- 1Department of Biology, Lomonosov Moscow State University, Moscow, Russia
- 2Chokan Limited Liability Partnership, Almaty, Kazakhstan
The 90-kDa heat shock proteins (HSP90s) are molecular chaperones essential for folding, unfolding, degradation and activity of a wide range of client proteins. HSP90s and their cognate co-chaperones are subject to various post-translational modifications, functional consequences of which are not fully understood in cancer. Intracellular and extracellular HSP90 family members (HSP90α, HSP90β, GRP94 and TRAP1) promote cancer by sustaining various hallmarks of cancer, including cell death resistance, replicative immortality, tumor immunity, angiogenesis, invasion and metastasis. Given the importance of HSP90 in tumor progression, various inhibitors and HSP90-based vaccines were developed for the treatment of cancer. Further understanding of HSP90 functions in cancer may provide new opportunities and novel therapeutic strategies for the treatment of cancer.
1 Introduction
Heat shock protein 90 (HSP90) chaperone machinery plays a critical role in protein folding, unfolding, degradation and maturation processes (1, 2). HSP90 chaperones interact with a large and diverse group of client proteins, many of which are important regulators of tumorigenesis, immune suppression, invasion and metastasis (3). HSP90s are primarily located in cytosol, endoplasmic reticulum, and mitochondria (4), but also have been found in the extracellular space associated with tumor progression and unfavorable clinical outcome (5). Overexpression of HSP90s has been implicated in survival and proliferation of tumor cells (6), which was further supported by the finding that HSP90s are upregulated in response to apoptotic stimuli, such as UV, sodium arsenite and doxorubicin (6–8). In addition, Kruta et al. demonstrated that ex vivo culture stress and aging also induce heat shock response by activating heat shock factor -1 (HSF-1) (9–11).
HSP90 family is composed of several members, including cytosolic stress-inducible HSP90α/HSP90AA1 and constitutive HSP90β/HSP90AB1, mitochondrial HSP90 called tumor necrosis factor receptor-associated protein 1 (TRAP1) and HSP90 member in endoplasmic reticulum (ER) called glucose-regulated protein 94 (GRP94/HSP90B1/gp96/ERp99/Endoplasmin) (4, 12). Different HSP90 homologs have distinct intracellular functions. For example, GRP94 is primarily responsible for the unfolded protein response whereas TRAP1 is involved in mitochondrial bioenergetics [reviewed in (12)].
In this Review, we focus on the role of HSP90 chaperone machinery in sustaining various hallmarks of cancer and exploring the potential of HSP90 as anti-cancer therapeutic targets.
2 The HSP90 structure and conformational cycle
Each HSP90 monomer consists of amino-terminal domain (NTD) that is connected to a middle domain (MD) by a linker, and a C-terminal domain (CTD) (Figure 1) (13). In the absence of ATP, HSP90 mainly adopts an open V-shaped conformation (13). ATP binding leads to the conformational change in NTD involving closure of the lids, which is followed by the NTD dimerization and twisting of HSP90 monomers for the efficient ATP hydrolysis (closed conformation) (Figure 1) (13–16). Various co-chaperones assist HSP90 throughout conformational cycle (13). HSP70/HSP90-organizing protein (HOP), also known as stress-inducible phosphoprotein 1 (STIP1) and cell division cycle 37 homologue (CDC37) inhibit HSP90 structural changes, whereas activator of HSP90 ATPase homologue 1 (Aha1) accelerates the formation of closed ATP-bound conformation (13, 16). Prostaglandin E synthase 3 (PTGES3/p23) acts as a co-chaperone slowing the ATPase cycle by stabilizing the closed conformation that is committed to ATP hydrolysis (13, 17) (Figure 1).
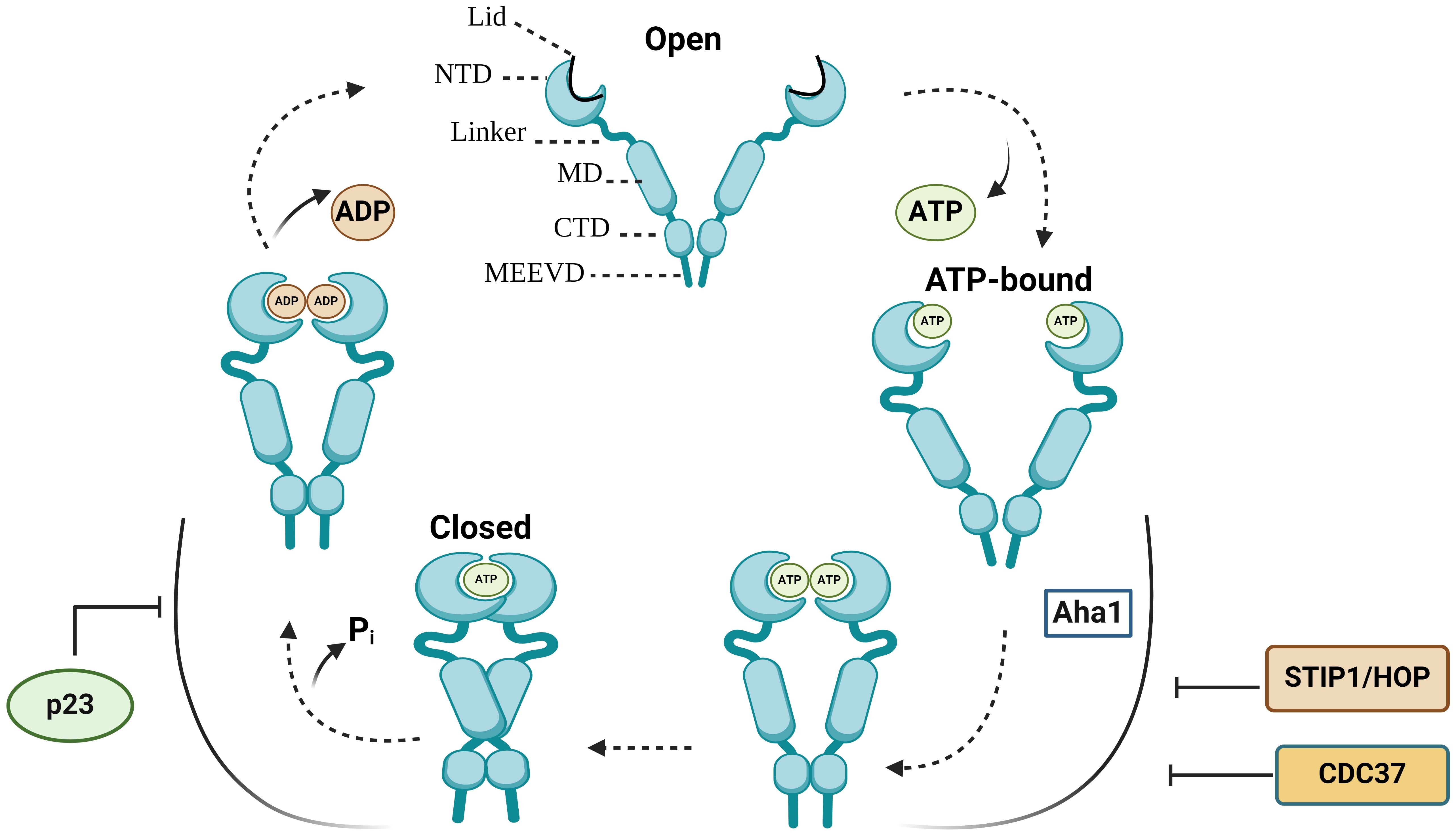
Figure 1 The HSP90 conformational cycle. HSP90 homodimer mainly adopts an open V-shaped conformation. ATP binding to the N-terminal domain (NTD) shifts HSP90 from an open conformation to a ‘closed and twisted’ conformation. Co-chaperones, such as p23, HSP70/HSP90-organizing protein (HOP/STIP1) and cell division cycle 37 homologue (CDC37) associate with specific HSP90 conformations. NTD, N-terminal domain; MD, middle domain; CTD, C-terminal domain; Pi, inorganic phosphate; p23, Prostaglandin E synthase 3.
3 HSP90 post-translational modifications
One of the main challenges in studying the function of HSP90 chaperone machinery in cancer is to understand the consequences of HSP90 and co-chaperone post-translational modifications (18). Indeed, HSP90s undergo various post-translational modifications, including phosphorylation, acetylation, oxidation, ubiquitination, SUMOylation, S-nitrosylation and methylation (18). Tyrosine phosphorylation was shown to increase HSP90 interaction with endothelial nitric oxide synthase and ionotropic P2X7 receptors (19). Double-stranded DNA protein kinase (20), B-Raf (21), Akt (22), c-Src kinase (23), protein kinase A (PKA) (24), CK2 protein kinase (25, 26) have been shown to phosphorylate HSP90s, however the functional consequences of HSP90 phosphorylation are not yet fully determined (18). Kurokawa and colleagues demonstrated that by contrast to untransformed cells the HSP90β phosphorylation at Ser 226/Ser 255 was not identified in leukemic cells (26). The functions of HSP90 are also impacted by co-chaperone post-translational modifications. Several investigators showed that PP5/Ppt1 dephosphorylates Cdc37, affecting its interaction with HSP90 and its protein kinase clients (27, 28).
The chaperone activity of HSP90 is also modulated by histone deacetylase 6 (HDAC6) (18, 29–31). HDAC inhibitor depsipeptide (Romidepsin) induced acetylation of HSP90 and destabilized HSP90 interaction with several clients, including ErbB2, Raf-1, and mutant p53 in in non-small cell lung cancer cells (32). Interestingly, HDAC6 deficiency also associated with the degradation of another HSP90 client, the hypoxia-inducible factor 1α (HIF-1α) (18, 33). Additionally, HDAC6 reduction increases the acetylation of FOXP3 and HSP90, enhancing suppressive functions of T regs (34, 35). Apart from HDAC6, other HDACs are also able to deacetylate HSP90. For example, HDAC1 has been shown to deacetylate HSP90 in human breast cancer cells (36), HDAC9 in T regs (34), while both HDAC6 and HDAC10 are involved in HSP90-mediated regulation of vascular endothelial growth factor receptors (37). Thiol oxidation of HSP90 and HSP70 associates with the degradation of HSP90 client proteins, such as Cdk4, Raf-1, Akt, mutant p53 and cyclin D1 (38). Oxidative stress also causes lipid peroxidation leading to the accumulation of reactive aldehydes which in turn affect HSP90 chaperone function (12, 18, 39). HSP90 has also been reported to be ubiquitinated by CHIP (12, 40), leading to the degradation of HSP90 clients (41). In addition, S-nitrosylation, SUMOylation and methylation also affect HSP90 chaperone activity (38, 42–44).
4 HSP90 secretion into the extracellular milieu
Elevated HSP90 level was detected in plasma/serum in patients with cancer, including liver cancer (45), advanced staged colorectal cancer (46, 47), lung cancer (48), acute myeloid leukemia (49), hepatocellular carcinoma (50). Extracellular HSP90s may affect other cells by modulating intercellular signaling when released via EVs (51). EVs play important roles in intercellular communication, regulating a range of biological processes. Given the ability of EVs to carry and transfer tumorigenic factors between cells, EVs have been explored as therapeutic targets, novel drug delivery vehicles, biomarkers and standalone therapeutics in cancer research (52). HSP90s and their co-chaperones have been found in EVs isolated from patients with melanoma (53–55), glioblastoma (56), pancreatic cancer (57), prostate cancer (58), bladder cancer (59), lung cancer (60) and papillary thyroid cancer (61) [reviewed in (62)]. Lauwers and colleagues demonstrated that HSP90 in Drosophila regulates the membrane deformation and exosome release (63). Subsequent study demonstrated that HSP90α is located on the surface of exosomes and the monoclonal antibody against HSP90α inhibits the pro-motility activity of tumor-secreted exosomes (64).
