- 1Department of Occupational Health and Toxicology, School of Public Health, Jiangxi Medical College, Nanchang University, Nanchang, China
- 2Jiangxi Provincial Key Laboratory of Preventive Medicine, Jiangxi Medical College, School of Public Health, Nanchang University, Nanchang, China
- 3Department of Histology and Embryology, School of Basic Medicine Sciences, Nanchang University, Nanchang, China
- 4Jiangxi Provincial Key Laboratory of Interdisciplinary Science, Nanchang University, Nanchang, China
With the continuous development of nuclear technology, the radiation exposure caused by radiation therapy is a serious health hazard. It is of great significance to further develop effective radiation countermeasures. B cells easily succumb to irradiation exposure along with immunosuppressive response. The approach to ameliorate radiation-induced B cell damage is rarely studied, implying that the underlying mechanisms of B cell damage after exposure are eager to be revealed. Recent studies suggest that Notch signaling plays an important role in B cell-mediated immune response. Notch signaling is a critical regulator for B cells to maintain immune function. Although accumulating studies reported that Notch signaling contributes to the functionality of hematopoietic stem cells and T cells, its role in B cells is scarcely appreciated. Presently, we discussed the regulation of Notch signaling on B cells under radiation exposure to provide a scientific basis to prevent radiation-induced B cell damage.
1 Introduction
Delayed recovery of the immune system after radiotherapy (RT) is one of the main reasons for death in patients with malignant tumors. The higher the dose of ionizing radiation (IR) used in radiotherapy, the more severe the impairment of the functioning of the immune system. B cells are one of the highly sensitive cells to IR. B cells are derived from common lymphoid progenitor (CLP) which can be differentiated from hematopoietic stem and progenitor cells (HSPCs) in the bone marrow (BM). Even though Notch signaling is essential for HSPCs and lymphocyte development, it is still important to in-depth investigate how Notch signaling affects B cells injury and regeneration under irradiation. In the present review, we summarize the progression on the role of the Notch signaling pathway in regulating B cells, which may be applied to the immune system damage and recovery after ionizing radiation.
2 Development and radiosensitivity of B cells
With impressive outcomes, radiotherapy (RT) has been extensively utilized in the treatment of B-cell malignancies (1). The decrease of B cells counts in patients following radiotherapy has long been recognized as a concern. RT has the potential to have both immunostimulatory and immunosuppressive effects (2, 3). The relationship between IR and the immune system is complicated (4). The development and differentiation of B cells are regulated by genes and external factors in the spleen and BM (5). The spleen, as the largest immune organ, contains many B cells and subsets, which is essential for immune regulation (6). The process of B cell development in human is showed in Figure 1. In agreement with previous findings in human, HSPCs in the BM can differentiate into Preprogenitor B cells (Pre-pro-B cells), Progenitor B cells (Pro-B cells), Precursor B cells (Pre-B cells), and immature B cells expressing IgM through the rearrangement of immunoglobulin genes (7, 8), which is independent of antigenic stimulation, named antigen-independent stage (8). The immature B cells are drained from the BM to the peripheral and undergo differentiation into transitional B cells (8, 9).
