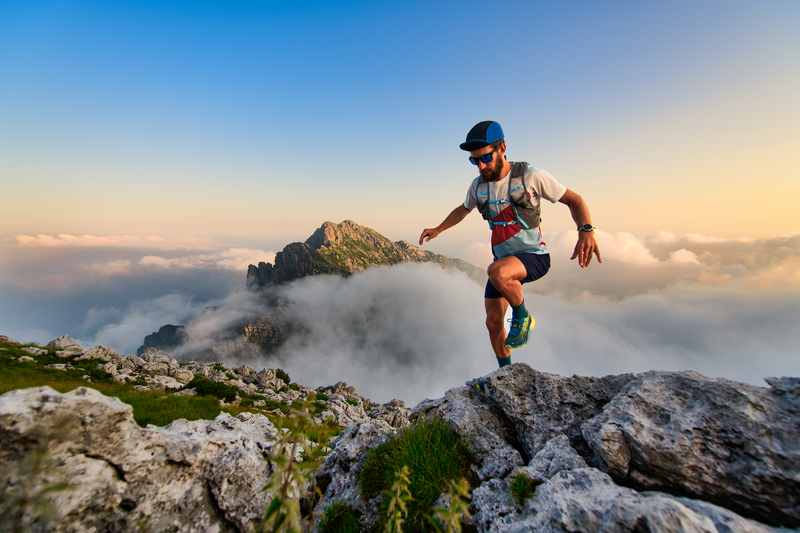
94% of researchers rate our articles as excellent or good
Learn more about the work of our research integrity team to safeguard the quality of each article we publish.
Find out more
REVIEW article
Front. Immunol. , 31 July 2024
Sec. Cancer Immunity and Immunotherapy
Volume 15 - 2024 | https://doi.org/10.3389/fimmu.2024.1452078
This article is part of the Research Topic Decoding Tumor Plasticity: Integrative Analysis of Epigenetic Regulation and Microenvironmental Adaptation View all 11 articles
Lung cancer (LC) is one of the most common cancer worldwide. Tumor-associated macrophages (TAMs) are important component of the tumor microenvironment (TME) and are closely related to the stages of tumor occurrence, development, and metastasis. Macrophages are plastic and can differentiate into different phenotypes and functions under the influence of different signaling pathways in TME. The classically activated (M1-like) and alternatively activated (M2-like) represent the two polarization states of macrophages. M1 macrophages exhibit anti-tumor functions, while M2 macrophages are considered to support tumor cell survival and metastasis. Macrophage polarization involves complex signaling pathways, and blocking or regulating these signaling pathways to enhance macrophages’ anti-tumor effects has become a research hotspot in recent years. At the same time, there have been new discoveries regarding the modulation of TAMs towards an anti-tumor phenotype by synthetic and natural drug components. Nanotechnology can better achieve combination therapy and targeted delivery of drugs, maximizing the efficacy of the drugs while minimizing side effects. Up to now, nanomedicines targeting the delivery of various active substances for reprogramming TAMs have made significant progress. In this review, we primarily provided a comprehensive overview of the signaling crosstalk between TAMs and various cells in the LC microenvironment. Additionally, the latest advancements in novel drugs and nano-based drug delivery systems (NDDSs) that target macrophages were also reviewed. Finally, we discussed the prospects of macrophages as therapeutic targets and the barriers to clinical translation.
Lung cancer (LC) is the most common malignant tumor with the highest incidence and mortality rates globally, seriously threatening human health (1). Tumors involve an ongoing struggle, adaptation, and evolution among tumor cells, surrounding normal cells, and various immune cells. Tumor microenvironment (TME) plays an important role in various stages of tumor (2). Additionally, the composition of the TME is rich and heterogeneous, including various cellular components and signaling molecules (3). Among patients with LC, these components can also serve as important biomarkers for auxiliary diagnosis, treatment efficacy evaluation, and prognosis assessment (4–7).
Tumor-associated macrophages (TAMs) mainly originate from peripheral blood monocytes and tissue-resident macrophages (TRMs) (8, 9). During embryonic development, precursor cells from the yolk sac or fetal liver migrate into various organ tissues, where they differentiate into tissue-resident macrophages. Resident macrophages in normal lung tissue include interstitial macrophages (IMs) and alveolar macrophages (AMs) (10). In adulthood, besides tissue-resident macrophages that persist long-term and self-renew, another major source of macrophages in tissues is peripheral blood monocytes (11, 12). In a mouse model of LC, both embryonically-derived TAMs and monocyte-derived TAMs cannot be strictly defined as either M1-like or M2-like macrophages, but rather appear to be a mixture of M1 and M2 phenotypes (13). Monocytes are recruited from the peripheral circulation to the TME through colony-stimulating factor-1 (CSF-1) secreted by tumor cells, and subsequently differentiate into macrophages (14). TAMs are important cellular components of the TME, exerting multiple supportive and inhibitory roles in LC growth and metastasis (15–17). TAMs possess at least seven functions, including promoting tumor growth, metastasis, therapy resistance, stemness maintenance, immune regulation, inflammation, and angiogenesis, which collectively influence tumor progression and treatment outcomes (18). Currently, two functionally distinct main phenotypes of macrophages have received extensive attention, namely the inflammatory or classically activated M1-like TAMs and the anti-inflammatory or alternatively activated M2-like TAMs. On the surface of M1 macrophages, there are expressed markers such as TLR2, TLR4, CD80, CD86, iNOS, and MHC-II, which are characteristic markers of M1 macrophages. In contrast, M2 macrophages express proteins including the mannose receptor, CD206, CD163, CD209, CD204, CD115, CD301, FIZZ1, and Ym1/2 on their surface (19, 20). With the advancement of biological detection technologies, more subtypes of TAMs have been defined. For instance, slight phenotypic variations have been observed within the M2 phenotype, leading to the classification of distinct M2 subgroups known as M2a, M2b, M2c, and M2d, based on the stimuli used for activation (21). However, this classification fails to explain the phenotypic range observed in macrophages under different homeostatic and pathological conditions. Additionally, genes associated with M1-like and M2-like TAM spectra are expressed concurrently in nearly all types of TAMs subgroups. Recently, research employing single-cell analysis identified five distinct TAM subgroups in various cancers: HES1 TAMs, C1Qhi TAMs, TREM2 TAMs, IL4I1 TAMs, and proliferative TAMs (22). TAMs interact with cancer cells, T cells, endothelial cells, B cells, fibroblasts, and NK cells through complex signaling pathways (23–26). In response to signaling crosstalk between TAMs and other cells, an immunosuppressive TME is formed, which promotes cancer cell proliferation, angiogenesis, lymphangiogenesis, and distant metastasis (27–29). Previous studies have shown that M2-like TAMs play three distinct roles in promoting tumor development and metastasis: (1) M2-like TAMs promote tumor cell entry into the vascular system and facilitate tumor spread through paracrine signaling loops (30); (2) M2-like TAMs can promote the formation of an immunosuppressive tumor microenvironment by secreting immunosuppressive factors including TGF-β, IL-10, arginase-1 (Arg-1), and NO, thereby facilitating tumor growth (31); (3) M2-like TAMs can enhance tumor angiogenesis, promoting tumor growth and post-therapeutic repair (32). In the progression of tumors, the immunosuppressive TME can further attract macrophages to accumulate, forming a vicious cycle (33, 34).
With the advancement of multi-omics technologies, various biomolecules have been identified as key signals mediating TAM polarization (34). Epigenetics has introduced novel concepts to comprehend genetics, garnering significant and profound research focus, particularly concerning tumor development. In the past, it was thought that new genetic phenotypes arose from alterations (or mutations) in DNA sequences inherited by the next generation. However, it is now known that epigenetic modifications can transmit new phenotypic traits to offspring without directly modifying the DNA sequence itself. In recent years, reprogramming macrophage plasticity and function through epigenetic mechanisms has been recognized as a key regulatory node upstream of transcriptomics, proteomics, and metabolic processes (2). At the same time, numerous epigenetic signaling pathways have been found to play crucial roles in regulating the TME in LC (35, 36). Drugs targeting macrophage have been shown to effectively inhibit cancer cells, including small molecule inhibitors and monoclonal antibodies (37–39). Additionally, an increasing number of natural drug compounds have been discovered to combat tumors by modulating the immune microenvironment (40–42). Nanomedicine is an emerging product of the combination of nanotechnology and medicine, used for the diagnosis, treatment, and prevention of various diseases including tumors and immune disorders. Nano-drug delivery systems (NDDSs) have the advantages of selectivity and controllability, and its effectiveness in enhancing anti-tumor responses by targeting macrophages has been widely demonstrated in vitro (43–47).
In this review, we primarily summarized the signaling pathways regulating TAM phenotype switching in LC. Based on TAM-related signaling targets, we reviewed the latest advances in NDDSs and novel drugs (Figure 1). At the same time, the future prospect of macrophages as therapeutic objects and the existing obstacles were discussed.
Figure 1 Origin and polarization-related signaling pathways of TAMs in the LC microenvironment. After entering the TME of LC, various biological or drug molecules promote or inhibit monocyte polarization into M1 or M2-like subtypes.
In the TME, TAMs are a principal cellular component, comprising more than half of the total immune cells in the tumor stroma and playing a significant role in tumor progression (48–50). According to the latest research, after macrophages senescence in the lungs, they not only persist, but also become pro-tumorigenic, suppressing the anti-cancer activity of cytotoxic T lymphocytes, ultimately promoting cancer development (51). Furthermore, researchers have found a close association between the senescence-associated secretory phenotype (SASP) secreted by senescent macrophages and KRAS-mutant NSCLC (52). In response to the influence of cytokines in the TME, macrophages differentiate into different types, mainly divided into M1-like macrophages and M2-like macrophages (26, 53). In early LC, the same TAMs can concurrently exhibit two distinct gene expression profiles, demonstrating that the expression of M1-like and M2-like gene characteristics at this stage is not mutually exclusive (54). And macrophages aggregate around cancer cells within the tissue, promoting cancer cell invasion (55). Simultaneously, macrophages induce an increase in regulatory T cells, aiding cancer cells in immune evasion (56). In the TME, the process by which macrophages differentiate into M1-like TAMs or M2-like TAMs is referred to as polarization (57). M1-like macrophages are typically regarded as tumoricidal macrophages, primarily involved in anti-tumor responses and immune promotion. Conversely, M2-like macrophages exhibit immunosuppressive properties, promoting tissue repair and tumor growth (Figure 2). According to reports, the polarization of TAMs in the TME is associated with different outcomes in LC patients, with a higher proportion of M2-like TAMs being correlated with a poorer survival time (6, 15, 58, 59). Additionally, TAM polarization leads to the widespread presence of M2 macrophages in the TME, which can induce LC cells to develop drug resistance (60, 61).
Figure 2 M1-like or M2-like macrophages and their functions (2). M1-like TAMs possess functions such as immune activation, tissue damage, phagocytosis of tumor cell, and promotion of tumor cell apoptosis. M2-like TAMs have functions including promoting tumor growth, metastasis, tissue remodeling/angiogenesis, and immune suppression.
With the development of technologies such as metabolomics and single-cell sequencing, more molecular signaling pathways regulating TAM polarization have been discovered, providing additional insights into the evolution of tumor cells and drug development.
