- 1Institute of Physiology, University of Zurich, Zurich, Switzerland
- 2Functional Genomics Center Zurich, Eidgenössische Technische Hochschule (ETH) Zurich and University of Zurich, Zurich, Switzerland
- 3Department of Neonatology, University Hospital Zurich and University of Zurich, Zurich, Switzerland
- 4Laboratory of Human Nutrition, Department of Health Sciences and Technology, Eidgenössische Technische Hochschule (ETH) Zurich, Zurich, Switzerland
- 5Medical Research Council (MRC) Translational Immune Discovery Unit, Medical Research Council (MRC) Weatherall Institute of Molecular Medicine, University of Oxford, Oxford, United Kingdom
- 6Department of Clinical Biochemistry, Hannover Medical School, Hannover, Germany
- 7Barcelona Institute for Global Health (ISGlobal), Hospital Clínic, University of Barcelona, Barcelona, Spain
- 8Centro de Investigación Biomédica en Red de Enfermedades Infecciosas (CIBERINFEC), Barcelona, Spain
Breast milk is a vital source of nutrients, prebiotics, probiotics, and protective factors, including antibodies, immune cells and antimicrobial proteins. Using bacterial lipopolysaccharide arrays, we investigated the reactivity and specificity of breast milk antibodies towards microbial antigens, comparing samples from rural Kenya and urban Switzerland. Results showed considerable variability in antibody reactivity both within and between these locations. Kenyan breast milk demonstrated broad reactivity to bacterial lipopolysaccharides, likely due to increased microbial exposure. Antibodies primarily recognized the O-antigens of lipopolysaccharides and showed strong binding to specific carbohydrate motifs. Notably, antibodies against specific Escherichia coli O-antigens showed cross-reactivity with parasitic pathogens like Leishmania major and Plasmodium falciparum, thus showing that antibodies reacting against lipopolysaccharide O-antigens can recognize a wide range of antigens beyond bacteria. The observed diversity in antigen recognition highlights the significance of breast milk in safeguarding infants from infections, particularly those prevalent in specific geographic regions. The findings also offer insights for potential immunobiotic strategies to augment natural antibody-mediated defense against diverse pathogens.
1 Introduction
Breast milk supplies infants with crucial nutrients, pro- and prebiotic elements such as complex oligosaccharides, and protective components such as antibodies, immune cells and antimicrobial proteins (1). The composition of breast milk evolves throughout lactation. Colostrum, which is only secreted within the first days after birth, contains the highest concentration of antibodies (2, 3). These antibodies, which predominantly belong to the IgA isotype, play a critical role in protecting the infant’s gastrointestinal tract against infections (4). Breast milk antibodies originate from maternal B-cells that mature in the gastrointestinal tract and migrate to the lactating mammary gland (5). Exposure to bacterial antigens in the gut stimulates these B-cells, leading to the production of antibodies secreted into breast milk. This process ensures that the milk contains diverse antibodies tailored to the mother’s gut microbiota, providing the infant with customized protection against potential pathogens (4, 6, 7). Breast milk antibodies work alongside maternal IgG antibodies transferred to the fetus during pregnancy, offering a comprehensive defense against potential infections (8). IgA antibodies in breast milk are particularly effective at neutralizing pathogens in the infant’s gastrointestinal tract, a common site of infections in newborns. Additionally, breast milk antibodies can recognize and bind to numerous pathogens, preventing their attachment to host cells and subsequent invasion. Overall, breast milk antibodies are critical components of an infant’s immune system and play an essential role in promoting their health and development (9–11).
Maternal B-cells in the mammary gland are primed to produce antibodies that recognize a wide range of antigens presented by gut microbes (12). These microbes, mainly gram-positive and -negative bacteria, are covered with carbohydrate antigens like peptidoglycan, teichoic acid, and lipopolysaccharide (LPS). Especially the large diversity of LPS O-antigens on gut bacteria represents a vast repertoire of carbohydrate antigens that stimulate the immune system (13). The precise mechanisms by which carbohydrate antigens stimulate B-cells and mediate Ig class switching in the gut are still being studied. In mucosal tissues, such as the intestinal lamina propria, B-cell activation and immunoglobulin class switching can occur independently of T-cells through the stimulatory effects of the TNF superfamily proteins BAFF and APRIL secreted by dendritic cells (14, 15). Because of the constant exposure of mucosal immune cells to gut bacteria, bacterial carbohydrate antigens are major stimuli for antibody production, thus leading to high titers of carbohydrate-specific antibodies in the gastrointestinal tract and blood circulation. Carbohydrate structures, which are prominently exposed on bacterial surfaces but absent on animal cells, elicit robust antibody responses. These carbohydrate antigens mainly consist of monosaccharides, such as rhamnose and galactofuranose (Galf), and disaccharides, such as the Gal(α1-3)Gal α-Gal antigen and GalNAc(α1-3)GalNAc Forssman antigen, which are not found on human cells. Antibodies directed towards ABO blood group antigens are also generated through the stimulation of B-cells by structurally similar carbohydrates exposed on gut bacteria (16).
Despite significant differences between prokaryotic and eukaryotic glycosylation pathways, specific carbohydrate structures are shared across taxonomic groups. Antibodies targeting bacterial glycans may therefore cross-react with similar epitopes presented on other organisms. For example, the presence of E. coli O86 in the gut stimulates the production of antibodies reacting with the α-Gal epitope occurring on its O-antigen, and which is also found on the surface of pathogens such as Plasmodium falciparum, thereby conferring protection towards malaria (17). However, the production of cross-reactive antibodies may also promote autoimmune responses when the recognized carbohydrate epitopes are also presented on host cells. Infection with Campylobacter jejuni leads to antibodies recognizing sialylated lipooligosaccharides on the bacterial surface, which resemble GM1 gangliosides presented on peripheral nerves. The production of antibodies cross-reacting with host gangliosides are associated with the development of Guillain-Barré syndrome characterized by immune-mediated peripheral neuropathy (18).
Although our understanding of the significance of breast milk antibodies has progressed, many questions remain unanswered. The range of antigens recognized by breast milk antibodies is not yet fully understood, nor is the functional relevance of cross-reactive protection. The specific repertoire of carbohydrate antigens recognized by milk antibodies also remains unknown, and it is unclear whether these antigens can cross-react with infectious pathogens relevant to different regions of the world. In our present study, we addressed these questions by examining the repertoire of antigens recognized by antibodies in breast milk collected from different geographical locations. Using an array of LPS antigens, we aimed to better understand the specific composition of breast milk antibodies and how they vary between lactating mothers and across different populations. Our research highlights the critical role of carbohydrate-specific antibodies in providing protection against different pathogens, emphasizing the importance of cross-reactivity in conferring an effective immune protection.
2 Results
Multiple factors such as genetics, diet, gut microbiota composition and the environmental exposure to pathogens influence mucosal antibody production. Considering the role of these factors in shaping the repertoire of antibodies secreted in breast milk, we compared the reactivity of breast milk antibodies from two geographical locations towards a panel of bacterial LPS antigens. The O-antigens at the tip of LPS represents a vast diversity of carbohydrate antigenic structures, characterized by oligosaccharide repeats (19, 20). These oligosaccharides consist of di- to octasaccharides comprising monosaccharides found in eukaryotic glycans and a broad range of monosaccharides unique to prokaryotes (16, 21). For our LPS arrays, we have selected a panel of 103 LPS representing a broad range of O-antigens, some of them including carbohydrate epitopes found on protist and animal cells such as α-Gal and the Forssman antigens (Supplementary Table 1). Rough mutant LPS featuring truncated glycan chains were also included on the array to assess the specificity of breast milk antibody towards carbohydrate O-antigens.
2.1 Validation of LPS microarrays
Pure LPS (Supplementary Figure 1A) were first spotted at four concentrations on NEXTERION® 3-D Hydrogel coated slides to evaluate the correlation between the quantity of printed antigen and signal intensity relevant to antibody recognition of these antigens. We noted a direct proportionality in antibody binding with increasing LPS concentrations for both O-antigen-specific immunoglobulin G (IgG) and breast milk immunoglobulin A (IgA) (Supplementary Figures 1B, C), thus confirming the suitability of the method to estimate antibody specificity to the LPS recognized. The method also allows estimating binding strength when testing pure antibodies. When testing antibody mixtures, binding to LPS does not allow to discriminate between low total levels of antibodies with high affinity and high total levels of antibodies with generally low affinity towards LPS. Because of the mass heterogeneity of LPS related to the variable degree of O-antigen polymerization, LPS were printed in a weight to volume ratio and not at a specific molarity. Consequently, the signals obtained for antibody binding to the printed LPS are semi-quantitative.
2.2 Breast milk IgA recognition of LPS O-antigens
To compare the antigenic specificity of breast milk antibodies originating from different geographical locations and environments, we investigated a cohort of mature breast milk samples collected from a rural region in Kenya (22) and mature breast milk samples collected from an urban environment in Switzerland. The average age of the donor mothers at delivery was 27 years for the Kenyan samples and 32 years for Swiss samples (Supplementary Table 2). In Kenyan breast milk, the concentrations of IgA, IgM, and IgG ranged from 0.25 to 0.74 mg/mL, 0.01 to 0.35 mg/mL and 0.02 to 0.10 mg/mL, respectively. In Swiss breast milk, the concentration range of IgA, IgM, and IgG were 0.24 to 1.47 mg/mL, 0.03 to 0.44 mg/mL and 0.01 to 0.15 mg/mL, respectively. IgM concentrations were higher in Swiss samples, whereas IgA and IgG concentrations were comparable in breast milk samples from both locations (Supplementary Figure 2). We first compared the reactivity of breast milk IgA, IgM and IgG from the 30 breast milk samples from Kenya towards the 103 LPS printed on the array. As expected, the strongest signals were measured for IgA, which is the most abundant antibody class in breast milk (Figure 1). Overall, a strong variability in the recognition of LPS antigens by IgA was observed within the breast milk samples tested. The strongest reactivities were detected towards LPS from the E. coli serotypes O13, O16, O55, O82, O85, O86, O111, O142, and K-235. The LPS of further Enterobacteriaceae including Shigella flexneri 2a and Klebsiella pneumoniae O1 were also strongly recognized, whereas low IgA reactivity was detected towards most of the Citrobacter LPS tested. The pattern of LPS recognized by IgA also significantly varied between individual breast milk samples, suggesting that LPS-specific IgA reflected previous exposure of the women’s immune system to bacteria presenting the corresponding epitopes. Given that IgM and IgG make up about 8% and 2% of antibodies in human breast milk, the reactivity of these Ig classes towards LPS was considerably weaker than the reactivity of IgA (Figure 1). When scanning the arrays at higher sensitivity, the signals measured for IgM and IgG binding pointed to differences in the recognition of individual LPS against IgA reactivity (Supplementary Figure 3), suggesting that individual LPS do not lead to the production of similar antibody responses across Ig classes. For all LPS investigated, the respective reactivity of IgA and IgM did not correlate significantly (Supplementary Figure 4). The same lack of correlation was observed between the reactivity of IgA and IgG towards individual LPS (Supplementary Figure 5).
