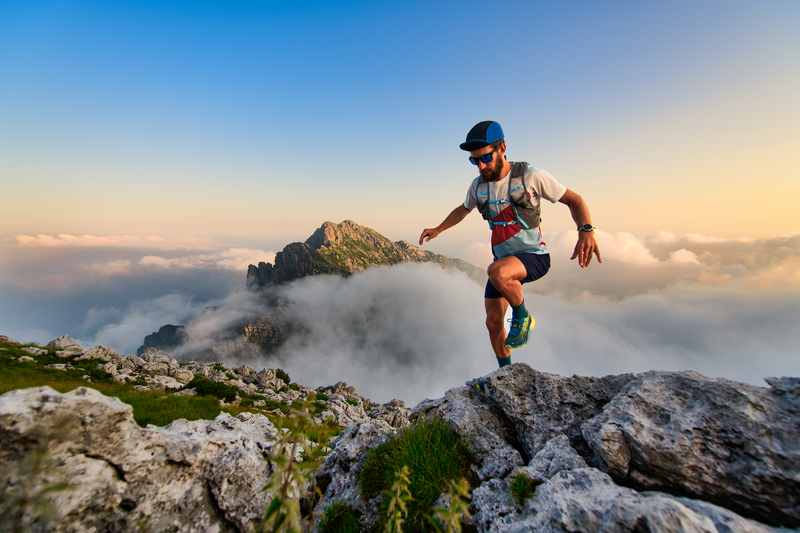
94% of researchers rate our articles as excellent or good
Learn more about the work of our research integrity team to safeguard the quality of each article we publish.
Find out more
REVIEW article
Front. Immunol. , 01 February 2024
Sec. Cancer Immunity and Immunotherapy
Volume 15 - 2024 | https://doi.org/10.3389/fimmu.2024.1343450
Non–small cell lung cancer (NSCLC) accounts for 80–85% of all lung cancers. In recent years, treatment with immune checkpoint inhibitors (ICIs) has gradually improved the survival rate of patients with NSCLC, especially those in the advanced stages. ICIs can block the tolerance pathways that are overexpressed by tumor cells and maintain the protective activity of immune system components against cancer cells. Emerging clinical evidence suggests that gut microbiota may modulate responses to ICIs treatment, possibly holding a key role in tumor immune surveillance and the efficacy of ICIs. Studies have also shown that diet can influence the abundance of gut microbiota in humans, therefore, dietary interventions and the adjustment of the gut microbiota is a novel and promising treatment strategy for adjunctive cancer therapy. This review comprehensively summarizes the effects of gut microbiota, antibiotics (ATBs), and dietary intervention on the efficacy of immunotherapy in NSCLC, with the aim of informing the development of novel strategies in NSCLC immunotherapy.
Lung cancer currently has an extremely high incidence and mortality rate, with non–small cell lung cancer (NSCLC) accounting for 80–85% of all lung cancers. Therefore, clarifying the pathogenesis and treatment of NSCLC is a critical healthcare need. The programmed cell death 1 (PD-1) and programmed cell death ligand 1 (PD-L1) axis play a key role in physiological immune homeostasis and can serve as a means for cancer cells to evade the immune system (1). PD-L1 binds its major receptor PD-1 in trans and cis (2, 3) by antagonizing T-cell receptors and CD28 costimulatory signals (4) to suppress antitumor PD-1–positive T-cell function (5). The PD-1 axis also inhibits the lytic activity against activated cells, including B cells and natural killer (NK) cells (6, 7). More importantly, PD-1 is highly expressed in regulatory T cells (Tregs), which can be activated and proliferated in the presence of the ligand (8). It can also suppress the immune response through increasing the expression of forkhead transcription factor FOXP3, muting the expression of effector cytokines such as interferon-gama (IFN-γ), and producing inhibitory cytokines such as transforming growth factor-beta (TGF-β), interleukin (IL)-10, and IL-35. Anti–PD-1/PD-L1 therapy is an effective treatment for patients with metastatic NSCLC lacking sensitizing EGFR or ALK mutations (9–13). The primary anticancer mechanism of anti–PD-L1 or anti-PD-1 antibody is thought to prevent PD-1 antitumor T cells from being inhibited by cell surface–expressed PD-L1, leading to T-cell resuscitation (14), reduced T-cell depletion or death, and increased T-cell memory and intratumoral antitumor immune cell infiltration (15–19). PD-L1 expressed on tumor cells is considered to be a biomarker for predicting immune checkpoint inhibitors (ICIs) efficacy in patients with NSCLC (20).
Overall and objective response rates improve significantly in some patients after immunotherapy (21). However, due to individual differences among patients (22), only a minority of patients show benefit from ICIs (23). Emerging evidence indicates that the efficacy of ICIs therapy is related to the characteristics of the host gut microbiota (24, 25). The gut microbiota is being increasingly recognized as an important factor associated with tumor development and efficacy of antitumor therapies (26). Various studies have confirmed that the gut microbiota can significantly influence ICIs treatment (27–30), and research indicates that complete gut microbiota is essential to improving the efficacy of ICIs and cancer treatment (27, 31–33). Patients with a favorable gut microbiota show enhanced memory T cell and NK cell signatures in the peripheral blood (34). The gut microbiota is emerging as an attractive therapeutic target for cancer. Using antibiotics (ATBs) has been shown to impair the efficacy of immunotherapy in mice and human (27, 31), an effect which may be attributable to a dramatic reduction in gut dysbiosis and beneficial microbial subpopulations. Therefore, it is important to strictly control the use of ATBs during immunotherapy in patients with tumor.
In addition, it has been proven that dietary intervention can regulate gut microbiota and affect the efficacy of immunotherapy. Studies have shown that probiotics use is associated with favorable clinical outcomes in patients with advanced or recurrent NSCLC receiving anti-PD-1 monotherapy. In patients with NSCLC receiving ICIs, combining probiotic therapy has been reported to result in significantly longer progression-free survival (PFS) and overall survival (OS) (35–37). Reasonable use of probiotics, prebiotics, and dietary intervention to target the gut microbiota may be a potential strategy for promoting the clinical efficacy of ICI treatment (38). Although the gastrointestinal tract and respiratory tract are physically distant, they share the same embryonic origin, and they are highly similar structurally (39). Recent findings attesting to the many pathways involving their respective microbiota support the presence of the gut–lung axis (GLA) (40), suggesting that gut microbiota can influence the development of lung cancer.
In this review, we focus on the “favorable” and “unfavorable” gut microbiota in preclinical and clinical studies related to NSCLC immunotherapy, particularly anti-PD-1/PD-L1 therapy, and the impact of ATBs and dietary interventions on the efficacy of NSCLC immunotherapy (Figure 1).
Figure 1 Dietary intervention and gut microbiota regulate the efficacy of immunotherapy for NSCLC. Dietary interventions and antibiotics can influence the efficacy of anti-PD-1/PD-L1 treatment by influencing the abundance of gut microbiota—which includes the synergistic and antagonistic effect of microbiota on PD-1/PD-L1 therapy—by modulating innate and/or adaptive immunity. NSCLC, Non–small Cell Lung Cancer; PD-L1, Programmed cell death ligand 1; PD-1, Programmed cell death 1.
The complex gut microbial ecosystem plays a role in human health and disease, and recent evidence suggests that the microbiota is linked to the efficacy and toxicity of different cancer treatments (41). The gut microbiota has emerged as a key factor in shaping tumor immune surveillance and determining the efficacy of ICIs (Table 1).
Table 1 Gut microbiota targeting innate and adaptive immune cells to affect the efficacy of anti-PD-1/PD-L1 treatment in NSCLC.
The human gut contains certain probiotics, and rational use of anti-PD-1/PD-L1 therapy combined with probiotics can significantly improve the efficacy of anti-PD-1/PD-L1 therapy in NSCLC, providing more opportunities for the prolonging of PFS and OS in those with NSCLC (Figure 2).
