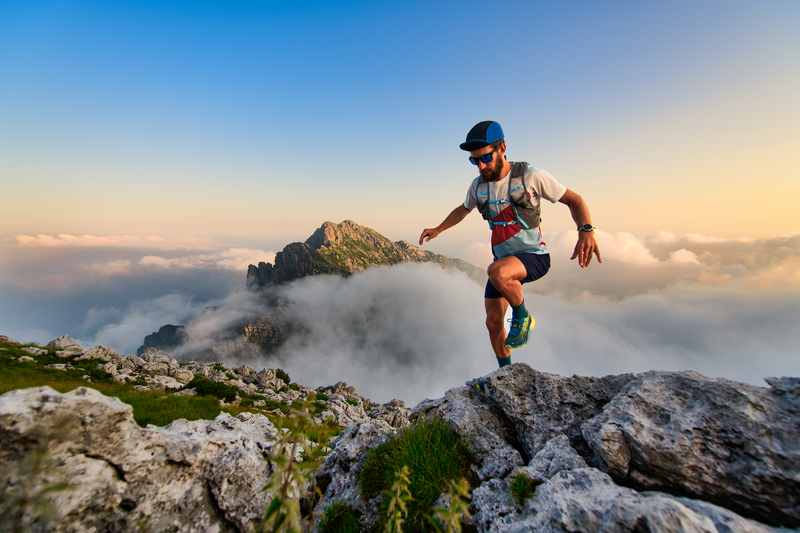
94% of researchers rate our articles as excellent or good
Learn more about the work of our research integrity team to safeguard the quality of each article we publish.
Find out more
ORIGINAL RESEARCH article
Front. Immunol. , 30 April 2024
Sec. Nutritional Immunology
Volume 15 - 2024 | https://doi.org/10.3389/fimmu.2024.1374425
This article is part of the Research Topic Immunomodulation by Food Components Via Dendritic Cells View all 7 articles
Various gut bacteria, including Lactobacillus plantarum, possess several enzymes that produce hydroxy fatty acids (FAs), oxo FAs, conjugated FAs, and partially saturated FAs from polyunsaturated FAs as secondary metabolites. Among these derivatives, we identified 10-oxo-cis-6,trans-11-octadecadienoic acid (γKetoC), a γ-linolenic acid (GLA)-derived enon FA, as the most effective immunomodulator, which inhibited the antigen-induced immunoactivation and LPS-induced production of inflammatory cytokines. The treatment with γKetoC significantly suppressed proliferation of CD4+ T cells, LPS-induced activation of bone marrow-derived dendritic cells (BMDCs), and LPS-induced IL-6 release from peritoneal cells, splenocytes, and CD11c+ cells isolated from the spleen. γKetoC also inhibited the release of inflammatory cytokines from BMDCs stimulated with poly-I:C, R-848, or CpG. Further in vitro experiments using an agonist of GPR40/120 suggested the involvement of these GPCRs in the effects of γKetoC on DCs. We also found that γKetoC stimulated the NRF2 pathway in DCs, and the suppressive effects of γKetoC and agonist of GPR40/120 on the release of IL-6 and IL-12 were reduced in Nrf2-/- BMDCs. We evaluated the role of NRF2 in the anti-inflammatory effects of γKetoC in a dextran sodium sulfate-induced colitis model. The oral administration of γKetoC significantly reduced body weight loss, improved stool scores, and attenuated atrophy of the colon, in wild-type C57BL/6 and Nrf2+/- mice with colitis. In contrast, the pathology of colitis was deteriorated in Nrf2-/- mice even with the administration of γKetoC. Collectively, the present results demonstrated the involvement of the NRF2 pathway and GPCRs in γKetoC-mediated anti-inflammatory responses.
In the intestines, various secondary metabolites are produced by intestinal bacteria using food ingredient-derived materials as substrates. Several bacteria metabolites exert beneficial effects on the host body, such as short-chain fatty acids (FAs) produced from dietary fibers by Clostridium, which are involved in the maintenance of homeostasis and prevention of immune-related inflammatory diseases by modulating the function of both hematopoietic cells and non-hematopoietic cells. Although polyunsaturated FAs (PUFAs) are catalyzed by enzymes in host cells to achieve various bioactivities and their relationships with inflammatory diseases have been vigorously studied with a focus on the ω3/ω6 balance (1), a recent study revealed that PUFAs are also converted to derivatives, including hydroxy FAs, oxo FAs, conjugated FAs, and partially saturated FAs, through the catalysis of enzymes identified in the gut lactic acid bacterium, Lactobacillus plantarum (2). The PUFA metabolite 10-hydroxy-cis-12-octadecenoic acid (HYA), a hydroxy FA derived from linoleic acid (LA), regulates glucose homeostasis by activating GPR40 and GPR120, and increases resistance to obesity (3). The HYA-mediated activation of GPR40 has also been shown to accelerate the recovery of an impaired intestinal epithelial barrier (4) and disrupted gingival epithelial barrier (5). The metabolite 10-oxo-cis-12-octadecenoic acid (KetoA), an oxo FA derived from LA, enhances energy metabolism by activating TRPV1 in adipose tissue and exerts anti-obesity effects on the host body (6). KetoA is also involved in the regulation of host energy metabolism by accelerating adipocyte differentiation, adiponectin production, and glucose uptake through the activation of PPARγ (7). Another LA derivative 10-oxo-trans-11-octadecenoic acid (KetoC), an enon FA, was found to regulate the function of monocytes (8) and epithelial cells (9) via GPR120 signaling. Although accumulating evidence has demonstrated the beneficial effects of the bacteria metabolites of PUFAs on the host body, the roles of these metabolites in immune-related events remain unclear.
In the present study, we examined the effects of the bacteria-generated FAs on antigen (Ag)-induced immunoresponses and revealed that enon FAs suppressed the proliferation of T cells and the activation of dendritic cells (DCs). Detailed analyses focusing on 10-oxo-cis-6,trans-11-octadecadienoic acid (γKetoC), an enon FA derived from γ-linolenic acid (GLA), demonstrated that the release of inflammatory cytokines from DCs upon stimulation by TLR ligands was inhibited by γKetoC. To reveal the molecular mechanisms underlying the immunoregulatory effects of γKetoC, we investigated the involvement of GPCRs and the NF-E2-related factor 2 (NRF2) pathway. In addition, we utilized colitis model to wild-type (WT) mice and Nrf2 knockout (KO) mice to evaluate the effects of γKetoC intake on the regulation of inflammatory responses in vivo.
