- Commonwealth Scientific and Industrial Research Organisation (CSIRO) Australian Centre for Disease Preparedness, Geelong, VIC, Australia
Enhancing livestock biosecurity is critical to safeguard the livelihoods of farmers, global and local economies, and food security. Vaccination is fundamental to the control and prevention of exotic and endemic high-priority infectious livestock diseases. Successful implementation of vaccination in a biosecurity plan is underpinned by a strong understanding of correlates of protection—those elements of the immune response that can reliably predict the level of protection from viral challenge. While correlates of protection have been successfully characterized for many human viral vaccines, for many high-priority livestock viral diseases, including African swine fever and foot and mouth disease, they remain largely uncharacterized. Current literature provides insights into potential correlates of protection that should be assessed during vaccine development for these high-priority mammalian livestock viral diseases. Establishment of correlates of protection for biosecurity purposes enables immune surveillance, rationale for vaccine development, and successful implementation of livestock vaccines as part of a biosecurity strategy.
1 Introduction
Biosecurity involves implementation of measures to minimize the risk of introducing and spreading pathogens within and between farms, between humans and animals, and within the environment (1, 2). The development of biosecurity programs requires a strong scientific basis and the use of risk assessment to evaluate and implement appropriate measures without unnecessarily hindering commerce and trade (1, 2). Many measures are utilized to prevent and control exotic and endemic high-priority viral animal diseases ranging from animal husbandry practices to culling of infected animals or herds (1, 2). Livestock vaccines can further bolster biosecurity strategies and contribute significantly to the overall health and productivity of animal populations (Figure 1) (3, 4). While the immune response to infection with many high-priority viral livestock diseases is well characterized, the protective immune response to a subsequent infection and the relationship to vaccine-mediated protection are incomplete. In this review, we discuss current knowledge of protective immunity and vaccine-induced correlates of protection (CoPs) for high-priority mammalian livestock viral diseases in the context of biosecurity (Table 1). We selected six examples of viral diseases notifiable to the World Organization for Animal Health (WOAH), namely, foot and mouth disease (FMD), African swine fever (ASF), lumpy skin disease (LSD), bluetongue (BT), porcine reproductive and respiratory syndrome (PRRS), and classical swine fever (CSF) (Table 1). These specific diseases were selected as they are high-consequence viral diseases in mammalian livestock, for which vaccination is or aims to be employed as part of a biosecurity and control strategy. Furthermore, these diseases represent diversity in the presentation of disease and the immune responses associated with protection and include both RNA and DNA viruses.
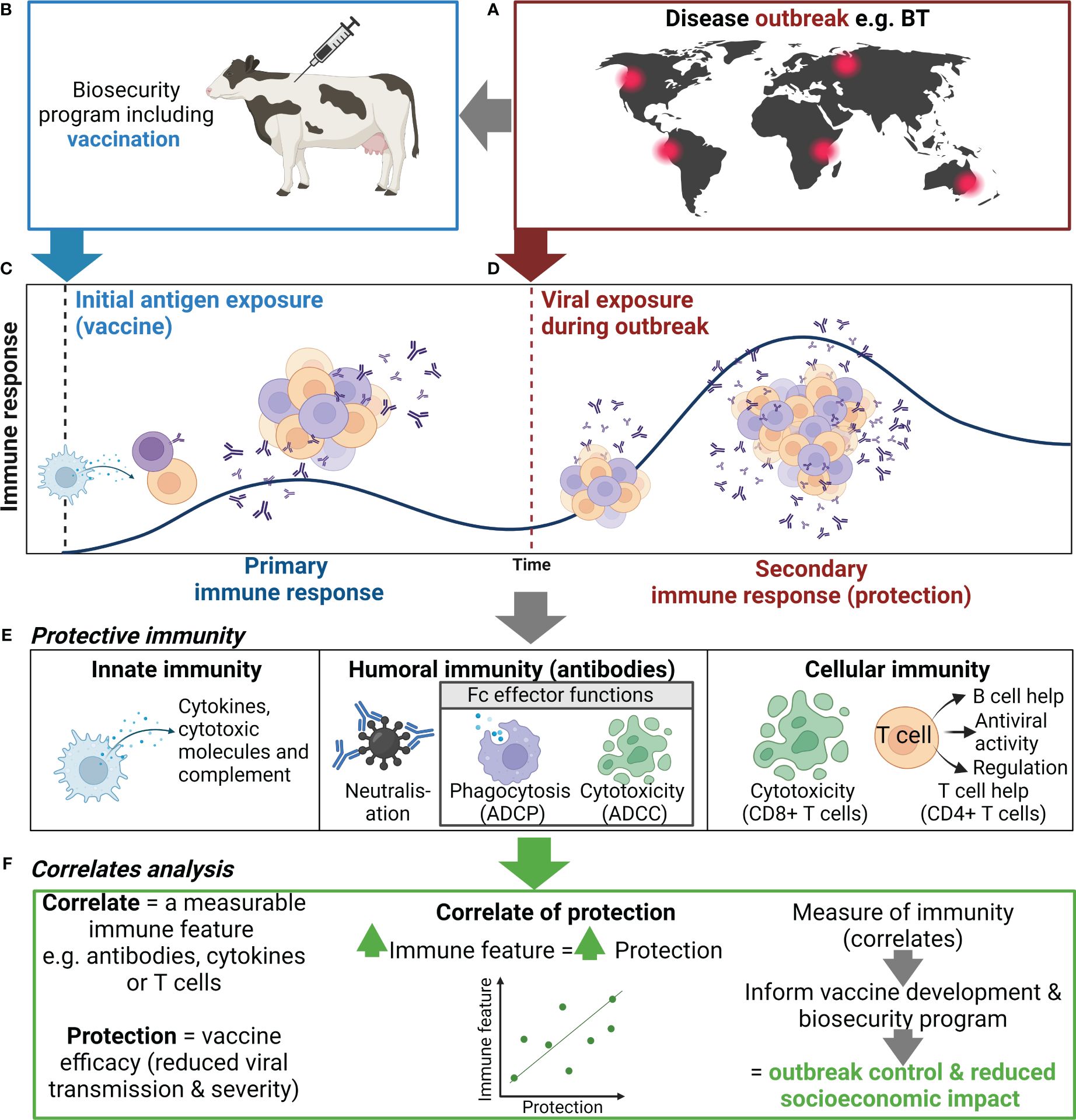
Figure 1 Use of correlates of protection to inform biosecurity programs. (A) An outbreak of an important viral livestock disease, e.g., bluetongue (BT), (B) triggers the implementation of a biosecurity program that may include vaccination. (C) Initial exposure to antigen via vaccination or natural infection will generate a primary immune response to the target virus, including activation, proliferation, and differentiation of innate (e.g., NK cells and DCs) and adaptive immune cells (e.g., B and T cells), and the production of antibody and generation of immune memory. (D) When immune animals encounter a subsequent infection, immune memory rapidly activates and expands to generate an efficient secondary immune response. (E) The antiviral immune response elicits various functions including cytokine secretion, antibody-mediated neutralization, and antibody Fc effector functions {i.e., antibody-dependent cellular phagocytosis (ADCP), cytotoxicity of infected cells [antibody-dependent cellular cytotoxicity (ADCC)], and CD8+ T cell mediated}, which collectively controls viral replication and clears infection, thus reducing disease severity and ongoing viral transmission. (F) Correlating these immune features can help identify indicators of protection to inform vaccine development and the use of vaccination in biosecurity programs. Created with BioRender.com.
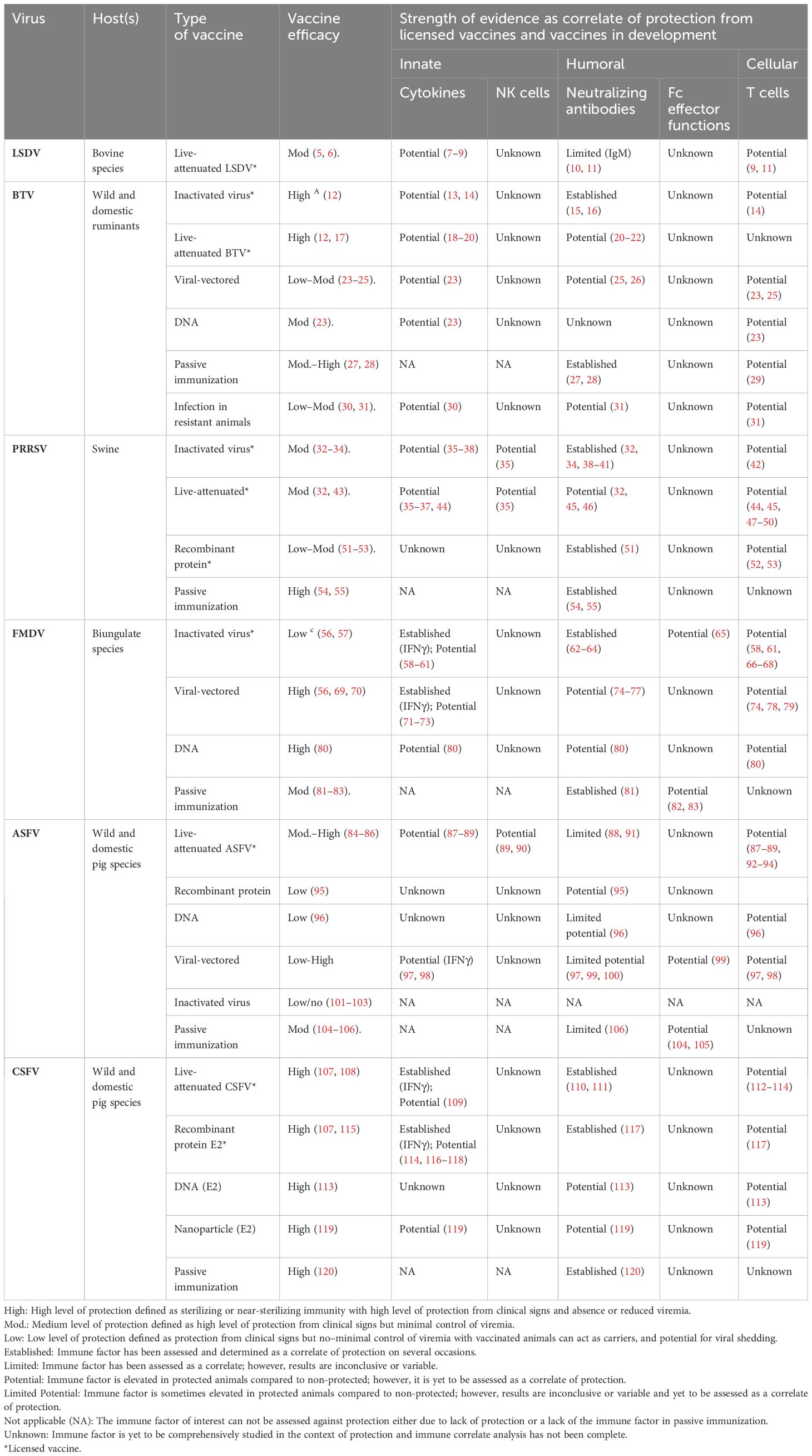
Table 1 Summary table of evidence for innate, humoral, and cellular immune features as correlates of protection against viruses causing disease of livestock.
2 Vaccination in response to high-priority livestock viral diseases
Viral vaccines typically comprise a part of the virus (e.g., sub-unit or recombinant protein), whole viral particles with reduced virulence [e.g., live-attenuated viral (LAV) vaccine], or inactivated viral particles (inactivated vaccine) (121). For the example diseases discussed here, licensed vaccines are most commonly LAVs or inactivated vaccines; however, subunit vaccines for PRRSV and CSFV have also been licensed (Table 1). LAVs generally induce strong cellular and humoral immune responses compared to inactivated and subunit vaccines (13, 122, 123). Inactivated and subunit vaccines generally confer protection through humoral immunity; however, inclusion of adjuvants, appropriate antigen selection, and additional doses can induce cell-mediated immunity (CMI) (13, 122, 123) (Table 1). Vaccination can be applied as part of a response to an incursion of a high-priority viral disease to limit the spread and eventually eradicate the disease (Figures 1A, B) (124, 125). For example, cattle plague caused by rinderpest virus led to an approximately 20% loss of dairy cattle in Europe during the 20th century (126). The elimination of rinderpest virus was achieved in 2011 through multi-organizational campaigns implementing vaccines as a prevention measure as part of a global biosecurity plan (126). Furthermore, control of FMD outbreaks has been achieved through vaccination programs in South Africa and Uruguay (127, 128). Livestock vaccination is an effective biosecurity measure to reduce the economic impact of high-priority livestock diseases (Figure 1). However, the implementation of such vaccines varies between countries and is contingent on various factors including cost, vaccine availability, epidemiological factors, regulatory considerations, and host distribution, and may require vaccine matching to the outbreak strain. For example, a LAV ASFV vaccine has recently been approved for use in Vietnam to help control this devastating virus (129). However, in ASF-free countries such as Australia, this vaccine is not approved due to the risks associated with the use of an ASFV LAV (e.g., reversion to virulence) and is not currently part of Australia’s emergency response plan to an ASF outbreak (130). A major advantage of non-LAV or inactivated vaccine platforms is that these vaccines can be designed in parallel with DIVA diagnostic assays to help inform the culling strategy of an outbreak in an endemic area and facilitate trade.
