- 1The Second Clinical Medical School, Lanzhou University, Lanzhou, China
- 2Department of Neurosurgery, Lanzhou University Second Hospital, Lanzhou, China
- 3Key Laboratory of Neurology of Gansu Province, Lanzhou University Second Hospital, Lanzhou, China
Glioma is a malignant tumor of the central nervous system (CNS). Currently, effective treatment options for gliomas are still lacking. Neutrophils, as an important member of the tumor microenvironment (TME), are widely distributed in circulation. Recently, the discovery of cranial-meningeal channels and intracranial lymphatic vessels has provided new insights into the origins of neutrophils in the CNS. Neutrophils in the brain may originate more from the skull and adjacent vertebral bone marrow. They cross the blood-brain barrier (BBB) under the action of chemokines and enter the brain parenchyma, subsequently migrating to the glioma TME and undergoing phenotypic changes upon contact with tumor cells. Under glycolytic metabolism model, neutrophils show complex and dual functions in different stages of cancer progression, including participation in the malignant progression, immune suppression, and anti-tumor effects of gliomas. Additionally, neutrophils in the TME interact with other immune cells, playing a crucial role in cancer immunotherapy. Targeting neutrophils may be a novel generation of immunotherapy and improve the efficacy of cancer treatments. This article reviews the molecular mechanisms of neutrophils infiltrating the central nervous system from the external environment, detailing the origin, functions, classifications, and targeted therapies of neutrophils in the context of glioma.
1 Introduction
Glioma, with insidious onset and terrible prognosis, is the most frequent aggressive primary brain tumor in adults. Glioblastoma (GBM) as the grade 4 glioma comprises only IDH wild-type (wt) tumors and is the most notoriously hard to treat. The standard of care (SOC) for GBM involves a combination of maximal surgical resection, as well as concurrent administration of temozolomide (TMZ) chemotherapy and radiotherapy (1). But it is still ineffective in preventing relapse or progress of GBM. Recently, the discovery of the cranial bone-dura mater channels and intracranial lymphatic vessels has brought significant attention to the role of immune cells in brain tumors. Immunotherapies targeting the TME may become a novel treatment.
Glioma TME is a complex system that undergoes dynamic changes based on genetic and epigenetic alterations in gliomas. The glioma TME consists of a variety of non-tumor cells in addition to tumor cells, mainly including immune cells, vascular cells, stromal cells, and extracellular matrix (2, 3). These constituents engage in intimate interaction which is crucial to the development of tumors. In the immune cell population of the TME, innate immune cells are predominant, primarily comprising Tumor-Associated Macrophages (TAMs), Tumor-Associated Neutrophils (TANs), Natural Killer (NK) cells, and Myeloid-Derived Suppressor Cells (MDSCs). Among these, TAMs can constitute 30-50% of the cellular composition in gliomas, leading researchers to concentrate more on macrophages that dominate the TME and to pay insufficient attention to neutrophils in gliomas. The ratio of mononuclear cells to neutrophils in GBM is approximately 1:7 to 1:10, which is opposite to their ratio of 7:1 in circulation (4). Neutrophils are incapable of proliferation and possess a highly contested lifespan that varies from 19 hours to 5.4 days (5–7). The rarity of neutrophils within the central nervous system, alongside their uncertain lifespan, leads to the underestimation of their role in cancer. However, with the deepening of glioma research, neutrophils have been discovered to be crucial in glioma. Increasing evidence shows that the ratio of neutrophils to lymphocytes (NLR) in glioma patients has diagnostic and prognostic value (8). Neutrophils play a significant role in anti-bacteria and inflammation via phagocytosis, degranulation, neutrophil extracellular traps (NETs) release, and antigen presentation (9, 10). Moreover, similar to the polarization states of macrophages M1/M2, there is also a classification method of N1/N2 in neutrophils, which respectively play anti-tumor and pro-tumor roles in the TME. They show complex and dual functions in different stages of cancer progression (11–13). Various chemokines and their receptors mediate the migration of neutrophils from the periphery to the brain parenchyma. They contribute to carcinogenesis by promoting tumor proliferation, invasion, migration, angiogenesis, immunosuppression, and enhancing tumor drug resistance (14).Targeting TANs may serve as a novel generation of immunotherapy and enhance the beneficial effects of cancer therapies (15).
2 The development, recruitment and migration of neutrophils
The most common kind of innate immune cell in human beings are neutrophils. They make up about 70% of all white blood cells in circulation and are the most frequent type of white blood cells, acting as the frontline of immune system protection against bacterial and fungal diseases (16). The understanding of the origin of neutrophils has been evolving. It is well known that white blood cells originate from red bone marrow. In infants and young children, most of their bone marrow consists of red marrow. As age increases, some areas of red marrow undergo fatty transformation and become yellow marrow. Therefore, adult red marrow is mainly found in the medullary cavities of long bones (such as the humerus and femur) and the sparse bony trabeculae in flat bones (such as the ilium). Previous views suggested that immune cells in the circulation enter the cerebrospinal fluid and meninges, serving as a source of immune cells in that region. Whereas, some evidence indicates that red marrow can also be found in the skull, and the infiltration of white blood cells in the brain is more likely coming from the skull (Figure 1). Exploring the cranial bone cavity may provide a new direction for immunotherapy of intracranial tumors. It has been reported that some white blood cells are released into the circulation from the bone marrow through the nasal sinuses (17). As research deepens, researchers have discovered microscopic channels between the cranial bone marrow cavity and the dura mater through studies on mouse skulls and human craniectomy specimens. Neutrophils migrate to the brain through these “shortcuts.” Furthermore, it has been observed that the cranial bone marrow contributes significantly more neutrophils to the brain than the tibial bone marrow (18). In 2021, Andrea Cugurra et al. demonstrated that neutrophils originated from cranial and vertebral bone marrow can migrate to the meninges and parenchyma via ossified channels containing blood vessels (19, 20). In 2023, Meeki Ld et al. further confirmed the recruitment of TANs from the skull in GBM (21). They proposed that the role of the skull in supplying immune cells to the GBM TME is pronounced, and that systemic bone marrow sites could not adequately compensate for the absence of skull marrow. In addition, there are lymphatic pathways parallel to the dural venous sinuses, which directly connect the brain to deep cervical lymph nodes (22, 23). These lymphatic channels may contain dendritic cells and neutrophil nests associated with antigen presentation in the peripheral immune system, making them potential targets for future treatments (24, 25). The extent to which these pathways are involved in brain tumors is still unclear.
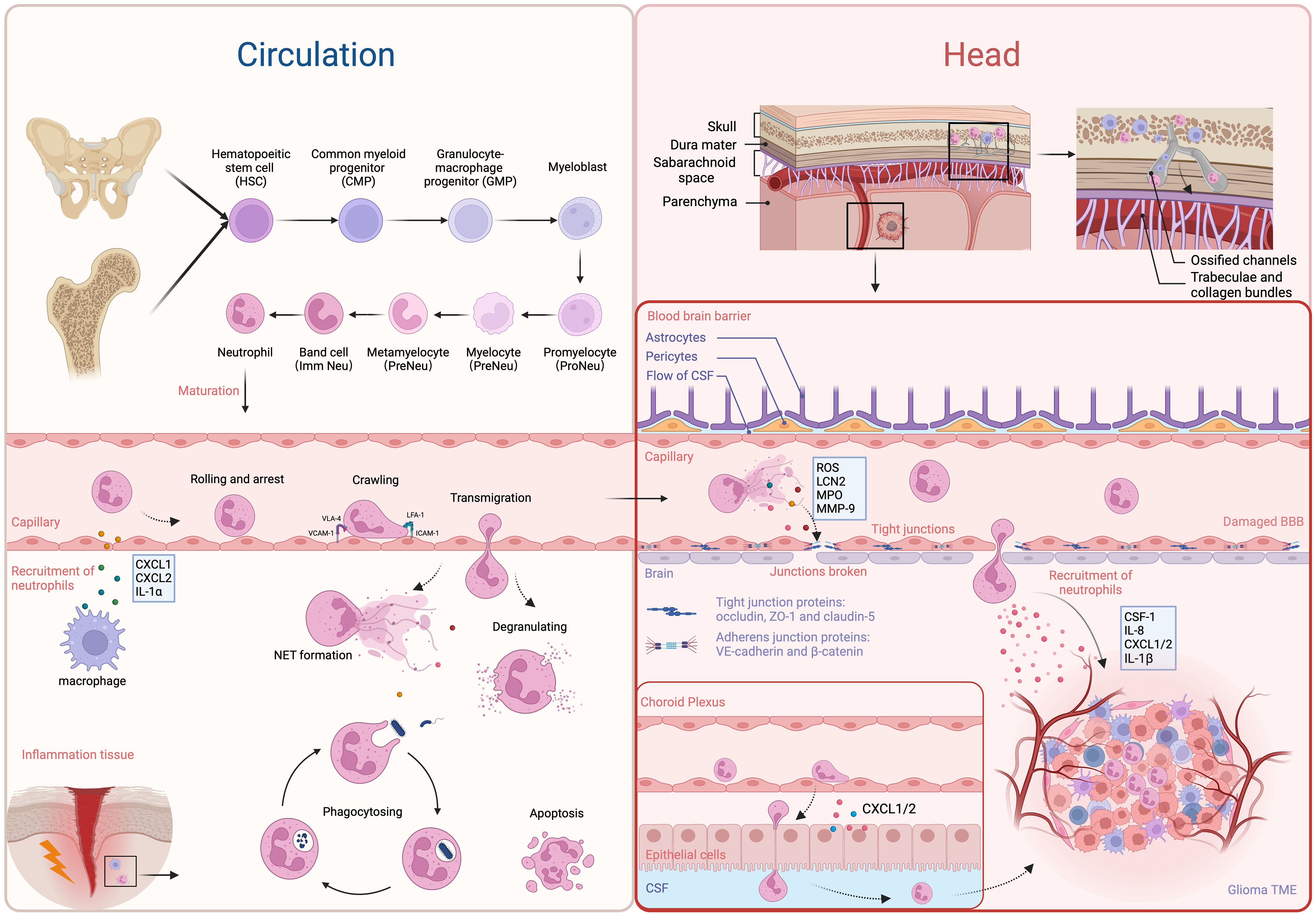
Figure 1 The development, recruitment and migration of neutrophils. The most majority of neutrophils in circulation are derived from bone marrow in long bones and the ilium. Starting from HSCs, they undergo several steps of development to become mature neutrophils, which are then released into the circulation. The resident macrophages and epithelial cells secrete chemoattractants to attract neutrophils (e.g. CXCL1, CXCL2, and IL-1α). Through the receptors LFA-4, ICAM-1, VCAM-1 and VLA-4, they migrate to sites of inflammation and exert their anti-bacteria and pathogen-clearing effects. Neutrophils in the brain primarily originate from bone marrow in the skull and adjacent vertebrae, with a smaller proportion derived from circulation. They only cross the BBB or choroid plexus and enter the brain parenchyma under pathological conditions, attracted by chemotactic factors in the TME. Furthermore, factors (e.g. ROS, LCN2, MPO, and MMP9) released by NETs can disrupt the integrity of the BBB, facilitating the infiltration of neutrophils into the brain parenchyma. On the other side, neutrophils also infiltrate the TME through chP under the attraction of CXCL1/2. In the TME, TANs are predominantly found in the central necrotic zone, while TAMs tend to accumulate at the periphery of the tumor. Created with BioRender.com.
The granulocyte-monocyte progenitors (GMPs) are produced by hematopoietic stem cells (HSCs) in the bone marrow. GMPs are considered to be the upstream precursor cells for all neutrophils in hematopoiesis. Regulated by granulocyte colony-stimulating factor (G-CSF), GMPs subsequently undergo several stages, including myeloblasts, promyelocytes, myelocytes, metamyelocytes, and band cells, finally developing into mature neutrophils (26). Neutrophils generally release more from the bone marrow and enter the circulation when functional CXC chemokine receptor 4 (CXCR4) gets lost and CXCR2 is expressed on neutrophils (27, 28). During emergency granulopoiesis, band cells complete maturation in injured tissue (29). The recruitment of neutrophils involves interactions between them and endothelial cells. During tissue damage or infection, neutrophils undergo a series of actions, including rolling, arrest, crawling, and transmigration, ultimately crossing the endothelial cells to enter inflammatory tissue under the influence of chemoattractants secreted by resident macrophages and epithelial cells, such as CXC-chemokine ligand 1 (CXCL1), CXCL2, and interleukin 1α (IL-1α) (30, 31). Endothelial intercellular adhesion molecule-1 (ICAM-1) and vascular adhesion molecule-1 (VCAM-1) regulate the arrest phase, respectively, through linking to neutrophil integrins lymphocyte functional antigen-1 (LFA-1) and very late antigen-4 (VLA-4) (31). Neutrophils then eliminate invading microorganisms through phagocytosis, degranulation (releasing granules containing cytotoxic substances), or in the form of NETs by releasing DNA-microbial protein complexes (32).
Under normal physiological conditions, immune cells are rarely found in the brain parenchyma. Those cells are mostly found in the organs surrounding the brain ventricles and choroid plexus, the meninges, and the perivascular space (33). The blood-brain barrier (BBB) and blood-cerebrospinal fluid barrier (BCSFB) constitute unique structures in the brain, which hinder the entry of immune cells and molecules into the brain, leading to immune responses different from the peripheral system (34). The BBB is an intricate system primarily formed by endothelial cells tightly connected through junctional molecules, astrocytic end-feet, and pericytes. Through tight junction (TJ), adherens junctions (AJ), and gap junctions (GJ) proteins, BBB restricts serum molecules and blood cells to entering the brain, and maintains the stability of the intracranial environment (35). Molecules such as occludin, claudin-5, and zonula occluden-1 (ZO-1) play crucial roles in regulating BBB permeability. Claudins are key factors in maintaining the “tightness” of the seal between adjacent endothelial cells (ECs) (36). Specifically, claudin-5 is the dominant transmembrane TJ protein on the BBB, maintaining vascular stability and regulating free molecular exchange (37). Furthermore, the BCSFB is responsible for isolating blood from cerebrospinal fluid and is frequently linked to the arachnoid mater and choroid plexus (ChP) (38). The presence of junctional molecules in the epithelial lining of the BCSFB at the choroid plexus allows for the selective permeability of blood components. However, due to its low expression of claudin-5, it is more permeable to small/large molecules and immune cells (39, 40). Neutrophils migrate into the brain under the attraction of CXCL1/2 chemokines secreted by the ChP epithelium in traumatic brain injury (TBI) models (41). Similarly, in the model of stroke, neutrophils also infiltrate the brain through the ChP (42). Although the BBB and BCSFB share functional similarities, more research has been conducted on the BBB due to its involvement in central nervous system pathology (31).
