- 1Department of Medical Genetics, School of Biomedical Engineering, University of British Columbia, Vancouver, BC, Canada
- 2Department of Biology and Biochemistry, University of Bath, Bath, United Kingdom
Eosinophils are a type of granulocyte named after the presence of their eosin-stained granules. Traditionally, eosinophils have been best known to play prominent roles in anti-parasitic responses and mediating allergic reactions. Knowledge of their behaviour has expanded with time, and they are now recognized to play integral parts in the homeostasis of gastrointestinal, respiratory, skeletal muscle, adipose, and connective tissue systems. As such, they are implicated in a myriad of pathologies, and have been the target of several medical therapies. This review focuses on the lifespan of eosinophils, from their origins in the bone marrow, to their tissue-resident role. In particular, we wish to highlight the functions of eosinophils in non-mucosal tissues with skeletal muscle and the adipose tissues as examples, and to discuss the current understanding of their participation in diseased states in these tissues.
1 Introduction
The eosinophil was discovered by Paul Erlich in, 1879, when he observed the distinctive properties of a particular subset of blood leukocytes that exhibited a pink hue when stained with eosin dye (1).
Eosinophils originate in the bone marrow from multipotent hematopoietic stem cells (HSC) that differentiate down the myeloid lineage (2). The common myeloid progenitor (CMP) gives rise to myeloblasts, which are capable of entering granulopoiesis towards one of three types of cells: neutrophils, basophils, and eosinophils (3). The differentiation and maturation of eosinophils along the eosinophilic lineage are dependent on the timely expression and presence of several transcription factors and cytokines (2, 4–6). Mature eosinophils are tissue-resident and distributed among the circulatory and lymphatic system and several organs in the body (2). Within the peripheral blood, eosinophils typically make up less than five percent of all peripheral white blood cells (7–9). Relative to other cell types, eosinophils predominate within the lamina propria of the gastrointestinal tract; the lung parenchyma; the cortico-medullary region of the thymus (in close proximity of CD4/CD8 negative thymocytes); the bulbous end of developing terminal end buds of mammary glands; the endometrium of the uterus; and finally, within the interstitial space of the adipose tissue and skeletal muscle (Figure 1). In response to infection or allergen, Type 2 inflammatory (Th2) cells in proximity to the stressor produce high levels of interleukine (IL)-5 which triggers eosinophil infiltration into tissues (11, 12). Pathogenic tissue hyper-eosinophilia arises in a number of diseased states, including atopic dermatitis, allergic airway inflammation, asthma, eosinophilic esophagitis, cancer, and some myopathies such as Duchenne Muscular Dystrophy (DMD) and eosinophilic myositis (13–17).
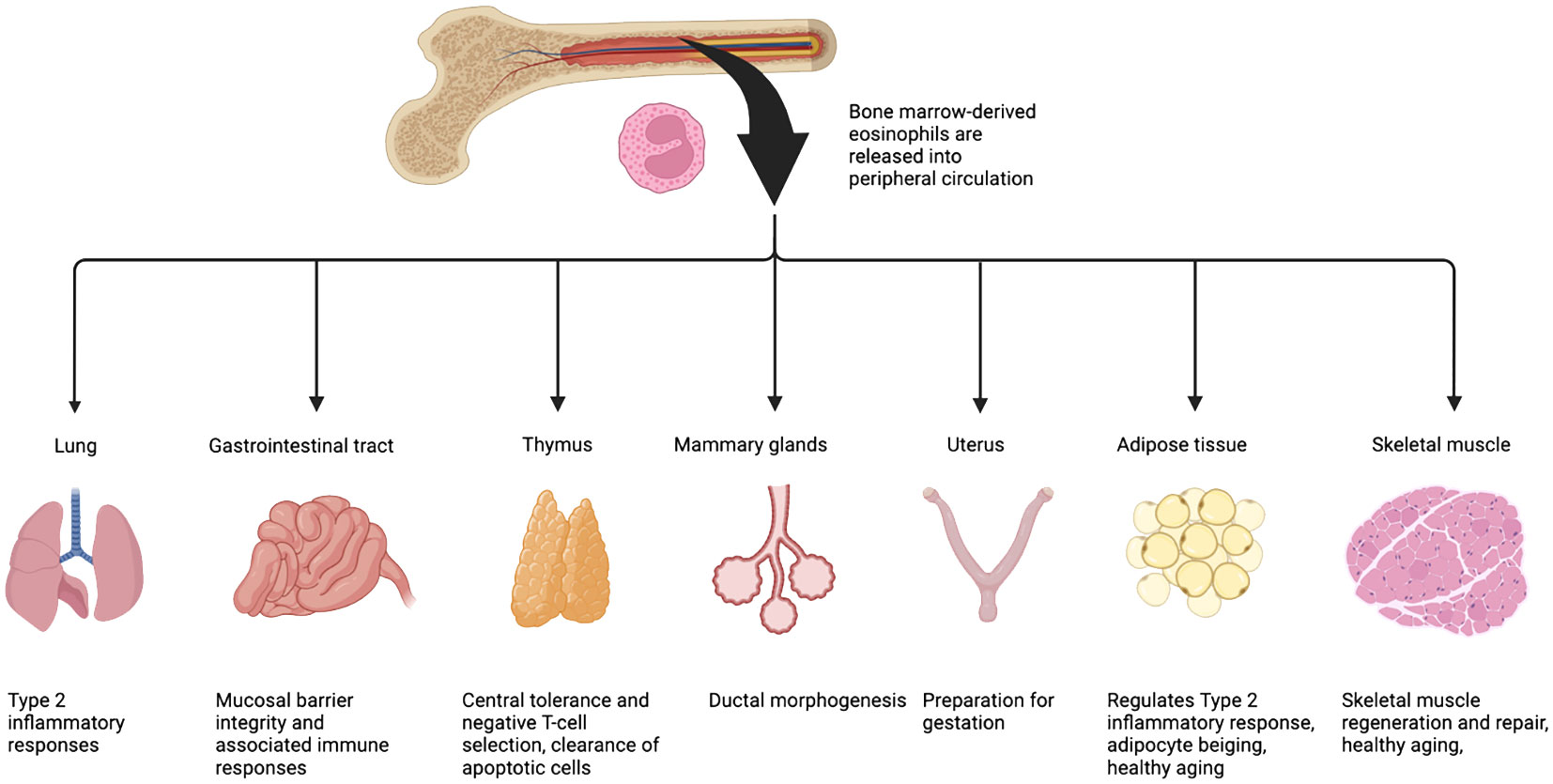
Figure 1 Eosinophils are present in most -if not all- type of tissues. Once fully differentiated, eosinophils exit the bone marrow, enter and patrol the circulation. Upon stimulation (damage, cytokine/chemokine gradient), they migrate to various tissues where they home into the interstitial space and, with the support of other immune cells, provide a Th2 micro-environment that will participate to maintain tissue homeostasis. Eosinophils predominate within the lamina propria of the gastrointestinal tract; the lung parenchyma; the cortico-medullary region of the thymus; the bulbous end of developing terminal end buds of mammary glands; the endometrium of the uterus; and finally, within the interstitial space of the adipose tissue and skeletal muscle. Figure adapted from Marichal et al. (10).
Mature eosinophils contain granules rich in major basic protein (MBP), eosinophil cationic protein (ECP), eosinophil peroxidase (EPO/EPX), and eosinophil-derived neurotoxin (EDN) (18, 19). Eosinophil granules are cytotoxic and are released in response to bacterial, viral, and parasitic infection as well as allergen exposure (19, 20). Other cell surface markers such as SiglecF (CD170) and IL-5Rα (CD125) allow the study of eosinophils at homeostasis and in diseased states. In human, eosinophils populations are characterized with SIGLEC8, CD62L, CD101 (21), but also CD15 and CD66b (22, 23). However, extensive attempts to study eosinophils across different tissues and diseases have uncovered that these cells are more heterogeneous than initially assumed, both in terms of spatial and temporal expression of various markers and functions (24). Particularly in the lung, blood-circulating eosinophils infiltrate the lung after house dust mite (HDM) stimulation. These eosinophils express higher level of SiglecF, CD34, lower level of CD125, and similar levels of F4/80 and CCR3 compared to resident eosinophils. They also have a highly segmented nucleus and a higher density of granules, suggesting some level of activation. Interestingly, these eosinophil also express CD101, shown to be a marker of non-classical activation, thus they are categorized as inflammatory eosinophils (25). More studies have described different profiles of infiltrating and resident eosinophils after damage, however these are mostly focused on mucosal tissues (21, 26) and very little is known about these subsets in skeletal muscle and adipose tissue.
To resolve this issue, higher-resolution techniques such as single-cell RNA sequencing (RNAseq) have been employed to better understand eosinophil heterogeneity and function. However, due to their poor survival after extraction eosinophils were absent from human and murine single cell RNAseq datasets until recently (27). As a result, the compendium of knowledge on eosinophil function and heterogeneity, particularly those belonging to non-mucosal tissues, is scattered or lacking altogether. The aim of this review is to summarize the origins of eosinophils and discuss the existing evidence for their function in two non-mucosal tissues at homeostasis and in disease.
2 Mouse models to study eosinophils
Several mouse models are available to study the role of eosinophils at homeostasis and in disease (Table 1). It is important to highlight that these models have systemic changes in eosinophil counts through their lifespan and might have stronger phenotypes than when inducing hyper eosinophilia with IL-5 injection, or when reducing their number with blocking antibody for IL-5.
2.1 Eosinophil-deficient models
One of the most common models it the ΔdblGATA mice in which a mutation has been inserted in the double GATA-site 21 base pair upstream of the first exon of the Gata-1 gene (28). GATA-1 is also needed for the development of red blood cells, megakaryocytes and mast cells, which are not affected in this model due to the specificity of the expression of the targeted exon. It is important to note that this mouse model can be found in two different backgrounds and that it should be compared to its correct control (BALB/c or C57BL/6). Indeed, in comparison to the C57BL/6 mice, BALB/c mice are more sensitive to infectious diseases and allergic reactions, which are both classified as Th2 immune responses. Hence, results coming from either of the backgrounds must be carefully considered.
The IL-5 deficient mouse (C57BL/6-Il5tm1Kopf/J) can also be used to study the absence of eosinophil in diseases. Interestingly, this mouse model does not have a defect in basal eosinophil production, suggesting that IL-5 is not needed for the production of eosinophil progenitors (EOPs) and blood circulating eosinophils. The production of other Th2 cytokines is also not affected, as the levels of IL-4 and IL-13 remain similar to WT controls (29).
A transgenic mouse model expressing diphtheria toxin A (DTA) behind the EPO/EPX promotor can also be used to study the role of eosinophils (PHIL, B6.Cg-Tg(Epo-DTA)#Nal/JleeJ). This results in eosinophil cell death prior to their full maturation and bone marrow exit. In practical terms, this mouse model allows for the depletion of EOPs and mature eosinophils, useful for elucidating the consequences of eosinophil deficiency in development, homeostasis, and disease (30).
Lastly, the interferon consensus sequence-binding protein (Icsbp)-deficient mouse model exhibits reduced bone marrow eosinophil production due to a reduction in the expression of GATA-1 (31). This model is unable to respond to IL-5 stimulation (38).
2.2 Eosinophil-abundant models
The IL5-Tg mouse model is used to study the effect of hyper-eosinophilia. In these mice, the gene coding for IL-5 has been placed under a different promotor or contain multiple copies of the Il5 gene. For example, IL-5 can be overexpressed using regulatory elements from the CD3delta gene to drive T cell expression of IL-5 (B6.Cg-Tg(Cd3d-Il5)NJ.1638Nal/JleeJ) (32), which results in a concentration of 400-800 pg/ml of IL-5 in the serum while it should be undetectable in normal conditions. The sequence coding for Il5 can also be placed under the dominant control region of the human CD2 promotor (33). Of interest, four independent eosinophilic transgenic lines were established: Tg5C1, Tg5C2, Tg5C3, and Tg5C4 (33). These transgenic lines contain between 8 and 49 transgene copies. Furthermore, IL-5 has been over-expressed under the rat Clara cell secretory protein regulatory elements, expressed in the lung epithelium (B6.Cg-Tg(Scgb1a1-Il5)NJ.1726Nal/JleeJ) (34).
