- 1Centre for Infectious Disease Control, National Institute for Public Health and the Environment (RIVM), Bilthoven, Netherlands
- 2Infectious Diseases and Immunology, Department of Biomolecular Health Sciences, Faculty of Veterinary Medicine, Utrecht University, Utrecht, Netherlands
- 3Department of Medical Microbiology and Infection Prevention, Virology and Immunology Research Group, University Medical Center Groningen, Groningen, Netherlands
Background: To date, it is still not clear why during the COVID-19 pandemic children generally developed no or milder symptoms compared to adults. As innate immune responses are crucial in the early defense against pathogens, we aimed at profiling these responses from both adults and children with a primary SARS-CoV-2 infection.
Methods: In the first months of the pandemic, PBMCs and serum were collected from peripheral blood of adults and children at different time points after testing SARS-CoV-2 PCR positive (PCR+). The levels of SARS-CoV-2 Spike-specific IgG were measured in serum. The cells were cultured for 24 hours in medium only, with heat inactivated SARS-CoV-2 (iSARS-CoV-2) or toll-like receptor (TLR) ligands. The levels of secreted cytokines/chemokines as well as monocyte phenotype were determined.
Results: Few days after testing PCR+, PBMCs from PCR+ children secreted higher levels of cytokines/chemokines compared to PCR+ adults, after these cells were incubated either in medium only or after stimulation with iSARS-CoV-2 or TLR ligands. Furthermore, PBMCs from children stimulated with iSARS-CoV-2 secreted significantly higher levels of IL-10 and GM-CSF compared to PBMCs from control children. In contrast, PBMCs from the PCR+ adults secreted lower levels of IL-8 compared to adult controls. Phenotypic analysis of monocytes indicates a smaller proportion non-classical monocytes for adults compared to children. The distinct cytokine profiles, symptom severity, and the proportion of non-classical monocytes correlated to each other. The levels of Spike-specific IgG overtime did not significantly differ between children and adults.
Conclusions: Within the first week after testing PCR+, children showed a stronger inflammatory innate immune profile and experienced less severe symptoms compared to adults. Our data implies correlations between the secretion of cytokines/chemokines, proportion of non-classical monocytes, and symptoms severity. These findings enhance our understanding of the distinct pediatric and adult innate immune profile after SARS-CoV-2 infection and contributes to the knowledge necessary to improve future prevention strategies.
Introduction
Towards the end of 2019, a novel coronavirus, designated as SARS-CoV-2, emerged in Wuhan, China, causing coronavirus disease-2019 (COVID-19) which spread worldwide and was declared in March 2020 by the WHO as a global pandemic (1, 2). Millions of cases and deaths were reported globally and different control and prevention measures were introduced (3). At present COVID-19 no longer represents a global health emergency, however, due to cases with long-term symptoms as well as breakthrough infections and the decline of vaccine-induced immunity, it still remains crucial to better understand the immune response to infection with this virus.
One of the remarkable aspects during the COVID-19 pandemic was that the symptoms were generally less frequent in children and if any, these were milder compared to those in adults (4–6). Although it is not fully understood why there are differences in the clinical manifestations between adults and children, several studies point towards the crucial role of the antiviral-immune response early after SARS-CoV-2 infection (7–10). Several PRRs, like the endosomal Toll-like-receptors (TLR) (TLR7 and TLR8) and RIG-I like receptors (RLR) (RIG-I and MDA5), can be triggered by SARS-CoV-2 RNA (11). Extracellular TLRs, usually recognize bacterial lipoproteins and lipopolysaccharides, respectively, although different viral proteins, like the SARS-CoV-2 spike protein, have been shown to activate these PRRs as well (8). Epithelial and immune cells from children have higher cell-intrinsic antiviral capacity and express higher levels of various PRRs and are therefore posed to be better primed for viral sensing, compared to adults (9, 10, 12). Age intrinsic differences in susceptibility to SARS-CoV-2 were also demonstrated using bronchial epithelial cell cultures form children and adults, showing a much more attenuated inflammatory response to SARS-CoV-2 by cells from children (13). The exact innate immune mechanisms that limit SARS-CoV-2 infection and COVID-19 symptoms remains to be determined.
The aim of this study was to determine differences between the innate immune profiles from children and adults with a SARS-CoV-2 infection and if these correlated with symptom severity and whether these profiles changed over time. To this end, peripheral blood mononuclear cells (PBMCs) and serum samples were collected from parents and children from the same household that had tested PCR positive for SARS-CoV-2 (PCR+) early in the pandemic. Next to the development of SARS-CoV-2 specific antibodies, the cytokine and chemokine secretion profiles along with the phenotype of monocytes upon ex-vivo stimulation with heat-inactivated SARS-CoV-2 (iSARS-CoV-2) and different TLR ligands were examined.
Materials and methods
Ethical statement
Samples used in this study derive from three different studies and were all approved by the Medical-Ethical Review Committee of the University Medical Center Utrecht (SARS-CoV-2 transmission study: NL13529.041.06, NVI-255 study: NL29241.000.09, and IMMFACT study: NL46795.094.13). Written informed consent was obtained from all participants and all trial-related activities were conducted according to Good Clinical Practice, including the provisions of the Declaration of Helsinki.
Population and sample collection
Participants of the previously described SARS-CoV-2 transmission study (14) included persons 18 years and older with a positive SARS-CoV-2 PCR test who had at least one child in their household. All household contacts (adults and children) were enrolled in the study, except for contacts below one year of age. This study was carried out in the Netherlands early in the COVID-19 pandemic in the period of March-April 2020. During home visits blood samples were collected for serum and PBMC isolation. In order to study the early immune responses, index case participants were selected with a first blood collection home visit (T1), 3 to 7 days, after testing PCR+ during routine COVID19 surveillance (Table 1). At time the index case participant was sampled, their household members also provided samples. Household members who tested PCR+ in either nose, throat, saliva, or feces were included in this study. The two follow-up time points took place 2-3 weeks (T2), 4-6 weeks (T3) after T1. For children, no PBMCs were collected at T3. PBMCS from healthy adults (NVI-255 study) (15) and healthy children (IMMFACT study) (16), both collected several years before the COVID-19 pandemic, were also included as SARS-CoV-2 unexposed controls.
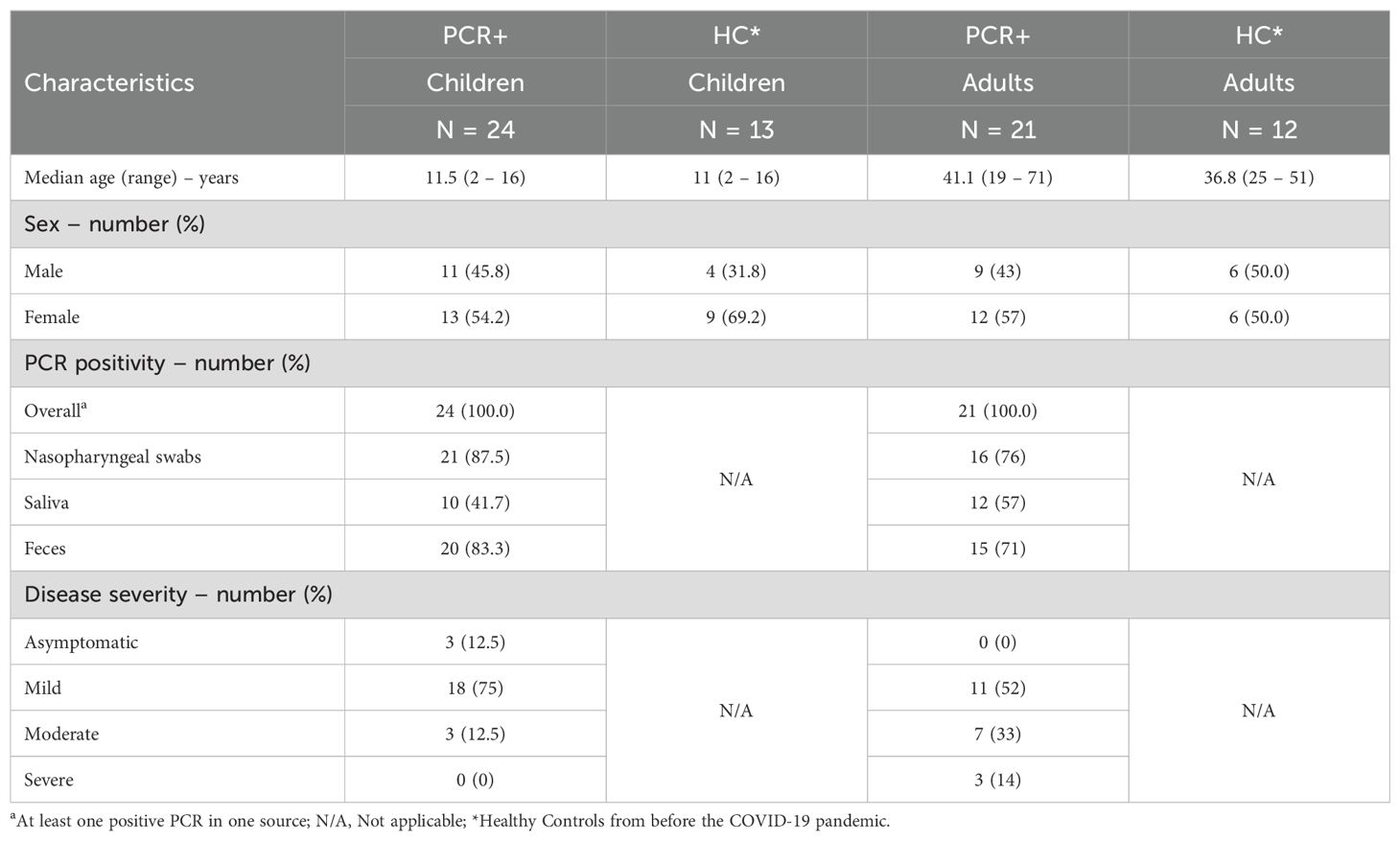
Table 1. Demographic and clinical characteristics of unexposed healthy controls and the PCR confirmed participants with a SARS-CoV-2 infection.
