- 1Aten Porus Lifesciences Pvt. Ltd., Bengaluru, India
- 2Avammune Therapeutics, Philadelphia, PA, United States
Immunotherapy has proved to be a breakthrough in cancer treatment. So far, a bulk of the approved/late-stage cancer immunotherapy are antibody-based. Although these antibody-based drugs have demonstrated great promise, a majority of them are limited due to their access to extracellular targets, lack of oral bioavailability, tumor microenvironment penetration, induction of antibody dependent cytotoxicity etc. In recent times, there has been an increased research focus on the development of small molecule immunomodulators since they have the potential to overcome the aforementioned limitations posed by antibodies. Furthermore, while most biologics based therapeutics that are in clinical use are limited to modulating the adaptive immune system, very few clinically approved therapeutic modalities exist that modulate the innate immune system. The innate immune system, which is the body’s first line of defense, has the ability to turn cold tumors hot and synergize strongly with existing adaptive immune modulators. In preclinical studies, small molecule innate immune modulators have demonstrated synergistic efficacy as combination modalities with current standard-of-care immune checkpoint antibodies. In this review, we highlight the recent advances made by small molecule innate immunomodulators in cancer immunotherapy.
Introduction
Immunotherapy is a type of therapy which uses a patient’s own immune system to fight the disease (1, 2). Recent advances in cancer therapy have highlighted the emergence and frequent use of immunotherapy as an alternative for hard to treat, refractory/relapse cases (3). Current immuno-oncology therapeutics are based on antibodies that target various proteins and receptors. These act as immune checkpoints to block immune activation in the tumor microenvironment (TME) (3–5).
Several monoclonal antibodies that target immune checkpoints are clinically approved for cancer therapy (6). Antibodies targeting immune checkpoints such as programmed cell death-1 (PD-1) and its ligand PD-L1 are now approved for various advanced cancers (7–10). In general, the immunoglobulin backbone poses PK challenges such as long half-life (9-21 days), natural killer mediated antibody-dependent cellular cytotoxicity which increases the chances of adverse events and poor bioactivity (11–14). Lack of oral bioavailability and limited solubility leads to feasibility challenges like maximal administrable volume for the intravenous (IV), intramuscular (IM), subcutaneous (SC) or intratumor (IT) delivery (13, 15). Apart from these concerns, antibody therapy is challenged by poor anti-tumor efficacy in some solid tumors due to lack of cell permeability resulting in the inability to recognize intracellular targets (16).
In contrast to therapeutic antibodies, small molecule immunomodulators have superior drug-like characteristics in terms of pharmacokinetic properties such as oral bioavailability, reasonable half-life, and membrane permeability (17–19). Apart from this, patient adherence is trickier with antibody therapy due to the intravenous administration while small molecules are often administered orally as a pill or tablet form allowing for better patient compliance. Interestingly, various preclinical and clinical studies have reported that small molecule immunomodulators when used as combination modalities with antibody therapy demonstrates synergistic anti-tumor efficacy and also used as adjuvants in cancer treatment (20–23). Surprisingly, majority of the focus has so far been towards harnessing the adaptive immune system by inhibiting the immunosuppressive signaling with immune checkpoint inhibitors. Tumor microenvironment (TME) comprises of multiple components such as the adaptive immune cells like (T and B cells) and innate immune cells like macrophages, dendritic cells, myeloid-derived suppressor cells, neutrophils, natural killer cells. Cell types such as macrophages, dendritic cells and natural killer cells that perform the routine surveillance are the first to encounter cancer cells (24, 25). In this review, we summarize the recent advances in small molecules innate immunomodulators being developed for treating cancer.
Innate immune system in cancer
Innate immune cells detects and processes cancer antigens and can directly eradicate tumors by its effector responses such as cytotoxicity and phagocytosis (26–31). Various pathways are involved in triggering the innate immune pathways (Figure 1). Antigen presenting cells such as dendritic cells and macrophages utilizes a specific type of DNA sensor known as cGAS/STING pathway. The cGAS/STING pathway senses chromosomal instabilities, double stranded breaks and fragmented DNA in cancer cells (32–34). Tumor derived DNA fragments resulting from genomic instability, mitochondrial dysfunction, and oxidative stress are sensed by the cGAS protein. cGAS converts ATP and GTP into a cyclic dinucleotide (CDN) known as 2’3’-cGAMP, a secondary messenger which acts as a ligand for STING protein and results in activation of the pathway (35–39). Various studies have demonstrated that tumor derived 2′3′-cGAMP molecules act as an immune activator and directly trigger anti-tumor immunity (40–44). Ecto-nucleotide pyrophosphatase/phosphodiesterase-1 (ENPP1/NPP1), a membrane-bound nucleotide hydrolase hydrolyzes 2’3’-cGAMP and dampen the STING-dependent anti-tumor innate immune response (Figure 1). ENPP1 acts as a negative regulator for the STING pathway (45). Interestingly, higher expression of ENPP1 has been directly linked with cancer progression, metastasis, and poor response to immunotherapy (46–48).
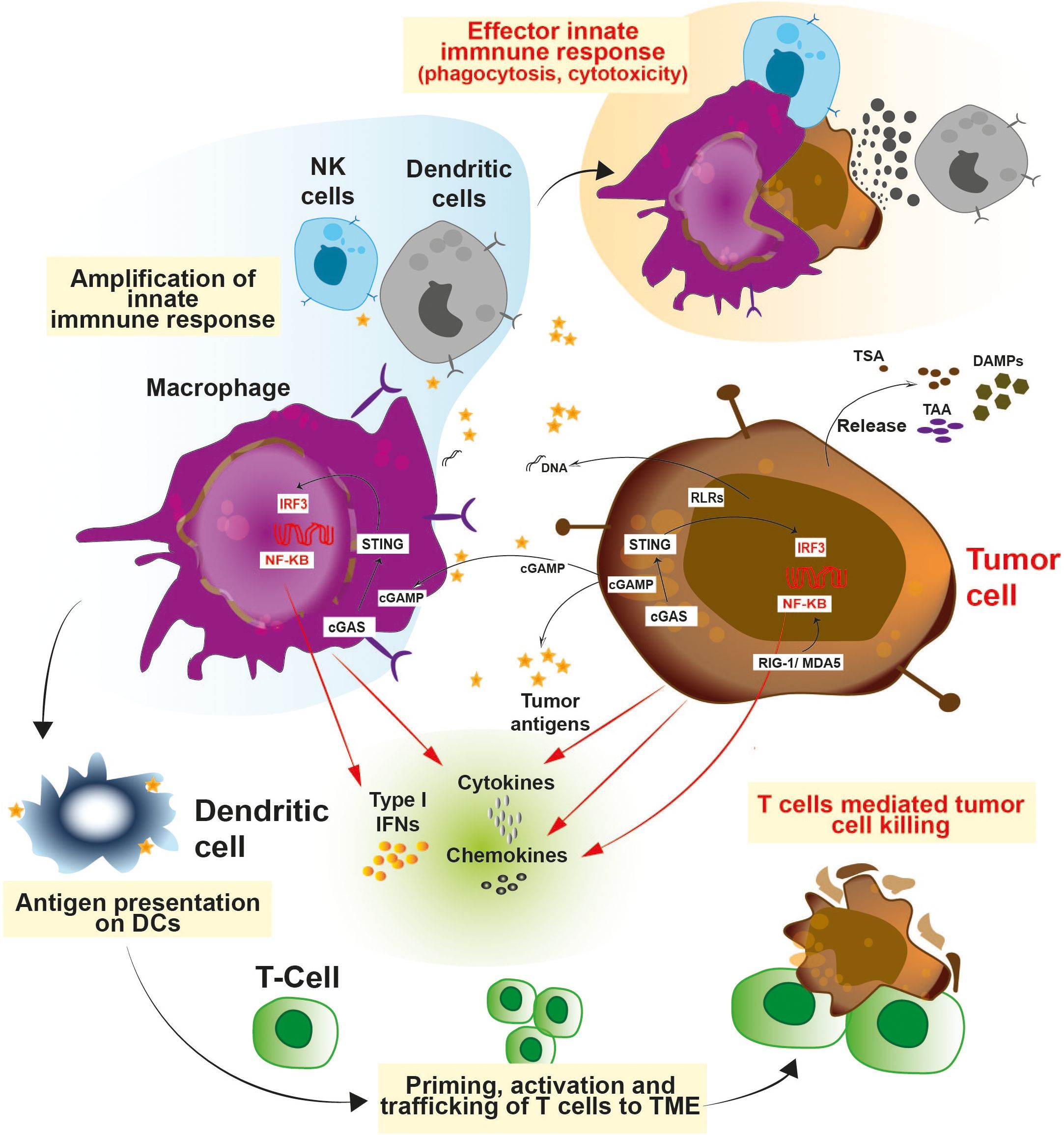
Figure 1. Schematic representation of the various innate immune pathways: Innate immune cells consists of a battery of sensors which equip them to fight against infection and cancer. Activation of STING and RIG-I pathways exerts a robust interferon response which allows the immune system to act against cancer and also curtail infection. Release of type 1 interferons, cytokines and chemokines facilitates the trafficking of the T cells to the TME and primes their activation. The cross-talk between innate and adaptive immune cells enables a potent anti-tumor immunity.