C57BL/6 mice were purchased from Japan SLC (Hamamatsu, Japan). OT-II mice purchased from The Jackson Laboratory (USA) and previously generated Nrf2-/- mice (10) were maintained on the C57BL/6 background. Mice were housed in a specific pathogen-free facility, and all animal experiments were performed in accordance with the guidelines of the Institutional Review Board of Tokyo University of Science. The present study was approved by the Animal Care and Use Committees of Tokyo University of Science: K22005, K21004, K20005, K19006, K18006, K17009, and K17012.
Bone marrow-derived DCs (BMDCs) generated as previously described (11), were stimulated with 100 ng/mL LPS (#L3024, Fujifilm Wako Chemicals Co., Ltd., Japan), 25 μg/mL poly-I:C (#P0913, Sigma-Aldrich), 1 μg/mL R-848 (#AG-CR1-3582-M005, AdipoGen), 1 μg/mL CpG (#tlrl-1826, InvivoGen). GW9508 (#10008907, Cayman Chemical, Ann Arbor, MI, USA) and YM-254890 (#257-00631, Fujifilm Wako Chemicals Co., Ltd.) were used as an agonist of GPR40 and GPR120 and an inhibitor of Gq, respectively. Ovalbumin (OVA) peptide 323-339 (POV-3636-PI, Peptide Institute Inc., Osaka, Japan) was added to the culture medium of whole spleen cells prepared from OT-II mice to induce the antigen-presenting cell (APC)-dependent activation of CD4+ T cells. The MojoSort Mouse Naïve CD4+ T cell Isolation Kit (#480040, BioLegend), anti-CD3ε antibody (Ab) (clone 145-2C11, BioLegend), and anti-CD28 Ab (clone 37.51, BioLegend) were used for the isolation and stimulation of CD4+ T cells, respectively, as previously described (12). Th1 polarization was induced by the supplementation of 10 ng/mL mIL-12p70 (#577002, BioLegend) and 10 μg/mL anti-mouse IL-4 Ab (clone 11B11, BioLegend) to the culture media of CD4+ T cells. For Th2 polarization, 20 ng/mL mIL-4 (#574306, BioLegend) and 10 μg/mL anti-mouse IL-12/23p40 Ab (clone C17.8, BioLegend) were added. CD11c MicroBeads UltraPure, mouse (#130-125-835, Miltenyi Biotec) was used to isolate CD11c+ cells from the spleen.
Hydroxy, oxo, and enon FAs were prepared from LA, α-linolenic acid (ALA), and GLA, using the conversion enzymes isolated from L. plantarum AKU1009 (2). LA (#126-06571), and ALA (#122-05831) were purchased from Fujifilm Wako Chemicals and GLA (#L0152) from Tokyo Chemical Industry Co., Ltd. (Tokyo, Japan).
The concentrations of mouse cytokines were measured using ELISA kits purchased from BioLegend (#431004 for IL-2, #431315 for IL-6, #430915 for TNF-α, and #431604 for IL-12p40, respectively).
CFSE (eBioscience Inc., San Diego, CA, USA) was used to monitor the proliferation of T cells. Surface MHC class II and CD86 on BMDCs were stained with anti-I-A/I-E-PerCP (clone M5/114.15.2, BioLegend) and anti-CD86-PE (clone GL-1, BioLegend), respectively. Fluorescence was detected by a MACS Quant Analyzer (Miltenyi Biotech) and analyzed with FlowJo (Tomy Digital Biology Co., Ltd., Tokyo, Japan).
The extraction of total RNA, synthesis of cDNA, and quantitative PCR were performed as previously described (13, 14). The nucleotide sequences of the primer sets used for qPCR are listed in Table 1.
A Western blot analysis was performed with anti-NRF2 Ab (clone D1Z9C, Cell Signaling) and anti-β-actin Ab (clone AC-15, Sigma-Aldrich) as previously described (15).
To induce colitis, mice were administered 2.5% (w/v) DSS (#160110, MP Biomedicals, Santa Ana, USA) in their drinking water. γKetoC (15 mg/kg/day) or vehicle (100 μl soybean oil) was orally administered using a sonde (#5202K, Fuchigami, Kyoto, Japan). The colons, which were collected from mice just after euthanasia, were fixed with 4% paraformaldehyde for 2h at 4°C and immersed in 30% sucrose overnight at 4°C. The colons were embedded in optimal cutting temperature compound and frozen at -80°C prior to cryosectioning. Sections of the colons with 8 μm thickness were stained with H&E for histological analysis using a light microscope.
A two-tailed Student’s t-test was used for comparisons of two samples. To compare more than three samples, a one-way ANOVA-followed by the Tukey-Kramer multiple comparison test or Dunnett’s multiple comparison test was used. Area-under-curve (AUC) formatted data in DSS-induced colitis were calculated by GraphPad Prism 7.04. P values <0.05 were considered to be significant.
To examine the effects of bacteria metabolites of PUFAs on Ag-induced immunoresponses, we incubated OVA-stimulated OT-II spleen cells in the presence or absence of 50 μM of each metabolite for 48 h. The treatments with KetoC, αKetoC, γKetoA, and γKetoC markedly reduced the concentration of IL-2 in culture media, whereas those with HYA, αHYA, and γHYA did not (Figure 1A). We then compared the suppressive effects of enon FAs on IL-2 production with those of the original PUFAs without conversion, and found that KetoC, αKetoC, and γKetoC significantly and dose-dependently suppressed IL-2 production, whereas apparent effects were not observed in LA, ALA, and GLA (Figure 1B).
