- 1Department of Surgery, Section of Pediatric Surgery, Indiana University School of Medicine, Indianapolis, IN, United States
- 2Riley Hospital for Children at Indiana University Health, Indianapolis, IN, United States
Necrotizing enterocolitis (NEC) is a destructive gastrointestinal disease primarily affecting preterm babies. Despite advancements in neonatal care, NEC remains a significant cause of morbidity and mortality in neonatal intensive care units worldwide and the etiology of NEC is still unclear. Risk factors for NEC include prematurity, very low birth weight, feeding with formula, intestinal dysbiosis and bacterial infection. A review of the literature would suggest that supplementation of prebiotics and probiotics prevents NEC by altering the immune responses. Innate T cells, a highly conserved subpopulation of T cells that responds quickly to stimulation, develops differently from conventional T cells in neonates. This review aims to provide a succinct overview of innate T cells in neonates, encompassing their phenotypic characteristics, functional roles, likely involvement in the pathogenesis of NEC, and potential therapeutic implications.
Introduction
Necrotizing enterocolitis (NEC) is a devastating disease that affects neonates born prematurely. Approximately 10% of infants are born pre-term, and about 7% of them develop NEC (1). While etiology of NEC is unknown, several risk factors have been previously reported: prematurity, very low birth weight, formula feeding, microbial dysbiosis and bacterial infection (2–7). The current treatment of NEC includes cessation of enteral feeding, use of broad-spectrum antibiotics, and parenteral administration of nutrition. About 50% of infants with NEC progress to requiring surgical intervention due to intestinal ischemia and necrosis, which is often associated with high mortality and long-term complications including intestinal stricture, short-gut syndrome, and neurodevelopmental delays (1, 8). Discovering new approaches to treat NEC medically is imperative to avoid disease progression and surgical interventions.
The diagnosis, classification, management, outcome, and complications of NEC have been summarized in many great review articles (2–6). This review focuses on exploring the possibility therapeutic role of innate T cells for NEC.
Unique characteristics of neonatal immune response
Tolerogenic nature
The neonatal immune system can be characterized as tolerogenic, immature, and naïve. During pregnancy, microchimerism occurs, leading to the presence of maternal cells in the fetuses and vice versa. As a result, fetal immune cells are tolerant towards maternal antigens, while maternal immune cells and antibodies are vertically transferred to offspring (9, 10). Due to this tolerogenic nature and inherent bias toward Th2-cell polarization, the developing immune system in neonates can be more susceptible to infections (11, 12).
Immaturity and naivety
Neonatal immune cells are quantitatively fewer and qualitatively different from their adult immune cells (12, 13). As a result, maternal immune cells and antibodies circulate in offspring long after birth and impact neonatal immune responses (13–15). While maternal IgG is critical in preventing bacterial infection in neonates (16), the presence of maternal antibodies worsen the intrinsic defect of the infant’s primary antibody response but does not appear to affect their T cell response (13). Though neonatal T cells are mostly recent thymic emigrants, less antigen-experienced, and produce less IL-2 and IFN-γ (17), these cells may be more sensitive to cytokines than to antigen stimulations due to higher expression of cytokine receptors on their cell surface (18).
Impact of microchimerism
The adaptive immune system in neonates is naïve and defective due to limited exposure to antigens and the tolerogenic environment in utero (19), therefore innate immunity is important in providing protection from infections (12, 20) despite its immaturity and hyporesponsiveness to stimulations (11, 21). A recent study showed that maternal microchimeric cells are enriched in fetal bone marrow and favor fetal monocyte differentiation (22), suggesting maternal/offspring microchimerism also affects neonatal innate immunity.
Neonatal T cells have full potential
Many investigations suggest that fetuses and neonates are capable of mounting robust T cell responses (13, 19). Early in life, neonates experience rapid growth and may allocate energy towards growth rather than mounting an immune response, leading to environmental enteric dysfunction (EED). Neonates need to maintain a balance between host defense against pathogens and other essential physiological processes (17).
While significant knowledge in neonatal immunity has been acquired, little is known about neonatal innate T cells and their role in the immune responses in neonatal diseases.
Innate T cells in neonates
Characteristics of innate T cells
Conventional T cells express highly diverse T-cell receptors (TCRs) and respond to peptide antigens presented by polymorphic MHC class I or II molecules. In contrast, innate T cells often have limited TCR diversity and predominantly recognize non-peptide antigens presented by monomorphic non-MHC molecules (23). Many of the non-peptide antigens that activate innate T cells are microbially derived (24–31). Therefore, their development and function are changed by the microbiome (32–34).
There are three subsets of innate T cells: Natural Killer T (NKT) cells, Mucosal-Associated Invariant T (MAIT) cells and Gamma Delta (γδ) T cells. NKT and MAIT cells are mostly semi-invariant αβ T cells. All three types of innate T cells develop in the thymus and localize in non-lymphoid tissues such as the liver, lung, and intestine (24, 35).
As shown in Table 1, innate T cells share some common characteristics, such as their ability to bridge the innate and adaptive immune systems by quickly responding to antigens and producing large amounts of pro- and anti-inflammatory cytokines (23). The development of all three types of innate T cells is regulated by the same transcription factor, PLZF (promyelocytic leukemia zinc finger; ZBTB16) (35, 53–55). Transcriptomic analyses have demonstrated that both NKT and MAIT cells are more similar with one another compared to conventional T cells (56, 57). While all innate T cells express IL-12 receptor (IL-12R), IL-18R and other surface markers (24, 51), both NKT and MAIT cells also express NK and T cell markers (51). The similar transcriptomic profiles of NKT and MAIT cells are likely acquired by their residence in the thymus (56).
Early in life, all three types of innate T cells seem to be more responsive and mature than conventional T cells in responding to stimulation (24, 42–44). These innate T cells are speculated to play important roles in tissue homeostasis and fighting against infection during early life when conventional T cells are still naïve and immature (58).
Neonatal NKT cells
NKT cells are innate T cells that can be activated by lipid antigens, with CD1d, an MHC class I-like molecule, acting as the antigen presenting molecule (59–62). The most potent lipid antigen for NKT cells is alpha-Galactosylceramide (a-GalCer, KRN7000), which is a synthetic glycolipid derived from the marine sponge Agelas mauritianus (63, 64). NKT cells can also be activated by various endogenous and microbial lipid antigens such as iGb3, sulfatide, and α-glucuronosylceramide (GSL-1) (28, 40, 65, 66). Activated NKT cells may be utilized in vaccine development and the treatment of conditions like autoimmune diseases, graft-versus-host disease, infections, neurological diseases, and cancer (67–77).
NKT cells are either CD4+ or CD4-CD8- T cells. Based on TCR usage, NKT cells can be further categorized as Type I (invariant TCR) and the much less studied Type II (variable CD1d-restricted TCR) NKT cells. In this review only type I NKT cells related work is discussed.
Studies have shown that a low number of neonatal NKT cells are present in cord blood (45, 78) and they are less responsive to stimulation compared to adult NKT cells (45). However, neonatal NKT cells were more responsive to stimulation compared to neonatal conventional T cells (45). The population of neonatal NKT cells was higher in the blood of day 14 preterm infants compared to those from age-matched full-term infants. In the subsequent 2-3 weeks, however, that higher proportion of NKT cells decreases to a level similar to that of full-term infants. This is likely due to gestational development because the proportion of CD3+ T cells expands and positively correlates with gestational age (52). The proportion of NKT cells in the blood should expand as infants grow older since the proportion of NKT cells in adult blood is much higher than that in cord blood (45).
