- 1Institute of Anatomy, First Faculty of Medicine, Charles, University, Prague, Czechia
- 2BIOCEV, First Faculty of Medicine, Charles University, Vestec, Czechia
- 3Department Dermatovenereology, First Faculty of Medicine, Charles University and General University Hospital, Prague, Czechia
- 4Laboratory of Genomics and Bioinformatics, Institute of Molecular Genetics of the Czech Academy of Sciences, Prague, Czechia
- 5Department of Pharmacology, Faculty of Medicine, Pavol Jozef Šafárik University in Košice, Košice, Slovakia
- 6Department of Biomedical Research, East-Slovak Institute of Cardiovascular Diseases Inc., Košice, Slovakia
- 7Prague Burn Centre, Third Faculty of Medicine, Charles University and University Hospital Královské Vinohrady, Prague, Czechia
- 8Department of Pharmacognosy and Botany, Faculty of Pharmacy, Comenius University, Bratislava, Slovakia
Wound healing represents a complex and evolutionarily conserved process across vertebrates, encompassing a series of life-rescuing events. The healing process runs in three main phases: inflammation, proliferation, and maturation/remodelling. While acute inflammation is indispensable for cleansing the wound, removing infection, and eliminating dead tissue characterised by the prevalence of neutrophils, the proliferation phase is characterised by transition into the inflammatory cell profile, shifting towards the prevalence of macrophages. The proliferation phase involves development of granulation tissue, comprising fibroblasts, activated myofibroblasts, and inflammatory and endothelial cells. Communication among these cellular components occurs through intercellular contacts, extracellular matrix secretion, as well as paracrine production of bioactive factors and proteolytic enzymes. The proliferation phase of healing is intricately regulated by inflammation, particularly interleukin-6. Prolonged inflammation results in dysregulations during the granulation tissue formation and may lead to the development of chronic wounds or hypertrophic/keloid scars. Notably, pathological processes such as autoimmune chronic inflammation, organ fibrosis, the tumour microenvironment, and impaired repair following viral infections notably share morphological and functional similarities with granulation tissue. Consequently, wound healing emerges as a prototype for understanding these diverse pathological processes. The prospect of gaining a comprehensive understanding of wound healing holds the potential to furnish fundamental insights into modulation of the intricate dialogue between cancer cells and non-cancer cells within the cancer ecosystem. This knowledge may pave the way for innovative approaches to cancer diagnostics, disease monitoring, and anticancer therapy.
Introduction
Globally, the elderly population is experiencing significant growth, attributed to enhanced management of chronic diseases (1). In 2004, there were 461 million individuals aged 65 and older, and projections suggest this number will increase to 2 billion by 2050, posing unprecedented challenges for healthcare planning and delivery (2). Recognising ageing as a key factor in chronic conditions (3), the growing elderly population’s socio-economic impact has forced research to focus on the aspects of extending human health span (4).
Wound healing is a fundamental biological response, an intricate orchestration of events, offering a unique lens through which we can unravel the complexities of autoimmune disorders, ageing-related conditions, and the intricate landscape of cancer. The present review aims to underscore the important role of wound healing as a model for understanding these overarching biological processes. Autoimmunity, ageing, and cancer, seemingly different biological events, reveal interconnected threads that converge in the epicentre of wound healing. Central to this convergence is the orchestrating influence of inflammation, with interleukin-6 (IL-6) emerging as a key regulatory player. The structural parallels between tumours and wound healing have been recognised for decades, notably illustrated in the seminal work by Dvorak in 1986 (5). Recent insights emphasise the striking resemblance between granulation tissue, pannus-like tissue from chronic inflammation (such as progressive arthritis), and tissues attacked by viral infections, with the solid cancer stroma (6). These pathologies result in fibrotic tissue, also observed in conditions such as tissue fibrosis in the lungs of COVID-19 patients or the stroma of pancreatic ductal adenocarcinoma (PDAC) (6, 7).
We will delve into the stages of wound healing, exploring the dynamic interplay of cellular and molecular components. By unravelling the parallels between wound healing and autoimmune responses, investigating the impact of ageing on tissue regeneration, and scrutinising the intricate relationship between wound healing and cancer ecosystems, we aspire to elucidate the profound implications that a comprehensive understanding of wound healing holds for these complex biological phenomena. The spotlight on inflammation, especially IL-6, serves as the central key point, guiding our exploration where wound healing serves not only as a metaphor but also as a model for broader biological processes.
Wound healing
Wound healing is a dynamic process influenced by various factors, including the size and extent of the wound, microbial contamination, and the overall health and age of the individual. Failure to navigate this intricate process can result in chronic wounds or formation of hypertrophic/keloid scars (8–10). An intriguing aspect lies in the regenerative capacity of the interfollicular epidermis in humans, capable of full regeneration. In contrast, the repair of the dermal architecture in humans is a more intricate process leading to scar formation. This dermal repair unfolds through three/four distinct phases: blood clotting/haemostasis (potentially part of the inflammatory response), inflammation, proliferation, and maturation/remodelling. Epidermal regeneration occurs concurrently with dermal healing, and the restoration of the barrier integrity is completed during the process of re-epithelisation, while dermal remodelling continues for several weeks or months after (11). Despite the complexity and precision inherent in wound healing, this orchestrated process is not immune to interruptions and failures. Such disruptions can result in the development of non-healing/chronic wounds or pathological scars, underscoring the delicate balance required for successful tissue repair and regeneration (8). The vulnerability of the wound healing process to deviations emphasises the need for a nuanced understanding of its intricacies to develop effective interventions and therapies. The key events in the wound healing sequence have been described in detail previously (8, 12) and are summarised in Table 1 and Figure 1.
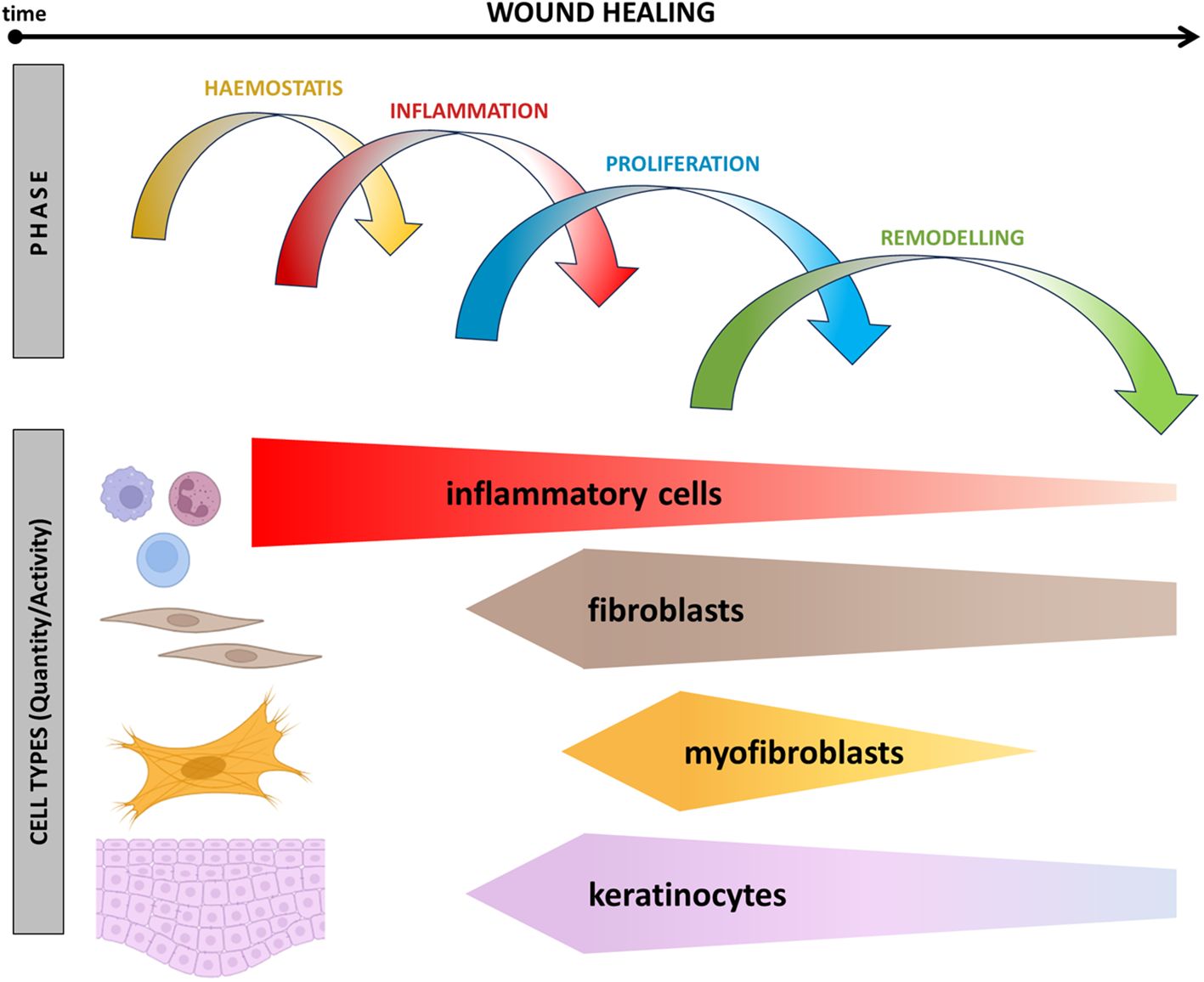
Figure 1. Schematic presentation of events associated with wound healing over time. The relationship between the presence of inflammatory cells, fibroblasts, myofibroblasts, and reepithelisation across different phases of wound healing is shown.
Inflammatory phase
Traumatic tissue injury often initiates with haemorrhage, leading to the formation of a stable haemostatic clot composed of polymerised fibrin. This essential process, known as haemostasis, is crucial in preventing major blood loss. The resulting coagulum, which temporarily fills the wound defect and forms a protective crust, serves as a provisional barrier, safeguarding deeper tissues. Both platelets and clotting factors participate in haemostasis, with the intrinsic and extrinsic pathways distinguished by the upstream factors that activate the coagulation cascade, ensuring swift establishment of a temporary protective barrier (12). Subsequent to haemostasis, vessel dilatation and permeabilisation facilitate the migration of leucocytes to the injury site, marking the onset of the inflammatory phase. The primary goal of infiltrating leucocytes is to eliminate pathogens, resolve inflammation, and clear necrotic cells/tissue (13). Key immune cell players include granulocytes (neutrophils and eosinophils), macrophages, mast cells, natural killer (NK) cells, B lymphocytes, and various T lymphocyte subsets. Neutrophils, the initial and predominant immune cells at the wound site, play a pivotal role in the removal of debris and pathogens (14). Macrophages play a central role throughout all phases of wound healing. M1-activated macrophages are crucial for orchestrating the onset of the inflammatory phase, while their reprogramming into M2-polarised macrophages is essential for resolving inflammation and supporting the subsequent phases of proliferation and remodelling. The ultimate functional and morphologic outcome of wound healing relies heavily on the successful resolution of inflammation (15). Persistent inflammation may disrupt keratinocyte and fibroblast differentiation, potentially leading to excessive scarring and, in severe cases, formation of hypertrophic/keloid scars (16).
To orchestrate the inflammatory activity across the wound site, immune cells release a diverse array of bioactive factors. These encompass transforming growth factor (TGF)-β, tumour necrosis factor (TNF)-α, interferon (IFN)-γ, vascular endothelial growth factor A (VEGFA), basic fibroblast growth factor (bFGF), interleukins (IL-4, -6, -9 -13, -17, -23), chemokines (CXCL-8, CXCL-12), and numerous proteases (8). Notably, certain factors exhibit pleiotropy, being produced by multiple immune cell types. For instance, IL-6 is synthesised by neutrophils, macrophages, mast cells, and T lymphocytes. Similarly, members of the TGF-β family are widely produced by immune cells, influencing various cells in the wound microenvironment (WME) simultaneously. Moreover, these cytokines can influence local fibroblasts, keratinocytes, and endothelial cells. These cytokines can also enter systemic circulation, making them measurable indicators for monitoring large wound healing (17). Hyperactivation of the immune system, although rare, has been observed in large, infected wounds or extensive burn injuries, leading anecdotally to the so-called cytokine storm syndrome (18).
Proliferation phase
Successful resolution of inflammation marks the transition to the proliferation phase, typically observed 2–4 days post-injury (19). Central to this phase is formation of granulation tissue, a provisional structure comprising persisting recruited inflammatory/immune cells, sprouting capillary endothelium, and fibroblasts actively engaged in extracellular matrix (ECM) production. Initially, fibroblasts generate a loose ECM, forming a temporary scaffold vital for the migration and proliferation of other cells involved in the healing process (20). Remarkably, dermal fibroblasts constitute a remarkably heterogeneous population of mesenchymal cells, with two principal subgroups - papillary and reticular - identified histologically. These subsets differ in proliferative capacity, wound contraction ability, and expression profiles. Recent single-cell sequencing has unveiled up to six functionally distinct subsets of dermal fibroblasts, among which the CD26-expressing population stands out as a key contributor to ECM production and, consequently, new tissue formation during the wound healing (21). The resulting scar’s characteristics are intricately linked to the fibroblast subset predominant at the wound site (22).
[note: Origin of fibroblasts - where do they come from? The intriguing diversity among fibroblasts may also stem from their developmental origin. In the craniofacial region, dermal fibroblasts may have a dual embryonic origin, originating from both cranial neural crest (facial fibroblasts) and cephalic mesoderm. Dorsal skin fibroblasts trace back to the somites, while those in the ventral flank and limb dermis arise from the lateral plate mesoderm (23). Notably, fibroblasts or fibroblast-like cells can emerge from various cell populations, including epithelial cells, through epithelial-to-mesenchymal transition (EMT) (24), epidermal stem cells (25), bone marrow-derived mesenchymal stem cells (26), and circulating fibrocytes (27). Additionally, endothelial-to-mesenchymal transition (EndMT) offers another pathway for the generation of fibroblast-like cells (28).]
