- 1Reproductive Immunology Research Center, Avicenna Research Institute, ACECR, Tehran, Iran
- 2Monoclonal Antibody Research Center, Avicenna Research Institute, ACECR, Tehran, Iran
- 3Department of Immunology, School of Public Health, Tehran University of Medical Sciences, Tehran, Iran
- 4Department of Biochemistry, School of Medical Sciences, Alborz University of Medical Sciences, Karaj, Iran
- 5Department of Health Science and Technology, Aalborg University, Aalborg, Denmark
- 6Clinical Cancer Research Center, Aalborg University Hospital, Aalborg, Denmark
The transition from oviparity to viviparity and the establishment of feto-maternal communications introduced the placenta as the major anatomical site to provide nutrients, gases, and hormones to the developing fetus. The placenta has endocrine functions, orchestrates maternal adaptations to pregnancy at different periods of pregnancy, and acts as a selective barrier to minimize exposure of developing fetus to xenobiotics, pathogens, and parasites. Despite the fact that this ancient organ is central for establishment of a normal pregnancy in eutherians, the placenta remains one of the least studied organs. The first step of pregnancy, embryo implantation, is finely regulated by the trophoectoderm, the precursor of all trophoblast cells. There is a bidirectional communication between placenta and endometrium leading to decidualization, a critical step for maintenance of pregnancy. There are three-direction interactions between the placenta, maternal immune cells, and the endometrium for adaptation of endometrial immune system to the allogeneic fetus. While 65% of all systemically expressed human proteins have been found in the placenta tissues, it expresses numerous placenta-specific proteins, whose expression are dramatically changed in gestational diseases and could serve as biomarkers for early detection of gestational diseases. Surprisingly, placentation and carcinogenesis exhibit numerous shared features in metabolism and cell behavior, proteins and molecular signatures, signaling pathways, and tissue microenvironment, which proposes the concept of “cancer as ectopic trophoblastic cells”. By extensive researches in this novel field, a handful of cancer biomarkers has been discovered. This review paper, which has been inspired in part by our extensive experiences during the past couple of years, highlights new aspects of placental functions with emphasis on its immunomodulatory role in establishment of a successful pregnancy and on a potential link between placentation and carcinogenesis.
Introduction
“Yet, in spite of being the star of the show, the placenta has never quite managed to gain the attention it deserves. Compared to other organs of the body, the placenta comes very low down the list when measured on the scale of public awareness. It is not the placenta’s fault that it has languished in such obscurity. No one has bothered to speak up on its behalf and put it firmly into the public domain.” (1)
- Y. W. Loke
Placental evolution in mammals
The way in which mammals reproduce is the major focus of evolutionary biology, and understanding these processes underpins much of the diversity in our lives. After many years of evolution from the egg-laying ancestor of vertebrates, viviparity converges and involves numerous anatomical, behavioral, genetic, and physiological changes to support internally-incubated embryos. The transition from oviparity to viviparity and the establishment of feto-maternal communications to provide nutrients, gases, and hormones to the developing fetuses introduced the ‘placenta’ as the anatomical mediator of exchange between fetal membranes. The time and approaches that viviparous mammals, marsupials, and eutherians invest in birth differ from one another. The brain size of the neonates in each species is considered one factor that may influence the type of maternal investment (1).
Egg-laying monotremes like the Platypus and Echidna lack a placenta and other reproductive compartments such as the uterus; however, marsupials like kangaroos, koalas, bandicoots, and opossums have a functional placenta. The gestation time in marsupials is as short as 12 days, so much of maternal investment focuses on lactation for the better development of the little joeys. It has been shown that the mammary glands of marsupials function as an alternative to the eutherian placenta, and the composition of milk gradually changes as the offspring grows (2). Delving into the transcriptome changes in the placenta of a small Australian marsupial, the tammar wallaby, researchers have found that marsupials have a eutherian-like placenta with similarly differentially expressed genes as the eutherian placenta in the early stages of pregnancy (3). This suggests that placental functions are the same in the two species, and the difference might lie in the way of compartmentalization and the type of maternal investment in each species.
Much of our knowledge on placenta development comes from animals in the eutherian lineage, which comprises over 5000 vertebrates? species and 20 phylogenetic orders. The different sizes, gross shapes, and histological structures of layers that separate maternal and fetal circulations result in various types of placentas. Regarding size and shape, placentas are classified as diffuse, cotyledonary, zonary, and discoid/bidiscoid types (Figure 1). In the diffuse type, as seen in horses and pigs, the entire surface of the allantochorion is involved in the formation of the placenta. The interaction of multiple patches of allantochorion with the endometrium results in cotyledonary placenta, observed in ruminants. Zonary is a complete or incomplete band of tissue that surrounds the fetus, found in carnivores. The last type is discoid/bidiscoid, confined to a roughly circular area and seen in primates, rodents, and rabbits (4).
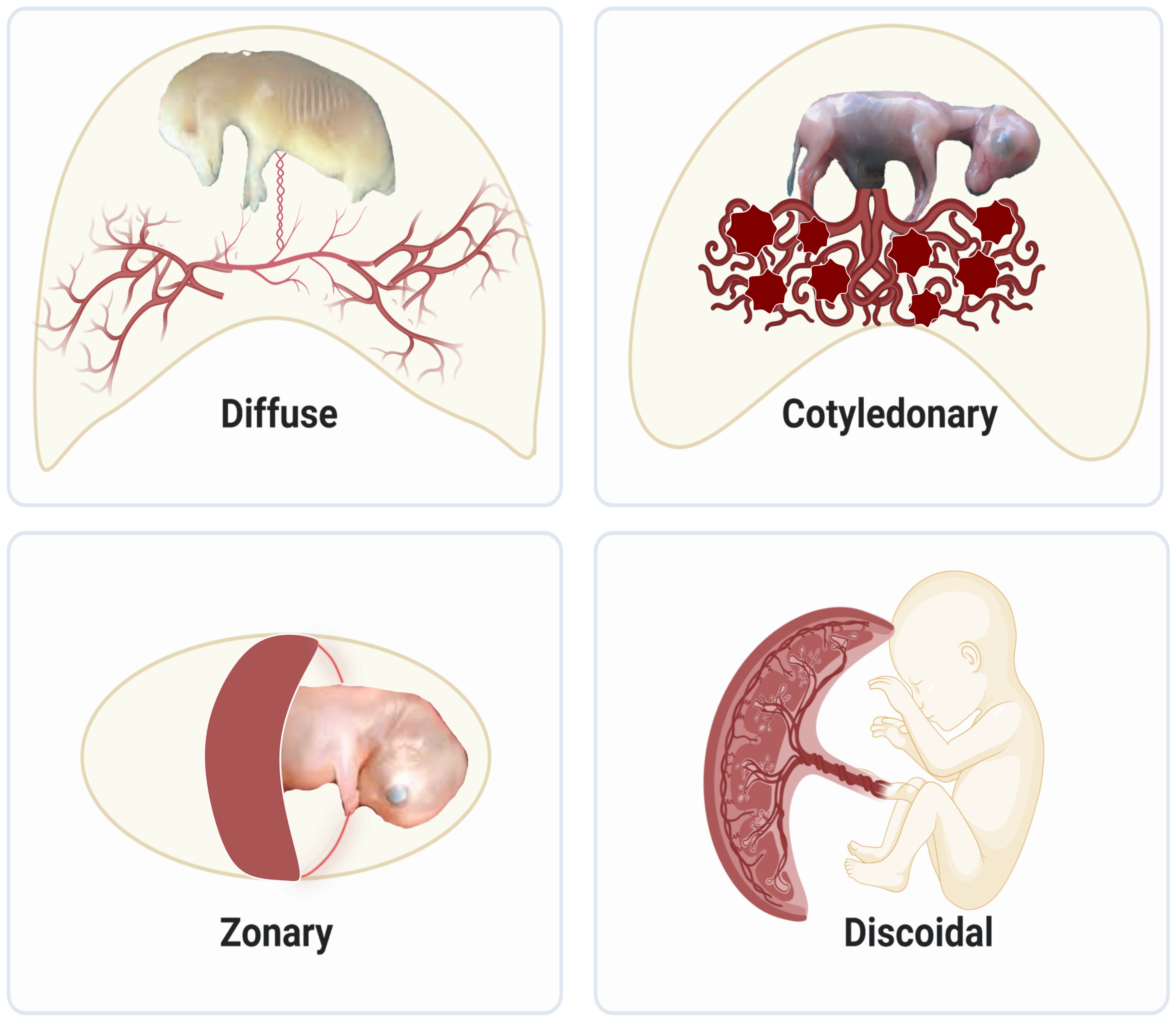
Figure 1 Structural diversity of placentas in mammals: Diffuse placentas have a uniform distribution of chorionic villi covering the chorion’s surface and are seen in horses and pigs. Cotyledonary placentas have numerous button-like structures called cotyledon that join together using vascularized intervening areas to form placentome. This type of structure is seen in sheep, goat, and cattle. Zonary placentas are like a belt created around the chorionic sac and are seen in carnivores like dogs, cats, and seals, omnivores like bears, and herbivores like elephants. Discoidal placentas form disc-like structures and this placental structure is seen in rodents and primates like humans.
Based on histological structure, four types of placentation have emerged, namely epitheliochorial, endotheliochorial, haemochorial, and synepitheliochorial (Figure 2). In species with non-invasive epitheliochorial placentation, such as horses and pigs, pockets of columnar trophoblasts loosely attach to the endometrial epithelium. Endotheliochorial placentation involves a medium degree of invasion, seen in carnivores. The most invasive type is haemochorial, as seen in humans, rats, and mice, in which the trophoblast is in direct contact with maternal blood. In synepitheliochorial placentation, trophoblast cells fuse with uterine epithelial cells to form syncytium. This type is observed in ruminants; however, some developmental biologists believe it has evolved from haemochorial placentation and named it secondary epitheliochorial placentation (5). The change in the type of placentation in ruminants might result from a positive pleiotropic effect, as described first for the relationship between placentation and cancer malignancy (see below for more information).
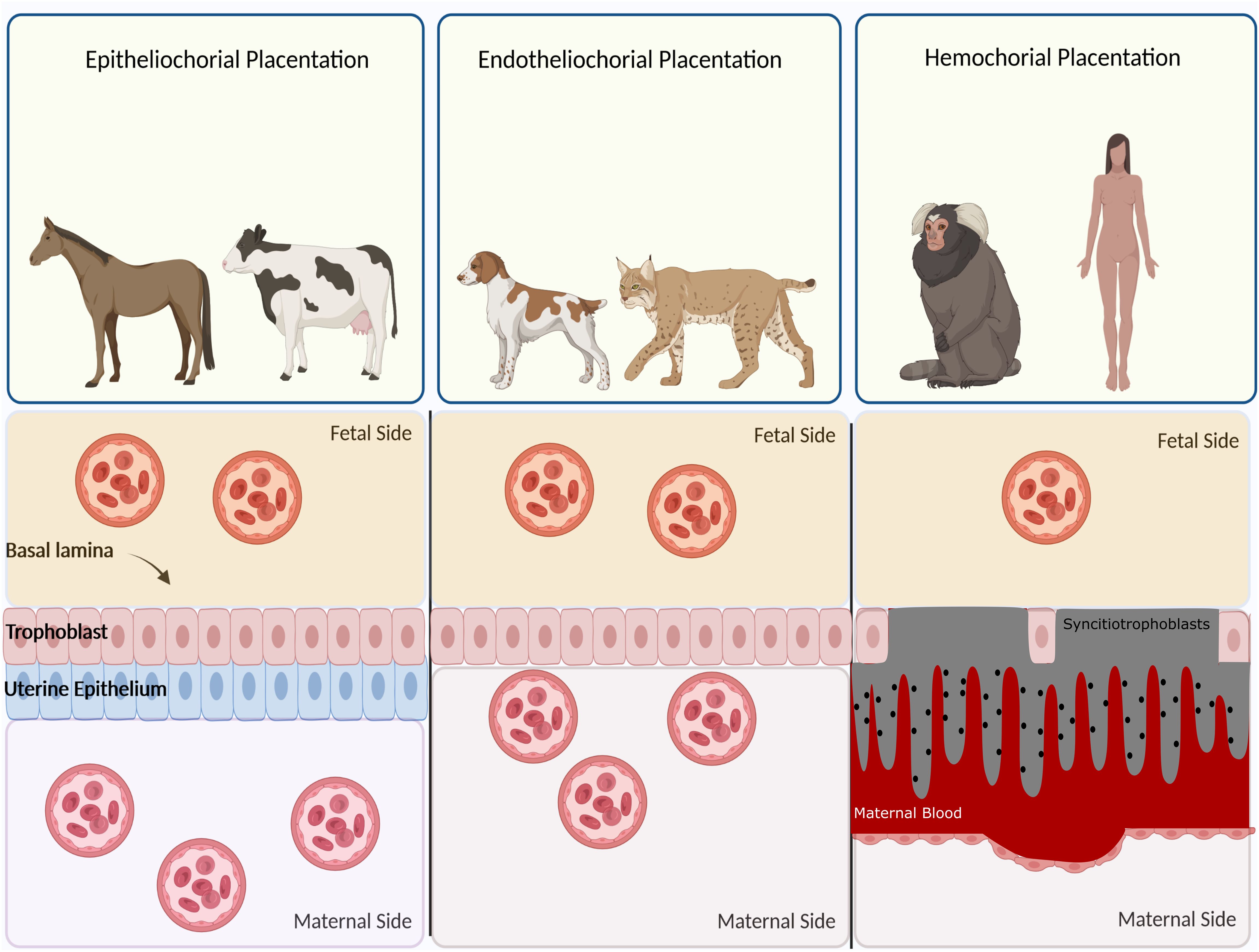
Figure 2 Classification of placentas based on cell layers comprising the maternal-fetal interface. In epitheliochorial placentation, as seen in cow, horse, and ruminants, the least intimate interactions occur between fetal tissue and maternal blood since a barrier containing maternal vascular endothelium and uterine epithelium exists. In endotheliochorial placentation, as seen in dogs and cats, trophoblasts invade the uterine epithelium and contact with maternal endothelium. In hemochorial placentation, as seen in primates and humans, the most intimate contact occurs between fetal trophoblasts and maternal circulation since both the epithelium and endothelium degrade and trophoblasts are soaked in the maternal blood.
Some researchers posit that marsupials possess a non-invasive placenta; however, recent hypotheses have indicated that the placenta of marsupials is also invasive. The challenge lies in the brief gestation period, which precludes the observation of changes related to placental invasiveness. This limitation has been compensated for by a relatively longer lactation period to better nourish the joeys (6).
In addition to the gestational period, maternal homeostatic mechanisms that cope with embryo-induced inflammation also determine the degree of invasiveness. This means that the short period of fetus implantation in marsupials is followed by parturition. In contrast, in eutherians, severe inflammation associated with deep placental invasion is compensated for by anti-inflammatory mechanisms (7). Therefore, deep placental invasion occurs in species that have evolved mechanisms to handle severe inflammation. Among the most important compensatory mechanisms is the platelet/megakaryocyte system responsible for hemostasis. The degree of invasion is much more related to maternal balancing systems than the nature of the placenta itself. Hemostatic mechanisms are needed to repair the destruction of blood vessels upon hemochorial placentation in eutherians (8). The evolution of the megakaryocyte platelet system emerged before the evolution of deep placentation. This suggests that deep invasion occurs in species that have learned how to deal with undesired outcomes. This idea echoes the words of the evolutionary biologist Theodosius Dobzhansky: ‘Nothing in biology makes sense except in the light of evolution.’”
An overview on placental development in humans and rodents
While human and mouse placentas share similar overall structures and molecular mechanisms underlying development, there are notable differences in their gross structures. Assuming the formation of the vaginal plug as day 0.5 of mouse pregnancy (9, 10), placental development begins at 3.5 days post conception (dpc) when the inner cell mass and outer trophoectoderm layers are established (10, 11). At the time of implantation (4.5 dpc), trophoblasts, corresponding to the area opposite and farthest from the inner cell mass, differentiate into polyploid trophoblast giant cells analogous to human extravillous cytotrophoblast cells (EVT) (12). Trophoectoderm cells adjacent to the inner cell mass, polar trophoectoderm, differentiate into the two main diploid cell types: the extraembryonic ectoderm and the ectoplacental cone (11).
In later stages of mouse placental development, the extraembryonic ectoderm forms the chorion layer and later the labyrinth. The ectoplacental cone differentiates into the spongiotrophoblasts, which form a compact layer between the labyrinth and the outer giant cell layer and is analogous to the column cytotrophoblast of the human placenta (10, 12). Subsequently, glycogen trophoblast cells are formed within the spongiotrophoblast layer (11). The allantois, formed at the posterior end of the developing embryo (8 dpc), is the origin of the vascular portion of the placenta (11).
The fusion of chorionic mesothelium and allantois occurs at 8.5 dpc, followed by the folding of the chorionic mesothelium, creating spaces into which fetal blood vessels grow from the allantois (11). This event coincides with the differentiation of chorionic trophoblast cells into two labyrinth cell types. In a process quite similar to human placental development, trophoblast cells of the labyrinth layer adjacent to the fetal endothelium fuse with each other, forming multinucleated syncytiotrophoblast cells (STB). The second cell type differentiated from labyrinth trophoblast cells is a mononuclear trophoblast cell that lines the maternal blood sinuses. Fetal vessels and labyrinth trophoblasts generate branched villi, analogous to human chorionic villi, which continue to grow and branch until 18.5-19.5 dpc. Notably, maternal and fetal blood circulate in opposite directions, maximizing nutrient transport (11).
Human placental development begins 6-7 days after conception, around the attachment of the blastocyst to the uterine wall (13). In humans, the polar trophoectoderm adheres to the endometrial epithelium, while in mice, it is the mural trophoectoderm that contacts the endometrium (14). Once attached, the polar trophoectoderm rapidly proliferates and forms a regional bilayer trophoblast, consisting of an inner layer of rapidly proliferating cytotrophoblasts (CTB) and an outer layer of multinucleated STB, differentiated by CTB fusion (15). Although STB are non-proliferative in essence, they can combine with the CTB basal cell layer and continue to proliferate throughout pregnancy (16). Trophoblast invasion into the maternal decidua is an essential step for the initiation of subsequent fetal development, including vasculature remodeling and crosstalk with endometrial decidual stromal cells (DSC) and immune cells residing in the decidua (17).
The development of human placental villi starts around 8 dpc through the proliferation of CTB and folding of the outer primitive STB layer into the decidua. The first villus mesenchymal cores are formed by the invasion of extraembryonic mesenchyme into the villi at around 12 dpc Endothelial cells can be identified within the villus core from 15 dpc. Similar to mouse villi, endothelial cords within human placenta villi are in close contact with the trophoblast basement membrane, implying that trophoblast cells may contribute to villous vascular network development through a paracrine manner. At around 18-20 dpc, the first fetal capillaries and placental macrophages (Hofbauer cells) appear in the stroma of placental villi. The development of placental villi capillaries continues, and at around 22 dpc, branching vessels are developed by connecting endothelial cell cords to each other and elongating parallel to the villus axis. The villi blood vessels connect to the fetal circulation via the umbilical cord around 32 dpc (18).
Villi structure in humans and mice is structurally similar, surrounded by a trophoblastic bilayer consisting of mononuclear trophoblasts and an overlying multinucleated STB layer. STB is in close contact with maternal blood and is the major site for the absorption of nutrients and oxygen and waste exchange. STB actively involves the production of human chorionic gonadotropin (hCG) and progesterone, necessary for fetal development. Around 15 dpc, CTB at the tips of anchoring villi rapidly proliferate, detach from villi columns, and differentiate into EVT (19). Two types of EVT can be identified: interstitial CTB (iCTB), which is highly invasive and invade decidual stroma, playing a fundamental role in shaping endometrial immune regulation (see below). Endovascular CTBs (eCTB) invade uterine spiral artery lumens and replace endothelial cells to ensure adequate placental perfusion as pregnancy proceeds. eCTB form trophoblastic plugs during the first weeks of pregnancy until around 7 weeks of gestation and prevent blood flow in the spiral arteries. This event provides a hypoxic microenvironment and is thought to play a major role in placental development (18). In the second and third trimesters of pregnancy, placental villi continue to mature, characterized by a densely packed stroma. In parallel, placental villi vasculature undergoes extensive expansion through branching angiogenesis. In the third trimester, vascular branching stops, and capillary loops are formed, further increasing exchange between maternal and fetal circulations.