3 Defining correlates of protection
In addition to clinical signs, protection can be defined by duration and levels of viremia, viral load in tissues, shedding, and transmission to naive or unvaccinated bystander animals (131). Notably, induction of sterilizing immunity (i.e., preventing replication of the virus) following vaccination is the gold standard level of protection (132). Measurable immune features or correlates, including antibodies, cytokines, or T cells, which are statistically associated with and can reliably predict protection, are defined as CoPs (Figures 1E, F) (131). Many factors influence the contribution of an immune feature to protection, including the vaccine delivery platform, antigen(s), host genetics, and the target viral disease (133). CoP analysis assesses the strength of the relationship between the immune feature and protection using animals with varying levels of protection—either immunized/vaccinated animals or naturally resistant animals (131, 134). Single or multiple immune features may be associated with protection for a given disease or vaccine.
Studying the levels of protection achieved through active and passive (transfer of immune sera or antibodies) immunization (134) can provide key insights into the immune response associated with protection and can enable identification of CoPs (Table 1). Notably, much of what is known currently about protective immunity against the focus diseases in this review has come from research on vaccine candidates currently in development, with the exception of commercial vaccines listed in Table 1.
4 Innate immune features as correlates of protection
4.1 Antigen-presenting cell function and counts
Depletion of key antigen-presenting cells including macrophages, monocytes, and/or DCs transiently occurs in animals during infection with ASFV, PRRSV, BTV, FMDV, LSDV, and CSFV (135–138). Depletion of these immune cell subsets during acute infection ultimately hinders the development of protective immunity. Furthermore, downregulation of MHC class I and/or MHC class II expression during FMDV (139), ASFV (140), and PRRSV (141) infection hampers antigen presentation and impairs T-cell responses. Therefore, measuring the number, viability, and MHC expression of these cells could act as an early indicator of vaccine success or failure (Figures 1C-E). However, such changes in cell counts can vary with strain and virulence, as seen in ASFV infection of pigs (142).
4.2 Natural killer cells
Natural killer (NK) cells elicit cytotoxic functions through secretion of cytotoxic molecules (perforin and granzyme) and cytokines, including interferon gamma (IFNγ) and tissue necrosis factor alpha (TNFα) (143). NK cells mediate cytotoxicity directly via cytotoxic molecules or indirectly via antibodies (see Section 5.2) (Figure 1E). NK responses are suppressed following infection with ASFV, FMDV, and PRRSV; however, vaccination can retain NK cell activity (35, 90, 144). Notably, protected pigs showed elevated NK cell cytotoxicity and cytokine secretion compared to non-protected pigs following PRRSV LAV vaccination (Table 1) (35). Similarly, enhanced NK cell activity has also been observed in pigs immunized with a low virulent strain of ASFV upon subsequent challenge with a highly virulent strain, compared to unimmunized pigs (Table 1) (90). Finally, NK cells have shown high levels of cytotoxicity in vitro in bovine and porcine cells infected with FMDV (144, 145). Collectively, the literature suggests that the functional response and frequency of NK cells could be assessed as an indicator of vaccine-mediated protection in the host for ASFV, FMDV, and PRRSV. However, the contribution of NK cells in protective immunity remains to be assessed for CSFV, LSDV, and BTV (Table 1).
4.3 Type I interferon
Viral proteins frequently inhibit the host’s Type I interferon (IFN) production during natural infection via various mechanisms (146–151). Type I IFNs, including IFNα and IFNβ, are anti-viral cytokines secreted by monocytes, macrophages, DCs, and NK cells. In vitro studies where virus-infected cells were treated with Type I IFNs have highlighted the potential for these cytokines to inhibit viral replication of BTV (152), ASFV (153), PRRSV (154), CSFV (155), and FMDV (156). Similarly, elevated Type I IFNs have been implicated in protection from homologous challenge following vaccination for BTV (13, 23) and PRRSV (157). Type I IFN adjuvanted vaccines have protected animals from clinical signs and viremia early post-vaccination in the absence of humoral immunity for FMDV (71, 72) and CSFV (116), providing strong evidence for the contribution of Type I IFN in early protective immunity. Interestingly, the contribution of Type I IFN to protection against FMDV appears to be host specific. Elevated levels of protection have been demonstrated in pigs in contrast to cattle using Adenovirus (Ad5) vectored vaccination adjuvanted with host-specific Type I IFN (71, 72). Notably, elevated levels of Type I IFNs following vaccination—either generated by the vaccine or the adjuvant—can enhance the antibody response, which can subsequently promote a long-lived adaptive immune response (116, 158). The potential role of Type I IFNs in early protection from infection with BTV, PRRSV, CSFV, and FMDV is well established (Table 1). As for ASFV, IFNα may play a role in vaccine-induced recall response to challenge (87), although the definitive role of IFN and other cytokines in protection from ASFV requires further investigation in protected animals. Similarly, the role of Type I IFN in protection from LSDV has not been assessed and should be investigated further.
4.4 Balancing immunopathology and protective immunity
Clinical signs associated with systemic disease caused by ASFV (159–161), BTV (30, 162, 163), PRRSV (146, 164), LSDV (165), and CSV (166, 167) are commonly associated with a “cytokine storm” where overproduction of pro-inflammatory cytokines occurs, leading to extensive immunopathology. The “cytokine storm” has not been reported for FMDV (168). Infection of natural hosts with virulent strains of these viruses have been associated with high levels of cytokines including IL-6, TNFα, IL-1β, IL-8, IFNγ, and/or Type I IFNs (169–178). However, when pro-inflammatory cytokines are generated in a regulated manner to these viruses, they have anti-viral potential (13, 30, 73, 117, 173, 179, 180) and are essential to orchestrating the adaptive protective immune response (87, 181) (Figure 1E) (Table 1). Measurement of cytokines, such as interleukin-2 (IL-2) or IFN gamma (IFNγ), are commonly used as a surrogate measure of CMI and vaccine immunogenicity (182–184). Most notably, protection from FMDV and CSFV has been correlated to IFNγ secretion by T cells (58, 73, 109). Similarly, IFNγ secretion by T cells has also been linked to protection from PRRSV, but does not fully predict protection (36). Furthermore, elevated levels of these cytokines post-vaccination are not always a reliable indicator of protection. Several candidate ASFV vaccines are considered immunogenic and induce a robust IFNγ response but are not protective (88, 160, 185). Owing to secretion of IFNγ by multiple immune cell subsets (various T cells, macrophages, NK cells, etc.), caution must be taken when making conclusions about the role of specific subsets in protection based on such data. Importantly, protection elicited by the innate immune response is not strain specific and, thus, may generate cross-protection to viruses with high antigenic diversity, including BTV, ASFV, and FMDV, and should be studied using vaccinated protected natural hosts (14, 87). The role of cytokines in protection from LSDV remains a large gap in knowledge and, thus, should be studied further (7–9). It is important to note that many different cytokines have a role in protective immunity for these diseases, and therefore, research should aim to investigate cytokines beyond IFNγ as CoPs (30, 37, 44, 59, 60, 118).
5 Humoral immune features as correlates of protection
Following vaccination against a virus of interest, B cells will become activated and differentiate into long-lived memory B cells or short-lived antibody secreting cells called plasmablasts. Antibodies generated from these cells recognize specific epitopes of viral antigens that are exposed on the virion or expressed on the cell surface of infected cells. Antibodies can occur as neutralizing or non-neutralizing antibodies and can elicit various protective immune functions.
5.1 Neutralizing antibodies
The most-utilized CoPs are neutralizing antibody (Nab) titers, with higher antibody titers commonly linked to increased protection (Figures 1E, F). Reduced viral loads and the absence of clinical disease have consistently been associated with higher Nab titers following passive immunization or vaccination against FMDV (sheep, cattle, and pigs) (62–64, 81), PRRSV (15, 32, 51, 54, 55), BTV (15, 26, 27, 31), and CSFV (107, 110, 111, 117, 120) (Table 1). Sterilizing immunity is associated with high Nab titers for PRRSV (54, 186), CSFV (187), and BTV (188, 189). Notably, the longevity of the vaccine-induced Nab response can vary among different vaccines for different diseases (189, 190). While strong Nab responses to PRRSV, FMDV, and BTV can induce strong protection to homologous strains, vaccine-induced protection mediated by Nabs is often strain/genotype specific, due to high levels of antigenic diversity. However, induction of broadly reactive Nabs following active or passive immunization can confer high levels of cross-protection against different strains of PRRSV and CSFV (55, 120, 191). It is also important to note that vaccination against PRRSV, FMDV, and BTV in several instances can elicit protection in the absence of Nabs (192–197).
The role of Nabs in protection from ASFV is a topic of contention. While protection from lethal ASFV infection has been correlated to Nab titers by Silva et al. (2022) (91) following LAV vaccination, many other studies have reported that Nabs were not sufficient for protection following vaccination, for example, with subunit vaccines (Table 1) (198, 199). Similarly, while Nabs can protect cattle against LSDV infection, Nabs are not a reliable indicator of protection following vaccination against LSDV as not all vaccinated animals that are fully protected against LSDV seroconvert (10, 11) (Table 1). This highlights the risk of assessing Nab titers as the sole CoP in all contexts.
5.2 Non-neutralizing antibodies and Fc effector functions
Antibodies bound to viral particles or viral antigens expressed on infected cells engage with Fc receptors (FcRs) on immune cells (e.g., macrophages, DCs, monocytes, and NK cells), resulting in the activation of Fc effector functions. Fc effector functions include antibody-dependent cellular phagocytosis (ADCP) and antibody-dependent cellular cytotoxicity (ADCC), which clear viral particles and kill infected cells to reduce disease severity (Figure 1E) (200, 201). Furthermore, Fc effector functions can enhance cross-protection due to the combined breadth of antibody targets recognized by neutralizing and non-Nabs (202, 203). While the mechanism of protection mediated by non-Nabs is poorly understood, phagocytosis has been shown to play a role. Phagocytosis of FMDV by macrophages was observed in mice that received FMDV non-neutralizing monoclonal antibody treatment and were protected from FMDV challenge, compared to untreated mice that succumbed to infection (65, 82, 83). ADCC has been identified as a potential CoP for ASFV. Elevated levels of ADCC were observed in surviving pigs following passive transfer of anti-ASFV immune sera and following serial immunization with attenuated ASFV compared to non-immunized susceptible pigs (99, 104, 105). However, the contribution of ASFV-specific ADCC to protection is likely vaccine dependent (204).
In rare instances, vaccine-induced antibodies may play a role in antibody-dependent enhancement (ADE) of infections where viral immune complexes are phagocytosed by immune cells and lead to a productive infection (205). ADE in livestock viral infections has been speculated for ASFV, PRRSV, and FMDV (206–208). Most literature surrounding ADE stems from in vitro assays where enhanced levels of viral replication have been detected in the presence of serum antibodies (206). Although well described for dengue virus (209), ADE remains controversial for these livestock diseases as the small number of in vivo studies reported have shown limited evidence of ADE occurring (206, 210).
Research on Fc effector functions such as CoPs has been limited or absent for ASFV, CSFV, BTV, LSDV, PRRSV, and, to a lesser extent, FMDV (Table 1). Thus, there is a need to characterize the role of vaccine-induced Fc effector functions in protection using in vivo models for these livestock diseases to make meaningful conclusions.
6 Cellular immune features as correlates of protection
Cytotoxic or killer T cells (CD8+ T cells) and T helper (Th) cells (CD4+ T cells) play a major role in viral immunity (Figure 1E). The function of T cells for livestock vaccine studies is commonly measured using IFNγ ELISpot, proliferation assays, and flow cytometry. T-cell response can target both structural and non-structural viral proteins, compared to antibody responses, which are generally more effective against structural proteins.
6.1 T cell-dependent antibody response
CD4+ T cells have a key role in generating strong protective humoral immunity through the development of T cell-dependent antibody responses against BTV (211), ASFV (212), and FMDV (213) and should be investigated for CSFV, PRRSV, and LSDV (Table 1). Notably, the depletion of CD4+ helper T cells in sheep enhanced clinical signs of BTV primary infection and impaired the development of Nabs, thus highlighting the importance of these cells in the development of protective antibody-mediated immunity (31). The level of T-cell dependency for generating an antibody response is likely antigen dependent (31). However, while BTV-specific CD4+ helper T-cell responses appear to be crucial in the development of a primary antibody response, CD4+ helper T cells were not activated/expanded in vitro following stimulation (180), and therefore may play a limited role in the memory response to challenge. Measurement of CD4+ T cells could provide a strong indicator of vaccine failure during vaccine development.