Within the intricate landscape of fibroblasts, a distinctive subpopulation emerges, characterised by the active ACTA2 gene, encoding α-smooth muscle actin. This distinctive fibroblast subpopulation plays a significant role in wound contraction, also facilitating the subsequent process of re-epithelisation (29, 30). While playing a central role in the wound contraction, myofibroblasts demonstrate a dual nature. In addition, these cells are potent producers of a diverse array of bioactive factors. Notably, among these factors are those with inflammation-supporting activity, such as IL-6 and IL-8, not only contributing to inflammation but also exhibiting pro-fibrotic effects. Multiple stimulators, including cytokines (TGF-β1), growth factors (CTGF, FGF, PDGF, IGF), and galectins (galectin-1), have been identified to regulate fibroblast and myofibroblast activities (31–35). TGF-β1, in particular, stands out as a recurrently reported critical factor driving fibroblast differentiation into myofibroblasts (36) (Figure 1). This intricate interplay between diverse fibroblast subsets and their regulatory factors forms the foundation for understanding the complexities of wound healing and scar formation.
Simultaneously with the proliferation phase, the critical process of angiogenesis unfolds, fostering the formation of new blood vessels to meet the escalating demands of rapidly proliferating cells (37). The vascular endothelial growth factor (VEGF) stands at the forefront of pro-angiogenic molecules during wound healing. Recognised as a vascular endothelial cell mitogen and a regulator of endothelial integrin expression during vessel sprouting, VEGF extends its influence as a chemokine for macrophages, activating them directly via the VEGF receptor (VEGFR) (38). Consequently, VEGF assumes the role of an indirect pro-inflammatory cytokine, potentially contributing to excessive scar formation.
Re-epithelisation stands as the ultimate process in the establishment of a protective barrier, shielding the body’s interior from potential microbial threats and minimising the loss of essential fluids and crystalloids (8). Keratinocytes, particularly those residing in the wound margins and hair follicles, emerge as a cellular base for re-epithelisation. This intricate process involves a harmonious interplay of biological events encompassing cell proliferation, epithelial-mesenchymal transition, and migration (39, 40). Underpinning re-epithelisation is the intrinsic genetic programme of epithelial cells, orchestrated in conjunction with the active involvement of granulation tissue cells and their secretory products that influence the epithelium (41). The interaction between the epithelium and granulation tissue cells is bidirectional, as the completion of wound closure marks the cessation of ECM, growth factor, cytokine, and chemokine production by granulation tissue cells, initiating the subsequent phase of scar remodelling (41, 6). This dynamic interplay ensures the seamless progression from wound closure to scar maturation, contributing to the overall integrity and functionality of the healed tissue.
Maturation/Remodelling phase
Regeneration in postnatal mammals is a rare phenomenon, with the human liver being a notable exception. In the majority of tissues, wound healing culminates in scar formation. Following wound closure, a critical phase of remodelling ensues, predominantly impacting the granulation tissue. This intricate process necessitates the degradation of the ECM and its subsequent reconstruction in the scar. Proteolytic enzymes play a remarkable role in wound healing, specifically in the context of tissue remodelling (42, 43). The degradation of the non-matured ECM is instrumental in facilitating the establishment of the matured matrix, important for achieving the correct size of the scar while ensuring sufficient strength to prevent secondary wounding due to mechanical stress (8, 44). Any misstep in these interactions can result in suboptimal healing outcomes, with scars that may be inconspicuous in neonates but more prominent in adults (45). It has been suggested that neonatal fibroblasts preserve distinct properties of mesenchymal stem cells (46), accompanied by the deregulation of TGF-β signalling and low expression of the TGF-β II receptor (47). Despite being a well-documented clinical phenomenon, the complex mechanisms underlying the age-dependent variation in healing capacity remain a subject of ongoing exploration. As the granulation tissue formation gradually halts through cell apoptosis, the wound transforms into an almost avascular and acellular scar (8).
In cases of complicated wounds with prolonged inflammatory response, for example, due to contamination by microorganisms or debris, scarring can become intricate, potentially leading to hypertrophic or keloid scars (48, 49). There is evidence suggesting a genetic predisposition to keloid formation, with ethnicity also playing a role. The highest incidence of keloids is reported in Africa (5-10%), with Asia following closely (up to 1%) (50). However, our understanding of the genetics underlying keloid formation is still evolving, and further research is needed in this area (51). Keloid formation is a complex process, sharing similarities with tumour development, particularly in the context of stromal characteristics (52). Inflammation is a key player in the aetiopathogenesis of keloids and hypertrophic scars, evident in the infiltration of leucocytes into the scar tissue (8, 53, 54). This pro-inflammatory microenvironment supports the presence of myofibroblasts, aligning with their frequent occurrence. In contrast to tumour stroma, keloid scars lack elastin and hyaluronic acid (55), further distinguishing their unique composition. Unravelling the intricate mechanisms underlying keloid formation holds promise for developing targeted interventions to modulate scar outcomes and improve patient outcomes.
Organ fibrosis
Connective tissue, containing the intricate molecules of the ECM, is a universal component found in all organs. The deposition of ECM plays an irreplaceable role in the healing process. However, an imbalance between deposition and degradation can lead to organ fibrosis, resulting in scar formation, and significantly affecting the organ function (56). Inflammation further contributes to the formation of a pro-fibrotic microenvironment, with immune cells producing an array of cytokines, chemokines, and bioactive factors such as IL-4, IL-6, IL-13, TGF-β, and TNF-α. These factors activate fibroblasts and also shift their phenotype from α-smooth muscle actin-negative (α-SMA-, fibroblast-like) to α-smooth muscle actin-positive (α-SMA+, myofibroblast-like), which, in turn, contributes to ECM deposition and perpetuation of inflammation (57).
Organ fibrosis is a severe condition often leading to fatal outcomes, exemplified in complications of COVID-19 lung injury due to SARS-CoV-2 infection. The massive occurrence of myofibroblasts, coupled with cytokine dysregulation during the cytokine storm, particularly accompanied by elevated levels of IL-6, has systemic effects on the patients (6, 58, 59). Monitoring IL-6 levels in severe COVID-19 cases has been recommended for assessing the health status and adjusting therapeutic protocols to prevent exacerbation of complications (60). Therapeutic interventions targeting the IL-6 signalling pathway, such as anti-IL-6 receptor antibodies tocilizumab or sarilumab, have shown promise in managing these complications (61). In parallel, immune infiltration and the presence of myofibroblasts are common in autoimmune diseases such as the terminal stage of systemic sclerosis or Hashimoto’s thyroiditis (62, 63). The association of systemic sclerosis and other autoimmune diseases with cytokine dysregulation, including hyperproduction of IL-6, has been well-established (64, 65).
Desmoplastic stroma characterises the microenvironment of certain tumours, such as pancreatic ductal adenocarcinoma (PDAC), resembling scar-like fibrosis with numerous myofibroblasts playing a significant role (66, 67, 7). The controlling role of myofibroblasts, particularly in producing IL-6, underscores their potential significance in the stromal desmoplasia of tumours. This intricate interplay between immune response, fibrosis, and tumour microenvironment (TME) highlights the interconnectedness of these biological processes, offering novel avenues for therapeutic exploration.
Autoimmunity
Autoimmune diseases, characterised by the immune system targeting self-antigens, result in chronic inflammation-mediated tissue injury. Coined by immunologist Paul Ehrlich, the term “horror autotoxicus” captures the phenomenon of immune-mediated self-destruction (Ehrlich, 1900). Despite the immune system’s diverse mechanisms causing tissue damage in autoimmune diseases, fibrosis and impaired function frequently emerge as common features in these deleterious scenarios, demonstrating scleroderma and rheumatoid arthritis as extemporary examples (6).
Scleroderma, also known as systemic sclerosis, is a complex autoimmune disease characterised by fibrotic changes affecting both the skin and visceral organs (68). It leads to increased mortality, particularly due to cardiac disease, pulmonary fibrosis, and pulmonary hypertension. The disease progression is not effectively prevented by current immunosuppressive treatments, as they are only partially successful in halting fibrotic tissue accumulation. Histological examination reveals myofibroblasts as key drivers of fibrosis in scleroderma, and their resistance to apoptosis contributes to abundant collagen overproduction, elevated ECM stiffness, and heightened pro-fibrotic cytokine levels (69, 70). TGF-β (71) and IL-6 (72) are implicated in scleroderma pathogenesis, with IL-6 acting as a pro-fibrotic factor and correlating with disease severity. Inhibition of IL-6 has shown promise in preventing early lung disease progression in patients with systemic sclerosis. Combining different immunotherapies, such as CD47 and IL-6 blockade, has demonstrated efficacy in a murine model, hinting at potential benefits for patients (73).
Rheumatoid arthritis, another autoimmune inflammatory disease, is characterised by joint pain, swelling, and stiffness. Activated mesenchymal cells, particularly fibroblast-like synoviocytes, contribute to pathological tissue repair, leading to pannus formation and joint destruction (74). Extra-articular manifestations, including lung fibrosis, are common in rheumatoid arthritis, and elevated levels of IL-6 are frequently associated with the disease (75). While IL-6 participates in controlling articular and extra-articular pathologies, it is important to note that experiencing the cytokine storm, characterised by elevated IL-6 levels, is relatively rare in these patients (76). However, the autoimmune progression in rheumatoid arthritis can lead to cachexia and severe psychiatric issues, and anti-IL-6 therapy has shown efficacy in treating affected joints, alleviating extra-articular manifestations, stabilising lung fibrosis, and reducing cachexia (77). Notably, drugs such as tocilizumab and sarilumab have demonstrated good efficacy and tolerability in rheumatoid arthritis patients with a poor response to conventional treatments.
Ageing
Inflammation is a natural process for tissue repair, but chronic inflammation, termed “inflammageing” in ageing individuals, can have adverse effects (78). Immunosenescence, age-related changes in the immune system, and increased cytokine secretion by adipose tissue contribute to chronic inflammation. Elevated levels of IL-6, IL-1, TNF-α, and C-reactive protein in older individuals are linked to higher morbidity and mortality (79), with TNF-α and IL-6 serving as frailty markers. In this context, chronic wounds often stall at the inflammatory stage, where pro-inflammatory cytokines such as TNF-α, IL-1 (α and β), and IL-6 play important roles in signalling immune cell recruitment, angiogenesis, and epithelisation (80). These cytokines, primarily secreted by immune cells but also by epithelial cells, fibroblasts, and endothelial cells, are major components of the senescent secretome. IL-1α, in particular, can stimulate the expression of other cytokines in senescent cells, reinforcing the pro-inflammatory senescence-associated secretory phenotype. The chronic presence of senescent cells in the WME may enhance inflammation and hinder its resolution, contributing to impaired wound healing if the inflammatory stage persists. Understanding this interplay is essential for developing effective interventions in chronic wound management.
In addition to the inflammageing issue, proper wound healing involves transient senescence marked by up-regulated cell cycle arrest proteins (p16, p21, p53) and SASP (81, 82). Studies in mice demonstrate impaired wound healing upon selective elimination of senescent cells, while senescent fibroblasts and endothelial cells, expressing PDGF-AA as part of the senescence-associated secretory phenotype, actively promote wound healing (81). In ageing, the altered senescence response contributes to delayed wound healing, with studies showing improved outcomes in aged mice by inhibiting p21 expression (83). Chronic wounds, such as venous and diabetic ulcers, exhibit senescent fibroblasts, and their presence correlates with decreased healing rates (84). Persistent senescent cells can lead to chronic wounds, emphasising the importance of transient senescence. Additionally, senescent fibroblasts, induced by oxidative stress, regulate the fibrotic response by inhibiting proliferation and matrix synthesis (85), with CCN1 triggering senescence and anti-fibrotic gene expression (86). This complex interplay of senescence dynamics plays a crucial role in regulating the trajectory of wound healing, scarring, and fibrosis.
Cancer ecosystem: understanding the tumour as an organ
Organogenesis, an intricate ballet of prenatal events encompassing cell proliferation, differentiation, migration, ECM deposition, intercellular interactions, and programmed cell death, orchestrates the correct formation and function of organs. Any deviations during this developmental course can significantly affect the architecture and subsequent function of an organ. In a conceptual shift, malignant solid tumours can be likened to organs, orchestrated by genetically aberrant cancer cells. Their interaction with non-cancer cells, including connective tissue cells, inflammatory cells, and blood vessels, plays a significant role in shaping their aberrant function and subsequent dissemination (87). The connective tissue component, often referred to as the stroma, is a dynamic entity within malignant tumours. It houses fibroblasts producing ECM, growth factors, chemokines, cytokines, matrix metalloproteinases (MMPs), as well as vessels supplying nutrition and oxygen to the tumour (88).
The stroma, far from being a passive bystander, actively participates in the tumour’s dynamic processes. The ECM influences cancer cell adhesion and migration, with proteolytic enzymes remodelling it to create channels facilitating cancer cell migration (11, 89). Furthermore, the stroma acts as a platform for immune cell infiltration into the tumour site. Despite cancer cells expressing numerous neo-antigens due to frequent mutations, the anticancer activity of immune cells is often down-regulated by products of various cell types in the cancer ecosystem. For instance, tumour-associated macrophages may paradoxically support cancer cell growth and spread (90). Accordingly, the intricate dialogue between immune cells and cancer cells opens new horizons in anticancer therapy. Therapeutic manipulation of this dialogue, exemplified by immune checkpoint inhibitors, proves to be a powerful anticancer strategy that enhances the quality of life and life expectancy for many oncological patients (91). Understanding the complexity of the tumour as an organ and deciphering the interplay between its cellular components paves the way for innovative approaches in cancer treatment and management.
