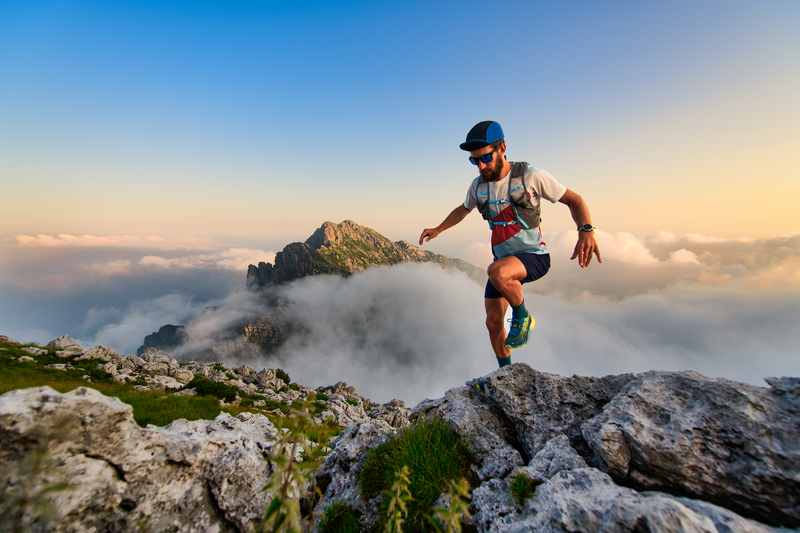
94% of researchers rate our articles as excellent or good
Learn more about the work of our research integrity team to safeguard the quality of each article we publish.
Find out more
MINI REVIEW article
Front. Immunol. , 26 April 2024
Sec. Alloimmunity and Transplantation
Volume 15 - 2024 | https://doi.org/10.3389/fimmu.2024.1396759
This article is part of the Research Topic Biomimetics and Immuno-informed Biomaterials: A Functional Role in Immune Response and In vivo Reprogramming View all 5 articles
Guided bone regeneration (GBR) is one of the most widely used and thoroughly documented alveolar bone augmentation surgeries. However, implanting GBR membranes inevitably triggers an immune response, which can lead to inflammation and failure of bone augmentation. It has been shown that GBR membranes may significantly improve in vivo outcomes as potent immunomodulators, rather than solely serving as traditional barriers. Macrophages play crucial roles in immune responses and participate in the entire process of bone injury repair. The significant diversity and high plasticity of macrophages complicate our understanding of the immunomodulatory mechanisms underlying GBR. This review provides a comprehensive summary of recent findings on the potential role of macrophages in GBR for bone defects in situ. Specifically, macrophages can promote osteogenesis or fibrous tissue formation in bone defects and degradation or fibrous encapsulation of membranes. Moreover, GBR membranes can influence the recruitment and polarization of macrophages. Therefore, immunomodulating GBR membranes are primarily developed by improving macrophage recruitment and aggregation as well as regulating macrophage polarization. However, certain challenges remain to be addressed in the future. For example, developing more rational and sophisticated sequential delivery systems for macrophage activation reagents; addressing the interference of bone graft materials and dental implants; and understanding the correlations among membrane degradation, macrophage responses, and bone regeneration.
Guided bone regeneration (GBR) is one of the most widely used alveolar bone augmentation surgeries owing to its ease of operation and reliable outcomes (1). In GBR, a barrier membrane is placed above the bone defect. This isolates fast-growing soft tissue cells, allowing slow-growing osteoblasts to preferentially occupy the defect and promote bone regeneration (2). Originally, the GBR membrane solely served as a physical barrier to prevent soft tissue penetration and provide space for osteogenesis (3, 4). However, increasing evidence suggests that GBR membranes are biologically active in bone regeneration, affecting osteoblast differentiation and stem cell recruitment (5, 6). Previous studies on GBR membrane materials mostly focused on their physical properties, antibacterial activity, and direct effects on osteoblasts and stem cells, or emphasized materials that do not elicit immune system reactions to ensure “immune safety” (7). However, inconsistent results between in vitro and in vivo conditions indicate a lack of comprehensive consideration of the immune response (8). Implantation of GBR membranes inevitably triggers a material-dependent inflammatory response, which is a significant determinant of regenerative outcomes that can lead to surgical failure (9). Bone-forming and immune cells interact within a shared microenvironment (10), with the local microenvironment, particularly the immune microenvironment, playing a key role in regulating osteogenesis (11). Therefore, it is crucial to consider immunomodulatory properties in the design of GBR membranes.
Macrophages play a crucial role in the immune response to biomaterials and exhibit significant diversity and high plasticity (12, 13). They can directly influence tissue regeneration by secreting cytokines and chemokines and participate in the entire process of damage repair (7, 14). The reduction or depletion of macrophage infiltration in GBR membranes significantly impedes bone regeneration (6, 15), and the status and sequence of macrophage polarization markedly determine the outcomes of GBR (7, 16, 17). Moreover, GBR membranes can influence the recruitment and polarization of macrophages (5, 6, 16), potentially serving as potent immunomodulators rather than traditional barrier, thereby significantly enhancing in vivo outcomes (16). However, the precise role of macrophages in GBR and the modulation of macrophages by GBR membranes remain unclear.