The role of placenta in maintenance of pregnancy
The placenta performs numerous vital biological functions, including nutrient and waste transfer, gas exchange, and the transmission of maternal immunoglobulins to the fetus, providing passive immunity (20). Additionally, it contributes to fetal development through hormone secretion (21). The proximity of the placenta and decidual blood arteries is carefully regulated to meet the developing fetus’s higher metabolic needs by adjusting the endometrial circulation appropriately (22). It’s noteworthy that the human placenta possesses safeguards preventing the passage of potentially harmful compounds. Export pumps in the STB’s maternal-facing membrane, along with the expression of cytochrome P450 (CYP) genes involved in xenobiotic metabolism during the first trimester of pregnancy, contribute to these protective characteristics (23, 24).
The placenta also acts as a safeguard against the vertical transmission of infectious agents. While few pathogens can infect the placenta and fetus in humans, maternal infection doesn’t guarantee placental or fetal infection (25). The placenta employs physical and immunological mechanisms to resist infection. The STB layer, consisting of almost 60 billion nuclei, lacks cell junctions, forming a barrier against microbial attachment and invasion. The microvasculature of fetal blood vessels restricts access of microorganisms to fetal blood. The maternal decidua, rich in leukocytes, serves as an effective first-line immunological defense. Various cells, such as decidual natural killer (dNK) cells and DSCs, contribute to placental innate immune defenses. The maternal decidua is a leukocyte-rich layer and constitutes an effective first-line immunological defense at the maternal–fetal interface. As an example, dNK cells could transfer granulysin to trophoblasts and confer resistance without actively killing these cells. DSCs, as the most frequent decidual cells, have a major role in placental innate immune defenses. Villous trophoblast, in addition to providing a physical defense, also acts as a chemical barrier to the vertical transmission of microorganisms. For instance, human trophoblasts secrete antiviral interferons (type III) that restrict infection in both an autocrine and a paracrine manner. Interestingly, placental extracellular vesicles (EV) contained antiviral microRNAs with broad antiviral activity. Inflammasome-associated cytokines such as Interleukin (IL)-1β, IL-18, and IL-1α have been reported to protect placental cell infection with L. monocytogenes (25). As an example of an effective function of the placenta against infection, it is noteworthy that only 1.5-2% of pregnancies in HIV-positive women appear to result in the vertical transmission of HIV across the placenta (26).
Additionally, the placenta is a source of autocrine, paracrine, and endocrine factors. Placental hormones can be broadly categorized into those acting at the feto-maternal interface, mediating communication between the mother and developing fetus, and those exerting regulatory effects in remote targets in maternal compartments. These regulatory effects are essential for maternal physiological adaptations during pregnancy, such as changes in maternal circulation, development of lactating glands, provision of hemotroph to the embryo, and parturition. Sex hormones, including estrogen and progesterone, play a crucial role in various functions such as endometrial differentiation, myometrial quiescence, cervical closure, local immunotolerance in the pregnant uterus (27–29), trophoblast differentiation, autoregulation of placental steroidogenesis, regulation of the maternal cardiovascular system, uteroplacental blood flow, placental neovascularization and mammary gland development (30). Growth hormone (GH) and prolactin (PRL) are well-studied placental hormones with significant activity in mediating maternal metabolic adaptations to pregnancy. Placental hormones also impact maternal neuroendocrine organs, including the brain, hypothalamus, and pituitary glands, facilitating maternal adaptation to pregnancy and maintaining homeostasis. Neuroactive hormones like melatonin, serotonin, and oxytocin are involved in these activities. Other placental hormones, such as activins, relaxin, leptin, and parathyroid hormone-related protein (PTHrP), play roles in mediating changes in maternal vascular function and metabolism (26). The immunoregulatory role of leptin in the human placenta has been discussed further in the next sections.
Placenta function in decidualization and implantation
In species with hemochorial placentation, the placenta must invade the endometrial spiral arteries to create sufficient blood supply for nourishing the developing embryo. This invasion, completed at the end of the first trimester, is regulated by the local decidual environment (31). According to an evolutionary hypothesis, known as the precondition hypothesis, the uterus undergoes various cellular and molecular alterations to be adapted for potential pregnancy. One mechanism for such adaptations is the extensive structural and molecular changes in endometrial stromal cells under the control of ovarian steroid hormones, collectively referred to as decidualization (32, 33). Decidualization occurs in the mid-to-late secretory phase of the menstrual cycle in menstruating species; however, in non-menstruating species, it occurs only after implantation. The decidual environment consists of DSCs, immune cells, and extracellular matrix (34).
Decidualization serves to protect the endometrium from oxidative stress and hyper-inflammation during the deep invasion of the placenta in hemochorial placentation (32, 34). In humans, decidualization coincides with the recruitment of uterine natural killer cells (uNK) to the uterus via local expression of chemokines such as CCL4, CXCL9, and CXCL10. This cell type is a rich source of growth and angiogenic factors and is the most frequent immune cells of the uterus that modulate T cell functions through the expression of glycodelin-A and galectin-1 (33). The endometrial immune system plays a fundamental role in immune-based uterine preconditioning necessary for blastocyst implantation. The activation of redox-sensitive signaling pathways, resistance to cell death, and the expression of angiogenic factors such as vascular endothelial cell growth factor (VEGF) are among the most important mechanisms for the immune-based uterine preconditioning process (32).
Decidualization has both protective and supportive roles in placental development. It provides cytokines and growth factors necessary for remodeling the implantation site, while simultaneously limiting the degree of placental invasion to prevent complications such as placenta accreta. The depth of trophoblast invasion in mammals, which differs according to the type of placentation, correlates with the extent of decidualization (35).
The connection between the placenta and decidua is bidirectional, with the secreted factors of each tissue regulating the function of the other tissue (Figure 3). For example, a recently-discovered small peptide secreted by viable embryos called pre-implantation factor (PIF) enhances decidualization of Endometrial Stromal Cells (ESCs) (36). In addition, Profilin-1 (PFN1), an actin-binding protein secreted by EVT, enhances the proliferation and decidualization of ESCs in vitro. HCG, produced by trophoblasts, maintains progesterone production by ovaries, which is necessary for decidualization. Reciprocally, conditioned media from DSCs increased the secretion of PFN1 by EVTs (37). Interestingly, EVs derived from endometrial stromal cells promote not only the decidualization process but also the differentiation of trophoblast stem cells into the EVT lineage. The increased expression of human leukocyte antigen (HLA)-G, matrix metalloproteinase 2 (MMP2), and integrin alpha (ITGAV) as markers of EVT differentiation validate the effect of stromal-derived EV (38). Moreover, these EVs could upregulate the expression of N-cadherin in trophoblasts, which is a critical marker of invasion (39).
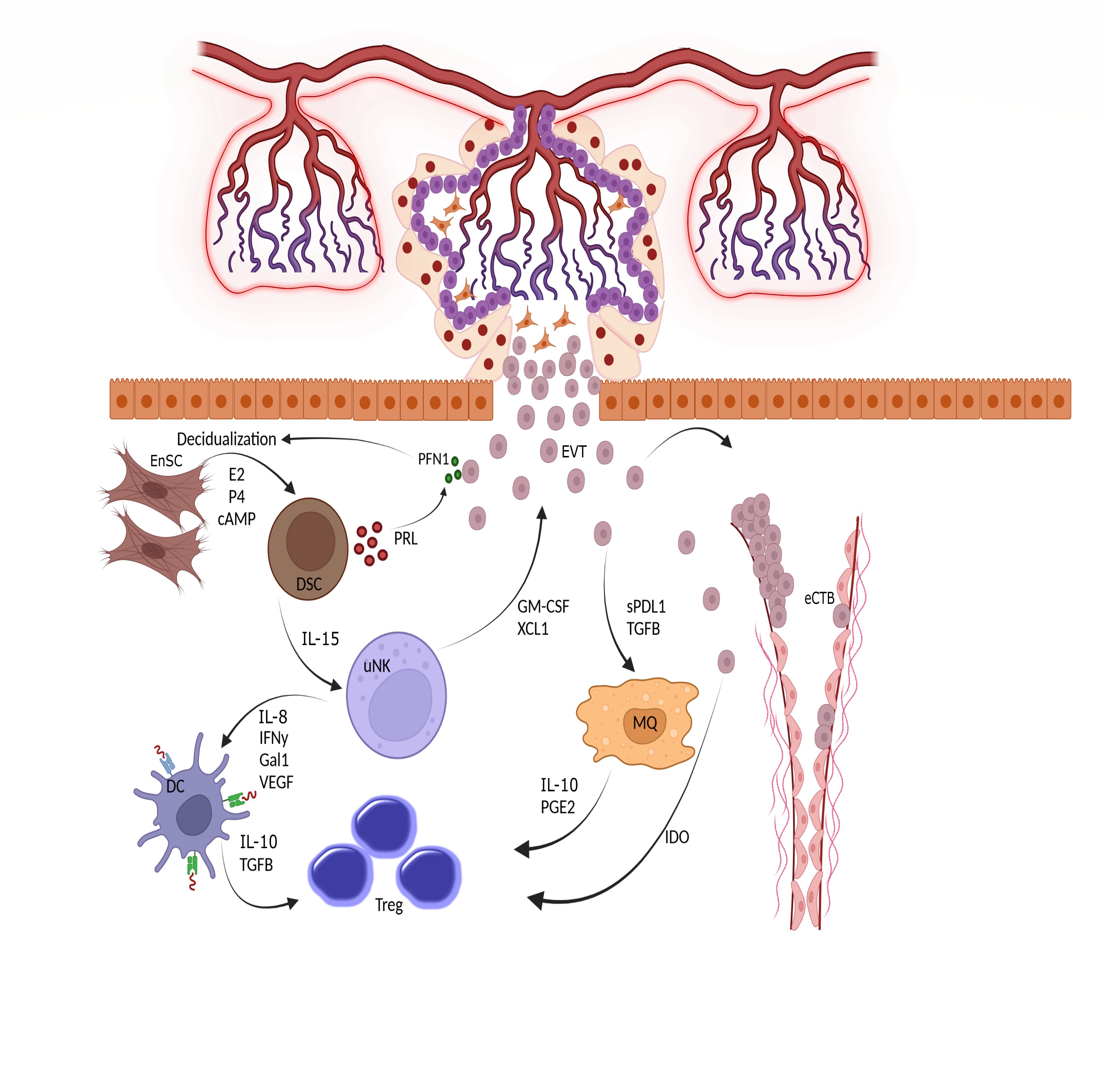
Figure 3 Triad interactions between trophoblast, decidual stromal cells and immune cells. Following implantation, the placental cytotrophoblasts in anchoring villi fuse together to form multinucleated syncitiotrophoblasts, which further differentiated to invasive extravillous trophoblasts (EVTs). The EVTs then invade the uterine tissues up to inner third of the myometrium. Some of the EVTs replace the endothelial cells and are called endovascular cytotrophoblasts (eCTBs). Secreted products from the invaded trophoblasts then modulate the function of endometrial stromal and immune cells. For example, Profilin-1 (PFN1) secreted from trophoblasts enhances the differentiation of endometrial stromal cells (EnSCs) into the epitheloid secretory decidual stromal cells (DSCs) in a process called decidualization through the action of estrogen (E2), progesterone (P4) and cyclic adenosine monophosphate (cAMP). Prolactin (PRL) is an important secreted product of DSCs and increases the production of PFN1 by trophoblasts. DSCs are master regulator of endometrial immune cells and help the function and survival of uterine natural killer cells (uNK), the most frequent immune cells in endometrium, by producing IL-15. The uNKs then produce factors such as IFN-γ, IL-18, Galectin-1, and VEGF to induce a tolerogenic phenotype in dendritic cells (DCs). Tolerogenic DCs are responsible for induction of regulatory T cells (Tregs) to maintain a pregnancy- friendly environment in the endometrium. These Tregs can also be induced by macrophages (MQs) under the influence of soluble products secreted from trophoblasts. Trophoblasts can directly induce Tregs using indoleamine 2,3-dioxygenase (IDO), an enzyme that degrades tryptophan. The available in vitro data has shown that the immune cells like uNKs can enhance the migration of trophoblasts through the secretion of chemokines. Hence, interaction between trophoblasts, EnSCs and uterine immune cells form the endometrial environment, in which each cell type modulate the function of the others. GM-CSF, Granulocyte-macrophage colony stimulating factor; XCL1, X-C motif chemokine ligand 1; IL, Interleukin; IFN, Interferon; Gal1, Galectin 1; VEGF, Vascular endothelial growth factor; TGF, Transforming growth factor; PGE2, Prostaglandin E2.
Regardless of decidualization, ESCs themselves could regulate the invasion and migration of trophoblasts. Recent evidence has pointed out that the inflammatory environment generated by ESCs promotes the migration and invasive capacity of trophoblasts in a process modulated by tumor necrosis factor (TNF)-α signaling (40). The process of trophoblast invasion, as directed by DSCs, is a necessary step for blastocyst implantation. The implantation process consists of three stages: apposition, adhesion, and invasion. All phases of implantation are controlled by trophoblasts in different stages of differentiation. The apposition and adhesion phases are promoted by the stable connections between STBs and uterine epithelium. Then, with the penetration of STBs through the epithelium, the invasion phase initiates; however, successful implantation is not solely controlled by the trophoblast itself. It requires interconnections between the trophoblasts and uterine cells. These dialogs include several molecules and mediators. Among them are integrins, which are critical for the attachment of trophoblast to uterine epithelium. Like endometrial cells that express integrins on their surfaces, trophoblasts express α3, α5, β1, β3, β4, and β5 integrins, and their repertoire is modulated during invasion and differentiation of trophoblast cells (41–43). The process of trophoblast attachment to the uterine epithelium is also modulated through their interaction with extracellular matrix (ECM) components. Evidence suggests that, in addition to uterine cells, trophoblasts also produce ECM components like laminin, fibronectin, and vitronectin to increase their production of MMP, which is necessary for their invasion in an autocrine manner (44). Growth factors like epidermal growth factor (EGF) are also produced by trophoblasts to induce their invasion, differentiation, and proliferation (45). Transforming growth factor (TGF)-β, expressed by trophoblasts, was found to inhibit trophoblast invasion and proliferation through reducing MMP-9 and stimulating MMP inhibitors like tissue inhibitor of metalloproteinase (TIMP) (46). Trophoblasts also produce hCG, which increases MMP-9 and activates adenylate cyclase to produce cyclic adenosine monophosphate (cAMP) to boost their invasiveness (44). cAMP is also necessary to initiate endometrial stromal decidualization (47). In addition to the critical role of trophoblasts in implantation, the successful development of the placenta is dependent on implantation and decidualization. Taken together, decidualization, placentation, and implantation are three sides of a triangle that are necessary for maintaining a successful pregnancy.
Bidirectional crosstalk between endometrial immune system and placenta
The coexistence of two genetically distinct individuals throughout gestation was pointed out by Peter Medawar following his studies on skin grafts. This scientific view of pregnancy, known as ‘Nature’s transplant,’ helped researchers better understand the interaction between the fetus and the maternal immune system (48). As an interface between fetal and maternal circulations, the placenta modulates the endometrial immune system. Placenta-derived factors help shape the function of immune cells in the uterus, and postnatal immune outcomes have also been associated with placental immune functions (49). Conversely, placental development and function are regulated by the local maternal immune system.
The first evidence for the regulation of placentation by the immune system comes from studies on one of the pregnancy complications known as preeclampsia (PE). Shallow trophoblast invasion in this syndrome causes maternal endothelial cell dysfunction and hypoxia in the developing fetus. Li and Wi, reported that women who have experienced preeclampsia in a previous pregnancy might exhibit protection when conceiving with a new partner. This finding could be aligned with the typical attributes of the immune system and highlight the central role of immunological specificity and memory against paternal antigens during pregnancy (50, 51).
There are two sites where fetal cells meet decidual immune cells: the large contact area between STB and maternal blood and the site of EVT-maternal decidua interaction. Endometrial immune cells have evolved mechanisms to simultaneously tolerate fetal antigens and regulate the invasion of trophoblasts (48). Bidirectional crosstalk between maternal immune cells and the placenta is exemplified by the unique functions of uNKs, the dominant leukocyte population in the first few weeks of pregnancy. Activation of Killer Immunoglobulin-like Receptor (KIR)-DS1 on uNKs by HLA-C2 on trophoblasts stimulates the production of soluble Granulocyte-Macrophage Colony Stimulating Factor (GM-CSF) and XCL1 from uNKs leading to increased migration of trophoblasts in vitro (52). This interaction also stimulates uNKs to secrete pro-inflammatory, angiogenic, and proteolytic mediators to help trophoblast invasion. Recent evidence has shown that extracellular matrix proteins such as fibronectin and the pro-inflammatory cytokine Interferon, (IFN)- γ, upregulate the expression of non-classical HLA molecules on trophoblasts. Interestingly, only first-trimester trophoblasts but not term trophoblasts respond to this stimulation (53). uNK cells can also orchestrate hemochorial placentation by regulating hypoxia, the key intrinsic regulator of placentation. In uNK-depleted mice, decreased oxygen tension stabilized hypoxia-inducible factor (HIF)-1 protein causing trophoblasts to become more invasive (54). Moreover, uNKs regulate the development of uterine spiral arteries by the production of VEGF (55). Mutually, the placenta also shapes the uterine immune system, and in this regard, uNKs play a crucial role. Classical HLA-C, and non-classical HLA-E and HLA-G are among the HLA molecules expressed by trophoblasts; however, trophoblasts do not express classical HLA-A and B (56). Both HLA-E and HLA-C can inhibit the cytotoxic response of NK cells to the trophoblasts. HLA-G in the soluble form induces a senescent phenotype with low cytotoxicity in NK cells (57).
It has been reported that HLA-G can be induced by leptin, a placental protein which is expressed by human trophoblasts (58). Recent evidence has shown that in addition to trophoblasts, leptin induces HLA-G expression on myocytes and inhibits contraction and differentiation of these cells. Moreover, leptin at low concentration can inhibit the production of reactive oxygen species from macrophages. This tocolytic effect of leptin can be used in prevention of preterm labor (59). Leptin level has been increased during the human pregnancy. Placenta is the second leptin-producing tissue after adipose tissue in humans during pregnancy (58). In humans, leptin is induced by beta-hCG, estradiol, and insulin and mediates immune tolerance in placenta (60–62). Although leptin has also a crucial role in rodent pregnancy, the main trigger for leptin induction in mice is unknown. In pregnant mice, adipose tissue is the main source that produces leptin and placenta is not responsible for producing this hormone; however, its receptor named OB-R or leptin receptor is expressed in placenta of both species. It is reported that mice lacking leptin receptor have fewer pups and adverse parturition outcomes (63). This implies that leptin expression with its broad immunoregulatory and functional roles has an evolutionary advantage in humans and mice as well as other primates.
