 NadA and OMV ID45 NHBA-2 at a total protein concentration of 20 μg led to a titre of 16,384. In contrast, SBAs tested against N. meningitidis M17 240102 which expresses fHbp subfamily B displayed a titre of <8 in all groups, including sera from animals immunised with OMV-ID100 NadA. It is possible that higher levels of IgG against fHbpID100 are required to elicit bactericidal activity against test strains expressing these antigens. Future work will therefore involve improving the expression levels of fHbp and NHBA on mOMVs by using alternative promoters, testing alternative adjuvants and broadening the number of MenB strains to be evaluated in SBA assays.
Although NHBA-2 alone may not stimulate high IgG levels, its presence on vesicles may still serve a purpose at enhancing the immunogenicity of fHbp in a synergistic pattern. In previous studies, mice were injected with either a formulation of recombinant fHbpID1 or NHBA-2 and obtained sera to act as a source of IgG in bactericidal studies. Treatment against a meningococcal strain with a high amino acid similarity for both antigens, alongside human serum complement source, resulted in far higher bactericidal killing when incubated with 1:1 preparation of both mouse groups, compared to treatment of just one of these sera groups (15, 60).
In conclusion, our study indicates that it is possible to deliver multiple Neisseria antigens using mOMV combinations and to elicit immune responses against these antigens. We also showed that NadA is highly immunogenic and vaccines containing this antigen induce sera with high SBA against NadA-expressing meningococcal isolates. Other Neisseria antigens can be presented in combination, and induce serum antibody responses and SBA. Further optimization is needed to enhance the functional activity of the immune sera against all variants of fHbp and to broaden strain coverage.
Data availability statement
The original contributions presented in the study are included in the article/supplementary material. Further inquiries can be directed to the corresponding author.
Ethics statement
The animal study was approved by University of Cambridge, under the appropriate Home Office licences and Ethical Review Committee approval. The study was conducted in accordance with the local legislation and institutional requirements.
Author contributions
SM: Writing – original draft, Writing – review & editing, Investigation, Validation, Formal analysis, Visualization. TF: Writing – original draft, Writing – review & editing, Investigation, Formal analysis, Validation. SP: Writing – review & editing, Investigation, Validation. PM: Writing – review & editing, Conceptualization, Funding acquisition, Investigation, Supervision.
Funding
The author(s) declare that financial support was received for the research, authorship, and/or publication of this article. The work was funded by internal funding of the University of Cambridge (Cambridge, UK).
Acknowledgments
We would like to thank the MIRA staff for carrying out the bleed of mice at the MIRA building (University of Cambridge, UK). We would also like to acknowledge the contribution of the Cambridge Advanced Imaging Centre for the use of the transmission electron microscopy (University of Cambridge, UK) and the Department of Biochemistry (University of Cambridge, UK) for the use of the dynamic light scattering. We would like to thank the Vaccine Evaluation Unit (UK Health Security Agency) for carrying out the serum bactericidal activity assay.
Conflict of interest
The authors declare that the research was conducted in the absence of any commercial or financial relationships that could be construed as a potential conflict of interest.
Publisher’s note
All claims expressed in this article are solely those of the authors and do not necessarily represent those of their affiliated organizations, or those of the publisher, the editors and the reviewers. Any product that may be evaluated in this article, or claim that may be made by its manufacturer, is not guaranteed or endorsed by the publisher.
Supplementary material
The Supplementary Material for this article can be found online at: https://www.frontiersin.org/articles/10.3389/fimmu.2024.1473064/full#supplementary-material
References
1. Pace D, Pollard AJ. Meningococcal disease: Clinical presentation and sequelae. Vaccine. (2012) 30:B3–9. doi: 10.1016/j.vaccine.2011.12.062
2. Harrison LH, Shutt KA, Schmink SE, Marsh JW, Harcourt BH, Wang X, et al. Population structure and capsular switching of invasive Neisseria meningitidis isolates in the pre-meningococcal conjugate vaccine Era - United States 2000-2005. J Infect Dis. (2010) 201:1208–24. doi: 10.1086/651505
3. Wyle FA, Artenstein MS, Brandt BL, Tramont EC, Kasper DL, Altieri PL, et al. Immunologic response of man to group B meningococcal polysaccharide vaccines. J Infect Dis. (1972) 126:514–22. https://academic.oup.com/jid/article/126/5/514/924707.
