- 1Department of Emergency and Critical Care Center, Beijing Friendship Hospital, Capital Medical University, Beijing, China
- 2Department of Emergency Medicine, Qilu Hospital of Shandong University, Jinan, China
Efferocytosis, the process of engulfing and removing apoptotic cells, plays an essential role in preserving tissue health and averting undue inflammation. While macrophages are primarily known for this task, dendritic cells (DCs) also play a significant role. This review delves into the unique contributions of various DC subsets to efferocytosis, highlighting the distinctions in how DCs and macrophages recognize and handle apoptotic cells. It further explores how efferocytosis influences DC maturation, thereby affecting immune tolerance. This underscores the pivotal role of DCs in orchestrating immune responses and sustaining immune equilibrium, providing new insights into their function in immune regulation.
1 Introduction
DCs are unequivocally central to the regulation of host immune responses. Recent advancements have highlighted the pivotal role of DCs in efferocytosis—a finely tuned mechanism where apoptotic cells are orderly cleared without triggering inflammatory responses. Dysregulation of efferocytosis is closely linked to the progression of various diseases, including autoimmune disorders (1), cancer (2), and chronic inflammation (3). While numerous studies have focused on the role of DCs in antigen presentation and the initiation of immune responses (4, 5), their unique contributions to efferocytosis and its profound impact on immune modulation remain to be fully elucidated.
This review synthesizes recent discoveries in DC-mediated efferocytosis, highlighting the distinct immunological functions and traits of DCs in this process. We explore the involvement of various DC subsets in efferocytosis, identifying and analyzing the ‘eat me’ signal molecules involved in DC-mediated efferocytosis. It is acknowledged that not all ‘eat me’ signals facilitate this process universally across phagocytes. The review distinguishes between signals that mediate efferocytosis in both macrophages and DCs, those unique to DC efferocytosis, and those exclusively involved in macrophage-driven efferocytosis, thereby shedding light on the selective mechanisms of efferocytosis in DCs. Additionally, we examine how DCs, through the efferocytosis process and subsequent antigen cross-presentation, contribute to the modulation of immune responses. The review further delves into how DC-mediated efferocytosis can cultivate immune tolerance by influencing DC maturation, thereby underscoring the critical role of DCs in fine-tuning immune responses and maintaining immunological balance.
2 Efficient clearance of apoptotic cells
In the human body, a remarkable process unfolds daily where hundreds of billions of cells undergo programmed cell death, leading to the generation of numerous apoptotic cells (6). This occurrence, despite its vast scale, remains rarely observed within the body due to the efficient clearance by phagocytes (7), a phenomenon aptly described by scientists as the “burial” of apoptotic cells, a process known as efferocytosis. The term “efferocytosis” was coined in 2006 by Peter Henson and his team, described the specialized phagocytosis of apoptotic cells (8). The cells responsible for efferocytosis encompass not only the professional phagocytes like macrophages and DCs but also include ‘non-professional’ phagocytes such as retinal pigment epithelial cells (9).
This process is distinct from the broader spectrum of phagocytosis, particularly in its mechanisms and biological implications. Traditional phagocytosis involves the identification and engulfment of pathogens by phagocytes through Toll-like receptors (TLRs) that recognize pathogen-associated molecular patterns (PAMPs), or through complement and Fc receptors when pathogens are opsonized. In contrast, efferocytosis employs specific receptors on phagocytes that detect “eat me” signals presented on the surface of apoptotic cells, such as members of the TIM and TAM receptor families (10).
From a biological standpoint, efferocytosis is characterized by its non-inflammatory and non-immunogenic nature, contrasting with the inflammatory response triggered by conventional phagocytosis of pathogens. The engulfment of apoptotic cells through efferocytosis does not incite the production of pro-inflammatory cytokines; rather, it results in the secretion of anti-inflammatory mediators that facilitate the resolution of inflammation (11).
Morphologically, the process of efferocytosis is distinguished by the active deformation of the phagocyte’s cell membrane and the creation of membrane ruffles, which serve to envelop and ingest apoptotic cells (12, 13). This contrasts with the engulfment of pathogens via complement receptors and FcRs, where the pathogens appear to “sink into” the phagocytes (14).
2.1 Molecular mechanisms and phases
Efferocytosis plays a pivotal role in maintaining tissue homeostasis and immune response regulation. This highly orchestrated process involves several critical steps: the identification, recognition, engulfment, and degradation of apoptotic cells. Below, we present a refined discussion of these steps, emphasizing the complex molecular interactions and signaling pathways involved.
2.2 Recognition and binding
In the initial phase, apoptotic cells release “Find-me” signals to attract phagocytes. These signals comprise a range of molecules such as sphingosine-1-phosphate (S1P) (15), CX3C chemokine ligand 1 (CX3CL1) (16), lysophosphatidylcholine (LPC) (17), and nucleotides including ATP and UTP (18). The collaborative effect of these signals effectively recruits phagocytes, facilitating the prompt removal of apoptotic cells.
Concurrently, apoptotic cells present “Eat Me” signals on their surface, promoting efferocytosis. Predominant among these signals is phosphatidylserine (PtdSer), complemented by molecules like calreticulin, ICAM-3, complement proteins, and alterations in cell surface charge and glycosylation patterns (18, 19). PtdSer’s presence is particularly notable as it marks a significant change on the surface of dying cells, distinguishing them from healthy cells, which exhibit “Do not eat me” signals such as CD47, CD24, CD31, CD46, and MHC class I molecules to avoid efferocytosis (20–24).
Phagocytes detect apoptotic cells via “Eat Me” ligands recognized by specific receptors on their surface. Key receptors include the CD300 family (CD300a、CD300b and CD300f), T cell immunoglobulin and mucin domain (TIM) receptors (TIM-1, TIM-3, and TIM-4), Stabilins (stabilin-1 and stabilin-2), brain angiogenesis inhibitor 1 (BAI1), receptor for advanced glycation end products (RAGE) (25), and scavenger receptors (SR-AI,SR-B1,CD36) (26). Additionally, some receptors like the TAM receptor tyrosine kinases (Tyro3, Axl, and Mertk) and integrins family require bridging molecules such as Growth-arrest-specific protein 6 (Gas6), Protein S, and Milk fat globule-EGF factor 8 (MFG-E8) for indirect recognition of the “eat me” signals Bridging molecules play a crucial role in the recognition process, selectively binding to receptors on phagocytes. Gas6, for instance, interacts with Tyro3, Axl, and Mertk, whereas Protein S only binds to Tyro3 and Mertk. Mfge8 and its homolog Developmental Endothelial Locus-1 (Del-1) target integrins to facilitate the recognition of PtdSer, further ensuring the efficient clearance of apoptotic cells (27, 28).
2.3 Engulfment
Phagocytes can internalize apoptotic cells whether through direct or indirect binding. This is because efferocytosis receptors, upon recognizing and binding apoptotic cells, can trigger various signaling pathways to activate small GTPases, including key members of the Rho family such as RhoA, Rac1, and CDC42. These GTPases play pivotal roles in modulating the actin cytoskeleton, as they alternate between their GDP-bound inactive states and GTP-bound active states. This alternation dictates the dynamics of actin filament organization, which is essential for phagocytosis. For example, αVβ5 can activate the p103cas-CRK-DOCK180 pathway, thereby triggering the activation of Rac1 (29). Mfge8 and Mertk can enhance the activation of Rac1 by αVβ5 (30, 31), while the trimeric complex formed by BAI1 with ELMO and DOCK180 can directly recruit Rac1, promoting the uptake of apoptotic cells (32). Additionally, SR-BI can activate PI3K and Rac1 by promoting Src phosphorylation and recruitment to the plasma membrane (33), and both LRP1 and stabilin-2 activate Rac1 through the adaptor protein GULP (34, 35). These interactions initiate cytoskeletal reorganization, thereby initiating cytoskeletal reorganization to facilitate the formation of efferosomes.
The level of GTP-bound Rac1 gradually increases during the recognition process of apoptotic cells, and Rac1 promotes the engulfment of apoptotic cells by phagocytic cells (29, 36–38). The activation pathway comprising CRKII, ELMO1, and DOCK180 serves as a pivotal conduit for Rac1 activation (38–41), Upon activation, Rac1 can interact with the WAVE regulatory complex, which in turn activates the actin nucleation complex Arp2/3, promoting the formation of branched actin network (42).— a critical event for actin polymerization. This polymerization is fundamental for phagocytic cup (the area where phagocytic cells contact apoptotic cells) formation (43–45), the nexus of phagocyte- apoptotic cells interaction, thereby facilitating the ultimate sequestration of apoptotic cells.
After the phagocytic binds to the apoptotic cells, RhoA is transiently upregulated just before the closure of the phagocytic cup, thereby delaying the phagocytic process (46). When RhoA is activated, it enhances the kinase activity of the Rho-associated coiled-coil-containing protein kinase (ROCK), leading to the phosphorylation of the myosin light chain (MLC) and promoting cellular contraction (47). This contraction may inhibit the extension of pseudopods and the closure of the phagocytic cup, which are crucial for the early stages of efferocytosis (47). The transient activation of RhoA ensures that the process does not proceed too quickly, thus giving the cell enough time to confirm whether the target cell truly needs to be engulfed. This mechanism not only ensures that cells only engulf those targets that need to be cleared but also prevents phagocytes from “overeating” (46, 48). RhoA also induces actin polymerization and the extension of pseudopods through distinct pathways that involve mDia and PI(4)P5K, along with further activation of ROCK, leading to the activation of Adducin. This regulates the stability of actin filaments, further supporting the formation of the phagocytic cup. As the process progresses, the level of RhoA gradually decreases, ensuring that phagocytosis can proceed normally, as inhibiting RhoA activity in this process can promote the phagocytosis of apoptotic cells (36, 37, 49). The fine regulation and dynamic changes of RhoA are key mechanisms in ensuring the effective clearance of apoptotic cells.
Cdc42 also contributes to actin polymerization and promotes the phagocytosis of apoptotic cells (50, 51). Cdc42 activates the effector proteins WASP (Wiskott-Aldrich syndrome protein) and N-WASP, which in turn promote the activation of the Arp2/3 complex, thereby inducing actin branching. This activation process leads to the formation of cellular pseudopodia (52–54) thereby enhancing cell migration capabilities and interaction with the environment. Studies utilizing overexpression of a dominant-negative form of Cdc42 and mice lacking the Cdc42 effector WASP have suggested a positive role for Cdc42 in the uptake of apoptotic cells (37, 55, 56).
2.4 Efferosomes processing
After actin polymerization forms the phagocytic cup and internalizes the apoptotic body, it then enters the degradation phase of the efferosomes. This degradation pathway parallels that of phagosomes, necessitating a sequential fusion with early endosomes, followed by late endosomes and ultimately lysosomes (57). Rab GTPases (Rab5, Rab7, and Rab17) and the autophagy-related protein LC3 play important roles in the formation and maturation of efferosomes. After phagocytic cup closes, actin disassembles, followed by the extension of microtubules. The microtubule-tip-associating protein, EB1, transports the guanine nucleotide exchange factor (GEF) Gapex-5 to the efferosomal membrane, increasing the ratio of GEF to GAP activity on the membrane, thereby activating and recruiting more Rab5 (58) Additionally, before the internalization of apoptotic cells, once phagocytes come into contact with apoptotic cells, they recruit Dynamin and Vps34 to the interface between the apoptotic cells and efferocytes. The interaction between Dynamin and Vps34 also facilitates the recruitment and activation of Rab5 to the efferosomes (59). Rab5-GTP further promotes the activation of Vps34, leading to the production of PtdIns(3)P on the surface of the efferosome (59). This PtdIns(3)P, in turn, recruits the effector protein Eea1 (Early Endosome Antigen 1), thereby enhancing the fusion of the efferosomes with early endosomes (58). At this point, the efferosomes begins to acidify (59). The next step involves the Mon1-Ccz1 complex, CED-1, and DYN-1 recruiting Rab7 to the efferosome and activating it (60, 61); Rab7, in turn, mediates the fusion of efferosomes with late endosomes and lysosomes (62, 63). The Rab7 effector protein Rab-interacting lysosomal protein (RILP) can interact with multiple subunits of the HOPS complex, including Vps41 and Vps39, and recruit them to late endosomes (59, 64, 65), triggering SNARE-mediated membrane fusion (66, 67). At this stage, the efferosome becomes increasingly acidic, leading to the activation of acidic proteases and nucleases, which then degrade the contents of the apoptotic cells (68). Additionally, Rab7 coordinates traffic between late endosomes and the lysosome, interacts with RILP to recruit the dynein-dynactin motor complex, and transports late endosomes towards centrosomes and the lysosome (62). leading to the formation of effero-lysosomes.
Microtubule-associated protein light chain 3 (LC3) also participates in the fusion of efferosomes with lysosomes, thereby promoting the maturation of efferosomes and more thorough degradation of their contents. Specifically, After the efferosome is formed, the LC3-II protein is recruited by autophagy family members and, following lipidation, becomes anchored to the efferosome membrane, a process known as LC3-associated phagocytosis (LAP). After the LC3-II protein binds to the membrane of the efferosome, the efferosome is typically renamed as a LAPosome. LAP is triggered by the recruitment of the class III PI3K complex, which includes components such as Rubicon, VPS34, Beclin-1, and VPS15 (69, 70), that facilitate the localization of PI3P to the efferosome. The localization of PI3P stabilizes the NOX2 complex to produce ROS. Both ROS and PI(3)P are necessary for LC3-II lipidation, thereby enabling its successful decoration on the efferosome. Subsequently, the LAPosome fuses with lysosomes, further enhancing the degradation of the contents (70).
Different from phagosome degradation, effero-lysosome degradation incorporates the involvement of Rab17 (71), which is a distinction in the mechanistic pathway. Propelled by the small GTPase Rab17, effero-lysosomes undergo division into smaller vesicles. Rab17 directs these Exosome-Derived Vesicles (EDVs) from the cell’s central to its peripheral regions. At the periphery, EDVs integrate with recycling endosomal compartments. This strategic relocation segregates EDVs from the Major Histocompatibility Complex (MHC) class II antigen-loading compartments (72). Consequently, this segregation prevents the exhibition of antigens originating from apoptotic cells, emphasizing a critical immunoregulatory mechanism. Pathways of Apoptotic Cell Recognition, Internalization, and Degradation are depicted in Figure 1.
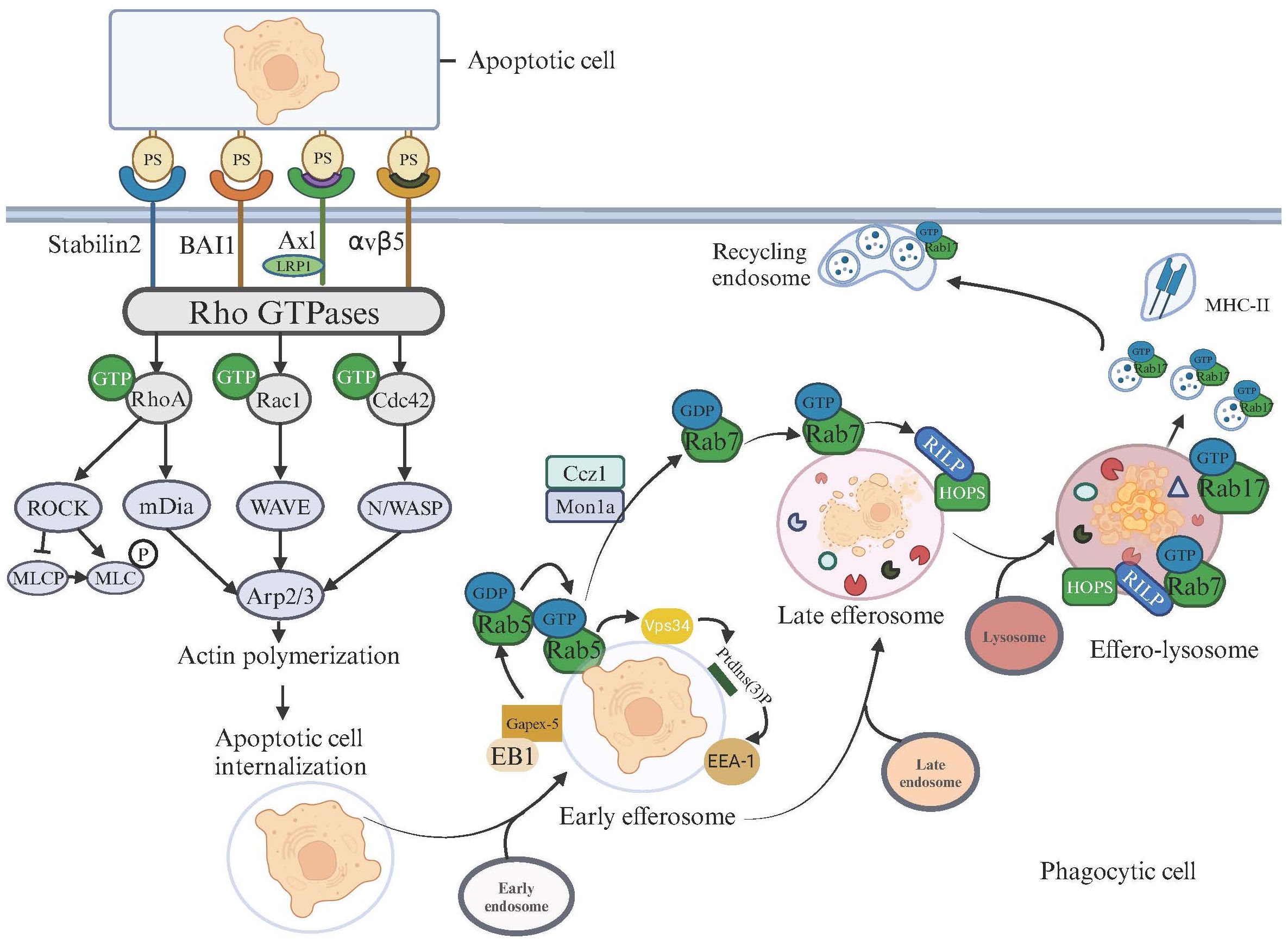
Figure 1 Apoptotic Cell Recognition, Internalization, and Degradation Pathways in Phagocytic Cells. This illustration depicts the signaling pathways activated for cytoskeletal rearrangement and apoptotic cell degradation following the recognition of apoptotic cells on the surfaces of phagocytic cells. Upon the binding of phosphatidylserine (PS) on apoptotic cells, surface receptors such as Stabilin2, BAI1, AXL, and integrin αvβ5 on phagocytic cells initiate signaling cascades. These cascades activate Rho GTPases, including RhoA, Rac1, and Cdc42, which subsequently trigger various downstream effectors. Activation of RhoA leads to the ROCK-mediated phosphorylation of MLC via MLCP, influencing actin polymerization, which is crucial for the formation of the phagocytic cup. Rac1 and Cdc42 facilitate actin assembly through mDia and the WAVE/Arp2/3 complex, respectively, enhancing the engulfment and internalization of apoptotic cells. After internalizing apoptotic cells, the maturation and degradation pathways commence. The microtubule-tip-associated protein EB1 transports the GEF Gapex-5 to the efferosome membrane, activating Rab5-GDP. The activated Rab5-GTP further promotes the activation of Vps34, leading to the production of PtdIns(3)P on the efferosome surface. This PtdIns(3)P subsequently recruits the effector protein Eea1 (Early Endosome Antigen 1), enhancing the fusion of efferosomes with early endosomes. At this point, the efferosome begins to acidify. The next step involves the Mon1-Ccz1 complex recruiting and activating Rab7 on the efferosome. Rab7 recruits the effector protein RILP, which in turn recruits and interacts with the HOPS complex, facilitating the sequential fusion of the early efferosome with late endosomes and lysosomes, ultimately forming the effero-lysosome. At this stage, the efferosome becomes increasingly acidic, activating acidic proteases and nucleases, beginning the degradation of apoptotic cell contents. Rab17 drives the division of effero-lysosomes into smaller vesicles and directs these vesicles to merge with recycling endosomes near the cell membrane, promoting material recycling and distancing from MHC-II.
2.5 Residue removal and resolution of response
The fusion of EDVs with recycling endosomal compartments enables the recycling of cellular components such as carbohydrates, amino acids, lipids, and nucleotides. These metabolites and other cellular components derived from the engulfed apoptotic cell can modulate functional responses in phagocytes (73–78).The digestive degradation mechanism of effero-lysosomes is a highly complex biological process involving the acidification of lysosomes and endosomes; their acidic environment is maintained by the continuous pumping of protons (H+) from the cytosol into the lysosomes or endosomes by V-ATPases (79, 80). This acidic environment is crucial for activating the various hydrolases required to break down cellular debris within effero-lysosomes. This enzymatic activity not only helps decompose cell fragments into reusable biomolecules, aiding in cellular maintenance and growth, but is also essential to prevent undigested cargo from improperly triggering intracellular signaling pathways, such as the cGAS-STING pathway triggered by undigested DNA, which in turn produces type I interferons (81), or the activation of lipid sensors of the peroxisome proliferator-activated receptors (PPAR) and liver X receptors (LXR) families (75, 76, 78) that orchestrate immune-tolerance associated programs. In murine macrophages, it has been observed that the identity of the engulfed cell has a significant impact on the functional programs triggered by efferocytosis, underscoring the highly precise decoding machinery underlying efferocytosis (82). Given the critical nature of this process in health and disease, future research efforts should focus on elucidating the transport and degradation mechanisms of efferosomes.