Inflammation and tumors share some common mechanisms for recruiting neutrophils. Research has shown that numerous cytokines and chemokines are associated with neutrophil recruitment, such as G-CSF, IL-1β, CXCL1, CXCL2, CC-chemokine ligand 3 (CCL3), and ICAM1 (43). G-CSF, in particular, is a major cytokine that regulates neutrophil recruitment and development (44, 45). Besides G-CSF, other factors that can enhance neutrophil proliferation include stem cell factor, IL-6, and granulocyte-macrophage colony-stimulating factor (GM-CSF) (46–48). The GBM-expressed long non-coding RNA (lncRNA) LINC01116 interacts with the transcriptional regulator DDX5, enhancing the transcriptional expression of IL-1β, thereby promoting the recruitment of neutrophils (49). DDX5 interacts with NF-kB p50, increasing the expression of p50 and promoting the growth of gliomas (50). Additionally, high expression of CD133 in gliomas facilitates the recruitment of neutrophils by modulating the regulation of IL-1β and its downstream chemotactic factors (51). Furthermore, the number of neutrophils around the necrotic areas is notably higher. Mesenchymal (MES) cellular state could be induced in glioma stem cells (GSCs) by IL-1β, which may also greatly improve the self-renewal capacity of GSCs (52, 53). Furthermore, it has been demonstrated that a number of other cancer cell-derived factors, including IL-8, CXCL3, CXCL5, and osteopontin (OPN), are efficient neutrophil chemoattractants (54, 55). IL-8 is produced by GBM via various pathways that can be induced by FasL and high mobility group protein 1 (HMGB1) (54, 56). It was first discovered that neutrophils expressed the chemokine receptor CXCR2, which is the receptor for IL-8 (57). CXCR2 was found to be expressed in GBM and significantly associated with tumor recurrence (58). Subsequently, it was found that CXCR2 also serves as a common chemokine receptor for CXCL3 and CXCL5. OPN is highly expressed in the necrotic areas of gliomas under the influence of hypoxia-inducible factor 1α (HIF-1α). OPN promotes the migration of neutrophils in vitro and co-localizes with them (55). These findings indicate that neutrophils are recruited to necrotic areas under the influence of OPN, CD133, and IL-1β. Neutrophils are mainly restricted to the necrotic core, which is the central area of the TME. Moreover, Patricia P. Ye et al. have shown that neutrophils are attracted to tissue damage sites by certain tumoral insults, such as ischemia that occurs in the early phases of tumor progression (59). This recruitment establishes a positive feedback loop that significantly amplifies necrosis development in GBM. TANs are associated with necrosis in the TME spatially and temporally. TANs correlate with the extent of tumor necrosis positively and predict lower survival rates in GBM patients (59). Monocytes and macrophages, are located around blood vessels and the peri necrotic area, while most microglia are found at the invasive edge of the tumor (60, 61).
Activated astrocytes and microglia release pro-inflammatory cytokines that upregulate adhesion molecules such as ICAM-1 on brain microvascular endothelial cells (BMECs), promoting neutrophil adhesion (62). Binding of neutrophil integrins and other stimuli leads to NETs forming and inflammatory chemicals releasing, containing reactive oxygen species (ROS), matrix metalloproteinase-9 (MMP-9), myeloperoxidase (MPO), and lipocalin-2 (LCN2). These neutrophil-derived substances help break down the BBB, increasing permeability, and reducing levels of tight junction proteins (claudin-5, occludin, and ZO-1) (35, 63). Therefore, neutrophils are more easily able to enter the brain parenchyma through the damaged BBB. The current understanding of the mechanisms underlying neutrophil recruitment in GBM is still limited. Further experiments are needed to explore precise mechanisms of signal transduction.
3 Neutrophil heterogeneity and plasticity
Different terms are used to classify neutrophils, such as N1/N2 neutrophils, TANs, and polymorphonuclear myeloid-derived suppressor cells (PMN-MDSCs) (64, 65). Mature neutrophils express specific cell surface proteins, including CD14-, CD15+, CD66b+, and CD16+ (66). Interestingly, the surface markers of PMN-MDSCs are similar to neutrophils,which includes CD14-, CD15+, CD66b+,CD11b+, CD33+, and HLA-DR- (67, 68). They both have similar morphology and phenotype, so some studies equate the two. However, they have some functional differences (65). Both PMN-MDSCs and mature neutrophils are capable of suppressing immune responses, but neutrophils are limited to cell activation. PMN-MDSC overlaps functionally with neutrophils and actually describes a subset of neutrophils (69). To distinguish them, these neutrophils are commonly named immunosuppressive neutrophils. The similarities and differences between immunosuppressive neutrophils and PMN-MDSCs are discussed in detail in the review by Benedict et al. (69).
Fridlender et al. (2009) categorized anti-tumor and pro-tumor TAN types, designating them as N1 (anti-tuomr) and N2 (pro-tumor), respectively (70). Interferon-beta (IFN-β) and transforming growth factor-beta (TGF-β) are responsible for polarizing neutrophils into N1 and N2, respectively (71, 72). The N1/N2 concept has now evolved to encompass broader aspects including neutrophil phenotypes, specialized morphologies, and particular functions. N1 neutrophils exhibit enhanced antigen presentation, greater phagocytic activity, stronger cytotoxicity, and an increased production of cytokines beneficial for antitumor immune responses (69). Conversely, N2 neutrophils show the opposite traits. Generally, N1 neutrophil has a hypersegmented nucleus, while N2 neutrophil has a circular nucleus. The N1 neutrophil phenotype is associated with high expression of Fas, TNF-α, and ICAM-1, while N2 expresses CXCR4, arginase1 (ARG1), VEGF (vascular endothelial growth factor), and MMP-9. Depletion of N1 was found to promote tumor growth (70, 73). Although there are functional differences, surface markers to distinguish N1 and N2 TAN have not yet been identified. Currently, the cellular state of neutrophils can be indirectly determined based on the expression of relevant genes and molecules (Table 1). The cellular state is uncertain, and the changes described above should only be interpreted as indicative of a general tendency. They provide a benchmark for reference but do not definitively determine that N1/N2 will invariably follow this pattern. Nonetheless, knowledge about neutrophil subgroups is still incomplete and controversial, and there is still a lack of specific molecular markers, unified research methods, and authoritative expertise.
Based on density, neutrophils can be further subdivided into three groups: immature low-density neutrophils (LDN), mature LDN, and mature high-density neutrophils (HDN) (80–82). Immature LDN has low levels of CD16, CD11b, and high CXCR2 expression (81). The phenotype of normal or HDN is similar to that of anti-tumor N1, while the LDN show impaired function and immunosuppressive characteristics (80, 81, 83). HDN can switch to LDN depending on factors in the TME. Theoretically, there is a middle state, the N0 state. It lies between N1 and N2, which has a neutral effect on tumors. One study indicated that IFN-β might decrease the pro-tumor function of N2 instead of driving the N2-N1 switch (71). This is similar with the MDSC-like and tumor-promoting activities frequently observed in TAN in the B16 model (84–86). Therefore, “N1-N2” refers to a continuum of distinct neutrophil activities, and anti-tumor polarization may be understood as a decrease in activity that promotes tumor growth.
4 Functions of neutrophils after activation
Neutrophil activation occurs prior to their infiltration (87).Under the stimulus or influence of chemokines and cytokines, neutrophils priming happens to get ready to immune response (88). Following activation, they participate in immune regulation through mechanisms such as NET formation, degranulation, and phagocytosis (9).
4.1 NET and NETosis in neutrophils
NET formation is a unique function of neutrophils. Several granular proteins and molecules are involved in the process of NET formation (89). Initially, the released MPO, cathepsin G, and NE from neutrophils enter the cytosol, where they begin to breakdown the nuclear lamina (90–92). Subsequently, peptidyl-arginine deaminase 4 (PAD4) gets into the nucleus and induces citrullination of histones, resulting in chromatin decondensation (93). After passing through the cellular and nuclear membranes, chromatin, along with its associated granular proteins, is ultimately secreted into the extracellular space. NETs promote the occurrence and invasion of GBM by expressing NET-related proteins (including elastase, protease-3, and protease G) (94–96). Neutrophil-derived NETs in cancer can act as a physical blockade, preventing immune cell infiltration and restricting the interaction between T cells and cancer cells (97). Certain proteins within the NETs can cause an immunosuppressive effect. An experiment revealed that the mesh-like structure of NETs is modified by HMGB1 (54). The externalized HMGB1 interacts with receptor for advanced glycation end products (RAGE) on GBM cells, resulting in the activation of the transcription factor NF-κB, which in turn enhances the expression and secretion of IL-8. The released IL-8 interacts with CXCR2 on neutrophils, triggering the generation of ROS and additional NET formation. This creates a positive feedback loop that promotes the progression of GBM (54).
Neutrophill cell death can be caused by NET formation, but this is not always the case. This regulated cell death that depends on NETs is called NETosis (98). NETosis occurs in two forms: suicidal and vital NETosis (99). Suicidal NETosis occurs in the presence of NADPH oxidase activity, leading to the release of neutrophil extracellular traps (NETs) and concurrent neutrophil death. In contrast, vital NETosis is characterized by the survival of neutrophils post-NET release, with intact nuclear and plasma membranes that retain inflammatory functions. The mechanisms underlying NETosis have been extensively discussed in other reviews (98, 100). NETosis is not limited to neutrophils. Cell direct interaction and receptor activation appear to be the catalysts for NETosis (99, 101, 102). NETosis also occurs in neutrophils within brain parenchyma. Subsequent neuronal injury and microglial activation amplify neuroinflammation and lead to neuronal loss (103). NETosis has shown great potential and may have important implications for future therapeutic and preventive strategies (104).
4.2 Degranulation in neutrophils
Degranulation is the process by which the contents of neutrophil granules are secreted into the extracellular space via exocytosis (105). Degranulation plays a role in various stages of neutrophil function. Primary (azurophilic granules), secondary (specific granules, tertiary (gelatinase), and secretory vesicles are the four main granule types found in neutrophils (87). Degranulation typically involves the secretion of contents from the first two types of granules, while the granules of the latter two types are often secreted periodically (106). Similar to exocytosis, degranulation is regulated by small Ras associated binding protein (Rab) GTPases, particularly the Rab27/Munc13-4 pathway (107).
4.3 Phagocytosis in neutrophils
Phagocytosis is another crucial function of neutrophils, responsible for effectively eliminating pathogens and/or debris (108, 109). However, the discovery of the phagocytic function of neutrophils in neuroscience has been relatively lagging. Phagocytic activity of cell debris is often thought to be caused by microglia in the CNS or macrophages in the peripheral nervous system (PNS) (110). Microglia and macrophages share a similar phagocytic function with neutrophils. To internalize different pathogens, neutrophils with fragment crystallizable (Fc) γ receptors (FcγRI, FcγRII, and FcγRIII) interact with IgG and other complement-mediated particles (111). This process results in the formation of phagosomes, which subsequently merge with lysosomes, generating an acidic environment for the enzymatic degradation of contents (112, 113).
5 Roles of neutrophils in glioma immunity
Immune infiltration is a characteristic of chronic inflammation, which can cause tissue damage and ultimately lead to tumor progression (64). Neutrophil infiltration begins early in tumorigenesis and persists throughout tumor progression (114). Like two sides of the same coin, neutrophils exhibit a complex dual function in different stages of cancer progression they possess an anti- or pro-tumoral phenotype through various molecular mechanisms of interaction with tumor cells, as well as modulating the development of tumor cells by regulating other immune cells (115, 116).
5.1 Glioma-neutrophils crosstalk
Multiple studies are currently exploring the correlation between neutrophils and cancer. Tumor cells secrete G-CSF, which promotes the proliferation of myeloid cells, resulting in a rise of neutrophils and an elevation of the neutrophil-lymphocyte ratio (NLR) in patients with GBM (117–119). In many types of tumors, NLR has emerged as a prognostic factor for predicting survival, such as colorectal cancer, liver cancer, breast tumor, and GBM (8, 120–122). In GBM patients undergoing radiotherapy or chemotherapy, an increase in neutrophils is associated with higher tumor grades and poor prognosis (123, 124). An NLR>4 is associated with poor prognosis, while an NLR<4 is linked to a better prognosis in GBM patients with wild-type IDH1 (125). In a zebrafish GBM model, neutrophils were observed to be recruited early in the tumorigenesis process, and their presence increased tumor cell proliferation due to the release of ROS, that could lead to DNA damage and trigger tumorigenesis in surrounding cells (126). Another in vivo experiment demonstrated that a higher percentage of Ki-67-positive cells (an antigen that marks cell proliferation status) in GBM is related to a higher concentration of neutrophils in peripheral blood. A high concentration of neutrophils appears to promote GBM proliferation and is more pronounced in high-grade GBM (127). Compared with non-GBM tumors, the Ki-67 positivity rate in high-grade GBM increases nearly two-fold, consistent with a higher NLR (>3).
