- 1Department of Urology, Jiujiang University Clinic College/Hospital, Jiujiang, Jiangxi, China
- 2Department of Urology, Lanxi People’s Hospital, Jinhua, Zhejiang, China
- 3The First Clinical College, Gannan Medical University, Ganzhou, Jiangxi, China
- 4Department of Urology, Jingdezhen Second People’s Hospital, Jingdezhen, Jiangxi, China
The emerging extracellular vesicles technologies is an advanced therapeutic approach showing promising potential for addressing inflammatory diseases. These techniques have been proven to have positive effects on immune modulation and anti-inflammatory responses. With these advancements, a comprehensive review and update on the role of extracellular vesicles in inflammatory diseases have become timely. This review aims to summarize the research progress of extracellular vesicle technologies such as plant-derived extracellular vesicles, milk-derived extracellular vesicles, mesenchymal stem cell-derived extracellular vesicles, macrophage-derived extracellular vesicles, etc., in the treatment of inflammatory diseases. It elucidates their potential significance in regulating inflammation, promoting tissue repair, and treating diseases. The goal is to provide insights for future research in this field, fostering the application and development of extracellular vesicle technology in the treatment of inflammatory diseases.
1 Introduction
Epidemiological studies indicate a significant increase in the incidence of inflammatory diseases over the past 20 years (1). Consequently, the total number of patients taking immunosuppressive drugs continues to rise (2). Prolonged use of immunosuppressive drugs inevitably increases the risk of infections and malignancies as antimicrobial and antitumor immunity remains suppressed (3). When the normal inflammatory process fails to resolve, chronic inflammatory pain ensues, leading to an excess of pro-inflammatory cytokines and chemotactic factors, ultimately resulting in central sensitization (4–6). Therefore, there is an urgent need for new therapeutic drugs in the treatment of autoimmune and inflammatory diseases, capable of suppressing detrimental immune responses without inducing life-threatening immunosuppression.
Extracellular vesicles (EVs) are a class of small cellular products with sizes in the nanometer range and are found to possess a bilayer membrane structure. These small cellular products are released by all types of cells under both normal and pathological conditions (7)(Figure 1). EVs contain a rich array of biomolecules such as proteins, lipids, RNA, cellular metabolites, as well as active molecules like growth factors and cytokines (8). Simultaneously, EVs as nanocarriers offer advantages over other systems due to their ability to carry various endogenous biomolecules, exhibiting biocompatibility, natural targeting capabilities, and evasion from clearance (9, 10) (Figure 2). Recently, increasing attention has been focused on the therapeutic role of emerging extracellular vesicle technology in inflammatory diseases. Recent studies have highlighted the prospects of extracellular vesicle technology in the treatment of inflammatory diseases. This emerging technology has garnered widespread attention not only for disease treatment but also for its involvement in immune system regulation. By exploring the potential role of extracellular vesicles in immune modulation, we may break the constraints of conventional treatments, presenting new opportunities for the diagnosis and treatment of inflammatory diseases. These findings offer unprecedented possibilities for the exploration of future therapeutic tools and approaches.
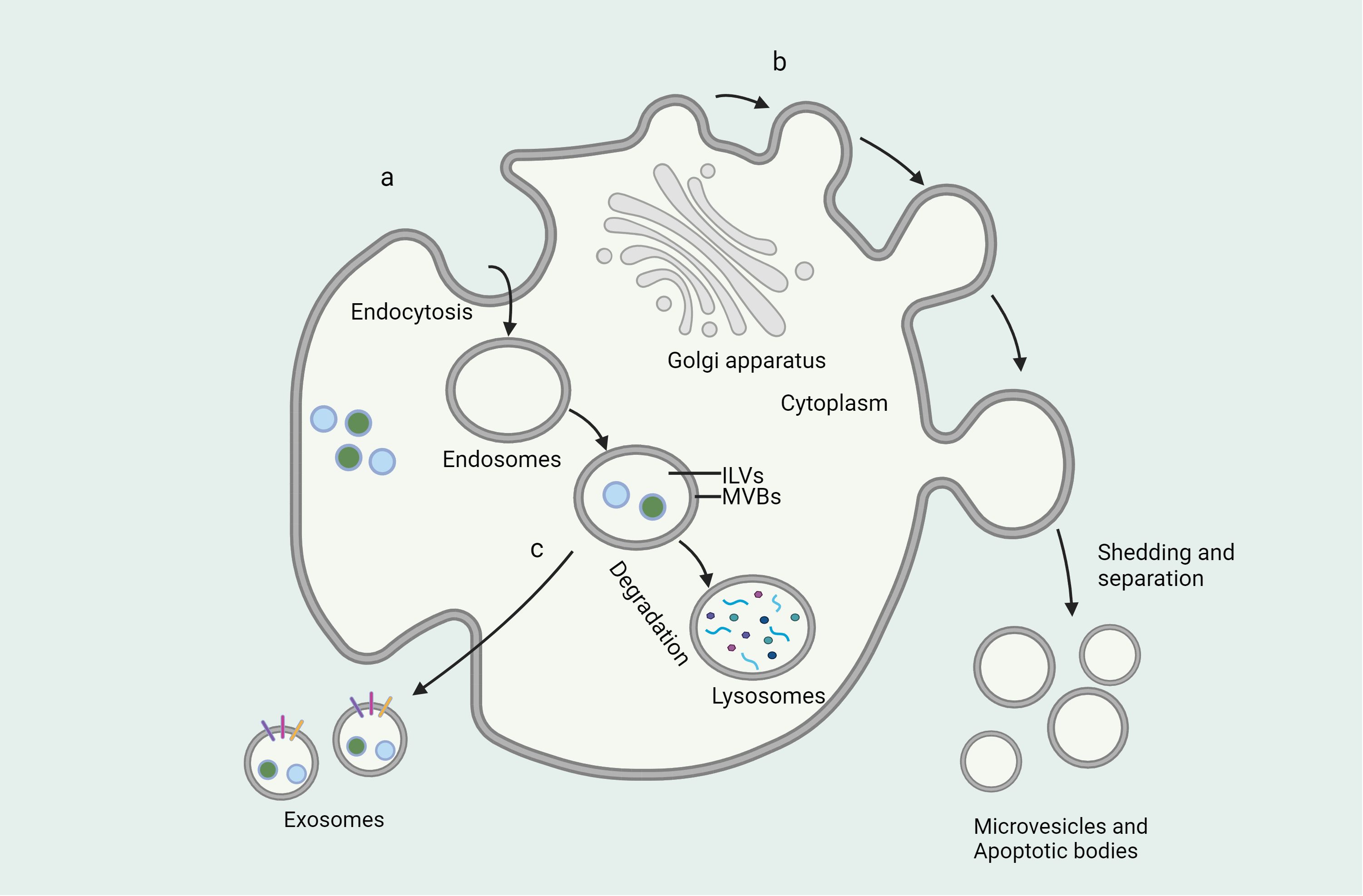
Figure 1 Formation of extracellular vesicles and mode of secretion. (A) Endosomes are vesicles formed by the cell membrane surrounding a substance and formed by endocytosis, where the substance is broken down by fusion with lysosomes or released outside the cell by direct fusion with the cell membrane. (B) Microvesicles are vesicles formed by the direct shedding of cell membranes through shedding and separation of cell membranes. (C) Exosomes are vesicles produced through the endoplasmic reticulum and Golgi apparatus that fuse with the cell membrane and release them outside the cell.
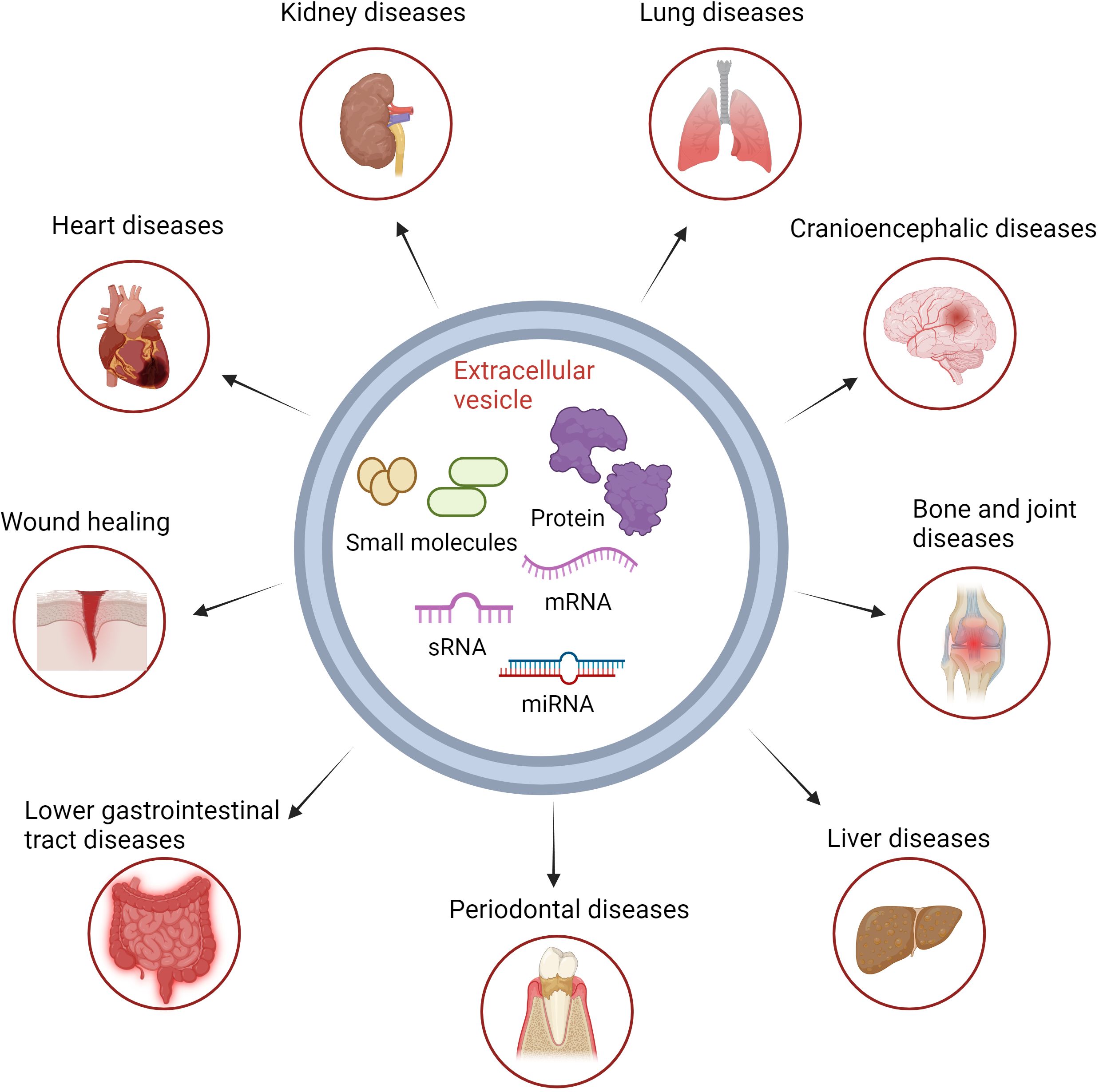
Figure 2 Basic structure of extracellular vesicles and the treatment of immune-related diseases. Biomolecules in extracellular vesicles can be directly transported by vesicle targeting to the target cells, resulting in therapeutic effects for inflammatory diseases.