Macrophages are the second most frequent immune cell population in the decidua. Modulation of macrophage polarization and function by trophoblasts is conducted via paracrine-secreted factors or cell-cell contacts. There are two subpopulations of macrophages: M1 and M2. M1 macrophages participate in extensive tissue remodeling via the secretion of matrix metalloproteinases upon trophoblast invasion, while M2 macrophages are activated by secreted factors from trophoblasts such as placental-specific glycoproteins (PSGs), M-CSF, TGF-β, IL-10, IL-34, and sHLA-G5 and support angiogenesis through the secretion of VEGF. In addition, M2 macrophages are involved in tissue repair and immune tolerance to the developing fetus (64). Recent evidence has pointed out that the phenotype of the macrophages in decidua basalis and parietalis, two anatomically different parts of the placenta, are different from each other and the secreted products of trophoblasts are responsible for this event. Macrophages of basalis layer highly express CD11c and are efficient inducers of regulatory T cells (Tregs) which secrete high levels of CXCL1, CXCL5, M-CSF, and IL-10. Whereas, macrophages of parietalis layer are motile and characterized by induced expression HLA class II for activation of T cell. It has shown that the conditioned media that collected from the culture of trophoblasts can convert the phenotype of decidua parietalis-associated macrophages into the decidua basalis phenotype (65).
Trophoblasts lack Major histocompatibility complex (MHC)-II molecules and are not able to prime maternal T cell activation; instead, decidual myeloid cells uptake EVT antigens and present them to maternal T cells in uterine draining lymph nodes (56). To avoid maternal T cell activation against fetal antigens, trophoblasts regulate T cell function by expressing indoleamine 2, 3 dioxygenases (IDO), an enzyme that inhibits T cell proliferation by depleting tryptophan (66–68). Similarly, trophoblasts control uterine T cells by Programmed cell Death Ligand (PDL)1 and PDL2 expression. This interaction is crucial for maternal tolerance during pregnancy since the blocking of PD1-PDL1 interaction in the decidua results in the expansion of T helper (Th)-1 and Th17 subtypes in favor of embryo resorption (69).
Trophoblasts also secrete TGF-β, which induces the differentiation of T cells to Treg. Moreover, placental TGF-β, IL-10, TNF-related apoptosis-inducing ligand (TRAIL) as well as galectin-1 help Tregs to expand (70). Progesterone produced by the early placenta is critical for the development of DSCs and differentiation of Tregs (71). Other examples of uterine immune stem modulation by trophoblasts are the expression of HLA-G dimers by trophoblasts which induce a tolerogenic phenotype in dendritic cells (DC) (72).
Besides trophoblasts, amniotic epithelial cells (AEC) also have a profound effect on modulation of immune cells. Human AEC (hAEC) exerted an inhibitor effect on proliferation of naive CD4+ T cells and production of Th1 and Th17 cytokines, while induced production of Th2 cytokines and augmented differentiation of Tregs and production of TGF-β1 and IL-10 (73, 74).
There is an increasing body of evidence suggesting a noticeable link between a pro-senescent decidual response in peri-implantation endometrium and recurrent pregnancy loss (75). Defective decidualization has been postulated to impair proper modulation of endometrial immune cells in a pregnancy-friendly manner, which could potentially lead to implantation failure or miscarriage (76). This finding shows that the endometrial stromal cells are one of the main elements of the regulation of the endometrial immune system and seriously challenges the immune system defect as the primary cause of repeated miscarriage and infertility (77–79). There is a growing body of evidence showing that mesenchymal stem cell therapy of female CBA/J mice protects fetuses from resorption in CBA/J x DBA/2 abortion model and induces Treg and Th2 type cytokines profile (80–82).Considering that highly regenerative endometrial stroma harbors multipotent endometrial stem cells with properties and phenotype similar to bone marrow and adipose tissue MSC and possibly are responsible for regenerating the endometrial stroma in each menstrual cycle (83), it would be of utmost interest to investigate whether mesenchymal stem cell therapy in CBA/J mice could potentially affect decidualization.
In the past decade, exosomes have emerged as novel mediators of intercellular communication by transfer of signaling molecules (84). All the aforementioned functions of the placenta can be mediated by exosomes. Placental exosomes can be detected in maternal blood as early as 6 weeks after conception and gradually increase until peaking at term (85). Evidence suggests that placental exosomes can be internalized by NK cells in vitro and in vivo and have diverse functions such as apoptosis induction and cytotoxicity reduction (86). These placental exosomes also educate classical phagocytic monocytes to the phenotype with impaired migratory and pro-inflammatory capacities (87). Placental exosomes can also affect apoptosis, differentiation, and cytotoxicity of maternal T cells. For instance, placental exosomes carrying NKG2D ligands such as MHC class I chain-related (MIC) and UL-16 binding protein (ULBP) as their cargo can decrease the cytotoxic action of CD8+ and γδ T cells (88).
Although, with a default conception in mind of the immunoregulatory activity of trophoblasts, most researchers have come to the conclusion that these cells exert suppressive activity on the uterine immune system, interpretation of these results should be made with caution. Pregnancy is a continuous spectrum starting from implantation and ending with parturition and involves different phases with inflammatory or anti-inflammatory natures. Many cell types and mediators from maternal origin are involved and work in concert in uterine immune modulation (89–95). In this regard, the immunoregulatory activity of trophoblasts could not tell us the whole story of endometrial immune regulation. There are three-direction interactions between the placenta, maternal immune cells, and the endometrium that shape the uterine immune fate during pregnancy and, in this regard, endometrial stromal cells are among the master regulators of uterine immune system (93).
The story of the link between placenta development and carcinogenesis
The concept of evolutionary similarities between cancer and the placenta originates from a hypothesis proposed by Scottish embryologist John Beard. He characterized cancer as ectopic trophoblastic cells (96). While not entirely comprehensive and lacking robust evidence, this hypothesis has spurred significant research in the field. Placentation and carcinogenesis exhibit numerous shared features, broadly categorized into four levels: metabolism and cell behavior, proteins and molecular signatures, signaling pathways, and tissue microenvironment (Table 1) (6, 144–146). The ability to invade the surrounding stroma is a crucial characteristic shared by trophoblasts and cancer cells. This similarity has led to the hypothesis that metastasis is a negative consequence of invasive placentation, termed “antagonistic pleiotropy.” Advocates of this theory argue that cancer occurs late in a human’s lifetime, making this negative consequence tolerable. Furthermore, the advantages of invasive placentation, particularly in nourishing the fetus, outweigh its negative costs (5).
The observation that marsupials’ placentas lack the hallmarks of mammalian invasive placentation but still develop invasive skin cancer later in life challenges the accuracy of antagonistic pleiotropy. To address this discrepancy, an alternative hypothesis called “positive pleiotropy” suggests that it is not the nature of the trophoblast itself that influences invasiveness, but rather the endometrium that controls trophoblast invasion. According to this view, animals like ruminants with less-invasive placentation have evolved to suppress trophoblast invasion, reducing the risk of malignancy (5); however, the reliability of this hypothesis is questioned by shared molecular mechanisms with other biological phenomena such as wound healing. A more detailed theory, the Evolved Level of Invasibility (ELI) theory, emphasizes the role of endometrial fibroblast cells. ELI proposes that the permissiveness or resistance of endometrial stromal fibroblasts to placental invasion in each species determines the level of malignancy and metastasis. Contrary to human embryonic stem cells (ESCs), bovine ESCs resist trophoblast invasion. Supporting this hypothesis, similar behavior is observed in skin fibroblasts of each species in response to cancer cell invasion. Tissue fibroblasts in each species, with different types of placentation, have evolved to respond to invasive cancer cells similarly to how their endometrial fibroblasts react to placental invasion (147).
As trophoblasts undergo differentiation into their lineages, they undergo an epithelial-mesenchymal transition (EMT)-like process and cell-cell fusion, akin to processes observed in cancer. Differentiation of CTB into EVT and the formation of STB from CTB involve EMT and cell-cell fusion, respectively. EMT is considered a crucial step for metastasis and invasion of tumor cells. Additionally, tumor cells respond to therapeutic intervention-induced cell damage through cell-cell fusion (148, 149).
Immune escape is another aspect of similarity between trophoblasts and tumor cells. Among the shared mechanisms of immune escape is the expression of cell surface antigens to induce immune tolerance. Proliferation, vasculogenic mimicry (VM), and angiogenesis are other aspects of similarity between placentation and carcinogenesis (144). Both tumor cells and trophoblasts are highly proliferative due to high telomerase activity, overexpression of anti-apoptotic proteins such as survivin, and activation of the insulin-like growth factor (IGF) signaling pathway (150, 151). In the VM process, tumor cells and trophoblasts form vascular structures upon invasion, providing an additional blood supply different from endothelial-dependent vasculature (132, 133). Both cell types use the same genes and signaling pathways in this process, including the overexpression of matrix glycoprotein-binding galectin-3 and Mig-7, key factors for the development of an endothelial phenotype (127). Apart from VM, the expression of key angiogenic factors such as angiopoietins, VEGF, and Placental Growth Factor (PGF), which are crucial for spiral artery remodeling in placentation and tumor cell growth, is a critical step for increasing blood supply (144).
The microenvironment of cancer and the placenta exerts profound effects on the behavior of cancer cells and trophoblasts. The decidua, as the maternal side of the placenta, affects gene expression reprogramming in trophoblasts. Similarly, the tumor microenvironment (TME) can induce epigenetic alterations in cancer cells, affecting the behavior of cancer cells, such as the promotion of cancer stemness. Immune regulation mechanisms are also shared in decidua and TME. The expression of HLA-G by both trophoblasts and tumor cells to prevent NK-mediated cytolysis, production of immunosuppressive factors to inhibit the function of cytotoxic immune cells, and the recruitment of immunosuppressive cells such as Treg and myeloid-derived suppressor cells (MDSC) are among these mechanisms. The use of the same ECM proteins and cell-secreted products and the induction of hypoxia have been observed in both environments. MMPs secreted by trophoblasts and cancer cells can shape the ECM, triggering its remodeling and facilitating invasion and the recruitment of immune cells. On the other hand, hypoxia induces EMT and invasion in CTB and VM, invasion, and angiogenesis in tumor cells (152).
Pregnancy and cancer are associated with profound systemic metabolic changes. Examples of these metabolic changes include increased blood volume and hyperglycemia to meet energy requirements during pregnancy, and abnormal metabolism in a process named cachexia, which involves the loss of skeletal muscle in cancer. In both conditions, insulin resistance (IR), defined by the inefficient function of insulin to promote glucose uptake, takes place. Inflammatory mediators, including TNF-α, IL-1, and IL-6, are key factors in the induction of IR. Nevertheless, the potential signaling pathways behind this IR remain to be further elucidated. After delivery or surgical removal of the tumor, IR gradually disappears (144).
Based on the aforementioned similarities between carcinogenesis and placentation, it could be inferred that the placenta can be considered a physiological tumor (125). In this context, the expression of placenta-specific antigens in different types of cancers has motivated many researchers to target them for cancer therapy (153–158). Moreover, biological products derived from the placenta can remarkably hinder the growth, proliferation, and progression of cancers (159, 160). For example, conditioned media from the term placenta can induce apoptosis and reduce proliferation in non-small cell lung cancer. The cellular and molecular mechanisms behind this observation involve the up-regulation of caspase 3 and suppression of the proliferation marker ki67 in tumor tissues and cell lines (161). It has also been shown that placental lysate extracts are able to suppress proliferation and induce differentiation of tumor cells in vitro (162). Besides in vitro experiments, immunization with human placenta-derived antigens has been used as an effective approach for preventive and therapeutic aims in animal cancer models (157, 163).
The idea of “immunoplacental therapy” dates back to the 1970s when a Muscovite oncoimmunologist, Valentin I. Govallo, immunized cancer patients with extracts of term placental chorionic villi. He found his therapeutic approach effective in solid tumors and published his findings in the book “Immunology of Pregnancy and Cancer.” According to Govallo’s opinion, cancer vaccination creates an immunological state similar to spontaneous abortion in pregnant women, in which immunologically foreign placental tissue would be rejected. In patients receiving placental extracts selected from various types of cancers, the overall three-, five-, and ten-year survival rates significantly increased, and metastasis was also resolved. Since Govallo’s observations, researchers have focused on using placental antigens for cancer vaccination (164).
The application of placenta-derived antigens in designing prophylactic cancer vaccines has been demonstrated in research on placental heat shock protein, glycoprotein-96 (gp96), for cancer immunotherapy. Immunization of mice with placental gp96, one week prior to challenge with melanoma cells, decreased tumor volume to 45% for about one month after the injection. In addition, higher cytotoxicity of CD8+ cells was observed against melanoma cells after immunization. Similar results were reported in gp96-immunized mice after a challenge with breast cancer cells. Comparing the tumor inhibitory effects of placental gp96 vaccine with tumor cell lysate-loaded DC-based vaccine has shown similar results in melanoma and breast cancer mouse models (163). The underlying idea of using placental antigens as effective cancer vaccines led to our recent observation that immunization with placenta-specific 1 (PLAC1), a membrane-associated protein, delays tumor onset and increases survival in a mouse model of melanoma. This immunization increased humoral and cellular immune responses, as shown by higher titers of antibodies and increased killing capacity of plac1-specific CD107a+ cytotoxic T cells (157). Using an anti-PLAC1 antibody-drug conjugate has also been shown to be effective in prostate cancer immunotherapy (158).
The anti-cancer effects of placenta-derived cells, such as hAEC, have also been the focus of much research. In a mouse model of colorectal cancer, an expanded population of systemic and splenic cytotoxic T cells and reduced tumor burden were observed after vaccination with hAEC (165). In addition to hAEC itself, secreted products derived from these cells exerted similar cytotoxic and anti-proliferative effects against cancer cell lines in vitro. Vaccination with hAEC-derived EVs can also induce apoptosis in cancer cells by triggering the Warburg effect and arginine consumption (160).
While the antigenic similarity between placenta and cancer could be viewed as a framework for effective targeted cancer immunotherapy, lessons from other aspects of similarity are no less important for controlling cancer cell growth. Shared cell behavior, signaling pathways, metabolic activity, and, most importantly, a common network of immunosuppressive microenvironment shed light on the future direction in the field of cancer immunotherapy. Nonetheless, the central role of the endometrium in controlling trophoblast invasion should not be ignored. According to the positive pleiotropy hypothesis, it is the endometrium and its controlling mechanisms that determine the degree of trophoblast invasion and dictate the type of placentation in eutherian mammals (5). In this context, a comprehensive view of the placenta and pregnancy decidua could better shape our understanding of the pathogenesis of cancer development and provide new clues for cancer treatment.
Placental development and pregnancy-related diseases
The placenta plays crucial protective roles for the fetus, but its structure and function are also associated with pregnancy complications such as IR, preeclampsia, and eclampsia (166–168). Malfunctioning placenta can affect the fetus, leading to preterm birth, fetal growth issues (169), and neurodevelopmental abnormalities (170, 171). Research on fetal nutrition and its essential role in healthy fetal development (172, 173) has laid the groundwork for the concept that many fetal and adult disorders originate in the placenta. In fact, future cardiovascular disorders may be both predicted and caused by placental function (174, 175).
Insufficient placental function, as reported, can influence early growth and obesity, which are associated with an earlier age at menarche. Additionally, measurements of the fetus’s size at 35 weeks of gestation closely correspond with the rate of placental growth between 17 and 20 weeks of gestation (176). Compared to infants of normal weight, the placentas of newborns with intrauterine growth restriction have smaller diameters, higher placental coefficients, and lower weights and volumes (177). Intrahepatic cholestasis of pregnancy (ICP), a liver disease during pregnancy, typically affects women in their third trimester. ICP is linked to a higher risk of spontaneous preterm birth, fetal distress, and sudden intrauterine death, causing complications for both mother and fetus. Although the exact origin of the disease is presently unknown, research suggests that maternal bile acid levels play a role. Studies indicate that high bile acid concentrations are linked to placental apoptosis. Additionally, the placenta’s function in protecting the fetus from the negative effects of potentially harmful endogenous compounds involves mitigating the effects of bile acids on the fetus (178).
Placental disorders are often categorized into ischemic and nonischemic types. Preeclampsia, and intrauterine growth retardation (IUGR), fall under conditions where the fetus lacks sufficient blood perfusion and are thus categorized as ischemic placental disorders. Although preterm labor (PTL) was once classified outside this category (179), about one-third of preterm births are associated with changes in placental ultrastructure typically seen in ischemic disease processes (180). This finding suggests a significant overlap in the mechanisms responsible for each type of pregnancy complication (22).Critical in determining life expectancy are patterns of intrauterine development and size at delivery, impacting adult rates of morbidity and mortality as well as neonatal viability. Low birth weight, according to human epidemiological studies, is linked to an increased risk of adult-onset cardiovascular and metabolic diseases such as hypertension, coronary heart disease, obesity, and type 2 diabetes, as well as a higher incidence of reproductive and neurological disorders (170, 171, 174, 175, 181, 182). Changes in the placenta’s surface area, affecting both diffusion and transporter-mediated processes, directly influence the capacity for nutrition transfer (183). In many species, there is a positive correlation between fetal and placental weight (22, 184–187). In human IUGR cases, the placental volume is on average 92.66 cm2 smaller in comparison to the placenta in normal pregnancy (188).
Placenta proteome in health and disease
Placental proteins in normal pregnancy
Transcriptome analysis indicates that 65% of all human proteins are expressed in the placenta. Within this subset, the placenta exhibits higher expression levels for 354 of these genes, the majority of which encode secreted proteins (189).
Placental proteins are not only fundamental for pregnancy maintenance and development of the fetus, but also exerts critical function in bidirectional crosstalk with endometrial immune system and establishment of maternal tolerance during pregnancy; however, our knowledge of placental proteome is still limited and these aspects must be studied in more detail to understand the dynamics of proteins in pregnancy, fetal growth and complications during pregnancy. Indeed, this information is central for our understanding of molecular mechanisms involved in placental and gestational disorders. In this regard, proteomics technology has fundamentally advanced our knowledge of placental proteins. In this context, we recently compared normal first-trimester and full-term human placenta proteomes. A total of 120 differentially expressed proteins were identified in the first trimester versus term placenta. Among these, 20 proteins with expression fold changes higher than 2 or less than 0.5 were analyzed. Accordingly, GRP78, PDIA3, ENOA, ECH1, PRDX4, ERP29, and ECHM vs. TRFE, ALBU, K2C1, ACTG, CSH2, PRDX2, FABP5, FABP4, K2C8, MESD, K1C9, MYDGF, HBG1 had significantly higher expression in the first trimester and full-term placenta, respectively. Interestingly, we found two proteins (MESD, MYDGF) that were exclusively expressed in the first-trimester placenta. These proteins play significant roles in biological quality control, programmed cell death, hemostasis and catabolic processes, protein folding, cellular oxidant detoxification, coagulation, and retinal homeostasis. They also play essential roles in reactions to chemical stimuli and stress (190). In a comprehensive study conducted by Mushahary et al., 117 proteins in the term placenta were reported. The functions of the reported proteins were categorized into eight distinct groups, including metabolism, cell stress, cytoskeletal, transport, signal transduction, nuclear proteins, translation, cell cycle, and growth (191). In a recent study, an anatomical approach was employed to unravel the proteome profile of different sub-anatomical regions of the human placenta (192). The results showed 1081, 1086, and 1101 proteins in maternal, middle, and fetal sub-anatomical regions respectively with 374 differentially expressed proteins between sample site locations and sub-anatomical regions. Notably, proteins with the anti-senescent function were decreased in the maternal sub-anatomical region, while proteins associated with a switch from ATP to fatty acid consumption were increased in the middle and fetal sub-anatomical regions.