Neonates have a Th-2 biased immunity with neonatal NKT cells producing more IL-4 than IFN-γ (46, 47). Interestingly, NKT cells are enriched in the fetal small intestine. These small intestinal NKT cells, different from NKT cells from other fetal organs, express mature markers and IFN-γ upon stimulation, resembling adult NKT cells (44).
As shown in Table 1, not much is known about the frequency of neonatal NKT cells in mice.
Neonatal MAIT cells
MAIT cells predominantly recognize non-peptide microbial antigens presented by monomorphic MHC class I-like molecule (MR1) (23, 30, 79). MAIT cells express limited TCR diversity (Vα19 in mice, Vα7.2 in humans with limited variation of TCR-β chains). The research of MAIT cell antigens experienced a breakthrough when 5-(2-oxopropylideneamino)-6-D-ribitylaminouracil (5-OP-RU) was identified and remains the most potent MAIT cell agonist to date (31, 41, 80). MAIT cells are mostly CD8+ or CD4-CD8- T cells. Emerging research demonstrates that MAIT cells are involved in many conditions, such as infection, cancer, tissue repair, autoimmunity, inflammation, and metabolic diseases (81–89).
Data on how gestational age impacts MAIT cell levels is conflicting. One study suggests that the proportion of neonatal MAIT cells is low and does not seem to be affected by gestational age ranged 23 to 28 weeks (52). However, another study using cord blood from broader range of gestational ages (24 weeks to full term) showed that the proportion of MAIT cells in CD3+ T cells negatively correlated with gestational age (36). Currently, there are two ways to identify human MAIT cells: CD3+Vα7.2+ CD161high T and CD3+MR1:5-OP-RU tetramer+ cells. In adult blood, these two populations almost fully overlap. However, in cord blood, only a small portion of CD3+Vα7.2+ CD161high T cells are also MR1:5-OP-RU tetramer+ (36, 53). This is likely due to specific expansion after encountering microbial antigens. Cord blood-derived MAIT cells consistently are more capable to proliferate upon stimulation compared to adult MAIT cells (36). Allogeneic hematopoietic cell transplantation study showed expansion of MAIT cells in recipients after cord blood transplantation but not in adult bone-marrow or peripheral blood stem cell transplantations, supporting the high proliferative capacity of neonatal MAIT cells (90).
Mouse MAIT cell studies have been lagging due to the scarcity of mouse MAIT cells. To solve this problem, a wild-derived inbred CAST/EiJ mouse model was discovered with frequencies of MAIT cells 20 times more than those in C57BL/6J mice (38). MAIT cells also increase significantly in the transgenic mice expressing the TCR Vα19, but its application is limited due to high non-specific binding of MR1:5-OP-RU tetramer in other T cells (91–93). Like human MAIT cells, mouse MAIT cells are almost undetectable at birth but expand significantly after encountering the developing microbiome (38).
Neonatal γδ T cells
Most mammalian T cells express αβ TCR. A small population of T cells express gamma and delta (γδ) TCR and these cells are called γδ T cells. The antigen presenting molecule for γδ T cells is not known. γδ TCR may interact with antigens in an antibody/antigen binding fashion (24, 94, 95). The functions of γδ T cells include immune surveillance, thermogenesis, and tissue homeostasis (96). γδ T cells are known to be important for maintaining mucosal tolerance (97, 98). Although similar numbers of γδ T cells can be found in the intestine of germ-free and specific pathogen-free mice (99), the crosstalk between microbiome and γδ T cells is important for the effector function of γδ T cells (32). Removal of gut microbiome by antibiotic treatment in drinking water impairs oral tolerance and also transiently removes intestinal γδ T cells.
Neonatal γδ T cells are Th2-prone and more naïve than adult γδ T cells, but more Th1-prone compared to neonatal αβ T cells. Thus, it seems reasonable to hypothesize that neonatal γδ T cells may be key providers of immunoprotection and immunomodulation in the perinatal period (42). γδ intraepithelial lymphocytes (IEL) are the first T-cell subset present in the intestine during embryogenesis (39, 100). Neonatal mouse γδ IELs were found to produce higher levels of cytokines, such as IFN-γ and IL-10, as compared to neonatal αβ IELs and adult γδ IELs, indicating enhanced activity of γδ IELs during early life (39).
Neonatal γδ T cells are more diverse compared to adult γδ T cells. The dominant Vγ9Vδ2 subset in human adult blood is due to the post-natal expansion of cells expressing unique CDR3 formed in response to encountering phosphor-antigens derived from the microbe-specific isoprenoid synthesis pathway. During mouse embryonic development, there are waves of γδ T cell development that start as early as day 15 of gestation so most peripheral tissues are colonized by long-lived γδ T cells early in life (96). The first wave of mouse γδ T cells are dendritic epidermal T cells (DETCs). These DETCs migrate to mouse skin and proliferate there during fetal development (24, 96). While some γδ T cells can be restored in 2 weeks in adult mice, fetal γδ T cells cannot be regenerated in the adult thymus (24).
Similar to neonatal NKT cells, the proportion of neonatal γδ T cells are larger in the blood from preterm infants than that from full-term infants, and the proportion of γδ T cells decreases to a similar level as that from full-term infants in the next 2-4 weeks (52). This is likely due to the expansion of CD3+ T cells in late gestational stages.
The role of innate T cells in NEC
Gut microbial community perturbations are the most consequential risk factor for NEC (101). The intestinal microbiome of preterm infants is distinct and less diverse than that of term-born infants. Interestingly, the gut microbiome in preterm infants seems to have an orderly progression where the bacterial classes switch from Bacilli to Gammaproteobacteria to Clostridia, and is minimally influenced by mode of delivery, antibiotics, or feeds (101, 102).
It is not clear how the bacterial class switch in preterm infants increases their risk of developing NEC but analysis of gene expression analysis in NEC tissues does reveal an altered immune response (48, 103–106). The microbial dysbiosis in NEC likely alters the development of innate T cells given the microbiome’s known influence on innate T cell maturation, activation, and expansion via changes in microbial antigens and modulation of the mucosal microenvironment (32, 81, 107, 108). Immune cell development needs microbial exposure, but there seems to be a “window of opportunity” (17, 58, 109). Using mouse models, several groups have demonstrated that exposure to certain microbiome early in life defines hosts’ T cell functions in adulthood (17, 58, 109). It is reasonable to speculate that the microbial dysbiosis in NEC impacts not only neonatal immunity but also long-term immunity beyond when the disease is resolved.
Studies about the relationship between innate T cells and NEC are sparse. A recent report has shown that more MAIT cells accumulate in the intestine of NEC patients compared to control infants. However, these MAIT cells within NEC intestine are mostly CD4-CD8-, while MAIT cells from healthy intestine are mainly CD8αα+ MAIT cells (49). CD8αα+ MAIT cells are known to be more mature than CD4-CD8- or CD8αβ+ MAIT cells (43, 110). These results suggest that there are more immature MAIT cells residing in NEC intestines. Weitkamp et al. discovered significantly lower CD8 + γδ IEL in preterm infants with NEC compared to control infants, suggesting that γδ IELs depletion occurs during the development of NEC (39).