2 Novel extracellular vesicles in inflammatory diseases
The role of extracellular vesicles in the study of inflammatory diseases has become a hot topic in biomedical research in recent years. They are capable of carrying and delivering proteins, lipids, RNA, and DNA and thus play a role in many physiological and pathological processes, including inflammatory response, immune regulation, and disease progression (11). Compared to conventional EVs, these emerging EVs can be engineered with specific markers to improve their targeting, allowing them to more effectively reach and act on inflamed or damaged tissues (7). Based on their unique origin and biological activity, these emerging EVs offer new possibilities for personalized and tailored therapies, especially in the treatment of inflammatory diseases (12, 13). The development of these emerging extracellular vesicle technologies not only extends our understanding of the role of EVs in physiological and pathological processes, but also provides new therapeutic tools and strategies, especially in the treatment of inflammatory diseases (14). With further research, these technologies are expected to overcome the limitations in the application of traditional EVs and realize more effective and safer therapies. For example, plant extracellular vesicles, breast milk extracellular vesicles, mesenchymal stem cell extracellular vesicles, macrophage extracellular vesicles, and other novel extracellular vesicle technologies. The respective advantages of these EVs and their potential value in inflammatory diseases may help to revolutionize and solve the current dilemma of treating immune diseases. Therefore, it is necessary to discuss in detail the research and challenges of these vesicles in inflammatory diseases with a view to promoting the clinical utilization of novel extracellular vesicles.
3 Macrophage-derived extracellular vesicles
3.1 Overview
Macrophage-derived extracellular vesicles (Mφ-EVs) play a unique role in immune responses, holding potential therapeutic applications for various diseases (15). These vesicles alter the phenotype and function of target cells by carrying a rich cargo of proteins, lipids, and genetic information, although their composition may vary with different phenotypes of macrophages or microenvironments (16). Mφ-EVs modulate communication between local and systemic cells, temporally and spatially regulating molecular events in recipient cells, such as promoting the resolution of inflammation and alleviating inflammation-induced allergic reactions (17). The surface of Mφ-EVs is enriched with immune molecules like cluster of differentiation 47 (CD47), enabling them to evade immune surveillance and evade immune attacks (18). Compared to larger macrophages, extracellular vesicles are smaller, more easily circulated, and possess the capability to traverse biological barriers, thus can be further engineered for potential drug delivery systems (19).
3.2 Applications as therapeutic agents
The RNA molecules (20, 21), protein components (22, 23), soluble mediators (such as enzymes and cytokines) (24, 25), and lipids (26) found in EVs secreted by macrophages can stimulate pro-inflammatory signal transduction and activate receptor immune cells, resulting in the formation of a local immune-stimulating microenvironment (17, 27, 28). They exhibit a strong inclination and high affinity for tumors and inflammatory tissues (29, 30). Mφ-EVs have three main phenotypes: unpolarized M0-derived EVs (M0-EVs), M1-derived EVs (M1-EVs), and M2-derived EVs (M2-EVs). Their biological functions differ based on the characteristics of the parent cells (31). M1-type macrophage-derived extracellular vesicles (M1-EVs) play a crucial role in heart repair and remodeling by transporting miR-222 and miR-155, regulating stem cell apoptosis, inflammatory responses, and vascular regeneration abilities (32–36). In contrast, M2-type macrophage-derived extracellular vesicles (M2-EVs) execute cardiac repair functions by transferring miR-1271-5p, inhibiting cardiomyocyte apoptosis (37). In ischemia-reperfusion injury (IRI), M1-type macrophage-derived extracellular vesicles (M1-EVs) promote cardiomyocyte necrosis through miR-29a, resulting in cardiac dysfunction, while M2-type macrophage-derived extracellular vesicles (M2-EVs) alleviate IRI by miR-148a, suppressing the activity of specific signaling pathways to mitigate cardiac injury (38, 39). M1-type macrophage-derived extracellular vesicles (M1-EVs) play a critical role in chronic low-grade tissue inflammation-induced insulin resistance, where their cargo miRNAs (such as miR-212-5p, miR-155, and miR-29a) target specific genes or modulate signaling pathways, limiting insulin secretion, impairing insulin’s suppression of glucose, potentially contributing to pancreatic β-cell failure, and obesity-induced insulin resistance (40–42). Both M1 and M2-type macrophage-derived extracellular vesicles have distinct roles in diabetic complications. M1-EVs aid in accelerating wound healing, modulating Mφ phenotypes, inhibiting osteogenesis, while potentially inducing inflammatory reactions (43–45). On the other hand, M2-EVs alleviate foot lesions, promote mesangial cell proliferation in glomeruli, yet are associated with inflammation and mitochondrial dysfunction (46–48). This underscores the intricate, sometimes contradictory, roles of EVs in the pathophysiology of diabetes. However, due to the complex and mixed phenotypes typically exhibited by macrophages in different diseases or stages within a disease, even within the same disease, identifying their exact subgroups of EVs (such as M1 or M2) is challenging.
3.3 Applications as therapeutic carrier
Due to the inherent ability of macrophage-derived EVs to traverse natural barriers within the body, they can selectively deliver payloads to hard-to-reach sites while minimizing side effects on healthy tissues (49, 50). Zhang et al. isolated EVs from umbilical cord blood of M1Mφ and M2Mφ loaded with cisplatin (CDDP). Results showed that compared to M2-EVs, M1-EVs exhibited higher cytotoxicity in drug-resistant cisplatin-resistant ovarian cancer cell line (A2780/DDP) cells, suggesting their potential as drug delivery tools in drug-resistant environments (51). Moreover, Mφ-EVs transport neuroprotective factors across the blood-brain barrier, demonstrating neuroprotective effects (49). Novel Mφ-EV delivery systems exhibit inhibitory effects on oxidative stress and inflammation, potentially aiding in the treatment of neurological disorders (52). Furthermore, Mφ-EVs carrying specific drugs show potential in enhancing drug targeting and promoting drug bioavailability in the brain, holding promise in reducing neuronal damage and promoting neuronal protection (53, 54). Gao et al. discovered that M2-EVs delivering berberine drugs enhanced drug targeting, prolonged duration, and impacted inflammation and cell apoptosis, prompting a shift of Mφ from an inflammatory to a healing phenotype (55). Similarly, Mφ-EVs loaded with baicalin improved drug solubility and brain targeting, providing significant neuroprotection for ischemic stroke patients (56). These findings offer new therapeutic strategies utilizing Mφ-EVs as drug delivery systems, opening new avenues for the treatment of neurological disorders.
4 Plant-derived extracellular vesicles
4.1 Overview
Plant-derived extracellular vesicles are nanosized vesicles secreted by plant cells, sharing similar characteristics to extracellular vesicles from animal cells. They originate from various edible plants and play roles in various applications through different mechanisms (57). One significant application is in the treatment of inflammatory diseases, where they can enhance the immune system’s resistance to diseases and serve as nanocarriers for therapeutics (58). Engineered plant-derived extracellular vesicles exhibit excellent targeting, nanoscale dimensions, low off-target effects, low toxicity, and have the potential for large-scale production, offering advantages in the field of drug delivery (59–61). However, due to significant variations in the quantity, size distribution, antioxidant activity, and other characteristics of plant-derived extracellular vesicles among different plant species, and influenced by the chemical properties of the source plants, separate evaluations are needed for the safety and effectiveness of each type of extracellular vesicle (62).
4.2 Applications as therapeutic agents
When discussing treatment strategies for inflammatory diseases, plant-derived extracellular vesicles have garnered significant attention as an effective therapeutic agent. They possess the ability to exert anti-inflammatory effects through various mechanisms such as modulating the immune system, inhibiting inflammatory factors, and clearing oxidative stress. Their role in the treatment of colitis, in particular, has been confirmed by numerous studies (63–67).
As an example, plant-derived extracellular vesicles have demonstrated significant therapeutic effects in numerous experiments concerning colitis. Nanoparticles derived from broccoli can inhibit colitis in mice by activating dendritic cell adenosine monophosphate-activated protein kinase (AMPK) (68). Grape-derived extracellular vesicles promote proliferation and differentiation of intestinal stem cells, facilitating intestinal epithelial regeneration and protecting mice from dextran sulfate sodium (DSS)-induced colitis (57). Ginseng-derived extracellular vesicles suppress the nuclear factor Kappa-Light-Chain-Enhancer of activated B cells (NF-κB) pathway, reduce inflammatory factor levels, and improve gut microbiota composition, effectively treating DSS-induced colitis in mouse models (69, 70). Lemon-derived extracellular vesicles manipulate probiotics (C. diff) to inhibit Clostridioides difficile infection, a major cause of antibiotic-associated colitis (71, 72). Moreover, ginger and ginger rhizome-derived extracellular vesicles not only inhibit colitis but also exhibit inhibitory effects on nucleotide-binding oligomerization domain (NOD)-like receptor protein 1 (NLRP1) inflammasome activation (59, 73, 74). Mulberry bark-derived extracellular vesicles also protect mice from colitis by activating the Aryl hydrocarbon receptor (AhR) signaling pathway mediated by heat shock protein family A member 8 (HSPA8) (75).
