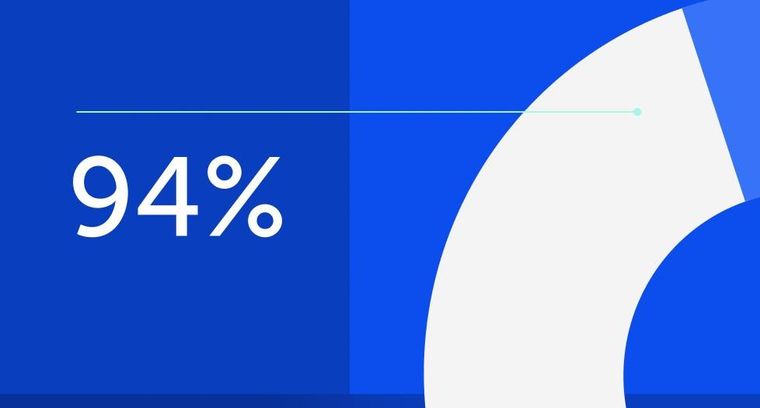
94% of researchers rate our articles as excellent or good
Learn more about the work of our research integrity team to safeguard the quality of each article we publish.
Find out more
REVIEW article
Front. Immunol., 08 February 2024
Sec. Cytokines and Soluble Mediators in Immunity
Volume 15 - 2024 | https://doi.org/10.3389/fimmu.2024.1355845
This article is part of the Research TopicThe immunological regulation of extracellular vesicles on chronic diseasesView all 12 articles
A correction has been applied to this article in:
Corrigendum: Mesenchymal stromal cell derived extracellular vesicles as a therapeutic tool: immune regulation, MSC priming, and applications to SLE
Systemic lupus erythematosus (SLE) is a chronic autoimmune disease characterized by a dysfunction of the immune system. Mesenchymal stromal cell (MSCs) derived extracellular vesicles (EVs) are nanometer-sized particles carrying a diverse range of bioactive molecules, such as proteins, miRNAs, and lipids. Despite the methodological disparities, recent works on MSC-EVs have highlighted their broad immunosuppressive effect, thus driving forwards the potential of MSC-EVs in the treatment of chronic diseases. Nonetheless, their mechanism of action is still unclear, and better understanding is needed for clinical application. Therefore, we describe in this review the diverse range of bioactive molecules mediating their immunomodulatory effect, the techniques and possibilities for enhancing their immune activity, and finally the potential application to SLE.
Systemic lupus erythematosus (SLE) is a chronic autoimmune disease, associated with multiorgan damage and variable clinical manifestations (1). SLE is a multifactorial disorder, in which the phenotype is modulated by a combination of genetic, epigenetic, environmental, hormonal and immunoregulatory factors. SLE is characterized by a dysfunction of the immune system, notably a presence of autoreactive T cells and hyperactive B cells, leading to a loss of tolerance, production of autoantibodies against self-antigens formation and deposition of immune complexes, as well as a sustained systemic inflammation. SLE is classified as interferonopathy, since type I interferons (IFN-I) play a crucial role in the development of the disease (2). The chronic production of IFN-I, especially IFN-α, is a key characteristic of SLE and contributes to the autoimmune process (3). IFN-I stimulates the activation of interferon producing cells, such as plasmacytoid dendritic cells (pDCs), which are responsible for elevated levels of IFN-α in blood plasma and organs (4). The excessive signaling of IFN-I results in the increased expression of various pro-inflammatory cytokines, chemokines, and markers of immune cell activation. This, in turn, contributes to the dysregulation of the immune system and the generation of autoantibodies. Additionally, prolonged exposure to IFN-I can boost the activation and survival of autoreactive B cells and encourage the differentiation of T cells into pro-inflammatory subsets, sustaining the autoimmune response (5). SLE manifests through periods of flares and remissions, with symptoms showing considerable variation among individuals. Treatment objectives revolve around managing symptoms, mitigating inflammation, and safeguarding against organ damage. Due to its clinical heterogeneity and complex pathogenesis, SLE remains hard to diagnose and the available treatments show limited efficiency.
Long considered as platelet debris, EVs have gained critical interest from the scientific community in the recent years (6). EVs are released by all cellular organisms and can be defined by their heterogeneity. Indeed, apart from their structural definition, a cell secreted particle enclosed by a lipidic bilayer, EVs differ from their sources, biogenesis, biophysical, biochemical characteristics and functional activity (7). A standard classification can be established based on the biogenesis of EVs: exosomes are generally small sized extracellular vesicles, from endosomal origin, released by multivesicular bodies (MVBs). Ectosomes can be defined as extracellular vesicles stemming from the plasma membrane. EV biogenesis pathways and mechanisms of interaction with target cells have been extensively reviewed (8, 9). EVs are heterogenous in size and have a diameter varying in the range of the nanometer. However, EVs are not the only nanometer-sized particles secreted by cells and there is still no specific markers allowing an efficient separation of EVs. Thus, guidelines have been set by the international community regarding ways of purifying and characterizing EVs. Transmembranal proteins such as tetraspanins, cytosolic proteins such as TSG101, and other cell-dependent proteins have been used to demonstrate the EV nature and to some extent the degree of purity of an EV preparation (10). EVs have been linked with a variety of cellular functions, and can affect other cells with their surface proteins or the cargo encapsulated by EVs. EVs carry diverse proteins, nucleic acids and lipids from their parent cell, which can in turn be delivered to the recipient cell. EVs have a fundamental role in the immune system and in immune-related diseases, highlighting their potential either as a new biomarker or a therapeutic tool (7).
In the field of mesenchymal stromal cells (MSCs), EVs have emerged as a potential avenue for cell-free therapy. MSCs have been used for their immunosuppressive capabilities, with utilization in clinical trials and availability on the market. Nonetheless, the use of MSCs can carry some drawbacks and EVs have been looked into as a cell-free alternative. More specifically, as EVs carry the same biological molecules as their parent cells, the effect of MSCs is partially mediated by them with paracrine actions. EVs have shown to have the same immunomodulatory and regenerative potential than their parent cells, and have thus become a promising alternative to MSCs themselves for therapies (11, 12). More broadly, the secretome embraces not only the previously mentioned EVs but also a variety of proteins, lipids, and nucleic acids. These components may be either inside or adsorbed to EVs, or freely present in the secretome (13). This added complexity of which fractions holds the therapeutical activity and the issues of standardization regarding means of production, concentration, and characterization in the field of EVs make any conclusion towards the effect of MSC derived EVs or MSC derived secretome a complex equation.
In order to understand which fractions hold the immunomodulatory potential, the next part focuses on having a critical look over the many parameters influencing MSC-EV immune activity. Some scientific papers use the term exosomes to describe their fractions, while they could be more accurately defined as EVs or even secretome. Various downstream processes such as size exclusion chromatography (SEC), polyethylene glycol (PEG) and ultracentrifugation broadly used by the scientific community separate selectively the content of the secretome (14), and alter the composition of the protein corona of EVs (15). The protein corona is a set of proteins and other molecules adsorbed to the EVs. These proteins can be bioactive and mediate part of the immunomodulatory potential (16, 17). All of this heterogeneity of methods brings at the same time strength to the immunomodulatory response of EVs as a global effect, but brings hardship as to determine what is driving this specific effect. A number of studies showed the difference of potency between the secretome, the EV free fraction, and the fractions containing EVs. Papait et al. showed that amniotic MSCs derived conditioned media (e.g secretome) and the EV free fraction (meaning the supernatant after ultracentrifugation) maintained their immunomodulatory potential by inhibition of T cell, promotion of Tregs, shifted monocytes towards M2 instead of M1, but also reduction of the maturation of dendritic cells. The EV fraction collected after ultracentrifugation had no effect even though these EVs were uptaken (18). Another study has found no effect of the three fractions on the inhibition of T cell proliferation (19). These differences in results might be from methodological differences, specifically in the dose parameter (e.g. protein amount in ug or particles concentration measured by Nanoparticles Tracking Analysis (NTA)), cell sources of EVs, or downstream processes. A recent study has shown that the immunomodulatory potential of MSCs is independent of EVs, which runs counter to most results in the literature using EV-enriched fractions (20). González-Cubero et al. showed that EVs and soluble fractions from conditioned media promote an in vitro anti-inflammatory modulation in intervertebral disc degeneration in a “highly synergistic way”, thus highlighting that the use of the whole secretome rather than isolated EVs might be more beneficial for therapy (21). While it is still hard to understand what exactly mediates the immunomodulatory potential, the effect might come from a synergistic combination of both the soluble factors from the secretome and the EVs.
Other factors have also been shown to have an impact on the immunomodulatory potential of EVs or secretome. Indeed, the donor of primary cells, the source of MSCs, the passage doubling number, and the production method (which will be addressed later on) can influence the secretome content and thus the potency of EVs (22–25). Immortalized MSCs might be a solution for reproducible batches of secretome/EVs for therapeutic use (26).
The characterization of the secretome of MSCs shows the presence of both pro and anti-inflammatory molecules. While the secretome of MSC-EVs has shown to have high levels of IL-6 and IL-8 (27), a recent study showed that the conditioned media derived from umbilical cord (UC)-MSC promoted anti-inflammatory macrophages polarization. This despite a mostly pro-inflammatory profile of cytokines, though the authors have only investigated surface markers of M2 macrophages (28). MSC-EVs contain nucleic acids, which may bind to TLR7 and 9 as foreign nucleic acids and trigger a pro-inflammatory cascade. However, this review hasn’t found any reports of inflammation induced by MSC-EV-associated DNA (29).
The methods to assess EV immune potency are also critical. The dose of EV is differently calculated, either with particles concentration, protein concentration, or even EVs per receptor cells, which may result in some disparities in results (30). Some studies tend to isolate a specific cell type for their in vitro potency, though immune cells do not uptake homogenously EVs. Monocytes seem to uptake the highest proportion of MSC-EVs (18, 31, 32). Nonetheless, even though EVs are not uptaken by lymphocytes, studies on MSC-EVs and on EV-free fractions added to PBMCs have shown an immunosuppressive effect on T cells, thus independent of the uptake (18, 33, 34). Others have shown that MSC-EVs failed to suppress lymphocyte proliferation (35). The immunomodulatory effect of MSCs on B cells is independent of secreted EVs (36). This reinforces that the global immunomodulatory effect might not be entirely mediated by EVs, but also through soluble factors of the secretome. The effect of EVs on T cells could also be mediated in an indirect way, through the actions on EV-uptaking immune cells (37, 38).
Regarding the immunoregulatory effect of MSC-EVs on immune cells, some reviews have already discussed it thoroughly, either as a comprehensive overview (39), or more precisely in the case of SLE (40). Thus, in the next part, this review will focus on the immunomodulatory factors in the secretome of MSCs.
The immunomodulatory potential of MSC-EVs could be driven by a broad range of bioactive molecules, including proteins, nucleic acids, and lipids. Many evidences suggest the importance of specific bioactive proteins mediating the immunomodulatory effect of MSC-EVs. This review focuses on the supposed localization of the bioactive molecules, though it is important to remember that soluble proteins secreted by MSCs are also probably carried by EVs. The lack of studies comparing EV-free conditioned media and purified EV fractions makes the localization of all bioactive molecules a hard task. (Figure 1) is a proposition of the immunomodulatory bioactive molecules of MSC-derived secretome and EVs.
The immune regulation of MSCs can be mediated by soluble proteins secreted by MSCs.
IL-10 is an anti-inflammatory cytokine secreted by immune cells such as macrophages, dendritic cells, Th and Tregs, amongst others. IL-10 induces a strong immunosuppressive response in immune cells, targeting specific genes, cytokines and chemokines production. An in-depth focus on IL-10 and its effects has already been reviewed (41). IL-10 is also secreted by MSCs and mediates part of MSC-EVs immunomodulatory activity. Jiang et al. showed cardioprotective effects of MSC-EVs using in vivo models of pigs, which were “largely blunted” after IL-10 knockdown (IL10KD). Using in vitro models, IL10KD MSC-EVs achieved less inhibition of T-cell proliferation than control MSC-EVs (42). These results are in accordance with Eirin et al. observation on their model of kidney inflammation, showing an IL-10 dependent immunomodulation of MSC-EVs (43). MSC-EVs derived from MSC overexpressing IL-10 showed higher concentrations of IL-10, enhanced the suppressive effect of these EVs on Th1 and Th17 and upregulated Tregs in vitro (44). IL-10 thus mediates the immunosuppressive effect of MSCs.
Hepatocyte growth factor (HGF) is secreted by MSCs, which can mediate immunosuppressive effects. The effect of HGF in the scope of MSCs has already been described (45, 46). Chen et al. showed that knockdown of HGF secretion by MSCs abrogate the suppression of T cell proliferation, and monocytes cultured with HGF alone or MSCs can secrete high levels of IL-10 through ERK1/2 pathway (47). In the case of MSC-EVs, treatment with MSC derived “microvesicles” reduced IL-6 production and increased IL-10 production in the conditioned media of endothelial cells, which was reverted after knockdown of HGF in MSCs. Notably, MSC-derived conditioned media had a higher regulative activity than microvesicles (48).
Tumor necrosis factor-inducible gene 6 protein (TSG-6) is a protein implicated in the immunomodulatory effects of MSCs. TSG-6 is a multifunctional protein with anti-inflammatory properties, and can interact with a broad variety of ligands such as chemokines. TSG-6 is overexpressed in a pro-inflammatory environment (49). Chaubey et al. showed the presence of TSG-6 in UC-MSC-EVs was linked to the therapeutic efficiency in their model of mouse lung disease (50). TSG-6 in canine MSC-EVs played a key role in the downregulation of pro-inflammatory cytokines, the polarization of M1 to M2 and the increase of Tregs in the colon (51). Human bone marrow(BM)-MSC derived EVs containing TSG-6 decreased pro-inflammatory cytokines in scar tissues, inhibited collagen deposition, thus suppressing scar formation. This effect was enhanced using BM-MSC modified to overexpress TSG-6, and reverted to the normal after knockdown, showing a TSG-6 dependent effect of MSC-EVs (52). Lu et et al. showed that AD-MSC derived EVs had therapeutic effects in their model of spinal cord ischemia reperfusion injury by transmitting TSG-6 (53). Other studies have shown the importance of TSG-6 in MSC conditioned media for immunomodulatory activity (54, 55).