Interestingly, the siglecF-null mice (C.129(Cg)-Siglecftm1.2Avrk/J) display elevated eosinophil infiltration in lung with bone marrow and blood hyper-eosinophilia after ovalbumin (OVA)-induced lung allergy, suggesting that siglecF participates in a negative feedback loop, reducing eosinophil function, and inducing eosinophil apoptosis (36). However McMillan and colleagues showed that the phenotype observed could be due to the experimental procedure (intranasal versus aerosolised OVA) and might be due the extent of the damage induced (39).
2.3 Other models
Eosinophil tracking is possible using a GFP reporter under IL-4 promotor: IL-4/GFP-enhanced transcript (4-Get) mouse model (C.129-Il4tm1Lky/J) (35). In order to study the cell-signaling process in eosinophils, a EPX-cre model (eoCRE) was designed by the team of Dr. James J Lee (37). This mouse is a knock-in, with a reduced production of EPX. However, the levels and properties of eosinophils from the eoCRe mice remain unchanged (37).
With multiple mouse models available, eosinophils are now easily targeted and studied. Nevertheless, the choice of model and its background, as well as the type of damage must be taken in account when designing the study.
3 Origin of eosinophils: from the bone marrow to the tissue
Once released into the circulation, eosinophils have a very short half-life that can vary from 8 -18 hours in the blood, and up to 6 -7 days once infiltrated in a homeostatic tissue (i.e. non-damaged). For example, in the thymus, eosinophils increase after birth and peak at 2 weeks, after which they fall and rise again during thymic involution (40). In the uterus, eosinophils follow the menstrual cycle as they infiltrate the endometrium adjacent to the myometrium 1 day prior, during, and 1 day after estrus. While these eosinophils are thought to contribute to preparing the uterus for pregnancy, their loss does not impact the fertility of mice (41). In mammary glands, eosinophils are present in and contribute to ductal morphogenesis from 3 - 8 weeks of age (42, 43).
The lifespan of eosinophils can be increased using IL-5, IL-3, or GM-CSF in vitro, suggesting that these molecules could also play a role in the viability of eosinophils, as they are released during fundamental processes like tissue remodeling. The infiltration and homing of eosinophils into tissues are driven by several factors (such as eotaxins). IL-5 the eosinophil chemoattractant and pro-survival factor, is necessary for the development and the migration of eosinophils from the bone marrow to the blood (4, 44, 45). Eosinophils are drawn into peripheral tissues via local chemokine attractants (46–48). For example, in homeostatic conditions, Eotaxin-1 (CCL11) is produced by local stromal cells and controls eosinophil migration via circulation to tissues such as the gastrointestinal tract, uterus, mammary glands, and thymus (Figure 1) (49–52).
3.1 Embryonic development
The role of eosinophils during mouse embryonic development is unclear, as mice lacking eosinophils are viable into adulthood. Moreover, there is very little evidence showing when eosinophils are first produced during embryogenesis. Using the 4-Get mouse model (IL-4 eGFP reporter mouse model) Voehringer et al., showed that a population of GFP+ cells appeared at E14.5, and that cells expressing a putative eosinophil marker pattern such as c-Kit- Sca-1- CCR3- SiglecF+ IL4-eGFP+ arise from the fetal liver at E16.5 (53). While these cells do not express CD34 (expressed by adult bone marrow EOPs), they were able to repopulate the bone marrow after transplantation. In vitro, eosinophils can be differentiated from murine embryonic cells cultivated on a feeding layer of OP9 fibroblasts with IL-5, and either IL-3, GM-CSF, or eotaxin (54).
Are eosinophils produced by the Aorta-Gonad-Mesonephros (AGM) or only by the fetal liver? During development, does their appearance follow that of circulating monocytes? While eosinophils are not present in the lung at birth but gradually increase in numbers to reach maximal density by day 7 after birth (25) they are already found in the gut tract before birth. We could then question if there is a sub-population of tissue-resident eosinophils, similar to tissue resident macrophages derived from the yolk sac. The timeline of eosinophil infiltration in non-mucosal tissues such as in skeletal muscle or adipose is unknown, and it would be compelling to see if it corresponds to the development of the microbiota, as suggested for lung infiltration (25).
3.2 Bone marrow hematopoiesis
During adulthood, eosinophils are released from the bone marrow into the circulation and their production depends on both transcription factor activity and cytokine stimulation (Figure 2).
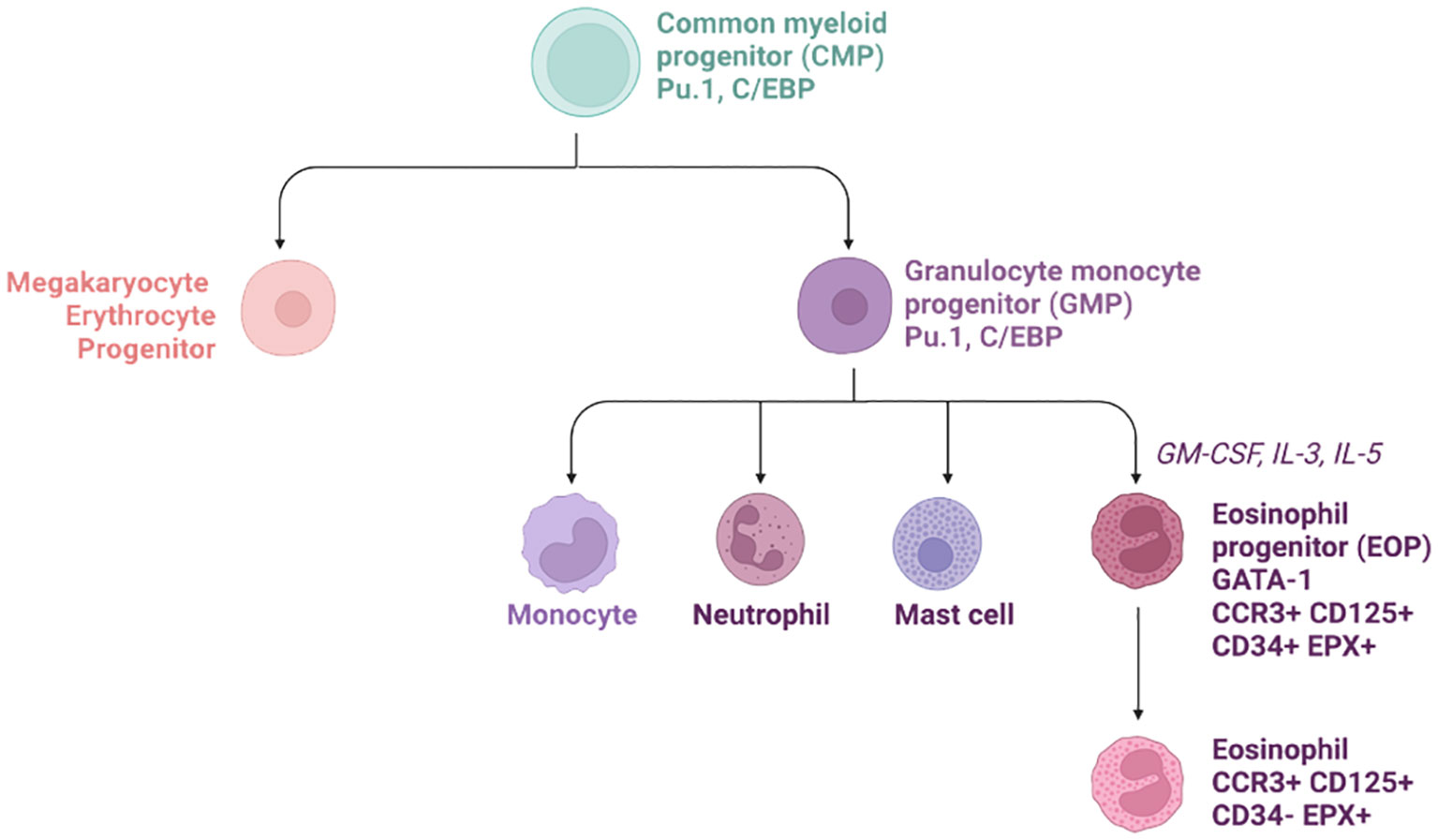
Figure 2 Hierarchical tree of eosinophil progenitor formation in the bone marrow. The common myeloid progenitor (CMP) give rise to both megakaryocyte Erythrocytes Progenitors (MEP) and Granulocyte monocyte progenitor (GMP). The latest then produce the eosinophil progenitors thanks to various chemokines such as GM-CSF, IL-3, and IL-5. Later on, these cells down-regulate CD34 and leave the bone marrow for the circulation.
A key transcription factor required for eosinophil production is PU.1. PU.1 is an ETS family member and is only expressed in hematopoietic cells. It is involved in regulating the balance between the lymphoid and myeloid lineage. PU.1-deficient mice lack B cells and dendritic cells, but also all cells belonging to the myeloid lineage such as monocytes and neutrophils. PU.1-deficient mice die within the first 48 hours post birth due to the absence of the wide range of immune cells (55). C/EBP (CCAAT/enhancer-binding protein) is expressed in CMPs and its activation leads to increased myeloid and eosinophil differentiation (56). Interestingly, deletion of C/EBPα will only affect the myeloid lineage, suggesting there are differential roles of C/EBPα/β in eosinophil development (56). GATA-1 (a zinc finger family member) is expressed at a later stage of the eosinophil differentiation as it regulates the expression of CCR3, CD125 (IL-5Rα) and other mature granule protein genes such as MBP, EPX, ECP, EDN, and Charcot-Leyden crystal protein (CLC, Galectin-10) (28).
Interestingly, ICSBP (an IFN-g induced transcription factor) has also been shown to be involved in eosinophil differentiation as it regulates GATA-1 expression. In vitro, HSC from ICSBP-KO mice do not respond to IL-5 stimulation, leading to a defect in the production of eosinophil progenitors (38).
Id proteins are also implicated in eosinophil development. Id proteins are composed of 4 isoforms and are basic helix-loop-helix transcription factors that lack a basic DNA binding domain. Their function is essential for stem cell fate, as they can block the differentiation of progenitors, promoting their proliferation and delaying senescence. In regard to eosinophil differentiation, Id1 and Id2 have opposing roles. Id1 inhibits eosinophil development whereas Id2 accelerates the final maturation of eosinophils. The roles of Id3 and Id4 in eosinophil production have not been described (57)
Finally, Friend of GATA (FOG) acts as a repressor of the eosinophil lineage. FOG is also part of the zinc finger family and binds to the N-terminal finger motif of GATA-1, inhibiting its ability to activate the transcription of Mbp (58).
Once committed to the eosinophilic lineage, the final differentiation step requires the synergic stimulation of IL-3, GM-CSF, and finally, IL-5. While IL-3 and GM-CSF enhance myeloid differentiation, IL-5 is specific to EOPs due to its unique affinity to CD125 (IL-5Rα). Elevated levels of IL-5, either from intravenous cytokine injection, or in a genetic mouse model over-expressing IL-5 under the CD3 promoter, induce blood hyper-eosinophilia (Table 1). Fully differentiated eosinophils are released from the bone marrow into the circulatory system, until they reach their tissue of interest.
3.3 Eosinophil homing and homeostasis
Eosinophils do not remain in the blood to patrol for pathogenic organisms, but rather use it as an expressway to go from the bone marrow to tissues of interest. These include primarily mucosal tissues such as the lung or gut. To enter tissues, eosinophils primarily rely on a CCL11 concentration gradient across epithelial cells, endothelial cells, or fibroblasts, as well as other chemokines such as eotaxin-2 and 3 (CCL24 and CCL26), via CCR3. While these cytokines are the main regulators of eosinophil infiltration, it is interesting to note that CCR3, the receptor for CCL11, has been shown to induce a negative feedback loop on eosinophil response to inflammatory stimuli. For example, pre-treatment with the chemokine Monokine induced by gamma interferon (Mig, also known as CXCL9) inhibits eosinophil responses by a CCR3-Rac2 dependent mechanism (59).
