- 1Human Longevity Program, IRCCS San Raffaele Roma, Rome, Italy
- 2Department of Molecular Medicine, Sapienza University of Rome, Rome, Italy
- 3Department of Neuroscience, Università Cattolica del Sacro Cuore, Rome, Italy
- 4Fondazione Policlinico Universitario A. Gemelli IRCCS, Rome, Italy
- 5Department of Human Sciences and Quality of Life Promotion, Università telematica San Raffaele, Rome, Italy
In recent years, significant advancements have been made in utilizing nanoparticles (NPs) to modulate immune responses within the central nervous system (CNS), offering new opportunities for nanotherapeutic interventions in neurological disorders. NPs can serve as carriers for immunomodulatory agents or platforms for delivering nucleic acid-based therapeutics to regulate gene expression and modulate immune responses. Several studies have demonstrated the efficacy of NP-mediated immune modulation in preclinical models of neurological diseases, including multiple sclerosis, stroke, Alzheimer’s disease, and Parkinson’s disease. While challenges remain, advancements in NPs engineering and design have led to the development of NPs using diverse strategies to overcome these challenges. The nano-bio interface with the immune system is key in the conceptualization of NPs to efficiently act as nanotherapeutics in the CNS. The biomolecular corona plays a pivotal role in dictating NPs behavior and immune recognition within the CNS, giving researchers the opportunity to optimize NPs design and surface modifications to minimize immunogenicity and enhance biocompatibility. Here, we review how NPs interact with the CNS immune system, focusing on immunosurveillance of NPs, NP-induced immune reprogramming and the impact of the biomolecular corona on NPs behavior in CNS immune responses. The integration of NPs into CNS nanotherapeutics offers promising opportunities for addressing the complex challenges of acute and chronic neurological conditions and pathologies, also in the context of preventive and rehabilitative medicine. By harnessing the nano-bio immune interface and understanding the significance of the biomolecular corona, researchers can develop targeted, safe, and effective nanotherapeutic interventions for a wide range of CNS disorders to improve treatment and rehabilitation. These advancements have the potential to revolutionize the treatment landscape of neurological diseases, offering promising solutions for improved patient care and quality of life in the future.
1 Introduction
The central nervous system (CNS) has traditionally been considered a privileged immune system due to the presence of the blood-brain barrier (BBB) and the absence of traditional lymphatic drainage (1). However, it is now recognized that the CNS harbors a complex immune system comprising resident microglia, astrocytes, and infiltrating immune cells (2). These cells play essential roles in immune surveillance, neuroprotection, and neuroinflammation, influencing both physiological and pathological processes in the brain (3). Understanding the neuroimmune system is critical, as it underlies various physiological processes and plays a central role in health and disease (4).
Nanotherapeutics, the application of nanotechnology in therapeutic interventions, has emerged as a promising approach for addressing the complexities of treating CNS disorders (5, 6). The unique challenges inherent in delivering therapeutic agents to the CNS, such as the BBB’s selective permeability and the delicate microenvironment of neural tissues, have long hindered traditional drug delivery methods (7). Nanoparticles (NPs), with their customizable properties and nanoscale dimensions, offer a solution to this challenge (8). Engineered to carry therapeutic payloads across biological barriers and precisely target diseased cells or tissues, NPs hold great potential for revolutionizing CNS therapeutics (8). Their ability to navigate through the BBB, achieve sustained drug release, and minimize systemic side effects presents a paradigm shift in the treatment of CNS disorders (8, 9).
Understanding the intricate interplay between the immune system and NPs is paramount for the development of effective nanotherapeutic interventions (7, 8). The immune system in the CNS presents both challenges and opportunities for tailored nanotherapeutic approaches that account for the unique immunological landscape of the CNS (6, 7, 10). By elucidating the intricacies of immune responses within the CNS and their implications for NPs interactions at the nano-bio interface, it is possible to develop strategies aiming at enhancing drug delivery efficiency, minimizing immune-mediated clearance, and modulating immune responses for therapeutic benefit (7).
The nano-bio interface in the CNS is critical for developing NP engineering strategies that exploit or evade the immune system to enact therapeutic benefits (11, 12). Personalized NP engineering offers promise in precision medicine for CNS disorders, enabling targeted drug delivery, immunomodulation, and neural repair (13). By exploiting immune cell targeting pathways, NPs can selectively deliver therapeutic agents to diseased CNS tissues, enhancing therapeutic efficacy (14). Biomimetic NPs, mimicking natural extracellular vesicles like exosomes, can evade immune surveillance, facilitating efficient delivery of therapeutic payloads within the CNS (14). Studies have shown that NPs designed to evade immune detection or specifically target immune cells can significantly alter the inflammatory landscape in the CNS, impacting both therapeutic outcomes and potential side effects (15–20).
Within this frame, we summarized the key pathways involved in brain immune system activation emphasizing the crosstalk among different cell types (Figure 1). Additionally, we examine the dynamic interactions between NPs and the CNS immune system, including how NP properties influence immune recognition and surveillance and immune system reprogramming. We further investigate the formation and impact of the biomolecular corona of NPs, assessing its effects on NP behavior, and the nano-bio interface in the immune responses within the CNS. Through these objectives, we provide the latest insights that are more crucial for developing effective nanotherapies for the CNS.
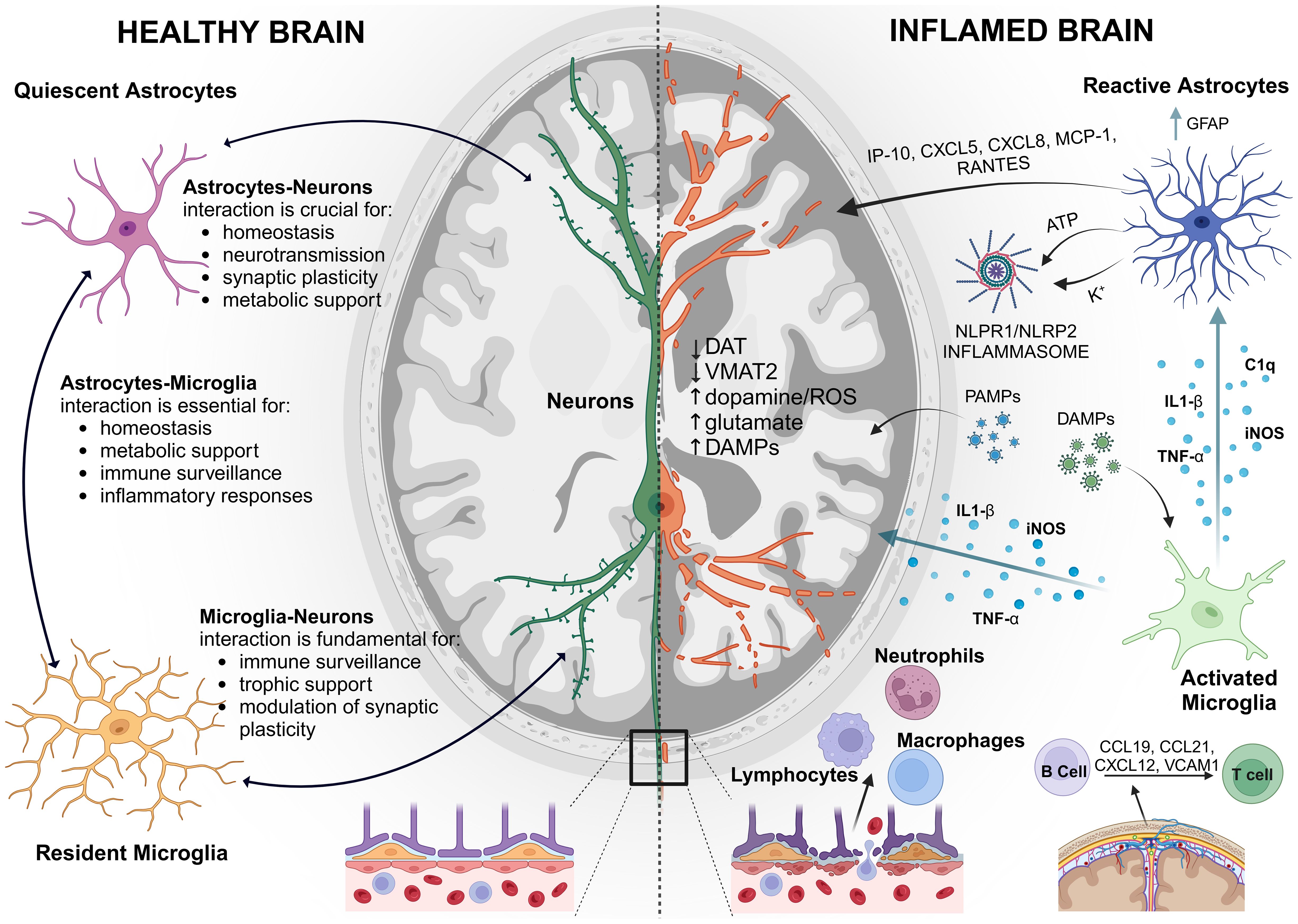
Figure 1. Schematic representation of the Neuroimmune System: On the left, a healthy brain with balanced immune activity. On the right, an “inflamed brain” showing activated microglia and astrocytes and exacerbated immune response, highlighting the brain’s defense mechanisms against injury and disease. GFAP, glial fibrillary acidic protein; IP-10, interferon-c-Inducible Protein; MCP-1, Monocyte chemotactic protein 1; K+, potassium ion; DAT, dopamine transporter; VMAT1, vesicular monoamine transporter type 1; VMAT2, vesicular monoamine transporter type 2; ROS, reactive oxygen species; C1q, complement component subunit 1q; iNOS, inducible nitric oxide synthase; TNF-α, tumor necrosis factor-α; IL-1β, interleukin-1 β; NLRP1/NLRP2, NOD-like receptors. Created with BioRender.com.
2 Nanoparticles and the immune system in the CNS
2.1 The immune context of the CNS
2.1.1 The blood-brain barrier and cellular constituents of the CNS immune system
The BBB is a dynamic and complex interface that separates the circulating blood from the brain parenchyma and regulates the exchange of molecules between the bloodstream and the brain. At its core are the brain microvascular endothelial cells, which form the primary barrier interface. Surrounding the endothelial cells are pericytes, which provide structural support and regulate blood flow. Astrocytic endfeet ensheath the microvessels and release signaling molecules that modulate BBB integrity and function. Additionally, the basement membrane, composed of extracellular matrix proteins, provides structural support and contributes to barrier properties (21).
A variety of cellular and molecular mechanisms adapt BBB to changing physiological conditions and protect the brain from insults. Crosstalk between the BBB and neuroimmune system plays a crucial role in orchestrating immune responses within the CNS and regulating BBB integrity under physiological and pathological conditions (22). In response to immune challenges or neuroinflammation, the BBB undergoes dynamic alterations, facilitating immune cell recruitment and cytokine signaling. Microglia, the resident immune cells of the CNS, survey brain parenchyma, monitoring for signs of injury, infection, or aberrant neuronal activity. Microglia exhibit a spectrum of activation states, ranging from surveillant to pro-inflammatory or anti-inflammatory phenotypes, depending on the microenvironmental cues (23). Astrocytes, traditionally viewed as support cells, also contribute to CNS immunity by releasing cytokines, chemokines, and growth factors in response to immune stimuli (24). In addition to resident cells, the CNS immune system includes infiltrating immune cells, such as T cells, B cells, and monocytes, which can enter the CNS during inflammatory or pathological conditions (25).
On the other side, immune mediators released by activated microglia or astrocytes can modulate BBB permeability and endothelial function, affecting CNS homeostasis (26). Dysfunction of the BBB has been implicated in the pathogenesis of various neurological disorders, including neurodegenerative diseases, cerebrovascular diseases and neuroinflammatory disorders. In Alzheimer’s disease (AD), disruption of the BBB leads to the accumulation of amyloid-beta plaques and neuroinflammation, contributing to disease progression (27). In stroke, ischemic injury and inflammation compromise BBB integrity, exacerbating neuronal damage and edema formation. In multiple sclerosis, immune-mediated damage to the BBB allows infiltration of autoreactive lymphocytes into the CNS, leading to demyelination and neurodegeneration (28). In neuroinflammation induced by low-dose lipopolysaccharide crosstalk between neutrophils and microglia occurs, through the brain blood vessel (29).
2.1.2 Microglia: the resident immune sentinels
Microglia are the resident immune cells of the CNS, comprising approximately 5-10% of the total glial cell population. Historically viewed as passive responders to injury and infection, microglia are now recognized as dynamic and multifunctional cells that actively survey the CNS microenvironment and respond to various stimuli. In addition to their immune surveillance role, microglia actively participate in synaptic pruning, neurogenesis, and synaptic plasticity, contributing to the refinement of neuronal circuits during development and adulthood (30, 31). Microglia exhibit a ramified morphology characterized by a small soma and highly dynamic processes that continuously survey the surrounding microenvironment. Under homeostatic conditions, microglia establish a non-overlapping territory within the CNS, allowing them to efficiently monitor their local environment for signs of damage, infection, or aberrant neuronal activity. Under pathological conditions, microglia undergo activation and morphological changes, retracting their processes and adopting an amoeboid-like shape to migrate towards the site of injury or inflammation (32). Microglia express a diverse array of pattern recognition receptors (PRRs), including Toll-like receptors (TLRs), NOD-like receptors (NLRs), and RIG-I-like receptors (RLRs), which recognize pathogen-associated molecular patterns (PAMPs) or damage-associated molecular patterns (DAMPs) (33). Upon ligand binding, these receptors trigger intracellular signaling cascades, leading to the activation of transcription factors such as nuclear factor kappa B (NF-κB) and interferon regulatory factors (IRFs), and the subsequent production of pro-inflammatory cytokines, chemokines, and reactive oxygen species (ROS) (34). The NF-κB-pathway is a central regulator of inflammatory gene expression, promoting the transcription of pro-inflammatory cytokines, such as tumor necrosis factor-α (TNF-α), interleukin-1 β (IL-1β) and inducible nitric oxide synthase (iNOS). Similarly, the mitogen-activated protein kinase (MAPK) pathway, including ERK, JNK and p38 MAPK, mediates microglial activation and cytokine production in response to extracellular stimuli (35). Moreover, microglial inflammasome activation has been implicated in the pathogenesis of neuroinflammatory diseases, contributing to neurodegeneration and neuronal dysfunction. Dysregulated inflammasome signaling may result from mitochondrial dysfunction, ROS generation, or the accumulation of misfolded proteins (36).
Dysregulated microglial activation is also a hallmark of various neurological disorders, including AD, Parkinson’s disease (PD), and multiple sclerosis, where sustained neuroinflammation exacerbates neuronal damage and contributes to disease progression (37, 38).
2.1.3 Astrocytes: guardians of CNS homeostasis
Astrocytes, the most abundant glial cells in the CNS are involved in several aspects of brain physiology and pathology, given their prominent role in homeostatic control of brain milieu (39). As such, although they are not considered immune cells, their role in the inflammatory response is well recognized.
Reactive astrogliosis is generally associated with microglial reactivity and leukocyte recruitment, under several pathological conditions characterized by neuroinflammation, including stroke (40), AD (41), PD (42) and brain senescence (43).
Reactive astrogliosis is a complex process which, depending on the severity of the injury, includes variable changes in gene expression, increased cellular proliferation and hypertrophy, and in severe cases leads to scar formation; the process initially can gain many beneficial functions in order to support neural tissue recovery, restore brain homeostasis, and limit tissue damage, but it can also have detrimental effects on neuron survival and axon regeneration.
Increased expression of glial fibrillary acidic protein (GFAP) and hypertrophy are prominent features of reactive astrocytes, yet not sufficient to unequivocally define the reactive state or phenotype. Seemingly, the dichotomy “A1” as neurotoxic and “A2” as neuroprotective phenotype has been gradually replaced by a more complex and dynamic feature, which implies molecular expression patterns and functional changes that might be even different depending on brain region and pathology (44).
In the context of neuroinflammation, activated microglia secrete pro-inflammatory mediators among which, TNF-α, IL-1α and complement component subunit 1q (C1q), which have been involved in astrocytes activation through the NF-κB signaling pathway, upregulating wide array of inflammatory response genes, with a crucial role attributed to complement component 3 (C3) (45, 46). A major pro-inflammatory pathway that seems to be activated in microglia-primed reactive astrocytes, involves the phosphorylation of IκBα promoting its dissociation from its complex with p50 and p65 allowing their phosphorylation and translocation from the cytosol to the nucleus to influence expression pro-inflammatory transcripts (47). Indeed, secretory astrocytes release pro-inflammatory chemokines such as interferon-c-Inducible Protein (IP)-10, CXCL5, CXCL8, Monocyte chemotactic protein1 (MCP-1), GRO-a, and RANTES, and cytokines such as IL-6, IL-1β, and TNF-α, granulocyte and macrophage colony stimulating factor (GMCSF), which, in turn, reinforce microglia to remain in an activated state in the context of bi-directional pro-inflammatory communication (48).
It has been demonstrated that also astrocytes express PRRs and other receptors involved in the detection of DAMPs, released by injured tissue, making them capable of detecting signals of brain damage (49), as canonical effectors cells of innate immunity. It is known that the activation of the different PRRs leads to transcriptional (cytokines and interferon genes) and non-transcriptional innate immune responses such as the induction of phagocytosis, autophagy, cell death, and cytokine processing (50). Specifically, astrocytes express an inflammasome that comprises the PRR, NOD-like receptors NLRP1/NALP2, the adaptor protein ASC and caspase-1 (51), which is necessary for enzymatic cleavage and maturation of the precursor cytokines pro-IL-1β and pro-IL-18 (52).
A model of inflammasome activation in the CNS has been proposed with ATP being released from dying cells after and activating P2X4. Once activated P2X4 would release potassium causing the opening of the pannexin-1 channel in neurons and astrocytes, leading to further release of ATP, contributing to the opening of the P2X7 receptor and subsequent activation of inflammasomes (49, 53).