5 HSP90 functions in the hallmarks of cancer
Being abundantly expressed in cancer, HSP90s promote growth and survival of tumor cells by regulating a wide range of processes. Here, we will explore HSP90 involvement in the hallmarks of cancer – a model of multi-step cancer development established by Hanahan and Weinberg (65, 66) (Figure 2).
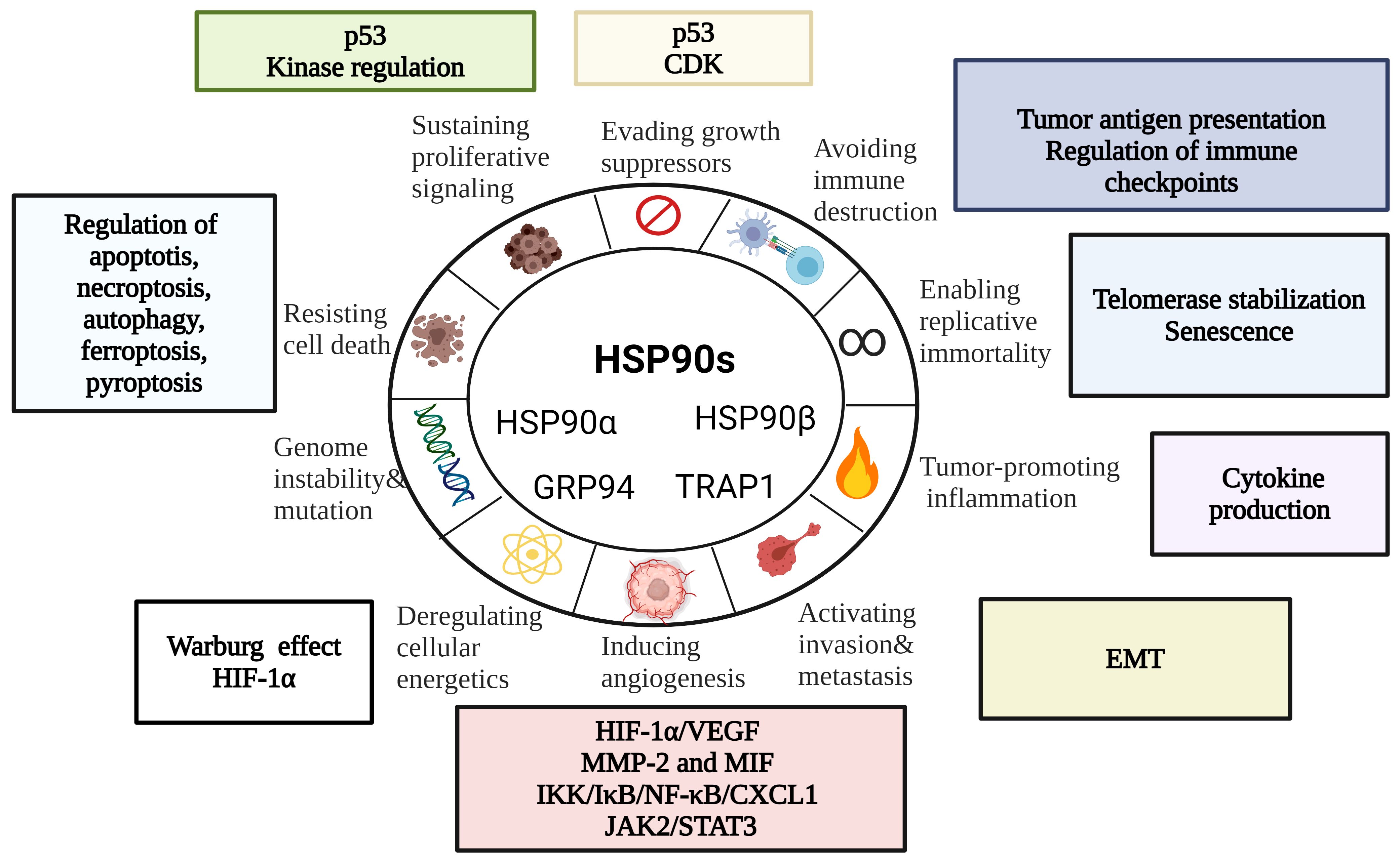
Figure 2 HSP90 in the Hallmarks of Cancer [modified from (67)]. HSP90s regulate cell death mechanisms, replicative immortality, tumor immunity, angiogenesis and metastasis. EMT, epithelial-mesenchymal transition; CDK, cyclin-dependent kinase; HIF-1α, hypoxia-inducible factor 1α; MIF, macrophage migration inhibitory factor; MMP-2, matrix metalloproteinase 2.
5.1 HSP90 and tumor immunity
In 1986 Ullrich and colleagues identified HSP90 as a highly abundant cytosolic and surface tumor-transplantation antigen in methylcholanthrene-induced tumors (Meth A) (68). At the same time Srivastava et al. isolated tumor rejection antigens from the membrane and cytosol fractions of Meth A and CMS5 which was later recognized as ER HSP90 homolog, glucose-regulated protein 94 (GRP94/HSP90B1/gp96/ERp99/Endoplasmin) (69, 70). HSP90s isolated from tumors have been shown to elicit potent anti-tumor response (3, 71–73). Mechanistically, tumor-isolated HSP90-peptide complexes interact with scavenger receptor expressed by endothelial cells (SREC-I) on APCs, leading to their cross-presentation via MHC class I or more standard MHC class II antigen presentation pathway (2, 74). This is also supported by the finding that downregulation of heat shock factor (HSF-1) or HSP90 associates with a defective cross-presentation by DCs (75). Furthermore, it has been shown that HSP90 inhibitor reduces the translocation of antigens into the cytosol whereas HSP90AA1 knockdown leads to a loss of proteolytic intermediates and reduced presentation of peptide-MHC I complexes on the cell surface (76, 77). Subsequent studies demonstrated that low-level inhibition of HSP90 diversifies the peptide MHC class I repertoire on tumor cells (78). HSP90 inhibitor also showed to decrease MHC II antigen presentation by IFNγ-treated APCs (79). Altogether, these data show that HSP90 is critical for MHC I and MHC II class antigen presentation.
Apart from antigen presentation, HSP90 is also critical for the phenotype and functional activity of immune cells. In this regard, Bae and colleagues demonstrated that HSP90 inhibitor downregulates CD3, CD8, CD25, CD28, CD40L and αβ on the surface of T cells and activating receptors, including CD2, CD11a, CD94, NKp30, NKp44, NKp46, KARp50.3 on NK cells (80). We and others show that HSP90 deficiency impairs NK and T cell proliferation, cytotoxicity and IFNγ production (80–83). By contrast, HSP90 ER homolog GRP94 stimulates NK cells indirectly via APCs (84). On DCs, GRP94 acts via Toll-like receptor 2 (TLR-2) and TLR-4 inducing the expression of CD86 and IL-12 and TNF-α production (85, 86). In T regs, GRP94 upregulates Foxp3, IL-10 and TGF-β1 via TLR-2/4-mediated NF-κB activation (87). Interaction of GRP94 with TLR is critical for the activation of cytotoxic T cells response (88). Additionally, GRP94 also induces NLRP3 inflammasome activation and IL-1β production in murine APCs via K+ efflux (89). HSP90α on the tumor-cell released autophagosomes (TRAPs) stimulate IL-6 release by CD4+ T cells via TLR2-MyD88-NF-κB pathway (90). Autocrine IL-6 further promotes the production of IL-10 and IL-21 by CD4+ T cells via STAT3, enhancing metastasis (90). It has also been shown that the production of HSP90α, IL-8 and IL-6 by macrophages induces JAK2-STAT3 pathway, supporting invasion and migration in pancreatic ductal epithelial cells (91). On the other hand, cytokines may also induce HSP90 expression, which further enhance their pro and anti-inflammatory activities (Figure 3) (92). Unlike HSP90AA1, HSP90AB1 and HSP90B1, TRAP1 could only be induced by IL-18 in NK cells and IL-3 in conventional DC2 (cDC2) cells (92). Collectively, these studies show that there is an important interplay between HSP90 and cytokines, which should be further explored in the context of cancer.
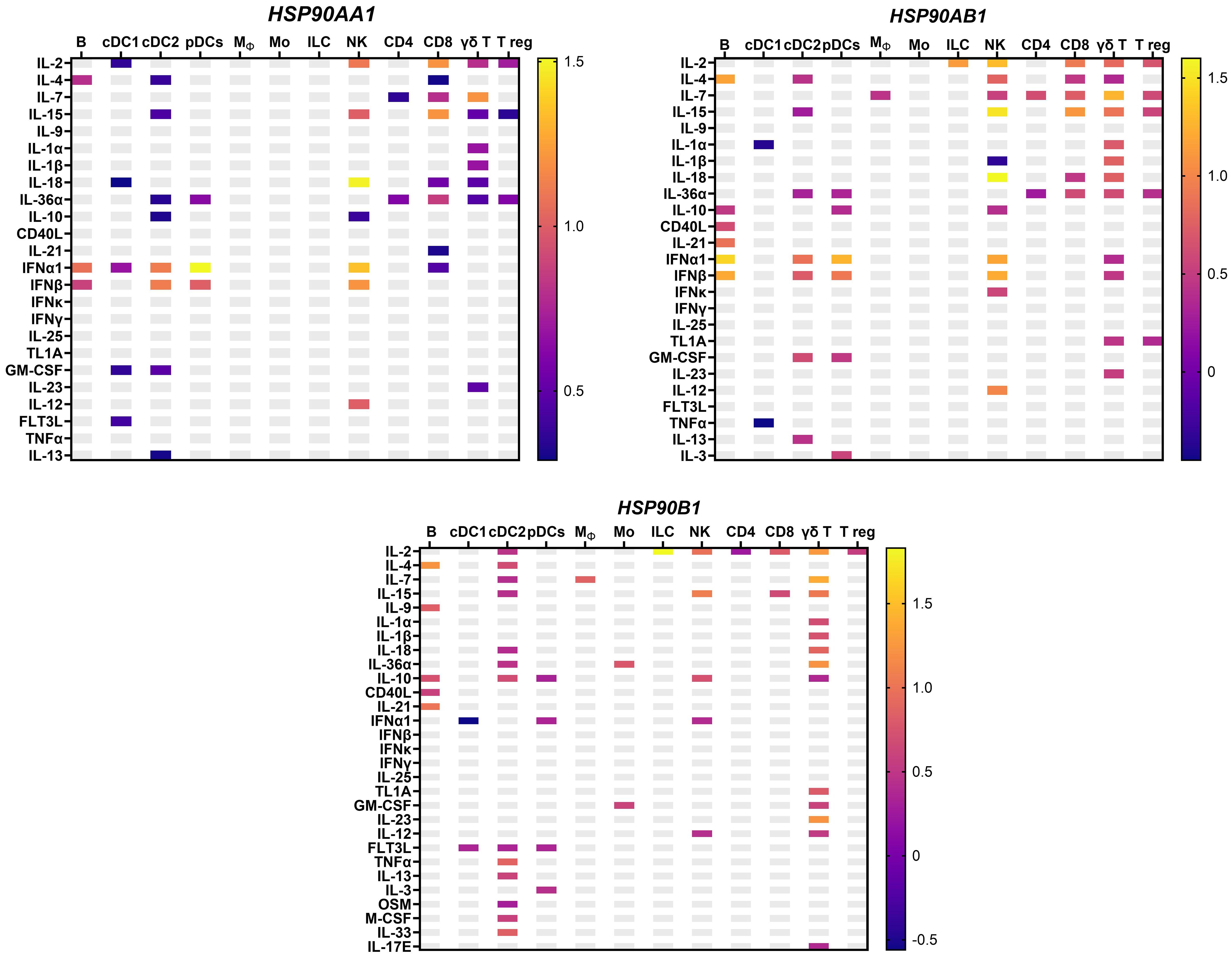
Figure 3 HSP90 gene expression in response to cytokines in murine lymph nodes in vivo from an independent dataset (92), with the mean log2 fold change. HSP90AA1 – cytoplasmic stress-inducible HSP90 homolog; HSP90AB1-cytoplasmic constitutive HSP90; HSP90B1 – ER-resident HSP90; Mϕ, macrophages; pDC, plasmacytoid dendritic cells; B, B cell; T reg, T regulatory cells; NK, natural killer cells; Mo, monocytes; ILC, innate lymphoid cells.