LC cells and TAMs exhibit complex signal crosstalk (62). LC is prone to genetic mutations, and many signaling pathways related to macrophage polarization have been discovered among the common types of gene mutations (63). Cellular senescence is a process characterized by cell cycle arrest accompanied by changes in gene expression, but with normal cell viability and metabolism, associated with cancer and aging (64, 65). M2-like TAMs are associated with high expression of IL-10, IL-1β, VEGF, and matrix metalloproteinases (MMPs) in vivo. What’s more, they also express abundant scavenger receptors, contributing to debris clearance, promotion of angiogenesis, tissue remodeling and repair, as well as facilitating tumor initiation and progression (66). Among these, VEGF exerts the strongest and most specific effects, not only promoting proliferation and neovascularization of endothelial cells but also increasing vascular permeability, facilitating the extravasation of tumor cells (67). Furthermore, TAMs can promote the formation of collagen fibers, facilitating rapid migration of LC cells along these fibers towards blood vessels, thereby enhancing the metastatic efficiency of LC cells (68). M2-like macrophages enhance cancer cell drug resistance by producing and releasing mediators that modulate the PI3K/Akt, JAK/STAT, MAPK, Notch, Hippo, and other related pathways (69). Extracellular vesicles derived from M2-TAMs promote aerobic glycolysis in LC cells via the miR-3679–5p/NEDD4L/c-Myc signaling pathway, thereby inhibiting tumor cell apoptosis and inducing cisplatin resistance (70). The TF-mTORC2 axis plays a role in negative regulation of macrophage phagocytic capacity, contributing to KRAS-driven lung adenocarcinoma (LUAD) resistance (71). M2-like TAMs promote osimertinib resistance in NSCLC through extracellular vesicles that regulate the miR-6386–5p/MAPK8IP3 axis (72).
KRAS-driven LC is characterized by senescent endothelial cells and macrophages (51, 52). Meanwhile, due to activation of the AKT-mTOR signaling pathway, ubiquitin-specific protease-12 (USP12) is typically downregulated, promoting both cancer cells growth and macrophages recruitment (73). In addition, in KRAS-mutant LC, activation of the PI3K/STAT3 signaling pathway can lead to increased CD47 expression, thereby reducing macrophage phagocytic capability (74). In KRAS-mutated tumor cells, the mechanistic study indicates that endogenous anti-silencing function protein 1 homolog A (Asf1a) deficiency induces polarization of M1-like macrophages by upregulating GM-CSF expression (75). In KRAS-mutant LUAD, LKB1 deficiency leads to increased expression of the MCT4 transporter protein, ultimately promoting lactate production and secretion (76). Fascin actin-bundling protein 1 (FSCN-1) activates the PI3K-AKT and JAK-STAT signaling pathways in LUAD cells, leading to increased lactate production (77). In acidic environments, macrophages become more polarized towards an M2-like phenotype (76–78). Additionally, low levels of lactate promote TAM maturation, which is regulated by the Notch/Hes1/MCT2 signaling pathway (79). In LC with activated epidermal growth factor receptor (EGFR) mutation, overexpression of immunoglobulin-like transcript (ILT) 4 in tumor cells induces recruitment of TAMs and M2-like polarization (80). Another study confirmed that the activation of the STAT3/CD47-SIRPα signaling axis promotes an increase in M2-like macrophages, ultimately leading to acquired resistance to EGFR-TKIs (61). In small cell lung cancer (SCLC), the Slit2/Robo1 signaling pathway inhibits the polarization of M2-like macrophages in the TME by suppressing the TGF-β1 signaling pathway (81).
The traditional view holds that cancer originates from the accumulation of somatic cell mutations. However, in recent years, many studies have found that the occurrence and susceptibility of cancer are also closely related to extensive epigenetic changes in the genome (82–84). Researchers have confirmed for the first time in model organism that transient epigenetic changes are sufficient to induce irreversible cancer transformation, which is associated with the irreversible derepression of certain cancer-driving genes (85). At present, three major epigenetic codes have been extensively studied, including DNA methylation, non-coding RNA (ncRNA) and histone modification (Figure 3). Many cancer-related gene mutations occur predominantly in non-coding regions. Restricting research focus to the minority of protein-coding genes would severely limit our understanding of cancer initiation and evolution mechanisms. In recent years, increasing evidence has also demonstrated the close association between non-coding RNAs (ncRNAs) and cancer (87). Epigenetic alterations can serve as biomarkers for cancer and even become targets for cancer therapy (88). In LC, the tumor-derived exosomes (TDEs) carrying epigenetic factors also play a significant role in the interaction between cancer cells and macrophages, especially ncRNAs (36, 78). MiRNAs in ncRNAs have gained more attention due to their relative stability, small size, and high conservation. At the same time, miRNAs are abundantly present and stably expressed in human serum, plasma, and sputum, and their detection in peripheral blood of tumor patients has become a hot topic (89, 90). In NSCLC researches, numerous signaling pathways related to miRNAs have been found to be involved in macrophage polarization. Cancer cells secrete exosomes containing miR-19b-3p, which targets the protein tyrosine phosphatase receptor type D (PTPRD) in TAMs, inhibiting the dephosphorylation of STAT, thereby leading to M2-like polarization (91). Additionally, exosomes secreted by LAUD can activate the c-Jun N-terminal kinase (JNK) signaling pathway through miR-3153, thereby inducing M2 macrophage polarization (92). Exosomes derived from LC promote M2-like macrophage polarization through the miR-124–3p/EZH2 signaling pathway mediated by circPVT1 (93). In vitro study of LC, circular RNA (circATP9A), when bound to HuR, induces M2-like polarization of macrophages by affecting downstream pathways mediated by the PI3K/AKT/mTOR axis (94). Hypoxic LUAD cells activate the STAT3 signaling pathway via miR-1290 to promote M2-like macrophage polarization (95). Morrissey et al. demonstrated in mouse models of LC that TDEs polarize macrophages into an immunosuppressive phenotype (M2-like) through NF-κB signaling (78). Liang et al. found that exosomes (TRIM59) can convert macrophages into M2-like phenotype by regulating ABHD5-mediated proteasomal degradation (96). In poorer prognosis SCLC, cancer cell-derived exosomes induce M2-like macrophages polarization through the NLRP6/NF-κB signaling axis (97).
Figure 3 Epigenetic modifications including DNA methylation, histone modifications and non-coding RNA (86). 36126898 Tumor cells can regulate TAMs towards M2-like polarization by transporting ncRNAs (such as miR-315 and miR-19b-3p) via secretion of exosomes.
Furthermore, in vivo or in vitro experiments, additional signaling pathways involved in regulating macrophages polarization have also been discovered. A study has shown that LC cells release succinate into microenvironment, activating succinate receptor (SUCNR1) signaling, which polarizes macrophages into the M2-like (98). Rab37, dependent on GTPase, regulates macrophage IL-6 secretion, thereby inducing M2-like polarization (99). Human endogenous retrovirus-H long terminal repeat-associating protein 2 (HHLA2) is upregulated in various tumors (100, 101). In NSCLC, HHLA2 can promote M2 polarization by regulating IL-10 (102). NADPH oxidase 4 (NOX 4) promotes NSCLC cell growth by recruiting immunosuppressive macrophages through ROS/PI3K signaling to produce cytokines (103). Estrogen receptor alpha (ERα) can activate the CCL2/CCR2 signaling pathway, promoting macrophage infiltration and M2-like polarization (104). Upregulation of the JAK-STAT and NF-κB signaling pathways can lead to the aggregation of M2-like macrophages (60). The expression of placental growth factor (PIGF) in LC cells can bind to the fms-like tyrosine kinase-1 (Flt-1), promoting the generation of M2-like macrophages (105). Altered expression of solute carrier family 3 member 2 (SLC3A2) changes the levels of various metabolites in the TME, including arachidonic acid. More importantly, in LC, arachidonic acid is responsible for mediating SLC3A2-induced polarization of macrophages to the M2-like (106). Gamma-aminobutyric acid (GABA) originating from LC cells inhibits both the NF-κB and STAT3 pathways, halting the polarization of M1-like phenotype. Simultaneously, it promotes macrophage M2-like polarization by activating the STAT2 pathway (107).
In view of the above, we can design different drugs against tumors or reduce drug resistance by targeting these molecular signaling pathways that influence macrophage polarization. Besides, epigenetic signaling pathways are involved in the polarization of macrophages, particularly miRNAs. It might be a feasible approach to treat LC by selectively regulating the aberrantly expressed miRNAs, which exist in various cancers in differential expression levels. Meanwhile, we can utilize exosomes as biomarkers, vaccines and drug delivery vehicles for LC diagnosis and treatment, but it remains a challenge that identifying, isolating and quantifying exosomes accurately, efficiently and selectively.
New findings have also been made regarding the signaling molecules that regulate polarization within macrophages. STAT protein family, comprised of transcription factors, is associated with the development, progression, metastasis, survival, and drug resistance of human cancers (108–111). Specifically, STAT3 and STAT6 are significant in cancer biology (108, 112, 113). In the preceding context, many STAT-related signaling pathways involved in macrophage polarization have already been summarized. In TAMs, the upregulation of the epigenetic modulator JMJD6 can be mediated by the STAT3/IL-10 signaling pathway, which can promote the emergence of an immunosuppressive phenotype in TAMs (17). In CD11b cells, the IL-4/STAT6 signaling axis promotes LC progression by increasing M2-like macrophages (114). Furthermore, the AKT/STAT6 signaling pathway can increase the expression of macrophage SLC7A11, promoting TAMs’ M2-like polarization (112). Zhao and colleagues demonstrated that Takeda G protein-coupled receptor 5 (TGR5) promotes the pro-tumoral M2-like polarization in TME by activating the cAMP-STAT3/STAT6 signaling pathway (115). N6-adenosine methylation is one of the most common modifications in RNA. YTHDF2 serves as a primary m6A reader, mediating mechanisms that promote the formation of TAMs (116). N6-methyladenosine (m6A) sequencing results in macrophages show that the absence of METTL3 promotes the activation of NF-kB and STAT3 via the ERK pathway, resulting in the polarization of M2-like macrophages (117). Zhang et al. discovered that GRP78 in macrophages promotes M2-like polarization through the JK/STAT6 pathway, and that the expression of insulin-like growth factor 1 (IGF 1) within macrophages has a similar effect (118). In various malignant tumors and immune cells, the transcription factor β-catenin signaling is often highly expressed (119, 120). In NSCLC, TAM-specific Wnt/β-catenin signaling plays a role in immune evasion by mediating the transformation of M1-like TAMs to M2-like TAMs (121). Research by Sarode et al. also demonstrated that β-catenin-mediated transcriptional activation of FOS-like antigen 2 (FOSL2) drives gene regulation that shifts from M1-like TAMs to M2-like TAM phenotypes. Similarly, the inhibition of the AT-rich interaction domain 5A (ARID5A) has a similar effect on the regulation of macrophage polarization (121). In SCLC, SLIT2 promotes M1 polarization by regulating the Tgf-β1/GSK3/β-catenin signaling pathway in TAMs (81). EGFR/Wnt/β-catenin upregulation of CD55/CD59 expression negatively correlates with the infiltration of M1 macrophages (122). In NSCLC, the transcription factor c-Maf is expressed in TAMs, where it controls oxidative phosphorylation and the N-glycan synthesis pathway, thereby reprogramming macrophages to an M2-like phenotype (123).
