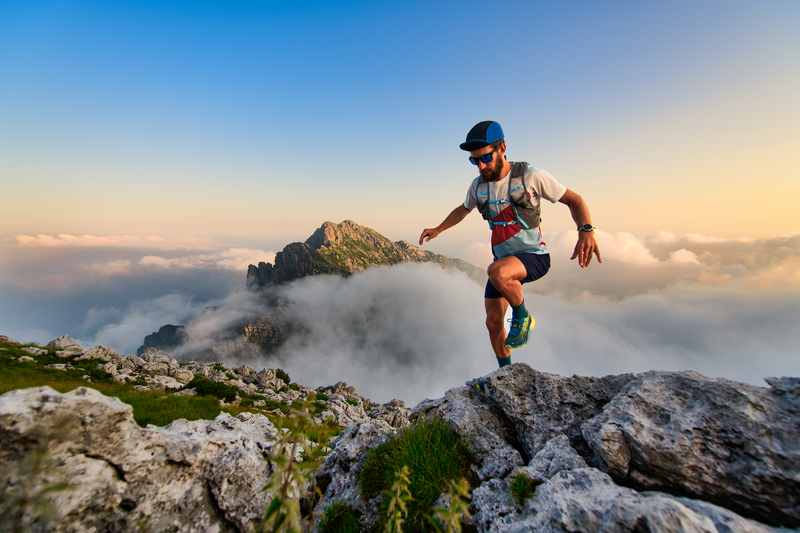
94% of researchers rate our articles as excellent or good
Learn more about the work of our research integrity team to safeguard the quality of each article we publish.
Find out more
REVIEW article
Front. Immunol. , 14 February 2024
Sec. Comparative Immunology
Volume 15 - 2024 | https://doi.org/10.3389/fimmu.2024.1349428
The midgut, a vital component of the digestive system in arthropods, serves as an interface between ingested food and the insect’s physiology, playing a pivotal role in nutrient absorption and immune defense mechanisms. Distinct cell types, including columnar, enteroendocrine, goblet and regenerative cells, comprise the midgut in insects and contribute to its robust immune response. Enterocytes/columnar cells, the primary absorptive cells, facilitate the immune response through enzyme secretions, while regenerative cells play a crucial role in maintaining midgut integrity by continuously replenishing damaged cells and maintaining the continuity of the immune defense. The peritrophic membrane is vital to the insect’s innate immunity, shielding the midgut from pathogens and abrasive food particles. Midgut juice, a mixture of digestive enzymes and antimicrobial factors, further contributes to the insect’s immune defense, helping the insect to combat invading pathogens and regulate the midgut microbial community. The cutting-edge single-cell transcriptomics also unveiled previously unrecognized subpopulations within the insect midgut cells and elucidated the striking similarities between the gastrointestinal tracts of insects and higher mammals. Understanding the intricate interplay between midgut cell types provides valuable insights into insect immunity. This review provides a solid foundation for unraveling the complex roles of the midgut, not only in digestion but also in immunity. Moreover, this review will discuss the novel immune strategies led by the midgut employed by insects to combat invading pathogens, ultimately contributing to the broader understanding of insect physiology and defense mechanisms.
In insects, the gastrointestinal tract, despite variations in its morphology (due to differences in feeding habits), comprises a monolayer of epithelial cells surrounded by visceral muscles. This intricate anatomical structure is divided into three discrete regions - the fore, mid, and hindgut (Figure 1), each characterized by distinct attributes, roles, and embryonic origin (1). In Drosophila and silkworms, the midgut is a complex tissue bearing a striking resemblance to its mammalian counterpart (2). Its multifaceted functions include digestion, immune responses, the regeneration of the aged cells, and recovery of the infected luminal tract.
Figure 1 Schematic representation of midgut cell types. (A) Mature larvae, (B) The midgut, (C) Different types of midgut cells in silkworm (D) Different roles of enterocyte due to the presence of various organelles. Secretory enterocytes in anterior midgut contain secretory organelles such as endoplasmic reticulum and lysosomes whereas, absorptive enterocytes located in the posterior midgut have absorptive organelles like microvilli and infoldings to support the absorption of the nutrients.
The second-largest organ within the insect body is involved in digestion-related activities and holds critical importance in the insect’s defense against pathogens (3). It serves as the initial line of defense against invading pathogens. One of the most conspicuous aspects of the midgut’s immune function lies in its ability to produce antimicrobial peptides (AMPs) and reactive oxygen species (ROS) (4, 5). These molecular weapons are integral components of the insect’s defense arsenal to combat and neutralize potential threats from invading pathogens. The production of AMPs is a particularly significant aspect of this defense mechanism, as these small yet potent molecules possess the ability to target a wide array of invading microorganisms (4). Researchers have dedicated significant efforts to unravel the intricate mechanisms underlying AMP production and to isolate novel AMPs (6).
Beyond its role in AMP production, the midgut also plays a crucial part in maintaining the intricate balance of gut microbes. To maintain this delicate equilibrium, the midgut expels disease-related microbes and pathogens (7), preserving a beneficial microbial community. The unique immunological features of the midgut render it a subject of considerable scientific interest and investigation. This ongoing pursuit of knowledge concerning the midgut’s immune functions is invaluable, as it contributes to a deeper understanding of silkworm’s immunity. Ultimately, these insights not only enhance our comprehension of silkworm biology but also hold the potential to inform broader discussions on immunity across various biological systems.
Further, the utilization of Bomby mori as a model organism offers numerous advantages for scientific research (8). Notably, a straightforward and well-characterized genome sequence has facilitated genetic manipulations and studies on gene functions and regulations (9). The short life cycle, progressing through stages in a matter of weeks and big progeny size, enables rapid experimentation and observation (Figure 2). Silkworms are easy to handle and can be maintained in laboratory conditions with a simple diet of mulberry leaves. Additionally, the large size of silkworm eggs and the transparency of their pupal stage enhance experimental accessibility (10). Their well-defined developmental stages, shared biological processes with other insects, and certain similarities to mammals further elucidate the value of silkworms as versatile model organisms across various research disciplines (11).
The advent of single-cell RNA sequencing technology (scRNA-seq) has revolutionized our understanding of gene regulation at the single-cell level by scrutinizing the gene expression profiles of thousands of individual cells, thus facilitating the identification of previously unknown cell types and their unique physiological states (12). Moreover, it has provided a means to unravel the intricate developmental trajectories of cells, shedding light on their origins and evolutionary pathways (12). Recent transcriptomic investigations have unveiled different cell types in the midgut in Drosophila (2, 13) mosquitoes (14) and silkworms (15), and a compelling narrative, revealing that the insect midgut, exemplified by Drosophila and silkworms, closely related to the mammalian small intestine in terms of the cell types present and their associated marker gene expression patterns (2). Table 1 summarizes well-established cell type markers from scRNA-seq analysis of midgut tissues in Drosophila, mosquitoes, silkworms and mice. In the model insect Drosophila, the midgut undergoes renewal at regular intervals of one to two weeks, facilitated by specialized regenerative cells known as intestinal stem cells (ISCs) (17). These ISCs exhibit similarities with mammalian transit-amplifying cells (TACs) in the G2 phase of the cell cycle. Additionally, within the midgut milieu in insects, there are endocrine cells, enterocytes, and enteroblasts, all of which share akin marker gene expression patterns when compared with their counterparts in mammals (2). In Drosophila, enteroendocrine cells (EEs) mirror their mammalian counterparts, while goblet cells found in lepidopteran midguts resemble mammalian goblet cells. Furthermore, cardia cells within the Drosophila midgut exhibit marked similarities in marker expression profiles with the goblet cells of mammals (2). Similar observations were reported by Xia et al. in a recent study, wherein diverse cell types identified in the silkworm midgut exhibited nearly identical patterns of marker gene expression (15). This convergence extends to the functional domain, where homologous marker genes underscore numerous facets of midgut function. These findings shed light on the remarkable conservation and convergent evolution of midgut biology across diverse taxa, underscoring its significance in understanding insect physiology and potential implications for our comprehension of mammalian gastrointestinal biology.
Regarding the origin of different types of cells, recent studies have revealed that the self-renewable ISCs similar to TACs in mammals raise enteroblasts (EBs) or enteroendocrine progenitor cells (EEPs), and the notch signaling pathway controls this determination. Heightened notch signaling pathway directs ISCs toward a developmental trajectory culminating in the formation of absorptive enterocytes (ISC → EB → EC) (18, 19). In contrast, when notch signaling is maintained at a lower level, ISCs follow an alternative route, leading to the generation of hormone-secreting enteroendocrine cells (ISC → EEP → EEs) (20). This finely regulated process of cellular fate determination plays a critical role in regulating the composition and functional dynamics of the insect midgut.
This review will discuss a concise yet comprehensive overview of the cell-level functionalities of the insect midgut, with a particular focus on the silkworm, from the insights derived from advanced transcriptomic studies. Additionally, we discuss the role of various midgut cell types in the immune defense mechanisms against pathogens. This integrated approach aims to provide readers with a coherent and deep understanding of the immunologically active organ, the insect midgut.
