- 1Laboratory of Nephrology and Transplantation, Vall d’Hebron Institut de Recerca (VHIR), Universitat Autònoma de Barcelona, Barcelona, Spain
- 2Vall d’Hebron for Solid Organ Transplantation Research Group, Vall d’Hebron Institut de Recerca (VHIR), Universitat Autònoma de Barcelona, Barcelona, Spain
- 3Laboratory of Pneumology, Vall d’Hebron Institut de Recerca (VHIR), Universitat Autònoma de Barcelona, Barcelona, Spain
- 4Ciber Enfermedades Respiratorias (CIBERES), Madrid, Spain
- 5Lung Transplant Unit, Pneumology Department, Vall d’Hebron Hospital Universitari, Universitat Autònoma de Barcelona, Barcelona, Spain
- 6Kidney Transplant Unit, Nephrology Department, Vall d’Hebron Hospital Universitari, Universitat Autònoma de Barcelona, Barcelona, Spain
- 7Liver Unit, Vall d'Hebron Hospital Universitari, Universitat Autònoma de Barcelona, Barcelona, Spain
- 8Centro de Investigación Biomédica en Red de Enfermedades Hepáticas y Digestivas (CIBERehd), Instituto de Salud Carlos III, Madrid, Spain
- 9Microbiology Department, Vall d’Hebron Hospital Universitari, Universitat Autònoma de Barcelona, Barcelona, Spain
- 10Pediatric Hepatology and Liver Transplant Department, Vall d’Hebron Hospital Universitari, Universitat Autònoma de Barcelona, Barcelona, Spain
- 11Department of Pediatric Nephrology, Vall d’Hebron Hospital Universitari, Universitat Autònoma de Barcelona, Barcelona, Spain
- 12Department of Preventive Medicine and Epidemiology, Bellvitge University Hospital, Barcelona, Spain
- 13Advanced Heart Failure and Heart Transplant Unit, Department of Cardiology, Hospital Universitari de Bellvitge, BIOHEART-Cardiovascular Diseases Research Group, Bellvitge Biomedical Research Institute (IDIBELL), Universitat de Barcelona, Ciber Cardiovascular (CIBERCV), Barcelona, Spain
- 14Liver Transplant Unit, Bellvitge University Hospital, Barcelona, Spain
- 15Kidney Transplant Unit, Bellvitge University Hospital, Barcelona, Spain
- 16Kidney Transplant Unit, Nephrology department, Germans Trias i Pujol Hospital, Badalona, Spain
- 17Equipo de Atención Primaria Sant Rafael, Servei d'Atenció Primària (SAP) Muntanya, Gerència Territorial de Barcelona Ciutat, Institut Català de la Salut, Barcelona, Spain
- 18Infectious Diseases Department, Vall d’Hebron Institut de Recerca (VHIR), Universitat Autònoma de Barcelona, Barcelona, Spain
- 19Department of Infectious Diseases, Vall d’Hebron Hospital Universitari, Universitat Autònoma de Barcelona, Barcelona, Spain
- 20Urology Department, Vall d’Hebron Hospital Universitari, Vall d’Hebron Institut de Recerca (VHIR), Universitat Autònoma de Barcelona, Barcelona, Spain
- 21Department of Preventive Medicine and Epidemiology, Vall d’Hebron Hospital Universitari, Vall d’Hebron Institut de Recerca (VHIR), Universitat Autònoma de Barcelona, Barcelona, Spain
Introduction: Solid organ transplant (SOT) recipients display weak seroconversion and neutralizing antibody (NAb) responses after severe acute respiratory syndrome coronavirus 2 (SARS-CoV-2) vaccination and remain at risk of severe coronavirus disease 2019 (COVID-19). While B-cell memory is the hallmark of serological immunity, its role in driving successful vaccine responses and providing immune protection in SOT patients remains unclear.
Methods: We investigated the function and interplay of SARS-CoV-2-specific memory B cells (mBc), different cytokineproducing T cells, and cross-reactive NAb in driving seroconversion and protection against COVID-19 in two cohorts. First, we studied a large cohort of 148 SOT recipients and 32 immunocompetent individuals who underwent several vaccinations. Subsequently, we assessed 25 SOT patients participating in a randomized controlled trial to compare two different immunosuppressive strategies for allowing successful seroconversion and memory-cell responses after booster vaccination.
Results: We corroborate previous findings that B- and T-cell memory responses are weaker and more delayed in SOT patients than in immunocompetent (IC) individuals; however, within the SOT cohort, we found that these responses are relatively stronger and more robust in patients not receiving mycophenolate mofetil (MMF)-based therapies. Anti- spike IgG titers strongly correlated with RBD-specific IgG-producing mBc, with both displaying broad viral cross reactivity. Prebooster SARS-CoV-2-specific mBc and IL-2- producing T cells accurately predicted Nab seroconversion (AUC, 0.828) and protection against severe COVID-19. While switching unresponsive SOT patients from calcineurin inhibitors (CNI)/MMF to a low-exposure CNI/mTOR-i regimen favored wider SARS-CoV-2-specific immune responses after a fourth booster vaccination, preformed RBD-specific mBc predicted NAb seroconversion.
Discussion: Our study adds new insights into the pathobiology of immune memory and highlights the pivotal role of SARS-CoV-2-specific mBc in promoting immune protection inSOT patients.
1 Introduction
With the extraordinarily fast development and implementation of active immunization with new vaccines against severe acute respiratory syndrome coronavirus 2 (SARS-CoV-2), a profound decline in coronavirus disease 2019 (COVID-19), especially the more severe clinical forms, has been achieved worldwide (1–4). Despite the global protective effect of SARS-CoV-2 vaccination (5, 6), high-risk patient populations, such as solid organ transplantation (SOT) recipients, remain significantly exposed to recurrent and more severe SARS-CoV-2 breakthrough infections (BTI) (7–12). This is due to markedly impaired and short- lived humoral and cellular immune responses following booster vaccination compared to immunocompetent (IC) individuals (13–18).
While serological immunity, characterized by neutralizing antibodies (NAb) specific to SARS-CoV- 2, is considered the hallmark of immune protection from symptomatic COVID-19 (19–21), different cellular immune compartments, such as SARS-CoV-2-specific cytotoxic T cells, play a complementary role by providing protection through the rapid expansion and elimination of virus-infected cells (22).
Importantly, a key feature of humoral immunity is the generation of antigen-specific B cells that harbor highly specific and affine B-cell receptors, which are the source of the outstanding adaptability of these immune cells. Subsequently, after clonal selection and somatic hypermutation, these B cells eventually give rise to plasma cells that produce high-affinity antibodies (23). Indeed, recent studies in mice and healthy individuals have highlighted the role of SARS-CoV-2-specific memory B cells (mBc) in driving successful seroconversion with NAb and protection against COVID-19 BTI after booster vaccination (24–28). However, we and others have shown the significantly poorer capacity of SOT to develop long-lasting T and especially B-cell memory responses after primary infection (29–32), as well as after several booster mRNA-based vaccinations (33–35).
To avoid allograft rejection, SOT patients receive different long-lasting immunosuppressive treatments, including calcineurin inhibitors (CNI; cyclosporine or tacrolimus), mammalian target of rapamycin (mTOR) inhibitors (rapamycin, everolimus), antimetabolites such as inosine monophosphate dehydrogenase Inhibitors (mycophenolate acids, mycophenolate mofetil (MMF)), and corticosteroids. While all of them abrogate alloreactive immune responses at different cellular levels (36), the lack of antigen-specific targeting function similarly inhibits protective antiviral immune responses. Interestingly, while high CNI trough levels and the use of MMF seem to not favor the development of antiviral humoral and cellular responses after infection or vaccination (37, 38), the use of mTOR inhibitor (mTOR-i) has been shown to boost antiviral cellular immune responses after viral infection or vaccination in different settings (13, 39, 40). Therefore, assessing the impact of current immunosuppressive regimens on SARS-CoV-2-specific humoral and cellular immune responses after repeated vaccinations, identifying robust independent immune correlates of successful immunization, and investigating safe immunosuppressive strategies favoring antiviral protective immunity are highly warranted for this high-risk patient population.
Herein, we aimed to thoroughly characterize the role and cross-reactivity of SARS-CoV-2-specific humoral and memory-cell responses in a large cohort of SOT patients receiving multiple booster vaccinations. We sought to decipher their role in predicting successful immunization and protection from severe forms of COVID-19. Additionally, we designed a mechanistic randomized controlled trial (RCT) to assess whether switching from CNI/MMF to a low-exposure CNI/mTOR-i immunosuppressive regimen would favor protective humoral and cellular immunity after a fourth booster vaccination among SOT unresponsive to three previous vaccine doses.
2 Materials and methods
2.1 Patients of the study and clinical definitions
The study included two distinct cohorts of SOT recipients; (i) a first large, multicenter, prospective observational cohort of SOT and IC patients who underwent three consecutive SARS-CoV-2 booster vaccinations and were monitored for distinct serological and cellular immune responses specific to the virus, and (ii) a second cohort of SOT group who, despite receiving three previous vaccine doses, did not have neutralizing SARS-CoV-2-specific IgG antibodies (NAb). These patients participated in an interventional RCT to assess the achievement of NAb and cellular immune memory after a fourth vaccine dose, comparing a standard- of-care immunosuppressive regimen based on CNI/MMF (MMF/mycophenolate sodium (MPS)) with a regimen switched to a CNI/mTOR -i-based immunosuppressive therapy (Figure 1).
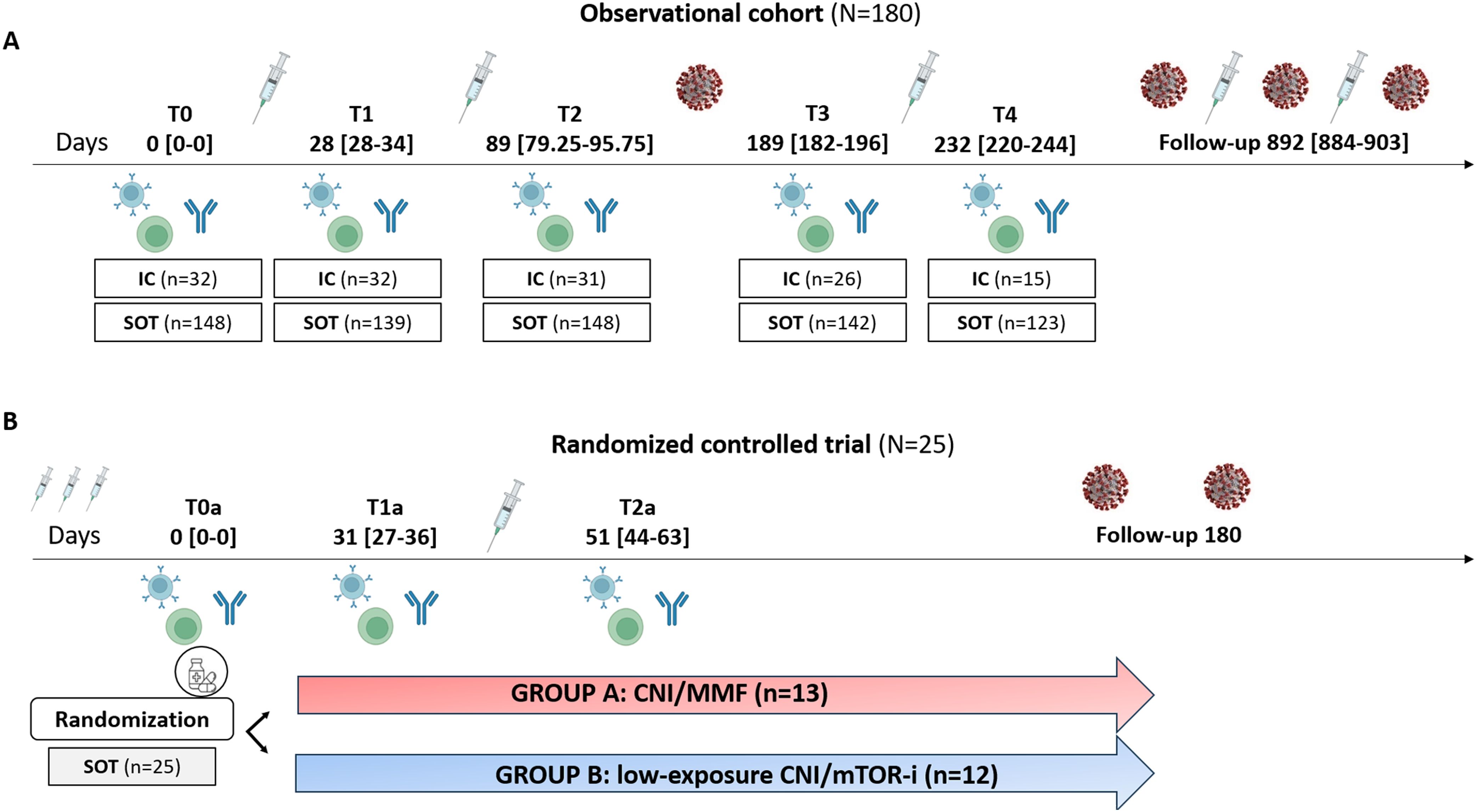
Figure 1. Flowchart of the study. (A) Observational cohort. (B) Randomized controlled trial. IC, immunocompetent; SOT, solid organ transplant; CNI, calcineurin inhibitors; MMF, micophenolate mofetil; mTOR-i, mTOR inhibitors.