4. Finne J, Leinonen M, Makela PH. Antigenic similarities between brain components and bacteria causing meningitis: implications for vaccine development and pathogenesis. Lancet. (1983) 322:355–7. doi: 10.1016/S0140-6736(83)90340-9
5. Jan AT. Outer Membrane Vesicles (OMVs) of gram-negative bacteria: A perspective update. Front Microbiol. (2017) 8:1053. doi: 10.3389/fmicb.2017.01053
6. Tan LKK, Carlone GM, Borrow R, Path FRC. Advances in the Development of Vaccines against Neisseria meningitidis. New Engl J Med. (2010) 362:1511–20. doi: 10.1056/NEJMra0906357
7. Christodoulides M, Heckels J. Novel approaches to Neisseria meningitidis vaccine design. Pathog Disease. (2017) 75:1–16. doi: 10.1093/femspd/ftx033
8. Shirley M, Taha MK. MenB-FHbp meningococcal group B vaccine (Trumenba®): A review in active immunization in individuals aged ≥ 10 years. Drugs. (2018) 78:257–68. doi: 10.1007/s40265-018-0869-7
9. Gorringe AR, Pajon R. Bexsero: A multicomponent vaccine for prevention of meningococcal disease. Hum Vaccines Immunotherapeutics. (2012) 8:174–83. doi: 10.4161/hv.18500
10. Parikh SR, Andrews NJ, Beebeejaun K, Campbell H, Ribeiro S, Ward C, et al. Effectiveness and impact of a reduced infant schedule of 4CMenB vaccine against group B meningococcal disease in England: a national observational cohort study. Lancet. (2016) 388:2775–82. doi: 10.1016/S0140-6736(16)31921-3
11. Bishai DM, Champion C, Steele ME, Thompson L. Product development partnerships hit their stride: Lessons from developing a meningitis vaccine for Africa. Health Affairs. (2011) 30:1058–64. doi: 10.1377/hlthaff.2011.0295
12. Serruto D, Spadafina T, Ciucchi L, Lewis LA, Ram S, Tontini M, et al. Neisseria meningitidis GNA2132, a heparin-binding protein that induces protective immunity in humans. Proc Natl Acad Sci United States America. (2010) 107:3770–5. doi: 10.1073/pnas.0915162107
13. Schneider MC, Exley RM, Ram S, Sim RB, Tang CM. Interactions between Neisseria meningitidis and the complement system. Trends Microbiol. (2007) 15:233–40. doi: 10.1016/j.tim.2007.03.005
14. Murphy E, Andrew L, Lee KL, Dilts DA, Nunez L, Fink PS, et al. Sequence diversity of the factor H binding protein vaccine candidate in epidemiological relevant strains of serogroup B Neisseria meningitidis. J Infect Dis. (2009) 200:379–89. doi: 10.1086/600141
15. Mubaiwa TD, Hartley-Tassell LE, Semchenko EA, Day CJ, Jennings MP, Seib KL. The Bexsero Neisseria meningitidis serogroup B vaccine antigen NHBA is a high-affinity chondroitin sulfate binding protein. Sci Rep. (2018) 8:1–9. doi: 10.1038/s41598-018-24639-x
16. Vacca I, Del Tordello E, Gasperini G, Pezzicoli A, Di Fede M, Paccani SR, et al. Neisserial heparin binding antigen (NHBA) contributes to the adhesion of Neisseria meningitidis to human epithelial cells. PloS One. (2016) 11:1–17. doi: 10.1371/journal.pone.0162878
17. Arenas J, Nijland R, Rodriguez FJ, Bosma TNP, Tommassen J. Involvement of three meningococcal surface-exposed proteins, the heparin-binding protein NhbA, the α-peptide of IgA protease and the autotransporter protease NalP, in initiation of biofilm formation. Mol Microbiol. (2013) 87:254–68. doi: 10.1111/mmi.12097
18. Capecchi B, Adu-Bobie J, Di Marcello F, Ciucchi L, Masignani V, Taddei A, et al. Neisseria meningitidis NadA is a new invasin which promotes bacterial adhesion to and penetration into human epithelial cells. Mol Microbiol. (2005) 55:687–98. doi: 10.1111/j.1365-2958.2004.04423.x
19. Bambini S, De Chiara M, Muzzi A, Mora M, Lucidarme J, Brehony C, et al. Neisseria adhesin A variation and revised nomenclature scheme. Clin Vaccine Immunol. (2014) 21:966–71. doi: 10.1128/CVI.00825-13
