- Department of Melanoma Medical Oncology, The University of Texas MD Anderson Cancer Center, Houston, TX, United States
The gut microbiome (GMB) plays a substantial role in human health and disease. From affecting gut barrier integrity to promoting immune cell differentiation, the GMB is capable of shaping host immunity and thus oncogenesis and anti-cancer therapeutic response, particularly with immunotherapy. Dietary patterns and components are key determinants of GMB composition, supporting the investigation of the diet-microbiome-immunity axis as a potential avenue to enhance immunotherapy response in cancer patients. As such, this review will discuss the role of the GMB and diet on anti-cancer immunity. We demonstrate that diet affects anti-cancer immunity through both GMB-independent and GMB-mediated mechanisms, and that different diet patterns mold the GMB’s functional and taxonomic composition in distinctive ways. Dietary modulation therefore shows promise as an intervention for improving cancer outcome; however, further and more extensive research in human cancer populations is needed.
Introduction
The gut microbiome (GMB), defined as the collective genome of microorganisms inhabiting the intestinal tract, is capable of exerting significant influence in maintaining health and modulating disease susceptibility. Emerging evidence has illuminated its potential involvement in cancer development, progression, and therapeutic response (1). For instance, dysbiosis—characterized by alterations in GMB composition and function—has been associated with promotion of pro-tumorigenic environments, impairment of immune surveillance mechanisms, and increased risk and progression of certain cancers, such as colorectal cancer (1, 2). Moreover, the GMB plays a role in modulating systemic inflammation, metabolism of dietary components, and production of microbial metabolites, all of which are postulated to contribute to cancer development and progression (3). Mounting evidence also highlights the bidirectional relationship between the GMB and cancer treatment outcomes, with microbial communities potentially shaping drug metabolism, efficacy, and toxicity (2–9). Understanding the intricate interplay between the GMB and cancer thus holds immense potential for novel therapeutic strategies in cancer prevention and treatment. Patient outcomes in many cancers have improved dramatically with the approval of immune checkpoint blockade (ICB) (2–7). Immune checkpoints, such as CTLA-4 and PD-1, are proteins expressed by immune cells, and they function in promoting self-tolerance to prevent damage to healthy host tissue. However, in the context of cancer, these checkpoint pathways can inhibit anti-tumor activity by limiting T cell function and activation (10). Tumor cells can also exploit immune checkpoints by expressing partner proteins like PD-L1, further facilitating cancer immune evasion (11). ICB addresses these issues by inhibiting the binding of immune checkpoints to their partner proteins, thereby releasing limits on T lymphocyte activity and increasing recognition and destruction of cancer cells. For instance, anti-CTLA-4 (e.g., ipilimumab), anti-PD-1 (e.g., nivolumab), and anti-PD-L1 (e.g., atezolizumab) therapies are all ICB treatments that have demonstrated remarkable efficacy across various malignancies. By harnessing a patient’s own immune system and inducing immunologic memory, ICB offers the potential for long-term, durable response and, in some patients, cure. Despite this promise, clinical responses remain heterogeneous (12–16), indicating the complexity of factors influencing treatment outcomes, and actionable strategies to enhance ICB efficacy are urgently needed. Prior investigations have predominantly focused on tumor signaling pathways and the role of the tumor microenvironment (TME) in shaping ICB response. However, there is growing recognition of the pivotal role of host-related factors, including modifiable ones, in shaping systemic and anti-tumor immunity. Diet is one such factor, with recent research contributing to its emergence as an intriguing potential modulator of ICB response.
As well-reviewed in other literature, there are multiple mechanisms through which diet has been shown to impact carcinogenesis as well as mucosal and systemic immunity in both physiologic and pathogenic states (17–20). There is now emerging preclinical and observational evidence that diet may also impact anti-tumor immunity, particularly in the context of immunotherapy. Of particular interest is the interaction between diet, the GMB, and immunity, a well-defined interplay that has supported biological plausibility of how diet could play a major role in shaping immunity—especially as it is now well-established that the GMB can affect response to ICB in cancers (2–7).
Diet is a key determinant of GMB structure. The metabolic output of the GMB (i.e., its function) is also strongly shaped by diet, and is a key mechanism underlying the GMB’s influence on immunotherapy efficacy. There is thus a strong rationale for examining the diet-microbiome-immunity axis as a potential approach to improve immunotherapy response. Understanding of interactions between diet, the microbiome, and immunity is gaining clarity among other populations and settings (21–23), but this was previously largely unexplored in the setting of cancer outcomes and in ICB treatment specifically. In this review, we will discuss the impact of overall dietary patterns (e.g., Mediterranean diet), specific dietary regimens (e.g., ketogenic diet and fasting-mimicking diets), and dietary components (e.g., fiber) on the GMB and immunity. While this review is anchored in the diet/GMB/anti-tumor immunity interaction, it should be noted that diet is complex, and that nutrition could shape immune response via both GMB-dependent and GMB-independent mechanisms. For example, even within the context of dietary patterns that can shape the GMB, such as a plant-based diet vs. Western diet, immune-modulating metabolites can be host-, nutrient-, and/or GMB-derived.
The potential for diet as a low-cost, low-risk strategy to improve outcomes is appealing to both patients and physicians. Much work remains to be done to further establish causality, mechanism, and to refine rational therapeutic strategies. In this review, we will summarize the emerging evidence, current gaps, and future directions in our understanding of diet and anti-tumor immunity, with a central focus on the microbiome.
GMB and ICB response
Given the proximity of the GMB to gut-associated lymphoid tissue, which constitutes the largest component of the human immune system, it is no surprise that the GMB has the potential to heavily influence immune (24). The GMB is crucial for maintaining gut mucosal barrier integrity and homeostasis, and shapes both innate and adaptive immune response at local and systemic levels (25). The bidirectional interplay between the GMB and immunity has been well-studied in the context of gastrointestinal and autoimmune disorders. More recently, the GMB has also been implicated in response to cancer therapies, most notably ICB (2–4, 8, 9, 26, 27). First studied in mice, the diversity, composition, and function of the GMB have now also been associated with response to ICB in humans. Importantly, the GMB is not only a biomarker of response but also a potential therapeutic target.
Transplantation of favorable/pro-ICB-response microbiota from either mice or ICB-responding patients into mouse models can enhance response to ICB, supporting a causal link between the microbiome and immunotherapy response (2–4, 8). More recently, two early phase clinical trials of FMT + anti-PD1 in anti-PD1 refractory melanoma have demonstrated that microbiome modulation in this setting is feasible, safe, and a promising approach to overcome immunotherapy resistance (27, 28), though larger studies powered for disease outcome are needed. However, there are many challenges with scaling FMT as a therapeutic approach, including availability, procedural risks, and donor selection and recruitment (29, 30). Thus, investigation of other methods to modulate the microbiome are urgently needed. Of particular relevance is a need for further investigation into diet-microbiome-immunity axis in the context of immunotherapy as a scalable, low risk approach to microbiome modulation.
There are various possible mechanisms through which the GMB can modulate the immune system and consequently immunotherapy efficacy, many of which are potentially modifiable by diet (31). In preclinical studies, favorable GMBs have been shown to promote dendritic cell (DC) and CD8+ T cell maturation, function, and response; increase frequency of activated T cells; induce cytokine secretion, and decrease peripheral T regulatory cells (Tregs) (3, 4, 8, 9). As to how the GMB induces these immune effects, there are multiple mechanisms. First, microbial activation of pattern-recognition receptors via pathogen-associated molecular patterns like lipopolysaccharides (LPS) and peptidoglycan, can alter the maturation and priming of immune cells such as DCs and T cells (32). Interestingly, though the emphasis has been on responder microbial signatures, there are also non-responder phenotypes shared across cohorts such as LPS-expressing gram-negative bacteria, which induce innate inflammatory pathways via TLR4 (6). As such, the reduction of potentially deleterious taxa may be as important a goal as enhancing beneficial bacteria, and dietary changes like reducing animal fat intake may be an avenue to accomplish this (23, 33–35). Another putative mechanism of GMB-induced immunomodulation includes molecular mimicry, wherein microbial antigens trigger antigen-specific immune responses due to resembling other antigens (31, 36). Finally, metabolites produced by the GMB, including byproducts of the GMB’s role in harvesting host-consumed nutrients, can have effects on both mucosal and systemic immunity (31, 36).
Microbial metabolites
Microbial-derived metabolism is intimately connected with our own dietary intake. For example, riboflavin (vitamin B2) —found in dairy, meats, cereals and grains, nuts, and green vegetables (37, 38) —is metabolized by the GMB to produce derivatives that can promote expansion of mucosal-associated invariant T cells, which are seen to be activated to a greater extent in responders to PD-1 ICB (39). However, while several microbial metabolites have been linked to improvements in immunity and immunotherapy response, the same metabolites are often also implicated in cancer formation. For instance, microbial metabolism of primary bile acids from the consumption of animal fat/proteins produces secondary bile acids (SBAs), which have been implicated in hepatocellular carcinoma carcinogenesis. Despite this, the SBA ursodeoxycholic acid has been found to reduce cancer immunosuppression by inducing TGF-β degradation (40).
The role of microbial tryptophan metabolism is also controversial, with some studies suggesting indoles can act as aryl hydrocarbon receptor (AhR) agonists, promoting immune evasion in the tumor microenvironment (TME), and others showing these metabolites can promote anti-tumor immunity (41–44). One study observed that selective antibiotic targeting of Lactobacilli, which are indole-producers, reduced pancreatic tumor growth and increased T cell infiltration. Conversely, provision of L. reuteri resulted in increased tumor growth and reduction in intratumoral CD8+ T cells (45). On the other hand, other studies have found that microbial tryptophan metabolites can promote chemotherapy and ICB efficacy (43, 44). Recently, Bender et al. demonstrated that L. reuteri was able to translocate from the intestine to the tumor bed and produce indole-3-aldehyde in situ which promoted antitumor immunity and ICB response in an AhR-dependent, Tc1-cell-inducing manner (43, 44). Notably, cruciferous vegetables like broccoli, cabbage, and brussels sprouts possess dietary indole compound precursors that can activate AhR yet demonstrate reduced cancer risk in a potentially dose- and context-dependent manner (46–51).
However, the GMB metabolites that have garnered the most attention as potential mediators of ICB response are short chain fatty acids (SCFAs), which will be discussed in detail below. Many of the bacteria associated with ICB efficacy and response across studies are involved in fiber fermentation and the production of SFCAs and/or support other fiber-fermenting bacteria (2, 3, 6, 7, 52, 53). Ultimately, further investigation into the function and metabolic output of the GMB rather than its taxonomical constituents may overcome some of the lack of concordance observed across studies of microbiome and ICB response given functional redundancy of the GMB (6, 7).
Diet, the GMB, and ICB response
Environment plays a much larger role than genetics in determining GMB composition (33, 54). One of the most important potentially modifiable factors that shapes the GMB is habitual diet, which may underlie many of the regional differences observed in GMB profiles as well as the lack of concordance in defining pro-ICB response associated taxa around the globe (6, 7, 54). Multiple pre-clinical and interventional trials have reported that specific nutrients and diet components can impact the GMB (6, 55–58). However, individuals consume foods rather than isolated nutrients, and the contribution of foods to the post-digestion substrate received by the GMB may be far more variable than the nutrient content suggests—thus, examining the impact of dietary patterns on the GMB, rather than the impact of specific macro/micronutrients, may provide a better understanding of the mechanisms of diet-associated health benefits and risk.
Healthy dietary patterns emphasizing plant-based foods support favorable GMB profiles with a higher abundance of species capable of carbohydrate fermentation and an increase in SCFA producers, which in turn have been linked to improved host metabolic profile and lower cancer risk (59). In addition to stimulating production of favorable metabolites, dietary fiber can also influence cancer processes by binding with and/or absorbing carcinogenic bile acids (60, 61). Conversely, “unhealthy” diets, such as those enriched in red meat, saturated fats, processed carbohydrates, added sugars, and lower fiber, may promote the outgrowth of inflammatory bacteria implicated in carcinogenesis and encourage microbial expression of genes encoding pro-inflammatory cytokines and transcription factors associated with worse cancer outcome, including with ICB (7, 62).
Diets high in animal protein are associated with higher abundance of harmful Bacteroides spp. and lower abundance of beneficial species like E. rectale and microbes in the Lactobacillus and Roseburia genera (33). Consumption of red and/or processed meat also introduces carnitine and choline into the GMB, whose eventual metabolic product trimethylamine N-oxide (TMAO) has been linked to inflammation, type II diabetes, and obesity (63, 64). However, it should be noted that TMAO abundance has been found to be associated with improved immunotherapy response in triple-negative breast cancer, indicating a need for deeper exploration of TMAO’s oncologic impact (65). Consumption of excess animal and/or saturated fats also increases LPS that promote a colonocyte pro-inflammatory gene signature (7, 23, 34, 35, 66, 67). However, high-fat diet patterns should be distinguished from ketogenic diets, which may have very different effects on the GMB and immunity as discussed in the corresponding section.
Significant intake of ultra-processed foods (UPFs) has also been associated with GMB disruptions and increased cancer risk. Made from substances extracted or derived from whole foods, UPFs are typically high in sugar, unhealthy fats, and salt, but low in protein, fiber, vitamins, and minerals. UPFs usually also contain artificial substances like sweeteners, emulsifiers, and preservatives (68, 69). The low vitamin, mineral, and fiber content of UPFs have been shown to decrease GMB diversity, richness, abundance of fiber-fermenting bacteria, and SCFA production (70–74). Moreover, emulsifiers found in UPFs, such carboxymethyl cellulose (CMC) and polysorbate 80 (P80), may promote bacterial translocation across the mucosal barrier, increase LPS synthesis, and enhance colon carcinogenesis (75–77). Observational studies have also found correlations between high UPF intake and overall cancer risk, as well as risk of specific conditions like breast, colorectal, and pancreatic cancer. Thus, while the direct, negative impact of UPFs on immunity has yet to be decisively proven, their consumption has been shown to be associated with dysbiosis and metabolome perturbations, which may thereby affect anti-cancer immunity.
While habitual diet is a major determinant of the microbiome, an important question is whether diet change can change the microbiome, i.e., whether it can be used therapeutically. In a landmark study by David et al, participants consumed one of two diets ad libitum over the course of 5 days: an animal-based diet, which consisted of meats, eggs, and cheeses, or a plant-based diet, which was based on grains, legumes, fruits, and vegetables (78). After the study, those in the animal-based diet arm were found to have increased abundance of bile-tolerant microbes, potentially increasing the carcinogenic burden of compounds like SBAs. The animal-based diet also increased bacterial gene expression for microbial bile salt hydrolases, which are prerequisites for the production of deoxycholic acid by the GMB, a potentially carcinogenic metabolite. In contrast, participants who consumed a plant-based diet had higher abundance of beneficial fiber-fermenting Firmicutes bacteria, such as Roseburia spp., E. rectale, and R. bromii (78).