Figure 2 A variety of gut microbiota can affect the efficacy of immunotherapy for NSCLC through multiple immune mechanisms, such as Bifidobacteria, Bifidobacterium longum, Bifidobacterium breve, LGG, AKK, Enterococcus bacteriophage, Nissle 1917. AKK, Akkermansia muciniphila; LGG, Lactobacillus rhamnosus GG; TNFα, tumor necrosis factor alpha; KbSIY, a model neoantigen, SIYRYYGL; CRISPR/Cas9, clustered regularly interspaced short palindromic repeat; ROS, reactive oxygen species; ICD, immunogenic cell death; IDO1, indolea-mine 2,3-dioxygenase-1; TMP1, the epitope-tail length measurement protein 1; PSMβ-4, proteasome subunit beta-4.
Bifidobacteria are key intestinal beneficial microorganisms, which as a type of physiologically beneficial bacteria, can exert an antitumor effect. Bifidobacterium species have been shown to be strongly associated with ICIs efficacy (57, 61), mainly by promoting the activation of dendritic cells (DCs) under homeostasis, which in turn improves the effector function of tumor-specific CD8+ T cells (29). Sivan (29) et al. showed that the therapeutic effect of Bifidobacterium was abolished in mice lacking CD8+ T cells, suggesting that the mechanism was not direct but exerted through the host antitumor T-cell response. Only specific Bifidobacterium strains (i.e., B. HIF-K57, B. HIF-K18, and B. HIF-MG731 [B. HIF-M31]) act synergistically with anti–PD-1 therapy to reduce tumor growth, while other Bifidobacterium bifidum strains (i.e., B. bifidum_B06, B. bifidum_R71 and B. bifidum CKDB001 (B. Bifidum_C01)) show no synergistic effect with anti-PD-1 therapy (62). It can be surmised that even different strains of the same bacteria have different effects on the efficacy of immunotherapy. Wu et al. (42) showed that Bifidobacterium longum (B. longum) had exert an antitumor effect but also had an antagonistic effect against pembrolizumab treatment. In addition, flow cytometry showed that the proportion of CD45+ cells in peripheral blood significantly changes after B. longum treatment (42). Another study found that in a mouse model of B16.SIY tumor, B. longum increased production of proinflammatory cytokine tumor necrosis factor alpha (TNFα) and significantly improved tumor control after anti-PD-L1 treatment (43). Moreover, studies have reported that Bifidobacterium breve (B. breve) in the gut significantly prolong the median PFS (mPFS) of patients with NSCLC receiving anti–PD-1 immunotherapy combined with chemotherapy, and B. breve may be an effective biomarker for predicting its clinical benefit (44). B. breve exposure can promote the ability of H2-Kb SIY (a model neoantigen, SIYRYYGL) (KbSIY) complex reactive cells to expand, resulting in an increase in CD8+ T cells, a higher affinity response, and greater KbSIY cross-reactivity, with targeted KbSVY (an epitope called SVYRYYGL) therapy being shown to slow tumor progression (43). These findings suggest that bifidobacteria are beneficial for antitumor immune responses. Despite the above mentioned findings, further identification of the effects of different Bifidobacterium strains on immunotherapy and their molecular mechanisms remains a challenge that requires continued research.
Lactobacillus rhamnosus GG (LGG), a species belonging to the Lactobacillus genus, is a Gram-positive, anaerobic, inactive, non–spore-forming, rod-shaped microbe that is one of the most widely studied probiotics in humans. LGG can penetrate the hypoxia tumor center, allowing efficient delivery of the clustered regularly interspaced short palindromic repeat (CRISPR/Cas9) system to the tumor region. The CRISPR/Cas9 nanosystem can generate abundant reactive oxygen species (ROS) under the ultrasound irradiation, resulting in immunogenic cell death (ICD), while the produced ROS can induce endosomal/lysosomal rupture and then releasing Cas9/sgRNA to knock down the indolea-mine 2,3-dioxygenase-1 (IDO1) gene to lift immunosuppression (45). Recent studies have found that Lactobacillus rhamnosus Probio-M9 can effectively restore the diversity and structure of intestinal microbes in mice, but ProbioM9 alone has no significant effect on tumor inhibition. However, in anti-PD-1 therapy, Probio-M9 inhibits harmful bacteria by enhancing beneficial bacteria, thus promoting an antitumor immune response to anti-PD-1 therapy (46, 47).
Akkermansia muciniphila (AKK) is a Gram-negative and anaerobic bacterium that is a minor component of the gut microbiota. It is generally acquired from the mother, is usually abundant in children, and decreases with age and disease. The relative abundance of AKK has been associated with human responses to anti-PD-1 or anti-PD-L1 therapy (29, 48, 63). Patients with AKK in the gut respond better to treatment (49). And in the NEOSTAR trial, Neoadjuvant ipilimumab+nivolumab (Ipi+Nivo) and nivolumab+chemotherapy (Nivo+CT) induce greater major pathologic response (MPR) rates than CT alone in patients with operable NSCLC. MPR rates were 32.1% in the Nivo + CT arm and 50% in the Ipi+Nivo+CT arm. Although the MPR was different between the two groups, both groups were AKK-rich in the fecal microbiota at baseline (64). However, the abundance of AKK is reduced in PD-1 nonresponders (50). Hypermucotropic AKK has been associated with durable clinical benefit in patients with advanced lung cancer (51).
The proportion of AKK is higher in patients with stable disease and partial response to immunotherapy compared with patients with progressive disease (49, 52). In one study, the objective response rate (ORR) was greater in AKK-positive patients than in AKK-negative patients receiving immunotherapy alone in first-line treatment. Moreover, 59% percent of the AKK-positive patients were still alive after 12 months, whereas only 35% of AKK-negative individuals were long-term survivors. For the entire cohort of patients (regardless of treatment regimen), the median OS (mOS) in the AKK-positive group was greater than in the AKK-negative group (63).
Routy et al. showed that oral supplementation with AKK in nonresponders restored the efficacy of PD-1 blockade in an IL-12-dependent manner by increasing the recruitment of CCR9+, CXCR3+, and CD4+ T lymphocytes to tumor beds in mice (27). Teng et al. reported that HYR-2, which is composed of Salvia miltiorrhiza, ginseng, and licorice at half the dose of the Ze Qi decoction, may exert its anti–lung cancer effect by downregulating PD-L1 and upregulating AKK mucotrophism. HYR-2 may down-regulate PD-L1 expression in vivo by inhibiting the PI3K/Akt signaling pathway (53). Thus, AKK can be used as a supportive marker of response to immunotherapy in NSCLC.
Evidence from clinical trials suggests that Firmicutes and Actinomyces are enriched in responders receiving intestinal flora transplantation (FMT) combined with PD-1 blockade (54). The epitope-tail length measurement protein 1 (TMP1) in the genome of Enterococcus bacteriophage is highly similar to the proteasome subunit beta-4 (PSMβ-4) tumor antigen. They can simultaneously activate CD8+ T cells and improve the efficacy of PD-1 blockade (55). Two butyrate-producing gut bacteria (Agathobacter M104/1 and Blautia SR1/5) were found to favorably regulate the host immune response, and the presence of these 2 substances in the stool could significantly prolong PFS (65). In one study, Granulicatella showed a significant association with responders to anti-PD-1 therapy and thus could be used as a biomarker for prognosis and diagnosis (56). Other studies have shown that the presence of Alistipes is related to better results in ICIs treatment (57), and Alistipes putredinis is reduced in patients with advanced NSCLC who respond to anti-PD-1 immunotherapy (34). Moreover, the overexpression of Alistipes indistinctus in NSCLC responders was found to be effective in restoring the efficacy of ICIs therapy (27). A high level of Alistipes onderdonkii has been associated with durable clinical benefit (DCB) (51). The engineered probiotic Escherichia coli Nissle 1917 strain can colonize the tumor site and continuously convert the metabolite ammonia to L-arginine in the tumor bed. Intertumoral injection of this strain in mice was shown to increase intracellular L-arginine concentrations, trigger intratumoral CD4+ and CD8+ T-cell infiltration, and exert a synergistic antitumor effect when combined with anti-PD-L1 therapy (58).