Research on neutrophils in glioma is still in its early stages. In-depth study of the inherent characteristics of tumor cells, including genetic and epigenetic changes, can help explore the role of neutrophils in the TME (128). The activation of K-ras and the loss of Tp53 in pancreatic tumors promote the release of CXCR2 ligands (129), while PTEN and TP53 deficiency in prostate cancer lead to the release of CXCL17, both of them enhance neutrophil recruitment and contribute to TME immune suppression (130). In gliomas, genetic backgrounds and molecular states are correlated with neutrophil infiltration. For instance, IDH mutations are a favorable prognostic indicator for glioma patients, and neutrophil infiltration is less common in IDH-mutated gliomas compared to wild-type GBMs due to suppression of genes linked to chemotaxis and lack of immunosuppressive effects (131–133). TERT mutations promote neutrophil infiltration (134). GBM is categorized into three subtypes by the Cancer Genome Atlas (TCGA) initiative: mesenchymal (MES) subtype indicated by NF1 and PTEN loss; proneural (PN) subtype linked to PDGFRA amplification/mutation or CDKN2A homozygous deletion; and classical (CL) subtype defined by EGFR amplification/mutation (135, 136). In mouse models, tumors with NF1 downregulation infiltrate more neutrophils and microglia but less monocytes (137). A research about GBM heterogeneity suggested that MES GBM subtype is mainly composed of MES-like cells with NF1 loss (138). Furthermore, MES GBMs have more necrosis and increased number of macrophages and neutrophils than other TCGA subtypes (135, 139, 140). The mechanisms responsible for their recruitment remain to be elucidated (136).
Neutrophils are thought to conduct their anti-tumor effects through many ways. Neutrophils have the ability to suppress the growth of early-stage tumors (141–143). Neutrophils may eliminate tumor cells by directly contacting them and producing ROS (70, 143, 144). Specifically, the H2O2 secreted by neutrophils induces lethal Ca2+ influx mediated by TRMP2 channels, ultimately killing tumor cells. Additionally, a mechanism involving tumor cell apoptosis mediated by Fas ligand/Fas interaction has been discovered (145). Hepatocyte growth factor (HGF) present in the TME induces the recruitment of neutrophils and the production of nitric oxide (NO) (116). In gliomas, superphysiologic levels of NO result in the killing of tumor cells (146). Neutrophils can exert anti-tumor effects through a recognized mechanism called antibody-dependent cellular cytotoxicity (ADCC). Studies have shown that the receptors involved in neutrophil ADCC include Fc and Mac-1 (147, 148). Extensive literature suggests that the FcγRIIa receptor plays a major role in ADCC and shows polymorphic variants in different tumors (148, 149). Monoclonal antibodies bind to activating Fc receptors on the surface of neutrophils to initiate indirect-mediated cell death. The precise mechanism of cell death mediated by ADCC remains unclear and may be associated with “trogoptosis”. Neutrophils take a “bite” out of the cancer cell membrane, causing membrane damage and resulting in necrotic cell death (150). Neutrophils also show antitumor activity through TNF related apoptosis-inducing ligand (TRAIL), binding to the TRAIL receptors on tumor cells to induce cytotoxicity (151).
As previously mentioned, neutrophils are scarce in brain parenchyma; however, gliomas release various chemokines and cytokines (e.g., CSF-1, IL-8, IL-1β, CXCL1/2) that facilitate the recruitment of neutrophils within the TME.Cancer cells modulate the ratio of pro-tumor and anti-tumor neutrophils depending on the context (80). The depletion of neutrophils in the early stages of tumor development suggests a compromised anti-tumor capacity as the tumor progresses. Mariana R. Aubin et al. demonstrated that neutrophils attack tumor cells and reduce their viability within the first 24 hours of contact with GBM cells (152). With prolonged contact, tumor cells successfully reprogram the functionality of neutrophils into a pro-tumor phenotype, highlighting the heterogeneity of tumor-infiltrating neutrophils depending on their actual time within the tumor microenvironment. Notably, TANs associated with brain tumors show significant differences compared to neutrophils in the circulation. In syngeneic mouse models, neutrophils from healthy mice inhibit the tumorigenicity of GSCs, whereas neutrophils from tumor-bearing mice promote tumor progression and immune suppression (114). TANs have an extended lifespan, immune suppression, and pro-angiogenic potential (153). In vitro experiments have shown that IL-6 and IL-8 produced by glioma cells prolong the lifespan of neutrophils, indicating that neutrophils and glioma cells interact reciprocally (154). A study analyzing clinical samples from over 190 different human brain tumors confirmed the tissue-specific presence of neutrophils in brain tissue, showing a distinct inflammatory phenotype compared to primary brain lymphomas. TNF-α and ceruloplasmin (CP) are soluble inflammatory mediators that may be responsible for this inflammatory phenotype (153). The glioma TME inhibits the production of ROS in neutrophils and facilitates their polarization from an antitumoral N1 phenotype to a protumoral N2 phenotype. However, the precise mechanisms affecting neutrophil phenotypic changes within the TME remain unclear (153).
Neutrophils promote proliferation, angiogenesis, and invasion of tumor cells through various mechanisms (155). There have been reports that neutrophils release factors that promote GBM growth by increasing oxidative stress and releasing NET (94). HMGB1 released by NET can activate the TLR9 de-peptide pathway, thereby maintaining tumor cell growth (156). By cleaving laminin-111, neutrophil-secreted MMP9 and neutrophil elastase (NE) activate integrin signaling, which in turn promotes the growth of cancer cells (157). Histones in NETs disrupt the adhesion and tight junctions of vascular endothelial cells, while MMP-9 degrades the basement membrane of type IV collagen (103). Both actions increase the permeability of the BBB, making it easier for neutrophils to enter the TME and thereby promote tumor progression. NE contributes to the destruction of brain tissue and facilitates glioma invasion (158). Roeltje R. Maas and colleagues’ research indicated that in gliomas, TANs showed higher expression of various pro-angiogenic genes (such as VEGFA, THBD, and ICAM1). Compared to neutrophils in peripheral blood, TANs were enriched with the angiogenesis-related factor S100A9 and MMP9 (153). Neutrophil-released BV8, S100A8/9, and MMP9 are key factors in activating VEGF-A, thereby promoting angiogenesis (159). Furthermore, Neutrophils can induce drug resistance in gliomas. Anti-angiogenic therapy (e.g., bevacizumab) increases neutrophil infiltration, which activates the S100A4 signaling pathway, upregulating GSC self-renewal and mesenchymal transition. This leads to acquired drug resistance in tumor cells, thereby diminishing the efficacy of the treatment (160). Similarly, in animal models, Ly6G+ neutrophils and myeloid-derived suppressor cells (MDSCs) promote the transformation of cancer cells into GSCs through the regulation of the NOS2-NO-ID4 signaling axis (161).
5.2 Neutrophils-other immune cells crosstalk
The crosstalk between neutrophils and other immune cells is also complex, and their functions are not singular. TAMs and tumor-infiltrating lymphocytes (TILs) are key elements in the TME. By modulating the recruitment, characteristics, and phenotypes of these immune cells, neutrophils can have an impact on tumor growth. Neutrophils attract T cells and other leukocytes by producing chemokines, such as CCL2, CCL3, CXCL1, CXCL2, and CXCL10 (141, 162, 163). Neutrophils participate in T cell-dependent anti-tumor immune networks. They express ARG1, ROS, and NO when G-CSF and TGFβ exist in the TME, which inactivates T cells (164). In the presence of GM-CSF and IFNγ, neutrophils tend to mature and acquire antigen-presenting cell (APC) functions, and they can stimulate T cell proliferation through interactions with relevant ligands (141, 142). The binding of galectin-9 from neutrophils to TIM3 on lymphocytes initiates a process that results in the death of T cells (165). TANs also induce apoptosis in unactivated CD8+ T cells through the production of NO and TNF-α (166). In breast cancer, IL17-producing γδ T cells transform neutrophils into immunosuppressive type to promote tumor cells metastasis (167). Neutrophils express immune checkpoint receptors PDL1 and VISTA. The direct contact between checkpoint ligands on neutrophils and immune checkpoints on T cells can hinder anti-tumor immune responses, potentially leading to T cell-mediated apoptosis or T cell exhaustion (168, 169). The suppression of T cells is associated with direct contact between the integrin Mac-1 on neutrophils and T cells (170). Furthermore, degranulated neutrophils in the peripheral blood of patients with GBM were found to be correlated with increased serum ARG1 levels and decreased expression of T-cell CD3ζ. These neutrophils can induce T cell suppression, and it could be reversed by inhibiting ARG1 or supplementing with arginine (171). It has been reported that H2O2 can inhibit T cell activation and proliferation by reducing NF-κB activation, inhibiting the TCR: CD3ζ chain, and suppressing cytokine production (26).
Additionally, neutrophils interact with macrophages and unconventional T cell (UTC) subsets, specifically CD4-CD8-TCRαβ+ double-negative UTCs (UTCαβ), which are crucial for effective anti-tumor immunity (172). Neutrophils enhance macrophage production of IL-12, promoting IFNγ generation and polarization of UTCαβ toward a type 1 immune response (172). In bladder cancer, NETs enhance the antitumor effect by increasing macrophage infiltration (173). Neutrophil-derived microvesicles (NDMVs) enhance the polarization of anti-inflammatory macrophages, while neutrophil-derived trails (NDTRs) induce the polarization of pro-inflammatory macrophages. Therefore, neutrophils exhibit a complex dual regulatory role within the TME with macrophages, requiring further experimental verification of the specific mechanisms involved (174).
In the crosstalk between neutrophils and DCs, prolonged contact with NETs leads to DC apoptosis, with NETs obstructing DC responses and suppressing antitumor immunity (175). Conversely, DCs secrete factors that activate DNase1L3, playing a role in NET degradation and thus hindering NET-induced recruitment of pro-tumorigenic neutrophils (176). Moreover, neutrophils induce DC maturation through TNF-α and ICAM3 and, via cell-cell contact, promote IL-12 production, ultimately driving T cell proliferation (177). In glioma TME, DCs are typically in an immature state, leading to reduced activation of effector T cells. Research has shown that overexpression of Nrf in DCs inhibits their maturation, but suppression of Nrf2 can restore DC activity (178). Activated DCs can secrete high levels of bioactive IL-12p70, enhancing NK cell activity and initiating CD8+ T cell immunity, thereby activating the collaborative antitumor capacity of immune cells (179). Interestingly, another study demonstrated the opposite result, where neutrophil activation in glioma patients correlated with elevated plasma IL12p70 levels, which is indicative of more aggressive glioma progression (180). The mechanisms of interaction between DCs and neutrophils in the glioma TME require further exploration. In mouse models, neutrophils can suppress NK cell activation in tumor-bearing mice, thereby inhibiting NK cell cytotoxicity, weakening antitumor effects, and enhancing tumor cell metastatic capabilities (181–184).
Finally, neutrophils influence the immunosuppressive environment by affecting the development of regulatory T cells (Tregs) (185). The reduced sensitivity of Tregs to oxidative stress in the TME leads to Treg enrichment, and neutrophils also recruit Tregs by secreting CCL17 (186–188). Additionally, a positive feedback loop mediated by the anti-inflammatory cytokine IL-10 between neutrophils and Tregs supports immunosuppression (189, 190).
6 Metabolism in neutrophils
Similar to all other cells, metabolism is essential to the functioning of neutrophils (Figure 2). Neutrophils primarily rely on glycolysis and minimal mitochondrial respiration to regulate and maintain ATP production, which facilitates chemotaxis, phagocytosis, cytokine expression, ROS generation, degranulation, and NET formation in circulation and peripheral tissues (191). In response to stimulation, neutrophils switch from glycolysis to the mitochondria-mediated pentose phosphate pathway (PPP) to improve oxidative burst (192). PPP can generate NADPH, and then NADPH oxidase oxidizes it to produce ROS, which in turn causes the production of NETs. ROS generation in neutrophils is significantly influenced by mitochondrial activity. The high glycolytic potential of neutrophils contributes to lactate production in the TME. NET formation depends on lactate formation (191). This acidic environment supports tumor survival, progression, and inhibition of immune activity near tumor cells (14). When there is inflammation and local oxygen levels are low, the preference for glycolysis improves neutrophil survival rates (193). This partly explains why neutrophils tend to concentrate in hypoxic areas at the center of the TME. Mechanistically, activated mature neutrophils induce lipid peroxidation in tumor cells by transferring granules containing MPO, which increases lipid-based ROS in these cells. This process promotes ferroptosis and tumor necrosis, which indicates poor prognosis in GBM (59). During neutrophil oxidative bursts, MPO granules produce hypochlorous acid (HOCl), which is crucial for NETosis (194). The NETosis pathway requires the production of ROS and an increase in intracellular Ca2+ (195). This is mediated by mitochondrial ATP production, which maintains a positive feedback loop by activating purinergic receptors such as P2Y2 (196).