Similar to the study by David et al, O’Keefe et al. performed a 2-week diet exchange between African-Americans and rural Africans. The African-American diet generally consisted of high fat, high animal protein, and low fiber content, while the rural African diet was plant-based, low fat, and high fiber content. Upon switching to the African-American diet, rural African participants displayed increased mucosal epithelial proliferation rates and greater inflammatory markers in tissue (e.g. CD68+ lamina propria macrophages). Increased SBA synthesis was also observed, with fecal SBAs increasing by almost 400% in African participants after consuming the African-American diet. Meanwhile, African-American participants consuming the rural African diet exhibited an almost 70% decrease in fecal SBAs, lower colonic mucosal inflammation and proliferation rates, and increased saccharolytic fermentation and butyrogenesis. Study participants’ reciprocal changes in mucosal/gut metabolome cancer risk biomarkers as a result of the diet swap suggest that the diet-microbiome interaction may play a key role in the higher rates of colorectal cancer found in industrialized countries (79).
Fiber, SCFAs, and immunity
A common feature shared by plant-based diets is the possession of high amounts of dietary fiber, which is defined as nondigestible carbohydrates and lignin that are intrinsic and intact in plants (80–82). In preclinical models, fiber deprivation lowers microbial diversity (83) and decreases the abundance of beneficial fiber-fermenting bacteria such as F. prausnitzii (bacteria associated with response to ICB). Insufficient fiber can also cause some bacterial taxa to begin degrading host mucin glycans, thus thinning the mucus layer; promoting inflammation, bacterial translocation, and oncogenic signaling pathways; and inducing greater tumor development as a result (83, 84).
SCFAs, i.e., butyrate, acetate, and propionate, are the product of dietary fiber fermentation by gut microbiota and a major mediator of the effects of plant-based diets. SCFAs serve as the main energy source for intestinal epithelial cells (IECs) (85), ensuring mucosal barrier integrity and preventing bacterial translocation (86–89), and also have both indirect and direct effects on immunity. In vitro administration of SCFAs to IECs activates G protein coupled receptors (GPCRs), increases the production of anti-inflammatory cytokines like IL-10 (85), and can also abrogate neutrophil recruitment, regulating the production of inflammatory mediators like TNF-a and IL-17 and thus playing an important role in recruiting leukocytes and activating T lymphocytes (88). SCFAs can also induce plasma cell production of IgA, blocking virulence and bacterial adherence to epithelial cells (90, 91). Perhaps most relevant for ICB response is that SCFAs can also enhance T cell activity. For example, acetate has been shown to enhance IFN-γ production from CD8+ and intratumoral cytotoxic T cells (CTLs) via the mammalian target of rapamycin pathway (92) as well as memory CD8+ T cell responses via GAPDH enzyme activity and glycolysis (93). Meanwhile, the role of the SCFA butyrate in suppressing carcinogenic pathways, such as the Wnt signaling pathway, has been well-studied in colonocytes (94, 95). More recent studies have demonstrated that butyrate can also impact immune cell function via histone deacetylation inhibition (88), which upregulates IFN-γ and granzyme B expression while suppressing IL-17A production in CTLs, thus facilitating an increase in the switch of Tc17 cells to a CTL phenotype (92).
However, although multiple studies have demonstrated the potential benefits of fiber and SCFAs for cancer immunity, there is also evidence for their negative impact. For instance, one study found that incorporating the fiber inulin into mice’s diets induced hepatocellular carcinoma via a microbiota-dependent mechanism (96). Another experiment detected an increase in mouse colonic tumor load when the amount of fiber—consisting of either inulin, cellulose, or brewers spent grain—was increased in their diets (97). Other studies, both human and preclinical, have also found an association between fiber/SCFAs and aggravation of factors promoting cancer risk/development, such as greater production of cancer-associated cytokines (88, 98–101). These seemingly discrepant results may be driven by the specific sources of dietary fiber incorporated, types of fiber, and differences in study populations, including cancer type (102). Isolated fiber may also have different effects than whole-food-derived fiber, i.e., it is possible that the combination of fiber and the micronutrients found in whole-food/natural dietary fiber sources is required in order for patients to reap the potential cancer-inhibiting benefits of a high-fiber diet. Furthermore, specific types of fiber may elicit differential biological effects (97, 103, 104), with one murine study observing a significantly lower tumor load in inulin-fed mice than in mice fed cellulose or brewers spent grain (97). It thus remains to be seen how we may optimize fiber-related dietary interventions targeted at improving cancer immunity in a microbiota-dependent manner. However, given the enormous amount of data supporting the health benefits of a whole-food, high-fiber diet and the context-dependent factors that may have led to the negative effects observed in other studies, it is reasonable to recommend increasing fiber consumption through natural sources while continuing to research its impact on cancer immunity.
Given the existing literature on the immunomodulatory role of SCFAs and the known role in fiber fermentation of many pro-ICB-response bacteria, there was a strong rationale to examine the potential link between dietary fiber consumption and immunotherapy response. Indeed, a 2021 study by Spencer et al. incorporated dietary screener questionnaires into their observational microbiome cohort and found that habitual higher dietary fiber consumption was associated with significantly improved progression-free survival in melanoma patients undergoing ICB (105). Similarly, a 2022 multicenter cohort study observed that a Mediterranean diet pattern—which features high intake of plant-based fiber alongside omega-3 fatty acids and various micronutrients—was positively associated with ICB response, progression-free survival, and less immunotoxicity among patients with advanced melanoma (106). As expected, dietary fiber intake is positively correlated with pro-ICB-response, fiber-fermenting bacteria (58). These findings were recapitulated in murine melanoma models, where high-fiber diet fed mice displayed improved treatment response to anti-PD-1 ICB—with increased CD4+ T cell infiltration in tumors, and greater expression of genes linked to T-cell activation and interferon response in CD45+ TILs —compared to anti-PD1 low-fiber fed mice (105).
Rapid shifts in the GMB and lower stool concentrations of the SCFA propionate are also observed with fiber deprivation in preclinical models. Importantly, synergy between ICB and dietary fiber were not seen in germ-free mice, supporting that the effects were indeed microbiome-mediated. In another preclinical study, a high-fiber diet modulated the GMB in a manner that triggered this intratumoral IFN-1/natural killer cell (NKC)/DC axis, improving ICB efficacy and programming TME mononuclear phagocytes toward immunostimulatory phenotypes (107). Given these observational and preclinical data supporting the role of fiber in modulating the microbiome and immunity, human interventional studies (NCT03950635 and NCT04645680) are now ongoing that investigate high-fiber diet as a GMB-focused strategy to improve anti-cancer immunity. Be that as it may, future microbiome-centered dietary interventions will likely require tailoring to individual patients. Recent findings suggest that GMB compositions associated with high fiber intake—namely, high-diversity GMBs with high relative Ruminococcaceae, butryogen, and methanogen abundance—are associated with ICB response, but that modulating taxa composition alone may not be enough to improve outcome. For instance, having higher relative abundance of merely one putatively beneficial taxon, such as F. prausnitzii, may be insufficient to induce response, and was in fact observed to pose no further advantage for ICB outcome in patients already possessing high-fiber-associated GMBs prior to ICB (58). Employing high-fiber diets in the context of immunotherapy may thus be an intervention better suited for patients with a GMB composition shaped by habitual low-fiber intake [i.e., low-diversity, Bacteroides-dominated microbiomes (58)], but even then, tumor-intrinsic, systemic, and other factors also need to be considered.
Dietary probiotics
Probiotics are live microorganisms with putative health benefits when consumed. They are found in fermented foods such as kimchi, kefir, sauerkraut, etc., which are “produced through controlled microbial growth … and the conversion of food components through enzymatic action” (108). As such, probiotic foods contain both live microorganisms and food sources for said microorganisms, such as non-digestible carbohydrates like inulin-type fructans and galacto-oligosaccharides (109, 110). This allows probiotic foods to support a beneficial GMB composition by encouraging the growth of bacteria like F. prausnitzii while pulling resources from and thus suppressing the growth of pathogenic bacteria.
These naturally occurring dietary probiotics, however, stand in contrast to commercial probiotic supplements, which are generally not targeted/rationally designed, but instead frequently composed of only aerobic, easy-to-culture bacteria in order to minimize production costs (111, 112). Commercial probiotics also lack the beneficial nutrients inherent to probiotic foods, such as essential amino acids, polyphenols, different types of fibers, and other fermentable bioactive compounds (113, 114). In fact, over-the-counter probiotic supplements have been shown to lower overall GMB diversity and worsen ICB response in preclinical models and were also associated with worse outcomes with ICB in an observational study (105). The negative impacts of unselected probiotics on GMB homeostasis has also been demonstrated after antibiotic use, wherein probiotics inhibited the restoration of normal gut ecology after antibiotics (105, 111). Thus, the use of probiotics outside the context of a clinical trial should be discouraged at this time.
Conversely, observational data suggests a beneficial impact of dietary probiotics on the GMB and immunity. Natural yogurt consumers have been found to display healthier metabolic profiles, with lower levels of inflammation and serum lipid peroxidation and higher fecal concentrations of Akkermansia (115, 116). In a preclinical study by Woo et al., mice with DSS-induced colitis were fed with a fermented barley and soybean mixture which led to increased intestinal barrier function and decreased levels of inflammatory cytokines in colonic tissue. The fermented mixture was also found to suppress bacterial translocation to mLNs and promote growth of the anti-inflammatory bacterial genera Lactobacilli and Bacteroides, the latter of which has been demonstrated to be an important contributor to anti-CTLA-4 ICB efficacy (9, 117).
A recent human interventional study substantiated these health-promoting effects of dietary probiotics. Wastyk et al. conducted a high-fermented-food diet intervention in healthy individuals targeting consumption of 6 servings of fermented foods daily. Post-intervention, participants exhibited increased GMB diversity; importantly, beneficial taxa that were enriched were in large part not derived from the microbes found in the fermented foods consumed, suggesting that dietary probiotics can influence the GMB composition both directly and indirectly and seem to support the overall ecosystem. They further examined the effects of this diet on circulating immunocytes, finding increased effector memory CD4+ T cells with decreased non-classical monocytes and circulating inflammatory cytokines in the post-intervention specimens (21).
Ketogenic diets
Ketogenic Diets (KD), characterized by low carbohydrate and high fat content, aim to reduce carbohydrate intake to the point of ketosis, where ketone bodies are produced and metabolized as an energy source rather than glucose, the usual main source of energy. The premise of using the ketogenic diet in cancer is based on the “Warburg effect”, an observation that cancer cells rely primarily on aerobic glycolysis rather than mitochondrial oxidative phosphorylation (118). However, it has subsequently been shown that cancer cells are much more metabolically plastic than originally thought (119). Nonetheless, the ketogenic diet has been shown to limit cancer growth in many preclinical models via lowering of insulin and IGF-1, and intriguingly has shown synergy in combination with cancer therapies that disrupt the insulin/IGF-1/PI3K axis in host organs (120).
Recent studies have examined the impact of the KD on anti-tumor immunity and on the GMB. In murine models, Ferrere et al. found that KD inhibited tumor growth and cancer progression and promoted ICB efficacy, at least in part via the ketone body 3-hydroxybutyrate (3HB) in a T-cell dependent manner (121). Notably, systemic administration of 3HB mimicked the antitumor effects of KD, enhancing ICB efficacy and promoting CXCR3+ T cell expansion. In terms of the effects on the microbiome, KD led to an increased abundance of A. muciniphila and other immunogenic bacteria. The tumor-suppressive effects of KD and 3HB were attenuated by antibiotics, indicating that the microbiome may mediate the KD’s anti-cancer effects (121). Other murine studies have similarly found that KD can increase potentially beneficial bacteria like A. muciniphila and Lactobacillus while decreasing putative inflammatory taxa, such as Desulfovibrio and Turicibacter (122).
However, implementation of KD in humans, especially cancer patients, requires close supervision by registered dietitians, as hypoglycemia, weight loss, and electrolyte imbalances may occur (123). Further, KD limits fruit, vegetables, whole grains, and fiber ingestion, and thus the effects of this diet on the human microbiome need to be carefully considered. Even so, clinical data has shown some promise. In a human diet intervention study by Link et al, participants sequentially consumed KD and vegan diets for 2 weeks each and in randomized order. Both KD and vegan diet induced differential changes on host immunity and GMB composition: the KD increased activated Treg and CD16+ NKC abundance as well as T cell activation; meanwhile, the vegan diet increased activated T helper and NKCs and upregulated innate-immunity-associated pathways (124). Further investigation is needed to elucidate the potential implications for anti-cancer immunity.
Calorie restriction/fasting-mimicking diet
Calorie restriction (CR) is defined as consuming 50-90% of usual ad libitum calorie intake without going to the extent of malnutrition. On the other hand, a fasting-mimicking-diet (FMD) reduces calorie intake to a lesser extent and is specifically low in protein and sugar yet high in unsaturated fat content (125–129). Multiple preclinical studies have demonstrated that CR and/or fasting-like conditions reduce cancer risk, incidence, progression, and mortality, though data in humans remains limited (107, 130–133). Like the KD, a major target of CR and FMD is insulin and IGF-1 levels. High serum IGF-1 has been associated with increased risk of multiple cancers (134). In rodents, long-term CR reduces serum IGF-1 concentration by 30-40%, and fasting in humans markedly decreases serum IGF-1 concentration provided that protein intake is not excessively high (135). In terms of immune effects, preclinical studies have found that CR alters NKC maturation and function; meanwhile, fasting-like conditions have been shown in pre-clinical models to increase cytotoxic CD8 T cell frequency and reduce Treg tumors in the TME while also impacting NKC maturation (107, 136–138).
FMD has been shown to enhance chemotherapy efficacy in murine breast cancer models by promoting T-cell-mediated tumor immunogenicity and cytotoxicity (107, 136–138). More specifically, FMD administration induced greater cytotoxic CD8+ T cell recruitment, greater levels of common lymphoid progenitor cells and circulating/tumor-infiltrating CD8+ lymphocytes, and decreased activation and frequency of Treg cells. These effects were mediated at least in part by a decrease in expression of the heme oxygenase-1 (HO-1) enzyme in cancer cells. This downregulation of HO-1 expression served to selectively sensitize cancer cells to chemotherapy while bypassing normal cells, whose HO-1 expression levels were not impacted (137, 139, 140).