Symptoms and severity of COVID-19
As previously described (14) severity of COVID-19 was classified into mild, moderate and severe, which were indicated by severity indexes 1, 2 and 3, respectively. Symptoms reported by participants included sore throat, cough, respiratory difficulties, fever, chills, headache, muscle pain, joint ache, diarrhea, nausea, vomiting, loss of appetite or fatigue. Participants with mild disease presented any of the clinical symptoms described above. Those with moderate COVID-19 reported having pneumonia, including dyspnea, and those with severe disease reported dyspnea and have consulted a healthcare professional or have been admitted to the hospital. The overall disease severity for each participant was calculated, by adding the severity indexes of each participant during the first three home visits which resulted in: No Symptoms (0), Mild (1-2) Moderate (3-4) and Severe disease (>5).
Inactivated SARS-CoV-2 virus preparation
A SARS-CoV-2 heat-inactivated viral suspension (iSARS-CoV-2) was used in the present study. The SARS-CoV-2 isolate (hCoV-19/Netherlands/ZuidHolland_10004/2020) was obtained from a Dutch patient (Centre for Infectious Disease Control, RIVM). The virus was grown on VERO-E6 cells as previously described (17). Heat-inactivation was performed by incubating the virus at 60°C for 2 hours after which the samples were stored at −80°C.
PBMCS isolation, stimulation, cytokine determination and flow cytometry
PBMCS were isolated from whole blood using Ficoll–Paque density centrifugation and cryopreserved following standardized protocols. Thawed PBMCS (2x105 cells/well) were stimulated for 24 h in the presence of inactivated (i)SARS-CoV-2 (MOI=3) or different PRR-ligands: 107 cells/mL heat killed Listeria monocytogenes (HKLM, TLR2 ligand), 2.5 mg/mL R848 (TLR7/8 ligand) or 25 ng/mL Lipopolysaccharide (TLR4 ligand) (Invivogen).
After 24 h, supernatants were collected and analyzed for cytokine production by Legendplex, measuring IL-1β, IL-6, TNFα, CXCL10, IFNλ1, IL-8, IFNα2, GM-CSF, IFNβ, IL-10, IFNγ, IL-12-p70, IFNλ2/3 (LEGENDplex™ Human anti-Virus response Panel (13-plex), BioLegend) following manufacturer’s instructions. IL-12-p70 levels were below lower limit of detection and were therefore excluded from further analysis.
After collection of the supernatants, the stimulated cells were analyzed for different monocyte subset markers by flow cytometry. For flow cytometry, cells were washed with 0.5%BSA/PBS and incubated 5 min with Trustain (10x in 0.5%BSA/PBS, Biolegend), thereafter a mixture of antibodies (all from Biolegend, unless stated otherwise) was added for 20 min: CD14-FITC (clone HCD14), CD16-APC (clone 3G8), CD3-AlexaFluor700 (clone HIT3a), CD4-AlexaFluor700 (clone RPA-T4), CD19-AlexaFluor700 (clone HIB19), CD56-AlexaFluor700 (clone HCD56), CD15-AlexaFluor700 (clone HI98), NKp56-AlexaFluor700 clone 9E2), Fixable Viability Stain780 (BD Biosciences), HLA-DR-BrilliantViolet510 (clone L243), CCR5-BrilliantViolet421 (clone J418F1), CCR2-BrilliantViolet605 (clone 036C2), CX3CR1-PE/Dazzle594 (clone 2A9-1), CD11c-PE (clone 3.9) and CD11b-BrilliantUV737 (clone M1/70, BD Biosciences). After washing, the cells were acquired on a FACS Symphony (BD) and total monocytes were gated using FlowJo Software (V10.6.2). To identify the specific monocyte populations, preprocessing and clustering of the FACS datasets was performed in R v4.2.0 (18) and RStudio (v2022) with packages flowCore (19) and CATALYST (20, 21) (Supplementary Figure 1).
SARS-CoV-2 multiplex immunoassay
SARS-CoV-2 multiplex immunoassays (MIA) were carried out to determine specific serum antibody IgG levels towards SARS-CoV-2 Spike S1 (Sino Biological, 40591-V08H) as described previously (22). In short, the serum was incubated with antigen-coupled beads for 45 minutes in the dark, followed by a 30-minute incubation with Goat-anti-human IgG. Samples were incubated in SM01 (Surmodics) and washing steps after each incubation were carried out with PBS. Antibody binding to antigen-coupled beads was determined with FM3D (Luminex) and antibody levels were expressed as binding antibody units (BAU)/ml for S1. The threshold for seropositivity was set at 10.1 BAU/mL for Spike S1, as previously standardized for the Wuhan (vaccine) strain against the NIBSC/WHO COVID-19 reference serum 20/136 (23).
Statistical analysis
Statistical analysis was performed using non-parametric Kruskal-Wallis test for unpaired analysis or Friedmans test for paired samples over time, both followed by Dunn’s multiple comparison test, or multiple Mann-Whitney test with Bonferroni-Dunn multiple comparison test, as indicated, all in Graphpad Prism version 9.5.1. Differences between groups were considered statistically significant at adjusted p-value <0.05. Spearman’s correlation analysis was used between cytokine levels and the proportion of monocyte subsets, or disease severity. A correlation was considered significant at adjusted p-value <0.05. Kruskal-Wallis Chi-squared test was used to analyze proportion of monocyte subsets with disease severity, p-value <0.05 was considered significant.
Results
PBMCs from PCR+ children stimulated with inactivated SARS-CoV-2 secrete higher levels of cytokines and chemokines compared to adults
PBCMs taken from children and adults within the first week after testing PCR+ for SARS-CoV-2 early in the pandemic were stimulated in vitro with the iSARS-CoV-2 preparation and the cytokine levels were measured 24 hours after incubation. PBMCs from the PCR+ children secreted significantly higher levels of IFNβ and CXCL10 compared to the PBMCs from PCR+ adults, after iSARS-CoV-2 stimulation (Figure 1). Compared to the corresponding unexposed healthy controls, stimulated PBMCs from the PCR+ children produced significant higher levels of IL-10 and GM-CSF (Figure 1). In contrast, PBMCs from the PCR+ adults secreted lower levels of IL-8 compared those from adult healthy controls (Figure 1). No significant differences were observed between children and adults in the healthy control groups. These data indicate different innate immune responses between PCR+ children and adults as well as between age-matched PCR+ and healthy individuals.