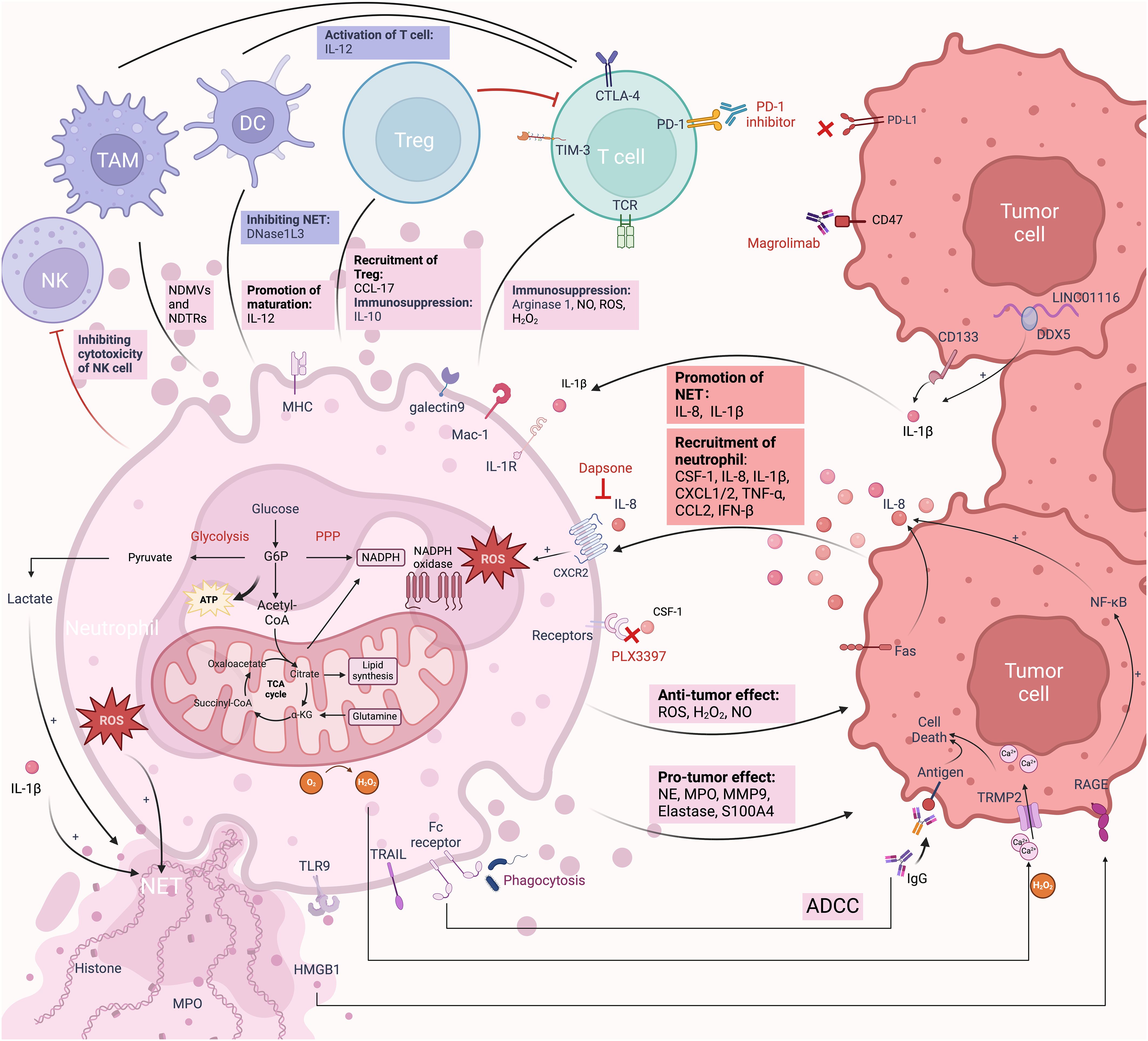
Figure 2 The immune responses of TANs in the TME. TANs and their interactions with tumor cells and immune cells constitute a complex TME. Tumor cells attract neutrophils into the TME through various chemokines and secretions. In the early stages of neutrophil entry into the TME, the secretion of NO, MPO, and ROS mediates tumor cell killing. Neutrophils also promote tumor cell death through ADCC and the secretion of H2O2. Over time, under the influence of chemokines and secretions in the TME, TANs transition from an anti-inflammatory to a pro-inflammatory phenotype. For example, IL-8 and IL-1β secreted by gliomas promote the formation of NETs through different mechanisms. ROS and lactate secreted by TANs also promote the formation of NETs. TANs associated secretion of NE, MMP9, elastase, and S100A4 plays crucial roles in tumor proliferation, invasion, and angiogenesis. Within the TME, TANs engage in complex interactions with TAMs, NK cells, DCs, T cells, and Tregs. Targeting these receptors is a form of neutrophil-mediated immunotherapy. Neutrophils themselves rely on glycolysis for energy supply and promote the generation of an acidic environment that favors tumor growth. Created with BioRender.com.
7 Neutrophils-targeted immunotherapy
Neutrophils-targeted therapeutic strategies can draw inspiration from macrophages, which also originate from myeloid cells. These strategies mainly involve inhibiting recruitment, reprogramming, and depleting neutrophils.
Due to the pro-tumor effects of neutrophils, blocking the recruitment of immunosuppressive neutrophils can help alleviate tumor progression to some extent. Given the powerful recruitment effects of G-CSF on myeloid cells, it is considered an ideal target for exploration (197). The anti-tumor therapy agent Pexidartinib (PLX3397) targeting CSF1R has completed a Phase II clinical trial for recurrent GBM (NCT01349036). Unfortunately, this treatment did not provide survival benefits to patients. In targeting IL-8/CXCR2-mediated neutrophil recruitment, Karpel-Massler et al. achieved certain efficacy by using dapsone to block IL-8-mediated neutrophil infiltration in gliomas (198, 199). A Phase I clinical trial (NCT03161431) investigating the use of CXCR2 inhibitor SX-682 for the treatment of melanoma cancer is currently recruiting participants.
Reprogramming neutrophils to the N1 phenotype and restoring their phagocytic function is also a promising strategy. TGFβ, as a polarization factor for N2 neutrophils, targeting its receptor presents a promising approach. The TGFβ receptor I kinase inhibitor Galunisertib (LY2157299) is currently under clinical trials in glioma patients. It is undergoing a Phase I clinical trial (NCT01682187) and a Phase II clinical trial (NCT01582269) for patients with recurrent GBM. Neutrophil-mediated tumor cell death is inhibited by CD47-SIRPα signaling (200). Targeting CD47-SIRPα can enhance the ability of neutrophils to eliminate tumors through ADCC (201). Currently, anti-CD47 and anti-SIRPα monoclonal antibodies (mAb) have shown preliminary efficacy in some solid tumors and have entered clinical trial stages, such as Hu5F9-G4 (anti-CD47, NCT02216409) and TTI-621 (anti-CD47, NCT02890368), and BI765063 (anti-SIRPα, NCT03990233). The anti-CD47 antibody Magrolimab, developed to target the CD47 receptor, has been tested in a Phase I clinical trial for glioma (NCT05169944). Anti-CD47 antibodies enhance the phagocytic activity of TAMs. In GBM mouse model, these antibodies show synergistic effects when combined with TMZ, PD1 antibodies, and activation of TLR3 and TLR9 (202–204). The involvement of neutrophils in this synergistic effect remains to be elucidated and could provide insights into therapeutic strategies. Exogenous administration of recombinant TRAIL or agonistic TRAIL-R antibodies can induce apoptosis in tumor cells and immunosuppressive cells (such as TAMs and Tregs), leading to an increase in cytotoxic T lymphocyte (CTL) numbers and enhanced phagocytic capabilities of neutrophils, monocytes, and macrophages (151).
Furthermore, reducing neutrophils by anti-Ly6G antibodies is a potential therapeutic strategy (59, 205). However, the use of anti-Ly6G antibody in experiments has not achieved consistent reduction in neutrophil levels, further research to explore more durable approaches is still needed. Inhibiting NET can weaken the pro-tumor effect of neutrophils. Experiments have found DNase I, PAD inhibitors, neonatal NET-inhibitory factor, metformin, and anti-HMGB antibodies are effective in reducing NET (206–210). The use of oncolytic herpes simplex virus (oHSV) for the treatment of glioma results in the upregulation of IGF2BP3 and increased NET formation in mouse models. BET inhibitors can block IGF2BP3-induced NETosis and inhibit NET formation, thereby enhancing the lytic activity of oHSV and improving therapeutic efficacy (211). The formation of NETs may impede the efficacy of DC vaccines by disrupting the antitumor responses induced by NK and T cells. Combining DC vaccines with NET inhibitors could be a promising strategy to enhance antitumor responses (175).
In addition, neutrophil engineering technologies that involve rational modifications of neutrophils have shown promising clinical prospects. For example, neutrophils loaded with paclitaxel (PTX)-loaded liposomes and neutrophil-derived exosome (NEs-Exos) loaded with doxorubicin have demonstrated the ability to effectively penetrate the BBB and target tumors (212, 213). Other therapeutic strategies, including neutrophil-associated nanoparticles (NPs), liposomes, and viral delivery systems, are extensively discussed in another review (214).
8 Conclusion
Neutrophils, as an important component of the immune system, are gradually revealing their roles in brain tumors. It has been demonstrated that there is a correlation between neutrophils and gliomas, and future attention should be paid to how the dual role of neutrophils can switch. Efforts are still required to distinguish neutrophils from PMN-MDSCs, N1, and N2 types, as a comprehensive understanding of the molecular targets of different immune cells is crucial for subsequent targeted therapies based on these insights. Current research on neutrophils primarily utilizes murine orthotopic transplant tumor models, but future studies should extend to human gliomas. The types of immune cell infiltrates vary across different grades of gliomas and different genetic types of GBM, necessitating extensive experimentation to identify the trends that lead to specific types of immune cell infiltrates, in order to design effective therapies applicable to various subtypes or cellular states. Some important processes, such as NET formation, metabolic changes, and neutrophil-T cell crosstalk, are also research focuses. The interactions between neutrophils and other immune cells in the TME are still in the early stages of research. Beyond the crosstalk between neutrophils, tumor cells, and immune cells, the connections between neutrophils and non-immune cells remain unexplored. Neutrophils are among the more fragile immune cells, how can they be extracted with minimal damage to surface proteins? Moreover, during the separation process of tissue samples, spatial information about TANs within the tumor is lost. Addressing these technical challenges and reducing costs are also critical. In addition, advancing the progress of neutrophils in gliomas, targeting the recruitment, reprogramming, depletion of neutrophils, and exploring new directions in neutrophil engineering to enhance drug utilization efficiency are important. The application of neutrophils in cancer immunotherapy should be promoted to provide more effective treatment options for cancer patients.
Author contributions
CS: Writing – original draft. SW: Writing – original draft. ZM: Writing – original draft. JZ: Writing – original draft. ZD: Writing – original draft. YP: Writing – review & editing. GY: Writing – review & editing.
Funding
The author(s) declare financial support was received for the research, authorship, and/or publication of this article. This study was supported by grants from the National Natural Science Foundation of China (82360566/81960541/82060455).
Acknowledgments
All figures are created with BioRender.com.
Conflict of interest
The authors declare that the research was conducted in the absence of any commercial or financial relationships that could be construed as a potential conflict of interest.
Publisher’s note
All claims expressed in this article are solely those of the authors and do not necessarily represent those of their affiliated organizations, or those of the publisher, the editors and the reviewers. Any product that may be evaluated in this article, or claim that may be made by its manufacturer, is not guaranteed or endorsed by the publisher.
References
1. Lim M, Xia Y, Bettegowda C, Weller M. Current state of immunotherapy for glioblastoma. Nat Rev Clin Oncol. (2018) 15:422–42. doi: 10.1038/s41571-018-0003-5
2. Quail DF, Joyce JA. The microenvironmental landscape of brain tumors. Cancer Cell. (2017) 31:326–41. doi: 10.1016/j.ccell.2017.02.009
3. Xuan W, Lesniak MS, James CD, Heimberger AB, Chen P. Context-dependent glioblastoma–macrophage/microglia symbiosis and associated mechanisms. Trends Immunol. (2021) 42:280–92. doi: 10.1016/j.it.2021.02.004
4. Doeing DC, Borowicz JL, Crockett ET. Gender dimorphism in differential peripheral blood leukocyte counts in mice using cardiac, tail, foot, and saphenous vein puncture methods. BMC Clin Pathol. (2003) 3:3. doi: 10.1186/1472-6890-3-3
5. Lahoz-Beneytez J, Elemans M, Zhang Y, Ahmed R, Salam A, Block M, et al. Human neutrophil kinetics: modeling of stable isotope labeling data supports short blood neutrophil half-lives. Blood. (2016) 127:3431–8. doi: 10.1182/blood-2016-03-700336
6. Pillay J, Den Braber I, Vrisekoop N, Kwast LM, De Boer RJ, Borghans JAM, et al. In vivo labeling with 2H2O reveals a human neutrophil lifespan of 5.4 days. Blood. (2010) 116:625–7. doi: 10.1182/blood-2010-01-259028
7. Summers C, Rankin SM, Condliffe AM, Singh N, Peters AM, Chilvers ER. Neutrophil kinetics in health and disease. Trends Immunol. (2010) 31:318–24. doi: 10.1016/j.it.2010.05.006
8. Gomes Dos Santos A, De Carvalho RF, De Morais ANLR, Silva TM, Baylão VMR, Azevedo M, et al. Role of neutrophil-lymphocyte ratio as a predictive factor of glioma tumor grade: A systematic review. Crit Rev Oncology/Hematol. (2021) 163:103372. doi: 10.1016/j.critrevonc.2021.103372
9. Rosales C. Neutrophil: A cell with many roles in inflammation or several cell types? Front Physiol. (2018) 9:113. doi: 10.3389/fphys.2018.00113
10. Li Y, Wang W, Yang F, Xu Y, Feng C, Zhao Y. The regulatory roles of neutrophils in adaptive immunity. Cell Commun Signal. (2019) 17:147. doi: 10.1186/s12964-019-0471-y
11. Yin H, Gao S, Chen Q, Liu S, Shoucair S, Ji Y, et al. Tumor-associated N1 and N2 neutrophils predict prognosis in patients with resected pancreatic ductal adenocarcinoma: A preliminary study. MedComm. (2022) 3:e183. doi: 10.1002/mco2.183
12. Chen Q, Yin H, Liu S, Shoucair S, Ding N, Ji Y, et al. Prognostic value of tumor-associated N1/N2 neutrophil plasticity in patients following radical resection of pancreas ductal adenocarcinoma. J Immunother Cancer. (2022) 10:e005798. doi: 10.1136/jitc-2022-005798
13. St. Paul M, Ohashi PS. The roles of CD8+ T cell subsets in antitumor immunity. Trends Cell Biol. (2020) 30:695–704. doi: 10.1016/j.tcb.2020.06.003
