- 1College of Life Science, Yangtze University, Jingzhou, China
- 2Department of Chemistry, Faculty of Science, University of Hradec Králové, Hradec Králové, Czechia
- 3Department of Chemistry and Biochemistry, Mendel University in Brno, Brno, Czechia
- 4Faculty of Chemical and Food Technology, Slovak University of Technology, Bratislava, Slovakia
- 5Andalusian Research Institute in Data Science and Computational Intelligence (DaSCI), University of Granada, Granada, Spain
Aging is generally regarded as an irreversible process, and its intricate relationship with the immune system has garnered significant attention due to its profound implications for the health and well-being of the aging population. As people age, a multitude of alterations occur within the immune system, affecting both innate and adaptive immunity. In the realm of innate immunity, aging brings about changes in the number and function of various immune cells, including neutrophils, monocytes, and macrophages. Additionally, certain immune pathways, like the cGAS-STING, become activated. These alterations can potentially result in telomere damage, the disruption of cytokine signaling, and impaired recognition of pathogens. The adaptive immune system, too, undergoes a myriad of changes as age advances. These include shifts in the number, frequency, subtype, and function of T cells and B cells. Furthermore, the human gut microbiota undergoes dynamic changes as a part of the aging process. Notably, the interplay between immune changes and gut microbiota highlights the gut’s role in modulating immune responses and maintaining immune homeostasis. The gut microbiota of centenarians exhibits characteristics akin to those found in young individuals, setting it apart from the microbiota observed in typical elderly individuals. This review delves into the current understanding of how aging impacts the immune system and suggests potential strategies for reversing aging through interventions in immune factors.
1 Introduction
Ever since Roy Walford first proposed the idea in 1962 that aging might be intricately linked to the immune system’s histoincompatibility response (1), the connection between aging and immune functionality has remained a prominent focus within gerontological research. As individuals age, their biological systems undergo an irreversible decline in normal physiological functions, such as memory deterioration (2), reduced secretion of pertinent hormones (3), degenerative changes in tissues and organs (4), and a weakened immune defense (5).
Aging is accompanied by a compromised immune response, while the bone marrow, thymus, and secondary lymphoid organs exhibit degeneration in the elderly when compared to the young. Within the bone marrow, hematopoietic stem cells can differentiate into myeloid cells (such as granulocytes, monocytes, and dendritic cells) as well as lymphoid cells (such as T cells, B cells, and natural killer (NK) cells) (6, 7). However, with age, the density of human bone marrow and angiogenesis decrease (8), which are coupled with a reduction of hematopoietic stem cell regeneration potential and the alteration of its epigenetic markers (9, 10). These changes might contribute to age-related immunodeficiency. Thymus serves as the primary site for T cell development (11), and the precisely organized structure of secondary lymphoid organs plays a crucial role in establishing distinct T and B cell compartments (12). The involution of these organs with advancing age may have a negative impact on the spatial and temporal interactions between stromal cells and lymphocytes, thus can jeopardize the survival of naïve T cells and impede the effective elimination of autoreactive lymphocytes (13–15). These age-related immune system changes, including both the innate and adaptive branches, are primarily manifested as a diminished immune response to exogenous and endogenous antigens, a reduced capacity to react to novel antigens, delayed responsiveness to the protective effects of vaccines, and weakened immune memory (16, 17). Consequently, the overall ability of the individual to fend off infectious diseases, combat tumors, and clear out senescent cells wanes with age (18). This age-related impairment in immune function is coined as “immunosenescence.”
Immunosenescence encompasses a comprehensive array of age-related alterations within the immune system, including both innate and adaptive immunity, along with imbalances between these two components (19). Notable examples include shifts in the quantity and functionality of neutrophils (20), monocytes (21), macrophages (22), NK cells (23), mast cells (24), and related cytokines in innate immunity; alteration in the number and function of T cells (25) and B cells (26) in adaptive immunity. Current studies have highlighted a more pronounced influence of aging on the adaptive immune system compared to the innate counterpart (27), and the impairment of immune surveillance will accelerate the accumulation of senescent cells and further expedite the aging process (28). However, whether in the context of health or disease, aging and immunosenescence are closely related but not equivalent concepts. Most aging individuals experience immunosenescence, but the degree of immune function decline varies greatly from individual to individual. At the same time, certain diseases may induce immune system changes resembling those seen in immunosenescence even in young patients (29). Therefore, the intricate interplay between “aging” and “immunity” necessitates nuanced analysis considering different categories, properties, and immune backgrounds. Most of the research on the immune system and organismal aging has been established at the level of immune cells, but the exploration of the cGAS-STING pathway illuminates the mechanisms through which DNA triggers innate immunity. In addition, investigations into alterations in gut microbiota during aging and the potential for gut microbiota rejuvenation in long-lived elderly individuals offer promising avenues to unravel the complex relationship between immunity and aging.
This review explores the effects of aging on both the innate and adaptive immune systems. We delve into the dynamic changes observed in the gut microbiota as humans age, highlighting the distinct gut microbiota found in long-lived elderly individuals. Furthermore, the review addresses contemporary clinical approaches to anti-aging, specifically focusing on strategies aimed at prolonging healthy lifespan by rejuvenating the immune system in the elderly.
2 Aging and the innate immune system
Aging is an irreversible natural development process of organisms. Consisting of different cells and factors, innate immunity (also known as nonspecific immunity or congenital immunity) is the first line of defense against infection (30, 31). Organisms directly resist the invasion of microorganisms to the host by identifying pathogens. Many aspects of the effector functions of innate immune cells, including neutrophils, monocytes, macrophages, natural killer cells, mast cells, and dendritic cells, change with age (32) (Figure 1).
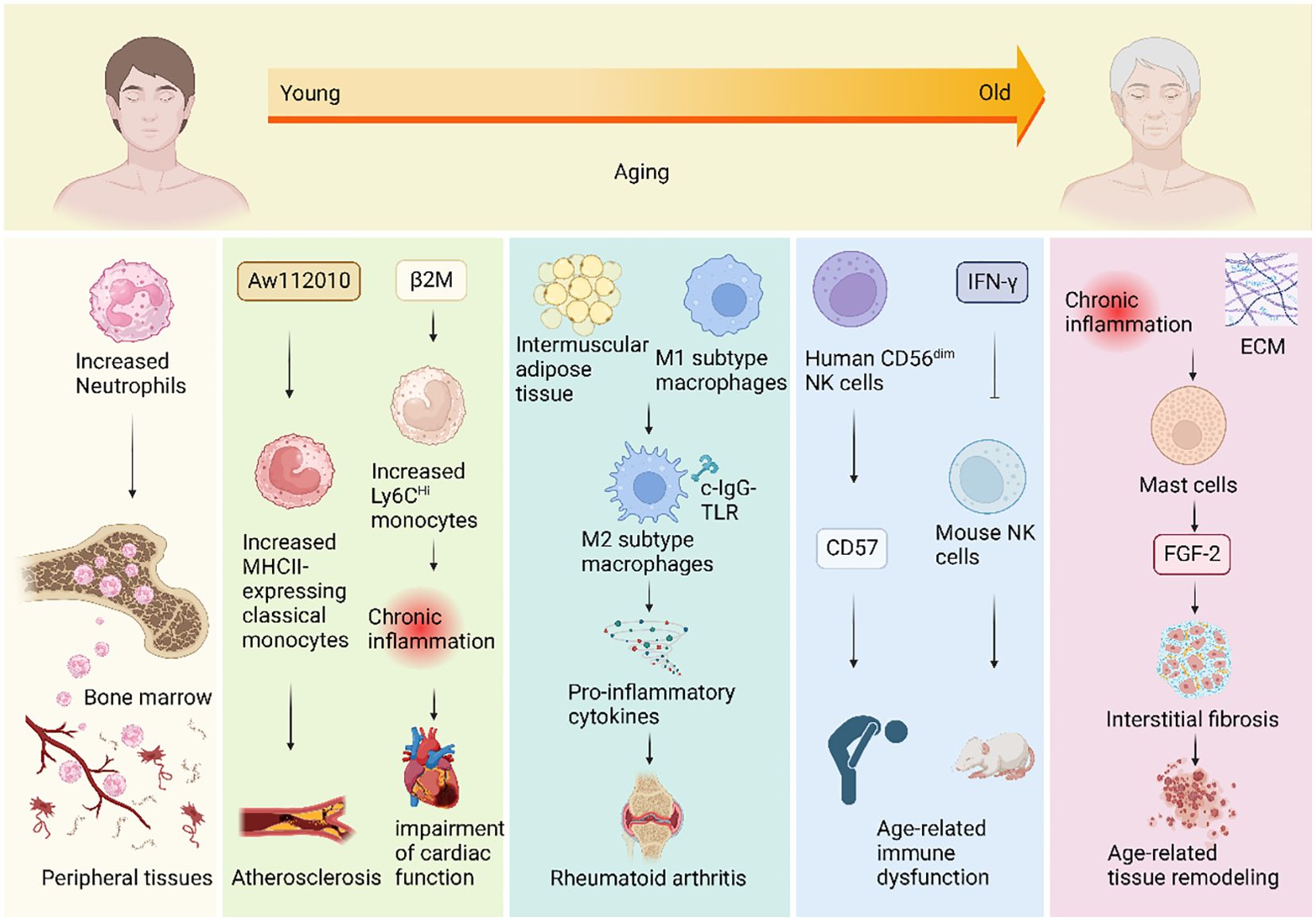
Figure 1. Mechanisms of changes in innate immunity during human aging. During the aging process, the innate immune system undergoes changes that impact various immune cells, giving rise to a spectrum of aging-related diseases. Neutrophil counts increase with age and they migrate from the bone marrow to infiltrate peripheral tissues. Additionally, an upregulation of the inflammatory regulator gene Aw112010 results in an elevated number of classical monocytes expressing the gene MHCII in aging mice, potentially triggering atherosclerosis. The aging-related rise in beta-2 microglobulin (β2M) levels is positively correlated with age and may contribute to an increase in circulating Ly6CHi monocytes. This elevation could lead to chronic inflammation, adversely affecting cardiac function. Moreover, aging induces the polarization of macrophages from the M1 subtype to the M2 subtype by expanding intermuscular adipose tissue in skeletal muscles. When subjected to complexed immunoglobulin G Toll-like receptor (c-IgG-TLR) stimulation, anti-inflammatory M2 macrophages can paradoxically produce pro-inflammatory cytokines, potentially contributing to conditions like rheumatoid arthritis. In the elderly, the number of CD56dim NK cells significantly increases, accompanied by heightened expression of the inhibitory receptor CD57, which may underlie age-related immune dysfunction. Furthermore, chronic inflammation in old age, coupled with increased extracellular matrix (ECM) levels, may contribute to elevated mast cell numbers. Concomitant with the rise in mast cell numbers, the expression level of FDF-2 in rats also increases, suggesting mast cells’ potential involvement in age-related tissue remodeling by promoting fibrosis.
2.1 Aging and neutrophils
Formed from stem cells in the bone marrow (33, 34), neutrophils, the most abundant types of granulocytes in human leukocyte population, are an important part of the innate immune system (34–36). When infection occurs, neutrophils are the first to reach the inflammatory site to kill pathogens through phagocytosis and degranulation (37–39). In addition, neutrophils recruit other immune cells by releasing cytokines and chemokines and exert an important function in coordinating innate and adaptive immune responses through antigen presentation (40, 41). Therefore, neutrophils play an important role in inflammation. However, the relationship between neutrophils and aging is presently not very clear. In the process of aging, neutrophils will migrate from bone marrow and infiltrate into peripheral tissues, such as white and brown adipose tissue, liver tissue, and so on (42). Previous studies have suggested that the number of neutrophils remains stable in healthy elderly (43–45). Yet, recent studies have found that the number of neutrophils in the elderly increases relatively with age and shows dysfunctional phagocytosis and chemotaxis (46–48), which may be linked to the decrease of apoptosis caused by neutrophils activated by chronic inflammation (49). CD11b and HLA-DR are markers indicating neutrophil activation. In experiments using CD11b++ and HLA-DR+ neutrophils, it was found that the increase of age can also lead to the upregulation of inflammation-related circulating products tumor necrosis factor (TNF) and circulating mitochondrial DNA (mtDNA) (50), which may also be a major reason for the increase in neutrophils with age (48). Compared with the young, the proliferation of neutrophil precursors in the bone marrow of the elderly is significantly reduced, so the decrease in neutrophils is considered to be the main reason for the increase in infectious events in the elderly (51). Aging is also a factor leading to the decrease of neutrophils, which is often associated with neutropenia (52–55). Previous studies have suggested that ≥65 years old is one of the triggers for neutropenia (54, 56–58). Other studies have found that there is a significant correlation between age and the incidence of febrile neutropenia in patients with small cell carcinoma treated with cisplatin plus etoposide and carboplatin plus etoposide (52).
Some studies suggested that neutrophils may induce senescence by causing telomere damage in vitro and ex vivo, which is ROS-dependent and may be related to organismal aging. For example, when neutrophils were co-cultured with fibroblasts, the ROS released by the neutrophils significantly reduced the telomere FISH intensity in the fibroblasts and markedly increased their telomere-associated foci, thereby inducing senescence. However, these effects could be prevented by adding catalase (59). In addition, the number of hepatocytes with telomere dysfunction increased with age in aged mice (60). Exposing precision-cut liver slices (PCLS) to wild-type neutrophils revealed increased expression of the senescence markers p21CIP1 and p16INK4A in PCLS; however, this effect was abolished when neutrophils from transgenic mice overexpressing human catalase in mitochondria were added (59).
Overall, these findings shed light on the complex dynamics that govern the connection between age and neutrophils, highlighting the multifaceted nature of this relationship (Table 1). The numbers of neutrophils can either decrease, increase, or remain stable with age, depending on a range of influential factors. These factors include chronic inflammation, upregulation of TNF and mtDNA, increased risk of comorbidities, race, low-performance status, male gender, and previous radiotherapy. The interplay of these elements collectively shapes the impact of age on neutrophil levels. In addition, neutrophils may play a role in triggering organismal aging, primarily through an indirect pathway involving the induction of cellular senescence. This process is initiated by oxidative damage, which in turn leads to telomere dysfunction. Therefore, although neutrophils are recognized for their pivotal role in microbial killing and inflammation, their involvement in the aging process and potential long-term negative effects on the body should not be overlooked.
2.2 Aging, monocytes, and macrophages
Aging is a complex process that involves multiple biological and biochemical mechanisms. Monocytes and macrophages, two key cell types in the innate immune system, also play important roles in the aging process. Both monocytes and macrophages belong to phagocytes. Monocytes are generally considered to be the precursors of macrophages, but current studies suggest that most macrophages are embryonic-derived (61, 62). With the aging of the organism, the phenotype and function of monocytes and macrophages in the elderly may change.
As the body ages, monocytes will change. In both aged mice and humans, the total number of circulating monocytes increases with age across classical, intermediate, and non-classical subsets (21, 63), while the percentage of classical monocytes relative to the total monocyte population decreases in the elderly (63). Plasma beta-2 microglobulin (β2M) is mainly derived from platelets (64) and positively correlated with age (65). An increase in β2M partially leads to an increase in circulating Ly6CHi monocytes. When platelet-specific β2M was knocked out (Plt-β2M-/-), aged mice had significantly fewer circulating Ly6CHi monocytes but more pro-reparative genes Il10, Il27, and Cxcl12, exhibiting an anti-inflammatory phenotype (66). This seems to suggest that aging can be delayed by reducing Ly6CHi pro-inflammatory monocytes. However, aged mice exhibited more aging phenotypes, such as reduced heart function (64). Inflammation, while harmful in some cases, can also help maintain heart function in others (67). Therefore, it seems that people should center more attention on maintaining immune homeostasis than preventing chronic inflammation. Furthermore, the number of classical monocytes with MHCII genes increased during aging in mice, which may be due to the increased expression of inflammatory regulator Aw112010 (21). In addition to quantitative changes, aging can also lead to changes in the function of monocytes. Aged monocytes are known to have reduced cellular effector capacity. The main manifestation is the impaired phagocytosis of monocytes, which leads to telomere shortening under the stimulation of Toll-like receptor (TLR) 4, significantly increased levels of intracellular tumor necrosis factor (TNF)-α level (63, 68), and decreased production of interleukin (IL)-1 (69) and IL-6 (63). Further, aging also decreased the expression of co-inhibitory molecules 2B4 (CD244), T-cell immunoglobulin domain and mucin domain 3 (TIM-3), CD200R, T-cell immunoglobulin and ITIM domain (TIGIT), and B and T lymphocyte attenuator (BTLA), while increased the expression of immune activation markers human leukocyte antigen-DR (HLA-DR), cluster of differentiation molecule 11b (CD11b), and L-selectin (CD62L) (63).
Compared with monocytes, much more effort has been devoted to studying the effects of aging on macrophages. The total number of macrophages remains stable in aged skeletal muscle, most of which are the anti-inflammatory M2 subtype, proportional to age, and a small part are M1 subtypes, which are inversely proportional to age (70). This seems to contradict the pro-inflammatory state of aging. In fact, M2 macrophages can also produce pro-inflammatory cytokines in response to complexed immunoglobulin G (c-IgG)-TLR stimulation (71), and macrophages are not the only cells that cause systemic inflammation due to aging (72). The reason why M2 becomes the main subtype of macrophages in skeletal muscle in the elderly may be intertwined with the increase of intermuscular adipose tissue in aged skeletal muscle (70). Aging can also lead to hepatic iron deposition and the accumulation of M1 and M2 hepatic macrophages, but no changes in the phenotype and number of macrophages were observed by administering iron dextran to mice, signifying that iron may not be the cause of the increase in liver macrophages due to aging (73). Paradoxically, iron can both promote the production of pro-inflammatory cytokines such as IL-6 and TNF-α, thereby increasing the number of macrophages (74), and accumulate continuously with age in monocytes, thus differentiating into macrophages (75). The reason for this contradictory phenomenon may lie in the different types of cellular iron. Malnutrition is one of the predisposing problems in the elderly population (76), and studies have linked macrophages to the disease. Compared with young malnourished animals, the number of F4/80+ CD11b+ macrophages was significantly decreased in aged malnourished animals, but there was no significant difference compared with aged control animals (77). CD86, one of the most representative surface markers of M1 macrophages (78), was also found to be reduced in aged animals, but it was not associated with malnutrition (77), which partly confirms that macrophages in aged animals are polarized to M2 subtype. TLR-4, the receptor of lipopolysaccharide (LPS) (79), plays a critical role in regulating the innate immune system (80). The expression of this receptor is decreased in the malnourished population, but this decrease is not implicated in age (81). Through this experiment, we can find that aging may lead to the decrease of M1 macrophages, but this decrease has nothing to do with malnutrition. Malnutrition may lead to a decrease in TLR-4 expression, but this decrease has nothing to do with aging (81). Therefore, based on the above findings, we conclude that aging or malnutrition induces the polarization of macrophages from pro-inflammatory M1 to anti-inflammatory M2 subtypes, adversely affecting the immune response. However, it is impossible to determine from this review if there are any interactions between aging and malnutrition. Albumin, a biological indicator of malnutrition, has been found to decrease with age in the elderly population (82), which directly links aging with malnutrition. Future research can utilize albumin as a medium to explore the connections among aging, malnutrition, and macrophages, thereby exploring the immune mechanism in greater depth.
In summary, the number of monocytes tends to increase with age. However, the knockout of platelet-specific β2M can inhibit the pro-inflammatory differentiation of monocytes in aged mice, suggesting that inflammation may not be entirely harmful to the human body. In fact, acute inflammation might be beneficial in some ways. Acute inflammation is the body’s response to infection and injury, aiding in the healing process. For example, when a person is injured or contracts a virus such as a cold or flu, the immune system releases white blood cells to the affected area. These cells surround and protect the injured site, playing a crucial role in the body’s healing mechanism. Nevertheless, aging can still lead to monocyte dysfunction and shortened telomere length, affecting their functionality. Regarding macrophages, their numbers typically remain stable in skeletal muscle as individuals age. The accumulation of hepatic macrophages in aging may not be directly linked to iron deposition, but previous studies have shown that iron can indirectly promote the increase of macrophages by generating pro-inflammatory cytokines such as IL-6 and TNF-α and accumulating in monocytes. However, the impact of long-term changes in iron metabolism on macrophages requires further investigation. Moreover, the high expression of IL-6 and TNF-α can also lead to a decrease in albumin (83), a biological indicator of malnutrition in elderly individuals. This finding might explain why the elderly, considered a high-risk group for malnutrition, experience reduced M1 macrophages after a high-fat diet. In conclusion, we shed light on the complex relationships between monocytes, macrophages, and aging (Table 1). Further research is necessary to fully understand these interactions and their implications for human health.
2.3 Aging and natural killer cells
NK cells are a group of toxic lymphocytes defined by CD3-, CD16+, and CD56+ phenotypes, which belong to a type of lymphocytes and can directly kill virus-infected cells, tumor cells, and abnormal cells. With age, the frequency and composition of NK cells may change. Previous studies found that the count of NK cells remains constant with age. For instance, the number of CD56dim NK cells was independent of aging (84). Recent studies have found that the frequency and absolute number of total NK cells and CD56dim NK cell subset were stable in the elderly compared to adults. In contrast, the frequency of CD56bright NK cell subsets was strikingly reduced in the elderly, although the absolute number of CD56bright NK cell subsets were stable when compared with adults (85, 86). The two experiments mentioned above have reached different conclusions regarding “the impact of aging on the absolute number and frequency of NK cells”. This discrepancy may be due to several factors: the different definitions of the age span of adults (the former is 20-40 years old; the latter is 19-59 years old), the distinct health status of the subjects and the limitations of techniques. For mice, since CD56 is not expressed, subset classification cannot be performed based on CD56, but can be divided according to CD27 (87). Aging leads to significant changes in the distribution of NK cells in different tissues of mice. Aged mice show a significant decrease in the frequency and number of NK cells in their blood, spleen, and liver compared to young mice (88), indicative of a decline in the immune function of aged mice, as NK cells play an important role in defending against viral infections and cancer. Although the proportion of NK cells in the immune system of the lung is altered in aged mice, the total number of NK cells in the lung remains stable (88). Moreover, the frequency and number of NK cells in the lymph nodes of aged mice tend to increase, albeit not significantly (88). This may suggest that the immune system of aged mice is compensating for the decline in NK cells in other organs by increasing the number of NK cells in lymph nodes. Memory-like NK cells (NK2, NKG2C+ CD122low) exhibit distinct characteristics, including lower expression of FcϵRγ (FCER1G), SYK (SYK), EAT-2 (SH2D1B), PLZF (ZBTB16) and higher NKG2C (KLRC2) compared with other NK cell subpopulations (89, 90). These cells have garnered significant attention for their robust response to viruses and their tendency to accumulate with age (91). However, the development process, cell life of memory-like NK cells and which subset of mature NK cells they are transformed from await further studies to be clarified.