HSP90 family members also play important roles in the regulation of immune checkpoints. Zavareh and colleagues demonstrated that HSP90 inhibitors downregulate PD-L1 mRNA level and surface expression by suppressing HSP90 clients c-Myc and signal transducer and activator of transcription 3 (STAT3) (93). Another HSP90 client nucleophosmin/anaplastic lymphoma kinase (NPM/ALK) showed to induce PD-L1 via STAT3 activation in T cell lymphoma cells (94). It has been also shown that the spliced isoform of HSP90 co-chaperone FKBP51 regulates the expression of glycosylated PD-L1 in glioma cells (95). Combination of HSP90 inhibitor ganetespib and anti-CTLA-4 associated with an increase in the frequency of CD8+ T cells in mice and decrease in T regs (96). Mechanistically, HSP90 inhibitor upregulates interferon response genes, leading to T cell-mediated killing of melanoma cells (96).
Using mass spectrometry-based proteome profiling several studies showed that various types of immune cells, including NK, T, dendritic cells, platelets, and neutrophils can secrete HSP90s and their cognate co-chaperones in EVs (summarized in Figure 4) (62). Overexpression of HSP90 in hypoxic macrophage-derived exosomes inhibited Hippo signaling pathway, leading to colorectal cancer progression (102). Heat shock and anti-cancer drugs significantly upregulate exosomes release (103). Exosomes secreted by mouse B cell lymphoma cells after heat shock showed elevated expression of HSP90, HSP60 and MHC I, MHC II, CD40, CD86, RANTES and IL-1β (104, 105). These exosomes stimulate DC maturation and more potently induce CTL responses (104). It has also been shown that HSP-bearing exosomes secreted by human hepatocellular carcinoma cells stimulate NK cell cytotoxicity and granzyme B secretion (103). Triple deletion of CDC37, HSP90α and HSP90β diminished EV-driven malignancy progression and macrophage M2 polarization (106).
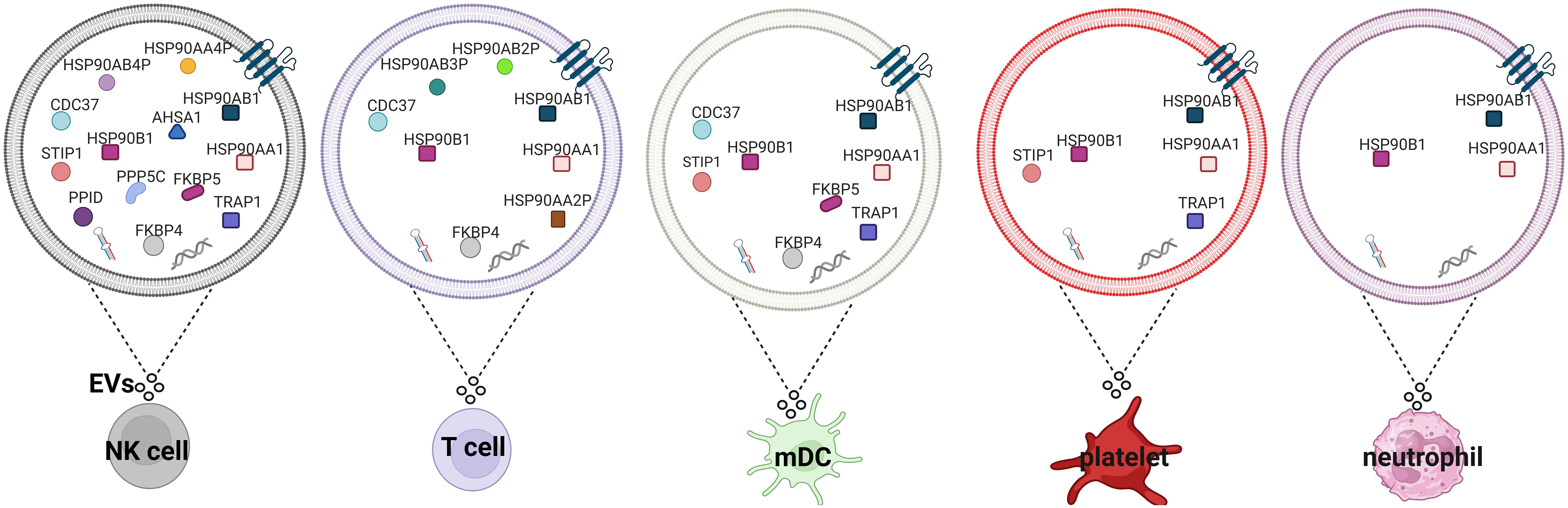
Figure 4 HSP90s and their co-chaperones in extracellular vesicles secreted by different types of immune cells. Extracellular vesicles secreted by NK cells (97), T cells (98), mDCs (99), platelets (100) and neutrophils (101) isolated from healthy donors. EVs, extracellular vesicles; mDCs, monocyte-derived dendritic cells.
5.2 HSP90 in tumor resistance to cell death
HSP90 regulates both intrinsic and extrinsic apoptotic pathways. In intrinsic pathway, HSP90 is implicated in the conformational change of Bax and the release of cytochrome c (107, 108). Moreover, HSP90 also interacts with Apaf-1, inhibiting pro-caspase-9 and pro-caspase-3 activation (6). HSP90 inhibition downregulates STAT3, survivin, cyclin D1 and upregulates cytochrome c, caspase-9 and caspase-3 (109). Results also showed that TRAP1 inhibitor gamitrinib containing triphenylphosphine induces cyclophilin D-dependent mitochondrial permeability transition in tumor cells, leading to apoptosis (108, 110, 111). In extrinsic pathway, FLICE-like inhibitory proteins (c-FLIP) is required for inhibiting apoptosis at the death inducing signaling complex (DISC) (108, 112). HSP90 inhibitors induced c-FLIPL degradation in human lung cancer cells mediated by C-terminus of HSP70-interacting protein (CHIP) (112).
HSP90 is also involved in the modulation of another form of regulated cell death necroptosis (108, 113). Jacobsen and co-workers demonstrated that HSP90 inhibitors block necroptosis by downregulating MLKL expression and membrane translocation (113). Several studies reported that HSP90 inhibitors impact RIP1 stability and function (114–117). A complex consisting of HSP90 and CDC37 is required for RIP3 activation during necroptosis (118).
Apart from apoptosis and necroptosis, HSP90 is implicated in autophagy. HSP90 is essential for the lysosome-associated membrane protein type 2A (LAMP-2A) stability (119). Moreover, HSP90 inhibition leads to the IκB kinase (IKK) degradation by autophagy while Atg5 or autophagy inhibition can reverse IKK degradation, suggesting that there is a molecular link between HSP90, NF-κB and autophagy (108, 120). In addition, HSP90/CDC37 stabilizes and activates ULK1, which is required for Atg13 phosphorylation and release. Subsequently, Atg13 is recruited to damaged mitochondria for efficient clearance (121). HSP90 inhibition downregulates Atg7 and upregulates caspase 9 in KRAS- mutant non-small cell lung cancer cells (122). HSP90 inhibition also leads to Beclin 1 proteasomal degradation, suppressing TLR3- and TLR4-mediated autophagy (123).
In addition, HSP90 is involved in ferroptosis facilitating the degradation of glutathione peroxidase 4 (GPX4) by chaperone-mediated autophagy (117, 124). It is interesting to note that HSP90 inhibitor 2-amino-5-chloro-N,3-dimethylbenzamide (CDDO) can block both necroptosis and ferroptosis, suggesting that HSP90 may be a common regulatory mechanism in necroptosis and ferroptosis (117). HSP90 is also implicated in pyroptosis by regulating priming and activation of NLRP3 inflammasome and subsequent IL-1β production (125–127).
5.3 HSP90 in sustained proliferation
Recent studies have reported that HSP90 regulates the activity of tumor suppressor p53 by interacting with its DNA binding domain (128). HSP90 stabilizes mutant p53 in cancer cells leading to uncontrolled proliferation of tumor cells (129, 130). HSP90 also stabilizes the epidermal growth factor receptor (EGFR) in tumor cells (129). HSP90 inhibition decreases total and phosphorylated EGFR and suppresses the proliferation of resistant cancer cells (131). In addition, HSP90 activity is essential for ErbB2/HER, v-Src, c-Src, BCR-ABL, Raf1, and other kinases which are known to promote proliferation and survival of cancer cells (132).
5.4 HSP90 in the deregulation of cellular energetics
HSP90 homolog TRAP1 is a critical regulator of mitochondrial bioenergetics (12). TRAP1 interacts and suppresses the activity of succinate dehydrogenase (SDH), promoting Warburg phenotype (133). Results also showed that TRAP1 decreases cell oxygen consumption rate and OXPHOS-dependent ATP synthesis (133). Furthermore, TRAP1 deficiency enhances mitochondrial respiration and inhibits glycolysis (134). These TRAP1-deficient cells also express increased levels of ATP, ROS and cytochrome c oxidase (complex IV) (134). Mitochondrial HSP90 homolog TRAP1, but not cytosolic HSP90, binds and stabilizes succinate dehydrogenase-B (SDHB) contributing to HIF-1α-mediated cancer progression in patients carrying SDHB mutations (135).
5.5 HSP90 in replicative immortality
Holt and colleagues demonstrated that HSP90 and its co-chaperone p23 associate with human telomerase reverse transcriptase and are required for efficient assembly of functional telomerase (136). HSP90 inhibitor geldanamycin inhibited the assembly of active telomerase in vitro and in vivo (136). Further biochemical studies demonstrated that HSP90 is critical for hTERT folding and stabilization of the assembled telomerase complex (137). HSP90 is also important for the maintenance of telomere length as overexpression of HSP90 associates with telomere shortening (138). In addition, HSP90 promotes telomerase DNA binding (139). Telomere dysfunction may also induce senescence (140). Indeed, Zhong and colleagues demonstrated that an increase in extracellular HSP90α promotes fibroblast senescence by activating TGFβ (141). HSP90 inhibitors downregulate phosphorylated form of AKT, leading to apoptosis of senescent cells (142). These data suggest that HSP90 favors tumor growth by modulating telomerase and senescence.
