- 1Department of Immunology, Key Laboratory of Immune Mechanism and Intervention on Serious Disease in Hebei Province, Hebei Medical University, Immunology Department of Hebei Medical University, Shijiazhuang, China
- 2Hebei Medical University-National University of Ireland Galway Stem Cell Research Center, Hebei Medical University, Shijiazhuang, Hebei, China
The tumor microenvironment (TME) contains cells that regulate medication response and cancer growth in a major way. Tumor immunology research has been rejuvenated and cancer treatment has been changed by immunotherapy, a rapidly developing therapeutic approach. The growth patterns of tumor cells in vivo and the heterogeneity, complexity, and individuality of tumors produced from patients are not reflected in traditional two-dimensional tumor cell profiles. On the other hand, an in vitro three-dimensional (3D) model called the organoid model is gaining popularity. It can replicate the physiological and pathological properties of the original tissues in vivo. Tumor cells are the source of immune organoids. The TME characteristics can be preserved while preserving the variety of tumors by cultivating epithelial tumor cells with various stromal and immunological components. In addition to having genetic and physical similarities to human diseases and the ability to partially reconstruct the complex structure of tumors, these models are now widely used in research fields including cancer, developmental biology, regenerative mechanisms, drug development, disease modeling, and organ transplantation. This study reviews the function of organoids in immunotherapy and the tumor immune milieu. We also discuss current developments and suggest translational uses of tumor organoids in immuno-oncology research, immunotherapy modeling, and precision medicine.
Introduction
The TME is an intricate system. It comprises not only tumor cells but also a variety of other cell types, such as blood vessels, activated fibroblasts, invading immune cells, and the extracellular matrix, that are involved in the growth of tumors (Figure 1). Understanding carcinogenesis, tumor growth, and tumor metastasis depends on understanding the TME, which is regulated by a range of cellular, hormonal, and inflammatory responses. As a result, this topic has long been a goal of cancer research and plays a significant role in the prevention, diagnosis, and treatment of cancers. Research has demonstrated that the TME can affect the course of tumors and how well immunotherapy drugs work (1).
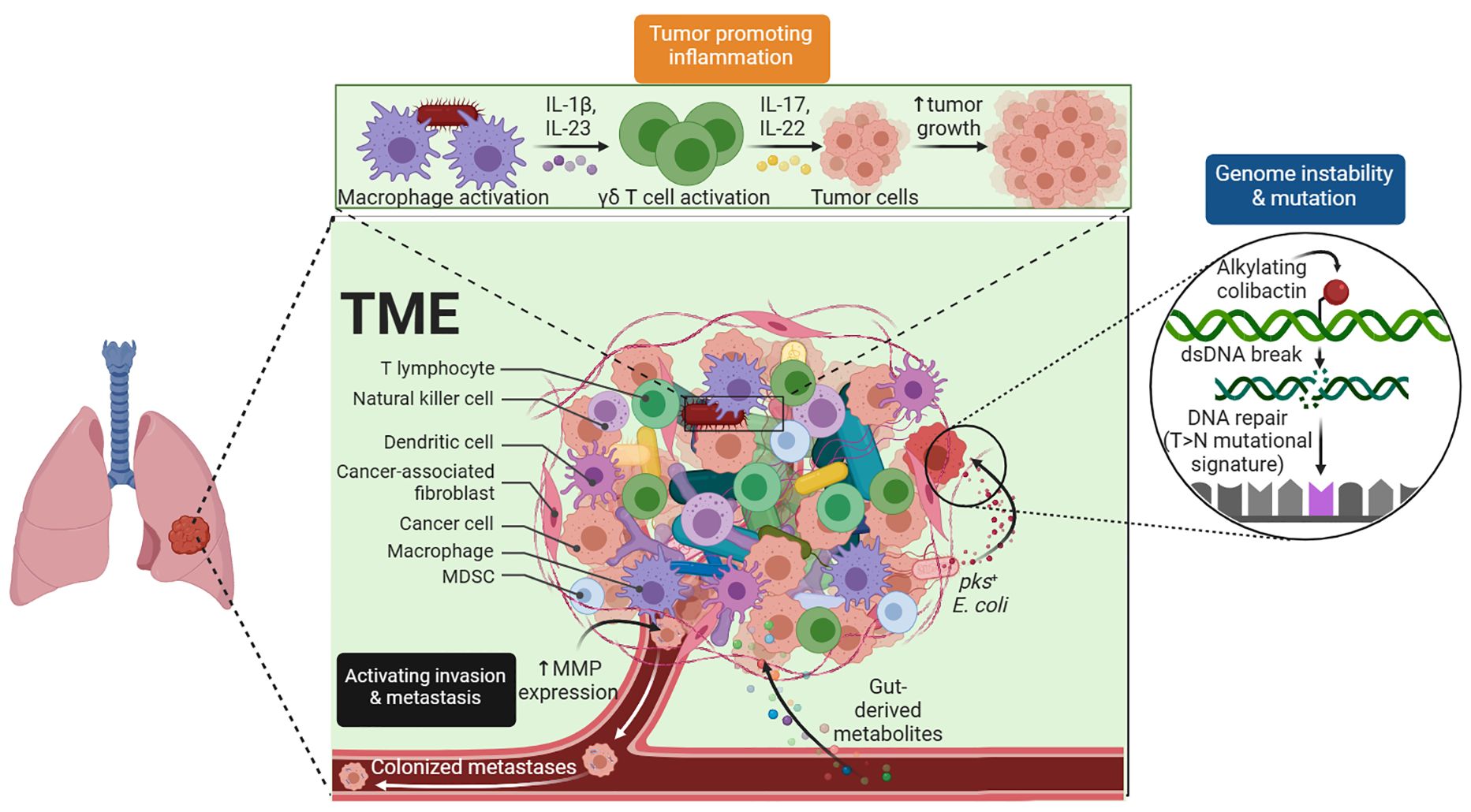
Figure 1 Tumor Microenvironment. The complex TME, which includes tumor cells, blood vessels, immune cells, and stromal components, is critical for tumor development and treatment response.
Coculturing two-dimensional tumor cells with foreign immune cells has become a standard technique for preclinical trials of pertinent immunotherapeutic medicines as well as fundamental tumor immunology research. It has a large variety of assays and well-established evaluation methodologies in addition to allowing straightforward gene editing and medication intervention. Tumor cell profiling, however, does not capture the in vivo growth pattern of tumor cells. Traditional two-dimensional tumor cell cultures have significant limitations; they fail to accurately represent the growth patterns, heterogeneity, complexity, and individuality of patient-derived tumors observed in vivo. The 2D culture model cannot accurately reflect the three-dimensional growth patterns of tumor cells or the spatial and temporal distribution characteristics and interaction modes between tumor and immune cells in vivo. Additionally, 2D cultures cannot realistically reproduce the tumor microenvironment, including interactions with stromal and immune cells, often leading to a loss of cellular heterogeneity. Long-term passaging culture may result in genetic mutations and phenotypic variance in additional immune cells, as they are unable to replicate the temporal and spatial properties and interaction patterns of the TME (2).
Inflammatory and immunodeficient conditions have historically been linked to cancer susceptibility, indicating the central role of immunity in carcinogenesis. Immunotherapy-based revolutionary methods for cancer treatment have recently been shown to be efficacious. Both medicines and cells are known to have immunomodulatory effects. These immunotherapies have the ability to locally modify the tumor’s immunological milieu while also methodically enhancing the body’s immunity. Tumor antigen-targeted monoclonal antibodies, vaccines, therapies targeting pattern recognition receptors (PRRs), and other nonspecific small molecules, such as interleukins (ILs), interferons, and colony-stimulating factors, are among the immunotherapies that have been employed in clinical settings (3, 4). Adoptive cell therapy (ACT) and immune checkpoint inhibitors (ICIs) are two examples of immunotherapy that have altered the conventional paradigm for treating tumors (5–8). However, the number of patients available for participation in these studies is still quite limited. The primary cause is that patients can be inherently resistant to immunotherapy due to a variety of factors, including genetic mutations, the presence of certain biomarkers, variations in the tumor microenvironment, and differences in immune system functioning. This inherent resistance is difficult to demonstrate using conventional immunological research techniques, which do not adequately capture the complex interactions between tumor cells. To conduct basic and clinical translational research on tumor immunity, it is therefore vital to design new preclinical models that accurately replicate the human tumor immune milieu (9, 10).
Immune organoids are three-dimensional culture systems derived from immune cells or containing immune cell components. They are designed to mimic the structure and function of the immune system or its specific parts. Organoid culture systems have become important resources for researching the interactions between cancer cells and other elements of the tumor immunological microenvironment and for simulating the TME (11). For instance, polymorphonuclear myeloid-derived suppressor cells (PMN-MDSCs) have been demonstrated to increase tumor growth and limit the proliferation of cytotoxic T lymphocytes (CTLs), hence reducing the efficiency of checkpoint inhibition in murine and human-derived autologous organoid/immune cell cocultures (12). To mimic tumor-specific immune responses and prevent alloreactivity, autologous tumor-infiltrating lymphocytes (TILs) have been added to 3D organoid cultures obtained from patient-derived xenografts (PDXs) (13). Knowing how dendritic cells (DCs) and colorectal cancer (CRC) cells interact in the immunosuppressive TME may help researchers discover important pathways involved in tumor growth and dissemination for treatment (14). Organoid technologies for mimicking cancer have advanced significantly. One option is to use forward genetic techniques, which involve modifying induced pluripotent stem cells (iPSCs) or cells produced from wild-type tissues to have oncogenic or cancer-suppressive mutations (15, 16). However, the strong dispersion of patient-derived human tumor biopsies (PDOs) is now made possible via organoid techniques. The widespread use of 3D-PDO cultures has significantly influenced the field of in vitro cancer biology, resulting in the establishment of extensive tumor libraries that encompass the genetic and histological diversity of human malignancies. This paper reviews the function of organoids in immunotherapy and the tumor immune microenvironment.
Organoid research in reconstructing the tumor immune microenvironment
In organoids, the tumor immune microenvironment can be affected by two different methods (Figure 2). In reconstituted models, organoids containing exclusively tumor cells, often from physically and enzymatically separated tissues, are cultivated in extracellular matrix domes (e.g., Matrigel or BME-2) and submerged in tissue culture media. After being separated, exogenous immune cells—such as those from autologous peripheral blood or tumors—are cocultured with developed organoids. Without reconstitution, the intrinsic immunological milieu of tumor specimens is retained in holistic native TME models in addition to the tumor cells themselves. It is possible to combine collagen and tumor spheroids from digested tumor tissues for injection into microfluidic culture apparatuses. As an alternative, collagen gels are implanted in inner transwell dishes containing minced primary tissue pieces comprising both tumor cells and immunological components in air–liquid interface (ALI) culture. Because the top of the collagen gel is open to the air, cells can obtain enough oxygen to function. The effects of different culture models on the function and phenotype of various immune cell populations are shown in Table 1.