Cancer is a genetic disease, grounded in fundamental gene alterations that drive its onset. The molecular scrutiny of individual cancer cells constituting the bulk has unequivocally revealed the heterogeneous nature of tumours, with metastatic cells often displaying significant distinctions from their counterparts in primary tumours (92, 93). Cancer cells, rather than existing in isolation, intricately collaborate with non-cancer cells, which play indispensable roles in fostering cancer cell growth and systemic dissemination. These non-cancer cells actively contribute to shaping a cancer-supporting microenvironment, typically characterised by pro-inflammatory conditions.
Central to the orchestration of tissues and organs are adult tissue stem cells, reliant on specific microenvironments to sustain their stemness. Extending this hypothesis to cancer stem cells suggests that non-cancer cells within the cancer ecosystem and their products actively participate in establishing the niche supportive of cancer stem cell maintenance (94, 95). As cancer progresses, the tumour evolves under the selective pressures exerted by endogenous mechanisms, including the microenvironment, immunity, and exogenous factors such as anticancer therapies (96).
Tumour-associated macrophages (TAM), cancer-associated fibroblasts (CAFs), and various types of lymphocytes are significant components within the cancer ecosystem, each contributing distinctive elements to the intricate milieu (97). Given the scope of this review, our focus will delve into the roles of TAMs and CAFs.
Tumour-associated macrophages
Macrophages, components of innate immunity originating from the bone marrow, play a critical role in shaping the intricate landscape of the cancer ecosystem. Their plasticity allows for polarisation into distinct subtypes: M1 pro-inflammatory macrophages and M2 alternatively activated macrophages associated with the resolution of inflammation. While M1 macrophages fuel Th1-dependent stimulation, fostering CD8-positive T-cell proliferation and exerting anticancer activity, M2 macrophages orchestrate an immunosuppressive microenvironment and drive tissue remodelling that supports tumour growth and formation of metastasis (98). The shift from M1 to M2 is orchestrated by interleukins IL-4 and IL-13, secreted by various cells within the cancer ecosystem, including cancer cells themselves (99–101). Intriguingly, the recruitment of macrophages to the tumour and their subsequent M2 polarisation fall under the regulatory influence of CAFs (102). Acting as chemical factories, TAMs unleash a diverse array of bioactive factors, including EGF, VEGFA/D, TGF-β, TNF-α, IL-1β, IL-6, IL-24, CXCL1/5/8, CCL2/8, MMP2/9, PDL1, and various non-coding RNAs. These factors exert a multifaceted impact on the tumour ecosystem, stimulating cancer cell proliferation, enhancing invasion, promoting angiogenesis, and concurrently suppressing the immune system’s anticancer activity (103, 104). Unfortunately, the prevailing expression of cancer-supporting factors tends to overshadow the inhibitory signals. For example, it is widely recognised that the inflammatory cytokine IL-1β is a key player in cancer-related inflammation (105). Exploring the therapeutic avenues, the manipulation of TAMs emerges as a suitable target for anticancer interventions, currently in the developmental stage. Propelling M1 cells as carriers of therapeutic cargo to cancer sites presents a promising strategy for the treatment of solid tumours (106).
Cancer-associated fibroblasts
Traditionally seen as a structural scaffold for organ development, connective tissue and its architects, fibroblasts, have evolved into dynamic contributors to organ homeostasis. These cells produce not only the ECM but also a diverse array of bioactive factors. CAFs often dominate the stromal landscape over cancer cells themselves. In contrast to the historical perception of fibroblasts as uniform entities, recent methodological advancements, particularly single-cell sequencing, have unravelled several fibroblast subtypes. Remarkably, these subtypes exhibit striking inter- and intra-organ heterogeneity and localisze to discrete anatomical positions, offering novel predictions about physiological functions (107). Myofibroblasts, characteriszed by the presence of α-SMA, make a ubiquitous appearance in the pathological scenarios discussed herein - ranging from wound healing and autoimmune disorders to organ fibrosis, including post-infectious conditions. Myofibroblasts, true conductors of the inflammatory orchestra, actively produce inflammation-supporting factors, notably IL-6, thereby shaping a microenvironment conducive to inflammation (6). Unravelling the mysteries surrounding myofibroblasts opens doors to therapeutic possibilities and deeper insights into various pathological conditions.
Origin of CAFs: Where do they come from?
Previously, the primary origin of CAFs was attributed to the activation of local fibroblasts by TGF-β factors, often involving human endogenous lectin galectin-1 (34); extensive research has revealed a more complex landscape of CAF precursors. Mesenchymal stem cells, emerging from adipose tissue migration (99) or bone marrow recruitment (108), stand out as strong contenders. These mesenchymal stem cells, reprogrammed by cancer cells, acquire CAF functionalities, albeit retaining some characteristics of their original phenotype (109). Despite these observations, the notion of local fibroblasts as the primary source for CAF formation persists, supported by experimental evidence demonstrating that CD26-positive fibroblasts, acting as CAF precursors, exhibit enhanced protumorigenic characteristics compared to their CD26-negative counterparts (110). Further cell types which may also contribute to the CAF pool include stellate cells, adipocytes, mesothelial cells, circulating fibrocytes, pericytes, smooth muscle cells, haematopoietic stem cells, and endothelial cells (111). Multiple factors, such as IL-1β, IL-12, FGF, PDGF, SDF1, HDGF, IFN-γ, and TNF-α, participate in CAF formation from various precursors. However, TGF-β cytokine family factors consistently play an active role, regardless of the precursor source of CAFs (112).
Remarkably, cancer cells undergoing EMT have been identified as potential contributors to the pool of CAFs, a phenomenon observed for example in breast and pancreatic cancers (113, 114). However, a cloud of controversy hangs over this observation, casting doubt on its universality. Experiments injecting human cancer cells into nu/nu mice have unveiled tumours exhibiting a stroma originating from the recipient mouse cells rather than EMT-induced CAFs (115). This discrepancy calls for meticulous examination, necessitating further experiments to untangle the intricacies surrounding the reliability of the utilised model and the true origin of CAFs. Resolving these lingering questions is inevitable for a comprehensive understanding of CAF genesis and its implications in cancer progression.
Distinguishing CAFs: a challenge in cellular identification resulting from heterogeneity
Vimentin, an intermediate filament, is expressed in fibroblasts, yet its presence in various cell types complicates the task of distinguishing CAFs definitively. Despite efforts, no specific marker exclusive to CAFs has been identified, contributing to the challenge of differentiation. The expression of α-SMA is commonly considered a CAF marker, but its limitations arise from a substantial subset of these cells being negative for this marker. Furthermore, α-SMA is shared with smooth muscle cells, adding to the complexity of the specificity issue (112). CAFs exhibit a diverse array of expressed proteins, including type I collagen, tenascin C, periostin, podoplanin, CCL2, RAB3B, S100A4, CD31, CD74, CD90, DDR2, FSP-1, CXCL12, calponin-1, PDGFR-α, FAP, ASC-1, TGF-β2, and NG2 (112). However, the absence of a singular, distinctive marker underscores the necessity of employing a combination of markers for accurate identification.
In in vitro experiments, determining the negativity for proteins associated with other cell types – e.g., keratins (epithelium), CD45 (leucocytes), CD31 (endothelium), and melanocyte markers such as MELAN-A or HMB45 – proves useful for characterising and isolating CAFs (116). The pursuit of more specific markers remains crucial for advancing our ability to identify CAFs in the cellular milieu.
CAFs display heterogeneity, appearing morphologically homogeneous, but further analysis reveals subcategories. MyCAFs, identified by α-SMA positivity, coexist with negative cells. Single-cell analysis delves deeper, identifying distinct subtypes. In experimental settings, dermal fibroblasts in 3D spheroids with melanoma cells differentiate into ECM producers, inflammation-supporting iCAFs, and ID gene-rich cells influenced by TGF-β family proteins, likely representing precursor CAFs (117). Prognostically, a prevalence of inflammation-supporting iCAFs is associated with poorer outcomes (118, 119). Directly isolated tumour CAFs exhibit further subdivision, including antigen-presenting apCAFs expressing MHC class II (120). Their anticancer effects vary with CAF origin (121). These subtypes also differ in metabolic profiles, with iCAFs favouring glycolysis and myCAFs exhibiting elevated tricarboxylic acid cycle markers (122). The functional implications of these metabolic distinctions are actively investigated.
Functions of CAFs
Summarising the impact of CAFs on cancer cells, they play a multifaceted role in stimulating cancer cell proliferation, EMT, ECM remodelling, anchorage-independent growth, dissemination of metastasis, immunosuppression, angiogenesis, chemoresistance, and radioresistance (123). Notably, iCAFs, particularly through IL-6 and IL-8 production, significantly influence the dialogue within the tumour ecosystem for various malignancies (124, 125). iCAFs’ stimulatory effects, driven by exosomes released by cancer cells, impact hepatocellular, breast and colorectal cancers (126–128). Elevated serum levels of IL-6 and IL-8 are associated with multiple tumour types, including melanoma, lung, oral (squamous), and gastric cancers (129–132). iCAFs also contribute to the pro-inflammatory microenvironment by increasing serum levels of factors such as IFN-α/γ, IL-1β, IL-2, IL-4, IL-5, IL-10, IL-12P70, IL-17A, and TNF-α (133–136).
Several of these factors participate in the premetastatic niche formation, supporting tumour spread (137). Normal dermal fibroblasts from unaffected skin in patients with metastatic melanoma exhibit CAF-like features, suggesting a role in facilitating malignant cell docking (138).
When CAFs express PDL1, they contribute to the immunosuppressive microenvironment, affecting patient prognosis (139). IL-6-induced STAT3 signalling in CAFs is implicated in muscle protein breakdown and cachexia, a severe systemic complication of cancer (140). IL-6, TNF-α, and IL-8 from CAFs influence adipose tissue loss, organ fibrosis, and appetite reduction, associated with cancer wasting and cachexia (141, 142). Additionally, neuropsychological symptoms, including depression in cancer patients, are linked to high levels of IL-6 and TNF-α (143–145).
CAFs secrete ECM molecules, including collagens, elastin, proteoglycans, periostin, hyaluronic acid, and heparan sulphate, influencing cancer cell proliferation and migration (146). Lysyl oxidase produced by CAFs crosslinks collagen strongly via exosomes (147). ECM components such as tenascin-C and fibronectin are up-regulated in tumour stroma, and the signal-specific information promotes tissue stiffness, facilitating cancer growth and dissemination (148).
Interestingly, the activity of CAFs may not be strictly specific to the tumour type. As we have shown previously, CAFs from one cancer type can influence the phenotype of cells from other cancers (115, 149). Active genes, including IL6, VEGFA, and MFGE8, appear to be common among CAFs across various origins and have a significant impact on cancer cell phenotypes (150). While most CAFs are known to promote cancer progression, local aggressiveness, and metastasis, recent findings indicate that certain subpopulations may exhibit anticancer effects, particularly those associated with MHC II expression and markers such as meflin or CD146 (151–154). Ongoing research aims to unravel the complexities of CAF function and its diverse roles in cancer biology. Differences between normal fibroblasts and those associated with wound healing, hypertrophic/keloid scars, autoimmunity, and cancer are summarised in Figure 2. These fibroblasts differ in their expression of α-SMA in some cells, their elevated production of extracellular matrix components, and their secretion of inflammation-supporting factors.
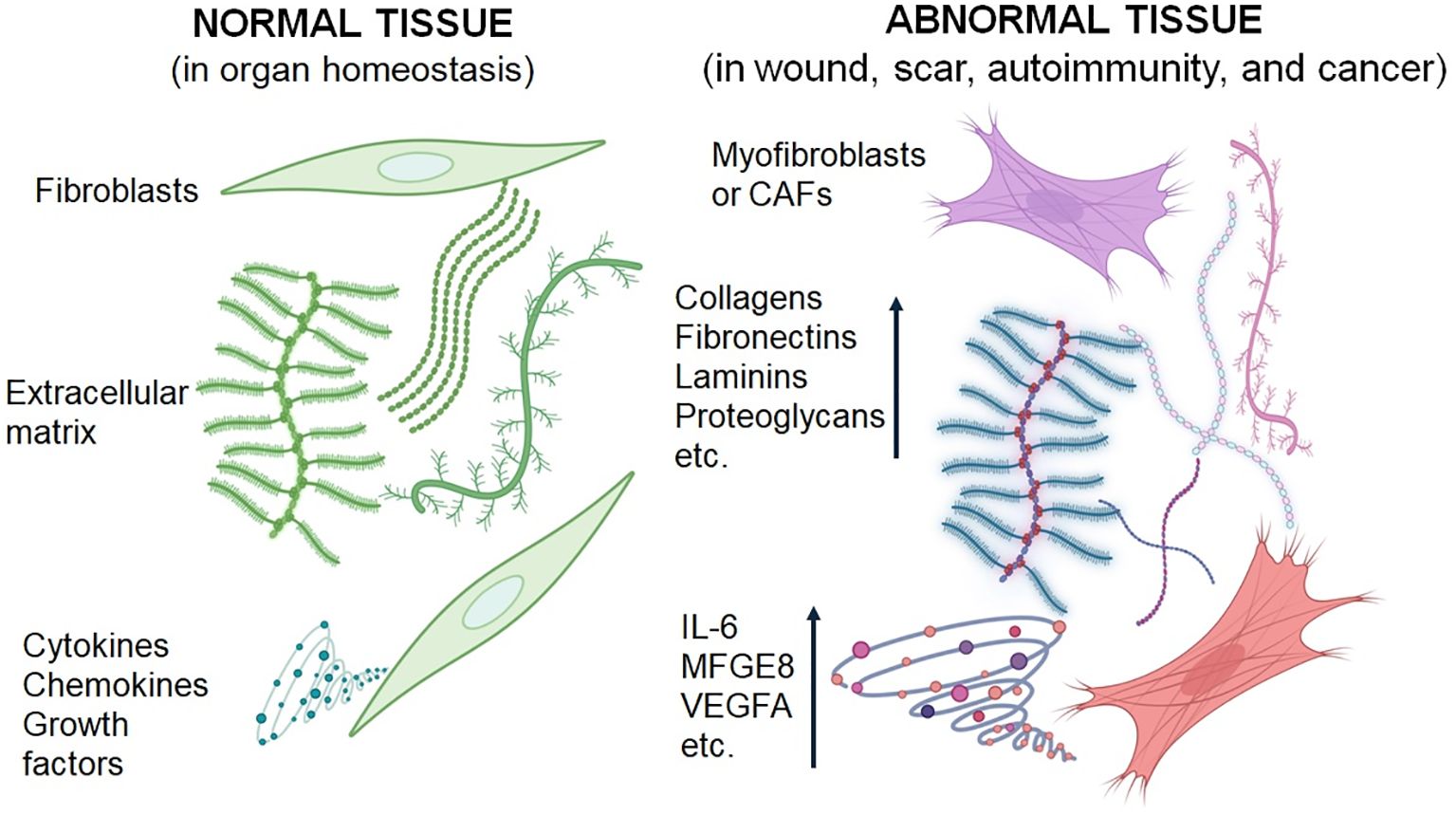
Figure 2. Schematic presentation of the differences between normal fibroblasts and activated fibroblasts associated with wound healing, scar formation, autoimmunity, and cancer. Fibroblasts from pathological conditions exhibit similar characteristics.
