- School of Edaphic Stress Management (SESM), ICAR-National Institute of Abiotic Stress Management, Baramati, India
The recent trend of global warming poses a significant threat to ecosystems worldwide. This global climate change has also impacted the pollution levels in aquatic ecosystems, subsequently affecting human health. To address these issues, an experiment was conducted to investigate the mitigating effects of iron nanoparticles (Fe-NPs) on arsenic and ammonia toxicity as well as high temperature stress (As+NH3+T). Fe-NPs were biologically synthesized using fish waste and incorporated into feed formulations at 10, 15, and 20 mg kg-1 diet. A total of 12 treatments were designed in triplicate following a completely randomized design involving 540 fish. Fe-NPs at 15 mg kg-1 diet notably reduced the cortisol levels in fish exposed to multiple stressors. The gene expressions of HSP 70, DNA damage-inducible protein (DDIP), and DNA damage were upregulated by stressors (As+NH3+T) and downregulated by Fe-NPs. Apoptotic genes (Cas 3a and 3b) and detoxifying genes (CYP 450), metallothionein (MT), and inducible nitric oxide synthase (iNOS) were downregulated by Fe-NPs at 15 mg kg-1 diet in fish subjected to As+NH3+T stress. Immune-related genes such as tumor necrosis factor (TNFα), immunoglobulin (Ig), and interleukin (IL) were upregulated by Fe-NPs, indicating enhanced immunity in fish under As+NH3+T stress. Conversely, Toll-like receptor (TLR) expression was notably downregulated by Fe-NPs at 15 mg kg-1 diet in fish under As+NH3+T stress. Immunological attributes such as nitro blue tetrazolium chloride, total protein, albumin, globulin, A:G ratio, and myeloperoxidase (MPO) were improved by dietary Fe-NPs at 15 mg kg-1 diet in fish, regardless of stressors. The antioxidant genes (CAT, SOD, and GPx) were also strengthened by Fe-NPs in fish. Genes associated with growth performance, such as growth hormone regulator (GHR1 and GHRβ), growth hormone (GH), and insulin-like growth factor (IGF 1X and IGF 2X), were upregulated, enhancing fish growth under stress, while SMT and MYST were downregulated by Fe-NPs in the diet. Various growth performance indicators were improved by dietary Fe-NPs at 15 mg kg-1 diet. Notably, Fe-NPs also enhanced arsenic detoxification and reduced the cumulative mortality after a bacterial infection. In conclusion, this study highlights that dietary Fe-NPs can effectively mitigate arsenic and ammonia toxicity as well as high temperature stress by modulating gene expression in fish.
1 Introduction
The recent dramatic change in ecosystems has been witnessed to affect all living organisms, including humans, animals, and fish. Climate change, pollution, and degraded water quality are affecting the aquatic systems, resulting in the species extinction of aquatic organisms including fish (1, 2). With fish reared under a degraded environment, this results in changes at the gene and cellular levels. The contamination reaches up to cellular level of the aquatic organism, and the final product is contaminated, which increases the chances of deadly diseases occurring in consumers such as humans. Climate change and pollution can also lead to occurrences of diseases in all ecosystems. While climate change and pollution are distinct factors, they often work together to degrade the food chain and web. In aquatic systems like aquaculture and fisheries, ammonia emerges as a critical abiotic factor affecting the production and survival of aquatic animals, including fish (1). The adverse effect of abiotic factors such as arsenic (As), ammonia (NH3), and high temperature has weakened the immunity of aquatic organisms, which results in decreases in the efficiency of gene regulations involved in the detoxification of contamination. Moreover, arsenic pollution broadly covers the globe, including Asia, America, Europe, African countries, etc. Asian countries such as Bangladesh and the northeastern parts of India and China are also badly affected. Almost 200 million people are at a high risk (3, 4), of which 43,000 people die annually in Bangladesh due to arsenic pollution (4). As per the International Agency for Research on Cancer (IARC), it is also considered a class I carcinogenic (5). Arsenic is widely used for agriculture, veterinary drugs, medicines, metal alloy manufacturing, microelectronics, glassware, and wood preservatives (6, 7). Furthermore, the toxicity of ammonia (NH3) in aquatic ecosystems is crucial, resulting in mass mortality in fish. NH3 mainly originates from high fish protein diet, fish waste, and metabolic process of the aquatic organism, resulting in NH3 toxicity in aquatic systems (8, 9). The breakdown of amino acids, pyrimidines, and purines also generates ammonia (10), which exists in two forms: unionized ammonia (NH3) and ionized ammonium (NH4+) (11). Ammonia toxicity can lead to noticeable reductions in growth performance (12), immunity, tissue erosion, neurotoxicity, and oxidative stress and ultimately result in high mortality (13). Similarly, elevated temperature also alters the fish physiology as fish are poikilothermic animals.
Iron (Fe) is an essential nutrient which has an important role in oxygen transport and cellular respiration in fish (14). Moreover, the fish can absorb Fe using its gills and intestinal mucosa (14). In this study, multiple abiotic factors (As, NH3, and high temperature—T) were employed for stress which induces a weakened immunity in fish, resulting in alterations of the gene regulations involved in the immunity of fish, although immunity has been indicated and reflected from primary stress response to tertiary stress response. In the case of weak immunity, the natural kappa factor (NFkB) signaling pathway is inhibited due to multiple abiotic factor stress. Moreover, the dietary iron nanoparticles (Fe-NPs) improve the immunity of the fish using the NFkB signaling pathway (15, 16). The nano-form of iron sulfate is highly bioavailable to fish compared to other forms of iron (17). Therefore, the supplementation of Fe-NPs diet can be maximized as enhancer of immunity, anti-oxidant status, and the growth performance of the fish (18). The gene responsible for apoptosis and programmed cell death, cytokines, NF-κB pathway, immune genes, and anti-oxidant defense genes are important for regulatory mechanism (19, 20), although cytokines are vital signaling molecules released during various conditions, modulating inflammatory responses and maintaining barrier integrity (21). Therefore, Fe-NPs control the gene regulation involved in the abovementioned process. Neurotoxicity using acetylcholine esterase (AChE) was also inhibited using multiple abiotic stresses, although the dietary Fe-NPs enhanced the AChE activities (22). Fe-NPs supplementation could enhance the growth performance in the fish. It has advantage over bulk Fe as Fe-NPs have higher bioavailability and better absorption, which can promote fish growth (18). The Fe-NPs also decrease alanine amino transferase (ALT) and aspartate amino transferase (AST) and promote good fish health (18).
A mechanistic study of multiple stresses is required to understand the gene regulation involved in such process. However, Pangasianodon hypophthalmus is the fish species best suited to study the impact of multiple stresses and different gene regulation pathways (23, 24). P. hypophthalmus has high demand due to its medicinal and taste characteristics, and it has potential for diversification as an aquaculture species (25). The aims of the present study are dealt with by two major objectives, namely (1): to understand the mechanistic role of Fe-NPs in mitigating multiple stresses (abiotic and biotic) and (2) to elucidate the role of gene regulation involved in the response to multiple stress, such as a low dose of arsenic and ammonia toxicity as well as high temperature in P. hypophthalmus.
2 Materials and methods
2.1 Ethics statement
The institute aquaculture wet lab facilities were registered under the Committee for the Purpose of Control and Supervision of Experiments on Animals (CCSEA)—2190/GO/RReBi/SL/2022/CCSEA. The study was approved by institute PME as 7–1(PME) 2024–04. This study was in compliance with Animal Research: Reporting of In Vivo Experiments (ARRIVE) guidelines.
2.2 Experimental animals and design
The fish were taken from the National Institute of Abiotic Stress Management’s Farm Pond. The fish weighed an average of 6.02 ± 0.24 g and measured 5.12 ± 0.18 cm in size. The fish were kept in a 150-L rectangular plastic aquarium. The fish were placed in quarantine with potassium permanganate (KMnO4) and a 1% dip salt solution. A total of 12 treatments were designed for this experiment: iron nanoparticles supplied to the group at 10, 15, and 20 mg kg-1 diet with or without stressors: arsenic (As); ammonia (NH3); arsenic and ammonia (As+NH3); and arsenic, ammonia, and high temperature (As+NH3+T). Table 1 presents the details of the treatments.
Fe-NPs diet was fed to the fish twice a day, at 9:00 AM and 5:00 PM. Every day, the uneaten food and excrement were removed by siphoning. The APHA method (26) was used to periodically analyze the water quality parameters, and the results were recorded within the typical range for this fish’s culture (27). Every other day, the water was physically changed (2/3rd of water), and arsenic (sodium arsenite, NaAsO2) and (NH4)2SO4 were added as sources of ammonia toxicity (NH3) and to provide constant aeration using a compressed air pump. The As, As+NH3, As+NH3+T, and NH3 stressor groups were kept together by ammonium sulfate [1/10th of LC50 2.0 mg L-1 of (NH4)2SO4] (12) and arsenic (1/10th of LC50 2.68 mg L-1) (28) and high temperature (34°C) maintained with thermostatic heaters. Four experimental diets of iso-nitrogenous (35% crude protein) and iso-caloric type were prepared. Different feed ingredients were used, such as fish meal, groundnut meal, soybean meal, wheat flour, carboxymethyl cellulose (CMC), cod liver oil, lecithin, and vitamin C. The Fe-NPs free mineral mixture was prepared manually for inclusion in the diets. The heat-labile ingredients were mixed after heating the feed ingredient. In terms of proximate analysis of the diets, these were analyzed using the method of AOAC (29). Crude protein was analyzed using nitrogen content, ether extract (EE) using solvent extraction, and ash estimation using muffle furnace (550°C) (Table 2). Total carbohydrate % was calculated by using the following equation:
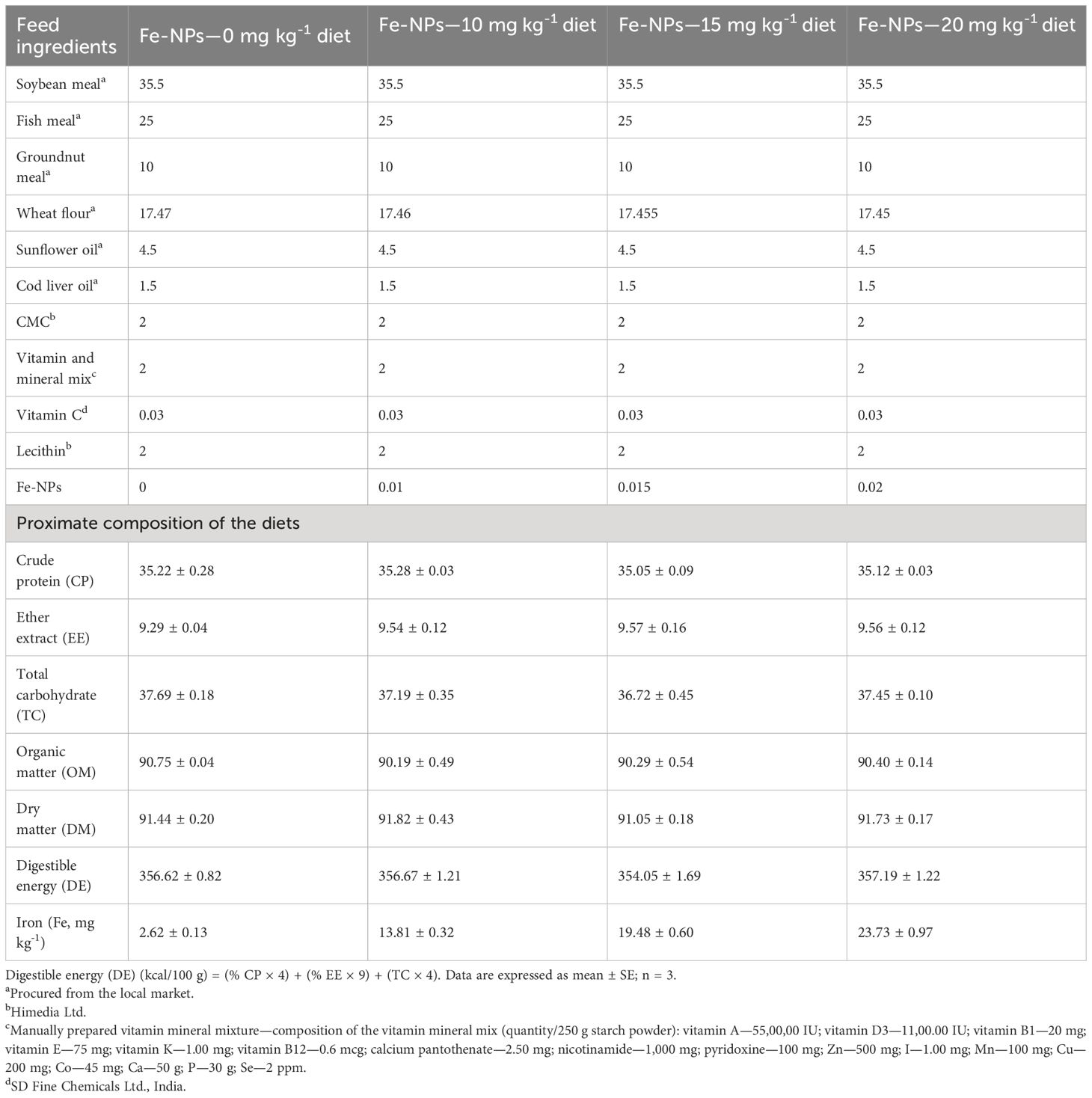
Table 2 Ingredient composition and proximate analysis of experimental diets (% dry matter) of iron nanoparticles (Fe-NPs) fed to Pangasianodon hypophthalmus for 90 days.
The gross energy of the diets was calculated using the method described by Halver (30).
2.3 Synthesis of iron nanoparticles using the green approach
2.3.1 Preparation of fish tissue extract
Fish waste (gill tissue) was used to synthesize Fe-NPs. The tissues were cut open, and blood and dust were washed away with running water. After tissue homogenization (SCILOGEX D160, Serial number-DA198AB0000585; 1275 Cromwell Ave., Suite 122; Rocky Hill, CT, USA), the supernatant was recovered by centrifuging (Thermoscientific, SORVALL, Legend MICRO 21 R) at 5,000–6,000 rpm. Whatman paper (0.45-μm pore size) was then used as filter to get the gill extract (31, 32).
2.3.2 Preparation and characterization of iron nanoparticles
Ferrous sulfate (0.4 M) was combined with the gill extract. After that, this was stirred at room temperature for 30 min. After that, the magnetic stirrer was maintained at 60C for 2.5–4 h while gradually adding 0.8 M NaOH drop by drop until a reddish-brown solution is achieved. Following the acquisition of a reddish-brown hue in the solution, a spectrophotometer [UV1900i; Shimadzu (Asia Pacific) Pte Ltd., Cintech IV, Science Park I, Singapore 118264] was used to measure the peak value at (216–600 nm), where a high absorption peak was found at 250 nm. After centrifuging it and washing it three times in distilled water, pellet was obtained. The pellet was then allowed to dry in the concentrator. Iron nanoparticles (Fe-NPs) are obtained as a reddish-brown powder after drying. Additionally, the synthesized Fe-NP formulations were combined with Milli-Q water and subjected to particle size characterization (Particle Analyser, Litesizer 500, Anton Paar, Austria). The mean size and zeta potential were obtained as 130.17 nm and 53.3 mV, respectively (Figures 1A, B).