Figure 1 Effects of bacteria metabolites of PUFAs on the activation of T cells and DCs in vitro. (A, B) IL-2 concentrations in the culture media of splenocytes incubated in the presence or absence of OVA and FAs. In total, 1.0 x 105/200 μL of OT-II spleen-derived single cell-suspended cells were stimulated by 2.5 μg/mL OVA with or without 50 μM bacteria metabolites of PUFAs or vehicle (ethanol) for 48 h (A). The indicated concentrations of enon FAs or their starting PUFAs were added to the culture media of OT-II spleen-derived cells with OVA during a 48-h incubation (B). (C) The proliferation of CD4+ T cells stimulated with plate-coated anti-CD3 and anti-CD28 Abs. CD4+ T cells, which were isolated from the C57BL/6 spleen and were stained with CFSE, were incubated in Abs-coated dishes in the presence of 50 μM enon FAs for 72 h. A result of one experiment performed with triplicate samples is shown, and a similar result was obtained in the other experiment (Supplementary Figure S1). (D) The frequencies of Th1 and Th2 cells that developed from naïve CD4+ T cells by stimulation with anti-CD3 and anti-CD28 Abs under polarizing conditions in the presence or absence of 25 μM γKetoC. Naïve CD4+ T cells were incubated in Abs-coated dishes in culture media supplemented with cytokines and neutralizing Abs for polarization to Th1 or Th2 (described in Materials and Methods) for 72 h. (E) Cell surface expression levels of MHC class II and CD86 in LPS-stimulated DCs. In total, 5.0 x 106/2 mL of BMDCs were stimulated by 100 ng/mL LPS for 24 h in the presence or absence of 50 μM enon FAs. MFIs were shown as a ratio to that of LPS-stimulated BMDCs without FAs. (F) Concentrations of cytokines in the culture media of LPS-stimulated DCs. In total, 5.0 x 106/2 mL of BMDCs were stimulated by 100 ng/mL LPS for 24 h in the presence or absence of 50 μM enon FAs. Data represent the mean ± SEM of three independent experiments (A, B, D–F), and the mean ± SD of a typical data of triplicate samples from two independent experiments (C). The Dunnett’s test (A-C), Student’s t-test (D), and the Tukey-Kramer test (E, F) were used. *p < 0.05, **p < 0.01, ***p < 0.005, ****p < 0.0001, ns, not significant.
These results indicate that converted FAs carrying the enon structure acquired immunosuppressive effects, which were not observed in hydroxy FAs and were moderately induced in oxo FAs.
To identify the cells in splenocytes that were regulated by the enon FAs, we examined the proliferation of T cells and the activation of DCs in the presence of enon FAs. The proliferation of naïve CD4+ T cells, which was induced by the treatment with plate-coated anti-CD3 and anti-CD28 Abs independent of APC, was suppressed by all three FAs at 50 μM (Figure 1C and Supplementary Figure S1). We also found that the development of Th1 and Th2 were not affected by γKetoC (Figure 1D). The pretreatment with 50 μM enon FAs also inhibited the up-regulation of MHC class II and CD86 on DCs (Figure 1E) and the release of TNF-α and IL-6 from DCs (Figure 1F) 24 h after the LPS stimulation.
These results demonstrate that enon FAs inhibited the activation of DCs and proliferation of T cells, which are involved in the suppression of Ag-induced IL-2 production in OT-II splenocyte, whereas development of Th1 and Th2 was not modulated by enon FA.
To elucidate the mechanisms underlying the anti-inflammatory effects of enon FAs, we performed further analyses with a focus on γKetoC as the strongest suppressor among the three enon FAs. We confirmed that γKetoC significantly suppressed the LPS-induced production of IL-6 in peritoneal cells and whole leukocytes isolated from the spleen (Figure 2A). The inhibition of IL-6 production by γKetoC was also observed in CD11c+ cells purified from the spleen (Figure 2B). We also stimulated DCs with poly-I:C, R-848, or CpG to investigate the effect of γKetoC on other stimulants-mediated activation of DCs. The measurement of cytokine concentrations revealed that the production of TNF-α, IL-6, IL-12p40 from BMDCs, which were induced by a stimulation via TLR3, TLR7/8, or TLR9, were significantly suppressed by γKetoC (Figure 2C).
Figure 2 The suppressive effects of γKetoC on inflammatory cytokine producing cells stimulated with various TLR ligands. (A, B) IL-6 release from LPS-stimulated peritoneal cells (A left), spleen cells (A right), and CD11c+ cells isolated from the spleen (B) was reduced by a treatment with γKetoC. Spleen cells (5.0 x 106/mL), peritoneal cells (4.0 x 105/mL), and CD11c+ cells (1.0 x 105/200 μL) were stimulated with 100 ng/mL LPS for 24 h with or without 50 μM γKetoC. (C) Concentrations of cytokines in the culture media of PAMPs-stimulated BMDCs. In total, 5.0 x 105/500 μL of BMDCs were stimulated by 25 μg/mL poly-I:C, 1 μg/mL R-848, or 1 μg/mL CpG, for 24 h in the presence or absence of 50 μM enon FAs. Data represent the mean ± SEM of three independent experiments (A–C). The Tukey-Kramer (A, C) and Dunnett’s (B) multiple comparison test were used. *p < 0.05, **p < 0.01, ***p < 0.005, ****p < 0.001, ns, not significant.
Measurements of the mRNA levels of cytokines in LPS-stimulated BMDCs revealed that the inhibitory effects of γKetoC on transactivation was marked in the Il12b, and significant in Il6 and Tnf (Figure 3A). To clarify the molecular mechanisms by which γKetoC inhibited the PAMPs-induced transactivation of inflammatory cytokine genes in DCs, we first took notice of GPCRs based on the observation obtained in previous studies including ours. Briefly, KetoC inhibited the LPS-induced activation of the monocyte cell line RAW264.7 with binding to GPR120 (8), and an agonist of Gq-GPCR mimicked inhibitory effects of γKetoC on LPS-induced IL-6 production in BM macrophages (16). As in a previous report showing that GPR120 is expressed in adipocytes, macrophages, and DCs (17), GPR120 mRNA was detected in the BMDCs generated under our experimental conditions (data not shown). To examine the involvement of GPR120 in the γKetoC-mediated suppression of DCs, we treated BMDCs with GW9508, an agonist common to GPR40/GPR120, and revealed that GW9508 inhibited the LPS-induced release of inflammatory cytokines in a dose-dependent manner (Figure 3B), suggesting that the stimulation of GPR120 suppressed the LPS-induced activation of DCs. Furthermore, the suppressive effects of γKetoC and GW9508 on LPS-induced production of TNF-α were abrogated by the pretreatment by YM-254890, an inhibitor of Gαq protein, whereas YM-254890 did not alter the production of IL-6 and IL-12p40 (Figure 3C).
