- 1Molecular Signaling and Cell Death Unit, VIB-UGent, Center for Inflammation Research, Flanders Institute for Biotechnology, Ghent, Belgium
- 2Department of Biomedical Molecular Biology, Ghent University, Ghent, Belgium
- 3Laboratory of Immunoregulation and Mucosal Immunology, VIB-UGent Center for Inflammation Research, Ghent, Belgium
The thymus plays a pivotal role in generating a highly-diverse repertoire of T lymphocytes while preventing autoimmunity. Thymus seeding progenitors (TSPs) are a heterogeneous group of multipotent progenitors that migrate to the thymus via CCR7 and CCR9 receptors. While NOTCH guides thymus progenitors toward T cell fate, the absence or disruption of NOTCH signaling renders the thymus microenvironment permissive to other cell fates. Following T cell commitment, developing T cells undergo multiple selection checkpoints by engaging with the extracellular matrix, and interacting with thymic epithelial cells (TECs) and other immune subsets across the different compartments of the thymus. The different selection checkpoints assess the T cell receptor (TCR) performance, with failure resulting in either repurposing (agonist selection), or cell death. Additionally, environmental cues such as inflammation and endocrine signaling induce acute thymus atrophy, contributing to the demise of most developing T cells during thymic selection. We discuss the occurrence of acute thymus atrophy in response to systemic inflammation. The thymus demonstrates high plasticity, shaping inflammation by abrogating T cell development and undergoing profound structural changes, and facilitating regeneration and restoration of T cell development once inflammation is resolved. Despite the challenges, thymic selection ensures a highly diverse T cell repertoire capable of discerning between self and non-self antigens, ultimately egressing to secondary lymphoid organs where they complete their maturation and exert their functions.
1 Introduction
1.1 A short history of the thymus
The term “thymus”, derived from the ancient Greek “θυμός (thumos)”, meaning “soul/spirit”, possibly believed to be the soul’s dwelling place for its central location just above the heart. For centuries the function of the thymus remained enigmatic, and until the 1950 no apparent immune function could be attributed to the thymus due to; 1) the apparent absence of health problems in thymectomized adult individuals 2) the lack of germinal centers as compared with the spleen, 2) the lack of antibody-producing cells post-immunization, 4) the insufficient immune responses following thymus lymphocyte transfer to immunocompromised recipients, 4) the misconception about the reduced thymus size in individuals that succumbed to infectious diseases versus young healthy individuals (1, 2).
It wasn’t until a series of pioneering studies by Jaques Miller and colleagues between 1959 and 1957 that the thymus function was elucidated, the last among the major internal organs to be discovered (3–7). Performing either thymectomy or bursectomy in chickens Miller’s team demonstrated the thymus’s essential role in cellular immunity and, in conjunction with the bursa for the cellular and humoral responses (5, 7–9). Despite these experiments and firm conclusions, one of the leading immunologists of that period, Peter Medawar (1915 – 1987) stated: “We shall come to regard the presence of lymphocytes in the thymus as an evolutionary accident of no very great significance” (10). Nevertheless, Miller’s discoveries contributed to enduring paradigms in immunology still valid to this day: 1) The thymus is not only a source for T cells but also the site where T cell progenitors are “trained” for self-tolerance and develop immunocompetence through interactions with thymic epithelial cells and other professional antigen-presenting cells (APCs); 2) Due to the role of T cells mediating allograft rejection, repressing T cells is a valuable therapeutic approach for transplantation; 3) B and T cells are different lineages that adopt their fate in different organs and; 4) Effective immune response requires a collaboration from both B and T cells. These paradigms laid the foundation of T cell biology, profoundly impacting modern medicine in fields like autoimmune and inflammatory diseases, oncology, and organ transplantation.
1.2 Thymus origin in evolution
The thymus is evolutionary conserved across species in jawed vertebrates (gnathostomes) (11). The anatomical position, the number of thymic lobes per animal, and its organogenesis can vary between species (12). The presence of a thymus-like organ has been suggested in the cyclostomes (lampreys) (13). Nevertheless, Chondrichthyes (cartilaginous fish, i.e. sharks and rays) are considered the oldest thymus-bearing taxon (12). Parts of the genetic network underlying thymopoiesis was already present before the thymus originated (14) from an ancient gut-associated lymphoid tissue (GALT) (12, 15). The prevailing view is that as the cellular immune system developed, the thymus evolved in relation to its role in immune tolerance to cope with the increased potential for self-reactive T cells (16). As the evolutionary origin of the thymus has been linked to the evolution of jaws in the cartilaginous fishes, its origin might also be related to their wider access to food sources increasing the need of a more specific immune responses (16).
1.3 Thymus organogenesis
The thymic organogenesis differs across species in terms of timing and the number of pharyngeal pouches required for its development (12). In mammals, thymus organogenesis is a multi-step process involving 1) thymus fate determination from the common thymus-parathyroid primordium, 2) detachment from the parathyroid, 3) migration to the thorax cavity and subsequently, 4) multipotent progenitors (MPP) colonization. In humans, the thymus continues growing during postnatal development until reaching its peak in cellularity few weeks after birth, followed by a decline starting before puberty (17). These kinetics are similar in mice, humans, equines, and zebrafish (18). The thymus derives from the pharyngeal pouches of the endodermal gut tube around embryonic day 9.5 (E9.5, “E#” hereafter referring to day of murine embryonic development), in a process regulated by HOX3, PAX1, PAX9, FGF8, and FOXN1 (the earliest thymus-specific marker, detected at E11) (15). By E11.5, the third pharyngeal pouch has formed the thymus and parathyroid structures, preparing for their separation, guided by the Pax-Eya-Six gene cascade. Surrounded by a mesenchymal capsule from neural crest cells, these structures then detach from the pharynx around E12.5 and begin migrating to the anterior thoracic cavity (19). The dissociation of the thymus from the parathyroid, potentially influenced by FOXN1 and GCM2, is followed by the thymus’s outgrowth regulated by HOX and FGF family members (19, 20). By E15 (8-10 weeks of gestation in humans) (21, 22), the thymus is positioned in its final anatomical location, coinciding with the colonization of hematopoietic precursors necessary for lymphopoiesis (12, 23).
The vasculature and lymphatic systems are developed in parallel in the embryo. The blood vascular system is one of the first functional systems formed in the body during embryonic development (starting from E8.5) (24) while the lymphatic system is initiated from day E9.5-10.5 (25, 26). The thymus vascularization starts around E15, while the onset of the lymphatic wiring remains unknown (27). Unlike other lymphoid organs, the thymus does not contain afferent lymphatic vessels entering it. It only contains efferent lymphatic vessels from where thymocytes egress to the periphery (28). Notably, the migration of multipotent progenitors (MPPs) from the different niches (yolk sac, fetal liver, and bone marrow) to the thymus starts prior formation of vasculature and lymphatic system (29). This process is crucial as a first step of T-cell development and it will be discussed in the coming sections.
2 The thymic landscape: architecture and compartments
The compartmentalized architecture of the thymus is essential for allowing the correct development and selection of T cells. In mammals, the thymus is a bi-lobulated organ separated by an interlobular septum and linked by connective tissue. The thymus morphology and cellular composition is highly conserved across species (30). Its microanatomy comprises connective tissue, extracellular matrix components, epithelial cells, and various immune cells. The immune cell compartment is predominantly formed by developing T lymphocytes, but also includes minor populations of dendritic cells (DCs), macrophages, monocytes, neutrophils, eosinophils, natural killer (NK) cells, innate-lymphoid cells (ILCs), and B cells (31–36). Structurally, the thymus is organized into four distinct morphological and histological layers: the capsula, cortex, corticomedullary junction, and medulla, arranged from the outermost to the innermost layer (37), as illustrated in Figure 1.
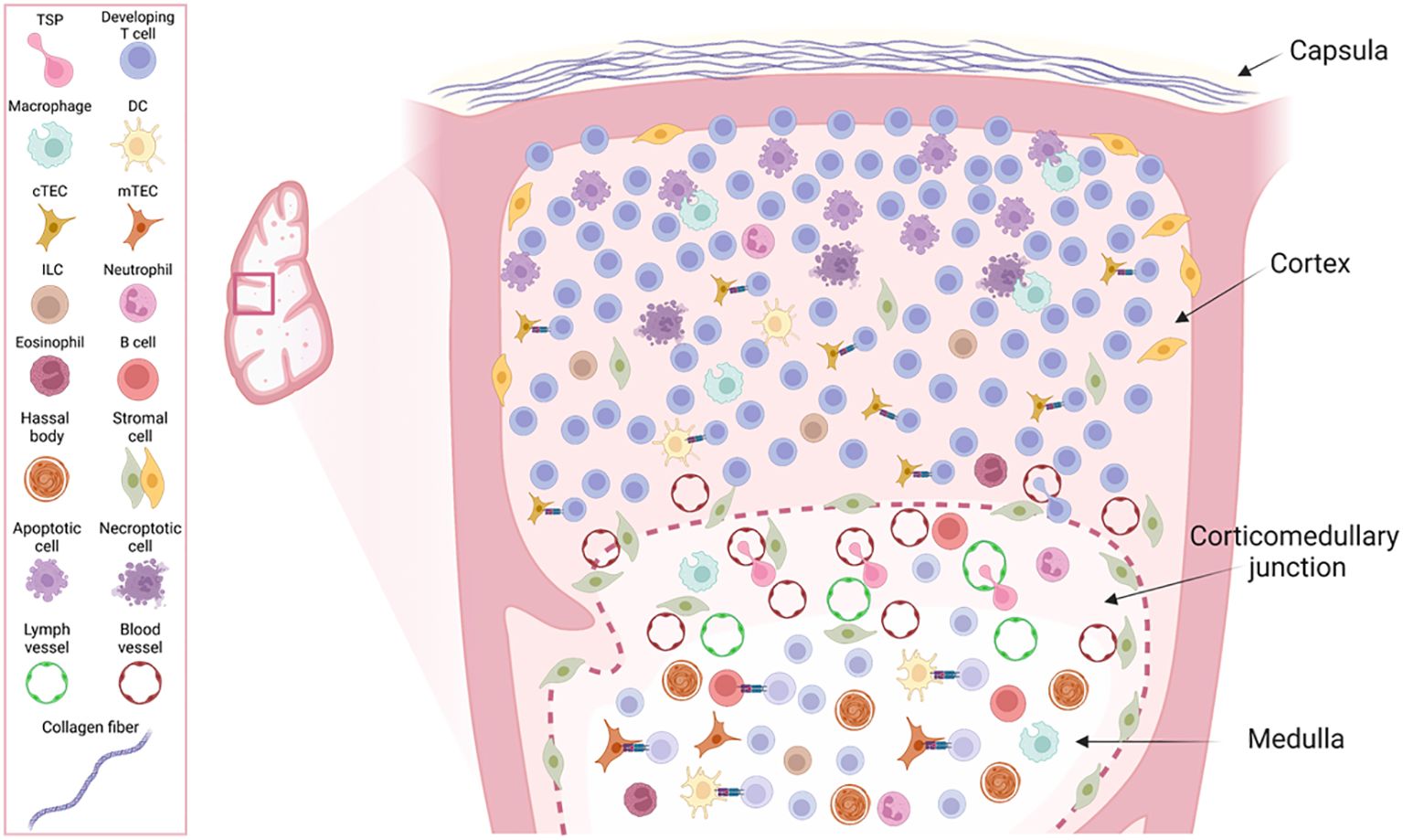
Figure 1. Overview of the structural layers and cellular composition of the thymus lobe. The figure illustrates a zoom view of the four different morphological layers from the outer to the inner: capsula, cortex, corticomedullary junction (CMJ) and medulla and the diversity of cell types contained within those layers with their reported anatomical localization within the thymus. The schematic does not represent their relative numbers within the thymus tissue.
2.1 Capsula
The thymus is encased in a connective tissue layer known as “capsula”, which envelops both lobes. The capsula comprises an outer and inner layer made up of collagen and reticular fibers. The inner layer can invaginate to form septa, from which thin trabeculae that contain vascularized capillaries extend towards the center of the thymus lobe. Approximately 5% of the developing thymocytes are found in the subcapsular area, region proximate to the outer cortex (21).
2.2 Cortex
The cortex is the next distinct histological region of the thymus, characterized by a high concentration of immature lymphocytes which outnumber epithelial cells, macrophages, and dendritic cells that support T-cell development. Noteworthy, there is a gradient of less mitotically active cells towards the inner part of the thymus getting close to the corticomedullary junction, that reflects the different stages of the development of pre-T cells, directly correlated with their anatomical position within the thymus (21). The cortex is the most cellular dense region in the thymus, accounting for about 70-80% of them, and is the primary site of positive selection (21). The cortex harbors the cortical thymic epithelial cells (cTECs) that play a role in the positive selection of developing thymocytes.
2.3 Corticomedullary junction
The inner cortex borders the corticomedullary junction, a layer characterized by a high density of blood and lymph vessels, supported by connective tissue (23). The thymus only contains efferent lymphatic vessels from where thymocytes egress into circulation. As a consequence, unlike lymph nodes, the thymus does not swell during an infection (28). Arteries supply the organ through the corticomedullary region, branching into capillaries that extend into the medulla and cortex. Generally, cortical capillaries are less fenestrated, resulting in limited circulation of antigens, whereas medullary capillaries are fenestrated, facilitating antigen flow (38). Nerve fibers innervate the thymus, following the vasculature within the capsule and septum adjacent to the corticomedullary junction (38). The areas surrounding large blood vessels in the CMJ are called perivascular spaces (PVS) (39). PVS primarily contain recently infiltrated early thymic progenitors (ETPs), SP CD4+, and SP CD8+ cells, but also plasma cells are present in the PSV (40, 41). Thymus seeding progenitors (TSPs) enter the thymus through large venules located at the corticomedullary junction and once they become naïve CD4+ or CD8+ T cells re-enter the circulation through the post-capillary venules. This area thus becomes a hub for both incoming immature lymphocytes and mature lymphocytes preparing to exit the thymus (23).
2.4 Medulla
The medulla forms the central layer of the thymus and continues between adjacent lobules, often extending deep into the cortex, near the capsular region. This region, less dense than the cortex, contains mature T cells, epithelial cells, Hassall’s corpuscles, macrophages, dendritic cells, B cells, and other myeloid cells (23). Approximately 10-15% of all thymocytes, predominantly single positive (SP) CD4+ or CD8+, are located in the medullary region, undergoing negative selection (Expanded in Section 3.2). The thymus medulla hosts various antigen-presenting cells (APCs) including medullary thymic epithelial cells (mTECs), DCs, macrophages, and B cells, which present self-peptides via major histocompatibility complexes I (MHC-I) and II (MHC-II) (42). The affinity between TCR and MHC-self peptide is a crucial determinant for the selection fate of developing thymocytes (expanded in Section 3.2). Those that have strong TCR affinity are negatively selected by programmed cell death (PCD). Thymocytes that successfully overcome the positive and negative selection steps, become mature and can eventually egress from the thymic medulla blood vessels into circulation. Localizing in the medulla, Hassall’s corpuscles, are thought to be involved in the clearance of dying thymocytes (43), and are an important source of thymic stromal lymphopoietin (TSLP) involved in dendritic cell instruction and regulatory T cells (Treg) induction in the thymus (44).
The compartmentalization of the thymus is critical for enabling the correct development and selection of developing T cells as specialized processes occur in different morphological layers that contain diverse immune-, and stromal- cells that orchestrate T cell development and survival. The precise location of each subset of developing T cells in the different structural layers will be described in the following sections.
3 How to become a T cell
Functioning as a primary lymphoid organ, the thymus provides the essential microenvironment to support the generation of a highly-diverse and self-tolerant T cell repertoire. The thymus is a dynamic organ that contains a high number of different cell types working together to allow the complex process of T-cell development. T cell development is a well-orchestrated, tightly-regulated process that encompasses a set of steps including: colonization by multipotent progenitors (45, 46), T cell specification, commitment, selection (47–51), and tolerance instruction (52).
3.1 Migration of multipotent progenitors to the thymus
Self-renewing hematopoietic stem cells (HSCs) have not been shown in the thymus (53) and therefore, the thymus is believed to rely on continuous seeding of multipotent progenitors (MPPs) from the aorta-gonad mesonephros (AGM), yolk-sac, fetal liver, or fetal bone marrow during embryogenesis and bone marrow throughout adult life (54) (Figure 2). Notably, not all circulating MPPs are able to successfully settle in the thymus. Thymus-homing MMPs exhibit specific molecular characteristics that enable them to settle in the thymus: 1) To migrate and enter the thymus they express CCR7 and CCR9 (55, 56), and 2) they should be responsive to NOTCH signaling (54), ultimately these thymus-homing MMPs are known as thymus seeding progenitors (TSPs). TSPs are a heterogeneous group of multipotent, non-committed progenitors that selectively home to the thymus (57). Multiple candidates have been proposed as TSPs, including multipotent progenitors (MPPs), lympho-myeloid primed progenitors (LMPPs), common-lymphoid progenitors (CLPs) or T cell-lineage committed progenitors (58). Identifying TSPs is challenging due to rapid changes in cell-surface phenotypes, transcripts, and the extremely limited numbers of these progenitors in circulation and the adult thymus (58–60). Further research is required to establish a consensus on the various subsets constituting the group of TSPs, as well as to develop a unified nomenclature for clarity in the field (see Table 1).
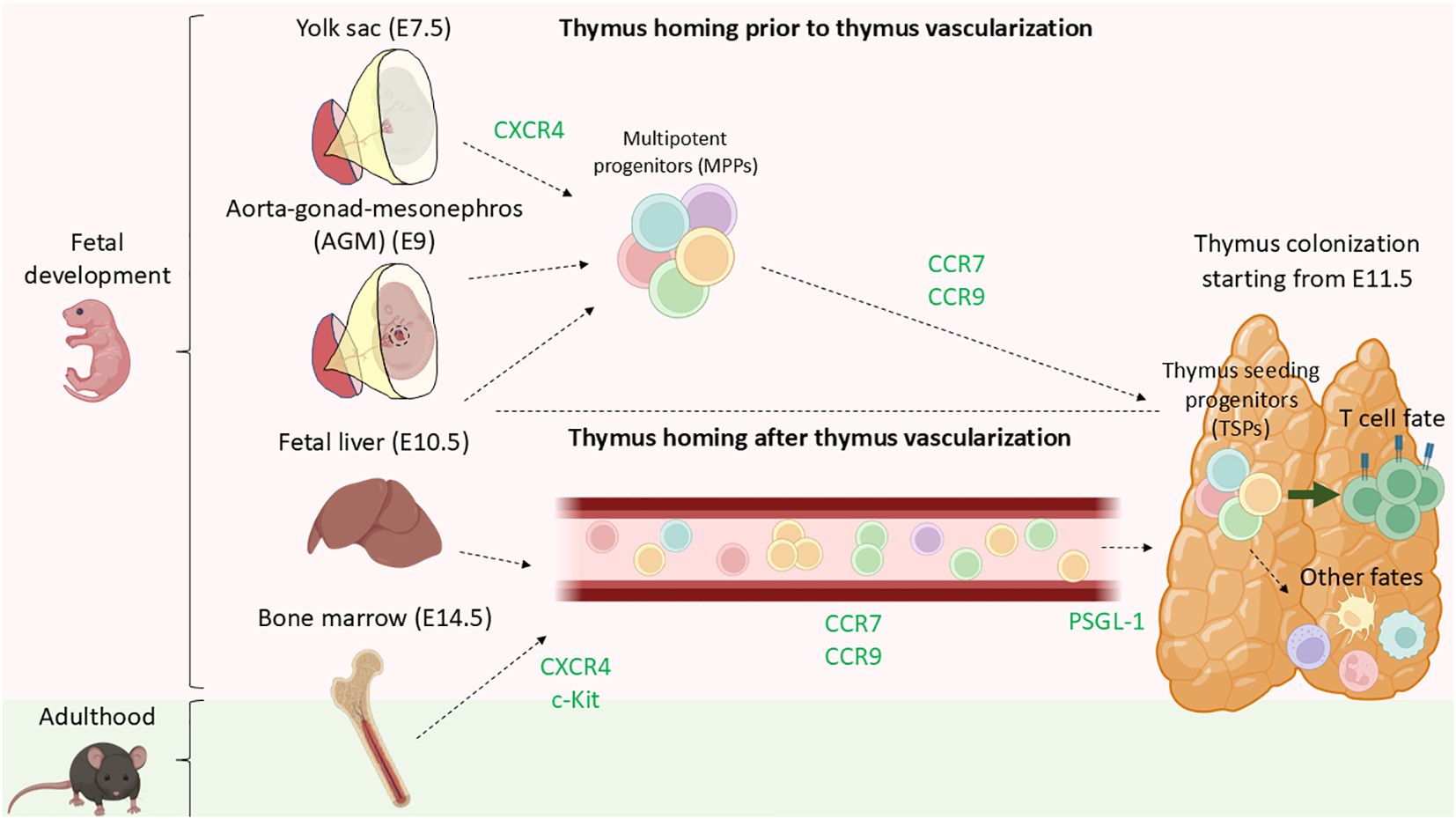
Figure 2. Current model of thymus colonization by MPPs during fetal development and thorough adulthood. During fetal development, CXCR4 plays a role in aiding MMPs being mobilized from either AGM, yolk sac, or fetal liver even prior complete formation of the blood circulation system. MPPs, primarily LMPPs and CLPs migrate to the thymus in a CCR9-, CCR7-dependent manner. Shortly after birth, the bone marrow becomes the primary site of MPPs residency and export. Analogous to during fetal development, progenitors are mobilized into circulation via CXCR4 and c-Kit and later guide towards the thymus by CCL21 and CCL25 (CCR7 and CCR9 ligands) by chemotaxis. Lastly PSGL-1 plays a role in the transmigration of the TSPs for entering the thymus.