2.1.1 Observational cohort
This large multicenter patient cohort consisted of 836 serial peripheral blood samples from 148 adult and adolescent (15–18 years old) SOT patients from three different transplant centers (Vall d’Hebron, Bellvitge, and Germans Trias I Pujol University Hospitals) as well as 32 IC individuals who received early vaccination according to national health policies. These individuals were used as controls and were all prospectively evaluated at five different time points, both prior and after three consecutive mRNA-based vaccine doses against SARS-CoV-2 (Figure 1). SOT patients received different immunosuppressive regimens: 85 were on CNI (tacrolimus or cyclosporine) plus MMF or MPS, eight were on MMF monotherapy, 33 were only on CNI, and 20 received a CNI plus a mTOR inhibitor (Table 1). Main clinical, demographic, and immunological patient characteristics were recorded. The study was approved by each ethical review board (PR115/20), and patient recruitment was done after obtaining a signed informed consent.
2.1.1.1 SARS-CoV-2 vaccination
All patients tested negative for serology against nucleoprotein and spike SARS-CoV-2 antigens prior to the first vaccination and had no recorded history of a positive nasopharyngeal PCR swab. A total of 146 (81.11%) patients received the mRNA-1273 (Moderna Inc., Massachussetts, US) vaccine and 34 (18.89%) the BNT162b2 (Pfizer-BioNTech Inc, New York, US). The first two doses were administered between March and June 2021, the third between September and December 2021, and the fourth and fifth doses were given between April 2022 and May 2023. All patients in the study received three doses; however, one SOT and one IC patients received only two doses. Among the patients, 74.32% (110/148) SOT recipients and 46.88% (15/32) of IC recipients received four doses, while only 23.65% (32/148) of SOT recipients, and none of the IC recipients, received a fifth dose. All patients were followed up for at least 3 months after the fifth dose (July 2023) (Table 1).
2.1.1.2 COVID-19 breakthrough infections
During the study period, all positive SARS-CoV-2 PCR tests were automatically reported to our regional Ministry of Health, ensuring accurate control of viral infection s. All patients were followed up by our different teams to record symptoms and clinical evolution. BTI occurred in four cases after the second dose, 49 cases after the third dose (44 [89.6%] SOT and 5 [10.4%] IC patients), 22 cases after the fourth dose (21 [95.45%] SOT and 1 [4.55%] IC), and 12 SOT patients after the fifth dose. BTI were classified according to World Health Organization guidelines as follows: severe if hospitalized with clinical signs of pneumonia plus oxygen supply requirements; mild when there is no evidence of viral pneumonia or hypoxia but showed COVID-19-related symptoms; and asymptomatic if only tested positive for SARS-CoV-2 by RT-PCR with no clinical or biological symptoms (e.g., lymphopenia, elevated PCR) (41). The main SARS-CoV-2 strain responsible for BTI after the second vaccine dose was predominantly the Delta variant, whereas all BTI following the third, fourth, and fifth doses were caused by Omicron subvariants, including BA.1, BA.2, BA.5, BQ. 1, and XBB.1 strains.
2.1.2 Randomized controlled trial (Tor-vax)
In view of the results obtained from the previous study, we designed a multicenter randomized controlled interventional trial to assess the value of switching to a CNI/mTOR-i-based regimen from a CNI/MMF-based IS, to achieve protective immunity based on SARS-CoV-2 NAb and cellular immune memory responses after a fourth mRNA-based booster vaccination in previously unresponsive SOT (Figure 1) (Eudract 2022-000617-13). Patients were randomized in a 1:1 ratio into two groups: group A (control group: CNI/MMF) and group B (conversion group: CNI/mTOR-i). Randomization was stratified according to the type of transplanted organ and donor age. To qualify for recruitment, all candidates had to be receiving chronic maintenance immunosuppression with a CNI/MMF-based immunosuppressive regimen.
As illustrated in Supplementary Figure S1, of the 50 patients enrolled, 12 were infected right before randomization; thus 38/50 (76%) were randomized to remain on the same immunosuppressive regimen (group A; n = 17, 44.7%) or switch to a mTOR-i-based regimen (group B; n = 21, 55.3%). After receiving the fourth vaccine dose, nine patients from the mTOR-i group and four from the control group also dropped out, mainly because of COVID-19 BTI. Consequently, 25/50 (50%) of the SOT patients remained on protocol at month 1 after vaccination (T2a). Due to the high incidence of COVID-19 BTI during the recruitment process, the primary endpoint of the study could not be assessed with sufficient statistical power. Consequently, a descriptive analysis between groups of patients remaining on protocol was performed.
Inclusion and exclusion criteria are depicted in detail in the Supplementary Materials. Briefly, the trial included stable adult SOT recipients who had not IgG developed NAb against SARS-CoV-2 after three previous mRNA-based vaccine doses, had no previous COVID-19 infection (absence of anti-N IgG Ab), and had available baseline peripheral blood samples.
2.1.2.1 Main endpoints of the study and sample size
The primary endpoint was to evaluate the capacity of each treatment group to achieve a protective serological immune response, defined as the development of SARS-CoV-2-specific IgG NAb, 1 month after receiving a fourth (T2a) SARS-CoV-2 mRNA-based vaccine. Secondary endpoints included comparing the percentage of seroconversion rates, frequencies of SARS-CoV- 2-specific IgG-producing mBc and cytokine-secreting T cells, and the number and severity of COVID-19 breakthrough infections between the two groups.
To detect a 20% superiority margin in NAb rates after switching to an mTOR-i-based immunosuppressive regimen, a total of 50 patients were needed to achieve statistical differences with an 80% statistical power, including 10% drop-out rates.
2.1.2.2 Inclusion criteria
Clinically stable adult SOT recipients, at least 12 months after transplantation, from two different transplant centers in Barcelona, Spain (Vall d’Hebron and Bellvitge University Hospitals), who lacked IgG NAb against SARS-CoV-2 after receiving three previous mRNA-based vaccine doses, were eligible to participate.
2.1.2.3 Exclusion criteria
Exclusion criteria included the presence of NAb against SARS-CoV-2, unavailability of baseline peripheral blood samples, previous COVID-19 infection (absence of anti-N IgG Ab), previous diagnosis of T-cell or antibody-mediated rejection within the previous 2 years, and the presence of donor-specific-HLA antibodies.
2.2 Collection and management of biological samples
Peripheral blood mononuclear cells (PBMC) and serum samples were obtained at the following specific time points for the descriptive cohort: prevaccination (T0), after the first dose (T1: 28 days; interquartile range [IQR], 28–34), 2 months after second dose (T2: 89 days; IQR, 79.25–95.75), 5 months after second dose (T3: 189 days; IQR 182–196), and 1 month after the third dose (T4: 232 days; IQR, 220–244).
In the randomized controlled trial whole blood and serum samples were obtained at the following prespecified time-points: at the time of randomization (T0a) (being one month prior to the fourth booster vaccination); at the time of the fourth dose (in case of Group B patients, being 1 month after mTOR-i conversion) (T1a) and at 1 month after fourth dose (T2a).
Peripheral blood mononuclear cells (PBMCs) were isolated from the patient’s blood by Ficol density gradient centrifugation and subsequently frozen in liquid nitrogen until their use in functional analyses. Serum samples were isolated by centrifugation and stored at – 20°C.
2.3 Assessment of SARS-CoV-2-specific adaptive immune memory
2.3.1 SARS-CoV-2-specific humoral memory
2.3.1.1 SARS-CoV-2-specific serological memory
2.3.1.1.1 SARS-CoV-2-specific serum IgG antibodies
SARS-CoV-2-specific IgG antibodies were assessed against two main SARS-CoV-2 antigens using two ELISA platforms (Elecsys anti-SARS-CoV-2 for nucleoprotein and LIAISON Sars-CoV-2 TrimericS for spike glycoprotein). Detailed information on the methodology and interpretation is provided in the Supplementary Material. Antinucleoprotein IgG antibodies were assessed only at T0 to ensure that patients had not been previously infected.
2.3.1.1.2 SARS-CoV-2-specific neutralizing antibodies
Neutralizing antibodies against the spike protein of SARS-CoV-2 from the Wuhan (D614G variant) and Omicron (BA.5 variant) strains were assessed using a plasma neutralization assay with a pseudotyped VSV-SARS-CoV-2 spike expressing luciferase (VSV-ΔG-Luc-S) (42). Detailed information on the methodology and interpretation is provided in the Supplementary Material.
As illustrated in Supplementary Figure S1, we confirmed previous reports (43, 44) showing that a cutoff of 143 BAU/mL for anti- spike IgG titers is an appropriate threshold for differentiating between patients with high antiviral neutralization activity. Notably, IgG levels and neutralizing antibody activity showed a positive correlation of R = 0.411 (p = 0.046).
2.3.1.2 RBD-specific spike IgG-producing memory B cells
Circulating SARS-CoV-2-specific IgG-producing mBc frequencies were assessed against the receptor-binding domain of SARS-CoV-2 spike (RBD-S) protein using a colorimetric B-cell ELISPOT assay (45). A thorough description of the method of this assay is depicted in the Supplementary Material.
2.3.2 SARS-CoV-2-reactive cytokine-producing memory T cells
Five distinct cytokine-producing Th1 and Th2 T-cell frequencies were simultaneously detected at all time points: effector (IFN-γ), proliferative (IL-2), central (IFN-γ/IL-2) Th1, proliferative (IL-21) Th2 and Th17, and stimulating (IL-5) Th2. Precise information is depicted in the Supplementary Methods in Supplementary Data Sheet 1.
2.3.3 Analysis of immune memory compartments
We also assessed and defined the response of the two main immune memory compartments in a qualitative manner: the B-cell compartment, which includes serological NAb and memory B-cell responses, and the Th1 T-cell compartment, which includes IFN-γ and/or IL-2 T-cell responses. Further interpretation of the different responses is provided in the Supplementary Material.
2.4 Statistics
All statistical analyses were performed using SPSS version 26 software and R package version 1.0.12, and graphs were generated using GraphPad Prism version 8.0 software (GraphPad Software, San Diego, CA, USA). Continuous variables were expressed as median [IQR] and categorical variables as number of total (n) and percentage (%). Comparison s between groups was performed using Pearson’s χ2 test for categorical data. Continuous measurements were compared among groups using the Kruskal–Wallis and Mann–Whitney U tests for nonnormally distributed data, and ANOVA and t-tests were used when data were normally distributed. p-values of < 0.05 were considered statistically significant. To assess longitudinal data, considering the missing values and the changes over time, a two/linear mixed model, considering study time points and transplant status as fixed effects, was used. A correlation analysis of repeated measurements (46) was performed. p-values were adjusted for multiple comparisons using the Benjamini–Hochberg method. Univariate logistic regression models were used to investigate the influence of clinical and immunological covariates by means of odds ratios (OR) with 95% confidence intervals.