The continuous research uncovering the mechanism of astrocyte activation in the complex landscape of neuroinflammation is of the utmost importance to pave the way for novel therapeutic approaches designed against astrocyte-specific targets.
2.1.4 Neurons: orchestrators of immune responses
Traditionally viewed as the primary mediators of electrical signaling in the nervous system, neurons are now recognized also as active participants in immune regulation within the CNS. Neurons express a diverse array of immune-related molecules, including cytokine receptors, PRRs, and major histocompatibility complex (MHC) proteins, enabling them to detect and respond to immune signals (54). For instance, neurons can sense the presence of pro-inflammatory cytokines released by activated microglia and astrocytes, triggering intracellular signaling cascades that modulate neuronal excitability and synaptic transmission (55). Additionally, the expression of MHC class I molecules on neurons allows them to present antigens to cytotoxic T cells, thereby participating in immune surveillance and immune-mediated neuroprotection (56).
Another important aspect of the neurons is the profound effects on glial cell function, influencing their activation states and inflammatory responses. The recruitment and activation of glial cells during neuroinflammation are intricately regulated by the release of neurotransmitters from neurons, such as glutamate, GABA, and extracellular nucleotides like ATP and UDP (57). In fact, GABA and glycine have been shown to decrease the secretion of several cytokines induced by lipopolysaccharide and to attenuate the phagocytic activity of microglia (58). Glutamate release from neurons can exert dual effects on microglial activation; it can either inhibit microglia activation via metabotropic receptors or mediate glial activation through AMPA and kainate ionotropic receptors (59). Furthermore, activation of α7 nicotinic acetylcholine receptors has been shown to downregulate the release of pro-inflammatory cytokines such as IL-6 and TNF-α (60). Additionally, it promotes the increased expression of glial cell-derived neurotrophic factor (GDNF) (61) and enhances glutamate uptake via the glutamate/aspartate transporter (GLAST) (62), suggesting a neuroprotective role for acetylcholine through the inhibition of microglia. Acetylcholine has been also found to elevate intracellular Ca2+ levels, thereby triggering glutamate release, which subsequently modulates GABAergic transmission in astrocytes (63), influencing network oscillations. Moreover, cholinergic transmission impacts satellite glia, enabling them to support and enwrap sensory peripheral neurons that were previously lacking nerve growth factor (64). In turn, glial cells can modulate neuronal excitability and synaptic transmission through the release of inflammatory mediators, highlighting the dynamic interplay between neurons and glia in CNS immune regulation (65). Dysregulation of neuronal-immune interactions has been implicated in the pathogenesis of neuroinflammatory diseases, including multiple sclerosis (MS), AD, and PD (66).
2.1.5 Peripheral immune cells: infiltrators and regulators. Leukocyte movement, surveillance, and penetration of the CNS macrophages and other myeloid cells
The migration of myeloid cells into the CNS occurs primarily in the context of injury or inflammation. Monocyte-derived cells are among the most abundant infiltrators in murine models of neuroinflammation, with their number correlating with axonal damage also in the brain biopsy of multiple sclerosis patients. These cells enter the CNS by rolling along activated endothelium, firmly adhering to cerebral vessels, then migrating into the subarachnoid space and parenchyma at sites of damage or neuroinflammation. Upon entering the subarachnoid space, activated monocytes differentiate into macrophages, presenting antigens to T cells, which in turn secrete cytokines, further activating brain inflammation. The phenotype of infiltrating macrophages evolves during neuroinflammation, transitioning from an inflammatory phase to a resolution phase. Immune surveillance of the brain by these monocytes is proposed to involve integrin LFA-1 for rolling and chemokines CCR2 and CX3CR1 for rapid tissue invasion (67). Mice lacking MCP-1, a ligand for CCR2, showed significantly reduced inflammation in EAE. CX3CR1 is vital not only for monocyte and myeloid cell trafficking into the CNS but also highly expressed on natural killer (NK) cells and lymphocytes entering the CNS. However, little is known about myeloid cell entry sites into the CNS, their source, and the mechanisms establishing residency at homeostasis (68).
Recent studies reveal that many monocytes and neutrophils residing in the brain and spinal cord originate not from blood but from CNS-associated bone marrow. These cells migrate from cranial bone marrow to meninges through microscopic vascular channels. Their differentiation resulted from local populations and not from peripheral tissue progenitors. CNS-associated bone marrow-derived monocytes exhibit fewer inflammatory traits compared to blood-derived ones, suggesting intrinsic differences and specialization for CNS function (69).
2.1.5.1 B Cells
The significance of B cell interaction in neuroinflammatory diseases is evident in successful B cell depletion therapy for treating CNS diseases (70). In MS the number of B cells increases in CNS compartments with evidence of B cell migration and ectopic lymphoid tissue formation via the CXCL13-CXCR5 axis (71). The CXCL13 expression in intrameningeal follicles, active lesions, and cerebrospinal fluid (CSF) attracts CXCR5-expressing B cells to form ectopic lymphoid follicles. Other chemokines like CCL19, CCL21, CXCL12, and adhesion molecules VCAM1 are induced during inflammation, aiding B cell trafficking into ectopic lymphoid tissues. The B cells reactivate auto-reactive T cells by cytokine secretion or act as antigen-presenting cells to induce T cell differentiation into pathological condition. Abnormal cytokine production by B cells from MS patients suggests their immunomodulatory role via cytokine signaling.
Less is known about B cell trafficking into non-diseased brain and meninges. Recent studies indicate that meningeal B cells in mice primarily derive from local bone marrow and minimally from blood. Specialized vascular channels facilitate their migration from cranial bone marrow to meninges. Dura B cells exhibit tissue-resident characteristics, with evidence of response to neuroinflammation. Gut microbiota influences autoreactive B and T cell recruitment in EAE, suggesting a gut-brain axis in CNS inflammatory disease regulation (72).
2.1.5.2 T Cells
Tissue-resident T cells are found in meninges, CSF, and parenchyma in humans and rodents. These T cells exhibit unique transcriptional profiles and increased functional capacity for certain cytokines, influencing neurological function. CNS T cells contribute to CNS homeostasis and can modulate neurotransmitter precursor availability. Meningeal T cells, for instance, affect social behavior and memory formation via cytokine signaling. CD4 and CD8 T cells in the brain express tissue-resident markers and cytokines, with potential roles in modulating synaptic plasticity and social behavior (73). Human parenchymal T cells also display a tissue-resident phenotype, with implications for CNS immunity and neuroregulation (74). The interactions between CNS T cells and microglia are vital for neurodevelopment and homeostasis, although mechanisms remain unclear.
2.2 Nanoparticles in the immune nano-bio interface
2.2.1 Nanoparticles
NPs are synthetic structures that possess unique physical and chemical properties due to their high surface area to volume ratio (75). Recently, NPs have become a significant trend in drug delivery, particularly as nanotherapeutics, due to their ability to enhance the solubility, stability, and bioavailability of drugs. They offer targeted delivery, controlled release, and reduced side effects, making them ideal for treating various diseases. NPs show great promise for treating CNS disorders due to their ability to cross the BBB, a major obstacle in delivering therapeutics to the brain (9, 76, 77). The BBB restricts the entry of various large molecules and therapeutic agents into the brain, but NPs can traverse this barrier through various mechanisms, including by passive transport, such as paracellular pathways between tight junctions and transcellular pathways, and by active transport, such as carrier-mediated transcytosis, receptor-mediated transcytosis, adsorptive mediated transcytosis and disruptive mediated transport (78). This capability offers potential breakthroughs in treating conditions such as AD, PD, and brain tumors (9, 76, 77). By successfully crossing the BBB, NPs can reach the CNS and interact with the neuroimmune environment, to improve rehabilitation and treatment of various acute and chronic CNS conditions to restore proper function.
Many types of organic and inorganic materials can be employed to construct NPs for medical applications, including lipids, polymers, metals and carbon according to the specific applications (75). Synthetic NPs include metallic and polymeric varieties. Polymeric NPs, for example, offer versatility in both design and functionality. Protein-based NPs, such as those constructed from ferritin, albumin, or collagen, can be engineered to display surface ligands that specifically target and interact with immune cells (79). Metallic NPs, such as those based on gold or silver, possess unique optical and physicochemical properties, making them useful in drug delivery and diagnostic applications (75, 80).
On the other hand, biomimetic NPs, such as lipid-based liposomes, cell-membrane-derived, and ghost NPs, mimic natural biological structures (81, 82). Extracellular vesicles (EVs) are natural lipid vesicles secreted by cells into the extracellular space (83, 84). There are three major types of EVs, including exosomes, microvesicles, and apoptotic bodies, which are distinguished based upon their biogenesis, release mechanisms, size, and function (83–85). Among these membrane vesicles, the role of exosomes in theragnostic research has been rapidly growing over the last two decades due to their small size (between 30 and 150 nm), their biocompatibility and inherent targeting capabilities. Inspired by these natural structures, biomimetic NPs offer unique advantages for immunomodulation (81, 86, 87). For example, lipid-based NPs, including liposomes, are capable of encapsulating and delivering various immunomodulatory agents, such as cytokines, antibodies, or small interfering RNAs (88, 89).
Furthermore, cell-derived NPs can be derived from the membranes of various cell types, such as immune cells, stem cells, or even cancer cells (90). They can carry specific surface proteins and signaling molecules that can interact with and modulate the function of immune cells. By incorporating biomimetic cues and surface modifications, polymeric NPs can target specific immune cells and modulate their activity (75, 91). Another class of biomimetic NPs includes Nano-Ghosts, which are technologically reconstructed using the cytoplasmic membranes, such as of mesenchymal stem cells, and are able to maintain the orientation composition, and function of the mesenchymal stem cell membrane as an inert particle. Notably, these Nano-Ghosts maintain the ability to selectively target and penetrate tissues of interest while being quickly cleared from other organs, with minimal off-target effects (92–95).
2.2.2 Immunosurveillance of nanoparticles in the CNS
2.2.2.1 Immunosurveillance of nanoparticles
In the CNS, NPs encounter a sophisticated immunosurveillance system involving all the immune cells previously described (Figure 2) (10, 96). Microglia, as the resident immune cells of the CNS, play a crucial role in monitoring the brain environment for foreign substances, including NPs, through pattern recognition receptors like Toll-like receptors (TLRs) (97). Upon NP entry into the CNS, microglia can recognize and phagocytose NPs, influencing their clearance and distribution within the CNS (97). Astrocytes also contribute significantly to immunosurveillance by detecting NPs through various receptors and triggering cellular responses like cytokine release (45, 98). Neurons, although not traditional immune cells, interact with NPs, impacting neuronal health and function through the modulation of electrical activity and synaptic transmission (99, 100). Finally, infiltrating immune cells like macrophages, dendritic cells, and lymphocytes participate in the immunosurveillance of NPs within the CNS, recognizing NPs through receptors like TLRs and scavenger receptors (101).
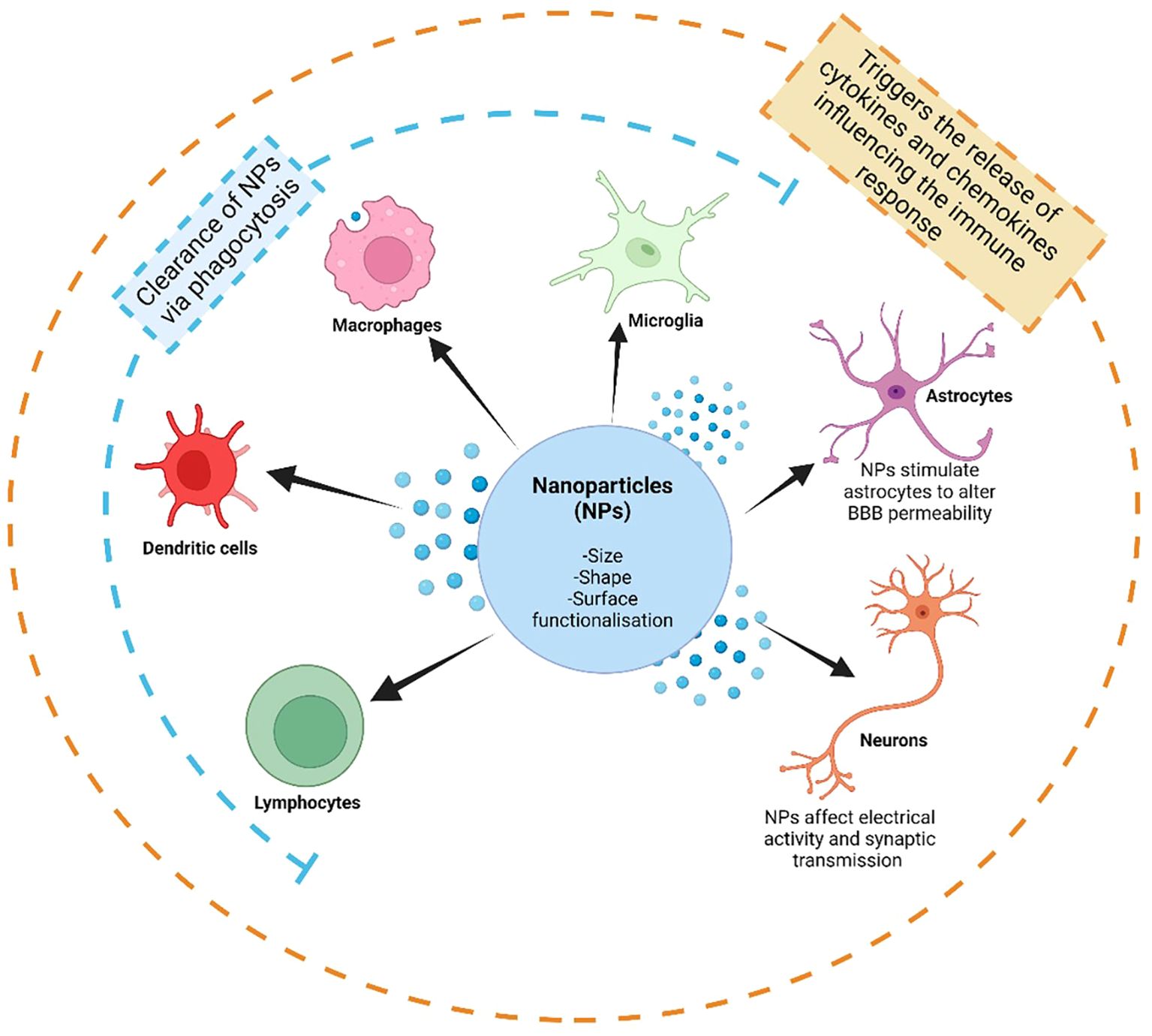
Figure 2. The immunosurveillance of nanoparticles (NPs) by the immune system in the central nervous system (CNS). The immune microenvironment in the CNS is dictated by microglia, the resident immune cell in the CNS, and interactions with astrocytes and neurons, as well as by infiltrating peripheral immune cells, including lymphocytes (such as T-cells and B-cells), dendritic cells and macrophages. The interactions between NPs and the immune system are influenced by the size, shape and surface functionalization and modifications of NPs. These interactions may cause the clearance of NPs via phagocytosis by certain immune cell types, as well as by stimulating many cell types to trigger further interactions, such as the release of cytokines and chemokines. These interactions, especially with astrocytes, may affect the regulation of blood brain barrier (BBB) permeability, and in turn further influencing the amount of infiltrating peripheral immune cells. Created with BioRender.com.
The immunosurveillance of NPs in the CNS poses challenges to their efficiency, affecting their fate, biodistribution, and immunomodulatory effects (10, 96). Infiltrating immune cells like macrophages, dendritic cells, and lymphocytes can recognize NPs through various receptors, leading to phagocytosis and clearance, especially during inflammation or injury when the BBB becomes permeable (10, 102, 103). Phagocytosis, a process executed by immune cells like macrophages, neutrophils, and monocytes, is influenced by particle geometry, with anisotropic shapes resisting phagocytosis longer than spherical ones (104). The physicochemical properties of NPs, such as size, shape, and surface functionalization, play a crucial role in determining their interactions with immune cells and subsequent immunomodulatory effects (10, 105, 106).
Size and shape are important factors governing NP immunosurveillance (11). Smaller NPs tend to evade immune detection, and those smaller than 200 nm are considered theoretically small enough to cross the BBB (11, 107). Moreover, the shape of NPs has been shown to affect their interactions with the immune system (108–110). For example, gold nanorods are more efficiently internalized by macrophages than nanospheres due to the generation of large vesicles that facilitate entry through macropinocytosis (111). Moreover, nanorods, of aluminum oxyhydroxide nanoparticles, were found to activate the NLRP3 inflammasome, leading to IL-1β production in THP-1 cells and bone marrow-derived dendritic cells (112). Nanorods with higher aspect ratios also reduced phagocytosis and enhanced cytokine secretion, such as IL-6 and IFN-γ (113). Furthermore, it has been shown that while spherical and star-like gold NPs accumulated similarly in the liver, only the star-shaped NPs were able to localize in the lungs (114). Moreover, TiO2 microparticles adorned with nanospikes affects the interaction of the NPs with the innate immune system. During phagocytosis, the nanospikes exert mechanical stress on cell membranes, triggering potassium efflux and inflammasome activation in a caspase-1- and NLRP3-dependent manner. This mechanical stress, combined with TLR4 pathway activation, enhances dendritic cell maturation and significantly boosts both T-cell and humoral immune responses (109).
2.2.2.2 Strategies to overcome NP sequestration by the immune system
As summarized in Table 1, multiple strategies have been developed in order to prevent NP sequestration by the immune system. Size, shape and surface optimization of NPs can minimize immune recognition and maximize tissue penetration, enabling efficient delivery of therapeutic payloads (142), either evading immune recognition and clearance, or prolonging circulation time and tissue accumulation within the CNS (143). Functionalizing NP surface with biocompatible polymers like polyethylene glycol (PEG) is widely regarded as the most effective strategy for protecting NPs from immune clearance and enhancing their bioavailability in the CNS (115). However, undesirable immune-related adverse effects have been linked to the presence of PEG on drug delivery vectors (144). For example, activation of the complement system, hypersensitivity reactions, and accelerated blood clearance (ABC) upon repeated administration are believed to be triggered by the generation of anti-PEG antibodies (116, 145). These antibodies subsequently facilitate the recognition of PEGylated NPs by the immune system (146).