IL-1 receptor antagonist (IL-1RA) has also been found in the secretome of MSCs. IL-1α and IL-1β, potent inflammatory cytokines can bind to IL-1R, eliciting a MyD88-dependent inflammatory cascade. On the other hand, IL-1RA can bind without triggering any downstream signaling, therefore acting as a potent antagonist of IL-1a and IL-1β and shutting down immune responses. An in-depth report of the actions of MSC-derived IL-1RA can be found (56). Kou et al. showed that IL-1RA was found in the supernatant of cultured mouse MSCs after centrifugation at 3,000 g and 20,000 g, and not in the pelleted fraction representing the bigger EVs, but was found in was in the EV pelleted fraction they call “small EVs” after ultracentrifugation at 120 000g. Using various methods of microscopy, they showed that IL-1RA was carried by EVs on their surface. They subsequently demonstrated that IL-1RA associated EV release was controlled by Fas through binding with Fap-1 and Cav-1 and upregulated when the MSCs were treated with TNF-α (57).
Transforming Growth Factor Beta (TGF-β) is a cytokine carried by MSC-EVs (58). TGF-β can bind on its receptor TGF-βRII, which triggers a downstream cascade targeting a variety of growth factors and inflammatory cytokines. TGF-β1 can be found either bounded to the plasma membrane, or in soluble form (59). TGF-β has a pleiotropic function on the regulation of immune cells. TGF-β suppresses T cells while promoting Tregs, regulates B cell activation, promotes expression of IDO in pDCs, inhibits DC function, amongst other actions (60, 61). TGF-β has been studied in MSC-EVs regarding its immunosuppressive activity. Alvarez et al. showed that TGF-β1 was primarily present in MSC-EV fraction, compared to EV free supernatant and that the immunomodulatory activity of MSC-EVs on CD4+ T cells is partially mediated by TGF-β1 (62). This same conclusion has been advanced by another group in a canine model (34). Kim et al. showed that amongst other molecules, TGF-β had a significant influence on the immunomodulatory properties of MSC-EVs in their model of cornea. Silencing of TGF-β1 resulted in the loss of MSC-EV suppressive effects, while overexpression resulted in more effective EVs in the suppression of T-cell receptor IL-2 and IFN-γ secretion in activated splenocytes (25). Song et al. used MSC-EVs produced in 3D with exogenous TGF-β3, which resulted in higher levels of TGF-β1 compared to non-treated 3D MSC-EVs, and higher immunomodulatory activity of treated EVs (63).
CD73 is one of the three conventional surface markers to identify MSCs (64). But it is also one of the enzyme of purinergic signaling, responsible for transforming Adenosine monophosphate (AMP) into Adenosine (Ado), a nucleoside known for its immunosuppressive role on T cells and Th17 cells (65). Indeed, CD73+ engineered UC-MSC derived EVs reduced concentration of ATP while increasing the levels of adenosine compared to non-engineered EVs. These engineered EVs improved the functional recovery after spinal cord injury, improving the polarization from M1 to M2 phenotype, but also downregulated more the pro-inflammatory cytokines after spinal cord injury compared to the native EVs, while it also upregulated more the anti-inflammatory cytokines such as IL-10 (66). Another study has shown that conditioning of MSCs with pro-inflammatory cytokines promoted the expression of CD73 in EVs, and that these EVs reprogram macrophages from M1 to M2 phenotype (67). Although MSCs and hence their EVs express CD39 (at a low level) and CD73, a study has shown that efficient adenosine production from ATP requires cooperation with activated T-cells expressing CD39 (68). The co-culture in the previous study significantly increased the expression of CD39 in MSCs and of CD73 in T-cells, supporting the previous findings of Saldanha-Araujo et al. (69). In the case of SLE, patients show a silenced activity of CD73 and CD38 in B cells, resulting in decrease of production of anti-inflammatory adenosine (70). MSC-EVs may thus constitute a potential therapeutic approach for the treatment of SLE due to their high expression of CD73. Other methods of overexpressing this enzyme could be also used to further improve the treatment.
Cytotoxic T lymphocyte antigen 4 (CTLA-4) is an important regulator of T cell activation (71). It can bind to CD80 and CD86, resulting in an inhibition response rather than a stimulatory one by CD28. Furthermore, CTLA-4 can also bind to DCs, resulting in a downregulation of CD80 and CD86 (72). CTLA-4 expressed on Tregs stabilizes the interaction with T cells allowing for the Treg mediated suppression of T cell (73). CTLA-4 has been shown to be expressed by MSCs under different isoforms. CTLA-4 can be found as a transmembrane protein or can also be secreted. Secreted CTLA-4 by BM-MSCs has been significantly increased under hypoxic conditions. The authors have also shown a CTLA-4 mediated inhibitory effect of the secretion of TNF-α induced by PHA of PBMCs (74). As far as we know, there are no studies directly showing the presence of CTLA-4 in the membrane of MSC-EVs. As CTLA-4 is expressed in MSCs, their derived EVs could potentially carry it.
On the contrary, PD-L1 has been found on the surface of MSC-EVs. PD-L1 binds to the receptor programmed death-1 (PD-1), expressed on T cell surface, leading to an inhibition of their activation (75, 76). Wu et al. have shown that PD-L1 overexpressing MSC-EVs have enhanced therapeutic activity compared to native EVs in a model of LPS-induced pneumonia in mice (77). Other teams also used PD-L1 overexpressing MSC-EVs, showing an increased therapeutic activity in their in vitro and in vivo models. Notably, the use of anti-PD-L1 antibody reverted the effect to the level of wild type MSC-EVs, suggesting a PD-L1/PD-1 dependent immunosuppression (76–79). Pro-inflammatory and hypoxia MSC conditioning resulted in higher levels of IDO and PD-L1 in EVs, resulting in higher immunomodulatory activity (80). In regard to SLE, CD4+/CD25+/Foxp3+ Tregs from SLE patients expressed significantly lower amounts of PD-L1 compared to healthy patients (81).
HLA-G is a non-classic major histocompatibility complex (MHC) molecule which can mediate immunosuppression. HLA-G can bind to receptors expressed in immune cells, such as CD158d, CD85j, CD85d, CD8 and CD160, and can polarize macrophages towards an M2 phenotype, inhibits the proliferation of T cells, induces Tregs, inhibits the maturation of DCs amongst other actions. There are seven different isoforms, with four membrane bound (HLA-G1, G2, G3, and G4), and three soluble isoforms (HLA-G5, G6, and G7) (82–85). A limited number of studies have examined the impact of human leukocyte antigen G (HLA-G) in MSC-EVs. Selmani et al. showed that HLA-G5 can be found in the secretome of BM-MSCs. BM-MSCs secreted HLA-G5 in an IL-10 dependent manner. Interestingly, they noted a decrease of HLA-G expression over passages, but HLA-G5 content in supernatants was not affected. They further demonstrated that HLA-G5 is necessary for the suppression of allogenic T-cell and the expansion of Tregs, and the inhibition of “NK-cell mediated cytolysis and interferon-y secretion” (86). HLA-G has been found in “high levels” of MSC-EVs of four bone marrow donors, purified by PEGylation and ultracentrifugation (87). This could indicate that HLA-G can indeed be found in the secretome, but also linked with MSC-EVs. In the same vein, HLA-G, in both its membrane and soluble isoforms, has also been identified in UC-MSCs, without specifying their EVs (88).
FasL or CD178 or CD95 Ligand induces apoptotic death upon binding with its receptor Fas. Interestingly, FasL induces cell death only in its membrane-bound form (mFasL) while its soluble form (sFasL) binds to Fas without induction of the proapoptotic signaling pathway, thus competing with its membrane-bound form (89). sFasL is found after cleavage of mFasL by metalloproteinases (MMPs). sFasL can trigger inflammatory pathways such as NF-κB (90). As a matter of fact, MMPs are found in the secretome of MSCs after priming with IL-1β (91), and might cleave FasL into sFasL at the surface of MSCs and their EVs, which might in turn compete with FasL. It is not known in the detail in the case of MSCs and EVs how this dynamic between pro-inflammatory sFasL, MMPs and pro-apoptotic mFasL plays out regarding the immunomodulatory potential of MSC-EVs. Some studies have shown a FasL dependent apoptotic effect using MSCs, but little has been done with MSC-EVs. Vacaru et al. used modified MSCs overexpressing FasL which showed improved death induction in CD4+ and CD8+ T cells, but has not looked into the effect of EVs (92). Akiyama et al. showed that bone marrow MSCs induced T-cell apototosis via Fas/FasL pathway. Recruitment of T cells was induced by secretion of Fas-regulated monocyte chemotactic protein 1 (MCP-1) (93). Wang et al. induced apoptotic EVs in mouse MSCs, and showed that these EVs used FasL to induce apoptosis in Multiple myeloma cells in vivo in mice (94).
Indoleamine 2,3-dioxygenase (IDO), is an immunosuppressive enzyme leading to the degradation of tryptophan to kynurenine and other metabolites, resulting in stopping T-cell proliferation, induction and activation of regulatory T cells (95). Kynurenine produced by DCs can be taken up by T cells through the large neutral amino acid transporter (LAT-1), and induces FoxP3 expression, resulting in Tregs differentiation. Kynurenine also inhibits the expression of retinoic acid receptor-related orphan receptor-γt (ROR-γt), thus suppressing the differentiation of Th17 cells. Kynurenine can also induce tolerogenic phenotype in DCs (96). IDO activation in plasmacytoid dendritic cells (pDC) induce immune tolerance and inhibition of IFN-I production (97, 98). As SLE is characterized by an enhanced IFN-I production by pDC, IDO could be a key immune modulator of MSC-EVs in SLE treatment. In MSCs, IDO is present in MSC-EVs and in cells, but only after IFN-y pre conditioning. Increased levels of IDO expression result in higher levels of Kynurenine, both in the cell and in the EVs. EVs unprimed with IFN-γ showed no immunomodulatory properties, while primed EVs suppressed T-cell and induced Treg cells (99). MSC-EVs from overexpressing IDO MSCs have shown to activate M2 polarization in an IDO dependent manner (100). Another study showed an effect of unprimed MSC-EVs on inhibition of PBMC proliferation, but no statistically significant increase of Tregs proportion. Treatment with MSC-EVs primed with IFN-γ and TGF-β showed a higher increase in the proportion of Tregs compared to conditions primed with only one molecule, or unprimed. This increased immunomodulatory activity was linked with a higher concentration of IDO and IL-10 (101). However, Serejo et al. showed that while IFN-y pre-treatment of MSCs did increase the expression of IDO in both cells and EVs, it did not result in increased T-cell suppression of proliferation (102). Another study found that conditioned media from primed cells successfully suppressed T cell proliferation, while primed MSC-EVs, and unprimed CM and EVs had no effect (103). The authors hypothesize that the absence of immunomodulatory effect could be due to a difference in experimental methodology. These studies show that IFN-γ priming is needed for IDO expression, which could mediate the immunosuppressive effect of MSC-EVs.
Regarding the iNOS-NO axis, the immunosuppressive activity of MSCs from human, monkey and pig is mostly mediated through IDO rather than iNOS, whereas MSCs derived from rat, hamster and rabbit mostly use iNOS (104). For that reason, we are redirecting to another review which has already described in-depth the role of iNOS in the immunomodulatory potential of MSCs and their EVs (105).
The immunomodulatory potential of MSC-EVs could also be mediated through miRNAs. miRNA are non-coding small nucleic acid of around twenty nucleotides which interfere with mRNA translation. As such, when delivered to the immune cells, miRNAs can influence their function through the inhibition of transcription factors or targeting of specific pathways regarding maturation, activation of the immune cells (106). miRNAs can be transported and delivered to other cells by extracellular vesicles, which make them a potent molecule responsible for the immunomodulatory potential of MSC-EVs. MSC-EVs have been found to be enriched in various miRNAs, with differentially expressed miRNAs depending on the source of the MSC-EVs (107). This should be kept in mind looking at potential immunomodulatory mediated miRNAs action as they could be weakly enriched in a specific source of MSC-EVs.
Kim et al. identified let-7b-5p and miR-21-5p as key microRNAs mediating the immunomodulatory effect of MSC-EVs (25). miR-21-5p, identified as one of the most enriched miRNA in MSC-EVs, targets CCR7 resulting in attenuated DC migration and function (108). miR-146a has been shown by Song et al. to promote M1-M2 transition and plays a protective role in sepsis (109). miR-181c in UC-MSC-EVs repressed inflammation by suppressing TLR4 (110). miR-155 has been shown to significantly reduce the proliferation of activated PBMCs, by targeting miR-221 as a potential inflammation mediator (111). miR-223 in MSC-EVs could also restrain adhesion and migration of T cells (112). A lot of other miRNAs have been identified as a potential mediator of MSC-EVs immunomodulatory effects (113). A few studies have highlighted limitations of current RNA-sequencing methods, which could raise concerns over the reproducibility and comparability of sequencing data across library preparation platforms (114, 115). Nonetheless, Srinivasan et al. indicate that technical variability is smaller than biological variability regarding the use of small RNAs in EVs (116). miRNAs have thus shown to mediate a broad Immunosuppressive effect of MSC-EVs.
While the scientific community has been mainly focused on the immunomodulatory impact of miRNAs and various proteins, some bioactive lipids have been coming in light.
Lipid mediators are lipids derived from polyunsaturated fatty acid (PUFAs) who can promote inflammation or resolution. PUFAs can be modified by different types of enzymes into a diversity of bioactive lipids. Among them, specialized pro resolving mediators (SPMs) such as resolvins or maresins are known to be anti-inflammatory and play a key role in the resolution of inflammation. The regulatory effect of SPMs on immune cells has already been described extensively (117, 118). Regulatory lipid mediators have been found in MSC-EVs (119–121), and their concentration can be increased by priming MSCs with PUFAs, or even with pro-inflammatory cytokines (121, 122). Enzymes of the many lipid mediators pathways are carried by MSC and their EVs. Cardiac MSCs and their EVs carry 5-LO and 15-LO, enzymes which play a role in transforming PUFAs in SPMs (123). Thus, these enzymes are able to modify dynamically the concentration in various SPMs inside EVs. PUFA supplementation of MSCs to improve the immunomodulatory potential has already been described in the literature (124, 125). Secretome of primed MSC with different types of PUFA promoted an immunoregulatory phenotype in macrophages (126). While lipid mediators may play a role in the immunomodulatory effect of MSC-EVs, little research has been done about them.