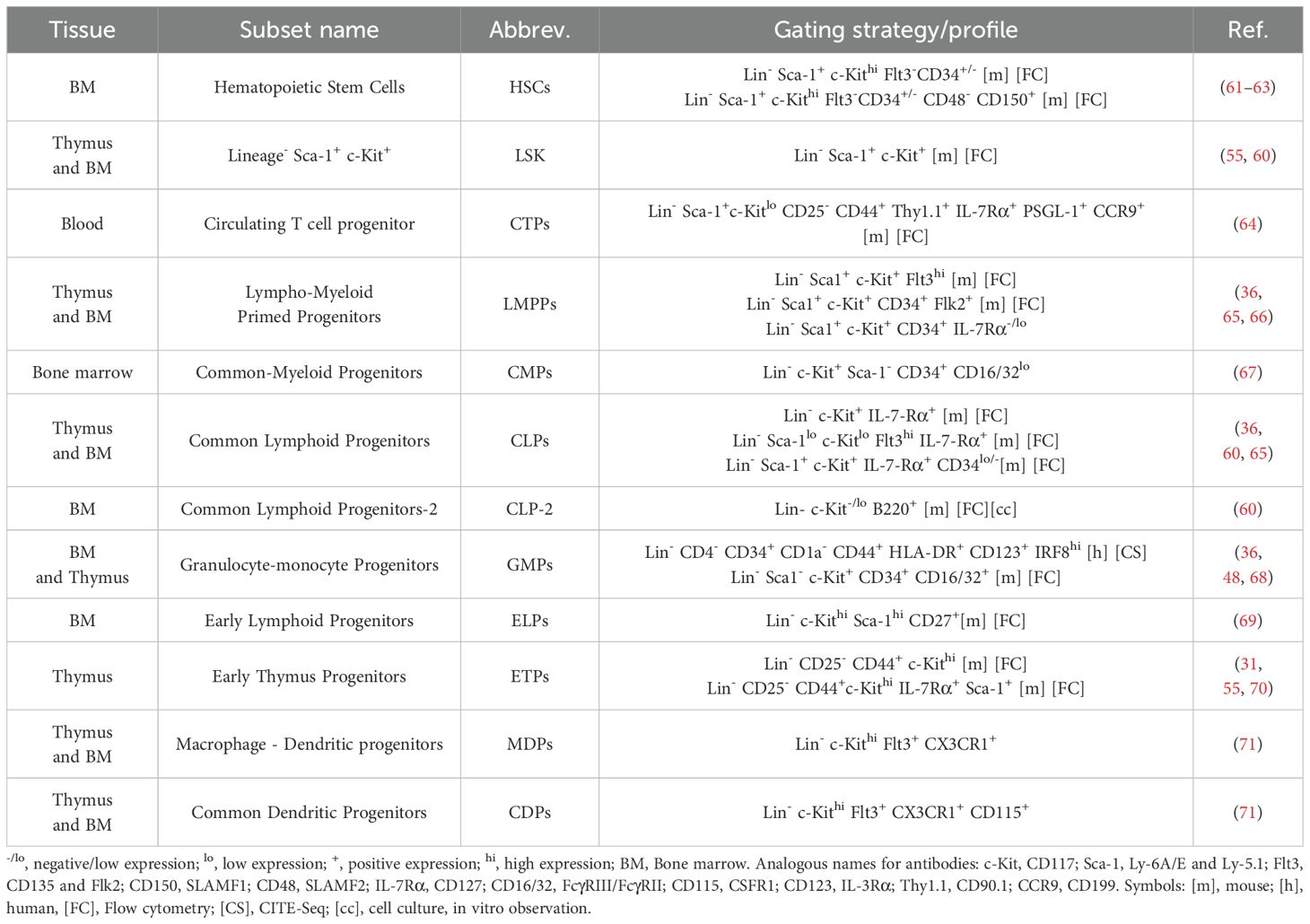
Table 1. Compilation of the different progenitors subsets identified as TSPs candidates in the bone marrow or blood and the progenitors subsets identified in the thymus with their corresponding flow cytometric profile.
TSPs consist of lympho-myeloid primed progenitors (LMPPs) (57, 72), common lymphoid progenitors (CLPs) (65, 73), and granulocyte-monocyte progenitors (GMPs) (36, 48, 68). Shortly after entry, CCR9 is downregulated by NOTCH signaling (74). The environmental cues by the thymus stroma influence TSPs, leading them to adopt the phenotype of early thymus progenitors (ETPs) (54). Murine ETPs have a great proliferative potential (75), with a single progenitor being able to give rise to more than 105 thymocytes in 12 days of fetal thymic organ cultures (FTOCs), implying a doubling time of 34 h on average (76). The doubling time of human ETPs has not been well characterized, likely due to technical limitations (59, 77). Constitutive expression of Notch1 in bone marrow progenitors leads to loss of B cell potential and aberrant T cell development (49). The presence of NK/T-biased (B-cell deficient) progenitors in the fetal liver and fetal blood suggests that NOTCH signaling might occur extrathymically prior thymus colonization (49). More recently, it has been shown that bone marrow LMPPs undergo NOTCH signaling resulting in inhibition their myeloid potential and skewing toward T cell fate (72). Furthermore, DN1a/b cells from mice RBPJ-inducible mice (unresponsive to NOTCH signaling) exhibited a myeloid bias (72). These findings demonstrate that NOTCH signaling pre-primes TSP progenitors extrathymically and is required before thymus colonization (72).
Throughout development, particularly in fetal and neonatal stages, there are different reservoirs of HSCs. The yolk-sac (YS) is the earliest niche of HSCs, exporting progenitors from E7.5 (78, 79). Subsequently, the aorta–gonad–mesonephros (AGM) contributes to the circulating HSCs pool from day E9. Progenitors from the YS and AGM seed the fetal liver that actively export HSCs to peripheral organs from E10.5, a process that gradually decreases during development (78, 79). Eventually, the bone marrow becomes the primary HSC reservoir from E14.5 onwards, dominating HSC export by E17 (78, 79) (Figure 2). The earliest stage of fetal thymus colonization in mice is detected at day E11.5 (29). Progenitor cells migrating from various hematopoietic niches to the thymus are guided by G protein-coupled receptors (GPCRs), responding to chemokine gradients towards the thymus (80). The first step of mobilization of hematopoietic progenitors from the bone marrow into circulation is regulated by the CXCR4-CXCL12 axis (54). Cxcr4-/- mice exhibited impaired migration from the bone marrow to the fetal liver (81). Additionally, c-Kit - stem cell factor (SCF) axis was also identified being involved in the mobilization of bone marrow progenitors into circulation (54). The fetal thymus produces CCL21, CCL25, and CXCL12, chemoattractants for TSPs (54). Blockage of CCL21 and CCL25 but not CXCL12 leads to reduced colonization of TSPs progenitors in the thymus (79). The number of fetal thymocytes is reduced in CCR7 knock-outs (receptor for CCL21) (79) and CCR9 knock-outs (receptor for CCL25) (82), while CXCR4 (receptor for CXCL12) knock-out mice were not defective in fetal thymus colonization. Various studies employing CCR7/CCR9 double knock-outs independently demonstrated that CCR7-CCL21 and CCR9-CCL25 are essential in regulating thymus homing of uncommitted progenitors towards the thymus prior and after vascularization (29, 56).
3.2 T-cell development and thymic selection in space and time
TSP progenitors enter the thymus at the CMJ region from the blood by transmigration and extravasation (46), a process regulated by P-selectin glycoprotein ligand-1 (PSGL-1), CCR7, and CCR9 (83) (Figure 2). Once in the thymus, most of the TSPs are instructed by the thymus microenvironment becoming ETPs and mostly being directed towards T cell fate. However, ETPs comprise a heterogeneous group of non-committed progenitors that can adopt granulocyte- (36, 84), monocyte/macrophage- (45, 85), dendritic- (86, 87), ILCs- (31, 73), B- (88) and, NK- (35, 89) fates. Following initial contact with thymus milieu and the thymic stroma, the TSPs become early thymic progenitors (ETPs), and remain uncommitted towards T cell fate (46, 53). Downstream ETPs, double negative (DN) thymocytes, characterized by the lack of CD4 and CD8 surface markers, comprise the different maturation stages of developing T cells. DN cells undergo a stepwise differentiation pathway orchestrated by the thymic stroma and supportive immune cells that consist in two main phases; specification and commitment (51). DN1 (Lin- CD44+ CD25-) are located close to the entry site in the corticomedullary junction. DN1 survival is promoted by IL-7 - IL7R and stem cell factor (SCF) - c-KIT interactions (90, 91), while Delta-like ligand 1 (DLL1) – NOTCH1 axis promotes differentiation (92), and CCR7 aid their progressive migration toward the cortex and subcapsular zone (93, 94). As they move through the medulla, they transition into the DN2 stage (Lin-CD44+CD25+). CCR7 deficient mice accumulate DN1-2 cells in the corticomedullary junction, unveiling that CCR7 is essential for controlling the migration of DN1-2 cells from the corticomedullary junction to the cortex (93). Additionally, CXCR4 deficient mice show an arrest in T cell development at DN1 stage, revealing the importance of CXCR4 in facilitating the migration of DN1 cells to the cortex (93) (Figure 3). Despite the roles of CCR7, CCR9, CXCR4, our understanding of the environmental cues that direct the migration of early DN thymocytes from the CMJ to the cortex remains uncomplete (96). DN1 thymocytes are multipotent, while DN2 thymocytes are uncommitted to T cell lineage but their fate is restricted to B-, T-, NK-, and DCs- lineages (94).
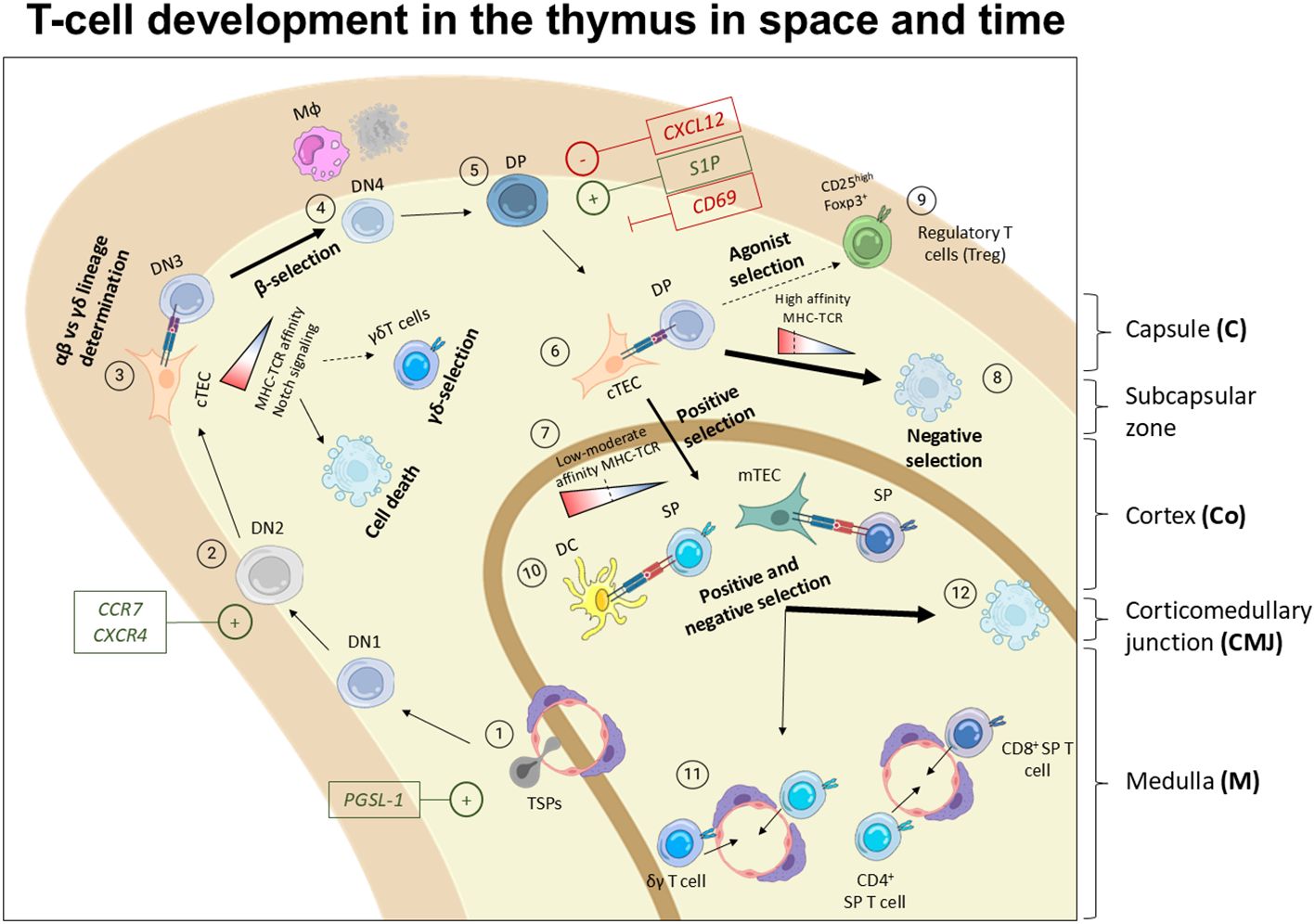
Figure 3. Spatiotemporal model of T cell development in the thymus. 1) TSPs enter the thymus through blood vessels typically irrigating the thymus in the medulla and CMJ in a process mediated by PGSL-1. Then, the thymus microenvironment instructs them to become ETPs or DN1 entering the T cell differentiation path. 2) DN1 move towards the cortex where they become DN2 which proliferate robustly. This migration through the cortex is mediated by chemokine gradients promoting the migration of the developing thymocytes via CCR7 and CXCR4. 3) In the outer cortex, DN3 undergo TCR rearrangement, thereby irreversibly committing towards T cell fate. 4) Next, DN thymocytes encounter αβ- vs γδ- lineage choice based on the MHC-TCR interaction strength, and NOTCH signaling among other factors. In this step, their TCR is tested in a process known as β- or γδ- selection, where cells must interact with MHC to receive a survival signal. Failure to engage MHC results in programmed cell death at the DN3 stage. 5) Later on, DN3 move forward in the development and become DN4 and DP, staying quiescent for several days. At this point, the polarization of the migration reverses. This inward migration of DP is regulated by S1P, CXCL12 and CD69. 6) DP interact with cTECs and undergo positive selection based on the TCR-MHC strength and affinity interactions. 7) Cells displaying low-moderate TCR-MHC interaction are positively selected. 8) Cells with high TCR-MHC affinity mostly undergo cell death, 9) while a small fraction that recognize self-antigens are rescue to become regulatory T cells (Tregs) or other types of unconventional T-cells (95). 10) Positively selected cells undergo another selection round by interacting with APCs, either DCs or mTECs. 11) Successful interactions lead to their maturation into SP CD4+ or CD8+ thymocytes and naïve T cells that are ready to egress to the periphery and complete their development in secondary lymphoid organs.
The commitment towards T cell fate starts at DN2a stage by upregulation of IL-7R, and components of the TCR complex (97). At DN2b stage, the progenitors experience an upregulation of transcription factors and genes linked to the rearrangement of the TCR, leading to irreversible commitment to T cell fate (92, 97, 98). DN2 thymocytes proliferate extensively before TCR rearrangement (99). Mice deficient for RAG1 or RAG2 recombinases show a complete block in T cell development at DN3 stage (100). In the subcapsular zone, where DN3 cells reside, part of the subset undergoes β-selection (99) where the strength of the TCRβ chain is tested in combination with a surrogate “pre-TCR” before the TCRα chain is created (101). With no lysine kinase 1 (WNK1) is serine/threonine-specific kinase is expressed in developing T cells that can regulate the activity of solute carrier transporters (SLCs) ultimately promoting cell motility. Notably, both TCR- and CCR7- signaling results in WNK1 upregulation that negatively controls LFA-1-mediated ICAM adhesion, promoting chemokine-mediated cortical migration of β-selected DN3 thymocytes (102). At the same time, fewer DN3 cells undergo γδ-selection where the newly form γ- and δ- chains are functionally tested (51).
Notably, γδ T cells constitute less than 0.5% of the total murine thymocyte population (103). DN cells expressing TCRγδ commit to the γδ-lineage, typically without entering the DP stage of T cell development (104). Whereas most γδ T cells remain DN and become mature γδ T cells prior to egressing the thymus, some acquire CD4 and CD8 markers suggesting that they undergo a similar DP-to-SP development route as αβ T cells (104). The αβ vs γδ lineage choice is currently debated between by two different models. Firstly, the classical model includes a pre-commitment selection where the lineage fate is determined before TCR-rearrangement. Secondly, the alternative model emphasizes the TCR signal strength and NOTCH signaling, rather than TCR identity, to dictate αβ vs γδ lineage fate, with strong TCR signal favoring γδ-skewing (103, 104). DN3a thymocytes, characterized by low expression of CD27 can differentiate into TCRγδ T cells in the absence of NOTCH/Delta signaling while the absence of NOTCH stimulation in DN3a cells reduce the number of TCR-αβ-lineage thymocytes (105). In mice, the NOTCH ligand JAG2, promotes γδ T cell development, while DLL1 and DLL4 contribute to αβ lineage development (104). OP9-DL1 cultured human Il2GFP DN3b thymocytes preferentially adopt γδ lineage over αβ (105). This subset was characterized as CD27+ CD5+ CD2+ CD62L+ in accordance with the expression pattern of differentiated γδ T cells (105). This study revealed differential gene expression of Runx3, Egr-2, Egr-3, Id3, Ikaros, Bcl-2, Helios and Aiolos in murine γδ T cells compared to other thymocyte subsets. Other studies showed increased NR4A1-3, ETV5, KLF2, RELB, HES1, and ZBTB16 in human γδ T cells (106, 107). Nonetheless, the identity of the supposed γδ-precursor within the DN fraction is still unknown. In mice, a DN1 subset expressing high levels of IL-7 and SOX13 preferentially adopt γδ fate (108, 109). Collectively, the two prevailing developmental models are not opposed to each other as both pre-TCR progenitor identity, TCR-signal strength, and NOTCH signaling seem to contribute to γδ-lineage development (110).