Ubiquitination and deubiquitination processes participate in various cellular events through immune regulation, signal transduction (124, 125). Among them, USP7 is identified as a highly expressed gene in M2 macrophages, where it inhibits the polarization of M2-like macrophages by activating the p38 MAPK pathway. Simultaneously, targeting USP7 can significantly promote the polarization of TAMs towards M1-like (126). Inactivation of EGLN3 in macrophages inhibits their migration, phagocytosis, and polarization towards the M2-like phenotype (127). Overexpression of lncRNA ADPGK-AS1 in TAMs upregulates the tricarboxylic acid cycle and promotes mitochondrial fission, indicating a shift towards an M2-like phenotype (128). Therefore, silencing ADPGK-AS1 in TAMs may provide a novel therapeutic strategy for LC. Li et al. identified a novel Mincle/Syk/NF-κB signaling pathway in TAMs, the blockade of which promotes an M1-like phenotype (129). TAMs-associated TMSB10 enhances the transformation and proliferation of M2 TAMs via the PI3K/Akt signaling pathway (130). Transcription factor 21 (TCF21) exerts its effects via the Notch signaling pathway, and its overexpression promotes polarization of TAMs towards M1 macrophages (131). JMJD6-induced M2 polarization in TAMs is mediated by STAT3/IL-10 signaling transduction (17). The 78 kilodalton glucose regulated protein (GRP78) reduces macrophage M1 polarization and promotes M2 polarization through JAK/IGF-1 (118). Histone deacetylase 2 (HDAC2) in macrophages regulates the M2-like phenotype through acetylation of histone H3 and transcription factor SP1 (15).
In the microenvironment of LC, there is complex signaling crosstalk between macrophages and non-tumor cells (132, 133). It has been demonstrated that cancer-associated fibroblasts (CAFs) are derived from macrophages of the M2-like, which are known as macrophage-to-mesenchyme transitions (MMTs). Meanwhile, Smad3 has been found to play a crucial role in MMT of LC (134). Wu et al. confirmed that CAFs secrete CXCL12 to mediate M2-polarized macrophages (135). Interleukin-11 (IL-11) secreted by CAF triggers the AXL-STAT3 signaling pathway, which can differentiate TAMs into a phenotype reminiscent of “M2-like” (136). Additionally, in heavy smokers, miR-320a secreted by neutrophils downregulates STAT4 upon entering macrophages, promoting the accumulation of M2-like pro-tumorigenic phenotypes (137). Upregulating the expression of signal regulatory protein-alpha (SIRPα) in neutrophil-like cells regulates the SHP-1/p38/MAPK/STAT3 signaling cascade, reducing M1-like macrophages, while lacking SIRPα decreases the polarization of M2-like macrophages (138). IL-17A/F secreted by Th17 cells promotes LC metastasis by inducing polarization of macrophages towards an M2-like phenotype (139). However, TNFSF15 secreted by vascular endothelial cells inhibits STAT6, which leads to macrophages towards M1-like (140).
Due to the impact of TAMs on tumor progression, drug development targeting TAMs has gained a lot of attention. To date, the therapeutic strategies targeting TAMs in the TME are primarily divided into four types (Figure 4). Drug development targeting macrophage phenotypes regulation is a promising direction. In terms of synthetic some studies have found that the TKIs imatinib and gefitinib can prevent macrophage M2 polarization by inhibiting STAT6 phosphorylation (142, 143). Polarized M2 macrophages can respond to external stimuli such as cytokines to repolarize into an M1 phenotype. Wei and colleagues found that thymosin alpha1 can reprogram TAMs towards an M1 phenotype through interaction with TLR7 and TLR8 on the lysosomal membrane (144). Additionally, more natural biological compounds have been discovered to be associated with macrophage polarization (Table 1). With the development of biotechnology, comprehensive analyses of the temporal changes in cell metabolism, function, and signaling pathways during the induction stages of M1 and M2 polarization have been facilitated. Integrating multidimensional datasets, new mechanisms of macrophage polarization have been proposed (154). This also provides more target options for drug development.
Figure 4 The strategies in TAM-targeted therapy include inhibiting recruitment of macrophages, reprogramming TAMs, depleting TAMs, and blocking the CD47-SIRPα pathway (141). Nanomedicines, natural drug components, and synthetic drugs can each reprogram TAMs through the cGAS-STING, IL-6R/JAK, HDGF/IL-4/JAK1/STAT3, and STAT6 signaling pathways.
In summary, developing drugs to regulate macrophages for anti-tumor phenotypes is a crucial work with significant clinical application prospects. However, substantial efforts are still needed for drug target screening and further basic research. Advancements in biotechnology are advantageous for promoting drug synthesis and target screening.
At present, nanotechnology is a focal point in the field of drug delivery systems, particularly prominent in targeted therapies. Nanoparticles (NPs) possess modifiable features such as shape, size, and functional properties (155). In the characteristics of NPs, the mechanism of interaction with macrophages has been extensively studied in terms of density. In summary, the high density of NPs engulfed by macrophages leads to reduced mobility of the cells themselves (156). NPs can collaborate with drugs, indicating that NDDSs have the potential to propel drug therapy into the era of precision medicine (157). In various types of diseases, there is substantial evidence that NDDSs improve treatment outcomes by modulating the phenotype of immune cells (158–160). NDDSs induce the repolarization of anti-inflammatory M2 macrophages to pro-inflammatory M1 phenotype, which enhances the anti-tumor effect (161, 162). NDDSs based on various nanomaterials have greatly transformed the field of TAM-related immunotherapy (53, 163).
A novel type of silver nano-cluster (AgNCs) can initiate M1-like polarization of macrophages through the toll-like receptor 4 (TLR-4) signaling axis (164). Nano-Doxorubicin (Nano-DOX) is a drug that has been shown to stimulate the immunogenicity of tumor cells, effectively reactivating TAMs into an anti-tumor M1 phenotype (165). Another type of NPs extracted from cuttlefish ink (CINPs), which can effectively reprogram TAMs from an immunosuppressive M2-like phenotype to an anti-tumor M1-like phenotype (166). Furthermore, plant-derived mitochondrial DNA (mtDNA), encapsulated in nanovesicles derived from artemisinin (ADNV), when internalized into TAMs, induces the major effector molecules of the cGAS-STING pathway, driving the M1-like polarization (167).
Furthermore, due to the direct communication of the lung with the external environment, inhalable NDDSs have also garnered significant attention. One inhalable nanoscale formulation, DFHC (Dex@Fe-HMSNs@Ct), interacts with the CD206 receptor highly expressed in M2-like macrophages, facilitating its uptake by TAMs. Subsequently, through endogenous iron metabolism, DFHC triggers iron death in cancer stem cells (CSCs) (168). The liposome-based NPs (AeroNP-CDN) effectively alleviate the immunosuppressive TME by reprogramming TAMs from the M2 to M1 phenotype (169).
Macrophage-based NDDSs represent an emerging cancer treatment strategy. Also, it has the potential to overcome various clinical challenges and pave the way for a new era of drug therapy. Unfortunately, there are still some barriers and challenges that limit its clinical application. For example, tracking and effectively delivering drugs to the TME remains difficult, necessitating a deeper understanding of the dynamic changes in the microenvironment and the exploration of novel nanomaterials. Furthermore, due to the plasticity of macrophages and the discovery of more subtypes, controlling their states also presents various challenges.
Macrophages are an important component of the TME in LC, and their infiltration levels depend on the severity and staging of the disease. Once inside the TME, macrophages are exposed to a variety of signals and stimuli that shape their phenotype and function. Depending on the dynamic balance of these signals, macrophages can exist in different activation states ranging from M1-like to M2-like. Generally, M1 macrophages have anti-tumor functions, while M2 macrophages promote tumor functions. Additionally, the signaling molecules associated with TAM polarization can serve as potential prognostic biomarkers for LC. Blocking or enhancing signaling pathways that polarize TAMs can enhance the body’s anti-tumor capabilities and have a synergistic effect with drug therapy. Due to the unique heterogeneity of TAMs, the timing and process of macrophage recruitment and polarization are not yet clear. The complexity and diversity of macrophage polarization make it challenging to find drugs that are effective against specific types of tumors. Therefore, further research is needed to effectively design drugs targeted at macrophages. Simultaneously, the upstream and downstream pathways can also serve as therapeutic targets to regulate the function of macrophages. Particularly, utilizing genetic engineering to genetically reprogram macrophages, transforming anti-tumor phenotypes. In both in vitro and in vivo models, an increasing number of natural plant ingredients have been confirmed to regulate macrophage phenotype. Due to the advantages of diverse types and low toxicity of natural plant ingredients, they have attracted attention. However, some metabolic mechanisms remain unclear, requiring further in-depth basic research. Promoting the polarization of TAMs towards an anti-tumor phenotype represents a very promising research direction. Epigenetics plays a crucial role in controlling the phenotypes of macrophages in TEM. Therefore, drugs that target epigenetic signaling pathways show promising potential for cancer treatment. Precision medicine is essential for advancing epigenetic therapies in the future. With hundreds of potential targets, epigenetic changes are prevalent abnormalities in cancer, occurring either early as foundational mutations or later to drive distinct subpopulations. Understanding how these alterations interact can aid in identifying mutations suitable for targeted therapies. The similarity of normal cell receptors can lead to the failure of targeting immune-suppressive macrophages, resulting in side effects and toxicity. It is essential to further decipher the functional characteristics of TAMs and the signaling crosstalk with various types of cells, which aids in identifying specific targets. Due to advancements in artificial intelligence and molecular biology, more specific functional subtypes of TAMs have been defined. Drugs designed specifically for these functionally distinct macrophage subtypes may be more advantageous for the treatment of LC.
Nanomedicine is a promising therapeutic approach and is continually being improved and developed. Nanomedicine strategies often exploit the increased expression of receptors on TAMs, which can change as the tumor progresses. Though NDDSs have shown some effectiveness in cancer immunotherapy and drug delivery, numerous challenges still need addressing. Critical factors such as the uptake of NPs by macrophages and the metabolic processes are essential mechanisms that require further clarification. Due to the characteristics of catalytic activity and modifiability of NPs, they bring unlimited possibilities for solving complex clinical problems. Given the complexity of the TME, cancer immunotherapies that solely focus on targeting TAM receptors are not enough to completely eradicate tumors. Chimeric antigen receptor (CAR) is a modified receptor protein that endows immune cells with the ability to recognize specific antigens on the surface of cancer cells, thereby stimulating immune cells to eliminate cancer cells (170). Macrophages can activate T-cells by presenting antigens, activating the adaptive immune response, and enhancing the anti-tumor effect. In terms of safety, macrophages have a limited time in circulation, and the risk of graft-versus-host disease (GvHD) is relatively low. Therefore, macrophages have the potential to be developed into chimeric antigen receptor macrophage (CAR-M) therapy, serving as a form of anti-tumor immune cell therapy (171). CAR-M has been demonstrated to have promising anti-tumor effects in vitro cell and animal experiments (172, 173). In comparison to CAR-T, CAR-M offers several advantages. CAR-M can reduce the proportion of TAMs, alter TAMs’ cellular phenotypes, and positively impact tumor treatment (172). In addition to its role in engulfing tumor cells, CAR-M also enhances antigen presentation capabilities and promotes T-cell cytotoxicity (174). The circulation time of CAR-M is limited, resulting in lower side effects on other organs (172). Recently, Shen and his colleagues developed an efficient monolayer culture system capable of producing approximately 6000 macrophages from a single human pluripotent stem cell (hPSC) within a 3-week period. Utilizing a CAR structure screening approach, the research team in vitro generated macrophages with stable CAR expression and potent tumor-killing activity (175). Zhang et al. designed antigen-dependent polarization of second-generation iPSC-derived CAR-M, elucidating their antigen-dependent polarization and activation mechanisms, as well as their tumor-killing mechanism through “phagoptosis” (176). These findings provide a more solid theoretical foundation for the application of CAR-M in immunotherapy for solid tumors, supporting its translation into clinical applications. Integrating these therapies with other immunotherapeutic approaches, like PD-L1 or CAR therapies, presents an effective strategy to tackle this problem. Epigenetic factors can be used to express antigens, gene editing, or regulate disease-related proteins. Among them, mRNA nanomedicine can directly produce the required proteins in the body, avoiding the complex process of drug development and production. NDDSs introduce innovative approaches for precise targeting and combination therapies. Developing combination therapies that leverage the properties of NPs and the TME to achieve synergistic effects and minimize adverse reactions is a pressing challenge. To overcome these obstacles, it’s crucial to design NPs and drugs with even greater targeting capabilities, further transforming macrophages into powerful tools against solid tumors. At the same time, conducting interdisciplinary basic experiments is necessary, as it will be more conducive to understanding various mechanisms.