In addition to the effect on the frequency of NK cells and circulating NK cell pool, aging also has an impact on NK cell phenotype and function. CD16, also known as FcγRIII, is an Fc receptor that can bind antibodies and virus particles to activate the killing effect of NK cells. It was found that the expression of CD16 in elderly donors has a slight but not significant extension compared with young controls (86, 92). NKG2D is another surface marker of NK cells, which can bind to a variety of protein ligands, including MICA/B (major histocompatibility complex class I chain-related molecules A/B) and ULBP (UL16-binding protein), to activate the killing effect of NK cells (93, 94). The effect of age on the expression of NKG2D in NK cells is controversial. Despite the expression of NKG2D in CD3-CD56dim NK cells only significantly increased in neonatal and middle-aged populations (92), other studies have also found that the expression of NKG2D decreased with age (95–97). In addition to CD16 and NKG2D, aging also affects the expression of other receptors on NK cells. For example, the expression of CD57, an inhibitory receptor, increases with age in CD56dim NK cells (86, 92, 97–99). CD57 is thought to reflect the accumulation of highly differentiated, senescent NK cells, which may contribute to age-related immune dysfunction. The expression of KLRG1 in CD3-CD56dim and CD3-CD56bright NK cells was negatively correlated with age (100). Withal, the expression of inhibitory receptors called killer-cell immunoglobulin-like receptor (KIR) superfamily on NK cells is known to be stable (92, 98) or elevated (in CD56bright NK cells) (85) when comparing adult controls with old subjects. It is important to note that the expression of KIR receptors can be influenced by various factors, the type and content of fatty acids in the plasma of the elderly may be the main influencing factors. Recent studies have found that arachidonic acid (AA) can inhibit the expression of KIR2DL1/S1 and KIR2DL5 in aged NK cells, while DHA and EPA can promote the expression of KIR2DL3 and KIR3DL1 (96). However, the NK cell responses of young adults and healthy elderly recipients in this experiment were similar, and the control experiment and paired Student’s t-test were not performed for statistical analysis of young and old recipients after fatty acid administration, thus it is impossible to determine whether the promotion or inhibition is caused by impaired cell function. Furthermore, aging also affects the functional capabilities of NK cells. NK cells, which are known to eliminate diseased cells through natural killer cell cytotoxicity (NKCC) and secretion of cytokines or chemokines (101, 102), were also found to be affected by aging. As people age, the ability of NK cells to lyse specific cells is significantly attenuated, but no age-related statistical changes have been found in the binding ability of NK cells to target cells, which indicates that the level of NKCC gradually declines, and the post-binding defect of target cells is responsible for the decrease of NK cell killing ability (86). Further, the decrease of perforin release from NK cells is responsible for the reduced NKCC in the elderly population (86). A recent study has used NKCC, rather than therapeutics such as senolytics or senomorphics to eliminate senescent cells (103). The researchers found that 40-50% of senescent cells were killed when the effector-target ratio was 1:1 and the co-culture time was 16 hours (103), which suggests that NKCC could be an effective way to eliminate senescent cells and potentially prevent or treat age-related diseases. In addition to the weakening of NKCC, aging also leads to the decrease of IFN-γ, perforin, and granzyme, yet the increase of TNF-α secreted by NK cells (104, 105), which induces NK cell dysfunction or exhaustion. However, some studies disagree with the above point of view (86, 92). NK cells are the main cells that can exert antibody-dependent cellular cytotoxicity (ADCC). As previously mentioned, research indicates that aging does not have a statistically significant impact on the expression of CD16 (86, 92) or CD16-induced cytotoxicity (106) in NK cells. Therefore, the prevailing belief remains that ADCC, which is a measure of CD16 signal transduction, is not affected by the aging process (106–108). However, aging leads to a shift in the composition of human lymphocytes, with CD57+ NK cells becoming the dominant cells (99), which are known to mediate more potent ADCC (109).
In conclusion, the effects of advancing age on NK cell phenotypes vary among different organisms (Table 1). In human NK cells, CD56 is an important surface marker. Upon aging, the number of CD56dim NK cells in the blood increases significantly, while the number of CD56bright NK cells remains stable. Due to species differences in the phenotype of NK cells, mouse NK cells are commonly classified into distinct subsets based on the expression of CD27, CD11b, and other markers. The distribution and development of NK cells in most tissues of aged mice have age-related defects, which may be attributed to the reduction of terminal mature CD11b+CD27− NK cells (88). Other markers of NK cells, such as CD16 and NKG2D have the effect of activating NK cell killing. CD57 is expressed on senescent NK cells, whereas KLRG1 is notably reduced in NK cell subsets of the elderly. Beyond the impact of aging on NK cells, these cells may in turn have an impact on immunosenescence and healthy aging by clearing senescent cells (110). Granule exocytosis of NK cells plays a crucial role in the elimination of senescent cells (111, 112). For instance, perforin-mediated exocytosis is a primary mechanism by which NK cells clear senescent cells. NK-92 cells have been found to eliminate senescent LX2 cells through the activation of NKG2D and its ligand MICA, ULBP2, as well as robust NKCC and granule exocytosis (112). Overall, understanding the varied effects of aging on NK cell phenotypes and their role in immunosenescence and healthy aging could provide valuable insights into potential therapeutic strategies for age-related conditions.
2.4 Aging and mast cells
Mast cells, first discovered by Paul Ehrlich in 1878, are a type of immune cell (113). Based on the tissue distribution of cells, they can be divided into connective tissue mast cells (CTMCs) and mucosal mast cells (MMCs) (114, 115). The origin of mast cells has been controversial, but it is currently believed to vary from the stage of development (116). For example, in humans and mice, mast cells first originate from primitive hematopoiesis in the yolk sac during the embryonic period (117–119), whereas they originate from hematopoietic stem cells in the bone marrow in adults (120). Mast cells play a crucial role as effector cells in the innate immune system. However, the impact of age on their cytobiology is not commonly explored. Therefore, this section aims to review the effects of physiological aging on the quantity, phenotype, and function of mast cells.
Studies on the effect of age on the number of mast cells have found that, as a kind of important effector and regulatory immune cells in the skin (121), the count and frequency of mast cells often increase with age. It was found that the dermis of male fetuses and young men had a low proportion of mast cells, whereas the percentage of mast cells in fibroblast-like cells was increased in the dermis of a 66-year-old man by immunohistochemical staining and spatial morphometry (24). The results of this experiment are similar to those of another experiment on photoprotected skin. In this experiment, mast cells were more numerous in aged vs. young skin by 40%, most of which were located in the papillary dermis, with a lower incidence of degranulation (122). However, some studies have found a significant increase in degranulated mast cells in the skin of aged rats by observing the changes in mast cells in albino rats at different ages (123). To explain this contradiction, we put forth a speculation that the increased coefficient of association between vasoactive intestinal peptide-positive nerve fibers and mast cells in aged skin could be responsible for the lower incidence of degranulation (124, 125). However, it should be noted that the paracrine effect of activated mast cells can trigger adjacent mast cells to undergo degranulation, leading to an increase in the incidence of degranulation (126). Intriguingly, although there are changes in the number of mast cells in the aging papillary dermis, the expressions of several mast cell phenotypes, specifically tryptase and chymase, were not found to be altered (122) (Table 1). As individuals age, the number of mast cells increases significantly in various organs, such as epididymis (127), ear skin (127), peritoneal cavity (127), intestine (128, 129), brain (129), heart (130), and kidney (130), not just in the skin. The increase in the number and frequency of mast cells may be due to chronic inflammation during the aging process (24, 128). As the extracellular matrix (ECM) accumulates in aging tissues (131, 132), it triggers the release of proinflammatory and cytotoxic compounds such as chymotrypsin, tryptase, CXCL1, TNF-α, and histamine from ECM-attached mast cells (127). On one hand, ECM may serve as a cell connector (133, 134), contributing to the accumulation of mast cells in aging organs, which may be due to the low apoptosis rate of senescent mast cells resulting in high survival rate (127). On the other hand, the toxic compounds released from mast cells may contribute to a reduction in the number of fibroblasts, which in turn promotes the frequency of mast cells (24).
Age may also affect the phenotype of mast cells. For example, CD45 is present in virtually all bone marrow-derived cells (135), including mast cells, and its expression is increased in the dermis of the elderly (24). Although many studies have examined the impact of age on mast cells, the majority have primarily focused on changes in mast cell numbers. There are comparatively fewer studies that have investigated the effects of age on mast cell surface markers such as CD117, FcϵRI, and T1/ST2 (119). Fibroblast growth factor-2 (FGF-2, or basic fibroblast growth factor, bFGF), intertwined with aging (136) and angiogenesis (137, 138), has been found to affect the function of mast cells. In line with the increased number of mast cells, the expression level of FGF-2 was also upregulated in adult rats compared with young rats, indicating that mast cells may play a role in the aging process by fostering fibrosis (130). Additionally, mast cells also have the ability to trigger lipolysis by releasing histamine (139). It has been observed that the secretory activity of mast cells is heightened and there is a substantial increase in the release of histamine in the skin of aged rats (123). The level of histamine can also be upregulated by stroke in an age-dependent fashion, which was found to be correlated with the significant increase of plasma pro-inflammatory cytokines such as IL-6, granulocyte colony-stimulating factor (G-CSF), TNF-α and IFN-γ in aged stroke mice (129). However, this study failed to identify the source of the pro-inflammatory cytokines, therefore, whether these pro-inflammatory factors are produced by mast cells remains to be further proved by subsequent experiments.
In conclusion, chronic inflammation can result in an increased number and frequency of mast cells in various organs and tissues with age. Firstly, ECM can be used as a local cell docking substrate, helping to reduce the apoptosis rate of mast cells in aging organs. This, in turn, contributes to the accumulation of mast cells. Secondly, cytotoxic products attached to ECM can reduce the number of fibroblasts, which indirectly leads to an increase in the percentage of mast cells. Regarding mast cell degranulation, there are conflicting findings. On the one hand, vasoactive intestinal peptide has been shown to inhibit mast cell degranulation, while on the other hand, activated mast cells can initiate degranulation of nearby mast cells in an autocrine or paracrine manner through the histamine pathway and the tryptase pathway effect. Studies investigating the effects of aging on mast cell phenotype have predominantly focused on CD45, a surface marker of mast cells. The expression of CD45 is found to be higher in the dermis of the elderly, suggesting a potential link between aging and mast cell behavior. Additionally, it’s worth noting that mast cells themselves are also involved in aging and lipolysis pathways, which are induced by age-dependent elevation of FGF-2 and histamine, respectively.
2.5 Aging and cGAS-STING signaling
Cyclic guanosine monophosphate (GMP)-adenosine monophosphate (AMP) (cGAMP) synthase (cGAS), which was first reported in 2013 as a cytoplasmic DNA recognition receptor, is also believed to activate innate immune pathways by binding to exogenous pathogenic DNA or cytosolic DNA escaped from the nucleus or mitochondria (140, 141). Stimulator of interferon genes (STING, a.k.a. MITA, MPYS, or ERIS), as a downstream signaling ligand of cGAS (140, 141), was discovered in 2008 in the study of antiviral immunity and found to be critical for the innate immune response induced by intracellular DNA (142–145). Since 2017, when people first began to recognize the significance of the cGAS-STING pathway in cellular senescence (146), the role of this innate immune pathway in the organismal aging has gradually been explored.
Cellular senescence, a fundamental process associated with aging, has emerged as a prominent mechanism in recent years and serves as a hallmark of the aging process (147–149). Yes-associated protein (YAP) and transcriptional co-activator with PDZ-binding motif (TAZ) serve as downstream transcriptional complexes in the protein kinase Hippo signaling pathway, activated in response to robust mechanical signals such as high cytoskeletal forces elicited by stiff ECM (150). When the ECM is flawed and experiences a reduction in mechanical force, the nuclear YAP/TAZ is devoid, consequently inhibiting the expression of downstream target genes such as Lamin B1 and actin related protein 2 (Actr2). As a result, the integrity of the nuclear membrane is compromised, and the DNA becomes exposed to the cytoplasm. Subsequently, cGAS identifies the exposed DNA and generates the second messenger cGAMP, which activates STING and its subsequent signaling pathways. This activation ultimately leads to the release of senescence-associated secretory phenotype (SASP) and the expression of senescence-associated β-galactosidase (SA-β-gal). Consequently, this cascade of events results in cellular senescence and aging phenotypes (151). Phosphatase and tensin homolog (PTEN), an extensively researched tumor suppressor gene (152), not only plays a crucial role in diverse signal transduction pathways and cell cycle regulation (153, 154), but also holds significant importance in various physiological activities, including cell adhesion, differentiation, senescence, and apoptosis (155–158). It is worth noting that the inactivation of PTEN lipid phosphatase can upregulate the expression of YAP/TAZ and facilitate the translocation of YAP into the nucleus, thereby inducing cell proliferation and migration (153). However, further studies are required to determine whether this process effectively safeguards the integrity of the nuclear envelope, thereby preventing DNA leakage from the nucleus. Such leakage could potentially activate the cGAS-STING pathway, leading to the promotion of aging. Mitochondrial dysregulation is a condition that induces the activation of the cGAS-STING pathway (159). The loss of PTEN-induced kinase 1 (PINK1) protein, which leads to mitochondrial dysfunction, promotes the activation of the cGAS-STING pathway and consequently results in pathological changes in the kidney, particularly renal tubular aging (160).
Humans and viruses share a co-evolutionary relationship. Endogenous retroviruses (ERVs), also known as long terminal repeat (LTR) retrotransposons (161), are remnants of retroviruses that invaded and integrated into the human genome in ancient times, accounting for 7-8% of the human genome sequence and playing a significant role as genetic memories (162–164). The Pol protein encoded by human endogenous retrovirus-K (HERV-K) performs the reverse transcription of RNA to DNA (165, 166), resulting in the addition of extra DNA to the cytoplasm of human mesenchymal progenitor cells (hMPCs). The DNA sensor cGAS recognizes this DNA and activates the innate immune system, inducing cellular senescence and organismal aging (167). Likewise, cGAS-STING pathway in the brain is also activated with advancing age (168). The inhibition of B-type lamin expression, along with the subsequent resurgence of ERVs, emerged as the primary trigger in the cascade of neuronal senility when human neurons were cultured for prolonged durations. By employing a siRNA gene silencing system that specifically targets the ERV or cGAS pathway, the suppression of aging in human neurons has been successfully achieved (169). However, the study into the aging mechanism focused solely on an in vitro model. To fully comprehend the mechanism of ERV-induced cellular aging through the cGAS-STING pathway, it is imperative to conduct future in vivo experiments, which will provide further insights and a clearer understanding of the intricate process.
Various neurological diseases including frontotemporal dementia (170), Alzheimer’s disease (171), Parkinson’s disease (172), Huntington’s disease (173), ischemic brain injury (174, 175), amyotrophic lateral sclerosis (176), and ataxia telangiectasia (177) are also germane to aging. These diseases are characterized by an acute or chronic neuroinflammatory response triggered by the cGAS-STING pathway, which significantly contributes to the pathological progression of the conditions (178, 179). Recent studies have not only linked the activation of the cGAS-STING pathway to neurological degeneration (180) and cardiac dysfunction (181) caused by aging but have also shed light on its association with age-related endothelial dysfunction (182). The aging-induced decline in endothelium-dependent vasodilation was significantly inhibited by knocking down cGAS using siRNA or by injecting the cGAS inhibitor RU.521 (182, 183). Consistently, comparable outcomes were observed when using si-STING or the STING inhibitor H-151 (182, 184).
Overall, the cGAS-STING pathway induces aging primarily indirectly through cellular senescence (Figure 2). Under the action of external factors such as extracellular matrix defects, reduced mechanical force, loss of PINK1 protein, and the resurrection of ERV, DNA is released into the cytoplasm to activate cGAS and generate 2’,3’-cGAMP. This molecule binds to STING on the endoplasmic reticulum, resulting in the production of the inflammatory molecule SASP. Ultimately, SASP leads to the death of senescent cells and senescence of adjacent cells, culminating in the aging process. In addition, the cGAS-STING pathway is also involved in the process of considerable aging-related diseases, including neurodegenerative diseases and cardiovascular diseases. Understanding the intricate interplay between the cGAS-STING pathway and these age-associated disorders may pave the way for novel therapeutic interventions and strategies to combat the challenges posed by aging populations.
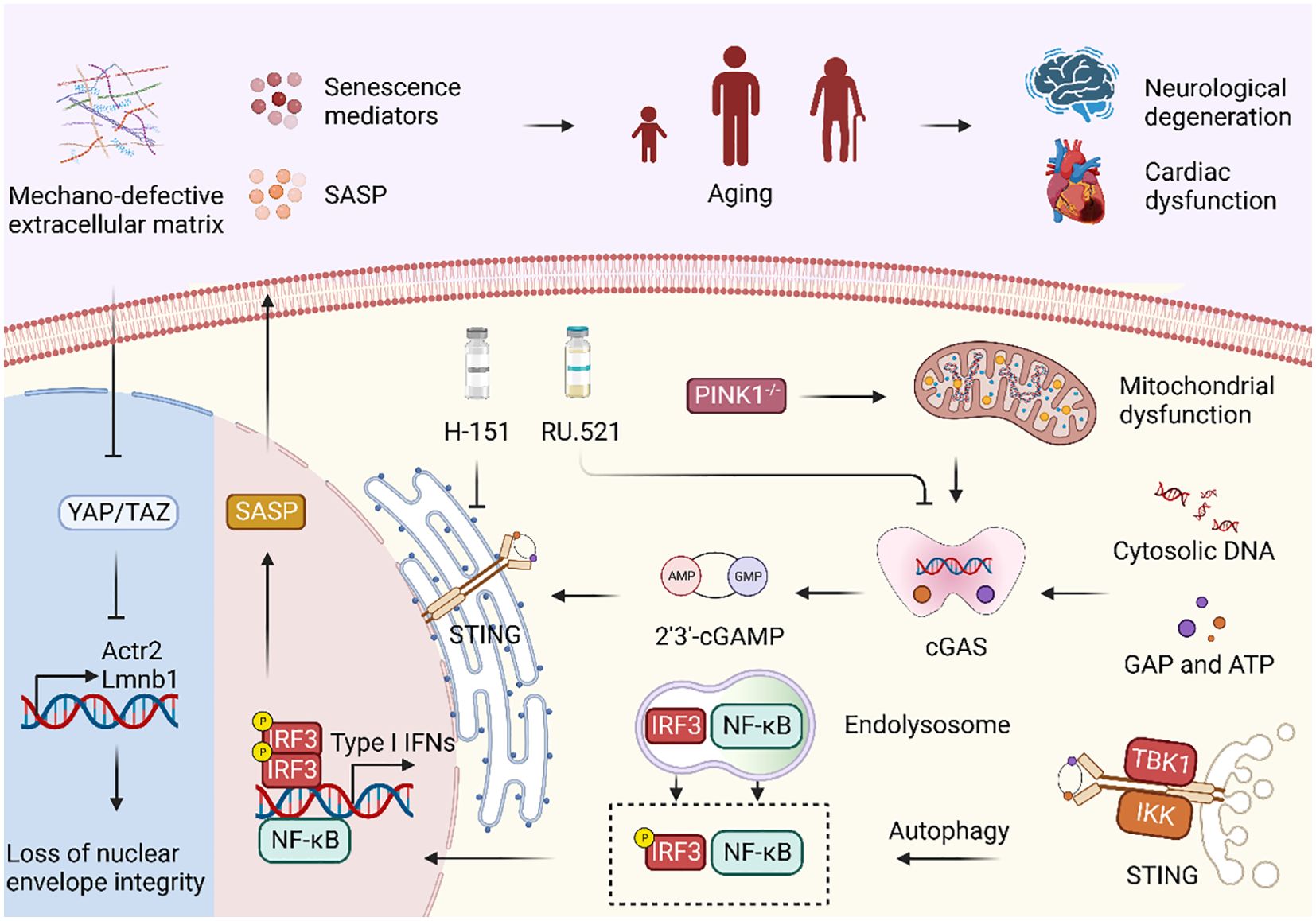
Figure 2. cGAS-STING signaling pathway regulates immune system in aging. Mechano-defective extracellular matrix (ECM) triggers the inactivation of nuclear YAP/TAZ, resulting in diminished expression of ACTR2 and LaminB1, along with the loss of the actin cap and nuclear deformity. Subsequent exposure to cytoplasmic DNA and its binding to cGAS facilitate cGAS-STING activation, ultimately causing cellular senescence and the manifestation of aging phenotypes. Moreover, PINK1 deficiency is correlated with mitochondrial dysfunction, which can also activate the cGAS-STING signaling pathway. Treatment with the STING inhibitor H-151 and the cGAS inhibitor RU.521 both demonstrated a reduction in the expression of senescence signaling mediators and SASP, thereby highlighting their potential as therapeutic interventions.
3 Aging and the adaptive immune system
When a pathogen manages to breach the first lines of defense and enters an organism, the adaptive immune system, also known as the specific or acquired immune system, is triggered and begins fighting off the infection (185, 186). Immunosenescence is not only associated with the adaptive immune system, but also with the innate immune system, as already stated above (187–189). These changes during immunosenescence involve a series of age-related modifications in both T cells and B cells (Figure 3).