6.2 Cytotoxic T lymphocyte response and cell-mediated immunity
Both CD4+ T cells and/or CD8+ T cells can perform antiviral functions through the release of cytotoxic molecules (perforin and granzymes) and cytokines (IFNγ, TNFα, and IL-2), which trigger apoptosis of the infected cell (214). Double-positive CD4+CD8+ T cells are considered a porcine effector memory subset of CD4+ T cells and are strong inducers of cytotoxicity and secretion of IFNγ, and are a major feature in the memory/recall response to vaccination (215). Polyfunctional T-cell responses have been described as important for protective immunity and cross-protection post-vaccination, including proliferation, cytokine secretion, and cytotoxicity for BTV (14, 31), ASFV (87, 92, 93, 182), FMDV (58, 61, 66–68, 216), CSFV (112–114), and PRRSV (42, 47, 217) (Table 1). Intriguingly, unlike the other viruses discussed in this review where Nabs—to homologous strains—are likely to be the primary CoP, CMI appears to be the primary CoP for ASFV (Table 1) (89, 218, 219). Following vaccination against LSDV, T-cell responses increase; however, the functional role of these cells is yet to be studied (9, 11). The importance of CD8+ T cells in protection from ASFV was highlighted through depletion of CD8+ cells (T cells and NK cells) from pigs immunized against ASFV, resulting in complete loss of protection in these pigs to ASFV challenge, compared to total protection observed in pigs immunized with CD8+ cells (92). Finally, early protection elicited post-vaccination is likely to be cell mediated as observed with the CSFV C-strain LAV, which induced protective immunity in the absence of Nabs, associated with CD4+ T-cell proliferation and IFNγ production (220). While total T-cell responses are commonly assessed, antigen-specific T-cell responses have been rarely characterized against high-priority viral diseases of livestock discussed in this review, and the protective potential of other T-cell subsets remains poorly characterized; for example, γδ (gamma delta) T cells and NK T cells.
6.3 T regulatory responses as markers of vaccine failure
It is important to note that some subsets of T cells elicit regulatory functions (221). Known as Tregs, these cells secrete high concentrations of the immunoregulatory IL-10, and excess induction of these responses has been associated with vaccine failure for ASFV (218) and poor clinical outcomes during infection with PRRSV (222, 223). Upregulation of IL-10 following PRRSV vaccination also resulted in reduced induction of an immune response to CSFV vaccination (223). Therefore, secretion of IL-10 by Tregs may act as an indicator of vaccine failure for ASFV and PRRSV. This relationship has not been observed or well characterized following vaccination against FMDV, CSFV, BTV, or LSDV. Further research is needed to elucidate the role of Tregs in protective immunity for disease discussed in this review.
7 Discussion
Substantial progress has been made in identifying protective immune features to various vaccine formulations for the high-consequence viral livestock diseases addressed in this review. Nabs are an established CoP for many of these viruses, and a wealth of research has also implicated Type I IFNs and CMI in early vaccine-mediated protection and protection against heterotypic strains of these viruses (i.e., cross-protection) (Table 1). However, direct analysis of the correlation to the level of protection for many of these immune features has not been fully established (Table 1). Furthermore, the quantitative level of protection for most immune features—except for protective Nab titers against CSFV, FMDV, and PRRSV (Table 1)—is not established. The role of an immune feature in protection can be cemented by performing vaccine dose escalation studies in the natural host and correlating level of protection with the immune feature (Figure 1). Furthermore, research into the protective role of antibody Fc effector functions, antigen-specific T-cell responses, and cytokine responses to new and existing vaccines is limited. CoP studies in the context of high-priority livestock viral diseases is restricted by reagent availability, lack of protective animal models, and limits to the scale; such experiments can typically be performed due to the practical constraints of large animal studies, compared to human vaccine clinical trials or mice pre-clinical vaccine work. While these limitations are a hurdle for livestock vaccine development, inspiration can be derived from human immune assay development and assessment of vaccine CoPs. Such lessons can be drawn from SARS-CoV-2 research (203, 224–230).
Identification of CoPs has three key applications within a biosecurity system, and these are discussed below. Firstly, identified CoPs can be used to guide the rationale for vaccine development (Figure 1F). This can be observed in the abundance of vaccine candidates being developed to generate CMI using DNA or viral vector vaccines against the viruses discussed in this review (122) (Table 1). Furthermore, because of high levels of antigenic diversity of strains/genotypes for these high-priority livestock diseases, vaccines are being designed to induce CMI to more conserved proteins to improve cross-protection (87, 231, 232). CoPs in combination with international vaccine standards, which set the minimum requirements for a vaccine, and DIVA capacity should be considered from the beginning of the vaccine development pipeline (233). Secondly, identification of CoPs facilitates selection of an appropriate immune feature to measure for immune surveillance. For example, Nab titers are easy to quantify and protective thresholds can be defined for vaccines that have established Nab titers as a CoP. Nab titers ≥50 following CSFV vaccination in pigs are sufficient to stop virus transmission from wild-type virus challenge, while titers <32 are inadequate to prevent transmission and clinical signs (110). Immune surveillance can be performed to determine the immune status of animals to be transported or exported during an outbreak, identify vaccine failures, and identify at-risk animals that need to be vaccinated (Figure 1F). Immune surveillance can be paired with assays to differentiate infected from vaccinated animals to further ensure biosecurity is maintained during trade with regions free of the virus. Finally, a general understanding of the CoP and the level of protection can inform the implementation of a vaccine program (Figure 1). For example, the Nab titer of current FMDV vaccines is a reliable indicator of protection against matched strains; however, the correlation between FMDV Nab titers and protection is less predictive of protection to non-matched or heterologous strains (62, 234), thus highlighting the importance of correctly matching the vaccine strain to circulating strains for effective vaccination. This is especially important to consider in regions where multiple virus genotypes or serotypes are circulating (235, 236).
7.1 Conclusion
With the emergence, re-emergence, and global movement of high-priority viral diseases of mammalian livestock, understanding CoPs and their applications to biosecurity programs is critical. Identification of CoPs can provide an evidence-based scientific framework to guide next-generation vaccine development and immune surveillance and maintain biosecurity in the livestock industry. Furthermore, a comprehensive understanding of CoPs can facilitate the identification of sub-optimal vaccines or vaccine failures, thus ensuring resilience of livestock populations, promoting global trade, protecting public health, and contributing to sustainable agricultural practices. However, for many important livestock viral diseases, an in-depth understanding of CoPs is lacking, making it difficult to develop improved next-generation vaccines and implement effective vaccination programs for biosecurity. Livestock research needs to take a holistic and comprehensive approach to identifying CoPs using current and novel technologies to drive vaccine development for successful implementation of vaccines in biosecurity strategies.
Author contributions
SD: Conceptualization, Data curation, Investigation, Project administration, Visualization, Writing – original draft, Writing – review & editing. FJ: Data curation, Investigation, Writing – original draft, Writing – review & editing. QW: Data curation, Investigation, Writing – original draft, Writing – review & editing. MI: Data curation, Investigation, Writing – original draft, Writing – review & editing. AB: Writing – review & editing. DL: Supervision, Writing – review & editing. DW: Supervision, Writing – review & editing. SL: Supervision, Writing – review & editing.
Funding
The author(s) declare financial support was received for the research, authorship, and/or publication of this article. This work was funded by the CSIRO Immune Resilience Future Science Platform (IR-FSP).
Acknowledgments
We would like to acknowledge the broader ASF research team and IR-FSP who have provided helpful discussion and valuable insights into this topic and review.
Conflict of interest
The authors declare that the research was conducted in the absence of any commercial or financial relationships that could be construed as a potential conflict of interest.
Publisher’s note
All claims expressed in this article are solely those of the authors and do not necessarily represent those of their affiliated organizations, or those of the publisher, the editors and the reviewers. Any product that may be evaluated in this article, or claim that may be made by its manufacturer, is not guaranteed or endorsed by the publisher.
References
1. Burnette RN. Redefining Biosecurity by Application in Global Health, Biodefense, and Developing Technologies. In: Applied Biosecurity: Global Health, Biodefense, and Developing Technologies, vol. 24. Springer Nature Switzerland: Springer (2021). p. 1–12.
2. Peng H, Bilal M, Iqbal HM. Improved biosafety and biosecurity measures and/or strategies to tackle laboratory-acquired infections and related risks. Int J Environ Res Public Health. (2018) 15:2697. doi: 10.3390/ijerph15122697
3. Stokstad M, Klem TB, Myrmel M, Oma VS, Toftaker I, Østerås O, et al. Using biosecurity measures to combat respiratory disease in cattle: The Norwegian control program for bovine respiratory syncytial virus and bovine coronavirus. Front Veterinary Sci. (2020) 7:167. doi: 10.3389/fvets.2020.00167
4. Youssef DM, Wieland B, Knight GM, Lines J, Naylor NR. The effectiveness of biosecurity interventions in reducing the transmission of bacteria from livestock to humans at the farm level: a systematic literature review. Zoonoses Public Health. (2021) 68:549–62. doi: 10.1111/zph.12807
5. Tuppurainen E, Dietze K, Wolff J, Bergmann H, Beltran-Alcrudo D, Fahrion A, et al. Vaccines and vaccination against lumpy skin disease. Vaccines. (2021) 9:1136. doi: 10.3390/vaccines9101136
6. Haegeman A, De Leeuw I, Mostin L, Campe WV, Aerts L, Venter E, et al. Comparative evaluation of lumpy skin disease virus-based live attenuated vaccines. Vaccines. (2021) 9:473. doi: 10.3390/vaccines9050473
7. Gari G, Abie G, Gizaw D, Wubete A, Kidane M, Asgedom H, et al. Evaluation of the safety, immunogenicity and efficacy of three capripoxvirus vaccine strains against lumpy skin disease virus. Vaccine. (2015) 33:3256–61. doi: 10.1016/j.vaccine.2015.01.035
8. Varshovi HR, Norian R, Azadmehr A, Afzal Ahangaran N. Immune response characteristics of Capri pox virus vaccines following emergency vaccination of cattle against lumpy skin disease virus. Iranian J Veterinary Sci Technol. (2017) 9:33–40. doi: 10.22067/VETERINARY.V9I2.65381
9. Suwankitwat N, Bhakha K, Molee L, Songkasupa T, Puangjinda K, Chamchoy T, et al. Long-term monitoring of immune response to recombinant lumpy skin disease virus in dairy cattle from small-household farms in western Thailand. Comp Immunol Microbiol Infect Diseases. (2023) 99:102008. doi: 10.1016/j.cimid.2023.102008
10. Milovanović M, Dietze K, Milićević V, Radojičić S, Valčić M, Moritz T, et al. Humoral immune response to repeated lumpy skin disease virus vaccination and performance of serological tests. BMC veterinary Res. (2019) 15:1–9. doi: 10.1186/s12917-019-1831-y
11. Fay PC, Wijesiriwardana N, Munyanduki H, Sanz-Bernardo B, Lewis I, Haga IR, et al. The immune response to lumpy skin disease virus in cattle is influenced by inoculation route. Front Immunol. (2022) 13:6947. doi: 10.3389/fimmu.2022.1051008
12. McVey DS, MacLachlan NJ. Vaccines for prevention of bluetongue and epizootic hemorrhagic disease in livestock: A North American perspective. Vector-Borne Zoonotic Diseases. (2015) 15:385–96. doi: 10.1089/vbz.2014.1698
13. Bitew M, Ravishankar C, Chakravarti S, Kumar Sharma G, Nandi S. Comparative evaluation of T-cell immune response to BTV infection in sheep vaccinated with pentavalent btv vaccine when compared to un-vaccinated animals. Veterinary Med Int. (2019) 2019:8762780. doi: 10.1155/2019/8762780
14. Umeshappa CS, Singh KP, Pandey AB, Singh RP, Nanjundappa RH. Cell-mediated immune response and cross-protective efficacy of binary ethylenimine-inactivated bluetongue virus serotype-1 vaccine in sheep. Vaccine. (2010) 28:2522–31. doi: 10.1016/j.vaccine.2010.01.039
15. Oura C, Wood J, Sanders A, Bin-Tarif , Henstock M, Edwards L, et al. Seroconversion, neutralising antibodies and protection in bluetongue serotype 8 vaccinated sheep. Vaccine. (2009) 27:7326–30. doi: 10.1016/j.vaccine.2009.09.070
16. Breard E, Belbis G, Viarouge C, Nomikou K, Haegeman A, De Clercq K, et al. Evaluation of adaptive immune responses and heterologous protection induced by inactivated bluetongue virus vaccines. Vaccine. (2015) 33:512–8. doi: 10.1016/j.vaccine.2014.11.053
17. Van Rijn PA. Prospects of next-generation vaccines for bluetongue. Front Veterinary Sci. (2019) 6:407. doi: 10.3389/fvets.2019.00407
18. Erasmus B. Virus infections of ruminants. Amsterdam: Bluetongue Virus: Elsevier Ltd (1990) p. 227–37.
19. Dungu B, Gerdes T, Smit T. The use of vaccination in the control of bluetongue in southern Africa. Vet Ital. (2004) 40:616–22.
