- 1Third Department of Breast Surgery, The Third Affiliated Hospital of Kunming Medical University, Yunnan Cancer Hospital, Kunming, China
- 2Guizhou Hospital of the First Affiliated Hospital, Sun Yat-sen University, Guizhou, China
- 3Department of Breast Surgery, The Third Affiliated Hospital of Kunming Medical University, Yunnan Cancer Hospital, Kunming, China
- 4International Education School of Kunming Medical University, Kunming, China
- 5Department of Gynecology, The Third Affiliated Hospital of Kunming Medical University, Yunnan Cancer Hospital, Kunming, China
- 6Department of Breast Surgery, The First Affiliated Hospital of Kunming Medical University, Kunming, China
Immune checkpoint inhibitors (ICIs) represent a groundbreaking advance in the treatment of malignancies such as melanoma and non-small cell lung cancer, showcasing substantial therapeutic benefits. Nonetheless, the efficacy of ICIs is limited to a small subset of patients, primarily benefiting those with “hot” tumors characterized by significant immune infiltration. The challenge of converting “cold” tumors, which exhibit minimal immune activity, into “hot” tumors to enhance their responsiveness to ICIs is a critical and complex area of current research. Central to this endeavor is the activation of the cGAS-STING pathway, a pivotal nexus between innate and adaptive immunity. This pathway’s activation promotes the production of type I interferon (IFN) and the recruitment of CD8+ T cells, thereby transforming the tumor microenvironment (TME) from “cold” to “hot”. This review comprehensively explores the cGAS-STING pathway’s role in reconditioning the TME, detailing the underlying mechanisms of innate and adaptive immunity and highlighting the contributions of various immune cells to tumor immunity. Furthermore, we delve into the latest clinical research on STING agonists and their potential in combination therapies, targeting this pathway. The discussion concludes with an examination of the challenges facing the advancement of promising STING agonists in clinical trials and the pressing issues within the cGAS-STING signaling pathway research.
1 Introduction
Tumor initiation, progression, and metastasis are intimately linked to the multifaceted tumor microenvironment (TME) (1). This network, enveloping the tumor within the body, comprises tumor cells and a variety of immune cells including T and B lymphocytes, dendritic cells (DCs), natural killer (NK) cells, and tumor-associated macrophages (TAMs), as well as stromal cells such as tumor-associated fibroblasts, mesenchymal stromal cells, and endothelial cells (2, 3). These cells engage in intricate interactions through signaling pathways, secreting a myriad of growth factors, cytokines, chemokines, and other biological factors, thereby fostering a complex tumor microecology that dictates the rapid proliferation and diversification of tumor cells (4). Thus, the tumor and its microenvironment are co-dependent, with their relationship characterized by mutual reinforcement as well as antagonism. The phenomenon of tumor immune escape is intricately associated with the TME’s heterogeneity, potentially affecting the responsiveness to immunotherapy (5, 6).
Based on the spatial distribution of CD8+ cytotoxic T lymphocytes (CTLs) within the TME, tumors have been categorized into three primary immune phenotypes: immune-inflamed, immune-excluded, and immune-desert (7, 8). In immune-inflamed tumors, CD8+ T cells penetrate the tumor parenchyma, whereas in immune-excluded tumors, they gather around the tumor parenchyma without infiltrating it, and are completely absent in immune-desert tumors and their peripheries (9, 10). Immune-inflamed tumors, termed “hot” tumors, demonstrate a notable response to treatment with immune checkpoint inhibitors (ICIs) (11, 12), characterized by significant T cell infiltration, increased inflammatory signaling (notably interferon-γ), heightened PD-L1 expression, and a substantial mutational burden (11, 13). Conversely, “cold” tumors, which include immune-excluded and immune-desert variants, are marked by a scant tumor mutational load, diminished expression of major histocompatibility complex (MHC)-class I molecules, decreased PD-L1 expression, and inadequate T cell infiltration (11, 13). Additionally, the presence of immunosuppressive cells such as myeloid-derived suppressor cells (MDSCs), TAMs, and regulatory T cells (Tregs) within cold tumors contributes to tumor immune evasion, obstructing the activation, proliferation, and cytotoxic function of CD8+ T cells (13) (Figure 1). Tumors with limited antigenic diversity and minimal inflammation are inherently more resistant to immunotherapy, attributed to their lack of innate and adaptive immune characteristics (7).
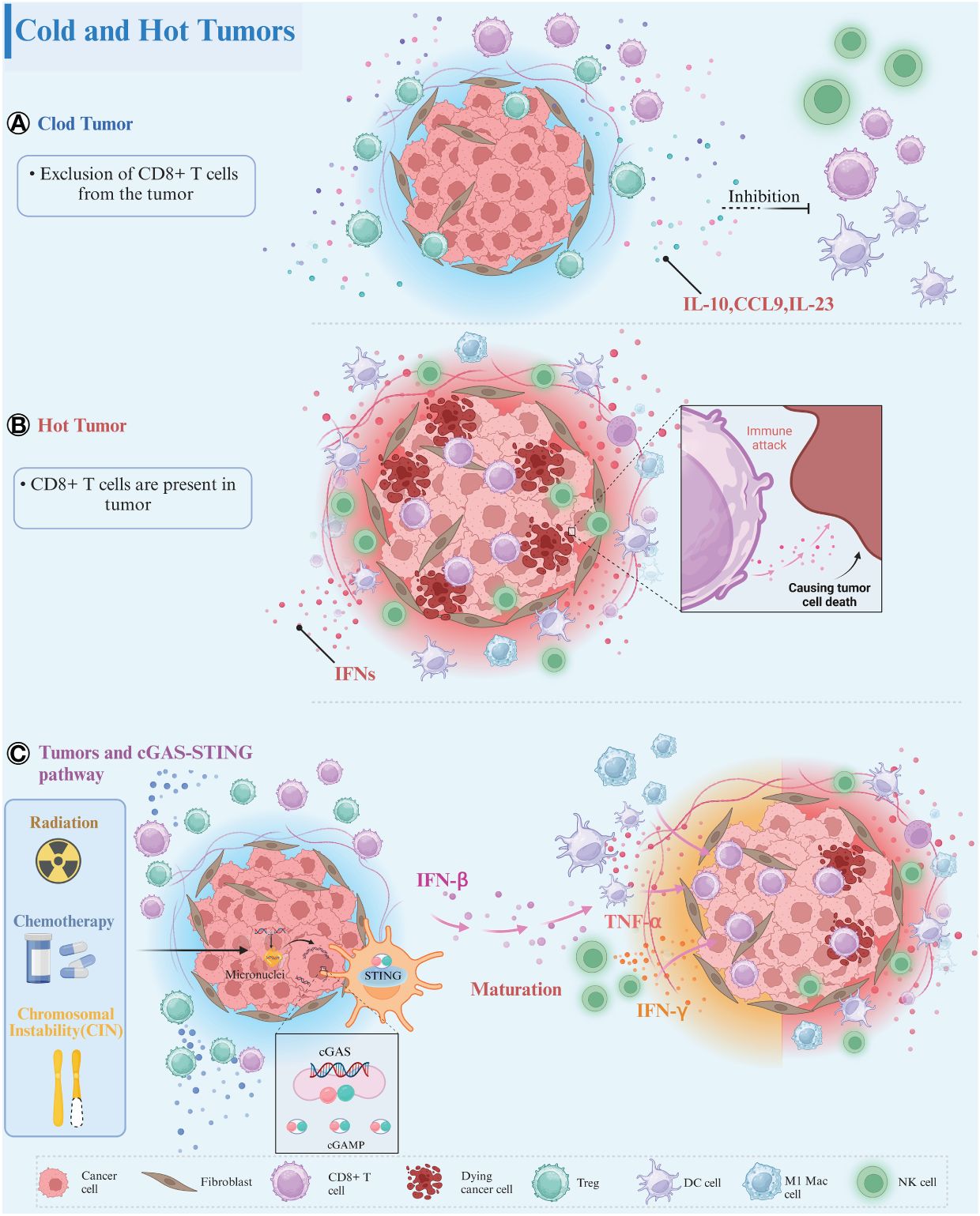
Figure 1 Tumor immune phenotypes. (A) Cold Tumors: In cold tumors, the absence of CD8+ T cells within the tumor parenchyma or its vicinity is remarkable. These tumors foster an immunosuppressive microenvironment characterized by the presence of immune-inhibitory cell populations, such as Treg cells. These cells secrete various immunosuppressive factors, including IL-10, CCL9, and IL-23, which impede the maturation of immune cells and contribute to the establishment of an immunosuppressive milieu conducive to tumor progression. (B) Hot Tumors: Conversely, in hot tumors, infiltration of CD8+ T cells into the tumor parenchyma is evident. These tumors display robust interferon signaling in the peritumoral region and harbor a diverse array of mature immune cells within the tumor parenchyma. (C) Tumors and cGAS-STING Signaling Pathway: CIN as well as radiotherapy/chemotherapy can induce nuclear DNA breaks in tumor cells, activating the cGAS-STING signaling pathway to produce large amounts of IFN-β. IFN-β can mature and activate a variety of immune cells, including DCs, NK cells, M1 macrophages, induce cytokine release, and recruit more CD8+ T cells into the tumor parenchyma. The crosstalk between cold and hot tumors is mediated through the engagement of the cGAS-STING signaling axis. Created with BioRender.com.
Immunosurveillance, the immune system’s capacity to detect, eliminate, and swiftly clear mutant cells, acts as a critical defense against tumor development. The ability to circumvent immune surveillance is essential for tumor progression, as malignant tumors evolve in the shadow of immune vigilance (14, 15). Mechanisms facilitating immune evasion include tumor antigen loss, resistance to apoptosis, antigenic modulation, restricted expression of MHC class I molecules, aberrant costimulatory signaling, and the recruitment of inhibitory immune cells by tumor cells to secrete immunosuppressive factors (16). The innate immune response serves as the primary defense mechanism against emerging tumors, while adaptive immune responses deliver more targeted effects via cytotoxic T lymphocytes and TH1 cells (14, 17). ICIs target inhibitory signals from receptors such as PD-1 or CTLA-4, commonly exploited by cancer cells on tumor-infiltrating lymphocytes (TILs), thereby restoring CTL-mediated immunity (18, 19).
The Stimulator of Interferon Genes (STING) pathway, alongside cyclic guanosine-adenosine triphosphate synthase (cGAS), is widely expressed across various cell types, including immune, non-immune, and cancer cells (20–22). STING, functioning as one of the cytoplasmic DNA sensors, and cGAS, its upstream molecule, form a 2:2 complex upon DNA binding, triggering a conformational change and leading to the synthesis of cyclic GMP-AMP (cGAMP) through a process catalyzed by ATP and GTP (23–28). This cGAMP, serving as a second messenger, binds to STING on endoplasmic reticulum (ER) membranes, inducing conformational alterations in STING and the formation of oligomers (29–31). Upon activation, STING is translocated from the ER to the Golgi apparatus, where two cysteines (Cys88 and Cys91) undergo palmitoylation, facilitating the recruitment and binding of the kinase TANK-binding kinase 1 (TBK1), subsequently phosphorylating the transcription factor IRF3 (32). Phosphorylated IRF3 dimerizes and translocates into the nucleus, regulating the expression of type I interferon-beta (IFN-β) (Figure 2). Additionally, STING activates IKK kinases (TBK1 and IKKϵ), phosphorylating the IκB family, thereby releasing NF-κB, resulting in the expression of IFN and inflammatory cytokines (34–40). The cGAS-STING signaling pathway serves as the primary effector for cells to sense and respond to cytoplasmic abnormal double-stranded DNA (dsDNA), establishing an innate immune response with high efficiency by stimulating the expression and secretion of type I IFNs and interferon-stimulated genes, crucial components of host defense mechanisms in organisms (41, 42). Chromosomal segregation errors during mitosis lead to chromosomal instability (CIN), a phenomenon exacerbated by radiotherapy or chemotherapy, resulting in DNA double-strand breaks (DSBs) and the formation of extranuclear micronuclei within cancer cells (43). This persistent accumulation of cytosolic DNA has the potential to activate the cGAS-STING pathway (44–46).