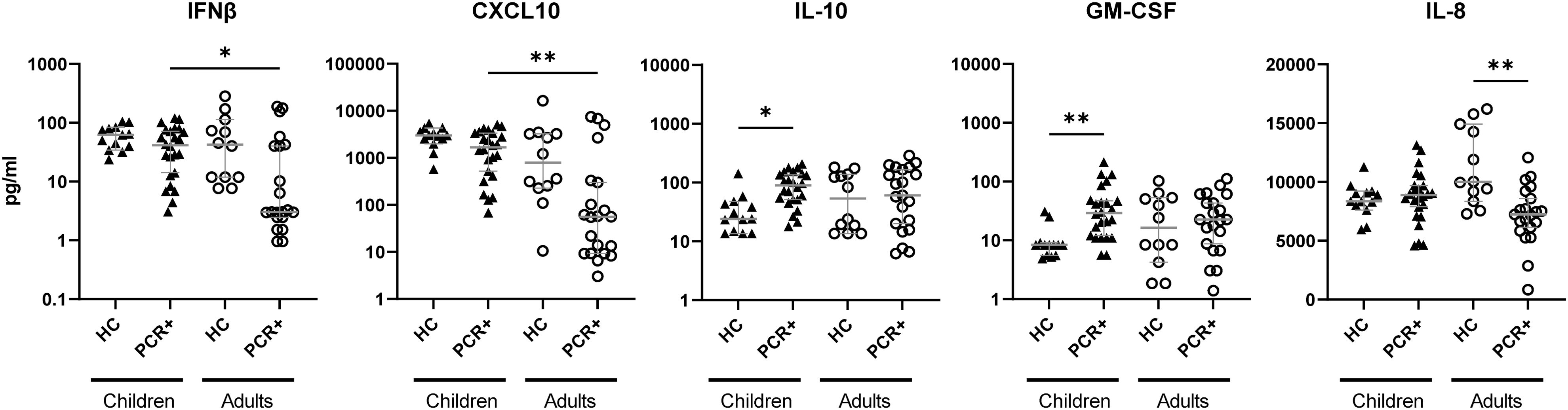
Figure 1. Divergent cytokine and chemokine profile induced by iSARS-CoV-2 in PBMCs from healthy control and recently infected children and adults. Cytokine and chemokine levels were measured in culture supernatant from PBMCs after overnight stimulation with iSARS-CoV-2 (MOI=3). Kruskal-Wallis non-parametric test was used to compare healthy control (HC) to PCR+ individuals among children (▲) or adults (○), as well as HC or PCR+ children to their counterparts in adults, after which Dunn’s multiple comparison test was executed. Error bars indicate median value with 95% CI. *p-adj<0.05. **p-adj-<0.01.
The levels of cytokines and chemokines secreted after iSARS-CoV2 stimulation do not correlate with the Spike-specific IgG antibodies dynamics
The development of specific antibody response after primary SARS-CoV-2 infection was measured at the different time points <7 days (T1), 2-3 weeks (T2) and 4-6 weeks (T3) after testing PCR+. For 22/24 children there were T2 and/or T3 serum sample available to determine SARS-CoV-2 Spike-specific IgG antibody levels. Four out of the 22 children did not show Spike-specific IgG levels at T2 and/or T3 (Figure 2A), whereas all the adults did develop Spike-specific IgG antibodies at T3 (Figure 2B). The Spike-specific antibody levels did not significantly differ between children and adults at any given timepoint. Next, we assessed whether the innate responsiveness of PBMCs at T1 stimulated with iSARS-CoV-2 could predict the development of the spike-specific IgG titers at T2 and T3. However, we did not find any significant association between the levels of cytokines and chemokines secreted by PBMCs after iSARS-CoV-2 stimulation and the Spike-specific IgG levels at T2 and T3.
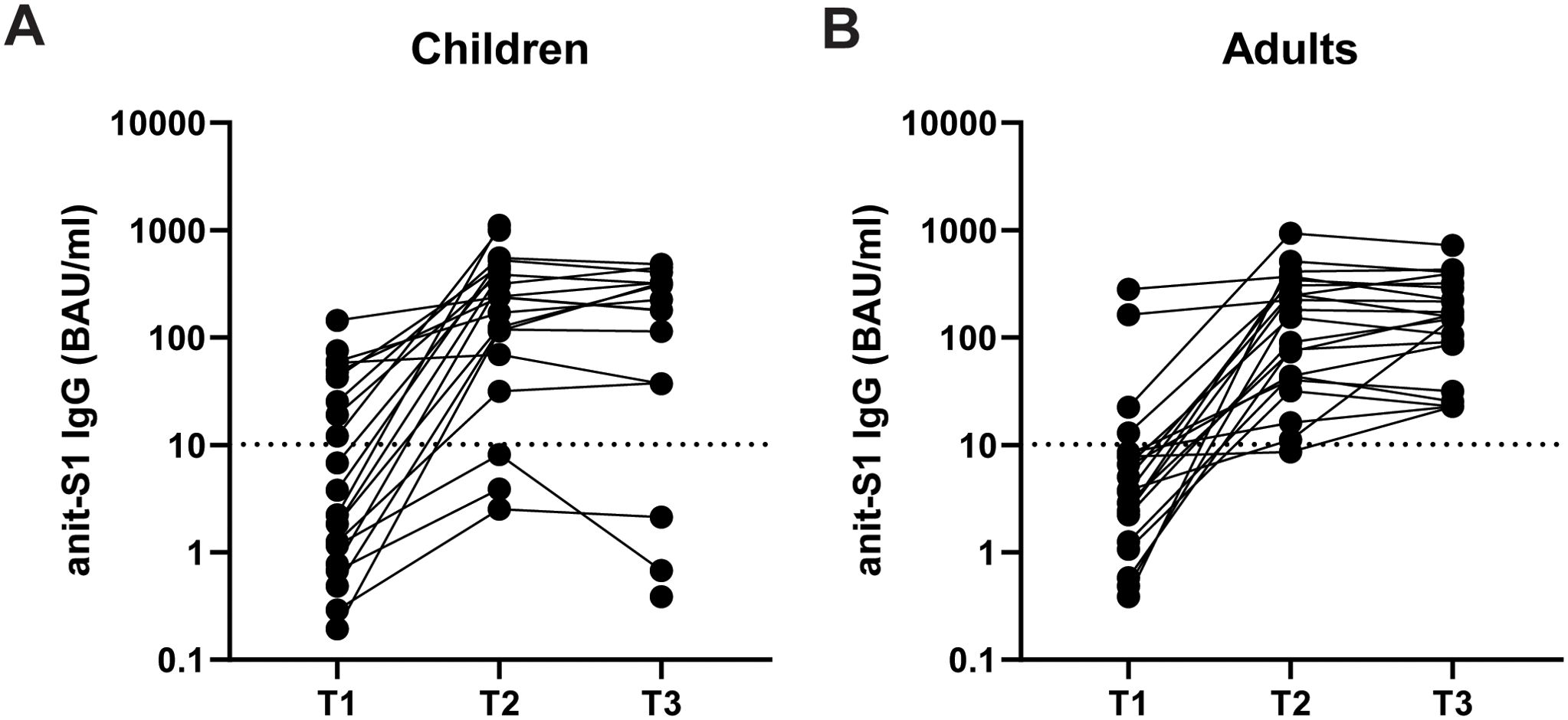
Figure 2. The development of anti-SARS-CoV-2 Spike-specific antibodies over time in PCR+ children and adults. SARS-CoV-2 Spike-specific IgG levels were measured within <7 days (T1), 2-3 weeks (T2), 4-6 weeks (T3) after testing PCR+ in serum of children (A) and adults (B). IgG levels are expressed as binding antibody units (BAU)/ml. Dotted line indicates seropositivity threshold of 10.1 BAU/ml.
The levels of IL-10, GM-CSF and IL-8 secreted by PBMCs after iSARS-CoV-2 stimulation correlate with symptom severity
Since the levels of some of the cytokine and chemokines secreted by PBMCs from PCR+ adults and children were highly variable after iSARS-CoV-2 stimulation, we questioned whether this could be related to the clinical status of the participants. Indeed, correlation analysis indicated a relation between the levels of IL-8 and the clinical status in adults (r=-0,527 p=0.0016). In children the levels of GM-CSF and IL-10 correlated with symptom severity (r=0.466, p=0.0036; r=0.621, p<0.001, respectively). Compared to healthy adults, the levels of IL-8 were significantly lower for adults with moderate symptoms (Figure 3A). No significant correlation was found between the levels of IFN-β or CXCL10 and symptom severity for adults (data not shown). For PBMCs from children with mild symptoms significantly higher levels of GM-CSF and IL-10 were found to be secreted after iSARS-CoV-2 stimulation, compared to their healthy counterparts (Figure 3B). Furthermore, the levels of IL-10 were also significantly higher for children with moderate symptoms (Figure 3B). These findings indicate distinct associations between cytokine responses and symptom severity in children and adults.
