- 1Clinical Immunology Translational Medicine Key Laboratory of Sichuan Province, Center of Organ Transplantation, Sichuan Academy of Medical Science and Sichuan Provincial People’s Hospital, Chengdu, China
- 2Department of Thyroid, Sichuan Second Hospital of Traditional Chinese Medicine, Chengdu, Sichuan, China
- 3School of Medicine, University of Electronic Science and Technology of China, Chengdu, China
- 4Department of Clinical Medicine, The First Clinical Medical College of Norman Bethune University of Medical Sciences, Jilin, China
- 5Department of Neuroscience, Baylor College of Medicine, Houston, TX, United States
- 6Department of Pharmacy, Guangxi University of Chinese Medicine, Nanning, China
- 7Clinical Immunology Translational Medicine Key Laboratory of Sichuan Province, Sichuan Academy of Medical Sciences and Sichuan Provincial People’s Hospital, School of Medicine, University of Electronic Science and Technology of China, Chengdu, China
Liver failure represents a critical medical condition with a traditionally grim prognosis, where treatment options have been notably limited. Historically, liver transplantation has stood as the sole definitive cure, yet the stark disparity between the limited availability of liver donations and the high demand for such organs has significantly hampered its feasibility. This discrepancy has necessitated the exploration of hepatocyte transplantation as a temporary, supportive intervention. In light of this, our review delves into the burgeoning field of hepatocyte transplantation, with a focus on the latest advancements in maintaining hepatocyte function, co-microencapsulation techniques, xenogeneic hepatocyte transplantation, and the selection of materials for microencapsulation. Our examination of hepatocyte microencapsulation research highlights that, to date, most studies have been conducted in vitro or using liver failure mouse models, with a notable paucity of experiments on larger mammals. The functionality of microencapsulated hepatocytes is primarily inferred through indirect measures such as urea and albumin production and the rate of ammonia clearance. Furthermore, research on the mechanisms underlying hepatocyte co-microencapsulation remains limited, and the practicality of xenogeneic hepatocyte transplantation requires further validation. The potential of hepatocyte microencapsulation extends beyond the current scope of application, suggesting a promising horizon for liver failure treatment modalities. Innovations in encapsulation materials and techniques aim to enhance cell viability and function, indicating a need for comprehensive studies that bridge the gap between small-scale laboratory success and clinical applicability. Moreover, the integration of bioengineering and regenerative medicine offers novel pathways to refine hepatocyte transplantation, potentially overcoming the challenges of immune rejection and ensuring the long-term functionality of transplanted cells. In conclusion, while hepatocyte microencapsulation and transplantation herald a new era in liver failure therapy, significant strides must be made to translate these experimental approaches into viable clinical solutions. Future research should aim to expand the experimental models to include larger mammals, thereby providing a clearer understanding of the clinical potential of these therapies. Additionally, a deeper exploration into the mechanisms of cell survival and function within microcapsules, alongside the development of innovative encapsulation materials, will be critical in advancing the field and offering new hope to patients with liver failure.
1 Introduction
1.1 The evolution of liver transplantation
The liver, one of the human body’s largest and most versatile organs, is incredible for being able to detoxificate, metabolize, and maintain complex interactions with other organs like the kidney and spleen. Despite its critical role, individuals suffering from liver-based metabolic disorders (LBMD), hepatocellular carcinoma, fulminant liver failure, and end-stage liver diseases often face limited treatment options. The landscape of liver disease treatment underwent a significant transformation in 1963 when Thomas E. Starzl and his team pioneered the first clinical trials of orthotopic liver transplantation (LT) in three patients (1). This groundbreaking procedure offered a new lease on life for patients with severe liver conditions, improving their lifespan and quality of life. The procedure’s advantages include the liver’s remarkable regenerative ability, which minimizes donor risk, and an overall increase in population survival rates. However, LT is not without its drawbacks, including surgical complications, high costs, and the requirement for lifelong immunosuppression. Above all, the chronic shortage of available organs has been a persistent hurdle, underscoring the need for more feasible treatment alternatives.
1.2 The advent and progress of hepatocyte transplantation
The growing discrepancy between the demand for liver transplants and the available supply, as highlighted in recent reports by the Organ Procurement and Transplantation Network (OPTN) and the Scientific Registry of Transplant Recipients (SRTR), underscores the urgent need for alternative liver tissue sources. Hepatocytes, or liver cells, possess distinct characteristics that make them particularly appealing for transplantation; they retain functional capabilities even when isolated, and cryopreserved hepatocytes can be rapidly deployed for urgent therapeutic needs. This realization sparked interest in the potential of hepatocyte transplantation (HT) as a viable alternative to LT for managing LBMD and acute liver failure (ALF). Although hepatocyte transplantation is limited by many obstacles in clinical practice, researchers are constantly working to overcome them. Tasks remains to solve include scarce supply of reliable and high-quality hepatocytes, sub-optimal survival and regeneration after hepatocyte transplantation with transient phenotype, and urgent need of more effective immunosuppressive protocols to reduce rejection (2). Demonstrated to functionally mimic the liver to a certain extent, especially in acute cases, HT presents several advantages over traditional LT. One donor liver could potentially benefit multiple patients, depending on the yield of viable cells obtained and the specific needs of each patient (3). HT offers a less invasive approach compared to LT, eliminating the need for major surgery. Additionally, the ability to repeat hepatocyte infusions and preserve cells for future use means that patients on the liver transplant waitlist can maintain some liver function until a suitable organ match is found. Furthermore, the lower costs, reduced risks, and fewer complications associated with HT have contributed to its growing popularity as a promising treatment alternative. The various encapsulation methods discussed herein are summarized in Figure 1, providing a concise overview of the innovative approaches in hepatocyte transplantation.
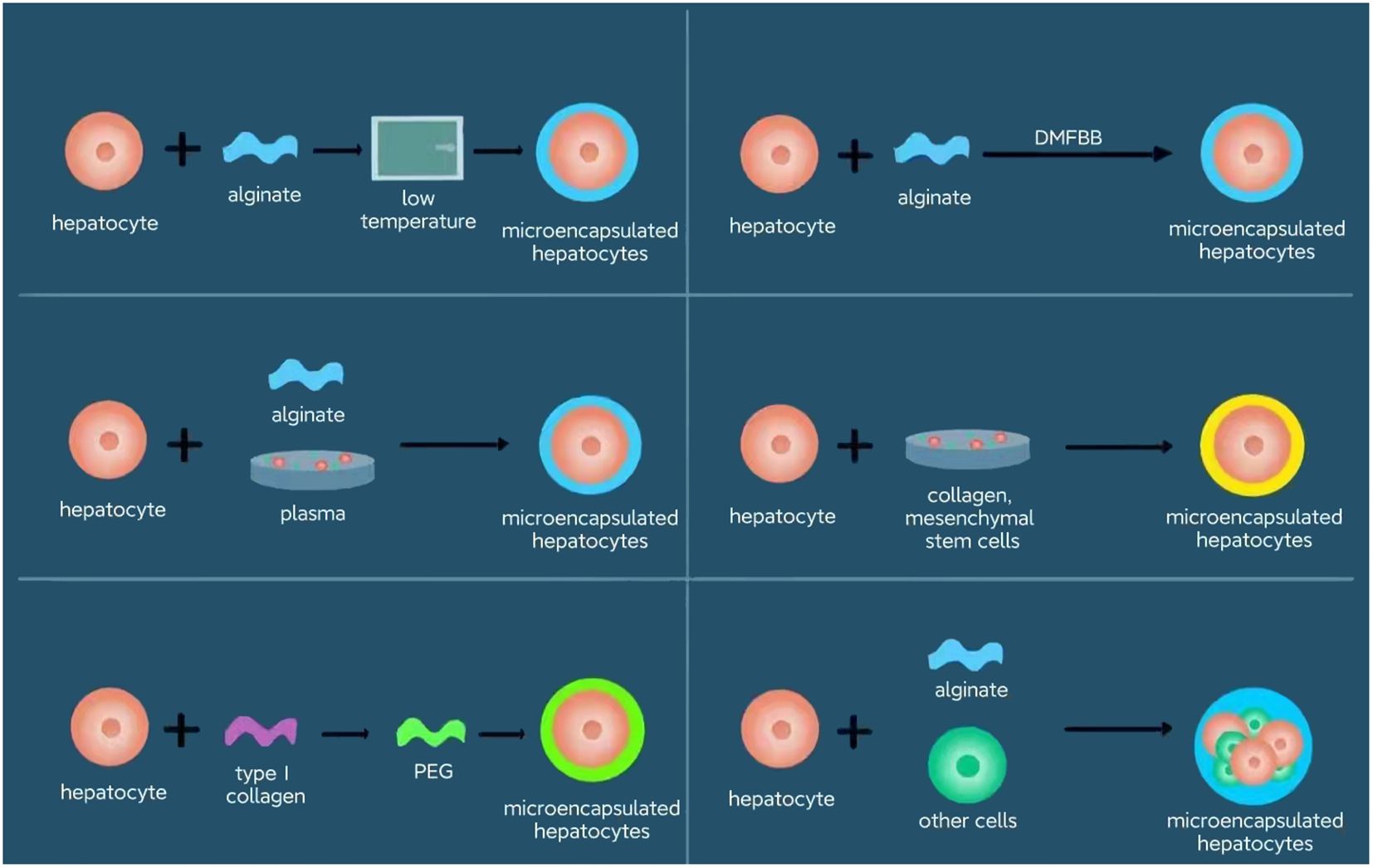
Figure 1 Encapsulation approaches for hepatocytes. The various encapsulation methods discussed herein are summarized, providing a concise overview of the innovative approaches in hepatocyte transplantation. The approaches include alginate plus low temperature, alginate plus plasma, type 1 collagen and PEG, alginate plus diversion-type microcapsule-suspension fluidized bed bioreactor (DMFBB), collagen plus mesenchymal stem cells, and alginate plus other cells.
2 Advancements in encapsulation materials for hepatocyte transplantation
2.1 Factors and mechanism related
Hepatocyte Transplantation demonstrates several important factors. The location of encapsulated hepatocytes within recipients can influence their viability and functionality. Existing literature and experiments suggest that the implantation site plays a crucial role in the success of hepatocyte transplantation. For instance, encapsulated hepatocytes transplanted into the peritoneal cavity may benefit from the rich blood supply and immune-privileged status, which could enhance cell survival and function (4). The microenvironment at the transplantation site, including factors like oxygenation and nutrient availability, can significantly impact the encapsulated hepatocytes’ ability to maintain their liver-specific functions.
