- Institute of Molecular Medicine and Experimental Immunology, University Hospital Bonn of the Rheinische Friedrich-Wilhelms-University, Bonn, Germany
Dendritic cells (DCs) are major antigen-presenting cells that connect innate and adaptive immunity. Hepatic DCs are less activated and contribute to maintain the tolerogenic environment of the liver under steady state. Several studies indicated DCs in metabolic dysfunction-associated steatohepatitis (MASH), representing a substantial burden on healthcare systems due to its association with liver-related morbidity and mortality. Studies highlighted the potential disease-promoting role of liver DCs in the development of MASH while other experimental systems suggested their protective role. This review discusses this controversy and the current understanding of how DCs affect the pathogenesis of MASH.
Introduction
Metabolic dysfunction-associated steatohepatitis (MASH) has recently become a significant health issue, presenting as a progressive and potentially severe form of metabolic dysfunction-associated fatty liver disease (MAFLD) (1, 2). This intricate condition is characterized by hepatic inflammation and liver cell damage and association with liver-related morbidity and mortality (3). During the progression of MAFLD caused by liver steatosis, patients are at risk for multiple complications, including hypertension, atherosclerosis, and other diseases (4). In addition, MASH has the potential to progress to a more advanced form of liver disease, such as cirrhosis and hepatocellular carcinoma (HCC) (5).
The pathogenesis of MASH is a multifaceted process involving hepatocyte triglyceride accumulation followed by additional triggering factors, including oxidative stress, lipotoxicity, mitochondrial dysfunction, and pro-inflammatory cytokine release, that contribute to the worsening of the condition (6). Hepatic lobular inflammation is a defining characteristic of the transition from simple steatosis to steatohepatitis. Presently, it is recognized as the primary factor driving the progression of MAFLD to MASH (7), as well as the development of cirrhosis and its specific contribution to extrahepatic complications associated with the disease (5, 8). Prolonged inflammation aggravates tissue damage and may lead to an abnormal response in tissue healing, thereby contributing to the emergence of MASH and liver fibrosis. In this context, as a response to metabolic-related stress, both the innate and adaptive immune system become activated. Understanding the interaction between immune and parenchymal cells is the key challenge in deciphering the pathogenesis of the disease (6, 9).
Liver as a multipurpose organ with complex immune and dendritic cell network
The liver is well known for its complex metabolic function and its role in the maintenance of homeostasis. Structurally, the liver is composed of repeating functional units that are defined by the vascular supply structures. These structures form a network of sinusoidal vessels that provide blood to the metabolic cellular units of the liver: hepatocytes and cholangiocytes (10). The hepatocytes are separated from the bloodstream by non-parenchymal cells, including a layer of fenestrated liver sinusoidal endothelial cells (LSECs) that lack a basement membrane. Hepatic stellate cells (HSCs) are also present in the small space of Dissé between LSECs and hepatocytes (10). Besides these parenchymal cells, the liver is home for multiple subsets of immune cells, e.g., macrophages, dendritic cells (DCs), classical T and B lymphocytes, and innate lymphoid cells (10–12). Recent years of scRNAseq and multiparameter flow cytometry analyses revealed diverse immune cell types present in the liver, but more importantly, it highlighted the manifold subset variety that could be identified (13–15). Based on extensive single-cell analyses, novel tools have also been developed such as the Liver Cell Atlas that are available for scientists to survey their molecule of interest (13, 14). It is becoming more evident that the presence of such complex immune network forms an interconnected functional unit with the metabolic and parenchymal liver tissue under both physiological and pathological conditions.
Among DCs, we distinguish different DC subtypes based on their origin and phenotypic criteria (16). Conventional DCs (cDCs) develop from hematopoietic stem cells in the bone marrow via intermediate stages as common DC progenitor (CDP) and pre-cDCs. This process is dependent on Flt3L–Flt3 interaction (17). Pre-DCs leave the bone marrow and colonize lymphoid and non-lymphoid organs and become conventional DC type 1 (cDC1) and conventional DC type 2 (cDC2) cells. The two subsets depend on different transcription factors for their development. cDC1s need, e.g., Irf8, Batf3, Id2, and Nfil3 while cDC2s need Irf4 and Irf2 (18). They also differ in their surface markers used to identify and distinguish these cells (Table 1). cDC1s in murine lymphoid organs express CD8α, but in non-lymphoid organs, they are rather positive for CD103 or CD24. Murine cDC2s are more abundant than cDC1s but represent a very heterogeneous cell population (16, 18). Using scRNAseq analyses, studies identified DCs expressing BDCA-3 (blood dendritic cell antigens 3) and XCR1 (X-C motif chemokine receptor 1) as cDC1s in humans (19–21). Human cDC2s lack the above canonical markers and exhibit heterogeneous populations with different gene expression profiles across tissues (19–23). High-resolution scRNAseq identified further putative cell clusters that may correspond to additional human cDC subsets (16, 22, 24). A recent study demonstrated DC3 population derived from Ly6C+ monocyte-dendritic cell progenitors representing a novel DC subtype (24). Further studies are necessary to clarify these clusters and how do they relate to other DC subsets.
Resting DCs receive environmental signals (e.g., type I IFNs) that maintain their homeostasis and survival (25, 26). cDCs are able to sense the environment via innate immune receptors such as pathogen-associated molecular patterns (PAMPs) and danger-associated molecular patterns (DAMPs) or respond to various cytokines (e.g., Il1β) (27, 28). Response to such danger signals is key in the initiation of immune responses (29). DCs upon danger signal become activated, a process referring to the ability of DCs to become capable of delivering information to other immune and/or non-immune cells (16). DCs are sampling the environment and able to migrate to draining lymph nodes to present antigens themselves on MHC-I or on MHC-II molecules or hand over antigens to resident cDCs for presentation (30, 31). Based on their processing machinery, cDC1s were considered to be superior in CD8 T-cell priming and specialized in cross-presentation while cDC2s favored CD4 T-cell priming (32). Several studies indicated that these differences are not due to intrinsic ability but rather differences in location and antigen access and both subsets of cDCs are able to activate both types of T cells (33, 34).
