- 1Department of Internal Medicine, Hospital of the University of Pennsylvania, Philadelphia, PA, United States
- 2Abramson Cancer Center and the Division of Hematology and Oncology, Hospital of the University of Pennsylvania, Philadelphia, PA, United States
Allogeneic hematopoietic cell transplantation (HCT) has transformed over the past several decades through enhanced supportive care, reduced intensity conditioning (RIC), improved human leukocyte antigen (HLA) typing, and novel graft-versus-host disease (GVHD)-prevention and treatment strategies. Most notably, the implementation of post-transplantation cyclophosphamide (PTCy) has dramatically increased the safety and availability of this life-saving therapy. Given reductions in nonrelapse mortality (NRM) with these advances, the HCT community has placed even greater emphasis on developing ways to reduce relapse - the leading cause of death after HCT. When using RIC HCT, protection from relapse relies predominantly on graft-versus-leukemia (GVL) reactions. Donor lymphocyte infusion (DLI), adoptive cellular therapy, checkpoint inhibition, and post-HCT maintenance strategies represent approaches under study that aim to augment or synergize with the GVL effects of HCT. Optimizing donor selection algorithms to leverage GVL represents another active area of research. Many of these strategies seek to harness the effects of T cells, which for decades were felt to be the primary mediators of GVL and the focus of investigation in relapse reduction. However, there is growing interest in capitalizing on the ability of natural killer (NK) cells to yield potent anti-tumor effects. A potential advantage of NK cell-based approaches over T cell-mediated is the potential to reduce NRM in addition to relapse. By decreasing infection, without increasing the risk of GVHD, NK cells may mitigate NRM, while still yielding relapse reduction through identification and clearance of cancer cells. Most T cell-focused relapse-prevention strategies must weigh the benefits of relapse reduction against the increased risk of NRM from GVHD. In contrast, NK cells have the potential to reduce both, potentially tipping the scales significantly in favor of survival. Here, we will review the role of NK cells in GVL, optimization of NK cell match or mismatch, and burgeoning areas of research in NK cell therapy such as adoptive transfer and chimeric antigen receptor (CAR) NK cells.
Introduction
Improved conditioning regimens and enhanced HLA typing have made allogeneic hematopoietic cell transplantation (HCT) safer. Whatsmore, the advent of modern GVHD prophylactic regimens has made HCT accessible to nearly every patient in need. In particular, PTCy has expanded the donor pool to safely include HLA-mismatched related (haplo) (1) and HLA-mismatched unrelated (MMUD) donors (2, 3). With reductions in nonrelapse mortality (NRM) through GVHD prevention, relapse remains the leading cause of HCT failure and the major unmet need in HCT (4). Unfortunately, therapeutic options to treat post-HCT relapse are limited and outcomes are generally poor (5). For instance, relapse after HCT accounts for 30% to 40% of deaths (6) and 1-year overall survival (OS) after acute myeloid leukemia (AML) relapse is only 23% (7). In HCT with PTCy, 1-year OS after relapse is between 37% to 46% (5). Typically, remission-inducing chemotherapy followed by DLI or second HCT has been associated with the best OS after post-HCT relapse and yet only 8-25% of patients achieve long-term survival with these therapies (8–10). Given these poor outcomes, it is important to better understand the biology of post-HCT relapse in order to both prevent and treat it.
There are multiple factors that have been associated with higher risk of post-HCT relapse, including lack of complete hematologic remission (i.e. CR) or presence of minimal residual disease (MRD) at time of HCT, high-risk cytogenetic or molecular characteristics, use of T cell-depleted (TCD) HCT platforms, and reduced-intensity conditioning (7, 11, 12). Currently, interventions to prevent post-HCT relapse span the pre-HCT, peri-HCT, and post-HCT settings. In the pre-HCT setting, efforts include novel therapies to achieve deeper remissions prior to HCT, better identification of MRD, and improvements in HCT conditioning (11, 13, 14). In the peri-HCT setting, strategies include optimization of graft cell dose, use of sirolimus rather than tacrolimus in post-HCT immunosuppression, and early and/or rapid immunosuppression taper (15–17). Finally, in the post-HCT setting, efforts are focused on pharmacologic or immunotherapeutic interventions such as maintenance chemotherapy or prophylactic DLI (8, 11, 14).
Acute myeloid leukemia (AML) is the most frequent indication for HCT because of the potency of the graft-versus-leukemia (GVL) effect mediated by donor-derived immune cells, chiefly natural killer (NK) and T cells, exerting anti-leukemic immune reactions (18). GVL was first described by Barnes et al. in 1956, when reporting in murine models cure of leukemia after total body irradiation (TBI) and HCT (19, 20). The clinical existence of GVL was then further demonstrated in a large registry study conducted by the International Bone Marrow Transplant Registry (IBMTR) (21). In that study, recipients of non-TCD allografts who developed acute GVHD (aGVHD), chronic GVHD (cGVHD), or both had decreased rates of relapse compared to recipients who never developed GVHD (21). A dose effect was demonstrated with increased severity of GVHD or presence of both acute and chronic GVHD being associated with the lowest relapse rates (21). These findings indicate that alloreactivity enhances GVL and illustrate the intimate relationship between GVHD and GVL, implicating cells involved in GVHD pathogenesis in mediation of GVL. In contrast to T cells, the main mediators of acute GVHD, NK cells also play a role in GVL, albeit without a significant association with GVHD development. Here, we review the immunobiology of GVL, with a focus on NK cells, and the current understanding of how NK cells might be harnessed to augment GVL to prevent post-HCT relapse.
NK cell biology and role in the graft-versus-leukemia effect
NK cells are innate immune lymphocytes that identify and eliminate stressed cells (i.e. malignant or viral-infected cells) via direct lysis or production of proinflammatory cytokines. They account for 5 to 15% of peripheral blood lymphocytes and are the third most abundant lymphocyte lineage in the peripheral blood (22–26). NK cells can identify stressed cells through their expression of ligands associated with cellular stress (NKG2D or DNAM-1) or through absence of expression of HLA class 1 molecules (27, 28). NK cell activation is mediated by the balance of activating and inhibitory signals received upon contact with host or foreign cells: either via a lack of interaction between a NK cell inhibitory receptor and its cognate ligand, an interaction between a NK cell activating receptor and its cognate ligand, or a predominance of activating over inhibitory signals (27, 29, 30). The inhibitory receptors found on NK cells, which include inhibitory killer immunoglobulin-like receptors (iKIRs), CD94/NKG2A, and LILRB1 among many others (Table 1), interact with HLA class I molecules and promote self-tolerance, preventing the initiation of NK cell cytotoxicity (30). NK activation and cellular cytotoxicity occurs when activating receptors such as activating killer immunoglobulin-like receptors (aKIRs), NKp46, and NKG2D (Table 1), bind to their respective cognate ligands (27, 29). Human NK cells express KIRs, whereas murine NK cells express members of the Ly49 family of the C-type lectin type II transmembrane proteins, but these receptors are functionally analogous (31).
NK cells are typically phenotypically defined by the expression of CD56 and lack of expression of CD3 (22). Within CD56 expressing cells, two subsets exist, defined based on level of CD56 expression: CD56dim and CD56bright (22). Development occurs in the bone marrow with IL-3, IL-7, c-kit ligand, and flt3 ligand coordinating to signal to CD34+ hematopoietic progenitor cells to commit to the NK cell lineage (Figure 1) (26). Then, IL-15 is needed for differentiation to CD56+ NK cells, which then further differentiate to CD56brightCD16-KIR- then to CD56dimCD16+KIR- then to CD56dimCD16+KIR+ subsets (Figure 1) (26). As compared to CD56bright NK cells, CD56dim NK cells are more cytotoxic likely in part due to higher expression of aKIRs and Ig-like transcripts (ILTs), which enable enhanced cytotoxic properties (22). However, the CD56bright NK cell is thought to be a more potent producer of cytokines, compared to the CD56dim NK cell (32). Differences in the level of expression of CD16, i.e. CD16bright versus CD16dim, have also been shown to have important functional consequences. CD56dimCD16bright are thought to be more cytotoxic, as CD16 mediates antibody-dependent cell-mediated cytotoxicity (22). The CD56dimCD16dim NK cell subset degranulates at a higher level than the CD56dimCD16bright cell, however, it is difficult to characterize where this population fits in the maturation spectrum of NK cells (33). Importantly, there is no murine homolog of CD56 expressed on mouse NK cells (22), but differential expression of the Ly49 family or CD27high versus CD27low expression has been used to functionally define murine NK cells (31, 34).
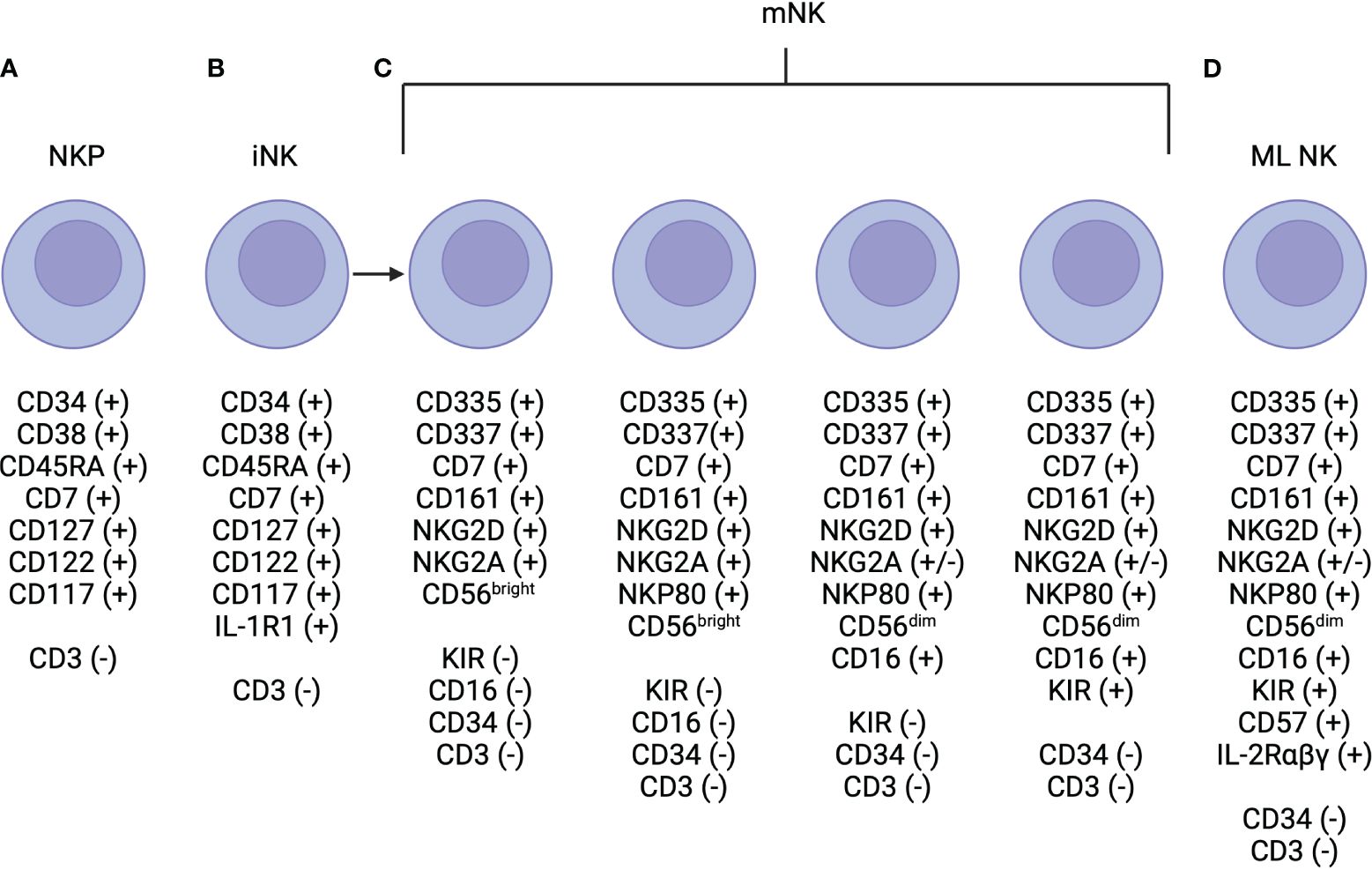
Figure 1 NK Cell Development. (A) Natural killer progenitor (NKP) cells are defined by are defined by expression of CD7, CD127, CD117 (c-Kit) and this marks the beginning of commitment to the NK cell lineage. (B) NKP cells progress to immature NK (iNK) cells, which are defined by higher expression of IL-R1. Expression of NKG2D, CD335, CD337, and CD161 are initiated and may be expressed at low levels near the end of this life-stage. (C) Mature NK (mNK) cells are defined by the expression of CD56 and maximal expression of NKG2D, CD335, CD337, and CD161. There are multiple subtypes of mature NK cells, which have unique functional properties, that are defined primarily via the level of expression of CD56 (i.e. CD56bright vs CD56dim), and CD16 and KIR positivity. (D) Finally, memory-like (ML) NK cells have also been described and are defined by high levels of expression of the high affinity IL-2αβγ receptor.