5.6 HSP90 in angiogenesis
Song and colleagues reported that HSP90α promotes angiogenesis via stabilizing activated matrix metalloproteinase 2 (MMP-2) (143). Further studies showed that HSP90 also stabilizes macrophage migration inhibitory factor (MIF), which acts as an angiogenesis promoting factor during neoplastic transformation (144, 145). Dong et al. demonstrated that breast cancer cells secrete HSP90α to survive under hypoxia (146). HSP90 inhibitor AT-533 has been reported to inhibit growth and angiogenesis by suppressing the HIF-1α/VEGF pathway in hypoxic breast cancer cells (147). These cells also secrete a splice variant VEGF90K which binds HSP90 on the surface of microvesicles further promoting angiogenesis (148). HSP90/phosphorylated IKK-rich extracellular vesicles from hypoxic melanoma activate pro-angiogenic melanoma-associated fibroblasts (MAFs) via the NF-κB/CXCL1 axis (149). Furthermore, C-terminal HSP90 inhibitor SL-145 has been shown to inhibit growth and angiogenesis by dysregulating JAK2/STAT3 signaling pathway in triple negative breast cancer cells (150).
5.7 HSP90 in invasion and metastasis
Extracellular HSP90 interacts with LRP1 (also known as CD91) to induce ERK and MMP-2/9 activation, leading to E-cadherin inhibition and the initiation of EMT in prostate cancer cells (51, 151). Furthermore, extracellular HSP90 secreted by these cells upregulates the expression of stem-like markers, promoting self-renewal (152). HSP90 interaction with LRP1 leads to the increased expression of phosphorylated IKKα/β and NF-κB resulting in the induction of TCF12, which in turn decreases E-cadherin and promotes colorectal cancer EMT, migration and invasion (153). HSP90β also associates with LRP5, promoting EMT via Akt and Wnt/β-catenin signaling (12, 154). In metastatic breast cancer cells, HIF-1α downregulation inhibits HSP90α secretion and invasion (155). GRP94, an ER paralog of HSP90 may also promote invasion and metastasis via the regulation of its client GARP, which is critical for the membrane expression of TGFβ (156).
6 HSP90 therapies targeting cancer
6.1 HSP90 inhibitors in cancer clinical trials
Owing to the importance of HSP90 in cancer, it has become an attractive target for anti-cancer therapies. HSP90 inhibitors in clinical trials are summarized in Table 1. Several clinical trials assessed HSP90 inhibitor-linked to verteporfin (HS-201, NCT03906643) or near infrared red probe (HS-196, NCT03333031) for imaging and detection of solid tumors. Currently, there are no FDA-approved HSP90 inhibitors. The low effectiveness of HSP90 inhibitors in clinical trials may be attributed to drug-related toxicity and limited efficacy. Insufficient isoform selectivity has been considered as one of the main reasons for these failures.
6.2 HSP90 vaccines
The ability of HSP90-peptide complexes to activate both CD8+ and CD4 +T cells led to the development of HSP90-based vaccines (3, 157). Innovative approach was proposed by Yamazaki and colleagues who generated a secretory form of ER HSP90 where HSP90 ER (gp96) KDEL retention signal was deleted and replaced with the Fc portion of IgG1, thus imitating necrotic cell death release of HSPs (158). Immunization of mice with tumor cells secreting gp96-Ig resulted in tumor rejection in vivo which was primarily dependent on CD8+ T cells (158, 159). Gp96-Ig vaccine, also called Viagenpumatucel-L or HS-110 was further assessed in phase I (NCT00503568) and phase II (NCT02117024) clinical trials in patients with non-small cell lung carcinoma. Gp96-Ig was also assessed in combination with anti-PD-1inhibitor Nivolumab (NCT02439450) and has shown to be well-tolerated and improve overall survival of PD-L1+ patients with advanced lung cancer (160, 161).
Crane and colleagues prepared autologous gp96-peptide complexes to immunize patients with recurrent glioblastoma in phase I trial (162). Re-stimulation of peripheral blood leukocytes with autologous gp96 led to increase in IFNγ (162). Autologous gp96 prepared from resected tumors in combination with standard radiation and chemotherapy improved overall survival in glioblastoma patients with low expression of PD-L1+ on peripheral myeloid CD45+ CD11b+ cells (163). Interestingly, dendritic cells (DCs) pulsed with tumor-derived gp96 showed anti-tumor effect which was significantly dependent on NK and CD8 T cells (164). Multi-chaperone vaccine called “chaperone-rich cell lysate” (CRCL) contains several chaperones, including HSP70, HSP90, gp96 and calreticulin showed to activate DCs and upregulate the expression of CD40, MHC II, IL-12, CD70, iNOS and NF-κB and enhance the phosphorylation of STAT1,STAT5, ERK1/2 and AKT (165, 166). CRCL-stimulated DCs and macrophages resisted the suppressive activity of T regulatory cells (167). Notably, depletion of chaperones from CRCL led to the decrease in IFNγ production by splenocytes (165). Similar to T cells, CRCL has also been shown to stimulate IFNγ, TNFα, RANTES production and the activation of STAT1 and NF-κB by NK cells (168).
Immunization of mice with another multi-chaperone vaccine purified from the mouse sarcoma cell line S180 containing the mixture of HSP60, HSP70, HSP110 and gp96 (mHSP/peptide vaccine) in combination with cyclophosphamide and IL-12 suppressed tumor growth and improved long-term survival (169). Further studies have shown that mHSP/peptide vaccine containing HSP70, HSP90 and gp96 showed superior anti-tumor effect than gp96/peptide vaccine (170). PD-L1 inhibitor in combination with tumor-derived mHSP/peptide vaccine induced the section of IFNγ, TNFα, IL-10 and IL-2 on day 14th whereas on day 28th combinational treatment led to decrease production of IFNγ, IL-2 and IL-10 (170).
7 Conclusion
HSP90 molecular chaperones are abundantly expressed in cancer, leading to tumor growth and survival via the modulation of various hallmarks of cancer, including sustained proliferation, deregulation of cellular energetics, unlimited replicative potential, tumor immunity, angiogenesis, metastasis and invasion. Given HSP90 ability to promote growth and survival of tumor cells by regulating a wide range of processes and enabling hallmarks of cancer, various HSP90 inhibitors entered clinical trials. Based on the ability of HSP90 to elicit anti-tumor response, several HSP90-based immunotherapies were developed. Further elucidating the complex role of HSP90 in cancer may provide new opportunities for the diagnosis and treatment of cancer patients.
Author contributions
ZA: Writing – review & editing, Writing – original draft, Visualization, Resources, Project administration, Investigation, Conceptualization.
Funding
The author(s) declare that no financial support was received for the research, authorship, and/or publication of this article.
Acknowledgments
The Figures were created with Biorender.com.
Conflict of interest
ZA was employed by Chokan Limited Liability Partnership.
Publisher’s note
All claims expressed in this article are solely those of the authors and do not necessarily represent those of their affiliated organizations, or those of the publisher, the editors and the reviewers. Any product that may be evaluated in this article, or claim that may be made by its manufacturer, is not guaranteed or endorsed by the publisher.
References
1. Pearl LH, Prodromou C. Structure and mechanism of the hsp90 molecular chaperone machinery. Annu Rev Biochem. (2006) 75:271–94. doi: 10.1146/annurev.biochem.75.103004.142738
2. Murshid A, Gong J, Calderwood SK. Hsp90-peptide complexes stimulate antigen presentation through the class II pathway after binding scavenger receptor SREC-I. Immunobiology. (2014) 219:924–31. doi: 10.1016/j.imbio.2014.08.001
3. Graner MW. Chapter eight - HSP90 and immune modulation in cancer. In: Isaacs J, Whitesell L, editors. Advances in cancer research, vol 129. Academic Press (2016). p. 191–224. doi: 10.1016/bs.acr.2015.10.001
4. Kampinga HH, Hageman J, Vos MJ, Kubota H, Tanguay RM, Bruford EA, et al. Guidelines for the nomenclature of the human heat shock proteins. Cell Stress Chaperones. (2009) 14:105–11. doi: 10.1007/s12192-008-0068-7
5. Wong DS, Jay DG. Chapter six - emerging roles of extracellular hsp90 in cancer. In: Isaacs J, Whitesell L, editors. Advances in cancer research, vol 129. Academic Press (2016). p. 141–63. doi: 10.1016/bs.acr.2016.01.001
6. Pandey P, Saleh A, Nakazawa A, Kumar S, Srinivasula SM, Kumar V, et al. Negative regulation of cytochrome c-mediated oligomerization of Apaf-1 and activation of procaspase-9 by heat shock protein 90. EMBO J. (2000) 19:4310–22. doi: 10.1093/emboj/19.16.4310
7. Bertram J, Palfner K, Hiddemann W, Kneba M. Increase of P-glycoprotein-mediated drug resistance by hsp 90b. Anti-Cancer Drugs. (1996) 7:838–45. doi: 10.1097/00001813-199611000-00004
8. Ali A, Krone PH, Pearson DS, Heikkila JJ. Evaluation of stress-inducible hsp90 gene expression as a potential molecular biomarker in Xenopus laevis. Cell Stress Chaperones. (1996) 1:62–9. doi: 10.1379/1466-1268(1996)001<0062:eosihg>2.3.co;2
9. Kruta M, Sunshine MJ, Chua BA, Fu Y, Chawla A, Dillingham CH, et al. Hsf1 promotes hematopoietic stem cell fitness and proteostasis in response to ex vivo culture stress and aging. Cell Stem Cell. (2021) 28:1950–1965.e1956. doi: 10.1016/j.stem.2021.07.009
10. Prodromou C. Mechanisms of hsp90 regulation. Biochem J. (2016) 473:2439–52. doi: 10.1042/bcj20160005
