- 1Department of Anesthesiology, Union Hospital, Tongji Medical College, Huazhong University of Science and Technology, Wuhan, China
- 2Institute of Anesthesia and Critical Care Medicine, Union Hospital, Tongji Medical College, Huazhong University of Science and Technology, Wuhan, China
- 3Key Laboratory of Anesthesiology and Resuscitation (Huazhong University of Science and Technology), Ministry of Education, Wuhan, China
- 4Department of Pain, Union Hospital, Tongji Medical College, Huazhong University of Science and Technology, Wuhan, China
Sepsis is a life-threatening syndrome of organ dysfunction, characterized by uncontrolled inflammatory response and immune dysregulation, often leading to multiple organ failure and even death. Specialized pro-resolving mediators (SPMs), which are typically thought to be formed via consecutive steps of oxidation of polyenoic fatty acids, have been shown to suppress inflammation and promote timely resolution of inflammation. They are mainly divided into four categories: lipoxins, resolvins, protectins, and maresins. The SPMs may improve the prognosis of sepsis by modulating the immune and inflammatory balance, thereby holding promise for clinical applications. However, their biosynthetic and pharmacological properties are very complex. Through a literature review, we aim to comprehensively elucidate the protective mechanisms of different SPMs in sepsis and its organ damage, in order to provide sufficient theoretical basis for the future clinical translation of SPMs.
1 Introduction
Sepsis is a life-threatening organ dysfunction syndrome resulting from a dysregulated host response to infection, and is a major public health problem affecting the general level of human health (1). Epidemiological surveys have shown that there are nearly 50 million cases of sepsis globally each year, of which approximately 11 million die, with the attendant healthcare costs of tens of billions of dollars (2). An important pathophysiological process in sepsis is uncontrolled inflammatory immunity, which is frequently accompanied by a confluence of over-activation of inflammatory hyperactivation and immunosuppression. In this scenario, pathogens evade the host’s defense mechanisms to multiply continuously, stimulating and damaging host cells ultimately leading to an inability to regain homeostasis, which then results multi-organ dysfunction (3, 4). Despite the great progress in anti-infective, fluid resuscitation and organ function support techniques, they do not intervene at the source of immune disorders. It is urgent to explore ways to regulate inflammatory immune disorders to improve the prognosis of patients in sepsis.
Specialized pro-resolving mediators (SPMs) are a class of lipid mediators derived from polyunsaturated fatty acids with anti-inflammatory and pro-resolving effects (5). In 1984, Serhan et al. (6) identified a new class of substances called lipoxins (LX) in the metabolism of arachidonic acid (AA). Besides AA derivatives, resolvins, protectins and maresins are found among the metabolites of n-3 polyunsaturated fatty acids (PUFAs). Resolvins are classified into E-series (RvE) and D-series (RvD) based on the usage of (eicosapentaenoic acid) EPA and (docosahexaenoic acid) DHA as substrate, respectively. Maresin (MaR), first identified in macrophages, mainly includes MaR1 and MaR2. In-depth reviews have been published on the biosynthesis, structure, and function of SPMs (7, 8). Noteworthy is the considerable debate on the formation of specific SPMs in the human body as well as in general. There seems to be a recent consensus that there is no significant formation of trihydroxylated SPMs in biological samples (9, 10). Several receptors are currently reported to bind SPMs, such as LXA4/formyl peptide receptor 2 (ALX/FPR2), RvD1/G protein-coupled receptor 32 (GPR32), RvD2/GPR18, RvE1/Chemerin Receptor 1 (ChemR23), and MaR1/leucine-rich repeat-containing G protein-coupled receptor 6 (LGR6) (5, 11). However, the functional validation of these receptors is still a subject of debate. Despite initial reports, not all SPM/receptor bindings have been consistently reproduced, and also functional responses such as those mediated by FPR2 or ChemR23 to their respective SPMs could not be reproduced (9, 12, 13). The field is evolving, and further research is needed to fully understand the role of these receptors in mediating the effects of SPMs.
A large number of studies have demonstrated the protective effects of SPMs against different sepsis-induced organ dysfunction (14–16). In our review, the protective effects of SPMs in sepsis-induced organ dysfunction are summarized (Tables 1–5), aiming to provide new ideas for the treatment of sepsis, as well as more evidence for the development and clinical application of SPMs.
2 Pharmacological effects of SPMs in sepsis-induced acute lung injury
Sepsis frequently impacts the lungs, often resulting in acute lung injury (ALI)/acute respiratory distress syndrome (ARDS), with over 200,000 cases annually in the United States (17). Shi Y et al. (18) reported that 48.6% of septic patients in China developed ALI in the ICU. Despite advancements in ALI/ARDS management, the mortality rate for sepsis-induced cases remains high, underscoring the severity of ALI/ARDS in sepsis (19). Sepsis-induced ALI (SALI) involves complex mechanisms such as imbalanced inflammatory responses, endothelial barrier disruption and alveolar epithelial damage (20–22). Current treatments for SALI include aggressive antibiotics, respiratory and nutritional support and fluid management (23, 24), yet an effective therapy is still lacking. Recent studies focusing on SPMs in preclinical animal models have shown promise (Table 1), emphasizing the need for further research into SPMs’ therapeutic mechanisms in SALI.
LXs have demonstrated therapeutic potential in SALI. LXA4 has been shown to enhance alveolar fluid clearance and reduce pulmonary edema, as indicated by a decreased wet/dry weight (W/D) ratio. LXA4 also diminishes bronchoalveolar lavage fluid (BALF) cell counts, particularly polymorphonuclear neutrophils, and reduced levels of TNF-ɑ and IL-6, along with myeloperoxidase (MPO) activity, contributing to improved 7-day survival rates in SALI rats, The p38/MAPK pathway is implicated in LXA4’s protective effects (25). Aspirin-triggered lipoxin A4 (AT-LXA4) mitigated lung injury by inhibiting neutrophil-platelet aggregation, a key factor in SALI’s inflammatory response (26). Furthermore, in a mouse model of LPS-induced lung injury, LPS damaged vascular endothelial cells leading to increased perivascular stiffness and stimulated the expression of extracellular matrix (ECM) proteins fibronectin, collagen I and ECM crosslinker enzyme, lysyl oxidase. In contrast, LXA4 mitigated vascular endothelial damage, reduced the associated inflammatory response, restored the lung compliance (27). Resolvins are lipid mediators derived from EPA and DHA. RvD1 exerted anti-inflammatory effects in a cecal ligation and puncture (CLP) mouse model by reducing levels of TNF-ɑ, IL-1β and IL-6, enhancing silent information regulator 1 (SIRT1) expression and inhibiting the activation of NF-κB, STAT3, ERK and p38, improved survival (28). The combination of RvD1 with Xuebijing diminished MPO activity and intercellular adhesion molecule 1 (ICAM-1) expression, thereby reducing leukocyte adhesion and lung injury in septic mice (29). In a 2-hit model of sepsis with secondary lung infection, RvD2 enhanced myeloid-derived suppressor cells (MDSC) accumulation in the spleen and increased the presence of non-inflammatory alveolar macrophages, facilitating bacterial clearance from both blood and lungs (30). Additionally, RvD2 significantly lowered the concentration of IL-23 in lung lavage fluid, a cytokine critical to the Th-17 inflammatory pathway, contributing to the protective effect against secondary pulmonary infections. Protectins also had a protective effect on SALI (31–33). PDX has been shown to decrease pro-inflammatory cytokines IL-1β, IL-6, TNF-ɑ, and MCP-1, and increase the anti-inflammatory factor IL-10 in BALF. This modulation is associated with PPARγ upregulation and inhibiting NF-κB p65 phosphorylation, leading to reduced lung inflammation and improved lung permeability. Protectin conjugates in tissue regeneration 1 (PCTR1) restored endothelial glycocalyx loss by downregulating heparanase (HPA) and upregulating exostatin-1 (EXT-1), enhancing lung function and the survival rate of SALI mice. Additionally, PCTR1 mitigated mitochondrial damage and reactive oxygen species (ROS) production by decreasing Fe2+ and prostaglandin-endoperoxide synthase 2 (PTGS2) levels and by increasing glutathione (GSH) and glutathione peroxidase 4 (GPX4), thereby protecting against LPS-induced ALI through the inhibition of ferroptosis. Maresins is a newly discovered family of lipid mediators synthesized from DHA by macrophages. MaR1 reduced levels of IL-6, TNF-ɑ and IL-1β, decreased bacterial load, and attenuated lung injury in CLP mice (34), partly through the inhibition of NF-κB pathway activation. Additionally, MaR1 mitigated lung injury in CLP mice by attenuating mitochondrial dysfunction through regulating ROS production (35). Maresin conjugates in tissue regeneration 1 (MCTR1) attenuated endothelial glycocalyx injury and improved survival in SALI mice in addition to decreasing serum levels of inflammatory cytokines (TNF-ɑ, IL-1β and IL-6). The mechanism may be related to MCTR1 upregulating the expression SIRT1 and reducing the phosphorylation of NF-κB p65, thereby downregulating HPA (36). The authors suggested that ALX is associated with the protective effect, but its role has not been confirmed using ALX knockout mice (35, 36). SPMs hold significant therapeutic potential for addressing SALI through various key mechanisms. Advancing our knowledge in this area can deepen the understanding of the therapeutic significance of SPMs and expand their potential applications in a range of lung diseases.