It is worth noting that a unique population of IELs, called innate CD8alpha (iCD8α) cells, that expresses the CD8αα homodimer and may be involved with NEC pathogenesis. Though neither T cells nor dendritic cells, they are IL-12R positive and responsive to stimulation by IL-12 and PMA/Ionomycin. iCD8α cells show capacity in antigen processing/presentation and protection from bacterial infection (111). iCD8α cells are also reduced in NEC patients compared to control infants, consistent with reduced CD8αα+ MAIT and CD8+ γδ T cells in NEC (39, 49, 111). These observations indicate mucosal CD8+ lymphocytes, either TCR+ (MAIT and γδ T cells) or TCR- (innate lymphoid cells) may be important in preventing NEC.
Deficiency of MR1 in neonatal mice renders protection from NEC pathogenesis (112) while TCRδ-deficient neonatal mice develop worse NEC disease compared to WT controls (39). These data suggest that innate T cells, probably altered by microbial dysbiosis, play a role in NEC pathogenesis (Figure 1A). Little is known how innate T cells may contribute to the pathogenesis of NEC. Innate T cells are known to bridge the innate and adaptive immune system and can mediate immune tolerance (113–116). It is speculated that the function of innate T cells may be altered with reduced immune tolerance due to microbial dysbiosis and immaturity in preterm infants. Another possible factor is IL-17 production that plays a critical role in pathogenesis of NEC (117). Innate T cells are known to produce IL-17 (118–120). Innate T cells from preterm infants may produce more IL-17 due to immaturity and microbial dysbiosis, contributing to NEC pathogenesis.
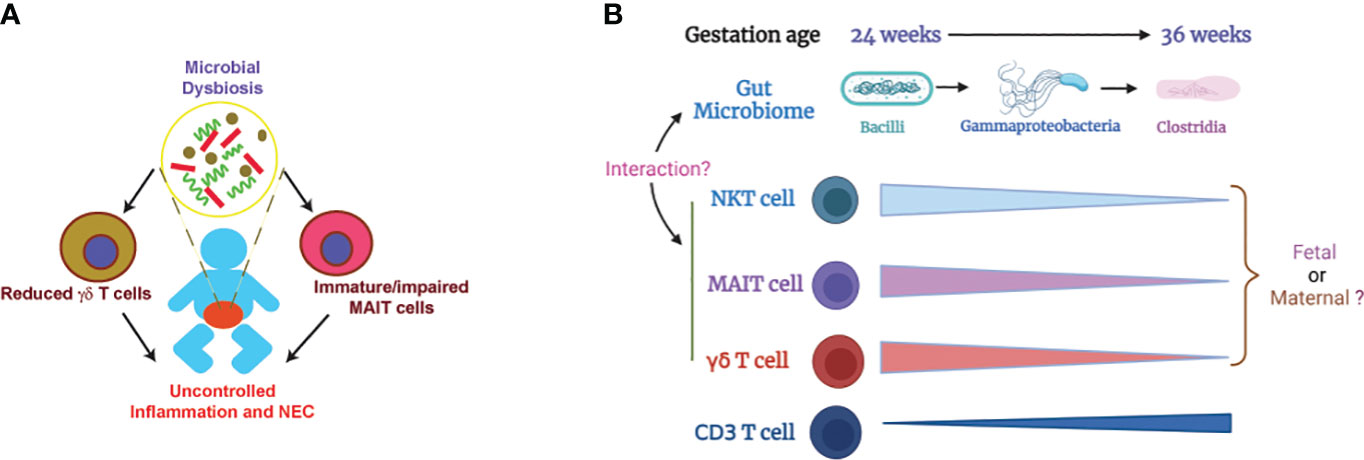
Figure 1 Illustration of how microbial dysbiosis and immaturity of innate T cells may affect NEC pathogenesis. (A) Immature/impaired MAIT cells and/or reduced γδ T cells, possibly caused by microbial dysbiosis, contribute to NEC pathogenesis (39, 48, 49, 112). (B) Change of gut microbiome and innate T cells in preterm infants based on gestation age. The proportions of innate T cells negatively correlate with gestational age. Little is known about whether the innate T cells in preterm infants are of fetal or maternal origin. It is also unclear how the transition of gut microbiome affects the development and function of innate T cells (36, 52, 101, 102). .
Conclusions and future direction
The current standard treatment regimen of NEC includes cessation of enteral feeding, institution of parenteral nutrition, initiating broad-spectrum antibiotics, respiratory support, and surgical intervention as needed (1). Human breastmilk has long been utilized as a way to reduce NEC (1). The expansion of Bifidobacteriaceae in gut microbiome after birth also decreases the risk of NEC (3, 100, 121). Human milk oligosaccharide (HMO) is important to bifidobacterial colonization, consistent with the observation that breast-feeding lowers the incidence of NEC (100). Prebiotics (e.g. human milk oligosaccharide) and probiotics (e.g. Bifidobacteria) are being investigated as potential preventative and therapeutics approaches for NEC (3, 7, 121, 122). A few acting mechanisms of probiotics in preventing NEC have been proposed (123), but little is known about how prebiotics and probiotics therapies may change innate T cells in NEC.
Because of their limited TCR diversity and monomorphism, innate T cells would be an off-the-shelf cell-based therapy with minimal graft-versus-host disease (124). Innate T cells can quickly respond to antigens and produce large amounts of pro- and anti-inflammatory cytokines to bridge the innate and adaptive immune systems (23, 51, 125–127). Innate T cells can also acquire effector T cell characteristics and accumulate in mucosal tissues early in life (42–44). IL-22 has been shown to alleviate NEC (128) and innate T cells are capable of producing IL-22 (43, 118). Genetic engineering technologies, such as CRISPR/Cre and CAR-T cells (76, 129–131), may facilitate the production of IL-22-producing γδ T and/or MAIT cells, even iCD8α IELs, for cell-based immunotherapy for NEC.
Contrarily, the accumulation of immature innate T cells in preterm infants may lead to the development of NEC. Blocking the activation of immature innate T cells may reduce the incidence of NEC. Antibodies for CD1d and MR1, the antigen presenting molecules for NKT and MAIT cells respectively, are effective in suppressing NKT and MAIT cell activation (132–134). These MR1 and CD1d specific antibodies may also be a potential avenue for further investigations in NEC immunotherapy.
Preterm infants are not developmentally primed to be colonized by microorganisms and the normal neonatal microbial adaptation may be hazardous in preterm infants (135). It is well-established that the development and maturation of innate T cells are influenced by the microbiome. The levels of innate T cells in preterm infants negatively correlates with gestational age (Figure 1B, Table 1) but the mechanism is unknown. Because the mother and offspring form a microchimer, innate T cells in fetus and preterm infants can be from fetal or maternal origin. The gut microbiome in preterm infants is transitional and different from term infants, but it is unclear exactly how the innate T cells in preterm infants are influenced by the altered gut microbiome. Future work should focus on identifying the origin of fetal and neonatal innate T cells and how their development and function are impacted by beneficial or pathogenic microbiome. The knowledge gained from this work will help facilitate the development of a novel innate T cell-based therapy for NEC.
Author contributions
JL: Writing – original draft, Writing – review & editing. KM: Writing – review & editing. SJ: Writing – review & editing. JL: Writing – review & editing. JB: Writing – review & editing. CS: Writing – review & editing. TM: Writing – review & editing.
Funding
The author(s) declare that no financial support was received for the research, authorship, and/or publication of this article.
Conflict of interest
The authors declare that the research was conducted in the absence of any commercial or financial relationships that could be construed as a potential conflict of interest.
