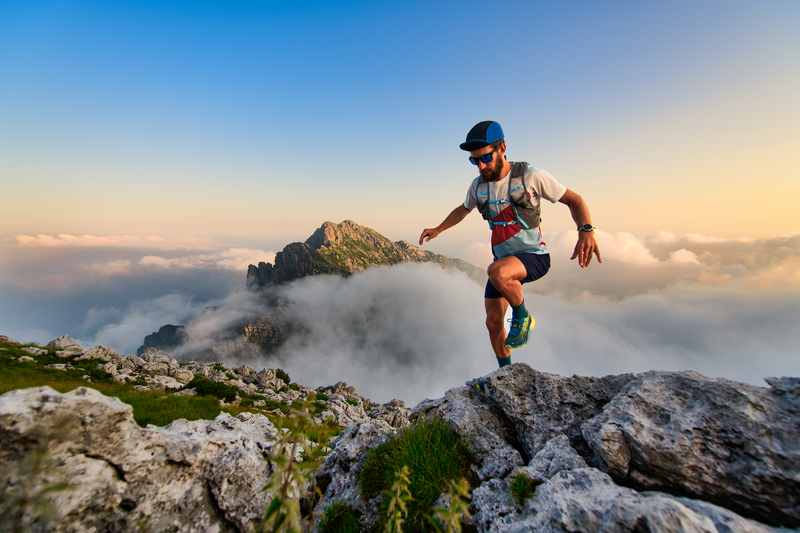
95% of researchers rate our articles as excellent or good
Learn more about the work of our research integrity team to safeguard the quality of each article we publish.
Find out more
REVIEW article
Front. Immunol. , 26 August 2024
Sec. Inflammation
Volume 15 - 2024 | https://doi.org/10.3389/fimmu.2024.1456244
Inflammatory diseases compromise a clinically common and diverse group of conditions, causing detrimental effects on body functions. Gasdermins (GSDM) are pore-forming proteins, playing pivotal roles in modulating inflammation. Belonging to the GSDM family, gasdermin D (GSDMD) actively mediates the pathogenesis of inflammatory diseases by mechanistically regulating different forms of cell death, particularly pyroptosis, and cytokine release, in an inflammasome-dependent manner. Aberrant activation of GSDMD in different types of cells, such as immune cells, cardiovascular cells, pancreatic cells and hepatocytes, critically contributes to the persistent inflammation in different tissues and organs. The contributory role of GSDMD has been implicated in diabetes mellitus, liver diseases, cardiovascular diseases, neurodegenerative diseases, and inflammatory bowel disease (IBD). Clinically, alterations in GSDMD levels are potentially indicative to the occurrence and severity of diseases. GSDMD inhibition might represent an attractive therapeutic direction to counteract the progression of inflammatory diseases, whereas a number of GSDMD inhibitors have been shown to restrain GSDMD-mediated pyroptosis through different mechanisms. This review discusses the current understanding and future perspectives on the role of GSDMD in the development of inflammatory diseases, as well as the clinical insights of GSDMD alterations, and therapeutic potential of GSDMD inhibitors against inflammatory diseases. Further investigation on the comprehensive role of GSDM shall deepen our understanding towards inflammation, opening up more diagnostic and therapeutic opportunities against inflammatory diseases.
As an evolutionarily conserved biological response, inflammation primarily confers host protection against pathogen insults and promotes tissue repair by activating both immune and non-immune cells. An unfavorable, prolonged, and persistent inflammation, either locally or systemically, characterizes inflammatory diseases (1). Inflammatory diseases are recognized as one of the leading causes of mortality worldwide today, where nearly 50% of all-cause mortality is attributed to inflammation-associated complications, like carcinomas, ischemic heart disease, diabetes mellitus, autoimmune diseases, and neurodegenerative disorders (2).
The gasdermin (GSDM) family is constituted by five members (GSDMA to GSDME), representing a group of lipid-binding and membrane pore-forming proteins. Primarily characterized as mediators of pyroptotic cell death, GSDMs are shown to also mediate vital cell functions, non-lytic release of inflammatory cytokines, and bactericidal action (3). GSDMs are constituted by N-terminal domains (NTDs), which can initiate cytolysis by forming membrane pores, and C-terminal domains (CTDs), which can inhibit cell death via intramolecular domain association (4). The predicted structures of human GSDMs are displayed in Figure 1. Belonging to the GSDM family, gasdermin D (GSDMD) is the most extensively studied GSDM, which is a critical modulator of inflammasome-dependent pyroptosis and diverse downstream immunological activities (5). Both human and murine GSDMDs are composed of NTDs and CTDs, interconnected by a partially disordered linker region. Suggested by crystal structure study, no gross structural alterations were noted across both NTDs and CTDs from human and murine GSDMDs (6).
Figure 1. Predicted protein structures of human GSDMs. The predicted structures of GSMD family (GSDMA to GSDME) are displayed at orient axes. The predicted protein structures are generated by AlphaFold 3. AlphaFold produces a per-residue model confidence score (pLDDT) between 0 and 100.
Briefly, activated by various inflammasomes, GSDMD is tightly involved in the execution of pyroptosis, downstream inflammatory signaling cascades, and the release of inflammatory cytokines. Aberrant GSDMD activation triggers persistent inflammation and has been linked to the development of numerous inflammatory diseases, such as diabetes mellitus, liver diseases, cardiovascular diseases, neurodegenerative diseases, inflammatory bowel disease (IBD), and sepsis (7). This review article discusses the relationship between GSDMD and cell death, the mechanisms of GSDMD-related inflammatory signal transduction, and the role of GSDMD in different cell types. In addition, the role of GSDMD in different inflammatory diseases, clinical insights of alterations in GSDMD levels, and the therapeutic potential of GSDMD inhibitors are also addressed.
Although GSDMD is primarily considered as the critical gatekeeper for igniting pyroptotic cell death (Figure 2), GSDMD also participates in the mediation of other types of cell death, namely apoptosis, necroptosis and NETosis.
Figure 2. Canonical and noncanonical pathways of GSDMD-mediated pyroptosis. In canonical pathway, sensing DAMPs and PAMPs by inflammasome triggers downstream activation of caspase 1, which cleaves GSDMD and pro-IL-1β/-18. In noncanonical pathway, LPS from Gram-negative bacteria activates caspase 4/5/11, which subsequently cleaves GSDMD. Upon the action of caspases, GSDMD is cleaved into fragments containing NTD and CTD (GSDMD-N and GSDMD-C). GSDMD-N assembles to cause pore formation on plasma membrane, leading to the release of interleukins.
Pyroptosis is considered an inflammatory form of programmed cell death, ignited by host factors or pathogenic infections (8). Pyroptosis can be initiated by canonical or noncanonical pathway, where GSDMD serves as a common downstream effector for both pathways (Figure 2) (9). In canonical pyroptotic pathway, pattern recognition receptors (PRRs) are intracellularly activated by damage-associated molecular patterns (DAMPs) and pathogen-associated molecular patterns (PAMPs) in cytoplasm, where activated PRRs assemble apoptosis-associated speck-like protein (ASC) and pro-caspase 1 to form a multi-protein complex termed inflammasome. The activated caspase 1 is responsible for the cleavage of GSDMD and pro-IL-1β/IL-18 to their mature forms (10). In noncanonical pathway, intracellular lipopolysaccharides (LPS) from Gram-negative bacteria activate pro-caspase 4/5 (in human) or pro-caspase 11 (in murine) (11). The active caspase 4/5/11 subsequently cleaves the linker region in GSDMD to produce NTD- and CTD-containing fragments (GSDMD-N and GSDMD-C) for pyroptosis initiation (12). The NTD-containing GSDMD fragment (GSDMD-N) oligomerizes to form transmembrane pore by selectively interacting with membrane lipids, resulting in the release of danger signals and cellular contents, especially inflammatory cytokines (13). Crosstalk between canonical and noncanonical pyroptotic pathways exists through NOD-like receptor family pyrin domain containing 3 (NLRP3) (14), which aggravates inflammation by activating caspase 1 for cleavage of pro-IL-1β/IL-18 into their mature forms (14, 15).
Additional to caspases, GSDMD can be cleaved by other proteases, such as neutrophil serine proteases (NSPs) (e.g. elastase and cathepsin G), and main protease (Mpro) from SARS-CoV-2 virus. In activated neutrophils, GSDMD can be cleaved by neutrophil elastase, where cleaved GSDMD plays a regulatory role in the generation of neutrophil extracellular traps (NETs), neutrophil pyroptosis, and inflammasome activation in neutrophils (16). Meanwhile, GSDMD can be cleaved by cathepsin G, another NSP. Cathepsin G cleaves GSDMD at leucine 274 to form the specific nitrogen-terminal domain p30, which is believed to play a critical role in regulating inflammatory responses and cell death pathways in neutrophils and monocytes (17). Mpro and papain-like protease (PLpro) are highly conserved viral proteases critical to the replication of SARS-COV-2. Recently, it was shown that Mpro can cleave porcine GSDMD at the glutamine 193-glycine 194 junction to generate two fragments, which are unable to cause pyroptosis. In other words, Mpro can cleave pore-forming p30 fragment of porcine GSDMD to antagonize GSDMD-mediated pyroptosis, and to facilitate the replication of coronaviruses (18). Further studies shall investigate the effects of other SARS-COV-2-derived proteases, like PLpro, on the structure and function of GSDMD.
GSDMs (GSDMB to GSDME) are generally considered as negative regulators of apoptosis that the presence of their active forms generally switches apoptosis to pyroptosis to elicit inflammation. In other words, low levels of these GSDMs generally facilitate apoptosis over pyroptosis (19). GSDMD has been implicated to switch apoptosis to pyroptosis in numerous disease models. Caspase-1 is responsible for the cleavage of GSDMD to GSDMD-N and GSDMD-C fragments to induce pyroptosis. Nevertheless, caspase-1 was shown to be pro-pyroptotic in GSDMD-sufficient cells, but pro-apoptotic in GSDMD-deficient macrophages through the Bid-caspase-9-caspase-3 axis (20). In GSDMD-deficient macrophages, inflammasome activation was also shown to activate apoptotic caspase-3 and caspase-7 in a caspase-1/8-dependent manner (21).