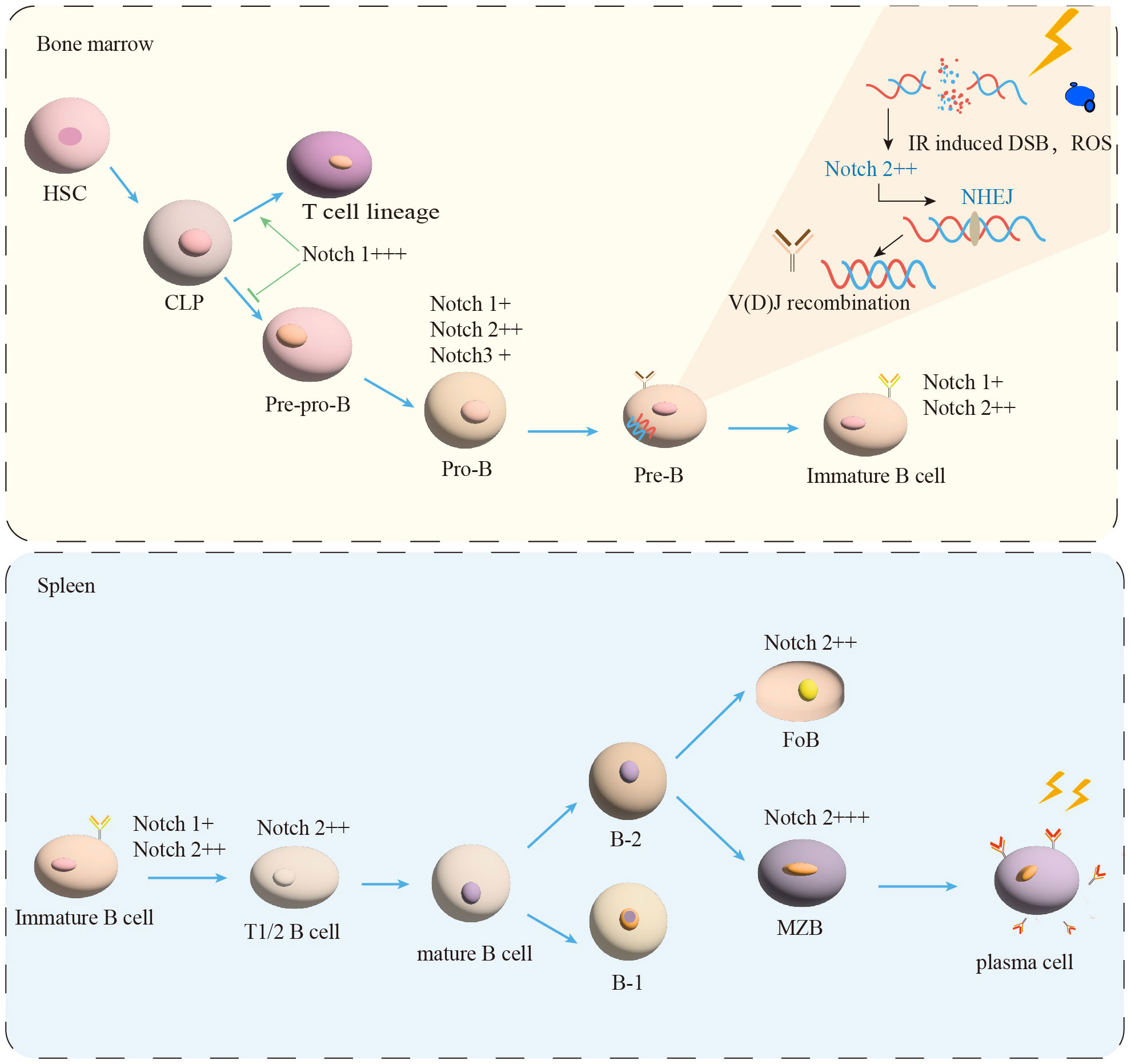
Figure 1 B-cell maturation and differentiation. In the BM, the B-lymphocyte lineage originates from hematopoietic stem cells (HSC) and progressively differentiates into pre-pro-B, pro-B, and pre-B cells. Immature B cells leave the BM and transfer to the spleen for further development into transitional B cells (T1/2). Mature B cells are composed of B-1 and B-2 cells. B-2 cells are classified into FoB and MZB cells. MZB cells continue to develop into plasma cells. IR induced DSB and ROS production. NHEJ is essential for the process of V(D)J recombination in Pre-B cells. Notch signal (+) expression, (++) moderated expression, and (+++) high expression.
Transitional B cells play a key role in linking BM immature and peripheral mature B cells (9). Human transitional B cells are subdivided into two populations: transitional B cells of type 1 (T1) and type 2 (T2) (10–12). It has been demonstrated that in the adult spleen T1 B cells develop into T2 B cells in 2 days (11–13). After passing the transitional stage, they become mature B cells (12, 14). The transitional B cells development in mice is similar to human (8, 12, 14). It is currently believed that peripherally developed mature B cells can be divided into two types of B cells: B-1 and B-2 (9, 15). B-2 B cells further differentiate into follicular B cells (FoB) and marginal zone B cells (MZB) in the human (12, 16, 17), as shown in Figure 1. The multiple critical phases from the BM of the central immune organ to the development of mature B cells in the peripheral immune organs are considered to be important targets for shaping the mature B cells pool (18).
