- 1Shuguang Hospital Affiliated to Shanghai University of Traditional Chinese Medicine, Shanghai, China
- 2Shanghai University of Traditional Chinese Medicine, Shanghai, China
- 3Key Laboratory of Liver and Kidney Diseases, Institute of Liver Diseases, Shuguang Hospital Affiliated to Shanghai University of Traditional Chinese Medicine, Shanghai, China
The non-natriuretic-dependent glutamate/cystine inverse transporter-system Xc- is composed of two protein subunits, SLC7A11 and SLC3A2, with SLC7A11 serving as the primary functional component responsible for cystine uptake and glutathione biosynthesis. SLC7A11 is implicated in tumor development through its regulation of redox homeostasis, amino acid metabolism, modulation of immune function, and induction of programmed cell death, among other processes relevant to tumorigenesis. In this paper, we summarize the structure and biological functions of SLC7A11, and discuss its potential role in tumor therapy, which provides a new direction for precision and personalized treatment of tumors.
Introduction
Tumors, as abnormal growths in the body, often demonstrate a heightened metabolic state that fuels their relentless expansion. This amplified metabolic activity is crucial for their survival and growth, as they voraciously consume nutrients to maintain their proliferative e momentum. To achieve this, tumors enhance their uptake of essential nutrients, ensuring a steady supply for their rapid cellular division and growth. This altered metabolic behavior is a hallmark of malignancy, reflecting the tumor’s adaptive response to meet its energetic and biosynthetic demands. Consequently, this heightened metabolic rate not only underscores the aggressive nature of the disease but also offers potential therapeutic targets for disrupting tumor growth (1–3). The unique amino acid transporter-Solute Carrier Family 7 Member 11(SLC7A11) has been found to be significantly upregulated in various tumor types, with its expression levels closely associated with tumor cell proliferation, invasion, metastasis, and the tumor microenvironment (4–6). Additionally, SLC7A11 has been linked to resistance to radiation and conventional chemotherapeutic agents (7, 8).Thus, SLC7A11 may serve as a promising biomarker for the diagnosis and prognostication of clinical tumors (9).
The significant abundance of SLC7A11 suggests its potential as a promising target for tumor therapy (10). SLC7A11 plays a crucial role in facilitating the import of cystine into cells for the synthesis of glutathione, which is essential for maintaining intracellular glutathione (GSH) levels and protecting cells from oxidative stress-induced damage (5). This process is intricately linked to the initiation of ferroptosis. The SLC7A11/GSH/GPx4 axis serves as the central defense mechanism against ferroptosis, and downregulating the expression and activity of SLC7A11 has been shown to enhance the sensitivity of tumor cells to ferroptosis (11). Moreover, the elevated intracellular GSH levels induced by SLC7A11 confer inherent resistance to oxidative stress therapy in cells. A novel approach in the realm of cancer treatment involves the use of immunotherapy-activated CD8+T cells, which release IFN-γ to enhance tumor cell ferroptosis via PD-L1 inhibition (12, 13). IFN-γ secretion decreases SLC7A11 expression, suggesting that combining SLC7A11 inhibitors with immunotherapy could improve cancer treatment (13, 14). The recent study revealed a significant reliance on NADPH and glucose in tumor cells expressing high levels of SLC7A11, thereby questioning the conventional understanding of SLC7A11 as a promoter of cancer (15). Suppression of glucose uptake in the presence of elevated SLC7A11 expression leads to intracellular disulfide stress, ultimately resulting in cell death (16).
Given the pivotal role of SLC7A11 in cancer treatment, this paper provides an overview of its structure and biological functions, as well as its involvement in oxidative stress, tumor metabolism, immune modulation, and cell death (Figure 1).
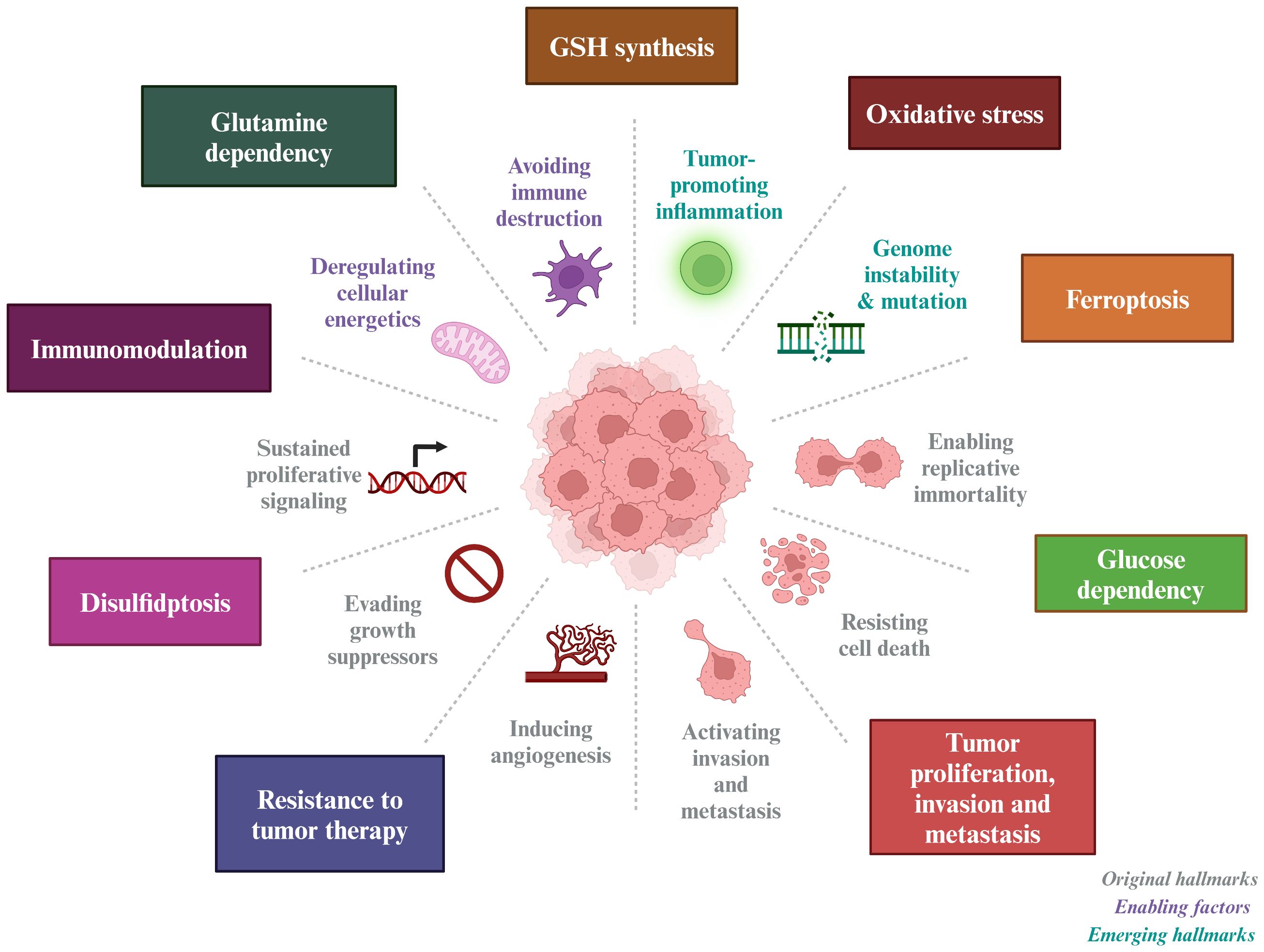
Figure 1 Tumor-based characterization of the interventional role of SLC7A11. SLC7A11 can improve tumor resistance to radiotherapy and inhibit tumor proliferation, invasion and metastasis by affecting GSH synthesis, inducing oxidative stress, causing glucose and glutamine dependence in tumor cells, and inducing ferroptosis and disulfidptosis in tumor cells.
Structure and function of SLC7A11
System Xc- is composed of two subunits: the light chain functional subunit SLC7A11, also referred to as xCT, and the heavy chain structural subunit SLC3A2, also known as CD98 or 4F2hc (17, 18).
In humans, the gene encoding SLC7A11 is situated on chromosome 4 and comprises 14 exons, resulting in a protein sequence of 502 amino acids. SLC7A11 is a transmembrane protein with 12 transmembrane domains, with both its N- and C-termini located intracellularly (19). This protein is abundantly expressed in various tissues and cells throughout the human body and is evolutionarily conserved among vertebrates. In contrast, SLC3A2 is a type 2 membrane glycoprotein characterized by a single transmembrane structural domain, with its N-terminal end intracellular and its C-terminal end extracellular and heavily glycosylated. SLC7A11 is linked to SLC3A2 through a disulfide bond formed between the conserved residue Cys 158 of SLC7A11 and Cys 109 of SLC3A2 (20). Notably, SLC3A2 serves as the chaperone protein for various members of the light subunits of heterodimeric amino acid transporters (LSHAT) family. Consequently, the determination of substrate specificity in system Xc-is primarily influenced by SLC7A11, while SLC3A2 plays a role in facilitating the transportation of SLC7A11 into the intracellular compartment or potentially enhancing the stability of the SLC7A11 protein (21). Furthermore, CD44 has been identified as capable of interacting with and stabilizing SLC7A11 on the membrane of cancer cells (22) (Figure 2).
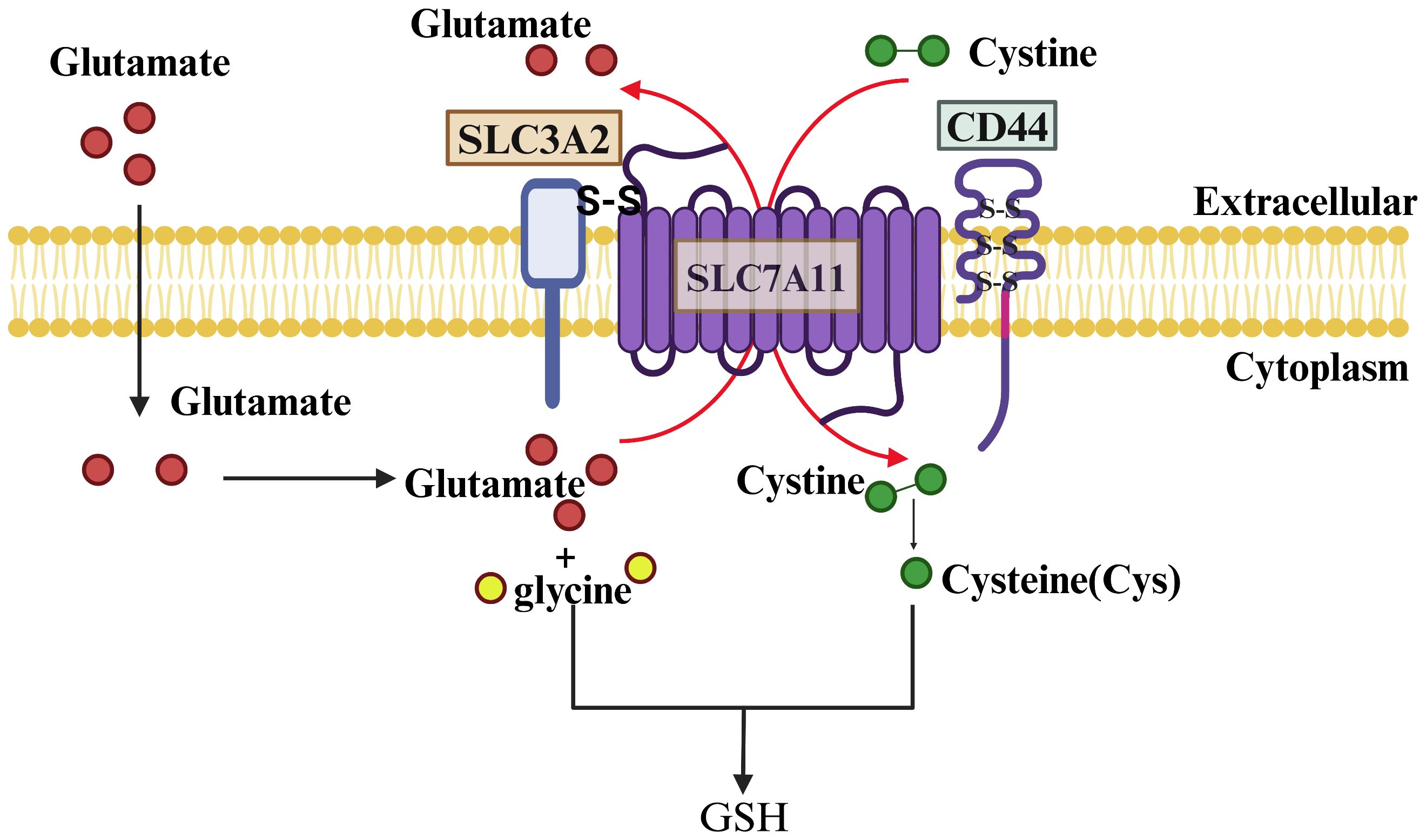
Figure 2 Structure and Function of SLC7A11. SLC7A11 and SLC3A2 collectively constitute the glutamate/cystine reverse transporter, with SLC7A11 being a 12-transmembrane-spanning protein featuring intracellular N- and C-termini, and SLC3A2 being a single-transmembrane-spanning protein with an extracellular N-terminus and an intracellular C-terminus. The two proteins are linked by a disulfide bond and function to facilitate the cellular uptake of cystine and the extrusion of glutamate. Cystine is rapidly reduced to cysteine inside the cell and combined with a molecule of glutamate and glycine to synthesize GSH under the action of GCL and GSS enzymes.