The dose or number of transplanted hepatocytes is a critical factor affecting transplant efficiency and the therapeutic outcome, which together with the place of transplantation, will largely decide the condition of hepatocytes (5). Determining the optimal number of hepatocytes for transplantation remains a challenge and is subject to ongoing research. The efficiency of transplantation and subsequent liver function recovery is likely dose-dependent, requiring a balance between sufficient cell mass for therapeutic effect and the host’s capacity to integrate and support the transplanted cells. Studies have suggested that a higher number of transplanted hepatocytes may improve the functional recovery in liver failure models, but this must be balanced against the risk of potential complications such as portal hypertension or embolization (6). Future research should aim to establish standardized protocols for dosing and to explore the mechanisms underlying dose-dependent effects on transplantation outcomes.
The detailed discussion on the underlying mechanisms of encapsulated hepatocytes is limited. Potential mechanisms behind the improved viability and function of encapsulated hepatocytes involve several aspects, including enhanced protection from immunological rejection, improved microenvironmental control within the capsules, and the supportive effects of co-encapsulated cells (7). For instance, encapsulation materials like alginate provide a semi-permeable barrier that can protect hepatocytes from the host’s immune response while allowing the exchange of nutrients and metabolic products (8). Additionally, co-microencapsulation with supportive cell types, such as mesenchymal stem cells, may provide trophic support and promote a more physiologically relevant microenvironment that enhances hepatocyte function (9).
2.2 Addressing immune rejection challenges
Addressing challenges such as immune rejection after hepatocyte transplantation, encapsulation emerges as a straightforward, economical, and effective strategy. The critical aspects of this approach—material selection, encapsulation technique optimization, and culture environment adaptation—are key to successful hepatocyte encapsulation. The choice of encapsulation material is particularly crucial as it directly impacts the encapsulated hepatocytes’ functionality and viability by influencing oxygen and nutrient transfer.
2.3 Alginate’s role in hepatocyte microencapsulation
Alginate, a material favored for its biocompatibility, ease of gel formation, and unique physicochemical properties, stands out in the realm of hepatocyte microencapsulation. Miranda et al. observed that alginate-encapsulated hepatocyte aggregates exhibited significantly enhanced albumin production, urea synthesis, and enzymatic activities such as 7-ethoxycoumarin O-deethylase and uridine diphosphate glucuronosyltransferase (UGT) compared to non-encapsulated controls (8). Notably, these encapsulated hepatocytes demonstrated improved functional outcomes when cultured in a bioreactor system, maintaining performance over three weeks.
In a novel exploration, Nhu-Mai et al. revealed that alginate hydrogel could shield human hepatoma-derived cells (Huh-7), the most commonly used cell line recently with high permissiveness, from Hepatitis C Virus (HCV) infection (10). This protective effect, dependent on the concentration and duration of culture, suggests alginate hydrogel’s broader viral defense capability, irrespective of encapsulation.
2.4 Optimization and comparative studies
Further research by Lan et al. compared the survival and metabolic function of hepatocytes encapsulated in different alginate compositions, SLM100 and SLG100, demonstrating sustained viability, enzyme secretion, and antioxidant activity under 3D culture conditions, albeit with reduced proliferation rates (11). Saeed Azandeh et al. investigated the impact of alginate hydrogel concentrations on Human Wharton’s Jelly-derived Mesenchymal Stem Cells (HWJ-MSCs) (12). They discovered that a 1.5% alginate concentration was more conducive to cell proliferation and urea production than a 2.5% concentration, highlighting the importance of finding the optimal alginate concentration for hepatocyte viability. Jitraruch et al. proposed an optimized protocol for producing alginate-encapsulated human hepatocytes, which demonstrated superior mechanical stability and ideal bead size for enhanced cell viability (13). Similarly, Pasqua et al. developed a technique for culturing hepatocytes in 1.5% alginate beads, facilitating the autonomous formation of spheroids with maintained liver functions over two weeks (14). Durkut et al. evaluated the viability and metabolism of primary rat hepatocytes encapsulated in various matrices and subjected to different cryopreservation conditions (15). Their findings indicated that cryopreservation in liquid nitrogen (LN2) best preserved hepatic functions and viability, with ACA-encapsulated hepatocytes maintaining nearly 90% of their metabolic activity post-thaw. These studies collectively underscore the significance of material selection and encapsulation conditions in enhancing the therapeutic potential of hepatocyte transplantation, paving the way for innovative approaches to liver disease treatment. The quest to mitigate immune rejection reactions post hepatocyte transplantation has steered research towards hepatocyte encapsulation as a viable, cost-effective solution. Key to this endeavor is the meticulous selection of encapsulation materials, microencapsulation methodologies, and the fine-tuning of the culture environment, all of which are pivotal for the successful encapsulation of hepatocytes.
2.5 Novel encapsulation materials and techniques
Stephanie H. Capone and associates explored various material combinations for cell microencapsulation, including alginate alone, alginate combined with type I collagen, with or without poly-L-lysine and alginate coatings (16). They discovered that incorporating collagen and polylysine enhanced the mechanical resilience of the beads but compromised vitamin B12 mass transfer kinetics. Alginate-collagen beads notably enhanced HepG2/C3A viability with increased metabolism rate. Upon subcutaneous implantation in mice, they also mitigated inflammation, spotlighting the crucial balance between mechanical strength, cell behavior, and biocompatibility. Subhas C. and his team innovated silk sericin–alginate–chitosan microcapsules, creating a sericin and alginate microbead core with a chitosan outer shell (17). These microcapsules, characterized by their spherical shape and glossy surface, demonstrated high cell viability and uniform encapsulation under confocal microscopy, indicating an optimized living microenvironment for the encapsulated cells.
2.6 Cell source considerations for clinical transplantation
A significant challenge in hepatocyte transplantation research has been sourcing cells that are both functional and safe for clinical use. Traditionally, hepatocellular carcinoma (HCC) cell lines such as HepG2, HepaRG, and HepG2/C3A have been extensively utilized in research due to their ease of propagation and maintenance. However, these cells are derived from liver cancers and, as such, are not suitable for clinical transplantation purposes. Their immortal nature, potential for uncontrolled proliferation, and inferior functionality compared to primary hepatocytes limit their applicability in therapeutic contexts. Recognizing these limitations, the field is increasingly turning towards human pluripotent stem cells (hPSCs) as a potential source of hepatocyte-like cells (HLCs) for transplantation. hPSCs, including both embryonic stem cells (ESCs) and induced pluripotent stem cells (iPSCs), possess the capacity for unlimited self-renewal and the potential to differentiate into any cell type, including hepatocyte-like cells. This differentiation is achieved through the mimicking of liver development stages in vitro, leading to the generation of cells that exhibit key hepatocyte functions such as albumin secretion, urea production, and drug-metabolizing enzyme activity.
The use of hPSC-derived HLCs presents a promising avenue for overcoming the cell source limitation in hepatocyte transplantation. These cells could provide a renewable, ethically accessible, and potentially customizable source of hepatocytes for therapeutic applications. Furthermore, advancements in differentiation protocols and three-dimensional culture systems are enhancing the functional maturation of HLCs, bringing them closer to the functionality of primary human hepatocytes. However, challenges remain in the clinical application of hPSC-derived HLCs, including ensuring the efficiency and consistency of differentiation protocols, the purity and safety of the cell populations (e.g., eliminating the risk of tumorigenicity), and the long-term functionality and integration of the transplanted cells in vivo. Addressing these challenges requires ongoing research and collaboration between stem cell biologists, tissue engineers, and clinical researchers. While HCC cell lines have provided valuable insights into liver biology and disease, the transition towards clinical transplantation necessitates the exploration of alternative cell sources like hPSC-derived HLCs. The promise of these cells in providing a viable, ethical, and functional source for hepatocyte transplantation underscores the importance of continued research and development in this exciting area of regenerative medicine.
2.7 Enhancing Liver-specific functions
Meng Tian et al. developed Galactosylated alginate (GA)–chitosan oligomer microcapsules, adjusting membrane porosity and thickness to balance mechanical stability and permeability (18). This selective permeability effectively transported human serum albumin while blocking immunoglobulin G, enhancing liver-specific functions within the microcapsules. In the study by Ying He et al., Cytodex 3 microcarriers formed the core of the microcapsules, enveloped by an alginic acid-chitosan-alginate (ACA) polyelectrolyte layer (19). Utilizing an aqueous two-phase emulsification technique, L02 cells on Cytodex-3 microcarriers were encased within a thin conformable layer, facilitating equitable transport of nutrients and wastes. These microcapsules consistently produced urea and human albumin in vitro and demonstrated the capability to stabilize serum markers in acetaminophen-damaged rats post-transplantation. Christian Siltanen et al. utilized a coaxial flow-focused droplet microfluidics approach to craft microcapsules with liquid cores and polyethylene glycol (PEG) shells (20). This encapsulation facilitated rapid aggregation of primary hepatocytes into dense globules, preserving liver function leaped from normally 1-2 days to 10 days. The technique also offered the flexibility to tailor the mechanical properties and permeability of the gel, making it adaptable for further experimental investigations. Shahla Khodabakhsh Aghdam et al. explored the incorporation of galactosylchitosan (GC) and collagen (COL) into alginate microcapsules, subsequently coated with chitosan to produce alginate-galactosylated chitosan-collagen/chitosan (AGCCol/C) microcapsules (21). This addition significantly influenced the hydrogels’ physical properties, enhancing the proliferation of HepG2 cells, and up-regulating the expression of P450 and albumin mRNA, demonstrating improved biocompatibility. anhong Zhang et al. adopted a one-step spray method to fabricate microcapsules using hyaluronic acid (HA)/sodium alginate (SA) as the core and chitosan (CS) as the shell (22). This method not only ensured high viability of C3A cells in vitro but also enhanced urea and albumin synthesis, highlighting HA’s role in promoting CYP450 gene expressions. Such advancements suggest a promising direction in hepatocyte microencapsulation techniques for liver transplantation applications. Further details and comparative analyses of these methodologies are summarized in Table 1, offering a comprehensive overview of the state-of-the-art in hepatocyte microencapsulation. Hyaluronic acid (HA) has been observed to enhance the expression of genes related to the cytochrome P450 family after a duration of three days. Utilizing an encapsulation technique, it was noted that the functionality of hepatocytes was markedly preserved, providing an improved habitat conducive to their survival. This method is seen as a potential option for the transplantation of hepatocyte microcapsules. Chan et al. explored an alternative approach by employing Double-emulsion (DE) droplets to generate microencapsulated homotypic or heterotypic hepatocyte spheroids within an alginate-collagen composite hydrogel, moving away from the sole use of alginate (42). Their microfluidics-based technique, which eliminates the necessity for spheroid loading and allows for the control over spheroid characteristics, has shown to enhance hepatocyte performance. This includes increased albumin and urea secretion, as well as improved cytochrome P450 activity. Moreover, hepatocyte function was further enhanced when co-cultured with endothelial progenitor cells at an optimal ratio of 5 to 1 in alginate-collagen. In contrast, Lee et al. experimented with hybrid hydrogels of varying stiffness to encapsulate HepaRG cells, either individually or with support cells. They utilized tissue engineering approaches to fabricate three-dimensional (3-D) liver models in vitro (43). When the elasticity of these 3D liver models was adjusted to closely match the range of 2.3-5.9 kPa, there was a notable increase in hepatic gene expression, albumin secretion, cytochrome p450-3A4 activity, and drug metabolism capabilities. This model also demonstrated the ability to extend the viability and functionality of hepatocytes over extended culture periods. Further contributing to this field, Cui and colleagues demonstrated that utilizing gelatin methacryloyl (GelMA) hydrogel as a base for constructing 3D lobule-like microtissues offers advantages for hepatocyte functionality (44). The GelMA hydrogel, shaped by a digital micromirror device (DMD)-based microfluidic channel, allows for the encapsulation of hepatocytes within micromodules featuring a central radial-type hole. After prolonged co-culture, hepatocytes encapsulated alongside fibroblasts showed an increase in albumin secretion and maintained over 90% cell survival rate. Chang et al. opted for volvox sphere microbeads to encapsulate hepatocytes, providing a dual-layer three-dimensional environment for the cells (45). This innovative approach contributes to the growing body of research focused on improving hepatocyte culture methods and transplantation strategies. Dynamic bioreactor cultures of AML12 hepatocytes together with rat mesenchymal stem cells (MSCs) demonstrated significant enhancements, with MSCs evolving into hepatocyte-like cells, doubling albumin (ALB) secretion, and increasing cytokeratin 18 expression by 2.5 times. In models of CCl4-induced liver damage in rats, encapsulation of MSCs and hepatocytes within volvox spheres markedly reduced AST and ALT levels, aiding liver repair and new tissue formation (46). Kim’s investigation into a three-dimensional heparin-based hydrogel scaffold for hepatocyte culture revealed that such encapsulated hepatocytes maintained high-level functionality, including albumin and urea synthesis, for up to three weeks. The addition of hepatocyte growth factor (HGF) into the hydrogel further enhanced these synthesis processes (47).