3 Efferocytosis by specialized DC subsets
3.1 Distribution and classification of DCs
DCs are widely distributed in various organs and tissues of the human body except for the brain and eyeballs, commonly found in blood, skin mucosa, and lymphoid tissues, accounting for less than 1% of the total peripheral blood white cells (83). DCs are differentiated from lympho-myeloid hematopoietic stem cells. DCs are composed of several subsets, which include conventional DCs (cDCs, further distinguished as cDC1 and cDC2) and plasmacytoid DCs (pDCs) (84). DC subsets along with Their Markers and Functions are illustrated in Figure 2.
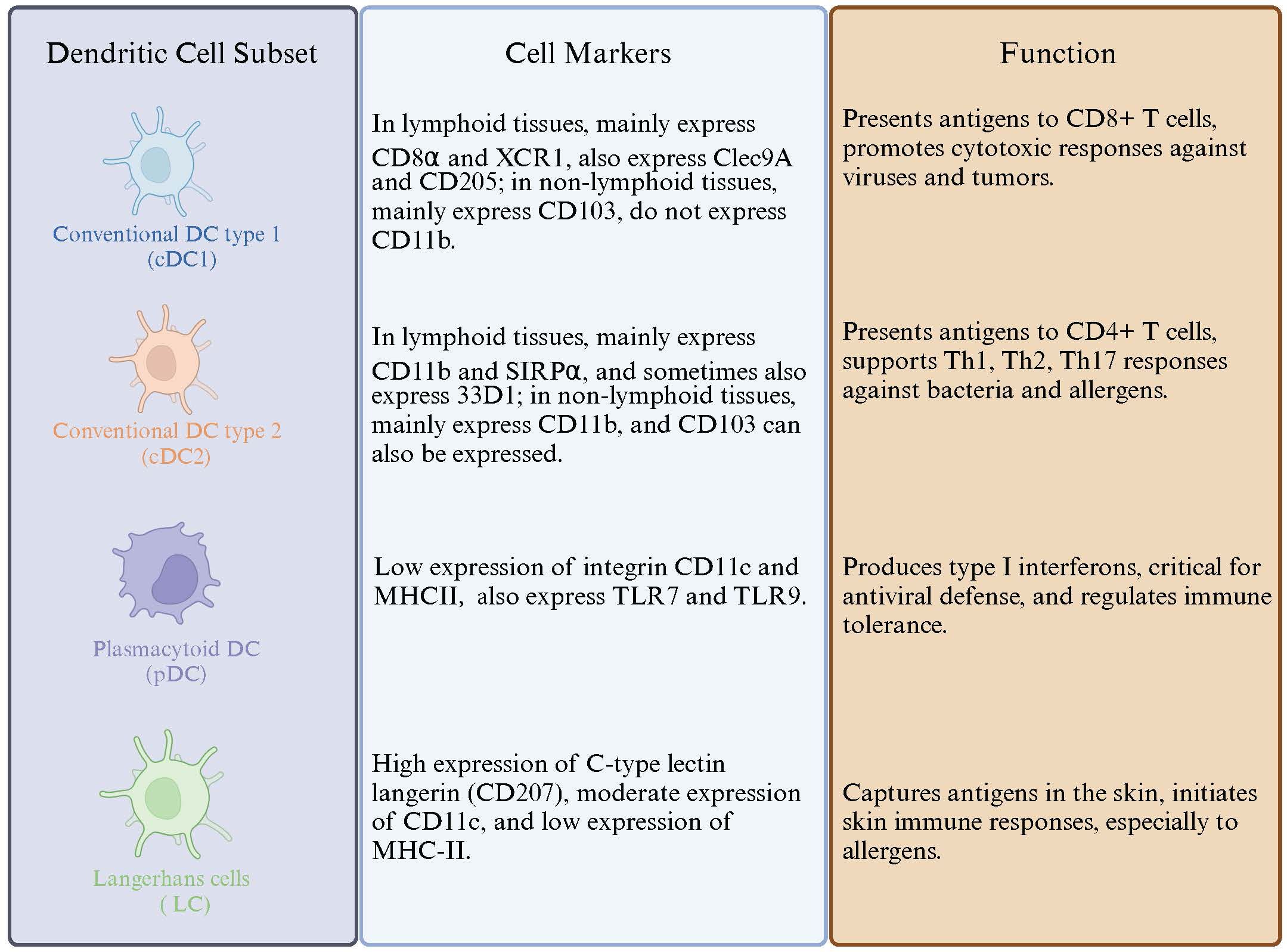
Figure 2 Dendritic Cell Subtypes and Their Markers and Functions. This figure illustrates the distinct subsets of dendritic cells, detailing their specific cell markers and functional roles in immune responses.
3.2 CD8+ cDC1
The cDC1 subset is distinguished by its capacity to cross-present exogenous antigens, facilitating the activation of CD8+ T cells (85). The genesis of cDC1 is regulated by the transcription factors BATF3 and IRF8 (86).
Further advancements in our understanding were propelled by the investigative efforts of Albert’s research team, which unveiled an additional functional facet of cDC1 subsets—their ability to engulf apoptotic cells (87). This phagocytic competence adds a new layer to the functional repertoire of cDC1, positioning them as pivotal players in maintaining immune homeostasis and in the orchestration of immune responses against pathogens.
The mechanism of action predominantly employed by cDC1 in the presentation of antigens from apoptotic cells involves MHC class I molecules (88). This discovery has spurred a series of investigative endeavors aimed at elucidating the processes governing DCs phagocytosis of apoptotic cells and the subsequent antigen presentation (89–92). Among these, a notable study conducted by Tomonori Iyoda et al. in Japan employed CFSE-labeled apoptotic spleen cells in mice to trace the phagocytic activity of DCs in vivo (93). The findings revealed a selective efferocytosis capacity within the DC subsets; while both CD8+ cDC1 and CD8- cDC2 subsets in the spleen were capable of engulfing latex beads, only the CD8+ cDC1 subset was proficient in phagocytosing the CFSE-labeled apoptotic spleen cells. This significant finding emphasizes that CD8+ cDC1 subsets have the superior capacity to phagocytose apoptotic cells compared to other types of DCs. Interestingly, this phenomenon is mirrored in the human immune system, where CD141+ DCs, akin to the murine CD8α+ cDC1 subset, exhibit the ability to phagocytose necrotic cells (94), further highlighting the conserved functional attributes across species. It is important to note that the majority of evidence concerning the efferocytic capabilities of DCs originates from studies conducted on mice.
Subsequent inquiries delved into the underlying reasons for the differential phagocytic abilities observed between CD8+DCs and CD8-DCs. A hypothesis emerged suggesting a potential linkage to the distinct efferocytosis receptors expressed by the respective DC subsets. In the murine spleen, DCs segregate into two primary subsets: CD8α+DEC205+DCs, localized within the T cell zones, and CD8α-33D1+DCs, situated in the red pulp and marginal zones (95). Notably, the CD8+ cDC1 subset is characterized by elevated levels of DEC205 expression, in contrast to the CD8-cDC2 subset, which lacks DEC205 expression (96–98). This led to investigations into DEC205’s role in mediating phagocytosis of apoptotic cells. However, the outcomes from studies using DEC205 gene knockout mice indicated that the absence of DEC205 did not impede the ability of the CD8+ cDC1 subset to phagocytose apoptotic cells (93), suggesting that despite its high expression, DEC205 is not the crucial factor in the efferocytosis activity of this subset. DEC205 acts as an endocytic receptor for phagocytosis, rather than as an efferocytic receptor for efferocytosis. It promotes the internalization and subsequent presentation of antigens (rather than apoptotic cells as antigens) among immune cells, thereby regulating immune responses (99). Subsequent studies using RT-PCR and microarray analysis have found that the expression of CD36 on CD8+DCs is significantly higher than on CD8-DCs, which may be a key factor contributing to the differences in their ability to engulf apoptotic cells (93, 100). The latest reliable evidence further confirms that CD36 indeed enhances the phagocytic capacity of DCs towards apoptotic cells (101).
3.3 CD103+ cDC1
A fraction of cDCs found in lymphoid and non lymphoid organs expresses the E-cadherin–binding integrin αE (CD103) (102, 103). The exploration of the ability of DC subsets to phagocytose apoptotic cells, has significantly advanced with contributions from the research teams led by Chun-Hong Qiu and Yasunobu Miyake (100). A notable discovery in this realm is the unique behavior and localization of CD103+CD207+DCs within the CD8α+cDC1 group in the spleen. Contrary to the conventional localization of CD8α+cDC1 subsets within T cell zones, these specific DCs exhibit a propensity to migrate to the marginal zones following the phagocytosis of blood-derived apoptotic cells.
This migratory pattern facilitates a specialized function of the CD103+DC subset in the spleen, where they preferentially engulf blood-derived apoptotic cells. Post-phagocytosis, these cells relocate to the T cell zones to engage in the cross-presentation of cell-associated antigens, thereby promoting antigen-specific immune responses. This behavior underscores the specialized role of CD103+DC subsets in the immune surveillance and response mechanisms, particularly in the context of apoptotic cell clearance and antigen presentation.
Further investigations have expanded the understanding of CD103+DCs beyond the spleen, revealing a genetic homology between lung tissue CD103+DCs and spleen CD8α+cDC1 (104, 105). This homology underpins the classification of lung CD103+DCs within the cDC1 subset, emphasizing the broader relevance of these cells across different anatomical sites. In the absence of the transcription factor BATF3, a notable reduction in the presence of CD103+DCs in the lungs and CD8α+ cDC1 in the spleen has been observed.
DCs in lung tissue can be divided into two major subsets: pDC and cDC. The cDC subset can be further divided into XCR1+CD103+cDC1 and SIRPα+CD103− cDC2 (106–110). The functional capabilities of lung CD103+DCs have been a focal point of research, particularly their proficiency in cross-presenting antigens via MHC Class I molecules, which is pivotal for the activation of cytotoxic T lymphocytes (CTLs) during viral infections. In vitro and in vivo studies have elucidated the role of lung CD103+ DCs in CTL activation (109, 111), highlighting their ability to capture viruses and phagocytose virus-infected apoptotic cells for efficient antigen presentation (105, 112, 113).
CD103+ DCs can phagocytose apoptotic cells not only in the spleen (100) and skin (114), but also in lung tissue (115), further confirming to their specialized phagocytic apoptotic cells function across different tissues. Research led by A. Nicole Desch investigated the selective phagocytic apoptotic cells behavior of lung tissue CD103+cDC1 subsets, Subsequent research further unveiled their reliance on the Mertk receptor for the engulfment of apoptotic cells (116). This specificity distinguishes CD103+cDC1 subsets from other DC subsets, such as CD103-cDC2, in their ability to recognize and phagocytose apoptotic cells.
Extending the exploration to the intestinal, studies have confirmed that CD103+ cDC1 subsets in the inherent layer of the intestines also have the ability to engulf apoptotic cells (117, 118). further validating the concept that specific DC subsets are endowed with distinct functionalities tailored to their anatomical and immunological context.
This growing body of evidence highlights the intricate specialization within the DC lineage, emphasizing the importance of understanding these nuances to unravel the complexities of the immune system’s orchestration of responses to pathogens, tumors, and self-antigens.
3.4 pDCs
pDCs are a unique type of DC, known for their ability to produce large amounts of type I interferons, such as interferon-alpha and interferon-beta, particularly in response to viral infections (119). pDCs play a crucial role in the immune system, not only in rapidly responding to viral infections but also in regulating autoimmune responses and other inflammatory reactions (120).
While pDCs do not directly engage in the phagocytosis of apoptotic cells. they play a crucial role in the induction of regulatory CD4+ T cells mediated by efferocytosis. This process is primarily facilitated through the interaction of pDCs with TGF-β, a cytokine released by macrophages that have phagocytosed apoptotic cells. If pDCs are absent, efferocytosis cannot effectively induce the differentiation of regulatory CD4+ T cells, highlighting the indirect yet vital role of pDCs in immune regulation (121).
4 Unveiling the receptors: recognition of apoptotic cells by DCs during efferocytosis
In the intricate process of efferocytosis, phagocytes rely on specific surface receptors to identify and ingest dying cells. Numerous systems capable of recognizing phosphatidylserine exist, but a specific phagocytic cell might not always express or utilize all of these systems (35, 122). Although extensive research has delineated various efferocytosis receptors in macrophages, our comprehension of the receptors and underlying molecular mechanisms within DCs remains significantly limited. The comprehensive list of efferocytosis receptors found in DCs and macrophages, along with their corresponding ligands, is detailed in Table 1.
4.1 TAM receptors in DC efferocytosis
The TAM receptor tyrosine kinase family, comprising Tyro3, Axl, and Mertk, plays a pivotal role in the recognition of apoptotic cells. These receptors, through their ligands GAS6 or Protein S, indirectly detect phosphatidylserine on apoptotic cells, facilitating efferocytosis. However, the contribution of TAM family members to efferocytosis varies across phagocytes. In macrophages, Mertk deletion completely impairs apoptotic cell clearance, while Axl or Tyro3 deletion, individually or combined, decreases efferocytosis efficiency by approximately 50%. In contrast, bone marrow-derived dendritic cells (BMDCs) and splenic CD11c+DCs predominantly utilize Axl and Tyro3 for apoptotic cell recognition, retaining clearance capability even in Mertk’s absence (127). Notably, a unique DCs subset in the lungs, identified from birth to two weeks, depends on Mertk to effectively clear apoptotic lung epithelial cells (116). This subpopulation, classified as cDC1, expresses intermediate CD103 levels and exhibits a limited capacity for CD8+ T cell proliferation and IFN-γ production induction.
Contradictory findings regarding Axl, Mertk, and Tyro3 expression across DC subpopulations further complicate our understanding. Some studies report Axl and Mertk expression in BMDCs and splenic CD11c+ DCs, excluding Tyro3 (153), while others have identified Tyro3 in a specific BMDC subpopulation marked by CD11c+PDL2+ (154).
The distinct engagement of TAM receptors in DCs compared to macrophages might stem from their unique responses to TAM-specific ligands, Gas6 and Protein S. While Mertk and Tyro3 can be activated by both ligands, Axl responds solely to Gas6. Macrophages, capable of expressing both Gas6 and Protein S, activate all TAM receptors, unlike DCs, which minimally express Gas6. This disparity could underpin the less efficient efferocytosis observed in DCs, though recombinant Gas6 supplementation in mice has been shown to boost efferocytosis by activating Axl (155).
The selective receptor usage may reflect the divergent functional roles within the immune system: macrophages as proficient phagocytes and DCs as specialized antigen-presenting cells, highlighting the complexity and specificity of immune responses during apoptotic cell clearance.
4.2 TIM receptors in DC efferocytosis
Beyond the TAM receptor family, the T cell immunoglobulin and mucin domain (TIM) gene family members, including TIM-1, TIM-3, and TIM-4, are instrumental in DC efferocytosis through their ability to bind PtdSer on apoptotic cells, facilitating their clearance (123–125). TIM-3 and TIM-4, in particular, are significant for their efferocytosis roles in DCs. TIM-3, expressed on Th1 and Tc1 cells, is also found on specific DC subpopulations such as CD8+ DCs. TIM-4, both expressed on DCs and macrophages, plays a crucial role in the engulfment of apoptotic cells (156, 157).
Antibody-mediated TIM-3 blockade results in an accumulation of apoptotic cells within mouse splenic follicles and an increase in serum levels of dsDNA antibodies, suggesting a disruption in normal efferocytosis and potential implications for autoimmunity. The interaction between HMGB1 and TIM-3 is pivotal for DCs’ internalization of extracellular dsDNA (158).
The interference in TIM-4 and PtdSer interaction, particularly following dexamethasone-induced thymocyte apoptosis, compromises DC efferocytosis and may predispose mice to autoimmune disorders (125). This indicates the critical role of TIM-4 in maintaining immune tolerance through efficient apoptotic cell clearance. Notably, TIM-4’s recognition of PtdSer does not trigger downstream signaling pathways (159), emphasizing its primary function in the physical engulfment of apoptotic cells rather than initiating intracellular signaling cascades.
4.3 Integrin, scavenger and CD300 receptors in DC efferocytosis
DC efferocytosis involves a diverse array of receptors beyond the TAM and TIM families, including integrins αvβ5, the scavenger receptor family member F1 (SR-F1) CD36, and CD300f from the CD300 family. These receptors play distinct roles in the identification and engulfment of apoptotic cells, reflecting the nuanced regulatory mechanisms of efferocytosis in the immune system.
Integrins αvβ5 and CD36 are predominantly expressed in immature DCs, with their expression levels diminishing as DCs mature (89). The αvβ5 integrin, through its interaction with Thrombospondin-1(TSP-1), uniquely contributes to DC efferocytosis (29). The β2 integrin family members αMβ2 and αXβ2 are also involved in the recognition and uptake of apoptotic cells by DCs. This process is achieved through their binding to the complement fragment iC3b on the surface of apoptotic cells (131). The role of αvβ3 integrin in DC efferocytosis has been contested (29, 132), with initial findings suggesting its involvement being later questioned due to the complexity of receptor interactions and the low expression levels of αvβ3 on DCs. Current views suggest that the αvβ3 integrin may only be involved in the efferocytosis of macrophages (160). This specificity underlines the tailored engagement of various integrins in apoptotic cell clearance across different cell types.
Despite CD36 high expression in CD8+ DCs, its necessity for apoptotic cell engulfment remains debatable. Comparative studies of efferocytosis rates between wild-type and CD36-deficient mice DCs show negligible differences (134), suggesting that CD36 may not be critical for DC efferocytosis. This observation contrasts with the role of CD36 in macrophages (135). However, subsequent research has found that CD36 plays a significant role in the efficiency of efferocytosis in DCs (101). With increased CD36 expression, the ability of DCs to engulf apoptotic cells significantly enhances. This finding not only provides new insights into the function of CD36 in the efferocytosis process of DCs but also corroborates its known role in promoting the clearance of apoptotic cells in macrophages (135), indicating that CD36 has a consistent functional across different types of phagocytes.
SR-F1, shared between macrophages and DCs, facilitates DC efferocytosis by recognizing PtdSer through C1q. The absence of SCARF1 leads to efferocytosis impairment, accumulation of apoptotic cells, autoantibody production, and lupus-like disease development in mice (161, 162), indicating its crucial role in maintaining immune homeostasis.
The CD300 family member CD300f, capable of recognizing phosphatidylserine (PS), exhibits divergent effects in different phagocytes—enhancing efferocytosis in macrophages while inhibiting it in DCs (138, 163). This dichotomy further illustrates the complex regulatory landscape of efferocytosis and its dependence on the specific cellular context.
5 Interconnected roles of DC efferocytosis and antigen cross-presentation
DCs, as pivotal antigen-presenting cells (APCs), orchestrate the immune response by presenting antigens to T cells. Direct presentation involves APCs displaying exogenous antigens from pathogens like bacteria, viruses, and fungi through MHC class II molecules to CD4+ T cells, and endogenous antigens through MHC class I to CD8+ T cells. Contrarily, cross-presentation allows APCs, predominantly DCs, to present exogenous antigens on MHC class I molecules (164), a critical function for activating CTLs against virus-infected or neoplastic cells.
Efferocytosis is intricately linked to antigen cross-presentation (165). DCs are adept at cross-presenting antigens from apoptotic cells (166), requiring minimal cell quantities and brief exposure periods (3-12 hours) to form MHC-antigen complexes that activate CTLs (87). Furthermore, apoptotic cells serve not only as a rich antigen source for DCs cross-presentation but also as preferred targets (87, 90, 165, 167, 168), emphasizing the significance of efferocytosis in adaptive immunity.
Two recognized pathways facilitate antigen cross-presentation (169): the vacuolar pathway, where antigen processing and MHC class I loading occur within APC phagosomes-endosomes (170–172), and the proteasome-dependent cytosolic pathway, involving exogenous antigen translocation to the cytoplasm for proteasomal processing (173, 174). DCs, especially when compared to macrophages, exhibit superior efficiency in processing and presenting antigens from apoptotic cells via cross-presentation (87), attributed to the unique properties of cDC1 and DNGR-1 receptor actions. The relatively low concentration of lysosomal proteases in DCs limits their ability to degrade proteins, resulting in a significant slowdown of acidification in the effero-lysosomes within DCs compared to macrophages (175). This prolongs the maturation time of effero-lysosomes, creating conditions for long-term antigen retention and effective presentation (176). DNGR-1 (also known as CLEC9A) is a specialized receptor for antigen cross-presentation. It preferentially locates and accumulates in poorly degradable early phagosomes (including effero-lysosomes), without affecting phagosome maturation. DNGR-1 can recognize F-actin-myosin complexes exposed in the effero-lysosomes cavity, and further activate SYK kinase and NADPH oxidase, leading to effero-lysosomal membrane damage and phagosome rupture (177). This process causes the contents of the phagosome to leak into the cytosol, and antigens can be processed and presented via the MHC class I pathway (178). A study identified that effero-lysosomes in macrophages lack MHC class II molecules and related molecules responsible for transporting them from the endoplasmic reticulum-Golgi complex to lysosomes (72), using liquid chromatography-mass spectrometry technology (this study did not perform mass spectrometry analysis on DCs’ effero-lysosomes). However, another study targeting DCs revealed that Rab39a exists in DCs (179). This molecule can transport MHC-I molecules from the endoplasmic reticulum to effero-lysosomes and promote effero-lysosomes to transform into the peptide loading area of MHC-I molecules.