Besides the cGAS/STING pathway, other immunomodulatory mechanisms involved in cancer include Toll-like receptor (TLRs), RIG-I, NLRP3, and CD47-SIRPα pathways (Figure 1) (49–51). TLRs are damage or pattern recognition molecules responsible for immune activation. For e.g., TLR-3, TLR-7 and TLR-9 are widely explored for their role in dendritic cell maturation (52). TLR-7 agonists have demonstrated significant immunostimulatory and anti-tumor efficacy in various cancer models (53). Alternative strategies for igniting the innate immune response and warming up cold tumors include RIG-I and NLRP3 (54, 55). MDA5 and RIG-I have different preferences for RNA ligands, while having comparable structures and causes upregulation of type I interferon signaling (54, 55). Endogenous RNAs are modified to escape the response triggered by the RNA sensors present in the cytosol. Adenine to Inosine conversion is one such modification catalyzed by the protein Adenosine deaminase acting on RNA (ADAR) (56). Tumors with high interferon signature are ADAR1 dependent, and transcripts induced by interferon contributes to accumulation of dsRNA in the cytoplasm (57). ADAR1 depletion resulted in upregulation of interferon stimulated genes (ISGs) in a RIG-1, MDA5 dependent manner (58, 59). Further, deletion of ADAR1 also rendered these cells more prone to immuno-therapy and overcame resistance to immune checkpoint blockade (60), demonstrating ADAR1 as a new immuno-oncology target. Apart from the RIG-I pathway, the NLRP3 inflammasome is a pattern recognition receptor which is activated by both exogenous and endogenous stimuli, leading to a downstream inflammatory response (61, 62). Reports have shown that NLRP3 inflammasomes are necessary for anti-tumor effect during radiation (61). Upon activation, NLRP3 triggers IL-1 mediated maturation of dendritic cells resulting in cross-priming and T-cell immune response (63). Cancer cells escape the innate immune response by means of SIRPα-CD47 interaction which provides a ‘‘don’t-eat-me’’ signal to the effector immune cells like neutrophils and macrophages (64). Increasing evidence suggests that SIRPα-CD47 immune checkpoint blockade enhances the efficacy of cancer immunotherapy (65–68). In this review, we discuss about various small molecule modulators (Summarized in Table 1) of targets such as STING/ENPP1, TLR, NLRP3, and SIRPα-CD47 which are key players in the innate immune system working against cancer.
Small molecule STING agonists
STING agonists activate the STING pathway, leading to the production of type I interferon and proinflammatory cytokines, resulting in anti-tumor immunity (112, 113). Vadimezan (DMXAA) – A vascular disrupting agent was the first STING agonist extensively evaluated pre-clinically. DMXAA demonstrated a potent anti-tumor immune response in murine tumor models when administered intratumorally (114). However, the lack of translation of this efficacy in clinical trials led to the termination of the trial (115). A detailed study later showed that DMXAA is a direct ligand for murine STING but does not bind to human STING (116). Several cyclic dinucleotides based (CDN) compounds such as ADU-S100, TAK-676, MK1454, BMS-986301, SB11285, BI-1387446, IMSA-101, etc. were the first generation of STING agonist being developed (Table 1). These CDN compounds progressed to clinical trials in accessible solid tumors amenable to intratumoral/intravascular/intramuscular delivery. However, their poor ADME properties and stability, lack of substantial anti-tumor effects in the clinical trials and risks of cytokine release syndrome led to discontinuation of several of the trials (117). Recent years have witnessed the development of several small molecule non-CDN based STING agonists (Table 1). These possess novel pharmacophores to address the ADME, PK and stability concerns associated with the first-generation CDN based compounds (113). Ramanjulu et al. group reported the amidobenzimidazole (ABZI) as a non CDN based STING agonist with systemic activity in mice (75). ABZI binds in the cGAMP binding pocket with two bound molecules per STING dimer with each ABZI molecule close in space, projected across the STING dimer interface and lacked interactions with the protein. Subsequently, SAR modification at N1-hydroxyphenethyl moiety with a linker between the two molecules to identify a dimeric amidobenzimidazole ligand (diABZI), which showed substantial increase in binding affinity. diABZI induced dose dependent activation of STING and secretion of IFN-β (EC50 = 130 nM). Compound 3 demonstrated satisfactory plasma concentrations and significant antitumor efficacy at a dose of 1.5 mg/kg intravenously in a CT-26 tumor model (75). Pan et al. group identified MSA-2 (benzothiophene- oxobutanoic acid) as an orally bioavailable non-nucleotidic human STING agonist. MSA-2 showed dose dependent anti-tumor effect by intratumoral, subcutaneous or oral administration in-vivo which synergized with anti-PD1 therapy (76). Molecular mechanism of action studies revealed that in solution, MSA-2 exists in equilibrium as a non-covalent dimer of MSA-2. Therefore, a series of covalently linked MSA-2 dimers were synthesized and compound 3 has shown enhanced binding affinity to STING (IC50 = 23 ± 7 nM; cGAMP displacement assay) and (EC50: 70 ± 50 nM; Secretion of IFN- β) (76). Chin et al. group identified SR-717 as a non-nucleotide STING agonist (EC50 = 2.1 µM) that demonstrated potent antitumor activity and promoted the activation of CD8+ T, NK and dendritic cells in vivo (77). Co-crystal structure revealed that SR-717 induced cGAMP mimetic closed conformation with STING. Moreover, SR-717 also induced the expression of clinically relevant targets, including PD-L1 in a STING-dependent manner (77). Banerjee et al. recently discovered CRD-5500 (Bicyclic benzamides; Structure not available) as a non-nucleotide STING agonist that activates major human STING variants and generates a strong pro-inflammatory response (78). CRD-5500 demonstrated antitumor activity in-vivo and a synergistic effect with anti-PD1 (78). Several other groups have reported Non-CDN based small molecule STING agonists (Table 1)). However, systemic administration of STING agonists causes cytokine release syndrome (immunotoxicity) due to the ubiquitous expression of STING in normal and tumor tissues which impedes its clinical translation. In order to minimize the risk of toxicity, tumor targeted delivery of STING agonists using Antibody- drug conjugates (ADC) are being explored as potential cancer immunotherapeutics. Chen et al. group reported STING ADC (αEGFR-172ADC) for their molecule IMSA172 that demonstrated potent antitumor efficacy in-vivo and promoted activation of dendritic cells, T cells, natural killer cells, natural killer T cells, as well as promotion of M2 to M1 polarization of tumor-associated macrophages. The STING ADC also showed synergistic effect with anti-PD-L1 (80). ADCs have emerged as a promising alternative to address cytokine related toxicity concerns, and extensive work is currently ongoing which is beyond the scope of this review.
ENPP1 inhibitors
ENPP1 has emerged as a promising therapeutic target in cancer immunotherapy and inhibition of ENPP1 augments anticancer immunity via STING-mediated innate immune activation (46, 101, 118–120). Nucleotide-based ENPP1 inhibitors have been developed (Table 1); however, these possess low oral bioavailability, high acidity of the phosphate backbone, moderate stability and potential off-target biological effects (119). To address the existing concerns several groups have identified and developed series of non-nucleotide based ENPP1 inhibitors (Table 1). An isoquinoline derivative based ENPP1 inhibitor has been reported by Ausekle et al. with IC50 values of 0.36 μM using p-NP-TMP as a substrate (97). Chang et al. reported a thioacetamide inhibitor with Ki value of 5 nM against human ENPP1 using p-Nph-5’-TMP as substrate (98). Patel et al. reported a quinazoline-piperidine-sulfamide ENPP1 inhibitor (QS1) with an IC50 of 36 nM using ATP as a substrate (121). Carozza et al. designed a phosphonate analog of QS1, STF-1084 with Ki,app = 110 nM. A quinazoline-4-piperidine sulfamide compound, QPS1 with Ki = 59 ± 5 nM is reported by Patel and coworkers as a selective non-competitive ENPP1 inhibitor (121). Using QPS1 as the lead compound, Forcellini et al. developed quinazoline-4-piperidine sulfamide analogues based ENPP1 inhibitors with Ki < 105 nM against human ENPP1 (100). STF-1084 is a potent, specific, and cell impermeable ENPP1 inhibitor (42). Another cell-impermeable, nontoxic specific ENPP1 inhibitor is STF-1623 (Ki,app = 16 nM in an in vitro assay) which delayed tumor growth in Panc02 syngeneic, pancreatic tumor model. STF-1623 resulted in a decreased rate of locoregional failure of breast cancer models treated with surgery and radiation (122).
Other small molecule ENPP1 inhibitors with promising preclinical results have been reported (Table 1), however, the structure for these inhibitors have not been disclosed yet. MV-626 is a selective ENPP1 inhibitor developed by Mavupharma (Ki: 5 nM) with 100% oral bioavailability in rats and mice. In-vivo, MV-626 in combination with radiation treatment delayed tumor growth in syngeneic Panc02 tumor model. Further, the combination of MV-626 with anti-PD-L1 enhanced overall survival in the MC38 murine colon carcinoma model (102). Another preclinical candidate AVA-NP-695, from Avammune Therapeutics is a selective and highly potent ENPP1 inhibitor, which apart from its immunomodulatory effect also modulates cancer metastasis. AVA-NP-695 has demonstrated strong synergy with PARPi, resulting in superior tumor growth inhibition and reduced metastasis in syngeneic 4T1 breast cancer mouse models (103, 123). SR-8314 is a potent ENPP1 inhibitor with a Ki value of 0.079 µM, which increases IFNβ, ISG15 and CXCL10 expression in THP1 cells. In-vivo efficacy of SR-8314 was confirmed in syngeneic murine tumor model and increased levels of CD3, CD4, and CD8 T cells were observed in SR-8314 treated tumors (104). ENPP1 inhibitor, ZXP-8202 with EC50 value of 20 nM in cell based-assay and ZX-8177 with IC50 of 9.5 nM are reported with 37-60% tumor growth inhibition (TGI) for ZX-8177 in CT26 syngeneic mouse model. Synergistic tumor inhibition of ZX-8177 in combination with anti-PD-L1 in MC38 syngeneic mouse model (TGI of anti-PD-L1 antibody vs. combo treatment is 53% vs. 75%) was observed as well. (106). ISM5939 is a selective inhibitor of ENPP1 with IC50 of 0.63 nM in the enzymatic assay with ISM5939 monotherapy (30 mg/kg, p.o. BID) having a 67% TGI in MC38 model (107).