Not all gut microbiota play an auxiliary role in anti-PD-1/PD-L1 therapy in NSCLC, and some gut microbiota have been shown to be associated with poor response to ICIs treatment and poor survival.
Gammaproteobacteria are the most diverse Gram-negative bacteria. The presence of Gammaproteobacteria is associated with low PD-L1 expression and poor response to checkpoint-based immunotherapy, resulting in poor survival (59). Gammaproteobacteria appear to be enriched in lung cancer, and their abundance in the tumor environment is associated with low PD-L1 expression and a tendency for low PFS and worse OS in ICIs therapy (59).
Helicobacter pylori is a typical spiral or arc bacteria located on the surface of gastric mucosal epithelial cells, appearing mainly on the mucosa near the pylorus and antrum of the stomach. In the Dijon cohort study, H. pylori seropositivity was found to be associated with reduced survival in patients with NSCLC treated with anti-PD-1 therapy. H. pylori–seropositive patients had a median survival of 6.7 months, compared with 15.4 months for seronegative patients. In addition, H. pylori seropositivity was found to be associated with significantly reduced PFS in patients with NSCLC cancer treated with anti-PD-1 therapy (60). H. pylori inhibits antitumor CD8+ T-cell responses by altering the cross-presenting activity of DCs and inhibits both innate and adaptive immune responses in infected hosts. Although H. pylori does not colonize the gut, based on the data we have available, it is critical to eradicate it when possible during anti-PD-1/PD-L1 therapy in patients with NSCLC. It has been discovered that the gut microbiota is a key factor affecting the efficacy of ICIs in NSCLC and can be used as a biomarker to predict the efficacy of ICIs treatment. However, the specific mechanism of action needs to be further studied. It is expected that other clinically significant intestinal flora will be discovered to provide a basis for targeting the advantaged population in NSCLC immunotherapy.
There are conflicting opinions concerning the effect of ATBs use on ICIs treatment. It has been shown that ATBs use is an independent predictor of shorter PFS and OS in patients with advanced cancer treated with ICIs (31, 66–72). In addition, several meta-analyses also demonstrated that ATB use was significantly associated with adverse OS and PFS in cancer patients receiving ICI immunotherapy (33, 73, 74). This negative antitumor effect is significantly enhanced with the cumulative use of ATBs (66). Schett et al. reported that patients with NSCLC who received ATBs within 60 days before ICIs had significantly shorter PFS and OS than did patients who did not receive ATBs before ICIs (70). Other studies indicate that at 60 days before ICIs initiation, ATBs have no effect in terms of objective response or PFS but may still be significantly associated with shorter OS (31, 75). Furthermore, the recent study indicated that the downregulation of mucosal addressin cell adhesion molecule 1 (MAdCAM-1) in the ileum, following ATBs treatment, led to gut recolonization by Enterocloster species. This, in turn, induced the emigration of enterotropic α4β7+CD4+ regulatory T 17 cells into the tumor. In independent cohorts of lung cancer patients, it was observed that low serum levels of soluble MAdCAM-1 had an adverse prognostic impact (76).
However, other studies did not find any difference in PFS and OS between patients treated with ATBs and those without treatment. Galli et al. propose that the duration of ATBs use associated with immunotherapy may be the most relevant factor in modulating PFS and OS, and not the type of ATBs prescribed or the time between the start of ATBs use and the start of immunotherapy. In their study, patients with high ATBs–immunotherapy exposure ratio (AIER) during the full immunotherapy period had worse PFS and OS compared to other patients (77). Previous studies have proposed a measure called ATBs exposure (AE), which is defined as the number of ATBs days divided by ICIs days. In a comparison of patients who received ATBs treatment and those who did not within a 4-week period before or after starting ICIs therapy, there were no differences in PFS or OS. However, it was observed that patients with higher AE had a significantly lower PFS and OS than did those with a median AE. This suggests that the cumulative use of ATBs, rather than its use within a specific time frame, may negatively impact the effectiveness of immunotherapy (78). Kaderbhai et al. conducted a study on patients with NSCLC and found that ATB-induced changes in the microbiota did not seem to affect the efficacy of nivolumab in terms of PFS (79).
Regarding the type of ATBs used, Lu et al. reported that that patients receiving fluoroquinolones exhibited worse OS than did those treated with other ATBs, and patients who received beta-lactamase inhibitors (BLBLI), fluoroquinolones, or sulfonamides 30 or 60 days prior to ICIs treatment had shorter PFS and OS. In addition, the effect of ATBs use during the first 60 days of ICIs therapy was less pronounced than during the first 30 days (72). In a study by Kim et al., patients treated with BLBLI showed a tendency toward longer PFS and OS compared to patients treated with other types of ATBs (68). Although there are differences between the results of the studies, we can see that the use of most ATBs is inversely related to the efficacy of immunotherapy. Therefore, it is clinically recommended that the use of ATBs should be strictly controlled during immunotherapy in patients with cancer.
Dietary intervention or prebiotics supplementation for patients with NSCLC receiving anti-PD-1/PD-L1 therapy may be a more promising approach for the general population, as it is not only less harmful but also more tolerable. Studies have shown that diet can alter gut microbiota abundance in humans, with short-term consumption of diets consisting exclusively of animal or plant products altering interindividual differences in microbial community structure and microbial gene expression (80). Dietary supplements may influence the gut microbiota and response to anti-PD-1 immunotherapy (Figure 3).
Figure 3 The effect of dietary interventions for the treatment of NSCLC immune. a. GPs increases the antitumor response to anti-PD-1 antibodies by regulating bacteria such as Muribaculum. b. RESV can improve the tumor immunosuppressive microenvironment through multiple mechanisms and promote immune cell-mediated immunotherapy response. c. Castalagin increases the anti-tumor response of anti-PD-1 antibodies by modulating bacteria such as Ruminococcus. d. SFRE combined with pembrolizumab enhanced the anti-PD-L1 immune response of tumor cells and improved the efficacy of immunotherapy. e. SV improves the immunosuppressive microenvironment of tumors by promoting multiple immune response pathways. f. Dietary intervention combined with immunotherapy improves immunotherapy efficacy and prolongs overall survival in patients with NSCLC. GPs, Ginseng polysaccharides; RESV, Resveratrol; SFRE, Supercritical extraction of rosemary; SV, Specific vegetable extracts.
Ginseng polysaccharides (GPs) are one of the most abundant components in ginseng, which can exert key immunomodulatory and antitumor effects. One study found that GPs increased the antitumor response to anti-PD-1 monoclonal antibodies by increasing the microbial metabolite valine and decreasing the ratio of L-kynuridine and the ratio of kynurenine to tryptophan, which helped suppress Tregs and induced effector T cells after combination therapy. Combination therapy with GPs and anti-PD-1 monoclonal antibodies reshaped the composition of the gut microbiota and increased the amount of short-chain fatty-acid–producing bacteria, Muribaculum, to sensitize the antitumor effect of anti-PD-1 therapy, restoring the composition of microbes in fecal samples from nonresponders to anti-PD-1 therapy. In another study, combination therapy increased the production of functional cytokines IFNγ, TNFα, and granzyme B by CD8+ T cells in peripheral blood and tumor tissue (80). These data suggest that combination therapy enhances the antitumor immunity effect.
Resveratrol (RESV), a nonflavonoid polyphenol organic compound, is an antitoxin produced by many plants when stimulated. The pharmacology of RESV in lung cancer cells enables dose-dependent upregulation of PD-L1 expression in the concentration range and is critical for suppressing T cell–mediated immune responses. Furthermore, the Wnt pathway mediates the upregulation of PD-L1 induced by RESV. Mechanistically, RESV activates Sirt 1 deacetylase to deacetylate and stabilize transcription factor Snail. Snail in turn represses Axin 2 transcription, which leads to disassembly of the destruction complex and enhanced binding of β-catenin/TCF to the PD-L1 promoter (81). In addition, RESV may affect vitamin D signaling in lung cancer cells. The vitamin D–active metabolite PRI-2191 was shown to cause a significant upregulation of PD-L1 expression in HCC 827 and NCI-H358 cells, and only when PRI-2191 was used with RESV was PD-L1 expression significantly increased (82). RESV can upregulate the cytotoxic effect of CD8+ T cells and improve the tumor immunosuppressive microenvironment, and the combination of RESV with other immunotherapy drugs may be a more effective treatment (83). RESV is considered to be a module of the inhibitory function of medullary-derived suppressor cells (MDSC), a novel booster of tumor immunotherapy. RESV has been shown to ameliorate tumor development by decreasing granulocytic MDSC (G‐MDSC) accumulation, impairing its suppressive ability on CD8+T cells and promoting monocytic MDSC (M‐MDSC) differentiation into CD11c+ and F4/80+ cells (84).