Specific stimuli within the TME can induce macrophages activation and differentiation into various functional subgroups. This process is associated with extensive epigenetic modifications, transcriptional reprogramming, and metabolic changes. Macrophages are a prevalent component of the TME and engage in complex interactions with cancer cells, the matrix, and immune active cells. Modulating the macrophage phenotype is an important direction in the development of anti-tumor drugs targeting TAMs. Macrophage polarization is a complex process of multifactorial interactions, regulated by various intracellular signaling molecules and their pathways. Drugs are categorized based on their effects on macrophage phenotypes, mainly including inhibitors of M2-like macrophage polarization, inducers of M2-like to M1-like macrophage transformation, and promoters of M1-like macrophage polarization. Significant progress has been made in drug development targeting macrophages. Natural plant ingredients as immunomodulatory drugs targeting macrophages have promising prospects for application. However, numerous obstacles remain, such as exploring specific target drugs in complex microenvironments, mitigating the adverse effects on other immune cells. Moreover, the adverse reactions and mechanisms in macrophage-targeted therapy and the rational combination of drugs require further research. The catalytic and modifiability of NPs often provide solutions to various clinical problems. NDDSs have created conditions for the precise delivery of drugs, but a deeper investigation is needed for drugs to better match with NPs. In summary, macrophages present a promising therapeutic target, especially by enhancing the anti-tumor effect through altering their phenotype. The complexity of the TME presents obstacles for drug development, with more mechanistic studies awaiting completion.
WL: Investigation, Project administration, Visualization, Writing – original draft. QY: Investigation, Project administration, Visualization, Writing – original draft. ML: Writing – original draft. XH: Writing – original draft. CS: Writing – review & editing. YRL: Writing – review & editing. YT: Writing – review & editing. YLi: Conceptualization, Formal analysis, Funding acquisition, Writing – review & editing. ZD: Conceptualization, Funding acquisition, Supervision, Validation, Writing – review & editing. YLu: Conceptualization, Investigation, Supervision, Validation, Visualization, Writing – review & editing.
The author(s) declare financial support was received for the research, authorship, and/or publication of this article. This work was funded by the Zigong Academy of Medical Sciences 2022 (ZGYKY22KF008, ZGYKY22KF009 and ZGYKY22KF005); Zigong Municipal Bureau of Science and Technology 2023 (2023YKY07); Science and Technology Foundation of Sichuan Province, China (2024NSFSC1922 and 2024NSFSC1540); Post-Doctor Research Project, West China Hospital, Sichuan University (2021HXBH009); Sichuan University Postdoctoral Interdisciplinary Innovation Fund.
The authors thank to M.S. Qian Huang from Dazhou Dachuan District People’s Hospital (Dazhou Third People’s Hospital) for her assistance with figure illustration in the manuscript.
The authors declare that the research was conducted in the absence of any commercial or financial relationships that could be construed as a potential conflict of interest.
All claims expressed in this article are solely those of the authors and do not necessarily represent those of their affiliated organizations, or those of the publisher, the editors and the reviewers. Any product that may be evaluated in this article, or claim that may be made by its manufacturer, is not guaranteed or endorsed by the publisher.
1. Leiter A, Veluswamy RR, Wisnivesky JP. The global burden of lung cancer: current status and future trends. Nat Rev Clin Oncol. (2023) 20:624–39. doi: 10.1038/s41571-023-00798-3
2. Kloosterman DJ, Akkari L. Macrophages at the interface of the co-evolving cancer ecosystem. Cell. (2023) 186:1627–51. doi: 10.1016/j.cell.2023.02.020
3. Yuan S, Almagro J, Fuchs E. Beyond genetics: driving cancer with the tumour microenvironment behind the wheel. Nat Rev Cancer. (2024) 24:274–86. doi: 10.1038/s41568-023-00660-9
4. Weeden CE, Gayevskiy V, Marceaux C, Batey D, Tan T, Yokote K, et al. Early immune pressure initiated by tissue-resident memory T cells sculpts tumor evolution in non-small cell lung cancer. Cancer Cell. (2023) 41:837–52. doi: 10.1016/j.ccell.2023.03.019
5. Jie X, Chen Y, Zhao Y, Yang X, Xu Y, Wang J, et al. Targeting KDM4C enhances CD8+T cell mediated antitumor immunity by activating chemokine CXCL10 transcription in lung cancer. J Immunother Cancer. (2022) 10:e003716. doi: 10.1136/jitc-2021-003716
6. Li Z, Zhou B, Zhu X, Yang F, Jin K, Dai J, et al. Differentiation-related genes in tumor-associated macrophages as potential prognostic biomarkers in non-small cell lung cancer. Front Immunol. (2023) 14:1123840. doi: 10.3389/fimmu.2023.1123840
7. Zhang H, Liu Z, Wen H, Guo Y, Xu F, Zhu Q, et al. Immunosuppressive TREM2(+) macrophages are associated with undesirable prognosis and responses to anti-PD-1 immunotherapy in non-small cell lung cancer. Cancer Immunol Immunother. (2022) 71:2511–22. doi: 10.1007/s00262-022-03173-w
8. Yang L, Zhang Y. Tumor-associated macrophages: from basic research to clinical application. J Hematol Oncol. (2017) 10:58. doi: 10.1186/s13045-017-0430-2
9. Huang R, Kang T, Chen S. The role of tumor-associated macrophages in tumor immune evasion. J Cancer Res Clin Oncol. (2024) 150:238. doi: 10.1007/s00432-024-05777-4
10. Franklin RA, Liao W, Sarkar A, Kim MV, Bivona MR, Liu K, et al. The cellular and molecular origin of tumor-associated macrophages. Science. (2014) 344:921–5. doi: 10.1126/science.1252510
11. Shi Q, Shen Q, Liu Y, Shi Y, Huang W, Wang X, et al. Increased glucose metabolism in TAMs fuels O-GlcNAcylation of lysosomal Cathepsin B to promote cancer metastasis and chemoresistance. Cancer Cell. (2022) 40:1207–22. doi: 10.1016/j.ccell.2022.08.012
12. Mantovani A, Marchesi F, Jaillon S, Garlanda C, Allavena P. Tumor-associated myeloid cells: diversity and therapeutic targeting. Cell Mol Immunol. (2021) 18:566–78. doi: 10.1038/s41423-020-00613-4
13. Xiang X, Wang J, Lu D, Xu X. Targeting tumor-associated macrophages to synergize tumor immunotherapy. Signal Transduct Target Ther. (2021) 6:75. doi: 10.1038/s41392-021-00484-9
14. Cassetta L, Fragkogianni S, Sims AH, Swierczak A, Forrester LM, Zhang H, et al. Human Tumor-associated macrophage and monocyte transcriptional landscapes reveal cancer-specific reprogramming, biomarkers, and therapeutic targets. Cancer Cell. (2019) 35:588–602. doi: 10.1016/j.ccell.2019.02.009
15. Zheng X, Sarode P, Weigert A, Turkowski K, Chelladurai P, Gunther S, et al. The HDAC2-SP1 axis orchestrates protumor macrophage polarization. Cancer Res. (2023) 83:2345–57. doi: 10.1158/0008-5472.CAN-22-1270
16. Huang M, Xia Y, Li K, Shao F, Feng Z, Li T, et al. Carcinogen exposure enhances cancer immunogenicity by blocking the development of an immunosuppressive tumor microenvironment. J Clin Invest. (2023) 133:e166494. doi: 10.1172/JCI166494
17. Chen S, Wang M, Lu T, Liu Y, Hong W, He X, et al. JMJD6 in tumor-associated macrophage regulates macrophage polarization and cancer progression via STAT3/IL-10 axis. Oncogene. (2023) 42:2737–50. doi: 10.1038/s41388-023-02781-9
18. Qian BZ, Pollard JW. Macrophage diversity enhances tumor progression and metastasis. Cell. (2010) 141:39–51. doi: 10.1016/j.cell.2010.03.014
19. Bosco MC. Macrophage polarization: Reaching across the aisle? J Allergy Clin Immunol. (2019) 143:1348–50. doi: 10.1016/j.jaci.2018.12.995
20. Wang N, Wang S, Wang X, Zheng Y, Yang B, Zhang J, et al. Research trends in pharmacological modulation of tumor-associated macrophages. Clin Transl Med. (2021) 11:e288. doi: 10.1002/ctm2.288
21. Bian Z, Gong Y, Huang T, Lee CZW, Bian L, Bai Z, et al. Deciphering human macrophage development at single-cell resolution. Nature. (2020) 582:571–6. doi: 10.1038/s41586-020-2316-7
22. Ma RY, Black A, Qian BZ. Macrophage diversity in cancer revisited in the era of single-cell omics. Trends Immunol. (2022) 43:546–63. doi: 10.1016/j.it.2022.04.008
23. Pittet MJ, Michielin O, Migliorini D. Clinical relevance of tumour-associated macrophages. Nat Rev Clin Oncol. (2022) 19:402–21. doi: 10.1038/s41571-022-00620-6
24. Pittet MJ, Michielin O, Migliorini D. Author Correction: Clinical relevance of tumour-associated macrophages. Nat Rev Clin Oncol. (2022) 19:424. doi: 10.1038/s41571-022-00632-2
25. Chen D, Zhang X, Li Z, Zhu B. Metabolic regulatory crosstalk between tumor microenvironment and tumor-associated macrophages. Theranostics. (2021) 11:1016–30. doi: 10.7150/thno.51777
26. Park JV, Chandra R, Cai L, Ganguly D, Li H, Toombs JE, et al. Tumor cells modulate macrophage phenotype in a novel in vitro co-culture model of the NSCLC tumor microenvironment. J Thorac Oncol. (2022) 17:1178–91. doi: 10.1016/j.jtho.2022.06.011