A variety of amino acid transporters present on the cell surface facilitate the uptake of amino acids by cells, with system Xc- being the main known amino acid transport complex responsible for the transportation of cystine (23). This complex operates by importing cystine into the cell and exporting glutamate out of the cell in a 1:1 ratio, without the need for sodium ions. Once inside the cell, cystine is promptly converted to cysteine, which plays a crucial role as the limiting factor in the synthesis of glutathione (24). Therefore, the expression level and activity of SLC7A11 are the main factors affecting GSH content.
Expression of SLC7A11 in tumors and prognosis
Since its initial identification, research has revealed that SLC7A11 exhibits a tissue-specific distribution, with varying levels of mRNA abundance across 27 different tissues. The findings indicate that SLC7A11 is most prominently expressed in the brain, followed by the thyroid, stomach, appendix, bladder, and gallbladder, while demonstrating lower expression levels in the kidney, heart, and liver. Given the specific subcellular localization of SLC7A11 and its recognized functional significance, it is not unexpected that a multitude of studies have consistently demonstrated the involvement of SLC7A11 in various neurodegenerative, ocular, and immune disorders (25, 26).
It is noteworthy that SLC7A11 is prominently expressed in numerous tumors and exerts influence on tumor progression, invasion, metastasis, and unfavorable prognosis. Elevated levels of SLC7A11 expression have been demonstrated in a diverse array of tumor types, such as lung, liver, pancreatic, breast, ovarian, prostate, bladder, colorectal, melanoma, and leukemia, in comparison to healthy tissues (9, 27–30). Particularly in oncology patients who exhibit insensitivity to initial therapeutic agents and demonstrate resistance to radiotherapy and chemotherapy interventions (31). Table 1 lists the expression and prognosis of SLC7A11 in different tumors.
Mechanically, tumor tissues tend to enhance their own antioxidant defenses in response to high levels of oxidative stress by up-regulating SLC7A11 expression, while SLC7A11-mediated synthesis of GSH acts as a defense against the cytotoxic effects of radiotherapy or certain drugs, which further reduces the sensitivity of tumor cells to treatment.
Role of SLC7A11 in cancer therapy
SLC7A11 expression causes cellular ferroptosis or enhances tumor killing by immune cells by affecting oxidative status or nutrient and energy metabolism in the tumor microenvironment (TME). Here we summarize the potential role of SLC7A11 in cancer.
SLC7A11 and oxidative stress
Oxidative stress is cellular and tissue damage caused by the production of reactive oxygen species (ROS) in the organism exceeding their removal.
ROS are products of normal physiological activities and are a general term for a class of chemically active molecules and ions with high oxidative activity, which play important roles in cell signaling and tissue homeostasis (32). Essentially, ROS are partially reduced oxygen-containing molecules, including superoxide anion (O2-), peroxides (H2O2 and LOOH), and free radicals (HO· and LO·). Mitochondria are the main site of ROS production, of which more than 90% are produced by mitochondria in the normal metabolism and energy supply of the electron transport chain (33). Also, ROS can be produced by cytochrome P450, NADPH oxidase (NOX), xanthine oxidase (XO), and peroxidase in the microsomes (34). Of course, the body also exists a ROS scavenging system to maintain homeostasis, which is mainly categorized into enzymatic antioxidants and non-enzymatic antioxidants.
Non-enzymatic antioxidants scavenge free radicals by interacting directly with them. Such substances include glutathione, vitamin A, vitamin C, vitamin E, and coenzyme Q10. Enzymatic antioxidants, on the other hand, act as antioxidants by catalyzing the degradation of ROS. The main ones are Super Oxide Dismutase(SOD), Trx system and Gpx family (35).
The Gpx family of glutathione peroxidases is an evolutionarily highly conserved group of enzymes containing eight main isoenzymes, among which Glutathione Peroxidase 4(GPx4) utilizes GSH as a cofactor to convert lipid hydroperoxides into nontoxic lipids alcohols and reduce free radical accumulation (36). Inhibition of GPx4 can lead to an increase in ROS whereas increased GPx4 expression can lead to a decrease in ROS content, and GPx4 performs this function dependent on GSH (37), which is an important component of the body’s endogenous antioxidant system, and is derived from glutamate, glycine, and cysteine by dehydration condensation, and its intracellular level is affected by the ability of SLC7A11 to transporter cystine (38). Studies have shown that pharmacological inhibition of SLC7A11 or knockdown of SLC7A11 reduces intracellular cysteine concentration, thereby affecting GSH concentration (39). erastin, a selective inhibitor of SLC7A11, inhibits cystine uptake, and salicylsulfonylpyrimidines and glutamate can also reduce intracellular cysteine concentration and deplete intracellular GSH by inhibiting SLC7A11 (40), depleting GSH in cells. thereby inducing cellular oxidative stress and death (Figure 3).
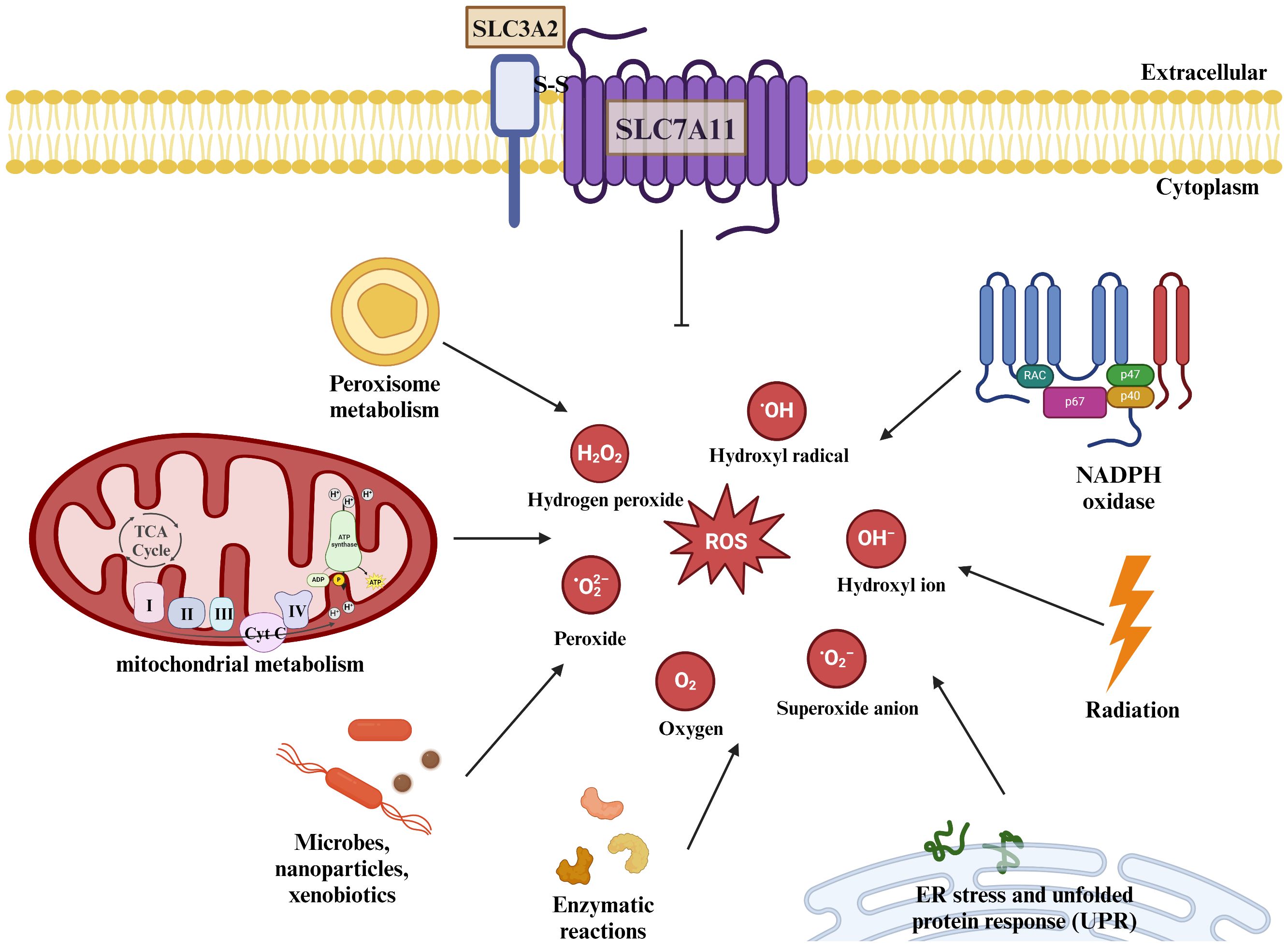
Figure 3 SLC7A11 regulates cellular redox homeostasis. ROS are present intracellularly in a variety of forms: including superoxide anions, peroxides, and oxygen radicals. ROS are present intracellularly in a variety of forms: including superoxide anions, peroxides, and oxygen radicals. Mitochondria produce large amounts of ROS through the electron transport chain, and the metabolism of NADPH and peroxisomes also increases intracellular ROS levels, as do some microorganisms or misfolded abnormal proteins, and studies have shown that radiation therapy also produces some ROS. The human body can scavenge excess ROS under normal physiological conditions through SLC7A11-mediated production of GSH. The body can remove excessive ROS accumulation through SLC7A11-mediated GSH production under normal physiological conditions to maintain redox homeostasis.
In conclusion, SLC7A11 has a pro-survival effect, and SLC7A11-mediated cystine uptake can help cells re-establish redox homeostasis in response to oxidative stress, whereas inhibition of SLC7A11 can lead to depletion of cellular GSH and thus make the cells more sensitive to chemotherapy or radiotherapy.
SLC7A11 and tumor metabolism
Beyond its well-documented antioxidant functions, SLC7A11 emerges as a pivotal metabolic regulator that profoundly influences intracellular nutrient processing and energy metabolism within cancer cells. A fascinating aspect of SLC7A11’s role in tumorigenesis is its ability to modulate the uptake and conversion of cystine. Cancer cells with elevated levels of SLC7A11 expression exhibit an increased affinity for cystine, which they rapidly convert into cysteine. This biochemical transformation, however, comes at an energetic cost (41).