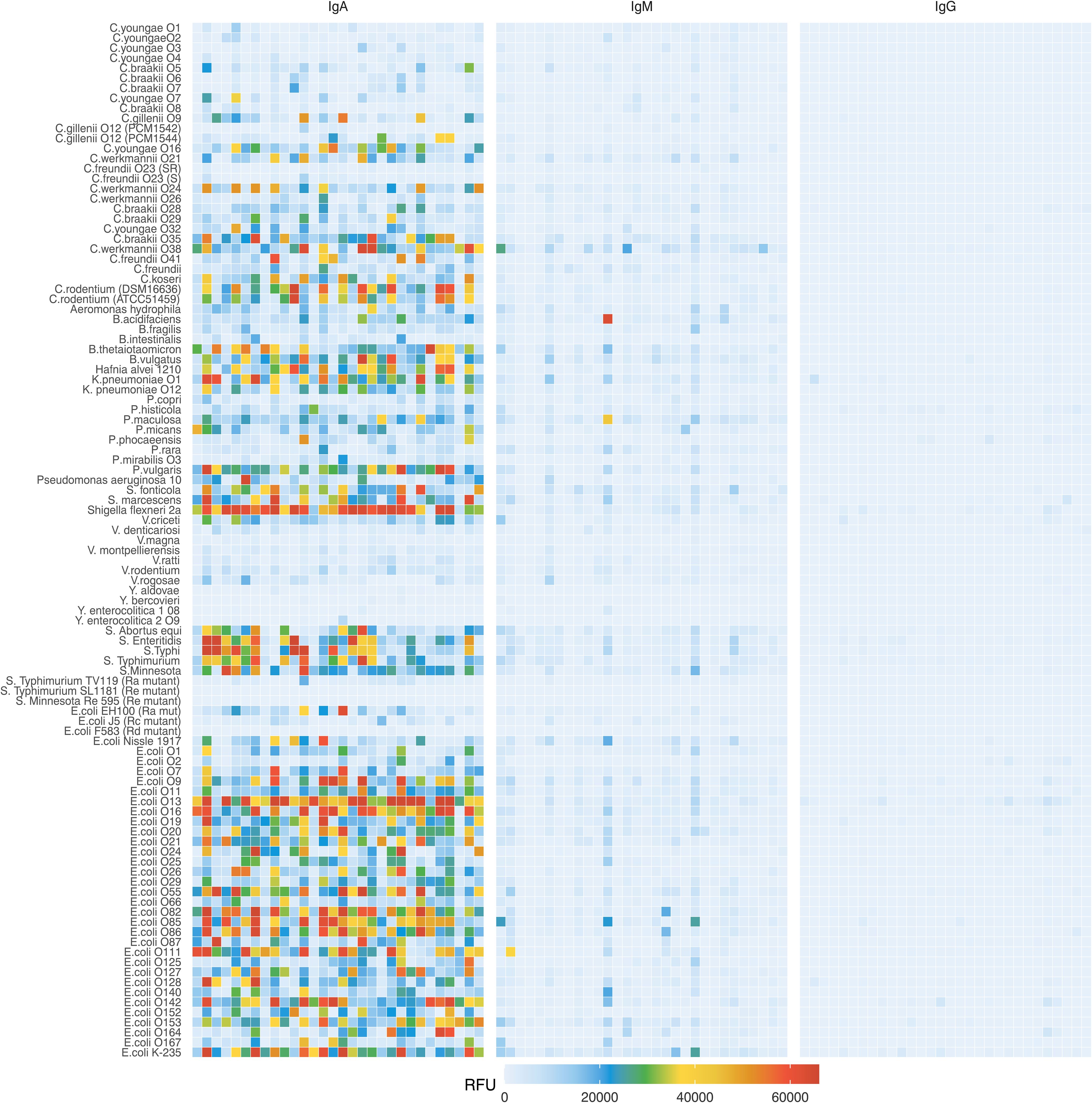
Figure 1. IgA, IgM and IgG reactivity to LPS in Kenyan breast milk samples (n=30). Breast milk samples were normalized to an IgA concentration of 10 μg/mL and applied to LPS arrays. Antibody binding to LPS was detected using either an anti-human IgA and IgM Cy3 or anti-human IgG AlexaFluor647 labelled antibody. Fluorescence signals were recorded at a PMT-gain of 190V. Data are shown as net mean RFU values of 9-replicate dots.
The incorporation of rough mutant LPS on the array allowed assessing which LPS moiety was mainly recognized by antibodies. A series of mutant LPS lacking only the O-antigen (Ra) or the O-antigen plus portions of the core oligosaccharide (Rc, Rd, Re, Figure 2A) confirmed that the O-antigen was the main epitope recognized by breast milk IgA, IgM and IgG. Reactivity towards the Ra and Rc mutant LPS of E. coli tested was on average 10-fold lower than towards smooth LPS featuring O-antigens (Figures 2B, C). Similar differences were measured between the smooth LPS from Salmonella enterica containing O-antigens and the rough mutant LPS lacking portions of the carbohydrate moiety (Figures 2D, E).
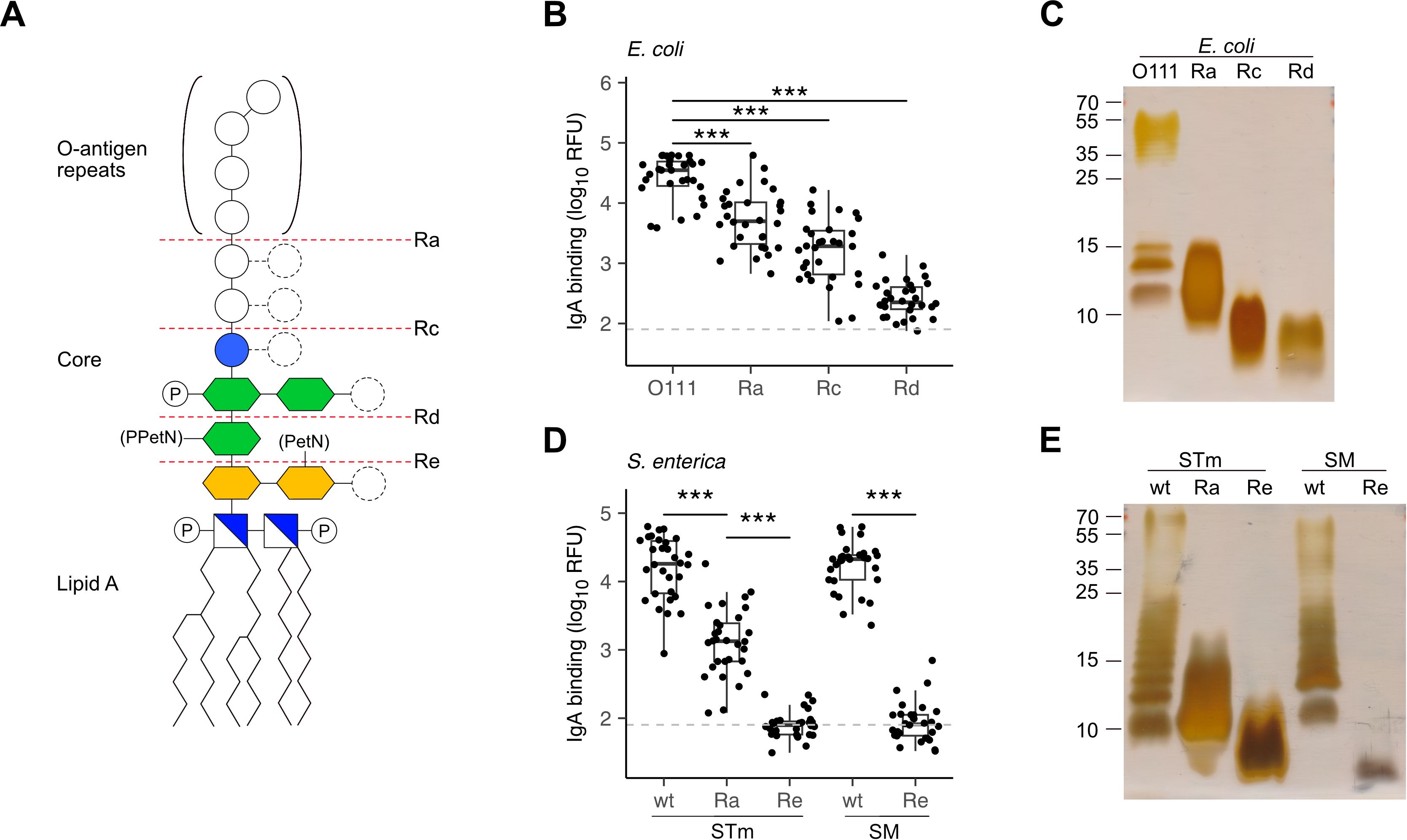
Figure 2. Recognition of O-antigens by breast milk IgA. (A) Schematic structure of LPS consisting of lipid A, a phosphorylated (P) glucosamine disaccharide (blue/white square), a glycan core of 2-keto-3-deoxy-D-mannooctanoic acid (KDO, yellow hexagon), heptose (green hexagon), glucose (blue circle) and repeats of O-antigen oligosaccharides. Distinct serotypes vary in sugar composition (empty circles) and number of sugars (dashed circles) and can be modified with phosphoethanolamine (PetN) or pyrophosphorylethanolamine (PPEtN). E. coli and S. enterica rough mutants lack the O-antigen (Ra) or variable regions of the glycan core (Rc, Rd and Re). (B) IgA binding intensity to E. coli smooth LPS (O111) and rough LPS (Ra, Rc, Rd). (C) Silver stain of E. coli smooth LPS (O111) and rough LPS mutants (Ra, Rc, Rd) separated by SDS-PAGE. (D) IgA binding intensity to S. enterica Typhimurium (STm) and S. Minnesota (SM) wildtype (wt) smooth LPS and Ra, Re rough LPS mutants. (E) Silver stain of STm and SM wt smooth LPS and rough LPS (Ra, Re) separated by SDS-PAGE. P values were determined by unpaired Wilcoxon test and indicated by asterisks. P-values <0.001 (***) were considered statistically significant. The grey dotted line marks the threshold of detection (TOD).