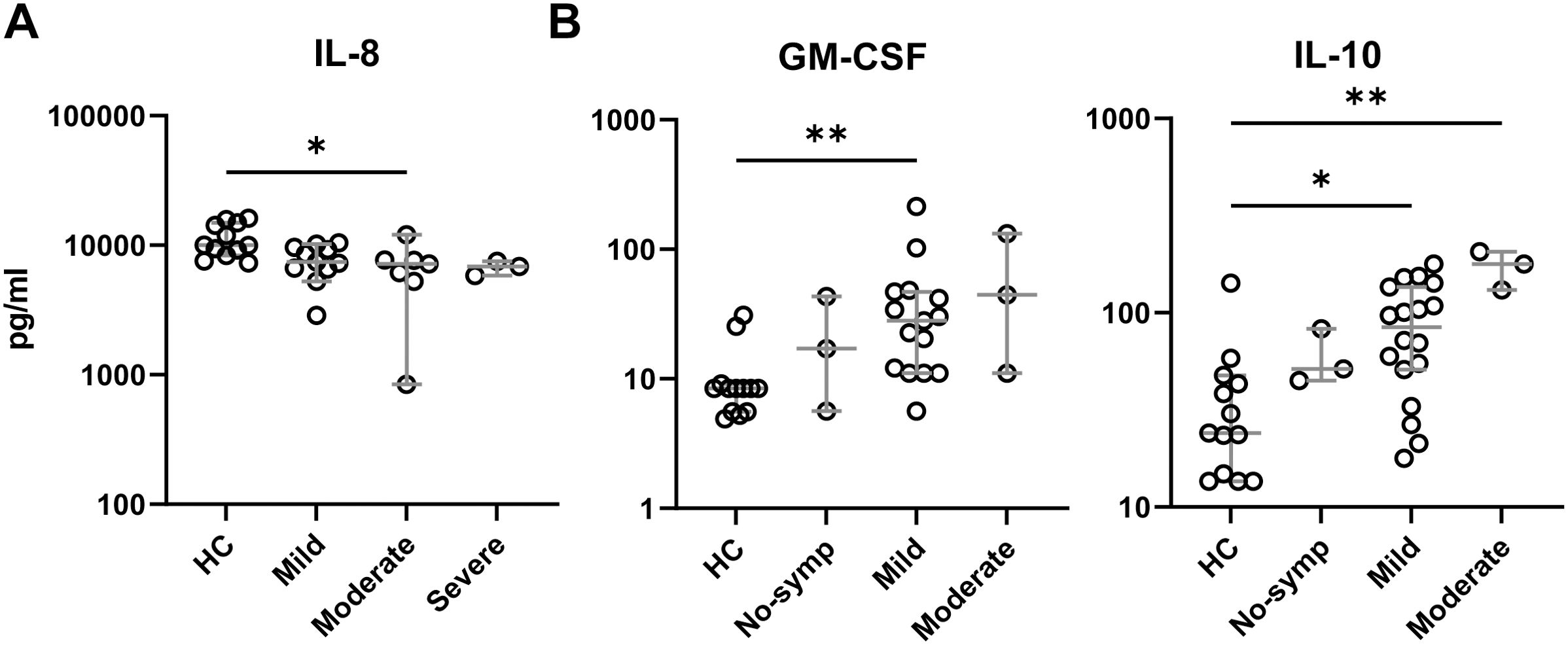
Figure 3. Symptom severity correlates with cytokine/chemokine production. Cytokine and chemokine levels delineated by symptom severity groups in adults (A) and children (B). Kruskal-Wallis non-parametric with Dunn’s multiple comparison test was performed comparing each group to each other. Error bars indicate median value with 95% CI. *p-adj<0.05. **p-adj<0.01.
Monocyte subset proportions differ between PCR+ adults and children, and associate with cytokine responses
Monocytes are the most abundant PRR expressing cells in the PBMCs compartment and may determine the outcome of the PBMCs interaction with iSARS-COV-2 (24). Phenotypic analysis indicates that upon in vitro iSARS-CoV-2 stimulation, the classical monocytes in PBMCs of PCR+ adults were the most abundant subset, which was significantly higher compared to PBMCs from PCR+ children (Figure 4). In contrast, iSARS-CoV-2 stimulated PBMCs from PCR+ children presented significantly larger proportion of intermediate and non-classical monocytes compared to those in adults (Figure 4). In adults the proportion of non-classical monocytes was significantly smaller in PCR+ compared to healthy individuals (Figure 4). Over time the proportion of monocyte subsets in PCR+ adults changed to levels similar to those of healthy controls with smaller proportion of classical, and larger proportion of non-classical monocytes at T2 and T3 (Supplementary Figure 2).
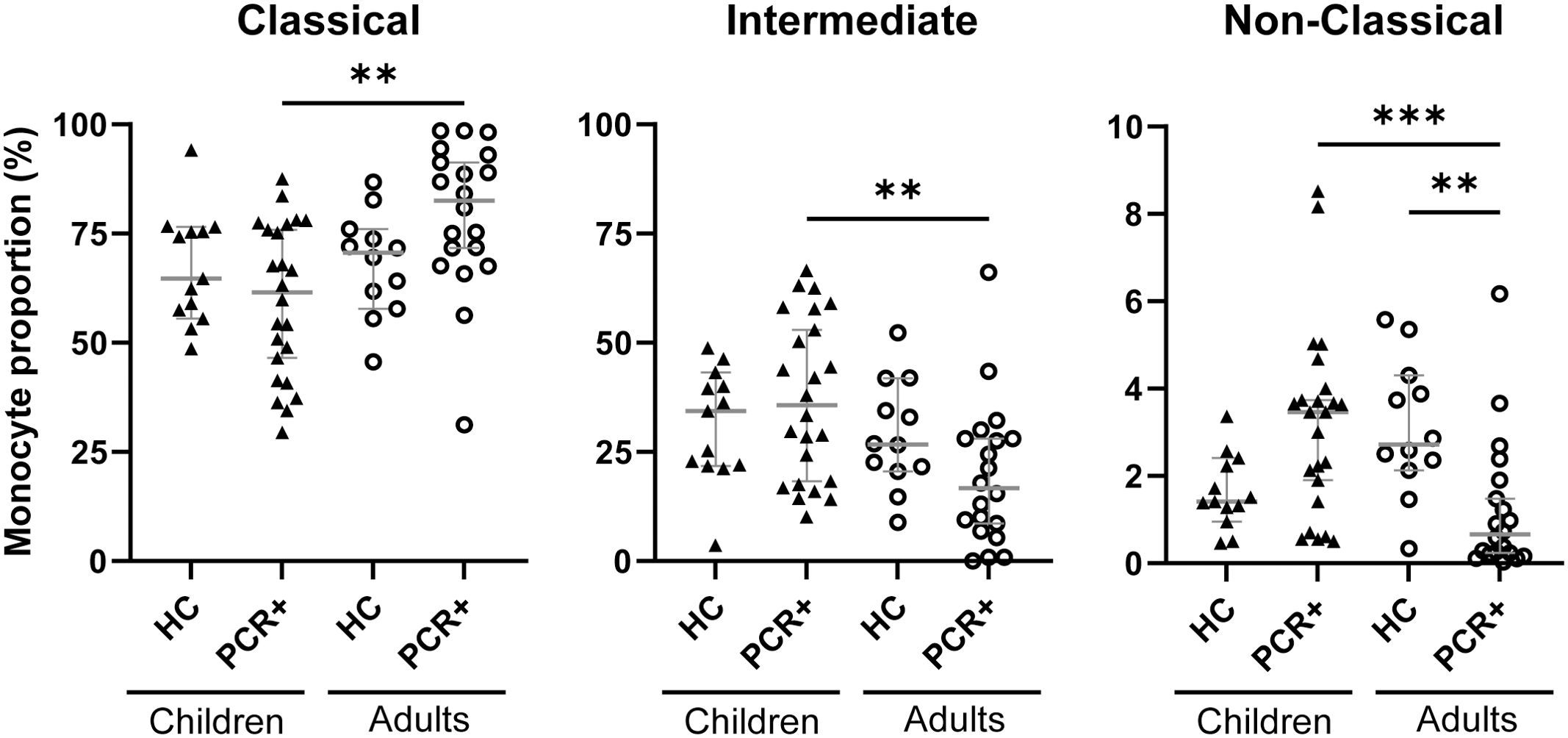
Figure 4. Monocyte subset proportions differ between PCR+ children and adults. Flow cytometric analysis of monocytes within PBMCS after iSARS-CoV-2 stimulation. Proportions of classical monocytes (CD14+CD16-), intermediate monocytes (CD14+CD16+), and non-classical monocytes (CD14lowCD16+) among total monocytes in PBMCs. Kruskal-Wallis non-parametric test was used to compare HC to PCR+ individuals among children (▲) or adults (○), as well as HC or PCR+ children to their counterparts in adults, after which Dunn’s multiple comparison test was executed. Error bars indicate median value with 95% CI. **p-adj-<0.01. ***p-adj<0.001.