In vitro studies have shown DCs capable of cross-presenting antigens from apoptotic cells infected with various pathogens, thereby activating CD8+ T cells (87). This phenomenon has been corroborated by in vivo studies, specifically, after injecting apoptotic vesicles generated from BCG-OVA infection into the footpads of mice, researchers observed significant proliferation of specific CD8+ T cells in the cervical lymph nodes of these mice (180). Further in vitro studies expanded upon these findings, showing that DCs also cross-present antigens from cells apoptotic due to infections with a range of pathogens including Salmonella enterica serovar Typhi, human cytomegalovirus, vaccinia virus, Mycobacterium tuberculosis, herpes simplex virus (HSV), and Human Immunodeficiency Virus Type 1 (90, 167, 181–184). These studies illustrate the breadth of the cross-presentation phenomenon and stress its significance in immune defense mechanisms.
Progress has been made in understanding the mechanism of antigen cross-presentation following phagocytosis of apoptotic cells. The phagocytic receptors on DCs, Axl, in conjunction with low-density lipoprotein receptor-related protein 1 (LRP1) and RAN binding protein 9 (RANBP9), collectively participate in the internalization of cells undergoing apoptosis post-HSV-1 infection. highlighting the role of phagocytic receptors like Axl, LRP1, and RANBP9 in facilitating antigen cross-presentation post-apoptotic cell engulfment (145). In addition, Annexin1, a protein on the membrane of apoptotic cells, TIM3 (124) and αvβ5 (89) also plays a pivotal role in regulating the efferocytosis and antigen cross-presentation process of DCs (180). The absence of Annexin1 leads to a reduction in LC3-II levels, thereby affecting the processing of antigens within lysosomes and restricting the loading of antigens onto MHC-I. This limitation weakens the capability of DCs to cross-present antigens (180). Tim-3 suppresses MHC-I expression through inhibiting the STAT1-NLRC5 signaling pathway, an effect that has been validated in macrophages (185). However, in a mouse model of acute graft-versus-host disease, Tim-3 expression is notably upregulated in splenic and hepatic T cells, DCs, and macrophages. Although the original studies did not directly explore the relationship between Tim-3 and antigen cross-presentation, its role in modulating the activity of CD8+ T cells suggests that it might indirectly enhance DC-mediated antigen cross-presentation by affecting the functions of T cells and DCs (186). As for integrin αvβ5, while it has been proven essential in the cross-presentation process of DCs (89), the specific mechanisms involved remain unclear and require further investigation.
In addition, the mechanisms by which certain pathogens evade host immune attacks are related to the efferocytosis of DCs. Research led by Kaufmann and his colleagues has shown that when DCs clear apoptotic bodies produced by macrophages infected with Mycobacterium tuberculosis or the BCG vaccine through efferocytosis, they can activate CD8+ T cells, thereby eliciting an adaptive immune response against pulmonary tuberculosis (184, 187). However, Mycobacterium tuberculosis can evade the efferocytic action of DCs by inhibiting the apoptosis of infected macrophages and promoting their necrosis, thus evading the host’s immune response (188–190). Studies have demonstrated that increasing the number of macrophages infected by Mycobacterium tuberculosis and undergoing apoptosis can enhance the efferocytosis of DCs, thereby triggering an early protective immune response against tuberculosis in the body (191).
In the physiological state, the cross-presentation of self-antigens by DCs leads to abortive proliferation of CD8+ T cells (192–196), whose quantity and function are crucial for maintaining the organism’s peripheral tolerance (197).
In conclusion, DC efferocytosis and antigen cross-presentation are fundamental processes that bridge innate and adaptive immunity. Understanding the intricate mechanisms underlying these processes provides insights into the development of novel immunotherapies and vaccines.
6 Impact of efferocytosis on DC maturation and function
The maturation of DCs is pivotal for their ability to perform immune functions effectively. Initially, in their immature form, DCs roam the organism, scouting for PAMPs and damage-associated molecular patterns (DAMPs), using their surface pattern recognition receptors (PRRs), such as TLRs. The recognition of these molecular patterns activates DCs and initiates their maturation process (198). During maturation, DCs enhance the expression of major histocompatibility complex (MHC) molecules and co-stimulatory molecules (like CD80 and CD86) on their surface, and they release substantial quantities of pro-inflammatory cytokines, such as interleukin-12 (IL-12), interleukin-6 (IL-6), and tumor necrosis factor-alpha (TNF-α). These cytokines are vital for T cell activation, preparing the immune system to respond to pathogens.
Nevertheless, the journey of DC maturation, especially following the efferocytosis of apoptotic cells, introduces a layer of complexity in their functional dynamics. This process involves nuanced mechanisms that not only influence T cell activation but also play a crucial role in determining whether an immune response will be activated or whether tolerance will be induced. The interplay between efferocytosis and DC maturation is intricate, reflecting the sophisticated regulation within the immune system to maintain a balance between defense and tolerance.
6.1 Inhibitory effects of efferocytosis on DC maturation and function: a self-limiting mechanism
The detailed information on the impact of efferocytosis on DC maturation involved in this review is exhibited in Table 2. Research shows that under steady-state conditions, the maturation of iDCs is inhibited after they engulf apoptotic cells, primarily characterized by reduced expression of key co-stimulatory molecules such as CD40, CD86, and MHC-II. Even when subsequently stimulated with high concentrations of LPS, these DCs are still difficult to induce to mature (131, 194, 199, 206) indicating that the efferocytosis process can inhibit not only the maturation of DCs but also inflammatory responses.
Moreover, this inhibitory effect is limited to those DCs that directly engulf apoptotic cells and does not involve surrounding DCs (201), further emphasizing that it is the direct contact with apoptotic cells that inhibits the maturation of DCs. The latest research corroborates this, showing that after DCs engulf apoptotic cells or lipid-rich nanoparticles, the activation of the LXR pathway, which promotes cholesterol efflux within the cell, plays a significant role in inducing the maturation of steady-state DCs into tolerogenic DCs (78). In studies of autoimmune diseases, it has been found that PGE2 produced by DCs after engulfing apoptotic pancreatic cells significantly inhibits the maturation of DCs and also significantly reduces their ability to stimulate T cell proliferation, thereby preventing the occurrence of autoimmune diseases (200).
Research indicates that prostaglandin E2 (PGE2) not only inhibits the activity and proliferation of CD4+ and CD8+ T cells by promoting the expression of co-inhibitory molecules PD-1 and TIM-3 on T cells (207–209), but also regulates the differentiation and activation of Th17 cells through prostaglandin receptor EP2- and EP4-mediated signaling, cAMP pathways, and by inhibiting the function of transcription factor IRF4 (208, 210). PGE2, through its dual regulation of DCs and T cells, not only reduces the production of inflammatory mediators but also decreases T cell-mediated inflammatory responses, thereby helping to maintain immune homeostasis and prevent the overactivation of autoimmune responses.
Furthermore, surface molecules on apoptotic cells, such as thrombospondin-1 (TSP-1) (199), cytokines secreted by apoptotic cells, such as high mobility group box 1 (HMGB1) (211), and complement fragments like iC3b produced after the activation of the complement system by apoptotic cells (131, 212, 213), also play significant roles in inhibiting the maturation of DCs and promoting immune tolerance.
The immaturity of DCs implies that they are unable to activate specific T cell immune responses or effectively promote T cell proliferation. Although studies have indeed shown that DCs that have phagocytized apoptotic cells can promote the proliferation of CD8+ T cells, more critically, these CD8+ T cells do not show an upregulation of CD25 (a marker indicative of T cell activation) nor a downregulation of CD62L (a molecule crucial for lymphocyte homing and migration). This indicates that despite the observed proliferation of T cells, these CD8+ T cells are neither activated nor prepared to migrate to sites of inflammation or lymphoid tissues. Additionally, although T cell proliferation is observed within the initial 2-3 days, by the 10th day, without additional stimuli to further their maturation, the numbers of these proliferating T cells gradually dwindle (194).
Meanwhile, although the maturation of DCs is inhibited by efferocytosis, they are still able to migrate to secondary lymph nodes after engulfing apoptotic cells in a steady state. After efferocytosis, there is a significant increase in the expression of CCR7 on the surface of DCs. CCR7, a crucial chemokine receptor responsible for guiding DCs to draining lymph nodes, sees increased expression following DC efferocytosis, promoting the migration of steady-state DCs, closely associating with the induction of self-antigen tolerance (214).
The inability of DCs to mature even when stimulated with LPS following efferocytosis indicates that this process plays a role in regulating TLR-mediated inflammatory responses. Normally, TLR activation by various ligands triggers signaling cascades involving the MAP kinase pathway, NF-κB pathway, and activation of interferon regulatory factors (IRFs), leading to the production of pro-inflammatory cytokines such as IL-6, IL-12, TNF-α, and type I interferons (IFNs) (215). However, DC efferocytosis has been shown to alleviate TLR-mediated inflammatory responses (201, 216) (Figure 3), characterized by a reduction in pro-inflammatory cytokine secretion (217). Specifically, DC efferocytosis inhibits the activation of the NF-κB pathway (161, 212), which is central to the inflammatory response and includes members such as p65 (RelA), RelB, cRel, p50, and p52. Under resting conditions, NF-κB is inhibited by IκB proteins, which prevent its nuclear translocation. Upon activation by LPS stimulation, the IKK complex phosphorylates IκB, leading to its degradation and subsequent nuclear translocation of NF-κB to initiate transcription of inflammatory genes. BMDCs pre-treated with apoptotic cells show significant inhibition of IκK activation and IκB degradation upon LPS stimulation, ultimately resulting in restricted nuclear translocation of the nuclear factors cRel, p50, and p65 (212, 218). This inhibitory effect has been shown to depend on the TAM receptors, and in experimental conditions lacking the Mertk gene or employing an antagonistic antibody targeting Mertk, the inhibitory effect of efferocytosis on the NF-κB pathway is absent. The inhibitory role of TAM receptors on inflammation also includes their suppression of the ubiquitination of key TLR signaling components such as TRAF3/6 (153), which is crucial for activating the MAPK and NF-κB pathways in DCs (219).
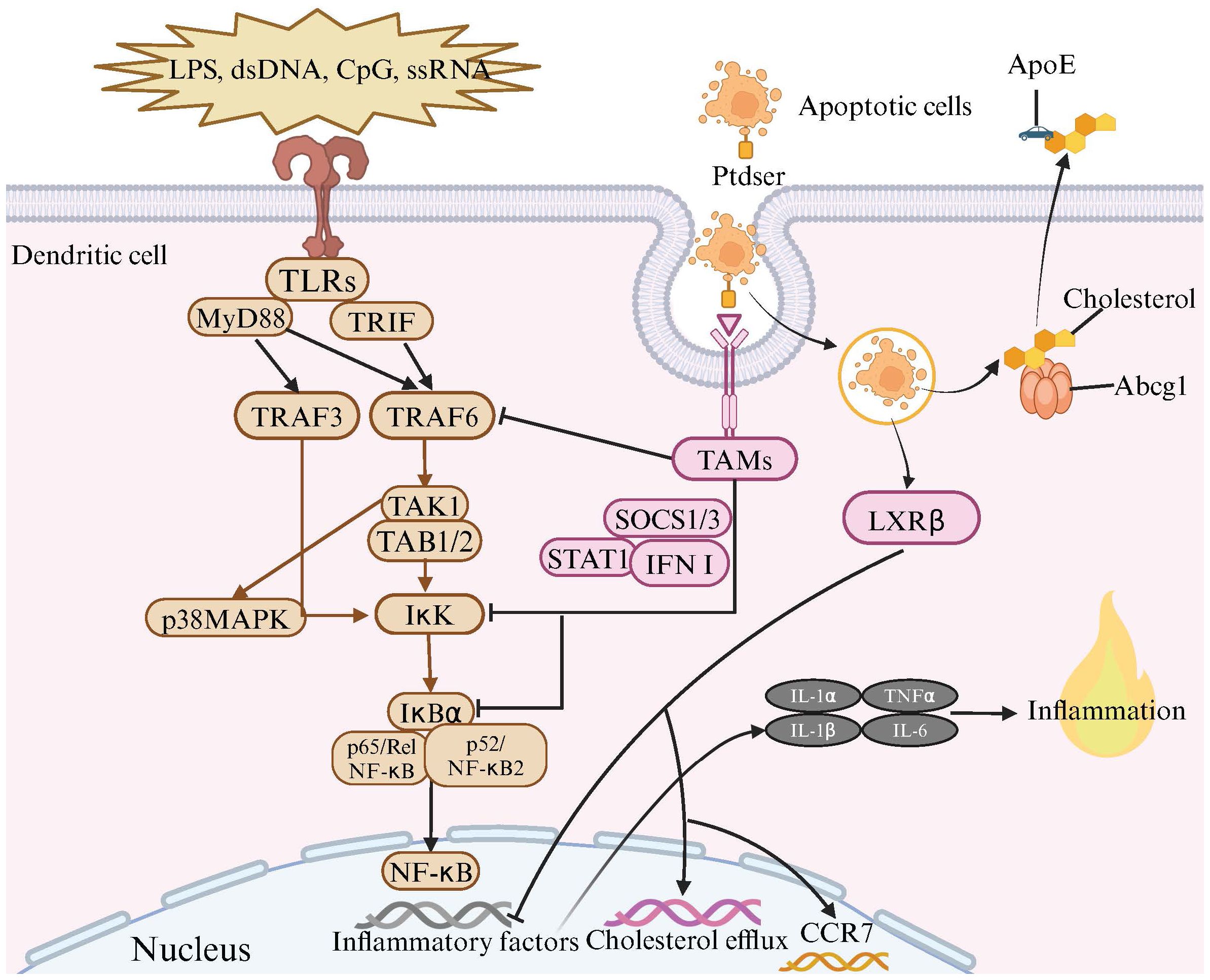
Figure 3 The Anti-Inflammatory Effects of TAM Receptor and LXR Signaling Pathways in Dendritic Cell Efferocytosis. Toll-like receptors (TLRs) are located on the cell membrane and are capable of recognizing various molecules such as lipopolysaccharides (LPS), double-stranded DNA (dsDNA), CpG, and single-stranded RNA (ssRNA). The binding of these molecules to TLRs triggers a series of signal transduction pathways, activating a set of proteins including MyD88, TRIF, TRAF3, TRAF6, TAK1, TAB1/2, and IκK. During this process, the IκK complex phosphorylates IκB protein, leading to its degradation and the release of NF-κB. Subsequently, NF-κB enters the cell nucleus and initiates the transcription of inflammatory factors. Meanwhile, when dendritic cells (DCs) recognize and bind to apoptotic cells through TAM receptors and bridging molecules, TAMs receptors are activated. The activated TAMs receptors, with the assistance of SOCS1/3, STAT1, and type I interferons, inhibit the inflammatory signaling induced by TLRs activation. This includes suppressing the activation of TRAF3/6 and IκK, as well as the degradation of IκBα, thereby inhibiting the NF-κB signaling pathway and preventing excessive inflammation. Upon the engulfment of apoptotic cells by DCs, the LXRβ is activated, leading to the upregulation of genes involved in cholesterol efflux, notably Abcg1 and ApoE. This upregulation facilitates the efflux of cholesterol from the engulfed apoptotic cells to the extracellular matrix. This mechanism not only mitigates the accumulation of cholesterol but also suppresses the transcription of pro-inflammatory cytokines. Additionally, activation of the LXR signaling pathway promotes the transcription of CCR7, enhancing the migration capabilities of tolerogenic dendritic cells, thus contributing to the maintenance of immune homeostasis.
In mice, the lack of Tyro3, Axl, and Mertk genes leads to an enhanced reactivity of DCs to TLR agonists, resulting in the overproduction of type I interferons and excessive activation of the immune system, causing the mice to die early from systemic inflammatory responses (153). The use of TAM receptor agonists can extensively inhibit cytokine production triggered by TLR 3, 4, and 9, effectively blocking the TLR-induced signaling cascade, particularly the phosphorylation of key signaling molecules ERK1/2 and p38 MAP kinase, as well as the degradation of IκBα and IκBβ (153).
This is attributed to TAM receptors promoting the production of cytokine signaling suppressor proteins SOCS1 and SOCS3 (153). In the presence of STAT1 and type I interferons, these SOCS proteins can effectively limit the IFNγ signaling pathway and reduce the transcriptional activity of NF-κB (220, 221).
By studying how DCs activate specific T lymphocyte responses through efferocytosis, we discovered that DCs do not necessarily need to be fully mature to activate effector T cells (222), challenging the traditional view that directly links maturation state with activation capacity. For instance, comparisons between LPS-treated and untreated DCs on the proliferation of OT1/OT2 T cells in vivo demonstrate that while LPS-treated DCs more effectively promote T cell proliferation, untreated DCs also have a significant effect (223). Further research indicates that under normal conditions, Langerhans cells (LCs) can present self-antigens to lymph nodes, which triggers the expansion of antigen-specific CD8+ T cells, rather than fostering tolerance (224). Additionally, Langerhans cells from the skin of K14-mOVA transgenic mice can significantly induce OT-I T cell proliferation even without additional antigen processing (225). Other studies show that CD11c+ CD8α− DCs continuously capture and present gastric self-antigens, such as H+/K+-ATPase, in the absence of an overt inflammatory response, yet fail to completely eliminate or suppress all autoreactive T cells (226), highlighting deficiencies in the establishment and maintenance of self-tolerance. These findings collectively demonstrate the immunological activation capabilities of immature DCs under specific conditions, challenging the previous notion that immature DCs are primarily associated with immune tolerance.
Interestingly, in some cases, mature DCs may also induce T cell tolerance (117, 227), adding complexity to the role of efferocytosis in immune regulation. A comparison of the effects of long-term and short-term exposure to LPS and IFN-γ on human monocyte-derived dendritic cells (moDCs) reveals significant differences in cellular responses. Under long-term activation, DCs not only begin to undergo apoptosis, but also, through involving their surface TAM receptors and efferocytosis, significantly increase their production of IL-10. In contrast, during short-term activation, levels of IL-12p70 and IL-10 are positively correlated, whereas a reverse correlation is evident with long-term activation. Short-term activation sees DCs as the main producers of IL-12p70, but with prolonged activation, DCs become the primary cells undergoing apoptosis and releasing IL-10. Furthermore, DCs that are long-term activated lose their ability to activate allogeneic IFNγ-responsive T cells found in the peripheral blood mononuclear cells of healthy volunteers. Additionally, a multiplex analysis of protein patterns in DC supernatants identifies a specific pattern associated with apoptosis, cancer, and chronic inflammation. This pattern partly overlaps with the well-documented non-functional, tumor-promoting, and anti-inflammatory phenotype of DCs (228).
In summary, DCs that are activated over the long-term exhibit significant functional shifts, evolving towards a non-functional, tumor-promoting, and anti-inflammatory phenotype. These findings underscore the hypothesis that in some cases, mature DCs may also induce T cell tolerance. This nuanced understanding of DC functionality suggests a delicate balance in the immune system, where the context and nature of DC maturation and activation play crucial roles in dictating the outcome of immune responses.
6.2 Promotive effects of efferocytosis on DC maturation and function: an enhancing mechanism
As mentioned above, apoptotic cells generally do not possess immunogenicity and their ingestion by DCs usually results in the suppression of DC maturation and triggers an immune tolerance response. However, when DCs ingest apoptotic cells infected with bacteria or viruses, they are often induced to mature (87, 204, 205). This is because when cells undergo apoptosis due to pathogen infection, components of the pathogen (including PAMPs) may adhere to or be included within these apoptotic cells. When DCs ingest apoptotic cells containing PAMPs, they not only take up the cellular debris but also the pathogenic PAMPs (180). DCs recognize these PAMPs through their surface pattern recognition receptors, initiating a cascade of signaling events that lead to DC maturation. However, we have observed that DCs’ tolerance to some apoptotic tumor cells is lost, inducing DC maturation and activating tumor-specific CD8+ T cell immune responses. This finding differs from the usual induction of immune tolerance by apoptotic cells under steady-state conditions. For instance, in the absence of PAMPs, after ingesting apoptotic melanoma cells, BMDCs undergo a series of changes, including increased expression of co-stimulatory molecules (MHC- II, CD40, CD86), pro-inflammatory cytokines (IL-1β, IL-6, TNF-α), and the chemokine receptor CCR7, which promote DC maturation and effectively activate specific T cell responses against melanoma (203). Additionally, studies have compared the effectiveness of co-culturing DCs with apoptotic tumor cells, tumor cell lysates, and supernatants of apoptotic tumor cells in inducing strong anti-tumor immune responses. The results show that co-culturing with apoptotic tumor cells significantly outperforms the other methods in eliciting robust anti-tumor immune responses (229).
This leads us to explore two critical questions: First, why can apoptotic cells from tumors induce DC maturation even without additional stimuli? Second, why can tumor cells in an apoptotic form stimulate DCs to trigger a stronger anti-tumor T cell immune response?
To address the first question, we must consider the unique properties of apoptotic cells from tumors. The enhanced immunogenicity of these cells is believed to be the key reason for the loss of DCs’ tolerance. This is primarily due to the translocation of calreticulin to their surface, increased secretion of HMGB1, and elevated expression of heat shock proteins (HSPs) (230–233).