A previous review only summarized the role of collagen membranes in immune regulation (12), and most references in the literature are limited to in vitro experiments or certain subcutaneous and intramuscular implantation experiments, which may not accurately replicate the actual conditions of bone defect repair in situ. Therefore, in this review, we comprehensively summarize the effects of macrophages on bone regeneration in GBR, the modulation of macrophages by GBR membranes in bone defects in situ, and the current experimental progress in the development of immunomodulating GBR membranes. Additionally, we discuss future directions that may offer new insights into alternative strategies for accelerating bone healing in GBR. For instance, developing more rational and sophisticated sequential delivery systems for macrophage activation reagents, modulating the immune microenvironment by regulating the degradation of GBR membranes, and integrating the immune properties of dental implants and bone graft materials to synergistically promote bone healing.
Innate immunity is activated after implanting GBR membranes, with macrophages being one of its most important functional components (18–20). Macrophages play a pivotal role in maintaining physiological bone homeostasis and modulating foreign body reactions to biomaterials (12, 21), and their polarization and spatiotemporal distribution may reflect the inflammatory status (8, 12). We hypothesize that the spatiotemporal expression profile of macrophages may indicate the outcomes of GBR (Figure 1).
Figure 1 Schematic illustration of the potential roles of macrophages in guided bone regeneration of bone defects. A barrier membrane is used to prevent fibroblasts from growing into the defect but its implantation inevitably triggers an immune response. The chemical composition, surface properties, loaded drugs and ions of the GBR membrane affect the recruitment and polarization of macrophages, and macrophages may play a critical role in membrane degradation. The sequential polarization of macrophages is pivotal for the outcome of GBR. In particular, M1 macrophage polarization contributes to phagocytizing bacteria, recruiting MSCs and additional immune cells, promoting osteogenic differentiation and vascular budding and growth, and mediate osteoclastogenesis,. Polarized M2 macrophages participate in bone repair by promoting the recruitment and osteogenic differentiation of MSCs and the maturation and remodeling of neovascularization. Moreover, they also contribute to fibrous encapsulation formation. An effective and timely M1–M2 switch can enhance bone formation. By contrast, the prolonged activation of M1 macrophages will lead to chronic inflammation without osteoanagenesis; premature M2 macrophage polarization and prolonged presence may result in fibrous encapsulation around the GBR membrane, which blocks the interaction between the GBR membrane and bone tissue, thus inhibiting bone healing.
Macrophages are heterogeneous and highly plastic cells that can be broadly characterized into two phenotypes: M1 and M2 (8). Classically activated M1 macrophages are generally considered proinflammatory, mediating inflammatory responses and osteoclastogenesis. In contrast, selectively activated M2 macrophages, known as anti-inflammatory or proregenerative macrophages, are responsible for immunomodulation and tissue remodeling (8, 12, 22).
Macrophages affect bone formation mainly by secreting various cytokines and chemokines. Within 24 h of biomaterial implantation, inflammation reaches its peak, during which M1 macrophages contribute to defending against microbes, amplifying inflammation, and attracting additional immune cells through phagocytosis and secretion of proinflammatory factors (23). It was traditionally believed that proinflammatory M1 macrophages and inflammatory reactions were detrimental to bone regeneration (8). However, increasing evidence suggests that early inflammatory stages and transient M1 macrophage polarization are crucial for bone regeneration in GBR (24, 25). Proinflammatory cytokines secreted by M1 macrophages recruit mesenchymal stem cells (MSCs) to the injury site and promote their proliferation and differentiation, initiating osteoanagenesis (26). Furthermore, M1 macrophages can recruit vascular endothelial progenitor cells to the wound site (16, 27) and promote vascular budding and growth by secreting several angiogenic factors (e.g., vascular endothelial growth factor [VEGF]), initiating angiogenesis and providing nutrients and oxygen for bone regeneration (28–30).
As the tissue insult resolves, M2 macrophages contribute to bone regeneration and remodeling by secreting anti-inflammatory factors, recruiting MSCs to bone defects, and promoting osteogenic differentiation (6). Additionally, M2a macrophages secrete platelet-derived growth factor BB to facilitate endothelial anastomoses of neovascularization and maintain the stability of neovascularization. Meanwhile, M2c macrophages participate in vascular remodeling by secreting matrix metalloproteinase 9 (28–30).
Despite the recognized pro-healing effects, premature M2 macrophage polarization and prolonged presence may be harmful to bone repair. Activated too early, M2 macrophages may actually inhibit healing and hinder vascularization by blocking VEGF function (24). Furthermore, premature or prolonged M2 macrophages secrete a large amount of fibrogenic cytokines, promoting the recruitment and proliferation of fibroblasts. This results in fibrous encapsulation around the GBR membrane, obstructing the interaction between the GBR membrane and bone tissue (25).
Notably, M1 macrophages must transition into M2 macrophages at the appropriate time. Effective and timely phenotype conversion of M1 macrophages can lead to the release of osteogenesis-enhancing cytokines by M2 macrophages, thus enhancing bone formation (8). Conversely, if M1 macrophages fail to switch to M2 macrophages in time, prolonged activation of M1 macrophages will continue to secrete proinflammatory cytokines, resulting in chronic inflammation without osteoanagenesis (24, 31). Furthermore, prolonged M1 polarization may increase the release of fibrosis-enhancing cytokines from M2 macrophages, contributing to the formation of fibrous encapsulation (8).