2.4 RNA isolation and quantification
Total RNA was isolated from P. hypophthalmus liver and muscle tissues using the TRIzol technique. The liver and muscle (MYST and SMT) tissues were homogenized using liquid nitrogen. After adding chloroform to the homogenized samples for phase separation, they were incubated for 5 min. Following centrifugation, the RNA-containing aqueous phase was separated into a 1.5-mL tube, and isopropanol was used to precipitate the RNA. After air-drying the RNA pellet, it was dissolved in RNAse-free water after the precipitated RNA had been cleaned with 75% ethanol. For later use, RNA was kept in storage at -80°C. Furthermore, 1.0% agarose gel was used to confirm the integrity of the RNA. It was made by melting agarose in 1X TAE buffer to the necessary amount. A gel documentation system (ChemiDocTM MP imaging system, Bio-Rad) was used to visualize the RNA bands. The RNA was quantified using a nano-drop spectrophotometer (Thermo-scientific).
2.5 cDNA synthesis and quantitative PCR
Revert Aid First Strand cDNA synthesis kit (Thermo-scientific) was used to synthesize cDNA from total extracted RNA. Prior to the creation of cDNA, trace quantities of DNA were eliminated using DNase I. A volume of 12 µL contained the reaction mixture, including 15 pmol of oligo dT primers and 100 ng of RNA. In the PCR, the reaction mixture was heated to 65°C for 5 min before being cooled on ice. With that, the chilled mixture was centrifuged for a brief period of time with the addition of 1 µL Ribo Lock RNase Inhibitor (20 U/µL), 1.0 µL of reverse transcriptase enzyme, 5X reaction buffer (4.0 µL), and 2 µL dNTP Mix (10 mM). Subsequently, the mixture was incubated for 42 min at 60°C and for 5 min at 70°C. Through the use of β-actin PCR, the generated cDNA was verified. Quantitative PCR (real-time PCR) was conducted using gene-specific primers and the SYBR green PCA master mix (Bio-Rad). SYBR Green Master Mix (1X), primer (1 µL), and 1 µL of cDNA were included in the quantification samples. The reaction cycle was set up as follows: 10 min of initial denaturation at 95°C, 39 cycles of cDNA amplification, followed by 15 s of denaturation at 95°C and 1 min of annealing at 60°C (33). Table 3 has the primers’ specifics listed.
2.6 Growth performance
The study examined the growth performance parameters, including relative feed intake (RFI), weight gain (%), feed conversion ratio (FCR), specific growth rate, thermal growth coefficient (TGC), protein efficiency ratio (PER), and daily growth index (DGI). For a total of 90 days, the fish’s weight was recorded every 15 days.
2.7 Gene study
The different genes were investigated in liver tissues in this study, viz., superoxide dismutase (SOD), catalase (CAT), glutathione-s-transferase (GST), heat shock protein (HSP 70), nitric oxide synthase (iNOS), cytochrome P450 (CYP 450), caspase 3a (CAS 3a and 3b), metallothionine (MT), tumor necrosis factor (TNFα), Toll-like receptor (TLR), growth hormone receptor (Ghr1 and Ghrb), interleukin (IL), immunoglobulin (Ig), growth hormone (GH), and insulin like growth factor 1 and 2 (IGF1 and IGF 2), and in muscle tissue, somatostatin (SMT) and myostatin (MYST) were studied for real-time quantification.
2.8 Cortisol
Cortisol was determined using ELISA kit (commercially available Cortisol EIA kit, catalog no. 500360, Cayman Chemicals, USA). The assay was performed as per the instruction provided with the kit. The final reading was obtained using an ELISA plate reader (Biotek India Pvt. Ltd.).
2.9 Arsenic and iron analysis from fish tissues, feed, and experimental water
To measure the levels of arsenic, samples were taken from the kidney, brain, gills, liver, and muscle. On the other hand, the concentration of Fe was determined in the fish muscle and diet. Using inductively coupled plasma mass spectrometry (ICP-MS) (Agilent 7700 series, Agilent Technologies, USA) in a microwave digestion system (Microwave Reaction System, Multiwave PRO, Anton Paar GmbH, Austria, Europe), the tissues and diets were processed in accordance with the method of Kumar et al. (31, 34).
2.10 Alkaline single-cell gel electrophoresis/comet assay
With a slight modification (32), the alkaline single-cell gel electrophoresis/comet assay of Ali et al. (35) was used to assess DNA damage in kidney tissue. The kidney tissue (50 mg) was placed in an ice-cold homogenization buffer [20 mM EDTA; 1-X Hanks’ balanced salt solution; 10% dimethyl sulfoxide (DMSO), pH 7.0–7.5] after having been cleaned twice with chilled phosphate buffer saline (Ca2+- and Mg2+-free). The tissue was used to create a single-cell suspension, which was then suspended in phosphate saline buffer after having been centrifuged for 5 min at 4°C and 3,000 rpm to extract the cell pellets. Trypan Blue Exclusion Test was also utilized for the cell viability test (35). After coating the glass slide with 200 µL of 1% regular agarose, it was combined with 85 µL of 0.5% low melting point agarose which was added to 15 µL of cell suspension (about 20,000 cells), which was then covered with a coverslip. Furthermore, following the removal of the cover slip, 100 µL of low melting point agarose was once more applied to the slides. The slides were then left in the lysing solution (100 mM Na2 EDTA, 2.5 M NaCl, 10 mM Tris, pH 10, with fresh additions of 1% Triton X-100 and 10% DMSO) for an entire night at 4°C. After that, the slides were put in a horizontal gel electrophoresis unit using electrophoresis buffer (1 Mm Na2EDTA, 300 mM NaOH, and 0.2% DMSO, pH >13.5), and the electrophoresis unit was run for 20 min at 4°C using 15 V (0.8 V cm-1) and 300 mA. After that, the slides were cleaned three times using 0.4 in neutralizing buffer using Tris buffer, pH 7.5, at 0.4 M. To visualize the DNA damage, 75 µL of ethidium bromide (20 µg mL-1) was applied to the slides for 5 min. After that, the slides were examined under a fluorescence microscope (Leica Microsystems Ltd., DM 2000, Heerbrugg, Switzerland), and pictures were taken and examined using an Open Comet image analysis system. According to the software calculated, the metric chosen for quantifying DNA damage was percent tail DNA (i.e., % tail DNA = 100% head DNA).
2.11 Challenge study with Aeromonas hydrophila
After 90 days of feeding trial, nine fishes per replicate (total of three replicates, 27 fish per treatment) were challenged with virulent A. hydrophila (Hi-Media, Mumbai, India, lot no. 637–51-5 and Ref 0637 P), grown in nutrient broth for 24 h at 37°C in a BOD incubator, and harvested by centrifuging the culture broth at 10,000 rpm for 10 min at 4°C. The cells were then washed thrice in sterile PBS (pH 7.2), and the final concentration was maintained at 108 CFU mL-1. The fishes were intraperitoneally injected with 0.15 mL of bacterial suspension in each treatment group. The fish mortality in each treatment group was recorded up to 7 days of the challenge study. The tissues were dissected out from dead fish for confirmation of A. hydrophila as a causative agent of death.
2.12 Statistics
The Statistical Package for the Social Sciences (SPSS) version 16 program has been used to process the statistical analysis of the experimental data. Using Duncan’s multiple-range tests and a one-way ANOVA (analysis of variance), the significance and treatment impact have been examined. After analysis, the data was determined to be significant at p<0.05.
3 Results
3.1 Cortisol
The results of the serum cortisol levels in P. hypophthalmus reared under ammonia and arsenic toxicity as well as high temperature stress are illustrated in Figure 2A. The serum cortisol level exhibited a noticeable elevation (p = 0.0017) when exposed concurrently to ammonia and arsenic toxicity along with high temperature stress. This elevation was followed by concurrent exposure to ammonia and arsenic toxicity alone and then by exposure to ammonia and arsenic alone when compared to control and Fe-NP-supplemented groups. Additionally, dietary supplementation of Fe-NPs at 15 mg kg-1 diet, with or without stressors (As+NH3+T) and subsequent supplementation at 10 mg kg-1 diet, significantly reduced the cortisol levels compared to the control and other experimental groups.
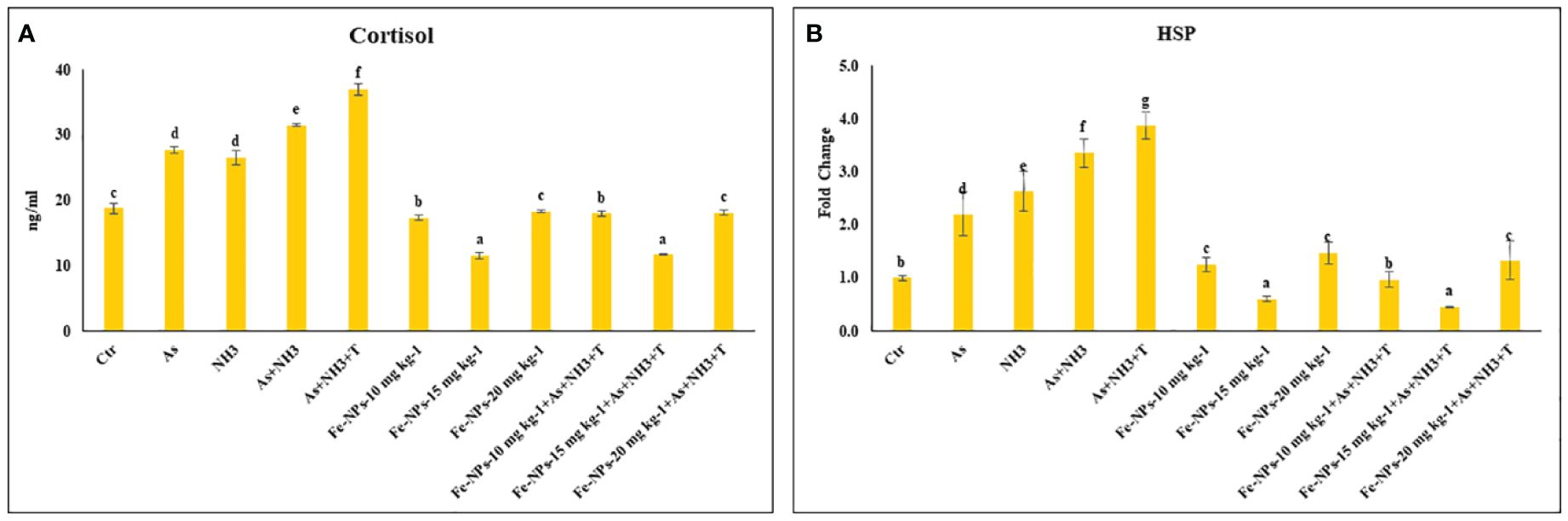
Figure 2 (A, B) Effect of dietary iron nanoparticles (Fe-NPs) on cortisol and HSP 70 against multiple stress in fish. Within endpoints and groups, bars with different superscripts differ significantly (a-g). Data expressed as Mean ± SE (n=3).
3.2 Heat shock protein (HSP 70)
The expression of the HSP 70 gene in liver tissue was significantly upregulated (p = 0.0022) by concurrent exposure to ammonia and arsenic toxicity, followed by exposure to arsenic combined with ammonia, ammonia alone, and arsenic alone, when compared to the control and other experimental groups. Interestingly, dietary supplementation of Fe-NPs at 15 mg kg-1 diet, with or without stressors, notably downregulated the expression of the HSP 70 gene compared to the control and other experimental groups. Moreover, groups fed with Fe-NPs at 10 and 20 mg kg-1 diet, with or without stressors, showed a significantly lower expression of the HSP 70 gene compared to all groups exposed to stressors (As, NH3, As+NH3, and As+NH3+T) (Figure 2B).
3.3 DNA damage inducible protein and DNA damage
The gene expression of DNA damage inducible protein (DDIP) was notably upregulated (p = 0.0013) by concurrent exposure to ammonia and arsenic toxicity as well as high temperature stress, followed by exposure to arsenic combined with ammonia, ammonia alone, and arsenic alone, when compared to the control and Fe-NP-supplemented groups. Moreover, the gene expression of DDIP was significantly downregulated in the group fed with Fe-NPs at 15 mg kg-1 diet, with or without stressors (As+NH3+T), compared to the control and other experimental groups. Groups fed with Fe-NPs at 10 and 20 mg kg-1 diet, without stressors, exhibited lower gene regulation of DDIP compared to groups exposed to stressors (arsenic, ammonia, arsenic combined with ammonia, and arsenic combined with ammonia and high temperature) (Figure 3A).
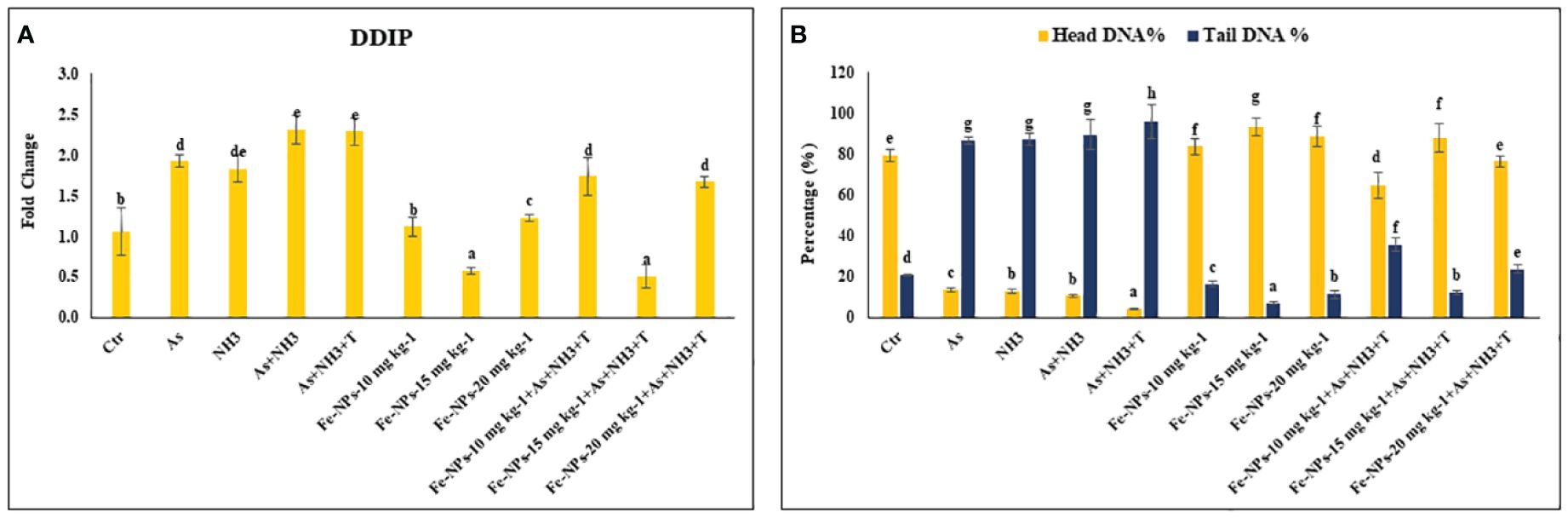
Figure 3 (A, B) Effect of dietary iron nanoparticles (Fe-NPs) on gene expression of DNA damage inducible protein (DDIP) and DNA damage against multiple stress in fish. Within endpoints and groups, bars with different superscripts differ significantly (a-g). Data expressed as Mean ± SE (n=3).