20. Comanducci M, Bambini S, Caugant DA, Mora M, Brunelli B, Capecchi B, et al. NadA diversity and carriage in Neisseria meningitidis. Infection Immun. (2004) 72:4217–23. doi: 10.1128/IAI.72.7.4217-4223.2004
21. Bertoldi I, Faleri A, Galli B. Exploiting chimeric human antibodies to characterize a protective epitope of one of the Bexsero vaccine components. FASEB J. (2016) 30:93–101. doi: 10.1096/fj.15-273813
22. Toh ZQ, Higgins RA, Mazarakis N, Abbott E, Nathanielsz J, Balloch A, et al. Evaluating functional immunity following encapsulated bacterial infection and vaccination. Vaccines. (2021) 9:1–8. doi: 10.3390/vaccines9060677
23. McIntosh EDG, Bröker M, Wassil J, Welsch JA, Borrow R. Serum bactericidal antibody assays - The role of complement in infection and immunity. Vaccine. (2015) 33:4414–21. doi: 10.1016/j.vaccine.2015.07.019
24. Borrow R, Carlone GM, Rosenstein N, Blake M, Feavers I, Martin D, et al. Neisseria meningitidis group B correlates of protection and assay standardization-International Meeting Report Emory University, Atlanta, Georgia, United States, 16-17 March 2005. Vaccine. (2006) 24(24):5093–107. doi: 10.1016/j.vaccine.2006.03.091
25. Nicholson A, Lepow IH. Host defense against neisseria meningitidis requires a complement-dependent bactericidal activity. Science. (1979) 205:298–9. doi: 10.1126/science.451601
26. Goldschneider I, Gotschlich EC, Artenstein MS. Human immunity to the meningococcus I. Role Of Humoral Antibodies. (1969) 129(6):1307–26. Available online at: http://rupress.org/jem/article-pdf/129/6/1307/1653127/1307.pdf.
27. Chen DJ, Osterrieder N, Metzger SM, Buckles E, Doody AM, DeLisa MP, et al. Delivery of foreign antigens by engineered outer membrane vesicle vaccines. Proc Natl Acad Sci United States America. (2010) 107:3099–104. doi: 10.1073/pnas.0805532107
28. Ellis TN, Kuehn MJ. Virulence and immunomodulatory roles of bacterial outer membrane vesicles. Microbiol Mol Biol Rev. (2010) 74:81–94. doi: 10.1128/mmbr.00031-09
29. Gerritzen MJH, Martens DE, Wijffels RH, van der Pol L, Stork M. Bioengineering bacterial outer membrane vesicles as vaccine platform. Biotechnol Advances. (2017) 35:565–74. doi: 10.1016/j.bioteChadv.2017.05.003
30. Irene C, Fantappiè L, Caproni E, Zerbini F, Anesi A, Tomasi M, et al. Bacterial outer membrane vesicles engineered with lipidated antigens as a platform for Staphylococcus aureus vaccine. Proc Natl Acad Sci United States America. (2019) 116:21780–8. doi: 10.1073/pnas.1905112116
31. Kesty NC, Kuehn MJ. Incorporation of Heterologous Outer Membrane and Periplasmic Proteins into Escherichia coli Outer Membrane Vesicles. J Biol Chem. (2004) 279:2069–76. doi: 10.1074/jbc.M307628200
32. Piliou S, Farman TA, Marini A, Manoharan S, Mastroeni P. Commensal Neisseria cinerea outer membrane vesicles as a platform for the delivery of meningococcal and gonococcal antigens to the immune system. Vaccine. (2023) 41:7671–81. doi: 10.1016/j.vaccine.2023.11.034
33. Scorza FB, Colucci AM, Maggiore L, Sanzone S, Rossi O, Ferlenghi I, et al. High yield production process for Shigella outer membrane particles. PloS One. (2012) 7:e35616. doi: 10.1371/journal.pone.0035616
34. Ladhani SN, Campbell H, Parikh SR, Saliba V, Borrow R, Ramsay M. The introduction of the meningococcal B (MenB) vaccine (Bexsero®) into the national infant immunisation programme - New challenges for public health. J Infection. (2015) 71:611–4. doi: 10.1016/j.jinf.2015.09.035