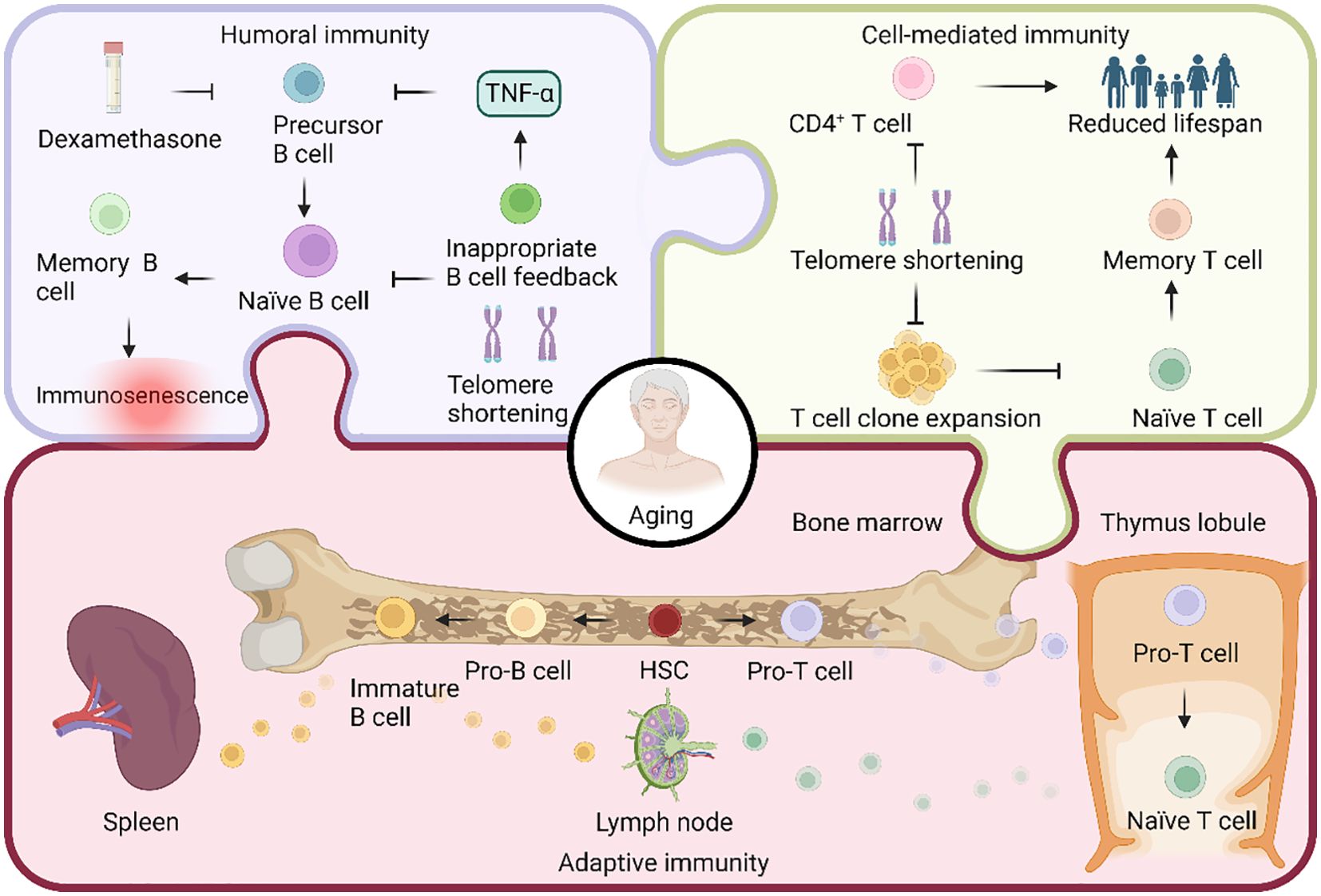
Figure 3. Aging in the regulation of adaptive immunity. Both T cells and B cells are pluripotent stem cells, differentiated from hematopoietic stem cells (HSCs). In the bone marrow, HSCs undergo differentiation, giving rise to progenitor T (pro-T) and pro-B cells. Subsequently, pro-T cells migrate to the thymus, where they undergo somatic recombination to transform into naïve T cells. These naïve T cells then migrate to the lymph nodes. On the other hand, pro-B cells undergo somatic cell recombination and V(D)J recombination, transitioning into immature B cells. These immature B cells migrate to the lymph nodes and spleen to actively participate in the immune response. Adaptive immunity comprises two types: humoral and cell-mediated. B cells mediate humoral immunity, and the reduction in telomere length due to telomerase deficiency during aging hinders B cell production. Additionally, the apoptosis-inducing drug dexamethasone, along with improper feedback from aged age-associated B cells producing TNF-α, inhibits precursor B cell production, ultimately decreasing the overall B cell count. Cell-mediated immunity, on the other hand, is orchestrated by T cells. Age-related telomere shortening results in a significant decline in the expansion ability of T cell clones, leading to a generational reduction in CD4+ T cells and, consequently, a decrease in lifespan.
3.1 Aging and T lymphocytes
During the natural aging process, the immune system undergoes dysregulation, resulting in the development of various immune-related diseases (190). T cells, which are a crucial component of the adaptive immune system, play a significant role in the process of immunosenescence (16). At the same time, they are also subject to age-related effects.
Thymus serves as the primary location for T cell differentiation and maturation, and plays a crucial role in the process of adaptive immunity (11). It is well-established that in humans, the thymus undergoes development during infancy and gradually involutes and atrophies during adolescence (191, 192). The reason for the large size of the thymus in infancy is possibly as a way to prepare for the establishment of the initial T cell repertoire. The involution of the thymus that occurs with age is primarily characterized by the deterioration of tissue structure, a decrease in thymocytes, and a decline in thymus mass. While the precise causes of thymus involution are not fully investigated, recent research has shed some light on the matter. Malnutrition, resulting from either insufficient or excessive nutrition, is often linked to inflammation, increasing the risk of infections and weakening the immune system (193). In the elderly, thymus, the primary immune organ, undergoes histological and functional decline due to aging and nutritional deficiencies, with disorganization of the corticomedullary boundary being a key feature of its involution (194). Nutrient deficiencies frequently occur in the elderly with age (76), and the histology of the aging thymus changes mainly in the destruction of the corticomedullary boundary, where T cells with different developmental stages are separated into cortical and medullary compartments (194). This separation is crucial for proper immune function, but when it fails, central tolerance is compromised, and autoimmune phenomena may develop (195, 196). Further, since the thymus naturally shrinks in size as we age, dietary restriction doesn’t have a robust impact on its size in older adults. Of note, studies have shown that the rate of thymic involution in mice seems to be slower than that of humans (197). However, the mentioned test on corticomedullary boundary of the thymus in young and old age was constructed using a mouse model, and as of now, there is insufficient evidence to suggest that thymic involution caused by nutritional deficiency will manifest similarly in humans. Some soluble molecules are also thought to be associated with age-related thymic atrophy. By establishing an infant thymic organoid model of thymocyte loss to simulate age-related thymus involution, it was observed that the viability and functional marker CCL21 of thymic epithelial cells was significantly increased along with the reduction of thymocytes (192). Conversely, L-selectin, a biomarker considered to be present in living thymocytes, was found to be significantly reduced in thymic organ cultures in vitro, but this change was not seen during aging in vivo (192).
In addition to the belief that thymic atrophy leads to the decrease of T cell production and the loss of T cell receptor (TCR) diversity (198), there have been new views on age-related T cell changes recently. Aside from heritability and early-life environment (199), aging is the primary factor that has a significant impact on telomere length (200). Developing a model of telomere length-dependent T-cell clonal expansion capacity with age, individuals with average hematopoietic cell telomere length at twenty years of age maintained maximal T cell clone expansion ability until their sixth decade of life. After this point, this capacity declines exponentially. Additionally, the generation of new naïve T cells and the capacity of naïve T cells to produce memory T cells were also impaired (201). Although naïve T cells possess telomerase capable of reversing telomere length (202), it is clear that this degree of telomere elongation is far from compensating for age-dependent telomere shortening. The telomere length of young mice is longer than that of newborn humans [~50kb (203) vs. ~9.5kb (204)] and telomerase has high activity in somatic cells (205), which may suggest that telomere length-mediated replicative aging is not a concern in the short lifespan of mice. However, the rate of telomere shrinkage is 100-fold faster in mice than in humans (203), which may contribute to their shorter lifespan. Therefore, it is crucial to investigate whether a correlation exists between telomere length and T cell repertoire in aged mice. A study conducted recently used mice deficient in telomerase (mTerc-/-) as a model to explore the effects of aging on adaptive immunity (206). The findings showed that in mTerc-/- mice, the number of CD4+ T cells in both blood and secondary lymphoid organs (like the spleen and mesenteric lymph nodes) tended to decrease with each successive generation when compared to the control counterpart. The impact of telomerase on naïve CD4+ T cells was especially significant. In the third generation of mTerc-/- mice, the frequency of naïve CD4+ T cells was significantly reduced in the thymus and spleen and relatively decreased in the blood compared to the control group. In addition, there was no significant difference in the expression of costimulatory molecules CD27 and CD28 (206), which are necessary for antigen activation of immature CD4+ T cells through TCR (207, 208), in different generations of mTerc-/- mice. This is different from the up-regulation of co-suppressor molecules CD244 and CD160 in CD8+ T cells of the elderly, where high levels of CD244 are also related to the aging of CD8+ T cells (209).
Apart from the negative alterations mentioned earlier, aging also causes an elevation in the number of T cells. For example, in aged mice, there was a notable decrease in the output of naïve T cells, while the proportion of memory T cells significantly increased (210). Also, in the subventricular zone of healthy and neurodegenerative elderly people, a substantial rise in the number of CD3+ and CD8+ T cells was found (211). However, the precise mechanism behind this increase in T cell count remains unclear.
Extensive research has been conducted on the impact of aging on the diminished T-cell repertoire, primarily attributed to thymic involution. Nonetheless, emerging evidence suggests that aging further alters the mechanical characteristics of cells and the internal organization of their diverse components. Various types of cells (212), including T cells (213), experience cellular stiffening as a result of aging. This means that T cells become less deformable with age. Cell deformation is a critical step for T cell activation and migration (214). When T cells become less deformable, their ability to migrate is reduced as well. Recent studies have confirmed that T cells are not exempt from age-related cellular stiffening, as evidenced by increased relative size of nuclei and reduced myosin content, indicating that aging reduces their ability to migrate effectively (213).
Taken altogether, T cells undergo a process of differentiation from naïve to mature cells during development (Table 2). T cell recruitment is associated with the generation of new T cells in the thymus. However, as individuals age, the thymus gradually shrinks and becomes involuted due to factors such as malnutrition, resulting in a continuous reduction of naïve T cells. Furthermore, during age-related in vitro thymic involution, researchers observe a decrease in L-selectin-labeled viable thymocytes. Surprisingly, despite this decrease in thymocyte content, the thymus actually produces more of the thymocyte chemoattractant CCL21. This suggests that the loss of thymocytes may activate homeostatic mechanisms attempting to counteract the underlying atrophy by enhancing the recruitment of T cell precursors. Unfortunately, these efforts prove unsuccessful in the context of aging. In addition to thymic atrophy and involution, shortened telomere length, decreased telomerase activity, and accumulation of the co-inhibitory molecule CD244 in the elderly may all lead to changes in the number of certain T cell subsets. The function of T cells will also gradually become dysregulated with age, which is specifically reflected in the reduced migration ability caused by T cell stiffening. Nevertheless, it’s worth noting that the effect of aging on the number of T cells is not always negative. The reduction of naïve T cells is often accompanied by an increase in the number of memory T cells and effector T cells. CD3+ and CD8+ T cells are also significantly increased in the subventricular zone of the elderly. At present, ongoing studies are focusing on artificial thymic organoids (215), presenting a promising new tool for investigating T cell differentiation and reconstruction. Concurrently, it is imperative to gain a deeper understanding of the interactions between T cells and other cell types, their crucial role in maintaining immune balance, and their effectiveness in combating diseases. By delving into these studies, we can advance the prevention and treatment of immune aging, while also devising more effective therapeutic strategies to improve human health.
3.2 Aging and B lymphocytes
B lymphocytes derive from progenitor B (pro-B) cells in the bone marrow, mature in the spleen, and then migrate into peripheral body fluids. Once stimulated by antigen, B cells undergo proliferation and differentiation, ultimately producing a large spectrum of plasma cells. These plasma cells produce specific antibodies, which play a critical role in the immune response within body fluids (216, 217).
Both the percentage and absolute number of B cells are known to decline during aging, which may be coupled with improper feedback from age-associated B cells (ABCs) (218) and telomere shortening caused by telomerase deficiency in the elderly population (206). With old age, aged ABCs secrete TNF-α, which hinders the production of precursor B (pre-B) cells (218). A recent study examined the effects of knocking out the Myc-interacting zinc finger protein 1 (Miz-1) gene in mice on the number and frequency of different types of immune cells as they age. The results showed that, compared to the control group of mice, there was no significant difference in the frequency of pro-B cells between the two groups as they aged. However, the frequencies of B cells and pre-B cells in the bone marrow were significantly lower in the mice lacking the Miz-1 gene (219). Administering dexamethasone, an apoptosis-inducing drug, to young adult mice observed a loss of pre-B cells similar to that seen spontaneously in aged mice, suggesting that aging-induced apoptosis may drive the loss of pre-B cells (220). In addition, a decrease in the expression of rag2 and recombinase activity in pro-B cells during aging may also lead to attrition of pro-B cells during passaging, resulting in a decline in pre-B cells (221). IL-7 plays a crucial role in the transition from pro-B to pre-B cells (222). Therefore, it is possible that the reduction of IL-7 levels during aging may also contribute to changes in the number of pre-B cells (223).
Naïve B cells are developed in the bone marrow from pre-B cells (216, 217), and their numbers also decline with age. This age-dependent reduction may be related to exogenous pathogen infection and deletion of certain genes in addition to pre-B cells. For example, when human B cell lines were infected with Epstein Barr virus, B cells underwent a switch from IgM-producing naïve B cells to IgA- and IgG-producing B cells, this phenomenon is particularly prevalent in B cells from the elderly (224). Additionally, it was found that the depletion of the Miz-1 in mice led to a significant decrease in the population of naïve B cells (219). However, inconsistent results have sometimes been obtained as to the effect of aging on the percentage of naïve B cells. For example, the proportion of circulating CD27- B cells, regarded as naïve B cells, in the blood was significantly higher in older donors than in their younger counterparts (225), which may be due to the fact that the decline in CD27+ B cells is more prominent than that of CD27- B cells with increasing age. On the other hand, research conducted in Malawians discovered that the frequency of naïve B cells was greatest in newborns and then decreased as they age (226).
In the aged immune system, memory B cells play a crucial role and can be distinguished from naïve B cells by differences in phenotype, the responses they exhibit after exposure to activating stimuli, and differential expression of CD27 particularly in humans (227). As a memory B cell subset, ABCs persist in the immune response of the body to external infection (228). In the experiments on mice, the number and proportion of ABCs in the spleen of C57BL/6 mice gradually increased with age, while the count and percentage of ABCs in the bone marrow were variable, with a peak at 24 months old, followed by a gradual decrease (218). Additionally, it has been observed that the frequency and number of peripheral memory B cells with CD27 as a surface marker are higher in young people but lower in older individuals (225). Carsetti’s group has also reported a similar trend (229). As individuals age, the number of memory B cells in the spleen also steadily rises, and these memory B cells of the elderly can make up as much as 60% of all B cells in the spleen (230). Nevertheless, contradictory results were found in the frequency of memory B cells in elderly subjects in Malawi, in line with the previously reviewed naïve B cells, suggesting that the frequency of CD19+CD27+ memory B cells increases with age (226). The reasons for these contradictory findings could potentially be intertwined with ethnicity (231, 232). In humans, marginal zone B cells are believed to be equivalent to the long-lived IgM memory B cells found in mice (233). Along with follicular B cells, both of which are part of the B2 cell lineage (234, 235). Aging also has an effect on these B cell populations. The frequency of follicular and marginal zone B cells in the spleen increased significantly in 12- or 15-month-old female C57BL/6J mice respectively, but decreased at later ages. For absolute numbers, when comparing 2-month-old and 18-month-old mice, there was a slight upward trend but no significant change (236). However, another experiment in female BALB/c mice showed that the percentage of marginal zone B cells in the spleens of older mice was about 40% lower than that of younger mice (237). This contradictory phenomenon may be related mainly to the different strains of mice used in the experiments. Similarly, this age-dependent reduction was also observed in human IgDhighCD27neg splenic marginal zone B cells (238).
In total, changes in B cells during aging can be reflected in three aspects: cell number, cell subsets, and secretory function (Table 2). As we age, the development of B cells in the bone marrow becomes impaired, leading to reduced production of B cells. Additionally, the aging process also influences the composition of B cell subsets in the body. One notable change is the decrease in the number of pre-B cells during aging, which has been associated with factors such as TNF-α secretion, loss of Miz-1, increased apoptosis, decreased rag2 expression and recombinase activity, and lower levels of IL-7 in ABCs. A significant hallmark of immunosenescence is the decline in naïve B cells and the simultaneous increase in memory B cells (239). In humans, these two cell subtypes are often differentiated by the expression of CD27. The loss of pre-B cells and the susceptibility to pathogen infections are the primary factors contributing to the reduced tolerance of naïve B cells in older individuals. However, the frequency of naïve B cells during human aging sometimes shows variation across experiments, which could be due to the influence of other B cell subtypes counteracting the changes in naïve B cells, as well as differences in ethnicity. On the other hand, memory B cells tend to increase with age and exhibit heightened production of cytokines like IL-1, IL-6, and TNF-α (240), which may contribute to the formation and maintenance of a chronic inflammatory state in the body. Similar to naïve B cells, changes in the number and frequency of memory B cells during aging can also vary slightly between individuals. Understanding the changes in B cells during aging is essential for comprehending age-related alterations in the immune system. Further research is required to shed light on the precise mechanisms that underlie these changes and their impact on overall health and immunity in the elderly population.
4 Aging and gut microbiota
Since the proposal of the nine hallmarks of aging in 2013 (147), recent studies have extended our understanding by identifying gut microbiota dysbiosis as a novel hallmark of aging (241). As an integral part of the dynamic organism, throughout our lifetime, the gut microbiota co-evolves with age, drives the maturation of the host’s immune system and thus contribute to host health (Table 3).
Maternal gut microbiota may influence the maturation of fetal immune cells. Pups born to mothers who were transiently colonized with the Escherichia coli HA107 strain during pregnancy showed significant increases in innate lymphocytes and F4/80+CD11c+ mononuclear cells in their gut (242). Thus, the maternal microbiota may play an important role in shaping the offspring’s immune system. Furthermore, feeding pregnant mothers a high-fiber diet resulted in a predominance of Bacteroidetes in their gut microbiota and increased levels of short-chain fatty acids (SCFAs). This intrauterine stimulation may suppress allergic respiratory diseases in offspring, possibly by increasing SCFAs, which induce regulatory T cells (243). Delivery mode strongly influences neonatal early microbial exposure. Moreover, the abnormal colonization of gut microbiota in infants delivered by caesarean section can prolong postnatal immune immaturity and hinder normal immune development, thereby increasing the risk of future immune diseases (244). Breastfeeding, the first and most natural source of nutrition for newborns, profoundly shapes the infant’s gut microbiota and immune system development. Although a baby’s immune system is not fully mature at birth, the complement components in breast milk can partially compensate for this deficiency, helping the baby resist pathogen invasions (245, 246). Bifidobacterium, which belongs to Actinobacteria phyla, was the predominant genus in the intestinal tract of breastfed infants (247), while non-breast-fed infants are predominantly colonized by Bacteroidaceae (248).Interestingly, disrupting the gut microbiota early in life seems to increase the risk of autoimmune and inflammatory diseases later on, which suggests that early antibiotic use may have long-lasting consequences (249). The notion that pets offer immune benefits to human health is rooted in the hygiene hypothesis. First proposed by David Strachan in 1989, this hypothesis suggests that an overly hygienic environment increases the risk of allergic diseases (250).
As the babies grow older, their dietary structure gradually changes, from exclusive breast milk and formula feeding to the addition of complementary foods. This transition makes the baby’s gut microbiota undergo a sharp change, known as weaning reaction (251). During this period, the Bacilli class, and in particular the Lactobacillales order, experience a rapid contraction in the gut, while the Clostridia undergo a significant expansion, co-dominate with Bacteroidia, ultimately reaching adult levels (252, 253). In addition, Ruminococcaceae and Faecalibacterium also increased significantly (248). The expanding microbiota at weaning triggers a strong immune response, inducing RORγ+ regulatory T cells, whose perturbation can lead to increased susceptibility to immune pathology later in life (252). Nonetheless, the effect of early weaning (≤4 months) on the gut microbiota did not differ at the phylum level while may be distinct at the family level (248). Early introduction of complementary foods was associated with a lower relative abundance of Bifidobacterium at 3 months of age. However, it is important to note that prematurely reducing the abundance of Bifidobacterium by introducing supplementary foods too soon may hinder their interaction with the immune system, potentially leading to higher levels of inflammation (254).
The gut microbiota of adults is relatively stable (255, 256). The initial colonization of the mammalian gut is pivotal for the maturation of the host’s immune system. The innate immune receptor Toll-like receptor 5 (TLR5) functions as a sensor for bacterial flagellin. In murine models, the TLR5-mediated counter-selection of colonizing flagellated bacteria is limited to the neonatal period. Despite this temporal restriction, the process is crucial in determining the composition of gut microbiota, which subsequently influences immune homeostasis and overall health during adulthood (257). Firmicutes and Bacteroidetes were the dominant bacterial phyla in the intestinal tract during the stable phase (258–260), however, there were significant differences in families and genera between children and adults. The largest distinction came from Blautia in the Lachnospiraceae (more abundant in the adult cohort) and Bacteroides in the Bacteroidaceae family (more abundant in the child cohort), respectively (260).
Although the gut microbiota maintains a relatively stable composition throughout adulthood, it tends to decline as individuals age (261, 262). Changes in the gut microbiota may elevate the expression of pro-inflammatory factors, contributing to chronic age-related inflammation (263). For example, in the absence of adequate SCFA production, the permeability of the intestinal mucosa increases, allowing intestinal bacteria to pass through and enter the bloodstream. Pathogen-associated molecular patterns of invading bacteria, such as LPS, bind to pattern recognition receptors expressed by immune cells and adipocytes and trigger the production of proinflammatory cytokines, leading to chronic inflammation (264, 265).
