- 1Guangdong Provincial Key Laboratory of Medical Molecular Diagnostics, Guangdong Medical University, Dongguan, Guangdong, China
- 2Institute of Laboratory Medicine, School of Medical Technology, Guangdong Medical University, Dongguan, Guangdong, China
- 3The Marine Biomedical Research Institute, Guangdong Medical University, Zhanjiang, Guangdong, China
The mammalian gastrointestinal tract quickly becomes densely populated with foreign microorganisms shortly after birth, thereby establishing a lifelong presence of a microbial community. These commensal gut microbiota serve various functions, such as providing nutrients, processing ingested compounds, maintaining gut homeostasis, and shaping the intestinal structure in the host. Dysbiosis, which is characterized by an imbalance in the microbial community, is closely linked to numerous human ailments and has recently emerged as a key factor in health prognosis. Tuberculosis (TB), a highly contagious and potentially fatal disease, presents a pressing need for improved methods of prevention, diagnosis, and treatment strategies. Thus, we aim to explore the latest developments on how the host’s immune defenses, inflammatory responses, metabolic pathways, and nutritional status collectively impact the host’s susceptibility to or resilience against Mycobacterium tuberculosis infection. The review addresses how the fluctuations in the gut microbiota not only affect the equilibrium of these physiological processes but also indirectly influence the host’s capacity to resist M. tuberculosis. This work highlights the central role of the gut microbiota in the host–microbe interactions and provides novel insights for the advancement of preventative and therapeutic approaches against tuberculosis.
1 Introduction
Tuberculosis (TB) is a highly contagious disease caused by Mycobacterium tuberculosis (Mtb) (1). Mtb evades the host immune system and develops drug resistance, complicating treatment. While the Bacillus Calmette-Guérin (BCG) is widely used to protect against TB, it provides limited protection in adults against pulmonary TB (2). Furthermore, mutations in genes that regulate Mtb growth and division during drug exposure can lead to antibiotic resistance, making TB difficult to treat and increasing susceptibility to lung inflammation (3, 4). Therefore, currently, preventing and treating Mtb infections remains a significant challenge in patients with TB.
The gut microbiota, which consists of microbes living in our digestive tract, plays a crucial role in various bodily functions, such as metabolism, immunity, digestion, and other aspects of the body (5). The gut microbiota influences host immunity and the penetrability and growth rate of the mucosal mucus layer, thereby becoming the first line of defense against foreign antigens (6, 7). Recent research has shown that imbalances in the gut microbiota, known as dysbiosis, can impact immune responses and lung infections (8–12). Several studies have reported that gut dysbiosis in extraintestinal diseases demonstrates the potential of indigenous gut bacteria to influence cross-organ diseases, particularly TB (3, 5, 8–13). It has been suggested that the gut microbiota communicates with the lungs, regulating lung function, managing inflammation, and providing protection against Mtb infection.
Given the link between gut microbiota and TB, it is crucial to understand this connection. This timely review discusses recent research on the involvement of gut microbiota in controlling or managing TB. We explore the role of the microbiota in the immune response, genetic regulation, and inflammation, all of which contribute to TB development. We also review practical implications from clinical studies and the potential relationship between TB and gut microbes. Furthermore, we examine the factors affecting gastrointestinal microbiota diversity, including drug usage, dietary patterns, host genetic variations, and microbial transplantation. Since gut microbiota plays a key role in maintaining microecological homeostasis, it could be a promising novel therapeutic target for TB treatment.
2 Gut microbiota–lung interaction
Symbiotic bacteria play an essential role in maintaining optimal lung immune function. A recent study showed that when dams were treated with polymyxin B during gestation, the gut microbiota of the pups altered (14). This treatment also induced a possible immune deficiency in these pups, as indicated by fewer splenic CD4+ T cells producing interleukin-2 after BCG vaccination, leading to their increased susceptibility to Mtb invasion (14). Additionally, an imbalance in the gut microbiota and mucus can cause pathological bacterial translocation, which can easily affect the extraintestinal organs (15, 16). The disruption in mucus secretion and stratification leads to “leaky gut” conditions, where intestinal permeability is increased, allowing extensive lipopolysaccharides (LPS) produced by Gram-negative bacteria (during dysbiosis) to enter the circulation (15). This process enhances lung inflammation by raising the levels of inflammatory cytokines such as IL-1β, IL-6, and TNF-α (17). A reduction in key mucins and tight junctions enhances lymphatic circulation, highlighting the enhanced crosstalk between the gut microbiota and the lungs.
The gut microbiota is recognized as a stimulator of the host immune cell repertoire. Gut bacteria can support the growth of innate immune cells by promoting hematopoiesis and regulating metabolism (18, 19). Dendritic cells (DCs) play a key role in bridging innate and adaptive immunity and primarily respond to antigen presentation among natural immune cells. The gut microbiota helps maintain the function of lung DCs, as it enables robust recognition and defense against Mtb infections (20–22). Through the secretion of microbial metabolites, the induction of inflammatory responses, and the regulation of immune reactions, the influence of gut microbial activity extends to the lungs via circulation (23). This process affects how immune cells respond and how well they can fight off pathogenic respiratory bacteria.
Interactions among the gut, microbes, and lungs help maintain the microecological balance of the body to fight against Mtb. Short-chain fatty acids (SCFAs), produced by the gut microbiota, have been implicated in the construction of the intestinal barrier and respiratory immunity. For example, succinate, a precursor of SCFAs, promotes pulmonary alveolar macrophage polarization and alveolar epithelial cell apoptosis during intestinal ischemia–reperfusion lung injury (24). Thus, succinate helps defend against pathogens. Butyrate, a SCFA, promotes the polarization of M2 macrophages, which helps reduce ongoing Mtb infection (25, 26). Research indicates that when M2 macrophages are primed by butyrate, the expression of goblet cell marker genes, including mucin2 (MUC2) and SPDEF, significantly increases in these immune cells (25, 26). Importantly, this process results in increased amounts of mucus, demonstrating the promising effects of microbial metabolites in repairing the mucosal barrier and pathogen clearance. SCFAs produced by the microbiota also enable signal transduction, thereby preventing excessive inflammation and tissue damage during TB infection. This process involves the promotion and proliferation of innate lymphoid cells (e.g., ILC3) via the metabolite-sensing Ffar2 receptor, which increases the expression of alveolar macrophages and enhances pathogen-killing against Mtb infection (27, 28).
These findings suggest that targeting the gut microbiota likely enhances the host immunity and reduces susceptibility to disease. The gut microbiome impacts overall health, with gut–lung interactions highlighting the need for interdisciplinary teamwork across microbiology, immunology, and respirology. However, more research is needed to fully understand the regulatory mechanisms associated with microbial stimuli.
3 Gut microbiota in TB infection
In patients with active TB infection or latent TB infection, two prominent gut phyla—Firmicutes and Bacteroidetes—show correlation with inflammatory biomarkers like IL-1β and IL-4 (29). Specifically, patients with active TB show a lower Firmicutes-to-Bacteroidetes (F/B) ratio, indicating a potential connection between inflammation, gut microbes, and TB development (30). Moreover, Mtb infection disrupts microbial metabolic pathways, leading to an elevated likelihood of inflammation and worsening TB. Compared to healthy individuals, patients with TB exhibit decreased biosynthesis of amino acids and fatty acids (31, 32). Notably, nucleotide metabolism pathways are significantly more active in patients who are not on antibiotics (the antibiotic-free TB group), with the greatest fold changes in related genera, such as Enterococcus, Clostridioides, and Rothia (32). These metabolic changes indicate altered energy transformation in patients with TB, which may be restored by sustaining certain prebiotics.
During the inflammatory response, the microbial metabolite like SCFAs regulate signaling pathways and influence metabolic switching mechanisms. Upon stimulation by the Mtb antigen, SCFAs inhibit the production of IFN-γ and IL-17, which modifies the microenvironment of the lung microbiota (33). While proinflammatory cytokines such as IFN-γ and IL-17 normally help limit inflammation by activating immune cells and controlling the pathogen spread, their reduced levels during Mtb infection impair the early immune response against TB (34, 35). This observation is consistent with the increased abundance of SCFA-producing anaerobes (36). Increased levels of SCFAs are also associated with the activation of TB-induced regulatory T cells (Tregs), which impede Mtb invasion and reduce lung lesions and bacterial load, helping to prevent excessive inflammation and promote immune tolerance (37). Mechanistically, SCFAs interact with cell-surface receptors like G-protein-coupled receptor 43 (GPR43), profoundly affect the inflammatory response, and improve recovery from inflammation (38).
Accordingly, the inflammatory trajectory of a disease may be determined by the type and quantity of gut flora that confer great benefits. Notably, some studies have suggested that an increase in the relative abundance of the genus Bacteroides is the main cause of gut microbiota dysbiosis in patients with pulmonary TB (39, 40). High levels of Bacteroidetes are positively associated with polymorphic neutrophils in the blood of patients with active TB (29). For example, Bacteroides fragilis, a prominent species of the Bacteroides genus, and its capsular polysaccharide-A (CPS-A) were shown to inhibit pulmonary inflammation by promoting IL-10 production by CPS-A-responsive T cells and antigen-presenting cell-mediated CPS-A processing (41). Moreover, the administration of B. fragilis or polysaccharide (PSA) to germ-free mice can restore the Th1/Th2 cytokine balance by boosting the production of Th1 cytokines, including IFN-γ and IL-2 (42). Specific microorganisms that exhibit conspicuous alterations in inflammation can interfere with the disease progression.
Anaerobes are found in higher levels in the sputum and stool of patients with pulmonary TB and are correlated with the activation of proinflammatory immune pathways, such as interferon and Nur77 signaling, as well as inflammasome pathways (8). Certain pathways have shown a positive correlation with the presence of the genus Anaerostipes bacteria in the gut (43, 44). In recent years, there has been a growing interest in the role of Akkermansia muciniphila in inflammatory diseases. Metabolites originating from A. muciniphila, such as the tripeptide Arg-Lys-His, the outer protein Amuc_1100, and palmitoleic acid, have been shown to have positive effects on mice by regulating metabolism and cellular signaling (45–47). Furthermore, a decrease in the abundance of A. muciniphila in the gut microbiota of patients with active TB is negatively correlated with low-grade inflammation, indicating the involvement of regulatory T cells and Toll-like receptors in mediating the immune responses (47, 48).
TB recurrence is often linked to immune fatigue and the suppression of inflammation. Compared to healthy individuals, patients with TB specifically exhibit reductions in beneficial bacterial genera, such as A. muciniphila and Bifidobacterium, and an increase in potentially harmful bacteria within the Proteobacteria and Actinobacteria phyla in the gut (32, 40, 47). However, changes in microbial populations can vary based on disease states and dietary habits, and changes in the abundance of microbial populations are not necessarily absolute (49, 50).
Overall, gut dysbiosis in patients with TB represents a complex stalemate between Mtb invasion, immune activation, and gut microbiota (Figure 1). Gut microbiota and their metabolites affect different immune cells, mediating pro- or anti-inflammatory signaling, disease development, and recovery. They play a significant role in the occurrence and development of diseases, not only influencing the diagnosis and treatment of diseases but also being capable of predicting clinical outcomes and prognosis and providing novel immunotherapies. Further studies on the types of metabolites produced by the gut microbiota and the underlying mechanisms could clarify the role of the microbiota–immune axis in TB management.
