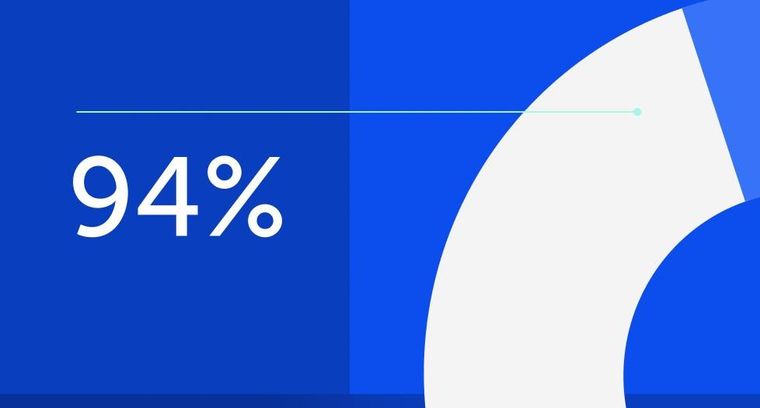
94% of researchers rate our articles as excellent or good
Learn more about the work of our research integrity team to safeguard the quality of each article we publish.
Find out more
EDITORIAL article
Front. Immunol., 18 June 2024
Sec. Multiple Sclerosis and Neuroimmunology
Volume 15 - 2024 | https://doi.org/10.3389/fimmu.2024.1442322
This article is part of the Research TopicTrends in Neuroimmunology: cross-talk between brain-resident and peripheral immune cells in both health and diseaseView all 12 articles
Editorial on the Research Topic
Trends in neuroimmunology: cross-talk between brain-resident and peripheral immune cells in both health and disease
The functional anatomy of organisms is maintained by the coordination of different systems, which often rely on interactions between specialized cells and between macromolecules. The immune system works with the circulatory and lymphatic systems to protect most of the organs. However, some organs are considered immune privileged due to the presence of highly selective and regulated barriers, such as the blood-brain barrier (BBB) within the brain (1). The BBB controls periphery-brain molecule exchange and prevents immune effector cells from entering the homeostatic brain. BBB-associated elements, such as endothelial cells, pericytes, astrocytes, and microglia, potentially can function as antigen-presenting cells (APC). Pathological scenarios that induce dysfunction of the BBB and its associated cells may lead to the infiltration of lymphocytes, crossing over from the blood to the brain. Similarly, traumas can also enable B and T lymphocytes to pass bidirectionally between the central nervous system (CNS) and the periphery, via the meningeal lymphatic vessels, which drain into the cervical lymph nodes. Research in animals and humans has revealed that B and T cells are involved in the progression of neurological diseases (NDs). It has been shown that under certain conditions, T cells establish themselves and become resident in the brain, from where they can exert either beneficial or detrimental effects on brain function. Amazing efforts have been made to further comprehend interactions between brain-specific cells and peripheral immune cells, especially their roles and impact on the onset, progression, and eventual resolution of diverse brain pathologies (2–4). The Research Topic discussed herein represents an effort of Frontiers Media S.A. and the authors of this Editorial to develop another special volume related to the healthy and diseased brain (5–8). This Research Topic, which is available for the scientific community and the public, focuses on understanding the complexity of central immune cells and peripheral immune cells, and their cross-talk mechanisms in diverse CNS pathologies. Eleven peer-reviewed manuscripts including four original articles, six reviews, and one systematic review, encompass this special volume. Seventy-five authors from research laboratories located in six countries: Australia, China, Germany, Japan, United Kingdom, and United States took part in this initiative.
Among the interesting contributions, an in vitro study on primary murine glia by Li et al. showed differential substrate-dependent and time-dependent phagocytic behavior and phenotypic plasticity among M0-like (unstimulated), M1-like (pro-inflammatory) and M2-like (anti-inflammatory) microglia subtypes. Although the application of M1/M2 terminology in the microglia field has been dismissed, the coexistence of pro-inflammatory and anti-inflammatory microglial states has been documented, including in circumventricular organs (9–12). Li et al. differentiated cultured glial cells into M1-like and M2-like microglia subtypes by treating them either with granulocyte colony stimulating factor and interferon-gamma (GM-CSF/IFNγ), or with macrophage colony-stimulating factor and interleukin-4 (M-CSF/IL-4), respectively. No supplements were added to obtain M0-like microglia. Phagocytosis assays using E. coli-rhodamine particles or IgG-FITC beads revealed different preferences and dynamics for the substrates among the microglia subtypes. M1-like microglial cells engulfed more bacteria particles than beads after 3 hours. The opposite behavior was observed with the anti-inflammatory subtype, where M0-like microglia internalized both substrates equally. The authors reported further differences among the three differentiated microglial phenotypes during incubation with both substrates for 16 hours. M2-like microglia showed discontinuous phagocytosis after 8 hours, while M0-like and M1-like microglial cells continuously internalized substrates with different profiles. One interesting observation after a prolonged exposure for 5 days to either E. coli particles or IgG-opsonized beads, was that M1-like states and M0/2-M1 transitions were both enhanced, indicating phenotypic plasticity like it occurs in neurodegenerative conditions (13, 14). The study by Li et al. complements the existing knowledge about microglia diversity and plasticity (15, 16), and it opens therapeutic avenues to intervene in microglia-mediated inflammation and neurodegeneration.