35. Serruto D, Bottomley MJ, Ram S, Giuliani MM, Rappuoli R. The new multicomponent vaccine against meningococcal serogroup B, 4CMenB: Immunological, functional and structural characterization of the antigens. Vaccine. (2012) 30:B87–97. doi: 10.1016/j.vaccine.2012.01.033
36. Rossi O, Caboni M, Negrea A, Necchi F, Alfini R, Micoli F, et al. Toll-like receptor activation by generalized modules for membrane antigens from lipid A mutants of salmonella enterica serovars typhimurium and enteritidis. Clin Vaccine Immunol. (2016) 23:304–14. doi: 10.1128/CVI.00023-16
37. Gerke C, Colucci AM, Giannelli C, Sanzone S, Vitali CG, Sollai L, et al. Production of a Shigella sonnei vaccine based on generalized modules for membrane antigens (GMMA), 1790GAHB. PloS One. (2015) 10:e0134478. doi: 10.1371/journal.pone.0134478
38. O’Dwyer CA, Reddin K, Martin D, Taylor SC, Gorringe AR, Hudson MJ, et al. Expression of heterologous antigens in commensal Neisseria spp.: Preservation of conformational epitopes with vaccine potential. Infection Immun. (2004) 72:6511–8. doi: 10.1128/IAI.72.11.6511-6518.2004
39. Gorringe A, Halliwell D, Matheson M, Reddin K, Finney M, Hudson M. The development of a meningococcal disease vaccine based on. Neisseria lactamica outer membrane vesicles. Vaccine. (2005) 23:2210–3. doi: 10.1016/j.vaccine.2005.01.055
40. Sardiñas G, Reddin K, Pajon R, Gorringe A. Outer membrane vesicles of Neisseria lactamica as a potential mucosal adjuvant. Vaccine. (2006) 24:206–14. doi: 10.1016/j.vaccine.2005.07.064
41. Nolfi-Donegan D, Konar M, Vianzon V, MacNeil J, Cooper J, Lurie P, et al. Fatal nongroupable Neisseria meningitidis disease in vaccinated patient receiving eculizumab. Emerging Infect Dis. (2018) 24:1561–4. doi: 10.3201/eid2408.180228
42. Kellogg DS, Peacock WL, Deacon WE, Brown L, Pirkle CI. Neisseria gonorrhoeae. Virulence genetically linked to clonal variation. J Bacteriology. (1963) 85:1274–9. doi: 10.1128/jb.85.6.1274-1279.1963
43. Beernink PT, Welsch JA, Bar-Lev M, Koeberling O, Comanducci M, Granoff DM. Fine antigenic specificity and cooperative bactericidal activity of monoclonal antibodies directed at the meningococcal vaccine candidate factor H-binding protein. Infection Immun. (2008) 76:4232–40. doi: 10.1128/IAI.00367-08
44. Lucidarme J, Louth J, Townsend-Payne K, Borrow R. “Meningococcal serogroup A, B, C, W, X, and Y serum bactericidal antibody assays. In: Seib KL, Peak IR, editors. Neisseria meningitidis. Methods in molecular biology. New York, NY: Springer New York (2019). p. 169–80. doi: 10.1007/978-1-4939-9202-7
45. Muzzi C, Mora A, Pizza M, Rappuoli M, Donati R. Conservation of meningococcal antigens in the genus. Neisseria. mBio. (2013) 4:163–76. doi: 10.1128/mBio.00163
46. Pajon R, Fergus AM, Granoff DM. Mutant native outer membrane vesicles combined with a serogroup A polysaccharide conjugate vaccine for prevention of meningococcal epidemics in africa. PloS One. (2013) 8:e66536. doi: 10.1371/journal.pone.0066536
47. Schwechheimer C, Kuehn MJ. Outer-membrane vesicles from Gram-negative bacteria: Biogenesis and functions. Nat Rev Microbiol. (2015) 13:605–19. doi: 10.1038/nrmicro3525
48. Kulp A, Kuehn MJ. Biological Functions and biogenesis of secreted bacterial outer membrane vesicles. Annu Rev Microbiol. (2010) 64:163–84. doi: 10.1146/annurev.micro.091208.073413
49. Van Der Ley P, Van Den Dobbelsteen G. Next-generation outer membrane vesicle vaccines against Neisseria meningitidis based on nontoxic LPS mutants. Hum Vaccines. (2011) 7:886–9. doi: 10.4161/hv.7.8.16086
50. Van Der Ley P, Steeghs L, Hamstra HJ, Ten Hove J, Zomer B, Van Alphen L. Modification of lipid a biosynthesis in Neisseria meningitidis lpxL mutants: Influence on lipopolysaccharide structure, toxicity, and adjuvant activity. Infection Immun. (2001) 69:5981–90. doi: 10.1128/IAI.69.10.5981-5990.2001
