- 1Biotherapy Center & Cancer Center, the First Affiliated Hospital of Zhengzhou University, Zhengzhou, China
- 2School of Life Sciences, Zhengzhou University, Zhengzhou, China
- 3State Key Laboratory of Esophageal Cancer Prevention & Treatment, Zhengzhou University, Zhengzhou, China
- 4School of Public Health, Zhengzhou University, Zhengzhou, China
Metal ions play an essential role in regulating the functions of immune cells by transmitting intracellular and extracellular signals in tumor microenvironment (TME). Among these immune cells, we focused on the impact of metal ions on T cells because they can recognize and kill cancer cells and play an important role in immune-based cancer treatment. Metal ions are often used in nanomedicines for tumor immunotherapy. In this review, we discuss seven metal ions related to anti-tumor immunity, elucidate their roles in immunotherapy, and provide novel insights into tumor immunotherapy and clinical applications.
1 Introduction
Cancer is a multifaceted disease characterized with abnormal proliferation of malignant cells. High morbidity and mortality rates as well as restricted treatment options pose a serious threat to public health. Consequently, innovative cancer treatments, such as immunotherapy and photothermal therapy, are emerging in clinical settings and trials (1). Cancer immunotherapy has been successful because of its ability to mobilize immune activation and memory, which results in the rapid and effective control of tumor growth and prevention of recurrence (2). The complex tumor microenvironment (TME) is the main reason for limiting immunotherapy, including immunosuppressive cytokines and cells, such as transforming growth factor-beta (TGF-β), interleukin (IL-10), regulatory T cells (Tregs) and myeloid-derived suppressor cells (MDSCs) (3, 4). With continuous research on immunomodulatory factors, the role of metal ions in tumor immunotherapy has gradually been emphasized (5).
Ion signals are transmitted by various transcellular and intracellular signaling cascades that regulate immunological memory and immune responses. Different metal ions play various roles in signal transmission, including calcium (Ca2+), zinc (Zn2+), manganese (Mn2+), magnesium (Mg2+), potassium (K+), iron (Fe2+/3+), and copper (Cu+/2+) (6). Cell membrane is the main barrier limiting free penetration in the process of metal ion transport. The transport of metal ions through active means results in the establishment of concentrations and electric gradients across the membranes. Various integral pore-forming membrane proteins facilitate this process by enabling the movement of ions across membranes, allowing the conversion of different signals through alterations in ion permeability. Currently, nanotechnology provides new methods for clinical tumor immunotherapy and has more advantages than traditional immunotherapy nanocarriers. Nanometallic materials have garnered attention as a promising delivery medium due to their nanocrystalline strengthening, high photoabsorptivity, heightened surface energy, and unique single magnetic domain characteristics (7). Metal ions are important components of nanoparticles to improve their anti-tumor effects (8, 9). Because metal-based nanomaterials are comparable in size to deoxyribonucleic acid (DNA), proteins, viruses, and biomolecules, there are distinctive interactions that occur between metal-based nanoparticles and proteins found in the matrix (10). Additionally, metal-based nanomaterials have the ability to enter the bloodstream and penetrate the TME, enabling them to be absorbed and transported by tumor cells. Crucially, nanomaterials of the appropriate size exhibit a unique enhanced permeability and retention (EPR) effect in tumor sites, significantly enhancing their utilization rate and tumor treatment efficacy (11). In this review, we discuss the role played by different metal ions in anti-tumor immunotherapy and how to use metal ions to improve the efficacy of tumor immunotherapy.
2 Metal ions in anti-cancer immunity
2.1 Calcium
Ca2+ is the most abundant metal ion involved in the proliferation and cycling of immune cells. Currently, Ca2+ is the most studied metal ion and widely recognized as a second messenger in immune responses (12). This section will discuss the transport and effect of Ca2+ in lymphocytes.
The concentration gradient of Ca2+ is involved in release and storage and dependent on Ca2+ release-activated Ca2+ (CRAC) channels, transient receptor potential (TRP) channels, P2X and Cav channels. After the engagement of immunoreceptors, the mitogenic signal emanating from the IS induces the breakdown of PIP2 to inositol trisphosphate (IP3) binding to the IP3 receptors resulting in the release of Ca2+ from the endoplasmic reticulum (ER), and activates stromal interaction molecules (STIM1/2) to bind to the N- and C-termini of ORAI1 which form the subunit of the CRAC channel (13). The CRAC channel exhibits a notable preference for Ca2+ and possesses remarkably low single-channel conductance (14, 15). The influx of Ca2+ into intracellular organelles is mediated by store-operated Ca2+ entry (SOCE) (16, 17) (Figure 1).
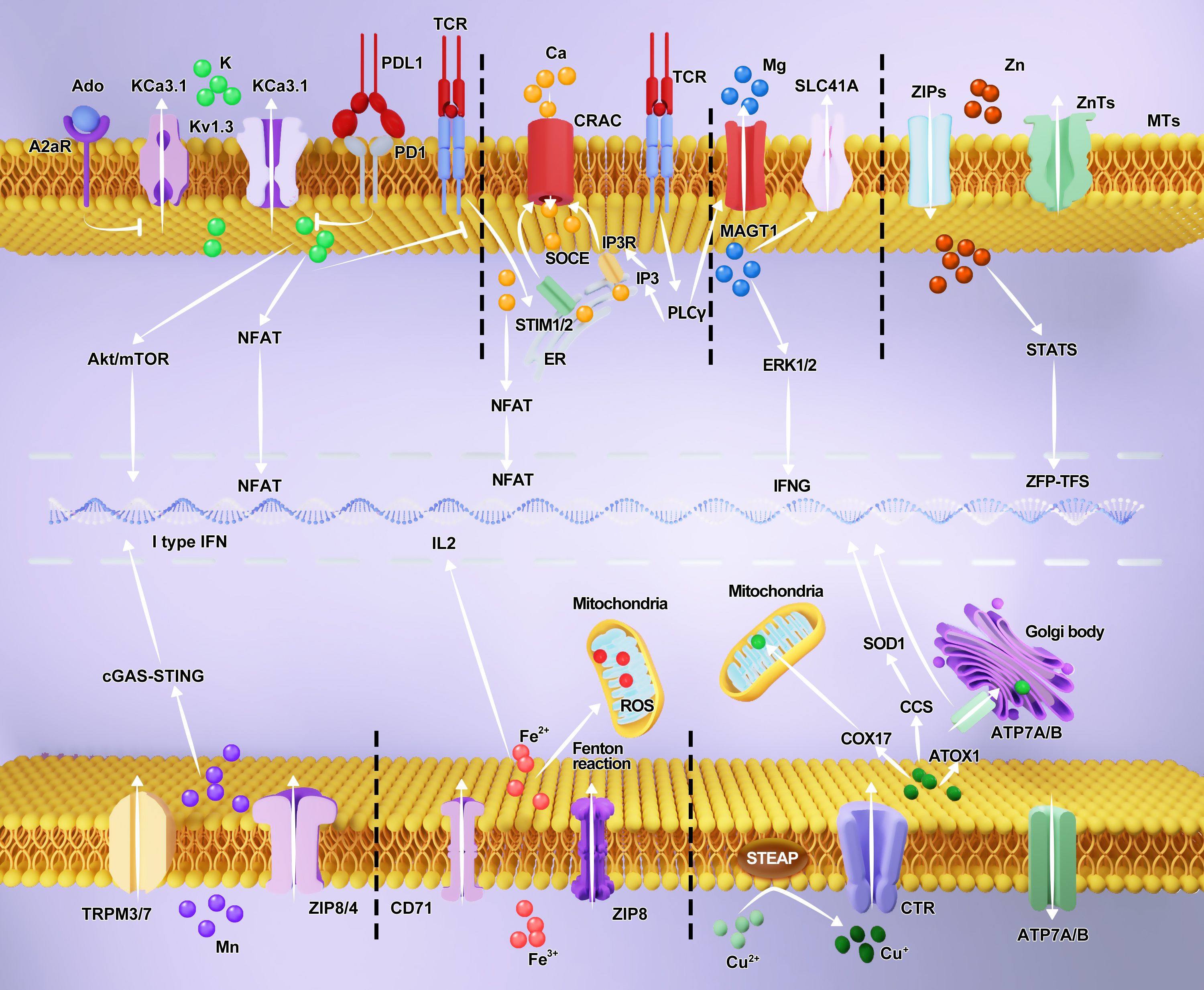
Figure 1 Role of metal ions in T cells. Various metal ion channels or transporters are present on the surface of T cells. They are mainly used to maintain the entry and exit of metal ions. In addition, some metal ions or transporters can regulate the transport of metal ions within the cytoplasm to various organelles and nuclei, thereby altering the function of related organelles or the transcription of target genes, thus regulating the cell function.
T cells activated by tumor antigens in an MHC-I-restricted manner begin to proliferate and release cytokines. During this progression, signal transduction considerably relies on key receptors and signaling molecules, including evocation and termination (18). IP3 binds to the IP3 receptor, resulting in Ca2+ influx to activate the calcineurin-nuclear factor of activated T (NFAT) pathway, which enhances the cellular processes, for example, polarization, proliferation, and cytokines release including interleukin 2 (IL-2), interferon-γ (IFNγ), tumor necrosis factor (TNF), perforin, and granzyme (19, 20). Abolishment of humanT cell function is caused by deletion of STIM1 or ORAI1 to inhibit Ca2+ influx in vitro (21). Functional CRAC channels and elevated levels of cytoplasmic Ca2+ may activate leukocyte function-associated antigen 1 (LFA-1), contributing to the adhesion of cytotoxic lymphocytes (CTLs) to the target cells both in vitro and in vivo (22–24). Co-receptor CD5 co-cross-linking with the TCR-CD3 complex down-regulated the TCR-CD3-increased Ca2+ mobilization to inhibit T cell function (25). Programmed death-1 (PD1) blockade increases Ca2+ signaling to enhance the migratory ability of CTLs in neck cancer (26). P2X, a type of Ca2+ channels, enhances T cell function and T cell receptor (TCR) signaling both in human and mouse model (27, 28). TRP and Cav channels promote rodent and human T cells differentiation and proliferation (29–32) (Table 1).
Tregs protect organisms from autoimmune reactions by maintaining T cell tolerance (60). Inhibition or depletion of Tregs enhances the efficacy of cancer immunotherapy (33). Deficiency of either STIM1 or STIM2 in T cells leads to differentiation to Treg, Th1and Th17 cells suggesting that STIM is vital for their development and function (34).
Natural killer (NK) cells originate from bone marrow stem cells, undergo two differentiation and maturation pathways in the bone marrow and thymus, and are widely distributed in the main organs. NK cells selectively kill target cells, such as viral infections or tumors, and play an important role in anti-tumor immunity (35, 36, 61, 62). The ablation of either RAI1 or STIM1 in NK cells severely impairs defective store-operated Ca2+ entry but not cytotoxic granule polarization (63, 64). After interaction with target cells, the release of cytokines, such as TNF-α, granulocyte-macrophage colony stimulating factor (GM-CSF), and interferons, by NK cells is increased via the calcineurin-NFAT-dependent pathway (65, 66). Increasing the intracellular Ca2+ concentration of dendritic cells (DCs) boosts autophagy and promotes antigen presentation (67). High intracellular Ca2+ levels inhibit the formation of WIP/WASP droplets, allowing PKC-θ to easily access and phosphorylate WIP, thereby increasing the actin polarization of macrophages and phagocytosis of tumor cells (68) (Figure 2).