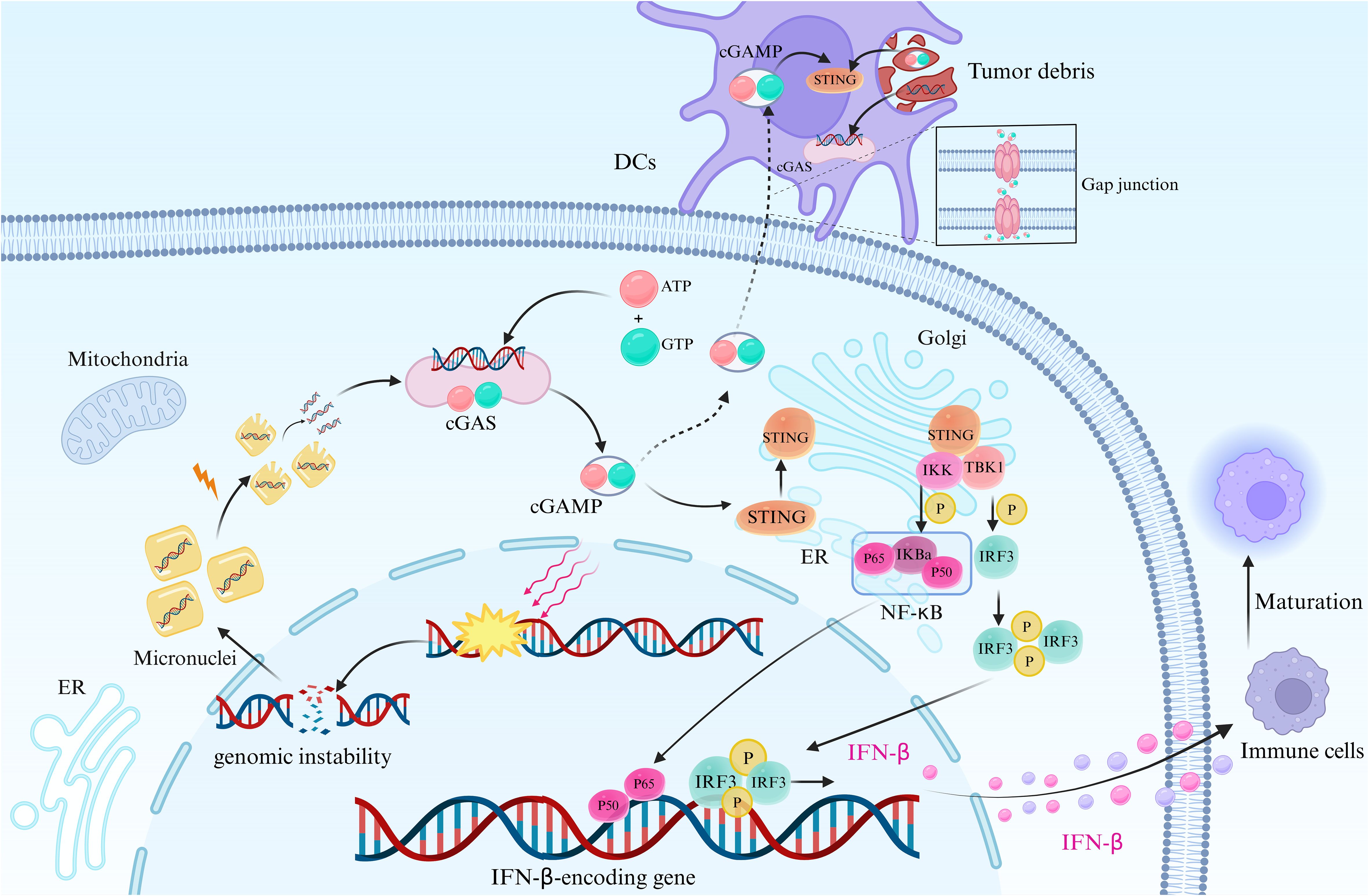
Figure 2 cGAS-STING signaling pathway in tumor cells. This figure depicts the cGAS-STING signaling pathway in tumor cells, initiated by genomic instability or DNA repair failure. This leads to nuclear DNA leakage and the formation of extranuclear micronuclei, facilitating the recognition of cytoplasmic DNA by cGAS upon micronucleus rupture. Subsequently, conformational changes occur in cGAS, and the synthesis of cGAMP is catalyzed by ATP and GTP. cGAMP then binds to STING, activating its transfer from the ER to the Golgi apparatus. This recruitment of STING leads to the binding of TBK1, which phosphorylates the transcription factor IRF3 (33). Phosphorylated IRF3 forms dimers that translocate into the nucleus, where they regulate the expression of IFN-β. Additionally, STING activation leads to the phosphorylation of IKK kinase, resulting in the release of NF-κB, which translocates into the nucleus. This induces the expression of IFN-β and inflammatory cytokines, along with IRF3 and other transcription factors. The synthesis and release of IFN-β stimulate the maturation of innate immune cells and promote the formation of the tumor inflammatory microenvironment. DCs possess the capacity to engulf cGAMP originating from tumor cells or neighboring cells, facilitated through mechanisms like intercellular communication via gap junctions and phagocytosis of tumor debris, which contains dsDNA as well. Following internalization, cGAMP activates the intracellular STING signaling pathway within DCs, prompting the production of IFN-β. Created with BioRender.com.
STING-deficient mice have demonstrated increased susceptibility to various malignancies and reduced survival rates, underscoring the essential role of a robust STING-mediated immune response in tumor suppression (47–50). Type I IFNs significantly contribute to restraining tumor growth and extending host survival rates by mounting a specific immune response against tumors (51). Cancer cells expressing cGAS poses the ability to recognize cytoplasmic DNA and generate cGAMP, which in turn stimulates the secretion of IFN-β and tumor necrosis factor alpha (TNF-α) through the STING pathway (17). Simultaneous stimulation of IFN-β and TNF-α signaling induces significant necrosis of tumor cells (52). Numerous research studies have linked a poor prognosis in human cancers to the inhibition of the cGAS-STING pathway, as the lack of cytosolic DNA detection contributes to immune escape in cancer cells (53). The p53-p21 pathway facilitates cGAS-STING to promote cancer cell senescence (54), while cGAS-STING-mediated autophagy during a mitotic crisis in normal cells prevents their transformation into cancer cells, highlighting the pathway’s role in cellular homeostasis (55). To prevent an excessive response to the cGAS-STING pathway, cGAS-STING is digested in autophagy lysosomes following transient activation of downstream signaling (56).
In summary, enhancing tumor antigen specificity and immune cell effector functions is paramount for improving tumor immunity. This review focuses on the molecular mechanism of the cGAS-STING signaling pathway, analyzing its relationship to tumor immunity, and discussing how the innate immune pathway cGAS-STING transforms “cold” tumors into “hot” tumors, thereby further enhancing the therapeutic effect of ICIs.
2 Mechanisms of cGAS-STING-mediated antitumor effects
2.1 cGAS-STING: a nexus of innate and adaptive immunity
Innate immunity and adaptive immunity represent two interrelated subtypes of the immune response. The latter encompasses humoral and cell-mediated immunity, orchestrating the self-activation, proliferation, and differentiation of T and B lymphocytes into effector cells upon antigenic stimulation in vivo, thereby instigating a cascade of biological events, including antigen clearance, among others (57). Innate immunity, evolving over the course of evolutionary history, serves as the body’s initial defense against pathogenic intrusion, comprising monocytes/macrophages, DCs, granulocytes, NK cells, and NKT cells (58, 59).
The activation of the cGAS-STING pathway serves as a pivotal link between innate and adaptive immunity. Upon activation, this pathway induces the production of type I IFN, which, in conjunction with STING, has been demonstrated to foster the development of innate immune cells such as DCs and NK cells (60, 61), thereby facilitating adaptive immune responses against tumors. These findings underscore the crucial role of the host STING pathway in the innate immune recognition of immunogenic tumors. This cascade of events culminates in the activation of antigen-presenting cells (APCs), the production of IFN-β, and the initiation of CD8+ T cell responses against tumor antigens (48). TAMs represent a critical class of tumor-infiltrating immune cells intricately involved in tumor growth and metastasis by modulating tumor immune surveillance. Within this spectrum, M1 and M2 macrophages delineate two opposing functional continua, representing antitumor and immunosuppressive phenotypes, respectively (62). Recent studies have elucidated the role of the cGAS-STING signaling pathway in orchestrating TAM polarization towards the M1 phenotype (63, 64).
This pathway not only fosters the secretion of inflammatory factors and chemokines but also facilitates the recruitment and activation of T lymphocytes. Moreover, it promotes the differentiation of both CD4+ and CD8+ T cells, thereby establishing a robust connection between innate and adaptive immunity (62).
2.2 The role of type I IFNs in antitumor immunity
Type I IFNs play a pivotal role in fostering protective antitumor immunity, particularly in the context of immunogenic or “hot” tumors characterized by heightened T lymphocyte infiltration and improved response to ICIs compared to “cold” tumors (65, 66). These IFNs, downstream products of the cGAS-STING pathway, exert multifaceted effects on both innate and adaptive immunity.
Upon activation, type I IFNs facilitate the maturation of macrophages, NK cells (67, 68) and DCs (69). They orchestrate a cascade of events that encompass direct suppression of tumor cells and indirect antitumor effects. This includes stimulating the maturation and activation of DCs and macrophages, enhancing the production of granzymes and perforins in CTLs and NK cells (70, 71), and bolstering the proportion of memory T cells (72, 73). Activated NK cells secrete cytokines such as IFN-γ and TNF-α, which synergistically activate DC cells, fostering robust proliferation of CD8+ T cells. Notably, the abundant IFN-γ produced by NK cells induces high expression of mbIL-15 in a subset of bystander DC cells, thereby promoting antigen-specific CD8+ T-cell responses and eliciting adaptive immunity against cancer cells (74, 75).
Moreover, type I IFNs directly facilitate the proliferation of CD8+ T cells and the differentiation of T-helper (TH)-1 cells while suppressing TH2 cell development (76–78). Thus, among innate cytokines, type I IFNs emerge as crucial signals that shape the repertoires of effector and memory T cells.
Evidence from studies, such as that conducted by Steven Lohard et al., highlights the synergistic effect of type I IFNs and TNF-α in inducing enhanced apoptosis in breast cancer recipient cells, underscoring the multifaceted role of these factors in promoting antitumor responses (52).
2.3 cGAS-STING and immune cells dynamics
A myriad of immune cells, including NK cells, CD4+ T cells, CD8+ T cells, DCs, and macrophages, respond to the antineoplastic effects induced by STING activation (41). Activation of STING in endothelial cells within the TME may contribute to tumor vascular remodeling, positively influencing tumor regression (71, 79). DCs are highly proficient APCs. DCs, as proficient APCs, tend to engulf tumor-derived DNA or cGAMP, subsequently inducing type I IFN production, which relies on STING presence (65, 80, 81). These DCs serve as a significant source of type I IFNs and TNF-α, both contributing to the promotion of an inflammatory TME (52). Moreover, tumor-derived cGAMP triggers a STING-mediated interferon response in non-tumor cells and activates NK cells, mediating the clearance of CD8+ T cell-resistant tumors in response to STING agonists, underscoring the broad applicability of STING activation in promoting antitumor responses across various tumor contexts (70, 71, 82, 83).
Tumors exhibiting cGAS positivity are characterized by elevated concentrations of T-cell-derived effector cytokines, such as IFN-γ and TNF-α, and demonstrate enhanced responsiveness to ICIs, indicative of a “hot” tumor phenotype (65). Notably, CD8+ T cells are predominantly found in regions expressing cGAS, suggesting a direct role for cGAS in driving CD8+ T cell infiltration, especially in tumors with heterogeneous cGAS expression (65). Additionally, the absence of cGAS expression in cancer cells, coupled with its presence in adjacent non-diseased tissues, implies a potential immune evasion mechanism (65).
Activation of the cGAS-STING cascade supports the maintenance of stem cell-like properties post T-cell differentiation (84). In xenograft models, STING agonists facilitate the generation of stem cell-like central memory CD8+ T cells, augmenting the antitumor response to chimeric antigen receptor (CAR) T cell therapy in cancer patients (84). Moreover, in triple-negative breast cancer (TNBC) with BRCA-deficiency, PARP inhibitor therapy relies on the recruitment of CD8+ T cells, mediated through the intratumoral STING pathway (85, 86). Furthermore, intratumoral administration of exogenous cGAMP, which enhances STING activity, significantly enhances anti-tumor CD8+ T cell responses, effectively controlling both injected and contralateral tumors (87).