11. Maiti S, Bhattacharya K, Wider D, Hany D, Panasenko O, Bernasconi L, et al. Hsf1 and the molecular chaperone Hsp90 support a “rewiring stress response” leading to an adaptive cell size increase in chronic stress. eLife. (2023) 12:1–41. doi: 10.7554/eLife.88658.3
12. Albakova Z, Mangasarova Y, Albakov A, Gorenkova L. HSP70 and HSP90 in cancer: cytosolic, endoplasmic reticulum and mitochondrial chaperones of tumorigenesis. Front Oncol. (2022) 12:829520. doi: 10.3389/fonc.2022.829520
13. Schopf FH, Biebl MM, Buchner J. The HSP90 chaperone machinery. Nat Rev Mol Cell Biol. (2017) 18:345–60. doi: 10.1038/nrm.2017.20
14. Trepel J, Mollapour M, Giaccone G, Neckers L. Targeting the dynamic HSP90 complex in cancer. Nat Rev Cancer. (2010) 10:537–49. doi: 10.1038/nrc2887
15. Lackie RE, Maciejewski A, Ostapchenko VG, Marques-Lopes J, Choy W-Y, Duennwald ML, et al. The hsp70/hsp90 chaperone machinery in neurodegenerative diseases. Front Neurosci. (2017) 11:254. doi: 10.3389/fnins.2017.00254
16. Hessling M, Richter K, Buchner J. Dissection of the ATP-induced conformational cycle of the molecular chaperone Hsp90. Nat Struct Mol Biol. (2009) 16:287–93. doi: 10.1038/nsmb.1565
17. Verkhivker GM. Conformational dynamics and mechanisms of client protein integration into the hsp90 chaperone controlled by allosteric interactions of regulatory switches: perturbation-based network approach for mutational profiling of the hsp90 binding and allostery. J Phys Chem B. (2022) 126:5421–42. doi: 10.1021/acs.jpcb.2c03464
18. Mollapour M, Neckers L. Post-translational modifications of Hsp90 and their contributions to chaperone regulation. Biochim Biophys Acta (BBA) - Mol Cell Res. (2012) 3):648–55. doi: 10.1016/j.bbamcr.2011.07.018
19. Brouet A, Sonveaux P, Dessy C, Moniotte S, Balligand J-L, Feron O. Hsp90 and caveolin are key targets for the proangiogenic nitric oxide–mediated effects of statins. Circ Res. (2001) 89:866–73. doi: 10.1161/hh2201.100319
20. Lees-Miller SP, Anderson CW. The Human Double-stranded DNA-activated Protein Kinase Phosphorylates the 90-kDa Heat-shock Protein, hsp90α at Two NH2-terminal Threonine Residues. J Biol Chem. (1989) 264:17275–80. doi: 10.1016/S0021-9258(18)71488-9
21. Old WM, Shabb JB, Houel S, Wang H, Couts KL, Yen C-Y, et al. Functional proteomics identifies targets of phosphorylation by B-Raf signaling in melanoma. Mol Cell. (2009) 34:115–31. doi: 10.1016/j.molcel.2009.03.007
22. Barati MT, Rane MJ, Klein JB, McLeish KR. A proteomic screen identified stress-induced chaperone proteins as targets of akt phosphorylation in mesangial cells. J Proteome Res. (2006) 5:1636–46. doi: 10.1021/pr0502469
23. Duval M, Bœuf FL, Huot J, Gratton J-P. Src-mediated phosphorylation of hsp90 in response to vascular endothelial growth factor (VEGF) is required for VEGF receptor-2 signaling to endothelial NO synthase. Mol Biol Cell. (2007) 18:4659–68. doi: 10.1091/mbc.e07-05-0467
24. Wang X, Song X, Zhuo W, Fu Y, Shi H, Liang Y, et al. The regulatory mechanism of Hsp90α secretion and its function in tumor Malignancy. Proc Natl Acad Sci. (2009) 106:21288–93. doi: 10.1073/pnas.0908151106
25. Lees-Miller SP, Anderson CW. Two human 90-kDa heat shock proteins are phosphorylated in vivo at conserved serines that are phosphorylated in vitro by casein kinase II. J Biol Chem. (1989) 264:2431–7. doi: 10.1016/S0021-9258(19)81631-9
26. Kurokawa M, Zhao C, Reya T, Kornbluth S. Inhibition of apoptosome formation by suppression of hsp90β Phosphorylation in tyrosine kinase-induced leukemias. Mol Cell Biol. (2008) 28:5494–506. doi: 10.1128/MCB.00265-08
27. Vaughan CK, Mollapour M, Smith JR, Truman A, Hu B, Good VM, et al. Hsp90-dependent activation of protein kinases is regulated by chaperone-targeted dephosphorylation of cdc37. Mol Cell. (2008) 31:886–95. doi: 10.1016/j.molcel.2008.07.021
28. Wandinger SK, Suhre MH, Wegele H, Buchner J. The phosphatase Ppt1 is a dedicated regulator of the molecular chaperone Hsp90. EMBO J. (2006) 25:367–376-376. doi: 10.1038/sj.emboj.7600930
29. Bali P, Pranpat M, Bradner J, Balasis M, Fiskus W, Guo F, et al. Inhibition of histone deacetylase 6 acetylates and disrupts the chaperone function of heat shock protein 90: A NOVEL BASIS FOR ANTILEUKEMIA ACTIVITY OF HISTONE DEACETYLASE INHIBITORS*. J Biol Chem. (2005) 280:26729–34. doi: 10.1074/jbc.C500186200
30. Kovacs JJ, Murphy PJM, Gaillard S, Zhao X, Wu J-T, Nicchitta CV, et al. HDAC6 regulates hsp90 acetylation and chaperone-dependent activation of glucocorticoid receptor. Mol Cell. (2005) 18:601–7. doi: 10.1016/j.molcel.2005.04.021
31. Kekatpure VD, Dannenberg AJ, Subbaramaiah K. HDAC6 modulates hsp90 chaperone activity and regulates activation of aryl hydrocarbon receptor signaling *. J Biol Chem. (2009) 284:7436–45. doi: 10.1074/jbc.M808999200
32. Yu X, Guo ZS, Marcu MG, Neckers L, Nguyen DM, Chen GA, et al. Modulation of p53, erbB1, erbB2, and raf-1 expression in lung cancer cells by depsipeptide FR901228. JNCI: J Natl Cancer Institute. (2002) 94:504–13. doi: 10.1093/jnci/94.7.504
33. Zhang D, Li J, Costa M, Gao J, Huang C. JNK1 mediates degradation HIF-1α by a VHL-independent mechanism that involves the chaperones hsp90/hsp70. Cancer Res. (2010) 70:813–23. doi: 10.1158/0008-5472.Can-09-0448
34. de Zoeten EF, Wang L, Butler K, Beier UH, Akimova T, Sai H, et al. Histone deacetylase 6 and heat shock protein 90 control the functions of foxp3+ T-regulatory cells. Mol Cell Biol. (2011) 31:2066–78. doi: 10.1128/MCB.05155-11
35. Beier UH, Wang L, Han R, Akimova T, Liu Y, Hancock WW. Histone deacetylases 6 and 9 and sirtuin-1 control foxp3+ Regulatory T cell function through shared and isoform-specific mechanisms. Sci Signaling. (2012) 5:ra45–5. doi: 10.1126/scisignal.2002873
36. Zhou Q, Agoston AT, Atadja P, Nelson WG, Davidson NE. Inhibition of histone deacetylases promotes ubiquitin-dependent proteasomal degradation of DNA methyltransferase 1 in human breast cancer cells. Mol Cancer Res. (2008) 6:873–83. doi: 10.1158/1541-7786.Mcr-07-0330
37. Park J-H, Kim S-H, Choi M-C, Lee J, Oh D-Y, Im S-A, et al. Class II histone deacetylases play pivotal roles in heat shock protein 90-mediated proteasomal degradation of vascular endothelial growth factor receptors. Biochem Biophys Res Commun. (2008) 368:318–22. doi: 10.1016/j.bbrc.2008.01.056
38. Retzlaff M, Stahl M, Eberl HC, Lagleder S, Beck J, Kessler H, et al. Hsp90 is regulated by a switch point in the C-terminal domain. EMBO Rep. (2009) 10:1147–53. doi: 10.1038/embor.2009.153
39. Carbone DL, Doorn JA, Kiebler Z, Ickes BR, Petersen DR. Modification of heat shock protein 90 by 4-hydroxynonenal in a rat model of chronic alcoholic liver disease. J Pharmacol Exp Ther. (2005) 315:8–15. doi: 10.1124/jpet.105.088088
40. Kundrat L, Regan L. Identification of residues on hsp70 and hsp90 ubiquitinated by the cochaperone CHIP. J Mol Biol. (2010) 395:587–94. doi: 10.1016/j.jmb.2009.11.017
41. Blank M, Mandel M, Keisari Y, Meruelo D, Lavie G. Enhanced ubiquitinylation of heat shock protein 90 as a potential mechanism for mitotic cell death in cancer cells induced with hypericin. Cancer Res. (2003) 63:8241–7.
42. Wolmarans A, Kwantes A, LaPointe P. A novel method for site-specific chemical SUMOylation: SUMOylation of Hsp90 modulates co-chaperone binding in vitro. Biol Chem. (2019) 400:487–500. doi: 10.1515/hsz-2018-0251
43. Rehn A, Lawatscheck J, Jokisch M-L, Mader SL, Luo Q, Tippel F, et al. A methylated lysine is a switch point for conformational communication in the chaperone Hsp90. Nat Commun. (2020) 11:1219. doi: 10.1038/s41467-020-15048-8
44. Martínez-Ruiz A, Villanueva L, de Orduña CG, López-Ferrer D, Higueras MÁ, Tarín C, et al. S-nitrosylation of Hsp90 promotes the inhibition of its ATPase and endothelial nitric oxide synthase regulatory activities. Proc Natl Acad Sci. (2005) 102:8525–30. doi: 10.1073/pnas.0407294102
45. Fu Y, Xu X, Huang D, Cui D, Liu L, Liu J, et al. Plasma heat shock protein 90alpha as a biomarker for the diagnosis of liver cancer: an official, large-scale, and multicenter clinical trial. eBioMedicine. (2017) 24:56–63. doi: 10.1016/j.ebiom.2017.09.007
46. Kasanga M, Liu L, Xue L, Song X. Plasma heat shock protein 90-alpha have an advantage in diagnosis of colorectal cancer at early stage. Biomarkers Med. (2018) 12:881–90. doi: 10.2217/bmm-2018-0155
47. Chen J-S, Hsu Y-M, Chen C-C, Chen L-L, Lee C-C, Huang T-S. Secreted heat shock protein 90α induces colorectal cancer cell invasion through CD91/LRP-1 and NF-κB-mediated integrin αV expression *. J Biol Chem. (2010) 285:25458–66. doi: 10.1074/jbc.M110.139345
48. Shi Y, Liu X, Lou J, Han X, Zhang L, Wang Q, et al. Plasma levels of heat shock protein 90 alpha associated with lung cancer development and treatment responses. Clin Cancer Res. (2014) 20:6016–22. doi: 10.1158/1078-0432.Ccr-14-0174
49. Fredly H, Reikvam H, Gjertsen BT, Bruserud Ø. Disease-stabilizing treatment with all-trans retinoic acid and valproic acid in acute myeloid leukemia: Serum hsp70 and hsp90 levels and serum cytokine profiles are determined by the disease, patient age, and anti-leukemic treatment. Am J Hematol. (2012) 87:368–76. doi: 10.1002/ajh.23116
50. Sun Y, Zang Z, Xu X, Zhang Z, Zhong L, Zan W, et al. Differential proteomics identification of HSP90 as potential serum biomarker in hepatocellular carcinoma by two-dimensional electrophoresis and mass spectrometry. Int J Mol Sci. (2010) 11:1423–33. doi: 10.3390/ijms11041423
51. Seclì L, Fusella F, Avalle L, Brancaccio M. The dark-side of the outside: how extracellular heat shock proteins promote cancer. Cell Mol Life Sci: CMLS. (2021) 78:4069–83. doi: 10.1007/s00018-021-03764-3