A few ENPP1 small molecule inhibitors have advanced to early clinical stages for potential use as cancer immunotherapeutics that enhance the STING-mediated immune response (Table 1). The structures of most of these compounds have not been disclosed yet. In 2022, the first Phase I clinical trial was initiated for an orally available small molecule ENPP1 inhibitor, RBS2418 from Riboscience in combination with pembrolizumab or as a monotherapy for advanced unresectable, recurrent or metastatic tumors (108, 109). TXN10128 is another orally available ENPP1 inhibitor in Phase 1 currently being evaluated for solid tumors (NCT05978492). The IC50 value of selective ENPP1 inhibitor TXN10128 with cGAMP as substrate was 4 nM. TXN10128 in combination with anti-PD-L1 antibody inhibited tumor growth in MC38 syngeneic mouse model (110). SR-8541A is an oral ENPP1 inhibitor candidate from Stingray Therapeutics in Phase 1, being evaluated in subjects with advanced/metastatic solid tumors (NCT06063681). In vitro SR-8541A triggers strong immune responses, with increased expression of IFN-β, ISG15 and CXCL10 and showed decreased tumor growth in a CT26 colon cancer model (124).
Small molecule TLR agonists
Toll-like receptors (TLRs) are pattern recognition receptors (PRRs) involved in the regulation of innate immunity. TLRs play a pivotal role in recognizing pathogen-associated molecular patterns (PAMPs) or damage-associated molecular patterns (DAMPs) and initiating immune responses. The activation of specific TLRs like TLR1/2, TLR3, TLR5, TLR7/8 and TLR9 have previously demonstrated antitumor immune responses. Moreover, TLR agonists like Diprovocim (TLR1/2) (125), 1V270 (TLR7) (22) and SD-101 (TLR9) (126) have shown synergistic effects with immune checkpoint inhibitors thus enhancing the tumor immunogenicity (Table 1). Therefore, TLR agonists present a promising strategy for cancer immunotherapy (127, 128). Imiquimod is a small molecule TLR7 agonist being studied in clinical trials for various tumors such as basal cell carcinoma (NCT00314756) and melanoma (NCT01720407) (129). Several other TLR7 agonists are at different stages of clinical development including Guretolimod (DSP-0509; NCT03416335; Phase I/II) for the treatment of solid tumors administered as monotherapy and in combination with pembrolizumab (130). Others include JNJ-64794964 (TQ-A3334; NCT04273815; Phase IB) for the treatment of advanced non-small cell lung cancer (131) and LHC-165 (NCT03301896; Phase I/IB; Terminated) as monotherapy and in combination with PDR001 in patients with advanced malignancies (132). Telratolimod (MEDI-9197; NCT02556463) is a TLR7/8 agonist being studied in solid tumors as monotherapy or in combination with durvalumab and/or palliative radiation therapy but failed (Table 1) (133). Motolimod (VTX-2337; NCT02431559; Phase I/II) is a TLR8 agonist being evaluated in combination with durvalumab and pegylated liposomal doxorubicin for recurrent, platinum-resistant ovarian cancer (Table 1) (134). GSK1795091 (CRX-601; NCT02798978; Phase I) is a TLR4 agonist being evaluated for safety, tolerability, pharmacodynamic (PD), and pharmacokinetics (PK) profile determination in healthy subjects (135). Wang et al. reported compound 24e (pyrido[3,2-d]pyrimidine-based) as TLR 7/8 dual agonists with EC50 of 24 nM hTLR 7 and 10 nM hTLR 8 (92). Compound 24e induced the secretion of IFN-α, IFN-γ, TNF-α, IL-1β, IL-12p40, and IP-10 in human peripheral blood mononuclear cell assays. Compound 24e also showed significant tumor growth inhibition as monotherapy in the CT-26 mouse model which led to complete tumor regression when combined with anti-PD-L1 antibody (92). Zhang et al. reported compound 17 e (CU-CPT17e) as multi-TLR agonist that shown to activate TLR 3, 8 and 9. It induces a strong immune response via the release of various cytokines in THP-1 cells. It has also inhibited the growth of HeLa cancer cells in their in vitro assay (93). Chen et al., reported SMU-C80 as TLR1/2 dual agonist with an EC50 of 31 nM. SMU-C80 is reported to act through the MyD88 and NF-κB pathways and mediated cytokine release and the activation of immune cells to produce antitumor effect in vitro (94).
CD47-SIRPα interaction blocker
Overexpression of CD47 enables tumor cells to evade immune surveillance via the blockade of phagocytic mechanisms and is associated with poor survival in various cancer (136, 137). Therefore, inhibition of CD47-SIRPα axis has a significant impact on tumor immunotherapy (138). NCGC00138783 is a small-molecule inhibitor that directly blocks the binding of CD47 to SIRPα with an IC50 of ~50 μM (Table 1) (81, 139). Further optimization of its physicochemical properties as well as its druggability led to the discovery of its analogues, NCGC00138419 and NCGC00138430 (139). Small-molecule inhibitors that inhibit CD47 expression at the transcriptional and translational levels are RRx-001, 4-methylumbelliferone (4-Mu), JQ1, and gefitinib. RRx-001 suppressed CD47 expression in tumor cells through the inhibition of c-MYC, a positive regulator of CD47 (140, 141). 4-Methylumbelliferone (4Mu) is a hyaluronan synthesis inhibitor that downregulated the expression of CD47 and promoted phagocytosis of intraperitoneal macrophages of hepatocellular carcinoma (HCC) cells (84). Combination of 4Mu with adenovirus encoding IL-12 (AdIL-12) significantly reduced tumor volume (TGI ∼80%) and largely improved overall survival in an orthotopic HCC mice model (84). JQ1 is a small-molecule inhibitor of bromodomain and extra-terminal (BET) proteins. JQ1 suppresses expression of CD47 and PD-L1 in acute lymphoblastic leukemia, melanoma, and non-small cell lung cancer (NSCLC) cells through a c-MYC-mediated pathway (85, 142). An interesting report had highlighted that the role of Glutaminyl cyclase isoenzyme (QPCTL) is critical for pyroglutamate formation on CD47 at the SIRPα binding site (87) and inhibition of QPCTL activity enhances antibody-dependent cellular phagocytosis and cellular cytotoxicity of tumor cells. QPCTL inhibitors, SEN177 (IC50 = 0.013 μM for QPCTL) and PQ912 have been reported for their impact on the CD47-SIRPα interaction (87, 143). In silico Medicine in collaboration with Fosun Pharma developed a first-in-class orally available small molecule ISM8207 (IC50 < 0.5 nM in enzymatic assay) inhibitor of QPCTL. ISM8207 has advanced to Phase I clinical trials for the treatment of triple-negative breast cancer (TNBC) and B-cell non-Hodgkin lymphoma (88).
NLRP3 modulators
Large cytosolic multiprotein complexes known as inflammasomes are involved in the host defence against microbial infections by mediating important inflammatory innate immune responses. NLRP3, the best-studied NLRP, is expressed by DCs, lymphocytes, macrophages, and non-immune populations such as epithelial cells. In various preclinical and clinical studies, the role of NLRP3 has been extensively explored and a correlation between lower expression of NLRP3 and advanced cancer has been established. Interestingly, both NLRP3 agonists and inhibitors have shown anti-tumor efficacy (Table 1). The novel NLRP3 agonist BMS-986299 is currently undergoing evaluation in a phase I clinical trial, both as a standalone treatment and in combination with nivolumab and ipilimumab, for advanced solid tumors [NCT03444753] (144). In a murine model of HNSCC, a novel NLRP3 inhibitor known as MCC950 demonstrated the ability to delay tumor growth, decrease levels of MDSCs, Tregs, and TAMs, while also enhancing T cell infiltration within the tumor microenvironment (TME). Additionally, Douguet et al. demonstrated an alternate way to activate the NLRP3 inflammasome is via P2RX7’s activation (145). HEI3090, a pyrrolidin-2-one derivative is a positive modulator of the P2RX7 receptor that potentiates anti-PD-1 treatment to effectively control the growth of lung tumors. This demonstrated complete tumor regression in 80% of LLC tumor-bearing mice, works by activating the NLRP3 pathway (90). A small molecule andrographolide (Andro) protected mice against colitis-associated cancer (CAC) in the mouse model of (AOM)-dextran sulphate sodium (DSS) through inhibiting NLRP3 inflammasome activation in macrophages. The tumor size was reduced by Andro in a dose-dependent manner and the average tumor load was significantly decreased in the Andro-treated group (91).