In the case of HCT and GVL, donor derived NK cells may identify leukemic cells that have downregulated or lack cognate HLA class I molecules to donor iKIRs (35–38) or those with surface markers that interact with NK cell activation receptors (18). After activation, NK cells conduct cell-killing through either release of cytotoxic granules loaded with perforin and granzymes, leading to target cell lysis, or through induction of Fas-L- or TRAIL- mediated target cell lysis (27, 38). NK cells also secrete pro-inflammatory cytokines (IFN-γ, TNF-α, IL-6), which contribute to both innate and adaptive immunity in the GVL reaction (38).
The kinetics of NK cell recovery after HCT highlight the importance of NK cells in early GVL reactions. NK cells are the first non-neutrophil white blood cell to recover after HCT, doing so during the period of severe lymphopenia following conditioning (39–41). This has been shown to be true with both non-PTCy and PTCy-based GVHD prophylaxis regimens (39–42). Two studies from the National Institutes of Health examined immunological recovery post-HCT in patients receiving TCD HCT from HLA-identical siblings (40, 41). In these studies, early NK recovery was noted to be the main predictor for molecular remission in patients with CML (41) and also was found to be associated with decreased relapse in patients with acute myeloid leukemia (AML), but not in patients with acute lymphocytic leukemia (ALL) (40). Importantly, early recovering NK cells after PTCy-based HCT may display unique phenotypic properties, as compared to those that recover after non-PTCy based GVHD HCT (39, 42, 43). Roberto et al. identified a population of CD56dimCD16neg NK cells that are detected in high frequency early post-HCT with PTCy and display defective cytotoxicity secondary to increased anergy associated with increased surface levels of CD94/NKG2A, an iKIR (43). Russo et al. and Rambaldi et al. both demonstrated that early NK cells recovering after PTCy had an immature phenotype and demonstrated impaired in-vitro antileukemic activity (39, 42). These studies allude to the potential utility of adoptive NK cell therapy in PTCy platforms to compensate for post-HCT NK cell immaturity.
One of the earliest studies demonstrating the importance of NK cells in GVL was published by Ruggeri et al. in 2002 (37). In this analysis of 92 patients, the Perugia group compared the outcomes after TCD haplo HCT for recipients with and without KIR ligand incompatibility (37). The findings were striking. In HCT from NK cell alloreactive donors, there were no cases of graft rejection or grades II-IV GVHD, compared to 15.5% and 13.7% incidence respectively in patients who received HCT from NK cell non-alloreactive donors (37). Moreover, no patients with AML had relapsed at 5 years in the NK cell alloreactive donor group (37). The group then supplemented their clinical data with in vivo murine mechanistic studies. NOD/SCID mice with AML were transfused with either human alloreactive, non-alloreactive, or no NK cells (37). Only mice that received alloreactive NK cells survived, while mice that received either non-alloreactive or no NK cells died over a period of about three weeks (37). Interestingly, when mice were conditioned with TBI and subsequently infused with escalating doses of alloreactive T cells, concomitant infusion of alloreactive NK cells protected against the fatal GVHD that was seen with alloreactive T cell infusions alone (37). However, in chimeric mice, in whom recipient antigen presenting cells (APCs) were engineered to be resistant to the donor alloreactive NK cells, the protective benefit of alloreactive NK cell infusion was eliminated and the mice succumbed to fatal GVHD (37). The authors postulated that alloreactive NK cells are a primary mediator of GVL and could, in the future, be used as a form of cell therapy for host immune suppression, GVHD prevention (potential mechanisms for which are discussed below), and leukemia ablation (37).
NK cell role in reducing non-relapse mortality after allogeneic HCT
Aside from their role in the GVL effect, NK cells have also been shown to be important in many additional post-HCT outcomes including protection against infection. After HCT, the recipient experiences many vulnerable months of profound immunosuppression (44). This period of immunosuppression is characterized by a high risk of bacterial and viral infections, including reactivation of opportunistic viruses like cytomegalovirus (CMV) (44). NK cells have an established role in anti-viral immunity, as demonstrated by the overwhelming viral infections associated with disorders marked by a congenital absence of NK cells (45). NK cells, as part of the innate immune system, play an important role in CMV control (44, 46). For example, in a murine model, induction and activation of NK cells via macrophage colony-stimulating factor (M-CSF) administration post-HCT led to protection against lethal CMV (44). Recipients of haplo HCT who experienced CMV reactivation have been noted to have higher proportions of mature CD56dimCD16+KIR+ NK cells (42) and faster acquisition of the activating NKG2C receptor (39), when compared to those who do not experience CMV reactivation. In fact, patients with a higher ratio of CD16- NK cells to CD16+ NK cells at one- and two-months post HCT developed CMV reactivation at higher rates, suggesting that delayed NK cell maturation may make patients more susceptible to CMV reactivation (42). Certain KIR haplotypes have also been shown to be associated with better post-HCT control of CMV (46).
NK cells may also play an important role in the suppression and prevention of GVHD through lysis of responsible alloreactive T cells (45–48). Low total numbers of NK cells have been linked to poor GVHD outcomes including worse OS (45, 49). However, there is also data that NK cell-mediated production of cytokines may contribute to the inflammatory cascade responsible for GVHD (45). Interestingly, subtypes of NK cells have been identified in murine intestines that may play a role in protection against bacterial infections associated with development of gastrointestinal GVHD, through both cytokine-induced defenses and regeneration of the gut epithelium (47).
NK cell-mediated prevention of GVHD and infection translates to reduced NRM in patients with better NK cell recovery (50). The relationship of NK cells with protection from NRM is also suggested by the beneficial effects of HLA-B leader matching, which has been associated with less NRM and improved OS after MUD and haplo HCT (51, 52). HLA-B leader refers to the peptide encoded by exon 1 of the 7 exons that comprise the HLA-B gene (53, 54). The leader sequence of HLA class-I molecules is presented by the non-classical class I molecule, HLA-E, and stabilizes HLA-E expression on the cell surface, enhancing binding to receptors on NK cells (53, 54). It is hypothesized that HLA-B presentation by HLA-E and subsequent recognition by NK cells may contribute to improvements in NRM that have been demonstrated in several HCT settings and has opened another avenue of research to understand NK alloreactivity through HLA-matching (53, 54).
Optimization of NK cell match and genotype
Fortunately, given safe HLA-mismatched HCT facilitated by PTCy, we now exist in an age of HCT where nearly every patient has a donor. In fact, many patients have multiple donors from whom to choose. These may include matched sibling donors (MSDs), matched unrelated donor (MUDs), MMUDs, HLA-haploidentical donors, or umbilical cord blood (UCB) donors. In this age of donor choice, there is increasing focus on refinement of selection algorithms to improve outcomes. This consists of optimization of HLA-matching, donor age, familial relationship, presence of donor specific antibodies (DSAs), CMV serostatus, and ABO match – among other factors. Much research has been conducted on how each of the aforementioned donor characteristics may differentially influence HCT outcomes. The creation of complex guidelines and algorithms that physicians may use for donor selection is still underway and how to prioritize these factors is still uncertain (52).
However, even less is known about optimization of NK cell alloreactivity between donor and recipient to facilitate amplified GVL and subsequently decrease risk of post-HCT relapse. What is known has been primarily studied in non-PTCy platforms, however, more data in PTCy-based HCT is emerging. Importantly, NK cell alloreactivity is probably more readily harnessed in the HLA-mismatched settings (55). However, the current body of literature (Table 2) regarding donor selection and NK cell alloreactivity is complex and in flux and there are indications that NK cells, regardless of alloreactivity, are critical in relapse prevention in all PTCy platforms (50). Despite the current discordant data, which will be examined below and is summarized in Table 2, the European Group for Blood and Marrow Transplantation (EBMT) includes NK cell alloreactivity as a criterion of interest in the 2019 Consensus Recommendations for Haploidentical Donor Selection (68).
In the case of donor optimization in HCT, KIRs are of specific interest because they are polymorphic, whereas CD94/NKG2 are not (35). Additionally, given that major histocompatibility complex (MHC) and KIR are located on chromosomes 6 and 19, respectively, they segregate and are thus inherited independently, which provides additional opportunity for donor optimization aside from HLA (35). For example, among unrelated donors and recipients who are HLA-identical it is rare to be KIR identical and only 25% of HLA-identical siblings will also be KIR identical (69).
KIR proteins are classified by their extracellular domain and the length of their cytoplasmic tail, which determines their ligand specificity and functionality, respectively (35). The nomenclature of KIRs follow this convention: KIR2Dxx and KIR3Dxx have two and three extracellular domains respectively, and KIRxDSx and KIRxDLx having short activating or long inhibitory cytoplasmic tails, respectively (35). MHC molecules act as interacting ligands for KIRs and each KIR has a cognate MHC molecule (35). These MHC molecules include HLA-A, HLA-Bw4, HLA-C, and HLA-E (29). Typing of KIR and MHC prior to HCT would provide information regarding which donor/recipient pairs possess KIR/MHC combinations that lead to alloreactivity in an effort to harness the NK GVL effect. However, KIR typing is not routinely performed at most centers or unrelated donor registries, limiting widespread study of its influences and utilization of any resulting data in clinical practice.
Several models have been proposed to predict KIR compatibility between recipient and donor post-HCT (Figure 2). Each model places emphasis on a different component of NK cell immunobiology and the interaction between donor NK cells and recipient cells: i.e. whether the onus of the mismatch lies in interface of the receptors or the ligands. These include: the ligand-ligand mismatch model (29, 35–38), receptor-ligand/missing-ligand model (27, 35, 37), receptor-receptor mismatch/haplotype model (60), educational/missing licensing proof model (71), and NK cell genotyping (66, 67). Ultimately, the multitude of models that exist in the literature to predict NK cell alloreactivity in HCT illustrates the complexity of NK cell alloreactivity and the need for unifying research to increase its applicability.
