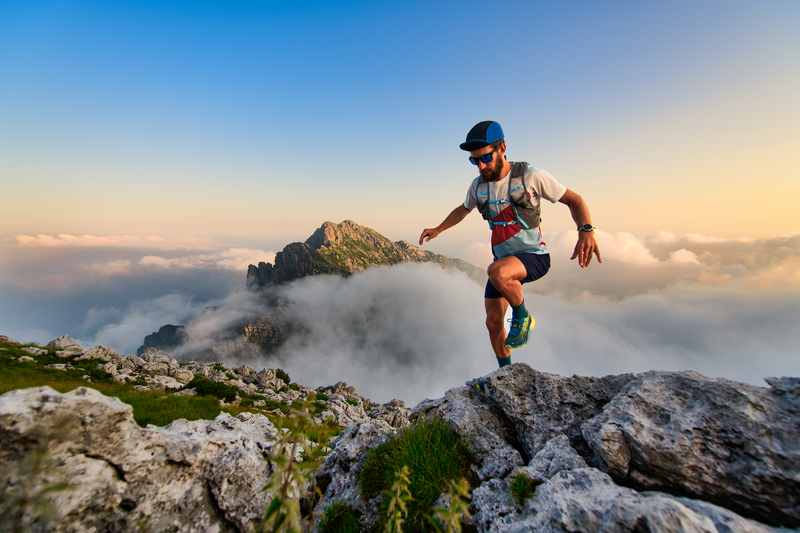
95% of researchers rate our articles as excellent or good
Learn more about the work of our research integrity team to safeguard the quality of each article we publish.
Find out more
REVIEW article
Front. Immunol. , 10 April 2024
Sec. Cancer Immunity and Immunotherapy
Volume 15 - 2024 | https://doi.org/10.3389/fimmu.2024.1363185
This article is part of the Research Topic Exosome-Derived Cargos in Immune Microenvironment View all 9 articles
Extracellular vesicles (EVs) have important roles as mediators of cell-to-cell communication, with physiological functions demonstrated in various in vivo models. Despite advances in our understanding of the biological function of EVs and their potential for use as therapeutics, there are limitations to the clinical approaches for which EVs would be effective. A primary determinant of the biodistribution of EVs is the profile of proteins and other factors on the surface of EVs that define the tropism of EVs in vivo. For example, proteins displayed on the surface of EVs can vary in composition by cell source of the EVs and the microenvironment into which EVs are delivered. In addition, interactions between EVs and recipient cells that determine uptake and endosomal escape in recipient cells affect overall systemic biodistribution. In this review, we discuss the contribution of the EV donor cell and the role of the microenvironment in determining EV tropism and thereby determining the uptake and biological activity of EVs.
Extracellular vesicles (EVs) are observed in most biological fluids and are notable for their wide range of sizes, payloads, and biological activities. As defined by the MISEV 2023 guidelines, we focus here primarily on small EVs that are under 200 nm (1–5). EVs convey protein, lipid, and RNA payloads to surrounding or distant recipient cells (6–8). EV-mediated physiological processes affect not only recipient cells but also the surrounding microenvironment, depending on the heterogeneity of EVs and, consequently, regulate many physiological systems including the immune system (9, 10). EVs that are secreted by both non-immune cells and immune cells mediate immune stimulation or suppression. Tumor-derived EVs contain specific markers, which induce phenotypic changes in recipient cells. For example, EVs derived from immune cells, specifically antigen-presenting cells (APCs), carry cargos that can directly induce the peripheral immune response (11). Studies of EV-mediated immune regulation provide insights for therapeutic approaches to treat cancer (12, 13), aging (14) as well as inflammatory diseases (15, 16). These reports demonstrate the potential of EVs as physiologically relevant therapeutics that modulate recipient cells through luminal or transmembrane payload delivery.
This review focuses on the specific challenges of engineering EVs to express essential surface molecules to direct tropism. There have been several excellent recent reviews that provide details on EVs as therapeutics in wound healing (17). EVs are naturally occurring nanoparticles that are generally biocompatible and efficient therapeutics in various systems that provide a foundation to design and deliver novel cargoes for next-generation therapeutics. This review addresses the importance of engineering tropism into the design of EVs that are relevant for biodistribution that we argue is important because there few clinical studies with engineered EVs.
Along with the potential for EVs to deliver therapeutic cargo, several engineering strategies have been reported for luminal loading or surface display of functional proteins or RNA. EVs can be engineered with known or screened cargo and enhance the therapeutic efficacy in disease models (18–21). Here, we review cell and organ tropism of EVs depending on the source and cellular target, and engineering strategies for EV surface display for targeting delivery.
In addition to differences in EV payloads, which include proteins (i.e., enzymes, cytokines, growth factors, and their receptors) and nucleic acids (mRNA, miRNA, and DNAs), the lipid composition of EVs is determined by the cell source (22). While many studies identify novel proteins that mediate tropism, existing datasets like those available from ExoCarta (ExoCarta.org) can be mined to establish relevance from various tissues, cell types, and experimental models. These molecular signatures provide insight into the interactions of EVs with target cells and its biological activity. For example, EVs from different cell sources produce specific transcriptional responses in recipient cells. Specifically, mesenchymal stem cell (MSC)–derived EVs induce wound healing, epithelial cell–derived EVs up-regulate cell adhesion, immune cell–derived EVs activate inflammatory genes, and most EVs associate with recipient fibroblasts (18). EV composition is determined largely by the type and physiological state of the producer cells (19). EVs show a similar lipid profile as the cell that produced them, including glycosphingolipid, phospholipid, and cholesterol composition (20, 21, 23). Proteins displayed on the surface of nearly all EVs include Alix, tetraspanins (CD9, CD63, and CD81), heat shock protein 70 (24, 25), and other proteins that are often associated with specific EV subsets (24, 26, 27).
Protein interactions at the interface between EVs and recipient cells provide the basis for EV uptake and tropism. For example, enzymatic depletion of heparan sulfate proteoglycans (HSPGs) on the cell surface or inhibition of endogenous proteoglycan synthesis attenuated uptake of cancer cell–derived EVs (28). These reports show that EV uptake is mediated by protein-protein interactions between EVs and recipient cells, including immune cells (29, 30). Digestion of surface protein by proteinase K shows significantly decreased EV uptake (31–33) providing examples of how surface proteins mediate interactions with recipient cells.
Integrin and tetraspanin proteins, canonical components of EVs, mediate the interaction of the EV with recipient cell membranes (Figure 1A and Table 1). Alpha and beta subunits in the heterodimeric integrin complex determine the specificity for binding to the extracellular matrix (45, 46). There are over 18 alpha-subunit variants and eight beta-subunit variants in the integrin family. Circulating platelet–derived EVs interact with integrin αM (CD11b) and Tyro3, Axl, and Mer (TAM) tyrosine kinase receptors on the monocytes and granulocytes in a Ca2+-dependent manner (34). Exosomal integrin αvβ6 can regulate the M2 inflammatory response of CD14+ monocytes (35). Colorectal cancer cells release integrin β-like 1-rich EVs to the circulation in the blood stream to activate resident fibroblasts in remote organs. These activated fibroblasts induce formation of the pre-metastatic niche and promote metastasis by secreting pro-inflammatory cytokines (36). Exosomal integrins α6β4, α6β1, and αvβ5 bind lung fibroblasts, epithelial cells, and liver-resident macrophages, respectively, and promote inflammatory responses in recipient cells (37). Tropism of palmitic acid–stimulated hepatocyte-derived EVs to hepatic stellate cells is an example of a biochemical modification that promotes EV tropism based on increased expression of integrins αvβ3 and α8β1 (47). EVs derived from bone-tropic breast cancer show a high expression of the integrin-binding sialoprotein. These stimulated EVs have tropism to osteoclasts and promote bone metastasis (38). The integrin αv-VAMP complex–associated protein A (VAPA) on hepatocellular carcinoma–derived EVs promotes bone metastasis. EV-mediated integrin–VAPA complexes are activated in the plasma membrane of recipient osteoclasts and result in actin cytoskeleton formation in osteoclasts (39).
Figure 1 Schematic illustration of representative EV tropism and modifications based on surface display (created with BioRender.com). (A) Surface display depending on the cell source determines EVs tropism. (B) Surface display targeting EV tropism by endogenous expression. (C) Summary of engineering methods for EV surface modification.
Tetraspanins function as integral membrane proteins and are widely studied as canonical EV markers that also provide insights into how they may be biologically active. For example, tetraspanin 8 (Tspan8), a member of the tetraspanin family of proteins, induces cellular tropism by forming a complex with other tetraspanins (CD9 and CD81) or integrins (α4β4) on the EV membrane. EVs expressing the Tspan8–integrin α4 complex show selectivity for CD54 as a major ligand on endothelial and pancreatic cells (40). CD49d (integrin α4) and CD106 (vascular cell adhesion molecule 1) on EVs recruited by Tspan8 induce cellular uptake by aortic endothelial cells, and CD151 and CD49c (integrin α3) are implicated in EV uptake by lung-resident fibroblast (41). Uptake of EVs by spleen-resident dendritic cells (DCs) is mediated via tetraspanins (CD9 and CD81), milk fat globule (MFG)–E8/lactadherin, and phosphatidylserine on the EVs and integrin αvβ3, integrin αL (CD11a), and CD54 (intercellular adhesion molecule 1) on DCs (42). Suppressing CD9 action with function-blocking antibodies decreases EV uptake and cargo transfer to recipient by endocytosis (48). Cell surface CD9 also can mediate the aggressiveness of pancreatic tumor cell interactions with annexin A6+ (ANXA6+) EVs. These EVs are derived from cancer-associated stromal fibroblasts, which induce the MAPK pathway, cell migration, and epithelial-to-mesenchymal transition (43). In contrast, lack of CD9 expression on EVs produced by colorectal cancer cells enhanced interaction between integrin αvβ1 and its ligand ADAM17 and uptake by recipient cells (44). These reports suggest that integrins, tetraspanins, and their ligands are involved in the tropism of EVs. In the context of understanding the functional role of tetraspanins in the biogenesis of EVs, several reports have shown that increased expression of tetraspanins increases the number of EVs formed (49), whereas knockdown of tetraspanins has led to minimal reductions in EV formation (50, 51). Therefore, more studies are required to understand the function of specific tetraspanins in inducible and cell-type–specific genetic systems to better define their role in intercellular signaling vs. EV biogenesis (52).