Neumaier et al. reviewed current knowledge and therapeutic potential of midkine (MDK), which is a neurotrophic growth factor with dual functions in the healthy and diseased CNS and periphery (17, 18). Due to its multi-functionality, MDK has been involved in the progression or suppression of numerous CNS-related pathologies including autoimmune disorders, such as multiple sclerosis (MS), brain tumors, acute injuries, and other conditions that imply neuroinflammation and neurodegeneration. In the CNS, MDK is spatio-temporally expressed by oligodendrocytes, astrocytes, and neuronal lineages, and maybe also by microglia in response to inflammatory stimuli. In the periphery, hematopoietic and non-hematopoietic cells can produce MDK. This regulator acts through multimolecular receptor complexes, with protein tyrosine phosphatase ζ (PTPζ) as one of the most established components. In addition, multiple signaling pathways are involved depending on the cellular context, thereby facilitating MDK’s multifaceted functions. Interestingly, the authors discussed the role of MDK as a mediator of the neuro-immune cell-to-cell cross-talk in CNS inflammatory scenarios that involve a dysfunctional or leaky BBB. These conditions facilitate the infiltration of MDK-expressing immune cells from the periphery. The recruitment of peripheral immune actors such as macrophages and T cells, and the impact of MDK-signaling events on CNS-resident cells are also addressed within the context of neoplastic diseases (19). The findings included in this review support the importance of MDK as a mediator of tumorigenesis and inflammatory disorders, irrespective of the tissue and cell type, and they emphasize the need for further research to better understand its mechanisms and biomarker potential in neurodegenerative diseases, such as Parkinson’s and Alzheimer’s diseases (PD and AD, respectively).
Neuroinflammation and neurodegeneration are associated with traumatic spinal cord injuries (SCI), which are highly debilitating pathologies. SCI progresses through various phases: acute (up to 3 days post-injury; dpi), subacute (3–14 dpi), and chronic (more than 14 dpi) stages. Yao et al. investigated differential gene expression profiles and pathways in macrophages and microglia across these SCI phases to pinpoint potential therapeutic targets for SCI. The authors applied bioinformatic analysis to the existing scRNA-seq dataset GSE159638 (total 30,958 cells; https://www.ncbi.nlm.nih.gov/geo/query/acc.cgi?acc=GSE159638), which was generated in a mouse model of thoracic contusion SCI (20). Then, they validated the results in a mouse model of cervical SC hemi-contusion injury [wild type and APOE-/- mice; (21)]. They identified apolipoprotein E (APOE) as a central gene of interest in both macrophages and microglia during the subacute and chronic phases of SCI. These cells exhibited high activity, suggesting a crucial role in regulating SCI-associated inflammation. On the other hand, APOE has been linked to pathways related to debris and dead cell clearance (phagocytosis), lipid metabolism, and lysosomal function (22–24). Subsequent experiments demonstrated that APOE knockout (KO) in mice exacerbated neurological deficits, increased neuroinflammation, and worsened white matter loss after SCI at the cervical level. Following SCI, ultrastructural analysis of the KO mice revealed myelin uptake and accumulation of lipid droplets, lysosomes, and needle-like cholesterol crystals in macrophages and microglia. APOE is vital for cholesterol homeostasis within the CNS (25). Together, these results make APOE and its associates promising therapeutic targets for reducing neuroinflammation and for enhancing recovery after SCI.
Zhang et al. contributed to this Research Topic with a comprehensive review of the impact of inflammation and the involvement of infiltrated regulatory T cells (Treg cells) on neuropathic pain (NP) following spinal cord injury (SCI), as well as the potential of cellular therapeutic interventions in SCI-related conditions. The authors discussed the mechanisms behind inflammation and NP after SCI, which include a plethora of cells and mediators. Glial cells, including astrocytes and microglia, and infiltrated immune cells, such as monocytes, macrophages, B cells, and T cells, are involved in these scenarios. These cells, when activated, release inflammatory mediators including chemokines (e.g., CXCL1 and CXCL2) and cytokines (e.g., TNF-α, IL-1β, and IL-6) (26). These molecules affect neurons through multiple signaling pathways, leading to neurotransmitter and ion channel imbalance, increased neuronal excitability, decreased neuronal inhibition, and boosted pain transmission (27). In particular, the authors reviewed the mechanisms by which astrocytes, microglia, and immunosuppressive Treg cells intervene in the pathogenesis of SCI and the subsequent NP. In fact, the inflammatory response following SCI has been tightly linked to a reduction in the number of Treg cells (28). Finally, this review provides a framework for thinking about strategies and challenges (i.e., cell purity, stability, and functionality) in the application of Treg cell therapy in SCI patients who suffer from neuropathic pain.
Chronic inflammation has been associated with different neurological disorders (NDs). Cumulative evidence showed that the recruitment of peripheral immune cells into the CNS is a common characteristic in various NDs (29–32). Among these neuroinflammatory cells, T helper (Th) 17 lymphocytes play an active role in the pathogenesis of CNS-related diseases. The biology of this CD4+ Th cell subtype in NDs is addressed in this volume by Shi et al. Th17 cells and their cytokines (e.g., IL-17A, IL-23, IL-21, IL-6, and IFN-γ) contribute to the disruption of the BBB, promote the infiltration of other immune cells into the CNS, excessively activate microglia, and can cause direct cytotoxic damage to neurons (33). The authors described Th17 lymphocytes, including the signaling pathways that induce their differentiation. They also introduced ND-linked environmental factors that may induce the pathogenic potential of Th17 cells, such as peripheral inflammation, enhanced oxidative stress, and changes in the microbiota or diet that affect the gut-brain axis. Shi et al. further discussed the possible immunopathological mechanisms of Th17 cells in AD, PD, MS, amyotrophic lateral sclerosis (ALS), and major depressive disorder (MDD). Finally, therapeutic strategies targeting Th17 lymphocytes, their associated cytokines, and Th17-related molecular mechanisms to treat neurodegenerative diseases, are also addressed.
