- 1Department of Microbiology, Department of Biomedicine & Health Sciences, College of Medicine, The Catholic University of Korea, Seoul, Republic of Korea
- 2Department of Biomedical Sciences, Department of Medicine, Seoul National University College of Medicine, Seoul, Republic of Korea
- 3Transplantation Research Institute, Seoul National University Hospital, Seoul, Republic of Korea
Germinal center (GC) responses are essential for establishing protective, long-lasting immunity through the differentiation of GC B cells (BGC) and plasma cells (BPC), along with the generation of antigen-specific antibodies. Among the various pathways influencing immune responses, the STING (Stimulator of Interferon Genes) pathway has emerged as significant, especially in innate immunity, and extends its influence to adaptive responses. In this study, we examined how the STING ligand cGAMP can modulate these key elements of the adaptive immune response, particularly in enhancing GC reactions and the differentiation of BGC, BPC, and follicular helper T cells (TFH). Employing in vivo models, we evaluated various antigens and the administration of cGAMP in Alum adjuvant, investigating the differentiation of BGC, BPC, and TFH cells, along with the production of antigen-specific antibodies. cGAMP enhances the differentiation of BGC and BPC, leading to increased antigen-specific antibody production. This effect is shown to be type I Interferon-dependent, with a substantial reduction in BPC frequency upon interferon (IFN)-β blockade. Additionally, cGAMP’s influence on TFH differentiation varies over time, which may be critical for refining vaccine strategies. The findings elucidate a complex, antigen-specific influence of cGAMP on T and B cell responses, providing insights that could optimize vaccine efficacy.
Introduction
The orchestration of adaptive immunity involves a complex interplay between various cells and molecular signals that ensure effective protection against pathogens. Germinal center (GC) response, including the differentiation of GC B cells (BGC) and plasma cells (BPC), and subsequent the production of antigen-specific antibodies, is critical for protective and long-term immunity (1, 2). The germinal center reaction is a pivotal process in the adaptive immune response, leading to the selection of B cells with high-affinity receptors. BGC cells then differentiate into plasma cells or memory B cells, which are essential for producing high-affinity antibodies and conferring long-lasting immunity (1, 3, 4). Follicular helper T cell (TFH), a specialized B cell help CD4 T cell subset, is indispensable for the GC reaction, supporting the differentiation of BGC cells and the affinity maturation (5–7).
The STING (Stimulator of Interferon Genes) pathway has emerged as a key player in innate immunity, influencing the adaptive immune branches as well (8). Recent studies have highlighted the significance of STING ligands, such as cyclic GMP-AMP (cGAMP) and its derivatives, in enhancing protective immunity against a range of pathogens, including SARS-CoV-2 and influenza viruses (9–12). These ligands have been shown to potentiate immune responses, yet their role in modulating the germinal center reaction remains to be fully elucidated. The specific impacts of STING activation on the differentiation of BGC cells and plasma cells, as well as the kinetics and quality of antigen-specific antibody responses, need further exploration. Moreover, the ability of cGAMP to modulate TFH cells poses intriguing questions about the broader implications of STING activation in vaccine and adjuvant designs.
In the present study, we aimed to dissect the influence of cGAMP on these critical components of the adaptive immune response. By employing various antigens and analyzing the differentiation and response kinetics of BGC, BPC, and TFH cells, and the production of antigen-specific antibodies in vivo models, we explored to elucidate the mechanisms by which STING ligands can enhance the efficacy of immune responses. Understanding these mechanisms is imperative for designing effective vaccine adjuvants and therapeutic strategies in relevant biomedical fields, including cancer vaccines.
Materials and methods
Mice
C57BL/6J mice were acquired from Orient Bio in Korea. CD45.1+ SMARTA mice were kindly provided by Youn Soo Choi at Seoul National University College of Medicine. Specific-pathogen-free male mice (6-8 weeks of age) were used for experiments. All procedures involving animals were conducted in compliance with the protocols approved by the Institutional Animal Care and Use Committee of the College of Medicine at The Catholic University of Korea (CUMC-2023-0164).
Adoptive cell transfer
Spleens from SMARTA mice were harvested, and naive CD4+ T cells were isolated using negative selection according to the manufacturer’s protocol (The EasySep™ Mouse CD4+ T Cell Isolation Kit, STEMCELL, #19852). The cells were counted, and the proportions of TCRVα2+ CD45.1+ CD4+ T cells were determined by flow cytometry. 5 x 104 (for day 8) or 10 x 104 (for day 15) naive SMARTA T cells (CD45.1+) were adoptively transferred into recipient mice (CD45.2+) via intravenous injection into the retroorbital sinus.
Protein immunization
For immunization, 5 μg of SARS-CoV-2 spike RBD (sRBD), 10 μg of KLH-NP, or 10 μg of KLH-gp61 were each mixed with Alum (Alhydrogel; Invivogen) alone or combined with 5 μg of 3′3′-cGAMP (Invivogen) to a final volume of 20 μL. These preparations were administered into each footpad of the mice. The chosen cGAMP concentration was based on its ability to induce BGC and BPC responses in dose-response trials using the KLH-NP antigen (1, 5, and 10 μg). A 5 μg cGAMP dosage reliably provoked BGC and BPC responses, with 10 μg showing no further impact. For IFN-β blockade, mice received 250 μg of anti-IFN-β blocking antibody or isotype control via intraperitoneal injection three times during the course of protein immunization.
Flow cytometry
Single-cell suspensions from popliteal or inguinal lymph nodes were stained with a panel of monoclonal antibodies for surface markers including CD4 (RM4-5, BV510), CD8 (53-6.7, BV605), SLAM1 (TC15-12F12.2, PepCP-Cy5.5), ICOS (C398.4A, FITC), IgD (11-26c.2a, PE), CD138 (281-2, APC), Streptavidin (BV421) (BioLegend), B220 (RA3-6B2, AF700), CD44 (IM7, PE.Cy7), PD1 (J43, PE), CD4 (RM4.5, APC-eF780), CD8 (53-6.7, APC-eF780), CD45.1 (A20, APC-eF780 or BV605), Fixable Viability Dye eF780 (eBioscience) and FAS (Jo2, BV510) (BD Biosciences) at 4°C for 30 min in PBS with 0.5% BSA.