Specifically, the conversion process requires a significant amount of NADPH, a crucial cofactor that primarily originates from the cytoplasmic glucose-pentose phosphate pathway. This pathway, in turn, plays a vital role in generating ribose-5-phosphate for nucleotide synthesis and NADPH for reductive biosynthesis and detoxification of reactive oxygen species. Consequently, cancer cells overexpressing SLC7A11 develop a heightened dependency on both the glucose and pentose phosphate pathways for their survival and proliferation (42). This enhanced reliance on glucose metabolism renders these cells particularly vulnerable to glucose deprivation. In the context of glioblastoma, for instance, glucose starvation can induce cell death more rapidly in cells with high SLC7A11 expression. This vulnerability presents a potential therapeutic window. By simultaneously targeting glucose transporter type 1 (GLUT1), a glucose transporter critical for glucose uptake, and glutathione synthesis, which is intimately linked to NADPH production and reactive oxygen species scavenging, it may be possible to deplete NADPH levels and cause a buildup of reactive oxygen species. This approach could potentially trigger synthetic lethal cell death specifically in cell lines that overexpress SLC7A11 and are thus sensitized to glucose deprivation, offering a promising avenue for targeted cancer therapies (43) (Figure 4).
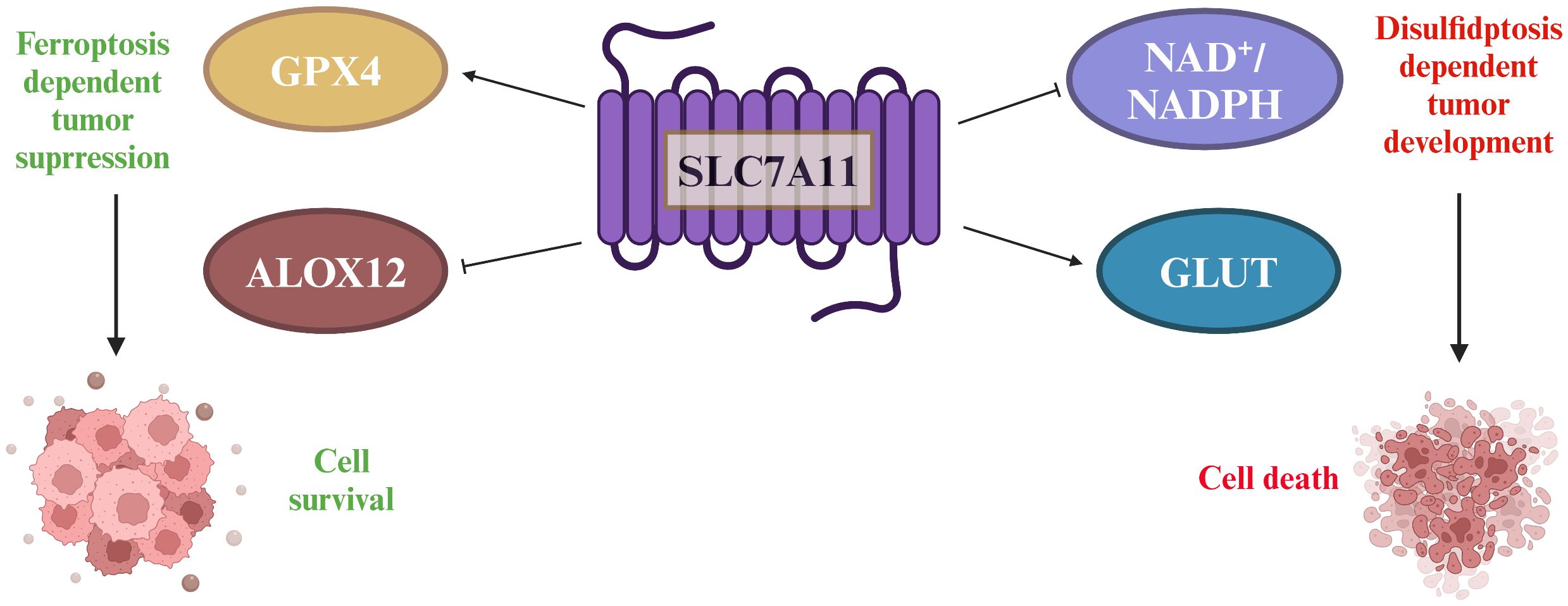
Figure 4 The double-edged sword played by SLC7A11 in tumors. SLC7A11 overexpression in tumors increases cysteine and GSH levels, which are important for reducing lipid peroxides through GPx4. SLC7A11 also suppresses ALOX12, decreasing lipid peroxides and promoting tumor cell survival. However, high SLC7A11 expression leads to cystine accumulation, requiring NADPH from the pentose phosphate pathway to convert it to cysteine. Inhibiting glucose transporter proteins depletes glucose, causing disulfide stress and cell death.
SLC7A11 also affects the nutrient dependence of tumor cells through glutamine backfilling and GLS dependence (43). SLC7A11-mediated glutamate transport may deplete the intracellular glutamate/α-KG pool and activate glutamine catabolism, leading to greater glutamine uptake (44).
SLC7A11 and immune regulation
Within the tumor microenvironment, interactions involving SLC7A11 between immune cells and tumor cells play a significant role in influencing tumor survival and proliferation. Specifically, cytokines released by immune cells have the potential to impact the expression of SLC7A11 within tumors. For instance, the secretion of interferon gamma (IFN-γ) by CD8+ T cells has been shown to down-regulate the expression of SLC3A2 and SLC7A11 in tumor cells, leading to a disruption in cystine uptake (45). This disruption ultimately promotes lipid peroxidation and iron-induced cell death within the tumor cells (46). Conversely, the interplay of cysteine competition and glutamate secretion among various immune cells, as well as between immune cells and tumor cells, significantly impacts the survival of tumors. Cysteine, a crucial amino acid for T-cell activation, is integral to tumor surveillance and cytotoxicity (12). T cells, lacking the functional SLC7A11 transporter protein and cystathionine beta-synthase enzyme, depend on neutral amino acid transporter proteins to uptake cysteine exported by antigen-presenting cells (APCs) (47).
Myeloid-derived suppressor cells (MDSCs) express the transporter SLC7A11, which selectively transports cystathionine but does not export cysteine. MDSCs compete with APCs for extracellular cystine, leading to a reduction in APC release of cysteine in the presence of MDSCs (45). This limitation of the extracellular pool of cysteine hinders T cell activation-mediated antitumor immunity. Additionally, SLC7A11-mediated glutamate release in dendritic cells inhibits metabotropic glutamate receptors, impairing T cell activation (48). The upregulation of SLC7A11 in glioblastoma (GBM) leads to heightened levels of extracellular glutamate, facilitating the proliferation, activation, and suppressive capabilities of regulatory T (Treg) cells, consequently fostering intratumoral immunosuppression (49). Metabolic alterations induced by T cells can influence the fate of GBM cells through SLC7A11, while tumor metabolism further contributes to immune evasion by impairing T cell activation via SLC7A11-mediated dysfunction (Figure 5).
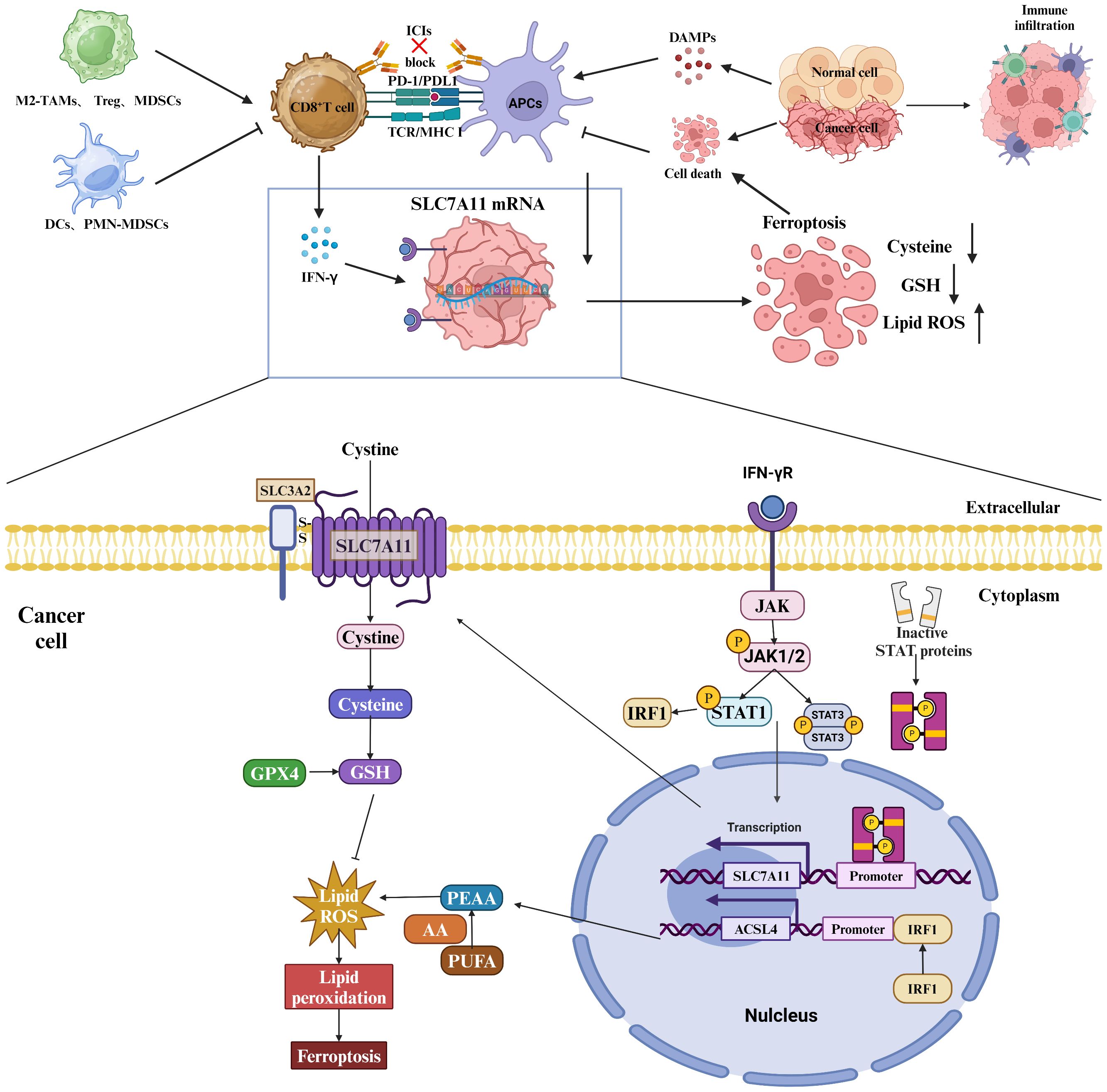
Figure 5 Role of SLC7A11 in regulating the tumor immune microenvironment. Myeloid-derived suppressor cells (MDSCs) express the transporter SLC7A11, which selectively transports cystathionine but does not export cysteine. MDSCs compete for extracellular cystathionine with antigen-presenting cells (APCs), resulting in a decrease in cysteine release from APCs in the presence of MDSCs. This limitation of the extracellular cysteine pool hinders T cell activation-mediated antitumor immunity. In addition, SLC7A11-mediated glutamate release in dendritic cells inhibits metabotropic glutamate receptors, thereby impairing T cell activation. Upregulation of SLC7A11 in glioblastoma (GBM) leads to elevated extracellular glutamate levels, which favors the proliferation, activation, and suppressive capacity of regulatory T (Treg) cells and thus promotes intratumorally immunosuppression. T-cell-induced metabolic alterations can affect the fate of GBM cells via SLC7A11, and tumor metabolism via SLC7A11-mediated dysfunction impairs T cell activation, which further promotes immune evasion. Specifically, IFN-γ affects the mRNA levels of SLC7A11 and ACSL4 through the JAK/STAT signaling pathway.