Columnar cells (CCs) are pivotal among the four primary cellular phenotypes within the insect midgut (Figure 1) (7). They are referred to as “enterocytes” by the feature of their akin absorptive functionalities to their mammalian small intestinal counterparts (21–23). Beyond their absorptive nature, these CCs prominently orchestrate the synthesis and secretion of digestive enzymes, thereby underscoring their indispensable contribution to digestive processes within the insect midgut environment (24). The distinct anatomical feature of CCs includes the centrally located nucleus accompanied by a prominently convoluted apical membrane, giving rise to microvilli with an inherent actin cytoskeletal structure (Figure 1) (25). In addition to the common characteristics inherent to CCs across insect species, there exist specialized ultrastructural features that are discernible within distinct regions of the midgut and feeding behaviors of insects (26–29). The ultrastructural composition of CCs within the midgut exhibits a transition along the longitudinal axis, displaying distinct roles in the shifting anatomical context (21). Structural evidence highlights that CCs in the anterior midgut are responsible for secreting an array of digestive enzymes encompassing exopeptidases, maltases, endopeptidases, amylases, lipases, and lysozymes (Figure 1) (24, 30). These enzymes are secreted into the midgut lumen via conventional exocytosis and through mechanisms involving apocrine or micro-apocrine modes of secretion (30). Conversely, CCs within the posterior midgut region are characterized by their involvement in synthesizing serine proteases (31–33). Given that digestive enzymes play a direct and crucial role in facilitating nutrient accessibility and development (34, 35), the production and activities of these enzymes are regulated by a rigorous regulatory framework. Multiple factors, encompassing the composition of nutrients, signals from endocrine and neuronal sources, and interactions with gut microorganisms, collectively contribute to the intricate modulation of enzymatic processes underlying nutrient digestion and absorption (36–39). Nutrient absorption within CCs is executed through the activity of transport proteins, which exhibit distinct distribution patterns upon the regionalization of the midgut (Figure 1) (32, 40). The Na+/K+ ATPase channel for absorption is absent in CCs, and the cotransporters of CCs capitalize on the electrochemical gradient of K+ rather than that of Na+ as a driving ion. The amino acids from protein digestion are translocated from the lumen into the intracellular cytoplasm, marking the specialized functionality of CCs in nutrient absorption (41, 42).
CCs play a multifaceted role in immunity and nutritional uptake. These cells secrete enzymes that have been demonstrated to have a significant role in immunity within various insect species. For instance, the lysozymes secreted by CCs regulate the microbial load across distinct midgut regions in synergy with the alkaline environment (43). A myriad of proteases is secreted into the lumen of the insect gut by CCs. Alongside their primary function of enzymatic breaking down of ingested food, they also play a critical role in degrading proteins linked to invading pathogens. Importantly, these proteases possess the ability to target the proteins of such pathogens. This includes the structural components of virions and their surface proteins crucial for initiating cellular infection (44, 45). Bm-SP142 is a 35 kDa protease mainly expressed in the middle part of the silkworm midgut, effectively impairs Bombyx mori nuclear polyhedrosis virus (BmNPV)’s ability to infect BmN cells. Furthermore, Bm-SP142 has demonstrated its efficacy in diminishing the propagation efficiency of both BmNPV and Bombyx mori bidensovirus (BmBDV) within silkworms, indicating the antiviral potential of proteases secreted by CCs (46). It was reported that a midgut serine protease, the alkaline digestive enzyme trypsin termed Alkaline A (BmTA), has antiviral potential against BmNPV. The proteomic analysis elucidated a substantially high level of BmTA in the resistant silkworm larvae compared to the susceptible. Viral analysis showed a decreased level of the Vp39 gene in the recombinant-BmTA-treated group (47).
The secretion of the signaling protein Hedgehog has been well-documented in both Drosophila and B. mori (48, 49). Following infection by Escherichia coli or Bacillus thuringiensis, a notable proliferation of midgut cells and upregulation of Hedgehog genes have been observed in the silkworm. When genes associated with the Hedgehog pathway were experimentally silenced, cell growth was suppressed, indicating the pathway’s regulatory role (49). These findings provide insights into the critical role of signaling proteins in immunity against pathogens. Notably, in Drosophila, these proteins exhibit a connection with nutritional availability and developmental process as these play a role in the delayed pupation observed in starved flies, attributed to their capacity to inhibit ecdy-streroid production due to a reduction in the transcription of genes linked to enzyme production related to molting (48).
Given the direct correlation between nutrient availability, growth, and development, which collectively underpin animal survival (50–52), it becomes evident that CCs from the midgut engage in communication to synchronize responses and evade the deleterious effects of invading pathogens. This interplay serves as a strategic mechanism to counteract the pathogenic influences. CCs’ the crucial components of midgut epithelium, have a dynamic role in insect health and survival, serving as a critical nexus between digestion and immunity.
Nutrient uptake and digestion are the critical functions performed by the insect midgut. Along with these vital physiological phenomena’s essential for growth and development, another critical function performed by the gut is the maintenance of gut homeostasis. The homeostasis is maintained through the complex gut-brain axis, forming a neurohumoral communication system. Thanks to midgut EEs, which secrete biologically active peptides responsible for the sophisticated interplay of communication within the organism (38, 53, 54). Table 2 summarize some important peptides secreted by the EEs. EEs discovered around three decades ago (64–66), have two distinct morphological forms, open and closed types (67), characterized by their peptidergic nature (64); the former have direct contact with the midgut lumen (67, 68), while the latter lacks such interaction as they do not extend through the epithelium (Figure 1) (33, 67). The functional application of recent genomic and proteomic approaches provides compelling evidence of the critical role of EEs within the insect midgut play in the physiology and developmental processes of insects (56, 69, 70). The EEs of silkworms were classified into four distinct subtypes according to their spatial distribution. Type I cells were identified across all three midgut regions, while type II, III and IV cells were localized in the anterior, middle, and posterior midgut, respectively (56). The spatial distribution of EEs across different midgut regions also depends on the specific peptide they produce (17, 71). The peptides are transformed into active states by cleavage and post-translation modification from protein precursors produced by EEs. In Drosophila, 9 major precursors were identified (58), whereas in silkworms, 18 distinct protein precursors were identified (56). In different insects, the peptides produced after cleavage are mainly involved in the regulation of food in the alimentary canal, from reduced ion transport to suppressed gut contractions (72, 73). In B. mori tachykinin peptides (Tk) secretions are regulated by B. mori gustatory receptor (BmGr4) expressed in some Tk-producing EEs in the anterior midgut when food and digestive products arrived after feeding began (60). The regulatory role of peptides secreted by EEs is evident in locusts and Drosophila. Notably, in the midgut of starved locusts, the levels of Tk diminish while their circulation in the hemolymph increases, suggesting a potential responsiveness to nutritional status for enhanced food uptake (74). Further insights have emerged from studies in Drosophila, demonstrating that the EE-produced Tks contributes to lipid metabolism through interaction with their receptor TKR99D situated in the brain (61). Targeted ablation of EEs expressing TKs resulted in the suppression of intestinal stem cell proliferation, indicating the developmental role of these peptides (37).
Few of the immunologically important peptides produced by the EEs are enlisted in the Table 2. Allatostatins, initially identified in cockroaches, are produced by insect brain and midgut EEs (75). The midgut of silkworms displays a pronounced enrichment of allatostatin receptors compared to the brain (76). These peptides play a crucial role in the development and secretion of juvenile hormone (55). Their involvement in immune functions has been documented in the well-studied model organism Drosophila (77). In red imported fire ants, an upregulation of allatostatin A2 expression was observed in response to Solenopsis invicta virus-3 (SINV-3) infection. The elevated expression of the peptide consequently leads to sharp production of the AMP cecropinA1, serving as an immune strategy (57). The midgut EEs produce another bioactive peptide known as diuretic hormone 31, which exhibits an immune response when faced with bacterial infection. ROS accumulates within the midgut lumen in a manner contingent on the transient receptor potential ankyrin 1 (TRPA1) receptor. This receptor activation enables EEs to release the diuretic hormone and its receptor in the neighboring visceral muscles, initiating the contraction of longitudinal visceral muscles. As a consequence, the expulsion of the pathogen from the midgut is facilitated.
EEs secrete peptides, which are also released from neurons (70, 78), posing a challenge in precisely attributing roles to EEs. Notably, midgut EEs are postulated to release peptides that exert paracrine effects on adjacent cells. While extensive research investigations are done to elaborate the EEs functions in development and immunity using model insects, a significant knowledge gap exists in comprehending the regulatory mechanisms governing EE-derived peptides in other insects, such as silkworms. Molecular and functional analyses utilizing cutting-edge techniques are imperative to elucidate the regulatory role of peptides comprehensively. These investigations can potentially unravel the intricate landscape of midgut physiology and insect homeostasis. Moreover, they offer insights into how the second-largest organ in insects contributes to immune defense against invading pathogens. This pursuit advances our fundamental understanding of endocrine activity from a molecular perspective and sheds light on broader physiological implications.
Lepidopteran insect’s midgut possesses a distinctive category of cells known as goblet cells (GCs) (21, 23, 79, 80). These GCs, in conjunction with the midgut’s CCs, play a pivotal role in the elevated pH of the midgut environment. These insects primarily feed on plant matter; their food encompasses secondary metabolites, such as tannins and phenols, which can be detrimental. The presence of these secondary metabolites diminishes nutrient accessibility, as they form insoluble complexes via cross-linking with enzymes and proteins. To counteract this constrained nutrient availability, the coordinated efforts of GCs and CCs come into play, preserving an elevated pH within the midgut lumen. GCs are characterized by a conspicuous chalice-shaped central cavity, which originates from the invagination of the apical membrane (Figure 1) (21, 22, 81, 82). Their distribution within the midgut varies according to their respective locations, resulting in heterogeneous concentrations (21). These GCs establish intricate connections with other midgut cells, employing septate and gap junction formations to facilitate intercellular communication and coordination (83). The chalice-shaped central cavity (Goblet cell cavity, GCC) is lined by small structures known as microvilli, and these microvilli are longer at the basal portion of GCC than the apical portion, providing extensive surface area. These microvilli are surrounded by actin. The GCC content is effectively separated from the intestinal lumen through a sophisticated apical structure called a valve. This valve consists of densely packed microvilli lacking mitochondria, which do not allow the free movement of the content (82). GCs play a pivotal role in preserving the distinctive characteristic of elevated alkaline pH within the midgut of these insects. This distinct milieu is upheld through the presence of the vacuolar-type proton pump (V-H+ ATPase) (82, 84, 85). This pump is actively engaged in the transportation of protons (H+) from the cytoplasm into the interior of GCCs (86, 87) at the expense of energy against the concentration gradient (82). Mitochondria located within the microvilli supply the requisite energy for this process. The active translocation of H+ is instrumental in the preservation of a transmembrane electrical potential of 150 mV (88). The V-H+ ATPase pump orchestrates an intricate exchange between H+ and K+, resulting in the net flow of K+ from the cytoplasm of GCs into the interior of the GCCs (81). This establishes an electrochemical gradient conducive to the efflux of K+ from the GCCs into the lumen of the midgut via a specialized valve. This transport phenomenon is augmented by the activity of GCs carbonic anhydrase, which facilitates the generation of a flux of HCO3- (81) This flux-causing K+ transport is essential in maintaining the elevated pH within the midgut.