2.4 Remodeling the cold tumor microenvironment via STING signaling
Activation of the STING pathway initiates a transformative process within the cold TME, leading to significant tumor regression and heightened immunity (41, 88). In a study focusing on STING agonists like DMXAA, conducted on murine models with cold pancreatic tumors, researchers observed a remarkable alteration in the immunologic landscape of the TME, resulting in prolonged survival rates (89, 90). Specifically, within the tumor, there was a notable increase in inflammatory chemokines and maturation markers of DCs and CTLs (89).
Furthermore, researchers identified a gain-of-function mutation variant of STING, known as STINGN153S, near the STING dimer site, extracted from patients with autoimmune diseases (19). Introducing this persistently active STING signal into cancer cells led to robust expression of chemokines, attracting infiltrating immune cells, particularly CD8+ T cells and NK cells, into the activated TME (19).
Moreover, studies have demonstrated that intratumoral injection of cGAMP, a second messenger of the STING pathway, induces potent antitumor responses by promoting the accumulation of activated macrophages within the TME, a process contingent on STING activation (91).
3 cGAS-STING related cancer therapy research areas
3.1 STING agonists
STING agonists have emerged as promising candidates for enhancing immune cell recruitment in the TME. They can be categorized into several classes, including cyclic dinucleotides (CDNs), small molecule agonists, bacterial carriers, agonists for in vivo delivery of antibody-coupled drugs, and nanovaccines (92). Vaccines utilizing STING agonists are developed based on the mechanism of the cGAS-STING pathway (93). In therapeutic cancer vaccines, CDNs act as effective adjuvants, robustly stimulating immune responses against tumors. This activation involves the activation of CTLs and NK cells, leading to consistent regression observed in various mouse tumor models (94). Direct intratumoral administration of selected CDNs in mouse models of B16 melanoma, CT26 colon cancer, and 4T1 breast cancer has resulted in rapid and significant tumor regression, with no apparent local or systemic toxicity. This intervention promotes persistent systemic T cell immunity targeting the relevant antigens (88). The wide-ranging potential applications and remarkable safety profile of CDNs have led to the initiation of clinical trials for STING agonists (Table 1). DMXAA, a mouse STING agonist, demonstrated strong antitumor activity in mice but failed in clinical trials due to its inability to activate human STING (95).
ADU-S100 (MIW815), a synthetic analogue of 2’,3’-cGAMP, an endogenous agonist, has been engineered to enhance its half-life, ensuring consistent activation of the STING pathway and highlighting its therapeutic potential (96). Outcomes from a Phase I clinical trial (NCT02675439) demonstrated the well-tolerated nature of MIW815 in patients with advanced or metastatic cancers, with an overall response rate (ORR) of 2.1% as monotherapy. Treatment-related adverse effects included fever, chills, injection site discomfort, and headache. Although the therapeutic efficacy of MIW815 as a standalone treatment appeared limited, indications of systemic immune activation have emerged, such as increased plasma cytokine levels and systemic T-cell clonal expansion (97). In a Phase Ib clinical trial (NCT03172936) investigating the safety and tolerability of combining MIW815 with spartalizumab in patients diagnosed with advanced/metastatic solid tumors or lymphomas, the combined therapy showed good tolerance. However, the observed ORR of 10.4% demonstrated mild anti-tumor effects (98).
MK-1454, a synthetic CDNs analog developed by Merck & Co., has been designed for the management of advanced or metastatic solid tumors and lymphoma. Initial findings from a phase I clinical trial (NCT03010176) showed that while MK-1454 monotherapy led to an increase in the release of pro-inflammatory cytokines, it did not result in a complete or partial response within the monotherapy cohort (n=20) (96). However, when administered in combination with pembrolizumab, the treatment achieved an ORR of 24%, accompanied by a median reduction of 83% in target tumors. Both the monotherapy and combination therapy cohorts reported treatment-related adverse events (TRAEs) at rates of 82.6% and 82.1%, respectively (96, 99). Although the study concluded in April 2022, the final results are still pending.
SYNB1891 is an engineered live strain capable of producing CDNs under hypoxic conditions to activate the STING pathway. Findings from the study NCT04167137 indicated that repeated intratumoral administration of SYNB1891, either alone or in combination with Atezolizumab, exhibited favorable safety and tolerability, along with evidence of activation of specific targets within the STING pathway (100). Concurrently, the study documented five cases of cytokine release syndrome in the monotherapy treatment group (100).
Mn2+ itself is a potent cGAS activator that increases the sensitivity of the DNA sensor cGAS and its downstream junction protein STING, which induces cellular production of type I IFN and cytokines in the absence of any infection in vivo (101). Mn2+ was found to significantly facilitate DC and macrophage maturation and antigen presentation in a cGAS-STING-dependent manner, enhance CD8+ T cell and NK cell activation, and increase the number of CD44hiCD8+ T cells. Mn2+ significantly promotes antitumor immunotherapy across diverse mouse models, and a phase 1 clinical trial completed in patients with advanced metastatic solid tumors yielded promising evidence affirming well-tolerated safety profile and expected antitumor effects of Mn2+ in patients. The clinical trial (NCT03991559) enrolled 22 patients with advanced metastatic solid tumors, and phase I clinical data demonstrated 45.5% (95% CI, 26.9-65.3) best objective response and 90.9% (95% CI, 72.2-97.5) best disease control rate. All 5 patients who had failed prior PD-1 therapy in combination with chemotherapy or radiotherapy demonstrated disease control, including three experiencing partial response (PR) while two maintained stable disease (SD) (95). Of these patients, 86% experienced any grade of treatment-related adverse event (ae), and 41% experienced serious treatment-related adverse events (grades 3-4). Grades 1-2 adverse events were well tolerated, while grades 3-4 adverse events were managed with supportive care measures. Additionally, five patients with widespread abdominal metastases developed acute suspicious local or systemic cytokine release syndrome (CRS). The simple and stable composition of Mn2+, the low cost, and wide accessibility of Mn2+ render this therapy appealing and highly assuring, and it is currently undergoing phase II clinical trials (95).
3.2 Radiotherapy and chemotherapy
Radiotherapy, as a first-line treatment for many solid tumors, exerts its cytotoxic effects mainly by inducing DNA damage in tumor cells (102–105). In addition to this, radiation therapy can also participate in anti-tumor activity by modulating the tumor microenvironment and promoting anti-tumor immune responses, one mechanism of which involves the excitation of the cGAS- STING pathway (105–107). Radiation therapy leads to accumulation of dsDNA in cancer cells, which can be monitored by cGAS as a cytosolic DNA sensor (108), leading to activation of cGAS/STING signaling. An optimal radiation dose could effectively activate the cGAS-STING signal pathway to prompt the production of IFN-β. Research demonstrated that administering nanoparticle-cGAMP through inhalation alongside radiotherapy induced deterioration of lung metastases through an effector CD8+ T-cell approach mediated by the STING signaling pathway (62, 109). The aforementioned results suggest that the antitumor immunity produced by radiotherapy alone or radiotherapy in combination with STING agonists is dependent on the modulation of CD8+ T-cell activation and differentiation by the cGAS-STING signaling pathway, and also point towards a potential usage of cGAS-STING signaling pathway agonists in conjunction with radiotherapy in oncology treatment (62). Clinical trial NCT03538314 is currently evaluating the impact of treatment through modulation of the cGAS-STING pathway on response to radiotherapy and ICIs for the treatment of patients with advanced solid tumors.
Chemotherapy induces DNA damage while inhibiting DNA repair. Damaged DNA activates cGAS-STING to enhance DC-mediated antigen presentation and T cell responses (44, 105, 110). For example, one of the mechanisms of cisplatin treatment is the initiation of the cGAS/STING pathway by upregulating the protein levels of cGAS and STING (53). The ability of the topoisomerase I inhibitor topotecan to restrict the proliferation of breast cancer cells in vivo was eliminated in mice deficient in STING (111). In addition, plethora of studies have indicated that cisplatin in combination with IFN, not only impede tumor growth effectively but also extend survival time in mice, compared with cisplatin alone (112–114). 5-Fluorouracil (5-FU) is a crucial chemotherapeutic agent that is predominantly involved in disrupting the DNA synthesis. Researchers found that the combination of cGAMP and 5-FU further enhanced the anti-tumor immune response elicited by 5-FU and effectively reduced the cytotoxic effects associated with 5-FU. The mechanism likely entails cGAMP activating the STING signaling pathway, subsequently inducing cytokine production activating DC. Activated DC not only induces cross priming of CD8+ T cells to generate anti-tumor responses, but also seems to reduce 5-FU-induced cytotoxicity (115). These outcomes suggest that chemotherapeutic agents may also elicit antitumor effects by activating the cGAS-STING signaling pathway, and that the combination of chemotherapeutic agents with agonists of the cGAS-STING signaling pathway represents a fruitful area of research in oncology therapy (62). Clinical trial NCT03410901 aims to investigate the efficacy and safety of the combination of cGAS-STING pathway activators and chemotherapy in patients with advanced solid tumors.
3.3 IR (irradiation)+ ATRI effectively induces activation of classical versus non-classical cGAS-STING signaling pathways
The study demonstrates that combining ATR inhibitors with radiotherapy effectively enhances the activation of both classical and non-classical cGAS-STING signaling pathways. Specifically, the addition of ATR inhibitors to radiotherapy significantly boosts STING signaling in mouse models of colorectal cancer, leading to heightened innate immunity and increased infiltration of TILs. This phenomenon has the potential to remodel the TME and enhance the efficacy of ICIs in anti-ICI tumors. During the investigation, inhibition of STING in CT26 cells resulted in a notable decrease in infiltrating CD8+ cells and levels of IFNs following treatment with IR+ ATRI, indicating that the immunomodulatory effects of IR+ ATRI are contingent upon STING signaling (52, 60).
3.4 G4 Binders
G-4 conjoined (G4s or G-quadruplexes) are unconventional nucleic acid configurations integral to biological mechanisms (116). They represent potential anticancer targets, with the pursuit of effective G4 binders allowing for the identification of cytotoxic ligands capable of interfering with selective G4 structures on oncogenes or telomeres. Notably, G4 binders such as pyridostigmine and PhenDC3 have been observed to elicit several effects, including an increase in micronuclei, activation of the cGAS-STING pathway in both human and mouse cancer cells, production of type I interferons, and activation of intrinsic immunity (116).
One such G-quadruplex stabilizer is CX-5461 (117) which induces severe cancer cell death by obstructing replication forks or generating single-stranded DNA gaps or breaks, particularly in cancer cells lacking functional homologous recombination (HR) and non-homologous end joining (NHEJ) mechanisms (117–119). CX-5461 initiates IRF-3-mediated type I interferon expression by activating the cGAS-STING pathway. Researchers have noted elevated levels of cGAMP and active interferon regulatory factor 3 (IRF3) in colorectal cancer (CRC) cells (118), followed by an increase in type I interferon, which triggers downstream signaling, including JAK1/TYK2-mediated phosphorylation of signal transducer and activator of transcription 1 (STAT1) on tyrosine residue 701 (Tyr701) to form STAT1-STAT1 homodimers (118). These dimers then translocate to the nucleus and bind to the interferon-γ activating site (GAS), initiating the transcription of numerous genes, including programmed death-ligand 1 (PD-L1), thereby mediating PD-L1 expression to indirectly kill CRC cells (120–122).
Furthermore, administration of CX-5461 alone or in combination with anti-PD-1 has been shown to augment cytotoxic T lymphocyte (CTL) count, while reducing the number of bone MDSCs in the spleen of mice (123). Therefore, the cGAS-STING pathway plays a crucial role in G4 conjoined-related drugs, and various drugs have the potential to convert “cold” tumors into “hot” tumors, providing a potential approach for combined immunotherapy in immune-resistant tumors to enhance the efficacy of ICI response (118).