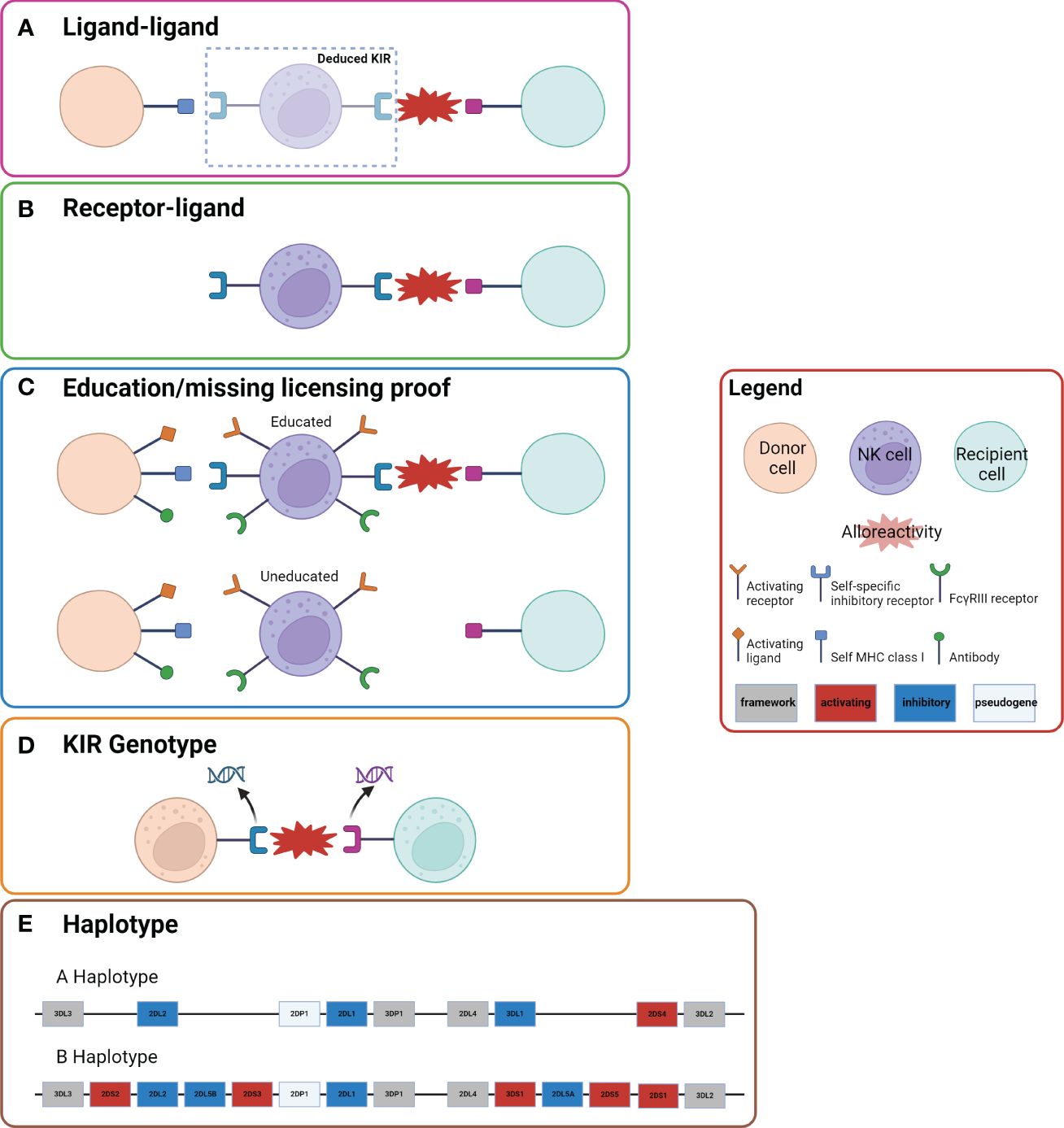
Figure 2 Five Models of NK Cell Alloreactivity. Representation of the five models of NK cell alloreactivity. Figure is adapted from Dhuyser et al. (36) and Boudrea et al. (70). (A) In the ligand-ligand model NK cell alloreactivity occurs when the recipient lacks a ligand that is present in the donor. (B) In the receptor-ligand model (missing-ligand model), if the KIR of the donor does not recognize the MHC molecule of the recipient there will be alloreactivity. (C) In the education/missing licensing proof model the process of NK cell licensing is considered. When a NK cell that has been “licensed” or “educated” based on a self-specific inhibitory receptor to a specific self MHC class I ligand encounters a recipient cell that does not have the same self MHC class I ligand, there is alloreactivity. (D) In the KIR genotype model certain mismatches of KIR genotypes result in alloreactivity. (E) The haplotype model examines the KIR haplotype of donor and recipient. Donors with KIR B haplotypes have been shown to have improved outcomes, which has been postulated to be due to more activating KIR genes.
Understanding NK cell licensing is critical to comprehending the complexities of each NK alloreactivity model. Just as T-cells undergo positive selection to prevent alloreactivity, NK cells also undergo a process so they may discriminate self from non-self called “licensing”. In a healthy person an NK cell will encounter the matching self-HLA-class I ligand for that NK cell’s specific inhibitory KIR and become “licensed” to refrain from attack on healthy self-antigens (72, 73). When licensed NK cells encounter a cell that does not express or is missing the appropriate KIR-ligand they are able to mount a response if there is also expression of ligands that interact with the aKIRs of the NK cell (72, 73). Unlicensed NK cells are not functionally competent and are hyporesponsive when compared to licensed NK cells (72, 73).
The ligand-ligand mismatch model was established in 2002 by Ruggeri et al. (29, 35–38). This model is derived primarily from the concept of “missing-self” or the activation of donor NK cells due to failure of donor iKIRs to bind to their cognate ligand on recipient target cells (29) (Figure 2A). This model postulates that donor NK cells will be alloreactive toward host cells when the recipient is lacking HLA class I ligands that are present in the donor (29, 35). Therefore, after HCT, donor-derived NK cells will encounter host cells that do not provide the requisite inhibitory signals, and absence of inhibitory signals leads to activation of NK cytotoxicity and a cascade of alloreactivity. As the name indicates, a comparison of the donor and recipient KIR ligands is conducted to assess alloreactivity in this model. As discussed in the proceeding section, reductions in relapse and absence of GVHD were seen in AML patients who underwent HCT from ligand-ligand mismatch donors (37).
The receptor-ligand mismatch/missing-ligand model was proposed by Leung et al. in 2004 (29, 35, 56) (Figure 2B). Defined by donor and recipient KIR ligand incompatibility, this model hypothesizes that if at least one KIR expressed in the donor does not recognize any of the HLA molecules of the recipient, then there will be a reduction in NK cell inhibition and therefore increased cytotoxic activity (35). An ideal donor would have at least one inhibitory KIR for which the recipient lacks a ligand, but also at least one cognate recipient ligand so that NK cells maintain some immunoregulatory function (35).
Prediction of NK cell alloreactivity based on donor KIR2DS1, KIR3DL1, and their cognate ligands has been proposed under the receptor-ligand mismatch model (57–59). KIR2DS1 is an activating KIR that has a role both in NK cell activation and tolerance via interaction with the HLA-C locus (57). More specifically, the interaction between KIR2DS1 and HLA-C1 versus HLA-C2 molecules drives NK responsiveness (57, 74–76). KIR2DS1-positive NK cells that have been exposed to HLA-C1 ligands secrete IFN-γ and are cytotoxic to target cells, especially those that express HLA-C2 (57). In a HLA-C2 homozygous host, KIR2DS1-positive NK cells are hyporesponsive– (i.e. the activating receptor KIR2DS1 causes limited NK cell function in the setting of exposure to self-ligand) (57).
In 2012, Venstrom et al. demonstrated that patients with AML who received allografts from donors who were positive for KIR2DS1 did indeed have a lower rate of relapse than those with allografts from donors who were negative for this KIR genotype (57). Additionally, recipients with an HLA-C2/C2 genotype who received allografts from KIR2DS1-postive donors did not benefit from relapse protection, while recipients with HLA-C1/C1 or C1/C2 genotypes had significantly reduced incidence of relapse (57). Similar findings were reported when KIR3DL1 and its cognate ligand HLA-Bw4 were examined (57, 58). Conversely, a more recent study in a more varied population had negative results when examining KIR2DS1, KIR3DL1, and their cognate ligands as predictors of NK alloreactivity (59). Importantly, patients in this more modern study underwent a variety of conditioning regimens intensities, peripheral blood stem cell (PBSC) grafts dominated, and anti-thymocyte globulin (ATG) was used as GVHD prophylaxis in the majority of patients (59). In contrast, the previously mentioned prior studies examined patients who in the majority received MAC, no ATG, and bone marrow (BM), less than 1/3 of which were TCD, grafts (57, 58).
Another model more recently proposed by Nowak et al. in 2014 is the education/missing licensing proof model (71) (Figure 2C). This model considers both donor and recipient HLA class I molecules, the KIR type of donor, and the education process required for NK cells to become competent or “licensed.” Specifically, for a NK cell to mature and possess the ability to become alloreactive, it must first become “licensed” via its iKIR interacting with its cognate ligand (35). In the case of HCT, alloreactivity is achieved when a licensed donor NK cell interacts with the recipient cells that lack its cognate ligand, therefore allowing for NK cytotoxicity and GVL effects (35).
Attempts in murine models have been made to understand whether donor or recipient MHC may play a more dominant role in NK cell alloreactivity or education (77–80). For instance, educated NK cells from wild-type mice transferred into MHC-deficient mice have been shown to be significantly less responsive in multiple studies (78, 79). On the other hand, when educated NK cells were depleted based on prediction from donor MHC haplotypes and transfused into a wild-type MHC-mismatched HCT murine model there was increased susceptibility to CMV infection (80). Human studies to tease out the respective effects of donor and recipient NK-cell education and alloreactivity have been mixed (63, 64, 81), with some indicating that donor HLA genotype may shape education (81) and others leaning towards host (63, 64) as driving NK cell alloreactivity. For instance, Zhao et al. showed that donor/recipient pairs that expressed all ligands for donor inhibitory KIRs had the lowest relapse rate after haplo-HCT, which they attributed to better education and function of NK cells (65). All in all, it is likely that both donor and recipient NK cells cooperate to maintain and adjust NK cell responsiveness and augment GVL effects.
KIR genotyping involves understanding whether a specific donor and/or recipient KIR gene, or mismatch between the two, may be associated with improved outcomes and reduced post-HCT relapse (Figure 2D). Data regarding the effects of KIR genotype on HCT outcomes is discrepant. Differences in iKIR gene content in haplo HCT were associated with improved OS, EFS, and lower relapse rates compared to pairs with identical iKIR gene content in both lymphoid and myeloid diseases in a study by the Johns Hopkins group (66). In contrast, another study found the inverse: that KIR matching in MSD HCT was associated with less relapse (67). As in many things, these results may be different due to the different GVHD prophylaxis strategies employed with PTCy in the former study and cyclosporine with methotrexate or mycophenolate mofetil in the latter. Thus, further analyses are required to fully elucidate the impact of KIR on HCT outcomes, as these effects are likely platform specific.
The last model we will discuss, the receptor-receptor mismatch/haplotype based model, was proposed by Gagne et al. in 2002 (82) (Figure 2E). This model is defined by donor KIR and recipient KIR incompatibility. Since KIR mismatch is closely related to the presence of B-haplotype activating genes, this model may alternatively be defined as a mismatch between donor and recipient for KIR B haplotypes (29). Briefly, KIR genes are organized and inherited via group A and group B haplotypes. The KIR B haplotype contains variable polymorphic gene content including one or more activating genes, while the KIR A haplotype contains preserved gene content, which does not include activating genes (60). Both haplotypes have distinctive centromeric (Cen) and telomeric (Tel) gene-content motifs that may be used in further classification (61).
