- 1Department of Pharmaceutical Sciences, School of Pharmacy and Nutrition, University of Navarra, Pamplona, Spain
- 2Navarra Medical Research Institute (IdiSNA), Pamplona, Spain
Macrophages play a pivotal role as host cells for Leishmania parasites, displaying a notable functional adaptability ranging from the proinflammatory, leishmanicidal M1 phenotype to the anti-inflammatory, parasite-permissive M2 phenotype. While macrophages can potentially eradicate amastigotes through appropriate activation, Leishmania employs diverse strategies to thwart this activation and redirect macrophages toward an M2 phenotype, facilitating its survival and replication. Additionally, a competition for iron between the two entities exits, as iron is vital for both and is also implicated in macrophage defensive oxidative mechanisms and modulation of their phenotype. This review explores the intricate interplay between macrophages, Leishmania, and iron. We focus the attention on the potential of iron oxide nanoparticles (IONPs) as a sort of immunotherapy to treat some leishmaniasis forms by reprogramming Leishmania-permissive M2 macrophages into antimicrobial M1 macrophages. Through the specific targeting of iron in macrophages, the use of IONPs emerges as a promising strategy to finely tune the parasite-host interaction, endowing macrophages with an augmented antimicrobial arsenal capable of efficiently eliminating these intrusive microbes.
1 Introduction
Flagellate protists of the genus Leishmania are the etiological agents of leishmaniasis, a vector-borne parasitic disease associated with a pronounced immune system dysfunction. The World Health Organization (WHO) classifies this condition as one of the twenty neglected tropical diseases (NTDs) that threaten more than 1.7 billion people and disproportionately affect the poorest strata of the population (1).
The term leishmaniasis encompasses a broad spectrum of clinical symptoms and pathologies, ranging from asymptomatic infections and self-healing skin lesions (cutaneous leishmaniasis, CL) to more severe forms such as mucocutaneous leishmaniasis (MCL) or visceral leishmaniasis (VL), which can be life-threatening if left untreated. This heterogeneity of clinical manifestations is the result of a complex interplay between the immune response and genetic characteristics of the host, and parasite characteristics such as infectivity and virulence (2).
The burden of this disease is immense, being endemic in 99 countries in tropical and subtropical areas worldwide. According to the WHO, there were approximately 250,000 new cases of leishmaniasis worldwide in 2022 although the actual burden of the disease is often underestimated due to under-reporting in many of the affected areas (3, 4). At the global level, there is a general increasing trend in the number of new cases, associated with environmental changes such as deforestation, the building of dams, irrigation schemes, and urbanization (4).
The unavailability of a human vaccine and effective vector control programs render chemotherapy the only option to control leishmaniasis. However, available treatments are expensive, impractical, toxic, and, subject to drug resistance that lessens their efficacy (1, 3). Importantly, drug resistance is the main cause of leishmanicidal chemotherapy failures, as the amazing genetic plasticity of the parasite allows it to adapt easily to new and challenging environments, such as drug pressure (5). Consequently, there is an urgent need for alternative therapeutic strategies that address these limitations.
Once in the host, and although Leishmania can infect different cells, macrophages are their preferred and final target, being indispensable for its survival, replication, and differentiation. In their interior, the extracellular forms of the parasite -promastigotes- differentiate into the intracellular ones -amastigotes. They divide by binary fission until reaching a high number, leading to the cellular lysis and its liberation into the blood flow. This release finally cause the infection of surrounding macrophages and thus expanding the infection (6).
Macrophages are phagocytic cells with enormous phenotypic plasticity that allows them to carry out a plethora of different functions, ranging from tissue repair to eliminating invasive microorganisms. A wide spectrum of distinct phenotypes exists, typically represented by the pro-inflammatory M1 macrophage and the anti-inflammatory M2 macrophage, that are determined by the presence of signals (e.g., cytokines) in the local microenvironment of the cell. This functional dichotomy includes the expression of specific markers, a particular cytokine pattern, and different types of energy metabolism (7).
Although the interior of a macrophage constitutes a hostile environment for any microbe or parasite, Leishmania adeptly manipulates host cell signaling, metabolism, and immune functions steering the macrophage toward an anti-inflammatory phenotype similar to M2 (8). This intricate interplay between macrophages and Leishmania unveils a dynamic relationship critical for determining the course of infection. The balance between M1/M2 phenotypes emerges as a pivotal factor in infection dynamics, as illustrated by studies in mouse models. C57BL/6 mice infected with L. major mice are resistant to infection, triggering a robust Th1 response, which is aligned with the pro-inflammatory M1 phenotype (interferon-gamma (IFN-γ) and interleukin-12 (IL-12) production). In contrast, the vulnerability of BALB/c mice is marked by a Th2 response, paralleling the anti-inflammatory M2 phenotype (9–11). This correlation underlines the importance of macrophage polarization in establishing a potent immune response capable of controlling Leishmania infection. Thus, addressing macrophage polarization either directly or indirectly emerges as a promising strategy for combating leishmaniasis, which is classified within host-directed therapies (HDT). These therapies utilize agents that are not microbicides per se, but rather their action is directed by modulating host cell immunity (12). This approach has the enormous advantage of being refractory to drug resistance that threatens the efficacy of current leishmanicidal therapies. Leveraging the sensitivity of Leishmania to endogenous microbicidal mechanisms of macrophages, various agents have been used to unlock the microbicidal functions that the parasite is able to encrypt, including toll-like receptors (TLR) agonist like Imiquimod and CpGs oligonucleotides (13–15).
One of the most intriguing battles between Leishmania and the macrophage is the competition for iron, an essential element for the survival and functions of both. On the one hand, Leishmania has developed sophisticated mechanisms to acquire the iron necessary for its proliferation. On the other hand, it must be very careful in its eagerness to increase macrophage iron levels due to the close relationship between cellular iron metabolism and antimicrobial mechanisms, such as the generation of reactive oxygen species (ROS) by the Fenton reaction (16).
With this review, we aim to provide insight into the intricate tug-of-war between Leishmania and the macrophage, with a particular emphasis on the battle for iron, recapitulating the works that use its modulation as a way to enhance the microbicidal response of the macrophage. In addition, we will focus on iron oxide nanoparticles (IONPs), already approved by the Food and Drug Administration (FDA) for other applications (17), as a host-targeted therapy against leishmaniasis. Our interest in IONPs is based on their ability to address three pivotal aspects crucial for treating a neglected disease like leishmaniasis: i) they influence the immunology of macrophages rather than directly targeting the parasite, which mitigates the risk of drug resistance development; ii) as nanoparticles (NPs), they offer the prospect of decreased toxicity and improved efficacy by selectively accumulating in macrophages, which mimics the final fate of the parasites; iii) their repurposing could significantly enhance patient accessibility by substantially lowering development and approval costs.
2 The Leishmania-macrophage tug-of-war. A tale of persuasion, manipulation, and exploitation
2.1 The macrophage: a lethal chamber for Leishmania
Once Leishmania is ingested by macrophages, it docks into the parasitophorous vacuole, which fuses with lysosomes, transforming into a phagolysosome with microbicidal properties. It is characterized by an acid pH (induced by vacuolar ATPase, which pumps protons into it) and many proteases (such as cathepsins) responsible for its proteolytic degradation (16, 18).
In addition to sequestering the parasite in an inhospitable terrain such as the interior of a phagolysosome, macrophages produce a variety of toxic compounds that help to destroy the phagocytosed microorganism: nitric oxide (NO), and ROS. Within the phagosome membrane, the orchestrated activity of the complex NADPH oxidase (Nox2) starts the respiratory burst, a pivotal phase in the macrophage’s offensive. Nox2 initiates the production of the superoxide ion from molecular oxygen, an elemental maneuver in the macrophage’s armamentarium against intruding pathogens. This superoxide ion is converted into hydrogen peroxide (H2O2) through the catalytic prowess of the enzyme superoxide dismutase (SOD). Simultaneously, additional reactions unfold, giving rise to toxic substances like hypochlorous acid (HOCl), further amplifying the macrophage’s arsenal in the ongoing cellular warfare (19).
Concomitantly, the macrophage orchestrates the induction of inducible nitric oxide synthase (iNOS). This catalytic maestro transforms L-arginine into L-citrulline with the generation of NO as a by-product. This enzymatic feat unfolds in the presence of immunomodulatory signals such as IFN-γ and tumor necrosis factor-alpha (TNF- α) (20). The dynamic interplay between NO and ROS ushers forth the generation of derivatives like peroxynitrite (OONO). These formidable agents of cellular damage covalently bind to DNA, inducing deamination of nucleotide bases and precipitating diverse alterations, that ultimately execute a lethal blow to the pathogens (21). Additionally, the liberation of transition metals such as iron from proteins in the phagosome can result in Fenton chemistry. It is a catalyzed oxidation reaction involving iron, resulting in the production of hydroxyl radicals in the presence of hydrogen peroxide (16).
This oxidative burst and NO generated by macrophages are key to restricting the intracellular growth of Leishmania. The inhibition of iNOS hampers this effect, which emphasize the indispensability of NO production in control infection (20, 22, 23). Clinical observations revealed an inverse correlation between iNOS expression and the severity of L. tropica infections (24). Additionally, studies inhibiting ROS production in L. braziliensis-infected monocytes underscored the crucial role of ROS, as their inhibition increases parasite survival (25). Collectively, NO and ROS stand as the main microbicide molecules orchestrating macrophage defenses, pivotal for effective Leishmania control.
In parallel with these microbicidal molecules, macrophages also secrete mediators to engage other types of immune cells and enhance the overall offense. Macrophages release proinflammatory cytokines such as TNF-α, IL-12, and IFN-γ, acting as alarm signals (26). These cytokines not only amplify the microbicidal activity of the macrophages themselves but also recruit additional immune cells, such as lymphocytes to participate in the defense, promoting a Th1 response. On the other end of the spectrum are IL-4 and IL-13, which are associated with a Th2 response and, therefore, with susceptibility to infection by not activating macrophages properly (27).
If none of these strategies succeed in controlling the infection, macrophages resort to an extreme measure: they induce their own sacrifice to prevent parasites’ spread and preserve the organism’s integrity. During the apoptotic process, signaling cascades are activated, leading to a controlled death, and avoiding the uncontrolled release of parasites into the surrounding environment (28, 29). This act of self-destruction represents a remarkable example of the immune system’s adaptive strategies in the face of persistent infections.
2.2 Survival strategies of Leishmania inside macrophages
Leishmania has evolved sophisticated ways to resist the array of microbicidal mechanisms generated by the macrophage, namely the oxidative stress, the recruitment of other immune cells and the process of apoptosis.
2.2.1 Quenching the flames: Leishmania´s control of oxidative stress
The oxidative burst is crucial to control Leishmania proliferation by the macrophage, so the main goal of the parasite is to protect itself from damage caused by these oxidative molecules (ROS and NO), which can eliminate it. Stopping this macrophage offensive is critical for this growth, as it represents a paradigmatic case of how the parasite exerts its manipulation on the host at all levels of the genetic decoding process (genome -> transcriptome -> proteome), underlining its significant importance (Figure 1).
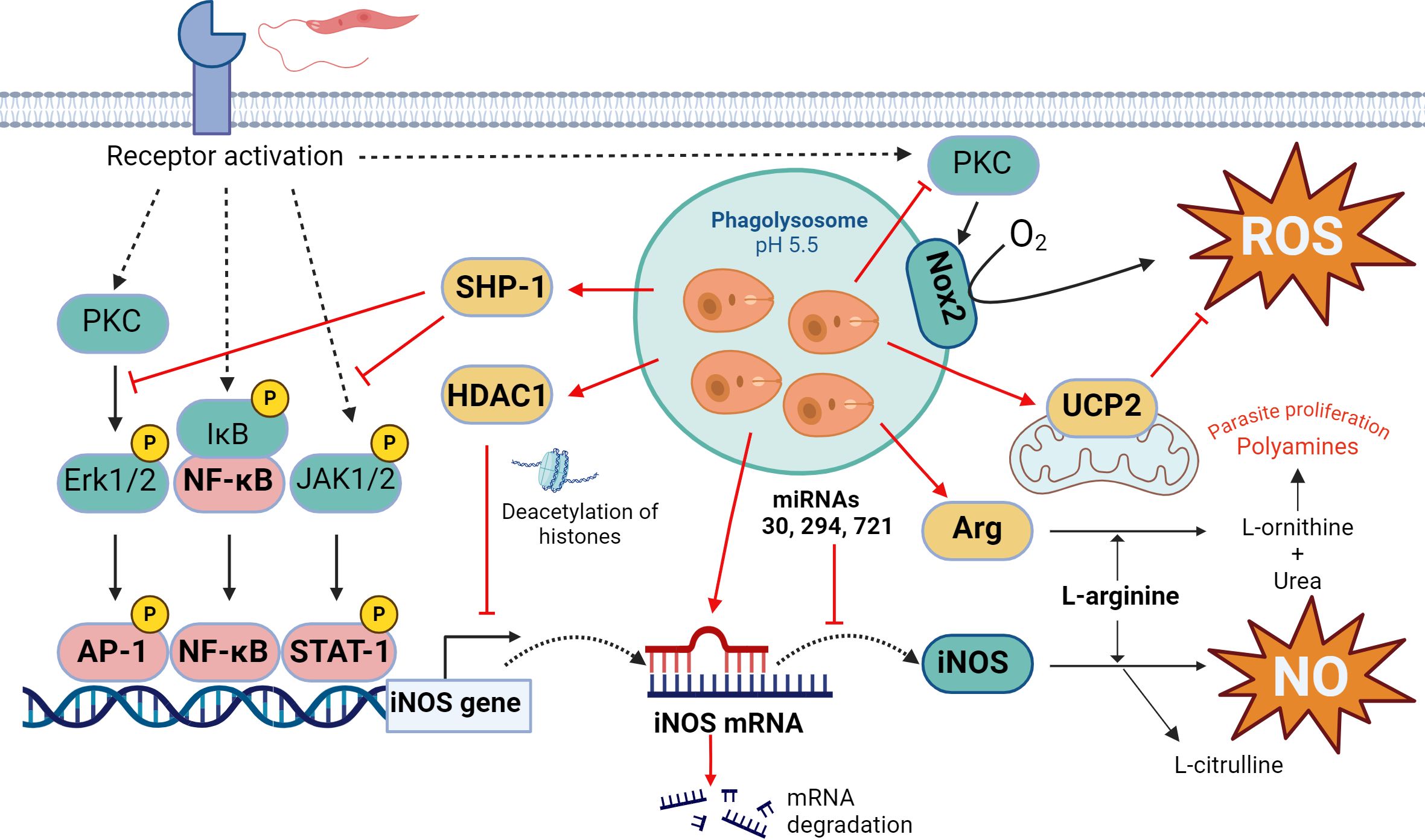
Figure 1. Oxidative response in Leishmania infection. The key leishmanicidal molecules produced by the macrophage are ROS and NO, although Leishmania parasite attempts to stop their generation at different levels. On the one hand, Leishmania can inhibit iNOS expression through multiple mechanisms, including JAK2-STAT1 and ERK1/2-AP1 signaling pathways interference, chromatin condensation through HDAC1 recruitment, negative regulation by microRNAs, and substrate competition through overexpression of the enzyme arginase. In the case of ROS, the parasite prevents Nox2 complex formation and PKC activation and induces mitochondrial UCP2. Actions that Leishmania triggers to counteract the production of ROS/NO by the host cell are indicated in red. Figure created with biorender.com.
First, Leishmania prevents transcription of the iNOS gene by interfering with the signaling pathway that leads to the binding of the transcription factor STAT-1 to its promoter. Thus, Leishmania inhibits Janus kinase 2-signal transducer and activator of transcription 2 (JAK2-STAT1) and extracellular-regulated kinase 1-activator protein 1 (ERK1/2-AP1) signaling cascades, thereby preventing iNOS expression (30, 31). Moreover, it activates the Src homology 2-containing phosphatase-1 (SHP-1) (32), which prevents phosphorylation of both JAK2 and ERK1/2 and downstream phosphorylation of the transcription factors STAT1 and AP1, which control enzyme expression (30, 33). Derivate of knockout mice for SHP1 showed a higher generation of NO and consequently, a higher efficiency in the control of the infection by L. donovani (34) (Figure 1).
Furthermore, even if these signaling pathways are activated, Leishmania can block access of the transcription machinery to the iNOS gene promoter. It induces chromatin condensation in that genomic region by modifying the expression and activity of histone deacetylase 1 (HDAC1) which methylates lysine 9 of histone 3 (35). The precise mechanism by which the parasite accomplishes this effect remains not fully understood, although competition for the activity of histone-modifying enzymes between histones released by the parasite could occurs. Additionally, these histones themselves are capable of independently altering chromatin structure (36, 37). A similar effect has been described for L. amazonensis, which, by activating the phosphatidylinositol 3-kinase/protein kinase B (PI3K/Akt) pathway, activates the p50/p50 transcriptional repressor of the nuclear factor kappa-light-chain-enhancer of activated B cells (NF-κB) family, which binds to the iNOS promoter and prevents its transcription (38).
The expression of iNOS is also controlled by microRNA (miRNAs), which are small non-codifying RNAs that interact with regions 3’ of the messengers. This leads to its degradation and, thus, prevent their translation. Leishmania infection has been shown to interfere with the regulation of miR-30, miR-294 and miR-721, which bind the iNOS messenger and reduce cellular levels of the enzyme (39, 40) (Figure 1).
Even if iNOS are produced, the parasite has another way to decrease NO production. Leishmania manages to upregulate arginase, an enzyme that competes for the same substrate as the iNOS, L-arginine. Wilkins-Rodríguez et al. attribute an increase in the virulence of L. mexicana strains in which arginase enzyme activity is higher compared to iNOS (41). In addition, arginase hydrolyzes L-arginine to generate urea and L-ornithine, a precursor in the biosynthesis of polyamines which is necessary for Leishmania proliferation (42), modifying the macrophage metabolite pool in its favor (Figure 1).