Furthermore, the results of DNA damage (comet) in the form of tail DNA % and head DNA % in the gill tissue of P. hypophthalmus are shown in Figure 3B. Head DNA (%) was notably lowered (p = 0.016), whereas tail DNA (%) was notably higher (p = 0.025) in the group exposed to concurrent arsenic, ammonia, and high temperature, followed by arsenic and ammonia, ammonia alone, and arsenic alone, compared to the control and other experimental groups. The group fed with dietary Fe-NPs at 15 mg kg-1 diet exhibited noticeably lower tail DNA (%) and higher head DNA (%), with or without stressors, compared to the control and other experimental groups.
3.4 Caspase, metallothionine, and cytochrome p450
In the present study, the gene expression of Cas 3a and 3b, MT, and CYP 450, respectively, was investigated in the liver tissue of P. hypophthalmus reared under multiple stress conditions (As, NH3, As+NH3, and As+NH3+T), and the results are presented in Figures 4A, B. Cas 3a gene expression was significantly upregulated (p = 0.017) by exposure to arsenic alone, followed by As+NH3+T, As+NH3, and NH3-alone groups. Conversely, Cas 3b expression was significantly upregulated (p = 0.0012) by concurrent exposure to arsenic, ammonia, and high temperature stress, followed by As+NH3, arsenic alone, and ammonia alone groups, compared to the control and other experimental groups. However, both Cas 3a and 3b were downregulated by Fe-NPs at 1.5 mg kg-1 diet, with or without stressors, compared to the control and other experimental groups (Figure 4A). MT gene expression was substantially upregulated (p = 0.0031) by As+NH3+T, followed by arsenic, ammonia, and As+NH3 exposure in P. hypophthalmus, compared to the control and Fe-NP-supplemented groups (Fe-NPs 10 and 15 mg kg-1 diet). Conversely, CYP 450 gene expression was notably (p = 0.016) highly upregulated by exposure to arsenic alone, followed by As+NH3+T, ammonia alone, and As+NH3 exposure, compared to the control and other experimental groups. Interestingly, MT and CYP 450 gene expressions were significantly downregulated by dietary Fe-NPs at 15 mg kg-1 diet compared to the control and other experimental groups (Figure 4B).
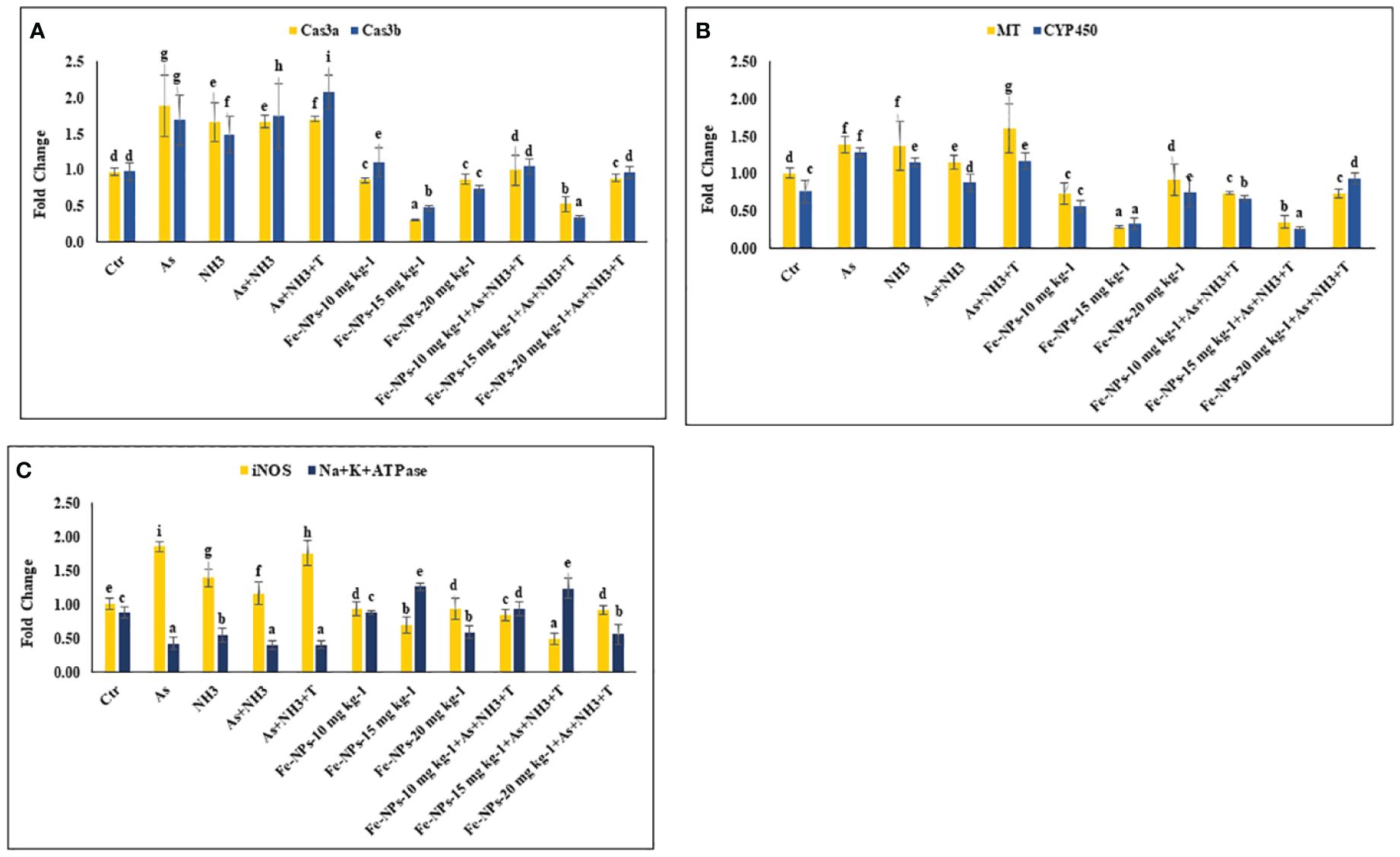
Figure 4 (A–C) Effect of dietary iron nanoparticles (Fe-NPs) on gene expression of Caspase 3a and 3b, metallothionine (MT), cytochrome P450 (CYP 450), inducible nitric oxide synthase (iNOS) and Na+K+ATPase against multiple stress in fish. Within endpoints and groups, bars with different superscripts differ significantly (a-g). Data expressed as Mean ± SE (n=3).
3.5 Inducible nitric oxide synthase and Na+K+ATPase
The gene expression of inducible nitric oxide synthase (iNOS) and Na+K+ATPase in the liver tissue of P. hypophthalmus reared under multiple stresses is depicted in Figure 4C. iNOS gene was substantially upregulated (p = 0.0071) by arsenic exposure, followed by As+NH3+T, ammonia alone, and As+NH3 exposure, compared to the control and Fe-NP-supplemented groups. Notably, the iNOS gene was significantly downregulated with Fe-NPs at 15 mg kg-1 diet, with or without stressors (As+NH3+T), followed by Fe-NPs at 10 and 20 mg kg-1 diet, with or without stressors, in comparison to the control and stressor-exposed groups. Furthermore, Na+K+ATPase gene expression was noticeably (p = 0.019) highly upregulated with dietary Fe-NPs at 15 mg kg-1 diet, with or without stressors, compared to the control and other experimental groups. Conversely, Na+K+ATPase was significantly downregulated with As+NH3+T, As+NH3, arsenic-alone, and ammonia-alone exposures, compared to the control and Fe-NP-supplemented groups (Figure 4C).
3.6 Cytokines and immunological genes: tumor necrosis factor, interleukin, immunoglobulin, and toll-like receptor
The gene expression of tumor necrosis factor (TNFα), interleukin (IL), and immunoglobulin (Ig), respectively, were determined in the liver tissue of P. hypophthalmus subjected to multiple stressors, and the results are presented in Figures 5A, B. TNFα gene expression was significantly downregulated (p = 0.0018) by As+NH3+T, followed by As+NH3, NH3, and As exposure, compared to the control and other experimental groups. Conversely, TNFα was noticeably upregulated by dietary Fe-NPs at 15 mg kg-1 diet, with or without stressors, followed by Fe-NPs at 10 and 20 mg kg-1 diet, compared to the control and other experimental groups (Figure 5A). Similarly, IL (p = 0.01) and Ig (p = 0.016) gene expressions were substantially downregulated by concurrent exposure to arsenic, ammonia, and high temperature, followed by As+NH3, NH3, and As, compared to the control and diet-supplemented groups. Conversely, both IL and Ig gene expression were significantly upregulated by dietary Fe-NPs at 15 mg kg-1 diet, with or without stressors, compared to the control, Fe-NP-fed groups (10 and 20 mg kg-1 diet), and stressor-exposed groups (Figure 5B). Furthermore, Toll-like receptor (TLR) gene expression was substantially upregulated by As+NH3, followed by As+NH3+T, NH3, and As-alone exposure, compared to the control and other experimental groups. Moreover, Fe-NPs at 15 mg kg-1 diet, with or without stressors, significantly downregulated TLR gene expression compared to the control and stressor-exposed groups. Dietary Fe-NPs at 10 and 20 mg kg-1 diet supplements were less effective in mitigating multiple stressors in fish (Figure 6A).
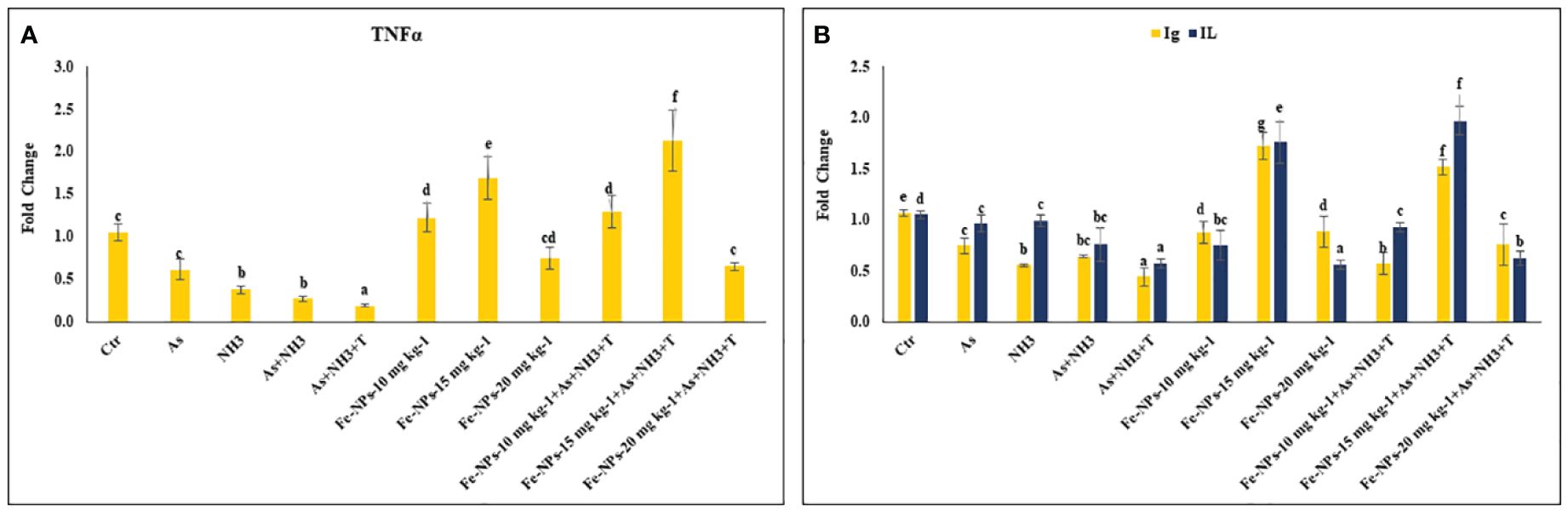
Figure 5 (A, B) Effect of dietary iron nanoparticles (Fe-NPs) on gene expression of tumor necrosis factor (TNFa), immunoglobin (Ig) and interleukin (IL) against multiple stress in fish. Within endpoints and groups, bars with different superscripts differ significantly (a-g). Data expressed as Mean ± SE (n=3).
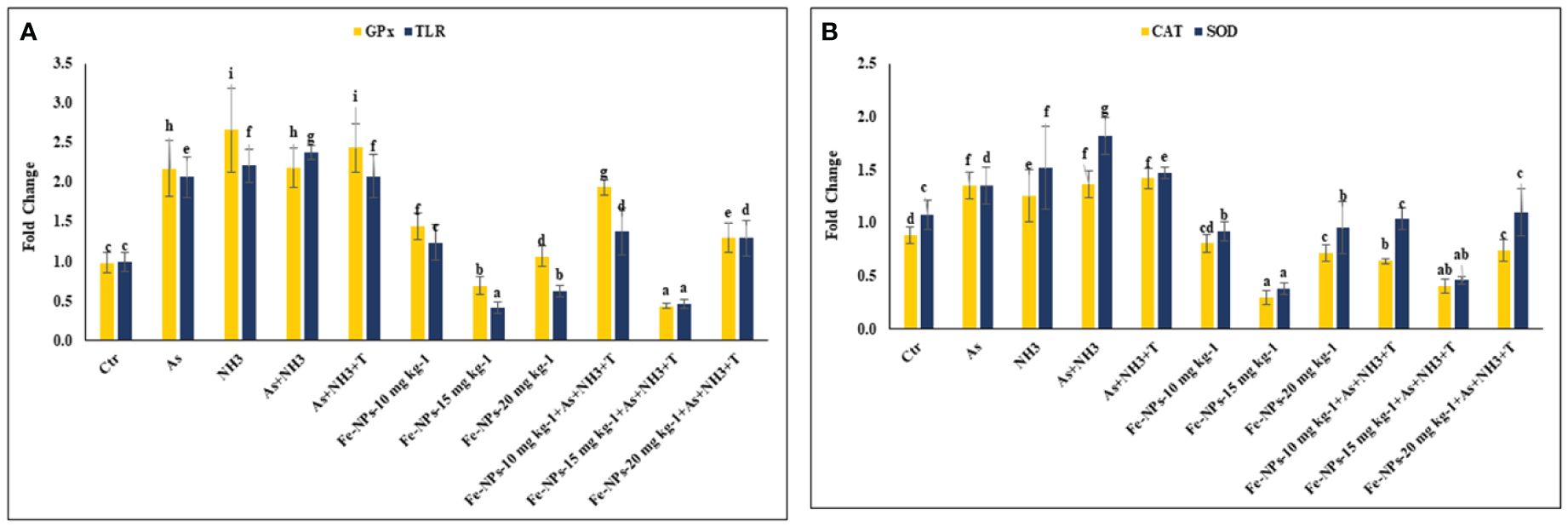
Figure 6 (A, B) Effect of dietary iron nanoparticles (Fe-NPs) on gene expression of GPx, toll like receptor (TLR), catalase (CAT) and superoxide dismutase (SOD) against multiple stress in fish. Within endpoints and groups, bars with different superscripts differ significantly (a-g). Data expressed as Mean ± SE (n=3).