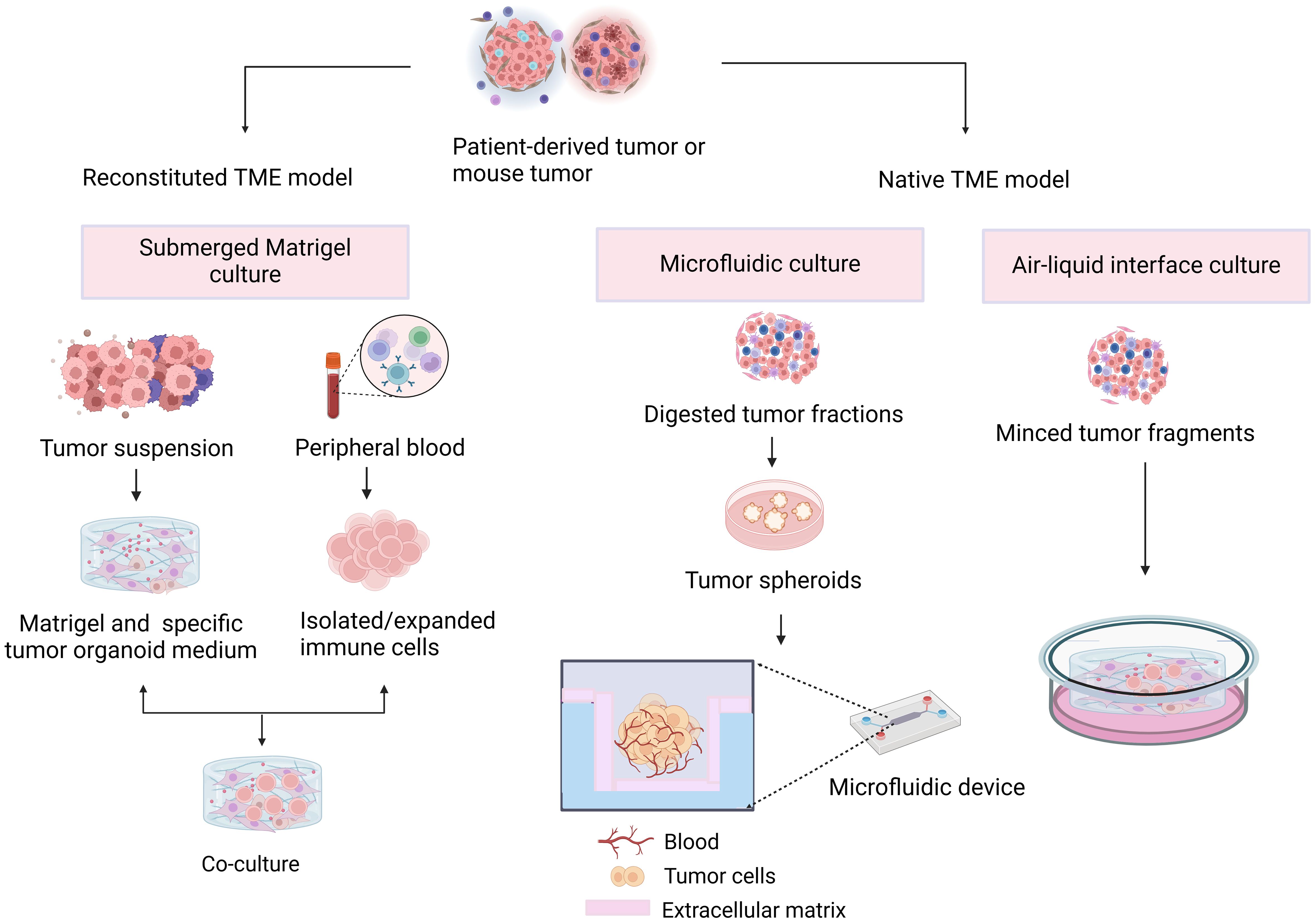
Figure 2 Different approaches to tumor immune microenvironment generation. In the reconstructed model, organoids containing tumor cells are cocultured with exogenous immune cells. In the nonreconstructed model, preservation of the inherent immune environment of the tumor specimen by injection or implantation of a collagen gel containing tumor cells and immune components.
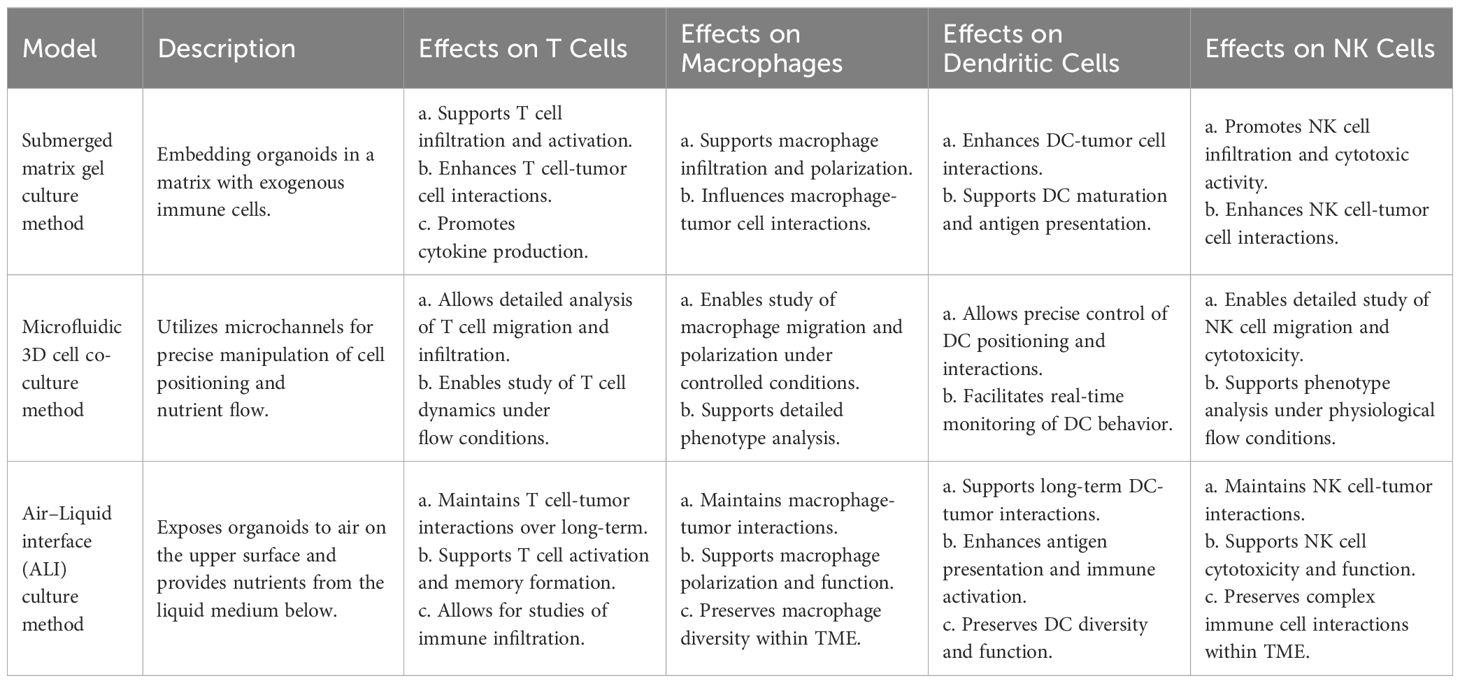
Table 1 Effects of different culture models on the function and phenotype of various immune cell populations.
3D bioprinting is also an advanced biocultural technology that uses layers of biomaterials to construct complex tissue and organ structures.
Coculture of tumor organoids with immune components
Organoid technology has progressed recently, offering more precise models to examine medication response, the TME, and tumor growth, revolutionizing research on cancer. In examining interactions between cells and between cancer cells within the TME, organoid models have proven to be especially useful (17). To investigate tumor-immune interactions and prospective therapeutic approaches for cancer treatment, coculture systems that include immune components and cancer organoids have become attractive.
By adding different elements, such as immune cells, cancer-associated fibroblasts, tumor vasculature, and other biological or chemical components, these organoid models seek to replicate the TME. Exogenous immune cells based on Matrigel deep culture must be added to immune organoid culture to model TMEs. The first method involves mixing organoids with the matrix and adding exogenous immune cells to the medium, which promotes indirect interactions. The second method is to first establish tumor organoids and, after a period of cultivation, dissociate the tumor organoids into single cells. At the same time, add lymphocytes extracted from peripheral blood to the dissociated tumor organoids, resuspend them in Matrigel, and re-embed them. The third method is to directly mix organoids and immune cells in the matrix and culture them in a dish (Figure 3). Additionally, the co-culture conditions need to be adjusted based on different tumor tissues and the added immune components (18). Table 2 provides a detailed comparison of the three methods for establishing tumor organoids with immune components, highlighting their respective advantages, disadvantages, and effectiveness in immune cell reconstruction.