To assess whether changes in monocyte subset proportions relate to cytokine and chemokine production after iSARS-CoV-2 stimulation early after infection (T1) we performed correlation analysis. The proportion of classical and intermediate monocytes in PBMCs from PCR+ adults showed a significant correlation with IL-1β, GM-CSF and IL-10 secretion (Table 2). The non-classical monocyte subset correlated with interferon (IFNλ1, IFNα2, and IFNβ), CXCL10, and IL-8 secretion. Proportion of non-classical monocytes in PBMCs from PCR+ children also showed a significant correlation with interferons (IFNα2 and IFNβ), CXCL10, and IL-8, albeit less strong as those in adults (Table 2). Comparing the proportion of non-classical monocyte between healthy control and PCR+ individuals we observed significantly lower values in adults, but not in children, with moderate symptoms (p-adj = 0.0088). The correlation between non-classical monocytes, symptom severity, and the production of interferons, CXCL10 and IL-8 after iSARS-CoV-2 stimulation indicates important differences in anti-viral innate immune response between PCR+ children and adults early after infection.
TLR activation results in increased cytokine and chemokine secretion by PBMCS from PCR+ children compared to adults
Several studies have highlighted the importance of PRR activation including that of TLR2, TLR4 and TLR7/8 in the innate immune response to SARS-CoV-2 (25–27). Therefore, we next measured the responsiveness of PBMCS from the PCR+ children and adults stimulated with pathogen-associated molecular patterns activating these PRR. Results indicate that PBMCS from the PCR+ children secrete significantly higher levels of IL-1β, IL-6, IFNλ1, IFNβ, TNFα, CXCL10 and GM-CSF after stimulation with TLR7/8 ligand R848 compared to adults (Figure 5). In addition, we observed that activation of TLR2 by HKLM resulted in significantly higher levels of IL-1β, IFNλ1, IFNγ, TNFα and GM-CSF for the PBMCS from the PCR+ children (Figure 5). Activation of TLR4 with LPS did not result in significant differences although a trend was observed for increased levels of several of these cytokines for the PCR+ children (Figure 5). Interestingly, when PBMCS from the PCR+ individuals were cultured overnight in medium only (Mock) the levels of secreted IL-6, IL-8, IL-10, and specially CXCL10 were already significantly higher for PCR+ children compared to PCR+ adults (Figure 5). For mock-stimulated PBMCs from healthy controls, higher levels of CXCL10 and IFNβ were also observed in children, but no increased levels of IL-6, IL-10 and IL-8 were found (Supplementary Figure 3). Also worth noticing is that while in healthy controls the levels of IL-8 after activation with the different TLR ligands were significantly higher for adults compared to children, this difference was reversed for the PCR+ adults. Altogether, these findings indicate that TLR activation, particularly TLR7/8, shows pronounced differences in the cytokine secretion profile between PCR+ children versus adults and that mock stimulated PBMCs already show some differences in the response between these two age groups.
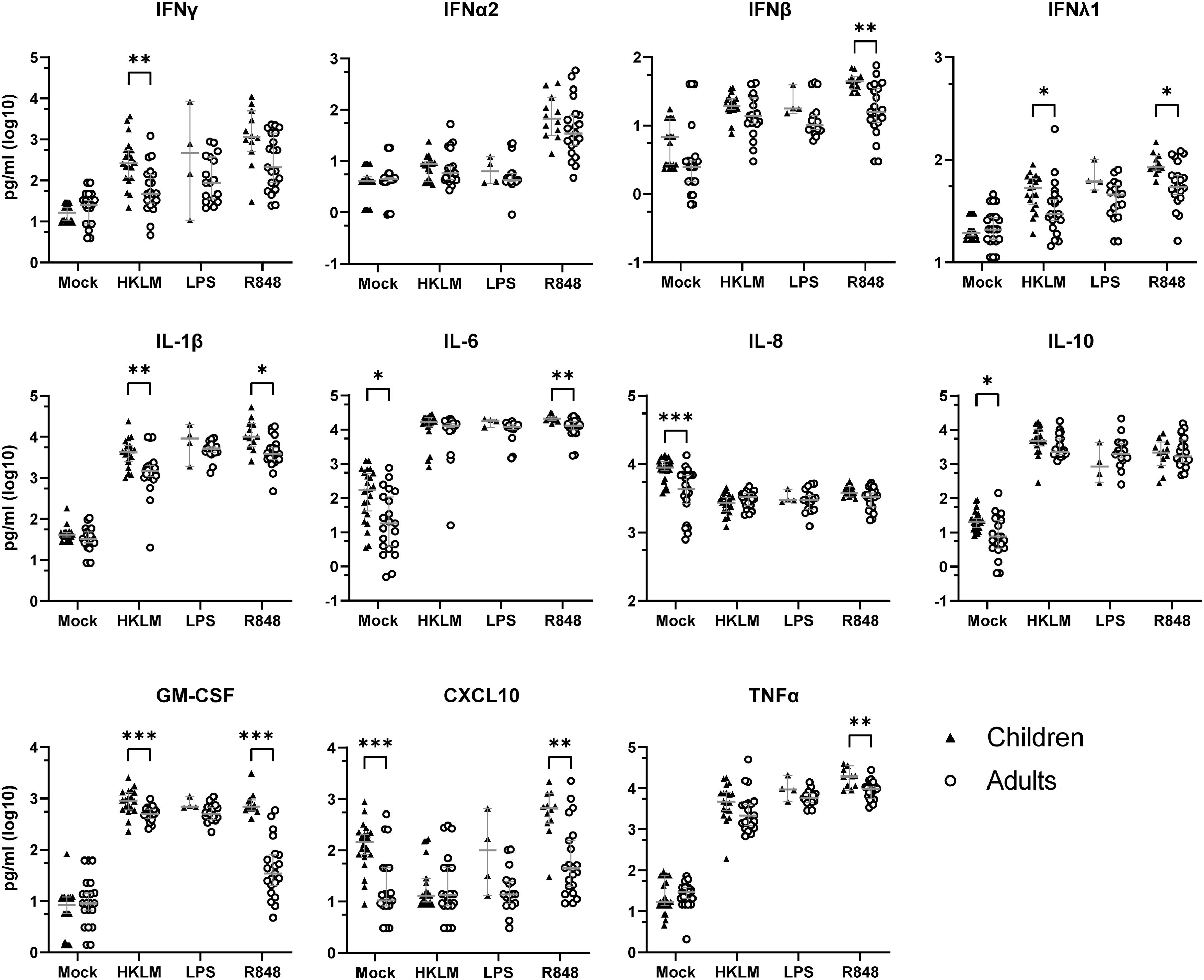
Figure 5. PBMCs from PCR+ children secret more cytokines and chemokines after TLR activation compared to adults. Cytokines and chemokines were measured in supernatant of PBMCs from PCR+ children (▲) and adults (○) after overnight stimulation of TLR2 with HKLM, TLR4 with lipopolysaccharide (LPS), or TLR7/8 with Resiquimod (R848). Values are shown as log10 pg/ml. Multiple Mann-Whitney test with Bonferroni-Dunn multiple comparison test were performed to compare response by children and adults for each stimulation or mock. Error bars indicate median value with 95% CI. *p-adj<0.05. **p-adj<0.01. ***p-adj<0.001.
Several weeks after testing SARS-CoV-2 PCR+ adults PBMCs show increased cytokine and chemokine secretion upon stimulation
To determine whether the cytokine secretion profile observed within a few days after testing PCR positive (T1) changed in time, we tested the responsiveness to iSARS-CoV-2 by PBMCs from the same adult individuals 2-3 weeks (T2) and 4-6 weeks (T3) later. The levels of CXCL10 after iSARS-CoV-2 stimulation of adult PBMCs were significantly higher at T2 and T3 compared to T1, and the levels of IFNγ and IFNα2 were significantly higher at T3 compared to T1 (Figure 6A). Notably, the levels of IL-8 remained lower compared to those of healthy controls up to six weeks post infection (Figures 1, 6A). Furthermore, adult PBMCs samples also produced higher levels of IFNγ at T2 and T3, compared to T1, after TLR2 stimulation (Figure 6B), TLR4 (Figure 6C), and TLR7/8 (Figure 6D) stimulation. In addition, TLR2 and TLR4, but not TLR7/8, stimulation also resulted in higher production of IL-1β in T2 and T3 samples compared to T1 samples (Figures 6B–D). The response to iSARS-CoV-2 or TLR stimulating agents by PBMCs from PCR+ children collected at T2 did not differ from T1 (data not shown). Together, these data indicate that at 2 and 6 weeks post infection adult PBMCs increase their responsiveness to iSARS-CoV-2 or PRR stimuli, whereas children retained stable response at T2.