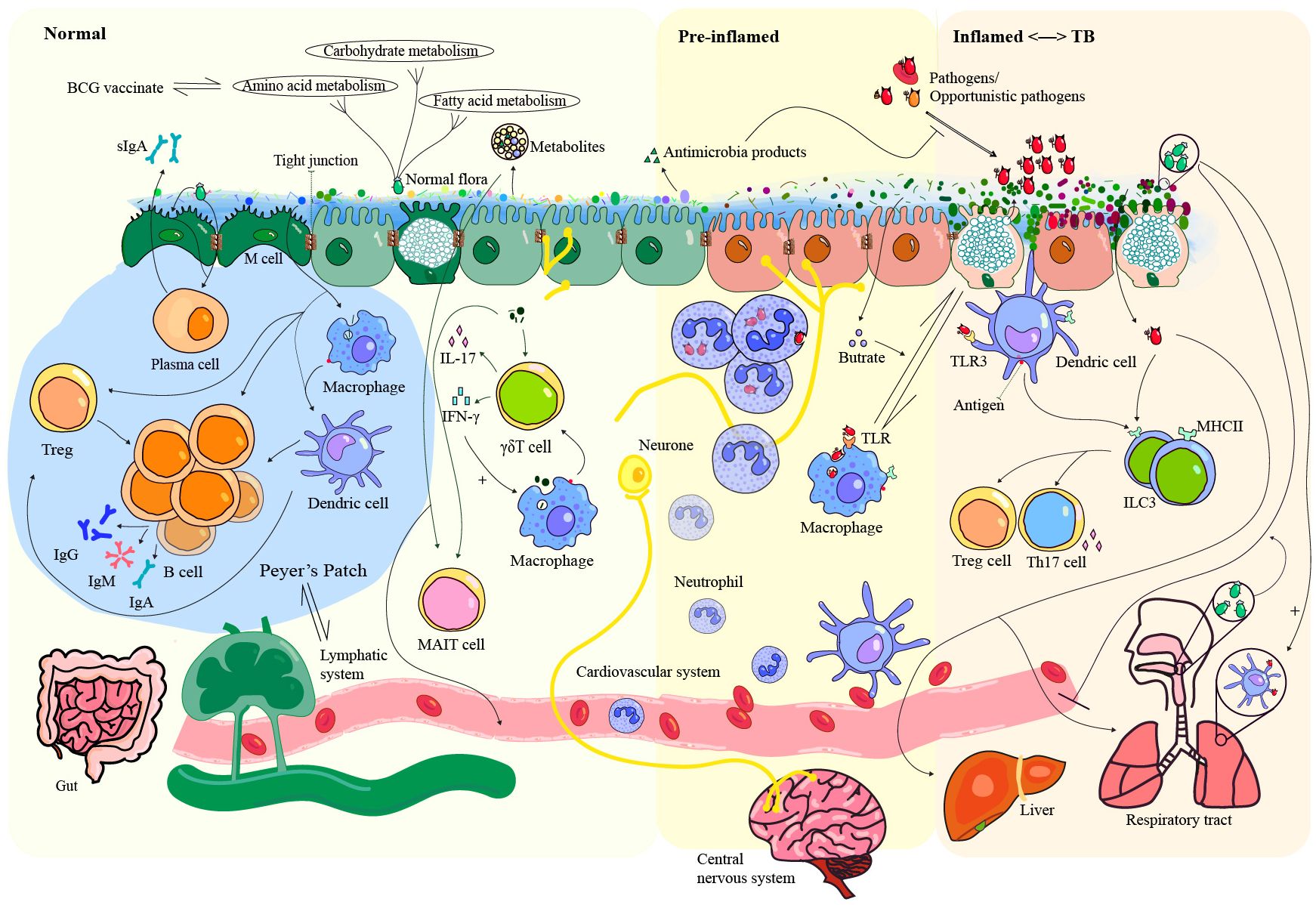
Figure 1. Interaction between microbiota, pathogens, and host immunity in health and TB-related inflammation. The gut microbiota plays a critical role in the construction of the mucosal barrier by interacting with immune cells, contributing to colonization resistance, and secreting metabolites that help maintain homeostasis. Close contact between luminal microbiota and epithelial cells triggers an immune response that can lead to inflammation and increase the likelihood of attack by opportunistic pathogens. Partial immune evasion by these pathogens can allow them to spread to organs such as the liver, lungs, and brain via the bloodstream or lymphatic circulation. Importantly, the commensal microbiota in both the gut and respiratory tract helps prevent lung infections by stimulating lung dendritic cells. The gut microbiota exhibits great potential as a reflector and modulator of TB. TLR, Toll-like receptors; Treg, regulatory T cell; MHCII, major compatibility complex II; ILC3, group 3 innate lymphoid cell; MAIT cell, mucosal-associated invariant T cell.
4 Gut microbiota in latent TB and drug-resistant TB
Latent tuberculosis infection (LTBI) is asymptomatic and poses a challenge for the timely detection of this condition. A healthy gut microbiota supports immunity and nutrient absorption, aiding the body to defend against TB. It also undergoes functional changes at different stages of the disease, which can influence TB pathogenesis. Dysregulation of the gut microbiota might be an early immune response to Mtb invasion, which likely occurs before inflammation begins. In a related study, patients with latent TB showed high levels of Bacteroidetes in their gut and an increased number of polymorphic neutrophils in their blood, though without inflammation (29). In addition, the abundance of Coriobacteriaceae appears to be associated with CD4+ T cells and the expression of IFN-γ (29). Therefore, a strategic approach for predicting the development of TB using microbiota with innate immunity-modulating properties holds promise. However, some studies reported no significant differences in gut microbiota composition between individuals with LTBI and healthy controls (49). Thus, further research is needed to understand the potential role of the gut microbiota in diagnosing latent TB.
Interestingly, changes in the gut microbiota have been reported by certain studies prior to the diagnosis of latent TB. Distinctive gut microbial signatures have been observed not only between LTBI patients and healthy individuals but also between individuals exposed to patients with TB and those who are not (49, 51). This study highlights the sensitivity of the gut microbiota to pathogen exposure and its potential for the early diagnosis of TB.
Interestingly, the composition and abundance of the gut microbiota appear to be an indicator of tuberculosis drug resistance. Multidrug-resistant TB (MDR-TB) is challenging to treat. A study conducted in patients with pre-extensive drug-resistant TB revealed higher levels of Enterobacteriales, Bifidobacteriales, Verrucomicrobiales, and Lactobacillales in the coliform flora (52). This microbial imbalance in the gut microbiome was linked to elevated de-novo fatty acid synthesis in patients with MDR-TB, with Bacteroides displaying significant correlations. Both drug resistance and immune evasion mechanisms contribute to the formation of biofilms by Mtb, contributing to its survival (53). Therefore, identifying the key factors that contribute to the increased susceptibility to multidrug-resistant TB, such as alterations in the gut microbiota and metabolic changes resulting from long-term antibiotic use, can help in predicting and treating this form of TB. The gut microbiota may affect the absorption, distribution, metabolism, and excretion of anti-TB drugs, thereby influencing the drug efficacy. By adjusting the composition of gut bacteria, theoretically, it is possible to change the intestinal microbial ecosystem, impacting the growth and reproduction of Mtb and even enhancing the drug activity against the bacteria.
5 Gut microbiota in TB control and prevention
5.1 Development of the TB vaccine
The gut microbiota significantly affects vaccine efficacy and plays a crucial role in preventing TB infections and fostering the development of a robust immune memory. BCG, a TB vaccine commonly employed in mass inoculations, offers cross-immunoprotection against Mtb infections in humans (54). However, its protective effect is limited in adults. A recent study found that the live BCG vaccine upregulated the expression of MHCII, TLR2, and genes involved in antigen presentation and processing in lung alveolar macrophages, compared with phosphate-buffered saline and heat-inactivated BCG (12). By spreading mycobacteria to proximal gut sites, parenteral administration of the BCG vaccine induces decreased microbial diversity, significant enrichment of Lactobacillaceae, irregular distribution of tight junction proteins, elevated levels of butyrate, and the development of mild colitis (12). Transplanting BCG-conditioned microbiota into naive mice elevated MHC II, IL-6, and TNF production in airway alveolar macrophages and triggered anti-TB-trained immunity, with similar effects observed in lung tissue (12). These findings shed light on the potential link between the vaccine response, trained immunity, and gut microbiota, providing valuable insights into the development of robust immune memory for future vaccine design.
Gut dysbacteriosis can reduce the efficacy of the BCG vaccines by inhibiting the function of Mtb-specific T cells, resulting in suboptimal pathogen clearance (55). However, trained immunity has valuable heterologous effects in TB vaccines (11). Reprofiling the gut microbiota can improve the trained and specific immune responses following vaccination. The gut microbiota and their metabolites can train cycling monocytes and modify the bactericidal function of alveolar macrophages, thereby affecting Mtb invasion (56). Both specific immunity and SCFAs produced by certain genera, such as Eggerthella lenta and Roseburia, are involved in immune regulation (57). For example, amplification of Roseburia levels has been shown to reduce the cytokine response and modify the phenylalanine pathway after BCG vaccination (57). These findings have established a direct link between BCG-altered microbiota and the activation of memory immune cells.
Immune cells exhibit the ability to respond to microbiota, suggesting that gut microbiota could help develop TB vaccines with enhanced immunogenicity (58). Identifying and cultivating the microbial species that can mediate and facilitate the interactions between innate and adaptive immune responses is crucial. Through their interaction with particular immune cells, certain gut microbiota help build a robust immune memory, which plays a key role in protection against TB.
5.2 How to prevent TB relapse
Research on gut microbiota in patients with recurrent tuberculosis is quite limited. One study demonstrated that patients with relapsing TB have higher gut microbiota diversity compared to that of healthy controls, distinguishing them from new-onset cases (40). Reduced abundance of Bacteroidetes and increased abundances of Actinobacteria and Proteobacteria are strongly associated with TB recurrence. To gain better insights into the potential use of microbiota in predicting TB recurrence, future studies should include larger sample sizes and exclude non-major factors.
Dysbiosis of the gut microbiota caused by antibiotic treatment does not resolve after disease resolution, leading to persistent symptoms and an increased likelihood of TB recurrence (10, 14, 59). Gut microbiota may significantly affect the occurrence and development of TB (49). Consequently, there is a growing focus on studying changes in the microbiota at different stages of disease progression. The influence of various factors such as geography, dietary habits, age, constitution, and coexisting diseases on the gut microbiota has been investigated through comprehensive microbiota assessments conducted across diverse populations (60–62). Additionally, long-term aggressive regimens have proven effective in reducing the risk of recurrence in patients with MDR-TB (63). Maintaining the homeostasis of gut microorganisms has positive implications for both host prognosis and the anticipation of the recurrence of Mtb infection. TB-related microbiota are shown in Figure 2.