In addition to their role as initiators of adaptive immunity, cDCs are involved in immune tolerance both in the thymus and at the periphery (35). Notably, tolerogenic DC activation was considered as a semi-activated state compared to the fully activated DCs evolved upon PAMP engagement. Growing lines of evidence suggest that tolerogenic DC activation represents not a different level of quantitative activation but an entirely complex gene expression profile enabling cDCs to use a different set of effector capacities to interact with the environment (36, 37).
One of the most distinguished ability of cDCs is the activation of naïve and memory T cells and thereby the initiation of adaptive immune responses. They also can directly present antigens to B cells, leading to humoral response and antibody production. Additionally, DCs interact with NK and NKT cells and connect innate and adaptive immunity (16, 38). Besides immune cells, DCs connect the immune and non-immune compartment to orchestrate the organ-specific responses. cDCs communicate with fibroblastic reticular cells and help adjust the stromal compartment during priming (39). They also produce VEGF or IL31 for endothelial and neuronal crosstalk (40, 41). Thus, cDCs represent a central component in the interconnective immune and non-immune network during immune responses.
Plasmacytoid DCs (pDCs) were long considered as a third subtype of DCs originating from a lymphoid progenitor distinct from the one that gives rise to cDCs (42). pDCs are capable of producing a large amount of type I IFNs upon infection, and their role in T-cell activation and tolerance is still debated. Recently, they have been suggested for re-classification as innate lymphocytes (43).
Monocyte-derived DCs (Mo-DCs) are positive for CD11c and MHCII and they were believed to serve as antigen-presenting cells especially during inflammation (44, 45). Mo-DCs express genes associated with inflammation, but their role in T-cell priming is questionable. A recent study demonstrated that highly purified Mo-DCs are unable to perform these tasks (46).
While DC physiology is well studied in lymphoid organs, it is less understood in the liver microenvironment.
Hepatic dendritic cells: functional dichotomy under steady state
Under steady state, hepatic DCs are relatively sparse in the liver (make up approximately 1% of the non-parenchymal cells) and mostly located in the periportal area. Here, they form an interconnected network where cDC1s mostly localized along the lymphatic vessels, while cDC2s surround the biliary tree (10, 47), suggesting functional division among subsets. DCs closely interact with the microenvironment in the portal area, respond to extracellular matrix (ECM) signals with maturation, and migrate to draining hepatic lymph node (48, 49). In this context, DCs as sentinels of the immune system play a central role in the initiation, modulation, and resolution of hepatic inflammation (10). Being strategically positioned within the liver allows liver DCs to survey and respond to changes in the hepatic microenvironment, making them indispensable contributors to the orchestration of immune responses in the liver. The liver, as most non-lymphoid organs, also possesses DC precursors with the capacity to differentiate towards all three DC subtypes (10, 50). Additionally, it has been reported that CD11c+ CX3CR1+ DCs, representing an additional DC subset in the liver, are located at the subcapsular area and replaced by monocytes from the circulation (51). What is the exact role of these cells and how they relate to other conventional DC subsets in liver homeostasis and pathology remain to be elucidated.
Liver cDCs under steady state have a lower capacity to endocytose antigen and are less efficient in allogenic T-cell activation than lymphoid DCs. They express immunosuppressive cytokines such as interleukin-10 (IL-10) and transforming growth factor-beta (TGF-β). To complement the liver tolerogenic environment, they respond less to LPS (and other TLR) stimuli: a phenomenon referred to as endotoxin tolerance (10, 50, 52, 53). pDCs, on the other hand, primarily promote Treg responses in the liver and are crucial in the tolerogenic response to oral antigens (10, 52, 53). Thus, overall, these specialized DC subsets in the liver act as APCs and modulate immune responses by promoting the generation/function of Tregs, by inhibiting pro-inflammatory T-cell responses, and by supporting the maintenance of immune tolerance under steady state via their unique cytokine profile.
MASH and the adaptive arm of the immune response
A large body of evidence suggests that the activation of adaptive immune components contributes significantly to the progression and modulation of inflammation in MASH, influencing the disease pathogenesis and outcomes. It is well acknowledged that patients with MASH exhibit notably elevated levels of hepatic cytotoxic CD8+ T cells in comparison to healthy individuals (54–56). This is accompanied by the elevated presence of Th17 and reduced numbers of Tregs in the liver, promoting a pro-inflammatory milieu (57, 58). Rag1KO animals that lack mature B cells, T cells, and NKT cells are unable to mount adaptive T-cell responses and have greatly attenuated MASH (59). Mice deficient in β2m, lacking CD8+ T cells, exhibit protection against both steatosis and MASH upon choline-deficient high-fat diet (CDHFD) challenge. This protection relates to a decrease in the production of LIGHT by CD8+ T cells and NKT cells and the consequently reduced hepatocyte damage (59). Accordingly, the increase in CD8+ T cells and the reduction in the CD4 T-cell compartment contribute not only to the progression of MASH but also to the transition of MASH to HCC (59, 60). In recent years, it became clear as well that CXCR6+granzyme-B+PD1+ effector/memory phenotype CD8 T cells accumulate in the liver during MASH (61). These cells became metabolically activated by acetate and extracellular ATP in the microenvironment. This leads to their autoaggressive behavior that resulted in hepatocyte death that was MHC-I independent and mediated via purinergic receptor signaling (P2X7) (61). Together, these data suggest that primed T cells homing to the liver are responsible for the liver damage and MASH. Metabolic activation of T cells is further promoted by B cells independent of TCR signaling (62). B cells become activated within the lamina propria independent of microbiota and home to the liver where they affect CD8 T cells and further promote damage and fibrosis via their IgA secretion (62). This phenomenon is accompanied by the increased presence of autoantibodies targeting oxidative stress-derived epitopes in approximately 40% of adults and in 60% of children with MASH (63). These lines of evidence suggest that MASH is highly mediated and dependent on the adaptive immune response. The question is how DCs, which are the major APCs linking innate and adaptive immunity, fit in this picture.