Prostaglandin E2 (PGE2) is a lipid mediator belonging to the prostaglandin family. PGE2 is produced by the enzymes COX-1 and COX-2 from the ω-3 PUFAs arachidonic acid (AA) and can bind on EP receptors expressed on the surface of immune cells (45). EP2 and EP4 receptors, upon binding, upregulates cAMP levels whereas EP3 downregulates it (127). PGE2 binds on EP2 and EP4 receptors and regulates T helper cells (128). PGE2 has shown to have a broad immunomodulatory effect. It promotes an anti-inflammatory phenotype in macrophages, has ambivalent effects on DCs depending on their development stage, suppresses T cell activation and promotes Treg cells (129, 130). PGE2 can also induce neutrophils to produce less pro-inflammatory lipid mediators and increase the production of anti-inflammatory lipid mediators such as Lipoxins (131). In prostate cancer cells, PGE2 has been found either to be carried with EVs but also secreted as a soluble factor (132). PGE2 is expressed in MSCs, carried by their EVs, and the cells can be primed with ω-3 PUFA to express more PGE2 (121). Conditioned media from human MSCs spheroids inhibited pro-inflammatory cytokines and increased the secretion of anti-inflammatory cytokines in LPS stimulated macrophages, in a PGE2 dependent manner, mediated by binding on the EP4 receptor (133). MSC-EVs from pluripotent stem cells isolated by anion-exchange chromatography were able to inhibit the activating effects of dendritic cells on group 2 innate lymphoid cell, mediated by PGE2 binding on EP2/EP4 (134). TNF-α and IFN-γ were reduced in activated splenocytes, partially through PGE2/COX2 (135). These studies show the importance of bioactive lipids in the therapeutic activity of MSC-EVs.
In the end, multiple mechanism of actions have been proposed, using in vitro and in vivo models. Taking all these studies into account, the immunomodulatory potential of MSC-EVs is probably mediated not by one specific pathway, but by a combination of all these bioactive molecules. Since these molecules are located in the secretome either as a soluble fraction, in the membrane of the EVs, or inside the EVs, it appears that managing to keep all these bioactive molecules as a part of the secretome downstream process will be key for keeping MSC-related potency.
Many studies discussed before used different techniques to enhance the quantity of immunomodulatory molecules in MSC-EVs. In the next part, we discuss the techniques to obtain MSC-EVs with a higher immunomodulatory potential.
MSCs have an important role in tissue repair and can be mobilized to the sites of tissue damage and inflammation. Thus, MSCs in vivo are often subject to inflammatory stimuli such as but not limited to, damage-associated molecular patterns (DAMPs), pathogen-associated molecular patterns (PAMPs), pro-inflammatory cytokines from activated immune cells, or hypoxia (136, 137). Attempts to recreate this environment in vitro have been used to increase the immunomodulatory potential of MSC-EVs. As a matter of fact, MSCs have high plasticity regarding their immunomodulatory potential: an anti-inflammatory environment might inhibit the immunosuppressive activity of MSCs, while a pro-inflammatory one will enhance it (138, 139). This can explain why some immunomodulatory molecules such as IDO are only produced under a pro-inflammatory environment. (Table 1) summarizes all the priming methods for MSC-EV enhanced immunomodulatory potential.
Table 1 MSC-priming for increased MSC derived secretome and EVs enhanced immunomodulatory potential.
Priming or conditioning MSCs with different methods have shown to induce different EV release, membrane markers, differential uptake and activation of T cell subsets (167). Priming also modifies the miRNA and protein EV cargo (168–170). Importantly, the source of MSCs and the inter-donor variability within the same source of MSCs has an impact on the response to priming and thus the improvement of immunosuppressive effect of MSC-EVs. Peltzer et al. showed that PCA failed to discriminate groups between MSC- EVs from 5 donors without priming, with IFN-γ priming and hypoxia, regarding their differential miRNA expression, showing that inter-individual variability was stronger, especially regarding their response to priming (171). Gorgun et al. showed that while priming had a significant effect on the secretome of MSCs, it did not majorly affect the miRNA in their EVs (172). A recent study was able to discriminate hypoxia and normoxia group looking at their miRNA, possibly underlying a donor disparity between studies (173). Jin et al. showed that priming overcame the MSC inter-donor variability by looking at gene expression (174). Priming with a cocktail of cytokines resulted in two different responses in different donors of MSCs (140). These studies show that priming MSC has significative changes on the biophysical, biochemical and bioactive properties of MSC-EVs, but the changes might be hidden by donor- and source-dependent differences.
One way to recreate the inflammatory conditions of tissue damage is to target Toll-Like Receptors (TLRs), which recognize various types of molecules such as DAMPs and PAMPs. LPS-preconditioned MSC-EVs were more efficient in converting THP-1 to M2 phenotype in vitro, and in relieving inflammation in vivo than untreated MSC-EVs, notably through miR-let-7b (141). LPS-primed MSC-EVs were significantly more efficient at increasing M2 and reducing M1 polarization in vitro, and were more effective in relieving post-infarction inflammation in mice (142). Hwang et al. showed that TLR-3 and 4 primed MSCs secretomes were more successful in reducing pro-inflammatory cytokines from LPS-induced macrophages. EVs were key in increasing the percentage of M2 (143). Notably, Kink et al. showed that the concentration of LPS for priming MSC resulted in EVs having different effects on macrophage receptors expression, which might explain the effect of the next studies (144). Indeed, some studies show that TLR-priming does not always result in enhanced immunosuppressive effect. Recent results from Cui et al. showed that LPS primed MSC-EVs induced a M1 and not a M2 phenotype (145). Previous results from Waterman et al. showed a pro-inflammatory phenotype MSCs after TLR-4 priming, and anti-inflammatory phenotype after TLR-3 priming (146). All in all, these results show that priming through TLRs might be an interesting method to prime MSC-EVs, though some optimization of concentration of priming might be important to achieve the highest enhanced immunosuppressive effect.
A second way to prime MSCs is to use pro-inflammatory cytokines. A study by Cheng et al. showed that priming with IFN-γ and TNF-α resulted with higher suppression of T cell proliferation and induced a different protein profile with higher levels of anti-inflammatory proteins such as TSG-6 and A20 (147). Ragni et al. showed that IFN-γ priming changes the proteins secreted by MSCs and the miRNA content of their EVs, resulting in a diminution of M1 polarization (148). Priming with IL-1β resulted in higher anti-inflammatory activity of EVs, with a significant increase of mir-147b, which partially mediated the immunomodulatory effect of primed MSC-EVs in osteoarthritis cells (149). IL-1β priming on mouse MSC resulted in an enhanced macrophage polarization to M2 in vivo and in vitro, and a better therapeutic effect on septic mice by MSC-EVs. Equivalent priming also induced higher expression of miR-21, which mediated the effect of MSC-EVs on sepsis (150). Nonetheless, conditioning does not always result in better activity: priming with IFN-γ did not induce a better therapeutic effect in T-cell modulation activity, and induced a loss of protection against ischemic acute kidney injury (151). A few teams have also tried cocktails of different pro-inflammatory molecules, compared to one-molecule priming. A combination of IFN-γ and TGF-β priming resulted in a higher proportion of Treg cells after treatment with EVs, but also elevated levels of IFN-γ, IL-10 and IDO within these EVs. The combination of priming resulted in better immunomodulatory effect than EVs derived from untreated MSC, or MSCs treated with only one of the molecules (101). Hackel et al. showed a variation of response between MSCs when treated with multicytokine combination of IFN-γ, TNF-α and IL-1β. One group of 3 donors secreted more PD-L1 with the full priming compared to two-cytokines priming, and the other group of 3 other donors responded equally with 3 and 2-cytokine priming. Nonetheless, priming still induced higher levels of PD-L1 and PD-L2 compared to non-primed EVs. Higher therapeutic effects of primed MSC-EVs were mediated by PD-1 ligands (140). Priming MSCs with pro-inflammatory components has been shown to modify the whole secretome, elevating the level of anti-inflammatory molecules in the secretome and in EVs, thus mediating higher potency and therapeutical effects of MSC-EVs. Priming with higher number of cytokines seems to induce a higher immunomodulatory effect of EVs.
Other molecules have also been used to prime MSCs. Conditioning with Atorvastatin resulted in a better cardioprotective effect on infarcted rat heart, with a better inhibition of TNF-α and Il-6 in the tissue of the infarct zone (152).
Genetic modifications of MSC producing EVs can improve the immunomodulatory potential of MSC-EVs. A few studies already described above have targeted specific immunomodulatory proteins such as IDO (100), IL-10 (44), TSG-6 (52), TGF-β, PTX3, miR-let7-5p and miR-21-5p (25), CD73 (66), PD-L1 (77–79), in order to secrete more of these specific proteins and induce a better immunoregulatory activity of MSC-EVs. Genetic modifications can also target the expression of bioactive miRNAs. Overexpression of miRNA-181a induced better inhibition of the inflammatory response, increased the percentage of Tregs among PBMCs, and delayed ischemic damage in vivo (153). Overexpression of miRNA-126 promoted functional recovery after stroke by suppressing microglia activation (154). Genetic modifications can also target molecules which are not constitutively expressed by MSCs. A study has shown that modified MSCs for the expression of TRAIL secrete EVs with TRAIL, and are able to induce apoptosis in various cell lines (155).
Conditioning of MSC in hypoxia has also been shown to improve the immunomodulatory potential of MSC-EVs. Hypoxic environment leads to increased levels of hypoxia-inducible factors, notably HIF-1a, which regulates many physiological pathways, including angiogenesis (175). The effect of hypoxia on the immune-modulatory properties of BM-MSCs has already been reviewed (176). Extracellular vesicles derived from hypoxia conditioned MSCs were more effective in decreasing levels of pro-inflammatory cytokines and in shifting microglia from M1 to M2 polarization, compared to non-treated MSC-derived EVs (156). Same results were observed by another group (157). Hypoxia was also shown to increase the expression of HGF (172), which we have already discussed the immunomodulatory effect. Gómez-Ferrer et al. showed that double-primed hypoxia + inflammation MSC-derived EVs were more efficient in repolarizing M1 to M2-like phenotype than single-primed inflammation MSC-EVs. These EVs promoted healing in a TNBS induced mouse colitis, partially through reduction of pro-inflammatory cytokines (80). Hypoxic UC-MSC-derived EVs inhibited more efficiently maturation of DCs (177). Hypoxia treated AD-MSC EVs were more efficient in reducing macrophage infiltration, reducing levels of Il-6, though MCP-1 levels were higher, compared to non-treated MSC-EVs, in renal tissue after ischemia reperfusion injury (158). Thus, hypoxia conditioning of MSCs could be a relevant technique to enhance the MSC-EV immunosuppressive functions for chronic diseases.
Finally, a few studies have reported higher potency with EVs derived from MSC cultured in 3D. 3D culture of MSCs englobes a great variety of techniques, including but not limited to microcarriers, diverse scaffolds, microgels, and spheroids, which can be then cultured in bioreactors or other types of vessels. 3D culture better mimics the natural cell conditions, and increases the levels of secreted angiogenic and immunomodulatory factors (178).
Bulati et al. were able to differentially cluster between IFN-γ primed and 3D spheroid cultured MSCs by looking at their miRNA EVs (179). Many studies have shown that MSCs have increased immunomodulatory potential after 3D spheroid culture (159–161). EVs derived from pro-inflammatory primed MSC spheroids had an enhanced anti-inflammatory effect by decreasing the expression of NF-κB, IL-8 and IL-6 in TNF-induced inflammation in HK2 cells, compared to 3D cultured only EVs and 2D cultured EVs (162). Nonetheless, Kusuma et al. have shown contrasting results where 3D spheroid cultured MSC-EVs produced significantly less IDO, and that overall, these EVs had a lower immunosuppressive and therapeutic potency than 2D MSC-EVs (163). Further studies are needed to understand the impact of MSC-EVs derived from spheroids.
Regarding MSC culture in bioreactors, 3D cultured in WAVE bioreactor MSC-EVs induced same decrease in M1 markers expression in macrophages than 2D MSC-EVS, but had enhanced suppression of CD8+ T cell proliferation (164). Hollow Fiber bioreactor systems have gained popularity for the 3D culture of MSC for EV research. 3D cultured MSC-EVs using this system were more efficient than 2D cultured MSC-EVs in alleviating acute kidney injury, notably by reducing inflammatory factors, repressing T cell and macrophage infiltration (165). Sun et al. also used the hollow fiber system, and found that 3D-MSC EVs exhibited a stronger anti-inflammatory effect on stimulated monocytes, but also in acute myocardial infarction rats (166). Thus, 3D culture in bioreactor seems to enhance the immune-modulatory potential of MSC-EVs.
Other than MSCs, other EVs have been showing immunosuppressive potential for a therapeutic approach. Namely, tumor cells like sarcoma cells evade the immune system, which is partially mediated by the release of EVs (180, 181). EVs derived from Ewing Sarcoma induced a pro-inflammatory response on myeloid cells, but impaired the maturation and function of dendritic cells (182). Droste et al. wrote an in-depth review about tumor derived EVs, their effect on immune cells and how in vivo animal models help understand the potential of these EVs (183).
Based on their broad immunosuppressive effects and the possibility to enhance them through a variety of techniques, MSC-EVs could be a promising therapeutic solution for the treatment of SLE.
Recent studies suggested that EVs open a new perspective for both diagnosis and treatment of SLE. Several teams reported differences between the EV profile of SLE patients and healthy controls. First, the number of total EV was found to vary. Most studies reported an increase of total EVs in SLE patients (184–189), while Nielsen et al. reported a decrease (190). Apart from the number of EV, their composition has also been shown to differ between SLE patients and healthy controls. Østergaard et al. outlined a decrease in the level of cytoskeletal, mitochondrial and organelle proteins contained in microparticles from SLE patients (191). Additionally, Chuang et al. recently reported an overexpression of Eosinophil Cationic Protein (ECP) in SLE T cell-derived EVs and demonstrated their pro-inflammatory property in a mouse model (192).