GCs within the insect alimentary tract are similar to their mammalian small intestinal counterparts, notably evidenced by large cavities embellished with microvilli (2, 89). The distinctive hallmark of the mammalian intestinal goblet cells is the production of mucus, forming a protective layer. This layer is critical in shielding the intestinal milieu from the abrasive action of ingested food and invading pathogens (90). Similar observations related to mucin secretion and the associated epithelial immune competence have been documented within fishes. Goblet cells encompass a substantial proportion of the epithelial constitution in fish (91). Notably, these cells evince an increase in size following exposure to microplastics (MPs), indicative of escalated mucus production. This phenomenon, in turn, confers enhanced epithelial protection against the abrasive effects exerted by MPs (92). The macromolecular composition of mucous secretions encompasses essential constituents like mucin proteins (93) alongside immunomodulatory proteins (94). This collective evidential paradigm underscores the multifarious contributions of goblet cells and their mucous effluents toward maintaining epithelial integrity and immune homeostasis (95). The histological resemblance observed among the GCs in mammals, fishes, and insects suggests a potential similarity in the functional roles attributed to these specialized insect cells. Several scientific investigations have revealed the significant contribution of GCs to immunity in mammals and fishes. Consequently, a conjecture arises that these cells might potentially serve an immunological role in insects, specifically lepidopteran insects. Despite relatively limited research, studies have begun to shed light on the involvement of GCs in immunity, exemplified by their response in instances such as that observed in Helicoverpa armigera. When the larvae of H. armigera were subjected to an infection by the cytoplasmic polyhedrosis virus, a typical pathogen targeting the midgut, a visible deformation in the midgut’s structural architecture emerged. This perturbation was accompanied by the emergence of anomalous microvilli projections subsequent to the viral infection (96).The GCs, historically linked with mucin secretion and maintenance of epithelial barrier function, might possess a multifaceted role beyond these established functions. The emergence of analogous histological features across diverse taxa sparks the inquiry into the potential conservation of immune functionalities associated with GCs. Given the intricate interplay between host organisms and pathogens, investigating the role of GCs in insect immunity represents a frontier of scientific inquiry that warrants further investigation. In the context of insects, such as H. armigera, the observed response of GCs to viral infection introduces a novel dimension to the understanding of insect immune defense mechanisms. The morphological alterations in the midgut’s microarchitecture following viral challenge allude to the potential involvement of GCs in orchestrating immune responses. Elucidating the precise molecular mechanisms that underpin this phenomenon remains an intriguing avenue for future research endeavors.
The gastrointestinal tract of insects faces numerous challenges, including the abrasive effects of ingested food’s movement along the alimentary canal interactions with gut microbiota, a primary barrier against ingested toxic compounds and invasive pathogens. ISCs of midgut play a pivotal role in these critical functions. ISCs are also critical in upholding gut integrity and homeostasis, even within the challenging environment of the digestive tract of insects (97). ISCs positioned along the basal side of the pseudostratified epithelial monolayer (Figure 1) exhibit resemblances to their mammalian counterparts as reported by the Huang et al. in Drosophila (2). Functioning as self-renewing and multipotent entities, these cells have the ability to give rise to diverse differentiated cell lineages constituting the intestinal tract (98, 99). ISCs have blast-like morphology characterized by fewer organelles and a cytoplasm of lighter density (22). Notably, recent research has unveiled the presence of storage entities, lipid droplets and glycogen granules within ISCs (100, 101). The division of ISCs depends upon the cellular function performed after division. Two types of cell divisions are observed in ISCs, i.e. asymmetric and symmetric. In the asymmetric division, to maintain the constant population of ISCs within the gastrointestinal milieu, ISCs generate a stem cell and a terminally differentiated counterpart, poised to undertake specific functional roles. In contrast, the symmetric division modality results in the formation of two daughter cells, both of which assume mature and functional phenotypes (40, 102, 103). Once the ISCs are divided and differentiated, they can’t revert to stem cells (104). This interplay between divisional strategies underscores the regenerative prowess of ISCs but also attests to their pivotal role in orchestrating tissue homeostasis and repair.
Various factors are responsible for the depletion of CCs, including gut microbes, pathogenic microorganisms and the production of AMPs as an immune strategy. The study of the model insect Drosophila has been instrumental in shaping our current knowledge regarding the involvement of ISCs in both immunity and tissue renewal. The efficient and rapid restoration of the lost cells is accomplished by a coordinated immune response with an epithelial renewal mechanism that facilitates the repair of the damage (37, 105). ISCs proliferation following infection contributes substantively to replenishing the depleted cell populations, with this process being intricately governed within the midgut milieu. The regulation of ISC activities, requisite to rebuild the cellular deficits, is elicited through the involvement of established canonical signaling pathways activated upon pathogenic invasion. Moreover, the phenomenon of epithelial renewal has been discerned as effective in countering the immune effects against oral viral infection (106). Hemocytes could also induce the proliferation of ISCs during systemic infections, resulting in increased epithelial renewal within the gut (107, 108). This process is significant due to its essential role in improved immunity and recovery during such infections. Central to this phenomenon is the increase of gut renewal orchestrated by the release of secreted ligands of the Upd family, which, in turn, engage the JAK/STAT pathway. During oral infections, hemocytes are employed in the midgut, which triggers the release of Dpp, orchestrating the stimulation of ISCs at an early infection phase and restraining this activation through the recovery stage (109). The intricate modulation of these ligands poses a complex regulatory puzzle, necessitating further exploration into the respective roles played by hemocytes, ISCs, and visceral muscles, which also partake in the regenerative cascade (110, 111). Recent studies have begun unrevealing the involvement of signaling pathways and transcription factors underlying the expression of Upd ligands within the midgut (112). The proliferation of ISCs following infection is regulated by diverse signaling cascades mediated by cell-autonomous and non-cell-autonomous mechanisms. Canonical pathways such as JAK-STAT, EGFR (103, 113, 114), and others, including Wnt/Wg (115), BMP (116), Hippo (117), JNK (118), and p38, play crucial roles in orchestrating the proliferative capacities of ISCs. Nonetheless, a comprehensive elucidation of their integrated regulation, ensuring a balanced proliferation of ISCs, remains elusive. Further investigations are necessary to understand these interactions and regulatory networks that underlie the intricate balance governing ISC proliferation, thereby advancing our understanding of intestinal homeostasis in the context of infection.
While ISCs aren’t directly involved in the immune response, their significance is evident in their pivotal role in expelling pathogens from the midgut and initiating the regeneration of the gut’s epithelium, which aids in the recovery of the insect after infection. Despite these insights, many uncertainties persist regarding the precise functions of ISCs in immunity. Considerable research efforts are required to investigate the full potential of these ever-young cells within the midgut in the context of immunity, particularly in lepidopteran models like silkworms. Such investigations promise not only to enhance our comprehension of the involvement of ISCs in immunity and epithelial renewal but also to unveil novel immune strategies employed by this multifunctional organ in insects.
In insects, an acellular structure known as the peritrophic membrane (PM) exists alongside various cell types within the midgut (Figure 1) (119–121). The PM, a semi-permeable membrane-like structure (Figure 1), is produced by epithelial cells (122). It possesses chitin as an essential component, cross-linked with glycoproteins to form a crucial component of the midgut’s defense and immune system (121). With a thickness ranging from 0.5 to 1.0 µm, the PM plays a pivotal role in selectively facilitating the transport of nutrients and ions while also shielding the midgut cells from abrasion caused by ingested food and potential harm from pathogens (121, 123). The composition of the PM matrix encompasses PM proteins (124), and numerous investigations have reported varying numbers of proteins within the PM matrix of different lepidopteran insects. For instance, there are reports of 305 proteins in silkworms (125) and 41 proteins in H.armigera (126) The extent of involvement of these proteins in PM thickness is contingent upon the composition of ingested food. It is hypothesized that these proteins are stored within the midgut cells and are subsequently released for PM synthesis in response to stimuli from ingested food (127). Although the thickness of PM during growth is evident in diverse insect species such as Ostrinia nubilalis (128), Manduca sexta (129), Anomala cuprea (130), Tribolium castaneum (131), Melipona quadrifasciata, and Apis mellifera (132). This thickening of the PM in response to the stimulus of food is not confined to Lepidoptera; it extends to other insects, such as Anopheles gambiae, where the post-blood feeding localization of Ag-Aper14 within the ectoperitrophic space has been documented (133). However, dietary conditions or starvation have no impact on the mRNA expression levels of peritrophins in Spodoptera litura (134) and insect intestinal mucins (IIMs) in M. configurata (135). The complex structural characteristics of the PM elucidate its specific biological role. Studies have reported that the disruption of PM structure facilitates the transport of the bacterial toxin into the midgut cells, enhancing microbial damage (136, 137). Disruption of chitin synthesis within the PM has been observed to impair the digestive processes in S. litura, resulting in reduced pupal weight and adult emergence rate (138). The complete inhibition of PM secretion by the chitin-binding reagent calcofluor has been found to induce larval developmental retardation, increased larval mortality rates, and a markedly increased susceptibility to baculoviral infection (139). Bm01504, one of many peritrophins discovered in silkworms, showed antiviral efficacy against BmNPV. The overexpression of Bm01504 results in decreased expression of the key viral gene p10. Conversely, the RNAi of Bm01504 resulted in increased expression of the p10 viral gene, suggesting the potential antiviral role of PM against BmNPV (140). The protective effects of the PM matrix are also demonstrated in the other model insects like T. castaneum. In this context, the silencing of two PM matrix proteins, TcPMP3 and TcPMP5-B, via RNA interference has been correlated with a depleted fat body component and growth-related effects, ultimately leading to increased mortality rates in T. castaneum (131). Chitin Synthetase B (CHSB), encoded by the CHS-2 gene, plays a pivotal role in chitin biosynthesis within the PM. RNAi targeting CHS2 has been instrumental in elucidating its function, resulting in structural alterations within the mosquito’s PM. These alterations encompass vacuolization, cell invagination, partial cell rupture, and the conspicuous disruption of PM architecture (141). Pathogenic microbes, especially viruses, have evolved strategies to exploit the unique properties of the PM. They produce enhancins, which, in turn, manipulate the PM’s permeability (142, 143). Notably, the viral protein Chitinase A shares analogous functional attributes with enhancins, contributing to the degradation of PM integrity and the emergence of structural perforations when administered to silkworm larvae (144, 145). The Chitinase enzyme identified within Spodoptera frugiperda nucleopolyhedrovirus (SfMNPV) showed insecticidal properties through its interaction with the host’s PM (146, 147). A similar phenomenon occurs with Dendrolimus kikuchii nucleopolyhedrovirus, where the secretion of chitinase results in insecticidal activity via the degradation of the chitinous framework comprising the peritrophic membrane (148). Overall, the non-cellular PM is critical in preserving midgut structural integrity, facilitating the digestive processes, and acting as the first line of defense against pathogenic microbes. A deeper exploration of the functional roles of structural proteins within the PM promises to enhance our understanding of this intricate midgut component. Comprehensive studies remain imperative to unravel the multifaceted roles of peritrophins, particularly their discernible antiviral effects. These endeavors not only hold the potential to illuminate context-specific responses of the midgut to microbial challenges but also to unveil novel windows for fortifying immunity in economically significant organisms such as the silkworm. Furthermore, such investigations offer a promising route for identifying innovative targets in developing insecticides designed to control insect pest populations.