It is important to note that the classification of macrophages represents a simplification of the complex in vivo scenario. The macrophage population comprises a mixture of macrophage phenotypes at any given time, with macrophages often expressing both M1 and M2 markers, making it challenging to distinguish between M1 and M2 phenotypes (32). This variability may be attributed to changes in stimuli. For instance, experiments have shown that the cytokines secreted by lipopolysaccharide (LPS)-stimulated macrophages may transition from proinflammatory to anti-inflammatory factors when the macrophages become Toll-like receptor-tolerant (33–35). Similarly, M2 macrophages can be induced to transition into M1 macrophages upon exposure to interferon-gamma (IFN-γ) or LPS. However, the mechanisms underlying macrophage phenotypic plasticity remain unclear. In summary, it is the pattern of macrophage switching that determines bone regeneration rather than a specific macrophage phenotype. Therefore, further studies are needed to better understand the pattern of macrophage transformation and its impact on GBR.
In GBR, macrophages aggregate within the membrane compartments and at the top of the bone defect, close to the membrane (27, 36). Therefore, we speculate that the behavior of macrophages is influenced by the GBR membrane. Indeed, it has been reported that the composition, surface properties, porosity, loaded drugs and ions of GBR membranes may modulate the aggregation, recruitment, and polarization of macrophages (Figure 2, Table 1) (5–7, 15–17, 37, 38).
Figure 2 GBR membranes promote bone regeneration by affecting macrophage behavior. The chemical composition, surface properties, porosity, loaded drugs and ions of GBR membranes may modulate the aggregation, recruitment, and polarization of macrophages, thereby improving GBR outcomes.
Table 1 Immunomodulating GBR membranes improve in-situ bone regeneration outcomes through modulating macrophage behavior.
In terms of chemical composition, the material source and processing method can influence the response of macrophages to the material. For example, as a representative of nonabsorbable membranes, macrophage infiltration has been rarely observed in polytetrafluoroethylene membranes (15). Conversely, as a representative of absorbable membranes, although macrophage infiltration is rare and thinly distributed in collagen membranes during the early stages, it is abundant with an M2-dominant profile when the membranes degrade into fragments (6). Furthermore, chemical crosslinking of collagen with carbodiimide has been shown to result in a shift from an M2-dominant profile to an M1-dominant profile in abdominal wall repair (39). This suggests that the processing method of materials should be considered in future research on osteoimmunomodulation.
with regard to surface morphology and roughness, the lattice surface is more conducive to macrophage recruitment and significantly upregulates the expression levels of M2 macrophage marker genes in the osteogenic microenvironment compared to random or aligned surface topologies (37). Surfaces resembling natural bone, with an ordered structure and greater surface roughness, promote macrophage polarization toward M2 during the early stages of bone repair (7).
In terms of porosity, 4D porous structures promote macrophage infiltration by providing more space for macrophages. Conversely, macrophages are only recruited to the surface of the electrospun film and scattered between the membrane and bone defect because of the dense internal structure (6). Additionally, an in vitro experiment showed that larger pore size and higher porosity promoted M2 polarization of macrophages. This finding may provide new insights for studying bone defect repair in situ (40).
With regard to loaded drugs, natural extracts, particles, or bioactive ions, the release of azithromycin promotes the timely transformation of macrophages into proregenerative M2 macrophages during the early stage of bone regeneration and exhibits a lower M1/M2 ratio during the late stage of bone repair (17). Dopamine coating on GBR membranes facilitates M2 macrophage transformation and inhibits M1 macrophage polarization (6). Chu et al. (5) modified collagen membranes with epigallocatechin gallate (EGCG) and discovered that it promotes M2 macrophage recruitment within the GBR membrane and bone defects. Loading of carbon nanohorns improves macrophage infiltration during early bone repair (15), and loading of MnO2 decreases M1 macrophage polarization in bone defects (38). IFN-γ is released in bursts from membranes and stimulates transient M1 macrophage polarization during the early phase, whereas the sustained release of strontium ions promotes M2 polarization during later stages, thus achieving sequential M1–M2 transitions (16).
As the importance of immunomodulatory properties in GBR membrane design has received increasing attention, developing immunomodulating GBR membranes to regulate a favorable immune environment has become a new potential strategy to accelerate bone healing (12, 41). Considering the role of macrophages in GBR, GBR membranes with immunomodulatory abilities are mainly developed by addressing two aspects. (Table 1).
One aspect involves the improvement of macrophage recruitment and aggregation. Kasai et al. (15) loaded carbon nanohorns onto polytetrafluoroethylene membranes to promote macrophage aggregation, which could aid in bone healing. Chu et al. (5) modified collagen membranes with EGCG and Jin et al. (37) prepared poly (lactate-co-glycolate)/fish collagen/nano-hydroxyapatite films with latticed surfaces to enhance the recruitment of M2 macrophages in bone defects. This facilitated growth factor secretion and osteoblast differentiation, thus inducing bone regeneration in vivo. The dense surfaces of electrospun membranes hinder macrophage infiltration. To overcome this disadvantage, Liu et al. (6) developed a GBR membrane with a 4D porous structure to provide more space for infiltrating cells, thereby enabling early and long-term recruitment of macrophages, which could actively participate in bone repair.