Furthermore, the potential applications of plant-derived extracellular vesicles in treating other inflammatory diseases are increasingly evident. For instance, extracellular vesicles from ginger exhibit significant efficacy in preventing and treating chronic periodontitis, primarily attributed to specific interactions between phosphatidic acid and miR-159a-3p in the vesicles and Hemin-binding protein 35 in P. gingivalis (76). Ginger-derived extracellular vesicles also target Severe Acute Respiratory Syndrome Coronavirus 2 nonstructural protein 12 (SARS-CoV-2 Nsp12) and spike genes to ameliorate lung inflammation and act through the Toll-like receptor 4 (TLR4)/TIR-domain-containing adapter-inducing interferon-β (TRIF)-dependent pathway in NF-E2-related factor 2 (Nrf2) induction, preventing alcohol-induced liver injury (77, 78). Extracellular vesicles from other sources like honey and shiitake mushrooms also inhibit the activation of the nucleotide-binding domain, leucine-rich-containing family, pyrin domain-containing 3 (NLRP3) inflammasome, reducing inflammation and liver damage in experimentally induced acute liver injury (79, 80). Currently, three plant-derived extracellular vesicles from grapes, ginger, and aloe vera are undergoing clinical trials related to inflammatory diseases (81). With more clinical trials underway, we may gain further insights into the role of plant-derived extracellular vesicles in the human body, aiding in a more accurate assessment of their potential and value in clinical applications.
4.3 Applications as therapeutic carrier
In recent years, plant-derived extracellular vesicles have been discovered to possess the capability of carrying drugs to target tissues, holding immense potential in treating inflammatory diseases. These vesicles are considered ideal nano-carriers as they contain common proteins, bioactive lipids, RNA, and other pharmacologically active molecules involved in transfer and transport (58, 82). Sung et al.’s research demonstrates that extracellular vesicles derived from ginger, when orally administered, effectively target the colon, reducing the incidence of acute colitis, enhancing intestinal repair capabilities, and preventing the onset of chronic colitis and colitis-associated cancers (83). Grapefruit-derived extracellular vesicles also exhibit similar abilities, efficiently delivering therapeutic agents to inflammatory tumor sites by activating leukocyte migration pathways (84, 85). More excitingly, when combining the anti-inflammatory drug methotrexate (MTX) with glucocorticoid-induced tumor necrosis factor receptor family-related protein (GNV), it significantly reduces the toxicity of MTX and enhances its therapeutic effects in inflammatory diseases (86). The advantages mentioned above endow plant extracellular vesicles with enormous potential as a novel drug delivery system. Researchers successfully utilized lipids extracted from extracellular vesicles sourced from ginger to prepare ‘natural’ nano-carriers. These carriers loaded with siRNA for treating ulcerative colitis demonstrated superior effects compared to traditional synthetic nanoparticles (74, 87, 88). Furthermore, a crucial characteristic of plant extracellular vesicles as drug delivery carriers is their capacity for personalized adjustments based on treatment targets. For instance, grape-derived extracellular vesicles can target intestinal cells, while grapefruit-derived extracellular vesicles can target macrophages (57, 86). This offers possibilities for personalized therapeutic approaches.
5 Mammary gland-derived extracellular vesicles
5.1 Overview
Mammary gland-derived extracellular vesicles (mEVs) are tiny vesicles originating from mammary epithelial cells, released into milk through endosomal pathways or directly from the cell membrane (89, 90). These mEVs contain a plethora of immune-related microRNAs and proteins, observed not to induce systemic toxicity or adverse immune reactions (91, 92). Breast milk is a rich source of miRNAs, where miRNAs play a crucial role in post-transcriptional gene regulation and may impact cellular gene expression through the presence of lactation-specific and immune-related proteins and miRNAs (93–95). These beneficial mEVs have been found not only in human milk but also in cow and goat milk (96). They carry beneficial miRNAs capable of entering cells and modulating biological functions such as promoting cell proliferation, regulating inflammatory responses, protecting cells from damage, and aiding in tissue functional recovery (97–99).
5.2 Applications as therapeutic agents
Mammary gland-derived extracellular vesicles carry unique RNA, proteins, lipids, and DNA, possessing anti-degradation, antioxidant, and anti-inflammatory biological properties (100). Studies have found these vesicles to play a crucial role in the treatment of intestinal inflammation and colitis. They can enhance the vitality and proliferation capacity of intestinal epithelial cells (97), improve intestinal barrier function (99), and inhibit inflammatory signal transduction (101). Additionally, mammary gland-derived extracellular vesicles can reduce intestinal epithelial damage, restore intestinal tight junction proteins, and regulate inflammation and cellular homeostasis, which holds significance for inflammatory bowel diseases such as necrotizing enterocolitis and inflammatory bowel disease (102, 103). The latest research indicates that mammary gland-derived extracellular vesicles can modulate lipid and amino acid metabolism in healthy mice and may alter the metabolomic characteristics of DSS-induced colitis in mice, particularly increasing levels of lipid anti-inflammatory metabolites and decreasing levels of fecal amino acids, which could be a primary driving force in alleviating colitis (100). Moreover, these vesicles have demonstrated potential applications in other inflammatory disease domains. Studies suggest their potential use in treating inflammatory lung diseases induced by agricultural dust exposure and alleviating cartilage degradation metabolism and inflammation processes in osteoarthritis (104, 105).
5.3 Applications as therapeutic carrier
Mammary gland-derived extracellular vesicles are considered potential drug delivery carriers due to their low immunogenicity, good biocompatibility, stability, and the ability to traverse the gastrointestinal barrier (106). Studies have found them particularly effective in the treatment of colitis (100). Furthermore, mammary gland-derived extracellular vesicles have shown potential as carriers for siRNA drug delivery. They can withstand harsh environments during digestion, improve intestinal permeability, and protect the payload (107). Because they can survive under the strongly acidic conditions of the stomach and the degrading conditions of the intestine and traverse biological barriers to reach targeted tissues, they are considered promising natural drug carrier tools for oral administration (108). These vesicles can also serve as delivery systems to enhance the bioavailability and efficacy of miRNA therapy. For instance, they can act as drug carriers for potential therapeutic miR-31-5p in diabetic wound healing (109). They can limit the degradation metabolism and inflammatory processes in cartilage by transferring growth factors and genetic modulators like miR-148a, thereby reducing cartilage damage in osteoarthritis patients (110). Another study assessed the use of mammary gland-derived extracellular vesicles as carriers for extracellular RNA therapeutics, finding breast milk to be a cost-effective source of extracellular vesicles suitable as nanocarriers for functional miRNA, potentially applicable in RNA-based therapies (111). However, the complex content and quality control of mammary gland-derived extracellular vesicles hinder their application in drug delivery (106). Further research should focus on developing novel purification and isolation techniques to address quality control issues, reduce batch-to-batch heterogeneity, and promote the application of mammary gland-derived extracellular vesicles in the field of drug delivery.
6 Mesenchymal stem cell-derived extracellular vesicles
6.1 Overview
Mesenchymal stem cells (MSCs), derived from various tissues, possess pluripotency, can differentiate into various tissues, and have regenerative capabilities (112). They not only treat tissue injuries but also exhibit immunomodulatory functions by migrating to inflammatory sites and utilizing extracellular vesicles to regulate immune responses (113). These EVs are small vesicles secreted by cells, facilitating intercellular communication through membrane transfer and various substances (114). Compared to MSCs, mesenchymal stem cell-derived extracellular vesicles (MSC-EVs) are easier to obtain and store. Furthermore, MSC-EVs are believed to pose no safety issues in cell-based therapies, such as the tumorigenic potential associated with cell administration (115, 116). Increasing evidence suggests that MSC-EVs play a significant role in immunoregulation. They contain various anti-inflammatory substances and regulate immune responses by interacting with immune effector cells (117).
6.2 Applications as therapeutic agents
MSC-EVs have been confirmed in numerous preclinical studies to have a positive impact on the treatment of liver diseases. Studies have shown that MSC-EVs improve liver inflammation and alleviate various liver diseases by modulating immune responses. MSC-EVs have demonstrated positive effects in the treatment of non-alcoholic fatty liver disease (NAFLD) (118–122), autoimmune hepatitis (123–125), acute liver failure (126–130), liver fibrosis (131–133), and liver ischemia-reperfusion injury (IRI) (134–136). They can regulate macrophage activation, alter cytokine expression, and influence related signaling pathways.
MSC-EVs in myocardial infarction reperfusion therapy can increase ATP levels, reduce oxidative stress, and activate the Phosphatidylinositol 3-kinase/protein kinase B (PI3K/Akt) pathway to enhance myocardial vitality and prevent adverse remodeling after myocardial ischemia/reperfusion injury (137). In the Ischaemia/Reperfusion (I/R) model of myocardial ischemia/reperfusion, injection of MSC-EVs significantly reduces cell apoptosis and myocardial infarct size while improving cardiac function (138). In the field of neuroscience, researchers found that extracellular vesicles from MSCs can restore synaptic dysfunction and regulate inflammatory responses by modulating miR-21, thus enhancing learning and memory abilities in amyloid precursor protein/presenilin 1 transgenic (APP/PS1) mice (139). In the treatment of colitis, extracellular vesicles secreted by bone marrow mesenchymal stem cells (BMSCs) exhibit a positive therapeutic effect in colitis. They promote macrophage proliferation and suppress inflammation, showing positive effects in improving symptoms of ulcerative colitis and reversing experimental colitis (140, 141). More specific studies have also revealed that MSC-EVs transfer various nucleic acids, proteins, and lipids from parent cells to recipient cells, participating in chronic inflammation and immune processes, playing a significant regulatory role in the pathogenesis of arthritis (142).
6.3 Applications as therapeutic carrier
Research on MSC-EVs as therapeutic carriers is continually making new strides. In the treatment of idiopathic pulmonary fibrosis (IPF), bone marrow mesenchymal stem cell-derived extracellular vesicles (BMSC-EVs) and their carried microRNAs (miRNAs) show promise. Particularly, miR-186 delivered by BMSC-EVs has been found to halt the progression of lung fibrosis by inhibiting fibroblast activation, downregulating SRY-box transcription factor 4 (SOX4) and Dickkopf-related protein 1 (DKK1), offering a novel therapeutic strategy for idiopathic pulmonary fibrosis (IPF) treatment (143). In the treatment of renal diseases, mesenchymal stem cells overexpressing miRNA-let7c electively localize to damaged kidneys and upregulate the expression of miR-let7c through their secreted extracellular vesicles, thereby alleviating renal injury and significantly downregulating various fibrotic genes (144). This provides a new direction for miRNA therapy targeting renal diseases. In skin wound healing, wingless-type MMTV integration site family member 4 (Wnt4) found in human umbilical cord mesenchymal stem cell-derived extracellular vesicles is discovered to promote nuclear translocation and activity of β-catenin, enhancing proliferation and migration of skin cells (145).