Moreover, GSDMD knockdown was found to promote apoptotic cell death and inhibit EGFR/Akt signaling in non−small cell lung cancer cells (22). Conversely, downstream to toll-like receptor 4 (TLR4), GSDMD upregulation was shown to elevate pyroptotic rate but reduce apoptotic rate in a proximal tubular cell to potentially aggravate tubular injury (23). Additionally, GSDMD deficiency was found to upregulate apoptosis during noninfectious liver injury (24). However, some recent findings have suggested controversial roles of GSDMD in mediating apoptosis (Figure 3). For instance, GSDMD overexpression was shown to enhance eIF2α phosphorylation and activate endoplasmic reticulum (ER) stress response to promote tumor cell apoptosis, rather than pyroptosis, during cisplatin chemotherapy (25). Another study even showed that GSDMD-induced pyroptosis is upstream to apoptosis in human neuroblastoma cells upon bisphenol A treatment, in a caspase-1-dependent manner (26). Further mechanistic studies are needed to investigate the crosstalk between pyroptosis and apoptosis, and the comprehensive role of GSDMD in relating inflammation, pyroptosis and apoptosis.
Figure 3. Role of GSDMD in different types of cell death. (A) GSDMD is suggested to be a negative regulator of apoptosis. However, GSDMD-induced pyroptosis can cause ER stress, which eventually triggers apoptosis. (B) GSDMD cleavage leads to pore formation on mitochondrial membrane to promote necroptosis. Meanwhile, GSDMD-mediated pyroptosis and inflammation are potentially upstream to necroptosis. (C) NETosis can be caused by GSDMD-dependent and -independent mechanisms. Active GSDMD (GSDMD-N) forms pores on nuclear membrane of neutrophil to the release of NETs and NETosis.
Necroptosis is another mode of programmed cell death that shares certain apoptotic and necrotic features, where receptor-interacting protein kinase 3 (RIPK3) and its substrate mixed lineage kinase domain like pseudokinase (MLKL) are crucial executors of this pathway (27, 28). Recent findings have suggested a linkage between GSDMD-mediated pyroptosis and necroptosis (Figure 3). Weindel et al. showed that upon perturbed mitochondrial homeostasis, increased mitochondrial ROS guides GSDMD to form pores on mitochondrial membranes, releasing mitochondrial ROS to the cytoplasm. The intracellular mitochondrial ROS then facilitates the switch from pyroptosis to RIPK3/MLKL-dependent necroptosis (29). These findings imply that GSDMD might be closely involved in mitochondrial homeostasis, and strategies that guide GSDMD away from plasma membrane might retard pyroptosis. Furthermore, another study indicates that GSDMD-mediated pyroptosis and inflammation potentially precede necroptosis during intestinal inflammation, contributory to the development of colitis (30). Therefore, GSDMD might potentially be an executor of different cell death pathways, which requires further extensive studies to confirm.
NETosis refers to a special type of programmed cell death, involving the formation and release of NETs by dying neutrophils to counteract pathogenic insults (31). GSDMD has been demonstrated to mediate the generation of NETs by neutrophils, in which neutrophil proteases cause proteolytical activation of GSDMD, where activated GSDMD in turn modulates protease activation and nuclear expansion in a feed-forward loop (32). GSDMD cleavage was previously shown to be upstream to NET release in neutrophils during acute respiratory distress syndrome, where extrinsic NETs could significantly reverse the protection of GSDMD inhibition against mouse lung injury (33). GSDMD-mediated NET formation was also found positively associated with infiltration of inflammatory cells, macrophage-to-myofibroblast transition, and renal fibrosis during obstructive nephropathy (34). Mechanistically, GSDMD-N can form pores on nuclear and plasma membranes of neutrophils (35), causing the release of NETs from neutrophils (36). However, some recent studies proposed that GSDMD is unessential for NETosis initiation. Chauhan et al. provided clues that phorbol ester-induced NETosis still occurs even in GSDMD-deficient neutrophils (37). In 2023, Stojkov et al. indicated that NETosis could be triggered by inflammasome-independent mechanisms as GSDMD cleavage is not necessarily a preceding event for NET formation. In other words, NETs can be formed by viable neutrophils upon inflammasome activation independent of GSDMD (38). Therefore, the previously suggested concept that pyroptosis is a prerequisite for NET generation need revisit. However, it is also reasonable to postulate that NETosis might occur in both GSDMD-dependent and GSDMD-independent mechanisms, urging further detailed investigation.
GSDMD-mediated pore formation, pyroptosis and inflammation have been implicated in different types of cells, such as immune cells, cardiovascular cells, epithelial cells, pancreatic β cells, and hepatocytes (Figure 4). Notably, GSDMD might elicit conflicting effects in different cell types, meaning that cell-specific therapies targeting GSDMD shall be taken into consideration in the context of different diseases.
Figure 4. Biological roles of GSDMD in different cell types. GSDMD plays mediatory roles in the functions of immune cells, cardiovascular cells, pancreatic β cells, hepatocytes and tumor cells. GSDMD can exert pleiotropic effects depending on cell types and stages.
GSDMD is actively involved in the mediation of pyroptosis in immune cells, particularly monocytes, macrophages, and neutrophils. GSDMD has been experimentally shown to modulate macrophage infiltration. Deletion of GSDMD remarkably retarded macrophage infiltration to the lesion sites in aortas during atherogenesis (39), hinting that GSDMD-mediated pyroptosis in aortic cells (e.g. resident immune cells and endothelial cells) might account for the release of chemokines. Besides, GSDMD is involved in the mediation of macrophage polarization towards M1 subtype during acute kidney injury (40). Whether GSDMD mediates macrophage polarization in a similar fashion in other tissues requires further investigation. Moreover, GSDMD is sensitive to mitochondrial ROS during mitochondrial dysfunction, where subsequent GSDMD oxidation represents a de novo mechanism driving macrophage pyroptosis (41). GSDMD-mediated pyroptosis and NETosis were found to coordinate antibacterial defense in macrophages (42). In addition to the pro-inflammatory cytokine IL-1β, GSDMD also mediates the secretion of fms-like tyrosine kinase 1 from macrophage/monocyte cell line THP-1, potentially contributory to preeclampsia pathology (43).
In a toll-like receptor (TLR)-dependent manner, GSDMD actively mediates the release of IL-1β from monocytes (44). Interestingly, monocytes can secrete extracellular vesicles to deliver active GSDMD to impact mesenchymal cell fate (45). As aforementioned, GSDMD is involved in the formation of NETs by neutrophils. Mechanistically, GSDMD can trigger mitochondrial pore formation in neutrophils, causing the leakage of mitochondrial DNA, which activates cGAS-STING signaling to stimulate NET formation (46). Additional to plasma membrane and mitochondria, GSDMD can migrate to azurophilic granules and autophagosomes of neutrophils to mediate IL-1β release (35). In aging neutrophils, cytoplasmic granules release neutrophil elastase, a neutrophil-specific serine protease, to cleave GSDMD at a distinct site for the induction of pore formation and death in neutrophils, resulting in anti-inflammatory effects (47). Meaningly, GSDMD can elicit pleiotropic effects (both pro- and anti-inflammatory effects), depending on the cleavage sites targeted by different proteases. Besides, GSDMD-mediated pyroptosis in macrophages facilities the secretion of microvesicles, encapsulating GSDMD-N-expressing mitochondria and mitochondrial ROS. These microvesicles in turn accelerate NET formation (48).
GSDMD, in a NLRP3/caspase-1-dependent manner, drives pyroptosis in endothelial cells. Histone deacetylase 11 promotes the NLRP3/caspase-1/GSDMD cascade by regulating E−26 transformation−specific−related gene (ERG) acetylation in human endothelial cells (49). Caspase-4/11-mediated GSDMD cleavage has been reported to contribute to pyroptosis, endothelial dysfunction, and subsequent pulmonary arterial hypertension (50). The longevity gene SIRT1 was found to be upstream to GSDMD-mediated pyroptosis in endothelial cells, where metformin treatment protects against LPS-induced endothelial pyroptosis and lung injury through SIRT1 upregulation (51). GSDMD was also shown to form mitochondrial pores in endothelial cells, causing the leakage of mitochondrial DNA, which activates cGAS Signaling and inhibits YAP-mediated endothelial regeneration (52). GSDMD has been implicated to modulate endothelial cell hyperactivation, a cell state facilitating the release of inflammatory factors. During vascular aging, protein kinase R activation promotes GSDMD cleavage, and hence endothelial pore formation and release of IL-1β and HMGB1, where these factors enhance phenotypic transformation of vascular smooth muscle cells (VSMCs) (53).
GSDMD-mediated pyroptosis in cardiomyocytes has been implicated in promoting myocardial ischemia/reperfusion (I/R) injury (54). GSDMD can also cause pore formation in cardiomyocyte mitochondria to mediate mitochondrial injury and mitophagy (55). During angiotensin II or pressure-overload-induced cardiac hypertrophy, GSDMD generates a positive feed-forward amplification loop through the mitochondria-STING axis. Briefly, GSDMD-N facilitates mitochondrial pore formation in cardiomyocytes, where the released mitochondrial DNA activates the STING/NLRP3/caspase-1 cascade to generate more GSDMD-N (56). Furthermore, GSDMD was found to form pores on ER membrane to activate ER stress by regulating the activity of FAM134B, an endoplasmic reticulum autophagy receptor, enhancing autophagy and apoptosis in doxorubicin-treated cardiomyocytes (57). The location of pore formation sites might be crucial for explaining the pleiotropic effects of GSDMD in different cell types.
MLKL-mediated necroptosis and GSDMD-mediated pyroptosis were observed in intestinal epithelial cells, where Fas-associated death domain (FADD), an adapter essential for caspase-8 activation, can inhibit both MLKL-mediated necroptosis and GSDMD-mediated pyroptosis to suppress intestinal inflammation (58). Besides, GSDMD plays a non-pyroptotic role by facilitating the secretion of IL-1β-encapsulating small extracellular vesicles from intestinal epithelial cells to mediate intestinal inflammation (59). Notably, GSDMD plays another non-pyroptotic role in intestinal epithelial cells by modulating mucus secretion in shaping intestinal homeostasis (60). Moreover, GSDMD modulates IL-33 secretion from mouse lung epithelial cells, where IL-33 plays a pivotal role in triggering airway inflammatory diseases (61). GSDMD is actively involved in mediating inflammation and epithelial-mesenchymal transition in pulmonary tissues (62). Recently, another less recognized N-terminal fragment of GSDMD (13 kD) has been identified, where such fragment results from caspase-3/7-mediated cleavage in intestinal epithelial cells upon exposure to dietary antigens. This 13-kD GSDMD fragment translocates into nucleus of intestinal epithelial cells to induce transcription of MHCII and CIITA for regulating immunity versus tolerance in small intestine (63). The presence of other less recognized GSDMD fragments and their potential biological functions require future extensive examination.