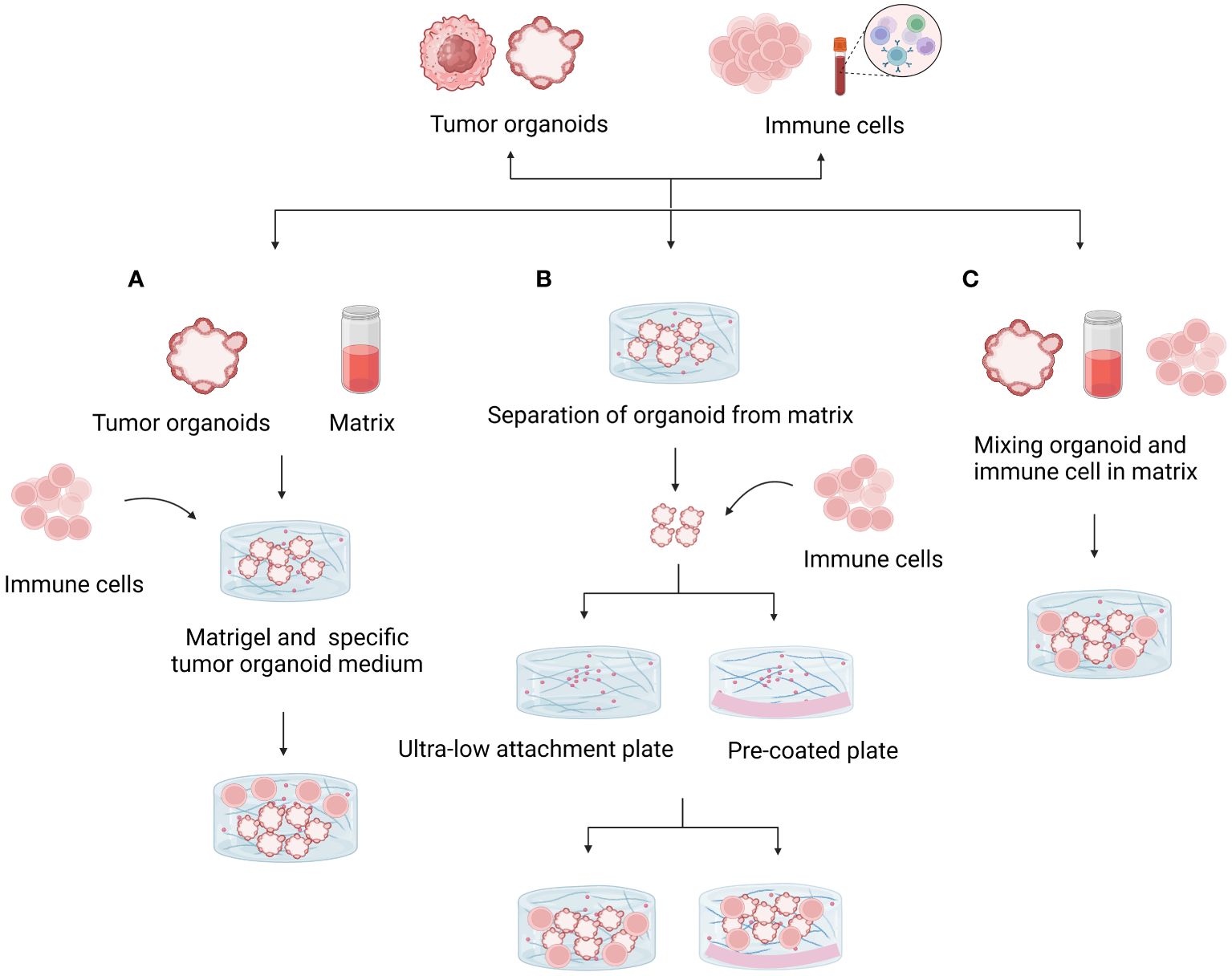
Figure 3 Methods for coculturing tumor organoids and immune cells. (A) Mixing the organoid and matrix and adding exogenous immune cells to the medium promoted indirect interactions. Matrix dilution facilitates immune cell infiltration and direct contact with tumor cells. (B) Isolated organoids were mixed with suspended immune cells to study direct interactions. The use of ultralow adhesion culture plates or plates precoated with substrate prevents organ adhesion and death. (C) Mixing organoids and immune cells directly in the matrix and culturing them in culture plates is a useful model for studying interactions.
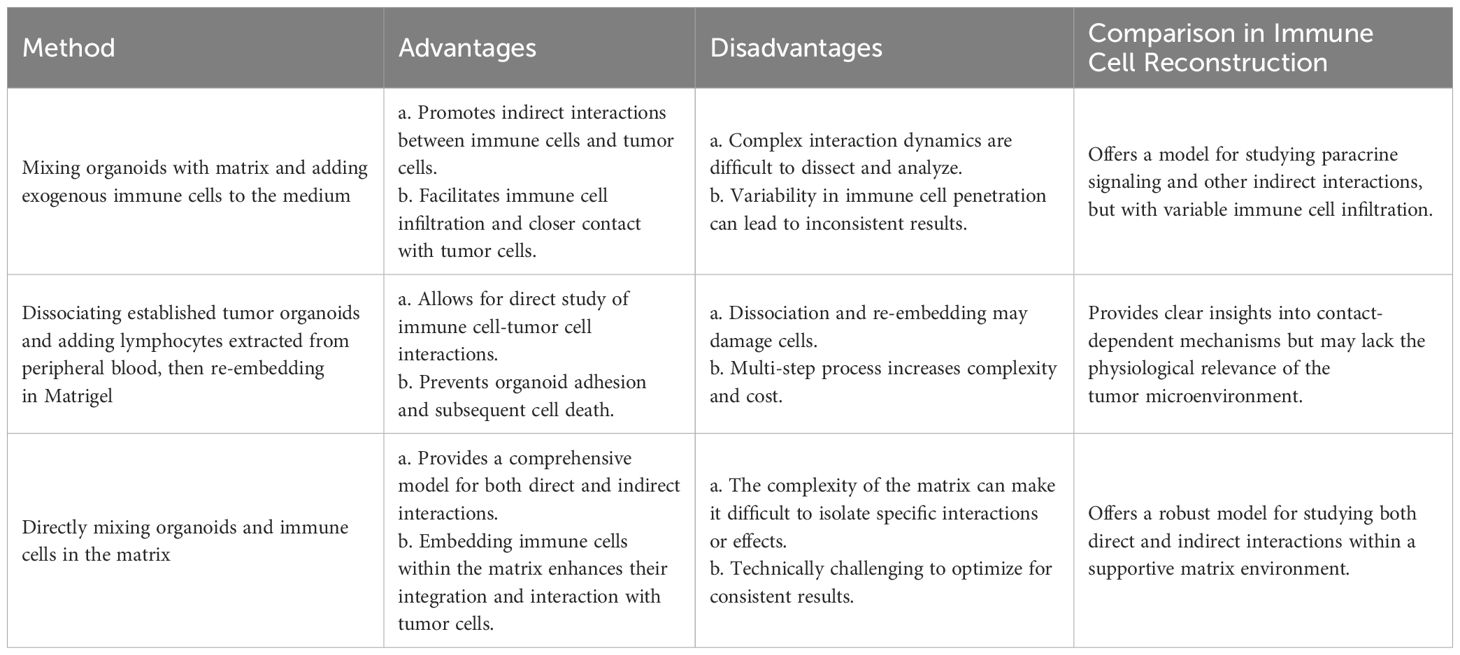
Table 2 Comparison of methods for establishing tumor organoids with immune components: advantages, disadvantages, and immune cell reconstruction.
The success of targeted therapeutics has been predicted using autologous pancreatic cancer organoid/immune cell cocultures produced from mice and humans, underscoring the importance of including immune components in preclinical models (12). Further insight into immunosuppression in cancer has come from the addition of immune cells to organoid models. The incorporation of immune cells and stromal components into three-dimensional organoid models has made it possible to gain a more thorough understanding of the TME and its function in immunosuppression (19). The significance of immune cell contacts in the progression of cancer has been highlighted by the characterization of angiocrine crosstalk and the generation of an inflammatory TME using coculture models of hepatocellular carcinoma organoids with endothelial cells (20). Additionally, the creation of coculture techniques utilizing immune cells and patient-derived cholangiocarcinoma organoids has demonstrated potential in simulating anticancer immunity in vitro (21). The significance of these organoid-immune coculture models in furthering oncoimmunology research is highlighted by their ability to clarify tumor-specific immune interactions and direct customized immunotherapies (22). To summary, the incorporation of immune components into cancer organoid coculture models has yielded significant insights into immunosuppressive mechanisms, tumor-immune interactions, and possible therapeutic approaches. These models could increase our knowledge of the biology of cancer and direct the creation of tailored immunotherapies to improve patient outcomes (23).
Microfluidic 3D culture
Microfluidic or 3D materials, which are more adaptable for imitating the TME, are placed into the channels and chambers of the microfluidic culture system (24). Controlling fluid flow and volume, as well as designing microchannels, can enhance the quality of organoids. It facilitates the mass manufacture of organoids and supports uniformity and controllability (25). Microfluidic chambers make up the microfluidic core. Every microfluidic chamber features a media conduit on both sides in addition to a center gel area. To create microspheres comprising tumor cells, immune cells, and stromal cells, fresh tumor tissue must first be chopped and beaten. Subsequently, the tumor microspheres are doped into a hydrogel made of collagen and injected into the center of the gel, strolling through the middle channel. Using a microfluidic device, Ahn et al. (26) created a bone-mimetic microenvironment to study the interactions between tumor cells and hydroxyapatite (HA) in a three-dimensional composite. The significance of building a three-dimensional TME for researching cell-cell interactions is emphasized by this work. The transition from planar to 3D cell culture on scaffolds, as well as the use of cell scaffolds in microfluidic devices, was covered by Castiaux et al. (27). Microfluidic platforms investigate a variety of scaffold types, including suspension culture, hydrogel scaffolds, paper-based scaffolds, and fiber-based scaffolds. Mulas et al. (28) presented a technique that uses hydrogel bead compartmentalization to encapsulate living cells in a 3D hydrogel matrix. This method highlights the versatility of 3D cell encapsulation in microfluidic devices by allowing retrieval for molecular and functional tests as well as monitoring of cellular dynamics. A quantitative image-based cell viability (QuantICV) assay was proposed by Ong et al. (29) for microfluidic 3D tissue culture applications. This assay method is a useful tool for drug testing applications because it overcomes the difficulties associated with conducting quantitative cell viability studies in microfluidic 3D tissue cultures. Specifically, the QuantICV assay employs advanced techniques such as high-throughput screening, which allows for the simultaneous automation of multiple assays to obtain precise and reliable data. It also utilizes fluorescent and luminescent dyes that bind to live or dead cells, enabling sensitive detection and accurate measurement of cell viability. Additionally, real-time monitoring through live-cell imaging systems provides dynamic data by tracking changes in cell viability over time. In summary, combining 3D cell culture methods with microfluidic technology is a viable strategy for examining cell behavior in a setting that is more physiologically appropriate.
Air–liquid interface culture
The technique of physically splitting tumors into tissue fragments and then growing them in a cell culture chamber coated with collagen gel is known as ALI culture. This paradigm allows tumor cells to proliferate properly and create tumors that mimic the original tumor, preserving the pathological features and genetic alterations of the original tumor, in contrast to the limitations of the coculture model in analyzing the TME. The top of the gel is open to the outside, and a permeable cell culture chamber allows the medium from the outer dish to permeate into the inner dish, forming an air-liquid interface that helps the cells obtain enough oxygen. This strategy maintains the intricate histological structure of the tumor parenchyma and mesenchyme in the TME, together with a range of naturally occurring immune cells, including functional TILs, and encourages tumor growth in its native state (30).
Initially, ALI organoids established from normal tissues of different sites, including the small intestine, colon, stomach, and pancreas, included both epithelial and stromal components. Subsequently, the ALI organoid method has been used to culture organoids from human biopsy tissues, such as melanoma, renal cell carcinoma, and non-small cell lung cancer (NSCLC), as well as syngeneic mouse tumor organoids with immune reactivity (30). ALI PDOs not only retain the genetic alterations of the original tumor but also preserve the complex cellular composition and structure of the TME. Both the tumor parenchyma and stroma are maintained, including fibroblasts and various endogenous infiltrating immune cell populations.