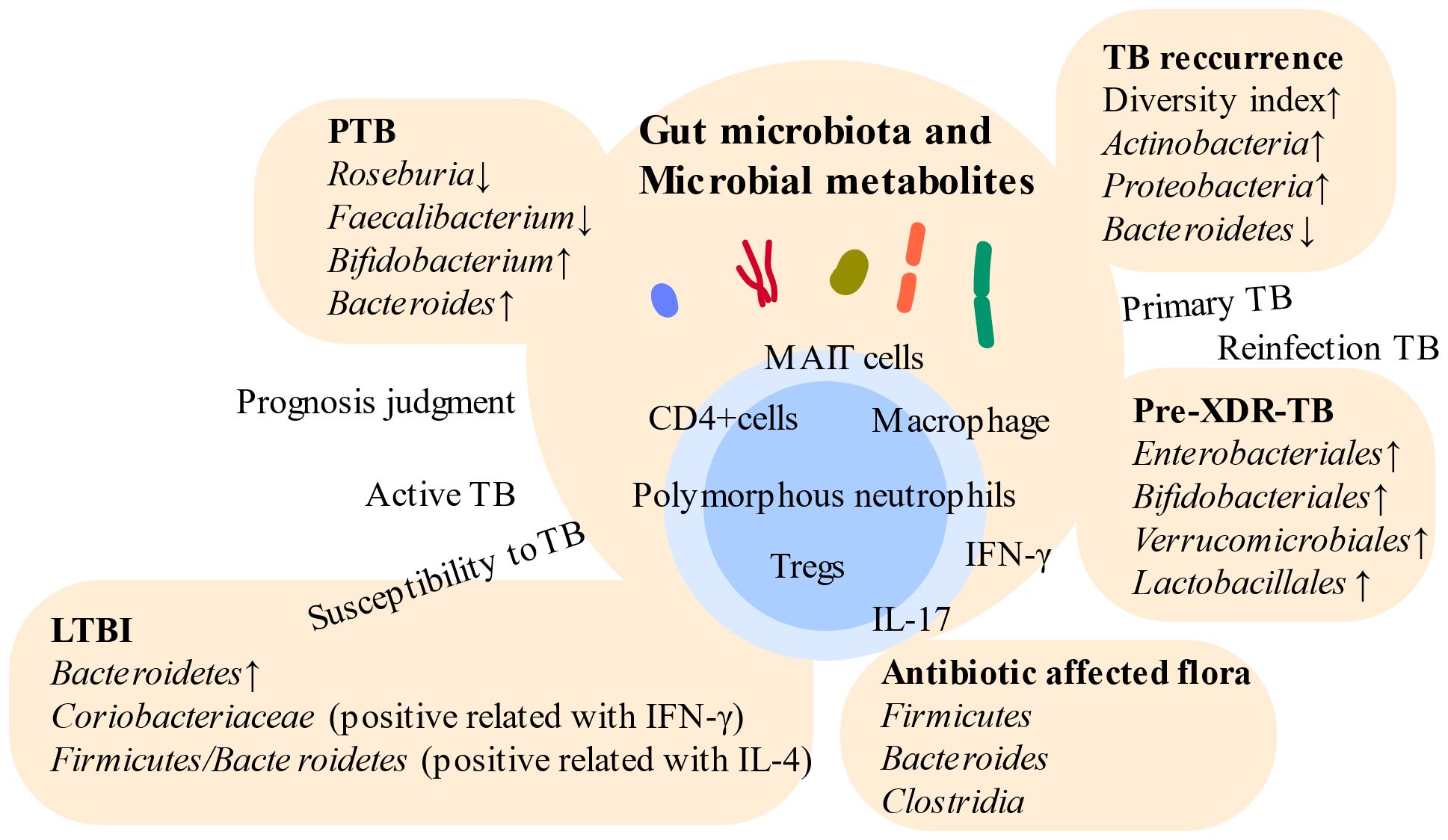
Figure 2. Relationships between gut microbiota and distinct TB. Gut microbiota vary across different stages of TB. Intestinal microbes become potential targets for indicating different states of TB and boosting immunity. However, high individual variation poses challenges in utilizing gut microbiota-based approaches for the diagnosis of TB. PTB, pulmonary tuberculosis; LTBI, latent tuberculosis infection; Pre-XDR-TB, pre-extensive drug-resistant tuberculosis; Tregs, regulatory T cells.
6 Considerations in the study of gut microecology in TB
6.1 Appropriate modeling
Selection of an appropriate model for studying the gut microbiota is crucial in TB research. The differences observed in the gut microbiota status between pulmonary and extrapulmonary tuberculosis, along with the severe pulmonary injury observed in Helicobacter hepaticus-colonized Mtb-infected mouse models, emphasize the importance of investigating multiple body sites and the varied outcomes associated with different commensal bacteria (64, 65). Notably, compared to pulmonary tuberculosis (PTB), extrapulmonary tuberculosis (EPTB) shows a greater association with drug resistance, underscoring the importance of examining gut microbiota in both forms of tuberculosis to elucidate the potential mechanisms underlying drug resistance (64).
Combined disease models may be helpful in revealing the TB characteristics of gut microecology. Typically, individuals who are highly susceptible to tuberculosis often have concurrent underlying health conditions. For example, diabetes mellitus is a critical risk factor for TB (51). Studies involving Mtb-infected mice with or without type 2 diabetes (T2D) have revealed distinct clusters in the gut microbiome, indicating the influence of metabolic disorders on the gut microbiota and their impact on respiratory disease susceptibility (66). Comorbid mouse models may provide insights into immune parameters, the metabolome, and the microbiome, thereby illuminating the complexities of the gut–lung axis. Further research is required to determine the validity and reliability of the gut microbiota as an indicator of host differences in various diseases and conditions.
6.2 Genetic calculations
Host genetics has been found to affect TB infection and lead to differences in microbiota characteristics (34). If strong correlations can be clearly established between host gene expression and the gut microbiota and between the genetic composition of the microbiota and desirable host traits, this could facilitate selective breeding for an optimum microbiome.
Gut microbes can influence genetic modifications by interacting with the genetic machinery of host cells (Figure 3). Disruption of the microbiota and its metabolites, especially SCFAs, may disrupt tight junctions and lead to a deficiency of Foxo1 in propria cells (67). SCFAs are involved in the regulation of gene expression, promotion of immune cell survival, and phagocytic activity enhancement, partly by inhibiting histone deacetylases (HDACs) (68). Among these metabolites, β-hydroxybutyrate, an inhibitor of HDACs, promotes intestinal cell differentiation and supports gut homeostasis by inhibiting mTOR signaling (69). SCFAs transmit signals into cells via G-protein-coupled receptors (GPCRs) and through transporters such as monocarboxylate transporter 1 (MCT1) and sodium-coupled monocarboxylate transporter 1 (SMCT1) (70). These receptors and transporters are highly expressed in colonocytes, but their potential involvement in regulating TB regulation requires further research.
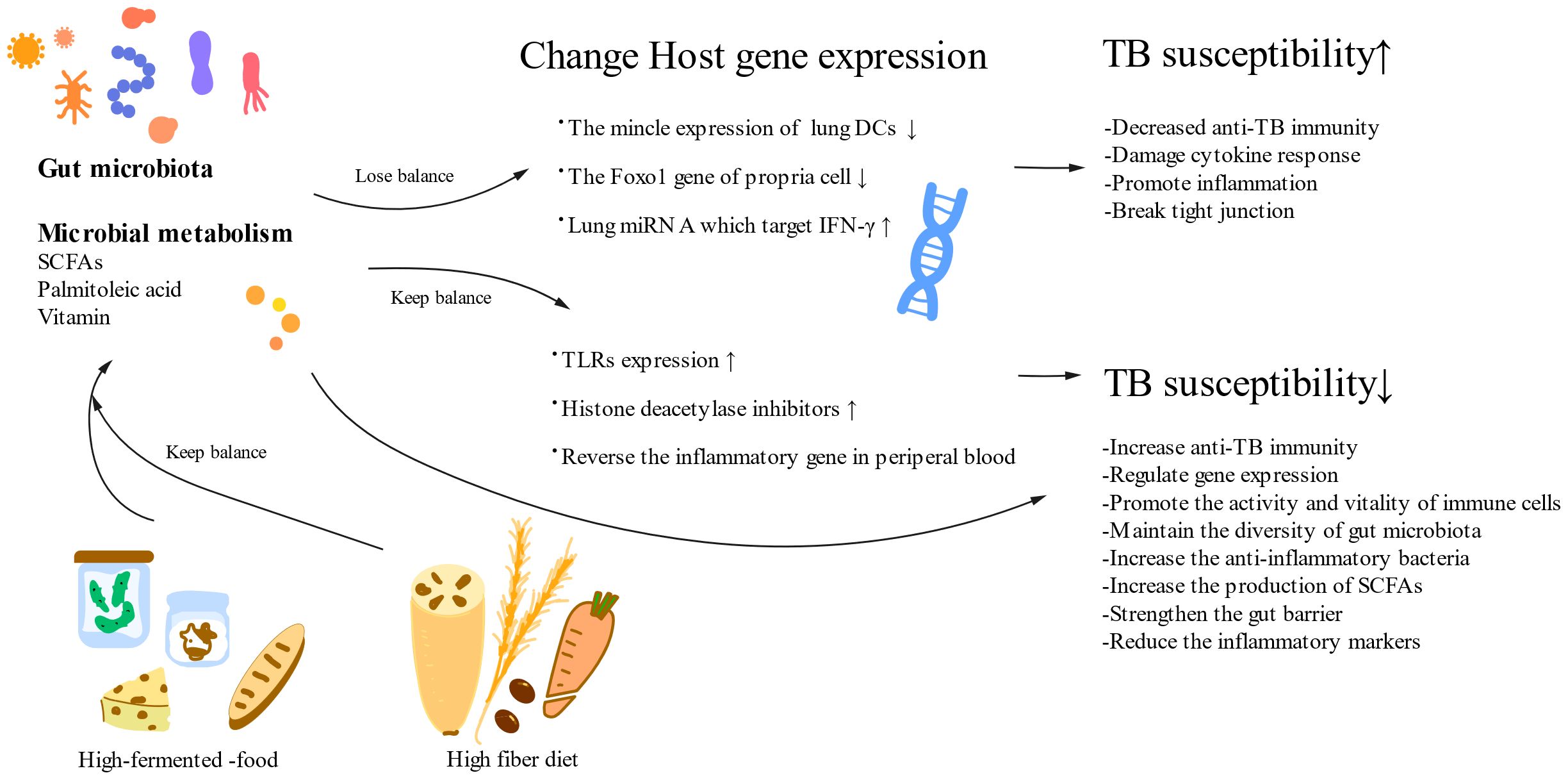
Figure 3. A healthy diet and normal gut microorganisms reduce TB susceptibility. A high-fermented food and a high-fiber diet can positively impact the gut microbiota and its metabolism, which can influence TB susceptibility through their effects on host genes. Gut microbiota changes anti-TB immunity by influencing host gene expression. SCFAs, short-chain fatty acids; DCs, dendric cells; TLRs, Toll-like receptors.
From a broader perspective, gut microbiota are involved in strengthening respiratory defenses against pathogens by activating antigen recognition. Specific bacteria can stimulate Nod-like receptors in the upper airway and gut, defending against lung infection via the activation of Nod2 and granulocyte-macrophage colony-stimulating factor (GM-CSF) (71). Mechanistically, the NOD2 gene encodes a host sensor of a bacterial cell wall component called muramyl dipeptide (MDP), which is positively associated with CD103+ DCs in the gut lamina propria and stimulates GM-CSF production (72). Additionally, certain gut microbiota can induce the expression of Toll-like receptors to recognize Mtb cell wall components and initiate innate immune responses (73). Mtb stimulates the expression of the Irg1 gene in macrophages either by activating the STING pathway to produce type I IFN through the release of Mtb products or by inducing signaling dependent on TLR-2, MyD88, and NF-κB. This finding implies the potential utility of probiotics in increasing self-resistance to tuberculosis through the gut–lung axis and their promising role as therapeutic agents for intervention.
MicroRNAs play crucial regulatory roles in cellular functions and are influenced by the gut microbiome. The burden of bacilli and pathological injury can be reduced by IRF7-mediated miRNA-31, which upregulates positive genes in the lungs (74). Additionally, both gut dysbiosis and M. tuberculosis infection upregulate the expression of lung miR-21 (75). Specifically, the expression of miR-21-3p has been found to impair anti-TB immunity by targeting IFN-γ mRNA and downregulating the levels of IFN-γ, as indicated by the commensal flora (75). A recent study identified B. fragilis as a direct regulator of protective immunity against TB through modulation of lncRNA-CGB (3). lncRNA-CGB functions as a negative regulator of H3K27 trimethylation (H3K27me3) epigenetic programming, ultimately enhancing IFN-γ expression by interacting with EZH2, thereby providing anti-TB immunity (3). Consequently, exploring the inhibition of such miRNAs by the commensal microbiota is a promising therapeutic approach for enhancing host protective immunity against TB. Actually, miRNA and gut microbiota interact with each other. Gut microbiota intervention may offer another way to overcome the technical challenges faced by miRNA therapy, such as low delivery efficiency and instability in the body.