The NLRP3/GSDMD axis was shown to modulate pyroptosis and inflammation in pancreatic β cells during hyperglycemia, implying the role of GSDMD in promoting the progression of diabetes mellitus (64). Pharmacological inhibition on NLRP3/caspase 1/GSDMD cascade by empagliflozin was shown to be beneficial against pancreatic damage during diabetes (65). GSDMD-mediated pyroptosis plays pivotal roles in hepatic homeostasis. GSDMD-mediated pyroptosis negatively modulates hepatocyte growth that GSDMD knockout significantly enhanced liver regeneration after 70% partial hepatectomy in mice (66). Direct GSDMD inhibition by phenethyl isothiocyanate, a natural compound present in cruciferous vegetables, can attenuate concanavalin A-induced hepatocyte pyroptosis and acute liver injury in mice (67). Pharmacological scavenging of mitochondrial ROS by quercetin, a dietary phytochemical presented in various vegetables, suppresses GSDMD-mediated pyroptosis in cultured human hepatocytes (68). Cleaved GSDMD was implied to accumulate on ER, leading to calcium leakage to the cytoplasm and resultant secretion of HMGB1-containing extracellular vesicles from hepatocytes (69). Meanwhile, GSDMD-mediated pyroptosis has been implicated in numerous types of cancer but with conflicting results (both pro- and anti-carcinogenic) depending on tumor cells used (70). Future extensive studies are required to elucidate the comprehensive role of GSDMD in different cancers at different stages for the development of potent anti-cancer therapies.
GSDMD plays a vital role in the onset and progression of various inflammatory diseases, including but not limited to diabetes mellitus, liver diseases, cardiovascular diseases, neurodegenerative disorders, intestinal diseases and blood infection. Pathophysiologically, GSDMD mediates pyroptosis to aggravate the progression of different inflammatory diseases (Figure 5).
Figure 5. GSDMD-mediated pyroptosis and inflammatory diseases. Under the action of inflammatory caspases, GSDMD is cleaved into fragments containing NTD and CTD (GSDMD-N and GSDMD-C). GSDMD-N assembles to form permeability pores in plasma membrane to promote pyroptosis. GSDMD-mediated pyroptosis contributes to the onset and progression of different inflammatory diseases.
GSDMD is involved in the pyroptosis-related inflammasome pathway to cause damage in pancreatic tissues during diabetes mellitus (65), where injured and dysfunctional pancreatic β cells are responsible for insulin deficiency and hence diabetes progression. Growing evidence has highlighted the roles of inflammation-associated pyroptosis and GSDMD in the pathogenesis of diabetes complications (71). Previous research reported that expression of pyroptosis-related proteins, such as GSDMD-N, NLRP3 inflammasome, caspase-1 and IL-1β, were upregulated in the cardiac tissues of diabetic mice (72). Moreover, higher levels of pyroptotic indicators, including GSDMD-N, caspase-1, caspase-11 and IL-1β were noted in doxorubicin-treated H9c2 cardiomyoblasts (73). Wang et al. has also suggested that the cleavage of GSDMD by caspase-1 is downstream to hyperglycemia-induced oxidative stress in H9c2 cardiomyoblasts (74). GSDMD-mediated pyroptosis was found downstream to mitochondrial dysfunction and ROS in diabetic cardiomyopathy mice in a cGAS/STING-dependent manner (75), however, we cannot exclude the possibility that GSDMD might generate a feedforward loop by forming pores on mitochondria. These findings implied the potential role of GSDMD in diabetic cardiomyopathy.
Diabetic nephropathy is one of the detrimental complications of diabetes mellitus and is considered as a sterile inflammatory disorder. The expression of pyroptosis-associated proteins, particularly GSDMD, caspase-1 and NLRP3, were found remarkably elevated in the renal tissues of diabetic mice (76). Notably, podocyte loss contributes to the progression of diabetic nephropathy (77). The expression of pyroptosis-associated proteins, such as GSDMD-N and NLRP3, were also found significantly augmented in podocytes upon hyperglycemic insult (78). Importantly, knockout of GSDMD attenuated podocyte loss in HFD/STZ-induced diabetic mice (79), hinting a pathophysiological role of GSDMD in the progression of diabetic nephropathy. Diabetic retinopathy causes severe visual damage and accumulated blindness in patients with diabetes mellitus. In human retinal pericytes, treatment with advanced glycation end product modified bovine serum albumin triggered the cleavage of caspase-1 and GSDMD, release of pro-inflammatory cytokines like IL-1β and IL-18, diminished cell viability and thereby progression of diabetic retinopathy (80). Moreover, hyperglycemia activated the NLRP3/caspase-1/GSDMD axis to cause pore formation, where GSDMD further aggravated pyroptosis in human retinal pericytes (81). These findings indicated the harmful role of GSDMD in retinal health.
Non-alcoholic fatty liver disease (NAFLD) results from fat accumulation in liver, which predisposes the patients to non-alcoholic steatohepatitis (NASH), fibrosis, cirrhosis, liver failure and liver cancer. The pathophysiological process is accompanied by chronic inflammation in liver tissues. GSDMD plays a pivotal role in mediating pyroptosis during NAFLD progression. The caspase-11/GSDMD cascade was shown to promote hepatic macrophage pyroptosis and inflammation to aggravate NAFLD progression (82). Notably, GSDMD and GSDMD-N were upregulated in the liver of patients with NAFLD/NASH. Additionally, GSDMD-N level was positively correlated to fibrosis (83). These findings imply that GSDMD-N is a potential biomarker for NASH diagnosis. Mechanistically, GSDMD activity contributes to NASH progression by promoting pore formation, and the subsequent cytokine secretion from liver (e.g. IL-1β, TNF-α, and MCP-1), macrophage infiltration, and persistent activation of NF-κB signaling pathway (84). GSDMD-mediated pyroptosis is upstream to the inflammation and fibrosis in liver tissues during the development of NASH (85). In mice with acute liver failure, GSDMD knockout significantly alleviated inflammatory damage and improved the survival rate (86). Furthermore, GSDMD and GSDMD-N were found highly expressed in the liver from patients with hepatocellular carcinoma and metastatic hepatocellular carcinoma. RNA sequencing demonstrated that GSDMD knockout altered cyclic GMP-AMP synthase and immune-related pathways (87). These findings highlight the role of GSDMD in different stages of liver diseases.
GSDMD participates in the development of multiple cardiovascular diseases by mediating pyroptosis in different cellular components of the cardiovascular system. In cardiomyocytes, cleavage of GSDMD causes the secretion of the pro-inflammatory cytokine IL-18 and augmented pyroptosis to promote myocardial I/R injury (54). Myocardial infarction refers to the decrease or shortage of blood flow to a heart portion, resulting in necrosis of heart muscles. During myocardial infarction, upregulated CXCR4 activates the NF-κB/GSDMD axis to cause damage to cardiomyocytes and impair heart function (88). Jiang et al. showed that GSDMD is required for the recruitment of neutrophils/monocytes to the infarcted mouse hearts, and GSDMD knockout dramatically attenuated myocardial injury after acute myocardial infarction (89). Heart failure refers to a chronic condition where the heart muscles fail to pump enough oxygen-rich blood to body parts. Myocardial remodeling is one of the major lesions in later stage of chronic heart failure. Pharmacological inhibition of GSDMD by the anti-inflammatory drug oridonin has been shown to lower the degree of cardiac remodeling both in vitro and in vivo (90). Cardiac hypertrophy often precedes the onset of heart failure. Pharmacological inhibition of GSDMD and GSDMD-mediated inflammation by DL-3-n-butylphthalide suppressed cardiac hypertrophy in the mouse model of transverse aortic constriction (91).
Atherosclerosis refers to the accumulation of lipid on artery walls and the chronic inflammation of arteries, underpinning ischemic heart disease and stroke (92). GSDMD knockout remarkably limited the development of atherosclerotic lesions in mice subjected to genetic inhibition of low density lipoprotein receptor (LDLR) (93). Recently, Puylaert et al. provided clues that GSDMD knockout did not alter the initiation of plaque formation in ApoE-/- mice (94). Instead, GSDMD knockout could facilitate a switch from pyroptosis to apoptosis in macrophages, hence restraining the transition of atherosclerotic plaques to a vulnerable and inflammatory phenotype (94). Moreover, GSDMD was shown to disrupt cholesterol efflux from macrophages, and thereby promoting foam cell formation and atherogenesis (93). Dysfunction of endothelial cells initiates atherogenesis, where GSDMD-mediated pyroptosis promotes endothelial dysfunction (95). In endothelial cells, TNF-α treatment upregulated the expression of histone deacetylase 11 (HDAC11), which subsequently ignited the NLRP3/caspase-1/GSDMD signaling cascade to mediate pyroptosis and inflammation (49). Cell deaths in the vasculature, particularly the VSMCs, promote atherosclerotic progression. In VSMCs, GSDMD has been found to mediate pyroptosis and inflammation in a melanoma 2-dependent manner (96). These findings indicate the contributory roles of GSDMD in different cellular components of cardiovascular system during atherosclerotic progression.
Substantial amount of evidence has identified the tight connection between pyroptosis and the development of neurodegenerative diseases, such as Alzheimer’s and Parkinson’s diseases (97). In the cerebrospinal fluids from patients with Alzheimer’s disease, the levels of GSDMD and T-Tau were significantly higher than those from healthy controls (98). One of the pathological features of Alzheimer’s disease is the formation of senile plaque due to the deposition of β‐amyloid in extracellular space. β‐amyloid has been demonstrated to induce pyroptosis in mouse cortical neurons, in which the expression levels of pyroptotic proteins like GSDMD, GSDMD-N, NLRP3 inflammasome and caspase-1 were upregulated (99). β‐amyloid-induced oxidative stress was found upstream to GSDMD-mediated pyroptosis in neuronal cells (100). Sevoflurane, the frequently used anesthetic, could activate the NLRP3/caspase-1/GSDMD cascade, and increase tau phosphorylation and β‐amyloid deposition, hence promoting the progression of Alzheimer’s disease (101). Like Alzheimer’s disease, GSDMD plays a pathological role in Parkinson’s disease. NLRP3/caspase-1/GSDMD pathway was shown to contribute to neuroinflammation in mouse model of Parkinson’s disease induced by N-methyl-4-phenyl-1,2,3,6-tetrahydropyridine (MPTP) (102). In a TLR4-dependent manner, MPTP activated NLRP3/caspase-1/GSDMD cascade in mouse model of Parkinson’s disease (103). In patients with Parkinson’s disease, GSDMD and GSDMD-N were found present in the extracellular vesicles isolated from plasma (104). These recent findings suggest the role of GSDMD in neurodegenerative diseases.