Organoid air-liquid interface cultures are being increasingly used in a variety of scientific fields. Ao et al. (31) created a microfluidic technology that includes perforable culture chambers and an air-liquid interface to simulate the effects of cannabis exposure during pregnancy on the development of the human brain. This platform enables the creation of many organoids without fusing or streamline fabrication processes. To better understand the pathophysiology of COVID-19, Lamers et al. (32) proposed a human 2D air-liquid interface culture system for studying SARS-CoV-2 infection of human alveolar type II-like cells. Sette et al. (33) investigated cystic fibrosis in vitro using air-liquid interface cultures and organoid models made from patient-derived nasal epithelial stem cells, and demonstrated that these models are appropriate for pharmacological testing of cystic fibrosis transmembrane conductance regulator (CFTR). The significance of three-dimensional culture for kidney organoids to better replicate in vivo conditions and adjust the extracellular matrix composition was emphasized by Geuens et al. (34). Using an air-liquid interface method, Cham et al. (35) reported the creation of a highly biomimetic organoid culture system that resembles native immature testis tissue, including the vasculature. Additionally, Boecking et al. (36) created human airway epithelial organoids with apical membranes oriented externally. These organoids and air-liquid interface cultures displayed identical gene expression profiles associated with bronchial development and ion transport. A different technique for growing airway organoids formed from nasal brushing from a 2D air-liquid interface culture was published by Amatngalim et al. (37), which made it possible to consistently detect CFTR modulator responses in patients with cystic fibrosis. Overall, research and treatment development can benefit greatly from the literature’s depiction of the adaptability and usefulness of organoid air-liquid interface culture in simulating a range of biological processes, illnesses, and pharmacological reactions.
3D bioprinting
The structuring of droplets on a surface coated with cells is the basis for the 3D tissue reconstruction technique known as bioprinting. A matrix of extracellular and tumor cells is present in these droplets. This concept has the advantage that the tissue can be fully rebuilt and that the droplets can be mechanically or chemically treated to acquire the appropriate tissue characteristics. As a result, bioprinting enables extremely accurate tissue and cell implantation. To enhance the printing of brain tumor organoids, Clark et al. (38) developed a bioprinting technique that includes monitoring the behavior of the supporting fluid. Frankowski et al. (39) addressed problems pertaining to the establishment of biological connections and the comprehension of tissue structure and discussed the use of 3D bioprinting to create human tissues and organoids for preclinical pharmacological investigations. The state of the art in organoid bioprinting was discussed by Cabral et al. (40), along with its possible applications in regenerative medicine, drug discovery, and tissue engineering.
Ultimately, Shrestha et al. (41) developed a novel microarray 3D bioprinting technique intended to generate human liver organoids in a repeatable way, resolving the technical drawbacks of conventional culture techniques and enabling a thorough assessment of the cumulative effects of hepatotoxicity. The body of research on organoid 3D bioprinting indicates notable advancements in printing technology, biolinking, and applications across a range of biological domains.
Organoid research in tumor immunotherapy
The microenvironment around tumor cells functions as a unit. The microenvironment engages in interactions and coevolution with tumor cells, exerting a significant influence on various aspects of carcinogenesis and development. As a result, research on the interactions between tumors and their microenvironment provides theoretical groundwork for the development of novel therapeutic targets and novel strategies for tumor immunotherapy and aids in our understanding of the biological behavior of tumors (Figure 4).
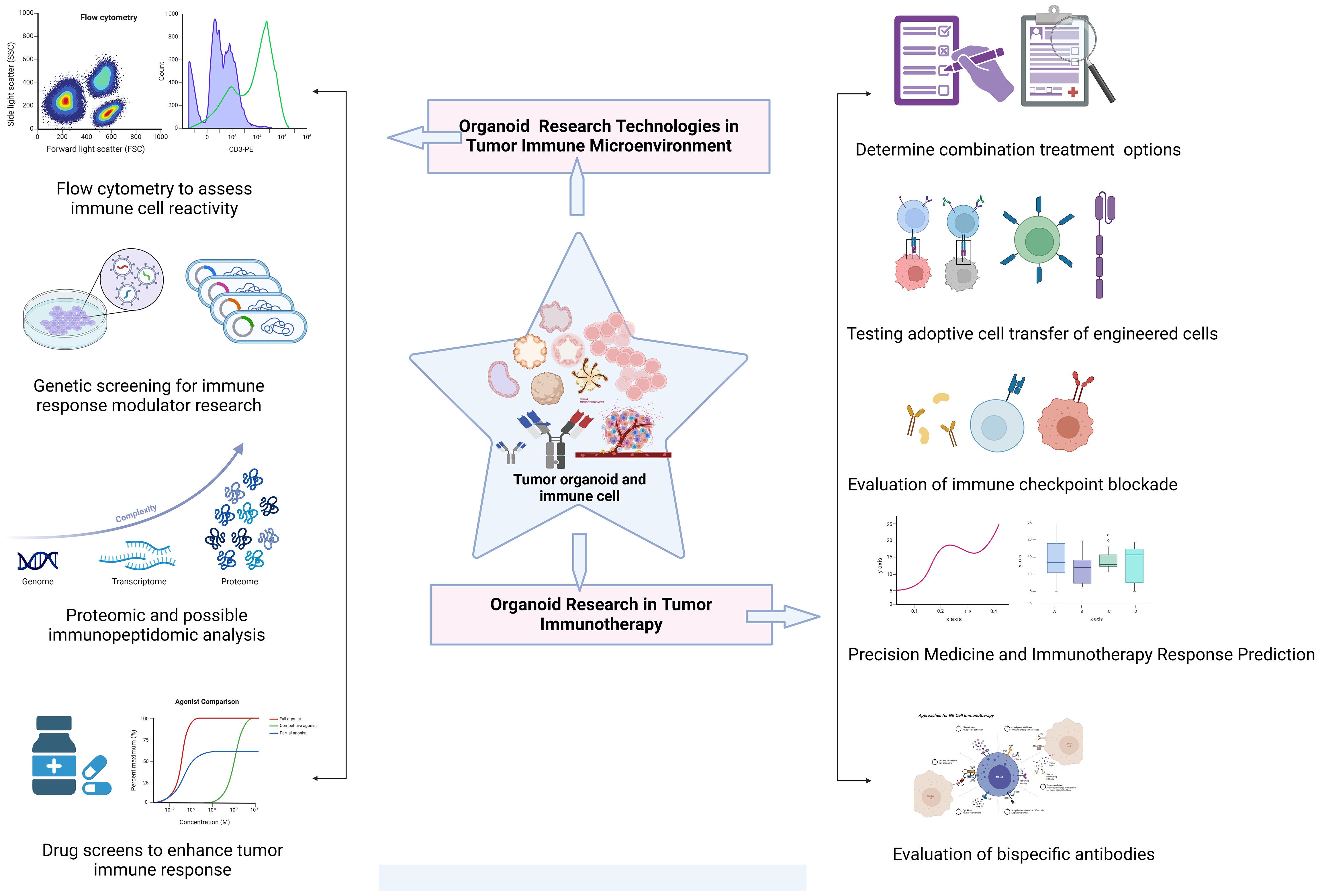
Figure 4 Exploration of common technologies and applications of organoid systems in tumor immunotherapy research. Tumor immunotherapy research using organoid systems expand the frontiers of personalized therapy and provides an important experimental platform for exploring novel immunotherapies.
Immunotherapy assessment
Numerous promising therapeutic outcomes of immunotherapy have been identified thus far due to intrinsic and acquired resistance, and it is evident that certain tumor tissues are immune checkpoint resistant. Organoids may be used as viable substitutes for in vivo techniques, enhancing the efficacy of already available immunotherapies and increasing the viability of novel strategies.
Preclinical testing of immunotherapy medications for lung cancer and other cancer types can be carried out by researchers by imitating immunotherapy responses using organoid culture platforms (42). Organoid models produced from patients have been especially useful in the evaluation of immunotherapies for melanoma (43). These models allow immune cells within the organoids or new tumor samples to be assessed, which offers important insights into how the immune system reacts to therapy. Furthermore, organoids have been effectively developed for the treatment of breast cancer and are used as in vitro models to assess immunological responses and medication sensitivity (44). Moreover, models of organoid culture have been created to facilitate the repetitive assessment of immune cell interactions and tests for killing in vitro (45). Organoid models have been used in the setting of lymphoma to replicate the death of cancer cells by T cells upon treatment with bispecific immunotherapies (46). Comparably, a tumor organoid platform that engages T cells has been created for pancreatic cancer immunotherapy, enabling monitoring of the immune profile and assessment of the effectiveness of the treatment (47). In conclusion, organoids produced from patients have proven to be useful instruments for assessing immunotherapies in different kinds of cancer.
Immunotherapy of engineered cells
Adoptive cell immunotherapy typically involves isolating immune cells from tumor patients, expanding and functionally characterizing them ex vivo, and then reinfusing them into the patient. Through genetic engineering, T cells can be combined with chimeric antigen receptors (CARs), and engineered chimeric antigen receptor T (CAR-T) cells can recognize tumor antigens, thereby achieving the goal of directly killing tumor cells or stimulating the body’s immune response to eliminate tumor cells.
Innovative organoid-T-cell coculture systems that offer a physiologic depiction of the interactions within the TME have been developed by Hubrecht Organoid Technology (HUB) (48). These HUB organoids, derived from both healthy and sick tissues, provide an excellent and repeatable platform that replicates the intricate features of the original parental tissue, such as functional properties and molecular heterogeneity. By evaluating T-cell cytotoxicity against tumor organoids and viewing and quantifying T-cell-tumor interactions, HUB’s organoid-T-cell coculture method facilitates the evaluation of novel therapeutics. This platform has promise as a robust instrument for the advancement and verification of cancer immunotherapy, encompassing immune checkpoint inhibition and bispecific antibody administration. Furthermore, TILs and CAR-engineered T cells may be able to function in cancer organoid-T-cell culture systems, providing prospects for preclinical development and customized therapeutic approaches. By offering a customized method for researching immune cell types and adoptive therapy, these organoid models may increase the effectiveness of immunotherapy treatments (49). Additionally, organoid models have been used to investigate adoptive cell immunotherapy and immune reconstitution, demonstrating their potential to advance cancer treatment approaches (50). In summary, organoid adoptive cellular immunotherapy is a potentially useful way to address the difficulties involved in converting preclinical research into viable cancer treatments.
Immune checkpoint inhibitor response
A group of molecules known as immune checkpoint molecules controls the immune system and suppresses immune cells. They are crucial for immune system induction, self-tolerance maintenance, and the prevention of autoimmune disorders. Nevertheless, malignant tissues can use these spots to bypass the immune system, meaning that immune checkpoint protein molecules are ineffective in assisting the body’s defense against cancer. ICIs, often referred to as immune system counterpoint inhibitors, are medications that inhibit the immune system with the dual goals of increasing a patient’s immunity against malignancies and facilitating the immune system’s ability to escape from them.