Distinct profiles of the gut microbiota are linked to inherent TB susceptibility, which is driven by host genetics. Risk alleles and age-at-onset play crucial roles in the progression of PTB. Genetic variations in humans, such as single nucleotide polymorphisms (SNPs), are correlated with the susceptibility to PTB (76). Genetic changes alter gut-colonizing microbes. For instance, in Th2 gene knockout mice, alterations in the structure of the gut microbiota have been observed, coexisting with enhanced fat metabolism through the increased expression of uncoupling protein 1 (UCP1) and GPRs (77). In addition, the Ifnar1 rs2257167 G allele in the host is associated with an increased susceptibility to TB, which is characterized by a decreased abundance of A. muciniphila in patients with active TB (47). Understanding the mechanisms underlying individual variations in immunity and the gut microbiota may help to evaluate TB susceptibility.
6.3 Dietary intake
Dietary adjustments have the potential to induce alterations in the gut microbiota, turning the gut into a bioreactor (78). The gut microbiota are influenced by long-term dietary habits, and they can rapidly respond to changes in diet, exhibiting unique communities related to metabolism and composition (79). Clinical trials have shown that the introduction of microbially driven complementary food and low-calorie Mediterranean and vegetarian diets can cause substantial changes in the composition of the gut microbiota at the genus level and affect various biochemical parameters (80, 81). Food has a positive impact on plasma proteomic biomarkers associated with growth, development, and immune function (81).
Food combinations are linked to the gut–lung axis via microbial metabolism. The expression of the aryl hydrocarbon receptor (AhR) in pulmonary endothelial cells is induced by foodborne ligands, such as indole and tryptophan (82). AhR is a ligand-activated transcription factor that maintains the stability of the pulmonary barrier and eliminates infections. However, a high-fat diet depletes tryptamine and indole-3-acetate levels (83). Metabolism of these metabolites is dependent upon the microbiota and may reduce the levels of proinflammatory cytokines induced by LPS in macrophages in an AhR-dependent manner (83). Dietary interventions hold great promise for the treatment of metabolic diseases. Moreover, infectious diseases commonly coexist with metabolic abnormalities, such as decreased levels of SCFAs, amino acids, and fatty acids, in patients with TB (32). These studies further emphasize the potential influence of diet on health and its possible interactions with the gut microbiota.
In addition, research suggests that the gut microbiota can either activate or deactivate host genes in response to dietary stimuli (Figure 3) (84). The establishment of immune tolerance to food antigens has been linked to the location, diversity, and metabolic functions of the gut microbiota (85). The ingestion of highly fermented foods enhances gut microbial diversity and reduces inflammatory markers, thereby offering benefits in the context of TB treatment (86). In summary, understanding the interplay between dietary patterns, gut microbiota equilibrium, and disease is vital for manipulating the gut microecology through dietary interventions, ultimately offering potential therapeutic benefits for TB.
6.4 Fungi and viruses
In addition to gut bacteria, the role of fungi and viruses in the gut in relation to tuberculosis is also worthy of in-depth investigation. Fungi provide several benefits to the body, including anti-inflammatory effects, barrier construction, and modulation of the gut microbiota composition (87). Yeast can be degraded by certain bacteria to release mannans, which helps to prevent infections and reduce the occurrence of immune diseases (88). A previous study revealed that the diminished network after anti-TB treatment may be rebuilt by the enrichment of fungal nodes (89). Implementing adjunctive therapies that focus on curbing fungal proliferation and fostering the growth of beneficial bacteria after anti-TB therapy is crucial for accelerating the recovery of patients with TB.
Host–bacterial–fungal interactions are strongly associated with inflammation. An intriguing study revealed the anti-inflammatory properties of sophorolipid, a glucolipid compound synthesized by yeast species such as Candida bombicola, competitively bind to TLR4/MD-2, outcompeting LPS and inhibiting IKKβ activation via hydrogen bonding and hydrophobic interactions (90). This mechanism effectively suppresses inflammatory signaling. Another yeast species, Candida albicans, secretes a peptide toxin called candidalysin during its transition to a pathogenic state, reflecting aggravated intestinal inflammation through IL-1β-dependent mechanisms (91). However, the fecal bacterial dysbiosis and local inflammation induced by Candida may be attenuated by certain bacteria, such as Lactobacillus rhamnosus L34 (92). In summary, fungi regulate inflammation and interact with bacteria in the gut, thereby participating in disease management.
Viruses are also involved in intestinal homeostasis. Antigen-presenting cells recognize the cytosolic RNA of commensal viruses via the MAVS–IRF1–IL-15 axis to maintain the balance of intestinal intraepithelial lymphocytes (IELs) (93). Additionally, both antiviral treatments and deficiency of RIG-I—a receptor for cytosolic viral RNA—alter gut bacteria in mice (93). Membrane vesicles derived from the gut microbiota can deliver DNA into distal host cells via circulation and prime antiviral immunity by activating cGAS-STING (94). The interactions among viruses, bacteria, and the immune system are worth exploring by researchers in terms of the role of commensal viruses in infectious diseases.
Intestinal phages target pathogens and reduce inflammation. Phages are abundant in the human gut; however, most remain uncultured (95). The oral administration of phages that target certain pathogens may potentially inhibit inflammation and disease exacerbation (96). Moreover, phage predation-induced alterations in the microbiota have a significant impact on the gut metabolome (97). By specifically targeting certain bacteria, phages not only control the growth of pathogens but also promote the proliferation of beneficial flora that are advantageous in combating TB, thereby offering a novel perspective for improving the intestinal microbiome of patients with TB.
Currently, the application of phages targets multiple aspects of TB infections, including vaccine development, TB diagnosis, and the detection of drug resistance (98, 99). Carrier-mediated phage delivery aids in the recognition of intracellular bacteria (100, 101). However, in the context of gut microbiome modulation, phages may exhibit several potential drawbacks such as excessive specificity, the potential spread of drug resistance, triggering of the host immune response, disruption of ecological balance, and unpredictability. In conclusion, under appropriate conditions and reasonable application, phages may also be beneficial regulatory tools for TB management.
7 Gut microbiota: blueprint for the fight against TB
7.1 The usage of antibiotics
Antibiotic treatment not only modulates inflammatory gene expression in the peripheral blood, but also disrupts the gut microbiome by reducing its biodiversity and altering the composition of the gut microbiota, leading to pronounced and sustained dysbiosis (34, 89). Anti-TB drug treatments affect various intestinal microorganisms, including those belonging to the phylum Firmicutes and the genus Bacteroides, particularly the class Clostridia among anaerobic Firmicutes, which are the major phyla disrupted by HRZE therapy, which consists of isoniazid, rifampicin, pyrazinamide, and ethambutol (39, 102). Conversely, extreme alterations in the microbiota caused by broad-spectrum antibiotics may directly or indirectly affect the efficacy of anti-TB drugs (103). A deficiency in the gut microbiota also disrupts host immunity and impacts the host’s response to antibiotics, including a decrease in inflammatory, IFN-α, and IFN-γ responses (102). After antibiotic cessation, the residual impact on microbiota can persist for up to 3 months, and a significant proportion of the altered microbial taxa is associated with modifications in immunological functions (104). In mice infected with Mtb, mucosal immune function is weakened by antibiotic treatment, as indicated by a reduction in MAIT cells (105). Notably, high susceptibility to severe infections can be induced by the broad immunological effects of antibiotics.
Antibiotic-induced dysbiosis appears to cause metabolic dysfunction. Exposure to antibiotics is associated with the depletion of microbes that produce SCFAs (32). Butyrate has been shown to influence the expression of anti-inflammatory and proinflammatory factors that regulate macrophage function in clearing Mtb (32). Specifically, the abundance of Actinomyces, which are involved in butyrate production through the modulation of its precursors such as acetate and succinate, decreased during the intensive and continuation phases of second-line anti-TB treatment (106). In addition, the abundances of certain Prevotella species and Megamonas hypermegale, which are associated with glucose metabolism and SCFA production, decreased. Broken early resistance in the lungs is commonly observed in Mtb-infected mice due to dysbiosis of the gut microbiota caused by broad-spectrum antibiotics (105). Adverse outcomes caused by dysregulated microbiota may contradict to the benefits of anti-TB treatment.
Inflammation recovery may be observed when the microecological damage caused by antibiotics is improved. In one study, the normalization of inflammation and clearance of Mtb were associated with increased numbers of cluster IV and XIVa Clostrida, along with reductions in Bacilli and Proteobacteria, which were triggered by antibiotic treatment (102). Recovery from dysbiosis caused by continuous drug treatment affects immune cell proliferation and autophagic processes, reflecting the outcomes in patients with TB. Moreover, although short-term antibiotic treatment may not significantly alter gut microbiota in all infections, its effects still require further consideration (13). The dynamic and long-term effects of antibiotics on gut microbiota require further study in a diverse and representative population.
7.2 Healthy lifestyle and dietary habits
Assessing species abundance is essential to determine whether a species plays a dominant role in the disease process. Notably, various factors, including but not limited to those mentioned previously, influence the modulation of the microbial community and the identification of specific disease-associated microorganisms within the ecosystem, highlighting the bidirectional relationship between habits and TB progression (Figure 4).
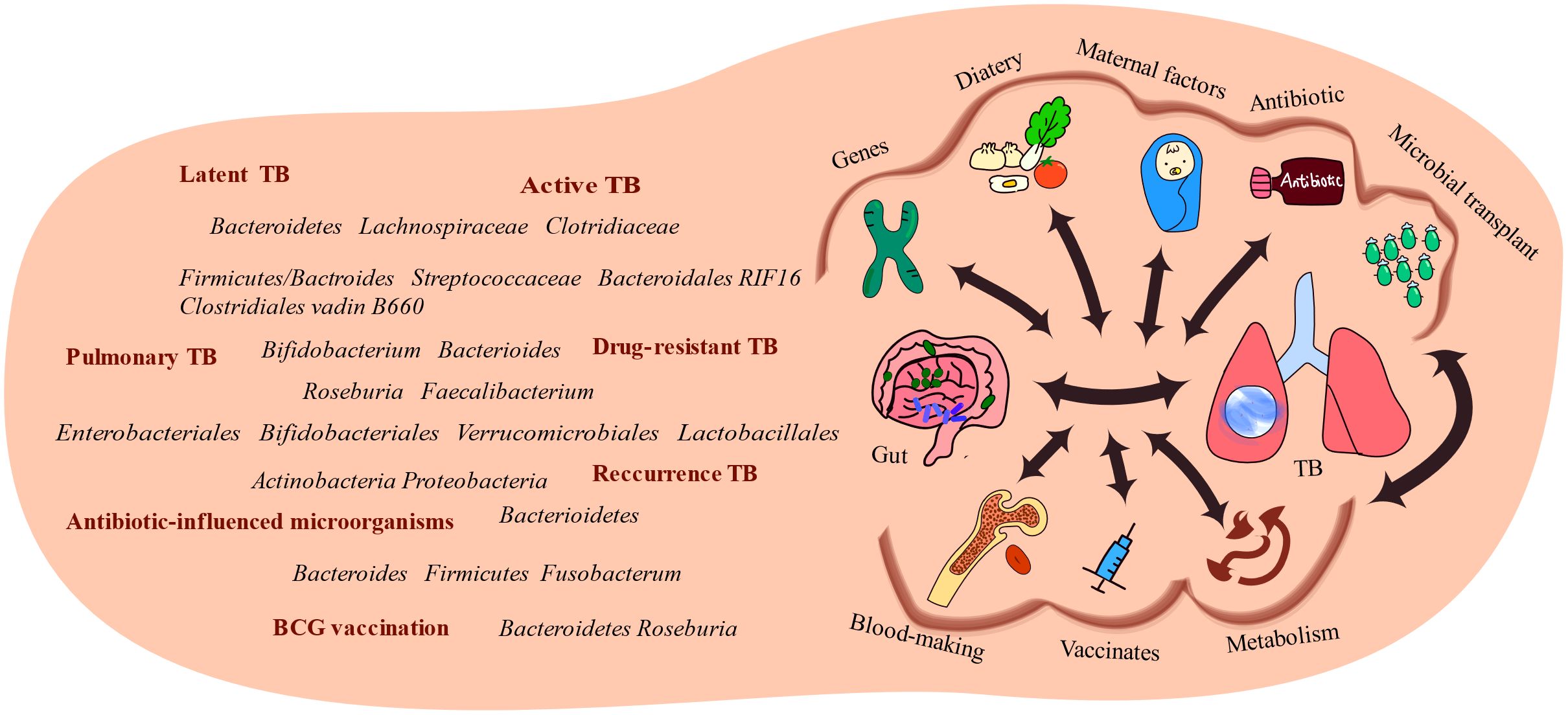
Figure 4. Several factors link the gut and the lungs. Alterations in gut microbiota have been observed in TB patients, highlighting the association between the gut microbiota and diseases. Several factors influence the interactions between gut microbiota and TB-infected organs, including genetic predispositions, dietary factors, material factors, antibiotic usage, and microbial transplantation. These factors influence the development and progression of TB.