Similarly, preclinical studies have shown that FMD elevates IFN-γ levels, increases CTLs and NKCs, promotes switching of CD8+ T cells to an activated/memory phenotype, and decreases immunosuppressive myeloid-derived suppressor cells (MDSCs) and Tregs (107). A periodic FMD has also been shown sensitize cancer cells to ICB, increasing anti-PD-L1 and anti-OX40 ICB efficacy against triple-negative breast tumors. In these murine subjects, FMD cycles were found to reactivate and expand early exhausted effector T cells and induce cancer cells to switch from respiratory to glycolytic metabolism (141). Meanwhile, human studies found that fasted cancer patients who adhered to a 5-day FMD (700 kcal consumed on day 1; 300 kcal consumed days 2-5) every 21-28 days displayed greater amounts of activated T cells and cytolytic NKCs in peripheral blood (142). In another study of heterogeneously treated (not ICB) cancer patients, FMD augmented intratumoral Th1/cytotoxic responses and reduced immunosuppressive MDSCs and Tregs. The study also noted greater amounts of CTLs and NKCs in patient blood; increased intratumoral populations of CD8+ T cells, DCs, NKCs, and effector memory T lymphocytes; a heightened switch of CD8+ cells toward a memory/activated phenotype; and increased levels of T cell stimulating cytokines (107). Another clinical trial observed that administration of hydroxycitrate, a CR mimetic, lead to depletion of Tregs and thus heightened anticancer immunosurveillance and decreased tumor burden (143).
In terms of the GMB, murine studies on CR/FMD have observed an increase in populations of putative protective/beneficial bacteria, such as SCFA-producing Bifidobacterium and Faecalibaculum as well as immunogenic A. muciniphila (144–147). In the same vein, preclinical models have noted a decrease in reputed inflammatory taxa, such as Helicobacter, Streptococcaceae, and Desulfovibrionaceae (144, 145, 148). Therefore, FMD and CR lead to marked systemic changes in the GMB, metabolism, and immunity in ways that substantiate the further investigation of FMD/CR. However, these types of diet interventions in patients with cancer should only be utilized under physician supervision and in the context of a clinical trial.
Conclusion
There is now a substantial body of evidence demonstrating that the GMB can impact both cancer risk and development as well as ICB response. However, mechanisms as well as the most effective and scalable strategies to modulate the microbiome to improve ICB outcomes remain to be active areas of investigation. Diet is a key determinant of the GMB and can impact immunity via both GMB-mediated mechanisms as well as GMB-independent pathways (78, 149).
Cohort studies support that a habitual high dietary fiber intake is associated with improved response to ICB (58, 105, 150), and that in preclinical models, dietary fiber manipulation can modulate ICB response via the GMB. However, it is unclear in humans whether the epidemiological association between dietary fiber and ICB response is truly from fiber specifically vs. from other beneficial nutrients found in a fiber-rich diet. Meanwhile, although diet patterns such as a plant-based vs. Western diet are captured in epidemiological dietary assessments, more “radical” diets such as the ketogenic diet and calorie restriction cannot be, yet they are much easier to study in rodent models. Thus, while there is ample literature supporting the anti-cancer effects of these diets in preclinical models, human data in cancer populations remain limited.
While diet is a relatively inexpensive, low-risk, scalable approach to potentially modulate the microbiome and improve ICB response, there are also several challenges. The exact mechanisms by which “beneficial” vs. “detrimental” dietary interventions produce their respective effects on cancer/immunotherapy need to be further defined (29). It also remains unclear if such effects are divided by tumor type: studies are limited by what model was used, and so it cannot be said with certainty that any observed effects of diet on immunity are tumor-specific. Therefore, until evidence emerges that a dietary intervention induces anti-tumor immunity in one cancer type but not another, future research should endeavor to study a diet’s effects across tumor models and patient populations to determine what effects are tumor-specific vs. agnostic. Another key question is whether the focus should be on specific nutrients/food components or on larger dietary patterns. For specific food components such as fiber, the “dose” needed to induce GMB changes has not been defined. Patient selection for microbiome-directed dietary interventions is another key question—should this be based on microbiome profiling, diet assessment, or a combination of the two? Then there is the heterogeneity and complexity of the GMB: the host factors and exposures that shape this ecosystem include ethnicity, body composition, metabolic phenotype, comorbidities, medication use, and still other lifestyle factors. In terms of timing, does the microbiome need to be primed prior to ICB initiation, and/or do diet changes need to be sustained throughout treatment? To begin addressing these questions, what is needed are rigorously and rationally designed interventional human studies with microbiome and disease endpoints. However, in addition to these questions regarding mechanism and mode, there is the additional challenge that behavior change is difficult, and supporting patients to make these changes will require provider education, increased access to dietitians or health coaches with a focus on diet optimization, tools such as apps and recipes, and ultimately public health measures.
In short, diet influences the GMB, which is potentially associated with clinical cancer outcomes and efficacy in ICB responses (Figures 1–3) via various possible mechanisms (Table 1). Although data is still emerging, based on published preclinical and human studies (Table 2), we recommend avoiding broad-spectrum antibiotic treatment before and during ICB and advise against therapies employing untargeted commercial probiotic supplements. We also recommend incorporating professional dietary assessments and counseling in patient consultations, and we encourage patients to consume a diverse diet rich in natural probiotics, plant species/plant-based foods, and dietary fiber. However, we advise patients to consider that more investigation is needed on dietary interventions such as KD and FMD, and that these diets should only be pursued under clinical supervision. The interactions between food components, gut microbes, the host immune system, and the genetic/immune characteristics unique to different cancers are complex and heterogeneous. Nevertheless, findings on the GMB-mediated effects of dietary interventions to improve antitumor immunity and ICB response support the pursuit of further, large-scale research to develop new interventions that could synergize with existing immunotherapy regimens to hopefully improve outcomes.
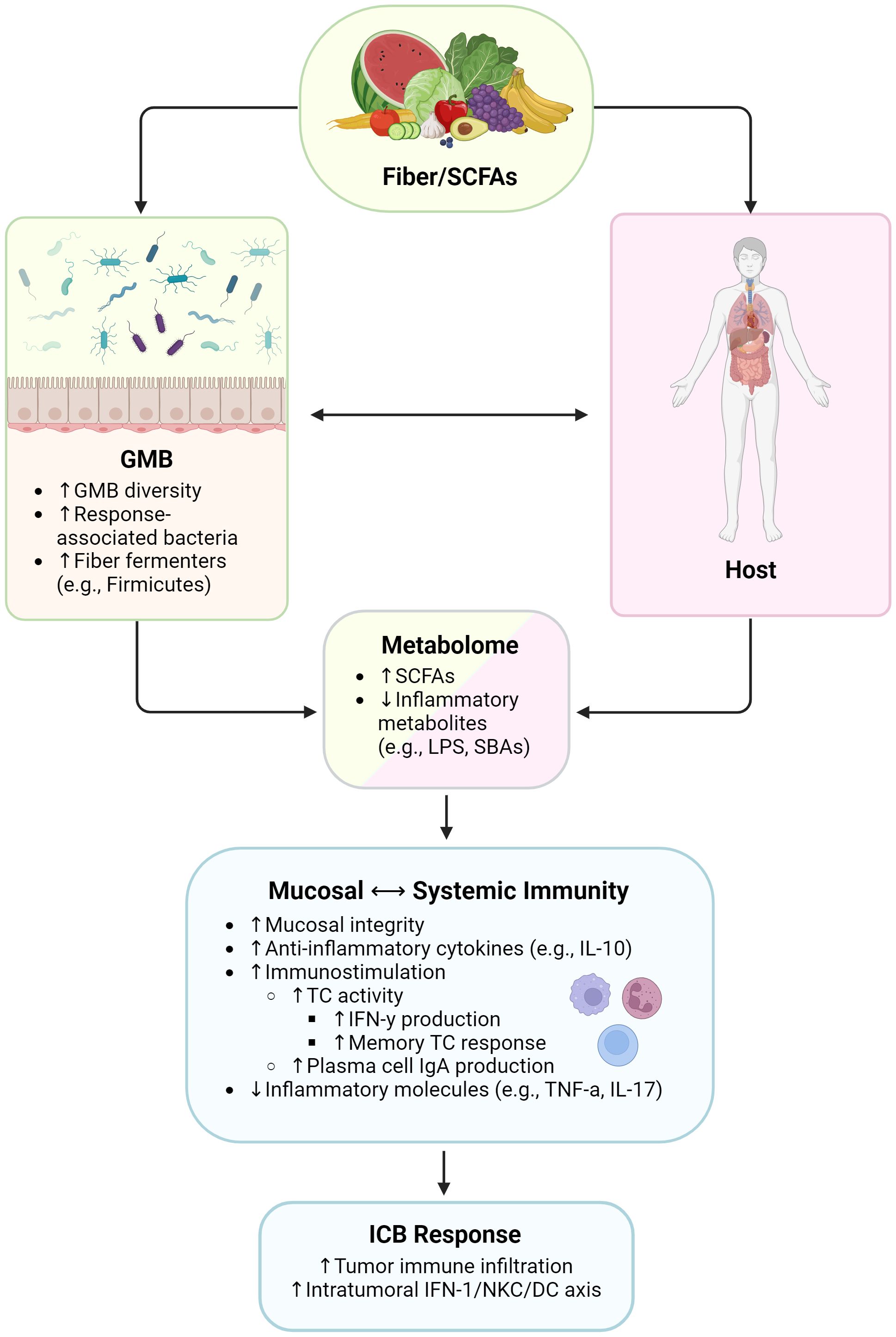
Figure 1 Influence of high-fiber diet and SCFAs on immunity and immunotherapy responses. SCFAs, short chain fatty acids; GMB, gut microbiome; LPS, lipopolysaccharides; SBAs, secondary bile acids; IL, interleukin; TC, T cell; IFN-γ, interferon gamma; IgA, immunoglobulin A; TNF-α, tumor necrosis factor alpha; IFN-1, type 1 interferon; NKC, natural killer cell; DC, dendritic cell. Created with BioRender.com.
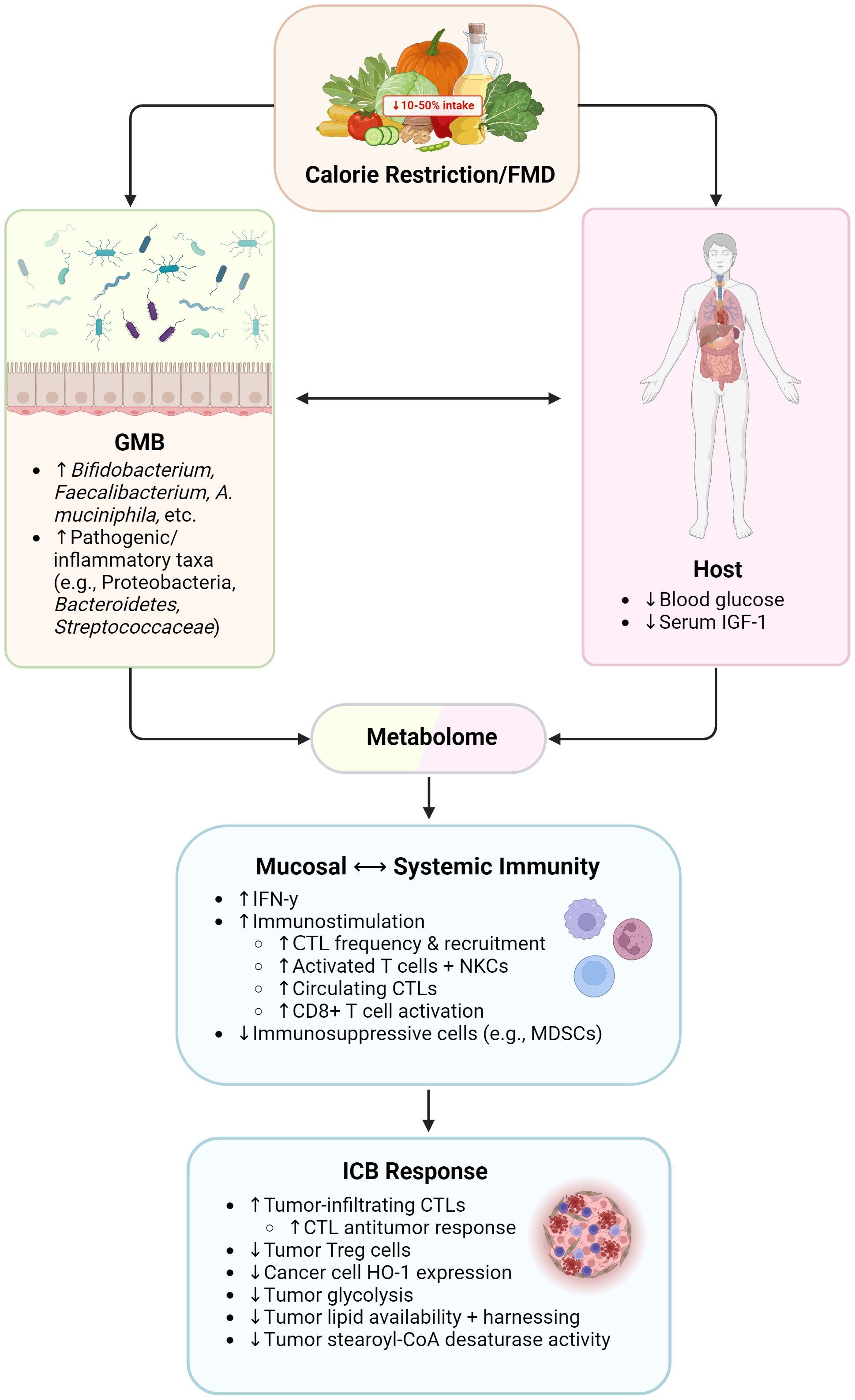
Figure 2 Influence of ketogenic diet on immunity and immunotherapy responses. GMB, gut microbiome. Created with BioRender.com.