The role of DCs in MASH: the cDC1 controversy
The role of DCs is highly controversial and still remain not well understood in MASH (Table 2). Increased DC (cDC1, cDC2, and pDCs) infiltration is a hallmark of human MASH (56, 68) and has been demonstrated in multiple animal models (64–66). The involvement of cDCs in MASH has been established in the study conducted by Henning et al., in which DCs exhibited a more activated phenotype and the ablation of DCs using BM chimeric CD11c.DTR mice resulted in a larger influx of inflammatory cells to the liver, increased production of various cytokines associated with hepatic injury, and accelerated hepatic fibrosis (64). These findings suggested that cDCs may have a protective role in the context of disease. This was further highlighted using animal model deficient in the transcription factor Batf3 (basic leucine zipper ATF-like transcription factor 3) that lacks CD103+ cDC1s. Batf3 KO animals demonstrated a switch towards MASH from steatosis under high sucrose diet (HSD) including an increased presence of inflammatory infiltrates, steatosis, and elevated hepatocellular damage compared to WT animals (65). CD11c+CD11b+ myeloid cells showed increased frequency in Batf3 KO HSD fed animals, and the production of cytokines including IL-1ra, CCL2, CXCL1, CCL5, and TNF was also elevated (65). In addition, upon adoptive transfer of bone marrow-derived CD103+ cDC1s, a notable reduction was observed in the influx of pro-inflammatory monocytes and TNF production by CD11c+ cells (65). Thus, both the above studies identified DCs that balance the pro- and anti-inflammatory environment and regulate the presence of the inflammatory infiltrates. Both studies, however, used systems that were not exclusively specific for DCs. CD11c is a marker that could also be expressed by, e.g., NK cells, inflammatory monocytes, and neutrophils, while Batf3 could be present in regulatory T cells, gamma/delta T cells, and type 9 helper T cells (16, 69, 70). Therefore, the effect of Batf3 ablation or depletion of CD11c+ cells could not be unequivocally regarded to DCs.
A recent study by Deczkowska et al. demonstrated the increased frequency of CD103+ XCR1+ cDC1 and CD11b+ cDC2s in the liver and showed elevated proliferation of DC-committed progenitors in the bone marrow, leading to higher frequencies of circulating pre-DCs in the bloodstream and in the liver (66). Importantly, blocking the infiltration of hepatic cDC1 by using XCL1 antibody in mice fed with CDHFD resulted in a moderate decrease in NASH pathology as compared to the isotype control-treated group (66). These changes included reduction in ALT and NAS score and reduced the presence of accumulated CD8 T cells with the activated/effector memory phenotype (66). Additionally, using a mouse model of XCR1-DTR mice, the loss of cDC1s attenuated immune rearrangements and MASH pathology in the liver (66). Notably, there was increased DC migration and priming activity in draining hepatic lymph node, which suggests superior adaptive T-cell activation that could feed the liver with effector/memory T cells that can become autoaggressive in the metabolically altered milieu. While the study is contradictory to the previous two DC studies, it still could not explain how DCs actually act in MASH. The XCR1-DTR model and DC ablation could simply affect draining LN priming or the lamina propria environment where priming of adaptive immune cells that home towards the liver is generated.
Besides cDCs, Mo-DCs are also increased in MASH liver, and their contribution is even less understood than cDC1s. Specific Mo-DCs expressing CX3CR1 seem to sustain hepatic inflammation by producing TNF. Additionally, ablation of CX3CR1 by hydrogen sulfide (H2S) donor NaHS alleviates parenchymal injury in MASH (67). Notably, the H2S donor and the lack of CX3CR1 affected bone marrow DC differentiation and precursor activity and questioned how this phenomenon could be directly related to the hepatic microenvironment.
Conclusions and outlook
The question “what role do DCs play in MASH?” remains. The answer is most likely a combination of multiple factors (Figure 1). Adaptive immune response is key for MASH, and DCs probably contribute (a) as antigen-presenting cells for generating the army of T cells that potentially can home to the liver and (b) in terms of providing CD4 T-cell help for B-cell activation. This would relate to the function of DCs from outside of the liver (in gut and draining LN). Migratory DCs coming from the liver could have significance in bringing oxidative stress-derived antigens and prime naïve T cells. It is also underlined by multiple animal and human studies where the frequency of DCs and their activation state increase (48, 64–66). Increased DC activation has been associated with elevated CCR7 levels, which are vital for DC migration to draining LN (16, 66). Within the liver microenvironment, specifically in the portal region, DCs could regulate the lymphoid aggregates resembling ectopic lymphoid structures as have been described in the lung during flu infection (71). Such structures could feed further T-cell priming without involvement of the lymph node. DCs positioned within or outside of these aggregates, e.g., DCs scattered within the parenchyma, could affect the balance in the inflammatory milieu via secreted molecules or extracellular vesicles. The release of EVs is a major hallmark of matured DCs (72), and secreted EVs have been demonstrated to play a role in liver pathology (73). This could also explain why a relatively low amount of cells (in the context of the total cellularity of the liver) could impede such substantial role on inflammation and disease outcome. Furthermore, DCs could influence innate cells such as NKT cells to affect MASH and liver pathology. It is also important to mention that we do not greatly understand the pre-DC phenotype observed in MASH in the liver. This could gain relevance in the light of other studies demonstrating immensely altered bone marrow hematopoiesis in obesity and during Western diet treatment (74).