Figure 3 Roles of Gq-GPCR-signaling in the effects on DCs. (A) mRNA levels of cytokine genes in LPS-stimulated DCs. 1.0 x 106/mL of BMDCs were stimulated by 100 ng/mL LPS for 4 h in the presence or absence of 50 μM enon FAs. (B, C) The amount of TNF-α, IL-6, and IL-12p40 released from LPS-stimulated DCs in the presence of the indicated concentrations of γKetoC or a Gq agonist (B) and those with a Gq inhibitor (C). BMDCs pretreated in the presence or absence of the indicated concentrations of γKetoC, GW9508, and/or YM-254890 for 24 h, were cultured with or without LPS for an additional 24 h. Data represent the mean ± SEM of three independent experiments (A–C). The Tukey-Kramer test (A, C) and Dunnett’s test (B) were used. *p < 0.05, **p < 0.01, ***p < 0.005, ****p < 0.001, ns, not significant.
Above-mentioned result indicating a partial involvement of GPCRs in the anti-inflammatory effects of γKetoC prompted us to analyze NRF2, a master transcription factor of antioxidant responses, as the other target of γKetoC. Previous studies reported that KetoC induced the expression of the antioxidant-related genes through the activation of NRF2, in the hepatic cell line HepG2 (18), and epithelial cell line Epi4 (9). In addition, a NRF2 deficiency enhanced the expression of IL-12p40 in stimulated DCs (14, 19). Therefore, to confirm whether γKetoC induced an antioxidant response via the activation of NRF2 in DCs, we examined NRF2 protein levels in γKetoC-treated DCs using Western blotting. The expression of NRF2 in BMDCs peaked at 1 h after the addition of γKetoC (Figure 4A). The mRNA levels of Hmox1, a target gene of NRF2, were also increased in γKetoC-treated DCs (Figure 4B). Furthermore, the suppressive effect of γKetoC on the LPS-induced IL-6 and IL-12p40 production were attenuated in Nrf2-/- DCs (Figure 4C). The effects of GW9508 on the production of IL-6 and IL-12p40 were also abrogated by the NRF2 deficiency (Figure 4D). On the other hand, the deficiency of NRF2 did not affect the production levels of TNF-α (Figures 4C, D).
Figure 4 Involvement of the NRF2 pathway in the suppressive effects of γKetoC on DCs. (A) NRF2 protein levels in γKetoC-treated DCs. BMDCs were cultured in the presence of 50 μM γKetoC for the indicated times, and aliquots of the whole cell lysate containing 10 μg of protein were applied to each lane of SDS-PAGE for Western blotting. (B) mRNA levels of Hmox1 in BMDCs derived from NRF2 deficient mice (Nrf2-/-) and its control (Nrf2+/+). 1.0 x 106/mL of BMDCs were stimulated by 100 ng/mL LPS for 24 h in the presence or absence of 50 μM γKetoC. (C, D) The amounts of cytokines released from NRF2-deficient DCs (Nrf2-/-) and their control DCs (Nrf2+/+). BMDCs derived from Nrf2+/+, or Nrf2-/- mice, which were pretreated with or without 50 μM γKetoC (C) or 100 μM GW9508 (D) for 24 h, were cultured in the presence or absence of 100 ng/mL LPS for an additional 24 h. Data represent the mean ± SEM of three independent experiments performed in triplicate (B–D). The Tukey-Kramer test was used. *p < 0.05, **p < 0.01, ***p < 0.005, ****p < 0.001, ns, not significant.
These results indicate that γKetoC stimulated the NRF2 pathway, which negatively regulated inflammatory cytokine production, and that GPR120-signaling suppressed LPS-induced IL-6 production in DCs in an NRF2-dependent manner.
We utilized a DSS-induced colitis model to examine the protective effects of γKetoC on inflammatory responses in vivo. In the first colitis experiment, wild-type C57BL/6J mice were orally administered γKetoC (Figure 5A). Although significant effect of γKetoC intake was not observed in the loss of body weight (Figure 5B), increases in the disease activity index (DAI) score was alleviated by the intake of γKetoC (Figure 5C). Fibrosis-mediated atrophy of the colon in mice with colitis was also significantly reduced in γKetoC-treated mice (Figure 5D). In the next experiment, we investigated the roles of NRF2 in the γKetoC-mediated amelioration of colitis by using Nrf2-/- mice after modifying the schedule to obtain a more significant effect of γKetoC in colitis. The administration of γKetoC was initiated 4 days earlier than that in the first experiment (Figure 5E) and the results revealed that the loss of body weight (Figure 5F) and increases in the DAI score (Figure 5G) were significantly suppressed by γKetoC in control (Nrf2+/+) mice. In addition, γKetoC-treated mice showed decreased epithelial cell disruption (focal erosion and ulcers) and inflammatory cell infiltration in the colon tissue and increased number of crypts (Figure 5H and Supplementary Figure S2), and γKetoC administration tended to decrease concentrations of TNF-α, IL-6, and IL-12p40 in serum (Figure 5I). Under the modified experimental condition (Figure 6A), DSS-induced body weight loss (Figure 6B) and DAI score increase (Figure 6C) in Nrf2+/- mice were significantly reduced by γKetoC administration, whereas the pathogeneses of colitis-induced Nrf2-/- mice were not improved by γKetoC administration. Atrophy of the colon in Nrf2+/- mice was significantly restored by the intake of γKetoC, with the length of the colon being similar with and without the administration of γKetoC in Nrf2-/- mice (Figure 6D).