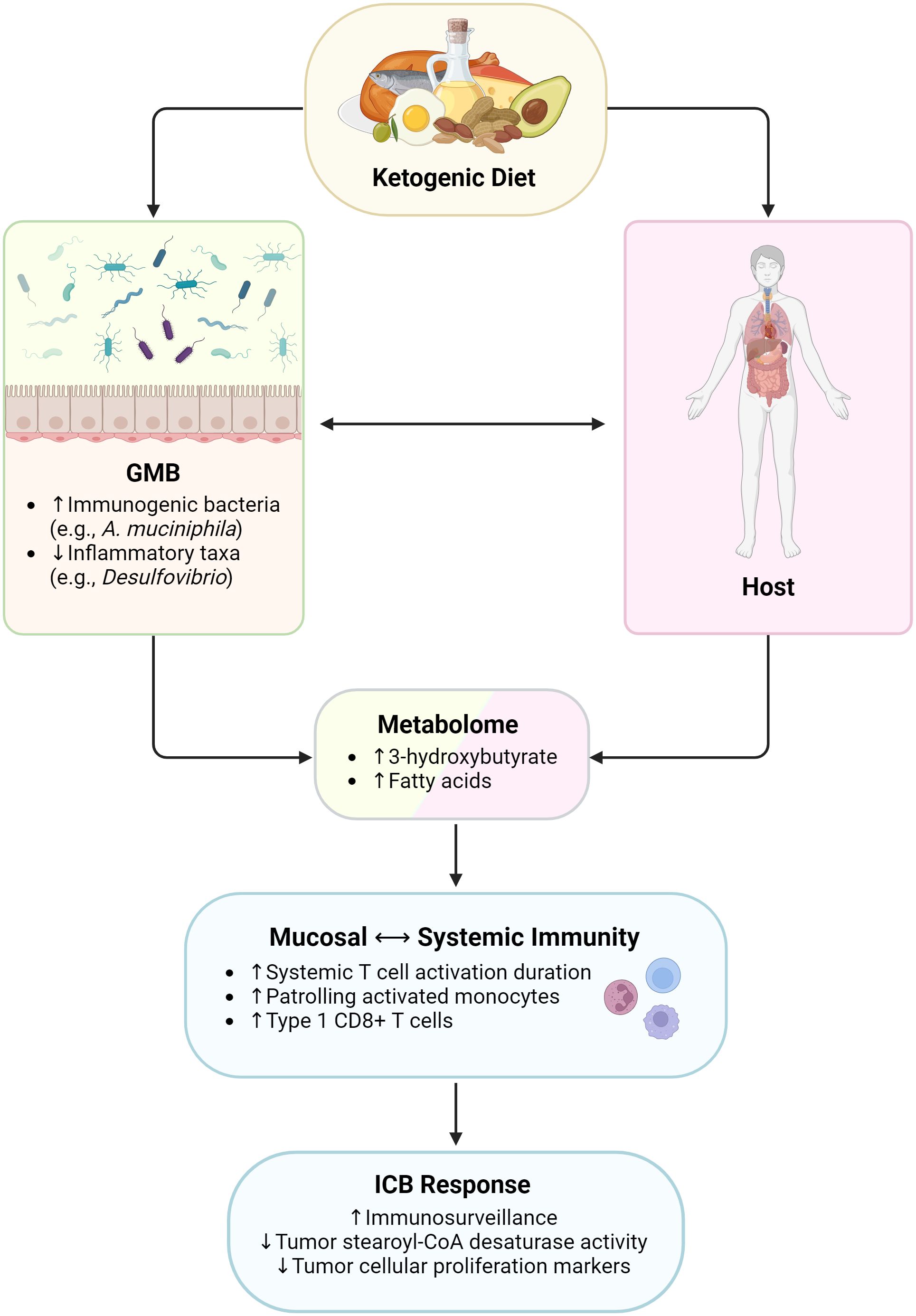
Figure 3 Influence of calorie restriction/fasting-mimicking diet on immunity and immunotherapy responses. FMD, fasting-mimicking diet; GMB, gut microbiome; IGF-1, insulin-like growth factor 1; IFN-γ, interferon gamma; CTL, cytotoxic T lymphocyte; NKCs, natural killer cells; MDSCs, myeloid-derived suppressor cells; Treg cells, regulatory T cells; HO-1, heme oxygenase 1. Created with BioRender.com.
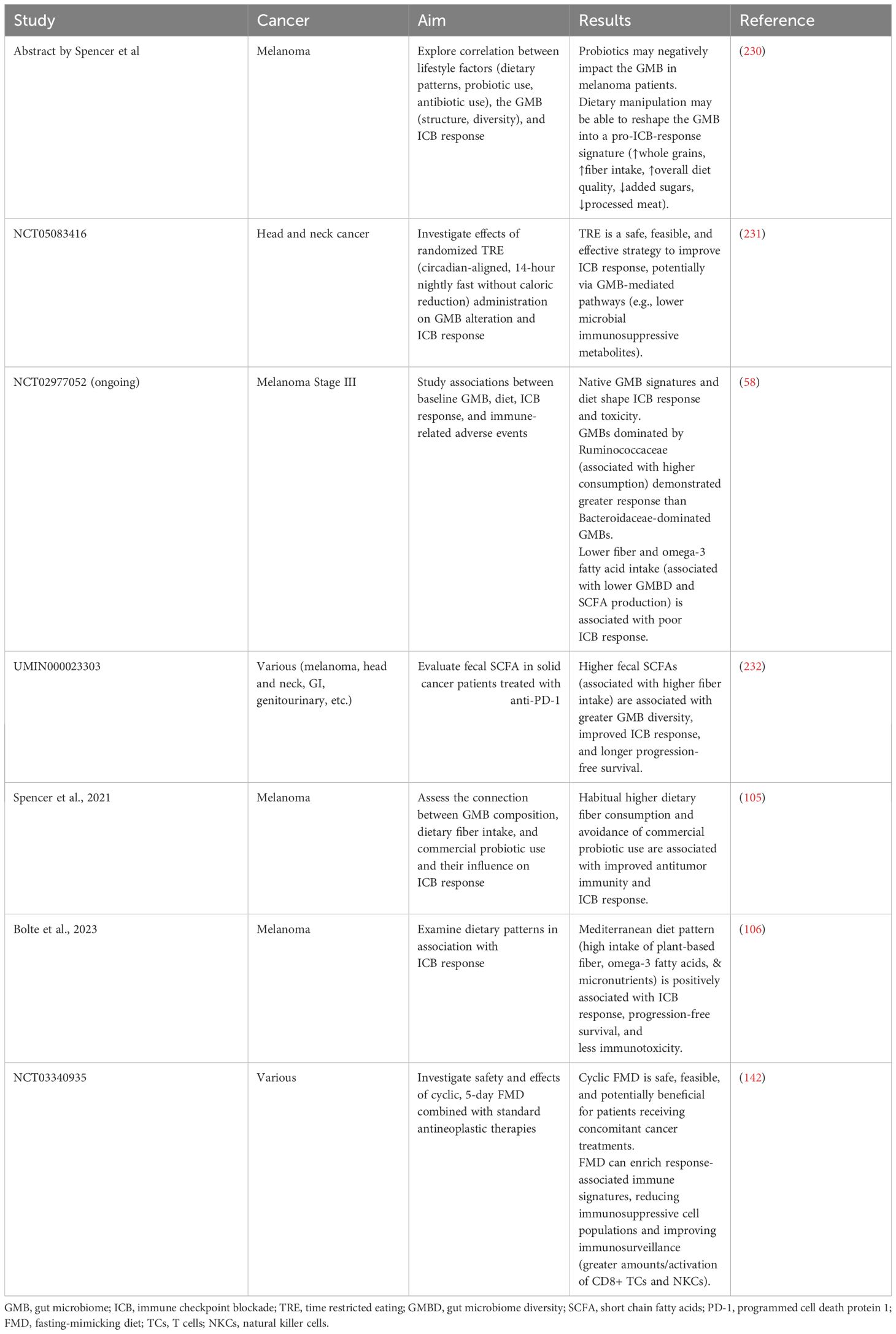
Table 2 Recommendations from published clinical studies investigating the role of diet/dietary components on immunotherapy in cancer patients.
Author contributions
N-TAN: Conceptualization, Visualization, Writing – original draft, Writing – review & editing. YJ: Conceptualization, Supervision, Visualization, Writing – review & editing. JM: Conceptualization, Funding acquisition, Supervision, Writing – review & editing.
Funding
The author(s) declare financial support was received for the research, authorship, and/or publication of this article. Financial support: This work was supported by American Society of Clinical Oncology and Conquer Cancer Foundation Young Investigator Award and Career Development Award to JM. JM also acknowledges the Transdisciplinary Research in Energetics and Cancer Research Training Workshop R25CA203650 and the MD Anderson Cancer Center (MDA) Center for Energy Balance in Cancer Prevention and Survivorship and is supported by the Melanoma Research Alliance, the Elkins Foundation, Seerave Foundation, Rising Tide Foundation, the Mark Foundation for Cancer Research, MDA Melanoma SPORE Developmental Research Program Award, MDA Physician Scientist Program and MDA Moonshot Program.
Conflict of interest
JM is a consultant for Bristol-Myers Squibb, Roche, and Merck.
The remaining authors declare that the research was conducted in the absence of any commercial or financial relationships that could be construed as a potential conflict of interest.
Publisher’s note
All claims expressed in this article are solely those of the authors and do not necessarily represent those of their affiliated organizations, or those of the publisher, the editors and the reviewers. Any product that may be evaluated in this article, or claim that may be made by its manufacturer, is not guaranteed or endorsed by the publisher.
Abbreviations
GMB, gut microbiome; ICB, immune checkpoint blockade; TME, tumor microenvironment; DC, dendritic cell; LPS, lipopolysaccharides; SBAs, secondary bile acids; AhR, aryl hydrocarbon receptor; TME, tumor microenvironment; SCFAs, short chain fatty acids; TMAO, trimethylamine N-oxide; IECs, intestinal epithelial cells; UPFs, ultra-processed foods; CMC, carboxymethyl cellulose; P80, polysorbate 80; GPCRs, G protein coupled receptors; CTLs, cytotoxic T cells; KD, Ketogenic Diets; 3HB, 3-hydroxybutyrate; CR, calorie restriction; FMD, fasting-mimicking-diet; HO-1, heme oxygenase-1; MDSCs, myeloid-derived suppressor cells; NKCs, natural killer cells.
References
1. Schwabe RF, Jobin C. The microbiome and cancer. Nat Rev Cancer. (2013) 13:800–12. doi: 10.1038/nrc3610
2. Gopalakrishnan V, Spencer CN, Nezi L, Reuben A, Andrews MC, Karpinets TV, et al. Gut microbiome modulates response to anti-pd-1 immunotherapy in melanoma patients. Science. (2018) 359:97–103. doi: 10.1126/science.aan4236
3. Routy B, Le Chatelier E, Derosa L, Duong CPM, Alou MT, Daillere R, et al. Gut microbiome influences efficacy of pd-1-based immunotherapy against epithelial tumors. Science. (2018) 359:91–7. doi: 10.1126/science.aan3706
4. Matson V, Fessler J, Bao R, Chongsuwat T, Zha Y, Alegre ML, et al. The commensal microbiome is associated with anti-pd-1 efficacy in metastatic melanoma patients. Science. (2018) 359:104–8. doi: 10.1126/science.aao3290
5. Dizman N, Meza L, Bergerot P, Alcantara M, Dorff T, Lyou Y, et al. Nivolumab plus ipilimumab with or without live bacterial supplementation in metastatic renal cell carcinoma: A randomized phase 1 trial. Nat Med. (2022) 28:704–12. doi: 10.1038/s41591-022-01694-6
6. Lee KA, Thomas AM, Bolte LA, Bjork JR, de Ruijter LK, Armanini F, et al. Cross-cohort gut microbiome associations with immune checkpoint inhibitor response in advanced melanoma. Nat Med. (2022) 28:535–44. doi: 10.1038/s41591-022-01695-5
7. McCulloch JA, Davar D, Rodrigues RR, Badger JH, Fang JR, Cole AM, et al. Intestinal microbiota signatures of clinical response and immune-related adverse events in melanoma patients treated with anti-pd-1. Nat Med. (2022) 28:545–56. doi: 10.1038/s41591-022-01698-2
8. Sivan A, Corrales L, Hubert N, Williams JB, Aquino-Michaels K, Earley ZM, et al. Commensal bifidobacterium promotes antitumor immunity and facilitates anti-pd-L1 efficacy. Science. (2015) 350:1084–9. doi: 10.1126/science.aac4255
9. Vetizou M, Pitt JM, Daillere R, Lepage P, Waldschmitt N, Flament C, et al. Anticancer immunotherapy by ctla-4 blockade relies on the gut microbiota. Science. (2015) 350:1079–84. doi: 10.1126/science.aad1329
10. Hahn AW, Gill DM, Pal SK, Agarwal N. The future of immune checkpoint cancer therapy after pd-1 and ctla-4. Immunotherapy. (2017) 9:681–92. doi: 10.2217/imt-2017-0024
11. Patel SP, Kurzrock R. Pd-L1 expression as a predictive biomarker in cancer immunotherapy. Mol Cancer Ther. (2015) 14:847–56. doi: 10.1158/1535-7163.MCT-14-0983
12. Larkin J, Chiarion-Sileni V, Gonzalez R, Grob JJ, Cowey CL, Lao CD, et al. Combined nivolumab and ipilimumab or monotherapy in untreated melanoma. N Engl J Med. (2015) 373:23–34. doi: 10.1056/NEJMoa1504030
13. Hamid O, Robert C, Daud A, Hodi FS, Hwu WJ, Kefford R, et al. Five-year survival outcomes for patients with advanced melanoma treated with pembrolizumab in keynote-001. Ann Oncol. (2019) 30:582–8. doi: 10.1093/annonc/mdz011
14. Tawbi HA, Chung C, Margolin K. Nivolumab and ipilimumab in melanoma metastatic to the brain. N Engl J Med. (2018) 379:2177–78. doi: 10.1056/NEJMc1812500
15. Larkin J, Chiarion-Sileni V, Gonzalez R, Grob JJ, Rutkowski P, Lao CD, et al. Five-year survival with combined nivolumab and ipilimumab in advanced melanoma. N Engl J Med. (2019) 381:1535–46. doi: 10.1056/NEJMoa1910836
16. Tawbi HA, SChadendorf D, Lipson EJ, Ascierto PA, Matamala L, Castillo Gutierrez E, et al. Relatlimab and nivolumab versus nivolumab in untreated advanced melanoma. N Engl J Med. (2022) 386:24–34. doi: 10.1056/NEJMoa2109970
17. Key TJ, Allen NE, Spencer EA, Travis RC. The effect of diet on risk of cancer. Lancet. (2002) 360:861–8. doi: 10.1016/S0140-6736(02)09958-0
18. Brown K, DeCoffe D, Molcan E, Gibson DL. Diet-induced dysbiosis of the intestinal microbiota and the effects on immunity and disease. Nutrients. (2012) 4:1095–119. doi: 10.3390/nu4081095
19. Childs CE, Calder PC, Miles EA. Diet and immune function. Nutrients. (2019) 11:1–9. doi: 10.3390/nu11081933
20. Donaldson MS. Nutrition and cancer: A review of the evidence for an anti-cancer diet. Nutr J. (2004) 3:19. doi: 10.1186/1475-2891-3-19
21. Wastyk HC, Fragiadakis GK, Perelman D, Dahan D, Merrill BD, Yu FB, et al. Gut-microbiota-targeted diets modulate human immune status. Cell. (2021) 184:4137–53 e14. doi: 10.1016/j.cell.2021.06.019
22. Ou J, Carbonero F, Zoetendal EG, DeLany JP, Wang M, Newton K, et al. Diet, microbiota, and microbial metabolites in colon cancer risk in rural Africans and African Americans. Am J Clin Nutr. (2013) 98:111–20. doi: 10.3945/ajcn.112.056689
23. Singh RK, Chang HW, Yan D, Lee KM, Ucmak D, Wong K, et al. Influence of diet on the gut microbiome and implications for human health. J Transl Med. (2017) 15:73. doi: 10.1186/s12967-017-1175-y
24. McDermott AJ, Huffnagle GB. The microbiome and regulation of mucosal immunity. Immunology. (2014) 142:24–31. doi: 10.1111/imm.12231
25. Coleman OI, Haller D. Microbe-mucus interface in the pathogenesis of colorectal cancer. Cancers (Basel). (2021) 13:616. doi: 10.3390/cancers13040616
26. Andrews MC, Duong CPM, Gopalakrishnan V, Iebba V, Chen WS, Derosa L, et al. Gut microbiota signatures are associated with toxicity to combined ctla-4 and pd-1 blockade. Nat Med. (2021) 27:1432–41. doi: 10.1038/s41591-021-01406-6
27. Baruch EN, Youngster I, Ben-Betzalel G, Ortenberg R, Lahat A, Katz L, et al. Fecal microbiota transplant promotes response in immunotherapy-refractory melanoma patients. Science. (2021) 371:602–9. doi: 10.1126/science.abb5920
28. Davar D, Dzutsev AK, McCulloch JA, Rodrigues RR, Chauvin JM, Morrison RM, et al. Fecal microbiota transplant overcomes resistance to anti-pd-1 therapy in melanoma patients. Science. (2021) 371:595–602. doi: 10.1126/science.abf3363
29. McQuade JL, Daniel CR, Helmink BA, Wargo JA. Modulating the microbiome to improve therapeutic response in cancer. Lancet Oncol. (2019) 20:e77–91. doi: 10.1016/S1470-2045(18)30952-5
30. McQuade JL, Ologun GO, Arora R, Wargo JA. Gut microbiome modulation via fecal microbiota transplant to augment immunotherapy in patients with melanoma or other cancers. Curr Oncol Rep. (2020) 22:74. doi: 10.1007/s11912-020-00913-y
31. Baruch EN, Wang J, Wargo JA. Gut microbiota and antitumor immunity: potential mechanisms for clinical effect. Cancer Immunol Res. (2021) 9:365–70. doi: 10.1158/2326-6066.CIR-20-0877
32. Lathrop SK, Bloom SM, Rao SM, Nutsch K, Lio CW, Santacruz N, et al. Peripheral education of the immune system by colonic commensal microbiota. Nature. (2011) 478:250–4. doi: 10.1038/nature10434
33. Turnbaugh PJ, Ley RE, Hamady M, Fraser-Liggett CM, Knight R, Gordon JI. The human microbiome project. Nature. (2007) 449:804–10. doi: 10.1038/nature06244
34. Ghoshal S, Witta J, Zhong J, de Villiers W, Eckhardt E. Chylomicrons promote intestinal absorption of lipopolysaccharides. J Lipid Res. (2009) 50:90–7. doi: 10.1194/jlr.M800156-JLR200
35. Beam A, Clinger E, Hao L. Effect of diet and dietary components on the composition of the gut microbiota. Nutrients. (2021) 13:2795. doi: 10.3390/nu13082795
36. Park EM, Chelvanambi M, Bhutiani N, Kroemer G, Zitvogel L, Wargo JA. Targeting the gut and tumor microbiota in cancer. Nat Med. (2022) 28:690–703. doi: 10.1038/s41591-022-01779-2