Castalagin is a polyphenol that enhances resistance to PD-1. In their study, Messaoudene et al. reported that oral supplementation with polyphenol-rich berry camu-camu (CC; Myrciaria dubia) altered the gut microbial composition, leading to antitumor activity and a stronger anti-PD-1 response. Castalagin improved the CD8+/FOXP3+CD4+ ratio in the tumor microenvironment. Moreover, castalagin induced metabolic changes, resulting in an increase level of taurine-conjugated bile acids. Ruminococcus-rich NSCLC responders were found to be able to metabolize castalagin. The increase in Ruminococcus after cancer treatment further suggests that castalagin has a propensity to interact with specific and beneficial bacteria (85).
Supercritical extraction of rosemary (SFRE) (12-16% composition of phenolic diterpenoid, carnosic acid, and carnol) works synergically with the standard treatment used in the clinic to inhibit the cell viability of NSCLC cells. SFRE was found to reduce the expression of PD-L1 and CX3CRl, which exert immunosuppressive effects in the tumor microenvironment, suggesting that SFRE may contribute to the reduction of immune evasion by lung cancer cells (86).
Specific vegetable extracts (SVs) have been shown to benefit the survival of patients with stage IIIb/IV NSCLC, and SV can regulate the antitumor efficacy of NK and adoptive T-cell immune responses. The antitumor effects of SV are also mediated by innate bone marrow cell function, which requires toll like receptor (TLR) and β-glucan signaling in a MyD88/TRIF– and Dectin-1–dependent manner, respectively. Furthermore, SV treatment reduced granulocytic MDSC infiltration into the tumor and limited monocytic MDSC toward the M2-like functional phenotype. SV treatment enhances antigen-specific immune responses by enhancing the activation and proliferation of antigen-specific T helper 1 (TH1)/TH 17 cells in secondary lymphoid organs and by reducing the Treg population in the tumor microenvironment. One study found that SVs mainly composed of mushrooms and green bean extract used alone or in combination with ICIs could exert significant antitumor effects in mice with lung cancer (87).
Studies have shown that a sugar-restricted diet improves the host pulmonary immune response and inhibits the tumor growth of experimental lung adenocarcinoma (LUAD). Thus, sugar-restricted diets have become a therapeutic approach for patients with LUAD (88). Methionine regulates tumor immunity by regulating the activity of cyclic GMP-AMP synthase (cGAS), so the tumor immune response can be improved by controlling dietary methionine intake (89). Patients should be advised to minimize their intake of animal meat and increase their intake of plants as much as possible, aiming for 30 plants per week. High fiber intake (>30 g/day) is thought to increase the chance of an ICIs response (90). In addition, alcohol intake has been shown to cause CD4+ T-lymphocyte depletion, allowing for in situ growth of LUAD xenografts in BALB/c mice. In addition, alcohol consumption reduces the ability of the compromised immune system to reject tumors. High intake of ethanol delays the recovery of the immune response after CD4+ T-lymphocyte depletion (91).
In recent years, a number of clinical trials have been carried out examining the efficacy of immunotherapy combined with dietary intervention. If dietary intervention is proven to have a positive auxiliary effect on immunotherapy, this will represent an unprecedented breakthrough in the treatment of lung cancer. One trial (NCT03700437) is investigating a plant-based fasting-mimicking diet (FMD) that provides ~300 calories/fasting day and includes all the food to be consumed during the dietary intervention, including supplements. Participants will start the diet 3 days prior to chemoimmunotherapy and continue on the first day of chemoimmunotherapy for the first 4 cycles of therapy. The aim of this study is to investigate the effect of FMD therapy on chemotherapy combined with immunotherapy for NSCLC. Another trial (NCT04924374) included an experimental group that pooled fecal microbiota capsules of 1 donor selected based on fecal abundance in Faecalibacterium prausnitzii, Bifidobacterium longum, AKK, and Fusobacterium spp. After the screening and metagenomic analysis of 10 donors with high-fiber diets (>30g/day) is performed, anti-PD-1 therapy will be administered every 1–2 weeks. In contrast, the control group will be only administered anti–PD-1 therapy every 2–3 weeks. The aim of this study is to investigate the effect of gut microbiota in patients with high-fiber diet on the efficacy of immunotherapy in patients with advanced NSCLC. In another study (NCT04175769), the experimental group will consist of participants who will orally consume 2 gelatin capsules of nutritional supplement (1 gram), 2 times per day with meals, and 1 capsule 1 additional meal or snack, totaling 5 capsules per day beginning the first day of treatment and continuing for the duration of their treatment. The control group will orally consume 2 gelatin capsules of placebo (1 gram), 2 times per day with meals, and 1 capsule at 1 additional meal or snack, totaling 5 capsules per day beginning the first day of treatment and continuing for the duration of treatment. The aim of this study is to investigate the effect of nutritional products on the response of patients to immunotherapy or immunotherapy combined with chemotherapy for NSCLC. Another trial (NCT05902260) will test whether high-energy and high-protein nutritional supplements can decrease protein clearance, including drug clearance in patients with NSCLC receiving anti-PD-1 ICIs; this in turn would positively affect anti-PD-1 drug bioavailability, leading to activation of the immune system and thereby an increased response to PD-1 therapy. Patients will start with the daily nutritional intervention prior to start of the first infusion of anti-PD-1 therapy (consuming two 200-mL bottles of study product per day) and will continue this nutritional support for 4 treatment cycles, corresponding with 12 weeks of treatment. Blood samples, questionnaires and fecal specimens will be collected on several time points during this treatment. The aim of this study is to investigate the effect of high-energy and high-protein nutritional supplements on anti-PD-1 therapy for NSCLC. In addition, ongoing clinical trials are investigating the correlation between probiotics and immunotherapy. These clinical trials involve a substantial number of participants, with well-designed and representative experimental and control groups. Upon reaching a conclusion that dietary intervention can affect immunotherapy, it will hold significant value in guiding clinical practices. For the latest and more detailed information, please refer to the clinicaltrials.gov database (Table 2).
Table 2 Ongoing clinical trials designed to investigate the effect of dietary and probiotics interventions on immunotherapy for NSCLC.
In recent years, trials on dietary intervention have been carried out in succession. Although many trials are still ongoing, it can be clearly seen that researchers are beginning to turn their attention to the combination of dietary intervention and immunotherapy for NSCLC treatment in order to maximize the efficacy of immunotherapy and maximize the benefit to patients.
In recent years, anti-PD-1/PD-L1 therapy for NSCLC has made impressive progress. Compared with conventional chemotherapy, anti-PD-1/PD-L1 therapy has shown better clinical efficacy in patients with advanced NSCLC. Therapeutic strategies that utilize the gut microbiota in combination with ICIs, including probiotics, prebiotics intake, fecal microbiota transplantation(FMT) and other dietary interventions, represent new possibilities in effective adjuvant for anti-PD-1/PD-L1 therapy. Further understanding of the synergistic mechanism between anti-PD-1/PD-L1 treatment and gut microbiota is expected to develop more effective combined treatment strategies for anti-PD-1/PD-L1 treatment and promote the development of precision medicine treatment strategies. However, there are still many issues associated with this combination therapy.