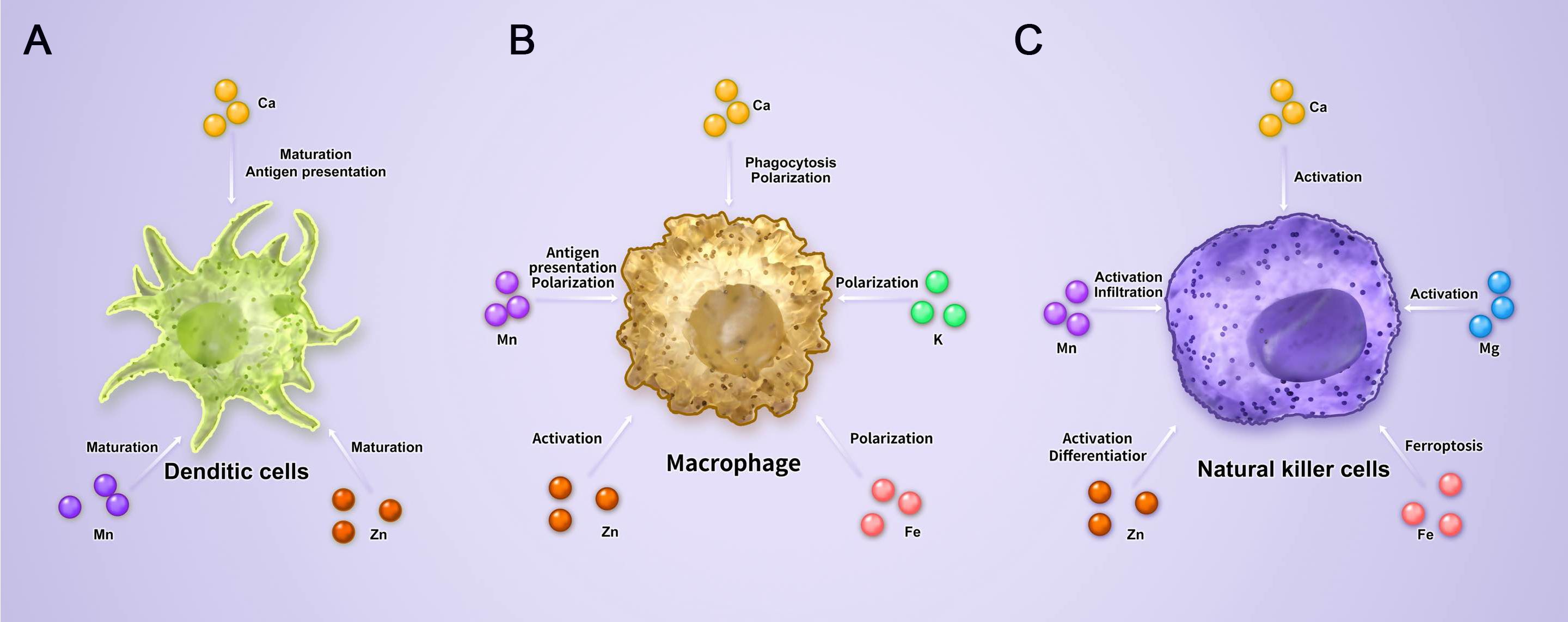
Figure 2 Role of metal ions in dendritic cells, macrophages, and natural killer cells. (A) The influence of various metal ions on DCs, the main antigen-presenting cells, is mainly reflected in antigen presentation and maturation. (B) Macrophages have both phagocytic and antigen presenting functions, as well as immunosuppressive effects. Therefore, the influence of metal ions on macrophages is mainly reflected in activation, antigen presentation, and polarization. (C) NK cells belong to innate immune cells and have strong anti-tumor effects. The main impact of metal ions on NK cells is to regulate their differentiation, activation, and infiltration.
Ca2+ can bind directly to anionic phospholipids, acting as a regulator for membrane protein functionality. A pH-responsive calcium carbonate nanoparticle (CaCO3/CAI@Liposome, CCL) was prepared by loading calcium carbonate nanoparticles with CAIX inhibitors (CAI) and coating with liposomes, which can accurately regulate the content of Ca2+ and pH in and out of tumor cells to promote DCs maturation and macrophage polarization towards the anti-tumor M1 type (69). Given the positive role of Ca2+ in immune cells, novel methods should be explored to utilize Ca to improve its anti-tumor effects.
2.2 Zinc
Zn2+ is a trace metal essential for several proteins and plays a vital role in cell proliferation, differentiation, oxidative stress, immune responses, and apoptosis by conjugating to zinc-binding proteins (37, 70, 71). Moreover, Zn2+ participates in various pathophysiological processes, including growth retardation, cognitive disorders, cardiovascular diseases, and cancer (72).
Zn2+ homeostasis is tightly regulated by two families of Zn2+ transporters: ZnTs and ZIPs (72). ZnTs contain10 members contributing to the export of Zn2+ from the intracellular space. ZIPs have 14 members that facilitate an increase in the cytosolic concentration of Zn2+ (73). Metal regulatory transcription factor 1 (MTF-1) is sensitive to Zn2+ and activated via cysteine2-histidine2 (Cys2-His2) Zn fingers binding to DNA to mediate the expression of ZnT1, ZnT2, and metallothioneins (MTs), which regulate the storage and release of intracellular Zn2+ (74, 75) (Figure 1).
In the T cell response, the MAPK, PI3K/Akt, and STAT3 pathways are the main signal target transducers of Zn2+ which regulate the expression of genes involved in differentiation, survival, proliferation, and apoptosis. ZIP6 expression is enhanced by the activation of the STAT3 signaling pathway to mediate cell migration (38, 39, 76). In sickle cell anemia, Zn2+ deficiency decreases CD73, a marker of cytotoxic T cells, resulting in suppressing T cell differentiation and maturation (77, 78). In addition, interaction between the domain of CD4 or CD8α with p56lck is also controlled by Zn2+ in regulating T cell activation and differentiation in vitro (79). Zap70, a TCR signaling downstream molecule, is phosphorylated through activating Zn2+ regulated kinase to sustain proliferation and activation in mouse spleen and thymus cells, as well as human Jurkat T cells. Moreover, Zn2+-dependent TCR signal amplification induces IL-2 receptor expression, leading to proliferation of activated T cells (76). In contrast, ZIP6 deletion impairs activation marker expression and cytokine production (80–82). Additionally, SLC39A6 indirectly promotes cell proliferation and cytokine production via TCR activation (83). Moreover, MTs and zinc-finger transcription factors are increased to regulate tumor infiltrating lymphocytes (TILs) in mouse model (40). TILs up-regulate GATA-3 and IKZF2 to mediate Zn2+ homeostasis inducing T-cell differentiation and exhaustion in the B16F10 murine melanoma model (84). The balance of T helper cell differentiation is altered by Zn2+ signaling. Zn2+ depletion decreases IFNγ and T-bet essential for Th1 differentiation in Con-A stimulated HUT-78 cells (41, 84).. TH17 and Treg cells formation is mediated by Zn2+ supplementation which remits the T cell-mediated allogeneic immune responses in the human mixed lymphocyte reaction (85, 86). GLI-similar 1 (GLIS1), a zinc finger protein, causes CD8+ T cell exhaustion via the SGK1-STAT3-PD1 pathway in hepatocellular carcinoma (HCC) (87). Zinc finger protein 64 (ZFP64) is a transcription factor for cytotoxic T-lymphocyte-associated protein 4 (CTLA-4) and anti- PD-1 in esophageal cancer (88, 89) (Table 1).
In addition to affecting T cells, Zn2+ can also regulate other immune cells. In mature DCs, the importers ZIP6/10 are decreased, whereas ZnT1/4/6 and MTs are increased to reduce intracellular Zn2+ (90). Unlike in DCs, an increase of Zn2+ activates macrophages and the recruitment of neutrophils (91). In NK cells, Zn2+ promotes the lytic activity and differentiation (92) (Figure 2).
Zn2+ is essential for innate and adaptive anti-tumor immunity leading to an increase of Zn2+-based nanometallic materials. ZnS@BSA (bovine serum albumin), a new zinc-based nanocluster, enhances the infiltration of CD8+ T cells and DCs via cGAS-STING signals in HCC (93). Moreover, a Zn-metal-organic skeleton vaccine (ZPM@OVA-CpG) achieved the targeted release of Zn2+ in DCs and the TME under acidic conditions. The vaccine actively promoted DCs maturation and antigen cross-presentation via cGAS-STING signaling, resulting in the activation of CD8+ T cells (94). Weichselbaum et al. investigated ZnCDA, a powerful tumor-targeting STING agonist, which enhances tumor accumulation by destroying endothelial cells in the tumor vascular system. ZnCDA preferentially targets tumor-associated macrophages to regulate antigen processing and presentation and subsequently triggers anti-tumor T cell responses. ZnCDA reinvigorates the anti-tumor activity of radiation therapy and immune checkpoint inhibitors in immunologically “cold” pancreatic and glioma tumor models (95). Based on the effects on different immune cells, Zn2+ is a double-edged sword. Therefore, these factors should be carefully considered before using Zn2+.
2.3 Manganese
Mn2+ is regarded as a second messenger in mammalian tissues and as an essential metal for intracellular processes, such as energy production, bone growth, reactive oxygen species (ROS) generation, and immune response (42, 96). The mobilization and distribution of Mn2+ is precisely mediated through gamma globulin, albumin, and Mn-transferrin complex. Mn2+ plays a key role in the activation of various enzymes, including glutamine synthetase (GS), isomerases, pyruvate carboxylase, arginase (ARG), lyases, and hydrolases (43, 44).
Usually, intracellular Mn2+ is stored in resting organelles, such as the mitochondria or Golgi apparatus. However, damage to mitochondrial activity and DNA can result in the presence of a large amount of intracellular Mn2+ (45, 97). Unlike Ca2+ and Zn2+, intracellular Mn2+ is transported using various transporters, such as divalent metal transporter A (DMT1), calcium-based proteins such as TRPM3/7, and metal-dependent proteins such as ZIP8/14 and ZnT10 (42, 98–100) (Figure 1).
The effect of Mn2+ on T cells is mainly reflected by an increase in the number, differentiation, and cytokine release of TILs by inducing type I interferon production in a murine orthotopic HCC model (101–103). Moreover, Mn2+ deficiency leads to a reduction of macrophage antigen presentation and DCs maturation, thereby mediating murine CD8+ T cell activation in vivo (104). Furthermore, Mn2+ treatment substantially increases the infiltration of murine NK cells and the release of cytokines to reduce tumor growth (105, 106). Mn2+ can change macrophage (M2) polarization to macrophage (M1) with anti-tumor effects (107). Mechanistically, the Mn2+-dependent cGAS-STING pathway plays a remarkable role in T and NK cell functions and DCs maturation. It was reported that NK cell activity increased by producing type I IFNs after adding MnCl2 (103). In addition, Mn2+ improved the clinical efficacy of PD-1/PD-L1 therapy (101, 108). Taken together, Mn2+ serves a vital function in the cross-presentation of immune cells, such as DCs, TILs, and NK cells (Figure 2).