Discussion
Developing small molecule immunomodulators for the innate immune system for the treatment of cancer is a fast growing and active area of research and development. The innate immune system plays a crucial role in recognizing and eliminating cancer cells. While small molecule immunomodulators offer several advantages, they also come with certain disadvantages and challenges. Small molecules may interact with unintended targets, leading to off-target effects. This lack of specificity can contribute to adverse reactions and limit the therapeutic index. For e.g., one challenge in the development of STING agonists is the potential for systemic toxicity. Activating the STING pathway can induce a robust immune response, and balancing this response to avoid excessive inflammation and off-target effects is critical. STING pathway hold significant potential in combination modalities like radiation and chemotherapies which induces double stranded DNA breaks. Alternatively, ENPP1 inhibition has much more potential to exert a significant anti-tumor activity with reduced autoimmune related toxicity concerns. Recent reports have also suggested that the loss of ENPP1 function in both cancer cells and normal tissues resulted in a reduced primary tumor initiation and growth. The phenomenon also hindered metastasis through a mechanism that relies on extracellular cGAMP and is dependent on STING (46). Notably, breast cancer patients exhibiting reduced ENPP1 expression demonstrate elevated levels of immune infiltration. These patients show enhanced responsiveness to therapeutics affecting cancer immunity at points preceding or following the cGAMP-STING pathway, such as PARP inhibitors and anti-PD1 treatments. Currently, three phase 1 clinical trials are ongoing with ENPP1 inhibitors and the potential of these inhibitors will be analyzed once the study completes. Apart from the STING pathway, other innate immune pathways also hold significant potential in cancer immunotherapy. TLR agonists are used as adjuvants to enhance the effectiveness of cancer vaccines or as standalone agents to stimulate the innate immune response against tumors.
Since, innate and adaptive immunity is highly interconnected, innate immune modulators can play a crucial role in overcoming immune tolerance and avoid resistance being developed against immune checkpoint inhibitors. Extensive research is currently ongoing to evaluate the combination of small-molecule immunotherapies with various anticancer modalities, as well as the monotherapy of these agents. For e.g. resistance to Immune checkpoint inhibitors (ICIs) therapy is frequent, and approximately only 30% to 40% of patients benefit from ICIs. ICIs, particularly monoclonal antibodies that specifically target CTLA-4 and PD-1/PD-L1, have greatly improved patient outcomes and progressed cancer treatment. Evidence suggests that combination of small molecule innate immune modulators with ICIs and other therapeutic approaches may greatly increase therapeutic efficacy. With the growing evidence of several immune checkpoint proteins, efforts need to be made towards development of small molecule inhibitors against these immune checkpoints. With growing research and early phase clinical trial along with biomarker driven study designs, novel pathways and combination strategies will be identified. Future developments in cancer immunotherapy appear to be greatly promising when it comes to exploiting these synergistic combination pathways that simultaneously engage innate and adaptive immunity.
Author contributions
AG: Conceptualization, Writing – original draft, Writing – review & editing. SG: Writing – review & editing, Writing – original draft. PK: Writing – original draft, Writing – review & editing. KS: Writing – original draft, Writing – review & editing. BD: Writing – original draft. AK: Conceptualization, Funding acquisition, Writing – review & editing.
Funding
The author(s) declare that no financial support was received for the research, authorship, and/or publication of this article.
Conflict of interest
AG, SG, PK, KS, BD, and AK are employees of Aten Porus Lifesciences APL. AK is the co-founder of both APL and Avammune Therapeutics USA.
Publisher’s note
All claims expressed in this article are solely those of the authors and do not necessarily represent those of their affiliated organizations, or those of the publisher, the editors and the reviewers. Any product that may be evaluated in this article, or claim that may be made by its manufacturer, is not guaranteed or endorsed by the publisher.
References
1. Waldman AD, Fritz JM, Lenardo MJ. A guide to cancer immunotherapy: from T cell basic science to clinical practice. Nat Rev Immunol. (2020) 20:651–68. doi: 10.1038/s41577-020-0306-5
2. Esfahani K, Roudaia L, Buhlaiga N, Del Rincon SV, Papneja N, Miller WH, et al. A review of cancer immunotherapy: from the past, to the present, to the future. Curr Oncol. (2020) 27:S87–97. doi: 10.3747/co.27.5223
3. Kruger S, Ilmer M, Kobold S, Cadilha BL, Endres S, Ormanns S, et al. Advances in cancer immunotherapy 2019 - latest trends. J Exp Clin Cancer research: CR. (2019) 38:268. doi: 10.1186/s13046-019-1266-0
4. Shiravand Y, Khodadadi F, Kashani SMA, Hosseini-Fard SR, Hosseini S, Sadeghirad H, et al. Immune checkpoint inhibitors in cancer therapy. Curr Oncol. (2022) 29:3044–60. doi: 10.3390/curroncol29050247
5. Pardoll DM. The blockade of immune checkpoints in cancer immunotherapy. Nat Rev Cancer. (2012) 12:252–64. doi: 10.1038/nrc3239
6. Liu C, Yang M, Zhang D, Chen M, Zhu D. Clinical cancer immunotherapy: Current progress and prospects. Front Immunol. (2022) 13:961805. doi: 10.3389/fimmu.2022.961805
7. Botticelli A, Cirillo A, Strigari L, Valentini F, Cerbelli B, Scagnoli S, et al. Anti-PD-1 and anti-PD-L1 in head and neck cancer: A network meta-analysis. Front Immunol. (2021) 12:705096. doi: 10.3389/fimmu.2021.705096
8. Sangro B, Sarobe P, Hervas-Stubbs S, Melero I. Advances in immunotherapy for hepatocellular carcinoma. Nat Rev Gastroenterol Hepatol. (2021) 18:525–43. doi: 10.1038/s41575-021-00438-0
9. Spisarova M, Melichar B, Vitaskova D, Studentova H. Pembrolizumab plus axitinib for the treatment of advanced renal cell carcinoma. Expert Rev Anticancer Ther. (2021) 21:693–703. doi: 10.1080/14737140.2021.1903321
10. Gong J, Chehrazi-Raffle A, Reddi S, Salgia R. Development of PD-1 and PD-L1 inhibitors as a form of cancer immunotherapy: a comprehensive review of registration trials and future considerations. J immunotherapy Cancer. (2018) 6:8. doi: 10.1186/s40425-018-0316-z
11. Schneider BJ, Naidoo J, Santomasso BD, Lacchetti C, Adkins S, Anadkat M, et al. Management of immune-related adverse events in patients treated with immune checkpoint inhibitor therapy: ASCO guideline update. J Clin oncology: Off J Am Soc Clin Oncol. (2021) 39:4073–126. doi: 10.1200/JCO.21.01440
12. Ryman JT, Meibohm B. Pharmacokinetics of monoclonal antibodies. CPT: pharmacometrics Syst Pharmacol. (2017) 6:576–88. doi: 10.1002/psp4.12224
13. Postel-Vinay S, Aspeslagh S, Lanoy E, Robert C, Soria JC, Marabelle A. Challenges of phase 1 clinical trials evaluating immune checkpoint-targeted antibodies. Ann oncology: Off J Eur Soc Med Oncol. (2016) 27:214–24. doi: 10.1093/annonc/mdv550
14. Tarhini A. Immune-mediated adverse events associated with ipilimumab ctla-4 blockade therapy: the underlying mechanisms and clinical management. Scientifica. (2013) 2013:857519. doi: 10.1155/2013/857519
15. Ovacik M, Lin K. Tutorial on monoclonal antibody pharmacokinetics and its considerations in early development. Clin Trans Sci. (2018) 11:540–52. doi: 10.1111/cts.12567
16. Wu Y, Yang Z, Cheng K, Bi H, Chen J. Small molecule-based immunomodulators for cancer therapy. Acta Pharm Sinica. B. (2022) 12:4287–308. doi: 10.1016/j.apsb.2022.11.007