Smoking affects disease susceptibility and alters intestinal microbiota composition. Cigarette smoking is a major global health threat that contributes to a significant number of deaths annually. In mice, smoking and cessation disrupted the gut microbiota community due to the entry of cigarette smoke-related metabolites into the intestine, leading to dysbiosis (107, 108). The connection between gut microbiota and weight gain associated with smoking cessation has been demonstrated through gut microbial transplantation, and similar effects are seen following microbiome depletion by antibiotics. Dysbiosis induced by smoking is accompanied by increased bile acid metabolites as well as elevated oncogenic MAPK/ERK signaling, resulting in impaired gut barrier function (108). Conversely, specific gut microbes also influence the risk of cigarette smoking. A previous study suggested that a decreased abundance of Actinobacteria due to smoking may worsen smoking status, potentially exacerbating the reduction in Actinobacteria abundance, indicating a potential positive feedback effect (109). The negative effects of smoking not only directly impact the lungs but also regulate susceptibility to further diseases by influencing gut microbiota.
It is important to recognize the wide individual variations in gut microbiota composition due to lifestyle, diet, and environment to identify disease-associated microbiota. In a study by Ivan Vujkovic-Cvijin et al., two host variables that appear to strongly affect the correlation between the gut microbiome and human diseases were identified: bowel movement quality and alcohol consumption (110). They proposed a profile of host variables; this profile serves as an insightful guide to minimize the “background” of individual differences in gut microorganisms and facilitate the identification of genuine disease states. Moreover, selecting specific time points for sample collection is crucial for minimizing the impact of circadian rhythm variations (111).
7.3 Fecal microbiota transplantation
Cutting-edge studies on fecal microbiota transplantation are offering valuable insights into disease management. Fecal microbial transplantation (FMT) has been utilized in various therapeutic applications to normalize the gut microbiota and improve physical function via the circulatory system to improve host metabolism, assist in immunomodulation, and address microecological imbalances caused by antibiotics, ultimately leading to positive outcomes (112, 113). Restoring a healthy gut microbial composition is a fundamental concept behind curing diseases.
The benefits of fecal bacterial transplantation further demonstrate the impact of the gut flora on extraintestinal organs. FMT, particularly with Bifidobacterium, can result in isoniazid-induced liver injury (23). However, it also confers benefits to overall health and reverses inflammation induced by specific bacteria, such as Citrobacter, in mice (77). This finding suggests a potential role for gut flora in modulating disease prognosis.
Microbial transplantation may not be suitable in all situations. In healthy individuals, there were no significant differences in the diversity and composition of gut microbiota before and after transplantation (112). Nonetheless, improvements in the gut microbiota were observed specifically in individuals with a low baseline microbial diversity (112). Low baseline microbiota diversity may partially reflect energy abnormalities without impairing the metabolic status. In the context of tuberculosis, it is important to consider the distinct benefits of short- and long-term microbial transplantation. In addition, autologous FMT has more benefits than heterologous FMT and may be a potential option in the future (114).
7.4 Microbial products
Maintaining the metabolic balance helps restore the microecology of the intestinal flora, thus preventing TB infection. Transplantation of more accurate and efficacious microbial components has been increasingly emphasized. Supplementation with Lactobacillus restores c-type lectin (mincle) expression in lung DCs, thereby enhancing immunity against Mtb (20). Moreover, Lactobacillus casei protect against anti-TB drug-induced liver injury by modulating the gut microflora and regulating intestinal inflammation via the TLR4–NF-κB–MyD88 pathways (115). In a tubercular mouse model, the production of palmitoleic acid by A. muciniphila strongly inhibited TB infection and was negatively correlated with the levels of tumor necrosis factors, such as TNF-α (47). Importantly, discontinuing anti-TNF therapy often leads to a paradoxical worsening of TB, particularly in patients with disseminated TB (116). However, oral administration of palmitoleic acid has been shown to remodel mucosal barriers and reduce inflammation by promoting the proliferation of anti-inflammatory bacteria such as A. muciniphila (117). The inverse relationship between palmitoleic acid and TNF suggests a molecular mechanism that differs from that of direct TNF blockade, implying that symbiotic bacterial products may offer alternative and gentler therapeutics to deliver anti-TB benefits to patients. Furthermore, specific gut microbiota may produce essential vitamins, such as B6, B9, and K, and also metabolize dietary compounds, such as flavonoids, which have been shown to have anti-Mtb effects (118, 119).
The use of probiotic and prebiotic products help reduce the duration and severity of inflammatory infections (120). Notably, gut bacterial metabolites, specifically those related to riboflavin, have been identified as potent stimulators of MAIT cells (58, 121–123). These T cells, which are imprinted by the microbiota, play a crucial role in immune development during early life and contribute significantly to the regulation of immune responses against pathogens. Butyrate, a beneficial SCFA, is currently under investigation as a potential therapeutic agent for its efficacy in the management of inflammatory diseases. Butyrate assists in regulating the integrity of the epithelial barrier and immune function, thereby facilitating interactions between macrophages and goblet cells (25). Reduced levels of butyrate, as evidenced by a significant reduction in the expression of the bcoA gene in the gut microbiota compared to that in healthy controls, have been linked to increased TB incidence (31, 124, 125). However, the accretion of acidic fermentation products, including SCFAs, reduces the luminal pH, leading to alterations in microbial composition and injury to the gut epithelium, with detrimental effects on health (126). These findings indicated that prebiotics may be counterproductive if not consumed in moderation.
8 Conclusion and perspectives
In conclusion, by elucidating the intricate interplay between gut microbiota and host immune systems, as well as their roles in modulating inflammation, metabolic pathways, and nutrient absorption, future research is poised to engineer more precise, personalized prevention strategies and treatment protocols. Several confounding factors, such as drug factors, living environment, lifestyle, immune status, and genetic variability, have been found to influence the diversity and composition of the gut microbiota (127). Targeting these factors can enhance human resistance against tuberculosis, thereby significantly curbing its incidence and dissemination.
Although investigating the gut microbiota as a potential adjunct to tuberculosis treatment is promising, high individual variation and the absence of a specific microbiota spectrum for TB pose significant challenges. These limitations preclude replacing traditional treatment approaches with gut microbiota-based therapies. Considering vaccine design, notably, researchers have uncovered a novel role of Mycobacteria, which may demonstrate protective effects and serve as strong non-specific immunopotentiators for the host (128). In the future, it may be possible to achieve tangible TB prevention through oral administration of specific beneficial microbes, thus leveraging the assistance of the gut microbiota. In addition to gut bacteria, the impact of fungi and viruses within the host intestinal tract on tuberculosis should not be ignored. Future research that integrates these analyses with other disease models will help us gain a more comprehensive understanding of the various factors that contribute to tuberculosis susceptibility.
Finally, we should be attentive to the delicate balance between maintaining the gut microbial equilibrium and combating tuberculosis with antibiotics to develop strategies that can effectively treat tuberculosis without disrupting the integrity of the gut microbiome. As our knowledge continues to expand, there is great potential to further improve our understanding of TB.
9 Method
The review was conducted in PubMed. The search strategy included the terms “gut microbiota,” “tuberculosis,” “lung,” “immunity,” and “antibiotic.” Studies were selected based on their relevance to the research question and publication date (within the last 10 years).
Author contributions
JL: Writing – review & editing, Writing – original draft, Software. DC: Writing – review & editing. YY: Writing – review & editing. JP: Writing – review & editing. JX: Writing – review & editing. LC: Writing – review & editing. BZ: Writing – review & editing.
Funding
The author(s) declare financial support was received for the research, authorship, and/or publication of this article. This research was supported by the National Natural Science Foundation of China (grant number: 82302537) and Doctoral Initial Funding of Guangdong Medical University (grant number: 4SG23186G).
Conflict of interest
The authors declare that the research was conducted in the absence of any commercial or financial relationships that could be construed as a potential conflict of interest.
The author(s) declared that they were an editorial board member of Frontiers, at the time of submission. This had no impact on the peer review process and the final decision.
Publisher’s note
All claims expressed in this article are solely those of the authors and do not necessarily represent those of their affiliated organizations, or those of the publisher, the editors and the reviewers. Any product that may be evaluated in this article, or claim that may be made by its manufacturer, is not guaranteed or endorsed by the publisher.