Grotemeyer et al. elaborated a detailed summary of the interconnected innate and adaptive immune responses in the context of Parkinson’s disease (PD), which is a ND characterized by neuroinflammation and dopaminergic neurodegeneration (34, 35). Interestingly, they propose a mechanism for how neuroinflammation is triggered in PD. They hypothesized that pathological forms of alpha-synuclein (pαSYN), the key protein in PD, might act as a damage-associated molecular pattern (DAMP) to induce and maintain a pro-inflammatory shift of the immune system, via pattern recognition receptor (PRR)-mediated processes (36). Central (e.g., microglia) and peripheral (e.g., T and B cells) immune cells and their mechanisms in the pathophysiology of PD in humans and animal models (e.g., MPTP, 6-OHDA, viral vector, and preformed fibrils), are discussed. Circulating and infiltrated CD4+ and CD8+ T cells are among the immune effector cells in PD, and their roles are both beneficial and detrimental. The authors also summarized current clinical trials on anti-inflammatory therapy in PD, focusing on the regulation of glucose metabolism, intestinal microbiota, and oxidative stress. Then, they discussed different signaling pathways associated with inflammation and neurodegeneration, such as the pentose phosphate pathway (PPP) (30) and the renin-angiotensin [-aldosterone] system (RA[A]S) (37), to use them as potential therapeutic targets. Finally, the authors suggested that dopaminergic neurodegeneration could be halted by administrating neuroprotective/anti-inflammatory agents early in the course of PD, before severe symptoms have developed.
The involvement of innate and adaptative immune cells in the pathophysiology of multiple sclerosis (MS), and their regulation by physical exercise, are addressed in this Research Topic by Zong et al. The pathogenesis of this neuroinflammatory and autoimmune disease is driven by the dysfunctional activity of immune cells, including those recruited from the periphery into the CNS (38). Aberrant immune responses damage oligodendrocytes and thus, cause severe demyelination, impaired remyelination, axonal degeneration, and altered neurotransmission (39, 40). This results in a spectrum of motor and non-motor symptoms. The disease has no cure and pharmacotherapy is considered the primary treatment. However, drugs have low efficacy, several side effects, and high costs. Alternative MS-modifying interventions, such as physical exercise, have gained attention as a new therapy to alleviate patients’ symptoms (41, 42). In summary, the authors present morphological, cellular, and molecular evidence from animal models (e.g., EAE and toxin and/or virus-induced demyelination models) and human studies of how this type of adjunctive intervention regulates innate and adaptive immune cells, reducing peripheral immune cell infiltration, and eventually leading to a reduction of the autoimmune responses and their concomitant negative effects in the CNS. The authors focused this review specifically on T cells (e.g., CD8+ and CD4+ cells, including Th17 and Treg cells), B cells, dendritic cells, neutrophils, microglia/macrophages, and astrocytes. Zong et al. also raised a critical view towards the need to conduct more studies in humans, stratifying patients by gender, disease stage, and type, duration, intensity, and cycle of exercise, to better understand the potential of the physical therapy in treating MS.
Beyond the immunological roles, immune cells can participate in other physiological responses that are essential to maintain the homeostasis of the organisms (43, 44). One instance of this is the interplay between enteric C1q-producing macrophages and the enteric nervous system to regulate neuronal and smooth muscle cell functions and thus, gastrointestinal motility and homeostasis (45). It was previously reported that bidirectional signaling between muscularis macrophages and enteric neurons is necessary to ensure gut peristalsis in healthy mice (46). Macrophage-derived bone morphogenetic protein 2 (BMP2) and neuronal colony stimulatory factor 1 (CSF1) are involved in this cross-talk mechanism. In this context, Yip et al. contributed to this Research Topic with an original article in which they studied the participation of CD163 intestinal macrophages and inhibitory interneurons of the myenteric plexus in the regulation of colonic motility. They used a conditional KO Cx3cr1 (chemokine receptor)-Dtr (diphtheria toxin receptor) rat model to transiently deplete resident macrophages in combination with the nitric oxide synthase (NOS) inhibitor NOLA (Nω-nitro-L-arginine), and ex vivo video imaging (47, 48). The authors showed that the resident intestinal macrophages are crucial in regulating colonic motility in the absence of the inhibitory neuronal input driven by NO. Whereas, under control conditions, these macrophages might not be relevant. They also showed that these immune cells are important in maintaining healthy intestinal structure. The authors highlighted CD163-positive intestinal macrophages as a potential therapeutic target for gastrointestinal disorders in which inhibitory neuronal input is impaired, such as gastroparesis and achalasia (49, 50). However, Yip et al. pointed out the need for further research to dissect the cell subtypes and to investigate the mechanisms of these functions.
Viengkhou and Hofer reviewed the dual pivotal roles of type I interferons (IFN-Is) in regulating cellular and molecular homeostasis within the CNS, as well as inflammation and immunity associated with diverse NDs, from chronic infections and autoimmune conditions to trauma, aging, and neurodegeneration. Some of these conditions are known as interferonopathies. The authors initially discussed mechanisms by which levels of IFN-Is are altered, especially those mediated by innate immune sensors (e.g., cyclic GMP-AMP synthase/STING signaling pathway), by genetic alterations (e.g., trisomy 21 and mutations in USP18 or ISG15), and by therapeutic interventions for diseases like chronic viral infections, MS, and certain cancers (51, 52). They further presented the canonical and non-canonical IFN-I signaling pathways that imply binding to cell surface receptors and activation of distinct response phases, including an early widespread protein phosphorylation stage and changes in the expression of several IFN-regulated genes (IRGs) (53). The classic path involves the interferon-stimulated gene factor 3 (ISGF3) complex (54), which consists of the transcription factors STAT1 (signal transducer and activator of transcription 1), STAT2, and interferon regulatory factor 9 (IRF9). Then, Viengkhou and Hofer focused the review on the specific responses to IFN-Is mounted by each cell type in the CNS, especially those mediated by neurons, glial cells, and BBB-associated cells. Understanding the diversity in cell responses has been facilitated by single-cell technologies. Moreover, it has been accepted that a diverse spectrum of cellular response states coexists within the diseased CNS, instead of a single prevalent response. Neurons respond to limit the impact of viral infections, but they can suffer neurotoxic effects from increased IFN-I signaling, including fewer dendrites, impaired neurogenesis, and altered neurotransmission (55). Although basal IFN-I signaling in astrocytes is crucial for brain health (56), its contribution to IFN-I neurotoxicity seems yet unclear. A small IFN-I-hyperresponsive microglia subset was identified by single-cell sequencing, which has been associated with age-dependent cognitive decline and synaptic stripping (57, 58). Due to that chronic inflammation has been related to NDs and that IFN-I therapy has been shown to have adverse effects, the authors finished their review discussing the implications and mechanisms of IFN-Is in cerebral interferonopathies, such as Aicardi-Goutières Syndrome (AGS) and chronic viral encephalopathies, as well as in aging, and in diseases with abnormal protein aggregation, including AD and PD (59–61). The authors pointed out that understanding the complexity of IFN-I responses in the CNS is critical for developing targeted therapies for neurological disorders that occur with IFN-I dysregulation. These therapies should consider factors such as cell type, signaling duration, and disease context.