Apart from high throughput proteomics approaches, there are some studies with a focus on isolated placental proteins. A specific placental protein, placental protein 13 (PP13), binds to β-galactoside residues on the cell surface, cytoskeleton, and extracellular matrix, generating various responses such as blood vessel adaptation, immune responses, and influencing functions like apoptosis and molecular recognition (193). PLAC1 is the product of a paternally imprinted X-linked gene (Plac1) with limited normal tissue expression, which plays fundamental roles in placental function and development. It is expressed in both human and mouse placenta and is necessary for placental and embryonic development. Knockout and heterozygous placentae of the Plac1-null allele inherited from the mother weighed roughly 100% more than wildtype placentae, though the corresponding embryos weighed 7-12% less (194, 195). Retrotransposon Gag-like-1 (RTL-1), also known as Peg11, is another placental protein with a known function in placenta and fetal development. Its expression during neonatal period has to be finely regulated. Inheritance of the Peg11/RTL1 KO allele from father causes late fetal lethality due to late fetal growth retardation, whereas maternal transmission leads to overexpression of Peg11/Rtl1 and neonatal lethality (196, 197). This protein is preferentially expressed in the labyrinth layer of the mouse placenta by the endothelial cells. In both the Peg11/Rtl1 paternal and maternal KO placenta, severe abnormalities of the fetal capillaries were observed. This finding is in favor of the critical role of PEG11/RTL1 protein in maintaining the integrity of the feto–maternal interface of the placenta during pregnancy (198). The placenta expresses approximately 100 placental-specific proteins. Table 2 provides examples of placental proteins and their related biological functions.
Modification of placental protein in preeclampsia
Dr. David Barker initially introduced the concept of fetal origins of adult disease (FOAD), which has garnered significant attention since its inception. The FOAD hypothesis posits that events during early development profoundly impact the risk of developing adult diseases in the future. Low birth weight, serving as a surrogate marker for poor fetal growth and nutrition, is associated with conditions such as coronary artery disease, hypertension, obesity, and IR (220–227). Epidemiological evidence now establishes a connection between an individual’s susceptibility to chronic diseases in adulthood and events during their intrauterine phase of development. Influences during this period can permanently alter the functional capacity and structure of organ systems, as well as the activity of enzyme systems and endocrine axes. These effects set the stage for a diverse array of diseases that may manifest many years later, often in response to secondary environmental stressors.
The foundation of fetal development lies in the placenta, which plays a crucial role in nutrient and oxygen delivery to the fetus. Impaired placental function or its inability to adapt may compromise fetal development (175). There is accumulating undeniable evidence in the past decade regarding the fundamental role of placental phenotype, structure, physiology, and function in a variety of postnatal diseases; however, we will specifically focus here on the modification of the placental proteome in preeclampsia, a leading cause of maternal and neonatal morbidity and mortality. This information is essential for establishing novel biomarkers for the non-invasive diagnosis of this disease.
Survivors of preeclampsia face reduced life expectancy with increased risks of diabetes, stroke, and cardiovascular diseases. Indeed, babies born from preeclamptic pregnancies are at higher risk of preterm birth, perinatal death, and postnatal diseases such as neurodevelopmental disability, and cardiovascular and metabolic diseases later in life. Preeclampsia is a syndrome driven by placental dysfunction causing maternal endothelial dysfunction and systemic inflammation (228).
Only a limited number of studies have delved into alterations in placental tissue proteins related to pregnancy-related disorders. Among a total of unique placental proteins reported by Mushahary et al. (191), twenty-five proteins (mainly cell stress proteins) have been associated with PE (229–235), early pregnancy loss [ubiquitin-conjugating enzyme E2 N, calgizzarin (S100 calcium-binding protein A11), galectin-1 (236), PTL (cytoskeletal proteins) (237) and assisted reproductive technology [fascin] (238).
In a systematic review, twelve studies employing mass spectrometry-based techniques were examined, comparing samples from preeclamptic and normotensive pregnancies. Across all studies, 401 proteins with significantly altered expression in PE were identified. Comparison between studies identified 52 proteins as significant in two or more studies, which were then enriched for 22 pathways, including those previously implicated in PE such as hemostasis, immune response, and lipid metabolism. In particular, the proteins complement component 4 and apolipoprotein E displayed abnormal expression at week 12 before the clinical diagnosis of PE, suggesting their value as clinical biomarkers (239). Downregulation of antioxidant proteins (peroxiredoxin two and peroxiredoxin 3) and altered expression of stress response proteins (Hsc) 70, Heat shock protein (Hsp) gp96, and protein disulfide isomerase) has also proposed in the pathogenesis of PE (235). To assess the effect of elevated levels of neurokinin B (NKB), a tachykinin linked to systemic symptoms of PE, on the placental proteome, the proteome profile of cultured human term CTB was assessed in response to NKB treatment. The results revealed a statistically significant decrease in 20 proteins, including those crucial in antioxidant defenses (240). Table 3 summarized alterations of placental proteins in women with PE.
Placental proteins as biomarkers for early diagnosis of Preeclampsia
There is a necessity to identify and establish gestational biomarkers that can be employed for monitoring pregnancies, and predicting adverse outcomes. While utilizing proteomic biomarkers in placental tissue is one approach for assessing health, nutritional status, and disease, it is practically challenging for the early diagnosis of gestational diseases. Therefore, recent trends primarily focus on the non-invasive examination of placental proteins in peripheral blood. Human placenta harbors numerous proteins, most of which are secreted to the maternal circulation during pregnancy. Notable examples of these secreted proteins include human chorionic gonadotropin, human placental lactogen (hPL), human GH variant or placental growth hormone, leptin, corticotropin-releasing hormone, placental growth factor (PlGF) all of which are secreted by the placenta into the mother’s bloodstream (31, 58, 178, 257–265).
The composition of maternal plasma proteome is steadily altered throughout gestation, which is in part due to the changes in the placental proteins secreted to the maternal circulation. This has offered a novel perspective for the precise timing of placental protein expression relative to gestational age, the “placental proteomic clock”. Keeping this concept in mind, several researchers have unveiled changes in the placental proteome during different gestational periods. In a cohort study, Degnet et al. identified five placenta-derived proteins capable of predicting conceptional age, including chorionic somatomammotropin (CSH1/2), biglycan (BGN), glypican 3 (GPC3), inter-alpha-trypsin inhibitor heavy chain H5 (ITIH5), and lysosomal alpha-glucosidase (GAA) (266). In this study, Degnes et.al., among nearly 5000 measured proteins in maternal circulation, described 256 placenta-derived proteins and 101 proteins absorbed by the placenta. Of these, 101 placenta-derived proteins were deemed placenta-specific, forming two clusters with distinct developmental patterns throughout gestation. This innovative concept may serve to monitor deviations in the developmental patterns of placenta-derived proteins across gestation, indicating potential placental dysfunction.
PlGF, a member of the VEGF family predominantly expressed in the placenta, is crucial for normal pregnancy. PlGF concentrations are initially low in the first trimester of an uncomplicated pregnancy, increasing from week 11 to 12 and peaking at week 30, followed by a decline. Decreased PlGF expression in the placenta is associated with disruptions in placental development, maturation, and the onset of conditions like PE. Since PlGF’s primary role outside the placenta is angiogenesis in response to pathological ischemia or injury, a low level of PlGF serves as an indicator of abnormal placentation. This is supported by observations that women without preeclampsia who give birth to small for gestational age babies also exhibit low PlGF levels early in pregnancy (267). Similarly, serum levels of inhibin A, a glycoprotein of human STB, have been explored for predicting and evaluating the severity of PE. The deficient trophoblastic invasion in PE leads to ischemic damage to the STB’s surface layer. This modification is suggested to contribute to the increased release of inhibin A into the maternal circulation. In comparison to the group without adverse pregnancy outcomes, elevated serum levels of inhibin A during the second trimester not only pose a risk for preeclampsia but also for gestational diabetes mellitus, preterm delivery, and low birth weight (268, 269).
PP13 has emerged as a biomarker for predicting preeclampsia. Lower levels of maternal serum PP13 in the first trimester are associated with the development of PE and intrauterine growth restriction (193, 270, 271).
Measurement of maternal serum levels of PlGF and Pregnancy-associated Plasma Protein-A (PAPP-A), combined with maternal risk factors (e.g., obesity, hypertension, maternal age, etc.) and uterine artery measures by Doppler ultrasound, is proposed to have sensitivity of about 95% for detection of early-onset PE (diagnosed < 34 weeks) (272). In addition to alterations in placental protein expression, there are reports indicating that placental stress can lead to maternal physiological maladaptation to pregnancy by causing abnormal glycosylation of placental-derived factors. Endoplasmic reticulum (ER) stress leads to diminished complexity and sialylation of trophoblast protein N-glycosylation, and aberrant glycosylation of VEGF leading to its reduced bioactivity. ER stress affects the expression of 66 out of 146 genes annotated with ‘protein glycosylation’ and diminishes the expression of sialyltransferases (273). Table 4 summarizes the serum proteins associated with early onset PE.
Of note, most of the evaluated markers have been found when the diagnosis of PE has been already established. Despite the identification of proteins that are differentially expressed in patients with PE, few studies have been conducted to find a specific and reliable marker for early prediction of the disease onset. Using a machine-learning approach, a recent study combined multi-omics datasets (transcriptome, proteome, etc.) of plasma, urine and vaginal swab, collected from pregnant women during the first 16 weeks of pregnancy to find a panel of markers for early diagnosis of PE. Plasma proteome and urine metabolome were the most accurate predictive models for PE and, in each model, top proteins and metabolites were identified. Adenine, isovalerylglutamic acid, uric acid ribonucleoside, 1,5-anhydroglucitol, dehydroepiandrosterone, sialyllactose, Nϵ-acetyl-L-lysine, and nonanoylcarnitine were the top urine metabolites that predict PE with high accuracy. Besides, serum proteins leptin (LEP), VEGF, L-selectin (SELL), E-selectin (SELE), IL-24, IL-22, tyrosine-protein kinase transmembrane receptor (ROR1), C-X-C motif chemokine ligand 10 (CXCL10), and SPARC-like 1 (SPARCL1) were found to have the top predictive value for PE. Along with the plasma proteome and urine metabolome, the immune cell dynamics of the single cells isolated from the patient’s blood between the first and second trimesters can also predict the onset of PE and correlated with the aforementioned markers in plasma and urine. Basal pSTAT1 (phosphorylated signal transducer and activator of transcription) and STAT5 signaling as well as basal NF-κB (Nuclear factor kappa B) levels in immune cells were the immune features that correlated with top predictive plasma proteins. There was also significant correlation between the top predictive proteins and metabolites with clinical data of the patients. Upon final univariate analysis of all the predictive features, the LEP, CCL23, and FAM3D had significant association with PE outcome (278).
Placental proteins as biomarkers for cancer diagnosis and target discovery
Based on investigations into differentially expressed proteins in cancer, certain proteins have been identified with high expression in the testis. Coined as “cancer-testes antigens” (CTAs) by Old and Chen in 1997 (279, 280), these proteins form over 70 families with more than 170 members, extensively documented in various cancer databases (281). Intriguingly, some of these proteins also exhibit elevated expression in placental tissues, leading to the emergence of a new term, “cancer placental antigens” (CPAs) or more comprehensively, “cancer testes placental antigens” (CTPAs). Figure 4 illustrates several CPAs, their cellular compartmentalization, and enrichment in diverse biological pathways. Certain markers, like cancer antigen 125 (CA-125) and carcinoembryonic antigen cellular adhesion molecule-5 (CEACAM-5), already serve as serum tumor markers for pancreatic and ovarian cancers (282, 283); however, their limited specificity and sensitivity hinder their reliability for accurate diagnoses (155). Notably, Melanoma-associated antigen 3 (MAGE-A3), a CTPA, has proven to be an attractive target in undifferentiated sarcoma (284).
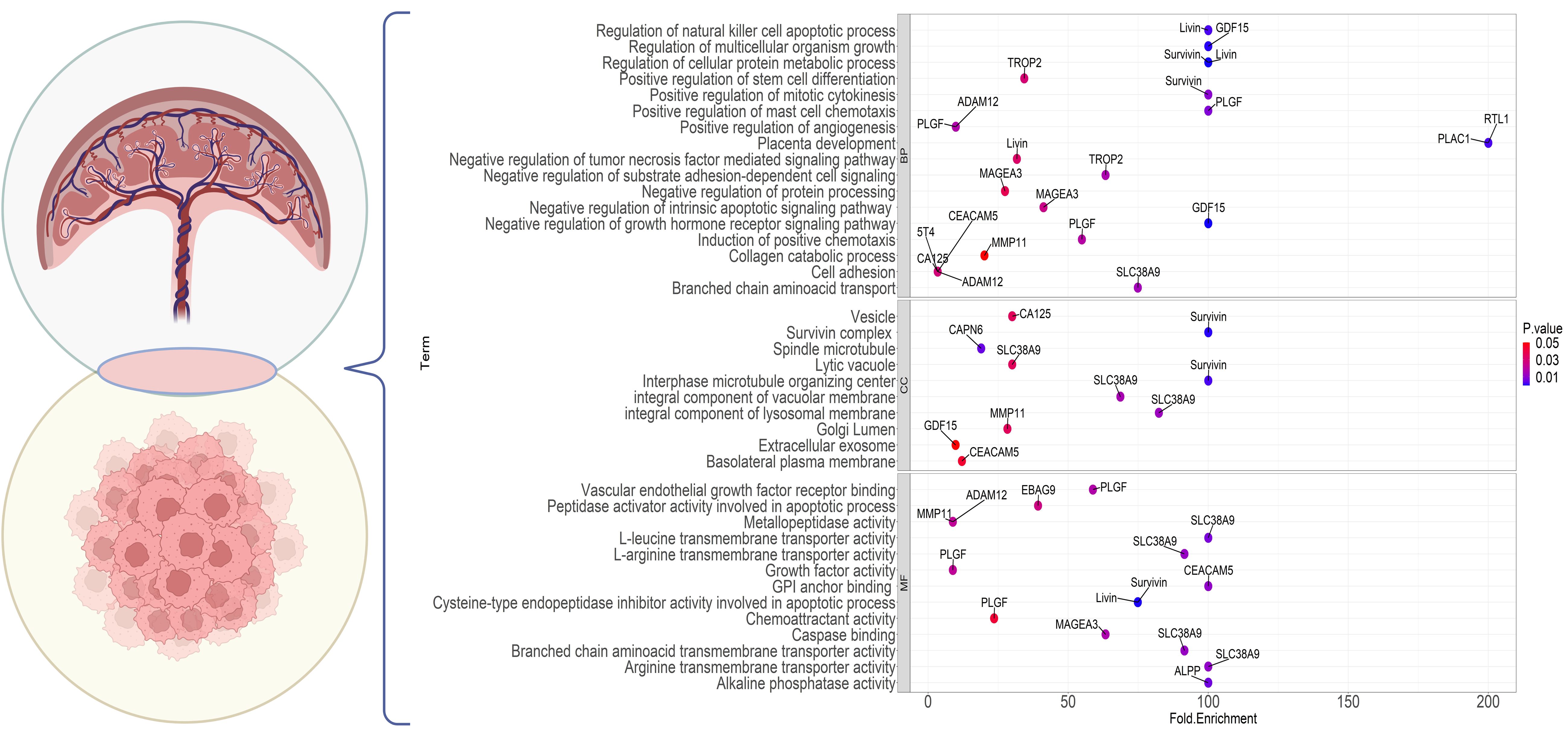
Figure 4 Gene Ontology enrichment analysis of the genes commonly expressed in cancer and placenta. The dot plot shows the top enrichment terms for the markers with a shared expression between the placenta and tumor cells. The color of each dot indicates the enrichment P value and the x-axis shows the enrichment fold. Each part points to one of the GO sections; BP, Biological Processes; CC, Cellular Component; MF, Molecular Function.
PlGF, utilized as a biomarker for predicting and diagnosing PE, has demonstrated overexpression in cancer and its associated stromal cells (104). Trophoblast surface glycoproteins TROP2 and 5T4 hold prognostic significance in at least 13 different types of cancers (285, 286). Antibodies against survivin, livin, and PLAC1 are detected in sera across various cancer types (287–290). Recent mining of transcriptomic databases has identified MMP11 and ADAM metallopeptidase domain 12 (ADAM12) as potential CPAs, with MMP11 exhibiting high transcript levels in 25 different types of cancers (155). PLAC1, an X-linked trophoblast antigen, is ectopically expressed in a large set of cancer cell lines and several cancers, including melanoma, breast, cervical, prostate, ovary, liver, and colorectal cancers (156, 158, 195, 291, 292). More recently, RTL1 has been introduced as a placenta-specific protein with ectopic expression in cancer, particularly evident in hepatocellular carcinoma (HCC) (293), melanoma (294), and breast cancer (295). In melanoma, RTL1 promotes cell proliferation through the Wnt/β-Catenin signaling pathway (294). Preliminary observations indicate high RTL1 expression in breast cancer cell lines and tissues, correlating significantly with histological grade and vascular invasion (295). Further research is required to uncover the cancer-modulatory effects of RTL1 in vivo.
Growth/differentiation factor-15 (GDF-15) is a placental protein, which is barely detected in most somatic tissues in humans. It is a member of the TGF-β superfamily and is highly expressed across various cancer types (296, 297). GDF-15 is induced under stress conditions to maintain cell and tissue homeostasis. Reportedly, high blood levels of GDF-15 are associated with inflammatory conditions, which is the hallmark of myocardial ischemia, and especially cancer (297).
Numerous studies have concentrated on tumor-associated autoantibodies as potential cancer biomarkers (298–301). Considering common antigens expressed in both placenta and various cancers (CPAs), autoantibodies against placenta proteins could be viewed as potential serologic cancer biomarkers. We recently reported that patients with breast, gastric, and colorectal cancers produce detectable levels of placenta-reactive autoantibodies. This was extremely the case for breast cancer, in which sera from early stage breast cancer patients contained antibodies reactive with the first-trimester placenta and reacted with a scattered subpopulation of cells, probably trophoblast stem cells (153).This approach, could be utilized for non-invasive cancer screening at early stages, paving the way for new strategies in targeted immunotherapy for cancer patients. Profiling of autoantigens by protein array technology for discovery and point-of-care type investigations are a promising approach for prognostics and diagnostics (302).
Concluding remarks
Despite the undeniable importance of the placenta in eutherian pregnancy, this vital organ has not been studied as much as it deserves. New findings show that the placenta, in addition to being the major anatomical site to provide nutrients to the developing fetus, has an undeniable role in regulating the microenvironment of the endometrium and preparing it for a successful pregnancy. Despite the conventional belief of the main role of maternal factors, placental cells, and its secretory factors exert a central role in regulating the maternal immune system and inducing immunological tolerance of pregnancy. Placental proteome and circulating levels of placental proteins are reliable sources for investigating and identifying gestational diseases. Interestingly, there is a very fascinating connection between placentation and carcinogenesis. Accordingly, the type of placentation in eutherian mammals and adaptation of endometrium to placental invasion are determining factors in the prevalence of the type of invasive cancers. In this context, a deep knowledge about the placenta and pregnancy decidua could better shape our understanding of the pathogenesis of cancer development and provide new clues for cancer treatment. In spite of being the star of the evolution of mammals, yet placenta is generally seen as a waste organ! It is not the placenta’s fault that it has languished in such obscurity. No one has bothered to speak up on its behalf.
Author contributions
SK-S: Writing – original draft, Writing – review & editing. NV: Software, Writing – review & editing. SS: Conceptualization, Data curation, Writing – review & editing. KZ: Writing – original draft, Formal analysis, Methodology. AS: Data curation, Formal analysis, Investigation, Writing – review & editing. MJ-T: Project administration, Supervision, Writing – review & editing. A-HZ: Project administration, Supervision, Validation, Writing – original draft, Writing – review & editing.
Funding
The author(s) declare financial support was received for the research, authorship, and/or publication of this article. This review paper was supported by the National Institute for Medical Research Development (NIMAD) (Grant No: 973140), Tehran, Iran, and in part by Iran National Science Foundation (INSF) (Grant No: 96013023), Tehran, Iran.
Conflict of interest
The authors declare that the research was conducted in the absence of any commercial or financial relationships that could be construed as a potential conflict of interest.
The author(s) declared that they were an editorial board member of Frontiers, at the time of submission. This had no impact on the peer review process and the final decision.