EVs have been demonstrated to improve cellular processes associated with wound healing, including proliferation, migration, and angiogenesis. Evidence of this progress is a phase I clinical trial of healthy volunteer adults that is currently underway to evaluate safety in the context of wound healing (53, 54). MSC-derived EVs have been shown to be involved in reduction of échelle d’évaluation clinique des cicatrices d’acné (ECCA) score, a measure of scarring, by about 12.6% more than a control group in a small sample set of 25 patients (53). Platelet-derived EVs show evidence for the safety and therapeutic utility (54). Several reports have focused on their role as growth factors in tissue repair and regeneration. Vascular endothelial growth factor A (VEGF-A), fibroblast growth factor 2 (FGF-2), hepatocyte growth factor, and platelet-derived growth factor are key growth factors that are known to promote wound healing in various animal models (55, 56). More recently, it has been proposed that EVs can deliver specific growth factors that may facilitate their biodistribution and overall pro-reparative activity (57). For example, it has been reported that EVs with growth factor payloads can improve tissue regeneration when displayed on the surface of EVs. FGF-2 bound EVs secreted from dermal fibroblasts activate the extracellular signal-regulated kinase (ERK) and signal transducers and activators of transcription (STAT) signaling pathways and enhance cell proliferation and migration in recipient cells (58). Endogenous expression of VEGF and transcription factor EB on EVs derived from endothelial cells improves vascularization, attenuates muscle injury, and improves recovery of limb function through VEGF/Vascular endothelial growth factor receptor (VEGFR) pathway and autophagy-lysosome pathway in critical limb ischemia mice model (59). In other studies, adipose tissue–derived EVs that overexpressed VEGF fused with CD63 on the surface showed increased tube formation, cell proliferation, and migration of lymphatic endothelial cells. Continuous EV treatment rescues lymphedema in a mouse model indicating controlled EV kinetics can be stable in vivo (60). Bone morphogenetic protein 2 displayed on EVs derived from bone marrow–derived MSC enhances osteogenic differentiation while maintaining the function of bone marrow MSC EVs (61). These studies are consistent with a role for growth factors on EVs in promoting tissue repair and regeneration and support the further refinement of the loading and display of specific growth factors targeting specific cell types relevant in injury and tissue repair (62).
In addition to the display and expression of known peptides like growth factors, phage display can be used for the discovery of novel peptides that are focused on naturally occurring peptides that can be displayed on EVs to target recipient cells. For example, expression of a fusion of the cardiomyocyte binding peptide with Lamp2b on EVs results in increased efficiency of EV uptake into cardiomyocytes and decreased cardiomyocyte apoptosis associated with higher cell retention (63). A fusion of the Lamp2b protein with the ischemic myocardium targeting peptide (CSTSMLKAC) expressed on MSC-derived EVs reduced fibrosis and enhanced vascular neogenesis, and cardiac function could be monitored in ischemic heart tissue (64). The therapeutic potential of EVs has been observed in skin repair (65) as well as in kidney (66). These studies show the promise in designing engineered EVs to produce a synergistic effect on wound healing and tissue regeneration by expression of growth factor EVs.
Expression of peptides on EVs has been explored as a mechanism to target EVs to tumor cells. For example, interleukin-3 (IL-3) expressed on the surface of EVs can be used to target the delivery of EVs to the IL-3 receptor that is highly expressed in chronic myelogenous leukemia cells compared with that in normal hematopoietic cells (67). High expression of EGFR on tumors of epithelial origin suggests that the EGF-binding peptide, nanobody, and aptamer (68–70) on the surface of EVs could be used to target the delivery of EVs for uptake by epithelial tumor cells. CD19 (71), CD20 (72), integrin (73), and other ligands are known to be highly expressed on tumor cells and could potentially be used to target EVs to these cells. In an alternative approach, EVs may be useful for modifying the immune microenvironment for cancer immunotherapy (74, 75). TNFR1 stimulation by tumor necrosis factor alpha (TNF-α)–decorated EVs can induce activation of necroptosis and cell death in cancer (76). Targeting the APCs via EVs provides specific antigens for cancer therapy. For example, APCs targeting EVs derived from tumor cells provide antigen to APCs cells, and it could enhance tumor recognition (77, 78). Reprogramming of immune cells by immune cell–targeting EVs shows the potential of EVs as a novel immunotherapeutic agent (79, 80). EVs derived from macrophages can modulate the immune microenvironment of tumors. Reprogramming of tumor-associated macrophages (TAMs) to a M1 macrophage via targeting TAMs or tumor cells by engineered M1-derived EVs decorating IL-4 receptor or CD47 via postinsertion of targeting peptide increased anti-tumor immunity (81, 82). These reports show the potential of cancer treatment through a direct tumor targeting strategy loaded with anti-cancer drug and control of the surrounding immune microenvironment through modification of EV surface display (Figure 1B).
EV-mediated cargo delivery and EV characteristics such as toxicity, immunogenicity, and engineerability are being tested in various diseases models as a cell-free therapy (83, 84). Exploring the feasibility of engineering the surface composition of EVs is important to enable cell or organ specific delivery and in vivo tracking (85, 86). Therapeutic approaches to treat cancer therapy based on cell source through engineered EV are also being explored (87). Several approaches to introduce targeting moieties are being applied directly to EVs or indirectly to cells (88).
In addition to the use of phage display to target cardiomyocytes, this approach can be used for various other cell types to screen for peptides that have cell-type–specific affinity. In the example of targeting ischemic cardiac tissue, phage display was performed with a fusion with Lamp2b protein on the surface of EVs (64). EVs engineered with silk fibroin patch (SFP)–biding peptides that were identified by phage display showed dose-dependent accumulation of the SFP and enhanced wound closured efficacy in the diabetic wound model (89). CD9 and CD63 are known as EVs markers, and CD63 is a well-known EV scaffold protein that is often used to endogenously express or decorate foreign peptide (29, 90). Endogenous display of target binding peptides via CD63 shows stable expression and specificity. For example, expression of glycosylation domains on the large extracellular loop of CD63 show specificity toward endothelial cells and DCs (62, 91). Screened peptides identified by phage display were decorated using CP05, which directly bind to the CD63 second loop (92). As another approach, transgenic mice expressing Eµ-myc were used to select for a Eµ-myc B lymphoma–specific phage sequence through several bio-panning cycles after intravenous injection. The malignant B cell–specific sequence recognizes cancer-derived exosomes (93). Together, several studies have shown the power of phage display to identify target-specific binding peptides that can be engineered onto EVs.
Direct modification of the EV surface (Figure 1C) using hydrophobic anchors, rather than endogenous expression, also exists. Cholesterol is also one component of EV membrane, which spontaneously inserts into the membrane via its hydrophobic moiety (94), and cholesterol-modified RNA structures can be displayed on the EV surface that improves the efficiency of delivery of the modified EVs (68). Similarly, ceramide and dioleoyl phosphatidylethanolamine (DOPE) are being explored for their lipid anchoring properties with DOPE showing the most efficient anchoring effect and stable retention (95).
Another approach to engineering EVs is to use functional moieties that can be engineered onto the surface of EVs that can then be functionalized by chemical reactions using a technology termed click reactions. Click reactions can introduce large molecules on the EV membrane, and a greater amount of quantitative decoration is possible than via endogenous expression (96). Over-modification of EV proteins with functional groups, which are necessary for click reactions, might inhibit their intrinsic surface properties (97). Another approach being explored uses a click reaction to attach a targeting moiety to polyethylene glycol (PEG), and the resulting PEG-anchored peptide inserted in the EVs to gives functionality (98). In this way, in addition to the properties of EVs such low toxicity, low immunogenicity, and functional cargo and surface proteins, EVs with added functionality through surface engineering are being tried in various disease models.
To address the translational progress of EV research to the clinic, we review here recent trials based on a literature search using the words “extracellular vesicles” in the ClinicalTrials.gov database. We reviewed a total of 81 registered studies and categorized them on the basis of the following: 1) biomarker and diagnosis accounted for 50 cases (62.5%); therapeutics-related studies accounted for 26 cases (32.1%) and 18 cases (22.2%) in phases 1 to 3 as summarized in Table 2. Various organs were the subject of these studies: lung (six cases), skin (three cases), gut (three cases), liver (two cases), heart (one case), eye (one case), ear (one case), and ovary (one case). Although EVs in clinical trials generally use unmodified MSC-derived EVs, the future of EV therapies will likely expand to include sources from other cell types and utilize engineered EVs (i.e., ones that enhance tropism and deliver therapeutic payloads).
Table 2 Clinical trials related to EVs as therapeutics (clinicaltrials.gov).
Classical EV tracking studies have relied on fluorescent membrane dyes, but several reports suggest that such labeling methods may negatively impact the sensitivity and/or not provide an accurate demonstration of the distribution in cells and tissues (99). Several tracers have been assessed to track the biodistribution of EVs (100), including their off-target distribution, which is important understand when assessing the therapeutic potential of EVs (Figure 2A) (101). mCherry red has been used for EV tracking in vivo, but its utility is limited by a poor signal to noise ratio. However, DiR [1,1′-dioctadecyl-3,3,3′,3′-tetramethylindotricarbocyanine iodide; DiIC18 (7)]–labeled EVs and radioisotope-labeled EV signals are monitored clearly and tend to accumulate in the liver and spleen (102).