17. Hao X, Chen Z, Li H, Wei M, Zuo Z, Su Q. Small-molecule drugs in immunotherapy. Mini Rev medicinal Chem. (2023) 23:1341–59. doi: 10.2174/1389557522666220930154527
18. Zhong L, Li Y, Xiong L, Wang W, Wu M, Yuan T, et al. Small molecules in targeted cancer therapy: advances, challenges, and future perspectives. Signal transduction targeted Ther. (2021) 6:201. doi: 10.1038/s41392-021-00572-w
19. van der Zanden SY, Luimstra JJ, Neefjes J, Borst J, Ovaa H. Opportunities for small molecules in cancer immunotherapy. Trends Immunol. (2020) 41:493–511. doi: 10.1016/j.it.2020.04.004
20. Singh S, Barik D, Arukha AP, Prasad S, Mohapatra I, Singh A, et al. Small molecule targeting immune cells: A novel approach for cancer treatment. Biomedicines. (2023) 11:2621. doi: 10.3390/biomedicines11102621
21. Ghaffari A, Peterson N, Khalaj K, Vitkin N, Robinson A, Francis JA, et al. STING agonist therapy in combination with PD-1 immune checkpoint blockade enhances response to carboplatin chemotherapy in high-grade serous ovarian cancer. Br J Cancer. (2018) 119:440–9. doi: 10.1038/s41416-018-0188-5
22. Sato-Kaneko F, Yao S, Ahmadi A, Zhang SS, Hosoya T, Kaneda MM, et al. Combination immunotherapy with TLR agonists and checkpoint inhibitors suppresses head and neck cancer. JCI Insight. (2017) 2:e93397. doi: 10.1172/jci.insight.93397
23. Wu X, Feng N, Wang C, Jiang H, Guo Z. Small molecule inhibitors as adjuvants in cancer immunotherapy: enhancing efficacy and overcoming resistance. Front Immunol. (2024) 15:1444452. doi: 10.3389/fimmu.2024.1444452
24. Lu C, Liu Y, Ali NM, Zhang B, Cui X. The role of innate immune cells in the tumor microenvironment and research progress in anti-tumor therapy. Front Immunol. (2022) 13:1039260. doi: 10.3389/fimmu.2022.1039260
25. Lei X, Lei Y, Li JK, Du WX, Li RG, Yang J, et al. Immune cells within the tumor microenvironment: Biological functions and roles in cancer immunotherapy. Cancer Lett. (2020) 470:126–33. doi: 10.1016/j.canlet.2019.11.009
26. Xu P, Luo H, Kong Y, Lai WF, Cui L, Zhu X. Cancer neoantigen: Boosting immunotherapy. Biomedicine pharmacotherapy = Biomedecine pharmacotherapie. (2020) 131:110640. doi: 10.1016/j.biopha.2020.110640
27. Efremova M, Finotello F, Rieder D, Trajanoski Z. Neoantigens generated by individual mutations and their role in cancer immunity and immunotherapy. Front Immunol. (2017) 8:1679. doi: 10.3389/fimmu.2017.01679
28. Ward JP, Gubin MM, Schreiber RD. The role of neoantigens in naturally occurring and therapeutically induced immune responses to cancer. Adv Immunol. (2016) 130:25–74. doi: 10.1016/bs.ai.2016.01.001
29. Woo SR, Corrales L, Gajewski TF. Innate immune recognition of cancer. Annu Rev Immunol. (2015) 33:445–74. doi: 10.1146/annurev-immunol-032414-112043
30. Vesely MD, Schreiber RD. Cancer immunoediting: antigens, mechanisms, and implications to cancer immunotherapy. Ann New York Acad Sci. (2013) 1284:1–5. doi: 10.1111/nyas.12105
31. Liu Y, Zeng G. Cancer and innate immune system interactions: translational potentials for cancer immunotherapy. J immunotherapy. (2012) 35:299–308. doi: 10.1097/CJI.0b013e3182518e83
32. Samson N, Ablasser A. The cGAS-STING pathway and cancer. Nat Cancer. (2022) 3:1452–63. doi: 10.1038/s43018-022-00468-w
33. Gan Y, Li X, Han S, Liang Q, Ma X, Rong P, et al. The cGAS/STING pathway: A novel target for cancer therapy. Front Immunol. (2021) 12:795401. doi: 10.3389/fimmu.2021.795401
34. Xia P, Wang S, Gao P, Gao G, Fan Z. DNA sensor cGAS-mediated immune recognition. Protein Cell. (2016) 7:777–91. doi: 10.1007/s13238-016-0320-3
35. Miller KN, Victorelli SG, Salmonowicz H, Dasgupta N, Liu T, Passos JF, et al. Cytoplasmic DNA: sources, sensing, and role in aging and disease. Cell. (2021) 184:5506–26. doi: 10.1016/j.cell.2021.09.034
36. Decout A, Katz JD, Venkatraman S, Ablasser A. The cGAS-STING pathway as a therapeutic target in inflammatory diseases. Nat Rev Immunol. (2021) 21:548–69. doi: 10.1038/s41577-021-00524-z
37. Baba T, Yoshida T, Tanabe Y, Nishimura T, Morishita S, Gotoh N, et al. Cytoplasmic DNA accumulation preferentially triggers cell death of myeloid leukemia cells by interacting with intracellular DNA sensing pathway. Cell Death Dis. (2021) 12:322. doi: 10.1038/s41419-021-03587-x
38. Reislander T, Groelly FJ, Tarsounas M. DNA damage and cancer immunotherapy: A STING in the tale. Mol Cell. (2020) 80:21–8. doi: 10.1016/j.molcel.2020.07.026
39. Talens F, Van Vugt M. Inflammatory signaling in genomically instable cancers. Cell Cycle. (2019) 18:1830–48. doi: 10.1080/15384101.2019.1638192
40. Falahat R, Berglund A, Perez-Villarroel P, Putney RM, Hamaidi I, Kim S, et al. Epigenetic state determines the in vivo efficacy of STING agonist therapy. Nat Commun. (2023) 14:1573. doi: 10.1038/s41467-023-37217-1
41. Shi J, Liu C, Luo S, Cao T, Lin B, Zhou M, et al. STING agonist and IDO inhibitor combination therapy inhibits tumor progression in murine models of colorectal cancer. Cell Immunol. (2021) 366:104384. doi: 10.1016/j.cellimm.2021.104384
42. Carozza JA, Böhnert V, Nguyen KC, Skariah G, Shaw KE, Brown JA, et al. Extracellular cGAMP is a cancer cell-produced immunotransmitter involved in radiation-induced anti-cancer immunity. Nat Cancer. (2020) 1:184–96. doi: 10.1038/s43018-020-0028-4
43. Khoo LT, Chen LY. Role of the cGAS-STING pathway in cancer development and oncotherapeutic approaches. EMBO Rep. (2018) 19:e46935. doi: 10.15252/embr.201846935
44. Marcus A, Mao AJ, Lensink-Vasan M, Wang L, Vance RE, Raulet DH. Tumor-derived cGAMP triggers a STING-mediated interferon response in non-tumor cells to activate the NK cell response. Immunity. (2018) 49:754–763 e754. doi: 10.1016/j.immuni.2018.09.016
45. Kato K, Nishimasu H, Oikawa D, Hirano S, Hirano H, Kasuya G, et al. Structural insights into cGAMP degradation by Ecto-nucleotide pyrophosphatase phosphodiesterase 1. Nat Commun. (2018) 9:4424. doi: 10.1038/s41467-018-06922-7
46. Wang S, Böhnert V, Joseph AJ, Sudaryo V, Skariah G, Swinderman JT, et al. ENPP1 is an innate immune checkpoint of the anticancer cGAMP-STING pathway in breast cancer. Proc Natl Acad Sci United States America. (2023) 120:e2313693120. doi: 10.1073/pnas.2313693120
47. Li J, Duran MA, Dhanota N, Chatila WK, Bettigole SE, Kwon J, et al. Metastasis and immune evasion from extracellular cGAMP hydrolysis. Cancer Discovery. (2021) 11:1212–27. doi: 10.1158/2159-8290.CD-20-0387
48. Lau WM, Doucet M, Stadel R, Huang D, Weber KL, Kominsky SL, et al. Enpp1: a potential facilitator of breast cancer bone metastasis. PloS One. (2013) 8:e66752. doi: 10.1371/journal.pone.0066752
49. Yi M, Li T, Niu M, Mei Q, Zhao B, Chu Q, et al. Exploiting innate immunity for cancer immunotherapy. Mol Cancer. (2023) 22:187. doi: 10.1186/s12943-023-01885-w
50. Workenhe ST, Pol J, Kroemer G. Tumor-intrinsic determinants of immunogenic cell death modalities. Oncoimmunology. (2021) 10:1893466. doi: 10.1080/2162402X.2021.1893466
51. Bai L, Li W, Zheng W, Xu D, Chen N, Cui J. Promising targets based on pattern recognition receptors for cancer immunotherapy. Pharmacol Res. (2020) 159:105017. doi: 10.1016/j.phrs.2020.105017
52. Kawai T, Akira S. The role of pattern-recognition receptors in innate immunity: update on Toll-like receptors. Nat Immunol. (2010) 11:373–84. doi: 10.1038/ni.1863
53. Chi H, Li C, Zhao FS, Zhang L, Ng TB, Jin G, et al. Anti-tumor activity of toll-like receptor 7 agonists. Front Pharmacol. (2017) 8:304. doi: 10.3389/fphar.2017.00304
54. Iurescia S, Fioretti D, Rinaldi M. The innate immune signalling pathways: turning RIG-I sensor activation against cancer. Cancers. (2020) 12(11):3158. doi: 10.3390/cancers12113158
55. Zhao C, Zhao W. NLRP3 inflammasome-A key player in antiviral responses. Front Immunol. (2020) 11:211. doi: 10.3389/fimmu.2020.00211