For cytokine detection, the cells were in vitro cultured with 10 μg/mL gp66-77 and 1 μg/mL brefeldin A for 5 hours. Intracellular staining for cytokines was performed with monoclonal antibodies for IL-4 (11B11, PE), IFN-γ (XMG1.2, AF700) (eBioscience), IL-2 (JES6-5H4, PE.Cy7) (BioLegend), and recombinant mouse IL-21 receptor Fc (R&D systems), followed by anti-human IgG (DyLight650) (Invitrogen). A Fixation/Permeabilization kit (BD Biosciences) was employed for permeabilization. Intracellular staining for T-bet transcription factor was performed with monoclonal antibody T-bet (4B10; PerCP.Cy5.5) using the Foxp3/Transcription Factor Staining Buffer Set (eBioscience). The cells were then analyzed using an LSR Fortessa (BD Biosciences) and FlowJo software v.10.8.
ELISA
Flat-bottom immuno plates (MaxiSorp) were coated with 1 μg/mL of various antigens (sRBD, BSA-gp61, OVAL-NP(17), BSA-NP(2) for high-affinity antibodies, and BSA-NP(36) for low-affinity antibodies) in PBS at 4°C overnight. Plates were washed with PBST (PBS + 0.1% Tween-20) and blocked with PBST-B (PBS with 0.05% Tween-20 and 0.5% BSA) for 90 min at room temperature. Mouse sera were then added in serial dilution and incubated for 90 min at room temperature. After washing, HRP-conjugated goat anti-mouse IgG (Thermo Fisher Scientific) was added in PBST-B (1:5,000), and the plates were incubated for another 90 min at room temperature. The enzymatic reaction was developed with a TMB solution (BioLegend). The reactions were stopped with 2N sulfuric acid, and the absorbance was measured at 450 nm with a microplate reader.
Results
cGAMP enhances the differentiation of germinal center B cells, plasma cells, and antigen-specific antibody production
STING ligands, such as 2’3’-cGAMP, 3’3’-cGAMP, and their derivatives, have been shown to enhance protective immunity against various pathogens, including SARS-CoV-2 and influenza (9–11). However, the impact of STING ligands on the germinal center reaction has remained somewhat unclear. To investigate the effect of STING ligands on the differentiation of BGC, BPC, and the generation of antigen-specific antibodies, we conducted experiments employing 3’3’-cGAMP (cGAMP) in response to various antigens. Mice were immunized with the SARS-CoV-2 spike RBD protein (sRBD) as an antigen, either in Alum alone or Alum+cGAMP as an adjuvant (Figure 1A). Mice immunized with Alum+cGAMP adjuvant exhibited a significant increase in frequencies of BGC (FashiGL7hi) cells and BPC (IgDloCD138hi) compared to the mice immunized with Alum alone on day 8 after immunization (Figure 1B). The kinetics of BGC and BPC differed significantly. The increase in BGC frequency induced by Alum+cGAMP persisted for 30 days with the peak response on day 15 after immunization. In contrast, the variance in BPC frequency peaked on day 8 and gradually declined over one month post-immunization (Supplementary Figure 1A). Consistent with a previous report (9), sRBD-specific antibody titers were significantly higher in mice treated with Alum+cGAMP compared to those treated with Alum alone (Figure 1C).
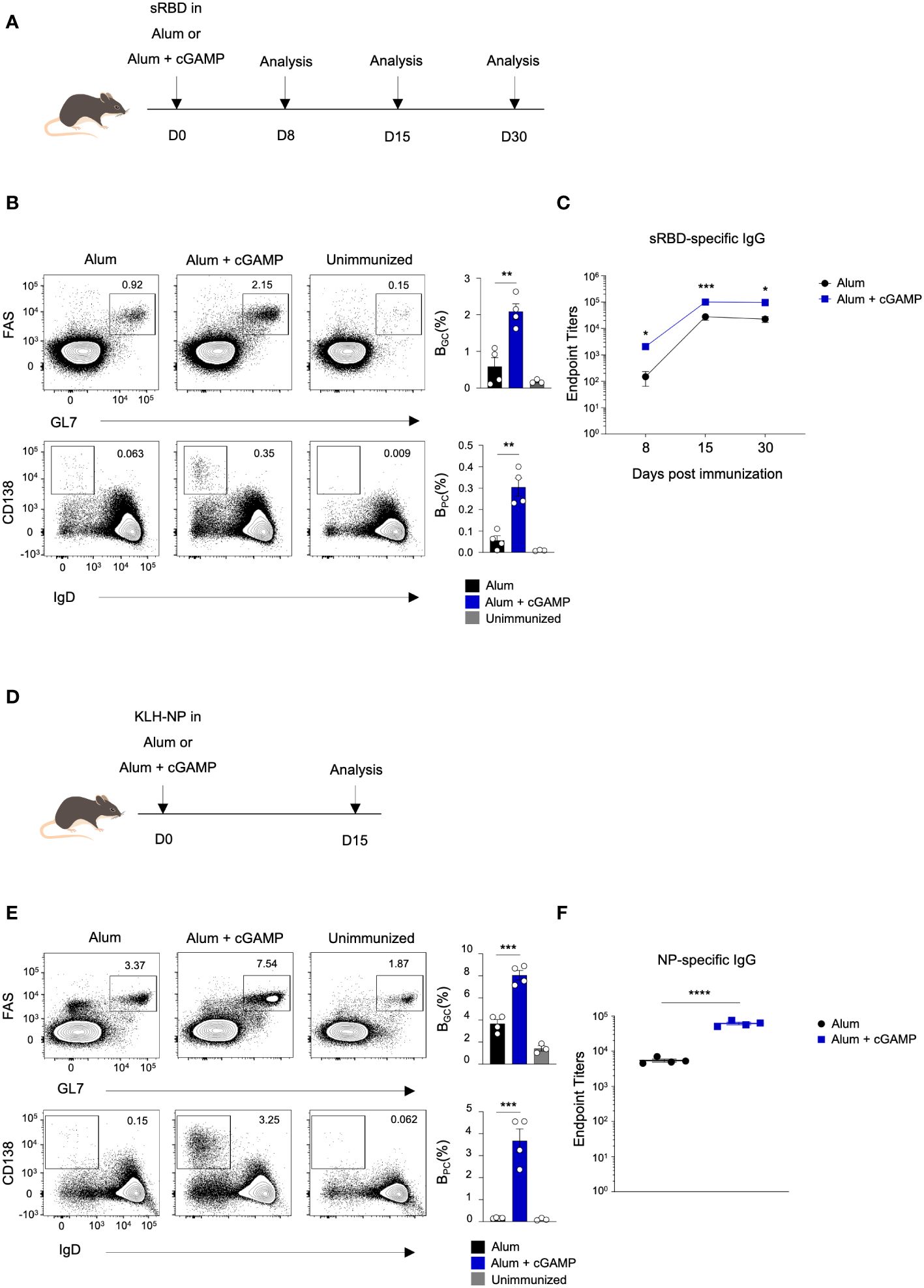