In addition, MSC-EVs have played a significant role in the treatment of liver and heart diseases. MSC-EVs can carry various RNAs, exert anti-fibrotic effects, reduce the deposition of extracellular matrix (ECM), and improve liver function (146–148). In the treatment of autoimmune hepatitis, MSC-EVs carrying dexamethasone can enhance the anti-inflammatory therapeutic effect of dexamethasone while reducing its side effects (149). In heart diseases, using extracellular vesicles derived from bone marrow mesenchymal stem cells (BM-MSCs) as carriers, miR-19a/19b has been proven to significantly inhibit apoptosis of cardiac HL-1 cells (150). In myocardial infarction (MI) models, Exo/miR-19a/19b combined with MSC transplantation can significantly enhance cardiac function recovery and reduce cardiac fibrosis (150). With technological advancements, the understanding and application of MSC-derived extracellular vesicles as therapeutic carriers will continue to deepen. It can be anticipated that they will play even more critical roles in future therapeutic research.
7 Expectations and challenges
Emerging EVs have received widespread attention for their potential application in the treatment of inflammatory diseases as an important tool for cellular communication. EVs from different sources have demonstrated their unique properties and potential therapeutic advantages, but have also revealed common challenges that need to be overcome before clinical applications can be promoted (Table 1). Macrophage EVs have significant immunomodulatory capabilities and are able to ameliorate the inflammatory state by directly acting at the site of inflammation (151). However, their effects may be affected by macrophage activation status, which requires detailed phenotypic and functional analyses prior to clinical application to ensure the desired therapeutic effect. Studies of plant EVs have provided new perspectives on EVs of non-mammalian origin, which demonstrate good biocompatibility and low toxicity, making them potentially safe therapeutic vectors. However, the composition and activity of plant EVs may vary depending on the plant species and growth conditions, requiring the establishment of standardized production and purification processes to ensure product quality (152). Lactic EVs have shown unique advantages in promoting gut health and immunomodulation, especially promising applications in neonatal development. However, access to milk EVs is limited by the availability of milk sources and individual differences in their composition, factors that may affect their consistency and widespread use as therapeutic tools (153). The ability of MSC EVs to promote tissue repair and anti-inflammation makes them a powerful tool in regenerative medicine. However, challenges remain for the large-scale production and clinical application of MSC EVs, including the diversity of cell sources, standardization of the production process, and evaluation of long-term safety.
Each of these EVs has its own advantages in treating inflammatory diseases, but they also face different challenges. Future research needs to address how to improve the targeting of EVs, enhance their therapeutic efficacy, optimize production and purification processes, and ensure safety and stability. In addition, an in-depth understanding of the distribution, metabolism and mechanism of action of EVs in vivo is essential for developing customized EVs-based therapeutics and enhancing their clinical application value. On this basis, interdisciplinary collaboration has become the key to promote the research and application of EVs, pooling the strengths of biology, materials science, engineering technology, and clinical medicine to explore and develop innovative EVs-based therapeutic strategies.
8 Discussion
This article reviews the research findings of various types of extracellular vesicles in the treatment of inflammatory diseases. Studies have indicated that these extracellular vesicles can exert therapeutic effects by modulating immune responses, inhibiting the release of inflammatory mediators, and promoting tissue repair (14, 17, 92, 151, 152). These results affirm the potential value of extracellular vesicle technology in the treatment of inflammatory diseases.
Despite the potential therapeutic efficacy demonstrated by emerging extracellular vesicle technology in treating inflammatory diseases, it faces several challenges in practical application. Research indicates therapeutic effects of different types of extracellular vesicles in inflammatory disease models, yet obstacles persist in clinical translation. Moreover, there remain controversies and uncertainties regarding the therapeutic mechanisms, optimal dosage, effective routes, and uncertainties surrounding extracellular vesicle treatment. Deeper understanding of the generation, release, targeting mechanisms of extracellular vesicles, and enhancing their efficacy as therapeutic tools are necessary to address challenges in large-scale production, stability, and targeting. Additionally, as a drug delivery system, extracellular vesicles still require solutions for effective drug loading, improved stability and specificity, assessment of safety and effectiveness, and differences in therapeutic effects from various sources.
Future research could focus on further exploring the characteristics and mechanisms of different types of extracellular vesicles to better understand their role in the treatment of inflammatory diseases. Simultaneously, there is a need to develop more efficient production methods and improve purification techniques to enhance the yield and quality of extracellular vesicles. Additionally, finding more suitable carriers and delivery routes and conducting further clinical trials to validate their safety and effectiveness are crucial directions for future research. More interestingly, the preparation of artificial extracellular vesicles with specific targeting effects, as in the case of liposomes, may be more conducive to the large-scale clinical use of nanovesicles. Of course, this would require a more complete study of the targeting and functional mechanisms of cell-derived vesicles.
Author contributions
KL: Writing – original draft, Writing – review & editing. HL: Investigation, Writing – original draft. XJ: Conceptualization, Writing – original draft. SF: Writing – original draft, Writing – review & editing.
Funding
The author(s) declare that no financial support was received for the research, authorship, and/or publication of this article.
Acknowledgments
All figures are created with BioRender.com.
Conflict of interest
The authors declare that the research was conducted in the absence of any commercial or financial relationships that could be construed as a potential conflict of interest.
Publisher’s note
All claims expressed in this article are solely those of the authors and do not necessarily represent those of their affiliated organizations, or those of the publisher, the editors and the reviewers. Any product that may be evaluated in this article, or claim that may be made by its manufacturer, is not guaranteed or endorsed by the publisher.
References
1. Ji J, Sundquist J, Sundquist K. Gender-specific incidence of autoimmune dis eases from national registers. J Autoimmun. (2016) 69:102–6. doi: 10.1016/j.jaut.2016.03.003
2. Schein CH. Repurposing approved drugs on the pathway to novel therapies. Med Res Rev. (2020) 40:586–605. doi: 10.1002/med.21627
3. McCaughan G. Molecular approaches to the side effects of immunosuppressive drugs. Transplantation. (2004) 78:1114–5. doi: 10.1097/01.TP.0000137263.30162.6B
4. Campbell JN, Meyer RA. Mechanisms of neuropathic pain. Neuron. (2006) 52:77–92. doi: 10.1016/j.neuron.2006.09.021
5. Chiu IM, von Hehn CA, Woolf CJ. Neurogenic inflammation and the peripheral nervous system in host defense and immunopathology. Nat Neurosci. (2012) 15:1063–7. doi: 10.1038/nn.3144
6. Nicotra L, Loram LC, Watkins LR, Hutchinson MR. Toll-like receptors in chronic pain. Exp Neurol. (2012) 234:316–29. doi: 10.1016/j.expneurol.2011.09.038
7. de Abreu RC, Fernandes H, da Costa Martins PA, Sahoo S, Emanueli C, Ferreira L. Native and bioengineered extracellular vesicles for cardiovascular therapeutics. Nat Rev Cardiol. (2020) 17:685–97. doi: 10.1038/s41569-020-0389-5
8. A. S,EL, Mäger I, Breakefield XO, Wood MJ. Extracellular vesicles: biology and emerging therapeutic opportunities. Nat Rev Drug Discovery. (2013) 12:347–57. doi: 10.1038/nrd3978
9. Lai RC, Yeo RW, Tan KH, Lim SK. Exosomes for drug delivery - a novel application for the mesenchymal stem cell. Biotechnol Adv. (2013) 31:543–51. doi: 10.1016/j.biotechadv.2012.08.008