3 Pharmacological effects of SPMs in sepsis-induced acute kidney injury
Sepsis-induced acute kidney injury (SAKI) is a common complication of sepsis, accounting for 26% to 50% of all AKI, and AKI is an independent risk factor for the increased risk of death in patients with sepsis (37). The pathogenesis of SAKI is complex and involves multiple factors, such as inflammation, renal tubular epithelial cell apoptosis, oxidative stress, and ferroptosis, etc., which can interact with each other and affect the progression of SAKI.
In recent years, research into the role of SPMs in SAKI has focused on their interaction with cytokines, chemokines, and ROS, as well as the exploration of their signaling pathways, including the IL-6, TLR4/NF-κB, and MAPK pathways. In a murine model of LPS-induced acute kidney injury, AT-RvD1 alleviated the inflammatory response and protected kidney function by reducing the expression of ICAM-1 and vascular cell adhesion protein 1 (VCAM-1), inhibiting IL-6 and IL-6-related inflammatory pathways (STAT3 and ERK phosphorylation), and reducing the phosphorylation and activation of NF-κB (38). Similarly, MaR1 also inhibited the NF-κB/STAT3/MAPK signaling pathway to suppress the inflammatory response, thereby dose-dependently mitigating SAKI (39). AT-RvD1 also stabilized the expression level of claudin-4, alleviated the barrier damage in the kidney, and further prevented the infiltration of inflammatory cells (38). RvD1 inhibited the entire pathway from TLR4, MyD88, NF-κB to TNF-α induced by LPS, reduced the inflammatory response and renal cell apoptosis, and thus protected against LPS-induced AKI (40). Mitochondria are the main source and primary target of intracellular ROS. NADPH oxidase 4 (NOX4), a major subtype in the kidney, is highly expressed and increasingly recognized as a major cause of elevated ROS levels, which play a crucial role in mediating mitochondrial dysfunction and cell apoptosis. MaR1 effectively inhibited the NOX4/ROS/NF-κB p65 signaling pathway and reduced kidney inflammation, cell apoptosis, oxidative stress and mitochondrial dysfunction, ultimately protecting against LPS-induced AKI (41).
Oxidative stress and inflammation can trigger senescence, also known as stress-induced premature senescence (SIPS). There is crosstalk between inflammation and senescence, forming a positive feedback loop. It is worth noting that premature cell senescence has become an important feature of AKI. Chen et al. (42) demonstrated that LXA4 inhibited NF-κB mediated inflammatory response and p53/p21 aging pathway in a PPAR-dependent manner, blocking the crosstalk between inflammation and premature senescence and exerting its renal protective effect.
Studies have shown that ferroptosis, a form of regulated cell death, is involved in various diseases, including tumors, neurodegenerative diseases, and ischemia-reperfusion injury. Ferroptosis is characterized by iron-dependent lipid peroxidation. GPX4 mediates the main cellular defense mechanism against it. Xiao et al. (14) first demonstrated that SAKI involved ferroptosis. After CLP, GPX4 expression in the kidney significantly decreased, while neutrophil gelatinase-associated lipocalin (NGAL) expression, a sensitive biomarker for AKI early diagnosis, significantly increased. However, pretreatment with ferroptosis inhibitor liproxstatin-1 or deferoxamine (DFO) reversed the increase in NGAL expression. It was further concluded that MCTR1 inhibited ferroptosis in CLP-induced AKI by upregulating nuclear factor erythroid 2-related factor 2 (Nrf2) expression.
Of note, sepsis survivors are also at significantly increased risk of progressing to chronic renal failure. AT-RvD1 has been shown to attenuate long-term renal dysfunction caused by a second insult after severe sepsis. This is based on finding that the levels of inflammatory factors such as IL-1β and TNF-α were reduced in septic-surviving mice treated with AT-RvD1 during a subsequent injury in a subacute AKI model. Additionally, pro-fibrotic markers such as transforming growth factor β (TGF-β), metalloproteinase (MMP)-3 and -9 were also significantly reduced after administration. It is well known that persistent inflammation, coupled with uncontrolled reparative processes, leads to excessive ECM deposition, resulting in interstitial fibrosis, which is a hallmark of progressive functional loss. AT-RvD1 confers renal protection against secondary injuries, including tubulointerstitial injuries and fibrosis induced by bovine serum albumin (BSA) after sepsis recovery (43). The protective mechanisms of SPMs against SAKI are detailed in Table 2.
4 Pharmacological effects of SPMs in sepsis-induced acute liver injury
The liver, with its roles in synthesis, metabolism, detoxification and immune defense, is susceptible to damage from various metabolites, microorganisms, and toxins. Sepsis-induced microcirculatory disorders can lead to intestinal mucosa ischemia, facilitating the translocation of endotoxins and bacteria via the portal vein, which in turn can impair liver function and trigger an inflammatory cascade. The pathogenesis of sepsis-induced acute liver injury likely involves alterations in hepatic blood flow and microcirculation, bacterial translocation, enhanced oxidative stress response, along with pro-inflammatory and immunosuppressive responses mediated by the liver.
D-galactosamine (D-GalN) has specific hepatotoxicity, and in LPS model of D-GalN-sensitized mice, the death of LPS/GalN mice was mainly caused by damage to the liver, but not to other organs, such as lungs. Studies indicated that SPMs, including LXA4 and RvD1, can mitigate apoptosis of liver parenchymal cell, decrease serum bacterial loads, and suppress inflammation by dose-dependently reducing levels of TNF-α, IL-6, IL-1β after LPS/D-GalN stimulation (34, 44, 45). Additionally, SPMs reduced the release of extracellular high mobility group box 1 (HMGB1), a critical late mediator in endotoxin shock. The anti-inflammatory action of SPMs involves dampening TLR4/NF-κB activation. Specifically, MaR1 upregulated the expression of Nrf2 and heme oxygenase-1 (HO-1) in a dose-dependent manner and inhibited phosphorylation of p38, ERK1/2, NF-κB p65 and IκBα, promoting an anti-inflammatory M2 macrophage phenotype in sepsis-induced acute liver injury mice. MaR1 exhibited anti-inflammatory and antioxidant properties (46).