3 Results
3.1 Patients and outcomes of the observational cohort
The first observational cohort consisted of 148 SOT patients who were compared to 32 IC individuals regarding serological and cellular immune memory responses triggered after three consecutive doses of SARS-CoV-2 mRNA-based vaccine s (Figure 1). Most patients were kidney (37%) and liver (28%) transplant recipients, receiving CNI-based immunosuppressive therapy combined with either MMF/MPS (n = 84) or an mTOR-i drug (n = 20). All patients received at least three booster doses. Among the 148 SOT patients, 110 (74.3%) received a fourth dose and 34 (23.7%) received a fifth booster dose. All patients were followed up for a median of 30 months (median, 892 days; IQR, 884–903) (Table 1). While COVID-19 BTI occurred after the third dose in 27.5% of patients (29.9% of SOT and 16.1% of IC patients) and in 17.6% of those who received a fourth dose (19.1% SOT and 6.7% IC), up to 34.3% of SOT patients who received a fifth dose developed COVID-19. Severe and mild COVID-19 only occurred among SOT in 12 (8.1%) and 33 (22.3%) patients, respectively. Asymptomatic COVID-19 was reported in 42 patients (36 (24.3%) SOT and six (18.8%) IC).
3.2 Strength and kinetics of adaptive immune memory after booster vaccination
As illustrated in Figure 2A, while IgG titers, as well as mBc and T-cell frequencies specific to SARS-CoV-2, significantly increased with the number of vaccine doses in both groups, SOT patients displayed significantly weaker immune memory responses compared to IC patients after each vaccine dose, except for Th1 cells producing IL-2 and Th2 cells producing IL-21 and IL-5 (Supplementary Tables S1, S2). After the second dose, both at T2 and T3, 41.9% (62/148) and 38.7% (55/142) of SOT patients developed NAb, whereas up to 96.8% (30/31) and 92.3% (24/26) of IC patients did, respectively. Notably, after the third dose (T4), 100% (15/15) of IC patients showed NAb, while only 67.7% (84/124) of SOT patients were NAb positive. There was generally a strong positive correlation between all distinct SARS-CoV-2-specific immune responses except for mBc and T-cell frequencies between T0 and T4 (Supplementary Table S3).
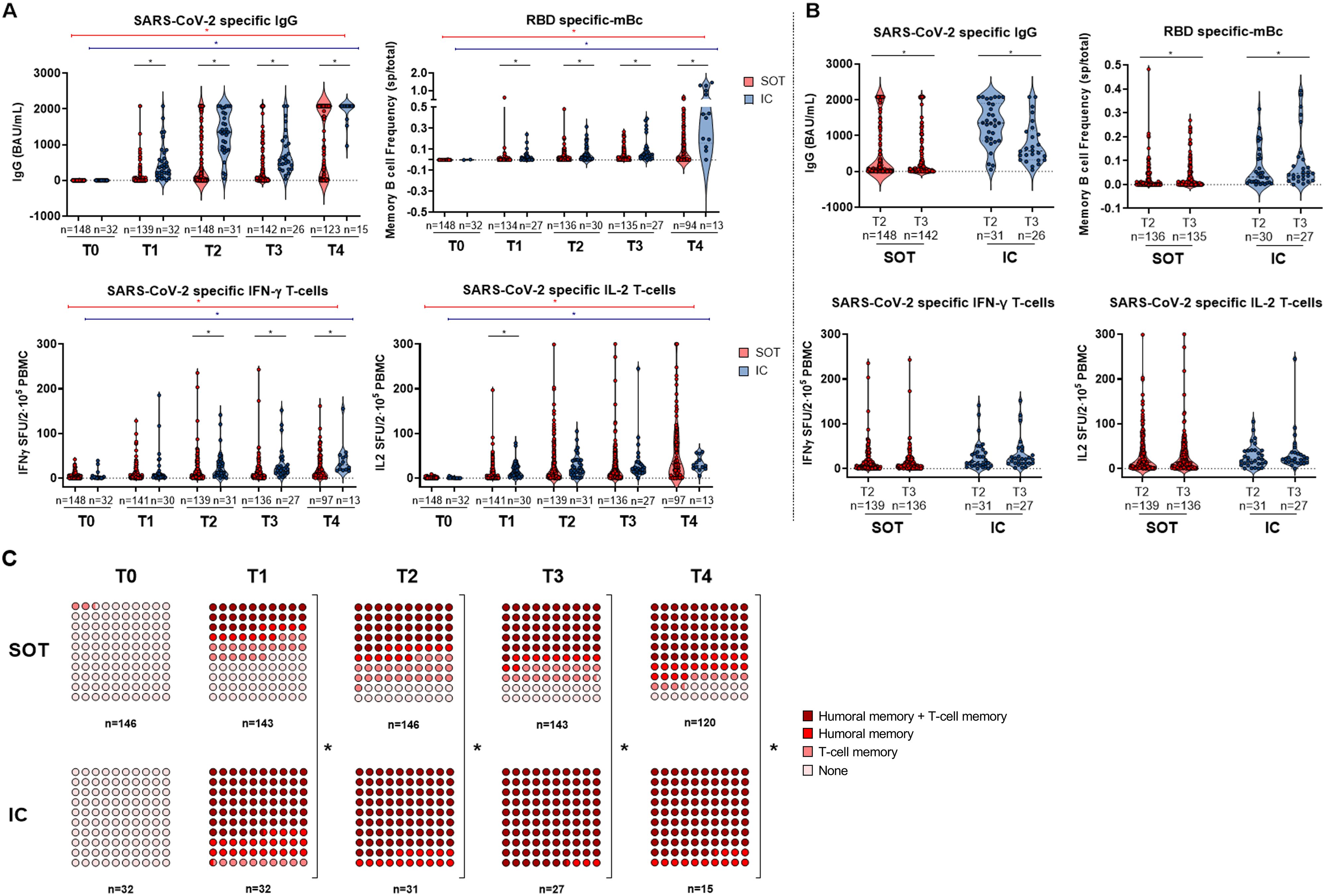
Figure 2. (A) SARS-CoV-2-specific serological and B- and T-cell memory immune responses between SOT and IC over time before and after booster vaccination. Significant intra- and intergroup differences are shown in Supplementary Tables S1, S2. (B) SARS-CoV-2-specific serological and memory-cell differences at 3 months postvaccination (two doses) and at 6 months postvaccination (two doses). (C) Global qualitative SARS-CoV-2-specific immune responses at main memory compartments between SOT and IC over time after booster vaccination. SOT, solid organ transplant; IC, immunocompetent; mBc, memory B cell; IFN-γ, interferon gamma; IL, interleukin; SFU, spot forming unit. *p < 0.05.
Notably, within the 3-month time frame between the second and third vaccine doses (T2 and T3), no major changes were observed in all SARS-CoV-2-specific T memory responses, and the antibody titers progressively decayed. However, RBD-specific IgG-producing mBc significantly increased over time in both SOT and IC individuals (Figure 2B).
When both B- and T-cell immune compartments were assessed together, IC individuals showed earlier detection of memory responses across all compartments compared to SOT patients (Figure 2C). Specifically, after two vaccine doses (T3), most IC patients (27/31; 87.1%) displayed broad immune memory responses, whereas only 45.2% (66/146) of SOT patients did (p < 0.001). Similarly, after the third dose (T4), only 63.6% (75/118) of SOT patients showed complete detectable immune responses, while this protection was seen in all IC patients (p < 0.001). Importantly, at this last time point, up to 15.3% (18/118) of SOT patients did not show any immune response, 11.9% (14/118) showed responses only within the T-cell immune compartment, and 9.3% (11/118) showed responses only within the humoral compartment.
3.3 Neutralizing cross-reactivity of serum and IgG-producing RBD-specific mBc
In all patient cohorts, a positive correlation between serum IgG titers and the frequencies of IgG-producing mBc was observed in both SOT and IC individuals at all time points of assessment (Supplementary Figure S2). We next assessed the presence of NAb against two different viral strains, Wuhan (D614g) and Omicron (BA.5), to evaluate the cross-reactivity of both serum IgG and those produced by mBc in SOT and IC patients after four vaccine doses. As shown in Figure 3A, 88% (22/25) of patients with high IgG titers (> 143 BAU/mL) showed NAb in the serum against the Wuhan strain, whereas only 20% (3/15) of patients with low IgG titers (< 143 BAU/mL) did. Similar results were observed for the Omicron BA.5 variant, with 60% (15/25) of patients with high IgG titers showing NAb, compared to only 6.7% (1/15) of patients with low titers. Of note, the assessment of the neutralizing capacity of IgG antibodies produced by polyclonally expanded mBc revealed that 50% (13/26) and 31.3% (5/16) of patients showed mBc with NAb against the Wuhan and the Omicron BA.5 variants, respectively (Figure 3B). As shown in Figure 3C, there was a high correlation between serum and mBc NAb for both viral strains.
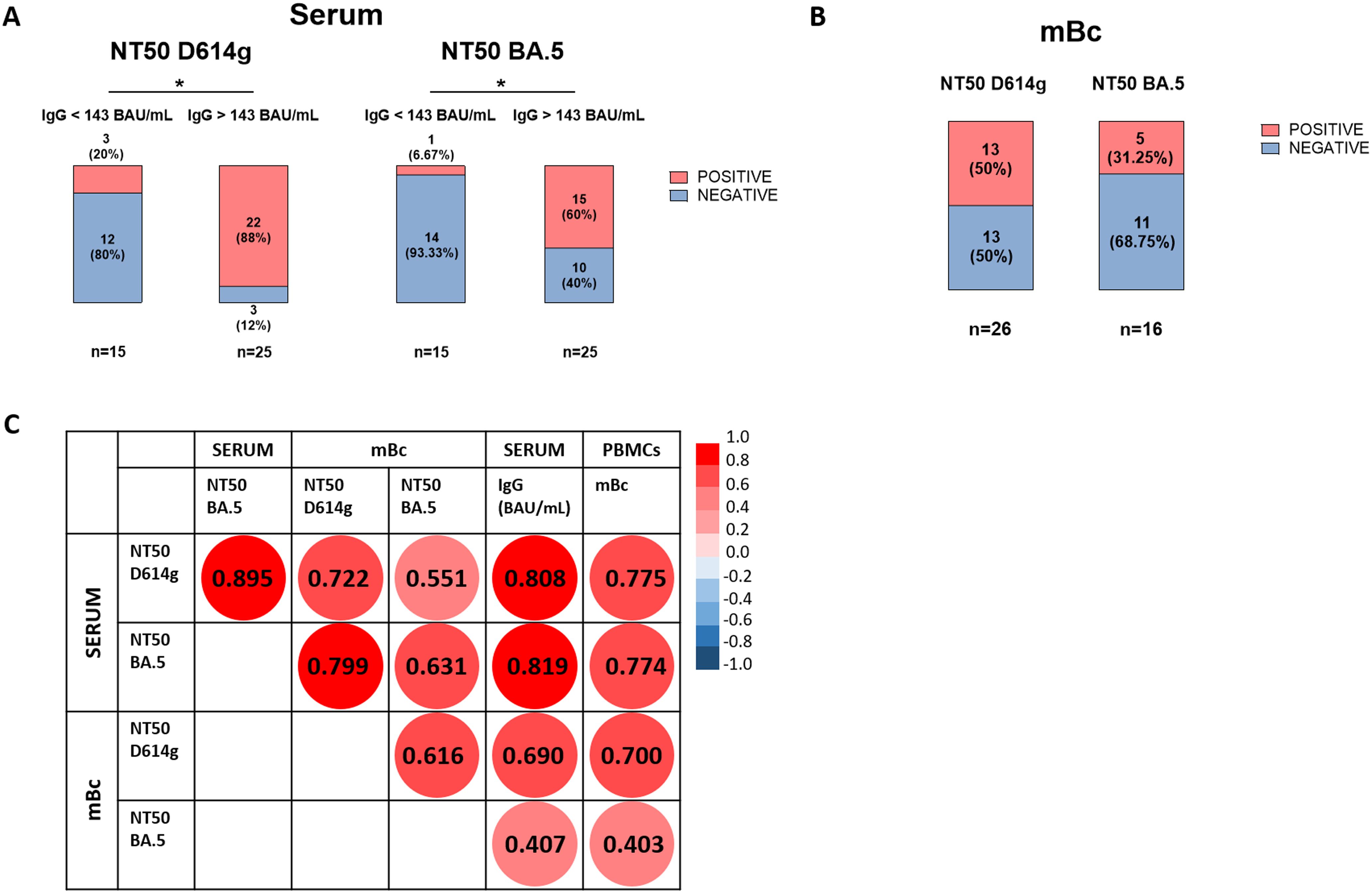
Figure 3. Neutralizing antibodies against SARS-CoV-2 in serum and supernatant samples of both SOT and IC patients. (A) Presence of neutralizing antibodies against Wuhan D614g and Omicron BA.5 strains in serum samples of patients with IgG levels < 143 BAU/mL and > 143 BAU/mL. *p < 0.05. (B) Presence of neutralizing antibodies against Wuhan D614g and Omicron BA.5 strains in supernatant samples of patients with detectable NAb in serum. (C) Spearman correlations between IgG levels in serum, frequencies of mBc, and neutralization titers against Wuhan D614g and Omicron BA.5 variants in both serum and supernatant from mBc samples. All correlations were statistically significant at p < 0.05.