2.3 Comparison of antibody reactivity between Switzerland and Kenya
The analysis of breast milk samples from Kenya revealed a wide inter-individual variability in the reactivity of breast milk antibodies to LPS (Figure 1). To evaluate the geographical specificity of the breast milk antibody response to LPS antigens, we have also analyzed the antibody reactivity of breast milk samples obtained from an urban environment in Switzerland. Generally, stronger and broader IgA reactivities to LPS were detected in the breast milk samples from Kenya (Figure 3). In particular, we detected higher IgA reactivities in Kenyan samples towards the LPS of bacteria causing diarrheal disease, such as E. coli, S. flexneri, and Salmonella enterica. We also observed that LPS containing specific mono- and disaccharide motifs, such as rhamnose, 3,6-dideoxysugars, Galf, α-Gal and Forssman epitopes resulted in strong IgA binding in Kenyan breast milk samples (Figure 4; Supplementary Figure 6). Rhamnose is a carbohydrate widely occurring in plants (23) and bacterial cell walls (24) but absent in animals (25), which explains the high titers of antibodies in humans recognizing rhamnose. The O-antigens of S. flexneri 2a and E. coli O13 (Figures 4A, B) include three α-linked rhamnose residues. Interestingly, the O-antigen of Citrobacter gillenii O9, which is made up of α-linked N-acetyl-rhamnosamine (RhaNAc), was about ten-fold less recognized than the rhamnosylated O-antigen of S. flexneri 2a and E. coli O13 (Figure 4C). Recognition of E. coli O86, which contains the α-Gal and Forssman disaccharide motifs was stronger for IgA from Kenyan samples than Swiss samples (Figure 4D). IgA reactivity towards LPS containing the 3,6-dideoxy sugars abequose, tyvelose and colitose was also stronger in Kenyan samples than Swiss samples (Figures 4E, F; Supplementary Figures 6D-G). These three 3,6-dideoxy sugars are commonly found in Salmonella enterica subsp. enterica serovar Typhimurium and Typhi, which are endemic in developing countries, cause non-typhoidal salmonellosis, and typhoidal fever, respectively (26). Salmonella enterica serovar Typhimurium (Figure 4E) and serovar Abortus equi (D) share similar O-antigens including abequose, while Salmonella enterica serovar Typhi shares a similar O-antigen but with tyvelose instead of abequose (Figure 4F). Abequose is also found in the O-antigen of C. werkmannii O38, which was strongly recognized by IgA in Kenyan samples (Supplementary Figure 6E). Colitose occurs in E. coli O55 and E. coli O111, which both elicited strong IgA responses in Kenyan samples (Supplementary Figures 6F, G). The enteropathogenic E. coli O111 serogroup is the primary cause of diarrhea in infants, which is particularly a problem in developing countries (27). Further LPS that were predominantly recognized by IgA in Kenyan breast milk samples were E. coli O86, O87, O127 and O142, which contain the α-Gal and/or Forssman disaccharide antigens (Figure 4D; Supplementary Figures 6H, I, L). Humans produce high antibody titers towards these carbohydrate antigens, which are absent on human cells (28, 29). Also, LPS containing Galf, a monosaccharide that is not found in humans (30) were strongly recognized by IgA in Kenyan samples (Supplementary Figures 6J-L). Altogether, Kenyan breast milk samples featured higher IgA reactivities towards LPS associated with disease in developing countries. The presence of such reactive antibodies in the breast milk certainly contributes to the protection of nursed infants towards a broad range of bacterial pathogens.
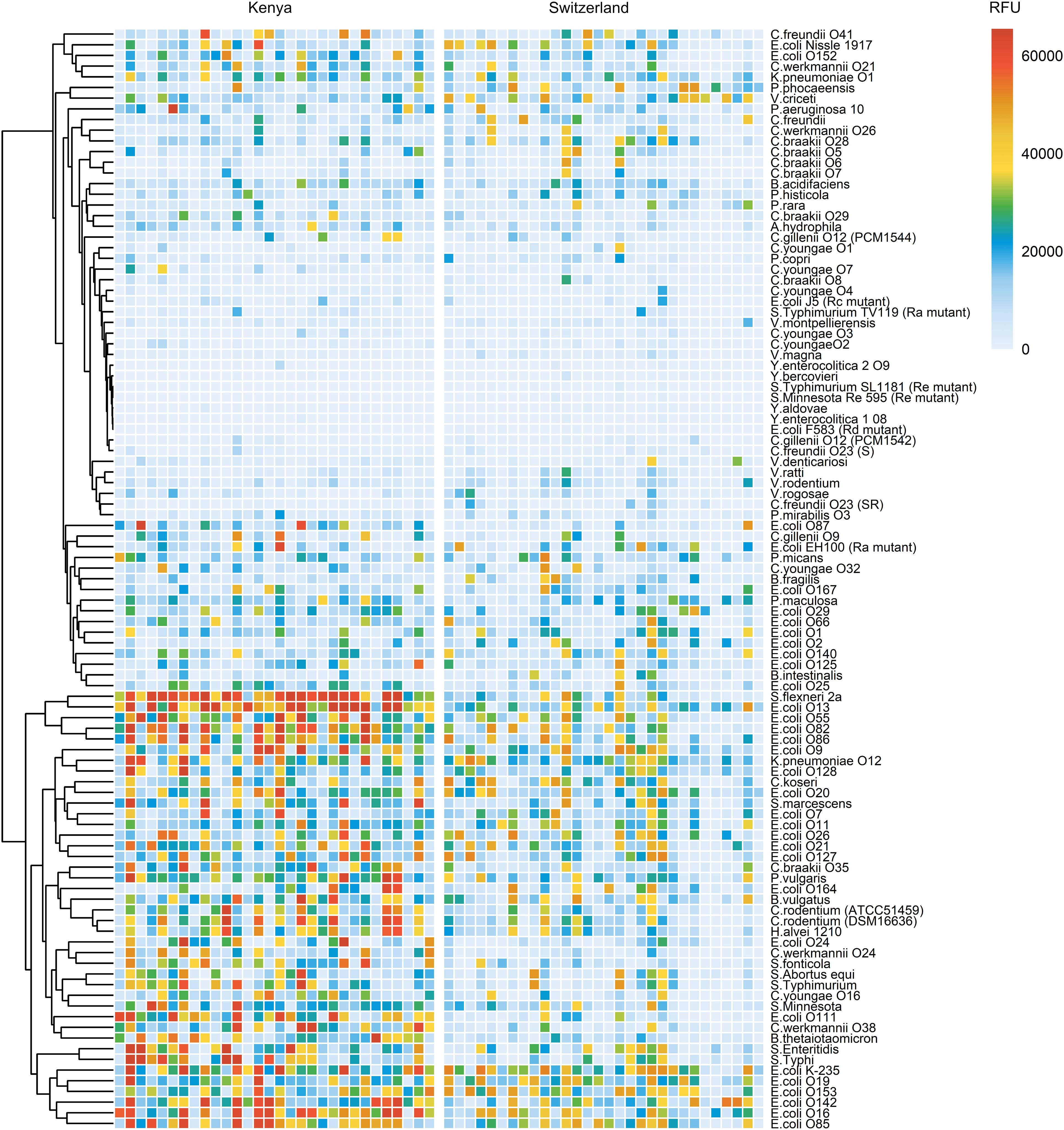
Figure 3. Comparison of IgA reactivity between Kenyan and Swiss breast milk samples. 30 breast milk samples from Kenya (left panel) and 30 samples from Switzerland (right panel) were normalized to an IgA concentration of 10 μg/mL and analyzed on LPS arrays. Antibody binding to LPS was detected using anti-human IgA secondary antibody coupled to Cy3. Fluorescence signals were recorded at a PMT-gain of 190V. The net mean RFU values of 9-replicate dots are shown. Hierarchical clustering of LPS binding signals was performed using the pheatmap package in R studio.