Once in the tissue, eosinophils protect against parasitic infections (in the gut), react to allergens (in the lung) or maintain a Th2 environment (in the adipose tissue). Their different functions suggest levels of heterogeneity within tissues, which have been difficult to study as eosinophils half-life can be a short as a few hours. The first scRNAseq eosinophil dataset was conducted by Gurtner et al., where the authors separated eosinophils from bone marrow, blood, spleen, stomach, small intestine, and colon into their progenitor, immature, circulating, basal, and active forms (27). Most importantly, they showed that two types of eosinophil exist within the GI tract as basal (i.e. non-activated) and activated populations. In the GI tract, these two populations can be separated by the expression of PD-L1 and CD80, with the activated population expressing both markers. PD-L1 and CD80 expression is regulated by NF-kB signaling, and in vitro IL-33 stimulation was sufficient to induce eosinophil activation. scRNAseq was also performed on the IL-5-Tg mice, which demonstrated that there was a population of pre-activated eosinophils in the absence of any prior damage or stimulus (27). The authors further validated their findings on WT C57BL/B6J mice. The role of this activated population has been further studied in colitis and inflammatory bowel disease, but not in other tissues thus far. It is imperative that the existence and the role of this population is confirmed in other eosinophil-rich tissues, such as in the lung and in some non-mucosal tissues.
4 Role of eosinophils in skeletal muscle homeostasis
Skeletal muscle is one of the three types of muscles found in the body and is the only type under voluntary control. This highly organised striated tissue is composed of multinucleated myofibers that produce contractile forces used to generate locomotion and stability (for review of its structure please read (60)). At homeostasis, skeletal muscle stem cell (also called satellite cells (SCs)) turnaround is slow to non-existent. However, after damage, skeletal muscle is able to regenerate and repair itself thanks to a complex cell-cell crosstalk. Muscle regeneration occurs through a multi-step process that includes: necrosis/degeneration, inflammation, maturation, and functional recovery (61). Skeletal muscle homeostasis and myofiber regeneration are affected in various pathological conditions such as in aging (sarcopenia), cancer (cachexia) and various myopathies such as Duchenne Muscular Dystrophy (DMD), Limb-girdle muscular dystrophies (LGMD), or eosinophilic myositis (for review read (62)). It is widely documented that eosinophils play an active role in Th2 immune responses in a variety of tissues and disease states, but the role that eosinophils play and the underlying mechanisms of activation and signalling in skeletal muscle tissue remains largely unknown.
4.1 Acute damage
Acute injury occurs in skeletal muscle either when the muscle experiences a traumatic or contraction-induced injury to the muscle fibres. Immune cells, and in particular macrophages have been shown to play a vital role in the repair process after acute injury (for review read (63)). In the first few hours after injury, tissue-resident cells produce CCL2, a chemoattractant to signal and recruit various inflammatory cells to the site of injury (64–66). At the site of injury, Ly-6Chi macrophages express pro-inflammatory cytokines, further promoting the recruitment of monocytes and eosinophils, which in turn, promotes myogenic cell proliferation (67, 68). Macrophages then transition to a pro-regenerative phenotype and down-regulate Ly-6C. Ly-6Clow macrophages produce TGF-β, IL-10, and IGF-1 which have been shown to stimulate myogenic cell differentiation and promote muscle growth (69–71). While macrophages have been studied for many years, it is not before 2013 that eosinophils started to be studied in the context of muscle regeneration. Heredia and colleagues found that the BALB/c ΔdblGATA mice (deficient in eosinophil, Table 1) exhibited delayed skeletal muscle regeneration, suggesting that factors secreted by eosinophils may play a role in skeletal muscle regeneration (72) (Figure 3). Further experiments show that after acute injury, a lack of Th2 cytokines (i.e. IL-4 and IL-13) showed significant delays in skeletal muscle regeneration, with the formation of adipocytes suggesting that eosinophil protect muscle against intramuscular adipose tissue (IMAT) (72). Following this, we have investigated the effects of hyper-eosinophilia on muscle repair in acute damage conditions by using the IL5-Tg mice. Significant decreases in the number of myonuclei were found at 7 days post injury, and decreased myofiber size at 14- and 28- days post injury, suggesting that skeletal muscle hyper-eosinophilia impacts the ability of skeletal muscle to regenerate (73). Furthermore, we have shown that during skeletal muscle regeneration eosinophils do not only produce Th2 cytokines but can also produce various molecules that have been shown to affect myofiber stability (please see below in DMD). Similar to macrophages during skeletal muscle regeneration, eosinophils need to be regulated at two different levels: number and inflammatory profile. Acute damage is often associated with pain. Of interest, IL-5 has been associated with pain in fibromyalgia. LPS stimulated PBMC from fibromyalgic women release more IL-5, along with IL-4 and IL-2, than pain free women (74). While no increase in IL-5 was observed in a mouse model of fibromyalgia (acidic saline injected in the gastrocnemius), i.v. injection of IL-5 improved the mechanical thresholds of the paw, sign of a decrease in pain (74). This treatment has since then been further explored with behavioral experiments, however only monocyte and T cell populations were analyzed and no eosinophil phenotyping was done on muscle tissue (75). When investigating intramuscular levels of cytokines in patient with jaw muscle pain, IL-5 was under the limit of detection (0.49 pg/mL) and removed from the study, along with IL-2, IL-4, IL-10 and IFNγ, suggesting that the increase in IL-5 associated with pain could be a systemic response (76).
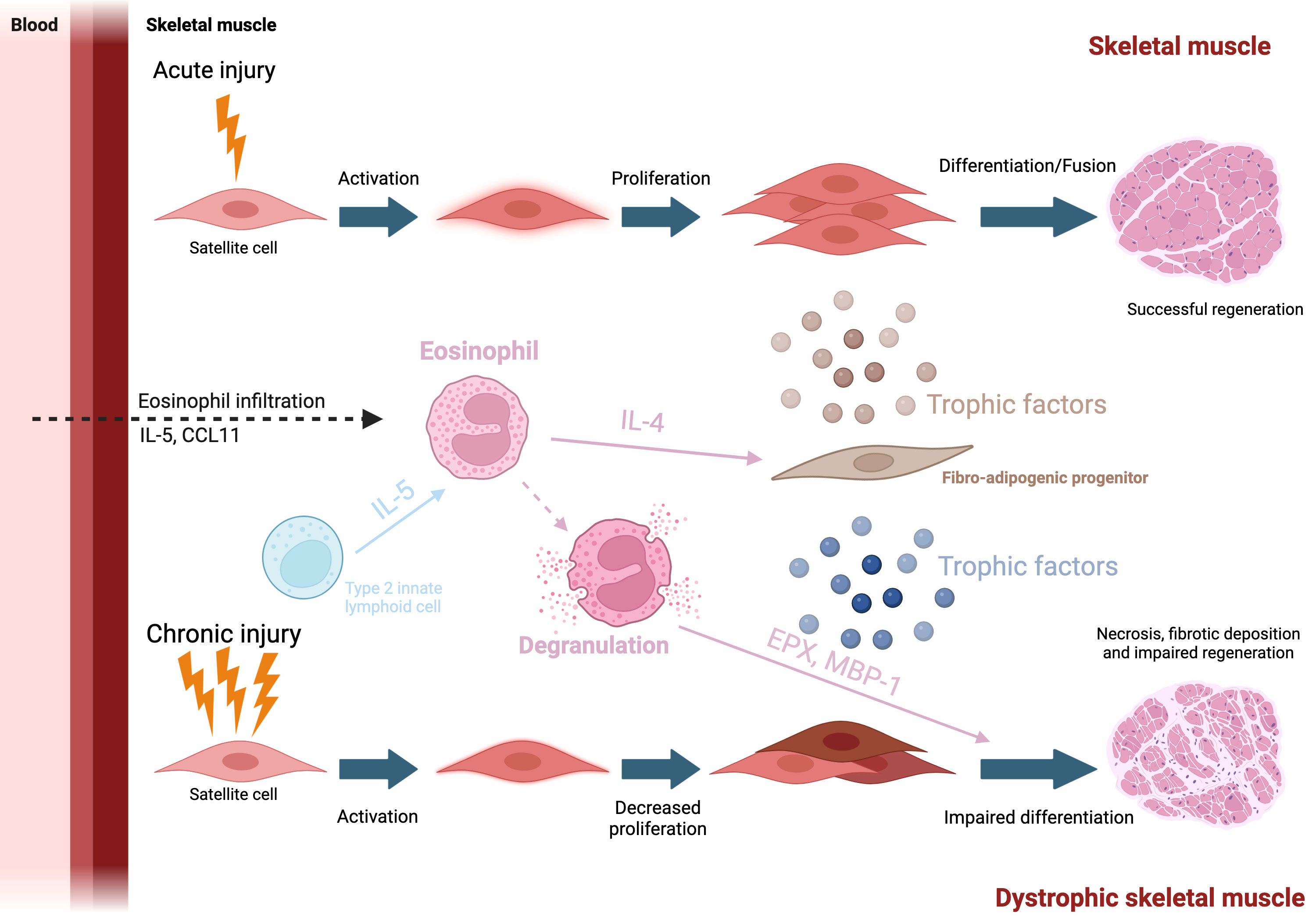
Figure 3 Eosinophil and muscle repair Top: After an injury, eosinophils are attracted to the site of damage via the production of CCL11 and IL-5. They then secrete various cytokine and in particular IL-4, which will support FAP trophic function toward myogenic cells. Bottom: In case of chronic injury, such as seen in dystrophic muscle, eosinophil will degranulate Major basic protein 1 will be released in the environment, lysing myofibers, participating into the degenerative phenotype.
4.2 Aging
The extent to which skeletal muscle can regenerate is dependent on the body’s ability to maintain its muscle-resident stem cell population. When the body ages, changes in the microenvironment and the niche of the SCs contribute to a decreased regenerative capacity of skeletal muscle (77). In addition, aging results in sarcopenia which is the involuntary loss of lean muscle mass (atrophy) with a reduction in force production and function. Sarcopenia is associated with a loss of SC activation, differentiation, and fusion, which consequently delays the capacity for muscle to repair itself. This can be exacerbated by other factors including muscle trauma and disease. An increase in blood eosinophil numbers have been associated with aging in the general population and in diseases such as asthma, type 2 diabetes (T2D), and cardiovascular diseases (49, 78, 79). However, with respect to aged skeletal muscle, research has mainly focused on age-related regenerative failures in both SCs and Fibro adipogenic progenitors (FAPs) (77, 80). FAPs are a type of tissue-resident mesenchymal stromal cell (MSC) that can be found by the SC niche and are known regulate muscle homeostasis and myogenesis (81, 82). Aberrant FAP activity may contribute to fibrotic deposition and adipogenesis (83). Some studies have looked at age-related changes in the inflammatory response to injury (inflammaging) but so far no study has explicitly researched the role of eosinophils in aged skeletal muscle (84). The Mathis laboratory has showed that during regeneration, FAPs are the main source of IL-33, a potent Th2 alarmin. In aged muscles (>6-month-old mice), IL-33 production is decreased, resulting in a decrease in regulatory T cell (Treg) accumulation, and a delay in muscle regeneration (85). Interestingly, the authors also showed a decrease in eosinophil number in aged muscle which could be also due to a decrease in IL-33 production, and potentially a decrease in type 2 innate lymphoid cell (ILC2) number, which has not been explored yet. As many cells express ST2 (IL-33R, Il1rl1), total KO display strong delay in muscle regeneration. In particular, the Kronke lab showed that IL-33 was required for macrophage skewing and proper alternative polarization during muscle regeneration (86). Neither the number of eosinophil nor their activation level were explored in this ST2-/- model. Age-related changes in eosinophils have been explored in other tissues than skeletal muscle. In asthma patients, aging has been associated with a decrease in eosinophil “effector” function which is implicated in physiological processes such as tissue remodelling, immunomodulation and cellular interactions, and degranulation (process of releasing granule proteins). This which can be toxic to tissue resulting in damage and increased inflammation (79). Recently, a study looked at age-related changes in eosinophils in skeletal muscle in T2D patients and investigated the relationship between inflammation markers (including eosinophils) and skeletal muscle mass in patients over 60 years of age (87). The study determined that eosinophils were positively associated with sarcopenia progression in older patients with T2D. Sarcopenia is a mortality risk factor for older individuals especially when comorbid with T2D, meaning sarcopenia prevention is an important therapeutic goal in human health (87). However, the study could not determine the precise role that eosinophils play during the development of sarcopenia. Furthermore, eosinophil number and muscle atrophy were not correlated in younger patients with T2D, suggesting that age is a key parameter in eosinophil function.