The gut microbiota can also influence the education and maturation of immune cells, enhancing their function and immunological plasticity (266, 267). For example, the gut microbiota can impact neutrophil production by regulating myelopoiesis in the bone marrow (266). When neutrophils were exposed to bacterial components (LPS), low doses (1 ng/mL) of small extracellular vesicles heightened their proinflammatory sensitivity, leading to elevated TNF-α, IL-6, ROS, and MCP-1 levels, along with enhanced migration and phagocytic activity (267). These findings suggest that small extracellular vesicles can prime neutrophils to respond more robustly to subsequent infections, a phenomenon known as trained immunity. This enhanced response helps defend against potential invading pathogens, thereby maintaining overall health and forming the basis for adaptive immune responses. Conversely, higher doses (28.1 µg/mL) induced a tolerant phenotype characterized by increased IL-10 production and decreased migration and phagocytosis. These effects were linked to changes in TLR2/MyD88 and TLR4/MyD88 signaling, which were associated with the activation of adaptive pathways in neutrophils in vitro (267). Microbiota-derived small extracellular vesicles were shown to modulate the function of mouse neutrophils, exhibiting memory-like features (267). With age, neutrophil proinflammatory activities—such as tissue infiltration, phagocytosis, and the formation of neutrophil extracellular traps—increase (266). However, this increase may not always be beneficial, particularly when not properly regulated (20). Changes in the microbiota can influence these neutrophil functions, potentially impacting immune responses in the elderly (266). Microglia are also innate immune cells with adaptive immune memory (268). Aging significantly alters how microglia respond to pathogen challenges by influencing their developmental state and the characteristics of immune memory (268). Neonatal microglia exhibit greater plasticity and can induce trained immunity in response to ultra-low doses of pathogen stimulation, whereas aged microglia are more likely to develop immune tolerance when exposed to high doses (268). This suggests that microglia may become more likely to develop suppressive immune responses with age, which could help reduce excessive tissue damage during repeated systemic inflammation.
Centenarians provide a valuable model for investigating the connection between longevity and gut microbiota. Negative changes in diverse gut microbiota caused by frailty with age lasted until ordinary old age, and was uncoupled from extreme longevity. Long-lived populations typically demonstrate a significant level of α-diversity and species richness (269). Centenarians, as the population with the longest life expectancy in humans, exhibit unique gut microbiota characteristics compared to elderly people. Previous research has indicated that Christensenellaceae could serve as a potential indicator of remarkable longevity in centenarians (270), recent studies have further explored additional microorganisms linked to prolonged lifespan. The original paper suggested minimal changes in the core gut microbiota with age, but certain bacterial taxa within the core microbiota decrease in abundance, particularly Ruminococcaceae, Lachnospiraceae, and Bacteroidaceae (270). Recent studies have shown that centenarians possess a unique composition and activity of immune cell types (271). However, few studies have explored the connection between their distinctive gut microbiota and the immune system. In the future, combining these two lines of research could provide a more comprehensive understanding of the mechanisms behind longevity in centenarians.
In short, as an integral part of the body, the gut microbiota develops alongside the immune system. During the growth and development of the human body from newborns to adults, and then gradually aging, the gut microbiota also undergoes dynamic changes of initial, transitional, stable, and ultimately declining states (Figure 4). Centenarians, the poster child for healthy aging, deviate from typical age-related gut microbiota trends, showcasing similarities to youth-associated patterns. Long-lived populations often exhibit heightened α-diversity and species richness. Studying the unique gut microbiota of centenarians offers insights into potential anti-aging strategies, such as fecal microbiota transplantation (FMT).
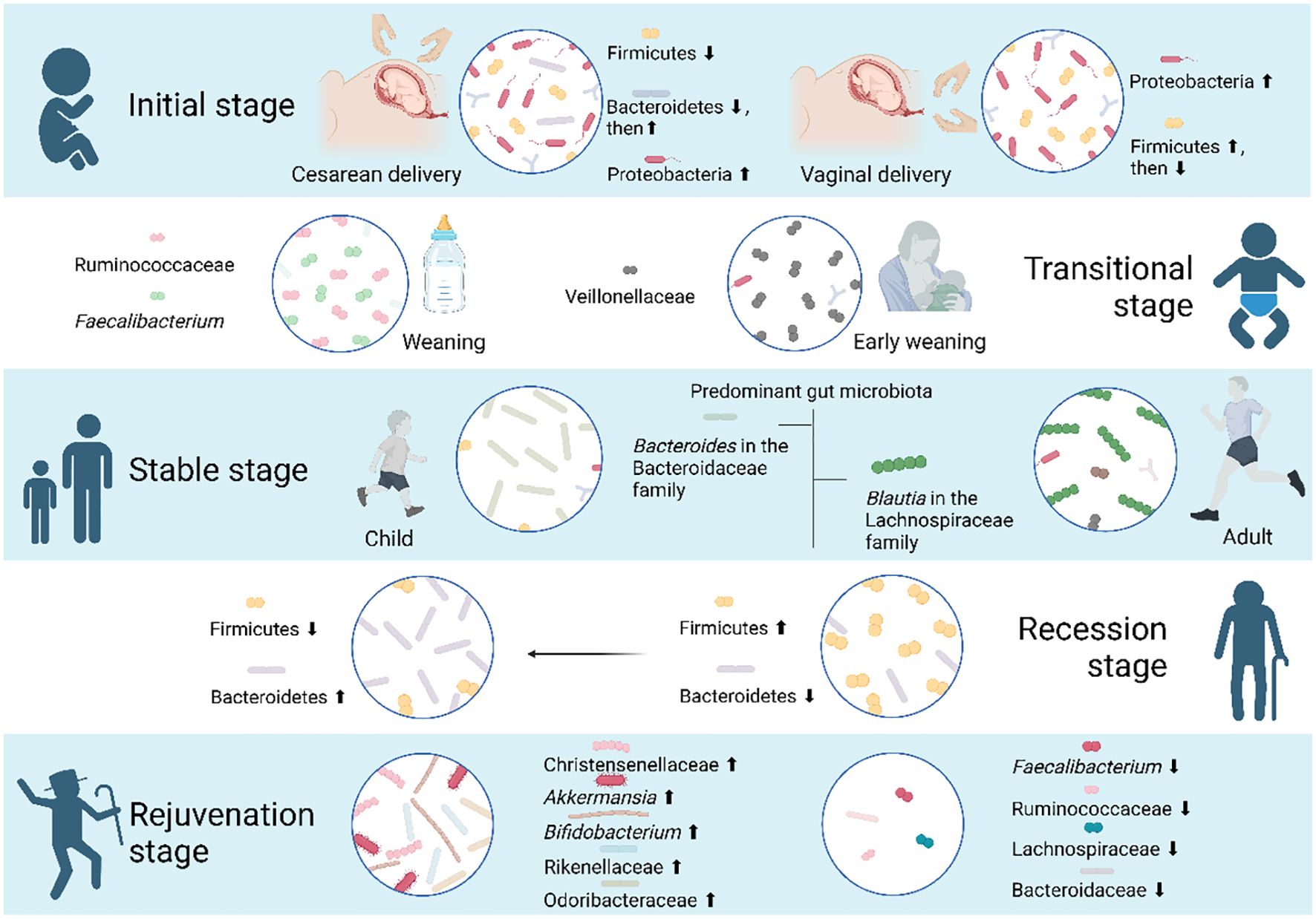
Figure 4. Five stages the gut microbiota goes through during human aging. As individuals age, the composition of the human gut microbiota undergoes distinct phases: an initial phase, a transitional phase, a stable phase, a recession phase, and a rejuvenation phase. In the initial stage, various factors impact the gut microbiota of newborns. Specifically, infants born via cesarean section experience a transition from Firmicutes to Bacteroidetes and Proteobacteria, while those born vaginally have Proteobacteria and Firmicutes as the predominant phyla. During the transitional phase, early weaning has a limited impact on the infant gut microbiota at the phylum level but leads to an enrichment of Veillonellaceae. Notably, Ruminococcaceae and Faecalibacterium show significant increases during the weaning stage. By the age of 3, toddlers experience a substantial increase in both the quantity and variety of their gut microbiota, reaching a level of maturity comparable to that of adults, entering a stable stage. However, distinctions persist between the gut microbiota of children and adults. Blautia in the Lachnospiraceae family is more abundant in the adult cohort, while Bacteroides in the Bacteroidaceae family is more prevalent in the child cohort. As individuals progress into the recession phase of aging, a decrease in the Firmicutes and Bacteroidetes ratio serves as an indicator. Centenarians, in particular, display unique gut microbiota profiles distinct from the average elderly population. Their microbiota undergoes a rejuvenation phase, suggesting that the distinctive microorganisms associated with longevity may play a role in maintaining youth and potentially reversing aging.
5 Clinical treatment of regulating immunity and aging
Aging, as an irreversible natural process, is closely linked to functional changes in the immune system and alterations in microbiota. These changes not only affect the body’s resistance to pathogens but may also exacerbate pathological conditions associated with aging. Therefore, studying how to regulate immune system function through clinical interventions to delay aging has become a significant focus in current scientific research. In this section, we will explore the latest developments in this field, aiming to provide new ideas and methods for the treatment and prevention of aging-related diseases in the future.
In recent years, a growing consensus among researchers underscores the escalating significance of immune factors in the processes of bodily degeneration and pathological changes, and the onset and progression of numerous diseases are intricately linked to the aging process. Consequently, the exploration of clinical interventions aimed at modulating immunity and retarding aging has emerged as a steadfast focus of ongoing investigation.
A prevalent type of malnutrition among older adults is the lack of essential micronutrients, including vitamins and minerals, which contributes to the gradual weakening of the immune system associated with aging (76). However, the administration of multi-vitamin and mineral supplements (MVM) to the elderly only resulted in a notable increase in the levels of immune-modulating micronutrients, namely vitamin C and zinc, there was no significant alteration observed in the immune function or immune status of older adults (272). Higher levels of micronutrients in the plasma of participants prior to the intervention, the limitations of dihydrorhodamine for measuring ROS (273), the rationality for the choice of specific immune markers (274), and the small sample size of the trial might have constrained the ability to detect significant changes. The beneficial impacts of simple caloric restriction on extending healthspan were initially showcased through rodent studies in 1935 (275), followed by human clinical trials (CALERIE study) in 2015 (276). Recent clinical studies on caloric restriction without malnutrition has also been shown to extend the lifespan of organisms and delay the onset of age-related diseases. For example, two years of caloric intake reduction in humans can rejuvenate the thymus and increase its ability to produce T cells, thereby ameliorating the age-related deterioration of immune function (277). In addition, an 800 kcal/day caloric diet exhibits the potential to postpone immune senescence by shaping the gut microbiota of humanized gnotobiotic mice and regulating immune cell types and proportions (278). Nevertheless, when caloric intake is drastically reduced by as much as 40%, it tends to impede the immune system, thereby increasing susceptibility to more severe infections (279, 280).
Certain senotherapeutic pharmacological agents, such as senolytics that eliminate senescent cells, and senostatics or senomorphics that prevent senescent cells from generating harmful cell-extrinsic effects, also exhibit immunomodulatory properties (281–283). In a groundbreaking in vitro experiment conducted in 2015, it was discovered for the first time that the combination of dasatinib and quercetin (D+Q) possesses the remarkable capability to enhance bodily function and extend the span of healthy life by eliminating senescent cells (284). Recent research has expanded upon this perspective. In a preclinical study conducted on the nonhuman primate cynomolgus macaques, the administration of a combination of D+Q (5 mg/kg + 50 mg/kg) for 3 months, the gene expressions of senescence markers p16INK4A and p21CIP1 exhibited significant reductions. At the 5-month mark, the expression of the apoptosis-related gene BAX showed a substantial increase, collectively indicating a notable improvement in the aging status of cynomolgus macaques (285). Aging is often accompanied by a decline in immune capacity. However, D+Q has also been found to possess anti-inflammatory properties. The number of immune cells in the experimental group of animals continued to decrease, allowing the animals to maintain healthier immune characteristics (285). Currently, D+Q combination therapy is being employed in Phase II clinical trials to address mental illnesses linked to accelerated aging (193). Fisetin, another senolytic compound, is abundantly present in various fruits and vegetables (286, 287). Preclinical studies have shown that it has the potential to decrease the number of damaged cells and senescent immune cells in mice, leading to improved health and an extended lifespan in elderly mice (288). These findings suggest that fisetin holds promise for further exploration in human clinical trials. At present, researchers are conducting clinical studies using fisetin as a potential means to target cellular senescence, with the aim of enhancing bone health in elderly individuals (289) and address frailty in the elderly (290). The immune system of the elderly gradually loses its vitality and becomes more susceptible to viruses. Clinical researchers have administered fisetin to elderly COVID-19 patients aged 65 and above to verify whether it can help enhance the immune response in older individuals and consequently reduce the mortality rate associated with the disease (291). In 2016, the senolytic efficacy of ABT-737 and ABT-263 (navitoclax), inhibitors of BCL-2 family proteins (including BCL-2, BCL-W, and BCL-XL), was first demonstrated, allowing senescent cells to initiate apoptosis (292–294). Senescent macrophages play a role in the development of lung cancer and tend to accumulate as individuals age (295). However, when young mice with tumorigenic lungs were treated with ABT-737, it led to the ablation of senescent macrophages, thereby enhancing the immunosurveillance process and effectively reducing tumor burden (296). However, due to technical limitations, the experiments fail to provide insights into the exact mechanism through which lung tumors trigger macrophage senescence, nor could it elucidate the reason behind the molecular similarities between senescent macrophages in the lungs of naturally aging mice and those in young mice with lung tumors. Furthermore, and the potential involvement of other macrophage or senescent cell populations in the process of tumorigenesis cannot be excluded. Regrettably, ABT-737’s limited oral bioavailability restricts its potential for therapeutic applications. On the other hand, ABT-263, while offering the advantage of oral bioavailability, presents a significant challenge due to its severe platelet toxicity in clinical settings, rendering it unsuitable for safe human use (297–299).
Age serves as an unalterable risk factor for the inflammation that forms the foundation of age-related conditions like type 2 diabetes mellitus (T2DM) (300, 301). Metformin, as a first-line medication for the prevention and treatment of T2DM in elderly individuals (302), its safety and efficacy have been guaranteed in more than 60 years of clinical use. This extended history of safe use suggests the potential for metformin to also function as an anti-inflammaging medication, promoting healthy aging. Oral metformin, on the one hand, has the ability to repair mitochondria, enabling them to supply energy to cells, restore autophagy function, and counteract the inflammatory response triggered by immune cell Th17. On the other hand, it enhances mitochondrial efficiency and reduces the generation of free radicals by activating the AMP-activated protein kinase (AMPK) pathway, effectively combating the aging process (303). Additionally, metformin has the potential to enhance the growth of beneficial gut bacteria known as Akkermansia muciniphila, improve the cognitive function of elderly mice by regulating inflammation-related pathways within the host and lowering the levels of the pro-inflammatory cytokine IL-6 (304). Nevertheless, it remains essential to confirm whether these effects will translate to humans through clinical trials. Ether lipids serve as essential intermediate substances for biguanide drugs, such as phenformin and metformin, which play a pivotal role in promoting life extension, and the biosynthetic state of it also affects dietary restriction, inhibition of mitochondrial electron transport chain, rapamycin, and other aging intervention strategies (305). Rapamycin is known as an immunomodulator (306, 307), and similar to metformin, it is also recognized as a well-established senomorphic. In 2009, the Interventions Testing Program (ITP) certified rapamycin as the first drug to significantly extend the lifespan of mammals, confirming its distinction among dozens of drugs tested for anti-aging research (308). Subsequent in vivo animal experiments have conclusively demonstrated that senescent immune cells can induce systemic tissue damage and lead to a shortened lifespan (309). Consequently, senescent immune cells have emerged as a pivotal therapeutic target for extending overall health and longevity. Notably, the administration of rapamycin has been shown to reduce senescence markers in peripheral T cells, boost anti-keyhole limpet hemocyanin (KLH) serum titers, increase white blood cell counts, and effectively reverse the processes associated with systemic aging (309). As individuals age, the deteriorative immune function leads to a diminished response to vaccinations (310). This decline is largely attributed to a reduced capacity of hematopoietic stem cells to generate naïve lymphocytes and an increase in the presence of PD-1 positive T cells. Rapamycin analog RAD001 has been shown to restore hematopoietic stem cell function, reduce the frequency of PD-1-positive CD4 and CD8 T cells, increase the production of naive lymphocytes, enhance influenza antibody titers, improve vaccination response, and extend lifespan (310). Moreover, the decline in immune function that accompanies aging increases the risk of infections, particularly respiratory infections (311). Low doses of RAD001 + BEZ235 (catalytic site mTOR inhibitor) combination synergistically inhibited multiple nodes downstream of TORC1 while avoiding TORC2 inhibition (311). This selective inhibition is significant as TORC2 inhibition is linked to adverse effects like a reduced lifespan in male mice (311). The combination of RAD001 + BEZ235 strengthened IFN-induced innate antiviral immunity, leading to a decrease in infection rates (311). This drug boosts the immune system by upregulating antiviral genes, which may offer broader protection against respiratory infections compared to treatments that target individual viruses. While the anti-aging potential of rapamycin has shown promise in numerous animal experiments (312–316), its transition to clinical applications has encountered significant hurdles. The primary challenge lies in the emergence of toxic side effects when used in humans. For example, rapamycin have been associated with adverse symptoms, including hyperglycemia, hyperlipidemia, nephrotoxicity, impaired wound healing, and immunosuppression (317). However, compared to oral and injectable administration routes, topical rapamycin has, for the first time, clinically demonstrated its ability to delay the aging of human skin tissue (318). This is evident in the increased collagen content observed in the skin of most participants who received rapamycin, as well as the decreased levels of skin cell senescence markers (319). Although rapamycin may affect local immune cell function, unfortunately, no immune cell infiltration was observed in any histological sections from this experiment. In addition, this experiment only observed significant changes in one senescence marker, p16INK4A, and future experiments should strive for a more comprehensive assessment of rapamycin’s influence on human tissue aging by examining a broader range of senescence markers.
Research in cell therapy represents a pivotal frontier in the field of medicine. In recent years, significant advancements have been achieved in cell therapy technology, with a focus on immune cells and stem cell regenerative medicine. The primary vehicle for chimeric antigen receptor (CAR) cell therapy is immune cells, originally employed to combat non-solid tumors such as leukemia, B-cell lymphoma, and multiple myeloma clinically (320). In addition to their role in targeting cancer cells, CAR T therapy has firstly found its way into the field of aging biology as a senolytic strategy in 2020 (321–323). In this capacity, animal experiments have shown that CAR T cells can target senescence-specific cell surface markers such as urokinase-type plasminogen activator receptor (uPAR) and natural killer group 2 member D ligands (NKG2DLs), with the potential to eliminate senescent cells, extend survival, and reinstate tissue homeostasis effectively (321, 324). Furthermore, as immune cell reinfusion therapy continues to evolve, T cell receptor-engineered T cell (TCR T) and CAR NK cell therapies are being developed and optimized, with expectations for their potential in anti-aging applications in clinical settings.
In general, current anti-aging interventions can be categorized by their risk levels, with caloric restriction, drugs, and cell therapy spanning from low to high risk. Additionally, other alternative approaches to reverse aging, like gene editing (325) and plasma exchange (326–328), have seen limited adoption primarily because of their cost and ethical concerns stemming from nascent technology. Consequently, there is an imperative to advance these technologies and conduct further clinical trials in the future to establish the safety and efficacy of diverse anti-aging strategies.
6 Concluding remarks and prospects
The intricate interplay between immunity and aging presents a captivating realm of study. It encompasses a physiological process entailing intricate mechanisms, encompassing manifold changes in both the phenotype and function of immune cells – both innate and adaptive. The waning vigor and diminishing numbers of these adaptive immune cells can precipitate a decline in the body’s capacity to combat infections and counter aberrant cells, thereby augmenting susceptibility to a spectrum of ailments. Moreover, innate immunocytes assume a pivotal role in regulating inflammatory responses and reparative processes, thereby potential alterations in their functionality could reverberate on the body’s retort to injuries and convalescence. Distinguishing aging from cellular senescence remains a controversial issue. Although cellular senescence is considered a hallmark of the aging process (147–149), some studies suggest that senescent cells in vitro and aged cells in vivo differ significantly in function and phenotype. For example, microglia cultured in vitro exhibit shortened telomeres, reduced cell proliferation, and increased levels of senescence marker proteins, such as p16INK4a, p21CIP1, and p53. In contrast, microglia acutely isolated from aged brains show only a moderate increase in p16INK4a, with no changes in telomere length or cell proliferation (329). This suggests that replicative senescence in vitro and aging in vivo may involve different mechanisms. In mice, aging in vivo appears to be primarily independent of telomeres.
In addition, the aging process is accompanied by dynamic changes in immune-related signaling pathways and gut microbiota. An increasingly robust body of research corroborates the intimate link between the gut and the immune system, underscoring the profound impact of the composition and function of the gut microbiota on immune regulation. With age, these microbial inhabitants of the gut may undergo transformations, thereby potentially exerting a cascading influence on the state and efficacy of the immune system.
Although current research has achieved pivotal strides, a deeper delve is imperative to unveil the intricate molecular underpinnings underpinning the nexus of immunity and aging, along with strategies to potentially stave off the aging process via immune system interventions. This endeavor not only augments our comprehension of the interface between immunity and aging, but also offers novel insights that could potentially shape the trajectory of therapeutic paradigms targeting age-related maladies. Continued in-depth exploration of this field will undoubtedly bring more hope for human health and longevity.
Author contributions
HG: Investigation, Methodology, Writing – original draft. EN: Investigation, Methodology, Writing – original draft. VA: Formal analysis, Supervision, Writing – review & editing. ZH: Funding acquisition, Methodology, Writing – review & editing. MV: Investigation, Supervision, Writing – review & editing. QW: Conceptualization, Funding acquisition, Methodology, Supervision, Writing – review & editing. KK: Conceptualization, Funding acquisition, Supervision, Writing – review & editing.