In patients with IBD and experimental colitis, GSDMD is highly expressed in intestinal epithelial cells. Notably, GSDMD was shown to guide the release of IL-1β-containing extracellular vesicles from intestinal epithelial cells, hinting the pathological role of GSDMD in intestinal inflammation (59). Dysregulation in microbiome, particularly commensal Escherichia coli, caused GSDMD activation to exacerbate colitis development by boosting IL-18 release from colon (105). GSDMD-mediated macrophage pyroptosis aggravates experimental colitis in mice (106). However, Ma et al. provided clues to suggest that GSDMD acts as a negative regulator of cyclic GMP–AMP synthase-dependent inflammation in macrophages, therefore conferring protective effect against colitis (107). Further study shall clarify the differential roles of GSDMD in different cell types. Sepsis refers to the severe organ dysfunction triggered by a dysregulated host response to infection, and a multifaceted disruption of the immunological balance between inflammation and anti-inflammation (108). GSDMD activity was observed in the neutrophils isolated from septic humans and mice. During sepsis, caspase-11/GSDMD pathway was activated to control the release of neutrophil extracellular traps by neutrophils (109), implying GSDMD as a therapeutic target against sepsis.
Various clinical studies have correlated GSDMD level to severity and progression of different diseases. Clinically, fluctuation in GSDMD levels might potentially be diagnostic and prognostic factors in inflammatory diseases and events (Table 1).
In patients exhibiting myocardial I/R injury, elevated serum levels of GSDMD were noted (54), implying GSDMD to be a potential biomarker and therapeutic target for the evaluation and treatment of myocardial I/R injury. Adult-onset Still’s disease (AOSD) refers to the rare systemic autoinflammatory disease, where the patients experience fevers, joint pain, and salmon-colored bumpy rash (110). In patients with AOSD, increased serum levels of GSDMD-N and IL-18 were detected, highlighting inflammasome activation and GSDMD-mediated pyroptosis in monocytes and macrophages (111). Anti-N-methyl-D-aspartate receptor (NMDAR) encephalitis refers to the rare autoimmune disorder in central nervous system, characterized by epilepsy, movement disorders, psychobehavioral changes and cognitive decline (112). Importantly, serum levels of GSDMD were remarkably higher in patients with anti-NMDAR encephalitis than those in healthy controls (113). A further 3-month follow-up evaluation revealed that serum GSDMD levels in patients with anti-NMDAR encephalitis decreased significantly post-treatment (113), implying that GSDMD might be a potential prognostic marker. In patients with Parkinson’s disease, GSDMD was also present in the extracellular vesicles isolated from plasma (104).
GSDMD levels in other tissues and body fluids might also serve as diagnostic and prognostic indicators. In patients with NAFLD/NASH, the levels of GSDMD and GSDMD-N were elevated in the liver tissues. Additionally, hepatic GSDMD-N levels were positively correlated to NAFLD activity score and fibrosis (83). Histological examination of GSDMD level in liver tissues might facilitate the severity assessment of liver diseases. Notably, in the cerebrospinal fluids from patients with Alzheimer’s disease, the levels of GSDMD was remarkably higher than those from healthy controls, implying that GSDMD might be a diagnostic biomarker for Alzheimer’s disease (98). As mentioned, GSDMD is upregulated in intestinal epithelial cells in patients with IBD and experimental colitis (59). In addition, GSDMD can be the in vivo clue indicating pyroptosis in spontaneous labor at term. In women who underwent spontaneous labor at term, GSDMD levels in the amniotic fluid and chorioamniotic membranes were higher than those without labor (114). Such finding might suggest that inflammasome-induced pyroptosis is involved in the physiological process of labor at term. Further study might uncover whether fluctuated GSDMD levels in amniotic fluid and chorioamniotic membranes are correlated to preterm labor and birth.
GSDMD inhibition shall be an attractive therapeutic strategy to counteract inflammation and hence inflammatory diseases. In recent years, a number of GSDMD inhibitors have been identified to restrain GSDMD-mediated pyroptosis through different mechanisms (Table 2).
Necrosulfonamide (NSA) is an inhibitor of necroptosis. NSA specifically blocks mixed-lineage kinase domain-like protein, which is the critical signaling molecule of necroptosis (115). Until recently, NSA has also been considered as a GSDMD inhibitor and NSA can potentially inhibit the oligomerization of GSDMD-N by docking to C191 amino acid (116). Bay 11-7082, a NF-κB inhibitor, and disulfiram, a drug commonly used against alcohol addiction, were found to be potential GSDMD inhibitors (16, 117). Similar to NSA, Bay 11-7082 and disulfiram also function as inhibitors of GSDMD oligomerization by binding to C191/192 residue (117, 118). More importantly, disulfiram covalently modifies C191/192 residue of GSDMD in both human and mice to block pore formation, hence limiting IL-1β release (117). However, Bay 11-7082 and disulfiram might target other pyroptosis-related proteins, implying the lack of specificity (119). Further efforts are needed to optimize the specificity of these two drugs before in vivo application.
LDC7559 is an inhibitor of neutrophil extracellular traps. In a large screening of library covering over 182,000 compounds, LDC7559 was identified to bind to GSDMD hindering NETosis (32). Mechanistically, LDC7559 can abrogate the toxicity of GSDMD-N in both human and mice, demonstrating a direct inhibitory effect of LDC7559 on GSDMD activity (32). Dimethyl fumarate (DMF), a Nrf2 activator used to treat multiple sclerosis, is also a potent GSDMD inhibitor. Humphries et al. provided evidence that DMF causes GSDMD succination by reacting with GASDMD at critical cysteine residues. Importantly, GSDMD succination hinders the interaction between GSDMD and caspases, restraining the oligomerization and pyroptosis-inducing capacity of GSDMD (120). In 2023, a new GSDMD inhibitor GI-Y1 identified by virtual and pharmacological screening was shown to inhibit GSDMD-mediated lipid-binding, pore formation and mitochondrial binding by targeting Arg7 residue of GSDMD-N in cardiomyocytes, implying the potentially cardioprotective effect of GI-Y1 against myocardial I/R injury (121).
Punicalagin, a phenolic compound reported to elicit antioxidant and anti-inflammatory effects, is another potent GSDMD inhibitor that prevents the insertion of GSDMD-N into plasma membrane of macrophages through its antioxidant effect on reactive thiols. Coherently, IL-1β release from macrophages were also decreased (122). However, whether punicalagin inhibits the cleavage of GSDMD, or elicits its effect independently of GSDMD, requires further study. Ac-FLTD-CMK, a selective inhibitor of caspases, has been suggested to be another potent GSDMD inhibitor. In macrophages, treatment with Ac-FLTD-CMK inhibited GSDMD cleavage, IL-1β release and pyroptosis (123). Nevertheless, whether these potent GSDMD inhibitors target pyroptosis in other non-immune cells to retard disease progression requires future extensive study. Further investigations with the aid of virtual and pharmacological screening tools shall facilitate the identification of more potent GSDMD inhibitors.
Certain studies have evaluated the potential of some therapeutic agents against GSDMD-mediated pyroptosis in different disease models. During progression of alcoholic steatohepatitis, GSDMD pore formation is critical to the excessive release of IL-1β from ethanol or acetaldehyde-stimulated macrophages (124). Luan et al. developed a hepatocyte-specific nanobiologics for prolonged expression of Interleukin-1 receptor antagonist and IL-1β blockade in liver (124). In other words, such nanobiologics counteracted GSDMD-mediated release of pro-inflammatory cytokine IL-1β to retard progression of alcoholic steatohepatitis. In addition, in mice with acute liver injury, 3-day administration of phenethyl isothiocyanate (PEITC), a natural compound found in cruciferous vegetables, alleviated hepatic pyroptosis and liver injury (67). Meanwhile, ellagic acid, a polyphenolic compound present in many fruits, was shown to protect mice against hepatic ischemia–reperfusion injury by targeting caspase-1/GSDMD pathway (125).
GSDMD can accumulate in ER to cause ER stress in pancreatic acinar cells. A recent study showed that diosgenin derivative D (Drug D) attenuated pancreatic inflammation by limiting GSDMD accumulation in ER of acinar cells (126). Furthermore, in vivo and in vitro studies showed that the anti-inflammatory drug oridonin can attenuate cardiac remodeling by suppressing GSDMD-mediated pyroptosis and inflammation in cardiomyocytes (90). In septic mice, administration of fudosteine, a cysteine derivative, could suppress pyroptosis by targeting the TXNIP/NLRP3/GSDMD signaling cascade (127). Helicobacter pylori (H. pylori) infection is closely related to the development of neuroinflammation and the progression of depression. Rapamycin treatment significantly lowered GSDMD expression in hippocampus and inhibited depression-like behavior in H. pylori-infected mice (128). However, further extensive clinical studies are urged to evaluate the safety and efficacy of these therapeutic agents in human patients. of virtual and pharmacological screening tools shall facilitate the identification of more potent GSDMD inhibitors.
GSDMD plays a critical role in the initiation and progression of various inflammatory diseases. GSDMD mediates pyroptosis and inflammation in multiple cell types during disease progression. Certain clinical studies have suggested GSDMD to be potential diagnostic and prognostic marker in different diseases. Certain preclinical studies have identified potential GSDMD inhibitors and other therapeutic agents to counteract pyroptosis and inflammation mediated by GSDMD.
However, a number of questions remain to be addressed in future investigation. Of note, certain GSDMD inhibitors, like NSA, Bay 11-7082 and disulfiram, target the C191/192 residue to prevent GSDMD oligomerization and pore formation, thereby limiting pyroptosis and inflammation. The exact role of the C191/192 residue of GSDMD requires further investigation. In addition, many preclinical and clinical studies are focusing on the pathophysiological role of GSDMD in immune cells, such as monocytes and macrophages. Future studies shall investigate the role of GSDMD in other non-immune cells in the context of different diseases. Serum GSDMD levels increase during myocardial I/R injury. It would be interesting to clinically correlate the elevation in serum GSDMD level with the progression of other atherosclerotic cardiovascular diseases, since GSDMD has been shown to contribute to atherosclerotic plaque formation.
Furthermore, the comprehensive role of GSDMD in the crosstalk between different types of cell death requires further extensive investigation. Different proteases cleave GSDMD at distinct sites, so future identification of the collection of GSDMD fragments and their biological functions shall benefit our understanding towards the mechanism of inflammatory diseases, and the development of more anti-inflammatory therapies. The sites of pore formation by GSDMD are also important information for understanding the mechanism of inflammatory diseases. Strategies that can reduce pore formation on membranous structures, including plasma and nuclear membranes, ER and mitochondria, or can shift the sites of perforation by GSDMD may provide new therapeutic opportunities. Identification of additional tissues and cells that could release GSDMD-containing extracellular vesicles shall broaden our understanding on the role of GSDMD in cell-cell communication during disease progression. GSDMD-mediated pyroptosis elicits pleiotropic roles in normal cells (both pro- and anti-inflammatory) and tumor cells (both pro- and anti-carcinogenic). Efforts are needed to develop specific GSDMD modulators to exert beneficial and protective effects on desired cells (Figure 6).