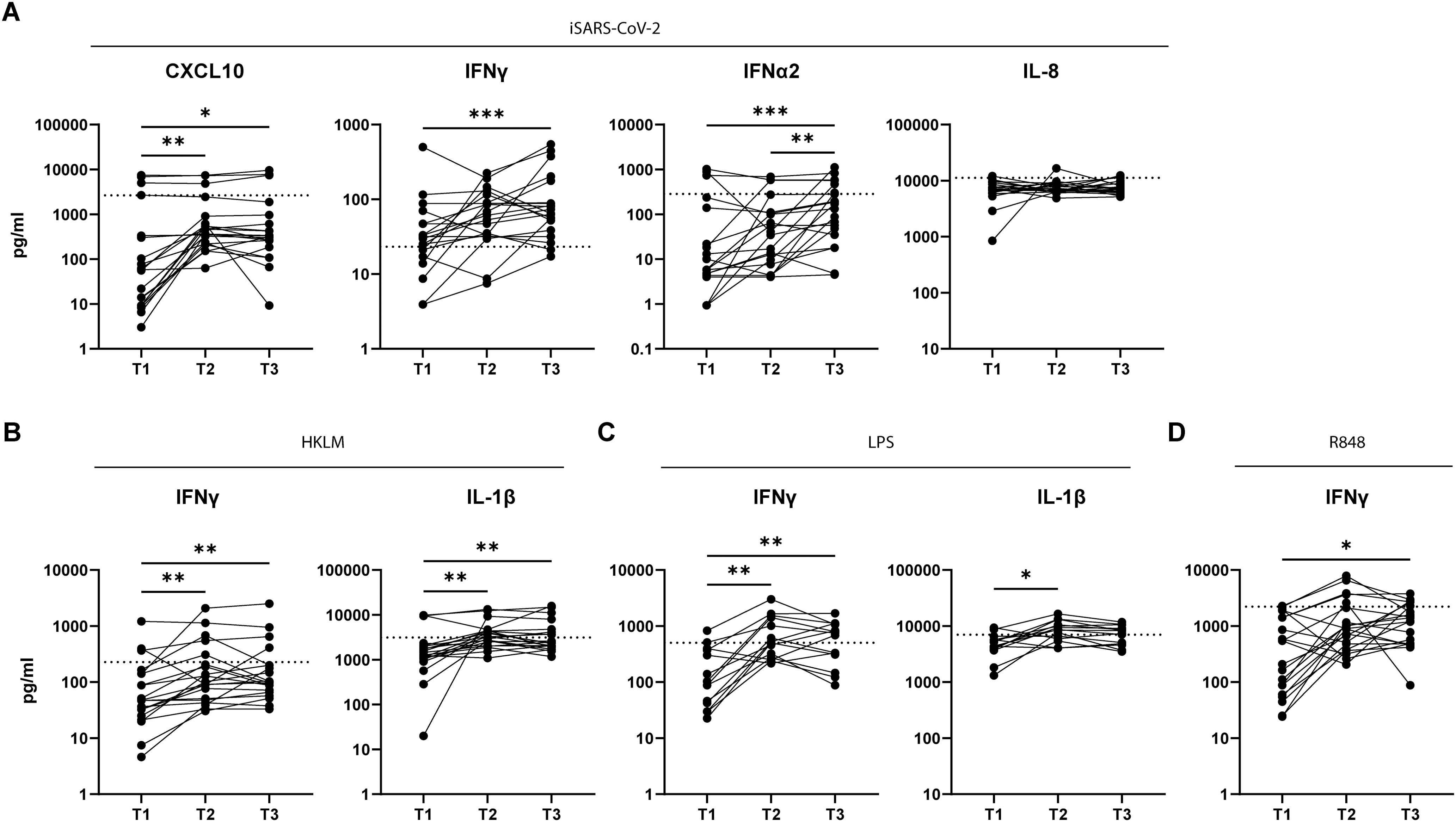
Figure 6. Adult PBMCs produce more cytokines and chemokines at later time points post infection. Cytokines and chemokines were measured in culture supernatant after iSARS-CoV-2 (A), HKLM (B), LPS (C), or R848 (D) stimulation of adult PBMCs collected at T1 (<7days), T2 (2-3 weeks), and T3 (4-6 weeks) post infection. Dotted line indicates average value of healthy control group. Friedmans test for non-parametric paired samples. followed by Dunn’s multiple comparison test was used to compare responses at each time point. *p-adj<0.05. **p-adj<0.01. ***p-adj<0.001.
Discussion
During the COVID-19 pandemic, children generally did not develop disease or when it occurred the severity, compared to adults, was relatively mild (5, 6). Several reports have argued that innate immune responses may determine the progression of COVID-19 (4, 7, 8, 28). In this study we showed that just a few days after primary infection, there are differences in the innate immune responses between PCR+ children and adults. The levels of SARS-CoV-2 Spike-specific IgG were also measured but no differences between children and adults were found.
SARS-CoV-2 has been described to suppress the innate arm of the immune responses during early stages of infection to evade the host directed anti-viral effect (29–31). In line with these observations, we observed that compared to the secretion profile of age matched unexposed healthy controls, adults showed early after testing PCR+ a reduced secretion of IL-8, which persisted for weeks. This was only observed in adults and not in children who on the contrary, showed enhanced innate immune response that did not change for two weeks after testing PCR+.
Previous studies have shown that the levels of IL-8 tended to decrease with more severe symptoms (32–34). Here we show that early after testing PCR+, PBMCs from adults with more severe COVID-19 symptoms produced less IL-8 upon iSARS-CoV-2 restimulation, corroborating previous findings. An interesting finding is that PBMC from PCR+ adults cultured in medium only secreted lower levels of IL-8 compared to PBMC from PCR+ children. Whether the observed differences are due to less production, downregulation, or upregulation of IL-8 receptors in the infected adults remains to be determined.
In our study, the levels of IL-6, IL-10, and CXCL10 production varied strongly for PBMCs from PCR+ adults and did not associate with symptom severity. PBMCs from PCR+ children did produce IL-10 after activation with iSARS-CoV-2, with higher levels in children with mild and moderate symptoms. Previous work showed that children resolved the innate immune response faster compared to adults, which could underly the difference in COVID-19 symptoms (10). Since the anti-inflammatory cytokine IL-10 did associate with symptoms severity in children, the exact role of this cytokine in controlling the immune response to SARS-CoV-2 remains to be determined.
When analyzing the innate cellular composition after iSARS-CoV-2 stimulation, no significant changes were observed in PCR+ children compared to healthy controls. Neeland MR et al. previously showed however that the overall monocyte count decreased during the acute phase of infection, which we are not able to deduce from our FACS analysis (35). What was clear from our findings is that in PCR+ adults the proportion of non-classical monocytes was significantly lower compared to the adult healthy controls and PCR+ children, which has been reported before (35). This decreased proportion of non-classical monocytes correlated with increased COVID-19 severity, a finding that has been previously reported by others (36, 37). A possible explanation for the smaller proportion could be that these non-classical monocytes migrate to tissues after infection. In fact, in a study using a single cell multi-omics approach, CD16+ blood monocytes were identified as potential source to replenish bronchoalveolar macrophages in the lungs of COVID-19 patients (38). Another interesting finding from our study is that the proportion of the non-classical monocytes correlated with the production of interferons, CXCL10 and IL-8. This fits with the notion that monocytes are a major source of CXCL10 in COVID-19 disease (39) and that non-classical monocytes after activation initiate an inflammatory response against viruses (40). In addition, our data show more production of CXCL10, IFNγ and IFNα2 by iSARS-CoV-2 stimulated PCR+ adult PBMCs at T2 and T3, which may in part can be explained by the observed increased proportion of non-classical and decreased proportion of classical monocytes at these time points. Altogether, the anti-viral response by PBMCs early after SARS-CoV-2 infection shows an important role for non-classical monocytes, chemokines CXCL10 and IL-8, as well as interferons.
The most relevant pattern recognition receptors recognizing SARS-CoV-2 are the RIG-I like receptors (RLR) (MDA5, RIG-I) and Toll like receptors (TLR) (TLR2, TLR4, TLR7 and TLR8) (27). Previous work has shown that myeloid cells from the nasal cavity in healthy and infected children express higher levels of RLR and TLRs compared to adults (12). This suggests that immune cells of children are primed for virus sensing resulting in stronger antiviral responses (9, 12). Our data, showed enhanced baseline cytokine/chemokine production by PBMCs from healthy and PCR+ children compared to adults. These differences were strengthened after iSARS-CoV-2 and PRR stimulation, indicating that this enhanced sensing and anti-viral state is also present systemically in children.