Figure 1 cGAMP enhances differentiation of BGC, BPC, and antibody production in response to sRBD and KLH-NP antigens. (A) Mice were immunized with sRBD in Alum or Alum+cGAMP. Draining lymph nodes (LNs) and blood were collected at 8, 15, and 30 days post-immunization (n=4). (B) Flow cytometry plots show the percentages of BGC (GL7hiFAShi) and BPC (IgDloCD138hi) on day 8. (C) sRBD-specific IgG endpoint titers were measured at 8, 15, and 30 days post-immunization. (D) Mice were immunized KLH-NP in Alum or Alum+cGAMP and were analyzed on day 15 (n=4). (E) Flow cytometry plots show the percentages of BGC and BPC on day 15. (F) NP-specific IgG endpoint titers were measured by ELISA. All experiments were performed twice, and each dot represents one mouse. Data are shown as mean ± SEM and were analyzed using an unpaired two-tailed Student’s t-test. *p < 0.05, **p < 0.01, ***p < 0.001, ****p < 0.0001.
We further investigated whether the effect of cGAMP on BGC and BPC is a general process in immunization with different antigens. Mice were immunized with keyhole limpet hemocyanin (KLH) conjugated with hapten 4-hydroxy-3-nitrophenyl acetyl (NP) (KLH–NP) as a model antigen, either in Alum alone or Alum+cGAMP (Figure 1D). Similarly, BGC and BPC responses were enhanced in mice treated with Alum+cGAMP in response to KLH–NP immunization (Figure 1E), along with an increase in NP-specific antibody production (Figure 1F). The increased frequency of both BGC and BPC was sustained for over one month when mice were immunized with Alum+cGAMP for priming (day0) and boosting (day14), suggesting that cGAMP treatment in primary and boost immunization can prolong the GC response and the differentiation of BPC (Supplementary Figures 1B,C). Moreover, the magnitude of high-affinity (NP(2); NP2-bound) antibody production was significantly greater in the Alum+cGAMP group compared to the control Alum alone group (Supplementary Figure 1D). These data demonstrate that cGAMP enhances the differentiation of GC B cells, plasma cells, and the production of antigen-specific antibodies.
Enhancement of plasma cell differentiation by cGAMP is type I Interferon-dependent
Ligation of cGAMP to STING triggers the production of type I interferon (IFN) (13). To explore whether cGAMP has the potential to enhance the germinal center response through the type I interferon pathway, we immunized mice with KLH–NP in Alum+cGAMP and administered either anti-IFN-β blocking antibodies or isotype control antibodies before (D-1) and after (D1 and D3) immunization (Figure 2A). Interestingly, there were no significant changes in the frequency of BGC in anti-IFN-β group. However, the frequency of BPC was significantly reduced upon blocking IFN-β (Figure 2B). Consistent with the BPC response, the production of NP-specific IgG was also decreased (Figure 2C). In summary, cGAMP plays a critical role in enhancing the differentiation of germinal center B cells and plasma cells, ultimately resulting in the production of antigen-specific antibodies. Specifically, cGAMP regulates plasma cell generation through the type I interferon pathway.