References
1. GBD 2021 Causes of Death Collaborators. Global burden of 288 causes of death and life expectancy decomposition in 204 countries and territories and 811 subnational locations, 1990-2021: a systematic analysis for the Global Burden of Disease Study 2021. Lancet. (2024) 403:2100–32. doi: 10.1016/S0140-6736(24)00367-2
2. Darrah PA, Zeppa JJ, Maiello P, Hackney JA, Wadsworth MH, Hughes TK, et al. Prevention of tuberculosis in macaques after intravenous BCG immunization. Nature. (2020) 577:95–102. doi: 10.1038/s41586-019-1817-8
3. Yang F, Yang Y, Chen L, Zhang Z, Liu L, Zhang C, et al. The gut microbiota mediates protective immunity against tuberculosis via modulation of lncRNA. Gut Microbes. (2022) 14:2029997. doi: 10.1080/19490976.2022.2029997
4. Liu Q, Zhu J, Dulberger CL, Stanley S, Wilson S, Chung ES, et al. Tuberculosis treatment failure associated with evolution of antibiotic resilience. Science. (2022) 378:1111–8. doi: 10.1126/science.abq2787
5. Ducarmon QR, Zwittink RD, Hornung BVH, van Schaik W, Young VB, Kuijper EJ. Gut microbiota and colonization resistance against bacterial enteric infection. Microbiol Mol Biol Rev. (2019) 83:e00007–19. doi: 10.1128/MMBR.00007-19
6. Schroeder BO, Birchenough GMH, Ståhlman M, Arike L, Johansson MEV, Hansson GC, et al. Bifidobacteria or fiber protects against diet-induced microbiota-mediated colonic mucus deterioration. Cell Host Microbe. (2018) 23:27–40.e7. doi: 10.1016/j.chom.2017.11.004
7. Corfield AP. The interaction of the gut microbiota with the mucus barrier in health and disease in human. Microorganisms. (2018) 6:78. doi: 10.3390/microorganisms6030078
8. Naidoo CC, Nyawo GR, Sulaiman I, Wu BG, Turner CT, Bu K, et al. Anaerobe-enriched gut microbiota predicts pro-inflammatory responses in pulmonary tuberculosis. EBioMedicine. (2021) 67:103374. doi: 10.1016/j.ebiom.2021.103374
9. Namasivayam S, Kauffman KD, McCulloch JA, Yuan W, Thovarai V, Mittereder LR, et al. Correlation between disease severity and the intestinal microbiome in mycobacterium tuberculosis-infected rhesus macaques. mBio. (2019) 10:e01018–19. doi: 10.1128/mBio.01018-19
10. Yeoh YK, Zuo T, Lui GC-Y, Zhang F, Liu Q, Li AY, et al. Gut microbiota composition reflects disease severity and dysfunctional immune responses in patients with COVID-19. Gut. (2021) 70:698–706. doi: 10.1136/gutjnl-2020-323020
11. Khader SA, Divangahi M, Hanekom W, Hill PC, Maeurer M, Makar KW, et al. Targeting innate immunity for tuberculosis vaccination. J Clin Invest. (2019) 129:3482–91. doi: 10.1172/JCI128877
12. Jeyanathan M, Vaseghi-Shanjani M, Afkhami S, Grondin JA, Kang A, D’Agostino MR, et al. Parenteral BCG vaccine induces lung-resident memory macrophages and trained immunity via the gut–lung axis. Nat Immunol. (2022) 23:1687–702. doi: 10.1038/s41590-022-01354-4
13. Grochowska M, Laskus T, Paciorek M, Pollak A, Lechowicz U, Makowiecki M, et al. Patients with infections of the central nervous system have lowered gut microbiota alpha diversity. CIMB. (2022) 44:2903–14. doi: 10.3390/cimb44070200
14. Nyangahu DD, Plumbee CR, Brown BP, Feng C, Havyarimana E, Cohen SB, et al. Antibiotic Treatment during Gestation Enhances Susceptibility to Mycobacterium tuberculosis in Offspring. Microbiol Spectr. (2022) 10. doi: 10.1128/spectrum.02491-22
15. Sorribas M, Jakob MO, Yilmaz B, Li H, Stutz D, Noser Y, et al. FXR modulates the gut-vascular barrier by regulating the entry sites for bacterial translocation in experimental cirrhosis. J Hepatol. (2019) 71:1126–40. doi: 10.1016/j.jhep.2019.06.017
16. Litvinova EA, Kozhevnikova EN, Achasova KM, Kontsevaya GV, Moshkin MP. Eradication of Helicobacter spp. in mucin2-deficient mice. Lab Anim. (2017) 51:311–4. doi: 10.1177/0023677216670687
17. Tang J, Xu L, Zeng Y, Gong F. Effect of gut microbiota on LPS-induced acute lung injury by regulating the TLR4/NF-kB signaling pathway. Int Immunopharmacol. (2021) 91:107272. doi: 10.1016/j.intimp.2020.107272
18. Schoeler M, Caesar R. Dietary lipids, gut microbiota and lipid metabolism. Rev Endocr Metab Disord. (2019) 20:461–72. doi: 10.1007/s11154-019-09512-0
19. Khosravi A, Yáñez A, Price JG, Chow A, Merad M, Goodridge HS, et al. Gut microbiota promote hematopoiesis to control bacterial infection. Cell Host Microbe. (2014) 15:374–81. doi: 10.1016/j.chom.2014.02.006
20. Negi S, Pahari S, Bashir H, Agrewala JN. Gut microbiota regulates mincle mediated activation of lung dendritic cells to protect against mycobacterium tuberculosis. Front Immunol. (2019) 10:1142. doi: 10.3389/fimmu.2019.01142
21. Uribe-Herranz M, Rafail S, Beghi S, Gil-de-Gómez L, Verginadis I, Bittinger K, et al. Gut microbiota modulate dendritic cell antigen presentation and radiotherapy-induced antitumor immune response. J Clin Invest. (2020) 130:466–79. doi: 10.1172/JCI124332
22. Yang W, Cong Y. Gut microbiota-derived metabolites in the regulation of host immune responses and immune-related inflammatory diseases. Cell Mol Immunol. (2021) 18:866–77. doi: 10.1038/s41423-021-00661-4
23. Liu N, Liu J, Zheng B, Zeng X, Ye Z, Huang X, et al. Gut microbiota affects sensitivity to immune-mediated isoniazid-induced liver injury. BioMed Pharmacother. (2023) 160:114400. doi: 10.1016/j.biopha.2023.114400
24. Wang Y-H, Yan Z-Z, Luo S-D, Hu J-J, Wu M, Zhao J, et al. Gut microbiota-derived succinate aggravates acute lung injury after intestinal ischaemia/reperfusion in mice. Eur Respir J. (2023) 61:2200840. doi: 10.1183/13993003.00840-2022
25. Liang L, Liu L, Zhou W, Yang C, Mai G, Li H, et al. Gut microbiota-derived butyrate regulates gut mucus barrier repair by activating the macrophage/WNT/ERK signaling pathway. Clin Sci (Lond). (2022) 136:291–307. doi: 10.1042/CS20210778
26. Piñeros AR, Campos LW, Fonseca DM, Bertolini TB, Gembre AF, Prado RQ, et al. M2 macrophages or IL-33 treatment attenuate ongoing Mycobacterium tuberculosis infection. Sci Rep. (2017) 7:41240. doi: 10.1038/srep41240
27. Chun E, Lavoie S, Fonseca-Pereira D, Bae S, Michaud M, Hoveyda HR, et al. Metabolite-sensing receptor ffar2 regulates colonic group 3 innate lymphoid cells and gut immunity. Immunity. (2019) 51:871–884.e6. doi: 10.1016/j.immuni.2019.09.014
28. Ardain A, Domingo-Gonzalez R, Das S, Kazer SW, Howard NC, Singh A, et al. Group 3 innate lymphoid cells mediate early protective immunity against tuberculosis. Nature. (2019) 570:528–32. doi: 10.1038/s41586-019-1276-2
29. Huang S-F, Yang Y-Y, Chou K-T, Fung C-P, Wang F-D, Su W-J. Systemic proinflammation after Mycobacterium tuberculosis infection was correlated to the gut microbiome in HIV-uninfected humans. Eur J Clin Invest. (2019) 49:e13068. doi: 10.1111/eci.13068
30. Yue S, Peng C, Zhao D, Xia X, Tan C, Wang Q, et al. Theabrownin isolated from Pu-erh tea regulates Bacteroidetes to improve metabolic syndrome of rats induced by high-fat, high-sugar and high-salt diet. J Sci Food Agric. (2022) 102:4250–65. doi: 10.1002/jsfa.11777
31. Khaliq A, Ravindran R, Afzal S, Jena PK, Akhtar MW, Ambreen A, et al. Gut microbiome dysbiosis and correlation with blood biomarkers in active-tuberculosis in endemic setting. PloS One. (2021) 16:e0245534. doi: 10.1371/journal.pone.0245534
32. Shi W, Hu Y, Ning Z, Xia F, Wu M, Hu YOO, et al. Alterations of gut microbiota in patients with active pulmonary tuberculosis in China: a pilot study. Int J Infect Dis. (2021) 111:313–21. doi: 10.1016/j.ijid.2021.08.064
33. He J, Zhang P, Shen L, Niu L, Tan Y, Chen L, et al. Short-chain fatty acids and their association with signalling pathways in inflammation, glucose and lipid metabolism. Int J Mol Sci. (2020) 21:6356. doi: 10.3390/ijms21176356
34. Namasivayam S, Diarra B, Diabate S, Sarro Y, Kone A, Kone B, et al. Patients infected with Mycobacterium africanum versus Mycobacterium tuberculosis possess distinct intestinal microbiota. PloS Negl Trop Dis. (2020) 14. doi: 10.1371/journal.pntd.0008230
35. Somboro AM, Diallo D, Holl JL, Maiga M. The role of the microbiome in inflammation during tuberculosis. EBioMedicine. (2021) 68:103435. doi: 10.1016/j.ebiom.2021.103435
36. Segal LN, Clemente JC, Li Y, Ruan C, Cao J, Danckers M, et al. Anaerobic bacterial fermentation products increase tuberculosis risk in antiretroviral-drug-treated HIV patients. Cell Host Microbe. (2017) 21:530–537.e4. doi: 10.1016/j.chom.2017.03.003
37. Dong Y, Yan H, Zhao X, Lin R, Lin L, Ding Y, et al. Gu-ben-fang-xiao decoction ameliorated murine asthma in remission stage by modulating microbiota-acetate-tregs axis. Front Pharmacol. (2020) 11:549. doi: 10.3389/fphar.2020.00549
38. Liu H, Nie H, Shi Y, Lai W, Bian L, Tian L, et al. Oil mistparticulate matter exposure induces hyperlipidemia-related inflammation via microbiota/SCFAs/GPR43 axis inhibition and TLR4/NF-κB activation. Environ pollut. (2024) 344:123331. doi: 10.1016/j.envpol.2024.123331
39. Hu Y, Yang Q, Liu B, Dong J, Sun L, Zhu Y, et al. Gut microbiota associated with pulmonary tuberculosis and dysbiosis caused by anti-tuberculosis drugs. J Infection. (2019) 78:317–22. doi: 10.1016/j.jinf.2018.08.006
40. Luo M, Liu Y, Wu P, Luo D-X, Sun Q, Zheng H, et al. Alternation of gut microbiota in patients with pulmonary tuberculosis. Front Physiol. (2017) 8:822. doi: 10.3389/fphys.2017.00822
41. Johnson JL, Jones MB, Cobb BA. Polysaccharide-experienced effector T cells induce IL-10 in FoxP3+ regulatory T cells to prevent pulmonary inflammation. Glycobiology. (2018) 28:50–8. doi: 10.1093/glycob/cwx093
42. Jiang F, Meng D, Weng M, Zhu W, Wu W, Kasper D, et al. The symbiotic bacterial surface factor polysaccharide A on Bacteroides fragilis inhibits IL-1β-induced inflammation in human fetal enterocytes via toll receptors 2 and 4. PloS One. (2017) 12:e0172738. doi: 10.1371/journal.pone.0172738
43. Liu C, Cheng Y, Guo Y, Qian H. Magnesium-L-threonate alleviate colonic inflammation and memory impairment in chronic-plus-binge alcohol feeding mice. Brain Res Bull. (2021) 174:184–93. doi: 10.1016/j.brainresbull.2021.06.009
44. Roberti MP, Yonekura S, Duong CPM, Picard M, Ferrere G, Tidjani Alou M, et al. Chemotherapy-induced ileal crypt apoptosis and the ileal microbiome shape immunosurveillance and prognosis of proximal colon cancer. Nat Med. (2020) 26:919–31. doi: 10.1038/s41591-020-0882-8