Funding
The author(s) declare financial support was received for the research, authorship, and/or publication of this article. This work was supported by the National Natural Science Foundation of China (Grant no. 32373073; 31972741); the Excelence project PrF UHK, Czech Republic (2208/2024-2025); Research Program of University of Granada; and Scientific Grant Agency (VEGA Project 1/0542/24).
Conflict of interest
The authors declare that the research was conducted in the absence of any commercial or financial relationships that could be construed as a potential conflict of interest.
Publisher’s note
All claims expressed in this article are solely those of the authors and do not necessarily represent those of their affiliated organizations, or those of the publisher, the editors and the reviewers. Any product that may be evaluated in this article, or claim that may be made by its manufacturer, is not guaranteed or endorsed by the publisher.
References
2. Dundee JM, Puigdellivol M, Butler R, Cockram TOJ, Brown GC. P2Y(6) receptor-dependent microglial phagocytosis of synapses mediates synaptic and memory loss in aging. Aging Cell. (2023) 22:e13761. doi: 10.1111/acel.13761
3. Pataky MW, Young WF, Nair KS. Hormonal and metabolic changes of aging and the influence of lifestyle modifications. Mayo Clin Proc. (2021) 96:788–814. doi: 10.1016/j.mayocp.2020.07.033
4. Schaum N, Lehallier B, Hahn O, Palovics R, Hosseinzadeh S, Lee SE, et al. Ageing hallmarks exhibit organ-specific temporal signatures. Nature. (2020) 583:596–602. doi: 10.1038/s41586-020-2499-y
5. Mogilenko DA, Shchukina I, Artyomov MN. Immune ageing at single-cell resolution. Nat Rev Immunol. (2022) 22:484–98. doi: 10.1038/s41577-021-00646-4
6. Mangel M, Bonsall MB. Stem cell biology is population biology: differentiation of hematopoietic multipotent progenitors to common lymphoid and myeloid progenitors. Theor Biol Med Model. (2013) 10:5. doi: 10.1186/1742-4682-10-5
7. Pietras EM. Inflammation: a key regulator of hematopoietic stem cell fate in health and disease. Blood. (2017) 130:1693–8. doi: 10.1182/blood-2017-06-780882
8. Stucker S, Chen J, Watt FE, Kusumbe AP. Bone angiogenesis and vascular niche remodeling in stress, aging, and diseases. Front Cell Dev Biol. (2020) 8:602269. doi: 10.3389/fcell.2020.602269
9. Florian MC, Klose M, Sacma M, Jablanovic J, Knudson L, Nattamai KJ, et al. Aging alters the epigenetic asymmetry of HSC division. PloS Biol. (2018) 16:e2003389. doi: 10.1371/journal.pbio.2003389
10. Hay SB, Ferchen K, ChEtal K, Grimes HL, Salomonis N. The Human Cell Atlas bone marrow single-cell interactive web portal. Exp Hematol. (2018) 68:51–61. doi: 10.1016/j.exphem.2018.09.004
11. Miller J. The function of the thymus and its impact on modern medicine. Science. (2020) 369(6503):522. doi: 10.1126/science.aba2429
12. Gomez Atria D, Gaudette BT, Londregan J, Kelly S, Perkey E, Allman A, et al. Stromal Notch ligands foster lymphopenia-driven functional plasticity and homeostatic proliferation of naive B cells. J Clin Invest. (2022) 132(13):e158885. doi: 10.1172/JCI158885
13. Ng YH, Chalasani G. Role of secondary lymphoid tissues in primary and memory T-cell responses to a transplanted organ. Transplant Rev (Orlando). (2010) 24:32–41. doi: 10.1016/j.trre.2009.09.003
14. Lefebvre JS, Maue AC, Eaton SM, Lanthier PA, Tighe M, Haynes L. The aged microenvironment contributes to the age-related functional defects of CD4 T cells in mice. Aging Cell. (2012) 11:732–40. doi: 10.1111/j.1474-9726.2012.00836.x
15. Becklund BR, Purton JF, Ramsey C, Favre S, Vogt TK, Martin CE, et al. The aged lymphoid tissue environment fails to support naive T cell homeostasis. Sci Rep. (2016) 6:30842. doi: 10.1038/srep30842
16. Lian J, Yue Y, Yu W, Zhang Y. Immunosenescence: a key player in cancer development. J Hematol Oncol. (2020) 13:151. doi: 10.1186/s13045-020-00986-z
17. Santoro A, Bientinesi E, Monti D. Immunosenescence and inflammaging in the aging process: age-related diseases or longevity? Ageing Res Rev. (2021) 71:101422. doi: 10.1016/j.arr.2021.101422
18. Campisi J. Senescent cells, tumor suppression, and organismal aging: good citizens, bad neighbors. Cell. (2005) 120:513–22. doi: 10.1016/j.cell.2005.02.003
19. Liu Z, Liang Q, Ren Y, Guo C, Ge X, Wang L, et al. Immunosenescence: molecular mechanisms and diseases. Signal Transduct Target Ther. (2023) 8:200. doi: 10.1038/s41392-023-01451-2
20. Van Avondt K, Strecker JK, Tulotta C, Minnerup J, Schulz C, Soehnlein O. Neutrophils in aging and aging-related pathologies. Immunol Rev. (2023) 314:357–75. doi: 10.1111/imr.13153
21. Barman PK, Shin JE, Lewis SA, Kang S, Wu D, Wang Y, et al. Production of MHCII-expressing classical monocytes increases during aging in mice and humans. Aging Cell. (2022) 21:e13701. doi: 10.1111/acel.13701
22. Esfahani NS, Wu Q, Kumar N, Ganesan LP, Lafuse WP, Rajaram MVS. Aging influences the cardiac macrophage phenotype and function during steady state and during inflammation. Aging Cell. (2021) 20:e13438. doi: 10.1111/acel.13438
23. Fionda C, Ruggeri S, Sciume G, Laffranchi M, Quinti I, Milito C, et al. Age-dependent NK cell dysfunctions in severe COVID-19 patients. Front Immunol. (2022) 13:1039120. doi: 10.3389/fimmu.2022.1039120
24. Gunin AG, Kornilova NK, Vasilieva OV, Petrov VV. Age-related changes in proliferation, the numbers of mast cells, eosinophils, and cd45-positive cells in human dermis. J Gerontol A Biol Sci Med Sci. (2011) 66:385–92. doi: 10.1093/gerona/glq205
25. Li M, Yao D, Zeng X, Kasakovski D, Zhang Y, Chen S, et al. Age related human T cell subset evolution and senescence. Immun Ageing. (2019) 16:24. doi: 10.1186/s12979-019-0165-8
26. Avivi I, Zisman-Rozen S, Naor S, Dai I, Benhamou D, Shahaf G, et al. Depletion of B cells rejuvenates the peripheral B-cell compartment but is insufficient to restore immune competence in aging. Aging Cell. (2019) 18:e12959. doi: 10.1111/acel.12959
27. Castelo-Branco C, Soveral I. The immune system and aging: a review. Gynecol Endocrinol. (2014) 30:16–22. doi: 10.3109/09513590.2013.852531
28. Ovadya Y, Landsberger T, Leins H, Vadai E, Gal H, Biran A, et al. Impaired immune surveillance accelerates accumulation of senescent cells and aging. Nat Commun. (2018) 9:5435. doi: 10.1038/s41467-018-07825-3
29. Barbe-Tuana F, Funchal G, Schmitz CRR, Maurmann RM, Bauer ME. The interplay between immunosenescence and age-related diseases. Semin Immunopathol. (2020) 42:545–57. doi: 10.1007/s00281-020-00806-z
30. Minasyan H, Flachsbart F. Blood coagulation: a powerful bactericidal mechanism of human innate immunity. Int Rev Immunol. (2019) 38:3–17. doi: 10.1080/08830185.2018.1533009
31. Turvey SE, Broide DH. Innate immunity. J Allergy Clin Immunol. (2010) 125:S24–32. doi: 10.1016/j.jaci.2009.07.016
32. Dranoff G. Cytokines in cancer pathogenesis and cancer therapy. Nat Rev Cancer. (2004) 4:11–22. doi: 10.1038/nrc1252
33. Borregaard N. Neutrophils, from marrow to microbes. Immunity. (2010) 33:657–70. doi: 10.1016/j.immuni.2010.11.011
34. Kraus RF, Gruber MA. Neutrophils-from bone marrow to first-line defense of the innate immune system. Front Immunol. (2021) 12:767175. doi: 10.3389/fimmu.2021.767175
35. Giovenzana A, Carnovale D, Phillips B, Petrelli A, Giannoukakis N. Neutrophils and their role in the aetiopathogenesis of type 1 and type 2 diabetes. Diabetes Metab Res Rev. (2022) 38:e3483. doi: 10.1002/dmrr.3483
36. Sionov RV. Leveling up the controversial role of neutrophils in cancer: when the complexity becomes entangled. Cells. (2021) 10(9):2486. doi: 10.3390/cells10092486
37. Amulic B, Cazalet C, Hayes GL, Metzler KD, Zychlinsky A. Neutrophil function: from mechanisms to disease. Annu Rev Immunol. (2012) 30:459–89. doi: 10.1146/annurev-immunol-020711-074942
38. Blanter M, Cambier S, De Bondt M, Vanbrabant L, Portner N, Abouelasrar Salama S, et al. Method matters: effect of purification technology on neutrophil phenotype and function. Front Immunol. (2022) 13:820058. doi: 10.3389/fimmu.2022.820058
39. Page AR, Good RA. A clinical and experimental study of the function of neutrophils in the inflammatory response. Am J pathol. (1958) 34:645.
40. Carnevale S, Ghasemi S, Rigatelli A, Jaillon S. The complexity of neutrophils in health and disease: Focus on cancer. Semin Immunol. (2020) 48:101409. doi: 10.1016/j.smim.2020.101409
41. Mantovani A, Cassatella MA, Costantini C, Jaillon S. Neutrophils in the activation and regulation of innate and adaptive immunity. Nat Rev Immunol. (2011) 11:519–31. doi: 10.1038/nri3024
42. Ma S, Sun S, Geng L, Song M, Wang W, Ye Y, et al. Caloric restriction reprograms the single-cell transcriptional landscape of rattus norvegicus aging. Cell. (2020) 180:984–1001 e22. doi: 10.1016/j.cell.2020.02.008
43. Chatta GS, Andrews RG, Rodger E, Schrag M, Hammond WP, Dale DC. Hematopoietic progenitors and aging: alterations in granulocytic precursors and responsiveness to recombinant human G-CSF, GM-CSF, and IL-3. J Gerontol. (1993) 48:M207–12. doi: 10.1093/geronj/48.5.M207
44. Chatta GS, Price TH, Stratton JR, Dale DC. Aging and marrow neutrophil reserves. J Am Geriatr Soc. (1994) 42:77–81. doi: 10.1111/j.1532-5415.1994.tb06077.x
45. De Martinis M, Modesti M, Ginaldi L. Phenotypic and functional changes of circulating monocytes and polymorphonuclear leucocytes from elderly persons. Immunol Cell Biol. (2004) 82:415–20. doi: 10.1111/j.0818-9641.2004.01242.x
46. Ferrando-Martinez S, Franco JM, Hernandez A, Ordonez A, Gutierrez E, Abad A, et al. Thymopoiesis in elderly human is associated with systemic inflammatory status. Age (Dordr). (2009) 31:87–97. doi: 10.1007/s11357-008-9084-x
47. Strindhall J, Nilsson BO, Lofgren S, Ernerudh J, Pawelec G, Johansson B, et al. No Immune Risk Profile among individuals who reach 100 years of age: findings from the Swedish NONA immune longitudinal study. Exp Gerontol. (2007) 42:753–61. doi: 10.1016/j.exger.2007.05.001
48. Verschoor CP, Loukov D, Naidoo A, Puchta A, Johnstone J, Millar J, et al. Circulating TNF and mitochondrial DNA are major determinants of neutrophil phenotype in the advanced-age, frail elderly. Mol Immunol. (2015) 65:148–56. doi: 10.1016/j.molimm.2015.01.015
49. Fülöp T, Dupuis G, Witkowski JM, Larbi A. The role of immunosenescence in the development of age-related diseases. Rev investigacion clinica. (2016) 68:84–91.
50. Pinti M, Cevenini E, Nasi M, De Biasi S, Salvioli S, Monti D, et al. Circulating mitochondrial DNA increases with age and is a familiar trait: Implications for “inflamm-aging. Eur J Immunol. (2014) 44:1552–62. doi: 10.1002/eji.201343921
51. Jackaman C, Tomay F, Duong L, Abdol Razak NB, Pixley FJ, Metharom P, et al. Aging and cancer: The role of macrophages and neutrophils. Ageing Res Rev. (2017) 36:105–16. doi: 10.1016/j.arr.2017.03.008
52. Fujiwara T, Kenmotsu H, Naito T, Kawamura T, Mamesaya N, Kotake M, et al. The incidence and risk factors of febrile neutropenia in chemotherapy-naive lung cancer patients receiving etoposide plus platinum. Cancer Chemother Pharmacol. (2017) 79:1229–37. doi: 10.1007/s00280-017-3324-7
53. Laskey RA, Poniewierski MS, Lopez MA, Hanna RK, Secord AA, Gehrig PA, et al. Predictors of severe and febrile neutropenia during primary chemotherapy for ovarian cancer. Gynecol Oncol. (2012) 125:625–30. doi: 10.1016/j.ygyno.2012.03.015
54. Lyman GH, Delgado DJ. Risk and timing of hospitalization for febrile neutropenia in patients receiving CHOP, CHOP-R, or CNOP chemotherapy for intermediate-grade non-Hodgkin lymphoma. Cancer. (2003) 98:2402–9. doi: 10.1002/cncr.11827
55. Salar A, Haioun C, Rossi FG, Duehrsen U, Pettengell R, Johnsen HE, et al. The need for improved neutropenia risk assessment in DLBCL patients receiving R-CHOP-21: findings from clinical practice. Leuk Res. (2012) 36:548–53. doi: 10.1016/j.leukres.2012.02.002
56. Chrischilles E, Delgado DJ, Stolshek BS, Lawless G, Fridman M, Carter WB, et al. Impact of age and colony-stimulating factor use on hospital length of stay for febrile neutropenia in CHOP-treated non-Hodgkin’s lymphoma. Cancer Control. (2002) 9:203–11. doi: 10.1177/107327480200900303
57. Lyman GH, Morrison VA, Dale DC, Crawford J, Delgado DJ, Fridman M, et al. Risk of febrile neutropenia among patients with intermediate-grade non-Hodgkin’s lymphoma receiving CHOP chemotherapy. Leuk Lymphoma. (2003) 44:2069–76. doi: 10.1080/1042819031000119262
58. Morrison VA, Picozzi V, Scott S, Pohlman B, Dickman E, Lee M, et al. The impact of age on delivered dose intensity and hospitalizations for febrile neutropenia in patients with intermediate-grade non-Hodgkin’s lymphoma receiving initial CHOP chemotherapy: a risk factor analysis. Clin Lymphoma. (2001) 2:47–56. doi: 10.3816/CLM.2001.n.011
59. Lagnado A, Leslie J, Ruchaud-Sparagano MH, Victorelli S, Hirsova P, Ogrodnik M, et al. Neutrophils induce paracrine telomere dysfunction and senescence in ROS-dependent manner. EMBO J. (2021) 40:e106048. doi: 10.15252/embj.2020106048
60. Hewitt G, Jurk D, Marques FD, Correia-Melo C, Hardy T, Gackowska A, et al. Telomeres are favoured targets of a persistent DNA damage response in ageing and stress-induced senescence. Nat Commun. (2012) 3:708. doi: 10.1038/ncomms1708
61. Bleriot C, Chakarov S, Ginhoux F. Determinants of resident tissue macrophage identity and function. Immunity. (2020) 52:957–70. doi: 10.1016/j.immuni.2020.05.014
62. Ginhoux F, Guilliams M. Tissue-resident macrophage ontogeny and homeostasis. Immunity. (2016) 44:439–49. doi: 10.1016/j.immuni.2016.02.024
63. Cao Y, Fan Y, Li F, Hao Y, Kong Y, Chen C, et al. Phenotypic and functional alterations of monocyte subsets with aging. Immun Ageing. (2022) 19:63. doi: 10.1186/s12979-022-00321-9
64. Hilt ZT, Pariser DN, Ture SK, Mohan A, Quijada P, Asante AA, et al. Platelet-derived β2M regulates monocyte inflammatory responses. JCI Insight. (2019) 4(5):e122943. doi: 10.1172/jci.insight.122943
65. Smith LK, He Y, Park J-S, Bieri G, Snethlage CE, Lin K, et al. [amp]]beta;2-microglobulin is a systemic pro-aging factor that impairs cognitive function and neurogenesis. Nat Med. (2015) 21:932–7. doi: 10.1038/nm.3898
66. Hilt ZT, Ture SK, Mohan A, Arne A, Morrell CN. Platelet-derived β2m regulates age related monocyte/macrophage functions. Aging. (2019) 11:11955–74. doi: 10.18632/aging.v11i24
67. Bartekova M, Radosinska J, Jelemensky M, Dhalla NS. Role of cytokines and inflammation in heart function during health and disease. Heart Failure Rev. (2018) 23:733–58. doi: 10.1007/s10741-018-9716-x
68. Hearps AC, Martin GE, Angelovich TA, Cheng WJ, Maisa A, Landay AL, et al. Aging is associated with chronic innate immune activation and dysregulation of monocyte phenotype and function. Aging Cell. (2012) 11:867–75. doi: 10.1111/j.1474-9726.2012.00851.x
69. McLachlan JA, Serkin CD, Morrey-Clark KM, Bakouche O. Immunological functions of aged human monocytes. Pathobiology. (1995) 63:148–59. doi: 10.1159/000163946
70. Cui CY, Driscoll RK, Piao Y, Chia CW, Gorospe M, Ferrucci L. Skewed macrophage polarization in aging skeletal muscle. Aging Cell. (2019) 18:e13032. doi: 10.1111/acel.13032
71. Vogelpoel LT, Hansen IS, Rispens T, Muller FJ, van Capel TM, Turina MC, et al. Fc gamma receptor-TLR cross-talk elicits pro-inflammatory cytokine production by human M2 macrophages. Nat Commun. (2014) 5:5444. doi: 10.1038/ncomms6444
72. Peake J, Della Gatta P, Cameron-Smith D. Aging and its effects on inflammation in skeletal muscle at rest and following exercise-induced muscle injury. Am J Physiol Regul Integr Comp Physiol. (2010) 298:R1485–95. doi: 10.1152/ajpregu.00467.2009
73. Bloomer SA. Hepatic macrophage abundance and phenotype in aging and liver iron accumulation. Int J Mol Sci. (2022) 23(12):6502. doi: 10.3390/ijms23126502
74. Handa P, Thomas S, Morgan-Stevenson V, Maliken BD, Gochanour E, Boukhar S, et al. Iron alters macrophage polarization status and leads to steatohepatitis and fibrogenesis. J Leukoc Biol. (2019) 105:1015–26. doi: 10.1002/JLB.3A0318-108R
75. Doulias PT, Vlachou C, Boudouri C, Kanavaros P, Siamopoulos KC, Galaris D. Flow cytometric estimation of ‘labile iron pool’ in human white blood cells reveals a positive association with ageing. Free Radic Res. (2008) 42:253–9. doi: 10.1080/10715760801911649
76. Norman K, Hass U, Pirlich M. Malnutrition in older adults-recent advances and remaining challenges. Nutrients. (2021) 13(8):2764. doi: 10.3390/nu13082764
77. Vivian GK, da Silva RO, Santos ACA, Hastreiter AA, Dias CC, Makiyama EN, et al. The interaction between aging and protein malnutrition modulates peritoneal macrophage function: An experimental study in male mice. Exp Gerontol. (2023) 171:112025. doi: 10.1016/j.exger.2022.112025
78. Orecchioni M, Ghosheh Y, Pramod AB, Ley K. Macrophage Polarization: Different Gene Signatures in M1(LPS+) vs. Classically and M2(LPS-) vs. Alternatively Activated Macrophages. Front Immunol. (2019) 10:1084. doi: 10.3389/fimmu.2019.01084
79. Vijay K. Toll-like receptors in immunity and inflammatory diseases: Past, present, and future. Int Immunopharmacol. (2018) 59:391–412. doi: 10.1016/j.intimp.2018.03.002
80. Haria D, Kiefel H, Katlinskaya Y, Jain S, Weinmaier T, Iwai S, et al. Abstract 1490: Novel microbiome-derived peptides activate the host innate immune system by regulation of TLR signaling. Cancer Res. (2019) 79:1490. doi: 10.1158/1538-7445.AM2019-1490
81. Song J, Farris D, Ariza P, Moorjani S, Varghese M, Blin M, et al. Age-associated adipose tissue inflammation promotes monocyte chemotaxis and enhances atherosclerosis. Aging Cell. (2023) 22:e13783. doi: 10.1111/acel.13783
82. Keller U. Nutritional laboratory markers in malnutrition. J Clin Med. (2019) 8(6):775. doi: 10.3390/jcm8060775
83. Cabrerizo S, Cuadras D, Gomez-Busto F, Artaza-Artabe I, Marin-Ciancas F, Malafarina V. Serum albumin and health in older people: Review and meta analysis. Maturitas. (2015) 81:17–27. doi: 10.1016/j.maturitas.2015.02.009
84. Chidrawar SM, Khan N, Chan YL, Nayak L, Moss PA. Ageing is associated with a decline in peripheral blood CD56bright NK cells. Immun Ageing. (2006) 3:10. doi: 10.1186/1742-4933-3-10
85. Almeida-Oliveira A, Smith-Carvalho M, Porto LC, Cardoso-Oliveira J, Ribeiro Ados S, Falcao RR, et al. Age-related changes in natural killer cell receptors from childhood through old age. Hum Immunol. (2011) 72:319–29. doi: 10.1016/j.humimm.2011.01.009
86. Hazeldine J, Hampson P, Lord JM. Reduced release and binding of perforin at the immunological synapse underlies the age-related decline in natural killer cell cytotoxicity. Aging Cell. (2012) 11:751–9. doi: 10.1111/j.1474-9726.2012.00839.x
87. Hayakawa Y, Huntington ND, Nutt SL, Smyth MJ. Functional subsets of mouse natural killer cells. Immunol Rev. (2006) 214:47–55. doi: 10.1111/j.1600-065X.2006.00454.x
88. Beli E, Duriancik DM, Clinthorne JF, Lee T, Kim S, Gardner EM. Natural killer cell development and maturation in aged mice. Mech Ageing Dev. (2014) 135:33–40. doi: 10.1016/j.mad.2013.11.007
89. Schlums H, Cichocki F, Tesi B, Theorell J, Beziat V, Holmes TD, et al. Cytomegalovirus infection drives adaptive epigenetic diversification of NK cells with altered signaling and effector function. Immunity. (2015) 42:443–56. doi: 10.1016/j.immuni.2015.02.008
90. Yang C, Siebert JR, Burns R, Gerbec ZJ, Bonacci B, Rymaszewski A, et al. Heterogeneity of human bone marrow and blood natural killer cells defined by single-cell transcriptome. Nat Commun. (2019) 10:3931. doi: 10.1038/s41467-019-11947-7
91. Guo C, Wu M, Huang B, Zhao R, Jin L, Fu B, et al. Single-cell transcriptomics reveal a unique memory-like NK cell subset that accumulates with ageing and correlates with disease severity in COVID-19. Genome Med. (2022) 14:46. doi: 10.1186/s13073-022-01049-3
92. Le Garff-Tavernier M, Beziat V, Decocq J, Siguret V, Gandjbakhch F, Pautas E, et al. Human NK cells display major phenotypic and functional changes over the life span. Aging Cell. (2010) 9:527–35. doi: 10.1111/j.1474-9726.2010.00584.x
93. Gannage M, Buzyn A, Bogiatzi SI, Lambert M, Soumelis V, Dal Cortivo L, et al. Induction of NKG2D ligands by gamma radiation and tumor necrosis factor-alpha may participate in the tissue damage during acute graft-versus-host disease. Transplantation. (2008) 85:911–5. doi: 10.1097/TP.0b013e31816691ef
94. Zhong J, Yang X, Chen J, He K, Gao X, Wu X, et al. Circular EZH2-encoded EZH2-92aa mediates immune evasion in glioblastoma via inhibition of surface NKG2D ligands. Nat Commun. (2022) 13:4795. doi: 10.1038/s41467-022-32311-2