Another aspect involves the modulation of the bone immune microenvironment by regulating macrophage polarization. One approach is to promote and/or inhibit M2 and M1 subtype polarization, respectively. Mathew et al. (17) loaded azithromycin onto polycaprolactone membranes, which promoted the early switch of macrophages into proregenerative M2 macrophages and maintained a lower M1/M2 ratio until the late stage of bone repair, resulting in improved bone repair outcomes. Xuan et al. (7) prepared a hierarchical intrafibrillarly mineralized collagen membrane with a nanostructured surface morphology similar to that of natural bone, regulating M2 macrophage polarization and thus improving GBR outcomes. MnO2, capable of catalyzing the decomposition of H2O2,was used to modify GBR membranes, decreasing M1 macrophage polarization in bone defects and improving bone repair outcomes (38). In another study, 4D porous GBR membranes were coated with dopamine, which simultaneously promoted M2 macrophage polarization and inhibited M1 macrophage polarization, thus accelerating angiogenesis and osteogenesis (6). The other approach is to promote sequential M1 and M2 polarization. IFN-γ released in bursts was used to stimulate brief and excessive M1 macrophage polarization during the early stage of bone repair, and sustained release of strontium ions was used to stimulate M2 macrophage polarization during the late stage of bone repair. This resulted in sequential M1–M2 transformation and a significantly higher M2/M1 ratio, strongly promoting vascularization and bone regeneration in situ (16).
Although all of the above methods promoted bone regeneration in GBR, it is worth noting that depletion of M1 macrophages can impair fracture healing (42), and excessive M2 polarization may lead to pathological fibrosis, delaying bone healing (25). Therefore, inducing an appropriate immune environment using biomaterials at a specific time is crucial for bone healing. Precise sequential macrophage polarization may be the focus of future research, rather than blindly promoting M2 macrophage or inhibiting M1 macrophage polarization.
Although immunomodulating GBR membranes are gradually gaining attention, many issues need to be addressed. First, current experiments are limited to small animal studies, necessitating further in vivo and in situ experiments to extrapolate the research findings to humans.
Second, in addition to the factors affecting the recruitment and polarization of macrophages mentioned above, the hydrophilicity and charge of biomaterials also influence macrophage behavior. Hydrophilic and negatively charged biomaterials promote M2 macrophage polarization, whereas hydrophobic and positively charged biomaterials promote M1 macrophage polarization (43). These findings indicate directions for potential strategies for developing immunoreactive GBR membranes.
Sequential polarization of macrophages is critical for the outcome of GBR (24). However, several key aspects remain unclear, including the optimal timing of the M1–M2 transformation, the appropriate M1/M2 ratio and duration for different stages of bone healing, and the mechanism underlying the M1–M2 transformation. Additionally, a major limitation of current sequential drug delivery systems is the lack of distinct separation between the release stages. Many systems that successfully achieve slow controlled delivery of M2 activation reagents do not entirely prevent their release within the initial days. This early release, even in small doses, may induce mixed phenotypes that impair crucial M1 activity (24). Therefore, there is a need for more rational and sophisticated sequential drug delivery systems.
Furthermore, the role of macrophages in GBR has been poorly studied in elderly patients and patients with systemic diseases (e.g., diabetes, systemic lupus erythematosus, and Sjogren’s syndrome) and thus dysfunctional immune systems (44–47). These vulnerable populations should be addressed in future studies.
Notably, when dental implant surgery and GBR are performed simultaneously, the barrier membrane, bone graft material, and dental implant are spatially very close, resulting in a more complex immune microenvironment. Certain materials may maintain macrophage homeostasis and promote bone regeneration, whereas others may elicit serious foreign body reactions, ultimately leading to bone integration failure (12, 48). Modifications of dental implants and bone graft materials can regulate the local immune microenvironment; for example, loading cytokines or altering the forms and surface morphologies of materials (49–52). Therefore, integrating the immune properties of dental implants and bone graft materials to synergistically promote bone healing may be an alternative strategy to improve the outcome of GBR.
Furthermore, the barrier function integrity of the absorbable GBR membrane must correspond to the rate of bone regeneration (53). If the GBR film degrades too quickly, its barrier function may be lost prematurely, thus impeding bone regeneration (6). Conversely, materials that degrade slowly may hinder macrophage spreading onto the surface, leading to the formation of foreign body giant cells (FBGCs) and subsequent fibrous encapsulation (54). This encapsulation prevents direct interactions between the GBR membrane and the surrounding environment. Although the material can persist in the body for sufficient periods, it also isolates the interaction between the GBR membrane and osteoblasts, ultimately impeding bone regeneration (12).