Figure 6. Future perspectives on GSDMD research. (A) The role of GSDMD in the crosstalk between different types of cell death requires further study. (B) Identification of other GSDMD fragments and their associated functions requires further efforts. (C) The comprehensive consequences downstream to GSDMD-mediated pore formation on recognized and other membranous organelles/structures need further investigation. (D) Potential cell sources and target cells of GSDMD-encapsulating extracellular vesicles remain largely unexplored. (E) The pleiotropic effects of GSDMD in different cell types at different cell states remain elusive.
Collectively, further efforts are still required to enhance our understanding towards the detailed mechanistic network of GSDMD-mediated pyroptosis and inflammation, and to narrow the gap between preclinical and clinical studies.
CC: Conceptualization, Visualization, Writing – original draft, Writing – review & editing. MY: Conceptualization, Writing – original draft. LW: Funding acquisition, Writing – review & editing. YH: Funding acquisition, Project administration, Resources, Supervision, Writing – review & editing.
The author(s) declare financial support was received for the research, authorship, and/or publication of this article. This work was supported by Hong Kong Research Grants Council (1103222, SRFS2021-4S04, T12-101/23-N, 14109720), and the City University of Hong Kong Start-up Fund. This work was also substantially supported by a fellowship award from the Research Grants Council of the Hong Kong Special Administrative Region, China (Project No. CityU PDFS2223-1S01).
We thank members of the Y.H. group for constructive discussion. Predicted protein structures were generated by AlphaFold 3. Some figure panels were created with BioRender.com.
The authors declare that the research was conducted in the absence of any commercial or financial relationships that could be construed as a potential conflict of interest.
All claims expressed in this article are solely those of the authors and do not necessarily represent those of their affiliated organizations, or those of the publisher, the editors and the reviewers. Any product that may be evaluated in this article, or claim that may be made by its manufacturer, is not guaranteed or endorsed by the publisher.
AOSD, Adult-onset Still’s disease; ASC, Apoptosis-associated speck-like protein; CTD, C-terminal domain; DAMP, Damage-associated molecular pattern; DMF, Dimethyl fumarate; Drug D, Diosgenin derivative D; ER, Endoplasmic reticulum; ERG, E−26 transformation−specific−related gene; FADD, Fas-associated death domain; GSDM, Gasdermin; GSDMD, Gasdermin D; GSDMD-C, CTD-containing GSDMD fragment; GSDMD-N, NTD-containing GSDMD fragment; HDAC11, Histone deacetylase 11; H. pylori, Helicobacter pylori; IBD, Inflammatory bowel disease; I/R, Ischemia/reperfusion; LDLR, Low density lipoprotein receptor; LPS, Lipopolysaccharides; MLKL, Mixed lineage kinase domain like pseudokinase; Mpro, Main protease; MPTP, N-methyl-4-phenyl-1,2,3,6-tetrahydropyridine; NAFLD, Non-alcoholic fatty liver disease; NASH, Non-alcoholic steatohepatitis; NET, Neutrophil extracellular trap; NLRP3, NOD-like receptor family pyrin domain containing 3; NMDAR, N-methyl-D-aspartate receptor; NSA, Necrosulfonamide; NSP, Neutrophil serine protease; NTD, N-terminal domain; PAMP, Pathogen-associated molecular pattern; PEITC, Phenethyl isothiocyanate; PLpro, Papain-like protease; PRR, Pattern recognition receptor; RIPK3, Receptor-interacting protein kinase 3; TLR, Toll-like receptor; TLR4, Toll-like receptor 4; VSMC, Vascular smooth muscle cell.
1. Fukuda D, Sata M. Frontiers of inflammatory disease research: inflammation in cardiovascular–cerebral diseases. Inflammation Regener. (2021) 41:10. doi: 10.1186/s41232-021-00160-z
2. Furman D, Campisi J, Verdin E, Carrera-Bastos P, Targ S, Franceschi C, et al. Chronic inflammation in the etiology of disease across the life span. Nat Med. (2019) 25:1822–32. doi: 10.1038/s41591-019-0675-0
3. Privitera G, Rana N, Armuzzi A, Pizarro TT. The gasdermin protein family: emerging roles in gastrointestinal health and disease. Nat Rev Gastroenterol Hepatol. (2023) 20:366–87. doi: 10.1038/s41575-023-00743-w
4. Wang C, Shivcharan S, Tian T, Wright S, Ma D, Chang JY, et al. Structural basis for GSDMB pore formation and its targeting by IpaH7.8. Nature. (2023) 616:590–7. doi: 10.1038/s41586-023-05832-z
5. Devant P, Kagan JC. Molecular mechanisms of gasdermin D pore-forming activity. Nat Immunol. (2023) 24:1064–75. doi: 10.1038/s41590-023-01526-w
6. Liu Z, Wang C, Yang J, Zhou B, Yang R, Ramachandran R, et al. Crystal structures of the full-length murine and human gasdermin D reveal mechanisms of autoinhibition, lipid binding, and oligomerization. Immunity. (2019) 51:43–49.e4. doi: 10.1016/j.immuni.2019.04.017
7. Dai Z, Liu WC, Chen XY, Wang X, Li JL, Zhang X. Gasdermin D-mediated pyroptosis: mechanisms, diseases, and inhibitors. Front Immunol. (2023) 14:1178662. doi: 10.3389/fimmu.2023.1178662
8. Rao Z, Zhu Y, Yang P, Chen Z, Xia Y, Qiao C, et al. Pyroptosis in inflammatory diseases and cancer. Theranostics. (2022) 12:4310–29. doi: 10.7150/THNO.71086
9. Li Z, Ji S, Jiang ML, Xu Y, Zhang CJ. The regulation and modification of GSDMD signaling in diseases. Front Immunol. (2022) 13:893912. doi: 10.3389/fimmu.2022.893912
10. Sborgi L, Rühl S, Mulvihill E, Pipercevic J, Heilig R, Stahlberg H, et al. GSDMD membrane pore formation constitutes the mechanism of pyroptotic cell death. EMBO J. (2016) 35:1766–78. doi: 10.15252/embj.201694696
11. Kayagaki N, Stowe IB, Lee BL, O’Rourke K, Anderson K, Warming S, et al. Caspase-11 cleaves gasdermin D for non-canonical inflammasome signalling. Nature. (2015) 526:666–71. doi: 10.1038/nature15541
12. Wright SS, Vasudevan SO, Rathinam VA. Mechanisms and consequences of noncanonical inflammasome-mediated pyroptosis. J Mol Biol. (2022) 434:167245. doi: 10.1016/j.jmb.2021.167245
13. Miao N, Wang Z, Wang Q, Xie H, Yang N, Wang Y, et al. Oxidized mitochondrial DNA induces gasdermin D oligomerization in systemic lupus erythematosus. Nat Commun. (2023) 14:872. doi: 10.1038/s41467-023-36522-z
14. Paik S, Kim JK, Silwal P, Sasakawa C, Jo EK. An update on the regulatory mechanisms of NLRP3 inflammasome activation. Cell Mol Immunol. (2021) 18:1141–60. doi: 10.1038/s41423-021-00670-3
15. Fujimura K, Karasawa T, Komada T, Yamada N, Mizushina Y, Baatarjav C, et al. NLRP3 inflammasome-driven IL-1β and IL-18 contribute to lipopolysaccharide-induced septic cardiomyopathy. J Mol Cell Cardiol. (2023) 180:58–68. doi: 10.1016/j.yjmcc.2023.05.003
16. Hu R, Liang J, Ding L, Zhang W, Wang Y, Zhang Y, et al. Gasdermin D inhibition ameliorates neutrophil mediated brain damage in acute ischemic stroke. Cell Death Discovery. (2023) 9:50. doi: 10.1038/s41420-023-01349-6
17. Burgener SS, Leborgne NGF, Snipas SJ, Salvesen GS, Bird PI, Benarafa C. Cathepsin G inhibition by serpinb1 and serpinb6 prevents programmed necrosis in neutrophils and monocytes and reduces GSDMD-driven inflammation. Cell Rep. (2019) 27:3646–3656.e5. doi: 10.1016/j.celrep.2019.05.065
18. Shi F, Lv Q, Wang T, Xu J, Xu W, Shi Y, et al. Coronaviruses nsp5 antagonizes porcine gasdermin D-mediated pyroptosis by cleaving pore-forming p30 fragment. mBio. (2022) 13:e0273921. doi: 10.1128/mbio.02739-21
19. Tsuchiya K. Switching from apoptosis to pyroptosis: Gasdermin-elicited inflammation and antitumor immunity. Int J Mol Sci. (2021) 22:426. doi: 10.3390/ijms22010426
20. Tsuchiya K, Nakajima S, Hosojima S, Thi Nguyen D, Hattori T, Manh Le T, et al. Caspase-1 initiates apoptosis in the absence of gasdermin D. Nat Commun. (2019) 10:2091. doi: 10.1038/s41467-019-09753-2
21. de Vasconcelos NM, Van Opdenbosch N, Van Gorp H, Martín-Pérez R, Zecchin A, Vandenabeele P, et al. An apoptotic caspase network safeguards cell death induction in pyroptotic macrophages. Cell Rep. (2020) 32:107959. doi: 10.1016/j.celrep.2020.107959
22. Gao J, Qiu X, Xi G, Liu H, Zhang F, Lv T, et al. Downregulation of GSDMD attenuates tumor proliferation via the intrinsic mitochondrial apoptotic pathway and inhibition of EGFR/Akt signaling and predicts a good prognosis in non-small cell lung cancer. Oncol Rep. (2018) 40:1971–84. doi: 10.3892/or.2018.6634
23. Yuan S, Wang Y, Li Z, Chen X, Song P, Chen A, et al. Gasdermin D is involved in switching from apoptosis to pyroptosis in TLR4-mediated renal tubular epithelial cells injury in diabetic kidney disease. Arch Biochem Biophys. (2022) 727:109347. doi: 10.1016/j.abb.2022.109347