Figure 5 Effects of γKetoC on colitis in mice. (A) Schematic of the oral administration schedule of γKetoC in the DSS-induced colitis model. C57BL/6J mice were orally administered 15 mg/kg/day of γKetoC in 100 μl soybean oil or vehicle. (B) Percent body weight change from that measured on day 0 (left), and percent body weight change from day 0 to day 10 in area-under-curve (AUC) format (right). (C) Disease activity index (DAI) scores (left), and DAI changes in AUC format (right). (D) Images (top) and length (bottom) of the large intestine. DSS-γKetoC-; without the DSS treatment (n=6), DSS+γKetoC-; with the DSS treatment (n=15), DSS+γKetoC+; administration of γKetoC with the DSS treatment (n=15) (A–D). (E) Schematics of the modified schedule of γKetoC administration. (F) Percent body weight changes. (G) DAI scores. (H) Histology of the colon tissue of colitis-induced mice. H&E staining photos of the colon of all tested individuals are shown in Supplementary Figure 2. (I) Concentrations of inflammatory cytokines in peripheral blood. DSS+γKetoC-; DSS treatment without γKetoC administration (n=4), DSS+γKetoC+; administration of γKetoC with the DSS treatment (n=5) (E–I). Data represent the mean ± SEM (B–D, F, G, I). The Tukey-Kramer test (B–D), Sidak’s multiple comparison test (F, G), and a two-tailed Student’s t-test (I) were used for statistical analyses. *p < 0.05, **p < 0.01, ***p < 0.001, ****p < 0.0001, ns, not significant.
Figure 6 Involvement of NRF2 in the effect of γKetoC on colitis. (A) Schematic of the schedule of administration of γKetoC (15 mg/kg/day) to colitis induced-Nrf2 gene targeted mice. (B) Percent body weight changes (left) and its AUC format (right). (C) DAI score (left) and its AUC format (right). (D) Atrophy levels of the colon. Nrf2+/- γKetoC-; colitis-induced Nrf2+/- mice (n=8), Nrf2+/- γKetoC+; γKetoC-treated colitis-induced Nrf2+/- mice (n=8), Nrf2-/- γKetoC+; colitis-induced Nrf2-/- mice (n=8), Nrf2-/- γKetoC+; γKetoC-treated colitis-induced Nrf2-/- mice (n=8) (A–D). The Tukey-Kramer test was used. Data are shown as the mean ± SEM. *p < 0.05, **p < 0.01, ns, not significant.
The gut microbiota metabolizes food ingredients, and the resulting compounds exert beneficial effects on homeostasis in the host body. PUFAs, which are positively associated with inflammatory diseases depending on the amount consumed and the ω3/ω6 ratio, were recently shown to be modified by the enzymes of gut bacteria (2). Although previous studies demonstrated the useful effects of the bacteria metabolites of PUFAs on host health, particularly the attenuation of metabolic disorders (3, 6, 7), their effects on immunoresponses remain unclear.
The present results revealed that enon FAs suppressed Ag-mediated immunoresponses, which were not observed for their precursors, namely, LA, ALA, and GLA, or hydroxy FAs. Further analyses with a focus on γKetoC indicated that γKetoC suppressed the release of inflammatory cytokines from LPS (and other PAMPs)-stimulated DCs, whole splenocytes, and peritoneal cells. KetoC has been shown to inhibit the expression of inflammatory cytokines in LPS-stimulated RAW264.7 cells, and this was mitigated by a GPR120 antagonist, but not a GPR40 antagonist (8). In contrast to GPR40, which is a receptor for long-chain FAs as well as GPR120, but is highly expressed in the pancreas and liver and is involved in metabolism, GPR120 has an anti-inflammatory role as an receptor for ω3 FAs (17). Since HYA, which activates GPR40 (3–5), did not suppress the production of IL-2 by OVA-stimulated OT-II splenocytes in the present study (Figure 1A), GPR40 might not play a prominent role in the regulation of inflammatory responses by immune-related cells. Based on the result showing that GW9508, a common agonist of GPR40 and GPR120, also reduced cytokine production by DCs, we speculate that GPR120 is involved in the anti-inflammatory effects of γKetoC as its receptor; however, we need to confirm this hypothesis in further experiments using a specific antagonist, siRNA, or KO mice. Although a previous study demonstrating high expression of GPR120 in DCs have shown low levels of Ffar1 mRNA (encoding GPR40) in DCs (17), which may support a primary contribution of GPR120, it is necessary to determine the protein levels of these GPCRs at least to rule out the involvement of GPR40 in regulation of DCs. Alternatively, the reporter assay of GPR40 and GPR120 would be useful to reveal the ligand activity of γKetoC and other FAs against GPR40 and GPR120. γKetoC, KetoC, and αKetoC are categorized as ω7, ω7, and ω3, respectively. The structure of a FA required for ligand activity against GPR120 may not be the location of the unsaturated bond, but rather other factors, which were increased in enon FAs. If the enon structure is essential for binding to GPR120, metabolism by bacteria confers anti-inflammatory effects on dietary PUFAs.
In a SV40-T-transformed human gingival epithelial cell line, KetoC induced ERK phosphorylation and the subsequent activation of the NRF2 pathway via GPR120 (9). Another previous study regarding ox-LDL-induced senescence also suggested that GW9508 activated NRF2 depending on GPR120 in human aortic endothelial cells (20). Under our experimental conditions, the GPR120 agonist did not induce Hmox1 transactivation in DCs (data not shown), whereas the suppressive effects of the GPR120 agonist on cytokine production in DCs were reduced by a NRF2 deficiency. These results suggest that γKetoC activated both the NRF2 pathway and GPR120 in DCs and also that the NRF2 pathway might modulate GPR120 activity, whereas the stimulation of GPR120 did not induce an antioxidant response in DCs. Given that γKetoC probably activates multiple pathways, further studies using various mice deficient one or more candidate targets of γKetoC, including GPR120, other GPCRs for FA, and nuclear receptors, in addition to Nrf2 KO, may clarify the complicated relationship between γKetoC, GPR120, and the NRF2 pathway.