Macrophages may play a crucial role in membrane degradation (55). When exposed to small particles (<5 μm), macrophages mainly participate in degradation through phagocytosis. However, when exposed to larger biomaterials (>10 μm), macrophages coalesce to form FBGCs. This process is induced by interleukin-13, primarily released by T helper 2 cells (9, 54–56). FBGCs exhibit enhanced phagocytic capability by expanding the contact area with the material (55). Moreover, they further degrade materials by releasing reactive oxygen species and degradative enzymes, including matrix metalloproteinases (57). In addition to macrophages, neutrophils are actively involved in membrane degradation by releasing hydrolytic enzymes and oxidative compounds (58).
Moreover, the degradation of collagen membranes may actively drive the transformation of proregenerative macrophages. Chemoattraction by products degraded from collagen could contribute to the recruitment and polarization of M2 macrophages (59, 60). When degradation is delayed or prevented by crosslinkers, polarization of M2 macrophages is inhibited, thereby impeding tissue regeneration (39). Therefore, modulating the immune microenvironment by regulating GBR membrane degradation emerges as a plausible strategy to manipulate GBR outcomes.
The implantation of GBR membranes inevitably activates innate immunity, in which macrophages play a vital role, participating in the entire process of bone healing. The composition, surface properties, loaded drugs, and ions of the GBR membrane affect the recruitment and polarization of macrophages. The sequential polarization of macrophages is pivotal for the outcome of GBR. In particular, early transient M1 macrophage polarization is critical for bone regeneration as it initiates acute inflammation and angiogenesis. M1 macrophages must transition into M2 macrophages at the appropriate time; otherwise, foreign body reactions become unbalanced, impeding bone healing. Polarized M2 macrophages participate in bone repair by promoting the recruitment and osteogenic differentiation of stem cells and the maturation and remodeling of neovascularization. However, the extent and duration of macrophage polarization and the precise timing of the M1–M2 macrophage switch remain unclear. Currently, the development of immunomodulating GBR membranes mainly focuses on regulating the recruitment and polarization of macrophages. Therefore, one of the future directions may involve developing more rational and sophisticated sequential delivery systems for macrophage activation reagents. Additionally, modulating the immune microenvironment by regulating GBR membrane degradation and integrating the immune properties of dental implants and bone graft materials to synergistically promote bone healing may be serve as the alternative strategies to improve the outcome of GBR. In summary, improvements in GBR membranes based on immunomodulation hold great potential for optimizing bone regeneration outcomes.
MG: Investigation, Writing – original draft. HW: Writing – original draft. HX: Writing – original draft, Writing – review & editing. HS: Funding acquisition, Writing – original draft, Writing – review & editing.
The author(s) declare that financial support was received for the research, authorship, and/or publication of this article. This study was supported by The Research Project of Sichuan Medical Association, No. S18012 (HS).
The authors declare that the research was conducted in the absence of any commercial or financial relationships that could be construed as a potential conflict of interest.
All claims expressed in this article are solely those of the authors and do not necessarily represent those of their affiliated organizations, or those of the publisher, the editors and the reviewers. Any product that may be evaluated in this article, or claim that may be made by its manufacturer, is not guaranteed or endorsed by the publisher.
1. Benic GI, Hammerle CH. Horizontal bone augmentation by means of guided bone regeneration. Periodontol 2000. (2014) 66:13–40. doi: 10.1111/prd.12039
2. Gou M, Huang YZ, Hu JG, Jiang YL, Zhang XZ, Su NC, et al. Epigallocatechin-3-gallate cross-linked small intestinal submucosa for guided bone regeneration. ACS Biomater Sci Eng. (2019) 5:5024–35. doi: 10.1021/acsbiomaterials.9b00920
3. Dahlin C, Linde A, Gottlow J, Nyman S. Healing of bone defects by guided tissue regeneration. Plast Reconstr Surg. (1988) 81:672–6. doi: 10.1097/00006534-198805000-00004
4. Retzepi M, Donos N. Guided Bone Regeneration: biological principle and therapeutic applications. Clin Oral Implants Res. (2010) 21:567–76. doi: 10.1111/j.1600-0501.2010.01922.x