95. Phan MT, Chun S, Kim SH, Ali AK, Lee SH, Kim S, et al. Natural killer cell subsets and receptor expression in peripheral blood mononuclear cells of a healthy Korean population: Reference range, influence of age and sex, and correlation between NK cell receptors and cytotoxicity. Hum Immunol. (2017) 78:103–12. doi: 10.1016/j.humimm.2016.11.006
96. De Sanctis JB, Dumut DC, Radzioch D, Hajduch M. Functionally relevant differences in plasma fatty acid composition and expression of cytotoxic and inhibitory NK cell receptors between healthy young and healthy elder adults. Nutrients. (2020) 12(12):3641. doi: 10.3390/nu12123641
97. Picard E, Armstrong S, Andrew MK, Haynes L, Loeb M, Pawelec G, et al. Markers of systemic inflammation are positively associated with influenza vaccine antibody responses with a possible role for ILT2(+)CD57(+) NK-cells. Immun Ageing. (2022) 19(1):26. doi: 10.1186/s12979-022-00284-x
98. Lutz CT, Karapetyan A, Al-Attar A, Shelton BJ, Holt KJ, Tucker JH, et al. Human NK cells proliferate and die in vivo more rapidly than T cells in healthy young and elderly adults. J Immunol. (2011) 186:4590–8. doi: 10.4049/jimmunol.1002732
99. Campos C, Pera A, Sanchez-Correa B, Alonso C, Lopez-Fernandez I, Morgado S, et al. Effect of age and CMV on NK cell subpopulations. Exp Gerontol. (2014) 54:130–7. doi: 10.1016/j.exger.2014.01.008
100. Hayhoe RPG, Henson SM, Akbar AN, Palmer DB. Variation of human natural killer cell phenotypes with age: Identification of a unique KLRG1-negative subset. Hum Immunol. (2010) 71:676–81. doi: 10.1016/j.humimm.2010.03.014
101. Hazeldine J, Lord JM. The impact of ageing on natural killer cell function and potential consequences for health in older adults. Ageing Res Rev. (2013) 12:1069–78. doi: 10.1016/j.arr.2013.04.003
102. Brauning A, Rae M, Zhu G, Fulton E, Admasu TD, Stolzing A, et al. Aging of the immune system: focus on natural killer cells phenotype and functions. Cells. (2022) 11(6):1017. doi: 10.3390/cells11061017
103. Kim K, Admasu TD, Stolzing A, Sharma A. Enhanced co-culture and enrichment of human natural killer cells for the selective clearance of senescent cells. Aging (Albany NY). (2022) 14:2131–47. doi: 10.18632/aging.v14i5
104. Camous X, Pera A, Solana R, Larbi A. NK cells in healthy aging and age-associated diseases. J BioMed Biotechnol. (2012) 2012:195956. doi: 10.1155/2012/195956
106. Lutz CT, Moore MB, Bradley S, Shelton BJ, Lutgendorf SK. Reciprocal age related change in natural killer cell receptors for MHC class I. Mech Ageing Dev. (2005) 126:722–31. doi: 10.1016/j.mad.2005.01.004
107. Fernandes G, Gupta S. Natural killing and antibody-dependent cytotoxicity by lymphocyte subpopulations in young and aging humans. J Clin Immunol. (1981) 1:141–8. doi: 10.1007/BF00922755
108. Mariani E, Mariani AR, Meneghetti A, Tarozzi A, Cocco L, Facchini A. Age-dependent decreases of NK cell phosphoinositide turnover during spontaneous but not Fc-mediated cytolytic activity. Int Immunol. (1998) 10:981–9. doi: 10.1093/intimm/10.7.981
109. Lopez-Verges S, Milush JM, Pandey S, York VA, Arakawa-Hoyt J, Pircher H, et al. CD57 defines a functionally distinct population of mature NK cells in the human CD56dimCD16+ NK-cell subset. Blood. (2010) 116:3865–74. doi: 10.1182/blood-2010-04-282301
110. Antonangeli F, Zingoni A, Soriani A, Santoni A. Senescent cells: Living or dying is a matter of NK cells. J Leukoc Biol. (2019) 105:1275–83. doi: 10.1002/JLB.MR0718-299R
111. Sagiv A, Biran A, Yon M, Simon J, Lowe SW, Krizhanovsky V. Granule exocytosis mediates immune surveillance of senescent cells. Oncogene. (2013) 32:1971–7. doi: 10.1038/onc.2012.206
112. Jin H, Jia Y, Yao Z, Huang J, Hao M, Yao S, et al. Hepatic stellate cell interferes with NK cell regulation of fibrogenesis via curcumin induced senescence of hepatic stellate cell. Cell Signal. (2017) 33:79–85. doi: 10.1016/j.cellsig.2017.02.006
113. Ehrlich P. Beiträge zur Theorie und Praxis der histologischen Färbung. Leipzig (SN): Leipzig University. (1878).
114. Irani AA, Schechter NM, Craig SS, DeBlois G, Schwartz LB. Two types of human mast cells that have distinct neutral protease compositions. Proc Natl Acad Sci U S A. (1986) 83:4464–8. doi: 10.1073/pnas.83.12.4464
115. Krystel-Whittemore M, Dileepan KN, Wood JG. Mast cell: A multi-functional master cell. Front Immunol. (2015) 6:620. doi: 10.3389/fimmu.2015.00620
116. St John AL, Rathore APS, Ginhoux F. New perspectives on the origins and heterogeneity of mast cells. Nat Rev Immunol. (2023) 23:55–68. doi: 10.1038/s41577-022-00731-2
117. Sonoda T, Hayashi C, Kitamura Y. Presence of mast cell precursors in the yolk sac of mice. Dev Biol. (1983) 97:89–94. doi: 10.1016/0012-1606(83)90066-0
118. Gentek R, Ghigo C, Hoeffel G, Bulle MJ, Msallam R, Gautier G, et al. Hemogenic endothelial fate mapping reveals dual developmental origin of mast cells. Immunity. (2018) 48:1160–71 e5. doi: 10.1016/j.immuni.2018.04.025
119. Li Z, Liu S, Xu J, Zhang X, Han D, Liu J, et al. Adult connective tissue-resident mast cells originate from late erythro-myeloid progenitors. Immunity. (2018) 49:640–53 e5. doi: 10.1016/j.immuni.2018.09.023
120. Chen CC, Grimbaldeston MA, Tsai M, Weissman IL, Galli SJ. Identification of mast cell progenitors in adult mice. Proc Natl Acad Sci U S A. (2005) 102:11408–13. doi: 10.1073/pnas.0504197102
121. Honda T, Keith YH. Novel insights into the immune-regulatory functions of mast cells in the cutaneous immune response. Front Immunol. (2022) 13:898419. doi: 10.3389/fimmu.2022.898419
122. Pilkington SM, Barron MJ, Watson REB, Griffiths CEM, Bulfone-Paus S. Aged human skin accumulates mast cells with altered functionality that localize to macrophages and vasoactive intestinal peptide-positive nerve fibres. Br J Dermatol. (2019) 180:849–58. doi: 10.1111/bjd.17268
123. Abdel Hafez SMN. Age related changes in the dermal mast cells and the associated changes in the dermal collagen and cells: A histological and electron microscopy study. Acta Histochem. (2019) 121:619–27. doi: 10.1016/j.acthis.2019.05.004
124. Tuncel N, Tore F, Sahinturk V, Ak D, Tuncel M. Vasoactive intestinal peptide inhibits degranulation and changes granular content of mast cells: a potential therapeutic strategy in controlling septic shock. Peptides. (2000) 21:81–9. doi: 10.1016/S0196-9781(99)00177-1
125. Song X, Pi S, Gao Y, Zhou F, Yan S, Chen Y, et al. The role of vasoactive intestinal peptide and mast cells in the regulatory effect of lactobacillus casei ATCC 393 on intestinal mucosal immune barrier. Front Immunol. (2021) 12:723173. doi: 10.3389/fimmu.2021.723173
126. He SH. Key role of mast cells and their major secretory products in inflammatory bowel disease. World J Gastroenterol. (2004) 10:309–18. doi: 10.3748/wjg.v10.i3.309
127. Barkaway A, Rolas L, Joulia R, Bodkin J, Lenn T, Owen-Woods C, et al. Age-related changes in the local milieu of inflamed tissues cause aberrant neutrophil trafficking and subsequent remote organ damage. Immunity. (2021) 54:1494–510 e7. doi: 10.1016/j.immuni.2021.04.025
128. Yu Y, Daly DM, Adam IJ, Kitsanta P, Hill CJ, Wild J, et al. Interplay between mast cells, enterochromaffin cells, and sensory signaling in the aging human bowel. Neurogastroenterol Motil. (2016) 28:1465–79. doi: 10.1111/nmo.12842
129. Blasco MP, Chauhan A, Honarpisheh P, Ahnstedt H, d’Aigle J, Ganesan A, et al. Age-dependent involvement of gut mast cells and histamine in post-stroke inflammation. J Neuroinflammation. (2020) 17:160. doi: 10.1186/s12974-020-01833-1
130. Stamenov N, Kotov G, Iliev A, Landzhov B, Kirkov V, Stanchev S. Mast cells and basic fibroblast growth factor in physiological aging of rat heart and kidney. Biotech Histochem. (2022) 97:504–18. doi: 10.1080/10520295.2021.2024251
131. Labat-Robert J. Cell-matrix interactions in aging: role of receptors and matricryptins. Ageing Res Rev. (2004) 3:233–47. doi: 10.1016/j.arr.2003.10.002
132. Kular JK, Basu S, Sharma RI. The extracellular matrix: Structure, composition, age-related differences, tools for analysis and applications for tissue engineering. J Tissue Eng. (2014) 5:2041731414557112. doi: 10.1177/2041731414557112
133. Kruger-Krasagakes S, Grutzkau A, Krasagakis K, Hoffmann S, Henz BM. Adhesion of human mast cells to extracellular matrix provides a co-stimulatory signal for cytokine production. Immunology. (1999) 98:253–7. doi: 10.1046/j.1365-2567.1999.00865.x
134. Oki T, Kitaura J, Eto K, Lu Y, Maeda-Yamamoto M, Inagaki N, et al. Integrin alphaIIbbeta3 induces the adhesion and activation of mast cells through interaction with fibrinogen. J Immunol. (2006) 176:52–60. doi: 10.4049/jimmunol.176.1.52
135. Tarnok A, Ulrich H, Bocsi J. Phenotypes of stem cells from diverse origin. Cytometry A. (2010) 77:6–10. doi: 10.1002/cyto.a.20844
136. Coutu DL, Galipeau J. Roles of FGF signaling in stem cell self-renewal, senescence and aging. Aging (Albany NY). (2011) 3:920–33. doi: 10.18632/aging.v3i10
137. Lappalainen H, Laine P, Pentikainen MO, Sajantila A, Kovanen PT. Mast cells in neovascularized human coronary plaques store and secrete basic fibroblast growth factor, a potent angiogenic mediator. Arterioscler Thromb Vasc Biol. (2004) 24:1880–5. doi: 10.1161/01.ATV.0000140820.51174.8d
138. Fan Z, Xu Z, Niu H, Sui Y, Li H, Ma J, et al. Spatiotemporal delivery of basic fibroblast growth factor to directly and simultaneously attenuate cardiac fibrosis and promote cardiac tissue vascularization following myocardial infarction. J Control Release. (2019) 311-312:233–44. doi: 10.1016/j.jconrel.2019.09.005
139. Finlin BS, Zhu B, Confides AL, Westgate PM, Harfmann BD, Dupont-Versteegden EE, et al. Mast cells promote seasonal white adipose beiging in humans. Diabetes. (2017) 66:1237–46. doi: 10.2337/db16-1057
140. Sun L, Wu J, Du F, Chen X, Chen ZJ. Cyclic GMP-AMP synthase is a cytosolic DNA sensor that activates the type I interferon pathway. Science. (2013) 339:786–91. doi: 10.1126/science.1232458
141. Wu J, Sun L, Chen X, Du F, Shi H, Chen C, et al. Cyclic GMP-AMP is an endogenous second messenger in innate immune signaling by cytosolic DNA. Science. (2013) 339:826–30. doi: 10.1126/science.1229963
142. Ishikawa H, Barber GN. STING is an endoplasmic reticulum adaptor that facilitates innate immune signalling. Nature. (2008) 455:674–8. doi: 10.1038/nature07317
143. Jin L, Waterman PM, Jonscher KR, Short CM, Reisdorph NA, Cambier JC. MPYS, a novel membrane tetraspanner, is associated with major histocompatibility complex class II and mediates transduction of apoptotic signals. Mol Cell Biol. (2008) 28:5014–26. doi: 10.1128/MCB.00640-08
144. Zhong B, Yang Y, Li S, Wang YY, Li Y, Diao F, et al. The adaptor protein MITA links virus-sensing receptors to IRF3 transcription factor activation. Immunity. (2008) 29:538–50. doi: 10.1016/j.immuni.2008.09.003
145. Sun W, Li Y, Chen L, Chen H, You F, Zhou X, et al. ERIS, an endoplasmic reticulum IFN stimulator, activates innate immune signaling through dimerization. Proc Natl Acad Sci U S A. (2009) 106:8653–8. doi: 10.1073/pnas.0900850106
146. Yang H, Wang H, Ren J, Chen Q, Chen ZJ. cGAS is essential for cellular senescence. Proc Natl Acad Sci U S A. (2017) 114:E4612–E20. doi: 10.1073/pnas.1705499114
147. Lopez-Otin C, Blasco MA, Partridge L, Serrano M, Kroemer G. The hallmarks of aging. Cell. (2013) 153:1194–217. doi: 10.1016/j.cell.2013.05.039
148. Childs BG, Gluscevic M, Baker DJ, Laberge RM, Marquess D, Dananberg J, et al. Senescent cells: an emerging target for diseases of ageing. Nat Rev Drug Discovery. (2017) 16:718–35. doi: 10.1038/nrd.2017.116
149. Lorenzo EC, Kuchel GA, Kuo CL, Moffitt TE, Diniz BS. Major depression and the biological hallmarks of aging. Ageing Res Rev. (2023) 83:101805. doi: 10.1016/j.arr.2022.101805
150. Dupont S, Morsut L, Aragona M, Enzo E, Giulitti S, Cordenonsi M, et al. Role of YAP/TAZ in mechanotransduction. Nature. (2011) 474:179–83. doi: 10.1038/nature10137
151. Sladitschek-Martens HL, Guarnieri A, Brumana G, Zanconato F, Battilana G, Xiccato RL, et al. YAP/TAZ activity in stromal cells prevents ageing by controlling cGAS-STING. Nature. (2022) 607:790–8. doi: 10.1038/s41586-022-04924-6
152. Li J, Yen C, Liaw D, Podsypanina K, Bose S, Wang SI, et al. PTEN, a putative protein tyrosine phosphatase gene mutated in human brain, breast, and prostate cancer. Science. (1997) 275:1943–7. doi: 10.1126/science.275.5308.1943
153. Xu W, Yang Z, Xie C, Zhu Y, Shu X, Zhang Z, et al. PTEN lipid phosphatase inactivation links the hippo and PI3K/Akt pathways to induce gastric tumorigenesis. J Exp Clin Cancer Res. (2018) 37:198. doi: 10.1186/s13046-018-0795-2
154. Bassi C, Fortin J, Snow BE, Wakeham A, Ho J, Haight J, et al. The PTEN and ATM axis controls the G1/S cell cycle checkpoint and tumorigenesis in HER2-positive breast cancer. Cell Death Differ. (2021) 28:3036–51. doi: 10.1038/s41418-021-00799-8
155. Haier J, Nicolson GL. PTEN regulates tumor cell adhesion of colon carcinoma cells under dynamic conditions of fluid flow. Oncogene. (2002) 21:1450–60. doi: 10.1038/sj.onc.1205213
156. Wu J, Gao H, Ge W, He J. Over expression of PTEN induces apoptosis and prevents cell proliferation in breast cancer cells. Acta Biochim Pol. (2020) 67:515–9. doi: 10.18388/abp.2020_5371
157. Kim SG, Sung JY, Kang YJ, Choi HC. Fisetin alleviates cellular senescence through PTEN mediated inhibition of PKCdelta-NOX1 pathway in vascular smooth muscle cells. Arch Gerontol Geriatr. (2023) 108:104927. doi: 10.1016/j.archger.2023.104927
158. Wang J, Cheng Y. The interaction of hsa_circ_0002594 and eIF4A3 promotes T-helper 2 cell differentiation by the regulation of PTEN. Clin Exp Med. (2023) 23:887–95. doi: 10.1007/s10238-022-00862-9
159. Smith JA. STING, the endoplasmic reticulum, and mitochondria: is three a crowd or a conversation? Front Immunol. (2020) 11:611347. doi: 10.3389/fimmu.2020.611347
160. Ha MH, Kim MS, An HJ, Sung MJ, Lee YH, Yang DH, et al. PTEN-induced kinase 1 is associated with renal aging, via the cGAS-STING pathway. Aging Cell. (2023) 22(7):e13865. doi: 10.1111/acel.13865
161. Friedli M, Trono D. The developmental control of transposable elements and the evolution of higher species. Annu Rev Cell Dev Biol. (2015) 31:429–51. doi: 10.1146/annurev-cellbio-100814-125514
162. Smit AF. Interspersed repeats and other mementos of transposable elements in mammalian genomes. Curr Opin Genet Dev. (1999) 9:657–63. doi: 10.1016/S0959-437X(99)00031-3
163. Bock M, Stoye JP. Endogenous retroviruses and the human germline. Curr Opin Genet Dev. (2000) 10:651–5. doi: 10.1016/S0959-437X(00)00138-6
164. Cordaux R, Batzer MA. The impact of retrotransposons on human genome evolution. Nat Rev Genet. (2009) 10:691–703. doi: 10.1038/nrg2640
165. Blikstad V, Benachenhou F, Sperber GO, Blomberg J. Evolution of human endogenous retroviral sequences: a conceptual account. Cell Mol Life Sci. (2008) 65:3348–65. doi: 10.1007/s00018-008-8495-2
166. Johnson WE. Origins and evolutionary consequences of ancient endogenous retroviruses. Nat Rev Microbiol. (2019) 17:355–70. doi: 10.1038/s41579-019-0189-2
167. Liu X, Liu Z, Wu Z, Ren J, Fan Y, Sun L, et al. Resurrection of endogenous retroviruses during aging reinforces senescence. Cell. (2023) 186:287–304 e26. doi: 10.1016/j.cell.2022.12.017
168. Xie X, Ma G, Li X, Zhao J, Zhao Z, Zeng J. Activation of innate immune cGAS-STING pathway contributes to Alzheimer’s pathogenesis in 5xFAD mice. Nat Aging. (2023) 3:202–12. doi: 10.1038/s43587-022-00337-2
169. Zhang H, Li J, Yu Y, Ren J, Liu Q, Bao Z, et al. Nuclear lamina erosion-induced resurrection of endogenous retroviruses underlies neuronal aging. Cell Rep. (2023) 42:112593. doi: 10.1016/j.celrep.2023.112593
170. Le Blanc G, Jette Pomerleau V, McCarthy J, Borroni B, van Swieten J, Galimberti D, et al. Faster cortical thinning and surface area loss in presymptomatic and symptomatic C9orf72 repeat expansion adult carriers. Ann Neurol. (2020) 88:113–22. doi: 10.1002/ana.25748
171. Saunders T, Gunn C, Blennow K, Kvartsberg H, Zetterberg H, Shenkin SD, et al. Neurogranin in Alzheimer’s disease and ageing: A human post-mortem study. Neurobiol Dis. (2023) 177:105991. doi: 10.1016/j.nbd.2023.105991
172. Chen Z, Wu B, Li G, Zhou L, Zhang L, Liu J. Age and sex differentially shape brain networks in Parkinson’s disease. CNS Neurosci Ther. (2023) 29(7):1907–22. doi: 10.1111/cns.14149
173. Rosenblatt A, Kumar BV, Mo A, Welsh CS, Margolis RL, Ross CA. Age, CAG repeat length, and clinical progression in Huntington’s disease. Mov Disord. (2012) 27:272–6. doi: 10.1002/mds.24024
174. Rosen CL, Dinapoli VA, Nagamine T, Crocco T. Influence of age on stroke outcome following transient focal ischemia. J Neurosurg. (2005) 103:687–94. doi: 10.3171/jns.2005.103.4.0687
175. Tan Z, Li X, Kelly KA, Rosen CL, Huber JD. Plasminogen activator inhibitor type 1 derived peptide, EEIIMD, diminishes cortical infarct but fails to improve neurological function in aged rats following middle cerebral artery occlusion. Brain Res. (2009) 1281:84–90. doi: 10.1016/j.brainres.2009.05.042
176. Borghero G, Pierri V, Vasta R, Ercoli T, Primicerio G, Pili F, et al. Incidence of amyotrophic lateral sclerosis in Sardinia, Italy: age-sex interaction and spatial-temporal variability. Amyotroph Lateral Scler Frontotemporal Degener. (2022) 23:585–91. doi: 10.1080/21678421.2022.2041670
177. Exley AR, Buckenham S, Hodges E, Hallam R, Byrd P, Last J, et al. Premature ageing of the immune system underlies immunodeficiency in ataxia telangiectasia. Clin Immunol. (2011) 140:26–36. doi: 10.1016/j.clim.2011.03.007
178. Decout A, Katz JD, Venkatraman S, Ablasser A. The cGAS-STING pathway as a therapeutic target in inflammatory diseases. Nat Rev Immunol. (2021) 21:548–69. doi: 10.1038/s41577-021-00524-z
179. Hou Y, Wei Y, Lautrup S, Yang B, Wang Y, Cordonnier S, et al. NAD(+) supplementation reduces neuroinflammation and cell senescence in a transgenic mouse model of Alzheimer’s disease via cGAS-STING. Proc Natl Acad Sci U.S.A. (2021) 118(37):e2011226118. doi: 10.1073/pnas.2011226118
180. Paul BD, Snyder SH, Bohr VA. Signaling by cGAS-STING in neurodegeneration, neuroinflammation, and aging. Trends Neurosci. (2021) 44:83–96. doi: 10.1016/j.tins.2020.10.008
181. Quan Y, Xin Y, Tian G, Zhou J, Liu X. Mitochondrial ROS-modulated mtDNA: A potential target for cardiac aging. Oxid Med Cell Longev. (2020) 2020:9423593. doi: 10.1155/2020/9423593
182. Yu H, Liao K, Hu Y, Lv D, Luo M, Liu Q, et al. Role of the cGAS-STING pathway in aging-related endothelial dysfunction. Aging Dis. (2022) 13:1901–18. doi: 10.14336/AD.2022.0316
183. Vincent J, Adura C, Gao P, Luz A, Lama L, Asano Y, et al. Small molecule inhibition of cGAS reduces interferon expression in primary macrophages from autoimmune mice. Nat Commun. (2017) 8:750. doi: 10.1038/s41467-017-00833-9
184. Haag SM, Gulen MF, Reymond L, Gibelin A, Abrami L, Decout A, et al. Targeting STING with covalent small-molecule inhibitors. Nature. (2018) 559:269–73. doi: 10.1038/s41586-018-0287-8
185. Iwasaki A, Medzhitov R. Regulation of adaptive immunity by the innate immune system. Science. (2010) 327:291–5. doi: 10.1126/science.1183021
186. Iwasaki A, Medzhitov R. Control of adaptive immunity by the innate immune system. Nat Immunol. (2015) 16:343–53. doi: 10.1038/ni.3123
187. Crooke SN, Ovsyannikova IG, Poland GA, Kennedy RB. Immunosenescence and human vaccine immune responses. Immun Ageing. (2019) 16:25. doi: 10.1186/s12979-019-0164-9
188. Oh SJ, Lee JK, Shin OS. Aging and the immune system: the impact of immunosenescence on viral infection, immunity and vaccine immunogenicity. Immune Netw. (2019) 19:e37. doi: 10.4110/in.2019.19.e37
189. Garnica M, Aiello A, Ligotti ME, Accardi G, Arasanz H, Bocanegra A, et al. How can we improve the vaccination response in older people? Part II: targeting immunosenescence of adaptive immunity cells. Int J Mol Sci. (2022) 23(17):9797. doi: 10.3390/ijms23179797
190. Linton PJ, Dorshkind K. Age-related changes in lymphocyte development and function. Nat Immunol. (2004) 5:133–9. doi: 10.1038/ni1033
191. Moore SE, Fulford AJ, Wagatsuma Y, Persson LA, Arifeen SE, Prentice AM. Thymus development and infant and child mortality in rural Bangladesh. Int J Epidemiol. (2014) 43:216–23. doi: 10.1093/ije/dyt232
192. Hale LP, Cheatham L, Macintyre AN, LaFleur B, Sanders B, Troy J, et al. T cell-depleted cultured pediatric thymus tissue as a model for some aspects of human age-related thymus involution. Geroscience. (2021) 43:1369–82. doi: 10.1007/s11357-020-00301-1
193. Dasatinib plus quercetin for accelerated aging in mental disorders . Available online at: https://classic.clinicaltrials.gov/show/NCT05838560. (accessed July 5, 2024).