Publisher’s note
All claims expressed in this article are solely those of the authors and do not necessarily represent those of their affiliated organizations, or those of the publisher, the editors and the reviewers. Any product that may be evaluated in this article, or claim that may be made by its manufacturer, is not guaranteed or endorsed by the publisher.
References
1. Rich BS, Dolgin SE. Necrotizing enterocolitis. Pediatr Rev (2017) 38(12):552–9. doi: 10.1542/pir.2017-0002
2. Markel TA, Engelstad H, Poindexter BB. Predicting disease severity of necrotizing enterocolitis: how to identify infants for future novel therapies. J Clin Neonatol. (2014) 3(1):1–9. doi: 10.4103/2249-4847.128717
3. Kim CS, Claud EC. Necrotizing enterocolitis pathophysiology: how microbiome data alter our understanding. Clin Perinatol. (2019) 46(1):29–38. doi: 10.1016/j.clp.2018.10.003
4. Fundora JB, Guha P, Shores DR, Pammi M, Maheshwari A. Intestinal dysbiosis and necrotizing enterocolitis: assessment for causality using Bradford Hill criteria. Pediatr Res (2020) 87(2):235–48. doi: 10.1038/s41390-019-0482-9
5. Neu J, Walker WA. Necrotizing enterocolitis. N Engl J Med (2011) 364(3):255–64. doi: 10.1056/NEJMra1005408
6. Alganabi M, Lee C, Bindi E, Li B, Pierro A. Recent advances in understanding necrotizing enterocolitis. F1000Res. (2019) 8:107. doi: 10.12688/f1000research.17228.1
7. Campos-Martinez AM, Exposito-Herrera J, Gonzalez-Bolivar M, Fernandez-Marin E, Uberos J. Evaluation of risk and preventive factors for necrotizing enterocolitis in premature newborns. A Systematic Rev Literature. Front Pediatr (2022) 10:874976. doi: 10.3389/fped.2022.874976
8. Drucker NA, McCulloh CJ, Li B, Pierro A, Besner GE, Markel TA. Stem cell therapy in necrotizing enterocolitis: Current state and future directions. Semin Pediatr Surg (2018) 27(1):57–64. doi: 10.1053/j.sempedsurg.2017.11.011
9. Kinder JM, Stelzer IA, Arck PC, Way SS. Immunological implications of pregnancy-induced microchimerism. Nat Rev Immunol (2017) 17(8):483–94. doi: 10.1038/nri.2017.38
10. Adams KM, Nelson JL. Microchimerism: an investigative frontier in autoimmunity and transplantation. Jama. (2004) 291(9):1127–31. doi: 10.1001/jama.291.9.1127
11. Levy O. Innate immunity of the newborn: basic mechanisms and clinical correlates. Nat Rev Immunol (2007) 7(5):379–90. doi: 10.1038/nri2075
12. Basha S, Surendran N, Pichichero M. Immune responses in neonates. Expert Rev Clin Immunol (2014) 10(9):1171–84. doi: 10.1586/1744666X.2014.942288
13. Adkins B, Leclerc C, Marshall-Clarke S. Neonatal adaptive immunity comes of age. Nat Rev Immunol (2004) 4(7):553–64. doi: 10.1038/nri1394
14. Touzot F, Dal-Cortivo L, Verkarre V, Lim A, Crucis-Armengaud A, Moshous D, et al. Massive expansion of maternal T cells in response to EBV infection in a patient with SCID-Xl. Blood. (2012) 120(9):1957–9. doi: 10.1182/blood-2012-04-426833
15. Maloney S, Smith A, Furst DE, Myerson D, Rupert K, Evans PC, et al. Microchimerism of maternal origin persists into adult life. J Clin Invest. (1999) 104(1):41–7. doi: 10.1172/JCI6611
16. Sanidad KZ, Amir M, Ananthanarayanan A, Singaraju A, Shiland NB, Hong HS, et al. Maternal gut microbiome-induced IgG regulates neonatal gut microbiome and immunity. Sci Immunol (2022) 7(72):eabh3816. doi: 10.1126/sciimmunol.abh3816
17. Torow N, Hand TW, Hornef MW. Programmed and environmental determinants driving neonatal mucosal immune development. Immunity. (2023) 56(3):485–99. doi: 10.1016/j.immuni.2023.02.013
18. Rudd BD. Neonatal T cells: A reinterpretation. Annu Rev Immunol (2020) 38:229–47. doi: 10.1146/annurev-immunol-091319-083608
19. Rackaityte E, Halkias J. Mechanisms of fetal T cell tolerance and immune regulation. Front Immunol (2020) 11:588. doi: 10.3389/fimmu.2020.00588
20. Kumar SK, Bhat BV. Distinct mechanisms of the newborn innate immunity. Immunol Lett (2016) 173:42–54. doi: 10.1016/j.imlet.2016.03.009
21. Yu JC, Khodadadi H, Malik A, Davidson B, Salles É DSL, Bhatia J, et al. Innate immunity of neonates and infants. Front Immunol (2018) 9:1759. doi: 10.3389/fimmu.2018.01759
22. Stelzer IA, Urbschat C, Schepanski S, Thiele K, Triviai I, Wieczorek A, et al. Vertically transferred maternal immune cells promote neonatal immunity against early life infections. Nat Commun (2021) 12(1):4706. doi: 10.1038/s41467-021-24719-z
23. Godfrey DI, Uldrich AP, McCluskey J, Rossjohn J, Moody DB. The burgeoning family of unconventional T cells. Nat Immunol (2015) 16(11):1114–23. doi: 10.1038/ni.3298
24. Vermijlen D, Prinz I. Ontogeny of innate T lymphocytes - some innate lymphocytes are more innate than others. Front Immunol (2014) 5:486. doi: 10.3389/fimmu.2014.00486
25. Wu D, Xing GW, Poles MA, Horowitz A, Kinjo Y, Sullivan B, et al. Bacterial glycolipids and analogs as antigens for CD1d-restricted NKT cells. Proc Natl Acad Sci U S A. (2005) 102(5):1351–6. doi: 10.1073/pnas.0408696102
26. Kinjo Y, Wu D, Kim G, Xing GW, Poles MA, Ho DD, et al. Recognition of bacterial glycosphingolipids by natural killer T cells. Nature. (2005) 434(7032):520–5. doi: 10.1038/nature03407
27. Mattner J, Debord KL, Ismail N, Goff RD, Cantu C 3rd, Zhou D, et al. Exogenous and endogenous glycolipid antigens activate NKT cells during microbial infections. Nature. (2005) 434(7032):525–9. doi: 10.1038/nature03408
28. Sriram V, Du W, Gervay-Hague J, Brutkiewicz RR. Cell wall glycosphingolipids of Sphingomonas paucimobilis are CD1d-specific ligands for NKT cells. Eur J Immunol (2005) 35(6):1692–701. doi: 10.1002/eji.200526157
29. Altincicek B, Moll J, Campos N, Foerster G, Beck E, Hoeffler JF, et al. Cutting edge: human gamma delta T cells are activated by intermediates of the 2-C-methyl-D-erythritol 4-phosphate pathway of isoprenoid biosynthesis. J Immunol (2001) 166(6):3655–8. doi: 10.4049/jimmunol.166.6.3655
30. Le Bourhis L, Martin E, Peguillet I, Guihot A, Froux N, Core M, et al. Antimicrobial activity of mucosal-associated invariant T cells. Nat Immunol (2010) 11(8):701–8. doi: 10.1038/ni.1890