Figure 2 Natural tropism of EV and potential applications (created with BioRender.com). (A) Representative EV donor cells in vitro. (B) Summary of EV biodistribution studies in mouse models. (C) Representative EV’s immune modulation. (D) Summary of applications of surface modified EVs.
More recent genetic approaches to the study of EV release and uptake have taken advantage of fusion proteins engineered between EV tetraspanins and fluorescent reporters like green fluorescent protein (GFP), tdTomato, and mCherry (103, 104). While there are some limitations, the ability to follow EV biogenesis with genetic tools is a powerful technique for in vitro and in vivo models. It is important to note that, while the expression of tagged tetraspanins have some limitations (105), recent reports show that luminescent tagging of CD63-Nluc can be used to their distribution to the lung and spleen (102). Using a GFP tag, CD63-EGFP–labeled EVs can be detected in the parenchyma of the liver and spleen after intravenous injection (105). In vivo EV labeling via transgenic inducible GFP EV reporter mouse that contain a Cre recombinase–inducible promoter-driven CD9 fused to GFP provides the insight for EV distribution that depends on cellular source (106). With the use of tissue-specific expression of Cre recombinase, transgenic mice can produce CD81-mNG EVs. These EV can then be used for mapping cell-type–specific contribution to blood exosome population (107). These studies show that fluorescent and luminescent reporters fused with tetraspanins are useful for tracking EV tropism.
EVs of different sizes have their own N-glycosylation, protein, lipid, and nucleic acid profiles and show different biodistribution patterns. At 24 h after intravenous injection, a wide range of EV sizes were taken up by various organs (84% by liver, 14% by spleen, and 1.6% by bone marrow). Although almost all EVs were taken up in the liver, large EVs displayed lymph node tropism (108). Previous reports have summarized the biodistribution of EVs (109), with many small EVs being cleared from the blood within 1 h. A recent study has examined EVs from multiple cell types and assessed their biodistribution. In this example, EVs derived from B16BL6 melanoma, C2C12 myoblast, NIH3T3 fibroblast, MAEC aortic endothelial cell, or RAW264.7 macrophage were administered via intravenous injection. This study showed efficient clearance from the circulation into the liver (110). These reports help understand the biodistribution of EVs in vivo and in vitro and help guide the design and testing of future engineered EVs (Figure 2B).
mNG (mNeon Green)–labeled HEK293 cell–derived EVs intravenously injected accumulate in hepatocytes. Differential expression analysis of recipient cells revealed that cells dosed with a low number of EVs showed gene expression involved in EV biogenesis, endocytosis, and homeostasis. In cells dosed with a high number of EV, expressed genes related to lysosome acidification, assembly, and localization (29). HEK293 cell lines are cells commonly used for transfection and are derived from human kidney. EVs derived from the human embryonic kidney cell line HEK293 accumulate in the liver and are used for liver diseases (111, 112). EVs derived from HEK 293 cells expressing CD9 and CD63 show a similar biodistribution trend toward the liver and spleen. In addition, the relative distribution of CD63-positive EVs was enhanced in the gastrointestinal tract and reduced in the lung (113).
MSC-derived EVs show injury-related organ tropism. MSC EVs show a homing effect in the lung and liver via integrin-mediated protein interaction (114, 115). In addition, MSC-EVs accumulate in ischemic kidney and proximal tubules and affect renal tubule repair (116). Placental EVs associate with lung interstitial macrophage and liver Kupffer cells in an integrin-mediated manner. Placental EVs target maternal immune cells and cause genetic alteration of maternal immune cells (Figure 2C) (117).
Cancer-derived EVs recognize early-stage neoplastic tissues with tumor-tropism mimicking the membrane structures of cancer cells and promote their uptake into tumor (118, 119). Tumor tropic cancer–derived EVs occur without specificity for donor cancer types (120). Cancer cells involved in metastasis produce EVs with tropism for the relevant organ. Lung metastatic hepatocellular carcinoma exhibits lung tropism. Solute carrier organic anion transporter family member 2A1, alanine aminopeptidase, and chloride intracellular channel 1 can be found to be involved in lung-tropic proteins of EVs (121, 122). These reports suggest organ distribution according to donor cell type, and most show tropism to the liver, spleen, lung, and kidney. Using this cell- or organ-tropism information, it may be possible to target delivery of EV cargos through direct or indirect strategies that take advantage of molecules that can be displayed on EVs to mediate tropism in the appropriate microenvironment (Figure 2D).
Cell-to-cell communication by EVs through cargo delivery begins with EV uptake by recipient cells (123, 124). EV uptake is mediated by various mechanisms that likely depend on physical properties such as size and protein and lipid composition of the membrane (125–127). Clathrin-mediated endocytosis and micropinocytosis are considered a main route of EV uptake through a specific pathway but not necessarily through a specific receptor. In this pathway, uptake occurs as early as 15 min after introduction in an energy-dependent manner (128–131). Additionally, depending on the characteristics of EVs, pathways such lipid raft-mediated endocytosis have also been reported (132–134). EVs also indirectly deliver their cargos by fusion with plasma membrane of recipient cells (32, 131, 135). Internalization of EVs is captured by integrin β3–interacting HSPGs. This internalization process is regulated by the integrin β3–focal adhesion kinase (FAK), which is activated by EVs in an integrin β3–dependent manner and required for endocytosis (136).
Endosomal escape of EVs in the recipient cells is an important intracellular step in the delivery of EV cargo necessary to complete their function. Internalized EVs form intracellular vesicles that fuse with endosomes to form early endosomes. These early endosomes contain EVs that often fuse with lysosomes followed by endo-lysosome acidification (137). Overall, EV cargos are thought to be rapidly digested by autophagy in recipient cells (32, 138). Using CD63-pHluo (pH-sensitive fluorescent)–labeled EV, acidification of endocytosed EVs occurred in a narrower time range, from 2 min to 11 min (139). However, internalized EVs that undergo endosomal escape, these EVs can deliver functional cargos into recipient cells (140). EV cargo exposure and/or release to the cytosol occurs when endosomes are acidified by fusion with lysosomes (129, 141). In this step, endosomal acidification is involved in the fusion of EV and endosomal membrane followed by cargo release (32, 129). Chloroquine, which improves endosomal acidification, results in unstable endosomes and increased release of EV cargo into the cytoplasm. Bafilomycin A inhibits the proton transfer of vacuolar type H+-ATPase (V-ATPase) resulting in accumulation of endosomal cargo and inhibition of releasing EV cargos (142). Advances in engineering approaches and drugs should lead to better efficiencies of endosomal escape of macromolecules, such as proteins, nucleic acids, and lipid nanoparticles (143–145). Recent examples include ligands such as glycans (146), fusogenic proteins (147–149), and endocytosis related proteins (150) that can enhance endosomal escape in recipient cells (151). A study was also reported to improve target delivery efficiency by depleting integrin β1 to avoid non-specific clearance (152).
Advances in the tracking EV release from cells have been largely guided by technology. In early EV studies, electron microscopy, along with immunogold staining, was the standard for high-resolution imaging (153, 154). More recently, genetic reporters such as GFP or luciferase have been expressed as fusion proteins with classic tetraspanins (155). These studies have greatly advanced the ability to identify which cells express EVs and, in some studies, assess the uptake of those EVs where advanced high-resolution imaging is used because single EVs are smaller than the resolution of standard light microscopy (156). In another example of advances in technology, to identify the release of EVs upon release, pHlourin reporters have been used because they are pH-dependent ratiometric reporters. With this kind of reporter, as an EV is trafficked to the neutral extracellular space, fluorescence is increased upon neutralization and thereby tracked in various in vitro models (139) and in vivo (156) models. We and others have recently shown how cell-type–specific promoters can be used to direct the expression of such fusion reporters (i.e., CD9-GFP) in specific cell types in mice (106, 107, 157). As these tools develop more sophisticated reporters and cell-type–specific promoters and access more advanced imaging increases, we anticipate that the visualization of engineered EVs will become more useful and prevalent.
EVs are known to regulate cell-to-cell communication in the microenvironment of injury and cancer, with immune cells having a major role in modulation of the inflammation responses associated with wound repair and blockade of tumor progression. Here, we focus on examples of tissue injury where macrophage-derived EVs regulate fibroblasts in the wound bed (157) and on changes in EVs draining in mesenteric lymph–regulating immune responses in sentinel lymph nodes (158, 159) and tumor microenvironments. In cancer, one of the earliest observations was that integrins had a major role in conditioning the metastatic niche (37). Additional examples included studies on the role of EVs derived from triple-negative breast cancer cell bearing CSF-1 that accelerated differentiation of monocyte to pro-inflammatory macrophages (160). Plant-derived EVs likely also have interesting but poorly defined surface proteins that were shown to promote the reprogramming of tumor-TAMs to induce the anti-tumorigenic infiltration of CD8+ T cells (161). The tropism of TAM-derived EVs can also be modified by display cyclic RGD peptides to deliver payloads that attenuate PD-L1 expression and enhance the activation of CD8+ T cells (162). Myeloid-derived suppressor cells (MDSCs) were also important regulators of the “cold” tumor microenvironment based on studies showing that MDSC-derived EVs dysregulated Wnt/β-catenin signaling pathway and decreased anti-tumoral responses (163).
Among the most well-defined immune cell crosstalk responses in immunology is the interaction between regulatory T cells (Treg), CD8+ cytotoxic T cells, and DCs. Therefore, as the EV field develops within the field of adaptive immunity, consideration of EV tropism, EV cell source, and EV uptake will need to be addressed in more detail. Interesting interactions include EV-mediated transfer of miRNA contents that regulate IL-10 and IL-6 production (164) and MSC-derived EVs suppressing antigen uptake by immature DCs (165). EV tropism may also influence dysfunctional immune responses based on tissue-specific microenvironments. Therefore, engineered EVs that reverse these responses may yield pro-reparative, anti-inflammatory, and anti-tumorigenic immune responses depending on the disease state (Figure 3).