Paraneoplastic syndromes
The onset of paraneoplastic syndromes may serve as an early warning sign, indicating an underlying health issue. These syndromes typically arise from chronic inflammation, with IL-6 playing a pivotal role and contributing to the dysregulation of blood plasma proteins (155). Many paraneoplastic syndromes exhibit significant biological activity, leading to systemic effects (130). Examples of syndromes not directly linked to cancer therapy are detailed in Table 2. Skin, muscles, joints, the nervous system, and endocrine glands appear particularly responsive to inflammation-associated dysregulation associated with cancer development (155). In addition to hormones produced by cancer cells, factors supporting inflammation seem implicated in many paraneoplastic syndromes. These syndromes are not strictly tumour type-specific and are observed in both leukaemias and solid tumours. Furthermore, cancer treatments, including immune checkpoint inhibitors (156), may exacerbate paraneoplastic syndromes, highlighting the potential role of cell disintegration in therapy-related manifestations (157). While the application of anti-IL-6 receptor antibody tocilizumab has a minimal impact on cancer growth, it can reduce the occurrence of paraneoplastic syndromes (158, 159).
Cancer and the elderly exhibit many systemic similarities
Cancer and ageing share several systemic similarities. Ageing is a complex process influenced by various endogenous and exogenous factors, with chronic inflammation being a hallmark (169, 170). In aged individuals, including humans, there is an elevated level of inflammation-supporting factors in the serum compared to younger counterparts (171–173). High levels of IL-6, both in absolute numbers and when expressed as a ratio to albumin, can predict mortality in seriously ill elderly individuals (174, 175) and may impact cognitive functions in the aged population (176). Similar to cancer cachexia, age-related sarcopoenia in the elderly is associated with increased inflammation-supporting factors (177). The mechanisms of age-related cachexia are not fully understood, but it is hypothesised that chronic inflammation in ageing is linked to the dysregulation of iron metabolism (178). Another explanation for elevated pro-inflammatory activity in the elderly may be differences in intestinal microbiota and intestinal permeability (179). Collectively, these age-dependent differences are referred to as inflammageing, as mentioned above, and can contribute to various age-related disorders (180). Given the negative impact of elevated cytokine levels on the physiological functions of the elderly, reducing inflammation could be a promising avenue to enhance the quality of life for seniors. Some approaches, such as incorporating dietary soy isoflavonoids and proteins, show promise (181). Monitoring inflammation-supporting factors in the serum of polymorbid older individuals is relevant in clinical medicine. The high levels of these cytokines associated with age-related frailty could serve as indicators to identify cancer patients who may not benefit from therapy and could even face a risk of reduced life quality (182).
IL-6: the key player from wound healing to cancer – therapeutic implications
It has been shown that the formation of wound-healing granulation tissue featuring α-SMA-rich myofibroblasts is a linking point of various pathological conditions such as wound healing, autoimmune inflammation, tissue fibrosis, post-serious infection status, cancer, and age-associated frailty. Central to these processes is the cytokine IL-6 and its signalling pathway (183, 184). IL-6, a glycoprotein of 184 amino acids (MW 23.7kDa), is produced by immune cells and, notably, by non-immune cells such as myofibroblasts. Its function hinges on receptor expression. The transmembrane IL-6 receptor interacts with the signal transducer (gp130) upon IL-6 binding, initiating signalling. However, the IL-6 receptor can also be found in biological fluids. When unoccupied, the IL-6 receptor undergoes enzymatic cleavage from its transmembrane domain, releasing it into the extracellular space. Once interacting with IL-6 and signal transducers, this soluble IL-6 receptor can then dock to the membrane and initiate signalling (185). The immune function, primarily initiation of the immune response, and other functions – spanning from wound healing to cancer – rely on the type of cells that express membrane receptors. This involves docking of the complex of soluble receptors with IL-6 to permissive cells. The non-immune functions encompass epithelial cell proliferation and migration, along with the production of ECM by fibroblasts and neovascularisation.
Given the IL-6’s impact on cancer-related processes, therapeutic modulation of its signalling axis holds promise. A range of drugs, from antibodies to small inhibitors targeting IL-6 signalling, have been developed. These interventions include blocking IL-6 synthesis and its receptor, neutralising IL-6, inhibiting its binding to the IL-6 receptor, and disrupting the interaction of the IL-6 receptor with gp130 (186). Anti-IL-6 therapy, exemplified by the widely used anti-IL-6 receptor humanised monoclonal antibody tocilizumab, is a common practice in rheumatology (187). Moreover, tocilizumab was successfully employed in treating COVID-19-induced cytokine storms in indicated patients (188). A similarly positive effect was also observed with the use of the anti-IL-6 antibody siltuximab, although it was not as commonly utilised as tocilizumab (189). Unfortunately, clinical studies attempting to influence IL-6 interaction with the receptor complex in anticancer treatment were not entirely successful (184). It is suggested that inhibition of Jak/Stat3, which is involved in the signalling pathways of various cytokines, chemokines, and growth factors, including IL-6, could be more effective (190, 186). A combination of anti-IL-6 signalling therapy with other anticancer drugs or drugs inhibiting chronic inflammation in the TME may be more appropriate (190, 191). Another potential approach could involve the use of drugs with dual effects, such as targeting both the IL-6 receptor and mitochondria, representing a promising model for complex therapy (192).
Of note, the potential systemic effects of IL-6 produced by the TME, including CAFs, on cancer wasting, cachexia, and paraneoplastic syndromes are detailed in the chapters “Functions of CAFs” and “Paraneoplastic syndromes”.
Conclusion
Inflammation influences wound healing, solid cancer progression and spreading, with cytokines, notably IL-6, playing a central role. During wound healing, macrophages and fibroblasts in the granulation tissue are the main contributors to IL-6 production, enhancing wound re-epithelisation. Closure of the wound prompts termination of new granulation tissue deposition, starting the maturation/remodelling phase. Complications such as bacterial infections or foreign body contamination may prolong healing, introducing inflammation and overproduction of myofibroblasts leading to hypertrophic scarring or keloid formation. This scenario mirrors inflammation seen in autoimmune disorders or viral infections (e.g., COVID-19), often resulting in fibrosis, which can be severe and fatal.
Solid tumours follow a similar narrative, as the cancer stroma, featuring CAFs, resembles the granulation tissue of healing, complete with myofibroblasts, akin to the proliferation phase of wound healing. However, in cancer, the granulation tissue-like stroma does not undergo shutdown, and the dialogue between cancer cells and stromal elements persists endlessly, facilitated by the unlimited proliferation of cancer cells (Figures 3A, B). Chronic wounds, autoimmune conditions, and cancer converge in their systemic impact on the patient, leading to frailty, wasting, and cachexia, ultimately compromising the quality of life and contributing to mortality.
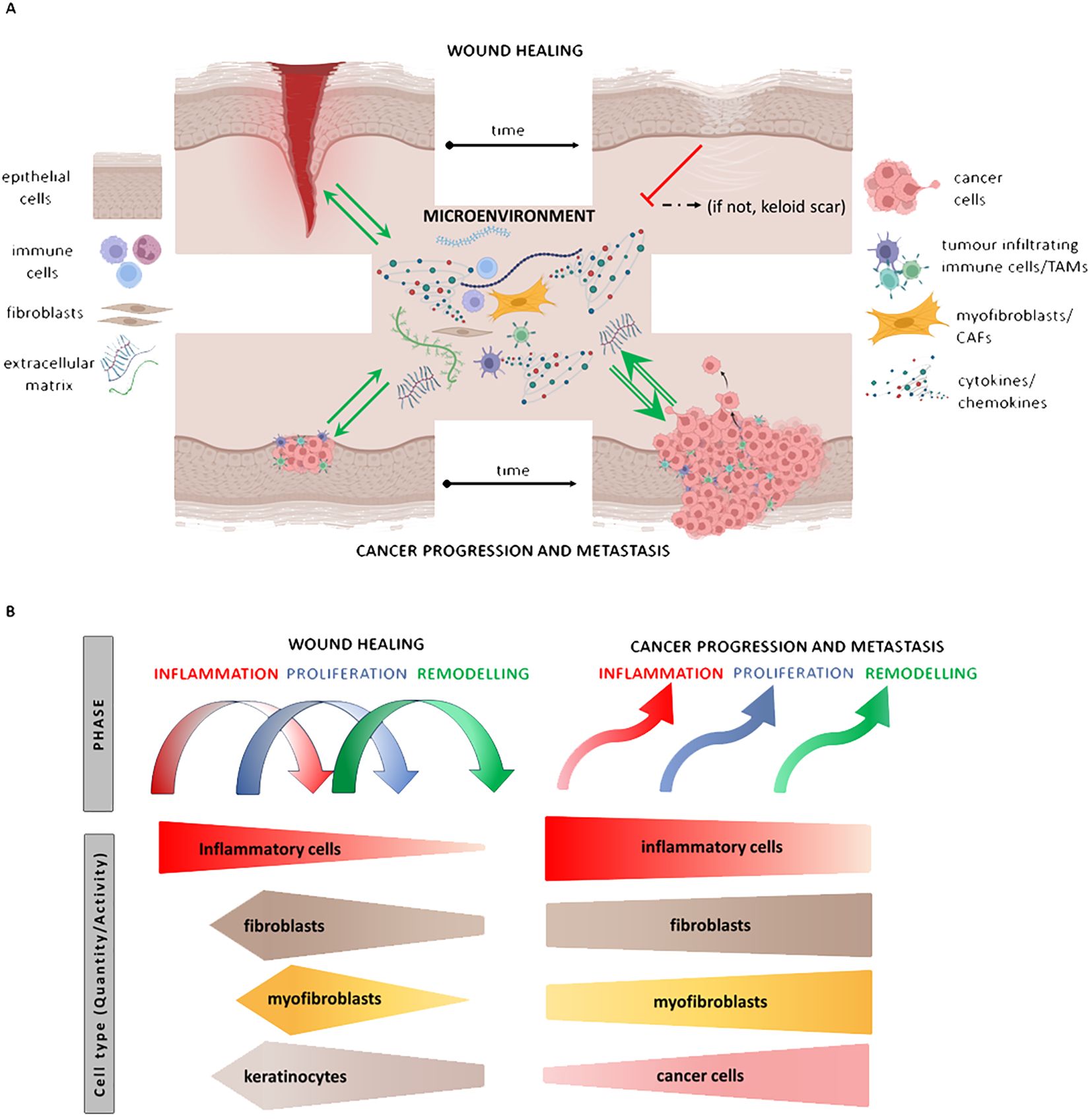
Figure 3. Illustrates the similarities between events in wound repair and cancer. The granulation tissue of the wound/tumour stroma is composed of fibroblasts/cancer-associated fibroblasts (CAFs), macrophages/tumour-associated macrophages (TAMs), and endothelial cells (ECs). Both granulation tissue and stromal elements communicate with normal/malignant epithelial cells through the production of extracellular matrix (ECM) and paracrine secretion of cytokines such as IL-6 and others. While the dialogue between cells of granulation tissue and the proliferating epithelium is switched off after reepithelisation, the unlimited proliferation of cancer cells stimulates further development of stromal tissue. This process leads to the progression of the tumour, its spreading, and subsequent wasting of patients (A). The hierarchy of the steps is disorganised in cancer compared to wound healing (B).
The present review weaves narratives under the theme of inflammation, orchestrated by the paracrine secretion of cytokines and chemokines, with IL-6 taking a central role – a ubiquitous cytokine with intricate effects. Wound healing, considered a primary event, is repurposed in autoimmunity and cancer, offering valuable insights into understanding cancer regulation and informing potential anticancer therapies. Although therapies targeting the IL-6 axis have not met all expectations, combining them with other anticancer approaches or employing drugs with multiple targets in cancer ecosystems may prove beneficial in the hopefully near future.
Author contributions
LL: Writing – original draft, Writing – review & editing, Data curation, Visualization. MK: Writing – original draft, Writing – review & editing, Data curation, Visualization. LP: Data curation, Writing – original draft, Writing – review & editing. PG: Writing – original draft, Writing – review & editing, Conceptualization, Formal analysis, Funding acquisition. KS: Writing – original draft, Writing – review & editing, Conceptualization, Formal analysis, Funding acquisition, Supervision.