14. Rice CM, Davies LC, Subleski JJ, Maio N, Gonzalez-Cotto M, Andrews C, et al. Tumour-elicited neutrophils engage mitochondrial metabolism to circumvent nutrient limitations and maintain immune suppression. Nat Commun. (2018) 9:5099. doi: 10.1038/s41467-018-07505-2
15. Gregory AD, McGarry Houghton A. Tumor-associated neutrophils: new targets for cancer therapy. Cancer Res. (2011) 71:2411–6. doi: 10.1158/0008-5472.CAN-10-2583
16. Wang J, Jia Y, Wang N, Zhang X, Tan B, Zhang G, et al. The clinical significance of tumor-infiltrating neutrophils and neutrophil-to-CD8+ lymphocyte ratio in patients with resectable esophageal squamous cell carcinoma. J Transl Med. (2014) 12:7. doi: 10.1186/1479-5876-12-7
17. Itkin T, Gur-Cohen S, Spencer JA, Schajnovitz A, Ramasamy SK, Kusumbe AP, et al. Distinct bone marrow blood vessels differentially regulate haematopoiesis. Nature. (2016) 532:323–8. doi: 10.1038/nature17624
18. Herisson F, Frodermann V, Courties G, Rohde D, Sun Y, Vandoorne K, et al. Direct vascular channels connect skull bone marrow and the brain surface enabling myeloid cell migration. Nat Neurosci. (2018) 21:1209–17. doi: 10.1038/s41593-018-0213-2
19. Cugurra A, Mamuladze T, Rustenhoven J, Dykstra T, Beroshvili G, Greenberg ZJ, et al. Skull and vertebral bone marrow are myeloid cell reservoirs for the meninges and CNS parenchyma. Science. (2021) 373:eabf7844. doi: 10.1126/science.abf7844
20. Brioschi S, Wang W-L, Peng V, Wang M, Shchukina I, Greenberg ZJ, et al. Heterogeneity of meningeal B cells reveals a lymphopoietic niche at the CNS borders. Science. (2021) 373:eabf9277. doi: 10.1126/science.abf9277
21. Lad BM, Beniwal AS, Jain S, Shukla P, Jung J, Shah SS, et al. Glioblastoma induces the recruitment and differentiation of hybrid neutrophils from skull bone marrow. BioRxiv [Preprint]. (2023), 2023.03.24.534105. doi: 10.1101/2023.03.24.534105
22. Louveau A, Smirnov I, Keyes TJ, Eccles JD, Rouhani SJ, Peske JD, et al. Structural and functional features of central nervous system lymphatic vessels. Nature. (2015) 523:337–41. doi: 10.1038/nature14432
23. Ringstad G, Valnes LM, Dale AM, Pripp AH, Vatnehol S-AS, Emblem KE, et al. Brain-wide glymphatic enhancement and clearance in humans assessed with MRI. JCI Insight. (2018) 3:e121537. doi: 10.1172/jci.insight.121537
24. Sas AR, Carbajal KS, Jerome AD, Menon R, Yoon C, Kalinski AL, et al. A new neutrophil subset promotes CNS neuron survival and axon regeneration. Nat Immunol. (2020) 21:1496–505. doi: 10.1038/s41590-020-00813-0
25. Mundt S, Mrdjen D, Utz SG, Greter M, Schreiner B, Becher B. Conventional DCs sample and present myelin antigens in the healthy CNS and allow parenchymal T cell entry to initiate neuroinflammation. Sci Immunol. (2019) 4:eaau8380. doi: 10.1126/sciimmunol.aau8380
26. Friedmann-Morvinski D, Hambardzumyan D. Monocyte-neutrophil entanglement in glioblastoma. J Clin Invest. (2023) 133:e163451. doi: 10.1172/JCI163451
27. Casanova-Acebes M, Nicolás-Ávila JA, Li JL, García-Silva S, Balachander A, Rubio-Ponce A, et al. Neutrophils instruct homeostatic and pathological states in naive tissues. J Exp Med. (2018) 215:2778–95. doi: 10.1084/jem.20181468
28. Eash KJ, Greenbaum AM, Gopalan PK, Link DC. CXCR2 and CXCR4 antagonistically regulate neutrophil trafficking from murine bone marrow. J Clin Invest. (2010) 120:2423–31. doi: 10.1172/JCI41649
29. Balog BM, Sonti A, Zigmond RE. Neutrophil biology in injuries and diseases of the central and peripheral nervous systems. Prog Neurobiol. (2023) 228:102488. doi: 10.1016/j.pneurobio.2023.102488
30. Prame Kumar K, Nicholls AJ. Wong CHY. Partners in crime: neutrophils and monocytes/macrophages in inflammation and disease. Cell Tissue Res. (2018) 371:551–65. doi: 10.1007/s00441-017-2753-2
31. Santos-Lima B, Pietronigro EC, Terrabuio E, Zenaro E, Constantin G. The role of neutrophils in the dysfunction of central nervous system barriers. Front Aging Neurosci. (2022) 14:965169. doi: 10.3389/fnagi.2022.965169
32. Uribe-Querol E, Rosales C. Neutrophils in cancer: two sides of the same coin. J Immunol Res. (2015) 2015:1–21. doi: 10.1155/2015/983698
33. Li Q, Barres BA. Microglia and macrophages in brain homeostasis and disease. Nat Rev Immunol. (2018) 18:225–42. doi: 10.1038/nri.2017.125
34. Engelhardt B, Vajkoczy P, Weller RO. The movers and shapers in immune privilege of the CNS. Nat Immunol. (2017) 18:123–31. doi: 10.1038/ni.3666
35. Zlokovic BV. The blood-brain barrier in health and chronic neurodegenerative disorders. Neuron. (2008) 57:178–201. doi: 10.1016/j.neuron.2008.01.003
36. Alahmari A. Blood-brain barrier overview: structural and functional correlation. Neural Plasticity. (2021) 2021:1–10. doi: 10.1155/2021/6564585
37. Greene C, Hanley N, Campbell M. Claudin-5: gatekeeper of neurological function. Fluids Barriers CNS. (2019) 16:3. doi: 10.1186/s12987-019-0123-z
38. Brøchner CB, Holst CB, Møllgård K. Outer brain barriers in rat and human development. Front Neurosci. (2015) 9:75. doi: 10.3389/fnins.2015.00075
39. Kratzer I, Vasiljevic A, Rey C, Fevre-Montange M, Saunders N, Strazielle N, et al. Complexity and developmental changes in the expression pattern of claudins at the blood–CSF barrier. Histochem Cell Biol. (2012) 138:861–79. doi: 10.1007/s00418-012-1001-9
40. Tietz S, Engelhardt B. Brain barriers: Crosstalk between complex tight junctions and adherens junctions. J Cell Biol. (2015) 209:493–506. doi: 10.1083/jcb.201412147
41. Szmydynger-Chodobska J, Strazielle N, Zink BJ, Ghersi-Egea J-F, Chodobski A. The role of the choroid plexus in neutrophil invasion after traumatic brain injury. J Cereb Blood Flow Metab. (2009) 29:1503–16. doi: 10.1038/jcbfm.2009.71
42. Otxoa-de-Amezaga A, Gallizioli M, Pedragosa J, Justicia C, Miró-Mur F, Salas-Perdomo A, et al. Location of neutrophils in different compartments of the damaged mouse brain after severe ischemia/reperfusion. Stroke. (2019) 50:1548–57. doi: 10.1161/STROKEAHA.118.023837
43. Furze RC, Rankin SM. Neutrophil mobilization and clearance in the bone marrow. Immunology. (2008) 125:281–8. doi: 10.1111/j.1365-2567.2008.02950.x
44. Liu F, Wu HY, Wesselschmidt R, Kornaga T, Link DC. Impaired production and increased apoptosis of neutrophils in granulocyte colony-stimulating factor receptor–deficient mice. Immunity. (1996) 5:491–501. doi: 10.1016/S1074-7613(00)80504-X
45. Richards MK, Liu F, Iwasaki H, Akashi K, Link DC. Pivotal role of granulocyte colony-stimulating factor in the development of progenitors in the common myeloid pathway. Blood. (2003) 102:3562–8. doi: 10.1182/blood-2003-02-0593
46. Seymour JF, Lieschke GJ, Grail D, Quilici C, Hodgson G, Dunn AR. Mice lacking both granulocyte colony-stimulating factor (CSF) and granulocyte-macrophage CSF have impaired reproductive capacity, perturbed neonatal granulopoiesis, lung disease, amyloidosis, and reduced long-term survival. Blood. (1997) 90:3037–49. doi: 10.1182/blood.V90.8.3037
47. Liu F, Poursine-Laurent J, Wu HY, Link DC. Interleukin-6 and the granulocyte colony-stimulating factor receptor are major independent regulators of granulopoiesis in vivo but are not required for lineage commitment or terminal differentiation. Blood. (1997) 90(7):2583–90.
48. Molineux G, Migdalska A, Szmitkowski M, Dexter TM. The effects on hematopoiesis of recombinant stenr cell factor (Ligand for c-kit) administered in vivo to mice either alone or in combination with granulocyte colony-stimulating factor. Blood. (1991) 78(4):961–6.
49. Wang T, Cao L, Dong X, Wu F, De W, Huang L, et al. LINC01116 promotes tumor proliferation and neutrophil recruitment via DDX5-mediated regulation of IL-1β in glioma cell. Cell Death Dis. (2020) 11:302. doi: 10.1038/s41419-020-2506-0
50. Wang R, Jiao Z, Li R, Yue H, Chen L. p68 RNA helicase promotes glioma cell proliferation in vitro and in vivo via direct regulation of NF- B transcription factor p50. Neuro-Oncology. (2012) 14:1116–24. doi: 10.1093/neuonc/nos131
51. Lee SY, Kim J-K, Jeon H-Y, Ham SW, Kim H. CD133 regulates IL-1β Signaling and neutrophil recruitment in glioblastoma. Mol Cells. (2017) 40:515–22. doi: 10.14348/molcells.2017.0089
52. Niklasson M, Bergström T, Jarvius M, Sundström A, Nyberg F, Haglund C, et al. Mesenchymal transition and increased therapy resistance of glioblastoma cells is related to astrocyte reactivity. J Pathol. (2019) 249:295–307. doi: 10.1002/path.5317
53. Wang L, Liu Z, Balivada S, Shrestha T, Bossmann S, Pyle M, et al. Interleukin-1β and transforming growth factor-β cooperate to induce neurosphere formation and increase tumorigenicity of adherent LN-229 glioma cells. Stem Cell Res Ther. (2012) 3:5. doi: 10.1186/scrt96
54. Zha C, Meng X, Li L, Mi S, Qian D, Li Z, et al. Neutrophil extracellular traps mediate the crosstalk between glioma progression and the tumor microenvironment via the HMGB1/RAGE/IL-8 axis. Cancer Biol Med. (2020) 17:154–68. doi: 10.20892/j.issn.2095-3941.2019.0353
55. Atai NA, Bansal M, Lo C, Bosman J, Tigchelaar W, Bosch KS, et al. Osteopontin is up-regulated and associated with neutrophil and macrophage infiltration in glioblastoma: Osteopontin is associated with leucocyte infiltration in glioblastoma. Immunology. (2011) 132:39–48. doi: 10.1111/j.1365-2567.2010.03335.x
56. Chio C-C, Wang Y-S, Chen Y-L, Lin S-J, Yang B-C. Down-regulation of Fas-L in glioma cells by ribozyme reduces cell apoptosis, tumour-infiltrating cells and liver damage but accelerates tumour formation in nude mice. Br J Cancer. (2001) 85:1185–92. doi: 10.1054/bjoc.2001.2055
57. Oppenheim JJ, Zachariae COC, Mukaida N, Matsushima K. Properties of the novel proinflammatory supergene “Intercrine” Cytokine family. Annu Rev Immunol. (1991) 9:617–48. doi: 10.1146/annurev.iy.09.040191.003153
58. Liu M, Yang L, Liu Z, Wu R, Gu Z, Yao Q. Correlation of C-X-C chemokine receptor 2 upregulation with poor prognosis and recurrence in human glioma. Onco Targets Ther. (2015) 8:3203–09. doi: 10.2147/OTT.S91626
59. Yee PP, Wei Y, Kim S-Y, Lu T, Chih SY, Lawson C, et al. Neutrophil-induced ferroptosis promotes tumor necrosis in glioblastoma progression. Nat Commun. (2020) 11:5424. doi: 10.1038/s41467-020-19193-y
60. Müller S, Kohanbash G, Liu SJ, Alvarado B, Carrera D, Bhaduri A, et al. Single-cell profiling of human gliomas reveals macrophage ontogeny as a basis for regional differences in macrophage activation in the tumor microenvironment. Genome Biol. (2017) 18:234. doi: 10.1186/s13059-017-1362-4
61. Darmanis S, Sloan SA, Croote D, Mignardi M, Chernikova S, Samghababi P, et al. Single-cell RNA-seq analysis of infiltrating neoplastic cells at the migrating front of human glioblastoma. Cell Rep. (2017) 21:1399–410. doi: 10.1016/j.celrep.2017.10.030
62. Greenwood J, Etienne-Manneville S, Adamson P, Couraud P-O. Lymphocyte migration into the central nervous system: implication of ICAM-1 signalling at the blood–brain barrier. Vasc Pharmacol. (2002) 38(6):315–22. doi: 10.1016/S1537-1891(02)00199-4
63. Li W, Chen Z, Chin I, Chen Z, Dai H. The role of VE-cadherin in blood-brain barrier integrity under central nervous system pathological conditions. CN. (2018) 16:1375–84. doi: 10.2174/1570159X16666180222164809
64. Giese MA, Hind LE, Huttenlocher A. Neutrophil plasticity in the tumor microenvironment. Blood. (2019) 133:2159–67. doi: 10.1182/blood-2018-11-844548
65. Veglia F, Sanseviero E, Gabrilovich DI. Myeloid-derived suppressor cells in the era of increasing myeloid cell diversity. Nat Rev Immunol. (2021) 21:485–98. doi: 10.1038/s41577-020-00490-y
66. Dumitru CA, Moses K, Trellakis S, Lang S, Brandau S. Neutrophils and granulocytic myeloid-derived suppressor cells: immunophenotyping, cell biology and clinical relevance in human oncology. Cancer Immunol Immunother. (2012) 61:1155–67. doi: 10.1007/s00262-012-1294-5