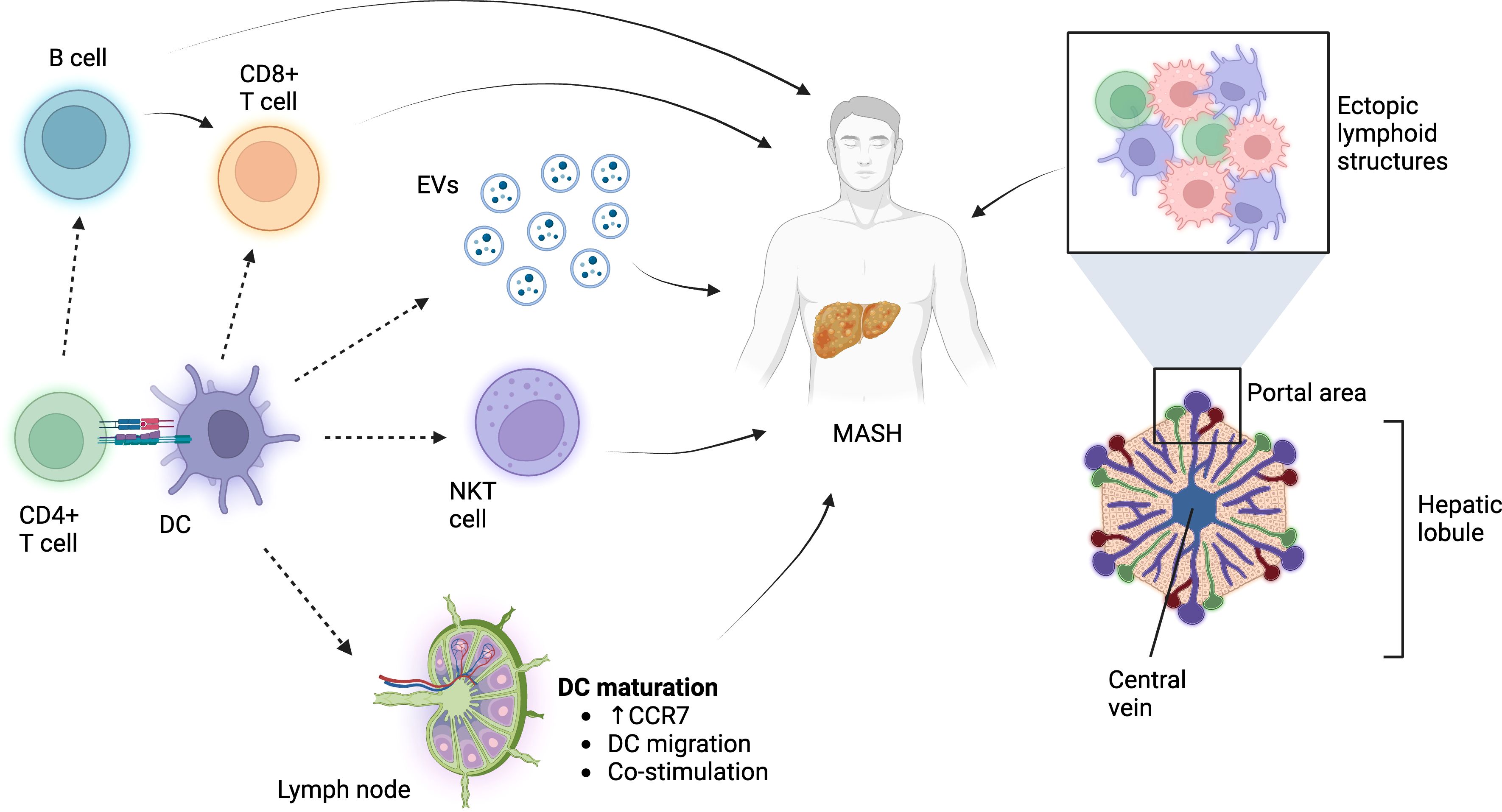
Figure 1 The role of DCs in MASH. DCs influence adaptive and innate immune cells in order to affect MASH pathology. Additionally, they might regulate the pro-anti-inflammatory milieu via soluble molecules, EVs, or immune processes within the ectopic lymphoid structures. Figure was prepared using BioRender.com.
Overall, the role of DCs in MASH is currently under investigation, and recent studies have highlighted the potential disease-promoting role of liver DCs in the progression of MASH while other experimental systems suggest their protective role. Further experiments are needed to clarify the exact and likely complex role of DCs in MASH. It will be necessary to use novel technologies such as spatial genomics, proteomics, and metabolomics to better understand the cell-specific effects and niche-specific reprogramming that can influence DCs and liver pathology. Clarifying the role of DCs in MASH holds promise for uncovering novel therapeutic targets and developing more effective treatment strategies for this complex and increasingly prevalent liver condition.
Author contributions
AP: Writing – original draft, Writing – review & editing, Visualization. VL-K: Writing – original draft, Writing – review & editing, Conceptualization.
Funding
The author(s) declare financial support was received for the research, authorship, and/or publication of this article. VL-K is funded by the Deutsche Forschungsgemeinschaft (DFG, German Research Foundation) under Germany’s Excellence Strategy – EXC 2151 – 390873048 and DFG Project numbers 411345524 and 432325352.
Conflict of interest
The authors declare that the research was conducted in the absence of any commercial or financial relationships that could be construed as a potential conflict of interest.