10. Pardridge WM. Drug transport across the blood-brain barrier. J Cereb Blood Flow Metab. (2012) 32:1959–72. doi: 10.1038/jcbfm.2012.126
11. Cheng L, Hill AF. Therapeutically harnessing extracellular vesicles. Nat Rev Drug Discovery. (2022) 21:379–99. doi: 10.1038/s41573-022-00410-w
12. Feng J, Xiu Q, Huang Y, Troyer Z, Li B, Zheng L. Plant-derived vesicle-like nanoparticles as promising biotherapeutic tools: present and future. Advanced materials (Deerfield Beach Fla.). (2023) 35:e2207826. doi: 10.1002/adma.202207826
13. Cai Q, Halilovic L, Shi T, Chen A, He B, Wu H, et al. Extracellular vesicles: cross-organismal RNA trafficking in plants, microbes, and mammalian cells. Extracellular vesicles circulating Nucleic Acids. (2023) 4:262–82. doi: 10.20517/evcna
14. Harrell CR, Jovicic N, Djonov V, Arsenijevic N, Volarevic V. Mesenchymal stem cell-derived exosomes and other extracellular vesicles as new remedies in the therapy of inflammatory diseases. Cells. (2019) 8(12):1605. doi: 10.3390/cells8121605
15. Aigner A, Fischer D, Merdan T, Brus C, Kissel T, Czubayko F. Delivery of unmodified bioactive ribozymes by an RNA-stabilizing polyethylenimine (LMW-PEI) efficiently down-regulates gene expression. Gene Ther. (2002) 9:1700–7. doi: 10.1038/sj.gt.3301839
16. Feinberg MW, Moore KJ. MicroRNA regulation of Atherosclerosis. Circ Res. (2016) 118:703–20. doi: 10.1161/CIRCRESAHA.115.306300
17. McDonald MK, Tian Y, Qureshi RA, Gormley M, Ertel A, Gao R, et al. Functional significance of macrophage-derived exosomes in inflammation and pain. Pain. (2014) 155:1527–39. doi: 10.1016/j.pain.2014.04.029
18. Kamerkar S, LeBleu VS, Sugimoto H, Yang S, Ruivo CF, Melo SA, et al. Exosomes facilitate therapeutic targeting of oncogenic KRAS in pancreatic cancer. Nature. (2017) 546:498–503. doi: 10.1038/nature22341
19. Formicola B, Cox A, Dal Magro R, Masserini M, Re F. Nanomedicine for the treatment of Alzheimer's disease. J Biomed nanotechnology. (2019) 15:1997–2024. doi: 10.1166/jbn.2019.2837
20. Lee KS, Lee J, Lee P, Kim CU, Kim DJ, Jeong YJ, et al. Exosomes released from Shiga toxin 2a-treated human macrophages modulate inflammatory responses and induce cell death in toxin receptor expressing human cells. Cell Microbiol. (2020) 22:e13249. doi: 10.1111/cmi.13249
21. Zhu Y, Chen X, Pan Q, Wang Y, Su S, Jiang C, et al. A comprehensive proteomics analysis reveals a secretory path- and status-dependent signature of exosomes released from tumor-associated macrophages. J Proteome Res. (2015) 14:4319–31. doi: 10.1021/acs.jproteome.5b00770
22. Wang G, Jin S, Ling X, Li Y, Hu Y, Zhang Y, et al. Proteomic profiling of LPS-induced macrophage-derived exosomes indicates their involvement in acute liver injury. Proteomics. (2019) 19:e1800274. doi: 10.1002/pmic.201800274
23. Huang R, Hao C, Wang D, Zhao Q, Li C, Wang C, et al. SPP1 derived from silica-exposed macrophage exosomes triggers fibroblast transdifferentiation. Toxicol Appl Pharmacol. (2021) 422:115559. doi: 10.1016/j.taap.2021.115559
24. Haque S, Sinha N, Ranjit S, Midde NM, Kashanchi F, Kumar S. Monocyte-derived exosomes upon exposure to cigarette smoke condensate alter their characteristics and show protective effect against cytotoxicity and HIV-1 replication. Sci Rep. (2017) 7:16120. doi: 10.1038/s41598-017-16301-9
25. Yang R, Liao Y, Wang L, He P, Hu Y, Yuan D, et al. Exosomes derived from M2b macrophages attenuate DSS-induced colitis. Front Immunol. (2019) 10:2346. doi: 10.3389/fimmu.2019.02346
26. Kadiu I, Narayanasamy P, Dash PK, Zhang W, Gendelman HE. Biochemical and biologic characterization of exosomes and microvesicles as facilitators of HIV-1 infection in macrophages. J Immunol. (2012) 189:744–54. doi: 10.4049/jimmunol.1102244
27. Reales-Calderón JA, Vaz C, Monteoliva L, Molero G, Gil C. Candida albicans modifies the protein composition and size distribution of THP-1 macrophage-derived extracellular vesicles. J Proteome Res. (2017) 16:87–105. doi: 10.1021/acs.jproteome.6b00605
28. Ismail N, Wang Y, Dakhlallah D, Moldovan L, Agarwal K, Batte K, et al. Macrophage microvesicles induce macrophage differentiation and miR-223 transfer. Blood. (2013) 121:984–95. doi: 10.1182/blood-2011-08-374793
29. Belli C, Trapani D, Viale G, D'Amico P, Duso BA, Della Vigna P, et al. Targeting the microenvironment in solid tumors. Cancer Treat Rev. (2018) 65:22–32. doi: 10.1016/j.ctrv.2018.02.004
30. Molinaro R, Martinez JO, Zinger A, De Vita A, Storci G, Arrighetti N, et al. Leukocyte-mimicking nanovesicles for effective doxorubicin delivery to treat breast cancer and melanoma. Biomater Sci. (2020) 8:333–41. doi: 10.1039/C9BM01766F
31. Funes SC, Rios M, Escobar-Vera J, Kalergis AM. Implications of macrophage polarization in autoimmunity. Immunology. (2018) 154:186–95. doi: 10.1111/imm.12910
32. Liu S, Chen J, Shi J, Zhou W, Wang L, Fang W, et al. M1-like macrophage-derived exosomes suppress angiogenesis and exacerbate cardiac dysfunction in a myocardial infarction microenvironment. Basic Res Cardiol. (2020) 115:22. doi: 10.1007/s00395-020-0781-7
33. Qi Y, Zhu T, Zhang T, Wang X, Li W, Chen D, et al. M1 macrophage-derived exosomes transfer miR-222 to induce bone marrow mesenchymal stem cell apoptosis. Lab Invest. (2021) 101:1318–26. doi: 10.1038/s41374-021-00622-5
34. Wang C, Zhang C, Liu L, A X, Chen B, Li Y, et al. Macrophage-Derived mir-155-Containing Exosomes Suppress Fibroblast Proliferation and Promote Fibroblast Inflammation during Cardiac Injury. Mol Ther. (2017) 25:192–204. doi: 10.1016/j.ymthe.2016.09.001
35. van der Vorst EPC, Weber C. Novel features of monocytes and macrophages in cardiovascular biology and disease. Arteriosclerosis thrombosis Vasc Biol. (2019) 39:e30–7. doi: 10.1161/ATVBAHA.118.312002
36. Richards J, Gabunia K, Kelemen SE, Kako F, Choi ET, Autieri MV. Interleukin-19 increases angiogenesis in ischemic hind limbs by direct effects on both endothelial cells and macrophage polarization. J Mol Cell Cardiol. (2015) 79:21–31. doi: 10.1016/j.yjmcc.2014.11.002
37. Long R, Gao L, Li Y, Li G, Qin P, Wei Z, et al. M2 macrophage-derived exosomes carry miR-1271-5p to alleviate cardiac injury in acute myocardial infarction through down-regulating SOX6. Mol Immunol. (2021) 136:26–35. doi: 10.1016/j.molimm.2021.05.006
38. Wang Y, Qiu Z, Yuan J, Li C, Zhao R, Liu W, et al. Hypoxia-reoxygenation induces macrophage polarization and causes the release of exosomal miR-29a to mediate cardiomyocyte pyroptosis. In vitro cellular & developmental biology. Animal. (2021) 57:30–41. doi: 10.1007/s11626-020-00524-8
39. Dai Y, Wang S, Chang S, Ren D, Shali S, Li C, et al. M2 macrophage-derived exosomes carry microRNA-148a to alleviate myocardial ischemia/reperfusion injury via inhibiting TXNIP and the TLR4/NF-κB/NLRP3 inflammasome signaling pathway. J Mol Cell Cardiol. (2020) 142:65–79. doi: 10.1016/j.yjmcc.2020.02.007
40. Liu T, Sun YC, Cheng P, Shao HG. Adipose tissue macrophage-derived exosomal miR-29a regulates obesity-associated insulin resistance. Biochem Biophys Res Commun. (2019) 515:352–8. doi: 10.1016/j.bbrc.2019.05.113
41. Ying W, Riopel M, Bandyopadhyay G, Dong Y, Birmingham A, Seo JB, et al. Adipose tissue macrophage-derived exosomal miRNAs can modulate in vivo and in vitro insulin sensitivity. Cell. (2017) 171:372–84.e12. doi: 10.1016/j.cell.2017.08.035
42. Qian B, Yang Y, Tang N, Wang J, Sun P, Yang N, et al. M1 macrophage-derived exosomes impair beta cell insulin secretion via miR-212-5p by targeting SIRT2 and inhibiting Akt/GSK-3β/β-catenin pathway in mice. Diabetologia. (2021) 64:2037–51. doi: 10.1007/s00125-021-05489-1
43. Zhang D, Wu Y, Li Z, Chen H, Huang S, Jian C, et al. MiR-144-5p, an exosomal miRNA from bone marrow-derived macrophage in type 2 diabetes, impairs bone fracture healing via targeting Smad1. J nanobiotechnology. (2021) 19:226. doi: 10.1186/s12951-021-00964-8
44. Li M, Wang T, Tian H, Wei G, Zhao L, Shi Y. Macrophage-derived exosomes accelerate wound healing through their anti-inflammation effects in a diabetic rat model. Artif cells nanomedicine Biotechnol. (2019) 47:3793–803. doi: 10.1080/21691401.2019.1669617
45. Kim H, Wang SY, Kwak G, Yang Y, Kwon IC, Kim SH. Exosome-guided phenotypic switch of M1 to M2 macrophages for cutaneous wound healing. Advanced Sci (Weinheim Baden-Wurttemberg Germany). (2019) 6:1900513. doi: 10.1002/advs.201900513
46. Zhu QJ, Zhu M, Xu XX, Meng XM, Wu YG. Exosomes from high glucose-treated macrophages activate glomerular mesangial cells via TGF-β1/Smad3 pathway in vivo and in vitro. FASEB J. (2019) 33:9279–90. doi: 10.1096/fj.201802427RRR
47. Tian F, Tang P, Sun Z, Zhang R, Zhu D, He J, et al. miR-210 in exosomes derived from macrophages under high glucose promotes mouse diabetic obesity pathogenesis by suppressing NDUFA4 expression. J Diabetes Res. (2020) 2020:6894684. doi: 10.1155/2020/6894684