Calreticulin is typically found in the endoplasmic reticulum of normal cells, where it assists in the proper folding of proteins. However, calreticulin is also abundantly expressed on the surface of tumor cells and further translocates to the cell surface during the apoptosis of tumor cells (231). Research has found that the recognition and uptake of apoptotic tumor cells by DCs depend on the binding of calreticulin on the apoptotic tumor cell surface to its receptor LRP1, which can activate tumor-specific CD8+ T cells (234). Although PtdSer is also present on the surface of apoptotic tumor cells and other efferocytosis receptors exist on DCs, such as the CD36-PtdSer pathway that mediates the recognition and uptake of apoptotic tumor cells, it is normally inhibited by the presence of the autophagy protein ATG5. This inhibition is actually beneficial for the immune attack against tumor cells (101) Blocking the calreticulin-LRP pathway, even when using anti-CD47 antibodies (which aim to block the “don’t eat me” signal on the surface of tumor cells, making them more susceptible to phagocytosis), impairs the phagocytosis of apoptotic tumor cells and significantly weakens or eliminates the tumor-specific CD8+ T cell immune response. This reveals the crucial role of the calreticulin-LRP pathway in promoting phagocyte uptake of apoptotic tumor cells and in the process of antigen cross-presentation (231). LRP1 also affects the intensity of the tumor-specific CD8+ T cell immune response by influencing the expression levels of the antigen cross-presentation-related gene MHC-I. Studies show that knocking out the LRP1 gene or inhibiting its function significantly reduces MHC-I expression levels, leading to a marked reduction in antigen-specific CD8+ T cell immune responses (235).
The increased secretion of HMGB1 following tumor cell apoptosis also promotes the activation of DCs and the presentation of tumor antigens. This is because HMGB1, acting as DAMPs, activates the TLR4-MyD88 signaling pathway in DCs, which not only promotes DC maturation but is also involved in the antigen processing process (236). Studies show that TLR4 can regulate the acidity of lysosomes, thereby affecting the antigen processing process. In DCs lacking TLR4, not only is the acidity within lysosomes increased, but the fusion rate between lysosomes and phagosomes is also accelerated (236–238), leading to lysosomes tending to degrade antigens rather than presenting them (238, 239). Breast cancer patients with dysfunctional TLR4 alleles experience faster recurrence after radiation and chemotherapy compared to those with normal TLR4 alleles (236, 239, 240), indicating a close association between TLR4 and anti-tumor immune responses.
The increased expression of HSPs on the surface of apoptotic tumor cells can significantly induce the maturation of DCs, thereby activating anti-tumor T cell immune responses (202). Inducing apoptosis in tumor cells and promoting the expression of HSPs on their surface is a target of many anti-cancer drugs or therapies. This is because HSPs, also acting as DAMPs, can bind to TLR2 and TLR4 and activate the MyD88/IRAK/NF-κB signaling pathway via a CD14-dependent mechanism, promoting the transcription of inflammatory factors and effectively activating DCs (241).
Whether apoptotic cells can stimulate the maturation of DCs and subsequently induce specific T cell responses not only depends on the apoptotic cells themselves but also on the local cellular and cytokine environment. For example, in experiments where DCs are co-cultured with apoptotic tumor cells, the expression of CCR7 is induced to increase, which aids in the migration of DCs and thereby promotes the formation of anti-tumor immune responses (242, 243), However, in some cases, despite DCs phagocytosing apoptotic tumor cells, their antigen presentation to CD8+ T cells is suboptimal. Yet, with the help of a mixture of cytokines such as IL-6, IL-1β, TNF-α, and PGE2, DCs can effectively activate CD8+ T cell immune responses (244). This suggests that the cytokine environment plays a key role in regulating DCs’ functions and promoting specific immune responses. Additionally, studies have found that macrophages co-localized with apoptotic tumor cells are predominantly of the anti-inflammatory type (245), which promote the production of anti-inflammatory cytokines, thereby driving DCs towards a tolerant phenotype and ultimately negatively impacting DC-mediated anti-tumor immunity (246).
When analyzing and comparing results from different studies, we must consider the variability in immunogenicity among different tumor cell lines, which mirrors the significant differences observed in human tumors in terms of differentiation, drug sensitivity, and prognosis. The variability in immunogenicity of tumor cell lines may affect the interpretation and conclusions of experiments, thereby impacting the accuracy and general applicability of the research (247). This also explains, to some extent, why in some studies, co-culturing apoptotic tumor cells with DCs did not induce DC maturation or effectively trigger tumor-specific T cell responses (244). Enhancing the immunogenicity of apoptotic tumor cells and restoring their apoptotic activity has become a current anti-cancer strategy (248, 249). The technique of loading specific tumor antigens into apoptotic cells to selectively activate T cell immune responses against those antigens has become an important method in studying tumor immune responses (250).
Turning to the second question, compared to tumor cell lysates, apoptotic tumor cells stimulate a more effective anti-tumor immune response in DCs (229). the ability of apoptotic tumor cells to stimulate a more robust anti-tumor T cell immune response is primarily due to their direct contact with DCs. This contact not only triggers the phagocytosis of these cells by DCs — aiding in their clearance — but also initiates significant immune responses. Specifically, direct contact between DCs and apoptotic tumor cells significantly increases the secretion of IL-12 and the expression of CCR7 (242) (251),. IL-12 is crucial for activating allogeneic T cells and natural killer (NK) cells (229). Moreover, CCR7 is essential for guiding DCs to lymph nodes, which are critical sites for initiating potent immune responses (242). While CD8+T cells are typically favored for targeting tumors, evidence suggests that after DCs phagocytose apoptotic tumor cells, the antigens can still be presented on MHC class II molecules, thereby activating tumor-specific CD4+ T cell responses (101). CD4+ T cells are also essential for anti-tumor responses, as they lead to DC “licensing” and initiation, thereby enhancing cross-presentation to CD8+ T cells (252, 253). Thus, these factors collectively amplify the effect of the anti-tumor immune response. Additionally, some researchers believe that the direct contact between DCs and apoptotic cells might mimic a more natural antigen presentation environment, with DC surfaces loaded with an appropriate density of tumor antigen peptides, which helps to activate CTLs with higher affinity and more specificity (244).
The use of apoptotic cells, loaded with specific tumor antigens on their surface to activate T-cell immune responses against targeted tumors, has emerged as a cutting-edge technology in cancer vaccine research (250). Currently, vaccines prepared from tumor cell lysates often fail to induce effective immune responses, partly because the physical form of antigens affects the efficiency of antigen presentation. Compared to soluble antigens, particulate antigens are more effectively cross-presented to MHC-I molecules (254, 255). Although tumor cell lysates contain various soluble antigens, these antigens are typically internalized through complement receptor-mediated phagocytosis rather than by efferocytosis. This mode of internalization may activate tumor-specific CD4+ or CD8+ T cells. However, the lack of cellular structural integrity and mechanical properties in these lysates prevents the formation of stable multimeric complexes with mechanoreceptors like integrins, which may limit the transmission of mechanical signals during the phagocytosis of tumor cell lysates (256), thereby potentially restricting the immune response to the tumor to a certain extent.
7 Efferocytosis on DCs: guardian of immune homeostasis
The immune responses activated by macrophages and DCs following efferocytosis exhibit marked differences. Macrophages exhibit a higher efficiency of efferocytosis compared to DCs and are capable of continuously engulfing and clearing apoptotic cells, often being a primary focus of efferocytosis research. Post-efferocytosis, macrophages secrete anti-inflammatory mediators such as IL-10 and TGF-β, as well as specialized pro-resolving molecules (257), and suppress pro-inflammatory cytokines including TNF-α, IL-1β, and IL-12. They also upregulate genes that enhance the internalization and degradation of apoptotic cells (117, 258, 259), thereby maintaining immune homeostasis. In contrast, DCs, upon undergoing efferocytosis, primarily activate anti-inflammatory pathways through the upregulation of genes involved in the differentiation of regulatory T cells (iTregs) and antigen presentation (117), thereby highlighting the unique anti-inflammatory mechanisms of DCs at the transcriptomic level following efferocytosis. All differences between DCs and macrophages are detailed in Table 3.
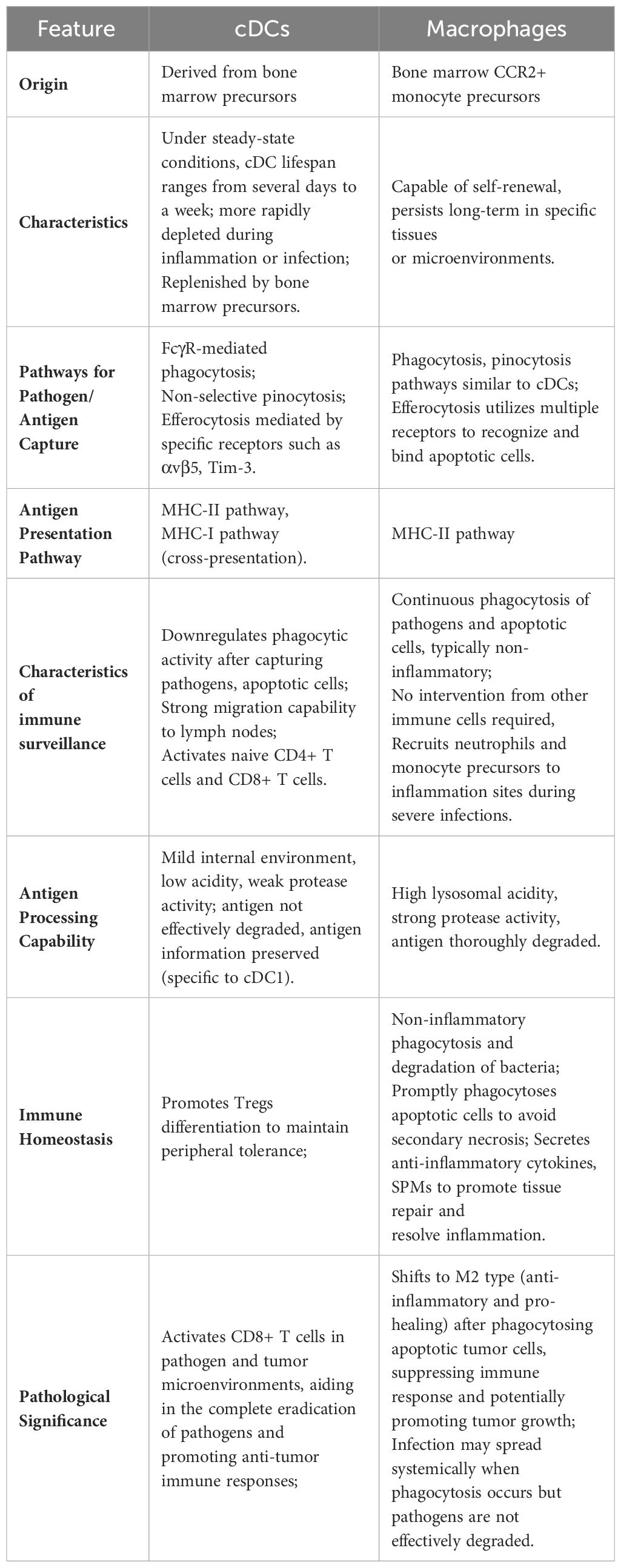
Table 3 Comparison of characteristics and functions between conventional dendritic cells and macrophages.
The phenomenon of DC mediated efferocytosis plays a pivotal role in fostering immune tolerance through the differentiation of iTregs. This process involves DCs engulfing apoptotic cells, subsequently processing and presenting the derived antigens, which is instrumental in establishing and maintaining peripheral T cell tolerance. Unlike central tolerance, which is achieved through the negative selection of autoreactive lymphocytes within the thymus and bone marrow (260, 261), peripheral tolerance is heavily reliant on CD4+ Tregs expressing the transcription factor Foxp3 (262, 263). These include thymus-derived naturally occurring Tregs (nTregs) and peripherally induced iTregs (264, 265).
7.1 Fostering immune tolerance and anti-inflammatory responses:enhancing Treg cell proliferation and function
Tregs are essential in dampening pro-inflammatory responses and aiding the resolution of inflammation. The expansion of Treg cells can boost the process of efferocytosis in a significant disease model characterized by both a reduction in Treg cells and impaired efferocytosis (266). Emerging evidence indicates that DC efferocytosis can promote the differentiation of Tregs (228, 267, 268) (Figure 4), This establishes an effective positive feedback loop. The mechanism by which DC efferocytosis facilitates Treg differentiation involves both TGF-beta and RA (269–274). DCs serve as significant producers of TGF-β (275). The absence of TGF-β results in the progressive depletion of iTregs within peripheral lymphoid structures (276). Conversely, exogenous TGF-β can trigger Foxp3 expression in specific subsets of T cells (277, 278), pivotal for iTregs formation (279). The importance of this pathway is underscored by observations that mice deficient in Foxp3 or TGF-βRII succumb to early death due to uncontrolled systemic inflammation (280, 281).
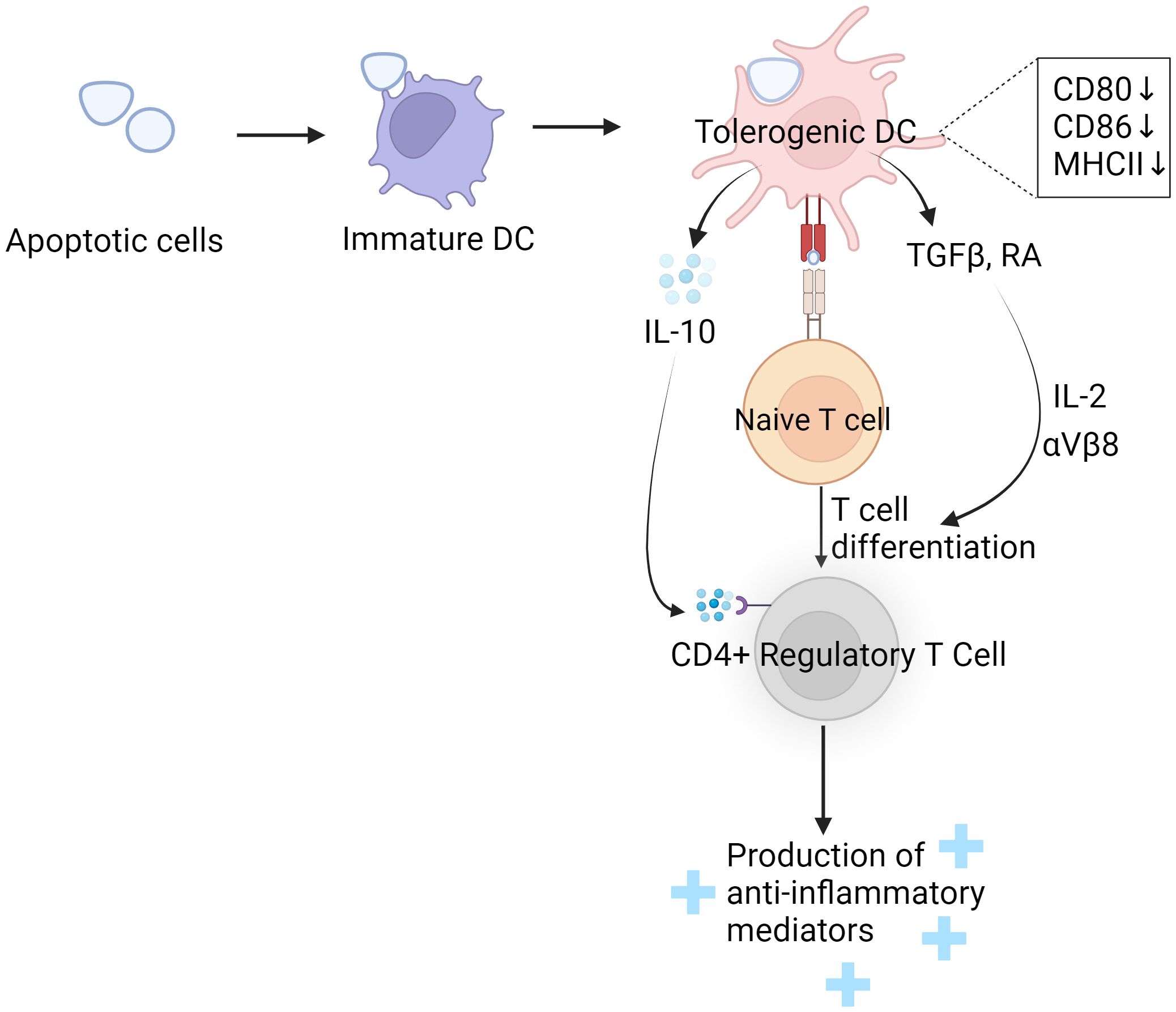
Figure 4 DC phagocytosis induces Treg differentiation and enhances its function. When immature dendritic cells (DCs) engulf apoptotic cells, they undergo a transformation into tolerogenic DCs. This transformation is marked by a reduction in the expression of co-stimulatory molecules. These tolerogenic DCs then produce and release immune-modulating substances such as IL10, TGF-beta, and retinoic acid (RA). The IL10 secreted by tolerogenic DCs binds to IL10 receptors on regulatory T cells (Tregs), which enhances the Tregs’ ability to function effectively. On the other hand, TGF-beta and RA work together in the presence of IL2 and integrins αvβ8 to drive the differentiation of naive T cells into regulatory T cells. These regulatory T cells play a crucial role in establishing and maintaining immune tolerance. They achieve this by releasing anti-inflammatory mediators, which help in preventing overactive immune responses that could lead to autoimmunity or chronic inflammation.
The generation of iTregs by TGF-β is highly dependent on the integrin αvβ8 (282–284). In the intestinal context, CD103+ DCs lacking this integrin fail to foster iTregs in vitro (285), leading to a marked decrease in iTregs numbers within the colonic mucosa (286). Moreover, RA, a metabolite of vitamin A produced by DCs, along with IL-2 from T cells, further augments TGF-β induced Foxp3 synthesis, enhancing iTreg differentiation (287, 288). This process also contributes to nTregs differentiation within the thymus, although TGF-β’s role in nTregs production was initially debated (289, 290). However, further studies revealed TGF-β’s protective role in safeguarding nTregs from apoptosis during thymic development (291).
In the intestinal mucosal layer, CD103+DCs, post-clearance of apoptotic intestinal epithelial cells, regulate a host of genes pivotal for iTregs proliferation, differentiation, recruitment, and migration, including Ccl22, Ccl17, Aldh1a2, IDO-1, GARP, Cd274 (117).
Additionally, DCs, upon phagocytosing apoptotic cells post-infection, produce substantial amounts of IL-10 (161, 204, 292), which acts through enhancing the suppressive function of Treg rather than promoting their proliferation and differentiation, as TGFβ does (293).
8 Conclusion and future perspectives
Despite the significant attention and progress that efferocytosis has garnered in recent research, our understanding remains limited regarding how DCs can precisely guide beneficial immune responses following the recognition and engulfment of apoptotic cells from diverse origins. For instance, in the field of oncology, the role of macrophage-mediated efferocytosis has been extensively documented to be closely associated with tumor immune evasion (128, 294, 295). In contrast, DCs are capable of initiating specific immune responses against tumor cells by engulfing apoptotic tumor cells (101). Compared to DCs, macrophages exhibit proficient efferocytosis and metabolic processing capabilities of cellular debris. However, during Mycobacterium tuberculosis infection, they may contribute to the pathogen’s resistance to host immune responses. In this context, the efferocytosis of apoptotic bodies released by infected macrophages by DCs triggers the host’s adaptive immunity against tuberculosis (184, 187).
This phenomenon underscores the irreplaceable and critical role of DCs efferocytosis in immune responses. But the efferocytosis by DCs is exceedingly complex within immune regulation, involving a multitude of signaling pathways, cooperative actions among different cell types, and diverse interactions between DCs and apoptotic cells. Although we have gained some understanding of how DCs clear apoptotic cells through efferocytosis to maintain tissue homeostasis and prevent uncontrolled inflammatory responses, the mechanisms by which DCs precisely regulate immune responses to promote immune tolerance or activate specific immune responses remain somewhat unclear. This review focuses on the pivotal role of DCs in efferocytosis and how they influence the balance of the immune system through this process. Several problems remain to be resolved in the future:
1. Some efferocytosis receptors, after accomplishing the task of recognizing and engulfing apoptotic cells, continue to participate in the subsequent antigen cross-presentation pathways of DCs, which may be one of the crucial mechanisms linking innate and adaptive immunity. Efferocytosis receptors are not merely facilitating the clearance of dying cells; they may also activate or modulate specific signaling pathways during efferocytosis, thereby influencing the maturation and functionality of DCs, and contributing to the formation of a comprehensive and effective immune response. Future research needs to delve deeper into the specific mechanisms of these efferocytosis receptors and their roles in regulating immune responses. Additionally, there is a need to discover unexplored signaling receptors to comprehensively explain the critical functions of DCs in immune surveillance and defense.
2. Integration of Efferocytosis with DCs’ Tolerance Induction: While the involvement of efferocytosis in antigen cross-presentation by DCs is acknowledged, its role in the induction of immune tolerance remains less understood. How do DCs, following efferocytosis, differentiate between contexts requiring immune activation versus tolerance induction? Identifying the cues and mechanisms that guide this decision-making process is crucial for understanding how efferocytosis contributes to maintaining immune homeostasis and preventing autoimmune diseases.
3. Impact of the Microenvironment on DC Efferocytosis: The microenvironment in which efferocytosis occurs can greatly influence the outcome of this process. Factors such as tissue-specific signals, the presence of inflammatory cytokines, and interactions with other immune cells can modulate DCs’ responses to engulfed apoptotic cells. Future research should explore how these microenvironmental factors affect efferocytosis by DCs and their subsequent immune functions. Additionally, understanding how DCs integrate these signals to orchestrate appropriate immune responses will shed light on their versatility and adaptability in diverse physiological and pathological contexts.