Pyroptosis, confirmed in recent years as a form of programmed cell death, is biochemically characterized primarily by the formation of inflammasomes, the activation of caspases and gasdermin, and the release of a plethora of pro-inflammatory cytokines. In LPS/D-GalN-induced liver injury, MaR1 significantly reduced the expression of P30-GSDMD (gasdermin D) and inhibited pyroptosis induced by NLR family pyrin domain containing 3 (NLRP3) inflammasome (46). Furthermore, MaR1 mitigated D-GalN/LPS -induced liver injury in mice by attenuating ferroptosis, characterized by increased hepatic lipid peroxidation. Compared with the D-GalN/LPS group, MaR1 treatment increased serum GSH level and GSH/GSSG ratio in liver tissue while lowering serum iron levels. MaR1 also countered the rise in ROS, a key ferroptosis activator, and upregulated GPX4 expression. These effects were associated with the activation of Nrf2/HO-1/GPX4 pathways (47). NMR-based metabolomics studies have identified disruptions in metabolites related to energy metabolism and inflammation in sepsis models. MaR1 treatment alleviated metabolic disorders in CLP-induced septic mice, particularly affecting pyruvate, alanine, aspartate, glutamate, and pulmonary taurine metabolism (48). However, MaR1’s influence was observed in serum and lungs, not liver, possibly due to its temporal effect on sepsis. The hepatic metabolic response to MaR1 may require a more extended period for observable effects (48). The protective mechanisms of SPMs against sepsis-induced acute liver injury are detailed in Table 3.
5 Pharmacological effects of SPMs in sepsis-induced heart injury
Sepsis-induced heart injury is prevalent in severe sepsis, with up to 60% of septic shock patients experiencing cardiac dysfunction within the initial three days, which is predictive of mortality (49). The pathogenesis of septic cardiomyopathy remains elusive, and no targeted therapies are available (50, 51). SPMs may offer a potential therapeutic avenue against myocardial injury in sepsis by reducing inflammation, oxidative stress, and mitochondrial dysfunction (Table 4).
In a septic heart injury model induced by intraperitoneal injection of LPS, RvD1 and RvE1 preconditioning significantly improved cardiac function, as evidenced by enhanced left ventricular ejection fraction, and decreased serum myocardial injury markers such as lactate dehydrogenase (LDH) and creatine kinase myocardial bound (CK-MB). These treatments also reduced cardiac apoptosis and neutrophils/macrophages infiltration, modulated macrophage polarization, and suppressed pro-inflammatory cytokines secretion, via the MAPK and NF-κB pathways (52, 53). Additionally, RvD1 and RvE1 mitigated systemic inflammation by diminishing neutrophils and pro-inflammatory M1 macrophages infiltration in the spleen. Unlike the suppression of macrophages infiltration in the heart and spleen, RvE1 uniquely increased M2 macrophages in the peritoneum, correlating with enhanced bacterial clearance (54). This suggests that RvE1 differentially modulates macrophage responses to protect organs in the absence of bacteria and enhance bacterial clearance in infected sites.
Cardiac fibroblasts (CFs) are pivotal in the inflammatory response following cardiac injury, critical for tissue repair. RvD1 and RvE1 have demonstrated activity in CFs, inhibited LPS-induced inflammation by reducing the expression of ICAM-1 and VCAM-1 protein levels and lessening spleen mononuclear cells adhesion to CFs (55). This action promotes the resolution of inflammation, counteracting the sustained leukocyte adhesion that can lead to the release of pro-inflammatory mediators, ROS production, and subsequent cardiac fibrosis and remodeling. Interestingly, RvD1 inhibited LPS-induced elevations of IL-6, MCP-1, TNF-α, and IL-10, whereas RvE1 did not (55). The differential effect may be attributed to the distinct molecular structures of RvD1 and RvE1 and their potential interactions with corresponding receptors or signaling pathways. In addition, the effect of RvE1 on cytokine secretion appears to be tissue and/or cell type dependent and mediated by differences in the mechanisms associated with the receptors.
IL-17A is a pivotal cytokine in neutrophil recruitment, driving the production of chemotactic factors, including granulocyte colony-stimulating factor (G-CSF), chemokine (C-X-C motif) ligand 1 protein (CXCL1) and CXCL2 (56). It stimulates G-CSF to enhance neutrophils production and maturation, while CXCL1 and CXCL2 guide neutrophils to sites of infection or injury. In a pioneering study by Yang et al. (57), neutralization of IL-17A or depletion of γδT cells was shown to reduce neutrophil recruitment and ameliorate cardiac dysfunction in an LPS-induced cardiac injury. Additionally, MCTR1 was identified to improve cardiac function by mitigating neutrophil infiltration via the γδT/IL-17A signaling pathway (57). However, clinical studies on IL-17’s role in sepsis have yielded inconsistent results, with some indicating a weak positive correlation with disease severity in pediatric sepsis and others suggesting a beneficial role in survival (58, 59). Further in-depth study of the mechanisms is needed in future research.
MaR1 mitigated oxidative stress by reducing serum malondialdehyde (MDA) levels and elevating superoxide dismutase (SOD) and GSH levels in mice, as well as upregulating the expression of NRF-2 and HO-1 in cardiac tissues (60). The NRF2/HO-1 pathway is known for its antioxidant and anti-inflammatory properties, crucial in mitigating mitochondrial damage and regulating cellular processes. Mitochondrial dysfunction is increasingly recognized as the pathophysiological core of sepsis-induced cardiac dysfunction. peroxisome proliferator-activated receptor (PPAR)-γ coactivator-1α (PGC-1α), a key regulator in mitochondrial biosynthesis, promotes the expression of NRF1, NRF2, and TFAM, enhancing mitochondrial function. SIRT1 has been implicated in modulating PGC-1α-mediated mitochondrial biogenesis. LPS-induced mitochondrial dysfunction was characterized by decreased membrane potential and reduced activity of mitochondrial complexes and ATP synthesis. Post-treatment of MCTR1 dose-dependently increased SIRT1 expression, leading to enhanced PGC-1α and its target factors, thereby improving mitochondrial biogenesis and function, and protecting against sepsis-induced cardiac dysfunction (61).
6 Pharmacological effects of SPMs in sepsis-induced brain injury
Sepsis-induced brain injury, also known as sepsis-associated encephalopathy (SAE), is characterized by diffuse brain dysfunction secondary to sepsis, manifesting as neurological symptoms such as dementia, delirium, and even long-term cognitive impairment. It typically lacks overt central nervous system infection or structural lesions. The etiology of SAE remains elusive, with uncertainty as to whether it arises from a cascading response to multi-organ dysfunction or is precipitated by inflammation caused by sepsis early (62). The pathophysiology of SAE is intricate, potentially involving blood-brain barrier disruption, neuroinflammation, mitochondrial dysfunction, and neurotransmitter (63).
Microglia, the brain’s innate immune cells, are implicated in the pathogenesis of SAE through their inflammatory response. Upon activation by inflammatory stimuli, these cells can protect neurons but may also induce neuronal death and tissue damage through excessive production of cytotoxic factors such as superoxide, nitric oxide, TNF-α, and IL-1β. In the LPS-stimulated BV-2 cells study, AT-LXA4 inhibited the production of NO, IL-1β and TNF-α in a concentration-dependent manner, at least partially through NF-κB, ERK, p38 MAPK and activator protein-1 (AP-1) pathways (64). Similarly, RvD1 was found to attenuate microglia activation in the hippocampus of SAE mice, thereby reducing inflammatory mediators such as TNF-α, IL-6, and IL-1β and enhancing cognitive functions. These effects were likely mediated by the suppression of NF-κB, MAPK, and STAT pathways (65). Interestingly, in an LPS-stimulated BV2 cell model, Rey et al. (66) demonstrated that RvD1 and RvE1 inhibited LPS-induced TNF-α, IL-6 and IL-1β gene expression and regulated microglia phenotype with distinct underlying mechanisms. RvE1 downregulated the NF-κB signaling pathway, while RvD1 regulated the expression of miRNAs. Following RvD1 treatment, the expression of miR-155, miR-21, and miR-146 was upregulated, while that of miR-219 was downregulated. miR-155 targeted proteins are involved in NF-κB activation, potentially controlling tissue damage associated with inflammation (67). The miR-21, a key player in inflammatory response, was crucial for the resolution of inflammation and the negative regulation of pro-inflammatory responses, particularly in macrophages (68). The miR-146, a microRNA with negative regulatory function, is implicated in the regulation of immune response (69). The mechanisms by which SPMs exert their influence on neuroinflammation in SAE remain to be fully elucidated and warrant further investigation. The protective mechanisms of SPMs against sepsis-induced brain injury are detailed in Table 5.