Research has indicated that coculturing immune cells and cancer organoids can improve our understanding of immune checkpoint blockade treatments. For example, coculturing immune cells treated with bispecific anti-PD-1/PD-L1 antibodies with high-grade serous ovarian cancer organoids showed improved efficacy compared with coculturing immune cells treated with monospecific antibodies (51). However, because each patient has a unique resistance mechanism, not every patient responds to this medication. Research has concentrated on understanding the molecular correlates and genetic context of the immune checkpoint inhibitor response in various cancer types. A study conducted on Merkel cell carcinoma (MCC) sought to determine clinical genomic indicators linked to the immune checkpoint inhibitor response as well as to define the molecular landscape (52). Furthermore, changes in PBRM1 have been confirmed to be a reliable indicator of the immune checkpoint inhibitor response in renal cell carcinoma (RCC) (53). One important element determining the response to ICIs and targeted therapy is the TME. To optimize checkpoint inhibitor-TKI combinations in RCC, an ex vivo 3D tumor organoid model has been used, which has shown differences in T-cell activation and tumor cell killing efficacy among patient samples (54). Additionally, research has investigated the use of cutting-edge strategies to strengthen the immune response to ICIs. To enhance anticancer immunity, for example, a transdermal administration of ICIs mediated by cold atmospheric plasma (CAP) and microneedles has been devised (55). Research has concentrated on identifying biomarkers and possible treatment targets to enhance the immune checkpoint inhibitor response, in addition to investigating the TME and innovative delivery strategies. By targeting sialoglycans that prevent immune cell activation, targeted glycan degradation has been suggested to enhance the anticancer immune response (56). Additionally, to surmount genetic heterogeneity and immune evasion that restrict the therapeutic response to ICIs, an organoid-based screen has been employed to find epigenetic inhibitors that enhance antigen presentation and amplify T-cell-mediated cytotoxicity in breast cancer (57). Overall, the creation of immune organoids as preclinical models has provided researchers with a platform to investigate the TME and direct precision oncology treatment approaches. Researchers intend to increase the effectiveness of ICIs and broaden their use in the treatment of cancer by comprehending the processes underlying resistance to ICIs and investigating cutting-edge tactics to boost the immune response (58).
Precision medicine and immunotherapy response prediction
Regarding immunotherapy response prediction, precision medicine is a rapidly developing discipline with enormous potential for tailored cancer treatment. The process of developing and implementing personalized immunotherapies is illustrated in Figure 5. Recent studies have highlighted the importance of biomarkers for predicting the efficacy of ICIs in cancer immunotherapy. One biomarker that has been proposed to be potentially significant in predicting the response to ICIs is the fecal microbiota (59). In individuals with recurrent or refractory classical Hodgkin lymphoma, circulating tumor DNA has also been found to be a predictive factor for therapeutic response (60).Finding predictive and prognostic biomarkers in the field of gastric cancer (GC) is now crucial for developing precision treatment plans (61). Given that GC is now understood to be a diverse disease, biomarkers are essential for determining which therapeutic strategy would work best for each patient.
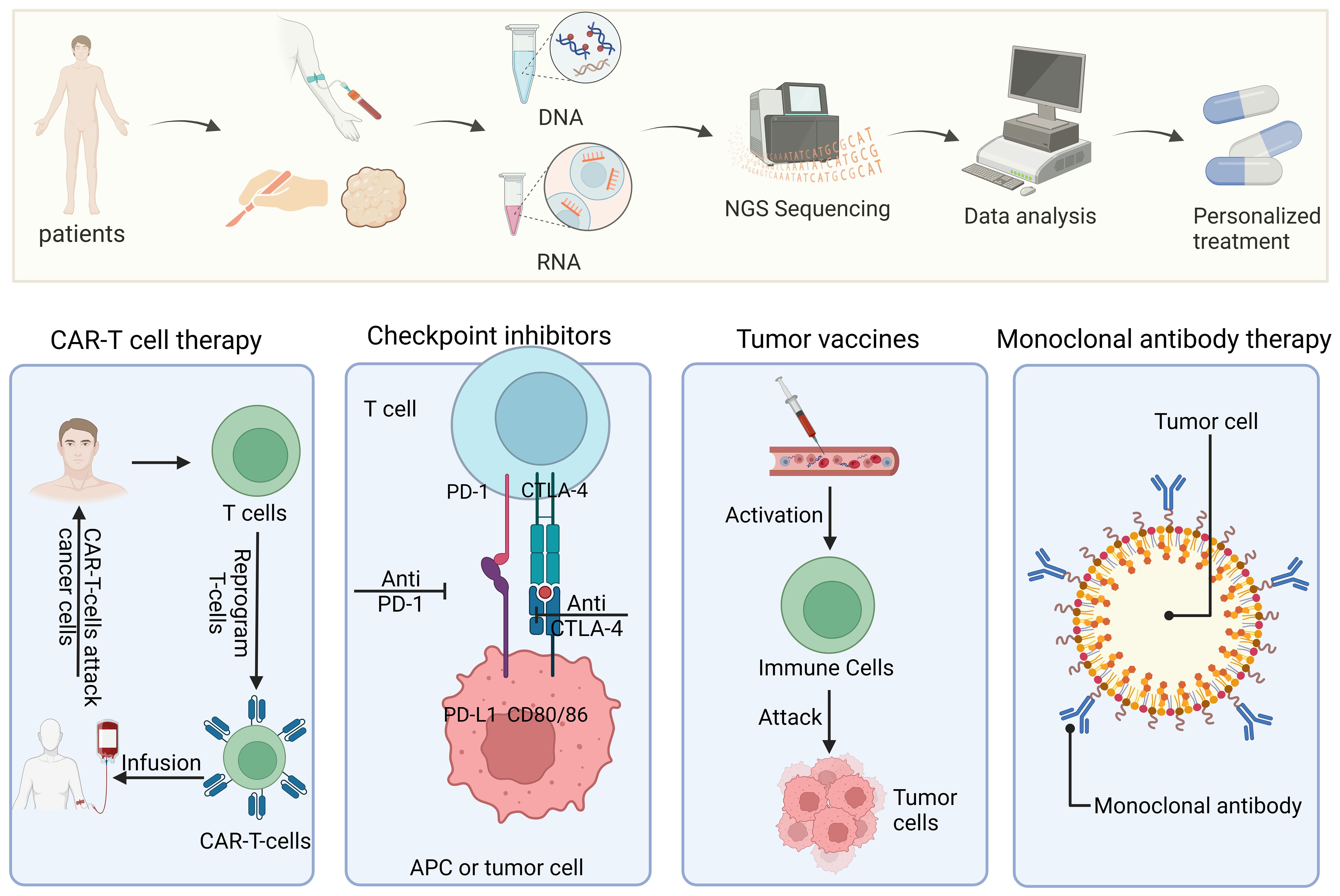
Figure 5 Precision medicine and personalized treatment process for immunotherapies. The diagram shows the entire process from patient sample collection and genome sequencing to personalized treatment plan formulation and implementation of different types of immunotherapies (such as CAR-T cell therapy, checkpoint inhibitors, tumor vaccines, and monoclonal antibody therapy).
Similarly, biomarkers have proven crucial in facilitating precise diagnosis and customized treatment regimens in allergic disorders and asthma (62). By offering insights into active tumor driving signal transduction pathways, developments in RNA-based diagnostic assays have also aided in the development of precision oncology techniques (63). These tests assist oncologists in selecting the most successful tailored therapy for patients by monitoring the activity of pertinent signaling pathways in tissue samples and circulating tumor cells. Using an in vitro system to culture numerous tumor tissues obtained from patients, Voabil et al. (64) introduced PD-1 antibodies to the culture system and measured the levels of four T-cell activation indicators, thirteen chemokines, and thirteen cytokines before and after adding the medication. The study revealed that the clinical responses of patients and cancer tissue response scores to therapy were quite similar, indicating that the model may be able to predict the early efficacy of PD-1 antibodies.
PDOs have become important instruments in precision oncology, enabling patients with hepatocellular carcinoma (HCC) to anticipate their response to treatment. Organoid-on-a-chip systems that are micro-engineered have been created to replicate the microenvironment of tumors and enable high-throughput drug screening for customized treatment approaches (65). The application of PDOs to predict patients’ individualized responses to conventional treatments is a hot research topic. Due to the difficulty in identifying biomarkers that can predict responses to immunotherapy, selecting the appropriate patient cohort to improve clinical response rates to ICIs remains challenging. However, comprehensive organoid culture systems, which include the TME, may have potential special roles.
In the patient-derived organotypic tumor spheroids (PDOTS) microfluidic culture containing tumor cells and autologous immune components, a one-week evaluation of T-cell-mediated tumor cell killing, and cytokine analysis showed that this model could predict or assess patients’ responses to ICI therapy (66). ALI organoids can also be used to assess T-cell function through flow cytometry, fluorescence staining, and tumor killing assays, thereby simulating responses to immune checkpoint inhibition. While the correlation of these predictive methods with clinical outcomes still needs to be validated, identifying cohorts with the best responses to immunotherapy through this platform provides substantial opportunities for clinical translation.
Oncolytic virus therapy
A particular type of virus known as an oncolytic virus is capable of selectively infecting and killing cancer cells. They can propagate viruses to neighboring cells, obliterate tumor cells directly, and inhibit tumor-free host cells. This can stimulate the immune system and cause the release of cytokines (67). Oncolytic measles virus therapy has been shown by Packiriswamy et al. to boost T-cell responses against tumor-associated antigens in multiple myeloma patients (68). The use of oncolytic herpes simplex virus type 1 (HSV-1) for hematological malignancies was also investigated by Ishino et al. (69), who successfully killed a variety of cell lines generated from hematological malignancies. According to Elsedawy et al. (70), developments in oncolytic viral therapy have resulted in the creation of tailored picornaviruses that are designed as synthetic infectious RNAs. Furthermore, Bots et al. (71) investigated the use of adenoviruses derived from NHPs as possible oncolytic agents, emphasizing the significance of comprehending the distinctions and similarities between adenoviruses derived from NHPs and humans for prospective therapeutic applications.
Additionally, as Sugawara et al. (72) have shown, the combination of immune checkpoint inhibitors with oncolytic viral therapy has shown encouraging results in increasing antitumor responses. The use of a triple-mutated oncolytic herpes virus to treat cancers that grow quickly and slowly was also studied by Fukuhara et al. (73), emphasizing the significance of creating effective and safe oncolytic viruses for various tumor types. In general, enhancing the effectiveness of oncolytic virus therapy in treating different forms of cancer requires an understanding of the mechanisms by which these viruses modulate the immune response as well as the combination therapies they employ with ICIs. Raimondi et al. (74) established normal pancreatic organoids and pancreatic ductal adenocarcinoma (PDAC) tumor organoids to test the feasibility of using organoids as a screening platform for oncolytic adenovirus therapy. The results showed that the oncolytic adenovirus exhibited good selectivity, replicating only in PDAC organoids. This study also demonstrated the cytotoxicity of the oncolytic adenovirus and the individual variability in its synergistic effect with standard chemotherapy, indicating that organoids can serve as an appropriate model for preclinical responses to oncolytic virus therapy.