The midgut juice, secreted by midgut cells (149) with a pH ranging from 9.2 to 11, has long been recognized for its digestive enzyme content essential for the digestion of food (24). Table 3 includes the proteins from the midgut juice having immune characteristics. Studies have revealed the immunological role of digestive juice, unveiling the presence of proteins harboring potent antiviral functions (150). The antimicrobial properties of midgut juice were first reported by Hayashiya et al. They documented that the silkworm midgut juice has a distinctive red fluorescence emitting substance (150). Subsequent investigations have elucidated that a membrane protein, P252, which, upon binding with chlorophyllide, forms a red fluorescent protein (RFP) complex, displaying robust antiviral, antibacterial, and antifungal properties (151). Different forms of RFPs exhibit unique antiviral activities against different viruses (157). Interestingly, different silkworm varieties, including both resistant and susceptible strains (158), showed varying numbers of related RFPs, implying their involvement in the resistance mechanisms against pathogens. Silkworm midgut juice also contains other potent antiviral factors, including BmNOX (152), Bmlipase-1 (153) and serine protease-2 (155), all of which demonstrate strong antiviral activities. A 33% increase in the survival rate of transgenic silkworms challenged with BmNPV indicated the antiviral potential of Bmlipase-1 (154). The digestive enzyme trypsin, alkaline A (BmTA) also showed antiviral potential against BmNPV (47). Transcriptomic and proteomic analyses further highlight the significance of these proteins in the context of antiviral defense, with differential expression patterns observed in resistant and susceptible silkworms. However, despite the strong efficacy of these purified proteins as antiviral agents, the intricate mechanisms governing their antiviral activities still need to be discovered. Although modern multi-omics techniques have advanced our comprehension of the antimicrobial functions of silkworm midgut juice, comprehensive functional studies are required to unravel the precise molecular mechanisms underpinning these processes. This deeper understanding holds promise for enhancing our understanding of immunity against pathogens and opens new avenues for developing robust silkworm varieties, ultimately benefiting sericulture practices.
The complex relationship between silkworm midgut cell types and immunity has emerged as a fascinating area of research with far-reaching implications. The diverse range of midgut cells, including goblet, columnar, and enteroendocrine cells, play pivotal roles in the silkworm’s immune response. These cells collectively contribute to recognizing, signaling, and defense mechanisms against invading pathogens. The peritrophic membrane not only acts as a barrier but also serves as a platform for displaying immune-related molecules, aiding in detecting and neutralizing pathogens within the midgut lumen. The involvement of the midgut in pattern recognition, immune signaling, and the production of antimicrobial peptides and enzymes further shed light on the antimicrobial role of the second-largest organ of the insect.
Further investigation into the specific molecular mechanisms underlying the immune functions of different midgut cell types will provide a deeper understanding of how silkworms combat pathogens. High-throughput omics approaches, such as transcriptomics and proteomics, can unveil novel immune effectors. Knowledge based on these modern omics about the different cell types of the midgut will help to modulate the silkworm’s immune response through genetic manipulation or bioengineering, leading to enhanced disease resistance in silkworm populations, which has implications for silk production and sericulture. The antiviral potential of midgut juice and its components could be a platform for developing novel antiviral therapies for agricultural and medical purposes. Investigating how silkworm midgut immunity influences interactions with other organisms, including pathogens and gut symbionts, can shed light on broader ecological processes.
In conclusion, the intricate interplay between silkworm midgut cell types, the peritrophic membrane, and midgut juice in immunity open up a world of possibilities for both scientific exploration and practical applications, offering a promising path toward a deeper understanding of insect immune defenses and their potential for biotechnological advancements in sericulture and beyond.
MA: Conceptualization, Writing – original draft. SF: Writing – original draft. JX: Writing – review & editing. MF: Conceptualization, Writing – review & editing. JS: Conceptualization, Funding acquisition, Supervision, Writing – review & editing.
The author(s) declare financial support was received for the research, authorship, and/or publication of this article. The study was supported by grants from Guangdong Basic and Applied Basic Research Foundation No. (2023A1515010133).
The authors declare that the research was conducted in the absence of any commercial or financial relationships that could be construed as a potential conflict of interest.
All claims expressed in this article are solely those of the authors and do not necessarily represent those of their affiliated organizations, or those of the publisher, the editors and the reviewers. Any product that may be evaluated in this article, or claim that may be made by its manufacturer, is not guaranteed or endorsed by the publisher.
1. Douglas AE. Alimentary canal, digestion and absorption. In: The Insects: Structure and Function. Cambridge: Cambridge University Press (2013). p. 46–80.
2. Hung RJ, Hu Y, Kirchner R, Liu Y, Xu C, Comjean A, et al. A cell atlas of the adult Drosophila midgut. Proc Natl Acad Sci (2020) 117(3):1514–23. doi: 10.1073/pnas.1916820117
3. Hakim RS, Baldwin K, Smagghe G. Regulation of midgut growth, development, and metamorphosis. Annu Rev Entomol (2010) 1:593–608. doi: 10.1146/annurev-ento-112408-085450
4. Wu Q, Patočka J, Kuča K. Insect antimicrobial peptides, a mini review. Toxins. (2018) 10(11):461. doi: 10.3390/toxins10110461
5. Wang RJ, Chen K, Xing LS, Lin Z, Zou Z, Lu Z. Reactive oxygen species and antimicrobial peptides are sequentially produced in silkworm midgut in response to bacterial infection. Dev Comp Immunol (2020) 110:110:103720. doi: 10.1016/j.dci.2020.103720
6. Mylonakis E, Podsiadlowski L, Muhammed M, Vilcinskas A. Diversity, evolution and medical applications of insect antimicrobial peptides. Philos Trans R Soc B Biol Sci (2016) 371(1695):20150290. doi: 10.1098/rstb.2015.0290
7. Castagnola A, Jurat–Fuentes JL. Intestinal regeneration as an insect resistance mechanism to entomopathogenic bacteria. Curr Opin Insect Sci (2016) 15:104–10. doi: 10.1016/j.cois.2016.04.008
8. Meng X, Zhu F, Chen K. Silkworm: A promising model organism in life science. J Insect Sci (2017) 17(5):97. doi: 10.1093/jisesa/iex064
9. Xia Q, Guo Y, Zhang Z, Li D, Xuan Z, Li Z, et al. Complete resequencing of 40 genomes reveals domestication events and genes in silkworm (Bombyx). Science. (2009) 326(5951):433–6. doi: 10.1126/science.1176620
10. Panthee S, Paudel A, Hamamoto H, Sekimizu K. Advantages of the silkworm as an animal model for developing novel antimicrobial agents. Front Microbiol [Internet] (2017) 8. doi: 10.3389/fmicb.2017.00373
11. Ashraf H, Qamar A. Silkworm Bombyx mori as a model organism: A review. Physiol Entomol (2023) 48(4):107–21. doi: 10.1111/phen.12421
12. Trapnell C. Defining cell types and states with single–cell genomics. Genome Res (2015) 25(10):1491–8. doi: 10.1101/gr.190595.115
13. Guo X, Yin C, Yang F, Zhang Y, Huang H, Wang J, et al. The cellular diversity and transcription factor code of Drosophila enteroendocrine cells. Cell Rep (2019) 29(12):4172–85. doi: 10.1016/j.celrep.2019.11.048
14. Cui Y, Franz AWE. Heterogeneity of midgut cells and their differential responses to blood meal ingestion by the mosquito, Aedes aEgypti. Insect Biochem Mol Biol (2020) 127:103496. doi: 10.1016/j.ibmb.2020.103496
15. Xia J, Fei S, Huang Y, Lai W, Yu Y, Liang L, et al. Single–nucleus sequencing of silkworm larval midgut reveals the immune escape strategy of BmNPV in the midgut during the late stage of infection. Insect Biochem Mol Biol (2023) 164:104043. doi: 10.2139/ssrn.4584877
16. Haber AL, Biton M, Rogel N, Herbst RH, Shekhar K, Smillie C, et al. A single–cell survey of the small intestinal epithelium. Nature. (2017) 551(7680):333–9. doi: 10.1038/nature24489
17. Chen J, Kim Sm, Kwon JY. A systematic analysis of Drosophila regulatory peptide expression in enteroendocrine cells. Mol Cells (2016) 39(4):358. doi: 10.14348/molcells.2016.0014
18. Perdigoto CN, Schweisguth F, Bardin AJ. Distinct levels of Notch activity for commitment and terminal differentiation of stem cells in the adult fly intestine. Development. (2011) 138(21):4585–95. doi: 10.1242/dev.065292
19. Biteau B, Jasper H. Slit/Robo signaling regulates cell fate decisions in the intestinal stem cell lineage of Drosophila. Cell Rep (2014) 7(6):1867–75. doi: 10.1016/j.celrep.2014.05.024
20. Zeng X, Hou SX. Enteroendocrine cells are generated from stem cells through a distinct progenitor in the adult Drosophila posterior midgut. Development. (2015) 142(4):644–53. doi: 10.1242/dev.113357
21. Cioffi M. Comparative ultrastructure of arthropod transporting epithelia. Am Zool (1984) 24(1):139–56. doi: 10.1093/icb/24.1.139
22. Billingsley PF, Lehane MJ. Structure and ultrastructure of the insect midgut. In: Biology of the insect midgut. Dordrecht, Netherlands: Springer (1996). p. 3–30.