7 SPMs in sepsis
SPMs appear to possess similar anti-inflammatory and pro-resolving effects in various sepsis-induced organ injuries, as evidenced by the current literature. Firstly, SPMs inhibit the production of pro-inflammatory cytokines and chemokines, which are key mediators in the inflammatory process, thereby mitigating tissue damage and organ dysfunction; Secondly, SPMs can accelerate the apoptosis and clearance of inflammatory cells, and also stimulate the repair and regeneration of damaged tissues. Thirdly, while reducing inflammation, SPMs also help to bolster the host’s defense mechanisms against pathogens by maintaining the function of immune cells and promoting their ability to eliminate invading microorganisms. Overall, SPMs exhibit anti-inflammatory activity, promote the resolution of inflammation, and modulate immune responses in septic organ damage, which helps to attenuate inflammatory damage and promote tissue repair. The relevant mechanisms involved are shown in Figure 1. Specifically, immunosuppression is frequently linked to mortality from sepsis. Unlike some anti-inflammatory drugs that carry a risk of immunosuppression, SPMs may be more appropriate for treating patients with sepsis because they suppress inflammation while enhancing host defense. Some studies suggested that SPMs may play a beneficial role in late-stage sepsis. RvD2 (administered late in an infection) improved bacterial clearance and increased survival in mice in a 2 - hit model of sepsis with secondary lung infection (70, 71). RvD2 was shown to directly increase macrophage phagocytosis and increase the number of M-MDSC in the spleens of CLP mice, which are the primary mechanisms for bacterial clearance in late-stage sepsis (30, 71). The ability to eradicate the source of infection while maintaining immunological balance provides an attractive therapeutic modality for sepsis. To clarify the precise role of SPMs during various stages of sepsis, more research would be worthwhile.
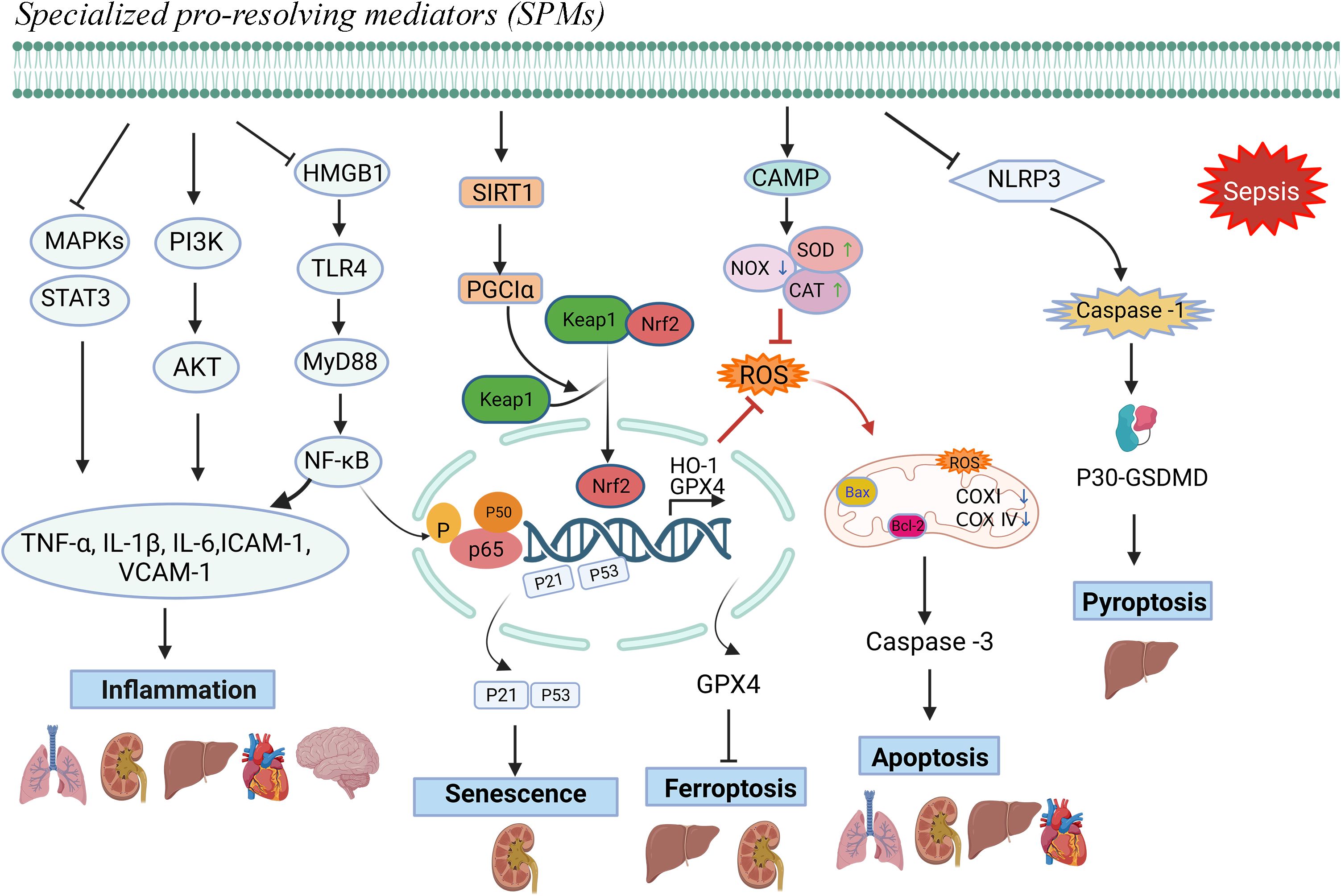
Figure 1. Protective mechanisms of SPMs against sepsis-induced organ dysfunction.Note: Specialized pro-resolving mediators (SPMs) can modulate inflammatory mediators, mitigate oxidative stress responses, and counteract cellular damage mechanisms, including apoptosis, ferroptosis, pyroptosis, and senescence, thereby facilitating the resolution of inflammation and preventing sepsis-induced organ damage.
There is some controversy about the specificity of detection methods for quantitative analysis of SPMs in biological samples. For example, Chen et al. (54) first reported that RvE1 levels were significantly reduced in SAHI mice (by~93-fold). However, this applied method has recently been seriously questioned (72). The LC-MS method in this paper (54) does not conform to internationally applied rules which use the S/N ratio for the LLOQ in LC-MS. Indeed, in another case, reanalysis of data obtained with this method by application of the generally accepted S/N ratio method revealed that the samples do not contain SPMs (73). The SPM profile in sepsis patients has also been studied in some human clinical trials. Dalli et al. (74) suggested that SPMs (RvE1, RvD5, and 17R-PD1) were detected at increased levels in the peripheral blood of those who succumbed to sepsis. The study also suggested that PDX at day 3 was more predictive of ARDS development than APACHE II scores (74). However, the same issue persists; the paper employed a nonconforming LC/MS method, and the data have to be interpreted with care. Plasma specimens from 18 cases of early septic shock (<12 h) revealed a trend towards higher SPMs in septic patients. Nevertheless, the authors concluded that this experiment did not reveal a significant correlation between SPMs levels and severity of sepsis, or days of survival (75). On the contrary, a study found that the SPM concentration in sepsis patients was lower than that in the healthy control group, but the measurement method used, ELISA, was clearly inappropriate (76). There is insufficient evidence to establish a correlation between endogenous SPM levels and clinical outcomes in sepsis. Clearly, the temporal distribution of SPMs levels in sepsis patients and their biological roles are complex. Furthermore, it is undeniable that consistently reported levels of SPMs are typically very low (77), and it is clearly challenging to reliably quantify SPMs levels in biological samples. The wide variation in SPM concentration within and between studies may be related to differences in sample storage, sample handling and analysis, but has not yet been fully explained. Accurate measurement techniques are critical to understanding the role of SPMs in sepsis and its potential clinical implications. Further research is needed to use highly reliable analytical methodology to determine the clinical relevance of SPMs to sepsis, as the current evidence is inconclusive.