Firstly, studies have shown that FMT has a certain auxiliary effect on tumor immunotherapy (27, 54, 92). However, the potential risks of disease transmission between donors and recipients, patient acceptance, undesirable outcomes, and uncertainties regarding effects on the immune system of recipients underscore the critical need for large-sample randomized controlled studies to comprehensively assess the effectiveness of FMT (93, 94). While significant progress has been made in understanding the human gut’s bacterial population in recent years, there remains limited knowledge about the composition, pathogenicity, and function of gut bacteria. Additionally, another uncertainty of FMT is the highly dynamic composition of live microbiota, which is sensitive to external factors such as diet and drugs (95). Therefore, future research should focus on identifying the gut microbiota, defining their functions, and further manipulating the gut microbiota more precisely. We will expect to personalize FMT for different patients based on different hosts and disease genotypes.
In addition, tools for diagnosing the gut microbiota in patients with cancer still need to be refined, and more research is needed to develop new diagnostic tools based on the gut microbiota of patients with cancer to predict the response and resistance to ICIs. Third, although many studies have provided an abundance of evidence, there is still controversy related to some conclusions. The design of additional experiments and tighter research controls are needed to support each conclusion. Finally, Some microbiota and diet-assisted therapies are still in their nascent stage. Researchers are still working hard to design robust clinical trials with the aim of overcoming issue related to variability and biases to verify and utilize the causal relationship between gut microbiota and the efficacy and safety of immunotherapy. In this way, a more rigorous, safe, and reliable, adjuvant treatment strategy in anti-PD-1/PD-L1 therapy for NSCLC can be developed. FMT and dietary intervention are expected to become powerful auxiliary tools in the anti-PD-1/PD-L1 therapy of NSCLC, raising the standard of lung cancer treatment to new heights.
YX: Writing – original draft. CGL: Writing – original draft. DZ: Writing – review & editing. JC: Writing – review & editing.
The author(s) declare financial support was received for the research, authorship, and/or publication of this article. This work was supported by the National Natural Science Foundation of China (82203056), the Natural Science Foundation of Liaoning Province (2023-BS-167), the Science and Technology.
The authors declare that the research was conducted in the absence of any commercial or financial relationships that could be construed as a potential conflict of interest.
All claims expressed in this article are solely those of the authors and do not necessarily represent those of their affiliated organizations, or those of the publisher, the editors and the reviewers. Any product that may be evaluated in this article, or claim that may be made by its manufacturer, is not guaranteed or endorsed by the publisher.
1. Sun C, Mezzadra R, Schumacher TN. Regulation and function of the PD-L1 checkpoint. Immunity (2018) 48(3):434–52. doi: 10.1016/j.immuni.2018.03.014
2. Lin DY, Tanaka Y, Iwasaki M, Gittis AG, Su HP, Mikami B, et al. The PD-1/PD-L1 complex resembles the antigen-binding Fv domains of antibodies and T cell receptors. Proc Natl Acad Sci U S A (2008) 105(8):3011–6. doi: 10.1073/pnas.0712278105
3. Zhao Y, Harrison DL, Song Y, Ji J, Huang J, Hui E. Antigen-presenting cell-intrinsic PD-1 neutralizes PD-L1 in cis to attenuate PD-1 signaling in T cells. Cell Rep (2018) 24(2):379–90.e6. doi: 10.1016/j.celrep.2018.06.054
4. Hui E, Cheung J, Zhu J, Su X, Taylor MJ, Wallweber HA, et al. T cell costimulatory receptor CD28 is a primary target for PD-1-mediated inhibition. Science (2017) 355(6332):1428–33. doi: 10.1126/science.aaf1292
5. Fernandes RA, Su L, Nishiga Y, Ren J, Bhuiyan AM, Cheng N, et al. Immune receptor inhibition through enforced phosphatase recruitment. Nature (2020) 586(7831):779–84. doi: 10.1038/s41586-020-2851-2
6. Terme M, Ullrich E, Aymeric L, Meinhardt K, Desbois M, Delahaye N, et al. IL-18 induces PD-1-dependent immunosuppression in cancer. Cancer Res (2011) 71(16):5393–9. doi: 10.1158/0008-5472.Can-11-0993
7. Fanoni D, Tavecchio S, Recalcati S, Balice Y, Venegoni L, Fiorani R, et al. New monoclonal antibodies against B-cell antigens: possible new strategies for diagnosis of primary cutaneous B-cell lymphomas. Immunol Lett (2011) 134(2):157–60. doi: 10.1016/j.imlet.2010.09.022
8. Francisco LM, Salinas VH, Brown KE, Vanguri VK, Freeman GJ, Kuchroo VK, et al. PD-L1 regulates the development, maintenance, and function of induced regulatory T cells. J Exp Med (2009) 206(13):3015–29. doi: 10.1084/jem.20090847
9. Vokes EE, Ready N, Felip E, Horn L, Burgio MA, Antonia SJ, et al. Nivolumab versus docetaxel in previously treated advanced non-small-cell lung cancer (CheckMate 017 and CheckMate 057): 3-year update and outcomes in patients with liver metastases. Ann Oncol (2018) 29(4):959–65. doi: 10.1093/annonc/mdy041
10. Borghaei H, Paz-Ares L, Horn L, Spigel DR, Steins M, Ready NE, et al. Nivolumab versus docetaxel in advanced nonsquamous non-small-cell lung cancer. N Engl J Med (2015) 373(17):1627–39. doi: 10.1056/NEJMoa1507643
11. Fehrenbacher L, Spira A, Ballinger M, Kowanetz M, Vansteenkiste J, Mazieres J, et al. Atezolizumab versus docetaxel for patients with previously treated non-small-cell lung cancer (POPLAR): a multicentre, open-label, phase 2 randomised controlled trial. Lancet (2016) 387(10030):1837–46. doi: 10.1016/s0140-6736(16)00587-0
12. Garon EB, Rizvi NA, Hui R, Leighl N, Balmanoukian AS, Eder JP, et al. Pembrolizumab for the treatment of non-small-cell lung cancer. N Engl J Med (2015) 372(21):2018–28. doi: 10.1056/NEJMoa1501824
13. Rittmeyer A, Barlesi F, Waterkamp D, Park K, Ciardiello F, von Pawel J, et al. Atezolizumab versus docetaxel in patients with previously treated non-small-cell lung cancer (OAK): a phase 3, open-label, multicentre randomised controlled trial. Lancet (2017) 389(10066):255–65. doi: 10.1016/s0140-6736(16)32517-x
14. Huang AC, Postow MA, Orlowski RJ, Mick R, Bengsch B, Manne S, et al. T-cell invigoration to tumour burden ratio associated with anti-PD-1 response. Nature (2017) 545(7652):60–5. doi: 10.1038/nature22079
15. Paterson AM, Brown KE, Keir ME, Vanguri VK, Riella LV, Chandraker A, et al. The programmed death-1 ligand 1:B7-1 pathway restrains diabetogenic effector T cells in vivo. J Immunol (2011) 187(3):1097–105. doi: 10.4049/jimmunol.1003496
16. Zou W, Wolchok JD, Chen L. PD-L1 (B7-H1) and PD-1 pathway blockade for cancer therapy: Mechanisms, response biomarkers, and combinations. Sci Transl Med (2016) 8(328):328rv4. doi: 10.1126/scitranslmed.aad7118