20. Zhugunissov K, Yershebulov Z, Barakbayev K, Bulatov Y, Taranov D, Amanova Z, et al. Duration of protective immunity after a single vaccination with a live attenuated bivalent bluetongue vaccine. Veterinary Res Commun. (2015) 39:203–10. doi: 10.1007/s11259-015-9643-4
21. van Gennip RG, van de Water SG, Maris-Veldhuis M, van Rijn PA. Bluetongue viruses based on modified-live vaccine serotype 6 with exchanged outer shell proteins confer full protection in sheep against virulent BTV8. PLoS One. (2012) 7(9). doi: 10.1371/journal.pone.0044619
22. Savini G, Monaco F, Calistri P, Panichi G, Ruiu A, Leone A, et al. Neutralising antibody response in cattle after vaccination with monovalent modified-live vaccine against bluetongue virus serotype 2. Vet Ital. (2004) 40:668–70.
23. Calvo-Pinilla E, Rodríguez-Calvo T, Sevilla N, Ortego J. Heterologous prime boost vaccination with DNA and recombinant modified vaccinia virus Ankara protects IFNAR (–/–) mice against lethal bluetongue infection. Vaccine. (2009) 28:437–45. doi: 10.1016/j.vaccine.2009.10.027
24. Wade-Evans A, Romero C, Mellor P, Takamatsu H, Anderson J, Thevasagayam J, et al. Expression of the major core structural protein (VP7) of bluetongue virus, by a recombinant capripox virus, provides partial protection of sheep against a virulent heterotypic bluetongue virus challenge. Virology. (1996) 220:227–31. doi: 10.1006/viro.1996.0306
25. Perrin A, Albina E, Breard E, Sailleau C, Promé S, Grillet C, et al. Recombinant capripoxviruses expressing proteins of bluetongue virus: evaluation of immune responses and protection in small ruminants. Vaccine. (2007) 25:6774–83. doi: 10.1016/j.vaccine.2007.06.052
26. Lobato ZIP, Coupar BEH, Gray CP, Lunt R, Andrew ME. Antibody responses and protective immunity to recombinant vaccinia virus-expressed bluetongue virus antigens. Veterinary Immunol Immunopathol. (1997) 59:293–309. doi: 10.1016/S0165-2427(97)00084-6
27. Letchworth GJ, Appleton JA. Passive protection of mice and sheep against bluetongue virus by a neutralizing monoclonal antibody. Infection Immunity. (1983) 39:208–12. doi: 10.1128/iai.39.1.208-212.1983
28. Jeggo M, Wardley R, Taylor W. Role of neutralising antibody in passive immunity to bluetongue infection. Res veterinary sci. (1984) 36:81–6. doi: 10.1016/S0034-5288(18)32007-1
29. Jeggo M, Wardley R, Brownlie J. Importance of ovine cytotoxic T cells in protection against bluetongue virus infection. Prog Clin Biol Res. (1985) 178:477–87.
30. Hardy A, Bakshi S, Furnon W, MacLean O, Gu Q, Varjak M, et al. The timing and magnitude of the type I interferon response are correlated with disease tolerance in arbovirus infection. mBio. (2023) 14:e00101–23. doi: 10.1128/mbio.00101-23
31. Newbrook K, Khan N, Fisher A, Chong K, Gubbins S, Davies WC, et al. Specific T-cell subsets have a role in anti-viral immunity and pathogenesis but not viral dynamics or onwards vector transmission of an important livestock arbovirus. Front Immunol. (2024) 15:1328820. doi: 10.3389/fimmu.2024.1328820
32. Ding Y, Wubshet AK, Ding X, Zhang Z, Li Q, Dai J, et al. Evaluation of four commercial vaccines for the protection of piglets against the highly pathogenic porcine reproductive and respiratory syndrome virus (hp-PRRSV) QH-08 strain. Vaccines. (2021) 9:1020. doi: 10.3390/vaccines9091020
33. Papatsiros V, Alexopoulos C, Kritas S, Koptopoulos G, Nauwynck H, Pensaert M, et al. Long-term administration of a commercial porcine reproductive and respiratory syndrome virus (PRRSV)-inactivated vaccine in PRRSV-endemically infected sows. J Veterinary Med Ser B. (2006) 53:266–72. doi: 10.1111/j.1439-0450.2006.00965.x
34. Geldhof MF, Vanhee M, Van Breedam W, Van Doorsselaere J, Karniychuk UU, Nauwynck HJ. Comparison of the efficacy of autogenous inactivated Porcine Reproductive and Respiratory Syndrome Virus (PRRSV) vaccines with that of commercial vaccines against homologous and heterologous challenges. BMC veterinary Res. (2012) 8:1–16. doi: 10.1186/1746-6148-8-182
35. Cao QM, Ni Y-Y, Cao D, Tian D, Yugo DM, Heffron CL, et al. Recombinant porcine reproductive and respiratory syndrome virus expressing membrane-bound interleukin-15 as an immunomodulatory adjuvant enhances NK and γδ T cell responses and confers heterologous protection. J Virol. (2018) 92:e00007-18. doi: 10.1128/JVI.00007-18
36. Zuckermann FA, Garcia EA, Luque ID, Christopher-Hennings J, Doster A, Brito M, et al. Assessment of the efficacy of commercial porcine reproductive and respiratory syndrome virus (PRRSV) vaccines based on measurement of serologic response, frequency of gamma-IFN-producing cells and virological parameters of protection upon challenge. Veterinary Microbiol. (2007) 123:69–85. doi: 10.1016/j.vetmic.2007.02.009
37. Lawson S, Lunney J, Zuckermann F, Osorio F, Nelson E, Welbon C, et al. Development of an 8-plex Luminex assay to detect swine cytokines for vaccine development: assessment of immunity after porcine reproductive and respiratory syndrome virus (PRRSV) vaccination. Vaccine. (2010) 28:5356–64. doi: 10.1016/j.vaccine.2010.05.016
38. Vreman S, Stockhofe-Zurwieden N, Popma-de Graaf DJ, Savelkoul HF, Barnier-Quer C, Collin N, et al. Immune responses induced by inactivated porcine reproductive and respiratory syndrome virus (PRRSV) vaccine in neonatal pigs using different adjuvants. Veterinary Immunol Immunopathol. (2021) 232:110170. doi: 10.1016/j.vetimm.2020.110170
39. Misinzo G, Delputte PL, Meerts P, Drexler C, Nauwynck HJ eds. Efficacy of an inactivated PRRSV vaccine: induction of virus-neutralizing antibodies and partial virological protection upon challenge. In: The Nidoviruses: Toward Control of SARS and other Nidovirus Diseases. Boston, MA: Springer.
40. Geldhof MF, Van Breedam W, De Jong E, Rodriguez AL, Karniychuk UU, Vanhee M, et al. Antibody response and maternal immunity upon boosting PRRSV-immune sows with experimental farm-specific and commercial PRRSV vaccines. Veterinary Microbiol. (2013) 167:260–71. doi: 10.1016/j.vetmic.2013.08.017
41. Vanhee M, Delputte PL, Delrue I, Geldhof MF, Nauwynck HJ. Development of an experimental inactivated PRRSV vaccine that induces virus-neutralizing antibodies. Veterinary Res. (2009) 40:63. doi: 10.1051/vetres/2009046
42. Piras F, Bollard S, Laval F, Joisel F, Reynaud G, Charreyre C, et al. Porcine Reproductive and Respiratory Syndrome (PRRS) virus-specific interferon-γ+ T-cell responses after PRRS virus infection or vaccination with an inactivated PRRS vaccine. Viral Immunol. (2005) 18:381–9. doi: 10.1089/vim.2005.18.381
43. Chae C. Commercial PRRS modified-live virus vaccines. Vaccines. (2021) 9:185. doi: 10.3390/vaccines9020185
44. Martelli P, Gozio S, Ferrari L, Rosina S, De Angelis E, Quintavalla C, et al. Efficacy of a modified live porcine reproductive and respiratory syndrome virus (PRRSV) vaccine in pigs naturally exposed to a heterologous European (Italian cluster) field strain: Clinical protection and cell-mediated immunity. Vaccine. (2009) 27:3788–99. doi: 10.1016/j.vaccine.2009.03.028
45. Diaz I, Darwich L, Pappaterra G, Pujols J, Mateu E. Different European-type vaccines against porcine reproductive and respiratory syndrome virus have different immunological properties and confer different protection to pigs. Virology. (2006) 351:249–59. doi: 10.1016/j.virol.2006.03.046
46. Jeong J, Kang I, Kim S, Park SJ, Park K, Oh T, et al. A modified-live porcine reproductive and respiratory syndrome virus (PRRSV)-1 vaccine protects late-term pregnancy gilts against heterologous PRRSV-1 but not PRRSV-2 challenge. Transboundary emerging diseases. (2018) 65:1227–34. doi: 10.1111/tbed.2018.65.issue-5
47. Li Y, Díaz I, Martín-Valls G, Beyersdorf N, Mateu E. Systemic CD4 cytotoxic T cells improve protection against PRRSV-1 transplacental infection. Front Immunol. (2023) 13:1020227. doi: 10.3389/fimmu.2022.1020227
48. Wang G, Yu Y, Zhang C, Tu Y, Tong J, Liu Y, et al. Immune responses to modified live virus vaccines developed from classical or highly pathogenic PRRSV following challenge with a highly pathogenic PRRSV strain. Dev Comp Immunol. (2016) 62:1–7. doi: 10.1016/j.dci.2016.04.019
49. Park C, Seo HW, Han K, Kang I, Chae C. Evaluation of the efficacy of a new modified live porcine reproductive and respiratory syndrome virus (PRRSV) vaccine (Fostera PRRS) against heterologous PRRSV challenge. Veterinary Microbiol. (2014) 172:432–42. doi: 10.1016/j.vetmic.2014.05.030
50. Madapong A, Saeng-Chuto K, Boonsoongnern A, Tantituvanont A, Nilubol D. Cell-mediated immune response and protective efficacy of porcine reproductive and respiratory syndrome virus modified-live vaccines against co-challenge with PRRSV-1 and PRRSV-2. Sci Rep. (2020) 10:1649. doi: 10.1038/s41598-020-58626-y
51. Oh T, Kim H, Park KH, Jeong J, Kang I, Yang S, et al. Effectiveness of a commercial porcine reproductive and respiratory syndrome virus (PRRSV) subunit vaccine against heterologous PRRSV-1 and PRRSV-2 challenge in late-term pregnant gilts. Can J Veterinary Res. (2019) 83:248–54.