Regarding ROS, Leishmania also controls their production at different levels. Leishmania prevents the assembly of Nox2 complex in the vacuole membrane, inhibiting the generation of ROS and favoring its survival (43). This protection is mediated by the metalloprotease gp63 present in their membrane, which directly cleaved the vesicle-associated membrane protein 8 (VAMP8), responsible for the recruitment of gp91phox- component of Nox2- to the phagolysosome (44). At the same time, through the lipophosphoglycan (LPG) and glycoprotein 63 (gp63), L. major interrupts the activation of protein kinase C (PKC) (42, 45), a kinase that stimulates the activity of Nox2 (46). Moreover, L. donovani induces the expression of the uncoupling protein 2 (UCP2), a protein of the mitochondria membrane that acts as a negative regulator of the production of ROS (47). In fact, silencing UCP2 by small interfering RNA (siRNA) increases ROS production and leads to reduced parasite survival (48) (Figure 1).
Apart from these ways in which Leishmania minimizes the production of ROS and NO, it also manages to enhance the host antioxidant response by upregulating the transcription factor NF-E2-related factor 2 (NRF2) (49, 50). This transcriptional factor (TF) binds to genes promoters of different antioxidant enzymes such as thioredoxin (TXN) or some glutathione S-transferases (GSTs) (51).
2.2.2 Interfering communications: Leishmania´s sabotage of antigen presentation and cytokine production
The collaboration between cells of the immune system is essential to mount a potent response to an invader. Thus, in addition to protecting itself from the microbicidal molecules of macrophages, Leishmania also prevents other cell types from entering the fray by interfering with antigen presentation and cytokine production - mediators responsible for intercellular communication (Figure 2) (52).
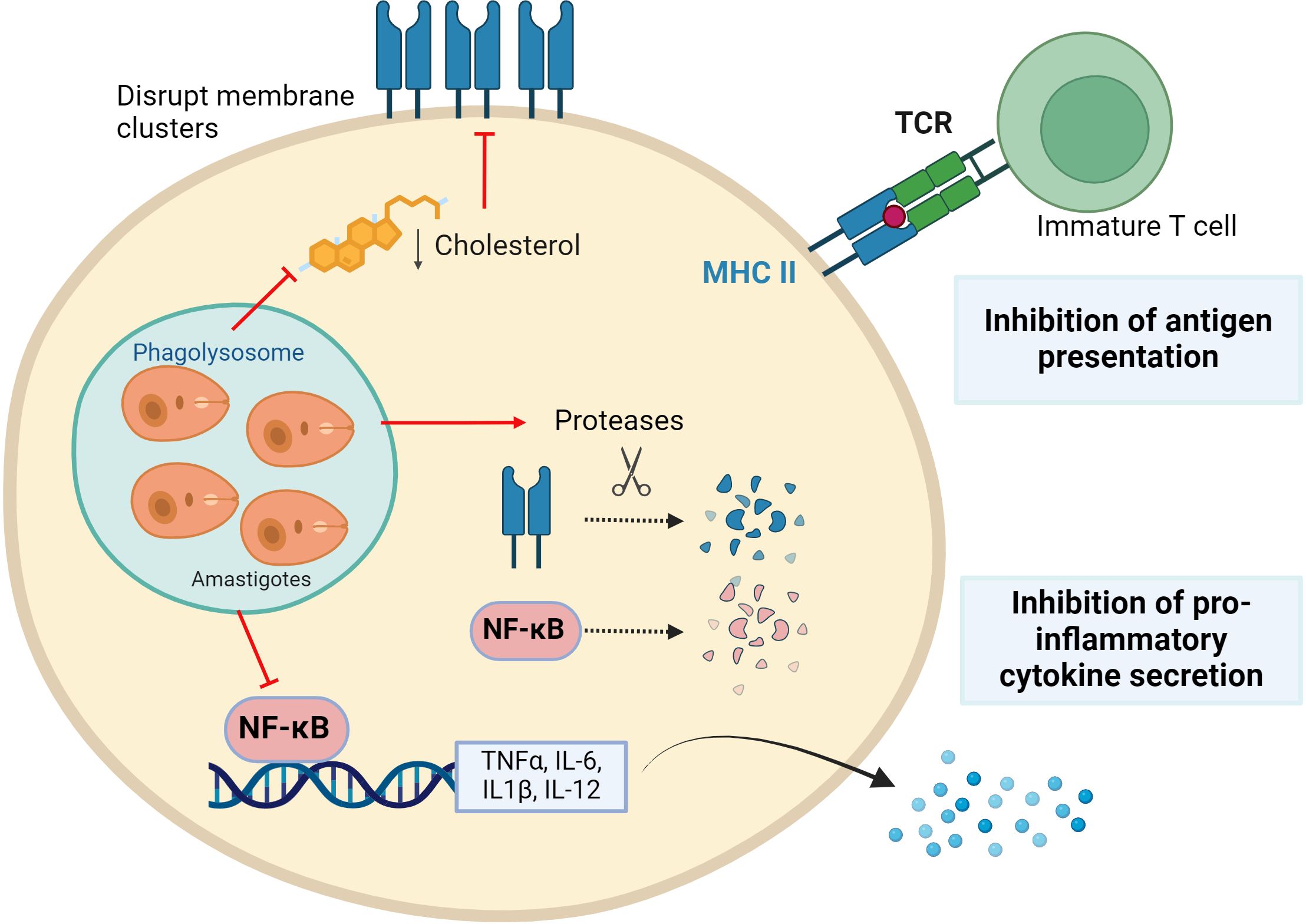
Figure 2. Leishmania parasites evading immune surveillance. Leishmania reduces antigenic presentation by preventing Major Histocompatibility Complex-II (MHCII) clusters formation by modifying membrane fluidity and inducing their protease-mediated degradation. In addition, the parasite induces proteases to degrade the transcription factor NF-kB, which is essential for the expression of pro-inflammatory cytokines. Figure created with biorender.com.
Antigens are presented less efficiently in Leishmania-infected macrophages than in uninfected macrophages (53), enabling evasion from T cell detection. This is accomplished by lowering the presence of major histocompatibility complex (MHC) molecules, especially MHC-II, on macrophage surfaces. In L. donovani-infected macrophages, expression of the MHC-II gene is blocked (54, 55). Furthermore, MHC-II protein is broken down by macrophage cysteine proteases, while some are internalized and degraded by parasitic cysteine proteases within amastigotes (56). Additionally, parasites disrupt the antigen processing and MHC binding pathway, which commence in the endoplasmic reticulum (57–59). The MHC II molecules are concentrated in membranes of antigen-presenting cells within lipid rafts, ensuring efficient T-cell activation (60). It has been described that Leishmania promotes a decrease in cholesterol in the macrophage membrane, increasing its fluidity and affecting antigen presentation. Thus, even if there are enough peptide-MHC complexes within the cell, they are unable to stimulate T cells due to their inability to form clusters in the lipid raft. Indeed, supplementation of Leishmania-infected macrophages with cholesterol restores membrane fluidity and therefore antigen presentation function (61–63).
Leishmania also modulates cytokine expression to promote an extracellular pro-parasite environment. The pattern of cytokines secreted by macrophages is very different depending on the infecting species, exhibiting sometimes dual functions. However, in general, all species of Leishmania tend to promote an anti-inflammatory phenotype, avoiding detection by surrounding immune cells (52, 64, 65). This is partly because the parasite alters the function of NF-κB, a master regulator that controls the transcription of pro-inflammatory cytokine genes (IL-12, IL-6, IL1-β, and TNF-α). Changes in methylation/acetylation of histones in promoters related to the NF-κB signaling pathway have been described. Concretely an increase in transcripts of pathway inhibitors such as TNF-α induced protein 3 (TNFAIP3) and a decrease in activators like myeloid differentiation primary response 88 (MYD88) and p65 (RelA) have been found. This is correlated with a diminished inflammatory response (37). Additionally, it has been observed that Leishmania proteolytically breaks down NF-kB through cysteine peptidase (CPB), affecting the transcription of the IL-12 gene, among others (66, 67).
2.2.3 Dancing with the death: the anti-apoptotic maneuvers of Leishmania
Another hurdle for Leishmania is to protect its niche because when macrophages, designed to eradicate pathogens, fail in its purpose, they sacrifice themselves by triggering apoptosis, a controlled form of cellular self-digestion. Thus, the parasite employs cunning strategies to inhibit the host’s self-destructive mechanisms.
A key feature of the apoptotic process is DNA fragmentation, often induced by antimicrobial oxidative stress (ROS are apoptosis inducers). In macrophages infected with Leishmania, both transcriptomic and proteomic analyses have revealed an increase in DNA repair enzymes (68). This DNA damage activates the p53 protein, recruiting Bax or Bad to the outer mitochondrial membrane, creating a pore and releasing cytochrome C (Cyt C) into the cytoplasm. Cyt C activates the apoptosome protein complex, which initiates the caspase cascade (3, 6, 9) and triggers the final stages of the death process (69).
A primary anti-apoptotic mechanism described in various Leishmania species involves the activation of the PI3K/Akt pathway (Figure 3). The parasite activates both Akt and PI3K, leading to the phosphorylation and inhibition of Bad, preventing the release of Cyt C into the cytosol (70–72). Furthermore, decreasing Akt levels through the application of siRNAs provoked incapacity of resisting apoptosis in infected macrophages (71).
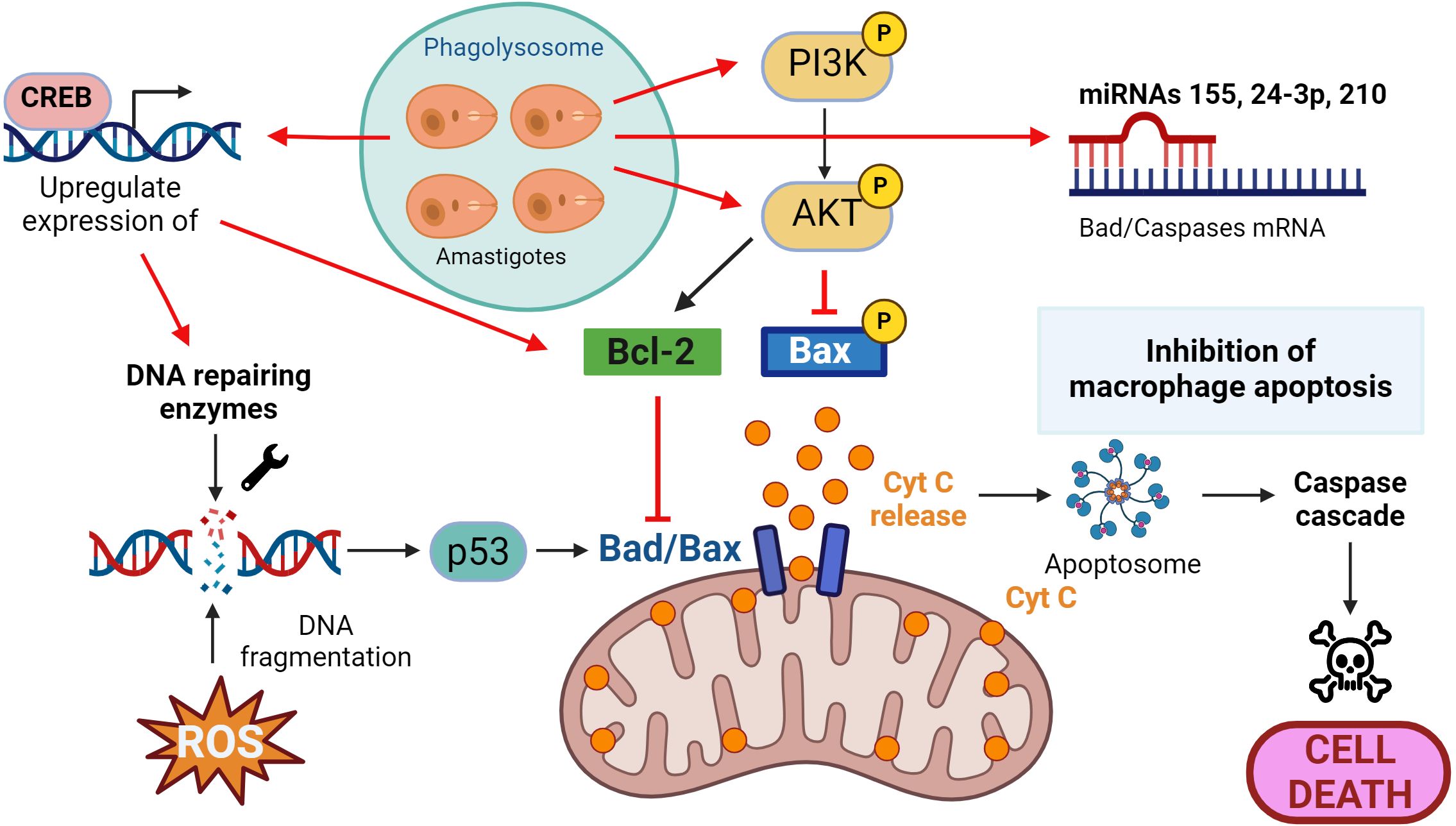
Figure 3. Leishmania and macrophage apoptosis. Macrophages activate the self-destruct mechanism in response to DNA fragmentation because of the infective process, and the parasite then implements strategies to inhibit this process. These include upregulation of DNA repair enzymes, activation of the PI3K/Akt pathway to prevent the release of cytochrome C (Cyt C) and promotion of anti-apoptotic proteins such as Bcl-2 and the inhibition of pro-apoptotic proteins such as Bad/Bax. Actions that Leishmania triggers to counteract the apoptotic process are presented in red. Figure created with biorender.com.
Like in the other “battlefronts” we’ve explored, Leishmania not only hinders the apoptotic process at the signaling cascade level (activating/deactivating phosphorylation processes, for instance) but also deploys its arsenal by interfering at transcriptional and post-transcriptional levels. The parasite promotes the expression of the anti-apoptotic protein B-cell lymphoma 2 (Bcl-2), directly antagonizing the effects of Bad/Bax on the mitochondria (73). Additionally, Leishmania acts on transcription factors that regulate the expression of pro-apoptotic genes, such as the cAMP response element-binding protein (CREB), controlling the expression of myeloid cell leukemia 1 (MCL-1), whose effect is similar to the aforementioned Bcl-2 (74). Finally, various studies have also shown that Leishmania upregulates miRNAs such as miR-24-3-p, miR-155, or miR-210, which bind to transcripts of caspases 3 and 7 or Bad, inhibiting the apoptotic process (75–77).
3 Macrophages and Leishmania: a quest for iron, the precious metal
Iron is a transition metal that exist in multiple oxidation states ranging from -2 to +7. This element is essential for living organisms as it serves as a cofactor for a multitude of proteins involved in numerous biological functions such as oxygen transport (hemoglobin), storage and use of oxygen in muscles (myoglobin), DNA synthesis (ribonucleotide reductase) or cellular respiration and electron transport (cytochromes), among others (78).
The key to its biological utility is the interconversion between its most common and biologically relevant species: the divalent ferrous iron (Fe2+) and the trivalent ferric ion (Fe3+), which allows it to act as a redox catalyst by easily accepting or donating electrons. However, this reactive property also makes it a dangerous element, as it participates in injurious ROS generation by forming part of the catalytic center of enzymes such as xanthine oxidase, Nox2, or lipoxygenases, or directly by the aforementioned Fenton reaction (79).
3.1 The importance of iron in macrophage biology: Its role in the polarization game and vice versa
Given the toxic potential of iron accumulation, iron levels must be finely regulated in the body and macrophages are the main cell type regulating iron homeostasis. Interestingly, in addition to their role in iron efflux at systemic level, macrophages have recently been described as “ferrostats”, capable of sensing and regulating iron availability at local level, assisting in tissue and cellular function (80).
At systemic level, macrophages play a crucial role in iron recycling, supporting the synthesis of hemoglobin required for the daily production of approximately 200 billion of red blood cells. Hemophagocytic macrophages are responsible for removing senescent red blood cells, facilitating iron reuse [detailed in (81, 82)]. Intracellularly, macrophages internalize iron through various receptors such as the transferrin receptor (TfR) and the hemoglobin-haptoglobin (CD163), and they also have transporters such as ferroportin (FPN) that export iron to the plasma. In addition, macrophages can either store iron, mostly complexed with ferritin, or released a free fraction in the cytoplasm, known as the labile iron pool (LIP). This free iron fraction, whose concentrations vary from nanomolar to millimolar, is metabolically accessible and is the key to macrophage metabolism and function (82, 83). Most of the intracellular iron is used by macrophages for its incorporation into iron-binding proteins, contributing to diverse effector functions such as mitochondrial respiration, DNA repair, or immune responses defense against pathogens (including the abovementioned Nox2 and iNOS, for example) (84–86).
In addition, iron is involved in the post-transcriptional regulation of a multitude of genes via the iron regulatory proteins/iron-responsive element (IRP/IRE) system. IRP recognize motifs known as IRE, which are loops that are in the 3’ and 5’ untranslatable regions of the mRNA. Binding of the IRP to the IRE occurs when there are low levels of iron in the cell, and depending on whether it is located in the 5’ or in the 3’ end, it will either prevent the translation machinery from binding or increase its stability (preventing its degradation), respectively (87). The IRP/IRE system regulates the expression of mRNAs coding for proteins related to different aspects of iron metabolism (internalization, storage, heme group synthesis, export), such as TfR, ferritin or FPN. However, in recent years, a multitude of mRNAs with IRE sequences have been described in many genes such as the tricarboxylic acid cycle (TCA) aconitase enzyme (ACO2) or hypoxia inducible factor (HIF), among others (88, 89). This broadens the regulatory functions of the IRP/IRE system and provides evidence of its physiological action what expands beyond the direct control of the cell’s iron status and about how iron connects to other target signaling pathways that would allow the cell to adapt to challenging environments, such as hypoxia, inflammation, or infection.
Therefore, given that iron is essential for the activity of many proteins and is also involved in the expression of many other genes by the IRP/IRE system, manipulation of iron homeostasis in a cell as plastic as the macrophage would significantly affect its function. Besides that, macrophages modify their phenotype to adapt their iron metabolism to specific situations (M1/M2), such as an infection.
There are numerous differences between M1 and M2 macrophages, including the pattern of secreted cytokines, the type of energy metabolism, and surface markers. Additionally, iron metabolism is also differentially regulated in macrophage polarization (90), leading to differences in intracellular and extracellular iron levels, which are appropriated to their specific function. It is estimated that about 60% of genes related to iron homeostasis are differentially expressed in late stages of macrophage polarization (91). In general, M1 macrophages have a phenotype of ferritinhigh, TfRhigh and FPNlow, so they tend to retain iron and remove it from the exterior, which has been shown to enhance their antimicrobial effector functions such as ROS production. In contrast, M2 macrophages show the opposite profile (ferritinlow, TfRlow and FPNhigh), being cells that tend to export iron, which is linked to their immunoregulatory and tissue repair functions (92, 93).