Publisher’s note
All claims expressed in this article are solely those of the authors and do not necessarily represent those of their affiliated organizations, or those of the publisher, the editors and the reviewers. Any product that may be evaluated in this article, or claim that may be made by its manufacturer, is not guaranteed or endorsed by the publisher.
References
1. Roberts RM, Green JA, Schulz LC. The evolution of the placenta. Reproduction. (2016) 152:R179–89. doi: 10.1530/rep-16-0325
2. Weisbecker V, Goswami A. Brain size, life history, and metabolism at the marsupial/placental dichotomy. Proc Natl Acad Sci. (2010) 107:16216–21. doi: 10.1073/pnas.0906486107
3. Guernsey MW, Chuong EB, Cornelis G, Renfree MB, Baker JC. Molecular conservation of marsupial and eutherian placentation and lactation. eLife. (2017) 6:e27450. doi: 10.7554/eLife.27450
4. Whittington CM, Van Dyke JU, Liang SQT, Edwards SV, Shine R, Thompson MB, et al. Understanding the evolution of viviparity using intraspecific variation in reproductive mode and transitional forms of pregnancy. Biol Rev. (2022) 97:1179–92. doi: 10.1111/brv.12836
5. D'Souza AW, Wagner GP. Malignant cancer and invasive placentation: A case for positive pleiotropy between endometrial and Malignancy phenotypes. Evolution medicine Public Health. (2014) 2014:136–45. doi: 10.1093/emph/eou022
6. Wagner GP, Kshitiz, Dighe A, Levchenko A. The coevolution of placentation and cancer. Annu Rev Anim Biosci. (2022) 10:259–79. doi: 10.1146/annurev-animal-020420-031544
7. Griffith OW, Chavan AR, Protopapas S, Maziarz J, Romero R, Wagner GP. Embryo implantation evolved from an ancestral inflammatory attachment reaction. Proc Natl Acad Sci U.S.A. (2017) 114:E6566–e75. doi: 10.1073/pnas.1701129114
8. Martin JF, Wagner GP. The origin of platelets enabled the evolution of eutherian placentation. Biol Lett. (2019) 15. doi: 10.1098/rsbl.2019.0374
9. Duan Y, Wang H, Mitchell-silbaugh K, Cai S, Fan F, Li Y, et al. Heat shock protein 60 regulates yolk sac erythropoiesis in mice. Cell Death Dis. (2019) 10:766. doi: 10.1038/s41419-019-2014-2
10. Fonseca BM, Correia-da-Silva G, Teixeira NA. The rat as an animal model for fetoplacental development: A reappraisal of the post-implantation period. Reprod Biol. (2012) 12:97–118. doi: 10.1016/s1642-431x(12)60080-1
11. Watson ED, Cross JC. Development of structures and transport functions in the mouse placenta. Physiol (Bethesda). (2005) 20:180–93. doi: 10.1152/physiol.00001.2005
12. Rossant J, Cross JC. Placental development: lessons from mouse mutants. Nat Rev Genet. (2001) 2:538–48. doi: 10.1038/35080570
13. Knöfler M, Haider S, Saleh L, Pollheimer J, Gamage T, James J. Human placenta and trophoblast development: key molecular mechanisms and model systems. Cell Mol Life Sci. (2019) 76:3479–96. doi: 10.1007/s00018-019-03104-6
14. Weberling A, Zernicka-Goetz M. Trophectoderm mechanics direct epiblast shape upon embryo implantation. Cell Rep. (2021) 34:108655. doi: 10.1016/j.celrep.2020.108655
15. Chang CW, Wakeland AK, Parast MM. Trophoblast lineage specification, differentiation and their regulation by oxygen tension. J Endocrinol. (2018) 236:R43–r56. doi: 10.1530/joe-17-0402
16. James JL, Lissaman A, Nursalim YNS, Chamley LW. Modelling human placental villous development: designing cultures that reflect anatomy. Cell Mol Life Sci. (2022) 79:384. doi: 10.1007/s00018-022-04407-x
17. Velicky P, Knöfler M, Pollheimer J. Function and control of human invasive trophoblast subtypes: intrinsic vs. Maternal control. Cell Adh Migr. (2016) 10:154–62. doi: 10.1080/19336918.2015.1089376
18. Boss AL, Chamley LW, James JL. Placental formation in early pregnancy: how is the centre of the placenta made? Hum Reprod Update. (2018) 24:750–60. doi: 10.1093/humupd/dmy030
19. Meakin C, Barrett ES, Aleksunes LM. Extravillous trophoblast migration and invasion: impact of environmental chemicals and pharmaceuticals. Reprod Toxicol. (2022) 107:60–8. doi: 10.1016/j.reprotox.2021.11.008
20. Ciobanu AM, Dumitru AE, Gica N, Botezatu R, Peltecu G, Panaitescu AM. Benefits and risks of igg transplacental transfer. Diagnostics (Basel). (2020) 10. doi: 10.3390/diagnostics10080583
21. Costa MA. The endocrine function of human placenta: an overview. Reprod BioMed Online. (2016) 32:14–43. doi: 10.1016/j.rbmo.2015.10.005
22. Maltepe E, Fisher SJ. Placenta: the forgotten organ. Annu Rev Cell And Dev Biol. (2015) 31:523–52. doi: 10.1146/annurev-cellbio-100814-125620
23. Kammala AK, Lintao RCV, Vora N, Mosebarger A, Khanipov K, Golovko G, et al. Expression of cyp450 enzymes in human fetal membranes and its implications in xenobiotic metabolism during pregnancy. Life Sci. (2022) 307:120867. doi: 10.1016/j.lfs.2022.120867
24. Joshi AA, Vaidya SS, St-Pierre MV, Mikheev AM, Desino KE, Nyandege AN, et al. Placental ABC transporters: biological impact and pharmaceutical significance. Pharm Res. (2016) 33:2847–78. doi: 10.1007/s11095-016-2028-8
25. Megli CJ, Coyne CB. Infections at the maternal–fetal interface: an overview of pathogenesis and defence. Nat Rev Microbiol. (2022) 20:67–82. doi: 10.1038/s41579-021-00610-y
26. Napso T, Yong HEJ, Lopez-Tello J, Sferruzzi-Perri AN. The role of placental hormones in mediating maternal adaptations to support pregnancy and lactation. Front Physiol. (2018) 9:1091. doi: 10.3389/fphys.2018.01091
27. Chwalisz K, Garfield RE. Regulation of the uterus and cervix during pregnancy and labor. Role of progesterone and nitric oxide. Ann N Y Acad Sci. (1997) 828:238–53. doi: 10.1111/j.1749-6632.1997.tb48545.x
28. Spencer TE, Johnson GA, Burghardt RC, Bazer FW. Progesterone and placental hormone actions on the uterus: insights from domestic animals. Biol Reprod. (2004) 71:2–10. doi: 10.1095/biolreprod.103.024133
29. Arck P, Hansen PJ, Mulac Jericevic B, Piccinni MP, Szekeres-Bartho J. Progesterone during pregnancy: endocrine-immune cross talk in mammalian species and the role of stress. Am J Reprod Immunol. (2007) 58:268–79. doi: 10.1111/j.1600-0897.2007.00512.x
30. Pepe GJ, Albrecht ED. Actions of placental and fetal adrenal steroid hormones in primate pregnancy. Endocr Rev. (1995) 16:608–48. doi: 10.1210/edrv-16-5-608
31. Soares MJ, Varberg KM, Iqbal K. Hemochorial placentation: development, function, and adaptations. Biol Reprod. (2018) 99:196–211. doi: 10.1093/biolre/ioy049
32. Evans J, Salamonsen LA, Winship A, Menkhorst E, Nie G, Gargett CE, et al. Fertile ground: human endometrial programming and lessons in health and disease. Nat Rev Endocrinol. (2016) 12:654–67. doi: 10.1038/nrendo.2016.116
33. Koopman LA, Kopcow HD, Rybalov B, Boyson JE, Orange JS, Schatz F, et al. Human decidual natural killer cells are a unique NK cell subset with immunomodulatory potential. J Exp Med. (2003) 198:1201–12. doi: 10.1084/jem.20030305
34. Brosens JJ, Parker MG, McIndoe A, Pijnenborg R, Brosens IA. A role for menstruation in preconditioning the uterus for successful pregnancy. Am J Obstet Gynecol. (2009) 200:615. doi: 10.1016/j.ajog.2008.11.037
35. Muter J, Kong C-S, Brosens JJ. The role of decidual subpopulations in implantation, menstruation and miscarriage. Front Reprod Health. (2021) 3:804921. doi: 10.3389/frph.2021.804921
36. Santos ED, Moindjie H, Sérazin V, Arnould L, Rodriguez Y, Fathallah K, et al. Preimplantation factor modulates trophoblastic invasion throughout the decidualization of human endometrial stromal cells. Reprod Biol Endocrinol. (2021) 19:96. doi: 10.1186/s12958-021-00774-5
37. Menkhorst EM, Van Sinderen ML, Rainczuk K, Cuman C, Winship A, Dimitriadis E. Invasive trophoblast promote stromal fibroblast decidualization via profilin 1 and alox5. Sci Rep. (2017) 7:8690. doi: 10.1038/s41598-017-05947-0
38. Ma Q, Beal JR, Bhurke A, Kannan A, Yu J, Taylor RN, et al. Extracellular vesicles secreted by human uterine stromal cells regulate decidualization, angiogenesis, and trophoblast differentiation. Proc Natl Acad Sci. (2022) 119:e2200252119. doi: 10.1073/pnas.2200252119
39. Liu M, Chen X, Chang QX, Hua R, Wei YX, Huang LP, et al. Decidual small extracellular vesicles induce trophoblast invasion by upregulating N-cadherin. Reprod (Cambridge England). (2020) 159:171–80. doi: 10.1530/rep-18-0616
40. You Y, Stelzl P, Joseph DN, Aldo PB, Maxwell AJ, Dekel N, et al. Tnf-Α Regulated endometrial stroma secretome promotes trophoblast invasion. Front Immunol. (2021) 12.
41. Cross JC, Werb Z, Fisher SJ. Implantation and the placenta: key pieces of the development puzzle. Science. (1994) 266:1508–18. doi: 10.1126/science.7985020
42. Male V. Medawar and the immunological paradox of pregnancy: in context. Oxford Open Immunol. (2021) 2:iqaa006. doi: 10.1093/oxfimm/iqaa006
43. Geldenhuys J, Rossouw TM, Lombaard HA, Ehlers MM, Kock MM. Disruption in the regulation of immune responses in the placental subtype of preeclampsia. Front Immunol. (2018) 9. doi: 10.3389/fimmu.2018.01659
44. Staun-Ram E, Goldman S, Gabarin D, Shalev E. Expression and importance of matrix metalloproteinase 2 and 9 (Mmp-2 and -9) in human trophoblast invasion. Reprod Biol Endocrinol. (2004) 2:59. doi: 10.1186/1477-7827-2-59
45. Bass KE, Morrish D, Roth I, Bhardwaj D, Taylor R, Zhou Y, et al. Human cytotrophoblast invasion is up-regulated by epidermal growth factor: evidence that paracrine factors modify this process. Dev Biol. (1994) 164:550–61. doi: 10.1006/dbio.1994.1223
46. Graham CH, Connelly I, MacDougall JR, Kerbel RS, Stetler-Stevenson WG, Lala PK. Resistance of Malignant trophoblast cells to both the anti-proliferative and anti-invasive effects of transforming growth factor-beta. Exp Cell Res. (1994) 214:93–9. doi: 10.1006/excr.1994.1237
47. Gellersen B, Brosens JJ. Cyclic decidualization of the human endometrium in reproductive health and failure. Endocr Rev. (2014) 35:851–905. doi: 10.1210/er.2014-1045
48. Moffett A, Shreeve N. Local immune recognition of trophoblast in early human pregnancy: controversies and questions. Nat Rev Immunol. (2022). doi: 10.1038/s41577-022-00777-2
49. Ding J, Maxwell A, Adzibolosu N, Hu A, You Y, Liao A, et al. Mechanisms of immune regulation by the placenta: role of type I interferon and interferon-stimulated genes signaling during pregnancy. Immunol Rev. (2022) 308:9–24. doi: 10.1111/imr.13077
50. Moffett A, Hiby SE, Sharkey AM. The role of the maternal immune system in the regulation of human birthweight. Philos Trans R Soc Lond B Biol Sci. (2015) 370:20140071. doi: 10.1098/rstb.2014.0071
51. Li DK, Wi S. Changing paternity and the risk of preeclampsia/eclampsia in the subsequent pregnancy. Am J Epidemiol. (2000) 151:57–62. doi: 10.1093/oxfordjournals.aje.a010122
52. Xiong S, Sharkey AM, Kennedy PR, Gardner L, Farrell LE, Chazara O, et al. Maternal uterine nk cell-activating receptor Kir2ds1 enhances placentation. J Clin Invest. (2013) 123:4264–72. doi: 10.1172/jci68991
53. Tilburgs T, Crespo ÂC, van der Zwan A, Rybalov B, Raj T, Stranger B, et al. Human hla-G+ Extravillous trophoblasts: immune-activating cells that interact with decidual leukocytes. Proc Natl Acad Sci U.S.A. (2015) 112:7219–24. doi: 10.1073/pnas.1507977112
54. Chakraborty D, Rumi MA, Konno T, Soares MJ. Natural killer cells direct hemochorial placentation by regulating hypoxia-inducible factor dependent trophoblast lineage decisions. Proc Natl Acad Sci U.S.A. (2011) 108:16295–300. doi: 10.1073/pnas.1109478108
55. Robson A, Harris LK, Innes BA, Lash GE, Aljunaidy MM, Aplin JD, et al. Uterine natural killer cells initiate spiral artery remodeling in human pregnancy. FASEB J. (2012) 26:4876–85. doi: 10.1096/fj.12-210310
56. Wang XQ, Li DJ. The mechanisms by which trophoblast-derived molecules induce maternal-fetal immune tolerance. Cell Mol Immunol. (2020) 17:1204–7. doi: 10.1038/s41423-020-0460-5
57. Rajagopalan S. Hla-G-mediated NK cell senescence promotes vascular remodeling: implications for reproduction. Cell Mol Immunol. (2014) 11:460–6. doi: 10.1038/cmi.2014.53
58. Henson MC, Castracane VD. Leptin in pregnancy: an update. Biol Reprod. (2006) 74:218–29. doi: 10.1095/biolreprod.105.045120
59. Wendremaire M, Lopez TE, Barrichon M, Zhang H, Hadi T, Ye XY, et al. Leptin-induced HLA-G inhibits myometrial contraction and differentiation. Cells. (2022) 11. doi: 10.3390/cells11060954
60. Maymó JL, Pérez Pérez A, Sánchez-Margalet V, Dueñas JL, Calvo JC, Varone CL. Up-regulation of placental leptin by human chorionic gonadotropin. Endocrinology. (2009) 150:304–13. doi: 10.1210/en.2008-0522
61. Gambino YP, Maymó JL, Pérez-Pérez A, Dueñas JL, Sánchez-Margalet V, Calvo JC, et al. 17beta-estradiol enhances leptin expression in human placental cells through genomic and nongenomic actions. Biol Reprod. (2010) 83:42–51. doi: 10.1095/biolreprod.110.083535
62. Pérez-Pérez A, Maymó J, Gambino Y, Guadix P, Dueñas JL, Varone C, et al. Insulin enhances leptin expression in human trophoblastic cells. Biol Reprod. (2013) 89:20. doi: 10.1095/biolreprod.113.109348
63. Pollock KE, Talton OO, Schulz LC. Morphology and gene expression in mouse placentas lacking leptin receptors. Biochem Biophys Res Commun. (2020) 528:336–42. doi: 10.1016/j.bbrc.2020.03.104
64. Ding J, Zhang Y, Cai X, Diao L, Yang C, Yang J. Crosstalk between trophoblast and macrophage at the maternal-fetal interface: current status and future perspectives. Front Immunol. (2021) 12:758281. doi: 10.3389/fimmu.2021.758281
65. Vondra S, Höbler AL, Lackner AI, Raffetseder J, Mihalic ZN, Vogel A, et al. The human placenta shapes the phenotype of decidual macrophages. Cell Rep. (2023) 42:111977. doi: 10.1016/j.celrep.2022.111977
66. Zarnani AH, Dokouhaki P, Jeddi-Tehrani M. Indoleamine 2,3-dioxygenase and immunological tolerance during pregnancy. Iranian J Immunol. (2004) 1:143–53.
67. Shayda H, Mahmood JT, Ebrahim T, Jamileh G, Golnaz Ensieh KS, Parivash D, et al. Indoleamine 2,3-dioxygenase (Ido) is expressed at feto-placental unit throughout mouse gestation: an immunohistochemical study. J Reprod Infertil. (2009) 10:177–83.
68. Nikoo S, Moazzeni S, Bozorgmehr M, Zarnani A. Effect of pregnant mouse serum on induction of indolamine 2, 3- dioxygenase in dendritic cells. J Reprod Infertility. (2006) 7:187–98.