17. Topalian SL, Taube JM, Anders RA, Pardoll DM. Mechanism-driven biomarkers to guide immune checkpoint blockade in cancer therapy. Nat Rev Cancer (2016) 16(5):275–87. doi: 10.1038/nrc.2016.36
18. Pauken KE, Godec J, Odorizzi PM, Brown KE, Yates KB, Ngiow SF, et al. The PD-1 pathway regulates development and function of memory CD8(+) T cells following respiratory viral infection. Cell Rep (2020) 31(13):107827. doi: 10.1016/j.celrep.2020.107827
19. Liu CG, Chen J, Goh RMW, Liu YX, Wang L, Ma Z. The role of tumor-derived extracellular vesicles containing noncoding RNAs in mediating immune cell function and its implications from bench to bedside. Pharmacol Res (2023) 191:106756. doi: 10.1016/j.phrs.2023.106756
20. Sacher AG, Gandhi L. Biomarkers for the clinical use of PD-1/PD-L1 inhibitors in non-small-cell lung cancer: A review. JAMA Oncol (2016) 2(9):1217–22. doi: 10.1001/jamaoncol.2016.0639
21. Almonte AA, Rangarajan H, Yip D, Fahrer AM. How does the gut microbiome influence immune checkpoint blockade therapy? Immunol Cell Biol (2021) 99(4):361–72. doi: 10.1111/imcb.12423
22. Wang Y, Ma R, Liu F, Lee SA, Zhang L. Modulation of gut microbiota: A novel paradigm of enhancing the efficacy of programmed death-1 and programmed death ligand-1 blockade therapy. Front Immunol (2018) 9:374. doi: 10.3389/fimmu.2018.00374
23. Havel JJ, Chowell D, Chan TA. The evolving landscape of biomarkers for checkpoint inhibitor immunotherapy. Nat Rev Cancer (2019) 19(3):133–50. doi: 10.1038/s41568-019-0116-x
24. Helmink BA, Khan MAW, Hermann A, Gopalakrishnan V, Wargo JA. The microbiome, cancer, and cancer therapy. Nat Med (2019) 25(3):377–88. doi: 10.1038/s41591-019-0377-7
25. Elkrief A, Derosa L, Zitvogel L, Kroemer G, Routy B. The intimate relationship between gut microbiota and cancer immunotherapy. Gut Microbes (2019) 10(3):424–8. doi: 10.1080/19490976.2018.1527167
26. Zitvogel L, Ma Y, Raoult D, Kroemer G, Gajewski TF. The microbiome in cancer immunotherapy: Diagnostic tools and therapeutic strategies. Science (2018) 359(6382):1366–70. doi: 10.1126/science.aar6918
27. Routy B, Le Chatelier E, Derosa L, Duong CPM, Alou MT, Daillère R, et al. Gut microbiome influences efficacy of PD-1-based immunotherapy against epithelial tumors. Science (2018) 359(6371):91–7. doi: 10.1126/science.aan3706
28. Gopalakrishnan V, Spencer CN, Nezi L, Reuben A, Andrews MC, Karpinets TV, et al. Gut microbiome modulates response to anti-PD-1 immunotherapy in melanoma patients. Science (2018) 359(6371):97–103. doi: 10.1126/science.aan4236
29. Sivan A, Corrales L, Hubert N, Williams JB, Aquino-Michaels K, Earley ZM, et al. Commensal Bifidobacterium promotes antitumor immunity and facilitates anti-PD-L1 efficacy. Science (2015) 350(6264):1084–9. doi: 10.1126/science.aac4255
30. Frankel AE, Coughlin LA, Kim J, Froehlich TW, Xie Y, Frenkel EP, et al. Metagenomic shotgun sequencing and unbiased metabolomic profiling identify specific human gut microbiota and metabolites associated with immune checkpoint therapy efficacy in melanoma patients. Neoplasia (2017) 19(10):848–55. doi: 10.1016/j.neo.2017.08.004
31. Derosa L, Hellmann MD, Spaziano M, Halpenny D, Fidelle M, Rizvi H, et al. Negative association of antibiotics on clinical activity of immune checkpoint inhibitors in patients with advanced renal cell and non-small-cell lung cancer. Ann Oncol (2018) 29(6):1437–44. doi: 10.1093/annonc/mdy103
32. Pinato DJ, Howlett S, Ottaviani D, Urus H, Patel A, Mineo T, et al. Association of prior antibiotic treatment with survival and response to immune checkpoint inhibitor therapy in patients with cancer. JAMA Oncol (2019) 5(12):1774–8. doi: 10.1001/jamaoncol.2019.2785
33. Huang XZ, Gao P, Song YX, Xu Y, Sun JX, Chen XW, et al. Antibiotic use and the efficacy of immune checkpoint inhibitors in cancer patients: a pooled analysis of 2740 cancer patients. Oncoimmunology (2019) 8(12):e1665973. doi: 10.1080/2162402x.2019.1665973
34. Jin Y, Dong H, Xia L, Yang Y, Zhu Y, Shen Y, et al. The diversity of gut microbiome is associated with favorable responses to anti-programmed death 1 immunotherapy in Chinese patients with NSCLC. J Thorac Oncol (2019) 14(8):1378–89. doi: 10.1016/j.jtho.2019.04.007
35. Wan L, Wu C, Wu Q, Luo S, Liu J, Xie X. Impact of probiotics use on clinical outcomes of immune checkpoint inhibitors therapy in cancer patients. Cancer Med (2023) 12(2):1841–9. doi: 10.1002/cam4.4994
36. Zhang L, Jin Q, Chai D, Kuang T, Li C, Guan Y, et al. The correlation between probiotic use and outcomes of cancer patients treated with immune checkpoint inhibitors. Front Pharmacol (2022) 13:937874. doi: 10.3389/fphar.2022.937874
37. Takada K, Shimokawa M, Takamori S, Shimamatsu S, Hirai F, Tagawa T, et al. Clinical impact of probiotics on the efficacy of anti-PD-1 monotherapy in patients with nonsmall cell lung cancer: A multicenter retrospective survival analysis study with inverse probability of treatment weighting. Int J Cancer (2021) 149(2):473–82. doi: 10.1002/ijc.33557
38. Gopalakrishnan V, Helmink BA, Spencer CN, Reuben A, Wargo JA. The influence of the gut microbiome on cancer, immunity, and cancer immunotherapy. Cancer Cell (2018) 33(4):570–80. doi: 10.1016/j.ccell.2018.03.015
39. Zhao Y, Liu Y, Li S, Peng Z, Liu X, Chen J, et al. Role of lung and gut microbiota on lung cancer pathogenesis. J Cancer Res Clin Oncol (2021) 147(8):2177–86. doi: 10.1007/s00432-021-03644-0
40. Enaud R, Prevel R, Ciarlo E, Beaufils F, Wieërs G, Guery B, et al. The gut-lung axis in health and respiratory diseases: A place for inter-organ and inter-kingdom crosstalks. Front Cell Infect Microbiol (2020) 10:9. doi: 10.3389/fcimb.2020.00009
41. Kroemer G, Zitvogel L. Cancer immunotherapy in 2017: The breakthrough of the microbiota. Nat Rev Immunol (2018) 18(2):87–8. doi: 10.1038/nri.2018.4
42. Wu C, Wang X, Shang H, Wei H. Construction of a humanized PBMC-PDX model to study the efficacy of a bacterial marker in lung cancer immunotherapy. Dis Markers (2022) 2022:1479246. doi: 10.1155/2022/1479246
43. Bessell CA, Isser A, Havel JJ, Lee S, Bell DR, Hickey JW, et al. Commensal bacteria stimulate antitumor responses via T cell cross-reactivity. JCI Insight (2020) 5(8):1–19. doi: 10.1172/jci.insight.135597
44. Zhao H, Li D, Liu J, Zhou X, Han J, Wang L, et al. Bifidobacterium breve predicts the efficacy of anti-PD-1 immunotherapy combined with chemotherapy in Chinese NSCLC patients. Cancer Med (2023) 12(5):6325–36. doi: 10.1002/cam4.5312
45. Yu J, Zhou B, Zhang S, Yin H, Sun L, Pu Y, et al. Design of a self-driven probiotic-CRISPR/Cas9 nanosystem for sono-immunometabolic cancer therapy. Nat Commun (2022) 13(1):7903. doi: 10.1038/s41467-022-35580-z
46. Gao G, Ma T, Zhang T, Jin H, Li Y, Kwok LY, et al. Adjunctive Probiotic Lactobacillus rhamnosus Probio-M9 Administration Enhances the Effect of Anti-PD-1 Antitumor Therapy via Restoring Antibiotic-Disrupted Gut Microbiota. Front Immunol (2021) 12:772532. doi: 10.3389/fimmu.2021.772532