If questioning the impact of iron on macrophage polarization, results are not always in accordance. Polarization pathways are complex, and it has been shown that iron can modulate the macrophage phenotype at different levels (signaling, metabolism, and epigenetics) [reviewed in (94)]. Although the effects of iron on the macrophage depend, among other things, on the iron source, concentration, exposure time and cellular context, studies generally show that iron supplementation induces a M1-like phenotype with pro-inflammatory cytokine production and ROS generation (90, 95–100). For example, a close relationship between NOS (M1 marker) and iron has been described. In addition to being an iron-requiring hemoprotein at its catalytic center, iron levels in the macrophage have been shown to control the transcriptional expression of the enzyme, and NO, in turn, modulates the expression of genes related to iron metabolism by promoting increased binding of IRP to IRE motifs (101–103).
On the contrary, and in general, iron deficiency has been shown to limit pro-inflammatory phenotype activation and promote an anti-inflammatory phenotype (104–106). In this regard, iron deprivation in macrophages by altering TCA has been shown to decrease the inflammatory response upon exposure to lipopolysaccharide (LPS) (107). This metabolic shift has been linked to iron acting as a cofactor for several mitochondrial electron transport chain complexes, hindering OXPHOS. Moreover, iron deprivation has been associated with reduced expression of proteins such as succinate dehydrogenase complex iron sulfur subunit B (SDHB), a subunit of the mitochondrial complex II, as its mRNA contains IRE motifs (106, 108).
Collectively, these findings suggest that iron levels within macrophages can elicit diverse immunomodulatory effects through the regulation of multiple signaling pathways, and vice versa, the polarization state of the macrophage determines its iron content to match its metabolism to its function. Thus, manipulation of iron levels has been proposed as a promising tool to modulate the polarization state of macrophages. With this strategy, and more specifically, with the administration of iron in the form of IONPs, macrophages have been re-educated in different scenarios, achieving significant advances in the field of cancer and infectious diseases.
3.2 Leishmania and macrophages: two organisms with the same goal, iron
Infecting a host provides pathogens with access to a nutrient-rich environment. Thus, hosts employ strategies to hinder microbial access to these resources, a phenomenon known as nutritional immunity (109). In the case of Leishmania, which is heme auxotroph and lack obvious iron storage systems, scavenging for host iron is an essential adaptation for survival and virulence (110).
In the membrane of phagolysosome there are transporters such as natural resistance-associated macrophage protein-1 (NRAMP-1) that are responsible for transporting iron to the cytoplasm, limiting the availability of iron to the pathogen (82). In fact, some of the determinants of susceptibility to leishmaniasis are certain polymorphisms in the NRAMP-1 transporter, reflecting the competition between the intracellular form of Leishmania and the host for the small amount of iron that enters the phagolysosome (111, 112). To counteract this access limitation to iron, Leishmania has evolved a multitude of strategies throughout its evolution to provide itself with the metal. For example, it has been described that L. donovani secretes a tryparedoxin peroxidase (TXNP), which downregulates NRAMP-1 activity and prevents iron levels in the phagolysosome from declining (113).
Leishmania tries to redirect the iron that reaches the macrophage to its cellular niche by altering iron trafficking pathways. Studies indicate that, when macrophages are infected with L. amazonensis, there is a remarkable fusion of endocytic vesicles containing transferrin bound to its receptor with the phagolysosome, delivering iron to the parasite (114). Additionally, various Leishmania species hinder the expression of FPN to inhibit the metal exit from the cell and increase its availability for incorporation into their own metabolism (115, 116). Attempts have been made to counteract this strategy by administering FPN-loaded NPs to L. major-infected mice, reducing the parasite load successfully (117).
Leishmania is also capable of altering and lowering the LIP, which is known to be crucial as an indicator of cellular iron demand as it is the fraction of metabolically available iron. When LIP levels are low, it indicates that the cell needs more iron. In this sense, L. donovani can deplete the iron pool to activate cellular iron sensors, triggering a response that increases the intracellular concentration of available iron. By inducing this response, the parasite gains access to more iron, which promotes its growth (118). Recently, it has been reported that L. donovani is also able to increase the fraction of available iron by cleaving poly(rC)-binding proteins (PCBPs), which are ferritin chaperones. What happens is that, as Leishmania degrades PCBPs, the loading of iron with ferritin is impeded, and the iron, instead of being stored in complexes, accumulates thus rendering available to enhance growth (119).
In addition to trying to maintain ferric levels both in the cytosol and within the phagolysosome, Leishmania must possess an iron transport system on its membrane in order to internalize this essential nutrient, as there is no evidence that trypanosomatids express siderophores like bacteria (120). First, since most of the iron within the parasitophorous vacuole is in its oxidated form (Fe3+), it needs ferric reductases to convert it to the soluble Fe2+ form, which can cross its membrane. Thus, Leishmania expresses the Leishmania ferric iron reductase 1 (LFR1) in their membrane and it is essential for its virulence. Without it, no matter how much iron is available, that it would not be able to use it (121, 122). The reduced iron is then transported into the parasite via the Leishmania Iron Transporter (LIT1). Interestingly, mutants lacking LIT1 can survive if LFR1 is overexpressed, suggesting the presence of alternative, lower-affinity iron transporters (120).
Leishmania also has transporters that allow it to internalize iron in the form of the heme group, which is already part of a multitude of enzymes in its metabolism and lacks the biosynthetic pathways of the heme group. Thus, it expresses Leishmania heme response 1 (LHR1) and the recently discovered Leishmania feline leukemia virus subgroup C receptor (LFLVCR), which is responsible for importing heme into its cytoplasm (123, 124). Moreover, Leishmania also expresses a hemoglobin receptor (HbR), as an alternative route for the acquisition of the heme group (125).
3.3 Iron, ally or enemy: the therapeutic use of iron in Leishmania infection
Competition between pathogens and hosts involves different war fronts, and the nutritional struggle plays a central role. Similar to what the host tries to do, and since iron availability is critical for the growth of Leishmania, the development of drugs aimed to limit access of iron to the parasite could be a strategy to control the infection. In this regard, different chelating agents have been tested to decrease the amount of iron accessible to Leishmania (126–131)(Table 1). For example, the use of quercetin as well as caffeic and rosmarinic acids, with described iron chelating activity, have been found to exert a leishmanicidal effect in different models (126–129). Furthermore, in mice infected with VL-causing L. chagasi and treated with desferrioxamine (DFO), a significant reduction of parasite load in the spleen and liver is observed after six weeks of infection (130).
However, it seems that there is a critical point at which the parasite manages to overcome this limitation to iron access, perhaps by up-regulating the systems it has developed in this parasite-host evolution, and the deposit of iron present in tissues is enough to sustain its growth. Thus, Bisti et al. found that DFO treatment of L. major-infected mice resulted in a delay in skin lesion development, but after about 12 weeks the lesion sizes were similar between the control and treated groups (131). Comparably, dietary iron restriction in mice, although significantly decreasing iron levels in the liver and spleen, did not affect the growth of L. infantum (133).
Thus, we suggest that the strategy of treating with iron may be more beneficial in this infective context, since, as we have described, iron can modulate the macrophage phenotype, enhancing the host defense response (Table 1). In contrast to other pathogens, where iron overload is associated with increased susceptibility, iron administration in murine models of CL limits parasite growth (132). Banerjee and Datta observed that infection with L. major in the footpad caused an increase in iron in the infected area while causing anemia-like symptoms (low systemic iron and hemoglobin levels). These observations prompted them to treat mice with ferric ammonium citrate (FAC), which resulted in infection control and restoration of iron balance at systemic level. The iron supplementation strategy was successful in restricting parasite growth by promoting a local oxidative response (ROS generation) (132).
Interestingly, Charleboir et al. studied the response of hemojuvelin (HJV) knockout mice to infection with L. major and L. infantum (134). HJV is a membrane receptor involved in regulating the expression of hepcidin - a central hormone to the regulation for iron metabolism regulation. Mutations in the gene coding for HJV correlate with low hepcidin expression and severe systemic iron overload, known as hemochromatosis (135). The reason is that hepcidin is responsible for controlling the efflux of iron at cellular level by FPN, promoting its internalization and degradation. In cases of low hepcidin levels, this regulation is absent, and when iron enters the cell, it is exported to the bloodstream via FPN, generating a pathological state with iron excess (136).
In HJV knockout mice, tissue macrophages overexpress FPN on their surface, so they are unable to retain iron and release all of it into the plasma (134). Therefore, unlike the iron overload model generated by FAC administration, in a situation of hemochromatosis, tissue iron levels are low, and macrophages become extremely iron depleted. All this means that, when infected mice with L. infantum, there is no difference in disease progression or parasite load in the spleen and liver between mutant mice and wild-type controls. However, in the case of CL, like what happened with chelating agents, although there is a delay in the development of skin involvement compared to wildtype controls, at longer times, wound sizes are similar (134).
Therefore, since Leishmania is an intracellular parasite, we concur with several authors who emphasize the importance of localizing iron overload within macrophages, where the battle between the immune system and the parasite takes place. One way to ensure that iron reaches macrophages is to deliver it via NPs.
4 Nanoparticles to increase drug-Leishmania confluency. Activation and reprogramming of macrophages with iron oxide nanoparticles
A major hurdle to overcome with current leishmanicidal drugs is their low specificity/selectivity for macrophages, causing them to be distributed throughout the body. This, coupled with the fact that they are often cytotoxic, leads to many adverse effects for patients. Therefore, there is a clear need to improve the delivery of anti-leishmanial molecules to their site of action (the macrophage) to enhance their efficacy while decreasing their toxicity, being nanomedicine the right tool to fulfill this purpose. The selective targeted drug delivery to the site of action within the body without affecting healthy organs and tissues results in improved efficacy and lower side effects (137).
Nanomedicine has other advantages: protecting the drug from rapid degradation in the organism, improving drug stability, ability to cross biological barriers and reach specific intracellular compartments within the site of action, and capacity to control drug release (138). Nanomedicine makes use of NPs, which are defined as particulate dispersions or solid particles with a size in the range of 10-1000 nm (139). The drug can be incorporated into the NP matrix by encapsulation, adsorption to its surface or covalent conjugation. There are NPs of very different composition and morphology, organic (polymeric, lipidic) and inorganic (gold, silica, iron oxides) (140).
The propensity of macrophages for phagocytic clearance offers a perfect scenario for diseases where, like leishmaniasis, macrophages play a central role (141). In essence, since both Leishmania parasites and NPs share a common fate, the use of nanosized delivery systems would enhance the convergence of parasites and drugs, thereby reducing their toxicity. Indeed, the introduction of a liposomal amphotericin B formulation (AmBisome®, Gilead Sciences) marked a significant advancement in the treatment of VL as the first nanomedicine to enter the market. This clinical success is justified because both VL parasites and AmBisome® are preferentially uptaken and accumulated in macrophages of the liver, spleen, and bone marrow. Moreover, AmBisome® creates a depot inside macrophages that slowly releases the drug in a way that avoids its hemolytic effects and nephrotoxicity (142, 143).
However, it is essential to recognize that while macrophages are the target cells in all clinical manifestations of leishmaniasis, the affected organs may vary. In VL, the spleen and liver serve as the primary affected organs, providing an ideal environment for NP treatment due to their role as main clearance organs (Figure 4). Nonetheless, addressing macrophages specifically in various CL forms poses challenges, as lesions are often distal and deep-seated in the skin, making the delivery of therapeutic agents difficult, whether locally or systemically (144). Indeed, AmBisome®, the first-line treatment for VL, has demonstrated reduced efficacy in treating cutaneous forms of leishmaniasis, likely because parasites primarily localize in dermal macrophages near the sandfly bite and draining lymph nodes (145). Inflammation at this site increases vascular permeability, allowing a fraction of liposomes to extravasate. However, the extravasated liposomes constitute a lower proportion of the total administered dose (146).
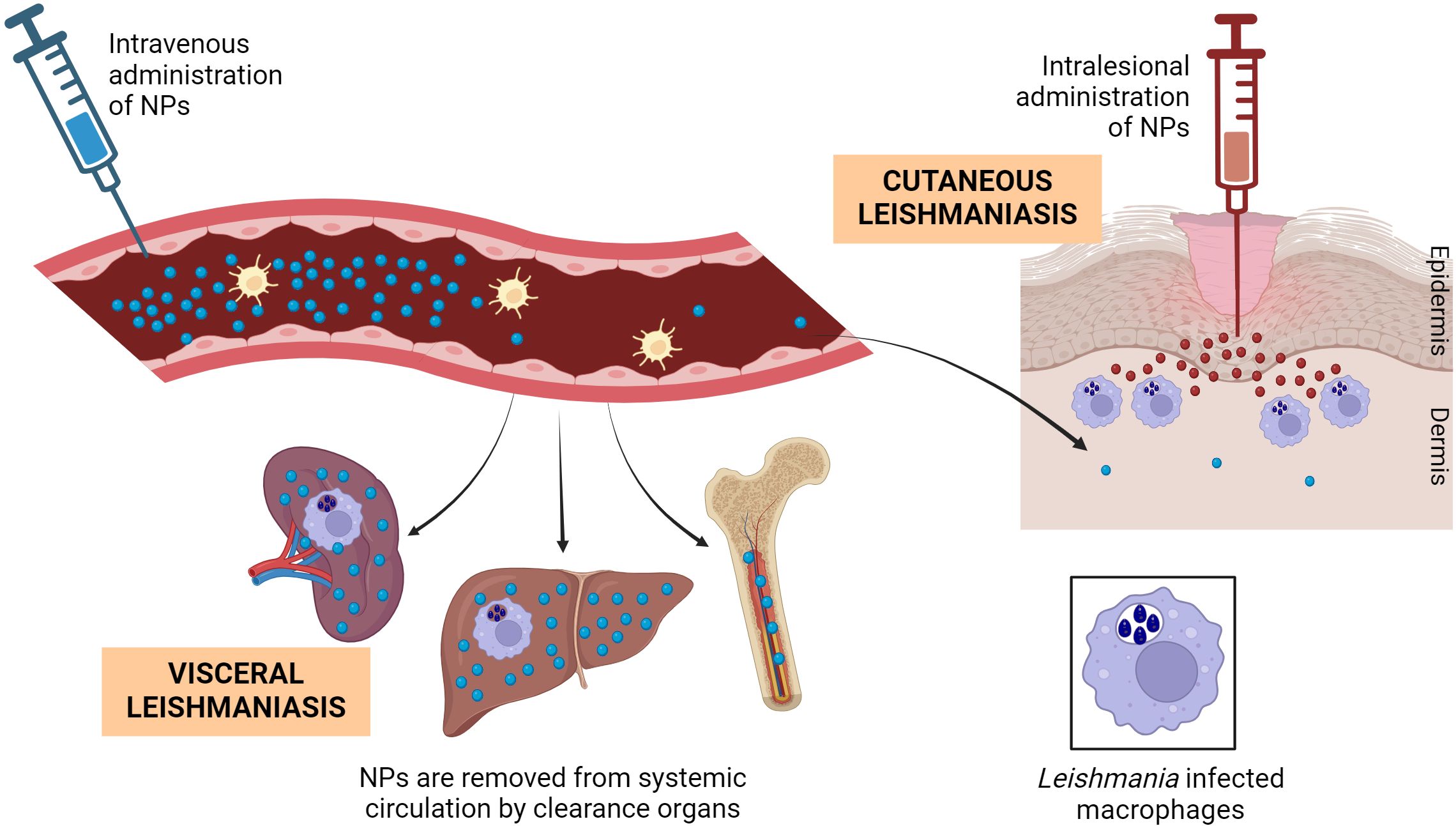
Figure 4. Biodistribution of NPs and encounter with Leishmania-infected macrophages. After their intravenous administration, NPs in general tend to accumulate in the macrophages of organs with fenestrated vasculature such as liver, spleen and bone marrow, also the major Leishmania hosts in VL. Only a small fraction of long-circulating NPs will get Leishmania-infected skin lesions. After topical administration and even in damaged skin, very small NPs have poor chance of arriving the dermal infected macrophages, making mandatory their intralesional (and uncomfortable) administration. Figure created with biorender.com.
To enhance the delivery of NPs to inflammation sites in CL, exploring alternative administration routes and tailoring NPs properties to evade rapid clearance by liver, spleen, and bone marrow macrophages is crucial. Consequently, significant efforts are being made to improve the formulation of amphotericin B liposomes, including modulation of composition and size. Generally, smaller liposomes prolong circulation time, thereby increasing accumulation at the lesion. Additionally, pegylation and functionalization of NPs with various ligands have been explored as strategies to prevent opsonization by plasma proteins and enable specific recognition by targeting tissues and cells (147).
4.1 Iron oxide nanoparticles
IONPs are composed of iron and oxygen atoms, forming a distinctive core-shell structure. The core is typically made of iron oxide (maghemite γ-Fe2O3, magnetite Fe3O4, hematite α-Fe2O3 and goethite FeO(OH)), while the shell can be functionalized with diverse compounds such as small molecules, metal ions, surfactants, and polymers (148). This unique composition grants IONPs remarkable properties that have found utility across various fields, including medicine, electronics, and environmental remediation (148–150).
In medicine, IONPs have garnered attention for their applications in diagnostics and therapies. They serve as contrast agents in magnetic resonance imaging (MRI), providing intricate details of tissues and disease conditions. Moreover, their versatility extends to drug delivery, where they have shown success in targeted delivery systems, augmenting treatment precision and efficacy while reducing adverse effects (17). Additionally, IONPs have been effectively utilized to induce photothermal or magnetic local hyperthermia, further expanding their therapeutic potential (151). Notably, the FDA has approved certain IONP-based products. For instance, Feridex®, which is composed of dextran-coated IONPs (ferumoxide), or Resovist® (ferucarbotran), comprised of IONPs coated with carboxydextran, are approved as imaging contrast agents for the detection of liver lesions. Another FDA-approved IONPs, Feraheme® (ferumoxytol), an IONP-based drug, has been sanctioned for the treatment of iron deficiency anemia in patients with chronic kidney disease or patients who do not tolerate oral iron supplementation (17).