27. Zhang C, Wei S, Dai S, Li X, Wang H, Zhang H, et al. The NR_109/FUBP1/c-Myc axis regulates TAM polarization and remodels the tumor microenvironment to promote cancer development. J Immunother Cancer. (2023) 11:e006230. doi: 10.1136/jitc-2022-006230
28. Wen D, Liang T, Chen G, Li H, Wang Z, Wang J, et al. Adipocytes encapsulating telratolimod recruit and polarize tumor-associated macrophages for cancer immunotherapy. Adv Sci (Weinh). (2023) 10:e2206001. doi: 10.1002/advs.202206001
29. Kersten K, Hu KH, Combes AJ, Samad B, Harwin T, Ray A, et al. Spatiotemporal co-dependency between macrophages and exhausted CD8+ T cells in cancer. Cancer Cell. (2022) 40:624–38. doi: 10.1016/j.ccell.2022.05.004
30. Harney AS, Arwert EN, Entenberg D, Wang Y, Guo P, Qian BZ, et al. Real-time imaging reveals local, transient vascular permeability, and tumor cell intravasation stimulated by TIE2hi macrophage-derived VEGFA. Cancer Discovery. (2015) 5:932–43. doi: 10.1158/2159-8290.CD-15-0012
31. Sica A, Bronte V. Altered macrophage differentiation and immune dysfunction in tumor development. J Clin Invest. (2007) 117:1155–66. doi: 10.1172/JCI31422
32. Chen L, Li J, Wang F, Dai C, Wu F, Liu X, et al. Tie2 expression on macrophages is required for blood vessel reconstruction and tumor relapse after chemotherapy. Cancer Res. (2016) 76:6828–38. doi: 10.1158/0008-5472.CAN-16-1114
33. Xiang C, Zhang M, Shang Z, Chen S, Zhao J, Ding B, et al. Single-cell profiling reveals the trajectory of FOLR2-expressing tumor-associated macrophages to regulatory T cells in the progression of lung adenocarcinoma. Cell Death Dis. (2023) 14:493. doi: 10.1038/s41419-023-06021-6
34. Chen S, Saeed A, Liu Q, Jiang Q, Xu H, Xiao GG, et al. Macrophages in immunoregulation and therapeutics. Signal Transduct Target Ther. (2023) 8:207. doi: 10.1038/s41392-023-01452-1
35. Lai CY, Yeh DW, Lu CH, Liu YL, Chuang YC, Ruan JW, et al. Epigenetic silencing of ubiquitin specific protease 4 by snail1 contributes to macrophage-dependent inflammation and therapeutic resistance in lung cancer. Cancers (Basel). (2020) 12:148. doi: 10.3390/cancers12010148
36. Gao J, Ao YQ, Zhang LX, Deng J, Wang S, Wang HK, et al. Exosomal circZNF451 restrains anti-PD1 treatment in lung adenocarcinoma via polarizing macrophages by complexing with TRIM56 and FXR1. J Exp Clin Cancer Res. (2022) 41:295. doi: 10.1186/s13046-022-02505-z
37. Wei K, Zhang H, Yang S, Cui Y, Zhang B, Liu J, et al. Chemo-drugs in cell microparticles reset antitumor activity of macrophages by activating lysosomal P450 and nuclear hnRNPA2B1. Signal Transduct Target Ther. (2023) 8:22. doi: 10.1038/s41392-022-01212-7
38. Eisinger S, Sarhan D, Boura VF, Ibarlucea-Benitez I, Tyystjarvi S, Oliynyk G, et al. Targeting a scavenger receptor on tumor-associated macrophages activates tumor cell killing by natural killer cells. Proc Natl Acad Sci U.S.A. (2020) 117:32005–16. doi: 10.1073/pnas.2015343117
39. Adams R, Osborn G, Mukhia B, Laddach R, Willsmore Z, Chenoweth A, et al. Influencing tumor-associated macrophages in Malignant melanoma with monoclonal antibodies. Oncoimmunology. (2022) 11:2127284. doi: 10.1080/2162402X.2022.2127284
40. Fan H, Xia S, Xiang J, Li Y, Ross MO, Lim SA, et al. Trans-vaccenic acid reprograms CD8+ T cells and anti-tumour immunity. Nature. (2023) 623:1034–43. doi: 10.1038/s41586-023-06749-3
41. Shang Q, Liu W, Leslie F, Yang J, Guo M, Sun M, et al. Nano-formulated delivery of active ingredients from traditional Chinese herbal medicines for cancer immunotherapy. Acta Pharm Sin B. (2024) 14:1525–41. doi: 10.1016/j.apsb.2023.12.008
42. Yao W, Ba Q, Li X, Li H, Zhang S, Yuan Y, et al. A Natural CCR2 Antagonist relieves tumor-associated macrophage-mediated immunosuppression to produce a therapeutic effect for liver cancer. EBioMedicine. (2017) 22:58–67. doi: 10.1016/j.ebiom.2017.07.014
43. Zhang W, Liu X, Cao S, Zhang Q, Chen X, Luo W, et al. Multifunctional redox-responsive nanoplatform with dual activation of macrophages and T cells for antitumor immunotherapy. ACS Nano. (2023) 17:14424–41. doi: 10.1021/acsnano.2c12498
44. Zhao YD, An HW, Mamuti M, Zeng XZ, Zheng R, Yang J, et al. Reprogramming hypoxic tumor-associated macrophages by nanoglycoclusters for boosted cancer immunotherapy. Adv Mater. (2023) 35:e2211332. doi: 10.1002/adma.202211332
45. Qiao G, Li S, Pan X, Xie P, Peng R, Huang X, et al. Surgical tumor-derived nanoplatform targets tumor-associated macrophage for personalized postsurgical cancer immunotherapy. Sci Adv. (2024) 10:eadk7955. doi: 10.1126/sciadv.adk7955
46. Feng S, Zhang Y, Gao Y, Liu Y, Wang Y, Han X, et al. A gene-editable palladium-based bioorthogonal nanoplatform facilitates macrophage phagocytosis for tumor therapy. Angew Chem Int Ed Engl. (2023) 62:e202313968. doi: 10.1002/anie.202313968
47. Tian HX, Mei J, Cao L, Song J, Rong D, Fang M, et al. Disruption of iron homeostasis to induce ferroptosis with albumin-encapsulated Pt(IV) nanodrug for the treatment of non-small cell lung cancer. Small. (2023) 19:e2206688. doi: 10.1002/smll.202206688
48. Jiang SH, Zhu LL, Zhang M, Li RK, Yang Q, Yan JY, et al. GABRP regulates chemokine signalling, macrophage recruitment and tumour progression in pancreatic cancer through tuning KCNN4-mediated Ca(2+) signalling in a GABA-independent manner. Gut. (2019) 68:1994–2006. doi: 10.1136/gutjnl-2018-317479
49. Jin Y, Kang Y, Wang M, Wu B, Su B, Yin H, et al. Targeting polarized phenotype of microglia via IL6/JAK2/STAT3 signaling to reduce NSCLC brain metastasis. Signal Transduct Target Ther. (2022) 7:52. doi: 10.1038/s41392-022-00872-9
50. Tie Y, Zheng H, He Z, Yang J, Shao B, Liu L, et al. Targeting folate receptor beta positive tumor-associated macrophages in lung cancer with a folate-modified liposomal complex. Signal Transduct Target Ther. (2020) 5:6. doi: 10.1038/s41392-020-0115-0
51. Prieto LI, Sturmlechner I, Graves SI, Zhang C, Goplen NP, Yi ES, et al. Senescent alveolar macrophages promote early-stage lung tumorigenesis. Cancer Cell. (2023) 41:1261–75. doi: 10.1016/j.ccell.2023.05.006
52. Haston S, Gonzalez-Gualda E, Morsli S, Ge J, Reen V, Calderwood A, et al. Clearance of senescent macrophages ameliorates tumorigenesis in KRAS-driven lung cancer. Cancer Cell. (2023) 41:1242–60. doi: 10.1016/j.ccell.2023.05.004
53. Park MD, Reyes-Torres I, LeBerichel J, Hamon P, LaMarche NM, Hegde S, et al. TREM2 macrophages drive NK cell paucity and dysfunction in lung cancer. Nat Immunol. (2023) 24:792–801. doi: 10.1038/s41590-023-01475-4
54. Xu R, Lee YJ, Kim CH, Min GH, Kim YB, Park JW, et al. Invasive FoxM1 phosphorylated by PLK1 induces the polarization of tumor-associated macrophages to promote immune escape and metastasis, amplified by IFITM1. J Exp Clin Cancer Res. (2023) 42:302. doi: 10.1186/s13046-023-02872-1
55. Taniguchi S, Matsui T, Kimura K, Funaki S, Miyamoto Y, Uchida Y, et al. In vivo induction of activin A-producing alveolar macrophages supports the progression of lung cell carcinoma. Nat Commun. (2023) 14:143. doi: 10.1038/s41467-022-35701-8
56. Casanova-Acebes M, Dalla E, Leader AM, LeBerichel J, Nikolic J, Morales BM, et al. Tissue-resident macrophages provide a pro-tumorigenic niche to early NSCLC cells. Nature. (2021) 595:578–84. doi: 10.1038/s41586-021-03651-8
57. Park MD, Silvin A, Ginhoux F, Merad M. Macrophages in health and disease. Cell. (2022) 185:4259–79. doi: 10.1016/j.cell.2022.10.007
58. Chen M, Ma P, Zhang Y, Wang D, Yu Z, Fu Y, et al. Divergent tumor and immune cell reprogramming underlying immunotherapy response and immune-related adverse events in lung squamous cell carcinoma. J Immunother Cancer. (2023) 11:e007305. doi: 10.1136/jitc-2023-007305
59. Hu Z, Jin X, Hong W, Sui Q, Zhao M, Huang Y, et al. Dissecting the single-cell transcriptome network of macrophage and identifies a signature to predict prognosis in lung adenocarcinoma. Cell Oncol (Dordr). (2023) 46:1351–68. doi: 10.1007/s13402-023-00816-7
60. Liu L, Wang C, Li S, Qu Y, Xue P, Ma Z, et al. ERO1L Is a Novel and potential biomarker in lung adenocarcinoma and shapes the immune-suppressive tumor microenvironment. Front Immunol. (2021) 12:677169. doi: 10.3389/fimmu.2021.677169
61. Lu J, Li J, Lin Z, Li H, Lou L, Ding W, et al. Reprogramming of TAMs via the STAT3/CD47-SIRPalpha axis promotes acquired resistance to EGFR-TKIs in lung cancer. Cancer Lett. (2023) 564:216205. doi: 10.1016/j.canlet.2023.216205
62. Wang X, Huang R, Lu Z, Wang Z, Chen X, Huang D. Exosomes from M1-polarized macrophages promote apoptosis in lung adenocarcinoma via the miR-181a-5p/ETS1/STK16 axis. Cancer Sci. (2022) 113:986–1001. doi: 10.1111/cas.15268
63. Huggins DN, LaRue RS, Wang Y, Knutson TP, Xu Y, Williams JW, et al. Characterizing macrophage diversity in metastasis-bearing lungs reveals a lipid-associated macrophage subset. Cancer Res. (2021) 81:5284–95. doi: 10.1158/0008-5472.CAN-21-0101
64. Salam R, Saliou A, Bielle F, Bertrand M, Antoniewski C, Carpentier C, et al. Cellular senescence in Malignant cells promotes tumor progression in mouse and patient Glioblastoma. Nat Commun. (2023) 14:441. doi: 10.1038/s41467-023-36124-9
65. Schmitt CA, Wang B, Demaria M. Senescence and cancer - role and therapeutic opportunities. Nat Rev Clin Oncol. (2022) 19:619–36. doi: 10.1038/s41571-022-00668-4