A limitation of our study is the use of an inactivated preparation of SARS-CoV-2. The heat treatment used may have denatured viral proteins, which may affect the measured immune response. Nevertheless, it is clear from our findings that stimulation with iSARS-CoV-2 did induce an anti-viral immune response.
In summary, we show that iSARS-COV-2 or TLR stimulation of PBMCS from individuals with a primary SARS-CoV-2 infection reveals a distinct innate immune profile for adults versus children. The lower levels of different chemokines/cytokines, including IL-8 and CXCL10, and a lower proportion of non-classical monocytes characterized the adult participants, particularly those with severe COVID-19. In contrast, the PCR+ children showed a clear inflammatory response and no or mild symptoms. The novelty of the data presented here reinforces the hypothesis that the quality of the innate immune response to SARS-CoV-2 is crucial for disease development and implicates an important role for PRR activation and their downstream signaling pathways.
Data availability statement
The raw data supporting the conclusions of this article will be made available by the authors, without undue reservation.
Ethics statement
The studies involving humans were approved by Medical-Ethical Review Committee of the University Medical Center Utrecht. The studies were conducted in accordance with the local legislation and institutional requirements. Written informed consent for participation in this study was provided by the participants’ legal guardians/next of kin.
Author contributions
MV: Data curation, Formal analysis, Visualization, Writing – original draft, Writing – review & editing. AM: Formal analysis, Methodology, Writing – original draft, Visualization. NN: Investigation, Writing – review & editing. RJ: Investigation, Writing – review & editing. AM: Investigation, Methodology, Writing – review & editing. DR: Conceptualization, Methodology, Writing – review & editing. JV: Conceptualization, Methodology, Resources, Writing – review & editing. CV: Resources, Writing – review & editing. DV: Writing – review & editing. NR: Conceptualization, Writing – review & editing. JD: Conceptualization, Supervision, Writing – review & editing. EP: Conceptualization, Supervision, Writing – original draft, Writing – review & editing.
Funding
The author(s) declare financial support was received for the research, authorship, and/or publication of this article. This study was funded by the Dutch Ministry of Health, Welfare and Sport (VWS), The Netherlands.
Acknowledgments
We are grateful to the members of the Dutch FFX-COVID-19 research group of the Centre for Infectious Disease Control, National Institute for Public Health and the Environment, the Netherlands, that includes Rianne van Gageldonk-Lafeber, Wim van der Hoek, Susan van den Hof, Chantal Reusken and Inge Roof for their contribution to the design and the conduct of the SARS-CoV-2-related clinical study. We thank the Public Health Service Utrecht for assistance in the recruitment of households. We thank Anneke Westerhof, Anne-Marie van den Brandt, Anoek Backx, Bas van der Veer, Elma Smeets-Roelofs, Elsa Porter, Elske Bijvank, Fion Brouwer, Francoise van Heiningen, Gabriel Goderski, Gert-Jan Godeke, Harry van Dijken, Helma Lith, Hinke ten Hulscher, Ilse Akkerman, Ilse Schinkel, Jeroen Hoeboer, Johan Reimerink, Jolanda Kool, Josien Lanfermeijer, Joyce Greeber, Kim Freriks, Kina Helm, Lidian Izeboud, Lisa Wijsman, Liza Tymchenko, Martijn Vos, Maarten Emmelot, Margriet Bisschoff, Marieke Hoogerwerf, Marit de Lange, Marit Middeldorp, Marjan Bogaard, Marjan Kuijer, Olga de Bruin, Rob van Binnendijk, Rogier Bodewes, Ruben Wiegmans, Sakinie Misiedjan, Saskia de Goede, Sharon van den Brink, Sophie van Tol, Teun Guichelaar, Titia Kortbeek, and Yolanda van Weert for support during the stages of this study, household visits, processing samples and laboratory analyses. From the Center for Infectious Disease Control, National Institute for Public Health and the Environment, the Netherlands we also thank Jorgen de Jonge for providing the inactivated SARS-CoV-2 preparation and Lisa Beckers for coordinating the processing of clinical samples for this study We thank Rutger Schepp, Gerco den Hartog, and Rob van Binnendijk for measuring the SARS-CoV-2 specific antibodies.
Conflict of interest
The authors declare that the research was conducted in the absence of any commercial or financial relationships that could be construed as a potential conflict of interest.
Publisher’s note
All claims expressed in this article are solely those of the authors and do not necessarily represent those of their affiliated organizations, or those of the publisher, the editors and the reviewers. Any product that may be evaluated in this article, or claim that may be made by its manufacturer, is not guaranteed or endorsed by the publisher.
Supplementary material
The Supplementary Material for this article can be found online at: https://www.frontiersin.org/articles/10.3389/fimmu.2024.1359993/full#supplementary-material
References
1. Sohrabi C, Alsafi Z, O’Neill N, Khan M, Kerwan A, Al-Jabir A, et al. World Health Organization declares global emergency: A review of the 2019 novel coronavirus (COVID-19). Int J Surg. (2020) 76:71–6. doi: 10.1016/j.ijsu.2020.02.034
2. Zhu N, Zhang D, Wang W, Li X, Yang B, Song J, et al. A novel coronavirus from patients with pneumonia in China, 2019. N Engl J Med. (2020) 382:727–33. doi: 10.1056/NEJMoa2001017
3. WHO coronavirus disease (COVID-19) dashboard 2020. Available at: https://data.who.int/dashboards/covid19/cases?n=c.
4. Mallapaty S. Kids and COVID: why young immune systems are still on top. Nature. (2021) 597:166–8. doi: 10.1038/d41586-021-02423-8
5. Irfan O, Li J, Tang K, Wang Z, Bhutta ZA. Risk of infection and transmission of SARS-CoV-2 among children and adolescents in households, communities and educational settings: A systematic review and meta-analysis. J Glob Health. (2021) 11:05013. doi: 10.7189/jogh.11.05013
6. Cui X, Zhao Z, Zhang T, Guo W, Guo W, Zheng J, et al. A systematic review and meta-analysis of children with coronavirus disease 2019 (COVID-19). J Med Virol. (2021) 93:1057–69. doi: 10.1002/jmv.26398
7. Paludan SR, Mogensen TH. Innate immunological pathways in COVID-19 pathogenesis. Sci Immunol. (2022) 7:eabm5505. doi: 10.1126/sciimmunol.abm5505
8. Schultze JL, Aschenbrenner AC. COVID-19 and the human innate immune system. Cell. (2021) 184:1671–92. doi: 10.1016/j.cell.2021.02.029
9. Yoshida M, Worlock KB, Huang N, Lindeboom RGH, Butler CR, Kumasaka N, et al. Local and systemic responses to SARS-CoV-2 infection in children and adults. Nature. (2022) 602:321–7. doi: 10.1038/s41586-021-04345-x
10. Vono M, Huttner A, Lemeille S, Martinez-Murillo P, Meyer B, Baggio S, et al. Robust innate responses to SARS-CoV-2 in children resolve faster than in adults without compromising adaptive immunity. Cell Rep. (2021) 37:109773. doi: 10.1016/j.celrep.2021.109773
11. Moreno-Eutimio MA, Lopez-Macias C, Pastelin-Palacios R. Bioinformatic analysis and identification of single-stranded RNA sequences recognized by TLR7/8 in the SARS-CoV-2, SARS-CoV, and MERS-CoV genomes. Microbes Infect. (2020) 22:226–9. doi: 10.1016/j.micinf.2020.04.009
12. Loske J, Rohmel J, Lukassen S, Stricker S, Magalhaes VG, Liebig J, et al. Pre-activated antiviral innate immunity in the upper airways controls early SARS-CoV-2 infection in children. Nat Biotechnol. (2022) 40:319–24. doi: 10.1038/s41587-021-01037-9
13. Beucher G, Blondot ML, Celle A, Pied N, Recordon-Pinson P, Esteves P, et al. Bronchial epithelia from adults and children: SARS-CoV-2 spread via syncytia formation and type III interferon infectivity restriction. Proc Natl Acad Sci U S A. (2022) 119:e2202370119. doi: 10.1073/pnas.2202370119
14. Reukers DFM, van Boven M, Meijer A, Rots N, Reusken C, Roof I, et al. High infection secondary attack rates of SARS-CoV-2 in Dutch households revealed by dense sampling. Clin Infect Dis. (2021) 74(1):52–8. doi: 10.1101/2021.01.26.21250512
15. Rosendahl Huber SK, Hendriks M, Jacobi RHJ, van de Kassteele J, Mandersloot-Oskam JC, van Boxtel RAJ, et al. Immunogenicity of influenza vaccines: evidence for differential effect of secondary vaccination on humoral and cellular immunity. Front Immunol. (2018) 9:3103. doi: 10.3389/fimmu.2018.03103
16. Kroes MM, van Vliet LC, Jacobi RHJ, Kuipers B, Pieren DKJ, Miranda-Bedate A, et al. Long lasting antibodies from convalescent pertussis patients induce ROS production and bacterial killing by human neutrophils. Front Cell Infect Microbiol. (2022) 12:888412. doi: 10.3389/fcimb.2022.888412
17. van de Ven K, van Dijken H, Wijsman L, Gomersbach A, Schouten T, Kool J, et al. Pathology and immunity after SARS-coV-2 infection in male ferrets is affected by age and inoculation route. Front Immunol. (2021) 12:750229. doi: 10.3389/fimmu.2021.750229
18. Team RC. A language and environment for statistical computing. Vienna, Austria: R Foundation for Statistical Computing (2021). Available at: https://www.R-project.org/.