45. Gu Z, Pei W, Shen Y, Wang L, Zhu J, Zhang Y, et al. Akkermansia muciniphila and its outer protein Amuc_1100 regulates tryptophan metabolism in colitis. Food Funct. (2021) 12:10184–95. doi: 10.1039/d1fo02172a
46. Xie S, Li J, Lyu F, Xiong Q, Gu P, Chen Y, et al. Novel tripeptide RKH derived from Akkermansia muciniphila protects against lethal sepsis. Gut. (2023) 73:78–91. doi: 10.1136/gutjnl-2023-329996
47. Chen L, Zhang G, Li G, Wang W, Ge Z, Yang Y, et al. Ifnar gene variants influence gut microbial production of palmitoleic acid and host immune responses to tuberculosis. Nat Metab. (2022) 4:359–73. doi: 10.1038/s42255-022-00547-3
48. Macchione IG, Lopetuso LR, Ianiro G, Napoli M, Gibiino G, Rizzatti G, et al. Akkermansia muciniphila: key player in metabolic and gastrointestinal disorders. Eur Rev Med Pharmacol Sci. (2019) 23:8075–83. doi: 10.26355/eurrev_201909_19024
49. Wang Y, Deng Y, Liu N, Chen Y, Jiang Y, Teng Z, et al. Alterations in the gut microbiome of individuals with tuberculosis of different disease states. Front Cell Infect Microbiol. (2022) 12:836987. doi: 10.3389/fcimb.2022.836987
50. Arias L, Goig GA, Cardona P, Torres-Puente M, Díaz J, Rosales Y, et al. Influence of gut microbiota on progression to tuberculosis generated by high fat diet-induced obesity in C3HeB/feJ mice. Front Immunol. (2019) 10:2464. doi: 10.3389/fimmu.2019.02464
51. Huang H-L, Luo Y-C, Lu P-L, Huang C-H, Lin K-D, Lee M-R, et al. Gut microbiota composition can reflect immune responses of latent tuberculosis infection in patients with poorly controlled diabetes. Respir Res. (2023) 24:11. doi: 10.1186/s12931-023-02312-w
52. Shi J, Gao G, Yu Z, Wu K, Huang Y, Wu L-P, et al. The Relevance of Host Gut Microbiome Signature Alterations on de novo Fatty Acids Synthesis in Patients with Multi-Drug Resistant Tuberculosis. Infect Drug Resist. (2022) 15:5589–600. doi: 10.2147/IDR.S372122
53. Chakraborty P, Bajeli S, Kaushal D, Radotra BD, Kumar A. Biofilm formation in the lung contributes to virulence and drug tolerance of Mycobacterium tuberculosis. Nat Commun. (2021) 12:1606. doi: 10.1038/s41467-021-21748-6
54. Peters JM, Irvine EB, Rosenberg JM, Wadsworth MH, Hughes TK, Sutton M, et al. Protective intravenous BCG vaccination induces enhanced immune signaling in the airways. bioRxiv. (2023). doi: 10.1101/2023.07.16.549208
55. Nadeem S, Maurya SK, Das DK, Khan N, Agrewala JN. Gut dysbiosis thwarts the efficacy of vaccine against mycobacterium tuberculosis. Front Immunol. (2020) 11:726. doi: 10.3389/fimmu.2020.00726
56. Sencio V, Barthelemy A, Tavares LP, MaChado MG, Soulard D, Cuinat C, et al. Gut Dysbiosis during Influenza Contributes to Pulmonary Pneumococcal Superinfection through Altered Short-Chain Fatty Acid Production. Cell Rep. (2020) 30:2934–2947.e6. doi: 10.1016/j.celrep.2020.02.013
57. Stražar M, Mourits VP, Koeken VACM, de Bree LCJ, Moorlag SJCFM, Joosten LAB, et al. The influence of the gut microbiome on BCG-induced trained immunity. Genome Biol. (2021) 22:275. doi: 10.1186/s13059-021-02482-0
58. Ivanov II, Tuganbaev T, Skelly AN, Honda K. T cell responses to the microbiota. Annu Rev Immunol. (2022) 40:559–87. doi: 10.1146/annurev-immunol-101320-011829
59. Taur Y, Coyte K, Schluter J, Robilotti E, Figueroa C, Gjonbalaj M, et al. Reconstitution of the gut microbiota of antibiotic-treated patients by autologous fecal microbiota transplant. Sci Transl Med. (2018) 10:eaap9489. doi: 10.1126/scitranslmed.aap9489
60. Song W, Sun L-Y, Zhu Z-J, Wei L, Qu W, Zeng Z-G, et al. Association of gut microbiota and metabolites with disease progression in children with biliary atresia. Front Immunol. (2021) 12:698900. doi: 10.3389/fimmu.2021.698900
61. Sun X, Pan CQ, Xing H. Effect of microbiota metabolites on the progression of chronic hepatitis B virus infection. Hepatol Int. (2021) 15:1053–67. doi: 10.1007/s12072-021-10230-6
62. Oresta B, Braga D, Lazzeri M, Frego N, Saita A, Faccani C, et al. The microbiome of catheter collected urine in males with bladder cancer according to disease stage. J Urol. (2021) 205:86–93. doi: 10.1097/JU.0000000000001336
63. Ahmad Khan F, Gelmanova IY, Franke MF, Atwood S, Zemlyanaya NA, Unakova IA, et al. Aggressive regimens reduce risk of recurrence after successful treatment of MDR-TB. Clin Infect Dis. (2016) 63:214–20. doi: 10.1093/cid/ciw276
64. Pang Y, An J, Shu W, Huo F, Chu N, Gao M, et al. Epidemiology of extrapulmonary tuberculosis among inpatients, China, 2008–2017. Emerg Infect Dis. (2019) 25:457–64. doi: 10.3201/eid2503.180572
65. Majlessi L, Sayes F, Bureau J-F, Pawlik A, Michel V, Jouvion G, et al. Colonization with Helicobacter is concomitant with modified gut microbiota and drastic failure of the immune control of Mycobacterium tuberculosis. Mucosal Immunol. (2017) 10:1178–89. doi: 10.1038/mi.2016.140
66. Sathkumara HD, Eaton JL, Field MA, Govan BL, Ketheesan N, Kupz A. A murine model of tuberculosis/type 2 diabetes comorbidity for investigating the microbiome, metabolome and associated immune parameters. Anim Model Exp Med. (2021) 4:181–8. doi: 10.1002/ame2.12159
67. Chen Z, Luo J, Li J, Kim G, Chen ES, Xiao S, et al. Foxo1 controls gut homeostasis and commensalism by regulating mucus secretion. J Exp Med. (2021) 218:e20210324. doi: 10.1084/jem.20210324
68. Fawad JA, Luzader DH, Hanson GF, Moutinho TJ, McKinney CA, Mitchell PG, et al. Histone deacetylase inhibition by gut microbe-generated short-chain fatty acids entrains intestinal epithelial circadian rhythms. Gastroenterology. (2022) 163:1377–1390.e11. doi: 10.1053/j.gastro.2022.07.051
69. Wang Q, Zhou Y, Rychahou P, Fan TW-M, Lane AN, Weiss HL, et al. Ketogenesis contributes to intestinal cell differentiation. Cell Death Differ. (2017) 24:458–68. doi: 10.1038/cdd.2016.142
70. Xu C, Marques FZ. How dietary fibre, acting via the gut microbiome, lowers blood pressure. Curr Hypertens Rep. (2022) 24:509–21. doi: 10.1007/s11906-022-01216-2
71. Sequeira RP, Clarke TB, Brown RL. The microbiota protects against respiratory infection via GM-CSF signaling. Nat Commun. (2017) 8:1512. doi: 10.1038/s41467-017-01803-x
72. Prescott D, Maisonneuve C, Yadav J, Rubino SJ, Girardin SE, Philpott DJ. NOD2 modulates immune tolerance via the GM-CSF-dependent generation of CD103+ dendritic cells. Proc Natl Acad Sci U.S.A. (2020) 117:10946–57. doi: 10.1073/pnas.1912866117
73. Bomfim CCB, Fisher L, Amaral EP, Mittereder L, McCann K, Correa AAS, et al. Mycobacterium tuberculosis induces irg1 in murine macrophages by a pathway involving both TLR-2 and STING/IFNAR signaling and requiring bacterial phagocytosis. Front Cell Infect Microbiol. (2022) 12:862582. doi: 10.3389/fcimb.2022.862582
74. Zhang Z, Mai Q, Yang L, Chen Y, Chen Z, Lin T, et al. MicroRNA-31 mediated by interferon regulatory factor 7 signaling facilitates control of Mycobacterium tuberculosis infection. Int J Med Microbiol. (2022) 312:151569. doi: 10.1016/j.ijmm.2022.151569
75. Yang F, Yang Y, Chen Y, Li G, Zhang G, Chen L, et al. MiR-21 is remotely governed by the commensal bacteria and impairs anti-TB immunity by down-regulating IFN-γ. Front Microbiol. (2021) 11:512581. doi: 10.3389/fmicb.2020.512581
76. Grant AV, Sabri A, Abid A, Rhorfi IA, Benkirane M, Souhi H, et al. A genome-wide association study of pulmonary tuberculosis in Morocco. Hum Genet. (2016) 135:299–307. doi: 10.1007/s00439-016-1633-2
77. Su CW, Chen C-Y, Jiao L, Long SR, Mao T, Ji Q, et al. Helminth-induced and th2-dependent alterations of the gut microbiota attenuate obesity caused by high-fat diet. Cell Mol Gastroenterol Hepatol. (2020) 10:763–78. doi: 10.1016/j.jcmgh.2020.06.010
78. Adithya KK, Rajeev R, Selvin J, Seghal Kiran G. Dietary influence on the dynamics of the human gut microbiome: prospective implications in interventional therapies. ACS Food Sci Technol. (2021) 1:717–36. doi: 10.1021/acsfoodscitech.0c00075
79. Sonnenburg JL, Bäckhed F. Diet–microbiota interactions as moderators of human metabolism. Nature. (2016) 535:56–64. doi: 10.1038/nature18846
80. Pagliai G, Russo E, Niccolai E, Dinu M, Di Pilato V, Magrini A, et al. Influence of a 3-month low-calorie Mediterranean diet compared to the vegetarian diet on human gut microbiota and SCFA: the CARDIVEG Study. Eur J Nutr. (2020) 59:2011–24. doi: 10.1007/s00394-019-02050-0
81. Gehrig JL, Venkatesh S, Chang H-W, Hibberd MC, Kung VL, Cheng J, et al. Effects of microbiota-directed foods in gnotobiotic animals and undernourished children. Science. (2019) 365:eaau4732. doi: 10.1126/science.aau4732
82. Major J, Crotta S, Finsterbusch K, Chakravarty P, Shah K, Frederico B, et al. Endothelial AHR activity prevents lung barrier disruption in viral infection. Nature. (2023) 621:813–20. doi: 10.1038/s41586-023-06287-y
83. Krishnan S, Ding Y, Saedi N, Choi M, Sridharan GV, Sherr DH, et al. Gut microbiota-derived tryptophan metabolites modulate inflammatory response in hepatocytes and macrophages. Cell Rep. (2018) 23:1099–111. doi: 10.1016/j.celrep.2018.03.109
84. Krautkramer KA, Kreznar JH, Romano KA, Vivas EI, Barrett-Wilt GA, Rabaglia ME, et al. Diet-microbiota interactions mediate global epigenetic programming in multiple host tissues. Mol Cell. (2016) 64:982–92. doi: 10.1016/j.molcel.2016.10.025
85. Bao R, Hesser LA, He Z, Zhou X, Nadeau KC, Nagler CR. Fecal microbiome and metabolome differ in healthy and food-allergic twins. J Clin Invest. (2021) 131:e141935. doi: 10.1172/JCI141935
86. Wastyk HC, Fragiadakis GK, Perelman D, Dahan D, Merrill BD, Yu FB, et al. Gut-microbiota-targeted diets modulate human immune status. Cell. (2021) 184:4137–53. doi: 10.1016/j.cell.2021.06.019
87. Wu T-R, Lin C-S, Chang C-J, Lin T-L, Martel J, Ko Y-F, et al. Gut commensal Parabacteroides goldsteinii plays a predominant role in the anti-obesity effects of polysaccharides isolated from Hirsutella sinensis. Gut. (2019) 68:248–62. doi: 10.1136/gutjnl-2017-315458
88. Cuskin F, Lowe EC, Temple MJ, Zhu Y, Cameron E, Pudlo NA, et al. Human gut Bacteroidetes can utilize yeast mannan through a selfish mechanism. Nature. (2015) 517:165–9. doi: 10.1038/nature13995
89. Cao D, Liu W, Lyu N, Li B, Song W, Yang Y, et al. Gut mycobiota dysbiosis in pulmonary tuberculosis patients undergoing anti-tuberculosis treatment. Microbiol Spectr. (2021) 9:e00615–21. doi: 10.1128/spectrum.00615-21