24. Yang C, Sun P, Deng M, Loughran P, Li W, Yi Z, et al. Gasdermin D protects against noninfectious liver injury by regulating apoptosis and necroptosis. Cell Death Dis. (2019) 10:481. doi: 10.1038/s41419-019-1719-6
25. Zhang Q, Huang Z, Rui X, Wang Y, Wang Y, Zhou Y, et al. GSDMD enhances cisplatin-induced apoptosis by promoting the phosphorylation of eIF2α and activating the ER-stress response. Cell Death Discovery. (2022) 8:114. doi: 10.1038/s41420-022-00915-8
26. Wang C, Wang L, Huang C, Liu Y, Liu J, Kuang H, et al. Involvement of NLRP3/Caspase-1/GSDMD-Dependent pyroptosis in BPA-Induced apoptosis of human neuroblastoma cells. Biochem Pharmacol. (2022) 200:115042. doi: 10.1016/j.bcp.2022.115042
27. Dhuriya YK, Sharma D. Necroptosis: A regulated inflammatory mode of cell death. J Neuroinflamm. (2018) 15:199. doi: 10.1186/s12974-018-1235-0
28. Weber K, Roelandt R, Bruggeman I, Estornes Y, Vandenabeele P. Nuclear RIPK3 and MLKL contribute to cytosolic necrosome formation and necroptosis. Commun Biol. (2018) 1:6. doi: 10.1038/s42003-017-0007-1
29. Weindel CG, Martinez EL, Zhao X, Mabry CJ, Bell SL, Vail KJ, et al. Mitochondrial ROS promotes susceptibility to infection via gasdermin D-mediated necroptosis. Cell. (2022) 185:3214–3231.e23. doi: 10.1016/j.cell.2022.06.038
30. Yang W, Tao K, Wang Y, Huang Y, Duan C, Wang T, et al. Necrosulfonamide ameliorates intestinal inflammation via inhibiting GSDMD-medicated pyroptosis and MLKL-mediated necroptosis. Biochem Pharmacol. (2022) 206:115338. doi: 10.1016/j.bcp.2022.115338
31. Rosazza T, Warner J, Sollberger G. NET formation – mechanisms and how they relate to other cell death pathways. FEBS J. (2021) 288:3334–50. doi: 10.1111/febs.15589
32. Sollberger G, Choidas A, Burn GL, Habenberger P, Lucrezia RD, Kordes S, et al. Gasdermin D plays a vital role in the generation of neutrophil extracellular traps. Sci Immunol. (2018) 3:eaar6689. doi: 10.1126/sciimmunol.aar6689
33. Xie J, Zhu CL, Wan XJ, Zhao ZZ, Meng Y, Li P, et al. GSDMD-mediated NETosis promotes the development of acute respiratory distress syndrome. Eur J Immunol. (2023) 53:e2250011. doi: 10.1002/eji.202250011
34. Wang Y, Li Y, Chen Z, Yuan Y, Su Q, Ye K, et al. GSDMD-dependent neutrophil extracellular traps promote macrophage-to-myofibroblast transition and renal fibrosis in obstructive nephropathy. Cell Death Dis. (2022) 13:693. doi: 10.1038/s41419-022-05138-4
35. Karmakar M, Minns M, Greenberg EN, Diaz-Aponte J, Pestonjamasp K, Johnson JL, et al. N-GSDMD trafficking to neutrophil organelles facilitates IL-1β release independently of plasma membrane pores and pyroptosis. Nat Commun. (2020) 11:2212. doi: 10.1038/s41467-020-16043-9
36. Tang L, Lu C, Zheng G, Burgering BM. Emerging insights on the role of gasdermins in infection and inflammatory diseases. Clin Transl Immunol. (2020) 9:e1186. doi: 10.1002/cti2.1186
37. Chauhan D, Demon D, Vande Walle L, Paerewijck O, Zecchin A, Bosseler L, et al. GSDMD drives canonical inflammasome-induced neutrophil pyroptosis and is dispensable for NETosis. EMBO Rep. (2022) 23:e54277. doi: 10.15252/embr.202154277
38. Stojkov D, Claus MJ, Kozlowski E, Oberson K, Schären OP, Benarafa C, et al. NET formation is independent of gasdermin D and pyroptotic cell death. Sci Signal. (2023) 16:eabm0517. doi: 10.1126/scisignal.abm0517
39. Huang B, Zou Z, Li Y, Chen H, Lai K, Yuan Y, et al. Gasdermin D-mediated pyroptosis promotes the development of atherosclerosis. Lab Invest. (2024) 104:100337. doi: 10.1016/j.labinv.2024.100337
40. Li N, Chen J, Geng C, Wang X, Wang Y, Sun N, et al. Myoglobin promotes macrophage polarization to M1 type and pyroptosis via the RIG-I/Caspase1/GSDMD signaling pathway in CS-AKI. Cell Death Discovery. (2022) 8:90. doi: 10.1038/s41420-022-00894-w
41. Wang Y, Shi P, Chen Q, Huang Z, Zou D, Zhang J, et al. Mitochondrial ROS promote macrophage pyroptosis by inducing GSDMD oxidation. J Mol Cell Biol. (2019) 11:1069–82. doi: 10.1093/jmcb/mjz020
42. Zhou L, Li Y, You J, Wu C, Zuo L, Chen Y, et al. Salmonella spvC gene suppresses macrophage/neutrophil antibacterial defense mediated by gasdermin D. Inflammation Res. (2024) 73:19–33. doi: 10.1007/s00011-023-01818-9
43. Tanaka H, Ozawa R, Henmi Y, Hosoda M, Karasawa T, Takahashi M, et al. Gasdermin D regulates soluble fms-like tyrosine kinase 1 release in macrophages. Reprod Biol. (2024) 24:100857. doi: 10.1016/j.repbio.2024.100857
44. Unterberger S, Mullen L, Flint MS, Sacre S. Multiple TLRs elicit alternative NLRP3 inflammasome activation in primary human monocytes independent of RIPK1 kinase activity. Front Immunol. (2023) 14:1092799. doi: 10.3389/fimmu.2023.1092799
45. Sarkar A, Das S, Bone H, DeVengencie I, Prasad J, Farkas D, et al. Regulation of mesenchymal cell fate by transfer of active gasdermin-D via monocyte-derived extracellular vesicles. J Immunol. (2023) 210:832–41. doi: 10.4049/jimmunol.2200511
46. Zhao C, Liang F, Ye M, Wu S, Qin Y, Zhao L, et al. GSDMD promotes neutrophil extracellular traps via mtDNA-cGAS-STING pathway during lung ischemia/reperfusion. Cell Death Discovery. (2023) 9:368. doi: 10.1038/s41420-023-01663-z
47. Kambara H, Liu F, Zhang X, Liu P, Bajrami B, Teng Y, et al. Gasdermin D exerts anti-inflammatory effects by promoting neutrophil death. Cell Rep. (2018) 22:2924–36. doi: 10.1016/j.celrep.2018.02.067
48. Kuang L, Wu Y, Shu J, Yang J, Zhou H, Huang X. Pyroptotic Macrophage-Derived Microvesicles Accelerate Formation of Neutrophil Extracellular Traps via GSDMD-N-expressing Mitochondrial Transfer during Sepsis. Int J Biol Sci. (2024) 20:733–50. doi: 10.7150/ijbs.87646
49. Yao F, Jin Z, Zheng Z, Lv X, Ren L, Yang J, et al. HDAC11 promotes both NLRP3/caspase-1/GSDMD and caspase-3/GSDME pathways causing pyroptosis via ERG in vascular endothelial cells. Cell Death Discovery. (2022) 8:112. doi: 10.1038/s41420-022-00906-9
50. Wu Y, Pan B, Zhang Z, Li X, Leng Y, Ji Y, et al. Caspase-4/11-mediated pulmonary artery endothelial cell pyroptosis contributes to pulmonary arterial hypertension. Hypertension. (2022) 79:536–48. doi: 10.1161/HYPERTENSIONAHA.121.17868
51. Zhang Y, Zhang H, Li S, Huang K, Jiang L, Wang Y. Metformin alleviates LPS-induced acute lung injury by regulating the SIRT1/NF-κB/NLRP3 pathway and inhibiting endothelial cell pyroptosis. Front Pharmacol. (2022) 13:801337. doi: 10.3389/fphar.2022.801337
52. Huang LS, Hong Z, Wu W, Xiong S, Zhong M, Gao X, et al. mtDNA activates cGAS signaling and suppresses the YAP-mediated endothelial cell proliferation program to promote inflammatory injury. Immunity. (2020) 52:475–486.e5. doi: 10.1016/j.immuni.2020.02.002
53. Peng Z, Tan X, Xie L, Li Z, Zhou S, Li Y. PKR deficiency delays vascular aging via inhibiting GSDMD-mediated endothelial cell hyperactivation. iScience. (2023) 26:105909. doi: 10.1016/j.isci.2022.105909
54. Shi H, Gao Y, Dong Z, Yang J, Gao R, Li X, et al. GSDMD-mediated cardiomyocyte pyroptosis promotes myocardial I/R injury. Circ Res. (2021) 129:383–96. doi: 10.1161/CIRCRESAHA.120.318629
55. Yu Z, Xiao Z, Guan L, Bao P, Yu Y, Liang Y, et al. Translocation of gasdermin D induced mitochondrial injury and mitophagy mediated quality control in lipopolysaccharide related cardiomyocyte injury. Clin Transl Med. (2022) 12:e1002. doi: 10.1002/ctm2.1002
56. Han J, Dai S, Zhong L, Shi X, Fan X, Zhong X, et al. GSDMD (Gasdermin D) mediates pathological cardiac hypertrophy and generates a feed-forward amplification cascade via mitochondria-STING (Stimulator of interferon genes) axis. Hypertension. (2022) 79:2505–18. doi: 10.1161/HYPERTENSIONAHA.122.20004
57. Qu Y, Gao R, Wei X, Sun X, Yang K, Shi H, et al. Gasdermin D mediates endoplasmic reticulum stress via FAM134B to regulate cardiomyocyte autophagy and apoptosis in doxorubicin-induced cardiotoxicity. Cell Death Dis. (2022) 13:901. doi: 10.1038/s41419-022-05333-3
58. Schwarzer R, Jiao H, Wachsmuth L, Tresch A, Pasparakis M. FADD and caspase-8 regulate gut homeostasis and inflammation by controlling MLKL- and GSDMD-mediated death of intestinal epithelial cells. Immunity. (2020) 52:978–993.e6. doi: 10.1016/j.immuni.2020.04.002
59. Bulek K, Zhao J, Liao Y, Rana N, Corridoni D, Antanaviciute A, et al. Epithelial-derived gasdermin D mediates nonlytic IL-1β release during experimental colitis. J Clin Invest. (2020) 130:4218–34. doi: 10.1172/JCI138103
60. Zhang J, Yu Q, Jiang D, Yu K, Yu W, Chi Z, et al. Epithelial Gasdermin D shapes the host-microbial interface by driving mucus layer formation. Sci Immunol. (2022) 7:eabk2092. doi: 10.1126/sciimmunol.abk2092