24. Holtof M, Lenaerts C, Cullen D, Vanden Broeck J. Extracellular nutrient digestion and absorption in the insect gut. Cell Tissue Res (2019) 377:397–414. doi: 10.1007/s00441-019-03031-9
25. Walski T, De Schutter K, Cappelle K, Van Damme EJ, Smagghe G. Distribution of glycan motifs at the surface of midgut cells in the cotton leafworm (Spodoptera littoralis) demonstrated by lectin binding. Front Physiol (2017) 8:1020. doi: 10.3389/fphys.2017.01020
26. Shanbhag S, Tripathi S. Epithelial ultrastructure and cellular mechanisms of acid and base transport in the Drosophila midgut. J Exp Biol (2009) 212(11):1731–44. doi: 10.1242/jeb.029306
27. Monteiro EC, Tamaki FK, Terra WR, Ribeiro AF. The digestive system of the “stick bug” Cladomorphus phyllinus (Phasmida, Phasmatidae): a morphological, physiological and biochemical analysis. Arthropod Struct Dev (2014) 43(2):123–34. doi: 10.1016/j.asd.2013.11.005
28. Rost–Roszkowska MM, Vilimova J, W\lodarczyk A, Sonakowska L, Kamińska K, Kaszuba F, et al. Investigation of the midgut structure and ultrastructure in Cimex lectularius and Cimex pipistrelli (Hemiptera: Cimicidae). Neotrop Entomol (2017) 46:45–57. doi: 10.1007/s13744-016-0430-x
29. Santos HP, Rost–Roszkowska M, Vilimova J, Serrão JE. Ultrastructure of the midgut in Heteroptera (Hemiptera) with different feeding habits. Protoplasma. (2017) 254:1743–53. doi: 10.1007/s00709-016-1051-2
30. Terra WR, Ferreira C, Baker JE. Compartmentalization of digestion. In: Biology of the insect midgut. Dordrecht, Netherlands: Springer (1996). p. 206–35.
31. Buchon N, Broderick NA, Lemaitre B. Gut homeostasis in a microbial world: insights from Drosophila melanogaster. Nat Rev Microbiol (2013) 11(9):615–26. doi: 10.1038/nrmicro3074
32. Buchon N, Osman D, David FP, Fang HY, Boquete JP, Deplancke B, et al. Morphological and molecular characterization of adult midgut compartmentalization in Drosophila. Cell Rep (2013) 3(5):1725–38. doi: 10.1016/j.celrep.2013.04.001
33. Bonelli M, Bruno D, Caccia S, Sgambetterra G, Cappellozza S, Jucker C, et al. Structural and functional characterization of Hermetia illucens larval midgut. Front Physiol (2019) 10:204. doi: 10.3389/fphys.2019.00204
34. Liu HW, Li YS, Tang X, Guo PC, Wang DD, Zhou CY, et al. A midgut–specific serine protease, BmSP36, is involved in dietary protein digestion in the silkworm, Bombyx mori. Insect Sci (2017) 24(5):753–67. doi: 10.1111/1744-7917.12369
35. Lee KS, Kim BY, Choo YM, Jin BR. Dual role of the serine protease homolog BmSPH–1 in the development and immunity of the silkworm Bombyx mori. Dev Comp Immunol (2018) 85:170–6. doi: 10.1016/j.dci.2018.04.011
36. Clissold FJ, Tedder BJ, Conigrave AD, Simpson SJ. The gastrointestinal tract as a nutrient–balancing organ. Proc R Soc B Biol Sci (2010) 277(1688):1751–9. doi: 10.1098/rspb.2009.2045
37. Amcheslavsky A, Song W, Li Q, Nie Y, Bragatto I, Ferrandon D, et al. Enteroendocrine cells support intestinal stem–cell–mediated homeostasis in Drosophila. Cell Rep (2014) 9(1):32–9. doi: 10.1016/j.celrep.2014.08.052
38. Caccia S, Casartelli M, Tettamanti G. The amazing complexity of insect midgut cells: types, peculiarities, and functions. Cell Tissue Res (2019) 377:505–25. doi: 10.1007/s00441-019-03076-w
39. Combe BE, Defaye A, Bozonnet N, Puthier D, Royet J, Leulier F. Drosophila microbiota modulates host metabolic gene expression via IMD/NF–κB signaling. PloS One (2014) 9(4):e94729. doi: 10.1371/journal.pone.0094729
40. Miguel–Aliaga I, Jasper H, Lemaitre B. Anatomy and physiology of the digestive tract of Drosophila melanogaster. Genetics (2018) 210(2):357–96. doi: 10.1534/genetics.118.300224
41. Casartelli M, Leonardi MG, Fiandra L, Parenti P, Giordana B. Multiple transport pathways for dibasic amino acids in the larval midgut of the silkworm Bombyx mori. Insect Biochem Mol Biol (2001) 31(6–7):621–32. doi: 10.1016/S0965-1748(00)00167-3
42. Leonardi MG, Caccia S, González–Cabrera J, Ferré J, Giordana B. Leucine transport is affected by Bacillus thuringiensis Cry1 toxins in brush border membrane vesicles from Ostrinia nubilalis Hb (Lepidoptera: Pyralidae) and Sesamia nonagrioides Lefebvre (Lepidoptera: Noctuidae) midgut. J Membr Biol (2006) 214:157–64. doi: 10.1007/s00232-006-0042-1
43. Bruno D, Bonelli M, Cadamuro AG, Reguzzoni M, Grimaldi A, Casartelli M, et al. The digestive system of the adult Hermetia illucens (Diptera: Stratiomyidae): morphological features and functional properties. Cell Tissue Res (2019) 378:221–38. doi: 10.1007/s00441-019-03025-7
44. Tellam RL, Wijffels G, Willadsen P. Peritrophic matrix proteins. Insect Biochem Mol Biol (1999) 29(2):87–101. doi: 10.1016/S0965-1748(98)00123-4
45. Marques JT, Imler JL. The diversity of insect antiviral immunity: insights from viruses. Curr Opin Microbiol (2016) 32:71–6. doi: 10.1016/j.mib.2016.05.002
46. Li G, Zhou Q, Qiu L, Yao Q, Chen K, Tang Q, et al. Serine protease Bm–SP142 was differentially expressed in resistant and susceptible Bombyx mori strains, involving in the defence response to viral infection. PloS One (2017) 12(4):e0175518. doi: 10.1371/journal.pone.0175518
47. Cao HH, Zhang SZ, Zhu LB, Wang J, Liu YX, Wang YL, et al. The digestive proteinase trypsin, alkaline A contributes to anti–BmNPV activity in silkworm (Bombyx mori). Dev Comp Immunol (2021) 119:104035. doi: 10.1016/j.dci.2021.104035
48. Rodenfels J, Lavrynenko O, Ayciriex S, Sampaio JL, Carvalho M, Shevchenko A, et al. Production of systemically circulating Hedgehog by the intestine couples nutrition to growth and development. Genes Dev (2014) 28(23):2636–51. doi: 10.1101/gad.249763.114
49. Zhu SQ, Zhang YJ, Abbas MN, Hao XW, Zhao YZ, Liang HH, et al. Hedgehog promotes cell proliferation in the midgut of silkworm, Bombyx mori. Insect Sci (2020) 27(4):697–707. doi: 10.1111/1744-7917.12672
50. Mirth C, Truman JW, Riddiford LM. The role of the prothoracic gland in determining critical weight for metamorphosis in Drosophila melanogaster. Curr Biol (2005) 15(20):1796–807. doi: 10.1016/j.cub.2005.09.017
51. Géminard C, Rulifson EJ, Léopold P. Remote control of insulin secretion by fat cells in Drosophila. Cell Metab (2009) 10(3):199–207. doi: 10.1016/j.cmet.2009.08.002
52. Delanoue R, Slaidina M, Léopold P. The steroid hormone ecdysone controls systemic growth by repressing dMyc function in Drosophila fat cells. Dev Cell (2010) 18(6):1012–21. doi: 10.1016/j.devcel.2010.05.007
53. Abou El Asrar R, Cools D, Vanden Broeck J. Role of peptide hormones in insect gut physiology. Curr Opin Insect Sci (2020) 41:71–8. doi: 10.1016/j.cois.2020.07.004
54. Khalid MZ, Ahmad S, Ngegba PM, Zhong G. Role of endocrine system in the regulation of female insect reproduction. Biology (2021) 10(7):614. doi: 10.3390/biology10070614