31. Kjer-Nielsen L, Patel O, Corbett AJ, Le Nours J, Meehan B, Liu L, et al. MR1 presents microbial vitamin B metabolites to MAIT cells. Nature. (2012) 491(7426):717–23. doi: 10.1038/nature11605
32. Papotto PH, Yilmaz B, Silva-Santos B. Crosstalk between gammadelta T cells and the microbiota. Nat Microbiol (2021) 6(9):1110–7. doi: 10.1038/s41564-021-00948-2
33. Su X, Gao Y, Yang R. Gut microbiota derived bile acid metabolites maintain the homeostasis of gut and systemic immunity. Front Immunol (2023) 14:1127743. doi: 10.3389/fimmu.2023.1127743
34. Jabeen MF, Hinks TSC. MAIT cells and the microbiome. Front Immunol (2023) 14:1127588. doi: 10.3389/fimmu.2023.1127588
35. Pellicci DG, Koay HF, Berzins SP. Thymic development of unconventional T cells: how NKT cells, MAIT cells and gammadelta T cells emerge. Nat Rev Immunol (2020) 20(12):756–70. doi: 10.1038/s41577-020-0345-y
36. Ben Youssef G, Tourret M, Salou M, Ghazarian L, Houdouin V, Mondot S, et al. Ontogeny of human mucosal-associated invariant T cells and related T cell subsets. J Exp Med (2018) 215(2):459–79. doi: 10.1084/jem.20171739
37. van der Heiden M, Bjorkander S, Rahman Qazi K, Bittmann J, Hell L, Jenmalm MC, et al. Characterization of the gammadelta T-cell compartment during infancy reveals clear differences between the early neonatal period and 2 years of age. Immunol Cell Biol (2020) 98(1):79–87. doi: 10.1111/imcb.12303
38. Cui Y, Franciszkiewicz K, Mburu YK, Mondot S, Le Bourhis L, Premel V, et al. Mucosal-associated invariant T cell-rich congenic mouse strain allows functional evaluation. J Clin Invest. (2015) 125(11):4171–85. doi: 10.1172/JCI82424
39. Weitkamp JH, Rosen MJ, Zhao Z, Koyama T, Geem D, Denning TL, et al. Small intestinal intraepithelial TCRgammadelta+ T lymphocytes are present in the premature intestine but selectively reduced in surgical necrotizing enterocolitis. PloS One (2014) 9(6):e99042. doi: 10.1371/journal.pone.0099042
40. Brutkiewicz RR. CD1d ligands: the good, the bad, and the ugly. J Immunol (2006) 177(2):769–75. doi: 10.4049/jimmunol.177.2.769
41. Corbett AJ, Awad W, Wang H, Chen Z. Antigen recognition by MR1-reactive T cells; MAIT cells, metabolites, and remaining mysteries. Front Immunol (2020) 11:1961. doi: 10.3389/fimmu.2020.01961
42. Gibbons DL, Haque SF, Silberzahn T, Hamilton K, Langford C, Ellis P, et al. Neonates harbour highly active gammadelta T cells with selective impairments in preterm infants. Eur J Immunol (2009) 39(7):1794–806. doi: 10.1002/eji.200939222
43. Leeansyah E, Loh L, Nixon DF, Sandberg JK. Acquisition of innate-like microbial reactivity in mucosal tissues during human fetal MAIT-cell development. Nat Commun (2014) 5:3143. doi: 10.1038/ncomms4143
44. Loh L, Ivarsson MA, Michaëlsson J, Sandberg JK, Nixon DF. Invariant natural killer T cells developing in the human fetus accumulate and mature in the small intestine. Mucosal Immunol (2014) 7(5):1233–43. doi: 10.1038/mi.2014.13
45. Swieboda D, Rice TF, Guo Y, Nadel S, Thwaites RS, Openshaw PJM, et al. Natural killer cells and innate lymphoid cells but not NKT cells are mature in their cytokine production at birth. Clin Exp Immunol ((2024)) 215(1):1–14. doi: 10.1093/cei/uxad094
46. Harner S, Noessner E, Nadas K, Leumann-Runge A, Schiemann M, Faber FL, et al. Cord blood Valpha24-Vbeta11 natural killer T cells display a Th2-chemokine receptor profile and cytokine responses. PloS One (2011) 6(1):e15714. doi: 10.1371/journal.pone.0015714
47. Kadowaki N, Antonenko S, Ho S, Rissoan MC, Soumelis V, Porcelli SA, et al. Distinct cytokine profiles of neonatal natural killer T cells after expansion with subsets of dendritic cells. J Exp Med (2001) 193(10):1221–6. doi: 10.1084/jem.193.10.1221
48. Weitkamp JH, Koyama T, Rock MT, Correa H, Goettel JA, Matta P, et al. Necrotising enterocolitis is characterised by disrupted immune regulation and diminished mucosal regulatory (FOXP3)/effector (CD4, CD8) T cell ratios. Gut. (2013) 62(1):73–82. doi: 10.1136/gutjnl-2011-301551
49. Tian J, Yan C, Jiang Y, Zhou H, Li L, Shen J, et al. Peripheral and intestinal mucosal-associated invariant T cells in premature infants with necrotizing enterocolitis. Front Pharmacol (2022) 13:1008080. doi: 10.3389/fphar.2022.1008080
50. Sproat T, Payne RP, Embleton ND, Berrington J, Hambleton S. T cells in preterm infants and the influence of milk diet. Front Immunol (2020) 11:1035. doi: 10.3389/fimmu.2020.01035
51. Treiner E, Lantz O. CD1d- and MR1-restricted invariant T cells: of mice and men. Curr Opin Immunol (2006) 18(5):519–26. doi: 10.1016/j.coi.2006.07.001
52. Rahman Qazi K, Jensen GB, van der Heiden M, Bjorkander S, Marchini G, Jenmalm MC, et al. Extreme prematurity and sepsis strongly influence frequencies and functional characteristics of circulating gammadelta T and natural killer cells. Clin Transl Immunol (2021) 10(6):e1294. doi: 10.1002/cti2.1294
53. Koay HF, Gherardin NA, Enders A, Loh L, Mackay LK, Almeida CF, et al. A three-stage intrathymic development pathway for the mucosal-associated invariant T cell lineage. Nat Immunol (2016) 17(11):1300–11. doi: 10.1038/ni.3565
54. Kovalovsky D, Uche OU, Eladad S, Hobbs RM, Yi W, Alonzo E, et al. The BTB-zinc finger transcriptional regulator PLZF controls the development of invariant natural killer T cell effector functions. Nat Immunol (2008) 9(9):1055–64. doi: 10.1038/ni.1641
55. Lu Y, Cao X, Zhang X, Kovalovsky D. PLZF controls the development of fetal-derived IL-17+Vgamma6+ gammadelta T cells. J Immunol (2015) 195(9):4273–81. doi: 10.4049/jimmunol.1500939
56. Salou M, Legoux F, Gilet J, Darbois A, du Halgouet A, Alonso R, et al. A common transcriptomic program acquired in the thymus defines tissue residency of MAIT and NKT subsets. J Exp Med (2019) 216(1):133–51. doi: 10.1084/jem.20181483
57. Gutierrez-Arcelus M, Teslovich N, Mola AR, Polidoro RB, Nathan A, Kim H, et al. Lymphocyte innateness defined by transcriptional states reflects a balance between proliferation and effector functions. Nat Commun (2019) 10(1):687. doi: 10.1038/s41467-019-08604-4