5. Chu C, Wang Y, Wang Y, Yang R, Liu L, Rung S, et al. Evaluation of epigallocatechin-3-gallate (EGCG) modified collagen in guided bone regeneration (GBR) surgery and modulation of macrophage phenotype. Mater Sci Eng C Mater Biol Appl. (2019) 99:73–82. doi: 10.1016/j.msec.2019.01.083
6. Liu X, Chen W, Shao B, Zhang X, Wang Y, Zhang S, et al. Mussel patterned with 4D biodegrading elastomer durably recruits regenerative macrophages to promote regeneration of craniofacial bone. Biomaterials. (2021) 276:120998. doi: 10.1016/j.biomaterials.2021.120998
7. Xuan Y, Li L, Ma M, Cao J, Zhang Z. Hierarchical intrafibrillarly mineralized collagen membrane promotes guided bone regeneration and regulates M2 macrophage polarization. Front Bioeng Biotechnol. (2022) 9:781268. doi: 10.3389/fbioe.2021.781268
8. Chen Z, Klein T, Murray RZ, Crawford R, Chang J, Wu C, et al. Osteoimmunomodulation for the development of advanced bone biomaterials. Mater Today. (2016) 19:304–21. doi: 10.1016/j.mattod.2015.11.004
9. Franz S, Rammelt S, Scharnweber D, Simon JC. Immune responses to implants - a review of the implications for the design of immunomodulatory biomaterials. Biomaterials. (2011) 32:6692–709. doi: 10.1016/j.biomaterials.2011.05.078
10. Vishwakarma A, Bhise NS, Evangelista MB, Rouwkema J, Dokmeci MR, Ghaemmaghami AM, et al. Engineering immunomodulatory biomaterials to tune the inflammatory response. Trends Biotechnol. (2016) 34:470–82. doi: 10.1016/j.tibtech.2016.03.009
11. Lin Z, Shen D, Zhou W, Zheng Y, Kong T, Liu X, et al. Regulation of extracellular bioactive cations in bone tissue microenvironment induces favorable osteoimmune conditions to accelerate in situ bone regeneration. Bioact Mater. (2021) 6:2315–30. doi: 10.1016/j.bioactmat.2021.01.018
12. Chu C, Deng J, Sun X, Qu Y, Man Y. Collagen membrane and immune response in guided bone regeneration: recent progress and perspectives. Tissue Eng Part B Rev. (2017) 23:421–35. doi: 10.1089/ten.TEB.2016.0463
13. Roy S, Sharma A, Ghosh S. Mechanistic crosstalk of extracellular calcium-mediated regulation of maturation and plasticity in human monocytes. Biochem Biophys Res Commun. (2023) 643:39–47. doi: 10.1016/j.bbrc.2022.12.054
14. Brancato SK, Albina JE. Wound macrophages as key regulators of repair: origin, phenotype, and function. Am J Pathol. (2011) 178:19–25. doi: 10.1016/j.ajpath.2010.08.003
15. Kasai T, Matsumura S, Iizuka T, Shiba K, Kanamori T, Yudasaka M, et al. Carbon nanohorns accelerate bone regeneration in rat calvarial bone defect. Nanotechnology. (2011) 22:65102. doi: 10.1088/0957-4484/22/6/065102
16. Yang L, Zhou J, Yu K, Yang S, Sun T, Ji Y, et al. Surface modified small intestinal submucosa membrane manipulates sequential immunomodulation coupled with enhanced angio- and osteogenesis towards ameliorative guided bone regeneration. Mater Sci Eng C Mater Biol Appl. (2021) 119:111641. doi: 10.1016/j.msec.2020.111641
17. Mathew A, Vaquette C, Hashimi S, Rathnayake I, Huygens F, Hutmacher DW, et al. Antimicrobial and immunomodulatory surface-functionalized electrospun membranes for bone regeneration. Adv Healthc Mater. (2017) 6. doi: 10.1002/adhm.201601345
18. Sridharan R, Cameron AR, Kelly DJ, Kearney CJ, O’Brien FJ. Biomaterial based modulation of macrophage polarization: a review and suggested design principles. Mater Today. (2015) 18:313–25. doi: 10.1016/j.mattod.2015.01.019
19. Venugopalan V, Vamsi AR, Shenoy SB, Ashok K, Thomas B. Guided bone regeneration-A comprehensive review. J Clin Diagn Res. (2021) 15:ZE01–4. doi: 10.7860/JCDR/2021/47728.14714
20. Michalski MN, McCauley LK. Macrophages and skeletal health. Pharmacol Ther. (2017) 174:43–54. doi: 10.1016/j.pharmthera.2017.02.017
21. Chang MK, Raggatt LJ, Alexander KA, Kuliwaba JS, Fazzalari NL, Schroder K, et al. Osteal tissue macrophages are intercalated throughout human and mouse bone lining tissues and regulate osteoblast function in vitro and in vivo. J Immunol. (2008) 181:1232–44. doi: 10.4049/jimmunol.181.2.1232
22. Majumder N, Roy S, Sharma A, Arora S, Vaishya R, Bandyopadhyay A, et al. Assessing the advantages of 3D bioprinting and 3D spheroids in deciphering the osteoarthritis healing mechanism using human chondrocytes and polarized macrophages. BioMed Mater. (2024) 19:025005. doi: 10.1088/1748-605X/ad1d18
23. Mosser DM, Edwards JP. Exploring the full spectrum of macrophage activation. Nat Rev Immunol. (2008) 8:958–69. doi: 10.1038/nri2448
24. O’Brien EM, Risser GE, Spiller KL. Sequential drug delivery to modulate macrophage behavior and enhance implant integration. Adv Drug Delivery Rev. (2019) 149-150:85–94. doi: 10.1016/j.addr.2019.05.005