Cooley et al. examined how donor KIR genotype would affect outcomes after TCR unrelated donor (URD) HCT in multiple consecutive studies (60–62). First, in 2009, they found a significantly improved 3-year relapse-free survival (RFS) when using donors who had at least one KIR B haplotype-defining loci (i.e. B/x) (60). These results were also replicated in later studies by the same (61) and other groups (83–85). Importantly, these studies include a variety of platforms: TCD grafts (83), PBSC grafts (84, 85), and varied conditioning regimens, including nonmyeloablative (NMA) (66) and RIC (84, 85). Interestingly, having multiple KIR B haplotype-defining loci provided no additional benefit beyond one KIR B-haplotype (60). When individual donor KIR genes were examined, KIR2DL2 (p=.07) and KIR2DS2 (p=.02) were shown to be independent predictors of OS (60). They also found that total centromeric and telomeric gene content were predictors of relapse and OS (61). Cen-B/homozygosity had the strongest independent effect and was associated with decreased relapse and improved OS (61). Expanding on their 2009 and 2010 findings, Cooley et al. examined the effect of the inhibitory C1 and C2 receptors, which are encoded by genes in the centromeric region, on the protection provided by donor KIR-B haplotypes in HCT (62). Relapse protection associated with donor KIR B was enhanced in recipients with one or more C1 bearing HLA-C allotypes (62). This suggests that the interaction between donor KIR B and recipient C1 may be a mechanistic explanation for protection against relapse.
A recently proposed model by a London group that has studied NK genotyping and its influence on chronic infection, suggested that the donor iKIR-ligand genotype measured by the count of iKIR-ligand gene pairs will impact relapse and GVHD in HCT (86). They posited that higher numbers of iKIR-ligand gene pairs will prolong memory CD8+ T cell survival and that this would prevent relapse, albeit possibly with an increase in risk of GVHD (86). This model puts the onus back on the T cells as the primary mediators of GVL and hypothesizes that the discrepant findings in prior studies that examined the effects of NK alloreactivity may be due to failure to account for this T cell interaction (86). While intriguing, this model has yet to be examined in HCT and we will await the forthcoming studies to review it further.
NK cell alloreactivity in PTCy
Most of the studies examining the prior NK alloreactivity models include a significant portion of patients who received ATG or TCD strategies – and therefore many of the aforementioned models and studies may not be as relevant in the modern age of HCT that is marked by increasing use of PTCy. Nevertheless, NK cell alloreactivity has been demonstrated to be associated with outcomes in several studies of HCT with PTCy (54, 66, 87, 88). While Shimoni et al. showed that KIR ligand mismatching was significantly associated with worse OS and a trend for higher incidence of relapse (89), the Georgia group showed that HCT with donor/recipient pairs who expressed at least one KIR receptor-ligand mismatch were associated with improved OS and reduced risk of relapse (87). Similarly, both the Georgia group and Symons et al. also showed that HCT from donor-recipient pairs with mismatched KIR haplotypes were associated with improved OS, EFS, and decreased relapse rates (66, 87). The Georgia group also showed that recipients with M+ genotype (-21M HLA-B) at the B-leader sequence had decreased relapse rates. They speculated that this was due to B-leader mediated expression of HLA-E that then recognizes NKG2A, thereby contributing to education of NK cells (54).
Symons et al. also examined the effects of KIR haplotypes in patients who received NMA haplo HCT with PTCy and found that recipients with KIR A haplotype who received grafts from KIR B haplotype donors had improved OS (66). In addition, recipients with iKIR mismatched haplotypes had significantly improved OS, EFS, and decreased relapse rate when compared to iKIR matched HCT (66). Illustrating the platform-dependent effects, Cooley et al. did not find a benefit of iKIR mismatching in patients receiving TCR HCT, in the absence of PTCy, despite demonstrating benefits in TCD HCT (60). These distinct outcomes may be a result of differences in expression of KIRs and in cytokine production between TCD and TCR grafts; for instance, NK cells after TCR grafts express fewer KIRs and produce more IFN-γ (90). Ultimately, NK cell alloreactivity may be more important in TCD HCT where T cells play less of a role in GVL (55). As such, all models must be tested in different platforms to determine their applicability and one model will likely not fit all.
Adopting the NK cell for enhanced GVL effects: an alternative to donor lymphocyte infusion?
While DLI, a major cellular treatment of post-HCT relapse, can be associated with remission rates of 10-15%, it also comes with a high risk of GVHD, which limits its use prophylactically (91–96). Prophylactic adoptive transfer of NK cells is an attractive alternative given its potential to increase GVL without significantly increasing the risk of GVHD. Haplo NK cell infusion was examined in the non-HCT setting where it induced remission in a few patients with poor-prognosis AML without significant toxicity (97). Moreover, adoptive transfer of NK cells has shown to be both feasible and safe in multiple HCT clinical trials, summarized in Table 3 (30, 37, 91). For instance, one group demonstrated safety of autologous NK cell infusion after HCT, but noted that there were no significant improvements in disease outcomes, which they attributed to a lack of KIR mismatching (97, 98). While adoptive transfer of donor NK cells has shown feasibility (99–102, 111) and efficacy (99), with reductions in progression of disease (101, 102) without increasing GVHD (100, 101), enhancing the GVL effects of adoptive NK cell transfer requires further optimization. For instance, in vitro activation (103, 104, 111) and expansion of NK cells in culture or via in vivo administration of IL-2, other cytokines, or adjuvant immunotherapies are avenues under study to increase NK cell expansion and/or persistence (97, 105, 106, 111, 112).
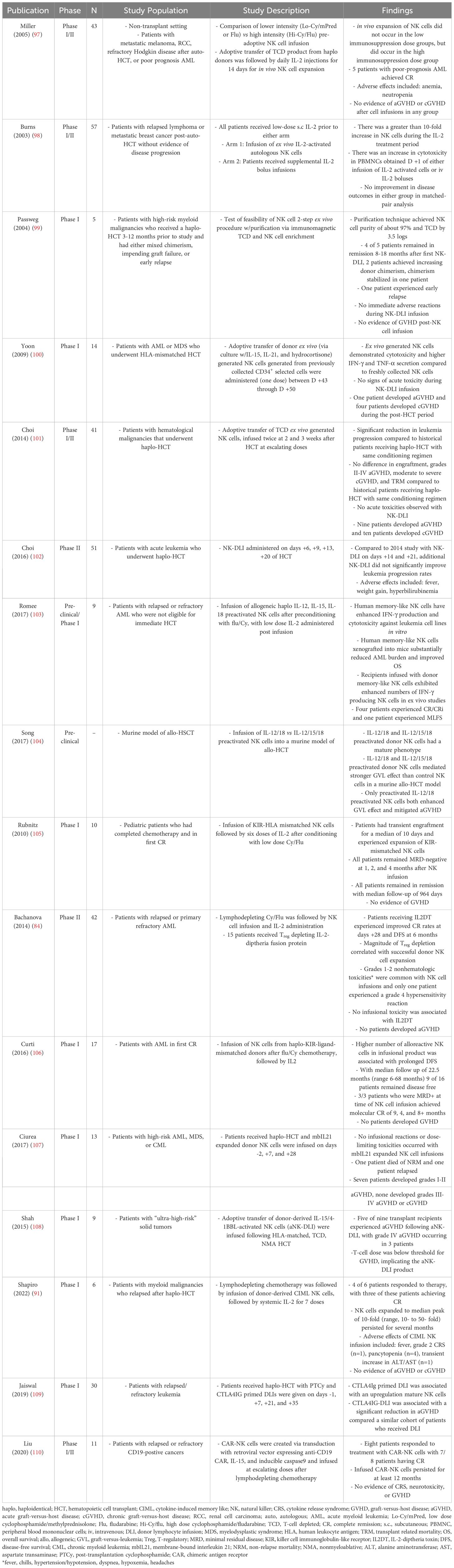
Table 3 Summary of studies examining the novel use of NK cells a therapeutic strategy for anti-tumor effects.
Exploration of methods to expand and/or activate NK cells have been numerous. IL-2 administration has been utilized to enhance NK cell activity and persistence in many studies (91, 97, 98, 103, 105, 106, 112), given that it augments NK cell activity (113) and is one of the second signals needed for CD56bright NK subset to product IFN-γ (32). Importantly, although IL-2 expands and activates NK cells it also expands T regulatory cells (Tregs), which have an immunosuppressive effect on NK cells (32). When Tregs were depleted in conjunction with NK cell infusion via IL-2 -diphtheria fusion protein, NK cell expansion was more successful, illustrating that manipulation of the immune milieu may be vital and infusion of IL-2 alone may be insufficient to improve effectiveness or persistence of adoptive NK cell therapy (84). Alternative NK cell infusion schedules, i.e. earlier and more frequent infusions to harness cytotoxic benefit, have been explored without additional benefits, but with increased infusional reactions (102). Additionally, alternative cytokines have been trialed either in vivo or ex vivo to enhance activation of NK cells, including IL-12, IL-15, and IL-18 (103, 104). In a murine model, IL-12/18 and IL-12/15/18-preactivated NK cells were shown to produce a primarily phenotypically mature NK cell and mediate stronger GVL effect as compared to non-activated NK cells (104). However, in a phase I study, NK cell pre-activation with IL-15/4-1BBL resulted in unacceptable rates of severe aGVHD (108). Alternatively, Miller et al. demonstrated that successful in vivo expansion of NK cells, even with subsequent IL-2 injections, may be dependent on lymphodepleting chemotherapy prior to NK cell infusion (97). Clearly, there are multiple strategies that may improve the effectiveness of adoptive NK cell therapy and further work will be needed to optimize these platforms.
NK cell adoptive transfer may be a particularly attractive strategy to prevent relapse after HCT with PTCy given the impairment in maturation and function of NK cells early after administration of Cy (39, 42, 50). A phase 1 clinical trial, conducted by Ciurea et al., explored the safety and feasibility of using NK cells expanded by stimulation with K562 feeder cells infused before or after haplo-HCT with PTCy to decrease the risk of leukemia relapse (107). NK cells examined at day +28 in were more active, as measured by secretion of TNF-α and IFN-y, and displayed a functional shift towards cytotoxicity (107). Importantly, there were no infusion reactions and no increased incidence of severe acute or chronic GVHD and a very low relapse rate when compared to historical controls (107).
Another attractive option to enhance GVL is cytokine-induced memory-like (CIML) NK cells, which can be generated via incubation with IL-12, IL-15, and IL-18 and have been shown to have enhanced cytotoxicity against target leukemia cells in preclinical studies (114). These CIML NK cells have also been shown to be capable of enhanced survival, expansion, and avoidance of anergy in murine studies (91, 103, 114). Given these encouraging pre-clinical findings, a phase I trial of CIML NK cells infused in patients with relapsed myeloid malignancies after haplo-HCT was conducted and demonstrated a reduction in disease burden in 4 out of 6 patients with sustained responses (91). No patient developed severe cytokine release syndrome (CRS), neurotoxicity, or any GVHD (91). Additionally, CIML NK cells were detected several months after infusion, perhaps making this strategy one of the most effective thus far to improve NK persistence (91). In all, these early studies of adoptive NK transfer after HCT are encouraging.
In another avenue of research, CTLA4 inhibition by abatacept has been employed to mitigate the risk of GVHD after traditional DLI and, in that setting, the efficacy of DLI may rely on NK cells (109, 115, 116). CTLA4Ig-IG binds to CD80/CD86 receptors on APCs, blocking T cell activation via CD28-B7 ligand interaction (109). In a melanoma murine model, CTLA4Ig infusion prolonged survival, but that benefit was lost when NK cells were depleted prior to CTLA4Ig infusion (116). Sequential co-infusions on days +7, +21, +35 of CTLA4-Ig and DLI in PTCy-based haplo-HCT was shown to be associated with significant surges of NK cells, a low incidence of GVHD and NRM, and failure to proliferate NK cells was associated with subsequent disease progression (115). These early results underscore that novel therapies may be able to enhance NK cell activity, while avoiding the risk of GVHD.