While γδ-selected DN3 thymocytes show little proliferation and remain double negative, DN3 thymocytes that underwent β-selection proliferate robustly and rapidly differentiate into DN4 cells (Lin-CD44-CD25-) and later acquire CD4 and CD8 surface markers becoming double-positive (DP) thymocytes (CD44-CD25-CD4+CD8+) in the subcapsular zone (51) (Figure 3). DP thymocytes remains quiescent for several days as the TCRα rearrangement process involves the creation of double-strand breaks in the DNA being incompatible with DNA replication (111, 112). At this stage, the polarity of the migration of DP T cells reverses, guiding them back from the cortex to the medulla (113). Gradients of CXCL12 (SDF-1) repel thymocytes, while S1P1 acts as a chemoattractant, aiding their migration across the thymus layers (113). CD69, which antagonizes S1P1, serves as a residency marker (113). In the cortex, DP T cells interact with cTEC, DCs, and B cells as antigen presenting cells (APCs) (114, 115). A weak TCR:MHC interaction is required to protect DP thymocytes from death by neglect, known as positive selection (116–118). Positively selected CD4+ or CD8+ SP thymocytes are relocated into the medulla. There, they engage with DCs, mTECs, and plasmacytoid dendritic cells (pDCS) for further screening. SP Thymocytes that show too strong TCR: MHC-self peptide interactions undergo negative selection and die predominantly by apoptosis (91, 115). Notably, SP thymocytes exhibiting high-affinity TCR:MHC-self peptide interactions, considered autoreactive, can be redirected to become regulatory T cells (Treg), invariant natural killer T cells (iNKT) or TCRαβ+ CD8αα+ intestinal intraepithelial lymphocytes (IELs), in a process known as agonist selection (91, 119).
A small fraction of thymic SP CD4+ T cells (∼1%) expressing the high-affinity IL-2 receptor alpha (IL-2Rα) or CD25 and displaying strong affinity for self-peptides, as revealed in Nur77GFP transgenic reporter mice, are agonist-selected to become Tregs (120, 121). Initial TCR stimulation causes upregulation of CD25, GITR, OX40 and TNFR2 among others (121). CD4+ CD25hi thymic Treg precursors subsequently acquire FOXP3 expression independently of further TCR engagement in the presence of IL-2 or IL-15 (122). FOXP3 expression is tightly regulated at the transcriptional level by four conserved non-coding sequences (CNS1-4) (95). Transcription factors bind these CNSs to control Foxp3 transcription. Notably, c-Rel binding to CNS3 is crucial for thymic Treg development, as evidenced by the dramatic decrease of thymic Tregs in c-Rel-/- mice (103). Interestingly, continuous endogenous production of IFN I and IFN III by AIRE+ mTECs is required to instruct APCs to promote Treg selection (42). While the thymus is the major site for Treg development, around ∼10–15% of peripheral CD4+ T cells can differentiate into Tregs instructed by environmental cues such as the presence of TGFβ (121, 123). Much less is known about the signals directing intrathymic Treg precursor migration, and the residency or egress of Tregs compared to their conventional T cell counterparts. Notably, the maintenance of immunological self-tolerance highly relies on Treg suppressive functions as unveiled by the lethality of Foxp3-/- murine model (124, 125) and the Foxp3 mutation in humas leading to immune dysregulation, polyendocrinopathy, enteropathy and X-linked (IPEX) syndrome ultimately resulting in death during infancy (126).
Ultimately, successfully selected naïve SP CD4+ and CD8+ T cells, CD4+ CD25+ FOXP3+ Tregs, and other unconventional T cells subsets egress the thymus via lymph and blood vessels and migrate to secondary lymphoid organs such as lymph node where they complete their maturation and exert their functions.
3.3 Hormone regulation of intra-thymic T-cell migration and survival
Beyond its crucial role as a primary lymphoid organ and its contribution to the establishment of the lifetime T cell pool, the thymus also functions as an endocrine gland (127, 128). Accordingly, the thymus is highly innervated by both sympathetic and parasympathetic nerves, facilitating neuroendocrine control and similar to the adenohypophysis, thyroid, adrenals releases hormones into the bloodstream (127, 129). Neurotransmitters such as norepinephrine (NE) and acetylcholine (ACh) are locally released and control the delivery and expression of other hormones (128).
The thymus produces several hormones like thymosin, thymopoietin, thymulin, thymic humoral factor (THF) and luteinizing and follicle-stimulating hormones, which are important in pre-thymocyte maturation (127, 129–132). Hormones play a critical role in influencing cell motility and contributing in the regulation of life versus death decisions of developing T cells. Thymulin is an important regulator of neuro-immune endocrine thymus axis (130). Paracrine signaling via thymopoietin specifically enhances the differentiation of T cells (131), and thymosin is essential for pre-thymocyte maturation (127, 129). Outside the thymus, thymosin, thymopoietin, and thymulin seem to be highly immunomodulatory but are also part of an extensive neuroendocrine network, affecting the production of other hormones, such as the production of pineal gland hormones affecting the circadian rhythm (133). Thymopoietin have been shown to directly bind to antigen-presenting cells on their MCH class II molecules, whereas thymulin and thymosin seem to retain anti-inflammatory functionalities (134–136).
Stress- and Age- related hormonal changes are known to influence thymic output (137–139). Endocrine hormone signaling significantly influences thymocyte development and output by regulating cell migration controlling the degradation or deposition of the thymus extracellular matrix components (ECM) thereby affecting chemokine-ECM intrathymic migration routes (137). For example, growth hormone (GH) and prolactin can boost thymocyte growth and movement (140, 141). Additionally, prolactin affects DCs-expressing prolactin receptors resulting in the increase production of proinflammatory cytokines leading to acute thymus atrophy induction (141). Thyroid hormones like triiodothyronine (T3) and thyroxine (T4) also play a role in T-cell development by affecting thymocyte adhesion and migration (137). Notably, glucocorticoids are known to trigger apoptosis in developing T cells (142), whereas leptin, primarily produced by adipose tissue crucially impact mTECs-expressing leptin receptor conferring protection against thymic stress and enhancing thymus output (143). Oxytocin (OT) and vasopressin (VP), mainly produced in the pituitary gland, can also be produced in the thymus and impact SP CD8+ thymocytes, leading to apoptosis and reduced proliferation (144).
In mammals, sex hormones like androgens and estrogens are hypothesized to contribute to thymic decline with age, as evidenced by the decline of thymus size and hormonal changes post-puberty (128). In line with this hypothesis, sex steroid hormones ablation in mice leads to increase expression of Delta-like 4 (Dll4) in TECs, ultimately improving thymic function (145). In rats, maternal protein deprivation during lactation resulted in increased endogenous leptin levels protecting thymocytes from apoptosis (143). Conversely, ghrelin enhances thymopoiesis in aged mice, and its diminished expression in the thymus with age suggests a protective role against age-related involution by increasing ETPs numbers in aged mice (139, 146).
Altogether, developing T cells migration, differentiation, maturation, proliferation, and survival are tightly regulated by a variety of hormones produced both locally and in other endocrine glands, critically impacting thymus homeostasis and output.
3.4 Regulation of thymocyte migration and survival by the thymus extracellular matrix
The thymus extracellular matrix (ECM) is a complex network of proteins, glycosaminoglycans, and other molecules that support and facilitates T cell development in combination with other thymus immune-, and stromal cells (147). Thymic stromal cells, including epithelial cells and fibroblasts, actively produce ECM components such as collagen. The main ECM components include: collagen I, collagen III (to a lesser extent), collagen IV, fibronectin, and laminins (148–150). Collagen I is predominantly found in the intracapsular and intraseptal fibers, while collagen IV, fibronectin, and laminin are defining the membranes in the capsula, septa, and perivascular spaces (151). These molecules are known to mediate adhesion, migration, and differentiation of thymocytes (152). Thymic myeloid cells express various metalloproteases (MMPs) that degrade collagen fibers, influencing the intrathymic migration routes, which are essential for interactions with thymic epithelial cells and developmental progression of T cells (153–155).
Thymocytes move through the inner thymus in an integrin-dependent manner with ECM components modulating their adhesion and spatially regulating thymocyte development. In vitro experiments showed that thymocyte-fibronectin specific interactions by recognizing the amino acid sequence Gly-Arg-Gly-Glu-Ser-Pro regulate the migration of developing thymocytes in a gradient-dependent manner (156). Developing thymocytes also engage the ECM via CD44, that binds hyaluronic acid and collagen (151), and adhere to laminin via α6β64 integrin. Additionally, VLA-4, -5, -6, and LFA-1 are important regulators of the rolling and adhesion motility of thymocytes to the ECM (151, 157). Laminins, primarily produced by thymic stromal cells form gradients and allow integrin-laminin specific interactions that create intrathymic routes for thymocyte development (158).
Notably, hormones also orchestrate ECM remodeling thereby controlling developing thymocytes migration and specification. Laminin production and secretion by TECs is regulated by growth hormone (GH) (158). Similarly, triiodothyronine (T3) treatment increased laminins and VLA-6, leading to an enhanced migration of thymocytes (158). Glucocorticoids (GCs) are steroid hormones that cross the plasma membrane and bind to an intracellular glucocorticoid receptor. Produced by thymic epithelial cells (TECs), GCs shapes thymocytes TCR repertoire by mitigating downstream TCR signaling events, thereby promoting thymocyte negative selection. For example, GC treatment independently inhibits the transcription factors Nur77 and Helios, which are upregulated in TCR-signaled thymocytes (159). Dexamethasone (Dex), a synthetic glucocorticoid commonly used in clinical settings, induces extensive thymocyte apoptosis, resulting in acute thymus atrophy. Dex administration triggers apoptosis through phosphoinositide-specific phospholipase C (PLC) and acidic sphingomyelinase (aSMase) signaling, leading to subsequent caspase-3 activation (160). Additionally, Dex treatment reduces NFAT, AP-1, and c-MYC activity in thymocytes and limits the expression of anti-apoptotic factors like BCL-2 by promoting proteasomal degradation, ultimately causing acute thymus atrophy (161). Additionally, GCs can stimulate the TECs leading to the accumulation of laminins between the cortex and medulla, therefore impairing DN4-DP migration leading to T cell development arrest and thymus atrophy (158, 162).
Taken together, the secretion, distribution, and breaking down of the ECM components are tightly regulated by thymus immune- and stromal subsets together with hormones, playing an active role in remodeling the thymus landscape, thereby promoting or limiting the developmental progression of pre-T cells.
3.5 Non-T cell thymic subsets contribution to thymus homeostasis and T cell development
Beyond developing T cells, the thymic immune landscape also contain other immune cell substes including B cells (114, 163), DCs (48, 68), eosinophils (32), macrophages (34), neutrophils (36, 164), and all three groups of ILCs (31, 165–168), each of them performing an essential role in maintaining thymus homeostasis and enabling T cell development. Thymic B cells participate in negative selection and tolerance acquisition by presenting autoantigens and contributing to the development of regulatory T cells (114, 163). Thymic DCs, together with thymic epithelial cells (TECs) engage in MHC-TCR interactions with developing T cells and present self-antigen playing a crucial role in the progression or clonal deletion of developing T cells (169, 170). Macrophages, neutrophils, and eosinophils act as scavengers eliminating the dying T cells in a very rapid process (117, 164, 171). Additionally, thymus macrophages functions extends beyond phagocytosis, as they can present antigen and induce cell death of self-reactive thymocytes (172). Although the functions of thymic ILCs during homeostasis remain largely unexplored, some studies suggest that ILCs can influence fate decisions of uncommitted thymus progenitors, and impact thymus epithelial cells that control the maturation and egress of developing T cells (165, 166).
3.6 Selection checkpoints: life and death of a developing T-cell
T cell development and selection is highly regulated to avoid self-reactivity and autoimmune disorders. During its most productive phase, the mouse thymus generates around 50 million of DP thymocytes each day that undergo a series of selection processes. In total, it is estimated that only 3-5% of developing thymocytes become mature CD4 or CD8 single positive (SP) T cells and exit the thymus (117). Programmed cell death (PCD) plays a crucial role in the elimination of a large number of Pre-T cells that do not overcome either β-, δ-, negative, positive or agonist selections to prevent autoimmunity (59). The specific molecular cell death pathways downstream the different T-cell development checkpoints have not been fully mapped (112, 173), although apoptosis is the default death modality during T cell development (174, 175).
Early during T cell development, DN1-2 survival is controlled by the expression of the cytokine receptors CD117 (c-KIT) and IL-7R that triggers anti-apoptotic signaling via Bcl-2 and the availability of their respective ligands SCF and IL-7 (176). After the DN2 stage, thymocyte survival depends on the correct expression of the TCRβ chain and the surrogate pre-TCRα chain in a process known as β-selection. BCL-2 is expressed both in DN and SP thymocytes, whereas BCL-XL is predominantly expressed in DP cells (177). Thymocytes that fail to bind self-MHC die by caspase-3 dependent apoptosis (178). NF-kB dependent activation of BCL-2-related protein A1 (BCL2A1) is required to inhibit caspase-3 dependent of DN thymocytes (178). At this stage, CXCR4 is required for the progression through β-selection as regulator of the localization of DN1-2 thymocytes and as a co-stimulator to promote the pre-TCR dependent activation of BCL-2 (179). Additionally, FAS-associated protein with death domain (FADD) plays a critical role in regulating survival and transition of DN2 and DN3 during β-selection by modulating NOTCH signaling (180).
The BCL-2 family member BIM is required for apoptosis of autoreactive SP CD4+ and CD8+ thymocytes. Indeed TCR ligation upregulated Bim expression and promoted interaction of BIM with BCL-XL, inhibiting its survival function (181). This demonstrates the engagement of the intrinsic mitochondrial pathway during negative selection. Bim-/- mice showed abnormal T cell development characterized by loss of DP T cells and accumulation of DN an SP subsets (182). In vivo deletion of Bid and Bim suppressed pre-TCR thymocyte cell death (183). DP thymocytes with appropriate TCR:MHC interaction strength are positively selected, becoming naïve SP CD4+ or SP CD8+ T cells (184). However, the majority of DP thymocytes (approximately 90%) are unresponsive to TCR:MHC engagement (184). These cells become sensitized to BIM-mediated intrinsic apoptosis due to BCL-2 downregulation, and ultimately undergo death by neglect (185). The MAPK pathway is upregulated in DP thymocytes that exhibit low TCR:MHC avidity, leading to their positive selection (186) while “strong” negatively selecting signals lead to Bim-mediated intrinsic apoptosis (181, 187).
Notably, deletion of Mcl-1 results in thymocyte cell death, a phenomenon rescued by additional deletion of Bak, but not by single deletion of Bak or Bim (188). This suggests that MCL-1 promotes thymocyte survival independently of BCL-2 by sequestering proapoptotic Bak (188). Interestingly, thymocytes from Bax-/- Bak-/- double-knock out mice were resistant to death-by-neglect (189). However, these mice displayed reduced thymopoiesis over time, suggesting that the elimination of apoptotic cells is needed for restoration of normal T cell development. Noteworthy, Bid-/- Bim-/- Puma-/- triple-knockout mice displayed a large thymus, and T cells were resistant to IL-7 deprivation (190). Mechanistically, the triple deletion of Bid, Bim, and Puma prevented the oligomerization of BAX and BAK, and subsequent cytochrome c–mediated release of caspases, thereby blocking intrinsic apoptosis (190).
Notably, many key contributors to the survival and developmental progression have been identified (Figure 4). While most studies emphasize the involvement of intrinsic apoptosis as the primary cell death mechanism through T cell selection, the death receptors tumor necrosis factor receptor 1 (TNFR1), death receptor 3 (DR3), and death receptor 5 (DR5) are expressed in DN3-4 thymocytes suggesting a role in thymic selection (191). Interestingly, RIPK1 expression is developmentally controlled and it is only expressed following positive selection (192). Deletion of Ikk1 and Ikk2 with the hCD2iCre (DN2 cells and downstream) or CD4Cre (DP cells and downstream) resulted in reduced numbers of SP CD4+ and CD8+ that were more sensitive to TNF-induced extrinsic apoptosis, while DP and DN numbers remain unaffected (192, 193). TNF-mediated NF-κB pathway and IKKs are key in controlling RIPK-kinase activity and promoting SP survival (192). IKKs control SP survival by repressing RIPK1-induced cell death independently of NF-κB signaling. Noteworthy, despite sensitizing CD4+ or CD8+ SP thymocytes to TNF-induced extrinsic apoptosis, deletion of RIPK1 in thymocytes, does not result in reduced numbers or frequencies of DN, DP, or CD4+ or CD8+ SP thymocytes in vivo (193). These results suggest that RIPK1 and RIPK1-kinase activity are not essential for the steady-state survival and developmental progression of thymocytes. Additional deletion of caspase-8 (Cd4Cre Ripk1-/- Casp8-/-) did not affect thymus development excluding engagement of RIPK1-mediated mechanisms (193). However, caspase-8 has been proposed to play a role in thymocyte development and elimination (194–196). Indeed, while caspase-8 activation in DP thymocytes was shown to be FAS-independent, the medullary CD4+ or CD8+ SP thymocytes that exhibit strong TCR:MHC interaction underwent FAS-dependent Caspase-8 mediated extrinsic apoptosis in a model of staphylococcal enterotoxin B (SEB), resulting in clonal elimination of SEB-reactive Vβ8+ cells (197).