3.4 Immunosuppression regimens and immune memory after vaccination
Distinct maintenance immunosuppressive (IS) regimens were associated with different humoral and cellular immune responses (Figure 4A). Patients on CNI monotherapy and those on a mTOR-i-based regimen displayed significantly higher immune responses after each vaccine dose, comparable to those of IC individuals. In contrast, patients on CNI/MMF and those few on MMF monotherapy showed the weakest responses, even after three vaccine doses (Supplementary Table S4). When both B- and T-cell immune memory compartments were analyzed together, patients on either CNI monotherapy or CNI/mTOR-i immunosuppression developed a global immune response significantly faster than those on CNI/MMF and MMF monotherapy (Figure 4B; Supplementary Table S5).
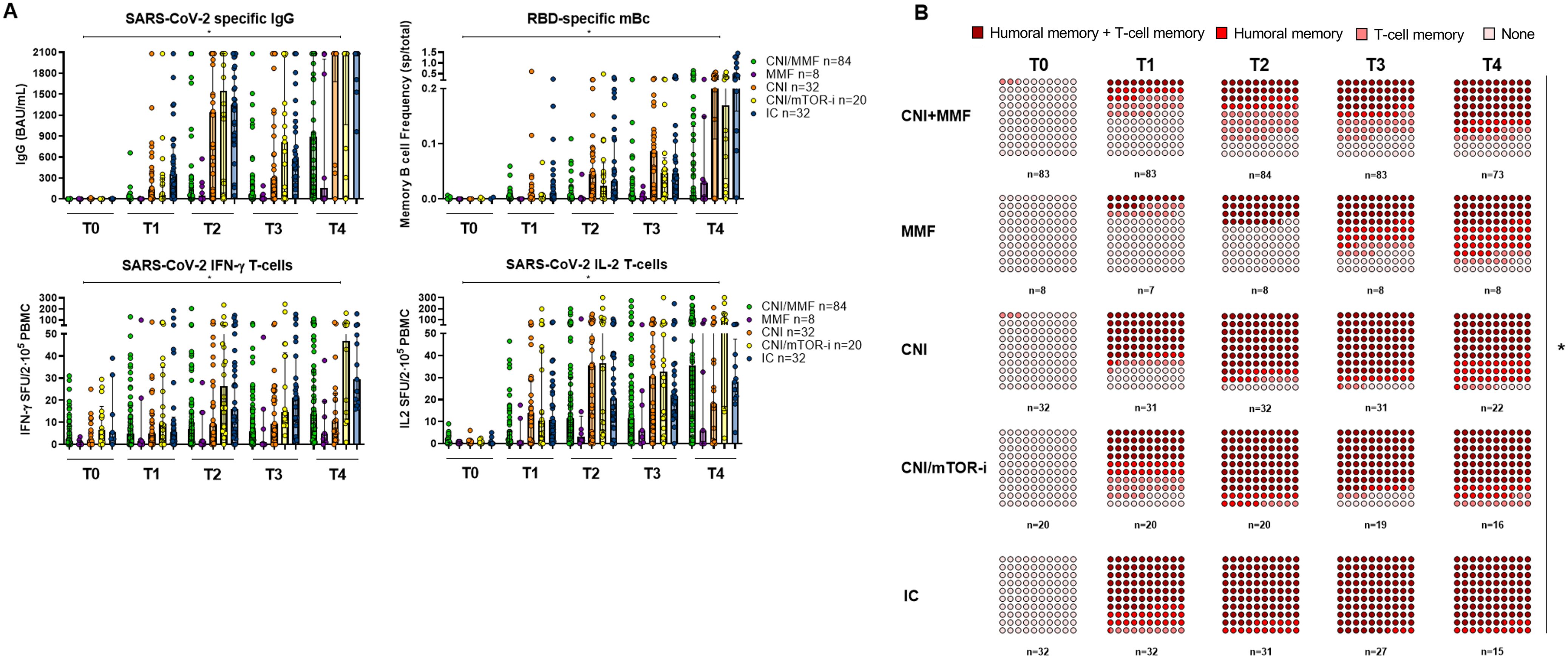
Figure 4. (A) SARS-CoV-2-specific serological and B- and T-cell memory immune responses between SOT with different IS protocols and IC over time before and after booster vaccination. Significant intra- and intergroup differences are shown in Supplementary Table S4. (B) Global qualitative SARS-CoV-2-specific immune responses at main memory compartments between SOT with different IS regimes and IC over time after booster vaccination. Significant intra- and intergroup differences are shown in Supplementary Table S5. *p < 0.05 is referred to the comparison made between all study groups.
3.5 RBD-specific memory B cells predict NAb seroconversion after booster vaccination and protection from severe COVID-19
Unsupervised hierarchical cluster analysis of all immune memory compartments prior to the third booster vaccination in all patients showed that the presence of prebooster cellular immune memory, especially RBD-specific IgG-producing mBc, predicted the successful development of NAb after the booster vaccination (Figure 5; Supplementary Table S6). Similarly, when only seronegative patients were analyzed (Figures 6A, B), those who developed NAb after a third booster vaccination displayed significantly higher IFN-γ, IL-2, and mBc frequencies prior to vaccination. Among these, RBD-specific mBc frequencies showed the best predictive capacity for the subsequent development of NAb (AUC, 0.758) (Figure 6C). Moreover, the combination of SARS-CoV-2-specific IL-2-producing T cells and RBD-specific mBc provided the best prediction for subsequent NAb (AUC, 0.828) development.
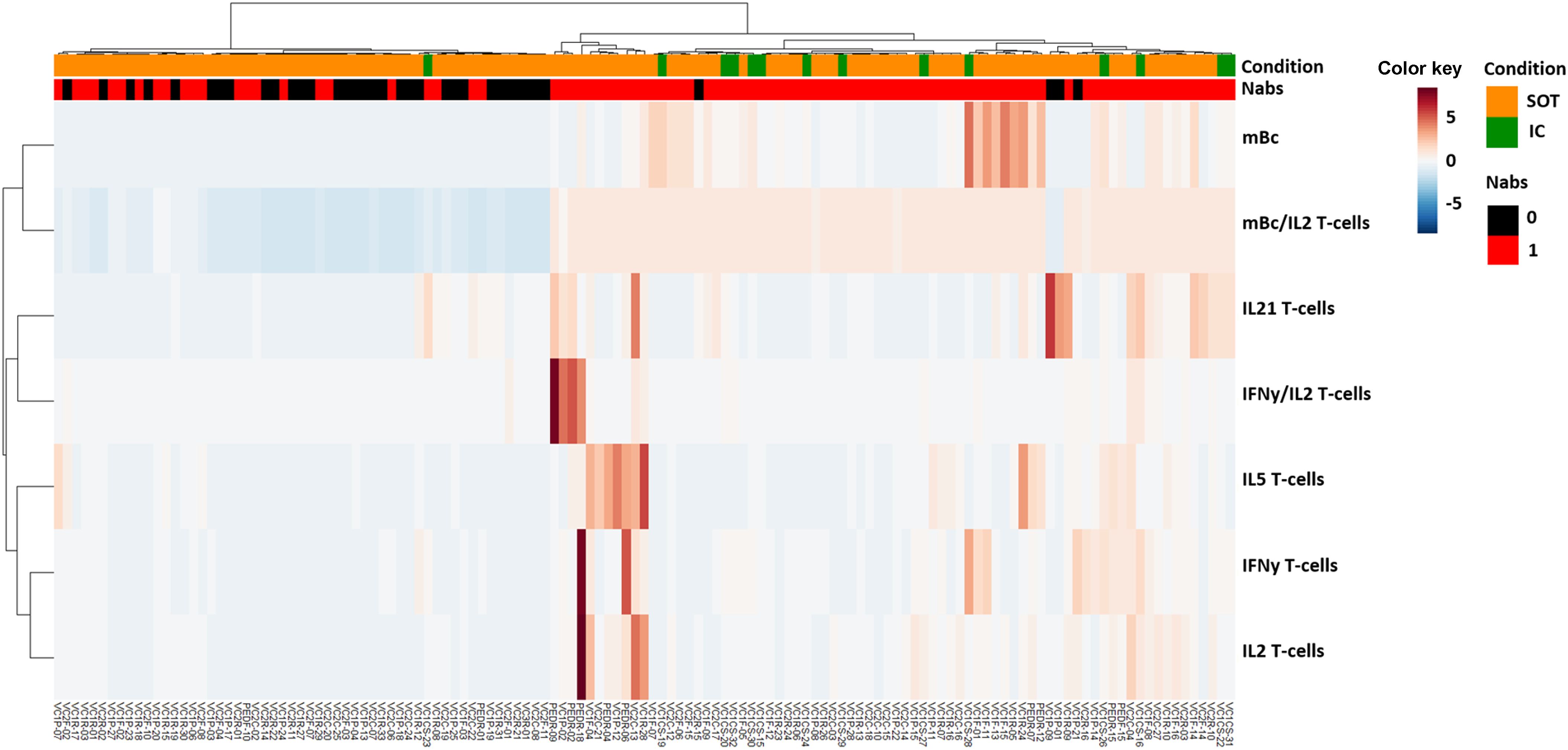
Figure 5. Heatmap generated by hierarchical clustering of different SARS-CoV-2-specific memory immune responses for SOT and IC patients, according to the development of SARS-CoV-2-specific neutralizing antibodies (NAb).