Aside from protein-containing EVs, attention was drawn to miRNA-containing EVs. Li et al. reported compared to healthy controls, an increase of miR-21 and miR-155, and a decrease of miR-146a in serum EVs (193). Additionally, the expression of miR-21 and miR-146a were negatively associated with respectively anti-SSA/RO antibodies and anti-dsDNA antibodies, which are important features of SLE pathogenesis. The decrease of miR-146a-containing exosomes in the serum of SLE patients was also demonstrated by Dong et al., who suggested that miR-146a is internalized into MSCs and contributes to MSC senescence in SLE patients by targeting the TRAF6/NF-κB pathway (194). Interestingly, Perez-Hernandez et al. had previously found an increase in the urinary miR-146a-containing exosomes in SLE patients (195), suggesting that the location of the EVs should be also taken into consideration. Furthermore, Tan et al. showed that exosomal miR-451a is downregulated in the serum of SLE patients and correlates with the SLE disease activity and renal damage, due to its implication in intercellular communication (196). It has also been demonstrated that microRNAs-containing exosomes, isolated from the plasma of SLE patients, can activate pDCs through the receptor TLR7 and induce excessive production of IFN-α, leading to a chronic state of inflammation in SLE (197). Overall, the EV profile of SLE patients seem to significantly differ from the one of healthy controls, which outlines them as prominent biomarkers for SLE. Moreover, their presence in various body fluids, such as blood, urine and saliva, guarantee a facilitated access for diagnosis and could replace the rather invasive biopsies traditionally used for monitoring the disease progression.
Allogenic MSCs have already been used as a potential treatment for SLE (198, 199). As the broad immunosuppressive effect of MSCs is mediated by the secretome and EVs, they represent a potential alternative for the treatment of SLE (200). Multiple studies have already been carried using MRL/lpr mice model. Xie et al. have tested the effect of human umbilical cord MSCs and their EVs on a classical animal model of SLE. They have shown that UC-MSCs exert immunoregulatory effects on SLE, partially mediated by their EVs. UC-MSC-EVs were able to inhibit CD4+ T cells in their model, but lower amounts of TGF-β and IL-17 were found in the supernatant (201). Another study also using UC-MSC-EVs has shown an amelioration of SLE after EV administration in MRL/lpr mice by inducing M2 macrophages polarization and increasing regulatory T cell (202). BM-MSC-EVs promoted anti-inflammatory phenotype of macrophages, and induced recruitment of Tregs in murine lupus nephritis model. Notably, they showed the importance of miR-16 and miR-21 in the polarization of macrophages (203). Another study has compared tooth MSCs and their EVs to treat SLE in the same MRL/lpr mice model. The administration of EVs exerted a therapeutic effect on this model by rescuing the immune microenvironment. Furthermore, they have shown a decreased effect with the presence of RNase, hinting at the importance of RNA in the immunomodulatory potential of EVs (204). BM-MSC derived apoptotic vesicles ameliorated lupus in the same model, by suppressing activated CD4+ T cells (205).
Dou et al. showed that MSC-EVs reduced the expression of pro-inflammatory cytokines and promoted M2 polarization of macrophages, notably through tsRNA-21109. This same RNA is downregulated in SLE patients, which sheds light on possible means of action of MSC-EVs in SLE (206). Chen et al. investigated the effect of MSC-EVs in diffuse alveolar hemorrhage (DAH) mice, an uncommon but fatal complication of SLE. EVs alleviated symptoms of DAH, decreased the expression of pro-inflammatory factors and enhanced M2 polarization (207).
Tu et al. showed a lower expression of miR-19b, an imbalance between Th17 and Tregs, a much higher expression of pro-inflammatory cytokines in PBMCs from SLE patients. UC-MSC EVs treatment increased the expression of miR-19b, regulated the Th17/Tregs balance and reduced the expression of pro-inflammatory factors (208). The amount of B cells in SLE patients is significantly upregulated. UC-MSC-EVs promoted B cell apoptosis, inhibited overactivation and decreased the levels of pro-inflammatory cytokines, possibly through regulation of the upregulated miR-155 in SLE patients (209). Type I IFN release by pDCs is closely related to the severity of SLE. While MSCs have shown to reduce the release of IFN-α and inhibit the function of pDCs (210, 211), no studies regarding the impact of MSC-EVs have been carried. Overall, these studies show the therapeutic potential of MSC-EVs for the treatment of SLE.
The emerging field of EVs presents a promising avenue for therapeutic treatment. EVs carry a variety of membrane and soluble proteins, and play a key role in immune processes. Thus, EVs could be used for diagnostic as a biomarker, or a therapeutic tool. More specifically, MSC-EVs mediate a broad immunosuppressive effect, showcasing their potential as a cell-free therapy for SLE. Further techniques such as pre-conditioning of MSCs, genetic modification or EV engineering could enhance their immunomodulatory activity and could be applied to further therapeutic applications of EVs.
However, a more specific understanding of whether the immunoregulatory activity is mediated by EV-associated bioactive molecules, soluble factors, or both is needed. The impact of the many sources of heterogeneity in EV studies on these immune mediators should be investigated. The EV field still suffers from barriers such as standardization of isolation and characterization methods, Good Manufacturing Practice (GMP)-compliant large scale production, or specific guidelines for validation of EVs as a therapeutic tool, which in turn hinders the use of EVs for the treatment of SLE. Furthermore, regarding SLE, the role of EVs on pDC activation should be further investigated to understand their potential role on chronic production of IFN-I in SLE. Similarly, a fine characterization of pDC derived EVs content should be carried out prior considering the use of EVs as therapeutic strategy. The effect of MSC-EVs regarding the regulation of interferon production of pDCs should be investigated. Finally, methodological studies on the dosage and administration interval of MSC-EVs in SLE are still essential to advance their therapeutic development.
CW: Writing – original draft, Writing – review & editing. IS: Writing – original draft, Writing – review & editing. FG: Writing – original draft, Writing – review & editing. J-PH: Writing – original draft, Writing – review & editing. TF: Writing – original draft, Writing – review & editing.
The author(s) declare financial support was received for the research, authorship, and/or publication of this article. CW is a student from the FIRE PhD program funded by the Bettencourt Schueller foundation and the EURIP graduate program (ANR-17-EURE-0012). IS is a student from the MTCI PhD program, and this work was supported by the Fondation pour la Recherche Médicale, grant number ECO202306017404.
All figures were created using Biorender.com.
CW and TF are employed by EVerZom.
The remaining authors declare that the research was conducted in the absence of any commercial or financial relationships that could be construed as a potential conflict of interest.
All claims expressed in this article are solely those of the authors and do not necessarily represent those of their affiliated organizations, or those of the publisher, the editors and the reviewers. Any product that may be evaluated in this article, or claim that may be made by its manufacturer, is not guaranteed or endorsed by the publisher.
1. Tsokos GC. Systemic lupus erythematosus. New Engl J Med (2011) 365(22):2110–21. doi: 10.1056/NEJMra1100359
2. Kaul A, Gordon C, Crow MK, Touma Z, Urowitz MB, Van Vollenhoven R, et al. Systemic lupus erythematosus. Nat Rev Dis Primers. (2016) 2(1):16039. doi: 10.1038/nrdp.2016.39
3. Banchereau J, Pascual V. Type I interferon in systemic lupus erythematosus and other autoimmune diseases. Immunity (2006) 25(3):383–92. doi: 10.1016/j.immuni.2006.08.010
4. Rodero MP, Decalf J, Bondet V, Hunt D, Rice GI, Werneke S, et al. Detection of interferon alpha protein reveals differential levels and cellular sources in disease. J Exp Med (2017) 214(5):1547–55. doi: 10.1084/jem.20161451
5. Dieudonné Y, Gies V, Guffroy A, Keime C, Bird AK, Liesveld J, et al. Transitional B cells in quiescent SLE: An early checkpoint imprinted by IFN. J Autoimmunity. (2019) 102:150–8. doi: 10.1016/j.jaut.2019.05.002
6. Couch Y, Buzàs EI, Vizio DD, Gho YS, Harrison P, Hill AF, et al. A brief history of nearly EV-erything – The rise and rise of extracellular vesicles. J Extracell Vesicles. (2021) 10(14):e12144. doi: 10.1002/jev2.12144
7. Buzas EI. The roles of extracellular vesicles in the immune system. Nat Rev Immunol (2023) 23(4):236–50. doi: 10.1038/s41577-022-00763-8
8. Meldolesi J. Exosomes and ectosomes in intercellular communication. Curr Biol (2018) 28(8):R435–44. doi: 10.1016/j.cub.2018.01.059
9. van Niel G, D’Angelo G, Raposo G. Shedding light on the cell biology of extracellular vesicles. Nat Rev Mol Cell Biol (2018) 19(4):213–28. doi: 10.1038/nrm.2017.125
10. Théry C, Witwer KW, Aikawa E, Alcaraz MJ, Anderson JD, Andriantsitohaina R, et al. Minimal information for studies of extracellular vesicles 2018 (MISEV2018): a position statement of the International Society for Extracellular Vesicles and update of the MISEV2014 guidelines. J Extracell Vesicles. (2018) 7(1):1535750. doi: 10.1080/20013078.2018.1535750
11. Witwer KW, Van Balkom BWM, Bruno S, Choo A, Dominici M, Gimona M, et al. Defining mesenchymal stromal cell (MSC)-derived small extracellular vesicles for therapeutic applications. J Extracell Vesicles. (2019) 8(1):1609206. doi: 10.1080/20013078.2019.1609206
12. Rani S, Ryan AE, Griffin MD, Ritter T. Mesenchymal stem cell-derived extracellular vesicles: toward cell-free therapeutic applications. Mol Ther (2015) 23(5):812–23. doi: 10.1038/mt.2015.44
13. Eleuteri S, Fierabracci A. Insights into the secretome of mesenchymal stem cells and its potential applications. Int J Mol Sci (2019) 20(18):4597. doi: 10.3390/ijms20184597
14. Sidhom K, Obi PO, Saleem A. A review of exosomal isolation methods: is size exclusion chromatography the best option? Int J Mol Sci (2020) 21(18):6466. doi: 10.3390/ijms21186466
15. Wolf M, Poupardin RW, Ebner-Peking P, Andrade AC, Blöchl C, Obermayer A, et al. A functional corona around extracellular vesicles enhances angiogenesis, skin regeneration and immunomodulation. J Extracellular Vesicles. (2022) 11(4):e12207. doi: 10.1002/jev2.12207
16. Heidarzadeh M, Zarebkohan A, Rahbarghazi R, Sokullu E. Protein corona and exosomes: new challenges and prospects. Cell Communication Signaling (2023) 21(1):64. doi: 10.1186/s12964-023-01089-1
17. Tóth EÁ, Turiák L, Visnovitz T, Cserép C, Mázló A, Sódar BW, et al. Formation of a protein corona on the surface of extracellular vesicles in blood plasma. J Extracell Vesicles. (2021) 10(11):e12140. doi: 10.1002/jev2.12140
18. Papait A, Ragni E, Cargnoni A, Vertua E, Romele P, Masserdotti A, et al. Comparison of EV-free fraction, EVs, and total secretome of amniotic mesenchymal stromal cells for their immunomodulatory potential: a translational perspective. Front Immunol (2022) 13:960909. doi: 10.3389/fimmu.2022.960909
19. Skovronova R, Scaccia E, Calcat-i-Cervera S, Bussolati B, O’Brien T, Bieback K. Adipose stromal cells bioproducts as cell-free therapies: manufacturing and therapeutic dose determine in vitro functionality. J Trans Med (2023) 21(1):723. doi: 10.1186/s12967-023-04602-9
20. Carceller MC, Guillén MI, Gil ML, Alcaraz MJ. Extracellular vesicles do not mediate the anti-inflammatory actions of mouse-derived adipose tissue mesenchymal stem cells secretome. Int J Mol Sci (2021) 22(3):1375. doi: 10.3390/ijms22031375
21. González-Cubero E, González-Fernández ML, Olivera ER, Villar-Suárez V. Extracellular vesicle and soluble fractions of adipose tissue-derived mesenchymal stem cells secretome induce inflammatory cytokines modulation in an in vitro model of discogenic pain. Spine J (2022) 22(7):1222–34. doi: 10.1016/j.spinee.2022.01.012