52. Tian D, Subramaniam S, Heffron CL, Mahsoub HM, Sooryanarain H, Wang B, et al. Construction and efficacy evaluation of novel swine leukocyte antigen (SLA) class I and class II allele-specific poly-T cell epitope vaccines against porcine reproductive and respiratory syndrome virus. J Gen Virol. (2020) 101:1191–201. doi: 10.1099/jgv.0.001492
53. DT DUY, Kim H, Jeong J, Park KH, Yang S, Oh T, et al. Comparative evaluation of the efficacy of commercial and prototype PRRS subunit vaccines against an HP-PRRSV challenge. J Veterinary Med Sci. (2018) 80:1463–7. doi: 10.1292/jvms.17-0583
54. Lopez OJ, Oliveira MF, Garcia EA, Kwon BJ, Doster A, Osorio FA. Protection against Porcine Reproductive and Respiratory Syndrome Virus (PRRSV) Infection through Passive Transfer of PRRSV-Neutralizing Antibodies Is Dose Dependent. Clin Vaccine Immunol. (2007) 14:269–75. doi: 10.1128/CVI.00304-06
55. Robinson SR, Rahe MC, Gray DK, Martins KV, Murtaugh MP. Porcine reproductive and respiratory syndrome virus neutralizing antibodies provide in vivo cross-protection to PRRSV1 and PRRSV2 viral challenge. Virus Res. (2018) 248:13–23. doi: 10.1016/j.virusres.2018.01.015
56. Kamel M, El-Sayed A, Castañeda Vazquez H. Foot-and-mouth disease vaccines: recent updates and future perspectives. Arch virol. (2019) 164:1501–13. doi: 10.1007/s00705-019-04216-x
57. Elnekave E, Li Y, Zamir L, Even-Tov B, Hamblin P, Gelman B, et al. The field effectiveness of routine and emergency vaccination with an inactivated vaccine against foot and mouth disease. Vaccine. (2013) 31:879–85. doi: 10.1016/j.vaccine.2012.12.011
58. Oh Y, Fleming L, Statham B, Hamblin P, Barnett P, Paton DJ, et al. Interferon-γ induced by in vitro re-stimulation of CD4+ T-cells correlates with in vivo FMD vaccine induced protection of cattle against disease and persistent infection. PLoS One. (2012) 7(9):. doi: 10.1371/journal.pone.0044365
59. Barnard AL, Arriens A, Cox S, Barnett P, Kristensen B, Summerfield A, et al. Immune response characteristics following emergency vaccination of pigs against foot-and-mouth disease. Vaccine. (2005) 23:1037–47. doi: 10.1016/j.vaccine.2004.07.034
60. Mingala CN, Konnai S, Venturina FA, Onuma M, Ohashi K. Quantification of water buffalo (Bubalus bubalis) cytokine expression in response to inactivated foot-and-mouth disease (FMD) vaccine. Res veterinary sci. (2009) 87:213–7. doi: 10.1016/j.rvsc.2009.02.008
61. Mu S, Chen L, Dong H, Li S, Zhang Y, Yin S, et al. Enhanced antigen-specific CD8 T cells contribute to early protection against FMDV through swine DC vaccination. J Virol. (2024) 98:e02002–23. doi: 10.1128/jvi.02002-23
62. Gubbins S, Paton DJ, Dekker A, Ludi AB, Wilsden G, Browning CFJ, et al. Predicting cross-protection against foot-and-mouth disease virus strains by serology after vaccination. Front Veterinary Sci. (2022) 9. doi: 10.3389/fvets.2022.1027006
63. Rieder E, Baxt B, Lubroth J, Mason PW. Vaccines prepared from chimeras of foot-and-mouth disease virus (FMDV) induce neutralizing antibodies and protective immunity to multiple serotypes of FMDV. J virol. (1994) 68:7092–8. doi: 10.1128/jvi.68.11.7092-7098.1994
64. Pay T, Hingley P. Foot and mouth disease vaccine potency test in cattle: the interrelationship of antigen dose, serum neutralizing antibody response and protection from challenge. Vaccine. (1992) 10:699–706. doi: 10.1016/0264-410X(92)90092-X
65. Summerfield A, Gerber H, Schmitt R, Liniger M, Grazioli S, Brocchi E. Relationship between neutralizing and opsonizing monoclonal antibodies against foot-and-mouth disease virus. Front Veterinary Sci. (2022) 9:1033276. doi: 10.3389/fvets.2022.1033276
66. Naessens J, Scheerlinck J-P, De Buysscher EV, Kennedy D, Sileghem M. Effective in vivo depletion of T cell subpopulations and loss of memory cells in cattle using mouse monoclonal antibodies. Veterinary Immunol immunopathol. (1998) 64:219–34. doi: 10.1016/S0165-2427(98)00138-X
67. Guzman E, Taylor G, Charleston B, Skinner MA, Ellis SA. An MHC-restricted CD8+ T-cell response is induced in cattle by foot-and-mouth disease virus (FMDV) infection and also following vaccination with inactivated FMDV. J Gen Virol. (2008) 89:667–75. doi: 10.1099/vir.0.83417-0
68. Zhang Z, Wang D, Yao Y, Yang J, Yang Z, Yang Y. Characterization of T-cell subsets in response to foot-and-mouth disease bivalent inactivated vaccine in Chinese Holstein cows. Microbiol Spectrum. (2023) 11:e01029–23. doi: 10.1128/spectrum.01029-23
69. Hardham JM, Krug P, Pacheco JM, Thompson J, Dominowski P, Moulin V, et al. Novel foot-and-mouth disease vaccine platform: formulations for safe and DIVA-compatible FMD vaccines with improved potency. Front Veterinary Sci. (2020) 7. doi: 10.3389/fvets.2020.554305
70. Grubman MJ, Diaz-San Segundo F, Dias CCA, Moraes MP, Perez-Martin E, De Los Santos T. Use of replication-defective adenoviruses to develop vaccines and biotherapeutics against foot-and-mouth disease. Future Virol. (2012) 7:767–78. doi: 10.2217/fvl.12.65
71. Chinsangaram J, Moraes MP, Koster M, Grubman MJ. Novel viral disease control strategy: adenovirus expressing alpha interferon rapidly protects swine from foot-and-mouth disease. J virol. (2003) 77:1621–5. doi: 10.1128/JVI.77.2.1621-1625.2003
72. Dias CC, Moraes MP, Segundo FD-S, De los Santos T, Grubman MJ. Porcine type I interferon rapidly protects swine against challenge with multiple serotypes of foot-and-mouth disease virus. J Interferon Cytokine Res. (2011) 31:227–36. doi: 10.1089/jir.2010.0055
73. Diaz-San Segundo F, Moraes MP, de Los Santos T, Dias CC, Grubman MJ. Interferon-induced protection against foot-and-mouth disease virus infection correlates with enhanced tissue-specific innate immune cell infiltration and interferon-stimulated gene expression. J virol. (2010) 84:2063–77. doi: 10.1128/JVI.01874-09
74. Romanutti C, D’Antuono A, Palacios C, Quattrocchi V, Zamorano P, La Torre J, et al. Evaluation of the immune response elicited by vaccination with viral vectors encoding FMDV capsid proteins and boosted with inactivated virus. Veterinary Microbiol. (2013) 165:333–40. doi: 10.1016/j.vetmic.2013.04.017
75. Pacheco JM, Brum MC, Moraes MP, Golde WT, Grubman MJ. Rapid protection of cattle from direct challenge with foot-and-mouth disease virus (FMDV) by a single inoculation with an adenovirus-vectored FMDV subunit vaccine. Virology. (2005) 337:205–9. doi: 10.1016/j.virol.2005.04.014
76. Barrera J, Brake DA, Schutta C, Ettyreddy D, Kamicker BJ, Rasmussen MV, et al. Versatility of the adenovirus-vectored foot-and-mouth disease vaccine platform across multiple foot-and-mouth disease virus serotypes and topotypes using a vaccine dose representative of the AdtA24 conditionally licensed vaccine. Vaccine. (2018) 36:7345–52. doi: 10.1016/j.vaccine.2018.10.031
77. Moraes M, Mayr G, Mason P, Grubman M. Early protection against homologous challenge after a single dose of replication-defective human adenovirus type 5 expressing capsid proteins of foot-and-mouth disease virus (FMDV) strain A24. Vaccine. (2002) 20:1631–9. doi: 10.1016/S0264-410X(01)00483-2
78. Su C, Duan X, Zheng J, Liang L, Wang F, Guo L. IFN-α as an adjuvant for adenovirus-vectored FMDV subunit vaccine through improving the generation of T follicular helper cells. PloS One. (2013) 8:e66134. doi: 10.1371/journal.pone.0066134
79. Moraes MP, Diaz-San Segundo F, Dias CC, Pena L, Grubman MJ. Increased efficacy of an adenovirus-vectored foot-and-mouth disease capsid subunit vaccine expressing nonstructural protein 2B is associated with a specific T cell response. Vaccine. (2011) 29:9431–40. doi: 10.1016/j.vaccine.2011.10.037
80. Jin H, Xiao W, Xiao C, Yu Y, Kang Y, Du X, et al. Protective immune responses against foot-and-mouth disease virus by vaccination with a DNA vaccine expressing virus-like particles. Viral Immunol. (2007) 20:429–40. doi: 10.1089/vim.2007.0031
81. Barrionuevo F, Di Giacomo S, Bucafusco D, Ayude A, Schammas J, Miraglia MC, et al. Systemic antibodies administered by passive immunization prevent generalization of the infection by foot-and-mouth disease virus in cattle after oronasal challenge. Virology. (2018) 518:143–51. doi: 10.1016/j.virol.2018.02.012
82. McCullough K, Parkinson D, Crowther J. Opsonization-enhanced phagocytosis of foot-and-mouth disease virus. Immunology. (1988) 65:187.
83. McCullough K, Crowther J, Butcher R, Carpenter W, Brocchi E, Capucci L, et al. Immune protection against foot-and-mouth disease virus studied using virus-neutralizing and non-neutralizing concentrations of monoclonal antibodies. Immunology. (1986) 58:421.
84. Borca MV, Ramirez-Medina E, Silva E, Vuono E, Rai A, Pruitt S, et al. Development of a highly effective African swine fever virus vaccine by deletion of the I177L gene results in sterile immunity against the current epidemic Eurasia strain. J Virol. (2020) 94:e02017-19. doi: 10.1128/JVI.02017-19
85. O'Donnell V, Holinka LG, Gladue DP, Sanford B, Krug PW, Lu X, et al. African swine fever virus Georgia isolate harboring deletions of MGF360 and MGF505 genes is attenuated in swine and confers protection against challenge with virulent parental virus. J virol. (2015) 89:6048–56. doi: 10.1128/JVI.00554-15
86. Deutschmann P, Carrau T, Sehl-Ewert J, Forth JH, Viaplana E, Mancera JC, et al. Taking a promising vaccine candidate further: efficacy of ASFV-G-ΔMGF after intramuscular vaccination of domestic pigs and Oral vaccination of wild boar. Pathogens. (2022) 11:996. doi: 10.3390/pathogens11090996
87. Bosch-Camós L, Alonso U, Esteve-Codina A, Chang C-Y, Martín-Mur B, Accensi F, et al. Cross-protection against African swine fever virus upon intranasal vaccination is associated with an adaptive-innate immune crosstalk. PloS Pathogens. (2022) 18:e1010931. doi: 10.1371/journal.ppat.1010931
88. Carlson J, O’Donnell V, Alfano M, Velazquez Salinas L, Holinka LG, Krug PW, et al. Association of the host immune response with protection using a live attenuated African swine fever virus model. Viruses. (2016) 8:291. doi: 10.3390/v8100291
89. Attreed SE, Silva C, Abbott S, Ramirez-Medina E, Espinoza N, Borca MV, et al. A highly effective African swine fever virus vaccine elicits a memory T cell response in vaccinated swine. Pathogens. (2022) 11:1438. doi: 10.3390/pathogens11121438
90. Leitão A, Cartaxeiro C, Coelho R, Cruz B, Parkhouse R, Portugal FC, et al. The non-haemadsorbing African swine fever virus isolate ASFV/NH/P68 provides a model for defining the protective anti-virus immune response. J Gen Virol. (2001) 82:513–23. doi: 10.1099/0022-1317-82-3-513
91. Silva EB, Krug PW, Ramirez-Medina E, Valladares A, Rai A, Espinoza N, et al. The Presence of Virus Neutralizing Antibodies Is Highly Associated with Protection against Virulent Challenge in Domestic Pigs Immunized with ASFV live Attenuated Vaccine Candidates. Pathogens. (2022) 11:1311. doi: 10.3390/pathogens11111311