Gevaert et al. compared HepG2 cell encapsulation effects between galactosylated gelatin and Methacrylamide modified gelatin, finding that methacrylamide modification had little impact on viability, whereas galactosylated gelatin significantly enhanced specific gene expression over long-term culture (>21 days) (48). Lee and co-researchers discovered that hepatic function in primary human hepatocytes (PHHs) encapsulated within biodegradable hydrogel systems was best maintained with hydrogels of intermediate initial degradability, outperforming Matrigel in cytochrome P450 functional assays (49). Wang et al. introduced a one-step synthesis method for creating composite hydrogel capsules (CHCs) characterized by uniformity, biocompatibility, stability, and high-throughput capabilities, showing that hepatocytes encapsulated in CHCs exhibited enhanced viability, growth, and liver-specific functions (50). Tirella’s study presented a protein/hydrogel formulation as a novel encapsulation choice, enhancing nutrient exchange and providing a 3D adhesive framework for cells. This study also included encapsulating ratiometric optical nanosensors within hepatocytes to monitor microenvironmental pH changes under stress, noting improved albumin secretion and urea production in encapsulated hepatocytes compared to controls (51). Khanal et al. developed a method for creating polymeric nanofiber-integrated alginate (PNA) hydrogel microcapsules using a Nano-in-micro (NIM) system, with PNA-10 showing optimal support for HepG2 cell growth and maintenance of liver-specific metabolic functions (52). Zheng and colleagues’ research on self-bonding real-time shape-programmable microcapsules via photo-induced electrodeposition (PIED) of cell-laden alginate hydrogel found that pre-coating with fibroblasts led to robust assembly through fibroblast-ECM interactions, closely mimicking tissue morphogenesis. HepG2 cells encapsulated in these new microcapsules showed nearly double the albumin and urea secretion compared to non-fibroblast-coated encapsulations (53). Yu et al. experimented with microcapsules of various inner structures and deformability, finding that hepatocyte viability was consistent across different types, but cell activity was significantly reduced in capsules with lower deformability (54). Cui’s work on spatially assembling gear-like microstructures from photo-crosslinkable poly (ethylene glycol) diacrylate (PEGDA) hydrogel, which co-encapsulated hepatocytes and fibroblasts, resulted in 3D lobule-like micro-architectures with high cell viability and proliferation, significantly enhancing albumin secretion and urea synthesis (55). Liu and colleagues’ study on encapsulated rat liver (RLC-18) cells forming hepatic lobule-shaped microtissue (HLSM) reported superior hepatic-specific functions in these structures compared to normal cell spheroids after 14 days of culture in poly-L-lysine-alginate microcapsules (56). Moriyama et al. developed a method for producing hydrogel microbeads using an octa-thiolated PEG derivative (8-arm PEGSH), which maintained higher levels of specific functions including albumin secretion and urea production when HepG2 cells were encapsulated (57). Agarwal and team’s application of decellularized Caprine liver ECM (CLECM) derived hydrogel for 2D and 3D hepatocyte cultures showed significantly enhanced functions, including albumin, urea, glycogen, and GAGs synthesis, and the formation of bile canaliculi-like structures and better expression of mature hepatocyte markers compared to collagen coatings (58). Zhang et al. investigated the effects of 2-(1’H-indole-3’-carbonyl)-thiazole-4-carboxylic acid methyl ester (ITE) on Huh7 cells/C3A cells in both monolayer cultures and microspheres, noting significant improvements in protein levels and metabolic activities of major cytochrome P450 enzymes (59). Ravichandran et al. explored two methods to generate photocrosslinkable methacrylated liver extracellular matrix (LivMA) hydrogels, finding better cytocompatibility for encapsulated hepatocytes despite different mechanical properties (60). Zhang and colleagues compared hepatocytes encapsulated in lupeol liposomes and Gal-lupeol liposomes, with the latter showing higher cell-uptake and apoptotic efficiency in HepG2 cells, along with reduced expressions of AKT/mTOR-related proteins and markers in vitro and in vivo, demonstrating liver targeting effects in FVB mice (61). Leroux introduced a novel hybrid alginate microcapsule using an aqueous stable titania precursor (TiBALDH) and a cationic polyamine (PDDAC), leading to increased mechanical stability and maintained hepatocyte functions for up to 43 days (62). Sk’s novel synthesis of photo-crosslinkable glycidyl methacrylate (GMA) functionalized gelatins (Gelatin-GMA) enhanced cell growth and cellular functions in Huh-7.5 cells encapsulated in 3D hydrogel scaffolds.
The cultivation environment’s materials for microencapsulated cells significantly impact cell function preservation. Tostoes et al. discovered that liver-specific functions such as urea production, phase I drug metabolizing activity, and oxygen uptake in hepatocytes encapsulated within ultra-high viscous alginate spheroids were substantially improved under a continuous perfusion system compared to a traditional 50% medium change routine, tripling the performance. However, albumin output remained consistent across both feeding methods (63). Sofia P. et al. proposed a three-dimensional culture strategy for HepaRG cells in alginate microcapsules without dimethyl sulfoxide (DMSO), enhancing hepatocyte differentiation significantly over 2D cultures. This approach yielded a higher prevalence of hepatocyte-like over biliary-like cells, alongside improved protein secretion and ammonia detoxification, despite some variance in basal gene expression levels (64).
2.8 Challenges of translating encapsulation materials to clinical use
The diversity of materials used for the microencapsulation of hepatocytes presents a spectrum of opportunities and challenges for clinical translation. Among these, photo-crosslinkable methacrylate-based materials have gained attention for their versatility, tunability, and the precision with which they can be manipulated using light. However, translating such advanced materials into clinical use encompasses several hurdles, particularly concerning safety, efficacy, and regulatory approval.
2.8.1 Safety and biocompatibility
The primary concern with any biomaterial intended for clinical use is its safety and biocompatibility. Photo-crosslinkable methacrylates, while useful in creating stable and customizable encapsulation systems, must be rigorously tested to ensure they do not elicit adverse immune responses, cause inflammation, or release toxic degradation products within the body. Long-term biocompatibility studies are essential to assess the risks of using these materials in humans.
2.8.2 Degradation and clearance
Understanding the degradation behavior of methacrylate-based materials in vivo is crucial. The materials must degrade at a rate that is compatible with tissue healing and regeneration processes without causing obstruction or toxicity. Moreover, the degradation products must be safely metabolizable or excretable by the human body.
2.8.3 Regulatory approval
Gaining regulatory approval for new biomaterials can be a complex and lengthy process. Regulatory bodies, such as the U.S. Food and Drug Administration (FDA) and the European Medicines Agency (EMA), require comprehensive data on the manufacturing process, quality control, safety, and efficacy of the biomaterials. For photo-crosslinkable methacrylates and other novel encapsulation materials, demonstrating compliance with these requirements involves extensive preclinical and clinical testing.
2.8.4 Scalability and consistency
Translating laboratory-scale encapsulation processes to clinical-scale production presents challenges in ensuring scalability, consistency, and cost-effectiveness of the material synthesis and encapsulation procedures. Ensuring that the properties of photo-crosslinkable methacrylates remain consistent across batches is critical for maintaining the reliability of the encapsulation system.
2.8.5 Ethical and legal considerations
The use of synthetic materials in medicine also raises ethical and legal considerations, particularly regarding long-term outcomes and patient consent. Patients must be fully informed of the benefits and risks associated with the use of such materials in treatments. While photo-crosslinkable methacrylate-based materials and other innovative encapsulation strategies offer significant potential for enhancing hepatocyte transplantation therapies, their path to clinical application is paved with challenges. Addressing these requires a multidisciplinary effort, combining insights from materials science, biology, medicine, and regulatory science to ensure that the benefits of these advanced materials can be safely and effectively realized in clinical settings.