3.7 Immunological attributes
The immunological attributes, such as nitroblue tetrazolium (NBT), blood glucose, total protein (TP), albumin (A), globulin (G), A:G ratio, and myeloperoxidase (MPO), were determined in P. hypophthalmus subjected to multiple stressors. The data are presented in Table 4. The NBT (p = 0.016), TP (p = 0.012), and globulin (p = 0.0022) levels were significantly decreased by concurrent exposure to arsenic, ammonia, and high temperature, followed by As+NH3, NH3, and As groups, compared to the control and Fe-NP-supplemented groups. Moreover, NBT, TP, and globulin levels were significantly elevated with dietary supplementation of Fe-NPs at 15 mg kg-1 diet, with or without stressors, compared to the control and other experimental groups. Furthermore, blood glucose was noticeably (p = 0.0027) elevated by stressors (As+NH3+T, As+NH3, NH3, and As) compared to the control and dietary Fe-NP-supplemented groups. Conversely, dietary Fe-NPs at 15 mg kg-1 diet significantly reduced the blood glucose levels in fish under both control and stressor conditions. Moreover, the A:G ratio showed similar trends to blood glucose levels, as Fe-NP supplementation improved the A:G ratio. Additionally, concurrent exposure to arsenic, ammonia, and high temperature significantly lowered the MPO levels, followed by NH3-alone and As-alone exposures. Interestingly, dietary Fe-NPs at 15 mg kg-1 diet noticeably enhanced (p = 0.0013) the MPO levels. The other supplemented groups of Fe-NPs at 10 and 20 mg kg-1 diet showed similar MPO levels to the control.
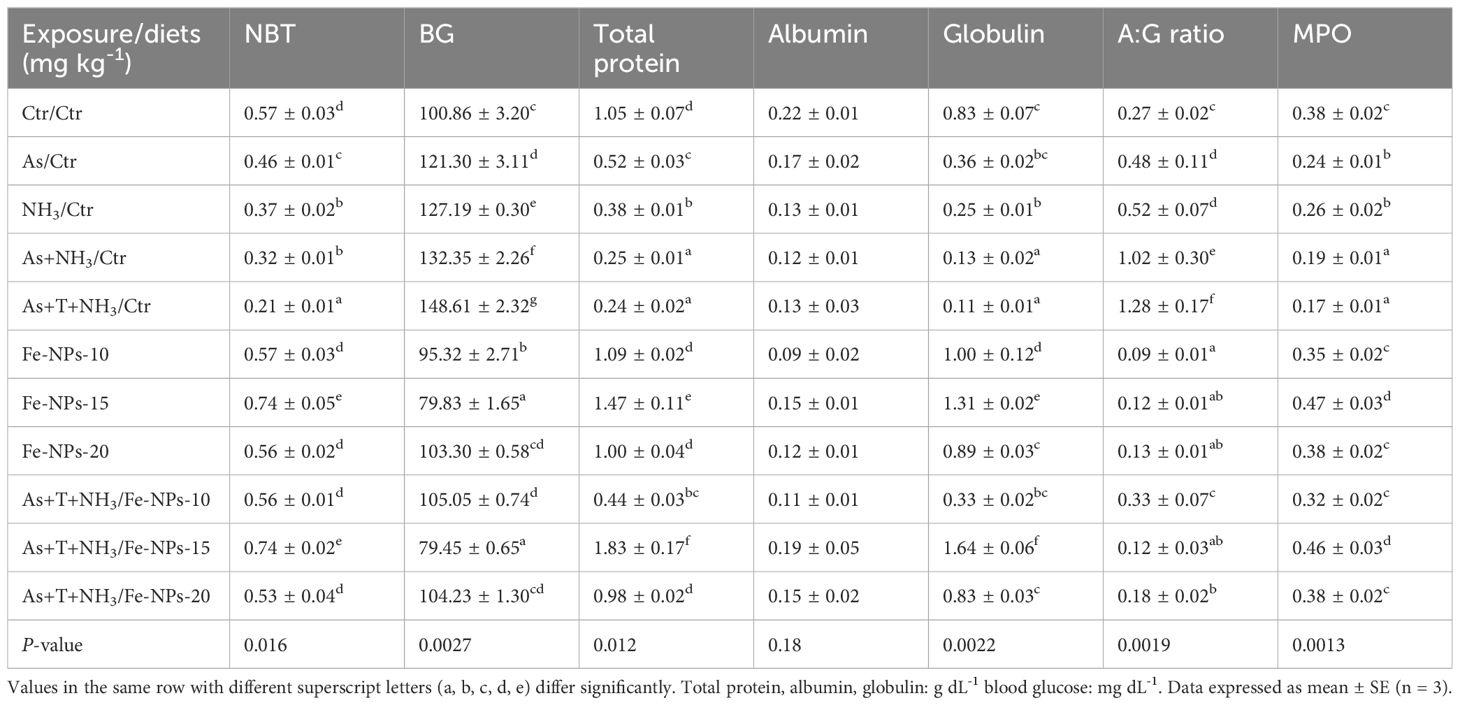
Table 4 Effect of dietary iron nanoparticles (Fe-NPs) on NBT, BG, total protein, albumin, globulin, A:G ratio, and MPO against multiple stress in fish.
3.8 Gene related to anti-oxidative status
The gene expression of GPx, SOD, and CAT in the liver tissue of P. hypophthalmus subjected to multiple stressors is documented in Figures 6A, B. Exposure to As+NH3+T, NH3, As+NH3, and As significantly upregulated the gene expression of GPx (p = 0.011), SOD (p = 0.002), and CAT (p = 0.001) compared to the control and other experimental groups. Interestingly, dietary Fe-NPs at 15 mg kg-1 diet were effective in downregulating the gene expression of GPx, SOD, and CAT compared to the control and other experimental groups. The dietary supplementation of Fe-NPs at 10 and 20 mg kg-1 diet showed similar gene expression levels of GPx, SOD, and CAT as the control group.
3.9 Growth-related gene expression
Figures 7A, B display the results of MYST and SMT gene regulation in the muscle tissue of P. hypophthalmus subjected to multiple stresses. MYST (p = 0.0065) and SMT (p = 0.0037) were substantially upregulated by concurrent exposure to As+NH3+T, followed by arsenic alone, As+NH3, and NH3 exposure, compared to the control and Fe-NP-supplemented groups. Moreover, MYST gene regulation was significantly downregulated by dietary Fe-NP at 15 mg kg-1 diet, with or without stressors, followed by Fe-NPs at 20 mg kg-1 diet, with or without stressors, and Fe-NPs at 10 mg kg-1 diet, compared to the control and other experimental groups. Similarly, SMT gene expression was significantly downregulated with Fe-NPs at 15 mg kg-1 diet, with or without stressors, followed by Fe-NPs at 10 mg kg-1 diet, with or without stressors, and Fe-NPs at 20 mg kg-1 diet without stressors, compared to the control and other experimental groups.
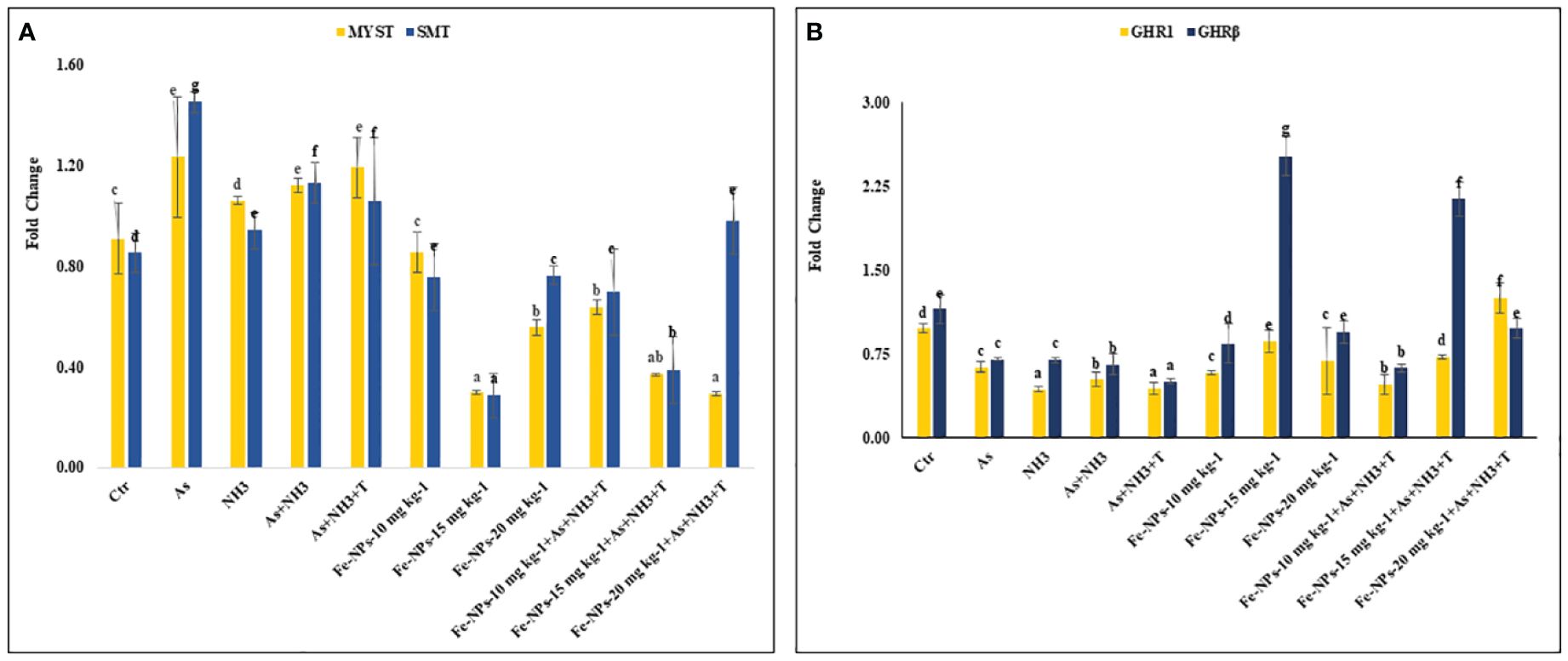
Figure 7 (A, B) Effect of dietary iron nanoparticles (Fe-NPs) on gene expression of myostatin (MYST), somatostatin (SMT), and growth hormone regulator (GHR1 and GHRβ) against multiple stress in fish. Within endpoints and groups, bars with different superscripts differ significantly (a-g). Data expressed as Mean ± SE (n=3).
3.10 Growth hormone regulator 1 and growth hormone regulator β
GHR1 (p = 0.0017) and GHR β (p = 0.0042) gene regulations were significantly downregulated in response to stressors (As+NH3+T, As+NH3, NH3, and As) compared to the control and other experimental groups. The GHR1 gene expression was significantly upregulated by Fe-NPs at 20 mg kg-1 diet with stressors, followed by Fe-NPs at 15 mg kg-1 diet without stressors, compared to the control and other experimental groups. Similarly, GHR β was significantly upregulated by Fe-NPs at 15 mg kg-1 diet, with or without stressors, compared to the control and other experimental groups (Figure 7B).
3.11 Growth hormone and insulin-like growth factors (IGF1X and IGF2X)
The gene regulations of GH, IGF1X, and IGF2X in the liver tissue of P. hypophthalmus subjected to stressors are presented in Figures 8A, B. GH was substantially upregulated (p = 0.018) by dietary Fe-NPs at 15 mg kg-1 diet, with or without stressors, compared to the control and stressor-exposed groups. Conversely, GH gene expression was significantly downregulated by As+NH3+T, followed by As+NH3, NH3, and As exposures, compared to the control and supplemented-diet groups. Furthermore, IGF1X and IGF2X gene regulations were noticeably downregulated by concurrent exposure to As+NH3+T, followed by As+NH3, NH3, and As-alone groups, compared to the control and Fe-NP-supplemented groups. Dietary Fe-NPs at 15 mg kg-1 diet, with or without stressors, substantially upregulated the IGF1X and IGF2X genes compared to the control and stressor-exposed groups. Additionally, other dietary groups, such as Fe-NPs at 10 and 20 mg kg-1 diet, were also efficient in upregulating IGF1X and IGF2X gene regulation against arsenic, ammonia, and high temperature stress (Figure 8B).