92. Oura CA, Denyer M, Takamatsu H, Parkhouse R. In vivo depletion of CD8+ T lymphocytes abrogates protective immunity to African swine fever virus. J Gen Virol. (2005) 86:2445–50. doi: 10.1099/vir.0.81038-0
93. Denyer MS, Wileman TE, Stirling CM, Zuber B, Takamatsu H-H. Perforin expression can define CD8 positive lymphocyte subsets in pigs allowing phenotypic and functional analysis of natural killer, cytotoxic T, natural killer T and MHC un-restricted cytotoxic T-cells. Veterinary Immunol immunopathol. (2006) 110:279–92. doi: 10.1016/j.vetimm.2005.10.005
94. Pedrera M, Soler A, Simón A, Casado N, Pérez C, García-Casado MA, et al. Characterization of the protective cellular immune response in pigs immunized intradermally with the live attenuated African swine fever virus (ASFV) lv17/WB/rie1. Vaccines. (2024) 12:443. doi: 10.3390/vaccines12040443
95. Gómez-Puertas P, Rodríguez F, Oviedo JM, Brun A, Alonso C, Escribano JM. The African swine fever virus proteins p54 and p30 are involved in two distinct steps of virus attachment and both contribute to the antibody-mediated protective immune response. Virology. (1998) 243:461–71. doi: 10.1006/viro.1998.9068
96. Argilaguet JM, Pérez-Martín E, Nofrarías M, Gallardo C, Accensi F, Lacasta A, et al. DNA vaccination partially protects against African swine fever virus lethal challenge in the absence of antibodies. PLoS One. (2012) 7(9):. doi: 10.1371/journal.pone.0040942
97. Zajac MD, Sangewar N, Lokhandwala S, Bray J, Sang H, McCall J, et al. Adenovirus-vectored African swine fever virus pp220 induces robust antibody, IFN-γ, and CTL responses in pigs. Front Veterinary Sci. (2022) 9:921481. doi: 10.3389/fvets.2022.921481
98. Liu W, Li H, Liu B, Lv T, Yang C, Chen S, et al. A new vaccination regimen using adenovirus-vectored vaccine confers effective protection against African swine fever virus in swine. Emerging Microbes Infections. (2023) 12:2233643. doi: 10.1080/22221751.2023.2233643
99. Noll JCG, Rani R, Butt SL, Fernandes MHV, do Nascimento GM, Martins M, et al. Identification of an Immunodominant B-Cell Epitope in African Swine Fever Virus p30 Protein and Evidence of p30 Antibody-Mediated Antibody Dependent Cellular Cytotoxicity. Viruses. (2024) 16:758. doi: 10.3390/v16050758
100. Zajac MD, Trujillo JD, Yao J, Kumar R, Sangewar N, Lokhandwala S, et al. Immunization of pigs with replication-incompetent adenovirus-vectored African swine fever virus multi-antigens induced humoral immune responses but no protection following contact challenge. Front Veterinary Sci. (2023) 10:1208275. doi: 10.3389/fvets.2023.1208275
101. Pikalo J, Porfiri L, Akimkin V, Roszyk H, Pannhorst K, Kangethe RT, et al. Vaccination with a gamma irradiation-inactivated African swine fever virus is safe but does not protect against a challenge. Front Immunol. (2022) 13:832264. doi: 10.3389/fimmu.2022.832264
102. Cadenas-Fernández E, Sánchez-Vizcaíno JM, van Den Born E, Kosowska A, van Kilsdonk E, Fernández-Pacheco P, et al. High doses of inactivated African swine fever virus are safe, but do not confer protection against a virulent challenge. Vaccines. (2021) 9:242. doi: 10.3390/vaccines9030242
103. Blome S, Gabriel C, Beer M. Modern adjuvants do not enhance the efficacy of an inactivated African swine fever virus vaccine preparation. Vaccine. (2014) 32:3879–82. doi: 10.1016/j.vaccine.2014.05.051
104. Wardley R, Norley S, Wilkinson P, Williams S. The role of antibody in protection against African swine fever virus. Veterinary Immunol immunopathol. (1985) 9:201–12. doi: 10.1016/0165-2427(85)90071-6
105. Norley S, Wardley R. Effector mechanisms in the pig. Antibody-dependent cellular cytolysis of African swine fever virus infected cells. Res veterinary sci. (1983) 35:75–9. doi: 10.1016/S0034-5288(18)32207-0
106. Onisk D, Borca M, Kutish S, Kramer E, Irusta P, Rock D. Passively transferred African swine fever virus antibodies protect swine against lethal infection. Virology. (1994) 198:350–4. doi: 10.1006/viro.1994.1040
107. Li F, Li B, Niu X, Chen W, Li Y, Wu K, et al. The development of classical swine fever marker vaccines in recent years. Vaccines. (2022) 10:603. doi: 10.3390/vaccines10040603
108. Luo Y, Ji S, Lei J-L, Xiang G-T, Liu Y, Gao Y, et al. Efficacy evaluation of the C-strain-based vaccines against the subgenotype 2.1 d classical swine fever virus emerging in China. Veterinary Microbiol. (2017) 201:154–61. doi: 10.1016/j.vetmic.2017.01.012
109. Suradhat S, Intrakamhaeng M, Damrongwatanapokin S. The correlation of virus-specific interferon-gamma production and protection against classical swine fever virus infection. Veterinary Immunol immunopathol. (2001) 83:177–89. doi: 10.1016/S0165-2427(01)00389-0
110. Terpstra C, Wensvoort G. The protective value of vaccine-induced neutralising antibody titres in swine fever. Veterinary Microbiol. (1988) 16:123–8. doi: 10.1016/0378-1135(88)90036-3
111. De Smit A, Bouma A, Van Gennip H, De Kluijver E, Moormann R. Chimeric (marker) C-strain viruses induce clinical protection against virulent classical swine fever virus (CSFV) and reduce transmission of CSFV between vaccinated pigs. Vaccine. (2001) 19:1467–76. doi: 10.1016/S0264-410X(00)00347-9
112. Franzoni G, Kurkure NV, Edgar DS, Everett HE, Gerner W, Bodman-Smith KB, et al. Assessment of the phenotype and functionality of porcine CD8 T cell responses following vaccination with live attenuated classical swine fever virus (CSFV) and virulent CSFV challenge. Clin Vaccine Immunol. (2013) 20:1604–16. doi: 10.1128/CVI.00415-13
113. Ganges L, Barrera M, Núñez JI, Blanco I, Frias MT, Rodríguez F, et al. A DNA vaccine expressing the E2 protein of classical swine fever virus elicits T cell responses that can prime for rapid antibody production and confer total protection upon viral challenge. Vaccine. (2005) 23:3741–52. doi: 10.1016/j.vaccine.2005.01.153
114. Graham SP, Haines FJ, Johns HL, Sosan O, La Rocca SA, Lamp B, et al. Characterisation of vaccine-induced, broadly cross-reactive IFN-γ secreting T cell responses that correlate with rapid protection against classical swine fever virus. Vaccine. (2012) 30:2742–8. doi: 10.1016/j.vaccine.2012.02.029
115. Gong W, Li J, Wang Z, Sun J, Mi S, Xu J, et al. Commercial E2 subunit vaccine provides full protection to pigs against lethal challenge with 4 strains of classical swine fever virus genotype 2. Veterinary Microbiol. (2019) 237:108403. doi: 10.1016/j.vetmic.2019.108403
116. Toledo JR, Barrera M, Farnós O, Gómez S, Rodríguez MP, Aguero F, et al. Human αIFN co-formulated with milk derived E2-CSFV protein induce early full protection in vaccinated pigs. Vaccine. (2010) 28:7907–14. doi: 10.1016/j.vaccine.2010.09.073
117. Zhang H, Wen W, Zhao Z, Wang J, Chen H, Qian P, et al. Enhanced protective immunity to CSFV E2 subunit vaccine by using IFN-γ as immunoadjuvant in weaning piglets. Vaccine. (2018) 36:7353–60. doi: 10.1016/j.vaccine.2018.10.030
118. Renson P, Le Dimna M, Gabriel C, Levai R, Blome S, Kulcsar G, et al. Cytokine and immunoglobulin isotype profiles during CP7_E2alf vaccination against a challenge with the highly virulent Koslov strain of classical swine fever virus. Res Veterinary Sci. (2014) 96:389–95. doi: 10.1016/j.rvsc.2014.01.002
119. Song H, Abdullah SW, Pei C, Shi X, Chen X, Ma Y, et al. Self-assembling E2-based nanoparticles improve vaccine thermostability and protective immunity against CSFV. Int J Mol Sci. (2024) 25:596. doi: 10.3390/ijms25010596
120. Xu H, Han G, Lu Y, Liu Z, Tao L, He F. Broad neutralization of CSFV with novel monoclonal antibodies in vivo. Int J Biol Macromolecules. (2021) 173:513–23. doi: 10.1016/j.ijbiomac.2021.01.142
121. Siegrist C-A, Lambert P-H. How vaccines work. In: The vaccine book, 2nd ed. London, United Kingdom: Elsevier (2016). p. 33–42.
122. Ura T, Takeuchi M, Kawagoe T, Mizuki N, Okuda K, Shimada M. Current vaccine platforms in enhancing T-cell response. Vaccines. (2022) 10:1367. doi: 10.3390/vaccines10081367
123. Ghattas M, Dwivedi G, Lavertu M, Alameh M-G. Vaccine technologies and platforms for infectious diseases: Current progress, challenges, and opportunities. Vaccines. (2021) 9:1490. doi: 10.3390/vaccines9121490
124. Roth JA. Veterinary vaccines and their importance to animal health and public health. Proc Vaccinol. (2011) 5:127–36. doi: 10.1016/j.provac.2011.10.009
125. Williams R. The use of vaccination in emergency animal disease responses. Veterinaria Italiana. (2007) 43:225–35.
126. Morens DM, Holmes EC, Davis AS, Taubenberger JK. Global rinderpest eradication: lessons learned and why humans should celebrate too. J Infect Diseases. (2011) 204:502–5. doi: 10.1093/infdis/jir327
127. Ugarte R. Strategy for the control of foot-and-mouth disease in Uruguay. Developments biologicals. (2004) 119:415–21.
128. Brückner G, Vosloo W, Cloete M, Dungu B, Du Plessis B. Foot-and-mouth disease control using vaccination: South African experience. Developments biologicals. (2004) 119:51–62.
129. Tran XH, Le TTP, Nguyen QH, Do TT, Nguyen VD, Gay CG, et al. African swine fever virus vaccine candidate ASFV-G-ΔI177L efficiently protects European and native pig breeds against circulating Vietnamese field strain. Transboundary Emerging Diseases. (2022) 69:e497–504. doi: 10.1111/tbed.14329
130. AusVetPlan. Response strategy African swine fever Animal Health Australia Website2024 . Available online at: https://animalhealthAustralia.com.au//wp-content/uploads/2023/05/AUSVETPLAN-Response-strategy-African-swine-fever.pdf.
131. Plotkin SA, Plotkin SA. Correlates of vaccine-induced immunity. Clin Infect Diseases. (2008) 47:401–9. doi: 10.1086/589862
132. Wahl I, Wardemann H. Sterilizing immunity: understanding COVID-19. Immunity. (2022) 55:2231–5. doi: 10.1016/j.immuni.2022.10.017
133. Zimmermann P, Curtis N. Factors that influence the immune response to vaccination. Clin Microbiol Rev. (2019) 32:e00084-18. doi: 10.1128/CMR.00084-18
134. Hedegaard CJ, Heegaard PM. Passive immunisation, an old idea revisited: Basic principles and application to modern animal production systems. Veterinary Immunol Immunopathol. (2016) 174:50–63. doi: 10.1016/j.vetimm.2016.04.007
135. McColl K, Gould A. Bluetongue virus infection in sheep: haematological changes and detection by polymerase chain reaction. Aust veterinary J. (1994) 71:97–101. doi: 10.1111/j.1751-0813.1994.tb03346.x
136. Bautista EM, Ferman GS, Golde WT. Induction of lymphopenia and inhibition of T cell function during acute infection of swine with foot and mouth disease virus (FMDV). Veterinary Immunol immunopathol. (2003) 92:61–73. doi: 10.1016/S0165-2427(03)00004-7
137. Ma S-m, Mao Q, Yi L, Zhao M-q, Chen J-d. Apoptosis, autophagy, and pyroptosis: immune escape strategies for persistent infection and pathogenesis of classical swine fever virus. Pathogens. (2019) 8:239. doi: 10.3390/pathogens8040239
138. Shilpa DA, Halmandge S, Kasaralikar VR, Ravindra B, Bhagavantappa B, Mallinath K, et al. Study on clinical, haemato-biochemical changes in lumpy skin disease affected cattle in Bidar. Pharma Innovation J. (2022) 11:2170–80.