2.9 Future directions in hepatocyte encapsulation
Despite the promising attributes of embryonic stem cells, such as high proliferation, renewability, and pluripotency, their differentiation into hepatocytes faces technical challenges, requiring an optimal culture microenvironment. Tim Maguire et al. explored how an alginate-based microenvironment supports cell viability, promotes differentiation, and enhances the functionality of embryonic stem cell-derived hepatocytes, demonstrating urea and albumin synthesis as key functional indicators. This finding suggests a viable alternative for sourcing human hepatocytes for transplantation (65). MacPherson and colleagues designed a 3D scaffold from a non-fibrous hydrogel, emphasizing mechanical properties and nanofiber morphology to enhance hepatocyte culture. Their findings showed stable maintenance of primary human hepatocytes’ viability and functionality, outperforming Matrigel in cytochrome P450 assays (66). Chen et al. assessed the stability of various alginate-based microcapsules in plasma, finding that alginate-α-poly (L-lysine)-alginate (α-APA) microcapsules demonstrated superior stability over alginate-ϵ-poly (L-lysine)-alginate (ϵ-APA) and alginate–chitosan–alginate (ACA) capsules. The stability of these capsules was influenced by different factors, with heparin significantly affecting α-APA microcapsules, while HCO3- and H2PO4-/HPO42- impacted ϵ-APA and ACA capsules, respectively (67). Liu introduced a method for creating porous alginate beads (PABs) using an aqueous two-phase system (ATPS) emulsion technique, blending a cell/dextran (Dex) mixture with an alginate (Alg)/polyethylene glycol (PEG) mixture. This approach allowed for control over the pore size, improving cell activity, proliferation, and function of encapsulated HeLa and human liver cancer cells compared to those in general alginate beads (GABs) (68).
Shogo Nagata et al. devised a technique for encapsulating cells within nucleocapsid hydrogel microfibers, creating a fibrous 3D ECM-rich microenvironment suitable for in vitro liver tissue formation. Induced pluripotent stem cell-derived hepatocytes (iPSC-hepatocytes) in this setup displayed liver-specific characteristics, including albumin secretion and liver marker gene expression, and maintained structural stability, indicating their potential for liver failure rescue. Transplantation of these microfibers into immunodeficient mice showed human albumin presence in peripheral blood after three days, confirming their viability and function as implants (69). Further details are presented in Table 1.
3 Enhancing hepatocyte viability and functionality
Ensuring high activity levels in hepatocytes is crucial for their ability to substitute for failing liver functions. However, hepatocytes are notably delicate, with even minor damages potentially leading to cell death and loss of activity. For microencapsulated liver cells to fulfill their intended roles post-transplantation, preserving their viability and functionality becomes a critical concern.
3.1 Hepatocyte cryopreservation
The primary strategy for the long-term storage of microencapsulated hepatocytes is cryopreservation. The effectiveness of this method and the ability of hepatocytes to resume their functions upon thawing are areas of active research. Mai and colleagues demonstrated that primary rodent hepatocytes could retain their synthetic functions temporarily through encapsulation and cryopreservation as early as 2005 (70). Despite the influence of immortalization on certain hepatocyte-specific functions remains questionable, which is to remove the upper limit of cell proliferation set by telomerase by either gene reactivation or deactivation, their findings suggested that both naïve and genetically modified hepatocytes could maintain metabolic functions and improve survival rates in xenogeneic recipients with liver failure when encapsulated, cryopreserved, and then transplanted, marking a significant advancement in hepatocyte therapy.
A subsequent study by Hang focused on the functional recovery of hepatocytes after cryopreservation (71). Results showed that pre-incubation at 4°C for 12–24 hours, followed by encapsulation in alginate–poly-L-lysine–alginate microcapsules, significantly enhanced hepatocyte functions, including mRNA and protein levels, as well as albumin and urea secretion post-thawing. The morphology and albumin production of post-thaw hepatocytes closely matched those of directly cultured groups over several days, underscoring the reliability of cryopreservation for hepatocyte storage despite potential risks to cell viability and functionality.
Kilbride et al.’s research revealed that alginate-encapsulated HepG2 liver microcapsules subjected to cryopreservation and subsequent short-term exposure to temperatures below 10°C from 1 to 90 minutes showed increased cell proliferation during 7-16 days of culture (72). This method presents a more efficient and cost-effective approach to achieving higher cell densities (73).
Recent work by Jitraruch et al. identified a pan-caspase inhibitor (ZVAD) that enhances the ultrastructure of cryopreserved hepatocyte microbeads and reduces cell apoptosis when combined with other cytoprotectants such as des-feroxamine (DFO), and human serum albumin (HSA) in the cryopreservation process (74). This improved cryopreservation technique optimizes the use of hepatocytes for emergency applications.
3.2 Alternative strategies for sustaining hepatocyte function
The liver’s metabolic capacity is immense, capable of processing nutrients as well as detoxifying substances and drugs. Koizumi et al. were the first to demonstrate that primary rat hepatocytes retain their drug metabolism and transport activities post-cryopreservation when encapsulated (75). Activities of a specific drug-metabolizing enzyme (CYP3A2) and drug transport for several substrates were maintained up to 120 days using a novel cryopreservation technique developed by the researchers.
Encapsulation aims to shield hepatocytes from the host’s immune system, yet the release of bioactive molecules from hepatocytes can potentially trigger immune responses (31). The extent of this reaction depends on the encapsulation material’s permeability and the host’s sensitivity. In animal studies, Baldini et al. showed that long-term cryopreserved encapsulated porcine hepatocytes maintained significant activity and viability when transplanted into rats without immunosuppression (4). Although the ultrastructure and morphological activity of encapsulated hepatocytes were maintained post-explant, albumin synthesis was adversely affected, indicating a need for further improvements in maintaining bio-activity post-transplantation. Maximizing the use of donor livers involves isolating hepatocytes for propagation, making the retention of their functional capabilities post-isolation critically important.
Another investigation intended to test efficacy of bioartificial liver device in 2014. In this investigation, the evaluation of Alginate-chitosan microencapsulated hepatocytes’ bioactivity was based on several metrics: cell proliferation, efficiency in ammonia detoxification, albumin production, and the rate of diazepam metabolism. The findings highlighted that, with the exception of cell proliferation which remained constant, immortalized human hepatocytes (HepLL) groups demonstrated superior performance in ammonia detoxification, albumin production, and diazepam metabolism compared to the HepG2 groups across all time points (29). Additionally, the viability of hepatocytes in spinner cultures showed variability over time, with day 10 marking the peak of cell growth, metabolic activity, and functionality.
Yamada et al. introduced a culture and encapsulation technique utilizing a Thermo-reversible gelation polymer (TGP), which transitions from solid to liquid states with temperature changes. This study revealed that hepatocytes encapsulated in TGP maintained over 70% viability after being cryopreserved in liquid nitrogen. Post-transplantation into the rat spleen, these hepatocytes were capable of performing liver-specific functions and secreting albumin (23).
Li and colleagues devised a method for hepatocyte encapsulation that involved micropatterning on collagen I to direct cell–cell interactions in two dimensions, followed by the formation of stable aggregates through collagenase digestion for three-dimensional encapsulation in polyethylene glycol (PEG) diacrylate. This configuration preserved the encapsulated hepatocytes’ specific functions for up to 50 days (27).
Lu and associates developed an innovative diversion-type microcapsule-suspension fluidized bed bioreactor (DMFBB), offering several enhancements over the traditional fluidized bed bioreactor (FBB), especially under conditions of high perfusion velocity. The research noted a significant reduction in the void volume of alginate/chitosan microcapsules and lower damage rates during the fluidization process in the DMFBB. It was observed that encapsulated C3A cells exhibited higher survival rates and activities of CYP1A2 and CYP3A4 in the DMFBB, though improvements in albumin and urea synthesis were modest. Additionally, there was a notable upregulation in the transcription levels of various CYP450-related genes and an albumin-related gene in C3A cells within the DMFBB (24).
3.3 Enhancing hepatocyte function through co-microencapsulation
As hepatocyte transplantation emerges as a viable option for treating acute liver failure, the sustained activity and functionality of individual hepatocytes until the point of liver transplantation remain challenges. Recognizing that the liver comprises not only hepatocytes but also non-parenchymal derivatives, which provide essential structural, biochemical support, and nutrients, underscores the pivotal role mesenchymal cells play in vivo. Their presence is crucial for supporting the physiological activities of hepatocytes. This understanding has spurred interest in the co-culture and co-microencapsulation of hepatocytes with various mesenchymal cells to augment hepatocyte survival and functionality, particularly for cell transplantation applications primarily investigated in animal models. While co-culture and co-microencapsulation differ significantly, insights from hepatocyte co-culture studies offer valuable perspectives for advancing co-microencapsulation strategies. Initial investigations by Rahman et al. demonstrated the protective effects of co-encapsulating HepG2 cells with Sertoli cells in animal models of acute hepatic failure (AHF), achieving localized immunosuppression and enhancing HepG2 cell survival post-intraperitoneal injection in rats (76). This approach suggested a novel strategy for cell transplantation, potentially reducing rejection risks by locally generating immunosuppressive environments.
Zheng et al. conducted further research to ascertain the efficacy of co-microencapsulating Sertoli cells with HepG2 cells in a rat model, aiming to establish a method of local immunosuppression facilitated by the unique immunoprivileged nature of Sertoli cells (30). Their findings indicated that such co-microencapsulation could enhance the function and bioactivity of hepatocytes in models of acute liver failure, offering a more effective solution than either mixed or solely microencapsulated hepatocytes and Sertoli cells.
Moreover, Liu and collaborators explored the potential of co-encapsulating hepatocytes with bone marrow stem cells using a novel two-step cell encapsulation technique. This method proved to enhance hepatocyte viability and support liver function in models of acute liver failure (34). Compared to traditional single-step encapsulation, this innovative approach resulted in extended hepatocyte viability beyond four months post-transplantation, with a noticeable reduction in host reaction and improved hepatocyte function due to the synergistic effects of co-encapsulation with bone marrow cells. Further investigations confirmed the superior viability and functionality of this co-encapsulation strategy both in vitro and in vivo, demonstrating its capacity to ameliorate conditions like hyperbilirubinemia in Gunn rats post-transplantation (36).
Isoda et al. identified bone marrow stromal cells (BMSCs) as another promising candidate for hepatocyte co-culture, showing significant support for differentiated hepatocyte functions, notably in enhancing urea synthesis and albumin secretion (37). Their sandwich-like co-culture model, comprising a monolayer of BMSCs, a semi-permeable membrane, and freshly isolated hepatocytes, revealed the critical role of interleukin-6 in maintaining these key hepatocyte functions.