Funding
The author(s) declare financial support was received for the research, authorship, and/or publication of this article. This study was supported by the project National Institute for Cancer Research (Programme EXCELES, ID Project No. LX22NPO5102) funded by the European Union – Next Generation EU. The Ministry of Health of the Czech Republic project No. NU22-03-00318, Charles University project COOPERATIO-Onco, and Charles University Grant Agency project No. 342522 also supported this study. The authors also appreciate the Grant Agency of the Ministry of Education, Science, Research and Sport of the Slovak Republic (under contracts Nos. 1/0455/22 and 1/0262/24), and the Slovak Research and Development Agency (under contracts Nos. APVV-20-0017 and APVV-22-0006) for support. This research was also partly supported by the European Union under the Next Generation EU recovery plan (under contract No. 09I03-03-V04-00075). The project “Center for Tumor Ecology - Research of the Cancer Microenvironment Supporting Cancer Growth and Spread” (reg. No. CZ.02.1.01/0.0/0.0/16_019/0000785) supported by the Operational Programme Research, Development and Education in the regimen of sustainability is also appreciated.
Acknowledgments
The authors are also grateful to Šárka Takáčová for the English revision.
Conflict of interest
The remaining authors declare that the research was conducted in the absence of any commercial or financial relationships that could be construed as a potential conflict of interest.
Publisher’s note
All claims expressed in this article are solely those of the authors and do not necessarily represent those of their affiliated organizations, or those of the publisher, the editors and the reviewers. Any product that may be evaluated in this article, or claim that may be made by its manufacturer, is not guaranteed or endorsed by the publisher.
References
1. Shvedova M, Samdavid Thanapaul RJR, Thompson EL, Niedernhofer LJ, Roh DS. Cellular senescence in aging, tissue repair, and regeneration. Plast Reconstr Surg. (2022) 150:4S–11S. doi: 10.1097/PRS.0000000000009667
2. Brivio P, Paladini MS, Racagni G, Riva MA, Calabrese F, Molteni R. From healthy aging to frailty: in search of the underlying mechanisms. Curr Med Chem. (2019) 26:3685–701. doi: 10.2174/0929867326666190717152739
3. Marengoni A, Angleman S, Melis R, Mangialasche F, Karp A, Garmen A, et al. Aging with multimorbidity: A systematic review of the literature. Ageing Res Rev. (2011) 10:430–9. doi: 10.1016/j.arr.2011.03.003
4. Kennedy BK, Berger SL, Brunet A, Campisi J, Cuervo AM, Epel ES, et al. Geroscience: linking aging to chronic disease. Cell. (2014) 159:709–13. doi: 10.1016/j.cell.2014.10.039
5. Dvorak HF. Tumors: wounds that do not heal. Similarities between tumor stroma generation and wound healing. N Engl J Med. (1986) 315:1650–9. doi: 10.1056/NEJM198612253152606
6. Gal P, Brabek J, Holub M, Jakubek M, Sedo A, Lacina L, et al. Autoimmunity, cancer and covid-19 abnormally activate wound healing pathways: critical role of inflammation. Histochem Cell Biol. (2022) 158:415–34. doi: 10.1007/s00418-022-02140-x
7. Novak S, Kolar M, Szabo A, Vernerova Z, Lacina L, Strnad H, et al. Desmoplastic crosstalk in pancreatic ductal adenocarcinoma is reflected by different responses of panc-1, miapaca-2, patu-8902, and capan-2 cell lines to cancer-associated/normal fibroblasts. Cancer Genomics Proteomics. (2021) 18:221–43. doi: 10.21873/cgp.20254
8. Coma M, Frohlichova L, Urban L, Zajicek R, Urban T, Szabo P, et al. Molecular changes underlying hypertrophic scarring following burns involve specific deregulations at all wound healing stages (Inflammation, proliferation and maturation). Int J Mol Sci. (2021) 22:897. doi: 10.3390/ijms22020897
9. Khalid KA, Nawi AFM, Zulkifli N, Barkat MA, Hadi H. Aging and wound healing of the skin: A review of clinical and pathophysiological hallmarks. Life (Basel). (2022) 12:2142. doi: 10.3390/life12122142
10. Matijevic T, Talapko J, Mestrovic T, Matijevic M, Eric S, Eric I, et al. Understanding the multifaceted etiopathogenesis of foot complications in individuals with diabetes. World J Clin cases. (2023) 11:1669–83. doi: 10.12998/wjcc.v11.i8.1669
11. Patras L, Paul D, Matei IR. Weaving the nest: extracellular matrix roles in pre-metastatic niche formation. Front Oncol. (2023) 13:1163786. doi: 10.3389/fonc.2023.1163786
12. Reinke JM, Sorg H. Wound repair and regeneration. Eur Surg Res. (2012) 49:35–43. doi: 10.1159/000339613
13. Ellis S, Lin EJ, Tartar D. Immunology of wound healing. Curr Dermatol Rep. (2018) 7:350–8. doi: 10.1007/s13671-018-0234-9
14. de Oliveira S, Rosowski EE, Huttenlocher A. Neutrophil migration in infection and wound repair: going forward in reverse. Nat Rev Immunol. (2016) 16:378–91. doi: 10.1038/nri.2016.49
15. Wilgus TA, Wulff BC. The importance of mast cells in dermal scarring. Adv Wound Care (New Rochelle). (2014) 3:356–65. doi: 10.1089/wound.2013.0457
16. Landen NX, Li D, Stahle M. Transition from inflammation to proliferation: A critical step during wound healing. Cell Mol Life Sci. (2016) 73:3861–85. doi: 10.1007/s00018-016-2268-0
17. Avazi DO, Awasum AC, Hassan AZ, Ayo JO, Aluwong T, Muhammed ST, et al. Evaluation of levels of interleukin-6, interleukin-8 and some haematologic parameters of dogs with cutaneous wounds. Cytokine. (2019) 113:128–38. doi: 10.1016/j.cyto.2018.06.024
18. Adamik B, Zimecki M, Wlaszczyk A, Kubler A. Immunological status of septic and trauma patients. I. High tumor necrosis factor alpha serum levels in septic and trauma patients are not responsible for increased mortality; a prognostic value of serum interleukin 6. Arch Immunol Ther Exp (Warsz). (1997) 45:169–75.
19. Wokalek H, Ruh H. Time course of wound healing. J Biomater Appl. (1991) 5:337–62. doi: 10.1177/088532829100500405
20. Tracy LE, Minasian RA, Caterson EJ. Extracellular matrix and dermal fibroblast function in the healing wound. Adv Wound Care (New Rochelle). (2016) 5:119–36. doi: 10.1089/wound.2014.0561
21. Vorstandlechner V, Laggner M, Kalinina P, Haslik W, Radtke C, Shaw L, et al. Deciphering the functional heterogeneity of skin fibroblasts using single-cell rna sequencing. FASEB J. (2020) 34:3677–92. doi: 10.1096/fj.201902001RR
22. Griffin MF, desJardins-Park HE, Mascharak S, Borrelli MR, Longaker MT. Understanding the impact of fibroblast heterogeneity on skin fibrosis. Dis Model Mech. (2020) 13:dmm044164. doi: 10.1242/dmm.044164
23. Wong CE, Paratore C, Dours-Zimmermann MT, Rochat A, Pietri T, Suter U, et al. Neural crest-derived cells with stem cell features can be traced back to multiple lineages in the adult skin. J Cell Biol. (2006) 175:1005–15. doi: 10.1083/jcb.200606062
24. Thiery JP, Acloque H, Huang RY, Nieto MA. Epithelial-mesenchymal transitions in development and disease. Cell. (2009) 139:871–90. doi: 10.1016/j.cell.2009.11.007
25. Li H, Yao Z, He W, Gao H, Bai Y, Yang S, et al. P311 induces the transdifferentiation of epidermal stem cells to myofibroblast-like cells by stimulating transforming growth factor B1 expression. Stem Cell Res Ther. (2016) 7:175. doi: 10.1186/s13287-016-0421-1
26. Saikia P, Crabb JS, Dibbin LL, Juszczak MJ, Willard B, Jang GF, et al. Quantitative proteomic comparison of myofibroblasts derived from bone marrow and cornea. Sci Rep. (2020) 10:16717. doi: 10.1038/s41598-020-73686-w
27. Quan TE, Cowper SE, Bucala R. The role of circulating fibrocytes in fibrosis. Curr Rheumatol Rep. (2006) 8:145–50. doi: 10.1007/s11926-006-0055-x
28. Piera-Velazquez S, Li Z, Jimenez SA. Role of endothelial-mesenchymal transition (Endomt) in the pathogenesis of fibrotic disorders. Am J Pathol. (2011) 179:1074–80. doi: 10.1016/j.ajpath.2011.06.001
29. Hinz B. The role of myofibroblasts in wound healing. Curr Res Transl Med. (2016) 64:171–7. doi: 10.1016/j.retram.2016.09.003
30. Chitturi RT, Balasubramaniam AM, Parameswar RA, Kesavan G, Haris KT, Mohideen K. The role of myofibroblasts in wound healing, contraction and its clinical implications in cleft palate repair. J Int Oral health: JIOH. (2015) 7:75–80.
31. Bonner JC, Badgett A, Osornio-Vargas AR, Hoffman M, Brody AR. Pdgf-stimulated fibroblast proliferation is enhanced synergistically by receptor-recognized alpha 2-macroglobulin. J Cell Physiol. (1990) 145:1–8. doi: 10.1002/jcp.1041450102
32. Desmouliere A, Geinoz A, Gabbiani F, Gabbiani G. Transforming growth factor-beta 1 induces alpha-smooth muscle actin expression in granulation tissue myofibroblasts and in quiescent and growing cultured fibroblasts. J Cell Biol. (1993) 122:103–11. doi: 10.1083/jcb.122.1.103
33. Grazul-Bilska AT, Luthra G, Reynolds LP, Bilski JJ, Johnson ML, Adbullah SA, et al. Effects of basic fibroblast growth factor (Fgf-2) on proliferation of human skin fibroblasts in type ii diabetes mellitus. Exp Clin Endocrinol Diabetes. (2002) 110:176–81. doi: 10.1055/s-2002-32149
34. Dvořánková B, Szabo P, Lacina L, Gal P, Uhrova J, Zima T, et al. Human galectins induce conversion of dermal fibroblasts into myofibroblasts and production of extracellular matrix: potential application in tissue engineering and wound repair. Cells Tissues Organs. (2011) 194:469–80. doi: 10.1159/000324864
35. Hung CF, Rohani MG, Lee SS, Chen P, Schnapp LM. Role of igf-1 pathway in lung fibroblast activation. Respir Res. (2013) 14:102. doi: 10.1186/1465-9921-14-102
36. Evans RA, Tian YC, Steadman R, Phillips AO. Tgf-beta1-mediated fibroblast-myofibroblast terminal differentiation-the role of smad proteins. Exp Cell Res. (2003) 282:90–100. doi: 10.1016/s0014-4827(02)00015-0
37. Shi Z, Yao C, Shui Y, Li S, Yan H. Research progress on the mechanism of angiogenesis in wound repair and regeneration. Front Physiol. (2023) 14:1284981. doi: 10.3389/fphys.2023.1284981
38. Barleon B, Sozzani S, Zhou D, Weich HA, Mantovani A, Marmé D. Migration of human monocytes in response to vascular endothelial growth factor (Vegf) is mediated via the vegf receptor flt-1. Blood. (1996) 87:3336–43. doi: 10.1182/blood.V87.8.3336.bloodjournal8783336
39. Santoro MM, Gaudino G. Cellular and molecular facets of keratinocyte reepithelization during wound healing. Exp Cell Res. (2005) 304:274–86. doi: 10.1016/j.yexcr.2004.10.033
40. Vu R, Dragan M, Sun P, Werner S, Dai X. Epithelial-mesenchymal plasticity and endothelial-mesenchymal transition in cutaneous wound healing. Cold Spring Harbor Perspect Biol. (2023) 15:a041237. doi: 10.1101/cshperspect.a041237
41. Werner S, Krieg T, Smola H. Keratinocyte-fibroblast interactions in wound healing. J Invest Dermatol. (2007) 127:998–1008. doi: 10.1038/sj.jid.5700786
42. Gill SE, Parks WC. Metalloproteinases and their inhibitors: regulators of wound healing. Int J Biochem Cell Biol. (2008) 40:1334–47. doi: 10.1016/j.biocel.2007.10.024
43. Rohani MG, Parks WC. Matrix remodeling by mmps during wound repair. Matrix Biol. (2015) 44-46:113–21. doi: 10.1016/j.matbio.2015.03.002
44. Barnes LA, Marshall CD, Leavitt T, Hu MS, Moore AL, Gonzalez JG, et al. Mechanical forces in cutaneous wound healing: emerging therapies to minimize scar formation. Adv Wound Care (New Rochelle). (2018) 7:47–56. doi: 10.1089/wound.2016.0709
45. Krejčí E, Kodet O, Szabo P, Borský J, Smetana K Jr., Grim M, et al. In vitro differences of neonatal and later postnatal keratinocytes and dermal fibroblasts. Physiol Res. (2015) 64:561–9. doi: 10.33549/physiolres.932893