48. Huang H, Liu H, Tang J, Xu W, Gan H, Fan Q, et al. M2 macrophage-derived exosomal miR-25-3p improves high glucose-induced podocytes injury through activation autophagy via inhibiting DUSP1 expression. IUBMB Life. (2020) 72:2651–62. doi: 10.1002/iub.2393
49. Yuan D, Zhao Y, Banks WA, Bullock KM, Haney M, Batrakova E, et al. Macrophage exosomes as natural nanocarriers for protein delivery to inflamed brain. Biomaterials. (2017) 142:1–12. doi: 10.1016/j.biomaterials.2017.07.011
50. Haney MJ, Zhao Y, Jin YS, Li SM, Bago JR, Klyachko NL, et al. Macrophage-derived extracellular vesicles as drug delivery systems for triple negative breast cancer (TNBC) therapy. J Neuroimmune Pharmacol. (2020) 15:487–500. doi: 10.1007/s11481-019-09884-9
51. Zhang X, Liu L, Tang M, Li H, Guo X, Yang X. The effects of umbilical cord-derived macrophage exosomes loaded with cisplatin on the growth and drug resistance of ovarian cancer cells. Drug Dev Ind Pharm. (2020) 46:1150–62. doi: 10.1080/03639045.2020.1776320
52. Haney MJ, Klyachko NL, Zhao Y, Gupta R, Plotnikova EG, He Z, et al. Exosomes as drug delivery vehicles for Parkinson's disease therapy. J Controlled release. (2015) 207:18–30. doi: 10.1016/j.jconrel.2015.03.033
53. Li F, Zhao L, Shi Y, Liang J. Edaravone-loaded macrophage-derived exosomes enhance neuroprotection in the rat permanent middle cerebral artery occlusion model of stroke. Mol pharmaceutics. (2020) 17:3192–201. doi: 10.1021/acs.molpharmaceut.0c00245
54. Huo Q, Shi Y, Qi Y, Huang L, Sui H, Zhao L. Biomimetic silibinin-loaded macrophage-derived exosomes induce dual inhibition of Aβ aggregation and astrocyte activation to alleviate cognitive impairment in a model of Alzheimer's disease. Materials Sci engineering. C Materials Biol Appl. (2021) 129:112365. doi: 10.1016/j.msec.2021.112365
55. Gao ZS, Zhang CJ, Xia N, Tian H, Li DY, Lin JQ, et al. Berberine-loaded M2 macrophage-derived exosomes for spinal cord injury therapy. Acta biomaterialia. (2021) 126:211–23. doi: 10.1016/j.actbio.2021.03.018
56. Huang Z, Guo L, Huang L, Shi Y, Liang J, Zhao L. Baicalin-loaded macrophage-derived exosomes ameliorate ischemic brain injury via the antioxidative pathway. Materials Sci engineering. C Materials Biol Appl. (2021) 126:112123. doi: 10.1016/j.msec.2021.112123
57. Ju S, Mu J, Dokland T, Zhuang X, Wang Q, Jiang H, et al. Grape exosome-like nanoparticles induce intestinal stem cells and protect mice from DSS-induced colitis. Mol Ther. (2013) 21:1345–57. doi: 10.1038/mt.2013.64
58. Kim J, Li S, Zhang S, Wang J. Plant-derived exosome-like nanoparticles and their therapeutic activities. Asian J Pharm Sci. (2022) 17:53–69. doi: 10.1016/j.ajps.2021.05.006
59. Teng Y, Ren Y, Sayed M, Hu X, Lei C, Kumar A, et al. Plant-derived exosomal microRNAs shape the gut microbiota. Cell Host Microbe. (2018) 24:637–52. doi: 10.1016/j.chom.2018.10.001
60. Zhang M, Viennois E, Xu C, Merlin D. Plant derived edible nanoparticles as a new therapeutic approach against diseases. Tissue barriers. (2016) 4:e1134415. doi: 10.1080/21688370.2015.1134415
61. Kim SQ, Kim KH. Emergence of edible plant-derived nanovesicles as functional food components and nanocarriers for therapeutics delivery: potentials in human health and disease. Cells. (2022) 11(14):2232. doi: 10.3390/cells11142232
62. Viršilė A, Samuolienė G, Laužikė K, Šipailaitė E, Balion Z, Jekabsone A. Species-specific plant-derived nanoparticle characteristics. Plants (Basel Switzerland). (2022) 11(22):3139. doi: 10.3390/plants11223139
63. Bruno SP, Paolini A, D'Oria V, Sarra A, Sennato S, Bordi F, et al. Extracellular vesicles derived from Citrus sinensis modulate inflammatory genes and tight junctions in a human model of intestinal epithelium. Front Nutr. (2021) 8:778998. doi: 10.3389/fnut.2021.778998
64. De Robertis M, Sarra A, D'Oria V, Mura F, Bordi F, Postorino P, et al. Blueberry-derived exosome-like nanoparticles counter the response to TNF-α-induced change on gene expression in EA.hy926 cells. Biomolecules. (2020) 10(5):742. doi: 10.3390/biom10050742
65. Trentini M, Zanotti F, Tiengo E, Camponogara F, Degasperi M, Licastro D, et al. An apple a day keeps the doctor away: potential role of miRNA 146 on macrophages treated with exosomes derived from apples. Biomedicines. (2022) 10(2):415. doi: 10.3390/biomedicines10020415
66. Kahroba H, Davatgaran-Taghipour Y. Exosomal Nrf2: From anti-oxidant and anti-inflammation response to wound healing and tissue regeneration in aged-related diseases. Biochimie. (2020) 171-172:103–9. doi: 10.1016/j.biochi.2020.02.011
67. Mu J, Zhuang X, Wang Q, Jiang H, Deng ZB, Wang B, et al. Interspecies communication between plant and mouse gut host cells through edible plant derived exosome-like nanoparticles. Mol Nutr Food Res. (2014) 58:1561–73. doi: 10.1002/mnfr.201300729
68. Deng Z, Rong Y, Teng Y, Mu J, Zhuang X, Tseng M, et al. Broccoli-derived nanoparticle inhibits mouse colitis by activating dendritic cell AMP-activated protein kinase. Mol Ther. (2017) 25:1641–54. doi: 10.1016/j.ymthe.2017.01.025
69. Yi YS. New mechanisms of ginseng saponin-mediated anti-inflammatory action via targeting canonical inflammasome signaling pathways. J Ethnopharmacol. (2021) 278:114292. doi: 10.1016/j.jep.2021.114292
70. Kim J, Zhang S, Zhu Y, Wang R, Wang J. Amelioration of colitis progression by ginseng-derived exosome-like nanoparticles through suppression of inflammatory cytokines. J Ginseng Res. (2023) 47(5):627–37. doi: 10.1016/j.jgr.2023.01.004
71. Gerding DN, Johnson S, Peterson LR, Mulligan ME, Silva J Jr. Clostridium difficile-associated diarrhea and colitis. Infect Control Hosp Epidemiol. (1995) 16:459–77. doi: 10.1086/648363
72. Lei C, Mu J, Teng Y, He L, Xu F, Zhang X, et al. Lemon Exosome-like Nanoparticles-Manipulated Probiotics Protect Mice from C. d iff Infection. iScience. (2020) 23:101571. doi: 10.1016/j.isci.2020.101571
73. Chen X, Zhou Y, Yu J. Exosome-like nanoparticles from ginger rhizomes inhibited NLRP3 inflammasome activation. Mol Pharm. (2019) 16:2690–9. doi: 10.1021/acs.molpharmaceut.9b00246
74. Zhang M, Viennois E, Prasad M, Zhang Y, Wang L, Zhang Z, et al. Edible ginger-derived nanoparticles: A novel therapeutic approach for the prevention and treatment of inflammatory bowel disease and colitis-associated cancer. Biomaterials. (2016) 101:321–40. doi: 10.1016/j.biomaterials.2016.06.018
75. Sriwastva MK, Deng ZB, Wang B, Teng Y, Kumar A, Sundaram K, et al. Exosome-like nanoparticles from Mulberry bark prevent DSS-induced colitis via the AhR/COPS8 pathway. EMBO Rep. (2022) 23:e53365. doi: 10.15252/embr.202153365
76. Sundaram K, Miller DP, Kumar A, Teng Y, Sayed M, Mu J, et al. Plant-derived exosomal nanoparticles inhibit pathogenicity of porphyromonas gingivalis. iScience. (2019) 21:308–27. doi: 10.1016/j.isci.2019.10.032
77. Teng Y, Xu F, Zhang X, Mu J, Sayed M, Hu X, et al. Plant-derived exosomal microRNAs inhibit lung inflammation induced by exosomes SARS-CoV-2 Nsp12. Mol Ther. (2021) 29:2424–40. doi: 10.1016/j.ymthe.2021.05.005
78. Zhuang X, Deng ZB, Mu J, Zhang L, Yan J, Miller D, et al. Ginger-derived nanoparticles protect against alcohol-induced liver damage. J Extracell Vesicles. (2015) 4:28713. doi: 10.3402/jev.v4.28713
79. Chen X, Liu B, Li X, An TT, Zhou Y, Li G, et al. Identification of anti-inflammatory vesicle-like nanoparticles in honey. J Extracell Vesicles. (2021) 10:e12069. doi: 10.1002/jev2.12069
80. Liu B, Lu Y, Chen X, Muthuraj PG, Li X, Pattabiraman M, et al. Protective role of shiitake mushroom-derived exosome-like nanoparticles in D-galactosamine and lipopolysaccharide-induced acute liver injury in mice. Nutrients. (2020) 12(2):477. doi: 10.3390/nu12020477
81. Cong M, Tan S, Li S, Gao L, Huang L, Zhang HG, et al. Technology insight: Plant-derived vesicles-How far from the clinical biotherapeutics and therapeutic drug carriers? Advanced Drug delivery Rev. (2022) 182:114108. doi: 10.1016/j.addr.2021.114108
82. Dad HA, Gu TW, Zhu AQ, Huang LQ, Peng LH. Plant exosome-like nanovesicles: emerging therapeutics and drug delivery nanoplatforms. Mol Ther. (2021) 29:13–31. doi: 10.1016/j.ymthe.2020.11.030
83. Sung J, Yang C, Viennois E, Zhang M, Merlin D. Isolation, purification, and characterization of ginger-derived nanoparticles (GDNPs) from ginger, rhizome of Zingiber officinale. Bio Protoc. (2019) 9(19):e3390. doi: 10.21769/BioProtoc.3390
84. Wang Q, Zhuang X, Mu J, Deng ZB, Jiang H, Zhang L, et al. Delivery of therapeutic agents by nanoparticles made of grapefruit-derived lipids. Nat Commun. (2013) 4:1867. doi: 10.1038/ncomms2886