A concerning issue is the biodistribution of IONPs, which is influenced by various factors such as their size, shape, surface coating, and charge, as well as the dose and route of administration [reviewed in (152)]. When administered intravenously, IONPs have a half-life ranging from 40 minutes to 24 hours and tend to accumulate in macrophages of the liver and spleen. However, when these organs are saturated, IONPs can also be distributed to other tissues such as the lungs, kidneys, and heart. Regarding their excretion, it is suggested that larger IONPs are eliminated via feces, while smaller ones are primarily excreted by the kidneys through urine (153, 154).
In addition, the effect of IONPs at cellular level will depend directly on their degradation by the cell, i.e. the release of the iron product. This contrasts with the administration of iron in salt form, where the effect is more rapid due to the instantaneous availability of iron ions for cellular use. Thus, within macrophages, IONPs enter via the endocytic pathway and accumulate in lysosomes. Here, the acidic pH triggers their degradation and subsequent release of iron into the cytoplasm. This delay in iron availability and cell use probably explains why, in vitro, iron in the form of salt can metabolically activate macrophages more than IONPs within 24-72 hours at any concentration (155). In addition, the behavior of IONPs compared to iron salts is significantly different in both in vivo and in vitro models, with salts exhibiting greater toxicity. This discrepancy is influenced by factors such as stability and aggregation capacity (155, 156).
4.2 IONPs pull the rope: macrophages reprogramming in cancer
Considering the intricate molecular and functional machinery that iron orchestrates in macrophages, IONPs have become a valuable tool to influence the immune response of this cell type. Thus, owing to their ability to accumulate preferentially in macrophages, IONPs offer promising prospects for novel therapeutic strategies, intending to harness the immune system’s strength to combat diseases such as cancer or infections.
In the field of cancer treatment, IONPs have been used to immunologically modulate the tumor microenvironment, in particular macrophages (157). Recent studies have outlined that utilizing IONPs in cancer treatment holds the potential to diminish tumor cell growth by reprogramming or re-educating tumor-associated macrophages (TAMs) from an M2-like pro-tumoral into an M1-like anti-tumoral phenotype.
For example, the FDA-approved IONP compound ferumoxytol significantly inhibited the growth of subcutaneous adenocarcinomas and prevented hepatic metastasis in mice. The effect was be accompanied by increased presence of pro-inflammatory M1 macrophages that attacked cancer cells in tumor tissues (158). Since then, the antitumor effect of ferumoxytol (alone or in combination with other immunomodulatory drugs) has also been reported for other types of cancer in in vivo models (159–162).
In addition to ferumoxytol, alternative types of IONPs have been investigated as potential antitumoral agents. For instance, NPs referred to as cross-linked iron oxide (CLIO) or IONPs coated with an anti-CD206 antibody (for targeting M2 macrophages) have demonstrated the capacity to attenuate tumor dimensions through their influence on macrophage polarization (163, 164). To augment this effect on the polarization of TAMs, Wu et al. loaded IONPs with L-arginine, with the aim of elevating NO secretion to induce tumor cell death. This NO-centered therapy was evaluated in a breast cancer model, revealing heightened NO levels and increased M1 markers, accompanied by an apoptotic antitumoral impact (165).
Following an innovative approach, Li et al. (166) employed IONPs to reprogram macrophages in vitro and subsequently used them as a therapeutic product, a strategy known as cellular therapy. In this study, murine RAW 246.7 macrophages were treated with IONPs coated with hyaluronic acid. After confirming their polarization into a pro-inflammatory phenotype and the enhancement of their innate functions (ROS and cytokine production), these modified macrophages were administered to mice bearing 4T1 tumors, resulting in a significant suppression of tumor growth. Remarkably, in addition to their cancer cell elimination abilities, the introduced macrophages polarized resident M2 tumor-associated macrophages into a M1-like phenotype in a paracrine-like manner. Moreover, the administered macrophages were guided to the tumor site using a magnetic field, leading to increased accumulation and a more pronounced antitumoral effect (166).
It’s essential to consider that, while IONPs have the ability to polarize macrophages towards an M1 phenotype in a tumor context, under certain circumstances, they can inhibit this inflammatory response by inducing polarization towards an M2 phenotype (167–169). Indeed, several studies have shown that when macrophages are exposed to IONPs together with LPS, a change in the pattern of gene expression occurs, with an up-regulation of M2-related genes and a reduction in the secretion of NO (170, 171). This is explained by the competition and regulation of the TLR4 receptor, the target of both LPS and IONPs. This difference in the phenotype induced by IONPs on macrophages highlights the complexity of the process, which is dependent on system composition, shape, size, coating and dose administered. However, in general, it can be concluded that in most cases and independently of their physicochemical characteristics, IONPs promote an M1 phenotype in macrophages, both in tumor and non-tumor tissues, even in the presence of a pro-M2 stimulus such as IL-4 (157).
4.3 How do IONPs manage to reprogram macrophages? A tangled skein of interactions
IONPs exert a crucial role in modulating the immunological functions of macrophages. Indeed, exposure of primary mouse macrophages to such naked IONPs has identified more than 1,000 differentially expressed genes compared to untreated controls, most of them linked to inflammatory response and oxidative stress (172). This wide variation in gene expression underscores the complexity of the biological responses triggered by the interaction between particles and macrophages and raises the challenge of understanding which cell signaling pathways orchestrate this enormous effect on transcription.
In general, and as we have seen in some examples within in a tumor context, there is evidence that IONPs promote an M1 profile in macrophages, with increased expression of M1 markers and decreased expression of M2 markers (173). However, the mechanisms of how IONPs favor a pro-inflammatory phenotype are not uniform. Until now, the molecular basis of IONPs and macrophage interactions have remained enigmatic due to the heterogeneous chemical composition and diverse physical properties of NPs (size, charge, and morphology), as well as the array of in vitro and in vivo models employed.
The first step in the IONPs-macrophage relationship is their interaction and internalization, which depends on the coating of the particle and also on the so-called protein corona complex (PC) that forms on the surface of the particle when in contact with biological fluids. The composition of the PC critically affects the interaction with the macrophage, and a recent proteomic study has shown that its composition depends more on the origin of the biological fluid (animal species) than on the coating of the particle (169). Furthermore, they studied whether this difference in PC composition affects its ability to modulate the macrophage phenotype in vitro. Interestingly, in the case of IONPs coated with dimercaptosuccinic acid (DMSA), they promoted in RAW264.7 macrophages a phenotype more similar to M2 when using a medium supplemented with mouse serum and a phenotype closer to M1 when using fetal bovine serum, tangling the skein a little more (169).
It is believed that IONPs interact with TLRs on the macrophage surface, primarily with TLR4. Clinically approved IONPs (ferucarbotran and ferumoxytol) have been shown to stimulate the production of pro-inflammatory cytokines (IL-1β, IL-12, TNF-α, IL-2, and IL-10) by binding to and activating TLR4 (174). Furthermore, pretreatment of macrophages with CLI-095 (a TLR4 inhibitor) prior to IONPs treatment significantly reduces the expression and levels of secreted pro-inflammatory cytokines. This implies that induction of the M1 phenotype is hindered by TLR4 blockade, highlighting its relevant role in macrophage interaction and activation (174, 175).
Another discovery supporting the IONPs-TLR4 relationship is that while polyethylene glycol (PEG)-coated IONPs exhibit a pro-inflammatory effect on macrophages per se, co-exposure of these particles with LPS attenuates the inflammatory response typically induced by this bacterial molecule. Since LPS is recognized by TLR4, it suggests that IONPs might compete for binding to the same receptor as LPS, thus blocking its binding and limiting its stronger pro-inflammatory effect (170). In addition to the central role of TLR4, small IONPs (10-60 nm) also interact and activate other surface TLRs such as TLR2 and TLR6, as well as the intracellular TLR8 (176, 177).
Upon binding of IONPs to TLRs, particularly TLR4, a cascade of intracellular signaling is triggered (Figure 5). The interaction of macrophages with IONPs has been associated with the activation of various signaling molecules within the canonical TLR pathway. Notably, the impact of IONPs on the ubiquitination of TNF receptor-associated factor 6 (TRAF6), a key adaptor protein in mediating TLR signal transduction, has been confirmed. TRAF6’s autoubiquitination is essential for subsequent steps in the signaling cascade and is facilitated by the presence of iron (178). In in vitro experiments involving macrophages, co-localization of IONPs with TRAF6 and ubiquitin was observed (179). Increased ubiquitination of TRAF6 leads to elevated interferon regulatory factor 5 (IRF5) expression, which has been linked to the induction of a more pronounced M1 phenotype in macrophages upon exposure to IONPs (179, 180).
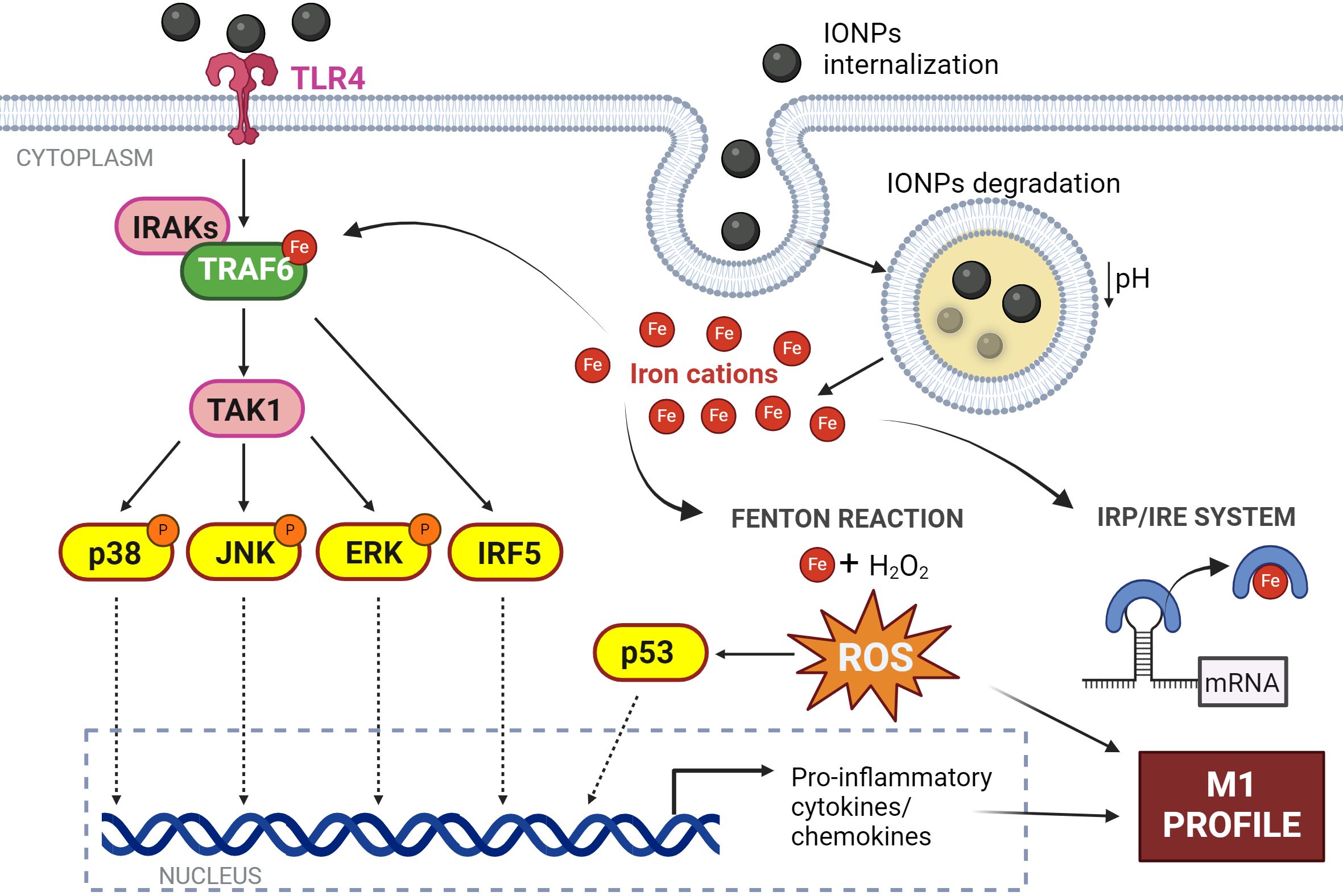
Figure 5. Effect of IONPs on macrophages. Interaction of IONPs with TLR4 initiates the activation of IRF5 and several MAPKs culminating in the expression of inflammatory response genes. In addition, IONPs are internalized by macrophages and are biodegraded in the phagolysosome resulting in the release of iron cations into the cytosol. This free iron induces ROS production by the Fenton reaction; and modulates protein activity by acting as a cofactor, and protein expression by the IRE system. All this converges in the induction of an M1 profile in the macrophage. Figure created with biorender.com.
TRAF6 acts as a platform for the downstream activation of several protein kinases such as the IkappaB kinase (IKK) complex or different members of the mitogen-activated protein kinases family (MAPK), pathways that lead to the activation of transcription factors linked to inflammatory responses and immune activation (181). Thus, numerous articles have described that the secretion of pro-inflammatory cytokines as a result of this IONPs-TLR4 interaction is dependent on the phosphorylation of p38/JNK/ERK kinases (174, 182–185) and subsequent activation of transcription factors such as NF-κB, AP-1, or the aforementioned IRF5 (159, 179, 186) (Figure 5).
IONPs not only exert their effect on the macrophage through interaction with cell surface receptors but are also efficiently taken up by macrophages as cells specialized in engulfing foreign particles. Different mechanisms of internalization of IONPs at cellular level have been proposed, such as clathrin-mediated endocytosis, caveolae-mediated endocytosis or receptor-mediated endocytosis, among others (169, 187–189).
Once inside the macrophage, IONPs localize to endocytic vesicles, which fuse with lysosomes (Figure 5). Exposure to this acidic environment (pH~5) induces their degradation and the release of iron cations from the NP core (183, 190). This free iron stimulates the production of ROS by the Fenton reaction leading to exacerbated oxidative stress. It has been shown that macrophages produce ROS in a concentration-dependent manner depending on the concentration of IONPs to which they are exposed (191, 192). This ROS generation resulting from treatment with IONPs has been linked to the overexpression of the p53 protein (193). This protein plays a crucial role in the regulation of ferroptosis, a type of cell death that depends on iron concentration (194). Thus, the presence of high levels of iron within the macrophage promotes the acetylation and subsequent activation of p53, which has been linked to the establishment of the M1 response (96). In addition, this free iron can modulate the expression of genes regulated under the IRE system (185) and the activity of numerous proteins that use it as a cofactor such as TRAF6, as we have described (Figure 5).
In short, IONPs trigger a complex and intricate network of molecular signaling in macrophages, a puzzle with missing pieces that is essential to understanding their impact on immune function.
4.4 IONPs against leishmaniasis: a promising therapy
The remarkable versatility of iron in modulating the effector functions of macrophages situates this metal as a promising weapon against leishmaniasis. In this context, IONPs offer a means to achieve targeted iron overload in macrophages, a vital aspect given that both co-localization with the parasite and appropriate concentrations are crucial for their effectiveness. The diversity in their application, with distinct sizes, charges, and coatings, reflects the adaptability of IONPs as nanotechnological weapons against leishmaniasis, although difficulties to draw conclusions on their use as a therapy. Some studies focus on their intrinsic leishmanicidal activity, while others use them as vehicles to administer specific drugs, employing IONPs as carriers of therapeutic agents to specific cells to enhance selectivity (Table 2).
The initial investigations involving IONPs in the context of leishmaniasis were conducted by Bisti et al. in the early years of this century (131, 195, 196). They employed the topical application of IONPs-dextran as therapy in murine models of CL. Subsequently, this type of colloidal system has also been applied in VL models (133). In both scenarios, the leishmanicidal effect of iron relies on the localized generation of ROS and NO within macrophages, which are key effector molecules for infection control. Vale-Costa et al., compared the effect of iron administration in knockout mice for iNOS and for the p47phox subunit of NOX2 with wildtype mice, and they found that parasite load falls was reduced in wildtype mice but not in mutants (133). Similarly in the CL model, Bisti et al. observed that the protective effect of iron overload was diminished when co-treating with diphenyleneiodonium (DPI), a NOX inhibitor (195). Furthermore, the same authors described that iron-mediated containment of infection was associated with an activation of NF-κB TF and the consequent pro-inflammatory response. Interestingly, they also observed increased recruitment of CD4+ T cells to the draining lymph node, inducing a sustained Th1 response and a protective state against Leishmania, as mice were resistant to reinfection with parasites 12 weeks after the first administration (196).
Abazari et al. also discussed the immunomodulatory potential of IONPs in the context of leishmaniasis. Following topical application of IONPs, there was an increase in IFN-γ secretion and a decrease in IL-4 levels in the lesions of infected mice, indicating the induction of a Th1 response that effectively controls the infection (202). As can be observed, all treatments based on IONPs for the cutaneous form of the disease have been tested through topical administration. It’s worth noting at this point that all formulations of IONPs that have been approved for clinical use are administered intravenously or orally (203). Regarding studies on skin penetration of IONPs, similar to other types of NPs, particles size and composition (flexibility) are determining parameters for their ability to penetrate into the skin as they enter primarily through the stratum corneum via the intercellular route. Thus, it has been observed that small IONPs ranging from 10-15 nm can penetrate the epidermis but are unable to reach the dermal layer (204–206), where infected macrophages are located. Despite the skin damage resulting from the infection, it has been demonstrated that no type of NPs can reach the areas where macrophages infected with Leishmania reside (207). Consequently, the proven leishmanicidal effect of IONPs in topical treatments suggests two possible mechanisms: either the free iron resulting from the degradation of the particles reaches the infected areas and act on these macrophages, or the key molecules for parasite control, such as ROS and NO, are generated in more superficial layers and diffuse towards the areas where the parasite is located.
Turning to the activity of IONPs, interestingly, the production of ROS/NO, which imparts their pro-inflammatory effect, can be amplified under exposure to light (photons). The photocatalytic prowess of IONPs is unveiled through light absorption, transferring energy to molecular oxygen and water, thereby boosting ROS production in the presence of light. Significantly, the study by Islam et al. with iron oxide nanorods (IONPs with elongated morphology) reveals an enhanced anti-amastigote effect of IONPs under exposure to LED light in vitro (200). Another leishmanicidal application of IONPs involves their use in photothermal therapy, generating heat when exposed to an alternating magnetic field. This technology has proven capable of raising the temperature in the NP-occupied area to 45°C, eliminating parasites effectively. Observations indicate success in killing axenic amastigotes of L. mexicana using this approach (198).