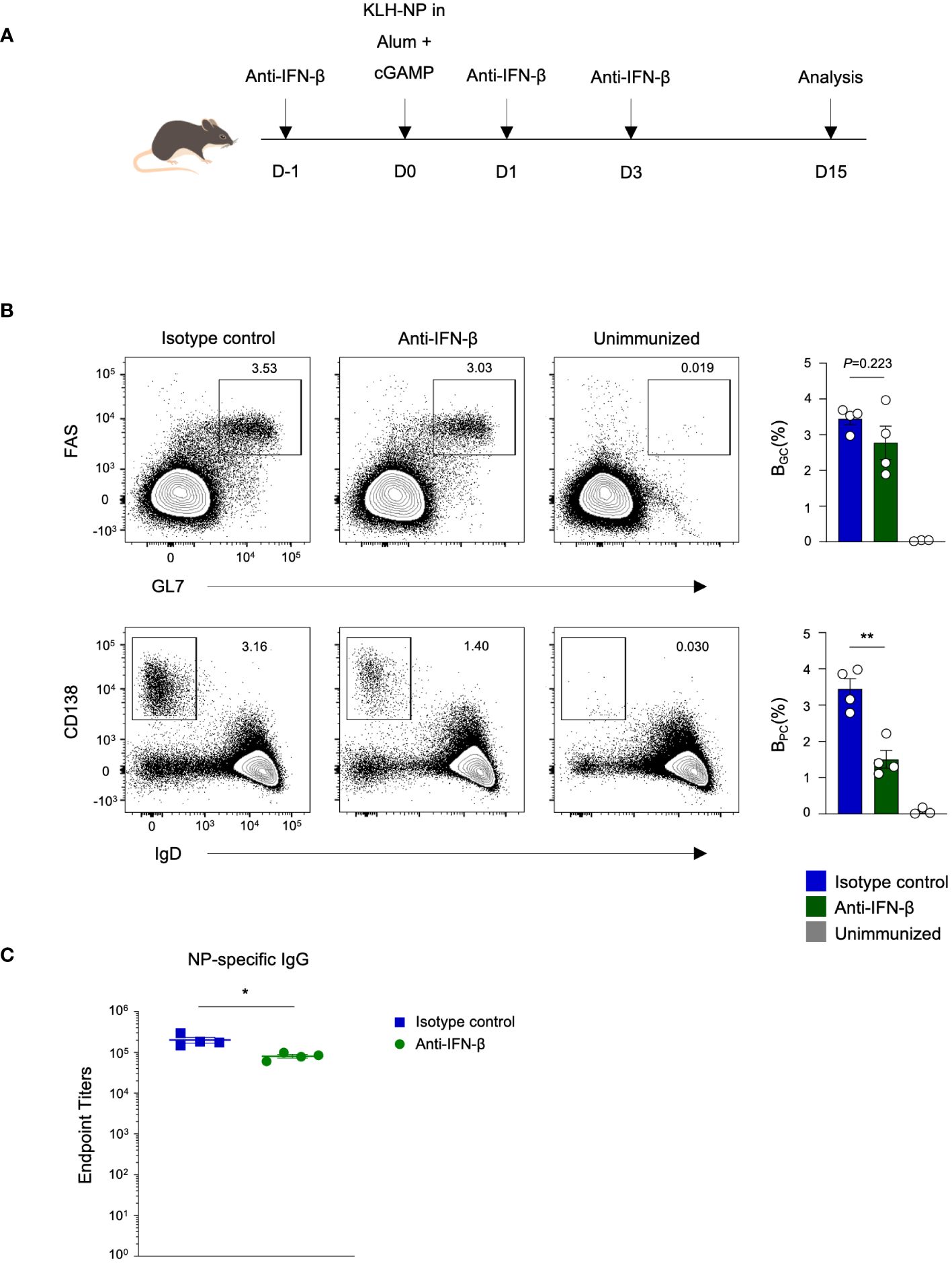
Figure 2 cGAMP augments antigen-specific antibody production and BPC differentiation via a type I interferon-dependent mechanism. (A) Mice were immunized with KLH-NP in Alum or Alum+cGAMP and received 250 μg of anti-IFN-β blocking antibody or isotype control treatments three times. Analyses were conducted on day 15 (n=4). (B) Flow cytometry plots show the percentages of BGC and BPC on day 15. (C) NP-specific IgG endpoint titers were measured by ELISA. All experiments were performed twice, and each dot represents one mouse. Data are shown as mean ± SEM and were analyzed by using an unpaired two-tailed Student’s t-test. *p < 0.05, **p < 0.01.
cGAMP differently impacts TFH differentiation in response to various antigens
TFH, a subset of CD4 T cells, plays a crucial role in GC formation, B cell differentiation and affinity maturation, and the production of high-affinity antibodies by producing plasma cells (1). We explored the potential of cGAMP to promote TFH differentiation. Notably, cGAMP significantly enhanced the frequency of GC TFH (CXCR5hiPD1hi) following sRBD immunization (Figures 1A, 3A). Furthermore, cGAMP markedly upregulated the expression of inducible T-cell costimulator (ICOS), a critical costimulatory molecule for TFH differentiation and synapse formation with B cells (Figure 3B). This upregulation was also observed in mice immunized with KLH-NP antigen, where cGAMP increased the frequency of GC TFH cells compared to immunization with Alum alone (Figures 1D , 3C). Importantly, the presence of GC TFH cells was sustained for over a month when mice received prime-boost immunization with KLH-NP in Alum+cGAMP, unlike those immunized with Alum alone (Figure 3D and Supplementary Figure 1B), indicating the capacity of cGAMP to induce TFH differentiation.
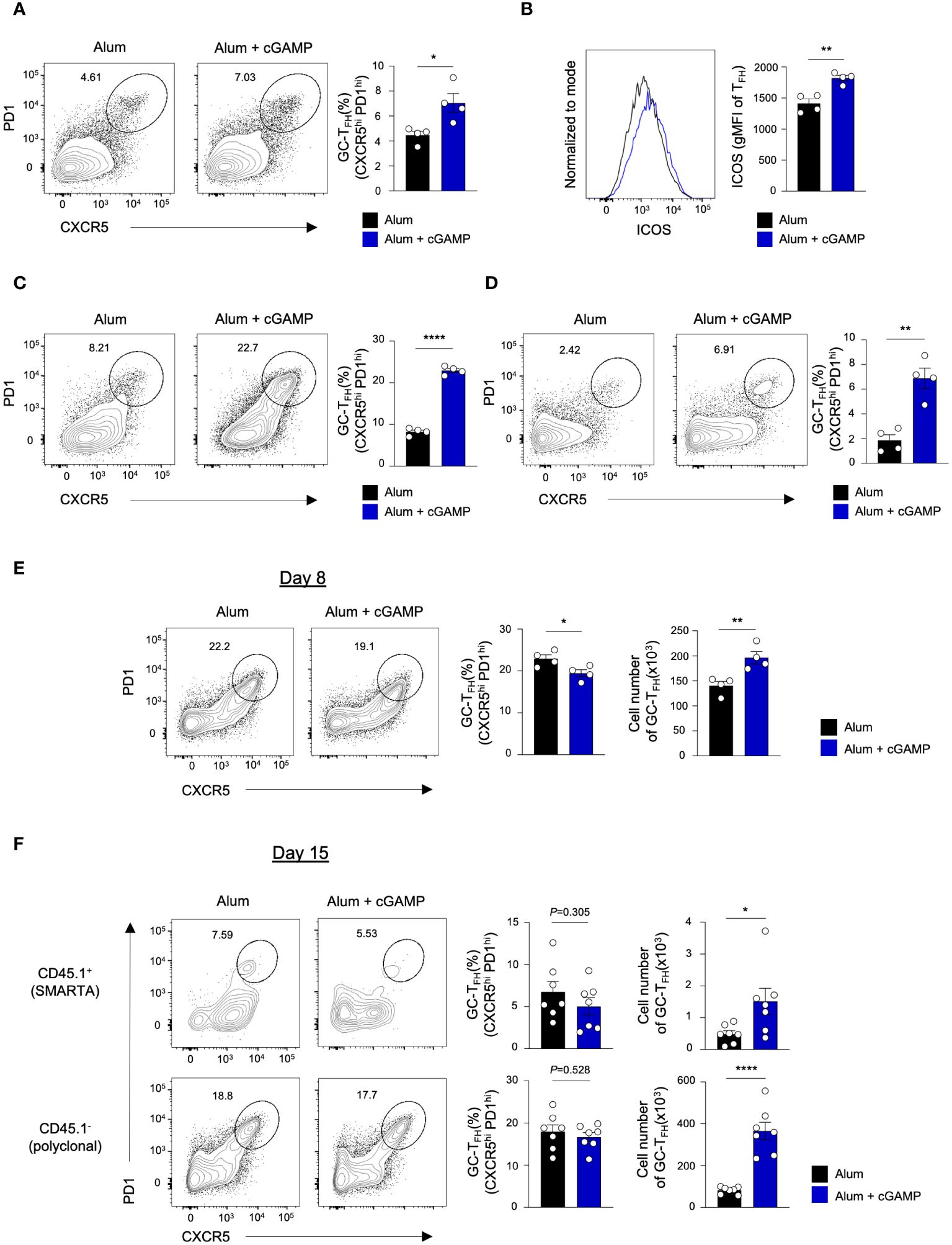
Figure 3 cGAMP promotes differentiation of GC-TFH in response to various antigens. (A, B) Mice were immunized with sRBD in Alum alone or Alum+cGAMP. GC-TFH population and ICOS expression levels on the CXCR5+ TFH population were analyzed by flow cytometry on day 15 (n=4). (C) Mice were immunized with KLH-NP in Alum alone or Alum+cGAMP. GC-TFH population was analyzed by flow cytometry on day 15 (n=4). (D) Mice underwent priming on day 0 and a booster on day 14 with KLH-NP in Alum alone or Alum+cGAMP. GC-TFH population was analyzed by flow cytometry 20 days after the booster immunization (day 34, n=4). (E) Mice were immunized with KLH-gp61 in Alum alone or Alum+cGAMP. GC-TFH population was analyzed by flow cytometry on day 8 (n=4). (F) SMARTA CD4+ T cells were adoptively transferred to mice, followed by KLH-gp61 immunization in Alum or Alum+cGAMP. Frequencies and cell number of GC-TFH in endogenous polyclonal CD4 T cells (CD45.1-) and adoptively transferred SMARTA cells (CD45.1+) were analyzed by flow cytometry on day 15 (n=7). All experiments were performed twice, and each dot represents one mouse. Data are shown as mean ± SEM and were analyzed by using an unpaired two-tailed Student’s t-test. *p < 0.05, **p < 0.01, ****p < 0.0001.