3.5 Anti-cancer effects exerted by inhibition of the cGAS-STING pathway
The anti-cancer effects resulting from inhibition of the cGAS-STING pathway have garnered increasing attention in recent research. While previous studies have primarily focused on the anti-tumor effects of STING agonists, emerging evidence suggests that STING inhibitors also possess inhibitory effects on tumor activity. Li et al. demonstrated that tumor CIN modifies the TME and stimulates the cGAS-STING pathway (124). Specifically, in the presence of a functional immune system, chromosomally unstable tumor cells activate STING, triggering ER stress and promoting tumor cell metastasis (125–127). CIN, prevalent in advanced cancers, disrupts the normal cell division process, contributing significantly to cancer’s treatment resistance, spread, and metastasis (128, 129). The study introduced a novel technique called Contact Tracing for single-cell RNA sequencing data analysis, revealing that chronic CIN-induced activation of the cGAS-STING pathway fosters downstream signaling reconnection in cancer cells, thereby establishing a pro-metastatic TME. This reconnection involves a rapid type I IFNs response selectively mediated by STING, along with an associated increase in ER stress response from cancer cells. Conversely, reversing CIN, eliminating cancer cell STING, or inhibiting ER stress response signaling abolished the TME-dependent effects of CIN reversal and suppressed tumor metastasis in immunocompetent environments, although these interventions had no effect in severely immunocompromised environments (124).
Similarly, Sirui et al. reported comparable findings, indicating that STING may impede the anti-cancer capabilities of NK cells through the IRF3-induced regulatory B cells (Bregs) pathway, contributing to the limited efficacy of STING monotherapy (130). This study identified the STING/IRF3/IL-35 signaling axis as the underlying cause of the limited efficacy of STING agonists, leading to immune evasion by inducing Bregs to secrete IL-35, independent of type I IFNs. Combining STING agonists with anti-IL-35 treatments showed promising efficacy in preclinical models of pancreatic and lung cancer, offering a new avenue for tumor immunotherapy (99). As a pivotal signal transduction molecule involved in the intrinsic immune response in vivo, STING significantly regulates type I IFNs and influences the body’s anti-tumor immune response (55). While STING agonists have been extensively studied and hold high expectations in tumor immunotherapy, the consistent lack of success in related studies suggests the presence of a potential negative immunomodulatory mechanism associated with STING activation.
4 Discussion
Epigenetic suppression of cGAS in cancer cells curtails the innate immune response to cytoplasmic DNA accumulation, fostering immune evasion (23, 83). Luis A. Martinez et al. elucidated that WTp53 facilitates the degradation of the cytoplasmic DNA exonuclease TREX1, leading to cytoplasmic DNA buildup and subsequent activation of the cGAS/STING pathway. Their findings imply a novel mechanism through which the tumor suppressor TP53 exploits the pathogen recognition receptor cGAS to suppress tumors, thereby activating the innate immune response to impede tumor growth. Elevated cytoplasmic DNA levels in cancer cells are a hallmark, contributing to a persistent basal activation of the cGAS/STING pathway, which can potentially be induced (45, 55, 131). Conversely, evidence suggests that in genetically unstable cells, the cGAS/STING pathway may facilitate metastatic progression. However, in this specific context, the activation of IRF3 appears notably absent (45, 125). The precise mechanism responsible for the attenuation of the signaling pathway to IRF3 from cGAS/STING, despite the existence of cytoplasmic DNA in tumor cells, remains poorly understood (45). Studies have demonstrated that Mtp53 disrupts the functionality of the cytoplasmic DNA sensing machinery, particularly the cGAS-STING-TBK1-IRF3 pathway, crucial for initiating the innate immune response. By interacting with TBK1, Mtp53 (but not wildtype p53) impedes the assembly of the trimeric complex involving TBK1-STING-IRF3, essential for IRF3 activation, nuclear translocation, and subsequent transcriptional activity (132). The restoration of TBK1 function holds promise for reactivating immunosurveillance and eliminating mtp53 tumors (132).
In addition to genomic instability, factors such as the release of heterologous DNA and mitochondrial damage caused by pathogen invasion and replication can lead to abnormal leakage of dsDNA within the body. This dsDNA is then recognized by the DNA receptor cGAS, consequently activating the cGAS-STING signaling pathway (41). Therefore, the cGAS-STING mechanism constitutes a comprehensive surveillance system in response to tissue damage and pathogen invasion, as abnormalities in this mechanism can result in infection (41). Stringent regulatory mechanisms are paramount to uphold cellular and tissue homeostasis under normal conditions owing to the non-specified activity of cGAS on DNA. SPSB3 has been identified as a cGAS-targeted substrate receptor that binds to the cullin-RING ubiquitin ligase 5 (CRL5) complex, facilitating the attachment of ubiquitin to nuclear cGAS (133). Apart from removing nucleosome-bound cGAS, SPSB3 may also assist in disassembling DNA from cGAS. This broad binding capacity and the irreversible nature of the degradation process contribute to an efficient mechanism for cGAS inactivation. This study elucidates the mechanism by which the ubiquitin proteasome system (UPS) degrades nuclear cGAS in circulating cells (133). It has been demonstrated that the binding of the MRE11-RAD50-NBN complex to nucleosome fragments is necessary to enable the release of cGAS from acid patch-mediated segregation, facilitating its mobilization and activation by dsDNA. Consequently, MRE11 is crucial for cGAS activation in response to oncogenic stress, cytoplasmic dsDNA, and ionizing radiation (134).
In clinical trials, the anticipated efficacy observed in mouse models for combating tumors was not replicated, except with Manganese. Based on the relevant literature, we have consolidated viewpoints regarding key aspects: 1. Age Factor: While age was tightly controlled in experimental mouse models, clinical trials, including NCT02675439, involved participants with a diverse age range, with a median age of 61 (94, 97). The response to vaccine administration may decline with advancing age (135, 136). Consequently, similar to many vaccines, the adjuvant potential of CDN vaccines is negatively affected by the aging process (137). Future animal research should explore the influence of mouse age on the efficacy of CDN vaccines and utilize mice that reflect the target age demographic, which may yield more precise experimental data for guiding clinical trials. 2. Tumor Heterogeneity: Current clinical trials include diverse patient cohorts with advanced/metastatic solid tumors or lymphomas, representing over ten distinct cancer types, such as melanoma, breast cancer, sarcoma, pancreatic cancer, etc. (96–98, 100). The varied immune profiles within different TMEs, along with variations in the genomic presence of the STING pathway, inherently impact immune modulation and clinical responses (97). Additionally, differences in tumor types may result in fluctuations in injection pressures during intratumoral delivery, causing inconsistencies in drug exposure and potentially influencing experimental outcomes (97). 3. Disparities in the STING gene: The human STING gene exhibits significant heterogeneity and shows population stratification (138, 139). There are over five allele variants of the human STING gene, including R232 (wild-type), H232, HAQ (R71H-G230A-R293Q), AQ (G230A-R293Q), and Q293, with a population frequency exceeding 1% (94). Notably, the HAQ-STING variant is prevalent in East Asian populations but rare in Africa, while the AQ and Q293 variants are exclusive to African populations (138, 139). The presence of these STING allele variants within the population can significantly impact the response to CDN vaccines (94) Substantial evidence suggests compromised functionality of the HAQ and H232 allele variants in STING (94). Therefore, the development of a CDN capable of effectively activating H232 or HAQ-STING seems essential (94). In the mentioned clinical trials, there was a significant range of ethnicities among the participants, including Black, Caucasian, Asian, and others (97). 4. Drug Resistance: It is crucial to acknowledge that the participants in current clinical trials primarily consist of individuals with advanced cancer who have experienced disease progression after undergoing multiple lines of therapies. This trend contributes to a heightened prevalence of multi-drug resistance, and the presence of unexplained heterogeneity poses challenges in effectively demonstrating the efficacy of STING agonists. 5. Drug Concentration: The need for repeated tissue sampling in participants has resulted in insufficient pharmacokinetic data for intratumoral injections (NCT02675439). Consequently, it remains uncertain whether the concentration of locally injected MIW815 corresponds to the concentration required for activity, as observed in preclinical models (97). Therefore, further research is needed to refine pathways for delivering drugs to the TME. Gathering relevant administration data, such as injection techniques, tumor tissue pressure, drug residence time at the injection site, and duration of tumor tissue exposure, could be crucial for future investigations into the intratumoral administration of small molecule immune agonists (97). Additionally, it is important to recognize that while most clinical trials have reported favorable evaluations regarding the safety and tolerability of STING agonists (96–98, 100), instances of serious treatment-related adverse reactions, including cytokine release syndrome, have been documented (100). The central concern associated with immune stimulatory therapy lies in the potential for a “cytokine storm,” as prolonged activation of STING can result in excessive cytokine production, leading to severe toxicity and potential fatality. However, the clinical understanding of how to effectively terminate the activated signaling to prevent this remains limited (96).
The clinical efficacy of STING agonists is constrained by several factors, including inadequate cytoplasmic delivery, swift immune clearance, lack of specific cellular targeting, and systemic inflammatory responses (140). The progression in STING agonist development has reached a critical impasse, akin to many contemporary immunotherapeutic agents (140). Consequently, the current studies have pivoted towards the utilization of varied vectors for STING agonist administration (140). The advent of nanotechnology offers a promising avenue for targeted delivery to specific tissues and cells, enhancing the absorption of immunomodulatory agents. This approach is anticipated to bolster cancer immunotherapy while mitigating adverse effects. Current research into lipid-based nanostructures for STING agonist conveyance is expanding, with Hao et al. assessing the capacity of different lipid nanoparticles (NPs) to amplify the impact of STING agonist treatments (140). Liposomal formulations of STING agonists, when used in conjunction with therapies such as radiotherapy, chemotherapy, vaccines, monoclonal antibodies (mAbs), or immune checkpoint inhibitors (ICIs), demonstrate significant potential in eliciting synergistic therapeutic effects (140). Li et al. have elaborated on a novel tumor microenvironment-responsive DNA-based nanomedicine encapsulated within dendritic mesoporous organosilica nanoparticles (DMONs). This formulation, as a standalone treatment, yielded a tumor growth inhibition (TGI) rate of 51.0% in the murine B16-F10 melanoma model. Furthermore, when combined with an anti-PD-L1 antibody, it significantly bolstered anti-tumor efficacy, culminating in near-total tumor elimination (141). Antibody Drug Conjugate (ADC) is a complex consisting of a cytotoxic drug attached to a tumor-targeting monoclonal antibody, which combines the characteristics of a targeted drug with those of a chemotherapeutic drug to achieve precision therapy. Applying the ADC strategy to the development of STING agonism, Merck Sharp & Dohme developed a STING ADC drug, XMT-205614, an ADC platform for STING agonists that employs a potent acyclic dinucleotide STING agonist, a cleavable ester-based linker, and a hydrophilic PEG8 bisglucosamine scaffold (142, 143). Tumor-targeted ADCs constructed using the resulting STING agonist platform have potent and long-lasting antitumor activity and exhibit high stability and good pharmacokinetics in non-clinical species. α epidermal growth factor receptor (EGFR)-172 ADC is a tumor-targeted ADC constructed with the cGAMP analog IMSA172 linked to EGFR antibody via a cleavable Mc-Val-Cit-PABC linker. EGFR antibody to generate ADCs, which showed potent antitumor efficacy in a synthetic mouse tumor model and exhibited good tolerability (144). It not only has further synergistic effects with anti-PD-L1 antibodies to achieve superior anti-tumor efficacy but also promotes multiple aspects of innate and adaptive anti-tumor immune responses. Mixing this small molecule ADC drug with human serum for up to 24 hours does not reduce its activity, and the payload IMSA172 also shows good stability in the same serum for 24 hours, which is not comparable to cGAMP drugs. STING ADC drugs have become the direction of development in the field of STING (144).