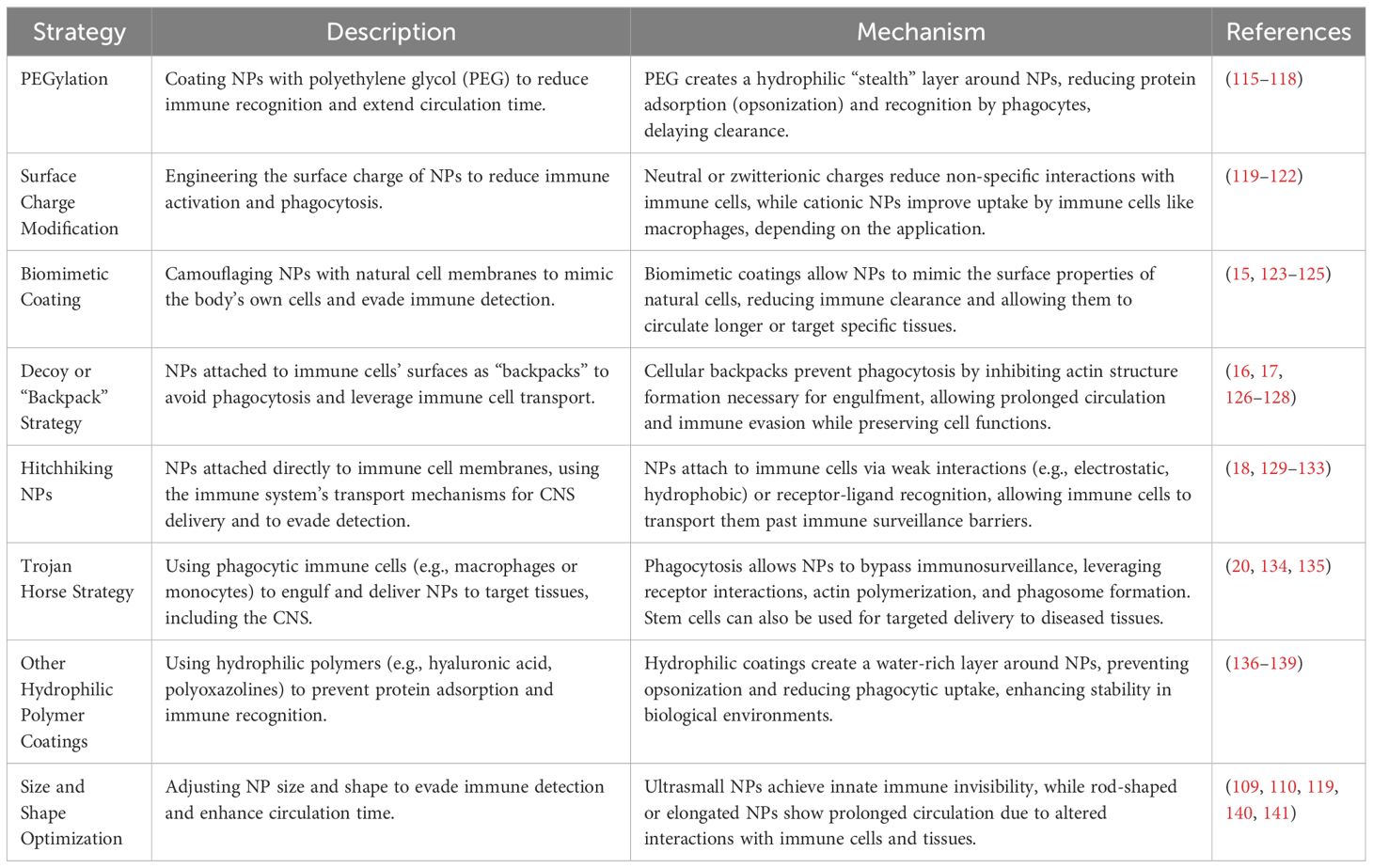
Table 1. Summary of the strategies used to prevent nanoparticle (NP) sequestration by the immune system.
Of note, biomimetic NPs have attracted substantial interest for their potential to evade immune clearance, prolong circulation time, and improve targeting (82). NPs coated with cell membranes (CM-NPs) can disguise themselves as native cells, thus avoiding detection by the immune system (147). These nanocarriers exploit the natural properties of cell membranes combined with engineered cores. The first biomimetic CM-NPs were created using leukocytes coating of a silicon core (123, 124), or red blood cells (RBCs) membrane coating a poly(lactic-co-glycolic acid) (PLGA) core (14). This innovation greatly increased circulation time compared to PEG modification due to the natural identity provided by leukocytes and RBCs which reduced mononuclear phagocyte system sequestration. Since then, CM-NPs have been developed using a variety of cells, including platelets (148), white blood cells (149), cancer cells (150), bacteria (151), and neurons (87) each providing unique benefits. Additionally, these cell membranes have been functionalized with targeting ligands to improve delivery to specific sites, enhancing both effectiveness and safety, and have been explored as nanotherapeutic options in the CNS (15). This practice has further developed into the use of specific cell membrane proteins directly into the structure of NPs, to improve targeting in the CNS. Neuron targeting by NPs was improved in this manner, through the use of cell membrane proteins, such as from human pluripotent stem-cell-derived neurons to make humanized biomimetic nanovesicles for neuron targeting (87, 125, 152).
Other strategies have been developed whereby immune cells may act as carriers of NPs hitchhiking to the cells as “backpacks”, or hiding in the cytoplasm as Trojan horses (153) to avoid immunosurveillance in the CNS, and evade immune detection. Cellular backpacks, typically composed of polymer layers measuring up to 10 μm and exhibiting anisotropic shapes, can adhere to cells and avoid immediate phagocytic uptake (16, 17, 126, 127) because their design inhibits the formation of the actin structures necessary for phagocytosis (104). These backpacks comprise a cell-adhesive region, a payload region, and a release region, which degrades rapidly under specific conditions like low pH, exposing the payload directly to the cellular environment (128). Ensuring that the cell-adhesive region properly anchors the structure to the cell membrane is key to avoid impairing the cells’ functions, such as brain migration and immune regulation (126).
Hitchhiking NPs attach directly to the membranes of carrier cells, takes advantage of the immune system’s transport mechanisms to evade CNS immunosurveillance (18, 129). These NPs use receptor-ligand recognition, chemical bonding, or physical adhesion for attachment (129). Physical adhesion methods, including electrostatic interactions, hydrophobic interactions, van der Waals forces, and hydrogen bonding, require minimal modification (130–133). Although these weak interactions result in limited stability during circulation, the abundance of receptors on cell carriers provides a reliable attachment method. Ligand modifications on NPs can enable them to attach to various cell types, potentially increasing their utility as CNS delivery vehicles. However, the numerous receptors may lead to non-specific attachment, and receptor-ligand interactions could disrupt cell functions (129, 154). Cell surface proteins provide active groups, such as amines and thiols, for NP anchoring (155, 156).
The Trojan horse strategy can exploit the natural phagocytic ability of immune cells, particularly macrophages and monocytes, to deliver NPs to the CNS (134), as these cells are ideal candidates due to their significant phagocytic capacity (19), but has also been further developed using stem cells such as mesenchymal stromal cells (135). The process involves receptor interactions, cytoskeleton rearrangement, actin polymerization, and phagosome formation (20). NP properties, such as size, shape, surface chemistry, and mechanical characteristics, influence their uptake by macrophages. Hydrophobic NPs are more easily phagocytosed compared to hydrophilic ones, and cationic NPs are more readily engulfed due to the negative charge of macrophage membranes. This method utilizes the immune system’s natural processes to ensure efficient delivery of therapeutic agents to the CNS, bypassing typical immunosurveillance mechanisms.
Finally, NP size has the power to shield NPs from immune detection, where smaller NPs may prevent immune detection (140, 141). Zhu and colleagues (140) developed a size-tunable method of developing spherical ultra small gold NPs, of sizes ranging from 3 to 15nm, with significant benefits for scaling up production while minimizing batch variability. The ultra-small gold NPs showed excellent biocompatibility and immunocompatibility, with no observed toxicity to monocytes or macrophages. They also exhibited very low thrombogenicity and evaded detection by the complement system. Of note, ultrasmall NPs of roughly 2nm in size have been shown to cross the BBB (157, 158).
2.2.3 Nanoparticle-induced reprogramming of the immune system
NPs possess properties that enable precise interaction with immune cells, leading to immunomodulation and potential neuroprotection (14, 159). For example, NPs can reprogram immune cell populations within the CNS to induce beneficial shifts in immune function (Figure 3) either by delivering immunomodulatory agents or targeting specific immune cell receptors, which modulate immune responses and promote immune tolerance (105, 106).
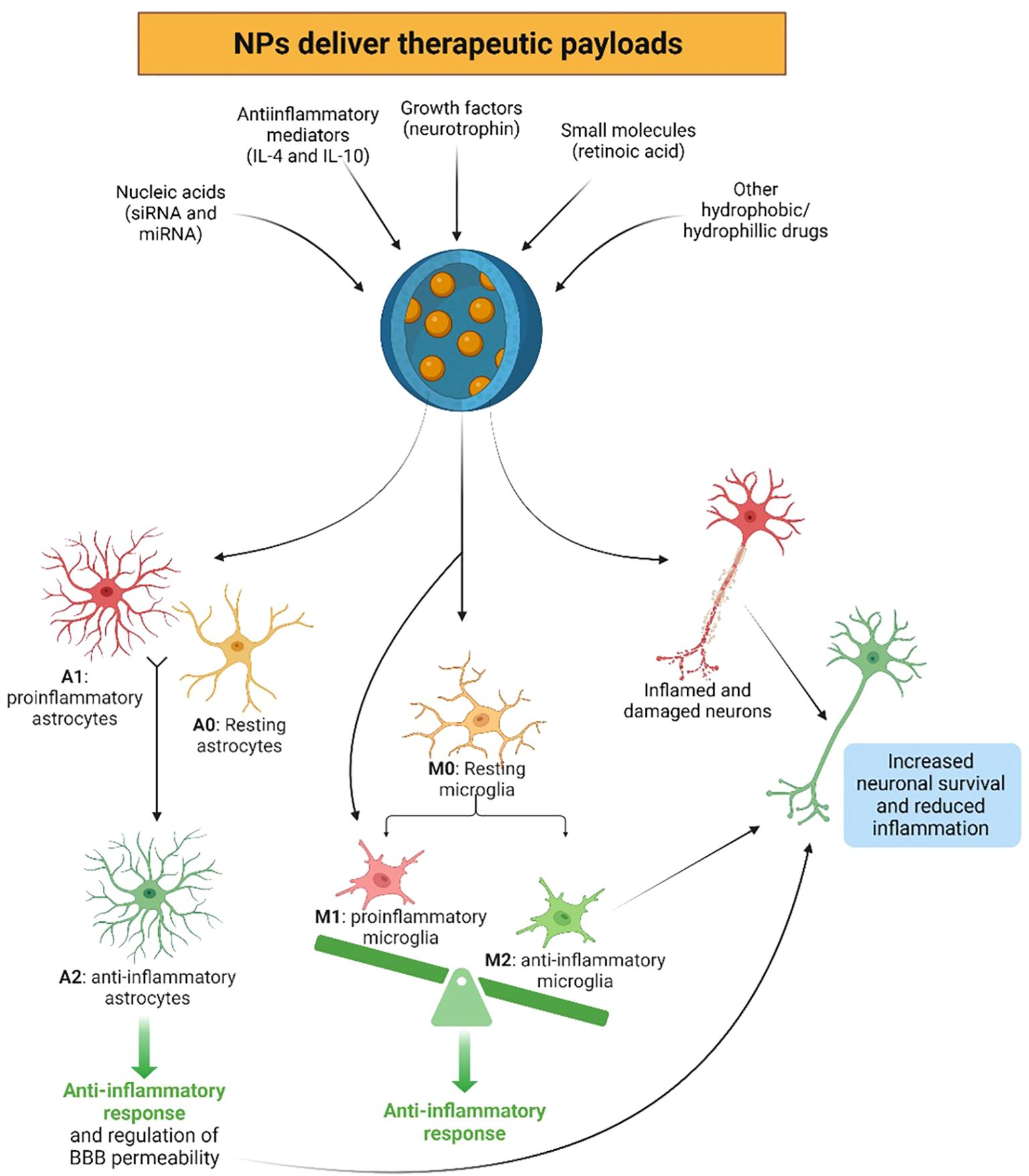
Figure 3. Nanoparticles (NPs) have the ability to beneficially reprogram the immune system in the central nervous system (CNS) by delivering therapeutic payloads, such as anti-inflammatory mediators, growth factors, nucleic acids, small molecules, as well as various hydrophobic and hydrophilic drugs. Due to NP uptake, resting (A0) or proinflammatory (A1) astrocytes may be reprogrammed to the anti-inflammatory and neuroprotective phenotype (A2), exerting anti-inflammatory responses in the CNS. Similarly, microglia can be programmed to the anti-inflammatory phenotype (M2) via the uptake of NPs, initiating anti-inflammatory responses, contrary to the resting (M0) and proinflammatory (M1) phenotypes. These NPs can also directly benefit neural survival, as well as through the beneficial anti-inflammatory effects exerted by the newly reprogrammed A2 astrocytes and M2 microglia. Created with BioRender.com.
As previously stated, one key mechanism involves the functionalization of NP surfaces with specific ligands to target receptors or antigens on immune cells such as microglia, astrocytes, and infiltrating lymphocytes. For example, modifying NPs with antibodies targeting microglial receptors like CD11b or TREM2 enables precise interaction, enabling much higher microglial internalization than control NPs, which resulted in the reduction of both pro-inflammatory cytokines and ROS in microglia, allowing for the rapid inhibition of microglial activation (14, 159, 160). Additionally, NPs can be engineered to carry molecules that alter the activation, proliferation, or cytokine production of immune cells, thereby shifting the immune response balance in the CNS (9, 105, 106). Also, NPs can induce immune tolerance, reducing autoimmune reactions or inflammatory responses (14, 159, 161). For instance, NPs engineered to release growth factors or anti-inflammatory mediators promote the repair and regeneration of damaged neural tissue (105, 162, 163). Furthermore, NPs can also serve as carriers for nucleic acids like siRNA or miRNA, which modulate the expression of immune regulatory genes, dampening pro-inflammatory responses and promoting immune tolerance (14, 159, 164), delivering therapeutic payloads directly into the cytoplasm of immune cells, influencing gene expression, protein synthesis, and cellular signaling pathways (105, 106).
Microglia, the resident immune cells of the CNS, are key targets for NPs in modulating neuroinflammation. NPs can reprogram microglial polarization from a pro-inflammatory (M1) to an anti-inflammatory (M2) phenotype, attenuating neuroinflammation and promoting tissue repair (105, 162). For instance, NPs loaded with anti-inflammatory cytokines such as IL-4 or IL-10 can polarize microglia towards an M2 phenotype (105, 159, 162). Retinoic acid-loaded NPs have shown effectiveness in reducing nitric oxide release and promoting the production of IL-4, enhancing neuronal survival and function (165). Moreover, astrocytes, which regulate CNS homeostasis and neuroinflammatory responses, can also be modulated by NPs. NPs loaded with neurotrophic factors or anti-inflammatory agents can attenuate astrocyte activation and promote neuroprotection in CNS diseases (105, 162).
One major challenge in using NPs for immune reprogramming in the CNS is their potential immunogenicity, which can trigger adverse immune reactions, inflammation, and tissue damage, compromising therapeutic efficacy (166). Another challenge is the risk of off-target effects, where NPs inadvertently modulate immune responses in unintended cell populations (167). To address these challenges, several strategies are employed. Functionalizing NPs to selectively target specific immune cells, such as microglia or infiltrating lymphocytes, can enhance specificity and reduce off-target effects (9, 168). Additionally, using NPs to encapsulate and deliver immunomodulatory agents or nucleic acids can exert precise control over immune cell activation, polarization, and cytokine production, which is crucial for addressing neuroinflammatory conditions, autoimmune disorders, and neurodegenerative diseases (77, 166).
Liposomes, with their aqueous core encased in a phospholipid bilayer, are versatile carriers for both hydrophobic and hydrophilic drugs (169). Currently, Phase III clinical trials are evaluating liposomes loaded with cytarabine for treating neoplastic meningitis. These liposomal NPs have demonstrated the ability to maintain therapeutic levels of cytarabine in the cerebrospinal fluid (CSF) for up to 14 days after administration (170). Another study utilized cationic nanoliposomes with transferrin receptor (TfR)-affinity ligands to deliver oligonucleotides and siRNA to the brain within six hours following intravenous injection. This approach effectively reduced neuroinflammation by targeting TNF-α with siRNA (171). Innovative NPs formulations are continually being developed for CNS applications. For instance, biodegradable PEGylated selenium NPs, conjugated with anti-TfR monoclonal antibody (OX26), have been shown to suppress pathological inflammation and oxidative metabolism linked to cerebral stroke (172). Additionally, inorganic gold NPs with diverse surface ligands hold potential for treating CNS bacterial infections due to their inherent bactericidal properties and the ability to conjugate antibiotics (80). These advancements highlight the ongoing efforts to exploit and evade the immune system for effective CNS drug delivery. By taking advantage of these strategies, researchers can develop targeted approaches to modulate immune responses within the CNS, offering new avenues for treating a wide range of neurological disorders.