The effect of cGAMP on TFH response to sRBD and KLH-NP antigens corresponds with the enhanced formation of BGC and BPC. Intriguingly, despite cGAMP’s known role in promoting TH1 differentiation and IFN-γ production through STING activation in CD4 T cells, it can also augment TFH differentiation (14). We previously noted an increased frequency of antigen-specific CXCR5loBlimp1+ non-TFH cells and a decreased CXCR5+ TFH population upon immunization with KLH conjugated with lymphocytic choriomeningitis virus (LCMV) glycoprotein 61–80 peptide (KLH–gp61) in Alum+cGAMP compared to Alum alone on day 7 (15). To explore whether cGAMP elicits variant CD4 T cell responses to different antigens, mice were immunized with the KLH-gp61 antigen in Alum alone or Alum+cGAMP. Consistent with our previous findings (15), cGAMP substantially reduced the frequency of GC TFH cells on day 8, which is considered the peak TFH response (Figure 3E and Supplementary Figure 2A). Although the frequency of GC TFH cells was lower in the Alum+cGAMP, the absolute number of GC TFH cells was higher. Furthermore, BPC differentiation post-KLH-gp61 immunization on day 8 was elevated compared to that with Alum alone (Supplementary Figure 2B). This indicates that cGAMP’s influence on BPC generation may not solely rely on the TFH to non-TFH ratio but also on the number of TFH cells present during this stage.
To determine whether the disparity in TFH frequency in response to the KLH-gp61 antigen was time-dependent, we evaluated the TFH response on day 15 post-immunization. In this experiment, we performed adoptive transfer of SMARTA CD4 T cells to examine both antigen-specific and endogenous polyclonal CD4 T cell responses (Supplementary Figure 2C). On day 15, the frequency of CD45.1+ gp-specific GC TFH was comparable between the Alum+cGAMP and Alum alone groups in the KLH-gp61-immunized mice (Figure 3F; upper panel). Intriguingly, while Alum+cGAMP markedly increased the frequency of GC TFH cells in response to KLH-NP on day 15 (Figure 3C), the frequency of GC TFH in the polyclonal CD4 T cell population (CD45.1-) in KLH-gp61-immunized mice treated with Alum+cGAMP was similar to that in the Alum alone group (Figure 3F; lower panel). These findings suggest that different conjugations to carrier proteins may induce varied T-dependent immune responses. Although the frequency of GC TFH was lower on day 8 or similar on day 15 in the Alum+cGAMP group, the total number of GC TFH cells was significantly higher in Alum+cGAMP-treated mice than those given Alum alone (Figures 3E, F). These observations suggest that cGAMP may initially favor non-TFH differentiation over TFH differentiation, especially in response to the KLH-gp61 antigen, yet it appears to support sustained TFH responses over time, influencing both the proportion and absolute number of TFH cells.