46. Mateu R, Živicová V, Krejčí ED, Grim M, Strnad H, Vlček Č, et al. Functional differences between neonatal and adult fibroblasts and keratinocytes: donor age affects epithelial-mesenchymal crosstalk in vitro. Int J Mol Med. (2016) 38:1063–74. doi: 10.3892/ijmm.2016.2706
47. Živicová V, Lacina L, Mateu R, Smetana K Jr., Kavková R, Drobná Krejčí E, et al. Analysis of dermal fibroblasts isolated from neonatal and child cleft lip and adult skin: developmental implications on reconstructive surgery. Int J Mol Med. (2017) 40:1323–34. doi: 10.3892/ijmm.2017.3128
48. Tan S, Khumalo N, Bayat A. Understanding keloid pathobiology from a quasi-neoplastic perspective: less of a scar and more of a chronic inflammatory disease with cancer-like tendencies. Front Immunol. (2019) 10:1810. doi: 10.3389/fimmu.2019.01810
49. Ogawa R. Keloid and hypertrophic scars are the result of chronic inflammation in the reticular dermis. Int J Mol Sci. (2017) 18:606. doi: 10.3390/ijms18030606
50. Louw L. Keloids in rural black South Africans. Part 1: general overview and essential fatty acid hypotheses for keloid formation and prevention. Prostaglandins leukotrienes essential Fatty Acids. (2000) 63:237–45. doi: 10.1054/plef.2000.0207
51. Nyika DT, Khumalo NP, Bayat A. Genetics and epigenetics of keloids. Adv Wound Care (New Rochelle). (2022) 11:192–201. doi: 10.1089/wound.2021.0094
52. Ud-Din S, Bayat A. Keloid scarring or disease: unresolved quasi-neoplastic tendencies in the human skin. Wound Repair Regener. (2020) 28:422–6. doi: 10.1111/wrr.12793
53. Ud-Din S, Bayat A. Controlling inflammation pre-emptively or at the time of cutaneous injury optimises outcome of skin scarring. Front Immunol. (2022) 13:883239. doi: 10.3389/fimmu.2022.883239
54. Bell RE, Shaw TJ. Keloid tissue analysis discredits a role for myofibroblasts in disease pathogenesis. Wound Repair Regener. (2021) 29:637–41. doi: 10.1111/wrr.12923
55. Zhang M, Chen H, Qian H, Wang C. Characterization of the skin keloid microenvironment. Cell Commun Signal. (2023) 21:207. doi: 10.1186/s12964-023-01214-0
56. Yin H, Liu N, Zhou X, Chen J, Duan L. The advance of ccn3 in fibrosis. J Cell Commun Signal. (2023) 17:1219–27. doi: 10.1007/s12079-023-00778-3
57. Rosendahl AH, Schönborn K, Krieg T. Pathophysiology of systemic sclerosis (Scleroderma). Kaohsiung J Med Sci. (2022) 38:187–95. doi: 10.1002/kjm2.12505
58. Brábek J, Jakubek M, Vellieux F, Novotný J, Kolář M, Lacina L, et al. Interleukin-6: molecule in the intersection of cancer, ageing and covid-19. Int J Mol Sci. (2020) 21:7937. doi: 10.3390/ijms21217937
59. Krygier A, Szmajda-Krygier D, Świechowski R, Pietrzak J, Wosiak A, Wodziński D, et al. Molecular pathogenesis of fibrosis, thrombosis and surfactant dysfunction in the lungs of severe covid-19 patients. Biomolecules. (2022) 12:1845. doi: 10.3390/biom12121845
60. Arturi F, Melegari G, Giansante A, Giuliani E, Bertellini E, Barbieri A. Covid-19 biomarkers for critically ill patients: A compendium for the physician. Neurol Int. (2023) 15:881–95. doi: 10.3390/neurolint15030056
61. Peng R, Yang T, Tong Y, Wang J, Zhou H, Yang M, et al. Efficacy and safety of interleukin-6 receptor antagonists in adult patients admitted to intensive care unit with covid-19: A systematic review and meta-analysis of randomized controlled trials. Prev Med Rep. (2023) 34:102276. doi: 10.1016/j.pmedr.2023.102276
62. Bale S, Verma P, Yalavarthi B, Scarneo SA, Hughes P, Amin MA, et al. Pharmacological inhibition of tak1 prevents and induces regression of experimental organ fibrosis. JCI Insight. (2023) 8:e165358. doi: 10.1172/jci.insight.165358
63. Zhang QY, Ye XP, Zhou Z, Zhu CF, Li R, Fang Y, et al. Lymphocyte infiltration and thyrocyte destruction are driven by stromal and immune cell components in hashimoto's thyroiditis. Nat Commun. (2022) 13:775. doi: 10.1038/s41467-022-28120-2
64. Alves NRM, Kurizky PS, da Mota LMH, de Albuquerque CP, Esper JT, Campos ASC, et al. Elevated serum il-6 levels predict treatment interruption in patients with moderate to severe psoriasis: A 6-year real-world cohort study. Anais brasileiros dermatologia. (2023) 99:34–42. doi: 10.1016/j.abd.2023.03.002
65. Piroozmand A, Zamani B, Haddad Kashani H, Amini Mahabadi J. Serum interleukin-6 level and its association with pulmonary involvement in progressive systemic sclerosis; a case-control study. Clin Mol allergy: CMA. (2023) 21:7. doi: 10.1186/s12948-023-00188-1
66. Cannon A, Thompson CM, Bhatia R, Armstrong KA, Solheim JC, Kumar S, et al. Molecular mechanisms of pancreatic myofibroblast activation in chronic pancreatitis and pancreatic ductal adenocarcinoma. J Gastroenterol. (2021) 56:689–703. doi: 10.1007/s00535-021-01800-4
67. Mandys V, Popov A, Gürlich R, Havránek J, Pfeiferová L, Kolář M, et al. Expression of selected mirnas in normal and cancer-associated fibroblasts and in bxpc3 and mia paca-2 cell lines of pancreatic ductal adenocarcinoma. Int J Mol Sci. (2023) 24:3617. doi: 10.3390/ijms24043617
68. van Caam A, Vonk M, van den Hoogen F, van Lent P, van der Kraan P. Unraveling ssc pathophysiology; the myofibroblast. Front Immunol. (2018) 9:2452. doi: 10.3389/fimmu.2018.02452
69. Van Praet JT, Smith V, Haspeslagh M, Degryse N, Elewaut D, De Keyser F. Histopathological cutaneous alterations in systemic sclerosis: A clinicopathological study. Arthritis Res Ther. (2011) 13:R35. doi: 10.1186/ar3267
70. Ferreli C, Gasparini G, Parodi A, Cozzani E, Rongioletti F, Atzori L. Cutaneous manifestations of scleroderma and scleroderma-like disorders: A comprehensive review. Clin Rev Allergy Immunol. (2017) 53:306–36. doi: 10.1007/s12016-017-8625-4
71. Ramirez AM, Shen Z, Ritzenthaler JD, Roman J. Myofibroblast transdifferentiation in obliterative bronchiolitis: tgf-beta signaling through smad3-dependent and -independent pathways. Am J Transplant. (2006) 6:2080–8. doi: 10.1111/j.1600-6143.2006.01430.x
72. Distler O, Assassi S, Cottin V, Cutolo M, Danoff SK, Denton CP, et al. Predictors of progression in systemic sclerosis patients with interstitial lung disease. Eur Respir J. (2020) 55:1902026. doi: 10.1183/13993003.02026-2019
73. Streets AJ, Prosseda PP, Ong AC. Polycystin-1 regulates arhgap35-dependent centrosomal rhoa activation and rock signaling. JCI Insight. (2020) 5:e135385. doi: 10.1172/jci.insight.135385
74. Schuster R, Rockel JS, Kapoor M, Hinz B. The inflammatory speech of fibroblasts. Immunol Rev. (2021) 302:126–46. doi: 10.1111/imr.12971
75. Jarlborg M, Gabay C. Systemic effects of il-6 blockade in rheumatoid arthritis beyond the joints. Cytokine. (2022) 149:155742. doi: 10.1016/j.cyto.2021.155742
76. Mehta P, Cron RQ, Hartwell J, Manson JJ, Tattersall RS. Silencing the cytokine storm: the use of intravenous anakinra in haemophagocytic lymphohistiocytosis or macrophage activation syndrome. Lancet Rheumatol. (2020) 2:e358–e67. doi: 10.1016/s2665-9913(20)30096-5
77. Yip RML, Yim CW. Role of interleukin 6 inhibitors in the management of rheumatoid arthritis. J Clin rheumatology: Pract Rep rheumatic musculoskeletal Dis. (2021) 27:e516–e24. doi: 10.1097/rhu.0000000000001293
78. Michaud M, Balardy L, Moulis G, Gaudin C, Peyrot C, Vellas B, et al. Proinflammatory cytokines, aging, and age-related diseases. J Am Med Directors Assoc. (2013) 14:877–82. doi: 10.1016/j.jamda.2013.05.009
79. Giovannini S, Onder G, Liperoti R, Russo A, Carter C, Capoluongo E, et al. Interleukin-6, C-reactive protein, and tumor necrosis factor-alpha as predictors of mortality in frail, community-living elderly individuals. J Am Geriatr Soc. (2011) 59:1679–85. doi: 10.1111/j.1532-5415.2011.03570.x
80. Xiao T, Yan Z, Xiao S, Xia Y. Proinflammatory cytokines regulate epidermal stem cells in wound epithelialization. Stem Cell Res Ther. (2020) 11:232. doi: 10.1186/s13287-020-01755-y
81. Demaria M, Ohtani N, Youssef SA, Rodier F, Toussaint W, Mitchell JR, et al. An essential role for senescent cells in optimal wound healing through secretion of pdgf-aa. Dev Cell. (2014) 31:722–33. doi: 10.1016/j.devcel.2014.11.012
82. Chia CW, Sherman-Baust CA, Larson SA, Pandey R, Withers R, Karikkineth AC, et al. Age-associated expression of P21and P53 during human wound healing. Aging Cell. (2021) 20:e13354. doi: 10.1111/acel.13354
83. Jiang D, de Vries JC, Muschhammer J, Schatz S, Ye H, Hein T, et al. Local and transient inhibition of P21 expression ameliorates age-related delayed wound healing. Wound Repair Regener. (2020) 28:49–60. doi: 10.1111/wrr.12763
84. Pulido T, Velarde MC, Alimirah F. The senescence-associated secretory phenotype: fueling a wound that never heals. Mech Ageing Dev. (2021) 199:111561. doi: 10.1016/j.mad.2021.111561
85. Blazić TM, Brajac I. Defective induction of senescence during wound healing is a possible mechanism of keloid formation. Med Hypotheses. (2006) 66:649–52. doi: 10.1016/j.mehy.2005.09.033
86. Jun JI, Lau LF. The matricellular protein ccn1 induces fibroblast senescence and restricts fibrosis in cutaneous wound healing. Nat Cell Biol. (2010) 12:676–85. doi: 10.1038/ncb2070
87. Egeblad M, Nakasone ES, Werb Z. Tumors as organs: complex tissues that interface with the entire organism. Dev Cell. (2010) 18:884–901. doi: 10.1016/j.devcel.2010.05.012
88. Oey O, Sunjaya AF, Khan Y, Redfern A. Stromal inflammation, fibrosis and cancer: an old intuition with promising potential. World J Clin Oncol. (2023) 14:230–46. doi: 10.5306/wjco.v14.i7.230
89. Mustafa S, Koran S, AlOmair L. Insights into the role of matrix metalloproteinases in cancer and its various therapeutic aspects: A review. Front Mol Biosci. (2022) 9:896099. doi: 10.3389/fmolb.2022.896099
90. Budi HS, Farhood B. Targeting oral tumor microenvironment for effective therapy. Cancer Cell Int. (2023) 23:101. doi: 10.1186/s12935-023-02943-5
91. Sun Q, Hong Z, Zhang C, Wang L, Han Z, Ma D. Immune checkpoint therapy for solid tumours: clinical dilemmas and future trends. Signal transduction targeted Ther. (2023) 8:320. doi: 10.1038/s41392-023-01522-4
92. Thakur S, Haider S, Natrajan R. Implications of tumour heterogeneity on cancer evolution and therapy resistance: lessons from breast cancer. J Pathol. (2023) 260:621–36. doi: 10.1002/path.6158
93. Kavan S, Kruse TA, Vogsen M, Hildebrandt MG, Thomassen M. Heterogeneity and tumor evolution reflected in liquid biopsy in metastatic breast cancer patients: A review. Cancer metastasis Rev. (2022) 41:433–46. doi: 10.1007/s10555-022-10023-9
94. Che J, Yu S. Ecological niches for colorectal cancer stem cell survival and thrival. Front Oncol. (2023) 13:1135364. doi: 10.3389/fonc.2023.1135364
95. Zhou H, Tan L, Liu B, Guan XY. Cancer stem cells: recent insights and therapies. Biochem Pharmacol. (2023) 209:115441. doi: 10.1016/j.bcp.2023.115441
96. Lacina L, Coma M, Dvorankova B, Kodet O, Melegova N, Gal P, et al. Evolution of cancer progression in the context of darwinism. Anticancer Res. (2019) 39:1–16. doi: 10.21873/anticanres.13074
97. Lacina L, Kodet O, Dvořánková B, Szabo P, Smetana K Jr. Ecology of melanoma cell. Histol Histopathol. (2018) 33:247–54. doi: 10.14670/hh-11-926
98. Storz P. Roles of differently polarized macrophages in the initiation and progressionof pancreatic cancer. Front Immunol. (2023) 14:1237711. doi: 10.3389/fimmu.2023.1237711
99. Xue J, Sharma V, Hsieh MH, Chawla A, Murali R, Pandol SJ, et al. Alternatively activated macrophages promote pancreatic fibrosis in chronic pancreatitis. Nat Commun. (2015) 6:7158. doi: 10.1038/ncomms8158