51. Marri PR, Paniscus M, Weyand NJ, Rendón MA, Calton CM, Hernández DR, et al. Genome sequencing reveals widespread virulence gene exchange among human. Neisseria species. PloS One. (2010) 5:e11835. doi: 10.1371/journal.pone.0011835
52. Calder A, Menkiti CJ, Çağdaş A, Santos JL, Streich R, Wong A, et al. Virulence genes and previously unexplored gene clusters in four commensal Neisseria spp. Isolated from the human throat expand the neisserial gene repertoire. Microbial Genomics. (2020) 6:1–19. doi: 10.1099/mgen.0.000423
53. Vu DM, Wong TT, Granoff DM. Cooperative serum bactericidal activity between human antibodies to meningococcal factor H binding protein and Neisserial heparin binding antigen. Vaccine. (2011) 29:1968–73. doi: 10.1016/j.vaccine.2010.12.075
54. Rossi R, Beernink PT, Giuntini S, Granoff DM. Susceptibility of meningococcal strains responsible for two serogroup b outbreaks on U.S. university campuses to serum bactericidal activity elicited by the menb-4c vaccine. Clin Vaccine Immunol. (2015) 22:1227–34. doi: 10.1128/CVI.00474-15
55. Partridge E, Lujan E, Giuntini S, Vu DM, Granoff DM. The role of anti-NHba antibody in bactericidal activity elicited by the meningococcal serogroup B vaccine, MenB-4C. Vaccine. (2017) 35:4236–44. doi: 10.1016/j.vaccine.2017.06.020
56. Pizza M, Scarlato V, Masignani V, Giuliani MM, Aricò B, Comanducci M, et al. Identification of vaccine candidates against serogroup B meningococcus by whole-genome sequencing. Science. (2000) 287:1816–20. doi: 10.1126/science.287.5459.1816
57. Fagnocchi L, Biolchi A, Ferlicca F, Boccadifuoco G, Brunelli B, Brier S, et al. Transcriptional regulation of the nadA gene in Neisseria meningitidis impacts the prediction of coverage of a multicomponent meningococcal serogroup b vaccine. Infection Immun. (2013) 81:560–9. doi: 10.1128/IAI.01085-12
58. Kaparakis-Liaskos M, Ferrero RL. Immune modulation by bacterial outer membrane vesicles. Nat Rev Immunol. (2015) 15:375–87. doi: 10.1038/nri3837
59. Matthias KA, Reveille A, Connolly KL, Jerse AE, Gao YS, Bash MC. Deletion of major porins from meningococcal outer membrane vesicle vaccines enhances reactivity against heterologous serogroup B. Neisseria meningitidis strains. Vaccine. (2020) 38:2396–405. doi: 10.1016/j.vaccine.2020.01.038
Keywords: modified outer membrane vesicles, mOMVs, Neisseria cinerea, meningitidis, fHbp, NHBA, NadA, Neisseria meningitidis
Citation: Manoharan S, Farman TA, Piliou S and Mastroeni P (2024) Characterisation and Immunogenicity of Neisseria cinerea outer membrane vesicles displaying NadA, NHBA and fHbp from Neisseria meningitidis serogroup B. Front. Immunol. 15:1473064. doi: 10.3389/fimmu.2024.1473064
Received: 30 July 2024; Accepted: 04 September 2024;
Published: 24 September 2024.
Edited by:
Roberto Adamo, GlaxoSmithKline, ItalyReviewed by:
Sanjay Ram, University of Massachusetts Medical School, United StatesPeter Van Der Ley, Intravacc, Netherlands
Copyright © 2024 Manoharan, Farman, Piliou and Mastroeni. This is an open-access article distributed under the terms of the Creative Commons Attribution License (CC BY). The use, distribution or reproduction in other forums is permitted, provided the original author(s) and the copyright owner(s) are credited and that the original publication in this journal is cited, in accordance with accepted academic practice. No use, distribution or reproduction is permitted which does not comply with these terms.
*Correspondence: Shathviga Manoharan, c20yNjkwQGNhbS5hYy51aw==