Publisher’s note
All claims expressed in this article are solely those of the authors and do not necessarily represent those of their affiliated organizations, or those of the publisher, the editors and the reviewers. Any product that may be evaluated in this article, or claim that may be made by its manufacturer, is not guaranteed or endorsed by the publisher.
Abbreviations
MAFLD, Metabolic dysfunction-associated fatty liver disease; MASH, Metabolic dysfunction-associated steatohepatitis; HCC Hepatocellular carcinoma; ILCs, Innate lymphoid cells; LSECs, Liver sinusoidal endothelial cells; HSCs, Hepatic stellate cells; DCs, Dendritic cells; cDCs, Conventional dendritic cells.; pDCs, Plasmocytoid dendritic cells; ECM, Extracellular matrix; HSD, High-sucrose diet; CDHFD, Choline-deficient high-fat diet; IL-10, Interleukin-10; TGF-β Transforming growth factor-beta; BATF3, Basic leucine zipper ATF-like transcription factor 3; H2S, Hydrogen sulfide; LN, Lymph node; EVs, Extracellular vesicles.
References
1. Eslam M, Sanyal AJ, George J, International Consensus P. MAFLD: A consensus-driven proposed nomenclature for metabolic associated fatty liver disease. Gastroenterology. (2020) 158:1999–2014.e1991. doi: 10.1053/j.gastro.2019.11.312
2. Rinella ME, Lazarus JV, Ratziu V, Francque SM, Sanyal AJ, Kanwal F, et al. A multisociety Delphi consensus statement on new fatty liver disease nomenclature. Hepatology. (2023) 78:1966–86. doi: 10.1097/HEP.0000000000000520
3. Younossi Z, Anstee QM, Marietti M, Hardy T, Henry L, Eslam M, et al. Global burden of NAFLD and NASH: trends, predictions, risk factors and prevention. Nat Rev Gastroenterol Hepatol. (2018) 15:11–20. doi: 10.1038/nrgastro.2017.109
4. Lonardo A, Nascimbeni F, Maurantonio M, Marrazzo A, Rinaldi L, Adinolfi LE. Nonalcoholic fatty liver disease: Evolving paradigms. World J Gastroenterol. (2017) 23:6571–92. doi: 10.3748/wjg.v23.i36.6571
5. Anstee QM, Targher G, Day CP. Progression of NAFLD to diabetes mellitus, cardiovascular disease or cirrhosis. Nat Rev Gastroenterol Hepatol. (2013) 10:330–44. doi: 10.1038/nrgastro.2013.41
6. Friedman SL, Neuschwander-Tetri BA, Rinella M, Sanyal AJ. Mechanisms of NAFLD development and therapeutic strategies. Nat Med. (2018) 24:908–22. doi: 10.1038/s41591-018-0104-9
7. Ibrahim SH, Hirsova P, Gores GJ. Non-alcoholic steatohepatitis pathogenesis: sublethal hepatocyte injury as a driver of liver inflammation. Gut. (2018) 67:963–72. doi: 10.1136/gutjnl-2017-315691
8. Armstrong MJ, Hazlehurst JM, Hull D, Guo K, Borrows S, Yu J, et al. Abdominal subcutaneous adipose tissue insulin resistance and lipolysis in patients with non-alcoholic steatohepatitis. Diabetes Obes Metab. (2014) 16:651–60. doi: 10.1111/dom.12272
9. Peiseler M, Schwabe R, Hampe J, Kubes P, Heikenwalder M, Tacke F. Immune mechanisms linking metabolic injury to inflammation and fibrosis in fatty liver disease - novel insights into cellular communication circuits. J Hepatol. (2022) 77:1136–60. doi: 10.1016/j.jhep.2022.06.012
10. Kubes P, Jenne C. Immune responses in the liver. Annu Rev Immunol. (2018) 36:247–77. doi: 10.1146/annurev-immunol-051116-052415
11. Guilliams M, Scott CL. Liver macrophages in health and disease. Immunity. (2022) 55:1515–29. doi: 10.1016/j.immuni.2022.08.002
12. Krenkel O, Hundertmark J, Abdallah AT, Kohlhepp M, Puengel T, Roth T, et al. Myeloid cells in liver and bone marrow acquire a functionally distinct inflammatory phenotype during obesity-related steatohepatitis. Gut. (2020) 69:551–63. doi: 10.1136/gutjnl-2019-318382
13. Guilliams M, Bonnardel J, Haest B, Vanderborght B, Wagner C, Remmerie A, et al. Spatial proteogenomics reveals distinct and evolutionarily conserved hepatic macrophage niches. Cell. (2022) 185:379–396.e338. doi: 10.1016/j.cell.2021.12.018
14. Remmerie A, Martens L, Thone T, Castoldi A, Seurinck R, Pavie B, et al. Osteopontin expression identifies a subset of recruited macrophages distinct from kupffer cells in the fatty liver. Immunity. (2020) 53:641–657.e614. doi: 10.1016/j.immuni.2020.08.004
15. Su Q, Kim SY, Adewale F, Zhou Y, Aldler C, Ni M, et al. Single-cell RNA transcriptome landscape of hepatocytes and non-parenchymal cells in healthy and NAFLD mouse liver. iScience. (2021) 24:103233. doi: 10.1016/j.isci.2021.103233
16. Cabeza-Cabrerizo M, Cardoso A, Minutti CM, Pereira da Costa M, Reis e Sousa C. Dendritic cells revisited. Annu Rev Immunol. (2021) 39:131–66. doi: 10.1146/annurev-immunol-061020-053707
17. Waskow C, Liu K, Darrasse-Jeze G, Guermonprez P, Ginhoux F, Merad M, et al. The receptor tyrosine kinase Flt3 is required for dendritic cell development in peripheral lymphoid tissues. Nat Immunol. (2008) 9:676–83. doi: 10.1038/ni.1615