cGAMP alters antigen-specific TFH and non-TFH cell profiles following immunization
We further examined the characteristics of antigen-specific CD4 T cells on day 8 after immunization. The mice were adoptively transferred with SMARTA CD4 T cells, followed by immunization with KLH-gp61 in Alum alone or Alum+cGAMP (Figure 4A). Similar to the observation from polyclonal CD4 T cell responses on day 8, frequency of CXCR5hiPD1hi GC TFH cells decreased significantly in the Alum+cGAMP group compared to the Alum alone group (Figure 4B). Interestingly, the expression level of ICOS markedly increased in the Alum+cGAMP group, while those of CXCR5 was similar (Figure 4C). These suggest that although the frequency of GC TFH was lower on day 8, the ICOS+ TFH cells might have the potential for further differentiation into GC TFH at a later time point. Moreover, Alum+cGAMP induced the production of IL-21, an important cytokine for the BGC differentiation, in CXCR5+ TFH cells (Figure 4D). Similarly, IL-2-producing TFH cells also increased in the Alum+cGAMP group. In contrast, IL-4-producing TFH cells were substantially decreased by cGAMP treatment, while the expression of IFN-γ was increased in TFH population by cGAMP (Figure 4D). Furthermore, the majority population of CXCR5low non-TFH SMARTA cells was IFN-γ+ cells in mice treated with Alum+cGAMP, emphasizing that cGAMP enhanced TH1 response and repressed TH2 and TFH responses at earlier immune response (Figure 4E). Correspondingly, the non-TFH population expressed higher levels of the T-bet transcription factor in the Alum+cGAMP group (Figure 4F). Taken together, these results demonstrate that cGAMP downregulates GC TFH differentiation in response to KLH-gp61 on day 8, but it improves its regulatory function over time.
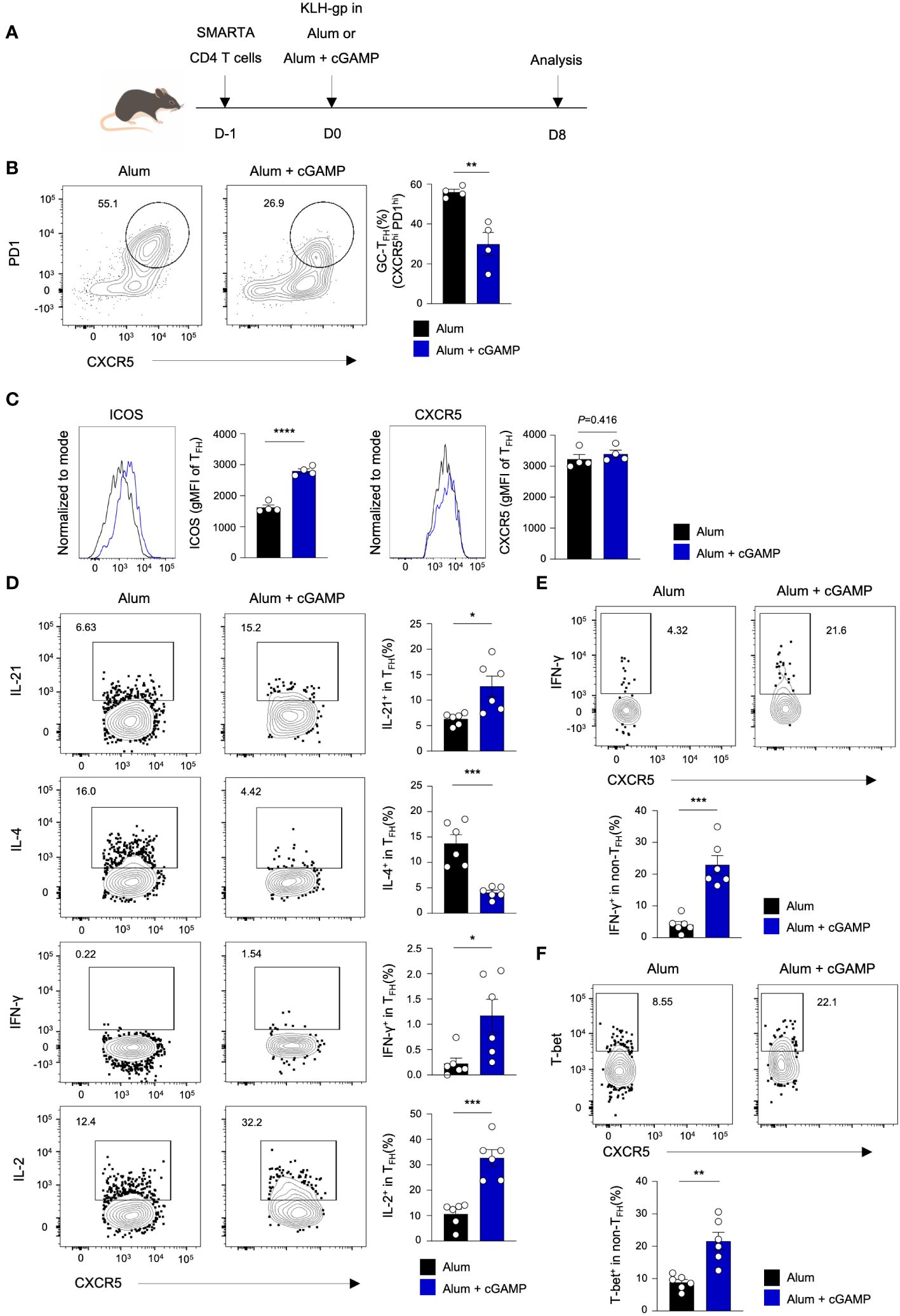
Figure 4 cGAMP alters antigen-specific TFH and non-TFH cell profiles following immunization. (A) SMARTA CD4+ T cells were adoptively transferred to mice followed by KLH-gp61 immunization in Alum alone or Alum+cGAMP, and analyzed after 8 days (n=4). (B) GC-TFH populations were analyzed by flow cytometry. (C) ICOS and CXCR5 expression levels in the TFH population are shown. (D) Flow cytometry analyses of gp66-restimulated IL-21+, IL-4+, IFN-γ+, and IL-2+ SMARTA cells in the TFH population from KLH-gp61 immunized mice are shown (n=6). (E) IFN-γ+ SMARTA cells in the non-TFH population are shown. (F) T-bet+ SMARTA cells in the non-TFH population are shown. All experiments were performed twice, and each dot represents one mouse. Data are shown as mean ± SEM and were analyzed by using an unpaired two-tailed Student’s t-test. *p < 0.05, **p < 0.01, ***p < 0.001, ****p < 0.0001.