Manganese oxide (MnO2) nanomaterials are typically used as enhancers to increase the immunotherapy efficiency of photothermal agents by catalyzing the conversion of H2O2 into O2 (109). Mn@CaCO3/ICG nanoparticles serve as vectors for loading small interfering RNA (siRNA) targeting PD-L1 (109). Hou et al. designed MnO nanoparticles combined with the tumor-homing peptide iRGD (CRGDKGPD) to effectively activate the cGAS-STING signaling pathway. Manganese also assisted α-PD-1 to inhibit tumor growth and metastasis (110). Therefore, Mn2+ is a potential therapeutic adjuvant for the treatment of tumors.
2.4 Magnesium
Mg2+ is the second-most abundant metal ion (111, 112). Mg2+ is normally complexed with ATP or ADP or acts as a cofactor, which is essential in the regulation of biological activities, such as the cell cycle, apoptosis, division, and differentiation (46, 113), as well as biochemical processes, including oxidative phosphorylation, glycolysis, DNA repair, metabolism, and synthesis (114–116).
Mg2+ distribution is different among the extracellular and intracellular spaces, which determines its various functions. Extracellular Mg2+ binds to plasma proteins to transmit activating or inhibitory signals, whereas intracellular Mg2+ interacts with nucleotides, nucleic acids, or ATP to mediate biochemical processes (117). The Mg2+ transport is tightly regulated by intake and excretion via Mg transporter 1 (MAGT1), TRP cation channel subfamily M member 7 (TRPM7), SLC41A1, and Na+ exchange; MAGT1 is a highly selective Mg2+ channel (47, 118, 119) (Figure 1).
Mg2+ is regarded as a second messenger that regulates proliferation, development, and function through MAGT1, TRPM7, and SLC41A1 transporters in T cells (48, 120),. After TCR stimulation or PLCγ1 activation, Mg2+ and Ca2+ enter T cells in an MAGT1-dependent manner (121). The deletion of MAGT1 decreases the capacity response to TCR and Ca2+ entry into human T cells in vitro (48, 122). In contrast, reducing the concentration of Mg2+ causes TCR signal suppression, resulting in human T cell exhaustion, decreased Ca2+ influx, proliferation, CD69 and CD25 expression both in vitro and vivo (120, 123). In addition, TRPM7, a non-selective Mg2+ channel, modulates Mg2+ concentration and T cell development and maturation (124, 125) and is fundamental for anti-tumor immunity (20, 126). Mouse TRPM7 deficiency did not affect acute uptake of Mg2+ or the maintenance of total cellular Mg2+ but suppresses T cell maturation (20, 49). Mechanistically, the binding between Mg2+ and metal-ion-dependent adhesion sites causes TCR signal stimulation, resulting in conformational changes in LFA-1, which is essential for T cell activation by mediating proximal and distal signaling activities, such as focal adhesion kinase phosphorylation and extracellular signal-regulated protein kinase 1/2 (ERK1/2) phosphorylation (50, 127–129). Lotscher et al. measured serum Mg2+ levels in 100 patients with leukemia and 67 patients with lung cancer and found that patients with low serum Mg2+ levels had lower survival rates to CAR-T therapy (128) (Table 1). In addition, activation and cytotoxicity are increased in intratumor CD8+ T cells and NK cells after intraperitoneal injection of MgCl2 (127) (Figure 2).
2.5 Potassium
K+ is an important inorganic electrolyte used to maintain normal physiological functions in the human body. K+ is involved in glucose, protein, and energy metabolism. K+ is the main cation in the cells and involved in maintaining osmotic pressure, myocardial function, and the acid-base balance of the intracellular and extracellular fluids (51, 130).
Similar to other cations, the balance of K+ within cells is finely controlled by several ion channels, including Kv1.3, KCa3.1, K2p3.1, K2p5.1, and K2p9.1 (131). Kv1.3 and KCa3.1 act as the main channels. The activation of Kv1.3- and KCa3.1-dependent membrane depolarization and release of Ca2+ and calmodulin, respectively, results in K+ efflux (52).
K+ has a crucial role in modulating T cell function and is associated with Ca2+ signaling (17, 132). TCR activation signals increase Ca2+ entry, resulting in intracellular Ca2+ release and membrane depolarization that causes Kv1.3 and KCa3.1 activation to increase K+ efflux, which in turn restores the membrane state, resulting in Ca2+ entry to induce NFAT expression, IL-2 release, T cell migratory capacity, and T cell subsets, including Th1, Th2, Th17, and Treg cells (133–135). In contrast, Ca2+ signaling is repressed if Kv1.3 and KCa3.1 are blocked upon TCR stimulation, indicating that the K+ gradient and Kv1.3 and KCa3.1 channels are essential for modulating T-cell activation (136). Moreover, reducing intracellular K+ via overexpression of KCa3.1 can increase the production of IFNγ in T cells to inhibit tumor growth and prolong survival, which is proved in patients with head and neck cancer (26, 53). In anti-tumor T-cell responses, large amounts of K+ produced by necrotizing tumor cells lead to a functional decline in CD8+ T cells owing to increased intracellular K+ affecting the Akt-mTOR pathway. Adenosine (Ado) and PD-1 signaling inhibit KCa3.1channel activity to limit K+ efflux leading to restrain the ability of CD8+ T cells from cancer patients (51) (Figure 1).
Another effect of K+ on T cells is the reprogramming of T cell metabolism. Higher extracellular K+ levels lead to autophagy and mitochondrial-based energy metabolism, causing changes in the expression of genes associated with activation, stemness, or exhaustion in T cells (137). In TILs isolated from B16 tumors, increasing extracellular K+ level induces central memory T cells, whereas reducing effector memory T cells and exhaustion markers, such as PD1, 2B4, and Tim-3 to enhance cancer immunotherapy. Hexokinase II also requires K+ as a cofactor to mediate anti-tumor immunity (137). In contrast, CD19-CAR-T cells express higher memory and lower effector genes after culturing in a medium containing a low glucose level and K+ (138) (Table 1). The anti-tumor effect of high K+ level was reported. The underlying mechanism was attributed to inhibition of tumor-associated macrophages (TAM), and the Kir2.1 channel was identified as the central regulator of TAM functional polarization in the high K+ TME (139) (Figure 2).
2.6 Iron
Fe2+/3+ has several biological functions in living organisms. Iron ions are important components of hemoglobin and myoglobin, which are used to maintain normal respiratory function (140). Second, iron ions participate in the catalytic reactions of various enzymes in the body, including fatty acid metabolism and oxidases. Iron ions also regulate various important metabolic processes, such as immune responses, neurotransmission, and DNA synthesis (141, 142).
Given the importance of Fe2+, its transport is finely regulated. Transferrin protein (Tf) is regarded as the primary transporter that forms a complex with Fe2+ and internalized by the transferrin receptor (CD71) (54, 143). In addition, DMT-1 and ZIP-8 are used to transport Fe2+ in a nonspecific manner (143).
T cell function and activation are impaired under conditions of defective Tf-receptors or decreased intracellular Fe2+ levels, such as reduced expression of CD25 and impaired to IL-2 receptor signaling (144). In contrast, Fe2+ supplementation in the culture medium can restore the biogenesis of T cell (145). Ferroptosis is an iron-dependent form of regulated cell death in both T and tumor cells. Fe3+ usually binds to transferrin in the form of trivalent iron, enters cells through transferrin channels, and is reduced to Fe2 + by the metal reductase STEAP3, resulting in a Fenton’s reaction that releases reactive oxygen species (ROS) represented by hydroxyl radicals. Accumulated ROS-mediated peroxidation of membrane lipids leads to damage to cell function and ferroptosis. At present, ferroptosis is regarded as a method of inhibiting tumor growth, and a close correlation has been found between ferroptosis and anti-tumor immunity (55, 146). In a recent study, CD36 expression decreased cytokine release and inhibited the anti-tumor activity of B16 tumor-infiltrating CD8+ T cells owing to CD36-mediated lipid peroxidation and ferroptosis (147). In contrast, CD8+ T cells can induce tumor ferroptosis via lipid peroxide accumulation triggered by CD8+ T cells-secreted IFNγ to increase STAT1 and suppress cystine/glutamate antiporter system Xc in B16 beard mouse model (148). Peng Liao et al. found that arachidonic acid and IFN-γ secreted by CD8+ T cells in the TME can induce MC38 and B16F10 cell ferroptosis (149) (Figure 1).
According to a previous report, Fe2+ is released by other immune cells, such as TAMs and tumor-associated neutrophils (TANs), to sustain cancer progression (150). Fe2+ transporters and metabolism play an essential role in macrophage polarization; for example, M1 macrophages tend to reduce the level of Fe2+, whereas M2 macrophages are inclined to recirculate free Fe2+ (151). Kim et al. reported that myeloid-derived immunosuppressive cells (PMN-MDSCs) in the TME spontaneously induce oxidized lipid release, causing repression of T cell activity. In mice with normal immune function, suppressing ferroptosis inhibits the immunosuppressive activity of PMN-MDSCs and can be combined with immune checkpoint inhibitors to inhibit tumor growth (152). In HCC, ferroptosis triggers tumor invasion of MDSCs via high mobility group protein B1 (153). In gastric cancer, NK cell levels are negatively correlated with the abundance of tumor-associated fibroblasts (CAF). CAF impair the anti-tumor ability of NK cells by inducing ferroptosis (154) (Figure 2).
Magnetic hyperthermia is a tumor therapy triggered by iron oxide nanoparticles, enabling an increase in antigen presentation, maturation of DCs, T lymphocyte recruitment, and a reduction in Tregs (155). Biocompatible PEG-coated ferrihydrite nanoparticles (PEG-Fns) mediate TAM polarization from M2 to M1 (156). Zhang et al. assembled quercetin and iron ions to prepare the nano-photosensitization agent, QFN. When QFN was decomposed, the released quercetin reduced programmed death ligand 1 (PD-L1) in tumor cells by decreasing the activation of JAK2 and STAT3 and reshaping the extracellular matrix (ECM) (157). In summary, these data indicate that iron plays a dual role in regulating immune and tumor cells.
2.7 Copper
Cu+/2+ is an important trace element and an essential component of many biochemical reactions. There are two types, Cu+ and Cu2+ in living organisms (158). Within cells, Cu2+ undergo redox reactions and metabolic pathways with other metabolites, such as cytochrome c redox reactions and intracellular respiration (159). In addition, Cu2+ is important components of many enzymatic reactions, such as intracellular oxygen site-catalyzed oxidation reactions. Finally, Cu2+ participates in the expression of many transcription factors that regulate various physiological reactions, such as nuclear factor-kappa B (NF-кB) and activator protein-1 (AP-1) (160–162).