139. Sanz-Parra A, Sobrino F, Ley V. Infection with foot-and-mouth disease virus results in a rapid reduction of MHC class I surface expression. J Gen virol. (1998) 79:433–6. doi: 10.1099/0022-1317-79-3-433
140. Zhu JJ, Ramanathan P, Bishop EA, O’Donnell V, Gladue DP, Borca MV. Mechanisms of African swine fever virus pathogenesis and immune evasion inferred from gene expression changes in infected swine macrophages. PloS One. (2019) 14:e0223955. doi: 10.1371/journal.pone.0223955
141. Wang X, Eaton M, Mayer M, Li H, He D, Nelson E, et al. Porcine reproductive and respiratory syndrome virus productively infects monocyte-derived dendritic cells and compromises their antigen-presenting ability. Arch virol. (2007) 152:289–303. doi: 10.1007/s00705-006-0857-1
142. Avagyan H, Hakobyan S, Baghdasaryan B, Arzumanyan H, Poghosyan A, Bayramyan N, et al. Pathology and clinics of naturally occurring low-virulence variants of African swine fever emerged in domestic pigs in the South caucasus. Pathogens. (2024) 13:130. doi: 10.3390/pathogens13020130
143. Krijgsman D, Hokland M, Kuppen PJ. The role of natural killer T cells in cancer—a phenotypical and functional approach. Front Immunol. (2018) 9:367. doi: 10.3389/fimmu.2018.00367
144. Patch JR, Dar PA, Waters R, Toka FN, Barrera J, Schutta C, et al. Infection with foot-and-mouth disease virus (FMDV) induces a natural killer (NK) cell response in cattle that is lacking following vaccination. Comp Immunol Microbiol Infect Diseases. (2014) 37:249–57. doi: 10.1016/j.cimid.2014.07.004
145. Toka FN, Nfon CK, Dawson H, Estes DM, Golde WT. Activation of porcine natural killer cells and lysis of foot-and-mouth disease virus infected cells. J Interferon Cytokine Res. (2009) 29:179–92. doi: 10.1089/jir.2008.0058
146. Gómez-Laguna J, Salguero FJ, Pallarés FJ, Carrasco L. Immunopathogenesis of porcine reproductive and respiratory syndrome in the respiratory tract of pigs. Veterinary J. (2013) 195:148–55. doi: 10.1016/j.tvjl.2012.11.012
147. Ayanwale A, Trapp S, Guabiraba R, Caballero I, Roesch F. New insights in the interplay between African swine fever virus and innate immunity and its impact on viral pathogenicity. Front Microbiol. (2022) 13:958307. doi: 10.3389/fmicb.2022.958307
148. Goraya MU, Ziaghum F, Chen S, Raza A, Chen Y, Chi X. Role of innate immunity in pathophysiology of classical swine fever virus infection. Microbial pathogenesis. (2018) 119:248–54. doi: 10.1016/j.micpath.2018.04.020
149. Nfon CK, Ferman GS, Toka FN, Gregg DA, Golde WT. Interferon-α production by swine dendritic cells is inhibited during acute infection with foot-and-mouth disease virus. Viral Immunol. (2008) 21:68–77. doi: 10.1089/vim.2007.0097
150. Nfon CK, Toka FN, Kenney M, Pacheco JM, Golde WT. Loss of plasmacytoid dendritic cell function coincides with lymphopenia and viremia during foot-and-mouth disease virus infection. Viral Immunol. (2010) 23:29–41. doi: 10.1089/vim.2009.0078
151. Liang Z, Wang S, Yao K, Ren S, Cheng P, Qu M, et al. Lumpy skin disease virus ORF127 protein suppresses type I interferon responses by inhibiting K63-linked ubiquitination of tank binding kinase 1. FASEB J. (2024) 38:e23467. doi: 10.1096/fj.202301987RR
152. Rodríguez-Calvo T, Rojas J-M, Martín V, Sevilla N. Type I interferon limits the capacity of bluetongue virus to infect hematopoietic precursors and dendritic cells in vitro and in vivo. J Virol. (2014) 88:859–67. doi: 10.1128/JVI.02697-13
153. Fan W, Jiao P, Zhang H, Chen T, Zhou X, Qi Y, et al. Inhibition of African swine fever virus replication by porcine type I and type II interferons. Front Microbiol. (2020) 11:1203. doi: 10.3389/fmicb.2020.01203
154. Overend C, Mitchell R, He D, Rompato G, Grubman M, Garmendia A. Recombinant swine beta interferon protects swine alveolar macrophages and MARC-145 cells from infection with Porcine reproductive and respiratory syndrome virus. J Gen virol. (2007) 88:925–31. doi: 10.1099/vir.0.82585-0
155. Xia C, Dan W, Wen-Xue W, Jian-Qing W, Li W, Tian-Yao Y, et al. Cloning and expression of interferon-α/γ from a domestic porcine breed and its effect on classical swine fever virus. Veterinary Immunol immunopathol. (2005) 104:81–9. doi: 10.1016/j.vetimm.2004.10.005
156. Zixiang Z, Du Xiaoli LP, Xiangle Z, Fan Y, Weijun C, Hong T, et al. Early growth response gene-1 suppresses foot-and-mouth disease virus replication by enhancing type I interferon pathway signal transduction. Front Microbiol. (2018) 9. doi: 10.3389/fmicb.2018.02326
157. Ke H, Yoo D. The viral innate immune antagonism and an alternative vaccine design for PRRS virus. Veterinary Microbiol. (2017) 209:75–89. doi: 10.1016/j.vetmic.2017.03.014
158. Chabalgoity JA, Baz A, Rial A, Grille S. The relevance of cytokines for development of protective immunity and rational design of vaccines. Cytokine Growth factor Rev. (2007) 18:195–207. doi: 10.1016/j.cytogfr.2007.01.016
159. Gómez-Villamandos J, Bautista M, Sánchez-Cordón P, Carrasco L. Pathology of African swine fever: the role of monocyte-macrophage. Virus Res. (2013) 173:140–9. doi: 10.1016/j.virusres.2013.01.017
160. Takamatsu H-H, Denyer MS, Lacasta A, Stirling CM, Argilaguet JM, Netherton CL, et al. Cellular immunity in ASFV responses. Virus Res. (2013) 173:110–21. doi: 10.1016/j.virusres.2012.11.009
161. Lacasta A, Monteagudo PL, Jiménez-Marín Á, Accensi F, Ballester M, Argilaguet J, et al. Live attenuated African swine fever viruses as ideal tools to dissect the mechanisms involved in viral pathogenesis and immune protection. Veterinary Res. (2015) 46:1–16. doi: 10.1186/s13567-015-0275-z
162. Maclachlan NJ, Drew C, Darpel K, Worwa G. The pathology and pathogenesis of bluetongue. J Comp pathol. (2009) 141:1–16. doi: 10.1016/j.jcpa.2009.04.003
163. Maclachlan NJ, Henderson C, Schwartz-Cornil I, Zientara S. The immune response of ruminant livestock to bluetongue virus: from type I interferon to antibody. Virus Res. (2014) 182:71–7. doi: 10.1016/j.virusres.2013.09.040
164. Zhang WT, Guo YY, Huang JH, Zhang L. Progress in research on inflammation and oxidative stress induced by PRRSV. Hubei Agric Sci. (2021) 60:9.
165. Deepa P, Koppu Vasavi RS, Sravathi V, Rather MM. Immune response to lumpy skin disease virus: Host-virus interactions and immunopathogenesis. Parma Innovation J. (2023) 12:711–4.
166. Barman N. Immunopathogy of classical swine fever. J Immunol Immunopathol. (2018) 20:1–15. doi: 10.5958/0973-9149.2018.00002.3
167. Summerfield A, Ruggli N. Immune responses against classical swine fever virus: between ignorance and lunacy. Front Veterinary Sci. (2015) 2:10. doi: 10.3389/fvets.2015.00010
168. Peng J, Yi J, Yang W, Ren J, Wen Y, Zheng H, et al. Advances in foot-and-mouth disease virus proteins regulating host innate immunity. Front Microbiol. (2020) 11:2046. doi: 10.3389/fmicb.2020.02046
169. Badr Y, Noreldin AE, Elewa YHA, Ahmed MS, Inoshima Y, Baker NM, et al. Cellular infiltration, cytokines, and histopathology of skin lesions associated with different clinical forms and stages of naturally occurring lumpy skin disease in cattle. Comp Immunol Microbiol Infect Diseases. (2022) 90:101894. doi: 10.1016/j.cimid.2022.101894
170. Wang S, Zhang J, Zhang Y, Yang J, Wang L, Qi Y, et al. Cytokine storm in domestic pigs induced by infection of virulent African swine fever virus. Front veterinary sci. (2021) 7:601641. doi: 10.3389/fvets.2020.601641
171. Zuo X, Peng G, Zhao J, Zhao Q, Zhu Y, Xu Y, et al. Infection of domestic pigs with a genotype II potent strain of ASFV causes cytokine storm and lymphocyte mass reduction. Front Immunol. (2024) 15:1361531. doi: 10.3389/fimmu.2024.1361531
172. Thanawongnuwech R, Young TF, Thacker BJ, Thacker EL. Differential production of proinflammatory cytokines: in vitro PRRSV and Mycoplasma hyopneumoniae co-infection model. Veterinary Immunol immunopathol. (2001) 79:115–27. doi: 10.1016/S0165-2427(01)00243-4
173. Royaee AR, Husmann RJ, Dawson HD, Calzada-Nova G, Schnitzlein WM, Zuckermann FA, et al. Deciphering the involvement of innate immune factors in the development of the host response to PRRSV vaccination. Veterinary Immunol immunopathol. (2004) 102:199–216. doi: 10.1016/j.vetimm.2004.09.018
174. Bensaude E, Turner JL, Wakeley PR, Sweetman DA, Pardieu C, Drew TW, et al. Classical swine fever virus induces proinflammatory cytokines and tissue factor expression and inhibits apoptosis and interferon synthesis during the establishment of long-term infection of porcine vascular endothelial cells. J Gen virol. (2004) 85:1029–37. doi: 10.1099/vir.0.19637-0
175. von Rosen T, Lohse L, Nielsen J, Uttenthal Å. Classical swine fever virus infection modulates serum levels of INF-α, IL-8 and TNF-α in 6-month-old pigs. Res veterinary sci. (2013) 95:1262–7. doi: 10.1016/j.rvsc.2013.09.011
176. DeMaula CD, Leutenegger CM, Bonneau KR, MacLachlan NJ. The role of endothelial cell-derived inflammatory and vasoactive mediators in the pathogenesis of bluetongue. Virology. (2002) 296:330–7. doi: 10.1006/viro.2002.1476
177. Channappanavar R, Singh K, Singh R, Umeshappa C, Ingale S, Pandey A. Enhanced proinflammatory cytokine activity during experimental bluetongue virus-1 infection in Indian native sheep. Veterinary Immunol immunopathol. (2012) 145:485–92. doi: 10.1016/j.vetimm.2011.10.013
178. El-Karim DRG, El-Amrawi GA, Salama AR. Serum concentration of some inflammatory cytokines, chemokines and proteins in holstein dairy cows affected with FMDV. J Advanced Veterinary Res. (2022) 12:686–9.
179. de Carvalho Ferreira H, Weesendorp E, Elbers A, Bouma A, Quak S, Stegeman J, et al. African swine fever virus excretion patterns in persistently infected animals: A quantitative approach. Veterinary Microbiol. (2012) 160:327–40. doi: 10.1016/j.vetmic.2012.06.025
180. Rojas J-M, Rodríguez-Calvo T, Sevilla N. Recall T cell responses to bluetongue virus produce a narrowing of the T cell repertoire. Veterinary Res. (2017) 48:1–12. doi: 10.1186/s13567-017-0444-3
181. Tanaka T, Narazaki M, Kishimoto T. IL-6 in inflammation, immunity, and disease. Cold Spring Harbor Perspect Biol. (2014) 6:a016295. doi: 10.1101/cshperspect.a016295
182. Schäfer A, Franzoni G, Netherton CL, Hartmann L, Blome S, Blohm U. Adaptive cellular immunity against African swine fever virus infections. Pathogens. (2022) 11:274. doi: 10.3390/pathogens11020274
183. Tian Y, Wang D, He S, Hao X, Zhu J, Shang S. Immune cell early activation, apoptotic kinetic, and T-cell functional impairment in domestic pigs after ASFV CADC_HN09 strain infection. Front Microbiol. (2024) 15:1328177. doi: 10.3389/fmicb.2024.1328177
184. Huang G, Liu X, Tang X, Du L, Feng W, Hu X, et al. Increased neutralizing antibody production and interferon-γ secretion in response to porcine reproductive and respiratory syndrome virus immunization in genetically modified pigs. Front Immunol. (2017) 8:1110. doi: 10.3389/fimmu.2017.01110
185. Franzoni G, Pedrera M, Sánchez-Cordón PJ. African swine fever virus infection and cytokine response in vivo: an update. Viruses. (2023) 15:233. doi: 10.3390/v15010233
186. Osorio FA, Galeota J, Nelson E, Brodersen B, Doster A, Wills R, et al. Passive transfer of virus-specific antibodies confers protection against reproductive failure induced by a virulent strain of porcine reproductive and respiratory syndrome virus and establishes sterilizing immunity. Virology. (2002) 302:9–20. doi: 10.1006/viro.2002.1612
187. Wang Q, Liu H, Xu L, Li J, Wu H, Yang C, et al. Different clinical presentations of subgenotype 2.1 strain of classical swine fever infection in weaned piglets and adults, and long-term cross-protection conferred by a C-strain vaccine. Veterinary Microbiol. (2021) 253:108915. doi: 10.1016/j.vetmic.2020.108915
188. Boone JD, Balasuriya UB, Karaca K, Audonnet J-C, Yao J, He L, et al. Recombinant canarypox virus vaccine co-expressing genes encoding the VP2 and VP5 outer capsid proteins of bluetongue virus induces high level protection in sheep. Vaccine. (2007) 25:672–8. doi: 10.1016/j.vaccine.2006.08.025
189. Ries C, Beer M, Hoffmann B. BTV antibody longevity in cattle five to eight years post BTV-8 vaccination. Vaccine. (2019) 37:2656–60. doi: 10.1016/j.vaccine.2019.03.082
190. Alexandersen S, Zhang Z, Donaldson AI. Aspects of the persistence of foot-and-mouth disease virus in animals—the carrier problem. Microbes infection. (2002) 4:1099–110. doi: 10.1016/S1286-4579(02)01634-9
191. Martínez-Lobo FJ, Díez-Fuertes F, Simarro I, Castro JM, Prieto C. The ability of porcine reproductive and respiratory syndrome virus isolates to induce broadly reactive neutralizing antibodies correlates with in vivo protection. Front Immunol. (2021) 12:691145. doi: 10.3389/fimmu.2021.691145
192. Sabarth N, Howard MK, Savidis-Dacho H, van Maurik A, Barrett PN, Kistner O. Comparison of single, homologous prime-boost and heterologous prime-boost immunization strategies against H5N1 influenza virus in a mouse challenge model. Vaccine. (2010) 28:650–6. doi: 10.1016/j.vaccine.2009.10.105
193. Stott J, Barber T, Osburn B. Immunologic response of sheep to inactivated and virulent bluetongue virus. Am J veterinary Res. (1985) 46:1043–9.