Figure 3 Regulation of immune microenvironment by EVs mediated immune cell crosstalk (Created with BioRender.com).
EVs are key players in cell-to-cell communication, mediating various physiological signals in the body. Recent advances in the field have focused on understanding the biological activity of EVs and utilizing their internal cargo to apply EVs as therapeutics. However, a lack of understanding of tropism limits their clinical utility. Understanding the EVs tropism, from their surface composition by cell source and microenvironment to cellular uptake and systemic biodistribution, provides a pivotal junction between EV applications and clinical approaches. Depending on the EV source and recipient cells, there is an emerging literature that supports the translational potential of engineered EVs that consider the natural specificity of uptake pathways and surface display technologies.
WC: Conceptualization, Investigation, Visualization, Writing – original draft, Writing – review & editing. DP: Writing – review & editing. BE: Conceptualization, Funding acquisition, Project administration, Supervision, Writing – review & editing.
The author(s) declare financial support was received for the research, authorship, and/or publication of this article. This work was supported by the National Institutes of Health grant (1R01-GM140137) (BE).
The authors declare that the research was conducted in the absence of any commercial or financial relationships that could be construed as a potential conflict of interest.
All claims expressed in this article are solely those of the authors and do not necessarily represent those of their affiliated organizations, or those of the publisher, the editors and the reviewers. Any product that may be evaluated in this article, or claim that may be made by its manufacturer, is not guaranteed or endorsed by the publisher.
1. Welsh JA, Goberdhan DC, O'Driscoll L, Buzas EI, Blenkiron C, Bussolati B, et al. Minimal information for studies of extracellular vesicles (MISEV2023): From basic to advanced approaches. J extracellular vesicles. (2024) 13:e12404. doi: 10.1002/jev2.12416
2. Cheng L, Hill AF. Therapeutically harnessing extracellular vesicles. Nat Rev Drug Discovery. (2022) 21:379–99. doi: 10.1038/s41573-022-00410-w
3. Cocucci E, Meldolesi J. Ectosomes and exosomes: shedding the confusion between extracellular vesicles. Trends Cell Biol. (2015) 25:364–72. doi: 10.1016/j.tcb.2015.01.004
4. Nam GH, Choi Y, Kim GB, Kim S, Kim SA, Kim IS. Emerging prospects of exosomes for cancer treatment: from conventional therapy to immunotherapy. Advanced Materials. (2020) 32:2002440. doi: 10.1002/adma.202002440
5. Holm MM, Kaiser J, Schwab ME. Extracellular vesicles: multimodal envoys in neural maintenance and repair. Trends Neurosciences. (2018) 41:360–72. doi: 10.1016/j.tins.2018.03.006
6. Zierden HC, Marx-Rattner R, Rock KD, Montgomery KR, Anastasiadis P, Folts L, et al. Extracellular vesicles are dynamic regulators of maternal glucose homeostasis during pregnancy. Sci Rep. (2023) 13:4568. doi: 10.1038/s41598-023-31425-x
7. Yates AG, Pink RC, Erdbrügger U, Siljander PRM, Dellar ER, Pantazi P, et al. In sickness and in health: The functional role of extracellular vesicles in physiology and pathology in vivo: Part I: Health and Normal Physiology. J extracellular vesicles. (2022) 11:e12151. doi: 10.1002/jev2.12151
8. Takeuchi T, Suzuki M, Fujikake N, Popiel HA, Kikuchi H, Futaki S, et al. Intercellular chaperone transmission via exosomes contributes to maintenance of protein homeostasis at the organismal level. Proc Natl Acad Sci. (2015) 112:E2497–506. doi: 10.1073/pnas.1412651112
9. Buzas EI. The roles of extracellular vesicles in the immune system. Nat Rev Immunol. (2023) 23:236–50. doi: 10.1038/s41577-022-00763-8
10. Robbins PD, Morelli AE. Regulation of immune responses by extracellular vesicles. Nat Rev Immunol. (2014) 14:195–208. doi: 10.1038/nri3622
11. Marar C, Starich B, Wirtz D. Extracellular vesicles in immunomodulation and tumor progression. Nat Immunol. (2021) 22:560–70. doi: 10.1038/s41590-021-00899-0
12. Chen J, Yang J, Wang W, Guo D, Zhang C, Wang S, et al. Tumor extracellular vesicles mediate anti-PD-L1 therapy resistance by decoying anti-PD-L1. Cell Mol Immunol. (2022) 19:1290–301. doi: 10.1038/s41423-022-00926-6
13. Hou P-p, Chen H-z. Extracellular vesicles in the tumor immune microenvironment. Cancer Letters. (2021) 516:48–56. doi: 10.1016/j.canlet.2021.05.032
14. Yin Y, Chen H, Wang Y, Zhang L, Wang X. Roles of extracellular vesicles in the aging microenvironment and age-related diseases. J Extracellular Vesicles. (2021) 10:e12154. doi: 10.1002/jev2.12154
15. Shen Q, Huang Z, Yao J, Jin Y. Extracellular vesicles-mediated interaction within intestinal microenvironment in inflammatory bowel disease. J advanced Res. (2022) 37:221–33. doi: 10.1016/j.jare.2021.07.002
16. Xu F, Fei Z, Dai H, Xu J, Fan Q, Shen S, et al. Mesenchymal stem cell-derived extracellular vesicles with high PD-L1 expression for autoimmune diseases treatment. Advanced Materials. (2022) 34:2106265. doi: 10.1002/adma.202106265
17. Prasai A, Jay JW, Jupiter D, Wolf SE, El Ayadi A. Role of exosomes in dermal wound healing: a systematic review. J Invest Dermatol. (2022) 142:662–678.e8. doi: 10.1016/j.jid.2021.07.167
18. Mancini L, Guirao B, Ortica S, Labusch M, Cheysson F, Bonnet V, et al. Apical size and deltaA expression predict adult neural stem cell decisions along lineage progression. Sci Adv. (2023) 9:eadg7519. doi: 10.1126/sciadv.adg7519
19. György B, Hung ME, Breakefield XO, Leonard JN. Therapeutic applications of extracellular vesicles: clinical promise and open questions. Annu Rev Pharmacol toxicology. (2015) 55:439–64. doi: 10.1146/annurev-pharmtox-010814-124630
20. Fyfe J, Casari I, Manfredi M, Falasca M. Role of lipid signalling in extracellular vesicles-mediated cell-to-cell communication. Cytokine Growth Factor Rev. (2023). doi: 10.1016/j.cytogfr.2023.08.006
21. Fyfe J, Malhotra P, Falasca M. Modified lipidomic profile of cancer-associated small extracellular vesicles facilitates tumorigenic behaviours and contributes to disease progression. Adv Biol Regulation. (2023) 87:100935. doi: 10.1016/j.jbior.2022.100935
22. Kooijmans SA, Schiffelers RM, Zarovni N, Vago R. Modulation of tissue tropism and biological activity of exosomes and other extracellular vesicles: New nanotools for cancer treatment. Pharmacol Res. (2016) 111:487–500. doi: 10.1016/j.phrs.2016.07.006
23. Su H, Rustam YH, Masters CL, Makalic E, McLean CA, Hill AF, et al. Characterization of brain-derived extracellular vesicle lipids in Alzheimer's disease. J extracellular vesicles. (2021) 10:e12089. doi: 10.1002/jev2.12089
24. Karimi N, Dalirfardouei R, Dias T, Lötvall J, Lässer C. Tetraspanins distinguish separate extracellular vesicle subpopulations in human serum and plasma–Contributions of platelet extracellular vesicles in plasma samples. J extracellular vesicles. (2022) 11:e12213. doi: 10.1002/jev2.12213
25. Nolan JP, Duggan E. Analysis of individual extracellular vesicles by flow cytometry. Flow cytometry Protoc. (2018) 1678:79–92. doi: 10.1007/978-1-4939-7346-0_5
26. Cheng AN, Cheng L-C, Kuo C-L, Lo YK, Chou H-Y, Chen C-H, et al. Mitochondrial Lon-induced mtDNA leakage contributes to PD-L1–mediated immunoescape via STING-IFN signaling and extracellular vesicles. J immunotherapy cancer. (2020) 8:e001372. doi: 10.1136/jitc-2020-001372
27. Sun N, Zhang C, Lee YT, Tran BV, Wang J, Kim H, et al. HCC EV ECG score: an extracellular vesicle-based protein assay for detection of early-stage hepatocellular carcinoma. Hepatology. (2023) 77:774–88. doi: 10.1002/hep.32692
28. Christianson HC, Svensson KJ, Van Kuppevelt TH, Li J-P, Belting M. Cancer cell exosomes depend on cell-surface heparan sulfate proteoglycans for their internalization and functional activity. Proc Natl Acad Sci. (2013) 110:17380–5. doi: 10.1073/pnas.1304266110
29. Hagey DW, Ojansivu M, Bostancioglu BR, Saher O, Bost JP, Gustafsson MO, et al. The cellular response to extracellular vesicles is dependent on their cell source and dose. Sci advances. (2023) 9:eadh1168. doi: 10.1126/sciadv.adh1168
30. Czernek L, Chworos A, Duechler M. The uptake of extracellular vesicles is affected by the differentiation status of myeloid cells. Scandinavian J Immunol. (2015) 82:506–14. doi: 10.1111/sji.12371