The present study suggested that the suppressive effects of γKetoC on TNF-α production were mediated by Gq-GPCR (probably GPR120), and the effects of γKetoC on production of IL-6 and IL-12p40 was largely dependent on NRF2. These differences might reflect the promoter specific roles of NRF2 and/or GPR120. A vigorous study revealed the direct binding of NRF2 to the Il6 promoter inhibits the transcription of the Il6 gene in macrophages (21). It is also known the anti-inflammatory effects of DHA is caused by the GPR120-dependent activation of β-arrestin (17). Further detailed analyses regarding GPR120-mediated signal transduction and NRF2 recruitment toward chromosomal DNA in γKetoC-treated DCs are required to uncover the molecular mechanisms of anti-inflammatory effects of γKetoC. In addition, the roles of γKetoC in T cell function were still unclear in the present study, even though DC-independent proliferation of T cells were significantly suppressed by γKetoC. Although γKetoC did not have apparent effect on differentiation of Th1 and Th2 at least, we have not been able to obtain further reliable results regarding T cells, including Treg development. Further research on T cells has not been carried out, since it is difficult to grow purified CD4+ T cells in the presence of γKetoC. The experiments for Th1 and Th2 differentiation in the present study were performed with the lower concentration (25 μM) of γKetoC than that (50 μM) in a T cell division assay. Obviously, if the suppression of T cell proliferation is not a result of artificial, then it is a crucial factor for immunosuppressive impact. We need to perform the experiments investigating the effects of γKetoC on various immuno-related cells, to clarify overall immunoregulatory effects of γKetoC.
γKetoC increased NRF2 protein and Hmox1 mRNA levels in DCs, and NRF2 deficiency reduced the anti-inflammatory effects of γKetoC both in vitro and in vivo. NRF2 is a ubiquitous transcription factor, and Nrf2 KO mice exhibit severe inflammation in various immune-related diseases, including contact hypersensitivity, autoimmune disease, colitis, and psoriasis (22–27). Therefore, γKetoC and other enon FAs have the potential to prevent and/or treat immune-related diseases. In addition, since we recently demonstrated that γKetoC suppressed osteoclast development and macrophage activation (16), it may also attenuate rheumatoid arthritis.
According to a previous study showing the intestinal concentrations of PUFA derivatives, high fat diet and/or germ-free condition reduced the concentrations of LA-derived FAs such as HYA in the intestine (3). In addition, the dysbiosis-mediated decrease of HYA was partially recovered by administration of HYA or HYA-producing Lactobacillus. Although GLA-related FAs were not mentioned in that study, the decrease of concentrations of KetoC and αKetoC was observed. Further studies investigating the association between enon FA concentrations and pathogenesis of inflammatory diseases may reveal the circumstances under which γKetoC may be effective.
Although we focused on the roles of the NRF2 pathway and GPR120 in the anti-inflammatory effects of γKetoC, we never exclude the involvement of other receptors including PPARγ and TRPV1, which have been identified as receptors of other PUFAs. It is well known that PPARγ agonists inhibit TLRs-mediated activation (28) and T cell priming activity of DCs (29) and in a study using reporter assay not only KetoA but also KetoC exerted agonistic activity of PPARγ (7). Inhibitory role of TRPV1-signaling in differentiation and activation of DCs is also reported (30), whereas the effect of γKetoC on TRPV1-signaling has not been investigated in a study identifying KetoA as the strongest activator of TRPV1 (6).
We identified γKetoC as the anti-inflammatory compound through a screening, which exerted protective effect on DSS-induced colitis by activating the NRF2 pathway. In contrast, we did not compare the activity and still do not know which PUFA metabolites exhibit the high activity against the NRF2 pathway. We are going to perform further investigation to evaluate the effects of PUFA metabolites on the NRF2 pathway in vitro, and on the pathogenesis of colitis and other disease model mice in vivo.
The present study showed that several bacteria metabolites of PUFAs, particularly enon FAs, were involved in the regulation of immunoresponses, which were not observed for their precursors. The NRF2 pathway and GPR120, both of which play important roles in anti-inflammatory responses, appear to be involved in the effects of γKetoC. The intake of γKetoC ameliorated colitis in mice in a NRF2-dependent manner. Based on these results, we conclude that gut bacteria and their metabolites of PUFAs exert beneficial effects on immune homeostasis in the host body.
The raw data supporting the conclusions of this article will be made available by the authors, without undue reservation.
The animal study was approved by Institutional Review Board of Tokyo University of Science Animal Care and Use Committees of Tokyo University of Science. The study was conducted in accordance with the local legislation and institutional requirements.
MA: Investigation, Validation, Writing – review & editing. KN: Data curation, Investigation, Methodology, Validation, Writing – review & editing. RT: Investigation, Validation, Writing – review & editing. NI: Investigation, Writing – review & editing. SN: Investigation, Writing – review & editing. NM: Investigation, Writing – review & editing. NK: Investigation, Writing – review & editing. AY: Writing – review & editing, Investigation. TY: Investigation, Writing – review & editing. MH: Writing – review & editing. GI: Resources, Writing – review & editing. SK: Resources, Writing – review & editing. MY: Resources, Writing – review & editing. JO: Resources, Writing – review & editing. CN: Conceptualization, Funding acquisition, Project administration, Supervision, Writing – original draft, Writing – review & editing.
The author(s) declare financial support was received for the research, authorship, and/or publication of this article. This work was supported by a Grant-in-Aid for Scientific Research (B) 23H02167 (CN) and 20H02939 (CN); a Research Fellowship for Young Scientists DC2 and a Grant-in-Aid for JSPS Fellows 21J12113 (KN); a Scholarship for a Doctoral Student in Immunology (from Japanese Society for Immunology to NI); a Tokyo University of Science Grant for President’s Research Promotion (CN); the Tojuro Iijima Foundation for Food Science and Technology (CN); a Research Grant from the Mishima Kaiun Memorial Foundation (CN); and a Research Grant from the Takeda Science Foundation (CN).
We thank the members of the Laboratory of Molecular Biology and Immunology, Department of Biological Science and Technology, Tokyo University of Science for constructive discussions and technical support. We greatly appreciate the consideration and support from Dr. Kimihiko Yasuda, Dr. Masako Yasuda, and the late Ms. Yayoi Yasuda.