69. Meggyes M, Miko E, Szigeti B, Farkas N, Szereday L. The importance of the PD-1/PD-L1 pathway at the maternal-fetal interface. BMC Pregnancy Childbirth. (2019) 19:74. doi: 10.1186/s12884-019-2218-6
70. Papuchova H, Kshirsagar S, Xu L, Bougleux Gomes HA, Li Q, Iyer V, et al. Three types of HLA-G+ Extravillous trophoblasts that have distinct immune regulatory properties. Proc Natl Acad Sci U.S.A. (2020) 117:15772–7. doi: 10.1073/pnas.2000484117
71. Yang Q, Li M, Zhao M, Lu F, Yu X, Li L, et al. Progesterone modulates CD4(+) CD25(+) FoxP3(+) regulatory T cells and TGF-Β1 in the maternal-fetal interface of the late pregnant mouse. Am J Reprod Immunol. (2022) 88:e13541. doi: 10.1111/aji.13541
72. Liang S, Ristich V, Arase H, Dausset J, Carosella ED, Horuzsko A. Modulation of dendritic cell differentiation by HLA-G and ILT4 requires the IL-6–STAT3 signaling pathway. Proc Natl Acad Sci U.S.A. (2008) 105:8357–62. doi: 10.1073/pnas.0803341105
73. Motedayyen H, Zarnani AH, Tajik N, Ghotloo S, Rezaei A. Immunomodulatory effects of human amniotic epithelial cells on naive CD4(+) T cells from women with unexplained recurrent spontaneous abortion. Placenta. (2018) 71:31–40. doi: 10.1016/j.placenta.2018.06.008
74. Motedayyen H, Rezaei A, Zarnani AH, Tajik N. Human amniotic epithelial cells inhibit activation and pro-inflammatory cytokines production of naive CD4+ T cells from women with unexplained recurrent spontaneous abortion. Reprod Biol. (2018) 18:182–8. doi: 10.1016/j.repbio.2018.04.002
75. Lucas ES, Vrljicak P, Muter J, Diniz-da-Costa MM, Brighton PJ, Kong C-S, et al. Recurrent pregnancy loss is associated with a pro-senescent decidual response during the peri-implantation window. Commun Biol. (2020) 3:37. doi: 10.1038/s42003-020-0763-1
76. Xu L, Li Y, Sang Y, Li D-J, Du M. Crosstalk between trophoblasts and decidual immune cells: the cornerstone of maternal-fetal immunotolerance. Front Immunol. (2021) 12:642392. doi: 10.3389/fimmu.2021.642392
77. Idali F, Rezaii-Nia S, Golshahi H, Fatemi R, Naderi MM, Goli LB, et al. Adoptive cell therapy with induced regulatory T cells normalises the abortion rate in abortion-prone mice. Reprod Fertil Dev. (2021) 33:220–8. doi: 10.1071/rd20063
78. Wang W-J, Liu F-J, Xin L, Hao C-F, Bao H-C, Qu Q-L, et al. Adoptive transfer of pregnancy-induced CD4+CD25+ Regulatory T cells reverses the increase in abortion rate caused by interleukin 17 in the CBA/J×BALB/c mouse model. Hum Reprod. (2014) 29:946–52. doi: 10.1093/humrep/deu014
79. Bonney EA, Brown SA. To drive or be driven: the path of a mouse model of recurrent pregnancy loss. Reproduction. (2014) 147:R153–67. doi: 10.1530/rep-13-0583
80. Salek Farrokhi A, Zarnani AH, Rezaei Kahmini F, Moazzeni SM. Mesenchymal stem cells induce expansion of regulatory T cells in abortion-prone mice. Reproduction. (2021) 161:477–87. doi: 10.1530/rep-20-0320
81. Salek Farrokhi A, Zarnani AH, Moazzeni SM. Mesenchymal stem cells therapy protects fetuses from resorption and induces Th2 type cytokines profile in abortion prone mouse model. Transpl Immunol. (2018) 47:26–31. doi: 10.1016/j.trim.2018.01.002
82. Sadighi-Moghaddam B, Salek Farrokhi A, Namdar Ahmadabad H, Barati M, Moazzeni SM. Mesenchymal stem cell therapy prevents abortion in CBA/J × DBA/2 mating. Reprod Sci. (2018) 25:1261–9. doi: 10.1177/1933719117737848
83. Gargett CE, Masuda H. Adult stem cells in the endometrium. Mol Hum Reprod. (2010) 16:818–34. doi: 10.1093/molehr/gaq061
84. Claridge B, Kastaniegaard K, Stensballe A, Greening DW. Post-translational and transcriptional dynamics – regulating extracellular vesicle biology. Expert Rev Proteomics. (2019) 16:17–31. doi: 10.1080/14789450.2019.1551135
85. Mitchell MD, Peiris HN, Kobayashi M, Koh YQ, Duncombe G, Illanes SE, et al. Placental exosomes in normal and complicated pregnancy. Am J Obstet Gynecol. (2015) 213:S173–81. doi: 10.1016/j.ajog.2015.07.001
86. Czernek L, Düchler M. Exosomes as messengers between mother and fetus in pregnancy. Int J Mol Sci. (2020) 21. doi: 10.3390/ijms21124264
87. Bai K, Lee C-L, Liu X, Li J, Cao D, Zhang L, et al. Human placental exosomes induce maternal systemic immune tolerance by reprogramming circulating monocytes. J Nanobiotechnology. (2022) 20:86. doi: 10.1186/s12951-022-01283-2
88. Hedlund M, Stenqvist AC, Nagaeva O, Kjellberg L, Wulff M, Baranov V, et al. Human placenta expresses and secretes NKG2D ligands via exosomes that down-modulate the cognate receptor expression: evidence for immunosuppressive function. J Immunol. (2009) 183:340–51. doi: 10.4049/jimmunol.0803477
89. Ghanavatinejad A, Bozorgmehr M, Shokri M-R, Aleahmad M, Tavakoli M, Shokri F, et al. Menscs exert a supportive role in establishing a pregnancy-friendly microenvironment by inhibiting TH17 polarization. J Reprod Immunol. (2021) 144:103252. doi: 10.1016/j.jri.2020.103252
90. Shokri M-R, Bozorgmehr M, Ghanavatinejad A, Falak R, Aleahmad M, Kazemnejad S, et al. Human menstrual blood-derived stromal/stem cells modulate functional features of natural killer cells. Sci Rep. (2019) 9:10007. doi: 10.1038/s41598-019-46316-3
91. Aleahmad M, Ghanavatinejad A, Bozorgmehr M, Shokri MR, Nikoo S, Tavakoli M, et al. Menstrual blood-derived stromal stem cells augment CD4+ T cells proliferation. Avicenna J Med Biotechnol. (2018) 10:183–91.
92. Bozorgmehr M, Moazzeni SM, Salehnia M, Sheikhian A, Nikoo S, Zarnani AH. Menstrual blood-derived stromal stem cells inhibit optimal generation and maturation of human monocyte-derived dendritic cells. Immunol Lett. (2014) 162:239–46. doi: 10.1016/j.imlet.2014.10.005
93. Bozorgmehr M, Gurung S, Darzi S, Nikoo S, Kazemnejad S, Zarnani AH, et al. Endometrial and menstrual blood mesenchymal stem/stromal cells: biological properties and clinical application. Front Cell Dev Biol. (2020) 8:497. doi: 10.3389/fcell.2020.00497
94. Hosseini S, Shokri F, Tokhmechy R, Savadi-Shiraz E, Jeddi-Tehrani M, Rahbari M, et al. Menstrual blood contains immune cells with inflammatory and anti-inflammatory properties. J Obstet Gynaecol Res. (2015) 41:1803–12. doi: 10.1111/jog.12801
95. Hosseini S, Shokri F, Pour SA, Khoshnoodi J, Jeddi-Tehrani M, Zarnani AH. Diminished frequency of menstrual and peripheral blood NKT-like cells in patients with unexplained recurrent spontaneous abortion and infertile women. Reprod Sci. (2019) 26:97–108. doi: 10.1177/1933719118766261
96. Ross CA. The trophoblast model of cancer. Nutr Cancer. (2015) 67:61–7. doi: 10.1080/01635581.2014.956257
97. Barbour LA, Shao J, Qiao L, Pulawa LK, Jensen DR, Bartke A, et al. Human placental growth hormone causes severe insulin resistance in transgenic mice. Am J Obstet Gynecol. (2002) 186:512–7. doi: 10.1067/mob.2002.121256
98. Masi T, Patel BM. Altered glucose metabolism and insulin resistance in cancer-induced cachexia: A sweet poison. Pharmacol Rep. (2021) 73:17–30. doi: 10.1007/s43440-020-00179-y
99. Zhu Y, Liu Q, Liao M, Diao L, Wu T, Liao W, et al. Overexpression of lncRNA EPB41L4A-AS1 induces metabolic reprogramming in trophoblast cells and placenta tissue of miscarriage. Mol Ther Nucleic Acids. (2019) 18:518–32. doi: 10.1016/j.omtn.2019.09.017
100. Liberti MV, Locasale JW. The warburg effect: how does it benefit cancer cells? Trends Biochem Sci. (2016) 41:211–8. doi: 10.1016/j.tibs.2015.12.001
101. Rajagopalan S, Bryceson YT, Kuppusamy SP, Geraghty DE, van der Meer A, Joosten I, et al. Activation of NK cells by an endocytosed receptor for soluble HLA-G. PloS Biol. (2006) 4:e9. doi: 10.1371/journal.pbio.0040009
102. Lin A, Yan WH. Human leukocyte antigen-G (Hla-G) expression in cancers: roles in immune evasion, metastasis and target for therapy. Mol Med. (2015) 21:782–91. doi: 10.2119/molmed.2015.00083
103. Nejabati HR, Latifi Z, Ghasemnejad T, Fattahi A, Nouri M. Placental growth factor (Plgf) as an angiogenic/inflammatory switcher: lesson from early pregnancy losses. Gynecol Endocrinol. (2017) 33:668–74. doi: 10.1080/09513590.2017.1318375
104. Kim KJ, Cho CS, Kim WU. Role of placenta growth factor in cancer and inflammation. Exp Mol Med. (2012) 44:10–9. doi: 10.3858/emm.2012.44.1.023
105. Staun-Ram E, Shalev E. Human trophoblast function during the implantation process. Reprod Biol Endocrinol. (2005) 3:56. doi: 10.1186/1477-7827-3-56
106. Jankowska A, Szczerba A. Chapter 4.2 - human chorionic gonadotropin in cancer—Where are we? In: Cole LA, Butler SA, editors. 100 years of human chorionic gonadotropin. Elsevier (2020). p. 255–60.
107. Kudo Y, Koh I, Sugimoto J. Localization of indoleamine 2,3-dioxygenase-1 and indoleamine 2,3-dioxygenase-2 at the human maternal-fetal interface. Int J Tryptophan Res. (2020) 13:1178646920984163. doi: 10.1177/1178646920984163
108. Meireson A, Devos M, Brochez L. Ido expression in cancer: different compartment, different functionality? Front Immunol. (2020) 11:531491. doi: 10.3389/fimmu.2020.531491
109. Jonescheit H, Oberg HH, Gonnermann D, Hermes M, Sulaj V, Peters C, et al. Influence of indoleamine-2,3-dioxygenase and its metabolite kynurenine on Γδ T cell cytotoxicity against ductal pancreatic adenocarcinoma cells. Cells. (2020) 9. doi: 10.3390/cells9051140
110. Balogh A, Toth E, Romero R, Parej K, Csala D, Szenasi NL, et al. Placental galectins are key players in regulating the maternal adaptive immune response. Front Immunol. (2019) 10:1240. doi: 10.3389/fimmu.2019.01240
111. Chou FC, Chen HY, Kuo CC, Sytwu HK. Role of galectins in tumors and in clinical immunotherapy. Int J Mol Sci. (2018) 19. doi: 10.3390/ijms19020430
112. Desoye G, Hartmann M, Jones CJ, Wolf HJ, Kohnen G, Kosanke G, et al. Location of insulin receptors in the placenta and its progenitor tissues. Microsc Res Tech. (1997) 38:63–75.
113. Malaguarnera R, Belfiore A. The insulin receptor: A new target for cancer therapy. Front Endocrinol (Lausanne). (2011) 2:93. doi: 10.3389/fendo.2011.00093
114. Shyu MK, Lin MC, Liu CH, Fu YR, Shih JC, Lee CN, et al. Muc1 expression is increased during human placental development and suppresses trophoblast-like cell invasion in vitro. Biol Reprod. (2008) 79:233–9. doi: 10.1095/biolreprod.108.067629
115. Nath S, Mukherjee P. Muc1: A multifaceted oncoprotein with a key role in cancer progression. Trends Mol Med. (2014) 20:332–42. doi: 10.1016/j.molmed.2014.02.007
116. Rattila S, Dunk CEE, Im M, Grichenko O, Zhou Y, Yanez-Mo M, et al. Interaction of pregnancy-specific glycoprotein 1 with integrin Α5β1 is a modulator of extravillous trophoblast functions. Cells. (2019) 8. doi: 10.3390/cells8111369
117. Hou J, Yan D, Liu Y, Huang P, Cui H. The roles of integrin Α5β1 in human cancer. Onco Targets Ther. (2020) 13:13329–44. doi: 10.2147/ott.S273803
118. Lin Z, Shi JL, Chen M, Zheng ZM, Li MQ, Shao J. Ccl2: an important cytokine in normal and pathological pregnancies: A review. Front Immunol. (2022) 13:1053457. doi: 10.3389/fimmu.2022.1053457
119. O'Connor T, Heikenwalder M. Ccl2 in the tumor microenvironment. Adv Exp Med Biol. (2021) 1302:1–14. doi: 10.1007/978-3-030-62658-7_1
120. Chuva de Sousa Lopes SM, Alexdottir MS, Valdimarsdottir G. The TGFβ Family in human placental development at the fetal-maternal interface. Biomolecules. (2020) 10. doi: 10.3390/biom10030453
121. Drabsch Y, ten Dijke P. TGF-β Signaling in breast cancer cell invasion and bone metastasis. J Mammary Gland Biol Neoplasia. (2011) 16:97–108. doi: 10.1007/s10911-011-9217-1
122. Nordor AV, Nehar-Belaid D, Richon S, Klatzmann D, Bellet D, Dangles-Marie V, et al. The early pregnancy placenta foreshadows DNA methylation alterations of solid tumors. Epigenetics. (2017) 12:793–803. doi: 10.1080/15592294.2017.1342912
123. Keighley LM, Lynch-Sutherland CF, Almomani SN, Eccles MR, Macaulay EC. Unveiling the hidden players: the crucial role of transposable elements in the placenta and their potential contribution to pre-eclampsia. Placenta. (2023) 141:57–64. doi: 10.1016/j.placenta.2023.05.017
124. Jang HS, Shah NM, Du AY, Dailey ZZ, Pehrsson EC, Godoy PM, et al. Transposable elements drive widespread expression of oncogenes in human cancers. Nat Genet. (2019) 51:611–7. doi: 10.1038/s41588-019-0373-3
125. Burki TK. Placenta can provide new insights on the genetics of cancer. Lancet Oncol. (2021) 22:591. doi: 10.1016/s1470-2045(21)00208-4
126. Alfieri F, Caravagna G, Schaefer MH. Cancer genomes tolerate deleterious coding mutations through somatic copy number amplifications of wild-type regions. Nat Commun. (2023) 14:3594. doi: 10.1038/s41467-023-39313-8
127. Holtan SG, Creedon DJ, Haluska P, Markovic SN. Cancer and pregnancy: parallels in growth, invasion, and immune modulation and implications for cancer therapeutic agents. Mayo Clin Proc. (2009) 84:985–1000. doi: 10.1016/s0025-6196(11)60669-1
128. DaSilva-Arnold S, James JL, Al-Khan A, Zamudio S, Illsley NP. Differentiation of first trimester cytotrophoblast to extravillous trophoblast involves an epithelial–mesenchymal transition. Placenta. (2015) 36:1412–8. doi: 10.1016/j.placenta.2015.10.013
129. Georgakopoulos-Soares I, Chartoumpekis DV, Kyriazopoulou V, Zaravinos A. Emt factors and metabolic pathways in cancer. Front Oncol. (2020) 10:499. doi: 10.3389/fonc.2020.00499
130. Renaud SJ, Jeyarajah MJ. How Trophoblasts Fuse: An in-Depth Look into Placental Syncytiotrophoblast Formation. Cell Mol Life Sci. (2022) 79:433. doi: 10.1007/s00018-022-04475-z
131. Wang HF, Xiang W, Xue BZ, Wang YH, Yi DY, Jiang XB, et al. Cell fusion in cancer hallmarks: current research status and future indications. Oncol Lett. (2021) 22:530. doi: 10.3892/ol.2021.12791
132. Rai A, Cross JC. Development of the hemochorial maternal vascular spaces in the placenta through endothelial and vasculogenic mimicry. Dev Biol. (2014) 387:131–41. doi: 10.1016/j.ydbio.2014.01.015
133. Hendrix MJ, Seftor EA, Hess AR, Seftor RE. Vasculogenic mimicry and tumour-cell plasticity: lessons from melanoma. Nat Rev Cancer. (2003) 3:411–21. doi: 10.1038/nrc1092
134. Zhang XH, Liang X, Liang XH, Wang TS, Qi QR, Deng WB, et al. The mesenchymal-epithelial transition during in vitro decidualization. Reprod Sci. (2013) 20:354–60. doi: 10.1177/1933719112472738
135. Tang PC, Chung JY, Xue VW, Xiao J, Meng XM, Huang XR, et al. Smad3 promotes cancer-associated fibroblasts generation via macrophage-myofibroblast transition. Adv Sci (Weinh). (2022) 9:e2101235. doi: 10.1002/advs.202101235
136. Genbacev O, Joslin R, Damsky CH, Polliotti BM, Fisher SJ. Hypoxia alters early gestation human cytotrophoblast differentiation/invasion in vitro and models the placental defects that occur in preeclampsia. J Clin Invest. (1996) 97:540–50. doi: 10.1172/JCI118447
137. Carmeliet P, Dor Y, Herbert J-M, Fukumura D, Brusselmans K, Dewerchin M, et al. Role of hif-1α in hypoxia-mediated apoptosis, cell proliferation and tumour angiogenesis. Nature. (1998) 394:485–90. doi: 10.1038/28867
138. Tersigni C, Meli F, Neri C, Iacoangeli A, Franco R, Lanzone A, et al. Role of human leukocyte antigens at the feto-maternal interface in normal and pathological pregnancy: an update. Int J Mol Sci. (2020) 21. doi: 10.3390/ijms21134756
139. DhatChinamoorthy K, Colbert JD, Rock KL. Cancer immune evasion through loss of mhc class I antigen presentation. Front Immunol. (2021) 12:636568. doi: 10.3389/fimmu.2021.636568
140. Veras E, Kurman RJ, Wang TL, Shih IM. Pd-L1 expression in human placentas and gestational trophoblastic diseases. Int J Gynecol Pathol. (2017) 36:146–53. doi: 10.1097/pgp.0000000000000305
141. Han Y, Liu D, Li L. Pd-1/pd-L1 pathway: current researches in cancer. Am J Cancer Res. (2020) 10:727–42.
142. Favaro R, Abrahamsohn PA, Zorn MT. 11 - decidualization and endometrial extracellular matrix remodeling. In: Croy BA, Yamada AT, DeMayo FJ, Adamson SL, editors. The guide to investigation of mouse pregnancy. Academic Press, Boston (2014). p. 125–42.
143. Deng B, Zhao Z, Kong W, Han C, Shen X, Zhou C. Biological role of matrix stiffness in tumor growth and treatment. J Trans Med. (2022) 20:540. doi: 10.1186/s12967-022-03768-y
144. Pang H, Lei D, Guo Y, Yu Y, Liu T, Liu Y, et al. Three categories of similarities between the placenta and cancer that can aid cancer treatment: cells, the microenvironment, and metabolites. Front Oncol. (2022) 12:977618. doi: 10.3389/fonc.2022.977618
145. Lala PK, Nandi P, Hadi A, Halari C. A crossroad between placental and tumor biology: what have we learnt? Placenta. (2021) 116:12–30. doi: 10.1016/j.placenta.2021.03.003
146. Costanzo V, Bardelli A, Siena S, Abrignani S. Exploring the links between cancer and placenta development. Open Biol. (2018) 8:180081. doi: 10.1098/rsob.180081
147. Kshitiz, Afzal J, Maziarz JD, Hamidzadeh A, Liang C, Erkenbrack EM, et al. Evolution of placental invasion and cancer metastasis are causally linked. Nat Ecol Evol. (2019) 3:1743–53. doi: 10.1038/s41559-019-1046-4
148. Dittmar T, Hass R. Intrinsic signalling factors associated with cancer cell-cell fusion. Cell Communication Signaling. (2023) 21:68. doi: 10.1186/s12964-023-01085-5
149. ED J, Pollheimer J, Yong HE, Kokkinos MI, Kalionis B, Knöfler M, et al. Epithelial-mesenchymal transition during extravillous trophoblast differentiation. Cell Adh Migr. (2016) 10:310–21. doi: 10.1080/19336918.2016.1170258
150. Fest S, Brachwitz N, Schumacher A, Zenclussen ML, Khan F, Wafula PO, et al. Supporting the hypothesis of pregnancy as a tumor: survivin is upregulated in normal pregnant mice and participates in human trophoblast proliferation. Am J Reprod Immunol. (2008) 59:75–83. doi: 10.1111/j.1600-0897.2007.00557.x
151. Lala PK, Lee BP, Xu G, Chakraborty C. Human placental trophoblast as an in vitro model for tumor progression. Can J Physiol Pharmacol. (2002) 80:142–9. doi: 10.1139/y02-006
152. Krstic J, Deutsch A, Fuchs J, Gauster M, Gorsek Sparovec T, Hiden U, et al. (Dis)Similarities between the decidual and tumor microenvironment. Biomedicines. (2022) 10. doi: 10.3390/biomedicines10051065
153. Khorami Sarvestani S, Shojaeian S, Sarrami-Forooshani R, Yekaninejad MS, Gilany K, Ghaderi A, et al. Cancer is associated with the emergence of placenta-reactive autoantibodies. Biomedicines. (2023) 11. doi: 10.3390/biomedicines11020316
154. Jasti S, Farahbakhsh M, Nguyen S, Petroff BK, Petroff MG. Immune response to a model shared placenta/tumor-associated antigen reduces cancer risk in parous mice. Biol Reprod. (2017) 96:134–44. doi: 10.1095/biolreprod.116.144907
155. Bradley SD, Talukder AH, Lai I, Davis R, Alvarez H, Tiriac H, et al. Vestigial-like 1 is a shared targetable cancer-placenta antigen expressed by pancreatic and basal-like breast cancers. Nat Commun. (2020) 11:5332. doi: 10.1038/s41467-020-19141-w
156. Gholami P, Asgarian-Omran H, Yaghmaie M, Mahmudian J, Kianersi S, Salari S, et al. Investigation of expression profile of placenta-specific 1 (Plac1) in acute myeloid and lymphoid leukemias. Avicenna J Med Biotechnol. (2023) 15:167–72. doi: 10.18502/ajmb.v15i3.12926
157. Rahdan S, Razavi SA, Shojaeian S, Shokri F, Amiri MM, Zarnani A-H. Immunization with placenta-specific 1 (Plac1) induces potent anti-tumor responses and prolongs survival in a mouse model of melanoma. Adv Med Sci. (2022) 67:338–45. doi: 10.1016/j.advms.2022.08.002
158. Nejadmoghaddam M-R, Zarnani A-H, Ghahremanzadeh R, Ghods R, Mahmoudian J, Yousefi M, et al. Placenta-specific1 (Plac1) is a potential target for antibody-drug conjugate-based prostate cancer immunotherapy. Sci Rep. (2017) 7:13373. doi: 10.1038/s41598-017-13682-9
159. Babajani A, Manzari-Tavakoli A, Jamshidi E, Tarasi R, Niknejad H. Anti-cancer effects of human placenta-derived amniotic epithelial stem cells loaded with paclitaxel on cancer cells. Sci Rep. (2022) 12:18148. doi: 10.1038/s41598-022-22562-w
160. Bolouri MR, Ghods R, Zarnani K, Vafaei S, Falak R, Zarnani AH. Human amniotic epithelial cells exert anti-cancer effects through secretion of immunomodulatory small extracellular vesicles (Sev). Cancer Cell Int. (2022) 22:329. doi: 10.1186/s12935-022-02755-z
161. Marwitz S, Zeiser T, Schultz H, Kähler D, Abdullah M, Hauber HP, et al. The human placenta releases substances that drive lung cancer into apoptosis. Diagn Pathol. (2009) 4:27. doi: 10.1186/1746-1596-4-27
162. Marleau AM, McDonald G, Koropatnick J, Chen CS, Koos D. Reduction of tumorigenicity by placental extracts. Anticancer Res. (2012) 32:1153–61.