56. Liddicoat BJ, Piskol R, Chalk AM, Ramaswami G, Higuchi M, Hartner JC, et al. RNA editing by ADAR1 prevents MDA5 sensing of endogenous dsRNA as nonself. Science. (2015) 349:1115–20. doi: 10.1126/science.aac7049
57. Song B, Shiromoto Y, Minakuchi M, Nishikura K. The role of RNA editing enzyme ADAR1 in human disease. Wiley Interdiscip Rev RNA. (2022) 13:e1665. doi: 10.1002/wrna.1665
58. Guo X, Liu S, Sheng Y, Zenati M, Billiar T, Herbert A, et al. ADAR1 Zalpha domain P195A mutation activates the MDA5-dependent RNA-sensing signaling pathway in brain without decreasing overall RNA editing. Cell Rep. (2023) 42:112733. doi: 10.1016/j.celrep.2023.112733
59. Lamers MM, van den Hoogen BG, Haagmans BL. ADAR1: "Editor-in-chief" of cytoplasmic innate immunity. Front Immunol. (2019) 10:1763. doi: 10.3389/fimmu.2019.01763
60. Ishizuka JJ, Manguso RT, Cheruiyot CK, Bi K, Panda A, Iracheta-Vellve A, et al. Loss of ADAR1 in tumours overcomes resistance to immune checkpoint blockade. Nature. (2019) 565:43–8. doi: 10.1038/s41586-018-0768-9
61. Barsoumian HB, He K, Hsu E, Bertolet G, Sezen D, Hu Y, et al. NLRP3 agonist enhances radiation-induced immune priming and promotes abscopal responses in anti-PD1 resistant model. Cancer Immunol Immunother. (2023) 72:3003–12. doi: 10.1007/s00262-023-03471-x
62. Kelley N, Jeltema D, Duan Y, He Y. The NLRP3 inflammasome: an overview of mechanisms of activation and regulation. Int J Mol Sci. (2019) 20:3328. doi: 10.3390/ijms20133328
63. Jo EK, Kim JK, Shin DM, Sasakawa C. Molecular mechanisms regulating NLRP3 inflammasome activation. Cell Mol Immunol. (2016) 13:148–59. doi: 10.1038/cmi.2015.95
64. Chao MP, Weissman IL, Majeti R. The CD47-SIRPalpha pathway in cancer immune evasion and potential therapeutic implications. Curr Opin Immunol. (2012) 24:225–32. doi: 10.1016/j.coi.2012.01.010
65. Liu J, Xavy S, Mihardja S, Chen S, Sompalli K, Feng D, et al. Targeting macrophage checkpoint inhibitor SIRPalpha for anticancer therapy. JCI Insight. (2020) 5:e134728. doi: 10.1172/jci.insight.134728
66. Iribarren K, Buque A, Mondragon L, Xie W, Lévesque S, Pol J, et al. Anticancer effects of anti-CD47 immunotherapy. vivo. Oncoimmunology. (2019) 8:1550619. doi: 10.1080/2162402X.2018.1550619
67. Ring NG, Herndler-Brandstetter D, Weiskopf K, Shan L, Volkmer JP, George BM, et al. Anti-SIRPalpha antibody immunotherapy enhances neutrophil and macrophage antitumor activity. Proc Natl Acad Sci United States America. (2017) 114:E10578–85. doi: 10.1073/pnas.1710877114
68. Petrova PS, Viller NN, Wong M, Pang X, Lin GH, Dodge K, et al. TTI-621 (SIRPalphaFc): A CD47-blocking innate immune checkpoint inhibitor with broad antitumor activity and minimal erythrocyte binding. Clin Cancer research: an Off J Am Assoc Cancer Res. (2017) 23:1068–79. doi: 10.1158/1078-0432.CCR-16-1700
69. Meric-Bernstam F, Sweis RF, Hodi FS, Messersmith WA, Andtbacka RHI, Ingham M, et al. Phase I dose-escalation trial of MIW815 (ADU-S100), an intratumoral STING agonist, in patients with advanced/metastatic solid tumors or lymphomas. Clin Cancer Res. (2022) 28:677–88. doi: 10.1158/1078-0432.ccr-21-1963
70. Meric-Bernstam F, Sandhu SK, Hamid O, Spreafico A, Kasper S, Dummer R, et al. Phase Ib study of MIW815 (ADU-S100) in combination with spartalizumab (PDR001) in patients (pts) with advanced/metastatic solid tumors or lymphomas. J Clin Oncol. (2019) 37:2507–7. doi: 10.1200/JCO.2019.37.15_suppl.2507
71. Cooper BT, Chmura SJ, Luke JL, Shiao SL, Basho RK, Iams WT, et al. TAK-676 in combination with pembrolizumab after radiation therapy in patients (pts) with advanced non–small cell lung cancer (NSCLC), triple-negative breast cancer (TNBC), or squamous-cell carcinoma of the head and neck (SCCHN): Phase 1 study design. J Clin Oncol. (2022) 40:TPS2698–TPS2698. doi: 10.1200/JCO.2022.40.16_suppl.TPS2698
72. Harrington KJ, Brody J, Ingham M, Strauss J, Cemerski S, Wang M, et al. Preliminary results of the first-in-human (FIH) study of MK-1454, an agonist of stimulator of interferon genes (STING), as monotherapy or in combination with pembrolizumab (pembro) in patients with advanced solid tumors or lymphomas. Ann Oncol. (2018) 29:viii712. doi: 10.1093/annonc/mdy424.015
73. Janku F, Strauss J, Karim R, Olszanski AJ, Luke JL, Leach K, et al. A phase Ia/Ib dose-escalation study of intravenously administered SB 11285 alone and in combination with nivolumab in patients with advanced solid tumors. J Clin Oncol. (2020) 38:TPS3162–TPS3162. doi: 10.1200/JCO.2020.38.15_suppl.TPS3162
74. Kim D-S, Endo A, Fang FG, Huang KC, Bao X, Choi HW, et al. E7766, a macrocycle-bridged stimulator of interferon genes (STING) agonist with potent pan-genotypic activity. ChemMedChem. (2021) 16:1740–3. doi: 10.1002/cmdc.202100068
75. Ramanjulu JM, Pesiridis GS, Yang J, Concha N, Singhaus R, Zhang SY, et al. Design of amidobenzimidazole STING receptor agonists with systemic activity. Nature. (2018) 564:439–43. doi: 10.1038/s41586-018-0705-y
76. Pan BS, Perera SA. An orally available non-nucleotide STING agonist with antitumor activity. Science. (2020) 369:eaba6098. doi: 10.1126/science.aba6098
77. Chin EN, Yu C. Antitumor activity of a systemic STING-activating non-nucleotide cGAMP mimetic. Science. (2020) 369:993–9. doi: 10.1126/science.abb4255
78. Banerjee M, Basu S, Middya S, Shrivastava R, Ghosh R, Pryde DC, et al. Abstract LB-061: CRD5500: A versatile small molecule STING agonist amenable to bioconjugation as an ADC. Cancer Res. (2019) 79:LB–061-LB-061. doi: 10.1158/1538-7445.am2019-lb-061
79. Song C, Liu D, Liu S, Li D, Horecny I, Zhang X, et al. SHR1032, a novel STING agonist, stimulates anti-tumor immunity and directly induces AML apoptosis. Sci Rep. (2022) 12:8579. doi: 10.1038/s41598-022-12449-1
80. Wu Y-T, Fang Y, Wei Q, Shi H, Tan H, Deng Y, et al. Tumor-targeted delivery of a STING agonist improves cancer immunotherapy. Proc Natl Acad Sci. (2022) 119:e2214278119. doi: 10.1073/pnas.2214278119
81. Burgess TL, Amason JD, Rubin JS, Duveau DY, Lamy L, Roberts DD, et al. A homogeneous SIRPα-CD47 cell-based, ligand-binding assay: Utility for small molecule drug development in immuno-oncology. PloS One. (2020) 15:e0226661. doi: 10.1371/journal.pone.0226661
82. Oronsky B, Carter CA, Caroen S, Scribner C, Oronsky A, Reid TR. RRx-001, a first-in-class small molecule inhibitor of MYC and a downregulator of CD47, is an "erythrophagoimmunotherapeutic. Oncoimmunology. (2020) 9:1746172. doi: 10.1080/2162402x.2020.1746172
83. Tan W, Tang H, Jiang X, Ye F, Huang L, Shi D, et al. Metformin mediates induction of miR-708 to inhibit self-renewal and chemoresistance of breast cancer stem cells through targeting CD47. J Cell Mol Med. (2019) 23:5994–6004. doi: 10.1111/jcmm.14462
84. Rodríguez MM, Fiore E, Bayo J, Atorrasagasti C, García M, Onorato A, et al. 4Mu decreases CD47 expression on hepatic cancer stem cells and primes a potent antitumor T cell response induced by interleukin-12. Mol therapy: J Am Soc Gene Ther. (2018) 26:2738–50. doi: 10.1016/j.ymthe.2018.09.012
85. Casey SC, Tong L, Li Y, Do R, Walz S, Fitzgerald KN, et al. MYC regulates the antitumor immune response through CD47 and PD-L1. Science. (2016) 352:227–31. doi: 10.1126/science.aac9935
86. Nigro A, Ricciardi L, Salvato I, Sabbatino F, Vitale M, Crescenzi MA, et al. Enhanced expression of CD47 is associated with off-target resistance to tyrosine kinase inhibitor gefitinib in NSCLC. Front Immunol. (2019) 10:3135. doi: 10.3389/fimmu.2019.03135
87. Logtenberg MEW, Jansen JHM, Raaben M, Toebes M, Franke K, Brandsma AM, et al. Glutaminyl cyclase is an enzymatic modifier of the CD47- SIRPα axis and a target for cancer immunotherapy. Nat Med. (2019) 25:612–9. doi: 10.1038/s41591-019-0356-z
88. Rao S, Fu Y, Zhang M, Qin L, Bavadekar S, Cui H, et al. 45P Therapeutic potential of ISM8207: A novel QPCTL inhibitor, in triple-negative breast cancer and B-cell non-Hodgkin lymphoma. Ann Oncol. (2023) 34:S201. doi: 10.1016/j.annonc.2023.09.1547