4.3 Duchenne Muscular Dystrophy
Duchenne Muscular Dystrophy (DMD) is a X-linked genetic disease characterised by muscle weakness and progressive degeneration due to a mutation found on the DMD gene that encodes for the DYSTROPHIN protein (Hoffman et al., 1988). Mutations in the DMD gene can either result in the formation of an incomplete, unstable form of DYSTROPHIN, to a complete absence or to the expression of very low levels of DYSTROPHIN as seen in BMD (Becker Muscular Dystrophy). Other forms of myopathies (limb-girdle) are linked to mutations in the components that form a protein complex associated with Dystrophin (Blake et al., 2002). In the early, 1980s, the single KO DMDmdx (mdx) mouse was discovered from a C57BL/10ScSn colony. It showed elevated muscle creatine kinase levels and histological characteristics of muscular dystrophy: increase in necrotic muscle tissue, phagocytosis and fibrosis (88). The initial round of muscle degeneration is first observed around the 3-4 week mark in mdx models (89, 90). In contrast, fibrosis, a hallmark of the disease, may take longer to develop, and deposit are first seen in the diaphragm. Hence the mdx model is not able to replicate all symptomatic aspects of DMD progression in humans. To improve upon this, the model was crossed with other genetic backgrounds and with other mutations (for example with the Utrophin-/+ mice) to better mimic the phenotypic characteristics that are seen in the progression of DMD in humans (91–93). In recent years, mdx models lacking eosinophils (mdx:PHIL) or inducing hyper-eosinophilia (mdx:IL-5tg) have been developed to understand their role in DMD. While eosinophils are one of the lesser studied leukocytes in skeletal muscle, they have been shown to infiltrate skeletal muscle tissues in both human DMD patients and mdx mice (15, 94, 95) Once infiltrated, eosinophils degranulate and release cytotoxic granule proteins (including EPX and MBP-1) that lyse myofibers causing additional damage in mdx mice (94) (Figure 3). Interestingly, eosinophil depletion (using anti-CCR3 antibody) reduced muscle damage in mdx mice, however, the genetic ablation of MBP-1 in mdx mice yielded mixed phenotype as it reduced collagen deposition, but did not improve skeletal muscle repair. These conflicting results suggest both MBP-1 dependent and independent mechanisms (96). The authors suggest a mechanism in with depletion of MBP-1 increases cytotoxic CD8 T cells activity and a possible switch of the inflammation towards a strongly Th1 response (although with no changes in the expression of Nos2, Tnfa, and Ifng). Moreover, treating mdx mice with prednisolone, an FDA approved corticosteroid used to treat DMD in humans, strongly decreases eosinophil number in mdx skeletal muscle. Interestingly Sek and colleagues observed slight changes in levels of IL-4 in skeletal muscle in mice strains with affected eosinophil infiltration (mdx, mdx:PHIL, and mdx:IL5Tg) but found no correlation between the amount of IL-4 and the extent of eosinophil infiltration (97) suggesting a potential role for another IL-4 producing cell, such as ILC2s. The authors also found no differences in muscle repair between eosinophil-sufficient (mdx) and eosinophil-deficient (mdx:PHIL) mice at an early age (97). In contrast, we found that hyper-eosinophilia had a significant effect on muscle fibrotic deposition and muscle repair (73). Notably, technical differences can be found between the studies, such as the background of the mice (C57BL/6J vs DBA) or the experimental endpoint (4 weeks vs 6-12 months), which could explain the conflicting results. In particular for the mdx:IL-5Tg mouse model, long term systemic hyper-eosinophilia could have led to confounding effects from other tissues such as the lungs. Of note, it has been recently shown that ILC2s present in skeletal muscle may regulate eosinophils in muscular dystrophy. Kastenschmidt and colleagues found elevated levels of eosinophils and IL-5 in both DMD patients and mdx mice (15). The authors also showed that by depleting IL-13-producing cells (which include ILC2s) in mdx at 4 weeks of age, IL-5 levels were decreased and muscle eosinophilia was diminished, suggesting that IL-13 is required for eosinophil infiltration (15). This phenomenon is well known in other tissues such as in the gut or adipose tissue but was not described in the muscle until then (98, 99). Collectively, these studies suggest that eosinophils may play a more important role in the later stages of DMD, proposing an alternative method of skeletal muscle eosinophilia via ILC2 regulation.
5 Role of eosinophils in adipose tissue
Adipose tissue, like skeletal muscle, is highly vascularized, permitting access for circulating immune cells, such as eosinophils, to maintain adipose tissue homeostasis (49, 100). Both circulating and tissue-resident eosinophils play a role in regulating the typical functions of adipose tissue, energy storage, metabolism, and endocrine function (49, 101). Shah et al. have performed mRNA sequencing from blood eosinophils, and resident eosinophils from two white adipose tissue (sub-cutaneous and gonadal) (102). While major changes were observed between the blood eosinophils and the tissue resident populations, such as upregulation in the IL-4/IL-13 signaling pathways, very little transcriptomic changes were observed between the two types of adipose tissue, and no pathways were significantly changed (102). This suggest that white adipose resident eosinophils do not differ between locations and that very little heterogeneity is observed within different white adipose tissues. Interestingly, while anti-IL5 therapy (using Mepolizumab, Reslizumab, or Benralizumab) in patients with asthma decreased their BMI (103), blood hyper-eosinophilia was associated with a decreased risk of T2D in an adult cohort composed of both women and men in China (104). Suggesting that the role of eosinophils in human health seems to depend on the disease category.
Eosinophil functions are thought to be established by a MSC-ILC2-eosinophil axis, whereby adipose tissue MSCs produce IL-33 to maintain ILC2 and eosinophils, in turn, ILC2s and MSCs respectively secrete IL-5 and CCL11 to maintain the eosinophil population (Figure 4A) (105). Among eosinophil secreted factors, IL-4 and IL-13 are important for adipose tissue macrophage polarization. Alternatively, activated macrophages induced by these factors release catecholamines that contributes to thermoregulation through promoting lipolysis of white adipocytes and promoting the expression Ucp1 in brown adipocytes. These changes contribute to an elevated energy expenditure and increased thermogenesis (49, 99, 106–111). Furthermore, eosinophil-produced IL-4 can minimize local and systemic age-related changes by attenuating elevations in Th1 cytokines such as IL1-β in aged mice, favouring a Th2 environment (Figure 4B) (49). In contrast, Th1 cytokines are also elevated in adipose tissue during obesity, and an Th2 inflammatory environment has been linked to the promotion of the lean/non-obese state (49, 109, 112–114). Due to their role in adipose tissue homeostasis and as orchestrators of the Th2 response, eosinophils and their immune responses have been investigated in hopes of unveiling a mechanism whereby they may confer protective effects against metabolic diseases like obesity and diabetes.
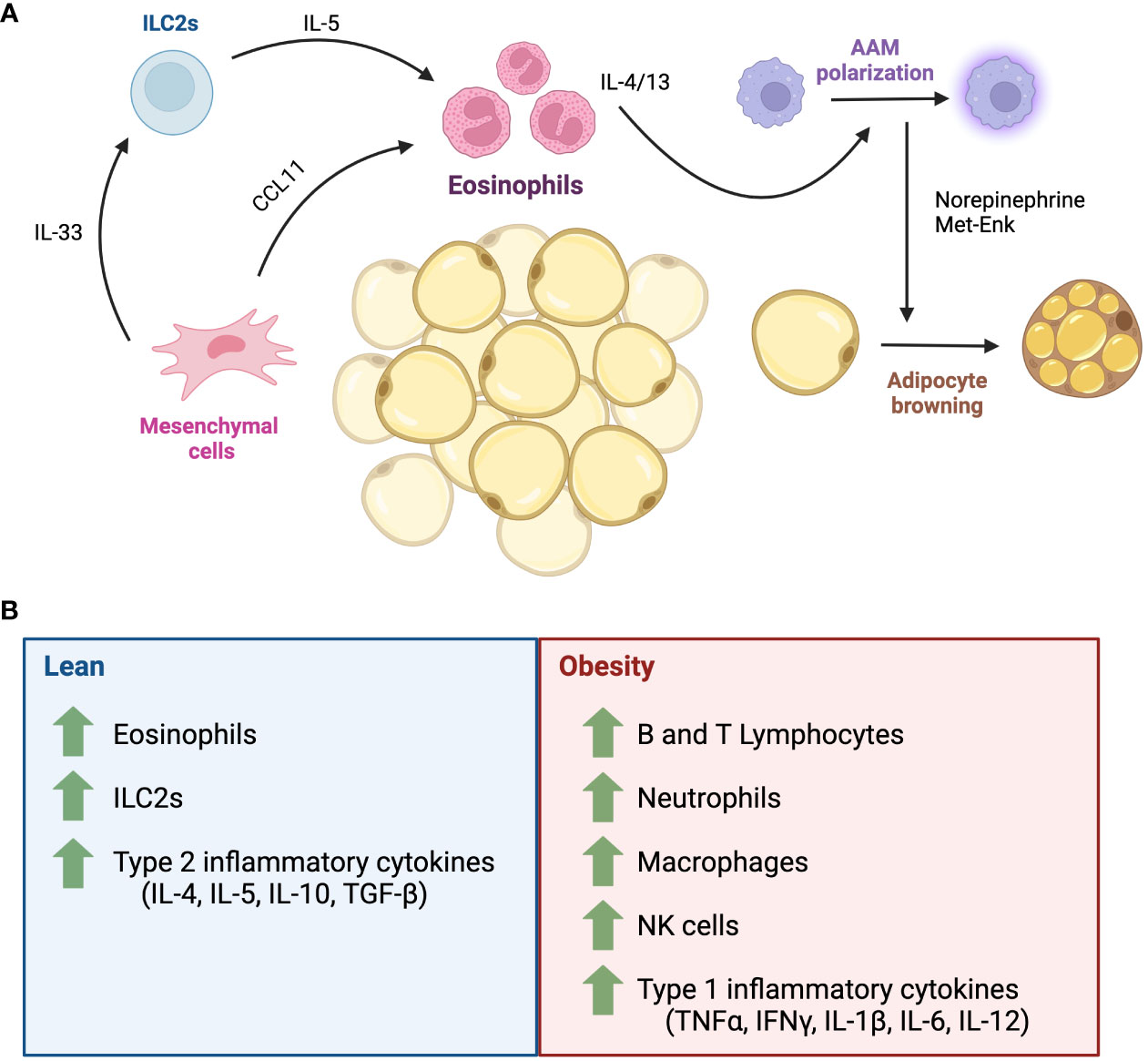
Figure 4 The role of eosinophils in adipose tissue homeostasis. (A) Adipose tissue is composed of a rich network of adipocytes, stromal cells and immune cells. In adipose tissue homeostasis, the eosinophil population is maintained by signals from mesenchymal stromal cells and ILC2s. Eosinophil-secreted IL-4 and IL-13 maintains alternatively activated macrophage polarization, which produce catecholamines that promote thermogenesis via the biogenesis of beige adipocytes. (B) Major inflammatory cells in lean and obese states. AAM, alternatively activated macrophages; CCL11, eotaxin-1; IL, interleukin; ILC2, Type 2 innate lymphoid cell; IFNγ, interferon gamma; Met-Enk, Met-enkephalin; NK, natural killer cells; TNFα, tumour necrosis factor alpha; TGF-β, transforming growth factor beta.