85. Wang Q, Ren Y, Mu J, Egilmez NK, Zhuang X, Deng Z, et al. Grapefruit-derived nanovectors use an activated leukocyte trafficking pathway to deliver therapeutic agents to inflammatory tumor sites. Cancer Res. (2015) 75:2520–9. doi: 10.1158/0008-5472.CAN-14-3095
86. Wang B, Zhuang X, Deng ZB, Jiang H, Mu J, Wang Q, et al. Targeted drug delivery to intestinal macrophages by bioactive nanovesicles released from grapefruit. Mol Ther. (2014) 22:522–34. doi: 10.1038/mt.2013.190
87. Zhang M, Xiao B, Wang H, Han MK, Zhang Z, Viennois E, et al. Edible ginger-derived nano-lipids loaded with doxorubicin as a novel drug-delivery approach for colon cancer therapy. Mol Ther. (2016) 24:1783–96. doi: 10.1038/mt.2016.159
88. Zhang M, Wang X, Han MK, Collins JF, Merlin D. Oral administration of ginger-derived nanolipids loaded with siRNA as a novel approach for efficient siRNA drug delivery to treat ulcerative colitis. Nanomedicine (Lond). (2017) 12:1927–43. doi: 10.2217/nnm-2017-0196
89. Alsaweed M, Lai CT, Hartmann PE, Geddes DT, Kakulas F. Human milk miRNAs primarily originate from the mammary gland resulting in unique miRNA profiles of fractionated milk. Sci Rep. (2016) 6:20680. doi: 10.1038/srep20680
90. van der Pol E, Böing AN, Harrison P, Sturk A, Nieuwland R. Classification, functions, and clinical relevance of extracellular vesicles. Pharmacol Rev. (2012) 64:676–705. doi: 10.1124/pr.112.005983
91. Benmoussa A, Gotti C, Bourassa S, Gilbert C, Provost P. Identification of protein markers for extracellular vesicle (EV) subsets in cow's milk. J Proteomics. (2019) 192:78–88. doi: 10.1016/j.jprot.2018.08.010
92. Munagala R, Aqil F, Jeyabalan J, Gupta RC. Bovine milk-derived exosomes for drug delivery. Cancer Lett. (2016) 371:48–61. doi: 10.1016/j.canlet.2015.10.020
93. Benmoussa A, Provost P. Milk microRNAs in health and disease. Compr Rev Food Sci Food Saf. (2019) 18:703–22. doi: 10.1111/1541-4337.12424
94. Pieters BC, Arntz OJ, Bennink MB, Broeren MG, van Caam AP, Koenders MI, et al. Commercial cow milk contains physically stable extracellular vesicles expressing immunoregulatory TGF-β. PloS One. (2015) 10:e0121123. doi: 10.1371/journal.pone.0121123
95. Baier SR, Nguyen C, Xie F, Wood JR, Zempleni J. MicroRNAs are absorbed in biologically meaningful amounts from nutritionally relevant doses of cow milk and affect gene expression in peripheral blood mononuclear cells, HEK-293 kidney cell cultures, and mouse livers. J Nutr. (2014) 144:1495–500. doi: 10.3945/jn.114.196436
96. Golan-Gerstl R, Elbaum Shiff Y, Moshayoff V, Schecter D, Leshkowitz D, Reif S. Characterization and biological function of milk-derived miRNAs. Mol Nutr Food Res. (2017) 61(10). doi: 10.1002/mnfr.201700009
97. Hock A, Miyake H, Li B, Lee C, Ermini L, Koike Y, et al. Breast milk-derived exosomes promote intestinal epithelial cell growth. J Pediatr Surg. (2017) 52:755–9. doi: 10.1016/j.jpedsurg.2017.01.032
98. Martin C, Patel M, Williams S, Arora H, Brawner K, Sims B. Human breast milk-derived exosomes attenuate cell death in intestinal epithelial cells. Innate Immun. (2018) 24:278–84. doi: 10.1177/1753425918785715
99. Reif S, Elbaum Shiff Y, Golan-Gerstl R. Milk-derived exosomes (MDEs) have a different biological effect on normal fetal colon epithelial cells compared to colon tumor cells in a miRNA-dependent manner. J Trans Med. (2019) 17:325. doi: 10.1186/s12967-019-2072-3
100. Du C, Quan S, Zhao Y, Nan X, Chen R, Tang X, et al. Bovine milk-derived extracellular vesicles prevent gut inflammation by regulating lipid and amino acid metabolism. Food Funct. (2023) 14:2212–22. doi: 10.1039/D2FO03975C
101. Melnik BC, Stremmel W, Weiskirchen R, John SM, Schmitz G. Exosome-derived microRNAs of human milk and their effects on infant health and development. Biomolecules. (2021) 11(6):851. doi: 10.3390/biom11060851
102. He S, Liu G, Zhu X. Human breast milk-derived exosomes may help maintain intestinal epithelial barrier integrity. Pediatr Res. (2021) 90:366–72. doi: 10.1038/s41390-021-01449-y
103. Mecocci S, Ottaviani A, Razzuoli E, Fiorani P, Pietrucci D, De Ciucis CG, et al. Cow milk extracellular vesicle effects on an in vitro model of intestinal inflammation. Biomedicines. (2022) 10(3):570. doi: 10.3390/biomedicines10030570
104. Nordgren TM, Heires AJ, Zempleni J, Swanson BJ, Wichman C, Romberger DJ. Bovine milk-derived extracellular vesicles enhance inflammation and promote M1 polarization following agricultural dust exposure in mice. J Nutr Biochem. (2019) 64:110–20. doi: 10.1016/j.jnutbio.2018.10.017
105. Liu Q, Hao H, Li J, Zheng T, Yao Y, Tian X, et al. Oral administration of bovine milk-derived extracellular vesicles attenuates cartilage degeneration via modulating gut microbiota in DMM-induced mice. Nutrients. (2023) 15(3):747. doi: 10.3390/nu15030747
106. Zhong Y, Wang X, Zhao X, Shen J, Wu X, Gao P, et al. Multifunctional milk-derived small extracellular vesicles and their biomedical applications. Pharmaceutics. (2023) 15(5):1418. doi: 10.3390/pharmaceutics15051418
107. Shandilya S, Rani P, Onteru SK, Singh D. Small interfering RNA in milk exosomes is resistant to digestion and crosses the intestinal barrier. In Vitro. J Agric Food Chem. (2017) 65:9506–13. doi: 10.1021/acs.jafc.7b03123
108. Zhong J, Xia B, Shan S, Zheng A, Zhang S, Chen J, et al. High-quality milk exosomes as oral drug delivery system. Biomaterials. (2021) 277:121126. doi: 10.1016/j.biomaterials.2021.121126
109. Yan C, Chen J, Wang C, Yuan M, Kang Y, Wu Z, et al. Milk exosomes-mediated miR-31-5p delivery accelerates diabetic wound healing through promoting angiogenesis. Drug Delivery. (2022) 29:214–28. doi: 10.1080/10717544.2021.2023699
110. Pieters BCH, Arntz OJ, Aarts J, Feitsma AL, van Neerven RJJ, van der Kraan PM, et al. Bovine milk-derived extracellular vesicles inhibit catabolic and inflammatory processes in cartilage from osteoarthritis patients. Mol Nutr Food Res. (2022) 66:e2100764. doi: 10.1002/mnfr.202100764
111. Del Pozo-Acebo L, Hazas MLL, Tomé-Carneiro J, Gil-Cabrerizo P, San-Cristobal R, Busto R, et al. Bovine milk-derived exosomes as a drug delivery vehicle for miRNA-based therapy. Int J Mol Sci. (2021) 22(3):1105. doi: 10.3390/ijms22031105
112. Pittenger MF, Discher DE, Péault BM, Phinney DG, Hare JM, Caplan AI. Mesenchymal stem cell perspective: cell biology to clinical progress. NPJ Regenerative Med. (2019) 4:22. doi: 10.1038/s41536-019-0083-6
113. Shi Y, Du L, Lin L, Wang Y. Tumour-associated mesenchymal stem/stromal cells: emerging therapeutic targets. Nature reviews. Drug Discovery. (2017) 16:35–52. doi: 10.1038/nrd.2016.193
114. Raposo G, Stoorvogel W. Extracellular vesicles: exosomes, microvesicles, and friends. J Cell Biol. (2013) 200:373–83. doi: 10.1083/jcb.201211138
115. Lou G, Chen Z, Zheng M, Liu Y. Mesenchymal stem cell-derived exosomes as a new therapeutic strategy for liver diseases. Exp Mol Med. (2017) 49:e346. doi: 10.1038/emm.2017.63
116. Cho BS, Kim JO, Ha DH, Yi YW. Exosomes derived from human adipose tissue-derived mesenchymal stem cells alleviate atopic dermatitis. Stem Cell Res Ther. (2018) 9:187. doi: 10.1186/s13287-018-0939-5
117. Bazzoni R, Takam Kamga P, Tanasi I, Krampera M. Extracellular vesicle-dependent communication between mesenchymal stromal cells and immune effector cells. Front Cell Dev Biol. (2020) 8:596079. doi: 10.3389/fcell.2020.596079
118. Ohara M, Ohnishi S, Hosono H, Yamamoto K, Yuyama K, Nakamura H, et al. Extracellular vesicles from amnion-derived mesenchymal stem cells ameliorate hepatic inflammation and fibrosis in rats. Stem Cells Int. (2018) 2018:3212643. doi: 10.1155/2018/3212643
119. Watanabe T, Tsuchiya A, Takeuchi S, Nojiri S, Yoshida T, Ogawa M, et al. Development of a non-alcoholic steatohepatitis model with rapid accumulation of fibrosis, and its treatment using mesenchymal stem cells and their small extracellular vesicles. Regener Ther. (2020) 14:252–61. doi: 10.1016/j.reth.2020.03.012
120. Kataru RP, Jung K, Jang C, Yang H, Schwendener RA, Baik JE, et al. Critical role of CD11b+ macrophages and VEGF in inflammatory lymphangiogenesis, antigen clearance, and inflammation resolution. Blood. (2009) 113:5650–9. doi: 10.1182/blood-2008-09-176776
121. Zhao H, Shang Q, Pan Z, Bai Y, Li Z, Zhang H, et al. Exosomes from adipose-derived stem cells attenuate adipose inflammation and obesity through polarizing M2 macrophages and Beiging in white adipose tissue. Diabetes. (2018) 67:235–47. doi: 10.2337/db17-0356
122. El-Derany MO, AbdelHamid SG. Upregulation of miR-96-5p by bone marrow mesenchymal stem cells and their exosomes alleviate non-alcoholic steatohepatitis: Emphasis on caspase-2 signaling inhibition. Biochem Pharmacol. (2021) 190:114624. doi: 10.1016/j.bcp.2021.114624