Sun et al. conducted a Mendelian randomization (MR) study to explore the causal relationship between immune cell surface antigens and post-stroke functional outcomes, and to identify novel biomarkers and therapeutic targets for ischemic stroke. The authors employed Genome-Wide Association Studies (GWAS) summary statistics for a two-sample MR analysis, followed by several alternative methods and sensitive approaches. They sourced genetic variants linked to immune cell surface antigens (measured by median fluorescence intensities, MFIs) from the publicly available GWAS catalog (62); outcome data from the Genetics of Ischemic Stroke Functional Outcome (GISCOME) network (63, 64), and statistics about the risk of ischemic stroke from the MEGASTROKE consortium (65). The cohorts were primarily of European ancestry, aged 18 and above. A total of 389 MFIs with surface antigens were included in seven panels (maturation stages of T cell, Treg cell, TBNK, DC, B cell, monocyte, and myeloid cell, respectively). The authors identified genetic variants including single nucleotide polymorphisms (SNPs) associated with MFIs of immune cell surface markers, as measured from samples of peripheral blood. They meticulously selected SNPs that were strongly linked to markers and less likely influenced by non-genetic factors like lifestyle, and they treated them as instrumental variables (IVs) for the MR analysis (66, 67). After a comprehensive analysis, Sun et al. identified 13 suggestive immune cell surface antigens that appear to be associated with post-stroke outcomes. Notably, elevated levels of CD20 on switched memory B cells and of PDL-1 on monocytes appeared to be linked to worse stroke outcomes and severity. In contrast, surface antigen CD25 on CD39+ resting Treg cells was found to be associated with favorable post-stroke functional outcomes, possibly due to enhanced Treg cell survival supported by IL2 affinity (68). CD39 was highlighted for its immunosuppressive role, which may be crucial for long-term immune balance after stroke (69). The authors discussed limitations of their analysis including those related to the nature of the sourced data. Overall, this study uncovers potential novel biomarkers and therapeutic strategies targeting immune cell surface antigens to enhance post-stroke recovery, and it warrants further exploration and validation across diverse populations and stroke subtypes.
Considering that research in Treg cells in NDs continues to be a topic of interest (70, 71), Gao et al. contributed to this Research Topic with a bibliometric analysis of the field, spanning from 1991 to 2023, and including 2,739 documents between articles and review articles from the Web of Science Core Collection. The authors used Tableau Public, VOSviewer, and CiteSpace software to perform the study. The research course was categorized into three phases: 1991–2003 (early stage), 2004–2019 (rapid expansion period), and 2020–2023 (fluctuating yet productive phase). A total of 85 countries/regions investigating Treg cells in NDs were identified with the United States, China, and Germany leading in document output. Collaboration among countries/regions was widespread, again with the United States cooperating most (with 57 countries/regions). Notably, Harvard Medical School showed exceptional productivity, citations, link strength, and centrality, reflecting its prolific research and collaborations. Studies examining Treg cells in NDs were published in 859 journals. Among them, the top 11 journals contributed 618 documents, with Frontiers in Immunology, Journal of Immunology, and Journal of Neuroinflammation as the most prominent publishers. The associations of high-frequency keywords, such as “multiple sclerosis”, “inflammation”, and “regulatory T cells”, were found to change throughout the research evolution. Initially, they appeared linked with neuroprotection, neuroimmunology, and immunoregulation (2014), and currently, they shifted towards ischemic stroke, gut microbiota, and the gut-brain axis. Gao et al. identified the top 10 most-cited documents, with three emphasizing the roles of cytokines in autoimmune neurological diseases (72–74), and others examining gut microbiota impact on immune responses and the influence of tumor microenvironment in tumorigenesis (75). Although the United States has led in document output and citations, China emerged as a significant contributor, rising to the forefront in 2022. The study conducted by Gao et al. acknowledges limitations such as language barriers and publication bias, but it emphasizes the need for continual updates to reflect ongoing scientific avenues. This review provides valuable insights for shaping future research directions and therapeutic strategies in this dynamic field.
Overall, the original research and review articles on this Research Topic illustrate the complexity behind the participation of immune cells in the healthy and diseased central nervous system. We expect this Research Topic will encourage researchers to continue their efforts to further investigate immunity and the brain, with the ultimate hope of finding not only new knowledge but also potential clinical interventions to prevent or ameliorate the devastating consequences of neurological diseases.
SM: Writing – review & editing, Writing – original draft, Conceptualization. JB: Writing – review & editing, Writing – original draft. SB: Writing – review & editing, Writing – original draft. VM: Writing – review & editing. RM: Writing – review & editing. EM: Writing – review & editing, Writing – original draft, Conceptualization.