163. Zhao B, Wang Y, Wu B, Liu S, Wu E, Fan H, et al. Placenta-derived gp96 as a multivalent prophylactic cancer vaccine. Sci Rep. (2013) 3:1947. doi: 10.1038/srep01947
164. Harandi A. Immunoplacental therapy, a potential multi-epitope cancer vaccine. Med Hypotheses. (2006) 66:1182–7. doi: 10.1016/j.mehy.2005.12.011
165. Tabatabaei M, Mosaffa N, Ghods R, Nikoo S, Kazemnejad S, Khanmohammadi M, et al. Vaccination with human amniotic epithelial cells confer effective protection in a murine model of colon adenocarcinoma. Int J Cancer. (2018) 142:1453–66. doi: 10.1002/ijc.31159
166. Kampmann U, Knorr S, Fuglsang J, Ovesen P. Determinants of maternal insulin resistance during pregnancy: an updated overview. J Diabetes Res. (2019) 2019:5320156. doi: 10.1155/2019/5320156
167. Skeith L, Blondon M, Ní Áinle F. Understanding and preventing placenta-mediated pregnancy complications. Hamostaseologie. (2020) 40:356–63. doi: 10.1055/a-1184-8388
168. Kozlosky D, Barrett E, Aleksunes LM. Regulation of placental efflux transporters during pregnancy complications. Drug Metab Dispos. (2022) 50:1364–75. doi: 10.1124/dmd.121.000449
169. Morgan TK. Role of the placenta in preterm birth: A review. Am J Perinatol. (2016) 33:258–66. doi: 10.1055/s-0035-1570379
170. Kundu S, Maurer SV, Stevens HE. Future horizons for neurodevelopmental disorders: placental mechanisms. Front Pediatr. (2021) 9:653230. doi: 10.3389/fped.2021.653230
171. Leon RL, Mir IN, Herrera CL, Sharma K, Spong CY, Twickler DM, et al. Neuroplacentology in congenital heart disease: placental connections to neurodevelopmental outcomes. Pediatr Res. (2022) 91:787–94. doi: 10.1038/s41390-021-01521-7
172. Thayer ZM, Rutherford J, Kuzawa CW. The maternal nutritional buffering model: an evolutionary framework for pregnancy nutritional intervention. Evol Med Public Health. (2020) 2020:14–27. doi: 10.1093/emph/eoz037
173. Harding JE. The nutritional basis of the fetal origins of adult disease. Int J Epidemiol. (2001) 30:15–23. doi: 10.1093/ije/30.1.15
174. Thornburg KL, O'Tierney PF, Louey S. Review: the placenta is a programming agent for cardiovascular disease. Placenta. (2010) 31 Suppl:S54–9. doi: 10.1016/j.placenta.2010.01.002
175. Burton GJ, Fowden AL, Thornburg KL. Placental origins of chronic disease. Physiol Rev. (2016) 96:1509–65. doi: 10.1152/physrev.00029.2015
176. Thame M, Osmond C, Bennett F, Wilks R, Forrester T. Fetal growth is directly related to maternal anthropometry and placental volume. Eur J Clin Nutr. (2004) 58:894–900. doi: 10.1038/sj.ejcn.1601909
177. Oliveira LH, Xavier CC, Lana AM. [Changes in placental morphology of small for gestational age newborns]. J Pediatr (Rio J). (2002) 78:397–402. doi: 10.2223/JPED.887
178. Arrese M, Reyes H. Intrahepatic cholestasis of pregnancy: A past and present riddle. Ann Hepatol. (2006) 5:202–5. doi: 10.1016/S1665-2681(19)32012-5
179. Gyamfi-Bannerman C, Ananth CV. Trends in spontaneous and indicated preterm delivery among singleton gestations in the United States, 2005-2012. Obstet Gynecol. (2014) 124:1069–74. doi: 10.1097/aog.0000000000000546
180. Romero R, Dey SK, Fisher SJ. Preterm labor: one syndrome, many causes. Science. (2014) 345:760–5. doi: 10.1126/science.1251816
181. Grillo MA, Mariani G, Ferraris JR. Prematurity and low birth weight in neonates as a risk factor for obesity, hypertension, and chronic kidney disease in pediatric and adult age. Front Med (Lausanne). (2021) 8:769734. doi: 10.3389/fmed.2021.769734
182. Malhotra A, Allison BJ, Castillo-Melendez M, Jenkin G, Polglase GR, Miller SL. Neonatal morbidities of fetal growth restriction: pathophysiology and impact. Front Endocrinol (Lausanne). (2019) 10:55. doi: 10.3389/fendo.2019.00055
183. Fowden AL, Ward JW, Wooding FP, Forhead AJ, Constancia M. Programming placental nutrient transport capacity. J Physiol. (2006) 572:5–15. doi: 10.1113/jphysiol.2005.104141
184. Panja S, Paria BC. Development of the mouse placenta. Adv Anat Embryol Cell Biol. (2021) 234:205–21. doi: 10.1007/978-3-030-77360-1_10
185. Aguilera N, Salas-Pérez F, Ortíz M, Álvarez D, Echiburú B, Maliqueo M. Rodent models in placental research. Implications for fetal origins of adult disease. Anim Reprod. (2022) 19:e20210134. doi: 10.1590/1984-3143-ar2021-0134
186. Ishikawa H, Seki R, Yokonishi S, Yamauchi T, Yokoyama K. Relationship between fetal weight, placental growth and litter size in mice from mid- to late-gestation. Reprod Toxicol. (2006) 21:267–70. doi: 10.1016/j.reprotox.2005.08.002
187. Michael K, Ward BS, Moore WM. Relationship of fetal to placental size: the pig model. Eur J Obstet Gynecol Reprod Biol. (1983) 16:53–62. doi: 10.1016/0028-2243(83)90220-4
188. Pomorski M, Zimmer M, Florjanski J, Michniewicz J, Wiatrowski A, Fuchs T, et al. Comparative analysis of placental vasculature and placental volume in normal and IUGR pregnancies with the use of three-dimensional power doppler. Arch Gynecol Obstet. (2012) 285:331–7. doi: 10.1007/s00404-011-1968-9
189. Uhlén M, Fagerberg L, Hallström BM, Lindskog C, Oksvold P, Mardinoglu A, et al. Tissue-based map of the human proteome. Science. (2015) 347:1260419. doi: 10.1126/science.1260419
190. Khorami Sarvestani S, Shojaeian S, Vanaki N, Ghresi-Fard B, Amini M, Gilany K, et al. Proteome profiling of human placenta reveals developmental stage-dependent alterations in protein signature. Clin Proteomics. (2021) 18:18. doi: 10.1186/s12014-021-09324-y
191. Mushahary D, Gautam P, Sundaram CS, Sirdeshmukh R. Expanded protein expression profile of human placenta using two-dimensional gel electrophoresis. Placenta. (2013) 34:193–6. doi: 10.1016/j.placenta.2012.11.015
192. Manna S, Scheel J, Noone A, McElwain CJ, Scaife C, Gupta S, et al. A proteomic profile of the healthy human placenta. Clin Proteomics. (2023) 20:1. doi: 10.1186/s12014-022-09388-4
193. Huppertz B, Meiri H, Gizurarson S, Osol G, Sammar M. Placental protein 13 (Pp13): A new biological target shifting individualized risk assessment to personalized drug design combating pre-eclampsia. Hum Reprod Update. (2013) 19:391–405. doi: 10.1093/humupd/dmt003
194. Jackman SM, Kong X, Fant ME. Plac1 (Placenta-specific 1) is essential for normal placental and embryonic development. Mol Reprod Dev. (2012) 79:564–72. doi: 10.1002/mrd.22062
195. Mahmoudian J, Ghods R, Nazari M, Jeddi-Tehrani M, Ghahremani MH, Ghaffari-Tabrizi-Wizsy N, et al. Plac1: biology and potential application in cancer immunotherapy. Cancer Immunol Immunother. (2019) 68:1039–58. doi: 10.1007/s00262-019-02350-8
196. Sekita Y, Wagatsuma H, Nakamura K, Ono R, Kagami M, Wakisaka N, et al. Role of retrotransposon-derived imprinted gene, Rtl1, in the feto-maternal interface of mouse placenta. Nat Genet. (2008) 40:243–8. doi: 10.1038/ng.2007.51
197. Kitazawa M, Tamura M, Kaneko-Ishino T, Ishino F. Severe damage to the placental fetal capillary network causes mid- to late fetal lethality and reduction in placental size in Peg11/Rtl1 ko mice. Genes to Cells. (2017) 22:174–88. doi: 10.1111/gtc.12465
198. Kaneko-Ishino T, Ishino F. Retrovirus-derived RTL/SIRH: their diverse roles in the current eutherian developmental system and contribution to eutherian evolution. Biomolecules. (2023) 13. doi: 10.20944/preprints202308.1012.v1
199. Li CM, Margolin AA, Salas M, Memeo L, Mansukhani M, Hibshoosh H, et al. Peg10 is a C-myc target gene in cancer cells. Cancer Res. (2006) 66:665–72. doi: 10.1158/0008-5472.Can-05-1553
200. Chen H, Sun M, Zhao G, Liu J, Gao W, Si S, et al. Elevated expression of peg10 in human placentas from preeclamptic pregnancies. Acta Histochem. (2012) 114:589–93. doi: 10.1016/j.acthis.2011.11.003
201. Salgia R, Jolly MK, Dorff T, Lau C, Weninger K, Orban J, et al. Prostate-associated gene 4 (Page4): leveraging the conformational dynamics of a dancing protein cloud as a therapeutic target. J Clin Med. (2018) 7. doi: 10.3390/jcm7060156
202. Marsh B, Zhou Y, Kapidzic M, Fisher S, Blelloch R. Regionally distinct trophoblast regulate barrier function and invasion in the human placenta. Elife. (2022) 11. doi: 10.7554/eLife.78829
203. Shiura H, Kitazawa M, Ishino F, Kaneko-Ishino T. Roles of retrovirus-derived PEG10 and PEG11/RTL1 in mammalian development and evolution and their involvement in human disease. Front Cell Dev Biol. (2023) 11:1273638. doi: 10.3389/fcell.2023.1273638
204. Spencer TE. Biological roles of uterine glands in pregnancy. Semin Reprod Med. (2014) 32:346–57. doi: 10.1055/s-0034-1376354
205. Wei J, Lau SY, Blenkiron C, Chen Q, James JL, Kleffmann T, et al. Trophoblastic debris modifies endothelial cell transcriptome in vitro: A mechanism by which fetal cells might control maternal responses to pregnancy. Sci Rep. (2016) 6:30632. doi: 10.1038/srep30632
206. Babwah AV. Uterine and placental kiss1 regulate pregnancy: what we know and the challenges that lie ahead. Reproduction. (2015) 150:R121–8. doi: 10.1530/rep-15-0252
207. Nash KT, Welch DR. The kiss1 metastasis suppressor: mechanistic insights and clinical utility. Front Biosci. (2006) 11:647–59. doi: 10.2741/1824
208. Jeyarajah MJ, Jaju Bhattad G, Kelly RD, Baines KJ, Jaremek A, Yang FP, et al. The multifaceted role of gcm1 during trophoblast differentiation in the human placenta. Proc Natl Acad Sci U.S.A. (2022) 119:e2203071119. doi: 10.1073/pnas.2203071119
209. Wu YH, Lo HF, Chen SH, Chen H. Caspase-14 suppresses gcm1 acetylation and inhibits placental cell differentiation. FASEB J. (2013) 27:2818–28. doi: 10.1096/fj.12-224279
210. Fant M, Farina A, Nagaraja R, Schlessinger D. Plac1 (Placenta-specific 1): A novel, X-linked gene with roles in reproductive and cancer biology. Prenat Diagn. (2010) 30:497–502. doi: 10.1002/pd.2506
211. Chang WL, Wang H, Cui L, Peng NN, Fan X, Xue LQ, et al. Plac1 is involved in human trophoblast syncytialization. Reprod Biol. (2016) 16:218–24. doi: 10.1016/j.repbio.2016.07.001
212. Wang HY, Zhang Z, Yu S. Expression of pappa2 in human fetomaternal interface and involvement in trophoblast invasion and migration. Genet Mol Res. (2016) 15. doi: 10.4238/gmr.15038075
213. Wang J, Qiu Q, Haider M, Bell M, Gruslin A, Christians JK. Expression of pregnancy-associated plasma protein A2 during pregnancy in human and mouse. J Endocrinol. (2009) 202:337–45. doi: 10.1677/joe-09-0136
214. Zhang ET, Hannibal RL, Badillo Rivera KM, Song JHT, McGowan K, Zhu X, et al. PRG2 and AQPEP are misexpressed in fetal membranes in placenta previa and percreta†. Biol Reprod. (2021) 105:244–57. doi: 10.1093/biolre/ioab068
215. Hackmon R, Pinnaduwage L, Zhang J, Lye SJ, Geraghty DE, Dunk CE. Definitive class I human leukocyte antigen expression in gestational placentation: HLA-F, HLA-E, HLA-C, and HLA-G in extravillous trophoblast invasion on placentation, pregnancy, and parturition. Am J Reprod Immunol. (2017) 77. doi: 10.1111/aji.12643
216. Apps R, Gardner L, Moffett A. A critical look at HLA-G. Trends Immunol. (2008) 29:313–21. doi: 10.1016/j.it.2008.02.012
217. Ishikawa T, Takizawa T, Iwaki J, Mishima T, Ui-Tei K, Takeshita T, et al. Fc gamma receptor IIb participates in maternal IgG trafficking of human placental endothelial cells. Int J Mol Med. (2015) 35:1273–89. doi: 10.3892/ijmm.2015.2141
218. Ali A, Bouma GJ, Anthony RV, Winger QA. The role of LIN28-let-7-ARID3B pathway in placental development. Int J Mol Sci. (2020) 21. doi: 10.3390/ijms21103637
219. Yu Y, He JH, Hu LL, Jiang LL, Fang L, Yao GD, et al. Placensin is a glucogenic hormone secreted by human placenta. EMBO Rep. (2020) 21:e49530. doi: 10.15252/embr.201949530
220. Malassiné A, Frendo JL, Evain-Brion D. A comparison of placental development and endocrine functions between the human and mouse model. Hum Reprod Update. (2003) 9:531–9. doi: 10.1093/humupd/dmg043
221. Sardet C, Paix A, Prodon F, Dru P, Chenevert J. From oocyte to 16-cell stage: cytoplasmic and cortical reorganizations that pattern the ascidian embryo. Dev Dyn. (2007) 236:1716–31. doi: 10.1002/dvdy.21136
222. Eckersley-Maslin MA, Alda-Catalinas C, Reik W. Dynamics of the epigenetic landscape during the maternal-to-zygotic transition. Nat Rev Mol Cell Biol. (2018) 19:436–50. doi: 10.1038/s41580-018-0008-z
223. Rayon T, Stamataki D, Perez-Carrasco R, Garcia-Perez L, Barrington C, Melchionda M, et al. Species-specific pace of development is associated with differences in protein stability. Science. (2020) 369. doi: 10.1126/science.aba7667
224. Serman A, Serman L. Development of placenta in a rodent–model for human placentation. Front Biosci (Elite Ed). (2011) 3:233–9. doi: 10.2741/e238
225. Welsh AO, Enders AC. Trophoblast-decidual cell interactions and establishment of maternal blood circulation in the parietal yolk sac placenta of the rat. Anat Rec. (1987) 217:203–19. doi: 10.1002/ar.1092170213
226. Pereda J, Monge JI, Niimi G. Two different pathways for the transport of primitive and definitive blood cells from the yolk sac to the embryo in humans. Microsc Res Tech. (2010) 73:803–9. doi: 10.1002/jemt.20823
227. Calkins K, Devaskar SU. Fetal origins of adult disease. Curr Probl Pediatr Adolesc Health Care. (2011) 41:158–76. doi: 10.1016/j.cppeds.2011.01.001
228. Dimitriadis E, Rolnik DL, Zhou W, Estrada-Gutierrez G, Koga K, Francisco RPV, et al. Pre-eclampsia. Nat Rev Dis Primers. (2023) 9:8. doi: 10.1038/s41572-023-00417-6
229. Hoang VM, Foulk R, Clauser K, Burlingame A, Gibson BW, Fisher SJ. Functional proteomics: examining the effects of hypoxia on the cytotrophoblast protein repertoire. Biochemistry. (2001) 40:4077–86. doi: 10.1021/bi0023910
230. Sun LZ, Yang NN, De W, Xiao YS. Proteomic analysis of proteins differentially expressed in preeclamptic trophoblasts. Gynecologic Obstetric Invest. (2007) 64:17–23. doi: 10.1159/000098399
231. Ishioka S, Ezaka Y, Umemura K, Hayashi T, Endo T, Saito T. Proteomic analysis of mechanisms of hypoxia-induced apoptosis in trophoblastic cells. Int J Med Sci. (2006) 4:36–44. doi: 10.7150/ijms.4.36
232. Hu R, Jin H, Zhou S, Yang P, Li X. Proteomic analysis of hypoxia-induced responses in the syncytialization of human placental cell line bewo. Placenta. (2007) 28:399–407. doi: 10.1016/j.placenta.2006.07.005
233. Vorum H, Østergaard M, Hensechke P, Enghild JJ, Riazati M, Rice GE. Proteomic analysis of hyperoxia-induced responses in the human choriocarcinoma cell line jeg-3. Proteomics. (2004) 4:861–7. doi: 10.1002/pmic.200300639
234. Webster RP, Pitzer BA, Roberts VH, Brockman D, Myatt L. Differences in the proteome profile in placenta from normal term and preeclamptic preterm pregnancies. Proteomics Clin Appl. (2007) 1:446–56. doi: 10.1002/prca.200600745
235. Gharesi-Fard B, Zolghadri J, Kamali-Sarvestani E. Proteome differences of placenta between pre-eclampsia and normal pregnancy. Placenta. (2010) 31:121–5. doi: 10.1016/j.placenta.2009.11.004
236. Liu AX, Jin F, Zhang WW, Zhou TH, Zhou CY, Yao WM, et al. Proteomic analysis on the alteration of protein expression in the placental villous tissue of early pregnancy loss. Biol Reprod. (2006) 75:414–20. doi: 10.1095/biolreprod.105.049379
237. Butt RH, Lee MW, Pirshahid SA, Backlund PS, Wood S, Coorssen JR. An initial proteomic analysis of human preterm labor: placental membranes. J Proteome Res. (2006) 5:3161–72. doi: 10.1021/pr060282n
238. Zhang Y, Zhang YL, Feng C, Wu YT, Liu AX, Sheng JZ, et al. Comparative proteomic analysis of human placenta derived from assisted reproductive technology. Proteomics. (2008) 8:4344–56. doi: 10.1002/pmic.200800294