8 Discussion
As we mentioned previously, concerns have been raised about some of the key data emerging from studies of SPMs. The biosynthetic pathway of most SPMs involves 5-LOX (ALOX5) as well as 12/15-LOX activity. Biochemical studies with purified lipoxygenases have shown that their ability to form trihydroxylated SPMs is extremely low, whereas the ability to form dihydroxylated SPMs is much lower than the rate of classical inflammatory response products (e.g. leukotrienes). Furthermore, to the best of our knowledge, knockout of ALOX12 or ALOX15 in mice which prevents SPMs formation does not lead to significant defects in inflammation resolution. Elimination or blockade of 12/15-lipoxygenase reduced neutrophil recruitment and improves survival in a mouse model of ALI (78). A loss-of-function variant in ALOX15 protected against nasal polyps and chronic rhinosinusitis in humans (79). However, anti-inflammatory effects of this enzyme have also been demonstrated in several reports. Corneal wound healing was delayed in eosinophile-specific 12/15-LOX knockout mice compared to wild-type mice (80). In the study by Pernet et al. (81), ALOX15-/- mice exhibited a heightened inflammatory response in alveolar macrophages and more severe lung inflammation and injury following LPS stimulation compared with wild-type mice. Notably, the authors attributed this effect to the formation of 12-HETE, not SPMs. These observation underscore the complexity of lipid mediators in the inflammatory process and highlights the need for a nuanced understanding of their roles in inflammation. The absence of its putative biosynthetic enzymes fails to provide evidence consistent with a role for SPM in resolving inflammation. At the same time, there is also no clear evidence that any of the described biological effects caused by lipoxygenase knockout are due to disruption in SPMs formation. Evidence for endogenous and pharmacological properties of SPMs remains controversial and incomplete.
As we have summarized from the literature, SPMs appear to have tremendous potential in alleviating organ damage in sepsis. The wide range of drug dosages used across studies, as well as the different routes of administration, are also noteworthy. It is indeed a valid concern that the concentrations used, such as 1 ng/mouse, may seem insufficient to induce a biological effect. Given the findings that suggest certain SPM receptors may not be functional, it is crucial to critically assess whether these low concentrations can indeed exert a significant effect on inflammation. Moreover, considering the nature of oxylipins and the potential for rapid metabolism, the persistence and bioavailability of SPMs at these low doses become even more questionable. It is crucial to consider the possibility that the observed effects may be due to indirect actions, alternative receptors, or other yet unidentified mechanisms. Future studies should aim to clarify the dose-response relationship of SPMs and validate their functional receptors to ensure that the concentrations used are biologically relevant and can effectively contribute to inflammation resolution. Furthermore, it is necessary to conduct further experiments, including pharmacokinetic analysis and tissue distribution studies. These data will help us to more accurately estimate the SPM concentration required to produce therapeutic effects in vivo and may guide us in adjusting the dosage to optimize therapeutic efficacy. Addressing these concerns will be crucial in advancing the field of resolution pharmacology and translating these findings into effective clinical applications.
It remains to be identified that the concentration of SPMs formed in the body correlates with the resolution of inflammation. Studies detecting these molecules within the human body typically report only low concentrations, potentially insufficient to significantly advance the resolution of inflammation, especially when juxtaposed with the notably higher levels of pro-inflammatory mediators (9). Evidence for the formation of biologically active concentrations of SPMs in humans to promote inflammation resolution remains to be provided.
However, the current consensus, at least with respect to SPMs, is that high concentrations of SPMs chemical entities do have a role in modulating the inflammatory response, and even though natural concentrations of SPMs do not eliminate inflammation, this has not deterred drug developers from synthesizing SPMs molecules and exploring their clinical applications (82). At least three biotech companies are currently planning clinical trials with SPMs synthetic molecules meant to restore or boost the body’s natural ability to end inflammation, which can wreak havoc if prolonged. There is already a phase I clinical trial of a lipoxin-based mouthwash, which has been shown to be safe for patients with periodontal disease and has also shown some efficacy, while Thetis Pharmaceuticals is planning to conduct clinical trials of a synthetic resolvin for the treatment of inflammatory bowel disease and cancer (82).
The results cited in our review were obtained mainly on the basis of preclinical modelling studies. The models of sepsis used in most studies are the LPS model and the CLP model. The LPS model reflects a systemic inflammatory response induced by a single pathogen component, primarily through LPS-induced activation of immune cells leading to a massive release of inflammatory factors. In contrast, the CLP model involves multiple bacterial infections and a more complex host response, including local and systemic inflammation, and subsequent immune modulation. The CLP model is better able to simulate the multi-organ damage and dysfunction of sepsis, including the lungs, kidneys, liver and heart. These differences are equally important for understanding the potential role of SPMs in sepsis treatment. Although these models are valuable in exploring mechanisms, they are poor predictors of potential clinical applications. SPMs have been used as pharmacological tools for decades, but their clinical development has been relatively slow, which may reflect the potential limitations of these compounds in clinical applications. More basic and clinical studies are needed in the future to answer and enrich the mode of action of SPMs in sepsis.
9 Conclusion
Sepsis is often characterized by an uncontrolled inflammatory response and immunosuppression. The resolution of inflammation is now recognized as an active process. SPMs exhibit anti-inflammatory, pro-resolving and immunomodulatory properties. A large body of evidence suggests that it may be a potential treatment for sepsis-induced organ dysfunction. However, evidence for endogenous and pharmacological properties of SPMs remains controversial and incomplete. Therefore further studies need to be conducted to clarify the role of SPMs in different stages of sepsis. As the field progresses, the translation of SPMs into clinical applications may provide new therapeutic strategies for sepsis, focusing not only on controlling inflammation but also on restoring immune homeostasis and organ function.
Author contributions
SS: Software, Data curation, Conceptualization, Writing – original draft. DY: Writing – original draft, Methodology, Funding acquisition. JL: Writing – review & editing, Software, Methodology, Data curation. HX: Writing – review & editing, Software, Resources. ZM: Writing – review & editing, Methodology. XC: Writing – review & editing, Supervision, Conceptualization, Funding acquisition. YG: Conceptualization, Writing – original draft.
Funding
The author(s) declare financial support was received for the research, authorship, and/or publication of this article. This study was supported by the 2022 Freedom Innovation Pre-research Fund (No. F016.01003.22003.121), the Hubei Provincial Natural Science Foundation (No. 2023AFB761) and the Health Commission Hubei Provice scientific research project (HBJG-220038). The sponsor will not be involved in study design; collection, management, analysis, or interpretation of data; writing of the report; or the decision to submit the report for publication.
Acknowledgments
Pictures are prepared by BioRender (https://biorender.com/).
Conflict of interest
The authors declare that the research was conducted in the absence of any commercial or financial relationships that could be construed as a potential conflict of interest.
Publisher’s note
All claims expressed in this article are solely those of the authors and do not necessarily represent those of their affiliated organizations, or those of the publisher, the editors and the reviewers. Any product that may be evaluated in this article, or claim that may be made by its manufacturer, is not guaranteed or endorsed by the publisher.