Furthermore, nanozymes are NPs with catalytic properties that mimic natural enzymes, enabling biochemical reactions (173, 174). Platinum-based nanozymes (PtNZs) demonstrate effective catalytic activity, particularly in reducing inflammation through ROS scavenging (173). Studies have shown their biocompatibility and potential in treating inflammation, including neuroinflammatory conditions. For instance, PtNZs administered to mice following ischemic stroke were able to cross the damaged BBB, reduce matrix metalloproteinase-9 (MMP-9), and improve motor function. These particles show promise for future therapies targeting brain inflammation and neurodegenerative diseases (173, 175–178). Additionally, trimetallic nanozymes (PtPdMo-NZs), designed for enhanced catalytic efficiency, demonstrated high selectivity in neutral pH environments, further supporting their potential in treating chronic neuroinflammatory conditions like AD (173, 179, 180).
2.2.4 The role of the biomolecular corona in the nano-bio immune interface
2.2.4.1 Impact of the biomolecular corona on the nano-immune interactions
The nano-bio interface represents a pivotal junction to understand the intricate interplay between NPs and the biology of the CNS microenvironment (181). At this interface, NPs encounter a dynamic milieu characterized by the presence of various immune cells, soluble factors, and extracellular matrix components, all of which influence NPs behavior and outcome (182, 183). Understanding the nano-bio immune interface is fundamental since these complex interactions are the key to designing and optimizing therapeutic interventions targeting CNS disorders.
A widely recognized approach to enhance the efficacy of nanomedicine involves targeting NPs to specific sites for improved diagnosis or therapy. However, less than 1.0% of a given nanomedicine reaches this intent, leading to inefficient drug delivery and hampering clinical translation (184, 185). To tackle this issue, it is crucial to comprehend the interaction between NPs and the human body, particularly focusing on what is known as the “biomolecular corona (BC) effect”.
In fact, the original physical-chemical properties defining the synthetic identity of NPs undergo significant alterations upon exposure to biological environments. This is related to the spontaneous interaction between the biomolecules of the biofluids and NPs surfaces and the subsequent formation of a protein-enriched layer, indeed the BC (186). As a result, the formed NP-BC complexes acquire a distinct and novel biological identity leading to a reprogramming of the biomedical and physiological characteristics of the NPs.
Actually, BC represents the biological interface mediating NP-cell interactions, as it is involved in manifold biological processes and ultimately impacts on NPs’ biodistribution, targeting efficiency and immunogenicity (187). Furthermore, the BC can activate the immune system triggering inflammatory responses, NP clearance from the body, and cellular toxicity (188). Understanding the correlation between NP properties, BC formation, and its reaction at the nano-bio interface is crucial for monitoring the fate of the NP in biological compartments and predicting physiological responses. For the past decade, researchers have been diligently investigating the primary factors influencing the formation of the BC in vivo, with the underlying assumption that this understanding could facilitate the deliberate manipulation of BC formation and composition through strategic NP design (189, 190).
It has been discovered that BC equilibrium composition and structure is due to the interplay of shaping factors belonging to (i) the physicochemical properties of NPs (e.g., size, surface chemistry, shape, charge, aggregation after synthesis, (ii) environmental factors (e.g., incubation time, temperature, and shear stress), and (iii) the protein source and concentration (186, 191–193). Among these factors, the protein source (e.g., human vs. mouse serum) is the primary contributor to the enrichment of the BC with immunogenic proteins such as immunoglobulins and complement proteins (194). Variations in immunogenic protein profiles can markedly influence the pharmacokinetic behavior of NPs in the bloodstream, thereby casting doubt on the efficacy of animal testing in predicting physiological responses in humans. Furthermore, even disparate protein sources originating from the same animal species can elicit divergent responses to NP administration, as instance by triggering or mitigating the immune responses (191, 195). In fact, as the BC changes the inherent properties of NPs, it dictates how immune cells recognize and interact with the particle, both by non-specific and molecular recognition mechanisms.
At a first level, BC-induced alterations of NP chemical-physical features regulate non-specific processes of immune system activation (188). Those processes are not directly related to the BC composition but rather to a dynamic interplay of manifold factors, such as size, surface charge, and aggregation state of NP-BC complexes. Enlargement of NP size in biological environment is firstly due to the steric hindrance of the formed BC and depends on NP type. Indeed, BC thickness has been reported to range from about 20 nm [for 30–50 nm citrate-stabilized gold NPs (196)] to 35 nm [for 200 nm PSOSO3 NPs (197)]. As most plasma proteins have hydrodynamic diameters in the range of 3–15 nm, the measured values of BC thickness are compatible with a multi-layered structure, in which ‘primary binders’ recognize the nanomaterial surface directly, and ‘secondary binders’ associate with the primary binders via protein–protein interactions (198, 199). Of note, size increase of NPs is not only ascribable the steric hindrance of this multi-layered structure, but also to the formation of NP-BC aggregates (197, 200). This process occurs as the adsorbed proteins neutralize the surface charge of NPs, diminishing the mutual electrostatic repulsion and promoting the formation of short-range van der Waals bonds (188, 201). One of the main consequences of NP clustering in large aggregates is their immediate recognition by immune systems cells and the subsequent clearance from the bloodstream, principally by splenic filtration and phagocytosis by mononuclear phagocyte system (MPS) in the liver (202). Apart from activating the immune system via non-specific processes, the BC can also trigger molecular recognition mechanisms (Figure 4).
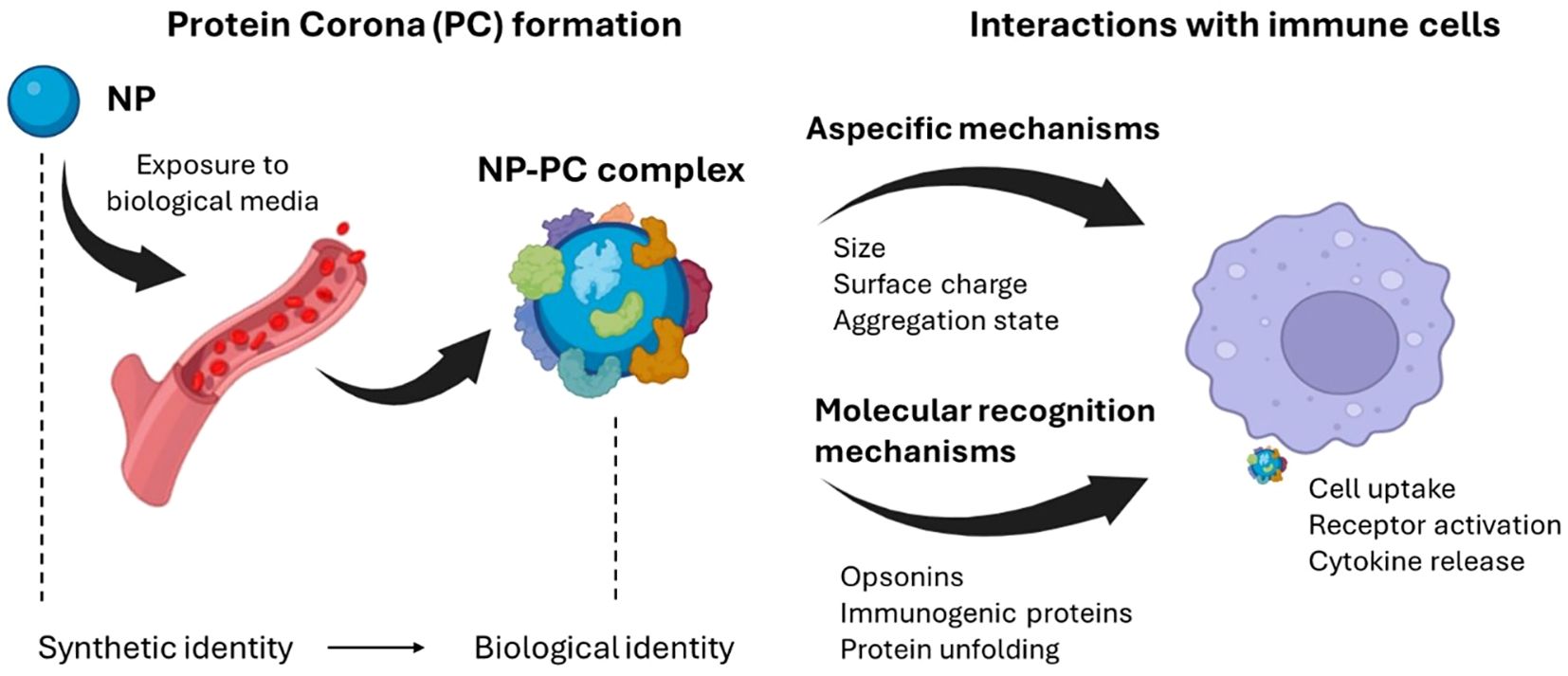
Figure 4. Exposure of nanomaterials to the biological environment alters the synthetic identity of the material, generating a protein-enriched layer known as the protein corona (PC). This new biological identity significantly impacts the physiological response, including the immune response. Among the biological effects induced by the PC is the activation of the immune system through nonspecific mechanisms, often leading to clearance from the circulatory system, or molecular recognition mechanisms. For instance, when the PC is enriched with specific proteins (e.g., opsonins), it may induce receptor-mediated inflammatory processes, degradation by phagocytes, or activation of the complement system. Created with BioRender.com.
This generally happens when the BC is enriched with opsonins, i.e. extracellular proteins that, when bound to foreign substances, make them more susceptible to the action of phagocytes and thus accelerate their degradation (187, 188, 203). Fibrinogen, immunoglobulins, and components of the complement system represent the most abundant opsonins found in the BC of NPs. Fibrinogen has a pivotal role in leukocyte activation and this process is notably intensified when fibrinogen adopts an unfolded state. Interestingly, unfolded fibrinogen in the BC of (PAA)-conjugated Au-NPs binds to the integrin receptor MAC-1, thus triggering an inflammatory response through the activation of the NF-κB signaling pathway (204, 205). This resulted in increased immunotoxicity of the systems. Furthermore, the BC has the capability to induce activation of the complement system, either by classical, alternative or lectin pathways. For example, Vu et al. elucidated that only a small number of surface-bound immunoglobulin molecules are required to initiate complement activation and opsonization (206). Tavano et al. demonstrated that NPs conjugated with poly(2-methyl-2-oxazoline), upon exposure to human serum, activate the classical complement pathway. In that work, C1q was identified as the initiating molecule capable of directly binding to NPs and promoting a rapid opsonization via the complement protein C3. Consequently, NPs were recognized and internalized by human polymorphonuclear granulocytes and monocyte-derived macrophages (207). In turn, dextran-coated superparamagnetic iron oxide core-shell nanoworms, when incubated in human serum and plasma, undergo rapid opsonization with the third complement component (C3) through the alternative pathway (208). Moreover, other pattern-recognition molecules have been recognized. Among these, mannose-binding lectin (MBL), ficolins and collectin detect various carbohydrate ligands on NPs and have been found to trigger the lectin pathway by activating MBL-associated serine proteases (MASPs) (209).
2.2.4.2 Engineering the biomolecular corona to control the immune response
Since adsorbed proteins can induce complement processes that culminate in inflammation, nanomaterials should be engineered to mitigate such immune toxicity and enhance safety. Surface modification emerged as a possible strategy toward this goal. In this regard, grafting stealth components, e.g. polyethylene glycol (PEG), to the surface of NPs may enhance colloidal stability through steric repulsion (210), reduce aggregation, phagocytosis, and thus prolong systemic circulation (117). It is now firmly established that the formation of a BC is an unavoidable process for any nanomaterial, whether stealth-modified (e.g. PEG-functionalized) or not (188). The recognition of the BC as an inevitable aspect of NP interactions with biological systems, along with the potential benefits of proteins that improve NP pharmacokinetics, has stimulated interest in modifying the composition of the BC rather than developing materials that evade BC formation (211). Therefore, an emerging approach involves pre-coating nanomaterials with an artificial BC, providing a natural shield against immune cell clearance (Figure 5).
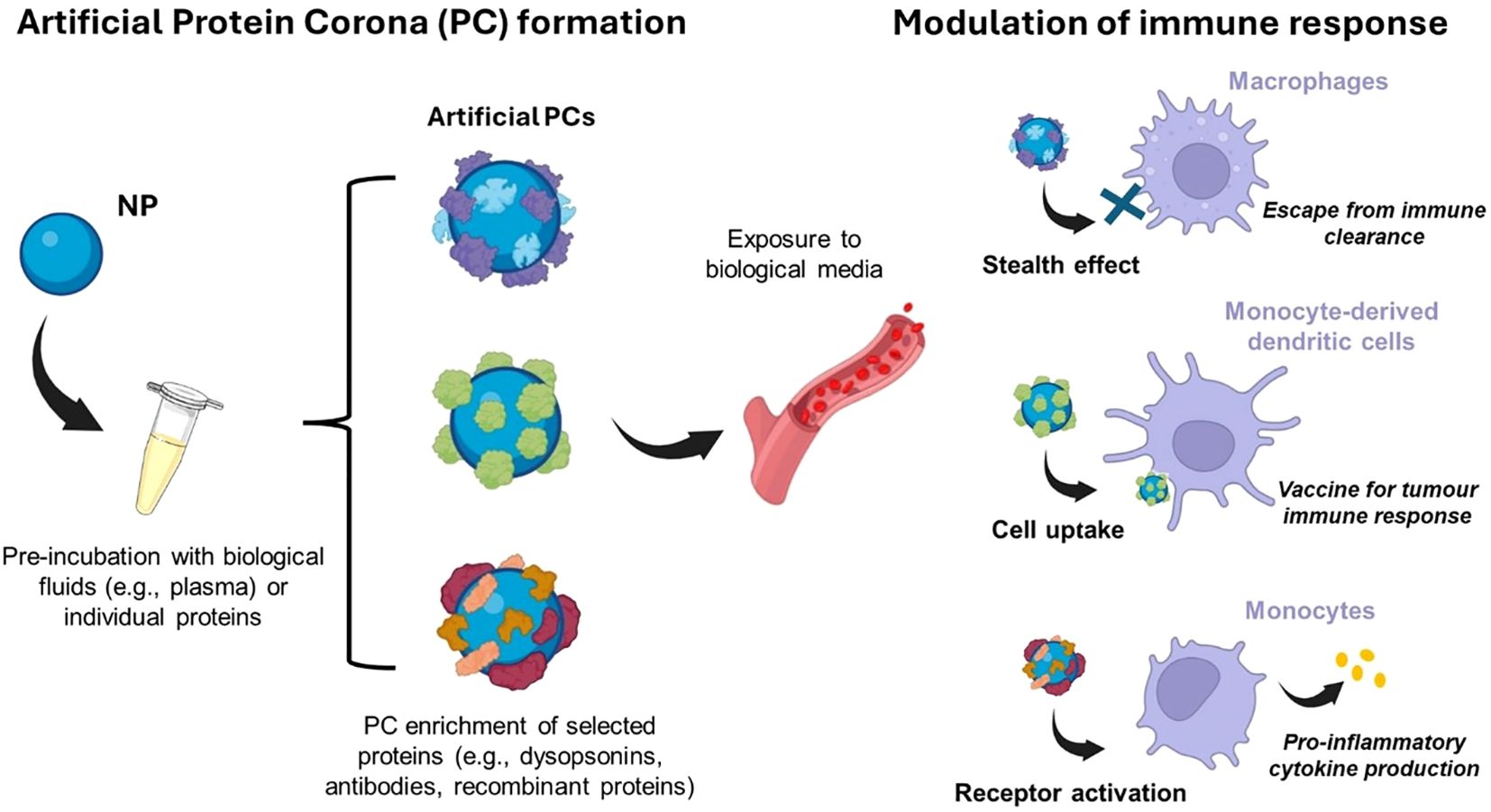
Figure 5. An emerging approach to modulate the immune system response is to pre-coat nanomaterials with an artificial protein corona (PC) before exposure to the biological environment. This artificial PC is generally exploited as a natural shield against immune system clearance or to activate immune cells for various purposes (e.g., pro-inflammatory cytokine production). Created with BioRender.com.
If the functional epitopes of corona proteins are not recognized by immune cell receptors, the BC itself could exhibit this shielding capacity. Artificial BC has been developed for brain targeting (212), and it involved the non-covalent functionalization of NPs with individual proteins. Recent studies have shown that non-covalent binding of the desired ligands is more efficient compared to other types of conjugation methods (213). In this context, Giulimondi et al. exploited the electric charge of DNA to create a lipid-based gene delivery system with a negatively charged surface that, upon exposure to human plasma, becomes coated with a BC enriched in dysopsonins, resulting in a biomimetic NP type with inherent stealth properties (214). In accordance, Tonigoldi et al., supported the beneficial of pre-coating NPs with artificial BC for enhancing NPs targeting ability and monitoring immune response (213). The authors selected the CD63 antibody, which binds to antigens on target cells such as monocyte-derived dendritic cells (moDCs), playing significant roles in the immune system. The study showed that NPs with covalently coupled antibodies lost their targeting ability, whereas those with pre-adsorbed antibodies remained highly functional in terms of targeting efficiency. Another study demonstrated that artificial coronas offer a means to precisely modulate the immune response, paving the way for innovative strategies in disease prevention and treatment (215). In particular, coating cationic liposomes with gelsolin, a key plasma protein involved in macrophage activation and modulation of inflammatory cytokine expression, triggers the activation of immune response in terms of uptake on innate immune THP-1 cells by modulating the pro-inflammatory cytokine production. On the other hand, gelsolin-coated DOTAP exposed to human plasma to mimic in vivo condition, showed a massive uptake by immune cells with phagocyte activity (i.e., monocytes and granulocytes) and retains the capability to induce tumor necrosis factor alpha (TNFα) production by monocytes. Yet, it was also determined that understanding the composition of the corona alone is insufficient to accurately predict immune cell capture. For precise prediction, it is essential to decipher the presentation of functional motifs at the interface. Dawson and colleagues developed effective methods for mapping protein binding sites on the BC using antibody-labeled gold NPs, differential centrifugal sedimentation, and imaging techniques (216). In line with this, Oh et al. engineered a nano-bio complex using recombinant proteins, forming a functional BC that served as a targeting moiety and a protein shield with minimal reactivity to other proteins when immersed in biological fluids. The NPs’ surface was pre-modified with a target linker capable of binding to a specific site on the recombinant protein. During the protein-NP interaction process, the recombinant proteins rapidly formed a stable protein layer on the NP surface. This layer hindered the clearance by the MPS facilitating the targeting to specific tumor tissue (217). Finally, activating immune cells by BC is emerging as a promising approach in innovative medical applications. Tumor-associated macrophages (TAMs) have been recognized as a crucial component of the tumor microenvironment. Pre-coating NPs with antibodies is a widely employed strategy for active targeting of TAMs (218). However, despite their specificity for certain cells and macrophages, their effectiveness is compromised by interactions with other cells expressing the same receptors and by significant nonspecific uptake by macrophages via Fc recognition. For instance, while CD206, a pattern recognition receptor, is upregulated on M2-like macrophages, it is also expressed by tissue-resident macrophages and dendritic cells. Although the mechanisms governing the interaction between TAMs and cancer cells remain incompletely understood, strategies employing modulation of BC for TAMs targeting to potentially restrain tumor advancement and eliminate metastases are still being explored (219). As an instance, it has been found that conformational alterations in proteins bound to nanomaterials can incite inflammatory reactions. Interactions with the surface of NPs drive alterations in the tertiary structure of proteins. These changes can uncover functional groups typically hidden within the hydrophobic core of the protein, promoting protein aggregation and amyloid fiber formation (220). Amyloid fiber formation, in turn, triggers inflammatory responses through receptor-mediated recognition, activating the immune cascade. Recently, the concept of an “amyloid BC” has also been exploited to develop novel sensing and mitigation strategies against amyloid-associated diseases, such as AD (220).