Furthermore, a series of studies have highlighted that the cGAS-STING signaling pathway promotes tumor growth. For instance, in cases where DSBs occur within cancer cells, cGAS relocates to the nucleus, thereby impeding the formation of PARP1–TIMELESS complexes, which inhibit homologous recombination (HR) repair and sustain CIN, thereby facilitating tumor evolution. Research has also indicated that upon recognition of CIN, cGAS activates non-canonical NF-κB, contributing to a program that enhances tumor metastasis (125). Treatments involving mutated 7,12-dimethylbenzanthracene (DMBA), cisplatin, and etoposide have been reported to facilitate skin carcinogenesis by triggering inflammatory cytokines and phagocytes that are STING-dependent (53). In the context of brain metastases, cyclic GMP-AMPs (cGAMPs) that metastasize to neighboring cells, such as astrocytes, can lead to the production of IFN-β and TNF-α within the TME. However, in this particular scenario, the response tends to promote tumor growth and contribute to resistance to chemotherapy (145). A deep understanding of the dual role of STING pathway activation in halting cancer progression is imperative. Clearer treatment protocols and the identification of selective biomarkers for specific tumor types, which can predict the therapeutic benefits of STING activation, are urgently needed (140). Benguigui et al. recently identified a novel biomarker: interferon-stimulated Ly6Ehi neutrophils, whose presence in both mice and human blood strongly correlates with successful outcomes in immunotherapy across various cancer types (146). These neutrophils are generated through tumor-specific activation of the STING pathway and can sensitize tumors unresponsive to anti-PD1 therapy, partly via IL12b-mediated activation of cytotoxic T cells (146). Wang et al. have developed PolySTING nanoparticles, incorporating cGAMP within a micelle nanoparticle formed from the STING-activating polymer polyethylene oxide-b-PSC7A (147). Employing a ‘shock-and-lock’ dual activation approach, they demonstrated that conventional type I dendritic cells (cDC1) are crucial for the STING-mediated elimination of established and metastatic murine tumors (147). Through multiplex immunohistochemistry analysis, they categorized non-small cell lung cancer patients into groups with high and low STING-activating cDC1 levels. Patients with high cDC1 activation showed prolonged progression-free and overall survival rates in both neoadjuvant chemotherapy and neoadjuvant chemotherapy plus Pembrolizumab settings, highlighting the potential of STING-activating cDC1 signatures (XCR1+STING+CXCL9+) as prognostic biomarkers for therapy response (147). In personalized medicine, predictive biomarkers are vital for tailoring treatments to individual patients and their specific tumor characteristics, yet reliable biomarkers for immune checkpoint inhibitor outcomes across different cancer types are still needed (146).
The mucosal immune system (MIS), or mucosa-associated lymphoid tissue (MALT), comprises immune tissues, cells, and molecules within the mucosal linings of the respiratory, gastrointestinal, and genitourinary tracts, exerting a critical function in the immune defense. The mucosal immunity pathway equips T cells with a mucosal homing program, enhancing their recruitment to initial activation sites (148). Evidence suggests that mucosal immunity more effectively induces resident memory T cells (Trm) at mucosal tumor locations compared to traditional systemic administration (149, 150). These Trm cells, a subset of long-lived memory T cells, reside in tissues, particularly at mucosal surfaces exposed to the environment, and do not recirculate (151). Intranasal vaccination with a mucosal vector in a head and neck cancer model showed induced local Trm and tumor suppression, correlating high Trm levels with improved overall survival in lung cancer patients (151). Thus, inducing persistent Trm is proposed as a novel target for developing effective therapeutic cancer vaccines (151). Manganese (Mn2+), a robust stimulator of type I interferon, activates the cGAS-STING pathway even without infection, showcasing its potential in immunotherapy. Mn2+ is actively and efficiently transported into cells via diverse transporters, such as divalent metal transporter (DMT1), Zn2+ and Ca2+ transporters (152, 153). Nasally administered Mn2+ produces immune responses akin to those triggered by cholera toxin B, including prolonged induction of IgA antibodies in lungs, saliva, and serum (154). The antitumor effects of Mn2+ stem from its promotion of monocyte-derived DC differentiation, DC maturation, and antigen presentation, alongside chemokine production that facilitates immune cell recruitment and germinal center formation, heavily reliant on the cGAS-STING pathway’s bridging role between innate and adaptive immunity (154). Research using a melanoma model in mice indicated that CD103+ dendritic cells (DCs) are unique in delivering intact antigens to tumor-draining lymph nodes, activating tumor-specific CD8+ T cells (155). Enhancing the myeloid lineage commitment to CD103+ DCs and their activation within tumors can significantly improve responses to checkpoint and BRAF inhibitors (155). Furthermore, the efficacy of intratumoral CD8+ T cell activation is linked to the presence of CD103+ DCs, suggesting their key role in overcoming limited T cell infiltration in certain tumors (156). Consequently, mucosal delivery emerges as a promising vaccine administration route, necessitating innovative formulation strategies for broader application.
5 Conclusion
The synergistic potential of combining the cGAS-STING signaling pathway with ICI therapy in the treatment of malignant tumors presents a promising avenue for clinical translation. However, before the successful integration of STING agonists into clinical practice can be achieved, several challenges must be addressed. Similar to the inflammation induced by cGAS-STING, inflammatory responses against tumors stimulated by cGAS-STING may display time sensitivity. While transient activation of cGAS-STING within innate immune cells could enhance antitumor efficacy, prolonged activation may lead to immune tolerance (81), thereby promoting tumor progression. Consequently, the impact of cGAS-STING on cancer outcomes depends on various factors, including tumor type, the host’s immune profile, specific cell types activated, therapeutic approach employed, and the degree of cGAS-STING activation. Further research is crucial to define the precise role of cGAS-STING in oncology and to elucidate both its distinct benefits and potential drawbacks associated with targeting the cGAS-STING pathway in cancer therapeutics.
Author contributions
MH: Methodology, Visualization, Writing – original draft, Writing – review & editing, Supervision, Validation. ZC: Supervision, Validation, Writing – original draft, Writing – review & editing. RL: Data curation, Writing – original draft. ML: Software, Writing – review & editing. NG: Supervision, Writing – review & editing. TK: Validation, Writing – review & editing. FG: Funding acquisition, Resources, Writing – original draft, Writing – review & editing. WC: Conceptualization, Writing – review & editing, Funding acquisition, Writing – original draft.
Funding
The author(s) declare financial support was received for the research, authorship, and/or publication of this article. This research was supported by grants from the National Natural Science Foundation of China (82060538, 82060543), the Wu Jieping Medical Foundation Special Fund for Clinical Research (320.6750.2022-19-56), the Middle-aged and Young Academic and Technical Leader Reserve Talent Program of Yunnan Province Science and Technology Department (202205AC160008), the Key Project of Yunnan Applied Basic Research (202401AS070023) awarded to WC, and the 535 Talent Project of the First Affiliated Hospital of Kunming Medical University (2023535D10) awarded to FG.
Conflict of interest
The authors declare that the research was conducted in the absence of any commercial or financial relationships that could be construed as a potential conflict of interest.
Publisher’s note
All claims expressed in this article are solely those of the authors and do not necessarily represent those of their affiliated organizations, or those of the publisher, the editors and the reviewers. Any product that may be evaluated in this article, or claim that may be made by its manufacturer, is not guaranteed or endorsed by the publisher.
References
1. Gao W, Wang X, Zhou Y, Wang X, Yu Y. Autophagy, ferroptosis, pyroptosis, and necroptosis in tumor immunotherapy. Signal Transduct Target Ther. (2022) 7:196. doi: 10.1038/s41392-022-01046-3
2. Duan S, Guo W, Xu Z, He Y, Liang C, Mo Y, et al. Natural killer group 2D receptor and its ligands in cancer immune escape. Mol Cancer. (2019) 18:29. doi: 10.1186/s12943-019-0956-8
3. Guillerey C. NK cells in the tumor microenvironment. Adv Exp Med Biol. (2020) 1273:69–90. doi: 10.1007/978-3-030-49270-0_4
4. Paerewijck O, Lamkanfi M. The human inflammasomes. Mol Aspects Med. (2022) 88:101100. doi: 10.1016/j.mam.2022.101100
5. Jiang X, Wang J, Deng X, Xiong F, Ge J, Xiang B, et al. Role of the tumor microenvironment in PD-L1/PD-1-mediated tumor immune escape. Mol Cancer. (2019) 18:10. doi: 10.1186/s12943-018-0928-4
6. Chen H, Yao J, Bao R, Dong Y, Zhang T, Du Y, et al. Cross-talk of four types of RNA modification writers defines tumor microenvironment and pharmacogenomic landscape in colorectal cancer. Mol Cancer. (2021) 20:29. doi: 10.1186/s12943-021-01322-w
7. Chen DS, Mellman I. Elements of cancer immunity and the cancer-immune set point. Nature. (2017) 541:321–30. doi: 10.1038/nature21349
8. Wang KW, Wang MD, Li ZX, Hu BS, Huang JF, Wu JJ, et al. Systematic analysis of the cuprotosis in tumor microenvironment and prognosis of gastric cancer. Heliyon. (2023) 9:e13831. doi: 10.1016/j.heliyon.2023.e13831
9. Hegde PS, Chen DS. Top 10 challenges in cancer immunotherapy. Immunity. (2020) 52:17–35. doi: 10.1016/j.immuni.2019.12.011
10. Huang ZD, Liu ZZ, Liu YY, Fu YC, Lin LL, Hu C, et al. Molecular subtypes based on cell differentiation trajectories in head and neck squamous cell carcinoma: differential prognosis and immunotherapeutic responses. Front Immunol. (2021) 12:791621. doi: 10.3389/fimmu.2021.791621
11. Liu YT, Sun ZJ. Turning cold tumors into hot tumors by improving T-cell infiltration. Theranostics. (2021) 11:5365–86. doi: 10.7150/thno.58390
12. Galon J, Bruni D. Approaches to treat immune hot, altered and cold tumours with combination immunotherapies. Nat Rev Drug Discovery. (2019) 18:197–218. doi: 10.1038/s41573-018-0007-y