The author(s) declare financial support was received for the research, authorship, and/or publication of this article. SM is an Assistant Professor in the Department of Zoology, University of Delhi, New Delhi, India. Supported by grants from the Indian Council of Medical Research (India; ICMR; EMDR/SG/11/2023–1452; https://www.icmr.gov.in), and the Institution of Eminence, University of Delhi (India; IOE/2021/12/FRP; https://www.ioe.du.ac.in). VM is a Professor in the Department of Pathology and Laboratory Medicine, UC Davis School of Medicine, Sacramento, USA. Supported by grants from NIMH and NINDS (USA; RO1 MH094681; https://www.nimh.nih.gov; RO1 NS107131; ttps://www.ninds.nih.gov). EM is a member of the CONICET scientific career at IHEM-UNCuyo-CONICET, Mendoza, Argentina. Supported by grants from ANPCyT (Argentina; PICT 2021–314; http://www.agencia.mincyt.gob.ar), and CONICET (Argentina; PUE 2017; PIP 2023–2025 GI: 112–202201–00410; http://www.conicet.gov.ar).
We thank Sundararajan Jayaraman (University of Illinois, United States), Rui Li (University of Pennsylvania, United States), Amy Lovett-Racke (The Ohio State University, United States), Serge Nataf (Université Claude Bernard Lyon 1, France), and Pedro A. Reche (Complutense University of Madrid, Spain), who edited five of the eleven articles included in this RT. We also thank Raymond D. Astrue for editing the Editorial.
The authors declare that the research was conducted in the absence of any commercial or financial relationships that could be construed as a potential conflict of interest.
The author(s) declared that they were an editorial board member of Frontiers, at the time of submission. This had no impact on the peer review process and the final decision.
All claims expressed in this article are solely those of the authors and do not necessarily represent those of their affiliated organizations, or those of the publisher, the editors and the reviewers. Any product that may be evaluated in this article, or claim that may be made by its manufacturer, is not guaranteed or endorsed by the publisher.
1. Daneman R, Prat A. The blood-brain barrier. Cold Spring Harb Perspect Biol. (2015) 7:a020412. doi: 10.1101/cshperspect.a020412
2. Dantzer R. Neuroimmune interactions: from the brain to the immune system and vice versa. Physiol Rev. (2018) 98:477–504. doi: 10.1152/physrev.00039.2016
3. Norris GT, Kipnis J. Immune cells and CNS physiology: Microglia and beyond. J Exp Med. (2019) 216:60–70. doi: 10.1084/jem.20180199
4. Chu C, Artis D, Chiu IM. Neuro-immune interactions in the tissues. Immunity. (2020) 52:464–74. doi: 10.1016/j.immuni.2020.02.017
5. Muñoz EM, de Souza FSJ, Rath MF, Martínez-Cerdeño V. Editorial: Transcription regulation – Brain development and homeostasis – A finely tuned and orchestrated scenario in physiology and pathology. Front Mol Neuroscience Section Neuroplasticity Dev. (2022). doi: 10.3389/fnmol.2021.834607/full
6. Muñoz EM, Martínez-Cerdeño V, De Souza FSJ, Rath MF. Transcription regulation – brain development and homeostasis – A finely tuned and orchestrated scenario in physiology and pathology. Lausanne: Front Media SA. (2022). doi: 10.3389/978–2-88974–457-2
7. Muñoz EM, Martínez-Cerdeño V. Editorial: Transcription regulation – Brain development and homeostasis - A finely tuned and orchestrated scenario in physiology and pathology, volume II. Front Mol Neurosci. (2023) 16:1280573. doi: 10.3389/fnmol.2023.1280573
8. Muñoz EM, Martínez-Cerdeño V. Transcription regulation – Brain development and homeostasis - A finely tuned and orchestrated scenario in physiology and pathology, volume II. Lausanne: Front Media SA. (2023). doi: 10.3389/978–2-8325–3381-9
9. Ransohoff RM. A polarizing question: do M1 and M2 microglia exist? Nat Neurosci. (2016) 19:987–91. doi: 10.1038/nn.4338
10. Muñoz EM. Microglia-precursor cell interactions in health and in pathology. Biocell. (2018) 42:41–5. doi: 10.32604/biocell.2018.07011
11. Farias Altamirano LE, Freites CL, Vásquez E, Muñoz EM. Signaling within the pineal gland: A parallelism with the central nervous system. Semin Cell Dev Biol. (2019) 95:151–9. doi: 10.1016/j.semcdb.2018.11.004
12. Muñoz EM. Microglia in circumventricular organs: the pineal gland example. ASN Neuro. (2022) 14:17590914221135697. doi: 10.1177/17590914221135697
13. Gomes-Leal W. Microglial physiopathology: how to explain the dual role of microglia after acute neural disorders? Brain Behav. (2012) 2:345–56. doi: 10.1002/brb3.51
14. Wendimu MY, Hooks SB. Microglia phenotypes in aging and neurodegenerative diseases. Cells. (2022) 11:2091. doi: 10.3390/cells11132091
15. Ibañez Rodriguez MP, Noctor S, Muñoz EM. Cellular basis of pineal gland development: emerging role of microglia as phenotype regulator. PloS One. (2016) 11:e0167063. doi: 10.1371/journal.pone.0167063