Cu2+ deficiency or excess can lead to oxidative stress and toxicity. Intracellular Cu2+ is delivered to various cellular compartments to modulate intracellular processes (163). Consequently, Cu2+ homeostasis is closely modulated by solute carrier family 31 member 1/2 (CTR1/2) and SLC11A2/DMT1, as well as ATPase copper transporting α (ATP7A) and β (ATP7B), MT, glutathione (GSH), Cu2+ chaperone for superoxide dismutase (CCS), antioxidant protein 1 (Atox1), and cytochrome c oxidase copper chaperone 17 (COX17). Extracellular Cu2+ is reduced to Cu+ by binding to metal reductases of the STEAP family and is transported into cells via SLC31A1 or combined with Cu2+ chaperone protein antioxidant-1 (ATOX1) to the Golgi network via ATP7A/B (164, 165). Within the cytoplasm, excess Cu2+ is excreted via ATP7A/B and transferred from the Golgi apparatus to other organelles to mediate cellular copper homeostasis (56). CCS1 delivers Cu2+ to superoxide dismutase 1 (SOD1) in the cytoplasm (57, 166). Cu+ in the mitochondrial membrane is regulated by COX17 during transport (167). CCS and ATOX1 are important transporters of Cu+ into the nucleus (168, 169). Furthermore, elesclomol, ATN-224, tetrathiomolybdate, disulfiram (DSF), pyrithione, and chloroquine are considered copper ionophores (58).
Cu2+ potentially is involved in the activation and regulation of T cells. Cu2+ deficiency impairs CTL capacity to kill target cells and reduces T cell proliferation both in vitro and in vivo (170, 171). Bala et al. found that the infiltration of CD4+ and CD8+ T cells decreased in splenic monocytes from copper-deficient rats (59). Both the protein and mRNA expression of IL-2 are reversibly decreased in human T lymphocytes, as well as superoxide anion production by neutrophils after Cu2+ deficiency (172–174). Moreover, the effect of Cu2+ on helper T cells is consistent with CTLs including their proliferation and function (175, 176). Voli et al. demonstrated that intracellular Cu2+ regulates PD-L1 expression to inhibit the function of T lymphocytes in brain tumors. Further exploration revealed that copper chelators decrease PD-L1 expression by suppressing the epidermal growth factor receptor (EGFR) and transcriptional activator protein (STAT) signaling pathways to increase the number of T lymphocytes and NK cells (177) (Figure 1).
Like other ions, Cu2+ also regulate other immune cells. Chatterjee et al. demonstrated that CuNG (a copper chelate) reprogrammed TAMs from M2 to M1, activating the T cell function (178, 179). Blood neutrophils are reduced in copper deficiency by decreasing the superoxide anions (180) (Table 1).
Cuproptosis is a unique mode of cell death and first reported by Tsvetkov et al. in 2022 (181–184). The classical mechanism of cuproptosis involves the binding of elesclomol to extracellular Cu2+ and its transport to the cellular compartment. Ferredoxin 1 (FDX1) reduces Cu2+ to Cu+ and inhibits iron-sulfur protein cluster (Fe-S) synthesis. Together, these abnormal processes lead to protein toxicity, ultimately leading to cell death. Wang et al. showed that chimeric antigen receptor T (CAR T) cells were reprogrammed by exposing DSF/Cu plus ionizing irradiation (IR). This method can reverse the immunosuppressive TME and increase the anti-tumor function of CAR-T cells (185, 186).
A nanoparticle drug, NP@ESCu, was developed to induce cancer cell apoptosis and simultaneously reprogram the immunosuppressive TME. When combined with αPD-L1 blockade, the therapeutic effect on cancer was obviously increased, which contributes to a broad range of clinical applications (187). BSO-CAT@MOF-199 @DDM (BCMD) nanomediated copper proliferation can induce immunogenic cell death (ICD) and enhance DC activation and T cell infiltration (188). In summary, although copper plays a key role in the activity of various enzymes, cytotoxicity can occur at higher concentrations.
3 Conclusion
Tumor immunotherapy has become one of the most promising treatment methods besides surgery, radiotherapy, and chemotherapy. However, tumor immunotherapy is influenced by various factors. The metabolism of metal ions in the tumor microenvironment, particularly their association with anti-tumor immunity, has been well-demonstrated. Metal ions can improve the tumor microenvironment and promote the activation and proliferation of immune cells. On the one hand, they activate the innate immune system by enhancing the presentation of DCs and the cytotoxicity of macrophages and NK cells to augment their anti-tumor effects. On the other hand, metal ions can also stimulate the activation and proliferation of adaptive immune cells, especially T cells that recognize and kill tumors to improve the effectiveness of tumor immunotherapy. With the continuous deepening of research, it has been found that metal ions can also directly induce tumor cell death, such as ferroptosis and cuproptosis, which mainly induce tumor cell death by changing their metabolic mode. Finally, metal ions are also used to prepare nanomedicines that can directly induce tumor death or exert anti-tumor effects by loading anti-tumor drugs and small RNAs to stimulate the immune cells. Although the application of metal ions appears to be a promising method for improving the efficacy of tumor immunotherapy based on existing results, making it a potential immune system agonist requires more attention regarding its safety.
Author contributions
YG: Formal Analysis, Investigation, Resources, Supervision, Writing – original draft, Writing – review & editing. SL: Funding acquisition, Methodology, Project administration, Supervision, Writing – original draft. YH: Methodology, Project administration, Resources, Supervision, Writing – original draft. FL: Methodology, Supervision, Writing – review & editing. YZ: Conceptualization, Funding acquisition, Supervision, Writing – review & editing.
Funding
The author(s) declare financial support was received for the research, authorship, and/or publication of this article. This work was supported by grants from the National Key Research and Development Program Intergovernmental Key Project for International Science and Technology Innovation Cooperation (No. 2022YFE0141000), Key Science and Technology Program of Henan Province (No. 242102310344), Natural Science Foundation of China (No. 82203548), China Postdoctoral Science Foundation Project (No. 2022M712894), and Major Science and Technology Project of Henan Province (No. 221100310100).
Conflict of interest
The authors declare that the research was conducted in the absence of any commercial or financial relationships that could be construed as a potential conflict of interest.
Publisher’s note
All claims expressed in this article are solely those of the authors and do not necessarily represent those of their affiliated organizations, or those of the publisher, the editors and the reviewers. Any product that may be evaluated in this article, or claim that may be made by its manufacturer, is not guaranteed or endorsed by the publisher.
References
1. Tsimberidou AM, Fountzilas E, Nikanjam M, Kurzrock R. Review of precision cancer medicine: Evolution of the treatment paradigm. Cancer Treat Rev. (2020) 86:102019. doi: 10.1016/j.ctrv.2020.102019
2. Kennedy LB, Salama AKS. A review of cancer immunotherapy toxicity. CA Cancer J Clin. (2020) 70:86–104. doi: 10.3322/caac.21596
3. Finn OJ. Immuno-oncology: understanding the function and dysfunction of the immune system in cancer. Ann Oncol. (2012) 23:viii6–9. doi: 10.1093/annonc/mds256
4. Lindau D, Gielen P, Kroesen M, Wesseling P, Adema GJ. The immunosuppressive tumor network: myeloid-derived suppressor cells, regulatory T cells and natural killer T cells. Immunology. (2013) 138:105–15. doi: 10.1111/imm.12036
5. Ni K, Luo T, Nash GT, Lin W. Nanoscale metal-organic frameworks for cancer immunotherapy. Acc Chem Res. (2020) 53:1739–48. doi: 10.1021/acs.accounts.0c00313
6. Pradeu T, Vivier E. The discontinuity theory of immunity. Sci Immunol. (2016) 1:AAG0479. doi: 10.1126/sciimmunol.aag0479
7. Sun Y, Ma X, Hu H. Application of nano-drug delivery system based on cascade technology in cancer treatment. Int J Mol Sci. (2021) 22:5698. doi: 10.3390/ijms22115698
8. Wu MX, Yang YW. Metal-organic framework (MOF)-based drug/cargo delivery and cancer therapy. Adv Mater. (2017) 29. doi: 10.1002/adma.201606134
9. Riley RS, June CH, Langer R, Mitchell MJ. Delivery technologies for cancer immunotherapy. Nat Rev Drug Discovery. (2019) 18:175–96. doi: 10.1038/s41573-018-0006-z
10. Delfi M, Sartorius R, Ashrafizadeh M, Sharifi E, Zhang Y, De Berardinis P, et al. Self-assembled peptide and protein nanostructures for anti-cancer therapy: Targeted delivery, stimuli-responsive devices and immunotherapy. Nano Today. (2021) 38:101119. doi: 10.1016/j.nantod.2021.101119
11. Bhandari C, Guirguis M, Savan NA, Shrivastava N, Oliveira S, Hasan T, et al. What NIR photodynamic activation offers molecular targeted nanomedicines: Perspectives into the conundrum of tumor specificity and selectivity. Nano Today. (2022) 36:101052. doi: 10.1016/j.nantod.2020.101052