37. Powers HJ. Riboflavin (Vitamin B-2) and health. Am J Clin Nutr. (2003) 77:1352–60. doi: 10.1093/ajcn/77.6.1352
39. Legoux F, Bellet D, Daviaud C, El Morr Y, Darbois A, Niort K, et al. Microbial metabolites control the thymic development of mucosal-associated invariant T cells. Science. (2019) 366:494–9. doi: 10.1126/science.aaw2719
40. Shen Y, Lu C, Song Z, Qiao C, Wang J, Chen J, et al. Ursodeoxycholic acid reduces antitumor immunosuppression by inducing chip-mediated tgf-beta degradation. Nat Commun. (2022) 13:3419. doi: 10.1038/s41467-022-31141-6
41. Hubbard TD, Murray IA, Perdew GH. Indole and tryptophan metabolism: endogenous and dietary routes to ah receptor activation. Drug Metab Dispos. (2015) 43:1522–35. doi: 10.1124/dmd.115.064246
42. Wyatt M, Greathouse KL. Targeting dietary and microbial tryptophan-indole metabolism as therapeutic approaches to colon cancer. Nutrients. (2021) 13:1189. doi: 10.3390/nu13041189
43. Tintelnot J, Xu Y, Lesker TR, Schonlein M, Konczalla L, Giannou AD, et al. Microbiota-derived 3-iaa influences chemotherapy efficacy in pancreatic cancer. Nature. (2023) 615:168–74. doi: 10.1038/s41586-023-05728-y
44. Bender MJ, McPherson AC, Phelps CM, Pandey SP, Laughlin CR, Shapira JH, et al. Dietary tryptophan metabolite released by intratumoral lactobacillus reuteri facilitates immune checkpoint inhibitor treatment. Cell. (2023) 186:1846–62 e26. doi: 10.1016/j.cell.2023.03.011
45. Hezaveh K, Shinde RS, Klotgen A, Halaby MJ, Lamorte S, Ciudad MT, et al. Tryptophan-derived microbial metabolites activate the aryl hydrocarbon receptor in tumor-associated macrophages to suppress anti-tumor immunity. Immunity. (2022) 55:324–40 e8. doi: 10.1016/j.immuni.2022.01.006
46. Bjeldanes LF, Kim JY, Grose KR, Bartholomew JC, Bradfield CA. Aromatic hydrocarbon responsiveness-receptor agonists generated from indole-3-carbinol in vitro and in vivo: comparisons with 2,3,7,8-tetrachlorodibenzo-P-dioxin. Proc Natl Acad Sci U.S.A. (1991) 88:9543–7. doi: 10.1073/pnas.88.21.9543
47. Dosz EB, Ku KM, Juvik JA, Jeffery EH. Total myrosinase activity estimates in brassica vegetable produce. J Agric Food Chem. (2014) 62:8094–100. doi: 10.1021/jf501692c
48. Ito S, Chen C, Satoh J, Yim S, Gonzalez FJ. Dietary phytochemicals regulate whole-body cyp1a1 expression through an arylhydrocarbon receptor nuclear translocator-dependent system in gut. J Clin Invest. (2007) 117:1940–50. doi: 10.1172/JCI31647
49. Loub WD, Wattenberg LW, Davis DW. Aryl hydrocarbon hydroxylase induction in rat tissues by naturally occurring indoles of cruciferous plants. J Natl Cancer Instit. (1975) 54:985–8.
50. Luang-In V, Narbad A, Nueno-Palop C, Mithen R, Bennett M, Rossiter JT. The metabolism of methylsulfinylalkyl- and methylthioalkyl-glucosinolates by a selection of human gut bacteria. Mol Nutr Food Res. (2014) 58:875–83. doi: 10.1002/mnfr.201300377
51. Shapiro TA, Fahey JW, Wade KL, Stephenson KK, Talalay P. Chemoprotective glucosinolates and isothiocyanates of broccoli sprouts: metabolism and excretion in humans. Cancer Epidemiol Biomarkers Prev. (2001) 10:501–8.
52. Chaput N, Lepage P, Coutzac C, Soularue E, Le Roux K, Monot C, et al. Baseline gut microbiota predicts clinical response and colitis in metastatic melanoma patients treated with ipilimumab. Ann Oncol. (2017) 28:1368–79. doi: 10.1093/annonc/mdx108
53. Frankel AE, Coughlin LA, Kim J, Froehlich TW, Xie Y, Frenkel EP, et al. Metagenomic shotgun sequencing and unbiased metabolomic profiling identify specific human gut microbiota and metabolites associated with immune checkpoint therapy efficacy in melanoma patients. Neoplasia. (2017) 19:848–55. doi: 10.1016/j.neo.2017.08.004
54. Rothschild D, Weissbrod O, Barkan E, Kurilshikov A, Korem T, Zeevi D, et al. Environment dominates over host genetics in shaping human gut microbiota. Nature. (2018) 555:210–5. doi: 10.1038/nature25973
55. Dahl WJ, Rivero Mendoza D, Lambert JM. Diet, nutrients and the microbiome. Prog Mol Biol Transl Sci. (2020) 171:237–63. doi: 10.1016/bs.pmbts.2020.04.006
56. Wu GD, Chen J, Hoffmann C, Bittinger K, Chen YY, Keilbaugh SA, et al. Linking long-term dietary patterns with gut microbial enterotypes. Science. (2011) 334:105–8. doi: 10.1126/science.1208344
57. McDonald D, Hyde E, Debelius JW, Morton JT, Gonzalez A, Ackermann G, et al. American gut: an open platform for citizen science microbiome research. mSystems. (2018) 3:e00031–18. doi: 10.1128/mSystems.00031-18
58. Simpson RC, Shanahan ER, Batten M, Reijers ILM, Read M, Silva IP, et al. Diet-driven microbial ecology underpins associations between cancer immunotherapy outcomes and the gut microbiome. Nat Med. (2022) 28:2344–52. doi: 10.1038/s41591-022-01965-2
59. O’Keefe SJD. Plant-based foods and the microbiome in the preservation of health and prevention of disease. Am J Clin Nutr. (2019) 110:265–6. doi: 10.1093/ajcn/nqz127
60. Koleva PT, Valcheva RS, Sun X, Ganzle MG, Dieleman LA. Inulin and fructo-oligosaccharides have divergent effects on colitis and commensal microbiota in hla-B27 transgenic rats. Br J Nutr. (2012) 108:1633–43. doi: 10.1017/S0007114511007203
61. Grasten SM, Juntunen KS, Poutanen KS, Gylling HK, Miettinen TA, Mykkanen HM. Rye bread improves bowel function and decreases the concentrations of some compounds that are putative colon cancer risk markers in middle-aged women and men. J Nutr. (2000) 130:2215–21. doi: 10.1093/jn/130.9.2215
62. Statovci D, Aguilera M, MacSharry J, Melgar S. The impact of western diet and nutrients on the microbiota and immune response at mucosal interfaces. Front Immunol. (2017) 8:838. doi: 10.3389/fimmu.2017.00838
63. Chhibber-Goel J, Gaur A, Singhal V, Parakh N, Bhargava B, Sharma A. The complex metabolism of trimethylamine in humans: endogenous and exogenous sources. Expert Rev Mol Med. (2016) 18:e8. doi: 10.1017/erm.2016.6
64. Schugar RC, Shih DM, Warrier M, Helsley RN, Burrows A, Ferguson D, et al. The tmao-producing enzyme flavin-containing monooxygenase 3 regulates obesity and the beiging of white adipose tissue. Cell Rep. (2017) 20:279. doi: 10.1016/j.celrep.2017.06.053
65. Wang H, Rong X, Zhao G, Zhou Y, Xiao Y, Ma D, et al. The microbial metabolite trimethylamine N-oxide promotes antitumor immunity in triple-negative breast cancer. Cell Metab. (2022) 34:581–94 e8. doi: 10.1016/j.cmet.2022.02.010
66. Wu X, Qian S, Zhang J, Feng J, Luo K, Sun L, et al. Lipopolysaccharide promotes metastasis via acceleration of glycolysis by the nuclear factor-kappab/snail/hexokinase3 signaling axis in colorectal cancer. Cancer Metab. (2021) 9:23. doi: 10.1186/s40170-021-00260-x
67. Cani PD, Amar J, Iglesias MA, Poggi M, Knauf C, Bastelica D, et al. Metabolic endotoxemia initiates obesity and insulin resistance. Diabetes. (2007) 56:1761–72. doi: 10.2337/db06-1491
68. Moodie R, Stuckler D, Monteiro C, Sheron N, Neal B, Thamarangsi T, et al. Profits and pandemics: prevention of harmful effects of tobacco, alcohol, and ultra-processed food and drink industries. Lancet. (2013) 381:670–9. doi: 10.1016/S0140-6736(12)62089-3
69. Gearhardt AN, Bueno NB, DiFeliceantonio AG, Roberto CA, Jimenez-Murcia S, Fernandez-Aranda F. Social, clinical, and policy implications of ultra-processed food addiction. BMJ. (2023) 383:e075354. doi: 10.1136/bmj-2023-075354
70. Reed S, Neuman H, Moscovich S, Glahn RP, Koren O, Tako E. Chronic zinc deficiency alters chick gut microbiota composition and function. Nutrients. (2015) 7:9768–84. doi: 10.3390/nu7125497
71. Spencer M, Gupta A, Dam LV, Shannon C, Menees S, Chey WD. Artificial sweeteners: A systematic review and primer for gastroenterologists. J Neurogastroenterol Motil. (2016) 22:168–80. doi: 10.5056/jnm15206
72. Suez J, Korem T, Zeevi D, Zilberman-Schapira G, Thaiss CA, Maza O, et al. Artificial sweeteners induce glucose intolerance by altering the gut microbiota. Nature. (2014) 514:181–6. doi: 10.1038/nature13793
73. Lopez-Legarrea P, Fuller NR, Zulet MA, Martinez JA, Caterson ID. The Influence of Microbiota and Small Intestinal Physiology before and after the Onset of Obesity. Biochimie. (2014) 121:97–106. doi: 10.1016/j.nut.2019.110609
74. Travinsky-Shmul T, Beresh O, Zaretsky J, Griess-Fishheimer S, Rozner R, Kalev-Altman R, et al. Ultra-processed food impairs bone quality, increases marrow adiposity and alters gut microbiome in mice. Foods. (2021) 10(12):3107. doi: 10.3390/foods10123107
75. Glade MJ, Meguid MM. A glance at … Dietary emulsifiers, the human intestinal mucus and microbiome, and dietary fiber. Nutrition. (2016) 32:609–14. doi: 10.1016/j.nut.2015.12.036
76. Chassaing B, Van de Wiele T, De Bodt J, Marzorati M, Gewirtz AT. Dietary emulsifiers directly alter human microbiota composition and gene expression ex vivo potentiating intestinal inflammation. Gut. (2017) 66:1414–27. doi: 10.1136/gutjnl-2016-313099
77. Viennois E, Merlin D, Gewirtz AT, Chassaing B. Dietary emulsifier-induced low-grade inflammation promotes colon carcinogenesis. Cancer Res. (2017) 77:27–40. doi: 10.1158/0008-5472.CAN-16-1359
78. David LA, Maurice CF, Carmody RN, Gootenberg DB, Button JE, Wolfe BE, et al. Diet rapidly and reproducibly alters the human gut microbiome. Nature. (2014) 505:559–63. doi: 10.1038/nature12820
79. O’Keefe SJ, Li JV, Lahti L, Ou J, Carbonero F, Mohammed K, et al. Fat, fibre and cancer risk in african americans and rural africans. Nat Commun. (2015) 6:6342. doi: 10.1038/ncomms7342
80. Dahl WJ, Stewart ML. Position of the academy of nutrition and dietetics: health implications of dietary fiber. J Acad Nutr Diet. (2015) 115:1861–70. doi: 10.1016/j.jand.2015.09.003
81. Cui J, Lian Y, Zhao C, Du H, Han Y, Gao W, et al. Dietary fibers from fruits and vegetables and their health benefits via modulation of gut microbiota. Compr Rev Food Sci Food Saf. (2019) 18:1514–32. doi: 10.1111/1541-4337.12489
82. Institute of Medicine (US) Panel on the Definition of Dietary Fiber and the Standing Committee on the Scientific Evaluation of Dietary Reference Intakes. Dietary Reference Intakes Proposed Definition of Dietary Fiber. In: Dietary Reference Intakes Proposed Definition of Dietary Fiber. Washington, DC: National Academies Press (US) (2001).