13. Hegde PS, Karanikas V, Evers S. The where, the when, and the how of immune monitoring for cancer immunotherapies in the era of checkpoint inhibition. Clin Cancer research: an Off J Am Assoc Cancer Res. (2016) 22:1865–74. doi: 10.1158/1078-0432.Ccr-15-1507
14. Katopodi T, Petanidis S, Charalampidis C, Chatziprodromidou I, Eskitzis P, Tsavlis D, et al. Tumor-infiltrating dendritic cells: decisive roles in cancer immunosurveillance, immunoediting, and tumor T cell tolerance. Cells. (2022) 11. doi: 10.3390/cells11203183
15. Wang G, Tai R, Wu Y, Yang S, Wang J, Yu X, et al. The expression and immunoregulation of immune checkpoint molecule VISTA in autoimmune diseases and cancers. Cytokine Growth Factor Rev. (2020) 52:1–14. doi: 10.1016/j.cytogfr.2020.02.002
16. Ferlazzo G. Natural killer and dendritic cell liaison: recent insights and open questions. Immunol Lett. (2005) 101:12–7. doi: 10.1016/j.imlet.2005.04.015
17. Garland KM, Sheehy TL, Wilson JT. Chemical and biomolecular strategies for STING pathway activation in cancer immunotherapy. Chem Rev. (2022) 122:5977–6039. doi: 10.1021/acs.chemrev.1c00750
18. Doroshow DB, Bhalla S, Beasley MB, Sholl LM, Kerr KM, Gnjatic S, et al. PD-L1 as a biomarker of response to immune-checkpoint inhibitors. Nat Rev Clin Oncol. (2021) 18:345–62. doi: 10.1038/s41571-021-00473-5
19. Vornholz L, Isay SE, Kurgyis Z, Strobl DC, Loll P, Mosa MH, et al. Synthetic enforcement of STING signaling in cancer cells appropriates the immune microenvironment for checkpoint inhibitor therapy. Sci Adv. (2023) 9:eadd8564. doi: 10.1126/sciadv.add8564
20. Gong Y, Chang C, Liu X, He Y, Wu Y, Wang S, et al. Stimulator of interferon genes signaling pathway and its role in anti-tumor immune therapy. Curr Pharm Des. (2020) 26:3085–95. doi: 10.2174/1381612826666200610183048
21. Temizoz B, Ishii KJ. Type I and II interferons toward ideal vaccine and immunotherapy. Expert Rev Vaccines. (2021) 20:527–44. doi: 10.1080/14760584.2021.1927724
22. Mabbott NA, Baillie JK, Brown H, Freeman TC, Hume DA. An expression atlas of human primary cells: inference of gene function from coexpression networks. BMC Genomics. (2013) 14:632. doi: 10.1186/1471-2164-14-632
23. Sun L, Wu J, Du F, Chen X, Chen ZJ. Cyclic GMP-AMP synthase is a cytosolic DNA sensor that activates the type I interferon pathway. Science. (2013) 339:786–91. doi: 10.1126/science.1232458
24. Zhang X, Wu J, Du F, Xu H, Sun L, Chen Z, et al. The cytosolic DNA sensor cGAS forms an oligomeric complex with DNA and undergoes switch-like conformational changes in the activation loop. Cell Rep. (2014) 6:421–30. doi: 10.1016/j.celrep.2014.01.003
25. Li X, Shu C, Yi G, Chaton CT, Shelton CL, Diao J, et al. Cyclic GMP-AMP synthase is activated by double-stranded DNA-induced oligomerization. Immunity. (2013) 39:1019–31. doi: 10.1016/j.immuni.2013.10.019
26. Kranzusch PJ, Lee AS, Berger JM, Doudna JA. Structure of human cGAS reveals a conserved family of second-messenger enzymes in innate immunity. Cell Rep. (2013) 3:1362–8. doi: 10.1016/j.celrep.2013.05.008
27. Gao P, Ascano M, Wu Y, Barchet W, Gaffney BL, Zillinger T, et al. Cyclic [G(2’,5’)pA(3’,5’)p] is the metazoan second messenger produced by DNA-activated cyclic GMP-AMP synthase. Cell. (2013) 153:1094–107. doi: 10.1016/j.cell.2013.04.046
28. Long ZJ, Wang JD, Xu JQ, Lei XX, Liu Q. cGAS/STING cross-talks with cell cycle and potentiates cancer immunotherapy. Mol Ther. (2022) 30:1006–17. doi: 10.1016/j.ymthe.2022.01.044
29. Kato K, Omura H, Ishitani R, Nureki O. Cyclic GMP-AMP as an endogenous second messenger in innate immune signaling by cytosolic DNA. Annu Rev Biochem. (2017) 86:541–66. doi: 10.1146/annurev-biochem-061516-044813
30. Zhang X, Shi H, Wu J, Zhang X, Sun L, Chen C, et al. Cyclic GMP-AMP containing mixed phosphodiester linkages is an endogenous high-affinity ligand for STING. Mol Cell. (2013) 51:226–35. doi: 10.1016/j.molcel.2013.05.022
31. Diner EJ, Burdette DL, Wilson SC, Monroe KM, Kellenberger CA, Hyodo M, et al. The innate immune DNA sensor cGAS produces a noncanonical cyclic dinucleotide that activates human STING. Cell Rep. (2013) 3:1355–61. doi: 10.1016/j.celrep.2013.05.009
32. Dobbs N, Burnaevskiy N, Chen D, Gonugunta VK, Alto NM, Yan N. STING activation by translocation from the ER is associated with infection and autoinflammatory disease. Cell Host Microbe. (2015) 18:157–68. doi: 10.1016/j.chom.2015.07.001
33. Oh JE, Lee HK. Pattern recognition receptors and autophagy. Front Immunol. (2014) 5:300. doi: 10.3389/fimmu.2014.00300
34. Tanaka Y, Chen ZJ. STING specifies IRF3 phosphorylation by TBK1 in the cytosolic DNA signaling pathway. Sci Signal. (2012) 5:ra20. doi: 10.1126/scisignal.2002521
35. Fitzgerald KA, McWhirter SM, Faia KL, Rowe DC, Latz E, Golenbock DT, et al. IKKepsilon and TBK1 are essential components of the IRF3 signaling pathway. Nat Immunol. (2003) 4:491–6. doi: 10.1038/ni921
36. Sharma S, tenOever BR, Grandvaux N, Zhou GP, Lin R, Hiscott J. Triggering the interferon antiviral response through an IKK-related pathway. Science. (2003) 300:1148–51. doi: 10.1126/science.1081315
37. Abe T, Barber GN. Cytosolic-DNA-mediated, STING-dependent proinflammatory gene induction necessitates canonical NF-κB activation through TBK1. J Virol. (2014) 88:5328–41. doi: 10.1128/jvi.00037-14
38. Balka KR, Louis C, Saunders TL, Smith AM, Calleja DJ, D’Silva DB, et al. TBK1 and IKKϵ Act redundantly to mediate STING-induced NF-κB responses in myeloid cells. Cell Rep. (2020) 31:107492. doi: 10.1016/j.celrep.2020.03.056
39. Li Q, Tian S, Liang J, Fan J, Lai J, Chen Q. Therapeutic development by targeting the cGAS-STING pathway in autoimmune disease and cancer. Front Pharmacol. (2021) 12:779425. doi: 10.3389/fphar.2021.779425
40. Gao M, He Y, Tang H, Chen X, Liu S, Tao Y. cGAS/STING: novel perspectives of the classic pathway. Mol Biomed. (2020) 1:7. doi: 10.1186/s43556-020-00006-z
41. Zhang Z, Zhou H, Ouyang X, Dong Y, Sarapultsev A, Luo S, et al. Multifaceted functions of STING in human health and disease: from molecular mechanism to targeted strategy. Signal Transduct Target Ther. (2022) 7:394. doi: 10.1038/s41392-022-01252-z
42. Zuo Z, He S, Qiu Y, Guo R, He Y, Jiao C, et al. Salvianolic acid A prevents UV-induced skin damage by inhibiting the cGAS-STING pathway. Int Immunopharmacol. (2024) 132:111971. doi: 10.1016/j.intimp.2024.111971
43. Ke X, Hu T, Jiang M. cGAS-STING signaling pathway in gastrointestinal inflammatory disease and cancers. FASEB J. (2022) 36:e22029. doi: 10.1096/fj.202101199R
44. Mackenzie KJ, Carroll P, Martin CA, Murina O, Fluteau A, Simpson DJ, et al. cGAS surveillance of micronuclei links genome instability to innate immunity. Nature. (2017) 548:461–5. doi: 10.1038/nature23449
45. Bakhoum SF, Cantley LC. The multifaceted role of chromosomal instability in cancer and its microenvironment. Cell. (2018) 174:1347–60. doi: 10.1016/j.cell.2018.08.027
46. Zhang H, You QD, Xu XL. Targeting stimulator of interferon genes (STING): A medicinal chemistry perspective. J Med Chem. (2020) 63:3785–816. doi: 10.1021/acs.jmedchem.9b01039
47. Ohkuri T, Ghosh A, Kosaka A, Zhu J, Ikeura M, David M, et al. STING contributes to antiglioma immunity via triggering type I IFN signals in the tumor microenvironment. Cancer Immunol Res. (2014) 2:1199–208. doi: 10.1158/2326-6066.Cir-14-0099
48. Woo SR, Fuertes MB, Corrales L, Spranger S, Furdyna MJ, Leung MY, et al. STING-dependent cytosolic DNA sensing mediates innate immune recognition of immunogenic tumors. Immunity. (2014) 41:830–42. doi: 10.1016/j.immuni.2014.10.017
49. Kitajima S, Ivanova E, Guo S, Yoshida R, Campisi M, Sundararaman SK, et al. Suppression of STING associated with LKB1 loss in KRAS-driven lung cancer. Cancer Discovery. (2019) 9:34–45. doi: 10.1158/2159-8290.Cd-18-0689
50. Wheeler OPG, Unterholzner L. DNA sensing in cancer: Pro-tumour and anti-tumour functions of cGAS-STING signalling. Essays Biochem. (2023) 67:905–918. doi: 10.1042/ebc20220241
51. Galluzzi L, Buqué A, Kepp O, Zitvogel L, Kroemer G. Immunogenic cell death in cancer and infectious disease. Nat Rev Immunol. (2017) 17:97–111. doi: 10.1038/nri.2016.107
52. Lohard S, Bourgeois N, Maillet L, Gautier F, Fétiveau A, Lasla H, et al. STING-dependent paracriny shapes apoptotic priming of breast tumors in response to anti-mitotic treatment. Nat Commun. (2020) 11:259. doi: 10.1038/s41467-019-13689-y
53. Ahn J, Xia T, Konno H, Konno K, Ruiz P, Barber GN. Inflammation-driven carcinogenesis is mediated through STING. Nat Commun. (2014) 5:5166. doi: 10.1038/ncomms6166
54. Coussens LM, Werb Z. Inflammation and cancer. Nature. (2002) 420:860–7. doi: 10.1038/nature01322
55. Kwon J, Bakhoum SF. The cytosolic DNA-sensing cGAS-STING pathway in cancer. Cancer Discovery. (2020) 10:26–39. doi: 10.1158/2159-8290.Cd-19-0761
56. Gonugunta VK, Sakai T, Pokatayev V, Yang K, Wu J, Dobbs N, et al. Trafficking-mediated STING degradation requires sorting to acidified endolysosomes and can be targeted to enhance anti-tumor response. Cell Rep. (2017) 21:3234–42. doi: 10.1016/j.celrep.2017.11.061
57. Vesely MD, Kershaw MH, Schreiber RD, Smyth MJ. Natural innate and adaptive immunity to cancer. Annu Rev Immunol. (2011) 29:235–71. doi: 10.1146/annurev-immunol-031210-101324
58. Linares-Fernández S, Lacroix C, Exposito JY, Verrier B. Tailoring mRNA vaccine to balance innate/adaptive immune response. Trends Mol Med. (2020) 26:311–23. doi: 10.1016/j.molmed.2019.10.002
59. Feng Y, Chen Z, Tu SQ, Wei JM, Hou YL, Kuang ZL, et al. Role of interleukin-17A in the pathomechanisms of periodontitis and related systemic chronic inflammatory diseases. Front Immunol. (2022) 13:862415. doi: 10.3389/fimmu.2022.862415
60. Liu C, Wang X, Qin W, Tu J, Li C, Zhao W, et al. Combining radiation and the ATR inhibitor berzosertib activates STING signaling and enhances immunotherapy via inhibiting SHP1 function in colorectal cancer. Cancer Commun (Lond). (2023) 43:435–54. doi: 10.1002/cac2.12412
61. Vasiyani H, Wadhwa B, Singh R. Regulation of cGAS-STING signalling in cancer: Approach for combination therapy. Biochim Biophys Acta Rev Cancer. (2023) 1878:188896. doi: 10.1016/j.bbcan.2023.188896
62. Ou L, Zhang A, Cheng Y, Chen Y. The cGAS-STING pathway: A promising immunotherapy target. Front Immunol. (2021) 12:795048. doi: 10.3389/fimmu.2021.795048
63. Noy R, Pollard JW. Tumor-associated macrophages: from mechanisms to therapy. Immunity. (2014) 41:49–61. doi: 10.1016/j.immuni.2014.06.010
64. Zhang Y, Sun Z, Pei J, Luo Q, Zeng X, Li Q, et al. Identification of α-mangostin as an agonist of human STING. ChemMedChem. (2018) 13:2057–64. doi: 10.1002/cmdc.201800481