22. Kordelas L, Schwich E, Dittrich R, Horn PA, Beelen DW, Börger V, et al. Individual immune-modulatory capabilities of MSC-derived extracellular vesicle (EV) preparations and recipient-dependent responsiveness. Int J Mol Sci (2019) 20(7):1642. doi: 10.3390/ijms20071642
23. Madel RJ, Börger V, Dittrich R, Bremer M, Tertel T, Phuong NNT, et al. Independent human mesenchymal stromal cell-derived extracellular vesicle preparations differentially attenuate symptoms in an advanced murine graft-versus-host disease model. Cytotherapy (2023) 25(8):821–36. doi: 10.1016/j.jcyt.2023.03.008
24. Turlo AJ, Hammond DE, Ramsbottom KA, Soul J, Gillen A, McDonald K, et al. Mesenchymal stromal cell secretome is affected by tissue source and donor age. Stem Cells (2023) 41(11):1047–59. doi: 10.1093/stmcls/sxad060
25. Kim H, Lee MJ, Bae EH, Ryu JS, Kaur G, Kim HJ, et al. Comprehensive molecular profiles of functionally effective MSC-derived extracellular vesicles in immunomodulation. Mol Ther (2020) 28(7):1628–44. doi: 10.1016/j.ymthe.2020.04.020
26. Ramos YFM, Tertel T, Shaw G, Staubach S, de Almeida RC, Suchiman E, et al. Characterizing the secretome of licensed hiPSC-derived MSCs. Stem Cell Res Ther (2022) 13(1):434. doi: 10.1186/s13287-022-03117-2
27. Kiselevskii MV, Vlasenko RY, Stepanyan NG, Shubina I, Sitdikova SM, Kirgizov KI, et al. Secretome of mesenchymal bone marrow stem cells: is it immunosuppressive or proinflammatory? Bull Exp Biol Med (2021) 172(2):250–3. doi: 10.1007/s10517-021-05371-5
28. Peshkova M, Korneev A, Suleimanov S, Vlasova II, Svistunov A, Kosheleva N, et al. MSCs’ conditioned media cytokine and growth factor profiles and their impact on macrophage polarization. Stem Cell Res Ther (2023) 14(1):142. doi: 10.1186/s13287-023-03381-w
29. Park KS, Bandeira E, Shelke GV, Lässer C, Lötvall J. Enhancement of therapeutic potential of mesenchymal stem cell-derived extracellular vesicles. Stem Cell Res Ther (2019) 10:288. doi: 10.1186/s13287-019-1398-3
30. Gupta D, Zickler AM, El Andaloussi S. Dosing extracellular vesicles. Advanced Drug Delivery Rev (2021) 178:113961. doi: 10.1016/j.addr.2021.113961
31. Eitan E, Green J, Bodogai M, Mode NA, Bæk R, Jørgensen MM, et al. Age-related changes in plasma extracellular vesicle characteristics and internalization by leukocytes. Sci Rep (2017) 7(1):1342. doi: 10.1038/s41598-017-01386-z
32. Khare D, Or R, Resnick I, Barkatz C, Almogi-Hazan O, Avni B. Mesenchymal stromal cell-derived exosomes affect mRNA expression and function of B-lymphocytes. Front Immunol (2018) 9:3053. doi: 10.3389/fimmu.2018.03053
33. Del Fattore A, Luciano R, Pascucci L, Goffredo BM, Giorda E, Scapaticci M, et al. Immunoregulatory effects of mesenchymal stem cell-derived extracellular vesicles on T lymphocytes. Cell Transplant. (2015) 24(12):2615–27. doi: 10.3727/096368915X687543
34. Crain SK, Robinson SR, Thane KE, Davis AM, Meola DM, Barton BA, et al. Extracellular vesicles from wharton’s jelly mesenchymal stem cells suppress CD4 expressing T cells through transforming growth factor beta and adenosine signaling in a canine model. Stem Cells Dev (2019) 28(3):212–26. doi: 10.1089/scd.2018.0097
35. Gouveia de Andrade AV, Bertolino G, Riewaldt J, Bieback K, Karbanová J, Odendahl M, et al. Extracellular vesicles secreted by bone marrow- and adipose tissue-derived mesenchymal stromal cells fail to suppress lymphocyte proliferation. Stem Cells Dev (2015) 24(11):1374–6. doi: 10.1089/scd.2014.0563
36. Carreras-Planella L, Monguió-Tortajada M, Borràs FE, Franquesa M. Immunomodulatory effect of MSC on B cells is independent of secreted extracellular vesicles. Front Immunol (2019) 10:1288. doi: 10.3389/fimmu.2019.01288
37. Zhang B, Yeo RWY, Lai RC, Sim EWK, Chin KC, Lim SK. Mesenchymal stromal cell exosome–enhanced regulatory T-cell production through an antigen-presenting cell–mediated pathway. Cytotherapy (2018) 20(5):687–96. doi: 10.1016/j.jcyt.2018.02.372
38. Du YM, Zhuansun YX, Chen R, Lin L, Lin Y, Li JG. Mesenchymal stem cell exosomes promote immunosuppression of regulatory T cells in asthma. Exp Cell Res (2018) 363(1):114–20. doi: 10.1016/j.yexcr.2017.12.021
39. Xie M, Xiong W, She Z, Wen Z, Abdirahman AS, Wan W, et al. Immunoregulatory effects of stem cell-derived extracellular vesicles on immune cells. Front Immunol (2020) 11:13. doi: 10.3389/fimmu.2020.00013
40. Yang C, Sun J, Tian Y, Li H, Zhang L, Yang J, et al. Immunomodulatory effect of MSCs and MSCs-derived extracellular vesicles in systemic lupus erythematosus. Front Immunol (2021) 12:714832. doi: 10.3389/fimmu.2021.714832
41. Saraiva M, Vieira P, O’Garra A. Biology and therapeutic potential of interleukin-10. J Exp Med (2019) 217(1):e20190418. doi: 10.1084/jem.20190418
42. Jiang Y, Hong S, Zhu X, Zhang L, Tang H, Jordan KL, et al. IL-10 partly mediates the ability of MSC-derived extracellular vesicles to attenuate myocardial damage in experimental metabolic renovascular hypertension. Front Immunol (2022) 13:940093. doi: 10.3389/fimmu.2022.940093
43. Eirin A, Zhu XY, Puranik AS, Tang H, McGurren KA, van Wijnen AJ, et al. Mesenchymal stem cell–derived extracellular vesicles attenuate kidney inflammation. Kidney Int (2017) 92(1):114–24. doi: 10.1016/j.kint.2016.12.023
44. Li Y, Ren X, Zhang Z, Duan Y, Li H, Chen S, et al. Effect of small extracellular vesicles derived from IL-10-overexpressing mesenchymal stem cells on experimental autoimmune uveitis. Stem Cell Res Ther (2022) 13(1):100. doi: 10.1186/s13287-022-02780-9
45. Jiang W, Xu J. Immune modulation by mesenchymal stem cells. Cell Proliferation. (2020) 53(1):e12712. doi: 10.1111/cpr.12712
46. Volarevic V, Gazdic M, Simovic Markovic B, Jovicic N, Djonov V, Arsenijevic N. Mesenchymal stem cell-derived factors: Immuno-modulatory effects and therapeutic potential. BioFactors (2017) 43(5):633–44. doi: 10.1002/biof.1374
47. Chen PM, Liu KJ, Hsu PJ, Wei CF, Bai CH, Ho LJ, et al. Induction of immunomodulatory monocytes by human mesenchymal stem cell-derived hepatocyte growth factor through ERK1/2. J Leukocyte Biol (2014) 96(2):295–303. doi: 10.1189/jlb.3A0513-242R
48. Wang H, Zheng R, Chen Q, Shao J, Yu J, Hu S. Mesenchymal stem cells microvesicles stabilize endothelial barrier function partly mediated by hepatocyte growth factor (HGF). Stem Cell Res Ther (2017) 8(1):211. doi: 10.1186/s13287-016-0450-9
49. Day AJ, Milner CM. TSG-6: A multifunctional protein with anti-inflammatory and tissue-protective properties. Matrix Biol (2019) 78–79:60–83. doi: 10.1016/j.matbio.2018.01.011
50. Chaubey S, Thueson S, Ponnalagu D, Alam MA, Gheorghe CP, Aghai Z, et al. Early gestational mesenchymal stem cell secretome attenuates experimental bronchopulmonary dysplasia in part via exosome-associated factor TSG-6. Stem Cell Res Ther (2018) 9(1):173. doi: 10.1186/s13287-018-0903-4
51. An JH, Li Q, Ryu MO, Nam AR, Bhang DH, Jung YC, et al. TSG-6 in extracellular vesicles from canine mesenchymal stem/stromal is a major factor in relieving DSS-induced colitis. PloS One (2020) 15(2):e0220756. doi: 10.1371/journal.pone.0220756
52. Jiang L, Zhang Y, Liu T, Wang X, Wang H, Song H, et al. Exosomes derived from TSG-6 modified mesenchymal stromal cells attenuate scar formation during wound healing. Biochimie (2020) 177:40–9. doi: 10.1016/j.biochi.2020.08.003
53. Lu X, Lv C, Zhao Y, Wang Y, Li Y, Ji C, et al. TSG-6 released from adipose stem cells-derived small extracellular vesicle protects against spinal cord ischemia reperfusion injury by inhibiting endoplasmic reticulum stress. Stem Cell Res Ther (2022) 13(1):291. doi: 10.1186/s13287-022-02963-4
54. Song WJ, Li Q, Ryu MO, Ahn JO, Ha Bhang D, Chan Jung Y, et al. TSG-6 secreted by human adipose tissue-derived mesenchymal stem cells ameliorates DSS-induced colitis by inducing M2 macrophage polarization in mice. Sci Rep (2017) 7(1):5187. doi: 10.1038/s41598-017-04766-7
55. Jha KA, Pentecost M, Lenin R, Gentry J, Klaic L, Del Mar N, et al. TSG-6 in conditioned media from adipose mesenchymal stem cells protects against visual deficits in mild traumatic brain injury model through neurovascular modulation. Stem Cell Res Ther (2019) 10:318. doi: 10.1186/s13287-019-1436-1
56. Harrell CR, Markovic BS, Fellabaum C, Arsenijevic N, Djonov V, Volarevic V. The role of Interleukin 1 receptor antagonist in mesenchymal stem cell-based tissue repair and regeneration. BioFactors (2020) 46(2):263–75. doi: 10.1002/biof.1587
57. Kou X, Xu X, Chen C, Sanmillan ML, Cai T, Zhou Y, et al. The Fas/Fap-1/Cav-1 complex regulates IL-1RA secretion in mesenchymal stem cells to accelerate wound healing. Sci Transl Med (2018) 10(432):eaai8524. doi: 10.1126/scitranslmed.aai8524
58. Han T, Song P, Wu Z, Xiang X, Liu Y, Wang Y, et al. MSC secreted extracellular vesicles carrying TGF-beta upregulate Smad 6 expression and promote the regrowth of neurons in spinal cord injured rats. Stem Cell Rev Rep (2022) 18(3):1078–96. doi: 10.1007/s12015-021-10219-6
59. Nolte M, Margadant C. Controlling immunity and inflammation through integrin-dependent regulation of TGF-β. Trends Cell Biol (2020) 30(1):49–59. doi: 10.1016/j.tcb.2019.10.002
60. Lodyga M, Hinz B. TGF-β1 – A truly transforming growth factor in fibrosis and immunity. Semin Cell Dev Biol (2020) 101:123–39. doi: 10.1016/j.semcdb.2019.12.010
61. Sanjabi S, Oh SA, Li MO. Regulation of the immune response by TGF-β: from conception to autoimmunity and infection. Cold Spring Harb Perspect Biol (2017) 9(6):a022236. doi: 10.1101/cshperspect.a022236
62. Álvarez V, Sánchez-Margallo FM, Macías-García B, Gómez-Serrano M, Jorge I, Vázquez J, et al. The immunomodulatory activity of extracellular vesicles derived from endometrial mesenchymal stem cells on CD4+ T cells is partially mediated by TGFbeta. J Tissue Eng Regenerative Med (2018) 12(10):2088–98. doi: 10.1002/term.2743
63. Song K, Dayem AA, Lee S, Choi Y, Lim KM, Kim S, et al. Superior therapeutic activity of TGF-β-induced extracellular vesicles against interstitial cystitis. J Controlled Release. (2022) 348:924–37. doi: 10.1016/j.jconrel.2022.06.045
64. Dominici M, Le Blanc K, Mueller I, Slaper-Cortenbach I, Marini F, Krause D, et al. Minimal criteria for defining multipotent mesenchymal stromal cells. Int Soc Cell Ther position statement. Cytotherapy. (2006) 8(4):315–7. doi: 10.1080/14653240600855905
65. Ferretti E, Horenstein AL, Canzonetta C, Costa F, Morandi F. Canonical and non-canonical adenosinergic pathways. Immunol Lett (2019) 205:25–30. doi: 10.1016/j.imlet.2018.03.007
66. Zhai X, Chen K, Yang H, Li B, Zhou T, Wang H, et al. Extracellular vesicles derived from CD73 modified human umbilical cord mesenchymal stem cells ameliorate inflammation after spinal cord injury. J Nanobiotechnology. (2021) 19(1):274. doi: 10.1186/s12951-021-01022-z
67. Watanabe Y, Fukuda T, Hayashi C, Nakao Y, Toyoda M, Kawakami K, et al. Extracellular vesicles derived from GMSCs stimulated with TNF-α and IFN-α promote M2 macrophage polarization via enhanced CD73 and CD5L expression. Sci Rep (2022) 12(1):13344. doi: 10.1038/s41598-022-17692-0
68. Kerkelä E, Laitinen A, Räbinä J, Valkonen S, Takatalo M, Larjo A, et al. Adenosinergic immunosuppression by human mesenchymal stromal cells requires co-operation with T cells. Stem Cells (2016) 34(3):781–90. doi: 10.1002/stem.2280
69. Saldanha-Araujo F, Ferreira FIS, Palma PV, Araujo AG, Queiroz RHC, Covas DT, et al. Mesenchymal stromal cells up-regulate CD39 and increase adenosine production to suppress activated T-lymphocytes. Stem Cell Res (2011) 7(1):66–74. doi: 10.1016/j.scr.2011.04.001
70. Hesse J, Siekierka-Harreis M, Steckel B, Alter C, Schallehn M, Honke N, et al. Profound inhibition of CD73-dependent formation of anti-inflammatory adenosine in B cells of SLE patients. EBioMedicine (2021) 73:103616. doi: 10.1016/j.ebiom.2021.103616
71. Halpert MM, Konduri V, Liang D, Chen Y, Wing JB, Paust S, et al. Dendritic cell-secreted cytotoxic T-lymphocyte-associated protein-4 regulates the T-cell response by downmodulating bystander surface B7. Stem Cells Dev (2016) 25(10):774–87. doi: 10.1089/scd.2016.0009
72. Bourque J, Hawiger D. Immunomodulatory bonds of the partnership between dendritic cells and T cells. Crit Rev Immunol (2018) 38(5):379–401. doi: 10.1615/CritRevImmunol.2018026790