67. Damuzzo V, Pinton L, Desantis G, Solito S, Marigo I, Bronte V, et al. Complexity and challenges in defining myeloid-derived suppressor cells. Cytometry. (2014) 88(2):77–91. doi: 10.1002/cytob.21206
68. Gustafson MP, Lin Y, Maas ML, Van Keulen VP, Johnston PB, Peikert T, et al. A method for identification and analysis of non-overlapping myeloid immunophenotypes in humans. PloS One. (2015) 10:e0121546. doi: 10.1371/journal.pone.0121546
69. Antuamwine BB, Bosnjakovic R, Hofmann-Vega F, Wang X, Theodosiou T, Iliopoulos I, et al. N1 versus N2 and PMN-MDSC: A critical appraisal of current concepts on tumor-associated neutrophils and new directions for human oncology. Immunol Rev. (2023) 314:250–79. doi: 10.1111/imr.13176
70. Fridlender ZG, Sun J, Kim S, Kapoor V, Cheng G, Ling L, et al. Polarization of tumor-associated neutrophil phenotype by TGF-β: “N1” versus “N2” TAN. Cancer Cell. (2009) 16:183–94. doi: 10.1016/j.ccr.2009.06.017
71. Jablonska J, Leschner S, Westphal K, Lienenklaus S, Weiss S. Neutrophils responsive to endogenous IFN-β regulate tumor angiogenesis and growth in a mouse tumor model. J Clin Invest. (2010) 120:1151–64. doi: 10.1172/JCI37223
72. Jablonska J, Wu C -F, Andzinski L, Leschner S, Weiss S. CXCR2-mediated tumor-associated neutrophil recruitment is regulated by IFN-β. Intl J Cancer. (2014) 134:1346–58. doi: 10.1002/ijc.28551
73. Andzinski L, Kasnitz N, Stahnke S, Wu C, Gereke M, Von Köckritz-Blickwede M, et al. Type I IFN s induce anti-tumor polarization of tumor associated neutrophils in mice and human. Intl J Cancer. (2016) 138:1982–93. doi: 10.1002/ijc.29945
74. Piccard H, Muschel RJ, Opdenakker G. On the dual roles and polarized phenotypes of neutrophils in tumor development and progression. Crit Rev Oncology/Hematol. (2012) 82:296–309. doi: 10.1016/j.critrevonc.2011.06.004
75. Ohms M, Möller S, Laskay T. An attempt to polarize human neutrophils toward N1 and N2 phenotypes in vitro. Front Immunol. (2020) 11:532. doi: 10.3389/fimmu.2020.00532
76. Tyagi A, Sharma S, Wu K, Wu S-Y, Xing F, Liu Y, et al. Nicotine promotes breast cancer metastasis by stimulating N2 neutrophils and generating pre-metastatic niche in lung. Nat Commun. (2021) 12:474. doi: 10.1038/s41467-020-20733-9
77. Fridlender ZG, Albelda SM. Tumor-associated neutrophils: friend or foe? Carcinogenesis. (2012) 33:949–55. doi: 10.1093/carcin/bgs123
78. Masucci MT, Minopoli M, Carriero MV. Tumor associated neutrophils. Their role in tumorigenesis, metastasis, prognosis and therapy. Front Oncol. (2019) 9:1146. doi: 10.3389/fonc.2019.01146
79. Qi M, Xia Y, Wu Y, Zhang Z, Wang X, Lu L, et al. Lin28B-high breast cancer cells promote immune suppression in the lung pre-metastatic niche via exosomes and support cancer progression. Nat Commun. (2022) 13:897. doi: 10.1038/s41467-022-28438-x
80. Sagiv JY, Michaeli J, Assi S, Mishalian I, Kisos H, Levy L, et al. Phenotypic diversity and plasticity in circulating neutrophil subpopulations in cancer. Cell Rep. (2015) 10:562–73. doi: 10.1016/j.celrep.2014.12.039
81. Brandau S, Trellakis S, Bruderek K, Schmaltz D, Steller G, Elian M, et al. Myeloid-derived suppressor cells in the peripheral blood of cancer patients contain a subset of immature neutrophils with impaired migratory properties. J Leukocyte Biol. (2010) 89:311–7. doi: 10.1189/jlb.0310162
82. Ley K, Hoffman HM, Kubes P, Cassatella MA, Zychlinsky A, Hedrick CC, et al. Neutrophils: New insights and open questions. Sci Immunol. (2018) 3:eaat4579. doi: 10.1126/sciimmunol.aat4579
83. Lang S, Bruderek K, Kaspar C, Höing B, Kanaan O, Dominas N, et al. Clinical relevance and suppressive capacity of human myeloid-derived suppressor cell subsets. Clin Cancer Res. (2018) 24:4834–44. doi: 10.1158/1078-0432.CCR-17-3726
84. Glodde N, Bald T, Van Den Boorn-Konijnenberg D, Nakamura K, O’Donnell JS, Szczepanski S, et al. Reactive neutrophil responses dependent on the receptor tyrosine kinase c-MET limit cancer immunotherapy. Immunity. (2017) 47:789–802.e9. doi: 10.1016/j.immuni.2017.09.012
85. Zhu YP, Padgett L, Dinh HQ, Marcovecchio P, Blatchley A, Wu R, et al. Identification of an early unipotent neutrophil progenitor with pro-tumoral activity in mouse and human bone marrow. Cell Rep. (2018) 24:2329–2341.e8. doi: 10.1016/j.celrep.2018.07.097
86. Uyanik B, Goloudina AR, Akbarali A, Grigorash BB, Petukhov AV, Singhal S, et al. Inhibition of the DNA damage response phosphatase PPM1D reprograms neutrophils to enhance anti-tumor immune responses. Nat Commun. (2021) 12:3622. doi: 10.1038/s41467-021-23330-6
87. Salken I, Provencio JJ, Coulibaly AP. A potential therapeutic target: The role of neutrophils in the central nervous system. Brain Behav Immun - Health. (2023) 33:100688. doi: 10.1016/j.bbih.2023.100688
88. Linas SL, Whittenburg D, Parsons PE, Repine JE. Mild renal ischemia activates primed neutrophils to cause acute renal failure. Kidney Int. (1992) 42:610–6. doi: 10.1038/ki.1992.325
89. Burn GL, Foti A, Marsman G, Patel DF, Zychlinsky A. The neutrophil. Immunity. (2021) 54:1377–91. doi: 10.1016/j.immuni.2021.06.006
90. Vorobjeva NV. Neutrophil extracellular traps: new aspects. Moscow Univ BiolSci Bull. (2020) 75:173–88. doi: 10.3103/S0096392520040112
91. Papayannopoulos V, Metzler KD, Hakkim A, Zychlinsky A. Neutrophil elastase and myeloperoxidase regulate the formation of neutrophil extracellular traps. J Cell Biol. (2010) 191:677–91. doi: 10.1083/jcb.201006052
92. Vorobjeva NV, Chernyak BV. NETosis: molecular mechanisms, role in physiology and pathology. Biochem Moscow. (2020) 85:1178–90. doi: 10.1134/S0006297920100065
93. Li P, Li M, Lindberg MR, Kennett MJ, Xiong N, Wang Y. PAD4 is essential for antibacterial innate immunity mediated by neutrophil extracellular traps. J Exp Med. (2010) 207:1853–62. doi: 10.1084/jem.20100239
94. Manda-Handzlik A, Demkow U. The brain entangled: the contribution of neutrophil extracellular traps to the diseases of the central nervous system. Cells. (2019) 8:1477. doi: 10.3390/cells8121477
95. Dumitru CA, Lang S, Brandau S. Modulation of neutrophil granulocytes in the tumor microenvironment: Mechanisms and consequences for tumor progression. Semin Cancer Biol. (2013) 23:141–8. doi: 10.1016/j.semcancer.2013.02.005
96. Shamamian P, Schwartz JD, Pocock BJZ, Monea S, Whiting D, Marcus SG, et al. Activation of progelatinase A (MMP-2) by neutrophil elastase, cathepsin G, and proteinase-3: A role for inflammatory cells in tumor invasion and angiogenesis. J Cell Physiol. (2001) 189:197–206. doi: 10.1002/jcp.10014
97. Teijeira Á, Garasa S, Gato M, Alfaro C, Migueliz I, Cirella A, et al. CXCR1 and CXCR2 chemokine receptor agonists produced by tumors induce neutrophil extracellular traps that interfere with immune cytotoxicity. Immunity. (2020) 52:856–871.e8. doi: 10.1016/j.immuni.2020.03.001
98. Huang J, Hong W, Wan M, Zheng L. Molecular mechanisms and therapeutic target of NETosis in diseases. MedComm. (2022) 3:e162. doi: 10.1002/mco2.162
99. Kaplan MJ, Radic M. Neutrophil extracellular traps: double-edged swords of innate immunity. J Immunol. (2012) 189:2689–95. doi: 10.4049/jimmunol.1201719
100. Chen Y, Zhang H, Hu X, Cai W, Ni W, Zhou K. Role of NETosis in central nervous system injury. Oxid Med Cell Longevity. (2022) 2022:1–15. doi: 10.1155/2022/3235524
101. Rodrigues DAS, Prestes EB, Gama AMS, Silva LDS, Pinheiro AAS, Ribeiro JMC, et al. CXCR4 and MIF are required for neutrophil extracellular trap release triggered by Plasmodium-infected erythrocytes. PloS Pathog. (2020) 16:e1008230. doi: 10.1371/journal.ppat.1008230
102. Yipp BG, Kubes P. NETosis: how vital is it? Blood. (2013) 122:2784–94. doi: 10.1182/blood-2013-04-457671
103. Shafqat A, Noor Eddin A, Adi G, Al-Rimawi M, Abdul Rab S, Abu-Shaar M, et al. Neutrophil extracellular traps in central nervous system pathologies: A mini review. Front Med. (2023) 10:1083242. doi: 10.3389/fmed.2023.1083242
104. Vandenabeele P, Galluzzi L, Vanden Berghe T, Kroemer G. Molecular mechanisms of necroptosis: an ordered cellular explosion. Nat Rev Mol Cell Biol. (2010) 11:700–14. doi: 10.1038/nrm2970
105. Sheshachalam A, Srivastava N, Mitchell T, Lacy P, Eitzen G. Granule protein processing and regulated secretion in neutrophils. Front Immunol. (2014) 5:448. doi: 10.3389/fimmu.2014.00448
106. Rawat K, Syeda S, Shrivastava A. Neutrophil-derived granule cargoes: paving the way for tumor growth and progression. Cancer Metastasis Rev. (2021) 40:221–44. doi: 10.1007/s10555-020-09951-1
107. Ramadass M, Catz SD. Molecular mechanisms regulating secretory organelles and endosomes in neutrophils and their implications for inflammation. Immunol Rev. (2016) 273:249–65. doi: 10.1111/imr.12452
108. DeFrancesco-Lisowitz A, Lindborg JA, Niemi JP, Zigmond RE. The neuroimmunology of degeneration and regeneration in the peripheral nervous system. Neuroscience. (2015) 302:174–203. doi: 10.1016/j.neuroscience.2014.09.027
109. Amulic B, Cazalet C, Hayes GL, Metzler KD, Zychlinsky A. Neutrophil function: from mechanisms to disease. Annu Rev Immunol. (2012) 30:459–89. doi: 10.1146/annurev-immunol-020711-074942
110. Gaudet AD, Popovich PG, Ramer MS. Wallerian degeneration: gaining perspective on inflammatory events after peripheral nerve injury. J Neuroinflamm. (2011) 8:110. doi: 10.1186/1742-2094-8-110
111. Lee WL, Harrison RE, Grinstein S. Phagocytosis by neutrophils. Microbes Infect. (2003) 5:1299–306. doi: 10.1016/j.micinf.2003.09.014
112. Richards DM, Endres RG. The mechanism of phagocytosis: two stages of engulfment. Biophys J. (2014) 107:1542–53. doi: 10.1016/j.bpj.2014.07.070
113. Lindborg JA, Mack M, Zigmond RE. Neutrophils are critical for myelin removal in a peripheral nerve injury model of wallerian degeneration. J Neurosci. (2017) 37:10258–77. doi: 10.1523/JNEUROSCI.2085-17.2017
114. Magod P, Mastandrea I, Rousso-Noori L, Agemy L, Shapira G, Shomron N, et al. Exploring the longitudinal glioma microenvironment landscape uncovers reprogrammed pro-tumorigenic neutrophils in the bone marrow. Cell Rep. (2021) 36:109480. doi: 10.1016/j.celrep.2021.109480
115. Shan Z, Chen J, Liu J, Zhang J, Wang T, Teng Y, et al. Activated neutrophils polarize protumorigenic interleukin-17A-producing T helper subsets through TNF-α-B7-H2-dependent pathway in human gastric cancer. Clin Trans Med. (2021) 11:e484. doi: 10.1002/ctm2.484
116. Finisguerra V, Di Conza G, Di Matteo M, Serneels J, Costa S, Thompson AAR, et al. MET is required for the recruitment of anti-tumoural neutrophils. Nature. (2015) 522:349–53. doi: 10.1038/nature14407
117. Nitta T, Sato K, Allegretta M, Brocke S, Lim M, Mitchell DJ, et al. Expression of granulocyte colony stimulating factor and granulocyte-macrophage colony stimulating factor genes in human astrocytoma cell lines and in glioma specimens. Brain Res. (1992) 571:19–25. doi: 10.1016/0006-8993(92)90505-4
118. Albulescu R, Codrici E, Popescu ID, Mihai S, Necula LG, Petrescu D, et al. Cytokine patterns in brain tumour progression. Mediators Inflammation. (2013) 2013:1–7. doi: 10.1155/2013/979748
119. Gabrusiewicz K, Rodriguez B, Wei J, Hashimoto Y, Healy LM, Maiti SN, et al. Glioblastoma-infiltrated innate immune cells resemble M0 macrophage phenotype. JCI Insight. (2016) 1(2):e85841. doi: 10.1172/jci.insight.85841
120. Li M-X, Liu X-M, Zhang X-F, Zhang J-F, Wang W-L, Zhu Y, et al. Prognostic role of neutrophil-to-lymphocyte ratio in colorectal cancer: A systematic review and meta-analysis: Neutrophil-to-Lymphocyte Ratio in Colorectal Cancer. Int J Cancer. (2014) 134:2403–13. doi: 10.1002/ijc.28536