194. Nakayama K, Kondo M, Okuno T, Razali N, Hasegawa H. Different properties of involuted thymus upon nutritional deficiency in young and aged mice. Biol Pharm Bull. (2023) 46:464–72. doi: 10.1248/bpb.b22-00842
195. Ueno T, Saito F, Gray DH, Kuse S, Hieshima K, Nakano H, et al. CCR7 signals are essential for cortex-medulla migration of developing thymocytes. J Exp Med. (2004) 200:493–505. doi: 10.1084/jem.20040643
196. Kurobe H, Liu C, Ueno T, Saito F, Ohigashi I, Seach N, et al. CCR7-dependent cortex-to-medulla migration of positively selected thymocytes is essential for establishing central tolerance. Immunity. (2006) 24:165–77. doi: 10.1016/j.immuni.2005.12.011
197. den Braber I, Mugwagwa T, Vrisekoop N, Westera L, Mogling R, de Boer AB, et al. Maintenance of peripheral naive T cells is sustained by thymus output in mice but not humans. Immunity. (2012) 36:288–97. doi: 10.1016/j.immuni.2012.02.006
198. Majumdar S, Nandi D. Thymic atrophy: experimental studies and therapeutic interventions. Scand J Immunol. (2018) 87:4–14. doi: 10.1111/sji.12618
199. Hjelmborg JB, Dalgard C, Moller S, Steenstrup T, Kimura M, Christensen K, et al. The heritability of leucocyte telomere length dynamics. J Med Genet. (2015) 52:297–302. doi: 10.1136/jmedgenet-2014-102736
200. Huang Z, Liu C, Ruan Y, Guo Y, Sun S, Shi Y, et al. Dynamics of leukocyte telomere length in adults aged 50 and older: a longitudinal population-based cohort study. Geroscience. (2021) 43:645–54. doi: 10.1007/s11357-020-00320-y
201. Anderson JJ, Susser E, Arbeev KG, Yashin AI, Levy D, Verhulst S, et al. Telomere-length dependent T-cell clonal expansion: A model linking ageing to COVID-19 T-cell lymphopenia and mortality. EBioMedicine. (2022) 78:103978. doi: 10.1016/j.ebiom.2022.103978
202. Yang L, Mailloux A, Rollison DE, Painter JS, Maciejewski J, Paquette RL, et al. Naive T-cells in myelodysplastic syndrome display intrinsic human telomerase reverse transcriptase (hTERT) deficiency. Leukemia. (2013) 27:897–906. doi: 10.1038/leu.2012.300
203. Vera E, Bernardes de Jesus B, Foronda M, Flores JM, Blasco MA. The rate of increase of short telomeres predicts longevity in mammals. Cell Rep. (2012) 2:732–7. doi: 10.1016/j.celrep.2012.08.023
204. Factor-Litvak P, Susser E, Kezios K, McKeague I, Kark JD, Hoffman M, et al. Leukocyte telomere length in newborns: implications for the role of telomeres in human disease. Pediatrics. (2016) 137(4):e20153927. doi: 10.1542/peds.2015-3927
205. Gorbunova V, Seluanov A. Coevolution of telomerase activity and body mass in mammals: from mice to beavers. Mech Ageing Dev. (2009) 130:3–9. doi: 10.1016/j.mad.2008.02.008
206. Matthe DM, Thoma OM, Sperka T, Neurath MF, Waldner MJ. Telomerase deficiency reflects age-associated changes in CD4+ T cells. Immun Ageing. (2022) 19:16. doi: 10.1186/s12979-022-00273-0
207. Luckheeram RV, Zhou R, Verma AD, Xia B. CD4(+)T cells: differentiation and functions. Clin Dev Immunol. (2012) 2012:925135. doi: 10.1155/2012/925135
208. Tanaskovic S, Price P, French MA, Fernandez S. Impaired upregulation of the costimulatory molecules, CD27 and CD28, on CD4(+) T cells from HIV patients receiving ART is associated with poor proliferative responses. AIDS Res Hum Retroviruses. (2017) 33:101–9. doi: 10.1089/aid.2015.0327
209. Wang X, Wang D, Du J, Wei Y, Song R, Wang B, et al. High levels of CD244 rather than CD160 associate with CD8(+) T-cell aging. Front Immunol. (2022) 13:853522. doi: 10.3389/fimmu.2022.853522
210. Kawata K, Suzuki T, Ozawa K, Sekiguchi M. Features of T-cell subset composition in a D-galactose-induced senescence mouse model. Exp Anim. (2021) 70:284–92. doi: 10.1538/expanim.20-0095
211. Moreno-Valladares M, Moreno-Cugnon L, Silva TM, Garces JP, Saenz-Antonanzas A, Alvarez-Satta M, et al. CD8(+) T cells are increased in the subventricular zone with physiological and pathological aging. Aging Cell. (2020) 19:e13198. doi: 10.1111/acel.13198
212. Bajpai A, Li R, Chen W. The cellular mechanobiology of aging: from biology to mechanics. Ann N Y Acad Sci. (2021) 1491:3–24. doi: 10.1111/nyas.14529
213. Gonzalez-Bermudez B, Kobayashi H, Abarca-Ortega A, Corcoles-Lucas M, Gonzalez-Sanchez M, de la Fuente M, et al. Aging is accompanied by T-cell stiffening and reduced interstitial migration through dysfunctional nuclear organization. Immunology. (2022) 167:622–39. doi: 10.1111/imm.13559
214. Sorokin L. The impact of the extracellular matrix on inflammation. Nat Rev Immunol. (2010) 10:712–23. doi: 10.1038/nri2852
215. Guo R, Li W, Li Y, Li Y, Jiang Z, Song Y. Generation and clinical potential of functional T lymphocytes from gene-edited pluripotent stem cells. Exp Hematol Oncol. (2022) 11:27. doi: 10.1186/s40164-022-00285-y
216. Hardy RR, Hayakawa K. B cell development pathways. Annu Rev Immunol. (2001) 19:595–621. doi: 10.1146/annurev.immunol.19.1.595
217. de Mol J, Kuiper J, Tsiantoulas D, Foks AC. The dynamics of B cell aging in health and disease. Front Immunol. (2021) 12:733566. doi: 10.3389/fimmu.2021.733566
218. Ratliff M, Alter S, Frasca D, Blomberg BB, Riley RL. In senescence, age-associated B cells secrete TNFalpha and inhibit survival of B-cell precursors. Aging Cell. (2013) 12:303–11. doi: 10.1111/acel.12055
219. Piskor EM, Ross J, Moroy T, Kosan C. Myc-interacting zinc finger protein 1 (Miz-1) is essential to maintain homeostasis and immunocompetence of the B cell lineage. Biol (Basel). (2022) 11(4):504. doi: 10.3390/biology11040504
220. Wilson EL, King AM, Sherwood EM, Riley RL. Pre-B cell loss in senescence coincides with preferential development of immature B cells characterized by partial activation and altered Vh repertoire. Exp Gerontol. (2005) 40:67–79. doi: 10.1016/j.exger.2004.11.005
221. Labrie JE 3rd, Sah AP, Allman DM, Cancro MP, Gerstein RM. Bone marrow microenvironmental changes underlie reduced RAG-mediated recombination and B cell generation in aged mice. J Exp Med. (2004) 200:411–23. doi: 10.1084/jem.20040845
222. Fleming HE, Paige CJ. Pre-B cell receptor signaling mediates selective response to IL-7 at the pro-B to pre-B cell transition via an ERK/MAP kinase-dependent pathway. Immunity. (2001) 15:521–31. doi: 10.1016/S1074-7613(01)00216-3
223. Miller JP, Allman D. The decline in B lymphopoiesis in aged mice reflects loss of very early B-lineage precursors. J Immunol. (2003) 171:2326–30. doi: 10.4049/jimmunol.171.5.2326
224. Fujiki T, Matsumoto SE, Kishihara K, Katakura Y. Age-related functional decline of human B cells. Cytotechnology. (2022) 74:319–27. doi: 10.1007/s10616-021-00513-z
225. Chong Y, Ikematsu H, Yamaji K, Nishimura M, Nabeshima S, Kashiwagi S, et al. CD27(+) (memory) B cell decrease and apoptosis-resistant CD27(-) (naive) B cell increase in aged humans: implications for age-related peripheral B cell developmental disturbances. Int Immunol. (2005) 17:383–90. doi: 10.1093/intimm/dxh218
226. Mandala WL, Longwe H. Variation of B cell subsets with age in healthy Malawians. PloS One. (2021) 16:e0254320. doi: 10.1371/journal.pone.0254320
227. Tangye SG, Hodgkin PD. Divide and conquer: the importance of cell division in regulating B-cell responses. Immunology. (2004) 112:509–20. doi: 10.1111/j.1365-2567.2004.01950.x
228. Cancro MP. Age-associated B cells. Annu Rev Immunol. (2020) 38:315–40. doi: 10.1146/annurev-immunol-092419-031130
229. Ciocca M, Zaffina S, Fernandez Salinas A, Bocci C, Palomba P, Conti MG, et al. Evolution of human memory B cells from childhood to old age. Front Immunol. (2021) 12:690534. doi: 10.3389/fimmu.2021.690534
230. Kibler A, Budeus B, Homp E, Bronischewski K, Berg V, Sellmann L, et al. Systematic memory B cell archiving and random display shape the human splenic marginal zone throughout life. J Exp Med. (2021) 218(4):e20201952. doi: 10.1084/jem.20201952
231. Mandala WL, Ananworanich J, Apornpong T, Kerr SJ, MacLennan JM, Hanson C, et al. Control lymphocyte subsets: can one country’s values serve for another’s? J Allergy Clin Immunol. (2014) 134:759–61 e8. doi: 10.1016/j.jaci.2014.06.030
232. Noren Hooten N, Longo DL, Evans MK. Age- and race-related changes in subpopulations of peripheral blood lymphocytes in humans. Handb Immunosenescence. (2018), 1–30. doi: 10.1007/978-3-319-64597-1_85-1
233. Reynaud CA, Descatoire M, Dogan I, Huetz F, Weller S, Weill JC. IgM memory B cells: a mouse/human paradox. Cell Mol Life Sci. (2012) 69:1625–34. doi: 10.1007/s00018-012-0971-z
234. Kurosaki T, Kometani K, Ise W. Memory B cells. Nat Rev Immunol. (2015) 15:149–59. doi: 10.1038/nri3802
235. Hoffman W, Lakkis FG, Chalasani G. B cells, antibodies, and more. Clin J Am Soc Nephrol. (2016) 11:137–54. doi: 10.2215/CJN.09430915
236. Turner VM, Mabbott NA. Ageing adversely affects the migration and function of marginal zone B cells. Immunology. (2017) 151:349–62. doi: 10.1111/imm.12737
237. Birjandi SZ, Ippolito JA, Ramadorai AK, Witte PL. Alterations in marginal zone macrophages and marginal zone B cells in old mice. J Immunol. (2011) 186:3441–51. doi: 10.4049/jimmunol.1001271
238. Kibler A, Budeus B, Kuppers R, Seifert M. The splenic marginal zone in children is characterized by a subpopulation of CD27-negative, lowly IGHV-mutated B cells. Front Immunol. (2022) 13:825619. doi: 10.3389/fimmu.2022.825619
239. Salminen A. Immunosuppressive network promotes immunosenescence associated with aging and chronic inflammatory conditions. J Mol Med (Berl). (2021) 99:1553–69. doi: 10.1007/s00109-021-02123-w
240. Agrawal S, Gupta S. TLR1/2, TLR7, and TLR9 signals directly activate human peripheral blood naive and memory B cell subsets to produce cytokines, chemokines, and hematopoietic growth factors. J Clin Immunol. (2011) 31:89–98. doi: 10.1007/s10875-010-9456-8
241. Lopez-Otin C, Blasco MA, Partridge L, Serrano M, Kroemer G. Hallmarks of aging: An expanding universe. Cell. (2023) 186:243–78. doi: 10.1016/j.cell.2022.11.001
242. Gomez de Aguero M, Ganal-Vonarburg SC, Fuhrer T, Rupp S, Uchimura Y, Li H, et al. The maternal microbiota drives early postnatal innate immune development. Science. (2016) 351:1296–302. doi: 10.1126/science.aad2571
243. Thorburn AN, McKenzie CI, Shen S, Stanley D, Macia L, Mason LJ, et al. Evidence that asthma is a developmental origin disease influenced by maternal diet and bacterial metabolites. Nat Commun. (2015) 6:7320. doi: 10.1038/ncomms8320
244. Ly NP, Ruiz-Perez B, Onderdonk AB, Tzianabos AO, Litonjua AA, Liang C, et al. Mode of delivery and cord blood cytokines: a birth cohort study. Clin Mol Allergy. (2006) 4:13. doi: 10.1186/1476-7961-4-13
245. Nobs SP, Elinav E. Complement(ing) the microbiome in infants through breastmilk. Cell Res. (2024) 34(7):469–70. doi: 10.1038/s41422-024-00944-1
246. Xu D, Zhou S, Liu Y, Scott AL, Yang J, Wan F. Complement in breast milk modifies offspring gut microbiota to promote infant health. Cell. (2024) 187:750–63 e20. doi: 10.1016/j.cell.2023.12.019
247. Ma J, Li Z, Zhang W, Zhang C, Zhang Y, Mei H, et al. Comparison of gut microbiota in exclusively breast-fed and formula-fed babies: a study of 91 term infants. Sci Rep. (2020) 10:15792. doi: 10.1038/s41598-020-72635-x
248. Vacca M, Raspini B, Calabrese FM, Porri D, De Giuseppe R, Chieppa M, et al. The establishment of the gut microbiota in 1-year-aged infants: from birth to family food. Eur J Nutr. (2022) 61:2517–30. doi: 10.1007/s00394-022-02822-1
249. Aires J. First 1000 days of life: consequences of antibiotics on gut microbiota. Front Microbiol. (2021) 12:681427. doi: 10.3389/fmicb.2021.681427
250. Strachan DP. Hay fever, hygiene, and household size. BMJ. (1989) 299:1259–60. doi: 10.1136/bmj.299.6710.1259
251. Cowan CSM, Dinan TG, Cryan JF. Annual Research Review: Critical windows - the microbiota-gut-brain axis in neurocognitive development. J Child Psychol Psychiatry. (2020) 61:353–71. doi: 10.1111/jcpp.13156
252. Al Nabhani Z, Dulauroy S, Marques R, Cousu C, Al Bounny S, Dejardin F, et al. A weaning reaction to microbiota is required for resistance to immunopathologies in the adult. Immunity. (2019) 50:1276–88 e5. doi: 10.1016/j.immuni.2019.02.014
253. Guzzardi MA, La Rosa F, Campani D, Cacciato Insilla A, De Sena V, Panetta D, et al. Maturation of the visceral (Gut-adipose-liver) network in response to the weaning reaction versus adult age and impact of maternal high-fat diet. Nutrients. (2021) 13(10):3438. doi: 10.3390/nu13103438
254. Differding MK, Benjamin-Neelon SE, Hoyo C, Ostbye T, Mueller NT. Timing of complementary feeding is associated with gut microbiota diversity and composition and short chain fatty acid concentrations over the first year of life. BMC Microbiol. (2020) 20:56. doi: 10.1186/s12866-020-01723-9
255. Rajilic-Stojanovic M, Heilig HG, Tims S, Zoetendal EG, de Vos WM. Long-term monitoring of the human intestinal microbiota composition. Environ Microbiol. (2012) 15(4):1146–59. doi: 10.1111/1462-2920.12023
256. Faith JJ, Guruge JL, Charbonneau M, Subramanian S, Seedorf H, Goodman AL, et al. The long-term stability of the human gut microbiota. Science. (2013) 341:1237439. doi: 10.1126/science.1237439
257. Zheng D, Liwinski T, Elinav E. Interaction between microbiota and immunity in health and disease. Cell Res. (2020) 30:492–506. doi: 10.1038/s41422-020-0332-7
258. Mariat D, Firmesse O, Levenez F, Guimaraes V, Sokol H, Dore J, et al. The Firmicutes/Bacteroidetes ratio of the human microbiota changes with age. BMC Microbiol. (2009) 9:123. doi: 10.1186/1471-2180-9-123
259. Koenig JE, Spor A, Scalfone N, Fricker AD, Stombaugh J, Knight R, et al. Succession of microbial consortia in the developing infant gut microbiome. Proc Natl Acad Sci U S A. (2011) 108 Suppl 1:4578–85. doi: 10.1073/pnas.1000081107
260. Radjabzadeh D, Boer CG, Beth SA, van der Wal P, Kiefte-De Jong JC, Jansen MAE, et al. Diversity, compositional and functional differences between gut microbiota of children and adults. Sci Rep. (2020) 10:1040. doi: 10.1038/s41598-020-57734-z
261. O’Toole PW, Jeffery IB. Gut microbiota and aging. Science. (2015) 350:1214–5. doi: 10.1126/science.aac8469
262. Wilmanski T, Diener C, Rappaport N, Patwardhan S, Wiedrick J, Lapidus J, et al. Gut microbiome pattern reflects healthy ageing and predicts survival in humans. Nat Metab. (2021) 3:274–86. doi: 10.1038/s42255-021-00348-0
263. Wu YL, Xu J, Rong XY, Wang F, Wang HJ, Zhao C. Gut microbiota alterations and health status in aging adults: From correlation to causation. Aging Med (Milton). (2021) 4:206–13. doi: 10.1002/agm2.12167
264. Kim CH. Complex regulatory effects of gut microbial short-chain fatty acids on immune tolerance and autoimmunity. Cell Mol Immunol. (2023) 20:341–50. doi: 10.1038/s41423-023-00987-1
265. Di Vincenzo F, Del Gaudio A, Petito V, Lopetuso LR, Scaldaferri F. Gut microbiota, intestinal permeability, and systemic inflammation: a narrative review. Intern Emerg Med. (2024) 19:275–93. doi: 10.1007/s11739-023-03374-w
266. Lajqi T, Poschl J, Frommhold D, Hudalla H. The role of microbiota in neutrophil regulation and adaptation in newborns. Front Immunol. (2020) 11:568685. doi: 10.3389/fimmu.2020.568685
267. Lajqi T, Kostlin-Gille N, Hillmer S, Braun M, Kranig SA, Dietz S, et al. Gut microbiota-derived small extracellular vesicles endorse memory-like inflammatory responses in murine neutrophils. Biomedicines. (2022) 10(2):442. doi: 10.3390/biomedicines10020442
268. Lajqi T, Stojiljkovic M, Williams DL, Hudalla H, Bauer M, Witte OW, et al. Memory-like responses of brain microglia are controlled by developmental state and pathogen dose. Front Immunol. (2020) 11:546415. doi: 10.3389/fimmu.2020.546415
269. Sepp E, Smidt I, Roop T, Stsepetova J, Koljalg S, Mikelsaar M, et al. Comparative analysis of gut microbiota in centenarians and young people: impact of eating habits and childhood living environment. Front Cell Infect Microbiol. (2022) 12:851404. doi: 10.3389/fcimb.2022.851404
270. Biagi E, Franceschi C, Rampelli S, Severgnini M, Ostan R, Turroni S, et al. Gut microbiota and extreme longevity. Curr Biol. (2016) 26:1480–5. doi: 10.1016/j.cub.2016.04.016
271. Karagiannis TT, Dowrey TW, Villacorta-Martin C, Montano M, Reed E, Belkina AC, et al. Multi-modal profiling of peripheral blood cells across the human lifespan reveals distinct immune cell signatures of aging and longevity. EBioMedicine. (2023) 90:104514. doi: 10.1016/j.ebiom.2023.104514