Discussion
Adjuvants are crucial for augmenting immunogenicity, particularly in protein subunit vaccines, significantly amplifying immune responses (16). Currently, various pathogen recognition receptor agonists are being developed to enhance the immunogenicity and efficacy of vaccines (17). The STING pathway, in particular, has been recognized for its role in both innate and adaptive immunity (9–11, 14, 18–21). cGAMP, a STING agonist, and its derivatives are being explored for use in conjunction with other adjuvants to combat a range of pathogens, including pan-sarbecoviruses and influenza (9, 10, 21). Our study adds the pivotal role of cGAMP in modulating the immune response, specifically by promoting the differentiation of BGC and BPC. The enhancement of BGC and BPC populations, coupled with sustained antigen-specific antibody production following cGAMP administration, highlights the potential of the STING agonist as a potent vaccine adjuvant. The extended germinal center reaction and BPC differentiation following cGAMP treatment indicate the establishment of a strong and lasting immune memory, a critical feature for sustained protective immunity.
In line with prior research on the effects of cGAMP on B cell activation (22), our results provide further insight into a type I Interferon-dependent mechanism by which cGAMP facilitates the differentiation of BPC. The reduction in BPC frequency upon IFN-β blockade further confirms the importance of this pathway in immunoenhancing effects mediated by cGAMP. The involvement of IFN-β provides insights into the complex interaction between STING activation and the type I interferon pathway, essential for driving an effective BPC immune response. The distinct response timings of BPC and BGC seem to be potentially influenced by IFN-β activity. Notably, the impact of cGAMP on BPC peaked on day 8, after which it gradually diminished to levels comparable to the control group treated with Alum alone in response to the sRBD. This suggests that sustaining BPC responses may necessitate extended innate signals. Indeed, boost immunization with cGAMP enhanced BPC response on day 34 in response to KLH-NP. It also raises the possibility that the initial BPC response could originate from extrafollicular B cells instead of BGC-derived cells. Furthermore, it is yet to be ascertained whether cGAMP’s influence on B cells, BPC, and CD4 T cells is attributable to direct intrinsic effects on each cell type or through indirect pathways. Addressing these questions will be an important focus for future studies.
Additionally, our study reveals a subtle yet significant influence of cGAMP on the differentiation of TFH, which is critical for GC reaction and the generation of high-affinity antibodies. The initial decline in GC TFH cells on day 8 post-immunization with KLH-gp61, followed by later enhancement of TFH function, offers a unique perspective on how cGAMP modulates the immune response over time. Although cGAMP appears to promote TH1 differentiation over TFH responses initially, it may also facilitate sustained TFH responses, likely advantageous for continuous antibody production. This biphasic effect indicates a sophisticated regulatory role of cGAMP, which could inform the optimization of vaccine approaches, particularly for diseases requiring a strong TH1 response.
In conclusion, our study not only corroborates the potent immunomodulatory properties of cGAMP but also highlights its complex, antigen-specific effects on T and B cell responses. These insights set the stage for subsequent studies into the mechanisms of cGAMP’s activity and its potential to enhance the efficacy of vaccines and precision immunotherapies.
Data availability statement
The original contributions presented in the study are included in the article/Supplementary Material. Further inquiries can be directed to the corresponding author.
Ethics statement
The animal study was approved by Institutional Animal Care and Use Committee of the College of Medicine at The Catholic University of Korea. The study was conducted in accordance with the local legislation and institutional requirements.
Author contributions
MY: Data curation, Formal analysis, Investigation, Visualization, Writing – original draft, Writing – review & editing. YC: Investigation, Writing – review & editing. TW: Investigation, Writing – review & editing. YSC: Conceptualization, Resources, Writing – review & editing. JC: Conceptualization, Data curation, Formal analysis, Funding acquisition, Investigation, Methodology, Resources, Supervision, Validation, Visualization, Writing – original draft, Writing – review & editing.
Funding
The author(s) declare financial support was received for the research, authorship, and/or publication of this article. This work was supported by the National Research Foundation of Korea (NRF) grant funded by the Korea government (MSIT) No. 2021R1F1A1060347, 2023R1A2C1007319, and RS-2023-00258956 to JC) and by the Catholic Medical Center Research Foundation made in the program years of 2021, 2022 and 2023 (JC).
Acknowledgments
We thank the staff of the animal facility and flow cytometry facility for their support.
Conflict of interest
The authors declare that the research was conducted in the absence of any commercial or financial relationships that could be construed as a potential conflict of interest.
Publisher’s note
All claims expressed in this article are solely those of the authors and do not necessarily represent those of their affiliated organizations, or those of the publisher, the editors and the reviewers. Any product that may be evaluated in this article, or claim that may be made by its manufacturer, is not guaranteed or endorsed by the publisher.