An intriguing finding is that IONPs seem, in some cases, to affect the survival of both promastigotes and amastigotes (199, 202, 208). However, recent research, exemplified by Vercoza et al., indicates that the effectiveness of IONPs is confined to the intracellular stage, emphasizing their immunomodulatory influence on macrophages rather than a direct impact on the parasite. The explanation does not stem from a variance in IONP penetration into promastigotes and amastigotes, as both life forms were observed to successfully accumulate these particles (201).
Among the studies using IONPs as a carrier, the vehiculization of AmB, the reference drug for VL, stands out. The use of IONPs as a vehicle in this context led to a two-fold reduction in parasite load compared to the administration of the drug alone, thus reaffirming its capacity for selective accumulation within infected macrophages (209). Concerning coatings, IONPs have been decorated, for example, with polyethyleneimine (PEI) and with pyroctone oleamine, for their proven cytolytic effect by embedding in cell membranes. In both cases, their leishmanicidal capacity has been tested both in vitro and in vivo, and the coatings enhance their efficacy to act compared to bare particles (197, 199). This duality of direct and vehicle strategies offers an immense range of possibilities to address the complexity of infection, maximizing therapeutic efficacy and selectivity of action against the parasite.
These findings highlight the multiple properties of IONPs, which make them a promising tool to revolutionize the treatment of leishmaniasis. Their versatile nature opens up diverse avenues for impressive therapeutic strategies, marking an important step forward in the search for effective and targeted interventions against this parasitic disease.
5 Limitations of IONPs as macrophage-directed therapies for treating leishmaniasis
Due to the ability of IONPs to selectively accumulate in macrophages, where Leishmania parasites live, and considering that intracellular iron balance is crucial for regulating macrophage polarization status, IONPs emerge as a promising strategy for treating leishmaniasis. This review has shown that IONP-challenged macrophages initiate a series of processes that trigger an activation towards an antimicrobial M1 phenotype (inflammatory response), capable of eliminating Leishmania. The proinflammatory effect would be mainly mediated by IONP interaction with TLR4 and iron overload.
However, this strategy has limitations. Apart from the accessibility of IONP to infected macrophages (limited in the cutaneous lesions) either after parenteral or local administration, a questionable issue is the targeting of macrophages as an immunomodulatory approach for the treatment of leishmaniasis versus other immune cells. In fact, the targeting of TAM in cancer is well suitable for those types of tumors with a high proportion of these immune cells whose polarization decide the overall tumoral microenvironment. The situation could be similar in DCL (diffuse cutaneous leishmaniasis) and PKDL (post kala-azar dermal leishmaniasis). The skin lesions of these clinical manifestations are characterized by a high number of heavily M2-like infected macrophages due to a lack of Th1 response and high levels of TGF-β and IL-10 (Regulatory T-cells) (210). Th bias would be similar in VL although the macrophages would not be the most abundant immune cells. However, in MCL, there is a low proportion of poorly infected macrophages. IFN-γ and TNF-α producing Th1 cells and cytotoxic CD8+ cells are predominant while levels of IL-10 are low, leading to exacerbated inflammation and tissue destruction (Figure 6) (210). Thus, in this clinical manifestation, Leishmania-infected macrophages phenotype would most closely resemble M1-macrophages, despite which parasites survive (211). IONPs would be a suitable strategy for VL, DCL or PKDL. All of them would also benefit from the blockage of immune-checkpoints such as TGF-β, IL-10, programmed cell death protein 1 (PD-1), programmed cell death ligand 1 (PDL-1) or cytotoxic T lymphocyte antigen 4 (CTLA4) (212, 213). On the contrary, IONP would be even contraindicated as MCL immunotherapy (Figure 6). The most suitable for this clinical manifestation would be the combination between leishmanicidal drugs (for clearance of the parasite) with immunomodulatory strategies addressed to avoid tissue destruction (207), such as the inhibition of TNF-α (with pentoxifylline), the cytolytic activity (Tofacitinib) or inflammasome activation (214).
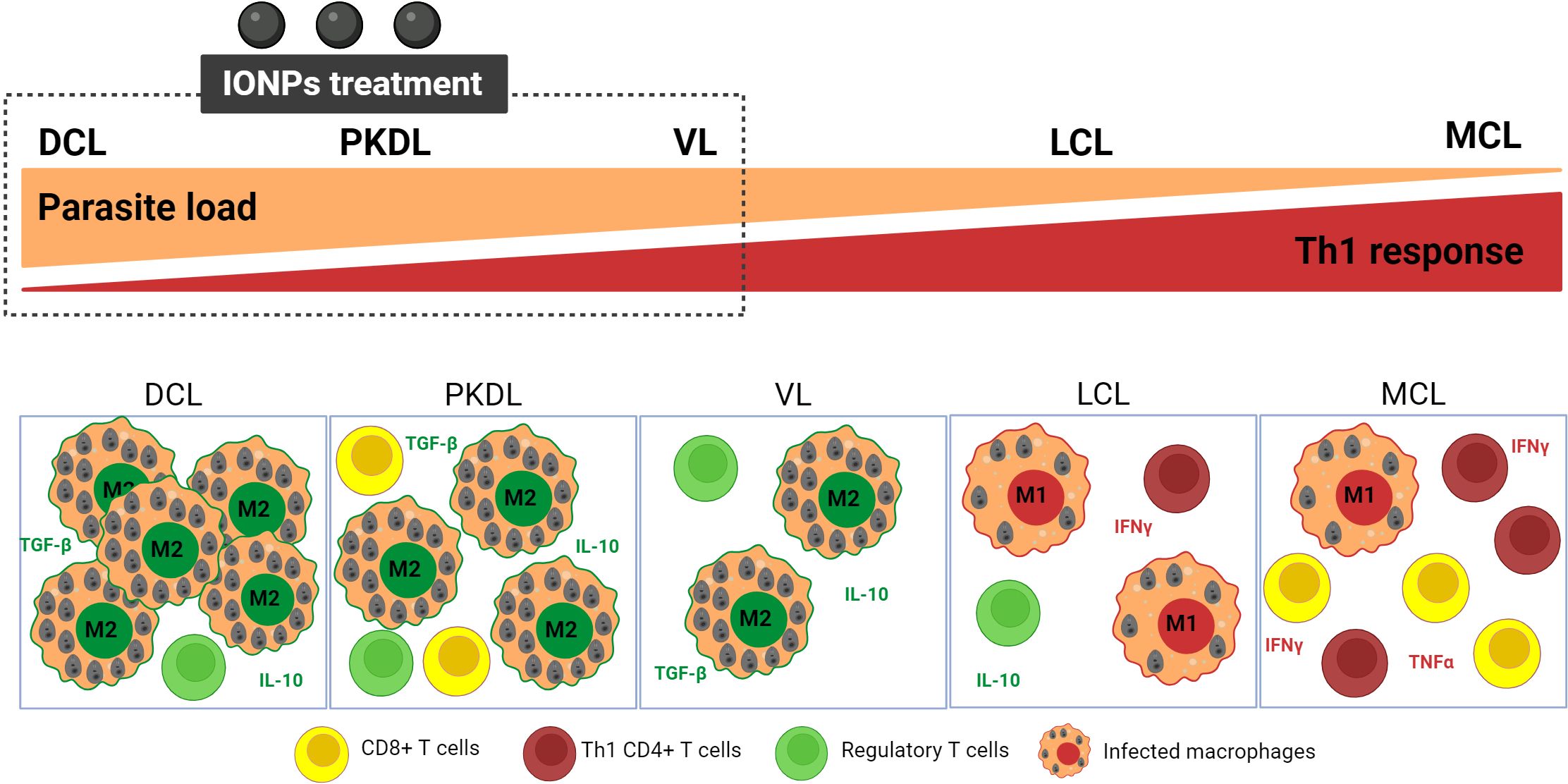
Figure 6. Scheme of immune profiles of the main clinical manifestations of leishmaniasis and suitability of IONPs. The balance between Th and macrophage responses highlights the suitability of IONP as a potential therapeutic strategy for VL, MCL, and PKDL, where macrophage abundance and/or pronounced T-Regulatory response are prominent features. DCL,Diffuse Cutaneous Leishmaniasis; PKDL, Post-Kala-Azar Dermal Leishmaniasis; VL, Visceral Leishmaniasis; LCL, Localized Cutaneous Leishmaniasis; MCL, Mucocutaneous Leishmaniasis. Figure created with biorender.com.
IONPs could also have an effect on T cell status and bias, although it should be indirectly produced by extracellular IONPs derived products (Fe or redox) or macrophage-mediated. The targeting of T cells with NPs is very limited by physiological barriers (position within organs) and the non-phagocytic nature of these cells, unlike macrophages, which are very well positioned for NP sampling and have very active and diverse endocytic pathways.
Another aspect to consider is the critical role that macrophage polarization status has not only the parasite clearance but also in tissue remodeling. In-depth, the persistence of M1-macrophages hampers the correct process of wound healing and tissue remodeling. Thus, the stimulus prone to produce M1-bias should be temporal and be removed after parasite clearance. In line with this, we cannot forget the dark side or immunotoxicity produced by IONPs mainly through their effect on oxidative stress and ongoing inflammation or cellular components alterations (215). Some IONPs such as Ferumoxide and Ferucarbotran initially approved in the USA and Europe for liver imaging were withdrawn from the market due to different toxic effects. Others such as Ferumoxytol remain approved for iron deficiency treatment for patients with chronic kidney disease and have recently been shown to be suitable for contrast aging (216). It is necessary a better comprehension of the relationships between IONP properties (iron release kinetics, stability, biodistribution as drivers of oxidative stress effects) and biological effects to delineate safer IONPs (215).
Many other stimuli could be proposed to induce M1 polarization in leishmaniasis immunotherapy (217). They should be associated with nanocarriers to favor their selective delivery to macrophages. IONPs emerge as an all-in-one solution as the carriers themselves produce M1 bias to a greater or lesser extent depending on the immune microenvironment.
Author contributions
CP-C: Conceptualization, Writing – original draft, Writing – review & editing. EM: Writing – review & editing. JI: Writing – review & editing. SE: Writing – review & editing, Conceptualization, Funding acquisition, Supervision, Writing – original draft.
Funding
The author(s) declare financial support was not received for the research, authorship, and/or publication of this article. CP-C is a beneficiary of a pre-doctoral fellowship from the Institute of Tropical Health of the University of Navarra (ISTUN).
Conflict of interest
The authors declare that the research was conducted in the absence of any commercial or financial relationships that could be construed as a potential conflict of interest.
Publisher’s note
All claims expressed in this article are solely those of the authors and do not necessarily represent those of their affiliated organizations, or those of the publisher, the editors and the reviewers. Any product that may be evaluated in this article, or claim that may be made by its manufacturer, is not guaranteed or endorsed by the publisher.
References
1. World Health Organization. Ending the neglect to attain the sustainable development goals: a road map for neglected tropical diseases 2021–2030 (2020). Available online at: https://www.who.int/publications/i/item/9789240010352 (Accessed May 15, 2024).
2. Georgiadou SP, Makaritsis KP, Dalekos GN. Leishmaniasis revisited: Current aspects on epidemiology, diagnosis and treatment. J Transl Int Med. (2015) 3:43–50. doi: 10.1515/jtim-2015-0002
3. Ruiz-Postigo JA, Jain S, Madjou S, Virrey Agua JF, Maia-Elkhoury AN, Valadas S, et al. Global leishmaniasis surveillance, 2022: assessing trends over the past 10 years (2023). Available online at: https://www.who.int/publications/i/item/who-wer9840-471-487 (Accessed May 15, 2024).
4. eBioMedicine. Leishmania: an urgent need for new treatments. EBioMedicine. (2023) 87:104440. doi: 10.1016/j.ebiom.2023.104440
5. Kamran M, Bhattacharjee R, Das S, Mukherjee S, Ali N. The paradigm of intracellular parasite survival and drug resistance in leishmanial parasite through genome plasticity and epigenetics: Perception and future perspective. Front Cell Infect Microbiol. (2023) 13:1001973. doi: 10.3389/fcimb.2023.1001973
6. Kaye P, Scott P. Leishmaniasis: complexity at the host–pathogen interface. Nat Rev Microbiol. (2011) 9:604–15. doi: 10.1038/nrmicro2608
7. Gordon S, Taylor PR. Monocyte and macrophage heterogeneity. Nat Rev Immunol. (2005) 5:953–64. doi: 10.1038/nri1733
8. Fortéa J, de la Llave E, Regnault B, Coppée J-Y, Milon G, Lang T, et al. Transcriptional signatures of BALB/c mouse macrophages housing multiplying Leishmania amazonensis amastigotes. BMC Genomics. (2009) 10:119. doi: 10.1186/1471-2164-10-119
9. Hurdayal R, Brombacher F. Interleukin-4 receptor alpha: from innate to adaptive immunity in murine models of cutaneous leishmaniasis. Front Immunol. (2017) 8:1354. doi: 10.3389/fimmu.2017.01354
10. Alexander J, Brombacher F. T helper1/T helper2 cells and resistance/susceptibility to leishmania infection: is this paradigm still relevant? Front Immunol. (2012) 3:80. doi: 10.3389/fimmu.2012.00080
11. Sacks D, Noben-Trauth N. The immunology of susceptibility and resistance to Leishmania major in mice. Nat Rev Immunol. (2002) 2:845–58. doi: 10.1038/nri933
12. Zumla A, Rao M, Wallis RS, Kaufmann SHE, Rustomjee R, Mwaba P, et al. Host-directed therapies for infectious diseases: current status, recent progress, and future prospects. Lancet Infect Dis. (2016) 16:e47–63. doi: 10.1016/S1473-3099(16)00078-5
13. El Hajj R, Bou Youness H, Lachaud L, Bastien P, Masquefa C, Bonnet P-A, et al. EAPB0503: An Imiquimod analog with potent in vitro activity against cutaneous leishmaniasis caused by Leishmania major and Leishmania tropica. PLoS Negl Trop Dis. (2018) 12:e0006854. doi: 10.1371/journal.pntd.0006854
14. Kaushik D, Granato JT, Macedo GC, Dib PRB, Piplani S, Fung J, et al. Toll-like receptor-7/8 agonist kill Leishmania amazonensis by acting as pro-oxidant and pro-inflammatory agent. J Pharm Pharmacol. (2021) 73:1180–90. doi: 10.1093/jpp/rgab063
15. Thacker SG, McWilliams IL, Bonnet B, Halie L, Beaucage S, Rachuri S, et al. CpG ODN D35 improves the response to abbreviated low-dose pentavalent antimonial treatment in non-human primate model of cutaneous leishmaniasis. PLoS Negl Trop Dis. (2020) 14:e0008050. doi: 10.1371/journal.pntd.0008050
16. Flannagan R, Heit B, Heinrichs D. Antimicrobial mechanisms of macrophages and the immune evasion strategies of staphylococcus aureus. Pathogens. (2015) 4:826–68. doi: 10.3390/pathogens4040826
17. Dadfar SM, Roemhild K, Drude NI, von Stillfried S, Knüchel R, Kiessling F, et al. Iron oxide nanoparticles: Diagnostic, therapeutic and theranostic applications. Adv Drug Deliv Rev. (2019) 138:302–25. doi: 10.1016/j.addr.2019.01.005
18. Szulc-Dąbrowska L, Bossowska-Nowicka M, Struzik J, Toka FN. Cathepsins in bacteria-macrophage interaction: defenders or victims of circumstance? Front Cell Infect Microbiol. (2020) 10:601072. doi: 10.3389/fcimb.2020.601072
19. Vermot A, Petit-Härtlein I, Smith SME, Fieschi F. NADPH oxidases (NOX): an overview from discovery, molecular mechanisms to physiology and pathology. Antioxidants (Basel). (2021) 10(6). doi: 10.3390/antiox10060890
20. Gantt KR, Goldman TL, McCormick ML, Miller MA, Jeronimo SMB, Nascimento ET, et al. Oxidative responses of human and murine macrophages during phagocytosis of Leishmania chagasi. J Immunol. (2001) 167:893–901. doi: 10.4049/jimmunol.167.2.893
21. Yang Y, Qi PK, Yang ZL, Huang N. Nitric oxide based strategies for applications of biomedical devices. Biosurf Biotribol. (2015) 1:177–201. doi: 10.1016/j.bsbt.2015.08.003
22. Assreuy J, Cunha FQ, Epperlein M, Noronha-Dutra A, O’Donnell CA, Liew FY, et al. Production of nitric oxide and superoxide by activated macrophages and killing ofLeishmania major. Eur J Immunol. (1994) 24:672–6. doi: 10.1002/eji.1830240328
23. Liew FY, Millott S, Parkinson C, Palmer RM, Moncada S. Macrophage killing of Leishmania parasite in vivo is mediated by nitric oxide from L-arginine. J Immunol. (1990) 144:4794–7.