83. Schroeder BO, Birchenough GMH, Stahlman M, Arike L, Johansson MEV, Hansson GC, et al. Bifidobacteria or fiber protects against diet-induced microbiota-mediated colonic mucus deterioration. Cell Host Microbe. (2018) 23:27–40 e7. doi: 10.1016/j.chom.2017.11.004
84. Kaur K, Saxena A, Debnath I, O’Brien JL, Ajami NJ, Auchtung TA, et al. Antibiotic-mediated bacteriome depletion in apc(Min/+) mice is associated with reduction in mucus-producing goblet cells and increased colorectal cancer progression. Cancer Med. (2018) 7:2003–12. doi: 10.1002/cam4.1460
85. Zhang X, Browman G, Siu W, Basen-Engquist KM, Hanash SM, Hoffman KL, et al. The be gone trial study protocol: A randomized crossover dietary intervention of dry beans targeting the gut microbiome of overweight and obese patients with a history of colorectal polyps or cancer. BMC Cancer. (2019) 19:1233. doi: 10.1186/s12885-019-6400-z
86. Earle KA, Billings G, Sigal M, Lichtman JS, Hansson GC, Elias JE, et al. Quantitative imaging of gut microbiota spatial organization. Cell Host Microbe. (2015) 18:478–88. doi: 10.1016/j.chom.2015.09.002
87. Desai MS, Seekatz AM, Koropatkin NM, Kamada N, Hickey CA, Wolter M, et al. A dietary fiber-deprived gut microbiota degrades the colonic mucus barrier and enhances pathogen susceptibility. Cell. (2016) 167:1339–53 e21. doi: 10.1016/j.cell.2016.10.043
88. Correa-Oliveira R, Fachi JL, Vieira A, Sato FT, Vinolo MA. Regulation of immune cell function by short-chain fatty acids. Clin Transl Immunol. (2016) 5:e73. doi: 10.1038/cti.2016.17
89. Armet AM, Deehan EC, O’Sullivan AF, Mota JF, Field CJ, Prado CM, et al. Rethinking healthy eating in light of the gut microbiome. Cell Host Microbe. (2022) 30:764–85. doi: 10.1016/j.chom.2022.04.016
90. Pabst O. New concepts in the generation and functions of iga. Nat Rev Immunol. (2012) 12:821–32. doi: 10.1038/nri3322
91. Mantis NJ, Rol N, Corthesy B. Secretory Iga’s complex roles in immunity and mucosal homeostasis in the gut. Mucosal Immunol. (2011) 4:603–11. doi: 10.1038/mi.2011.41
92. Luu M, Weigand K, Wedi F, Breidenbend C, Leister H, Pautz S, et al. Regulation of the effector function of cd8(+) T cells by gut microbiota-derived metabolite butyrate. Sci Rep. (2018) 8:14430. doi: 10.1038/s41598-018-32860-x
93. Balmer ML, Ma EH, Bantug GR, Grahlert J, Pfister S, Glatter T, et al. Memory cd8(+) T cells require increased concentrations of acetate induced by stress for optimal function. Immunity. (2016) 44:1312–24. doi: 10.1016/j.immuni.2016.03.016
94. Bachem A, Makhlouf C, Binger KJ, de Souza DP, Tull D, Hochheiser K, et al. Microbiota-derived short-chain fatty acids promote the memory potential of antigen-activated cd8(+) T cells. Immunity. (2019) 51:285–97 e5. doi: 10.1016/j.immuni.2019.06.002
95. Bordonaro M, Lazarova DL, Sartorelli AC. Butyrate and wnt signaling: A possible solution to the puzzle of dietary fiber and colon cancer risk? Cell Cycle. (2008) 7:1178–83. doi: 10.4161/cc.7.9.5818
96. Singh V, Yeoh BS, Chassaing B, Xiao X, Saha P, Aguilera Olvera R, et al. Dysregulated microbial fermentation of soluble fiber induces cholestatic liver cancer. Cell. (2018) 175:679–94.e22. doi: 10.1016/j.cell.2018.09.004
97. Moen B, Henjum K, Mage I, Knutsen SH, Rud I, Hetland RB, et al. Effect of dietary fibers on cecal microbiota and intestinal tumorigenesis in azoxymethane treated a/J min/+ Mice. PloS One. (2016) 11:e0155402. doi: 10.1371/journal.pone.0155402
98. Zumbrun SD, Melton-Celsa AR, Smith MA, Gilbreath JJ, Merrell DS, O’Brien AD. Dietary choice affects shiga toxin-producing escherichia coli (Stec) O157:H7 colonization and disease. Proc Natl Acad Sci U.S.A. (2013) 110:E2126–33. doi: 10.1073/pnas.1222014110
99. Zumbrun SD, Melton-Celsa AR, O’Brien AD. When a healthy diet turns deadly. Gut Microbes. (2014) 5:40–3. doi: 10.4161/gmic.26263
100. Belcheva A, Irrazabal T, Robertson SJ, Streutker C, Maughan H, Rubino S, et al. Gut microbial metabolism drives transformation of msh2-deficient colon epithelial cells. Cell. (2014) 158:288–99. doi: 10.1016/j.cell.2014.04.051
101. Trapecar M, Communal C, Velazquez J, Maass CA, Huang YJ, Schneider K, et al. Gut-liver physiomimetics reveal paradoxical modulation of ibd-related inflammation by short-chain fatty acids. Cell Syst. (2020) 10:223–39 e9. doi: 10.1016/j.cels.2020.02.008
102. Song H, Chu Q, Yan F, Yang Y, Han W, Zheng X. Red pitaya betacyanins protects from diet-induced obesity, liver steatosis and insulin resistance in association with modulation of gut microbiota in mice. J Gastroenterol Hepatol. (2016) 31:1462–9. doi: 10.1111/jgh.13278
103. Fischer F, Romero R, Hellhund A, Linne U, Bertrams W, Pinkenburg O, et al. Dietary cellulose induces anti-inflammatory immunity and transcriptional programs via maturation of the intestinal microbiota. Gut Microbes. (2020) 12:1–17. doi: 10.1080/19490976.2020.1829962
104. Reddy BS, Engle A, Simi B, Goldman M. Effect of dietary fiber on colonic bacterial enzymes and bile acids in relation to colon cancer. Gastroenterology. (1992) 102:1475–82. doi: 10.1016/0016-5085(92)91704-8
105. Spencer CN, McQuade JL, Gopalakrishnan V, McCulloch JA, Vetizou M, Cogdill AP, et al. Dietary fiber and probiotics influence the gut microbiome and melanoma immunotherapy response. Science. (2021) 374:1632–40. doi: 10.1126/science.aaz7015
106. Bolte LA, Lee KA, Björk JR, Leeming ER, Campmans-Kuijpers MJ, de Haan JJ, et al. Association of a mediterranean diet with outcomes for patients treated with immune checkpoint blockade for advanced melanoma. JAMA Oncol. (2023) 9:705–9. doi: 10.1001/jamaoncol.2022.7753
107. Vernieri C, Fuca G, Ligorio F, Huber V, Vingiani A, Iannelli F, et al. Fasting-mimicking diet is safe and reshapes metabolism and antitumor immunity in patients with cancer. Cancer Discovery. (2022) 12:90–107. doi: 10.1158/2159-8290.CD-21-0030
108. Dimidi E, Cox SR, Rossi M, Whelan K. Fermented foods: definitions and characteristics, impact on the gut microbiota and effects on gastrointestinal health and disease. Nutrients. (2019) 11:1806. doi: 10.3390/nu11081806
109. Johnstone N, Dart S, Knytl P, Nauta A, Hart K, Cohen Kadosh K. Nutrient intake and gut microbial genera changes after a 4-week placebo controlled galacto-oligosaccharides intervention in young females. Nutrients. (2021) 13(12):4384. doi: 10.3390/nu13124384
110. Rios-Covian D, Gueimonde M, Duncan SH, Flint HJ, de los Reyes-Gavilan CG. Enhanced butyrate formation by cross-feeding between faecalibacterium prausnitzii and bifidobacterium adolescentis. FEMS Microbiol Lett. (2015) 362(21):fnv176. doi: 10.1093/femsle/fnv176
111. Suez J, Zmora N, Segal E, Elinav E. The pros, cons, and many unknowns of probiotics. Nat Med. (2019) 25:716–29. doi: 10.1038/s41591-019-0439-x
112. Elmer GW. Probiotics: “Living drugs”. Am J Health Syst Pharm. (2001) 58:1101–9. doi: 10.1093/ajhp/58.12.1101
113. Sonnenburg Erica D, Sonnenburg Justin L. Starving our microbial self: the deleterious consequences of a diet deficient in microbiota-accessible carbohydrates. Cell Metab. (2014) 20:779–86. doi: 10.1016/j.cmet.2014.07.003
114. Oliphant K, Allen-Vercoe E. Macronutrient metabolism by the human gut microbiome: major fermentation by-products and their impact on host health. Microbiome. (2019) 7:91. doi: 10.1186/s40168-019-0704-8
115. Derrien M, Belzer C, de Vos WM. Akkermansia muciniphila and its role in regulating host functions. Microb Pathog. (2017) 106:171–81. doi: 10.1016/j.micpath.2016.02.005
116. Gonzalez S, Fernandez-Navarro T, Arboleya S, de Los Reyes-Gavilan CG, Salazar N, Gueimonde M. Fermented dairy foods: impact on intestinal microbiota and health-linked biomarkers. Front Microbiol. (2019) 10:1046. doi: 10.3389/fmicb.2019.01046
117. Woo JK, Choi S, Kang JH, Kim DE, Hurh BS, Jeon JE, et al. Fermented barley and soybean (Bs) mixture enhances intestinal barrier function in dextran sulfate sodium (Dss)-induced colitis mouse model. BMC Complement Altern Med. (2016) 16:498. doi: 10.1186/s12906-016-1479-0
118. Warburg O. On the origin of cancer cells. Science. (1956) 123:309–14. doi: 10.1126/science.123.3191.309
119. Vander Heiden MG, DeBerardinis RJ. Understanding the intersections between metabolism and cancer biology. Cell. (2017) 168:657–69. doi: 10.1016/j.cell.2016.12.039
120. Hopkins BD, Pauli C, Du X, Wang DG, Li X, Wu D, et al. Suppression of insulin feedback enhances the efficacy of pi3k inhibitors. Nature. (2018) 560:499–503. doi: 10.1038/s41586-018-0343-4
121. Ferrere G, Tidjani Alou M, Liu P, Goubet AG, Fidelle M, Kepp O, et al. Ketogenic diet and ketone bodies enhance the anticancer effects of pd-1 blockade. JCI Insight. (2021) 6:e145207. doi: 10.1172/jci.insight.145207
122. Ma D, Wang AC, Parikh I, Green SJ, Hoffman JD, Chlipala G, et al. Ketogenic diet enhances neurovascular function with altered gut microbiome in young healthy mice. Sci Rep. (2018) 8:6670. doi: 10.1038/s41598-018-25190-5
123. Oliveira CLP, Mattingly S, Schirrmacher R, Sawyer MB, Fine EJ, Prado CM. A nutritional perspective of ketogenic diet in cancer: A narrative review. J Acad Nutr Diet. (2018) 118:668–88. doi: 10.1016/j.jand.2017.02.003
124. Link VM, Subramanian P, Cheung F, Han KL, Stacy A, Chi L, et al. Differential peripheral immune signatures elicited by vegan versus ketogenic diets in humans. Nat Med. (2024) 30:560–72. doi: 10.1038/s41591-023-02761-2
125. Chung HY, Kim DH, Bang E, Yu BP. Impacts of calorie restriction and intermittent fasting on health and diseases: current trends. Nutrients. (2020) 12:2948. doi: 10.3390/nu12102948
126. Varady KA. Intermittent versus daily calorie restriction: which diet regimen is more effective for weight loss? Obes Rev. (2011) 12:e593–601. doi: 10.1111/j.1467-789X.2011.00873.x
127. Fontana L, Klein S. Aging, adiposity, and calorie restriction. JAMA. (2007) 297:986–94. doi: 10.1001/jama.297.9.986
128. Fanti M, Mishra A, Longo VD, Brandhorst S. Time-restricted eating, intermittent fasting, and fasting-mimicking diets in weight loss. Curr Obes Rep. (2021) 10:70–80. doi: 10.1007/s13679-021-00424-2
129. Lee KA, Shaw HM, Bataille V, Nathan P, Spector TD. Role of the gut microbiome for cancer patients receiving immunotherapy: dietary and treatment implications. Eur J Cancer. (2020) 138:149–55. doi: 10.1016/j.ejca.2020.07.026
130. De Lorenzo MS, Baljinnyam E, Vatner DE, Abarzua P, Vatner SF, Rabson AB. Caloric restriction reduces growth of mammary tumors and metastases. Carcinogenesis. (2011) 32:1381–7. doi: 10.1093/carcin/bgr107
131. Mulrooney TJ, Marsh J, Urits I, Seyfried TN, Mukherjee P. Influence of caloric restriction on constitutive expression of nf-kappab in an experimental mouse astrocytoma. PloS One. (2011) 6:e18085. doi: 10.1371/journal.pone.0018085
132. Lee C, Raffaghello L, Brandhorst S, Safdie FM, Bianchi G, Martin-Montalvo A, et al. Fasting cycles retard growth of tumors and sensitize a range of cancer cell types to chemotherapy. Sci Transl Med. (2012) 4:124ra27. doi: 10.1126/scitranslmed.3003293
133. Colman RJ, Anderson RM, Johnson SC, Kastman EK, Kosmatka KJ, Beasley TM, et al. Caloric restriction delays disease onset and mortality in rhesus monkeys. Science. (2009) 325:201–4. doi: 10.1126/science.1173635
134. Renehan AG, Zwahlen M, Minder C, O’Dwyer ST, Shalet SM, Egger M. Insulin-like growth factor (Igf)-I, igf binding protein-3, and cancer risk: systematic review and meta-regression analysis. Lancet. (2004) 363:1346–53. doi: 10.1016/S0140-6736(04)16044-3
135. Longo VD, Fontana L. Calorie restriction and cancer prevention: metabolic and molecular mechanisms. Trends Pharmacol Sci. (2010) 31:89–98. doi: 10.1016/j.tips.2009.11.004
136. Lien EC, Westermark AM, Zhang Y, Yuan C, Li Z, Lau AN, et al. Low glycaemic diets alter lipid metabolism to influence tumour growth. Nature. (2021) 599:302–7. doi: 10.1038/s41586-021-04049-2