Moreover, in a study based on breast cancer (BC) organoids, researchers tested the efficacy of the measles vaccine virus and vaccinia virus for BC oncolytic therapy. The results suggested that all oncolytic viruses significantly inhibited the survival of BC organoids (75). The organoid platform can help in testing and designing oncolytic virus treatment regimens. However, the current research on oncolytic viruses mainly utilizes tumor organoid platforms. Tumor organoids have shown great potential in assessing the infectivity and cytotoxicity of oncolytic viruses, but there is a lack of experimental studies on the immune response elicited by oncolytic viruses. The application of immune organoids may bring new hope for oncolytic virotherapy.
Therapeutic application of organoids in recent advanced treatment
Considered to be a breakthrough moment with great potential for customized medicine and cancer biology as a preclinical human tumor model, PDO technology has lately surged. Ma et al. (76) demonstrated the potential of organoids in the treatment of disease by creating an epithelial and mesenchymal organoid model obtained from human induced pluripotent stem cells for Localized Scleroderma (LoS) therapy. Similarly, Sahoo et al. (77) highlighted the potential of organoids to predict patient-specific responses to therapy by demonstrating the use of PDOs as an ex vivo model for the treatment of urothelial carcinoma (UC). Furthermore, Zhao et al. (78) demonstrated the promise of organoids in developing drug delivery systems for cancer treatment by proposing the use of nanotechnology and organoids to create cancer nanomedicines. Furthermore, the development of epidermal organoids (EpiOs) from iPSCs for skin regeneration was described by Kwak et al. (79), highlighting the therapeutic potential of extracellular vesicles (EVs) formed from organoids in regenerative medicine. Future developments in technology may lead to the widespread use of PDO models in cancer diagnosis and treatment, and more patients may gain from the use of the functional PDO assay in precision radiotherapy.
Discussion
Organoids are emerging as a new technology in the field of antitumor immunology research, but they are still evolving. Immunocompetent cancer-on-chip (iCoC) systems with integrated immune components have surfaced in the last few years. The parameters of the sophisticated iCoC model TME may be precisely adjusted to more closely resemble the intricate relationships and dynamics that exist between immune system components and human tumor cells. The iCoC has been effectively applied in numerous immunotherapy studies. ICoC has been applied successfully in numerous immunotherapy studies (80).
Even while organoids show great promise for both clinical and basic cancer research, there are still a few difficult obstacles that need to be overcome. Initially, it is expensive to establish, maintain, and age organoids. Secondly, the organoids generated from the tissue samples are merely a tiny fraction of the whole tumor. Because tumors vary widely, it is unclear how reliable it is to use small portions of tumor tissue in place of the entire tumor. Translational cancer research can be reliably facilitated, and tumor heterogeneity better reflected by removing tissue from multiple regions of the same tumor. Thirdly, the complexity of immunological environments unique to each patient is difficult for present organoid technology to mimic. While co-culture systems containing immune and tumor-like cells have improved the modeling of tumor-immune interactions and their effect on therapy, several issues could prevent precise modeling and prediction of immunotherapy responses. For example, the immune components and cell counts of distinct tumor forms vary, which influences the immune cell composition in the early phases of tumor-like culture and the selection for preserving and growing these immune cells. While some tumor types only have immune cells in the surrounding stroma or do not have any immune cells at all, other tumor types do have numerous complicated types of immune cells.
There is no single model system that can perfectly replicate a tumor; instead, the tumor immune microenvironment is a complex, highly diverse, and dynamic system of numerous immune cells and stromal cells. Similar to the organoid/immune cell coculture model, there is a greater restriction on the kinds of immune cells that can be added externally. Additionally, the condition of the extraimmune cells varies greatly from that of the original TME and is not consistent with that of the original cells in the immune microenvironment. Additionally, immune cell survival and cellular activity may be hampered by the inability of organoid media to maintain immune cell function at its peak. On the other hand, in models of ALI or microfluidic culture, some of the initial immune cells that infiltrate the tumor remain within the organoid. These immune cell fractions are more likely to be lost during passaging or freeze-thawing and are only preserved for a brief period of time. As such, these techniques are limited to research that lasts a short time. More significantly, circulating immune cells interact intricately with tumor and tumor-infiltrating immune cells, and the tumor immunological microenvironment is constantly changing. These models do not account for circulating immune cells; instead, they consider only the local immunological profile of the TME. In immune organoid research, modeling susceptibility and resistance to immunotherapies—which include checkpoint inhibition and new pathways—represents a significant translational hurdle. Organoids can not only clarify possible resistance mechanisms but also support therapeutic endeavors such as drug screening and optimization of in vitro and cellular immunotherapies.
Multiple microenvironmental components could be integrated into the same system in future studies. Ultimately, the goal of the study should be to determine which modeling system is most appropriate, and the benefits and drawbacks of each system should be balanced. A greater technological investment should be made in TME components such as vascular and neural populations. Future improvements could be made to in vitro 3D models to improve tumor immunology research and clinical translation by more closely resembling the original tumor both physically and functionally.
Summary and future perspectives
In addition to retaining the genomic and genetic characteristics, heterogeneity, passage stability, and the ability to be massively expanded in vitro, tumor organoids can also undergo gene editing without ethical issues. Therefore, this platform has significant advantages in evaluating combined immunotherapy strategies for cancer patients, screening new immunotherapy methods, and identifying biomarkers, making personalized precision treatment for patients possible.
Furthermore, tumor organoids derived from liquid biopsies are not limited by tumor location and can be dynamically observed at different stages of tumor progression or before and after treatment. Organoid technology enables the visualization of tumor growth processes.
Combining organoid culture technology with organ-on-a-chip technology allows for the integration of multiple components of the TME, aiming to study the intercomponent communication within this microfluidic system. This approach ensures controllable and reproducible organoid cultures and, to some extent, achieves organoid vascularization. Utilizing 4D imaging technology allows for the dynamic monitoring of live cell interactions between different cell subsets, which is particularly important for studying immunodynamics in co-culture systems.
Immune organoids have great potential in simulating the effects of immunotherapy, studying resistance mechanisms, and developing new combination treatment strategies. With continuous optimization of culture protocols, immune organoids are expected to drive the clinical translation of tumor immunotherapy, ultimately achieving personalized immunotherapy. Current and future organoid methodologies will greatly advance basic science and clinical research in immuno-oncology. It is anticipated that this approach will provide significant therapeutic benefits for patients, paving the way for promising breakthroughs in human tumor immunotherapy.
Author contributions
YY: Writing – review & editing, Writing – original draft, Software, Resources, Project administration, Methodology, Formal analysis, Conceptualization. JC: Writing – review & editing, Visualization, Project administration, Investigation. YK: Writing – review & editing, Investigation, Conceptualization. YH: Writing – review & editing, Resources, Methodology. CM: Writing – review & editing, Software, Methodology, Investigation, Data curation.
Funding
The author(s) declare financial support was received for the research, authorship, and/or publication of this article. The work was supported by grants from the National Natural Science Foundation of China (No. 81971474), Hebei Key R&D Program Project Special Project for the Construction of Beijing-Tianjin-Hebei Collaborative Innovation Community (No. 22347702D), and Key Project of Natural Science Foundation of Hebei Province (C2021206011).
Acknowledgments
Springer Nature remains neutral regarding jurisdictional claims in published maps and institutional affiliations.
Conflict of interest
The authors declare that the research was conducted in the absence of any commercial or financial relationships that could be construed as a potential conflict of interest.
Publisher’s note
All claims expressed in this article are solely those of the authors and do not necessarily represent those of their affiliated organizations, or those of the publisher, the editors and the reviewers. Any product that may be evaluated in this article, or claim that may be made by its manufacturer, is not guaranteed or endorsed by the publisher.
Abbreviations
TME, tumor microenvironment; PRR, pattern recognition receptor; ILs, interleukins; ACT, adoptive cell therapy; ICIs, immune checkpoint inhibitors; PMN-MDSCs, polymorphonuclear myeloid-derived suppressor cells; CTLs, cytotoxic T lymphocytes; TILs, tumor-infiltrating lymphocytes; PDX, patient-derived xenografts; DCs, dendritic cells; CRC, colorectal cancer; PDOs, patient-derived human tumor biopsies; ALI, air–liquid interface; HA, hydroxyapatite; QuantICV, quantitative image-based cell viability; NSCLC, non-small cell lung cancer; CFTR, cystic fibrosis transmembrane conductance regulator; CARs, chimeric antigen receptors; CAR-T, chimeric antigen receptor T cells; HUB, Hubrecht Organoid Technology; MCC, Merkel Cell Carcinoma; RCC, renal cell carcinoma; CAP, cold atmospheric plasma; GC, gastric cancer; HCC, hepatocellular carcinoma; PDOTS, patient-derived organotypic tumor spheroids; HSV-1, herpes simplex virus type 1; NHPs, nonhuman primates; PDAC, pancreatic ductal adenocarcinoma; BC, breast cancer; UC, urothelial carcinoma; EpiOs, epidermal organoids; iPSCs, pluripotent stem cells; EVs, extracellular vesicles; iCoC, Immunocompetent cancer-on-chip.