66. Pan Y, Yu Y, Wang X, Zhang T. Tumor-associated macrophages in tumor immunity. Front Immunol. (2020) 11:583084. doi: 10.3389/fimmu.2020.583084
67. Lewis CE, Harney AS, Pollard JW. The multifaceted role of perivascular macrophages in tumors. Cancer Cell. (2016) 30:18–25. doi: 10.1016/j.ccell.2016.05.017
68. Bonnans C, Chou J, Werb Z. Remodelling the extracellular matrix in development and disease. Nat Rev Mol Cell Biol. (2014) 15:786–801. doi: 10.1038/nrm3904
69. Wang S, Wang J, Chen Z, Luo J, Guo W, Sun L, et al. Targeting M2-like tumor-associated macrophages is a potential therapeutic approach to overcome antitumor drug resistance. NPJ Precis Oncol. (2024) 8:31. doi: 10.1038/s41698-024-00522-z
70. Wang H, Wang L, Pan H, Wang Y, Shi M, Yu H, et al. Exosomes derived from macrophages enhance aerobic glycolysis and chemoresistance in lung cancer by stabilizing c-Myc via the inhibition of NEDD4L. Front Cell Dev Biol. (2020) 8:620603. doi: 10.3389/fcell.2020.620603
71. Zhang Y, Liu L, Pei J, Ren Z, Deng Y, Yu K. Tissue factor overexpression promotes resistance to KRAS-G12C inhibition in non-small cell lung cancer. Oncogene. (2024) 43:668–81. doi: 10.1038/s41388-023-02924-y
72. Wan X, Xie B, Sun H, Gu W, Wang C, Deng Q, et al. Exosomes derived from M2 type tumor-associated macrophages promote osimertinib resistance in non-small cell lung cancer through MSTRG.292666.16-miR-6836-5p-MAPK8IP3 axis. Cancer Cell Int. (2022) 22:83. doi: 10.1186/s12935-022-02509-x
73. Yang Z, Xu G, Wang B, Liu Y, Zhang L, Jing T, et al. USP12 downregulation orchestrates a protumourigenic microenvironment and enhances lung tumour resistance to PD-1 blockade. Nat Commun. (2021) 12:4852. doi: 10.1038/s41467-021-25032-5
74. Hu H, Cheng R, Wang Y, Wang X, Wu J, Kong Y, et al. Oncogenic KRAS signaling drives evasion of innate immune surveillance in lung adenocarcinoma by activating CD47. J Clin Invest. (2023) 133:e153470. doi: 10.1172/JCI153470
75. Li F, Huang Q, Luster TA, Hu H, Zhang H, Ng WL, et al. In vivo epigenetic CRISPR screen identifies Asf1a as an immunotherapeutic target in Kras-Mutant lung adenocarcinoma. Cancer Discovery. (2020) 10:270–87. doi: 10.1158/2159-8290.CD-19-0780
76. Qian Y, Galan-Cobo A, Guijarro I, Dang M, Molkentine D, Poteete A, et al. MCT4-dependent lactate secretion suppresses antitumor immunity in LKB1-deficient lung adenocarcinoma. Cancer Cell. (2023) 41:1363–80. doi: 10.1016/j.ccell.2023.05.015
77. Huang Y, Shan G, Yi Y, Liang J, Hu Z, Bi G, et al. FSCN1 induced PTPRF-dependent tumor microenvironment inflammatory reprogramming promotes lung adenocarcinoma progression via regulating macrophagic glycolysis. Cell Oncol (Dordr). (2022) 45:1383–99. doi: 10.1007/s13402-022-00726-0
78. Morrissey SM, Zhang F, Ding C, Montoya-Durango DE, Hu X, Yang C, et al. Tumor-derived exosomes drive immunosuppressive macrophages in a pre-metastatic niche through glycolytic dominant metabolic reprogramming. Cell Metab. (2021) 33:2040–58. doi: 10.1016/j.cmet.2021.09.002
79. Zhao JL, Ye YC, Gao CC, Wang L, Ren KX, Jiang R, et al. Notch-mediated lactate metabolism regulates MDSC development through the Hes1/MCT2/c-Jun axis. Cell Rep. (2022) 38:110451. doi: 10.1016/j.celrep.2022.110451
80. Chen X, Gao A, Zhang F, Yang Z, Wang S, Fang Y, et al. ILT4 inhibition prevents TAM- and dysfunctional T cell-mediated immunosuppression and enhances the efficacy of anti-PD-L1 therapy in NSCLC with EGFR activation. Theranostics. (2021) 11:3392–416. doi: 10.7150/thno.52435
81. Ahirwar DK, Peng B, Charan M, Misri S, Mishra S, Kaul K, et al. Slit2/Robo1 signaling inhibits small-cell lung cancer by targeting beta-catenin signaling in tumor cells and macrophages. Mol Oncol. (2023) 17:839–56. doi: 10.1002/1878-0261.13289
82. Jakab M, Lee KH, Uvarovskii A, Ovchinnikova S, Kulkarni SR, Jakab S, et al. Lung endothelium exploits susceptible tumor cell states to instruct metastatic latency. Nat Cancer. (2024) 5:716–30. doi: 10.1038/s43018-023-00716-7
83. Terekhanova NV, Karpova A, Liang WW, Strzalkowski A, Chen S, Li Y, et al. Epigenetic regulation during cancer transitions across 11 tumour types. Nature. (2023) 623:432–41. doi: 10.1038/s41586-023-06682-5
84. Pan Y, Gu Y, Liu T, Zhang Q, Yang F, Duan L, et al. Epitranscriptic regulation of HRAS by N(6)-methyladenosine drives tumor progression. Proc Natl Acad Sci U.S.A. (2023) 120:e2302291120. doi: 10.1073/pnas.2302291120
85. Parreno V, Loubiere V, Schuettengruber B, Fritsch L, Rawal CC, Erokhin M, et al. Transient loss of Polycomb components induces an epigenetic cancer fate. Nature. (2024) 629:688–96. doi: 10.1038/s41586-024-07328-w
86. Babar Q, Saeed A, Tabish TA, Pricl S, Townley H, Thorat N. Novel epigenetic therapeutic strategies and targets in cancer. Biochim Biophys Acta Mol Basis Dis. (2022) 1868:166552. doi: 10.1016/j.bbadis.2022.166552
87. Feinberg AP, Levchenko A. Epigenetics as a mediator of plasticity in cancer. Science. (2023) 379:eaaw3835. doi: 10.1126/science.aaw3835
88. Sun X, Yi J, Yang J, Han Y, Qian X, Liu Y, et al. An integrated epigenomic-transcriptomic landscape of lung cancer reveals novel methylation driver genes of diagnostic and therapeutic relevance. Theranostics. (2021) 11:5346–64. doi: 10.7150/thno.58385
89. Fehlmann T, Kahraman M, Ludwig N, Backes C, Galata V, Keller V, et al. Evaluating the use of circulating microRNA profiles for lung cancer detection in symptomatic patients. JAMA Oncol. (2020) 6:714–23. doi: 10.1001/jamaoncol.2020.0001
90. Sikosek T, Horos R, Trudzinski F, Jehn J, Frank M, Rajakumar T, et al. Early detection of lung cancer using small RNAs. J Thorac Oncol. (2023) 18:1504–23. doi: 10.1016/j.jtho.2023.07.005
91. Chen J, Zhang K, Zhi Y, Wu Y, Chen B, Bai J, et al. Tumor-derived exosomal miR-19b-3p facilitates M2 macrophage polarization and exosomal LINC00273 secretion to promote lung adenocarcinoma metastasis via Hippo pathway. Clin Transl Med. (2021) 11:e478. doi: 10.1002/ctm2.478
92. Xu L, Wang L, Yang R, Li T, Zhu X. Lung adenocarcinoma cell-derived exosomes promote M2 macrophage polarization through transmission of miR-3153 to activate the JNK signaling pathway. Hum Mol Genet. (2023) 32:2162–76. doi: 10.1093/hmg/ddad052
93. Liu Y, Li L, Song X. Exosomal circPVT1 derived from lung cancer promotes the progression of lung cancer by targeting miR-124-3p/EZH2 axis and regulating macrophage polarization. Cell Cycle. (2022) 21:514–30. doi: 10.1080/15384101.2021.2024997
94. Yao Y, Chen C, Wang J, Xuan H, Chen X, Li Z, et al. Circular RNA circATP9A promotes non-small cell lung cancer progression by interacting with HuR and by promoting extracellular vesicles-mediated macrophage M2 polarization. J Exp Clin Cancer Res. (2023) 42:330. doi: 10.1186/s13046-023-02916-6
95. Gu J, Yang S, Wang X, Wu Y, Wei J, Xu J. Hypoxic lung adenocarcinoma-derived exosomal miR-1290 induces M2 macrophage polarization by targeting SOCS3. Cancer Med. (2023) 12:12639–52. doi: 10.1002/cam4.5954
96. Liang M, Chen X, Wang L, Qin L, Wang H, Sun Z, et al. Cancer-derived exosomal TRIM59 regulates macrophage NLRP3 inflammasome activation to promote lung cancer progression. J Exp Clin Cancer Res. (2020) 39:176. doi: 10.1186/s13046-020-01688-7
97. Rao X, Zhou X, Wang G, Jie X, Xing B, Xu Y, et al. NLRP6 is required for cancer-derived exosome-modified macrophage M2 polarization and promotes metastasis in small cell lung cancer. Cell Death Dis. (2022) 13:891. doi: 10.1038/s41419-022-05336-0
98. Wu JY, Huang TW, Hsieh YT, Wang YF, Yen CC, Lee GL, et al. Cancer-derived succinate promotes macrophage polarization and cancer metastasis via succinate receptor. Mol Cell. (2020) 77:213–27. doi: 10.1016/j.molcel.2019.10.023
99. Kuo IY, Yang YE, Yang PS, Tsai YJ, Tzeng HT, Cheng HC, et al. Converged Rab37/IL-6 trafficking and STAT3/PD-1 transcription axes elicit an immunosuppressive lung tumor microenvironment. Theranostics. (2021) 11:7029–44. doi: 10.7150/thno.60040
100. Cheng H, Janakiram M, Borczuk A, Lin J, Qiu W, Liu H, et al. HHLA2, a new immune checkpoint member of the B7 family, is widely expressed in humanlung cancer and associated with EGFR mutational status. Clin Cancer Res. (2017) 23:825–32. doi: 10.1158/1078-0432.CCR-15-3071
101. Li Y, Lv C, Yu Y, Wu B, Zhang Y, Lang Q, et al. KIR3DL3-HHLA2 and TMIGD2-HHLA2 pathways: The dual role of HHLA2 in immune responses and its potential therapeutic approach for cancer immunotherapy. J Adv Res. (2023) 47:137–50. doi: 10.1016/j.jare.2022.07.013
102. Sun W, Li S, Tang G, Sun S, Luo Y, Bai R, et al. HHLA2 deficiency inhibits non-small cell lung cancer progression and THP-1 macrophage M2 polarization. Cancer Med. (2021) 10:5256–69. doi: 10.1002/cam4.4081
103. Zhang J, Li H, Wu Q, Chen Y, Deng Y, Yang Z, et al. Tumoral NOX4 recruits M2 tumor-associated macrophages via ROS/PI3K signaling-dependent various cytokine production to promote NSCLC growth. Redox Biol. (2019) 22:101116. doi: 10.1016/j.redox.2019.101116
104. He M, Yu W, Chang C, Miyamoto H, Liu X, Jiang K, et al. Estrogen receptor alpha promotes lung cancer cell invasion via increase of and cross-talk with infiltrated macrophages through the CCL2/CCR2/MMP9 and CXCL12/CXCR4 signaling pathways. Mol Oncol. (2020) 14:1779–99. doi: 10.1002/1878-0261.12701