12. Hsu WL, Noda M, Yoshioka T, Ito E. A novel strategy for treating cancer: understanding the role of Ca(2+) signaling from nociceptive TRP channels in regulating cancer progression. Explor Target Antitumor Ther. (2021) 2:401–15. doi: 10.37349/etat.2021.00053
13. Prakriya M, Feske S, Gwack Y, Srikanth S, Rao A, Hogan PG. Orai1 is an essential pore subunit of the CRAC channel. Nature. (2006) 443:230–3. doi: 10.1038/nature05122
14. Zweifach A, Lewis RS. Mitogen-regulated Ca2+ current of T lymphocytes is activated by depletion of intracellular Ca2+ stores. Proc Natl Acad Sci U.S.A. (1993) 90:6295–9. doi: 10.1073/pnas.90.13.6295
15. Lin TC, Chung PJ, Shen CA, Nguyen TMH, Lin YS, Lin SC, et al. Depletion of intracellular Ca(2+) induces FOXM1 SUMOylation and accumulation on the inner nuclear membrane and accelerates G2/M cell cycle transition. Eur J Cell Biol. (2023) 102:151332. doi: 10.1016/j.ejcb.2023.151332
16. Parekh AB, Putney JW Jr. Store-operated calcium channels. Physiol Rev. (2005) 85:757–810. doi: 10.1152/physrev.00057.2003
17. Cahalan MD, Chandy KG. The functional network of ion channels in T lymphocytes. Immunol Rev. (2009) 231:59–87. doi: 10.1111/j.1600-065X.2009.00816.x
18. Dustin ML, Depoil D. New insights into the T cell synapse from single molecule techniques. Nat Rev Immunol. (2011) 11:672–84. doi: 10.1038/nri3066
19. Zweifach A. Target-cell contact activates a highly selective capacitative calcium entry pathway in cytotoxic T lymphocytes. J Cell Biol. (2000) 148:603–14. doi: 10.1083/jcb.148.3.603
20. Feske S, Skolnik EY, Prakriya M. Ion channels and transporters in lymphocyte function and immunity. Nat Rev Immunol. (2012) 12:532–47. doi: 10.1038/nri3233
21. Szilagyi O, Boratko A, Panyi G, Hajdu P. The role of PSD-95 in the rearrangement of Kv1.3 channels to the immunological synapse. Pflugers Arch. (2013) 465:1341–53. doi: 10.1038/nri3233
22. Franciszkiewicz K, Le Floc'h A, Boutet M, Vergnon I, Schmitt A, Mami-Chouaib F. CD103 or LFA-1 engagement at the immune synapse between cytotoxic T cells and tumor cells promotes maturation and regulates T-cell effector functions. Cancer Res. (2013) 73:617–28. doi: 10.1158/0008–5472.CAN-12–2569
23. Quintana A, Pasche M, Junker C, Al-Ansary D, Rieger H, Kummerow C, et al. Calcium microdomains at the immunological synapse: how ORAI channels, mitochondria and calcium pumps generate local calcium signals for efficient T-cell activation. EMBO J. (2011) 30:3895–912. doi: 10.1038/emboj.2011.289
24. Pores-Fernando AT, Zweifach A. Calcium influx and signaling in cytotoxic T-lymphocyte lytic granule exocytosis. Immunol Rev. (2009) 231:160–73. doi: 10.1111/j.1600–065X.2009.00809.x
25. Freitas CMT, Johnson DK, Weber KS. T cell calcium signaling regulation by the co-receptor CD5. Int J Mol Sci. (2018) 19:1295. doi: 10.3390/ijms19051295
26. Newton HS, Gawali VS, Chimote AA, Lehn MA, Palackdharry SM, Hinrichs BH, et al. PD1 blockade enhances K(+) channel activity, Ca(2+) signaling, and migratory ability in cytotoxic T lymphocytes of patients with head and neck cancer. J Immunother Cancer. (2020) 8:e000844. doi: 10.1136/jitc-2020–000844
27. Schenk U, Westendorf AM, Radaelli E, Casati A, Ferro M, Fumagalli M, et al. Purinergic control of T cell activation by ATP released through pannexin-1 hemichannels. Sci Signal. (2008) 1:ra6. doi: 10.1126/scisignal.1160583
28. Junger WG. Immune cell regulation by autocrine purinergic signaling. Nat Rev Immunol. (2011) 11:201–12. doi: 10.1038/nri2938
29. Weber KS, Hildner K, Murphy KM, Allen PM. Trpm4 differentially regulates Th1 and Th2 function by altering calcium signaling and NFAT localization. J Immunol. (2010) 185:2836–46. doi: 10.4049/jimmunol.1000880
30. Acharya TK, Tiwari A, Majhi RK, Goswami C. TRPM8 channel augments T-cell activation and proliferation. Cell Biol Int. (2021) 45:198–210. doi: 10.1002/cbin.11483
31. Cabral MD, Paulet PE, Robert V, Gomes B, Renoud ML, Savignac M, et al. Knocking down Cav1 calcium channels implicated in Th2 cell activation prevents experimental asthma. Am J Respir Crit Care Med. (2010) 181:1310–7. doi: 10.1164/rccm.200907-1166OC
32. Omilusik K, Priatel JJ, Chen X, Wang YT, Xu H, Choi KB, et al. The Ca(v)1.4 calcium channel is a critical regulator of T cell receptor signaling and naive T cell homeostasis. Immunity. (2011) 35:349–60. doi: 10.1016/j.immuni.2011.07.011
33. Ruter J, Barnett BG, Kryczek I, Brumlik MJ, Daniel BJ, Coukos G, et al. Altering regulatory T cell function in cancer immunotherapy: a novel means to boost the efficacy of cancer vaccines. Front Biosci (Landmark Ed). (2009) 14:1761–70. doi: 10.2741/3338
34. Oh-Hora M, Komatsu N, Pishyareh M, Feske S, Hori S, Taniguchi M, et al. Agonist-selected T cell development requires strong T cell receptor signaling and store-operated calcium entry. Immunity. (2013) 38:881–95. doi: 10.1016/j.immuni.2013.02.008
35. Meng F, Zhang S, Xie J, Zhou Y, Wu Q, Lu B, et al. Leveraging CD16 fusion receptors to remodel the immune response for enhancing anti-tumor immunotherapy in iPSC-derived NK cells. J Hematol Oncol. (2023) 16:62. doi: 10.1186/s13045-023-01455-z
36. Khoshandam M, Soltaninejad H, Hamidieh AA, Hosseinkhani S. CRISPR, CAR-T, and NK: Current applications and future perspectives. Genes Dis. (2024) 11:101121. doi: 10.1016/j.gendis.2023.101121
37. Krezel A, Maret W. The biological inorganic chemistry of zinc ions. Arch Biochem Biophys. (2016) 611:3–19. doi: 10.1016/j.abb.2016.04.010
38. Yamasaki S, Sakata-Sogawa K, Hasegawa A, Suzuki T, Kabu K, Sato E, et al. Zinc is a novel intracellular second messenger. J Cell Biol. (2007) 177:637–45. doi: 10.1083/jcb.200702081
39. Supasai S, Aimo L, Adamo AM, Mackenzie GG, Oteiza PI. Zinc deficiency affects the STAT1/3 signaling pathways in part through redox-mediated mechanisms. Redox Biol. (2017) 11:469–81. doi: 10.1016/j.redox.2016.12.027
40. Singer M, Wang C, Cong L, Marjanovic ND, Kowalczyk MS, Zhang H, et al. A distinct gene module for dysfunction uncoupled from activation in tumor-infiltrating T cells. Cell. (2017) 171:1221–23. doi: 10.1016/j.cell.2017.11.006
41. Prasad AS. Effects of zinc deficiency on Th1 and Th2 cytokine shifts. J Infect Dis. (2000) 182 Suppl 1:S62–8. doi: 10.1086/315916
42. Horning KJ, Caito SW, Tipps KG, Bowman AB, Aschner M. Manganese is essential for neuronal health. Annu Rev Nutr. (2015) 35:71–108. doi: 10.1146/annurev-nutr-071714–034419
43. Nischwitz V, Berthele A, Michalke B. Speciation analysis of selected metals and determination of their total contents in paired serum and cerebrospinal fluid samples: An approach to investigate the permeability of the human blood-cerebrospinal fluid-barrier. Anal Chim Acta. (2008) 627:258–69. doi: 10.1016/j.aca.2008.08.018
44. Aschner JL, Aschner M. Nutritional aspects of manganese homeostasis. Mol Aspects Med. (2005) 26:353–62. doi: 10.1016/j.mam.2005.07.003
45. Carmona A, Roudeau S, Perrin L, Veronesi G, Ortega R. Environmental manganese compounds accumulate as Mn(II) within the Golgi apparatus of dopamine cells: relationship between speciation, subcellular distribution, and cytotoxicity. Metallomics. (2014) 6:822–32. doi: 10.1039/c4mt00012a
46. Walsh SB, Zdebik AA, Unwin RJ. Magnesium: the disregarded cation. Mayo Clin Proc. (2015) 90:993–5. doi: 10.1016/j.mayocp.2015.06.011
47. Kolisek M, Launay P, Beck A, Sponder G, Serafini N, Brenkus M, et al. SLC41A1 is a novel mammalian Mg2+ carrier. J Biol Chem. (2008) 283:16235–47. doi: 10.1074/jbc.M707276200
48. Li FY, Chaigne-Delalande B, Kanellopoulou C, Davis JC, Matthews HF, Douek DC, et al. Second messenger role for Mg2+ revealed by human T-cell immunodeficiency. Nature. (2011) 475:471–6. doi: 10.1038/nature10246
49. Schmitz C, Perraud AL, Johnson CO, Inabe K, Smith MK, Penner R, et al. Regulation of vertebrate cellular Mg2+ homeostasis by TRPM7. Cell. (2003) 114:191–200. doi: 10.1016/s0092–8674(03)00556–7
50. Al-Aghbar MA, Jainarayanan AK, Dustin ML, Roffler SR. The interplay between membrane topology and mechanical forces in regulating T cell receptor activity. Commun Biol. (2022) 5:40. doi: 10.1038/s42003–021-02995–1
51. Ong ST, Ng AS, Ng XR, Zhuang Z, Wong BHS, Prasannan P, et al. Extracellular K(+) dampens T cell functions: implications for immune suppression in the tumor microenvironment. Bioelectricity. (2019) 1:169–79. doi: 10.1089/bioe.2019.0016
52. Wulff H, Christophersen P, Colussi P, Chandy KG, Yarov-Yarovoy V. Antibodies and venom peptides: new modalities for ion channels. Nat Rev Drug Discovery. (2019) 18:339–57. doi: 10.1038/s41573–019-0013–8
53. Chimote AA, Hajdu P, Sfyris AM, Gleich BN, Wise-Draper T, Casper KA, et al. Kv1.3 channels mark functionally competent CD8+ Tumor-infiltrating lymphocytes in head and neck cancer. Cancer Res. (2017) 77:53–61. doi: 10.1158/0008–5472.CAN-16–2372
54. Mims MP, Prchal JT. Divalent metal transporter 1. Hematology. (2005) 10:339–45. doi: 10.1080/10245330500093419
55. Grant SM, Wiesinger JA, Beard JL, Cantorna MT. Iron-deficient mice fail to develop autoimmune encephalomyelitis. J Nutr. (2003) 133:2635–8. doi: 10.1093/jn/133.8.2635