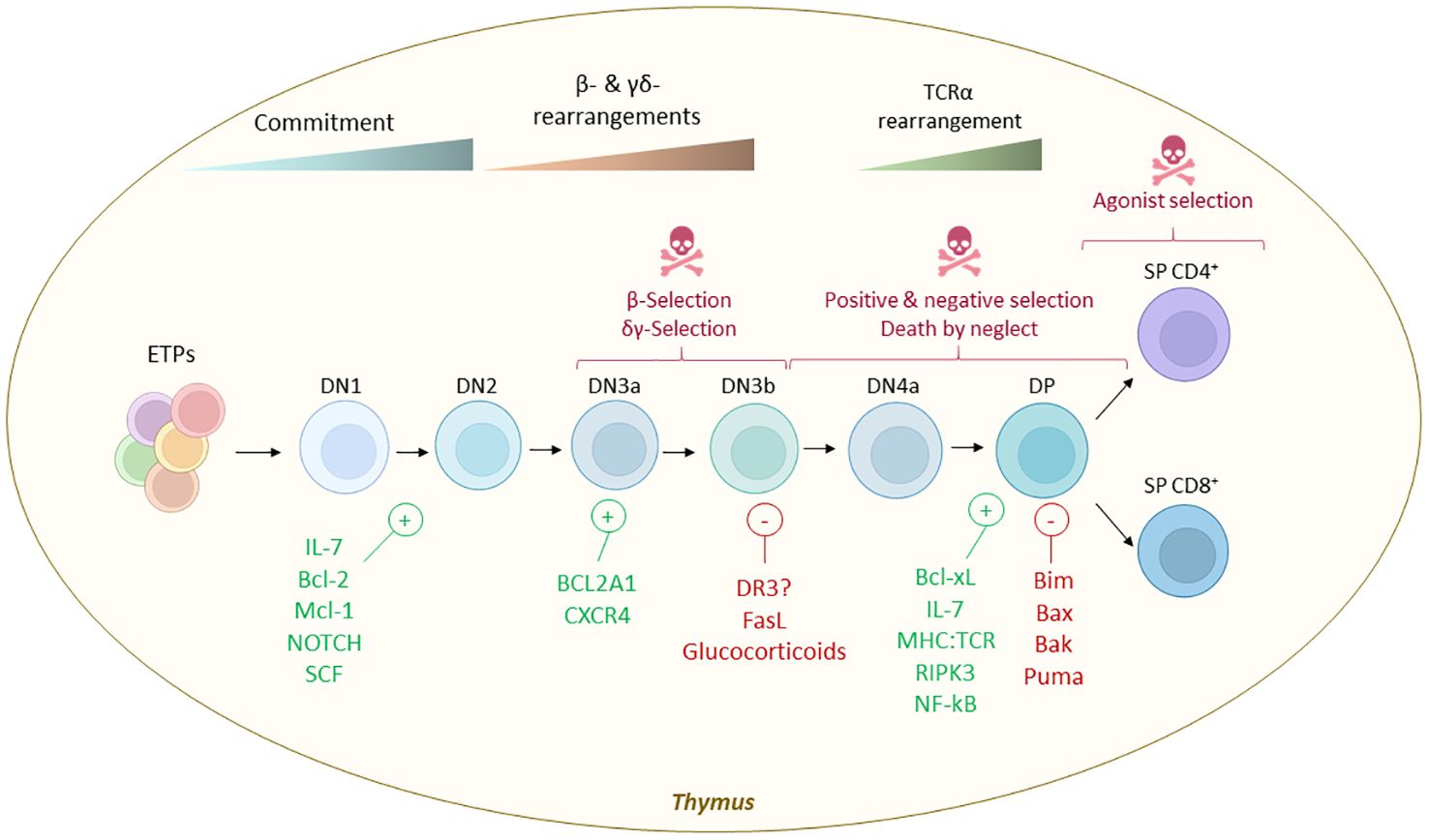
Figure 4. Cell death selection checkpoints during thymic T-cell development and molecules that control life vs death decisions. Early thymus progenitors (ETPs) are uncommited progenitors that enter the T cell differentiation path instructed by the thymus stroma. The earlier stage is known as DN1 (defined as Lin- CD44+ CD25- c-Kithi), later becoming DN2 (defined as Lin- CD44+ CD25+ c-Kit+). The survival of these progenitors is controlled mainly by IL-7 availability, SCF - c-KIT interactions, NOTCH signaling and BCL-2 and MCL-1. Later, when the machinery of TCR recombination these cells are irreversibly committed to the T cell fate and become DN3a (defined as Lin- CD44- CD25+ c-Kit-/lo CD27+ CD28-) and later DN3b (defined as Lin- CD44- CD25+ c-Kit- CD27+ CD28+). During this stage the TCR chain is tested and BCL2A1 and CXCR4 were reported to control the survival of DN3 while FASL and glucocorticoids promote cell death when the TCR is defective. Then, DN3 cells move forward in the development acquiring a DN4 phenotype (characterized as Lin- CD44- CD25- CD27+ CD28+) and ultimately a DP phenotype (characterized as CD4+ CD8+). These cells remain quiescent for some days and later undergo additional selection rounds where the TCR:MHC affinity is tested. TCR:MHC interaction, BCL-XL, IL-7, RIPK3 and NF-κB are some of the signals that dictates their survival, while BH3-only proteins BIM and PUMA and their mitochondrial pore forming targets BAX and BAK are implicated in the cell death execution when the TCR:MHC interaction is not successful. Lastly, DP thymocytes will adopt a SP CD4+ or CD8+ phenotype and will egress from the thymus to secondary lymphoid organs where they complete their maturation into different effector and helper subsets. A small fraction of SP CD4+ cells that show high TCR:MHC interaction can be rescued from cell death to become regulatory T cells (Tregs, defined as CD4+ FOXP3+ CD25+) or unconventional T cells (iNKT, MAIT, etc.).
Besides extrinsic apoptosis by death domain receptors, also the involvement of other cell death mechanisms such as RIPK3/MLKL-driven necroptosis in thymocyte development have been investigated. Thymus morphology and macrostructure remained largely unchanged in necroptosis deficient Mlkl-/- and Ripk3-/- mice compared to littermate controls (198). Further characterization of the RIPK3-deficient mice revealed abnormal proliferation of DP thymocytes, leading to thymus hyperplasia and an increased incidence of thymomas (199). This study suggests that RIPK3 may play a role in regulating the homeostasis of DP thymocytes independently of MLKL and the necroptotic signaling complex.
While intrinsic apoptosis seem to be the primary pathway regulating steady state thymic selection (197), systemic inflammation due to different triggers such as infections or glucocorticoids leads sudden thymocyte depletion, known as acute thymic atrophy (160, 178, 200). However, it remains unclear whether extrinsic apoptosis by death domain receptors or necrotic cell death modalities such as necroptosis, pyroptosis, or ferroptosis are involved in infection-induced acute thymus atrophy or other types of acute thymus atrophy.
4 Age-related thymic involution vs acute thymus atrophy
Despite its crucial role in maintaining immune system homeostasis, the thymus starts undergoing chronological regression from a year of life in a process known as age-related thymic involution (201). Age-related thymic involution is evolutionary conserved across jawed vertebrates (202–205). In humans, the rate of thymus regression is around 3% per year until middle age (35-45 years of age), after which this decreases to a rate of around 1% until death (206). In humans, age-related thymic involution takes place from infancy, but increases after puberty. The most acute phase of age-related thymic involution occurs at 30-40 years in humans and at 9-12 months of age in mice (207). While T cell production regresses throughout life, new naïve T cells can be detected even in individuals of advanced age (208).
Age-related thymic involution is characterized by shrinkage of the thymus, decreased thymus cellularity (18, 209), diminished thymus colonization by TSPs and lower number and proliferation capacity of ETPs (18, 207), reduced number of recent thymic emigrants (reflected by the number of signal joint T-cell excision circles (sjTREC) (210), replacement of lymphoid tissue and fibroblasts by adipose tissue (201, 211), changes in the thymus’s architecture and morphology (209, 212), and defects in the thymic epithelial cell (TECs) compartment (213, 214). Interestingly, memory B cells accumulate in the aging thymus of humans co-localizing with cytokeratin+ mTECs (41). Since the thymus cellularity declines with age, the PVS extend to a larger surface in aged individuals (41). As an overall result of age-related thymic involution, a reduced number of recent thymic emigrants can be detected in peripheral blood with age (208, 210). Related to this, no apparent differences in the DP percentage can be observed with age, whereas the decline in thymus cellularity is directly proportional with ETP numbers (215). However, the number of functional TSP/ETP niches does not diminish as we age, rather the pre-thymic progenitors in the blood and bone marrow reduces with age (215), showing that the thymus regression is not merely due to thymic morphologic changes. In addition to the previously described phenomena, the aged thymus exhibit increased reactive oxygen species (ROS), enhanced senescence of TECs and quiescence of TEC progenitors (214) and elevated thymocyte apoptosis (178).
Acute thymic atrophy fundamentally differs from age-related thymic involution (216). Acute thymic atrophy is a stress response and results in reduced thymic cellularity that disproportionally affects developing T cells (216). While age-related thymic involution is a progressive process that worsens throughout life, acute thymic atrophy is a transient state in which the thymus can recover its normal cellularity and tissue architecture. Similarly as age-related thymic involution, acute thymus atrophy is evolutionary conserved in vertebrates (202–205). Acute thymic atrophy can be induced by a wide range of triggers such as infectious diseases (155, 178, 217–223), sepsis (224), and pro-inflammatory cytokines (225, 226).
In this regard, specific pro-inflammatory cytokines have been shown to induce acute thymic atrophy, such as the type 1 cytokines IL-12, IL-18, and IFNγ (31, 227), or the type 2 cytokine IL-33 (225), among others. Duing type 1 immunity, such as infection with the mouse pathogen Salmonella typhimorium, ETPs/DN1 fraction remain largly unnaffected during the resultingthymic atrophy (221, 222) while DP thymocytes are espcially sensitive to acute inflammation (200, 221).
Acute thymic atrophy also occurs in response to other triggers, such as malnutrition (228–231), radiotherapy and chemotherapy (232–234), and pregnancy (235–238). Of note, steroid hormones, such as the sex hormones progesterone, androgens, and estrogens (239), as well as glucocorticoids (160, 162) are all capable of inducing thymus atrophy. Pregnancy-induced thymic atrophy is primarily mediated by progesterone, and can be inhibited by the use of progesterone receptor knock out mice (240). Although, steroid hormones, inhibit effector T cell functions (241), thymus atrophy induced by androgens and progesterone is mediated by directly affecting TECs (239). Castration studies revealed that androgens effect on thymic size, appear to be a result of reduced TEC proliferation (242). The steroid hormone progesterone affects TECs by reducing CCL25 production resulting in less ETP recruitment (242). Other studies have also shown that progesterone regulates Aire expression in mTECs, thereby affecting negative selection (242, 243).
The reduction of the thymus cellularity, disorganization of the thymus morphology and shrinkage of the thymus (in size and weight) are the general hallmarks for acute thymic atrophy (216). Interestingly, depending on the initial trigger, there are variations of which stage of T cell development that is blocked and specific morphological changes can be observed (see Table 2). The variety of different cytokine and hormonal triggers and their specific thymic effects indicates that that there are different ‘flavors’ of thymus atrophy. However, the different effects these various types of thymus atrophy have on the peripheral immune system remains to be elucidated. Despit various triggers, we have summarised some of the primary features of thymus atrophy (see Table 2 and Figure 5). Systemic inflammation can impact the thymus resulting in acute thymic atrophy.
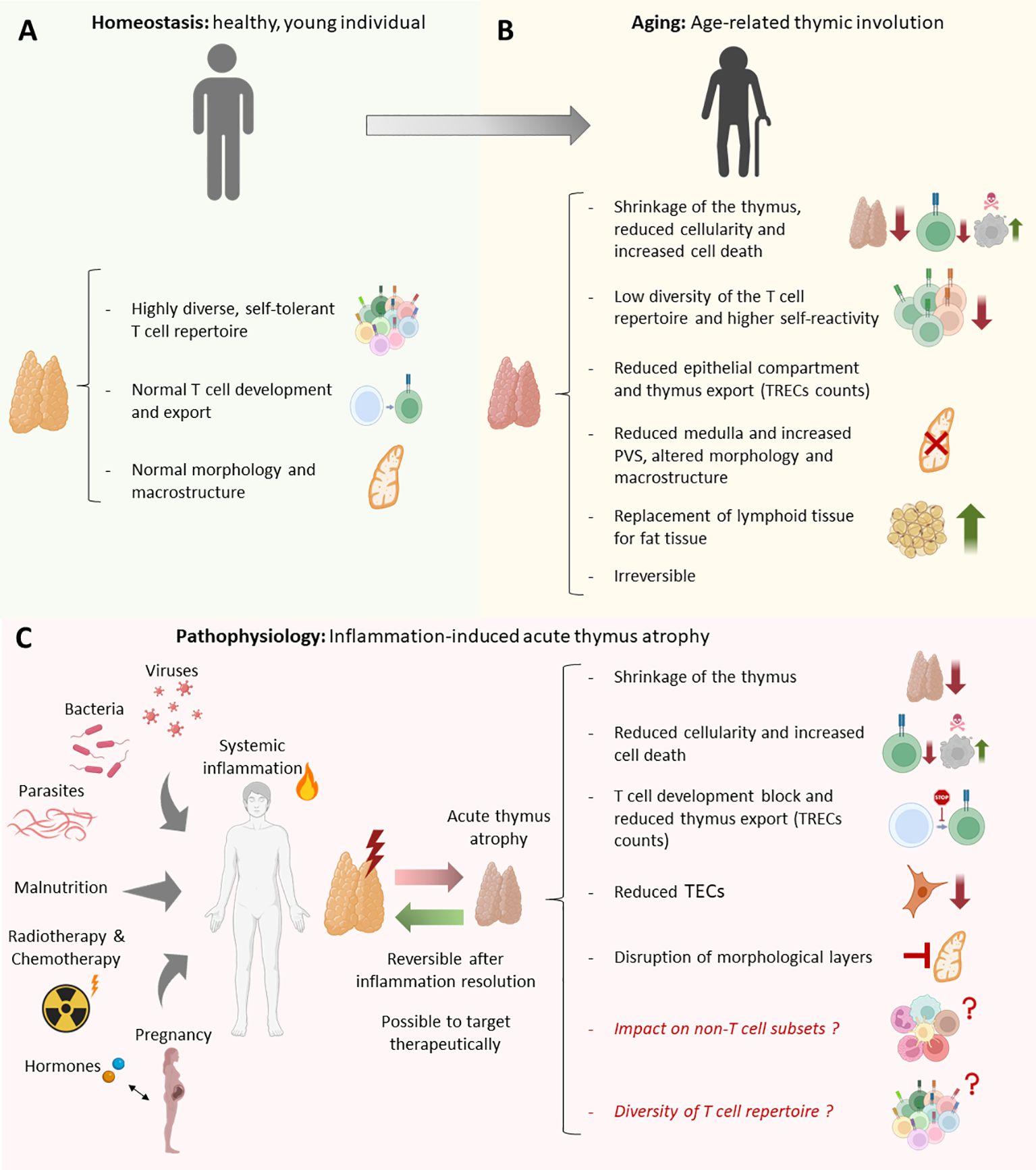
Figure 5. Anatomical, morphological, cellular and molecular differences between the healthy thymus, inflammation induced-acute thymus atrophy, and age-related thymus involution. (A) Morphological and cellular characteristics of a young-healthy thymus vs (B) aged-individual thymus. (C) Common morphological, cellular and molecular alterations produced in inflammation-induced acute thymus atrophy in response to different triggers such as infections, steroid hormones, malnutrition, irradiation, and pregnancy.
Generally, ETPs and DN1 fraction remain unnaffected during Salmonella typhimorium-induced thymic atrophy (221, 222), while DP thymocytes have been reported as one of the most sensitive subsets to acute inflammation (200, 221). Remarkably, most of the infectious triggers such as MCMV, Salmonella or Micobacterium avium do not specifically target the thymus (219, 221, 252), but it is rather the systemic inflammation that results in the cascade events characteristic of the thymus atrophy. Thus, some cells might be responsile of sensing systemic inflammation may trigger the local dowstream effect in the thymus ultimately leading to atrophy induction. Remarkably, plasma cells were reported to accumulate in the thymus perivascular spaces (PVS) and constitutively secrete immunoglobulin G and show reactivity to common viral proteins (41). Interestingly, beyond the impact of systemic inflammation on the thymus T cell counterpart, some immune subsets are specially resistant to acute inflammation (31, 33, 36, 249, 253). In particular, recent investigations have highlighted the critical role of thymic ILCs in driving the endogenous regeneration of the thymus by producing pro-repair cytokines such as IL-22 (33, 249). Recently, we showed that the type 1 cytokines IL-12 and IL-18, while inducing acute thymus atrophy in mice also enhance the production of neonatal thymic ILC1s, capable of migrating to peripheral organs (31). Additionally, dexamethasone-induced thymus atrophy promoted a type 2 immune response driven by thymic ILC2s, that resulted in tissue regeneration (33).
5 Physiological relevance and future perspectives on thymus function, development and atrophy
5.1 Physiological implications of the thymus function for human health
The thymus is critical for health and survival of individuals, both in early life and during adulthood. Newborns born with a genetic defect lacking a thymus suffer from a pathology known as severe combined immunodeficiency (SCID), which is lethal in the first years of life unless diagnosed and followed by allogeneic hematopoietic stem cell transplantation (254). Furthermore, a deletion on chromosome 22 results in the failure of thymus development, leading to a pathology known as DiGeorge syndrome (255). DiGeorge syndrome’s clinical manifestations include T-cell deficiency (due to thymic hypoplasia), hypoparathyroidism, cardiac malformations, facial abnormalities neurodevelopmental delay, behavioral, and psychiatric features (255). These patients show higher risk of suffering opportunistic infections (256). Interestingly, patients with partial DiGeorge Syndrome (pDGS) and Down Syndrome (DS) exhibit changes in thymus size (hypoplasia) and architecture, increasing their risk of developing autoimmunity (257). While pDGS patients displayed lower frequency of SP CD4+, SP CD8+ and Treg, DS-isolated Tregs showed impaired suppressive capacity (257). These results reveal a link between thymic abnormalities and the immune dysregulation observed in pDGS and DS patients. Myasthenia gravis (MG) is a rare autoimmune disease mediated by antibodies against proteins expressed in the neuro-muscular junction such as the acetylcholine receptor that can be linked to issues with the thymus gland such as increased risk of thymomas (258, 259). The thymus of MG patients shows increased production of inflammatory cytokines and chemokines, leading to B-cell recruitment and the aberrant development of germinal centers in the thymus (259). Thymic B cells secrete antibodies against the acetylcholine receptor. Furthermore, the elevated local inflammation dysregulates the function of thymic Tregs (259). The acetycholine receptor expression in human TECs is elevated by type I and II interferons contributing to a positive feedback loop aggravating the pathology (259). MG patients are frequently subjected to corticosteroid treatment or thymectomy in order to stop thymic B-cell development. Chronic graft-versus-host disease (GVHD) is a complex multiorgan disorder characterized by autoimmunity and immunodeficiency, which arises as a complication of allogeneic hematopoietic cell transplant (HCT) (260). Typically, some months after allogeneic HCT, donor-derived mature T cells cause local inflammation and damage epithelial cells in the skin, liver, and gastrointestinal tract often resulting in sclerosis and fibrosis in multiple organs (261, 262).
A recent study revealed that thymectomized adult patients following cardiac surgery showed increased cancer risk and overall mortality in compared to controls (263). This study confirmed previous reports indicating the importance of the thymus for the maintenance of the immune health in adult individuals (264, 265). Notably, adult individuals are less responsive to vaccination partly due to the age-related thymus involution (266, 267). Despite its importance for the human health, many aspects of thymus biology remain unknown, particularly regarding the cellular and molecular changes during atrophy and the functions of non-T cell subsets.
5.2 Future perspectives in thymus development, function and atrophy
Many models of sytemic inflammation leads to acute thymus atrophy heavily impacting the thymus immune landscape. Particularly, the medullary area decreases with age while the perivascular spaces are enlarged (41). However, while the impact of thymic atrophy in the T cell comparment has been thoroughly characterized, the changes in the non-T cell immune compartment, as well as the function of these cells during injury remain elusive. Some recent studies suggest potential roles of non-T cells as sensors of systemic inflammation, initiators of acute thymus atrophy, and conductors of the repair phase post-damage. For example, thymic DCs sense systemic inflammation and can inhibit mTECs proliferation via cell-cell contact in a JAGGED1-NOTCH3 axis dependent manner, ultimately resulting in massive death of developing T cells and acute thymus atropy (268). Notably, B cells are suggested to play a role in the humoral response of the thymus as a protective mechanism against viral infections (41, 269). Macrophages, neutrophils, and eosinophils are proportionally increased during acute thymus atrophy (32, 33, 36, 164). These cells act as phagocytes in response to increased thymocyte death (164, 171). Furthermore, thymus macrophages and neutrophils express high levels of metalloproteases, revealed important in the remodelling of the thymus ECM contributing to a repair response and reestablishment of thymus homeostasis (154, 155, 270). In addition, MafB+/GFP mice diplayed impaired thymic recovery following irradiation. As Mafb expression was mostly restricted to thymus macrophages and monocytes this study illustrates an important role of macrophages and monocytes in thymus repair. The removal of apoptotic cells in the thymus was proven essential to trigger the repair program and the production of BMP-4, IL-23, IL-22 by the stroma to restore thymus homeostasis (271).