16. Ibañez Rodriguez MP, Galiana MD, Rásmussen JA, Freites CL, Noctor S, Muñoz EM. Differential response of pineal microglia to surgical versus pharmacological stimuli. J Comp Neurol. (2018) 526:2462–81. doi: 10.1002/cne.24505
17. Herradón G, Pérez-García C. Targeting midkine and pleiotrophin signalling pathways in addiction and neurodegenerative disorders: recent progress and perspectives. Br J Pharmacol. (2014) 171:837–48. doi: 10.1111/bph.12312
18. Takada S, Sakakima H, Matsuyama T, Otsuka S, Nakanishi K, Norimatsu K, et al. Disruption of Midkine gene reduces traumatic brain injury through the modulation of neuroinflammation. J Neuroinflammation. (2020) 17:40. doi: 10.1186/s12974–020-1709–8
19. Guo X, Pan Y, Xiong M, Sanapala S, Anastasaki C, Cobb O, et al. Midkine activation of CD8+ T cells establishes a neuron-immune-cancer axis responsible for low-grade glioma growth. Nat Commun. (2020) 11:2177. doi: 10.1038/s41467–020-15770–3
20. Hamel R, Peruzzotti-Jametti L, Ridley K, Testa V, Yu B, Rowitch D, et al. Time-resolved single-cell RNAseq profiling identifies a novel Fabp5+ subpopulation of inflammatory myeloid cells with delayed cytotoxic profile in chronic spinal cord injury. Heliyon. (2023) 9:e18339. doi: 10.1016/j.heliyon.2023.e18339
21. Huang Z, Huang Z, Kong G, Lin J, Liu J, Yang Z, et al. Anatomical and behavioral outcomes following a graded hemi-contusive cervical spinal cord injury model in mice. Behav Brain Res. (2022) 419:113698. doi: 10.1016/j.bbr.2021.113698
22. Muth C, Hartmann A, Sepulveda-Falla D, Glatzel M, Krasemann S. Phagocytosis of Apoptotic cells is specifically upregulated in ApoE4 expressing microglia in vitro. Front Cell Neurosci. (2019) 13:181. doi: 10.3389/fncel.2019.00181
23. Lee H, Cho S, Kim M-J, Park YJ, Cho E, Jo YS, et al. ApoE4-dependent lysosomal cholesterol accumulation impairs mitochondrial homeostasis and oxidative phosphorylation in human astrocytes. Cell Rep. (2023) 42:113183. doi: 10.1016/j.celrep.2023.113183
24. Yang LG, March ZM, Stephenson RA, Narayan PS. Apolipoprotein E in lipid metabolism and neurodegenerative disease. Trends Endocrinol Metab. (2023) 34:430–45. doi: 10.1016/j.tem.2023.05.002
25. Cantuti-Castelvetri L, Fitzner D, Bosch-Queralt M, Weil MT, Su M, Sen P, et al. Defective cholesterol clearance limits remyelination in the aged central nervous system. Sci (New York N.Y.). (2018) 359:684–8. doi: 10.1126/science.aan4183
26. Gwak YS, Hulsebosch CE, Leem JW. Neuronal-glial interactions maintain chronic neuropathic pain after spinal cord injury. Neural Plast. (2017) 2017:2480689. doi: 10.1155/2017/2480689
27. Ellis A, Bennett DL. Neuroinflammation and the generation of neuropathic pain. Br J Anaesth. (2013) 111:26–37. doi: 10.1093/bja/aet128
28. Liu R, Li Y, Wang Z, Chen P, Xie Y, Qu W, et al. Regulatory T cells promote functional recovery after spinal cord injury by alleviating microglia inflammation via STAT3 inhibition. CNS Neurosci Ther. (2023) 29:2129–44. doi: 10.1111/cns.14161
29. Hickman S, Izzy S, Sen P, Morsett L, El Khoury J. Microglia in neurodegeneration. Nat Neurosci. (2018) 21:1359–69. doi: 10.1038/s41593-018-0242-x
30. Tu D, Gao Y, Yang R, Guan T, Hong JS, Gao HM. The pentose phosphate pathway regulates chronic neuroinflammation and dopaminergic neurodegeneration. J Neuroinflammation. (2019) 16:255. doi: 10.1186/s12974–019-1659–1
31. Williams GP, Schonhoff AM, Jurkuvenaite A, Gallups NJ, Standaert DG, Harms AS. CD4 T cells mediate brain inflammation and neurodegeneration in a mouse model of Parkinson’s disease. Brain. (2021) 144:2047–59. doi: 10.1093/brain/awab103
32. Asamu MO, Oladipo OO, Abayomi OA, Adebayo AA. Alzheimer’s disease: The role of T lymphocytes in neuroinflammation and neurodegeneration. Brain Res. (2023) 1821:148589. doi: 10.1016/j.brainres.2023.148589
33. Fu J, Huang Y, Bao T, Liu C, Liu X, Chen X. The role of Th17 cells/IL-17A in AD, PD, ALS and the strategic therapy targeting on IL-17A. J Neuroinflammation. (2022) 19:98. doi: 10.1186/s12974–022-02446–6
34. McGeer PL, Itagaki S, Boyes BE, McGeer EG. Reactive microglia are positive for HLA-DR in the substantia nigra of Parkinson’s and Alzheimer’s disease brains. Neurology. (1988) 38:1285–91. doi: 10.1212/wnl.38.8.1285
35. Zhang F, Chen B, Ren W, Yan Y, Zheng X, Jin S, et al. Association analysis of dopaminergic degeneration and the neutrophil-to-lymphocyte ratio in Parkinson’s disease. Front Aging Neurosci. (2024) 16:1377994. doi: 10.3389/fnagi.2024.1377994
36. Earls RH, Menees KB, Chung J, Barber J, Gutekunst CA, Hazim MG, et al. Intrastriatal injection of preformed alpha-synuclein fibrils alters central and peripheral immune cell profiles in non-transgenic mice. J Neuroinflammation. (2019) 16:250. doi: 10.1186/s12974–019-1636–8