31. Inder KL, Ruelcke JE, Petelin L, Moon H, Choi E, Rae J, et al. Cavin-1/PTRF alters prostate cancer cell-derived extracellular vesicle content and internalization to attenuate extracellular vesicle-mediated osteoclastogenesis and osteoblast proliferation. J extracellular vesicles. (2014) 3:23784. doi: 10.3402/jev.v3.23784
32. Bonsergent E, Grisard E, Buchrieser J, Schwartz O, Théry C, Lavieu G. Quantitative characterization of extracellular vesicle uptake and content delivery within mammalian cells. Nat Commun. (2021) 12:1864. doi: 10.1038/s41467-021-22126-y
33. Charoenviriyakul C, Takahashi Y, Morishita M, Nishikawa M, Takakura Y. Role of extracellular vesicle surface proteins in the pharmacokinetics of extracellular vesicles. Mol pharmaceutics. (2018) 15:1073–80. doi: 10.1021/acs.molpharmaceut.7b00950
34. Fendl B, Eichhorn T, Weiss R, Tripisciano C, Spittler A, Fischer MB, et al. Differential interaction of platelet-derived extracellular vesicles with circulating immune cells: Roles of TAM receptors, CD11b, and phosphatidylserine. Front Immunol. (2018) 2797. doi: 10.3389/fimmu.2018.02797
35. Lu H, Bowler N, Harshyne LA, Hooper DC, Krishn SR, Kurtoglu S, et al. Exosomal αvβ6 integrin is required for monocyte M2 polarization in prostate cancer. Matrix Biol. (2018) 70:20–35. doi: 10.1016/j.matbio.2018.03.009
36. Ji Q, Zhou L, Sui H, Yang L, Wu X, Song Q, et al. Primary tumors release ITGBL1-rich extracellular vesicles to promote distal metastatic tumor growth through fibroblast-niche formation. Nat Commun. (2020) 11:1211. doi: 10.1038/s41467-020-14869-x
37. Hoshino A, Costa-Silva B, Shen T-L, Rodrigues G, Hashimoto A, Tesic Mark M, et al. Tumour exosome integrins determine organotropic metastasis. Nature. (2015) 527:329–35. doi: 10.1038/nature15756
38. Wu K, Feng J, Lyu F, Xing F, Sharma S, Liu Y, et al. Exosomal miR-19a and IBSP cooperate to induce osteolytic bone metastasis of estrogen receptor-positive breast cancer. Nat Commun. (2021) 12:5196. doi: 10.1038/s41467-021-25473-y
39. Zhang S, Liao X, Chen S, Qian W, Li M, Xu Y, et al. Large oncosome-loaded VAPA promotes bone-tropic metastasis of hepatocellular carcinoma via formation of osteoclastic pre-metastatic niche. Advanced Science. (2022) 9:2201974. doi: 10.1002/advs.202201974
40. Rana S, Yue S, Stadel D, Zöller M. Toward tailored exosomes: the exosomal tetraspanin web contributes to target cell selection. Int J Biochem Cell Biol. (2012) 44:1574–84. doi: 10.1016/j.biocel.2012.06.018
41. Nazarenko I, Rana S, Baumann A, McAlear J, Hellwig A, Trendelenburg M, et al. Cell surface tetraspanin Tspan8 contributes to molecular pathways of exosome-induced endothelial cell activation. Cancer Res. (2010) 70:1668–78. doi: 10.1158/0008-5472.CAN-09-2470
42. Morelli AE, Larregina AT, Shufesky WJ, Sullivan ML, Stolz DB, Papworth GD, et al. Endocytosis, intracellular sorting, and processing of exosomes by dendritic cells. Blood. (2004) 104:3257–66. doi: 10.1182/blood-2004-03-0824
43. Nigri J, Leca J, Tubiana S-S, Finetti P, Guillaumond F, Martinez S, et al. CD9 mediates the uptake of extracellular vesicles from cancer-associated fibroblasts that promote pancreatic cancer cell aggressiveness. Sci Signaling. (2022) 15:eabg8191. doi: 10.1126/scisignal.abg8191
44. Cardeñes B, Clares I, Toribio V, Pascual L, López-Martín S, Torres-Gomez A, et al. Cellular integrin α5β1 and exosomal ADAM17 mediate the binding and uptake of exosomes produced by colorectal carcinoma cells. Int J Mol Sci. (2021) 22:9938. doi: 10.3390/ijms22189938
45. Jung JW, Kim JE, Kim E, Lee H, Lee H, Shin EA, et al. Liver-originated small extracellular vesicles with TM4SF5 target brown adipose tissue for homeostatic glucose clearance. J Extracellular Vesicles. (2022) 11:e12262. doi: 10.1002/jev2.12262
46. Edelmann MJ, Kima PE. Current understanding of extracellular vesicle homing/tropism. Zoonoses (Burlington Mass). (2022) 2. doi: 10.15212/ZOONOSES-2022-0004
47. Yamaguchi M, Kanazawa T, Morino S, Iioka S, Watanabe Y, Dohi N, et al. Increased tropism of extracellular vesicles derived from palmitic acid-treated hepatocytes to activated hepatic stellate cells. Membranes. (2022) 12:1023. doi: 10.3390/membranes12101023
48. Santos MF, Rappa G, Karbanová J, Vanier C, Morimoto C, Corbeil D, et al. Anti-human CD 9 antibody Fab fragment impairs the internalization of extracellular vesicles and the nuclear transfer of their cargo proteins. J Cell Mol Med. (2019) 23:4408–21. doi: 10.1111/jcmm.14334
49. Ma S, Mangala LS, Hu W, Bayaktar E, Yokoi A, Hu W, et al. CD63-mediated cloaking of VEGF in small extracellular vesicles contributes to anti-VEGF therapy resistance. Cell Rep. (2021) 36:109549. doi: 10.1016/j.celrep.2021.109549
50. Tognoli ML, Dancourt J, Bonsergent E, Palmulli R, de Jong OG, Van Niel G, et al. Lack of involvement of CD63 and CD9 tetraspanins in the extracellular vesicle content delivery process. Commun Biol. (2023) 6:532. doi: 10.1038/s42003-023-04911-1
51. Hurwitz SN, Conlon MM, Rider MA, Brownstein NC, Meckes DG Jr. Nanoparticle analysis sheds budding insights into genetic drivers of extracellular vesicle biogenesis. J extracellular vesicles. (2016) 5:31295. doi: 10.3402/jev.v5.31295
52. Rädler J, Gupta D, Zickler A, Andaloussi SE. Exploiting the biogenesis of EVs for bioengineering and therapeutic cargo loading. Mol Ther. (2023) 31:1231–50. doi: 10.1016/j.ymthe.2023.02.013
53. Kwon HH, Yang SH, Lee J, Park BC, Park KY, Jung JY, et al. Combination treatment with human adipose tissue stem cell-derived exosomes and fractional CO2 laser for acne scars: a 12-week prospective, double-blind, randomized, split-face study. Acta dermato-venereologica. (2020) 100:1–8. doi: 10.2340/00015555-3666
54. Johnson J, Law SQ, Shojaee M, Hall AS, Bhuiyan S, Lim MB, et al. First-in-human clinical trial of allogeneic, platelet-derived extracellular vesicles as a potential therapeutic for delayed wound healing. J Extracellular Vesicles. (2023) 12:12332. doi: 10.1002/jev2.12332
55. Ren X, Zhao M, Lash B, Martino MM, Julier Z. Growth factor engineering strategies for regenerative medicine applications. Front bioengineering Biotechnol. (2020) 7:469. doi: 10.3389/fbioe.2019.00469
56. Hoang DH, Nguyen TD, Nguyen H-P, Nguyen X-H, Do PTX, Dang VD, et al. Differential wound healing capacity of mesenchymal stem cell-derived exosomes originated from bone marrow, adipose tissue and umbilical cord under serum-and xeno-free condition. Front Mol biosciences. (2020) 7:119. doi: 10.3389/fmolb.2020.00119
57. Shaabani E, Sharifiaghdam M, Faridi-Majidi R, De Smedt SC, Braeckmans K, Fraire JC. Gene therapy to enhance angiogenesis in chronic wounds. Mol Ther Nucleic Acids. (2022) 29:871. doi: 10.1016/j.omtn.2022.08.020
58. Petit I, Levy A, Estrach S, Féral CC, Trentin AG, Dingli F, et al. Fibroblast growth factor-2 bound to specific dermal fibroblast-derived extracellular vesicles is protected from degradation. Sci Rep. (2022) 12:22131. doi: 10.1038/s41598-022-26217-8
59. Xing Z, Zhao C, Wu S, Yang D, Zhang C, Wei X, et al. Hydrogel loaded with VEGF/TFEB-engineered extracellular vesicles for rescuing critical limb ischemia by a dual-pathway activation strategy. Advanced healthcare materials. (2022) 11:2100334. doi: 10.1002/adhm.202100334
60. Li B, Yang J, Wang R, Li J, Li X, Zhou X, et al. Delivery of vascular endothelial growth factor (VEGFC) via engineered exosomes improves lymphedema. Ann Trans Med. (2020) 8, 1498. doi: 10.21037/atm
61. Huang C-C, Kang M, Lu Y, Shirazi S, Diaz JI, Cooper LF, et al. Functionally engineered extracellular vesicles improve bone regeneration. Acta biomaterialia. (2020) 109:182–94. doi: 10.1016/j.actbio.2020.04.017
62. Zheng W, He R, Liang X, Roudi S, Bost J, Coly PM, et al. Cell-specific targeting of extracellular vesicles though engineering the glycocalyx. J Extracellular Vesicles. (2022) 11:12290. doi: 10.1002/jev2.12290
63. Mentkowski KI, Lang JK. Exosomes engineered to express a cardiomyocyte binding peptide demonstrate improved cardiac retention in vivo. Sci Rep. (2019) 9:10041. doi: 10.1038/s41598-019-46407-1
64. Wang X, Chen Y, Zhao Z, Meng Q, Yu Y, Sun J, et al. Engineered exosomes with ischemic myocardium-targeting peptide for targeted therapy in myocardial infarction. J Am Heart Assoc. (2018) 7:e008737. doi: 10.1161/JAHA.118.008737