61. Chen W, Chen S, Yan C, Zhang Y, Zhang R, Chen M, et al. Allergen protease-activated stress granule assembly and gasdermin D fragmentation control interleukin-33 secretion. Nat Immunol. (2022) 23:1021–30. doi: 10.1038/s41590-022-01255-6
62. Peng L, Wen L, Shi Q-F, Gao F, Huang B, Meng J, et al. Scutellarin ameliorates pulmonary fibrosis through inhibiting NF-κB/NLRP3-mediated epithelial-mesenchymal transition and inflammation. Cell Death Dis. (2020) 11:978. doi: 10.1038/s41419-020-03178-2
63. He K, Wan T, Wang D, Hu J, Zhou T, Tao W, et al. Gasdermin D licenses MHCII induction to maintain food tolerance in small intestine. Cell. (2023) 186:3033–3048.e20. doi: 10.1016/j.cell.2023.05.027
64. Xu W, Wang H, Sun Q, Hua T, Bai J, Zhang Q, et al. TXNIP-NLRP3-GSDMD axis-mediated inflammation and pyroptosis of islet β-cells is involved in cigarette smoke-induced hyperglycemia, which is alleviated by andrographolide. Environ Toxicol. (2024) 39:1415–28. doi: 10.1002/tox.24046
65. Liu P, Zhang Z, Wang J, Zhang X, Yu X, Li Y. Empagliflozin protects diabetic pancreatic tissue from damage by inhibiting the activation of the NLRP3/caspase-1/GSDMD pathway in pancreatic β cells: in vitro and in vivo studies. Bioengineered. (2021) 12:9356–66. doi: 10.1080/21655979.2021.2001240
66. Lv X, Chen J, He J, Hou L, Ren Y, Shen X, et al. Gasdermin D-mediated pyroptosis suppresses liver regeneration after 70% partial hepatectomy. Hepatol Commun. (2022) 6:2340–53. doi: 10.1002/hep4.1973
67. Wang J, Shi K, An N, Li S, Bai M, Wu X, et al. Direct inhibition of GSDMD by PEITC reduces hepatocyte pyroptosis and alleviates acute liver injury in mice. Front Immunol. (2022) 13:825428. doi: 10.3389/fimmu.2022.825428
68. Zhao X, Wang C, Dai S, Liu Y, Zhang F, Peng C, et al. Quercetin protects ethanol-induced hepatocyte pyroptosis via scavenging mitochondrial ROS and promoting PGC-1α-regulated mitochondrial homeostasis in L02 cells. Oxid Med Cell Longev. (2022) 2022:4591134. doi: 10.1155/2022/4591134
69. Li W, Deng M, Loughran PA, Yang M, Lin M, Yang C, et al. LPS induces active HMGB1 release from hepatocytes into exosomes through the coordinated activities of TLR4 and caspase-11/GSDMD signaling. Front Immunol. (2020) 11:229. doi: 10.3389/fimmu.2020.00229
70. Fang Y, Tian S, Pan Y, Li W, Wang Q, Tang Y, et al. Pyroptosis: A new frontier in cancer. BioMed Pharmacother. (2020) 121:109595. doi: 10.1016/j.biopha.2019.109595
71. Mamun A, Wu Y, Nasrin F, Akter A, Taniya MA, Munir F, et al. Role of pyroptosis in diabetes and its therapeutic implications. J Inflammation Res. (2021) 14:2187–206. doi: 10.2147/JIR.S291453
72. Yang F, Qin Y, Lv J, Wang Y, Che H, Chen X, et al. Silencing long non-coding RNA Kcnq1ot1 alleviates pyroptosis and fibrosis in diabetic cardiomyopathy. Cell Death Dis. (2018) 9:1–13. doi: 10.1038/s41419-018-1029-4
73. Dargani ZT, Singla DK. Embryonic stem cell-derived exosomes inhibit doxorubicin-induced TLR4-NLRP3-mediated cell death-pyroptosis. Am J Physiol Heart Circ Physiol. (2019) 317:H460–71. doi: 10.1152/ajpheart.00056.2019
74. Wang X, Pan J, Liu H, Zhang M, Liu D, Lu L, et al. AIM2 gene silencing attenuates diabetic cardiomyopathy in type 2 diabetic rat model. Life Sci. (2019) 221:249–58. doi: 10.1016/j.lfs.2019.02.035
75. Yan M, Li Y, Luo Q, Zeng W, Shao X, Li L, et al. Mitochondrial damage and activation of the cytosolic DNA sensor cGAS-STING pathway lead to cardiac pyroptosis and hypertrophy in diabetic cardiomyopathy mice. Cell Death Discovery. (2022) 8:258. doi: 10.1038/s41420-022-01046-w
76. An X, Zhang Y, Cao Y, Chen J, Qin H, Yang L. Punicalagin protects diabetic nephropathy by inhibiting pyroptosis based on TXNIP/NLRP3 pathway. Nutrients. (2020) 12:1516. doi: 10.3390/nu12051516
77. Liapis H, Romagnani P, Anders HJ. New insights into the pathology of podocyte loss: mitotic catastrophe. Am J Pathol. (2013) 183:1364–74. doi: 10.1016/J.AJPATH.2013.06.033
78. Li F, Chen Y, Li Y, Huang M, Zhao W. Geniposide alleviates diabetic nephropathy of mice through AMPK/SIRT1/NF-κB pathway. Eur J Pharmacol. (2020) 886:173449. doi: 10.1016/j.ejphar.2020.173449
79. Cheng Q, Pan J, Zhou ZL, Yin F, Xie HY, Chen PP, et al. Caspase-11/4 and gasdermin D-mediated pyroptosis contributes to podocyte injury in mouse diabetic nephropathy. Acta Pharmacol Sin. (2021) 42:954–63. doi: 10.1038/s41401-020-00525-z
80. Yu X, Ma X, Lin W, Xu Q, Zhou H, Kuang HY. Long noncoding RNA MIAT regulates primary human retinal pericyte pyroptosis by modulating miR-342-3p targeting of CASP1 in diabetic retinopathy. Exp Eye Res. (2021) 202:108300. doi: 10.1016/j.exer.2020.108300
81. Gan J, Huang M, Lan G, Liu L, Xu F. High glucose induces the loss of retinal pericytes partly via NLRP3-caspase-1-GSDMD-mediated pyroptosis. BioMed Res Int. (2020) 2020:4510628. doi: 10.1155/2020/4510628
82. Drummer C, Saaoud F, Jhala NC, Cueto R, Sun Y, Xu K, et al. Caspase-11 promotes high-fat diet-induced NAFLD by increasing glycolysis, OXPHOS, and pyroptosis in macrophages. Front Immunol. (2023) 14:1113883. doi: 10.3389/fimmu.2023.1113883
83. Xu B, Jiang M, Chu Y, Wang W, Chen D, Li X, et al. Gasdermin D plays a key role as a pyroptosis executor of non-alcoholic steatohepatitis in humans and mice. J Hepatol. (2018) 68:773–82. doi: 10.1016/j.jhep.2017.11.040
84. Rodríguez-Antonio I, López-Sánchez GN, Uribe M, Chávez-Tapia NC, Nuño-Lámbarri N. Role of the inflammasome, gasdermin D, and pyroptosis in non-alcoholic fatty liver disease. J Gastroenterol Hepatol. (2021) 36:2720–7. doi: 10.1111/jgh.15561
85. Zhu Y, Zhao H, Lu J, Lin K, Ni J, Wu G, et al. Caspase-11-mediated hepatocytic pyroptosis promotes the progression of nonalcoholic steatohepatitis. Cell Mol Gastroenterol Hepatol. (2021) 12:653–64. doi: 10.1016/j.jcmgh.2021.04.009
86. Li H, Zhao XK, Cheng YJ, Zhang Q, Wu J, Lu S, et al. Gasdermin D-mediated hepatocyte pyroptosis expands inflammatory responses that aggravate acute liver failure by upregulating monocyte chemotactic protein 1/CC chemokine receptor-2 to recruit macrophages. World J Gastroenterol. (2019) 25:6527–40. doi: 10.3748/wjg.v25.i44.6527
87. Lv T, Xiong X, Yan W, Liu M, Xu H, He Q. Original research: Targeting of GSDMD sensitizes HCC to anti-PD-1 by activating cGAS pathway and downregulating PD-L1 expression. J Immunother Cancer. (2022) 10:e004763. doi: 10.1136/jitc-2022-004763
88. Hou J, Wang C, Ma D, Chen Y, Jin H, An Y, et al. The cardioprotective and anxiolytic effects of Chaihujialonggumuli granule on rats with anxiety after acute myocardial infarction is partly mediated by suppression of CXCR4/NF-κB/GSDMD pathway. Biomedicine Pharmacotherapy. (2021) 133:111015. doi: 10.1016/j.biopha.2020.111015
89. Jiang K, Tu Z, Chen K, Xu Y, Chen F, Xu S, et al. Gasdermin D inhibition confers antineutrophil-mediated cardioprotection in acute myocardial infarction. J Clin Invest. (2022) 132:e151268. doi: 10.1172/JCI151268
90. Lin S, Dai S, Lin J, Liang X, Wang W, Huang W, et al. Oridonin relieves angiotensin II-induced cardiac remodeling via inhibiting GSDMD-mediated inflammation. Cardiovasc Ther. (2022) 2022:3167959. doi: 10.1155/2022/3167959
91. Han B, Xu J, Shi X, Zheng Z, Shi F, Jiang F, et al. DL-3-n-butylphthalide attenuates myocardial hypertrophy by targeting gasdermin D and inhibiting gasdermin D mediated inflammation. Front Pharmacol. (2021) 12:688140. doi: 10.3389/fphar.2021.688140
92. Cheng CK, Lin X, Pu Y, Tse JKY, Wang Y, Zhang C-L, et al. SOX4 is a novel phenotypic regulator of endothelial cells in atherosclerosis revealed by single-cell analysis. J Adv Res. (2023) 43:187–203. doi: 10.1016/j.jare.2022.02.017
93. Opoku E, Traughber CA, Zhang D, Iacano AJ, Khan M, Han J, et al. Gasdermin D mediates inflammation-induced defects in reverse cholesterol transport and promotes atherosclerosis. Front Cell Dev Biol. (2021) 9:715211. doi: 10.3389/FCELL.2021.715211
94. Puylaert P, Van Praet M, Vaes F, Neutel CHG, Roth L, Guns P-J, et al. Gasdermin D deficiency limits the transition of atherosclerotic plaques to an inflammatory phenotype in apoE knock-out mice. Biomedicines. (2022) 10:1171. doi: 10.3390/biomedicines10051171
95. Ju J, Liu Y, Liang H, Yang B. The role of pyroptosis in endothelial dysfunction induced by diseases. Front Immunol. (2022) 13:1093985. doi: 10.3389/fimmu.2022.1093985
96. Pan J, Han L, Guo J, Wang X, Liu D, Tian J, et al. AIM2 accelerates the atherosclerotic plaque progressions in ApoE–/– mice. Biochem Biophys Res Commun. (2018) 498:487–94. doi: 10.1016/j.bbrc.2018.03.005