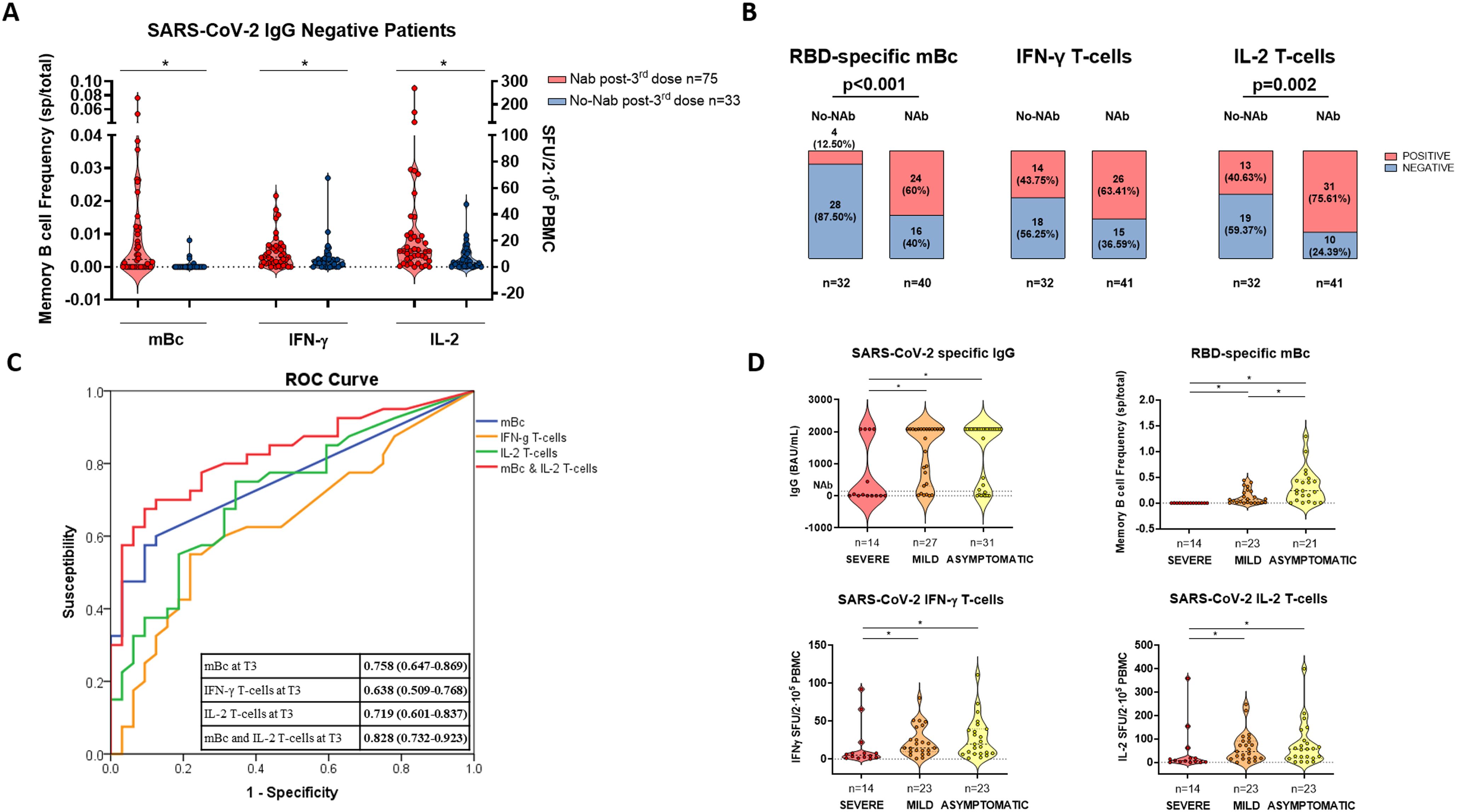
Figure 6. SARS-CoV-2-specific immune-memory responses predicting NAb and severity of breakthrough infection after third booster vaccination. (A) Pre- third vaccine dose frequencies of SARS-CoV-2-specific memory B and T cells in patients developing or not NAb after third booster vaccination in previously seronegative patients. (B) The presence of pre- third dose vaccine of SARS-CoV-2-specific memory T- and B-cell responses between patients developing or not NAb after third vaccination in seronegative patients. (C) ROC curve of SARS-CoV-2-specific immune-memory responses predicting the advent of NAb after vaccination in seronegative patients. (D) SARS-CoV-2-specific serological and memory-cell immune responses in patients developing distinct severity of SARS-CoV-2 breakthrough infection. Significant intra- and intergroup differences are shown in Supplementary Table S7. mBc, memory B cell; IFN-γ, interferon gamma; IL, interleukin; SFU, spot forming unit. *p < 0.05.
Multivariate analysis revealed that the main factors favoring the development of NAb were the presence of RBD-specific mBc (OR, 19.313 [95% CI, 3.721–100.24]; p < 0.001), lower age (OR, 0.920 [95% CI, 0.863–0.980]; p = 0.010), and CNI trough levels (OR, 0.771 [95% CI, 0.586–1.013]; p = 0.062). Similarly, for the development of RBD-specific mBc, the time after transplant (OR, 1.007 [95% CI, 1.002–1.013]; p = 0.012) and the absence of MMF-based therapy—with either the combination of CNI/mTOR-i (OR, 11.092 [95% CI, 2.084–59.029]; p = 0.005) or CNI monotherapy (OR, 4.224 [95% CI, 1.482–12.040]; p = 0.007)—were independent correlates.
When we analyzed the association between memory immune responses and subsequent COVID-19 BTI during the study period, significantly lower cytokine-producing T-cell frequencies and a lack of RBD-specific mBc were observed among patients who developed severe COVID-19 compared to those with mild or asymptomatic infections (Figure 6D; Supplementary Table S7). Multivariate analysis, considering both clinical and immunological variables predicting severe COVID- 19, showed that the only factor favoring severe BTI was the absence of RBD-specific mBc (OR, 0.097 [95% CI, 0.018–0.533]; p = 0.007) (Supplementary Table S8).
3.6 RBD-specific mBC and changes in immunosuppression favor successful immunization in nonresponders
A total of 25 SOT patients who did not develop NAb despite receiving three consecutive vaccine doses were subsequently evaluated in a RCT. This trial compared standard-of-care CNI/MMF immunosuppression (group A) with a low-exposure CNI/mTOR-i-based regimen (group B) to assess the development of anti-SARS-CoV-2 protective immunity at 1 month after the fourth vaccine dose (Supplementary Figure S1). The two groups did not differ in terms of main clinical, demographic, or immunological baseline characteristics (Table 2).
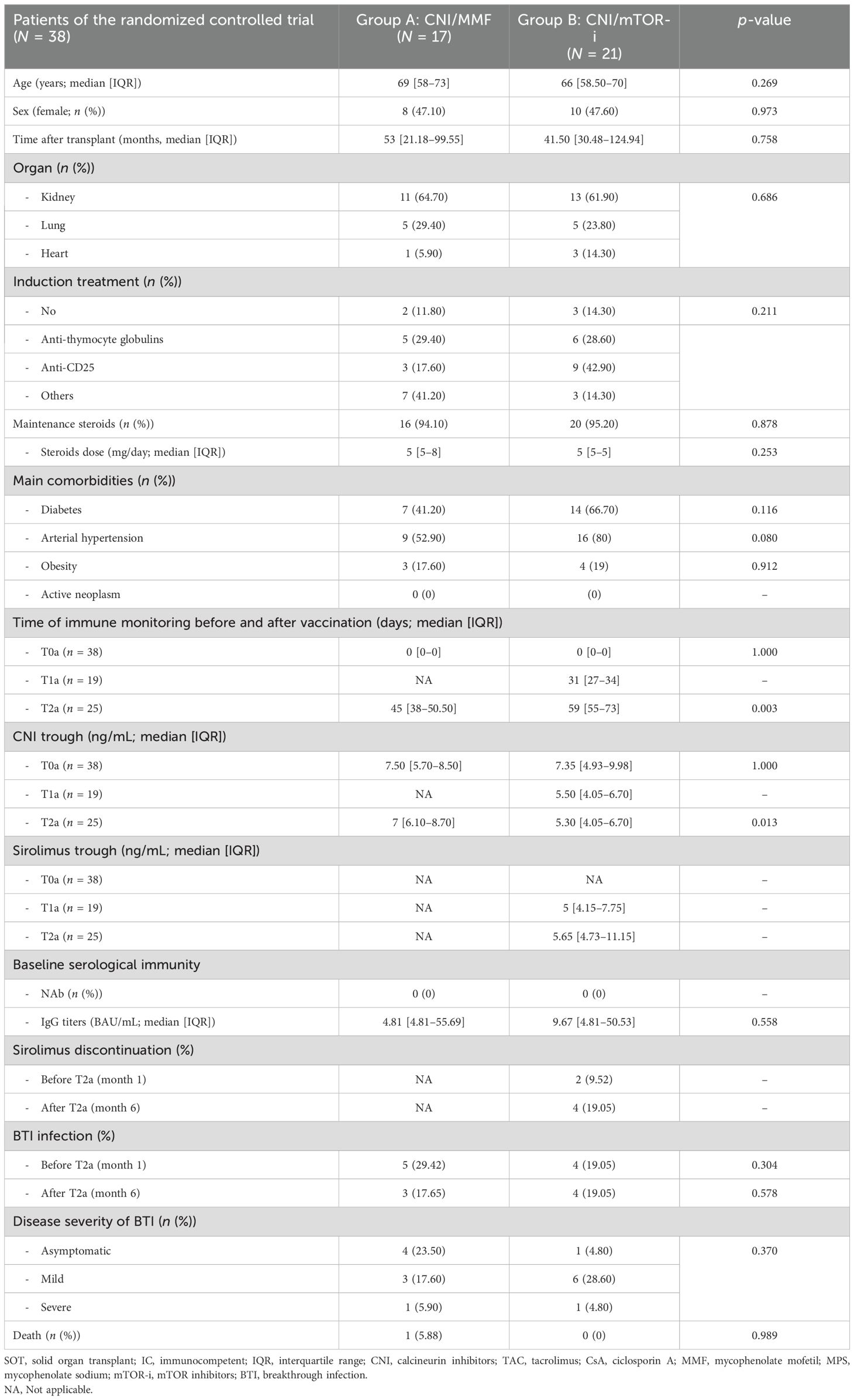
Table 2. Main demographic and clinical characteristics of the randomized controlled trial population.
Firstly, although none of the 12 CNI/mTOR-i patients developed NAb before the fourth vaccine booster, we observed that cellular immune responses had already appeared 1 month after conversion to CNI/mTOR-i and prior to vaccination (T1a) in some patients (RBD-specific mBc, two patients; IFN-γ- and IL-2-producing T cells, two and one patients, respectively) (Supplementary Figure S4). Secondly, 1 month after vaccination (T2a), 20% more patients developed NAb in group B compared to group A (8/12 [66.7%] vs. 6/13 [46.1%], p = 0.306, respectively) (Figure 7A). Additionally, the proportion of patients with detectable SARS-CoV-2-specific IgG-, IFN- γ- and IL-2-producing B- and T-cell responses, respectively, was also numerically higher in group B than in group A at T2a (9/12 [75%] vs. 7/13 [53.8%], p = 0.271 for RBD-specific mBc; 7/12 [58.3%] vs. 2/13 [15.4%], p = 0.025 for IFN-γ; and 4/12 [33.3%] vs. 5/13 [38.5%], p = 0.790 for IL-2-producing T cells, respectively) (Figure 7B). Despite the absence of NAb at baseline (T0a), some patients in each group displayed cellular responses; RBD-specific mBc in 12/25 (48%), IFN-γ-producing T cells in 6/25 (24%), and IL-2-producing T cells in 7/25 (28%). Although no clinical, demographic, or immunological variables predicted NAb seroconversion, prevaccine RBD-specific mBc was a significant predictor (OR, 33 [95% CI, 2.909–374.31]; p = 0.005) (Figure 7C). Similarly, in the observational cohort, there was a high correlation between serum IgG titers and IgG-producing mBc both after vaccination and between prevaccine mBc and postvaccine IgG titers (Supplementary Figure S5).