89. Chen L, Huang CF, Li YC, Deng WW, Mao L, Wu L, et al. Blockage of the NLRP3 inflammasome by MCC950 improves anti-tumor immune responses in head and neck squamous cell carcinoma. Cell Mol Life Sci. (2018) 75:2045–58. doi: 10.1007/s00018-017-2720-9
90. Douguet L, Janho Dit Hreich S, Benzaquen J, Seguin L, Juhel T, Dezitter X, et al. A small-molecule P2RX7 activator promotes anti-tumor immune responses and sensitizes lung tumor to immunotherapy. Nat Commun. (2021) 12:653. doi: 10.1038/s41467-021-20912-2
91. Guo W, Sun Y, Liu W, Wu X, Guo L, Cai P, et al. Small molecule-driven mitophagy-mediated NLRP3 inflammasome inhibition is responsible for the prevention of colitis-associated cancer. Autophagy. (2014) 10:972–85. doi: 10.4161/auto.28374
92. Wang Z, Gao Y, He L, Sun S, Xia T, Hu L, et al. Structure-based design of highly potent toll-like receptor 7/8 dual agonists for cancer immunotherapy. J Medicinal Chem. (2021) 64:7507–32. doi: 10.1021/acs.jmedchem.1c00179
93. Zhang L, Dewan V, Yin H. Discovery of small molecules as multi-toll-like receptor agonists with proinflammatory and anticancer activities. J Medicinal Chem. (2017) 60:5029–44. doi: 10.1021/acs.jmedchem.7b00419
94. Chen Z, Zhang L, Yang J, Zheng L, Hu F, Duan S, et al. Design, synthesis, and structure-activity relationship of N-aryl-N'-(thiophen-2-yl)thiourea derivatives as novel and specific human TLR1/2 agonists for potential cancer immunotherapy. J Medicinal Chem. (2021) 64:7371–89. doi: 10.1021/acs.jmedchem.0c02266
95. Lecka J, Ben-David G, Simhaev L, Eliahu S, Oscar J Jr, Luyindula P, et al. Nonhydrolyzable ATP analogues as selective inhibitors of human NPP1: a combined computational/experimental study. J medicinal Chem. (2013) 56:8308–20. doi: 10.1021/jm400918s
96. Nadel Y, Lecka J, Gilad Y, Ben-David G, Förster D, Reiser G, et al. Highly potent and selective ectonucleotide pyrophosphatase/phosphodiesterase I inhibitors based on an adenosine 5'-(α or γ)-thio-(α,β- or β,γ)-methylenetriphosphate scaffold. J medicinal Chem. (2014) 57:4677–91. doi: 10.1021/jm500196c
97. Ausekle E, Ejaz SA, Khan SU, Ehlers P, Villinger A, Lecka J, et al. New one-pot synthesis of N-fused isoquinoline derivatives by palladium-catalyzed C-H arylation: potent inhibitors of nucleotide pyrophosphatase-1 and -3. Organic biomolecular Chem. (2016) 14:11402–14. doi: 10.1039/c6ob02236g
98. Chang L, Lee SY, Leonczak P, Rozenski J, De Jonghe S, Hanck T, et al. Imidazopyridine- and purine-thioacetamide derivatives: potent inhibitors of nucleotide pyrophosphatase/phosphodiesterase 1 (NPP1). J medicinal Chem. (2014) 57:10080–100. doi: 10.1021/jm501434y
99. Shayhidin EE, Forcellini E, Boulanger MC, Mahmut A, Dautrey S, Barbeau X, et al. Quinazoline-4-piperidine sulfamides are specific inhibitors of human NPP1 and prevent pathological mineralization of valve interstitial cells. Br J Pharmacol. (2015) 172:4189–99. doi: 10.1111/bph.13204
100. Forcellini E, Boutin S, Lefebvre CA, Shayhidin EE, Boulanger MC, Rhéaume G, et al. Synthesis and biological evaluation of novel quinazoline-4-piperidinesulfamide derivatives as inhibitors of NPP1. Eur J medicinal Chem. (2018) 147:130–49. doi: 10.1016/j.ejmech.2018.01.094
101. Carozza JA, Brown JA, Böhnert V, Fernandez D, AlSaif Y, Mardjuki RE, et al. Structure-aided development of small-molecule inhibitors of ENPP1, the extracellular phosphodiesterase of the immunotransmitter cGAMP. Cell Chem Biol. (2020) 27:1347–1358 e1345. doi: 10.1016/j.chembiol.2020.07.007
102. Baird JD, Vincent F, Michael G, Clayton K, Joshua O, Marka; C, et al. MV-626, a potent and selective inhibitor of ENPP1 enhances STING activation and augments T-cell mediated anti-tumor activity in vivo. Soc Immunotherapy Cancer 2018 Annu Meeting Posters. (2018) 7. Available at: https://digitalcommons.psjhealth.org/sitc2018/7
103. Goswami A, Deb B, Goyal S, Gosavi A, Mali M, Martis AM, et al. AVA-NP-695 selectively inhibits ENPP1 to activate STING pathway and abrogate tumor metastasis in 4T1 breast cancer syngeneic mouse model. Molecules. (2022) 27:6721. doi: 10.3390/molecules27196721
104. Weston A, Thode T, Munoz R, Daniel S, Soldi R, Kaadige M, et al. Abstract 3077: Preclinical studies of SR-8314, a highly selective ENPP1 inhibitor and an activator of STING pathway. Cancer Res. (2019) 79:3077–7. doi: 10.1158/1538-7445.am2019-3077
105. Peng Z, Guo K, Li Y-Y, Kong D, Yang J, Qin X, et al. Abstract 1275: Potent ENPP1 inhibitors activating STING pathway in tumor microenvironment. Cancer Res. (2021) 81:1275–5. doi: 10.1158/1538-7445.am2021-1275
106. Li Y-Y, Peng Z, Sun S, Guo K, Kong D, Li X, et al. Abstract 5486: ENPP1 inhibitor ZX-8177 enhances anti-tumor activity of conventional therapies by modulating tumor microenvironment. Cancer Res. (2022) 82:5486–6. doi: 10.1158/1538-7445.am2022-5486
107. Chen C, Liu J, Wu J, Cheng X, Qin L, Bavadekar S, et al. 873 ISM5939, a novel, potent, orally available, selective ENPP1 inhibitor enhances the anti-tumor effects of immune checkpoint inhibitors in syngeneic murine cancer models. J immunotherapy Cancer. (2023) 11:A972–2. doi: 10.1136/jitc-2023-SITC2023.0873
108. Csiki I, Glenn J, Schanzer J, Tuan B, Huang N, Dong A, et al. 169P Immunomodulatory effects of RBS2418, an oral ENPP1 inhibitor in combination with pembrolizumab in checkpoint-refractory metastatic adrenal cancer. Immuno-Oncology Technol. (2022) 16:100281. doi: 10.1016/j.iotech.2022.100281
109. Marron TU, Misleh JG, Chen C, Wainberg ZA, Powderly J, Boccia R, et al. 1025MO Preliminary safety, pharmacokinetics and immunomodulatory activity of RBS2418, an oral ENPP1 inhibitor, alone and in combination with pembrolizumab in patients with solid tumors. Ann Oncol. (2023) 34:S623–4. doi: 10.1016/j.annonc.2023.09.2164
110. Kim S, Ali I, Yu A, Lee SW, Park SY, Choi JH, et al. Abstract LBA009: Orally available ENPP1 inhibitor, TXN10128, restores STING activation in tumor microenvironment and confers anti-tumor responses in combination with immune checkpoint blockade. Mol Cancer Ther. (2021) 20:LBA009–9. doi: 10.1158/1535-7163.targ-21-lba009
111. Weston AS, Thode T, Ng S, Ghosh-Halder T, Rheinschmidt S, Adamson S, et al. Abstract LB323: Inhibition of ENPP1 using small molecule, SR-8541A, enhances the effect of checkpoint inhibition in cancer models. Cancer Res. (2023) 83:LB323–3. doi: 10.1158/1538-7445.am2023-lb323
112. Kong X, Zuo H, Huang HD, Zhang Q, Chen J, He C, et al. STING as an emerging therapeutic target for drug discovery: Perspectives from the global patent landscape. J Advanced Res. (2023) 44:119–33. doi: 10.1016/j.jare.2022.05.006
113. Ding C, Song Z, Shen A, Chen T, Zhang A. Small molecules targeting the innate immune cGAS‒STING‒TBK1 signaling pathway. Acta Pharm Sin B. (2020) 10:2272–98. doi: 10.1016/j.apsb.2020.03.001
114. Corrales L, Glickman LH, McWhirter SM, Kanne DB, Sivick KE, Katibah GE, et al. Direct activation of STING in the tumor microenvironment leads to potent and systemic tumor regression and immunity. Cell Rep. (2015) 11:1018–30. doi: 10.1016/j.celrep.2015.04.031
115. Kanwar JR, Kanwar RK, Pandey S, Ching LM, Krissansen GW. Vascular attack by 5,6-dimethylxanthenone-4-acetic acid combined with B7.1 (CD80)-mediated immunotherapy overcomes immune resistance and leads to the eradication of large tumors and multiple tumor foci. Cancer Res. (2001) 61:1948–56. doi: 10.1136/jitc-2020-001095
116. Shih AY, Damm-Ganamet KL, Mirzadegan T. Dynamic structural differences between human and mouse STING lead to differing sensitivity to DMXAA. Biophys J. (2018) 114:32–9. doi: 10.1016/j.bpj.2017.10.027
117. Le Naour J, Zitvogel L. Trial watch: STING agonists in cancer therapy. Oncoimmunology. (2020) 9:1777624. doi: 10.1080/2162402x.2020.1777624
118. Ruiz-Fernandez de Cordoba B, Martinez-Monge R, Lecanda F. ENPP1 immunobiology as a therapeutic target. Clin Cancer research: an Off J Am Assoc Cancer Res. (2023) 29:2184–93. doi: 10.1158/1078-0432.CCR-22-1681