90. Xu R, Ma L, Chen T, Wang J. Sophorolipid suppresses LPS-induced inflammation in RAW264.7 cells through the NF-κB signaling pathway. Molecules. (2022) 27:5037. doi: 10.3390/molecules27155037
91. Li XV, Leonardi I, Putzel GG, Semon A, Fiers WD, Kusakabe T, et al. Immune regulation by fungal strain diversity in inflammatory bowel disease. Nature. (2022) 603:672–8. doi: 10.1038/s41586-022-04502-w
92. Panpetch W, Hiengrach P, Nilgate S, Tumwasorn S, Somboonna N, Wilantho A, et al. Additional Candida albicans administration enhances the severity of dextran sulfate solution induced colitis mouse model through leaky gut-enhanced systemic inflammation and gut-dysbiosis but attenuated by Lactobacillus rhamnosus L34. Gut Microbes. (2020) 11:465–80. doi: 10.1080/19490976.2019.1662712
93. Liu L, Gong T, Tao W, Lin B, Li C, Zheng X, et al. Commensal viruses maintain intestinal intraepithelial lymphocytes via noncanonical RIG-I signaling. Nat Immunol. (2019) 20:1681–91. doi: 10.1038/s41590-019-0513-z
94. Erttmann SF, Swacha P, Aung KM, Brindefalk B, Jiang H, Härtlova A, et al. The gut microbiota prime systemic antiviral immunity via the cGAS-STING-IFN-I axis. Immunity. (2022) 55:847–861.e10. doi: 10.1016/j.immuni.2022.04.006
95. Shen J, Zhang J, Mo L, Li Y, Li Y, Li C, et al. Large-scale phage cultivation for commensal human gut bacteria. Cell Host Microbe. (2023) 31:665–677.e7. doi: 10.1016/j.chom.2023.03.013
96. Federici S, Kredo-Russo S, Valdés-Mas R, Kviatcovsky D, Weinstock E, Matiuhin Y, et al. Targeted suppression of human IBD-associated gut microbiota commensals by phage consortia for treatment of intestinal inflammation. Cell. (2022) 185:2879–2898.e24. doi: 10.1016/j.cell.2022.07.003
97. Hsu BB, Gibson TE, Yeliseyev V, Liu Q, Lyon L, Bry L, et al. Dynamic modulation of the gut microbiota and metabolome by bacteriophages in a mouse model. Cell Host Microbe. (2019) 25:803–814.e5. doi: 10.1016/j.chom.2019.05.001
98. Talwar H, Hanoudi SN, Draghici S, Samavati L. Novel T7 phage display library detects classifiers for active mycobacterium tuberculosis infection. Viruses. (2018) 10:375. doi: 10.3390/v10070375
99. O’Donnell MR, Pym A, Jain P, Munsamy V, Wolf A, Karim F, et al. A novel reporter phage to detect tuberculosis and rifampin resistance in a high-HIV-burden population. J Clin Microbiol. (2015) 53:2188–94. doi: 10.1128/JCM.03530-14
100. Dedrick RM, Smith BE, Cristinziano M, Freeman KG, Jacobs-Sera D, Belessis Y, et al. Phage therapy of mycobacterium infections: compassionate use of phages in 20 patients with drug-resistant mycobacterial disease. Clin Infect Dis. (2023) 76:103–12. doi: 10.1093/cid/ciac453
101. Dedrick RM, Guerrero-Bustamante CA, Garlena RA, Russell DA, Ford K, Harris K, et al. Engineered bacteriophages for treatment of a patient with a disseminated drug-resistant Mycobacterium abscessus. Nat Med. (2019) 25:730–3. doi: 10.1038/s41591-019-0437-z
102. Wipperman MF, Bhattarai SK, Vorkas CK, Maringati VS, Taur Y, Mathurin L, et al. Gastrointestinal microbiota composition predicts peripheral inflammatory state during treatment of human tuberculosis. Nat Commun. (2021) 12:1141. doi: 10.1038/s41467-021-21475-y
103. Namasivayam S, Zimmerman M, Oland S, Wang H, Mittereder LR, Dartois V, et al. The dysbiosis triggered by first-line tuberculosis antibiotics fails to reduce their bioavailability. mBio. (2023) 14:e0035323. doi: 10.1128/mbio.00353-23
104. Namasivayam S, Maiga M, Yuan W, Thovarai V, Costa DL, Mittereder LR, et al. Longitudinal profiling reveals a persistent intestinal dysbiosis triggered by conventional anti-tuberculosis therapy. Microbiome. (2017) 5:71. doi: 10.1186/s40168-017-0286-2
105. Dumas A, Corral D, Colom A, Levillain F, Peixoto A, Hudrisier D, et al. The host microbiota contributes to early protection against lung colonization by mycobacterium tuberculosis. Front Immunol. (2018) 9:2656. doi: 10.3389/fimmu.2018.02656
106. Esquivel-Elizondo S, Ilhan ZE, Garcia-Peña EI, Krajmalnik-Brown R. Insights into Butyrate Production in a Controlled Fermentation System via Gene Predictions. mSystems. (2017) 2:e00051–17. doi: 10.1128/mSystems.00051-17
107. Fluhr L, Mor U, Kolodziejczyk AA, Dori-Bachash M, Leshem A, Itav S, et al. Gut microbiota modulates weight gain in mice after discontinued smoke exposure. Nature. (2021) 600:713–9. doi: 10.1038/s41586-021-04194-8
108. Bai X, Wei H, Liu W, Coker OO, Gou H, Liu C, et al. Cigarette smoke promotes colorectal cancer through modulation of gut microbiota and related metabolites. Gut. (2022) 71:2439–50. doi: 10.1136/gutjnl-2021-325021
109. Fan J, Zhou Y, Meng R, Tang J, Zhu J, Aldrich MC, et al. Cross-talks between gut microbiota and tobacco smoking: a two-sample Mendelian randomization study. BMC Med. (2023) 21:163. doi: 10.1186/s12916-023-02863-1
110. Vujkovic-Cvijin I, Sklar J, Jiang L, Natarajan L, Knight R, Belkaid Y. Host variables confound gut microbiota studies of human disease. Nature. (2020) 587:448–54. doi: 10.1038/s41586-020-2881-9
111. Shahzad M, Andrews SC, Ul-Haq Z. Exploring the role of Microbiome in Susceptibility, Treatment Response and Outcome among Tuberculosis Patients from Pakistan study protocol for a prospective cohort study (Micro-STOP). BMJ Open. (2022) 12:e058463. doi: 10.1136/bmjopen-2021-058463
112. Yu EW, Gao L, Stastka P, Cheney MC, Mahabamunuge J, Torres Soto M, et al. Fecal microbiota transplantation for the improvement of metabolism in obesity: The FMT-TRIM double-blind placebo-controlled pilot trial. PloS Med. (2020) 17:e1003051. doi: 10.1371/journal.pmed.1003051
113. Haifer C, Paramsothy S, Kaakoush NO, Saikal A, Ghaly S, Yang T, et al. Lyophilised oral faecal microbiota transplantation for ulcerative colitis (LOTUS): a randomised, double-blind, placebo-controlled trial. Lancet Gastroenterol Hepatol. (2022) 7:141–51. doi: 10.1016/S2468-1253(21)00400-3
114. Yu Y, Wang W, Zhang F. The next generation fecal microbiota transplantation: to transplant bacteria or virome. Adv Sci (Weinh). (2023):e2301097. doi: 10.1002/advs.202301097
115. Li Y, Zhao L, Sun C, Yang J, Zhang X, Dou S, et al. Regulation of gut microflora by lactobacillus casei zhang attenuates liver injury in mice caused by anti-tuberculosis drugs. Int J Mol Sci. (2023) 24:9444. doi: 10.3390/ijms24119444
116. Rivoisy C, Tubach F, Roy C, Nicolas N, Mariette X, Salmon D, et al. Paradoxical anti-TNF-associated TB worsening: Frequency and factors associated with IRIS. Joint Bone Spine. (2016) 83:173–8. doi: 10.1016/j.jbspin.2015.04.022
117. Chen Y, Mai Q, Chen Z, Lin T, Cai Y, Han J, et al. Dietary palmitoleic acid reprograms gut microbiota and improves biological therapy against colitis. Gut Microbes. (2023) 15:2211501. doi: 10.1080/19490976.2023.2211501
118. Tang M, Wang G, Li J, Wang Y, Peng C, Chang X, et al. Flavonoid extract from propolis alleviates periodontitis by boosting periodontium regeneration and inflammation resolution via regulating TLR4/MyD88/NF-κB and RANK/NF-κB pathway. J Ethnopharmacol. (2024) 319:117324. doi: 10.1016/j.jep.2023.117324
119. Wang M, Yu F, Zhang Y, Chang W, Zhou M. The effects and mechanisms of flavonoids on cancer prevention and therapy: focus on gut microbiota. Int J Biol Sci. (2022) 18:1451–75. doi: 10.7150/ijbs.68170
120. Bustamante M, Oomah BD, Oliveira WP, Burgos-Díaz C, Rubilar M, Shene C. Probiotics and prebiotics potential for the care of skin, female urogenital tract, and respiratory tract. Folia Microbiol (Praha). (2020) 65:245–64. doi: 10.1007/s12223-019-00759-3
121. Legoux F, Salou M, Lantz O. MAIT cell development and functions: the microbial connection. Immunity. (2020) 53:710–23. doi: 10.1016/j.immuni.2020.09.009
122. Constantinides MG, Link VM, Tamoutounour S, Wong AC, Perez-Chaparro PJ, Han S-J, et al. MAIT cells are imprinted by the microbiota in early life and promote tissue repair. Science. (2019) 366:eaax6624. doi: 10.1126/science.aax6624
123. Schmaler M, Colone A, Spagnuolo J, Zimmermann M, Lepore M, Kalinichenko A, et al. Modulation of bacterial metabolism by the microenvironment controls MAIT cell stimulation. Mucosal Immunol. (2018) 11:1060–70. doi: 10.1038/s41385-018-0020-9
124. Parada Venegas D, de la Fuente MK, Landskron G, González MJ, Quera R, Dijkstra G, et al. Short chain fatty acids (SCFAs)-mediated gut epithelial and immune regulation and its relevance for inflammatory bowel diseases. Front Immunol. (2019) 10:277. doi: 10.3389/fimmu.2019.00277
125. Wenzel TJ, Gates EJ, Ranger AL, Klegeris A. Short-chain fatty acids (SCFAs) alone or in combination regulate select immune functions of microglia-like cells. Mol Cell Neurosci. (2020) 105:103493. doi: 10.1016/j.mcn.2020.103493
126. Deleu S, Machiels K, Raes J, Verbeke K, Vermeire S. Short chain fatty acids and its producing organisms: An overlooked therapy for IBD? EBioMedicine. (2021) 66:103293. doi: 10.1016/j.ebiom.2021.103293
127. Huey SL, Yu EA, Finkelstein JL, Glesby MJ, Bonam W, Russell DG, et al. Nutrition, inflammation, and the gut microbiota among outpatients with active tuberculosis disease in India. Am J Trop Med Hyg. (2021) 105:1645–56. doi: 10.4269/ajtmh.21-0310
Keywords: dysbiosis, tuberculosis, gut microbiota, microecology, gut-lung axis
Citation: Lin J, Chen D, Yan Y, Pi J, Xu J, Chen L and Zheng B (2024) Gut microbiota: a crucial player in the combat against tuberculosis. Front. Immunol. 15:1442095. doi: 10.3389/fimmu.2024.1442095
Received: 01 June 2024; Accepted: 30 September 2024;
Published: 22 October 2024.
Edited by:
Maryam Dadar, Razi Vaccine and Serum Research Institute, IranReviewed by:
Le Liu, Southern Medical University, ChinaSwadha Anand, Tata Consultancy Services, India
Sweta Ghosh, University of Louisville, United States
Copyright © 2024 Lin, Chen, Yan, Pi, Xu, Chen and Zheng. This is an open-access article distributed under the terms of the Creative Commons Attribution License (CC BY). The use, distribution or reproduction in other forums is permitted, provided the original author(s) and the copyright owner(s) are credited and that the original publication in this journal is cited, in accordance with accepted academic practice. No use, distribution or reproduction is permitted which does not comply with these terms.
*Correspondence: Lingming Chen, Y2hlbmxtM0BtYWlsMy5zeXN1LmVkdS5jbg==; Biying Zheng, emJ5ODhrckBnZG11LmVkdS5jbg==