References
1. Palucka AK, Coussens LM. The basis of oncoimmunology. Cell. (2016) 164:1233–47. doi: 10.1016/j.cell.2016.01.049
2. Teicher BA. In vivo/ex vivo and in situ assays used in cancer research: A brief review. Toxicol Pathol. (2009) 37:114–22. doi: 10.1177/0192623308329473
3. Houot R, Kohrt HE, Marabelle A, Levy R. Targeting immune effector cells to promote antibody-induced cytotoxicity in cancer immunotherapy. Trends Immunol. (2011) 32:510–6. doi: 10.1016/j.it.2011.07.003
4. Demaria O, Cornen S, Daëron M, Morel Y, Medzhitov R, Vivier E. Harnessing innate immunity in cancer therapy. Nature. (2019) 574:45–56. doi: 10.1038/s41586-019-1593-5
5. Sharawy I. Neuroimmune crosstalk and its impact on cancer therapy and research. Discov Oncol. (2022) 13(1):80. doi: 10.1007/s12672-022-00547-5
6. Li J, Xuan S, Yu X, Xiang Z, Gao C, Li M, et al. Immunotherapy of hepatocellular carcinoma: recent progress and new strategy. Front Immunol. (2023) 14:1192506. doi: 10.3389/fimmu.2023.1192506
7. Cole K, Al-Kadhimi Z, Talmadge JE. Highlights into historical and current immune interventions for cancer. Int Immunopharmacol. (2023) 117:109882. doi: 10.1016/j.intimp.2023.109882
8. Velasco RM, García AG, Sánchez PJ, Sellart IM, Sánchez-Arévalo Lobo VJ. Tumour microenvironment and heterotypic interactions in pancreatic cancer. J Physiol Biochem. (2022) 79(1):179–92. doi: 10.1007/s13105-022-00875-8
9. Riedl A, Schlederer M, Pudelko K, Stadler M, Walter S, Unterleuthner D, et al. Comparison of cancer cells in 2D vs 3D culture reveals differences in AKT–mTOR–S6K signaling and drug responses. J Cell Sci. (2016) 130:203–18. doi: 10.1242/jcs.188102
10. Zhao Y, Shuen TWH, Toh TB, Chan XY, Liu M, Tan SY, et al. Development of a new patient-derived xenograft humanised mouse model to study human-specific tumour microenvironment and immunotherapy. Gut. (2018) 67:1845–54. doi: 10.1136/gutjnl-2017-315201
11. Fiorini E, Veghini L, Corbo V. Modeling cell communication in cancer with organoids: making the complex simple. Front Cell Dev Biol. (2020) 8:166. doi: 10.3389/fcell.2020.00166
12. Holokai L, Chakrabarti J, Lundy J, Croagh D, Adhikary P, Richards SS, et al. Murine- and human-derived autologous organoid/immune cell co-cultures as pre-clinical models of pancreatic ductal adenocarcinoma. Cancers. (2020) 12:3816. doi: 10.3390/cancers12123816
13. Kaushik G, Kamineny P, Wesa A. Abstract 2978: Unique 3D organoids autologous TIL coculture platform enables high throughput immune-oncology drug response studies. Cancer Res. (2021) 81:2978–8. doi: 10.1158/1538-7445.AM2021-2978
14. Subtil B, Cambi A, Tauriello DVF, de Vries IJM. The therapeutic potential of tackling tumor-induced dendritic cell dysfunction in colorectal cancer. Front Immunol. (2021) 12:724883. doi: 10.3389/fimmu.2021.724883
15. Li X, Nadauld L, Ootani A, Corney DC, Pai RK, Gevaert O, et al. Oncogenic transformation of diverse gastrointestinal tissues in primary organoid culture. Nat Med. (2014) 20:769–77. doi: 10.1038/nm.3585
16. Matano M, Date S, Shimokawa M, Takano A, Fujii M, Ohta Y, et al. Modeling colorectal cancer using CRISPR-Cas9–mediated engineering of human intestinal organoids. Nat Med. (2015) 21:256–62. doi: 10.1038/nm.3802
17. Xu R, Zhou X, Wang S, Trinkle C. Tumor organoid models in precision medicine and investigating cancer-stromal interactions. Pharmacol Ther. (2020) 218:107668. doi: 10.1016/j.pharmthera.2020.107668
18. Chan IS, Ewald AJ. Organoid co-culture methods to capture cancer cell-natural killer cell interactions. Methods Mol Biol (Clifton N.J.). (2022) 2463:235–50. doi: 10.1007/978-1-0716-2160-8_17
19. Ho T, Msallam R. Tissues and tumor microenvironment (TME) in 3D: models to shed light on immunosuppression in cancer. Cells. (2021) 10:831. doi: 10.3390/cells10040831
20. Lim JTC, Kwang LG, Ho NCW, Toh CCM, Too NSH, Hooi L, et al. Hepatocellular carcinoma organoid co-cultures mimic angiocrine crosstalk to generate inflammatory tumor microenvironment. Biomaterials. (2022) 284:121527–7. doi: 10.1016/j.biomaterials.2022.121527
21. Zhou G, Lieshout R, van Tienderen GS, de Ruiter V, van Royen ME, Boor PPC, et al. Modelling immune cytotoxicity for cholangiocarcinoma with tumour-derived organoids and effector T cells. Br J Cancer. (2022) 127:649–60. doi: 10.1038/s41416-022-01839-x
22. Magré L, Verstegen MMA, Buschow S, van der Laan LJW, Peppelenbosch M, Desai J. Emerging organoid-immune co-culture models for cancer research: from oncoimmunology to personalized immunotherapies. J Immunother Cancer. (2023) 11:e006290–e006290. doi: 10.1136/jitc-2022-006290
23. Xu H, Jiao D, Liu A, Wu K. Tumor organoids: applications in cancer modeling and potentials in precision medicine. J Hematol Oncol. (2022) 15(1):58. doi: 10.1186/s13045-022-01278-4
24. Xie H, Appelt JW, Jenkins RW. Going with the flow: modeling the tumor microenvironment using microfluidic technology. Cancers. (2021) 13:6052. doi: 10.3390/cancers13236052
25. Liu H, Wang Y, Wang H, Zhao M, Tao T, Zhang X, et al. A droplet microfluidic system to fabricate hybrid capsules enabling stem cell organoid engineering. Adv Sci. (2020) 7:1903739–1903739. doi: 10.1002/advs.201903739
26. Ahn J, Lim J, Jusoh N, Lee J, Park TE, Kim Y, et al. 3D microfluidic bone tumor microenvironment comprised of hydroxyapatite/fibrin composite. Front Bioeng Biotechnol. (2019) 7:168. doi: 10.3389/fbioe.2019.00168
27. Castiaux AD, Spence DM, Martin RS. Review of 3D cell culture with analysis in microfluidic systems. Anal Methods. (2019) 11:4220–32. doi: 10.1039/C9AY01328H
28. Mulas C, Hodgson AC, Kohler TN, Agley CC, Humphreys P, Kleine-Brüggeney H, et al. Microfluidic platform for 3D cell culture with live imaging and clone retrieval. Lab Chip (Online). (2020) 20:2580–91. doi: 10.1039/D0LC00165A
29. Ong LJY, Zhu L, Tan GJS, Toh Y-C. Quantitative image-based cell viability (QuantICV) assay for microfluidic 3D tissue culture applications. Micromachines. (2020) 11:669. doi: 10.3390/mi11070669
30. Neal JT, Li X, Zhu J, Giangarra V, Grzeskowiak CL, Ju J, et al. Organoid modeling of the tumor immune microenvironment. Cell. (2018) 175:1972–88.e16. doi: 10.1016/j.cell.2018.11.021
31. Ao Z, Cai H, Havert DJ, Wu Z, Gong Z, Beggs JM, et al. One-stop microfluidic assembly of human brain organoids to model prenatal cannabis exposure. Anal Chem. (2020) 92:4630–8. doi: 10.1021/acs.analchem.0c00205
32. Lamers MM, van der Vaart J, Knoops K, Riesebosch S, Breugem TI, Mykytyn AZ, et al. An organoid-derived bronchioalveolar model for SARS-CoV-2 infection of human alveolar type II-like cells. EMBO J. (2021) 40(5):e105912. doi: 10.15252/embj.2020105912
33. Sette G, Lo Cicero S, Blaconà G, Pierandrei S, Bruno SM, Salvati V, et al. Theratyping cystic fibrosis in vitro in ALI culture and organoid models generated from patient-derived nasal epithelial conditionally reprogrammed stem cells. Eur Respir J. (2021) 58:2100908. doi: 10.1183/13993003.00908-2021
34. Geuens T, Ruiter FAA, Schumacher A, Morgan FLC, Rademakers T, Wiersma LE, et al. Thiol-ene cross-linked alginate hydrogel encapsulation modulates the extracellular matrix of kidney organoids by reducing abnormal type 1a1 collagen deposition. Biomaterials. (2021) 275:120976. doi: 10.1016/j.biomaterials.2021.120976
35. Cham T-C, Ibtisham F, Fayaz MA, Honaramooz A. Generation of a highly biomimetic organoid, including vasculature, resembling the native immature testis tissue. Cells. (2021) 10:1696. doi: 10.3390/cells10071696
36. Boecking CA, Walentek P, Zlock LT, Sun DI, Wolters PJ, Ishikawa H, et al. A simple method to generate human airway epithelial organoids with externally orientated apical membranes. Am J Physiol Lung Cell Mol Physiol. (2022) 322:L420–37. doi: 10.1152/ajplung.00536.2020
37. Amatngalim GD, Rodenburg LW, Aalbers BL, Raeven HH, Aarts EM, Sarhane D, et al. Measuring cystic fibrosis drug responses in organoids derived from 2D differentiated nasal epithelia. Life Sci Alliance. (2022) 5:e202101320. doi: 10.26508/lsa.202101320
38. Clark C, Yoo KM, Sivakumar H, Strumpf K, Laxton A, Tatter SB, et al. Immersion bioprinting of hyaluronan and collagen bioink-supported 3D patient-derived brain tumor organoids. Biomed Mater. (Bristol. Print). (2022) 18:015014–4. doi: 10.1088/1748-605X/aca05d
39. Frankowski J, Kurzątkowska M, Sobczak M, Piotrowska U. Utilization of 3D bioprinting technology in creating human tissue and organoid models for preclinical drug research – State-of-the-art. Int J Pharm. (2023) 644:123313–3. doi: 10.1016/j.ijpharm.2023.123313
40. Cabral M, Cheng K, Zhu D. Three-dimensional bioprinting of organoids: past, present, and prospective. Tissue Eng Part A. (2024) 30(11-12):314–21. doi: 10.1089/ten.tea.2023.0209
41. Shrestha S, Lekkala VKR, Acharya P, Kang SY, Vanga MG, Lee MY. Reproducible generation of human liver organoids (HLOs) on a pillar plate platformviamicroarray 3D bioprinting. BioRxiv (Cold Spring Harbor Laboratory). (2024) 24:2747–61. doi: 10.1101/2024.03.11.584478
42. Tian H, Ren J, Mou R, Jia Y. Application of organoids in precision immunotherapy of lung cancer (Review). Oncol Lett. (2023) 26(5):484. doi: 10.3892/ol.2023.14071
43. Ou L, Liu S, Wang H, Guo Y, Guan L, Shen L, et al. Patient-derived melanoma organoid models facilitate the assessment of immunotherapies. EBioMedicine. (2023) 92:104614–4. doi: 10.1016/j.ebiom.2023.104614