3 Discussion
The use of NPs to modulate immune responses within the CNS offers new exciting opportunities for therapeutic interventions and rehabilitation strategies for CNS disorders (9, 211). Researchers have explored various strategies to harness the unique properties of NPs for immune modulation, aiming to mitigate neuroinflammation, enhance tissue repair, and restore CNS homeostasis.
One promising approach involves the use of NPs as carriers for immunomodulatory agents, such as cytokines, antibodies, or small molecules, to selectively target and modulate specific immune cell populations within the CNS (8, 77). By encapsulating these agents within NPs, researchers can achieve controlled release kinetics, prolonged circulation times, and enhanced bioavailability, thus maximizing their therapeutic effects while minimizing systemic side effects (221, 222).
Additionally, NPs can serve as platforms for delivering nucleic acid-based therapeutics, such as small interfering RNA (siRNA) or messenger RNA (mRNA), to regulate gene expression and modulate immune responses within the CNS (10, 223). Through precise targeting and delivery, NPs enable efficient intracellular delivery of nucleic acids to immune cells, allowing for the selective suppression or activation of immune pathways implicated in CNS disorders.
Several studies have demonstrated the efficacy of NP-mediated immune modulation in preclinical models of neurological diseases, including multiple sclerosis, stroke, AD, and PD (224–232). For example, NPs coated with myelin-derived antigens have been shown to induce antigen-specific tolerance and suppress autoimmune responses in experimental models of multiple sclerosis, capable of inducing robust tolerance and long-term comprehensive disease protection, offering a promising approach for treating autoimmune neuroinflammatory disorders (233, 234).
Furthermore, advancements in NPs engineering and design have led to the development of multifunctional nanoplatforms capable of simultaneously targeting multiple immune pathways or delivering combination therapies within the CNS. These multifunctional NPs offer synergistic therapeutic effects and enhanced therapeutic outcomes compared to single-agent approaches, paving the way for personalized and precision medicine in CNS immunotherapy (235–238).
Another factor driving the development of nanotherapeutic strategies tailored to address the unique challenges of CNS diseases is the potential for targeted and precise drug delivery to specific cell types or regions within the CNS. The nano-bio interface with the immune system is key in the conceptualization of NPs to selectively target immune cells involved in neuroinflammation, such as microglia and astrocytes, while sparing healthy neurons. This targeted delivery approach minimizes off-target effects and systemic toxicity, enhancing the safety and efficacy of CNS therapeutics (76, 236, 239, 240).
Moreover, the biomolecular corona plays a pivotal role in dictating NP behavior and immune recognition within the CNS, giving researchers the opportunity to optimize NP design and surface modifications to minimize immunogenicity and enhance biocompatibility (211, 217, 219). By tailoring NPs to evade immune clearance and enhance cellular uptake, researchers can improve drug delivery efficiency and therapeutic outcomes in CNS disorders.
The significance of these advancements extends beyond drug delivery to encompass immune modulation, neuroprotection, personalized medicine, and precision therapeutics within the CNS. Tailored NPs are promising tools for rehabilitation and treatment of CNS conditions such as AD, PD and stroke (224, 232). NPs offer a versatile platform for delivering immunomodulatory agents and neuroprotective factors directly to diseased tissues, thereby modulating immune responses, reducing neuroinflammation, and promoting tissue repair. This immune reprogramming capability holds promise for treating a wide range of neurological conditions, including neurodegenerative diseases, autoimmune disorders, and brain tumors. With advances in nanotechnology, biomarker discovery, and imaging modalities, clinicians can tailor treatment regimens to the immune profiles and disease characteristics of individual patients (77, 236, 240).
In conclusion, the integration of NPs into CNS nanotherapeutics offers promising opportunities for addressing the complex challenges of neurological conditions and pathologies. By harnessing the nano-bio immune interface and understanding the significance of the biomolecular corona, researchers can develop targeted, safe, and effective nanotherapeutic interventions for a wide range of CNS disorders. These advancements have the potential to revolutionize the treatment landscape and rehabilitation of neurological diseases, offering hope for improved patient care and quality of life in the future.
3.1 Future directions and implications
While there have been many advancements in the field, several key avenues emerge for future research aimed at optimizing immune-NP interactions and harnessing their potential for enhanced CNS therapeutics. One promising direction involves further elucidating the dynamic interplay between NPs and the immune system within the CNS microenvironment. Moreover, there is a pressing need to explore the therapeutic potential of immune-NP interactions in specific CNS disorders, including neuroinflammatory diseases, neurodegenerative disorders, and cancers. By tailoring NP properties to modulate immune responses associated with these conditions, researchers can develop personalized nanotherapeutic interventions that address the contextual pathophysiology and improve patient outcomes (217, 218).
Beyond the realm of CNS nanotherapeutics, understanding the nano-bio immune interface holds broader implications for the development of novel nanotherapeutic strategies across various disease contexts. By deciphering the principles governing immune-NP interactions, researchers can apply this knowledge to improve rehabilitation and design innovative and personalized nanomedicines for targeting systemic immune disorders, infectious diseases, and cancer.
Author contributions
CM: Visualization, Writing – original draft, Writing – review & editing. AB: Writing – original draft, Visualization. EQ: Visualization, Writing – original draft. LL: Visualization, Writing – original draft. LD: Visualization, Writing – original draft. MM: Writing – original draft, Visualization. GC: Visualization, Writing – review & editing. MVP: Visualization, Writing – review & editing. ET: Conceptualization, Writing – review & editing.
Funding
The author(s) declare financial support was received for the research, authorship, and/or publication of this article. This work was supported by the European Union – Next Generation EU – PNRR M6C2 – Investimento 2.1 Valorizzazione e potenziamento della Ricerca biomedica del SSN “Brain connectivity and complexity parameters to monitor disease progression in dementia patients and antiinflammatory nanotherapeutics in a preclinical model of Alzheimer’s disease” – PNRR-MAD-2022-12376667.
Acknowledgments
We would like to thank Cino e Giuse Cunial for their support of the Human Longevity Program.
Conflict of interest
The authors declare that the research was conducted in the absence of any commercial or financial relationships that could be construed as a potential conflict of interest.
Publisher’s note
All claims expressed in this article are solely those of the authors and do not necessarily represent those of their affiliated organizations, or those of the publisher, the editors and the reviewers. Any product that may be evaluated in this article, or claim that may be made by its manufacturer, is not guaranteed or endorsed by the publisher.
References
1. Ampie L, McGavern DB. Immunological defense of CNS barriers against infections. Immunity. (2022) 55:781–99. doi: 10.1016/j.immuni.2022.04.012
2. Morimoto K, Nakajima K. Role of the immune system in the development of the central nervous system. Front Neurosci. (2019) 13:916. doi: 10.3389/fnins.2019.00916
3. Kölliker-Frers R, Udovin L, Otero-Losada M, Kobiec T, Herrera MI, Palacios J, et al. Neuroinflammation: an integrating overview of reactive-neuroimmune cell interactions in health and disease. Mediators Inflammation. (2021) 2021:1–20. doi: 10.1155/2021/9999146
4. Ransohoff RM. How neuroinflammation contributes to neurodegeneration. Science. (2016) 353:777–83. doi: 10.1126/science.aag2590
5. Srikanth M, Kessler JA. Nanotechnology-novel therapeutics for CNS disorders. Nat Rev Neurol. (2012) 8:307–18. doi: 10.1038/nrneurol.2012.76
6. Ventola CL. Progress in nanomedicine: approved and investigational nanodrugs. P T Peer-Rev J Formul Manag. (2017) 42:742–55.
7. Achar A, Myers R, Ghosh C. Drug delivery challenges in brain disorders across the blood-brain barrier: novel methods and future considerations for improved therapy. Biomedicines. (2021) 9:1834. doi: 10.3390/biomedicines9121834
8. Teleanu DM, Negut I, Grumezescu V, Grumezescu AM, Teleanu RI. Nanomaterials for drug delivery to the central nervous system. Nanomater Basel Switz. (2019) 9:371. doi: 10.3390/nano9030371
9. Zhu F-D, Hu Y-J, Yu L, Zhou X-G, Wu J-M, Tang Y, et al. Nanoparticles: A hope for the treatment of inflammation in CNS. Front Pharmacol. (2021) 12:683935. doi: 10.3389/fphar.2021.683935
10. Liu J, Liu Z, Pang Y, Zhou H. The interaction between nanoparticles and immune system: application in the treatment of inflammatory diseases. J Nanobiotechnology. (2022) 20:127. doi: 10.1186/s12951-022-01343-7
11. Aljabali AA, Obeid MA, Bashatwah RM, Serrano-Aroca Á, Mishra V, Mishra Y, et al. Nanomaterials and their impact on the immune system. Int J Mol Sci. (2023) 24:2008. doi: 10.3390/ijms24032008
12. Bharti C, Gulati N, Nagaich U, Pal A. Mesoporous silica nanoparticles in target drug delivery system: A review. Int J Pharm Investig. (2015) 5:124. doi: 10.4103/2230-973X.160844
13. Feng L, Liu Z. Graphene in biomedicine: opportunities and challenges. Nanomed. (2011) 6:317–24. doi: 10.2217/nnm.10.158
14. Hu C-MJ, Zhang L, Aryal S, Cheung C, Fang RH, Zhang L. Erythrocyte membrane-camouflaged polymeric nanoparticles as a biomimetic delivery platform. Proc Natl Acad Sci. (2011) 108:10980–5. doi: 10.1073/pnas.1106634108
15. Li W, Cheng J, He F, Zhang P, Zhang N, Wang J, et al. Cell membrane-based nanomaterials for theranostics of central nervous system diseases. J Nanobiotechnology. (2023) 21:276. doi: 10.1186/s12951-023-02004-z
16. Klyachko NL, Polak R, Haney MJ, Zhao Y, Gomes Neto RJ, Hill MC, et al. Macrophages with cellular backpacks for targeted drug delivery to the brain. Biomaterials. (2017) 140:79–87. doi: 10.1016/j.biomaterials.2017.06.017
17. Shields CW, Evans MA, Wang LL-W, Baugh N, Iyer S, Wu D, et al. Cellular backpacks for macrophage immunotherapy. Sci Adv. (2020) 6:eaaz6579. doi: 10.1126/sciadv.aaz6579
18. Liu T, Gao C, Gu D, Tang H. Cell-based carrier for targeted hitchhiking delivery. Drug Delivery Transl Res. (2022) 12:2634–48. doi: 10.1007/s13346-022-01149-y
19. Batrakova EV, Li S, Reynolds AD, Mosley RL, Bronich TK, Kabanov AV, et al. A macrophage–nanozyme delivery system for parkinson’s disease. Bioconjug Chem. (2007) 18:1498–506. doi: 10.1021/bc700184b
20. Wróblewska A, Szczygieł A, Szermer-Olearnik B, Pajtasz-Piasecka E. Macrophages as promising carriers for nanoparticle delivery in anticancer therapy. Int J Nanomedicine. (2023) 18:4521–39. doi: 10.2147/IJN.S421173
21. Kadry H, Noorani B, Cucullo L. A blood–brain barrier overview on structure, function, impairment, and biomarkers of integrity. Fluids Barriers CNS. (2020) 17:69. doi: 10.1186/s12987-020-00230-3
22. Daneman R, Prat A. The blood–brain barrier. Cold Spring Harb Perspect Biol. (2015) 7:a020412. doi: 10.1101/cshperspect.a020412
23. Prinz M, Priller J. Microglia and brain macrophages in the molecular age: from origin to neuropsychiatric disease. Nat Rev Neurosci. (2014) 15:300–12. doi: 10.1038/nrn3722
24. Sofroniew MV, Vinters HV. Astrocytes: biology and pathology. Acta Neuropathol (Berl). (2010) 119:7–35. doi: 10.1007/s00401-009-0619-8
25. Yang Q, Wang G, Zhang F. Role of peripheral immune cells-mediated inflammation on the process of neurodegenerative diseases. Front Immunol. (2020) 11:582825. doi: 10.3389/fimmu.2020.582825
26. Takata F, Nakagawa S, Matsumoto J, Dohgu S. Blood-brain barrier dysfunction amplifies the development of neuroinflammation: understanding of cellular events in brain microvascular endothelial cells for prevention and treatment of BBB dysfunction. Front Cell Neurosci. (2021) 15:661838. doi: 10.3389/fncel.2021.661838
27. Zenaro E, Piacentino G, Constantin G. The blood-brain barrier in Alzheimer’s disease. Neurobiol Dis. (2017) 107:41–56. doi: 10.1016/j.nbd.2016.07.007
28. Archie SR, Al Shoyaib A, Cucullo L. Blood-brain barrier dysfunction in CNS disorders and putative therapeutic targets: an overview. Pharmaceutics. (2021) 13:1779. doi: 10.3390/pharmaceutics13111779
29. Kim YR, Kim YM, Lee J, Park J, Lee JE, Hyun Y-M. Neutrophils return to bloodstream through the brain blood vessel after crosstalk with microglia during LPS-induced neuroinflammation. Front Cell Dev Biol. (2020) 8:613733. doi: 10.3389/fcell.2020.613733
30. Schafer DP, Lehrman EK, Kautzman AG, Koyama R, Mardinly AR, Yamasaki R, et al. Microglia sculpt postnatal neural circuits in an activity and complement-dependent manner. Neuron. (2012) 74:691–705. doi: 10.1016/j.neuron.2012.03.026
31. Tay TL, Mai D, Dautzenberg J, Fernández-Klett F, Lin G, Sagar, et al. A new fate mapping system reveals context-dependent random or clonal expansion of microglia. Nat Neurosci. (2017) 20:793–803. doi: 10.1038/nn.4547
32. Wendimu MY, Hooks SB. Microglia phenotypes in aging and neurodegenerative diseases. Cells. (2022) 11:2091. doi: 10.3390/cells11132091
33. Kawai T, Akira S. The roles of TLRs, RLRs and NLRs in pathogen recognition. Int Immunol. (2009) 21:317–37. doi: 10.1093/intimm/dxp017
34. Barnabei L, Laplantine E, Mbongo W, Rieux-Laucat F, Weil R. NF-κB: at the borders of autoimmunity and inflammation. Front Immunol. (2021) 12:716469. doi: 10.3389/fimmu.2021.716469
35. Liu T, Zhang L, Joo D, Sun S-C. NF-κB signaling in inflammation. Signal Transduct Target Ther. (2017) 2:17023. doi: 10.1038/sigtrans.2017.23
36. Gao C, Jiang J, Tan Y, Chen S. Microglia in neurodegenerative diseases: mechanism and potential therapeutic targets. Signal Transduct Target Ther. (2023) 8:359. doi: 10.1038/s41392-023-01588-0
37. Colonna M, Butovsky O. Microglia function in the central nervous system during health and neurodegeneration. Annu Rev Immunol. (2017) 35:441–68. doi: 10.1146/annurev-immunol-051116-052358
38. Deczkowska A, Keren-Shaul H, Weiner A, Colonna M, Schwartz M, Amit I. Disease-associated microglia: A universal immune sensor of neurodegeneration. Cell. (2018) 173:1073–81. doi: 10.1016/j.cell.2018.05.003
39. Semyanov A, Verkhratsky A. Astrocytic processes: from tripartite synapses to the active milieu. Trends Neurosci. (2021) 44:781–92. doi: 10.1016/j.tins.2021.07.006
40. Nedergaard M, Dirnagl U. Role of glial cells in cerebral ischemia. Glia. (2005) 50:281–6. doi: 10.1002/glia.20205
41. Serrano-Pozo A, Mielke ML, Gómez-Isla T, Betensky RA, Growdon JH, Frosch MP, et al. Reactive Glia not only Associates with Plaques but also Parallels Tangles in Alzheimer’s Disease. Am J Pathol. (2011) 179:1373–84. doi: 10.1016/j.ajpath.2011.05.047
42. Hirsch EC, Breidert T, Rousselet E, Hunot S, Hartmann A, Michel PP. The role of glial reaction and inflammation in parkinson’s disease. Ann N Y Acad Sci. (2003) 991:214–28. doi: 10.1111/j.1749-6632.2003.tb07478.x
43. Lazic A, Balint V, Stanisavljevic Ninkovic D, Peric M, Stevanovic M. Reactive and senescent astroglial phenotypes as hallmarks of brain pathologies. Int J Mol Sci. (2022) 23:4995. doi: 10.3390/ijms23094995
44. Escartin C, Galea E, Lakatos A, O’Callaghan JP, Petzold GC, Serrano-Pozo A, et al. Reactive astrocyte nomenclature, definitions, and future directions. Nat Neurosci. (2021) 24:312–25. doi: 10.1038/s41593-020-00783-4
45. Liddelow SA, Barres BA. Reactive astrocytes: production, function, and therapeutic potential. Immunity. (2017) 46:957–67. doi: 10.1016/j.immuni.2017.06.006
46. Pahl HL. Activators and target genes of Rel/NF-κB transcription factors. Oncogene. (1999) 18:6853–66. doi: 10.1038/sj.onc.1203239
47. Xu X, Zhang A, Zhu Y, He W, Di W, Fang Y, et al. MFG-E8 reverses microglial-induced neurotoxic astrocyte (A1) via NF-κB and PI3K-Akt pathways. J Cell Physiol. (2019) 234:904–14. doi: 10.1002/jcp.26918