55. Felix RC, Trindade M, Pires IRP, Fonseca VG, Martins RS, Silveira H, et al. Unravelling the evolution of the allatostatin–type A, KISS and galanin peptide–receptor gene families in bilaterians: insights from anopheles mosquitoes. Wegener C editor. PloS One (2015) 10(7):e0130347. doi: 10.1371/journal.pone.0130347
56. Roller L, Daubnerová I, Mizoguchi A, Satake H, Tanaka Y, Stano M, et al. Expression analysis of peptidergic enteroendocrine cells in the silkworm Bombyx mori. Cell Tissue Res (2022) 389(3):385–407. doi: 10.1007/s00441-022-03666-1
57. Holmes VR, Johnston JS. Differential gene expression of innate immune response genes consequent to solenopsis invicta virus–3 infection. Genes. (2023) 14(1):188. doi: 10.3390/genes14010188
58. Reiher W, Shirras C, Kahnt J, Baumeister S, Isaac RE, Wegener C. Peptidomics and peptide hormone processing in the Drosophila midgut. J Proteome Res (2011) 10(4):1881–92. doi: 10.1021/pr101116g
59. Benguettat O, Jneid R, Soltys J, Loudhaief R, Brun–Barale A, Osman D, et al. The DH31/CGRP enteroendocrine peptide triggers intestinal contractions favoring the elimination of opportunistic bacteria. PloS Pathog (2018) 14(9):e1007279. doi: 10.1371/journal.ppat.1007279
60. Toyam T, Yamagishi T, Sato R. The roles of enteroendocrine cell distribution and gustatory receptor expression in regulating peptide hormone secretion in the midgut of Bombyx mori larvae. Arch Insect Biochem Physiol (2023) 114(1):e22032. doi: 10.1002/arch.22032
61. Song W, Veenstra JA, Perrimon N. Control of lipid metabolism by tachykinin in Drosophila. Cell Rep (2014) 9(1):40–7. doi: 10.1016/j.celrep.2014.08.060
62. Yamanaka N, Roller L, Žitňan D, Satake H, Mizoguchi A, Kataoka H, et al. Bombyx orcokinins are brain–gut peptides involved in the neuronal regulation of ecdysteroidogenesis. J Comp Neurol (2011) 519(2):238–46. doi: 10.1002/cne.22517
63. Huang Y, Crim JW, Nuss AB, Brown MR. Neuropeptide F and the corn earworm, Helicoverpa zea: A midgut peptide revisited. Peptides. (2011) 32(3):483–92. doi: 10.1016/j.peptides.2010.09.014
64. Fujita T, Yui R, Iwanaga T, Nishiitsutsuji–Uwo J, Endo Y, Yanaihara N. Evolutionary aspects of “brain–gut peptides”: an immunohistochemical study. Peptides. (1981) 2:123–31. doi: 10.1016/0196-9781(81)90023-1
65. Iwanaga T, Fujita T, Nishiitsutsuji–uwo J, Endo Y. Immunohistochemical demonstration of PP–, somatostatin–, enteroglucagon–and VIP–like immunoreactivities in the cockroach midgut. BioMed Res (1981) 2(2):202–7. doi: 10.2220/biomedres.2.202
66. Nishiitsutsuji–uwo J, Endo Y. Gut endocrine cells in insects: the ultrastructure of the endocrine cells in the cockroach midgut. BioMed Res (1981) 2(1):30–44. doi: 10.2220/biomedres.2.30
67. Sehnal F, Žitňan D. Midgut endocrine cells. In: Biology of The Insect Midgut. Dordrecht, Netherlands: Springer (1996). p. 55–85.
68. Pabla N, Lange AB. The distribution and myotropic activity of locust tachykinin–like peptides in locust midgut. Peptides. (1999) 20(10):1159–67. doi: 10.1016/S0196-9781(99)00119-9
69. Predel R, Neupert S, Garczynski SF, Crim JW, Brown MR, Russell WK, et al. Neuropeptidomics of the mosquito Aedes aEgypti. J Proteome Res (2010) 9(4):2006–15. doi: 10.1021/pr901187p
70. Wegener C, Veenstra JA. Chemical identity, function and regulation of enteroendocrine peptides in insects. Curr Opin Insect Sci (2015) 11:8–13. doi: 10.1016/j.cois.2015.07.003
71. Veenstra JA, Ida T. More Drosophila enteroendocrine peptides: Orcokinin B and the CCHamides 1 and 2. Cell Tissue Res (2014) 357:607–21. doi: 10.1007/s00441-014-1880-2
72. Lee KY, Horodyski FM, Chamberlin ME. Inhibition of midgut ion transport by allatotropin (Mas–AT) and Manduca FLRFamides in the tobacco hornworm Manduca sexta. J Exp Biol (1998) 201(22):3067–74. doi: 10.1242/jeb.201.22.3067
73. Matsumoto S, Ohara A, Nagai–Okatani C, Zhou YJ, Fujinaga D, Seike H, et al. Antagonistic effect of short neuropeptide F on allatotropin–inhibited feeding motivation of the silkworm larva, bombyx mori. Zoolog Sci (2019) 36(1):58–67. doi: 10.2108/zs180119
74. Winther AM, Nassel DR. Intestinal peptides as circulating hormones: release of tachykinin–related peptide from the locust and cockroach midgut. J Exp Biol (2001) 204(7):1269–80. doi: 10.1242/jeb.204.7.1269
75. Reichwald K, Unnithan GC, Davis NT, Agricola H, Feyereisen R. Expression of the allatostatin gene in endocrine cells of the cockroach midgut. Proc Natl Acad Sci (1994) 91(25):11894–8. doi: 10.1073/pnas.91.25.11894
76. Secher T, Lenz C, Cazzamali G, Sørensen G, Williamson M, Hansen GN, et al. Molecular cloning of a functional allatostatin gut/brain receptor and an allatostatin preprohormone from the silkworm bombyx mori *. J Biol Chem (2001) 276(50):47052–60. doi: 10.1074/jbc.M106675200
77. Bachtel ND, Hovsepian GA, Nixon DF, Eleftherianos I. Allatostatin C modulates nociception and immunity in Drosophila. Sci Rep (2018) 8(1):7501. doi: 10.1038/s41598-018-25855-1
78. Li S, Torre–Muruzabal T, Søgaard KC, Ren GR, Hauser F, Engelsen SM, et al. Expression patterns of the Drosophila neuropeptide CCHamide–2 and its receptor may suggest hormonal signaling from the gut to the brain. PloS One (2013) 8(10):e76131. doi: 10.1371/journal.pone.0076131
79. Anderson E, Harvey WR. Active transport by the Cecropia midgut: II. Fine structure of the midgut epithelium. J Cell Biol (1966) 31(1):107–34. doi: 10.1083/jcb.31.1.107
80. Pinheiro DO, Quagio–Grassiotto I, Gregório EA. Morphological regional differences of epithelial cells along the midgut in Diatraea saccharalis Fabricius (Lepidoptera: Crambidae) larvae. Neotrop Entomol (2008) 37:413–9. doi: 10.1590/S1519-566X2008000400009
81. Moffett DF, Koch A. The insect goblet cell: a problem in functional cytoarchitecture. Physiology (1992) 7(1):19–23. doi: 10.1152/physiologyonline.1992.7.1.19
82. Gomes FM, Carvalho DB, MaChado EA, Miranda K. Ultrastructural and functional analysis of secretory goblet cells in the midgut of the lepidopteran Anticarsia gemmatali. Cell Tissue Res (2013) 352(2):313–26. doi: 10.1007/s00441-013-1563-4
83. Baldwin KM, Hakim RS. Growth and differentiation of the larval midgut epithelium during molting in the moth, Manduca sexta. Tissue Cell (1991) 23(3):411–22. doi: 10.1016/0040-8166(91)90058-2
84. Schweikl H, Klein U, Schindlbeck M, Wieczorek H. A vacuolar–type ATPase, partially purified from potassium transporting plasma membranes of tobacco hornworm midgut. J Biol Chem (1989) 264(19):11136–42. doi: 10.1016/S0021-9258(18)60440-5
85. Levy SM, Falleiros AMF, Gregório EA, Arrebola NR, Toledo LA. The larval midgut of Anticarsia gemmatalis (Hübner)(Lepidoptera: Noctuidae): light and electron microscopy studies of the epithelial cells. Braz J Biol (2004) 64:633–8. doi: 10.1590/S1519-69842004000400010
86. Wieczorek H, Putzenlechner M, Zeiske W, Klein U. A vacuolar–type proton pump energizes K+/H+ antiport in an animal plasma membrane. J Biol Chem (1991) 266(23):15340–7. doi: 10.1016/S0021-9258(18)98621-7