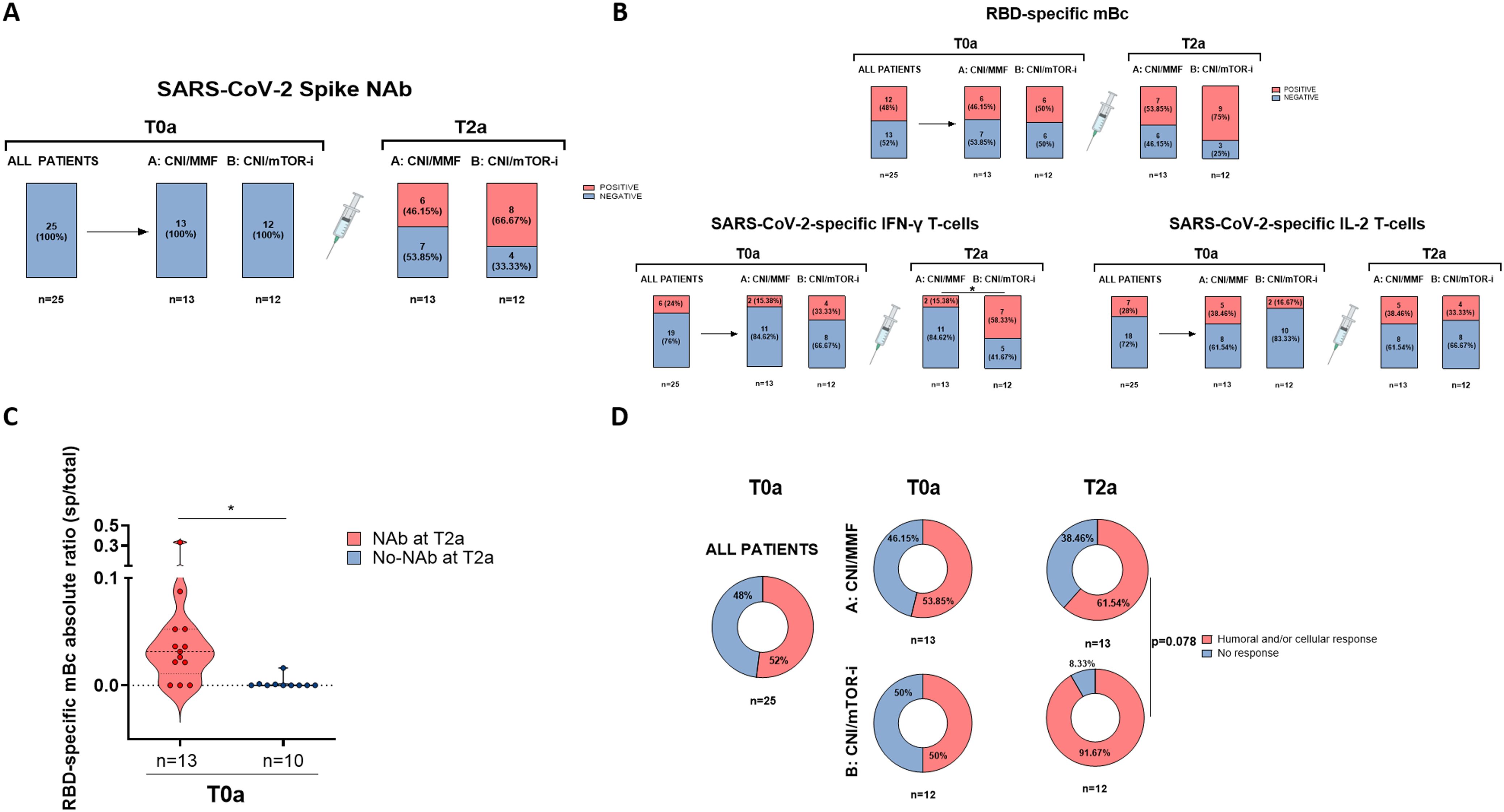
Figure 7. Results of the randomized controlled trial. (A) Presence of SARS-CoV-2-specific NAb (main end-point of the study) in patients from both groups of the trial after the fourth vaccine dose (T2a). (B) Presence of RBD-specific memory B - and memory T-cell responses in patients from both groups of the trial after the fourth vaccine dose (T2a). (C) Frequencies of RBD-specific mBc prior to the fourth dose (T0a) in patients with and without neutralizing antibodies after the fourth vaccine dose (T2a). Patients from both groups (A, B) were combined according to the presence of neutralizing antibodies at T2a. (D) Global qualitative SARS-CoV-2-specific immune responses at main memory compartments for both groups of study (A, B) before (T0a) and after the fourth dose (T2a). Humoral and/or cellular response means the presence of either NAb, mBc, and/or IFN-γ/IL-2 T-cells. *p < 0.05.
The proportion of patients receiving broader adaptive immune responses achieved after booster vaccination (NAb, RBD-specific mBc, and/or IFN-γ/IL-2-producing T cells) was higher in those switched to CNI/mTOR-i immunosuppression compared to those remaining on CNI/MMF (11/12 [91.7%] vs. 8/13 [61.5%], p = 0.078, respectively) (Figure 7D).
4 Discussion
In this study, we describe in a large cohort of SOT undergoing multiple mRNA-based vaccinations the impaired magnitude and kinetics of both humoral and functional memory T and B-cell responses specific to SARS-CoV-2 and the key role of cross-reactive RBD-specific mBc predicting successful neutralizing humoral responses to booster vaccination as well as protecting from severe clinical forms of COVID-19. Furthermore, in an RCT, we show that while the presence of circulating RBD-specific mBc in unresponsive SOT patients is key to predicting the development of NAb, regardless of the type of immunosuppression, switching from CNI/MMF to low-exposure CNI/mTOR-i immunosuppression appears to promote broader SARS-CoV-2-specific immune responses with the involvement of more immune compartments.
The first data from our study confirmed that both SOT and IC individuals display a progressive and generalized increase in detectable antiviral immune responses across major peripheral immune compartments (47–51). However, SOT patients display significantly weaker and more delayed humoral and cellular immunity compared to IC patients, with up to 35% of SOT patients lacking any functional immune response (such as NAb, RBD-specific IgG-producing mBc, and IFN-y and IL-2-producing T cells) despite receiving three vaccine doses. Conversely, despite being significantly older, IC patients in our study developed strong, detectable immune responses. Interestingly, despite a progressive decay in detectable serological titers between 2 and 5 months after vaccination, memory T- and B-cell frequencies were maintained, with RBD-specific mBc increasing over time. This finding underscores the long-lasting expansion and survival of mBc, in addition to serum antibodies, following active immunization, even among SOT recipients.
Despite the strong correlation between all functional immune memory responses at each time point and over time, illustrating a close biological interplay between different adaptive immune compartments, we found that detectable frequencies of IL-2-producing T cells, and especially of RBD-specific IgG-producing mBc, played a preponderant role in predicting successful vaccine responses. Indeed, patients with high frequencies of RBD-specific mBc and IL-2-producing T cells, even without detectable serum IgG titers, were more likely to develop NAb after booster vaccination. Furthermore, the absence of detectable frequencies of RBD-specific mBc strongly associated with a higher risk of developing severe forms of COVID-19 if infected. In this regard, we recently reported the importance of these two memory cell responses in providing long-lasting immune protection after natural SARS-CoV-2 infection and in distinguishing patients with better clinical outcomes when developing severe forms of COVID-19 (29, 30). To note, similar frequencies of T-cell responses were detected after natural infection compared to active immunization, whereas for RBD-specific mBc responses, patients with natural infection showed lower frequencies of mBc compared to those receiving three doses of the SARS-CoV-2 vaccine. Therefore, monitoring these two functional cellular immune responses could help identify SOT patients who might benefit from additional booster vaccinations and those who m ay require different preventive strategies to avoid COVID-19.
The relevance of SARS-CoV-2-specific mBc in contributing to serological memory is also illustrated by the strong correlation between serum antiviral IgG titers and those produced by circulating mBc thus, strongly suggesting a direct contribution of the peripheral mBc compartment to serum NAb. Interestingly, we also observed similar neutralizing cross-reactivity of both serum IgG and IgG-producing mBc against two different SARS-CoV-2 strains, Wuhan (D614g) and Omicron (BA.5), produced in response to either the vaccine or a natural BTI.
The development of broad immune responses, including humoral and distinct memory T- and B-cell compartments, was directly challenged by the time between transplant surgery and vaccination as well as the types of immunosuppression used. MMF-based regimens were the most clearly impaired, while CNI/mTOR-i displayed the most robust immune responses. In this regard, a recent report showed that a temporary MMF withdrawal from a CNI/MMF-based regimen in seronegative kidney transplant patients resulted in seroconversion in 76% of patients and a marked increase of RBD-specific B cells and plasmablasts after a fourth booster vaccination (38). Importantly, while this therapeutic approach may be safe for low immunological risk SOT patients (52–54), such a strategy may not be feasible for those who are recently transplanted (16) or at a high immunological risk (55). Furthermore, the withdrawal of MMF did not allow for the expansion of SARS-CoV-2-specific CD4+ cells, suggesting the need for an alternative strategy to foster broader antiviral immune compartments. To overcome these limitations, we designed an RCT to evaluate the efficacy of switching to a low-exposure CNI/mTOR-i-based regimen, considering both the safety of this immunosuppressive strategy (56) and the reported immunomodulatory effects of mTOR-i in promoting antiviral and vaccine-induced immune responses in other clinical settings (39, 57, 58). Interestingly, despite the number of study patients being lower than expected due to high infection rates during enrollment, we found an overall increase in SARS-CoV-2-specific immune responses in patients switched to CNI/mTOR-i as compared to those who remained on CNI/MMF. Indeed, a significantly higher proportion of patients switched to CNI/mTOR-i developed IFN-γ-producing T cells and had numerically higher levels of NAb and RBD-specific IgG-producing mBc compared to those remaining on CNI/MMF. Of note, 4 weeks after switching to CNI/mTOR-i but prior to the booster vaccination, some patients displayed novel detectable cellular responses, suggesting an immunomodulatory effect of this immunosuppressive strategy based on mTOR-i favoring SARS-CoV-2 vaccine-induced immunity. We did not perform any analysis 1 month after randomization in the group of patients who remained on the same immunosuppressive regimen, as no intervention was done and they just received the fourth vaccine dose. Nevertheless, while some patients displayed detectable SARS-CoV-2-specific T- or B-cell responses despite no NAb, only the presence of RBD-specific mBc predicted the advent of NAb after the fourth booster vaccination, regardless of the type of immunosuppressive regimen.
Our study has some limitations. While the inclusion of distinct SOT in this study could be considered a confounding factor, it actually allowed us to show that differences in immune responses are predominantly driven by different immunosuppressive regimens rather than by the types of SOT. Unfortunately, the sample size of patients analyzed in the trial was smaller than expected due to COVID-19 infections during the recruitment process. However, the results observed in the first observational study together with the overall improved humoral and cellular immune responses in the CNI/mTOR-i group of the RCT, strongly support the validity of these findings.
In summary, our work provides new evidence regarding the key role of SARS-CoV-2-specific mBc in facilitating successful responses to booster vaccination and in providing immune protection against severe forms of COVID-19 in SOT recipients. These data may have relevant clinical implications, as monitoring these cellular immune responses could guide decision-making on the type of preventive strategy to follow —either pursuing additional booster vaccinations or establishing alternative immunosuppressive strategies, such as low-exposure CNI/mTOR-i to promote broader SARS-CoV 2-vaccine-induced immunity. Additional interventional RCTs are highly warranted to further validate our findings and to guide the selection of the most suitable and safe immunosuppressive strategy for developing protective immunity after booster vaccinations.
Data availability statement
The raw data supporting the conclusions of this article will be made available by the authors, without undue reservation.
Ethics statement
The studies involving humans were approved by Ethical Review Board (PR115/20). The studies were conducted in accordance with the local legislation and institutional requirements. Written informed consent for participation in this study was provided by the participants’ legal guardians/next of kin.