CAR-NK cells
Anti-CD19 CAR-T cell therapy has been demonstrated to induce high rates of durable remissions in adult patients with lymphoma and chronic lymphocytic leukemia as well as in children with acute lymphoblastic leukemia (ALL) (117–126). However, in adults with ALL, CAR-T cell-induced remissions tend to be much less durable and require consolidative HCT to reduce subsequent relapse (127–129). Manufacturing autologous CAR-Ts for patients with acute leukemia is difficult in part due to the reduced lymphocyte counts associated with relapsed leukemia treated with multiple cycles of cytotoxic lymphodepleting chemotherapy (130). However, “off-the-shelf” NK cells, which do not require full HLA matching, may be collected from unrelated donors, and engineered to express anti-CD19 CAR, may be an avenue to circumvent the potential obstacle of a poor lymphocyte harvest when manufacturing autologous CAR-T cells (130). Just as in adoptive NK cells, co-culture with NK cell-stimulating interleukins like IL-2 or IL-15 is under study as a way to further augment CAR-NK persistence. MD Anderson conducted a phase I/II clinical trial of anti-CD19 CAR-NK cells, which also expressed IL-15 and an inducible caspase 9 safety switch in patients with relapsed CD19-positive cancers (130). They demonstrated that CAR-NK cells were not associated with development of CRS or GVHD, that they were capable of inducing CR in 7 of 11 examined patients, and that they persisted at 1-year (130). Similarly, pre-clinical data demonstrated that NK CARs expressing IL-15 and IL-15/IL-15Rα were associated with improved anti-tumor activity and persistence (97, 110). Attempts to expand CAR-NK cell platforms to other hematologic malignancies are underway (131). The absence of CRS or GVHD after infusion of CAR-NK cells makes them particularly appealing as a strategy to prevent relapse after HCT in high-risk patients.
Concluding thoughts
Decades of progress in HCT have heralded a modern age in which decreased incidences of graft failure, GVHD, and NRM have made HCT safer for patients with hematologic malignancies (4). Additionally, modern GVHD prophylaxis, including PTCy and abatacept, has expanded the donor pool allowing virtually all patients to have access to this life-saving therapy and unlocking an age of donor choice (132). As such, prevention and treatment of post-HCT relapse remains the biggest area of unmet clinical need (4, 6, 7). The GVL reaction, and particularly the role of NK cells in this reaction, is an exciting and dynamic area of research with broad potential application.
While NK alloreactivity has been associated with decreased leukemia relapse in many studies over many decades, it has yet to be implemented broadly and KIR genotyping is not routinely performed (133). Just as we are beginning to refine our selection of the optimal donor thru a deeper understanding of HLA (52), further research regarding the immunobiology of NK cells will one day allow us to define an ideal donor based on NK cell genotyping and match. Until that day is reached, we must examine NK alloreactivity in a platform-specific manner given that novel platforms, such as PTCy, will most likely influence the effects of NK alloreactivity, just as they have changed the implications of HLA-matching (35, 36, 39, 42, 50). We anticipate that harnessing the effects of allogeneic NK cells utilizing KIR mismatched donors will become increasingly important with the growing use of PTCy (134).
Beyond donor selection, newly emerging data regarding the safety and feasibility of adoptive NK cells for prevention and treatment of post-HCT relapse may represent yet another era in designer DLI with the potential to separate GVL from GVHD (91, 97, 98, 103, 105, 106, 112). Given that NK cells may exhibit functional differences after PTCy (39, 50), NK cell adoptive therapy may be leveraged to support maturation and function of NK cells in patients either at high-risk for relapse or with inadequate NK cell recovery or functional markers post-HCT. Moreover, changes to the PTCy platform to promote recovery of NK cells such as reducing the dose of PTCy or minimizing calcineurin inhibitor use are promising therapeutic options to augment the GVL reaction of HCT (135). Finally, allogeneic CAR-NK cells have the potential to overcome the manufacturing issues associated with autologous CAR-T, while lacking the risk of GVHD associated with allogeneic CAR-T cells and increasing their applicability to HCT platforms (110, 130).
NK cells provide a promising, but so far underutilized, platform for major improvements in HCT. As we define the ideal donor based on factors including KIR alloreactivity, we may soon be able to individualize donor selection algorithms and usher in the era of personalized HCT. In time, we anticipate that planned and preemptive adoptive NK and CAR-NK therapies will be commonplace, decreasing relapse and NRM, to improve post-HCT survival for the next generation of HCT recipients.
Author contributions
AH: Writing – review & editing, Writing – original draft, Conceptualization. SM: Writing – review & editing, Writing – original draft, Conceptualization.
Funding
The author(s) declare that no financial support was received for the research, authorship, and/or publication of this article.
Conflict of interest
The authors declare that the research was conducted in the absence of any commercial or financial relationships that could be construed as a potential conflict of interest.
Publisher’s note
All claims expressed in this article are solely those of the authors and do not necessarily represent those of their affiliated organizations, or those of the publisher, the editors and the reviewers. Any product that may be evaluated in this article, or claim that may be made by its manufacturer, is not guaranteed or endorsed by the publisher.
References
1. Luznik L, O'Donnell PV, Symons HJ, Chen AR, Leffell MS, Zahurak M, et al. HLA-haploidentical bone marrow transplantation for hematologic Malignancies using nonmyeloablative conditioning and high-dose, posttransplantation cyclophosphamide. Biol Blood Marrow Transplant. (2008) 14:641–50. doi: 10.1016/j.bbmt.2008.03.005
2. Shaw BE, Jimenez-Jimenez AM, Burns LJ, Logan BR, Khimani F, Shaffer BC, et al. National marrow donor program-sponsored multicenter, phase II trial of HLA-mismatched unrelated donor bone marrow transplantation using post-transplant cyclophosphamide. J Clin Oncol. (2021) 39:1971–82. doi: 10.1200/jco.20.03502
3. Kasamon YL, Bolaños-Meade J, Prince GT, Tsai HL, McCurdy SR, Kanakry JA, et al. Outcomes of nonmyeloablative HLA-haploidentical blood or marrow transplantation with high-dose post-transplantation cyclophosphamide in older adults. J Clin Oncol. (2015) 33:3152–61. doi: 10.1200/jco.2014.60.4777
4. Bolon YT AR, Allbee-Johnson M, Estrada-Merly N, Lee SJ. Current use and outcome of hematopoietic stem cell transplantation: CIBMTR summary slides. (2022).
5. McCurdy SR, Kasamon YL, Kanakry CG, Bolaños-Meade J, Tsai HL, Showel MM, et al. Comparable composite endpoints after HLA-matched and HLA-haploidentical transplantation with post-transplantation cyclophosphamide. Haematologica. (2017) 102:391–400. doi: 10.3324/haematol.2016.144139
6. Zuanelli Brambilla C, Lobaugh SM, Ruiz JD, Dahi PB, Goldberg AD, Young JW, et al. Relapse after allogeneic stem cell transplantation of acute myelogenous leukemia and myelodysplastic syndrome and the importance of second cellular therapy. Transplant Cell Therapy Off Publ Am Soc Transplant Cell Ther. (2021) 27:771.e1–.e10. doi: 10.1016/j.jtct.2021.05.011
7. Bejanyan N, Weisdorf DJ, Logan BR, Wang H-L, Devine SM, de Lima M, et al. Survival of patients with acute myeloid leukemia relapsing after allogeneic hematopoietic cell transplantation: A center for international blood and marrow transplant research study. Biol Blood Marrow Transplant. (2015) 21:454–9. doi: 10.1016/j.bbmt.2014.11.007
8. Schmid C, Labopin M, Nagler A, Bornhaüuser M, Finke J, Fassas A, et al. Donor lymphocyte infusion in the treatment of first hematological relapse after allogeneic stem-cell transplantation in adults with acute myeloid leukemia: a retrospective risk factors analysis and comparison with other strategies by the EBMT Acute Leukemia Working Party. J Clin Oncol. (2007) 25:4938–45. doi: 10.1200/JCO.2007.11.6053
9. Christopeit M, Kuss O, Finke J, Bacher U, Beelen DW, Bornhäuser M, et al. Second allograft for hematologic relapse of acute leukemia after first allogeneic stem-cell transplantation from related and unrelated donors: the role of donor change. J Clin Oncol. (2013) 31:3259–71. doi: 10.1200/JCO.2012.44.7961
10. Spyridonidis A, Labopin M, Schmid C, Volin L, Yakoub-Agha I, Stadler M, et al. Outcomes and prognostic factors of adults with acute lymphoblastic leukemia who relapse after allogeneic hematopoietic cell transplantation. An analysis on behalf of the Acute Leukemia Working Party of EBMT. Leukemia. (2012) 26:1211–7. doi: 10.1038/leu.2011.351
11. Kreidieh F, Abou Dalle I, Moukalled N, El-Cheikh J, Brissot E, Mohty M, et al. Relapse after allogeneic hematopoietic stem cell transplantation in acute myeloid leukemia: an overview of prevention and treatment. Int J Hematol. (2022) 116:330–40. doi: 10.1007/s12185-022-03416-7
12. Ossenkoppele GJ, Janssen JJ, van de Loosdrecht AA. Risk factors for relapse after allogeneic transplantation in acute myeloid leukemia. Haematologica. (2016) 101:20–5. doi: 10.3324/haematol.2015.139105
13. Schmid C, Labopin M, Nagler A, Niederwieser D, Castagna L, Tabrizi R, et al. Treatment, risk factors, and outcome of adults with relapsed AML after reduced intensity conditioning for allogeneic stem cell transplantation. Blood. (2012) 119:1599–606. doi: 10.1182/blood-2011-08-375840
14. Rautenberg C, Germing U, Haas R, Kobbe G, Schroeder T. Relapse of acute myeloid leukemia after allogeneic stem cell transplantation: prevention, detection, and treatment. Int J Mol Sci. (2019) 20:228. doi: 10.3390/ijms20010228
15. Kekre N, Kim HT, Thanarajasingam G, Armand P, Antin JH, Cutler C, et al. Efficacy of immune suppression tapering in treating relapse after reduced intensity allogeneic stem cell transplantation. Haematologica. (2015) 100:1222–7. doi: 10.3324/haematol.2015.129650
16. Nakamura R, Auayporn N, Smith DD, Palmer J, Sun JY, Schriber J, et al. Impact of graft cell dose on transplant outcomes following unrelated donor allogeneic peripheral blood stem cell transplantation: higher CD34+ cell doses are associated with decreased relapse rates. Biol Blood Marrow Transplant. (2008) 14:449–57. doi: 10.1016/j.bbmt.2008.02.005
17. Ho VT, Aldridge J, Kim HT, Cutler C, Koreth J, Armand P, et al. Comparison of Tacrolimus and Sirolimus (Tac/Sir) versus Tacrolimus, Sirolimus, and mini-methotrexate (Tac/Sir/MTX) as acute graft-versus-host disease prophylaxis after reduced-intensity conditioning allogeneic peripheral blood stem cell transplantation. Biol Blood Marrow Transplant. (2009) 15:844–50. doi: 10.1016/j.bbmt.2009.03.017
18. Chiad Z, Chojecki A. Graft versus leukemia in 2023. Best Pract Res Clin Haematology. (2023) 36:101476. doi: 10.1016/j.beha.2023.101476
19. Socié G, Blazar BR. CH 1 - Overview of the immune biology of allogeneic hematopoietic stem cell transplantation. In: Socié G, Blazar BR, editors. Immune biology of allogeneic hematopoietic stem cell transplantation. Academic Press, San Diego (2013). p. 1–17.