References
1. Singer M, Deutschman CS, Seymour CW, Shankar-Hari M, Annane D, Bauer M, et al. The third international consensus definitions for sepsis and septic shock (Sepsis-3). Jama. (2016) 315:801–10. doi: 10.1001/jama.2016.0287
2. Rudd KE, Johnson SC, Agesa KM, Shackelford KA, Tsoi D, Kievlan DR, et al. Global, regional, and national sepsis incidence and mortality, 1990-2017: analysis for the Global Burden of Disease Study. Lancet. (2020) 395:200–11. doi: 10.1016/s0140-6736(19)32989-7
3. Fleischmann-Struzek C, Mellhammar L, Rose N, Cassini A, Rudd KE, Schlattmann P, et al. Incidence and mortality of hospital- and ICU-treated sepsis: results from an updated and expanded systematic review and meta-analysis. Intensive Care Med. (2020) 46:1552–62. doi: 10.1007/s00134-020-06151-x
4. van der Poll T, Shankar-Hari M, Wiersinga WJ. The immunology of sepsis. Immunity. (2021) 54:2450–64. doi: 10.1016/j.immuni.2021.10.012
5. Sandhaus S, Swick AG. Specialized proresolving mediators in infection and lung injury. Biofactors. (2021) 47:6–18. doi: 10.1002/biof.1691
6. Serhan CN, Hamberg M, Samuelsson B. Lipoxins: novel series of biologically active compounds formed from arachidonic acid in human leukocytes. Proc Natl Acad Sci U.S.A. (1984) 81:5335–9. doi: 10.1073/pnas.81.17.5335
7. Dyall SC, Balas L, Bazan NG, Brenna JT, Chiang N, da Costa Souza F, et al. Polyunsaturated fatty acids and fatty acid-derived lipid mediators: Recent advances in the understanding of their biosynthesis, structures, and functions. Prog Lipid Res. (2022) 86:101165. doi: 10.1016/j.plipres.2022.101165
8. Jordan PM, Werz O. Specialized pro-resolving mediators: biosynthesis and biological role in bacterial infections. FEBS J. (2022) 289:4212–27. doi: 10.1111/febs.16266
9. Schebb NH, Kühn H, Kahnt AS, Rund KM, O’Donnell VB, Flamand N, et al. Formation, Signaling and Occurrence of Specialized Pro-Resolving Lipid Mediators-What is the Evidence so far? Front Pharmacol. (2022) 13:838782. doi: 10.3389/fphar.2022.838782
10. Kahnt AS, Schebb NH, Steinhilber D. Formation of lipoxins and resolvins in human leukocytes. Prostaglandins Other Lipid Mediat. (2023) 166:106726. doi: 10.1016/j.prostaglandins.2023.106726
11. Im DS. Maresin-1 resolution with RORα and LGR6. Prog Lipid Res. (2020) 78:101034. doi: 10.1016/j.plipres.2020.101034
12. Hanson J, Ferreirós N, Pirotte B, Geisslinger G, Offermanns S. Heterologously expressed formyl peptide receptor 2 (FPR2/ALX) does not respond to lipoxin A4. Biochem Pharmacol. (2013) 85:1795–802. doi: 10.1016/j.bcp.2013.04.019
13. Merlin J, Park J, Vandekolk TH, Fabb SA, Allinne J, Summers RJ, et al. Multipathway in vitro pharmacological characterization of specialized proresolving G protein-coupled receptors. Mol Pharmacol. (2022) 101:246–56. doi: 10.1124/molpharm.121.000422
14. Xiao J, Yang Q, Zhang Y, Xu H, Ye Y, Li L, et al. Maresin conjugates in tissue regeneration-1 suppresses ferroptosis in septic acute kidney injury. Cell Biosci. (2021) 11:221. doi: 10.1186/s13578-021-00734-x
15. Nijmeh J, Levy BD. Lipid-derived mediators are pivotal to leukocyte and lung cell responses in sepsis and ARDS. Cell Biochem Biophys. (2021) 79:449–59. doi: 10.1007/s12013-021-01012-w
16. Singer P, Calder PC. The role of omega-3 polyunsaturated fatty acids in the intensive care unit. Curr Opin Clin Nutr Metab Care. (2023) 26:129–37. doi: 10.1097/mco.0000000000000896
17. Jarczak D, Kluge S, Nierhaus A. Sepsis-pathophysiology and therapeutic concepts. Front Med (Lausanne). (2021) 8:628302. doi: 10.3389/fmed.2021.628302
18. Shi Y, Wang L, Yu S, Ma X, Li X. Risk factors for acute respiratory distress syndrome in sepsis patients: a retrospective study from a tertiary hospital in China. BMC Pulm Med. (2022) 22:238. doi: 10.1186/s12890-022-02015-w
19. Rubenfeld GD, Herridge MS. Epidemiology and outcomes of acute lung injury. Chest. (2007) 131:554–62. doi: 10.1378/chest.06-1976
20. Zhang F, Zheng X, Zhao F, Li L, Ren Y, Li L, et al. TFAM-Mediated mitochondrial transfer of MSCs improved the permeability barrier in sepsis-associated acute lung injury. Apoptosis. (2023) 28:1048–59. doi: 10.1007/s10495-023-01847-z
21. Miao RF, Tu J. LncRNA CDKN2B-AS1 interacts with LIN28B to exacerbate sepsis-induced acute lung injury by inducing HIF-1α/NLRP3-mediated pyroptosis. Kaohsiung J Med Sci. (2023) 39:883–95. doi: 10.1002/kjm2.12697
22. Ali FEM, Ahmed SF, Eltrawy AH, Yousef RS, Ali HS, Mahmoud AR, et al. Pretreatment with coenzyme Q10 combined with aescin protects against sepsis-induced acute lung injury. Cells Tissues Organs. (2021) 210:195–217. doi: 10.1159/000516192
23. Tongyoo S, Permpikul C, Mongkolpun W, Vattanavanit V, Udompanturak S, Kocak M, et al. Hydrocortisone treatment in early sepsis-associated acute respiratory distress syndrome: results of a randomized controlled trial. Crit Care. (2016) 20:329. doi: 10.1186/s13054-016-1511-2
24. Pontes-Arruda A, Martins LF, de Lima SM, Isola AM, Toledo D, Rezende E, et al. Enteral nutrition with eicosapentaenoic acid, γ-linolenic acid and antioxidants in the early treatment of sepsis: results from a multicenter, prospective, randomized, double-blinded, controlled study: the INTERSEPT study. Crit Care. (2011) 15:R144. doi: 10.1186/cc10267
25. Hu XH, Situ HL, Chen JP, Yu RH. Lipoxin A4 alleviates lung injury in sepsis rats through p38/MAPK signaling pathway. J Biol Regul Homeost Agents. (2020) 34:807–14. doi: 10.23812/20-108-a-20
26. Ortiz-Muñoz G, Mallavia B, Bins A, Headley M, Krummel MF, Looney MR. Aspirin-triggered 15-epi-lipoxin A4 regulates neutrophil-platelet aggregation and attenuates acute lung injury in mice. Blood. (2014) 124:2625–34. doi: 10.1182/blood-2014-03-562876
27. Meng F, Mambetsariev I, Tian Y, Beckham Y, Meliton A, Leff A, et al. Attenuation of lipopolysaccharide-induced lung vascular stiffening by lipoxin reduces lung inflammation. Am J Respir Cell Mol Biol. (2015) 52:152–61. doi: 10.1165/rcmb.2013-0468OC
28. Zhuo Y, Zhang S, Li C, Yang L, Gao H, Wang X. Resolvin D1 promotes SIRT1 expression to counteract the activation of STAT3 and NF-κB in mice with septic-associated lung injury. Inflammation. (2018) 41:1762–71. doi: 10.1007/s10753-018-0819-2
29. Zhang SK, Zhuo YZ, Li CX, Yang L, Gao HW, Wang XM. Xuebijing injection () and resolvin D1 synergize regulate leukocyte adhesion and improve survival rate in mice with sepsis-induced lung injury. Chin J Integr Med. (2018) 24:272–7. doi: 10.1007/s11655-017-2959-x
30. Sundarasivarao PYK, Walker JM, Rodriguez A, Spur BW, Yin K. Resolvin D2 induces anti-microbial mechanisms in a model of infectious peritonitis and secondary lung infection. Front Immunol. (2022) 13:1011944. doi: 10.3389/fimmu.2022.1011944
31. Xia H, Ge Y, Wang F, Ming Y, Wu Z, Wang J, et al. Protectin DX ameliorates inflammation in sepsis-induced acute lung injury through mediating PPARγ/NF-κB pathway. Immunol Res. (2020) 68:280–8. doi: 10.1007/s12026-020-09151-7
32. Wang XY, Li XY, Wu CH, Hao Y, Fu PH, Mei HX, et al. Protectin conjugates in tissue regeneration 1 restores lipopolysaccharide-induced pulmonary endothelial glycocalyx loss via ALX/SIRT1/NF-kappa B axis. Respir Res. (2021) 22:193. doi: 10.1186/s12931-021-01793-x
33. Lv Y, Chen D, Tian X, Xiao J, Xu C, Du L, et al. Protectin conjugates in tissue regeneration 1 alleviates sepsis-induced acute lung injury by inhibiting ferroptosis. J Transl Med. (2023) 21:293. doi: 10.1186/s12967-023-04111-9