65. Hao D, Lu L, Song H, Duan Y, Chen J, Carney R, et al. Engineered extracellular vesicles with high collagen-binding affinity present superior in situ retention and therapeutic efficacy in tissue repair. Theranostics. (2022) 12:6021. doi: 10.7150/thno.70448
66. Zhang K, Li R, Chen X, Yan H, Li H, Zhao X, et al. Renal endothelial cell-targeted extracellular vesicles protect the kidney from ischemic injury. Advanced Science. (2023) 10:2204626. doi: 10.1002/advs.202204626
67. Bellavia D, Raimondo S, Calabrese G, Forte S, Cristaldi M, Patinella A, et al. Interleukin 3-receptor targeted exosomes inhibit in vitro and in vivo Chronic Myelogenous Leukemia cell growth. Theranostics. (2017) 7:1333. doi: 10.7150/thno.17092
68. Pi F, Binzel DW, Lee TJ, Li Z, Sun M, Rychahou P, et al. Nanoparticle orientation to control RNA loading and ligand display on extracellular vesicles for cancer regression. Nat nanotechnology. (2018) 13:82–9. doi: 10.1038/s41565-017-0012-z
69. Kooijmans SA, Aleza CG, Roffler SR, van Solinge WW, Vader P, Schiffelers RM. Display of GPI-anchored anti-EGFR nanobodies on extracellular vesicles promotes tumour cell targeting. J extracellular vesicles. (2016) 5:31053. doi: 10.3402/jev.v5.31053
70. McDonald M, MacMullen C, Liu D, Leal S, Davis R. Genetic association of cyclic AMP signaling genes with bipolar disorder. Trans Psychiatry. (2012) 2:e169–9. doi: 10.1038/tp.2012.92
71. Xu Q, Zhang Z, Zhao L, Qin Y, Cai H, Geng Z, et al. Tropism-facilitated delivery of CRISPR/Cas9 system with chimeric antigen receptor-extracellular vesicles against B-cell Malignancies. J Controlled Release. (2020) 326:455–67. doi: 10.1016/j.jconrel.2020.07.033
72. Limongi T, Susa F, Dumontel B, Racca L, Perrone Donnorso M, Debellis D, et al. Extracellular vesicles tropism: A comparative study between passive innate tropism and the active engineered targeting capability of lymphocyte-derived evs. Membranes. (2021) 11:886. doi: 10.3390/membranes11110886
73. Geng T, Leung E, Chamley LW, Wu Z. Functionalisation of extracellular vesicles with cyclic-RGDyC potentially for glioblastoma targeted intracellular drug delivery. Biomaterials Advances. (2023) 149:213388. doi: 10.1016/j.bioadv.2023.213388
74. Shi X, Cheng Q, Hou T, Han M, Smbatyan G, Lang JE, et al. Genetically engineered cell-derived nanoparticles for targeted breast cancer immunotherapy. Mol Ther. (2020) 28:536–47. doi: 10.1016/j.ymthe.2019.11.020
75. Jung D, Shin S, Kang SM, Jung I, Ryu S, Noh S, et al. Reprogramming of T cell-derived small extracellular vesicles using IL2 surface engineering induces potent anti-cancer effects through miRNA delivery. J Extracellular Vesicles. (2022) 11:12287. doi: 10.1002/jev2.12287
76. Gulei D, Berindan-Neagoe I. Activation of necroptosis by engineered self tumor-derived exosomes loaded with CRISPR/Cas9. Mol Therapy-Nucleic Acids. (2019) 17:448–51. doi: 10.1016/j.omtn.2019.05.032
77. Lee H, Park H, Yu HS, Na K, Oh KT, Lee ES. Dendritic cell-targeted pH-responsive extracellular vesicles for anticancer vaccination. Pharmaceutics. (2019) 11:54. doi: 10.3390/pharmaceutics11020054
78. Matsumoto A, Takahashi Y, Ariizumi R, Nishikawa M, Takakura Y. Development of DNA-anchored assembly of small extracellular vesicle for efficient antigen delivery to antigen presenting cells. Biomaterials. (2019) 225:119518. doi: 10.1016/j.biomaterials.2019.119518
79. Yue M, Hu S, Sun H, Tuo B, Jia B, Chen C, et al. Extracellular vesicles remodel tumor environment for cancer immunotherapy. Mol Cancer. (2023) 22:203. doi: 10.1186/s12943-023-01898-5
80. Yang P, Peng Y, Feng Y, Xu Z, Feng P, Cao J, et al. Immune cell-derived extracellular vesicles–new strategies in cancer immunotherapy. Front Immunol. (2021) 12:771551. doi: 10.3389/fimmu.2021.771551
81. Nie W, Wu G, Zhang J, Huang LL, Ding J, Jiang A, et al. Responsive exosome nano-bioconjugates for synergistic cancer therapy. Angewandte Chemie Int Edition. (2020) 59:2018–22. doi: 10.1002/anie.201912524
82. Gunassekaran GR, Vadevoo SMP, Baek M-C, Lee B. M1 macrophage exosomes engineered to foster M1 polarization and target the IL-4 receptor inhibit tumor growth by reprogramming tumor-associated macrophages into M1-like macrophages. Biomaterials. (2021) 278:121137. doi: 10.1016/j.biomaterials.2021.121137
83. Li J, Li J, Peng Y, Du Y, Yang Z, Qi X. Dendritic cell derived exosomes loaded neoantigens for personalized cancer immunotherapies. J Controlled Release. (2023) 353:423–33. doi: 10.1016/j.jconrel.2022.11.053
84. Kanuma T, Yamamoto T, Kobiyama K, Moriishi E, Masuta Y, Kusakabe T, et al. CD63-mediated antigen delivery into extracellular vesicles via DNA vaccination results in robust CD8+ T cell responses. J Immunol. (2017) 198:4707–15. doi: 10.4049/jimmunol.1600731
85. Salunkhe S, Basak M, Chitkara D, Mittal A. Surface functionalization of exosomes for target-specific delivery and in vivo imaging & tracking: Strategies and significance. J Controlled Release. (2020) 326:599–614. doi: 10.1016/j.jconrel.2020.07.042
86. Richter M, Vader P, Fuhrmann G. Approaches to surface engineering of extracellular vesicles. Advanced Drug delivery Rev. (2021) 173:416–26. doi: 10.1016/j.addr.2021.03.020
87. Zhang M, Hu S, Liu L, Dang P, Liu Y, Sun Z, et al. Engineered exosomes from different sources for cancer-targeted therapy. Signal Transduction Targeted Ther. (2023) 8:124. doi: 10.1038/s41392-023-01382-y
88. Nieland L, Mahjoum S, Grandell E, Breyne K, Breakefield XO. Engineered EVs designed to target diseases of the CNS. J Controlled Release. (2023) 356:493–506. doi: 10.1016/j.jconrel.2023.03.009
89. Li Q, Hu W, Huang Q, Yang J, Li B, Ma K, et al. MiR146a-loaded engineered exosomes released from silk fibroin patch promote diabetic wound healing by targeting IRAK1. Signal Transduction Targeted Ther. (2023) 8:62. doi: 10.1038/s41392-022-01263-w
90. Zheng W, Rädler J, Sork H, Niu Z, Roudi S, Bost JP, et al. Identification of scaffold proteins for improved endogenous engineering of extracellular vesicles. Nat Commun. (2023) 14:4734. doi: 10.1038/s41467-023-40453-0
91. Stickney Z, Losacco J, McDevitt S, Zhang Z, Lu B. Development of exosome surface display technology in living human cells. Biochem Biophys Res Commun. (2016) 472:53–9. doi: 10.1016/j.bbrc.2016.02.058
92. Gao X, Ran N, Dong X, Zuo B, Yang R, Zhou Q, et al. Anchor peptide captures, targets, and loads exosomes of diverse origins for diagnostics and therapy. Sci Trans Med. (2018) 10:eaat0195. doi: 10.1126/scitranslmed.aat0195
93. Maisano D, Mimmi S, Dattilo V, Marino F, Gentile M, Vecchio E, et al. A novel phage display based platform for exosome diversity characterization. Nanoscale. (2022) 14:2998–3003. doi: 10.1039/D1NR06804K
94. Tréton G, Sayer C, Schürz M, Jaritsch M, Müller A, Matea C-T, et al. Quantitative and functional characterisation of extracellular vesicles after passive loading with hydrophobic or cholesterol-tagged small molecules. J Controlled Release. (2023) 361:694–716. doi: 10.1016/j.jconrel.2023.08.010
95. Zheng W, Schürz M, Wiklander RJ, Gustafsson O, Gupta D, Slovak R, et al. Surface display of functional moieties on extracellular vesicles using lipid anchors. J Controlled Release. (2023) 357:630–40. doi: 10.1016/j.jconrel.2023.04.033
96. Smyth T, Petrova K, Payton NM, Persaud I, Redzic JS, Graner MW, et al. Surface functionalization of exosomes using click chemistry. Bioconjugate Chem. (2014) 25:1777–84. doi: 10.1021/bc500291r
97. Musicò A, Zenatelli R, Romano M, Zendrini A, Alacqua S, Tassoni S, et al. Surface functionalization of extracellular vesicle nanoparticles with antibodies: a first study on the protein corona “variable”. Nanoscale Advances. (2023) 5:4703–17. doi: 10.1039/d3na00280b
98. Kooijmans S, Fliervoet L, van der Meel R, Fens M, Heijnen H, en Henegouwen P, et al. PEGylated and targeted extracellular vesicles display enhanced cell specificity and circulation time. J Controlled Release. (2016) 224:77–85. doi: 10.1016/j.jconrel.2016.01.009
99. Verweij FJ, Balaj L, Boulanger CM, Carter DR, Compeer EB, D’angelo G, et al. The power of imaging to understand extracellular vesicle biology in vivo. Nat Methods. (2021) 18:1013–26. doi: 10.1038/s41592-021-01206-3
100. Li Y-J, Wu J-Y, Wang J-M, Hu X-B, Xiang D-X. Emerging strategies for labeling and tracking of extracellular vesicles. J Controlled Release. (2020) 328:141–59. doi: 10.1016/j.jconrel.2020.08.056