121. Corbeau I, Jacot W, Guiu S. Neutrophil to lymphocyte ratio as prognostic and predictive factor in breast cancer patients: A systematic review. Cancers. (2020) 12:958. doi: 10.3390/cancers12040958
122. Arvanitakis K, Mitroulis I, Germanidis G. Tumor-associated neutrophils in hepatocellular carcinoma pathogenesis, prognosis, and therapy. Cancers. (2021) 13:2899. doi: 10.3390/cancers13122899
123. Bambury RM, Teo MY, Power DG, Yusuf A, Murray S, Battley JE, et al. The association of pre-treatment neutrophil to lymphocyte ratio with overall survival in patients with glioblastoma multiforme. J Neurooncol. (2013) 114:149–54. doi: 10.1007/s11060-013-1164-9
124. Mason M, Maurice C, McNamara MG, Tieu MT, Lwin Z, Millar B-A, et al. Neutrophil–lymphocyte ratio dynamics during concurrent chemo-radiotherapy for glioblastoma is an independent predictor for overall survival. J Neurooncol. (2017) 132:463–71. doi: 10.1007/s11060-017-2395-y
125. Wang P-F, Song H-W, Cai H-Q, Kong L-W, Yao K, Jiang T, et al. Preoperative inflammation markers and IDH mutation status predict glioblastoma patient survival. Oncotarget. (2017) 8:50117–23. doi: 10.18632/oncotarget.15235
126. Powell D, Lou M, Barros Becker F, Huttenlocher A. Cxcr1 mediates recruitment of neutrophils and supports proliferation of tumor-initiating astrocytes in vivo. Sci Rep. (2018) 8:13285. doi: 10.1038/s41598-018-31675-0
127. Xu G, Li C, Wang Y, Ma J, Zhang J. Correlation between preoperative inflammatory markers, Ki-67 and the pathological grade of glioma. Medicine. (2021) 100:e26750. doi: 10.1097/MD.0000000000026750
128. Duits DEM, De Visser KE. Impact of cancer cell-intrinsic features on neutrophil behavior. Semin Immunol. (2021) 57:101546. doi: 10.1016/j.smim.2021.101546
129. Blagih J, Zani F, Chakravarty P, Hennequart M, Pilley S, Hobor S, et al. Cancer-specific loss of p53 leads to a modulation of myeloid and T cell responses. Cell Rep. (2020) 30:481–496.e6. doi: 10.1016/j.celrep.2019.12.028
130. Bezzi M, Seitzer N, Ishikawa T, Reschke M, Chen M, Wang G, et al. Diverse genetic-driven immune landscapes dictate tumor progression through distinct mechanisms. Nat Med. (2018) 24:165–75. doi: 10.1038/nm.4463
131. Klemm F, Maas RR, Bowman RL, Kornete M, Soukup K, Nassiri S, et al. Interrogation of the microenvironmental landscape in brain tumors reveals disease-specific alterations of immune cells. Cell. (2020) 181:1643–1660.e17. doi: 10.1016/j.cell.2020.05.007
132. Amankulor NM, Kim Y, Arora S, Kargl J, Szulzewsky F, Hanke M, et al. Mutant IDH1 regulates the tumor-associated immune system in gliomas. Genes Dev. (2017) 31:774–86. doi: 10.1101/gad.294991.116
133. Alghamri MS, McClellan BL, Avvari RP, Thalla R, Carney S, Hartlage CS, et al. G-CSF secreted by mutant IDH1 glioma stem cells abolishes myeloid cell immunosuppression and enhances the efficacy of immunotherapy. Sci Adv. (2021) 7:eabh3243. doi: 10.1126/sciadv.abh3243
134. Gao M, Lin Y, Liu X, Zhao Z, Zhu Z, Zhang H, et al. TERT mutation is accompanied by neutrophil infiltration and contributes to poor survival in isocitrate dehydrogenase wild-type glioma. Front Cell Dev Biol. (2021) 9:654407. doi: 10.3389/fcell.2021.654407
135. Verhaak RGW, Hoadley KA, Purdom E, Wang V, Qi Y, Wilkerson MD, et al. Integrated genomic analysis identifies clinically relevant subtypes of glioblastoma characterized by abnormalities in PDGFRA, IDH1, EGFR, and NF1. Cancer Cell. (2010) 17:98–110. doi: 10.1016/j.ccr.2009.12.020
136. Wang Q, Hu B, Hu X, Kim H, Squatrito M, Scarpace L, et al. Tumor evolution of glioma-intrinsic gene expression subtypes associates with immunological changes in the microenvironment. Cancer Cell. (2017) 32:42–56.e6. doi: 10.1016/j.ccell.2017.06.003
137. Chen Z, Herting CJ, Ross JL, Gabanic B, Puigdelloses Vallcorba M, Szulzewsky F, et al. Genetic driver mutations introduced in identical cell-of-origin in murine glioblastoma reveal distinct immune landscapes but similar response to checkpoint blockade. Glia. (2020) 68:2148–66. doi: 10.1002/glia.23883
138. Neftel C, Laffy J, Filbin MG, Hara T, Shore ME, Rahme GJ, et al. An integrative model of cellular states, plasticity, and genetics for glioblastoma. Cell. (2019) 178:835–849.e21. doi: 10.1016/j.cell.2019.06.024
139. Naeini KM, Pope WB, Cloughesy TF, Harris RJ, Lai A, Eskin A, et al. Identifying the mesenchymal molecular subtype of glioblastoma using quantitative volumetric analysis of anatomic magnetic resonance images. Neuro-Oncology. (2013) 15:626–34. doi: 10.1093/neuonc/not008
140. Cooper LAD, Gutman DA, Chisolm C, Appin C, Kong J, Rong Y, et al. The tumor microenvironment strongly impacts master transcriptional regulators and gene expression class of glioblastoma. Am J Pathol. (2012) 180:2108–19. doi: 10.1016/j.ajpath.2012.01.040
141. Singhal S, Bhojnagarwala PS, O’Brien S, Moon EK, Garfall AL, Rao AS, et al. Origin and role of a subset of tumor-associated neutrophils with antigen-presenting cell features in early-stage human lung cancer. Cancer Cell. (2016) 30:120–35. doi: 10.1016/j.ccell.2016.06.001
142. Eruslanov EB, Bhojnagarwala PS, Quatromoni JG, Stephen TL, Ranganathan A, Deshpande C, et al. Tumor-associated neutrophils stimulate T cell responses in early-stage human lung cancer. J Clin Invest. (2014) 124:5466–80. doi: 10.1172/JCI77053
143. Blaisdell A, Crequer A, Columbus D, Daikoku T, Mittal K, Dey SK, et al. Neutrophils oppose uterine epithelial carcinogenesis via debridement of hypoxic tumor cells. Cancer Cell. (2015) 28:785–99. doi: 10.1016/j.ccell.2015.11.005
144. Mahiddine K, Blaisdell A, Ma S, Créquer-Grandhomme A, Lowell CA, Erlebacher A. Relief of tumor hypoxia unleashes the tumoricidal potential of neutrophils. J Clin Invest. (2019) 130:389–403. doi: 10.1172/JCI130952
145. Sun B, Qin W, Song M, Liu L, Yu Y, Qi X, et al. Neutrophil suppresses tumor cell proliferation via fas /fas ligand pathway mediated cell cycle arrested. Int J Biol Sci. (2018) 14:2103–13. doi: 10.7150/ijbs.29297
146. Tran AN, Boyd NH, Walker K, Hjelmeland AB. NOS expression and NO function in glioma and implications for patient therapies. Antioxid Redox Signaling. (2017) 26:986–99. doi: 10.1089/ars.2016.6820
147. Spicer JD, McDonald B, Cools-Lartigue JJ, Chow SC, Giannias B, Kubes P, et al. Neutrophils promote liver metastasis via mac-1–mediated interactions with circulating tumor cells. Cancer Res. (2012) 72:3919–27. doi: 10.1158/0008-5472.CAN-11-2393
148. Tamura K, Shimizu C, Hojo T, Akashi-Tanaka S, Kinoshita T, Yonemori K, et al. FcγR2A and 3A polymorphisms predict clinical outcome of trastuzumab in both neoadjuvant and metastatic settings in patients with HER2-positive breast cancer. Ann Oncol. (2011) 22:1302–7. doi: 10.1093/annonc/mdq585
149. Musolino A, Naldi N, Bortesi B, Pezzuolo D, Capelletti M, Missale G, et al. Immunoglobulin G fragment C receptor polymorphisms and clinical efficacy of trastuzumab-based therapy in patients with HER-2/ neu –positive metastatic breast cancer. JCO. (2008) 26:1789–96. doi: 10.1200/JCO.2007.14.8957
150. Matlung HL, Babes L, Zhao XW, Van Houdt M, Treffers LW, Van Rees DJ, et al. Neutrophils kill antibody-opsonized cancer cells by trogoptosis. Cell Rep. (2018) 23:3946–3959.e6. doi: 10.1016/j.celrep.2018.05.082
151. De Looff M, De Jong S, Kruyt FAE. Multiple interactions between cancer cells and the tumor microenvironment modulate TRAIL signaling: implications for TRAIL receptor targeted therapy. Front Immunol. (2019) 10:1530. doi: 10.3389/fimmu.2019.01530
152. Rubenich DS, De Souza PO, Omizzollo N, Aubin MR, Basso PJ, Silva LM, et al. Tumor-neutrophil crosstalk promotes in vitro and in vivo glioblastoma progression. Front Immunol. (2023) 14:1183465. doi: 10.3389/fimmu.2023.1183465
153. Maas RR, Soukup K, Fournier N, Massara M, Galland S, Kornete M, et al. The local microenvironment drives activation of neutrophils in human brain tumors. Cell. (2023) 186:4546–4566.e27. doi: 10.1016/j.cell.2023.08.043
154. Hor W-S, Huang W-L, Lin Y-S, Yang B-C. Cross-talk between tumor cells and neutrophils through the Fas (APO-1, CD95)/FasL system: human glioma cells enhance cell viability and stimulate cytokine production in neutrophils. J Leukocyte Biol. (2003) 73:363–8. doi: 10.1189/jlb.0702375
155. Furumaya C, Martinez-Sanz P, Bouti P, Kuijpers TW, Matlung HL. Plasticity in pro- and anti-tumor activity of neutrophils: shifting the balance. Front Immunol. (2020) 11:2100. doi: 10.3389/fimmu.2020.02100
156. Lin Y-J, Wei K-C, Chen P-Y, Lim M, Hwang T-L. Roles of neutrophils in glioma and brain metastases. Front Immunol. (2021) 12:701383. doi: 10.3389/fimmu.2021.701383
157. Albrengues J, Shields MA, Ng D, Park CG, Ambrico A, Poindexter ME, et al. Neutrophil extracellular traps produced during inflammation awaken dormant cancer cells in mice. Science. (2018) 361:eaao4227. doi: 10.1126/science.aao4227
158. Iwatsuki K-C, Kumara E, Yoshimine T-C, Nakagawa H-C, Sato M, Hayakawa T. Elastase expression by infiltrating neutrophils in gliomas. Neurol Res. (2000) 22:465–8. doi: 10.1080/01616412.2000.11740701
159. Lin Y-J, Wu CY-J, Wu JY, Lim M. The role of myeloid cells in GBM immunosuppression. Front Immunol. (2022) 13:887781. doi: 10.3389/fimmu.2022.887781
160. Liang J, Piao Y, Holmes L, Fuller GN, Henry V, Tiao N, et al. Neutrophils promote the Malignant glioma phenotype through S100A4. Clin Cancer Res. (2014) 20:187–98. doi: 10.1158/1078-0432.CCR-13-1279
161. Jeon H-Y, Ham SW, Kim J-K, Jin X, Lee SY, Shin YJ, et al. Ly6G+ inflammatory cells enable the conversion of cancer cells to cancer stem cells in an irradiated glioblastoma model. Cell Death Differ. (2019) 26:2139–56. doi: 10.1038/s41418-019-0282-0
162. Mantovani A, Cassatella MA, Costantini C, Jaillon S. Neutrophils in the activation and regulation of innate and adaptive immunity. Nat Rev Immunol. (2011) 11:519–31. doi: 10.1038/nri3024
163. Fridlender ZG, Sun J, Mishalian I, Singhal S, Cheng G, Kapoor V, et al. Transcriptomic analysis comparing tumor-associated neutrophils with granulocytic myeloid-derived suppressor cells and normal neutrophils. PloS One. (2012) 7:e31524. doi: 10.1371/journal.pone.0031524
164. Coffelt SB, Wellenstein MD, De Visser KE. Neutrophils in cancer: neutral no more. Nat Rev Cancer. (2016) 16:431–46. doi: 10.1038/nrc.2016.52
165. Dardalhon V, Anderson AC, Karman J, Apetoh L, Chandwaskar R, Lee DH, et al. Tim-3/galectin-9 pathway: regulation of th1 immunity through promotion of CD11b+Ly-6G+ Myeloid cells. J Immunol. (2010) 185:1383–92. doi: 10.4049/jimmunol.0903275
166. Michaeli J, Shaul ME, Mishalian I, Hovav A-H, Levy L, Zolotriov L, et al. Tumor-associated neutrophils induce apoptosis of non-activated CD8 T-cells in a TNFα and NO-dependent mechanism, promoting a tumor-supportive environment. OncoImmunology. (2017) 6:e1356965. doi: 10.1080/2162402X.2017.1356965
167. Coffelt SB, Kersten K, Doornebal CW, Weiden J, Vrijland K, Hau C-S, et al. IL-17-producing γδ T cells and neutrophils conspire to promote breast cancer metastasis. Nature. (2015) 522:345–8. doi: 10.1038/nature14282
168. Waldman AD, Fritz JM, Lenardo MJ. A guide to cancer immunotherapy: from T cell basic science to clinical practice. Nat Rev Immunol. (2020) 20:651–68. doi: 10.1038/s41577-020-0306-5
169. Dubinski D, Wölfer J, Hasselblatt M, Schneider-Hohendorf T, Bogdahn U, Stummer W, et al. CD4 + T effector memory cell dysfunction is associated with the accumulation of granulocytic myeloid-derived suppressor cells in glioblastoma patients. Neuro Oncol. (2016) 18:807–18. doi: 10.1093/neuonc/nov280
170. Pillay J, Kamp VM, Van Hoffen E, Visser T, Tak T, Lammers J-W, et al. A subset of neutrophils in human systemic inflammation inhibits T cell responses through Mac-1. J Clin Invest. (2012) 122:327–36. doi: 10.1172/JCI57990