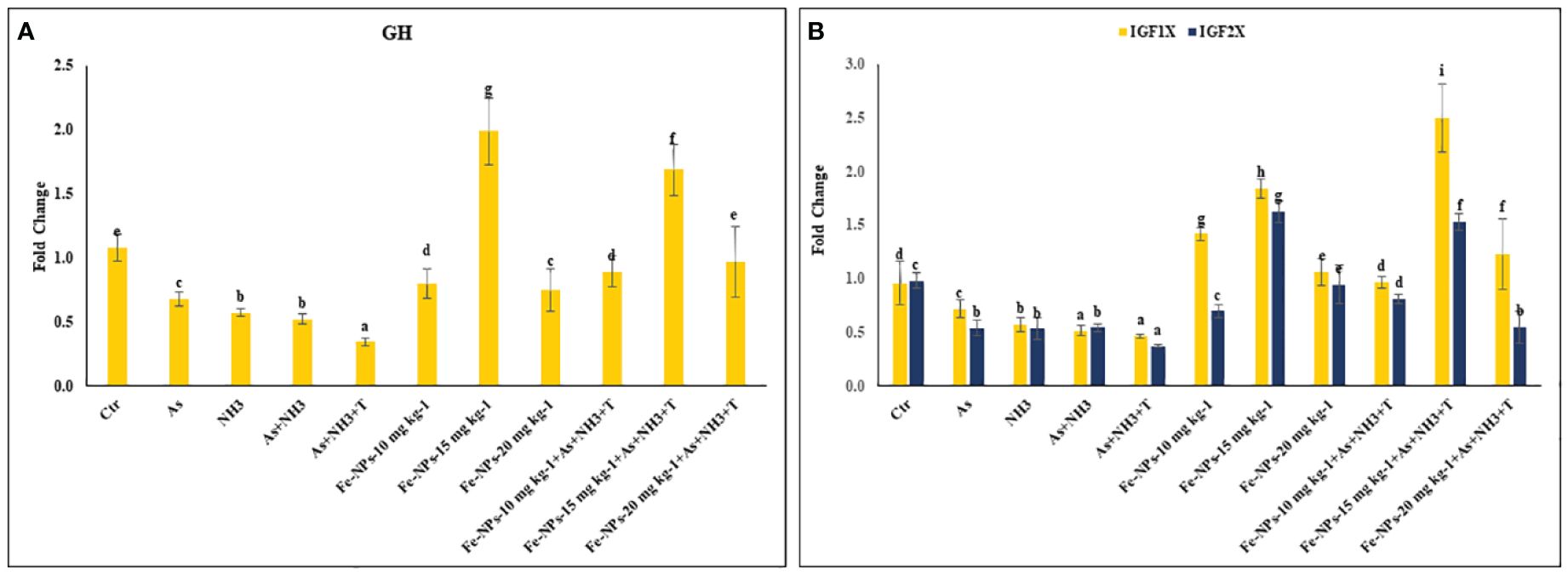
Figure 8 (A, B) Effect of dietary iron nanoparticles (Fe-NPs) on gene expression of growth hormone (GH), insulin like growth factor (IGF IX and IGF 2X) against multiple stress in fish. Within endpoints and groups, bars with different superscripts differ significantly (a-g). Data expressed as Mean ± SE (n=3).
3.12 Growth performance
Table 5 summarizes the results of growth performance indicators such as final weight gain %, food conversion ratio (FCR), specific growth rate (SGR), protein efficiency ratio (PER), daily growth index (DGI), and relative feed intake (RFI) of P. hypophthalmus reared under different stress conditions. The final weight gain % was noticeably enhanced by dietary Fe-NPs at 15 mg kg-1 diet, with (160%) or without stressors (162%), followed by Fe-NPs with (137%) or without stressors (147%), compared to the control and stressor-exposed groups. Conversely, the final weight gain % was significantly reduced by concurrent exposure to As+NH3+T (60%), As+NH3 (68%), NH3 (68%), and As (72%), compared to the control and Fe-NP-supplemented groups. Similarly, SGR, PER, DGI, and RFI followed the same pattern and were enhanced with dietary Fe-NPs at 15 mg kg-1 diet.
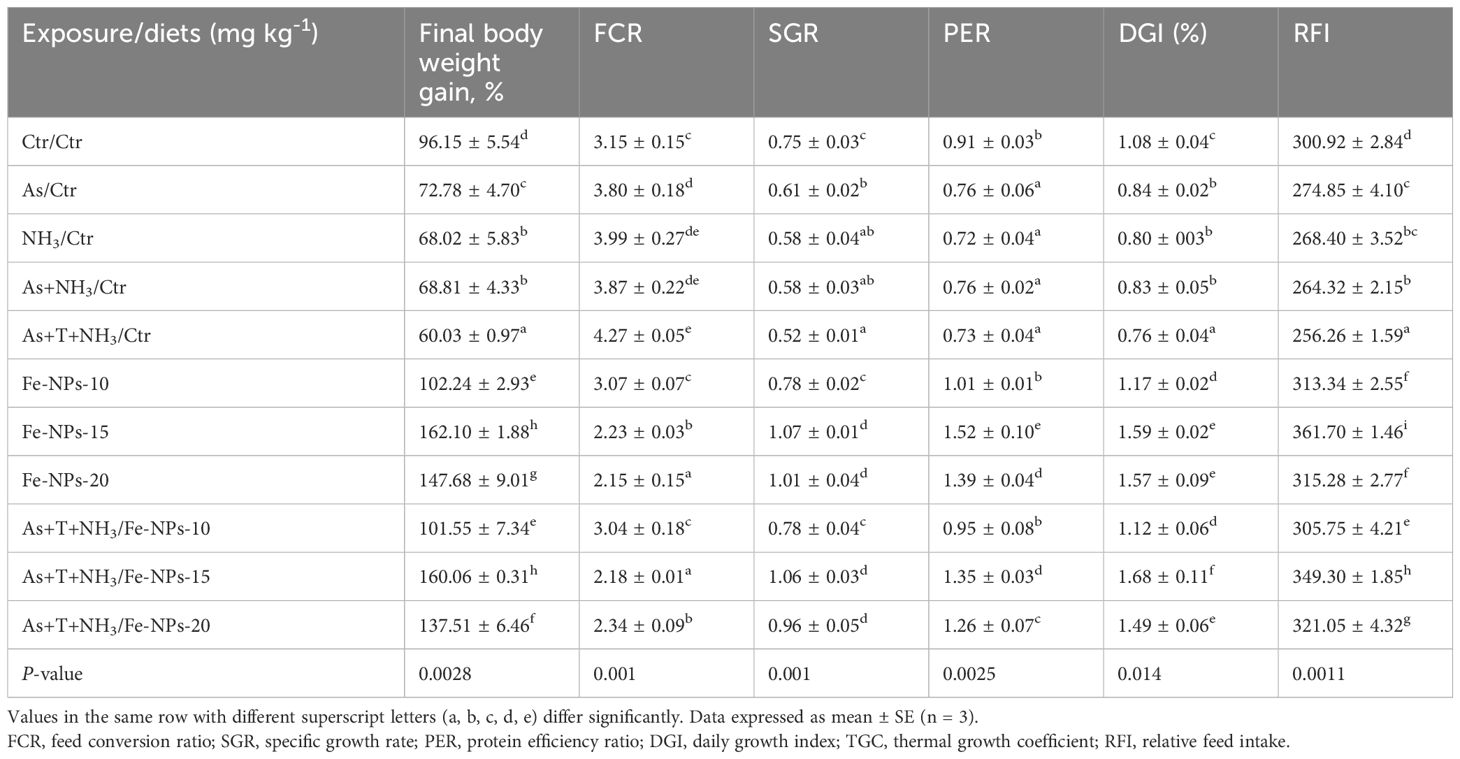
Table 5 Effect of dietary iron nano-particles (Fe-NPs) on growth performance, viz., final body weight gain (%), FCR, SGR, PER, DGI (%), TGC, and RFI against multiple stress in fish.
3.13 Detoxification of arsenic by Fe-NPs diet
The arsenic concentration in the experimental water varied as follows: control (0.02 mg kg-1 diet), As group (1931 µg L-1), NH3 group (0.01 µg L-1), As+NH3 (2160 µg L-1), As+NH3+T (2324 µg L-1), Fe-NPs at 10, 15, and 20 mg kg-1 diet (below detection limit), and Fe-NPs with As+NH3+T at 10 mg kg-1 diet (1730 µg L-1), 15 mg kg-1 diet (507 µg L-1), and 20 mg kg-1 diet (1925 µg L-1). However, the results showed that dietary Fe-NPs at 15 mg kg-1 diet with stressors (As+NH3+T) exhibited the lowest arsenic concentration. Moreover, the highest bioaccumulation was observed in the kidney, followed by the liver, gill, muscle, and brain in the group exposed to As+NH3+T. Dietary Fe-NPs at 15 mg kg-1 diet showed the highest detoxification of arsenic in all tissues (Table 6).
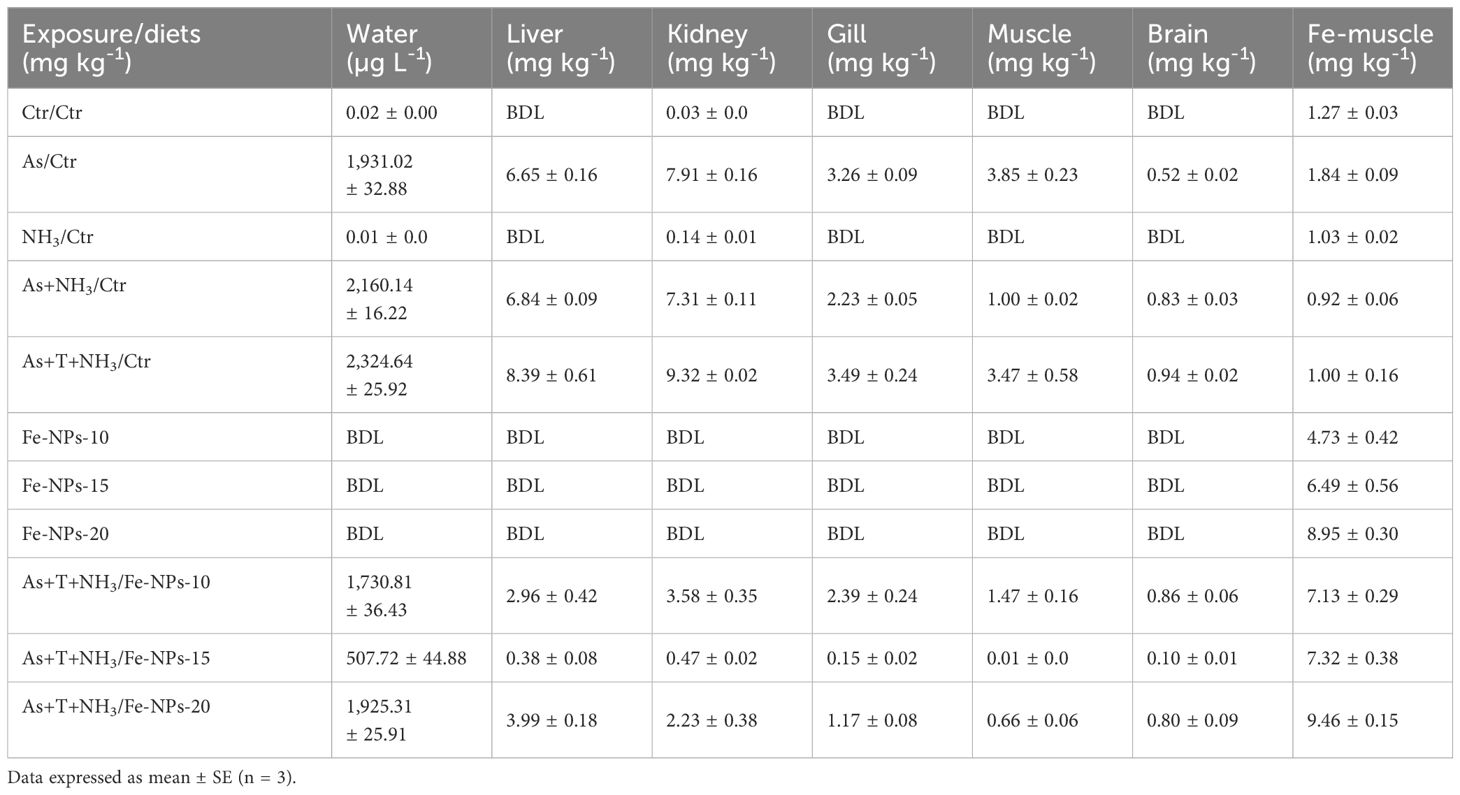
Table 6 Effect of dietary iron nanoparticles (Fe-NPs) on detoxification of arsenic in different fish tissues reared under control and multiple stress conditions.
3.14 Challenging test
The cumulative mortality and relative percentage survival were determined after a 90-day experimental trial. Cumulative mortality rates were observed as follows: 44%, 61%, 61%, 63.9%, 61%, 69%, 44%, 27%, 55%, 50%, and 38.9% in the control, As alone, NH3 alone, As+NH3, As+NH3+T, and Fe-NPs at 10, 15, and 20 mg kg-1 diet without stressors and with stressors, respectively. Similarly, the relative percentage survival varied as (–) 17% (–), 17% (–), 35% (–), 41%, 11%, 41%, 17%, 0%, 35%, and (–) 5.9% in the corresponding groups: control, As alone, NH3 alone, As+NH3, As+NH3+T, and Fe-NPs at 10, 15, and 20 mg kg-1 diet without stressors and with stressors, respectively (Figure 9).
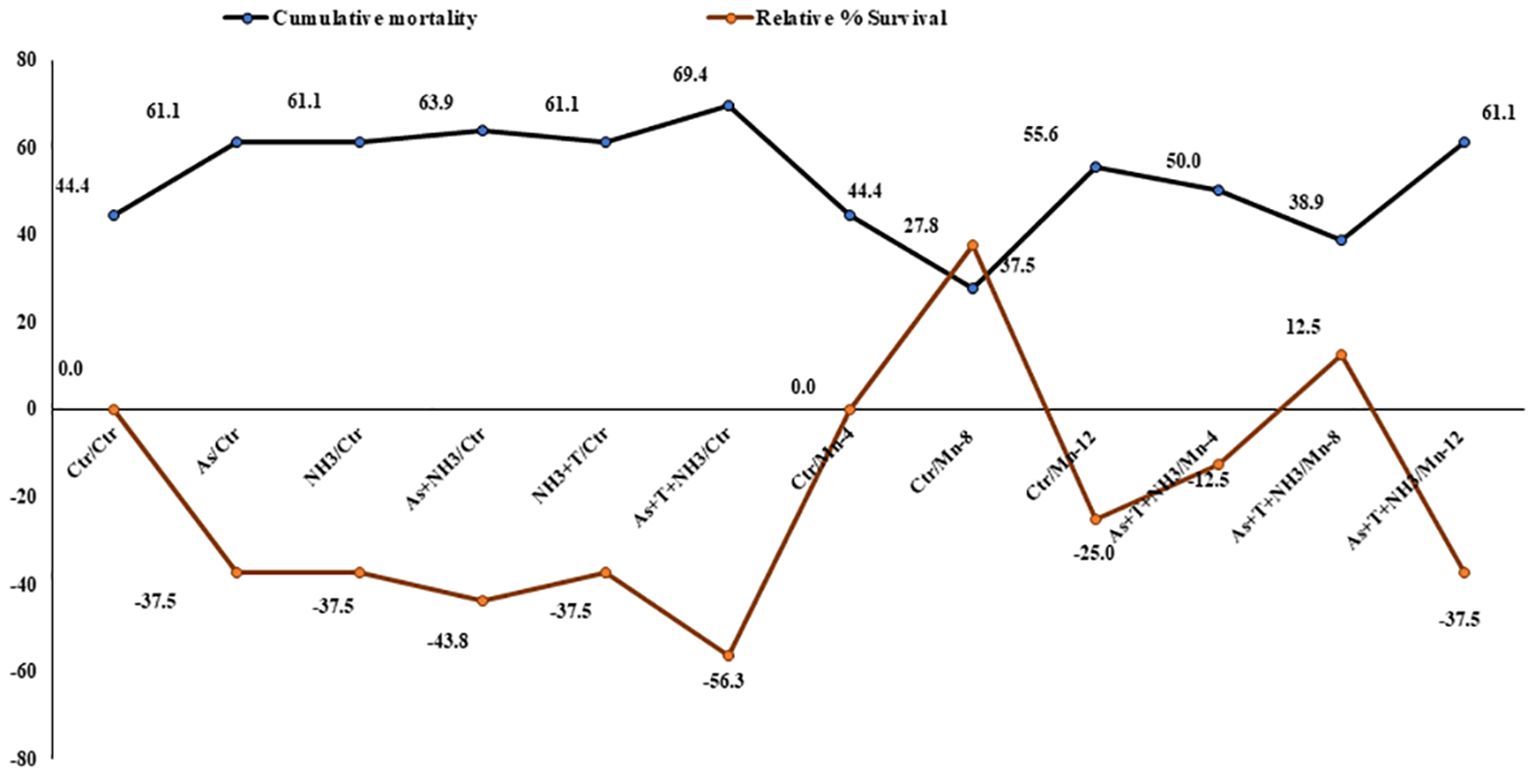
Figure 9 Effect of dietary iron nanoparticles (Fe-NPs) on cumulative mortality and relative percentage survival of fish reared under multiple stress after infected with pathogenic bacteria. Within endpoints and groups, bars with different superscripts differ significantly (a-g). Data expressed as Mean ± SE (n = 3).