The exploration of mesenchymal stem cells (MSCs), known for their limited self-renewal while multidirectional differentiation capabilities, has become a focal point of recent research (40). The minimal ethical and legal hurdles associated with MSCs, coupled with their easy extraction from various sources including umbilical cord, endometrial polyps, menstrual blood, bone marrow, and adipose tissue, make them a compelling option for experiments in hepatocyte co-encapsulation. Shi and colleagues highlighted the potential of bone marrow mesenchymal stem cells (BM-MSCs) in enhancing hepatocyte functionality when co-encapsulated, observing significant improvements in hepatocyte survival, liver function, and cellular changes post-transplantation using immunofluorescence microscopy. This study illustrated not only an increase in the specific functions of hepatocytes, such as albumin secretion and urea synthesis, but also an enhancement in cell cycle progression in vitro. Furthermore, hepatocyte transplantation strategies incorporating co-encapsulation demonstrated enhanced viability and bioactivity in rats models of acute liver failure, with MSCs potentially differentiating into hepatocyte-like cells and assuming liver metabolic functions (25).
Fitzpatrick et al. explored the advantages of coculturing human MSCs with hepatocytes, observing significant benefits in hepatocyte functions and viability when in direct or indirect contact with MSCs (26). This interaction notably increased albumin and urea production, with peak effects observed around day 15 for albumin. The study confirmed that coculturing hepatocytes with MSCs could enhance hepatocyte viability by up to 16%, suggesting a promising approach for cell transplantation.
Yang et al. developed a tissue engineering-based platform using cell-laden microbeads in a 3D printed tubular perfusion bioreactor, finding that co-encapsulation of human hepatocytes with collagen and MSCs resulted in improved cell activity and maintenance of parenchymal cell functions for up to 30 days (77). This setup facilitated better oxygen and medium diffusion, vital for sustaining cell vitality. Montanari and colleagues focused on the coculture and co-microencapsulation of porcine hepatocytes with human MSCs, identifying a beneficial role of MSCs in enhancing hepatocyte bioactivity and function (28). Their findings indicated that while hepatocyte viability may initially decrease, coencapsulation with MSCs led to sustained albumin secretion and diazepam metabolism, underlining the positive impact of MSCs on hepatocyte functionality.
Iansante et al. established a high-throughput system for cell encapsulation research, enabling the comparison of various conditions such as cell numbers, combinations, and alginate modifications (39). Their platform revealed that MSCs could notably improve the behavior and function of hepatocyte microcapsules. This enhancement was further validated through low-throughput analysis, underscoring the promising role of MSCs in boosting hepatocyte function.
Kong and colleagues demonstrated the therapeutic effects of co-encapsulating hepatocytes with HNF4α-overexpressing human umbilical cord MSCs (HNF4α-UMSCs) in models of acute liver failure (33). Their research showed that HNF4α-UMSCs could significantly enhance hepatocyte microbead functions and accelerate M2 macrophage polarization, potentially reducing the inflammatory response through the paracrine factor HB-EGF secreted by HNF4α-UMSCs. This study not only confirmed the functional benefits of co-encapsulation but also highlighted the underlying mechanisms contributing to improved outcomes in acute liver failure treatment.
Gao et al. explored co-encapsulation of hepatocytes with islets to create a bioartificial liver support system, showing a marked improvement in survival rates and biochemical parameters in ALF mice models (32). Takayama et al. discovered that co-culturing hepatocytes with human umbilical vein endothelial cells (HUVECs) enhances cellular functions, attributing this improvement to increased expression of certain genes linked to cell-to-cell communication (38). This insight into gene expression dynamics underlines the potential of co-culture systems in advancing liver tissue engineering.
Kim and colleagues developed a co-culture system based on cell sheets, demonstrating sustained albumin secretion and enhanced expression of hepatocyte-specific genes, thus significantly preserving hepatocyte functions (41). Nishikawa et al. showed that co-cultivating rat hepatocytes with NIH/3T3 fibroblasts on collagen-immobilized PDMS membranes enhances growth and function, notably albumin secretion, by providing ample oxygen (35).
Kukla et al. found that co-encapsulation of primary human hepatocytes with supportive fibroblasts significantly improves hepatic functions and gene expression, highlighting the benefits of incorporating 3T3-J2 murine embryonic fibroblasts or primary human hepatic stellate cells (HSCs) (78). Zhang et al.’s research on co-encapsulating hepatocytes with adipose-derived stem cells (ADSCs) demonstrated dramatic advantages in enhancing hepatocyte functions and viability, suggesting a potent cell-based therapy for liver failure (79). Teng and colleagues introduced a strategy employing rat hepatocytes and human fetal liver stromal cells (hFLSCs) for acute liver failure treatment, showing that the co-encapsulated approach significantly improves survival and hepatic function, partly due to the release of basic fibroblast growth factor (bFGF) (80). Qiu et al. confirmed the efficacy of co-encapsulating hepatocytes with HUVECs in treating fulminant hepatic failure (FHF), observing improved biochemical parameters and reduced mortality in rat models (81).
Liu’s study on co-encapsulating human hepatocyte-derived liver progenitor-like cells (HepLPCs) with HUVECs highlighted the potential of this approach in ameliorating liver injury in mice, facilitated by the secretion of glial cell line-derived neurotrophic factor (GDNF) from HUVECs (82). Song et al. demonstrated that co-encapsulation of human induced pluripotent stem cell-derived hepatocyte-like cells with stromal cells in hydrogel capsules maintains human albumin and α1-antitrypsin levels effectively in mouse sera, mirroring the performance of primary hepatocyte aggregates (83). Most recently, Xiang Yuan’s research on proliferating human hepatocytes (ProliHHs) revealed that Encapsulated ProliHHs could be engineered, intraperitoneally transplanted to those liver-failure animals, causing liver functions to reinforce though alleviated hyperammonemia and hypoglycemia, leading to less severe post-hepatectomy liver failure (PHLF) with minimal inflammatory response, adverse effects or tumorigenic (84).
These findings collectively underscore the vast potential of co-encapsulation strategies in enhancing hepatocyte functionality and viability, offering new avenues for liver failure treatment and tissue engineering. Further details are summarized in Table 2.
4 The impact of xenotransplantation
With hepatocyte transplantation emerging as a notable strategy for addressing acute liver diseases, the scarcity of human liver donations has prompted the exploration of xenotransplantation. This approach, involving the transplantation of hepatocytes from other species, offers a potential solution to the shortage of allogeneic hepatocytes. However, numerous challenges, including immunological rejection and the potential for anaphylactic reactions to xenoproteins, necessitate further investigation. Studies have shown that encapsulation can enhance the survival and function of both fresh and cryopreserved porcine hepatocytes in models of fulminant liver failure (85). These studies revealed two critical phases: an in vitro decline in metabolic functions over a week post-transplantation and an in vivo extension of survival rates and maintenance of metabolic functions in encapsulated hepatocytes compared to non-encapsulated controls. Sgrio et al. discovered that encapsulated human hepatocytes, immortalized to stabilize metabolic functions, could substantially support metabolism and mitigate liver regeneration inhibition in acute liver failure models by reducing inflammatory stress (86). This dual approach highlights the need for distinct research focuses on metabolic function and regeneration in acute liver failure studies. Furthermore, encapsulated transplantation was found to significantly reduce cytokine levels, illustrating a decrease in inflammatory stress and a restraint on the regeneration of remaining hepatocytes (87).
Investigations into porcine hepatocytes as a xenotransplantation source have identified potential limitations, including safety concerns related to porcine endogenous retroviruses. Despite these challenges, studies have demonstrated therapeutic effects of encapsulated porcine hepatocytes in rodent and non-human primate models of fulminant liver failure (88). The use of neonatal pig re-aggregated liver cells (NPRLCs) has shown promise in improving survival rates and metabolic function in acute liver failure models, suggesting an alternative to alleviate the human hepatocyte shortage (89).
Machaidze et al. explored the transplantation of encapsulated miniature swine hepatocytes in baboons with fulminant liver failure, revealing a temporary support to liver metabolism and a restoration of normal liver functions in the majority of the treated animals (82). This indicates a viable method for large mammal xenotransplantation. Varaa et al. examined the effects of umbilical cord stem cells (UCSCs) and UCSC-derived hepatocyte-like cells (HLCs) encapsulated in high mannuronic alginate scaffolds on acute liver failure models, showcasing significant improvements in liver function markers (90).
Xenotransplantation research suggests that while xenohepatocytes offer a readily available solution to hepatocyte scarcity, the immunorejection challenge remains significant. Therefore, less immunogenic transgenic pigs and innovative cell encapsulation techniques are being considered as future research directions to address these hurdles (91). This overview underscores the complexities and potential of xenotransplantation in hepatocyte therapy, emphasizing the need for ongoing investigation into improving viability and functionality through advanced encapsulation methods and genetic modifications. Details on these studies are summarized in Table 2.
5 Clinical applications and future prospects
Although encapsulated hepatocyte technology has demonstrated promising results in vitro and in animal models, its transition to clinical applications presents a horizon ripe with potential. The clinical implications of hepatocyte encapsulation span several critical areas in liver failure treatment and regenerative medicine.
One primary application envisaged for encapsulated hepatocytes is in the development of a bioartificial liver device (BAL). Such devices aim to provide temporary liver support for patients with acute liver failure, bridging the gap to liver regeneration or transplantation. Encapsulated hepatocytes within BALs offer a biocompatible and immunoprotected environment, which could enhance cell function and longevity, thus improving the therapeutic efficacy of these devices. Moreover, the potential for allogeneic or xenogeneic cell transplantation without the need for lifelong immunosuppression could revolutionize the treatment landscape for liver diseases. The microencapsulation technique acts as a barrier to immune cells while allowing the exchange of nutrients and metabolic waste, making it a promising approach for cell transplantation therapies.
Clinical trials exploring the efficacy and safety of encapsulated hepatocyte transplantation are crucial next steps. Such studies will help determine the optimal cell sources, encapsulation materials, and transplantation protocols. Additionally, understanding the long-term outcomes of these interventions, including the risk of potential complications and the durability of treatment effects, is essential. Furthermore, integrating advances in biomaterials and stem cell technology could enhance the clinical applicability of hepatocyte encapsulation. For instance, the use of stem cell-derived hepatocytes for encapsulation could overcome the limitations associated with donor cell availability. Innovations in encapsulation materials that mimic the liver extracellular matrix could further support hepatocyte function and integration post-transplantation. In conclusion, while encapsulated hepatocytes herald a promising frontier in liver failure therapy, significant efforts in clinical research and technology development are necessary to translate these experimental approaches into viable clinical solutions. The progression of encapsulated hepatocyte technology into clinical trials and ultimately clinical practice will require multidisciplinary collaboration among scientists, clinicians, and regulatory bodies to ensure safety, efficacy, and patient accessibility.