137. Di Biase S, Lee C, Brandhorst S, Manes B, Buono R, Cheng CW, et al. Fasting-mimicking diet reduces ho-1 to promote T cell-mediated tumor cytotoxicity. Cancer Cell. (2016) 30:136–46. doi: 10.1016/j.ccell.2016.06.005
138. Clinthorne JF, Beli E, Duriancik DM, Gardner EM. Nk cell maturation and function in C57bl/6 mice are altered by caloric restriction. J Immunol. (2013) 190:712–22. doi: 10.4049/jimmunol.1201837
139. Liu ZM, Chen GG, Ng EK, Leung WK, Sung JJ, Chung SC. Upregulation of heme oxygenase-1 and P21 confers resistance to apoptosis in human gastric cancer cells. Oncogene. (2004) 23:503–13. doi: 10.1038/sj.onc.1207173
140. Nitti M, Piras S, Marinari UM, Moretta L, Pronzato MA, Furfaro AL. Ho-1 induction in cancer progression: A matter of cell adaptation. Antioxid (Basel). (2017) 6:29. doi: 10.3390/antiox6020029
141. Cortellino S, Raveane A, Chiodoni C, Delfanti G, Pisati F, Spagnolo V, et al. Fasting renders immunotherapy effective against low-immunogenic breast cancer while reducing side effects. Cell Rep. (2022) 40:111256. doi: 10.1016/j.celrep.2022.111256
142. de Braud F, Vernieri C, Huber V, Cova A, Squarcina P, Milano M, et al. Abstract B022: metabolic and immunologic effects of the fasting mimicking diet in cancer patients. Mol Cancer Ther. (2018) 17:B022–B. doi: 10.1158/1535-7163.TARG-17-B022
143. Pietrocola F, Pol J, Vacchelli E, Rao S, Enot DP, Baracco EE, et al. Caloric restriction mimetics enhance anticancer immunosurveillance. Cancer Cell. (2016) 30:147–60. doi: 10.1016/j.ccell.2016.05.016
144. Wang S, Huang M, You X, Zhao J, Chen L, Wang L, et al. Gut microbiota mediates the anti-obesity effect of calorie restriction in mice. Sci Rep. (2018) 8:13037. doi: 10.1038/s41598-018-31353-1
145. Zhang C, Li S, Yang L, Huang P, Li W, Wang S, et al. Structural modulation of gut microbiota in life-long calorie-restricted mice. Nat Commun. (2013) 4:2163. doi: 10.1038/ncomms3163
146. Zheng X, Wang S, Jia W. Calorie restriction and its impact on gut microbial composition and global metabolism. Front Med. (2018) 12:634–44. doi: 10.1007/s11684-018-0670-8
147. Rangan P, Choi I, Wei M, Navarrete G, Guen E, Brandhorst S, et al. Fasting-mimicking diet modulates microbiota and promotes intestinal regeneration to reduce inflammatory bowel disease pathology. Cell Rep. (2019) 26:2704–19 e6. doi: 10.1016/j.celrep.2019.02.019
148. Zhou ZL, Jia XB, Sun MF, Zhu YL, Qiao CM, Zhang BP, et al. Neuroprotection of fasting mimicking diet on mptp-induced parkinson’s disease mice via gut microbiota and metabolites. Neurotherapeutics. (2019) 16:741–60. doi: 10.1007/s13311-019-00719-2
149. Sekirov I, Russell SL, Antunes LC, Finlay BB. Gut microbiota in health and disease. Physiol Rev. (2010) 90:859–904. doi: 10.1152/physrev.00045.2009
150. Lam KC, Araya RE, Huang A, Chen Q, Di Modica M, Rodrigues RR, et al. Microbiota triggers sting-type I ifn-dependent monocyte reprogramming of the tumor microenvironment. Cell. (2021) 184:5338–56 e21. doi: 10.1016/j.cell.2021.09.019
151. Bibbo S, Ianiro G, Giorgio V, Scaldaferri F, Masucci L, Gasbarrini A, et al. The role of diet on gut microbiota composition. Eur Rev Med Pharmacol Sci. (2016) 20:4742–9.
152. Agus A, Clement K, Sokol H. Gut microbiota-derived metabolites as central regulators in metabolic disorders. Gut. (2021) 70:1174–82. doi: 10.1136/gutjnl-2020-323071
153. Yang J, Yu J. The association of diet, gut microbiota and colorectal cancer: what we eat may imply what we get. Protein Cell. (2018) 9:474–87. doi: 10.1007/s13238-018-0543-6
154. Zhu W, Gregory JC, Org E, Buffa JA, Gupta N, Wang Z, et al. Gut microbial metabolite tmao enhances platelet hyperreactivity and thrombosis risk. Cell. (2016) 165:111–24. doi: 10.1016/j.cell.2016.02.011
155. Tanoue T, Honda K. Induction of treg cells in the mouse colonic mucosa: A central mechanism to maintain host-microbiota homeostasis. Semin Immunol. (2012) 24:50–7. doi: 10.1016/j.smim.2011.11.009
156. Kamada N, Kim YG, Sham HP, Vallance BA, Puente JL, Martens EC, et al. Regulated virulence controls the ability of a pathogen to compete with the gut microbiota. Science. (2012) 336:1325–9. doi: 10.1126/science.1222195
157. Soldati L, Di Renzo L, Jirillo E, Ascierto PA, Marincola FM, De Lorenzo A. The influence of diet on anti-cancer immune responsiveness. J Transl Med. (2018) 16:75. doi: 10.1186/s12967-018-1448-0
158. Backhed F, Manchester JK, Semenkovich CF, Gordon JI. Mechanisms underlying the resistance to diet-induced obesity in germ-free mice. Proc Natl Acad Sci U.S.A. (2007) 104:979–84. doi: 10.1073/pnas.0605374104
159. Ieronymaki E, Daskalaki MG, Lyroni K, Tsatsanis C. Insulin signaling and insulin resistance facilitate trained immunity in macrophages through metabolic and epigenetic changes. Front Immunol. (2019) 10:1330. doi: 10.3389/fimmu.2019.01330
160. Algire C, Amrein L, Bazile M, David S, Zakikhani M, Pollak M. Diet and tumor lkb1 expression interact to determine sensitivity to anti-neoplastic effects of metformin in vivo. Oncogene. (2011) 30:1174–82. doi: 10.1038/onc.2010.483
161. Christ A, Lauterbach M, Latz E. Western diet and the immune system: an inflammatory connection. Immunity. (2019) 51:794–811. doi: 10.1016/j.immuni.2019.09.020
162. Shamshiev AT, Ampenberger F, Ernst B, Rohrer L, Marsland BJ, Kopf M. Dyslipidemia inhibits toll-like receptor-induced activation of cd8alpha-negative dendritic cells and protective th1 type immunity. J Exp Med. (2007) 204:441–52. doi: 10.1084/jem.20061737
163. Angeli V, Llodra J, Rong JX, Satoh K, Ishii S, Shimizu T, et al. Dyslipidemia associated with atherosclerotic disease systemically alters dendritic cell mobilization. Immunity. (2004) 21:561–74. doi: 10.1016/j.immuni.2004.09.003
164. Chassaing B, Koren O, Goodrich JK, Poole AC, Srinivasan S, Ley RE, et al. Dietary emulsifiers impact the mouse gut microbiota promoting colitis and metabolic syndrome. Nature. (2015) 519:92–6. doi: 10.1038/nature14232
165. Wu C, Yosef N, Thalhamer T, Zhu C, Xiao S, Kishi Y, et al. Induction of pathogenic th17 cells by inducible salt-sensing kinase sgk1. Nature. (2013) 496:513–7. doi: 10.1038/nature11984
166. Kleinewietfeld M, Manzel A, Titze J, Kvakan H, Yosef N, Linker RA, et al. Sodium chloride drives autoimmune disease by the induction of pathogenic th17 cells. Nature. (2013) 496:518–22. doi: 10.1038/nature11868
167. Duewell P, Kono H, Rayner KJ, Sirois CM, Vladimer G, Bauernfeind FG, et al. Nlrp3 inflammasomes are required for atherogenesis and activated by cholesterol crystals. Nature. (2010) 464:1357–61. doi: 10.1038/nature08938
168. Chassaing B, Compher C, Bonhomme B, Liu Q, Tian Y, Walters W, et al. Randomized controlled-feeding study of dietary emulsifier carboxymethylcellulose reveals detrimental impacts on the gut microbiota and metabolome. Gastroenterology. (2022) 162:743–56. doi: 10.1053/j.gastro.2021.11.006
169. Martinez-Medina M, Denizot J, Dreux N, Robin F, Billard E, Bonnet R, et al. Western diet induces dysbiosis with increased E coli in ceabac10 mice, alters host barrier function favouring aiec colonisation. Gut. (2014) 63:116–24. doi: 10.1136/gutjnl-2012-304119
170. Hildebrandt MA, Hoffmann C, Sherrill-Mix SA, Keilbaugh SA, Hamady M, Chen YY, et al. High-fat diet determines the composition of the murine gut microbiome independently of obesity. Gastroenterology. (2009) 137:1716–24 e1-2. doi: 10.1053/j.gastro.2009.08.042
171. Bertrand A, Kostine M, Barnetche T, Truchetet ME, Schaeverbeke T. Immune related adverse events associated with anti-ctla-4 antibodies: systematic review and meta-analysis. BMC Med. (2015) 13:211. doi: 10.1186/s12916-015-0455-8
172. Burcelin R, Garidou L, Pomie C. Immuno-microbiota cross and talk: the new paradigm of metabolic diseases. Semin Immunol. (2012) 24:67–74. doi: 10.1016/j.smim.2011.11.011
173. Cani PD, Bibiloni R, Knauf C, Waget A, Neyrinck AM, Delzenne NM, et al. Changes in gut microbiota control metabolic endotoxemia-induced inflammation in high-fat diet-induced obesity and diabetes in mice. Diabetes. (2008) 57:1470–81. doi: 10.2337/db07-1403
174. Poppitt SD, Keogh GF, Lithander FE, Wang Y, Mulvey TB, Chan YK, et al. Postprandial response of adiponectin, interleukin-6, tumor necrosis factor-alpha, and C-reactive protein to a high-fat dietary load. Nutrition. (2008) 24:322–9. doi: 10.1016/j.nut.2007.12.012
175. Kien CL, Bunn JY, Fukagawa NK, Anathy V, Matthews DE, Crain KI, et al. Lipidomic evidence that lowering the typical dietary palmitate to oleate ratio in humans decreases the leukocyte production of proinflammatory cytokines and muscle expression of redox-sensitive genes. J Nutr Biochem. (2015) 26:1599–606. doi: 10.1016/j.jnutbio.2015.07.014
176. Beyaz S, Mana MD, Roper J, Kedrin D, Saadatpour A, Hong SJ, et al. High-fat diet enhances stemness and tumorigenicity of intestinal progenitors. Nature. (2016) 531:53–8. doi: 10.1038/nature17173
177. Shi H, Kokoeva MV, Inouye K, Tzameli I, Yin H, Flier JS. Tlr4 links innate immunity and fatty acid-induced insulin resistance. J Clin Invest. (2006) 116:3015–25. doi: 10.1172/JCI28898
178. Snodgrass RG, Huang S, Choi IW, Rutledge JC, Hwang DH. Inflammasome-mediated secretion of il-1beta in human monocytes through tlr2 activation; modulation by dietary fatty acids. J Immunol. (2013) 191:4337–47. doi: 10.4049/jimmunol.1300298
179. Erbay E, Babaev VR, Mayers JR, Makowski L, Charles KN, Snitow ME, et al. Reducing endoplasmic reticulum stress through a macrophage lipid chaperone alleviates atherosclerosis. Nat Med. (2009) 15:1383–91. doi: 10.1038/nm.2067
180. Paik J, Fierce Y, Treuting PM, Brabb T, Maggio-Price L. High-fat diet-induced obesity exacerbates inflammatory bowel disease in genetically susceptible mdr1a-/- male mice. J Nutr. (2013) 143:1240–7. doi: 10.3945/jn.113.174615
181. Reddy KVK, Naidu KA. Oleic acid, hydroxytyrosol and N-3 fatty acids collectively modulate colitis through reduction of oxidative stress and il-8 synthesis; in vitro and in vivo studies. Int Immunopharmacol. (2016) 35:29–42. doi: 10.1016/j.intimp.2016.03.019
182. Hermetet F, Buffiere A, Aznague A, Pais de Barros JP, Bastie JN, Delva L, et al. High-fat diet disturbs lipid raft/tgf-beta signaling-mediated maintenance of hematopoietic stem cells in mouse bone marrow. Nat Commun. (2019) 10:523. doi: 10.1038/s41467-018-08228-0
183. Agus A, Denizot J, Thevenot J, Martinez-Medina M, Massier S, Sauvanet P, et al. Western diet induces a shift in microbiota composition enhancing susceptibility to adherent-invasive E. Coli Infect Intest Inflamm Sci Rep. (2016) 6:19032. doi: 10.1038/srep19032
184. Klement RJ, Pazienza V. Impact of different types of diet on gut microbiota profiles and cancer prevention and treatment. Med (Kaunas). (2019) 55:84. doi: 10.3390/medicina55040084
185. Hills RD Jr., Pontefract BA, Mishcon HR, Black CA, Sutton SC, Theberge CR. Gut microbiome: profound implications for diet and disease. Nutrients. (2019) 11:1613. doi: 10.3390/nu11071613
186. Zhang D, Jin W, Wu R, Li J, Park SA, Tu E, et al. High glucose intake exacerbates autoimmunity through reactive-oxygen-species-mediated tgf-beta cytokine activation. Immunity. (2019) 51:671–81 e5. doi: 10.1016/j.immuni.2019.08.001
187. Gerriets VA, Kishton RJ, Johnson MO, Cohen S, Siska PJ, Nichols AG, et al. Foxp3 and toll-like receptor signaling balance treg cell anabolic metabolism for suppression. Nat Immunol. (2016) 17:1459–66. doi: 10.1038/ni.3577
188. Sanchez A, Reeser JL, Lau HS, Yahiku PY, Willard RE, McMillan PJ, et al. Role of sugars in human neutrophilic phagocytosis. Am J Clin Nutr. (1973) 26:1180–4. doi: 10.1093/ajcn/26.11.1180
189. Sorensen LB, Raben A, Stender S, Astrup A. Effect of sucrose on inflammatory markers in overweight humans. Am J Clin Nutr. (2005) 82:421–7. doi: 10.1093/ajcn.82.2.421