5.1 Obesity
One of the characteristics of obesity is a low-grade systemic inflammation, where the abnormal expansion of hypertrophic adipocytes results in the secretion of adipocyte-derived metabolic factors, infiltration of Th1 inflammatory immune cells (Figure 4B) such as Ly-6C+ monocytes/macrophages, neutrophils, Natural Killer cells and T Helper 1 cells (112). These cells release pro-inflammatory cytokines such as IFNγ, TNFα, IL-6, IL-12, among others, which can lead to adipose tissue damage and abnormal function (99, 112, 115). Whether eosinophils and Th2 response can oppose this process and promote a metabolically “lean” state has been a controversial topic of discussion. Indeed, high fat diet (HFD)-treated mice have elevated levels of IFNγ (116), which can repress IL-33-mediated ILC2 activation (117) and IL-33-/- mice spontaneously gain weight (118). C57BL/6 mice fed on an 10-14-week HFD, or those genetically predisposed (C57BL/6 ob/ob) also have reduced levels of perigonadal adipose eosinophils (101). Eosinophil-deficient BALB/c mice (ΔdblGATA) fed on a 15-week HFD gained more weight than eosinophil-competent mice, which was attributed to an increase in perigonadal adipose tissue mass. Meanwhile, hyper-eosinophilic IL-5Tg mice on a BALB/c background have smaller depots of visceral adipose tissue (101). IL-5 deficient mice fed on an 18-20 week HFD gained more weight, have increased total body adiposity, and perigonadal adipose weight compared to IL-5 sufficient mice (98). Contrary to these findings, Lee et al., 2018 showed that C.129S1(B6) ΔdblGATA mice fed on a HFD gained less weight and showed reduced body fat, smaller enlargement of adipocytes and decreased expression of adipogenic genes such as Retnlg, Alox15, and Drd2 (119). However, ΔdblGATA mice (C.129S1(B6)-Gata1tm6Sho/J) were more insulin resistant, with increased lipid storage in the liver compared to BALB/c WT mice (119). Furthermore, they found that eosinophils migrate towards adipocytes in a CCL11-dependent process in vitro. This was supported in vivo with the WAT of BALB/c WT HFD mice having an increased frequency of infiltrating eosinophils, in concordance with an increased expression of WAT Ccr3 and Ccl11 (119). These obesity studies reported that male mice were used to conduct experiments, herein lies an area where new knowledge can be unveiled with study of female mice. Indeed, it has been demonstrated that female BALB/c mice exhibit a stronger STAT6-dependent Th2 response and increased eosinophilia to triggers such as ovalbumin (120), compared to male mice. Thus, future studies should examine whether sex influences the impact of eosinophils and Th2 environment on obesity. Moreover, all the mice were not all from the same background (BALB/c or C57BL/6) which could have contributed to the conflicting results between Molofsky and Lee. In humans, the role of eosinophils in adipose tissue health and its metabolic regulation remains largely conflicting. According to Brigger et al., the number of eosinophils in adipose tissue is negatively correlated with age (49). Other studies have reported a positive correlation between blood eosinophil count and body mass index in obesity, however this was not as sticking as with the number of neutrophils (121), while metabolic syndrome was associated with higher eosinophil count in subcutaneous white adipose tissue (122). The overall profile (inflammatory vs resident) of eosinophils in these studies was not assessed.
5.2 Metabolism
Brown and beige adipose tissues can counteract obesity by metabolizing sugars and fats, releasing the excess energy as heat (123). However, an obese state has been shown to promote the transition of adipocytes from beige-to-white, impacting energy balance and promoting weight gain (123, 124). Eosinophils have been demonstrated to regulate beige adipocyte formation though a variety of mechanisms, including white adipocyte lipolysis and brown adipocyte thermogenesis, which has implications for energy metabolism and weight gain (109, 117, 125). Indeed, eosinophils produce IL-4 abundantly in subcutaneous and perigonadal visceral white adipose tissues (109). Qiu et al. showed that the loss of eosinophils (in the 4-Get- ΔdblGATA mice), or IL-4/13 signaling (BALB/cJ IL-4/13-/-), impaired cold-induced biogenesis of beige fat from subcutaneous white adipose tissue in mice, as assessed by Ucp1 expression and UCP1 protein quantification (109). In addition, administration of exogenous IL-4 to thermoneutral mice increased beige fat mass, and eosinophil-derived IL-4, along with IL-13 from ILC2s was demonstrated to directly promote the differentiation of PDGFRα+ adipocyte precursors (MSC-like cell) to beige adipocytes, rather than white adipocytes (109). Exposure to cold temperature also induces the production of fibroblast growth factor 21 (FGF21), which act as an autocrine loop to induce CCL11 secretion by fibroblast and promoting eosinophil infiltration in WAT (117). Alternatively, eosinophil-derived IL-4/IL-13 has been linked to the polarization of adipose tissue macrophages towards a Th2 (Ly-6C-) responsive phenotype. Such macrophages increased tyrosine hydroxylase expression and increased catecholamine synthesis, which in turn promoted the formation of beige adipocytes (109, 111, 126) although this has been debated (110). In addition, eosinophils themselves have been explored as synthesizers of catecholamines which also could promote beige fat formation (127).
Thus, existing evidence withstanding the contributions of eosinophils in adipose tissue metabolism has yet to be fully understood. Future studies will be required to unravel their precise role.
5.3 Type 2 diabetes
Obesity and T2D are frequently co-morbid, with obesity being the leading risk factor for developing T2D (128). The chronic excess intake of glucose and fats can stimulate the release of Th1 cytokines (such as TNF-α, and IL-1β) and activate Th1 responsive inflammatory cells in adipose and pancreatic tissues such as Ly-6C+ macrophages and Th1 helper T cells (115, 129, 130). Whether eosinophils and their role in Th2 inflammation could counteract the development of T2D is a question posed by some researchers. As reported by Lee et al., BALB/c ΔdblGATA mice fed on an HFD have been observed to be insulin resistant and have poor glycemic control (119). In a study done by Bolus et al., recombinant IL-5 administered to obese mice on an 8-week HFD did not rescue metabolic parameters such as blood glucose, plasma triglycerides, free fatty acids, or cholesterol (131). On the other hand, numerous studies have examined the effect of the Th2 response on metabolic parameters in obese mice fed an HFD. In order to induce a Th2 response, Hams et al., injected HFD-fed C57BL/6J mice with recombinant helminth egg-derived w1 protein. Overall these mice did not gain weight as much as control, had smaller adipocyte hypertrophy, and displayed adipose tissue hyper-eosinophilia and increased numbers of other Th2 cells (such as ILC2s) and IL-33 (132). Another study showed comparable results in white adipose tissue (133). The role of eosinophils in diabetes in humans has been less studied. However, in a study on Chinese populations, T2D prevalence and blood eosinophils were inversely correlated (104), confirming that eosinophils might have a protective role against the disease. On the other hand, Sokolova et al. reported in, 2017 that non-obese patients with T2D exhibited higher levels of Th2 cytokines (IL-4, IL-5) (134).
A positive correlation between increased levels of Th2 cytokines and eosinophil number is not always true. Interestingly, Brigger et al. showed that while aged C57BL/6J mice (18-22 months) produced higher levels of the chemokine CCL11 in epididymal fat, the number of adipose tissue eosinophils decreased (49). This was complemented by increases in Th1 cytokines TNF-α, IL6, and IL1-β in aged mice. Furthermore, when aged mice were connected to young (2-3 months) mice of the same background by parabiosis, eosinophil number and Th1 cytokines were restored to WT levels, with eosinophils from the young donors taking residence in the epididymal fat of their older partner (49). Given that the prevalence of obesity and diabetes increase with age (135) perhaps the ability of adipose tissue eosinophils to regulate inflammation associated with metabolic diseases like obesity and diabetes is diminished over time and at a systemic level.
Overall, while our understanding of the role circulating and tissue-resident eosinophils play in maintaining adipose tissue homeostasis is well-established, the exact role of eosinophils in metabolic diseases such as obesity and diabetes demands further investigation before we can begin working towards eosinophil-based therapies.
6 Discussion
Overall, eosinophils are a very well-studied cell type, in particular in the lung and in the intestine where two populations of eosinophil have been described after damage: the inflammatory eosinophil (iEos) and the resident eosinophils (rEos). Knowing that the half-life of eosinophil in the tissue is 2 to 6 days, we could ask whether rEos are true tissue-resident cells like macrophages. Circulating eosinophils are thought to infiltrate after stimuli/damage and participate into the inflammatory response. However, most of the studies have been carried out in an acute setting with very little return to homeostasis by the experimental end-point, which could potentially show that inflammatory eosinophils eventually become similar to pre-damaged resident eosinophils. In that case, we could question whether these differences represent true heterogeneity, or if they are rather similar to the relationship between monocytes and macrophages. Longer experiments, associated with bone-marrow transplantation or parabiosis to follow their infiltration may help answering these questions. Moreover, eosinophil function and heterogeneity in muscle homeostasis is still to debate. Indeed, as eosinophils do not seem be to principal actors of muscular diseases, the comprehension of their behaviour is not the focus of the muscle community. However, as any immune cell, their function can be modulated by cues and tuning their level of activation could have positive effect on muscle resident cells such as FAPs, myogenic cells, and myofibers. Furthermore, are there skeletal resident eosinophils? What is their half-life? Do infiltrating eosinophils display a similar profile to iEos in the lung or the gut? Flow cytometry coupled with scRNAseq or CITE-seq at various time point after injury on parabiotic pairs would be a way to answer these questions. In the adipose tissue, eosinophils have been more studied due to the obvious change in number during weight gain, systemic inflammation, age, and T2D and are now proposed as key players when it comes to adipocyte health. As skeletal muscle health is also affected by the various parameters cited above, it would not be surprising if eosinophils present in skeletal muscle act similarly. In particular, the role of eosinophil in the development of skeletal muscle insulin resistance has not been investigated. As of now, the results within the muscle community are conflicting and a better characterization of these cells would help to understand their function, with potentially better clinical applications.
Author contributions
KD: Writing – original draft, Writing – review & editing. LR: Writing – original draft, Writing – review & editing. FR: Writing – review & editing, Resources. MT: Conceptualization, Supervision, Writing – original draft, Writing – review & editing.
Funding
The author(s) declare financial support was received for the research, authorship, and/or publication of this article. MT and FR are supported by the Canadian Institutes of Health Research (472535 to MT and FDN-159908 to FR).
Acknowledgments
Figures were made using BioRender.
Conflict of interest
The authors declare that the research was conducted in the absence of any commercial or financial relationships that could be construed as a potential conflict of interest.
Publisher’s note
All claims expressed in this article are solely those of the authors and do not necessarily represent those of their affiliated organizations, or those of the publisher, the editors and the reviewers. Any product that may be evaluated in this article, or claim that may be made by its manufacturer, is not guaranteed or endorsed by the publisher.