56. Polishchuk EV, Concilli M, Iacobacci S, Chesi G, Pastore N, Piccolo P, et al. Wilson disease protein ATP7B utilizes lysosomal exocytosis to maintain copper homeostasis. Dev Cell. (2014) 29:686–700. doi: 10.1016/j.devcel.2014.04.033
57. Suzuki Y, Ali M, Fischer M, Riemer J. Human copper chaperone for superoxide dismutase 1 mediates its own oxidation-dependent import into mitochondria. Nat Commun. (2013) 4:2430. doi: 10.1038/ncomms3430
58. Gao W, Huang Z, Duan J, Nice EC, Lin J, Huang C. Elesclomol induces copper-dependent ferroptosis in colorectal cancer cells via degradation of ATP7A. Mol Oncol. (2021) 15:3527–44. doi: 10.1002/1878–0261.13079
59. Bala S, Failla ML, Lunney JK. Alterations in splenic lymphoid cell subsets and activation antigens in copper-deficient rats. J Nutr. (1991) 121:745–53. doi: 10.1093/jn/121.5.745
60. Zou W. Regulatory T cells, tumor immunity and immunotherapy. Nat Rev Immunol. (2006) 6:295–307. doi: 10.1038/nri1806
61. Di Vito C, Coianiz N, Calvi M, Terzoli S, Zaghi E, Puccio S, et al. Persistence of KIR(neg) NK cells after haploidentical hematopoietic stem cell transplantation protects from human cytomegalovirus infection/reactivation. Front Immunol. (2024) 14:1266051. doi: 10.3389/fimmu.2023.1266051
62. Landolina N, Mariotti FR, Pelosi A, D'Oria V, Ingegnere T, Alicata C, et al. The anti-inflammatory cytokine IL-37 improves the NK cell-mediated anti-tumor response. Oncoimmunology. (2024) 13:2297504. doi: 10.1080/2162402X.2023.2297504
63. Maul-Pavicic A, Chiang SC, Rensing-Ehl A, Jessen B, Fauriat C, Wood SM, et al. ORAI1-mediated calcium influx is required for human cytotoxic lymphocyte degranulation and target cell lysis. Proc Natl Acad Sci U.S.A. (2011) 108:3324–9. doi: 10.1073/pnas.1013285108
64. Hess SD, Oortgiesen M, Cahalan MD. Calcium oscillations in human T and natural killer cells depend upon membrane potential and calcium influx. J Immunol. (1993) 150:2620–33. doi: 10.4049/jimmunol.150.7.2620
65. Tassi I, Cella M, Presti R, Colucci A, Gilfillan S, Littman DR, et al. NK cell-activating receptors require PKC-theta for sustained signaling, transcriptional activation, and IFN-gamma secretion. Blood. (2008) 12:4109–16. doi: 10.1182/blood-2008–02-139527
66. Wang H, Grzywacz B, Sukovich D, McCullar V, Cao Q, Lee AB, et al. The unexpected effect of cyclosporin A on CD56+CD16- and CD56+CD16+ natural killer cell subpopulations. Blood. (2007) 110:1530–9. doi: 10.1182/blood-2006–10-048173
67. An J, Zhang K, Wang B, Wu S, Wang Y, Zhang H, et al. Nanoenabled disruption of multiple barriers in antigen cross-presentation of dendritic cells via calcium interference for enhanced chemo-immunotherapy. ACS Nano. (2020) 14:7639–50. doi: 10.1021/acsnano.0c03881
68. Li J, Ye Y, Liu Z, Zhang G, Dai H, Li J, et al. Macrophage mitochondrial fission improves cancer cell phagocytosis induced by therapeutic antibodies and is impaired by glutamine competition. Nat Cancer. (2022) 3:453–70. doi: 10.1038/s43018–022-00354–5
69. Qian R, Yi X, Liu T, Chen H, Wang Y, Hu L, et al. Regulation of ion homeostasis for enhanced tumor radio-immunotherapy. Adv Sci (Weinh). (2023) 10:e2304092. doi: 10.1002/advs.202304092
70. Haase H, Rink L. Multiple impacts of zinc on immune function. Metallomics. (2014) 6:1175–80. doi: 10.1039/c3mt00353a
71. Haase H, Rink L. Zinc signals and immune function. Biofactors. (2014) 40:27–40. doi: 10.1002/biof.1114
72. Wessels I, Maywald M, Rink L. Zinc as a gatekeeper of immune function. Nutrients. (2017) 9:1826. doi: 10.3390/nu9121286
73. Kim B, Lee WW. Regulatory role of zinc in immune cell signaling. Mol Cells. (2021) 44:335–41. doi: 10.14348/molcells.2021.0061
74. Khan AA, Ham SJ, Yen LN, Lee HL, Huh J, Jeon H, et al. A novel role of metal response element binding transcription factor 2 at the Hox gene cluster in the regulation of H3K27me3 by polycomb repressive complex 2. Oncotarget. (2018) 9:26572–85. doi: 10.18632/oncotarget.25505
75. Guo L, Lichten LA, Ryu MS, Liuzzi JP, Wang F, Cousins RJ. STAT5-glucocorticoid receptor interaction and MTF-1 regulate the expression of ZnT2 (Slc30a2) in pancreatic acinar cells. Proc Natl Acad Sci U.S.A. (2010) 107:2818–23. doi: 10.1073/pnas.0914941107
76. Kaltenberg J, Plum LM, Ober-Blobaum JL, Honscheid A, Rink L, Haase H. Zinc signals promote IL-2-dependent proliferation of T cells. Eur J Immunol. (2010) 40:1496–503. doi: 10.1002/eji.200939574
77. Beck FW, Kaplan J, Fine N, Handschu W, Prasad AS. Decreased expression of CD73 (ecto-5'-nucleotidase) in the CD8+ subset is associated with zinc deficiency in human patients. J Lab Clin Med. (1997) 130:147–56. doi: 10.1016/s0022–2143(97)90091–3
78. Saha AR, Hadden EM, Hadden JW. Zinc induces thymulin secretion from human thymic epithelial cells in vitro and augments splenocyte and thymocyte responses in vivo. Int J Immunopharmacol. (1995) 17:729–33. doi: 10.1016/0192–0561(95)00061–6
79. Rudd CE. How the discovery of the CD4/CD8-p56(lck) complexes changed immunology and immunotherapy. Front Cell Dev Biol. (2021) 9:626095. doi: 10.3389/fcell.2021.626095
80. Yu M, Lee WW, Tomar D, Pryshchep S, Czesnikiewicz-Guzik M, Lamar DL, et al. Regulation of T cell receptor signaling by activation-induced zinc influx. J Exp Med. (2011) 208:775–85. doi: 10.1084/jem.20100031
81. Colomar-Carando N, Meseguer A, Company-Garrido I, Jutz S, Herrera-Fernandez V, Olvera A, et al. Zip6 transporter is an essential component of the lymphocyte activation machinery. J Immunol. (2019) 202:441–50. doi: 10.4049/jimmunol.1800689
82. Aydemir TB, Liuzzi JP, McClellan S, Cousins RJ. Zinc transporter ZIP8 (SLC39A8) and zinc influence IFN-gamma expression in activated human T cells. J Leukoc Biol. (2009) 86:337–48. doi: 10.1189/jlb.1208759
83. Lee K, Sung C, Kim BG, Hahn JS. Activation of Aro80 transcription factor by heat-induced aromatic amino acid influx in Saccharomyces cerevisiae. Biochem Biophys Res Commun. (2013) 438:43–7. doi: 10.1016/j.bbrc.2013.07.019
84. Bao S, Liu MJ, Lee B, Besecker B, Lai JP, Guttridge DC, et al. Zinc modulates the innate immune response in vivo to polymicrobial sepsis through regulation of NF-kappaB. Am J Physiol Lung Cell Mol Physiol. (2010) 298:L744–54. doi: 10.1152/ajplung.00368.2009
85. Stoye D, Schubert C, Goihl A, Guttek K, Reinhold A, Brocke S, et al. Zinc aspartate suppresses T cell activation in vitro and relapsing experimental autoimmune encephalomyelitis in SJL/J mice. Biometals. (2012) 25:529–39. doi: 10.1007/s10534-012-9532-z
86. Kulik L, Maywald M, Kloubert V, Wessels I, Rink L. Zinc deficiency drives Th17 polarization and promotes loss of Treg cell function. J Nutr Biochem. (2019) 63:11–8. doi: 10.1016/j.jnutbio.2018.09.011
87. Rong D, Wang Y, Liu L, Cao H, Huang T, Liu H, et al. GLIS1 intervention enhances anti-PD1 therapy for hepatocellular carcinoma by targeting SGK1-STAT3-PD1 pathway. J Immunother Cancer. (2023) 11:e005126. doi: 10.1136/jitc-2022–005126
88. Qiu G, Deng Y. ZFP64 transcriptionally activates PD-1 and CTLA-4 and plays an oncogenic role in esophageal cancer. Biochem Biophys Res Commun. (2022) 622:72–8. doi: 10.1016/j.bbrc.2022.06.011
89. Ping Y, Shan J, Liu Y, Liu F, Wang L, Liu Z, et al. Taurine enhances the antitumor efficacy of PD-1 antibody by boosting CD8(+) T cell function. Cancer Immunol Immunother. (2023) 72:1015–27. doi: 10.1007/s00262-022-03308-z
90. Liu MJ, Bao S, Galvez-Peralta M, Pyle CJ, Rudawsky AC, Pavlovicz RE, et al. ZIP8 regulates host defense through zinc-mediated inhibition of NF-kappaB. Cell Rep. (2013) 3:386–400. doi: 10.1016/j.celrep.2013.01.009
91. Brieger A, Rink L, Haase H. Differential regulation of TLR-dependent MyD88 and TRIF signaling pathways by free zinc ions. J Immunol. (2013) 191:1808–17. doi: 10.4049/jimmunol.1301261
92. Muzzioli M, Stecconi R, Donnini A, Re F, Provinciali M. Zinc improves the development of human CD34+ cell progenitors towards Natural Killer cells and induces the expression of GATA-3 transcription factor. Int J Biochem Cell Biol. (2007) 39:955–65. doi: 10.1016/j.biocel.2007.01.011
93. Cen D, Ge Q, Xie C, Zheng Q, Guo J, Zhang Y, et al. ZnS@BSA nanoclusters potentiate efficacy of cancer immunotherapy. Adv Mater. (2021) 33:e2104037. doi: 10.1002/adma.202104037
94. Ding L, Liang M, Li Y, Zeng M, Liu M, Ma W, et al. Zinc-organometallic framework vaccine controlled-release zn(2+) regulates tumor extracellular matrix degradation potentiate efficacy of immunotherapy. Adv Sci (Weinh). (2023) 10:e2302967. doi: 10.1002/advs.202302967
95. Yang K, Han W, Jiang X, Piffko A, Bugno J, Han C, et al. Zinc cyclic di-AMP nanoparticles target and suppress tumors via endothelial STING activation and tumor-associated macrophage reinvigoration. Nat Nanotechnol. (2022) 17:1322–31. doi: 10.1038/s41565-022-01225-x
96. Chen P, Bornhorst J, Aschner M. Manganese metabolism in humans. Front Biosci (Landmark Ed). (2018) 23:1655–79. doi: 10.2741/4665
97. Morello M, Canini A, Mattioli P, Sorge RP, Alimonti A, Bocca B, et al. Sub-cellular localization of manganese in the basal ganglia of normal and manganese-treated rats An electron spectroscopy imaging and electron energy-loss spectroscopy study. Neurotoxicology. (2008) 29:60–72. doi: 10.1016/j.neuro.2007.09.001