24. Serarslan G, Atik E. Expression of inducible nitric oxide synthase in human cutaneous leishmaniasis. Mol Cell Biochem. (2005) 280:147–9. doi: 10.1007/s11010-005-8542-3
25. Carneiro PP, Conceição J, Macedo M, Magalhães V, Carvalho EM, Bacellar O. The role of nitric oxide and reactive oxygen species in the killing of leishmania Braziliensis by monocytes from patients with cutaneous leishmaniasis. PLoS One. (2016) 11:e0148084. doi: 10.1371/journal.pone.0148084
26. Almeida FS, Vanderley SER, Comberlang FC, Andrade AG, Cavalcante-Silva LHA, Silva EDS, et al. Leishmaniasis: immune cells crosstalk in macrophage polarization. Trop Med Infect Dis. (2023) 8(5). doi: 10.3390/tropicalmed8050276
27. Moulik S, Karmakar J, Joshi S, Dube A, Mandal C, Chatterjee M. Status of IL-4 and IL-10 driven markers in experimental models of Visceral Leishmaniasis. Parasite Immunol. (2021) 43(1):e12783. doi: 10.1111/pim.12783
28. Aghaei M, KhanAhmad H, Aghaei S, Ali Nilforoushzadeh M, Mohaghegh M-A, Hejazi SH. The role of Bax in the apoptosis of Leishmania-infected macrophages. Microb Pathog. (2020) 139:e103892. doi: 10.1016/j.micpath.2019.103892
29. Behar SM, Martin CJ, Booty MG, Nishimura T, Zhao X, Gan H-X, et al. Apoptosis is an innate defense function of macrophages against Mycobacterium tuberculosis. Mucosal Immunol. (2011) 4:279–87. doi: 10.1038/mi.2011.3
30. Forget G, Gregory DJ, Whitcombe LA, Olivier M. Role of host protein tyrosine phosphatase SHP-1 in Leishmania donovani -induced inhibition of nitric oxide production. Infect Immun. (2006) 74:6272–9. doi: 10.1128/IAI.00853-05
31. Blanchette J, Abu-Dayyeh I, Hassani K, Whitcombe L, Olivier M. Regulation of macrophage nitric oxide production by the protein tyrosine phosphatase Src homology 2 domain phosphotyrosine phosphatase 1 (SHP-1). Immunology. (2009) 127:123–33. doi: 10.1111/j.1365-2567.2008.02929.x
32. Nandan D, Reiner NE. Leishmania donovani engages in regulatory interference by targeting macrophage protein tyrosine phosphatase SHP-1. Clin Immunol. (2005) 114:266–77. doi: 10.1016/j.clim.2004.07.017
33. Pautz A, Art J, Hahn S, Nowag S, Voss C, Kleinert H. Regulation of the expression of inducible nitric oxide synthase. Nitric Oxide. (2010) 23:75–93. doi: 10.1016/j.niox.2010.04.007
34. Forget G, Siminovitch KA, Brochu S, Rivest S, Radzioch D, Olivier M. Role of host phosphotyrosine phosphatase SHP-1 in the development of murine leishmaniasis. Eur J Immunol. (2001) 31:3185–96. doi: 10.1002/1521-4141(200111)31:11<3185::AID-IMMU3185>3.0.CO;2-J
35. Calegari-Silva TC, Vivarini ÁC, Pereira R de MS, Dias-Teixeira KL, Rath CT, Pacheco ASS, et al. Leishmania amazonensis downregulates macrophage iNOS expression via Histone Deacetylase 1 (HDAC1): a novel parasite evasion mechanism. Eur J Immunol. (2018) 48:1188–98. doi: 10.1002/eji.201747257
36. Dacher M, Tachiwana H, Horikoshi N, Kujirai T, Taguchi H, Kimura H, et al. Incorporation and influence of Leishmania histone H3 in chromatin. Nucleic Acids Res. (2019) 47:11637–48. doi: 10.1093/nar/gkz1040
37. Lecoeur H, Prina E, Rosazza T, Kokou K, N’Diaye P, Aulner N, et al. Targeting macrophage histone H3 modification as a leishmania strategy to dampen the NF-κB/NLRP3-mediated inflammatory response. Cell Rep. (2020) 30:1870–82.e4. doi: 10.1016/j.celrep.2020.01.030
38. Calegari-Silva TC, Vivarini ÁC, Miqueline M, Dos Santos GR, Teixeira KL, Saliba AM, et al. The human parasite Leishmania amazonensis downregulates iNOS expression via NF-κB p50/p50 homodimer: role of the PI3K/Akt pathway. Open Biol. (2015) 5(9):150118. doi: 10.1098/rsob.150118
39. Fernandes JCR, Aoki JI, Maia Acuña S, Zampieri RA, Markus RP, Floeter-Winter LM, et al. Melatonin and Leishmania amazonensis Infection Altered miR-294, miR-30e, and miR-302d Impacting on Tnf, Mcp-1, and Nos2 Expression. Front Cell Infect Microbiol. (2019) 9:60. doi: 10.3389/fcimb.2019.00060
40. Muxel SM, Laranjeira-Silva MF, Zampieri RA, Floeter-Winter LM. Leishmania (Leishmania) amazonensis induces macrophage miR-294 and miR-721 expression and modulates infection by targeting NOS2 and L-arginine metabolism. Sci Rep. (2017) 7:1–15. doi: 10.1038/srep44141
41. Wilkins-Rodríguez AA, Pérez-Torres A, Escalona-Montaño AR, Gutiérrez-Kobeh L. Differential regulation of L-arginine metabolism through Arginase 1 during infection with Leishmania Mexicana isolates obtained from patients with localized and diffuse cutaneous Leishmaniasis. Infect Immun. (2020) 88(7). doi: 10.1128/IAI.00963-19
42. Gupta AK, Das S, Kamran M, Ejazi SA, Ali N. The pathogenicity and virulence of Leishmania - interplay of virulence factors with host defenses. Virulence. (2022) 13:903–35. doi: 10.1080/21505594.2022.2074130
43. Moradin N, Descoteaux A. Leishmania promastigotes: building a safe niche within macrophages. Front Cell Infect Microbiol. (2012) 2:121. doi: 10.3389/fcimb.2012.00121
44. Arango Duque G, Descoteaux A. Leishmania survival in the macrophage: where the ends justify the means. Curr Opin Microbiol. (2015) 26:32–40. doi: 10.1016/j.mib.2015.04.007
45. Shio MT, Christian JG, Jung JY, Chang K-P, Olivier M. PKC/ROS-mediated NLRP3 inflammasome activation is attenuated by Leishmania zinc-metalloprotease during infection. PLoS Negl Trop Dis. (2015) 9(6):e0003868. doi: 10.1371/journal.pntd.0003868
46. Inoguchi T, Sonta T, Tsubouchi H, Etoh T, Kakimoto M, Sonoda N, et al. Protein kinase C–dependent increase in reactive oxygen species (ROS) production in vascular tissues of diabetes. J Am Soc Nephrol. (2003) 14:S227–32. doi: 10.1097/01.ASN.0000077407.90309.65
47. Podinovskaia M, Descoteaux A. Leishmania and the macrophage: a multifaceted interaction. Future Microbiol. (2015) 10:111–29. doi: 10.2217/fmb.14.103
48. Basu Ball W, Kar S, Mukherjee M, Chande AG, Mukhopadhyaya R, Das PK. Uncoupling protein 2 negatively regulates mitochondrial reactive oxygen species generation and induces phosphatase-mediated anti-inflammatory response in experimental visceral Leishmaniasis. J Immunol. (2011) 187:1322–32. doi: 10.4049/jimmunol.1004237
49. Vivarini A de C, Lopes UG. The potential role of Nrf2 signaling in Leishmania infection outcomes. Front Cell Infect Microbiol. (2020) 9:453. doi: 10.3389/fcimb.2019.00453
50. Reverte M, Eren RO, Jha B, Desponds C, Snäkä T, Prevel F, et al. The antioxidant response favors Leishmania parasites survival, limits inflammation and reprograms the host cell metabolism. PLoS Pathog. (2021) 17(3):e1009422. doi: 10.1371/journal.ppat.1009422
51. Tonelli C, Chio IIC, Tuveson DA. Transcriptional regulation by nrf2. Antioxid Redox Signal. (2018) 29:1727–45. doi: 10.1089/ars.2017.7342
52. Bogdan C. Macrophages as host, effector and immunoregulatory cells in leishmaniasis: Impact of tissue micro-environment and metabolism. Cytokine X. (2020) 2(4):100041. doi: 10.1016/j.cytox.2020.100041
53. Antoine J-C, Prina E, Courret N, Lang T. Leishmania spp.: on the Interactions They Establish with Antigen-Presenting Cells of their Mammalian Hosts. Adv Parasitol (2004) 58:1–68. doi: 10.1016/S0065-308X(04)58001-6
54. Meier CL, Svensson M, Kaye PM. Leishmania -induced inhibition of macrophage antigen presentation analyzed at the single-cell level. J Immunol. (2003) 171:6706–13. doi: 10.4049/jimmunol.171.12.6706
55. Kwan WC, McMaster WR, Wong N, Reiner NE. Inhibition of expression of major histocompatibility complex class II molecules in macrophages infected with Leishmania donovani occurs at the level of gene transcription via a cyclic AMP-independent mechanism. Infect Immun. (1992) 60:2115–20. doi: 10.1128/iai.60.5.2115-2120.1992
56. Leao SDS, Lang T, Prina E, Hellio R, Antoine J-C. Intracellular Leishmania amazonensis amastigotes internalize and degrade MHC class II molecules of their host cells. J Cell Sci. (1995) 108:3219–31. doi: 10.1242/jcs.108.10.3219
57. Cecílio P, Pérez-Cabezas B, Santarém N, Maciel J, Rodrigues V, Cordeiro da Silva A. Deception and manipulation: the arms of leishmania, a successful parasite. Front Immunol. (2014) 5:480. doi: 10.3389/fimmu.2014.00480
58. Lamotte S, Späth GF, Rachidi N, Prina E. The enemy within: Targeting host–parasite interaction for antileishmanial drug discovery. PLoS Negl Trop Dis. (2017) 11(6):e0005480. doi: 10.1371/journal.pntd.0005480
59. Nyambura LW, Jarmalavicius S, Walden P. Impact of Leishmania donovani infection on the HLA I self peptide repertoire of human macrophages. PLoS One. (2018) 13(7):e0200297. doi: 10.1371/journal.pone.0200297
60. Anderson HA, Roche PA. MHC class II association with lipid rafts on the antigen presenting cell surface. Biochim Biophys Acta (BBA) - Mol Cell Res. (2015) 1853:775–80. doi: 10.1016/j.bbamcr.2014.09.019
61. Chakraborty D, Banerjee S, Sen A, Banerjee KK, Das P, Roy S. Leishmania donovani affects antigen presentation of macrophage by disrupting lipid rafts. J Immunol. (2005) 175:3214–24. doi: 10.4049/jimmunol.175.5.3214
62. Roy K, Mandloi S, Chakrabarti S, Roy S. Cholesterol corrects altered conformation of MHC-II protein in Leishmania donovani infected macrophages: implication in therapy. PLoS Negl Trop Dis. (2016) 10(5):e0004710. doi: 10.1371/journal.pntd.0004710
63. Pradhan S, Ghosh S, Hussain S, Paul J, Mukherjee B. Linking membrane fluidity with defective antigen presentation in leishmaniasis. Parasite Immunol. (2021) 43(7):e12835. doi: 10.1111/pim.12835
64. Bodhale N, Ohms M, Ferreira C, Mesquita I, Mukherjee A, André S, et al. Cytokines and metabolic regulation: A framework of bidirectional influences affecting Leishmania infection. Cytokine. (2021) 147:155267. doi: 10.1016/j.cyto.2020.155267
65. Dayakar A, Chandrasekaran S, Kuchipudi SV, Kalangi SK. Cytokines: key determinants of resistance or disease progression in visceral leishmaniasis: opportunities for novel diagnostics and immunotherapy. Front Immunol. (2019) 10:670. doi: 10.3389/fimmu.2019.00670
66. Mottram JC, Coombs GH, Alexander J. Cysteine peptidases as virulence factors of Leishmania. Curr Opin Microbiol. (2004) 7:375–81. doi: 10.1016/j.mib.2004.06.010
67. Cameron P, McGachy A, Anderson M, Paul A, Coombs GH, Mottram JC, et al. Inhibition of lipopolysaccharide-induced macrophage IL-12 production by Leishmania mexicana amastigotes: the role of cysteine peptidases and the NF-κB signaling pathway. J Immunol. (2004) 173:3297–304. doi: 10.4049/jimmunol.173.5.3297
68. Chaparro V, Graber TE, Alain T, Jaramillo M. Transcriptional profiling of macrophages reveals distinct parasite stage-driven signatures during early infection by Leishmania donovani. Sci Rep. (2022) 12(1):6369. doi: 10.1038/s41598-022-10317-6
69. Ichim G, Tait SWG. A fate worse than death: apoptosis as an oncogenic process. Nat Rev Cancer. (2016) 16:539–48. doi: 10.1038/nrc.2016.58
70. Gupta P, Srivastav S, Saha S, Das PK, Ukil A. Leishmania donovani inhibits macrophage apoptosis and pro-inflammatory response through AKT-mediated regulation of β-catenin and FOXO-1. Cell Death Differ. (2016) 23:1815–26. doi: 10.1038/cdd.2016.101
71. Ruhland A, Leal N, Kima PE. Leishmania promastigotes activate PI3K/Akt signalling to confer host cell resistance to apoptosis. Cell Microbiol. (2007) 9:84–96. doi: 10.1111/j.1462-5822.2006.00769.x
72. Ranatunga M, Rai R, Richardson SCW, Dyer P, Harbige L, Deacon A, et al. Leishmania aethiopica cell-to-cell spreading involves caspase-3, AkT, and NF-κB but not PKC-δ activation and involves uptake of LAMP-1-positive bodies containing parasites. FEBS J. (2020) 287:1777–97. doi: 10.1111/febs.15166
73. Pandey RK, Mehrotra S, Sharma S, Gudde RS, Sundar S, Shaha C. Leishmania donovani-induced increase in macrophage bcl-2 favors parasite survival. Front Immunol. (2016) 7:456. doi: 10.3389/fimmu.2016.00456
74. Giri J, Srivastav S, Basu M, Palit S, Gupta P, Ukil A. Leishmania donovani exploits myeloid cell leukemia 1 (MCL-1) protein to prevent mitochondria-dependent host cell apoptosis. J Biol Chem. (2016) 291:3496–507. doi: 10.1074/jbc.M115.672873
75. Gholamrezaei M, Rouhani S, Mohebali M, Mohammadi-Yeganeh S, Haji Molla Hoseini M, Haghighi A, et al. MicroRNAs expression induces apoptosis of macrophages in response to leishmania major (MRHO/IR/75/ER): an in-vitro and in-vivo study. Iran J Parasitol. (2020) 15(4):475–87. doi: 10.18502/ijpa.v15i4.4851
76. Kumar V, Kumar A, Das S, Kumar A, Abhishek K, Verma S, et al. Leishmania donovani Activates Hypoxia Inducible Factor-1α and miR-210 for Survival in Macrophages by Downregulation of NF-κB Mediated Pro-inflammatory Immune Response. Front Microbiol. (2018) 9:385. doi: 10.3389/fmicb.2018.00385
77. Lasjerdi Z, Ghanbarian H, Mohammadi-Yeganeh S, Seyyed Tabaei SJ, Mohebali M, Taghipour N, et al. Comparative Expression Profile Analysis of Apoptosis-Related miRNA and Its Target Gene in Leishmania major Infected Macrophages. Iran J Parasitol. (2020) 15(3):332–40. doi: 10.18502/ijpa.v15i3.4197
78. Cairo G, Bernuzzi F, Recalcati S. A precious metal: Iron, an essential nutrient for all cells. Genes Nutr. (2006) 1:25–39. doi: 10.1007/BF02829934
79. Dixon SJ, Stockwell BR. The role of iron and reactive oxygen species in cell death. Nat Chem Biol. (2014) 10:9–17. doi: 10.1038/nchembio.1416
80. Winn NC, Volk KM, Hasty AH. Regulation of tissue iron homeostasis: the macrophage “ferrostat”. JCI Insight. (2020) 5(2). doi: 10.1172/jci.insight.132964
81. Muckenthaler MU, Rivella S, Hentze MW, Galy B. A red carpet for iron metabolism. Cell. (2017) 168:344–61. doi: 10.1016/j.cell.2016.12.034
82. Soares MP, Hamza I. Macrophages and iron metabolism. Immunity. (2016) 44:492–504. doi: 10.1016/j.immuni.2016.02.016
83. Sukhbaatar N, Weichhart T. Iron regulation: macrophages in control. Pharmaceuticals (Basel). (2018) 11(4). doi: 10.3390/ph11040137
84. Behmoaras J. The versatile biochemistry of iron in macrophage effector functions. FEBS J. (2021) 288:6972–89. doi: 10.1111/febs.15682
85. Puig S, Ramos-Alonso L, Romero AM, Martínez-Pastor MT. The elemental role of iron in DNA synthesis and repair. Metallomics. (2017) 9:1483–500. doi: 10.1039/C7MT00116A
86. Ganz T, Nemeth E. Iron homeostasis in host defence and inflammation. Nat Rev Immunol. (2015) 15:500–10. doi: 10.1038/nri3863
87. Hentze MW, Kühn LC. Molecular control of vertebrate iron metabolism: mRNA-based regulatory circuits operated by iron, nitric oxide, and oxidative stress. Proc Natl Acad Sci. (1996) 93:8175–82. doi: 10.1073/pnas.93.16.8175
88. Garza KR, Clarke SL, Ho Y-H, Bruss MD, Vasanthakumar A, Anderson SA, et al. Differential translational control of 5’ IRE-containing mRNA in response to dietary iron deficiency and acute iron overload. Metallomics. (2020) 12:2186–98. doi: 10.1039/d0mt00192a
89. Sanchez M, Galy B, Schwanhaeusser B, Blake J, Bähr-Ivacevic T, Benes V, et al. Iron regulatory protein-1 and -2: transcriptome-wide definition of binding mRNAs and shaping of the cellular proteome by iron regulatory proteins. Blood. (2011) 118:e168–79. doi: 10.1182/blood-2011-04-343541
90. Agoro R, Taleb M, Quesniaux VFJ, Mura C. Cell iron status influences macrophage polarization. PloS One. (2018) 13(5):e0196921. doi: 10.1371/journal.pone.0196921
91. Recalcati S, Locati M, Marini A, Santambrogio P, Zaninotto F, De Pizzol M, et al. Differential regulation of iron homeostasis during human macrophage polarized activation. Eur J Immunol. (2010) 40:824–35. doi: 10.1002/eji.200939889
92. Cairo G, Recalcati S, Mantovani A, Locati M. Iron trafficking and metabolism in macrophages: contribution to the polarized phenotype. Trends Immunol. (2011) 32:241–7. doi: 10.1016/j.it.2011.03.007
93. Fraternale A, Brundu S, Magnani M. Polarization and repolarization of macrophages. J Clin Cell Immunol. (2015) 6:1–10. doi: 10.4172/2155-9899.1000319
94. Xia Y, Li Y, Wu X, Zhang Q, Chen S, Ma X, et al. Ironing out the details: how iron orchestrates macrophage polarization. Front Immunol. (2021) 12:669566. doi: 10.3389/fimmu.2021.669566
95. Sindrilaru A, Peters T, Wieschalka S, Baican C, Baican A, Peter H, et al. An unrestrained proinflammatory M1 macrophage population induced by iron impairs wound healing in humans and mice. J Clin Invest. (2011) 121:985–97. doi: 10.1172/JCI44490
96. Zhou Y, Que K-T, Zhang Z, Yi ZJ, Zhao PX, You Y, et al. Iron overloaded polarizes macrophage to proinflammation phenotype through ROS/acetyl-p53 pathway. Cancer Med. (2018) 7:4012–22. doi: 10.1002/cam4.1670
97. Wang S, Cheng M, Peng P, Lou Y, Zhang A, Liu P. Iron Released after Cryo-Thermal Therapy Induced M1 Macrophage Polarization, Promoting the Differentiation of CD4+ T Cells into CTLs. Int J Mol Sci. (2021) 22:1–19. doi: 10.3390/ijms22137010
98. Bloomer SA. Hepatic macrophage abundance and phenotype in aging and liver iron accumulation. Int J Mol Sci. (2022) 23(12). doi: 10.3390/ijms23126502
99. Hu X, Cai X, Ma R, Fu W, Zhang C, Du X. Iron-load exacerbates the severity of atherosclerosis via inducing inflammation and enhancing the glycolysis in macrophages. J Cell Physiol. (2019) 234:18792–800. doi: 10.1002/jcp.28518
100. Handa P, Thomas S, Morgan-Stevenson V, Maliken BD, Gochanour E, Boukhar S, et al. Iron alters macrophage polarization status and leads to steatohepatitis and fibrogenesis. J Leukoc Biol. (2019) 105:1015–26. doi: 10.1002/JLB.3A0318-108R
101. Nairz M, Schleicher U, Schroll A, Sonnweber T, Theurl I, Ludwiczek S, et al. Nitric oxide–mediated regulation of ferroportin-1 controls macrophage iron homeostasis and immune function in Salmonella infection. J Exp Med. (2013) 210:855–73. doi: 10.1084/jem.20121946
102. Weiss G, Werner-Felmayer G, Werner ER, Grünewald K, Wachter H, Hentze MW. Iron regulates nitric oxide synthase activity by controlling nuclear transcription. J Exp Med. (1994) 180:969–76. doi: 10.1084/jem.180.3.969
103. Liu C, Liang MC, Soong TW. Nitric oxide, iron and neurodegeneration. Front Neurosci. (2019) 13:114. doi: 10.3389/fnins.2019.00114
104. Vinchi F, Costa da Silva M, Ingoglia G, Petrillo S, Brinkman N, Zuercher A, et al. Hemopexin therapy reverts heme-induced proinflammatory phenotypic switching of macrophages in a mouse model of sickle cell disease. Blood. (2016) 127:473–86. doi: 10.1182/blood-2015-08-663245
105. Mertens C, Akam EA, Rehwald C, Brüne B, Tomat E, Jung M. Intracellular iron chelation modulates the macrophage iron phenotype with consequences on tumor progression. PLoS One. (2016) 11(11):e0166164. doi: 10.1371/journal.pone.0166164
106. Pereira M, Chen T-D, Buang N, Olona A, Ko J-H, Prendecki M, et al. Acute iron deprivation reprograms human macrophage metabolism and reduces inflammation in vivo. Cell Rep. (2019) 28:498–511.e5. doi: 10.1016/j.celrep.2019.06.039
107. Perng V, Navazesh SE, Park J, Arballo JR, Ji P. Iron deficiency and overload modulate the inflammatory responses and metabolism of alveolar macrophages. Nutrients. (2022) 14(15). doi: 10.3390/nu14153100
108. Surdej P, Richman L, Kühn LC. Differential translational regulation of IRE-containing mRNAs in Drosophila melanogaster by endogenous IRP and a constitutive human IRP1 mutant. Insect Biochem Mol Biol. (2008) 38:891–4. doi: 10.1016/j.ibmb.2008.05.010
109. Hood MI, Skaar EP. Nutritional immunity: transition metals at the pathogen-host interface. Nat Rev Microbiol. (2012) 10:525–37. doi: 10.1038/nrmicro2836
110. Laranjeira-Silva MF, Hamza I, Pérez-Victoria JM. Iron and heme metabolism at the Leishmania–host interface. Trends Parasitol. (2020) 36:279–89. doi: 10.1016/j.pt.2019.12.010
111. Fattahi-Dolatabadi M, Mousavi T, Mohammadi-Barzelighi H, Irian S, Bakhshi B, Nilforoushzadeh M-A, et al. NRAMP1 gene polymorphisms and cutaneous leishmaniasis: An evaluation on host susceptibility and treatment outcome. J Vector Borne Dis. (2016) 53:257–63.