6 Summary and future directions
This review has delved into the advancements in hepatocyte encapsulation research, emphasizing the strides made in preserving hepatocyte viability, the innovative approach of co-microencapsulation, the exploration of xenotransplantation, and the development of novel encapsulation materials. Hepatocyte transplantation represents a promising avenue for mitigating the immune response challenges and addressing the scarcity of liver donations, offering a beacon of hope for numerous patients. The preservation of hepatocyte activity prior to transplantation is predominantly managed through encapsulation techniques, cryopreservation, enzyme inhibitors, and immunosuppressive agents. Future investigations could enhance these methods by fine-tuning temperature controls and elucidating the roles of specific enzymes in the longevity and functionality of transplanted hepatocytes.
The strategy of co-encapsulating hepatocytes with various cell types, such as Sertoli cells, bone marrow mesenchymal stem cells (MSCs), fibroblasts, adipose-derived stem cells, and human umbilical vein endothelial cells (HUVECs), has shown to enhance the survival and functionality of hepatocyte transplants. However, the underlying mechanisms of these co-encapsulation benefits remain to be fully understood and warrant further exploration. Xenotransplantation has emerged as a viable strategy to broaden the donor pool for hepatocyte transplantation. Research has predominantly focused on encapsulating and transplanting porcine or human liver cells into models of liver failure. These preclinical endeavors have demonstrated notable improvements in the functionality of transplanted liver cells. Advancements in the materials used for hepatocyte microencapsulation have also been significant, ranging from the optimization of traditional substances like alginate to the introduction of novel materials and structures for hepatocyte microcapsules, as well as refining the pre-encapsulation cell culture environments.
Last but not least, some of the accomplishments in animal and human-cell based studies are still in need of a more cautious attitude towards clinical achievement in reality. Many experiments take advantage of the marker serum albumin to check cell viability, which indeed has a long half-life does not fit in a lot. Investigation needs to be done on what actually happens to the liver cells from stem cells to its ultimate form due to their unique ability of regeneration, possibly using the Flow Cytometry techniques (92).
Looking ahead, hepatocyte microencapsulation research should aim to diversify the sources of transplantable cells, minimize the need for immunosuppression, and enhance the survival and functionality of transplanted hepatocytes. Such efforts will not only extend the applicability and safety of hepatocyte microencapsulation techniques but will also provide greater insights into liver cell biology and transplantation methodologies, ultimately benefiting a wider spectrum of patients with liver failure.
Author contributions
HW: Writing – original draft. FJ: Writing – original draft. PR: Writing – original draft. YY: Writing – review & editing. SS: Writing – review & editing. ZY: Funding acquisition, Writing – review & editing. YW: Conceptualization, Funding acquisition, Project administration, Writing – review & editing. LW: Writing – original draft.
Funding
The author(s) declare financial support was received for the research, authorship, and/or publication of this article. This research was supported by the National Natural Science Foundation of China (81802504), grants from the Sichuan Science and Technology Bureau (2021YFH0384 and 2023YFH0010), and a grant from the Guangxi Science and Technology Base and Talent Special Project (AD23023011).
Conflict of interest
The authors declare the research was conducted in the absence of any commercial or financial relationships that could be construed as a potential conflict of interest.
Publisher’s note
All claims expressed in this article are solely those of the authors and do not necessarily represent those of their affiliated organizations, or those of the publisher, the editors and the reviewers. Any product that may be evaluated in this article, or claim that may be made by its manufacturer, is not guaranteed or endorsed by the publisher.
References
1. Nguyen MP, Jain V, Iansante V, Mitry RR, Filippi C, Dhawan A. Clinical application of hepatocyte transplantation: current status, applicability, limitations, and future outlook. Expert Rev Gastroenterol Hepatol. (2020) 14:185–96. doi: 10.1080/17474124.2020.1733975
2. Sun Z, Yuan X, Wu J, Wang C, Zhang K, Zhang L, et al. Hepatocyte transplantation: The progress and the challenges. Hepatol Commun. (2023) 7(10):e0266. doi: 10.1097/HC9.0000000000000266
3. Hansel MC, Gramignoli R, Skvorak KJ, Dorko K, Marongiu F, Blake W, et al. The history and use of human hepatocytes for the treatment of liver diseases: the first 100 patients. Curr Protoc Toxicol. (2014) 62:14 12 1–23. doi: 10.1002/0471140856.tx1412s62
4. Baldini E, Cursio R, Sousa De G, Margara A, Honiger J, Saint-Paul M-C, et al. Peritoneal implantation of cryopreserved encapsulated porcine hepatocytes in rats without immunosuppression: viability and function. Transplant Proc. (2008) 40:2049–52. doi: 10.1016/j.transproceed.2008.05.038
5. Canaple L, Rehor A, Hunkeler D. Improving cell encapsulation through size control. J Biomater Sci Polym Ed. (2002) 13:783–96. doi: 10.1163/156856202760197410
6. Mao SA, Glorioso JM, Nyberg SL. Liver regeneration. Transl Res. (2014) 163:352–62. doi: 10.1016/j.trsl.2014.01.005
7. Fitzpatrick E, Filippi C, Jagadisan B, Shivapatham D, Anand H, Lyne M, et al. Intraperitoneal transplant of Hepatocytes co-Encapsulated with mesenchymal stromal cells in modified alginate microbeads for the treatment of acute Liver failure in Pediatric patients (HELP)-An open-label, single-arm Simon’s two stage phase 1 study protocol. PloS One. (2023) 18:e0288185. doi: 10.1371/journal.pone.0288185
8. Miranda JP, Rodrigues A, Tostões RM, Leite S, Zimmerman H, Carrondo MJT, et al. Extending hepatocyte functionality for drug-testing applications using high-viscosity alginate-encapsulated three-dimensional cultures in bioreactors. Tissue Eng Part C Methods. (2010) 16:1223–32. doi: 10.1089/ten.tec.2009.0784
9. Nino-Vasquez IA, Muñiz-Márquez D, Ascacio-Valdés JA, Contreras-Esquivel JC, Aguilar CN, Rodríguez-Herrera R, et al. Co-microencapsulation: a promising multi-approach technique for enhancement of functional properties. Bioengineered. (2022) 13:5168–89. doi: 10.1080/21655979.2022.2037363
10. Tran NM, Dufresne M, Helle F, Hoffmann TW, François C, Brochot E, et al. Alginate hydrogel protects encapsulated hepatic HuH-7 cells against hepatitis C virus and other viral infections. PloS One. (2014) 9:e109969. doi: 10.1371/journal.pone.0109969
11. Lan SF, Safiejko-Mroczka B, Starly B. Long-term cultivation of HepG2 liver cells encapsulated in alginate hydrogels: a study of cell viability, morphology and drug metabolism. Toxicol In Vitro. (2010) 24:1314–23. doi: 10.1016/j.tiv.2010.02.015
12. Azandeh S, Nejad DB, Bayati V, Shakoor F, Varaa N, Cheraghian B. High mannoronic acid containing alginate affects the differentiation of Wharton’s jelly-derived stem cells to hepatocyte-like cell. J Adv Pharm Technol Res. (2019) 10:9–15. doi: 10.4103/japtr.JAPTR_312_18
13. Jitraruch S, Dhawan A, Hughes RD, Filippi C, Soong D, Philippeos C, et al. Alginate microencapsulated hepatocytes optimised for transplantation in acute liver failure. PloS One. (2014) 9:e113609. doi: 10.1371/journal.pone.0113609
14. Pasqua M, Pereira U, Messina A, Lartigue C, Vigneron P, Dubart-Kupperschmitt A, et al. HepaRG self-assembled spheroids in alginate beads meet the clinical needs for bioartificial liver. Tissue Eng Part A. (2020) 26:613–22. doi: 10.1089/ten.tea.2019.0262
15. Durkut S, Elcin AE, Elcin YM. In vitro evaluation of encapsulated primary rat hepatocytes pre- and post-cryopreservation at -80 degrees C and in liquid nitrogen. Artif Cells Nanomed Biotechnol. (2015) 43:50–61. doi: 10.3109/21691401.2013.837476
16. Capone SH, Dufresne M, Rechel M, Fleury M-J, Salsac A-V, Paullier P, et al. Impact of alginate composition: from bead mechanical properties to encapsulated HepG2/C3A cell activities for in vivo implantation. PloS One. (2013) 8:e62032. doi: 10.1371/journal.pone.0062032
17. Nayak S, Dey S, Kundu SC. Silk sericin-alginate-chitosan microcapsules: hepatocytes encapsulation for enhanced cellular functions. Int J Biol Macromol. (2014) 65:258–66. doi: 10.1016/j.ijbiomac.2014.01.042
18. Tian M, Han B, Tan H, You C. Preparation and characterization of galactosylated alginate-chitosan oligomer microcapsule for hepatocytes microencapsulation. Carbohydr Polym. (2014) 112:502–11. doi: 10.1016/j.carbpol.2014.06.025
19. He Y, Liu C, Xia X, Liu L. Conformal microcapsules encapsulating microcarrier-L02 cell complexes for treatment of acetaminophen-induced liver injury in rats. J Mater Chem B. (2017) 5:1962–70. doi: 10.1039/C6TB03033E