65. SChadt L, Sparano C, Schweiger NA, Silina K, Cecconi V, Lucchiari G, et al. Cancer-cell-intrinsic cGAS expression mediates tumor immunogenicity. Cell Rep. (2019) 29:1236–48. doi: 10.1016/j.celrep.2019.09.065
66. Le Gouvello S, Bastuji-Garin S, Aloulou N, Mansour H, Chaumette MT, Berrehar F, et al. High prevalence of Foxp3 and IL17 in MMR-proficient colorectal carcinomas. Gut. (2008) 57:772–9. doi: 10.1136/gut.2007.123794
67. Denis M. Interferon-gamma-treated murine macrophages inhibit growth of tubercle bacilli via the generation of reactive nitrogen intermediates. Cell Immunol. (1991) 132:150–7. doi: 10.1016/0008-8749(91)90014-3
68. Gidlund M, Orn A, Wigzell H, Senik A, Gresser I. Enhanced NK cell activity in mice injected with interferon and interferon inducers. Nature. (1978) 273:759–61. doi: 10.1038/273759a0
69. Luft T, Pang KC, Thomas E, Hertzog P, Hart DN, Trapani J, et al. Type I IFNs enhance the terminal differentiation of dendritic cells. J Immunol. (1998) 161:1947–53. doi: 10.4049/jimmunol.161.4.1947
70. Nicolai CJ, Wolf N, Chang IC, Kirn G, Marcus A, Ndubaku CO, et al. NK cells mediate clearance of CD8(+) T cell-resistant tumors in response to STING agonists. Sci Immunol. (2020) 5. doi: 10.1126/sciimmunol.aaz2738
71. Marcus A, Mao AJ, Lensink-Vasan M, Wang L, Vance RE, Raulet DH. Tumor-derived cGAMP triggers a STING-mediated interferon response in non-tumor cells to activate the NK cell response. Immunity. (2018) 49:754–63. doi: 10.1016/j.immuni.2018.09.016
72. Dunn GP, Bruce AT, Sheehan KC, Shankaran V, Uppaluri R, Bui JD, et al. A critical function for type I interferons in cancer immunoediting. Nat Immunol. (2005) 6:722–9. doi: 10.1038/ni1213
73. Zitvogel L, Galluzzi L, Kepp O, Smyth MJ, Kroemer G. Type I interferons in anticancer immunity. Nat Rev Immunol. (2015) 15:405–14. doi: 10.1038/nri3845
74. Vitale M, Della Chiesa M, Carlomagno S, Pende D, Aricò M, Moretta L, et al. NK-dependent DC maturation is mediated by TNFalpha and IFNgamma released upon engagement of the NKp30 triggering receptor. Blood. (2005) 106:566–71. doi: 10.1182/blood-2004-10-4035
75. Morandi B, Mortara L, Carrega P, Cantoni C, Costa G, Accolla RS, et al. NK cells provide helper signal for CD8+ T cells by inducing the expression of membrane-bound IL-15 on DCs. Int Immunol. (2009) 21:599–606. doi: 10.1093/intimm/dxp029
76. Tough DF, Borrow P, Sprent J. Induction of bystander T cell proliferation by viruses and type I interferon in vivo. Science. (1996) 272:1947–50. doi: 10.1126/science.272.5270.1947
77. Huber JP, Farrar JD. Regulation of effector and memory T-cell functions by type I interferon. Immunology. (2011) 132:466–74. doi: 10.1111/j.1365-2567.2011.03412.x
78. van Vugt M, Parkes EE. When breaks get hot: inflammatory signaling in BRCA1/2-mutant cancers. Trends Cancer. (2022) 8:174–89. doi: 10.1016/j.trecan.2021.12.003
79. Yang H, Lee WS, Kong SJ, Kim CG, Kim JH, Chang SK, et al. STING activation reprograms tumor vasculatures and synergizes with VEGFR2 blockade. J Clin Invest. (2019) 129:4350–64. doi: 10.1172/jci125413
80. Cao X, Cordova AF, Li L. Therapeutic interventions targeting innate immune receptors: A balancing act. Chem Rev. (2022) 122:3414–58. doi: 10.1021/acs.chemrev.1c00716
81. Wan D, Jiang W, Hao J. Research advances in how the cGAS-STING pathway controls the cellular inflammatory response. Front Immunol. (2020) 11:615. doi: 10.3389/fimmu.2020.00615
82. Ramanjulu JM, Pesiridis GS, Yang J, Concha N, Singhaus R, Zhang SY, et al. Design of amidobenzimidazole STING receptor agonists with systemic activity. Nature. (2018) 564:439–43. doi: 10.1038/s41586-018-0705-y
83. Li T, Chen ZJ. The cGAS-cGAMP-STING pathway connects DNA damage to inflammation, senescence, and cancer. J Exp Med. (2018) 215:1287–99. doi: 10.1084/jem.20180139
84. Li W, Lu L, Lu J, Wang X, Yang C, Jin J, et al. cGAS-STING-mediated DNA sensing maintains CD8(+) T cell stemness and promotes antitumor T cell therapy. Sci Transl Med. (2020) 12. doi: 10.1126/scitranslmed.aay9013
85. Pantelidou C, Sonzogni O, De Oliveria Taveira M, Mehta AK, Kothari A, Wang D, et al. PARP inhibitor efficacy depends on CD8(+) T-cell recruitment via intratumoral STING pathway activation in BRCA-deficient models of triple-negative breast cancer. Cancer Discovery. (2019) 9:722–37. doi: 10.1158/2159-8290.Cd-18-1218
86. Schoonen PM, Kok YP, Wierenga E, Bakker B, Foijer F, Spierings DCJ, et al. Premature mitotic entry induced by ATR inhibition potentiates olaparib inhibition-mediated genomic instability, inflammatory signaling, and cytotoxicity in BRCA2-deficient cancer cells. Mol Oncol. (2019) 13:2422–40. doi: 10.1002/1878-0261.12573
87. Demaria O, De Gassart A, Coso S, Gestermann N, Di Domizio J, Flatz L, et al. STING activation of tumor endothelial cells initiates spontaneous and therapeutic antitumor immunity. Proc Natl Acad Sci U S A. (2015) 112:15408–13. doi: 10.1073/pnas.1512832112
88. Corrales L, Glickman LH, McWhirter SM, Kanne DB, Sivick KE, Katibah GE, et al. Direct activation of STING in the tumor microenvironment leads to potent and systemic tumor regression and immunity. Cell Rep. (2015) 11:1018–30. doi: 10.1016/j.celrep.2015.04.031
89. Jing W, McAllister D, Vonderhaar EP, Palen K, Riese MJ, Gershan J, et al. STING agonist inflames the pancreatic cancer immune microenvironment and reduces tumor burden in mouse models. J Immunother Cancer. (2019) 7:115. doi: 10.1186/s40425-019-0573-5
90. Liu Z, Jiang W, Nam J, Moon JJ, Kim BYS. Immunomodulating nanomedicine for cancer therapy. Nano Lett. (2018) 18:6655–9. doi: 10.1021/acs.nanolett.8b02340
91. Ohkuri T, Kosaka A, Nagato T, Kobayashi H. Effects of STING stimulation on macrophages: STING agonists polarize into “classically” or “alternatively” activated macrophages? Hum Vaccin Immunother. (2018) 14:285–7. doi: 10.1080/21645515.2017.1395995
92. Wang Y, Luo J, Alu A, Han X, Wei Y, Wei X. cGAS-STING pathway in cancer biotherapy. Mol Cancer. (2020) 19:136. doi: 10.1186/s12943-020-01247-w
93. Rameshbabu S, Labadie BW, Argulian A, Patnaik A. Targeting innate immunity in cancer therapy. Vaccines (Basel). (2021) 9. doi: 10.3390/vaccines9020138
94. Gogoi H, Mansouri S, Jin L. The age of cyclic dinucleotide vaccine adjuvants. Vaccines (Basel). (2020) 8. doi: 10.3390/vaccines8030453
95. Lv M, Chen M, Zhang R, Zhang W, Wang C, Zhang Y, et al. Manganese is critical for antitumor immune responses via cGAS-STING and improves the efficacy of clinical immunotherapy. Cell Res. (2020) 30:966–79. doi: 10.1038/s41422-020-00395-4
96. Ding C, Song Z, Shen A, Chen T, Zhang A. Small molecules targeting the innate immune cGAS−STING−TBK1 signaling pathway. Acta Pharm Sin B. (2020) 10:2272–98. doi: 10.1016/j.apsb.2020.03.001
97. Meric-Bernstam F, Sweis RF, Hodi FS, Messersmith WA, Andtbacka RHI, Ingham M, et al. Phase I dose-escalation trial of MIW815 (ADU-S100), an intratumoral STING agonist, in patients with advanced/metastatic solid tumors or lymphomas. Clin Cancer Res. (2022) 28:677–88. doi: 10.1158/1078-0432.Ccr-21-1963
98. Meric-Bernstam F, Sweis RF, Kasper S, Hamid O, Bhatia S, Dummer R, et al. Combination of the STING agonist MIW815 (ADU-S100) and PD-1 inhibitor spartalizumab in advanced/metastatic solid tumors or lymphomas: an open-label, multicenter, phase ib study. Clin Cancer Res. (2023) 29:110–21. doi: 10.1158/1078-0432.Ccr-22-2235
99. Zhao K, Huang J, Zhao Y, Wang S, Xu J, Yin K. Targeting STING in cancer: Challenges and emerging opportunities. Biochim Biophys Acta Rev Cancer. (2023) 1878:188983. doi: 10.1016/j.bbcan.2023.188983
100. Luke JJ, Piha-Paul SA, Medina T, Verschraegen CF, Varterasian M, Brennan AM, et al. Phase I Study of SYNB1891, an Engineered E. coli Nissle Strain Expressing STING Agonist, with and without Atezolizumab in Advanced Malignancies. Clin Cancer Res. (2023) 29:2435–44. doi: 10.1158/1078-0432.Ccr-23-0118
101. Wang C, Guan Y, Lv M, Zhang R, Guo Z, Wei X, et al. Manganese increases the sensitivity of the cGAS-STING pathway for double-stranded DNA and is required for the host defense against DNA viruses. Immunity. (2018) 48:675–87. doi: 10.1016/j.immuni.2018.03.017
102. Shen J, Zhao W, Ju Z, Wang L, Peng Y, Labrie M, et al. PARPi triggers the STING-dependent immune response and enhances the therapeutic efficacy of immune checkpoint blockade independent of BRCAness. Cancer Res. (2019) 79:311–9. doi: 10.1158/0008-5472.Can-18-1003
103. McLaughlin M, Patin EC, Pedersen M, Wilkins A, Dillon MT, Melcher AA, et al. Inflammatory microenvironment remodelling by tumour cells after radiotherapy. Nat Rev Cancer. (2020) 20:203–17. doi: 10.1038/s41568-020-0246-1
104. Formenti SC, Rudqvist NP, Golden E, Cooper B, Wennerberg E, Lhuillier C, et al. Radiotherapy induces responses of lung cancer to CTLA-4 blockade. Nat Med. (2018) 24:1845–51. doi: 10.1038/s41591-018-0232-2
105. Harding SM, Benci JL, Irianto J, Discher DE, Minn AJ, Greenberg RA. Mitotic progression following DNA damage enables pattern recognition within micronuclei. Nature. (2017) 548:466–70. doi: 10.1038/nature23470
106. Deng L, Liang H, Xu M, Yang X, Burnette B, Arina A, et al. STING-dependent cytosolic DNA sensing promotes radiation-induced type I interferon-dependent antitumor immunity in immunogenic tumors. Immunity. (2014) 41:843–52. doi: 10.1016/j.immuni.2014.10.019
107. Vanpouille-Box C, Alard A, Aryankalayil MJ, Sarfraz Y, Diamond JM, Schneider RJ, et al. DNA exonuclease Trex1 regulates radiotherapy-induced tumour immunogenicity. Nat Commun. (2017) 8:15618. doi: 10.1038/ncomms15618
108. Sheng H, Huang Y, Xiao Y, Zhu Z, Shen M, Zhou P, et al. ATR inhibitor AZD6738 enhances the antitumor activity of radiotherapy and immune checkpoint inhibitors by potentiating the tumor immune microenvironment in hepatocellular carcinoma. J Immunother Cancer. (2020) 8. doi: 10.1136/jitc-2019-000340
109. Liu Y, Crowe WN, Wang L, Lu Y, Petty WJ, Habib AA, et al. An inhalable nanoparticulate STING agonist synergizes with radiotherapy to confer long-term control of lung metastases. Nat Commun. (2019) 10:5108. doi: 10.1038/s41467-019-13094-5
110. Glück S, Guey B, Gulen MF, Wolter K, Kang TW, Schmacke NA, et al. Innate immune sensing of cytosolic chromatin fragments through cGAS promotes senescence. Nat Cell Biol. (2017) 19:1061–70. doi: 10.1038/ncb3586
111. Erdal E, Haider S, Rehwinkel J, Harris AL, McHugh PJ. A prosurvival DNA damage-induced cytoplasmic interferon response is mediated by end resection factors and is limited by Trex1. Genes Dev. (2017) 31:353–69. doi: 10.1101/gad.289769.116