20. Barnes DWH, Loutit JF. Treatment of murine leukaemia with X-rays and homologous bone marrow: II. Br J haematology. (1957) 3:241–52. doi: 10.1111/j.1365-2141.1957.tb05793.x
21. Horowitz M, Gale R, Sondel P, Goldman J, Kersey J, Kolb H, et al. Graft-versus-leukemia reactions after bone marrow transplantation. Blood. (1990) 75:555–62. doi: 10.1182/blood.V75.3.555.555
22. Cooper MA, Fehniger TA, Caligiuri MA. The biology of human natural killer-cell subsets. Trends Immunol. (2001) 22:633–40. doi: 10.1016/s1471-4906(01)02060-9
23. Shin E, Bak SH, Park T, Kim JW, Yoon SR, Jung H, et al. Understanding NK cell biology for harnessing NK cell therapies: targeting cancer and beyond. Front Immunol. (2023) 14:1192907. doi: 10.3389/fimmu.2023.1192907
24. Lanier LL, Le AM, Civin C, Loken M, Phillips J. The relationship of CD16 (Leu-11) and Leu-19 (NKH-1) antigen expression on human peripheral blood NK cells and cytotoxic T lymphocytes. J Immunol (Baltimore Md: 1950). (1986) 136:4480–6. doi: 10.4049/jimmunol.136.12.4480
25. Robertson MJ, Ritz J. Biology and clinical relevance of human natural killer cells. (1990) 76:2421–38. doi: 10.1182/blood.V76.12.2421.2421
26. Wang X, Zhao XY. Transcription factors associated with IL-15 cytokine signaling during NK cell development. Front Immunol. (2021) 12:610789. doi: 10.3389/fimmu.2021.610789
27. Zhang M, Lam KP, Xu S. Natural Killer Cell Engagers (NKCEs): a new frontier in cancer immunotherapy. Front Immunol. (2023) 14:1207276. doi: 10.3389/fimmu.2023.1207276
28. Antonangeli F, Zingoni A, Soriani A, Santoni A. Senescent cells: Living or dying is a matter of NK cells. J Leukoc Biol. (2019) 105:1275–83. doi: 10.1002/JLB.MR0718-299R
29. Zhang Y, Ye C, Zhu H, Zhuang Y, Chen S, Weng Y, et al. Association of iKIR-mismatch model and donor aKIRs with better outcome in haploidentical hematopoietic stem cell transplantation for acute myeloid leukemia. Front Immunol. (2023) 13:1091188. doi: 10.3389/fimmu.2022.1091188
30. Locatelli F, Pende D, Falco M, Della Chiesa M, Moretta A, Moretta L. NK cells mediate a crucial graft-versus-leukemia effect in haploidentical-HSCT to cure high-risk acute leukemia. Trends Immunol. (2018) 39:577–90. doi: 10.1016/j.it.2018.04.009
31. Zamora AE, Grossenbacher SK, Aguilar EG, Murphy WJ. Models to study NK cell biology and possible clinical application. Curr Protoc Immunol. (2015) 110:14.37.1–14.37.14. doi: 10.1002/0471142735.im1437s110
32. Caligiuri MA. Human natural killer cells. Blood. (2008) 112:461–9. doi: 10.1182/blood-2007-09-077438
33. Amand M, Iserentant G, Poli A, Sleiman M, Fievez V, Sanchez IP, et al. Human CD56(dim)CD16(dim) cells as an individualized natural killer cell subset. Front Immunol. (2017) 8:699. doi: 10.3389/fimmu.2017.00699
34. Hayakawa Y, Huntington ND, Nutt SL, Smyth MJ. Functional subsets of mouse natural killer cells. Immunol Rev. (2006) 214:47–55. doi: 10.1111/j.1600-065X.2006.00454.x
35. Dhuyser A, Aarnink A, Pérès M, Jayaraman J, Nemat-Gorgani N, Rubio MT, et al. KIR in allogeneic hematopoietic stem cell transplantation: need for a unified paradigm for donor selection. Front Immunol. (2022) 13:821533. doi: 10.3389/fimmu.2022.821533
36. Dhuyser A, Remen T, Pérès M, Chamberlain-Evans V, Nemat-Gorgani N, Campidelli A, et al. Comparison of NK alloreactivity prediction models based on KIR-MHC interactions in haematopoietic stem cell transplantation. Front Immunol. (2023) 14:1028162. doi: 10.3389/fimmu.2023.1028162
37. Ruggeri L, Capanni M, Urbani E, Perruccio K, Shlomchik WD, Tosti A, et al. Effectiveness of donor natural killer cell alloreactivity in mismatched hematopoietic transplants. Science. (2002) 295:2097–100. doi: 10.1126/science.1068440
38. Jennifer Zhang Q. Donor selection based on NK alloreactivity for patients with hematological Malignancies. Hum Immunol. (2022) 83:695–703. doi: 10.1016/j.humimm.2022.07.006
39. Russo A, Oliveira G, Berglund S, Greco R, Gambacorta V, Cieri N, et al. NK cell recovery after haploidentical HSCT with posttransplant cyclophosphamide: dynamics and clinical implications. Blood. (2018) 131:247–62. doi: 10.1182/blood-2017-05-780668
40. Savani B, Mielke S, Adams S, Uribe M, Rezvani K, Yong A, et al. Rapid natural killer cell recovery determines outcome after T-cell-depleted HLA-identical stem cell transplantation in patients with myeloid leukemias but not with acute lymphoblastic leukemia. Leukemia. (2007) 21:2145–52. doi: 10.1038/sj.leu.2404892
41. Savani BN, Rezvani K, Mielke S, Montero A, Kurlander R, Carter CS, et al. Factors associated with early molecular remission after T cell-depleted allogeneic stem cell transplantation for chronic myelogenous leukemia. Blood. (2006) 107:1688–95. doi: 10.1182/blood-2005-05-1897
42. Rambaldi B, Kim HT, Reynolds C, Chamling Rai S, Arihara Y, Kubo T, et al. Impaired T- and NK cell reconstitution after haploidentical HCT with posttransplant cyclophosphamide. Blood Adv. (2021) 5:352–64. doi: 10.1182/bloodadvances.2020003005
43. Roberto A, Di Vito C, Zaghi E, Mazza EMC, Capucetti A, Calvi M, et al. The early expansion of anergic NKG2A(pos)/CD56(dim)/CD16(neg) natural killer represents a therapeutic target in haploidentical hematopoietic stem cell transplantation. Haematologica. (2018) 103:1390–402. doi: 10.3324/haematol.2017.186619
44. Kandalla PK, Subburayalu J, Cocita C, de Laval B, Tomasello E, Iacono J, et al. M-CSF directs myeloid and NK cell differentiation to protect from CMV after hematopoietic cell transplantation. EMBO Mol Med. (2023) n/a:e17694. doi: 10.15252/emmm.202317694
45. Gail LM, Schell KJ, Łacina P, Strobl J, Bolton SJ, Steinbakk Ulriksen E, et al. Complex interactions of cellular players in chronic Graft-versus-Host Disease. Front Immunol. (2023) 14:1199422. doi: 10.3389/fimmu.2023.1199422
46. Vacca P, Montaldo E, Croxatto D, Moretta F, Bertaina A, Vitale C, et al. NK cells and other innate lymphoid cells in hematopoietic stem cell transplantation. Front Immunol. (2016) 7:188. doi: 10.3389/fimmu.2016.00188
47. Palmer JM, Rajasekaran K, Thakar MS, Malarkannan S. Clinical relevance of natural killer cells following hematopoietic stem cell transplantation. J Cancer. (2013) 4:25–35. doi: 10.7150/jca.5049
48. Ruggeri L, Capanni M, Casucci M, Volpi I, Tosti A, Perruccio K, et al. Role of natural killer cell alloreactivity in HLA-mismatched hematopoietic stem cell transplantation. Blood J Am Soc Hematol. (1999) 94:333–9. doi: 10.1182/blood.V94.1.333.413a31_333_339
49. Serpenti F, Lorentino F, Marktel S, Milani R, Messina C, Greco R, et al. Immune reconstitution-based score for risk stratification of chronic graft-versus-host disease patients. Front Oncol. (2021) 11:705568. doi: 10.3389/fonc.2021.705568
50. McCurdy SR, Radojcic V, Tsai H-L, Vulic A, Thompson E, Ivcevic S, et al. Signatures of GVHD and relapse after posttransplant cyclophosphamide revealed by immune profiling and machine learning. Blood. (2022) 139:608–23. doi: 10.1182/blood.2021013054
51. Petersdorf EW, Gooley T, Volt F, Kenzey C, Madrigal A, McKallor C, et al. Use of the HLA-B leader to optimize cord blood transplantation. Haematologica. (2020) 106:3107–14. doi: 10.3324/haematol.2020.264424
52. Fuchs EJ, McCurdy SR, Solomon SR, Wang T, Herr MR, Modi D, et al. HLA informs risk predictions after haploidentical stem cell transplantation with posttransplantation cyclophosphamide. Blood. (2022) 139:1452–68. doi: 10.1182/blood.2021013443
53. Sajulga R, Bolon YT, Maiers MJ, Petersdorf EW. Assessment of HLA-B genetic variation with an HLA-B leader tool and implications in clinical transplantation. Blood Adv. (2022) 6:270–80. doi: 10.1182/bloodadvances.2021004561
54. Solomon SR, Aubrey MT, Zhang X, Jackson KC, Roark CL, Freed BM, et al. Lineage-specific relapse prediction after haploidentical transplantation with post-transplant cyclophosphamide based on recipient HLA-B-leader genotype and HLA-C-group KIR ligand. Transplant Cell Ther. (2022) 28:601.e1–.e8. doi: 10.1016/j.jtct.2022.06.023
55. McCurdy SR. Harnessing allogeneic NK cells: improving outcomes with tailored donor lymphocyte infusion. J Clin Invest. (2022) 132. doi: 10.1172/JCI160584
56. Leung W, Iyengar R, Turner V, Lang P, Bader P, Conn P, et al. Determinants of antileukemia effects of allogeneic NK cells. J Immunol. (2004) 172:644–50. doi: 10.4049/jimmunol.172.1.644
57. Venstrom JM, Pittari G, Gooley TA, Chewning JH, Spellman S, Haagenson M, et al. HLA-C–dependent prevention of leukemia relapse by donor activating KIR2DS1. New Engl J Med. (2012) 367:805–16. doi: 10.1056/NEJMoa1200503
58. Boudreau JE, Giglio F, Gooley TA, Stevenson PA, Le Luduec JB, Shaffer BC, et al. KIR3DL1/HLA-B subtypes govern acute myelogenous leukemia relapse after hematopoietic cell transplantation. J Clin Oncol. (2017) 35:2268–78. doi: 10.1200/jco.2016.70.7059
59. Schetelig J, Baldauf H, Heidenreich F, Massalski C, Frank S, Sauter J, et al. External validation of models for KIR2DS1/KIR3DL1-informed selection of hematopoietic cell donors fails. Blood. (2020) 135:1386–95. doi: 10.1182/blood.2019002887
60. Cooley S, Trachtenberg E, Bergemann TL, Saeteurn K, Klein J, Le CT, et al. Donors with group B KIR haplotypes improve relapse-free survival after unrelated hematopoietic cell transplantation for acute myelogenous leukemia. Blood J Am Soc Hematol. (2009) 113:726–32. doi: 10.1182/blood-2008-07-171926
61. Cooley S, Weisdorf DJ, Guethlein LA, Klein JP, Wang T, Le CT, et al. Donor selection for natural killer cell receptor genes leads to superior survival after unrelated transplantation for acute myelogenous leukemia. Blood J Am Soc Hematol. (2010) 116:2411–9. doi: 10.1182/blood-2010-05-283051
62. Cooley S, Weisdorf DJ, Guethlein LA, Klein JP, Wang T, Marsh SG, et al. Donor killer cell Ig-like receptor B haplotypes, recipient HLA-C1, and HLA-C mismatch enhance the clinical benefit of unrelated transplantation for acute myelogenous leukemia. J Immunol. (2014) 192:4592–600. doi: 10.4049/jimmunol.1302517