ILCs produce cytokines that can alter the development and fate of developing T cells. Group-1 ILCs are relatively increased upon viral infection (31, 244), proinflammatory cytokine exposure (31), or following irradiation (226), resulting in increased production of IFN-γ and Perforin, among others (31, 244). These factors have been shown to drive acute thymus atrophy as unveiled in Ifnγ-/- (244) and Prf1-/- (226) mouse models. Additionally, targeting of NK1.1+ cells (majority of group-1 ILCs in the thymus) ameliorated irradiation induced-acute thymus atrophy (226). Notably, ILC2s were also relatively increased and activated in dexamethasone-induced thymus atrophy (33). Dexamethasone - activated ILC2s increased the expression of genes such as Csf2, Il13, Il5, or Areg known to impact thymus stroma, myeloid compartment and mTEC proliferation. In line with these findings, a study conducted in 2012, revealed that following irradiation, thymic DCs produce IL-23 leading to IL-22 secretion by thymus LTis resulting in enhance TECs proliferation and survival (249). Studies identifying the critical mediators responsible for inducing thymus atrophy and promoting thymus regeneration are crucial for understanding the most effective approaches to enhance thymus function. In the past few years, BMP4 was identified as a potent endogenous thymus regeneration factor by enhancing Foxn1 expression in TECs (253). These studies offer innovative strategies to achieve thymus rejuvenation.
In fact, some studies have shown that there are treatments that enhance thymus function and output. The treatment with ghrelin enhance the production and development of T cells and promotes the engraftment of T cells in SCID mice (146). Similarly, IL-7 plays a key, non-redundant role in the generation of the T-cell repertoire (272), and its administration enhances T cell development (273). Linked to this, a recent study has shown that CAR-T cell transplantation cultured with IL-7 and IL-15 improve the efficiency of the transplantation and reduced the exhaustion of the transplanted cells (274).
Altogether, non-T cell immune subsets play a crucial role in maintaining T cell homeostasis and creating the proper environment for T cell development. Simultaneously, they also play key roles in initiating acute thymus atrophy and facilitating repair processes after damage, thus restoring homeostasis. This may occur through various mechanisms such as (1) the release of proinflammatory cytokines, (2) direct cell-cell interactions with thymic epithelial cells (TECs) and thymocytes leading to cell death in neighboring cells, or (3) actively inducing morphological changes in thymus architecture through ECM remodeling, resulting in increased thymocyte death and developmental arrest.
Since the availability of human thymus samples is low, murine studies to investigate thymus changes induced by systemic inflammation are very relevant as, conforming a limitation for performing functional studies in humans. Instead, a recent study utilized spatial omics techniques in the human thymus (275). These emerging technologies including spatial transcriptomics resolution, combined with mouse genetic studies hold promise for elucidating the interactions between developing T cells and the stroma, potentially providing insights for interventions to either promote or inhibit T cell development according to disease requirements. Another promising alternative is the use of tissue-engineered artificial human thymus from human iPSCs (276), or the recently generated thymus organoids (277). These models may better resemble the physiological conditions in humans and help determine the extent to which findings from murine studies are applicable to humans.
Looking forward, it remains unclear whether extrinsic apoptosis by death domain receptors and necrotic cell death modalities such as necroptosis, pyroptosis, or ferroptosis are involved in acute thymus atrophy. Additionally, optimizing thymus function through the administration of cytokines or hormones to inhibit thymus atrophy may prove beneficial in certain immunotherapies against cancer, infectious diseases, or autoimmunity. However, further research into the various aspects of thymus atrophy and its regeneration are required understand when it would be most beneficial to support thymic T cell development.
Author contributions
MR: Conceptualization, Funding acquisition, Investigation, Visualization, Writing – original draft, Writing – review & editing. PV: Funding acquisition, Resources, Supervision, Writing – review & editing. PT: Funding acquisition, Supervision, Writing – review & editing.
Funding
The author(s) declare financial support was received for the research, authorship, and/or publication of this article. FWO Research Grants G.0B96.20N (PV, PT), G.0C76.18N (PV), G.0B71.18N (PV), G.0A93.22N (PV), Special Research Fund UGent (Methusalem grant BOF16/MET_V/007 (PV) BOF22/MET_V/007(PV), and iBOF ATLANTIS grant 20/IBF/039 (PV), EOS MODEL-IDI Grant (30826052) (PV), and EOS CD-INFLADIS (40007512) (PV), Foundation against Cancer (F/2016/865, F/2020/1505) (PV), FWO fundamental research fellowship PhD grant (MP) (11A7222N), Cancer Research Institute Ghent (CRIG) consortia, Ghent Gut Inflammation Group (GGIG) consortia, Flanders Institute for Biotechnology (VIB).
Acknowledgments
We thank Prof. Dr. Tom Taghon, Prof. Dr. Natalia Zietara, Prof. Dr. Sophia Maschalidi, and Dr. Helena Catharine Aegerter for their critical feedback on the manuscript draft. We used Biorender (academic subscription) to create the all the cartoons and figures displayed in the manuscript. We used grammarly (https://app.grammarly.com/) and ChatGPT4.0 (https://openai.com/chatgpt/) to check for misspelling, grammatical mistakes and language curation.
Conflict of interest
The authors declare that the research was conducted in the absence of any commercial or financial relationships that could be construed as a potential conflict of interest.
Publisher’s note
All claims expressed in this article are solely those of the authors and do not necessarily represent those of their affiliated organizations, or those of the publisher, the editors and the reviewers. Any product that may be evaluated in this article, or claim that may be made by its manufacturer, is not guaranteed or endorsed by the publisher.
Glossary
Ach: Acetylcholine
AGM: Aorta-gonad mesonephros
APC: Antigen presenting cell
APCs: Antigen-presenting cells
aSMase: Acidic Sphingomyelinase
BM: Bone Marrow
CAR-T: Chimeric Antigen Receptor T-cell
CD: Cluster of Differentiation
CDPs: Common Dendritic Progenitors
CLP: Cecal Ligation and Puncture
CLP: Common-lymphoid progenitor
CLP-2: Common Lymphoid Progenitors-2
CMJ: Corticomedullary junction
CMP: Common-Myeloid Progenitor
CNS: Conserved non-coding sequence
cTEC: Cortical thymic epithelial cell
CTP: Circulating T cell progenitor
DCs: Dendritic cells
Dex: Dexamethasone
Dll4: Delta-like 4
DN: Double negative
DP: Double positive
DR3: Death Receptor 3
DR5: Death Receptor 5
DS: Down syndrome
E#: Embryonic day
ECM: Extracellular Matrix
ELPs: Early Lymphoid Progenitors
ETP: Early Thymic Progenitor
FADD: FAS-Associated Protein with Death Domain
FOXP3: Forkhead box P3
GALT: Gut-associated lymphoid tissue
GH: Growth Hormone
GMP: Granulocyte-monocyte progenitor
GPCR: G protein-coupled receptor
GVHD: Graft-Versus-Host Disease
HCT: Hematopoietic cell transplant
HSC: Hematopoietic stem cell
IEL: Intestinal intraepithelial lymphocyte
ILC: Innate lymphoid cell
iNKT: Invariant natural killer T cell
IPEX: Immune dysregulation, polyendocrinopathy, enteropathy, X-linked syndrome
iPSC: Induced Pluripotent Stem Cell
LFA-1: Lymphocyte Function-Associated Antigen 1
LMPP: Lympho-myeloid primed progenitor
LPS: Lipopolysaccharide
LSK: Lineage-Sca-1+ c-Kit+
MAPK: Mitogen-Activated Protein Kinase
MCMV: Murine Cytomegalovirus
MDP: Macrophage-Dendritic Progenitor
MG: Myasthenia gravis
MHC-I: Major histocompatibility complex I
MHC-II: Major histocompatibility complex II
MLKL: mixed lineage kinase domain like
MPP: Multipotent progenitor
mTEC: Medullary thymic epithelial cell
NE: Norepinephrine
NK: Natural killer
OT: Oxytocin
pDGS: Partial DiGeorge syndrome
PCD: Programmed cell death
pDC: Plasmacytoid dendritic cell
PLC: Phosphoinositide-Specific Phospholipase C
PVM: Pneumonia Virus of Mice
PVS: Perivascular spaces
RIPK1: Receptor-Interacting Protein Kinase 1
RIPK3: Receptor-Interacting Protein Kinase 3
ROS: Reactive Oxygen Species
SCF: Stem Cell Factor
SCID: Severe Combined Immunodeficiency
sjTREC: Signal Joint T-Cell Receptor Excision Circle
SLC: Solute carrier transporter
SP: Single positive
T3: Triiodothyronine
T4: Thyroxine
TCR: T cell receptor
THF: Thymic Humoral Factor
TL1A: TNF-like ligand 1A
Treg: Regulatory T cell
TSLP: Thymic stromal lymphopoietin
TSP: Thymus seeding progenitor
VLA: Very Late Antigen
VP: Vasopressin
WNK1: With no lysine kinase 1
References
1. Miller JFAP. The function of the thymus and its impact on modern medicine. Science. (2020) 369:1–8. doi: 10.1126/science.aba2429
2. Miller JFAP. The discovery of thymus function and of thymus-derived lymphocytes. Immunol Rev. (2002) 185:7–14. doi: 10.1034/j.1600-065X.2002.18502.x
3. Miller JFAP. Fate of subcutaneous thymus grafts in thymectomized mice inoculated with leukæmic filtrate. Nature. (1959) 184:1809–10. doi: 10.1038/1841809a0
4. Miller JFAP. The thymus and the development of immunologic responsiveness. Sci (80- ). (1964) 144:1544–51. doi: 10.1126/science.144.3626.1544
5. Miller JFAP. Immunological function of the thymus. Lancet. (1961) 278:748–9. doi: 10.1016/S0140-6736(61)90693-6
6. Miller JFAP. The thymus, yesterday, today and tomorrow. Lancet. (1967) 290:1299–302. doi: 10.1016/S0140-6736(67)90407-2
7. Miller JFAP. Analysis of the thymus influence in leukaemogenesis. Nature. (1961) 191:248–9. doi: 10.1038/191248a0
8. Pancer Z, Amemiya CT, Ehrhardt GötzRA, Ceitlin J, Gartland GL, Cooper MD. Somatic diversification of variable lymphocyte receptors in the agnathan sea lamprey. Nature. (2004) 420:174–80. doi: 10.1038/nature02740
9. Garcia KC. Dual arms of adaptive immunity: division of labor and collaboration between B and T cells. Cell. (2019) 179:3–7. doi: 10.1016/j.cell.2019.08.022
10. Miller JFAP. Revisiting thymus function. Front Immunol. (2014) 187:703. doi: 10.3389/fimmu.2014.00411
11. Franchini A, Ottaviani E. Thymus: conservation in evolution. Gen Comp Endocrinol. (2017) 246:46–50. doi: 10.1016/j.ygcen.2017.03.011
12. Rodewald HR. Thymus organogenesis. Annu Rev Immunol. (2008) 26:355–88. doi: 10.1146/annurev.immunol.26.021607.090408
13. Bajoghli B, Guo P, Aghaallaei N, Hirano M, Strohmeier C, McCurley N, et al. A thymus candidate in lampreys. Nature. (2011) 470:90–5. doi: 10.1038/nature09655
14. Bajoghli B, Aghaallaei N, Hess I, Rode I, Netuschil N, Tay BH, et al. Evolution of genetic networks underlying the emergence of thymopoiesis in vertebrates. Cell. (2009) 138:186–97. doi: 10.1016/j.cell.2009.04.017
15. Ge Q, Zhao Y. Evolution of thymus organogenesis. Dev Comp Immunol. (2013) 39:85–90. doi: 10.1016/j.dci.2012.01.002
16. Boehm T, Bleul CC. The evolutionary history of lymphoid organs. Nat Immunol. (2007) 8:131–5. doi: 10.1038/ni1435
17. Rezzani R, Nardo L, Favero G, Peroni M, Rodella LF. Thymus and aging: Morphological, radiological, and functional overview. Age (Omaha). (2014) 36:313–51. doi: 10.1007/s11357-013-9564-5
18. Palmer DB, Senescence C. The effect of age on thymic function. Front Immunol. (2013) 4:1–6. doi: 10.3389/fimmu.2013.00316
19. Blackburn CC, Manley NR. Developing a new paradigm for thymus organogenesis. Nat Rev Immunol. (2004) 4:278–89. doi: 10.1038/nri1331
20. Gordon J, Manley NR. Mechanisms of thymus organogenesis and morphogenesis. Development. (2011) 138:3865–78. doi: 10.1242/dev.059998
21. Perez YE, Moran CA. The thymus : General concepts on embryology, anatomy, histology and immunohistochemistry. Semin Diagn Pathol. (2022) 39:86–91. doi: 10.1053/j.semdp.2021.06.003
22. Zeng Y, Liu C, Gong Y, Lan Y, Liu B, Zeng Y, et al. Single-cell RNA sequencing resolves spatiotemporal development of pre-thymic lymphoid progenitors and thymus organogenesis in human embryos. Immunity. (2019) 51:930–48. doi: 10.1016/j.immuni.2019.09.008
23. Pearse G. Normal structure, function and histology of the thymus. Toxicol Pathol. (2006) 34:504–14. doi: 10.1080/01926230600865549
24. McGrath KE, Koniski AD, Malik J, Palis J. Circulation is established in a stepwise pattern in the mammalian embryo. Blood. (2003) 101:1669–76. doi: 10.1182/blood-2002-08-2531
25. Butler MG, Isogai S, Weinstein BM. Lymphatic development. Birth defects Res. (2009) 231:222–31. doi: 10.1002/bdrc.20155
26. Oliver G, Alitalo K. The lymphatic vasculature : recent progress and paradigms. Annu Rev Cell Dev Biol. (2005) 21:457–83. doi: 10.1146/annurev.cellbio.21.012704.132338
27. Boehm T. Thymus development and function. Curr Opin Immunol. (2008) 20:178–84. doi: 10.1016/j.coi.2008.03.001
28. Odaka C, Morisada T, Oike Y, Suda T. Distribution of lymphatic vessels in mouse thymus: Immunofluorescence analysis. Cell Tissue Res. (2006) 325:13–22. doi: 10.1007/s00441-005-0139-3
29. Liu C, Saito F, Liu Z, Lei Y, Uehara S, Love P, et al. Coordination between CCR7- and CCR9-mediated chemokine signals in prevascular fetal thymus colonization. Blood. (2006) 108:2531–9. doi: 10.1182/blood-2006-05-024190
30. Haley PJ. Species differences in the structure and function of the immune system. Toxicology. (2003) 188:49–71. doi: 10.1016/S0300-483X(03)00043-X
31. Tougaard P, Ruiz Pérez M, Steels W, Huysentruyt J, Verstraeten B, Vetters J, et al. Type 1 immunity enables neonatal thymic ILC1 production. Sci Adv. (2024) 10:1–17. doi: 10.1126/sciadv.adh5520
32. Cosway EJ, White AJ, Parnell SM, Schweighoffer E, Jolin HE, Bacon A, et al. Eosinophils are an essential element of a type 2 immune axis that controls thymus regeneration. Sci Immunol. (2022) 7:1–12. doi: 10.1126/sciimmunol.abn3286
33. Nevo S, Frenkel N, Kadouri N, Gome T, Rosenthal N, Givony T, et al. Tuft cells and fibroblasts promote thymus regeneration through ILC2 mediated type 2 immune response. Sci Immunol. (2024) 9:1–15. doi: 10.1126/sciimmunol.abq6930
34. Zhou TA, Hsu HP, Tu YH, Cheng HK, Lin CY, Chen NJ, et al. Thymic macrophages consist of two populations with distinct localization and origin. Elife. (2022), 1–35. doi: 10.7554/eLife.75148
35. Ribeiro VSG, Hasan M, Wilson A, Boucontet L, Pereira P, Lesjean-Pottier S, et al. Cutting edge: thymic NK cells develop independently from T cell precursors. J Immunol. (2010) 185:4993–7. doi: 10.4049/jimmunol.1002273
36. Ruiz Pérez M, Maueröder C, Steels W, Verstraeten B, Lameire S, Xie W, et al. TL1A and IL-18 synergy promotes GM-CSF-dependent thymic granulopoiesis in mice. Cell Mol Immunol. (2024) 21:807–24. doi: 10.1038/s41423-024-01180-8
37. Pearse G. Histopathology of the thymus. Histopathology of the Thymus. (2006) 34:515–47. doi: 10.1080/01926230600978458
38. Ribatti D. The discovery of the blood – thymus barrier. Immunol Lett. (2015) 168:325–8. doi: 10.1016/j.imlet.2015.10.014
39. Ushiki T, Takeda M. Three-dimensional ultrastructure of the perivascular space in the rat thymus. Arch Histol Cytol. (1997) 60:89–99. doi: 10.1679/aohc.60.89
40. Mori K, Itoi M, Tsukamoto N, Kubo H, Amagai T. The perivascular space as a path of hematopoietic progenitor cells and mature T cells between the blood circulation and the thymic parenchyma. Int Immunol. (2007) 19:745–53. doi: 10.1093/intimm/dxm041
41. Nuñez S, Moore C, Gao B, Rogers K, Hidalgo Y, Del Nido PJ, et al. The human thymus perivascular space is a functional niche for viral-specific plasma cells. Sci Immunol. (2016) 1:1–10. doi: 10.1126/sciimmunol.aah4447
42. Ashby KM, Vobořil M, Salgado OC, Lee ST, Martinez RJ, Connor CHO, et al. Sterile production of interferons in the thymus affects T cell repertoire selection. Sci Immunol. (2024) 9:1–15. doi: 10.1126/sciimmunol.adp1139
43. Keogh EV, Walsh RJ. A phagocytic function of Hassall’s Corpuscles. Nature. (1965) 208:239–41. doi: 10.1038/208564a0
44. Watanabe N, Wang Yh, Lee HK, Ito T, Wang Yh, Cao W, et al. Hassall ‘ s corpuscles instruct dendritic cells to induce CD4+ CD25+ regulatory T cells in human thymus. Nat Lett. (2005) 436:1181–5. doi: 10.1038/nature03886
45. Bell JJ, Bhandoola A. The earliest thymic progenitors for T cells possess myeloid lineage potential. Nat Lett. (2008) 452:764–7. doi: 10.1038/nature06840
46. Zlotoff DA, Bhandoola A. Hematopoietic progenitor migration to the adult thymus. Ann N Y Acad Sci. (2011) 1217:122–38. doi: 10.1111/j.1749-6632.2010.05881.x
47. Koch U, Radtke F. Mechanisms of T cell development and transformation. Annu Rev Cell Dev Biol. (2011) 27):539–62. doi: 10.1146/annurev-cellbio-092910-154008