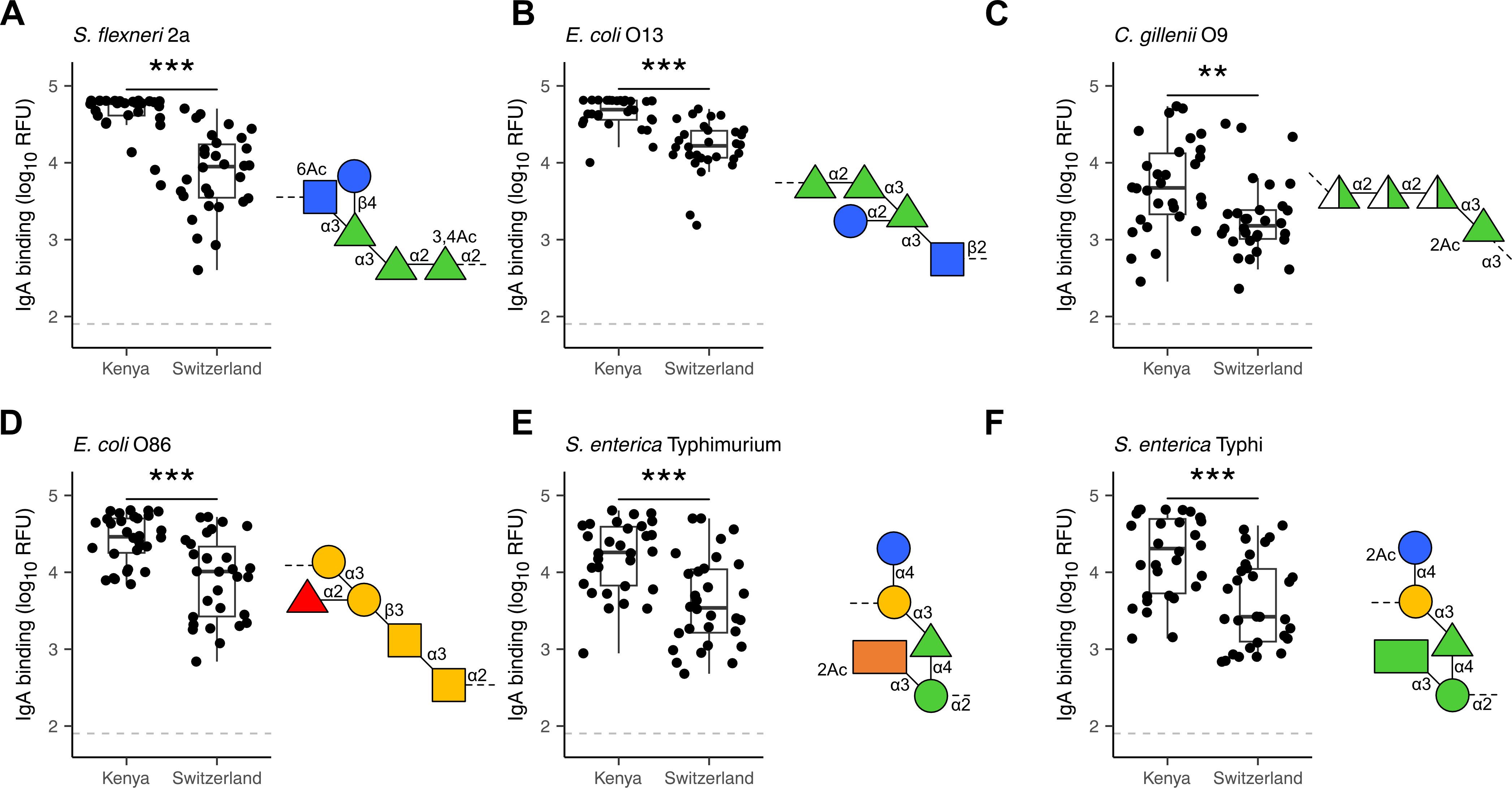
Figure 4. Differential recognition of specific LPS O-antigens by Kenyan and Swiss breast milk IgA. IgA binding to LPS O-antigens including the immunogenic epitopes rhamnose (green triangles) as found in (A) S. flexneri 2a; (B) E.coli O13 and (C) C. gillenii O9; the α-Gal (two yellow circles) and Forssman (two yellow squares) antigens as found in (D) E. coli O86; the dideoxy sugars abequose (orange rectangle) and tyvelose (green rectangle) as found in (E) S. enterica Typhimurium and (F) S. enterica Typhi. IgA binding was detected using anti-human IgA Cy3, scanned at a PMT-gain of 190V. The grey dotted line shows TOD. Comparisons of net mean RFU values between Kenyan (n=30) and Swiss (n=30) samples was done using an unpaired Wilcoxon test. P-values <0.001 (***), <0.01 (**) were considered statistically significant. The symbols used to display O-antigens is based on the standard symbolic nomenclature for glycans (52).
2.4 Cross-reactivity of LPS-specific antibodies
The vast diversity of O-antigens not only encompasses a multitude of distinct antigens but often gives rise to structurally similar antigens. Hierarchical clustering of our array analysis pointed to similar antibody responses towards LPS sharing similar O-antigen structures. For example, the similar O-antigens of E. coli O13 and S. flexneri 2a (Figures 4A, B) elicited cross-reactive antibody responses among the Kenyan breast milk samples analyzed (Figure 3). Likewise, the structurally overlapping O-antigens of S. Enteritis and S. Typhi (Figure 4F) were similarly recognized across the breast milk samples analyzed (Figure 3), thus suggesting recognition by cross-reacting antibodies. To confirm the cross-reactivity of antibodies between structurally similar O-antigens, we have analyzed the reactivity of a polyclonal antibody specific to the E. coli O-antigen O152. The same O-antigen is also expressed by Citrobacter rodentium ATCC51459 and similar O-antigens are found on Hafnia alvei 1210 and Klebsiella pneumoniae O12, which both contain the rhamnose(β1-4)N-acetylglucosamine (GlcNAc) motif (Figure 5A). Using immunoblotting, we confirmed that the anti-O152 antibody indeed recognized the purified LPS from C. rodentium and H. alvei (Figure 5B). The LPS of K. pneumoniae O12 remained undetected by the anti-O152 antibody, which may be due to the masking of β-rhamnose by the direct linkage to the next GlcNAc unit, which may prevent the recognition by the antibody.
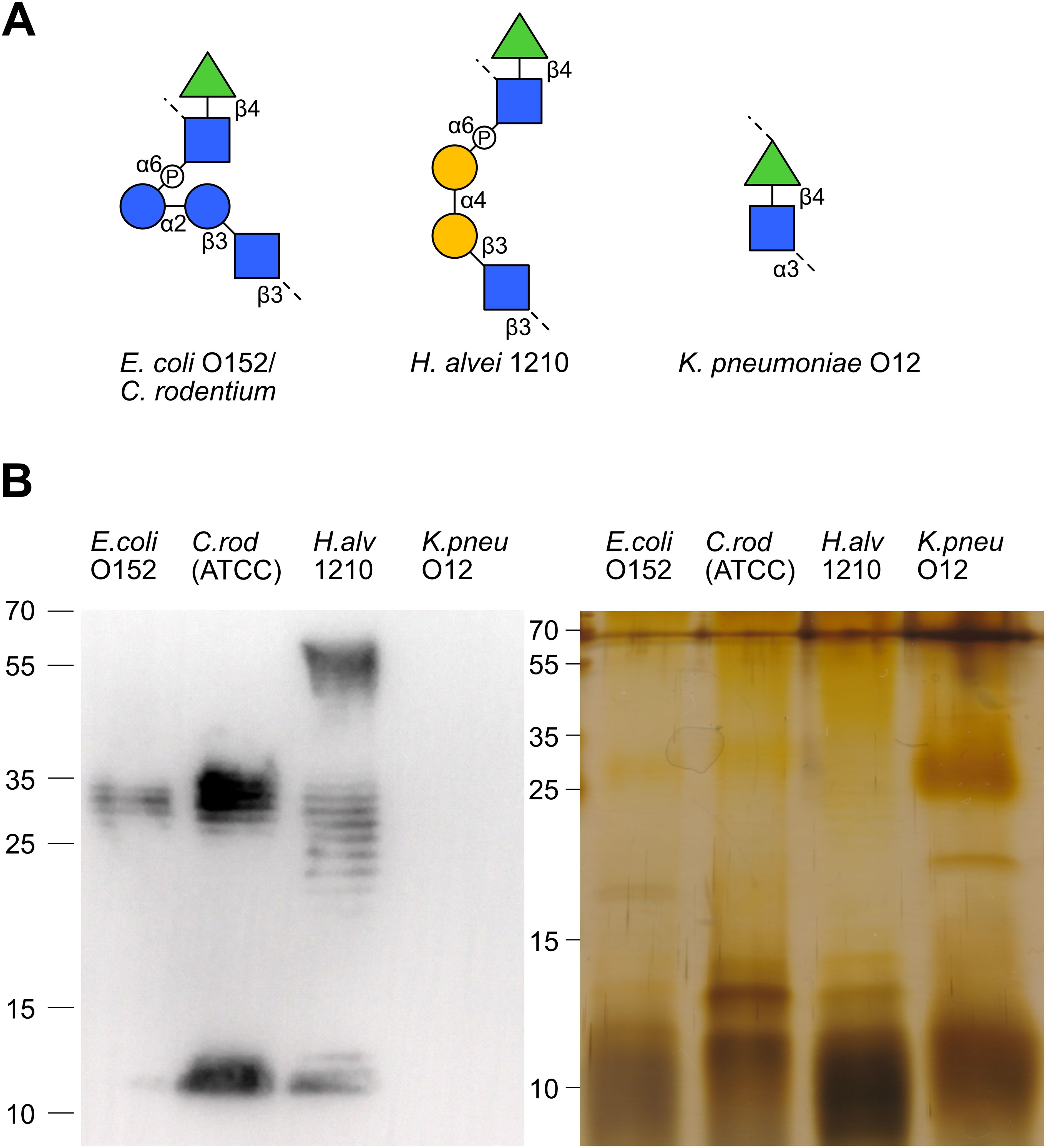
Figure 5. Antibody cross-reactivity to structurally similar LPS O-antigens. (A) The O-antigen structures of E. coli O152/C. rodentium (ATCC51459), H. alvei 1210 and K. pneumoniae O12. (B) Immunoblotting and silver stained SDS-PAGE of E. coli O152, C. rodentium, H.alvei 1210 and K. pneumoniae O12 LPS recognized by anti-O152 polyclonal antibody.