With the advancement of technologies such as spatial transcriptomics and advanced imaging techniques, we now have the opportunity to explore the spatial and molecular dimensions of DC efferocytosis with unprecedented detail and precision. These technologies can reveal how DCs recognize and engulf apoptotic cells within the tissue microenvironment and how this process impacts their antigen presentation and immune regulatory functions. Through spatially resolved transcriptomic analysis, we can gain a deeper understanding of the heterogeneity of DC efferocytosis across different tissues and pathological states. This will aid in uncovering novel regulatory mechanisms of efferocytosis receptors and how they interact with other immune cells and factors within the tissue microenvironment. Consequently, we are encouraged to develop new diagnostic tools and therapeutic strategies aimed at specifically modulating the efferocytosis function of DCs to treat immune-related diseases caused by aberrant efferocytosis.
Author contributions
YM: Writing – original draft. TJ: Writing – review & editing. XZ: Writing – review & editing. YX: Writing – review & editing. KW: Writing – review & editing. TZ: Writing – review & editing. MX: Writing – review & editing.
Funding
The author(s) declare that financial support was received for the research, authorship, and/or publication of this article. This research was supported by the Ministry of Science and Technology of China Project 1208 - China-Israel Emergency Response Training Center Construction.
Acknowledgments
Thanks are extended to the members of the laboratory at the Translational Medicine Research Center, Medical Innovation Research Division, and Fourth Medical Center of the Chinese PLA General Hospital for their discussion of this paper.
Conflict of interest
The authors declare that the research was conducted in the absence of any commercial or financial relationships that could be construed as a potential conflict of interest.
Publisher’s note
All claims expressed in this article are solely those of the authors and do not necessarily represent those of their affiliated organizations, or those of the publisher, the editors and the reviewers. Any product that may be evaluated in this article, or claim that may be made by its manufacturer, is not guaranteed or endorsed by the publisher.
References
1. Schneider K, Arandjelovic S. Apoptotic cell clearance components in inflammatory arthritis. Immunol Rev. (2023) 319:142–50. doi: 10.1111/imr.13256
2. Qiu H, Shao Z, Wen X, Liu Z, Chen Z, Qu D, et al. Efferocytosis: An accomplice of cancer immune escape. BioMed Pharmacother. (2023) 167:115540. doi: 10.1016/j.biopha.2023.115540
3. Zhang M, Wei J, Sun Y, He C, Ma S, Pan X, et al. The efferocytosis process in aging: Supporting evidence, mechanisms, and therapeutic prospects for age-related diseases. J Adv Res. (2024). doi: 10.1016/j.jare.2024.03.008
4. Belabed M, Mauvais FX, Maschalidi S, Kurowska M, Goudin N, Huang JD, et al. Kinesin-1 regulates antigen cross-presentation through the scission of tubulations from early endosomes in dendritic cells. Nat Commun. (2020) 11:1817. doi: 10.1038/s41467-020-15692-0
5. Koga MM, Engel A, Pigni M, Lavanchy C, Stevanin M, Laversenne V, et al. IL10- and IL35-secreting mutuDC lines act in cooperation to inhibit memory T cell activation through LAG-3 expression. Front Immunol. (2021) 12:607315. doi: 10.3389/fimmu.2021.607315
6. Bianconi E, Piovesan A, Facchin F, Beraudi A, Casadei R, Frabetti F, et al. An estimation of the number of cells in the human body. Ann Hum Biol. (2013) 40:463–71. doi: 10.3109/03014460.2013.807878
7. Henson PM, Hume DA. Apoptotic cell removal in development and tissue homeostasis. Trends Immunol. (2006) 27:244–50. doi: 10.1016/j.it.2006.03.005
8. Vandivier RW, Henson PM, Douglas IS. Burying the dead: the impact of failed apoptotic cell removal (efferocytosis) on chronic inflammatory lung disease. Chest. (2006) 129:1673–82. doi: 10.1378/chest.129.6.1673
9. Rieu Q, Bougouin A, Zagar Y, Chatagnon J, Hamieh A, Enderlin J, et al. Pleiotropic roles of scavenger receptors in circadian retinal phagocytosis: A new function for lysosomal SR-B2/LIMP-2 at the RPE cell surface. Int J Mol Sci. (2022) 23:3445–63. doi: 10.3390/ijms23073445
10. Savill J, Fadok V. Corpse clearance defines the meaning of cell death. Nature. (2000) 407:784–8. doi: 10.1038/35037722
11. Flemming A. Sepsis: DAMPening inflammation. Nat Rev Drug Discovery. (2011) 10:416. doi: 10.1038/nrd3468
12. Ogden CA, deCathelineau A, Hoffmann PR, Bratton D, Ghebrehiwet B, Fadok VA, et al. C1q and mannose binding lectin engagement of cell surface calreticulin and CD91 initiates macropinocytosis and uptake of apoptotic cells. J Exp Med. (2001) 194:781–95. doi: 10.1084/jem.194.6.781
13. Hoffmann PR, deCathelineau AM, Ogden CA, Leverrier Y, Bratton DL, Daleke DL, et al. Phosphatidylserine (PS) induces PS receptor-mediated macropinocytosis and promotes clearance of apoptotic cells. J Cell Biol. (2001) 155:649–59. doi: 10.1083/jcb.200108080
14. Aderem A, Underhill DM. Mechanisms of phagocytosis in macrophages. Annu Rev Immunol. (1999) 17:593–623. doi: 10.1146/annurev.immunol.17.1.593
15. Gude DR, Alvarez SE, Paugh SW, Mitra P, Yu J, Griffiths R, et al. Apoptosis induces expression of sphingosine kinase 1 to release sphingosine-1-phosphate as a "come-and-get-me" signal. FASEB J. (2008) 22:2629–38. doi: 10.1096/fj.08-107169
16. Truman LA, Ford CA, Pasikowska M, Pound JD, Wilkinson SJ, Dumitriu IE, et al. CX3CL1/fractalkine is released from apoptotic lymphocytes to stimulate macrophage chemotaxis. Blood. (2008) 112:5026–36. doi: 10.1182/blood-2008-06-162404
17. Lauber K, Bohn E, Krober SM, Xiao YJ, Blumenthal SG, Lindemann RK, et al. Apoptotic cells induce migration of phagocytes via caspase-3-mediated release of a lipid attraction signal. Cell. (2003) 113:717–30. doi: 10.1016/S0092-8674(03)00422-7
18. Doran AC, Yurdagul A Jr., Tabas I. Efferocytosis in health and disease. Nat Rev Immunol. (2020) 20:254–67. doi: 10.1038/s41577-019-0240-6
19. Gardai SJ, Bratton DL, Ogden CA, Henson PM. Recognition ligands on apoptotic cells: a perspective. J Leukoc Biol. (2006) 79:896–903. doi: 10.1189/jlb.1005550
20. Oldenborg PA, Zheleznyak A, Fang YF, Lagenaur CF, Gresham HD, Lindberg FP. Role of CD47 as a marker of self on red blood cells. Science. (2000) 288:2051–4. doi: 10.1126/science.288.5473.2051
21. Barkal AA, Brewer RE, Markovic M, Kowarsky M, Barkal SA, Zaro BW, et al. CD24 signalling through macrophage Siglec-10 is a target for cancer immunotherapy. Nature. (2019) 572:392–6. doi: 10.1038/s41586-019-1456-0
22. Poon IK, Lucas CD, Rossi AG, Ravichandran KS. Apoptotic cell clearance: basic biology and therapeutic potential. Nat Rev Immunol. (2014) 14:166–80. doi: 10.1038/nri3607
23. Elward K, Griffiths M, Mizuno M, Harris CL, Neal JW, Morgan BP, et al. CD46 plays a key role in tailoring innate immune recognition of apoptotic and necrotic cells. J Biol Chem. (2005) 280:36342–54. doi: 10.1074/jbc.M506579200
24. Barkal AA, Weiskopf K, Kao KS, Gordon SR, Rosental B, Yiu YY, et al. Engagement of MHC class I by the inhibitory receptor LILRB1 suppresses macrophages and is a target of cancer immunotherapy. Nat Immunol. (2018) 19:76–84. doi: 10.1038/s41590-017-0004-z
25. He M, Kubo H, Morimoto K, Fujino N, Suzuki T, Takahasi T, et al. Receptor for advanced glycation end products binds to phosphatidylserine and assists in the clearance of apoptotic cells. EMBO Rep. (2011) 12:358–64. doi: 10.1038/embor.2011.28
26. Greenberg ME, Sun M, Zhang R, Febbraio M, Silverstein R, Hazen SL. Oxidized phosphatidylserine-CD36 interactions play an essential role in macrophage-dependent phagocytosis of apoptotic cells. J Exp Med. (2006) 203:2613–25. doi: 10.1084/jem.20060370
27. Vago JP, Amaral FA, van de Loo FAJ. Resolving inflammation by TAM receptor activation. Pharmacol Ther. (2021) 227:107893. doi: 10.1016/j.pharmthera.2021.107893
28. Kourtzelis I, Li X, Mitroulis I, Grosser D, Kajikawa T, Wang B, et al. DEL-1 promotes macrophage efferocytosis and clearance of inflammation. Nat Immunol. (2019) 20:40–9. doi: 10.1038/s41590-018-0249-1
29. Albert ML, Kim JI, Birge RB. alphavbeta5 integrin recruits the CrkII-Dock180-rac1 complex for phagocytosis of apoptotic cells. Nat Cell Biol. (2000) 2:899–905. doi: 10.1038/35046549
30. Wu Y, Singh S, Georgescu MM, Birge RB. A role for Mer tyrosine kinase in alphavbeta5 integrin-mediated phagocytosis of apoptotic cells. J Cell Sci. (2005) 118:539–53. doi: 10.1242/jcs.01632
31. Akakura S, Singh S, Spataro M, Akakura R, Kim JI, Albert ML, et al. The opsonin MFG-E8 is a ligand for the alphavbeta5 integrin and triggers DOCK180-dependent Rac1 activation for the phagocytosis of apoptotic cells. Exp Cell Res. (2004) 292:403–16. doi: 10.1016/j.yexcr.2003.09.011
32. Park D, Tosello-Trampont AC, Elliott MR, Lu M, Haney LB, Ma Z, et al. BAI1 is an engulfment receptor for apoptotic cells upstream of the ELMO/Dock180/Rac module. Nature. (2007) 450:430–4. doi: 10.1038/nature06329
33. Tao H, Yancey PG, Babaev VR, Blakemore JL, Zhang Y, Ding L, et al. Macrophage SR-BI mediates efferocytosis via Src/PI3K/Rac1 signaling and reduces atherosclerotic lesion necrosis. J Lipid Res. (2015) 56:1449–60. doi: 10.1194/jlr.M056689
34. Park SY, Kang KB, Thapa N, Kim SY, Lee SJ, Kim IS. Requirement of adaptor protein GULP during stabilin-2-mediated cell corpse engulfment. J Biol Chem. (2008) 283:10593–600. doi: 10.1074/jbc.M709105200
35. Hochreiter-Hufford A, Ravichandran KS. Clearing the dead: apoptotic cell sensing, recognition, engulfment, and digestion. Cold Spring Harb Perspect Biol. (2013) 5:a008748. doi: 10.1101/cshperspect.a008748
36. Nakaya M, Tanaka M, Okabe Y, Hanayama R, Nagata S. Opposite effects of rho family GTPases on engulfment of apoptotic cells by macrophages. J Biol Chem. (2006) 281:8836–42. doi: 10.1074/jbc.M510972200
37. Leverrier Y, Ridley AJ. Requirement for Rho GTPases and PI 3-kinases during apoptotic cell phagocytosis by macrophages. Curr Biol. (2001) 11:195–9. doi: 10.1016/S0960-9822(01)00047-1
38. Tosello-Trampont AC, Kinchen JM, Brugnera E, Haney LB, Hengartner MO, Ravichandran KS. Identification of two signaling submodules within the CrkII/ELMO/Dock180 pathway regulating engulfment of apoptotic cells. Cell Death Differ. (2007) 14:963–72. doi: 10.1038/sj.cdd.4402094
39. Gumienny TL, Brugnera E, Tosello-Trampont AC, Kinchen JM, Haney LB, Nishiwaki K, et al. CED-12/ELMO, a novel member of the CrkII/Dock180/Rac pathway, is required for phagocytosis and cell migration. Cell. (2001) 107:27–41. doi: 10.1016/S0092-8674(01)00520-7
40. Brugnera E, Haney L, Grimsley C, Lu M, Walk SF, Tosello-Trampont AC, et al. Unconventional Rac-GEF activity is mediated through the Dock180-ELMO complex. Nat Cell Biol. (2002) 4:574–82. doi: 10.1038/ncb824
41. Grimsley CM, Kinchen JM, Tosello-Trampont AC, Brugnera E, Haney LB, Lu M, et al. Dock180 and ELMO1 proteins cooperate to promote evolutionarily conserved Rac-dependent cell migration. J Biol Chem. (2004) 279:6087–97. doi: 10.1074/jbc.M307087200
42. Chen Z, Borek D, Padrick SB, Gomez TS, Metlagel Z, Ismail AM, et al. Structure and control of the actin regulatory WAVE complex. Nature. (2010) 468:533–8. doi: 10.1038/nature09623
43. Ding B, Yang S, Schaks M, Liu Y, Brown AJ, Rottner K, et al. Structures reveal a key mechanism of WAVE regulatory complex activation by Rac1 GTPase. Nat Commun. (2022) 13:5444. doi: 10.1038/s41467-022-33174-3
44. Heasman SJ, Ridley AJ. Mammalian Rho GTPases: new insights into their functions from in vivo studies. Nat Rev Mol Cell Biol. (2008) 9:690–701. doi: 10.1038/nrm2476
45. Evans IR, Ghai PA, Urbancic V, Tan KL, Wood W. SCAR/WAVE-mediated processing of engulfed apoptotic corpses is essential for effective macrophage migration in Drosophila. Cell Death Differ. (2013) 20:709–20. doi: 10.1038/cdd.2012.166
46. Kim SY, Kim S, Bae DJ, Park SY, Lee GY, Park GM, et al. Coordinated balance of Rac1 and RhoA plays key roles in determining phagocytic appetite. PloS One. (2017) 12:e0174603. doi: 10.1371/journal.pone.0174603
47. Riento K, Ridley AJ. Rocks: multifunctional kinases in cell behaviour. Nat Rev Mol Cell Biol. (2003) 4:446–56. doi: 10.1038/nrm1128
48. Erwig LP, Gordon S, Walsh GM, Rees AJ. Previous uptake of apoptotic neutrophils or ligation of integrin receptors downmodulates the ability of macrophages to ingest apoptotic neutrophils. Blood. (1999) 93:1406–12. doi: 10.1182/blood.V93.4.1406
49. Tosello-Trampont AC, Nakada-Tsukui K, Ravichandran KS. Engulfment of apoptotic cells is negatively regulated by Rho-mediated signaling. J Biol Chem. (2003) 278:49911–9. doi: 10.1074/jbc.M306079200
50. Lou Y, Han M, Liu H, Niu Y, Liang Y, Guo J, et al. Essential roles of S100A10 in Toll-like receptor signaling and immunity to infection. Cell Mol Immunol. (2020) 17:1053–62. doi: 10.1038/s41423-019-0278-1
51. Bonfim-Melo A, Ferreira ER, Mortara RA. Rac1/WAVE2 and Cdc42/N-WASP participation in actin-dependent host cell invasion by extracellular amastigotes of Trypanosoma cruzi. Front Microbiol. (2018) 9:360. doi: 10.3389/fmicb.2018.00360
52. Ho HY, Rohatgi R, Lebensohn AM, Le M, Li J, Gygi SP, et al. Toca-1 mediates Cdc42-dependent actin nucleation by activating the N-WASP-WIP complex. Cell. (2004) 118:203–16. doi: 10.1016/j.cell.2004.06.027
53. Jubrail J, Africano-Gomez K, Herit F, Mularski A, Bourdoncle P, Oberg L, et al. Arpin is critical for phagocytosis in macrophages and is targeted by human rhinovirus. EMBO Rep. (2020) 21:e47963. doi: 10.15252/embr.201947963
54. Rohatgi R, Ma L, Miki H, Lopez M, Kirchhausen T, Takenawa T, et al. The interaction between N-WASP and the Arp2/3 complex links Cdc42-dependent signals to actin assembly. Cell. (1999) 97:221–31. doi: 10.1016/S0092-8674(00)80732-1
55. Caron E, Hall A. Identification of two distinct mechanisms of phagocytosis controlled by different Rho GTPases. Science. (1998) 282:1717–21. doi: 10.1126/science.282.5394.1717
56. Leverrier Y, Lorenzi R, Blundell MP, Brickell P, Kinnon C, Ridley AJ, et al. Cutting edge: the Wiskott-Aldrich syndrome protein is required for efficient phagocytosis of apoptotic cells. J Immunol. (2001) 166:4831–4. doi: 10.4049/jimmunol.166.8.4831
57. Yin C, Heit B. Cellular responses to the efferocytosis of apoptotic cells. Front Immunol. (2021) 12:631714. doi: 10.3389/fimmu.2021.631714
58. Kitano M, Nakaya M, Nakamura T, Nagata S, Matsuda M. Imaging of Rab5 activity identifies essential regulators for phagosome maturation. Nature. (2008) 453:241–5. doi: 10.1038/nature06857
59. Kinchen JM, Doukoumetzidis K, Almendinger J, Stergiou L, Tosello-Trampont A, Sifri CD, et al. A pathway for phagosome maturation during engulfment of apoptotic cells. Nat Cell Biol. (2008) 10:556–66. doi: 10.1038/ncb1718
60. Kinchen JM, Ravichandran KS. Identification of two evolutionarily conserved genes regulating processing of engulfed apoptotic cells. Nature. (2010) 464:778–82. doi: 10.1038/nature08853
61. Yu X, Lu N, Zhou Z. Phagocytic receptor CED-1 initiates a signaling pathway for degrading engulfed apoptotic cells. PloS Biol. (2008) 6:e61. doi: 10.1371/journal.pbio.0060061
62. Harrison RE, Bucci C, Vieira OV, Schroer TA, Grinstein S. Phagosomes fuse with late endosomes and/or lysosomes by extension of membrane protrusions along microtubules: role of Rab7 and RILP. Mol Cell Biol. (2003) 23:6494–506. doi: 10.1128/MCB.23.18.6494-6506.2003
63. Johansson M, Lehto M, Tanhuanpaa K, Cover TL, Olkkonen VM. The oxysterol-binding protein homologue ORP1L interacts with Rab7 and alters functional properties of late endocytic compartments. Mol Biol Cell. (2005) 16:5480–92. doi: 10.1091/mbc.e05-03-0189
64. Rink J, Ghigo E, Kalaidzidis Y, Zerial M. Rab conversion as a mechanism of progression from early to late endosomes. Cell. (2005) 122:735–49. doi: 10.1016/j.cell.2005.06.043
65. Balderhaar HJ, Ungermann C. CORVET and HOPS tethering complexes - coordinators of endosome and lysosome fusion. J Cell Sci. (2013) 126:1307–16. doi: 10.1242/jcs.107805
66. Miller HE, Larson CL, Heinzen RA. Actin polymerization in the endosomal pathway, but not on the Coxiella-containing vacuole, is essential for pathogen growth. PloS Pathog. (2018) 14:e1007005. doi: 10.1371/journal.ppat.1007005
67. Wu Z, Thiyagarajan S, O'Shaughnessy B, Karatekin E. Regulation of exocytotic fusion pores by SNARE protein transmembrane domains. Front Mol Neurosci. (2017) 10:315. doi: 10.3389/fnmol.2017.00315
68. Lennon-Dumenil AM, Bakker AH, Maehr R, Fiebiger E, Overkleeft HS, Rosemblatt M, et al. Analysis of protease activity in live antigen-presenting cells shows regulation of the phagosomal proteolytic contents during dendritic cell activation. J Exp Med. (2002) 196:529–40. doi: 10.1084/jem.20020327
69. Backer JM. The intricate regulation and complex functions of the Class III phosphoinositide 3-kinase Vps34. Biochem J. (2016) 473:2251–71. doi: 10.1042/BCJ20160170
70. Martinez J, Malireddi RK, Lu Q, Cunha LD, Pelletier S, Gingras S, et al. Molecular characterization of LC3-associated phagocytosis reveals distinct roles for Rubicon, NOX2 and autophagy proteins. Nat Cell Biol. (2015) 17:893–906. doi: 10.1038/ncb3192
71. Yin C, Argintaru D, Heit B. Rab17 mediates intermixing of phagocytosed apoptotic cells with recycling endosomes. Small GTPases. (2019) 10:218–26. doi: 10.1080/21541248.2017.1308852
72. Yin C, Kim Y, Argintaru D, Heit B. Rab17 mediates differential antigen sorting following efferocytosis and phagocytosis. Cell Death Dis. (2016) 7:e2529. doi: 10.1038/cddis.2016.431
73. Yurdagul A Jr., Subramanian M, Wang X, Crown SB, Ilkayeva OR, Darville L, et al. Macrophage metabolism of apoptotic cell-derived arginine promotes continual efferocytosis and resolution of injury. Cell Metab. (2020) 31:518–33.e10. doi: 10.1016/j.cmet.2020.01.001