SLC7A11 and cell death
In the initial investigations into cell growth conditions, researchers observed that a lack of SLC7A11-mediated cystine transport proved fatal for certain cells (50, 51). Subsequent findings revealed that cells deficient in cystine exhibited diminished levels of intracellular GSH, a key antioxidant, and that supplementation with vitamin E effectively prevented this form of cell death induced by cystine deficiency (52–54). These results imply a strong association between this mode of cell death and oxidative stress. Further research identified this specific form of cell death as ferroptosis (55). Ferroptosis is characterized by the accumulation of lipid peroxidation products resulting from iron metabolism and ROS accumulation (56). The SLC7A11/GSH/GPx4 axis plays a central role in the cell’s defense against ferroptosis, with GPx4 utilizing glutathione (GSH) as a cofactor to reduce toxic lipid peroxides at the plasma membrane, thereby protecting the cell from ferroptosis. SLC7A11 is positioned at the initial stage of the ferroptosis pathway, and inhibitors targeting SLC7A11 are commonly used as inducers of ferroptosis in research studies (57)(Figure 6).
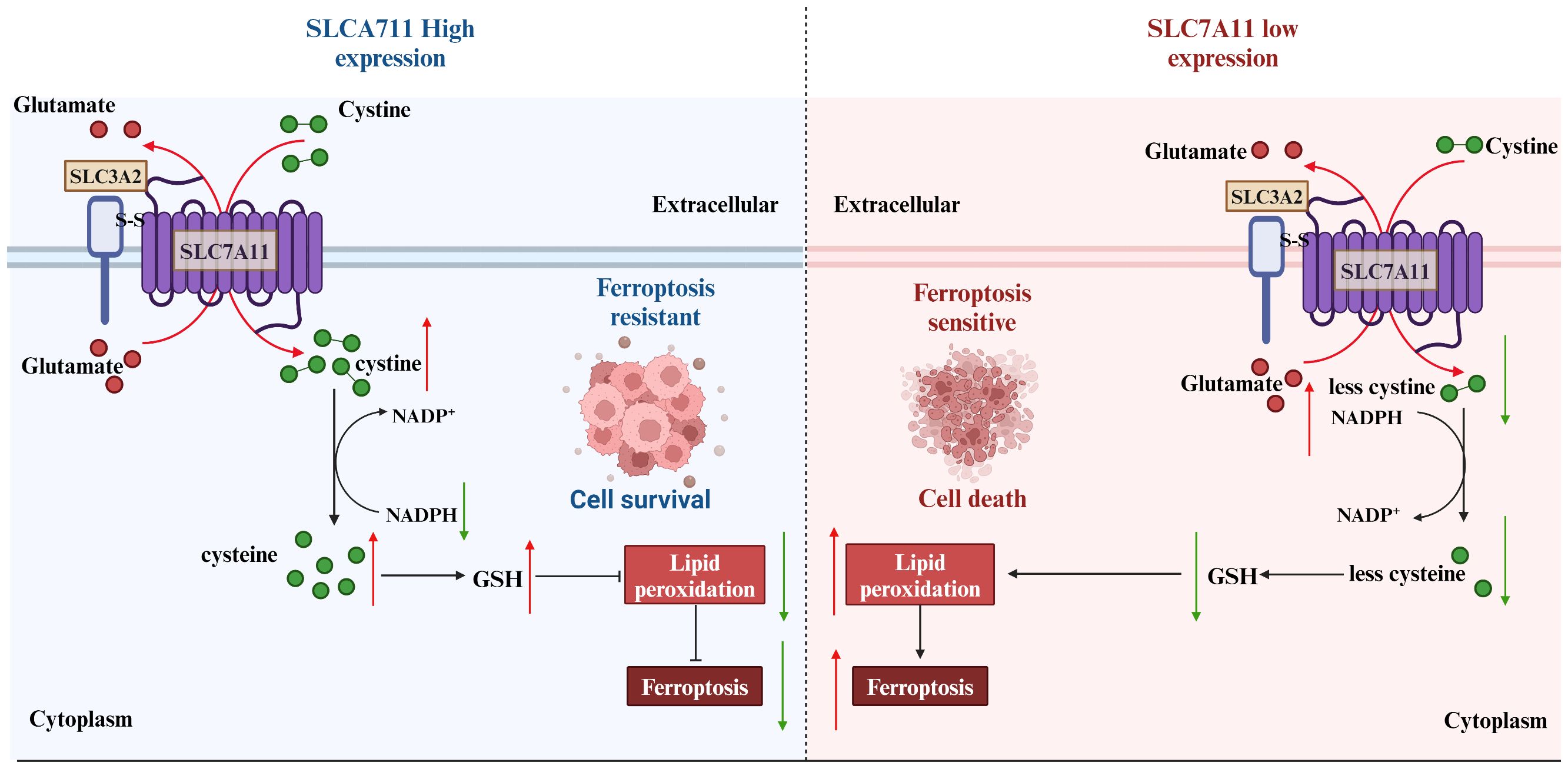
Figure 6 Strategies of SLC7A11 in mediating ferroptosis in the treatment of tumors. The expression levels of SLC7A11 in different cancers were characterized differently. For cells with low SLC7A11 expression, intracellular GSH deprivation leads to diminished lipid peroxide scavenging, resulting in tumor cells that are sensitive to ferroptosis. In contrast, tumor cells with high SLC7A11 expression would be naturally highly resistant to ferroptosis.
Contrary to the oncogenic implications outlined earlier, the upregulation of SLC7A11 can also induce apoptotic cell death in cancer cells under conditions. For instance, in glucose-deficient glioblastoma, heightened SLC7A11 expression leads to the generation of ROS and oxidative stress through the consumption of intracellular NADPH during the conversion of imported L-cystine to L-cysteine (58). Moreover, the addition of α-ketoglutarate (α-KG), a metabolite derived from glutamate, effectively restored the viability of cancer cells with elevated levels of SLC7A11 during glucose deprivation, indicating a potential role for exported glutamate in promoting cancer cell death (59). Subsequent research corroborated the significance of converting glutamate to α-KG for the survival of cancer cells in the absence of glucose, highlighting the regulatory function of SLC7A11 in modulating the metabolic adaptability of cancer cells (60). Cancer cells exhibiting elevated levels of SLC7A11 expression demonstrate a reliance on glucose metabolism, while cells with reduced SLC7A11 expression show heightened oxidative phosphorylation (OXPHOS) activity, as observed in lung cancer cell lines. This shift in metabolic preferences has been further validated in specific cell lines, such as A549 and H1299, where SLC7A11 knockdown led to an increase in OXPHOS and a decrease in glycolysis (5). Additionally, this phenomenon has been replicated in vivo, within a tumor microenvironment, where the metabolic reprogramming associated with altered SLC7A11 expression was evident (61). We underscore the credibility and reproducibility of the observed metabolic shifts in cancer cells with varying SLC7A11 expression levels (62). Given the limited scope of current experiments, additional research is necessary to explore potential underlying mechanisms or constraints contributing to this paradox. Factors such as the intensity and duration of ROS exposure may offer insight into the observed discrepancies. There appears to exist a toxicity threshold for ROS in the induction of tumor cell death (63). Cell death is only induced at high levels of ROS, while levels below this threshold have been shown to enhance tumor malignancy. This phenomenon has been attributed to the inadequate redox capacity in conditions of limited glucose supply, as well as the abnormal accumulation of cystine or other disulfide molecules in cells with high expression of SLC7A11. This accumulation leads to disulfide stress, ultimately resulting in cell death through a novel form of programmed cell death known as disulfidptosis (64). Subsequent investigations revealed that elevated levels of the SLC7A11 protein unexpectedly heightened the susceptibility of tumor cells to oxidative stressors, leading to increased rates of tumor cell apoptosis. Additionally, in vivo experiments demonstrated that heightened SLC7A11 expression facilitated localized tumor growth while impeding tumor migration. In summary, the molecular regulatory mechanism of disulfidptosis involves multiple aspects of cystine uptake, glucose metabolic pathways, disulfide stress, and altered cytoskeletal structure (Figure 7).
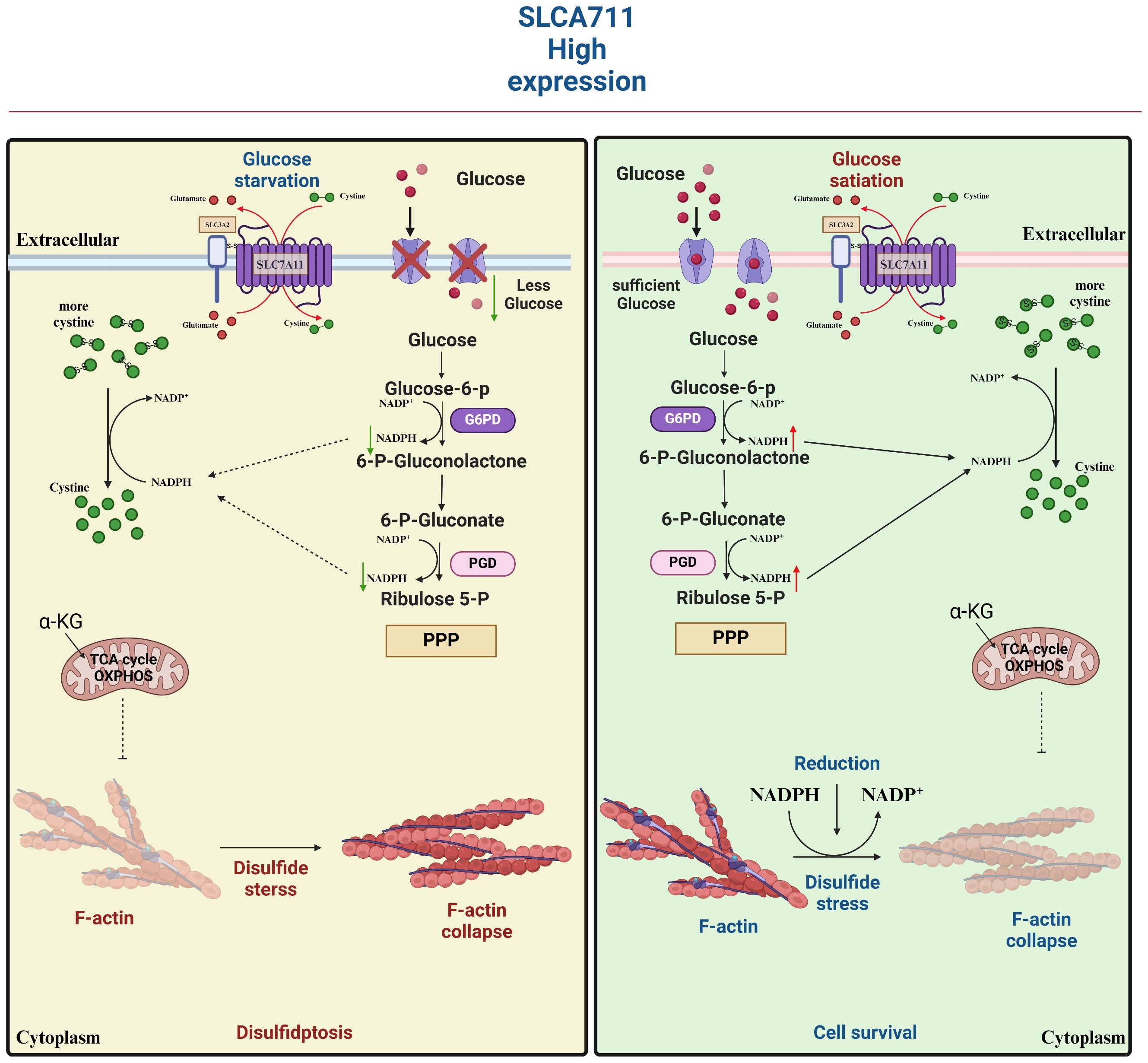
Figure 7 SLC7A11 is highly expressed in tumor cells in response to glucose-dependent induction of disulfidptosis. Disulfidptosis, a unique form of cell death, relies on redox reactions and disulfide bond formation. This process is controlled by cystine uptake and glucose metabolism, with SLC7A11 playing a key role in transporting cystine for glutathione synthesis. However, cystine can be toxic and must be quickly converted to cysteine to prevent harmful buildup. A shortage of NADPH during this conversion can lead to disulfide stress, causing cytoskeletal proteins to form disulfide bonds, contract, and detach from the membrane, disrupting cell function and leading to death.