100. Liou GY, Bastea L, Fleming A, Döppler H, Edenfield BH, Dawson DW, et al. The presence of interleukin-13 at pancreatic adm/panin lesions alters macrophage populations and mediates pancreatic tumorigenesis. Cell Rep. (2017) 19:1322–33. doi: 10.1016/j.celrep.2017.04.052
101. Wu J, Zhang L, Shi J, He R, Yang W, Habtezion A, et al. Macrophage phenotypic switch orchestrates the inflammation and repair/regeneration following acute pancreatitis injury. EBioMedicine. (2020) 58:102920. doi: 10.1016/j.ebiom.2020.102920
102. Yang Y, Ye YC, Chen Y, Zhao JL, Gao CC, Han H, et al. Crosstalk between hepatic tumor cells and macrophages via wnt/B-catenin signaling promotes M2-like macrophage polarization and reinforces tumor Malignant behaviors. Cell Death Dis. (2018) 9:793. doi: 10.1038/s41419-018-0818-0
103. Wang Y, Barrett A, Hu Q. Targeting macrophages for tumor therapy. AAPS J. (2023) 25:80. doi: 10.1208/s12248-023-00845-y
104. Li J, Sun J, Zeng Z, Liu Z, Ma M, Zheng Z, et al. Tumour-associated macrophages in gastric cancer: from function and mechanism to application. Clin Trans Med. (2023) 13:e1386. doi: 10.1002/ctm2.1386
105. Li M, Yang Y, Xiong L, Jiang P, Wang J, Li C. Metabolism, metabolites, and macrophages in cancer. J Hematol Oncol. (2023) 16:80. doi: 10.1186/s13045-023-01478-6
106. Shao Y, Wang Y, Su R, Pu W, Chen S, Fu L, et al. Dual identity of tumor-associated macrophage in regulated cell death and oncotherapy. Heliyon. (2023) 9:e17582. doi: 10.1016/j.heliyon.2023.e17582
107. Muhl L, Genové G, Leptidis S, Liu J, He L, Mocci G, et al. Single-cell analysis uncovers fibroblast heterogeneity and criteria for fibroblast and mural cell identification and discrimination. Nat Commun. (2020) 11:3953. doi: 10.1038/s41467-020-17740-1
108. Moratin H, Böhm S, Hagen R, Scherzad A, Hackenberg S. Influence of wound fluid on the transdifferentiation of human mesenchymal bone marrow stem cells into cancer-associated fibroblasts. Cells Tissues Organs. (2023) 212:304–16. doi: 10.1159/000525342
109. Frisbie L, Buckanovich RJ, Coffman L. Carcinoma-associated mesenchymal stem/stromal cells: architects of the pro-tumorigenic tumor microenvironment. Stem Cells. (2022) 40:705–15. doi: 10.1093/stmcls/sxac036
110. Houthuijzen JM, de Bruijn R, van der Burg E, Drenth AP, Wientjens E, Filipovic T, et al. Cd26-negative and cd26-positive tissue-resident fibroblasts contribute to functionally distinct caf subpopulations in breast cancer. Nat Commun. (2023) 14:183. doi: 10.1038/s41467-023-35793-w
111. Aden D, Zaheer S, Ahluwalia H, Ranga S. Cancer-associated fibroblasts: is it a key to an intricate lock of tumorigenesis? Cell Biol Int. (2023) 47:859–93. doi: 10.1002/cbin.12004
112. Vokurka M, Lacina L, Brabek J, Kolar M, Ng YZ, Smetana K Jr. Cancer-associated fibroblasts influence the biological properties of Malignant tumours via paracrine secretion and exosome production. Int J Mol Sci. (2022) 23:964. doi: 10.3390/ijms23020964
113. Aboussekhra A, Islam SS, Alraouji NN. Activated breast stromal fibroblasts exhibit myoepithelial and mammary stem cells features. Trans Oncol. (2023) 35:101721. doi: 10.1016/j.tranon.2023.101721
114. Adjuto-Saccone M, Soubeyran P, Garcia J, Audebert S, Camoin L, Rubis M, et al. Tnf-A Induces endothelial-mesenchymal transition promoting stromal development of pancreatic adenocarcinoma. Cell Death Dis. (2021) 12:649. doi: 10.1038/s41419-021-03920-4
115. Dvorankova B, Smetana K Jr., Rihova B, Kucera J, Mateu R, Szabo P. Cancer-associated fibroblasts are not formed from cancer cells by epithelial-to-mesenchymal transition in nu/nu mice. Histochem Cell Biol. (2015) 143:463–9. doi: 10.1007/s00418-014-1293-z
116. Dvorankova B, Lacina L, Smetana K Jr. Isolation of normal fibroblasts and their cancer-associated counterparts (Cafs) for biomedical research. Methods Mol Biol. (2019) 1879:393–406. doi: 10.1007/7651_2018_137
117. Novotný J, Strnadová K, Dvořánková B, Kocourková Š, Jakša R, Dundr P, et al. Single-cell rna sequencing unravels heterogeneity of the stromal niche in cutaneous melanoma heterogeneous spheroids. Cancers (Basel). (2020) 12:3324. doi: 10.3390/cancers12113324
118. Knipper K, Lyu SI, Quaas A, Bruns CJ, Schmidt T. Cancer-associated fibroblast heterogeneity and its influence on the extracellular matrix and the tumor microenvironment. Int J Mol Sci. (2023) 24:13482. doi: 10.3390/ijms241713482
119. Mou T, Zhu H, Jiang Y, Xu X, Cai L, Zhong Y, et al. Heterogeneity of cancer-associated fibroblasts in head and neck squamous cell carcinoma. Trans Oncol. (2023) 35:101717. doi: 10.1016/j.tranon.2023.101717
120. Liu W, Wang M, Wang M, Liu M. Single-cell and bulk rna sequencing reveal cancer-associated fibroblast heterogeneity and a prognostic signature in prostate cancer. Med (Baltimore). (2023) 102:e34611. doi: 10.1097/md.0000000000034611
121. Macy AM, Herrmann LM, Adams AC, Hastings KT. Major histocompatibility complex class ii in the tumor microenvironment: functions of nonprofessional antigen-presenting cells. Curr Opin Immunol. (2023) 83:102330. doi: 10.1016/j.coi.2023.102330
122. Hu B, Wu C, Mao H, Gu H, Dong H, Yan J, et al. Subpopulations of cancer-associated fibroblasts link the prognosis and metabolic features of pancreatic ductal adenocarcinoma. Ann Transl Med. (2022) 10:262. doi: 10.21037/atm-22-407
123. Raudenska M, Balvan J, Hanelova K, Bugajova M, Masarik M. Cancer-associated fibroblasts: mediators of head and neck tumor microenvironment remodeling. Biochim Biophys Acta Rev Cancer. (2023) 1878:188940. doi: 10.1016/j.bbcan.2023.188940
124. Jobe NP, Rösel D, Dvořánková B, Kodet O, Lacina L, Mateu R, et al. Simultaneous blocking of il-6 and il-8 is sufficient to fully inhibit caf-induced human melanoma cell invasiveness. Histochem Cell Biol. (2016) 146:205–17. doi: 10.1007/s00418-016-1433-8
125. Xiang X, Niu YR, Wang ZH, Ye LL, Peng WB, Zhou Q. Cancer-associated fibroblasts: vital suppressors of the immune response in the tumor microenvironment. Cytokine Growth Factor Rev. (2022) 67:35–48. doi: 10.1016/j.cytogfr.2022.07.006
126. Chuangchot N, Jamjuntra P, Yangngam S, Luangwattananun P, Thongchot S, Junking M, et al. Enhancement of pd-L1-attenuated car-T cell function through breast cancer-associated fibroblasts-derived il-6 signaling via stat3/akt pathways. Breast Cancer Res. (2023) 25:86. doi: 10.1186/s13058-023-01684-7
127. Mun K, Han J, Roh P, Park J, Kim G, Hur W, et al. Isolation and characterization of cancer-associated fibroblasts in the tumor microenvironment of hepatocellular carcinoma. J liver Cancer. (2023) 23:341–9. doi: 10.17998/jlc.2023.04.30
128. Uehara T, Sato K, Iwaya M, Asaka S, Nakajima T, Nagaya T, et al. Interleukin-6 stromal expression is correlated with epithelial-mesenchymal transition at tumor budding in colorectal cancer. Int J Surg Pathol. (2023) 32:10668969231177705. doi: 10.1177/10668969231177705
129. Li J, Xu L, Run ZC, Feng W, Liu W, Zhang PJ, et al. Multiple cytokine profiling in serum for early detection of gastric cancer. World J Gastroenterol. (2018) 24:2269–78. doi: 10.3748/wjg.v24.i21.2269
130. Kucera J, Strnadova K, Dvorankova B, Lacina L, Krajsova I, Stork J, et al. Serum proteomic analysis of melanoma patients with immunohistochemical profiling of primary melanomas and cultured cells: pilot study. Oncol Rep. (2019) 42:1793–804. doi: 10.3892/or.2019.7319
131. Rezaei F, Mozaffari HR, Tavasoli J, Zavattaro E, Imani MM, Sadeghi M. Evaluation of serum and salivary interleukin-6 and interleukin-8 levels in oral squamous cell carcinoma patients: systematic review and meta-analysis. J Interferon Cytokine research: Off J Int Soc Interferon Cytokine Res. (2019) 39:727–39. doi: 10.1089/jir.2019.0070
132. Zhao Y, Jia S, Zhang K, Zhang L. Serum cytokine levels and other associated factors as possible immunotherapeutic targets and prognostic indicators for lung cancer. Front Oncol. (2023) 13:1064616. doi: 10.3389/fonc.2023.1064616
133. Bao C, Gu J, Huang X, You L, Zhou Z, Jin J. Cytokine profiles in patients with newly diagnosed diffuse large B-cell lymphoma: il-6 and il-10 levels are associated with adverse clinical features and poor outcomes. Cytokine. (2023) 169:156289. doi: 10.1016/j.cyto.2023.156289
134. Brierly G, Celentano A, Breik O, Moslemivayeghan E, Patini R, McCullough M, et al. Tumour necrosis factor alpha (Tnf-A) and oral squamous cell carcinoma. Cancers (Basel). (2023) 15:1841. doi: 10.3390/cancers15061841
135. Wang W, Liu X, Yang S, Peng X, Ma Y, Xiong X, et al. Serum levels of soluble interleukin 2 receptor (Sil-2r), tumor necrosis factor-alpha (Tnf-A), and immunoglobulin M are correlated with the disease extent in childhood langerhans cell histiocytosis. J Cancer Res Clin Oncol. (2023) 149:11431–42. doi: 10.1007/s00432-023-04991-w
136. Zeng B, Wang X, Qin Y, Cao L, Zhang C, Meng F, et al. Differences in serum cytokine levels distinguish between clinically noninvasive lung adenocarcinoma and invasive lung adenocarcinoma: A cross-sectional study. Health Sci Rep. (2023) 6:e1522. doi: 10.1002/hsr2.1522
137. Kodet O, Kučera J, Strnadová K, Dvořánková B, Štork J, Lacina L, et al. Cutaneous melanoma dissemination is dependent on the Malignant cell properties and factors of intercellular crosstalk in the cancer microenvironment (Review). Int J Oncol. (2020) 57:619–30. doi: 10.3892/ijo.2020.5090
138. Kodet O, Dvořánková B, Bendlová B, Sýkorová V, Krajsová I, Štork J, et al. Microenvironment−Driven resistance to B−Raf inhibition in a melanoma patient is accompanied by broad changes of gene methylation and expression in distal fibroblasts. Int J Mol Med. (2018) 41:2687–703. doi: 10.3892/ijmm.2018.3448
139. Kawasaki K, Noma K, Kato T, Ohara T, Tanabe S, Takeda Y, et al. Pd-L1-expressing cancer-associated fibroblasts induce tumor immunosuppression and contribute to poor clinical outcome in esophageal cancer. Cancer Immunol Immunother. (2023) 72:3787–802. doi: 10.1007/s00262-023-03531-2
140. Shivnani P, Shekhawat S, Prajapati A. Cancer cachexia and breast cancer stem cell signalling - a crosstalk of signalling molecules. Cell Signal. (2023) 110:110847. doi: 10.1016/j.cellsig.2023.110847
141. Malla J, Zahra A, Venugopal S, Selvamani TY, Shoukrie SI, Selvaraj R, et al. What role do inflammatory cytokines play in cancer cachexia? Cureus. (2022) 14:e26798. doi: 10.7759/cureus.26798
142. Paval DR, Patton R, McDonald J, Skipworth RJE, Gallagher IJ, Laird BJ. A systematic review examining the relationship between cytokines and cachexia in incurable cancer. J Cachexia Sarcopenia Muscle. (2022) 13:824–38. doi: 10.1002/jcsm.12912
143. Amirkhanzadeh Barandouzi Z, Bruner DW, Miller AH, Paul S, Felger JC, Wommack EC, et al. Associations of inflammation with neuropsychological symptom cluster in patients with head and neck cancer: A longitudinal study. Brain behavior Immun - Health. (2023) 30:100649. doi: 10.1016/j.bbih.2023.100649
144. Kim HJ, Moon JH, Chung SW, Abraham I. The role of cytokines and indolamine-2.3 dioxygenase in experiencing a psycho-neurological symptom cluster in hematological cancer patients: il-1alpha, il-1beta, il-4, il-6, tnf-alpha, kynurenine, and tryptophan. J psychosomatic Res. (2023) 173:111455. doi: 10.1016/j.jpsychores.2023.111455
145. Madison AA, Andridge R, Kantaras AH, Renna ME, Bennett JM, Alfano CM, et al. Depression, inflammation, and intestinal permeability: associations with subjective and objective cognitive functioning throughout breast cancer survivorship. Cancers (Basel). (2023) 15:4414. doi: 10.3390/cancers15174414