112. Ghaffari A, Peterson N, Khalaj K, Vitkin N, Robinson A, Francis JA, et al. STING agonist therapy in combination with PD-1 immune checkpoint blockade enhances response to carboplatin chemotherapy in high-grade serous ovarian cancer. Br J Cancer. (2018) 119:440–9. doi: 10.1038/s41416-018-0188-5
113. Ethiraj P, Veerappan K, Samuel S, Sivapatham S. Interferon β improves the efficacy of low dose cisplatin by inhibiting NF-κB/p-Akt signaling on HeLa cells. BioMed Pharmacother. (2016) 82:124–32. doi: 10.1016/j.biopha.2016.04.058
114. Li Q, Kawamura K, Yang S, Okamoto S, Kobayashi H, Tada Y, et al. Interferon-β produces synergistic combinatory anti-tumor effects with cisplatin or pemetrexed on mesothelioma cells. PloS One. (2013) 8:e72709. doi: 10.1371/journal.pone.0072709
115. Li T, Cheng H, Yuan H, Xu Q, Shu C, Zhang Y, et al. Antitumor Activity of cGAMP via Stimulation of cGAS-cGAMP-STING-IRF3 Mediated Innate Immune Response. Sci Rep. (2016) 6:19049. doi: 10.1038/srep19049
116. Miglietta G, Russo M, Duardo RC, Capranico G. G-quadruplex binders as cytostatic modulators of innate immune genes in cancer cells. Nucleic Acids Res. (2021) 49:6673–86. doi: 10.1093/nar/gkab500
117. Marzano S, Miglietta G, Morigi R, Marinello J, Arleo A, Procacci M, et al. Balancing affinity, selectivity, and cytotoxicity of hydrazone-based G-quadruplex ligands for activation of interferon β Genes in cancer cells. J Med Chem. (2022) 65:12055–67. doi: 10.1021/acs.jmedchem.2c00772
118. Chung SY, Chang YC, Hsu DS, Hung YC, Lu ML, Hung YP, et al. A G-quadruplex stabilizer, CX-5461 combined with two immune checkpoint inhibitors enhances in vivo therapeutic efficacy by increasing PD-L1 expression in colorectal cancer. Neoplasia. (2023) 35:100856. doi: 10.1016/j.neo.2022.100856
119. Xu H, Di Antonio M, McKinney S, Mathew V, Ho B, O’Neil NJ, et al. CX-5461 is a DNA G-quadruplex stabilizer with selective lethality in BRCA1/2 deficient tumours. Nat Commun. (2017) 8:14432. doi: 10.1038/ncomms14432
120. Sadzak I, Schiff M, Gattermeier I, Glinitzer R, Sauer I, Saalmüller A, et al. Recruitment of Stat1 to chromatin is required for interferon-induced serine phosphorylation of Stat1 transactivation domain. Proc Natl Acad Sci U S A. (2008) 105:8944–9. doi: 10.1073/pnas.0801794105
121. Cheon H, Stark GR. Unphosphorylated STAT1 prolongs the expression of interferon-induced immune regulatory genes. Proc Natl Acad Sci U S A. (2009) 106:9373–8. doi: 10.1073/pnas.0903487106
122. Pilz A, Ramsauer K, Heidari H, Leitges M, Kovarik P, Decker T. Phosphorylation of the Stat1 transactivating domain is required for the response to type I interferons. EMBO Rep. (2003) 4:368–73. doi: 10.1038/sj.embor.embor802
123. van Weverwijk A, de Visser KE. Mechanisms driving the immunoregulatory function of cancer cells. Nat Rev Cancer. (2023) 23:193–215. doi: 10.1038/s41568-022-00544-4
124. Li J, Hubisz MJ, Earlie EM, Duran MA, Hong C, Varela AA, et al. Non-cell-autonomous cancer progression from chromosomal instability. Nature. (2023) 620:1080–8. doi: 10.1038/s41586-023-06464-z
125. Bakhoum SF, Ngo B, Laughney AM, Cavallo JA, Murphy CJ, Ly P, et al. Chromosomal instability drives metastasis through a cytosolic DNA response. Nature. (2018) 553:467–72. doi: 10.1038/nature25432
126. Hong C, Schubert M, Tijhuis AE, Requesens M, Roorda M, van den Brink A, et al. cGAS-STING drives the IL-6-dependent survival of chromosomally instable cancers. Nature. (2022) 607:366–73. doi: 10.1038/s41586-022-04847-2
127. Lanska DJ, Lanska MJ, Mendez MF. Brainstem auditory hallucinosis. Neurology. (1987) 37:1685. doi: 10.1212/wnl.37.10.1685
128. Nguyen B, Fong C, Luthra A, Smith SA, DiNatale RG, Nandakumar S, et al. Genomic characterization of metastatic patterns from prospective clinical sequencing of 25,000 patients. Cell. (2022) 185:563–575.e11. doi: 10.1016/j.cell.2022.01.003
129. Davoli T, Uno H, Wooten EC, Elledge SJ. Tumor aneuploidy correlates with markers of immune evasion and with reduced response to immunotherapy. Science. (2017) 355. doi: 10.1126/science.aaf8399
130. Li S, Mirlekar B, Johnson BM, Brickey WJ, Wrobel JA, Yang N, et al. STING-induced regulatory B cells compromise NK function in cancer immunity. Nature. (2022) 610:373–80. doi: 10.1038/s41586-022-05254-3
131. Vanpouille-Box C, Demaria S, Formenti SC, Galluzzi L. Cytosolic DNA sensing in organismal tumor control. Cancer Cell. (2018) 34:361–78. doi: 10.1016/j.ccell.2018.05.013
132. Ghosh M, Saha S, Bettke J, Nagar R, Parrales A, Iwakuma T, et al. Mutant p53 suppresses innate immune signaling to promote tumorigenesis. Cancer Cell. (2021) 39:494–508. doi: 10.1016/j.ccell.2021.01.003
133. Xu P, Liu Y, Liu C, Guey B, Li L, Melenec P, et al. The CRL5-SPSB3 ubiquitin ligase targets nuclear cGAS for degradation. Nature. (2024) 627:873–9. doi: 10.1038/s41586-024-07112-w
134. Cho MG, Kumar RJ, Lin CC, Boyer JA, Shahir JA, Fagan-Solis K, et al. MRE11 liberates cGAS from nucleosome sequestration during tumorigenesis. Nature. (2024) 625:585–92. doi: 10.1038/s41586-023-06889-6
135. Lefebvre JS, Haynes L. Aging of the CD4 T cell compartment. Open Longev Sci. (2012) 6:83–91. doi: 10.2174/1876326x01206010083
136. Maue AC, Haynes L. CD4+ T cells and immunosenescence–a mini-review. Gerontology. (2009) 55:491–5. doi: 10.1159/000214842
137. Gogoi H, Mansouri S, Katikaneni DS, Jin L. New moDC-targeting TNF fusion proteins enhance cyclic di-GMP vaccine adjuvanticity in middle-aged and aged mice. Front Immunol. (2020) 11:1674. doi: 10.3389/fimmu.2020.01674
138. Patel S, Blaauboer SM, Tucker HR, Mansouri S, Ruiz-Moreno JS, Hamann L, et al. The common R71H-G230A-R293Q human TMEM173 is a null allele. J Immunol. (2017) 198:776–87. doi: 10.4049/jimmunol.1601585
139. Patel S, Jin L. TMEM173 variants and potential importance to human biology and disease. Genes Immun. (2019) 20:82–9. doi: 10.1038/s41435-018-0029-9
140. Hao Y, Ji Z, Zhou H, Wu D, Gu Z, Wang D, et al. Lipid-based nanoparticles as drug delivery systems for cancer immunotherapy. MedComm. (2020) 4:e339. doi: 10.1002/mco2.339
141. Li J, Han X, Gao S, Yan Y, Li X, Wang H. Tumor microenvironment-responsive DNA-based nanomedicine triggers innate sensing for enhanced immunotherapy. J Nanobiotechnology. (2023) 21:382. doi: 10.1186/s12951-023-02132-6
142. Duvall JR, Thomas JD, Bukhalid RA, Catcott KC, Bentley KW, Collins SD, et al. Discovery and optimization of a STING agonist platform for application in antibody drug conjugates. J Med Chem. (2023) 66:10715–33. doi: 10.1021/acs.jmedchem.3c00907
143. Lu Y, You L, Li L, Kilgore JA, Liu S, Wang X, et al. Orthogonal hydroxyl functionalization of cGAMP confers metabolic stability and enables antibody conjugation. ACS Cent Sci. (2023) 9:2298–305. doi: 10.1021/acscentsci.3c01122
144. Wu YT, Fang Y, Wei Q, Shi H, Tan H, Deng Y, et al. Tumor-targeted delivery of a STING agonist improvescancer immunotherapy. Proc Natl Acad Sci U S A. (2022) 119:e2214278119. doi: 10.1073/pnas.2214278119
145. Chen Q, Boire A, Jin X, Valiente M, Er EE, Lopez-Soto A, et al. Carcinoma-astrocyte gap junctions promote brain metastasis by cGAMP transfer. Nature. (2016) 533:493–8. doi: 10.1038/nature18268
146. Benguigui M, Cooper TJ, Kalkar P, Schif-Zuck S, Halaban R, Bacchiocchi A, et al. Interferon-stimulated neutrophils as a predictor of immunotherapy response. Cancer Cell. (2024) 42:253–265.e12. doi: 10.1016/j.ccell.2023.12.005
147. Wang J, Li S, Wang M, Wang X, Chen S, Sun Z, et al. STING licensing of type I dendritic cells potentiates antitumor immunity. bioRxiv. (2024). doi: 10.1101/2024.01.02.573934
148. Nizard M, Diniz MO, Roussel H, Tran T, Ferreira LC, Badoual C, et al. Mucosal vaccines: novel strategies and applications for the control of pathogens and tumors at mucosal sites. Hum Vaccin Immunother. (2014) 10:2175–87. doi: 10.4161/hv.29269
149. Sun YY, Peng S, Han L, Qiu J, Song L, Tsai Y, et al. Local HPV recombinant vaccinia boost following priming with an HPV DNA vaccine enhances local HPV-specific CD8+ T-cell-mediated tumor control in the genital tract. Clin Cancer Res. (2016) 22:657–69. doi: 10.1158/1078-0432.Ccr-15-0234
150. Decrausaz L, Pythoud C, Domingos-Pereira S, Derré L, Jichlinski P, Nardelli-Haefliger D. Intravaginal live attenuated Salmonella increase local antitumor vaccine-specific CD8(+) T cells. Oncoimmunology. (2013) 2:e22944. doi: 10.4161/onci.22944
151. Nizard M, Roussel H, Diniz MO, Karaki S, Tran T, Voron T, et al. Induction of resident memory T cells enhances the efficacy of cancer vaccine. Nat Commun. (2017) 8:15221. doi: 10.1038/ncomms15221
152. Hooy RM, Massaccesi G, Rousseau KE, Chattergoon MA, Sohn J. Allosteric coupling between Mn2+ and dsDNA controls the catalytic efficiency and fidelity of cGAS. Nucleic Acids Res. (2020) 48:4435–47. doi: 10.1093/nar/gkaa084
153. Zhao Z, Ma Z, Wang B, Guan Y, Su XD, Jiang Z. Mn(2+) directly activates cGAS and structural analysis suggests mn(2+) induces a noncanonical catalytic synthesis of 2’3’-cGAMP. Cell Rep. (2020) 32:108053. doi: 10.1016/j.celrep.2020.108053
154. Zhang R, Wang C, Guan Y, Wei X, Sha M, Yi M, et al. Manganese salts function as potent adjuvants. Cell Mol Immunol. (2021) 18:1222–34. doi: 10.1038/s41423-021-00669-w
155. Salmon H, Idoyaga J, Rahman A, Leboeuf M, Remark R, Jordan S, et al. Expansion and activation of CD103(+) dendritic cell progenitors at the tumor site enhances tumor responses to therapeutic PD-L1 and BRAF inhibition. Immunity. (2016) 44:924–38. doi: 10.1016/j.immuni.2016.03.012
Keywords: cGAS-STING, hot tumor, tumor immunology, immune checkpoint inhibitor (ICI), tumor microenvironment
Citation: Huang M, Cha Z, Liu R, Lin M, Gafoor NA, Kong T, Ge F and Chen W (2024) Enhancing immunotherapy outcomes by targeted remodeling of the tumor microenvironment via combined cGAS-STING pathway strategies. Front. Immunol. 15:1399926. doi: 10.3389/fimmu.2024.1399926
Received: 12 March 2024; Accepted: 26 April 2024;
Published: 16 May 2024.
Edited by:
Alejandro López-Soto, University of Oviedo, SpainReviewed by:
Mengze Lyu, Cornell University, United StatesAnkita Leekha, University of Houston, United States
Copyright © 2024 Huang, Cha, Liu, Lin, Gafoor, Kong, Ge and Chen. This is an open-access article distributed under the terms of the Creative Commons Attribution License (CC BY). The use, distribution or reproduction in other forums is permitted, provided the original author(s) and the copyright owner(s) are credited and that the original publication in this journal is cited, in accordance with accepted academic practice. No use, distribution or reproduction is permitted which does not comply with these terms.
*Correspondence: Wenlin Chen, Y2hlbndlbmxpbjFAaG90bWFpbC5jb20=; Fei Ge, YWpxbmFkamRAaG90bWFpbC5jb20=
†These authors have contributed equally to this work