73. Matheu MP, Othy S, Greenberg ML, Dong TX, Schuijs M, Deswarte K, et al. Imaging regulatory T cell dynamics and CTLA4-mediated suppression of T cell priming. Nat Commun (2015) 6(1):6219. doi: 10.1038/ncomms7219
74. Gaber T, Schönbeck K, Hoff H, Tran CL, Strehl C, Lang A, et al. CTLA-4 mediates inhibitory function of mesenchymal stem/stromal cells. Int J Mol Sci (2018) 19(8):2312. doi: 10.3390/ijms19082312
75. Nirschl CJ, Drake CG. Molecular pathways: coexpression of immune checkpoint molecules: signaling pathways and implications for cancer immunotherapy. Clin Cancer Res (2013) 19(18):4917–24. doi: 10.1158/1078-0432.CCR-12-1972
76. Li M, Soder R, Abhyankar S, Abdelhakim H, Braun MW, Trinidad CV, et al. WJMSC-derived small extracellular vesicle enhance T cell suppression through PD-L1. J Extracellular Vesicles. (2021) 10(4):e12067. doi: 10.1002/jev2.12067
77. Wu Y, Wang H, Song A, Wang X, Ma Q, Yao C, et al. PD-L1-expressing extracellular vesicles for the treatment of pneumonia. ACS Biomater Sci Eng. (2023) 9(11):6464–71. doi: 10.1021/acsbiomaterials.3c01173
78. Ou Q, Dou X, Tang J, Wu P, Pan D. Small extracellular vesicles derived from PD-L1-modified mesenchymal stem cell promote Tregs differentiation and prolong allograft survival. Cell Tissue Res (2022) 389(3):465–81. doi: 10.1007/s00441-022-03650-9
79. Xu F, Fei Z, Dai H, Xu J, Fan Q, Shen S, et al. Mesenchymal stem cell-derived extracellular vesicles with high PD-L1 expression for autoimmune diseases treatment. Advanced Materials. (2022) 34(1):2106265. doi: 10.1002/adma.202106265
80. Gómez-Ferrer M, Amaro-Prellezo E, Dorronsoro A, Sánchez-Sánchez R, Vicente Á, Cosín-Roger J, et al. HIF-overexpression and pro-inflammatory priming in human mesenchymal stromal cells improves the healing properties of extracellular vesicles in experimental crohn’s disease. Int J Mol Sci (2021) 22(20):11269. doi: 10.3390/ijms222011269
81. Zhao L, Zhou X, Zhou X, Wang H, Gu L, Ke Y, et al. Low expressions of PD-L1 and CTLA-4 by induced CD4+CD25+ Foxp3+ Tregs in patients with SLE and their correlation with the disease activity. Cytokine (2020) 133:155119. doi: 10.1016/j.cyto.2020.155119
82. Li P, Wang N, Zhang Y, Wang C, Du L. HLA-G/sHLA-G and HLA-G-bearing extracellular vesicles in cancers: potential role as biomarkers. Front Immunol (2021) 12:791535. doi: 10.3389/fimmu.2021.791535
83. Rebmann V, König L, Nardi F da S, Wagner B, Manvailer LFS, Horn PA. The potential of HLA-G-bearing extracellular vesicles as a future element in HLA-G immune biology. Front Immunol (2016) 7:>173. doi: 10.3389/fimmu.2016.00173
84. Grange C, Camussi G. Immunosuppressive role of extracellular vesicles: HLA-G, an important player. Ann Trans Med (2017) 5(10):223–3. doi: 10.21037/atm.2017.03.61
85. Zoehler B, Fracaro L, Boldrini-Leite LM, da Silva JS, Travers PJ, Brofman PRS, et al. HLA-G and CD152 expression levels encourage the use of umbilical cord tissue-derived mesenchymal stromal cells as an alternative for immunosuppressive therapy. Cells (2022) 11(8):1339. doi: 10.3390/cells11081339
86. Selmani Z, Naji A, Zidi I, Favier B, Gaiffe E, Obert L, et al. Human leukocyte antigen-G5 secretion by human mesenchymal stem cells is required to suppress T lymphocyte and natural killer function and to induce CD4+CD25highFOXP3+ regulatory T cells. Stem Cells (2008) 26(1):212–22. doi: 10.1634/stemcells.2007-0554
87. Kordelas L, Rebmann V, Ludwig AK, Radtke S, Ruesing J, Doeppner TR, et al. MSC-derived exosomes: a novel tool to treat therapy-refractory graft-versus-host disease. Leukemia (2014) 28(4):970–3. doi: 10.1038/leu.2014.41
88. Ding DC, Chou HL, Chang YH, Hung WT, Liu HW, Chu TY. Characterization of HLA-G and related immunosuppressive effects in human umbilical cord stroma-derived stem cells. Cell Transplant. (2016) 25(2):217–28. doi: 10.3727/096368915X688182
89. Jang S, Krammer PH, Salgame P. Lack of proapoptotic activity of soluble CD95 ligand is due to its failure to induce CD95 oligomers. J Interferon Cytokine Res (2003) 23(8):441–7. doi: 10.1089/107999003322277856
90. Haymour L, Jean M, Smulski C, Legembre P. CD95 (Fas) and CD95L (FasL)-mediated non-canonical signaling pathways. Biochim Biophys Acta (BBA) - Rev Cancer. (2023) 1878(6):189004. doi: 10.1016/j.bbcan.2023.189004
91. Chen MS, Lin CY, Chiu YH, Chen CP, Tsai PJ, Wang HS. IL-1β-induced matrix metalloprotease-1 promotes mesenchymal stem cell migration via PAR1 and G-protein-coupled signaling pathway. Stem Cells Int (2018) 2018:e3524759. doi: 10.1155/2018/3524759
92. Vacaru AM, Dumitrescu M, Vacaru AM, Fenyo IM, Ionita R, Gafencu AV, et al. Enhanced suppression of immune cells in vitro by MSC overexpressing fasL. Int J Mol Sci (2020) 22(1):348. doi: 10.3390/ijms22010348
93. Akiyama K, Chen C, Wang D, Xu X, Qu C, Yamaza T, et al. Mesenchymal stem cell-induced immunoregulation involves fas ligand/fas-mediated T cell apoptosis. Cell Stem Cell (2012) 10(5):544–55. doi: 10.1016/j.stem.2012.03.007
94. Wang J, Cao Z, Wang P, Zhang X, Tang J, He Y, et al. Apoptotic extracellular vesicles ameliorate multiple myeloma by restoring fas-mediated apoptosis. ACS Nano. (2021) 15(9):14360–72. doi: 10.1021/acsnano.1c03517
95. Fallarino F, Grohmann U. Using an ancient tool for igniting and propagating immune tolerance: IDO as an inducer and amplifier of regulatory T cell functions. Curr Med Chem (2011) 18(15):2215–21. doi: 10.2174/092986711795656027
96. Stone TW, Williams RO. Modulation of T cells by tryptophan metabolites in the kynurenine pathway. Trends Pharmacol Sci (2023) 44(7):442–56. doi: 10.1016/j.tips.2023.04.006
97. Boasso A, Herbeuval JP, Hardy AW, Anderson SA, Dolan MJ, Fuchs D, et al. HIV inhibits CD4+ T-cell proliferation by inducing indoleamine 2,3-dioxygenase in plasmacytoid dendritic cells. Blood (2007) 109(8):3351–9. doi: 10.1182/blood-2006-07-034785
98. Chen W, Liang X, Peterson AJ, Munn DH, Blazar BR. The indoleamine 2,3-dioxygenase pathway is essential for human plasmacytoid dendritic cell-induced adaptive T regulatory cell generation1. J Immunol (2008) 181(8):5396–404. doi: 10.4049/jimmunol.181.8.5396
99. Romani R, Pirisinu I, Calvitti M, Pallotta MT, Gargaro M, Bistoni G, et al. Stem cells from human amniotic fluid exert immunoregulatory function via secreted indoleamine 2,3-dioxygenase1. J Cell Mol Med (2015) 19(7):1593–605. doi: 10.1111/jcmm.12534
100. Xie X, Yang X, Wu J, Tang S, Yang L, Fei X, et al. Exosome from indoleamine 2,3-dioxygenase-overexpressing bone marrow mesenchymal stem cells accelerates repair process of ischemia/reperfusion-induced acute kidney injury by regulating macrophages polarization. Stem Cell Res Ther (2022) 13:367. doi: 10.1186/s13287-022-03075-9
101. Zhang Q, Fu L, Liang Y, Guo Z, Wang L, Ma C, et al. Exosomes originating from MSCs stimulated with TGF-β and IFN-γ promote Treg differentiation. J Cell Physiol (2018) 233(9):6832–40. doi: 10.1002/jcp.26436
102. Serejo TRT, Silva-Carvalho AÉ, Braga LD de CF, Neves F de AR, Pereira RW, de Carvalho JL, et al. Assessment of the immunosuppressive potential of INF-γ Licensed adipose mesenchymal stem cells, their secretome and extracellular vesicles. Cells (2019) 8(1):22. doi: 10.3390/cells8010022
103. Torres Crigna A, Uhlig S, Elvers-Hornung S, Klüter H, Bieback K. Human adipose tissue-derived stromal cells suppress human, but not murine lymphocyte proliferation, via indoleamine 2,3-dioxygenase activity. Cells (2020) 9(11):2419. doi: 10.3390/cells9112419
104. Su J, Chen X, Huang Y, Li W, Li J, Cao K, et al. Phylogenetic distinction of iNOS and IDO function in mesenchymal stem cell-mediated immunosuppression in mammalian species. Cell Death Differ (2014) 21(3):388–96. doi: 10.1038/cdd.2013.149
105. Han Y, Yang J, Fang J, Zhou Y, Candi E, Wang J, et al. The secretion profile of mesenchymal stem cells and potential applications in treating human diseases. Sig Transduct Target Ther (2022) 7(1):1–19. doi: 10.1038/s41392-022-00932-0
106. Fernández-Messina L, Gutiérrez-Vázquez C, Rivas-García E, Sánchez-Madrid F, de la Fuente H. Immunomodulatory role of microRNAs transferred by extracellular vesicles. Biol Cell (2015) 107(3):61–77. doi: 10.1111/boc.201400081
107. Qiu G, Zheng G, Ge M, Wang J, Huang R, Shu Q, et al. Mesenchymal stem cell-derived extracellular vesicles affect disease outcomes via transfer of microRNAs. Stem Cell Res Ther (2018) 9(1):320. doi: 10.1186/s13287-018-1069-9
108. Reis M, Mavin E, Nicholson L, Green K, Dickinson AM, Wang XN. Mesenchymal stromal cell-derived extracellular vesicles attenuate dendritic cell maturation and function. Front Immunol (2018) 9:2538. doi: 10.3389/fimmu.2018.02538
109. Song Y, Dou H, Li X, Zhao X, Li Y, Liu D, et al. Exosomal miR-146a contributes to the enhanced therapeutic efficacy of interleukin-1β-primed mesenchymal stem cells against sepsis. Stem Cells (2017) 35(5):1208–21. doi: 10.1002/stem.2564
110. Li X, Liu L, Yang J, Yu Y, Chai J, Wang L, et al. Exosome derived from human umbilical cord mesenchymal stem cell mediates miR-181c attenuating burn-induced excessive inflammation. eBioMedicine (2016) 8:72–82. doi: 10.1016/j.ebiom.2016.04.030
111. Pers YM, Bony C, Duroux-Richard I, Bernard L, Maumus M, Assou S, et al. miR-155 contributes to the immunoregulatory function of human mesenchymal stem cells. Front Immunol (2021), 624024. doi: 10.3389/fimmu.2021.624024
112. Liu W, Zhou N, Liu Y, Zhang W, Li X, Wang Y, et al. Mesenchymal stem cell exosome-derived miR-223 alleviates acute graft-versus-host disease via reducing the migration of donor T cells. Stem Cell Res Ther (2021) 12(1):153. doi: 10.1186/s13287-021-02159-2
113. Liu H, Chen Y, Yin G, Xie Q. Therapeutic prospects of MicroRNAs carried by mesenchymal stem cells-derived extracellular vesicles in autoimmune diseases. Life Sci (2021) 277:119458. doi: 10.1016/j.lfs.2021.119458
114. Wright C, Rajpurohit A, Burke EE, Williams C, Collado-Torres L, Kimos M, et al. Comprehensive assessment of multiple biases in small RNA sequencing reveals significant differences in the performance of widely used methods. BMC Genomics (2019) 20(1):513. doi: 10.1186/s12864-019-5870-3
115. Godoy PM, Barczak AJ, DeHoff P, Srinivasan S, Etheridge A, Galas D, et al. Comparison of reproducibility, accuracy, sensitivity, and specificity of miRNA quantification platforms. Cell Rep (2019) 29(12):4212–4222.e5. doi: 10.1016/j.celrep.2019.11.078
116. Srinivasan S, Duval MX, Kaimal V, Cuff C, Clarke SH. Assessment of methods for serum extracellular vesicle small RNA sequencing to support biomarker development. J Extracellular Vesicles. (2019) 8(1):1684425. doi: 10.1080/20013078.2019.1684425
117. Perez-Hernandez J, Chiurchiù V, Perruche S, You S. Regulation of T-cell immune responses by pro-resolving lipid mediators. Front Immunol (2021) 12:768133. doi: 10.3389/fimmu.2021.768133
118. Pan G, Zhang P, Yang J, Wu Y. The regulatory effect of specialized pro-resolving mediators on immune cells. Biomedicine Pharmacotherapy. (2022) 156:113980. doi: 10.1016/j.biopha.2022.113980
119. Valkonen S, Holopainen M, Colas RA, Impola U, Dalli J, Käkelä R, et al. Lipid mediators in platelet concentrate and extracellular vesicles: Molecular mechanisms from membrane glycerophospholipids to bioactive molecules. Biochim Biophys Acta Mol Cell Biol Lipids. (2019) 1864(8):1168–82. doi: 10.1016/j.bbalip.2019.03.011
120. Sagini K, Costanzi E, Emiliani C, Buratta S, Urbanelli L. Extracellular vesicles as conveyors of membrane-derived bioactive lipids in immune system. Int J Mol Sci (2018) 19(4):1227. doi: 10.3390/ijms19041227
121. Pizzinat N, Ong-Meang V, Bourgailh-Tortosa F, Blanzat M, Perquis L, Cussac D, et al. Extracellular vesicles of MSCs and cardiomyoblasts are vehicles for lipid mediators. Biochimie (2020) 178:69–80. doi: 10.1016/j.biochi.2020.07.013
122. Holopainen M, Colas RA, Valkonen S, Tigistu-Sahle F, Hyvärinen K, Mazzacuva F, et al. Polyunsaturated fatty acids modify the extracellular vesicle membranes and increase the production of proresolving lipid mediators of human mesenchymal stromal cells. Biochim Biophys Acta Mol Cell Biol Lipids. (2019) 1864(10):1350–62. doi: 10.1016/j.bbalip.2019.06.010