112. Hernández-Rivera MP, Ramírez-Ramírez A, Chiñas-Pérez A, Monroy-Ostria A, Cancino-Díaz ME, Hernández-Montes O. NRAMP1 polymorphisms like susceptibility marker in Mexican focus of cutaneous leishmaniasis. BioMed Res Int. (2016) 2016:7951285. doi: 10.1155/2016/7951285
113. Singh N, Bajpai S, Kumar V, Gour JK, Singh RK. Identification and functional characterization of leishmania donovani secretory peroxidase: delineating its role in NRAMP1 regulation. PLoS One. (2013) 8(1):e53442. doi: 10.1371/journal.pone.0053442
114. Borges VM, Vannier-Santos MA, de Souza W. Subverted transferrin trafficking in Leishmania-infected macrophages. Parasitol Res. (1998) 84:811–22. doi: 10.1007/s004360050493
115. Ben-Othman R, Flannery AR, Miguel DC, Ward DM, Kaplan J, Andrews NW. Leishmania-mediated inhibition of iron export promotes parasite replication in macrophages. PLoS Pathog. (2014) 10(1):e1003901. doi: 10.1371/journal.ppat.1003901
116. Das NK, Sandhya S, G VV, Kumar R, Singh AK, Bal SK, et al. Leishmania donovani inhibits ferroportin translation by modulating FBXL5-IRP2 axis for its growth within host macrophages. Cell Microbiol. (2018) 20(7):e12834. doi: 10.1111/cmi.12834
117. Rafiee A, Riazi-rad F, Darabi H, Khaze V, Javadian S, Ajdary S, et al. Ferroportin-encapsulated nanoparticles reduce infection and improve immunity in mice infected with Leishmania major. Int J Pharm. (2014) 466:375–81. doi: 10.1016/j.ijpharm.2014.03.039
118. Das NK, Biswas S, Solanki S, Mukhopadhyay CK. Leishmania donovani depletes labile iron pool to exploit iron uptake capacity of macrophage for its intracellular growth. Cell Microbiol. (2009) 11:83–94. doi: 10.1111/j.1462-5822.2008.01241.x
119. Sen S, Bal SK, Yadav S, Mishra P, G VV, Rastogi R, et al. Intracellular pathogen Leishmania intervenes in iron loading into ferritin by cleaving chaperones in host macrophages as an iron acquisition strategy. J Biol Chem. (2022) 298(12):102646. doi: 10.1016/j.jbc.2022.102646
120. Mittra B, Andrews NW. IRONy OF FATE: role of iron-mediated ROS in Leishmania differentiation. Trends Parasitol. (2013) 29:489–96. doi: 10.1016/j.pt.2013.07.007
121. Flannery AR, Huynh C, Mittra B, Mortara RA, Andrews NW. LFR1 ferric iron reductase of Leishmania amazonensis is essential for the generation of infective parasite forms. J Biol Chem. (2011) 286:23266–79. doi: 10.1074/jbc.M111.229674
122. Wilson ME, Lewis TS, Miller MA, McCormick ML, Britigan BE. Leishmania chagasi: uptake of iron bound to lactoferrin or transferrin requires an iron reductase. Exp Parasitol. (2002) 100:196–207. doi: 10.1016/S0014-4894(02)00018-8
123. Miguel DC, Flannery AR, Mittra B, Andrews NW. Heme uptake mediated by LHR1 is essential for Leishmania amazonensis virulence. Infect Immun. (2013) 81:3620–6. doi: 10.1128/IAI.00687-13
124. Cabello-Donayre M, Orrego LM, Herráez E, Vargas P, Martínez-García M, Campos-Salinas J, et al. Leishmania heme uptake involves LmFLVCRb, a novel porphyrin transporter essential for the parasite. Cell Mol Life Sci. (2020) 77:1827–45. doi: 10.1007/s00018-019-03258-3
125. Rastogi R, Verma JK, Singh V, Krishnamurthy G, Sood C, Kapoor A, et al. Identification and characterization of the hemoglobin-binding domain of hemoglobin receptor in. Leishmania. FEBS Lett. (2021) 595:548–58. doi: 10.1002/1873-3468.14027
126. dos Santos RF, Da Silva T, Brito AC de S, Inácio JD, Ventura BD, Mendes MAP, et al. Therapeutic effect of oral quercetin in hamsters infected with Leishmania Viannia Braziliensis. Front Cell Infect Microbiol. (2023) 12:1059168. doi: 10.3389/fcimb.2022.1059168
127. Cataneo AHD, Tomiotto-Pellissier F, Miranda-Sapla MM, Assolini JP, Panis C, Kian D, et al. Quercetin promotes antipromastigote effect by increasing the ROS production and anti-amastigote by upregulating Nrf2/HO-1 expression, affecting iron availability. Biomed Pharmacother. (2019) 113:108745. doi: 10.1016/j.biopha.2019.108745
128. Antwi CA, Amisigo CM, Adjimani JP, Gwira TM. In vitro activity and mode of action of phenolic compounds on Leishmania donovani. PloS Negl Trop Dis. (2019) 13(2):e0007206. doi: 10.1371/journal.pntd.0007206
129. Bortoleti BT da S, Tomiotto-Pellissier F, Gonçalves MD, Miranda-Sapla MM, Assolini JP, Carloto AC, et al. Caffeic acid has antipromastigote activity by apoptosis-like process; and anti-amastigote by TNF-α/ROS/NO production and decreased of iron availability. Phytomedicine. (2019) 57:262–70. doi: 10.1016/j.phymed.2018.12.035
130. Malafaia G, Marcon L de N, Pereira L de F, Pedrosa ML, Rezende SA. Leishmania chagasi: Effect of the iron deficiency on the infection in BALB/c mice. Exp Parasitol. (2011) 127:719–23. doi: 10.1016/j.exppara.2010.11.010
131. Bisti S, Konidou G, Papageorgiou F, Milon G, Boelaert JR, Soteriadou K. The outcome of Leishmania major experimental infection in BALB/c mice can be modulated by exogenously delivered iron. Eur J Immunol. (2000) 30(12):3732–40. doi: 10.1002/1521-4141(200012)30:12<3732::AID-IMMU3732>3.0.CO;2-D
132. Banerjee S, Datta R. Localized Leishmania major infection disrupts systemic iron homeostasis that can be controlled by oral iron supplementation. J Biol Chem. (2023) 299(8):105064. doi: 10.1016/j.jbc.2023.105064
133. Vale-Costa S, Gomes-Pereira S, Teixeira CM, Rosa G, Rodrigues PN, Tomás A, et al. Iron Overload Favors the Elimination of Leishmania infantum from Mouse Tissues through Interaction with Reactive Oxygen and Nitrogen Species. PloS Negl Trop Dis. (2013) 7(2):e2061. doi: 10.1371/journal.pntd.0002061
134. Charlebois E, Li Y, Wagner V, Pantopoulos K, Olivier M. Genetic iron overload hampers development of cutaneous leishmaniasis in mice. Int J Mol Sci. (2023) 24(2). doi: 10.3390/ijms24021669
135. Babitt JL, Huang FW, Wrighting DM, Xia Y, Sidis Y, Samad TA, et al. Bone morphogenetic protein signaling by hemojuvelin regulates hepcidin expression. Nat Genet. (2006) 38:531–9. doi: 10.1038/ng1777
136. Ginzburg YZ. Hepcidin-ferroportin axis in health and disease. Vitam Horm. (2019), 110:17–45. doi: 10.1016/bs.vh.2019.01.002
137. Tewabe A, Abate A, Tamrie M, Seyfu A, Abdela Siraj E. Targeted drug delivery - from magic bullet to nanomedicine: principles, challenges, and future perspectives. J Multidiscip Healthc. (2021) 14:1711–24. doi: 10.2147/JMDH.S313968
138. Mitchell MJ, Billingsley MM, Haley RM, Wechsler ME, Peppas NA, Langer R. Engineering precision nanoparticles for drug delivery. Nat Rev Drug Discov. (2021) 20:101–24. doi: 10.1038/s41573-020-0090-8
139. Patra JK, Das G, Fraceto LF, Campos EVR, Rodriguez-Torres MDP, Acosta-Torres LS, et al. Nano based drug delivery systems: recent developments and future prospects. J Nanobiotechnol. (2018) 16(1):71. doi: 10.1186/s12951-018-0392-8
140. Ganta S, Devalapally H, Shahiwala A, Amiji M. A review of stimuli-responsive nanocarriers for drug and gene delivery. J Controlled Release. (2008) 126:187–204. doi: 10.1016/j.jconrel.2007.12.017
141. Moghimi SM, Parhamifar L, Ahmadvand D, Wibroe PP, Andresen TL, Farhangrazi ZS. Hunter AC. Particulate systems for targeting of macrophages: basic and therapeutic concepts. J Innate Immun. (2012) 4:509–28. doi: 10.1159/000339153
142. Sundar S, Chakravarty J, Agarwal D, Rai M, Murray HW. Single-dose liposomal amphotericin B for visceral leishmaniasis in India. N Engl J Med. (2010) 362:504–12. doi: 10.1056/NEJMoa0903627
143. Berman J. Amphotericin B formulations and other drugs for visceral leishmaniasis. Am J Trop Med Hyg. (2015) 92:471–3. doi: 10.4269/ajtmh.14-0743
144. Van Bocxlaer K, Croft SL. Pharmacokinetics and pharmacodynamics in the treatment of cutaneous leishmaniasis – challenges and opportunities. RSC Med Chem. (2021) 12:472–82. doi: 10.1039/D0MD00343C
145. Guery R, Henry B, Martin-Blondel G, Rouzaud C, Cordoliani F, Harms G, et al. Liposomal amphotericin B in travelers with cutaneous and muco-cutaneous leishmaniasis: Not a panacea. PLoS Negl Trop Dis. (2017) 11(11):e0006094. doi: 10.1371/journal.pntd.0006094
146. Wijnant G-J, Van Bocxlaer K, Yardley V, Harris A, Alavijeh M, Silva-Pedrosa R, et al. Comparative efficacy, toxicity and biodistribution of the liposomal amphotericin B formulations Fungisome® and AmBisome® in murine cutaneous leishmaniasis. Int J Parasitol Drugs Drug Resist. (2018) 8:223–8. doi: 10.1016/j.ijpddr.2018.04.001
147. Frézard F, Aguiar MMG, Ferreira LAM, Ramos GS, Santos TT, Borges GSM, et al. Liposomal amphotericin B for treatment of leishmaniasis: from the identification of critical physicochemical attributes to the design of effective topical and oral formulations. Pharmaceutics. (2022) 15(1). doi: 10.3390/pharmaceutics15010099
148. Lodhia J, Mandarano G, Ferris N, Eu P, Cowell S. Development and use of iron oxide nanoparticles (Part 1): Synthesis of iron oxide nanoparticles for MRI. BioMed Imaging Interv J. (2010) 6(2):e12. doi: 10.2349/biij.6.2.e12
149. Ajinkya N, Yu X, Kaithal P, Luo H, Somani P, Ramakrishna S. Magnetic iron oxide nanoparticle (IONP) synthesis to applications: present and future. Materials (Basel). (2020) 13(20). doi: 10.3390/ma13204644
150. Tao Z, Zhou Q, Zheng T, Mo F, Ouyang S. Iron oxide nanoparticles in the soil environment: Adsorption, transformation, and environmental risk. J Hazard Mater. (2023) 459:1–17. doi: 10.1016/j.jhazmat.2023.132107
151. Palzer J, Eckstein L, Slabu I, Reisen O, Neumann UP, Roeth AA. Iron oxide nanoparticle-based hyperthermia as a treatment option in various gastrointestinal Malignancies. Nanomaterials (Basel). (2021) 11(11). doi: 10.3390/nano11113013
152. Alphandéry E. Biodistribution and targeting properties of iron oxide nanoparticles for treatments of cancer and iron anemia disease. Nanotoxicology. (2019) 13:573–96. doi: 10.1080/17435390.2019.1572809
153. Nowak-Jary J, Machnicka B. In vivo biodistribution and clearance of magnetic iron oxide nanoparticles for medical applications. Int J Nanomed. (2023) 18:4067–100. doi: 10.2147/IJN.S415063
154. Kumar M, Kulkarni P, Liu S, Chemuturi N, Shah DK. Nanoparticle biodistribution coefficients: A quantitative approach for understanding the tissue distribution of nanoparticles. Adv Drug Deliv Rev. (2023) 194:114708. doi: 10.1016/j.addr.2023.114708
155. Janik-Olchawa N, Drozdz A, Ryszawy D, Pudełek M, Planeta K, Setkowicz Z, et al. Comparison of ultrasmall IONPs and Fe salts biocompatibility and activity in multi-cellular in vitro models. Sci Rep. (2020) 10(1):15447. doi: 10.1038/s41598-020-72414-8
156. Sayadi MH, Mansouri B, Shahri E, Tyler CR, Shekari H, Kharkan J. Exposure effects of iron oxide nanoparticles and iron salts in blackfish (Capoeta fusca): Acute toxicity, bioaccumulation, depuration, and tissue histopathology. Chemosphere. (2020) 247:125900. doi: 10.1016/j.chemosphere.2020.125900
157. Mulens-Arias V, Rojas JM, Barber DF. The use of iron oxide nanoparticles to reprogram macrophage responses and the immunological tumor microenvironment. Front Immunol. (2021) 12:693709. doi: 10.3389/fimmu.2021.693709
158. Zanganeh S, Hutter G, Spitler R, Lenkov O, Mahmoudi M, Shaw A, et al. Iron oxide nanoparticles inhibit tumor growth by inducing pro-inflammatory macrophage polarization in tumor tissues. Nat Nanotechnol. (2016) 11:986–94. doi: 10.1038/nnano.2016.168
159. zhao J, Zhang Z, Xue Y, Wang G, Cheng Y, Pan Y, et al. Anti-tumor macrophages activated by ferumoxytol combined or surface-functionalized with the TLR3 agonist poly (I : C) promote melanoma regression. Theranostics. (2018) 8:6307–21. doi: 10.7150/thno.29746
160. Wang G, Zhao J, Zhang M, Wang Q, Chen B, Hou Y, et al. Ferumoxytol and CpG oligodeoxynucleotide 2395 synergistically enhance antitumor activity of macrophages against NSCLC with EGFRL858R/T790M mutation. Int J Nanomed. (2019) 14:4503–15. doi: 10.2147/IJN.S193583
161. Zheng Y, Jiang B, Guo H, Zhang Z, Chen B, Zhang Z, et al. The combinational nano-immunotherapy of ferumoxytol and poly(I:C) inhibits melanoma via boosting anti-angiogenic immunity. Nanomedicine. (2023) 49:102658. doi: 10.1016/j.nano.2023.102658
162. Trujillo-Alonso V, Pratt EC, Zong H, Lara-Martinez A, Kaittanis C, Rabie MO, et al. FDA-approved ferumoxytol displays anti-leukaemia efficacy against cells with low ferroportin levels. Nat Nanotechnol. (2019) 14:616–22. doi: 10.1038/s41565-019-0406-1
163. Zhou Y, Que K-T, Tang H-M, Zhang P, Fu Q-M, Liu Z-J. Anti-CD206 antibody-conjugated Fe3O4-based PLGA nanoparticles selectively promote tumor-associated macrophages to polarize to the pro-inflammatory subtype. Oncol Lett. (2020) 20(6):298. doi: 10.3892/ol.2020.12161
164. Costa da Silva M, Breckwoldt MO, Vinchi F, Correia MP, Stojanovic A, Thielmann CM, et al. Iron induces anti-tumor activity in tumor-associated macrophages. Front Immunol. (2017) 8:1479. doi: 10.3389/fimmu.2017.01479
165. Wu X, Cheng Y, Zheng R, Xu K, Yan J, Song P, et al. Immunomodulation of tumor microenvironment by arginine-loaded iron oxide nanoparticles for gaseous immunotherapy. ACS Appl Mater Interfaces. (2021) 13:19825–35. doi: 10.1021/acsami.1c04638
166. Li CX, Zhang Y, Dong X, Zhang L, Liu MD, Li B, et al. Artificially reprogrammed macrophages as tumor-tropic immunosuppression-resistant biologics to realize therapeutics production and immune activation. Adv Mater. (2019) 31(15):e1807211. doi: 10.1002/adma.201807211
167. Vu HD, Huynh PT, Ryu J, Kang UR, Youn SW, Kim H, et al. Melittin-loaded iron oxide nanoparticles prevent intracranial arterial dolichoectasia development through inhibition of macrophage-mediated inflammation. Int J Biol Sci. (2021) 17:3818–36. doi: 10.7150/ijbs.60588