18. Satpathy AT, Wu X, Albring JC, Murphy KM. Re(de)fining the dendritic cell lineage. Nat Immunol. (2012) 13:1145–54. doi: 10.1038/ni.2467
19. Bachem A, Guttler S, Hartung E, Ebstein F, Schaefer M, Tannert A, et al. Superior antigen cross-presentation and XCR1 expression define human CD11c+CD141+ cells as homologues of mouse CD8+ dendritic cells. J Exp Med. (2010) 207:1273–81. doi: 10.1084/jem.20100348
20. Poulin LF, Reyal Y, Uronen-Hansson H, Schraml BU, Sancho D, Murphy KM, et al. DNGR-1 is a specific and universal marker of mouse and human Batf3-dependent dendritic cells in lymphoid and nonlymphoid tissues. Blood. (2012) 119:6052–62. doi: 10.1182/blood-2012-01-406967
21. See P, Dutertre CA, Chen J, Gunther P, McGovern N, Irac SE, et al. Mapping the human DC lineage through the integration of high-dimensional techniques. Science. (2017) 356(6342). doi: 10.1126/science.aag3009
22. Villani AC, Satija R, Reynolds G, Sarkizova S, Shekhar K, Fletcher J, et al. Single-cell RNA-seq reveals new types of human blood dendritic cells, monocytes, and progenitors. Science. (2017) 356(6335). doi: 10.1126/science.aah4573
23. Haniffa M, Shin A, Bigley V, McGovern N, Teo P, See P, et al. Human tissues contain CD141hi cross-presenting dendritic cells with functional homology to mouse CD103+ nonlymphoid dendritic cells. Immunity. (2012) 37:60–73. doi: 10.1016/j.immuni.2012.04.012
24. Liu Z, Wang H, Li Z, Dress RJ, Zhu Y, Zhang S, et al. Dendritic cell type 3 arises from Ly6C(+) monocyte-dendritic cell progenitors. Immunity. (2023) 56:1761–1777.e1766. doi: 10.1016/j.immuni.2023.07.001
25. Hanc P, Gonzalez RJ, Mazo IB, Wang Y, Lambert T, Ortiz G, et al. Multimodal control of dendritic cell functions by nociceptors. Science. (2023) 379:eabm5658. doi: 10.1126/science.abm5658
26. Schaupp L, Muth S, Rogell L, Kofoed-Branzk M, Melchior F, Lienenklaus S, et al. Microbiota-induced type I interferons instruct a poised basal state of dendritic cells. Cell. (2020) 181:1080–1096.e1019. doi: 10.1016/j.cell.2020.04.022
27. Hemont C, Neel A, Heslan M, Braudeau C, Josien R. Human blood mDC subsets exhibit distinct TLR repertoire and responsiveness. J Leukoc Biol. (2013) 93:599–609. doi: 10.1189/jlb.0912452
28. Segura E, Kapp E, Gupta N, Wong J, Lim J, Ji H, et al. Differential expression of pathogen-recognition molecules between dendritic cell subsets revealed by plasma membrane proteomic analysis. Mol Immunol. (2010) 47:1765–73. doi: 10.1016/j.molimm.2010.02.028
29. Matzinger P. Tolerance, danger, and the extended family. Annu Rev Immunol. (1994) 12:991–1045. doi: 10.1146/annurev.iy.12.040194.005015
30. Allan RS, Waithman J, Bedoui S, Jones CM, Villadangos JA, Zhan Y, et al. Migratory dendritic cells transfer antigen to a lymph node-resident dendritic cell population for efficient CTL priming. Immunity. (2006) 25:153–62. doi: 10.1016/j.immuni.2006.04.017
31. de Winde CM, Munday C, Acton SE. Molecular mechanisms of dendritic cell migration in immunity and cancer. Med Microbiol Immunol. (2020) 209:515–29. doi: 10.1007/s00430-020-00680-4
32. Dudziak D, Kamphorst AO, Heidkamp GF, Buchholz VR, Trumpfheller C, Yamazaki S, et al. Differential antigen processing by dendritic cell subsets in vivo. Science. (2007) 315:107–11. doi: 10.1126/science.1136080
33. Hawiger D, Inaba K, Dorsett Y, Guo M, Mahnke K, Rivera M, et al. Dendritic cells induce peripheral T cell unresponsiveness under steady state conditions in vivo. J Exp Med. (2001) 194:769–79. doi: 10.1084/jem.194.6.769
34. Soares H, Waechter H, Glaichenhaus N, Mougneau E, Yagita H, Mizenina O, et al. A subset of dendritic cells induces CD4+ T cells to produce IFN-gamma by an IL-12-independent but CD70-dependent mechanism in vivo. J Exp Med. (2007) 204:1095–106. doi: 10.1084/jem.20070176
35. Morante-Palacios O, Fondelli F, Ballestar E, Martinez-Caceres EM. Tolerogenic dendritic cells in autoimmunity and inflammatory diseases. Trends Immunol. (2021) 42:59–75. doi: 10.1016/j.it.2020.11.001
36. Ardouin L, Luche H, Chelbi R, Carpentier S, Shawket A, Montanana Sanchis F, et al. Broad and largely concordant molecular changes characterize tolerogenic and immunogenic dendritic cell maturation in thymus and periphery. Immunity. (2016) 45:305–18. doi: 10.1016/j.immuni.2016.07.019
37. Lukacs-Kornek V, Turley SJ. Self-antigen presentation by dendritic cells and lymphoid stroma and its implications for autoimmunity. Curr Opin Immunol. (2011) 23:138–45. doi: 10.1016/j.coi.2010.11.012