98. Crossgrove JS, Yokel RA. Manganese distribution across the blood-brain barrier III. The divalent metal transporter-1 is not the major mechanism mediating brain manganese uptake. Neurotoxicology. (2004) 25:451–60. doi: 10.1016/j.neuro.2003.10.005
99. Liuzzi JP, Aydemir F, Nam H, Knutson MD, Cousins RJ. Zip14 (Slc39a14) mediates non-transferrin-bound iron uptake into cells. Proc Natl Acad Sci U.S.A. (2006) 103:13612–7. doi: 10.1073/pnas.0606424103
100. Seo YA, Wessling-Resnick M. Ferroportin deficiency impairs manganese metabolism in flatiron mice. FASEB J. (2015) 29:2726–33. doi: 10.1096/fj.14–262592
101. Lv M, Chen M, Zhang R, Zhang W, Wang C, Zhang Y, et al. Manganese is critical for antitumor immune responses via cGAS-STING and improves the efficacy of clinical immunotherapy. Cell Res. (2020) 30:966–79. doi: 10.1038/s41422–020-00395–4
102. Rogers RR, Garner RJ, Riddle MM, Luebke RW, Smialowicz RJ. Augmentation of murine natural killer cell activity by manganese chloride. Toxicol Appl Pharmacol. (1983) 70:7–17. doi: 10.1016/0041–008x(83)90174–6
103. Smialowicz RJ, Rogers RR, Riddle MM, Luebke RW, Rowe DG, Garner RJ. Manganese chloride enhances murine cell-mediated cytotoxicity: effects on natural killer cells. J Immunopharmacol. (1984) 6:1–23. doi: 10.3109/08923978409026455
104. Yang G, Ji J, Liu Z. Multifunctional MnO2 nanoparticles for tumor microenvironment modulation and cancer therapy. Wiley Interdiscip Rev Nanomed Nanobiotechnol. (2021) 13:e1720. doi: 10.1002/wnan.1720
105. Yang G, Xu L, Chao Y, Xu J, Sun X, Wu Y, et al. Hollow MnO(2) as a tumor-microenvironment-responsive biodegradable nano-platform for combination therapy favoring antitumor immune responses. Nat Commun. (2017) 8:902. doi: 10.1038/s41467–017-01050–0
106. Song M, Liu T, Shi C, Zhang X, Chen X. Bioconjugated manganese dioxide nanoparticles enhance chemotherapy response by priming tumor-associated macrophages toward M1-like phenotype and attenuating tumor hypoxia. ACS Nano. (2016) 10:633–47. doi: 10.1021/acsnano.5b06779
107. Song Y, Liu Y, Teo HY, Hanafi ZB, Mei Y, Zhu Y, et al. Manganese enhances the antitumor function of CD8(+) T cells by inducing type I interferon production. Cell Mol Immunol. (2021) 18:1571–74. doi: 10.1038/s41423–020-00524–4
108. Zhang L, Zhao J, Hu X, Wang C, Jia Y, Zhu C, et al. A peritumorally injected immunomodulating adjuvant elicits robust and safe metalloimmunotherapy against solid tumors. Adv Mater. (2022) 34:e2206915. doi: 10.1002/adma.202206915
109. Liu Y, Pan Y, Cao W, Xia F, Liu B, Niu J, et al. A tumor microenvironment responsive biodegradable CaCO(3)/MnO(2)- based nanoplatform for the enhanced photodynamic therapy and improved PD-L1 immunotherapy. Theranostics. (2019) 9:6867–84. doi: 10.7150/thno.37586
110. Sun Z, Wang Z, Wang T, Wang J, Zhang H, Li Z, et al. Biodegradable mnO-based nanoparticles with engineering surface for tumor therapy: simultaneous fenton-like ion delivery and immune activation. ACS Nano. (2022) 16:11862–75. doi: 10.1021/acsnano.2c00969
111. Workinger JL, Doyle RP, Bortz J. Challenges in the diagnosis of magnesium status. Nutrients. (2018) 10:1202. doi: 10.3390/nu10091202
112. Romani AM. Cellular magnesium homeostasis. Arch Biochem Biophys. (2011) 512:1–23. doi: 10.1016/j.abb.2011.05.010
113. Jahnen-Dechent W, Ketteler M. Magnesium basics. Clin Kidney J. (2012) 5:i3–i14. doi: 10.1093/ndtplus/sfr163
114. Barbagallo M, Gupta RK, Dominguez LJ, Resnick LM. Cellular ionic alterations with age: relation to hypertension and diabetes. J Am Geriatr Soc. (2000) 48:1111–6. doi: 10.1111/j.1532-5415.2000.tb04788.x
115. Saris NE, Mervaala E, Karppanen H, Khawaja JA, Lewenstam A. Magnesium. An update on physiological, clinical and analytical aspects. Clin Chim Acta. (2000) 294:1–26. doi: 10.1016/s0009–8981(99)00258–2
116. Barbagallo M, Veronese N, Dominguez LJ. Magnesium in type 2 diabetes mellitus, obesity, and metabolic syndrome. Nutrients. (2022) 14:714. doi: 10.3390/nu14030714
117. Kroll MH, Elin RJ. Relationships between magnesium and protein concentrations in serum. Clin Chem. (1985) 31:244–6. doi: 10.1093/clinchem/31.2.244
118. Ravell J, Otim I, Nabalende H, Legason ID, Reynolds SJ, Ogwang MD, et al. Plasma magnesium is inversely associated with Epstein-Barr virus load in peripheral blood and Burkitt lymphoma in Uganda. Cancer Epidemiol. (2018) 52:70–4. doi: 10.1016/j.canep.2017.12.004
119. Dai Q, Shrubsole MJ, Ness RM, Schlundt D, Cai Q, Smalley WE, et al. The relation of magnesium and calcium intakes and a genetic polymorphism in the magnesium transporter to colorectal neoplasia risk. Am J Clin Nutr. (2007) 86:743–51. doi: 10.1093/ajcn/86.3.743
120. Kanellopoulou C, George AB, Masutani E, Cannons JL, Ravell JC, Yamamoto TN, et al. Mg(2+) regulation of kinase signaling and immune function. J Exp Med. (2019) 216:1828–42. doi: 10.1084/jem.20181970
121. Ravell JC, Chauvin SD, He T, Lenardo M. An update on XMEN disease. J Clin Immunol. (2020) 40:671–81. doi: 10.1007/s10875-020-00790-x
122. Brandao K, Deason-Towne F, Perraud AL, Schmitz C. The role of Mg2+ in immune cells. Immunol Res. (2013) 55:261–9. doi: 10.1007/s12026-012-8371-x
123. Leitzmann MF, Stampfer MJ, Wu K, Colditz GA, Willett WC, Giovannucci EL. Zinc supplement use and risk of prostate cancer. J Natl Cancer Inst. (2003) 95:1004–7. doi: 10.1093/jnci/95.13.1004
124. Bates-Withers C, Sah R, Clapham DE. TRPM7, the Mg(2+) inhibited channel and kinase. Adv Exp Med Biol. (2011) 704:173–83. doi: 10.1007/978–94-007–0265-3_9
125. Antunes TT, Callera GE, He Y, Yogi A, Ryazanov AG, Ryazanova LV, et al. Transient receptor potential melastatin 7 cation channel kinase: new player in angiotensin II-induced hypertension. Hypertension. (2016) 67:763–73. doi: 10.1161/HYPERTENSIONAHA.115.07021
126. Jin J, Desai BN, Navarro B, Donovan A, Andrews NC, Clapham DE. Deletion of Trpm7 disrupts embryonic development and thymopoiesis without altering Mg2+ homeostasis. Science. (2008) 322:756–60. doi: 10.1126/science.1163493
127. Bird L. Magnesium: essential for T cells. Nat Rev Immunol. (2022) 22:144–45. doi: 10.1038/s41577–022-00688–2
128. Lotscher J, Marti ILAA, Kirchhammer N, Cribioli E, Giordano Attianese GMP, Trefny MP, et al. Magnesium sensing via LFA-1 regulates CD8(+) T cell effector function. Cell. (2022) 185:585–602 e29. doi: 10.1016/j.cell.2021.12.039
129. Shan J, Jing W, Ping Y, Shen C, Han D, Liu F, et al. LFA-1 regulated by IL-2/STAT5 pathway boosts antitumor function of intratumoral CD8(+) T cells for improving anti-PD-1 antibody therapy. Oncoimmunology. (2024) 13:2293511. doi: 10.1080/2162402X.2023.2293511
130. Gurusamy D, Clever D, Eil R, Restifo NP. Novel "Elements" of immune suppression within the tumor microenvironment. Cancer Immunol Res. (2017) 5:426–33. doi: 10.1158/2326–6066.CIR-17–0117
131. Feske S, Wulff H, Skolnik EY. Ion channels in innate and adaptive immunity. Annu Rev Immunol. (2015) 33:291–353. doi: 10.1146/annurev-immunol-032414–112212
132. Hwang JR, Byeon Y, Kim D, Park SG. Recent insights of T cell receptor-mediated signaling pathways for T cell activation and development. Exp Mol Med. (2020) 52:750–61. doi: 10.1038/s12276–020-0435–8
133. Sim JH, Kim KS, Park H, Kim KJ, Lin H, Kim TJ, et al. Differentially expressed potassium channels are associated with function of human effector memory CD8(+) T cells. Front Immunol. (2017) 8:85. doi: 10.3389/fimmu.2017.0085
134. Chimote AA, Balajthy A, Arnold MJ, Newton HS, Hajdu P, Qualtieri J, et al. A defect in KCa3.1 channel activity limits the ability of CD8(+) T cells from cancer patients to infiltrate an adenosine-rich microenvironment. Sci Signal. (2018) 11:eaaq1616. doi: 10.1126/scisignal.aaq1616
135. Di L, Srivastava S, Zhdanova O, Ding Y, Li Z, Wulff H, et al. Inhibition of the K+ channel KCa3.1 ameliorates T cell-mediated colitis. Proc Natl Acad Sci U.S.A. (2010) 107:1541–6. doi: 10.1073/pnas.0910133107
136. Beeton C, Wulff H, Standifer NE, Azam P, Mullen KM, Pennington MW, et al. Kv1.3 channels are a therapeutic target for T cell-mediated autoimmune diseases. Proc Natl Acad Sci U.S.A. (2006) 103:17414–9. doi: 10.1073/pnas.0605136103
137. Vodnala SK, Eil R, Kishton RJ, Sukumar M, Yamamoto TN, Ha NH, et al. T cell stemness and dysfunction in tumors are triggered by a common mechanism. Science. (2019) 363:eaau0135. doi: 10.1126/science.aau0135
138. Wang X, Huynh C, Urak R, Weng L, Walter M, Lim L, et al. The cerebroventricular environment modifies CAR T cells for potent activity against both central nervous system and systemic lymphoma. Cancer Immunol Res. (2021) 9:75–88. doi: 10.1158/2326–6066.CIR-20–0236
139. Chen S, Cui W, Chi Z, Xiao Q, Hu T, Ye Q, et al. Tumor-associated macrophages are shaped by intratumoral high potassium via Kir2.1. Cell Metab. (2022) 34:1843–59 e11. doi: 10.1016/j.cmet.2022.08.016
140. Raza M, Chakraborty S, Choudhury M, Ghosh PC, Nag A. Cellular iron homeostasis and therapeutic implications of iron chelators in cancer. Curr Pharm Biotechnol. (2014) 15:1125–40. doi: 10.2174/138920101512141202111915