168. Guo W, Wu X, Wei W, Wang Y, Dai H. Mesoporous hollow Fe 3 O 4 nanoparticles regulate the behavior of neuro-associated cells through induction of macrophage polarization in an alternating magnetic field. J Mater Chem B. (2022) 10:5633–43. doi: 10.1039/D2TB00527A
169. Portilla Y, Mulens-Arias V, Daviu N, Paradela A, Pérez-Yagüe S, Barber DF. Interaction of iron oxide nanoparticles with macrophages is influenced distinctly by “Self” and “Non-self” Biological identities. ACS Appl Mater Interfaces. (2023) 15:35906–26. doi: 10.1021/acsami.3c05555
170. Chen Y, Zeng Z, Ying H, Wu C, Chen S. Superparamagnetic iron oxide nanoparticles attenuate lipopolysaccharide-induced inflammatory responses through modulation of toll-like receptor 4 expression. J Appl Toxicol. (2020) 40:1067–75. doi: 10.1002/jat.3967
171. Dalzon B, Torres A, Reymond S, Gallet B, Saint-Antonin F, Collin-Faure V. Influences of nanoparticles characteristics on the cellular responses: the example of iron oxide and macrophages. Nanomaterials (Basel). (2020) 10(2). doi: 10.3390/nano10020266
172. Kodali V, Littke MH, Tilton SC, Teeguarden JG, Shi L, Frevert CW, et al. Dysregulation of macrophage activation profiles by engineered nanoparticles. ACS Nano. (2013) 7:6997–7010. doi: 10.1021/nn402145t
173. Dias AMM, Courteau A, Bellaye P-S, Kohli E, Oudot A, Doulain P-E, et al. Superparamagnetic iron oxide nanoparticles for immunotherapy of cancers through macrophages and magnetic hyperthermia. Pharmaceutics. (2022) 14(11). doi: 10.3390/pharmaceutics14112388
174. Jin R, Liu L, Zhu W, Li D, Yang L, Duan J, et al. Iron oxide nanoparticles promote macrophage autophagy and inflammatory response through activation of toll-like Receptor-4 signaling. Biomaterials. (2019) 203:23–30. doi: 10.1016/j.biomaterials.2019.02.026
175. Deng D, Fu S, Cai Z, Fu X, Jin R, Ai H. Surface carboxylation of iron oxide nanoparticles brings reduced macrophage inflammatory response through inhibiting macrophage autophagy. Regen Biomater. (2022) 9:rbac018. doi: 10.1093/rb/rbac018
176. Wolf-Grosse S, Mollnes TE, Ali S, Stenvik J, Nilsen AM. Iron oxide nanoparticles enhance Toll-like receptor-induced cytokines in a particle size- and actin-dependent manner in human blood. Nanomedicine. (2018) 13:1773–85. doi: 10.2217/nnm-2017-0362
177. Vasilichin VA, Tsymbal SA, Fakhardo AF, Anastasova EI, Marchenko AS, Shtil AA, et al. Effects of metal oxide nanoparticles on toll-like receptor mRNAs in human monocytes. Nanomaterials (Basel). (2020) 10(1). doi: 10.3390/nano10010127
178. Zhong S, Xu J, Li P, Tsukamoto H. Caveosomal oxidative stress causes Src-p21 activation and lysine 63 TRAF6 protein polyubiquitination in iron-induced M1 hepatic macrophage activation. J Biol Chem. (2012) 287:32078–84. doi: 10.1074/jbc.M112.377358
179. Gu Z, Liu T, Tang J, Yang Y, Song H, Tuong ZK, et al. Mechanism of iron oxide-induced macrophage activation: the impact of composition and the underlying signaling pathway. J Am Chem Soc. (2019) 141:6122–6. doi: 10.1021/jacs.8b10904
180. Su Y, Yang F, Chen L, Cheung PCK. Mushroom carboxymethylated β- d -glucan functions as a macrophage-targeting carrier for iron oxide nanoparticles and an inducer of proinflammatory macrophage polarization for immunotherapy. J Agric Food Chem. (2022) 70:7110–21. doi: 10.1021/acs.jafc.2c01710
181. Duan T, Du Y, Xing C, Wang HY, Wang R-F. Toll-like receptor signaling and its role in cell-mediated immunity. Front Immunol. (2022) 13:812774. doi: 10.3389/fimmu.2022.812774
182. Mulens-Arias V, Rojas JM, Pérez-Yagüe S, Morales MP, Barber DF. Polyethylenimine-coated SPIONs trigger macrophage activation through TLR-4 signaling and ROS production and modulate podosome dynamics. Biomaterials. (2015) 52:494–506. doi: 10.1016/j.biomaterials.2015.02.068
183. Lunov O, Syrovets T, Büchele B, Jiang X, Röcker C, Tron K, et al. The effect of carboxydextran-coated superparamagnetic iron oxide nanoparticles on c-Jun N-terminal kinase-mediated apoptosis in human macrophages. Biomaterials. (2010) 31:5063–71. doi: 10.1016/j.biomaterials.2010.03.023
184. Wu Q, Miao T, Feng T, Yang C, Guo Y, Li H. Dextran-coated superparamagnetic iron oxide nanoparticles activate the MAPK pathway in human primary monocyte cells. Mol Med Rep. (2018) 18(1):564–70. doi: 10.3892/mmr.2018.8972
185. Rojas JM, Sanz-Ortega L, Mulens-Arias V, Gutiérrez L, Pérez-Yagüe S, Barber DF. Superparamagnetic iron oxide nanoparticle uptake alters M2 macrophage phenotype, iron metabolism, migration and invasion. Nanomedicine. (2016) 12:1127–38. doi: 10.1016/j.nano.2015.11.020
186. Murray AR, Kisin E, Inman A, Young S-H, Muhammed M, Burks T, et al. Oxidative stress and dermal toxicity of iron oxide nanoparticles in vitro. Cell Biochem Biophys. (2013) 67:461–76. doi: 10.1007/s12013-012-9367-9
187. Arsianti M, Lim M, Marquis CP, Amal R. Polyethylenimine based magnetic iron-oxide vector: the effect of vector component assembly on cellular entry mechanism, intracellular localization, and cellular viability. Biomacromolecules. (2010) 11:2521–31. doi: 10.1021/bm100748p
188. Gu J, Xu H, Han Y, Dai W, Hao W, Wang C, et al. The internalization pathway, metabolic fate and biological effect of superparamagnetic iron oxide nanoparticles in the macrophage-like RAW264.7 cell. Sci China Life Sci. (2011) 54:793–805. doi: 10.1007/s11427-011-4215-5
189. Lee SH, Park DJ, Yun WS, Park J-E, Choi JS, Key J, et al. Endocytic trafficking of polymeric clustered superparamagnetic iron oxide nanoparticles in mesenchymal stem cells. J Controlled Release. (2020) 326:408–18. doi: 10.1016/j.jconrel.2020.07.032
190. Arbab AS, Wilson LB, Ashari P, Jordan EK, Lewis BK, Frank JA. A model of lysosomal metabolism of dextran coated superparamagnetic iron oxide (SPIO) nanoparticles: implications for cellular magnetic resonance imaging. NMR BioMed. (2005) 18:383–9. doi: 10.1002/nbm.970
191. Ying H, Ruan Y, Zeng Z, Bai Y, Xu J, Chen S. Iron oxide nanoparticles size-dependently activate mouse primary macrophages via oxidative stress and endoplasmic reticulum stress. Int Immunopharmacol. (2022) 105:108533. doi: 10.1016/j.intimp.2022.108533
192. Yu B, Wang Z, Almutairi L, Huang S, Kim M-H. Harnessing iron-oxide nanoparticles towards the improved bactericidal activity of macrophage against Staphylococcus aureus. Nanomedicine. (2020) 24:102158. doi: 10.1016/j.nano.2020.102158
193. Wu C, Shen Z, Lu Y, Sun F, Shi H. p53 promotes ferroptosis in macrophages treated with Fe 3 O 4 nanoparticles. ACS Appl Mater Interfaces. (2022) 14:42791–803. doi: 10.1021/acsami.2c00707
194. Liu Y, Gu W. p53 in ferroptosis regulation: the new weapon for the old guardian. Cell Death Differ. (2022) 29:895–910. doi: 10.1038/s41418-022-00943-y
195. Bisti S, Konidou G, Boelaert J, Lebastard M, Soteriadou K. The prevention of the growth of Leishmania major progeny in BALB/c iron-loaded mice: a process coupled to increased oxidative burst, the amplitude and duration of which depend on initial parasite developmental stage and dose. Microbes Infect. (2006) 8:1464–72. doi: 10.1016/j.micinf.2006.01.014
196. Bisti S, Soteriadou K. Is the reactive oxygen species-dependent-NF-kappaB activation observed in iron-loaded BALB/c mice a key process preventing growth of Leishmania major progeny and tissue-damage? Microbes Infect. (2006) 8:1473–82. doi: 10.1016/j.micinf.2006.01.004
197. Kannan S, Harel Y, Levy E, Dolitzky A, Sagiv AE, Aryal S, et al. Nano-Leish-IL: A novel iron oxide-based nanocomposite drug platform for effective treatment of cutaneous leishmaniasis. J Controlled Release. (2021) 335:203–15. doi: 10.1016/j.jconrel.2021.05.019
198. Berry SL, Walker K, Hoskins C, Telling ND, Price HP. Nanoparticle-mediated magnetic hyperthermia is an effective method for killing the human-infective protozoan parasite Leishmania mexicana in vitro. Sci Rep. (2019) 9(1):1059. doi: 10.1038/s41598-018-37670-9
199. Albalawi AE, Khalaf AK, Alyousif MS, Alanazi AD, Baharvand P, Shakibaie M, et al. Fe3O4@piroctone olamine magnetic nanoparticles: Synthesize and therapeutic potential in cutaneous leishmaniasis. Biomed Pharmacother. (2021) 139:111566. doi: 10.1016/j.biopha.2021.111566
200. Islam A, Ain Q, Munawar A, Corrêa Junior JD, Khan A, Ahmad F, et al. Reactive oxygen species generating photosynthesized ferromagnetic iron oxide nanorods as promising antileishmanial agent. Nanomedicine (Lond). (2020) 15:755–71. doi: 10.2217/nnm-2019-0095
201. Verçoza BRF, Bernardo RR, de Oliveira LAS, Rodrigues JCF. Green SPIONs as a novel highly selective treatment for leishmaniasis: an in vitro study against Leishmania amazonensis intracellular amastigotes. Beilstein J Nanotechnol. (2023) 14:893–903. doi: 10.3762/bjnano.14.73
202. Abazari R, Mahjoub AR, Molaie S, Ghaffarifar F, Ghasemi E, Slawin AMZ, et al. The effect of different parameters under ultrasound irradiation for synthesis of new nanostructured Fe3O4@bio-MOF as an efficient anti-leishmanial in vitro and in vivo conditions. Ultrason Sonochem. (2018) 43:248–61. doi: 10.1016/j.ultsonch.2018.01.022
203. Musazzi UM, Santini B, Selmin F, Marini V, Corsi F, Allevi R, et al. Impact of semi-solid formulations on skin penetration of iron oxide nanoparticles. J Nanobiotechnol. (2017) 15(1):14. doi: 10.1186/s12951-017-0249-6
204. Baroli B, Ennas MG, Loffredo F, Isola M, Pinna R, Arturo López-Quintela M. Penetration of metallic nanoparticles in human full-thickness skin. J Invest Dermatol. (2007) 127:1701–12. doi: 10.1038/sj.jid.5700733
205. Jung E, Hui X, Zhu H, Zhang A, Wang W, Buchholz B, et al. Effect of iron and silica nanoparticles’ size on in vitro human skin binding and penetration. Toxicol Res Appl. (2019) 3. doi: 10.1177/2397847319893054
206. Lee SE, Choi KJ, Menon GK, Kim HJ, Choi EH, Ahn SK, et al. Penetration pathways induced by low-frequency sonophoresis with physical and chemical enhancers: iron oxide nanoparticles versus lanthanum nitrates. J Invest Dermatol. (2010) 130:1063–72. doi: 10.1038/jid.2009.361
207. Espuelas S, Schwartz J, Moreno E. Nanoparticles in the topical treatment of cutaneous leishmaniasis. In: Nanoscience in Dermatology. Elsevier (2016). p. 135–55. doi: 10.1016/B978-0-12-802926-8.00011-2
208. Khatami M, Alijani H, Sharifi I, Sharifi F, Pourseyedi S, Kharazi S, et al. Leishmanicidal activity of biogenic Fe3O4 Nanoparticles. Sci Pharm. (2017) 85(4). doi: 10.3390/scipharm85040036
209. Kumar R, Pandey K, Sahoo GC, Das S, Das V, Topno RK, et al. Development of high efficacy peptide coated iron oxide nanoparticles encapsulated amphotericin B drug delivery system against visceral leishmaniasis. Mater Sci Eng C Mater Biol Appl. (2017) 75:1465–71. doi: 10.1016/j.msec.2017.02.145
210. Nylén S, Eidsmo L. Tissue damage and immunity in cutaneous leishmaniasis. Parasite Immunol. (2012) 34:551–61. doi: 10.1111/pim.12007
211. Hartley M-A, Kohl K, Ronet C, Fasel N. The therapeutic potential of immune cross-talk in leishmaniasis. Clin Microbiol Infect. (2013) 19:119–30. doi: 10.1111/1469-0691.12095
212. Kumar R, Chauhan SB, Ng SS, Sundar S, Engwerda CR. Immune checkpoint targets for host-directed therapy to prevent and treat leishmaniasis. Front Immunol. (2017) 8:1492. doi: 10.3389/fimmu.2017.01492
213. Ikeogu NM, Akaluka GN, Edechi CA, Salako ES, Onyilagha C, Barazandeh AF, et al. Leishmania immunity: advancing immunotherapy and vaccine development. Microorganisms. (2020) 8(8). doi: 10.3390/microorganisms8081201
214. Novais FO, Amorim CF, Scott P. Host-directed therapies for cutaneous leishmaniasis. Front Immunol. (2021) 12:660183. doi: 10.3389/fimmu.2021.660183
215. Bi J, Mo C, Li S, Huang M, Lin Y, Yuan P, et al. Immunotoxicity of metal and metal oxide nanoparticles: from toxic mechanisms to metabolism and outcomes. Biomater Sci. (2023) 11:4151–83. doi: 10.1039/D3BM00271C
216. Shah A, Dobrovolskaia MA. Immunological effects of iron oxide nanoparticles and iron-based complex drug formulations: Therapeutic benefits, toxicity, mechanistic insights, and translational considerations. Nanomedicine. (2018) 14:977–90. doi: 10.1016/j.nano.2018.01.014
Keywords: leishmania, iron oxide nanoparticles, macrophages, host-directed therapies, target, targeted delivery
Citation: Palomino-Cano C, Moreno E, Irache JM and Espuelas S (2024) Targeting and activation of macrophages in leishmaniasis. A focus on iron oxide nanoparticles. Front. Immunol. 15:1437430. doi: 10.3389/fimmu.2024.1437430
Received: 23 May 2024; Accepted: 16 July 2024;
Published: 15 August 2024.
Edited by:
Maria Carolina Accioly Brelaz de Castro, Federal University of Pernambuco, BrazilReviewed by:
Fátima Ribeiro-Dias, Universidade Federal de Goiás, BrazilJunaid Jawed, Presidency University, India
Copyright © 2024 Palomino-Cano, Moreno, Irache and Espuelas. This is an open-access article distributed under the terms of the Creative Commons Attribution License (CC BY). The use, distribution or reproduction in other forums is permitted, provided the original author(s) and the copyright owner(s) are credited and that the original publication in this journal is cited, in accordance with accepted academic practice. No use, distribution or reproduction is permitted which does not comply with these terms.
*Correspondence: Socorro Espuelas, c2VzcHVlbGFzQHVuYXYuZXM=