Beyond cross-reactivity between O-antigens, antibodies recognizing bacterial carbohydrate antigens likely also recognize similar carbohydrate epitopes presented on eukaryotic cells. Previous studies have demonstrated the ability of antibodies targeting bacterial antigens to cross-react with parasitic antigens. The detection of strong IgA responses towards α-Gal and Galf-containing O-antigens in our analysis suggested that breast milk antibodies against these epitopes may cross-react with similar epitopes on parasitic pathogens endemic in Kenya, such as Plasmodium falciparum (31) and Leishmania major (32). To test this hypothesis, we have investigated the reactivity of O-antigen-specific antibodies to protein extracts of P. falciparum and L. major using immunofluorescence and immunoblotting. Antibodies specific to E. coli O142 strongly bound to blood stage P. falciparum, as well as weaker bindings by E. coli O152 (Figure 6) which is consistent with the results from immunoblotting, where they weakly reacted with an antigen of P. falciparum at asexual intraerythrocytic stages (Figures 6E, F). E. coli O55- and O85- specific antibodies poorly bound to P. falciparum (Figure 6). Antibodies specific to E. coli O55, O85, O142 and O152 were found to bind to L. major at the promastigote stage to various extent as detected by immunofluorescence (Figure 6B). We also tested reactivity of these O-antigen-specific antibodies to L. major wildtype and to a mutant strain lacking Galf (33) by Western blotting. The anti-O85 antibody recognized an antigen on both wildtype and Galf -deficient mutant L. major (Figure 6D), which was unexpected considering the presence of Galf in the O85 O-antigen. The anti-O142 antibody did not reveal any antigen binding of L. major on Western blot, whereas the E. coli O152 antibody recognized several antigens of L. major extracts (Figure 6D). By comparison, the anti-O55 antibody failed to cross-react with any antigen of P. falciparum and L. major on Western blot (Figure 6C) despite its strong binding to L. major detected by immunofluorescence (Figure 6B). The confirmation that O-antigen-specific antibodies indeed recognized epitopes on parasite extracts indicate that the vast repertoire of carbohydrate-specific cross-reactive antibodies in the breast milk could provide a broad protection of nursed infants, not just towards bacterial infections but also towards other endemic eukaryotic pathogens.
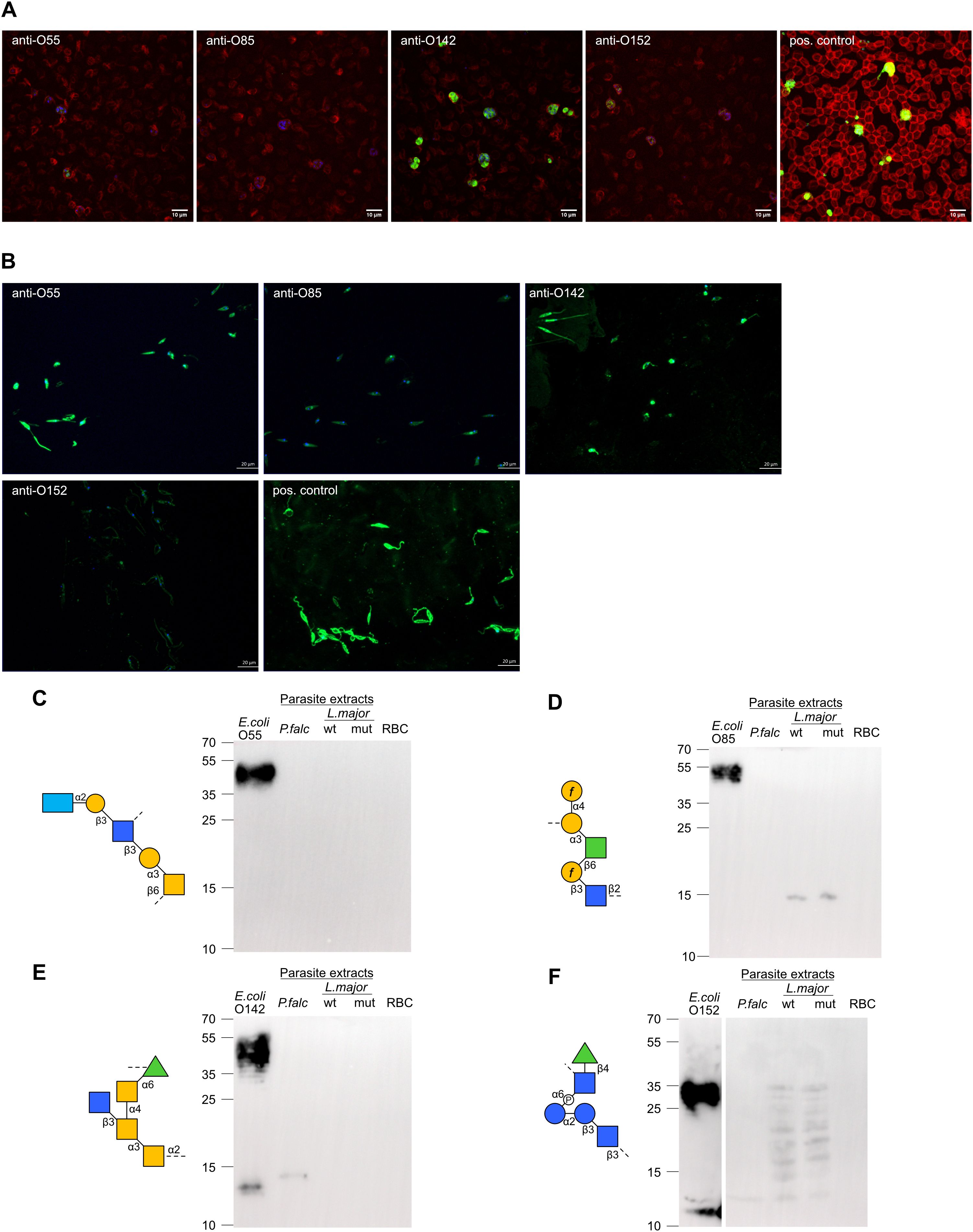
Figure 6. Cross-reactivity of O-antigen-specific antibodies to L. major and P. falciparum antigens. (A) Immunofluorescence staining of P. falciparum non-synchronized cultures with anti-O-antigen antibodies specific towards E. coli O55, O85, O142, O152 and with positive control antibody. (B) Immunofluorescence staining of L. major promastigotes with anti-O-antigen antibodies specific towards E. coli O55, O85, O142, O152 and with positive control antibody. (C-F) SDS-PAGE with E. coli LPS, parasite extracts from P. falciparum (P.falc) and L. major wildtype (wt) and Galf mutant (mut), and red blood cell (RBC) control. Immunoblotting using O-antigen specific sera from (C) E. coli O85, (D) (E) coli O142, (E) E. coli O152 and (F) E. coli O55.
3 Discussion
Our investigation of breast milk antibodies not only underlined the broad range of bacterial LPS antigens recognized, but also the evident variability in the reactivity of these antibodies between individual breast milk samples. The inter-individual variability in the reactivity of breast milk IgA toward bacterial antigens has been reported in previous studies (34, 35), which also pointed to differences in the recognition of bacteria by breast milk antibodies collected from various geographical locations (34). These studies however only surveyed few bacteria without discrimination of the antigens recognized. The application of an array of pure LPS antigens provided here a more precise mapping of the range and types of bacterial epitopes recognized by breast milk IgA, IgM and IgG. The resulting higher resolution of antibody specificities confirmed the variability of antigens recognized between individual breast milk samples and between samples collected in different geographical locations. These differences in antigen recognition likely reflect the microbial exposure of the mothers prior to lactation, as interactions of microbes with mucosal immunity in the gut are known to shape the range and amount of IgA secreted in the breast milk (36, 37). IgM concentrations in Kenyan breast milk samples were also significantly lower compared to IgM in Swiss samples. This difference in IgM levels may also be related to environmental or genetic factors affecting antibody production in the two geographical locations.
The broader range of LPS antigens recognized and the stronger IgA binding measured for the breast milk samples from Kenya likely reflect the prominent microbial exposure of these mothers living in rural regions with limited access to clean water, which increases their susceptibility to gastrointestinal infections (38). Whereas the elevated infection pressure represents a significant health risk especially for women during pregnancy, the increased exposure to microbes obviously leads to the generation of breast milk that is rich in protective antibodies towards a vast range of bacterial pathogens (39). The broad spectrum of breast milk antibodies against microbes therefore underscores the importance of breastfeeding to ensure the protection of infants, especially in locations with precarious environmental health conditions.
The application of pure LPS structures on the antigen arrays also allowed defining the epitopes that are mainly recognized by breast milk antibodies. The presence of rough mutant LPS on the array, which lack portions of the carbohydrate moiety, clearly showed that breast milk IgA, IgM and IgG predominantly recognize O-antigens. Re rough mutants featuring lipid A with only two KDO residues were hardly recognized by breast milk antibodies. Studies on the specificity of monoclonal antibodies cloned from intestinal IgA and lactating mammary gland IgA pointed to the polyreactivity of these antibodies to various microbial antigens such as LPS, capsular polysaccharides and flagellin (40). The polyreactivity of these IgA monoclonal antibodies was shown through their ability to bind to multiple bacterial targets (12). Although the mechanisms of such a polyreactivity are not completely clear, the phenomenon can be explained by the sharing of similar glycan epitopes across multiple bacterial taxa. The relevance of glycan epitope similarity in eliciting polyreactive IgA and IgM was confirmed in the case of cross-specific protective antibodies targeting LPS of various serotypes of Klebsiella pneumoniae and even structurally-similar LPS of non–Klebsiella pneumoniae species (41). Beyond the example of Klebsiella pneumoniae LPS, our study shows that O-antigen similarity leads to the development of antibodies that confer a broad recognition of bacterial antigens, thereby providing protection against a wide range of invading pathogens. While our study solely shows the ability of O-antigen specific antibodies to bind to Plasmodium falciparum and Leishmania major cells, the extent and functional significance of cross-reactivity of breast milk antibodies towards LPS and eukaryotic antigens still requires further investigation. Only the purification of antibodies targeting specific parasites and subsequent demonstration of their binding to bacterial O-antigens would clearly demonstrate cross-reactivity between antigens.