97. Yue M, Xiao L, Yan R, Li X, Yang W. Pyroptosis in neurodegenerative diseases: What lies beneath the tip of the iceberg? Int Rev Immunol. (2022) 21:1–16. doi: 10.1080/08830185.2022.2052064
98. Shen H, Han C, Yang Y, Guo L, Sheng Y, Wang J, et al. Pyroptosis executive protein GSDMD as a biomarker for diagnosis and identification of Alzheimer’s disease. Brain Behav. (2021) 11:e02063. doi: 10.1002/brb3.2063
99. Han C, Yang Y, Guan Q, Zhang X, Shen H, Sheng Y, et al. New mechanism of nerve injury in Alzheimer’s disease: β-amyloid-induced neuronal pyroptosis. J Cell Mol Med. (2020) 24:8078–90. doi: 10.1111/jcmm.15439
100. de Dios C, Abadin X, Roca-Agujetas V, Jimenez-Martinez M, Morales A, Trullas R, et al. Inflammasome activation under high cholesterol load triggers a protective microglial phenotype while promoting neuronal pyroptosis. Transl Neurodegener. (2023) 12:10. doi: 10.1186/s40035-023-00343-3
101. Tian D, Xing Y, Gao W, Zhang H, Song Y, Tian Y, et al. Sevoflurane aggravates the progress of alzheimer’s disease through NLRP3/caspase-1/gasdermin D pathway. Front Cell Dev Biol. (2022) 9:801422/BIBTEX. doi: 10.3389/FCELL.2021.801422/BIBTEX
102. Rui W, Li S, Xiao H, Xiao M, Shi J. Baicalein attenuates neuroinflammation by inhibiting NLRP3/caspase-1/GSDMD pathway in MPTP induced mice model of parkinson’s disease. Int J Neuropsychopharmacol. (2020) 23:762–73. doi: 10.1093/ijnp/pyaa060
103. Zhang X, Zhang Y, Li R, Zhu L, Fu B, Yan T. Salidroside ameliorates Parkinson’s disease by inhibiting NLRP3-dependent pyroptosis. Aging (Albany NY). (2020) 12:9405–26. doi: 10.18632/aging.103215
104. Anderson FL, von Herrmann KM, Andrew AS, Kuras YI, Young AL, Scherzer CR, et al. Plasma-borne indicators of inflammasome activity in Parkinson’s disease patients. NPJ Parkinsons Dis. (2021) 7:1–12. doi: 10.1038/s41531-020-00147-6
105. Gao H, Cao M, Yao Y, Hu W, Sun H, Zhang Y, et al. Dysregulated microbiota-driven gasdermin D activation promotes colitis development by mediating IL-18 release. Front Immunol. (2021) 12:750841. doi: 10.3389/fimmu.2021.750841
106. Liu X, Zhou M, Dai Z, Luo S, Shi Y, He Z, et al. Salidroside alleviates ulcerative colitis via inhibiting macrophage pyroptosis and repairing the dysbacteriosis-associated Th17/Treg imbalance. Phytother Res. (2023) 37:367–82. doi: 10.1002/ptr.7636
107. Chunmei M, Yang D, Wang B, Wu C, Wu Y, Li S, et al. Gasdermin D in macrophages restrains colitis by controlling cGAS-mediated inflammation. Sci Adv. (2020) 6:eaaz6717. doi: 10.1126/sciadv.aaz6717
108. Jarczak D, Kluge S, Nierhaus A. Sepsis-pathophysiology and therapeutic concepts. Front Med (Lausanne). (2021) 8:628302. doi: 10.3389/fmed.2021.628302
109. Silva CMS, Wanderley CWS, Veras FP, Sonego F, Nascimento DC, Gonçalves AV, et al. Gasdermin D inhibition prevents multiple organ dysfunction during sepsis by blocking NET formation. Blood. (2021) 138:2702–13. doi: 10.1182/blood.2021011525
110. Giacomelli R, Ruscitti P, Shoenfeld Y. A comprehensive review on adult onset Still’s disease. J Autoimmun. (2018) 93:24–36. doi: 10.1016/j.jaut.2018.07.018
111. Nagai H, Kirino Y, Nakano H, Kunishita Y, Henmi R, Szymanski AM, et al. Elevated serum gasdermin D N-terminal implicates monocyte and macrophage pyroptosis in adult-onset Still’s disease. Rheumatology. (2021) 60:3888–95. doi: 10.1093/rheumatology/keaa814
112. Dalmau J, Armangué T, Planagumà J, Radosevic M, Mannara F, Leypoldt F, et al. An update on anti-NMDA receptor encephalitis for neurologists and psychiatrists: mechanisms and models. Lancet Neurol. (2019) 18:1045–57. doi: 10.1016/S1474-4422(19)30244-3
113. Ma X, Chen C, Lu Y, Fang L, Cao B, Hu X, et al. Association of serum Gasdermin D with anti-N-methyl-D-aspartate receptor encephalitis. J Neurochem. (2021) 159:923–30. doi: 10.1111/jnc.15497
114. Gomez-Lopez N, Romero R, Panaitescu B, Miller D, Zou C, Gudicha DW, et al. Gasdermin D: in vivo evidence of pyroptosis in spontaneous labor at term. J Matern Fetal Neonatal Med. (2021) 34:569–79. doi: 10.1080/14767058.2019.1610740
115. Jiao J, Wang Y, Ren P, Sun S, Wu M. Necrosulfonamide ameliorates neurological impairment in spinal cord injury by improving antioxidative capacity. Front Pharmacol. (2020) 10:1538. doi: 10.3389/fphar.2019.01538
116. Rathkey JK, Zhao J, Liu Z, Chen Y, Yang J, Kondolf HC, et al. Chemical disruption of the pyroptotic pore-forming protein gasdermin D inhibits inflammatory cell death and sepsis. Sci Immunol. (2018) 3:eaat2738. doi: 10.1126/sciimmunol.aat2738
117. Orning P, Lien E, Fitzgerald KA. Gasdermins and their role in immunity and inflammation. J Exp Med. (2019) 216:2453–65. doi: 10.1084/jem.20190545
118. Hu JJ, Liu X, Xia S, Zhang Z, Zhang Y, Zhao J, et al. FDA-approved disulfiram inhibits pyroptosis by blocking gasdermin D pore formation. Nat Immunol. (2020) 21:736–45. doi: 10.1038/s41590-020-0669-6
119. Pandeya A, Li L, Li Z, Wei Y. Gasdermin D (GSDMD) as a new target for the treatment of infection. Medchemcomm. (2019) 10:660–7. doi: 10.1039/c9md00059c
120. Humphries F, Shmuel-Galia L, Ketelut-Carneiro N, Li S, Wang B, Nemmara VV, et al. Succination inactivates gasdermin D and blocks pyroptosis. Sci (1979). (2020) 369:1633–7. doi: 10.1126/science.abb9818
121. Zhong L, Han J, Fan X, Huang Z, Su L, Cai X, et al. Novel GSDMD inhibitor GI-Y1 protects heart against pyroptosis and ischemia/reperfusion injury by blocking pyroptotic pore formation. Basic Res Cardiol. (2023) 118:40. doi: 10.1007/s00395-023-01010-4
122. Martin-Sanchez F, Diamond C, Zeitler M, Gomez AI, Baroja-Mazo A, Bagnall J, et al. Inflammasome-dependent IL-1β release depends upon membrane permeabilisation. Cell Death Differ. (2016) 23:1219–31. doi: 10.1038/cdd.2015.176
123. Yang J, Liu Z, Wang C, Yang R, Rathkey JK, Pinkard OW, et al. Mechanism of gasdermin D recognition by inflammatory caspases and their inhibition by a gasdermin D-derived peptide inhibitor. Proc Natl Acad Sci U.S.A. (2018) 115:6792–7. doi: 10.1073/pnas.1800562115
124. Luan J, Chen W, Fan J, Wang S, Zhang X, Zai W, et al. GSDMD membrane pore is critical for IL-1β release and antagonizing IL-1β by hepatocyte-specific nanobiologics is a promising therapeutics for murine alcoholic steatohepatitis. Biomaterials. (2020) 227:119570. doi: 10.1016/j.biomaterials.2019.119570
125. Wang H, Miao F, Ning D, Shan C. Ellagic acid Alleviates hepatic ischemia–reperfusion injury in C57 mice via the Caspase-1-GSDMD pathway. BMC Vet Res. (2022) 18:229. doi: 10.1186/s12917-022-03326-0
126. Zhang C, Niu H, Wan C, Yu X, Xin G, Zhu Y, et al. Drug D, a Diosgenin Derive, Inhibits L-Arginine-Induced Acute Pancreatitis through Meditating GSDMD in the Endoplasmic Reticulum via the TXNIP/HIF-1alpha Pathway. Nutrients. (2022) 14:2591. doi: 10.3390/nu14132591
127. He G, Chen K, Wang H, Li X, Li W, Liu L, et al. Fudosteine attenuates acute lung injury in septic mice by inhibiting pyroptosis via the TXNIP/NLRP3/GSDMD pathway. Eur J Pharmacol. (2022) 926:175047. doi: 10.1016/j.ejphar.2022.175047
Keywords: cell death, gasdermin D, GSDMD inhibitor, inflammation, inflammatory diseases, necroptosis, NEtosis, pyroptosis
Citation: Cheng CK, Yi M, Wang L and Huang Y (2024) Role of gasdermin D in inflammatory diseases: from mechanism to therapeutics. Front. Immunol. 15:1456244. doi: 10.3389/fimmu.2024.1456244
Received: 28 June 2024; Accepted: 08 August 2024;
Published: 26 August 2024.
Edited by:
Lynn Xiaoling Qiang, Northwell Health, United StatesReviewed by:
Asha Varghese, Feinstein Institute for Medical Research, United StatesCopyright © 2024 Cheng, Yi, Wang and Huang. This is an open-access article distributed under the terms of the Creative Commons Attribution License (CC BY). The use, distribution or reproduction in other forums is permitted, provided the original author(s) and the copyright owner(s) are credited and that the original publication in this journal is cited, in accordance with accepted academic practice. No use, distribution or reproduction is permitted which does not comply with these terms.
*Correspondence: Yu Huang, eXUuaHVhbmdAY2l0eXUuZWR1Lmhr
†These authors have contributed equally to this work
Disclaimer: All claims expressed in this article are solely those of the authors and do not necessarily represent those of their affiliated organizations, or those of the publisher, the editors and the reviewers. Any product that may be evaluated in this article or claim that may be made by its manufacturer is not guaranteed or endorsed by the publisher.
Research integrity at Frontiers
Learn more about the work of our research integrity team to safeguard the quality of each article we publish.