47. Tan H, Zhai Q, Chen W. Investigations of Bacteroides spp. towards next-generation probiotics. Food Res Int (2019) 116:637–44. doi: 10.1016/j.foodres.2018.08.088
48. Newsome RC, Gharaibeh RZ, Pierce CM, da Silva WV, Paul S, Hogue SR, et al. Interaction of bacterial genera associated with therapeutic response to immune checkpoint PD-1 blockade in a United States cohort. Genome Med (2022) 14(1):35. doi: 10.1186/s13073-022-01037-7
49. Grenda A, Iwan E, Chmielewska I, Krawczyk P, Giza A, Bomba A, et al. Presence of Akkermansiaceae in gut microbiome and immunotherapy effectiveness in patients with advanced non-small cell lung cancer. AMB Express (2022) 12(1):86. doi: 10.1186/s13568-022-01428-4
50. Sarkar J, Cortes Gomez E, Oba T, Chen H, Dy GK, Segal BH, et al. Fluctuations in gut microbiome composition during immune checkpoint inhibitor therapy. World J Oncol (2023) 14(3):178–87. doi: 10.14740/wjon1587
51. Haberman Y, Kamer I, Amir A, Goldenberg S, Efroni G, Daniel-Meshulam I, et al. Gut microbial signature in lung cancer patients highlights specific taxa as predictors for durable clinical benefit. Sci Rep (2023) 13(1):2007. doi: 10.1038/s41598-023-29136-4
52. He D, Li X, An R, Wang L, Wang Y, Zheng S, et al. Response to PD-1-based immunotherapy for non-small cell lung cancer altered by gut microbiota. Oncol Ther (2021) 9(2):647–57. doi: 10.1007/s40487-021-00171-3
53. Teng L, Wang K, Chen W, Wang YS, Bi L. HYR-2 plays an anti-lung cancer role by regulating PD-L1 and Akkermansia muciniphila. Pharmacol Res (2020) 160:105086. doi: 10.1016/j.phrs.2020.105086
54. Davar D, Dzutsev AK, McCulloch JA, Rodrigues RR, Chauvin JM, Morrison RM, et al. Fecal microbiota transplant overcomes resistance to anti-PD-1 therapy in melanoma patients. Science (2021) 371(6529):595–602. doi: 10.1126/science.abf3363
55. Fluckiger A, Daillère R, Sassi M, Sixt B, Liu P, Loos F, et al. Cross-reactivity between tumor MHC class I-restricted antigens and an enterococcal bacteriophage. Pharmacol Res (2020) 369(6506):936–42. doi: 10.1126/science.aax0701
56. Vernocchi P, Gili T, Conte F, Del Chierico F, Conta G, Miccheli A, et al. Network analysis of gut microbiome and metabolome to discover microbiota-linked biomarkers in patients affected by non-small cell lung cancer. Int J Mol Sci (2020) 21(22):1–19. doi: 10.3390/ijms21228730
57. Dora D, Ligeti B, Kovacs T, Revisnyei P, Galffy G, Dulka E, et al. Non-small cell lung cancer patients treated with Anti-PD1 immunotherapy show distinct microbial signatures and metabolic pathways according to progression-free survival and PD-L1 status. Oncoimmunology (2023) 12(1):2204746. doi: 10.1080/2162402x.2023.2204746
58. Canale FP, Basso C, Antonini G, Perotti M, Li N, Sokolovska A, et al. Metabolic modulation of tumours with engineered bacteria for immunotherapy. Nature (2021) 598(7882):662–6. doi: 10.1038/s41586-021-04003-2
59. Boesch M, Baty F, Albrich WC, Flatz L, Rodriguez R, Rothschild SI, et al. Local tumor microbial signatures and response to checkpoint blockade in non-small cell lung cancer. Oncoimmunology (2021) 10(1):1988403. doi: 10.1080/2162402x.2021.1988403
60. Oster P, Vaillant L, Riva E, McMillan B, Begka C, Truntzer C, et al. Helicobacter pylori infection has a detrimental impact on the efficacy of cancer immunotherapies. Orig Res (2022) 71(3):457–66. doi: 10.1136/gutjnl-2020-323392
61. Wu Z, Zhang S, Li L, Huang Z, Huang D, Hu Y. The gut microbiota modulates responses to anti-PD-1 and chemotherapy combination therapy and related adverse events in patients with advanced solid tumors. Front Oncol (2022) 12:887383. doi: 10.3389/fonc.2022.887383
62. Lee S, Cho S, Yoon Y, Park C, Sohn J, Jeong J, et al. Bifidobacterium bifidum strains synergize with immune checkpoint inhibitors to reduce tumour burden in mice. Nat Microbiol (2021) 6(3):277–88. doi: 10.1038/s41564-020-00831-6
63. Derosa L, Routy B, Thomas AM, Iebba V, Zalcman G, Friard S, et al. Intestinal Akkermansia muciniphila predicts clinical response to PD-1 blockade in patients with advanced non-small-cell lung cancer. Nat Med (2022) 28(2):315–24. doi: 10.1038/s41591-021-01655-5
64. Cascone T, Leung CH, Weissferdt A, Pataer A, Carter BW, Godoy MCB, et al. Neoadjuvant chemotherapy plus nivolumab with or without ipilimumab in operable non-small cell lung cancer: the phase 2 platform NEOSTAR trial. Nat Med (2023) 29(3):593–604. doi: 10.1038/s41591-022-02189-0
65. Martini G, Ciardiello D, Dallio M, Famiglietti V, Esposito L, Corte CMD, et al. Gut microbiota correlates with antitumor activity in patients with mCRC and NSCLC treated with cetuximab plus avelumab. Int J Cancer (2022) 151(3):473–80. doi: 10.1002/ijc.34033
66. Tinsley N, Zhou C, Tan G, Rack S, Lorigan P, Blackhall F, et al. Cumulative antibiotic use significantly decreases efficacy of checkpoint inhibitors in patients with advanced cancer. Oncologist (2020) 25(1):55–63. doi: 10.1634/theoncologist.2019-0160
67. Huemer F, Rinnerthaler G, Westphal T, Hackl H, Hutarew G, Gampenrieder SP, et al. Impact of antibiotic treatment on immune-checkpoint blockade efficacy in advanced non-squamous non-small cell lung cancer. Oncotarget (2018) 9(23):16512–20. doi: 10.18632/oncotarget.24751
68. Kim H, Lee JE, Hong SH, Lee MA, Kang JH, Kim IH. The effect of antibiotics on the clinical outcomes of patients with solid cancers undergoing immune checkpoint inhibitor treatment: a retrospective study. BMC Cancer (2019) 19(1):1100. doi: 10.1186/s12885-019-6267-z
69. von Itzstein MS, Gonugunta AS, Sheffield T, Homsi J, Dowell JE, Koh AY, et al. Association between antibiotic exposure and systemic immune parameters in cancer patients receiving checkpoint inhibitor therapy. Cancers (Basel) (2022) 14(5):1–18. doi: 10.3390/cancers14051327
70. Schett A, Rothschild SI, Curioni-Fontecedro A, Krähenbühl S, Früh M, Schmid S, et al. Predictive impact of antibiotics in patients with advanced non small-cell lung cancer receiving immune checkpoint inhibitors : Antibiotics immune checkpoint inhibitors in advanced NSCLC. Cancer Chemother Pharmacol (2020) 85(1):121–31. doi: 10.1007/s00280-019-03993-1
71. Hakozaki T, Okuma Y, Omori M, Hosomi Y. Impact of prior antibiotic use on the efficacy of nivolumab for non-small cell lung cancer. Oncol Lett (2019) 17(3):2946–52. doi: 10.3892/ol.2019.9899
72. Lu PH, Tsai TC, Chang JW, Deng ST, Cheng CY. Association of prior fluoroquinolone treatment with survival outcomes of immune checkpoint inhibitors in Asia. J Clin Pharm Ther (2021) 46(2):408–14. doi: 10.1111/jcpt.13298
73. Yu Y, Zheng P, Gao L, Li H, Tao P, Wang D, et al. Effects of antibiotic use on outcomes in cancer patients treated using immune checkpoint inhibitors: A systematic review and meta-analysis. J Immunother (2021) 44(2):76–85. doi: 10.1097/cji.0000000000000346