These findings suggest the potential for inducing distinct forms of cell death in individuals with varying levels of SLC7A11 expression, although the interplay between ferroptosis and disulfidptosis remains an unresolved inquiry.
Tumor therapeutic strategies targeting SLC7A11
Several compounds have been identified as inhibitors of SLC7A11, including Erastin, IKE, sorafenib, DPI2, SAS, glutamate, and INF-γ. A high-throughput screening of synthetic compounds aimed at identifying substances capable of eliminating tumor cells led to the discovery of erastin, a compound exhibiting RAS-selective activity (65). Notably, erastin was observed to trigger a distinct form of cell death, non-apoptotic in nature. Subsequent investigations delved deeper into its mechanism, revealing that erastin directly inhibits Systems Xc-, thereby depleting GSH levels. The lethality of erastin may primarily stem from its quinazolinone backbone, while other chemical moieties could potentially enhance its inhibitory effects on Systems Xc-. Additionally, erastin targets the mitochondrial voltage-dependent anion system (VDAC) (66). Beyond its direct activation of ferroptosis in the treatment of hepatocellular carcinoma, erastin also potentiates the antitumor effects of certain conventional chemotherapeutic agents in hepatocellular carcinoma cell lines. Furthermore, erastin has the potential to augment the clinical efficacy of PD1/L1 by influencing the polarization of tumor-associated macrophages (TAM) (4). In another study, aspirin was found to elicit a pronounced ferroptosis response in HepG2 and Huh7 cells, an effect that was amplified by the ferroptosis-inducing properties of erastin (67). Given its established role as a classical ferroptosis inducer, erastin has become a benchmark for researchers evaluating novel compounds or assessing the ferroptosis-inducing potential of existing drugs (Table 2).
Although erastin demonstrates a potent inhibitory effect on Systems Xc-, its practical application in vivo is significantly hindered by its limited water solubility and metabolic instability. To address these challenges, Imidazolidinone (IKE) was developed as a structurally improved derivative of erastin. IKE not only enhances aqueous solubility but also boosts its anticancer properties. Specifically, IKE boasts a solubility that is three times higher than erastin, and it exhibits a remarkable 50-fold reduction in the Lethal Concentration 50 (LC50) for tumor cells, indicating a significantly enhanced antitumor potency (68).
DPI2, a FIN (ferroptosis-inducing compound), which does not inhibit GPx4 activity but specifically inhibits SLC7A11 expression, consumed 90% of the GSH in BjeLR cells compared to the untreated group. The observed effect of DPI2 was comparable to that of erastin, indicating that DPI2 might trigger cellular ferroptosis via a mechanism analogous to that employed by erastin (37).
Sorafenib, a multikinase inhibitor, has gained widespread use in the treatment of clinically advanced hepatocellular carcinoma, offering patients prolonged survival. Previous research attributed sorafenib’s therapeutic effect primarily to its multikinase inhibitory function, which halts cell proliferation, angiogenesis, and promotes tumor cell apoptosis (69, 70). However, recent discoveries indicate that sorafenib’s toxic impact on hepatocellular carcinoma cells relies partly on ferroptosis, rather than apoptosis. This effect isn’t tied to its kinase inhibitory activity but rather to its ability to trigger iron accumulation and lipid peroxidation stress in the cancer cells (71). Notably, depleting stored iron in these cells through iron chelating agents significantly diminishes sorafenib’s cytotoxic effects. Further investigations have uncovered that sorafenib prompts ferroptosis by impeding the activity of the SLC7A11 transporter on the cell membrane. This reduction leads to decreased cystine levels in the cancer cells, subsequently causing insufficient GSH synthesis and a decline in GPX4 activity. Clinically, hepatocellular carcinoma patients treated with sorafenib often develop drug resistance rapidly, influencing their prognosis. Nrf2, Rb, MT-1G, and SIR are involved in regulating the sensitivity to sorafenib-induced ferroptosis through various pathways, contributing to the emergence of drug resistance (66, 72–74). Adopting a fresh perspective, targeting specific inhibitor pathways to stimulate ferroptosis represents a promising strategy to enhance sorafenib’s drug resistance effectively.
Sulfasalazine (SAS) is a long-approved anti-inflammatory drug by the U.S. Food and Drug Administration, serving as a primary therapy for rheumatoid arthritis. SAS promotes ferroptosis through inhibiting the Xc-system, akin to erastin’s mechanism. Nevertheless, SAS is significantly less potent in triggering ferroptosis compared to erastin. Studies have demonstrated SAS’s ability to induce ferroptosis in diverse tumor cells, suggesting its potential as a combinatory treatment with other cancer therapies to enhance overall treatment effectiveness (75).
As an excitatory neurotransmitter that binds to both excitatory neurotransmitter binding sites and Cl-dependent cysteine- and cystine-inhibited transporter sites, the Xc-system facilitates the transfer of glutamate out of and cystine in. Glutamate-induced toxicity of cellular ferroptosis is proportional to its ability to inhibit cystine uptake, which is in effect a negative feedback mechanism. Exposure to glutamate leads to a decrease in GSH levels and an accumulation of intracellular peroxides, resulting in oxidative stress and cell death (76).
As talked about previously, tumor-associated immunotherapy studies have shown that CD8+ T cells enhance ferroptosis-specific lipid peroxidation in tumor cells. Specifically, INF-γ released by CD8+T cells downregulated the expression of SLC7A11 and SLC3A2, leading to a decrease in GSH and an increase in lipid peroxidation. Inhibition of the ferroptosis pathway eliminated the synergistic effect of INF-γ on ferroptosis in tumor cells in both in vivo and in vitro mouse models (46). And retrospective analysis showed that the expression of SLC7A11 was negatively correlated with CD8+ T cell signaling, INF-γ level and prognosis of tumor patients. Combining immunotherapy with SLC7A11 inhibitors produces a powerful therapeutic effect (77).
A powerful SLC7A11 inhibitor, HG106, has been discovered. This inhibitor notably decreases cystine uptake and disrupts intracellular glutathione biosynthesis. Furthermore, HG106 demonstrates targeted cytotoxicity specifically towards KRAS mutant cells. This effect is achieved by amplifying oxidative stress and triggering apoptosis mediated by endoplasmic reticulum (ER) stress. Remarkably, the administration of HG106 in KRAS mutant lung adenocarcinoma (LUAD) models significantly inhibited tumor growth and extended survival rates in multiple preclinical mouse models of lung cancer (78).
Lepadin H, a marine alkaloid, stands out as an effective inducer of ferroptosis. It demonstrates considerable cytotoxicity, stimulates p53 expression, elevates ROS generation and lipid peroxidation, while simultaneously reducing SLC7A11 and GPX4 levels. Additionally, Lepadin H upregulates ACSL4 expression. Remarkably, it exhibits minimal toxicity towards normal organs, highlighting its potential as a transformative ferroptosis inducer (79).
Certain small molecule monomers possess the ability to adjust SLC7A11 activity, thereby influencing intracellular GSH levels and potentially providing therapeutic advantages for treating specific tumors. This hints at the possibility of these monomers acting as innovative ferroptosis inducers by regulating GSH content. One such example is Pseudolaric acid B (PAB), a naturally occurring diterpene acid extracted from Kaempferia roots and bark. Studies have revealed that PAB can trigger ferroptosis in glioma cells by depleting GSH through SLC7A11 inhibition. By suppressing the expression or function of the Xc-system, PAB slows tumor growth in vivo, while also inhibiting cancer cell invasion and metastasis (80). This cancer-suppressing effect is primarily attributed to the rapid depletion of GSH caused by SLC7A11 transporter dysfunction, leading to lipid ROS buildup and ferroptosis induction.
Ursolic acid, a pentacyclic triterpenoid derived from traditional plants, significantly boosts ROS accumulation in a hepatocellular model when paired with sorafenib (81). This enhancement is likely due to the downregulation of SLC7A11 expression, causing a drop in intracellular GSH levels and compromising ROS scavenging abilities. As a result, lipid peroxidation occurs, inhibiting ferroptosis and offering notable therapeutic benefits in hepatic cell treatment (82).
Sodium butyrate demonstrates anti-cancer properties by modulating the GSH/GSSG ratio, intracellular ROS levels, and lipid peroxide content, thereby inducing ferroptosis in endometrial cancer cells. It also shows promise in suppressing osteosarcoma growth and metastasis through ferroptosis promotion. Pre-exposure to sodium butyrate intensifies erastin-induced changes in GSH depletion, lipid peroxidation, and mitochondrial morphology in CRC cells. Mechanistically, sodium butyrate down-regulates SLC7A11 transcription by modifying ATF3 expression (83).
Tirapazamine (TPZ), a hypoxic prodrug, is renowned for its antitumor effects in the hypoxic tumor microenvironment. TPZ effectively inhibits all three osteosarcoma cell lines tested. Additionally, TPZ enhances fluorescent staining of ferrous ions while reducing SLC7A11 and GPX4 expression, thus promoting ferroptosis and hindering the proliferation and migration of osteosarcoma cells (84).
Pientzehuang (PZH) demonstrates inhibitory effects on the diethylnitrosamine (DEN)-induced hepatocellular carcinoma (HCC) model in rats. The SLC7A11/GSH/GPX4 axis, associated with the ferroptosis response, is seen as a potential target for PZH in preventing the malignant transition from liver fibrosis to HCC (85).
Levobupivacaine, a renowned local anesthetic, exhibits promising anticancer capabilities. It triggers ferroptosis in gastric cancer cells by manipulating the miR-489–3p/SLC7A11 pathway, thereby hindering cancer cell proliferation (86).
Curcumin, a component derived from turmeric and used in traditional Chinese medicine, has been discovered to cause iron accumulation, GSH depletion, and lipid peroxidation in non-small cell lung cancer (NSCLC) cells. However, suppressing Fer-1 and IREB2—both inhibitors of ferroptosis—substantially diminishes curcumin’s anticancer and ferroptosis-inducing effects in A549 and H1299 cells (87).
β-elemene, a naturally occurring compound, has emerged as a novel inducer of ferroptosis. When combined with cetuximab, it demonstrates enhanced sensitivity towards KRAS-mutant colorectal cancer (CRC) cells by promoting ferroptosis, offering a potential therapeutic approach for such cancers (88).
Agrimonolide, extracted from Lungwort, possesses various biomedical properties, including anticancer activity. In ovarian cancer cell lines A2780 and SKOV-3, Agrimonolide not only restricts proliferation, migration, and invasion in a dose-dependent manner but also initiates apoptosis. Its ferroptosis-inducing effects in ovarian cancer cells are evident from increased ROS, total iron, and Fe2+ levels, coupled with reduced expression of ferroptosis markers SLC7A11 and GPX4 (89).
Cisplatin (DDP) stands as a frontline treatment for advanced NSCLC. Ginkgetin has been found to augment DDP’s cytotoxic effects in NSCLC cells by facilitating the accumulation of labile iron and lipid peroxidation. Studies confirm Ginkgetin’s role in mediating ferroptosis through significant reductions in SLC7A11 and GPX4 expression, along with alterations in the GSH/GSSG ratio (90).