4 Discussion
The present investigation highlights the critical role of dietary Fe-NPs in mitigating various stressors such as arsenic, ammonia, and high temperature (34°C) in P. hypophthalmus. The study primarily elucidates the mechanistic role of different gene regulations involved in immunity, antioxidative status, apoptosis, genotoxicity, growth performance, and more. It demonstrates that dietary Fe-NPs strengthen fish immunity through various gene regulations. In this study, Fe-NPs at 15 mg kg-1 diet substantially decreased the cortisol levels in fish reared under both control and stressor conditions. This reduction could be attributed to Fe-NPs’ activation of the hypothalamus–pituitary–interrenal (HPI) axis to control the stimulating cortisol synthesis in interrenal kidney tissue (36, 37).
Interestingly, dietary Fe-NPs reduced the cortisol levels possibly by suppressing the gene expression of CYP 450 in fish exposed to arsenic, ammonia toxicity, and high temperature stress (38). It is also plausible that non-heme proteins containing iron, present in the adrenal cortex and involved in cortisol synthesis, play a role (39). It was also found that deficiency and overdose of iron supplementation can lead to CYP impairment. Such report has been reflected in our study (40).
The expression of the HSP 70 gene significantly increased under stressors (As, NH3, As+NH3, and As+NH3+T), leading to diminished protein protection from oxidative damage, enhanced ROS production, protein misfolding, and exacerbated oxidative stress (23). Conversely, dietary Fe-NPs at 15 mg kg-1 diet downregulated HSP 70 gene expression, protecting against proteasomal degradation (41), promoting proper protein folding, preventing protein aggregation, degrading denatured proteins, and folding misfolded proteins (42). Surprisingly, dietary Fe-NPs downregulated the HSP 70 gene expression, and this could be due to activation of the HSP transcription factor, which reduces the binding activity of heat shock elements (35, 43).
Furthermore, dietary Fe-NPs at 15 mg kg-1 diet protected against DNA damage and downregulated DDIP gene expression. Even slight changes in the dose of dietary Fe-NPs induced genotoxicity, as demonstrated in the present study, although stressors (As, NH3, As+NH3, and As+NH3+T) induced a higher generation of reactive oxygen species, dysregulation of cell proliferation, apoptosis, diminished DNA repair, and aberrations in histone post-translational modification and DNA methylation (44).
The gene expression of Cas 3a and 3b was regulated by dietary Fe-NPs at 15 mg kg-1 diet, whereas stressors (As, NH3, As+NH3, and As+NH3+T) upregulated Cas 3a and 3b (45). Iron nanoparticles (Fe-NPs) possess the protein disulfide isomerase (PDI) multifunctional enzyme systems, which control apoptosis via Cas 3a and 3b (46, 47). The isomerization and rearrangement of disulfide bonds are the major functions of PDI, which has two active sites of thiol groups. It also has the capacity to bind zinc and copper at active thiol sites (48, 49).
The toxicity induced by arsenic, ammonia, and high temperature stress significantly upregulated MT gene expression. MT gene expression is commonly induced by metal toxicity and various environmental stressors in fish (50, 51). This upregulation might be mediated by the metal-responsive transcription factor 1 (MTF-1), a major activator of MT gene expression (52) primarily regulated by phosphorylation (53). It was reported that protein kinases such as c-Jun N-terminal kinase (JNK), phosphoinositide 3-kinase (PI3K), and protein kinase C (PKC) play roles in modifying the MTF-1 signaling pathway (53). Interestingly, dietary Fe-NPs at 15 mg kg-1 diet noticeably controlled and downregulated MT gene expression, potentially due to the essential role of iron in the erythropoietic system and the production of reticulocytes or other young cells with a basal level of MT-I much higher than that in mature erythrocytes (54).
Cytochrome P450 (CYP 450) is an important heme-thiolate protein involved in detoxifying xenobiotics, drugs, carcinogens, and other toxic substances (55). In this investigation, arsenic, ammonia, and high temperature stress upregulated CYP 450 gene expression in the liver tissue of P. hypophthalmus. This upregulation might be attributed to reactive oxygen species generated by stressors, leading to lipid peroxidation, cellular toxicity, and, ultimately, cell death. Additionally, it is involved in apoptosis and upregulation of the transcription of bcl2-associated X (Bax) (56), an important cell death-promoting gene in fish that induces the release of cytochrome c, leading to caspase activation (57). Interestingly, dietary Fe-NPs at 15 mg kg-1 diet controlled the gene expression of CYP 450 in the liver tissue of P. hypophthalmus.
The iNOS gene expression was significantly upregulated by stressors (As+NH3+T, As+NH3, NH3, and As). iNOS gene expression mainly depends on ammonia toxicity as it accumulates in tissues. Ammonia is converted into urea via the ornithine–urea cycle (OUC) and then into glutamine via glutamine synthetase, including non-essential amino acids (58). Similarly, blood carrying high ammonia concentrations affects the liver tissue (59). Moreover, nitric oxide provides protection to the cellular system against oxidative stress (59). Interestingly, dietary Fe-NPs at 15 mg kg-1 diet substantially downregulated iNOS gene expression. The interrelationship between iron and iNOS gene expression is profound, as iron nanoparticles prevent the accumulation of higher oxygen radicals through a NOS-dependent mechanism (60). Iron also triggers hypertrophy, inducing the expression of antiapoptotic proteins such as Bcl-2 and survivin and hypertrophic agents endothelin (ET-1) and leukemia inhibitory factor (LIF), thus decreasing H2O2-induced necrosis (61, 62). Furthermore, during stress conditions, the organism requires more energy in the form of ATPase; therefore, the gene expression of Na+K+ATPase was highly upregulated. Notably, Fe-NPs at 15 mg kg-1 diet aid in the formation of more ATPase and supply it to the fish reared under multiple stress conditions.
Furthermore, dietary Fe-NPs regulate the expression of cytokine genes such as TNFα. This regulation could be attributed to the role of iron in the regulation of ferritin levels in macrophages, enhancing the gene regulation of TNFα (63). In the present study, TNFα was substantially downregulated by stressors (As+NH3+T, As+NH3, NH3, and As). TNFα is also associated with the activation of B-cell-activating factor (BAFF), which aids in NFkB regulation (64). TNFα and IL are inflammation-related cytokines upregulated after exposure to ammonia, arsenic, and high temperature stress (65). IL promotes T-cell-induced inflammatory responses and releases pro-inflammatory cytokines for amplification. It binds to receptors and plays an important biological role using the nuclear factor kappa-B (NF-κB) and MAP kinase pathway (66). Additionally, TNFα is involved in apoptosis, inflammation, and cell proliferation, stimulating the immune system and activating the NF-κB pathway (67). Hence, exposure to ammonia, arsenic, and high temperature stress increases these gene regulations in fish. Moreover, dietary Fe-NPs at 15 mg kg-1 diet improve IL gene expression and upregulate its expression. This could be due to the potential of Fe-NPs to stimulate the production of hepcidin (68). The iron storage protein ferritin has two units, H-subunits (H-mRNA) and light subunits (L-mRNA), which remain unchanged, indicating that IL is regulated by translational mechanisms during infection and stress (69).
Furthermore, the expression of TLR genes was upregulated by arsenic, ammonia, and high temperature stress, whereas dietary Fe-NPs at 15 mg kg-1 diet downregulated TLR gene expression. These results could be due to heme and iron sequestration in response to inflammatory/infectious stimuli during stress (70). TLR also plays an important role in activating signaling pathways that enhance fish immunity and reduce stress using the NF-κB pathway. Interestingly, dietary Fe-NPs upregulated Ig gene expression in fish reared under control and stressor conditions (As, NH3, and high temperature). This strengthening of fish immunity via the upregulation of Ig gene expression by Fe-NPs diet could be due to enhanced immunoglobulin and T-cell levels in fish under control and stressor conditions (71, 72).
NBT, BG, total protein, albumin, globulin, A:G ratio, and MPO are also important immunological attributes whose levels indicate fish immunity. These immunological attributes were noticeably affected by exposure to arsenic, ammonia, and high temperature, leading to reduced immunity (23). This reduction could be attributed to the higher energy requirements of fish during stress conditions, resulting in significant decreases in these parameters (73). Albumin, crucial for transporting biomolecules such as drugs, hormones, vitamins, and bilirubin, also supports the regulation of hormones and fat metabolism (74). MPO produces hypochlorous acid through respiratory burst via hydrogen peroxide, exhibiting cytotoxic effects on mammals and bacteria (75). Despite higher NBT levels typically indicating better immunity, the present study observed a noticeable reduction in NBT levels under stressors (As, NH3, and T), reflecting compromised immunity in the fish. Surprisingly, dietary Fe-NPs at 15 mg kg-1 diet enhance fish immunity using these immunological attributes (71, 72). Dietary Fe-NPs help activate dendritic cells and enhance interleukin and cytokine production, accelerating the enhancement of fish immunity (76, 77).
Fe-NPs are essential nutrients for antioxidant enzymatic systems as they strengthen antioxidant gene regulation and enzymatic systems such as CAT, SOD, and GPx. In the present investigation, arsenic, ammonia, and high temperature stress substantially upregulated the gene regulation of SOD, CAT, and GPx in fish liver tissue (23). However, dietary Fe-NPs at 15 mg kg-1 noticeably downregulated the gene expression of SOD, CAT, and GPx, reducing reactive oxygen species (78, 79). This could be attributed to the role of Fe-NPs in maintaining oxidative stress by reducing reactive oxygen species (ROS) and acting as oxygen carrier proteins that regulate the antioxidant status of the fish (80). It is important to note that the antioxidant defense system is crucial to maintain overall health by safeguarding cells and tissues from oxidative stress damage caused by excessive hydroxyl radicals and reactive oxygen species (ROS) through scavenging them (81, 82). Moreover, Fe-NPs have many biological functions, including serving as an antioxidant defense system in fish by utilizing superoxide dismutase and glutathione peroxidase to prevent free radical production and lipid peroxidation (83). Interestingly, CAT, known as a heme-containing enzyme, acts together with SOD to counterbalance cellular ROS and improve the antioxidative status (83).
Growth performance indicators such as weight gain %, specific growth rate (SGR), protein efficiency ratio (PER), daily growth index (DGI), and relative feed intake (RFI) were noticeably reduced, and the feed conversion ratio (FCR) was enhanced with stressors (As, NH3, and high temperature). Conversely, dietary Fe-NPs improved the growth performance of the fish. Iron plays an important role in maintaining organismal iron homeostasis and enhances growth performance. Supplementation of Fe-NP diets enhances growth performance, possibly due to the nutrient ability of iron oxide nanoparticles and the enhancement of feed utilization in fish reared under control and stressor conditions (As, NH3, As+NH3, and As+NH3+T) (84). Additionally, Fe-NPs have smaller sizes and are efficiently utilized in dietary form (84). Fe-NPs diets also enhance digestive activities and increase physiological processes, which help in enhancing growth performance (85). Fe-NP diets also have higher bioavailability and better absorption, which enhances growth performance in fish reared under control and adverse conditions (86). Furthermore, genes related to growth performance such as MYST, SMT, GHR1, GHRβ, GH, IGF1X, and IGF2X were also altered by arsenic, ammonia, and high temperature stress. These genes were noticeably upregulated (GHR1, GHRβ, GH, IGF1X, and IGF2X) by dietary Fe-NPs at 15 mg kg-1. Dietary Fe-NPs at 15 mg kg-1 diet substantially downregulated MYST and SMT, possibly due to the role of MYST in decreasing myoblasts, resulting in terminal differentiation and fiber enlargement (87). Moreover, gene regulation of IGF1X1 and IGF1X2 is important for biomolecular regulation via lipid, carbohydrate, protein, and mineral metabolism and differentiation and proliferation of cells, ultimately influencing growth (18). As GH binds to the receptor in liver cells to stimulate the release and synthesis of IGF gene expression, dietary Fe-NPs aid in this process. Fe-NPs diet is responsible for growth enhancement and biomolecular function in the cells of aquatic organisms. Interestingly, Fe has a close relationship with the secretion and regulation of IGF, which directly affects growth performance using the central nervous system of fish reared under control and stressor conditions (88, 89). Ghrelin is also important for stimulating growth hormone from the pituitary gland, which controls the regulation of GH. It also stimulates the growth hormone (GH) releasing hormone and somatostatin axis, resulting in increased production of IGF-I in the liver (90).
Furthermore, the bioaccumulation of arsenic in the liver, kidney, gill, muscle, and brain was determined. The highest bioaccumulation of arsenic was observed in the kidney and liver tissues of the group concurrently exposed to arsenic, ammonia, and high temperature stress. Interestingly, in the group fed with Fe-NPs at 15 mg kg-1 diet with stressors, the arsenic levels were notably reduced. Arsenic levels below the detection limit were observed in the groups fed with Fe-NPs at 10, 15, and 20 mg kg-1 diet without stressors. These results could be attributed to the role of Fe-NPs in enhancing the detoxification of arsenic in kidney and liver tissues through CYP 450 gene expression, as shown in the results.
Moreover, the survival rate of the fish was higher in the groups fed with Fe-NPs at 10, 15, and 20 mg kg-1 diet with or without stressors after bacterial challenge. Dietary Fe-NPs possess strong antibacterial and phagocytic capacities, which enhance the immunity of the fish and reduce mortality after bacterial infection. Moreover, in the present study, the immunity-related genes have been improved by dietary Fe-NPs diet. Genes such as TNFα, TLR, Ig, and IL strongly support the immunity during stress conditions, especially during infection condition (12). Fe-NPs also enhance the fluidity of membranes of antibacterial compounds like lysozyme and reduce lipid peroxidation (91–93).