The authors declare that the research was conducted in the absence of any commercial or financial relationships that could be construed as a potential conflict of interest.
All claims expressed in this article are solely those of the authors and do not necessarily represent those of their affiliated organizations, or those of the publisher, the editors and the reviewers. Any product that may be evaluated in this article, or claim that may be made by its manufacturer, is not guaranteed or endorsed by the publisher.
The Supplementary Material for this article can be found online at: https://www.frontiersin.org/articles/10.3389/fimmu.2024.1374425/full#supplementary-material
1. Ishihara T, Yoshida M, Arita M. Omega-3 fatty acid-derived mediators that control inflammation and tissue homeostasis. Int Immunol. (2019) 31:559–67. doi: 10.1093/intimm/dxz001
2. Kishino S, Takeuchi M, Park SB, Hirata A, Kitamura N, Kunisawa J, et al. Polyunsaturated fatty acid saturation by gut lactic acid bacteria affecting host lipid composition. Proc Natl Acad Sci U.S.A. (2013) 110:17808–13. doi: 10.1073/pnas.1312937110
3. Miyamoto J, Igarashi M, Watanabe K, Karaki SI, Mukouyama H, Kishino S, et al. Gut microbiota confers host resistance to obesity by metabolizing dietary polyunsaturated fatty acids. Nat Commun. (2019) 10:4007. doi: 10.1038/s41467-019-11978-0
4. Miyamoto J, Mizukure T, Park SB, Kishino S, Kimura I, Hirano K, et al. A gut microbial metabolite of linoleic acid, 10-hydroxy-cis-12-octadecenoic acid, ameliorates intestinal epithelial barrier impairment partially via GPR40-MEK-ERK pathway. J Biol Chem. (2015) 290:2902–18. doi: 10.1074/jbc.M114.610733
5. Yamada M, Takahashi N, Matsuda Y, Sato K, Yokoji M, Sulijaya B, et al. A bacterial metabolite ameliorates periodontal pathogen-induced gingival epithelial barrier disruption via GPR40 signaling. Sci Rep. (2018) 8:9008. doi: 10.1038/s41598-018-27408-y
6. Kim M, Furuzono T, Yamakuni K, Li Y, Kim YI, Takahashi H, et al. (2017) 31(11):5036–48. doi: 10.1096/fj.201700151R. 10-oxo-12(Z)-octadecenoic acid, a linoleic acid metabolite produced by gut lactic acid bacteria, enhances energy metabolism by activation of TRPV1. FASEB J (2017) 31:5036–48. doi: 10.1096/fj.201700151R
7. Goto T, Kim YI, Furuzono T, Takahashi N, Yamakuni K, Yang HE, et al. 10-oxo-12(Z)-octadecenoic acid, a linoleic acid metabolite produced by gut lactic acid bacteria, potently activates PPARγ and stimulates adipogenesis. Biochem Biophys Res Commun. (2015) 459:597–603. doi: 10.1016/j.bbrc.2015.02.154
8. Sulijaya B, Takahashi N, Yamada M, Yokoji M, Sato K, Aoki-Nonaka Y, et al. The anti-inflammatory effect of 10-oxo-trans-11-octadecenoic acid (KetoC) on RAW 264.7 cells stimulated with Porphyromonas gingivalis lipopolysaccharide. J Periodontal Res. (2018) 53:777–84. doi: 10.1111/jre.12564
9. Yokoji-Takeuchi M, Takahashi N, Yamada-Hara M, Sulijaya B, Tsuzuno T, Aoki-Nonaka Y, et al. A bacterial metabolite induces Nrf2-mediated anti-oxidative responses in gingival epithelial cells by activating the MAPK signaling pathway. Arch Oral Biol. (2020) 110:104602. doi: 10.1016/j.archoralbio.2019.104602
10. Itoh K, Chiba T, Takahashi S, Ishii T, Igarashi K, Katoh Y, et al. An Nrf2/small Maf heterodimer mediates the induction of phase II detoxifying enzyme genes through antioxidant response elements. Biochem Biophys Res Commun. (1997) 236:313–22. doi: 10.1006/bbrc.1997.6943
11. Kanada S, Nishiyama C, Nakano N, Suzuki R, Maeda K, Hara M, et al. Critical role of transcription factor PU.1 in the expression of CD80 and CD86 on dendritic cells. Blood. (2011) 117:2211–22. doi: 10.1182/blood-2010-06-291898
12. Nagata K, Nagase H, Okuzumi A, Nishiyama C. Delta opioid receptor agonists ameliorate colonic inflammation by modulating immune responses. Front Immunol. (2021) 12:730706. doi: 10.3389/fimmu.2021.730706
13. Ito N, Sakata F, Hachisu M, Nagata K, Ito T, Nomura K, et al. The Ccl17 gene encoding TARC is synergistically transactivated by PU.1 and IRF4 driven by the mammalian common promoter in dendritic cells. Allergy. (2022) 77:1054–9. doi: 10.1111/all.15184
14. Kodama N, Okada H, Hachisu M, Ando M, Ito N, Nagata K, et al. A rose flavor compound activating the NRF2 pathway in dendritic cells ameliorates contact hypersensitivity in mice. Front Nutr. (2023) 10:1081263. doi: 10.3389/fnut.2023.1081263
15. Kitamura N, Yokoyama H, Yashiro T, Nakano N, Nishiyama M, Kanada S, et al. Role of PU.1 in MHC class II expression through transcriptional regulation of class II transactivator pI in dendritic cells. J Allergy Clin Immunol. (2012) 129:814–824.e6. doi: 10.1016/j.jaci.2011.10.019
16. Hagiwara S, Nagata K, Kasakura K, Sakata F, Kishino S, Ogawa J, et al. The anti-inflammatory effect of the gut lactic acid bacteria-generated metabolite 10-oxo-cis-6,trans-11-octadecadienoic acid on monocytes. Biochem Biophys Res Commun. (2020) 530:342–7. doi: 10.1016/j.bbrc.2020.07.006