Mustardine (SI) has been identified as a potent anti-NSCLC agent, inducing ferroptosis by elevating subferric iron, ROS, and lipid peroxidation. Additionally, SI treatment leads to SLC7A11-dependent downregulation of P53 (91).
Colorectal cancer stem cells (CCSC) significantly impact prognosis, chemotherapy resistance, and treatment outcomes in CRC. Remarkably, Vitamin D administration substantially inhibits CCSC proliferation and reduces tumor spheroid formation in vitro. Further analysis reveals that Vitamin D-treated CCSC exhibit elevated ROS levels, decreased cysteine and GSH levels, and thicker mitochondrial membranes, suggesting that SLC7A11 may be a specific target of Vitamin D’s action (92).
Tanshinone IIA, a bioactive compound extracted from Salvia miltiorrhiza, has been found to reduce the stem-like properties of gastric cancer cells. Its mechanism involves increasing lipid peroxidation and decreasing ferroptosis markers in these cells (93).
Ginsenoside Rh3 has proven effective in eliminating colorectal cancer (CRC) cells. It activates Gasdermin D (GSDMD)-dependent pyroptosis and inhibits SLC7A11 through the Stat3/p53/NRF2 pathway, thereby inducing ferroptosis (94).
Licorice chalcone A (Lico A), a key component of the traditional Chinese medicine Glycyrrhiza glabra, is a naturally occurring small molecule with various pharmacological effects. Both in vivo and in vitro studies have shown that Lico A promotes ferroptosis in hepatocellular carcinoma cells by suppressing SLC7A11 expression. This suppression leads to the inhibition of the GSH-GPX4 pathway and the activation of reactive oxygen species (ROS) (95).
Talaroconvolutin A (TalaA) has emerged as a new inducer of ferroptosis, exhibiting cytotoxicity against colorectal carcinoma cells in a dose- and time-dependent manner. TalaA significantly raises ROS levels to a point where ferroptosis is initiated. Additionally, it downregulates SLC7A11 channel protein expression while upregulating ALOX3, further promoting ferroptosis (56).
Multiple studies have also revealed that Saikosaponin A can induce ferroptosis in hepatocellular carcinoma (HCC) cells, both in laboratory settings and in living organisms. Through RNA sequencing analysis, it has been determined that Saikosaponin A primarily affects the glutathione metabolic pathway and suppresses the expression of the cystine transporter protein SLC7A11. Furthermore, Saikosaponin A has been observed to increase intracellular malondialdehyde (MDA) and iron levels while decreasing reduced glutathione levels in HCC cells. Interestingly, Deferoxamine (DFO), Fer-1, and GSH can reduce the cytotoxic effects of Saikosaponin A, while Z-VAD-FMK is ineffective in preventing Saikosaponin A-induced cell death in HCC (96).
Conclusions
SLC7A11, as the active subunit of the amino acid transporter on cell membranes, plays a wide range of biological functions in organisms. The regulation of SLC7A11 is affected by multiple dimensions, and also abnormal regulation of SLC7A11 leads to malignant tumors related to proliferation, invasion, metastasis and drug resistance. Given that SLC7A11 is often aberrantly expressed by tissues in tumors, it is expected to be an important biomarker for the diagnosis and prognosis of a wide range of tumors. SLC7A11 presents itself as a viable target for tumor therapy, with two primary strategies currently under consideration. The first involves the development of direct inhibitors targeting SLC7A11 to impede cystine uptake in cancer cells, thereby diminishing intracellular GSH levels and inducing cancer cell ferroptosis. The second strategy entails the utilization of inhibitors targeting glucose transporter proteins or glutaminase in the treatment of tumors overexpressing SLC7A11. This approach capitalizes on the heightened vulnerability of SLC7A11-overexpressing tumors to glucose and glutamine deprivation, ultimately leading to tumor cell death. Nevertheless, the efficacy of the therapeutic strategy is hindered by the inadequate induction of ferroptosis caused by SLC7A11 inhibitors in the presence of intracellular cysteine. Recent studies have demonstrated that combining highly specific SLC7A11 inhibitors with immune checkpoint inhibitors PD1/PDL1 may enhance therapeutic efficacy.
Prospects and perspectives
SLC7A11 appears to represent a potential Achilles heel for tumor targeting; however, several challenges still need to be addressed. First, most of the treatments for tumors based on SLC7A11 affecting ferroptosis are preclinical studies that are not yet supported by sufficient clinical evidence. Second, the determination of how to define a baseline SLC7A11 expression level is a critical core of tumor treatment strategies based on SLC7A11 expression levels. Differences in the expression levels of SLC7A11 in different tumor tissues, and differences in the expression levels of SLC7A11 between different patients may also be closely related to the temporal, spatial, and individual heterogeneity of tumor tissues. Finally, how to achieve tissue- and cell-targeted delivery of SLC7A11 to maximize the efficacy of the drug to minimize its toxic side effects.
Author contributions
YJ: Data curation, Methodology, Writing – original draft. MS: Funding acquisition, Resources, Supervision, Validation, Writing – review & editing.
Funding
The author(s) declare financial support was received for the research, authorship, and/or publication of this article. This work was supported by the Shandong Province Key R&D Program (Major Science and Technology Innovation Project, 2021CXGC010509), Key Laboratory of Chronic Deficiency Liver Disease of the State Administration of Traditional Chinese Medicine of the People’s Republic of China (20DZ2272200), Shanghai Key Specialty of Traditional Chinese Clinical Medicine (shslczdzk01201). State Administration of Traditional Chinese Medicine of The Peoples Republic of China(zyyzdxk-2023060).
Acknowledgments
We apologize to all the scientists whose work could not be discussed in the pages of this review. We thank all our lab partners for their continued help and advice on this manuscript. We want to express our gratitude for the drawing materials provided by BioRender. (https://www.biorender.com/).
Conflict of interest
The authors declare that the research was conducted in the absence of any commercial or financial relationships that could be construed as a potential conflict of interest.
Publisher’s note
All claims expressed in this article are solely those of the authors and do not necessarily represent those of their affiliated organizations, or those of the publisher, the editors and the reviewers. Any product that may be evaluated in this article, or claim that may be made by its manufacturer, is not guaranteed or endorsed by the publisher.
Abbreviations
ACC, Adrenocortical Carcinoma; BLCA, Bladder Urothelial Carcinoma; BRCA, Breast Invasive Carcinoma; CESC, Cervical Squamous Cell Carcinoma and Endocervical Adenocarcinoma; CHOL, Cholangiocarcinoma; COAD, Colon Adenocarcinoma; DLBC, Diffuse Large B-Cell Lymphoma; ESCA, Esophageal Carcinoma; GBM, Glioblastoma Multiforme; HNSC, Head and Neck Squamous Cell Carcinoma; KICH, Kidney Chromophobe; KIRC, Kidney Renal Clear Cell Carcinoma; KIRP, Kidney Renal Papillary Cell Carcinoma; LAML, Acute Myeloid Leukemia; LGG, Low Grade Glioma; LIHC, Liver Hepatocellular Carcinoma; LUAD, Lung Adenocarcinoma; LUSC, Lung Squamous Cell Carcinoma; MESO, Malignant Mesothelioma; OV, Ovarian Serous Cystadenocarcinoma; PAAD, Pancreatic Adenocarcinoma; PCPG, Pheochromocytoma and Paraganglioma; PRAD, Prostate Adenocarcinoma; READ, Rectum Adenocarcinoma; SARC, Soft Tissue Sarcoma; SKCM, Skin Cutaneous Melanoma; STAD, Stomach Adenocarcinoma; TGCT, Testicular Germ Cell Tumors; THCA, Thyroid Carcinoma; THYM, Thymoma; UCEC, Uterine Corpus Endometrial Carcinoma; UCS, Uterine Carcinosarcoma; UVM, Uveal Melanoma.
References
1. Koppula P, Zhuang L, Gan B. Cystine transporter SLC7A11/xct in cancer: ferroptosis, nutrient dependency, and cancer therapy. Protein Cell. (2021) 12(8):599–620. doi: 10.1007/s13238-020-00789-5
2. Hanahan D, Weinberg RA. Hallmarks of cancer: the next generation. Cell. (2011) 144(5):646–74. doi: 10.1016/j.cell.2011.02.013
3. Hanahan D. Hallmarks of cancer: New dimensions. Cancer Discovery. (2022) 12(1):31–46. doi: 10.1158/2159-8290.CD-21-1059
4. Badgley MA, Kremer DM, Maurer HC, Delgiorno KE, Lee HJ, Purohit V, et al. Cysteine depletion induces pancreatic tumor ferroptosis in mice. Science. (2020) 368(6486):85–9. doi: 10.1126/science.aaw9872
5. Yan Y, Teng H, Hang Q, Kondiparthi L, Lei G, Horbath A, et al. SLC7A11 expression level dictates differential responses to oxidative stress in cancer cells. Nat Commun. (2023) 14(1):3673. doi: 10.1038/s41467-023-39401-9
6. Ouyang S, Li H, Lou L, Huang Q, Zhang Z, Mo J, et al. Inhibition of STAT3-ferroptosis negative regulatory axis suppresses tumor growth and alleviates chemoresistance in gastric cancer. Redox Biol. (2022) 52:102317. doi: 10.1016/j.redox.2022.102317
7. Chen Q, Zheng W, Guan J, Liu H, Dan Y, Zhu L, et al. SOCS2-enhanced ubiquitination of SLC7A11 promotes ferroptosis and radiosensitization in hepatocellular carcinoma. Cell Death Differ. (2023) 30(1):137–51. doi: 10.1038/s41418-022-01051-7
8. Zheng H, Liu J, Cheng Q, Zhang Q, Zhang Y, Jiang L, et al. Targeted activation of ferroptosis in colorectal cancer via LGR4 targeting overcomes acquired drug resistance. Nat Cancer. (2024) 5(4):572–89. doi: 10.1038/s43018-023-00715-8
9. Lin Y, Dong Y, Liu W, Fan X, Sun Y. Pan-cancer analyses confirmed the ferroptosis-related gene SLC7A11 as a prognostic biomarker for cancer. Int J Gen Med. (2022) 15:2501–13. doi: 10.2147/IJGM.S341502