101. Yamashita T, Takahashi Y, Takakura Y. Possibility of exosome-based therapeutics and challenges in production of exosomes eligible for therapeutic application. Biol Pharm Bulletin. (2018) 41:835–42. doi: 10.1248/bpb.b18-00133
102. Laízaro-Ibaíñez E, Faruqu FN, Saleh AF, Silva AM, Tzu-Wen Wang J, Rak J, et al. Selection of fluorescent, bioluminescent, and radioactive tracers to accurately reflect extracellular vesicle biodistribution in vivo. ACS nano. (2021) 15:3212–27. doi: 10.1021/acsnano.0c09873
103. Carrillo Sanchez B, Hinchliffe M, Bracewell DG. GFP-tagging of extracellular vesicles for rapid process development. Biotechnol J. (2022) 17:2100583. doi: 10.1002/biot.202100583
104. Sung BH, Ketova T, Hoshino D, Zijlstra A, Weaver AM. Directional cell movement through tissues is controlled by exosome secretion. Nat Commun. (2015) 6:7164. doi: 10.1038/ncomms8164
105. Wiklander OP, Nordin JZ, O'Loughlin A, Gustafsson Y, Corso G, Mäger I, et al. Extracellular vesicle in vivo biodistribution is determined by cell source, route of administration and targeting. J extracellular vesicles. (2015) 4:26316. doi: 10.3402/jev.v4.26316
106. Neckles VN, Morton MC, Holmberg JC, Sokolov AM, Nottoli T, Liu D, et al. A transgenic inducible GFP extracellular-vesicle reporter (TIGER) mouse illuminates neonatal cortical astrocytes as a source of immunomodulatory extracellular vesicles. Sci Rep. (2019) 9:3094. doi: 10.1038/s41598-019-39679-0
107. Fordjour FK, Abuelreich S, Hong X, Chatterjee E, Lallai V, Ng M, et al. Exomap1 mouse: a transgenic model for in vivo studies of exosome biology. Extracellular Vesicle. (2023) 2:100030. doi: 10.1016/j.vesic.2023.100030
108. Zhang H, Freitas D, Kim HS, Fabijanic K, Li Z, Chen H, et al. Identification of distinct nanoparticles and subsets of extracellular vesicles by asymmetric flow field-flow fractionation. Nat Cell Biol. (2018) 20:332–43. doi: 10.1038/s41556-018-0040-4
109. Kang M, Jordan V, Blenkiron C, Chamley LW. Biodistribution of extracellular vesicles following administration into animals: A systematic review. J Extracellular Vesicles. (2021) 10:e12085. doi: 10.1002/jev2.12085
110. Charoenviriyakul C, Takahashi Y, Morishita M, Matsumoto A, Nishikawa M, Takakura Y. Cell type-specific and common characteristics of exosomes derived from mouse cell lines: Yield, physicochemical properties, and pharmacokinetics. Eur J Pharm Sci. (2017) 96:316–22. doi: 10.1016/j.ejps.2016.10.009
111. Wan T, Zhong J, Pan Q, Zhou T, Ping Y, Liu X. Exosome-mediated delivery of Cas9 ribonucleoprotein complexes for tissue-specific gene therapy of liver diseases. Sci Advances. (2022) 8:eabp9435. doi: 10.1126/sciadv.abp9435
112. Li Z, Zhou X, Wei M, Gao X, Zhao L, Shi R, et al. In vitro and in vivo RNA inhibition by CD9-HuR functionalized exosomes encapsulated with miRNA or CRISPR/dCas9. Nano letters. (2018) 19:19–28. doi: 10.1021/acs.nanolett.8b02689
113. Gupta D, Liang X, Pavlova S, Wiklander OP, Corso G, Zhao Y, et al. Quantification of extracellular vesicles in vitro and in vivo using sensitive bioluminescence imaging. J extracellular vesicles. (2020) 9:1800222. doi: 10.1080/20013078.2020.1800222
114. Takeda N, Tsuchiya A, Mito M, Natsui K, Natusi Y, Koseki Y, et al. Analysis of distribution, collection, and confirmation of capacity dependency of small extracellular vesicles toward a therapy for liver cirrhosis. Inflammation Regeneration. (2023) 43:48. doi: 10.1186/s41232-023-00299-x
115. Tieu A, Stewart DJ, Chwastek D, Lansdell C, Burger D, Lalu MM. Biodistribution of mesenchymal stromal cell-derived extracellular vesicles administered during acute lung injury. Stem Cell Res Ther. (2023) 14:250. doi: 10.1186/s13287-023-03472-8
116. Cao J-Y, Wang B, Tang T-T, Wen Y, Li Z-L, Feng S-T, et al. Exosomal miR-125b-5p deriving from mesenchymal stem cells promotes tubular repair by suppression of p53 in ischemic acute kidney injury. Theranostics. (2021) 11:5248. doi: 10.7150/thno.54550
117. Nguyen SL, Ahn SH, Greenberg JW, Collaer BW, Agnew DW, Arora R, et al. Integrins mediate placental extracellular vesicle trafficking to lung and liver in vivo. Sci Rep. (2021) 11:4217. doi: 10.1038/s41598-021-82752-w
118. Garofalo M, Villa A, Brunialti E, Crescenti D, Dell'Omo G, Kuryk L, et al. Cancer-derived EVs show tropism for tissues at early stage of neoplastic transformation. Nanotheranostics. (2021) 5:1. doi: 10.7150/ntno.47226
119. Kim SM, Yang Y, Oh SJ, Hong Y, Seo M, Jang M. Cancer-derived exosomes as a delivery platform of CRISPR/Cas9 confer cancer cell tropism-dependent targeting. J Controlled Release. (2017) 266:8–16. doi: 10.1016/j.jconrel.2017.09.013
120. Garofalo M, Villa A, Crescenti D, Marzagalli M, Kuryk L, Limonta P, et al. Heterologous and cross-species tropism of cancer-derived extracellular vesicles. Theranostics. (2019) 9:5681. doi: 10.7150/thno.34824
121. Rodrigues G, Hoshino A, Kenific CM, Matei IR, Steiner L, Freitas D, et al. Tumour exosomal CEMIP protein promotes cancer cell colonization in brain metastasis. Nat Cell Biol. (2019) 21:1403–12. doi: 10.1038/s41556-019-0404-4
122. Wu AYT, Sung YC, Chen YJ, Chou STY, Guo V, Chien JCY, et al. Multiresolution imaging using bioluminescence resonance energy transfer identifies distinct biodistribution profiles of extracellular vesicles and exomeres with redirected tropism. Advanced Science. (2020) 7:2001467. doi: 10.1002/advs.202001467
123. Gurung S, Perocheau D, Touramanidou L, Baruteau J. The exosome journey: From biogenesis to uptake and intracellular signalling. Cell Communication Signaling. (2021) 19:1–19. doi: 10.1186/s12964-021-00730-1
124. Mathieu M, Martin-Jaular L, Lavieu G, Théry C. Specificities of secretion and uptake of exosomes and other extracellular vesicles for cell-to-cell communication. Nat Cell Biol. (2019) 21:9–17. doi: 10.1038/s41556-018-0250-9
125. Rennick JJ, Johnston AP, Parton RG. Key principles and methods for studying the endocytosis of biological and nanoparticle therapeutics. Nat nanotechnology. (2021) 16:266–76. doi: 10.1038/s41565-021-00858-8
126. Mulcahy LA, Pink RC, Carter DRF. Routes and mechanisms of extracellular vesicle uptake. J extracellular vesicles. (2014) 3:24641. doi: 10.3402/jev.v3.24641
127. Bost JP, Barriga H, Holme MN, Gallud A, Maugeri M, Gupta D, et al. Delivery of oligonucleotide therapeutics: Chemical modifications, lipid nanoparticles, and extracellular vesicles. ACS nano. (2021) 15:13993–4021. doi: 10.1021/acsnano.1c05099
128. Nicholson C, Shah N, Ishii M, Annamalai B, Brandon C, Rodgers J, et al. Mechanisms of extracellular vesicle uptake in stressed retinal pigment epithelial cell monolayers. Biochim Biophys Acta (BBA)-Molecular Basis Disease. (2020) 1866:165608. doi: 10.1016/j.bbadis.2019.165608
129. Joshi BS, de Beer MA, Giepmans BN, Zuhorn IS. Endocytosis of extracellular vesicles and release of their cargo from endosomes. ACS nano. (2020) 14:4444–55. doi: 10.1021/acsnano.9b10033
130. Deng Z, Wang J, Xiao Y, Li F, Niu L, Liu X, et al. Ultrasound-mediated augmented exosome release from astrocytes alleviates amyloid-β-induced neurotoxicity. Theranostics. (2021) 11:4351. doi: 10.7150/thno.52436
131. Hirose H, Hirai Y, Sasaki M, Sawa H, Futaki S. Quantitative analysis of extracellular vesicle uptake and fusion with recipient cells. Bioconjugate Chem. (2022) 33:1852–9. doi: 10.1021/acs.bioconjchem.2c00307
132. Verdera HC, Gitz-Francois JJ, Schiffelers RM, Vader P. Cellular uptake of extracellular vesicles is mediated by clathrin-independent endocytosis and macropinocytosis. J Controlled Release. (2017) 266:100–8. doi: 10.1016/j.jconrel.2017.09.019
133. Wang W, Zhu N, Yan T, Shi Y-N, Chen J, Zhang C-J, et al. The crosstalk: Exosomes and lipid metabolism. Cell Communication Signaling. (2020) 18:1–12. doi: 10.1186/s12964-020-00581-2
134. Ghadami S, Dellinger K. The lipid composition of extracellular vesicles: applications in diagnostics and therapeutic delivery. Front Mol Biosciences. (2023) 10. doi: 10.3389/fmolb.2023.1198044
135. Morandi MI, Busko P, Ozer-Partuk E, Khan S, Zarfati G, Elbaz-Alon Y, et al. Extracellular vesicle fusion visualized by cryo-electron microscopy. PNAS nexus. (2022) 1:pgac156. doi: 10.1093/pnasnexus/pgac156