5 Conclusions
The present investigation revealed that nano-nutrients in the form of iron nanoparticles (Fe-NPs) mitigate multiple abiotic stresses such as ammonia and arsenic toxicity as well as high temperature stress in fish. Moreover, it is the first to report the mechanistic role of Fe-NPs in mitigating arsenic and ammonia toxicity as well as high temperature stress in fish. Fe-NPs regulate genes involved in growth performance, immunity, antioxidative capacity, genotoxicity, and stress responses in P. hypophthalmus. Additionally, Fe-NPs lead to the positive modulation of gene expression related to cortisol regulation, HSP 70 production, apoptosis inhibition, and genotoxicity prevention. Interestingly, Fe-NPs at 15 mg kg-1 diet enhance the detoxification of arsenic in fish tissues. Fe-NPs have also improved immunity against pathogenic bacteria and enhanced fish survival under multiple stresses. Importantly, these findings highlight the potential of Fe-NPs at 15 mg kg-1 in enhancing the wellbeing of fish species in the face of contemporary challenges posed by climate change and pollution.
Data availability statement
The datasets for this article are not publicly available due to concerns regarding participant/patient anonymity. Requests to access the datasets should be directed to the corresponding author.
Ethics statement
The animal study was approved by Prioritization, Monitoring and Evaluation (PME). The study was conducted in accordance with the local legislation and institutional requirements.
Author contributions
NK: Writing – review & editing, Writing – original draft, Visualization, Validation, Supervision, Software, Resources, Project administration, Methodology, Investigation, Funding acquisition, Formal analysis, Data curation, Conceptualization. ST: Writing – review & editing, Methodology, Formal analysis. MG: Writing – review & editing, Methodology. PK: Writing – review & editing, Validation, Methodology, Data curation. KR: Writing – review & editing, Supervision.
Funding
The author(s) declare financial support was received for the research, authorship, and/or publication of this article. The present work was supported by the Indian Council of Agricultural Research (ICAR), New Delhi, India, under institute project #IXX15014.
Acknowledgments
The authors sincerely acknowledge the director of ICAR-NIASM for providing the research facilities for this work.
Conflict of interest
The authors declare that the research was conducted in the absence of any commercial or financial relationships that could be construed as a potential conflict of interest.
Publisher’s note
All claims expressed in this article are solely those of the authors and do not necessarily represent those of their affiliated organizations, or those of the publisher, the editors and the reviewers. Any product that may be evaluated in this article, or claim that may be made by its manufacturer, is not guaranteed or endorsed by the publisher.
Abbreviations
Fe-NPs, Iron nanoparticles; As, Arsenic; NH3,Ammonia; T, High temperature; NFkB, Nuclear factor kappa B; iNOS, Inducible nitric oxide synthase; HSP70, Heat shock protein; MT, Metallothionine; CAT, Catalase; SOD, Superoxide dismutase; GPx, Glutathione peroxidase; CAS 3a, Caspase 3a; TNFα, Tumor necrosis factor; IL, Interleukin; TLR, Toll-like receptors; GH, Growth hormone; GHR1; GHRβ, Growth hormone regulator 1 and β; MYST, Myostatin; SMT, Somatostatin; NFkB, Natural kappa factor; IARC, International Agency for Research on Cancer; T, High temperature; IPCC, Intergovernmental Panel on Climate Change; TAN, Total ammonia nitrogen; RAC, Research Advisory Committee; PME, Prioritization, Monitoring and Evaluation; LC50, Lethal concentration; CMC, Carboxymethyl cellulose; CP, Crude protein; EE, Ether extract; FEC, Feed conversion efficiency; SGR, Specific growth rate; PER, Protein efficiency ratio; DGI, Daily growth index; TGC, Thermal growth coefficient; RFI, Relative feed intake; ICPMS, Inductively coupled plasma mass spectrometry.
References
1. Kumar N, Chandan NK, Bhushan S, Singh DK, Kumar S. Health risk assessment and metal contamination in fish, water and soil sediments in the East Kolkata Wetlands, India, Ramsar site. Sci Rep. (2023) 13:1546. doi: 10.1038/s41598-023-28801-y
2. Kumar N, Krishnani KK, Singh NP. Oxidative and cellular stress as bioindicators for metals contamination in freshwater mollusk Lamellidens marginalis. Environ Sci pollut Res. (2017) 24:16137–47. doi: 10.1007/s11356-017-9266-0
3. Ye Y, Gaugler B, Mohty M, Malard F. Old dog, new trick: trivalent arsenic as an immunomodulatory drug. Br J Pharmacol. (2020) 177:2199–214. doi: 10.1111/bph.15011
4. Shaji E, Santosh M, Sarath KV, Prakash P, Deepchand V, Divya BV. Arsenic contamination of groundwater: a global synopsis with focus on the Indian Peninsula. Geosci Front. (2021) 12:101079. doi: 10.1016/j.gsf.2020.08.015
5. IARC International Agency for Research on Cancer. IARC monographs on the evaluation of carcinogenic risks to humans: a review of human carcinogens: arsenic, metals, fibres, and dusts Vol. 100C. . Lyon, France: World Health Organization (2012).
6. Mohanty B.P. T, Ganguly S, Sarkar SD, Mahanty A. Curcumin has protective effect on the eye lens against arsenic toxicity, Biol. Trace Elem Res. (2020) 199(9):3354–59. doi: 10.1007/s12011–020-02448–6
7. Okoye E.A. B, Ruggieri F, Ezejiofor AN, Nwaogazie IL, Frazzoli C, Orish E, et al. Arsenic and toxic metals in meat and fish consumed in Niger delta, Nigeria: Employing the margin of exposure approach in human health risk assessment, Food and Chem. Toxicol. (2022) 159:112767. doi: 10.1016/j.fct.2021.112767
8. Ip YK, Ammonia production SF. excretion, toxicity, and defence in fish: a review. Front Physiol. (2010) 1:134. doi: 10.3389/fphys.2010.00134
9. YSI. Understanding ammonia in aquaculture ponds (2010). Available online at: http://www.ysi.com/media/pdfs/A585-Understanding-Ammonia-in-Aquaculture-Ponds.pdf.
10. Ruyet PLJ, Chartois H, Quemener L. Comparative acute ammonia toxicity in marine fish and plasma ammonia response. Aquaculture. (1995) 136:181–94. doi: 10.1016/0044-8486(95)01026-2
11. Randall DJ, Tsui TKN. Ammonia toxicity in fish. Mar pollut Bull. (2002) 45:17–23. doi: 10.1016/S0025-326X(02)00227-8
12. Kumar N, Kumar S, Singh AK, Gite A, Patole PB, Thorat ST. Exploring mitigating role of zinc nanoparticles on arsenic, ammonia and temperature stress using molecular signature in fish. J Trace Elements Med Biol. (2022) 74:127076. doi: 10.1016/j.jtemb.2022.127076
13. Li M, Yu N, Qin JG, Li E, Du Z, Chen L. Effects of ammonia stress, dietary linseed oil and Edwardsiella ictaluri challenge on juvenile darkbarbel catfish Pelteobagrus vachelli. Fish Shellfish Immunol. (2014) 38:158–65. doi: 10.1016/j.fsi.2014.03.015
14. National Research Council (NRC). Nutrient requirements of fish and shrimp. Washington, DC: National Academy Press (2011).
15. Guo Y.-L, Wu P, Jiang W-D, Liu Y, Kuang S-Y, Jiang J, et al. The impaired immune function and structural integrity by dietary iron deficiency or excess in gill of fish after infection with Flavobacterium columnare: regulation of NF-κB, TOR, JNK, p38MAPK, Nrf2 and MLCK signalling. Fish Shellfish Immunol. (2018) 74:593–608. doi: 10.1016/j.fsi.2018.01.027
16. Guo Y-L, Jiang W-D, Wu P, Liu Y, Zhou X-Q, Kuang S-Y, et al. The decreased growth performance and impaired immune function and structural integrity by dietary iron deficiency or excess are associated with TOR, NF-κB, p38MAPK, Nrf2 and MLCK signaling in head kidney, spleen and skin of grass carp (Ctenopharyngodon idella). Fish Shellfish Immunol. (2017) 65:145–68. doi: 10.1016/j.fsi.2017.04.009
17. Behera T, Swain P, Rangacharulu PV, Samanta M. Nano-Fe as feed additive improves the hematological and immunological parameters of fish, Labeo rohita H. Appl Nanosci. (2014) 4:687–94. doi: 10.1007/s13204-013-0251-8
18. Afshari A, Sourinejad I, Gharaei A, Johari SA, Ghasemi Z. The effects of diet supplementation with inorganic and nanoparticulate iron and copper on growth performance, blood biochemical parameters, antioxidant response and immune function of snow trout Schizothorax zarudnyi (Nikolskii, 1897). Aquaculture. (2021) 539:736638. doi: 10.1016/j.aquaculture.2021.736638
19. Zhang T, Ma C, Zhang Z, Zhang H, Hu H. NF-κB signaling in inflammation and cancer. Med Commun. (2020) 2:618–53. doi: 10.1002/mco2.104
20. Qian S, Wei Z, Yang W, Huang J, Yang Y, Jinghui Wang J. The role of BCL-2 family proteins in regulating apoptosis and cancer therapy. Front Oncol. (2022) 12:985363. doi: 10.3389/fonc.2022.985363
21. Christian F, Smith EL, Carmody RJ. The regulation of NF-κB subunits by phosphorylation. Cells. (2016) 5:12. doi: 10.3390/cells5010012
22. Zafar S, Faisal S, Jan H, Ullah R, Rizwan M, Abdullah, et al. Development of iron nanoparticles (FeNPs) using biomass of enterobacter: its characterization, antimicrobial, anti-alzheimer’s, and enzyme inhibition potential. Micromachines (Basel). (2022) 13:1259. doi: 10.3390/mi13081259
23. Kumar N, Thorat ST, Chavhan S, Reddy KS. Understanding the molecular mechanism of arsenic and ammonia toxicity and high-temperature stress in Pangasianodon hypophthalmus. Environ Sci Pollut Res. (2024) 31:15821–36. doi: 10.1007/s11356–024-32093–8
24. Kumar N, Thorat ST, Kochewad SA, Reddy KS. Manganese nutrient mitigates ammonia, arsenic toxicity and high temperature stress using gene regulation via NFkB mechanism in fish. Sci Rep. (2024) 14:1273. doi: 10.1038/s41598–024-51740–1
25. Singh AK, Lakra WS. Culture of Pangasianodon hypophthalmus in India: Impacts and present scenario. Pak J Biol Sci. (2012) 15:19–26. doi: 10.3923/pjbs.2012.19.26
26. APHA-AWWA-WEF. Standard methods for the estimation of water and waste water. 20th edn. Clesceri LS, Greenberg AE, Eaton AD, editors. Washington, DC: American Public Health Association, American Water Works Association, Water Environment Federation (1998).
27. Kumar N, Krishnani KK, Singh NP. Effect of dietary zinc-nanoparticles on growth performance, anti-oxidative and immunological status of fish reared under multiple stressors. Biol Trace Elem Res. (2018) 186:267–78. doi: 10.1007/s12011-018-1285-2
28. Kumar N, Gupta SK, Bhushan S, Singh NP. Impacts of acute toxicity of arsenic (III) alone and with high temperature on stress biomarkers, immunological status and cellular metabolism in fish. Aquat. Toxicol. (2019) 4:105233. doi: 10.1016/j.aquatox.2019.105233
29. AOAC. Official methods of analysis of the association of official analytical chemists. 16th edn. Arlington: AOAC International (1995) p. 31–65.
30. Halver JE. The nutritional requirements of cultivated warm water and cold water fish species. In Report of the FAO Technical Conference on Aquaculture, Kyoto, Japan, 26 May–2 June 1976. FAO Fisheries Report No. 188 FI/ R188 (En), p 9 (1976).