38. Wculek SK, Cueto FJ, Mujal AM, Melero I, Krummel MF, Sancho D. Dendritic cells in cancer immunology and immunotherapy. Nat Rev Immunol. (2020) 20:7–24. doi: 10.1038/s41577-019-0210-z
39. Acton SE, Farrugia AJ, Astarita JL, Mourao-Sa D, Jenkins RP, Nye E, et al. Dendritic cells control fibroblastic reticular network tension and lymph node expansion. Nature. (2014) 514:498–502. doi: 10.1038/nature13814
40. Menzel L, Hopken UE, Rehm A. Angiogenesis in lymph nodes is a critical regulator of immune response and lymphoma growth. Front Immunol. (2020) 11:591741. doi: 10.3389/fimmu.2020.591741
41. Xu J, Zanvit P, Hu L, Tseng PY, Liu N, Wang F, et al. The cytokine TGF-beta induces interleukin-31 expression from dermal dendritic cells to activate sensory neurons and stimulate wound itching. Immunity. (2020) 53:371–383.e375. doi: 10.1016/j.immuni.2020.06.023
42. Swiecki M, Colonna M. The multifaceted biology of plasmacytoid dendritic cells. Nat Rev Immunol. (2015) 15:471–85. doi: 10.1038/nri3865
43. Ziegler-Heitbrock L, Ohteki T, Ginhoux F, Shortman K, Spits H. Reclassifying plasmacytoid dendritic cells as innate lymphocytes. Nat Rev Immunol. (2023) 23:1–2. doi: 10.1038/s41577-022-00806-0
44. Tang-Huau TL, Gueguen P, Goudot C, Durand M, Bohec M, Baulande S, et al. Human in vivo-generated monocyte-derived dendritic cells and macrophages cross-present antigens through a vacuolar pathway. Nat Commun. (2018) 9:2570. doi: 10.1038/s41467-018-04985-0
45. Segura E, Amigorena S. Inflammatory dendritic cells in mice and humans. Trends Immunol. (2013) 34:440–5. doi: 10.1016/j.it.2013.06.001
46. Bosteels C, Neyt K, Vanheerswynghels M, van Helden MJ, Sichien D, Debeuf N, et al. Inflammatory Type 2 cDCs Acquire Features of cDC1s and Macrophages to Orchestrate Immunity to Respiratory Virus Infection. Immunity. (2020) 52:1039–1056.e1039. doi: 10.1016/j.immuni.2020.04.005
47. English K, Tan SY, Kwan R, Holz LE, Sierro F, McGuffog C, et al. The liver contains distinct interconnected networks of CX3CR1(+) macrophages, XCR1(+) type 1 and CD301a(+) type 2 conventional dendritic cells embedded within portal tracts. Immunol Cell Biol. (2022) 100:394–408. doi: 10.1111/imcb.12559
48. Barbier L, Tay SS, McGuffog C, Triccas JA, McCaughan GW, Bowen DG, et al. Two lymph nodes draining the mouse liver are the preferential site of DC migration and T cell activation. J Hepatol. (2012) 57:352–8. doi: 10.1016/j.jhep.2012.03.023
49. Matsuno K, Kudo S, Ezaki T. The liver sinusoids as a specialized site for blood-lymph translocation of rat dendritic cells. Adv Exp Med Biol. (1997) 417:77–81. doi: 10.1007/978-1-4757-9966-8_13
50. Lukacs-Kornek V, Schuppan D. Dendritic cells in liver injury and fibrosis: shortcomings and promises. J Hepatol. (2013) 59:1124–6. doi: 10.1016/j.jhep.2013.05.033
51. David BA, Rezende RM, Antunes MM, Santos MM, Freitas Lopes MA, Diniz AB, et al. Combination of mass cytometry and imaging analysis reveals origin, location, and functional repopulation of liver myeloid cells in mice. Gastroenterology. (2016) 151:1176–91. doi: 10.1053/j.gastro.2016.08.024
52. Eckert C, Klein N, Kornek M, Lukacs-Kornek V. The complex myeloid network of the liver with diverse functional capacity at steady state and in inflammation. Front Immunol. (2015) 6:179. doi: 10.3389/fimmu.2015.00179
53. Thomson AW, Knolle PA. Antigen-presenting cell function in the tolerogenic liver environment. Nat Rev Immunol. (2010) 10:753–66. doi: 10.1038/nri2858
54. Ghazarian M, Revelo XS, Nohr MK, Luck H, Zeng K, Lei H, et al. Type I interferon responses drive intrahepatic T cells to promote metabolic syndrome. Sci Immunol. (2017) 2(10):eaai7616. doi: 10.1126/sciimmunol.aai7616
55. Gadd VL, Skoien R, Powell EE, Fagan KJ, Winterford C, Horsfall L, et al. The portal inflammatory infiltrate and ductular reaction in human nonalcoholic fatty liver disease. Hepatology. (2014) 59:1393–405. doi: 10.1002/hep.26937
56. Kelly A, Fahey R, Fletcher JM, Keogh C, Carroll AG, Siddachari R, et al. CD141(+) myeloid dendritic cells are enriched in healthy human liver. J Hepatol. (2014) 60:135–42. doi: 10.1016/j.jhep.2013.08.007
57. Rau M, Schilling AK, Meertens J, Hering I, Weiss J, Jurowich C, et al. Progression from nonalcoholic fatty liver to nonalcoholic steatohepatitis is marked by a higher frequency of th17 cells in the liver and an increased th17/resting regulatory T cell ratio in peripheral blood and in the liver. J Immunol. (2016) 196:97–105. doi: 10.4049/jimmunol.1501175