O-antigens including carbohydrate motifs that are absent from human cells elicited strong IgA binding, as especially evidenced in breast milk samples from Kenya. Monosaccharides, such as rhamnose, colitose, abequose, tyvelose, Galf and the disaccharides α-Gal and Forssman antigens were associated with the strongest signals recorded for IgA binding on the LPS array. Some of these immunogenic carbohydrate antigens do not only occur on bacterial polysaccharides but are also found on eukaryotic cells including protists, fungi plants and animals. The 3,6-dideoxysugar tyvelose is part of an immunodominant surface antigen of the nematode Trichinella spiralis (42). Galf is also commonly found on of the glycans of the kinetoplastids Leishmania spp (43). and Trypanosoma spp (44).The α-Gal antigen also occurs on P. falciparum (17), while the presence of antibodies to this carbohydrate antigen has been correlated with protection against malaria transmission (45, 46). Interestingly, gut colonization with E. coli O86, which contains α-Gal antigen in its O-antigen, was shown to be associated with malaria protection in humans and in a mouse model (17). This protection was related to the production of anti-α-Gal IgM, whereas IgG to α-Gal did not appear to confer protection against P. falciparum infection. Beyond E. coli O86 colonization and antibodies towards the α-Gal antigen, our present work shows that further E. coli serotypes stimulate the production of antibodies, which cross-react with P. falciparum and L. major antigens. This cross-reactivity of anti-LPS antibodies to parasites indicates that the E. coli O-antigens may stimulate the production of antibodies conferring partial protection to malaria and leishmaniasis. In general, commensal bacteria featuring carbohydrate antigens shared with specific pathogens could be used in immunobiotic approaches to boost the natural antibody protection towards a broad range of pathogens, which would be especially relevant for infections where vaccines are still unavailable. The functional investigation of cross-reactive antibodies in respect to the neutralization of pathogens, such as P. falciparum and L. major, will contribute to establish the broad protective role of such antibodies.
In conclusion, breast milk antibodies targeting carbohydrate antigens on bacteria may have a protective effect against a wide range of pathogens beyond prokaryotes. In the context of Kenya, where endemic infections such as malaria and leishmaniasis impose significant burden on local populations, carbohydrate-reactive breast milk antibodies may contribute to protect infants from multiple infections. Furthermore, studying breast milk antibody specificity in additional geographical locations will contribute to better understand how various regional factors, such as diet and endemic infection diseases, influence the repertoire and protective functions of these antibodies.
4 Methods
4.1 Breast milk samples
Breast milk samples were collected at the University Hospital Zurich, Switzerland (n=30). Breast milk samples from Kenya (n=30) were collected in a hospital and health center in Kwale County, southern coastal Kenya (22) (Supplementary Table 2). Ethical approval was given by the Ethical review committees of ETH Zurich (EK 2019-N-59) and Jomo Kenyatta University of Agriculture and Technology, Nairobi, Kenya (JKU/2/4/896B). We only included mature breast milk samples from healthy donors in our study, which was defined by the time of sample collection after delivery. IgA, IgM and IgG levels in breast milk samples were measured using commercially available ELISA kits (Abnova, Taiwan) according to the manufacturer’s recommended protocols.
4.2 Bacterial cultures
Pure bacterial strains were purchased from the Leibniz Institute German Collection of
Microorganisms and Cell Cultures GmbH (DSMZ, Braunschweig, Germany), the Polish Collection of Microorganisms (PCM, Wroclaw, Poland), the National Collection of Type Cultures (NCTC, Salisbury, UK) and the American Type Culture Collection (ATCC, Manassas, USA). Bacteria were cultured under conditions recommended by the respective repositories (Supplementary Table 1). Obligate anaerobic bacteria were grown in an anaerobic workstation (Bugbox, Baker, Sanford).
4.3 LPS purification
LPS (Supplementary Table 1) was purified using the hot aqueous-phenol method (47). Briefly, bacteria were harvested from a 1 L culture at OD600 2.0, pelleted by centrifugation at 8,000 x g for 10 min, washed with 40 mL of ice-cold water and pelleted again. Pellets were resuspended in 20 mL of 2% SDS, 10% glycerol, 2% β-mercaptoethanol in 50 mM Tris-HCl, pH 6.8, boiled at 95°C for 30 min, then incubated twice with a final concentration of 50 μg/mL DNase I (Roche, Basel, Switzerland) and RNase A (Roche, Basel, Switzerland) at 37°C for 30 min, subsequently followed by another incubation with proteinase K (Thermo Scientific, Waltham, MA, USA) at a final concentration of 50 μg/mL at 55°C overnight. Equal volumes of phenol (Sigma-Aldrich, St. Louis, USA) were added to the samples, which were incubated for 15 min at 65°C after thorough mixing. A volume of 150 mL of diethyl ether was added, samples were vortexed for 30 s before centrifuging at 13,000 x g for 20 min. The upper phase was discarded, and phenol extraction was repeated using the lower phase. For precipitation, samples were supplemented with 0.5 M sodium acetate, along with 10-times the volume of 95% ethanol and were kept overnight at -20°C. After centrifugation at 3,000 x g at 4°C for 10 min, the supernatant was discarded. The pellet was resuspended in 20 mL deionized water and dialyzed using the Pur-A-Lyzer Mega 3500 Dialysis Kit (Sigma-Aldrich) and lyophilized. To ensure purity of the LPS fractions, phenol extractions and dialysis were repeated. Final products were stored at -20°C.
4.4 LPS arrays
LPS stocks were diluted to 250, 125, 62 and 31 μg/mL in 0.5 M phosphate buffer at pH 8.5 and spotted to NEXTERION® 3-D Hydrogel coated slides (Schott Nexterion, Jena, Germany) using a sciFLEXARRAYER S11 microarray spotter (Scienion, Berlin, Germany). For immobilization, the slides were stored for one to six days in a humidity chamber containing a saturated sodium chloride solution, setting 75% relative humidity. All following processing steps were performed in an automated hybridization station (HS4800, Tecan, Männedorf, Switzerland), which allows to control temperature and duration of all incubation and washing steps. The incubations with 40 mg/mL Blotting-Grade Blocker (BioRad, Hercules, CA, USA) as blocking buffer, breast milk samples, and secondary antibody were followed by washing steps consisting of a rinse for 1 min with PBS-T followed by an agitation of the stationary solution for 3 min. Typically, series of up to 3 repeated washing steps were conducted that were completed by a final 1 min PBS-T rinse. In a first step, slides were washed once with PBS-T to remove non-immobilized LPS and blocked with skim milk for 7 h. Following two washing steps, breast milk samples diluted to an IgA concentration of 10 μg/mL in blocking buffer were incubated for 16 h. The washing steps were repeated twice before the second antibody was added for 1 h incubation time. The secondary antibodies goat anti-human IgA Cy3 (109-165-011), donkey anti-human IgM Cy3 (709-165-073) and donkey anti-human IgG Alexa Fluor 647 (709-605-098) (all from Jackson ImmunoResearch, West Grove, PA, USA) were diluted 1:10,000 in blocking buffer. Washing steps were repeated twice followed by washing with 0.01% Tween 20 for 90 s. Finally, the slides were dried in the hybridization station HS4800 with nitrogen for 3.5 min at 30°C. The slides were scanned immediately after processing using an LS400 confocal microarray scanner (Tecan, Männedorf, Switzerland). Cy3 fluorescence was excited at 532 nm and detected at 590 nm, Alexa Fluor 647 at 633nm. Mean net intensities in relative fluorescence units (RFU) and standard deviation (SD) were calculated using the ArrayPro 4.5 software (Media Cybernetics, Rockville, MD, USA).
4.5 Leishmania lysates
L. major MHOM/SU/73/5ASKH and glf¯ derivative (33) were grown at 27°C in M199 medium (Invitrogen, Karlsruhe, Germany) supplemented with 10% fetal calf serum, 40 mM Hepes pH 7.5, 0.1 mM adenine, 0.0005% hemin, and 0.0002% biotin. Exponentially growing promastigotes were harvested by centrifugation at 1300 x g for 10 min, washed with PBS, and resuspended at a concentration of 109 parasites/mL in 50 mM Tris/HCl pH 8.0, 0.1% TritonX-100, 1 mM phenylmethylsulfonyl fluoride (PMSF), 2 µg/mL Leupeptin and 5 µg/mL pepstatin. The parasites were homogenized by sonification, centrifuged at 1,300 x g for 10 min and the supernatant was collected. The protein concentration was determined in triplicate with a PierceTM BCA assay kit according to the manufacturer instructions. Laemmli buffer was added to the lysate and heated at 95°C for 5 min.
4.6 Plasmodium lysates
P. falciparum strain 3D7 parasites were cultured with human B+ erythrocytes (3% hematocrit) in RPMI medium supplemented with Albumax and incubated at 37°C in an atmosphere of 92% N2, 3% O2 and 5% CO2 using standard methods (48). Human erythrocytes were purchased from the Banc de Sang i Teixits (Catalonia, Spain) after approval from the Comitè Ètic Investigació Clínica Hospital Clínic de Barcelona (HCB/2020/0051). Parasite growth was regularly monitored by counting the infected erythrocytes in Giemsa-stain blood smears by light microscopy. Asynchronous cultures at asexual intraerythrocytic stages with > 6-8% parasitemia were centrifuged for 5 min at 1,500 rpm and the pellets resuspended in 2 red blood cell (RBC) volumes of 0.2% saponin in PBS to disrupt RBCs membranes. The suspensions were incubated for 10 min on ice, then 10 mL of PBS were added and centrifuged for 8 min at 1,800 rpm at 4°C. Supernatant was removed and saponin lysis was repeated. Uninfected B+ RBCs were used as controls. After centrifugation, the pellet was washed with PBS, transferred to a 1.5 mL tube and centrifuged at 10,000 x g for 10 min at 4°C. Pellets were kept at −80°C before total protein extraction. Frozen pellets were thoroughly resuspended with 200-300 μL Laemmli buffer (depending on the total number of parasites) and sonicated on ice three times for 10 s. Suspensions were then centrifuged at 10,000 x g for 30 min at 4°C. Supernatants containing the extracted soluble proteins were stored at −80°C before Western blot analysis.