105. Kong X, Bu J, Chen J, Ni B, Fu B, Zhou F, et al. PIGF and Flt-1 on the surface of macrophages induces the production of TGF-beta1 by polarized tumor-associated macrophages to promote lung cancer angiogenesis. Eur J Pharmacol. (2021) 912:174550. doi: 10.1016/j.ejphar.2021.174550
106. Li Z, Chen S, He X, Gong S, Sun L, Weng L. SLC3A2 promotes tumor-associated macrophage polarization through metabolic reprogramming in lung cancer. Cancer Sci. (2023) 114:2306–17. doi: 10.1111/cas.15760
107. Dong Y, Wang G, Nie D, Xu Y, Bai X, Lu C, et al. Tumor-derived GABA promotes lung cancer progression by influencing TAMs polarization and neovascularization. Int Immunopharmacol. (2024) 126:111217. doi: 10.1016/j.intimp.2023.111217
108. Verhoeven Y, Tilborghs S, Jacobs J, De Waele J, Quatannens D, Deben C, et al. The potential and controversy of targeting STAT family members in cancer. Semin Cancer Biol. (2020) 60:41–56. doi: 10.1016/j.semcancer.2019.10.002
109. Shi JH, Liu LN, Song DD, Liu WW, Ling C, Wu FX, et al. TRAF3/STAT6 axis regulates macrophage polarization and tumor progression. Cell Death Differ. (2023) 30:2005–16. doi: 10.1038/s41418-023-01194-1
110. Li YJ, Zhang C, Martincuks A, Herrmann A, Yu H. STAT proteins in cancer: orchestration of metabolism. Nat Rev Cancer. (2023) 23:115–34. doi: 10.1038/s41568-022-00537-3
111. Wong GL, Manore SG, Doheny DL, Lo HW. STAT family of transcription factors in breast cancer: Pathogenesis and therapeutic opportunities and challenges. Semin Cancer Biol. (2022) 86:84–106. doi: 10.1016/j.semcancer.2022.08.003
112. Tang B, Wang Y, Xu W, Zhu J, Weng Q, Chen W, et al. Macrophage xCT deficiency drives immune activation and boosts responses to immune checkpoint blockade in lung cancer. Cancer Lett. (2023) 554:216021. doi: 10.1016/j.canlet.2022.216021
113. Yu T, Gan S, Zhu Q, Dai D, Li N, Wang H, et al. Modulation of M2 macrophage polarization by the crosstalk between Stat6 and Trim24. Nat Commun. (2019) 10:4353. doi: 10.1038/s41467-019-12384-2
114. Fu C, Jiang L, Hao S, Liu Z, Ding S, Zhang W, et al. Activation of the IL-4/STAT6 signaling pathway promotes lung cancer progression by increasing M2 myeloid cells. Front Immunol. (2019) 10:2638. doi: 10.3389/fimmu.2019.02638
115. Chen YC, Young MJ, Chang HP, Liu CY, Lee CC, Tseng YL, et al. Estradiol-mediated inhibition of DNMT1 decreases p53 expression to induce M2-macrophage polarization in lung cancer progression. Oncogenesis. (2022) 11:25. doi: 10.1038/s41389-022-00397-4
116. Ma S, Sun B, Duan S, Han J, Barr T, Zhang J, et al. YTHDF2 orchestrates tumor-associated macrophage reprogramming and controls antitumor immunity through CD8+ T cells. Nat Immunol. (2023) 24:255–66. doi: 10.1038/s41590-022-01398-6
117. Yin H, Zhang X, Yang P, Zhang X, Peng Y, Li D, et al. RNA m6A methylation orchestrates cancer growth and metastasis via macrophage reprogramming. Nat Commun. (2021) 12:1394. doi: 10.1038/s41467-021-21514-8
118. Zhang H, Wang SQ, Hang L, Zhang CF, Wang L, Duan CJ, et al. GRP78 facilitates M2 macrophage polarization and tumour progression. Cell Mol Life Sci. (2021) 78:7709–32. doi: 10.1007/s00018-021-03997-2
119. Nusse R, Clevers H. Wnt/beta-catenin signaling,disease, and emerging therapeutic modalities. Cell. (2017) 169:985–99. doi: 10.1016/j.cell.2017.05.016
120. Kramer ED, Tzetzo SL, Colligan SH, Hensen ML, Brackett CM, Clausen BE, et al. beta-Catenin signaling in alveolar macrophages enhances lung metastasis through a TNF-dependent mechanism. JCI Insight. (2023) 8:e160978. doi: 10.1172/jci.insight.160978
121. Sarode P, Zheng X, Giotopoulou GA, Weigert A, Kuenne C, Gunther S, et al. Reprogramming of tumor-associated macrophages by targeting beta-catenin/FOSL2/ARID5A signaling: A potential treatment of lung cancer. Sci Adv. (2020) 6:eaaz6105. doi: 10.1126/sciadv.aaz6105
122. Shao F, Gao Y, Wang W, He H, Xiao L, Geng X, et al. Silencing EGFR-upregulated expression of CD55 and CD59 activates the complement system and sensitizes lung cancer to checkpoint blockade. Nat Cancer. (2022) 3:1192–210. doi: 10.1038/s43018-022-00444-4
123. Liu M, Tong Z, Ding C, Luo F, Wu S, Wu C, et al. Transcription factor c-Maf is a checkpoint that programs macrophages in lung cancer. J Clin Invest. (2020) 130:2081–96. doi: 10.1172/JCI131335
124. Niu MY, Liu YJ, Shi JJ, Chen RY, Zhang S, Li CY, et al. The emerging role of ubiquitin-specific protease 36 (USP36) in cancer and beyond. Biomolecules. (2024) 14:572. doi: 10.3390/biom14050572
125. Shi ZY, Li CY, Chen RY, Shi JJ, Liu YJ, Lu JF, et al. The emerging role of deubiquitylating enzyme USP21 as a potential therapeutic target in cancer. Bioorg Chem. (2024) 147:107400. doi: 10.1016/j.bioorg.2024.107400
126. Dai X, Lu L, Deng S, Meng J, Wan C, Huang J, et al. USP7 targeting modulates anti-tumor immune response by reprogramming Tumor-associated Macrophages in Lung Cancer. Theranostics. (2020) 10:9332–47. doi: 10.7150/thno.47137
127. Jin Y, Pan Y, Zheng S, Liu Y, Xu J, Peng Y, et al. Inactivation of EGLN3 hydroxylase facilitates Erk3 degradation via autophagy and impedes lung cancer growth. Oncogene. (2022) 41:1752–66. doi: 10.1038/s41388-022-02203-2
128. Karger A, Mansouri S, Leisegang MS, Weigert A, Gunther S, Kuenne C, et al. ADPGK-AS1 long noncoding RNA switches macrophage metabolic and phenotypic state to promote lung cancer growth. EMBO J. (2023) 42:e111620. doi: 10.15252/embj.2022111620
129. Li C, Xue VW, Wang QM, Lian GY, Huang XR, Lee TL, et al. The Mincle/Syk/NF-kappaB signaling circuit is essential for maintaining the protumoral activities of tumor-associated macrophages. Cancer Immunol Res. (2020) 8:1004–17. doi: 10.1158/2326-6066.CIR-19-0782
130. Zeng J, Yang X, Yang L, Li W, Zheng Y. Thymosin beta10 promotes tumor-associated macrophages M2 conversion and proliferation via the PI3K/Akt pathway in lung adenocarcinoma. Respir Res. (2020) 21:328. doi: 10.1186/s12931-020-01587-7
131. Liu H, He R, Yang X, Huang B, Liu H. Mechanism of TCF21 downregulation leading to immunosuppression of tumor-associated macrophages in non-small cell lung cancer. Pharmaceutics. (2023) 15:2295. doi: 10.3390/pharmaceutics15092295
132. Singhal S, Stadanlick J, Annunziata MJ, Rao AS, Bhojnagarwala PS, O'Brien S, et al. Human tumor-associated monocytes/macrophages and their regulation of T cell responses in early-stage lung cancer. Sci Transl Med. (2019) 11:eaat1500. doi: 10.1126/scitranslmed.aat1500
133. Herzog BH, Baer JM, Borcherding N, Kingston NL, Belle JI, Knolhoff BL, et al. Tumor-associated fibrosis impairs immune surveillance and response to immune checkpoint blockade in non-small cell lung cancer. Sci Transl Med. (2023) 15:eadh8005. doi: 10.1126/scitranslmed.adh8005
134. Tang PC, Chung JY, Xue VW, Xiao J, Meng XM, Huang XR, et al. Smad3 promotes cancer-associated fibroblasts generation via macrophage-myofibroblast transition. Adv Sci (Weinh). (2022) 9:e2101235. doi: 10.1002/advs.202101235
135. Wu J, Liu X, Wu J, Lou C, Zhang Q, Chen H, et al. CXCL12 derived from CD248-expressing cancer-associated fibroblasts mediates M2-polarized macrophages to promote nonsmall cell lung cancer progression. Biochim Biophys Acta Mol Basis Dis. (2022) 1868:166521. doi: 10.1016/j.bbadis.2022.166521
136. Hung CN, Chen M, DeArmond DT, Chiu CH, Limboy CA, Tan X, et al. AXL-initiated paracrine activation of pSTAT3 enhances mesenchymal and vasculogenic supportive features of tumor-associated macrophages. Cell Rep. (2023) 42:113067. doi: 10.1016/j.celrep.2023.113067
137. Fortunato O, Borzi C, Milione M, Centonze G, Conte D, Boeri M, et al. Circulating mir-320a promotes immunosuppressive macrophages M2 phenotype associated with lung cancer risk. Int J Cancer. (2019) 144:2746–61. doi: 10.1002/ijc.31988
138. Pan L, Wang B, Chen M, Ma Y, Cui B, Chen Z, et al. Lack of SIRP-alpha reduces lung cancer growth in mice by promoting anti-tumour ability of macrophages and neutrophils. Cell Prolif. (2023) 56:e13361. doi: 10.1111/cpr.13361
139. Ferreira N, Mesquita I, Baltazar F, Silvestre R, Granja S. IL-17A and IL-17F orchestrate macrophages to promote lung cancer. Cell Oncol (Dordr). (2020) 43:643–54. doi: 10.1007/s13402-020-00510-y
140. Zhao CC, Han QJ, Ying HY, Gu XX, Yang N, Li LY, et al. TNFSF15 facilitates differentiation and polarization of macrophages toward M1 phenotype to inhibit tumor growth. Oncoimmunology. (2022) 11:2032918. doi: 10.1080/2162402X.2022.2032918
141. Xia Y, Rao L, Yao H, Wang Z, Ning P, Chen X. Engineering macrophages for cancer immunotherapy and drug delivery. Adv Mater. (2020) 32:e2002054. doi: 10.1002/adma.202002054
142. Yao Z, Zhang J, Zhang B, Liang G, Chen X, Yao F, et al. Imatinib prevents lung cancer metastasis by inhibiting M2-like polarization of macrophages. Pharmacol Res. (2018) 133:121–31. doi: 10.1016/j.phrs.2018.05.002
143. Tariq M, Zhang JQ, Liang GK, He QJ, Ding L, Yang B. Gefitinib inhibits M2-like polarization of tumor-associated macrophages in Lewis lung cancer by targeting the STAT6 signaling pathway. Acta Pharmacol Sin. (2017) 38:1501–11. doi: 10.1038/aps.2017.124