31. Kumar N, Chandan NK, Wakchaure GC, Singh NP. Synergistic effect of zinc nanoparticles and temperature on acute toxicity with response to biochemical markers and histopathological attributes in fish. Comp Biochem Physiol Part- C: Toxicol Pharmacol. (2020) 229:108678. doi: 10.1016/j.cbpc.2019.108678
32. Kumar N, Thorat ST, Gite A, Patole PB. Nano-copper enhances gene regulation of non-specific immunity and antioxidative status of fish reared under multiple stresses. Biol Trace Elem Res. (2023) 201:4926–50. doi: 10.1007/s12011-023-03575-6
33. Pfaf MW. A new mathematical model for relative quantification in real-time RT-PCR, Nucl. Acids Res. (2001) 29:e45. doi: 10.1093/nar/29.9.e45
34. Kumar N, Krishnani KK, Meena KK, Gupta SK, Singh NP. Oxidative and cellular metabolic stress of Oreochromis mossambicus as biomarkers indicators of trace element contaminants. Chemosphere. (2017) 171:265–74. doi: 10.1016/j.chemosphere.2016.12.066
35. Ali A, Bharadwaj S, O’Carroll R, Ovsenek N. HSP90 interacts with and regulates the activity of heat shock factor 1 in Xenopus oocytes. Mol Cell Biol. (1998) 18:4949–60. doi: 10.1128/MCB.18.9.4949
36. Hajirezaee S, Mohammadi G, Naserabad SS. The protective effects of vitamin C on common carp (Cyprinus carpio) exposed to titanium oxide nanoparticles (TiO2-NPs). Aquaculture. (2020) 518:734734. doi: 10.1016/j.aquaculture.2019.734734
37. Khoei AJ. Evaluation of potential immunotoxic effects of iron oxide nanoparticles (IONPs) on antioxidant capacity, immune responses and tissue bioaccumulation in common carp (Cyprinus carpio). Comp Biochem Physiol C Toxicol Pharmacol. (2021) 244:109005. doi: 10.1016/j.cbpc.2021.109005
38. Saad MJA, Morais SM, T.O. Saad STO. Reduced cortisol secretion in patients with iron deficiency. Ann Nutr Metab. (1991) 35(2):111–5. doi: 10.1159/000177633
39. Simpson ER, Mason JI. Molecular aspects of the biosynthesis of adrenal steroids. Pharmacol Ther Part B: Gen Systemat Pharmacol. (1976) 2:339–69. doi: 10.1016/S0306-039X(76)80012-8
40. Voss L, Yilmaz K, Burkard L, Vidmar J, Stock V, Hofmann U, et al. Impact of iron oxide nanoparticles on xenobiotic metabolism in Hepa RG cells. Arch Toxicol. (2020) 94:4023–35. doi: 10.1007/s00204-020-02904-1
41. Vos MJ, Hageman J, Carra S, Kampinga HH. Structural and functional diversities between members of the human HSPB, HSPH, HSPA, and DNAJ chaperone families. Biochemistry. (2008) 47:7001–11. doi: 10.1021/bi800639z
42. Desai NS, Agarwal AA, Uplap SS. HSP: evolved and conserved proteins, structure and sequence studies. Int J Bioinform Res. (2010) 2:67–87. doi: 10.9735/0975-3087
43. Zou J, Guo Y, Guettouche T, Smith DF, Voellmy R. Repression of heat shock transcription factor HSF1 by HSP90 (HSP90 complex) that forms a stress sensitive complex with HSF1. Cell. (1998) 94:471–80. doi: 10.1016/S0092-8674(00)81588-3
44. Hughes MF. Arsenic toxicity and potential mechanisms of action. Toxicol Lett. (2002) 133:1–16. doi: 10.1016/S0378-4274(02)00084-X
45. Kumar N, Singh DK, Chandan NK, Thorat ST, Patole PB, Gite A, et al. Nano−zinc enhances gene regulation of non−specific immunity and antioxidative status to mitigate multiple stresses in fish. Sci Rep. (2023) 13:5015. doi: 10.1038/s41598-023-32296-y
46. Ferrari DM, Soling HD. The protein disulphide-isomerase family: unravelling a string of folds. Biochem J. (1999) 339:1–10. doi: 10.1042/bj3390001
47. Wroblewski VJ, Masnyk M, Khambatta SS, Becker GW. Mechanisms involved in degradation of human insulin by cytosolic fractions of human, monkey, and rat liver. Diabetes. (1992) 41:539–47. doi: 10.2337/diabetes.41.4.539
48. Solovyov A, Gilbert HF. Zinc-dependent dimerization of the folding catalyst, protein disulfide isomerase. Protein Sci. (2004) 13:1902–7. doi: 10.1110/ps.04716104
49. Narindrasorasak S, Yao P, Sarkar B. Protein disulfide isomerase, a multifunctional protein chaperone, shows copper-binding activity. BBRC. (2003) 33:405–14. doi: 10.1016/j.bbrc.2003.09.226
50. Kumar N, Thorat ST, Chavhan S. Multifunctional role of dietary copper to regulate stress-responsive gene for mitigation of multiple stresses in Pangasianodon hypophthalmus. Sci Rep. (2024) 14:2252. doi: 10.1038/s41598-024-51170-z
51. Kumar N, Thorata ST, Gite A, Patole P. Synergistic effect of nickel and temperature on gene expression, multiple stress markers, and depuration: an acute toxicity in fish. Environ Sci pollut Res. (2023) 30:123729–50. doi: 10.1007/s11356–023-30996–6
52. Andrews GK. Regulation of metallothionein gene expression by oxidative stress and metal ions. Biochem Pharmacol. (2000) 59:95–104. doi: 10.1016/S0006-2952(99)00301-9
53. Saydam N, Adams TK, Steiner F, Schaffner W, Freedman JH. Regulation of metallothionein transcription by the metal-responsive transcription factor MTF-1: identification of signal transduction cascades that control metal-inducible transcription. J Biol Chem. (2002) 277:20438–45. doi: 10.1074/jbc.M110631200
54. Aileen R, James NM, Anne MW, Ian B. Effects of iron deficiency on metallothionein-I concentrations in blood and tissues of rats. J Nutr. (1989) 119:439–45. doi: 10.1093/jn/119.3.439
55. Zangar RC, Davydov DR, Verma S. Mechanisms that regulate the production of reactive oxygen species by cytochrome P450. Toxicol Appl Pharmacol. (2004) 199:316–31. doi: 10.1016/j.taap.2004.01.018
56. Zeng C, Sun H, Xie P, Wang J, Zhang G, Chen N, et al. The role of apoptosis in MCLR-induced developmental toxicity in zebrafish embryos. Gig. Sanit. (2014) 149:25–32. doi: 10.1016/j.aquatox.2014.01.021
57. Wei MC, Zong W, Cheng EH, Lindsten T, Panoutsakopoulou V, Ross AJ, et al. Proapoptotic BAX and BAK: a requisite gateway to mitochondrial dysfunction and death. Science. (2001) 292:727–30. doi: 10.1126/science.1059108
58. Banerjee B, Bhuyan G, Koner D, Saha N. Differential expression of multiple glutamine synthetase genes in airbreathing magur catfish, Clarias magur and their induction under hyper-ammonia stress. Gene. (2018) 671:85–95. doi: 10.1016/j.gene.2018.05.111
59. Vile GF, Tanew-Ilitschew A, Tyrrell RM. Activation of NFkappa B in human skin fibroblasts by the oxidative stress generated by UVA radiation. Photochem Photobiol. (1995) 62:463–8. doi: 10.1111/j.1751-1097.1995.tb02369.x
60. Dendorfer A, Heidbreder M, Hellwig-Burgel T, Johren O, Qadri F, Dominiak P. Deferoxamine induces prolonged cardiac preconditioning via accumulation of oxygen radicals. Free Radic Biol Med. (2005) 38:117–24. doi: 10.1016/j.freeradbiomed.2004.10.015
61. Hahn JY, Cho HJ, Bae JW, Yuk HS, Kim K, Park KW, et al. beta-Catenin overexpression reduces myocardial infarct size through differential effects on cardiomyocytes and cardiac fibroblasts. J Biol Chem. (2006) 281:30979–89. doi: 10.1074/jbc.M603916200
62. Zhao XS, Pan W, Bekeredjian R, Shohet RV. Endogenous endothelin-1 is required for cardiomyocyte survival in vivo. Circulation. (2006) 114:830–7. doi: 10.1161/CIRCULATIONAHA.105.577288
63. Laskar A, Eilertsen J, Li W, Yuan X-M. SPION primes THP1 derived M2 macrophages towards M1-like macrophages. Biochem Biophys Res Commun. (2013) 441:737–42. doi: 10.1016/j.bbrc.2013.10.115
64. Ai XG, Shen YF, Min C, Pang SY, Zhang JX, Zhang SQ, et al. Molecular structure, expression and bioactivity characterization of TNF13B (BAFF) gene in mefugu Takifugu obscurus. Fish Shellfish Immunol. (2011) 30:1265–74. doi: 10.1016/j.fsi.2011.03.020
65. Zhang TX, Yan ZG, Zheng X, Wang SP, Fan JT, Liu ZT. Effects of acute ammonia toxicity on oxidative stress, DNA damage and apoptosis in digestive gland and gill of Asian clam (Corbicula fluminea). Fish Shellfish Immunol. (2020) 99:514–25. doi: 10.1016/j.fsi.2020.02.046
66. Ding Y. J, Chen X. Comparative study of interleukin-17C (IL-17C) and IL-17D in large yellow croaker Larimichthys crocea reveals their similar but differential functional activity. Dev Comp Immunol. (2017) 76:34–44. doi: 10.1016/j.dci.2017.05.014
67. Lam W.S. SY, Lin SJ, Lin CC, Chen YM, Wang HC, Chen TY, et al. The expression of two novel orange-spotted grouper (Epinephelus coioides) TNF genes in peripheral blood leukocytes, various organs, and fish larvae. fish shellfish Immunol. (2011) 30:618–29. doi: 10.1016/j.fsi.2010.12.011
68. Nemeth E, Roetto A, Garozzo G, Ganz T, Camaschella C. Hepcidin is decreased in TFR2 hemochromatosis. Blood. (2005) 105:1803–6. doi: 10.1182/blood-2004-08-3042
69. Rogers JT. Ferritin translation by interleukin-1and interleukin-6: the role of sequences upstream of the start codons of the heavy and light subunit genes. Blood. (1996) 87:2525–37. doi: 10.1182/blood.V87.6.2525.bloodjournal8762525
70. Recalcati S, Locati M, Marini A, Santambrogio P, Zaninotto F, De Pizzol M, et al. Differential regulation of iron homeostasis during human macrophage polarized activation. Eur J Immunol. (2010) 40:824–35. doi: 10.1002/eji.200939889
71. Abdel Rahman AN, Mansour DA, Abd El-Rahman GI, Elseddawy NM, Zaglool AW, et al. Imidacloprid toxicity in Clarias gariepinus: protective role of dietary Hyphaene thebaica against biochemical and histopathological disruption, oxidative stress, immune genes expressions, and Aeromonas sobria infection. Aquaculture. (2022) 555:738170. doi: 10.1016/j.aquaculture.2022.738170
72. Elabd H, Youssuf H, Mahboub HH, Salem SMR, Husseiny WA, Khalid A, et al. Growth, hemato-biochemical, immune-antioxidant response, and gene expression in Nile tilapia (Oreochromis niloticus) received nano iron oxide-incorporated diets. Fish Shellfish Immunol. (2022) 128:574–81. doi: 10.1016/j.fsi.2022.07.051
73. Zhang YL, Wang GY, Zhang ZH, Xie YY, Jin H, Dong ZR. Partial amino acid metabolism and glutamine synthesis as the ammonia defensive strategies during aerial exposure in Chinese loach Paramisgurnus dabryanus. Front Physiol. (2019) 10:10–3389. doi: 10.3389/fphys.2019.00014
74. Uribe C, Folch H, Enriquez R, Moran G. Innate and adaptive immunity in teleost fish: A review. Vet Med. (2011) 56:486–503. doi: 10.17221/3294-VETMED
75. Beutler B. Innate immunity: An overview. Mol Immunol. (2004) 40:845–59. doi: 10.1016/j.molimm.2003.10.005
76. Chen P-J, Chih-Hsiang S, Chi-Yen T, Shih-Wei T, Chiung-Hsiang C. Toxicity assessments of nanoscale zerovalent iron and its oxidation products in medaka (Oryzias latipes) fish. Mar pollut Bull. (2011) 63:339–46. doi: 10.1016/j.marpolbul.2011.02.045
77. Goya GF, Marcos-Campos I, Fernandez-Pacheco R, Saez B, Godino J, As L, et al. Dendritic cell uptake of iron-based magnetic nanoparticles. Cell Bio Int. (2008) 32:1001–5. doi: 10.1016/j.cellbi.2008.04.001
78. He K, Huang R, Cheng L, Liu Q, Zhang Y, Yan H, et al. Effects of dietary nano-iron on growth, hematological parameters, immune antioxidant response, and hypoxic tolerance in juvenile Largemouth Bass (Micropterus salmoides). Aquacul Rep. (2023) 33:101759. doi: 10.1016/j.aqrep.2023.101759
79. Kripli B, Solyom B, Speier G, Kaizer J. Stability and catalase-like activity of a mononuclear non-heme oxoiron (IV) complex in aqueous solution. Molecules. (2019) 24:3236. doi: 10.3390/molecules24183236
80. Finazzi D, Arosio P. Biology of ferritin in mammals: an update on iron storage, oxidative damage and neurodegeneration. Arch Toxicol. (2014) 88:1787–802. doi: 10.1007/s00204-014-1329-0
81. Ismail M, Al-Naqeep G, Chan KW. Nigella sativa thymoquinone-rich fraction greatly improves plasma antioxidant capacity and expression of antioxidant genes in hypercholesterolemic rats. Free Radic Biol Med. (2010) 48:664–72. doi: 10.1016/j.freeradbiomed.2009.12.002
82. Nalage RR, Thorata ST, Chandramore K, Reddy KS, Kumar N. Dietary manganese nano-particles improves gene regulation and biochemical attributes for mitigation of lead and ammonia toxicity in fish. Comp Biochem Physiol Part C. (2023) 276:109818. doi: 10.1016/j.cbpc.2023.109818
83. Kumar N, Gupta S, Chandan NK, Aklakur Md, Pal AK, Jadhao SB. Lipotropes protect against pathogen-aggravated stress and mortality in low dose pesticide-exposed fish. PloS One. (2014) 9:e93499. doi: 10.1371/journal.pone.0093499
84. Behera T, Swain P, Rangacharulu PV, Samanta M. Nano-Fe as feed additive improves the hematological and immunological parameters of fish, Labeo rohita H. Appl Nanosci. (2014) 4:687–94. doi: 10.1007/s13204-013-0251-8
85. Vetrano AM, Heck DE, Mariano TM, Mishin V, Laskin DL, Laskin JD. Characterization of the oxidase activity in mammalian catalase. J Biol Chem. (2005) 280:35372–81. doi: 10.1074/jbc.M503991200
86. Thangapandiyan S, Alisha ASA, Anidha K. Growth performance, hematological and biochemical effects of iron oxide nanoparticles in Labeo rohita. Biocatalysis Agric Biotechnol. (2020) 25:101582. doi: 10.1016/j.bcab.2020.101582
87. Uzo-God OC, Agarwal A, Singh NB. Effects of dietary nano and macro iron oxide (Fe2O3) on the growth, biochemical, and hematological profiles of African catfish (Clarias gariepinus) fingerlings. J Appl Aquac. (2018) 31(2):153–71. doi: 10.1080/10454438.2018.1534704
88. Bass J, Oldham J, Sharma M, Kambadur R. Growth factors controlling muscle development, Domest. Anim. Endocrinol. (1999) 17:191–7. doi: 10.1016/S0739-7240(99)00036-3
89. Moriyama S, Ayson FG, Kawauchi H. Growth regulation by insulin-like growth factor-I in fish. Biosci Biotechnol Biochem. (2000) 64:1553–62. doi: 10.1271/bbb.64.1553
90. Prodanović R, Kirovski D, Vujanac I, Dodovski P, Jovanović L, Šamanc H. Relationship between serum iron and insulin-like growth factor-I concentrations in 10-day-old calves. Acta Vet Brno. (2014) 83:133–7. doi: 10.2754/avb201483020133
91. González EM, Contreras I, Estrada JA. Effect of iron deficiency on the expression of insulin-like growth factor-II and its receptor in neuronal and glial cells. Neurología (English Edition). (2014) 29:408–15. doi: 10.1016/j.nrleng.2013.10.017
92. Hashizume T, Horiuchi M, Tate N, Nonaka S, Mikami U, Kojima M. Effects of ghrelin on growth hormone secretion from cultured adenohypophysial cells in pigs. Domest. Anim. Endocrinol. (2003) 24:209–18. doi: 10.1016/S0739-7240(02)00240-0
Keywords: iron nanoparticles, toxicity, gene regulation, detoxification, fish
Citation: Kumar N, Thorat ST, Gunaware MA, Kumar P and Reddy KS (2024) Unraveling gene regulation mechanisms in fish: insights into multistress responses and mitigation through iron nanoparticles. Front. Immunol. 15:1410150. doi: 10.3389/fimmu.2024.1410150
Received: 31 March 2024; Accepted: 23 May 2024;
Published: 14 June 2024.
Edited by:
Elena Chaves-Pozo, Spanish Institute of Oceanography, SpainCopyright © 2024 Kumar, Thorat, Gunaware, Kumar and Reddy. This is an open-access article distributed under the terms of the Creative Commons Attribution License (CC BY). The use, distribution or reproduction in other forums is permitted, provided the original author(s) and the copyright owner(s) are credited and that the original publication in this journal is cited, in accordance with accepted academic practice. No use, distribution or reproduction is permitted which does not comply with these terms.
*Correspondence: Neeraj Kumar, bmVlcmFqX2pvdXJuYWxAbGl2ZS5pbg==