34. Li R, Wang Y, Ma Z, Ma M, Wang D, Xie G, et al. Maresin 1 mitigates inflammatory response and protects mice from sepsis. Mediators Inflammation. (2016) 2016:3798465. doi: 10.1155/2016/3798465
35. Gu J, Luo L, Wang Q, Yan S, Lin J, Li D, et al. Maresin 1 attenuates mitochondrial dysfunction through the ALX/cAMP/ROS pathway in the cecal ligation and puncture mouse model and sepsis patients. Lab Invest. (2018) 98:715–33. doi: 10.1038/s41374-018-0031-x
36. Li H, Hao Y, Yang LL, Wang XY, Li XY, Bhandari S, et al. MCTR1 alleviates lipopolysaccharide-induced acute lung injury by protecting lung endothelial glycocalyx. J Cell Physiol. (2020) 235:7283–94. doi: 10.1002/jcp.29628
37. Bagshaw SM, George C, Bellomo R. Early acute kidney injury and sepsis: a multicentre evaluation. Crit Care. (2008) 12:R47. doi: 10.1186/cc6863
38. Chen J, Shetty S, Zhang P, Gao R, Hu Y, Wang S, et al. Aspirin-triggered resolvin D1 down-regulates inflammatory responses and protects against endotoxin-induced acute kidney injury. Toxicol Appl Pharmacol. (2014) 277:118–23. doi: 10.1016/j.taap.2014.03.017
39. Sun S, Wang J, Wang J, Wang F, Yao S, Xia H. Maresin 1 mitigates sepsis-associated acute kidney injury in mice via inhibition of the NF-κB/STAT3/MAPK pathways. Front Pharmacol. (2019) 10:1323. doi: 10.3389/fphar.2019.01323
40. Zhao YL, Zhang L, Yang YY, Tang Y, Zhou JJ, Feng YY, et al. Resolvin D1 protects lipopolysaccharide-induced acute kidney injury by down-regulating nuclear factor-kappa B signal and inhibiting apoptosis. Chin(Engl). (2016) 129:1100–7. doi: 10.4103/0366-6999.180517
41. Li J, Zhang Z, Wang L, Jiang L, Qin Z, Zhao Y, et al. Maresin 1 attenuates lipopolysaccharide-induced acute kidney injury via inhibiting NOX4/ROS/NF-κB pathway. Front Pharmacol. (2021) 12:782660. doi: 10.3389/fphar.2021.782660
42. Chen C, Qiu R, Yang J, Zhang Q, Sun G, Gao X, et al. Lipoxin A4 restores septic renal function via blocking crosstalk between inflammation and premature senescence. Front Immunol. (2021) 12:637753. doi: 10.3389/fimmu.2021.637753
43. Silva J, Calcia TBB, Silva CP, Guilherme RF, Almeida-Souza F, Lemos FS, et al. ATRvD1 attenuates renal tubulointerstitial injury induced by albumin overload in sepsis-surviving mice. Int J Mol Sci. (2021) 22:11634. doi: 10.3390/ijms222111634
44. Murakami T, Suzuki K, Tamura H, Nagaoka I. Suppressive action of resolvin D1 on the production and release of septic mediators in D-galactosamine-sensitized endotoxin shock mice. Exp Ther Med. (2011) 2:57–61. doi: 10.3892/etm.2010.170
45. Jiang WW, Gao LL, Wu M, Zhao T, Shen DL. Effect of lipoxin A4 on the expression of Toll-like receptor 4 and TNF receptor-associated factor 6 in the liver of obese rats with sepsis. Zhongguo Dang Dai Er Ke Za Zhi. (2018) 20:578–84. doi: 10.7499/j.issn.1008-8830.2018.07.013
46. Yang W, Tao K, Zhang P, Chen X, Sun X, Li R. Maresin 1 protects against lipopolysaccharide/d-galactosamine-induced acute liver injury by inhibiting macrophage pyroptosis and inflammatory response. Biochem Pharmacol. (2022) 195:114863. doi: 10.1016/j.bcp.2021.114863
47. Yang W, Wang Y, Zhang C, Huang Y, Yu J, Shi L, et al. Maresin1 protect against ferroptosis-induced liver injury through ROS inhibition and nrf2/HO-1/GPX4 activation. Front Pharmacol. (2022) 13:865689. doi: 10.3389/fphar.2022.865689
48. Hao Y, Zheng H, Wang RH, Li H, Yang LL, Bhandari S, et al. Maresin1 alleviates metabolic dysfunction in septic mice: A (1)H NMR-based metabolomics analysis. Mediators Inflammation. (2019) 2019:2309175. doi: 10.1155/2019/2309175
49. Vieillard-Baron A, Caille V, Charron C, Belliard G, Page B, Jardin F. Actual incidence of global left ventricular hypokinesia in adult septic shock. Crit Care Med. (2008) 36:1701–6. doi: 10.1097/CCM.0b013e318174db05
50. Martin L, Derwall M, Al Zoubi S, Zechendorf E, Reuter DA, Thiemermann C, et al. The septic heart: current understanding of molecular mechanisms and clinical implications. Chest. (2019) 155:427–37. doi: 10.1016/j.chest.2018.08.1037
51. Furmanek S, Salunkhe V, Pahwa S, Samanapally H, Nathala P, Xu Q, et al. Association between echocardiographic features, troponin levels, and survival time in hospitalized COVID-19 patients with cardiovascular events. J Anesth Transl Med. (2024) 3:36–44. doi: 10.1016/j.jatmed.2024.05.001
52. Wang M, Liu M, Zhang J, Liu J, Ye J, Xu Y, et al. Resolvin D1 protects against sepsis-induced cardiac injury in mice. Biofactors. (2020) 46:766–76. doi: 10.1002/biof.1668
53. Zhang J, Wang M, Ye J, Liu J, Xu Y, Wang Z, et al. The anti-inflammatory mediator resolvin E1 protects mice against lipopolysaccharide-induced heart injury. Front Pharmacol. (2020) 11:203. doi: 10.3389/fphar.2020.00203
54. Chen J, Purvis GSD, Collotta D, Al Zoubi S, Sugimoto MA, Cacace A, et al. RvE1 attenuates polymicrobial sepsis-induced cardiac dysfunction and enhances bacterial clearance. Front Immunol. (2020) 11:2080. doi: 10.3389/fimmu.2020.02080
55. Salas-Hernández A, Espinoza-Pérez C, Vivar R, Espitia-Corredor J, Lillo J, Parra-Flores P, et al. Resolvin D1 and E1 promote resolution of inflammation in rat cardiac fibroblast. vitro. Mol Biol Rep. (2021) 48:57–66. doi: 10.1007/s11033-020-06133-8
56. Xu S, Cao X. Interleukin-17 and its expanding biological functions. Cell Mol Immunol. (2010) 7:164–74. doi: 10.1038/cmi.2010.21
57. Yang Y, Li XY, Li LC, Xiao J, Zhu YM, Tian Y, et al. [amp]]gamma;δ T/interleukin-17A contributes to the effect of maresin conjugates in tissue regeneration 1 on lipopolysaccharide-induced cardiac injury. Front Immunol. (2021) 12:674542. doi: 10.3389/fimmu.2021.674542
58. Angurana SK, Bansal A, Muralidharan J, Aggarwal R, Singhi S. Cytokine levels in critically ill children with severe sepsis and their relation with the severity of illness and mortality. J Intensive Care Med. (2021) 36:576–83. doi: 10.1177/0885066620912989
59. Razazi K, Boissier F, Surenaud M, Bedet A, Seemann A, Carteaux G, et al. A multiplex analysis of sepsis mediators during human septic shock: a preliminary study on myocardial depression and organ failures. Ann Intensive Care. (2019) 9:64. doi: 10.1186/s13613-019-0538-3
60. Li D, Wang M, Ye J, Zhang J, Xu Y, Wang Z, et al. Maresin 1 alleviates the inflammatory response, reduces oxidative stress and protects against cardiac injury in LPS-induced mice. Life Sci. (2021) 277:119467. doi: 10.1016/j.lfs.2021.119467
61. Yang Y, Zhu Y, Xiao J, Tian Y, Ma M, Li X, et al. Maresin conjugates in tissue regeneration 1 prevents lipopolysaccharide-induced cardiac dysfunction through improvement of mitochondrial biogenesis and function. Biochem Pharmacol. (2020) 177:114005. doi: 10.1016/j.bcp.2020.114005
62. Iacobone E, Bailly-Salin J, Polito A, Friedman D, Stevens RD, Sharshar T. Sepsis-associated encephalopathy and its differential diagnosis. Crit Care Med. (2009) 37:S331–336. doi: 10.1097/CCM.0b013e3181b6ed58
63. Ren C, Yao RQ, Zhang H, Feng YW, Yao YM. Sepsis-associated encephalopathy: a vicious cycle of immunosuppression. J Neuroinflamm. (2020) 17:14. doi: 10.1186/s12974-020-1701-3
64. Wang YP, Wu Y, Li LY, Zheng J, Liu RG, Zhou JP, et al. Aspirin-triggered lipoxin A4 attenuates LPS-induced pro-inflammatory responses by inhibiting activation of NF-κB and MAPKs in BV-2 microglial cells. J Neuroinflamm. (2011) 8:95. doi: 10.1186/1742-2094-8-95
65. Xu B, Li M, Cheng T, Xia J, Deng X, Hou J. Resolvin D1 protects against sepsis-associated encephalopathy in mice by inhibiting neuro-inflammation induced by microglia. Am J Transl Res. (2022) 14:6737–50.