119. Schlicher L, Green LG, Romagnani A, Renner F. Small molecule inhibitors for cancer immunotherapy and associated biomarkers - the current status. Front Immunol. (2023) 14:1297175. doi: 10.3389/fimmu.2023.1297175
120. Gangar M, Goyal S, Raykar D, Khurana P, Martis AM, Goswami A, et al. Design, synthesis and biological evaluation studies of novel small molecule ENPP1 inhibitors for cancer immunotherapy. Bioorganic Chem. (2022) 119:105549. doi: 10.1016/j.bioorg.2021.105549
121. Patel SD, Habeski WM, Cheng AC, de la Cruz E, Loh C, Kablaoui NM., et al. Quinazolin-4-piperidin-4-methyl sulfamide PC-1 inhibitors: alleviating hERG interactions through structure based design. Bioorganic medicinal Chem Lett. (2009) 19:3339–43. doi: 10.1016/j.bmcl.2009.04.006
122. Ruiz-Fernandez de Cordoba B, Moreno H, Valencia K, Perurena N, Ruedas P, Walle T, et al. Tumor ENPP1 (CD203a)/haptoglobin axis exploits myeloid-derived suppressor cells to promote post-radiotherapy local recurrence in breast cancer. Cancer Discovery. (2022) 12:1356–77. doi: 10.1158/2159-8290.CD-21-0932
123. Kulkarni A, Goswami A, Goyal S, Khurana P, Ghoshal I. Abstract 3244: AVA-NP-695, a potent and selective ENPP1 inhibitor, abrogates tumor metastasis in 4T1 syngeneic tumor model and demonstrates strong tumor regression when combined with radiation. Cancer Res. (2023) 83:3244–4. doi: 10.1158/1538-7445.am2023-3244
124. Weston A, Thode T, Ng S, Ghosh-Halder T, Gutowsky K, Durbin B, et al. 897-G SR-8541A, a small molecule inhibitor of ENPP1, enhances the effect of immune checkpoint inhibitors in a colorectal cancer model. J immunotherapy Cancer. (2023) 11:A1826–6. doi: 10.1136/jitc-2023-SITC2023.0897-G
125. Wang Y, Su L, Morin MD, Jones BT, Mifune Y, Shi H, et al. Adjuvant effect of the novel TLR1/TLR2 agonist Diprovocim synergizes with anti-PD-L1 to eliminate melanoma in mice. Proc Natl Acad Sci United States America. (2018) 115:E8698–e8706. doi: 10.1073/pnas.1809232115
126. Ribas A, Medina T, Kummar S, Amin A, Kalbasi A, Drabick JJ, et al. SD-101 in combination with pembrolizumab in advanced melanoma: results of a phase ib, multicenter study. Cancer Discovery. (2018) 8:1250–7. doi: 10.1158/2159-8290.cd-18-0280
127. Farooq M, Batool M, Kim MS, Choi S. Toll-like receptors as a therapeutic target in the era of immunotherapies. Front Cell Dev Biol. (2021) 9:756315. doi: 10.3389/fcell.2021.756315
128. Ding R, Jiao A, Zhang B. Targeting toll-like receptors on T cells as a therapeutic strategy against tumors. Int Immunopharmacol. (2022) 107:108708. doi: 10.1016/j.intimp.2022.108708
129. Williams HC, Bath-Hextall F, Ozolins M, Armstrong SJ, Colver GB, Perkins W, et al. Surgery versus 5% Imiquimod for nodular and superficial basal cell carcinoma: 5-year results of the SINS randomized controlled trial. J Invest Dermatol. (2017) 137:614–9. doi: 10.1016/j.jid.2016.10.019
130. Ota Y, Nagai Y, Hirose Y, Hori S, Koga-Yamakawa E, Eguchi K, et al. DSP-0509, a systemically available TLR7 agonist, exhibits combination effect with immune checkpoint blockade by activating anti-tumor immune effects. Front Immunol. (2023) 14:1055671. doi: 10.3389/fimmu.2023.1055671
131. Gane E, Pastagia M, Schwertschlag U, De Creus A, Schwabe C, Vandenbossche J, et al. Safety, tolerability, pharmacokinetics, and pharmacodynamics of oral JNJ-64794964, a TLR-7 agonist, in healthy adults. Antivir Ther. (2021) 26:58–68. doi: 10.1177/13596535211056581
132. Deane JA, Cortez GA, Li C, Eifler N, Kasibhatla S, Parikh N, et al. Abstract 4128: Identification and characterization of LHC165, a TLR7 agonist designed for localized intratumoral therapies. Cancer Res. (2019) 79:4128–8. doi: 10.1158/1538-7445.am2019-4128
133. Siu L, Brody J, Gupta S, Marabelle A. Safety and clinical activity of intratumoral MEDI9197 alone and in combination with durvalumab and/or palliative radiation therapy in patients with advanced solid tumors. J ImmunoTherapy Cancer. (2020) 8:e001095. doi: 10.1136/jitc-2020
134. Northfelt DW, Ramanathan RK, Cohen PA, Von Hoff DD, Weiss GJ, Dietsch GN, et al. A phase I dose-finding study of the novel Toll-like receptor 8 agonist VTX-2337 in adult subjects with advanced solid tumors or lymphoma. Clin Cancer research: an Off J Am Assoc Cancer Res. (2014) 20:3683–91. doi: 10.1158/1078-0432.ccr-14-0392
135. Hug BA, Matheny CJ, Burns O, Struemper H, Wang X, Washburn ML. Safety, pharmacokinetics, and pharmacodynamics of the TLR4 agonist GSK1795091 in healthy individuals: results from a randomized, double-blind, placebo-controlled, ascending dose study. Clin Ther. (2020) 42:1519–1534.e1533. doi: 10.1016/j.clinthera.2020.05.022
136. Willingham SB, Volkmer JP, Gentles AJ, Sahoo D, Dalerba P, Mitra SS, et al. The CD47-signal regulatory protein alpha (SIRPa) interaction is a therapeutic target for human solid tumors. Proc Natl Acad Sci United States America. (2012) 109:6662–7. doi: 10.1073/pnas.1121623109
137. Orozco-Morales M, Avilés-Salas A, Hernández-Pedro N, Catalán R. Clinicopathological and prognostic significance of CD47 expression in lung neuroendocrine tumors. J Immunol Res. (2021) 2021:6632249. doi: 10.1155/2021/6632249
138. Jiang Z, Sun H, Yu J, Tian W, Song Y. Targeting CD47 for cancer immunotherapy. J Hematol Oncol. (2021) 14:180. doi: 10.1186/s13045-021-01197-w
139. Miller TW, Amason JD, Garcin ED, Lamy L, Dranchak PK, Macarthur R, et al. Quantitative high-throughput screening assays for the discovery and development of SIRPα-CD47 interaction inhibitors. PloS One. (2019) 14:e0218897. doi: 10.1371/journal.pone.0218897
140. Oronsky B, Guo X, Wang X, Cabrales P, Sher D, Cannizzo L. Discovery of RRx-001, a myc and CD47 downregulating small molecule with tumor targeted cytotoxicity and healthy tissue cytoprotective properties in clinical development. J medicinal Chem. (2021) 64:7261–71. doi: 10.1021/acs.jmedchem.1c00599
141. Cabrales P. RRx-001 acts as a dual small molecule checkpoint inhibitor by downregulating CD47 on cancer cells and SIRP-α on monocytes/macrophages. Trans Oncol. (2019) 12:626–32. doi: 10.1016/j.tranon.2018.12.001
142. Stathis A, Bertoni F. BET proteins as targets for anticancer treatment. Cancer Discovery. (2018) 8:24–36. doi: 10.1158/2159-8290.cd-17-0605
143. Wu Z, Weng L, Zhang T, Tian H, Fang L, Teng H, et al. Identification of Glutaminyl Cyclase isoenzyme isoQC as a regulator of SIRPα-CD47 axis. Cell Res. (2019) 29:502–5. doi: 10.1038/s41422-019-0177-0
144. Nelson BE, Sheth R, Kelly K, Park H, Patel SP, McKinley D, et al. First in human phase I study of BMS-986299 as monotherapy and combined with nivolumab and ipilimumab in advanced solid tumors. J Clin Oncol. (2023) 41:e14584–4. doi: 10.1200/JCO.2023.41.16_suppl.e14584
Keywords: small molecule immunomodulators, cancer immunotherapy, innate immunity, immuno-oncology, small molecule
Citation: Goswami A, Goyal S, Khurana P, Singh K, Deb B and Kulkarni A (2024) Small molecule innate immune modulators in cancer therapy. Front. Immunol. 15:1395655. doi: 10.3389/fimmu.2024.1395655
Received: 05 March 2024; Accepted: 21 August 2024;
Published: 10 September 2024.
Edited by:
Zong Sheng Guo, University at Buffalo, United StatesReviewed by:
Hongfei Jiang, Qingdao University, ChinaCopyright © 2024 Goswami, Goyal, Khurana, Singh, Deb and Kulkarni. This is an open-access article distributed under the terms of the Creative Commons Attribution License (CC BY). The use, distribution or reproduction in other forums is permitted, provided the original author(s) and the copyright owner(s) are credited and that the original publication in this journal is cited, in accordance with accepted academic practice. No use, distribution or reproduction is permitted which does not comply with these terms.
*Correspondence: Aditya Kulkarni, YWRpdHlhQGF2YW1tdW5lLmNvbQ==