146. Rafaeva M, Jensen ARD, Horton ER, Zornhagen KW, Strøbech JE, Fleischhauer L, et al. Fibroblast-derived matrix models desmoplastic properties and forms a prognostic signature in cancer progression. Front Immunol. (2023) 14:1154528. doi: 10.3389/fimmu.2023.1154528
147. Liu X, Li J, Yang X, Li X, Kong J, Qi D, et al. Carcinoma-associated fibroblast-derived lysyl oxidase-rich extracellular vesicles mediate collagen crosslinking and promote epithelial-mesenchymal transition via P-fak/P-paxillin/yap signaling. Int J Oral Sci. (2023) 15:32. doi: 10.1038/s41368-023-00236-1
148. Pajic-Lijakovic I, Milivojevic M. Physics of collective cell migration. Eur biophysics journal: EBJ. (2023) 52:625–40. doi: 10.1007/s00249-023-01681-w
149. Trylcova J, Busek P, Smetana K Jr., Balaziova E, Dvorankova B, Mifkova A, et al. Effect of cancer-associated fibroblasts on the migration of glioma cells in vitro. Tumour Biol. (2015) 36:5873–9. doi: 10.1007/s13277-015-3259-8
150. Urban L, Novák Š, Čoma M, Dvořánková B, Lacina L, Šáchová J, et al. Unravelling heterogeneous effects of cancer−Associated fibroblasts on poor prognosis markers in breast cancer em−G3 cell line: in vitro−Targeted treatment (Anti−Il-6, anti−Vegf-a, anti−Mfge8) based on transcriptomic profiling. Oncol Rep. (2024) 51:3. doi: 10.3892/or.2023.8662
151. Kerdidani D, Aerakis E, Verrou KM, Angelidis I, Douka K, Maniou MA, et al. Lung tumor mhcii immunity depends on in situ antigen presentation by fibroblasts. J Exp Med. (2022) 219:e20210815. doi: 10.1084/jem.20210815
152. Tsoumakidou M. The advent of immune stimulating cafs in cancer. Nat Rev Cancer. (2023) 23:258–69. doi: 10.1038/s41568-023-00549-7
153. Miyai Y, Sugiyama D, Hase T, Asai N, Taki T, Nishida K, et al. Meflin-positive cancer-associated fibroblasts enhance tumor response to immune checkpoint blockade. Life Sci alliance. (2022) 5:e202101230. doi: 10.26508/lsa.202101230
154. Zhao Z, Li T, Yuan Y, Zhu Y. What is new in cancer-associated fibroblast biomarkers? Cell Commun Signal. (2023) 21:96. doi: 10.1186/s12964-023-01125-0
155. Aguilar-Cazares D, Chavez-Dominguez R, Marroquin-Muciño M, Perez-Medina M, Benito-Lopez JJ, Camarena A, et al. The systemic-level repercussions of cancer-associated inflammation mediators produced in the tumor microenvironment. Front Endocrinol. (2022) 13:929572. doi: 10.3389/fendo.2022.929572
156. Rajendram P, Torbic H, Duggal A, Campbell J, Hovden M, Dhawan V, et al. Critically ill patients with severe immune checkpoint inhibitor related neurotoxicity: A multi-center case series. J Crit Care. (2021) 65:126–32. doi: 10.1016/j.jcrc.2021.05.020
157. Duong SL, Prüss H. Paraneoplastic autoimmune neurological syndromes and the role of immune checkpoint inhibitors. Neurotherapeutics: J Am Soc Exp Neurother. (2022) 19:848–63. doi: 10.1007/s13311-022-01184-0
158. Eberst L, Cassier PA, Brahmi M, Tirode F, Blay JY. Tocilizumab for the treatment of paraneoplastic inflammatory syndrome associated with angiomatoid fibrous histiocytoma. ESMO Open. (2020) 5:e000756. doi: 10.1136/esmoopen-2020-000756
159. Sabe H, Inoue A, Nagata S, Imura Y, Wakamatsu T, Takenaka S, et al. Tocilizumab controls paraneoplastic inflammatory syndrome but does not suppress tumor growth of angiomatoid fibrous histiocytoma. Case Rep oncological Med. (2021) 2021:5532258. doi: 10.1155/2021/5532258
160. Anderson HJ, Huang S, Lee JB. Paraneoplastic pemphigus/paraneoplastic autoimmune multiorgan syndrome: part I. Clinical overview and pathophysiology. J Am Acad Dermatol. (2024) 91:1–10. doi: 10.1016/j.jaad.2023.08.020
161. Jimenez LF, Castellon EA, Marenco JD, Mejia JM, Rojas CA, Jimenez FT, et al. Chronic urticaria associated with lung adenocarcinoma - a paraneoplastic manifestation: A case report and literature review. World J Clin cases. (2022) 10:7553–64. doi: 10.12998/wjcc.v10.i21.7553
162. Wick MR, Patterson JW. Cutaneous paraneoplastic syndromes. Semin Diagn Pathol. (2019) 36:211–28. doi: 10.1053/j.semdp.2019.01.001
163. Gra M, Pham-Ledard A, Gerard E, Dutriaux C, Beylot-Barry M, Duval F, et al. Brief communication: lambert-eaton myasthenic paraneoplastic syndrome associated with merkel cell carcinoma successfully treated by immune checkpoint inhibitors: 2 cases. J Immunother. (2023) 46:276–8. doi: 10.1097/CJI.0000000000000480
164. Kisacik B, Albayrak F, Balci MA, Koc E. Paraneoplastic arthritis: A series of 92 cases. Rheumatol (Oxford). (2024) 63:1923–6. doi: 10.1093/rheumatology/kead500
165. Ferronato M, Lalanne C, Quarneti C, Cevolani M, Ricci C, Granito A, et al. Paraneoplastic anti-tif1-gamma autoantibody-positive dermatomyositis as clinical presentation of hepatocellular carcinoma recurrence. J Clin Transl Hepatol. (2023) 11:253–9. doi: 10.14218/JCTH.2021.00573
166. Milani R, Cannizzaro M, Arrigoni G, Filipello F, Cerri F, Filippi M. Subacute sensory neuronopathy associated with merkel cell carcinoma with unknown primary: A case report with literature review. J Neurol. (2022) 269:4080–8. doi: 10.1007/s00415-022-11116-9
167. Sardina Gonzalez C, Martinez Vivero C, Lopez Castro J. Paraneoplastic syndromes review: the great forgotten ones. Crit Rev Oncol Hematol. (2022) 174:103676. doi: 10.1016/j.critrevonc.2022.103676
168. Onyema MC, Drakou EE, Dimitriadis GK. Endocrine abnormality in paraneoplastic syndrome. Best Pract Res Clin Endocrinol Metab. (2022) 36:101621. doi: 10.1016/j.beem.2022.101621
169. Baechle JJ, Chen N, Makhijani P, Winer S, Furman D, Winer DA. Chronic inflammation and the hallmarks of aging. Mol Metab. (2023) 74:101755. doi: 10.1016/j.molmet.2023.101755
170. López-Otín C, Pietrocola F, Roiz-Valle D, Galluzzi L, Kroemer G. Meta-hallmarks of aging and cancer. Cell Metab. (2023) 35:12–35. doi: 10.1016/j.cmet.2022.11.001
171. Singh T, Newman AB. Inflammatory markers in population studies of aging. Ageing Res Rev. (2011) 10:319–29. doi: 10.1016/j.arr.2010.11.002
172. Soysal P, Stubbs B, Lucato P, Luchini C, Solmi M, Peluso R, et al. Inflammation and frailty in the elderly: A systematic review and meta-analysis. Ageing Res Rev. (2016) 31:1–8. doi: 10.1016/j.arr.2016.08.006
173. Logan S, Baier MP, Owen DB, Peasari J, Jones KL, Ranjit R, et al. Cognitive heterogeneity reveals molecular signatures of age-related impairment. PNAS nexus. (2023) 2:pgad101. doi: 10.1093/pnasnexus/pgad101
174. Adriaensen W, Matheï C, Vaes B, van Pottelbergh G, Wallemacq P, Degryse JM. Interleukin-6 as a first-rated serum inflammatory marker to predict mortality and hospitalization in the oldest old: A regression and cart approach in the belfrail study. Exp Gerontol. (2015) 69:53–61. doi: 10.1016/j.exger.2015.06.005
175. Lim KY, Shukeri W, Hassan W, Mat-Nor MB, Hanafi MH. The combined use of interleukin-6 with serum albumin for mortality prediction in critically ill elderly patients: the interleukin-6-to-albumin ratio. Indian J Crit Care medicine: peer-reviewed Off Publ Indian Soc Crit Care Med. (2022) 26:1126–30. doi: 10.5005/jp-journals-10071-24324
176. Ali NS, Hashem AHH, Hassan AM, Saleh AA, El-Baz HN. Serum interleukin-6 is related to lower cognitive functioning in elderly patients with major depression<Sup/>. Aging Ment Health. (2018) 22:655–61. doi: 10.1080/13607863.2017.1293005
177. Pan L, Xie W, Fu X, Lu W, Jin H, Lai J, et al. Inflammation and sarcopenia: A focus on circulating inflammatory cytokines. Exp Gerontol. (2021) 154:111544. doi: 10.1016/j.exger.2021.111544
178. Rosenblum SL. Inflammation, dysregulated iron metabolism, and cardiovascular disease. Front Aging. (2023) 4:1124178. doi: 10.3389/fragi.2023.1124178
179. Rashidah NH, Lim SM, Neoh CF, Majeed ABA, Tan MP, Khor HM, et al. Differential gut microbiota and intestinal permeability between frail and healthy older adults: A systematic review. Ageing Res Rev. (2022) 82:101744. doi: 10.1016/j.arr.2022.101744
180. Salvioli S, Basile MS, Bencivenga L, Carrino S, Conte M, Damanti S, et al. Biomarkers of aging in frailty and age-associated disorders: state of the art and future perspective. Ageing Res Rev. (2023) 91:102044. doi: 10.1016/j.arr.2023.102044
181. Gholami A, Baradaran HR, Hariri M. Can soy isoflavones plus soy protein change serum levels of interlukin-6? A systematic review and meta-analysis of randomized controlled trials. Phytother Res. (2021) 35:1147–62. doi: 10.1002/ptr.6881
182. Balducci L. Aging, frailty, and chemotherapy. Cancer control: J Moffitt Cancer Center. (2007) 14:7–12. doi: 10.1177/107327480701400102
183. Lacina L, Brábek J, Král V, Kodet O, Smetana K Jr. Interleukin-6: A molecule with complex biological impact in cancer. Histol Histopathol. (2019) 34:125–36. doi: 10.14670/hh-18-033
184. Španko M, Strnadová K, Pavlíček AJ, Szabo P, Kodet O, Valach J, et al. Il-6 in the ecosystem of head and neck cancer: possible therapeutic perspectives. Int J Mol Sci. (2021) 22:11027. doi: 10.3390/ijms222011027
185. Rose-John S, Jenkins BJ, Garbers C, Moll JM, Scheller J. Targeting il-6 trans-signalling: past, present and future prospects. Nat Rev Immunol. (2023) 23:666–81. doi: 10.1038/s41577-023-00856-y
186. Matsuda T. The physiological and pathophysiological role of il-6/stat3-mediated signal transduction and stat3 binding partners in therapeutic applications. Biol Pharm Bull. (2023) 46:364–78. doi: 10.1248/bpb.b22-00887
187. Aletaha D, Kerschbaumer A, Kastrati K, Dejaco C, Dougados M, McInnes IB, et al. Consensus statement on blocking interleukin-6 receptor and interleukin-6 in inflammatory conditions: an update. Ann rheumatic Dis. (2023) 82:773–87. doi: 10.1136/ard-2022-222784
188. Ascierto PA, Fu B, Wei H. Il-6 modulation for covid-19: the right patients at the right time? J Immunother Cancer. (2021) 9:e002285. doi: 10.1136/jitc-2020-002285
189. Villaescusa L, Zaragozá F, Gayo-Abeleira I, Zaragozá C. A new approach to the management of covid-19. Antagonists of il-6: siltuximab. Adv Ther. (2022) 39:1126–48. doi: 10.1007/s12325-022-02042-3
190. Rašková M, Lacina L, Kejík Z, Venhauerová A, Skaličková M, Kolář M, et al. The role of il-6 in cancer cell invasiveness and metastasis-overview and therapeutic opportunities. Cells. (2022) 11:3698. doi: 10.3390/cells11223698
191. Wen Y, Zhu Y, Zhang C, Yang X, Gao Y, Li M, et al. Chronic inflammation, cancer development and immunotherapy. Front Pharmacol. (2022) 13:1040163. doi: 10.3389/fphar.2022.1040163
Keywords: wound healing, granulation tissue, myofibroblasts, cancer-associated fibroblasts, IL-6
Citation: Lacina L, Kolář M, Pfeiferová L, Gál P and Smetana K Jr. (2024) Wound healing: insights into autoimmunity, ageing, and cancer ecosystems through inflammation and IL-6 modulation. Front. Immunol. 15:1403570. doi: 10.3389/fimmu.2024.1403570
Received: 19 March 2024; Accepted: 30 October 2024;
Published: 29 November 2024.
Edited by:
Yuanyuan Zhang, Broad Institute, United StatesReviewed by:
Slavko Mojsilovic, University of Belgrade, SerbiaChiara Porta, University of Eastern Piedmont, Italy
Copyright © 2024 Lacina, Kolář, Pfeiferová, Gál and Smetana. This is an open-access article distributed under the terms of the Creative Commons Attribution License (CC BY). The use, distribution or reproduction in other forums is permitted, provided the original author(s) and the copyright owner(s) are credited and that the original publication in this journal is cited, in accordance with accepted academic practice. No use, distribution or reproduction is permitted which does not comply with these terms.
*Correspondence: Peter Gál, Z2Fsb3ZjaUB5YWhvby5jb20=; Karel Smetana Jr., a2FyZWwuc21ldGFuYUBsZjEuY3VuaS5jeg==