17. Oh DY, Talukdar S, Bae EJ, Imamura T, Morinaga H, Fan W, et al. GPR120 is an omega-3 fatty acid receptor mediating potent anti-inflammatory and insulin-sensitizing effects. Cell. (2010) 142:687–98. doi: 10.1016/j.cell.2010.07.041
18. Furumoto H, Nanthirudjanar T, Kume T, Izumi Y, Park SB, Kitamura N, et al. 10-Oxo-trans-11-octadecenoic acid generated from linoleic acid by a gut lactic acid bacterium Lactobacillus plantarum is cytoprotective against oxidative stress. Toxicol Appl Pharmacol. (2016) 296:1–9. doi: 10.1016/j.taap.2016.02.012
19. Macoch M, Morzadec C, Génard R, Pallardy M, Kerdine-Römer S, Fardel O, et al. Nrf2-dependent repression of interleukin-12 expression in human dendritic cells exposed to inorganic arsenic. Free Radic Biol Med. (2015) 88:381–90. doi: 10.1016/j.freeradbiomed.2015.02.003
20. Liu R, Cheng F, Zeng K, Li W, Lan J. GPR120 agonist GW9508 ameliorated cellular senescence induced by ox-LDL. ACS Omega. (2020) 5:32195–202. doi: 10.1021/acsomega.0c03581
21. Kobayashi EH, Suzuki T, Funayama R, Nagashima T, Hayashi M, Sekine H, et al. Nrf2 suppresses macrophage inflammatory response by blocking proinflammatory cytokine transcription. Nat Commun. (2016) 7:11624. doi: 10.1038/ncomms11624
22. Helou DG, Noël B, Gaudin F, Groux H, El Ali Z, Pallardy M, et al. Cutting edge: nrf2 regulates neutrophil recruitment and accumulation in skin during contact hypersensitivity. J Immunol. (2019) 202:2189–94. doi: 10.4049/jimmunol.1801065
23. El Ali Z, Gerbeix C, Hemon P, Esser PR, Martin SF, Pallardy M, et al. Allergic skin inflammation induced by chemical sensitizers is controlled by the transcription factor Nrf2. Toxicol Sci. (2013) 134:39–48. doi: 10.1093/toxsci/kft084
24. Khor TO, Huang MT, Kwon KH, Chan JY, Reddy BS, Kong AN. Nrf2-deficient mice have an increased susceptibility to dextran sulfate sodium-induced colitis. Cancer Res. (2006) 66:11580–4. doi: 10.1158/0008-5472.CAN-06-3562
25. Ogawa T, Ishitsuka Y, Inoue S, Nakamura Y, Saito A, Okiyama N, et al. Nuclear factor erythroid 2-related factor 2 (Nrf2) regulates epidermal keratinization under psoriatic skin inflammation. Am J Pathol. (2020) 190:577–85. doi: 10.1016/j.ajpath.2019.10.022
26. Yoh K, Itoh K, Enomoto A, Hirayama A, Yamaguchi N, Kobayashi M, et al. Nrf2-deficient female mice develop lupus-like autoimmune nephritis. Kidney Int. (2001) 60:1343–53. doi: 10.1046/j.1523-1755.2001.00939.x
27. Johnson DA, Amirahmadi S, Ward C, Fabry Z, Johnson JA. The absence of the pro-antioxidant transcription factor Nrf2 exacerbates experimental autoimmune encephalomyelitis. Toxicol Sci. (2010) 114:237–46. doi: 10.1093/toxsci/kfp274
28. Appel S, Mirakaj V, Bringmann A, Weck MM, Grünebach F, Brossart P. PPAR-gamma agonists inhibit toll-like receptor-mediated activation of dendritic cells via the MAP kinase and NF-kappaB pathways. Blood. (2005) 106:3888–94. doi: 10.1182/blood-2004-12-4709
29. Klotz L, Dani I, Edenhofer F, Nolden L, Evert B, Paul B, et al. Peroxisome proliferator-activated receptor gamma control of dendritic cell function contributes to development of CD4+ T cell anergy. J Immunol. (2007) 178:2122–31. doi: 10.4049/jimmunol.178.4.2122
Keywords: colitis, dendritic cell, GPCR, inflammatory cytokine, NRF2, polyunsaturated fatty acid
Citation: Ando M, Nagata K, Takeshita R, Ito N, Noguchi S, Minamikawa N, Kodama N, Yamamoto A, Yashiro T, Hachisu M, Ichihara G, Kishino S, Yamamoto M, Ogawa J and Nishiyama C (2024) The gut lactic acid bacteria metabolite, 10-oxo-cis-6,trans-11-octadecadienoic acid, suppresses inflammatory bowel disease in mice by modulating the NRF2 pathway and GPCR-signaling. Front. Immunol. 15:1374425. doi: 10.3389/fimmu.2024.1374425
Received: 22 January 2024; Accepted: 05 April 2024;
Published: 30 April 2024.
Edited by:
Kosuke Nishi, Ehime University, JapanReviewed by:
Satoshi Tanaka, Kyoto Pharmaceutical University, JapanCopyright © 2024 Ando, Nagata, Takeshita, Ito, Noguchi, Minamikawa, Kodama, Yamamoto, Yashiro, Hachisu, Ichihara, Kishino, Yamamoto, Ogawa and Nishiyama. This is an open-access article distributed under the terms of the Creative Commons Attribution License (CC BY). The use, distribution or reproduction in other forums is permitted, provided the original author(s) and the copyright owner(s) are credited and that the original publication in this journal is cited, in accordance with accepted academic practice. No use, distribution or reproduction is permitted which does not comply with these terms.
*Correspondence: Chiharu Nishiyama, Y2hpbmlzaGlAcnMudHVzLmFjLmpw
†These authors have contributed equally to this work and share first authorship
Disclaimer: All claims expressed in this article are solely those of the authors and do not necessarily represent those of their affiliated organizations, or those of the publisher, the editors and the reviewers. Any product that may be evaluated in this article or claim that may be made by its manufacturer is not guaranteed or endorsed by the publisher.
Research integrity at Frontiers
Learn more about the work of our research integrity team to safeguard the quality of each article we publish.