52. Cheng L, Hill AF. Therapeutically harnessing extracellular vesicles. Nat Rev Drug Discov. (2022) 21:379–99. doi: 10.1038/s41573-022-00410-w
53. Crescitelli R, Lässer C, Jang SC, Cvjetkovic A, Malmhäll C, Karimi N, et al. Subpopulations of extracellular vesicles from human metastatic melanoma tissue identified by quantitative proteomics after optimized isolation. J Extracell Vesicles. (2020) 9:1722433. doi: 10.1080/20013078.2020.1722433
54. García-Silva S, Benito-Martín A, Sánchez-Redondo S, Hernández-Barranco A, Ximénez-Embún P, Nogués L, et al. Use of extracellular vesicles from lymphatic drainage as surrogate markers of melanoma progression and BRAFV600E mutation. J Exp Med. (2019) 216:1061–70. doi: 10.1084/jem.20181522
55. Peinado H, Alečković M, Lavotshkin S, Matei I, Costa-Silva B, Moreno-Bueno G, et al. Melanoma exosomes educate bone marrow progenitor cells toward a pro-metastatic phenotype through MET. Nat Med. (2012) 18:883–91. doi: 10.1038/nm.2753
56. Hallal S, Russell BP, Wei H, Lee MYT, Toon CW, Sy J, et al. Extracellular vesicles from neurosurgical aspirates identifies chaperonin containing TCP1 subunit 6A as a potential glioblastoma biomarker with prognostic significance. PROTEOMICS. (2019) 19:1800157. doi: 10.1002/pmic.201800157
57. An M, Lohse I, Tan Z, Zhu J, Wu J, Kurapati H, et al. Quantitative proteomic analysis of serum exosomes from patients with locally advanced pancreatic cancer undergoing chemoradiotherapy. J Proteome Res. (2017) 16:1763–72. doi: 10.1021/acs.jproteome.7b00024
58. Dhondt B, Geeurickx E, Tulkens J, Van Deun J, Vergauwen G, Lippens L, et al. Unravelling the proteomic landscape of extracellular vesicles in prostate cancer by density-based fractionation of urine. J Extracell Vesicles. (2020) 9:1736935. doi: 10.1080/20013078.2020.1736935
59. Hiltbrunner S, Mints M, Eldh M, Rosenblatt R, Holmström B, Alamdari F, et al. Urinary exosomes from bladder cancer patients show a residual cancer phenotype despite complete pathological downstaging. Sci Rep. (2020) 10:5960. doi: 10.1038/s41598-020-62753-x
60. Sun Y, Huo C, Qiao Z, Shang Z, Uzzaman A, Liu S, et al. Comparative proteomic analysis of exosomes and microvesicles in human saliva for lung cancer. J Proteome Res. (2018) 17:1101–7. doi: 10.1021/acs.jproteome.7b00770
61. Luo D, Zhan S, Xia W, Huang L, Ge W, Wang T. Proteomics study of serum exosomes from papillary thyroid cancer patients. Endocrine-Related Cancer. (2018) 25:879–91. doi: 10.1530/ERC-17-0547
62. Albakova Z, Siam MKS, Sacitharan PK, Ziganshin RH, Ryazantsev DY, Sapozhnikov AM. Extracellular heat shock proteins and cancer: New perspectives. Trans Oncol. (2021) 14:100995. doi: 10.1016/j.tranon.2020.100995
63. Lauwers E, Wang Y-C, Gallardo R, van der Kant R, Michiels E, Swerts J, et al. Hsp90 mediates membrane deformation and exosome release. Mol Cell. (2018) 71:689–702.e689. doi: 10.1016/j.molcel.2018.07.016
64. Tang X, Chang C, Guo J, Lincoln V, Liang C, Chen M, et al. Tumour-secreted hsp90α on external surface of exosomes mediates tumour - stromal cell communication via autocrine and paracrine mechanisms. Sci Rep. (2019) 9:15108. doi: 10.1038/s41598-019-51704-w
65. Hanahan D, Weinberg RA. The hallmarks of cancer. Cell. (2000) 100:57–70. doi: 10.1016/s0092-8674(00)81683-9
66. Hanahan D, Weinberg RA. Hallmarks of cancer: the next generation. Cell. (2011) 144:646–74. doi: 10.1016/j.cell.2011.02.013
67. Albakova Z, Armeev GA, Kanevskiy LM, Kovalenko EI, Sapozhnikov AM. HSP70 multi-functionality in cancer. Cells. (2020) 9:(3). doi: 10.3390/cells9030587
68. Ullrich SJ, Robinson EA, Law LW, Willingham M, Appella E. A mouse tumor-specific transplantation antigen is a heat shock-related protein. Proc Natl Acad Sci. (1986) 83:3121–5. doi: 10.1073/pnas.83.10.3121
69. Srivastava PK, DeLeo AB, Old LJ. Tumor rejection antigens of chemically induced sarcomas of inbred mice. Proc Natl Acad Sci. (1986) 83:3407–11. doi: 10.1073/pnas.83.10.3407
70. Maki RG, Old LJ, Srivastava PK. Human homologue of murine tumor rejection antigen gp96: 5’-regulatory and coding regions and relationship to stress-induced proteins. Proc Natl Acad Sci. (1990) 87:5658–62. doi: 10.1073/pnas.87.15.5658
71. Graner M, Raymond A, Romney D, He L, Whitesell L, Katsanis E. Immunoprotective activities of multiple chaperone proteins isolated from murine B-cell leukemia/lymphoma. Clin Cancer Res. (2000) 6:909–15.
72. Udono H, Srivastava PK. Comparison of tumor-specific immunogenicities of stress-induced proteins gp96, hsp90, and hsp70. J Immunol. (1994) 152:5398–403. doi: 10.4049/jimmunol.152.11.5398
73. Kang J, Lee HJ, Lee J, Hong J, Hong Kim Y, Disis ML, et al. Novel peptide-based vaccine targeting heat shock protein 90 induces effective antitumor immunity in a HER2+ breast cancer murine model. J Immunother Cancer. (2022) 10:(9). doi: 10.1136/jitc-2022-004702
74. Murshid A, Gong J, Calderwood SK. Heat shock protein 90 mediates efficient antigen cross presentation through the scavenger receptor expressed by endothelial cells-I. J Immunol. (2010) 185:2903–17. doi: 10.4049/jimmunol.0903635
75. Ichiyanagi T, Imai T, Kajiwara C, Mizukami S, Nakai A, Nakayama T, et al. Essential role of endogenous heat shock protein 90 of dendritic cells in antigen cross-presentation. J Immunol. (2010) 185:2693. doi: 10.4049/jimmunol.1000821
76. Kunisawa J, Shastri N. Hsp90α chaperones large C-terminally extended proteolytic intermediates in the MHC class I antigen processing pathway. Immunity. (2006) 24:523–34. doi: 10.1016/j.immuni.2006.03.015
77. Imai T, Kato Y, Kajiwara C, Mizukami S, Ishige I, Ichiyanagi T, et al. Heat shock protein 90 (HSP90) contributes to cytosolic translocation of extracellular antigen for cross-presentation by dendritic cells. Proc Natl Acad Sci. (2011) 108:201108372. doi: 10.1073/pnas.1108372108
78. Jaeger AM, Stopfer L, Lee S, Gaglia G, Sandel D, Santagata S, et al. Rebalancing protein homeostasis enhances tumor antigen presentation. Clin Cancer Res. (2019) 25:6392–405. doi: 10.1158/1078-0432.Ccr-19-0596
79. Rajagopal D, Bal V, Mayor S, George A, Rath S. A role for the Hsp90 molecular chaperone family in antigen presentation to T lymphocytes via major histocompatibility complex class II molecules. Eur J Immunol. (2006) 36:828–41. doi: 10.1002/eji.200535326
80. Bae J, Munshi A, Li C, Samur M, Prabhala R, Mitsiades C, et al. Heat shock protein 90 is critical for regulation of phenotype and functional activity of human T lymphocytes and NK cells. J Immunol (Baltimore Md. (2013) 1950) 190:1360–71. doi: 10.4049/jimmunol.1200593
81. Huyan T, Li Q, Dong D-D, Yang H, Zhang J, Huang Q-S, et al. Heat shock protein 90 inhibitors induce functional inhibition of human natural killer cells in a dose-dependent manner. Immunopharmacol Immunotoxicol. (2016) 38:77–86. doi: 10.3109/08923973.2015.1119159
82. Albakova Z, Mangasarova Y, Sapozhnikov A. Impaired heat shock protein expression in activated T cells in B-cell lymphoma. Biomedicines. (2022) 10:2747. doi: 10.3390/biomedicines10112747
83. Albakova Z, Mangasarova Y, Albakov A, Nikulina E, Kravchenko S, Sapozhnikov A. Aberrant HSP90 expression in lymphocytes and HSP90 response to anti-PD-1 therapy in lymphoma patients. Front Immunol. (2022) 13:893137. doi: 10.3389/fimmu.2022.893137
84. Sedlacek AL, Kinner-Bibeau LB, Binder RJ. Phenotypically distinct helper NK cells are required for gp96-mediated anti-tumor immunity. Sci Rep. (2016) 6:29889. doi: 10.1038/srep29889
85. Yang Y, Liu B, Dai J, Srivastava PK, Zammit DJ, Lefrançois L, et al. Heat shock protein gp96 is a master chaperone for toll-like receptors and is important in the innate function of macrophages. Immunity. (2007) 26:215–26. doi: 10.1016/j.immuni.2006.12.005
86. Vabulas RM, Braedel S, Hilf N, Singh-Jasuja H, Herter S, Ahmad-Nejad P, et al. The endoplasmic reticulum-resident heat shock protein gp96 activates dendritic cells via the toll-like receptor 2/4 pathway *. J Biol Chem. (2002) 277:20847–53. doi: 10.1074/jbc.M200425200
87. Li X, Liu Z, Yan X, Zhang X, Li Y, Zhao B, et al. Induction of regulatory T cells by high-dose gp96 suppresses murine liver immune hyperactivation. PloS One. (2013) 8:e68997. doi: 10.1371/journal.pone.0068997
88. Liu W, Chen M, Li X, Zhao B, Hou J, Zheng H, et al. Interaction of toll-like receptors with the molecular chaperone gp96 is essential for its activation of cytotoxic T lymphocyte response. PloS One. (2016) 11:e0155202. doi: 10.1371/journal.pone.0155202
89. Wang Y, Sedlacek AL, Pawaria S, Xu H, Scott MJ, Binder RJ. Cutting edge: the heat shock protein gp96 activates inflammasome-signaling platforms in APCs. J Immunol. (2018) 201:2209. doi: 10.4049/jimmunol.1800505
90. Chen Y-Q, Li P-C, Pan N, Gao R, Wen Z-F, Zhang T-Y, et al. Tumor-released autophagosomes induces CD4<sup<+</sup< T cell-mediated immunosuppression via a TLR2–IL-6 cascade. J Immunother Cancer. (2019) 7:178. doi: 10.1186/s40425-019-0646-5
91. Chen C-C, Chen L-L, Li C-P, Hsu Y-T, Jiang S-S, Fan C-S, et al. Myeloid-derived macrophages and secreted HSP90α induce pancreatic ductal adenocarcinoma development. Oncoimmunology. (2018) 7:e1424612–e1424612. doi: 10.1080/2162402X.2018.1424612
92. Cui A, Huang T, Li S, Ma A, Pérez JL, Sander C, et al. Dictionary of immune responses to cytokines at single-cell resolution. Nature. (2024) 625:377–84. doi: 10.1038/s41586-023-06816-9