190. Schutte S, Esser D, Hoevenaars FPM, Hooiveld G, Priebe MG, Vonk RJ, et al. A 12-wk whole-grain wheat intervention protects against hepatic fat: the graandioos study, a randomized trial in overweight subjects. Am J Clin Nutr. (2018) 108:1264–74. doi: 10.1093/ajcn/nqy204
191. Kopf JC, Suhr MJ, Clarke J, Eyun SI, Riethoven JM, Ramer-Tait AE, et al. Role of whole grains versus fruits and vegetables in reducing subclinical inflammation and promoting gastrointestinal health in individuals affected by overweight and obesity: A randomized controlled trial. Nutr J. (2018) 17:72. doi: 10.1186/s12937-018-0381-7
192. Foerster J, Maskarinec G, Reichardt N, Tett A, Narbad A, Blaut M, et al. The influence of whole grain products and red meat on intestinal microbiota composition in normal weight adults: A randomized crossover intervention trial. PloS One. (2014) 9:e109606. doi: 10.1371/journal.pone.0109606
193. De Filippis F, Pellegrini N, Vannini L, Jeffery IB, La Storia A, Laghi L, et al. High-level adherence to a mediterranean diet beneficially impacts the gut microbiota and associated metabolome. Gut. (2016) 65:1812–21. doi: 10.1136/gutjnl-2015-309957
194. Vanegas SM, Meydani M, Barnett JB, Goldin B, Kane A, Rasmussen H, et al. Substituting whole grains for refined grains in a 6-wk randomized trial has a modest effect on gut microbiota and immune and inflammatory markers of healthy adults. Am J Clin Nutr. (2017) 105:635–50. doi: 10.3945/ajcn.116.146928
195. Hidalgo M, Oruna-Concha MJ, Kolida S, Walton GE, Kallithraka S, Spencer JP, et al. Metabolism of anthocyanins by human gut microflora and their influence on gut bacterial growth. J Agric Food Chem. (2012) 60:3882–90. doi: 10.1021/jf3002153
196. Rafter J, Bennett M, Caderni G, Clune Y, Hughes R, Karlsson PC, et al. Dietary synbiotics reduce cancer risk factors in polypectomized and colon cancer patients. Am J Clin Nutr. (2007) 85:488–96. doi: 10.1093/ajcn/85.2.488
197. Clemente-Postigo M, Queipo-Ortuno MI, Boto-Ordonez M, Coin-Araguez L, Roca-Rodriguez MM, Delgado-Lista J, et al. Effect of acute and chronic red wine consumption on lipopolysaccharide concentrations. Am J Clin Nutr. (2013) 97:1053–61. doi: 10.3945/ajcn.112.051128
198. Roager HM, Vogt JK, Kristensen M, Hansen LBS, Ibrugger S, Maerkedahl RB, et al. Whole grain-rich diet reduces body weight and systemic low-grade inflammation without inducing major changes of the gut microbiome: A randomised cross-over trial. Gut. (2019) 68:83–93. doi: 10.1136/gutjnl-2017-314786
199. Jang SE, Kim KA, Han MJ, Kim DH. Doenjang, a fermented Korean soybean paste, inhibits lipopolysaccharide production of gut microbiota in mice. J Med Food. (2014) 17:67–75. doi: 10.1089/jmf.2013.3073
200. Cotillard A, Kennedy SP, Kong LC, Prifti E, Pons N, Le Chatelier E, et al. Dietary intervention impact on gut microbial gene richness. Nature. (2013) 500:585–8. doi: 10.1038/nature12480
201. Anhe FF, Nachbar RT, Varin TV, Vilela V, Dudonne S, Pilon G, et al. A polyphenol-rich cranberry extract reverses insulin resistance and hepatic steatosis independently of body weight loss. Mol Metab. (2017) 6:1563–73. doi: 10.1016/j.molmet.2017.10.003
202. Calinoiu LF, Vodnar DC. Whole grains and phenolic acids: A review on bioactivity, functionality, health benefits and bioavailability. Nutrients. (2018) 10:1615. doi: 10.3390/nu10111615
203. Venancio VP, Kim H, Sirven MA, Tekwe CD, Honvoh G, Talcott ST, et al. Polyphenol-rich mango (Mangifera indica L.) ameliorate functional constipation symptoms in humans beyond equivalent amount of fiber. Mol Nutr Food Res. (2018) 62:e1701034. doi: 10.1002/mnfr.201701034
204. Lindsay JO, Whelan K, Stagg AJ, Gobin P, Al-Hassi HO, Rayment N, et al. Clinical, microbiological, and immunological effects of fructo-oligosaccharide in patients with Crohn’s disease. Gut. (2006) 55:348–55. doi: 10.1136/gut.2005.074971
205. Welters CF, Heineman E, Thunnissen FB, van den Bogaard AE, Soeters PB, Baeten CG. Effect of dietary inulin supplementation on inflammation of pouch mucosa in patients with an ileal pouch-anal anastomosis. Dis Colon Rectum. (2002) 45:621–7. doi: 10.1007/s10350-004-6257-2
206. Wang LS, Mo YY, Huang YW, Echeveste CE, Wang HT, Chen J, et al. Effects of dietary interventions on gut microbiota in humans and the possible impacts of foods on patients’ Responses to cancer immunotherapy. eFood. (2020) 1:279–87. doi: 10.2991/efood.k.200824.002
207. Benus RF, van der Werf TS, Welling GW, Judd PA, Taylor MA, Harmsen HJ, et al. Association between faecalibacterium prausnitzii and dietary fibre in colonic fermentation in healthy human subjects. Br J Nutr. (2010) 104:693–700. doi: 10.1017/S0007114510001030
208. Vitaglione P, Mennella I, Ferracane R, Rivellese AA, Giacco R, Ercolini D, et al. Whole-grain wheat consumption reduces inflammation in a randomized controlled trial on overweight and obese subjects with unhealthy dietary and lifestyle behaviors: role of polyphenols bound to cereal dietary fiber. Am J Clin Nutr. (2015) 101:251–61. doi: 10.3945/ajcn.114.088120
209. May T, Mackie RI, Fahey GC Jr., Cremin JC, Garleb KA. Effect of fiber source on short-chain fatty acid production and on the growth and toxin production by clostridium difficile. Scand J Gastroenterol. (1994) 29:916–22. doi: 10.3109/00365529409094863
210. Blaak EE, Canfora EE, Theis S, Frost G, Groen AK, Mithieux G, et al. Short chain fatty acids in human gut and metabolic health. Benef Microbes. (2020) 11:411–55. doi: 10.3920/BM2020.0057
211. Raqib R, Sarker P, Mily A, Alam NH, Arifuzzaman AS, Rekha RS, et al. Efficacy of sodium butyrate adjunct therapy in shigellosis: A randomized, double-blind, placebo-controlled clinical trial. BMC Infect Dis. (2012) 12:111. doi: 10.1186/1471-2334-12-111
212. Zeng X, Sunkara LT, Jiang W, Bible M, Carter S, Ma X, et al. Induction of porcine host defense peptide gene expression by short-chain fatty acids and their analogs. PloS One. (2013) 8:e72922. doi: 10.1371/journal.pone.0072922
213. Sunkara LT, Jiang W, Zhang G. Modulation of antimicrobial host defense peptide gene expression by free fatty acids. PloS One. (2012) 7:e49558. doi: 10.1371/journal.pone.0049558
214. Iraporda C, Errea A, Romanin DE, Cayet D, Pereyra E, Pignataro O, et al. Lactate and short chain fatty acids produced by microbial fermentation downregulate proinflammatory responses in intestinal epithelial cells and myeloid cells. Immunobiology. (2015) 220:1161–9. doi: 10.1016/j.imbio.2015.06.004
215. Vinolo MA, Rodrigues HG, Hatanaka E, Sato FT, Sampaio SC, Curi R. Suppressive effect of short-chain fatty acids on production of proinflammatory mediators by neutrophils. J Nutr Biochem. (2011) 22:849–55. doi: 10.1016/j.jnutbio.2010.07.009
216. Donohoe DR, Collins LB, Wali A, Bigler R, Sun W, Bultman SJ. The warburg effect dictates the mechanism of butyrate-mediated histone acetylation and cell proliferation. Mol Cell. (2012) 48:612–26. doi: 10.1016/j.molcel.2012.08.033
217. Schauber J, Svanholm C, Termen S, Iffland K, Menzel T, Scheppach W, et al. Expression of the cathelicidin ll-37 is modulated by short chain fatty acids in colonocytes: relevance of signalling pathways. Gut. (2003) 52:735–41. doi: 10.1136/gut.52.5.735
218. Domokos M, Jakus J, Szeker K, Csizinszky R, Csiko G, Neogrady Z, et al. Butyrate-induced cell death and differentiation are associated with distinct patterns of ros in ht29-derived human colon cancer cells. Dig Dis Sci. (2010) 55:920–30. doi: 10.1007/s10620-009-0820-6
219. Chirakkal H, Leech SH, Brookes KE, Prais AL, Waby JS, Corfe BM. Upregulation of bak by butyrate in the colon is associated with increased sp3 binding. Oncogene. (2006) 25:7192–200. doi: 10.1038/sj.onc.1209702
220. Hinnebusch BF, Meng S, Wu JT, Archer SY, Hodin RA. The effects of short-chain fatty acids on human colon cancer cell phenotype are associated with histone hyperacetylation. J Nutr. (2002) 132:1012–7. doi: 10.1093/jn/132.5.1012
221. Zeng H, Briske-Anderson M. Prolonged butyrate treatment inhibits the migration and invasion potential of ht1080 tumor cells. J Nutr. (2005) 135:291–5. doi: 10.1093/jn/135.2.291
222. Lauder SN, Allen-Redpath K, Slatter DA, Aldrovandi M, O’Connor A, Farewell D, et al. Networks of enzymatically oxidized membrane lipids support calcium-dependent coagulation factor binding to maintain hemostasis. Sci Signal. (2017) 10:eaan2787. doi: 10.1126/scisignal.aan2787
223. Maeyama M, Tanaka K, Nishihara M, Irino Y, Shinohara M, Nagashima H, et al. Metabolic changes and anti-tumor effects of a ketogenic diet combined with anti-angiogenic therapy in a glioblastoma mouse model. Sci Rep. (2021) 11:79. doi: 10.1038/s41598-020-79465-x
224. Brand A, Singer K, Koehl GE, Kolitzus M, Schoenhammer G, Thiel A, et al. Ldha-associated lactic acid production blunts tumor immunosurveillance by T and nk cells. Cell Metab. (2016) 24:657–71. doi: 10.1016/j.cmet.2016.08.011
225. Ho PC, Bihuniak JD, Macintyre AN, Staron M, Liu X, Amezquita R, et al. Phosphoenolpyruvate is a metabolic checkpoint of anti-tumor T cell responses. Cell. (2015) 162:1217–28. doi: 10.1016/j.cell.2015.08.012
226. Cascone T, McKenzie JA, Mbofung RM, Punt S, Wang Z, Xu C, et al. Increased tumor glycolysis characterizes immune resistance to adoptive T cell therapy. Cell Metab. (2018) 27:977–87 e4. doi: 10.1016/j.cmet.2018.02.024
227. Ajona D, Ortiz-Espinosa S, Lozano T, Exposito F, Calvo A, Valencia K, et al. Short-term starvation reduces igf-1 levels to sensitize lung tumors to pd-1 immune checkpoint blockade. Nat Cancer. (2020) 1:75–85. doi: 10.1038/s43018-019-0007-9
228. Heemskerk VH, Daemen MA, Buurman WA. Insulin-like growth factor-1 (Igf-1) and growth hormone (Gh) in immunity and inflammation. Cytokine Growth Fact Rev. (1999) 10:5–14. doi: 10.1016/s1359-6101(98)00022-7
229. Madeo F, Pietrocola F, Eisenberg T, Kroemer G. Caloric restriction mimetics: towards a molecular definition. Nat Rev Drug Discov. (2014) 13:727–40. doi: 10.1038/nrd4391
230. Spencer CN, Gopalakrishnan V, McQuade J, Andrews MC, Helmink B, Khan MW, et al. The gut microbiome (Gm) and immunotherapy response are influenced by host lifestyle factors. Cancer Res. (2019) 79:2838. doi: 10.1158/1538-7445.SABCS18-2838
231. Sarfraz H, Bari S, Eysha M, Patel K, Dutta T, Gardell S, et al. Safety and efficacy of time restricted eating (Tre) in improving response to immunotherapy in patients with head and neck cancer (Hnscc). Am Soc Clin Oncol. (2023) 31:6051. doi: 10.1200/JCO.2023.41.16_suppl.6051
232. Nomura M, Nagatomo R, Doi K, Shimizu J, Baba K, Saito T, et al. Association of short-chain fatty acids in the gut microbiome with clinical response to treatment with nivolumab or pembrolizumab in patients with solid cancer tumors. JAMA Netw Open. (2020) 3:e202895. doi: 10.1001/jamanetworkopen.2020.2895
Keywords: diet, microbiome, immunotherapy, cancer, immunity
Citation: Nguyen N-TA, Jiang Y and McQuade JL (2024) Eating away cancer: the potential of diet and the microbiome for shaping immunotherapy outcome. Front. Immunol. 15:1409414. doi: 10.3389/fimmu.2024.1409414
Received: 30 March 2024; Accepted: 14 May 2024;
Published: 30 May 2024.
Edited by:
Giovanni Monteleone, University of Rome Tor Vergata, ItalyReviewed by:
Federica Laudisi, University of Rome Tor Vergata, ItalyIrene Marafini, Policlinico Tor Vergata, Italy
Copyright © 2024 Nguyen, Jiang and McQuade. This is an open-access article distributed under the terms of the Creative Commons Attribution License (CC BY). The use, distribution or reproduction in other forums is permitted, provided the original author(s) and the copyright owner(s) are credited and that the original publication in this journal is cited, in accordance with accepted academic practice. No use, distribution or reproduction is permitted which does not comply with these terms.
*Correspondence: Jennifer L. McQuade, am1jcXVhZGVAbWRhbmRlcnNvbi5vcmc=