123. Tamura R, Uemoto S, Tabata Y. Immunosuppressive effect of mesenchymal stem cell-derived exosomes on a concanavalin A-induced liver injury model. Inflammation Regener. (2016) 36:26. doi: 10.1186/s41232-016-0030-5
124. Lu FB, Chen DZ, Chen L, Hu ED, Wu JL, Li H, et al. Attenuation of experimental autoimmune hepatitis in mice with bone mesenchymal stem cell-derived exosomes carrying microRNA-223-3p. Mol Cells. (2019) 42:906–18. doi: 10.14348/molcells.2019.2283
125. Chen L, Lu FB, Chen DZ, Wu JL, Hu ED, Xu LM, et al. BMSCs-derived miR-223-containing exosomes contribute to liver protection in experimental autoimmune hepatitis. Mol Immunol. (2018) 93:38–46. doi: 10.1016/j.molimm.2017.11.008
126. Zhang S, Jiang L, Hu H, Wang H, Wang X, Jiang J, et al. Pretreatment of exosomes derived from hUCMSCs with TNF-α ameliorates acute liver failure by inhibiting the activation of NLRP3 in macrophage. Life Sci. (2020) 246:117401. doi: 10.1016/j.lfs.2020.117401
127. Shao M, Xu Q, Wu Z, Chen Y, Shu Y, Cao X, et al. Exosomes derived from human umbilical cord mesenchymal stem cells ameliorate IL-6-induced acute liver injury through miR-455-3p. Stem Cell Res Ther. (2020) 11:37. doi: 10.1186/s13287-020-1550-0
128. Jiang W, Tan Y, Cai M, Zhao T, Mao F, Zhang X, et al. Human umbilical cord MSC-derived exosomes suppress the development of CCl(4)-induced liver injury through antioxidant effect. Stem Cells Int. (2018) 2018:6079642. doi: 10.1155/2018/6079642
129. Zhao S, Liu Y, Pu Z. Bone marrow mesenchymal stem cell-derived exosomes attenuate D-GaIN/LPS-induced hepatocyte apoptosis by activating autophagy in vitro. Drug Des Devel Ther. (2019) 13:2887–97. doi: 10.2147/DDDT
130. Damania A, Jaiman D, Teotia AK, Kumar A. Mesenchymal stromal cell-derived exosome-rich fractionated secretome confers a hepatoprotective effect in liver injury. Stem Cell Res Ther. (2018) 9:31. doi: 10.1186/s13287-017-0752-6
131. Bruno S, Pasquino C, Herrera Sanchez MB, Tapparo M, Figliolini F, Grange C, et al. HLSC-derived extracellular vesicles attenuate liver fibrosis and inflammation in a murine model of non-alcoholic steatohepatitis. Mol Ther. (2020) 28:479–89. doi: 10.1016/j.ymthe.2019.10.016
132. Mardpour S, Hassani SN, Mardpour S, Sayahpour F, Vosough M, Ai J, et al. Extracellular vesicles derived from human embryonic stem cell-MSCs ameliorate cirrhosis in thioacetamide-induced chronic liver injury. J Cell Physiol. (2018) 233:9330–44. doi: 10.1002/jcp.26413
133. Li T, Yan Y, Wang B, Qian H, Zhang X, Shen L, et al. Exosomes derived from human umbilical cord mesenchymal stem cells alleviate liver fibrosis. Stem Cells Dev. (2013) 22:845–54. doi: 10.1089/scd.2012.0395
134. Zheng J, Lu T, Zhou C, Cai J, Zhang X, Liang J, et al. Extracellular vesicles derived from human umbilical cord mesenchymal stem cells protect liver ischemia/reperfusion injury by reducing CD154 expression on CD4+ T cells via CCT2. Adv Sci (Weinh). (2020) 7:1903746. doi: 10.1002/advs.201903746
135. Shen X, Wang Y, Gao F, Ren F, Busuttil RW, Kupiec-Weglinski JW, et al. CD4 T cells promote tissue inflammation via CD40 signaling without de novo activation in a murine model of liver ischemia/reperfusion injury. Hepatology. (2009) 50:1537–46. doi: 10.1002/hep.23153
136. Xie K, Liu L, Chen J, Liu F. Exosomes derived from human umbilical cord blood mesenchymal stem cells improve hepatic ischemia reperfusion injury via delivering miR-1246. Cell Cycle. (2019) 18:3491–501. doi: 10.1080/15384101.2019.1689480
137. Arslan F, Lai RC, Smeets MB, Akeroyd L, Choo A, Aguor EN, et al. Mesenchymal stem cell-derived exosomes increase ATP levels, decrease oxidative stress and activate PI3K/Akt pathway to enhance myocardial viability and prevent adverse remodeling after myocardial ischemia/reperfusion injury. Stem Cell Res. (2013) 10:301–12. doi: 10.1016/j.scr.2013.01.002
138. Liu L, Jin X, Hu CF, Li R, Zhou Z, Shen CX. Exosomes derived from mesenchymal stem cells rescue myocardial Ischaemia/reperfusion injury by inducing cardiomyocyte autophagy via AMPK and Akt pathways. Cell Physiol Biochem. (2017) 43:52–68. doi: 10.1159/000480317
139. Cui GH, Wu J, Mou FF, Xie WH, Wang FB, Wang QL, et al. Exosomes derived from hypoxia-preconditioned mesenchymal stromal cells ameliorate cognitive decline by rescuing synaptic dysfunction and regulating inflammatory responses in APP/PS1 mice. FASEB J. (2018) 32:654–68. doi: 10.1096/fj.201700600R
140. Cao L, Xu H, Wang G, Liu M, Tian D, Yuan Z. Extracellular vesicles derived from bone marrow mesenchymal stem cells attenuate dextran sodium sulfate-induced ulcerative colitis by promoting M2 macrophage polarization. Int Immunopharmacol. (2019) 72:264–74. doi: 10.1016/j.intimp.2019.04.020
141. Yang J, Liu XX, Fan H, Tang Q, Shou ZX, Zuo DM, et al. Extracellular Vesicles Derived from Bone Marrow Mesenchymal Stem Cells Protect against Experimental Colitis via Attenuating Colon Inflammation, Oxidative Stress and Apoptosis. PloS One. (2015) 10:e0140551. doi: 10.1371/journal.pone.0140551
142. Miao C, Wang X, Zhou W, Huang J. The emerging roles of exosomes in autoimmune diseases, with special emphasis on microRNAs in exosomes. Pharmacol Res. (2021) 169:105680. doi: 10.1016/j.phrs.2021.105680
143. Zhou J, Lin Y, Kang X, Liu Z, Zhang W, Xu F. microRNA-186 in extracellular vesicles from bone marrow mesenchymal stem cells alleviates idiopathic pulmonary fibrosis via interaction with SOX4 and DKK1. Stem Cell Res Ther. (2021) 12:96. doi: 10.1186/s13287-020-02083-x
144. Wang B, Yao K, Huuskes BM, Shen HH, Zhuang J, Godson C, et al. Mesenchymal stem cells deliver exogenous microRNA-let7c via exosomes to attenuate renal fibrosis. Mol Ther. (2016) 24:1290–301. doi: 10.1038/mt.2016.90
145. Zhang B, Wang M, Gong A, Zhang X, Wu X, Zhu Y, et al. HucMSC-exosome mediated-Wnt4 signaling is required for cutaneous wound healing. Stem Cells. (2015) 33:2158–68. doi: 10.1002/stem.1771
146. Lou G, Yang Y, Liu F, Ye B, Chen Z, Zheng M, et al. MiR-122 modification enhances the therapeutic efficacy of adipose tissue-derived mesenchymal stem cells against liver fibrosis. J Cell Mol Med. (2017) 21:2963–73. doi: 10.1111/jcmm.13208
147. Qu Y, Zhang Q, Cai X, Li F, Ma Z, Xu M, et al. Exosomes derived from miR-181-5p-modified adipose-derived mesenchymal stem cells prevent liver fibrosis via autophagy activation. J Cell Mol Med. (2017) 21:2491–502. doi: 10.1111/jcmm.13170
148. Tang M, Chen Y, Li B, Sugimoto H, Yang S, Yang C, et al. Therapeutic targeting of STAT3 with small interference RNAs and antisense oligonucleotides embedded exosomes in liver fibrosis. FASEB J. (2021) 35:e21557. doi: 10.1096/fj.202002777RR
149. Zhao J, Li Y, Jia R, Wang J, Shi M, Wang Y. Mesenchymal stem cells-derived exosomes as dexamethasone delivery vehicles for autoimmune hepatitis therapy. Front Bioeng Biotechnol. (2021) 9:650376. doi: 10.3389/fbioe.2021.650376
150. Wang S, Li L, Liu T, Jiang W, Hu X. miR-19a/19b-loaded exosomes in combination with mesenchymal stem cell transplantation in a preclinical model of myocardial infarction. Regener Med. (2020) 15:1749–59. doi: 10.2217/rme-2019-0136
151. Wu R, Fan X, Wang Y, Shen M, Zheng Y, Zhao S, et al. Mesenchymal stem cell-derived extracellular vesicles in liver immunity and therapy. Front Immunol. (2022) 13:833878. doi: 10.3389/fimmu.2022.833878
152. Yi Q, Xu Z, Thakur A, Zhang K, Liang Q, Liu Y, et al. Current understanding of plant-derived exosome-like nanoparticles in regulating the inflammatory response and immune system microenvironment. Pharmacol Res. (2023) 190:106733. doi: 10.1016/j.phrs.2023.106733
Keywords: extracellular vesicles, inflammatory, plant-derived EVs, milk-derived EVs, MSC-derived EVs, macrophage-derived EVs
Citation: Lou K, Luo H, Jiang X and Feng S (2024) Applications of emerging extracellular vesicles technologies in the treatment of inflammatory diseases. Front. Immunol. 15:1364401. doi: 10.3389/fimmu.2024.1364401
Received: 02 January 2024; Accepted: 04 March 2024;
Published: 13 March 2024.
Edited by:
Fabien Touzot, University of Montreal, CanadaReviewed by:
Sadiq Umar, University of Illinois Chicago, United StatesZhenhua Li, Brigham and Women’s Hospital and Harvard Medical School, United States
Copyright © 2024 Lou, Luo, Jiang and Feng. This is an open-access article distributed under the terms of the Creative Commons Attribution License (CC BY). The use, distribution or reproduction in other forums is permitted, provided the original author(s) and the copyright owner(s) are credited and that the original publication in this journal is cited, in accordance with accepted academic practice. No use, distribution or reproduction is permitted which does not comply with these terms.
*Correspondence: Shangzhi Feng, MTEzMTcxODI2NkBxcS5jb20=