10. Jiang Y, Yu Y, Pan Z, Glandorff C, Sun M. Ferroptosis: a new hunter of hepatocellular carcinoma. Cell Death Discovery. (2024) 10(1):136. doi: 10.1038/s41420-024-01863-1
11. Mou Y, Wang J, Wu J, He D, Zhang C, Duan C, et al. Ferroptosis, a new form of cell death: opportunities and challenges in cancer. J Hematol Oncol. (2019) 12(1):34. doi: 10.1186/s13045-019-0720-y
12. Tang B, Zhu J, Wang Y, Chen W, Fang S, Mao W, et al. Targeted xct-mediated ferroptosis and protumoral polarization of macrophages is effective against HCC and enhances the efficacy of the anti-PD-1/L1 response. Adv Sci (Weinh). (2023) 10(2):e2203973. doi: 10.1002/advs.202203973
13. Tang B, Wang Y, Xu W, Zhu J, Weng Q, Chen W, et al. Macrophage xct deficiency drives immune activation and boosts responses to immune checkpoint blockade in lung cancer. Cancer Lett. (2023) 554:216021. doi: 10.1016/j.canlet.2022.216021
14. Liu N, Zhang J, Yin M, Liu H, Zhang X, Li J, et al. Inhibition of xct suppresses the efficacy of anti-PD-1/L1 melanoma treatment through exosomal PD-L1-induced macrophage M2 polarization. Mol Ther. (2021) 29(7):2321–34. doi: 10.1016/j.ymthe.2021.03.013
15. Zheng P, Zhou C, Ding Y, Duan S. Disulfidptosis: a new target for metabolic cancer therapy. J Exp Clin Cancer Res. (2023) 42(1):103. doi: 10.1186/s13046-023-02675-4
16. Zheng T, Liu Q, Xing F, Zeng C, Wang W. Disulfidptosis: a new form of programmed cell death. J Exp Clin Cancer Res. (2023) 42(1):137. doi: 10.1186/s13046-023-02712-2
17. Liu M-R, Zhu W-T, Pei D-S. System xc–: a key regulatory target of ferroptosis in cancer. Investigational New Drugs. (2021) 39(4):1123–31. doi: 10.1007/s10637-021-01070-0
18. Fotiadis D, Kanai Y, Palacin M. The SLC3 and SLC7 families of amino acid transporters. Mol Aspects Med. (2013) 34(2-3):139–58. doi: 10.1016/j.mam.2012.10.007
19. Su Y, Zhao B, Zhou L, Zhang Z, Shen Y, Lv H, et al. Ferroptosis, a novel pharmacological mechanism of anti-cancer drugs. Cancer Lett. (2020) 483:127–36. doi: 10.1016/j.canlet.2020.02.015
20. Nachef M, Ali AK, Almutairi SM, Lee SH. Targeting SLC1A5 and SLC3A2/SLC7A5 as a potential strategy to strengthen anti-tumor immunity in the tumor microenvironment. Front Immunol. (2021) 12:624324. doi: 10.3389/fimmu.2021.624324
21. Digomann D, Linge A, Dubrovska A. SLC3A2/CD98hc, autophagy and tumor radioresistance: a link confirmed. Autophagy. (2019) 15(10):1850–1. doi: 10.1080/15548627.2019.1639302
22. Stockwell BR. Ferroptosis turns 10: Emerging mechanisms, physiological functions, and therapeutic applications. Cell. (2022) 185(14):2401–21. doi: 10.1016/j.cell.2022.06.003
23. Stockwell BR, Jiang X, Gu W. Emerging mechanisms and disease relevance of ferroptosis. Trends Cell Biol. (2020) 30(6):478–90. doi: 10.1016/j.tcb.2020.02.009
24. Chen X, Li J, Kang R, Klionsky DJ, Tang D. Ferroptosis: machinery and regulation. Autophagy. (2021) 17(9):2054–81. doi: 10.1080/15548627.2020.1810918
25. Cheng X, Wang Y, Liu L, Lv C, Liu C, Xu J. SLC7A11, a potential therapeutic target through induced ferroptosis in colon adenocarcinoma. Front Mol Biosci. (2022) 9:889688. doi: 10.3389/fmolb.2022.889688
26. Koppula P, Zhang Y, Zhuang L, Gan B. Amino acid transporter SLC7A11/xct at the crossroads of regulating redox homeostasis and nutrient dependency of cancer. Cancer Commun (Lond). (2018) 38(1):12. doi: 10.1186/s40880-018-0288-x
27. Lin Y, Dong Y, Liu W, Fan X, Sun Y. Pan-cancer analyses confirmed the ferroptosis-related gene SLC7A11 as a prognostic biomarker for cancer. Int J Gen Med. (2022) 15:2501–13. doi: 10.2147/IJGM.S341502
28. Nath P, Alfarsi LH, El-Ansari R, Masisi BK, Erkan B, Fakroun A, et al. The amino acid transporter SLC7A11 expression in breast cancer. Cancer Biol Ther. (2024) 25(1):2291855. doi: 10.1080/15384047.2023.2291855
29. He J, Ding H, Li H, Pan Z, Chen Q. Intra-tumoral expression of SLC7A11 is associated with immune microenvironment, drug resistance, and prognosis in cancers: A pan-cancer analysis. Front Genet. (2021) 12:770857. doi: 10.3389/fgene.2021.770857
30. Zheng Z, Hong X, Huang X, Jiang X, Jiang H, Huang Y, et al. Comprehensive analysis of ferroptosis-related gene signatures as a potential therapeutic target for acute myeloid leukemia: A bioinformatics analysis and experimental verification. Front Oncol. (2022) 12:930654. doi: 10.3389/fonc.2022.930654
31. Xu F, Guan Y, Xue L, Zhang P, Li M, Gao M, et al. The roles of ferroptosis regulatory gene SLC7A11 in renal cell carcinoma: A multi-omics study. Cancer Med. (2021) 10(24):9078–96. doi: 10.1002/cam4.4395
32. Chen X, Yu C, Kang R, Kroemer G, Tang D. Cellular degradation systems in ferroptosis. Cell Death Differ. (2021) 28(4):1135–48. doi: 10.1038/s41418-020-00728-1
33. Yang S, Lian G. ROS and diseases: role in metabolism and energy supply. Mol Cell Biochem. (2020) 467(1-2):1–12. doi: 10.1007/s11010-019-03667-9
34. Moloney JN, Cotter TG. ROS signalling in the biology of cancer. Semin Cell Dev Biol. (2018) 80:50–64. doi: 10.1016/j.semcdb.2017.05.023
35. D'autreaux B, Toledano MB. ROS as signalling molecules: mechanisms that generate specificity in ROS homeostasis. Nat Rev Mol Cell Biol. (2007) 8(10):813–24. doi: 10.1038/nrm2256
36. Raza MH, Siraj S, Arshad A, Waheed U, Aldakheel F, Alduraywish S, et al. ROS-modulated therapeutic approaches in cancer treatment. J Cancer Res Clin Oncol. (2017) 143(9):1789–809. doi: 10.1007/s00432-017-2464-9
37. Yang WS, Sriramaratnam R, Welsch ME, Shimada K, Skouta R, Viswanathan VS, et al. Regulation of ferroptotic cancer cell death by GPX4. Cell. (2014) 156(1-2):317–31. doi: 10.1016/j.cell.2013.12.010
38. Cheung EC, Vousden KH. The role of ROS in tumour development and progression. Nat Rev Cancer. (2022) 22(5):280–97. doi: 10.1038/s41568-021-00435-0
39. Ye Y, Chen A, Li L, Liang Q, Wang S, Dong Q, et al. Repression of the antiporter SLC7A11/glutathione/glutathione peroxidase 4 axis drives ferroptosis of vascular smooth muscle cells to facilitate vascular calcification. Kidney Int. (2022) 102(6):1259–75. doi: 10.1016/j.kint.2022.07.034
40. Li Y, Zeng X, Lu D, Yin M, Shan M, Gao Y. Erastin induces ferroptosis via ferroportin-mediated iron accumulation in endometriosis. Hum Reprod. (2021) 36(4):951–64. doi: 10.1093/humrep/deaa363
41. Li S, Lu Z, Sun R, Guo S, Gao F, Cao B, et al. The role of SLC7A11 in cancer: Friend or foe? Cancers (Basel). (2022) 14(13):3059. doi: 10.3390/cancers14133059
42. Liu X, Zhang Y, Zhuang L, Olszewski K, Gan B. NADPH debt drives redox bankruptcy: SLC7A11/xct-mediated cystine uptake as a double-edged sword in cellular redox regulation. Genes Dis. (2021) 8(6):731–45. doi: 10.1016/j.gendis.2020.11.010
43. Saito Y, Soga T. Amino acid transporters as emerging therapeutic targets in cancer. Cancer Sci. (2021) 112(8):2958–65. doi: 10.1111/cas.15006
44. Zou J, Du K, Li S, Lu L, Mei J, Lin W, et al. Glutamine metabolism regulators associated with cancer development and the tumor microenvironment: A pan-cancer multi-omics analysis. Genes (Basel). (2021) 12(9):1305. doi: 10.3390/genes12091305
45. He Q, Liu M, Huang W, Chen X, Zhang B, Zhang T, et al. IL-1beta-Induced elevation of solute carrier family 7 member 11 promotes hepatocellular carcinoma metastasis through up-regulating programmed death ligand 1 and colony-stimulating factor 1. Hepatology. (2021) 74(6):3174–93. doi: 10.1002/hep.32062
46. Wang W, Green M, Choi JE, Gijon M, Kennedy PD, Johnson JK, et al. CD8(+) t cells regulate tumour ferroptosis during cancer immunotherapy. Nature. (2019) 569(7755):270–4. doi: 10.1038/s41586-019-1170-y
47. Tang B, Zhu J, Li J, Fan K, Gao Y, Cheng S, et al. The ferroptosis and iron-metabolism signature robustly predicts clinical diagnosis, prognosis and immune microenvironment for hepatocellular carcinoma. Cell Commun Signal. (2020) 18(1):174. doi: 10.1186/s12964-020-00663-1
48. Long Y, Tao H, Karachi A, Grippin AJ, Jin L, Chang YE, et al. Dysregulation of glutamate transport enhances treg function that promotes VEGF blockade resistance in glioblastoma. Cancer Res. (2020) 80(3):499–509. doi: 10.1158/0008-5472.CAN-19-1577
49. Procaccini C, Garavelli S, Carbone F, Di Silvestre D, La Rocca C, Greco D, et al. Signals of pseudo-starvation unveil the amino acid transporter SLC7A11 as key determinant in the control of treg cell proliferative potential. Immunity. (2021) 54(7):1543–60.e6. doi: 10.1016/j.immuni.2021.04.014
50. Wang L, Hinoi E, Takemori A, Nakamichi N, Yoneda Y. Glutamate inhibits chondral mineralization through apoptotic cell death mediated by retrograde operation of the cystine/glutamate antiporter. J Biol Chem. (2006) 281(34):24553–65. doi: 10.1074/jbc.M600939200
51. Yonezawa M, Back SA, Gan X, Rosenberg PA, Volpe JJ. Cystine deprivation induces oligodendroglial death: rescue by free radical scavengers and by a diffusible glial factor. J Neurochem. (1996) 67(2):566–73. doi: 10.1046/j.1471-4159.1996.67020566.x
52. Stockwell BR, Jiang X. The chemistry and biology of ferroptosis. Cell Chem Biol. (2020) 27(4):365–75. doi: 10.1016/j.chembiol.2020.03.013
53. Hirschhorn T, Stockwell BR. Vitamin k: A new guardian against ferroptosis. Mol Cell. (2022) 82(20):3760–2. doi: 10.1016/j.molcel.2022.10.001
54. Abraham A, Kattoor AJ, Saldeen T, Mehta JL. Vitamin e and its anticancer effects. Crit Rev Food Sci Nutr. (2019) 59(17):2831–8. doi: 10.1080/10408398.2018.1474169
55. Chen X, Kang R, Kroemer G, Tang D. Broadening horizons: the role of ferroptosis in cancer. Nat Rev Clin Oncol. (2021) 18(5):280–96. doi: 10.1038/s41571-020-00462-0
56. Xia Y, Liu S, Li C, Ai Z, Shen W, Ren W, et al. Discovery of a novel ferroptosis inducer-talaroconvolutin a-killing colorectal cancer cells in vitro and in vivo. Cell Death Dis. (2020) 11(11):988. doi: 10.1038/s41419-020-03194-2
57. Xu T, Ding W, Ji X, Ao X, Liu Y, Yu W, et al. Molecular mechanisms of ferroptosis and its role in cancer therapy. J Cell Mol Med. (2019) 23(8):4900–12. doi: 10.1111/jcmm.14511