References
1. Kay AB. The early history of the eosinophil. Clin Exp Allergy. (2015) 45:575–82. doi: 10.1111/cea.12480
2. Fulkerson PC, Rothenberg ME. Origin, regulation and physiological function of intestinal oeosinophils. Best Pract Res Clin Gastroenterol. (2008) 22:411–23. doi: 10.1016/j.bpg.2007.10.023
3. Boyce JA, Friend D, Matsumoto R, Austen KF, Owen WF. Differentiation in vitro of hybrid eosinophil/basophil granulocytes: autocrine function of an eosinophil developmental intermediate. J Exp Med. (1995) 182:49–57. doi: 10.1084/jem.182.1.49
4. Nerlov C, McNagny KM, Döderlein G, Kowenz-Leutz E, Graf T. Distinct C/EBP functions are required for eosinophil lineage commitment and maturation. Genes Dev. (1998) 12:2413–23. doi: 10.1101/gad.12.15.2413
6. McNagny K, Graf T. Making Eosinophils through subtle shifts in transcription factor expression. J Exp Med. (2002) 195:F43–7. doi: 10.1084/jem.20020636
7. Klion AD, Ackerman SJ, Bochner BS. Contributions of eosinophils to human health and disease. Annu Rev Pathol Mech Dis. (2020) 15:179–209. doi: 10.1146/annurev-pathmechdis-012419-032756
8. Kato M, Kephart GM, Talley NJ, Wagner JM, Sarr MG, Bonno M, et al. Eosinophil infiltration and degranulation in normal human tissue. Anat Rec. (1998) 252:418–25. doi: 10.1002/(ISSN)1097-0185
9. Lee JJ, Jacobsen EA, Ochkur SI, McGarry MP, Condjella RM, Doyle AD, et al. Human versus mouse eosinophils: “That which we call an eosinophil, by any other name would stain as red”. J Allergy Clin Immunol. (2012) 130:572–84. doi: 10.1016/j.jaci.2012.07.025
10. Marichal T, Mesnil C, Bureau F. Homeostatic Eosinophils: Characteristics and Functions. Front. Med. (2017) 4:101. doi: 10.3389/fmed.2017.00101
11. Greenfeder S, Umland SP, Cuss FM, Chapman RW, Egan RW. Th2 cytokines and asthma The role of interleukin-5 in allergic eosinophilic disease. Respir Res. (2001) 2:71. doi: 10.1186/rr41
12. Rosenberg HF, Dyer KD, Foster PS. Eosinophils: changing perspectives in health and disease. Nat Rev Immunol. (2013) 13:9–22. doi: 10.1038/nri3341
13. Bain BJ, Fletcher SH. Chronic eosinophilic leukemias and the myeloproliferative variant of the hypereosinophilic syndrome. Immunol Allergy Clinics North America. (2007) 27(3):377–88. doi: 10.1016/j.iac.2007.06.001
14. Blanchard C, Wang N, Rothenberg M. Eosinophilic esophagitis: Pathogenesis, genetics, and therapy. J Allergy Clin Immunol. (2006) 118:1054–9. doi: 10.1016/j.jaci.2006.07.038
15. Kastenschmidt JM, Coulis G, Farahat PK, Pham P, Rios R, Cristal TT, et al. A stromal progenitor and ILC2 niche promotes muscle eosinophilia and fibrosis-associated gene expression. Cell Rep. (2021) 35:108997. doi: 10.1016/j.celrep.2021.108997
16. Shin J-H, Kim D-S. Focal eosinophilic myositis presenting with leg pain and tenderness. Ann Clin Neurophysiol. (2020) 22:125–8. doi: 10.14253/acn.2020.22.2.125
17. Simon D, Vassina E, Yousefi S, Kozlowski E, Braathen LR, Simon HU. Reduced dermal infiltration of cytokine-expressing inflammatory cells in atopic dermatitis after short-term topical tacrolimus treatment. J Allergy Clin Immunol. (2004) 114:887–95. doi: 10.1016/j.jaci.2004.05.066
18. Hamann KJ, Barker RL, Ten RM, Gleich GJ. The molecular biology of eosinophil granule proteins. Int Arch Allergy Immunol. (1991) 94:202–9. doi: 10.1159/000235362
19. Gleich GJ, Adolphson CR. The eosinophilic leukocyte: Structure and function. Adv Immunol. (1986) 39:177–253. doi: 10.1016/S0065-2776(08)60351-X
20. Ackerman SJ, Gleich GJ, Loegering DA, Richardson BA, Butterworth AE. Comparative toxicity of purified human eosinophil granule cationic proteins for schistosomula of schistosoma mansoni. Am J Trop Med Hyg. (1985) 34:735–45. doi: 10.4269/ajtmh.1985.34.735
21. Januskevicius A, Jurkeviciute E, Janulaityte I, Kalinauskaite-Zukauske V, Miliauskas S, Malakauskas K. Blood eosinophils subtypes and their survivability in asthma patients. Cells. (2020) 9(5):1248. doi: 10.3390/cells9051248
22. Curto E, Mateus-Medina ÉF, Crespo-Lessmann A, Osuna-Gómez R, Ujaldón-Miró C, García-Moral A, et al. Identification of two eosinophil subsets in induced sputum from patients with allergic asthma according to CD15 and CD66b expression. Int J Environ Res Public Health. (2022) 1919(20):13400. doi: 10.3390/ijerph192013400
23. Kanda A, Yun Y, Bui DV, Nguyen LM, Kobayashi Y, Suzuki K, et al. The multiple functions and subpopulations of eosinophils in tissues under steady-state and pathological conditions. Allergol Int. (2021) 70:9–18. doi: 10.1016/j.alit.2020.11.001
24. Dolitzky A, Shapira G, Grisaru-Tal S, Hazut I, Avlas S, Gordon Y, et al. Transcriptional profiling of mouse eosinophils identifies distinct gene signatures following cellular activation. Front Immunol. (2021) 12:1–15. doi: 10.3389/fimmu.2021.802839
25. Mesnil C, Raulier S, Paulissen G, Xiao X, Birrell MA, Pirottin D, et al. Lung-resident eosinophils represent a distinct regulatory eosinophil subset. J Clin Invest. (2016) 126:3279. doi: 10.1172/JCI85664
26. Li Y, Liu S, Zhou K, Wang Y, Chen Y, Hu W, et al. Neuromedin U programs eosinophils to promote mucosal immunity of the small intestine. Sci (80- ). (2023) 381:1189–96. doi: 10.1126/science.ade4177
27. Gurtner A, Borrelli C, Gonzalez-Perez I, Bach K, Acar IE, Núñez NG, et al. Active eosinophils regulate host defence and immune responses in colitis. Nature. (2023) 615:151–7. doi: 10.1038/s41586-022-05628-7
28. Yu C, Cantor AB, Yang H, Browne C, Wells RA, Fujiwara Y, et al. Targeted deletion of a high-affinity GATA-binding site in the GATA-1 promoter leads to selective loss of the eosinophil lineage in vivo. J Exp Med. (2002) 195:1387–95. doi: 10.1084/jem.20020656
29. Kopf M, Brombacher F, Hodgkin PD, Ramsay AJ, Milbourne EA, Dai WJ, et al. IL-5-deficient mice have a developmental defect in CD5+ B-1 cells and lack eosinophilia but have normal antibody and cytotoxic T cell responses. Immunity. (1996) 4:15–24. doi: 10.1016/S1074-7613(00)80294-0
30. Lee JJ, Dimina D, Macias MMP, Ochkur SI, McGarry MP, O’Neill KR, et al. Defining a link with asthma in mice congenitally deficient in eosinophils. Science. (2004) 305:1773–6. doi: 10.1126/science.1099472
31. Holtschke T, Löhler J, Kanno Y, Fehr T, Giese N, Rosenbauer F, et al. Immunodeficiency and chronic myelogenous leukemia-like syndrome in mice with a targeted mutation of the ICSBP gene. Cell. (1996) 87:307–17. doi: 10.1016/S0092-8674(00)81348-3
32. Lee NA, McGarry MP, Larson KA, Horton MA, Kristensen AB, Lee JJ. Expression of IL-5 in thymocytes/T cells leads to the development of a massive eosinophilia, extramedullary eosinophilopoiesis, and unique histopathologies. J Immunol. (1997) 158:1332–44. doi: 10.4049/jimmunol.158.3.1332
33. Dent LA, Strath M, Mellor AL, Sanderson CJ. Eosinophilia in transgenic mice expressing interleukin 5. J Exp Med. (1990) 172:1425–31. doi: 10.1084/jem.172.5.1425
34. Lee JJ, McGarry MP, Farmer SC, Denzler KL, Larson KA, Carrigan PE, et al. Interleukin-5 expression in the lung epithelium of transgenic mice leads to pulmonary changes pathognomonic of asthma. J Exp Med. (1997) 185(12):2143–56. doi: 10.1084/jem.185.12.2143
35. Mohrs M, Shinkai K, Mohrs K, Locksley RM. Analysis of type 2 immunity in vivo with a bicistronic IL-4 reporter. Immunity. (2001) 15:303–11. doi: 10.1016/S1074-7613(01)00186-8
36. Zhang M, Angata T, Jae YC, Miller M, Broide DH, Varki A. Defining the in vivo function of Siglec-F, a CD33-related Siglec expressed on mouse eosinophils. Blood. (2007) 109:4280–7. doi: 10.1182/blood-2006-08-039255
37. Doyle AD, Jacobsen EA, Ochkur SI, Willetts L, Shim K, Neely J, et al. Homologous recombination into the eosinophil peroxidase locus generates a strain of mice expressing Cre recombinase exclusively in eosinophils. J Leukoc Biol. (2013) 94:17. doi: 10.1189/jlb.0213089
38. Milanovic M, Terszowski G, Struck D, Liesenfeld O, Carstanjen D. IFN consensus sequence binding protein (Icsbp) is critical for eosinophil development. J Immunol. (2008) 181(7):5045–53. doi: 10.4049/jimmunol.181.7.5045
39. McMillan SJ, Richards HE, Crocker PR. Siglec-F-dependent negative regulation of allergen-induced eosinophilia depends critically on the experimental model. Immunol Lett. (2014) 160:11. doi: 10.1016/j.imlet.2014.03.008
40. Throsby M, Herbelin A, Pleíau J-M, Dardenne M. CD11c+ Eosinophils in the murine thymus: Developmental regulation and recruitment upon MHC class I-restricted thymocyte deletion. J Immunol. (2000) 165:1965–75. doi: 10.4049/jimmunol.165.4.1965
41. Rie Gouon-evans V, Pollard JW. Eotaxin is required for eosinophil homing into the stroma of the pubertal and cycling uterus. Endocrinology. (2001) 142(01):4515–21. doi: 10.1210/endo.142.10.8459
42. Gouon-Evans V, Lin EY, Pollard JW. Requirement of macrophages and eosinophils and their cytokines/chemokines for mammary gland development. Breast Cancer Res. (2002) 4:155. doi: 10.1186/bcr441
43. Gouon-Evans V, Rothenberg ME, Pollard JW. Postnatal mammary gland development requires macrophages and eosinophils. Development. (2000) 127:2269–82. doi: 10.1242/dev.127.11.2269
44. Collins PD, Marleau S, Griffiths-Johnson DA, Jose PJ, Williams TJ. Cooperation between interleukin-5 and the chemokine eotaxin to induce eosinophil accumulation in vivo. J Exp Med. (1995) 182:1169–74. doi: 10.1084/jem.182.4.1169
45. Foster PS, Hogan SP, Ramsay AJ, Matthaei KI, Young IG. Interleukin 5 deficiency abolishes eosinophilia, airways hyperreactivity, and lung damage in a mouse asthma model. J Exp Med. (1996) 183:195–201. doi: 10.1084/jem.183.1.195
46. Bochner BS, Schleimer RP. The role of adhesion molecules in human eosinophil and basophil recruitment. J Allergy Clin Immunol. (1994) 94:427–38. doi: 10.1016/0091-6749(94)90195-3
47. Artis D, Humphreys NE, Potten CS, Wagner N, Müller W, McDermott JR, et al. β7 integrin-deficient mice: delayed leukocyte recruitment and attenuated protective immunity in the small intestine during enteric helminth infection. Eur J Immunol. (2000) 30:1656–64. doi: 10.1002/1521-4141(200006)30:6<1656::AID-IMMU1656>3.0.CO;2-Z
48. Gonzalo JA, Lloyd CM, Kremer L, Finger E, Martinez-A C, Siegelman MH, et al. Eosinophil recruitment to the lung in a murine model of allergic inflammation. The role of T cells, chemokines, and adhesion receptors. J Clin Invest. (1996) 98:2332–45. doi: 10.1172/JCI119045