87. Azuma M, Harvey WR, Wieczorek H. Stoichiometry of K+/H+ antiport helps to explain extracellular pH 11 in a model epithelium. FEBS Lett (1995) 361(2–3):153–6. doi: 10.1016/0014-5793(95)00146-Z
88. Dow JA. pH gradients in lepidopteran midgut. J Exp Biol (1992) 172(1):355–75. doi: 10.1242/jeb.172.1.355
89. Crosnier C, Stamataki D, Lewis J. Organizing cell renewal in the intestine: stem cells, signals and combinatorial control. Nat Rev Genet (2006) 7(5):349–59. doi: 10.1038/nrg1840
90. Cone RA. Barrier properties of mucus. Adv Drug Deliv Rev (2009) 61(2):75–85. doi: 10.1016/j.addr.2008.09.008
91. Qiao R, Deng Y, Zhang S, Wolosker MB, Zhu Q, Ren H, et al. Accumulation of different shapes of microplastics initiates intestinal injury and gut microbiota dysbiosis in the gut of zebrafish. Chemosphere (2019) 236:124334. doi: 10.1016/j.chemosphere.2019.07.065
92. Huang JN, Wen B, Zhu JG, Zhang YS, Gao JZ, Chen ZZ. Exposure to microplastics impairs digestive performance, stimulates immune response and induces microbiota dysbiosis in the gut of juvenile guppy (Poecilia reticulata). Sci Total Environ (2020) 733:138929. doi: 10.1016/j.scitotenv.2020.138929
93. Kim YS, Ho SB. Intestinal goblet cells and mucins in health and disease: recent insights and progress. Curr Gastroenterol Rep (2010) 12:319–30. doi: 10.1007/s11894-010-0131-2
94. Bansil R, Turner BS. The biology of mucus: Composition, synthesis and organization. Adv Drug Delivery Rev (2018) 124:3–15. doi: 10.1016/j.addr.2017.09.023
95. Birchenough GMH, Johansson ME, Gustafsson JK, Bergström JH, Hansson GC. New developments in goblet cell mucus secretion and function. Mucosal Immunol (2015) 8(4):712–9. doi: 10.1038/mi.2015.32
96. Marzban R, He Q, Zhang Q, Liu XX. Histopathology of cotton bollworm midgut infected with Helicoverpa armigera cytoplasmic polyhedrosis virus. Braz J Microbiol (2013) 44:1231–6. doi: 10.1590/S1517-83822013000400029
97. Huang JH, Jing X, Douglas AE. The multi–tasking gut epithelium of insects. Insect Biochem Mol Biol (2015) 67:15–20. doi: 10.1016/j.ibmb.2015.05.004
98. Micchelli CA, Perrimon N. Evidence that stem cells reside in the adult Drosophila midgut epithelium. Nature. (2006) 439(7075):475–9. doi: 10.1038/nature04371
99. Ohlstein B, Spradling A. Multipotent drosophila intestinal stem cells specify daughter cell fates by differential notch signaling. Science. (2007) 315(5814):988–92. doi: 10.1126/science.1136606
100. Tettamanti G, Grimaldi A, Casartelli M, Ambrosetti E, Ponti B, Congiu T, et al. Programmed cell death and stem cell differentiation are responsible for midgut replacement in Heliothis virescens during prepupal instar. Cell Tissue Res (2007) 330:345–59. doi: 10.1007/s00441-007-0449-8
101. Franzetti E, Romanelli D, Caccia S, Cappellozza S, Congiu T, Rajagopalan M, et al. The midgut of the silkmoth Bombyx mori is able to recycle molecules derived from degeneration of the larval midgut epithelium. Cell Tissue Res (2015) 361:509–28. doi: 10.1007/s00441-014-2081-8
102. O’Brien LE, Soliman SS, Li X, Bilder D. Altered modes of stem cell division drive adaptive intestinal growth. Cell. (2011) 147(3):603–14. doi: 10.1016/j.cell.2011.08.048
103. Gervais L, Bardin AJ. Tissue homeostasis and aging: new insight from the fly intestine. Curr Opin Cell Biol (2017) 48:97–105. doi: 10.1016/j.ceb.2017.06.005
104. Lu Y, Li Z. No intestinal stem cell regeneration after complete progenitor ablation in Drosophila adult midgut. J Genet Genomics Yi Chuan Xue Bao (2015) 42(2):83–6. doi: 10.1016/j.jgg.2014.10.002
105. Jiang H, Edgar BA. EGFR signaling regulates the proliferation of Drosophila adult midgut progenitors. Development. (2009) 136(3):483–93. doi: 10.1242/dev.026955
106. Sansone CL, Cohen J, Yasunaga A, Xu J, Osborn G, Subramanian H, et al. Microbiota–dependent priming of antiviral intestinal immunity in drosophila. Cell Host Microbe (2015) 18(5):571–81. doi: 10.1016/j.chom.2015.10.010
107. Takeishi A, Kuranaga E, Tonoki A, Misaki K, Yonemura S, Kanuka H, et al. Homeostatic epithelial renewal in the gut is required for dampening a fatal systemic wound response in drosophila. Cell Rep (2013) 3(3):919–30. doi: 10.1016/j.celrep.2013.02.022
108. Chakrabarti S, Dudzic JP, Li X, Collas EJ, Boquete JP, Lemaitre B. Remote control of intestinal stem cell activity by haemocytes in drosophila. PloS Genet (2016) 12(5):e1006089. doi: 10.1371/journal.pgen.1006089
109. Ayyaz A, Li H, Jasper H. Haemocytes control stem cell activity in the Drosophila intestine. Nat Cell Biol (2015) 17(6):736–48. doi: 10.1038/ncb3174
110. Jiang H, Tian A, Jiang J. Intestinal stem cell response to injury: lessons from Drosophila. Cell Mol Life Sci (2016) 73(17):3337–49. doi: 10.1007/s00018-016-2235-9
111. Houtz P, Bonfini A, Liu X, Revah J, Guillou A, Poidevin M, et al. Hippo, TGF–β, and Src–MAPK pathways regulate transcription of the upd3 cytokine in Drosophila enterocytes upon bacterial infection. PloS Genet (2017) 13(11):e1007091. doi: 10.1371/journal.pgen.1007091
112. Zhai Z, Boquete JP, Lemaitre B. Cell–specific imd–NF–κB responses enable simultaneous antibacterial immunity and intestinal epithelial cell shedding upon bacterial infection. Immunity (2018) 48(5):897–910.e7. doi: 10.1016/j.immuni.2018.04.010
113. Boumard B, Bardin AJ. An amuse–bouche of stem cell regulation: Underlying principles and mechanisms from adult Drosophila intestinal stem cells. Curr Opin Cell Biol (2021) 73:58–68. doi: 10.1016/j.ceb.2021.05.007
114. Zhang P, Edgar BA. Insect gut regeneration. Cold Spring Harb Perspect Biol (2022) 14(2):a040915. doi: 10.1101/cshperspect.a040915
115. Tian A, Benchabane H, Wang Z, Ahmed Y. Regulation of stem cell proliferation and cell fate specification by wingless/wnt signaling gradients enriched at adult intestinal compartment boundaries. PloS Genet (2016) 12(2):e1005822. doi: 10.1371/journal.pgen.1005822
116. Tracy Cai X, Li H, Safyan A, Gawlik J, Pyrowolakis G, Jasper H. AWD regulates timed activation of BMP signaling in intestinal stem cells to maintain tissue homeostasis. Nat Commun (2019) 10(1):2988. doi: 10.1038/s41467-019-10926-2
117. Ren F, Wang B, Yue T, Yun EY, Ip YT, Jiang J. Hippo signaling regulates Drosophila intestine stem cell proliferation through multiple pathways. Proc Natl Acad Sci (2010) 107(49):21064–9. doi: 10.1073/pnas.1012759107
118. Biteau B, Hochmuth CE, Jasper H. JNK activity in somatic stem cells causes loss of tissue homeostasis in the aging drosophila gut. Cell Stem Cell (2008) 3(4):442–55. doi: 10.1016/j.stem.2008.07.024
119. Miller N, Lehane MJ. Peritrophic membranes, cell surface molecules and parasite tropisms within arthropod vectors. Parasitol Today (1993) 9(2):45–50. doi: 10.1016/0169-4758(93)90030-J
120. Lehane MJ. Peritrophic matrix structure and function. Annu Rev Entomol (1997) 42(1):525–50. doi: 10.1146/annurev.ento.42.1.525
121. Toprak U, Erlandson M. Toprak U, Erlandson M, Hegedus D. Peritrophic matrix proteins. Trends in Entomology. Trends Entomol (2010) 6:23–51.