136. Fuentes P, Sesé M, Guijarro PJ, Emperador M, Sánchez-Redondo S, Peinado H, et al. ITGB3-mediated uptake of small extracellular vesicles facilitates intercellular communication in breast cancer cells. Nat Commun. (2020) 11:4261. doi: 10.1038/s41467-020-18081-9
137. Maugeri M, Nawaz M, Papadimitriou A, Angerfors A, Camponeschi A, Na M, et al. Linkage between endosomal escape of LNP-mRNA and loading into EVs for transport to other cells. Nat Commun. (2019) 10:4333. doi: 10.1038/s41467-019-12275-6
138. Hung ME, Leonard JN. A platform for actively loading cargo RNA to elucidate limiting steps in EV-mediated delivery. J extracellular vesicles. (2016) 5:31027. doi: 10.3402/jev.v5.31027
139. Sung BH, von Lersner A, Guerrero J, Krystofiak ES, Inman D, Pelletier R, et al. A live cell reporter of exosome secretion and uptake reveals pathfinding behavior of migrating cells. Nat Commun. (2020) 11:2092. doi: 10.1038/s41467-020-15747-2
140. O’Brien K, Ughetto S, Mahjoum S, Nair AV, Breakefield XO. Uptake, functionality, and re-release of extracellular vesicle-encapsulated cargo. Cell Rep. (2022) 39:110651. doi: 10.1016/j.celrep.2022.110651
141. Polanco JC, Hand GR, Briner A, Li C, Götz J. Exosomes induce endolysosomal permeabilization as a gateway by which exosomal tau seeds escape into the cytosol. Acta neuropathologica. (2021) 141:235–56. doi: 10.1007/s00401-020-02254-3
142. Heath N, Osteikoetxea X, de Oliveria TM, Lázaro-Ibáñez E, Shatnyeva O, Schindler C, et al. Endosomal escape enhancing compounds facilitate functional delivery of extracellular vesicle cargo. Nanomedicine. (2019) 14:2799–814. doi: 10.2217/nnm-2019-0061
143. Smith SA, Selby LI, Johnston AP, Such GK. The endosomal escape of nanoparticles: toward more efficient cellular delivery. Bioconjugate Chem. (2018) 30:263–72. doi: 10.1021/acs.bioconjchem.8b00732
144. Brock DJ, Kondow-McConaghy HM, Hager EC, Pellois J-P. Endosomal escape and cytosolic penetration of macromolecules mediated by synthetic delivery agents. Bioconjugate Chem. (2018) 30:293–304. doi: 10.1021/acs.bioconjchem.8b00799
145. Varkouhi AK, Scholte M, Storm G, Haisma HJ. Endosomal escape pathways for delivery of biologicals. J Controlled Release. (2011) 151:220–8. doi: 10.1016/j.jconrel.2010.11.004
146. Williams C, Pazos R, Royo F, González E, Roura-Ferrer M, Martinez A, et al. Assessing the role of surface glycans of extracellular vesicles on cellular uptake. Sci Rep. (2019) 9:11920. doi: 10.1038/s41598-019-48499-1
147. Ilahibaks NF, Ardisasmita AI, Xie S, Gunnarsson A, Brealey J, Vader P, et al. TOP-EVs: Technology of Protein delivery through Extracellular Vesicles is a versatile platform for intracellular protein delivery. J Controlled Release. (2023) 355:579–92. doi: 10.1016/j.jconrel.2023.02.003
148. An SJ, Rivera-Molina F, Anneken A, Xi Z, McNellis B, Polejaev VI, et al. An active tethering mechanism controls the fate of vesicles. Nat Commun. (2021) 12:5434. doi: 10.1038/s41467-021-25465-y
149. Zhang X, Xu Q, Zi Z, Liu Z, Wan C, Crisman L, et al. Programmable extracellular vesicles for macromolecule delivery and genome modifications. Dev Cell. (2020) 55:784–801.e9. doi: 10.1016/j.devcel.2020.11.007
150. Liao Z, Liu H, Ma L, Lei J, Tong B, Li G, et al. Engineering extracellular vesicles restore the impaired cellular uptake and attenuate intervertebral disc degeneration. ACS nano. (2021) 15:14709–24. doi: 10.1021/acsnano.1c04514
151. Liang X, Gupta D, Xie J, Wonterghem EV, Hoecke LV, Hean J, et al. Multimodal engineering of extracellular vesicles for efficient intracellular protein delivery. bioRxiv. (2023). doi: 10.21203/rs.3.rs-3329019/v1
152. Zhang J, Song H, Dong Y, Li G, Li J, Cai Q, et al. Surface engineering of HEK293 cell-derived extracellular vesicles for improved pharmacokinetic profile and targeted delivery of IL-12 for the treatment of hepatocellular carcinoma. Int J Nanomedicine. (2023) 18:209–23. doi: 10.2147/IJN.S388916
153. Rikkert LG, Nieuwland R, Terstappen L, Coumans F. Quality of extracellular vesicle images by transmission electron microscopy is operator and protocol dependent. J extracellular vesicles. (2019) 8:1555419. doi: 10.1080/20013078.2018.1555419
154. Harding CV, Heuser JE, Stahl PD. Exosomes: looking back three decades and into the future. J Cell Biol. (2013) 200:367. doi: 10.1083/jcb.201212113
155. McNamara RP, Zhou Y, Eason AB, Landis JT, Chambers MG, Willcox S, et al. Imaging of surface microdomains on individual extracellular vesicles in 3-D. J Extracellular Vesicles. (2022) 11:e12191. doi: 10.1002/jev2.12191
156. Verweij FJ, Revenu C, Arras G, Dingli F, Loew D, Pegtel DM, et al. Live tracking of inter-organ communication by endogenous exosomes in vivo. Dev Cell. (2019) 48:573–589. e4. doi: 10.1016/j.devcel.2019.01.004
157. Park DJ, Choi W, Sayeed S, Dorschner RA, Rainaldi J, Ho K, et al. Defining the activity of pro-reparative extracellular vesicles in wound healing based on miRNA payloads and cell type-specific lineage mapping. Mol Ther. (2024). doi: 10.1016/j.ymthe.2024.02.019
158. Kojima M, Costantini TW, Eliceiri BP, Chan TW, Baird A, Coimbra R. Gut epithelial cell-derived exosomes trigger posttrauma immune dysfunction. J Trauma Acute Care Surgery. (2018) 84:257–64. doi: 10.1097/TA.0000000000001748
159. Kojima M, Gimenes-Junior JA, Langness S, Morishita K, Lavoie-Gagne O, Eliceiri B, et al. Exosomes, not protein or lipids, in mesenteric lymph activate inflammation: unlocking the mystery of post-shock multiple organ failure. J Trauma Acute Care Surgery. (2017) 82:42–50. doi: 10.1097/TA.0000000000001296
160. Tkach M, Thalmensi J, Timperi E, Gueguen P, Névo N, Grisard E, et al. Extracellular vesicles from triple negative breast cancer promote pro-inflammatory macrophages associated with better clinical outcome. Proc Natl Acad Sci. (2022) 119:e2107394119. doi: 10.1073/pnas.2107394119
161. Han X, Wei Q, Lv Y, Weng L, Huang H, Wei Q, et al. Ginseng-derived nanoparticles potentiate immune checkpoint antibody efficacy by reprogramming the cold tumor microenvironment. Mol Ther. (2022) 30:327–40. doi: 10.1016/j.ymthe.2021.08.028
162. Tian T, Liang R, Erel-Akbaba G, Saad L, Obeid PJ, Gao J, et al. Immune checkpoint inhibition in GBM primed with radiation by engineered extracellular vesicles. ACS nano. (2022) 16:1940–53. doi: 10.1021/acsnano.1c05505
163. Duarte-Sanmiguel S, Panic A, Dodd DJ, Salazar-Puerta A, Moore JT, Lawrence WR, et al. In Situ Deployment of Engineered Extracellular Vesicles into the Tumor Niche via Myeloid-Derived Suppressor Cells. Advanced healthcare materials. (2022) 11:2101619. doi: 10.1002/adhm.202101619
164. Tung SL, Boardman DA, Sen M, Letizia M, Peng Q, Cianci N, et al. Regulatory T cell-derived extracellular vesicles modify dendritic cell function. Sci Rep. (2018) 8:6065. doi: 10.1038/s41598-018-24531-8
Keywords: extracellular vesicles (EVs), EV tropism, EV surface engineering, EV ligands, targeting EV, adaptive immunity
Citation: Choi W, Park DJ and Eliceiri BP (2024) Defining tropism and activity of natural and engineered extracellular vesicles. Front. Immunol. 15:1363185. doi: 10.3389/fimmu.2024.1363185
Received: 30 December 2023; Accepted: 25 March 2024;
Published: 10 April 2024.
Edited by:
Li Fu, Shenzhen University, ChinaReviewed by:
Juan Antonio Fafian Labora, University of A Coruña, SpainCopyright © 2024 Choi, Park and Eliceiri. This is an open-access article distributed under the terms of the Creative Commons Attribution License (CC BY). The use, distribution or reproduction in other forums is permitted, provided the original author(s) and the copyright owner(s) are credited and that the original publication in this journal is cited, in accordance with accepted academic practice. No use, distribution or reproduction is permitted which does not comply with these terms.
*Correspondence: Brian P. Eliceiri, YmVsaWNlaXJpQGhlYWx0aC51Y3NkLmVkdQ==
Disclaimer: All claims expressed in this article are solely those of the authors and do not necessarily represent those of their affiliated organizations, or those of the publisher, the editors and the reviewers. Any product that may be evaluated in this article or claim that may be made by its manufacturer is not guaranteed or endorsed by the publisher.
Research integrity at Frontiers
Learn more about the work of our research integrity team to safeguard the quality of each article we publish.