66. Rey C, Nadjar A, Buaud B, Vaysse C, Aubert A, Pallet V, et al. Resolvin D1 and E1 promote resolution of inflammation in microglial cells. vitro. Brain Behav Immun. (2016) 55:249–59. doi: 10.1016/j.bbi.2015.12.013
67. Faraoni I, Antonetti FR, Cardone J, Bonmassar E. miR-155 gene: a typical multifunctional microRNA. Biochim Biophys Acta. (2009) 1792:497–505. doi: 10.1016/j.bbadis.2009.02.013
68. Sheedy FJ, O’Neill LA. Adding fuel to fire: microRNAs as a new class of mediators of inflammation. Ann Rheum Dis. (2008) 67 Suppl 3:iii50–55. doi: 10.1136/ard.2008.100289
69. O’Neill LA, Sheedy FJ, McCoy CE. MicroRNAs: the fine-tuners of Toll-like receptor signalling. Nat Rev Immunol. (2011) 11:163–75. doi: 10.1038/nri2957
70. Padovani CM, Yin K. Immunosuppression in sepsis: biomarkers and specialized pro-resolving mediators. Biomedicines. (2024) 12:175. doi: 10.3390/biomedicines12010175
71. Walker JM, Sundarasivarao PYK, Thornton JM, Sochacki K, Rodriguez A, Spur BW, et al. Resolvin D2 promotes host defense in a 2 - hit model of sepsis with secondary lung infection. Prostaglandins Other Lipid Mediat. (2022) 159:106617. doi: 10.1016/j.prostaglandins.2022.106617
72. O’Donnell VB, Schebb NH, Milne GL, Murphy MP, Thomas CP, Steinhilber D, et al. Failure to apply standard limit-of-detection or limit-of-quantitation criteria to specialized pro-resolving mediator analysis incorrectly characterizes their presence in biological samples. Nat Commun. (2023) 14:7172. doi: 10.1038/s41467-023-41766-w
73. Motwani MP, Newson J, Kwong S, Richard-Loendt A, Colas R, Dalli J, et al. Prolonged immune alteration following resolution of acute inflammation in humans. PloS One. (2017) 12:e0186964. doi: 10.1371/journal.pone.0186964
74. Dalli J, Colas RA, Quintana C, Barragan-Bradford D, Hurwitz S, Levy BD, et al. Human sepsis eicosanoid and proresolving lipid mediator temporal profiles: correlations with survival and clinical outcomes. Crit Care Med. (2017) 45:58–68. doi: 10.1097/ccm.0000000000002014
75. Kutzner L, Rund KM, Ostermann AI, Hartung NM, Galano JM, Balas L, et al. Development of an optimized LC-MS method for the detection of specialized pro-resolving mediators in biological samples. Front Pharmacol. (2019) 10:169. doi: 10.3389/fphar.2019.00169
76. Tsai WH, Shih CH, Yu YB, Hsu HC. Plasma levels in sepsis patients of annexin A1, lipoxin A4, macrophage inflammatory protein-3a, and neutrophil gelatinase-associated lipocalin. J Chin Med Assoc. (2013) 76:486–90. doi: 10.1016/j.jcma.2013.05.004
77. Calder PC. Eicosapentaenoic and docosahexaenoic acid derived specialised pro-resolving mediators: Concentrations in humans and the effects of age, sex, disease and increased omega-3 fatty acid intake. Biochimie. (2020) 178:105–23. doi: 10.1016/j.biochi.2020.08.015
78. Rossaint J, Nadler JL, Ley K, Zarbock A. Eliminating or blocking 12/15-lipoxygenase reduces neutrophil recruitment in mouse models of acute lung injury. Crit Care. (2012) 16:R166. doi: 10.1186/cc11518
79. Kristjansson RP, Benonisdottir S, Davidsson OB, Oddsson A, Tragante V, Sigurdsson JK, et al. A loss-of-function variant in ALOX15 protects against nasal polyps and chronic rhinosinusitis. Nat Genet. (2019) 51:267–76. doi: 10.1038/s41588-018-0314-6
80. Ogawa M, Ishihara T, Isobe Y, Kato T, Kuba K, Imai Y, et al. Eosinophils promote corneal wound healing via the 12/15-lipoxygenase pathway. FASEB J. (2020) 34:12492–501. doi: 10.1096/fj.202000483R
81. Pernet E, Sun S, Sarden N, Gona S, Nguyen A, Khan N, et al. Neonatal imprinting of alveolar macrophages via neutrophil-derived 12-HETE. Nature. (2023) 614:530–8. doi: 10.1038/s41586-022-05660-7
Keywords: specialized pro-resolving mediators (SPMs), sepsis, lipoxin, resolvin, protectin, maresins
Citation: Sun S, Yang D, Lv J, Xia H, Mao Z, Chen X and Gao Y (2024) Pharmacological effects of specialized pro-resolving mediators in sepsis-induced organ dysfunction: a narrative review. Front. Immunol. 15:1444740. doi: 10.3389/fimmu.2024.1444740
Received: 06 June 2024; Accepted: 30 August 2024;
Published: 20 September 2024.
Edited by:
Perenlei Enkhbaatar, University of Texas Medical Branch at Galveston, United StatesReviewed by:
Cassie Zhu, North Shore University Hospital, United StatesDieter Steinhilber, Goethe University Frankfurt, Germany
Copyright © 2024 Sun, Yang, Lv, Xia, Mao, Chen and Gao. This is an open-access article distributed under the terms of the Creative Commons Attribution License (CC BY). The use, distribution or reproduction in other forums is permitted, provided the original author(s) and the copyright owner(s) are credited and that the original publication in this journal is cited, in accordance with accepted academic practice. No use, distribution or reproduction is permitted which does not comply with these terms.
*Correspondence: Xiangdong Chen, eGlhbmdkb25nY2hlbjIwMTNAMTYzLmNvbQ==; Yafen Gao, Z2FveWFmZW4xMDMwQDE2My5jb20=
†These authors have contributed equally to this work