58. Constantinides MG, Belkaid Y. Early-life imprinting of unconventional T cells and tissue homeostasis. Science (2021) 374(6573):eabf0095. doi: 10.1126/science.abf0095
59. Cardell S, Tangri S, Chan S, Kronenberg M, Benoist C, Mathis D. CD1-restricted CD4+ T cells in major histocompatibility complex class II-deficient mice. J Exp Med (1995) 182(4):993–1004. doi: 10.1084/jem.182.4.993
60. Chen YH, Chiu NM, Mandal M, Wang N, Wang CR. Impaired NK1+ T cell development and early IL-4 production in CD1-deficient mice. Immunity. (1997) 6(4):459–67. doi: 10.1016/S1074-7613(00)80289-7
61. Bendelac A, Lantz O, Quimby ME, Yewdell JW, Bennink JR, Brutkiewicz RR. CD1 recognition by mouse NK1+ T lymphocytes. Science. (1995) 268:863–5. doi: 10.1126/science.7538697
62. Mendiratta SK, Martin WD, Hong S, Boesteanu A, Joyce S, Van Kaer L. CD1d1 mutant mice are deficient in natural T cells that promptly produce IL-4. Immunity. (1997) 6(4):469–77. doi: 10.1016/S1074-7613(00)80290-3
63. Morita M, Motoki K, Akimoto K, Natori T, Sakai T, Sawa E, et al. Structure-activity relationship of alpha-galactosylceramides against B16-bearing mice. J Med Chem (1995) 38(12):2176–87. doi: 10.1021/jm00012a018
64. Kawano T, Cui J, Koezuka Y, Toura I, Kaneko Y, Motoki K, et al. CD1d-restricted and TCR-mediated activation of Va14 NKT cells by glycosylceramides. Science. (1997) 278(5343):1626–9. doi: 10.1126/science.278.5343.1626
65. Zhou D, Mattner J, Cantu C 3rd, Schrantz N, Yin N, Gao Y, et al. Lysosomal glycosphingolipid recognition by NKT cells. Science. (2004) 306(5702):1786–9. doi: 10.1126/science.1103440
66. Blomqvist M, Rhost S, Teneberg S, Lofbom L, Osterbye T, Brigl M, et al. Multiple tissue-specific isoforms of sulfatide activate CD1d-restricted type II NKT cells. Eur J Immunol (2009) 39(7):1726–35. doi: 10.1002/eji.200839001
67. Wu L, Van Kaer L. Natural killer T cells in health and disease. Front Biosci (Schol Ed). (2011) 3(1):236–51. doi: 10.2741/s148
68. Wu X, Liu J, Li W, Khan MF, Dai H, Tian J, et al. CD1d-dependent neuroinflammation impairs tissue repair and functional recovery following a spinal cord injury. bioRxiv (2023). doi: 10.1101/2023.10.13.562047
69. Liu J, Renukaradhya GJ, Brutkiewicz RR. The Regulation of CD1d+ and CD1d- Tumors by NKT Cells: The roles of NKT cells in regulating CD1d+ and CD1d- tumor immunity. In: Terabe M, Berzofsky JA, editors. Natural killer T cells: balancing the regulation of tumor immunity. New York: Springer Science+Business Media, LLC (2011). p. 71–94.
70. Liu J, Gallo RM, Khan MA, Renukaradhya GJ, Brutkiewicz RR. Neurofibromin 1 impairs natural killer T-cell-dependent antitumor immunity against a T-cell lymphoma. Front Immunol (1901) 2018:8. doi: 10.3389/fimmu.2017.01901
71. Cui Y, Wan Q. NKT cells in neurological diseases. Front Cell Neurosci (2019) 13:245. doi: 10.3389/fncel.2019.00245
72. Jeong D, Woo YD, Chung DH. Invariant natural killer T cells in lung diseases. Exp Mol Med (2023) 55(9):1885–94. doi: 10.1038/s12276-023-01024-x
73. Miyake S, Yamamura T. NKT cells and autoimmune diseases: unraveling the complexity. In: Moody DB, editor. T cell activation by CD1 and lipid antigens, current topics in microbiology and immunology. Berlin, Heidelberg: Springer (2007).
74. Hadiloo K, Tahmasebi S, Esmaeilzadeh A. CAR-NKT cell therapy: a new promising paradigm of cancer immunotherapy. Cancer Cell Int (2023) 23(1):86. doi: 10.1186/s12935-023-02923-9
75. Ahmadi A, Fallah Vastani Z, Abounoori M, Azizi M, Labani-Motlagh A, Mami S, et al. The role of NK and NKT cells in the pathogenesis and improvement of multiple sclerosis following disease-modifying therapies. Health Sci Rep (2022) 5(1):e489. doi: 10.1002/hsr2.489
76. Heczey A, Courtney AN, Montalbano A, Robinson S, Liu K, Li M, et al. Anti-GD2 CAR-NKT cells in patients with relapsed or refractory neuroblastoma: an interim analysis. Nat Med (2020) 26(11):1686–90. doi: 10.1038/s41591-020-1074-2
77. Coman T, Rossignol J, D'Aveni M, Fabiani B, Dussiot M, Rignault R, et al. Human CD4- invariant NKT lymphocytes regulate graft versus host disease. Oncoimmunology. (2018) 7(11):e1470735. doi: 10.1080/2162402X.2018.1470735
78. Prabhu SB, Rathore DK, Nair D, Chaudhary A, Raza S, Kanodia P, et al. Comparison of human neonatal and adult blood leukocyte subset composition phenotypes. PloS One (2016) 11(9):e0162242. doi: 10.1371/journal.pone.0162242
79. Huang S, Martin E, Kim S, Yu L, Soudais C, Fremont DH, et al. MR1 antigen presentation to mucosal-associated invariant T cells was highly conserved in evolution. Proc Natl Acad Sci U S A. (2009) 106(20):8290–5. doi: 10.1073/pnas.0903196106
80. Corbett AJ, Eckle SB, Birkinshaw RW, Liu L, Patel O, Mahony J, et al. T-cell activation by transitory neo-antigens derived from distinct microbial pathways. Nature. (2014) 509(7500):361–5. doi: 10.1038/nature13160
81. Godfrey DI, Koay HF, McCluskey J, Gherardin NA. The biology and functional importance of MAIT cells. Nat Immunol (2019) 20(9):1110–28. doi: 10.1038/s41590-019-0444-8
82. Nel I, Bertrand L, Toubal A, Lehuen A. MAIT cells, guardians of skin and mucosa? Mucosal Immunol (2021) 14(4):803–14. doi: 10.1038/s41385-021-00391-w
83. Toubal A, Lehuen A. Role of MAIT cells in metabolic diseases. Mol Immunol (2021) 130:142–7. doi: 10.1016/j.molimm.2020.12.014
84. Kubica P, Lara-Velazquez M, Bam M, Siraj S, Ong I, Liu P, et al. MR1 overexpression correlates with poor clinical prognosis in glioma patients. Neurooncol Adv (2021) 3(1):vdab034. doi: 10.1093/noajnl/vdab034
85. Serriari NE, Eoche M, Lamotte L, Lion J, Fumery M, Marcelo P, et al. Innate mucosal-associated invariant T (MAIT) cells are activated in inflammatory bowel diseases. Clin Exp Immunol (2014) 176(2):266–74. doi: 10.1111/cei.12277
86. Constantinides MG, Link VM, Tamoutounour S, Wong AC, Perez-Chaparro PJ, Han SJ, et al. MAIT cells are imprinted by the microbiota in early life and promote tissue repair. Science (2019) 366(6464):eaax6624. doi: 10.1126/science.aax6624