Author contributions
LD: Methodology, Investigation, Formal analysis, Data curation, Conceptualization, Writing – original draft. SG-O: Data curation, Conceptualization, Writing – review & editing. FC: Software, Formal analysis, Data curation, Writing – review & editing. AT: Investigation, Writing – review & editing, Methodology. ML-M: Writing – review & editing, Funding acquisition, Data curation, Conceptualization. MB-P: Writing – review & editing, Methodology, Investigation. DK: Writing – review & editing, Data curation. EC: Writing – review & editing, Methodology, Investigation. CC-M: Writing – review & editing, Data curation. IC-V: Writing – review & editing, Data curation. LlC: Writing – review & editing, Data curation. MC: Writing – review & editing, Methodology, Investigation. JE: Writing – review & editing, Methodology, Investigation. CF-N: Writing – review & editing, Methodology. JQ: Writing – review & editing, Data curation. MM: Writing – review & editing, Data curation. FA: Writing – review & editing, Project administration, Data curation. JG-C: Writing – review & editing, Data curation. LL: Writing – review & editing, Data curation. ÀF: Writing – review & editing, Data curation. LaC: Writing – review & editing, Data curation. MH-C: Writing – review & editing, Data curation. MM: Writing – review & editing, Data curation. IT: Writing – review & editing, Data curation. MJ: Writing – review & editing, Methodology. FH: Writing – review & editing, Methodology, Investigation. MV: Writing – review & editing, Methodology. AR: Writing – review & editing, Methodology, Investigation. MB: Writing – review & editing, Methodology, Investigation. NT: Writing – review & editing, Data curation. JZ: Writing – review & editing, Data curation. VM: Writing – review & editing, Data curation. BS-G: Writing – review & editing, Data curation. OL: Writing – review & editing, Data curation. IA: Writing – review & editing, Data curation. EM: Writing – review & editing, Data curation. GA: Writing – review & editing, Data curation. EP: Writing – review & editing, Software, Data curation. XM: Writing – review & editing, Data curation. FM: Writing – review & editing, Data curation. OB: Writing – original draft, Supervision, Project administration, Investigation, Funding acquisition, Formal analysis, Data curation, Conceptualization.
Funding
The author(s) declare financial support was received for the research, authorship, and/or publication of this article. This work was supported by a grant from La Fundació La Marató de TV3 (Grant No. 202101 [64/198] to OB), by a European Union’s Horizon 2020 research and innovation program under Grant Agreement No. 754995 (EUTRAIN; OB), and three national research grants from Instituto de Salud Carlos III (Grant No. PI19/01710 to OB, No. PI22/01019 to OB, No. FI22/00252 to LD, and No. PI19/00330 to ICV), the European Union (ERDF/ESF)—A way to build Europe, and the Instituto de Salud Carlos III Redes de Investigación Cooperativa Orientadas a Resultados en Salud (RICORS) network.
Acknowledgments
We would like to acknowledge all the staff from the distinct kidney, heart, liver, and lung transplant units at the various centers, as well as the Sant Rafael primary health care team, for their support and care of the patients. We would also like to acknowledge all study coordinators from all transplant centers and all the patients who agreed to participate in this study.
Conflict of interest
The authors declare that the research was conducted in the absence of any commercial or financial relationships that could be construed as a potential conflict of interest.
The author(s) declared that they were an editorial board member of Frontiers, at the time of submission. This had no impact on the peer review process and the final decision.
Publisher’s note
All claims expressed in this article are solely those of the authors and do not necessarily represent those of their affiliated organizations, or those of the publisher, the editors and the reviewers. Any product that may be evaluated in this article, or claim that may be made by its manufacturer, is not guaranteed or endorsed by the publisher.
Supplementary material
The Supplementary Material for this article can be found online at: https://www.frontiersin.org/articles/10.3389/fimmu.2024.1463769/full#supplementary-material
Abbreviations
AUC, area under the curve; BTI, breakthrough infection; CNI, calcineurin inhibitors; COVID-19, coronavirus disease 2019; IC, immunocompetent; IFN-γ, interferon gamma; IL, interleukin; mBc, memory B cell; MMF, mycophenolate mofetil; MPS, mycophenolate sodium; mTOR, mammalian/mechanistic target of rapamycin; NAb, neutralizing antibodies; PBMC, peripheral blood mononuclear cells; SOT, solid organ transplant.
References
1. Dagan N, Barda N, Kepten E, Miron O, Perchik S, Katz MA, et al. BNT162b2 mRNA COVID-19 vaccine in a nationwide mass vaccination setting. N Engl J Med. (2021) 384:1412–23. doi: 10.1056/NEJMoa2101765
2. Marinaki S, Xagas E, Tsoutsoura P, Katsaros D, Korogiannou M, Boletis IN. Occurrence of severe SARS-CoV-2 infection in fully vaccinated solid organ transplant recipients. Transplant Proc. (2022) 54:1405–8. doi: 10.1016/j.transproceed.2021.12.012
3. Marinaki S, Tsiakas S, Korogiannou M, Grigorakos K, Papalois V, Boletis I. A systematic review of COVID-19 infection in kidney transplant recipients: A universal effort to preserve patients’ Lives and allografts. J Clin Med. (2020) 9:2986. doi: 10.3390/jcm9092986
4. European Centre for Disease Prevention and Control. Interim public health considerations for the provision of additional COVID-19 vaccine doses (2021). ECDC: Stockholm (Accessed 1 September 2021).
5. Baden RL, El Sahly HM, Essink B, Kotloff K, Frey S, Novak R, et al. Efficacy and safety of the mRNA-1273 SARS-CoV-2 vaccine. N Engl J Med. (2021) 384:403–16. doi: 10.1056/NEJMoa2035389
6. Bestard O, Jouve T, Castells Ll Lopez M, Muñoz M, Crespo E, et al. Reconciling short-term clinical and immunological outcomes of SARS-CoV-2 vaccination in solid organ transplant recipients. Am J Transplant. (2021) 22:673–5. doi: 10.1111/ajt.16855
7. Miele M, Busà R, Russelli G, Concetta Sorrentino M, Di Bella M, Timoneri F, et al. Impaired anti-SARS-CoV-2 humoral and cellular immune response induced by Pfizer-BioNTech BNT162b2 mRNA vaccine in solid organ transplanted patients. Am J Transplant. (2021) 21:2919–21. doi: 10.1111/ajt.16702
8. Caillard S, Chavarot N, Bertrand D, Kamar N, Thaunat O, Moal V, et al. Occurrence of severe COVID-19 in vaccinated transplant patients. Kidney Int. (2021) 100:477–9. doi: 10.1016/j.kint.2021.05.011
9. Qin CX, Moore LW, Anjan S, Rahamimov R, Sifri CD, Ali NM, et al. Risk of breakthrough SARS-CoV-2 infections in adult transplant recipients. Transplantation. (2021) 105:e265–6. doi: 10.1097/TP.0000000000003907
10. Yetmar ZA, Bhaimia E, Bierle DM, Ganesh R, Razonable RR. Breakthrough COVID-19 after SARS-CoV-2 vaccination in solid organ transplant recipients: An analysis of symptomatic cases and monoclonal antibody therapy. Transpl Infect Dis. (2022) 24:e13779. doi: 10.1111/tid.13779
11. Anjan S, Natori Y, Fernandez Betances AA, Agritelley MS, Mattiazzi A, Arosemena L, et al. Breakthrough COVID-19 infections after mRNA vaccination in solid organ transplant recipients in miami, florida. Transplantation. (2021) 105:e139–41. doi: 10.1097/TP.0000000000003902
12. Sun J, Zheng Q, Madhira V, Olex AL, Anzalone AJ, Vinson A, et al. Association between immune dysfunction and COVID 19 breakthrough infection after SARS-CoV-2 vaccination in the US. JAMA Intern Med. (2022) 182:153–62. doi: 10.1001/jamainternmed.2021.7024
13. Netti GS, Infante B, Troise D, Mercuri S, Panico M, Spadaccino F, et al. mTOR inhibitors improve both humoral and cellular response to SARS-CoV-2 messenger RNA BNT16b2 vaccine in kidney transplant recipients. Am J Transplant. (2022) 22:1475–82. doi: 10.1111/ajt.16958
14. Marion O, Del Bello A, Abravanel F, Couat C, Faguer S, Esposito L, et al. Safety and immunogenicity of anti-SARS-CoV-2 messenger RNA vaccines in recipients of solid organ transplants. Ann Intern Med. (2021) 174:1336–8. doi: 10.7326/M21-1341
15. Kamar N, Abravanel F, Marion O, Couat C, Izopet J, Del Bello A. Three doses of an mRNA covid-19 vaccine in solid-organ transplant recipients. N Engl J Med. (2021) 385:661–2. doi: 10.1056/NEJMc2108861
16. Boyarsky BJ, Werbel WA, Avery RK, Tobian AAR, Massie AB, Segev DL, et al. Antibody response to 2-dose SARS-CoV-2 mRNA vaccine series in solid organ transplant recipients. JAMA. (2021) 325:2204–6. doi: 10.1001/jama.2021.7489
17. Yanis A, Haddadin Z, Spieker AJ, Waqfi D, Rankin DA, Talj R, et al. Humoral and cellular immune responses to the SARS-CoV-2 BNT162b2 vaccine among a cohort of solid organ transplant recipients and healthy controls. Transpl Infect Dis. (2021) 24:e13772. doi: 10.1111/tid.13772
18. Campos-Varela I, Len O, Villagrasa A, Marquez-Algaba E, Esperalba J, Dopazo C, et al. Low seroprevalence of SARS-CoV-2 antibodies in a liver transplant cohort. Transpl Int. (2021) 34:1908–13. doi: 10.1111/tri.13946
19. Cromer D, Steain M, Reynaldi A, Schlub TE, Wheatley AK, Juno JA, et al. Neutralising antibody titres as predictors of protection against SARS-CoV-2 variants and the impact of boosting: a meta-analysis. Lancet Microbe. (2022) 3:e52–61. doi: 10.1016/S2666-5247(21)00267-6
20. Khoury SD, Cromer D, Reynaldi A, Schlub TE, Wheatley AK, Juno JA, et al. Neutralizing antibody levels are highly predictive of immune protection from symptomatic SARS-CoV-2 infection. Nat Med. (2021) 27:1205–11. doi: 10.1038/s41591-021-01377-8
21. Abebe EC, Dejenie TA. Protective roles and protective mechanisms of neutralizing antibodies against SARS-CoV-2 infection and their potential clinical implications. Front Immunol. (2023) 14:1055457. doi: 10.3389/fimmu.2023.1055457
22. Painter MM, Johnston TS, Lundgreen KA, Santos JJS, Qin JS, Goel RR, et al. Prior vaccination promotes early activation of memory T cells and enchances immune responses during SARS-CoV-2 breakthrough infection. Nat Immunol. (2023) 24:1711–24. doi: 10.1038/s41590-023-01613-y
23. Kaku CI, Bergeron AJ, Ahlm C, Normark J, Sakharkar M, Forsell MNE, et al. Recall of preexisting cross-reactive B cell memory after Omicron BA.1 breakthrough infection. Sci Immunol. (2022) 7:eabq3511. doi: 10.1126/sciimmunol.abq3511
24. Sokal A, Barba-Spaeth G, Hunault L, Fernandez I, Broketa M, Meola A, et al. SARS-CoV-2 Omicron BA.1 breakthrough infection drives late remodeling of the memory B cell repertoire in vaccinated individuals. Immunity. (2023) 56:2137–51. doi: 10.1016/j.immuni.2023.07.007