25. You J, Zhang Y, Zhou Y. Strontium functionalized in biomaterials for bone tissue engineering: A prominent role in osteoimmunomodulation. Front Bioeng Biotechnol. (2022) 10:928799. doi: 10.3389/fbioe.2022.928799
26. Wei F, Xiao Y. Modulation of the osteoimmune environment in the development of biomaterials for osteogenesis. Adv Exp Med Biol. (2018) 1077:69–86. doi: 10.1007/978-981-13-0947-2_5
27. Toledano M, Vallecillo C, Serrera-Figallo MA, Vallecillo-Rivas M, Gutierrez-Corrales A, Lynch CD, et al. Doped electrospinned material-guides high efficiency regional bone regeneration. Polymers. (2023) 15:1726. doi: 10.3390/polym15071726
28. Li T, Peng M, Yang Z, Zhou X, Deng Y, Jiang C, et al. 3D-printed IFN-gamma-loading calcium silicate-beta-tricalcium phosphate scaffold sequentially activates M1 and M2 polarization of macrophages to promote vascularization of tissue engineering bone. Acta Biomater. (2018) 71:96–107. doi: 10.1016/j.actbio.2018.03.012
29. Spiller KL, Nassiri S, Witherel CE, Anfang RR, Ng J, Nakazawa KR, et al. Sequential delivery of immunomodulatory cytokines to facilitate the M1-to-M2 transition of macrophages and enhance vascularization of bone scaffolds. Biomaterials. (2015) 37:194–207. doi: 10.1016/j.biomaterials.2014.10.017
30. Spiller KL, Anfang RR, Spiller KJ, Ng J, Nakazawa KR, Daulton JW, et al. The role of macrophage phenotype in vascularization of tissue engineering scaffolds. Biomaterials. (2014) 35:4477–88. doi: 10.1016/j.biomaterials.2014.02.012
31. Pajarinen J, Lin T, Gibon E, Kohno Y, Maruyama M, Nathan K, et al. Mesenchymal stem cell-macrophage crosstalk and bone healing. Biomaterials. (2019) 196:80–9. doi: 10.1016/j.biomaterials.2017.12.025
32. Brown BN, Ratner BD, Goodman SB, Amar S, Badylak SF. Macrophage polarization: an opportunity for improved outcomes in biomaterials and regenerative medicine. Biomaterials. (2012) 33:3792–802. doi: 10.1016/j.biomaterials.2012.02.034
33. Stout RD, Jiang C, Matta B, Tietzel I, Watkins SK, Suttles J. Macrophages sequentially change their functional phenotype in response to changes in microenvironmental influences. J Immunol. (2005) 175:342–9. doi: 10.4049/jimmunol.175.1.342
34. Foster SL, Hargreaves DC, Medzhitov R. Gene-specific control of inflammation by TLR-induced chromatin modifications. Nature. (2007) 447:972–8. doi: 10.1038/nature05836
35. Biswas SK, Lopez-Collazo E. Endotoxin tolerance: new mechanisms, molecules and clinical significance. Trends Immunol. (2009) 30:475–87. doi: 10.1016/j.it.2009.07.009
36. Alkildani S, Ren Y, Liu L, Rimashevskiy D, Schnettler R, Radenković M, et al. Analyses of the cellular interactions between the ossification of collagen-based barrier membranes and the underlying bone defects. Int J Mol Sci. (2023) 24:6833. doi: 10.3390/ijms24076833
37. Jin S, Yang R, Chu C, Hu C, Zou Q, Li Y, et al. Topological structure of electrospun membrane regulates immune response, angiogenesis and bone regeneration. Acta Biomater. (2021) 129:148–58. doi: 10.1016/j.actbio.2021.05.042
38. Liu S, Yang H, Zhang L, Bianco A, Ma B, Ge S. Multifunctional barrier membranes promote bone regeneration by scavenging H2O2, generating O2, eliminating inflammation, and regulating immune response. Colloids Surf B Biointerfaces. (2023) 222:113147. doi: 10.1016/j.colsurfb.2023.113147
39. Badylak SF, Valentin JE, Ravindra AK, McCabe GP, Stewart-Akers AM. Macrophage phenotype as a determinant of biologic scaffold remodeling. Tissue Eng Part A. (2008) 14:1835–42. doi: 10.1089/ten.tea.2007.0264
40. Garg K, Pullen NA, Oskeritzian CA, Ryan JJ, Bowlin GL. Macrophage functional polarization (M1/M2) in response to varying fiber and pore dimensions of electrospun scaffolds. Biomaterials. (2013) 34:4439–51. doi: 10.1016/j.biomaterials.2013.02.065
41. Chen Z, Chen L, Liu R, Lin Y, Chen S, Lu S, et al. The osteoimmunomodulatory property of a barrier collagen membrane and its manipulation: Via coating nanometer-sized bioactive glass to improve guided bone regeneration. Biomater Sci. (2018) 6:1007–19. doi: 10.1039/c7bm00869d
42. Hozain S, Cottrell J. CDllb+ targeted depletion of macrophages negatively affects bone fracture healing. Bone. (2020) 138:115479. doi: 10.1016/j.bone.2020.115479
43. Toledano-Osorio M, Manzano-Moreno FJ, Ruiz C, Toledano M, Osorio R. Testing active membranes for bone regeneration: A review. J Dent. (2021) 105:103580. doi: 10.1016/j.jdent.2021.103580
44. Louiselle AE, Niemiec SM, Zgheib C, Liechty KW. Macrophage polarization and diabetic wound healing. Transl Res. (2021) 236:109–16. doi: 10.1016/j.trsl.2021.05.006
45. Gibon E, Loi F, Córdova LA, Pajarinen J, Lin T, Lu L, et al. Aging affects bone marrow macrophage polarization: relevance to bone healing. Regener Eng Transl Med. (2016) 2:98–104. doi: 10.1007/s40883-016-0016-5