123. Ong-Meang V, Blanzat M, Savchenko L, Perquis L, Guardia M, Pizzinat N, et al. Extracellular vesicles produced by the cardiac microenvironment carry functional enzymes to produce lipid mediators in situ. Int J Mol Sci (2023) 24(6):5866. doi: 10.3390/ijms24065866
124. Abreu SC, Lopes-Pacheco M, da Silva AL, Xisto DG, de Oliveira TB, Kitoko JZ, et al. Eicosapentaenoic acid enhances the effects of mesenchymal stromal cell therapy in experimental allergic asthma. Front Immunol (2018) 9:1147. doi: 10.3389/fimmu.2018.01147
125. Silva JD, Lopes-Pacheco M, de Castro LL, Kitoko JZ, Trivelin SA, Amorim NR, et al. Eicosapentaenoic acid potentiates the therapeutic effects of adipose tissue-derived mesenchymal stromal cells on lung and distal organ injury in experimental sepsis. Stem Cell Res Ther (2019) 10(1):264. doi: 10.1186/s13287-019-1365-z
126. Holopainen M, Impola U, Lehenkari P, Laitinen S, Kerkelä E. Human mesenchymal stromal cell secretome promotes the immunoregulatory phenotype and phagocytosis activity in human macrophages. Cells (2020) 9(9):2142. doi: 10.3390/cells9092142
127. Jin K, Qian C, Lin J, Liu B. Cyclooxygenase-2-Prostaglandin E2 pathway: A key player in tumor-associated immune cells. Front Oncol (2023) 13:1099811. doi: 10.3389/fonc.2023.1099811
128. Wei J, Ouyang X, Tang Y, Li H, Wang B, Ye Y, et al. ER-stressed MSC displayed more effective immunomodulation in RA CD4+CXCR5+ICOS+ follicular helper-like T cells through higher PGE2 binding with EP2/EP4. Modern Rheumatol (2020) 30(3):509–16. doi: 10.1080/14397595.2019.1651446
129. Kulesza A, Paczek L, Burdzinska A. The role of COX-2 and PGE2 in the regulation of immunomodulation and other functions of mesenchymal stromal cells. Biomedicines (2023) 11(2):445. doi: 10.3390/biomedicines11020445
130. Kalinski P. Regulation of immune responses by prostaglandin E2. J Immunol (2012) 188(1):21–8. doi: 10.4049/jimmunol.1101029
131. Levy BD, Clish CB, Schmidt B, Gronert K, Serhan CN. Lipid mediator class switching during acute inflammation: signals in resolution. Nat Immunol (2001) 2(7):612–9. doi: 10.1038/89759
132. Salimu J, Webber J, Gurney M, Al-Taei S, Clayton A, Tabi Z. Dominant immunosuppression of dendritic cell function by prostate-cancer-derived exosomes. J Extracell Vesicles. (2017) 6(1):1368823. doi: 10.1080/20013078.2017.1368823
133. Ylöstalo JH, Bartosh TJ, Coble K, Prockop DJ. Human mesenchymal stem/stromal cells (hMSCs) cultured as spheroids are self-activated to produce prostaglandin E2 (PGE2) that directs stimulated macrophages into an anti-inflammatory phenotype. Stem Cells (2012) 30(10):2283–96. doi: 10.1002/stem.1191
134. Liu XQ, Peng YQ, Huang LX, Li CG, Kuang PP, Chen DH, et al. Dendritic cells mediated by small extracellular vesicles derived from MSCs attenuated the ILC2 activity via PGE2 in patients with allergic rhinitis. Stem Cell Res Ther (2023) 14(1):180. doi: 10.1186/s13287-023-03408-2
135. Harting MT, Srivastava AK, Zhaorigetu S, Bair H, Prabhakara KS, Toledano Furman NE, et al. Inflammation-stimulated mesenchymal stromal cell-derived extracellular vesicles attenuate inflammation. Stem Cells (2018) 36(1):79–90. doi: 10.1002/stem.2730
136. Szydlak R. Mesenchymal stem cells’ homing and cardiac tissue repair. Acta Biochim Pol (2019) 66(4):483–9. doi: 10.18388/abp.2019_2890
137. Najar M, Krayem M, Merimi M, Burny A, Meuleman N, Bron D, et al. Insights into inflammatory priming of mesenchymal stromal cells: functional biological impacts. Inflammation Res (2018) 67(6):467–77. doi: 10.1007/s00011-018-1131-1
138. Wang Y, Chen X, Cao W, Shi Y. Plasticity of mesenchymal stem cells in immunomodulation: pathological and therapeutic implications. Nat Immunol (2014) 15(11):1009–16. doi: 10.1038/ni.3002
139. Bernardo ME, Fibbe WE. Mesenchymal stromal cells: sensors and switchers of inflammation. Cell Stem Cell (2013) 13(4):392–402. doi: 10.1016/j.stem.2013.09.006
140. Hackel A, Vollmer S, Bruderek K, Lang S, Brandau S. Immunological priming of mesenchymal stromal/stem cells and their extracellular vesicles augments their therapeutic benefits in experimental graft-versus-host disease via engagement of PD-1 ligands. Front Immunol (2023) 14:1078551. doi: 10.3389/fimmu.2023.1078551
141. Ti D, Hao H, Tong C, Liu J, Dong L, Zheng J, et al. LPS-preconditioned mesenchymal stromal cells modify macrophage polarization for resolution of chronic inflammation via exosome-shuttled let-7b. J Transl Med (2015) 13:308. doi: 10.1186/s12967-015-0642-6
142. Xu R, Zhang F, Chai R, Zhou W, Hu M, Liu B, et al. Exosomes derived from pro-inflammatory bone marrow-derived mesenchymal stem cells reduce inflammation and myocardial injury via mediating macrophage polarization. J Cell Mol Med (2019) 23(11):7617–31. doi: 10.1111/jcmm.14635
143. Hwang S, Sung DK, Kim YE, Yang M, Ahn SY, Sung SI, et al. Mesenchymal Stromal Cells Primed by Toll-like Receptors 3 and 4 Enhanced Anti-Inflammatory Effects against LPS-Induced Macrophages via Extracellular Vesicles. Int J Mol Sci (2023) 24(22):16264. doi: 10.3390/ijms242216264
144. Kink JA, Forsberg MH, Reshetylo S, Besharat S, Childs CJ, Pederson JD, et al. Macrophages educated with exosomes from primed mesenchymal stem cells treat acute radiation syndrome by promoting hematopoietic recovery. Biol Blood Marrow Transplantation. (2019) 25(11):2124–33. doi: 10.1016/j.bbmt.2019.07.026
145. Cui S, Zhang Z, Cheng C, Tang S, Zhai M, Li L, et al. Small Extracellular Vesicles from Periodontal Ligament Stem Cells Primed by Lipopolysaccharide Regulate Macrophage M1 Polarization via miR-433-3p Targeting TLR2/TLR4/NF-κB. Inflammation (2023) 46(5):1849–58. doi: 10.1007/s10753-023-01845-y
146. Waterman RS, Tomchuck SL, Henkle SL, Betancourt AM. A new mesenchymal stem cell (MSC) paradigm: polarization into a pro-inflammatory MSC1 or an immunosuppressive MSC2 phenotype. PloS One (2010) 5(4):e10088. doi: 10.1371/journal.pone.0010088
147. Cheng A, Choi D, Lora M, Shum-Tim D, Rak J, Colmegna I. Human multipotent mesenchymal stromal cells cytokine priming promotes RAB27B-regulated secretion of small extracellular vesicles with immunomodulatory cargo. Stem Cell Res Ther (2020) 11(1):539. doi: 10.1186/s13287-020-02050-6
148. Ragni E, Perucca Orfei C, De Luca P, Mondadori C, Viganò M, Colombini A, et al. Inflammatory priming enhances mesenchymal stromal cell secretome potential as a clinical product for regenerative medicine approaches through secreted factors and EV-miRNAs: the example of joint disease. Stem Cell Res Ther (2020) 11(1):165. doi: 10.1186/s13287-020-01677-9
149. Kim M, Shin DI, Choi BH, Min BH. Exosomes from IL-1β-primed mesenchymal stem cells inhibited IL-1β- and TNF-α-mediated inflammatory responses in osteoarthritic SW982 cells. Tissue Eng Regener Med (2021) 18(4):525–36. doi: 10.1007/s13770-020-00324-x
150. Yao M, Cui B, Zhang W, Ma W, Zhao G, Xing L. Exosomal miR-21 secreted by IL-1β-primed-mesenchymal stem cells induces macrophage M2 polarization and ameliorates sepsis. Life Sci (2021) 264:118658. doi: 10.1016/j.lfs.2020.118658
151. Kilpinen L, Impola U, Sankkila L, Ritamo I, Aatonen M, Kilpinen S, et al. Extracellular membrane vesicles from umbilical cord blood-derived MSC protect against ischemic acute kidney injury, a feature that is lost after inflammatory conditioning. J Extracellular Vesicles. (2013) 2(1):21927. doi: 10.3402/jev.v2i0.21927
152. Huang P, Wang L, Li Q, Tian X, Xu J, Xu J, et al. Atorvastatin enhances the therapeutic efficacy of mesenchymal stem cells-derived exosomes in acute myocardial infarction via up-regulating long non-coding RNA H19. Cardiovasc Res (2020) 116(2):353–67. doi: 10.1093/cvr/cvz139
153. Wei Z, Qiao S, Zhao J, Liu Y, Li Q, Wei Z, et al. miRNA-181a over-expression in mesenchymal stem cell-derived exosomes influenced inflammatory response after myocardial ischemia-reperfusion injury. Life Sci (2019) 232:116632. doi: 10.1016/j.lfs.2019.116632
154. Geng W, Tang H, Luo S, Lv Y, Liang D, Kang X, et al. Exosomes from miRNA-126-modified ADSCs promotes functional recovery after stroke in rats by improving neurogenesis and suppressing microglia activation. Am J Transl Res (2019) 11(2):780–92.
155. Yuan Z, Kolluri KK, Gowers KHC, Janes SM. TRAIL delivery by MSC-derived extracellular vesicles is an effective anticancer therapy. J Extracell Vesicles. (2017) 6(1):1265291. doi: 10.1080/20013078.2017.1265291
156. Shao M, Jin M, Xu S, Zheng C, Zhu W, Ma X, et al. Exosomes from long noncoding RNA-gm37494-ADSCs repair spinal cord injury via shifting microglial M1/M2 polarization. Inflammation (2020) 43(4):1536–47. doi: 10.1007/s10753-020-01230-z
157. Liu W, Rong Y, Wang J, Zhou Z, Ge X, Ji C, et al. Exosome-shuttled miR-216a-5p from hypoxic preconditioned mesenchymal stem cells repair traumatic spinal cord injury by shifting microglial M1/M2 polarization. J Neuroinflammation. (2020) 17(1):47. doi: 10.1186/s12974-019-1653-7
158. Collino F, Lopes JA, Corrêa S, Abdelhay E, Takiya CM, Wendt CHC, et al. Adipose-derived mesenchymal stromal cells under hypoxia: changes in extracellular vesicles secretion and improvement of renal recovery after ischemic injury. Cell Physiol Biochem (2019) 52(6):1463–83. doi: 10.33594/000000102
159. Miceli V, Pampalone M, Vella S, Carreca AP, Amico G, Conaldi PG. Comparison of immunosuppressive and angiogenic properties of human amnion-derived mesenchymal stem cells between 2D and 3D culture systems. Stem Cells Int (2019) 2019:e7486279. doi: 10.1155/2019/7486279
160. Xie AW, Zacharias NA, Binder BYK, Murphy WL. Controlled aggregation enhances immunomodulatory potential of mesenchymal stromal cell aggregates. Stem Cells Trans Med (2021) 10(8):1184–201. doi: 10.1002/sctm.19-0414
161. Regmi S, Seo Y, Ahn JS, Pathak S, Acharya S, Nguyen TT, et al. Heterospheroid formation improves therapeutic efficacy of mesenchymal stem cells in murine colitis through immunomodulation and epithelial regeneration. Biomaterials (2021) 271:120752. doi: 10.1016/j.biomaterials.2021.120752
162. Kim JY, Rhim WK, Cha SG, Woo J, Lee JY, Park CG, et al. Bolstering the secretion and bioactivities of umbilical cord MSC-derived extracellular vesicles with 3D culture and priming in chemically defined media. Nano Convergence. (2022) 9(1):57. doi: 10.1186/s40580-022-00349-z
163. Kusuma GD, Li A, Zhu D, McDonald H, Inocencio IM, Chambers DC, et al. Effect of 2D and 3D culture microenvironments on mesenchymal stem cell-derived extracellular vesicles potencies. Front Cell Dev Biol (2022) 10:819726. doi: 10.3389/fcell.2022.819726
164. Yuan X, Sun L, Jeske R, Nkosi D, York SB, Liu Y, et al. Engineering extracellular vesicles by three-dimensional dynamic culture of human mesenchymal stem cells. J Extracell Vesicles. (2022) 11(6):e12235. doi: 10.1002/jev2.12235
165. Cao J, Wang B, Tang T, Lv L, Ding Z, Li Z, et al. Three-dimensional culture of MSCs produces exosomes with improved yield and enhanced therapeutic efficacy for cisplatin-induced acute kidney injury. Stem Cell Res Ther (2020) 11(1):206. doi: 10.1186/s13287-020-01719-2
166. Sun L, Ji Y, Chi B, Xiao T, Li C, Yan X, et al. A 3D culture system improves the yield of MSCs-derived extracellular vesicles and enhances their therapeutic efficacy for heart repair. Biomedicine Pharmacotherapy. (2023) 161:114557. doi: 10.1016/j.biopha.2023.114557
167. Andrews S, Maughon T, Marklein R, Stice S. Priming of MSCs with inflammation-relevant signals affects extracellular vesicle biogenesis, surface markers, and modulation of T cell subsets. J Immunol Regenerative Med (2021) 13:100036. doi: 10.1016/j.regen.2020.100036
168. Hyland M, Mennan C, Davies R, Wilson E, Tonge DP, Clayton A, et al. Extracellular vesicles derived from umbilical cord mesenchymal stromal cells show enhanced anti-inflammatory properties via upregulation of miRNAs after pro-inflammatory priming. Stem Cell Rev Rep (2023) 19(7):2391–406. doi: 10.1007/s12015-023-10586-2
169. Rozier P, Maumus M, Maria ATJ, Toupet K, Jorgensen C, Guilpain P, et al. Lung fibrosis is improved by extracellular vesicles from IFNγ-primed mesenchymal stromal cells in murine systemic sclerosis. Cells (2021) 10(10):2727. doi: 10.3390/cells10102727
170. Hyland M, Mennan C, Wilson E, Clayton A, Kehoe O. Pro-inflammatory priming of umbilical cord mesenchymal stromal cells alters the protein cargo of their extracellular vesicles. Cells (2020) 9(3):726. doi: 10.3390/cells9030726