25. Purtha WE, Tedder TF, Johnson S, Bhattacharya D, Diamond MS. Memory B cells, but not long-lived plasma cells, possess antigen specificities for viral escape mutants. J Exp Med. (2011) 208:2599–606. doi: 10.1084/jem.20110740
26. Weisel F, Shlomchik M. Memory B cells of mice and humans. Annu Rev Immunol. (2017) 35:255–84. doi: 10.1146/annurev-immunol-041015-055531
27. GeurtsvanKessel CH, Geers D, Schmitz KS, Mykytyn A, Lamers MM, Bogers S, et al. Divergent SARS-CoV-2 Omicron-reactive T and B cell responses in COVID-19 vaccine recipients. Sci Immunol. (2022) 7:eabo2202. doi: 10.1126/sciimmunol.abo2202
28. Siedner MJ, Boucau J, Gilbert RF, Uddin R, Luu J, Haneuse S, et al. Duration of viral shedding and culture positivity with postvaccination SARS-CoV-2 delta variant infections. JCI Insight. (2022) 7:e155483. doi: 10.1172/jci.insight.155483
29. Favà A, Donadeu L, Sabe N, Pernin V, Gonzalez-Costello J, Llado L, et al. SARS-CoV-2-specific serological and functional T cell immune responses during acute and early COVID-19 convalescence in solid organ transplant patients. Am J Transplant. (2021) 21:2749–61. doi: 10.1111/ajt.16570
30. Favà A, Donadeu L, Jouve T, Gonzalez-Costello J, Llado L, Santana C, et al. A comprehensive assessment of long-term SARS-CoV-2-specific adaptive immune memory in convalescent COVID-19 Solid Organ Transplant recipients. Kidney Int. (2022) 101:1027–38. doi: 10.1016/j.kint.2021.12.029
31. Zavaglio F, Frangipane V, Morosini M, Gabanti E, Zelini P, Sammartino JC, et al. Robust and persistent B- and T-cell responses after COVID-19 in immunocompetent and solid organ transplant recipient patients. Viruses. (2021) 13:2261. doi: 10.3390/v13112261
32. Sattler A, Angermair S, Stockmann H, Moira Heim K, Khadzhynov D, Treskatsch S, et al. SARS-CoV-2-specific T cell responses and correlations with COVID-19 patient predisposition. J Clin Invest. (2020) 130:6477–89. doi: 10.1172/JCI140965
33. Charmetant X, Espi M, Barba T, Ovize A, Morelon E, Mathieu C, et al. Predictive factors of a viral neutralizing humoral response after a third dose of COVID-19 mRNA vaccine. Am J Transplant. (2022) 22:1442–50. doi: 10.1111/ajt.16990
34. Rincon-Arevalo H, Choi M, Stefanski A, Halleck F, Weber U, Szelinski F, et al. Impaired humoral immunity to SARS-CoV-2 BNT162b2 vaccine in kidney transplant recipients and dialysis patients. Sci Immunol. (2021) 6:eabj1031. doi: 10.1126/sciimmunol.abj1031
35. Kared H, Alirezaylavasani A, Persgard Lund K, Chopra A, Tietze L, de Matos Kasahara T, et al. Hybrid and SARS-CoV-2-vaccine immunity in kidney transplant recipients. EBioMedicine. (2023) 97:104833. doi: 10.1016/j.ebiom.2023.104833
36. Halloran PF. Immunosupressive drugs for kidney transplantation. N Engl J Med. (2004) 351:2715–29. doi: 10.1056/NEJMra033540
37. Willicombe M, Thomas D, McAdoo S. COVID-19 and calcineurin inhibitors: should they get left out in the storm? J Am Soc Nephrol. (2020) 31:1145–6. doi: 10.1681/ASN.2020030348
38. Schrezenmeier E, Rincon-Arevalo H, Jens A, Stefanski A, Hammett C, Osmanodja B, et al. Temporary antimetabolite treatment hold boosts SARS-CoV-2 vaccination-specific humoral and cellular immunity in kidney transplant patients. JCI Insight. (2022) 7:e157836. doi: 10.1172/jci.insight.157836
39. Araki K, Turner AP, Oliva Shaffer V, Gangappa S, Keller SA, Bachmann MF, et al. mTOR regulates memory CD8 T-cell differentiation. Nature. (2009) 460:108–12. doi: 10.1038/nature08155
40. Berger SP, Sommerer C, Witzke O, Tedesco H, Chadban S, Mulgaonkar S, et al. Two-year outcomes in de novo renal transplant recipients receiving everolimus-facilitated calcineurin inhibitor reduction regimen from the TRANSFORM study. Am J Transplant. (2019) 19:3018–34. doi: 10.1111/ajt.15480
41. World Health Organization. Living guidance for clinical management of COVID-19: Living Guidance (Accessed 23 November 2021).
42. Grau-Expósito J, Perea D, Suppi M, Massana N, Vergara A, Soler MJ, et al. Evaluation of SARS-CoV-2 entry, inflammation and new therapeutics in human lung tissue cells. PLoS Pathog. (2022) 18:e1010171. doi: 10.1371/journal.ppat.1010171
43. Caillard S, Thaunat O, Benotmane I, Masset C, Blancho G. Antibody response to a fourth messenger RNA COVID-19 vaccine dose in kidney transplant recipients: A case series. Ann Intern Med. (2022) 175:455–6. doi: 10.7326/L21-0598
44. Gallais F, Gantner P, Bruel T, Velay A, Planas D, Wendling M, et al. Evolution of antibody responses up to 13 months after SARS-CoV-2 infection and risk of reinfection. EBioMedicine. (2021) 71:103561. doi: 10.1016/j.ebiom.2021.103561
45. Gorovits B, Azadeh M, Buchlis G, Fiscella M, Harrison T, Havert M, et al. Evaluation of cellular immune response to adeno-associated virus-based gene therapy. AAPS J. (2023) 25:47. doi: 10.1208/s12248-023-00814-5
46. Bland JM, Altman DGI. RCalculating correlation coefficients with repeated observations: Part 2—Correlation between subjects. BMJ. (1995) 310(6980):633. doi: 10.1136/bmj.310.6980.633
47. Sattler A, Schrezenmeier E, Weber UA, Potekhin A, Bachmann F, Straub/Hohenbleicher H, et al. Impaired humoral and cellular immunity after SARS-CoV-2 BNT162b2 (tozinameran) prime-boost vaccination in kidney transplant recipients. J Clin Invest. (2021) 131:e150175. doi: 10.1172/JCI150175
48. Grupper A, Rabinowich L, Schwartz D, Schwartz IF, Ben-Yehoyada M, Shashar M, et al. Reduced humoral response to mRNA SARS-CoV-2 BNT162b2 vaccine in kidney transplant recipients without prior exposure to the virus. Am J Transplant. (2021) 21:2719–26. doi: 10.1111/ajt.16615
49. Cucchiari D, Egri N, Bodro M, Herrera S, Del Risco-Zevallos J, Casals-Urquiza J, et al. Cellular and humoral response after MRNA-1273 SARS-CoV-2 vaccine in kidney transplant recipients. Am J Transplant. (2021) 21:2727–39. doi: 10.1111/ajt.16701
50. Boyarsky BJ, Werbel WA, Avery RK, Tobian AAR, Massie AB, Segev DL, et al. Immunogenicity of a single dose of SARS-CoV-2 messenger RNA vaccine in solid organ transplant recipients. JAMA. (2021) 325:1784–6. doi: 10.1001/jama.2021.4385
51. Christophorou E, Nilsson AC, Petersen I, Lindvig SO, Davidsen JR, Abazi R, et al. Humoral antibody response following mRNA vaccines against SARS-CoV-2 in solid organ transplant recipients; a status after a fifth and bivalent vaccine dose. Front Immunol. (2023) 14:1270814. doi: 10.3389/fimmu.2023.1270814
52. Vanrenterghem Y, van Hooff J, Squifflet J, Salmela K, Rigotti P, Jindal RM, et al. Minimization of immunosuppressive therapy after renal transplantation: results of a randomized controlled trial. Am J Transplant. (2005) 5:87–95. doi: 10.1111/j.1600-6143.2004.00638.x
53. Asberg A, Apeland T, Reisaeter AV, Foss A, Leivestad T, Heldal K, et al. Long-term outcomes after cyclosporine or mycophenolate withdrawal in kidney transplantation - results from an aborted trial. Clin Transplant. (2013) 27:e151–6. doi: 10.1111/ctr.12076
54. Baran DA, Zucker MJ, Arroyo LH, Camacho M, Goldschmidt ME, Nicholls SJ, et al. A prospective, randomized trial of single-drug versus dual-drug immunosupression in heart transplantation. Circulation: Heart Failure. (2011) 4:129–37. doi: 10.1161/CIRCHEARTFAILURE.110.958520
55. Bestard O, Meneghini M, Crespo E, Bemelman F, Koch M, Volk HD, et al. Preformed T cell alloimmunity and HLA eplet mismatch to guide immunosuppression minimization with tacrolimus monotherapy in kidney transplantation: Results of the CELLIMIN trial. Am J Transplant. (2021) 21:2833–45. doi: 10.1111/ajt.16563
56. Pascual J, Berger SP, Witzke O, Tedesco H, Mulgaonkar S, Qazi Y, et al. Everolimus with reduced calcineurin inhibitor exposure in renal transplantation. J Am Soc Nephrol. (2018) 29:1979–91. doi: 10.1681/ASN.2018010009
57. Mannick JB, del Fiudice F, Lattanzi M, Valiante NM, Praestgaard J, Huang B, et al. mTOR inhibition improves immune function in the elderly. Sci Transl Med. (2014) 6:268ra179. doi: 10.1126/scitranslmed.3009892
58. Pérez-Flores I, Juarez I, Aiffil Meneses AS, Lopez-Gomez A, Calvo Romero N, Rodriguez-Cubillo B, et al. Role of mTOR inhibitor in the celular and humoral immune response to a booster dose of SARS-CoV-2 mRNA-1273 vaccine in kidney transplant recipients. Front Immunol. (2023) 14:1111569. doi: 10.3389/fimmu.2023.1111569
Keywords: SARS-CoV-2, booster vaccination, solid organ transplantation, adaptive immunity, neutralizing antibodies
Citation: Donadeu L, Gomez-Olles S, Casanova F, Torija A, Lopez-Meseguer M, Boada-Pérez M, Kervella D, Crespo E, Carrera-Muñoz C, Campos-Varela I, Castells L, Cortese MF, Esperalba J, Fernández-Naval C, Quintero J, Muñoz M, Agüero F, Gonzalez-Costello J, Lladó L, Favà A, Cañas L, del Mar de la Hoz-Caballero M, Meneghini M, Torres IB, Juvé M, Hafkamp FMJ, Vila M, Robles AG, Buzón MJ, Toapanta N, Zúñiga JM, Monforte V, Saez-Giménez B, Len O, Arcos IL, Miret E, Ariceta G, Pardo E, Martínez X, Moreso F and Bestard O (2024) Role of SARS-CoV-2-specific memory B cells promoting immune protection after booster vaccination in solid organ transplantation. Front. Immunol. 15:1463769. doi: 10.3389/fimmu.2024.1463769
Received: 12 July 2024; Accepted: 09 September 2024;
Published: 08 October 2024.
Edited by:
Adriana Colovai, Albert Einstein College of Medicine, United StatesReviewed by:
Eva Stadler, University of New South Wales, AustraliaMaha Alenazy, King Saud University, Saudi Arabia
Tianling Ou, Broad Institute, United States
Sameer Kumar Malladi, Washington University in St. Louis, United States
Copyright © 2024 Donadeu, Gomez-Olles, Casanova, Torija, Lopez-Meseguer, Boada-Pérez, Kervella, Crespo, Carrera-Muñoz, Campos-Varela, Castells, Cortese, Esperalba, Fernández-Naval, Quintero, Muñoz, Agüero, Gonzalez-Costello, Lladó, Favà, Cañas, del Mar de la Hoz-Caballero, Meneghini, Torres, Juvé, Hafkamp, Vila, Robles, Buzón, Toapanta, Zúñiga, Monforte, Saez-Giménez, Len, Arcos, Miret, Ariceta, Pardo, Martínez, Moreso and Bestard. This is an open-access article distributed under the terms of the Creative Commons Attribution License (CC BY). The use, distribution or reproduction in other forums is permitted, provided the original author(s) and the copyright owner(s) are credited and that the original publication in this journal is cited, in accordance with accepted academic practice. No use, distribution or reproduction is permitted which does not comply with these terms.
*Correspondence: Oriol Bestard, b3Jpb2wuYmVzdGFyZEB2YWxsaGVicm9uLmNhdA==