87. Magalhaes I, Pingris K, Poitou C, Bessoles S, Venteclef N, Kiaf B, et al. Mucosal-associated invariant T cell alterations in obese and type 2 diabetic patients. J Clin Invest. (2015) 125(4):1752–62. doi: 10.1172/JCI78941
88. Liu J, Nan H, Brutkiewicz RR, Casasnovas J, Kua KL. Sex discrepancy in the reduction of mucosal-associated invariant T cells caused by obesity. Immun Inflammation Dis (2021) 9(1):299–309. doi: 10.1002/iid3.393
89. Eberhard JM, Hartjen P, Kummer S, Schmidt RE, Bockhorn M, Lehmann C, et al. CD161+ MAIT cells are severely reduced in peripheral blood and lymph nodes of HIV-infected individuals independently of disease progression. PloS One (2014) 9(11):e111323. doi: 10.1371/journal.pone.0111323
90. Konuma T, Kohara C, Watanabe E, Takahashi S, Ozawa G, Suzuki K, et al. Reconstitution of circulating mucosal-associated invariant T cells after allogeneic hematopoietic cell transplantation: its association with the riboflavin synthetic pathway of gut microbiota in cord blood transplant recipients. J Immunol (2020) 204(6):1462–73. doi: 10.4049/jimmunol.1900681
91. Reantragoon R, Corbett AJ, Sakala IG, Gherardin NA, Furness JB, Chen Z, et al. Antigen-loaded MR1 tetramers define T cell receptor heterogeneity in mucosal-associated invariant T cells. J Exp Med (2013) 210(11):2305–20. doi: 10.1084/jem.20130958
92. Rahimpour A, Koay HF, Enders A, Clanchy R, Eckle SB, Meehan B, et al. Identification of phenotypically and functionally heterogeneous mouse mucosal-associated invariant T cells using MR1 tetramers. J Exp Med (2015) 212(7):1095–108. doi: 10.1084/jem.20142110
93. Sakala IG, Kjer-Nielsen L, Eickhoff CS, Wang X, Blazevic A, Liu L, et al. Functional heterogeneity and antimycobacterial effects of mouse mucosal-associated invariant T cells specific for riboflavin metabolites. J Immunol (2015) 195(2):587–601. doi: 10.4049/jimmunol.1402545
94. Willcox CR, Pitard V, Netzer S, Couzi L, Salim M, Silberzahn T, et al. Cytomegalovirus and tumor stress surveillance by binding of a human gammadelta T cell antigen receptor to endothelial protein C receptor. Nat Immunol (2012) 13(9):872–9. doi: 10.1038/ni.2394
95. Zeng X, Wei YL, Huang J, Newell EW, Yu H, Kidd BA, et al. gammadelta T cells recognize a microbial encoded B cell antigen to initiate a rapid antigen-specific interleukin-17 response. Immunity. (2012) 37(3):524–34. doi: 10.1016/j.immuni.2012.06.011
96. Ribot JC, Lopes N, Silva-Santos B. gammadelta T cells in tissue physiology and surveillance. Nat Rev Immunol (2021) 21(4):221–32. doi: 10.1038/s41577-020-00452-4
97. Chen Y, Chou K, Fuchs E, Havran WL, Boismenu R. Protection of the intestinal mucosa by intraepithelial gamma delta T cells. Proc Natl Acad Sci U S A. (2002) 99(22):14338–43. doi: 10.1073/pnas.212290499
98. Rezende RM, Cox LM, Moreira TG, Liu S, Boulenouar S, Dhang F, et al. Gamma-delta T cells modulate the microbiota and fecal micro-RNAs to maintain mucosal tolerance. Microbiome. (2023) 11(1):32. doi: 10.1186/s40168-023-01478-1
99. Bandeira A, Mota-Santos T, Itohara S, Degermann S, Heusser C, Tonegawa S, et al. Localization of gamma/delta T cells to the intestinal epithelium is independent of normal microbial colonization. J Exp Med (1990) 172(1):239–44. doi: 10.1084/jem.172.1.239
100. Henrick BM, Rodriguez L, Lakshmikanth T, Pou C, Henckel E, Arzoomand A, et al. Bifidobacteria-mediated immune system imprinting early in life. Cell. (2021) 184(15):3884–98e11. doi: 10.1016/j.cell.2021.05.030
101. La Rosa PS, Warner BB, Zhou Y, Weinstock GM, Sodergren E, Hall-Moore CM, et al. Patterned progression of bacterial populations in the premature infant gut. Proc Natl Acad Sci U S A. (2014) 111(34):12522–7. doi: 10.1073/pnas.1409497111
102. Thanert R, Keen EC, Dantas G, Warner BB, Tarr PI. Necrotizing enterocolitis and the microbiome: current status and future directions. J Infect Dis (2021) 223(12 Suppl 2):S257–S63. doi: 10.1093/infdis/jiaa604
103. Tremblay É, Thibault MP, Ferretti E, Babakissa C, Bertelle V, Bettolli M, et al. Gene expression profiling in necrotizing enterocolitis reveals pathways common to those reported in Crohn's disease. BMC Med Genomics (2016) 9:6. doi: 10.1186/s12920-016-0166-9
104. Egozi A, Olaloye O, Werner L, Silva T, McCourt B, Pierce RW, et al. Single cell atlas of the neonatal small intestine with necrotizing enterocolitis. bioRxiv. (2022) 2022:03.01.482508. doi: 10.2139/ssrn.4032068
105. Olaloye OO, Liu P, Toothaker JM, McCourt BT, McCourt CC, Xiao J, et al. CD16+CD163+ monocytes traffic to sites of inflammation during necrotizing enterocolitis in premature infants. J Exp Med (2021) 218(9):e20200344. doi: 10.1084/jem.20200344
106. Egozi A, Olaloye O, Werner L, Silva T, McCourt B, Pierce RW, et al. Single-cell atlas of the human neonatal small intestine affected by necrotizing enterocolitis. PloS Biol (2023) 21(5):e3002124. doi: 10.1371/journal.pbio.3002124
107. Wingender G, Stepniak D, Krebs P, Lin L, McBride S, Wei B, et al. Intestinal microbes affect phenotypes and functions of invariant natural killer T cells in mice. Gastroenterology. (2012) 143(2):418–28. doi: 10.1053/j.gastro.2012.04.017
108. Olszak T, An D, Zeissig S, Vera MP, Richter J, Franke A, et al. Microbial exposure during early life has persistent effects on natural killer T cell function. Science. (2012) 336(6080):489–93. doi: 10.1126/science.1219328
109. Tabilas C, Iu DS, Daly CWP, Yee Mon KJ, Reynaldi A, Wesnak SP, et al. Early microbial exposure shapes adult immunity by altering CD8+ T cell development. Proc Natl Acad Sci U S A. (2022) 119(49):e2212548119. doi: 10.1073/pnas.2212548119
110. Dias J, Boulouis C, Gorin JB, van den Biggelaar R, Lal KG, Gibbs A, et al. The CD4(-)CD8(-) MAIT cell subpopulation is a functionally distinct subset developmentally related to the main CD8(+) MAIT cell pool. Proc Natl Acad Sci U S A. (2018) 115(49):E11513–E22. doi: 10.1073/pnas.1812273115
111. Van Kaer L, Algood HMS, Singh K, Parekh VV, Greer MJ, Piazuelo MB, et al. CD8alphaalpha(+) innate-type lymphocytes in the intestinal epithelium mediate mucosal immunity. Immunity. (2014) 41(3):451–64. doi: 10.1016/j.immuni.2014.08.010