44. Guan D, Liu X, Shi Q, He B, Zheng C, Meng X. Breast cancer organoids and their applications for precision cancer immunotherapy. World J Surg Oncol. (2023) 21(1):343. doi: 10.1186/s12957-023-03231-2
45. Ota H, Tanabe K, Saeki Y, Takemoto Y, Chikuie E, Sakamoto N, et al. Establishment of a novel overlay culture method that enables immune response assessment using gastric cancer organoids. Heliyon (Londen). (2024) 10:e23520–0. doi: 10.1016/j.heliyon.2023.e23520
46. Kastenschmidt JM, Schroers-Martin JG, Sworder BJ, Sureshchandra S, Khodadoust MS, Liu CL, et al. A human lymphoma organoid model for evaluating and targeting the follicular lymphoma tumor immune microenvironment. Cell Stem Cell. (2024) 31(3):410–20. doi: 10.1016/j.stem.2024.01.012
47. Zhou Z, van der Jeught K, Li Y, Sharma S, Yu T, Moulana I, et al. A T cell-engaging tumor organoid platform for pancreatic cancer immunotherapy. Adv Sci (Weinheim Baden-Wurttemberg Germany). (2023) 10:e2300548. doi: 10.1002/advs.202300548
48. Hombrink P, Mardpour S, Spaan E, Hanrath J, Pourfarzad F, Mullenders J, et al. Abstract 2977: Organoid-T-cell co-cultures for preclinical testing of adoptive cell and cancer immunotherapy. Cancer Res (Chicago Ill.). (2021) 81:2977–7. doi: 10.1158/1538-7445.AM2021-2977
49. Huang H, Pan Y, Huang J, Zhang C, Liao Y, Du Q, et al. Patient-derived organoids as personalized avatars and a potential immunotherapy model in cervical cancer. IScience. (2023) 26:108198–8. doi: 10.1016/j.isci.2023.108198
50. Yuki K, Cheng N, Nakano M, Kuo CJ. Organoid models of tumor immunology. Trends Immunol. (2020) 41:652–64. doi: 10.1016/j.it.2020.06.010
51. Wan C, Keany MP, Dong H, Al-Alem LF, Pandya UM, Lazo S, et al. Enhanced efficacy of simultaneous PD-1 and PD-L1 immune checkpoint blockade in high-grade serous ovarian cancer. Cancer Res. (2021) 81:158–73. doi: 10.1158/0008-5472.CAN-20-1674
52. Knepper TC, Montesion M, Russell JS, Sokol ES, Frampton GM, Miller VA, et al. The genomic landscape of merkel cell carcinoma and clinicogenomic biomarkers of response to immune checkpoint inhibitor therapy. Clin Cancer Res. (2019) 25:5961–71. doi: 10.1158/1078-0432.CCR-18-4159
53. Braun DA, Ishii Y, Walsh AM, Allen V, Wu CJ, Shukla SA, et al. Clinical validation of PBRM1 alterations as a marker of immune checkpoint inhibitor response in renal cell carcinoma. JAMA Oncol (Print). (2019) 5:1631–1. doi: 10.1001/jamaoncol.2019.3158
54. Ehrhart JC, Pabón MM, Agrawal V, Pastoor T, Kreahling JM, Altiok S. Abstract 935: Optimization of checkpoint inhibitor-TKI combinations in renal cell carcinoma using an ex vivo 3D tumor organoid model of fresh patient tissue with intact TME. Cancer Res. (2020) 80:935–5. doi: 10.1158/1538-7445.AM2020-935
55. Chen G, Chen Z, Wen D, Wang Z, Li H, Zeng Y, et al. Transdermal cold atmospheric plasma-mediated immune checkpoint blockade therapy. Proc Natl Acad Sci. (2020) 117:3687–92. doi: 10.1073/pnas.1917891117
56. Gray MA, Stanczak MA, Mantuano NR, Xiao H, Pijnenborg JFA, Malaker SA, et al. Targeted glycan degradation potentiates the anticancer immune response in vivo. Nat Chem Biol. (2020) 16:1376–84. doi: 10.1038/s41589-020-0622-x
57. Zhou Z, Van K, Fang Y, Yu T, Li Y, Ao Z, et al. An organoid-based screen for epigenetic inhibitors that stimulate antigen presentation and potentiate T-cell-mediated cytotoxicity. Nat Biomed Eng. (2021) 5:1320–35. doi: 10.1038/s41551-021-00805-x
58. Dao V, Yuki K, Lo Y-H, Nakano M, Kuo CJ. Immune organoids: from tumor modeling to precision oncology. Trends Cancer. (2022) 8:870–80. doi: 10.1016/j.trecan.2022.06.001
59. Otoshi T, Nagano T, Tachihara M, Nishimura Y. Possible biomarkers for cancer immunotherapy. Cancers. (2019) 11:935. doi: 10.3390/cancers11070935
60. Shi Y, Su H, Song Y, Jiang W, Sun X, Qian W, et al. Circulating tumor DNA predicts response in Chinese patients with relapsed or refractory classical hodgkin lymphoma treated with sintilimab. EBioMedicine. (2020) 54:102731. doi: 10.1016/j.ebiom.2020.102731
61. Petrillo A, Smyth EC. Biomarkers for precision treatment in gastric cancer. Visceral Med. (2020) 36:364–72. doi: 10.1159/000510489
62. Breiteneder H, Peng Y, Agache I, Diamant Z, Eiwegger T, Fokkens WJ, et al. Biomarkers for diagnosis and prediction of therapy responses in allergic diseases and asthma. Allergy. (2020) 75:3039–68. doi: 10.1111/all.14582
63. van de Stolpe A, Verhaegh W, Blay JY, Ma CX, Pauwels P, Pegram M, et al. RNA based approaches to profile oncogenic pathways from low quantity samples to drive precision oncology strategies. Front Genet. (2021) 11:598118. doi: 10.3389/fgene.2020.598118
64. Voabil P, de Bruijn M, Roelofsen LM, Hendriks SH, Brokamp S, van den Braber M, et al. An ex vivo tumor fragment platform to dissect response to PD-1 blockade in cancer. Nat Med. (2021) 27:1250–61. doi: 10.1038/s41591-021-01398-3
65. Zou Z, Lin Z, Wu C, Tan J, Zhang J, Peng Y, et al. Micro-engineered organoid-on-a-chip based on mesenchymal stromal cells to predict immunotherapy responses of HCC patients. Adv Sci (Weinh). (2023) 10(27):e2302640. doi: 10.1002/advs.202302640
66. Jenkins RW, Aref AR, Lizotte PH, Ivanova E, Stinson S, Zhou CW, et al. Ex vivo profiling of PD-1 blockade using organotypic tumor spheroids. Cancer Discov. (2018) 8:196–215. doi: 10.1158/2159-8290.CD-17-0833
67. Ylösmäki E, Cerullo V. Design and application of oncolytic viruses for cancer immunotherapy. Curr Opin Biotechnol. (2020) 65:25–36. doi: 10.1016/j.copbio.2019.11.016
68. Packiriswamy N, Upreti D, Zhou Y, Khan R, Miller A, Diaz RM, et al. Oncolytic measles virus therapy enhances tumor antigen-specific T-cell responses in patients with multiple myeloma. Leukemia. (2020) 34(12):3310–22. doi: 10.1038/s41375-020-0828-7
69. Ishino R, Kawase Y, Kitawaki T, Sugimoto N, Oku M, Uchida S, et al. Oncolytic virus therapy with HSV-1 for hematological Malignancies. Mol Ther. (2021) 29:762–74. doi: 10.1016/j.ymthe.2020.09.041
70. Elsedawy NB, Nace RA, Russell SJ, Schulze AJ. Oncolytic activity of targeted picornaviruses formulated as synthetic infectious RNA. Mol Ther Oncolytics. (2020) 17:484–95. doi: 10.1016/j.omto.2020.05.003
71. Bots STF, Hoeben RC. Non-human primate-derived adenoviruses for future use as oncolytic agents? Int J Mol Sci. (2020) 21:4821. doi: 10.3390/ijms21144821
72. Sugawara K, Iwai M, Ito H, Tanaka M, Seto Y, Todo T. Oncolytic herpes virus G47Δ works synergistically with CTLA-4 inhibition via dynamic intratumoral immune modulation. Mol Ther - Oncolytics. (2021) 22:129–42. doi: 10.1016/j.omto.2021.05.004
73. Fukuhara H, Takeshima Y, Todo T. Triple-mutated oncolytic herpes virus for treating both fast- and slow-growing tumors. Cancer Sci. (2021) 112:3293–301. doi: 10.1111/cas.14981
74. Raimondi G, Mato-Berciano A, Pascual-Sabater S, Rovira-Rigau M, Cuatrecasas M, Fondevila C, et al. Patient-derived pancreatic tumour organoids identify therapeutic responses to oncolytic adenoviruses. Ebiomedicine. (2020) 56:102786–6. doi: 10.1016/j.ebiom.2020.102786
75. Carter ME, Hartkopf AD, Wagner A, Volmer LL, Brucker SY, Berchtold S, et al. A three-dimensional organoid model of primary breast cancer to investigate the effects of oncolytic virotherapy. Front Mol Biosci. (2022) 9:826302. doi: 10.3389/fmolb.2022.826302
76. Ma J, Li W, Cao R, Gao D, Zhang Q, Li X, et al. Application of an iPSC-derived organoid model for localized scleroderma therapy. Adv Sci (Weinh). (2022) 9(16):e2106075. doi: 10.1002/advs.202106075
77. Sahoo DK, Mosichuk AP, Lucien F, Frank I, Cheville JC, Allenspach K, et al. Abstract 3092: Urine-derived urinary carcinoma organoids: A novel tool for providing new insights into human and canine bladder cancer treatment. Cancer Res. (2022) 82:3092–2. doi: 10.1158/1538-7445.AM2022-3092
78. Zhao DK, Liang J, Huang XY, Shen S, Wang J. Organoids technology for advancing the clinical translation of cancer nanomedicine. Wiley Interdiscip Rev Nanomed Nanobiotechnol. (2023) 15(5):e1892. doi: 10.1002/wnan.1892
79. Kwak S, Song CL, Lee J, Kim S, Nam S, Park YJ, et al. Development of pluripotent stem cell-derived epidermal organoids that generate effective extracellular vesicles in skin regeneration. Biomaterials. (2024) 307:122522. doi: 10.1016/j.biomaterials.2024.122522
Keywords: organoid, immunotherapy, tumor microenvironment, precision medicine, cancer
Citation: Yang Y, Cui J, Kong Y, Hou Y and Ma C (2024) Organoids: new frontiers in tumor immune microenvironment research. Front. Immunol. 15:1422031. doi: 10.3389/fimmu.2024.1422031
Received: 23 April 2024; Accepted: 15 July 2024;
Published: 29 July 2024.
Edited by:
Jeremy C. Simpson, University College Dublin, IrelandCopyright © 2024 Yang, Cui, Kong, Hou and Ma. This is an open-access article distributed under the terms of the Creative Commons Attribution License (CC BY). The use, distribution or reproduction in other forums is permitted, provided the original author(s) and the copyright owner(s) are credited and that the original publication in this journal is cited, in accordance with accepted academic practice. No use, distribution or reproduction is permitted which does not comply with these terms.
*Correspondence: Cuiqing Ma, bWFjdWlxaW5nQGhlYm11LmVkdS5jbg==