171. Peltzer J, Lund K, Goriot ME, Grosbot M, Lataillade JJ, Mauduit P, et al. Interferon-γ and hypoxia priming have limited effect on the miRNA landscape of human mesenchymal stromal cells-derived extracellular vesicles. Front Cell Dev Biol (2020) 8:581436. doi: 10.3389/fcell.2020.581436
172. Gorgun C, Ceresa D, Lesage R, Villa F, Reverberi D, Balbi C, et al. Dissecting the effects of preconditioning with inflammatory cytokines and hypoxia on the angiogenic potential of mesenchymal stromal cell (MSC)-derived soluble proteins and extracellular vesicles (EVs). Biomaterials (2021) 269:120633. doi: 10.1016/j.biomaterials.2020.120633
173. Zang H, Wang Z, Wu Q, Shi L, Chen G. Effect of hypoxia on the expression of microRNA in extracellular vesicles of human umbilical cord stem cells in vitro. Cell Tissue Bank. (2023) 24(4):769–78. doi: 10.1007/s10561-023-10095-z
174. Jin P, Zhao Y, Liu H, Chen J, Ren J, Jin J, et al. Interferon-γ and tumor necrosis factor-α Polarize bone marrow stromal cells uniformly to a th1 phenotype. Sci Rep (2016) 6(1):26345. doi: 10.1038/srep26345
175. Bai H, Wang Y, Zhao Y, Chen X, Xiao Y, Bao C. HIF signaling: A new propellant in bone regeneration. Biomaterials Advances. (2022) 138:212874. doi: 10.1016/j.bioadv.2022.212874
176. Fábián Z. The effects of hypoxia on the immune-modulatory properties of bone marrow-derived mesenchymal stromal cells. Stem Cells Int (2019) 2019:e2509606. doi: 10.1155/2019/2509606
177. Wu J, Huang QM, Liu Y, Zhou J, Tang WR, Wang XY, et al. Long-term hypoxic hUCMSCs-derived extracellular vesicles alleviates allergic rhinitis through triggering immunotolerance of their VEGF-mediated inhibition of dendritic cells maturation. Int Immunopharmacology. (2023) 124:110875. doi: 10.1016/j.intimp.2023.110875
178. Fuentes P, Torres MJ, Arancibia R, Aulestia F, Vergara M, Carrión F, et al. Dynamic culture of mesenchymal stromal/stem cell spheroids and secretion of paracrine factors. Front Bioeng Biotechnol (2022) 10:916229. doi: 10.3389/fbioe.2022.916229
179. Bulati M, Gallo A, Zito G, Busà R, Iannolo G, Cuscino N, et al. 3D culture and interferon-γ Priming modulates characteristics of mesenchymal stromal/stem cells by modifying the expression of both intracellular and exosomal microRNAs. Biology (2023) 12(8):1063. doi: 10.3390/biology12081063
180. Perut F, Roncuzzi L, Baldini N. The emerging roles of extracellular vesicles in osteosarcoma. Front Oncol (2019) 9:1342. doi: 10.3389/fonc.2019.01342
181. Evdokimova V, Gassmann H, Radvanyi L, Burdach SEG. Current state of immunotherapy and mechanisms of immune evasion in ewing sarcoma and osteosarcoma. Cancers (2023) 15(1):272. doi: 10.3390/cancers15010272
182. Gassmann H, Schneider K, Evdokimova V, Ruzanov P, Schober SJ, Xue B, et al. Ewing sarcoma-derived extracellular vesicles impair dendritic cell maturation and function. Cells (2021) 10(8):2081. doi: 10.3390/cells10082081
183. Droste M, Thakur BK, Eliceiri BP. Tumor-derived extracellular vesicles and the immune system—Lessons from immune-competent mouse-tumor models. Front Immunol (2020) 11:606859. doi: 10.3389/fimmu.2020.606859
184. Pereira J, Alfaro G, Goycoolea M, Quiroga T, Ocqueteau M, Massardo L, et al. Circulating platelet-derived microparticles in systemic lupus erythematosus: Association with increased thrombin generation and procoagulant state. Thromb Haemost. (2006) 95(01):94–9. doi: 10.1160/TH05-05-0310
185. Sellam J, Proulle V, Jüngel A, Ittah M, Miceli Richard C, Gottenberg JE, et al. Increased levels of circulating microparticles in primary Sjögren’s syndrome, systemic lupus erythematosus and rheumatoid arthritis and relation with disease activity. Arthritis Res Ther (2009) 11(5):R156. doi: 10.1186/ar2833
186. Duval A, Helley D, Capron L, Youinou P, Renaudineau Y, Dubucquoi S, et al. Endothelial dysfunction in systemic lupus patients with low disease activity: evaluation by quantification and characterization of circulating endothelial microparticles, role of anti-endothelial cell antibodies. Rheumatology (2010) 49(6):1049–55. doi: 10.1093/rheumatology/keq041
187. Mobarrez F, Vikerfors A, Gustafsson JT, Gunnarsson I, Zickert A, Larsson A, et al. Microparticles in the blood of patients with systemic lupus erythematosus (SLE): phenotypic characterization and clinical associations. Sci Rep (2016) 6(1):36025. doi: 10.1038/srep36025
188. Lee JY, Park JK, Lee EY, Lee EB, Song YW. Circulating exosomes from patients with systemic lupus erythematosus induce a proinflammatory immune response. Arthritis Res Ther (2016) 18(1):264. doi: 10.1186/s13075-016-1159-y
189. López P, Rodríguez-Carrio J, Martínez-Zapico A, Caminal-Montero L, Suárez A. Circulating microparticle subpopulations in systemic lupus erythematosus are affected by disease activity. Int J Cardiol (2017) 236:138–44. doi: 10.1016/j.ijcard.2017.02.107
190. Nielsen CT, Østergaard O, Johnsen C, Jacobsen S, Heegaard NHH. Distinct features of circulating microparticles and their relationship to clinical manifestations in systemic lupus erythematosus: Plasma Microparticles in SLE. Arthritis Rheumatism. (2011) 63(10):3067–77. doi: 10.1002/art.30499
191. Østergaard O, Nielsen CT, Iversen LV, Tanassi JT, Knudsen S, Jacobsen S, et al. Unique protein signature of circulating microparticles in systemic lupus erythematosus. Arthritis Rheumatism. (2013) 65(10):2680–90. doi: 10.1002/art.38065
192. Chuang H, Chen M, Chen Y, Ciou Y, Hsueh C, Tsai C, et al. Induction of interferon-γ and tissue inflammation by overexpression of eosinophil cationic protein in T cells and exosomes. Arthritis Rheumatol (2022) 74(1):92–104. doi: 10.1002/art.41920
193. Li W, Liu S, Chen Y, Weng R, Zhang K, He X, et al. Circulating exosomal microRNAs as biomarkers of systemic lupus erythematosus. Clinics (2020) 75:e1528. doi: 10.6061/clinics/2020/e1528
194. Dong C, Zhou Q, Fu T, Zhao R, Yang J, Kong X, et al. Circulating exosomes derived-miR-146a from systemic lupus erythematosus patients regulates senescence of mesenchymal stem cells. BioMed Res Int (2019) 2019:1–10. doi: 10.1155/2019/6071308
195. Perez-Hernandez J, Forner MJ, Pinto C, Chaves FJ, Cortes R, Redon J. Increased urinary exosomal microRNAs in patients with systemic lupus erythematosus. Alvarez ML editor. PloS One (2015) 10(9):e0138618. doi: 10.1371/journal.pone.0138618
196. Tan L, Zhao M, Wu H, Zhang Y, Tong X, Gao L, et al. Downregulated serum exosomal miR-451a expression correlates with renal damage and its intercellular communication role in systemic lupus erythematosus. Front Immunol (2021) 12:630112. doi: 10.3389/fimmu.2021.630112
197. Salvi V, Gianello V, Busatto S, Bergese P, Andreoli L, D’Oro U, et al. Exosome-delivered microRNAs promote IFN-α secretion by human plasmacytoid DCs via TLR7. JCI Insight (2018) 3(10):e98204. doi: 10.1172/jci.insight.98204
198. Wang D, Zhang H, Liang J, Wang H, Hua B, Feng X, et al. A long-term follow-up study of allogeneic mesenchymal stem/stromal cell transplantation in patients with drug-resistant systemic lupus erythematosus. Stem Cell Rep (2018) 10(3):933–41. doi: 10.1016/j.stemcr.2018.01.029
199. Wen L, Labopin M, Badoglio M, Wang D, Sun L, Farge-Bancel D. Prognostic factors for clinical response in systemic lupus erythematosus patients treated by allogeneic mesenchymal stem cells. Stem Cells Int (2019) 2019:e7061408. doi: 10.1155/2019/7061408
200. Sharma J, Hampton JM, Valiente GR, Wada T, Steigelman H, Young MC, et al. Therapeutic development of mesenchymal stem cells or their extracellular vesicles to inhibit autoimmune-mediated inflammatory processes in systemic lupus erythematosus. Front Immunol (2017) 8:526. doi: 10.3389/fimmu.2017.00526
201. Xie M, Li C, She Z, Wu F, Mao J, Hun M, et al. Human umbilical cord mesenchymal stem cells derived extracellular vesicles regulate acquired immune response of lupus mouse in vitro. Sci Rep (2022) 12(1):13101. doi: 10.1038/s41598-022-17331-8
202. Sun W, Yan S, Yang C, Yang J, Wang H, Li C, et al. Mesenchymal stem cells-derived exosomes ameliorate lupus by inducing M2 macrophage polarization and regulatory T cell expansion in MRL/lpr mice. Immunol Investigations. (2022) 51(6):1785–803. doi: 10.1080/08820139.2022.2055478
203. Zhang M, Johnson-Stephenson TK, Wang W, Wang Y, Li J, Li L, et al. Mesenchymal stem cell-derived exosome-educated macrophages alleviate systemic lupus erythematosus by promoting efferocytosis and recruitment of IL-17+ regulatory T cell. Stem Cell Res Ther (2022) 13(1):484. doi: 10.1186/s13287-022-03174-7
204. Sonoda S, Murata S, Kato H, Zakaria F, Kyumoto-Nakamura Y, Uehara N, et al. Targeting of deciduous tooth pulp stem cell–derived extracellular vesicles on telomerase-mediated stem cell niche and immune regulation in systemic lupus erythematosus. J Immunol (2021) 206(12):3053–63. doi: 10.4049/jimmunol.2001312
205. Wang R, Hao M, Kou X, Sui B, Sanmillan ML, Zhang X, et al. Apoptotic vesicles ameliorate lupus and arthritis via phosphatidylserine-mediated modulation of T cell receptor signaling. Bioactive Materials. (2023) 25:472–84. doi: 10.1016/j.bioactmat.2022.07.026
206. Dou R, Zhang X, Xu X, Wang P, Yan B. Mesenchymal stem cell exosomal tsRNA-21109 alleviate systemic lupus erythematosus by inhibiting macrophage M1 polarization. Mol Immunol (2021) 139:106–14. doi: 10.1016/j.molimm.2021.08.015
207. Chen X, Wei Q, Sun H, Zhang X, Yang C, Tao Y, et al. Exosomes derived from human umbilical cord mesenchymal stem cells regulate macrophage polarization to attenuate systemic lupus erythematosus-associated diffuse alveolar hemorrhage in mice. Int J Stem Cells (2021) 14(3):331–40. doi: 10.15283/ijsc20156
208. Tu J, Zheng N, Mao C, Liu S, Zhang H, Sun L. UC-BSCs Exosomes Regulate Th17/Treg Balance in Patients with Systemic Lupus Erythematosus via miR-19b/KLF13. Cells (2022) 11(24):4123. doi: 10.3390/cells11244123
209. Zhao Y, Song W, Yuan Z, Li M, Wang G, Wang L, et al. Exosome derived from human umbilical cord mesenchymal cell exerts immunomodulatory effects on B cells from SLE patients. J Immunol Res (2023) 2023:e3177584. doi: 10.1155/2023/3177584
210. Chen M, Peng J, Xie Q, Xiao N, Su X, Mei H, et al. Mesenchymal stem cells alleviate moderate-to-severe psoriasis by reducing the production of type I interferon (IFN-I) by plasmacytoid dendritic cells (pDCs). Stem Cells Int (2019) 2019:e6961052. doi: 10.1155/2019/6961052
Keywords: extracellular vesicles, secretome, immune regulation, mesenchymal stromal cell, priming, systemic lupus erythematosus
Citation: Wong C, Stoilova I, Gazeau F, Herbeuval J-P and Fourniols T (2024) Mesenchymal stromal cell derived extracellular vesicles as a therapeutic tool: immune regulation, MSC priming, and applications to SLE. Front. Immunol. 15:1355845. doi: 10.3389/fimmu.2024.1355845
Received: 14 December 2023; Accepted: 24 January 2024;
Published: 08 February 2024.
Edited by:
Chun Wai Mai, UCSI University, MalaysiaReviewed by:
Noa B Martin-Cofreces, Princess University Hospital, SpainCopyright © 2024 Wong, Stoilova, Gazeau, Herbeuval and Fourniols. This is an open-access article distributed under the terms of the Creative Commons Attribution License (CC BY). The use, distribution or reproduction in other forums is permitted, provided the original author(s) and the copyright owner(s) are credited and that the original publication in this journal is cited, in accordance with accepted academic practice. No use, distribution or reproduction is permitted which does not comply with these terms.
*Correspondence: Jean-Philippe Herbeuval, amVhbi1waGlsaXBwZS5oZXJiZXV2YWxAcGFyaXNkZXNjYXJ0ZXMuZnI=; Thibaut Fourniols, dGhpYmF1dC5mb3VybmlvbHNAZXZlcnpvbS5jb20=
†These authors have contributed equally to this work and share last authorship
Disclaimer: All claims expressed in this article are solely those of the authors and do not necessarily represent those of their affiliated organizations, or those of the publisher, the editors and the reviewers. Any product that may be evaluated in this article or claim that may be made by its manufacturer is not guaranteed or endorsed by the publisher.
Research integrity at Frontiers
Learn more about the work of our research integrity team to safeguard the quality of each article we publish.