48. López-Teros M, Alarcón-Aguilar A, López-Diazguerrero NE, Luna-López A, Königsberg M. Contribution of senescent and reactive astrocytes on central nervous system inflammaging. Biogerontology. (2022) 23:21–33. doi: 10.1007/s10522-022-09952-3
49. Kigerl KA, De Rivero Vaccari JP, Dietrich WD, Popovich PG, Keane RW. Pattern recognition receptors and central nervous system repair. Exp Neurol. (2014) 258:5–16. doi: 10.1016/j.expneurol.2014.01.001
50. Sofroniew MV. Astrocyte barriers to neurotoxic inflammation. Nat Rev Neurosci. (2015) 16:249–63. doi: 10.1038/nrn3898
51. Minkiewicz J, De Rivero Vaccari JP, Keane RW. Human astrocytes express a novel NLRP2 inflammasome. Glia. (2013) 61:1113–21. doi: 10.1002/glia.22499
52. Martinon F, Burns K, Tschopp J. The inflammasome. Mol Cell. (2002) 10:417–26. doi: 10.1016/S1097-2765(02)00599-3
53. Silverman WR, De Rivero Vaccari JP, Locovei S, Qiu F, Carlsson SK, Scemes E, et al. The pannexin 1 channel activates the inflammasome in neurons and astrocytes. J Biol Chem. (2009) 284:18143–51. doi: 10.1074/jbc.M109.004804
54. Ransohoff RM, Brown MA. Innate immunity in the central nervous system. J Clin Invest. (2012) 122:1164–71. doi: 10.1172/JCI58644
55. Sochocka M, Diniz BS, Leszek J. Inflammatory response in the CNS: friend or foe? Mol Neurobiol. (2017) 54:8071–89. doi: 10.1007/s12035-016-0297-1
56. Cebrián C, Loike JD, Sulzer D. Neuronal MHC-I expression and its implications in synaptic function, axonal regeneration and Parkinsonâ€TMs and other brain diseases. Front Neuroanat. (2014) 8:114. doi: 10.3389/fnana.2014.00114
57. Haynes SE, Hollopeter G, Yang G, Kurpius D, Dailey ME, Gan W-B, et al. The P2Y12 receptor regulates microglial activation by extracellular nucleotides. Nat Neurosci. (2006) 9:1512–9. doi: 10.1038/nn1805
58. Kuhn SA, Van Landeghem FKH, Zacharias R, Färber K, Rappert A, Pavlovic S, et al. Microglia express GABA B receptors to modulate interleukin release. Mol Cell Neurosci. (2004) 25:312–22. doi: 10.1016/j.mcn.2003.10.023
59. Taylor DL, Diemel LT, Cuzner ML, Pocock JM. Activation of group II metabotropic glutamate receptors underlies microglial reactivity and neurotoxicity following stimulation with chromogranin A, a peptide up-regulated in Alzheimer’s disease. J Neurochem. (2002) 82:1179–91. doi: 10.1046/j.1471-4159.2002.01062.x
60. Liu Y, Hu J, Wu J, Zhu C, Hui Y, Han Y, et al. [amp]]alpha;7 nicotinic acetylcholine receptor-mediated neuroprotection against dopaminergic neuron loss in an MPTP mouse model via inhibition of astrocyte activation. J Neuroinflamm. (2012) 9:98. doi: 10.1186/1742-2094-9-98
61. Takarada T, Nakamichi N, Kawagoe H, Ogura M, Fukumori R, Nakazato R, et al. Possible neuroprotective property of nicotinic acetylcholine receptors in association with predominant upregulation of glial cell line-derived neurotrophic factor in astrocytes. J Neurosci Res. (2012) 90:2074–85. doi: 10.1002/jnr.23101
62. Mashimo M, Okubo Y, Yamazawa T, Yamasaki M, Watanabe M, Murayama T, et al. Inositol 1,4,5-trisphosphate signaling maintains the activity of glutamate uptake in Bergmann glia. Eur J Neurosci. (2010) 32:1668–77. doi: 10.1111/j.1460-9568.2010.07452.x
63. Banerjee J, Alkondon M, Pereira EFR, Albuquerque EX. Regulation of GABAergic inputs to CA1 pyramidal neurons by nicotinic receptors and kynurenic acid. J Pharmacol Exp Ther. (2012) 341:500–9. doi: 10.1124/jpet.111.189860
64. Enes J, Haburčák M, Sona S, Gerard N, Mitchell AC, Fu W, et al. Satellite glial cells modulate cholinergic transmission between sympathetic neurons. PLoS One. (2020) 15:e0218643. doi: 10.1371/journal.pone.0218643
65. Donnelly CR, Andriessen AS, Chen G, Wang K, Jiang C, Maixner W, et al. Central nervous system targets: glial cell mechanisms in chronic pain. Neurotherapeutics. (2020) 17:846–60. doi: 10.1007/s13311-020-00905-7
66. Passaro AP, Lebos AL, Yao Y, Stice SL. Immune response in neurological pathology: emerging role of central and peripheral immune crosstalk. Front Immunol. (2021) 12:676621. doi: 10.3389/fimmu.2021.676621
67. Prinz M, Priller J. Tickets to the brain: role of CCR2 and CX3CR1 in myeloid cell entry in the CNS. J Neuroimmunol. (2010) 224:80–4. doi: 10.1016/j.jneuroim.2010.05.015
68. Herisson F, Frodermann V, Courties G, Rohde D, Sun Y, Vandoorne K, et al. Direct vascular channels connect skull bone marrow and the brain surface enabling myeloid cell migration. Nat Neurosci. (2018) 21:1209–17. doi: 10.1038/s41593-018-0213-2
69. Cugurra A, Mamuladze T, Rustenhoven J, Dykstra T, Beroshvili G, Greenberg ZJ, et al. Skull and vertebral bone marrow are myeloid cell reservoirs for the meninges and CNS parenchyma. Science. (2021) 373:eabf7844. doi: 10.1126/science.abf7844
70. Sabatino JJ, Pröbstel A-K, Zamvil SS. B cells in autoimmune and neurodegenerative central nervous system diseases. Nat Rev Neurosci. (2019) 20:728–45. doi: 10.1038/s41583-019-0233-2
71. Ahn JJ, Abu-Rub M, Miller RH. B cells in neuroinflammation: new perspectives and mechanistic insights. Cells. (2021) 10:1605. doi: 10.3390/cells10071605
72. Cekanaviciute E, Yoo BB, Runia TF, Debelius JW, Singh S, Nelson CA, et al. Gut bacteria from multiple sclerosis patients modulate human T cells and exacerbate symptoms in mouse models. Proc Natl Acad Sci. (2017) 114:10713–8. doi: 10.1073/pnas.1711235114
73. Amoriello R, Memo C, Ballerini L, Ballerini C. The brain cytokine orchestra in multiple sclerosis: from neuroinflammation to synaptopathology. Mol Brain. (2024) 17:4. doi: 10.1186/s13041-024-01077-7
74. Smolders J, Heutinck KM, Fransen NL, Remmerswaal EBM, Hombrink P, Ten Berge IJM, et al. Tissue-resident memory T cells populate the human brain. Nat Commun. (2018) 9:4593. doi: 10.1038/s41467-018-07053-9
75. Altammar KA. A review on nanoparticles: characteristics, synthesis, applications, and challenges. Front Microbiol. (2023) 14:1155622. doi: 10.3389/fmicb.2023.1155622
76. Annu, Sartaj A, Qamar Z, Md S, Alhakamy NA, Baboota S, et al. An insight to brain targeting utilizing polymeric nanoparticles: effective treatment modalities for neurological disorders and brain tumor. Front Bioeng Biotechnol. (2022) 10:788128. doi: 10.3389/fbioe.2022.788128
77. Cerqueira SR, Ayad NG, Lee JK. Neuroinflammation treatment via targeted delivery of nanoparticles. Front Cell Neurosci. (2020) 14:576037. doi: 10.3389/fncel.2020.576037
78. La Barbera L, Mauri E, D’Amelio M, Gori M. Functionalization strategies of polymeric nanoparticles for drug delivery in Alzheimer’s disease: Current trends and future perspectives. Front Neurosci. (2022) 16:939855. doi: 10.3389/fnins.2022.939855
79. Saif A, Anjum L, Faisal Z, Akram N, Shah YA, Irfan R, et al. Recent advances in protein-based nanoparticles and their applications in the delivery of bioactive compounds. Int J Food Prop. (2023) 26:2866–80. doi: 10.1080/10942912.2023.2261662
80. Rizvi SMD, Hussain T, Ahmed ABF, Alshammari TM, Moin A, Ahmed MQ, et al. Gold nanoparticles: A plausible tool to combat neurological bacterial infections in humans. BioMed Pharmacother. (2018) 107:7–18. doi: 10.1016/j.biopha.2018.07.130
81. Martinez JO, Molinaro R, Hartman KA, Boada C, Sukhovershin R, De Rosa E, et al. Biomimetic nanoparticles with enhanced affinity towards activated endothelium as versatile tools for theranostic drug delivery. Theranostics. (2018) 8:1131–45. doi: 10.7150/thno.22078
82. Han H, Bártolo R, Li J, Shahbazi M-A, Santos HA. Biomimetic platelet membrane-coated nanoparticles for targeted therapy. Eur J Pharm Biopharm. (2022) 172:1–15. doi: 10.1016/j.ejpb.2022.01.004
83. Yáñez-Mó M, Siljander PR-M, Andreu Z, Bedina Zavec A, Borràs FE, Buzas EI, et al. Biological properties of extracellular vesicles and their physiological functions. J Extracell Vesicles. (2015) 4:27066. doi: 10.3402/jev.v4.27066
84. Zabrodskaya Y, Plotnikova M, Gavrilova N, Lozhkov A, Klotchenko S, Kiselev A, et al. Exosomes released by influenza-virus-infected cells carry factors capable of suppressing immune defense genes in naïve cells. Viruses. (2022) 14:2690. doi: 10.3390/v14122690
85. Borges FT, Reis LA, Schor N. Extracellular vesicles: structure, function, and potential clinical uses in renal diseases. Braz J Med Biol Res. (2013) 46:824–30. doi: 10.1590/1414-431X20132964
86. Krishnan N, Fang RH, Zhang L. Engineering of stimuli-responsive self-assembled biomimetic nanoparticles. Adv Drug Delivery Rev. (2021) 179:114006. doi: 10.1016/j.addr.2021.114006
87. Zinger A, Cvetkovic C, Sushnitha M, Naoi T, Baudo G, Anderson M, et al. Humanized biomimetic nanovesicles for neuron targeting. Adv Sci. (2021) 8:2101437. doi: 10.1002/advs.202101437
88. Hoffman JR, Tasciotti E, Molinaro R. Microfluidic assembly of liposomes with tunable size and coloading capabilities. In: Heuck C, Weinhold N, editors. Multiple Myeloma. Methods in Molecular Biology. Springer New York, New York, NY (2018). p. 205–14. doi: 10.1007/978-1-4939-7865-6_15
89. Nsairat H, Khater D, Sayed U, Odeh F, Al Bawab A, Alshaer W. Liposomes: structure, composition, types, and clinical applications. Heliyon. (2022) 8:e09394. doi: 10.1016/j.heliyon.2022.e09394
90. Yurkin ST, Wang Z. Cell membrane-derived nanoparticles: emerging clinical opportunities for targeted drug delivery. Nanomed. (2017) 12:2007–19. doi: 10.2217/nnm-2017-0100
91. Daramola OO, Adara P, Adewuyi BO, Sadiku ER, Kupolati WK. Polymer nanoparticles (nanomedicine) for therapeutic applications. In: Polymeric Biomaterials for Healthcare Applications. Varaprasad, K., Ed. Sawston, UK: Woodhead Publishing. (2022). p. 71–123. doi: 10.1016/B978-0-323-85233-3.00003-3
92. Toledano Furman NE, Lupu-Haber Y, Bronshtein T, Kaneti L, Letko N, Weinstein E, et al. Reconstructed stem cell nanoghosts: A natural tumor targeting platform. Nano Lett. (2013) 13:3248–55. doi: 10.1021/nl401376w
93. Kaneti L, Bronshtein T, Malkah Dayan N, Kovregina I, Letko Khait N, Lupu-Haber Y, et al. Nanoghosts as a novel natural nonviral gene delivery platform safely targeting multiple cancers. Nano Lett. (2016) 16:1574–82. doi: 10.1021/acs.nanolett.5b04237
94. Timaner M, Letko-Khait N, Kotsofruk R, Benguigui M, Beyar-Katz O, Rachman-Tzemah C, et al. Therapy-educated mesenchymal stem cells enrich for tumor-initiating cells. Cancer Res. (2018) 78:1253–65. doi: 10.1158/0008-5472.CAN-17-1547
95. Oieni J, Levy L, Letko Khait N, Yosef L, Schoen B, Fliman M, et al. Nano-Ghosts: Biomimetic membranal vesicles, technology and characterization. Methods. (2020) 177:126–34. doi: 10.1016/j.ymeth.2019.11.013
96. Zhang S-S, Li R-Q, Chen Z, Wang X-Y, Dumont AS, Fan X. Immune cells: potential carriers or agents for drug delivery to the central nervous system. Mil Med Res. (2024) 11:19. doi: 10.1186/s40779-024-00521-y
97. Zhao N, Francis NL, Calvelli HR, Moghe PV. Microglia-targeting nanotherapeutics for neurodegenerative diseases. APL Bioeng. (2020) 4:030902. doi: 10.1063/5.0013178
98. Dai D, He L, Chen Y, Zhang C. Astrocyte responses to nanomaterials: Functional changes, pathological changes and potential applications. Acta Biomater. (2021) 122:66–81. doi: 10.1016/j.actbio.2020.12.013
99. Iversen T-G, Skotland T, Sandvig K. Endocytosis and intracellular transport of nanoparticles: Present knowledge and need for future studies. Nano Today. (2011) 6:176–85. doi: 10.1016/j.nantod.2011.02.003
100. Sousa de Almeida M, Susnik E, Drasler B, Taladriz-Blanco P, Petri-Fink A, Rothen-Rutishauser B. Understanding nanoparticle endocytosis to improve targeting strategies in nanomedicine. Chem Soc Rev. (2021) 50:5397–434. doi: 10.1039/d0cs01127d
101. Luo Y-H, Chang LW, Lin P. Metal-based nanoparticles and the immune system: activation, inflammation, and potential applications. BioMed Res Int. (2015) 2015:143720. doi: 10.1155/2015/143720
102. Muldoon LL, Alvarez JI, Begley DJ, Boado RJ, Del Zoppo GJ, Doolittle ND, et al. Immunologic privilege in the central nervous system and the blood-brain barrier. J Cereb Blood Flow Metab Off J Int Soc Cereb Blood Flow Metab. (2013) 33:13–21. doi: 10.1038/jcbfm.2012.153
103. Zou R, Gu R, Yu X, Hu Y, Yu J, Xue X, et al. Characteristics of infiltrating immune cells and a predictive immune model for cervical cancer. J Cancer. (2021) 12:3501–14. doi: 10.7150/jca.55970
104. Champion JA, Mitragotri S. Role of target geometry in phagocytosis. Proc Natl Acad Sci. (2006) 103:4930–4. doi: 10.1073/pnas.0600997103
105. Agarwal A, Lariya N, Saraogi G, Dubey N, Agrawal H, Agrawal G. Nanoparticles as novel carrier for brain delivery: A review. Curr Pharm Des. (2009) 15:917–25. doi: 10.2174/138161209787582057
106. Albanese A, Tang PS, Chan WCW. The effect of nanoparticle size, shape, and surface chemistry on biological systems. Annu Rev BioMed Eng. (2012) 14:1–16. doi: 10.1146/annurev-bioeng-071811-150124
107. Lee JH, Chapman DV, Saltzman WM. Nanoparticle targeting with antibodies in the central nervous system. BME Front. (2023) 4:12. doi: 10.34133/bmef.0012
108. Zhang W, Lopez H, Boselli L, Bigini P, Perez-Potti A, Xie Z, et al. A nanoscale shape-discovery framework supporting systematic investigations of shape-dependent biological effects and immunomodulation. ACS Nano. (2022) 16:1547–59. doi: 10.1021/acsnano.1c10074
109. Wang J, Chen H-J, Hang T, Yu Y, Liu G, He G, et al. Physical activation of innate immunity by spiky particles. Nat Nanotechnol. (2018) 13:1078–86. doi: 10.1038/s41565-018-0274-0
110. Albanese A, Sykes EA, Chan WCW. Rough around the edges: the inflammatory response of microglial cells to spiky nanoparticles. ACS Nano. (2010) 4:2490–3. doi: 10.1021/nn100776z
111. Bartneck M, Keul HA, Singh S, Czaja K, Bornemann J, Bockstaller M, et al. Rapid uptake of gold nanorods by primary human blood phagocytes and immunomodulatory effects of surface chemistry. ACS Nano. (2010) 4:3073–86. doi: 10.1021/nn100262h
112. Sun B, Ji Z, Liao Y-P, Wang M, Wang X, Dong J, et al. Engineering an effective immune adjuvant by designed control of shape and crystallinity of aluminum oxyhydroxide nanoparticles. ACS Nano. (2013) 7:10834–49. doi: 10.1021/nn404211j
113. Son YJ, Kim H, Leong KW, Yoo HS. Multifunctional nanorods serving as nanobridges to modulate T cell-mediated immunity. ACS Nano. (2013) 7:9771–9. doi: 10.1021/nn403275p
114. Talamini L, Violatto MB, Cai Q, Monopoli MP, Kantner K, Krpetić Ž, et al. Influence of size and shape on the anatomical distribution of endotoxin-free gold nanoparticles. ACS Nano. (2017) 11:5519–29. doi: 10.1021/acsnano.7b00497
115. Sercombe L, Veerati T, Moheimani F, Wu SY, Sood AK, Hua S. Advances and challenges of liposome assisted drug delivery. Front Pharmacol. (2015) 6:286. doi: 10.3389/fphar.2015.00286
116. Mohamed M, Abu Lila AS, Shimizu T, Alaaeldin E, Hussein A, Sarhan HA, et al. PEGylated liposomes: immunological responses. Sci Technol Adv Mater. (2019) 20:710–24. doi: 10.1080/14686996.2019.1627174
117. Suk JS, Xu Q, Kim N, Hanes J, Ensign LM. PEGylation as a strategy for improving nanoparticle-based drug and gene delivery. Adv Drug Delivery Rev. (2016) 99:28–51. doi: 10.1016/j.addr.2015.09.012
118. Ezhilarasan D, Shree Harini K. Nanodrug delivery: Strategies to circumvent nanoparticle trafficking by Kupffer cells in the liver. J Drug Delivery Sci Technol. (2023) 86:104731. doi: 10.1016/j.jddst.2023.104731
119. Deng J, Wang J, Shi J, Li H, Lu M, Fan Z, et al. Tailoring the physicochemical properties of nanomaterials for immunomodulation. Adv Drug Delivery Rev. (2022) 180:114039. doi: 10.1016/j.addr.2021.114039
120. Fromen CA, Rahhal TB, Robbins GR, Kai MP, Shen TW, Luft JC, et al. Nanoparticle surface charge impacts distribution, uptake and lymph node trafficking by pulmonary antigen-presenting cells. Nanomedicine Nanotechnol Biol Med. (2016) 12:677–87. doi: 10.1016/j.nano.2015.11.002
121. Jiang S, Cao Z. Ultralow-fouling, functionalizable, and hydrolyzable zwitterionic materials and their derivatives for biological applications. Adv Mater. (2010) 22:920–32. doi: 10.1002/adma.200901407
122. Li L, Wang H, Ye J, Chen Y, Wang R, Jin D, et al. Mechanism study on nanoparticle negative surface charge modification by ascorbyl palmitate and its improvement of tumor targeting ability. Molecules. (2022) 27:4408. doi: 10.3390/molecules27144408
123. Parodi A, Quattrocchi N, Van De Ven AL, Chiappini C, Evangelopoulos M, Martinez JO, et al. Synthetic nanoparticles functionalized with biomimetic leukocyte membranes possess cell-like functions. Nat Nanotechnol. (2013) 8:61–8. doi: 10.1038/nnano.2012.212
124. Quattrocchi N, Chiappini C, Cooper L, Ferrari M, Tasciotti E. A “leukolike” multistage delivery system to overcome biological barriers. (2010) 3.