74. Yurdagul A Jr. Metabolic consequences of efferocytosis and its impact on atherosclerosis. Immunometabolism. (2021) 3:e210017-1–22. doi: 10.20900/immunometab20210017
75. Mukundan L, Odegaard JI, Morel CR, Heredia JE, Mwangi JW, Ricardo-Gonzalez RR, et al. PPAR-delta senses and orchestrates clearance of apoptotic cells to promote tolerance. Nat Med. (2009) 15:1266–72. doi: 10.1038/nm.2048
76. Yoon YS, Kim SY, Kim MJ, Lim JH, Cho MS, Kang JL. PPARgamma activation following apoptotic cell instillation promotes resolution of lung inflammation and fibrosis via regulation of efferocytosis and proresolving cytokines. Mucosal Immunol. (2015) 8:1031–46. doi: 10.1038/mi.2014.130
77. Mota AC, Dominguez M, Weigert A, Snodgrass RG, Namgaladze D, Brune B. Lysosome-dependent LXR and PPARdelta activation upon efferocytosis in human macrophages. Front Immunol. (2021) 12:637778. doi: 10.3389/fimmu.2021.637778
78. Bosteels V, Marechal S, De Nolf C, Rennen S, Maelfait J, Tavernier SJ, et al. LXR signaling controls homeostatic dendritic cell maturation. Sci Immunol. (2023) 8:eadd3955. doi: 10.1126/sciimmunol.add3955
79. Forgac M. Vacuolar ATPases: rotary proton pumps in physiology and pathophysiology. Nat Rev Mol Cell Biol. (2007) 8:917–29. doi: 10.1038/nrm2272
80. Jefferies KC, Cipriano DJ, Forgac M. Function, structure and regulation of the vacuolar (H+)-ATPases. Arch Biochem Biophys. (2008) 476:33–42. doi: 10.1016/j.abb.2008.03.025
81. Ablasser A, Chen ZJ. cGAS in action: Expanding roles in immunity and inflammation. Science. (2019) 363:3621–9. doi: 10.1126/science.aat8657
82. Liebold I, Al Jawazneh A, Casar C, Lanzloth C, Leyk S, Hamley M, et al. Apoptotic cell identity induces distinct functional responses to IL-4 in efferocytic macrophages. Science. (2024) 384:eabo7027. doi: 10.1126/science.abo7027
83. Ohteki T, Kawamura S, Onai N. Commitment to dendritic cells and monocytes. Int Immunol. (2021) 33:815–9. doi: 10.1093/intimm/dxab031
84. Gardner A, de Mingo Pulido A, Ruffell B. Dendritic cells and their role in immunotherapy. Front Immunol. (2020) 11:924. doi: 10.3389/fimmu.2020.00924
85. Anderson DA 3rd, Dutertre CA, Ginhoux F, Murphy KM. Genetic models of human and mouse dendritic cell development and function. Nat Rev Immunol. (2021) 21:101–15. doi: 10.1038/s41577-020-00413-x
86. Bagadia P, Huang X, Liu TT, Durai V, Grajales-Reyes GE, Nitschke M, et al. An Nfil3-Zeb2-Id2 pathway imposes Irf8 enhancer switching during cDC1 development. Nat Immunol. (2019) 20:1174–85. doi: 10.1038/s41590-019-0449-3
87. Albert ML, Sauter B, Bhardwaj N. Dendritic cells acquire antigen from apoptotic cells and induce class I-restricted CTLs. Nature. (1998) 392:86–9. doi: 10.1038/32183
88. Schnorrer P, Behrens GM, Wilson NS, Pooley JL, Smith CM, El-Sukkari D, et al. The dominant role of CD8+ dendritic cells in cross-presentation is not dictated by antigen capture. Proc Natl Acad Sci U.S.A. (2006) 103:10729–34. doi: 10.1073/pnas.0601956103
89. Albert ML, Pearce SF, Francisco LM, Sauter B, Roy P, Silverstein RL, et al. Immature dendritic cells phagocytose apoptotic cells via alphavbeta5 and CD36, and cross-present antigens to cytotoxic T lymphocytes. J Exp Med. (1998) 188:1359–68. doi: 10.1084/jem.188.7.1359
90. Yrlid U, Wick MJ. Salmonella-induced apoptosis of infected macrophages results in presentation of a bacteria-encoded antigen after uptake by bystander dendritic cells. J Exp Med. (2000) 191:613–24. doi: 10.1084/jem.191.4.613
91. Subklewe M, Paludan C, Tsang ML, Mahnke K, Steinman RM, Munz C. Dendritic cells cross-present latency gene products from Epstein-Barr virus-transformed B cells and expand tumor-reactive CD8(+) killer T cells. J Exp Med. (2001) 193:405–11. doi: 10.1084/jem.193.3.405
92. Motta I, Andre F, Lim A, Tartaglia J, Cox WI, Zitvogel L, et al. Cross-presentation by dendritic cells of tumor antigen expressed in apoptotic recombinant canarypox virus-infected dendritic cells. J Immunol. (2001) 167:1795–802. doi: 10.4049/jimmunol.167.3.1795
93. Iyoda T, Shimoyama S, Liu K, Omatsu Y, Akiyama Y, Maeda Y, et al. The CD8+ dendritic cell subset selectively endocytoses dying cells in culture and in vivo. J Exp Med. (2002) 195:1289–302. doi: 10.1084/jem.20020161
94. Gu FF, Zhang K, Ma LL, Liu YY, Li C, Hu Y, et al. The superior ability of human BDCA3(+) (CD141(+)) dendritic cells (DCs) to cross-present antigens derived from necrotic lung cancer cells. Front Immunol. (2020) 11:1267. doi: 10.3389/fimmu.2020.01267
95. Dudziak D, Kamphorst AO, Heidkamp GF, Buchholz VR, Trumpfheller C, Yamazaki S, et al. Differential antigen processing by dendritic cell subsets in vivo. Science. (2007) 315:107–11. doi: 10.1126/science.1136080
96. Wakabayashi A, Shimizu M, Shinya E, Takahashi H. HMGB1 released from intestinal epithelia damaged by cholera toxin adjuvant contributes to activation of mucosal dendritic cells and induction of intestinal cytotoxic T lymphocytes and IgA. Cell Death Dis. (2018) 9:631. doi: 10.1038/s41419-018-0665-z
97. Bandyopadhyay A, Fine RL, Demento S, Bockenstedt LK, Fahmy TM. The impact of nanoparticle ligand density on dendritic-cell targeted vaccines. Biomaterials. (2011) 32:3094–105. doi: 10.1016/j.biomaterials.2010.12.054
98. Vremec D, Pooley J, Hochrein H, Wu L, Shortman K. CD4 and CD8 expression by dendritic cell subtypes in mouse thymus and spleen. J Immunol. (2000) 164:2978–86. doi: 10.4049/jimmunol.164.6.2978
99. Niezold T, Storcksdieck Genannt Bonsmann M, Maaske A, Temchura V, Heinecke V, Hannaman D, et al. DNA vaccines encoding DEC205-targeted antigens: immunity or tolerance? Immunology. (2015) 145:519–33. doi: 10.1111/imm.12467
100. Qiu CH, Miyake Y, Kaise H, Kitamura H, Ohara O, Tanaka M. Novel subset of CD8alpha+ dendritic cells localized in the marginal zone is responsible for tolerance to cell-associated antigens. J Immunol. (2009) 182:4127–36. doi: 10.4049/jimmunol.0803364
101. Oh DS, Lee HK. Autophagy protein ATG5 regulates CD36 expression and anti-tumor MHC class II antigen presentation in dendritic cells. Autophagy. (2019) 15:2091–106. doi: 10.1080/15548627.2019.1596493
102. del Rio ML, Bernhardt G, Rodriguez-Barbosa JI, Forster R. Development and functional specialization of CD103+ dendritic cells. Immunol Rev. (2010) 234:268–81. doi: 10.1111/j.0105-2896.2009.00874.x
103. Cabeza-Cabrerizo M, Cardoso A, Minutti CM, Pereira da Costa M, Reis e Sousa C. Dendritic cells revisited. Annu Rev Immunol. (2021) 39:131–66. doi: 10.1146/annurev-immunol-061020-053707
104. Hildner K, Edelson BT, Purtha WE, Diamond M, Matsushita H, Kohyama M, et al. Batf3 deficiency reveals a critical role for CD8alpha+ dendritic cells in cytotoxic T cell immunity. Science. (2008) 322:1097–100. doi: 10.1126/science.1164206
105. Edelson BT, Kc W, Juang R, Kohyama M, Benoit LA, Klekotka PA, et al. Peripheral CD103+ dendritic cells form a unified subset developmentally related to CD8alpha+ conventional dendritic cells. J Exp Med. (2010) 207:823–36. doi: 10.1084/jem.20091627
106. Jakubzick C, Helft J, Kaplan TJ, Randolph GJ. Optimization of methods to study pulmonary dendritic cell migration reveals distinct capacities of DC subsets to acquire soluble versus particulate antigen. J Immunol Methods. (2008) 337:121–31. doi: 10.1016/j.jim.2008.07.005
107. Vermaelen K, Pauwels R. Accurate and simple discrimination of mouse pulmonary dendritic cell and macrophage populations by flow cytometry: methodology and new insights. Cytometry A. (2004) 61:170–77. doi: 10.1002/cyto.a.20064
108. Sung SS, Fu SM, Rose CE Jr., Gaskin F, Ju ST, Beaty SR. A major lung CD103 (alphaE)-beta7 integrin-positive epithelial dendritic cell population expressing Langerin and tight junction proteins. J Immunol. (2006) 176:2161–72. doi: 10.4049/jimmunol.176.4.2161
109. del Rio ML, Rodriguez-Barbosa JI, Kremmer E, Forster R. CD103- and CD103+ bronchial lymph node dendritic cells are specialized in presenting and cross-presenting innocuous antigen to CD4+ and CD8+ T cells. J Immunol. (2007) 178:6861–6. doi: 10.4049/jimmunol.178.11.6861
110. GeurtsvanKessel CH, Lambrecht BN. Division of labor between dendritic cell subsets of the lung. Mucosal Immunol. (2008) 1:442–50. doi: 10.1038/mi.2008.39
111. Belz GT, Bedoui S, Kupresanin F, Carbone FR, Heath WR. Minimal activation of memory CD8+ T cell by tissue-derived dendritic cells favors the stimulation of naive CD8+ T cells. Nat Immunol. (2007) 8:1060–6. doi: 10.1038/ni1505
112. GeurtsvanKessel CH, Willart MA, van Rijt LS, Muskens F, Kool M, Baas C, et al. Clearance of influenza virus from the lung depends on migratory langerin+CD11b- but not plasmacytoid dendritic cells. J Exp Med. (2008) 205:1621–34. doi: 10.1084/jem.20071365
113. Kim TS, Braciale TJ. Respiratory dendritic cell subsets differ in their capacity to support the induction of virus-specific cytotoxic CD8+ T cell responses. PloS One. (2009) 4:e4204. doi: 10.1371/journal.pone.0004204
114. Bedoui S, Whitney PG, Waithman J, Eidsmo L, Wakim L, Caminschi I, et al. Cross-presentation of viral and self antigens by skin-derived CD103+ dendritic cells. Nat Immunol. (2009) 10:488–95. doi: 10.1038/ni.1724
115. Desch AN, Randolph GJ, Murphy K, Gautier EL, Kedl RM, Lahoud MH, et al. CD103+ pulmonary dendritic cells preferentially acquire and present apoptotic cell-associated antigen. J Exp Med. (2011) 208:1789–97. doi: 10.1084/jem.20110538
116. Silva-Sanchez A, Meza-Perez S, Liu M, Stone SL, Flores-Romo L, Ubil E, et al. Activation of regulatory dendritic cells by Mertk coincides with a temporal wave of apoptosis in neonatal lungs. Sci Immunol. (2023) 8:eadc9081. doi: 10.1126/sciimmunol.adc9081
117. Cummings RJ, Barbet G, Bongers G, Hartmann BM, Gettler K, Muniz L, et al. Different tissue phagocytes sample apoptotic cells to direct distinct homeostasis programs. Nature. (2016) 539:565–9. doi: 10.1038/nature20138
118. Kelsall B. Recent progress in understanding the phenotype and function of intestinal dendritic cells and macrophages. Mucosal Immunol. (2008) 1:460–9. doi: 10.1038/mi.2008.61
119. Sawai CM, Sisirak V, Ghosh HS, Hou EZ, Ceribelli M, Staudt LM, et al. Transcription factor Runx2 controls the development and migration of plasmacytoid dendritic cells. J Exp Med. (2013) 210:2151–9. doi: 10.1084/jem.20130443
120. Jahrsdorfer B, Vollmer A, Blackwell SE, Maier J, Sontheimer K, Beyer T, et al. Granzyme B produced by human plasmacytoid dendritic cells suppresses T-cell expansion. Blood. (2010) 115:1156–65. doi: 10.1182/blood-2009-07-235382
121. Bonnefoy F, Perruche S, Couturier M, Sedrati A, Sun Y, Tiberghien P, et al. Plasmacytoid dendritic cells play a major role in apoptotic leukocyte-induced immune modulation. J Immunol. (2011) 186:5696–705. doi: 10.4049/jimmunol.1001523
122. Wu Y, Tibrewal N, Birge RB. Phosphatidylserine recognition by phagocytes: a view to a kill. Trends Cell Biol. (2006) 16:189–97. doi: 10.1016/j.tcb.2006.02.003
123. Kobayashi N, Karisola P, Pena-Cruz V, Dorfman DM, Jinushi M, Umetsu SE, et al. TIM-1 and TIM-4 glycoproteins bind phosphatidylserine and mediate uptake of apoptotic cells. Immunity. (2007) 27:927–40. doi: 10.1016/j.immuni.2007.11.011
124. Nakayama M, Akiba H, Takeda K, Kojima Y, Hashiguchi M, Azuma M, et al. Tim-3 mediates phagocytosis of apoptotic cells and cross-presentation. Blood. (2009) 113:3821–30. doi: 10.1182/blood-2008-10-185884
125. Miyanishi M, Tada K, Koike M, Uchiyama Y, Kitamura T, Nagata S. Identification of Tim4 as a phosphatidylserine receptor. Nature. (2007) 450:435–9. doi: 10.1038/nature06307
126. Kuroiwa M, Yamaguchi SI, Kato Y, Hori A, Toyoura S, Nakahara M, et al. Tim4, a macrophage receptor for apoptotic cells, binds polystyrene microplastics via aromatic-aromatic interactions. Sci Total Environ. (2023) 875:162586. doi: 10.1016/j.scitotenv.2023.162586
127. Seitz HM, Camenisch TD, Lemke G, Earp HS, Matsushima GK. Macrophages and dendritic cells use different Axl/Mertk/Tyro3 receptors in clearance of apoptotic cells. J Immunol. (2007) 178:5635–42. doi: 10.4049/jimmunol.178.9.5635
128. Myers KV, Amend SR, Pienta KJ. Targeting Tyro3, Axl and MerTK (TAM receptors): implications for macrophages in the tumor microenvironment. Mol Cancer. (2019) 18:94. doi: 10.1186/s12943-019-1022-2
129. Guttenberg MA, Vose AT, Birukova A, Lewars K, Cumming RI, Albright MC, et al. Tissue-resident alveolar macrophages reduce ozone-induced inflammation via MerTK mediated efferocytosis. Am J Respir Cell Mol Biol. (2024). doi: 10.1165/rcmb.2023-0390OC
130. Rigoni TS, Vellozo NS, Guimaraes-Pinto K, Cabral-Piccin M, Fabiano-Coelho L, Matos-Silva TC, et al. Axl receptor induces efferocytosis, dampens M1 macrophage responses and promotes heart pathology in Trypanosoma cruzi infection. Commun Biol. (2022) 5:1421. doi: 10.1038/s42003-022-04401-w
131. Verbovetski I, Bychkov H, Trahtemberg U, Shapira I, Hareuveni M, Ben-Tal O, et al. Opsonization of apoptotic cells by autologous iC3b facilitates clearance by immature dendritic cells, down-regulates DR and CD86, and up-regulates CC chemokine receptor 7. J Exp Med. (2002) 196:1553–61. doi: 10.1084/jem.20020263
132. Rubartelli A, Poggi A, Zocchi MR. The selective engulfment of apoptotic bodies by dendritic cells is mediated by the alpha(v)beta3 integrin and requires intracellular and extracellular calcium. Eur J Immunol. (1997) 27:1893–900. doi: 10.1002/eji.1830270812
133. Mevorach D, Mascarenhas JO, Gershov D, Elkon KB. Complement-dependent clearance of apoptotic cells by human macrophages. J Exp Med. (1998) 188:2313–20. doi: 10.1084/jem.188.12.2313
134. Belz GT, Vremec D, Febbraio M, Corcoran L, Shortman K, Carbone FR, et al. CD36 is differentially expressed by CD8+ splenic dendritic cells but is not required for cross-presentation in vivo. J Immunol. (2002) 168:6066–70. doi: 10.4049/jimmunol.168.12.6066
135. Canavati C, Siam A, Labes S, Trabelsi N, Regev E, Parnasa E, et al. Pathogenic variants of scavenger receptor CD36 lead to decreased efferocytosis and predispose to myocarditis following vaccination with Pfizer-bioNTech BNT162b2 against coronavirus infection (COVID-19). Circulation. (2024) 149:270–3. doi: 10.1161/CIRCULATIONAHA.123.064884
136. Wicker-Planquart C, Dufour S, Tacnet-Delorme P, Bally I, Delneste Y, Frachet P, et al. Molecular and cellular interactions of scavenger receptor SR-F1 with complement C1q provide insights into its role in the clearance of apoptotic cells. Front Immunol. (2020) 11:544. doi: 10.3389/fimmu.2020.00544
137. Zhang Z, Jiang Y, Zhou Z, Huang J, Chen S, Zhou W, et al. Scavenger receptor A1 attenuates aortic dissection via promoting efferocytosis in macrophages. Biochem Pharmacol. (2019) 168:392–403. doi: 10.1016/j.bcp.2019.07.027
138. Lee HN, Tian L, Bouladoux N, Davis J, Quinones M, Belkaid Y, et al. Dendritic cells expressing immunoreceptor CD300f are critical for controlling chronic gut inflammation. J Clin Invest. (2017) 127:1905–17. doi: 10.1172/JCI89531
139. Murakami Y, Tian L, Voss OH, Margulies DH, Krzewski K, Coligan JE. CD300b regulates the phagocytosis of apoptotic cells via phosphatidylserine recognition. Cell Death Differ. (2014) 21:1746–57. doi: 10.1038/cdd.2014.86
140. Simhadri VR, Andersen JF, Calvo E, Choi SC, Coligan JE, Borrego F. Human CD300a binds to phosphatidylethanolamine and phosphatidylserine, and modulates the phagocytosis of dead cells. Blood. (2012) 119:2799–809. doi: 10.1182/blood-2011-08-372425
141. Kim S, Park SY, Kim SY, Bae DJ, Pyo JH, Hong M, et al. Cross talk between engulfment receptors stabilin-2 and integrin alphavbeta5 orchestrates engulfment of phosphatidylserine-exposed erythrocytes. Mol Cell Biol. (2012) 32:2698–708. doi: 10.1128/MCB.06743-11
142. Lee W, Park SY, Yoo Y, Kim SY, Kim JE, Kim SW, et al. Macrophagic stabilin-1 restored disruption of vascular integrity caused by sepsis. Thromb Haemost. (2018) 118:1776–89. doi: 10.1055/s-0038-1669477
143. Mirtschink P, Chavakis T. Stabilin-1-mediated efferocytosis protects against vascular leakage in sepsis: A novel therapeutic approach? Thromb Haemost. (2018) 118:1852–3. doi: 10.1055/s-0038-1673688
144. Park SY, Jung MY, Kim HJ, Lee SJ, Kim SY, Lee BH, et al. Rapid cell corpse clearance by stabilin-2, a membrane phosphatidylserine receptor. Cell Death Differ. (2008) 15:192–201. doi: 10.1038/sj.cdd.4402242
145. Subramanian M, Hayes CD, Thome JJ, Thorp E, Matsushima GK, Herz J, et al. An AXL/LRP-1/RANBP9 complex mediates DC efferocytosis and antigen cross-presentation in vivo. J Clin Invest. (2014) 124:1296–308. doi: 10.1172/JCI72051
146. Brophy ML, Dong Y, Tao H, Yancey PG, Song K, Zhang K, et al. Myeloid-specific deletion of epsins 1 and 2 reduces atherosclerosis by preventing LRP-1 downregulation. Circ Res. (2019) 124:e6–e19. doi: 10.1161/CIRCRESAHA.118.313028
147. Mueller PA, Kojima Y, Huynh KT, Maldonado RA, Ye J, Tavori H, et al. Macrophage LRP1 (Low-density lipoprotein receptor-related protein 1) is required for the effect of CD47 blockade on efferocytosis and atherogenesis-brief report. Arterioscler Thromb Vasc Biol. (2022) 42:e1–9. doi: 10.1161/ATVBAHA.121.316854
148. Yancey PG, Blakemore J, Ding L, Fan D, Overton CD, Zhang Y, et al. Macrophage LRP-1 controls plaque cellularity by regulating efferocytosis and Akt activation. Arterioscler Thromb Vasc Biol. (2010) 30:787–95. doi: 10.1161/ATVBAHA.109.202051
149. Davis K, Banerjee S, Friggeri A, Bell C, Abraham E, Zerfaoui M. Poly(ADP-ribosyl)ation of high mobility group box 1 (HMGB1) protein enhances inhibition of efferocytosis. Mol Med. (2012) 18:359–69. doi: 10.2119/molmed.2011.00203
150. Li K, Chen G, Luo H, Li J, Liu A, Yang C, et al. MRP8/14 mediates macrophage efferocytosis through RAGE and Gas6/MFG-E8, and induces polarization via TLR4-dependent pathway. J Cell Physiol. (2021) 236:1375–90. doi: 10.1002/jcp.29944
151. Mahida RY, Lax S, Bassford CR, Scott A, Parekh D, Hardy RS, et al. Impaired alveolar macrophage 11beta-hydroxysteroid dehydrogenase type 1 reductase activity contributes to increased pulmonary inflammation and mortality in sepsis-related ARDS. Front Immunol. (2023) 14:1159831. doi: 10.3389/fimmu.2023.1159831