4.7 Immunofluorescence
L. major promastigotes were pelleted at 1,500 x g for 10 min washed and resuspended in PBS. Parasites were deposited on polylysine-coated coverslip and fixed with 4% paraformaldehyde for 15 min at room temperature. Pre-incubation, antibody incubation and washes were conducted in PBS buffer containing 2% bovine serum albumin (BSA). Polyclonal antisera specific to E. coli O55, E. coli O85, E. coli O142 and E. coli O152 O-antigens were purchased from SSI Diagnostica (Hillerød, Denmark). The antisera and Alexa Fluor488 goat anti-rabbit IgG1 (Jackson ImmunoResearch, West Grove, PA) were diluted 1:10 and 1:2,000, respectively. Nuclei were stained with 2.5 µg/mL 4´,6-diamidino-2-phenylindole. Coverslips were analyzed under a Zeiss Axiovert 200M microscope equipped with ApoTome module, AxioCam MRm digital camera, and Axio Vision software (Zeiss, Oberkochen, Germany). P. falciparum cultures were pelleted at 800 x g for 30 s, washed and resuspended in PBS. The culture was distributed on Concanavalin A-coated Ibidi removable wells and fixed with 4% paraformaldehyde for 10 min at room temperature. Autofluorescence was reduced by quenching with 0.1 M glycine in PBS for 10 min. RBCs were permeabilized with 0.2% Triton X-100 in PBS for 20 min and blocked with 5% BSA in 0.2% Triton X-100 in PBS for 20 minutes at room temperature. Antibody incubation, diluted 1:10, and washes were conducted in 2% BSA and 0.2% Triton X-100 in PBS. The Alexa Fluor488 goat anti-rabbit IgG1 secondary antibody was diluted 1:500. A 2 µg/mL dilution of the polyclonal rabbit anti-PfHSP70 (StressMarq Bioscience, Canada) antibody was used as the positive control. Nuclei were stained with Hoechst 33342 and RBC surfaces with wheat germ agglutinin. Coverslips were analyzed under a Leica DMI4000 B microscope equipped with Leica CTR6500, Leica TCS SPE-II and LAS-AF Lite software (Leica, Wetzlar, Germany).
4.8 Gel electrophoresis and western blotting
LPS samples (15 µg) and parasite extracts (20 µg protein) were separated on 14% acrylamide gels at 80-120 V for up to 3 h. Gels were either stained with silver (49, 50) and colloidal Coomassie (51) or used for Western blotting. For silver staining, gels were oxidized in 40% ethanol, 5% acetic acid, 0.7% periodic acid in deionized water, followed by two washing steps of 20 min in 30% ethanol and one washing step with deionized water for 20 min. Gels were sensitized for one minute in 0.02% sodium thiosulfate in deionized water, washed three times for 20 s in deionized water before being stained with a 0.1% silver nitrate solution for 20 min at 4°C. Gels were washed three more times for 20 s in deionized water and finally developed in a solution containing 3% sodium carbonate, 0.05% formaldehyde in deionized water for around 5 min until bands appeared. After another washing step of 20 s with water, the reaction was stopped with 5% acetic acid. Gels were stored in a 1% acetic acid solution. For blotting, gels contents were transferred onto PDVF membranes at 250 mA for 1 h. Membranes were blocked overnight at 4°C in 5% skim milk solution in 1 x PBS-T supplemented with 0.1% Tween 20. Polyclonal antisera specific to O-antigens from E. coli O55, E. coli O85, E. coli O142 and E. coli O152 were diluted 1:100 in PBS-T and incubated overnight at 4°C. Membranes were washed 4-times for 10 min in 1 x PBS-T before 1 h incubation at room temperature with secondary anti-rabbit IgG coupled to horseradish peroxidase (Cell Signaling Technology, Danvers,MA, USA) diluted 1:1,000 in 1 x PBS-T. Membranes were washed for up to six times for 10 min with PBS-T, and antibody binding was detected using SuperSignal R West Pico PLUS chemiluminescence substrate (Thermo Fisher Scientific, Waltham, MA) and the FUSION FX imager (Vilber, Collégien, France).
4.9 Statistical analysis
All statistical analysis were done with R studio version 4.1.1 (2021-08-10). Heatmaps with hierarchical clustering were done using pheatmaps (v1.0.12), heatmaps without clustering were done with ggplot2 (v3.4.1). The significance of IgA-LPS reactivity in Kenyan vs. Swiss samples as well as the significance of IgA recognition between the rough mutants were determined using non-parametric and unpaired Wilcoxon test. Correlation between IgA/IgM or IgA/IgG binding towards certain LPS was tested using the Spearman correlation test. P-values ≤ 0.05 were considered statistically significant. The threshold of detection (TOD) at a RFU value of 88 was based on the signal intensity measured for empty samples and buffer controls.
Data availability statement
The raw data supporting the conclusions of this article will be made available by the authors, without undue reservation.
Ethics statement
The studies involving humans were approved by Ethical review committees of ETH Zurich (EK 2019-N-59) and Jomo Kenyatta University of Agriculture and Technology, Nairobi, Kenya (JKU/2/4/896B). The studies were conducted in accordance with the local legislation and institutional requirements. The participants provided their written informed consent to participate in this study.
Author contributions
LC: Writing – review & editing, Writing – original draft, Visualization, Validation, Methodology, Investigation, Formal Analysis, Data curation, Conceptualization. JS: Writing – review & editing, Writing – original draft, Validation, Methodology, Investigation, Formal Analysis. NM: Writing – review & editing, Writing – original draft, Methodology, Formal Analysis. TR: Writing – review & editing, Writing – original draft, Resources, Data curation. DB: Writing – review & editing, Writing – original draft, Resources, Data curation. DP: Writing – review & editing, Writing – original draft, Resources, Data curation. MZ: Writing – review & editing, Writing – original draft, Resources, Data curation. PZ: Writing – review & editing, Writing – original draft, Validation, Formal Analysis. FR: Writing – review & editing, Writing – original draft, Visualization, Validation, Supervision, Resources, Funding acquisition, Formal Analysis, Data curation. TR-U: Writing – review & editing, Writing – original draft, Validation, Formal Analysis. LI: Writing – review & editing, Writing – original draft, Visualization, Validation, Supervision, Resources, Funding acquisition, Formal Analysis, Data curation. TH: Writing – review & editing, Writing – original draft, Visualization, Validation, Supervision, Resources, Project administration, Methodology, Investigation, Funding acquisition, Data curation, Conceptualization.
Funding
The author(s) declare financial support was received for the research, authorship, and/or publication of this article. This work was supported by the Swiss National Foundation grant 310030_212231 and partially by the Spanish Ministry of Science and Innovation grant (PID2022-137031OB-I00) and the Program on the Molecular Mechanisms of Malaria (Fundación Ramón Areces) to LI.
Acknowledgments
We thank Miriam Ramírez for producing the Plasmodium falciparum extracts.
Conflict of interest
The authors declare that the research was conducted in the absence of any commercial or financial relationships that could be construed as a potential conflict of interest.
Publisher’s note
All claims expressed in this article are solely those of the authors and do not necessarily represent those of their affiliated organizations, or those of the publisher, the editors and the reviewers. Any product that may be evaluated in this article, or claim that may be made by its manufacturer, is not guaranteed or endorsed by the publisher.
Supplementary material
The Supplementary Material for this article can be found online at: https://www.frontiersin.org/articles/10.3389/fimmu.2024.1404192/full#supplementary-material
References
1. Carr LE, Virmani MD, Rosa F, Munblit D, Matazel KS, Elolimy AA, et al. Role of human milk bioactives on infants’ Gut and immune health. Front Immunol. (2021) 12:604080. doi: 10.3389/fimmu.2021.604080
2. Hennet T, Borsig L. Breastfed at tiffany’s. Trends Biochem Sci. (2016) 41:508–18. doi: 10.1016/j.tibs.2016.02.008
3. Lyons KE, Ryan CA, Dempsey EM, Ross RP, Stanton C. Breast milk, a source of beneficial microbes and associated benefits for infant health. Nutrients. (2020) 12:1039. doi: 10.3390/nu12041039
4. Zheng W, Zhao W, Wu M, Song X, Caro F, Sun X, et al. Microbiota-targeted maternal antibodies protect neonates from enteric infection. Nature. (2020) 577:543–8. doi: 10.1038/s41586-019-1898-4
5. Wilson E, Butcher EC. CCL28 controls immunoglobulin (Ig)A plasma cell accumulation in the lactating mammary gland and IgA antibody transfer to the neonate. J Exp Med. (2004) 200:805–9. doi: 10.1084/jem.20041069
6. Gopalakrishna KP, Macadangdang BR, Rogers MB, Tometich JT, Firek BA, Baker R, et al. Maternal IgA protects against the development of necrotizing enterocolitis in preterm infants. Nat Med. (2019) 25:1110–5. doi: 10.1038/s41591-019-0480-9
7. Durand D, Ochoa TJ, Bellomo SME, Contreras CA, Bustamante VH, Ruiz J, et al. Detection of secretory immunoglobulin A in human colostrum as mucosal immune response against proteins of the type III secretion system of salmonella, shigella and enteropathogenic escherichia coli. Pediatr Infect Dis J. (2013) 32:1122–6. doi: 10.1097/INF.0b013e318293306c
8. Castro-Dopico T, Clatworthy MR. IgG and fcγ Receptors in intestinal immunity and inflammation. Front Immunol. (2019) 10:805. doi: 10.3389/fimmu.2019.00805