171. Sippel TR, White J, Nag K, Tsvankin V, Klaassen M, Kleinschmidt-DeMasters BK, et al. Neutrophil degranulation and immunosuppression in patients with GBM: restoration of cellular immune function by targeting arginase I. Clin Cancer Res. (2011) 17:6992–7002. doi: 10.1158/1078-0432.CCR-11-1107
172. Ponzetta A, Carriero R, Carnevale S, Barbagallo M, Molgora M, Perucchini C, et al. Neutrophils driving unconventional T cells mediate resistance against murine sarcomas and selected human tumors. Cell. (2019) 178:346–360.e24. doi: 10.1016/j.cell.2019.05.047
173. Liu K, Sun E, Lei M, Li L, Gao J, Nian X, et al. BCG-induced formation of neutrophil extracellular traps play an important role in bladder cancer treatment. Clin Immunol. (2019) 201:4–14. doi: 10.1016/j.clim.2019.02.005
174. Youn Y-J, Shrestha S, Lee Y-B, Kim J-K, Lee JH, Hur K, et al. Neutrophil-derived trail is a proinflammatory subtype of neutrophil-derived extracellular vesicles. Theranostics. (2021) 11:2770–87. doi: 10.7150/thno.51756
175. Chan L, Wood GA, Wootton SK, Bridle BW, Karimi K. Neutrophils in dendritic cell-based cancer vaccination: the potential roles of neutrophil extracellular trap formation. IJMS. (2023) 24:896. doi: 10.3390/ijms24020896
176. Lazzaretto B, Fadeel B. Intra- and extracellular degradation of neutrophil extracellular traps by macrophages and dendritic cells. J Immunol. (2019) 203:2276–90. doi: 10.4049/jimmunol.1800159
177. Van Egmond M. Neutrophils in antibody-based immunotherapy of cancer. Expert Opin Biol Ther. (2008) 8:83–94. doi: 10.1517/14712598.8.1.83
178. Wang J, Liu P, Xin S, Wang Z, Li J. Nrf2 suppresses the function of dendritic cells to facilitate the immune escape of glioma cells. Exp Cell Res. (2017) 360:66–73. doi: 10.1016/j.yexcr.2017.07.031
179. Michielsen AJ, Hogan AE, Marry J, Tosetto M, Cox F, Hyland JM, et al. Tumour tissue microenvironment can inhibit dendritic cell maturation in colorectal cancer. PloS One. (2011) 6:e27944. doi: 10.1371/journal.pone.0027944
180. Rahbar A, Cederarv M, Wolmer-Solberg N, Tammik C, Stragliotto G, Peredo I, et al. Enhanced neutrophil activity is associated with shorter time to tumor progression in glioblastoma patients. OncoImmunology. (2016) 5:e1075693. doi: 10.1080/2162402X.2015.1075693
181. Suzuki E, Kapoor V, Jassar AS, Kaiser LR, Albelda SM. Gemcitabine selectively eliminates splenic gr-1+/CD11b+ Myeloid suppressor cells in tumor-bearing animals and enhances antitumor immune activity. Clin Cancer Res. (2005) 11:6713–21. doi: 10.1158/1078-0432.CCR-05-0883
182. Li H, Han Y, Guo Q, Zhang M, Cao X. Cancer-expanded myeloid-derived suppressor cells induce anergy of NK cells through membrane-bound TGF-β1. J Immunol. (2009) 182:240–9. doi: 10.4049/jimmunol.182.1.240
183. Sceneay J, Chow MT, Chen A, Halse HM, Wong CSF, Andrews DM, et al. Primary tumor hypoxia recruits CD11b+/ly6Cmed/ly6G+ Immune suppressor cells and compromises NK cell cytotoxicity in the premetastatic niche. Cancer Res. (2012) 72:3906–11. doi: 10.1158/0008-5472.CAN-11-3873
184. Spiegel A, Brooks MW, Houshyar S, Reinhardt F, Ardolino M, Fessler E, et al. Neutrophils suppress intraluminal NK cell–mediated tumor cell clearance and enhance extravasation of disseminated carcinoma cells. Cancer Discovery. (2016) 6:630–49. doi: 10.1158/2159-8290.CD-15-1157
185. Chaudhary B, Elkord E. Regulatory T cells in the tumor microenvironment and cancer progression: role and therapeutic targeting. Vaccines. (2016) 4:28. doi: 10.3390/vaccines4030028
186. Mougiakakos D, Johansson CC, Jitschin R, Böttcher M, Kiessling R. Increased thioredoxin-1 production in human naturally occurring regulatory T cells confers enhanced tolerance to oxidative stress. Blood. (2011) 117:857–61. doi: 10.1182/blood-2010-09-307041
187. Mougiakakos D, Johansson CC, Kiessling R. Naturally occurring regulatory T cells show reduced sensitivity toward oxidative stress–induced cell death. Blood. (2009) 113:3542–5. doi: 10.1182/blood-2008-09-181040
188. Mishalian I, Bayuh R, Eruslanov E, Michaeli J, Levy L, Zolotarov L, et al. Neutrophils recruit regulatory T-cells into tumors via secretion of CCL17—A new mechanism of impaired antitumor immunity. Intl J Cancer. (2014) 135:1178–86. doi: 10.1002/ijc.28770
189. Lewkowicz N, Klink M, Mycko MP, Lewkowicz P. Neutrophil – CD4+CD25+ T regulatory cell interactions: A possible new mechanism of infectious tolerance. Immunobiology. (2013) 218:455–64. doi: 10.1016/j.imbio.2012.05.029
190. Lewkowicz N, Mycko MP, Przygodzka P, Ćwiklińska H, Cichalewska M, Matysiak M, et al. Induction of human IL-10-producing neutrophils by LPS-stimulated Treg cells and IL-10. Mucosal Immunol. (2016) 9:364–78. doi: 10.1038/mi.2015.66
191. Thind MK, Uhlig HH, Glogauer M, Palaniyar N, Bourdon C, Gwela A, et al. A metabolic perspective of the neutrophil life cycle: new avenues in immunometabolism. Front Immunol. (2024) 14:1334205. doi: 10.3389/fimmu.2023.1334205
192. Britt EC, Lika J, Giese MA, Schoen TJ, Seim GL, Huang Z, et al. Switching to the cyclic pentose phosphate pathway powers the oxidative burst in activated neutrophils. Nat Metab. (2022) 4:389–403. doi: 10.1038/s42255-022-00550-8
193. Injarabian L, Devin A, Ransac S, Marteyn BS. Neutrophil metabolic shift during their lifecycle: impact on their survival and activation. IJMS. (2019) 21:287. doi: 10.3390/ijms21010287
194. Parker H, Winterbourn CC. Reactive oxidants and myeloperoxidase and their involvement in neutrophil extracellular traps. Front Immun. (2013) 3:424. doi: 10.3389/fimmu.2012.00424
195. Douda DN, Khan MA, Grasemann H, Palaniyar N. SK3 channel and mitochondrial ROS mediate NADPH oxidase-independent NETosis induced by calcium influx. Proc Natl Acad Sci USA. (2015) 112:2817–22. doi: 10.1073/pnas.1414055112
196. Rubenich DS, De Souza PO, Omizzollo N, Lenz GS, Sevigny J, Braganhol E. Neutrophils: fast and furious—the nucleotide pathway. Purinergic Signalling. (2021) 17:371–83. doi: 10.1007/s11302-021-09786-7
197. Aapro MS, Bohlius J, Cameron DA, Lago LD, Donnelly JP, Kearney N, et al. 2010 update of EORTC guidelines for the use of granulocyte-colony stimulating factor to reduce the incidence of chemotherapy-induced febrile neutropenia in adult patients with lymphoproliferative disorders and solid tumours. Eur J Cancer. (2011) 47:8–32. doi: 10.1016/j.ejca.2010.10.013
198. Karpel-Massler G, Kast RE, Siegelin MD, Dwucet A, Schneider E, Westhoff M-A, et al. Anti-glioma activity of dapsone and its enhancement by synthetic chemical modification. Neurochem Res. (2017) 42:3382–9. doi: 10.1007/s11064-017-2378-6
199. Alfaro C, Teijeira A, Oñate C, Pérez G, Sanmamed MF, Andueza MP, et al. Tumor-produced interleukin-8 attracts human myeloid-derived suppressor cells and elicits extrusion of neutrophil extracellular traps (NETs). Clin Cancer Res. (2016) 22:3924–36. doi: 10.1158/1078-0432.CCR-15-2463
200. Behrens LM, Van Den Berg TK, Van Egmond M. Targeting the CD47-SIRPα Innate immune checkpoint to potentiate antibody therapy in cancer by neutrophils. Cancers. (2022) 14:3366. doi: 10.3390/cancers14143366
201. Ring NG, Herndler-Brandstetter D, Weiskopf K, Shan L, Volkmer J-P, George BM, et al. Anti-SIRPα antibody immunotherapy enhances neutrophil and macrophage antitumor activity. Proc Natl Acad Sci USA. (2017) 114(49):E10578-85. doi: 10.1073/pnas.1710877114
202. Huang Y, Zhang Q, Lubas M, Yuan Y, Yalcin F, Efe IE, et al. Synergistic toll-like receptor 3/9 signaling affects properties and impairs glioma-promoting activity of microglia. J Neurosci. (2020) 40:6428–43. doi: 10.1523/JNEUROSCI.0666-20.2020
203. Von Roemeling CA, Wang Y, Qie Y, Yuan H, Zhao H, Liu X, et al. Therapeutic modulation of phagocytosis in glioblastoma can activate both innate and adaptive antitumour immunity. Nat Commun. (2020) 11:1508. doi: 10.1038/s41467-020-15129-8
204. Gholamin S, Mitra SS, Feroze AH, Liu J, Kahn SA, Zhang M, et al. Disrupting the CD47-SIRPa anti-phagocytic axis by a humanized anti-CD47 antibody is an efficacious treatment for Malignant pediatric brain tumors. Sci Trans Med. (2017) 9(381):eaaf2968. doi: 10.1126/scitranslmed.aaf2968
205. Moses K, Klein JC, Männ L, Klingberg A, Gunzer M, Brandau S. Survival of residual neutrophils and accelerated myelopoiesis limit the efficacy of antibody-mediated depletion of Ly-6G+ cells in tumor-bearing mice. J Leukocyte Biol. (2016) 99:811–23. doi: 10.1189/jlb.1HI0715-289R
206. Denorme F, Rustad JL, Portier I, Crandell JL, De Araujo CV, Cody MJ, et al. Neutrophil extracellular trap inhibition improves survival in neonatal mouse infectious peritonitis. Pediatr Res. (2023) 93:862–9. doi: 10.1038/s41390-022-02219-0
207. Yost CC, Schwertz H, Cody MJ, Wallace JA, Campbell RA, Vieira-de-Abreu A, et al. Neonatal NET-inhibitory factor and related peptides inhibit neutrophil extracellular trap formation. J Clin Invest. (2016) 126:3783–98. doi: 10.1172/JCI83873
208. Meurer M, Öhlmann S, Bonilla MC, Valentin-Weigand P, Beineke A, Hennig-Pauka I, et al. Role of Bacterial and Host DNases on Host-Pathogen Interaction during Streptococcus suis Meningitis. IJMS. (2020) 21:5289. doi: 10.3390/ijms21155289
209. Tadie J-M, Bae H-B, Jiang S, Park DW, Bell CP, Yang H, et al. HMGB1 promotes neutrophil extracellular trap formation through interactions with Toll-like receptor 4. Am J Physiol Lung Cell Mol Physiol. (2013) 304(5):L342–49. doi: 10.1152/ajplung.00151.2012
210. Menegazzo L, Scattolini V, Cappellari R, Bonora BM, Albiero M, Bortolozzi M, et al. The antidiabetic drug metformin blunts NETosis in vitro and reduces circulating NETosis biomarkers in vivo. Acta Diabetol. (2018) 55:593–601. doi: 10.1007/s00592-018-1129-8
211. Dai W, Tian R, Yu L, Bian S, Chen Y, Yin B, et al. Overcoming therapeutic resistance in oncolytic herpes virotherapy by targeting IGF2BP3-induced NETosis in Malignant glioma. Nat Commun. (2024) 15:131. doi: 10.1038/s41467-023-44576-2
212. Xue J, Zhao Z, Zhang L, Xue L, Shen S, Wen Y, et al. Neutrophil-mediated anticancer drug delivery for suppression of postoperative Malignant glioma recurrence. Nat Nanotech. (2017) 12:692–700. doi: 10.1038/nnano.2017.54
213. Wang J, Tang W, Yang M, Yin Y, Li H, Hu F, et al. Inflammatory tumor microenvironment responsive neutrophil exosomes-based drug delivery system for targeted glioma therapy. Biomaterials. (2021) 273:120784. doi: 10.1016/j.biomaterials.2021.120784
Keywords: glioma, neutrophil, tumor-associated neutrophils, immunotherapy, tumor microenvironment, neutrophil extracellular trap
Citation: Sun C, Wang S, Ma Z, Zhou J, Ding Z, Yuan G and Pan Y (2024) Neutrophils in glioma microenvironment: from immune function to immunotherapy. Front. Immunol. 15:1393173. doi: 10.3389/fimmu.2024.1393173
Received: 28 February 2024; Accepted: 25 April 2024;
Published: 08 May 2024.
Edited by:
Suprabhat Mukherjee, Kazi Nazrul University, IndiaReviewed by:
Mattia Laffranchi, Sapienza University of Rome, ItalyJozsef Dudas, Innsbruck Medical University, Austria
Copyright © 2024 Sun, Wang, Ma, Zhou, Ding, Yuan and Pan. This is an open-access article distributed under the terms of the Creative Commons Attribution License (CC BY). The use, distribution or reproduction in other forums is permitted, provided the original author(s) and the copyright owner(s) are credited and that the original publication in this journal is cited, in accordance with accepted academic practice. No use, distribution or reproduction is permitted which does not comply with these terms.
*Correspondence: Yawen Pan, cGFueWF3ZW42NjZAc29odS5jb20=; Guoqiang Yuan, eXVhbmdxMDhAbHp1LmVkdS5jbg==