48. Liang KL, Roels J, Lavaert M, Putteman T, Boehme L, Tilleman L, et al. Intrathymic dendritic cell-biased precursors promote human T cell lineage specification through IRF8-driven transmembrane TNF. Nat Immunol. (2023) 24:474–86. doi: 10.1038/s41590-022-01417-6
49. Schmitt TM, Ciofani M, Petrie HT, Zúñiga-pflücker JC. Maintenance of T cell specification and differentiation requires recurrent notch receptor – ligand interactions. J Exp Med. (2004) 200:469–79. doi: 10.1084/jem.20040394
50. Park Je, Botting RA, Conde CD, Popescu DM, Lavaert M, Kunz DJ, et al. A cell atlas of human thymic development defines T cell repertoire formation. Science. (2020) 367:1–11. doi: 10.1126/science.aay3224
51. Taghon T, Rothenberg EV. Molecular mechanisms that control mouse and human TCR-αβ and TCR-γδ T cell development. Semin Immunopathol. (2008) 30:383–98. doi: 10.1007/s00281-008-0134-3
52. Schwarz BA, B A. Trafficking from the bone marrow to the thymus : a prerequisite for thymopoiesis. Immunol Rev. (2006) 209:47–57. doi: 10.1111/j.0105-2896.2006.00350.x
53. Yang Q, Jeremiah Bell J, Bhandoola A. T-cell lineage determination. Immunol Rev. (2010) 238:12–22. doi: 10.1111/j.1600-065X.2010.00956.x
54. Zlotoff DA, Schwarz BA. The long road to the thymus : the generation , mobilization , and circulation of T-cell progenitors in mouse and man. Semin Immunopathol. (2008) 30:371–82. doi: 10.1007/s00281-008-0133-4
55. Krueger A, Willenzon S, Łyszkiewicz M, Kremmer E, Fo R. CC chemokine receptor 7 and 9 double-deficient hematopoietic progenitors are severely impaired in seeding the adult thymus. Blood. (2010) 115:1906–12. doi: 10.1182/blood-2009-07-235721
56. Zlotoff DA, Sambandam A, Logan TD, Bell JJ, Schwarz BA. CCR7 and CCR9 together recruit hematopoietic progenitors to the adult thymus. Blood. (2010) 115:1897–905. doi: 10.1182/blood-2009-08-237784
57. Cordes M, Canté-Barrett K, van den Akker EB, Moretti FA, Kiełbasa SM, Vloemans SA, et al. Single-cell immune profiling reveals thymus-seeding populations, T cell commitment, and multilineage development in the human thymus. Sci Immunol. (2022) 7:1–17. doi: 10.1126/sciimmunol.ade0182
58. Zietara N, Łyszkiewicz M, Puchałka J, Witzlau K, Reinhardt A, Förster R, et al. Multicongenic fate mapping quantification of dynamics of thymus colonization. J Exp Med. (2015) 212:1589–601. doi: 10.1084/jem.20142143
59. Krueger A, Ziętara N, Łyszkiewicz M. T cell development by the numbers. Trends Immunol. (2017) 38:128–39. doi: 10.1016/j.it.2016.10.007
60. Krueger A, Garbe AI, Von Boehmer H. Phenotypic plasticity of T cell progenitors upon exposure to Notch ligands. J Exp Med. (2006) 203:1977–84. doi: 10.1084/jem.20060731
61. Schwarz BA, Bhandoola A. Circulating hematopoietic progenitors with T lineage potential. Nat Immunol. (2004) 5:953–60. doi: 10.1038/ni1101
62. Sommerkamp P, Romero-Mulero MC, Narr A, Ladel L, Hustin L, Schönberger K, et al. Mouse multipotent progenitor 5 cells are located at the interphase between hematopoietic stem and progenitor cells. Blood. (2021) 137:3218–24. doi: 10.1182/blood.2020007876
63. Challen GA, Pietras EM, Wallscheid NC, Signer RAJ. Simplified murine multipotent progenitor isolation scheme: Establishing a consensus approach for multipotent progenitor identification. Exp Hematol. (2021) 104:55–63. doi: 10.1016/j.exphem.2021.09.007
64. Bhandoola A, von Boehmer H, Petrie HT, Zúñiga-Pflücker JC. Commitment and developmental potential of extrathymic and intrathymic T cell precursors: plenty to choose from. Immunity. (2007) 26:678–89. doi: 10.1016/j.immuni.2007.05.009
65. Sultana DA, Bell JJ, Zlotoff DA, Obaldia MEDe, Bhandoola A. Seminars in Immunology Eliciting the T cell fate with Notch. Semin Immunol. (2010) 22:254–60. doi: 10.1016/j.smim.2010.04.011
66. Schlenner SM, Rodewald HR. Early T cell development and the pitfalls of potential. Trends Immunol. (2010) 31:303–10. doi: 10.1016/j.it.2010.06.002
67. Fujimoto S, Ikawa T, Kina T, Yokota Y. Forced expression of Id2 in fetal thymic T cell progenitors allows some of their progeny to adopt NK cell fate. Int Immunol. (2007) 19:1175–82. doi: 10.1093/intimm/dxm085
68. Lavaert M, Liang KL, Vandamme N, Park JE, Roels J, Kowalczyk MS, et al. Integrated scRNA-seq identifies human postnatal thymus seeding progenitors and regulatory dynamics of differentiating immature thymocytes. Immunity. (2020) 52:1088–1104.e6. doi: 10.1016/j.immuni.2020.03.019
69. Igarashi H, Gregory SC, Yokota T, Sakaguchi N, Kincade PW. Transcription from the RAG1 locus marks the earliest lymphocyte progenitors in bone marrow. Immunity. (2002) 17:117–30. doi: 10.1016/S1074-7613(02)00366-7
70. Allman D, Sambandam A, Kim S, Miller JP, Pagan A, Well D, et al. Thymopoiesis independent of common lymphoid progenitors. Nat Immunol. (2003) 4:168–74. doi: 10.1038/ni878
71. Łyszkiewicz M, Zie¸ tN, Föhse L, Puchałka J, Diestelhorst J, Witzlau K, et al. Limited niche availability suppresses murine intrathymic dendritic-cell development from noncommitted progenitors. Blood. (2015) 125:457–64. doi: 10.1182/blood-2014-07-592667
72. Chen ELY, Thompson PK, Zúñiga-pflücker JC. RBPJ-dependent Notch signaling initiates the T cell program in a subset of thymus-seeding progenitors. Nat Immunol. (2019) 20:1456–68. doi: 10.1038/s41590-019-0518-7
73. Elsaid R, Meunier S, Burlen-Defranoux O, Soares-da-Silva F, Perchet T, Iturri L, et al. A wave of bipotent T/ILC-restricted progenitors shapes the embryonic thymus microenvironment in a time-dependent manner. Blood. (2021) 137:1024–36. doi: 10.1182/blood.2020006779
74. Krishnamoorthy V, Carr T, Pooter RF, Akinola EO, Gounari F, Kee BL. Repression of ccr9 transcription in mouse T lymphocyte progenitors by the notch signaling pathway. J Immunol. (2015) 194:3191–200. doi: 10.4049/jimmunol.1402443
75. Benz C, Bleul CC. A multipotent precursor in the thymus maps to the branching point of the T versus B lineage decision. J Exp Med. (2005) 202:21–31. doi: 10.1084/jem.20050146
76. Kingston R, Jenkinson EJ, Owen JJT. A single cell can recolonize and embryonic thymus, producing phenotypically distinct T-cell populations. Nature. (1985) 317:811–3. doi: 10.1038/317811a0
77. Martín-Gayo E, González-García S, García-León MJ, Murcia-Ceballos A, Alcain J, García-Peydró M, et al. Spatially restricted JAG1-Notch signaling in human thymus provides suitable DC developmental niches. J Exp Med. (2017) 214:3361–79. doi: 10.1084/jem.20161564
78. Orkin SH, Zon LI. Hematopoiesis: an evolving paradigm for stem cell biology. Cell. (2008) 132:631–44. doi: 10.1016/j.cell.2008.01.025
79. Ginhoux F, Greter M, Leboeuf M, Nandi S, See P, Gokhan S, et al. Fate mapping analysis reveals that adult microglia derive from primitive macrophages. Sci (80- ). (2010) 701:841–5. doi: 10.1126/science.1194637
80. Love PE, Bhandoola A. Signal integration and crosstalk during thymocyte migration and emigration. Nat Rev Immunol. (2011) 11:469–77. doi: 10.1038/nri2989
81. Yong-Rui Z, Andreas HK, Masahiko K, Ichiro T, Dan RL. Function of the chemokine receptor CXCR4 in heaematopolesis and in cerebellar development. Nature. (1998) 393:595–9. doi: 10.1038/31269
82. Wurbel MA, Malissen M, Guy-Grand D, Mettre E, Nussenzweig MC, Richelme M, et al. Mice lacking the CCR9 CC-chemokine receptor show a mild impairment of early T- and B-cell development and a reduction in T-cell receptor γδ+ gut intraepithelial lymphocytes. Blood. (2001) 98:2626–32. doi: 10.1182/blood.V98.9.2626
83. Rossi FMV, Corbel SY, Merzaban JS, Carlow DA, Gossens K, Duenas J, et al. Recruitment of adult thymic progenitors is regulated by P-selectin and its ligand PSGL-1. Nat Immunol. (2005) 6:626–34. doi: 10.1038/ni1203
84. De Obaldia ME, Bell JJ, Bhandoola A. Early T-cell progenitors are the major granulocyte precursors in the adult mouse thymus. Blood. (2013) 121:64–71. doi: 10.1182/blood-2012-08-451773
85. Schlenner SM, Madan V, Busch K, Tietz A, Läufle C, Costa C, et al. Fate mapping reveals separate origins of T cells and myeloid lineages in the thymus. Immunity. (2010) 32:426–36. doi: 10.1016/j.immuni.2010.03.005
86. Manz MG, Traver D, Miyamoto T, Weissman IL, Akashi K. Dendritic cell potentials of early lymphoid and myeloid progenitors. Blood. (2001) 97:3333–41. doi: 10.1182/blood.V97.11.3333
87. Wu L, D’Amico A, Hochrein H, O’Keeffe M, Shortman K, Lucas K. Development of thymic and splenic dendritic cell populations from different hemopoietic precursors. Blood. (2001) 98:3376–82. doi: 10.1182/blood.V98.12.3376
88. Luc S, Luis TC, Boukarabila H, Macaulay IC, Buza- N. The earliest thymic T cell progenitors sustain B cell and myeloid lineage potentials. Nat Immunol. (2012) 13:412–9. doi: 10.1038/ni.2255
89. Vosshenrich CAJ, García-Ojeda ME, Samson-Villéger SI, Pasqualetto V, Enault L, Goff ORLe, et al. A thymic pathway of mouse natural killer cell development characterized by expression of GATA-3 and CD127. Nat Immunol. (2006) 7:1217–24. doi: 10.1038/ni1395
90. Hong C, Luckey MA, Park JH. Intrathymic IL-7: The where, when, and why of IL-7 signaling during T cell development. Semin Immunol. (2012) 24:151–8. doi: 10.1016/j.smim.2012.02.002
91. Irla M. Instructive cues of thymic T cell selection. Annu Rev Immunol. (2022) 40:95–119. doi: 10.1146/annurev-immunol-101320-022432
92. Zúñiga-Pflücker JC. T-cell development made simple. Nat Rev Immunol. (2004) 4:67–72. doi: 10.1038/nri1257
93. Misslitz A, Pabst O, Hintzen G, Ohl L, Kremmer E, Petrie HT, et al. Thymic T cell development and progenitor localization depend on CCR7. J Exp Med. (2004) 200:481–91. doi: 10.1084/jem.20040383
94. Lind EF, Prockop SE, Porritt HE, Petrie HT. Mapping precursor movement through the postnatal thymus reveals specific microenvironments supporting defined stages of early lymphoid development. J Exp Med. (2001) 194:127–34. doi: 10.1084/jem.194.2.127
95. Lee W, Lee GR. Transcriptional regulation and development of regulatory T cells. Exp Mol Med. (2018) 50:e456–10. doi: 10.1038/emm.2017.313
96. Tong Q, Yao L, Su M, Yang YG, Sun L. Thymocyte migration and emigration. Immunol Lett. (2024) 267:106861. doi: 10.1016/j.imlet.2024.106861
97. Hosokawa H, Rothenberg EV. How transcription factors drive choice of the T cell fate. Nat Rev Immunol. (2021) 21:162–76. doi: 10.1038/s41577-020-00426-6
98. Yui MA, Feng N, Rothenberg EV. Fine-scale staging of T cell lineage commitment in adult mouse thymus. J Immunol. (2010) 185:284–93. doi: 10.4049/jimmunol.1000679
99. Rothenberg EV, Moore JE, Yui MA. Launching the T-cell-lineage developmental programme. Nat Rev Immunol. (2008) 8:9–21. doi: 10.1038/nri2232
100. Laurent J, Bosco N, Marche PN, Ceredig R. New insights into the proliferation and differentiation of early mouse thymocytes. Int Immunol. (2004) 16:1069–80. doi: 10.1093/intimm/dxh108
101. Dutta A, Zhao B, Love PE. New insights into TCR β-selection. Trends Immunol. (2021) 42:735–50. doi: 10.1016/j.it.2021.06.005
102. Kochl R, Vanes L, Sopena ML, Chakravarty P, Hartweger H, Fountain K, et al. Critical role of WNK1 in MYC-dependent early mouse thymocyte development. Elife. (2020) 9:1–32. doi: 10.7554/eLife.56934
103. Ruan Q, Kameswaran V, Tone Y, Li L, Liou HC, Greene MI, et al. Development of Foxp3+ Regulatory T cells is driven by the c-rel enhanceosome. Immunity. (2009) 31:932–40. doi: 10.1016/j.immuni.2009.10.006
104. Hu Y, Hu Q, Li Y, Lu L, Xiang Z, Yin Z, et al. γδ T cells: origin and fate, subsets, diseases and immunotherapy. Signal Transduct Target Ther. (2023) 8. doi: 10.1038/s41392-023-01653-8
105. Taghon T, Yui MA, Pant R, Diamond RA, Rothenberg EV. Developmental and molecular characterization of emerging β- and γδ-selected pre-T cells in the adult mouse thymus. Immunity. (2006) 24:53–64. doi: 10.1016/j.immuni.2005.11.012
106. Roels J, Kuchmiy A, De Decker M, Strubbe S, Lavaert M, Liang KL, et al. Distinct and temporary-restricted epigenetic mechanisms regulate human αβ and γδ T cell development. Nat Immunol. (2020) 21:1280–92. doi: 10.1038/s41590-020-0747-9
107. Sagar, Pokrovskii M, Herman JS, Naik S, Sock E, Zeis P, et al. Deciphering the regulatory landscape of fetal and adult γδ T-cell development at single-cell resolution. EMBO J. (2020) 39:1–20. doi: 10.15252/embj.2019104159
108. Spidale NA, Sylvia K, Narayan K, Miu B, Frascoli M, Melichar HJ, et al. Interleukin-17-producing γδ T cells originate from SOX13+ Progenitors that are independent of γδTCR signaling. Immunity. (2018) 49:857–872.e5. doi: 10.1016/j.immuni.2018.09.010
109. Melichar HJ, Narayan K, Der SO, Hiraoka Y, Gardiol N, Jeannet G, et al. Regulation of γδ versus αβ T lymphocyte differentiation by the transcription factor SOX13. Sci (80- ). (2007) 315:230–3. doi: 10.1126/science.1135344
110. Sumaria N, Martin S, Pennington DJ. Developmental origins of murine γδ T-cell subsets. Immunology. (2019) 156:299–304. doi: 10.1111/imm.13032
111. Xi H, Schwartz R, Engel I, Murre C, Kersh GJ. Interplay between RORγt, Egr3, and E proteins controls proliferation in response to pre-TCR signals. Immunity. (2006) 24:813–26. doi: 10.1016/j.immuni.2006.03.023
112. Hernandez JB, Newton RH, Walsh CM. Life and death in the thymus – cell death signaling during T cell development. Curr Opin Cell Biol. (2011) 22:865–71. doi: 10.1016/j.ceb.2010.08.003
113. Weinreich MA, Hogquist KA. Thymic emigration: when and how T cells leave home. J Immunol. (2008) 181:2265–70. doi: 10.4049/jimmunol.181.4.2265
114. Perera J, Meng L, Meng F, Huang H. Autoreactive thymic B cells are efficient antigen-presenting cells of cognate self-antigens for T cell negative selection. Proc Natl Acad Sci U S A. (2013) 110:17011–6. doi: 10.1073/pnas.1313001110
115. Klein L, Kyewski B, Allen PM, Hogquist KA. Positive and negative selection of the T cell repertoire: What thymocytes see (and don’t see). Nat Rev Immunol. (2014) 14:377–91. doi: 10.1038/nri3667
116. Marrack P, Kappler J. Positive selection of thymocytes bearing αβ T cell receptors. Curr Opin Immunol. (1997) 9:250–5. doi: 10.1016/S0952-7915(97)80144-6
117. Wang H, Zúñiga-Pflücker JC. Thymic microenvironment: interactions between innate immune cells and developing thymocytes. Front Immunol. (2022) 13:1–9. doi: 10.3389/fimmu.2022.885280
118. Kim S, Park GY, Park JS, Park J, Hong H, Lee Y. Regulation of positive and negative selection and TCR signaling during thymic T cell development by capicua. Elife. (2021) 10:1–25. doi: 10.7554/eLife.71769
119. Oh-Hora M, Komatsu N, Pishyareh M, Feske S, Hori S, Taniguchi M, et al. Agonist-selected T cell development requires strong T cell receptor signaling and store-operated calcium entry. Immunity. (2013) 38:881–95. doi: 10.1016/j.immuni.2013.02.008
120. Sakaguchi S, Sakaguchi N, Asano M, Itoh M, Toda M. Immunologic self-tolerance maintained by activated T cells expressing IL-2 receptor alpha-chains (CD25). Breakdown of a single mechanism of self-tolerance causes various autoimmune diseases. J Immunol. (1995) 155:1151–64. doi: 10.4049/jimmunol.155.3.1151
121. Owen DL, Sjaastad LE, Farrar MA. Regulatory T cell development in the thymus. J Immunol. (2019) 203:2031–41. doi: 10.4049/jimmunol.1900662
122. Lio CWJ, Hsieh CS. A two-step process for thymic regulatory T cell development. Immunity. (2008) 28:100–11. doi: 10.1016/j.immuni.2007.11.021
123. Chen WJ, Jin W, Hardegen N, Lei KJ, Li L, Marinos N, et al. Conversion of peripheral CD4+CD25- naive T cells to CD4+CD25+ Regulatory T cells by TGF-β Induction of transcription factor Foxp3. J Exp Med. (2003) 198:1875–86. doi: 10.1084/jem.20030152
124. Hori S, Nomura T, Sakaguchi S. Control of regulatory T cell development by the transcription factor Foxp3. Sci (80- ). (2003) 299:1057–61. doi: 10.1126/science.1079490
125. Fontenot JD, Gavin MA, Rudensky AY. Foxp3 programs the development and function of CD4+CD25+ regulatory T cells. Nat Immunol. (2003) 4:330–6. doi: 10.1038/ni904
126. d’Hennezel E, Ben-Shoshan M, Ochs HD, Torgerson TR, Russell LJ, Lejtenyi C, et al. FOXP3 forkhead domain mutation and regulatory T cells in the IPEX syndrome. N Engl J Med. (2009) 361:1710–3. doi: 10.1056/NEJMc0907093
127. Goldstein AL, Asanuma Y, White A. (1969). The thymus as an endocrine gland: properties of thymosin, a new thymus hormone. Recent Prog Horm Res. 26:505–38. doi: 10.1016/B978-0-12-571126-5.50016-9
128. Savino W, Mendes-da-cruz DA, Lepletier A, Dardenne M. Hormonal control of T-cell development in health and disease. Nat Rev Endocrinol. (2016) 12:77–89. doi: 10.1038/nrendo.2015.168
129. Mignini F, Sabbatini M, Mattioli L, Cosenza M, Artico M, Cavallotti C. Neuro-immune modulation of the thymus microenvironment. Int J Mol Med. (2014) 33:1392–400. doi: 10.3892/ijmm.2014.1709