19. Hahne F, LeMeur N, Brinkman RR, Ellis B, Haaland P, Sarkar D, et al. flowCore: a Bioconductor package for high throughput flow cytometry. BMC Bioinf. (2009) 10:106. doi: 10.1186/1471-2105-10-106
20. Crowell HL, Chevrier S, Jacobs A, Sivapatham S, Tumor Profiler C, Bodenmiller B, et al. An R-based reproducible and user-friendly preprocessing pipeline for CyTOF data. F1000Res. (2020) 9:1263. doi: 10.12688/f1000research
21. Crowell H, Zanotelli V, Chevrier S, Robinson M. CATALYST: Cytometry dATa anALYSis Tools (2021). Available online at: https://github.com/HelenaLC/CATALYST. (accessed December 20, 2023)
22. den Hartog G, Schepp RM, Kuijer M, GeurtsvanKessel C, van Beek J, Rots N, et al. SARS-coV-2-specific antibody detection for seroepidemiology: A multiplex analysis approach accounting for accurate seroprevalence. J Infect Dis. (2020) 222:1452–61. doi: 10.1093/infdis/jiaa479
23. van den Hoogen LL, Smits G, van Hagen CCE, Wong D, Vos ERA, van Boven M, et al. Seropositivity to Nucleoprotein to detect mild and asymptomatic SARS-CoV-2 infections: A complementary tool to detect breakthrough infections after COVID-19 vaccination? Vaccine. (2022) 40:2251–7. doi: 10.1016/j.vaccine.2022.03.009
24. Monaco G, Lee B, Xu W, Mustafah S, Hwang YY, Carre C, et al. RNA-seq signatures normalized by mRNA abundance allow absolute deconvolution of human immune cell types. Cell Rep. (2019) 26:1627–40.e7. doi: 10.1016/j.celrep.2019.01.041
25. Khan S, Shafiei MS, Longoria C, Schoggins JW, Savani RC, Zaki H. SARS-CoV-2 spike protein induces inflammation via TLR2-dependent activation of the NF-kappaB pathway. Elife. (2021) 6(10):e68563. doi: 10.7554/eLife.68563
26. Zhao Y, Kuang M, Li J, Zhu L, Jia Z, Guo X, et al. SARS-CoV-2 spike protein interacts with and activates TLR41. Cell Res. (2021) 31:818–20. doi: 10.1038/s41422-021-00495-9
27. Salvi V, Nguyen HO, Sozio F, Schioppa T, Gaudenzi C, Laffranchi M, et al. SARS-CoV-2-associated ssRNAs activate inflammation and immunity via TLR7/8. JCI Insight. (2021) 6(18):e150542. doi: 10.1172/jci.insight.150542
28. Diamond MS, Kanneganti TD. Innate immunity: the first line of defense against SARS-CoV-2. Nat Immunol. (2022) 23:165–76. doi: 10.1038/s41590-021-01091-0
29. Hadjadj J, Yatim N, Barnabei L, Corneau A, Boussier J, Smith N, et al. Impaired type I interferon activity and inflammatory responses in severe COVID-19 patients. Science. (2020) 369:718–24. doi: 10.1126/science.abc6027
30. Tian W, Zhang N, Jin R, Feng Y, Wang S, Gao S, et al. Immune suppression in the early stage of COVID-19 disease. Nat Commun. (2020) 11:5859. doi: 10.1038/s41467-020-19706-9
31. Minkoff JM, tenOever B. Innate immune evasion strategies of SARS-CoV-2. Nat Rev Microbiol. (2023) 21:178–94. doi: 10.1038/s41579-022-00839-1
32. Laing AG, Lorenc A, Del Molino Del Barrio I, Das A, Fish M, Monin L, et al. A dynamic COVID-19 immune signature includes associations with poor prognosis. Nat Med. (2020) 26:1623–35. doi: 10.1038/s41591-020-1038-6
33. Del Valle DM, Kim-Schulze S, Huang HH, Beckmann ND, Nirenberg S, Wang B, et al. An inflammatory cytokine signature predicts COVID-19 severity and survival. Nat Med. (2020) 26:1636–43. doi: 10.1038/s41591-020-1051-9
34. Lev S, Gottesman T, Sahaf Levin G, Lederfein D, Berkov E, Diker D, et al. Observational cohort study of IP-10’s potential as a biomarker to aid in inflammation regulation within a clinical decision support protocol for patients with severe COVID-19. PloS One. (2021) 16:e0245296. doi: 10.1371/journal.pone.0245296
35. Neeland MR, Bannister S, Clifford V, Dohle K, Mulholland K, Sutton P, et al. Innate cell profiles during the acute and convalescent phase of SARS-CoV-2 infection in children. Nat Commun. (2021) 12:1084. doi: 10.1038/s41467-021-21414-x
36. Silvin A, Chapuis N, Dunsmore G, Goubet AG, Dubuisson A, Derosa L, et al. Elevated calprotectin and abnormal myeloid cell subsets discriminate severe from mild COVID-19. Cell. (2020) 182:1401–18.e18. doi: 10.1016/j.cell.2020.08.002
37. Schulte-Schrepping J, Reusch N, Paclik D, Bassler K, Schlickeiser S, Zhang B, et al. Severe COVID-19 is marked by a dysregulated myeloid cell compartment. Cell. (2020) 182:1419–40.e23. doi: 10.1016/j.cell.2020.08.001
38. Stephenson E, Reynolds G, Botting RA, Calero-Nieto FJ, Morgan MD, Tuong ZK, et al. Single-cell multi-omics analysis of the immune response in COVID-19. Nat Med. (2021) 27:904–16. doi: 10.1038/s41591-021-01329-2
39. Cillo AR, Somasundaram A, Shan F, Cardello C, Workman CJ, Kitsios GD, et al. People critically ill with COVID-19 exhibit peripheral immune profiles predictive of mortality and reflective of SARS-CoV-2 lung viral burden. Cell Rep Med. (2021) 2:100476. doi: 10.1016/j.xcrm.2021.100476
Keywords: COVID-19, innate immune response, age, monocytes, toll like receptors
Citation: Van de Garde MDB, Miranda-Bedate A, Nanlohy NM, Jacobi RHJ, Meijer A, Reukers DFM, Van Beek J, Van Els CACM, Van Baarle D, Rots NY, De Wit J and Pinelli E (2024) Early immune profiling reveals distinct inflammatory responses between children and adults few days after primary SARS-CoV-2 infection. Front. Immunol. 15:1359993. doi: 10.3389/fimmu.2024.1359993
Received: 22 December 2023; Accepted: 24 October 2024;
Published: 18 November 2024.
Edited by:
Rüdiger Groß, Ulm University Medical Center, GermanyReviewed by:
Lisa A. Chakrabarti, Institut Pasteur, FranceRia Goswami, Cornell University, United States
Suresh D. Sharma, United States Department of Health and Human Services, United States
Copyright © 2024 Van de Garde, Miranda-Bedate, Nanlohy, Jacobi, Meijer, Reukers, Van Beek, Van Els, Van Baarle, Rots, De Wit and Pinelli. This is an open-access article distributed under the terms of the Creative Commons Attribution License (CC BY). The use, distribution or reproduction in other forums is permitted, provided the original author(s) and the copyright owner(s) are credited and that the original publication in this journal is cited, in accordance with accepted academic practice. No use, distribution or reproduction is permitted which does not comply with these terms.
*Correspondence: Martijn D. B. Van de Garde, bWFydGlqbi52YW4uZGUuZ2FyZGVAcml2bS5ubA==; Jelle De Wit, amVsbGUuZGUud2l0QHJpdm0ubmw=