46. Ahamada MM, Jia Y, Wu X. Macrophage polarization and plasticity in systemic lupus erythematosus. Front Immunol. (2021) 12:734008. doi: 10.3389/fimmu.2021.734008
47. Srivastava A, Makarenkova HP. Innate immunity and biological therapies for the treatment of sjögren’s syndrome. Int J Mol Sci. (2020) 21:9172. doi: 10.3390/ijms21239172
48. Chakraborty J, Roy S, Ghosh S. 3D printed hydroxyapatite promotes congruent bone ingrowth in rat load bearing defects. BioMed Mater. (2022) 17:035008. doi: 10.1088/1748-605X/ac6471
49. Fujioka-Kobayashi M, Marjanowski SD, Kono M, Katagiri H, Miron RJ, Schaller B. In vitro comparison of macrophage polarization and osteoblast differentiation potentials between granules and block forms of deproteinized bovine bone mineral. Mater (Basel). (2020) 13:2682. doi: 10.3390/ma13122682
50. He XT, Li X, Zhang M, Tian BM, Sun LJ, Bi CS, et al. Role of molybdenum in material immunomodulation and periodontal wound healing: Targeting immunometabolism and mitochondrial function for macrophage modulation. Biomaterials. (2022) 283:121439. doi: 10.1016/j.biomaterials.2022.121439
51. He Y, Gao Y, Ma Q, Zhang X, Zhang Y, Song W. Nanotopographical cues for regulation of macrophages and osteoclasts: emerging opportunities for osseointegration. J Nanobiotechnol. (2022) 20:510. doi: 10.1186/s12951-022-01721-1
52. Zhao DW, Ren B, Wang HW, Zhang X, Yu MZ, Cheng L, et al. 3D-printed titanium implant combined with interleukin 4 regulates ordered macrophage polarization to promote bone regeneration and angiogenesis. Bone Joint Res. (2021) 10:411–24. doi: 10.1302/2046-3758.107.BJR-2020-0334.R4
53. Rakhmatia YD, Ayukawa Y, Furuhashi A, Koyano K. Current barrier membranes: titanium mesh and other membranes for guided bone regeneration in dental applications. J Prosthodont Res. (2013) 57:3–14. doi: 10.1016/j.jpor.2012.12.001
54. Anderson JM, Rodriguez A, Chang DT. Foreign body reaction to biomaterials. Semin Immunol. (2008) 20:86–100. doi: 10.1016/j.smim.2007.11.004
55. Fang J, Liu R, Chen S, Liu Q, Cai H, Lin Y, et al. Tuning the immune reaction to manipulate the cell-mediated degradation of a collagen barrier membrane. Acta Biomater. (2020) 109:95–108. doi: 10.1016/j.actbio.2020.03.038
56. Xia Z, Triffitt JT. A review on macrophage responses to biomaterials. BioMed Mater. (2006) 1:R1–9. doi: 10.1088/1748-6041/1/1/R01
57. Luttikhuizen DT, van Amerongen MJ, de Feijter PC, Petersen AH, Harmsen MC, van Luyn MJ. The correlation between difference in foreign body reaction between implant locations and cytokine and MMP expression. Biomaterials. (2006) 27:5763–70. doi: 10.1016/j.biomaterials.2006.07.004
58. Labow RS, Meek E, Santerre JP. Neutrophil-mediated biodegradation of medical implant materials. J Cell Physiol. (2001) 186:95–103. doi: 10.1002/1097-4652(200101)186:1<95::AID-JCP1008>3.0.CO;2-0
59. Badylak SF, Gilbert TW. Immune response to biologic scaffold materials. Semin Immunol. (2008) 20:109–16. doi: 10.1016/j.smim.2007.11.003
Keywords: macrophage, guided bone regeneration, osteoimmunomodulation, innate immunity, macrophage polarization
Citation: Gou M, Wang H, Xie H and Song H (2024) Macrophages in guided bone regeneration: potential roles and future directions. Front. Immunol. 15:1396759. doi: 10.3389/fimmu.2024.1396759
Received: 06 March 2024; Accepted: 15 April 2024;
Published: 26 April 2024.
Edited by:
Subhadeep Roy, National Institute of Pharmaceutical Education and Research, IndiaReviewed by:
Ruby Sharma, Albert Einstein College of Medicine, United StatesCopyright © 2024 Gou, Wang, Xie and Song. This is an open-access article distributed under the terms of the Creative Commons Attribution License (CC BY). The use, distribution or reproduction in other forums is permitted, provided the original author(s) and the copyright owner(s) are credited and that the original publication in this journal is cited, in accordance with accepted academic practice. No use, distribution or reproduction is permitted which does not comply with these terms.
*Correspondence: Hongjie Song, c2hqODE4MjAwMkBzb2h1LmNvbQ==; Huiqi Xie, eGllaHVpcWlAc2N1LmVkdS5jbg==
Disclaimer: All claims expressed in this article are solely those of the authors and do not necessarily represent those of their affiliated organizations, or those of the publisher, the editors and the reviewers. Any product that may be evaluated in this article or claim that may be made by its manufacturer is not guaranteed or endorsed by the publisher.
Research integrity at Frontiers
Learn more about the work of our research integrity team to safeguard the quality of each article we publish.