125. Wu H, Zhang T, Li N, Gao J. Cell membrane-based biomimetic vehicles for effective central nervous system target delivery: Insights and challenges. J Control Release Off J Control Release Soc. (2023) 360:169–84. doi: 10.1016/j.jconrel.2023.06.023
126. Doshi N, Swiston AJ, Gilbert JB, Alcaraz ML, Cohen RE, Rubner MF, et al. Cell-based drug delivery devices using phagocytosis-resistant backpacks. Adv Mater. (2011) 23:H105–9. doi: 10.1002/adma.201004074
127. Polak R, Lim RM, Beppu MM, Pitombo RNM, Cohen RE, Rubner MF. Liposome-loaded cell backpacks. Adv Healthc Mater. (2015) 4:2832–41. doi: 10.1002/adhm.201500604
128. Swiston AJ, Cheng C, Um SH, Irvine DJ, Cohen RE, Rubner MF. Surface functionalization of living cells with multilayer patches. Nano Lett. (2008) 8:4446–53. doi: 10.1021/nl802404h
129. Anselmo AC, Mitragotri S. Cell-mediated delivery of nanoparticles: Taking advantage of circulatory cells to target nanoparticles. J Controlled Release. (2014) 190:531–41. doi: 10.1016/j.jconrel.2014.03.050
130. Anselmo AC, Gupta V, Zern BJ, Pan D, Zakrewsky M, Muzykantov V, et al. Delivering Nanoparticles to Lungs while Avoiding Liver and Spleen through Adsorption on Red Blood Cells. ACS Nano. (2013) 7:11129–37. doi: 10.1021/nn404853z
131. Chambers E, Mitragotri S. Prolonged circulation of large polymeric nanoparticles by non-covalent adsorption on erythrocytes. J Controlled Release. (2004) 100:111–9. doi: 10.1016/j.jconrel.2004.08.005
132. Chambers E, Mitragotri S. Long circulating nanoparticles via adhesion on red blood cells: mechanism and extended circulation. Exp Biol Med Maywood NJ. (2007) 232:958–66.
133. Udofa E, Zhao Z. In situ cellular hitchhiking of nanoparticles for drug delivery. Adv Drug Delivery Rev. (2024) 204:115143. doi: 10.1016/j.addr.2023.115143
134. Choi M-R, Stanton-Maxey KJ, Stanley JK, Levin CS, Bardhan R, Akin D, et al. A cellular trojan horse for delivery of therapeutic nanoparticles into tumors. Nano Lett. (2007) 7:3759–65. doi: 10.1021/nl072209h
135. Martinez JO, Evangelopoulos M, Brozovich AA, Bauza G, Molinaro R, Corbo C, et al. Mesenchymal stromal cell-mediated treatment of local and systemic inflammation through the triggering of an anti-inflammatory response. Adv Funct Mater. (2021) 31:2002997. doi: 10.1002/adfm.202002997
136. Chapman RG, Ostuni E, Takayama S, Holmlin RE, Yan L, Whitesides GM. Surveying for surfaces that resist the adsorption of proteins. J Am Chem Soc. (2000) 122:8303–4. doi: 10.1021/ja000774f
137. Fam SY, Chee CF, Yong CY, Ho KL, Mariatulqabtiah AR, Tan WS. Stealth coating of nanoparticles in drug-delivery systems. Nanomaterials. (2020) 10:787. doi: 10.3390/nano10040787
138. Gulati NM, Stewart PL, Steinmetz NF. Bioinspired shielding strategies for nanoparticle drug delivery applications. Mol Pharm. (2018) 15:2900–9. doi: 10.1021/acs.molpharmaceut.8b00292
139. Zhang Q, Deng C, Fu Y, Sun X, Gong T, Zhang Z. Repeated administration of hyaluronic acid coated liposomes with improved pharmacokinetics and reduced immune response. Mol Pharm. (2016) 13:1800–8. doi: 10.1021/acs.molpharmaceut.5b00952
140. Zhu GH, Azharuddin M, Islam R, Rahmoune H, Deb S, Kanji U, et al. Innate immune invisible ultrasmall gold nanoparticles—Framework for synthesis and evaluation. ACS Appl Mater Interfaces. (2021) 13:23410–22. doi: 10.1021/acsami.1c02834
141. Jiang X, Du B, Huang Y, Zheng J. Ultrasmall noble metal nanoparticles: breakthroughs and biomedical implications. Nano Today. (2018) 21:106–25. doi: 10.1016/j.nantod.2018.06.006
142. Bobo D, Robinson KJ, Islam J, Thurecht KJ, Corrie SR. Nanoparticle-based medicines: A review of FDA-approved materials and clinical trials to date. Pharm Res. (2016) 33:2373–87. doi: 10.1007/s11095-016-1958-5
143. Anselmo AC, Mitragotri S. Nanoparticles in the clinic: An update. Bioeng Transl Med. (2019) 4:e10143. doi: 10.1002/btm2.10143
144. de Vrieze J. Suspicions grow that nanoparticles in Pfizer’s COVID-19 vaccine trigger rare allergic reactions. (2020). doi: 10.1126/science.abg2359
145. Verhoef JJF, Carpenter JF, Anchordoquy TJ, Schellekens H. Potential induction of anti-PEG antibodies and complement activation toward PEGylated therapeutics. Drug Discovery Today. (2014) 19:1945–52. doi: 10.1016/j.drudis.2014.08.015
146. Tian Y, Gao Z, Wang N, Hu M, Ju Y, Li Q, et al. Engineering poly(ethylene glycol) nanoparticles for accelerated blood clearance inhibition and targeted drug delivery. J Am Chem Soc. (2022) 144:18419–28. doi: 10.1021/jacs.2c06877
147. Rahikkala A, Fontana F, Bauleth-Ramos T, Correia A, Kemell M, Seitsonen J, et al. Hybrid red blood cell membrane coated porous silicon nanoparticles functionalized with cancer antigen induce depletion of T cells. RSC Adv. (2020) 10:35198–205. doi: 10.1039/D0RA05900E
148. Hu Q, Sun W, Qian C, Wang C, Bomba HN, Gu Z. Anticancer platelet-mimicking nanovehicles. Adv Mater. (2015) 27:7043–50. doi: 10.1002/adma.201503323
149. Fontana F, Albertini S, Correia A, Kemell M, Lindgren R, Mäkilä E, et al. Bioengineered porous silicon nanoparticles macrophages cell membrane as composite platforms for rheumatoid arthritis. Adv Funct Mater. (2018) 28:1801355. doi: 10.1002/adfm.201801355
150. Fontana F, Shahbazi M, Liu D, Zhang H, Mäkilä E, Salonen J, et al. Multistaged nanovaccines based on porous silicon acetalated dextran cancer cell membrane for cancer immunotherapy. Adv Mater. (2017) 29:1603239. doi: 10.1002/adma.201603239
151. Gao W, Fang RH, Thamphiwatana S, Luk BT, Li J, Angsantikul P, et al. Modulating antibacterial immunity via bacterial membrane-coated nanoparticles. Nano Lett. (2015) 15:1403–9. doi: 10.1021/nl504798g
152. Zhang G, Yao M, Ma S, Zhang K, Wang Y, Wang Z, et al. Application of cell membrane-functionalized biomimetic nanoparticles in the treatment of glioma. J Mater Chem B. (2023) 11:7055–68. doi: 10.1039/D3TB00605K
153. Charabati M, Rabanel J-M, Ramassamy C, Prat A. Overcoming the brain barriers: from immune cells to nanoparticles. Trends Pharmacol Sci. (2020) 41:42–54. doi: 10.1016/j.tips.2019.11.001
154. Yu H, Yang Z, Li F, Xu L, Sun Y. Cell-mediated targeting drugs delivery systems. Drug Delivery. (2020) 27:1425–37. doi: 10.1080/10717544.2020.1831103
155. Hu Q, Sun W, Wang J, Ruan H, Zhang X, Ye Y, et al. Conjugation of haematopoietic stem cells and platelets decorated with anti-PD-1 antibodies augments anti-leukaemia efficacy. Nat BioMed Eng. (2018) 2:831–40. doi: 10.1038/s41551-018-0310-2
156. Tang L, Zheng Y, Melo MB, Mabardi L, Castaño AP, Xie Y-Q, et al. Enhancing T cell therapy through TCR-signaling-responsive nanoparticle drug delivery. Nat Biotechnol. (2018) 36:707–16. doi: 10.1038/nbt.4181
157. Kostka K, Sokolova V, El-Taibany A, Kruse B, Porada D, Wolff N, et al. The application of ultrasmall gold nanoparticles (2 nm) functionalized with doxorubicin in three-dimensional normal and glioblastoma organoid models of the blood-brain barrier. Mol Basel Switz. (2024) 29:2469. doi: 10.3390/molecules29112469
158. Sokolova V, Mekky G, van der Meer SB, Seeds MC, Atala AJ, Epple M. Transport of ultrasmall gold nanoparticles (2 nm) across the blood–brain barrier in a six-cell brain spheroid model. Sci Rep. (2020) 10:18033. doi: 10.1038/s41598-020-75125-2
159. Khan FA, Almohazey D, Alomari M, Almofty SA. Impact of nanoparticles on neuron biology: current research trends. Int J Nanomedicine. (2018) 13:2767–76. doi: 10.2147/IJN.S165675
160. Choi B, Soh M, Manandhar Y, Kim D, Han SI, Baik S, et al. Highly selective microglial uptake of ceria–zirconia nanoparticles for enhanced analgesic treatment of neuropathic pain. Nanoscale. (2019) 11:19437–47. doi: 10.1039/C9NR02648G
161. Maldonado RA, LaMothe RA, Ferrari JD, Zhang A-H, Rossi RJ, Kolte PN, et al. Polymeric synthetic nanoparticles for the induction of antigen-specific immunological tolerance. Proc Natl Acad Sci U.S.A. (2015) 112:E156–165. doi: 10.1073/pnas.1408686111
162. Bernacki J, Dobrowolska A, Nierwińska K, Małecki A. Physiology and pharmacological role of the blood-brain barrier. Pharmacol Rep PR. (2008) 60:600–22.