93. Zavareh RB, Spangenberg SH, Woods A, Martínez-Peña F, Lairson LL. HSP90 inhibition enhances cancer immunotherapy by modulating the surface expression of multiple immune checkpoint proteins. Cell Chem Biol. (2021) 28:158–168.e155. doi: 10.1016/j.chembiol.2020.10.005
94. Marzec M, Zhang Q, Goradia A, Raghunath PN, Liu X, Paessler M, et al. Oncogenic kinase NPM/ALK induces through STAT3 expression of immunosuppressive protein CD274 (PD-L1, B7-H1). Proc Natl Acad Sci USA. (2008) 105:20852–7. doi: 10.1073/pnas.0810958105
95. D’Arrigo P, Russo M, Rea A, Tufano M, Guadagno E, Del Basso De Caro ML, et al. A regulatory role for the co-chaperone FKBP51s in PD-L1 expression in glioma. Oncotarget. (2017) 8:68291–304. doi: 10.18632/oncotarget.19309
96. Mbofung RM, McKenzie JA, Malu S, Zhang M, Peng W, Liu C, et al. HSP90 inhibition enhances cancer immunotherapy by upregulating interferon response genes. Nat Commun. (2017) 8:451–1. doi: 10.1038/s41467-017-00449-z
97. Federici C, Shahaj E, Cecchetti S, Camerini S, Casella M, Iessi E, et al. Natural-killer-derived extracellular vesicles: immune sensors and interactors. Front Immunol. (2020) 11. doi:10.3389/fimmu.2020.00262. doi: 10.3389/fimmu.2020.00262
98. Perez-Hernandez D, Gutiérrez-Vázquez C, Jorge I, López-Martín S, Ursa A, Sánchez-Madrid F, et al. The intracellular interactome of tetraspanin-enriched microdomains reveals their function as sorting machineries toward exosomes *. J Biol Chem. (2013) 288:11649–61. doi: 10.1074/jbc.M112.445304
99. Kowal J, Arras G, Colombo M, Jouve M, Morath JP, Primdal-Bengtson B, et al. Proteomic comparison defines novel markers to characterize heterogeneous populations of extracellular vesicle subtypes. Proc Natl Acad Sci. (2016) 113:E968–77. doi: 10.1073/pnas.1521230113
100. Garcia BA, Smalley DM, Shabanowitz J, Ley K, Hunt DF. The platelet microparticle proteome. J Proteome Res. (2005) 4:1516–21. doi: 10.1021/pr0500760
101. Dalli J, Montero-Melendez T, Norling LV, Yin X, Hinds C, Haskard D, et al. Heterogeneity in neutrophil microparticles reveals distinct proteome and functional properties *. Mol Cell Proteomics. (2013) 12:2205–19. doi: 10.1074/mcp.M113.028589
102. Jiang J, Wang W, Zhu L, Shi B, Chen Y, Xia Y, et al. Unveiling the role of hypoxic macrophage-derived exosomes in driving colorectal cancer progression. Front Immunol. (2023) 14:1260638. doi: 10.3389/fimmu.2023.1260638
103. Lv L-H, Wan Y-L, Lin Y, Zhang W, Yang M, Li G-L, et al. Anticancer drugs cause release of exosomes with heat shock proteins from human hepatocellular carcinoma cells that elicit effective natural killer cell antitumor responses in vitro*. J Biol Chem. (2012) 287:15874–85. doi: 10.1074/jbc.M112.340588
104. Chen W, Wang J, Shao C, Liu S, Yu Y, Wang Q, et al. Efficient induction of antitumor T cell immunity by exosomes derived from heat-shocked lymphoma cells. Eur J Immunol. (2006) 36:1598–607. doi: 10.1002/eji.200535501
105. Jung I, Shin S, Baek M-C, Yea K. Modification of immune cell-derived exosomes for enhanced cancer immunotherapy: current advances and therapeutic applications. Exp Mol Med. (2024) 56:19–31. doi: 10.1038/s12276-023-01132-8
106. Ono K, Sogawa C, Kawai H, Tran MT, Taha EA, Lu Y, et al. Triple knockdown of CDC37, HSP90-alpha and HSP90-beta diminishes extracellular vesicles-driven Malignancy events and macrophage M2 polarization in oral cancer. J Extracell Vesicles. (2020) 9:1769373. doi: 10.1080/20013078.2020.1769373
107. Nimmanapalli R, O’Bryan E, Kuhn D, Yamaguchi H, Wang H-G, Bhalla KN. Regulation of 17-AAG—induced apoptosis: role of Bcl-2, Bcl-xL, and Bax downstream of 17-AAG—mediated down-regulation of Akt, Raf-1, and Src kinases. Blood. (2003) 102:269–75. doi: 10.1182/blood-2002-12-3718
108. Peng C, Zhao F, Li H, Li L, Yang Y, Liu F. HSP90 mediates the connection of multiple programmed cell death in diseases. Cell Death Dis. (2022) 13:929. doi: 10.1038/s41419-022-05373-9
109. Zhao X, Wang J, Xiao L, Xu Q, Zhao E, Zheng X, et al. Effects of 17-AAG on the cell cycle and apoptosis of H446 cells and the associated mechanisms. Mol Med Rep. (2016) 14:1067–74. doi: 10.3892/mmr.2016.5365
110. Yan C, Oh JS, Yoo SH, Lee JS, Yoon YG, Oh YJ, et al. The targeted inhibition of mitochondrial Hsp90 overcomes the apoptosis resistance conferred by Bcl-2 in Hep3B cells via necroptosis. Toxicol Appl Pharmacol. (2013) 266:9–18. doi: 10.1016/j.taap.2012.11.001
111. Kang BH, Plescia J, Song HY, Meli M, Colombo G, Beebe K, et al. Combinatorial drug design targeting multiple cancer signaling networks controlled by mitochondrial Hsp90. J Clin Invest. (2009) 119:454–64. doi: 10.1172/JCI37613
112. Wang Q, Sun W, Hao X, Li T, Su L, Liu X. Down-regulation of cellular FLICE-inhibitory protein (Long Form) contributes to apoptosis induced by Hsp90 inhibition in human lung cancer cells. Cancer Cell Int. (2012) 12:54. doi: 10.1186/1475-2867-12-54
113. Jacobsen AV, Lowes KN, Tanzer MC, Lucet IS, Hildebrand JM, Petrie EJ, et al. HSP90 activity is required for MLKL oligomerisation and membrane translocation and the induction of necroptotic cell death. Cell Death Dis. (2016) 7:e2051–1. doi: 10.1038/cddis.2015.386
114. Chen W-W, Yu H, Fan H-B, Zhang C-C, Zhang M, Zhang C, et al. RIP1 mediates the protection of geldanamycin on neuronal injury induced by oxygen-glucose deprivation combined with zVAD in primary cortical neurons. J Neurochem. (2012) 120:70–7. doi: 10.1111/j.1471-4159.2011.07526.x
115. Fearns C, Pan Q, Mathison JC, Chuang T-H. Triad3A regulates ubiquitination and proteasomal degradation of RIP1 following disruption of hsp90 binding *. J Biol Chem. (2006) 281:34592–600. doi: 10.1074/jbc.M604019200
116. Gentle IE, Wong WW-L, Evans JM, Bankovacki A, Cook WD, Khan NR, et al. In TNF-stimulated Cells, RIPK1 Promotes Cell Survival by Stabilizing TRAF2 and cIAP1, which Limits Induction of Non-canonical NF-κB and Activation of Caspase-8 *. J Biol Chem. (2011) 286:13282–91. doi: 10.1074/jbc.M110.216226
117. Wu Z, Geng Y, Lu X, Shi Y, Wu G, Zhang M, et al. Chaperone-mediated autophagy is involved in the execution of ferroptosis. Proc Natl Acad Sci. (2019) 116:2996–3005. doi: 10.1073/pnas.1819728116
118. Li D, Xu T, Cao Y, Wang H, Li L, Chen S, et al. A cytosolic heat shock protein 90 and cochaperone CDC37 complex is required for RIP3 activation during necroptosis. Proc Natl Acad Sci. (2015) 112:5017–22. doi: 10.1073/pnas.1505244112
119. Bandyopadhyay U, Kaushik S, Varticovski L, Cuervo AM. The chaperone-mediated autophagy receptor organizes in dynamic protein complexes at the lysosomal membrane. Mol Cell Biol. (2008) 28:5747–63. doi: 10.1128/MCB.02070-07
120. Qing G, Yan P, Xiao G. Hsp90 inhibition results in autophagy-mediated proteasome-independent degradation of IκB kinase (IKK). Cell Res. (2006) 16:895–901. doi: 10.1038/sj.cr.7310109
121. Joo Joung H, Dorsey Frank C, Joshi A, Hennessy-Walters Kristin M, Rose Kristie L, McCastlain K, et al. Hsp90-cdc37 chaperone complex regulates ulk1- and atg13-mediated mitophagy. Mol Cell. (2011) 43:572–85. doi: 10.1016/j.molcel.2011.06.018
122. Han J, Goldstein LA, Hou W, Chatterjee S, Burns TF, Rabinowich H. HSP90 inhibition targets autophagy and induces a CASP9-dependent resistance mechanism in NSCLC. Autophagy. (2018) 14:958–71. doi: 10.1080/15548627.2018.1434471
123. Xu C, Liu J, Hsu L-C, Luo Y, Xiang R, Chuang T-H. Functional interaction of heat shock protein 90 and Beclin 1 modulates Toll-like receptor-mediated autophagy. FASEB J. (2011) 25:2700–10. doi: 10.1096/fj.10-167676
124. Zhou Q, Meng Y, Li D, Yao L, Le J, Liu Y, et al. Ferroptosis in cancer: From molecular mechanisms to therapeutic strategies. Signal Transduct Target Ther. (2024) 9:55. doi: 10.1038/s41392-024-01769-5
125. Albakova Z, Mangasarova Y. The HSP immune network in cancer. Front Immunol. (2021) 12:796493. doi: 10.3389/fimmu.2021.796493
126. Nizami S, Arunasalam K, Green J, Cook J, Lawrence CB, Zarganes-Tzitzikas T, et al. Inhibition of the NLRP3 inflammasome by HSP90 inhibitors. Immunology. (2021) 162:84–91. doi: 10.1111/imm.13267
127. Spel L, Hou C, Theodoropoulou K, Zaffalon L, Wang Z, Bertoni A, et al. HSP90β controls NLRP3 autoactivation. Sci Adv. (2024) 10:eadj6289. doi: 10.1126/sciadv.adj6289
128. Wu H, Dyson HJ. Aggregation of zinc-free p53 is inhibited by Hsp90 but not other chaperones. Protein Sci. (2019) 28:2020–3. doi: 10.1002/pro.3726
129. Ahsan A, Ramanand SG, Whitehead C, Hiniker SM, Rehemtulla A, Pratt WB, et al. Wild-type EGFR is stabilized by direct interaction with HSP90 in cancer cells and tumors. Neoplasia. (2012) 14:670–IN671. doi: 10.1593/neo.12986
130. Lacey T, Lacey H. Linking hsp90’s role as an evolutionary capacitator to the development of cancer. Cancer Treat Res Commun. (2021) 28:100400. doi: 10.1016/j.ctarc.2021.100400
131. Watanabe S, Goto Y, Yasuda H, Kohno T, Motoi N, Ohe Y, et al. HSP90 inhibition overcomes EGFR amplification-induced resistance to third-generation EGFR-TKIs. Thorac Cancer. (2021) 12:631–42. doi: 10.1111/1759-7714.13839
132. Miyata Y, Nakamoto H, Neckers L. The therapeutic target Hsp90 and cancer hallmarks. Curr Pharm Des. (2013) 19:347–65. doi: 10.2174/138161213804143725
133. Sciacovelli M, Guzzo G, Morello V, Frezza C, Zheng L, Nannini N, et al. The mitochondrial chaperone TRAP1 promotes neoplastic growth by inhibiting succinate dehydrogenase. Cell Metab. (2013) 17:988–99. doi: 10.1016/j.cmet.2013.04.019