141. Doherty CP. Host-pathogen interactions: the role of iron. J Nutr. (2007) 137:1341–4. doi: 10.1093/jn/137.5.1341
142. Walker EM Jr., Walker SM. Effects of iron overload on the immune system. Ann Clin Lab Sci. (2000) 30:354–65.
143. Wang CY, Jenkitkasemwong S, Duarte S, Sparkman BK, Shawki A, Mackenzie B, et al. ZIP8 is an iron and zinc transporter whose cell-surface expression is up-regulated by cellular iron loading. J J Biol Chem. (2012) 287:34032–43. doi: 10.1074/jbc.M112.367284
144. Jabara HH, Boyden SE, Chou J, Ramesh N, Massaad MJ, Benson H, et al. A missense mutation in TFRC, encoding transferrin receptor 1, causes combined immunodeficiency. Nat Genet. (2016) 48:74–8. doi: 10.1038/ng.3465
145. Yarosz EL, Ye C, Kumar A, Black C, Choi EK, Seo YA, et al. Cutting edge: activation-induced iron flux controls CD4 T cell proliferation by promoting proper IL-2R signaling and mitochondrial function. J Immunol. (2020) 204:1708–13. doi: 10.4049/jimmunol.1901399
146. Kuvibidila SR, Porretta C. Iron deficiency and in vitro iron chelation reduce the expression of cluster of differentiation molecule (CD)28 but not CD3 receptors on murine thymocytes and spleen cells. Br J Nutr. (2003) 90:179–89. doi: 10.1079/bjn2003864
147. Ma X, Xiao L, Liu L, Ye L, Su P, Bi E, et al. CD36-mediated ferroptosis dampens intratumoral CD8(+) T cell effector function and impairs their antitumor ability. Cell Metab. (2021) 33:1001–12 e5. doi: 10.1016/j.cmet.2021.02.015
148. Wang W, Green M, Choi JE, Gijon M, Kennedy PD, Johnson JK, et al. CD8(+) T cells regulate tumor ferroptosis during cancer immunotherapy. Nature. (2019) 569:270–74. doi: 10.1038/s41586-019-1170-y
149. Liao P, Wang W, Wang W, Kryczek I, Li X, Bian Y, et al. CD8(+) T cells and fatty acids orchestrate tumor ferroptosis and immunity via ACSL4. Cancer Cell. (2022) 40:365–78 e6. doi: 10.1016/j.ccell.2022.02.003
150. Liu Y, Duan C, Dai R, Zeng Y. Ferroptosis-mediated crosstalk in the tumor microenvironment implicated in cancer progression and therapy. Front Cell Dev Biol. (2021) 9:739392. doi: 10.3389/fcell.2021.739392
151. Cairo G, Recalcati S, Mantovani A, Locati M. Iron trafficking and metabolism in macrophages: contribution to the polarized phenotype. Trends Immunol. (2011) 32:241–7. doi: 10.1016/j.it.2011.03.007
152. Kim R, Hashimoto A, Markosyan N, Tyurin VA, Tyurina YY, Kar G, et al. Ferroptosis of tumor neutrophils causes immune suppression in cancer. Nature. (2022) 612:338–46. doi: 10.1038/s41586–022-05443–0
153. Conche C, Finkelmeier F, Pesic M, Nicolas AM, Bottger TW, Kennel KB, et al. Combining ferroptosis induction with MDSC blockade renders primary tumors and metastases in liver sensitive to immune checkpoint blockade. Gut. (2023) 72:1774–82. doi: 10.1136/gutjnl-2022–327909
154. Yao L, Hou J, Wu X, Lu Y, Jin Z, Yu Z, et al. Cancer-associated fibroblasts impair the cytotoxic function of NK cells in gastric cancer by inducing ferroptosis via iron regulation. Redox Biol. (2023) 67:102923. doi: 10.1016/j.redox.2023.102923
155. Guo Y, Ran Y, Wang Z, Cheng J, Cao Y, Yang C, et al. Magnetic-responsive and targeted cancer nanotheranostics by PA/MR bimodal imaging-guided photothermally triggered immunotherapy. Biomaterials. (2019) 219:119370. doi: 10.1016/j.biomaterials.2019.119370
156. Wang Y, Chen J, Lu J, Xi J, Xu Z, Fan L, et al. Metal ions/nucleotide coordinated nanoparticles comprehensively suppress tumor by synergizing ferroptosis with energy metabolism interference. J Nanobiotechnol. (2022) 20:199. doi: 10.1186/s12951-022-01405-w
157. Li L, Zhang M, Liu T, Li J, Sun S, Chen J, et al. Quercetin-ferrum nanoparticles enhance photothermal therapy by modulating the tumor immunosuppressive microenvironment. Acta Biomater. (2022) 154:454–66. doi: 10.1016/j.actbio.2022.10.008
158. Ge EJ, Bush AI, Casini A, Cobine PA, Cross JR, DeNicola GM, et al. Connecting copper and cancer: from transition metal signaling to metalloplasia. Nat Rev Cancer. (2022) 22:102–13. doi: 10.1038/s41568–021-00417–2
159. Shanbhag VC, Gudekar N, Jasmer K, Papageorgiou C, Singh K, Petris MJ. Copper metabolism as a unique vulnerability in cancer. Biochim Biophys Acta Mol Cell Res. (2021) 1868:118893. doi: 10.1016/j.bbamcr.2020.118893
160. Kim BE, Nevitt T, Thiele DJ. Mechanisms for copper acquisition, distribution and regulation. Nat Chem Biol. (2008) 4:176–85. doi: 10.1038/nchembio.72
161. Czlonkowska A, Litwin T, Dusek P, Ferenci P, Lutsenko S, Medici V, et al. Wilson disease. Nat Rev Dis Primers. (2018) 4:21. doi: 10.1038/s41572–018-0018–3
162. Mao S, Huang S. Zinc and copper levels in bladder cancer: a systematic review and meta-analysis. Biol Trace Elem Res. (2013) 153:5–10. doi: 10.1007/s12011-013-9682-z
163. Zhang M, Shi M, Zhao Y. Association between serum copper levels and cervical cancer risk: a meta-analysis. Biosci Rep. (2018) 38. doi: 10.1042/BSR20180161
164. Ohgami RS, Campagna DR, McDonald A, Fleming MD. The Steap proteins are metalloreductases. Blood. (2006) 108:1388–94. doi: 10.1182/blood-2006–02-003681
165. Oosterheert W, van Bezouwen LS, Rodenburg RNP, Granneman J, Forster F, Mattevi A, et al. Cryo-EM structures of human STEAP4 reveal mechanism of iron(III) reduction. Nat Commun. (2018) 9:4337. doi: 10.1038/s41467–018-06817–7
166. Wang B, Dong D, Kang YJ. Copper chaperone for superoxide dismutase-1 transfers copper to mitochondria but does not affect cytochrome c oxidase activity. Exp Biol Med (Maywood). (2013) 238:1017–23. doi: 10.1177/1535370213497327
167. Leary SC, Kaufman BA, Pellecchia G, Guercin GH, Mattman A, Jaksch M, et al. Human SCO1 and SCO2 have independent, cooperative functions in copper delivery to cytochrome c oxidase. Hum Mol Genet. (2004) 13:1839–48. doi: 10.1093/hmg/ddh197
168. Feng W, Ye F, Xue W, Zhou Z, Kang YJ. Copper regulation of hypoxia-inducible factor-1 activity. Mol Pharmacol. (2009) 75:174–82. doi: 10.1124/mol.108.051516
169. Beaino W, Guo Y, Chang AJ, Anderson CJ. Roles of Atox1 and p53 in the trafficking of copper-64 to tumor cell nuclei: implications for cancer therapy. J Biol Inorg Chem. (2014) 19:427–38. doi: 10.1007/s00775–013-1087–0
170. Lukasewycz OA, Prohaska JR. Immunization against transplantable leukemia impaired in copper-deficient mice. J Natl Cancer Inst. (1982) 69:489–93.
171. McMurray DN. Cell-mediated immunity in nutritional deficiency. Prog Food Nutr Sci. (1984) 8:193–228
172. Hopkins RG, Failla ML. Copper deficiency reduces interleukin-2 (IL-2) production and IL-2 mRNA in human T-lymphocytes. J Nutr. (1997) 127:257–62. doi: 10.1093/jn/127.2.257
173. Hopkins RG, Failla ML. Transcriptional regulation of interleukin-2 gene expression is impaired by copper deficiency in Jurkat human T lymphocytes. J Nutr. (1999) 129:596–601. doi: 10.1093/jn/129.3.596
174. Bala S, Failla ML. Copper deficiency reversibly impairs DNA synthesis in activated T lymphocytes by limiting interleukin 2 activity. Proc Natl Acad Sci U.S.A. (1992) 89:6794–7. doi: 10.1073/pnas.89.15.6794
175. Lukasewycz OA, Prohaska JR, Meyer SG, Schmidtke JR, Hatfield SM, Marder P. Alterations in lymphocyte subpopulations in copper-deficient mice. Infect Immun. (1985) 48:644–7. doi: 10.1128/iai.48.3.644–647.1985
176. Flynn A, Yen BR. Mineral deficiency effects on the generation of cytotoxic T-cells and T-helper cell factors in vitro. J Nutr. (1981) 111:907–13. doi: 10.1093/jn/111.5.907
177. Voli F, Valli E, Lerra L, Kimpton K, Saletta F, Giorgi FM, et al. Intratumoral copper modulates PD-L1 expression and influences tumor immune evasion. Cancer Res. (2020) 80:4129–44. doi: 10.1158/0008–5472.CAN-20–0471
178. Mantovani A, Marchesi F, Malesci A, Laghi L, Allavena P. Tumor-associated macrophages as treatment targets in oncology. Nat Rev Clin Oncol. (2017) 14:399–416. doi: 10.1038/nrclinonc.2016.217
179. Chatterjee S, Mookerjee A, Basu JM, Chakraborty P, Ganguly A, Adhikary A, et al. A novel copper chelate modulates tumor associated macrophages to promote anti-tumor response of T cells. PloS One. (2009) 4:e7048. doi: 10.1371/journal.pone.0007048
180. Cordano A, Placko RP, Graham GG. Hypocupremia and neutropenia in copper deficiency. Blood. (1966) 28:280–3. doi: 10.1182/blood.V28.2.280.280
181. Tsvetkov P, Coy S, Petrova B, Dreishpoon M, Verma A, Abdusamad M, et al. Copper induces cell death by targeting lipoylated TCA cycle proteins. Science. (2022) 375:1254–61. doi: 10.1126/science.abf0529
182. Yip NC, Fombon IS, Liu P, Brown S, Kannappan V, Armesilla AL, et al. Disulfiram modulated ROS-MAPK and NFkappaB pathways and targeted breast cancer cells with cancer stem cell-like properties. Br J Cancer. (2011) 104:1564–74. doi: 10.1038/bjc.2011.126
183. Chen D, Cui QC, Yang H, Dou QP. Disulfiram, a clinically used anti-alcoholism drug and copper-binding agent, induces apoptotic cell death in breast cancer cultures and xenografts via inhibition of the proteasome activity. Cancer Res. (2006) 66:10425–33. doi: 10.1158/0008–5472.CAN-06–2126
184. Skrott Z, Mistrik M, Andersen KK, Friis S, Majera D, Gursky J, et al. Alcohol-abuse drug disulfiram targets cancer via p97 segregase adaptor NPL4. Nature. (2017) 552:194–99. doi: 10.1038/nature25016
185. Wang Y, Drum DL, Sun R, Zhang Y, Yu L, Jia L, et al. Stressed target cancer cells drive nongenetic reprogramming of CAR T cells and tumor microenvironment, overcoming multiple obstacles of CAR T therapy for solid tumors. Res Sq. (2023). doi: 10.21203/rs.3.rs-2595410/v1
186. Cheng F, Peng G, Lu Y, Wang K, Ju Q, Ju Y, et al. Relationship between copper and immunity: The potential role of copper in tumor immunity. Front Oncol. (2022) 12:1019153. doi: 10.3389/fonc.2022.1019153
187. Guo B, Yang F, Zhang L, Zhao Q, Wang W, Yin L, et al. Cuproptosis induced by ROS responsive nanoparticles with elesclomol and copper combined with alphaPD-L1 for enhanced cancer immunotherapy. Adv Mater. (2023) 35:e2212267. doi: 10.1002/adma.202212267
Keywords: metal ions, tumor microenvironment, tumor immunotherapy, nanomedicines, T cells
Citation: Gao Y, Liu S, Huang Y, Li F and Zhang Y (2024) Regulation of anti-tumor immunity by metal ion in the tumor microenvironment. Front. Immunol. 15:1379365. doi: 10.3389/fimmu.2024.1379365
Received: 31 January 2024; Accepted: 29 May 2024;
Published: 10 June 2024.
Edited by:
Steven Fiering, Dartmouth College, United StatesReviewed by:
Sunita Keshari, University of Texas MD Anderson Cancer Center, United StatesGuangyong Peng, Washington University in St. Louis, United States
Copyright © 2024 Gao, Liu, Huang, Li and Zhang. This is an open-access article distributed under the terms of the Creative Commons Attribution License (CC BY). The use, distribution or reproduction in other forums is permitted, provided the original author(s) and the copyright owner(s) are credited and that the original publication in this journal is cited, in accordance with accepted academic practice. No use, distribution or reproduction is permitted which does not comply with these terms.
*Correspondence: Yi Zhang, eWl6aGFuZ0B6enUuZWR1LmNu; Feng Li, bGlmZW5nMDFAbXNuLmNvbQ==
†These authors have contributed equally to this work