Supplementary material
The Supplementary Material for this article can be found online at: https://www.frontiersin.org/articles/10.3389/fimmu.2024.1340001/full#supplementary-material
References
1. Crotty S. T follicular helper cell biology: A decade of discovery and diseases. Immunity. (2019) 50:1132–48. doi: 10.1016/j.immuni.2019.04.011
2. Laidlaw BJ, Ellebedy AH. The germinal centre B cell response to SARS-CoV-2. Nat Rev Immunol. (2022) 22:7–18. doi: 10.1038/s41577-021-00657-1
3. Tas JMJ, Mesin L, Pasqual G, Targ S, Jacobsen JT, Mano YM, et al. Visualizing antibody affinity maturation in germinal centers. Science. (2016) 351:1048–54. doi: 10.1126/science.aad3439
4. Victora GD, Nussenzweig MC. Germinal centers. Annu Rev Immunol. (2022) 40:413–42. doi: 10.1146/annurev-immunol-120419-022408
5. Betzler AC, Ushmorov A, Brunner C. The transcriptional program during germinal center reaction - a close view at GC B cells, Tfh cells and Tfr cells. Front Immunol. (2023) 14:1125503. doi: 10.3389/fimmu.2023.1125503
6. Crotty S. Follicular helper CD4 T cells (TFH). Annu Rev Immunol. (2011) 29:621–63. doi: 10.1146/annurev-immunol-031210-101400
7. Crotty S. T follicular helper cell differentiation, function, and roles in disease. Immunity. (2014) 41:529–42. doi: 10.1016/j.immuni.2014.10.004
8. Zheng J, Mo J, Zhu T, Zhuo W, Yi Y, Hu S, et al. Comprehensive elaboration of the cGAS-STING signaling axis in cancer development and immunotherapy. Mol Cancer. (2020) 19:133. doi: 10.1186/s12943-020-01250-1
9. Liu Z, Zhou J, Xu W, Deng W, Wang Y, Wang M, et al. A novel STING agonist-adjuvanted pan-sarbecovirus vaccine elicits potent and durable neutralizing antibody and T cell responses in mice, rabbits and NHPs. Cell Res. (2022) 32:269–87. doi: 10.1038/s41422-022-00612-2
10. Vassilieva EV, Li S, Korniychuk H, Taylor DM, Wang S, Prausnitz MR, et al. cGAMP/saponin adjuvant combination improves protective response to influenza vaccination by microneedle patch in an aged mouse model. Front Immunol. (2021) 11:583251. doi: 10.3389/fimmu.2020.583251
11. Chauveau L, Bridgeman A, Tan TK, Beveridge R, Frost JN, Rijal P, et al. Inclusion of cGAMP within virus-like particle vaccines enhances their immunogenicity. EMBO Rep. (2021) 22:e52447. doi: 10.15252/embr.202152447
12. Herck SV, Feng B, Tang L. Delivery of STING agonists for adjuvanting subunit vaccines. Adv Drug Delivery Rev. (2021) 179:114020. doi: 10.1016/j.addr.2021.114020
13. Zhang X, Shi H, Wu J, Zhang X, Sun L, Chen C, et al. Cyclic GMP-AMP containing mixed phosphodiester linkages is an endogenous high-affinity ligand for STING. Mol Cell. (2013) 51:226–35. doi: 10.1016/j.molcel.2013.05.022
14. Benoit-Lizon I, Jacquin E, Vargas TR, Richard C, Roussey A, Zuffo LD, et al. CD4 T cell-intrinsic STING signaling controls the differentiation and effector functions of TH1 and TH9 cells. J Immunother Cancer. (2022) 10:e003459. doi: 10.1136/jitc-2021-003459
15. Choi J, Diao H, Faliti CE, Truong J, Rossi M, Bélanger S, et al. Bcl-6 is the nexus transcription factor of T follicular helper cells via repressor-of-repressor circuits. Nat Immunol. (2020) 21:777–89. doi: 10.1038/s41590-020-0706-5
16. Reed SG, Orr MT, Fox CB. Key roles of adjuvants in modern vaccines. Nat Med. (2013) 19:1597–608. doi: 10.1038/nm.3409
17. Gutjahr A, Papagno L, Nicoli F, Kanuma T, Kuse N, Cabral-Piccin MP, et al. The STING ligand cGAMP potentiates the efficacy of vaccine-induced CD8+ T cells. JCI Insight. (2019) 4:e125107. doi: 10.1172/jci.insight.125107
18. Jneid B, Bochnakian A, Hoffmann C, Delisle F, Djacoto E, Sirven P, et al. Selective STING stimulation in dendritic cells primes antitumor T cell responses. Sci Immunol. (2023) 8:eabn6612. doi: 10.1126/sciimmunol.abn6612
19. Kuhl N, Linder A, Philipp N, Nixdorf D, Fischer H, Veth S, et al. STING agonism turns human T cells into interferon-producing cells but impedes their functionality. EMBO Rep. (2023) 24:e55536. doi: 10.15252/embr.202255536
20. Calvet-Mirabent M, Claiborne DT, Deruaz M, Tanno S, Serra C, Delgado-Arévalo C, et al. Poly I:C and STING agonist-primed DC increase lymphoid tissue polyfunctional HIV-1-specific CD8+ T cells and limit CD4+ T-cell loss in BLT mice. Eur J Immunol. (2022) 52:447–61. doi: 10.1002/eji.202149502
21. Zhu W, Wei L, Dong C, Wang Y, Kim J, Ma Y, et al. cGAMP-adjuvanted multivalent influenza mRNA vaccines induce broadly protective immunity through cutaneous vaccination in mice. Mol Ther - Nucleic Acids. (2022) 30:421–37. doi: 10.1016/j.omtn.2022.10.024
Keywords: STING ligand, cGAMP, germinal center, follicular helper T cells, plasma cells
Citation: Yoon M, Choi Y, Wi T, Choi YS and Choi J (2024) The role of cGAMP via the STING pathway in modulating germinal center responses and CD4 T cell differentiation. Front. Immunol. 15:1340001. doi: 10.3389/fimmu.2024.1340001
Received: 17 November 2023; Accepted: 12 February 2024;
Published: 12 April 2024.
Edited by:
Bingdong Zhu, Lanzhou University, ChinaReviewed by:
Yanpu He, Massachusetts Institute of Technology, United StatesJune-Yong Lee, Yonsei University, Republic of Korea
Copyright © 2024 Yoon, Choi, Wi, Choi and Choi. This is an open-access article distributed under the terms of the Creative Commons Attribution License (CC BY). The use, distribution or reproduction in other forums is permitted, provided the original author(s) and the copyright owner(s) are credited and that the original publication in this journal is cited, in accordance with accepted academic practice. No use, distribution or reproduction is permitted which does not comply with these terms.
*Correspondence: Jinyong Choi, amNob2lAY2F0aG9saWMuYWMua3I=