74. Zhou J, Huang G, Wong WC, Hu DH, Zhu JW, Li R, et al. The impact of antibiotic use on clinical features and survival outcomes of cancer patients treated with immune checkpoint inhibitors. Front Immunol (2022) 13:968729. doi: 10.3389/fimmu.2022.968729
75. Yang M, Wang Y, Yuan M, Tao M, Kong C, Li H, et al. Antibiotic administration shortly before or after immunotherapy initiation is correlated with poor prognosis in solid cancer patients: An up-to-date systematic review and meta-analysis. Int Immunopharmacol (2020) 88:106876. doi: 10.1016/j.intimp.2020.106876
76. Fidelle M, Rauber C, Alves Costa Silva C, Tian AL, Lahmar I, de la Varende AM, et al. A microbiota-modulated checkpoint directs immunosuppressive intestinal T cells into cancers. Science (2023) 380(6649):eabo2296. doi: 10.1126/science.abo2296
77. Galli G, Triulzi T, Proto C, Signorelli D, Imbimbo M, Poggi M, et al. Association between antibiotic-immunotherapy exposure ratio and outcome in metastatic non small cell lung cancer. Lung Cancer (2019) 132:72–8. doi: 10.1016/j.lungcan.2019.04.008
78. Iglesias-Santamaría A. Impact of antibiotic use and other concomitant medications on the efficacy of immune checkpoint inhibitors in patients with advanced cancer. Clin Transl Oncol (2020) 22(9):1481–90. doi: 10.1007/s12094-019-02282-w
79. Kaderbhai C, Richard C, Fumet JD, Aarnink A, Foucher P, Coudert B, et al. Antibiotic use does not appear to influence response to nivolumab. Anticancer Res (2017) 37(6):3195–200. doi: 10.21873/anticanres.11680
80. David LA, Maurice CF, Carmody RN, Gootenberg DB, Button JE, Wolfe BE, et al. Diet rapidly and reproducibly alters the human gut microbiome. Nature (2014) 505(7484):559–63. doi: 10.1038/nature12820
81. Yang M, Li Z, Tao J, Hu H, Li Z, Zhang Z, et al. Resveratrol induces PD-L1 expression through snail-driven activation of Wnt pathway in lung cancer cells. J Cancer Res Clin Oncol (2021) 147(4):1101–13. doi: 10.1007/s00432-021-03510-z
82. Maj E, Maj B, Bobak K, Gos M, Chodyński M, Kutner A, et al. Differential response of lung cancer cells, with various driver mutations, to plant polyphenol resveratrol and vitamin D active metabolite PRI-2191. Int J Mol Sci (2021) 22(5):1–22. doi: 10.3390/ijms22052354
83. Shan G, Minchao K, Jizhao W, Rui Z, Guangjian Z, Jin Z, et al. Resveratrol improves the cytotoxic effect of CD8 +T cells in the tumor microenvironment by regulating HMMR/Ferroptosis in lung squamous cell carcinoma. J Pharm BioMed Anal (2023) 229:115346. doi: 10.1016/j.jpba.2023.115346
84. Zhao Y, Shao Q, Zhu H, Xu H, Long W, Yu B, et al. Resveratrol ameliorates Lewis lung carcinoma-bearing mice development, decreases granulocytic myeloid-derived suppressor cell accumulation and impairs its suppressive ability. Cancer Sci (2018) 109(9):2677–86. doi: 10.1111/cas.13720
85. Messaoudene M, Pidgeon R, Richard C, Ponce M, Diop K, Benlaifaoui M, et al. A natural polyphenol exerts antitumor activity and circumvents anti-PD-1 resistance through effects on the gut microbiota. Cancer Discov (2022) 12(4):1070–87. doi: 10.1158/2159-8290.Cd-21-0808
86. Bouzas A, Gómez de Cedrón M, Colmenarejo G, Laparra-Llopis JM, Moreno-Rubio J, Montoya JJ, et al. Phenolic diterpenes from Rosemary supercritical extract inhibit non-small cell lung cancer lipid metabolism and synergise with therapeutic drugs in the clinic. Front Oncol (2022) 12:1046369. doi: 10.3389/fonc.2022.1046369
87. Chen HM, Sun L, Pan PY, Wang LH, Chen SH. Nutrient supplements from selected botanicals mediated immune modulation of the tumor microenvironment and antitumor mechanism. Cancer Immunol Immunother (2021) 70(12):3435–49. doi: 10.1007/s00262-021-02927-2
88. Gähler A, Trufa DI, Chiriac MT, Tausche P, Hohenberger K, Brunst AK, et al. Glucose-restricted diet regulates the tumor immune microenvironment and prevents tumor growth in lung adenocarcinoma. Front Oncol (2022) 12:873293. doi: 10.3389/fonc.2022.873293
89. Fang L, Hao Y, Yu H, Gu X, Peng Q, Zhuo H, et al. Methionine restriction promotes cGAS activation and chromatin untethering through demethylation to enhance antitumor immunity. Cancer Cell (2023) 41(6):1118–33.e12. doi: 10.1016/j.ccell.2023.05.005
90. Lee KA, Shaw HM, Bataille V, Nathan P, Spector TD. Role of the gut microbiome for cancer patients receiving immunotherapy: Dietary and treatment implications. Eur J Cancer (2020) 138:149–55. doi: 10.1016/j.ejca.2020.07.026
91. Hunt JD, Robert EG, Zieske AW, Bautista AP, Bukara M, Lei D, et al. Orthotopic human lung carcinoma xenografts in BALB/c mice immunosuppressed with anti-CD4 monoclonal antibodies and chronic alcohol consumption. Cancer (2000) 88(2):468–79.
92. Routy B, Lenehan JG, Miller WH Jr., Jamal R, Messaoudene M, Daisley BA, et al. Fecal microbiota transplantation plus anti-PD-1 immunotherapy in advanced melanoma: a phase I trial. Nat Med (2023) 29(8):2121–32. doi: 10.1038/s41591-023-02453-x
93. McQuade JL, Ologun GO, Arora R, Wargo JA. Gut microbiome modulation via fecal microbiota transplant to augment immunotherapy in patients with melanoma or other cancers. Curr Oncol Rep (2020) 22(7):74. doi: 10.1007/s11912-020-00913-y
94. Porcari S, Benech N, Valles-Colomer M, Segata N, Gasbarrini A, Cammarota G, et al. Key determinants of success in fecal microbiota transplantation: From microbiome to clinic. Cell Host Microbe (2023) 31(5):712–33. doi: 10.1016/j.chom.2023.03.020
Keywords: non-small cell lung cancer, gut microbiota, PD-1/PD-L1, antibiotics, dietary intervention
Citation: Xin Y, Liu C-G, Zang D and Chen J (2024) Gut microbiota and dietary intervention: affecting immunotherapy efficacy in non–small cell lung cancer. Front. Immunol. 15:1343450. doi: 10.3389/fimmu.2024.1343450
Received: 23 November 2023; Accepted: 18 January 2024;
Published: 01 February 2024.
Edited by:
Maria Gazouli, National and Kapodistrian University of Athens, GreeceReviewed by:
Ming Yi, Zhejiang University, ChinaCopyright © 2024 Xin, Liu, Zang and Chen. This is an open-access article distributed under the terms of the Creative Commons Attribution License (CC BY). The use, distribution or reproduction in other forums is permitted, provided the original author(s) and the copyright owner(s) are credited and that the original publication in this journal is cited, in accordance with accepted academic practice. No use, distribution or reproduction is permitted which does not comply with these terms.
*Correspondence: Jun Chen, Y2hlbmp1bl9kbXVAMTI2LmNvbQ==
†These authors have contributed equally to this work
Disclaimer: All claims expressed in this article are solely those of the authors and do not necessarily represent those of their affiliated organizations, or those of the publisher, the editors and the reviewers. Any product that may be evaluated in this article or claim that may be made by its manufacturer is not guaranteed or endorsed by the publisher.
Research integrity at Frontiers
Learn more about the work of our research integrity team to safeguard the quality of each article we publish.