112. Liu J, Hunter C, Manohar K, Mesfin F, Shelley WC, Brokaw J, et al. MR1-deficiency in neonatal mice renders protection from necrotizing enterocolitis. J Immunol (2023) 210(1_Supplement):66.16–6. doi: 10.4049/jimmunol.210.Supp.66.16
113. Bharadwaj NS, Gumperz JE. Harnessing invariant natural killer T cells to control pathological inflammation. Front Immunol (2022) 13:998378. doi: 10.3389/fimmu.2022.998378
114. Kapp JA, Kapp LM, McKenna KC. Gammadelta T cells play an essential role in several forms of tolerance. Immunol Res (2004) 29(1-3):93–102. doi: 10.1385/IR:29:1-3:093
115. Giri S, Lal G. Differentiation and functional plasticity of gamma-delta (γδ) T cells under homeostatic and disease conditions. Mol Immunol (2021) 136:138–49. doi: 10.1016/j.molimm.2021.06.006
116. Giri S, Meitei HT, Mishra A, Lal G. Vγ2(+) γδ T cells in the presence of anti-CD40L control surgical inflammation and promote skin allograft survival. J Invest Dermatol (2022) 142(10):2706–14.e3. doi: 10.1016/j.jid.2022.03.016
117. Egan CE, Sodhi CP, Good M, Lin J, Jia H, Yamaguchi Y, et al. Toll-like receptor 4-mediated lymphocyte influx induces neonatal necrotizing enterocolitis. J Clin Invest. (2016) 126(2):495–508. doi: 10.1172/JCI83356
118. Ness-Schwickerath KJ, Morita CT. Regulation and function of IL-17A- and IL-22-producing gammadelta T cells. Cell Mol Life Sci (2011) 68(14):2371–90. doi: 10.1007/s00018-011-0700-z
119. Lee YJ, Holzapfel KL, Zhu J, Jameson SC, Hogquist KA. Steady-state production of IL-4 modulates immunity in mouse strains and is determined by lineage diversity of iNKT cells. Nat Immunol (2013) 14(11):1146–54. doi: 10.1038/ni.2731
120. Park KJ, Jin HM, Cho YN, Yoon JH, Kee SJ, Kim HS, et al. Altered frequency, activation, and clinical relevance of circulating innate and innate-like lymphocytes in patients with alcoholic liver cirrhosis. Immune Netw (2023) 23(3):e22. doi: 10.4110/in.2023.23.e22
121. Deshpande G, Rao S, Patole S, Bulsara M. Updated meta-analysis of probiotics for preventing necrotizing enterocolitis in preterm neonates. Pediatrics. (2010) 125(5):921–30. doi: 10.1542/peds.2009-1301
122. AlFaleh K, Anabrees J. Probiotics for prevention of necrotizing enterocolitis in preterm infants. Cochrane Database Syst Rev (2022) PUB4:CD005496. doi: 10.1002/14651858.CD005496.pub4
123. Patel RM, Underwood MA. Probiotics and necrotizing enterocolitis. Semin Pediatr Surg (2018) 27(1):39–46. doi: 10.1053/j.sempedsurg.2017.11.008
124. Godfrey DI, Le Nours J, Andrews DM, Uldrich AP, Rossjohn J. Unconventional T cell targets for cancer immunotherapy. Immunity. (2018) 48(3):453–73. doi: 10.1016/j.immuni.2018.03.009
125. Huang S. Targeting innate-like T cells in tuberculosis. Front Immunol (2016) 7:594. doi: 10.3389/fimmu.2016.00594
126. Huang W, He W, Shi X, He X, Dou L, Gao Y. The role of CD1d and MR1 restricted T cells in the liver. Front Immunol (2018) 9:2424. doi: 10.3389/fimmu.2018.02424
127. Stolk D, van der Vliet HJ, de Gruijl TD, van Kooyk Y, Exley MA. Positive & Negative roles of innate effector cells in controlling cancer progression. Front Immunol (2018) 9:1990. doi: 10.3389/fimmu.2018.01990
128. Mihi B, Gong Q, Nolan LS, Gale SE, Goree M, Hu E, et al. Interleukin-22 signaling attenuates necrotizing enterocolitis by promoting epithelial cell regeneration. Cell Rep Med (2021) 2(6):100320. doi: 10.1016/j.xcrm.2021.100320
129. Makkouk A, Yang XC, Barca T, Lucas A, Turkoz M, Wong JTS, et al. Off-the-shelf Vδ1 gamma delta T cells engineered with glypican-3 (GPC-3)-specific chimeric antigen receptor (CAR) and soluble IL-15 display robust antitumor efficacy against hepatocellular carcinoma. J Immunother Cancer (2021) 9(12):e003441. doi: 10.1136/jitc-2021-003441
130. Bohineust A, Tourret M, Derivry L, Caillat-Zucman S. Mucosal-associated invariant T (MAIT) cells, a new source of universal immune cells for chimeric antigen receptor (CAR)-cell therapy. Bull Cancer (2021) 108(10s):S92–s5. doi: 10.1016/j.bulcan.2021.07.003
131. Stadtmauer EA, Fraietta JA, Davis MM, Cohen AD, Weber KL, Lancaster E, et al. CRISPR-engineered T cells in patients with refractory cancer. Science (2020) 367(6481):eaba7365. doi: 10.1126/science.aba7365
132. Liu J, Brutkiewicz RR. The Toll-like receptor 9 signalling pathway regulates MR1-mediated bacterial antigen presentation in B cells. Immunology. (2017) 152(2):232–42. doi: 10.1111/imm.12759
133. Teng MW, Sharkey J, McLaughlin NM, Exley MA, Smyth MJ. CD1d-based combination therapy eradicates established tumors in mice. J Immunol (2009) 183(3):1911–20. doi: 10.4049/jimmunol.0900796
134. Tazawa H, Irei T, Tanaka Y, Igarashi Y, Tashiro H, Ohdan H. Blockade of invariant TCR-CD1d interaction specifically inhibits antibody production against blood group A carbohydrates. Blood. (2013) 122(15):2582–90. doi: 10.1182/blood-2012-02-407452
Keywords: immunity, innate T cells, NKT, MAIT, γδ T, neonates, preterm, necrotizing enterocolitis
Citation: Liu J, Joseph S, Manohar K, Lee J, Brokaw JP, Shelley WC and Markel TA (2024) Role of innate T cells in necrotizing enterocolitis. Front. Immunol. 15:1357483. doi: 10.3389/fimmu.2024.1357483
Received: 18 December 2023; Accepted: 16 January 2024;
Published: 08 February 2024.
Edited by:
Luc Van Kaer, Vanderbilt University Medical Center, United StatesReviewed by:
Danyvid Olivares-Villagómez, Vanderbilt University Medical Center, United StatesGuan Yang, City University of Hong Kong, Hong Kong SAR, China
Copyright © 2024 Liu, Joseph, Manohar, Lee, Brokaw, Shelley and Markel. This is an open-access article distributed under the terms of the Creative Commons Attribution License (CC BY). The use, distribution or reproduction in other forums is permitted, provided the original author(s) and the copyright owner(s) are credited and that the original publication in this journal is cited, in accordance with accepted academic practice. No use, distribution or reproduction is permitted which does not comply with these terms.
*Correspondence: Jianyun Liu, jealiu@iu.edu