152. Das S, Sarkar A, Ryan KA, Fox S, Berger AH, Juncadella IJ, et al. Brain angiogenesis inhibitor 1 is expressed by gastric phagocytes during infection with Helicobacter pylori and mediates the recognition and engulfment of human apoptotic gastric epithelial cells. FASEB J. (2014) 28:2214–24. doi: 10.1096/fj.13-243238
153. Rothlin CV, Ghosh S, Zuniga EI, Oldstone MB, Lemke G. TAM receptors are pleiotropic inhibitors of the innate immune response. Cell. (2007) 131:1124–36. doi: 10.1016/j.cell.2007.10.034
154. Chan PY, Carrera Silva EA, De Kouchkovsky D, Joannas LD, Hao L, Hu D, et al. The TAM family receptor tyrosine kinase TYRO3 is a negative regulator of type 2 immunity. Science. (2016) 352:99–103. doi: 10.1126/science.aaf1358
155. Tang J, Jin Y, Jia F, Lv T, Manaenko A, Zhang LF, et al. Gas6 promotes microglia efferocytosis and suppresses inflammation through activating Axl/Rac1 signaling in subarachnoid hemorrhage mice. Transl Stroke Res. (2023) 14:955–69. doi: 10.1007/s12975-022-01099-0
156. Zhu C, Anderson AC, Schubart A, Xiong H, Imitola J, Khoury SJ, et al. The Tim-3 ligand galectin-9 negatively regulates T helper type 1 immunity. Nat Immunol. (2005) 6:1245–52. doi: 10.1038/ni1271
157. Rodriguez-Manzanet R, DeKruyff R, Kuchroo VK, Umetsu DT. The costimulatory role of TIM molecules. Immunol Rev. (2009) 229:259–70. doi: 10.1111/j.1600-065X.2009.00772.x
158. de Mingo Pulido A, Hanggi K, Celias DP, Gardner A, Li J, Batista-Bittencourt B, et al. The inhibitory receptor TIM-3 limits activation of the cGAS-STING pathway in intra-tumoral dendritic cells by suppressing extracellular DNA uptake. Immunity. (2021) 54:1154–67.e7. doi: 10.1016/j.immuni.2021.04.019
159. Park D, Hochreiter-Hufford A, Ravichandran KS. The phosphatidylserine receptor TIM-4 does not mediate direct signaling. Curr Biol. (2009) 19:346–51. doi: 10.1016/j.cub.2009.01.042
160. Jun JI, Kim KH, Lau LF. The matricellular protein CCN1 mediates neutrophil efferocytosis in cutaneous wound healing. Nat Commun. (2015) 6:7386. doi: 10.1038/ncomms8386
161. Jorge AM, Lao T, Kim R, Licciardi S, El Khoury J, Luster AD, et al. SCARF1-induced efferocytosis plays an immunomodulatory role in humans, and autoantibodies targeting SCARF1 are produced in patients with systemic lupus erythematosus. J Immunol. (2022) 208:955–67. doi: 10.4049/jimmunol.2100532
162. Ramirez-Ortiz ZG, Pendergraft WF 3rd, Prasad A, Byrne MH, Iram T, Blanchette CJ, et al. The scavenger receptor SCARF1 mediates the clearance of apoptotic cells and prevents autoimmunity. Nat Immunol. (2013) 14:917–26. doi: 10.1038/ni.2670
163. Tian L, Choi SC, Lee HN, Murakami Y, Qi CF, Sengottuvelu M, et al. Enhanced efferocytosis by dendritic cells underlies memory T-cell expansion and susceptibility to autoimmune disease in CD300f-deficient mice. Cell Death Differ. (2016) 23:1086–96. doi: 10.1038/cdd.2015.161
164. Mantegazza AR, Magalhaes JG, Amigorena S, Marks MS. Presentation of phagocytosed antigens by MHC class I and II. Traffic. (2013) 14:135–52. doi: 10.1111/tra.12026
165. Albert ML. Death-defying immunity: do apoptotic cells influence antigen processing and presentation? Nat Rev Immunol. (2004) 4:223–31. doi: 10.1038/nri11308
166. Kovacsovics-Bankowski M, Clark K, Benacerraf B, Rock KL. Efficient major histocompatibility complex class I presentation of exogenous antigen upon phagocytosis by macrophages. Proc Natl Acad Sci U.S.A. (1993) 90:4942–6. doi: 10.1073/pnas.90.11.4942
167. Bosnjak L, Miranda-Saksena M, Koelle DM, Boadle RA, Jones CA, Cunningham AL. Herpes simplex virus infection of human dendritic cells induces apoptosis and allows cross-presentation via uninfected dendritic cells. J Immunol. (2005) 174:2220–7. doi: 10.4049/jimmunol.174.4.2220
168. Blachere NE, Darnell RB, Albert ML. Apoptotic cells deliver processed antigen to dendritic cells for cross-presentation. PloS Biol. (2005) 3:e185. doi: 10.1371/journal.pbio.0030185
169. Segura E, Villadangos JA. A modular and combinatorial view of the antigen cross-presentation pathway in dendritic cells. Traffic. (2011) 12:1677–85. doi: 10.1111/j.1600-0854.2011.01254.x
170. Cruz FM, Colbert JD, Merino E, Kriegsman BA, Rock KL. The biology and underlying mechanisms of cross-presentation of exogenous antigens on MHC-I molecules. Annu Rev Immunol. (2017) 35:149–76. doi: 10.1146/annurev-immunol-041015-055254
171. Gros M, Amigorena S. Regulation of antigen export to the cytosol during cross-presentation. Front Immunol. (2019) 10:41. doi: 10.3389/fimmu.2019.00041
172. Colbert JD, Cruz FM, Rock KL. Cross-presentation of exogenous antigens on MHC I molecules. Curr Opin Immunol. (2020) 64:1–8. doi: 10.1016/j.coi.2019.12.005
173. Rodriguez A, Regnault A, Kleijmeer M, Ricciardi-Castagnoli P, Amigorena S. Selective transport of internalized antigens to the cytosol for MHC class I presentation in dendritic cells. Nat Cell Biol. (1999) 1:362–8. doi: 10.1038/14058
174. Grotzke JE, Cresswell P. Are ERAD components involved in cross-presentation? Mol Immunol. (2015) 68:112–5. doi: 10.1016/j.molimm.2015.05.002
175. Delamarre L, Pack M, Chang H, Mellman I, Trombetta ES. Differential lysosomal proteolysis in antigen-presenting cells determines antigen fate. Science. (2005) 307:1630–4. doi: 10.1126/science.1108003
176. Erwig LP, McPhilips KA, Wynes MW, Ivetic A, Ridley AJ, Henson PM. Differential regulation of phagosome maturation in macrophages and dendritic cells mediated by Rho GTPases and ezrin-radixin-moesin (ERM) proteins. Proc Natl Acad Sci U.S.A. (2006) 103:12825–30. doi: 10.1073/pnas.0605331103
177. Canton J, Blees H, Henry CM, Buck MD, Schulz O, Rogers NC, et al. The receptor DNGR-1 signals for phagosomal rupture to promote cross-presentation of dead-cell-associated antigens. Nat Immunol. (2021) 22:140–53. doi: 10.1038/s41590-020-00824-x
178. Abdolmaleki F, Farahani N, Gheibi Hayat SM, Pirro M, Bianconi V, Barreto GE, et al. The role of efferocytosis in autoimmune diseases. Front Immunol. (2018) 9:1645. doi: 10.3389/fimmu.2018.01645
179. Cruz FM, Colbert JD, Rock KL. The GTPase Rab39a promotes phagosome maturation into MHC-I antigen-presenting compartments. EMBO J. (2020) 39:e102020. doi: 10.15252/embj.2019102020
180. Tzelepis F, Verway M, Daoud J, Gillard J, Hassani-Ardakani K, Dunn J, et al. Annexin1 regulates DC efferocytosis and cross-presentation during Mycobacterium tuberculosis infection. J Clin Invest. (2015) 125:752–68. doi: 10.1172/JCI77014
181. Arrode G, Boccaccio C, Lule J, Allart S, Moinard N, Abastado JP, et al. Incoming human cytomegalovirus pp65 (UL83) contained in apoptotic infected fibroblasts is cross-presented to CD8(+) T cells by dendritic cells. J Virol. (2000) 74:10018–24. doi: 10.1128/JVI.74.21.10018-10024.2000
182. Larsson M, Fonteneau JF, Lirvall M, Haslett P, Lifson JD, Bhardwaj N. Activation of HIV-1 specific CD4 and CD8 T cells by human dendritic cells: roles for cross-presentation and non-infectious HIV-1 virus. AIDS. (2002) 16:1319–29. doi: 10.1097/00002030-200207050-00003
183. Larsson M, Fonteneau JF, Somersan S, Sanders C, Bickham K, Thomas EK, et al. Efficiency of cross presentation of vaccinia virus-derived antigens by human dendritic cells. Eur J Immunol. (2001) 31:3432–42. doi: 10.1002/(ISSN)1521-4141
184. Schaible UE, Winau F, Sieling PA, Fischer K, Collins HL, Hagens K, et al. Apoptosis facilitates antigen presentation to T lymphocytes through MHC-I and CD1 in tuberculosis. Nat Med. (2003) 9:1039–46. doi: 10.1038/nm906
185. Wang Z, Li G, Dou S, Zhang Y, Liu Y, Zhang J, et al. Tim-3 promotes listeria monocytogenes immune evasion by suppressing major histocompatibility complex class I. J Infect Dis. (2020) 221:830–40. doi: 10.1093/infdis/jiz512
186. Oikawa T, Kamimura Y, Akiba H, Yagita H, Okumura K, Takahashi H, et al. Preferential involvement of Tim-3 in the regulation of hepatic CD8+ T cells in murine acute graft-versus-host disease. J Immunol. (2006) 177:4281–7. doi: 10.4049/jimmunol.177.7.4281
187. Winau F, Weber S, Sad S, de Diego J, Hoops SL, Breiden B, et al. Apoptotic vesicles crossprime CD8 T cells and protect against tuberculosis. Immunity. (2006) 24:105–17. doi: 10.1016/j.immuni.2005.12.001
188. Behar SM, Divangahi M, Remold HG. Evasion of innate immunity by Mycobacterium tuberculosis: is death an exit strategy? Nat Rev Microbiol. (2010) 8:668–74. doi: 10.1038/nrmicro2387
189. Divangahi M, Chen M, Gan H, Desjardins D, Hickman TT, Lee DM, et al. Mycobacterium tuberculosis evades macrophage defenses by inhibiting plasma membrane repair. Nat Immunol. (2009) 10:899–906. doi: 10.1038/ni.1758
190. Behar SM, Martin CJ, Nunes-Alves C, Divangahi M, Remold HG. Lipids, apoptosis, and cross-presentation: links in the chain of host defense against Mycobacterium tuberculosis. Microbes Infect. (2011) 13:749–56. doi: 10.1016/j.micinf.2011.03.002
191. Divangahi M, Desjardins D, Nunes-Alves C, Remold HG, Behar SM. Eicosanoid pathways regulate adaptive immunity to Mycobacterium tuberculosis. Nat Immunol. (2010) 11:751–8. doi: 10.1038/ni.1904
192. Heath WR, Belz GT, Behrens GM, Smith CM, Forehan SP, Parish IA, et al. Cross-presentation, dendritic cell subsets, and the generation of immunity to cellular antigens. Immunol Rev. (2004) 199:9–26. doi: 10.1111/j.0105-2896.2004.00142.x
193. Kurts C, Kosaka H, Carbone FR, Miller JF, Heath WR. Class I-restricted cross-presentation of exogenous self-antigens leads to deletion of autoreactive CD8(+) T cells. J Exp Med. (1997) 186:239–45. doi: 10.1084/jem.186.2.239
194. Liu K, Iyoda T, Saternus M, Kimura Y, Inaba K, Steinman RM. Immune tolerance after delivery of dying cells to dendritic cells in situ. J Exp Med. (2002) 196:1091–7. doi: 10.1084/jem.20021215
195. Redmond WL, Sherman LA. Peripheral tolerance of CD8 T lymphocytes. Immunity. (2005) 22:275–84. doi: 10.1016/j.immuni.2005.01.010
196. Luckashenak N, Schroeder S, Endt K, Schmidt D, Mahnke K, Bachmann MF, et al. Constitutive crosspresentation of tissue antigens by dendritic cells controls CD8+ T cell tolerance in vivo. Immunity. (2008) 28:521–32. doi: 10.1016/j.immuni.2008.02.018
197. Guo L, Shen S, Rowley JW, Tolley ND, Jia W, Manne BK, et al. Platelet MHC class I mediates CD8+ T-cell suppression during sepsis. Blood. (2021) 138:401–16. doi: 10.1182/blood.2020008958
198. Kawai T, Akira S. The role of pattern-recognition receptors in innate immunity: update on Toll-like receptors. Nat Immunol. (2010) 11:373–84. doi: 10.1038/ni.1863
199. Krispin A, Bledi Y, Atallah M, Trahtemberg U, Verbovetski I, Nahari E, et al. Apoptotic cell thrombospondin-1 and heparin-binding domain lead to dendritic-cell phagocytic and tolerizing states. Blood. (2006) 108:3580–9. doi: 10.1182/blood-2006-03-013334
200. Pujol-Autonell I, Ampudia RM, Planas R, Marin-Gallen S, Carrascal J, Sanchez A, et al. Efferocytosis promotes suppressive effects on dendritic cells through prostaglandin E2 production in the context of autoimmunity. PloS One. (2013) 8:e63296. doi: 10.1371/journal.pone.0063296
201. Stuart LM, Lucas M, Simpson C, Lamb J, Savill J, Lacy-Hulbert A. Inhibitory effects of apoptotic cell ingestion upon endotoxin-driven myeloid dendritic cell maturation. J Immunol. (2002) 168:1627–35. doi: 10.4049/jimmunol.168.4.1627
202. Feng H, Zeng Y, Whitesell L, Katsanis E. Stressed apoptotic tumor cells express heat shock proteins and elicit tumor-specific immunity. Blood. (2001) 97:3505–12. doi: 10.1182/blood.V97.11.3505
203. Chen Z, Moyana T, Saxena A, Warrington R, Jia Z, Xiang J. Efficient antitumor immunity derived from maturation of dendritic cells that had phagocytosed apoptotic/necrotic tumor cells. Int J Cancer. (2001) 93:539–48. doi: 10.1002/(ISSN)1097-0215
204. Penteado LA, Dejani NN, Verdan FF, Orlando AB, Nino VE, Dias FN, et al. Distinctive role of efferocytosis in dendritic cell maturation and migration in sterile or infectious conditions. Immunology. (2017) 151:304–13. doi: 10.1111/imm.12731
205. Torchinsky MB, Garaude J, Martin AP, Blander JM. Innate immune recognition of infected apoptotic cells directs T(H)17 cell differentiation. Nature. (2009) 458:78–82. doi: 10.1038/nature07781
206. Rodriguez-Fernandez S, Pujol-Autonell I, Brianso F, Perna-Barrull D, Cano-Sarabia M, Garcia-Jimeno S, et al. Phosphatidylserine-liposomes promote tolerogenic features on dendritic cells in human type 1 diabetes by apoptotic mimicry. Front Immunol. (2018) 9:253. doi: 10.3389/fimmu.2018.00253
207. Sreeramkumar V, Fresno M, Cuesta N. Prostaglandin E2 and T cells: friends or foes? Immunol Cell Biol. (2012) 90:579–86. doi: 10.1038/icb.2011.75
208. Valdez PA, Vithayathil PJ, Janelsins BM, Shaffer AL, Williamson PR, Datta SK. Prostaglandin E2 suppresses antifungal immunity by inhibiting interferon regulatory factor 4 function and interleukin-17 expression in T cells. Immunity. (2012) 36:668–79. doi: 10.1016/j.immuni.2012.02.013
209. Gorchs L, Fernandez Moro C, Bankhead P, Kern KP, Sadeak I, Meng Q, et al. Human pancreatic carcinoma-associated fibroblasts promote expression of co-inhibitory markers on CD4(+) and CD8(+) T-cells. Front Immunol. (2019) 10:847. doi: 10.3389/fimmu.2019.00847
210. Boniface K, Bak-Jensen KS, Li Y, Blumenschein WM, McGeachy MJ, McClanahan TK, et al. Prostaglandin E2 regulates Th17 cell differentiation and function through cyclic AMP and EP2/EP4 receptor signaling. J Exp Med. (2009) 206:535–48. doi: 10.1084/jem.20082293
211. Kazama H, Ricci JE, Herndon JM, Hoppe G, Green DR, Ferguson TA. Induction of immunological tolerance by apoptotic cells requires caspase-dependent oxidation of high-mobility group box-1 protein. Immunity. (2008) 29:21–32. doi: 10.1016/j.immuni.2008.05.013
212. Amarilyo G, Verbovetski I, Atallah M, Grau A, Wiser G, Gil O, et al. iC3b-opsonized apoptotic cells mediate a distinct anti-inflammatory response and transcriptional NF-kappaB-dependent blockade. Eur J Immunol. (2010) 40:699–709. doi: 10.1002/eji.200838951
213. Sohn JH, Bora PS, Suk HJ, Molina H, Kaplan HJ, Bora NS. Tolerance is dependent on complement C3 fragment iC3b binding to antigen-presenting cells. Nat Med. (2003) 9:206–12. doi: 10.1038/nm814
214. Ohl L, Mohaupt M, Czeloth N, Hintzen G, Kiafard Z, Zwirner J, et al. CCR7 governs skin dendritic cell migration under inflammatory and steady-state conditions. Immunity. (2004) 21:279–88. doi: 10.1016/j.immuni.2004.06.014
215. Kawai T, Akira S. Toll-like receptors and their crosstalk with other innate receptors in infection and immunity. Immunity. (2011) 34:637–50. doi: 10.1016/j.immuni.2011.05.006
216. Kool M, van Loo G, Waelput W, De Prijck S, Muskens F, Sze M, et al. The ubiquitin-editing protein A20 prevents dendritic cell activation, recognition of apoptotic cells, and systemic autoimmunity. Immunity. (2011) 35:82–96. doi: 10.1016/j.immuni.2011.05.013
217. Morelli AE, Larregina AT, Shufesky WJ, Zahorchak AF, Logar AJ, Papworth GD, et al. Internalization of circulating apoptotic cells by splenic marginal zone dendritic cells: dependence on complement receptors and effect on cytokine production. Blood. (2003) 101:611–20. doi: 10.1182/blood-2002-06-1769
218. Sen P, Wallet MA, Yi Z, Huang Y, Henderson M, Mathews CE, et al. Apoptotic cells induce Mer tyrosine kinase-dependent blockade of NF-kappaB activation in dendritic cells. Blood. (2007) 109:653–60. doi: 10.1182/blood-2006-04-017368
219. Hacker H, Redecke V, Blagoev B, Kratchmarova I, Hsu LC, Wang GG, et al. Specificity in Toll-like receptor signalling through distinct effector functions of TRAF3 and TRAF6. Nature. (2006) 439:204–7. doi: 10.1038/nature04369
220. Kinjyo I, Hanada T, Inagaki-Ohara K, Mori H, Aki D, Ohishi M, et al. SOCS1/JAB is a negative regulator of LPS-induced macrophage activation. Immunity. (2002) 17:583–91. doi: 10.1016/S1074-7613(02)00446-6
221. Nakagawa R, Naka T, Tsutsui H, Fujimoto M, Kimura A, Abe T, et al. SOCS-1 participates in negative regulation of LPS responses. Immunity. (2002) 17:677–87. doi: 10.1016/S1074-7613(02)00449-1
222. Labarriere N, Bretaudeau L, Gervois N, Bodinier M, Bougras G, Diez E, et al. Apoptotic body-loaded dendritic cells efficiently cross-prime cytotoxic T lymphocytes specific for NA17-A antigen but not for Melan-A/MART-1 antigen. Int J Cancer. (2002) 101:280–6. doi: 10.1002/ijc.10605
223. Chen M, Huang L, Wang J. Deficiency of Bim in dendritic cells contributes to overactivation of lymphocytes and autoimmunity. Blood. (2007) 109:4360–7. doi: 10.1182/blood-2006-11-056424
224. Mayerova D, Parke EA, Bursch LS, Odumade OA, Hogquist KA. Langerhans cells activate naive self-antigen-specific CD8 T cells in the steady state. Immunity. (2004) 21:391–400. doi: 10.1016/j.immuni.2004.07.019
225. Shibaki A, Sato A, Vogel JC, Miyagawa F, Katz SI. Induction of GVHD-like skin disease by passively transferred CD8(+) T-cell receptor transgenic T cells into keratin 14-ovalbumin transgenic mice. J Invest Dermatol. (2004) 123:109–15. doi: 10.1111/j.0022-202X.2004.22701.x
226. Scheinecker C, McHugh R, Shevach EM, Germain RN. Constitutive presentation of a natural tissue autoantigen exclusively by dendritic cells in the draining lymph node. J Exp Med. (2002) 196:1079–90. doi: 10.1084/jem.20020991
227. Joeris T, Gomez-Casado C, Holmkvist P, Tavernier SJ, Silva-Sanchez A, Klotz L, et al. Intestinal cDC1 drive cross-tolerance to epithelial-derived antigen via induction of FoxP3(+)CD8(+) T(regs). Sci Immunol. (2021) 6:1–14. doi: 10.1126/sciimmunol.abd3774
228. Carstensen LS, Lie-Andersen O, Obers A, Crowther MD, Svane IM, Hansen M. Long-term exposure to inflammation induces differential cytokine patterns and apoptosis in dendritic cells. Front Immunol. (2019) 10:2702. doi: 10.3389/fimmu.2019.02702
229. Schnurr M, Scholz C, Rothenfusser S, Galambos P, Dauer M, Robe J, et al. Apoptotic pancreatic tumor cells are superior to cell lysates in promoting cross-priming of cytotoxic T cells and activate NK and gammadelta T cells. Cancer Res. (2002) 62:2347–52.