130. Haddad JJ, Saadé NE, Safieh-Garabedian B. Thymulin: An emerging anti-inflammatory molecule. Curr Med Chem Anti-inflamm Anti-Allergy Agents. (2005) 4:333–8. doi: 10.2174/1568014054065195
131. Goldstein G. The isolation of thymopoietin (Thymin). Ann N Y Acad Sci. (1975) 249:177–85. doi: 10.1111/j.1749-6632.1975.tb29067.x
132. Rebar RW, Miyake A, Low TL, Goldstein AL. Thymosin stimulates secretion of luteinizing hormone-releasing factor. Science. (1981) 214:669–71. doi: 10.1126/science.7027442
133. Rezzani R, Franco C, Hardeland R, Rodella LF. Thymus-pineal gland axis: Revisiting its role in human life and ageing. Int J Mol Sci. (2020) 21:1–40. doi: 10.3390/ijms21228806
134. Liu Z, Wang J, Wang E. Direct binding of thymopentin to surface class II Major histocompatibility complex in living cells. J Phys Chem B. (2010) 114:638–42. doi: 10.1021/jp909017j
135. King R, Tuthill C. Immune modulation with thymosin alpha 1 treatment. Vitamins and Hormones. (2016) 102:151–78. doi: 10.1016/bs.vh.2016.04.003
136. Lunin SM, Khrenov MO, Novoselova TV, Parfenyuk SB, Glushkova OV, Fesenko EE, et al. Modulation of inflammatory response in mice with severe autoimmune disease by thymic peptide thymulin and an inhibitor of NF-kappaB signalling. Int Immunopharmacol. (2015) 25:260–6. doi: 10.1016/j.intimp.2015.01.021
137. Savino W. Intrathymic T cell migration is a multivectorial process under a complex neuroendocrine control. Neuroinmmunomodulation. (2010) 17:142–5. doi: 10.1159/000258708
138. Cepeda S, Griffith AV. Thymic stromal cells: Roles in atrophy and age-associated dysfunction of the thymus. Exp Gerontol. (2018) 105:113–7. doi: 10.3892/ijmm.2014.1709
139. Dixit VD, Smith RG, Taub DD, Dixit VD, Yang H, Sun Y, et al. Ghrelin promotes thymopoiesis during aging. J Clin Invest. (2007) 117:2778–90. doi: 10.1172/JCI30248
140. Savino W, Smaniotto S, Ar D. Growth hormone modulates migration of thymocytes and peripheral T cells. New York Acad Sci. (2012) 1261:49–54. doi: 10.1111/j.1749-6632.2012.06637.x
141. Carreño PC, Jiménez E, Sacedón R, Vicente AGZ Ángeles. Prolactin stimulates maturation and function of rat thymic dendritic cells. J Neuroimmunol. (2004) 153:83–90. doi: 10.1016/j.jneuroim.2004.04.020
142. Talabe G, Boldizsa F, Bartis D. Developmental shift in TcR-mediated rescue of thymocytes from glucocorticoid-induced apoptosis. Immunobiology. (2008) 213:39–50. doi: 10.1016/j.imbio.2007.06.004
143. Vargas S, Salama C, Renovato-martins M, Helal-neto E, Citelli M, Savino W, et al. Increased leptin response and inhibition of apoptosis in thymocytes of young rats offspring from protein deprived dams during lactation. PLoS One. (2013) 8:1–12. doi: 10.1371/journal.pone.0064220
144. Hansenne I, Rasier G, Pe C, Brilot F, Renard C, Breton C. Ontogenesis and functional aspects of oxytocin and vasopressin gene expression in the thymus network. J Neuroimmunol. (2005) 158:67–75. doi: 10.1016/j.jneuroim.2004.08.007
145. Velardi E, Tsai JJ, Holland AM, Wertheimer T, Yu VWC, Zakrzewski JL, et al. Sex steroid blockade enhances thymopoiesis by modulating Notch signaling. J Exp Med. (2014) 211:2341–9. doi: 10.1084/jem.20131289
146. Taub DD, Murphy WJ, Longo DL. Rejuvenation of the aging thymus : growth hormone-mediated and ghrelin-mediated signaling pathways. Curr Opin Pharmacol. (2010) 10:408–24. doi: 10.1016/j.coph.2010.04.015
147. Lins MP. Thymic extracellular matrix in the thymopoiesis : just a supporting? Biotech. (2022) 11:1–6. doi: 10.3390/biotech11030027
148. Asnaghi MA, Barthlott T, Gullotta F, Strusi V, Amovilli A, Hafen K, et al. Thymus extracellular matrix-derived scaffolds support graft-resident thymopoiesis and long-term in vitro culture of adult thymic epithelial cells. Adv Funct Mater. (2021) 31:2010747. doi: 10.1002/adfm.202010747
149. Hun M, Barsanti M, Wong K, Ramshaw J, Werkmeister J, Chidgey AP. Biomaterials Native thymic extracellular matrix improves in vivo thymic organoid T cell output , and drives in vitro thymic epithelial cell differentiation. Biomaterials. (2017) 118:1–15. doi: 10.1016/j.biomaterials.2016.11.054
150. Berrih S, Savino W, Cohen S. Extracellular matrix of the human thymus: Immunofluorescence studies on frozen sections and cultured epithelial cells. J Histochem Cytochem. (1985) 33:655–64. doi: 10.1177/33.7.3891843
151. Savino W, Villa-verde DMS, Lannes-vieira J. Extracellular matrix proteins in intrathymic T-cell migration and differentiation? Trends Immunol. (1993) 4):2–5. doi: 10.1016/0167-5699(93)90278-S
152. Savino W, Mendes-da-cruz DA, Silva S, Dardenne M, Cotta-de-almeida V. Intrathymic T-cell migration : a combinatorial interplay of extracellular matrix and chemokines? Trends Immunol. (2002) 23:305–13. doi: 10.1016/S1471-4906(02)02224-X
153. Brandt K, Mueller MS, Sadowski T, Brauer R, Beck IM. MMP19 is essential for T cell development and T cell- mediated cutaneous immune responses. PloS One. (2008) 3. doi: 10.1371/journal.pone.0002343
154. Odaka C, Tanioka M, Itoh T. Matrix metalloproteinase-9 in macrophages induces thymic neovascularization following thymocyte apoptosis. J Immunol. (2005) 174:846–53. doi: 10.4049/jimmunol.174.2.846
155. Lima ACD, Francelin C, Ferrucci DL, Stach-MaChado DR, Verinaud L. Thymic alterations induced by plasmodium berghei: Expression of matrix metalloproteinases and their tissue inhibitors. Cell Immunol. (2012) 279:53–9. doi: 10.1016/j.cellimm.2012.09.006
156. Cardarelli PM, Pierschbacher MD. T-lymphocyte differentiation and the extracellular matrix : Identification of a thymocyte subset that attaches specifically to fibronectin. Proc Natl Acad Sci. (1986) 83:2647–51. doi: 10.1073/pnas.83.8.2647
157. Chigaev A, Sklar LA. Aspects of VLA-4 and LFA-1 regulation that may contribute to rolling and firm adhesion. Front Immunol. (2012) 3:1–9. doi: 10.3389/fimmu.2012.00242
158. Savino W, Mendes-da-cruz DA, Cristina D, Golbert F, Fletcher AL. Laminin-mediated interactions in thymocyte migration and development. Front Immunol. (2015) 6. doi: 10.3389/fimmu.2015.00579
159. Mittelstadt PR, Taves MD, Ashwell JD. Glucocorticoids oppose thymocyte negative selection by inhibiting helios and Nur77. J Immunol. (2019) 203:2163–70. doi: 10.4049/jimmunol.1900559
160. Cifone MG, Migliorati G, Parroni R, Marchetti C, Millimaggi D, Santoni A, et al. Dexamethasone-induced thymocyte apoptosis: Apoptotic signal involves the sequential activation of phosphoinositide-specific phospholipase C, acidic sphingomyelinase, and caspases. Blood. (1999) 93:2282–96. doi: 10.1182/blood.V93.7.2282
161. Distelhorst CW. Recent insights into the mechanism of glucocorticosteroid-induced apoptosis. Cell Death Differ. (2002) 9:6–19. doi: 10.1038/sj.cdd.4400969
162. Lannes-Vieria J, Dardenne M, S W. Extracellular matrix components of the mouse thymus microenvironment: ontogenetic studies and modulation by glucocorticoid hormones. J Histochem Cytochem. (1991) 39:1539–46. doi: 10.1177/39.11.1918928
163. Martinez RJ, Breed ER, Worota Y, Ashby KM, Vobořil Matouš, Mathes T, et al. Type III interferon drives thymic B cell activation and regulatory T cell generation. Proc Natl Acad Sci. (2017) 120:2017. doi: 10.1073/pnas.2216830120
164. Kim HJ, Alonzo ES, Dorothee G, Pollard JW, Sant’Angelo DB. Selective depletion of eosinophils or neutrophils in mice impacts the efficiency of apoptotic cell clearance in the thymus. PLoS One. (2010) 5:1–15. doi: 10.1371/journal.pone.0011439
165. Cupedo T. ILC2: at home in the thymus. Eur J Immunol. (2018) 48:1441–4. doi: 10.1002/eji.201847779
166. Wang HC, Qian L, Zhao Y, Mengarelli J, Adrianto I, Montgomery CG, et al. Downregulation of E protein activity augments an ILC2 differentiation program in the thymus. J Immunol. (2017) 198:3149–56. doi: 10.4049/jimmunol.1602009
167. Jones R, Cosway EJ, Willis C, White AJ, Jenkinson WE, Fehling HJ, et al. Dynamic changes in intrathymic ILC populations during murine neonatal development. Eur J Immunol. (2018) 48:1481–91. doi: 10.1002/eji.201847511
168. Shin SB, McNagny KM. ILC-you in the thymus: A fresh look at innate lymphoid cell development. Front Immunol. (2021) 12:1–12. doi: 10.3389/fimmu.2021.681110
169. Vobo M, Brabec T, Dobeš J. A model of preferential pairing between epithelial and dendritic cells in thymic antigen transfer. Elife. (2022), 1–19. doi: 10.7554/eLife.71578
170. Breed ER, Vobořil M, Ashby KM, Martinez RJ, Qian L, Wang H, et al. Type 2 cytokines in the thymus activate Sirpα+ dendritic cells to promote clonal deletion. Nat Immunol. (2022) 23:1042–51. doi: 10.1038/s41590-022-01218-x
171. Tacke R, Hilgendorf I, Garner H, Waterborg C, Park K, Nowyhed H, et al. The transcription factor NR4A1 is essential for the development of a novel macrophage subset in the thymus. Sci Rep. (2015) 5:1–13. doi: 10.1038/srep10055
172. Kurd NS, Lutes LK, Yoon J, Chan SW, Dzhagalov IL, Hoover AR, et al. A role for phagocytosis in inducing cell death during thymocyte negative selection. Elife. (2019), 1–18. doi: 10.7554/eLife.48097
173. Daley SR, Teh C, Hu DY, Strasser A, Gray DHD. Cell death and thymic tolerance. Immunol Rev. (2017) 277:9–20. doi: 10.1111/imr.12532
174. Pozzesi N, Fierabracci A, Liberati AM, Martelli MP, Ayroldi E, Riccardi C, et al. Role of caspase-8 in thymus function. Cell Death Differ. (2014) 21:226–33. doi: 10.1038/cdd.2013.166
175. Zhang N, Hartig H, Dzhagalov I, Draper D, He YW. The role of apoptosis in the development and function of T lymphocytes. Cell Res. (2005) 15:749–69. doi: 10.1038/sj.cr.7290345
176. Hernandez JB, Newton RH, Walsh CM. Life and death in the thymus-cell death signaling during T cell development. Curr Opin Cell Biol. (2010) 22:865–71. doi: 10.1016/j.ceb.2010.08.003
177. Ma A, Pena JC, Chang B, Margosian E, Davidson L, Alt FW, et al. Bclx regulates the survival of double-positive thymocytes. Proc Natl Acad Sci U S A. (1995) 92:4763–7. doi: 10.1073/pnas.92.11.4763
178. Farias-de-Oliveira DA, Villa-Verde DMS, Nunes Panzenhagen PH, Silva dos Santos D, Berbert LR, Savino W, et al. Caspase-8 and caspase-9 mediate thymocyte apoptosis in Trypanosoma cruzi acutely infected mice. J Leukoc Biol. (2013) 93:227–34. doi: 10.1189/jlb.1211589
179. Trampont PC, Tosello-Trampont AC, Shen Y, Duley AK, Sutherland AE, Bender TP, et al. CXCR4 acts as a costimulator during thymic B-selection. Nat Immunol. (2010) 11:162–70. doi: 10.1038/ni.1830
180. Zhang X, Dong X, Wang H, Li J, Yang B, Zhang J, et al. FADD regulates thymocyte development at the β-selection checkpoint by modulating Notch signaling. Cell Death Dis. (2014) 5:1–11. doi: 10.1038/cddis.2014.198
181. Bouillet P, Purton JF, Godfrey DI, Zhang LC, Coultas L, Puthalakath H, et al. BH3-only Bcl-2 family member Bim is required for apoptosis of autoreactive thymocytes. Nature. (2002) 418:108. doi: 10.1038/nature00885
182. Labi V, Woess C, Tuzlak S, Erlacher M, Bouillet P, Strasser A, et al. Deregulated cell death and lymphocyte homeostasis cause premature lethality in mice lacking the BH3-only proteins Bim and Bmf. Blood. (2014) 123:2652–62. doi: 10.1182/blood-2013-11-537217
183. Mandal M, Crusio KM, Meng F, Liu S, Kinsella M, Clark MR, et al. Regulation of lymphocyte progenitor survival by the proapoptotic activities of Bim and Bid. Proc Natl Acad Sci U.S.A. (2008) 105:20840–5. doi: 10.1073/pnas.0807557106
184. Van Meerwijk JPM, Marguerat S, Lees RK, Germain RN, Fowlkes BJ, MacDonald HR. Quantitative impact of thymic clonal deletion on the T cell repertoire. J Exp Med. (1997) 185:377–83. doi: 10.1084/jem.185.3.377
185. Kondo M, Akashi K, Domen J, Sugamura K, Weissman IL. Bcl-2 rescues T lymphopoiesis, but not B or NK cell development, in common γ chain-deficient mice. Immunity. (1997) 7:155–62. doi: 10.1016/S1074-7613(00)80518-X
186. Daniels MA, Teixeiro E, Gill J, Hausmann B, Roubaty D, Holmberg K, et al. Thymic selection threshold defined by compartmentalization of Ras/MAPK signalling. Nature. (2006) 444:724–9. doi: 10.1038/nature05269
187. Gallo EM, Winslow MM, Canté-Barrett K, Radermacher AN, Ho L, McGinnis L, et al. Calcineurin sets the bandwidth for discrimination of signals during thymocyte development. Nature. (2007) 450:731–5. doi: 10.1038/nature06305
188. Dunkle A, Dzhagalov I, He YW. Mcl-1 promotes survival of thymocytes by inhibition of Bak in a pathway separate from Bcl-2. Cell Death Differ. (2010) 17:994–1002. doi: 10.1038/cdd.2009.201
189. Rathmell JC, Lindsten T, Zong WX, Cinalli RM, Thompson CB. Deficiency in Bak and Bax perturbs thymic selection and lymphoid homeostasis. Nat Immunol. (2002) 3:932–9. doi: 10.1038/ni834
190. Ren D, Tu HC, Kim H, Wang GX, Bean GR, Takeuchi O, et al. BID, BIM, and PUMA are essential for activation of the BAX- and BAK-dependent cell death program. Sci (80- ). (2010) 330:1390–3. doi: 10.1126/science.1190217
191. Newton K, Harris AW, Strasser A. FADD/MORT1 regulates the pre-TCR checkpoint and can function as a tumour suppressor. EMBO J. (2000) 19:931–41. doi: 10.1093/emboj/19.5.931
192. Webb LV, Barbarulo A, Huysentruyt J, Vanden Berghe T, Takahashi N, Ley S, et al. Survival of single positive thymocytes depends upon developmental control of RIPK1 kinase signaling by the IKK complex independent of NF-κB. Immunity. (2019) 50:348–361.e4. doi: 10.1016/j.immuni.2019.01.004
193. Huysentruyt J, Steels W, Perez MR, Verstraeten B, Vadi M, Divert T, et al. RIPK1 protects naive and regulatory T cells from TNFR1-induced apoptosis. Cell Death Differ. (2024) 31:820–32. doi: 10.1038/s41418-024-01301-w
194. Kishimoto H, Sprent J. A defect in central tolerance in NOD mice. Nat Immunol. (2001) 2:1025–31. doi: 10.1038/ni726
195. Huo J, Xu S, Guo K, Zeng Q, Lam KP. Genetic deletion of faim reveals its role in modulating c-FLIP expression during CD95-mediated apoptosis of lymphocytes and hepatocytes. Cell Death Differ. (2009) 16:1062–70. doi: 10.1038/cdd.2009.26
196. Leverrier S, Salvesen GS, Walsh CM. Enzymatically active single chain caspase-8 maintains T-cell survival during clonal expansion. Cell Death Differ. (2011) 18:90–8. doi: 10.1038/cdd.2010.69
197. Kishimoto H, Surh CD, Sprent J. A role for Fas in negative selection of thymocytes in vivo. J Exp Med. (1998) 187:1427–38. doi: 10.1084/jem.187.9.1427
198. Tovey Crutchfield EC, Garnish SE, Day J, Anderton H, Chiou S, Hempel A, et al. MLKL deficiency protects against low-grade, sterile inflammation in aged mice. Cell Death Differ. (2023) 30:1059–71. doi: 10.1038/s41418-023-01121-4
199. Hwang SM, Ha YJ, Koo GB, Noh HJ, Lee AY, Kim BJ, et al. LCK-mediated RIPK3 activation controls double-positive thymocyte proliferation and restrains thymic lymphoma by regulating the PP2A-ERK axis. Adv Sci. (2022) 9:1–16. doi: 10.1002/advs.202204522
200. Luo M, Xu L, Qian Z, Sun X. Infection-associated thymic atrophy. Front Immunol. (2021) 12:1–21. doi: 10.3389/fimmu.2021.652538
201. Barbouti A, Vasileiou PVS, Evangelou K, Vlasis KG, Papoudou-bai A, Gorgoulis VG. Implications of oxidative stress and cellular senescence in age-related thymus involution. Oxid Med Cell Longev. (2020). doi: 10.1155/2020/7986071
202. Di N, Ciriaco E, Pi PP. Age-related changes in the avian primary lymphoid organs (Thymus and bursa of fabricius). Microsc Res Tech. (2003) 487:482–7. doi: 10.1002/jemt.10416
203. Lam SH, Chua HL, Gong Z, Wen Z, Lam TJ, Sin YM. Morphologic transformation of the thymus in developing. Dev Dyn. (2002) 94:87–94. doi: 10.1002/dvdy.10127
204. Danilova N, Hohman VS, Sacher F, Ota T, Willett CE, Steiner LA. T cells and the thymus in developing zebrafish. Dev Comp Immunol. (2004) 28:755–67. doi: 10.1016/j.dci.2003.12.003
205. Plytycz B, Mika J, Bigaj J. Age-dependent changes in thymuses in the european common frog, rana temporaria. J Exp Zool. (1995) 460:451–60. doi: 10.1002/jez.1402730602
206. Steinmann GG, Klaus B, Müller-Hermelink H-K. The involution of the ageing human thymic epithelium is independent of puberty: A morphometric study. Scand J Immunol. (1985) 22:563–75. doi: 10.1111/j.1365-3083.1985.tb01916.x
207. Montecino-rodriguez E, Angeles L. Reduction in the developmental potential of intrathymic T cell progenitors with age. J Immunol. (2004) 173:245–50. doi: 10.4049/jimmunol.173.1.245
208. Arellano V, AORD O, Leal-noval SR, Molina-pinelo S, Andez ANAH, Vallejo A, et al. Thymic function-related markers within the thymus and peripheral blood : are they comparable? J Clin Immunol. (2006) 26:96–100. doi: 10.1007/s10875-006-7519-7
209. Zook EC, Krishack PA, Zhang S, Zeleznik-Le NJ, Firulli AB, Witte PL, et al. Overexpression of Foxn1 attenuates age-associated thymic involution and prevents the expansion of peripheral CD4 memory T cells. Blood. (2011) 118:5723–31. doi: 10.1182/blood-2011-03-342097
210. Gui J, Mustachio LM, Su DM, Craig RW. Thymus size and age-related thymic involution: Early programming, sexual dimorphism, progenitors and stroma. Aging Dis. (2012) 3:280–90.