63. Zhao X, Chang Y, Xu L, Zhang X, Liu K, Li D, et al. HLA and KIR genotyping correlates with relapse after T-cell-replete haploidentical transplantation in chronic myeloid leukaemia patients. Br J Cancer. (2014) 111:1080–8. doi: 10.1038/bjc.2014.423
64. Zhao XY, Chang YJ, Zhao XS, Xu LP, Zhang XH, Liu KY, et al. Recipient expression of ligands for donor inhibitory KIRs enhances NK cell function to control leukemic relapse after haploidentical transplantation. Eur J Immunol. (2015) 45:2396–408. doi: 10.1002/eji.201445057
65. Zhao X-Y, Yu X-X, Xu Z-L, Cao X-H, Huo M-R, Zhao X-S, et al. Donor and host coexpressing KIR ligands promote NK education after allogeneic hematopoietic stem cell transplantation. Blood Adv. (2019) 3:4312–25. doi: 10.1182/bloodadvances.2019000242
66. Symons HJ, Leffell MS, Rossiter ND, Zahurak M, Jones RJ, Fuchs EJ. Improved survival with inhibitory killer immunoglobulin receptor (KIR) gene mismatches and KIR haplotype B donors after nonmyeloablative, HLA-haploidentical bone marrow transplantation. Biol Blood Marrow Transplant. (2010) 16:533–42. doi: 10.1016/j.bbmt.2009.11.022
67. Sahin U, Dalva K, Gungor F, Ustun C, Beksac M. Donor-recipient killer immunoglobulin like receptor (KIR) genotype matching has a protective effect on chronic graft versus host disease and relapse incidence following HLA-identical sibling hematopoietic stem cell transplantation. Ann Hematol. (2018) 97:1027–39. doi: 10.1007/s00277-018-3274-0
68. Ciurea SO, Al Malki MM, Kongtim P, Fuchs EJ, Luznik L, Huang X-J, et al. The European Society for Blood and Marrow Transplantation (EBMT) consensus recommendations for donor selection in haploidentical hematopoietic cell transplantation. Bone marrow Transplant. (2020) 55:12–24. doi: 10.1038/s41409-019-0499-z
69. Shilling HG, Young N, Guethlein LA, Cheng NW, Gardiner CM, Tyan D, et al. Genetic control of human NK cell repertoire. J Immunol. (2002) 169:239–47. doi: 10.4049/jimmunol.169.1.239
70. Boudreau JE, Hsu KC. Natural killer cell education and the response to infection and cancer therapy: stay tuned. Trends Immunol. (2018) 39:222–39. doi: 10.1016/j.it.2017.12.001
71. Nowak J, Kościńska K, Mika-Witkowska R, Rogatko-Koroś M, Mizia S, Jaskuła E, et al. Donor NK cell licensing in control of Malignancy in hematopoietic stem cell transplant recipients. Am J Hematol. (2014) 89:E176–E83. doi: 10.1002/ajh.23802
72. Tu MM, Mahmoud AB, Makrigiannis AP. Licensed and unlicensed NK cells: differential roles in cancer and viral control. Front Immunol. (2016) 7:166. doi: 10.3389/fimmu.2016.00166
73. Kim S, Poursine-Laurent J, Truscott SM, Lybarger L, Song Y-J, Yang L, et al. Licensing of natural killer cells by host major histocompatibility complex class I molecules. Nature. (2005) 436:709–13. doi: 10.1038/nature03847
74. Biassoni R, Cantoni C, Pende D, Sivori S, Parolini S, Vitale M, et al. Human natural killer cell receptors and co-receptors. Immunol Rev. (2001) 181:203–14. doi: 10.1034/j.1600-065X.2001.1810117.x
75. Biassoni R, Pessino A, Malaspina A, Cantoni C, Bottino C, Sivori S, et al. Role of amino acid position 70 in the binding affinity of p50. 1 and p58. 1 receptors for HLA-Cw4 molecules. Eur J Immunol. (1997) 27:3095–9. doi: 10.1002/eji.1830271203
76. Stewart CA, Laugier-Anfossi F, Vély F, Saulquin X, Riedmuller J, Tisserant A, et al. Recognition of peptide–MHC class I complexes by activating killer immunoglobulin-like receptors. Proc Natl Acad Sci. (2005) 102:13224–9. doi: 10.1073/pnas.0503594102
77. Boudreau JE, Liu X-R, Zhao Z, Zhang A, Shultz LD, Greiner DL, et al. Cell-extrinsic MHC class I molecule engagement augments human NK cell education programmed by cell-intrinsic MHC class I. Immunity. (2016) 45:280–91. doi: 10.1016/j.immuni.2016.07.005
78. Elliott JM, Wahle JA, Yokoyama WM. MHC class I–deficient natural killer cells acquire a licensed phenotype after transfer into an MHC class I–sufficient environment. J Exp Med. (2010) 207:2073–9. doi: 10.1084/jem.20100986
79. Joncker NT, Shifrin N, Delebecque F, Raulet DH. Mature natural killer cells reset their responsiveness when exposed to an altered MHC environment. J Exp Med. (2010) 207:2065–72. doi: 10.1084/jem.20100570
80. Sungur CM, Tang-Feldman YJ, Zamora AE, Alvarez M, Pomeroy C, Murphy WJ. Murine NK cell licensing is reflective of donor MHC-I following allogeneic hematopoietic stem cell transplantation in murine cytomegalovirus responses. Blood J Am Soc Hematol. (2013) 122:1518–21. doi: 10.1182/blood-2013-02-483503
81. Dulphy N, Haas P, Busson M, Belhadj Sp, Peffault de Latour Rg, Robin M, et al. An unusual CD56brightCD16low NK cell subset dominates the early posttransplant period following HLA-matched hematopoietic stem cell transplantation1. J Immunol. (2008) 181:2227–37. doi: 10.4049/jimmunol.181.3.2227
82. Gagne K, Brizard G, Gueglio B, Milpied N, Herry P, Bonneville F, et al. Relevance of KIR gene polymorphisms in bone marrow transplantation outcome. Hum Immunol. (2002) 63:271–80. doi: 10.1016/S0198-8859(02)00373-7
83. Stringaris K, Adams S, Uribe M, Eniafe R, Wu CO, Savani BN, et al. Donor KIR Genes 2DL5A, 2DS1 and 3DS1 are associated with a reduced rate of leukemia relapse after HLA-identical sibling stem cell transplantation for acute myeloid leukemia but not other hematologic Malignancies. Biol Blood Marrow Transplant. (2010) 16:1257–64. doi: 10.1016/j.bbmt.2010.03.004
84. Bachanova V, Weisdorf DJ, Wang T, Marsh SG, Trachtenberg E, Haagenson MD, et al. Donor KIR B genotype improves progression-free survival of non-Hodgkin lymphoma patients receiving unrelated donor transplantation. Biol Blood Marrow Transplant. (2016) 22:1602–7. doi: 10.1016/j.bbmt.2016.05.016
85. Weisdorf D, Cooley S, Wang T, Trachtenberg E, Vierra-Green C, Spellman S, et al. KIR B donors improve the outcome for AML patients given reduced intensity conditioning and unrelated donor transplantation. Blood Adv. (2020) 4:740–54. doi: 10.1182/bloodadvances.2019001053
86. Zhang Y, Yan AW, Boelen L, Hadcocks L, Salam A, Gispert DP, et al. KIR-HLA interactions extend human CD8+ T cell lifespan in vivo. J Clin Invest. (2023) 133. doi: 10.1172/jci169496
87. Solomon SR, Aubrey MT, Zhang X, Piluso A, Freed BM, Brown S, et al. Selecting the best donor for haploidentical transplant: impact of HLA, killer cell immunoglobulin-like receptor genotyping, and other clinical variables. Biol Blood Marrow Transplant. (2018) 24:789–98. doi: 10.1016/j.bbmt.2018.01.013
88. Wanquet A, Bramanti S, Harbi S, Fürst S, Legrand F, Faucher C, et al. Killer cell immunoglobulin-like receptor–ligand mismatch in donor versus recipient direction provides better graft-versus-tumor effect in patients with hematologic Malignancies undergoing allogeneic T cell–replete haploidentical transplantation followed by post-transplant cyclophosphamide. Biol Blood Marrow Transplant. (2018) 24:549–54. doi: 10.1016/j.bbmt.2017.11.042
89. Shimoni A, Labopin M, Lorentino F, Van Lint MT, Koc Y, Gülbas Z, et al. Killer cell immunoglobulin-like receptor ligand mismatching and outcome after haploidentical transplantation with post-transplant cyclophosphamide. Leukemia. (2019) 33:230–9. doi: 10.1038/s41375-018-0170-5
90. Cooley S, McCullar V, Wangen R, Bergemann TL, Spellman S, Weisdorf DJ, et al. KIR reconstitution is altered by T cells in the graft and correlates with clinical outcomes after unrelated donor transplantation. Blood. (2005) 106:4370–6. doi: 10.1182/blood-2005-04-1644
91. Shapiro RM, Birch GC, Hu G, Vergara Cadavid J, Nikiforow S, Baginska J, et al. Expansion, persistence, and efficacy of donor memory-like NK cells infused for posttransplant relapse. J Clin Invest. (2022) 132. doi: 10.1172/JCI154334
92. Patriarca F, Sperotto A, Lorentino F, Oldani E, Mammoliti S, Isola M, et al. Donor lymphocyte infusions after allogeneic stem cell transplantation in acute leukemia: a survey from the Gruppo Italiano Trapianto Midollo Osseo (GITMO). Front Oncol. (2020) 10:572918. doi: 10.3389/fonc.2020.572918
93. Ghiso A, Raiola A, Gualandi F, Dominietto A, Varaldo R, Van Lint M, et al. DLI after haploidentical BMT with post-transplant CY. Bone marrow Transplant. (2015) 50:56–61. doi: 10.1038/bmt.2014.217
94. Zeidan AM, Forde PM, Symons H, Chen A, Smith BD, Pratz K, et al. HLA-haploidentical donor lymphocyte infusions for patients with relapsed hematologic Malignancies after related HLA-haploidentical bone marrow transplantation. Biol Blood Marrow Transplant. (2014) 20:314–8. doi: 10.1016/j.bbmt.2013.11.020
95. Frey NV, Porter DL. Graft-versus-host disease after donor leukocyte infusions: presentation and management. Best Pract Res Clin haematology. (2008) 21:205–22. doi: 10.1016/j.beha.2008.02.007
96. Toffalori C, Zito L, Gambacorta V, Riba M, Oliveira G, Bucci G, et al. Immune signature drives leukemia escape and relapse after hematopoietic cell transplantation. Nat Med. (2019) 25:603–11. doi: 10.1038/s41591-019-0400-z
97. Miller JS, Soignier Y, Panoskaltsis-Mortari A, McNearney SA, Yun GH, Fautsch SK, et al. Successful adoptive transfer and in vivo expansion of human haploidentical NK cells in patients with cancer. Blood. (2005) 105:3051–7. doi: 10.1182/blood-2004-07-2974
98. Burns LJ, Weisdorf DJ, DeFor TE, Vesole DH, Repka TL, Blazar BR, et al. IL-2-based immunotherapy after autologous transplantation for lymphoma and breast cancer induces immune activation and cytokine release: a phase I/II trial. Bone Marrow Transplant. (2003) 32:177–86. doi: 10.1038/sj.bmt.1704086
99. Passweg JR, Tichelli A, Meyer-Monard S, Heim D, Stern M, Kühne T, et al. Purified donor NK-lymphocyte infusion to consolidate engraftment after haploidentical stem cell transplantation. Leukemia. (2004) 18:1835–8. doi: 10.1038/sj.leu.2403524
100. Yoon SR, Lee YS, Yang SH, Ahn KH, Lee J-H, Lee J-H, et al. Generation of donor natural killer cells from CD34+ progenitor cells and subsequent infusion after HLA-mismatched allogeneic hematopoietic cell transplantation: a feasibility study. Bone Marrow Transplant. (2010) 45:1038–46. doi: 10.1038/bmt.2009.304