134. Yoshida S, Tsutsumi S, Muhlebach G, Sourbier C, Lee M-J, Lee S, et al. Molecular chaperone TRAP1 regulates a metabolic switch between mitochondrial respiration and aerobic glycolysis. Proc Natl Acad Sci USA. (2013) 110:E1604–12. doi: 10.1073/pnas.1220659110
135. Chae YC, Angelin A, Lisanti S, Kossenkov AV, Speicher KD, Wang H, et al. Landscape of the mitochondrial Hsp90 metabolome in tumours. Nat Commun. (2013) 4:2139–9. doi: 10.1038/ncomms3139
136. Holt SE, Aisner DL, Baur J, Tesmer VM, Dy M, Ouellette M, et al. Functional requirement of p23 and Hsp90 in telomerase complexes. Genes Dev. (1999) 13:817–26. doi: 10.1101/gad.13.7.817
137. Keppler BR, Grady AT, Jarstfer MB. The biochemical role of the heat shock protein 90 chaperone complex in establishing human telomerase activity *. J Biol Chem. (2006) 281:19840–8. doi: 10.1074/jbc.M511067200
138. Grandin N, Charbonneau M. Hsp90 levels affect telomere length in yeast. Mol Genet Genomics. (2001) 265:126–34. doi: 10.1007/s004380000398
139. Toogun OA, DeZwaan DC, Freeman BC. The hsp90 molecular chaperone modulates multiple telomerase activities. Mol Cell Biol. (2008) 28:457–67. doi: 10.1128/MCB.01417-07
140. Yaswen P, MacKenzie KL, Keith WN, Hentosh P, Rodier F, Zhu J, et al. Therapeutic targeting of replicative immortality. Semin Cancer Biol. (2015) 35:S104–28. doi: 10.1016/j.semcancer.2015.03.007
141. Zhong W, Chen W, Liu Y, Zhang J, Lu Y, Wan X, et al. Extracellular HSP90α promotes cellular senescence by modulating TGF-β signaling in pulmonary fibrosis. FASEB J. (2022) 36:e22475. doi: 10.1096/fj.202200406RR
142. Fuhrmann-Stroissnigg H, Ling YY, Zhao J, McGowan SJ, Zhu Y, Brooks RW, et al. Identification of HSP90 inhibitors as a novel class of senolytics. Nat Commun. (2017) 8:422. doi: 10.1038/s41467-017-00314-z
143. Song X, Wang X, Zhuo W, Shi H, Feng D, Sun Y, et al. The regulatory mechanism of extracellular hsp90α on matrix metalloproteinase-2 processing and tumor angiogenesis *. J Biol Chem. (2010) 285:40039–49. doi: 10.1074/jbc.M110.181941
144. Klemke L, De Oliveira T, Witt D, Winkler N, Bohnenberger H, Bucala R, et al. Hsp90-stabilized MIF supports tumor progression via macrophage recruitment and angiogenesis in colorectal cancer. Cell Death Dis. (2021) 12:155. doi: 10.1038/s41419-021-03426-z
145. Schulz R, Dobbelstein M, Moll UM. HSP90 inhibitor antagonizing MIF. Oncoimmunology. (2012) 1:1425–6. doi: 10.4161/onci.21173
146. Dong H, Zou M, Bhatia A, Jayaprakash P, Hofman F, Ying Q, et al. Breast cancer MDA-MB-231 cells use secreted heat shock protein-90alpha (Hsp90α) to survive a hostile hypoxic environment. Sci Rep. (2016) 6:20605. doi: 10.1038/srep20605
147. Zhang P-C, Liu X, Li M-M, Ma Y-Y, Sun H-T, Tian X-Y, et al. AT-533, a novel Hsp90 inhibitor, inhibits breast cancer growth and HIF-1α/VEGF/VEGFR-2-mediated angiogenesis in vitro and in vivo. Biochem Pharmacol. (2020) 172:113771. doi: 10.1016/j.bcp.2019.113771
148. Feng Q, Zhang C, Lum D, Druso JE, Blank B, Wilson KF, et al. A class of extracellular vesicles from breast cancer cells activates VEGF receptors and tumour angiogenesis. Nat Commun. (2017) 8:14450. doi: 10.1038/ncomms14450
149. Tang H, Zhou X, Zhao X, Luo X, Luo T, Chen Y, et al. HSP90/IKK-rich small extracellular vesicles activate pro-angiogenic melanoma-associated fibroblasts via the NF-κB/CXCL1 axis. Cancer Sci. (2022) 113:1168–81. doi: 10.1111/cas.15271
150. Kim JY, Cho T-M, Park JM, Park S, Park M, Nam KD, et al. A novel HSP90 inhibitor SL-145 suppresses metastatic triple-negative breast cancer without triggering the heat shock response. Oncogene. (2022) 41:3289–97. doi: 10.1038/s41388-022-02269-y
151. Hance MW, Dole K, Gopal U, Bohonowych JE, Jezierska-Drutel A, Neumann CA, et al. Secreted hsp90 is a novel regulator of the epithelial to mesenchymal transition (EMT) in prostate cancer *. J Biol Chem. (2012) 287:37732–44. doi: 10.1074/jbc.M112.389015
152. Nolan KD, Kaur J, Isaacs JS. Secreted heat shock protein 90 promotes prostate cancer stem cell heterogeneity. Oncotarget. (2016) 8:(12). doi: 10.18632/oncotarget.v8i12
153. Chen W-S, Chen C-C, Chen L-L, Lee C-C, Huang T-S. Secreted heat shock protein 90 (HSP90); induces nuclear factor-B-mediated TCF12 protein expression to down-regulate E-cadherin and to enhance colorectal cancer cell migration and invasion *. J Biol Chem. (2013) 288:9001–10. doi: 10.1074/jbc.M112.437897
154. Wang H, Deng G, Ai M, Xu Z, Mou T, Yu J, et al. Hsp90ab1 stabilizes LRP5 to promote epithelial-mesenchymal transition via activating of AKT and Wnt/β-catenin signaling pathways in gastric cancer progression. Oncogene. (2019) 38:1489–507. doi: 10.1038/s41388-018-0532-5
155. Sahu D, Zhao Z, Tsen F, Cheng C-F, Park R, Situ AJ, et al. A potentially common peptide target in secreted heat shock protein-90α for hypoxia-inducible factor-1α-positive tumors. Mol Biol Cell. (2012) 23:602–13. doi: 10.1091/mbc.E11-06-0575
156. Wu BX, Hong F, Zhang Y, Ansa-Addo E, Li Z. Chapter seven - GRP94/gp96 in cancer: biology, structure, immunology, and drug development. In: Isaacs J, Whitesell L, editors. Advances in cancer research, vol 129. Academic Press (2016). p. 165–90. doi: 10.1016/bs.acr.2015.09.001
157. Albakova Z. Heat shock proteins in cancer immunotherapy. In: Rezaei N, editor. Handbook of cancer and immunology. Springer International Publishing, Cham (2022). p. 1–15. doi: 10.1007/978-3-030-80962-1_213-1
158. Yamazaki K, Nguyen T, Podack ER. Cutting edge: tumor secreted heat shock-fusion protein elicits CD8 cells for rejection. J Immunol. (1999) 163:5178. doi: 10.4049/jimmunol.163.10.5178
159. Strbo N, Garcia-Soto A, Schreiber TH, Podack ER. Secreted heat shock protein gp96-Ig: next-generation vaccines for cancer and infectious diseases. Immunologic Res. (2013) 57:311–25. doi: 10.1007/s12026-013-8468-x
160. Morgensztern D, Harb W, Schalper K, Price M, Early B, Schreiber T. MA09.06 viagenpumatucel-L bolsters response to nivolumab therapy in advanced lung adenocarcinoma: preliminary data from the DURGA trial. J Thorac Oncol. (2017) 12:S394–5. doi: 10.1016/j.jtho.2016.11.447
161. Cohen RB, Peoples GE, Kawashima T, Arana B, Cui X, Bazhenova L, et al. Interim results of viagenpumatucel-L (HS-110) plus nivolumab in previously treated patients (pts) with advanced non-small cell lung cancer (NSCLC) in two treatment settings. J Clin Oncol. (2021) 39:9100–0. doi: 10.1200/JCO.2021.39.15_suppl.9100
162. Crane CA, Han SJ, Ahn B, Oehlke J, Kivett V, Fedoroff A, et al. Individual Patient-Specific Immunity against High-Grade Glioma after Vaccination with Autologous Tumor Derived Peptides Bound to the 96 KD Chaperone Protein. Clin Cancer Res. (2013) 19:205–14. doi: 10.1158/1078-0432.Ccr-11-3358
163. Bloch O, Lim M, Sughrue ME, Komotar RJ, Abrahams JM, O’Rourke DM, et al. Autologous heat shock protein peptide vaccination for newly diagnosed glioblastoma: impact of peripheral PD-L1 expression on response to therapy. Clin Cancer Res. (2017) 23:3575–84. doi: 10.1158/1078-0432.Ccr-16-1369
164. Shinagawa N, Yamazaki K, Tamura Y, Imai A, Kikuchi E, Yokouchi H, et al. Immunotherapy with dendritic cells pulsed with tumor-derived gp96 against murine lung cancer is effective through immune response of CD8+ cytotoxic T lymphocytes and natural killer cells. Cancer Immunol Immunother. (2008) 57:165–74. doi: 10.1007/s00262-007-0359-3
165. Zeng Y, Feng H, Graner MW, Katsanis E. Tumor-derived, chaperone-rich cell lysate activates dendritic cells and elicits potent antitumor immunity. Blood. (2003) 101:4485–91. doi: 10.1182/blood-2002-10-3108
166. Cantrell J, Larmonier C, Janikashvili N, Bustamante S, Fraszczak J, Herrell A, et al. Signaling pathways induced by a tumor-derived vaccine in antigen presenting cells. Immunobiology. (2010) 215:535–44. doi: 10.1016/j.imbio.2009.09.006
167. Larmonier N, Cantrell J, LaCasse C, Li G, Janikashvili N, Situ E, et al. Chaperone-rich tumor cell lysate-mediated activation of antigen-presenting cells resists regulatory T cell suppression. J Leukocyte Biol. (2008) 83:1049–59. doi: 10.1189/jlb.0907635
168. Zeng Y, Chen X, Larmonier N, Larmonier C, Li G, Sepassi M, et al. Natural killer cells play a key role in the antitumor immunity generated by chaperone-rich cell lysate vaccination. Int J Cancer. (2006) 119:2624–31. doi: 10.1002/ijc.22150
169. Guo Q-Y, Yuan M, Peng J, Cui X-M, Song G, Sui X, et al. Antitumor activity of mixed heat shock protein/peptide vaccine and cyclophosphamide plus interleukin-12 in mice sarcoma. J Exp Clin Cancer Res. (2011) 30:24. doi: 10.1186/1756-9966-30-24
Keywords: HSP90, cancer, extracellular HSP90, metastasis, angiogenesis, tumor immunity
Citation: Albakova Z (2024) HSP90 multi-functionality in cancer. Front. Immunol. 15:1436973. doi: 10.3389/fimmu.2024.1436973
Received: 22 May 2024; Accepted: 18 July 2024;
Published: 01 August 2024.
Edited by:
Julia Schueler, Charles River Discovery Research Services GmbH, GermanyReviewed by:
Wolfgang Sommergruber, Medical University of Vienna, AustriaYusuf Tutar, University of Health Sciences, Türkiye
Copyright © 2024 Albakova. This is an open-access article distributed under the terms of the Creative Commons Attribution License (CC BY). The use, distribution or reproduction in other forums is permitted, provided the original author(s) and the copyright owner(s) are credited and that the original publication in this journal is cited, in accordance with accepted academic practice. No use, distribution or reproduction is permitted which does not comply with these terms.
*Correspondence: Zarema Albakova, emFyZW1hLmFsYmFrb3ZhMTRAZ21haWwuY29t