58. Yu Y, Jiang Y, Glandorff C, Sun M. Exploring the mystery of tumor metabolism: Warburg effect and mitochondrial metabolism fighting side by side. Cell Signal. (2024) 120:111239. doi: 10.1016/j.cellsig.2024.111239
59. Okazaki S, Umene K, Yamasaki J, Suina K, Otsuki Y, Yoshikawa M, et al. Glutaminolysis-related genes determine sensitivity to xct-targeted therapy in head and neck squamous cell carcinoma. Cancer Sci. (2019) 110(11):3453–63. doi: 10.1111/cas.14182
60. Wang X, Zheng C, Yao H, Guo Y, Wang Y, He G, et al. Disulfidptosis: Six riddles necessitating solutions. Int J Biol Sci. (2024) 20(3):1042–4. doi: 10.7150/ijbs.90606
61. Yoshida GJ. The harmonious interplay of amino acid and monocarboxylate transporters induces the robustness of cancer cells. Metabolites. (2021) 11(1):27. doi: 10.3390/metabo11010027
62. Yang X, Cai Z, Wang C, Jiang C, Li J, Chen F, et al. Integrated multiomic analysis reveals disulfidptosis subtypes in glioblastoma: implications for immunotherapy, targeted therapy, and chemotherapy. Front Immunol. (2024) 15:1362543. doi: 10.3389/fimmu.2024.1362543
63. Liu X, Nie L, Zhang Y, Yan Y, Wang C, Colic M, et al. Actin cytoskeleton vulnerability to disulfide stress mediates disulfidptosis. Nat Cell Biol. (2023) 25(3):404–14. doi: 10.1038/s41556-023-01091-2
64. Shao D, Shi L, Ji H. Disulfidptosis: Disulfide stress mediates a novel cell death pathway via actin cytoskeletal vulnerability. Mol Cells. (2023) 46(7):414–6. doi: 10.14348/molcells.2023.0060
65. Yang WS, Stockwell BR. Synthetic lethal screening identifies compounds activating iron-dependent, nonapoptotic cell death in oncogenic-RAS-harboring cancer cells. Chem Biol. (2008) 15(3):234–45. doi: 10.1016/j.chembiol.2008.02.010
66. Dixon SJ, Patel DN, Welsch M, Skouta R, Lee ED, Hayano M, et al. Pharmacological inhibition of cystine-glutamate exchange induces endoplasmic reticulum stress and ferroptosis. Elife. (2014) 3:e02523. doi: 10.7554/eLife.02523
67. Sun X, Ou Z, Chen R, Niu X, Chen D, Kang R, et al. Activation of the p62-Keap1-NRF2 pathway protects against ferroptosis in hepatocellular carcinoma cells. Hepatology. (2016) 63(1):173–84. doi: 10.1002/hep.28251
68. Zhang Y, Tan H, Daniels JD, Zandkarimi F, Liu H, Brown LM, et al. Imidazole ketone erastin induces ferroptosis and slows tumor growth in a mouse lymphoma model. Cell Chem Biol. (2019) 26(5):623–33 e9. doi: 10.1016/j.chembiol.2019.01.008
69. Tang W, Chen Z, Zhang W, Cheng Y, Zhang B, Wu F, et al. The mechanisms of sorafenib resistance in hepatocellular carcinoma: theoretical basis and therapeutic aspects. Signal Transduct Target Ther. (2020) 5(1):87. doi: 10.1038/s41392-020-0187-x
70. Li X, Li Y, Lian P, Lv Q, Liu F. Silencing lncrna HCG18 regulates GPX4-inhibited ferroptosis by adsorbing mir-450b-5p to avert sorafenib resistance in hepatocellular carcinoma. Hum Exp Toxicol. (2023) 42:9603271221142818. doi: 10.1177/09603271221142818
71. Byun JK, Lee S, Kang GW, Lee YR, Park SY, Song IS, et al. Macropinocytosis is an alternative pathway of cysteine acquisition and mitigates sorafenib-induced ferroptosis in hepatocellular carcinoma. J Exp Clin Cancer Res. (2022) 41(1):98. doi: 10.1186/s13046-022-02296-3
72. Louandre C, Marcq I, Bouhlal H, Lachaier E, Godin C, Saidak Z, et al. The retinoblastoma (Rb) protein regulates ferroptosis induced by sorafenib in human hepatocellular carcinoma cells. Cancer Lett. (2015) 356(2 Pt B):971–7. doi: 10.1016/j.canlet.2014.11.014
73. Sun X, Niu X, Chen R, He W, Chen D, Kang R, et al. Metallothionein-1G facilitates sorafenib resistance through inhibition of ferroptosis. Hepatology. (2016) 64(2):488–500. doi: 10.1002/hep.28574
74. Bai T, Lei P, Zhou H, Liang R, Zhu R, Wang W, et al. Sigma-1 receptor protects against ferroptosis in hepatocellular carcinoma cells. J Cell Mol Med. (2019) 23(11):7349–59. doi: 10.1111/jcmm.14594
75. Sun S, Guo C, Gao T, Ma D, Su X, Pang Q, et al. Hypoxia enhances glioma resistance to sulfasalazine-induced ferroptosis by upregulating SLC7A11 via PI3K/AKT/HIF-1alpha axis. Oxid Med Cell Longev. (2022) 2022:7862430. doi: 10.1155/2022/7862430
76. Peng H, Wang Y, Luo W. Multifaceted role of branched-chain amino acid metabolism in cancer. Oncogene. (2020) 39(44):6747–56. doi: 10.1038/s41388-020-01480-z
77. Zhou X, Zou L, Liao H, Luo J, Yang T, Wu J, et al. Abrogation of hnrnp l enhances anti-PD-1 therapy efficacy via diminishing PD-L1 and promoting CD8(+) t cell-mediated ferroptosis in castration-resistant prostate cancer. Acta Pharm Sin B. (2022) 12(2):692–707. doi: 10.1016/j.apsb.2021.07.016
78. Hu K, Li K, Lv J, Feng J, Chen J, Wu H, et al. Suppression of the SLC7A11/glutathione axis causes synthetic lethality in KRAS-mutant lung adenocarcinoma. J Clin Invest. (2020) 130(4):1752–66. doi: 10.1172/JCI124049
79. Wang W, Ma F, Cheung YT, Zeng G, Zhou Y, Chen Z, et al. Marine alkaloid lepadins e and h induce ferroptosis for cancer chemotherapy. J Med Chem. (2023) 66(16):11201–15. doi: 10.1021/acs.jmedchem.3c00659
80. Wang Z, Ding Y, Wang X, Lu S, Wang C, He C, et al. Pseudolaric acid b triggers ferroptosis in glioma cells via activation of Nox4 and inhibition of xct. Cancer Lett. (2018) 428:21–33. doi: 10.1016/j.canlet.2018.04.021
81. Yang M, Hu C, Cao Y, Liang W, Yang X, Xiao T. Ursolic acid regulates cell cycle and proliferation in colon adenocarcinoma by suppressing cyclin B1. Front Pharmacol. (2020) 11:622212. doi: 10.3389/fphar.2020.622212
82. Li H, Yu Y, Liu Y, Luo Z, Law BYK, Zheng Y, et al. Ursolic acid enhances the antitumor effects of sorafenib associated with mcl-1-related apoptosis and SLC7A11-dependent ferroptosis in human cancer. Pharmacol Res. (2022) 182:106306. doi: 10.1016/j.phrs.2022.106306
83. Crawford RR, Prescott ET, Sylvester CF, Higdon AN, Shan J, Kilberg MS, et al. Human CHAC1 protein degrades glutathione, and mrna induction is regulated by the transcription factors ATF4 and ATF3 and a bipartite ATF/CRE regulatory element. J Biol Chem. (2015) 290(25):15878–91. doi: 10.1074/jbc.M114.635144
84. Shi Y, Gong M, Deng Z, Liu H, Chang Y, Yang Z, et al. Tirapazamine suppress osteosarcoma cells in part through SLC7A11 mediated ferroptosis. Biochem Biophys Res Commun. (2021) 567:118–24. doi: 10.1016/j.bbrc.2021.06.036
85. Yan X, Liu Y, Li C, Mao X, Xu T, Hu Z, et al. Pien-Tze-Huang prevents hepatocellular carcinoma by inducing ferroptosis via inhibiting SLC7A11-GSH-GPX4 axis. Cancer Cell Int. (2023) 23(1):109. doi: 10.1186/s12935-023-02946-2
86. Mao SH, Zhu CH, Nie Y, Yu J, Wang L. Levobupivacaine induces ferroptosis by mir-489-3p/SLC7A11 signaling in gastric cancer. Front Pharmacol. (2021) 12:681338. doi: 10.3389/fphar.2021.681338
87. Tang X, Ding H, Liang M, Chen X, Yan Y, Wan N, et al. Curcumin induces ferroptosis in non-small-cell lung cancer via activating autophagy. Thorac Cancer. (2021) 12(8):1219–30. doi: 10.1111/1759-7714.13904
88. Chen P, Li X, Zhang R, Liu S, Xiang Y, Zhang M, et al. Combinative treatment of beta-elemene and cetuximab is sensitive to KRAS mutant colorectal cancer cells by inducing ferroptosis and inhibiting epithelial-mesenchymal transformation. Theranostics. (2020) 10(11):5107–19. doi: 10.7150/thno.44705
89. Liu Y, Liu X, Wang H, Ding P, Wang C. Agrimonolide inhibits cancer progression and induces ferroptosis and apoptosis by targeting SCD1 in ovarian cancer cells. Phytomedicine. (2022) 101:154102. doi: 10.1016/j.phymed.2022.154102
90. Menezes J, Diederich MF. Bioactivity of natural biflavonoids in metabolism-related disease and cancer therapies. Pharmacol Res. (2021) 167:105525. doi: 10.1016/j.phrs.2021.105525
91. Sinha S, Doble M, Manju SL. 5-lipoxygenase as a drug target: A review on trends in inhibitors structural design, SAR and mechanism based approach. Bioorg Med Chem. (2019) 27(17):3745–59. doi: 10.1016/j.bmc.2019.06.040
92. Guo S, Zhao W, Zhang W, Li S, Teng G, Liu L. Vitamin d promotes ferroptosis in colorectal cancer stem cells via SLC7A11 downregulation. Oxid Med Cell Longev. (2023) 2023:4772134. doi: 10.1155/2023/4772134
93. Ni H, Ruan G, Sun C, Yang X, Miao Z, Li J, et al. Tanshinone IIA inhibits gastric cancer cell stemness through inducing ferroptosis. Environ Toxicol. (2022) 37(2):192–200. doi: 10.1002/tox.23388
94. Wu Y, Pi D, Zhou S, Yi Z, Dong Y, Wang W, et al. Ginsenoside Rh3 induces pyroptosis and ferroptosis through the Stat3/p53/NRF2 axis in colorectal cancer cells. Acta Biochim Biophys Sin (Shanghai). (2023) 55(4):587–600. doi: 10.3724/abbs.2023068
95. Ley-Martínez JS, Ortega-Valencia JE, García-Barradas O, Jiménez-Fernández M, Uribe-Lam E, Vencedor-Meraz CI, et al. Active compounds in zingiber officinale as possible redox inhibitors of 5-lipoxygenase using an in silico approach. Int J Mol Sci. (2022) 23(11):6093. doi: 10.3390/ijms23116093
Keywords: SLC7A11, redox homeostasis, tumor metabolism, immune, cell death
Citation: Jiang Y and Sun M (2024) SLC7A11: the Achilles heel of tumor? Front. Immunol. 15:1438807. doi: 10.3389/fimmu.2024.1438807
Received: 26 May 2024; Accepted: 25 June 2024;
Published: 08 July 2024.
Edited by:
Lian Xiang Luo, Guangdong Medical University, ChinaReviewed by:
Shuai Gong, University of Massachusetts Amherst, United StatesWanheng Zhang, Baylor College of Medicine, United States
Copyright © 2024 Jiang and Sun. This is an open-access article distributed under the terms of the Creative Commons Attribution License (CC BY). The use, distribution or reproduction in other forums is permitted, provided the original author(s) and the copyright owner(s) are credited and that the original publication in this journal is cited, in accordance with accepted academic practice. No use, distribution or reproduction is permitted which does not comply with these terms.
*Correspondence: Mingyu Sun, bXlzdW4yNDhAaG90bWFpbC5jb20=