163. Fathi-Achachelouei M, Knopf-Marques H, Ribeiro da Silva CE, Barthès J, Bat E, Tezcaner A, et al. Use of nanoparticles in tissue engineering and regenerative medicine. Front Bioeng Biotechnol. (2019) 7:113. doi: 10.3389/fbioe.2019.00113
164. Elzoghby AO, Samy WM, Elgindy NA. Protein-based nanocarriers as promising drug and gene delivery systems. J Controlled Release. (2012) 161:38–49. doi: 10.1016/j.jconrel.2012.04.036
165. MaChado-Pereira M, Santos T, Ferreira L, Bernardino L, Ferreira R. Anti-inflammatory strategy for M2 microglial polarization using retinoic acid-loaded nanoparticles. Mediators Inflammation. (2017) 2017:6742427. doi: 10.1155/2017/6742427
166. Quintana FJ, Pozo D. Editorial: nanoparticle-mediated signaling rewiring and reprogramming of immune responses. Front Immunol. (2022) 13:927733. doi: 10.3389/fimmu.2022.927733
167. Steichen SD, Caldorera-Moore M, Peppas NA. A review of current nanoparticle and targeting moieties for the delivery of cancer therapeutics. Eur J Pharm Sci. (2013) 48:416–27. doi: 10.1016/j.ejps.2012.12.006
168. Zhang F, Lin Y-A, Kannan S, Kannan RM. Targeting specific cells in the brain with nanomedicines for CNS therapies. J Control Release Off J Control Release Soc. (2016) 240:212–26. doi: 10.1016/j.jconrel.2015.12.013
169. Korshoj LE, Shi W, Duan B, Kielian T. The prospect of nanoparticle systems for modulating immune cell polarization during central nervous system infection. Front Immunol. (2021) 12:670931. doi: 10.3389/fimmu.2021.670931
170. Phuphanich S, Maria B, Braeckman R, Chamberlain M. A pharmacokinetic study of intra-CSF administered encapsulated cytarabine (DepoCyt®) for the treatment of neoplastic meningitis in patients with leukemia, lymphoma, or solid tumors as part of a phase III study. J Neurooncol. (2006) 81:201–8. doi: 10.1007/s11060-006-9218-x
171. Kim S-S, Rait A, Garrido-Sanabria ER, Pirollo KF, Harford JB, Chang EH. Nanotherapeutics for gene modulation that prevents apoptosis in the brain and fatal neuroinflammation. Mol Ther. (2018) 26:84–94. doi: 10.1016/j.ymthe.2017.10.003
172. Amani H, Habibey R, Shokri F, Hajmiresmail SJ, Akhavan O, Mashaghi A, et al. Selenium nanoparticles for targeted stroke therapy through modulation of inflammatory and metabolic signaling. Sci Rep. (2019) 9:6044. doi: 10.1038/s41598-019-42633-9
173. Bardi G, Boselli L, Pompa PP. Anti-inflammatory potential of platinum nanozymes: mechanisms and perspectives. Nanoscale. (2023) 15:14284–300. doi: 10.1039/D3NR03016D
174. Yu Z, Lou R, Pan W, Li N, Tang B. Nanoenzymes in disease diagnosis and therapy. Chem Commun. (2020) 56:15513–24. doi: 10.1039/D0CC05427E
175. Gulino M, Santos SD, Pêgo AP. Biocompatibility of Platinum Nanoparticles in Brain ex vivo Models in Physiological and Pathological Conditions. Front Neurosci. (2021) 15:787518. doi: 10.3389/fnins.2021.787518
176. Takamiya M, Miyamoto Y, Yamashita T, Deguchi K, Ohta Y, Ikeda Y, et al. Neurological and pathological improvements of cerebral infarction in mice with platinum nanoparticles. J Neurosci Res. (2011) 89:1125–33. doi: 10.1002/jnr.22622
177. Gasche Y, Copin J-C, Sugawara T, Fujimura M, Chan PH. Matrix metalloproteinase inhibition prevents oxidative stress-associated blood–brain barrier disruption after transient focal cerebral ischemia. J Cereb Blood Flow Metab. (2001) 21:1393–400. doi: 10.1097/00004647-200112000-00003
178. Takamiya M, Miyamoto Y, Yamashita T, Deguchi K, Ohta Y, Abe K. Strong neuroprotection with a novel platinum nanoparticle against ischemic stroke- and tissue plasminogen activator-related brain damages in mice. Neuroscience. (2012) 221:47–55. doi: 10.1016/j.neuroscience.2012.06.060
179. Mu X, Wang J, Li Y, Xu F, Long W, Ouyang L, et al. Redox trimetallic nanozyme with neutral environment preference for brain injury. ACS Nano. (2019) 13:1870–84. doi: 10.1021/acsnano.8b08045
180. Harry GJ, Kraft AD. Neuroinflammation and microglia: considerations and approaches for neurotoxicity assessment. Expert Opin Drug Metab Toxicol. (2008) 4:1265–77. doi: 10.1517/17425255.4.10.1265
181. Waheed S, Li Z, Zhang F, Chiarini A, Armato U, Wu J. Engineering nano-drug biointerface to overcome biological barriers toward precision drug delivery. J Nanobiotechnology. (2022) 20:395. doi: 10.1186/s12951-022-01605-4
182. Capjak I, Goreta SŠ, Jurašin DD, Vrček IV. How protein coronas determine the fate of engineered nanoparticles in biological environment. Arch Ind Hyg Toxicol. (2017) 68:245–53. doi: 10.1515/aiht-2017-68-3054
183. Nel AE, Mädler L, Velegol D, Xia T, Hoek EMV, Somasundaran P, et al. Understanding biophysicochemical interactions at the nano–bio interface. Nat Mater. (2009) 8:543–57. doi: 10.1038/nmat2442
184. Đorđević S, Gonzalez MM, Conejos-Sánchez I, Carreira B, Pozzi S, Acúrcio RC, et al. Current hurdles to the translation of nanomedicines from bench to the clinic. Drug Delivery Transl Res. (2022) 12:500–25. doi: 10.1007/s13346-021-01024-2
185. Wilhelm S, Tavares AJ, Dai Q, Ohta S, Audet J, Dvorak HF, et al. Analysis of nanoparticle delivery to tumours. Nat Rev Mater. (2016) 1:16014. doi: 10.1038/natrevmats.2016.14
186. Lundqvist M, Stigler J, Elia G, Lynch I, Cedervall T, Dawson KA. Nanoparticle size and surface properties determine the protein corona with possible implications for biological impacts. Proc Natl Acad Sci. (2008) 105:14265–70. doi: 10.1073/pnas.0805135105
187. Panico S, Capolla S, Bozzer S, Toffoli G, Dal Bo M, Macor P. Biological features of nanoparticles: protein corona formation and interaction with the immune system. Pharmaceutics. (2022) 14:2605. doi: 10.3390/pharmaceutics14122605
188. Palmieri V, Caracciolo G. Tuning the immune system by nanoparticle-biomolecular corona. Nanoscale Adv. (2022) 4:3300–8. doi: 10.1039/d2na00290f
189. Nguyen VH, Lee B-J. Protein corona: a new approach for nanomedicine design. Int J Nanomedicine. (2017) 12:3137–51. doi: 10.2147/IJN.S129300
190. Pinals RL, Chio L, Ledesma F, Landry MP. Engineering at the nano-bio interface: harnessing the protein corona towards nanoparticle design and function. Analyst. (2020) 145:5090–112. doi: 10.1039/D0AN00633E
191. Corbo C, Molinaro R, Parodi A, Toledano Furman NE, Salvatore F, Tasciotti E. The impact of nanoparticle protein corona on cytotoxicity, immunotoxicity and target drug delivery. Nanomed. (2016) 11:81–100. doi: 10.2217/nnm.15.188
192. Foroozandeh P, Aziz AA. Merging worlds of nanomaterials and biological environment: factors governing protein corona formation on nanoparticles and its biological consequences. Nanoscale Res Lett. (2015) 10:221. doi: 10.1186/s11671-015-0922-3
193. Tenzer S, Docter D, Kuharev J, Musyanovych A, Fetz V, Hecht R, et al. Rapid formation of plasma protein corona critically affects nanoparticle pathophysiology. Nat Nanotechnol. (2013) 8:772–81. doi: 10.1038/nnano.2013.181
194. Caracciolo G, Pozzi D, Capriotti AL, Cavaliere C, Piovesana S, La Barbera G, et al. The liposome–protein corona in mice and humans and its implications for in vivo delivery. J Mater Chem B. (2014) 2:7419–28. doi: 10.1039/C4TB01316F
195. Digiacomo L, Pozzi D, Palchetti S, Zingoni A, Caracciolo G. Impact of the protein corona on nanomaterial immune response and targeting ability. WIREs Nanomedicine Nanobiotechnology. (2020) 12:e1615. doi: 10.1002/wnan.1615
196. Dobrovolskaia MA, Patri AK, Zheng J, Clogston JD, Ayub N, Aggarwal P, et al. Interaction of colloidal gold nanoparticles with human blood: effects on particle size and analysis of plasma protein binding profiles. Nanomedicine Nanotechnol Biol Med. (2009) 5:106–17. doi: 10.1016/j.nano.2008.08.001
197. Monopoli MP, Walczyk D, Campbell A, Elia G, Lynch I, Baldelli Bombelli F, et al. Physical–chemical aspects of protein corona: relevance to in vitro and in vivo biological impacts of nanoparticles. J Am Chem Soc. (2011) 133:2525–34. doi: 10.1021/ja107583h
198. Simberg D, Park J-H, Karmali PP, Zhang W-M, Merkulov S, McCrae K, et al. Differential proteomics analysis of the surface heterogeneity of dextran iron oxide nanoparticles and the implications for their in vivo clearance. Biomaterials. (2009) 30:3926–33. doi: 10.1016/j.biomaterials.2009.03.056
199. Walkey CD, Chan WCW. Understanding and controlling the interaction of nanomaterials with proteins in a physiological environment. Chem Soc Rev. (2012) 41:2780–99. doi: 10.1039/c1cs15233e
200. Pozzi D, Caracciolo G, Digiacomo L, Colapicchioni V, Palchetti S, Capriotti AL, et al. The biomolecular corona of nanoparticles in circulating biological media. Nanoscale. (2015) 7:13958–66. doi: 10.1039/C5NR03701H
201. Zhdanov VP. Nanoparticles without and with protein corona: van der Waals and hydration interaction. J Biol Phys. (2019) 45:307–16. doi: 10.1007/s10867-019-09530-8
202. Alexis F, Pridgen E, Molnar LK, Farokhzad OC. Factors affecting the clearance and biodistribution of polymeric nanoparticles. Mol Pharm. (2008) 5:505–15. doi: 10.1021/mp800051m
203. Owens DE, Peppas NA. Opsonization, biodistribution, and pharmacokinetics of polymeric nanoparticles. Int J Pharm. (2006) 307:93–102. doi: 10.1016/j.ijpharm.2005.10.010
204. Cai R, Chen C. The crown and the scepter: roles of the protein corona in nanomedicine. Adv Mater. (2019) 31:1805740. doi: 10.1002/adma.201805740
205. Deng ZJ, Liang M, Monteiro M, Toth I, Minchin RF. Nanoparticle-induced unfolding of fibrinogen promotes Mac-1 receptor activation and inflammation. Nat Nanotechnol. (2011) 6:39–44. doi: 10.1038/nnano.2010.250
206. Vu VP, Gifford GB, Chen F, Benasutti H, Wang G, Groman EV, et al. Immunoglobulin deposition on biomolecule corona determines complement opsonization efficiency of preclinical and clinical nanoparticles. Nat Nanotechnol. (2019) 14:260–8. doi: 10.1038/s41565-018-0344-3
207. Tavano R, Gabrielli L, Lubian E, Fedeli C, Visentin S, Polverino De Laureto P, et al. C1q-mediated complement activation and C3 opsonization trigger recognition of stealth poly(2-methyl-2-oxazoline)-coated silica nanoparticles by human phagocytes. ACS Nano. (2018) 12:5834–47. doi: 10.1021/acsnano.8b01806
208. Chen F, Wang G, Griffin JI, Brenneman B, Banda NK, Holers VM, et al. Complement proteins bind to nanoparticle protein corona and undergo dynamic exchange in vivo. Nat Nanotechnol. (2017) 12:387–93. doi: 10.1038/nnano.2016.269
209. Moghimi SM, Simberg D, Skotland T, Yaghmur A, Hunter AC. The interplay between blood proteins, complement, and macrophages on nanomedicine performance and responses. J Pharmacol Exp Ther. (2019) 370:581–92. doi: 10.1124/jpet.119.258012
210. Shi L, Zhang J, Zhao M, Tang S, Cheng X, Zhang W, et al. Effects of polyethylene glycol on the surface of nanoparticles for targeted drug delivery. Nanoscale. (2021) 13:10748–64. doi: 10.1039/D1NR02065J
211. Rampado R, Crotti S, Caliceti P, Pucciarelli S, Agostini M. Recent advances in understanding the protein corona of nanoparticles and in the formulation of “Stealthy” Nanomaterials. Front Bioeng Biotechnol. (2020) 8:166. doi: 10.3389/fbioe.2020.00166
212. Dal Magro R, Albertini B, Beretta S, Rigolio R, Donzelli E, Chiorazzi A, et al. Artificial apolipoprotein corona enables nanoparticle brain targeting. Nanomedicine Nanotechnol Biol Med. (2018) 14:429–38. doi: 10.1016/j.nano.2017.11.008
213. Tonigold M, Simon J, Estupiñán D, Kokkinopoulou M, Reinholz J, Kintzel U, et al. Pre-adsorption of antibodies enables targeting of nanocarriers despite a biomolecular corona. Nat Nanotechnol. (2018) 13:862–9. doi: 10.1038/s41565-018-0171-6
214. Giulimondi F, Vulpis E, Digiacomo L, Giuli MV, Mancusi A, Capriotti AL, et al. Opsonin-deficient nucleoproteic corona endows unPEGylated liposomes with stealth properties. In Vivo. ACS Nano. (2022) 16:2088–100. doi: 10.1021/acsnano.1c07687
215. Giulimondi F, Digiacomo L, Vulpis E, Loconte L, Ferri G, Cardarelli F, et al. In vitro and ex vivo nano-enabled immunomodulation by the protein corona. Nanoscale. (2022) 14:10531–9. doi: 10.1039/D2NR01878K
216. Kelly PM, Åberg C, Polo E, O’Connell A, Cookman J, Fallon J, et al. Mapping protein binding sites on the biomolecular corona of nanoparticles. Nat Nanotechnol. (2015) 10:472–9. doi: 10.1038/nnano.2015.47
217. Oh JY, Kim HS, Palanikumar L, Go EM, Jana B, Park SA, et al. Cloaking nanoparticles with protein corona shield for targeted drug delivery. Nat Commun. (2018) 9:4548. doi: 10.1038/s41467-018-06979-4
218. Sylvestre M, Crane CA, Pun SH. Progress on modulating tumor-associated macrophages with biomaterials. Adv Mater Deerfield Beach Fla. (2020) 32:e1902007. doi: 10.1002/adma.201902007
219. Mahmoudi M, Bertrand N, Zope H, Farokhzad OC. Emerging understanding of the protein corona at the nano-bio interfaces. Nano Today. (2016) 11:817–32. doi: 10.1016/j.nantod.2016.10.005
220. Chen P, Ding F, Cai R, Javed I, Yang W, Zhang Z, et al. Amyloidosis inhibition, a new frontier of the protein corona. Nano Today. (2020) 35:100937. doi: 10.1016/j.nantod.2020.100937
221. Petros RA, DeSimone JM. Strategies in the design of nanoparticles for therapeutic applications. Nat Rev Drug Discovery. (2010) 9:615–27. doi: 10.1038/nrd2591
222. Takakura Y, Takahashi Y. Strategies for persistent retention of macromolecules and nanoparticles in the blood circulation. J Control Release Off J Control Release Soc. (2022) 350:486–93. doi: 10.1016/j.jconrel.2022.05.063
223. Chen D, Love KT, Chen Y, Eltoukhy AA, Kastrup C, Sahay G, et al. Rapid discovery of potent siRNA-containing lipid nanoparticles enabled by controlled microfluidic formulation. J Am Chem Soc. (2012) 134:6948–51. doi: 10.1021/ja301621z
224. Hu L, Tao Y, Jiang Y, Qin F. Recent progress of nanomedicine in the treatment of Alzheimer’s disease. Front Cell Dev Biol. (2023) 11:1228679. doi: 10.3389/fcell.2023.1228679
225. Jagaran K, Singh M. Lipid nanoparticles: promising treatment approach for parkinson’s disease. Int J Mol Sci. (2022) 23:9361. doi: 10.3390/ijms23169361
226. Khan NH, Mir M, Ngowi EE, Zafar U, Khakwani MMAK, Khattak S, et al. Nanomedicine: A promising way to manage alzheimer’s disease. Front Bioeng Biotechnol. (2021) 9:630055. doi: 10.3389/fbioe.2021.630055
227. Mir Najib Ullah SN, Afzal O, Altamimi ASA, Ather H, Sultana S, Almalki WH, et al. Nanomedicine in the management of alzheimer’s disease: state-of-the-art. Biomedicines. (2023) 11:1752. doi: 10.3390/biomedicines11061752
228. Monge-Fuentes V, Biolchi Mayer A, Lima MR, Geraldes LR, Zanotto LN, Moreira KG, et al. Dopamine-loaded nanoparticle systems circumvent the blood–brain barrier restoring motor function in mouse model for Parkinson’s Disease. Sci Rep. (2021) 11:15185. doi: 10.1038/s41598-021-94175-8
229. Song G, Zhao M, Chen H, Lenahan C, Zhou X, Ou Y, et al. The role of nanomaterials in stroke treatment: targeting oxidative stress. Oxid Med Cell Longev. (2021) 2021:8857486. doi: 10.1155/2021/8857486
230. Toljan K, Ashok A, Labhasetwar V, Hussain MS. Nanotechnology in stroke: new trails with smaller scales. Biomedicines. (2023) 11:780. doi: 10.3390/biomedicines11030780
231. Van Vliet EF, Knol MJ, Schiffelers RM, Caiazzo M, Fens MHAM. Levodopa-loaded nanoparticles for the treatment of Parkinson’s disease. J Controlled Release. (2023) 360:212–24. doi: 10.1016/j.jconrel.2023.06.026
232. Yuan J, Li L, Yang Q, Ran H, Wang J, Hu K, et al. Targeted treatment of ischemic stroke by bioactive nanoparticle-derived reactive oxygen species responsive and inflammation-resolving nanotherapies. ACS Nano. (2021) 15:16076–94. doi: 10.1021/acsnano.1c04753
233. Hunter Z, McCarthy DP, Yap WT, Harp CT, Getts DR, Shea LD, et al. A biodegradable nanoparticle platform for the induction of antigen-specific immune tolerance for treatment of autoimmune disease. ACS Nano. (2014) 8:2148–60. doi: 10.1021/nn405033r
234. Nuzzo D, Picone P. Multiple sclerosis: focus on extracellular and artificial vesicles, nanoparticles as potential therapeutic approaches. Int J Mol Sci. (2021) 22:8866. doi: 10.3390/ijms22168866
235. Cheng X, Xie Q, Sun Y. Advances in nanomaterial-based targeted drug delivery systems. Front Bioeng Biotechnol. (2023) 11:1177151. doi: 10.3389/fbioe.2023.1177151
236. Hersh AM, Alomari S, Tyler BM. Crossing the blood-brain barrier: advances in nanoparticle technology for drug delivery in neuro-oncology. Int J Mol Sci. (2022) 23:4153. doi: 10.3390/ijms23084153
237. Jia F, Liu X, Li L, Mallapragada S, Narasimhan B, Wang Q. Multifunctional nanoparticles for targeted delivery of immune activating and cancer therapeutic agents. J Controlled Release. (2013) 172:1020–34. doi: 10.1016/j.jconrel.2013.10.012
238. Peng X, Fang J, Lou C, Yang L, Shan S, Wang Z, et al. Engineered nanoparticles for precise targeted drug delivery and enhanced therapeutic efficacy in cancer immunotherapy. Acta Pharm Sin B. (2024) 8:3432–56. doi: 10.1016/j.apsb.2024.05.010
239. Gonzalez-Carter D, Liu X, Tockary TA, Dirisala A, Toh K, Anraku Y, et al. Targeting nanoparticles to the brain by exploiting the blood–brain barrier impermeability to selectively label the brain endothelium. Proc Natl Acad Sci. (2020) 117:19141–50. doi: 10.1073/pnas.2002016117
Keywords: nanoparticles, central nervous system, immunosurveillance, immune reprogramming, biomolecular corona, nanotherapeutics, molecular rehabilitation
Citation: Moulton C, Baroni A, Quagliarini E, Leone L, Digiacomo L, Morotti M, Caracciolo G, Podda MV and Tasciotti E (2024) Navigating the nano-bio immune interface: advancements and challenges in CNS nanotherapeutics. Front. Immunol. 15:1447567. doi: 10.3389/fimmu.2024.1447567
Received: 12 June 2024; Accepted: 21 October 2024;
Published: 12 November 2024.
Edited by:
Subhadeep Roy, National Institute of Pharmaceutical Education and Research, IndiaReviewed by:
Giorgia Moschetti, University of Milan, ItalyValentina Castagnola, Italian Institute of Technology (IIT), Italy
Copyright © 2024 Moulton, Baroni, Quagliarini, Leone, Digiacomo, Morotti, Caracciolo, Podda and Tasciotti. This is an open-access article distributed under the terms of the Creative Commons Attribution License (CC BY). The use, distribution or reproduction in other forums is permitted, provided the original author(s) and the copyright owner(s) are credited and that the original publication in this journal is cited, in accordance with accepted academic practice. No use, distribution or reproduction is permitted which does not comply with these terms.
*Correspondence: Ennio Tasciotti, ZW5uaW8udGFzY2lvdHRpQHNhbnJhZmZhZWxlLml0
†These authors have contributed equally to this work and share first authorship
‡These authors have contributed equally to this work and share last authorship