194. Martín V, Pascual E, Avia M, Pena L, Valcarcel F, Sevilla N. Protective efficacy in sheep of adenovirus-vectored vaccines against bluetongue virus is associated with specific T cell responses. PloS One. (2015) 10:e0143273. doi: 10.1371/journal.pone.0143273
195. Garcıá-Briones M, Blanco E, Chiva C, Andreu D, Ley V, Sobrino F. Immunogenicity and T cell recognition in swine of foot-and-mouth disease virus polymerase 3D. Virology. (2004) 322:264–75. doi: 10.1016/j.virol.2004.01.027
196. Sanz-Parra A, Jimenez-Clavero M, Garcıa-Briones M, Blanco E, Sobrino F, Ley V. Recombinant viruses expressing the foot-and-mouth disease virus capsid precursor polypeptide (P1) induce cellular but not humoral antiviral immunity and partial protection in pigs. Virology. (1999) 259:129–34. doi: 10.1006/viro.1999.9717
197. Nelson EA, Christopher-Hennings J, Benfield DA. Serum immune responses to the proteins of porcine reproductive and respiratory syndrome (PRRS) virus. J Veterinary Diagn Invest. (1994) 6:410–5. doi: 10.1177/104063879400600402
198. Escribano JM, Galindo I, Alonso C. Antibody-mediated neutralization of African swine fever virus: myths and facts. Virus Res. (2013) 173:101–9. doi: 10.1016/j.virusres.2012.10.012
199. Neilan JG, Zsak L, Lu Z, Burrage TG, Kutish GF, Rock DL. Neutralizing antibodies to African swine fever virus proteins p30, p54, and p72 are not sufficient for antibody-mediated protection. Virology. (2004) 319:337–42. doi: 10.1016/j.virol.2003.11.011
200. Gunn BM, Bai S. Building a better antibody through the Fc: advances and challenges in harnessing antibody Fc effector functions for antiviral protection. Hum Vaccines Immunotherapeutics. (2021) 17:4328–44. doi: 10.1080/21645515.2021.1976580
201. Richardson SI, Moore PL. Targeting Fc effector function in vaccine design. Expert Opin Ther Targets. (2021) 25:467–77. doi: 10.1080/14728222.2021.1907343
202. Richardson SI, Manamela NP, Motsoeneng BM, Kaldine H, Ayres F, Makhado Z, et al. SARS-CoV-2 Beta and Delta variants trigger Fc effector function with increased cross-reactivity. Cell Rep Med. (2022) 3:100510. doi: 10.1016/j.xcrm.2022.100510
203. Haycroft ER, Davis SK, Ramanathan P, Lopez E, Purcell RA, Tan LL, et al. Antibody Fc-binding profiles and ACE2 affinity to SARS-CoV-2 RBD variants. Med Microbiol Immunol. (2023) 212:291–305. doi: 10.1007/s00430-023-00773-w
204. Sereda AD, Kazakova AS, Namsrayn SG, Vlasov ME, Kolbasov DV. The attenuated ASFV strains MK-200 and FK-32/135 as possible models for investigation of protective immunity by ASFV infection. PloS One. (2022) 17:e0270641. doi: 10.1371/journal.pone.0270641
205. Narayan R, Tripathi S. Intrinsic ADE: the dark side of antibody dependent enhancement during dengue infection. Front Cell Infection Microbiol. (2020) 10. doi: 10.3389/fcimb.2020.580096
206. Cai H, Zhang H, Cheng H, Liu M, Wen S, Ren J. Progress in PRRSV infection and adaptive immune response mechanisms. Viruses. (2023) 15:1442. doi: 10.3390/v15071442
207. Robinson L, Windsor M, McLaughlin K, Hope J, Jackson T, Charleston B. Foot-and-mouth disease virus exhibits an altered tropism in the presence of specific immunoglobulins, enabling productive infection and killing of dendritic cells. J Virol. (2011) 85:2212–23. doi: 10.1128/JVI.02180-10
208. Sunwoo S-Y, Pérez-Núñez D, Morozov I, Sánchez EG, Gaudreault NN, Trujillo JD, et al. DNA-protein vaccination strategy does not protect from challenge with African swine fever virus Armenia 2007 strain. Vaccines. (2019) 7:12. doi: 10.3390/vaccines7010012
209. Dejnirattisai W, Jumnainsong A, Onsirisakul N, Fitton P, Vasanawathana S, Limpitikul W, et al. Cross-reacting antibodies enhance dengue virus infection in humans. Science. (2010) 328:745–8. doi: 10.1126/science.1185181
210. Zhu JJ. African swine fever vaccinology: the biological challenges from immunological perspectives. Viruses. (2022) 14:2021. doi: 10.3390/v14092021
211. Marín-López A, Otero-Romero I, de la Poza F, Menaya-Vargas R, Calvo-Pinilla E, Benavente J, et al. VP2, VP7, and NS1 proteins of bluetongue virus targeted in avian reovirus muNS-Mi microspheres elicit a protective immune response in IFNAR (–/–) mice. Antiviral Res. (2014) 110:42–51. doi: 10.1016/j.antiviral.2014.07.008
212. Casal I, Vinuela E, Enjuanes L. Synthesis of African swine fever (ASF) virus-specific antibodies in vitro in a porcine leucocyte system. Immunology. (1987) 62:207.
213. Carr BV, Lefevre EA, Windsor MA, Inghese C, Gubbins S, Prentice H, et al. CD4+ T-cell responses to foot-and-mouth disease virus in vaccinated cattle. J Gen Virol. (2013) 94:97–107. doi: 10.1099/vir.0.045732-0
214. Janeway CA Jr., Travers P, Walport M, Shlomchik MJ. T cell-mediated cytotoxicity. Immunobiology: The Immune System in Health and Disease 5th edition. Fith ed. New York, USA: Garland Science (2001).
215. Zuckermann FA, Husmann R. Functional and phenotypic analysis of porcine peripheral blood CD4/CD8 double-positive T cells. Immunology. (1996) 87:500.
216. Abubakar M, Manzoor S, Ahmed A. Interplay of foot and mouth disease virus with cell-mediated and humoral immunity of host. Rev Med Virol. (2018) 28:e1966. doi: 10.1002/rmv.1966
217. An T-Q, Li J-N, Su C-M, Yoo D. Molecular and cellular mechanisms for PRRSV pathogenesis and host response to infection. Virus Res. (2020) 286:197980. doi: 10.1016/j.virusres.2020.197980
218. Sánchez-Cordón PJ, Jabbar T, Chapman D, Dixon LK, Montoya M. Absence of long-term protection in domestic pigs immunized with attenuated African swine fever virus isolate OURT88/3 or BeninΔMGF correlates with increased levels of regulatory T cells and interleukin-10. J Virol. (2020) 94:e00350-20. doi: 10.1128/JVI.00350-20
219. Bosch-Camós L, López E, Collado J, Navas MJ, Blanco-Fuertes M, Pina-Pedrero S, et al. M448R and MGF505-7R: two african swine fever virus antigens commonly recognized by ASFV-specific T-cells and with protective potential. Vaccines. (2021) 9:508. doi: 10.3390/vaccines9050508
220. Xu L, Fan X-Z, Zhao Q-Z, Zhang Z-X, Chen K, Ning Y-b, et al. Effects of vaccination with the C-strain vaccine on immune cells and cytokines of pigs against classical swine fever virus. Viral Immunol. (2018) 31:34–9. doi: 10.1089/vim.2017.0010
221. Luckheeram RV, Zhou R, Verma AD, Xia B. CD4+ T cells: differentiation and functions. Clin Dev Immunol. (2012) 2012:925135. doi: 10.1155/2012/925135
222. Silva-Campa E, Mata-Haro V, Mateu E, Hernández J. Porcine reproductive and respiratory syndrome virus induces CD4+ CD8+ CD25+ Foxp3+ regulatory T cells (Tregs). Virology. (2012) 430:73–80. doi: 10.1016/j.virol.2012.04.009
223. Wang X, Mu G, Dang R, Yang Z. Up-regulation of IL-10 upon PRRSV vaccination impacts on the immune response against CSFV. Veterinary Microbiol. (2016) 197:68–71. doi: 10.1016/j.vetmic.2016.11.007
224. Lopez E, Haycroft ER, Adair A, Mordant FL, O’Neill MT, Pymm P, et al. Simultaneous evaluation of antibodies that inhibit SARS-CoV-2 variants via multiplex assay. JCI Insight. (2021) 6:e150012. doi: 10.1172/jci.insight.150012
225. Mariën J, Michiels J, Heyndrickx L, Nkuba-Ndaye A, Ceulemans A, Bartholomeeusen K, et al. Evaluation of a surrogate virus neutralization test for high-throughput serosurveillance of SARS-CoV-2. J Virological Methods. (2021) 297:114228. doi: 10.1016/j.jviromet.2021.114228
226. Tong X, McNamara R, Avendaño M, Serrano E, García-Salum T, Pardo-Roa C, et al. Waning and boosting of antibody Fc-effector functions upon SARS-CoV-2 vaccination. Nat Commun. (2023) 14:4174. doi: 10.1038/s41467-023-39189-8
227. Lee WS, Selva KJ, Davis SK, Wines BD, Reynaldi A, Esterbauer R, et al. Decay of Fc-dependent antibody functions after mild to moderate COVID-19. Cell Rep Med. (2021) 2:100296. doi: 10.1016/j.xcrm.2021.100296
228. Kaplonek P, Fischinger S, Cizmeci D, Bartsch YC, Kang J, Burke JS, et al. mRNA-1273 vaccine-induced antibodies maintain Fc effector functions across SARS-CoV-2 variants of concern. Immunity. (2022) 55:355–65.e4. doi: 10.1016/j.immuni.2022.01.001
229. Musicò A, Frigerio R, Mussida A, Barzon L, Sinigaglia A, Riccetti S, et al. SARS-CoV-2 epitope mapping on microarrays highlights strong immune-response to N protein region. Vaccines. (2021) 9:35. doi: 10.3390/vaccines9010035
230. Saini SK, Hersby DS, Tamhane T, Povlsen HR, Hernandez SPA, Nielsen M, et al. SARS-CoV-2 genome-wide T cell epitope mapping reveals immunodominance and substantial CD8+ T cell activation in COVID-19 patients. Sci Immunol. (2021) 6:eabf7550. doi: 10.1126/sciimmunol.abf7550
231. Glass E, Millar P. Induction of effective cross-reactive immunity by FMDV peptides is critically dependent upon specific MHC-peptide-T cell interactions. Immunology. (1994) 82:1–8.
232. Balz K, Trassl L, Härtel V, Nelson PP, Skevaki C. Virus-induced T cell-mediated heterologous immunity and vaccine development. Front Immunol. (2020) 11:513. doi: 10.3389/fimmu.2020.00513
233. WOAH. Manual of Diagnostic Tests and Vaccines for Terrestrial Animals, Twelfth ed. Paris, France: World Organisation for Animal Health (WOAH) (2023).
234. Paton D, Reeve R, Capozzo AV, Ludi A. Estimating the protection afforded by foot-and-mouth disease vaccines in the laboratory. Vaccine. (2019) 37:5515–24. doi: 10.1016/j.vaccine.2019.07.102
235. Mahapatra M, Parida S. Foot and mouth disease vaccine strain selection: current approaches and future perspectives. Expert Rev Vaccines. (2018) 17:577–91. doi: 10.1080/14760584.2018.1492378
Keywords: correlates of protection, livestock, biosecurity, vaccines, protection, immunity, antibodies, T cells
Citation: Davis SK, Jia F, Wright QG, Islam MT, Bean A, Layton D, Williams DT and Lynch SE (2024) Defining correlates of protection for mammalian livestock vaccines against high-priority viral diseases. Front. Immunol. 15:1397780. doi: 10.3389/fimmu.2024.1397780
Received: 08 March 2024; Accepted: 28 June 2024;
Published: 19 July 2024.
Edited by:
Pervaiz Dar, Sher-e-Kashmir University of Agricultural Sciences and Technology, IndiaReviewed by:
Sergei Raev, The Ohio State University, United StatesBasavaraj S. Mathapati, Indian Council of Medical Research (ICMR), India
Copyright © 2024 Davis, Jia, Wright, Islam, Bean, Layton, Williams and Lynch. This is an open-access article distributed under the terms of the Creative Commons Attribution License (CC BY). The use, distribution or reproduction in other forums is permitted, provided the original author(s) and the copyright owner(s) are credited and that the original publication in this journal is cited, in accordance with accepted academic practice. No use, distribution or reproduction is permitted which does not comply with these terms.
*Correspondence: Samantha K. Davis, c2FtYW50aGEuZGF2aXNAY3Npcm8uYXU=