101. Choi I, Yoon SR, Park S-Y, Kim H, Jung S-J, Jang YJ, et al. Donor-derived natural killer cells infused after human leukocyte antigen–haploidentical hematopoietic cell transplantation: A dose-escalation study. Biol Blood Marrow Transplant. (2014) 20:696–704. doi: 10.1016/j.bbmt.2014.01.031
102. Choi I, Yoon SR, Park S-Y, Kim H, Jung S-J, Kang Y-L, et al. Donor-derived natural killer cell infusion after human leukocyte antigen–haploidentical hematopoietic cell transplantation in patients with refractory acute leukemia. Biol Blood Marrow Transplant. (2016) 22:2065–76. doi: 10.1016/j.bbmt.2016.08.008
103. Romee R, Rosario M, Berrien-Elliott MM, Wagner JA, Jewell BA, Schappe T, et al. Cytokine-induced memory-like natural killer cells exhibit enhanced responses against myeloid leukemia. Sci Trans Med. (2016) 8:357ra123–357ra123. doi: 10.1126/scitranslmed.aaf2341
104. Song Y, Hu B, Liu Y, Jin Z, Zhang Y, Lin D, et al. IL-12/IL-18-preactivated donor NK cells enhance GVL effects and mitigate GvHD after allogeneic hematopoietic stem cell transplantation. Eur J Immunol. (2018) 48:670–82. doi: 10.1002/eji.201747177
105. Rubnitz JE, Inaba H, Ribeiro RC, Pounds S, Rooney B, Bell T, et al. NKAML: a pilot study to determine the safety and feasibility of haploidentical natural killer cell transplantation in childhood acute myeloid leukemia. J Clin Oncol. (2010) 28:955–9. doi: 10.1200/jco.2009.24.4590
106. Curti A, Ruggeri L, Parisi S, Bontadini A, Dan E, Motta MR, et al. Larger size of donor alloreactive NK cell repertoire correlates with better response to NK cell immunotherapy in elderly acute myeloid leukemia patients. Clin Cancer Res. (2016) 22:1914–21. doi: 10.1158/1078-0432.CCR-15-1604
107. Ciurea SO, Schafer JR, Bassett R, Denman CJ, Cao K, Willis D, et al. Phase 1 clinical trial using mbIL21 ex vivo–expanded donor-derived NK cells after haploidentical transplantation. Blood. (2017) 130:1857–68. doi: 10.1182/blood-2017-05-785659
108. Shah NN, Baird K, Delbrook CP, Fleisher TA, Kohler ME, Rampertaap S, et al. Acute GVHD in patients receiving IL-15/4-1BBL activated NK cells following T-cell–depleted stem cell transplantation. Blood. (2015) 125:784–92. doi: 10.1182/blood-2014-07-592881
109. Jaiswal SR, Chakrabarti S. Natural killer cell-based immunotherapy with CTLA4Ig-primed donor lymphocytes following haploidentical transplantation. Immunotherapy. (2019) 11:1221–30. doi: 10.2217/imt-2019-0037
110. Liu E, Tong Y, Dotti G, Shaim H, Savoldo B, Mukherjee M, et al. Cord blood NK cells engineered to express IL-15 and a CD19-targeted CAR show long-term persistence and potent antitumor activity. Leukemia. (2018) 32:520–31. doi: 10.1038/leu.2017.226
111. Hattori N, Nakamaki T. Natural killer immunotherapy for minimal residual disease eradication following allogeneic hematopoietic stem cell transplantation in acute myeloid leukemia. Int J Mol Sci. (2019) 20:2057. doi: 10.3390/ijms20092057
112. Bachanova V, Cooley S, Defor TE, Verneris MR, Zhang B, McKenna DH, et al. Clearance of acute myeloid leukemia by haploidentical natural killer cells is improved using IL-2 diphtheria toxin fusion protein. Blood. (2014) 123:3855–63. doi: 10.1182/blood-2013-10-532531
113. Henney CS, Kuribayashi K, Kern DE, Gillis S. Interleukin-2 augments natural killer cell activity. Nature. (1981) 291:335–8. doi: 10.1038/291335a0
114. Romee R, Schneider SE, Leong JW, Chase JM, Keppel CR, Sullivan RP, et al. Cytokine activation induces human memory-like NK cells. Blood J Am Soc Hematol. (2012) 120:4751–60. doi: 10.1182/blood-2012-04-419283
115. Jaiswal SR, Bhakuni P, Joy A, Kaushal S, Chakrabarti A, Chakrabarti S. CTLA4Ig primed donor lymphocyte infusion: A novel approach to immunotherapy after haploidentical transplantation for advanced leukemia. Biol Blood Marrow Transplant. (2019) 25:673–82. doi: 10.1016/j.bbmt.2018.12.836
116. Peng Y, Luo G, Zhou J, Wang X, Hu J, Cui Y, et al. CD86 is an activation receptor for NK cell cytotoxicity against tumor cells. PloS One. (2013) 8:e83913. doi: 10.1371/journal.pone.0083913
117. Maude SL, Laetsch TW, Buechner J, Rives S, Boyer M, Bittencourt H, et al. Tisagenlecleucel in children and young adults with B-cell lymphoblastic leukemia. New Engl J Med. (2018) 378:439–48. doi: 10.1056/NEJMoa1709866
118. Neelapu SS, Locke FL, Bartlett NL, Lekakis LJ, Miklos DB, Jacobson CA, et al. Axicabtagene ciloleucel CAR T-cell therapy in refractory large B-cell lymphoma. New Engl J Med. (2017) 377:2531–44. doi: 10.1056/NEJMoa1707447
119. Park JH, Rivière I, Gonen M, Wang X, Sénéchal B, Curran KJ, et al. Long-term follow-up of CD19 CAR therapy in acute lymphoblastic leukemia. New Engl J Med. (2018) 378:449–59. doi: 10.1056/NEJMoa1709919
120. Porter DL, Hwang W-T, Frey NV, Lacey SF, Shaw PA, Loren AW, et al. Chimeric antigen receptor T cells persist and induce sustained remissions in relapsed refractory chronic lymphocytic leukemia. Sci Trans Med. (2015) 7:303ra139–303ra139. doi: 10.1126/scitranslmed.aac5415
121. Porter DL, Levine BL, Kalos M, Bagg A, June CH. Chimeric antigen receptor–modified T cells in chronic lymphoid leukemia. New Engl J Med. (2011) 365:725–33. doi: 10.1056/NEJMoa1103849
122. Schuster SJ, Svoboda J, Chong EA, Nasta SD, Mato AR, Anak Ö, et al. Chimeric antigen receptor T cells in refractory B-cell lymphomas. New Engl J Med. (2017) 377:2545–54. doi: 10.1056/NEJMoa1708566
123. Turtle CJ, Hay KA, Hanafi L-A, Li D, Cherian S, Chen X, et al. Durable molecular remissions in chronic lymphocytic leukemia treated with CD19-specific chimeric antigen receptor–modified T cells after failure of ibrutinib. J Clin Oncol. (2017) 35:3010. doi: 10.1200/JCO.2017.72.8519
124. Brentjens RJ, Riviere I, Park JH, Davila ML, Wang X, Stefanski J, et al. Safety and persistence of adoptively transferred autologous CD19-targeted T cells in patients with relapsed or chemotherapy refractory B-cell leukemias. Blood J Am Soc Hematol. (2011) 118:4817–28. doi: 10.1182/blood-2011-04-348540
125. Davila ML, Riviere I, Wang X, Bartido S, Park J, Curran K, et al. Efficacy and toxicity management of 19-28z CAR T cell therapy in B cell acute lymphoblastic leukemia. Sci Trans Med. (2014) 6:224ra25–ra25. doi: 10.1126/scitranslmed.3008226
126. Lee DW, Kochenderfer JN, Stetler-Stevenson M, Cui YK, Delbrook C, Feldman SA, et al. T cells expressing CD19 chimeric antigen receptors for acute lymphoblastic leukaemia in children and young adults: a phase 1 dose-escalation trial. Lancet. (2015) 385:517–28. doi: 10.1016/S0140-6736(14)61403-3
127. Hay KA, Gauthier J, Hirayama AV, Voutsinas JM, Wu Q, Li D, et al. Factors associated with durable EFS in adult B-cell ALL patients achieving MRD-negative CR after CD19 CAR T-cell therapy. Blood J Am Soc Hematol. (2019) 133:1652–63. doi: 10.1182/blood-2018-11-883710
128. Frey NV, Shaw PA, Hexner EO, Pequignot E, Gill S, Luger SM, et al. Optimizing chimeric antigen receptor T-cell therapy for adults with acute lymphoblastic leukemia. J Clin Oncol. (2020) 38:415–22. doi: 10.1200/jco.19.01892
129. Yurkiewicz I, Craig J, Muffly L. Hematopoietic cell transplantation for philadelphia chromosome negative adult acute lymphoblastic leukemia in the modern era of immune therapy. Curr Hematol Malig Rep. (2020) 15:187–93. doi: 10.1007/s11899-020-00579-0
130. Liu E, Marin D, Banerjee P, Macapinlac HA, Thompson P, Basar R, et al. Use of CAR-transduced natural killer cells in CD19-positive lymphoid tumors. New Engl J Med. (2020) 382:545–53. doi: 10.1056/NEJMoa1910607
131. Xu J, Niu T. Natural killer cell-based immunotherapy for acute myeloid leukemia. J Hematol Oncol. (2020) 13:167. doi: 10.1186/s13045-020-00996-x
132. Auletta JJ, Kou J, Chen M, Bolon Y-T, Broglie L, Bupp C, et al. Real-world data showing trends and outcomes by race and ethnicity in allogeneic hematopoietic cell transplantation: A report from the center for international blood and marrow transplant research. Transplant Cell Ther. (2023) 29:346.e1–.e10. doi: 10.1016/j.jtct.2023.03.007
133. Weisdorf D, Cooley S, Wang T, Trachtenberg E, Haagenson MD, Vierra-Green C, et al. KIR donor selection: feasibility in identifying better donors. Biol Blood Marrow Transplant. (2019) 25:e28–32. doi: 10.1016/j.bbmt.2018.08.022
134. Gooptu M, Romee R, St Martin A, Arora M, Al Malki M, Antin JH, et al. HLA-haploidentical vs matched unrelated donor transplants with posttransplant cyclophosphamide-based prophylaxis. Blood. (2021) 138:273–82. doi: 10.1182/blood.2021011281
135. McAdams MJ, Dimitrova D, Sadler JL, Steinberg SM, Cuellar-Rodriguez J, Hughes TE, et al. 11 - phase I study de-intensifying exposure of post-transplantation cyclophosphamide (PTCy) after HLA-haploidentical hematopoietic cell transplantation (HCT) for hematologic Malignancies. Transplant Cell Ther. (2021) 27:S9–11. doi: 10.1016/S2666-6367(21)00037-3
Keywords: NK cell, graft-versus-leukemia, allogeneic transplant, PTCy, alloreactivity, KIR
Citation: Hadjis AD and McCurdy SR (2024) The role and novel use of natural killer cells in graft-versus-leukemia reactions after allogeneic transplantation. Front. Immunol. 15:1358668. doi: 10.3389/fimmu.2024.1358668
Received: 20 December 2023; Accepted: 28 March 2024;
Published: 16 May 2024.
Edited by:
Robert Zeiser, University of Freiburg, GermanyReviewed by:
Nana Talvard-Balland, University of Freiburg, GermanyBenedetta Rambaldi, Ospedale Papa Giovanni XXIII, Italy
Copyright © 2024 Hadjis and McCurdy. This is an open-access article distributed under the terms of the Creative Commons Attribution License (CC BY). The use, distribution or reproduction in other forums is permitted, provided the original author(s) and the copyright owner(s) are credited and that the original publication in this journal is cited, in accordance with accepted academic practice. No use, distribution or reproduction is permitted which does not comply with these terms.
*Correspondence: Shannon R. McCurdy, c2hhbm5vbi5tY2N1cmR5QHBlbm5tZWRpY2luZS51cGVubi5lZHU=