9. Rogier EW, Frantz AL, Bruno MEC, Wedlund L, Cohen DA, Stromberg AJ, et al. Secretory antibodies in breast milk promote long-term intestinal homeostasis by regulating the gut microbiota and host gene expression. Proc Natl Acad Sci. (2014) 111:3074–9. doi: 10.1073/pnas.1315792111
10. Mantis NJ, Rol N, Corthésy B. Secretory IgA’s complex roles in immunity and mucosal homeostasis in the gut. Mucosal Immunol. (2011) 4:603–11. doi: 10.1038/mi.2011.41
11. Atyeo C, Alter G. The multifaceted roles of breast milk antibodies. Cell. (2021) 184:1486–99. doi: 10.1016/j.cell.2021.02.031
12. Sterlin D, Fadlallah J, Adams O, Fieschi C, Parizot C, Dorgham K, et al. Human IgA binds a diverse array of commensal bacteria. J Exp Med. (2020) 217. doi: 10.1084/jem.20181635
13. Mostowy RJ, Holt KE. Diversity-generating machines: genetics of bacterial sugar-coating. Trends Microbiol. (2018) 26:1008–21. doi: 10.1016/j.tim.2018.06.006
14. Cerutti A. The regulation of IgA class switching. Nat Rev Immunol. (2008) 8:421–34. doi: 10.1038/nri2322
15. Pabst O, Slack E. IgA and the intestinal microbiota: the importance of being specific. Mucosal Immunol. (2020) 13:12–21. doi: 10.1038/s41385-019-0227-4
16. Kappler K, Hennet T. Emergence and significance of carbohydrate-specific antibodies. Genes Immun. (2020) 21:224–39. doi: 10.1038/s41435-020-0105-9
17. Yilmaz B, Portugal S, Tran TM, Gozzelino R, Ramos S, Gomes J, et al. Gut microbiota elicits a protective immune response against malaria transmission. Cell. (2014) 159:1277–89. doi: 10.1016/j.cell.2014.10.053
18. Latov N. Campylobacter jejuni infection, anti-ganglioside antibodies, and neuropathy. Microorganisms. (2022) 10:2139. doi: 10.3390/microorganisms10112139
19. Raetz CRH, Whitfield C. Lipopolysaccharide endotoxins. Annu Rev Biochem. (2002) 71:635–700. doi: 10.1146/annurev.biochem.71.110601.135414
20. Whitfield C, Williams DM, Kelly SD. Lipopolysaccharide O-antigens-bacterial glycans made to measure. J Biol Chem. (2020) 295:10593–609. doi: 10.1074/jbc.REV120.009402
22. Giorgetti A, Paganini D, Nyilima S, Kottler R, Frick M, Karanja S, et al. The effects of 2’-fucosyllactose and lacto-N-neotetraose, galacto-oligosaccharides, and maternal human milk oligosaccharide profile on iron absorption in Kenyan infants. Am J Clin Nutr. (2023) 117:64–72. doi: 10.1016/j.ajcnut.2022.10.005
23. Jiang N, Dillon FM, Silva A, Gomez-Cano L, Grotewold E. Rhamnose in plants - from biosynthesis to diverse functions. Plant science: an Int J Exp Plant Biol. (2021) 302:110687. doi: 10.1016/j.plantsci.2020.110687
24. Mistou M-Y, Sutcliffe IC, van Sorge NM. Bacterial glycobiology: rhamnose-containing cell wall polysaccharides in Gram-positive bacteria. FEMS Microbiol Rev. (2016) 40:464–79. doi: 10.1093/femsre/fuw006
25. Adibekian A, Stallforth P, Hecht M-L, Werz DB, Gagneux P, Seeberger PH. Comparative bioinformatics analysis of the mammalian and bacterial glycomes. Chem Sci. (2011) 2:337–44. doi: 10.1039/C0SC00322K
26. Smith SI, Seriki A, Ajayi A. Typhoidal and non-typhoidal Salmonella infections in Africa. Eur J Clin Microbiol Infect Dis. (2016) 35:1913–22. doi: 10.1007/s10096-016-2760-3
27. Nataro JP, Kaper JB. Diarrheagenic escherichia coli. Clin Microbiol Rev. (1998) 11:142–201. doi: 10.1128/CMR.11.1.142
28. Galili U, Rachmilewitz EA, Peleg A, Flechner I. A unique natural human IgG antibody with anti-alpha-galactosyl specificity. J Exp Med. (1984) 160:1519 – 31. doi: 10.1084/jem.160.5.1519
29. Oyelaran O, McShane LM, Dodd L, Gildersleeve JC. Profiling human serum antibodies with a carbohydrate antigen microarray. J Proteome Res. (2009) 8:4301–10. doi: 10.1021/pr900515y
30. Tefsen B, Ram AF, van Die I, Routier FH. Galactofuranose in eukaryotes: aspects of biosynthesis and functional impact. Glycobiology. (2011) 22:456–69. doi: 10.1093/glycob/cwr144
31. Njuguna P, Maitland K, Nyaguara A, Mwanga D, Mogeni P, Mturi N, et al. Observational study: 27 years of severe malaria surveillance in Kilifi, Kenya. BMC Med. (2019) 17:124. doi: 10.1186/s12916-019-1359-9
32. Ngere I, Gufu Boru W, Isack A, Muiruri J, Obonyo M, Matendechero S, et al. Burden and risk factors of cutaneous leishmaniasis in a peri-urban settlement in Kenya, 2016. PloS One. (2020) 15:e0227697. doi: 10.1371/journal.pone.0227697
33. Kleczka B, Lamerz A-C, van Zandbergen G, Wenzel A, Gerardy-Schahn R, Wiese M, et al. Targeted gene deletion of Leishmania major UDP-galactopyranose mutase leads to attenuated virulence. J Biol Chem. (2007) 282:10498–505. doi: 10.1074/jbc.M700023200
34. McGuire MK, Randall AZ, Seppo AE, Järvinen KM, Meehan CL, Gindola D, et al. Multipathogen analysis of IgA and IgG antigen specificity for selected pathogens in milk produced by women from diverse geographical regions: the INSPIRE study. Front Immunol. (2020) 11:614372. doi: 10.3389/fimmu.2020.614372
35. Takahashi T, Yoshida Y, Hatano S, Sugita-Konishi Y, Igimi S, Yajima M, et al. Reactivity of secretory IgA antibodies in breast milk from 107 Japanese mothers to 20 environmental antigens. Biol neonate. (2002) 82:238–42. doi: 10.1159/000065893
36. Bunker JJ, Bendelac A. IgA responses to microbiota. Immunity. (2018) 49:211–24. doi: 10.1016/j.immuni.2018.08.011
37. Donald K, Petersen C, Turvey SE, Finlay BB, Azad MB. Secretory IgA: Linking microbes, maternal health, and infant health through human milk. Cell Host Microbe. (2022) 30:650–9. doi: 10.1016/j.chom.2022.02.005
38. Yang K, LeJeune J, Alsdorf D, Lu B, Shum CK, Liang S. Global distribution of outbreaks of water-associated infectious diseases. PloS Negl Trop Dis. (2012) 6:e1483. doi: 10.1371/journal.pntd.0001483
39. Kalbermatter C, Fernandez Trigo N, Christensen S, Ganal-Vonarburg SC. Maternal microbiota, early life colonization and breast milk drive immune development in the newborn. Front Immunol. (2021) 12:683022. doi: 10.3389/fimmu.2021.683022
40. Bunker JJ, Erickson SA, Flynn TM, Henry C, Koval JC, Meisel M, et al. Natural polyreactive IgA antibodies coat the intestinal microbiota. Science. (2017) 358. doi: 10.1126/science.aan6619
41. Rollenske T, Szijarto V, Lukasiewicz J, Guachalla LM, Stojkovic K, Hartl K, et al. Cross-specificity of protective human antibodies against Klebsiella pneumoniae LPS O-antigen. Nat Immunol. (2018) 19:617–24. doi: 10.1038/s41590-018-0106-2
42. Goyal PK, Wheatcroft J, Wakelin D. Tyvelose and protective responses to the intestinal stages of Trichinella spiralis. Parasitol Int. (2002) 51:91–8. doi: 10.1016/S1383-5769(02)00002-8
43. Turco SJ, Orlandi PA Jr., Homans SW, Ferguson MA, Dwek RA, Rademacher TW. Structure of the phosphosaccharide-inositol core of the Leishmania donovani lipophosphoglycan. J Biol Chem. (1989) 264:6711–5. doi: 10.1016/S0021-9258(18)83487-1
44. Haynes PA, Ferguson MA, Cross GA. Structural characterization of novel oligosaccharides of cell-surface glycoproteins of Trypanosoma cruzi. Glycobiology. (1996) 6:869–78. doi: 10.1093/glycob/6.8.869
45. Cabezas-Cruz A, Mateos-Hernández L, Alberdi P, Villar M, Riveau G, Hermann E, et al. Effect of blood type on anti-α-Gal immunity and the incidence of infectious diseases. Exp Mol Med. (2017) 49:e301. doi: 10.1038/emm.2016.164
46. Aguilar R, Ubillos I, Vidal M, Balanza N, Crespo N, Jiménez A, et al. Antibody responses to α-Gal in African children vary with age and site and are associated with malaria protection. Sci Rep. (2018) 8:9999. doi: 10.1038/s41598-018-28325-w
47. Davis MR Jr., Goldberg JB. Purification and visualization of lipopolysaccharide from Gram-negative bacteria by hot aqueous-phenol extraction. J Vis Exp. (2012) 63. doi: 10.3791/3916
48. Trager W, Jensen JB. Human malaria parasites in continuous culture. Science. (1976) 193:673–5. doi: 10.1126/science.781840
49. Blum H, Beier H, Gross HJ. Improved silver staining of plant proteins, RNA and DNA in polyacrylamide gels. Electrophoresis. (1987) 8:93–9. doi: 10.1002/elps.1150080203
50. Fomsgaard A, Freudenberg MA, Galanos C. Modification of the silver staining technique to detect lipopolysaccharide in polyacrylamide gels. J Clin Microbiol. (1990) 28:2627–31. doi: 10.1128/jcm.28.12.2627-2631.1990
51. Dyballa N, Metzger S. Fast and sensitive colloidal coomassie G-250 staining for proteins in polyacrylamide gels. J Visualized Experiments: JoVE. (2009) 30. doi: 10.3791/1431
Keywords: glycan, O-antigen, lactation, infection, microarray, antigen mimicry, natural protection
Citation: Crone L, Sobek J, Müller N, Restin T, Bassler D, Paganini D, Zimmermann MB, Zarnovican P, Routier FH, Romero-Uruñuela T, Izquierdo L and Hennet T (2024) Inter-individual and inter-regional variability of breast milk antibody reactivity to bacterial lipopolysaccharides. Front. Immunol. 15:1404192. doi: 10.3389/fimmu.2024.1404192
Received: 20 March 2024; Accepted: 20 August 2024;
Published: 06 September 2024.
Edited by:
Mahavir Singh, LIONEX GmbH, GermanyReviewed by:
Gatien Lokossou, University of Abomey-Calavi, BeninSaidou Balam, University Medical Center Regensburg, Germany
Copyright © 2024 Crone, Sobek, Müller, Restin, Bassler, Paganini, Zimmermann, Zarnovican, Routier, Romero-Uruñuela, Izquierdo and Hennet. This is an open-access article distributed under the terms of the Creative Commons Attribution License (CC BY). The use, distribution or reproduction in other forums is permitted, provided the original author(s) and the copyright owner(s) are credited and that the original publication in this journal is cited, in accordance with accepted academic practice. No use, distribution or reproduction is permitted which does not comply with these terms.
*Correspondence: Thierry Hennet, VGhpZXJyeS5oZW5uZXRAdXpoLmNo