239. Wong F, Cox B. Proteomics analysis of preeclampsia, a systematic review of maternal and fetal compartments. J Proteomics Bioinform S. (2014) 10:2. doi: 10.4172/jpb
240. Sawicki G, Dakour J, Morrish DW. Functional proteomics of neurokinin B in the placenta indicates a novel role in regulating cytotrophoblast antioxidant defences. Proteomics. (2003) 3:2044–51. doi: 10.1002/pmic.200300537
241. Wang F, Shi Z, Wang P, You W, Liang G. Comparative proteome profile of human placenta from normal and preeclamptic pregnancies. PloS One. (2013) 8:e78025. doi: 10.1371/journal.pone.0078025
242. Zhang HH, Wang YP, Chen DB. Analysis of nitroso-proteomes in normotensive and severe preeclamptic human placentas. Biol Reprod. (2011) 84:966–75. doi: 10.1095/biolreprod.110.090688
243. Baig S, Kothandaraman N, Manikandan J, Rong L, Ee KH, Hill J, et al. Proteomic analysis of human placental syncytiotrophoblast microvesicles in preeclampsia. Clin Proteomics. (2014) 11:40. doi: 10.1186/1559-0275-11-40
244. Yang JI, Kong TW, Kim HS, Kim HY. The proteomic analysis of human placenta with pre-eclampsia and normal pregnancy. J Korean Med Sci. (2015) 30:770–8. doi: 10.3346/jkms.2015.30.6.770
245. Sun X, Qu T, He X, Yang X, Guo N, Mao Y, et al. Screening of differentially expressed proteins from syncytiotrophoblast for severe early-onset preeclampsia in women with gestational diabetes mellitus using tandem mass tag quantitative proteomics. BMC Pregnancy Childbirth. (2018) 18:437. doi: 10.1186/s12884-018-2066-9
246. Jin H, Ma KD, Hu R, Chen Y, Yang F, Yao J, et al. Analysis of expression and comparative profile of normal placental tissue proteins and those in preeclampsia patients using proteomic approaches. Anal Chim Acta. (2008) 629:158–64. doi: 10.1016/j.aca.2008.09.015
247. Shin JK, Baek JC, Kang MY, Park JK, Lee SA, Lee JH, et al. Proteomic analysis reveals an elevated expression of heat shock protein 27 in preeclamptic placentas. Gynecol Obstet Invest. (2011) 71:151–7. doi: 10.1159/000315162
248. Zhang W, Chen X, Yan Z, Chen Y, Cui Y, Chen B, et al. Detergent-insoluble proteome analysis revealed aberrantly aggregated proteins in human preeclampsia placentas. J Proteome Res. (2017) 16:4468–80. doi: 10.1021/acs.jproteome.7b00352
249. Mary S, Kulkarni MJ, Malakar D, Joshi SR, Mehendale SS, Giri AP. Placental proteomics provides insights into pathophysiology of pre-eclampsia and predicts possible markers in plasma. J Proteome Res. (2017) 16:1050–60. doi: 10.1021/acs.jproteome.6b00955
250. Jin X, Xu Z, Cao J, Shao P, Zhou M, Qin Z, et al. Proteomics analysis of human placenta reveals glutathione metabolism dysfunction as the underlying pathogenesis for preeclampsia. Biochim Biophys Acta Proteins Proteom. (2017) 1865:1207–14. doi: 10.1016/j.bbapap.2017.07.003
251. Xu Z, Jin X, Cai W, Zhou M, Shao P, Yang Z, et al. Proteomics analysis reveals abnormal electron transport and excessive oxidative stress cause mitochondrial dysfunction in placental tissues of early-onset preeclampsia. Proteomics Clin Appl. (2018) 12:e1700165. doi: 10.1002/prca.201700165
252. Ma K, Jin H, Hu R, Xiong Y, Zhou S, Ting P, et al. A proteomic analysis of placental trophoblastic cells in preeclampsia-eclampsia. Cell Biochem Biophys. (2014) 69:247–58. doi: 10.1007/s12013-013-9792-4
253. Shi Z, Long W, Zhao C, Guo X, Shen R, Ding H. Comparative proteomics analysis suggests that placental mitochondria are involved in the development of pre-eclampsia. PloS One. (2013) 8:e64351. doi: 10.1371/journal.pone.0064351
254. Johnstone ED, Sawicki G, Guilbert L, Winkler-Lowen B, Cadete VJ, Morrish DW. Differential proteomic analysis of highly purified placental cytotrophoblasts in pre-eclampsia demonstrates a state of increased oxidative stress and reduced cytotrophoblast antioxidant defense. Proteomics. (2011) 11:4077–84. doi: 10.1002/pmic.201000505
255. He A, Wang J, Yang X, Liu J, Yang X, Wang G, et al. Screening of differentially expressed proteins in placentas from patients with late-onset preeclampsia. Proteomics Clin Appl. (2022) 16:e2100053. doi: 10.1002/prca.202100053
256. Wang H, Shi Y, Ma J, Wang W, Gao J, Zhao L, et al. Integrated proteomic and N-glycoproteomic profiling of placental tissues of patients with preeclampsia. Int J Womens Health. (2023) 15:59–68. doi: 10.2147/ijwh.S387672
257. Kalousová M, Muravská A, Zima T. Pregnancy-associated plasma protein a (Papp-a) and preeclampsia. Adv Clin Chem. (2014) 63:169–209. doi: 10.1016/b978-0-12-800094-6.00005-4
258. Handwerger S, Freemark M. The roles of placental growth hormone and placental lactogen in the regulation of human fetal growth and development. J Pediatr Endocrinol Metab. (2000) 13:343–56. doi: 10.1515/jpem.2000.13.4.343
259. Alsat E, Guibourdenche J, Couturier A, Evain-Brion D. Physiological role of human placental growth hormone. Mol Cell Endocrinol. (1998) 140:121–7. doi: 10.1016/s0303-7207(98)00040-9
260. Alsat E, Guibourdenche J, Luton D, Frankenne F, Evain-Brion D. Human placental growth hormone. Am J Obstetrics Gynecology. (1997) 177:1526–34. doi: 10.1016/S0002-9378(97)70103-0
261. Sasaki A, Shinkawa O, Margioris AN, Liotta AS, Sato S, Murakami O, et al. Immunoreactive corticotropin-releasing hormone in human plasma during pregnancy, labor, and delivery. J Clin Endocrinol Metab. (1987) 64:224–9. doi: 10.1210/jcem-64-2-224
262. Burton GJ, Charnock-Jones DS, Jauniaux E. Regulation of vascular growth and function in the human placenta. Reproduction. (2009) 138:895–902. doi: 10.1530/rep-09-0092
263. Leguy MC, Brun S, Pidoux G, Salhi H, Choiset A, Menet MC, et al. Pattern of secretion of pregnancy-associated plasma protein-a (Papp-a) during pregnancies complicated by fetal aneuploidy, in vivo and in vitro. Reprod Biol Endocrinol. (2014) 12:129. doi: 10.1186/1477-7827-12-129
264. Degnes ML, Westerberg AC, Zucknick M, Powell TL, Jansson T, Henriksen T, et al. Placenta-derived proteins across gestation in healthy pregnancies-a novel approach to assess placental function? BMC Med. (2022) 20:227. doi: 10.1186/s12916-022-02415-z
265. Zhang T, Zhao C, Luo L, Xiang J, Cheng J, Wang T, et al. High concentraction of taurocholic acid induced apoptosis in HTR-8/SVneo cells via overexpression of ERp29 and activation of P38. Placenta. (2014) 35:496–500. doi: 10.1016/j.placenta.2014.03.023
266. Degnes M-HL, Westerberg AC, Zucknick M, Powell TL, Jansson T, Henriksen T, et al. Placenta-derived proteins across gestation in healthy pregnancies—a novel approach to assess placental function? BMC Med. (2022) 20:227. doi: 10.1186/s12916-022-02415-z
267. Chau K, Hennessy A, Makris A. Placental growth factor and pre-eclampsia. J Hum Hypertens. (2017) 31:782–6. doi: 10.1038/jhh.2017.61
268. Kim SY, Ryu HM, Yang JH, Kim MY, Ahn HK, Shin JS, et al. Maternal serum and amniotic fluid inhibin a levels in women who subsequently develop severe preeclampsia. J Korean Med Sci. (2006) 21:452–6. doi: 10.3346/jkms.2006.21.3.452
269. Yue CY, Zhang CY, Ni YH, Ying CM. Are serum levels of inhibin a in second trimester predictors of adverse pregnancy outcome? PloS One. (2020) 15:e0232634. doi: 10.1371/journal.pone.0232634
270. Khalil A, Cowans NJ, Spencer K, Goichman S, Meiri H, Harrington K. First trimester maternal serum placental protein 13 for the prediction of pre-eclampsia in women with a priori high risk. Prenat Diagn. (2009) 29:781–9. doi: 10.1002/pd.2287
271. Gonen R, Shahar R, Grimpel YI, Chefetz I, Sammar M, Meiri H, et al. Placental protein 13 as an early marker for pre-eclampsia: A prospective longitudinal study. Bjog. (2008) 115:1465–72. doi: 10.1111/j.1471-0528.2008.01902.x
272. Poon LC, Nicolaides KH. Early prediction of preeclampsia. Obstet Gynecol Int. (2014) 2014:297397. doi: 10.1155/2014/297397
273. Yung HW, Zhao X, Glover L, Burrin C, Pang PC, Jones CJP, et al. Perturbation of placental protein glycosylation by endoplasmic reticulum stress promotes maladaptation of maternal hepatic glucose metabolism. iScience. (2023) 26:105911. doi: 10.1016/j.isci.2022.105911
274. Liu LY, Yang T, Ji J, Wen Q, Morgan AA, Jin B, et al. Integrating multiple 'Omics' Analyses identifies serological protein biomarkers for preeclampsia. BMC Med. (2013) 11:236. doi: 10.1186/1741-7015-11-236
275. Ji Q, Zhang S, Jiang W, Wang J, Luan Y, Xin Q. Serum protein profile analysis via label-free quantitation proteomics in patients with early-onset preeclampsia. J Obstet Gynaecol. (2023) 43:2259982. doi: 10.1080/01443615.2023.2259982
276. Chen H, Aneman I, Nikolic V, Karadzov Orlic N, Mikovic Z, Stefanovic M, et al. Maternal plasma proteome profiling of biomarkers and pathogenic mechanisms of early-onset and late-onset preeclampsia. Sci Rep. (2022) 12:19099. doi: 10.1038/s41598-022-20658-x
277. Tu C, Tao F, Qin Y, Wu M, Cheng J, Xie M, et al. Serum proteins differentially expressed in early- and late-onset preeclampsia assessed using iTRAQ proteomics and bioinformatics analyses. PeerJ. (2020) 8:e9753. doi: 10.7717/peerj.9753
278. Marić I, Contrepois K, Moufarrej MN, Stelzer IA, Feyaerts D, Han X, et al. Early prediction and longitudinal modeling of preeclampsia from multiomics. Patterns. (2022) 3:100655. doi: 10.1016/j.patter.2022.100655
279. Chen ME, Lin SH, Chung LW, Sikes RA. Isolation and characterization of PAGE-1 and GAGE-7. New genes expressed in the LNCaP prostate cancer progression model that share homology with melanoma-associated antigens. J Biol Chem. (1998) 273:17618–25. doi: 10.1074/jbc.273.28.17618
280. Chen YT, Scanlan MJ, Sahin U, Türeci O, Gure AO, Tsang S, et al. A testicular antigen aberrantly expressed in human cancers detected by autologous antibody screening. Proc Natl Acad Sci U.S.A. (1997) 94:1914–8. doi: 10.1073/pnas.94.5.1914
281. Nin DS, Deng L-W. Biology of cancer-testis antigens and their therapeutic implications in cancer. Cells. (2023) 12. doi: 10.3390/cells12060926
282. Liu L, Xu HX, Wang WQ, Wu CT, Xiang JF, Liu C, et al. Serum CA125 is a novel predictive marker for pancreatic cancer metastasis and correlates with the metastasis-associated burden. Oncotarget. (2016) 7:5943–56. doi: 10.18632/oncotarget.6819
283. Blumenthal RD, Leon E, Hansen HJ, Goldenberg DM. Expression patterns of ceacam5 and ceacam6 in primary and metastatic cancers. BMC Cancer. (2007) 7:2. doi: 10.1186/1471-2407-7-2
284. Conley AP, Wang WL, Livingston JA, Ravi V, Tsai JW, Ali A, et al. Mage-A3 is a clinically relevant target in undifferentiated pleomorphic sarcoma/myxofibrosarcoma. Cancers (Basel). (2019) 11. doi: 10.3390/cancers11050677
285. Shvartsur A, Bonavida B. Trop2 and its overexpression in cancers: regulation and clinical/therapeutic implications. Genes Cancer. (2015) 6:84–105. doi: 10.18632/genesandcancer.40
286. Harrop R, O'Neill E, Stern PL. Cancer stem cell mobilization and therapeutic targeting of the 5T4 oncofetal antigen. Ther Adv Vaccines Immunother. (2019) 7:2515135518821623. doi: 10.1177/2515135518821623
287. Yagihashi A, Ohmura T, Asanuma K, Kobayashi D, Tsuji N, Torigoe T, et al. Detection of autoantibodies to survivin and livin in sera from patients with breast cancer. Clin Chim Acta. (2005) 362:125–30. doi: 10.1016/j.cccn.2005.06.009
288. Dong XY, Peng JR, Ye YJ, Chen HS, Zhang LJ, Pang XW, et al. Plac1 is a tumor-specific antigen capable of eliciting spontaneous antibody responses in human cancer patients. Int J Cancer. (2008) 122:2038–43. doi: 10.1002/ijc.23341
289. Silva WA Jr., Gnjatic S, Ritter E, Chua R, Cohen T, Hsu M, et al. Plac1, a trophoblast-specific cell surface protein, is expressed in a range of human tumors and elicits spontaneous antibody responses. Cancer Immun. (2007) 7:18.
290. Liu FF, Dong XY, Pang XW, Xing Q, Wang HC, Zhang HG, et al. The specific immune response to tumor antigen CP1 and its correlation with improved survival in colon cancer patients. Gastroenterology. (2008) 134:998–1006. doi: 10.1053/j.gastro.2008.01.029
291. Mahmoudian J, Ghods R, Nazari M, Jeddi-Tehrani M, Ghahremani MH, Ostad SN, et al. Expression profiling of plac1 in murine cancer cell lines. Exp Oncol. (2019) 41:7–13. doi: 10.32471/10.32471/exp-oncology.2312-8852.vol-41-no-1
292. Ghods R, Ghahremani MH, Madjd Z, Asgari M, Abolhasani M, Tavasoli S, et al. High placenta-specific 1/low prostate-specific antigen expression pattern in high-grade prostate adenocarcinoma. Cancer Immunol Immunother. (2014) 63:1319–27. doi: 10.1007/s00262-014-1594-z
293. Riordan JD, Keng VW, Tschida BR, Scheetz TE, Bell JB, Podetz-Pedersen KM, et al. Identification of rtl1, a retrotransposon-derived imprinted gene, as a novel driver of hepatocarcinogenesis. PloS Genet. (2013) 9:e1003441. doi: 10.1371/journal.pgen.1003441
294. Fan G, Ye D, Zhu S, Xi J, Guo X, Qiao J, et al. RTL1 promotes melanoma proliferation by regulating Wnt/Β-catenin signalling. Oncotarget. (2017) 8:106026–37. doi: 10.18632/oncotarget.22523
295. Mahmoudi AR, Ghods R, Madjd Z, Abolhasani M, Saeednejad Zanjani L, Safaei M, et al. Expression profiling of rtl1 in human breast cancer tissues and cell lines. Exp Mol Pathol. (2021) 121:104654. doi: 10.1016/j.yexmp.2021.104654
296. Welsh JB, Sapinoso LM, Kern SG, Brown DA, Liu T, Bauskin AR, et al. Large-scale delineation of secreted protein biomarkers overexpressed in cancer tissue and serum. Proc Natl Acad Sci. (2003) 100:3410–5. doi: 10.1073/pnas.0530278100
297. Wischhusen J, Melero I, Fridman WH. Growth/differentiation factor-15 (Gdf-15): from biomarker to novel targetable immune checkpoint. Front Immunol. (2020) 11:951. doi: 10.3389/fimmu.2020.00951
298. Yang R, Han Y, Yi W, Long Q. Autoantibodies as biomarkers for breast cancer diagnosis and prognosis. Front Immunol. (2022) 13:1035402. doi: 10.3389/fimmu.2022.1035402
299. Macdonald IK, Parsy-Kowalska CB, Chapman CJ. Autoantibodies: opportunities for early cancer detection. Trends Cancer. (2017) 3:198–213. doi: 10.1016/j.trecan.2017.02.003
300. Tan HT, Low J, Lim SG, Chung MC. Serum autoantibodies as biomarkers for early cancer detection. FEBS J. (2009) 276:6880–904. doi: 10.1111/j.1742-4658.2009.07396.x
301. Zaenker P, Ziman MR. Serologic autoantibodies as diagnostic cancer biomarkers–a review. Cancer Epidemiol Biomarkers Prev. (2013) 22:2161–81. doi: 10.1158/1055-9965.Epi-13-0621
Keywords: placenta, proteins, pregnancy, immunomodulation, embryo implantation, cancer, biomarker
Citation: Khorami-Sarvestani S, Vanaki N, Shojaeian S, Zarnani K, Stensballe A, Jeddi-Tehrani M and Zarnani A-H (2024) Placenta: an old organ with new functions. Front. Immunol. 15:1385762. doi: 10.3389/fimmu.2024.1385762
Received: 13 February 2024; Accepted: 08 April 2024;
Published: 19 April 2024.
Edited by:
Alicia E. Damiano, University of Buenos Aires, ArgentinaReviewed by:
Reggie García-Robles, Pontifical Javeriana University, ColombiaVictor Sánchez-Margalet, Virgen Macarena University Hospital, Spain
Copyright © 2024 Khorami-Sarvestani, Vanaki, Shojaeian, Zarnani, Stensballe, Jeddi-Tehrani and Zarnani. This is an open-access article distributed under the terms of the Creative Commons Attribution License (CC BY). The use, distribution or reproduction in other forums is permitted, provided the original author(s) and the copyright owner(s) are credited and that the original publication in this journal is cited, in accordance with accepted academic practice. No use, distribution or reproduction is permitted which does not comply with these terms.
*Correspondence: Amir-Hassan Zarnani, emFybmFuaWFAc2luYS50dW1zLmFjLmly; emFybmFuaWFAZ21haWwuY29t; Mahmood Jeddi-Tehrani, bWFoamVkQGF2aWNlbm5hLmFjLmly; bWFoamVkQHlhaG9vLmNvbQ==
†These authors have contributed equally to this work