37. Abiodun OA, Ola MS. Role of brain renin angiotensin system in neurodegeneration: An update. Saudi J Biol Sci. (2020) 27:905–12. doi: 10.1016/j.sjbs.2020.01.026
38. Liu R, Du S, Zhao L, Jain S, Sahay K, Rizvanov A, et al. Autoreactive lymphocytes in multiple sclerosis: Pathogenesis and treatment target. Front Immunol. (2022) 13:996469. doi: 10.3389/fimmu.2022.996469
39. Faissner S, Plemel JR, Gold R, Yong VW. Progressive multiple sclerosis: from pathophysiology to therapeutic strategies. Nat Rev Drug Discovery. (2019) 18:905–22. doi: 10.1038/s41573–019-0035–2
40. Charabati M, Wheeler MA, Weiner HL, Quintana FJ. Multiple sclerosis: Neuroimmune crosstalk and therapeutic targeting. Cell. (2023) 186:1309–27. doi: 10.1016/j.cell.2023.03.008
41. Faraclas E. Interventions to improve quality of life in multiple sclerosis: new opportunities and key talking points. Degener Neurol Neuromuscul Dis. (2023) 13:55–68. doi: 10.2147/DNND.S395733
42. Gitman V, Moss K, Hodgson D. A systematic review and meta-analysis of the effects of non-pharmacological interventions on quality of life in adults with multiple sclerosis. Eur J Med Res. (2023) 28:294. doi: 10.1186/s40001–023-01185–5
43. Thacker MA, Clark AK, Marchand F, McMahon SB. Pathophysiology of peripheral neuropathic pain: immune cells and molecules. Anesth Analg. (2007) 105:838–47. doi: 10.1213/01.ane.0000275190.42912.37
44. Ji RR, Chamessian A, Zhang YQ. Pain regulation by non-neuronal cells and inflammation. Sci (New York N.Y.). (2016) 354:572–7. doi: 10.1126/science.aaf8924
45. Pendse M, De Selle H, Vo N, Quinn G, Dende C, Li Y, et al. Macrophages regulate gastrointestinal motility through complement component 1q. eLife. (2023) 12:e78558. doi: 10.7554/eLife.78558
46. Muller PA, Koscsó B, Rajani GM, Stevanovic K, Berres ML, Hashimoto D, et al. Crosstalk between muscularis macrophages and enteric neurons regulates gastrointestinal motility. Cell. (2014) 158:300–13. doi: 10.1016/j.cell.2014.04.050
47. Jung S, Aliberti J, Graemmel P, Sunshine MJ, Kreutzberg GW, Sher A, et al. Analysis of fractalkine receptor CX(3)CR1 function by targeted deletion and green fluorescent protein reporter gene insertion. Mol Cell Biol. (2000) 20:4106–14. doi: 10.1128/MCB.20.11.4106–4114.2000
48. Yip JLK, Balasuriya GK, Spencer SJ, Hill-Yardin EL. Examining enteric nervous system function in rat and mouse: an interspecies comparison of colonic motility. Am J Physiol Gastrointest Liver Physiol. (2022) 323:G477–87. doi: 10.1152/ajpgi.00175.2022
49. Furuzawa-Carballeda J, Torres-Landa S, Valdovinos MÁ, Coss-Adame E, Martín Del Campo LA, Torres-Villalobos G. New insights into the pathophysiology of achalasia and implications for future treatment. World J Gastroenterol. (2016) 22:7892–907. doi: 10.3748/wjg.v22.i35.7892
50. Usai-Satta P, Bellini M, Morelli O, Geri F, Lai M, Bassotti G. Gastroparesis: New insights into an old disease. World J Gastroenterol. (2020) 26:2333–48. doi: 10.3748/wjg.v26.i19.2333
51. Schneider WM, Chevillotte MD, Rice CM. Interferon-stimulated genes: a complex web of host defenses. Annu Rev Immunol. (2014) 32:513–45. doi: 10.1146/annurev-immunol-032713–120231
52. Snell LM, McGaha TL, Brooks DG. Type I interferon in chronic virus infection and cancer. Trends Immunol. (2017) 38(8):542–57. doi: 10.1016/j.it.2017.05.005
53. Tan PH, Ji J, Hsing CH, Tan R, Ji RR. Emerging roles of type-I interferons in neuroinflammation, neurological diseases, and long-haul COVID. Int J Mol Sci. (2022) 23:14394. doi: 10.3390/ijms232214394
54. Kumar R, Korutla L. Induction of expression of interferon-stimulated gene factor-3 (ISGF-3) proteins by interferons. Exp Cell Res. (1995) 216:143–8. doi: 10.1006/excr.1995.1018
55. Lang R, Li H, Luo X, Liu C, Zhang Y, Guo S, et al. Expression and mechanisms of interferon-stimulated genes in viral infection of the central nervous system (CNS) and neurological diseases. Front Immunol. (2022) 13:1008072. doi: 10.3389/fimmu.2022.1008072
56. Hosseini S, Michaelsen-Preusse K, Grigoryan G, Chhatbar C, Kalinke U, Korte M. Type I Interferon receptor signaling in astrocytes regulates hippocampal synaptic plasticity and cognitive function of the healthy CNS. Cell Rep. (2020) 31:107666. doi: 10.1016/j.celrep.2020.107666
57. Aw E, Zhang Y, Carroll M. Microglial responses to peripheral type 1 interferon. J Neuroinflamm. (2020) 17:340. doi: 10.1186/s12974-020-02003-z
58. Roy E, Cao W. Glial interference: impact of type I interferon in neurodegenerative diseases. Mol Neurodegener. (2022) 17:78. doi: 10.1186/s13024–022-00583–3
59. Nazmi A, Field RH, Griffin EW, Haugh O, Hennessy E, Cox D, et al. Chronic neurodegeneration induces type I interferon synthesis via STING, shaping microglial phenotype and accelerating disease progression. Glia. (2019) 67:1254–76. doi: 10.1002/glia.23592
60. Cao W. IFN-aging: coupling aging with interferon response. Front Aging. (2022) 3:870489. doi: 10.3389/fragi.2022.870489