20. Siltanen C, Diakatou M, Lowen J, Haque A, Rahimian A, Stybayeva G, et al. One step fabrication of hydrogel microcapsules with hollow core for assembly and cultivation of hepatocyte spheroids. Acta Biomater. (2017) 50:428–36. doi: 10.1016/j.actbio.2017.01.010
21. Khodabakhsh Aghdam S, Khoshfetrat AB, Rahbarghazi R, Jafarizadeh-Malmiri H, Khaksar M. Collagen modulates functional activity of hepatic cells inside alginate-galactosylated chitosan hydrogel microcapsules. Int J Biol Macromol. (2020) 156:1270–8. doi: 10.1016/j.ijbiomac.2019.11.164
22. Zhang Y, Lu J, Li Z, Zhu D, Yu X, Li L. Enhanced cellular functions of hepatocytes in the hyaluronate-alginate-chitosan microcapsules. Int J Artif Organs. (2021) 44:340–9. doi: 10.1177/0391398820959345
23. Yamada K, Aoki T, Enami Y, Tashiro Y, Zehaou Z, Koizumi T, et al. An improved encapsulation method for cryopreserving hepatocytes for functional transplantation using a thermo-reversible gelation polymer. In Vivo. (2020) 34:2309–16. doi: 10.21873/invivo.12043
24. Lu J, Zhang X, Li J, Yu L, Chen E, Zhu D. A new fluidized bed bioreactor based on diversion-type microcapsule suspension for bioartificial liver systems. PloS One. (2016) 11:e0147376. doi: 10.1371/journal.pone.0147376
25. Shi XL, Zhang Y, Gu J-Y, Ding Y-T. Coencapsulation of hepatocytes with bone marrow mesenchymal stem cells improves hepatocyte-specific functions. Transplantation. (2009) 88:1178–85. doi: 10.1097/TP.0b013e3181bc288b
26. Fitzpatrick E, Wu Y, Dhadda P, Hughes RD, Mitry RR, Qin H, et al. Coculture with mesenchymal stem cells results in improved viability and function of human hepatocytes. Cell Transplant. (2015) 24:73–83. doi: 10.3727/096368913X674080
27. Li CY, Stevens KR, Schwartz RE, Alejandro BS, Huang JH, Bhatia SN. Micropatterned cell-cell interactions enable functional encapsulation of primary hepatocytes in hydrogel microtissues. Tissue Eng Part A. (2014) 20:2200–12. doi: 10.1089/ten.tea.2013.0667
28. Elisa M, Pimenta J, Szabó L, Noverraz F, Passemard S, Meier RPH, et al. Beneficial effects of human mesenchymal stromal cells on porcine hepatocyte viability and albumin secretion. J Immunol Res. (2018) 2018:1078547. doi: 10.1155/2018/1078547
29. Chen Y, Yu C, Lv G, Cao H, Yang S, Zhang Y, et al. Rapid large-scale culturing of microencapsulated hepatocytes: a promising approach for cell-based hepatic support. Transplant Proc. (2014) 46:1649–57. doi: 10.1016/j.transproceed.2014.03.002
30. Zheng MH, Lin H-L, Qiu L-X, Cui Y-L, Sun Q-F, Chen Y-P. Mixed microencapsulation of rat primary hepatocytes and Sertoli cells improves the metabolic function in a D-galactosamine and lipopolysaccharide-induced rat model of acute liver failure. Cytotherapy. (2009) 11:326–9. doi: 10.1080/14653240802582091
31. Satta S, Shahabipour F, Gao W, Lentz SR, Perlman S, Ashammakhi N, et al. Engineering viral genomics and nano-liposomes in microfluidic platforms for patient-specific analysis of SARS-CoV-2 variants. Theranostics. (2022) 12:4779–90. doi: 10.7150/thno.72339
32. Gao Y, Xu J, Sun B, Jiang H-C. Microencapsulated hepatocytes and islets as in vivo bioartificial liver support system. World J Gastroenterol. (2004) 10:2067–71. doi: 10.3748/wjg.v10.i14.2067
33. Kong D. Co-encapsulation of HNF4α overexpressing UMSCs and human primary hepatocytes ameliorates mouse acute liver failure. Stem Cell Res Ther. (2020) 11(1):449. doi: 10.1186/s13287-020-01962-7
34. Liu ZC, Chang T, I. Biotechnology. Increased viability of transplanted hepatocytes when hepatocytes are co-encapsulated with bone marrow stem cells using a novel method. Artif Cells Blood Substit Immobil Biotechnol. (2002) 30(2):99–112. doi: 10.1081/BIO-120003191
35. Nishikawa M, Kojima N, Komori K, Yamamoto T, Fujii T, Sakai Y. Enhanced maintenance and functions of rat hepatocytes induced by combination of on-site oxygenation and coculture with fibroblasts. J Biotechnol. (2008) 133:253–60. doi: 10.1016/j.jbiotec.2007.08.041
36. Liu ZC, Chang TMS. Coencapsulation of hepatocytes and bone marrow stem cells: in vitro conversion of ammonia and in vivo lowering of bilirubin in hyperbilirubemia Gunn rats. Int J Artif Organs. (2003) 26(6):491–7. doi: 10.1177/039139880302600607
37. Isoda K, Kojima M, Takeda M, Higashiyama S, Kawase M, Yagi K. Maintenance of hepatocyte functions by coculture with bone marrow stromal cells. J Biosci Bioengineering. (2004) 97:343–6. doi: 10.1263/jbb.97.343
38. Goh Takayama AT, Okano T. Identification of differentially expressed genes in hepatocyte/endothelial cell co-culture system. J Tissue Eng. (2007) 13:159. doi: 10.1089/ten.2007.13.159
39. Valeria I, Dhawan A, Masmoudi F, Lee CA, Fernandez-Dacosta R, Walker S, et al. A new high throughput screening platform for cell encapsulation in alginate hydrogel shows improved hepatocyte functions by mesenchymal stromal cells co-encapsulation. Front Med. (2018) 5:216. doi: 10.3389/fmed.2018.00216
40. Ding DC, Shyu WC, Lin SZ. Mesenchymal stem cells. Cell Transplant. (2011) 20:5–14. doi: 10.3727/096368910X
41. Kim K, Ohashi K, Utoh R, Kano K, Okano T. Preserved liver-specific functions of hepatocytes in 3D co-culture with endothelial cell sheets. Biomaterials. (2012) 33:1406–13. doi: 10.1016/j.biomaterials.2011.10.084
42. Chan HF, Zhang Y, Leong KW. Efficient one-step production of microencapsulated hepatocyte spheroids with enhanced functions. Small. (2016) 12:2720–30. doi: 10.1002/smll.201502932
43. Lee HJ, Son MJ, Ahn J, Oh SJ, Lee M, Kim A, et al. Elasticity-based development of functionally enhanced multicellular 3D liver encapsulated in hybrid hydrogel. Acta Biomater. (2017) 64:67–79. doi: 10.1016/j.actbio.2017.09.041
44. Cui J, Wang H, Shi Q, Sun T, Huang Q, Fukuda T. Multicellular co-culture in three-dimensional gelatin methacryloyl hydrogels for liver tissue engineering. Molecules. (2019) 24(9):1762. doi: 10.3390/molecules24091762
45. Chang SH, Huang HH, Kang PL, Wu YC, Chang M-H, Kuo Ming S. In vitro and in vivo study of the application of volvox spheres to co-culture vehicles in liver tissue engineering. Acta Biomater. (2017) 63:261–73. doi: 10.1016/j.actbio.2017.09.028
46. Kim M, Lee JY, Jones CN, Revzin A, Tae G. Heparin-based hydrogel as a matrix for encapsulation and cultivation of primary hepatocytes. Biomaterials. (2010) 31:3596–603. doi: 10.1016/j.biomaterials.2010.01.068
47. Gevaert E, Billiet T, Declercq H, Dubruel P, Cornelissen R. Galactose-functionalized gelatin hydrogels improve the functionality of encapsulated HepG2 cells. Macromol Biosci. (2014) 14:419–27. doi: 10.1002/mabi.201300320
48. Lee HJ, Ahn J, Jung C-R, Jeung Y-J, Cho H-S, Son MJ, et al. Optimization of 3D hydrogel microenvironment for enhanced hepatic functionality of primary human hepatocytes. Biotechnol Bioeng. (2020) 117:1864–76. doi: 10.1002/bit.27328
49. Wang Y, Liu H, Zhang M, Wang H, Chen W, Qin J. One-step synthesis of composite hydrogel capsules to support liver organoid generation from hiPSCs. Biomater Sci. (2020) 8:5476–88. doi: 10.1039/D0BM01085E
50. Tirella A, Marca La M, Brace L-A, Mattei G, Aylott JW, Ahluwalia A, et al. Nano-in-micro self-reporting hydrogel constructs. J BioMed Nanotechnol. (2015) 11:1451–60. doi: 10.1166/jbn.2015.2085
51. Khanal S, Bhattarai SR, Sankar J, Bhandari RK, Macdonald JM, Bhattarai N. Nano-fibre integrated microcapsules: A nano-in-micro platform for 3D cell culture. Sci Rep. (2019) 9:13951. doi: 10.1038/s41598-019-50380-0
52. Zheng Z, Wang H, Li J, Shi Q, Cui J, Sun T, et al. 3D construction of shape-controllable tissues through self-bonding of multicellular microcapsules. ACS Appl Mater Interfaces. (2019) 11:22950–61. doi: 10.1021/acsami.9b05108
53. Yu W, Zhang W, Chen Y, Song X, Tong W, Mao Z, et al. Cellular uptake of poly(allylamine hydrochloride) microcapsules with different deformability and its influence on cell functions. J Colloid Interface Sci. (2016) 465:149–57. doi: 10.1016/j.jcis.2015.11.065
54. Cui J, Wang H, Zheng Z, Shi Q, Sun T, Huang Q, et al. Fabrication of perfusable 3D hepatic lobule-like constructs through assembly of multiple cell type laden hydrogel microstructures. Biofabrication. (2018) 11:015016. doi: 10.1088/1758-5090/aaf3c9
55. Liu Z, Takeuchi M, Nakajima M, Hu C, Hasegawa Y, Huang Q, et al. Three-dimensional hepatic lobule-like tissue constructs using cell-microcapsule technology. Acta Biomater. (2017) 50:178–87. doi: 10.1016/j.actbio.2016.12.020
56. Moriyama K, Naito S, Wakabayashi R, Goto M, Kamiya N. Enzymatically prepared redox-responsive hydrogels as potent matrices for hepatocellular carcinoma cell spheroid formation. Biotechnol J. (2016) 11:1452–60. doi: 10.1002/biot.201600087
57. Agarwal T, Narayan R, Maji S, Ghosh SK, Maiti TK. Decellularized caprine liver extracellular matrix as a 2D substrate coating and 3D hydrogel platform for vascularized liver tissue engineering. J Tissue Eng Regener Med. (2018) 12:e1678–90. doi: 10.1002/term.2594
58. Zhang X, Lu J, He B, Tang L, Liu X, Zhu D, et al. A tryptophan derivative, ITE, enhances liver cell metabolic functions in vitro. Int J Mol Med. (2017) 39:101–12. doi: 10.3892/ijmm.2016.2825