122. Hegedus D, Erlandson M, Gillott C, Toprak U. New insights into peritrophic matrix synthesis, architecture, and function. Annu Rev Entomol (2009) 54:285–302. doi: 10.1146/annurev.ento.54.110807.090559
123. Erlandson MA, Toprak U, Hegedus DD. Role of the peritrophic matrix in insect–pathogen interactions. J Insect Physiol (2019) 117:103894. doi: 10.1016/j.jinsphys.2019.103894
124. Hegedus DD, Toprak U, Erlandson M. Peritrophic matrix formation. J Insect Physiol (2019) 117:103898. doi: 10.1016/j.jinsphys.2019.103898
125. Zhong X, Zhang L, Zou Y, Yi Q, Zhao P, Xia Q, et al. Shotgun analysis on the peritrophic membrane of the silkworm Bombyx mori. BMB Rep (2012) 45(11):665–70. doi: 10.5483/BMBRep.2012.45.11.261
126. Campbell PM, Cao AT, Hines ER, East PD, Gordon KH. Proteomic analysis of the peritrophic matrix from the gut of the caterpillar, Helicoverpa armigera. Insect Biochem Mol Biol (2008) 38(10):950–8. doi: 10.1016/j.ibmb.2008.07.009
127. Zha XL, Wang H, Sun W, Zhang HY, Wen J, Huang XZ, et al. Characteristics of the peritrophic matrix of the silkworm, bombyx mori and factors influencing its formation. Insects. (2021) 12(6):516. doi: 10.3390/insects12060516
128. Harper MS, Hopkins TL. Peritrophic membrane structure and secretion in european corn borer larvae (Ostrinia nubilalis). Tissue Cell (1997) 29(4):463–75. doi: 10.1016/S0040-8166(97)80032-8
129. Hopkins TL, Harper MS. Lepidopteran peritrophic membranes and effects of dietary wheat germ agglutinin on their formation and structure. Arch Insect Biochem Physiol (2001) 47(2):100–9. doi: 10.1002/arch.1040
130. Kawakita H, Miyamoto K, Wada S, Mitsuhashi W. Analysis of the ultrastructure and formation pattern of the peritrophic membrane in the cupreous chafer, Anomala cuprea (Coleoptera: Scarabaeidae). Appl Entomol Zool (2016) 51(1):133–42. doi: 10.1007/s13355-015-0382-3
131. Agrawal S, Kelkenberg M, Begum K, Steinfeld L, Williams CE, Kramer KJ, et al. Two essential peritrophic matrix proteins mediate matrix barrier functions in the insect midgut. Insect Biochem Mol Biol (2014) 49:24–34. doi: 10.1016/j.ibmb.2014.03.009
132. Oliveira AH, Fernandes KM, Gonçalves WG, Zanuncio JC, Serrão JE. A peritrophin mediates the peritrophic matrix permeability in the workers of the bees Melipona quadrifasciata and Apis mellifera. Arthropod Struct Dev (2019) 53:100885. doi: 10.1016/j.asd.2019.100885
133. Devenport M, Fujioka H, Donnelly–Doman M, Shen Z, Jacobs–Lorena M. Storage and secretion of Ag–Aper14, a novel peritrophic matrix protein, and Ag–Muc1 from the mosquito Anopheles Gambiae. Cell Tissue Res (2005) 320(1):175–85. doi: 10.1007/s00441-004-1067-3
134. Chen WJ, Huang LX, Hu D, Liu LY, Gu J, Huang LH, et al. Cloning, expression and chitin–binding activity of two peritrophin–like protein genes in the common cutworm, Spodoptera litura. Insect Sci (2014) 21(4):449–58. doi: 10.1111/1744-7917.12055
135. Toprak U, Baldwin D, Erlandson M, Gillott C, Hegedus DD. Insect intestinal mucins and serine proteases associated with the peritrophic matrix from feeding, starved and moulting Mamestra configurata larvae. Insect Mol Biol (2010) 19(2):163–75. doi: 10.1111/j.1365-2583.2009.00966.x
136. Kuraishi T, Binggeli O, Opota O, Buchon N, Lemaitre B. Genetic evidence for a protective role of the peritrophic matrix against intestinal bacterial infection in Drosophila melanogaster. Proc Natl Acad Sci (2011) 108(38):15966–71. doi: 10.1073/pnas.1105994108
137. Shibata T, Maki K, Hadano J, Fujikawa T, Kitazaki K, Koshiba T, et al. Crosslinking of a peritrophic matrix protein protects gut epithelia from bacterial exotoxins. PloS Pathog (2015) 11(10):e1005244. doi: 10.1371/journal.ppat.1005244
138. Rodríguez–de la Noval C, Rodríguez–Cabrera L, Izquierdo L, Espinosa LA, Hernandez D, Ponce M, et al. Functional expression of a peritrophin A–like SfPER protein is required for larval development in Spodoptera frugiperda (Lepidoptera: Noctuidae). Sci Rep (2019) 9(1):2630. doi: 10.1038/s41598-019-38734-0
139. Wang P, Granados RR. Molecular structure of the peritrophic membrane (PM): identification of potential PM target sites for insect control. Arch Insect Biochem Physiol Publ Collab Entomol Soc Am (2001) 47(2):110–8. doi: 10.1002/arch.1041
140. Zha XL, Yu XB, Zhang HY, Wang H, Huang XZ, Shen YH, et al. Identification of Peritrophins and Antiviral Effect of Bm01504 against BmNPV in the Silkworm, Bombyx mori. Int J Mol Sci (2020) 21(21):7973. doi: 10.3390/ijms21217973
141. Zhang C, Ding Y, Zhou M, Tang Y, Chen R, Chen Y, et al. RNAi–mediated CHS–2 silencing affects the synthesis of chitin and the formation of the peritrophic membrane in the midgut of Aedes albopictus larvae. Parasit Vectors (2023) 16(1):259. doi: 10.1186/s13071-023-05865-3
142. Peng J, Zhong J, Granados RR. A baculovirus enhancin alters the permeability of a mucosal midgut peritrophic matrix from lepidopteran larvae. J Insect Physiol (1999) 45(2):159–66. doi: 10.1016/S0022-1910(98)00110-3
143. Wang M, Hu Z. Cross–talking between baculoviruses and host insects towards a successful infection. Philos Trans R Soc B Biol Sci (2019) 374(1767):20180324. doi: 10.1098/rstb.2018.0324
144. Rao R, Fiandra L, Giordana B, de Eguileor M, Congiu T, Burlini N, et al. AcMNPV ChiA protein disrupts the peritrophic membrane and alters midgut physiology of Bombyx mori larvae. Insect Biochem Mol Biol (2004) 34(11):1205–13. doi: 10.1016/j.ibmb.2004.08.002
145. Di Maro A, Terracciano I, Sticco L, Fiandra L, Ruocco M, Corrado G, et al. Purification and characterization of a viral chitinase active against plant pathogens and herbivores from transgenic tobacco. J Biotechnol (2010) 147(1):1–6. doi: 10.1016/j.jbiotec.2010.03.005
146. Wang Y, Choi JY, Roh JY, Tao XY, Liu Q, Lee JH, et al. Insecticidal activity of the chitinase from the S podoptera litura nucleopolyhedrovirus. Entomol Res (2013) 43(1):63–9. doi: 10.1111/1748-5967.12004
147. Cuartas PE, Barrera GP, Belaich MN, Barreto E, Ghiringhelli PD, Villamizar LF. The complete sequence of the first Spodoptera frugiperda Betabaculovirus genome: a natural multiple recombinant virus. Viruses. (2015) 7(1):394–421. doi: 10.3390/v7010394
148. Wang Q, Qu L, Zhang Z, Wang Y, Zhang Y. Characterization of a novel chitinase, DkChi, from Dendrolimus kikuchii nucleopolyhedrovirus. Arch Virol (2013) 158:2523–30. doi: 10.1007/s00705-013-1775-7
149. Hu X, Zhu M, Wang S, Zhu L, Xue R, Cao G, et al. Proteomics analysis of digestive juice from silkworm during Bombyx mori nucleopolyhedrovirus infection. PROTEOMICS. (2015) 15(15):2691–700. doi: 10.1002/pmic.201400475
150. Hayashiya K, Nishida J, Matsubara F. The production of anti–viral substance, a red fluorescent protein, in the digestive juice of the silkworm larvae (Lepidoptera: bombycidae). Appl Entomol Zool (1969) 4(3):154–5. doi: 10.1303/aez.4.154
151. Hayashiya K, Nishida J, Uchida Y. The mechanism of formation of the red fluorescent protein in the digestive juice of silkworm larvae: the formation of chlorophyllide a. Jpn J Appl Entomol (1976) 20(1):1-8. doi: 10.1303/jjaez.20.37
152. Selot R, Kumar V, Shukla S, Chandrakuntal K, Brahmaraju M, Dandin SB, et al. Identification of a soluble NADPH oxidoreductase (BmNOX) with antiviral activites in the gut juice of Bombyx mori. Biosci Biotechnol Biochem (2007) 71(1):200–5. doi: 10.1271/bbb.60450
153. Ponnuvel KM, Nakazawa H, Furukawa S, Asaoka A, Ishibashi J, Tanaka H, et al. A lipase isolated from the silkworm Bombyx mori shows antiviral activity against nucleopolyhedrovirus. J Virol (2003) 77(19):10725–9. doi: 10.1128/JVI.77.19.10725-10729.2003
154. Jiang L, Wang G, Cheng T, Yang Q, Jin S, Lu G, et al. Resistance to Bombyx mori nucleopolyhedrovirus via overexpression of an endogenous antiviral gene in transgenic silkworms. Arch Virol (2012) 157:1323–8. doi: 10.1007/s00705-012-1309-8
155. Nakazawa H, Tsuneishi E, Ponnuvel KM, Furukawa S, Asaoka A, Tanaka H, et al. Antiviral activity of a serine protease from the digestive juice of Bombyx mori larvae against nucleopolyhedrovirus. Virology. (2004) 321(1):154–62. doi: 10.1016/j.virol.2003.12.011
156. Zhang SZ, Zhu LB, You LL, Wang J, Cao HH, Liu YX, et al. A Novel Digestive Proteinase Lipase Member H–A in Bombyx mori Contributes to Digestive Juice Antiviral Activity against B. mori Nucleopolyhedrovirus. Insects (2020) 11(3):154. doi: 10.3390/insects11030154
157. Sunagar SG, Lakkappan VJ, Ingalhalli SS, Savanurmath CJ, Hinchigeri SB. Characterization of the photochromic pigments in red fluorescent proteins purified from the gut juice of the silkworm Bombyx mori L. Photochem Photobiol (2008) 84(6):1440–4. doi: 10.1111/j.1751-1097.2008.00360.x
Keywords: columnar cells, goblet cells, regenerative cells, endocrine cells, immunity, peritrophic membrane, digestive juice
Citation: Awais MM, Fei S, Xia J, Feng M and Sun J (2024) Insights into midgut cell types and their crucial role in antiviral immunity in the lepidopteran model Bombyx mori. Front. Immunol. 15:1349428. doi: 10.3389/fimmu.2024.1349428
Received: 05 December 2023; Accepted: 18 January 2024;
Published: 14 February 2024.
Edited by:
Shoichiro Kurata, Tohoku University, JapanCopyright © 2024 Awais, Fei, Xia, Feng and Sun. This is an open-access article distributed under the terms of the Creative Commons Attribution License (CC BY). The use, distribution or reproduction in other forums is permitted, provided the original author(s) and the copyright owner(s) are credited and that the original publication in this journal is cited, in accordance with accepted academic practice. No use, distribution or reproduction is permitted which does not comply with these terms.
*Correspondence: Jingchen Sun, Y3lmekBzY2F1LmVkdS5jbg==; Min Feng, aHVuYW5mZW5nbWluQHNjYXUuZWR1LmNu
Disclaimer: All claims expressed in this article are solely those of the authors and do not necessarily represent those of their affiliated organizations, or those of the publisher, the editors and the reviewers. Any product that may be evaluated in this article or claim that may be made by its manufacturer is not guaranteed or endorsed by the publisher.
Research integrity at Frontiers
Learn more about the work of our research integrity team to safeguard the quality of each article we publish.