61. Liu A, Ying S. Aicardi-Goutières syndrome: A monogenic type I interferonopathy. Scand J Immunol. (2023) 98:e13314. doi: 10.1111/sji.13314
62. Orrù V, Steri M, Sidore C, Marongiu M, Serra V, Olla S, et al. Complex genetic signatures in immune cells underlie autoimmunity and inform therapy. Nat Genet. (2020) 52:1036–45. doi: 10.1038/s41588–020-0684–4
63. Maguire JM, Bevan S, Stanne TM, Lorenzen E, Fernandez-Cadenas I, Hankey GJ, et al. GISCOME – Genetics of Ischaemic Stroke Functional Outcome network: A protocol for an international multicentre genetic association study. Eur Stroke J. (2017) 2:229–37. doi: 10.1177/2396987317704547
64. Söderholm M, Pedersen A, Lorentzen E, Stanne TM, Bevan S, Olsson M, et al. Genome-wide association meta-analysis of functional outcome after ischemic stroke. Neurology. (2019) 92:e1271–83. doi: 10.1212/WNL.0000000000007138
65. Malik R, Chauhan G, Traylor M, Sargurupremraj M, Okada Y, Mishra A, et al. Multiancestry genome-wide association study of 520,000 subjects identifies 32 loci associated with stroke and stroke subtypes. Nat Genet. (2018) 50:524–37. doi: 10.1038/s41588–018-0058–3
66. Mizoo T, Taira N, Nishiyama K, Nogami T, Iwamoto T, Motoki T, et al. Effects of lifestyle and single nucleotide polymorphisms on breast cancer risk: a case-control study in Japanese women. BMC Cancer. (2013) 13:565. doi: 10.1186/1471–2407-13–565
67. Li Z, Wei H, Tang X, Liu T, Li S, Wang X. Blood metabolites mediate the impact of lifestyle factors on the risk of urolithiasis: a multivariate, mediation Mendelian randomization study. Urolithiasis. (2024) 52:44. doi: 10.1007/s00240–024-01545–8
68. Lykhopiy V, Malviya V, Humblet-Baron S, Schlenner SM. IL-2 immunotherapy for targeting regulatory T cells in autoimmunity. Genes Immun. (2023) 24:248–62. doi: 10.1038/s41435-023-00221-y
69. Timperi E, Barnaba V. CD39 regulation and functions in T cells. Int J Mol Sci. (2021) 22:8068. doi: 10.3390/ijms22158068
70. He F, Balling R. The role of regulatory T cells in neurodegenerative diseases. Wiley Interdiscip Rev Syst Biol Med. (2013) 5:153–80. doi: 10.1002/wsbm.1187
71. Duffy SS, Keating BA, Perera CJ, Moalem-Taylor G. The role of regulatory T cells in nervous system pathologies. J Neurosci Res. (2018) 96:951–68. doi: 10.1002/jnr.24073
72. Setoguchi R, Hori S, Takahashi T, Sakaguchi S. Homeostatic maintenance of natural Foxp3(+) CD25(+) CD4(+) regulatory T cells by interleukin (IL)-2 and induction of autoimmune disease by IL-2 neutralization. J Exp Med. (2005) 201:723–35. doi: 10.1084/jem.20041982
73. Scheller J, Chalaris A, Schmidt-Arras D, Rose-John S. The pro- and anti-inflammatory properties of the cytokine interleukin-6. Biochim Biophys Acta. (2011) 1813:878–88. doi: 10.1016/j.bbamcr.2011.01.034
74. Shi LZ, Wang R, Huang G, Vogel P, Neale G, Green DR, et al. HIF1alpha-dependent glycolytic pathway orchestrates a metabolic checkpoint for the differentiation of TH17 and Treg cells. J Exp Med. (2011) 208:1367–76. doi: 10.1084/jem.20110278
Keywords: neuroinflammation, neurodegeneration, microglia, T cells, B cells
Citation: Maurya SK, Borgonovo JE, Biswal S, Martínez-Cerdeño V, Mishra R and Muñoz EM (2024) Editorial: Trends in neuroimmunology: cross-talk between brain-resident and peripheral immune cells in both health and disease. Front. Immunol. 15:1442322. doi: 10.3389/fimmu.2024.1442322
Received: 01 June 2024; Accepted: 11 June 2024;
Published: 18 June 2024.
Edited and Reviewed by:
Hans-Peter Hartung, Heinrich Heine University, GermanyCopyright © 2024 Maurya, Borgonovo, Biswal, Martínez-Cerdeño, Mishra and Muñoz. This is an open-access article distributed under the terms of the Creative Commons Attribution License (CC BY). The use, distribution or reproduction in other forums is permitted, provided the original author(s) and the copyright owner(s) are credited and that the original publication in this journal is cited, in accordance with accepted academic practice. No use, distribution or reproduction is permitted which does not comply with these terms.
*Correspondence: Shashank K. Maurya, c21hdXJ5YTFAem9vbG9neS5kdS5hYy5pbg==; bWF1cnlhLnNoYXNoYW5ra3VtYXJAZ21haWwuY29t; Rajnikant Mishra, cm1pc2hyYWFAYmh1LmFjLmlu; Estela M. Muñoz, bXVub3ouZXN0ZWxhQGZjbS51bmN1LmVkdS5hcg==; ZW11bm96QG1lbmRvemEtY29uaWNldC5nb2IuYXI=
Disclaimer: All claims expressed in this article are solely those of the authors and do not necessarily represent those of their affiliated organizations, or those of the publisher, the editors and the reviewers. Any product that may be evaluated in this article or claim that may be made by its manufacturer is not guaranteed or endorsed by the publisher.
Research integrity at Frontiers
Learn more about the work of our research integrity team to safeguard the quality of each article we publish.