59. Ravichandran A, Murekatete B, Moedder D, Meinert C, Bray LJ. Photocrosslinkable liver extracellular matrix hydrogels for the generation of 3D liver microenvironment models. Sci Rep. (2021) 11:15566. doi: 10.1038/s41598-021-94990-z
60. Zhang J, Hu X, Zheng G, Yao H, Liang H. In vitro and in vivo antitumor effects of lupeol-loaded galactosylated liposomes. Drug Delivery. (2021) 28:709–18. doi: 10.1080/10717544.2021.1905749
61. Leroux G, Neumann M, Meunier CF, Fattaccioli A, Michiels C, Arnould T, et al. Hybrid alginate@TiO2 porous microcapsules as a reservoir of animal cells for cell therapy. ACS Appl Mater Interfaces. (2018) 10:37865–77. doi: 10.1021/acsami.8b15483
62. Sk MM, Das P, Panwar A, Tan LP. Synthesis and characterization of site selective photo-crosslinkable glycidyl methacrylate functionalized gelatin-based 3D hydrogel scaffold for liver tissue engineering. Mater Sci Eng C Mater Biol Appl. (2021) 123:111694. doi: 10.1016/j.msec.2020.111694
63. Tostoes RM, Leite SB, Miranda JP, Sousa M, Wang DIC, Carrondo MJT, et al. Perfusion of 3D encapsulated hepatocytes–a synergistic effect enhancing long-term functionality in bioreactors. Biotechnol Bioeng. (2011) 108:41–9. doi: 10.1002/bit.22920
64. Rebelo SP, Costa R, Estrada M, Shevchenko V, Brito C, Alves PM. HepaRG microencapsulated spheroids in DMSO-free culture: novel culturing approaches for enhanced xenobiotic and biosynthetic metabolism. Arch Toxicol. (2015) 89:1347–58. doi: 10.1007/s00204-014-1320-9
65. Maguire T, Novik E, Schloss R, Yarmush M. Alginate-PLL microencapsulation: effect on the differentiation of embryonic stem cells into hepatocytes. Biotechnol Bioeng. (2006) 93:581–91. doi: 10.1002/bit.20748
66. MacPherson D, Bram Y, Park J, Schwartz RE. Peptide-based scaffolds for the culture and maintenance of primary human hepatocytes. Sci Rep. (2021) 11:6772. doi: 10.1038/s41598-021-86016-5
67. Chen L, Zhang Y, Li S, Wang X, Li N, Wang Y. Effect of plasma components on the stability and permeability of microcapsule. J BioMed Mater Res A. (2014) 102:2408–16. doi: 10.1002/jbm.a.34907
68. Liu T, Yi S, Liu G, Hao X, Du T, Chen J, et al. Aqueous two-phase emulsions-templated tailorable porous alginate beads for 3D cell culture. Carbohydr Polym. (2021) 258:117702. doi: 10.1016/j.carbpol.2021.117702
69. Nagata S, Ozawa F, Nie M, Takeuchi S. 3D culture of functional human iPSC-derived hepatocytes using a core-shell microfiber. PloS One. (2020) 15:e0234441. doi: 10.1371/journal.pone.0234441
70. Mai G, Nguyen TH, Morel P, Mei J, Andres A, Bosco D, et al. Treatment of fulminant liver failure by transplantation of microencapsulated primary or immortalized xenogeneic hepatocytes. Xenotransplantation. (2005) 12:457–64. doi: 10.1111/j.1399-3089.2005.00248.x
71. Hang H, Shi X, Gu G, Wu Y, Gu J, Ding Y. In vitro analysis of cryopreserved alginate-poly-L-lysine-alginate-microencapsulated human hepatocytes. Liver Int. (2010) 30:611–22. doi: 10.1111/liv.2010.30.issue-4
72. Kilbride P, Mahbubani KT, Saeb-Parsy K, Morris GJ. Engaging cold to upregulate cell proliferation in alginate-encapsulated liver spheroids. Tissue Eng Part C Methods. (2017) 23:455–64. doi: 10.1089/ten.tec.2017.0131
73. Koizumi T, Aoki T, Kobayashi Y, Yasuda D, Izumida Y, Jin Z, et al. Long-term maintenance of the drug transport activity in cryopreservation of microencapsulated rat hepatocytes. Cell Transplant. (2007) 16:67–73. doi: 10.3727/000000007783464489
74. Jitraruch S, Dhawan A, Hughes RD, Filippi C, Lehec SC, Glover L, et al. Cryopreservation of hepatocyte microbeads for clinical transplantation. Cell Transplant. (2017) 26:1341–54. doi: 10.1177/0963689717720050
75. Lee JH, Lee DH, Park JK, Kim SK, Kwon CHD, Lee SK. Effect of fulminant hepatic failure porcine plasma supplemented with essential components on encapsulated rat hepatocyte spheroids. Transplant Proc. (2012) 44:1009–11. doi: 10.1016/j.transproceed.2012.01.106
76. Rahman TM, Diakanov I, Selden C, Hodgson H. Co-transplantation of encapsulated HepG2 and rat Sertoli cells improves outcome in a thioacetamide induced rat model of acute hepatic failure. Transpl Int. (2010) 18(8):1001–9. doi: 10.1111/j.1432-2277.2005.00156.x
77. Yang G, Mahadik B, Mollot T, Pinsky J, Jones A, Robinson A, et al. Engineered liver tissue culture in an in vitro tubular perfusion system. Tissue Eng Part A. (2020) 26:1369–77. doi: 10.1089/ten.tea.2020.0213
78. Kukla DA, Crampton AL, Wood DK, Khetani SR. Microscale collagen and fibroblast interactions enhance primary human hepatocyte functions in three-dimensional models. Gene Expr. (2020) 20:1–18. doi: 10.3727/105221620X15868728381608
79. Zhang Y, Chen XM, Sun DL. Effects of coencapsulation of hepatocytes with adipose-derived stem cells in the treatment of rats with acute-on-chronic liver failure. Int J Artif Organs. (2014) 37:133–41. doi: 10.5301/ijao.5000284
80. Teng Y, Wang Y, Li S, Wang W, Gu R, Guo X, et al. Treatment of acute hepatic failure in mice by transplantation of mixed microencapsulation of rat hepatocytes and transgenic human fetal liver stromal cells. Tissue Eng Part C Methods. (2010) 16(5):1125–34. doi: 10.1089/ten.tec.2009.0374
81. Liyuan, Wang J, Wen X, Wang H, Wang Y, Lin Q, et al. Transplantation of co-microencapsulated hepatocytes and HUVECs for treatment of fulminant hepatic failure. Int J Artif Organs. (2012) 35(6):458–65. doi: 10.5301/ijao.5000092
82. Liu WM, Zhou X, Chen C-Y, Lv D-D, Huang W-J, Peng Y, et al. Establishment of functional liver spheroids from human hepatocyte-derived liver progenitor-like cells for cell therapy. Front Bioeng Biotechnol. (2021) 9:738081. doi: 10.3389/fbioe.2021.738081
83. Song W, Lu Y-C, Frankel AS, An D, Schwartz RE, Ma M. Engraftment of human induced pluripotent stem cell-derived hepatocytes in immunocompetent mice via 3D co-aggregation and encapsulation. Sci Rep. (2015) 5:16884. doi: 10.1038/srep16884
84. Yuan X, Wu J, Sun Z, Cen J, Shu Y, Wang C, et al. Preclinical efficacy and safety of encapsulated proliferating human hepatocyte organoids in treating liver failure. Cell Stem Cell. (2024) 31(4):484–98.e5. doi: 10.1016/j.stem.2024.02.005
85. Mei J, Mai G, Baertschiger R, Gonelle-Gispert C, Serre-Beinier V, et al. Improved survival of fulminant liver failure by transplantation of microencapsulated cryopreserved porcine hepatocytes in mice. Cell Transplant. (2009) 18:101–10. doi: 10.3727/096368909788237168
86. Sgroi A, Mai G, Morel P, Baertschiger RM, Gonelle-Gispert C, Serre-Beinier V, et al. Transplantation of Encapsulated Hepatocytes during Acute Liver Failure Improves Survival without Stimulating Native Liver Regeneration. Cell Transplant. (2011) 20:1791–803. doi: 10.3727/096368911X564976
87. Meier RPH, Navarro-Alvarez N, Morel P, Schuurman H-J, Strom S, Bühler LH. Current status of hepatocyte xenotransplantation. Int J Surg. (2015) 23:273–9. doi: 10.1016/j.ijsu.2015.08.077
88. Ham DS, Song M-S, Park H-S, Rhee M, Yang HK, Lee S-H, et al. Successful xenotransplantation with re-aggregated and encapsulated neonatal pig liver cells for treatment of mice with acute liver failure. Xenotransplantation. (2015) 22:249–59. doi: 10.1111/xen.12177
89. Machaidze Z, Yeh H, Wei L, Schuetz C, Carvello M, Sgroi A, et al. Testing of microencapsulated porcine hepatocytes in a new model of fulminant liver failure in baboons. Xenotransplantation. (2017) 24(3). doi: 10.1111/xen.12297
90. Varaa N, Azandeh S, Khorsandi L, Nejad DB, Bayati V, Bahreini A. Ameliorating effect of encapsulated hepatocyte-like cells derived from umbilical cord in high mannuronic alginate scaffolds on acute liver failure in rats. Iran J Basic Med Sci. (2018) 21:928–35. doi: 10.22038/IJBMS.2018.27928.6847
91. Bonavita AG, Quaresma K, Cotta-de-Almeida V, Pinto MA, Saraiva RM, Alves LA. Hepatocyte xenotransplantation for treating liver disease. Xenotransplantation. (2010) 17:181–7. doi: 10.1111/xen.2010.17.issue-3
Keywords: hepatocyte encapsulation, microencapsulation, coculture, xenotransplantation, alginate
Citation: Wang H, Wen L, Jiang F, Ren P, Yang Y, Song S, Yang Z and Wang Y (2024) A comprehensive review of advances in hepatocyte microencapsulation: selecting materials and preserving cell viability. Front. Immunol. 15:1385022. doi: 10.3389/fimmu.2024.1385022
Received: 11 February 2024; Accepted: 28 March 2024;
Published: 17 April 2024.
Edited by:
Lisha Mou, Shenzhen Second People’s Hospital, ChinaReviewed by:
Lingyu Bao, National Institutes of Health (NIH), United StatesJin-Ming Zhang, Capital Medical University, China
Copyright © 2024 Wang, Wen, Jiang, Ren, Yang, Song, Yang and Wang. This is an open-access article distributed under the terms of the Creative Commons Attribution License (CC BY). The use, distribution or reproduction in other forums is permitted, provided the original author(s) and the copyright owner(s) are credited and that the original publication in this journal is cited, in accordance with accepted academic practice. No use, distribution or reproduction is permitted which does not comply with these terms.
*Correspondence: Yi Wang, d195aTIwMjJAMTYzLmNvbQ==; Zhengteng Yang, MzMwNTYwMTY3QHFxLmNvbQ==; Siyuan Song, c2kteXVhbi5zb25nQGJjbS5lZHU=
†These authors have contributed equally to this work