58. Rolla S, Alchera E, Imarisio C, Bardina V, Valente G, Cappello P, et al. The balance between IL-17 and IL-22 produced by liver-infiltrating T-helper cells critically controls NASH development in mice. Clin Sci (Lond). (2016) 130:193–203. doi: 10.1042/CS20150405
59. Wolf MJ, Adili A, Piotrowitz K, Abdullah Z, Boege Y, Stemmer K, et al. Metabolic activation of intrahepatic CD8+ T cells and NKT cells causes nonalcoholic steatohepatitis and liver cancer via cross-talk with hepatocytes. Cancer Cell. (2014) 26:549–64. doi: 10.1016/j.ccell.2014.09.003
60. Ma C, Kesarwala AH, Eggert T, Medina-Echeverz J, Kleiner DE, Jin P, et al. NAFLD causes selective CD4(+) T lymphocyte loss and promotes hepatocarcinogenesis. Nature. (2016) 531:253–7. doi: 10.1038/nature16969
61. Dudek M, Pfister D, Donakonda S, Filpe P, Schneider A, Laschinger M, et al. Auto-aggressive CXCR6(+) CD8 T cells cause liver immune pathology in NASH. Nature. (2021) 592:444–9. doi: 10.1038/s41586-021-03233-8
62. Kotsiliti E, Leone V, Schuehle S, Govaere O, Li H, Wolf MJ, et al. Intestinal B cells license metabolic T-cell activation in NASH microbiota/antigen-independently and contribute to fibrosis by IgA-FcR signalling. J Hepatol. (2023) 79:296–313. doi: 10.1016/j.jhep.2023.04.037
63. Albano E, Mottaran E, Vidali M, Reale E, Saksena S, Occhino G, et al. Immune response towards lipid peroxidation products as a predictor of progression of non-alcoholic fatty liver disease to advanced fibrosis. Gut. (2005) 54:987–93. doi: 10.1136/gut.2004.057968
64. Henning JR, Graffeo CS, Rehman A, Fallon NC, Zambirinis CP, Ochi A, et al. Dendritic cells limit fibroinflammatory injury in nonalcoholic steatohepatitis in mice. Hepatology. (2013) 58:589–602. doi: 10.1002/hep.26267
65. Heier EC, Meier A, Julich-Haertel H, Djudjaj S, Rau M, Tschernig T, et al. Murine CD103(+) dendritic cells protect against steatosis progression towards steatohepatitis. J Hepatol. (2017) 66:1241–50. doi: 10.1016/j.jhep.2017.01.008
66. Deczkowska A, David E, Ramadori P, Pfister D, Safran M, Li B, et al. XCR1(+) type 1 conventional dendritic cells drive liver pathology in non-alcoholic steatohepatitis. Nat Med. (2021) 27:1043–54. doi: 10.1038/s41591-021-01344-3
67. Sutti S, Bruzzi S, Heymann F, Liepelt A, Krenkel O, Toscani A, et al. CX(3)CR1 mediates the development of monocyte-derived dendritic cells during hepatic inflammation. Cells. (2019) 8(9):1099. doi: 10.3390/cells8091099
68. Haas JT, Vonghia L, Mogilenko DA, Verrijken A, Molendi-Coste O, Fleury S, et al. Transcriptional Network Analysis Implicates Altered Hepatic Immune Function in NASH development and resolution. Nat Metab. (2019) 1:604–14. doi: 10.1038/s42255-019-0076-1
69. Lee W, Kim HS, Hwang SS, Lee GR. The transcription factor Batf3 inhibits the differentiation of regulatory T cells in the periphery. Exp Mol Med. (2017) 49:e393. doi: 10.1038/emm.2017.157
70. Li J, Chen S, Xiao X, Zhao Y, Ding W, Li XC. IL-9 and Th9 cells in health and diseases-From tolerance to immunopathology. Cytokine Growth Factor Rev. (2017) 37:47–55. doi: 10.1016/j.cytogfr.2017.07.004
71. Pitzalis C, Jones GW, Bombardieri M, Jones SA. Ectopic lymphoid-like structures in infection, cancer and autoimmunity. Nat Rev Immunol. (2014) 14:447–62. doi: 10.1038/nri3700
72. Segura E, Nicco C, Lombard B, Veron P, Raposo G, Batteux F, et al. ICAM-1 on exosomes from mature dendritic cells is critical for efficient naive T-cell priming. Blood. (2005) 106:216–23. doi: 10.1182/blood-2005-01-0220
73. Wang G, Li J, Bojmar L, Chen H, Li Z, Tobias GC, et al. Tumour extracellular vesicles and particles induce liver metabolic dysfunction. Nature. (2023) 618:374–82. doi: 10.1038/s41586-023-06114-4
Keywords: dendritic cells, liver, MASH, inflammation, metabolic dysfunction associated fatty liver disease
Citation: Pinto AT and Lukacs-Kornek V (2024) The role of dendritic cells in MASH: friends or foes? Front. Immunol. 15:1379225. doi: 10.3389/fimmu.2024.1379225
Received: 30 January 2024; Accepted: 21 March 2024;
Published: 08 April 2024.
Edited by:
Beate E. Kehrel, University Hospital Münster, GermanyReviewed by:
Lukas Heger, University Hospital Erlangen, GermanyCopyright © 2024 Pinto and Lukacs-Kornek. This is an open-access article distributed under the terms of the Creative Commons Attribution License (CC BY). The use, distribution or reproduction in other forums is permitted, provided the original author(s) and the copyright owner(s) are credited and that the original publication in this journal is cited, in accordance with accepted academic practice. No use, distribution or reproduction is permitted which does not comply with these terms.
*Correspondence: Veronika Lukacs-Kornek, dmx1a2Fjc2tAdW5pLWJvbm4uZGU=