230. Lu X, Ding ZC, Cao Y, Liu C, Habtetsion T, Yu M, et al. Alkylating agent melphalan augments the efficacy of adoptive immunotherapy using tumor-specific CD4+ T cells. J Immunol. (2015) 194:2011–21. doi: 10.4049/jimmunol.1401894
231. Chao MP, Jaiswal S, Weissman-Tsukamoto R, Alizadeh AA, Gentles AJ, Volkmer J, et al. Calreticulin is the dominant pro-phagocytic signal on multiple human cancers and is counterbalanced by CD47. Sci Transl Med. (2010) 2:63ra94. doi: 10.1126/scitranslmed.3001375
232. Obeid M, Tesniere A, Ghiringhelli F, Fimia GM, Apetoh L, Perfettini JL, et al. Calreticulin exposure dictates the immunogenicity of cancer cell death. Nat Med. (2007) 13:54–61. doi: 10.1038/nm1523
233. Son KJ, Choi KR, Lee SJ, Lee H. Immunogenic cell death induced by ginsenoside Rg3: significance in dendritic cell-based anti-tumor immunotherapy. Immune Netw. (2016) 16:75–84. doi: 10.4110/in.2016.16.1.75
234. Leone P, Berardi S, Frassanito MA, Ria R, De Re V, Cicco S, et al. Dendritic cells accumulate in the bone marrow of myeloma patients where they protect tumor plasma cells from CD8+ T-cell killing. Blood. (2015) 126:1443–51. doi: 10.1182/blood-2015-01-623975
235. Fernandez-Castaneda A, Chappell MS, Rosen DA, Seki SM, Beiter RM, Johanson DM, et al. The active contribution of OPCs to neuroinflammation is mediated by LRP1. Acta Neuropathol. (2020) 139:365–82. doi: 10.1007/s00401-019-02073-1
236. Apetoh L, Ghiringhelli F, Tesniere A, Obeid M, Ortiz C, Criollo A, et al. Toll-like receptor 4-dependent contribution of the immune system to anticancer chemotherapy and radiotherapy. Nat Med. (2007) 13:1050–9. doi: 10.1038/nm1622
237. Shiratsuchi A, Watanabe I, Takeuchi O, Akira S, Nakanishi Y. Inhibitory effect of Toll-like receptor 4 on fusion between phagosomes and endosomes/lysosomes in macrophages. J Immunol. (2004) 172:2039–47. doi: 10.4049/jimmunol.172.4.2039
238. Delamarre L, Couture R, Mellman I, Trombetta ES. Enhancing immunogenicity by limiting susceptibility to lysosomal proteolysis. J Exp Med. (2006) 203:2049–55. doi: 10.1084/jem.20052442
239. Yamazaki T, Hannani D, Poirier-Colame V, Ladoire S, Locher C, Sistigu A, et al. Defective immunogenic cell death of HMGB1-deficient tumors: compensatory therapy with TLR4 agonists. Cell Death Differ. (2014) 21:69–78. doi: 10.1038/cdd.2013.72
240. Vacchelli E, Ma Y, Baracco EE, Sistigu A, Enot DP, Pietrocola F, et al. Chemotherapy-induced antitumor immunity requires formyl peptide receptor 1. Science. (2015) 350:972–8. doi: 10.1126/science.aad0779
241. Asea A, Rehli M, Kabingu E, Boch JA, Bare O, Auron PE, et al. Novel signal transduction pathway utilized by extracellular HSP70: role of toll-like receptor (TLR) 2 and TLR4. J Biol Chem. (2002) 277:15028–34. doi: 10.1074/jbc.M200497200
242. Hirao M, Onai N, Hiroishi K, Watkins SC, Matsushima K, Robbins PD, et al. CC chemokine receptor-7 on dendritic cells is induced after interaction with apoptotic tumor cells: critical role in migration from the tumor site to draining lymph nodes. Cancer Res. (2000) 60:2209–17.
243. Roberts EW, Broz ML, Binnewies M, Headley MB, Nelson AE, Wolf DM, et al. Critical role for CD103(+)/CD141(+) dendritic cells bearing CCR7 for tumor antigen trafficking and priming of T cell immunity in melanoma. Cancer Cell. (2016) 30:324–36. doi: 10.1016/j.ccell.2016.06.003
244. Jenne L, Arrighi JF, Jonuleit H, Saurat JH, Hauser C. Dendritic cells containing apoptotic melanoma cells prime human CD8+ T cells for efficient tumor cell lysis. Cancer Res. (2000) 60:4446–52.
245. Liang X, Luo M, Shao B, Yang JY, Tong A, Wang RB, et al. Phosphatidylserine released from apoptotic cells in tumor induces M2-like macrophage polarization through the PSR-STAT3-JMJD3 axis. Cancer Commun (Lond). (2022) 42:205–22. doi: 10.1002/cac2.12272
246. Igietseme JU, Ananaba GA, Bolier J, Bowers S, Moore T, Belay T, et al. Suppression of endogenous IL-10 gene expression in dendritic cells enhances antigen presentation for specific Th1 induction: potential for cellular vaccine development. J Immunol. (2000) 164:4212–9. doi: 10.4049/jimmunol.164.8.4212
247. Ravindranathan S, Nguyen KG, Kurtz SL, Frazier HN, Smith SG, Koppolu BP, et al. Tumor-derived granulocyte colony-stimulating factor diminishes efficacy of breast tumor cell vaccines. Breast Cancer Res. (2018) 20:126. doi: 10.1186/s13058-018-1054-3
248. Sun L, Chen C, Zhu A, Huang Y, Zhu H, Yi C. TRAIL mutant membrane penetrating peptide alike-MuR6-TR enhances the antitumor effects of TRAIL in pancreatic carcinoma both in vitro and in vivo. Int J Mol Med. (2017) 39:1468–76. doi: 10.3892/ijmm.2017.2968
249. Van Loenhout J, Flieswasser T, Freire Boullosa L, De Waele J, Van Audenaerde J, Marcq E, et al. Cold atmospheric plasma-treated PBS eliminates immunosuppressive pancreatic stellate cells and induces immunogenic cell death of pancreatic cancer cells. Cancers (Basel). (2019) 11:1597–612. doi: 10.3390/cancers11101597
250. Galea-Lauri J, Wells JW, Darling D, Harrison P, Farzaneh F. Strategies for antigen choice and priming of dendritic cells influence the polarization and efficacy of antitumor T-cell responses in dendritic cell-based cancer vaccination. Cancer Immunol Immunother. (2004) 53:963–77. doi: 10.1007/s00262-004-0542-8
251. Lovgren T, Sarhan D, Truxova I, Choudhary B, Maas R, Melief J, et al. Enhanced stimulation of human tumor-specific T cells by dendritic cells matured in the presence of interferon-gamma and multiple toll-like receptor agonists. Cancer Immunol Immunother. (2017) 66:1333–44.
252. Kurts C, Robinson BW, Knolle PA. Cross-priming in health and disease. Nat Rev Immunol. (2010) 10:403–14. doi: 10.1038/nri2780
253. Borst J, Ahrends T, Babala N, Melief CJM, Kastenmuller W. CD4(+) T cell help in cancer immunology and immunotherapy. Nat Rev Immunol. (2018) 18:635–47. doi: 10.1038/s41577-018-0044-0
254. Speidel K, Osen W, Faath S, Hilgert I, Obst R, Braspenning J, et al. Priming of cytotoxic T lymphocytes by five heat-aggregated antigens in vivo: conditions, efficiency, and relation to antibody responses. Eur J Immunol. (1997) 27:2391–9. doi: 10.1002/eji.1830270938
255. Tran KK, Shen H. The role of phagosomal pH on the size-dependent efficiency of cross-presentation by dendritic cells. Biomaterials. (2009) 30:1356–62. doi: 10.1016/j.biomaterials.2008.11.034
256. Jaumouille V, Cartagena-Rivera AX, Waterman CM. Coupling of beta(2) integrins to actin by a mechanosensitive molecular clutch drives complement receptor-mediated phagocytosis. Nat Cell Biol. (2019) 21:1357–69. doi: 10.1038/s41556-019-0414-2
257. Dalli J, Serhan CN. Specific lipid mediator signatures of human phagocytes: microparticles stimulate macrophage efferocytosis and pro-resolving mediators. Blood. (2012) 120:e60–72. doi: 10.1182/blood-2012-04-423525
258. Voll RE, Herrmann M, Roth EA, Stach C, Kalden JR, Girkontaite I. Immunosuppressive effects of apoptotic cells. Nature. (1997) 390:350–1. doi: 10.1038/37022
259. Fadok VA, Bratton DL, Konowal A, Freed PW, Westcott JY, Henson PM. Macrophages that have ingested apoptotic cells in vitro inhibit proinflammatory cytokine production through autocrine/paracrine mechanisms involving TGF-beta, PGE2, and PAF. J Clin Invest. (1998) 101:890–8. doi: 10.1172/JCI1112
260. McCarron MJ, Irla M, Serge A, Soudja SM, Marie JC. Transforming Growth Factor-beta signaling in alphabeta thymocytes promotes negative selection. Nat Commun. (2019) 10:5690. doi: 10.1038/s41467-019-13456-z
261. Horkova V, Drobek A, Mueller D, Gubser C, Niederlova V, Wyss L, et al. Dynamics of the coreceptor-LCK interactions during T cell development shape the self-reactivity of peripheral CD4 and CD8 T cells. Cell Rep. (2020) 30:1504–14.e7. doi: 10.1016/j.celrep.2020.01.008
262. Konkel JE, Jin W, Abbatiello B, Grainger JR, Chen W. Thymocyte apoptosis drives the intrathymic generation of regulatory T cells. Proc Natl Acad Sci U.S.A. (2014) 111:E465–73. doi: 10.1073/pnas.1320319111
263. Drashansky TT, Helm E, Huo Z, Curkovic N, Kumar P, Luo X, et al. Bcl11b prevents fatal autoimmunity by promoting T(reg) cell program and constraining innate lineages in T(reg) cells. Sci Adv. (2019) 5:eaaw0480. doi: 10.1126/sciadv.aaw0480
264. Wang L, Liu Y, Han R, Beier UH, Bhatti TR, Akimova T, et al. FOXP3+ regulatory T cell development and function require histone/protein deacetylase 3. J Clin Invest. (2015) 125:1111–23. doi: 10.1172/JCI77088
265. Tsai JJ, Velardi E, Shono Y, Argyropoulos KV, Holland AM, Smith OM, et al. Nrf2 regulates CD4(+) T cell-induced acute graft-versus-host disease in mice. Blood. (2018) 132:2763–74. doi: 10.1182/blood-2017-10-812941
266. Proto JD, Doran AC, Gusarova G, Yurdagul A Jr., Sozen E, Subramanian M, et al. Regulatory T cells promote macrophage efferocytosis during inflammation resolution. Immunity. (2018) 49:666–77 e6. doi: 10.1016/j.immuni.2018.07.015
267. Rescigno M, Di Sabatino A. Dendritic cells in intestinal homeostasis and disease. J Clin Invest. (2009) 119:2441–50. doi: 10.1172/JCI39134
268. Wang Y, Yu Z, Zhou Y, Zhu Y, Wang J, Fu J, et al. Different doses of ovalbumin exposure on dendritic cells determine their genetic/epigenetic regulation and T cell differentiation. Aging (Albany NY). (2020) 12:25432–51. doi: 10.18632/aging.v12i24
269. Evers BD, Engel DR, Bohner AM, Tittel AP, Krause TA, Heuser C, et al. CD103+ Kidney dendritic cells protect against crescentic GN by maintaining IL-10-producing regulatory T cells. J Am Soc Nephrol. (2016) 27:3368–82. doi: 10.1681/ASN.2015080873
270. Figueiredo CR, Azevedo RA, Mousdell S, Resende-Lara PT, Ireland L, Santos A, et al. Blockade of MIF-CD74 signalling on macrophages and dendritic cells restores the antitumour immune response against metastatic melanoma. Front Immunol. (2018) 9:1132. doi: 10.3389/fimmu.2018.01132
271. Paidassi H, Acharya M, Zhang A, Mukhopadhyay S, Kwon M, Chow C, et al. Preferential expression of integrin alphavbeta8 promotes generation of regulatory T cells by mouse CD103+ dendritic cells. Gastroenterology. (2011) 141:1813–20. doi: 10.1053/j.gastro.2011.06.076
272. Coombes JL, Siddiqui KR, Arancibia-Carcamo CV, Hall J, Sun CM, Belkaid Y, et al. A functionally specialized population of mucosal CD103+ DCs induces Foxp3+ regulatory T cells via a TGF-beta and retinoic acid-dependent mechanism. J Exp Med. (2007) 204:1757–64. doi: 10.1084/jem.20070590
273. Yamazaki S, Dudziak D, Heidkamp GF, Fiorese C, Bonito AJ, Inaba K, et al. CD8+ CD205+ splenic dendritic cells are specialized to induce Foxp3+ regulatory T cells. J Immunol. (2008) 181:6923–33. doi: 10.4049/jimmunol.181.10.6923
274. Sun CM, Hall JA, Blank RB, Bouladoux N, Oukka M, Mora JR, et al. Small intestine lamina propria dendritic cells promote de novo generation of Foxp3 T reg cells via retinoic acid. J Exp Med. (2007) 204:1775–85. doi: 10.1084/jem.20070602
275. Moreira AP, Cavassani KA, Ismailoglu UB, Hullinger R, Dunleavy MP, Knight DA, et al. The protective role of TLR6 in a mouse model of asthma is mediated by IL-23 and IL-17A. J Clin Invest. (2011) 121:4420–32. doi: 10.1172/JCI44999
276. Li MO, Flavell RA. Contextual regulation of inflammation: a duet by transforming growth factor-beta and interleukin-10. Immunity. (2008) 28:468–76. doi: 10.1016/j.immuni.2008.03.003
277. Chen W, Jin W, Hardegen N, Lei KJ, Li L, Marinos N, et al. Conversion of peripheral CD4+CD25- naive T cells to CD4+CD25+ regulatory T cells by TGF-beta induction of transcription factor Foxp3. J Exp Med. (2003) 198:1875–86. doi: 10.1084/jem.20030152
278. Fantini MC, Becker C, Monteleone G, Pallone F, Galle PR, Neurath MF. Cutting edge: TGF-beta induces a regulatory phenotype in CD4+CD25- T cells through Foxp3 induction and down-regulation of Smad7. J Immunol. (2004) 172:5149–53. doi: 10.4049/jimmunol.172.9.5149
279. Zheng Y, Rudensky AY. Foxp3 in control of the regulatory T cell lineage. Nat Immunol. (2007) 8:457–62. doi: 10.1038/ni1455
280. Brunkow ME, Jeffery EW, Hjerrild KA, Paeper B, Clark LB, Yasayko SA, et al. Disruption of a new forkhead/winged-helix protein, scurfin, results in the fatal lymphoproliferative disorder of the scurfy mouse. Nat Genet. (2001) 27:68–73. doi: 10.1038/83784
281. Fontenot JD, Gavin MA, Rudensky AY. Foxp3 programs the development and function of CD4+CD25+ regulatory T cells. Nat Immunol. (2003) 4:330–6. doi: 10.1038/ni904
282. Yang Z, Mu Z, Dabovic B, Jurukovski V, Yu D, Sung J, et al. Absence of integrin-mediated TGFbeta1 activation in vivo recapitulates the phenotype of TGFbeta1-null mice. J Cell Biol. (2007) 176:787–93. doi: 10.1083/jcb.200611044
283. Travis MA, Sheppard D. TGF-beta activation and function in immunity. Annu Rev Immunol. (2014) 32:51–82. doi: 10.1146/annurev-immunol-032713-120257
284. Aluwihare P, Mu Z, Zhao Z, Yu D, Weinreb PH, Horan GS, et al. Mice that lack activity of alphavbeta6- and alphavbeta8-integrins reproduce the abnormalities of Tgfb1- and Tgfb3-null mice. J Cell Sci. (2009) 122:227–32. doi: 10.1242/jcs.035246
285. Worthington JJ, Czajkowska BI, Melton AC, Travis MA. Intestinal dendritic cells specialize to activate transforming growth factor-beta and induce Foxp3+ regulatory T cells via integrin alphavbeta8. Gastroenterology. (2011) 141:1802–12. doi: 10.1053/j.gastro.2011.06.057
286. Travis MA, Reizis B, Melton AC, Masteller E, Tang Q, Proctor JM, et al. Loss of integrin alpha(v)beta8 on dendritic cells causes autoimmunity and colitis in mice. Nature. (2007) 449:361–5. doi: 10.1038/nature06110
287. Davidson TS, DiPaolo RJ, Andersson J, Shevach EM. Cutting Edge: IL-2 is essential for TGF-beta-mediated induction of Foxp3+ T regulatory cells. J Immunol. (2007) 178:4022–6. doi: 10.4049/jimmunol.178.7.4022
288. Zheng SG, Wang J, Wang P, Gray JD, Horwitz DA. IL-2 is essential for TGF-beta to convert naive CD4+CD25- cells to CD25+Foxp3+ regulatory T cells and for expansion of these cells. J Immunol. (2007) 178:2018–27. doi: 10.4049/jimmunol.178.4.2018
289. Li MO, Sanjabi S, Flavell RA. Transforming growth factor-beta controls development, homeostasis, and tolerance of T cells by regulatory T cell-dependent and -independent mechanisms. Immunity. (2006) 25:455–71. doi: 10.1016/j.immuni.2006.07.011
290. Marie JC, Liggitt D, Rudensky AY. Cellular mechanisms of fatal early-onset autoimmunity in mice with the T cell-specific targeting of transforming growth factor-beta receptor. Immunity. (2006) 25:441–54. doi: 10.1016/j.immuni.2006.07.012
291. Ouyang W, Beckett O, Ma Q, Li MO. Transforming growth factor-beta signaling curbs thymic negative selection promoting regulatory T cell development. Immunity. (2010) 32:642–53. doi: 10.1016/j.immuni.2010.04.012
292. Matharu KS, Mizoguchi E, Cotoner CA, Nguyen DD, Mingle B, Iweala OI, et al. Toll-like receptor 4-mediated regulation of spontaneous Helicobacter-dependent colitis in IL-10-deficient mice. Gastroenterology. (2009) 137:1380–90.e1-3. doi: 10.1053/j.gastro.2009.07.004
293. Ward NC, Yu A, Moro A, Ban Y, Chen X, Hsiung S, et al. IL-2/CD25: A long-acting fusion protein that promotes immune tolerance by selectively targeting the IL-2 receptor on regulatory T cells. J Immunol. (2018) 201:2579–92. doi: 10.4049/jimmunol.1800907
294. Tajbakhsh A, Gheibi Hayat SM, Movahedpour A, Savardashtaki A, Loveless R, Barreto GE, et al. The complex roles of efferocytosis in cancer development, metastasis, and treatment. BioMed Pharmacother. (2021) 140:111776. doi: 10.1016/j.biopha.2021.111776
Keywords: dendritic cell, apoptotic cell, efferocytosis, immune regulation, antigen cross-presentation, Treg differentiation
Citation: Ma Y, Jiang T, Zhu X, Xu Y, Wan K, Zhang T and Xie M (2024) Efferocytosis in dendritic cells: an overlooked immunoregulatory process. Front. Immunol. 15:1415573. doi: 10.3389/fimmu.2024.1415573
Received: 10 April 2024; Accepted: 09 May 2024;
Published: 21 May 2024.
Edited by:
Silvia Gregori, San Raffaele Telethon Institute for Gene Therapy (SR-Tiget), ItalyReviewed by:
Carla Guenther, Osaka University, JapanFernando Erra Díaz, University of Buenos Aires, Argentina
Copyright © 2024 Ma, Jiang, Zhu, Xu, Wan, Zhang and Xie. This is an open-access article distributed under the terms of the Creative Commons Attribution License (CC BY). The use, distribution or reproduction in other forums is permitted, provided the original author(s) and the copyright owner(s) are credited and that the original publication in this journal is cited, in accordance with accepted academic practice. No use, distribution or reproduction is permitted which does not comply with these terms.
*Correspondence: Miaorong Xie, xiemiao27@126.com