272. Fantacone ML, Lowry MB, Uesugi SL, Michels AJ, Choi J, Leonard SW, et al. The effect of a multivitamin and mineral supplement on immune function in healthy older adults: A double-blind, randomized, controlled trial. Nutrients. (2020) 12(8):2447. doi: 10.3390/nu12082447
273. Kalyanaraman B, Darley-Usmar V, Davies KJ, Dennery PA, Forman HJ, Grisham MB, et al. Measuring reactive oxygen and nitrogen species with fluorescent probes: challenges and limitations. Free Radic Biol Med. (2012) 52:1–6. doi: 10.1016/j.freeradbiomed.2011.09.030
274. Albers R, Bourdet-Sicard R, Braun D, Calder PC, Herz U, Lambert C, et al. Monitoring immune modulation by nutrition in the general population: identifying and substantiating effects on human health. Br J Nutr. (2013) 110 Suppl 2:S1–30. doi: 10.1017/S0007114513001505
275. McCay CM, Crowell MF, Maynard LA. The effect of retarded growth upon the length of life span and upon the ultimate body size. J Nutr. (1935) 10:63–79. doi: 10.1093/jn/10.1.63
276. Ravussin E, Redman LM, Rochon J, Das SK, Fontana L, Kraus WE, et al. A 2-year randomized controlled trial of human caloric restriction: feasibility and effects on predictors of health span and longevity. J Gerontol A Biol Sci Med Sci. (2015) 70:1097–104. doi: 10.1093/gerona/glv057
277. Spadaro O, Youm Y, Shchukina I, Ryu S, Sidorov S, Ravussin A, et al. Caloric restriction in humans reveals immunometabolic regulators of health span. Science. (2022) 375:671–7. doi: 10.1126/science.abg7292
278. Sbierski-Kind J, Grenkowitz S, Schlickeiser S, Sandforth A, Friedrich M, Kunkel D, et al. Effects of caloric restriction on the gut microbiome are linked with immune senescence. Microbiome. (2022) 10:57. doi: 10.1186/s40168-022-01249-4
279. Gardner EM. Caloric restriction decreases survival of aged mice in response to primary influenza infection. J Gerontol A Biol Sci Med Sci. (2005) 60:688–94. doi: 10.1093/gerona/60.6.688
280. Kristan DM. Chronic calorie restriction increases susceptibility of laboratory mice (Mus musculus) to a primary intestinal parasite infection. Aging Cell. (2007) 6:817–25. doi: 10.1111/j.1474-9726.2007.00345.x
281. Di Micco R, Krizhanovsky V, Baker D, d’Adda di Fagagna F. Cellular senescence in ageing: from mechanisms to therapeutic opportunities. Nat Rev Mol Cell Biol. (2021) 22:75–95. doi: 10.1038/s41580-020-00314-w
282. Zhu XY, Lerman LO. Senomorphic, senolytic, and rejuvenation therapies. Regenerative Nephrol. New York, USA: Academic Press. (2022), 405–17. doi: 10.1016/B978-0-12-823318-4.00025-1
283. Lorenzo EC, Torrance BL, Haynes L. Impact of senolytic treatment on immunity, aging, and disease. Front Aging. (2023) 4:1161799. doi: 10.3389/fragi.2023.1161799
284. Zhu Y, Tchkonia T, Pirtskhalava T, Gower AC, Ding H, Giorgadze N, et al. The Achilles’ heel of senescent cells: from transcriptome to senolytic drugs. Aging Cell. (2015) 14:644–58. doi: 10.1111/acel.12344
285. Ruggiero AD, Vemuri R, Blawas M, Long M, DeStephanis D, Williams AG, et al. term dasatinib plus quercetin effects on aging outcomes and inflammation in nonhuman primates: implications for senolytic clinical trial design. Geroscience. (2023) 45(5):2785–803. doi: 10.1007/s11357-023-00830-5
286. Grynkiewicz G, Demchuk OM. New perspectives for fisetin. Front Chem. (2019) 7:697. doi: 10.3389/fchem.2019.00697
287. Ravula AR, Teegala SB, Kalakotla S, Pasangulapati JP, Perumal V, Boyina HK. Fisetin, potential flavonoid with multifarious targets for treating neurological disorders: An updated review. Eur J Pharmacol. (2021) 910:174492. doi: 10.1016/j.ejphar.2021.174492
288. Yousefzadeh MJ, Zhu Y, McGowan SJ, Angelini L, Fuhrmann-Stroissnigg H, Xu M, et al. Fisetin is a senotherapeutic that extends health and lifespan. EBioMedicine. (2018) 36:18–28. doi: 10.1016/j.ebiom.2018.09.015
289. Targeting cellular senescence with senolytics to improve skeletal health in older humans. Available online at: https://classic.clinicaltrials.gov/show/NCT04313634. (accessed July 22, 2024).
290. Alleviation by fisetin of frailty, inflammation, and related measures in older adults. Available online at: https://classic.clinicaltrials.gov/show/NCT03675724. (accessed July 11, 2024).
291. COVID-FIS: pilot in COVID-19 (SARS-coV-2) of fisetin in older adults in nursing homes. Available online at: https://classic.clinicaltrials.gov/show/NCT04537299. (accessed July 12, 2024).
292. Chang J, Wang Y, Shao L, Laberge RM, Demaria M, Campisi J, et al. Clearance of senescent cells by ABT263 rejuvenates aged hematopoietic stem cells in mice. Nat Med. (2016) 22:78–83. doi: 10.1038/nm.4010
293. Yosef R, Pilpel N, Tokarsky-Amiel R, Biran A, Ovadya Y, Cohen S, et al. Directed elimination of senescent cells by inhibition of BCL-W and BCL-XL. Nat Commun. (2016) 7:11190. doi: 10.1038/ncomms11190
294. Zhu Y, Tchkonia T, Fuhrmann-Stroissnigg H, Dai HM, Ling YY, Stout MB, et al. Identification of a novel senolytic agent, navitoclax, targeting the Bcl-2 family of anti-apoptotic factors. Aging Cell. (2016) 15:428–35. doi: 10.1111/acel.12445
295. Prieto LI, Sturmlechner I, Graves SI, Zhang C, Goplen NP, Yi ES, et al. Senescent alveolar macrophages promote early-stage lung tumorigenesis. Cancer Cell. (2023) 41:1261–75 e6. doi: 10.1016/j.ccell.2023.05.006
296. Haston S, Gonzalez-Gualda E, Morsli S, Ge J, Reen V, Calderwood A, et al. Clearance of senescent macrophages ameliorates tumorigenesis in KRAS-driven lung cancer. Cancer Cell. (2023) 41:1242–60 e6. doi: 10.1016/j.ccell.2023.05.004
297. Tse C, Shoemaker AR, Adickes J, Anderson MG, Chen J, Jin S, et al. ABT-263: a potent and orally bioavailable Bcl-2 family inhibitor. Cancer Res. (2008) 68:3421–8. doi: 10.1158/0008-5472.CAN-07-5836
298. Wilson WH, O’Connor OA, Czuczman MS, LaCasce AS, Gerecitano JF, Leonard JP, et al. Navitoclax, a targeted high-affinity inhibitor of BCL-2, in lymphoid Malignancies: a phase 1 dose-escalation study of safety, pharmacokinetics, pharmacodynamics, and antitumour activity. Lancet Oncol. (2010) 11:1149–59. doi: 10.1016/S1470-2045(10)70261-8
299. Roberts AW, Seymour JF, Brown JR, Wierda WG, Kipps TJ, Khaw SL, et al. Substantial susceptibility of chronic lymphocytic leukemia to BCL2 inhibition: results of a phase I study of navitoclax in patients with relapsed or refractory disease. J Clin Oncol. (2012) 30:488–96. doi: 10.1200/JCO.2011.34.7898
300. Franceschi C, Campisi J. Chronic inflammation (inflammaging) and its potential contribution to age-associated diseases. J Gerontol A Biol Sci Med Sci. (2014) 69 Suppl 1:S4–9. doi: 10.1093/gerona/glu057
301. Franceschi C, Garagnani P, Parini P, Giuliani C, Santoro A. Inflammaging: a new immune-metabolic viewpoint for age-related diseases. Nat Rev Endocrinol. (2018) 14:576–90. doi: 10.1038/s41574-018-0059-4
302. ElSayed NA, Aleppo G, Aroda VR, Bannuru RR, Brown FM, Bruemmer D, et al. 13. Older adults: standards of care in diabetes-2023. Diabetes Care. (2023) 46:S216–S29. doi: 10.2337/dc23-S013
303. Bharath LP, Agrawal M, McCambridge G, Nicholas DA, Hasturk H, Liu J, et al. Metformin enhances autophagy and normalizes mitochondrial function to alleviate aging-associated inflammation. Cell Metab. (2020) 32:44–55 e6. doi: 10.1016/j.cmet.2020.04.015
304. Zhu X, Shen J, Feng S, Huang C, Wang H, Huo F, et al. Akkermansia muciniphila, which is enriched in the gut microbiota by metformin, improves cognitive function in aged mice by reducing the proinflammatory cytokine interleukin-6. Microbiome. (2023) 11:120. doi: 10.1186/s40168-023-01567-1
305. Cedillo L, Ahsan FM, Li S, Stuhr NL, Zhou Y, Zhang Y, et al. Ether lipid biosynthesis promotes lifespan extension and enables diverse pro-longevity paradigms in Caenorhabditis elegans. Elife. (2023) 12:e82210. doi: 10.7554/eLife.82210.sa2
306. Hurez V, Dao V, Liu A, Pandeswara S, Gelfond J, Sun L, et al. Chronic mTOR inhibition in mice with rapamycin alters T, B, myeloid, and innate lymphoid cells and gut flora and prolongs life of immune-deficient mice. Aging Cell. (2015) 14:945–56. doi: 10.1111/acel.12380
307. Blagosklonny MV. Rapamycin for longevity: opinion article. Aging (Albany NY). (2019) 11:8048–67. doi: 10.18632/aging.v11i19
308. Harrison DE, Strong R, Sharp ZD, Nelson JF, Astle CM, Flurkey K, et al. Rapamycin fed late in life extends lifespan in genetically heterogeneous mice. Nature. (2009) 460:392–5. doi: 10.1038/nature08221
309. Yousefzadeh MJ, Flores RR, Zhu Y, Schmiechen ZC, Brooks RW, Trussoni CE, et al. An aged immune system drives senescence and ageing of solid organs. Nature. (2021) 594:100–5. doi: 10.1038/s41586-021-03547-7
310. Mannick JB, Del Giudice G, Lattanzi M, Valiante NM, Praestgaard J, Huang B, et al. mTOR inhibition improves immune function in the elderly. Sci Transl Med. (2014) 6:268ra179. doi: 10.1126/scitranslmed.3009892
311. Mannick JB, Morris M, Hockey HP, Roma G, Beibel M, Kulmatycki K, et al. TORC1 inhibition enhances immune function and reduces infections in the elderly. Sci Transl Med. (2018) 10(449):eaaq1564. doi: 10.1126/scitranslmed.aaq1564
312. Partridge L, Fuentealba M, Kennedy BK. The quest to slow ageing through drug discovery. Nat Rev Drug Discovery. (2020) 19:513–32. doi: 10.1038/s41573-020-0067-7
313. Lu YX, Regan JC, Esser J, Drews LF, Weinseis T, Stinn J, et al. A TORC1-histone axis regulates chromatin organisation and non-canonical induction of autophagy to ameliorate ageing. Elife. (2021) 10:e62233. doi: 10.7554/eLife.62233.sa2
314. Ham DJ, Borsch A, Chojnowska K, Lin S, Leuchtmann AB, Ham AS, et al. Distinct and additive effects of calorie restriction and rapamycin in aging skeletal muscle. Nat Commun. (2022) 13:2025. doi: 10.1038/s41467-022-29714-6
315. Juricic P, Lu YX, Leech T, Drews LF, Paulitz J, Lu J, et al. Long-lasting geroprotection from brief rapamycin treatment in early adulthood by persistently increased intestinal autophagy. Nat Aging. (2022) 2:824–36. doi: 10.1038/s43587-022-00278-w
316. Shindyapina AV, Cho Y, Kaya A, Tyshkovskiy A, Castro JP, Deik A, et al. Rapamycin treatment during development extends life span and health span of male mice and Daphnia magna. Sci Adv. (2022) 8:eabo5482. doi: 10.1126/sciadv.abo5482
317. Mannick JB, Lamming DW. Targeting the biology of aging with mTOR inhibitors. Nat Aging. (2023) 3:642–60. doi: 10.1038/s43587-023-00416-y
318. Novel compositions for treating or preventing dermal disorders. Available online at: https://classic.clinicaltrials.gov/show/NCT03103893. (accessed Jun 22, 2024).
319. Chung CL, Lawrence I, Hoffman M, Elgindi D, Nadhan K, Potnis M, et al. Topical rapamycin reduces markers of senescence and aging in human skin: an exploratory, prospective, randomized trial. Geroscience. (2019) 41:861–9. doi: 10.1007/s11357-019-00113-y
320. Cappell KM, Kochenderfer JN. Long-term outcomes following CAR T cell therapy: what we know so far. Nat Rev Clin Oncol. (2023) 20:359–71. doi: 10.1038/s41571-023-00754-1
321. Amor C, Feucht J, Leibold J, Ho YJ, Zhu C, Alonso-Curbelo D, et al. Senolytic CAR T cells reverse senescence-associated pathologies. Nature. (2020) 583:127–32. doi: 10.1038/s41586-020-2403-9
322. Carney EF. Use of CAR T cells as senolytic agents. Nat Rev Nephrol. (2020) 16:485. doi: 10.1038/s41581-020-0324-3
323. Chaib S, Tchkonia T, Kirkland JL. Cellular senescence and senolytics: the path to the clinic. Nat Med. (2022) 28:1556–68. doi: 10.1038/s41591-022-01923-y
324. Yang D, Sun B, Li S, Wei W, Liu X, Cui X, et al. NKG2D-CAR T cells eliminate senescent cells in aged mice and nonhuman primates. Sci Transl Med. (2023) 15:eadd1951. doi: 10.1126/scitranslmed.add1951
325. Gill D, Parry A, Santos F, Okkenhaug H, Todd CD, Hernando-Herraez I, et al. Multi-omic rejuvenation of human cells by maturation phase transient reprogramming. Elife. (2022) 11:e71624. doi: 10.7554/eLife.71624
326. Conboy IM, Conboy MJ, Wagers AJ, Girma ER, Weissman IL, Rando TA. Rejuvenation of aged progenitor cells by exposure to a young systemic environment. Nature. (2005) 433:760–4. doi: 10.1038/nature03260
327. Kim D, Kiprov DD, Luellen C, Lieb M, Liu C, Watanabe E, et al. Old plasma dilution reduces human biological age: a clinical study. Geroscience. (2022) 44:2701–20. doi: 10.1007/s11357-022-00645-w
328. Mehdipour M, Amiri P, Liu C, DeCastro J, Kato C, Skinner CM, et al. Small-animal blood exchange is an emerging approach for systemic aging research. Nat Protoc. (2022) 17:2469–93. doi: 10.1038/s41596-022-00731-5
329. Stojiljkovic MR, Ain Q, Bondeva T, Heller R, Schmeer C, Witte OW. Phenotypic and functional differences between senescent and aged murine microglia. Neurobiol Aging. (2019) 74:56–69. doi: 10.1016/j.neurobiolaging.2018.10.007
330. Long G, Hu Y, Tao E, Chen B, Shu X, Zheng W, et al. The influence of cesarean section on the composition and development of gut microbiota during the first 3 months of life. Front Microbiol. (2021) 12:691312. doi: 10.3389/fmicb.2021.691312
331. Tun HM, Konya T, Takaro TK, Brook JR, Chari R, Field CJ, et al. Exposure to household furry pets influences the gut microbiota of infant at 3-4 months following various birth scenarios. Microbiome. (2017) 5:40. doi: 10.1186/s40168-017-0254-x
332. Panzer AR, Sitarik AR, Fadrosh D, Havstad SL, Jones K, Davidson B, et al. The impact of prenatal dog keeping on infant gut microbiota development. Clin Exp Allergy. (2023) 53(8):833–45. doi: 10.1111/cea.14303
333. Clark RI, Salazar A, Yamada R, Fitz-Gibbon S, Morselli M, Alcaraz J, et al. Distinct shifts in microbiota composition during drosophila aging impair intestinal function and drive mortality. Cell Rep. (2015) 12:1656–67. doi: 10.1016/j.celrep.2015.08.004
334. Marra A, Hanson MA, Kondo S, Erkosar B, Lemaitre B. Drosophila antimicrobial peptides and lysozymes regulate gut microbiota composition and abundance. mBio. (2021) 12:e0082421. doi: 10.1128/mBio.00824-21
335. Pang S, Chen X, Lu Z, Meng L, Huang Y, Yu X, et al. Longevity of centenarians is reflected by the gut microbiome with youth-associated signatures. Nat Aging. (2023) 3:436–49. doi: 10.1038/s43587-023-00389-y
336. Wang J, Qie J, Zhu D, Zhang X, Zhang Q, Xu Y, et al. The landscape in the gut microbiome of long-lived families reveals new insights on longevity and aging - relevant neural and immune function. Gut Microbes. (2022) 14:2107288. doi: 10.1080/19490976.2022.2107288
337. Ren M, Li H, Fu Z, Li Q. Succession analysis of gut microbiota structure of participants from long-lived families in Hechi, Guangxi, China. Microorganisms. (2021) 9(12):2524. doi: 10.3390/microorganisms9122524
338. Wang F, Yu T, Huang G, Cai D, Liang X, Su H, et al. Gut microbiota community and its assembly associated with age and diet in Chinese centenarians. J Microbiol Biotechnol. (2015) 25:1195–204. doi: 10.4014/jmb.1410.10014
339. Wu L, Zeng T, Zinellu A, Rubino S, Kelvin DJ, Carru C. A cross-sectional study of compositional and functional profiles of gut microbiota in Sardinian centenarians. mSystems. (2019) 4(4):e00325–19. doi: 10.1128/mSystems.00325-19
340. Sato Y, Atarashi K, Plichta DR, Arai Y, Sasajima S, Kearney SM, et al. Novel bile acid biosynthetic pathways are enriched in the microbiome of centenarians. Nature. (2021) 599:458–64. doi: 10.1038/s41586-021-03832-5
Keywords: aging, innate immunity, adaptive immunity, cGAS-STING, gut microbiota aging, gut microbiota
Citation: Gao H, Nepovimova E, Adam V, Heger Z, Valko M, Wu Q and Kuca K (2024) Age-associated changes in innate and adaptive immunity: role of the gut microbiota. Front. Immunol. 15:1421062. doi: 10.3389/fimmu.2024.1421062
Received: 21 April 2024; Accepted: 26 August 2024;
Published: 16 September 2024.
Edited by:
Trim Lajqi, Heidelberg University Hospital, GermanyReviewed by:
Christian Schmeer, University Hospital Jena, GermanyMatthew Yousefzadeh, Columbia University, United States
Copyright © 2024 Gao, Nepovimova, Adam, Heger, Valko, Wu and Kuca. This is an open-access article distributed under the terms of the Creative Commons Attribution License (CC BY). The use, distribution or reproduction in other forums is permitted, provided the original author(s) and the copyright owner(s) are credited and that the original publication in this journal is cited, in accordance with accepted academic practice. No use, distribution or reproduction is permitted which does not comply with these terms.
*Correspondence: Qinghua Wu, d3FoMjEyQGhvdG1haWwuY29t; Kamil Kuca, a2FtaWwua3VjYUB1aGsuY3o=