49. Brigger D, Riether C, van Brummelen R, Mosher KI, Shiu A, Ding Z, et al. Eosinophils regulate adipose tissue inflammation and sustain physical and immunological fitness in old age. Nat Metab. (2020) 2:688–702. doi: 10.1038/s42255-020-0228-3
50. Jose PJ, Griffiths-Johnson DA, Collins PD, Walsh DT, Moqbel R, Totty NF, et al. Eotaxin: a potent eosinophil chemoattractant cytokine detected in a Guinea pig model of allergic airways inflammation. J Exp Med. (1994) 179:881–7. doi: 10.1084/jem.179.3.881
51. Rankin SM, Conroy DM, Williams TJ. Eotaxin and eosinophil recruitment: implications for human disease. Mol Med Today. (2000) 6:20–7. doi: 10.1016/S1357-4310(99)01635-4
52. Fulkerson PC, Fischetti CA, McBride ML, Hassman LM, Hogan SP, Rothenberg ME. A central regulatory role for eosinophils and the eotaxin/CCR3 axis in chronic experimental allergic airway inflammation. Proc Natl Acad Sci. (2006) 103:16418–23. doi: 10.1073/pnas.0607863103
53. Voehringer D, van Rooijen N, Locksley RM. Eosinophils develop in distinct stages and are recruited to peripheral sites by alternatively activated macrophages. J Leukoc Biol. (2007) 81(6):1434–44. doi: 10.1189/jlb.1106686
54. Hamaguchi-Tsuru E, Nobumoto A, Hirose N, Kataoka S, Fujikawa-Adachi K, Furuya M, et al. Development and functional analysis of eosinophils from murine embryonic stem cells. Br J Haematol. (2004) 124(6):695–850. doi: 10.1111/j.1365-2141.2004.04850.x
55. McKercher SR, Torbett BE, Anderson KL, Henkel GW, Vestal DJ, Baribault H, et al. Targeted disruption of the PU.1 gene results in multiple hematopoietic abnormalities. EMBO J. (1996) 15:5647–58. doi: 10.1002/embj.1996.15.issue-20
56. Zhang DE, Zhang P, Wang ND, Hetherington CJ, Darlington GJ, Tenen DG. Absence of granulocyte colony-stimulating factor signaling and neutrophil development in CCAAT enhancer binding protein alpha-deficient mice. Proc Natl Acad Sci U.S.A. (1997) 94:569–74. doi: 10.1073/pnas.94.2.569
57. Buitenhuis M, Van Deutekom HWM, Verhagen LP, Castor A, Jacobsen SEW, Lammers JWJ, et al. Differential regulation of granulopoiesis by the basic helix-loop-helix transcriptional inhibitors Id1 and Id2. Blood. (2005) 105:4272–81. doi: 10.1182/blood-2004-12-4883
58. Yamaguchi Y, Nishio H, Kishi K, Ackerman SJ, Suda T. C/EBPβ and GATA-1 synergistically regulate activity of the eosinophil granule major basic protein Promoter: implication for C/EBPβ Activity in eosinophil gene expression. Blood. (1999) 94:1429–39. doi: 10.1182/blood.V94.4.1429
59. Fulkerson PC, Zhu H, Williams DA, Zimmermann N, Rothenberg ME. CXCL9 inhibits eosinophil responses by a CCR3- and Rac2-dependent mechanism. Blood. (2005) 106:436. doi: 10.1182/blood-2005-02-0489
60. Mukund K, Subramaniam S. Skeletal muscle: A review of molecular structure and function, in health and disease. WIREs Syst Biol Med. (2020) 12. doi: 10.1002/wsbm.1462
61. Schmidt M, Schüler SC, Hüttner SS, von Eyss B, von Maltzahn J. Adult stem cells at work: regenerating skeletal muscle. Cell Mol Life Sci. (2019) 76:2559–70. doi: 10.1007/s00018-019-03093-6
62. Johnson AL, Kamal M, Parise G. The role of supporting cell populations in satellite cell mediated muscle repair. Cells. (2023) 12:1968. doi: 10.3390/cells12151968
63. Bernard C, Zavoriti A, Pucelle Q, Chazaud B, Gondin J. Role of macrophages during skeletal muscle regeneration and hypertrophy—Implications for immunomodulatory strategies. Physiol Rep. (2022) 10. doi: 10.14814/phy2.15480
64. Chazaud B, Brigitte M, Yacoub-Youssef H, Arnold L, Gherardi RK, Sonnet C, et al. Muscle resident macrophages control the immune cell reaction in a mouse model of notexin-induced myoinjury. J Cell Sci. (2009) 119:18–22. doi: 10.1097/JES.0b013e318190ebdb
65. Brigitte M, Schilte C, Plonquet A, Baba-Amer Y, Henri A, Charlier C, et al. Muscle resident macrophages control the immune cell reaction in a mouse model of notexin-induced myoinjury. Arthritis Rheumatol. (2010) 62:268–79. doi: 10.1002/art.27183
66. Lu H, Huang D, Saederup N, Charo IF, Ransohoff RM, Zhou L. Macrophages recruited via CCR2 produce insulin-like growth factor-1 to repair acute skeletal muscle injury. FASEB J. (2011) 25(1):358–69. doi: 10.1096/fj.10-171579
67. Koike H, Manabe I, Oishi Y. Mechanisms of cooperative cell-cell interactions in skeletal muscle regeneration. Inflammation Regen. (2022) 42:48. doi: 10.1186/s41232-022-00234-6
68. Juban G, Saclier M, Yacoub-Youssef H, Kernou A, Arnold L, Boisson C, et al. AMPK activation regulates LTBP4-dependent TGF-β1 secretion by pro-inflammatory macrophages and controls fibrosis in duchenne muscular dystrophy. Cell Rep. (2018) 25:2163–2176.e6. doi: 10.1016/j.celrep.2018.10.077
69. Saclier M, Yacoub-Youssef H, Mackey AL, Arnold L, Ardjoune H, Magnan M, et al. Differentially activated macrophages orchestrate myogenic precursor cell fate during human skeletal muscle regeneration. Stem Cells. (2013) 31:384–96. doi: 10.1002/stem.1288
70. Mounier R, Théret M, Arnold L, Cuvellier S, Bultot L, Göransson O, et al. AMPKα1 regulates macrophage skewing at the time of resolution of inflammation during skeletal muscle regeneration. Cell Metab. (2013) 18:251–64. doi: 10.1016/j.cmet.2013.06.017
71. Wang X, Zhao W, Ransohoff RM, Zhou L. Infiltrating macrophages are broadly activated at the early stage to support acute skeletal muscle injury repair. J Neuroimmunol. (2018) 317:55–66. doi: 10.1016/j.jneuroim.2018.01.004
72. Heredia JE, Mukundan L, Chen FM, Mueller AA, Deo RC, Locksley RM, et al. Type 2 innate signals stimulate fibro/adipogenic progenitors to facilitate muscle regeneration. Cell. (2013) 153:376–88. doi: 10.1016/j.cell.2013.02.053
73. Theret M, Rempel L, Hashimoto J, Ritso M, Tung LW, Li FF, et al. Elevated numbers of infiltrating eosinophils accelerate the progression of Duchenne muscular dystrophy pathology in mdx mice. Development. (2022) 149. doi: 10.1242/dev.200112
74. Merriwether EN, Agalave NM, Dailey DL, Rakel BA, Kolker SJ, Lenert ME, et al. IL-5 mediates monocyte phenotype and pain outcomes in fibromyalgia. Pain. (2021) 162:1468–82. doi: 10.1097/j.pain.0000000000002089
75. Lenert ME, Szabo-Pardi TA, Burton MD. Regulatory T-cells and IL-5 mediate pain outcomes in a preclinical model of chronic muscle pain. Mol Pain. (2023) 19. doi: 10.1177/17448069221110691
76. Louca Jounger S, Christidis N, Svensson P, List T, Ernberg M. Increased levels of intramuscular cytokines in patients with jaw muscle pain. J Headache Pain. (2017) 18:30. doi: 10.1186/s10194-017-0737-y
77. Almada AE, Wagers AJ. Molecular circuitry of stem cell fate in skeletal muscle regeneration, ageing and disease. Nat Rev Mol Cell Biol. (2016) 17:267–79. doi: 10.1038/nrm.2016.7
78. Pongdee T, Manemann SM, Decker PA, Larson NB, Moon S, Killian JM, et al. Rethinking blood eosinophil counts: Epidemiology, associated chronic diseases, and increased risks of cardiovascular disease. J Allergy Clin Immunol Glob. (2022) 1:233–40. doi: 10.1016/j.jacig.2022.09.001
79. Mathur SK, Schwantes EA, Jarjour NN, Busse WW. Age-related changes in eosinophil function in human subjects. Chest. (2008) 133:412–9. doi: 10.1378/chest.07-2114
80. Lukjanenko L, Karaz S, Stuelsatz P, Gurriaran-Rodriguez U, Michaud J, Dammone G, et al. Aging disrupts muscle stem cell function by impairing matricellular WISP1 secretion from fibro-adipogenic progenitors. Cell Stem Cell. (2019) 24:433–46.e7. doi: 10.1016/j.stem.2018.12.014
81. Lemos DR, Babaeijandaghi F, Low M, Chang C-K, Lee ST, Fiore D, et al. Nilotinib reduces muscle fibrosis in chronic muscle injury by promoting TNF-mediated apoptosis of fibro/adipogenic progenitors. Nat Med. (2015) 21:786–94. doi: 10.1038/nm.3869
82. Wosczyna MN, Konishi CT, Perez Carbajal EE, Wang TT, Walsh RA, Gan Q, et al. Mesenchymal stromal cells are required for regeneration and homeostatic maintenance of skeletal muscle. Cell Rep. (2019) 27:2029–35.e5. doi: 10.1016/j.celrep.2019.04.074
83. Farup J, Just J, de Paoli F, Lin L, Jensen JB, Billeskov T, et al. Human skeletal muscle CD90+ fibro-adipogenic progenitors are associated with muscle degeneration in type 2 diabetic patients. Cell Metab. (2021) 33. doi: 10.1016/j.cmet.2021.10.001
84. Tidball JG, Flores I, Welc SS, Wehling-Henricks M, Ochi E. Aging of the immune system and impaired muscle regeneration: A failure of immunomodulation of adult myogenesis. Exp Gerontol. (2021) 145:111200. doi: 10.1016/j.exger.2020.111200
85. Kuswanto W, Burzyn D, Panduro M, Wang KK, Jang YC, Wagers AJ, et al. Poor repair of skeletal muscle in aging mice reflects a defect in local, interleukin-33-dependent accumulation of regulatory T cells. Immunity. (2016) 44:355–67. doi: 10.1016/j.immuni.2016.01.009
86. Faas M, Ipseiz N, Ackermann J, Culemann S, Grüneboom A, Schröder F, et al. IL-33-induced metabolic reprogramming controls the differentiation of alternatively activated macrophages and the resolution of inflammation. Immunity. (2021) 54:2531–2546.e5. doi: 10.1016/j.immuni.2021.09.010
87. Mastui T, Hashimoto Y, Okamura T, Kaji A, Sakai R, Kondo Y, et al. Relationship between eosinophils counts and muscle mass decline in older people with type 2 diabetes: A prospective study of the KAMOGAWA-DM cohort. Exp Gerontol. (2022) 159:111671. doi: 10.1016/j.exger.2021.111671
88. Bulfield G, Siller WG, Wight PA, Moore KJ. X chromosome-linked muscular dystrophy (mdx) in the mouse. Proc Natl Acad Sci. (1984) 81:1189–92. doi: 10.1073/pnas.81.4.1189
89. DiMario JX, Uzman A, Strohman RC. Fiber regeneration is not persistent in dystrophic (mdx) mouse skeletal muscle. Dev Biol. (1991) 148:314–21. doi: 10.1016/0012-1606(91)90340-9
90. Hoffman EP, Brown RH, Kunkel LM. Dystrophin: The protein product of the duchenne muscular dystrophy locus. Cell. (1987) 51:919–28. doi: 10.1016/0092-8674(87)90579-4
91. Yucel N, Chang AC, Day JW, Rosenthal N, Blau HM. Humanizing the mdx mouse model of DMD: the long and the short of it. NPJ Regener Med. (2018) 3:4. doi: 10.1038/s41536-018-0045-4
92. Fukada S, Morikawa D, Yamamoto Y, Yoshida T, Sumie N, Yamaguchi M, et al. Genetic background affects properties of satellite cells and mdx phenotypes. Am J Pathol. (2010) 176:2414–24. doi: 10.2353/ajpath.2010.090887
93. Mázala DAG, Hindupur R, Moon YJ, Shaikh F, Gamu IH, Alladi D, et al. Altered muscle niche contributes to myogenic deficit in the D2-mdx model of severe DMD. Cell Death Discovery. (2023) 9:224. doi: 10.1038/s41420-023-01503-0