144. Wei YT, Wang XR, Yan C, Huang F, Zhang Y, Liu X, et al. Thymosin alpha-1 reverses M2 polarization of tumor-associated macrophages during efferocytosis. Cancer Res. (2022) 82:1991–2002. doi: 10.1158/0008-5472.CAN-21-4260
145. Cui L, Yang G, Ye J, Yao Y, Lu G, Chen J, et al. Dioscin elicits anti-tumour immunity by inhibiting macrophage M2 polarization via JNK and STAT3 pathways in lung cancer. J Cell Mol Med. (2020) 24:9217–30. doi: 10.1111/jcmm.15563
146. Zhang Z, Bai L, Lu C, Li X, Wu Y, Zhang X, et al. Lapachol inhibits the growth of lung cancer by reversing M2-like macrophage polarization via activating NF-kappaB signaling pathway. Cell Signal. (2023) 112:110902. doi: 10.1016/j.cellsig.2023.110902
147. Fu JL, Hao HF, Wang S, Jiao YN, Li PP, Han SY. Marsdenia tenacissima extract disturbs the interaction between tumor-associated macrophages and non-small cell lung cancer cells by targeting HDGF. J Ethnopharmacol. (2022) 298:115607. doi: 10.1016/j.jep.2022.115607
148. Zhu M, Tang X, Zhu Z, Gong Z, Tang W, Hu Y, et al. STING activation in macrophages by vanillic acid exhibits antineoplastic potential. Biochem Pharmacol. (2023) 213:115618. doi: 10.1016/j.bcp.2023.115618
149. Xiao X, Li Y, Wang Y, Zhang Y, Chen J, Liu W, et al. Dihydroartemisinin inhibits Lewis Lung carcinoma progression by inducing macrophages M1 polarization via AKT/mTOR pathway. Int Immunopharmacol. (2022) 103:108427. doi: 10.1016/j.intimp.2021.108427
150. Park HJ, Chi GY, Choi YH, Park SH. The root bark of Morus alba L. regulates tumor-associated macrophages by blocking recruitment and M2 polarization of macrophages. Phytother Res. (2020) 34:3333–44. doi: 10.1002/ptr.6783
151. Han Z, Liu S, Lin H, Trivett AL, Hannifin S, Yang D, et al. Inhibition of murine hepatoma tumor growth by cryptotanshinone involves TLR7-dependent activation of macrophages and induction of adaptive antitumor immune defenses. Cancer Immunol Immunother. (2019) 68:1073–85. doi: 10.1007/s00262-019-02338-4
152. Cui Y, Luo Y, Qian Q, Tian J, Fang Z, Wang X, et al. Sanguinarine regulates tumor-associated macrophages to prevent lung cancer angiogenesis through the WNT/beta-catenin pathway. Front Oncol. (2022) 12:732860. doi: 10.3389/fonc.2022.732860
153. Xu F, Cui WQ, Wei Y, Cui J, Qiu J, Hu LL, et al. Astragaloside IV inhibits lung cancer progression and metastasis by modulating macrophage polarization through AMPK signaling. J Exp Clin Cancer Res. (2018) 37:207. doi: 10.1186/s13046-018-0878-0
154. He L, Jhong JH, Chen Q, Huang KY, Strittmatter K, Kreuzer J, et al. Global characterization of macrophage polarization mechanisms and identification of M2-type polarization inhibitors. Cell Rep. (2021) 37:109955. doi: 10.1016/j.celrep.2021.109955
155. Hu R, Chen X, Li Z, Zhao G, Ding L, Chen L, et al. Liquid nanoparticles for nanocatalytic cancer therapy. Adv Mater. (2023) 35:e2306469. doi: 10.1002/adma.202306469
156. Zhang C, Tang J, Xie W, Allioux FM, Cao Z, Biazik JM, et al. Mechanistic observation of interactions between macrophages and inorganic particles with different densities. Small. (2023) 19:e2204781. doi: 10.1002/smll.202204781
157. Mitchell MJ, Billingsley MM, Haley RM, Wechsler ME, Peppas NA, Langer R. Engineering precision nanoparticles for drug delivery. Nat Rev Drug Discovery. (2021) 20:101–24. doi: 10.1038/s41573-020-0090-8
158. Jin SM, Yoo YJ, Shin HS, Kim S, Lee SN, Lee CH, et al. A nanoadjuvant that dynamically coordinates innate immune stimuli activation enhances cancer immunotherapy and reduces immune cell exhaustion. Nat Nanotechnol. (2023) 18:390–402. doi: 10.1038/s41565-022-01296-w
159. Liu X, Chen B, Chen J, Wang X, Dai X, Li Y, et al. A cardiac-targeted nanozyme interrupts the inflammation-free radical cycle in myocardial infarction. Adv Mater. (2024) 36:e2308477. doi: 10.1002/adma.202308477
160. Zhu J, Xie R, Gao R, Zhao Y, Yodsanit N, Zhu M, et al. Multimodal nanoimmunotherapy engages neutrophils to eliminate Staphylococcus aureus infections. Nat Nanotechnol. (2024). doi: 10.1038/s41565-024-01648-8
161. Zanganeh S, Hutter G, Spitler R, Lenkov O, Mahmoudi M, Shaw A, et al. Iron oxide nanoparticles inhibit tumour growth by inducing pro-inflammatory macrophage polarization in tumour tissues. Nat Nanotechnol. (2016) 11:986–94. doi: 10.1038/nnano.2016.168
162. Hsieh CH, Hsieh HC, Shih FS, Wang PW, Yang LX, Shieh DB, et al. An innovative NRF2 nano-modulator induces lung cancer ferroptosis and elicits an immunostimulatory tumor microenvironment. Theranostics. (2021) 11:7072–91. doi: 10.7150/thno.57803
163. Sylvestre M, Crane CA, Pun SH. Progress on modulating tumor-associated macrophages with biomaterials. Adv Mater. (2020) 32:e1902007. doi: 10.1002/adma.201902007
164. Yan X, Qi Y, Ren L, Ma J, Xu M, Xia T, et al. Silver nanoclusters show advantages in macrophage tracing in vivo and modulation of anti-tumor immuno-microenvironment. J Control Release. (2022) 348:470–82. doi: 10.1016/j.jconrel.2022.06.006
165. Xu HZ, Li TF, Wang C, Ma Y, Liu Y, Zheng MY, et al. Synergy of nanodiamond-doxorubicin conjugates and PD-L1 blockade effectively turns tumor-associated macrophages against tumor cells. J Nanobiotechnology. (2021) 19:268. doi: 10.1186/s12951-021-01017-w
166. Deng RH, Zou MZ, Zheng D, Peng SY, Liu W, Bai XF, et al. Nanoparticles from cuttlefish ink inhibit tumor growth by synergizing immunotherapy and photothermal therapy. ACS Nano. (2019) 13:8618–29. doi: 10.1021/acsnano.9b02993
167. Liu J, Xiang J, Jin C, Ye L, Wang L, Gao Y, et al. Medicinal plant-derived mtDNA via nanovesicles induces the cGAS-STING pathway to remold tumor-associated macrophages for tumor regression. J Nanobiotechnology. (2023) 21:78. doi: 10.1186/s12951-023-01835-0
168. Feng Q, Fang W, Guo Y, Hu P, Shi J. Nebulized therapy of early orthotopic lung cancer by iron-based nanoparticles: macrophage-regulated ferroptosis of cancer stem cells. J Am Chem Soc. (2023) 145:24153–65. doi: 10.1021/jacs.3c08032
169. Liu Y, Crowe WN, Wang L, Petty WJ, Habib AA, Zhao D. Aerosolized immunotherapeutic nanoparticle inhalation potentiates PD-L1 blockade for locally advanced lung cancer. Nano Res. (2023) 16:5300–10. doi: 10.1007/s12274-022-5205-6
170. Mazinani M, Rahbarizadeh F. New cell sources for CAR-based immunotherapy. biomark Res. (2023) 11:49. doi: 10.1186/s40364-023-00482-9
171. Hadiloo K, Taremi S, Heidari M, Esmaeilzadeh A. The CAR macrophage cells, a novel generation of chimeric antigen-based approach against solid tumors. biomark Res. (2023) 11:103. doi: 10.1186/s40364-023-00537-x
172. Klichinsky M, Ruella M, Shestova O, Lu XM, Best A, Zeeman M, et al. Human chimeric antigen receptor macrophages for cancer immunotherapy. Nat Biotechnol. (2020) 38:947–53. doi: 10.1038/s41587-020-0462-y
173. Pan K, Farrukh H, Chittepu V, Xu H, Pan CX, Zhu Z. CAR race to cancer immunotherapy: from CAR T, CAR NK to CAR macrophage therapy. J Exp Clin Cancer Res. (2022) 41:119. doi: 10.1186/s13046-022-02327-z
174. Chen Y, Yu Z, Tan X, Jiang H, Xu Z, Fang Y, et al. CAR-macrophage: A new immunotherapy candidate against solid tumors. BioMed Pharmacother. (2021) 139:111605. doi: 10.1016/j.biopha.2021.111605
175. Shen J, Lyu S, Xu Y, Zhang S, Li L, Li J, et al. Activating innate immune responses repolarizes hPSC-derived CAR macrophages to improve anti-tumor activity. Cell Stem Cell. (2024) 31:1003–1019.e9. doi: 10.1016/j.stem.2024.04.012
Keywords: LC, TME, TAMs, polarization, NDDSs
Citation: Li W, Yuan Q, Li M, He X, Shen C, Luo Y, Tai Y, Li Y, Deng Z and Luo Y (2024) Research advances on signaling pathways regulating the polarization of tumor-associated macrophages in lung cancer microenvironment. Front. Immunol. 15:1452078. doi: 10.3389/fimmu.2024.1452078
Received: 20 June 2024; Accepted: 17 July 2024;
Published: 31 July 2024.
Edited by:
Guan-Jun Yang, Ningbo University, ChinaReviewed by:
Chen Gong, Huazhong University of Science and Technology, ChinaCopyright © 2024 Li, Yuan, Li, He, Shen, Luo, Tai, Li, Deng and Luo. This is an open-access article distributed under the terms of the Creative Commons Attribution License (CC BY). The use, distribution or reproduction in other forums is permitted, provided the original author(s) and the copyright owner(s) are credited and that the original publication in this journal is cited, in accordance with accepted academic practice. No use, distribution or reproduction is permitted which does not comply with these terms.
*Correspondence: Yao Luo, bHVveWFvQHNjdS5lZHUuY24=; Zhiping Deng, ZGVuZ3pwMTAxNkAxNjMuY29t; Yi Li, bGl5aTEyQHNjdS5lZHUuY24=
†These authors have contributed equally to this work and share first authorship
Disclaimer: All claims expressed in this article are solely those of the authors and do not necessarily represent those of their affiliated organizations, or those of the publisher, the editors and the reviewers. Any product that may be evaluated in this article or claim that may be made by its manufacturer is not guaranteed or endorsed by the publisher.
Research integrity at Frontiers
Learn more about the work of our research integrity team to safeguard the quality of each article we publish.