211. Dixit VD. Thymic fatness and approaches to enhance thymopoietic fitness in aging. Curr Opin Immunol. (2010) 22:521–8. doi: 10.1016/j.coi.2010.06.010
212. Savino W. The Thymus Is a Common Target Organ in infectious diseases. PLoS Pathog. (2006) 2. doi: 10.1371/journal.ppat.0020062
213. Kousa AI, Jahn L, Zhao K, Flores AE, Acenas D II, Lederer E, Argyropoulos KV, et al. Age-related epithelial defects limit thymic function and regeneration. Nat Immunol. (2023). doi: 10.1038/s41590-024-01915-9
214. Baran-gale J, Morgan MD, Maio S, Dhalla F, Calvo-asensio I, Deadman ME, et al. Ageing compromises mouse thymus function and remodels epithelial cell differentiation. Elife. (2020), 1–27. doi: 10.7554/eLife.56221
215. Srinivasan J, Vasudev A, Shasha C, Selden HJ, Perez E, LaFleur B, et al. The initial age-associated decline in early T-cell progenitors reflects fewer pre-thymic progenitors and altered signals in the bone marrow and thymus microenvironments. Aging Cell. (2023) 22:1–15. doi: 10.1111/acel.13870
216. Ansari AR, Liu H. Acute thymic involution and mechanisms for recovery. Arch Immunol Ther Exp (Warsz). (2017) 65:401–20. doi: 10.1007/s00005-017-0462-x
217. Liu B, Zhang X, Deng W, Liu J, Li H, Wen M, et al. Severe influenza A(H1N1) pdm09 infection induces thymic atrophy through activating innate CD8+ CD44hi T cells by upregulating IFN-γ. Cell Death Dis. (2014) 5):1–12. doi: 10.1038/cddis.2014.323
218. Lepletier A, de Almeida L, Santos L, da Silva Sampaio L, Paredes B, González FB, et al. Early double-negative thymocyte export in trypanosoma cruzi infection is restricted by sphingosine receptors and associated with human chagas disease. PloS Negl Trop Dis. (2014) 8. doi: 10.1371/journal.pntd.0003203
219. Price P, Olver SD, Gibbons AE, Teo HK, Shellam GR. Characterization of thymic involution induced by murine cytomegalovirus infection. Immunol Cell Biol. (1993) 71:155–65. doi: 10.1038/icb.1993.18
220. Leyva-rangel JP, Ortiz-navarrete V. Bacterial clearance reverses a skewed T-cell repertoire induced by Salmonella infection. Immune Inflammatory Diseases. (2015) 3:209–23. doi: 10.1002/iid3.60
221. Majumdar S, Deobagkar-Lele M, Adiga V, Raghavan A, Wadhwa N, Ahmed SM, et al. Differential susceptibility and maturation of thymocyte subsets during Salmonella Typhimurium infection: Insights on the roles of glucocorticoids and Interferon-gamma. Sci Rep. (2017) 7:1–15. doi: 10.1038/srep40793
222. Ross EA, Coughlan RE, Flores-Langarica A, Lax S, Nicholson J, Desanti GE, et al. Thymic function is maintained during salmonella -induced atrophy and recovery. J Immunol. (2012) 189:4266–74. doi: 10.4049/jimmunol.1200070
223. Meis J, Panzenhagen PHN, Maran N, Villa-verde MS, Morrot A, Savino W. Thymus atrophy and double-positive escape are common features in infectious diseases. J Parasitol Res. (2012). doi: 10.1155/2012/574020
224. Hospital D, Key B, Hospital T. Sepsis - induced thymic atrophy is associated with defects in early lymphopoiesis. Stem Cells. (2016) 34:2902–15. doi: 10.1002/stem.2464
225. Xu L, Wei C, Chen Y, Wu Y, Shou X, Chen W, et al. IL-33 induces thymic involution-associated naive T cell aging and impairs host control of severe infection. Nat Commun. (2022) 13:1–17. doi: 10.1038/s41467-022-34660-4
226. Granadier D, Cooper K, Kinsella S, Evandy C, Iovino L, DeRoos P, et al. Interleukin 18 suppresses regeneration of the thymus. Blood. (2022) 140:1169–70. doi: 10.1182/blood-2022-168432
227. Liu B, Zhang W, Deng W, Liu J, Li H, Wen M, et al. Severe influenza A(H1N1)pdm09 infection induces thymic atrophy through activating innate CD8+CD44hi T cells by upregulating IFN-c. Cell Death Dis. (2014) 5:1–12. doi: 10.1038/cddis.2014.323
228. Savino W, Dardenne M, Velloso LA, Silva-barbosa SD. The thymus is a common target in malnutrition and infection. Br J Nutr. (2007) 98. doi: 10.1017/S0007114507832880
229. Nabukeera-Barungi N, Lanyero B, Grenov B, Friis H, Namusoke H, Mupere E, et al. Thymus size and its correlates among children admitted with severe acute malnutrition: a cross-sectional study in Uganda. BMC Pediatr. (2021) 21:1–7. doi: 10.1186/s12887-020-02457-3
230. Savino W. The thymus gland is a target in malnutrition. Eur J Clin Nutr. (2002) 56:S46–9. doi: 10.1038/sj.ejcn.1601485
231. Losada-barragán M, Umaña-pérez A, Durães J, Cuervo-escobar S, Rodríguez-vega A, Ribeiro-gomes FL, et al. Thymic microenvironment is modified by malnutrition and leishmania infantum infection. Front Cell Infect Microbiol. (2019) 9:1–19. doi: 10.3389/fcimb.2019.00252
232. Choyke PL, Zeman RK, Gootenberg JE, Greenberg JN, Hoffer F, Frank JA. Thymic atrophy and regrowth in response to chemotherapy: CT evaluation. Am J Roentgenol. (1987) 149:269–72. doi: 10.2214/ajr.149.2.269
233. Qiu L, Zhao Y, Yang Y, Huang H, Cai Z, He J. Thymic rebound hyperplasia post-chemotherapy mistaken as disease progression in a patient with lymphoma involving mediastinum: a case report and reflection. BMC Surg. (2021) 21:1–6. doi: 10.1186/s12893-021-01048-y
234. Ito R, Hale LP, Geyer SM, Li J, Sornborger A, Kajimura J, et al. Late effects of exposure to ionizing radiation and age on human thymus morphology and function. Radiat Res. (2017) 187:589–98. doi: 10.1667/RR4554.1
235. Laan M, Haljasorg U, Kisand K, Salumets A, Peterson P. Pregnancy-induced thymic involution is associated with suppression of chemokines essential for T-lymphoid progenitor homing. Eur J Immunol. (2016) 46:2008–17. doi: 10.1002/eji.201646309
236. Ruitenberg EJ, Buys J. Thymus atrophy during early pregnancy and its effect on a trichinella spiralis infection in mice, including intestinal pathology and blood eosinophilia. Vet Immunol Immunopathol. (1980) 1:199–214. doi: 10.1016/0165-2427(80)90022-7
237. Zoller AL, Schnell FJ, Kersh GJ. Murine pregnancy leads to reduced proliferation of maternal thymocytes and decreased thymic emigration. Immunology. (2007) 121:207–15. doi: 10.1111/j.1365-2567.2006.02559.x
238. Swami S, Tong I, Bilodeau CC, Bourjeily G. Thymic involution in pregnancy: a universal finding? Obstet Med. (2012) 5:130–2. doi: 10.1258/om.2011.110077
239. Taves MD, Ashwell JD. Effects of sex steroids on thymic epithelium and thymocyte development. Front Immunol. (2022) 13:1–9. doi: 10.3389/fimmu.2022.975858
240. Tibbetts TA, DeMayo F, Rich S, Conneely OM, O’Malley BW. Progesterone receptors in the thymus are required for thymic involution during pregnancy and for normal fertility. Proc Natl Acad Sci U.S.A. (1999) 96:12021–6. doi: 10.1073/pnas.96.21.12021
241. Anderson AC, Acharya N. Steroid hormone regulation of immune responses in cancer. Immunometabolism (United States). (2022) 4:E00012. doi: 10.1097/IN9.0000000000000012
242. Gray DHD, Seach N, Ueno T, Milton MK, Liston A, Lew AM, et al. Developmental kinetics, turnover, and stimulatory capacity of thymic epithelial cells. Blood. (2006) 108:3777–85. doi: 10.1182/blood-2006-02-004531
243. Ahn SH, Nguyen SL, Kim TH, Jeong JW, Arora R, Lydon JP, et al. Nuclear progesterone receptor expressed by the cortical thymic epithelial cells dictates thymus involution in murine pregnancy. Front Endocrinol (Lausanne). (2022) 13:1–13. doi: 10.3389/fendo.2022.846226
244. Duan X, Lu J, Zhou K, Wang J, Wu J, Gao GF, et al. NK-cells are involved in thymic atrophy induced by influenza A virus infection. J Gen Virol. (2015) 96:3223–35. doi: 10.1099/jgv.0.000276
245. Huang H, Liu A, Wu H, Ansari AR, Wang J, Huang X, et al. Transcriptome analysis indicated that Salmonella lipopolysaccharide-induced thymocyte death and thymic atrophy were related to TLR4-FOS/JUN pathway in chicks. BMC Genomics. (2016) 17:1–11. doi: 10.1186/s12864-016-2674-6
246. Carbajosa S, Gea S, Chillón-marinas C, Poveda C, Maza C, Fresno M, et al. Altered bone marrow lymphopoiesis and interleukin-6- dependent inhibition of thymocyte differentiation contribute to thymic atrophy during Trypanosoma cruzi infection. Oncotarget. (2017) 8:17551–61. doi: 10.18632/oncotarget.v8i11
247. Linhares-lacerda L, Palu CC, Ribeiro-Alves M, Díaz-Paredes B, Morrot A, Garcia-Silva MR, et al. Differential expression of microRNAs in thymic epithelial cells from Trypanosoma cruzi acutely infected mice : putative role in thymic atrophy. Front Immunol. (2015) 6:1–12. doi: 10.3389/fimmu.2015.00428
248. Billard MJ, Gruver AL, Sempowski GD. Acute endotoxin-induced thymic atrophy is characterized by intrathymic inflammatory and wound healing responses. PloS One. (2011) 6. doi: 10.1371/journal.pone.0017940
249. Dudakov JA, Hanash AM, Jenq RR, Young LF, Ghosh A, Singer NV, et al. Interleukin-22 drives endogenous thymic regeneration in mice. Sci (80- ). (2012) 335:91–5. doi: 10.1126/science.1218004
250. Laan M, Haljasorg U, Kisand K, Salumets A, Peterson P. Pregnancy-induced thymic involution is associated with suppression of chemokines essential for T-lymphoid progenitor homing. Eur J Immunol. (2017) 46:2008–17. doi: 10.1002/eji.201646309
251. Webster NL, Zufferey C, Pane JA, Coulson BS. Alteration of the thymic T cell repertoire by rotavirus infection is associated with delayed type 1 diabetes development in non-obese diabetic mice. PLoS One. (2013) 8:1–12. doi: 10.1371/journal.pone.0059182
252. Barreira-Silva P, Melo-Miranda R, Nobrega C, Roque S, Serre-Miranda C, Borges M, et al. IFNγ and iNOS-Mediated Alterations in the Bone Marrow and Thymus and Its Impact on Mycobacterium avium-Induced Thymic Atrophy. Front Immunol. (2021) 12:1–17. doi: 10.3389/fimmu.2021.696415
253. Wertheimer T, Velardi E, Tsai J, Cooper K, Xiao S, Kloss CC, et al. Production of BMP4 by endothelial cells is crucial for endogenous thymic regeneration. Sci Immunol. (2018) 3. doi: 10.1126/sciimmunol.aal2736
254. Howley E, Soomann M, Kreins AY. Parental engagement in identifying information needs after newborn screening for families of infants with suspected athymia. J Clin Immunol. (2024) 44:1–10. doi: 10.1007/s10875-024-01678-w
255. Davies EG. Immunodeficiency in diGeorge syndrome and options for treating cases with complete athymia. Front Immunol. (2013) 4:1–9. doi: 10.3389/fimmu.2013.00322
256. Sharma H, Moroni L. Recent advancements in regenerative approaches for thymus rejuvenation. Adv Sci. (2021) 8:1–22. doi: 10.1002/advs.202100543
257. Marcovecchio GE, Bortolomai I, Ferrua F, Fontana E, Imberti L, Conforti E, et al. Thymic epithelium abnormalities in DiGeorge and Down syndrome patients contribute to dysregulation in T cell development. Front Immunol. (2019) 10:1–15. doi: 10.3389/fimmu.2019.00447
258. Levin N, Abramsky O, Lossos A, Karussis D, Siegal T, Argov Z, et al. Extrathymic Malignancies in patients with myasthenia gravis. J Neurol Sci. (2005) 237:39–43. doi: 10.1016/j.jns.2005.05.009
259. Berrih-Aknin S, Le Panse R. Thymectomy in myasthenia gravis: when, why, and how? Lancet Neurol. (2019) 18:225–6. doi: 10.1016/S1474-4422(18)30467-8
260. Sakoda Y, Hashimoto D, Asakura S, Takeuchi K, Harada M, Tanimoto M, et al. Donor-derived thymic-dependent T cells cause chronic graft-versus-host disease. Blood. (2007) 109:1756–64. doi: 10.1182/blood-2006-08-042853
261. Hassan MN, Waller EK. GVHD clears the Aire in thymic selection. Blood. (2015) 125:2593–5. doi: 10.1182/blood-2015-03-630871
262. Alawam AS, Cosway EJ, James KD, Lucas B, Bacon A, Parnell SM, et al. Failures in thymus medulla regeneration during immune recovery cause tolerance loss and prime recipients for auto-GVHD. J Exp Med. (2021) 219. doi: 10.1084/jem.20211239
263. Kooshesh KA, Foy BH, Sykes DB, Gustafsson K, Scadden DT. Health consequences of thymus removal in adults. N Engl J Med. (2023) 389:406–17. doi: 10.1056/NEJMoa2302892
264. Van Gent R, SChadenberg AWL, Otto SA, Nievelstein RAJ, Sieswerda GT, Haas F, et al. Long-term restoration of the human T-cell compartment after thymectomy during infancy: A role for thymic regeneration? Blood. (2011) 118:627–34. doi: 10.1182/blood-2011-03-341396
265. Palmer S, Albergante L, Blackburn CC, Newman TJ. Thymic involution and rising disease incidence with age. Proc Natl Acad Sci U.S.A. (2018) 115:1883–8. doi: 10.1073/pnas.1714478115
266. Prelog M, Wilk C, Keller M, Karall T, Orth D, Geiger R, et al. Diminished response to tick-borne encephalitis vaccination in thymectomized children. Vaccine. (2008) 26:595–600. doi: 10.1016/j.vaccine.2007.11.074
267. Ciabattini A, Nardini C, Santoro F, Garagnani P, Franceschi C, Medaglini D. Vaccination in the elderly: The challenge of immune changes with aging. Semin Immunol. (2018) 40:83–94. doi: 10.1016/j.smim.2018.10.010
268. Wu H, Wang H. Circulating mature dendritic cells homing to the thymus promote thymic epithelial cells involution via the Jagged1 / Notch3 axis. Cell Death Discovery. (2021) :1–10. doi: 10.1038/s41420-021-00619-5
269. Castañeda J, Hidalgo Y, Sauma D, Rosemblatt M, Bono MR, Núñez S. The multifaceted roles of B cells in the thymus: from immune tolerance to autoimmunity. Front Immunol. (2021) 12:1–14. doi: 10.3389/fimmu.2021.766698
270. Gounko NV, Martens E, Opdenakker G, Rybakin V. Thymocyte development in the absence of matrix metalloproteinase-9/gelatinase B. Sci Rep. (2016) 6:1–12. doi: 10.1038/srep29852
271. Kinsella S, Evandy CA, Cooper K, Iovino L, deRoos PC, Hopwo KS, et al. Attenuation of apoptotic cell detection triggers thymic regeneration after damage. Cell Rep. (2021) 37. doi: 10.1016/j.celrep.2021.109789
272. Von Freeden-Jeffry U, Vieira P, Lucian LA, McNeil T, Burdach SEG, Murray R. Lymphopenia in interleukin (IL)-7 gene-deleted mice identifies IL-7 as a nonredundant cytokine. J Exp Med. (1995) 181:1519–26. doi: 10.1084/jem.181.4.1519
273. El Kassar N, Lucas PJ, Klug DB, Zamisch M, Merchant M, Bare CV, et al. A dose effect of IL-7 on thymocyte development. Blood. (2004) 104:1419–27. doi: 10.1182/blood-2004-01-0201
274. Carrillo MA, Zhen A, Mu W, Rezek V, Martin H, Peterson CW, et al. Stem cell-derived CAR T cells show greater persistence , trafficking , and viral control compared to ex vivo transduced CAR T cells. Mol Ther. (2024) 32. doi: 10.1016/j.ymthe.2024.02.026
275. Yayon N, Kedlian VR, Boehme L, Suo C, Wachter B, Beuschel RT, et al. A spatial human thymus cell atlas mapped to a continuous tissue axis. bioRxiv. (2023). doi: 10.1101/2023.10.25.562925
276. Fan Y. A tissue-engineered artificial human thymus from human iPSCs to study T cell immunity. Nat Methods. (2022) 19:1191–2. doi: 10.1038/s41592-022-01584-2
Keywords: thymus organogenesis, thymus morphology, thymus colonization, T cell development, acute thymus atrophy, thymocyte cell death
Citation: Ruiz Pérez M, Vandenabeele P and Tougaard P (2024) The thymus road to a T cell: migration, selection, and atrophy. Front. Immunol. 15:1443910. doi: 10.3389/fimmu.2024.1443910
Received: 04 June 2024; Accepted: 08 August 2024;
Published: 27 August 2024.
Edited by:
Dominik Filipp, Institute of Molecular Genetics (ASCR), CzechiaReviewed by:
Yingkai Li, Duke University, United StatesNichole Danzl, Bristol Myers Squibb, United States
Copyright © 2024 Ruiz Pérez, Vandenabeele and Tougaard. This is an open-access article distributed under the terms of the Creative Commons Attribution License (CC BY). The use, distribution or reproduction in other forums is permitted, provided the original author(s) and the copyright owner(s) are credited and that the original publication in this journal is cited, in accordance with accepted academic practice. No use, distribution or reproduction is permitted which does not comply with these terms.
*Correspondence: Peter Vandenabeele, Peter.Vandenabeele@irc.vib-ugent.be; Peter Tougaard, Peter.Tougaard@irc.vib-ugent.be