94. Cai B, Spencer MJ, Nakamura G, Tseng-Ong L, Tidball JG. Eosinophilia of dystrophin-deficient muscle is promoted by perforin-mediated cytotoxicity by T cell effectors. Am J Pathol. (2000) 156:1789–96. doi: 10.1016/S0002-9440(10)65050-X
95. Podkalicka P, Mucha O, Bronisz-Budzyńska I, Kozakowska M, Pietraszek-Gremplewicz K, Cetnarowska A, et al. Lack of miR-378 attenuates muscular dystrophy in mdx mice. JCI Insight. (2020) 5(11):e135576. doi: 10.1172/jci.insight.135576
96. Wehling-Henricks M, Sokolow S, Lee JJ, Myung KH, Villalta SA, Tidball JG. Major basic protein-1 promotes fibrosis of dystrophic muscle and attenuates the cellular immune response in muscular dystrophy. Hum Mol Genet. (2008) 17:2280–92. doi: 10.1093/hmg/ddn129
97. Sek AC, Moore IN, Smelkinson MG, Pak K, Minai M, Smith R, et al. Eosinophils do not drive acute muscle pathology in the mdx mouse model of duchenne muscular dystrophy. J Immunol. (2019) 203:476–84. doi: 10.4049/jimmunol.1900307
98. Molofsky AB, Nussbaum JC, Liang H-E, Van Dyken SJ, Cheng LE, Mohapatra A, et al. Innate lymphoid type 2 cells sustain visceral adipose tissue eosinophils and alternatively activated macrophages. J Exp Med. (2013) 210:535–49. doi: 10.1084/jem.20121964
99. Rana BMJ, Jou E, Barlow JL, Rodriguez-Rodriguez N, Walker JA, Knox C, et al. A stromal cell niche sustains ILC2-mediated type-2 conditioning in adipose tissue. J Exp Med. (2019) 216:1999–2009. doi: 10.1084/jem.20190689
100. Gu P, Xu A. Interplay between adipose tissue and blood vessels in obesity and vascular dysfunction. Rev Endocr Metab Disord. (2013) 14:49–58. doi: 10.1007/s11154-012-9230-8
101. Wu D, Molofsky AB, Liang H-E, Ricardo-Gonzalez RR, Jouihan HA, Bando JK, et al. Eosinophils sustain adipose alternatively activated macrophages associated with glucose homeostasis. Sci (80- ). (2011) 332:243–7. doi: 10.1126/science.1201475
102. Shah M, Knights AJ, Vohralik EJ, Psaila AM, Quinlan KGR. Blood and adipose-resident eosinophils are defined by distinct transcriptional profiles. J Leukoc Biol. (2023) 113:191–202. doi: 10.1093/jleuko/qiac009
103. Kuruvilla M, Patrawala M, Levy JM, Shih J, Lee FE. Association of antieosinophil therapy with decreased body mass index in patients with severe asthma: A preliminary retrospective analysis. Ann Allergy Asthma Immunol. (2019) 122:649–50. doi: 10.1016/j.anai.2019.03.031
104. Zhu L, Su T, Xu M, Xu Y, Li M, Wang T, et al. Eosinophil inversely associates with type 2 diabetes and insulin resistance in chinese adults. PLoS One. (2013) 8:e67613. doi: 10.1371/journal.pone.0067613
105. Spits H, Mjösberg J. Heterogeneity of type 2 innate lymphoid cells. Nat Rev Immunol. (2022) 22:701–12. doi: 10.1038/s41577-022-00704-5
106. Lumeng CN, Liu J, Geletka L, Delaney C, Delproposto J, Desai A, et al. Aging is associated with an increase in T cells and inflammatory macrophages in visceral adipose tissue. J Immunol. (2011) 187:6208–16. doi: 10.4049/jimmunol.1102188
107. Mahlakõiv T, Flamar A-L, Johnston LK, Moriyama S, Putzel GG, Bryce PJ, et al. Stromal cells maintain immune cell homeostasis in adipose tissue via production of interleukin-33. Sci Immunol. (2019) 4:eaax0416. doi: 10.1126/sciimmunol.aax0416
108. Hashiguchi M, Kashiwakura Y, Kojima H, Kobayashi A, Kanno Y, Kobata T. IL-33 activates eosinophils of visceral adipose tissue both directly and via innate lymphoid cells. Eur J Immunol. (2015) 45:876–85. doi: 10.1002/eji.201444969
109. Qiu Y, Nguyen KD, Odegaard JI, Cui X, Tian X, Locksley RM, et al. Eosinophils and type 2 cytokine signaling in macrophages orchestrate development of functional beige fat. Cell. (2014) 157:1292–308. doi: 10.1016/j.cell.2014.03.066
110. Fischer K, Ruiz HH, Jhun K, Finan B, Oberlin DJ, van der Heide V, et al. Alternatively activated macrophages do not synthesize catecholamines or contribute to adipose tissue adaptive thermogenesis. Nat Med. (2017) 23:623–30. doi: 10.1038/nm.4316
111. Pirzgalska RM, Seixas E, Seidman JS, Link VM, Sánchez NM, Mahú I, et al. Sympathetic neuron–associated macrophages contribute to obesity by importing and metabolizing norepinephrine. Nat Med. (2017) 23:1309–18. doi: 10.1038/nm.4422
112. Wu H, Ballantyne CM. Metabolic inflammation and insulin resistance in obesity. Circ Res. (2020) 126:1549–64. doi: 10.1161/CIRCRESAHA.119.315896
113. Vohralik EJ, Psaila AM, Knights AJ, Quinlan KGR. EoTHINophils: Eosinophils as key players in adipose tissue homeostasis. Clin Exp Pharmacol Physiol. (2020) 47:1495–505. doi: 10.1111/1440-1681.13304
114. Brestoff JR, Kim BS, Saenz SA, Stine RR, Monticelli LA, Sonnenberg GF, et al. Group 2 innate lymphoid cells promote beiging of white adipose tissue and limit obesity. Nat. (2014) 519:242–6. doi: 10.1038/nature14115
115. Ellulu MS, Patimah I, Khaza’ai H, Rahmat A, Abed Y. Obesity and inflammation: the linking mechanism and the complications. Arch Med Sci. (2017) 4:851–63. doi: 10.5114/aoms.2016.58928
116. Wensveen FM, Jelenčić V, Valentić S, Šestan M, Wensveen TT, Theurich S, et al. NK cells link obesity-induced adipose stress to inflammation and insulin resistance. Nat Immunol. (2015) 16:376–85. doi: 10.1038/ni.3120
117. Huang Z, Zhong L, Tsz J, Lee H, Wang Y, Wong C-M, et al. The FGF21-CCL11 axis mediates beiging of white adipose tissues by coupling sympathetic nervous system to type 2 immunity. Cell Metab. (2017) 26:493–508.e4. doi: 10.1016/j.cmet.2017.08.003
118. Obi S, Shimokawa C, Katsuura M, Olia A, Imai T, Suzue K, et al. IL-33 is essential to prevent high-fat diet–induced obesity in mice infected with an intestinal helminth. Parasite Immunol. (2020) 42:e12700. doi: 10.1111/pim.12700
119. Lee E-H, Itan M, Jang J, Gu H-J, Rozenberg P, Mingler MK, et al. Eosinophils support adipocyte maturation and promote glucose tolerance in obesity. Sci Rep. (2018) 8:9894. doi: 10.1038/s41598-018-28371-4
120. Zhao H, Moarbes V, Gaudreault V, Shan J, Aldossary H, Cyr L, et al. Sex differences in IL-33-induced STAT6-dependent type 2 airway inflammation. Front Immunol. (2019) 10:421077. doi: 10.3389/fimmu.2019.00859
121. Sunadome H, Matsumoto H, Izuhara Y, Nagasaki T, Kanemitsu Y, Ishiyama Y, et al. Correlation between eosinophil count, its genetic background and body mass index: The Nagahama Study. Allergol Int. (2020) 69:46–52. doi: 10.1016/j.alit.2019.05.012
122. Moussa K, Gurung P, Adams-Huet B, Devaraj S, Jialal I. Increased eosinophils in adipose tissue of metabolic syndrome. J Diabetes Complications. (2019) 33:535–8. doi: 10.1016/j.jdiacomp.2019.05.010
123. Seale P, Lazar MA. Brown fat in humans: Turning up the heat on obesity. Diabetes. (2009) 58:1482–4. doi: 10.2337/db09-0622
124. Rabiee A. Beige fat maintenance; toward a sustained metabolic health. Front Endocrinol (Lausanne). (2020), 11. doi: 10.3389/fendo.2020.00634
125. Lee M-W, Odegaard JI, Mukundan L, Qiu Y, Molofsky AB, Nussbaum JC, et al. Activated type 2 innate lymphoid cells regulate beige fat biogenesis. Cell. (2015) 160:74–87. doi: 10.1016/j.cell.2014.12.011
126. Nguyen KD, Qiu Y, Cui X, Goh YPS, Mwangi J, David T, et al. Alternatively activated macrophages produce catecholamines to sustain adaptive thermogenesis. Nature. (2011) 480:104–8. doi: 10.1038/nature10653
127. Withers SB, Forman R, Meza-Perez S, Sorobetea D, Sitnik K, Hopwood T, et al. Eosinophils are key regulators of perivascular adipose tissue and vascular functionality. Sci Rep. (2017) 7:44571. doi: 10.1038/srep44571
128. Leitner DR, Frühbeck G, Yumuk V, Schindler K, Micic D, Woodward E, et al. Obesity and type 2 diabetes: Two diseases with a need for combined treatment strategies - EASO can lead the way. Obes Facts. (2017) 10:483–92. doi: 10.1159/000480525
129. Lumeng CN, DeYoung SM, Bodzin JL, Saltiel AR. Increased inflammatory properties of adipose tissue macrophages recruited during diet-induced obesity. Diabetes. (2007) 56:16–23. doi: 10.2337/db06-1076
130. Donath MY, Shoelson SE. Type 2 diabetes as an inflammatory disease. Nat Rev Immunol. (2011) 11:98–107. doi: 10.1038/nri2925
131. Bolus WR, Peterson KR, Hubler MJ, Kennedy AJ, Gruen ML, Hasty AH. Elevating adipose eosinophils in obese mice to physiologically normal levels does not rescue metabolic impairments. Mol Metab. (2018) 8:86–95. doi: 10.1016/j.molmet.2017.12.004
132. Hams E, Bermingham R, Wurlod FA, Hogan AE, O’Shea D, Preston RJ, et al. The helminth T2 RNase ω1 promotes metabolic homeostasis in an IL-33- and group 2 innate lymphoid cell-dependent mechanism. FASEB J. (2016) 30:824–35. doi: 10.1096/fj.15-277822
133. Hussaarts L, García-Tardón N, Van Beek L, Heemskerk MM, Haeberlein S, van der Zon GC, et al. Chronic helminth infection and helminth-derived egg antigens promote adipose tissue M2 macrophages and improve insulin sensitivity in obese mice. FASEB J. (2015) 29:3027–39. doi: 10.1096/fj.14-266239
134. Sokolova RN, Yankova RK, Abadjieva TI, Popova TA, Ivanovska MV, Murdjeva MA, et al. Association between type 2 diabetes, obesity and key immunological components of igE-mediated inflammation. Folia Med (Plovdiv). (2017) 59:159–64. doi: 10.1515/folmed-2017-0021
Keywords: skeletal muscle, tissue repair, immune cells, adipose tissue, eosinophil
Citation: Day KS, Rempel L, Rossi FMV and Theret M (2024) Origins and functions of eosinophils in two non-mucosal tissues. Front. Immunol. 15:1368142. doi: 10.3389/fimmu.2024.1368142
Received: 10 January 2024; Accepted: 26 February 2024;
Published: 22 March 2024.
Edited by:
Garry M. Walsh, University of Aberdeen, United KingdomReviewed by:
Heather Caslin, University of Houston, United StatesToshiyuki Murai, Osaka University, Japan
Svetoslav Chakarov, Shanghai Jiao Tong University, China
B. Hilvering, Amsterdam University Medical Center, Netherlands
Copyright © 2024 Day, Rempel, Rossi and Theret. This is an open-access article distributed under the terms of the Creative Commons Attribution License (CC BY). The use, distribution or reproduction in other forums is permitted, provided the original author(s) and the copyright owner(s) are credited and that the original publication in this journal is cited, in accordance with accepted academic practice. No use, distribution or reproduction is permitted which does not comply with these terms.
*Correspondence: Marine Theret, bXRoZXJldEBicmMudWJjLmNh
†These authors have contributed equally to this work