How to maintain the homeostasis of sufficient B cells in the BM and peripheral spleen B cell compartments is still unclear in response to exposure to IR. It is known that lymphocytes among blood cells are the most sensitive to radiation (19–22). B cells are susceptible to radiation-induced apoptosis (4, 20, 23–25). Through determining the frequency of apoptosis in different lymphocyte subpopulations of peripheral blood mononuclear cells (PBMCs) under irradiated (24 h, 2 Gy), the following order of radiosensitivity was observed: B cells > memory T cells > NK cells (26). According to previous studies, different subpopulations of B cells have different radiosensitivities (20, 26). For example, exposed to X-radiation(0, 1, 2, 3, 4, 5 Gy), the rank order of increasing sensitivity was pre-B>pro-B>mature B cells (27). Furthermore, previous data had shown that pre-B cells were ultra-sensitive to radiation and underwent apoptosis at very low levels of radiation exposure (28–30). Moreover, the population of T1 B cells in the spleen was severely decreased 24 hours after irradiation (2, 8, 20 Gy) while the population of T2 B cells was increased (27). To determine the radioresistance of the mature B cell subsets, purified splenic B-2 cells and peritoneal B-1 cells were exposed to 2 Gy of irradiation (27, 31). B-2 cells were found to rapidly undergo apoptosis following irradiation, whereas B-1 cells maintained viability (31). A deeper analysis of the sequence of the B cell receptor (BCR) has shown that radiation induces alterations in B cells repertoire and clonogenicity (32, 33). Radiation increases the differentiation of nuclear plasma cells from tumor-antigenic B cells (34). Immunosuppression and imbalance of immune homeostasis induced by IR may lead to inflammatory responses and death in exposed organisms (35). The spleen experiences histomorphologic changes following radiation at varying doses (36). These changes include a reduction in the splenic index, a shrinkage of the B cells follicular zone, a decrease in the area of the red medulla oblongata, dense and compact splenic trabeculae, aggravation of splenic white medulla atrophy, and a massive decrease in lymphocyte counts (36, 37).
Previous studies have shown that the main target of IR is intracellular genetic materials (38), including direct damage such as double-strand breaks (DSBs), single-strand breaks (SSBs), and inter-strand crosslinks (ICLs) (39, 40). The generation of DSBs induces replicative stress that disrupts the stability of the cellular genome (41). DNA repair can be carried out through pathways such as non-homologous end joining (NHEJ), which is the main approach to repair damaged DNA in mammalian cells and occurs throughout the cell cycle (42). NHEJ is required for the repair of DNA double-strand breaks associated with the normal physiological Rag endonuclease-related process of V(D)J recombination, which is important for B-cell development (42–44) (Figure 1). Once DNA repair defects are created, they will affect hematopoietic and immune regulation, leading to bone marrow failure (BMF) and immune system malignancies (45). X-ray irradiation has been shown to cause an increase in the number of micronuclei in mouse spleen and bone marrow cells, which is a major damage of SSB and DSB (46, 47). Radiation may interact with free or bound water (35) in the cell to generate reactive oxygen species (ROS) (35, 48). Additionally, excessive accumulation of ROS, a byproduct of normal oxidative metabolism in eukaryotic cells is the main factor causing indirect oxidative stress (49, 50). ROS damages to B cells by interfering with the structure and function of DNA, lipids (51), and proteins (48, 52). A previous study demonstrated that the overproduction of ROS after radiation exposure resulted in the formation of apoptotic nuclei leading to cellular apoptosis, inducing neutrophil accumulation and inflammatory response (53–55). Nuclear factor erythroid-2-related factor 2 (Nrf2) as the major effector of ROS in the cell regulates Notch activation to counteract the deleterious effects of ROS, such as DNA damage and apoptosis (56, 57). Paul has reported that ROS acts as a rheostat to regulate the Nrf2-Notch pathway (56). To further confirm ROS regulation of Nrf2-Notch, relevant studies have demonstrated that the delayed repair seen in the NRF2-/- airway after injury was rescued by activation of Notch (56, 58). NRF2 can expand HSPCs by activating Notch1 signaling in irradiated mice after ROS (57). In the case of oxidative stress after radiation, the large amount of ROS produced can activate the ROS-Nrf2-Notch pathway to regulate cell proliferation and thus reduce ROS level (56). To gain a better understanding of how Notch signals are involved in radiation response, correlated studies have found that knockdown of Notch1 or Notch2 increased the radiosensitivity of glioma stem cells (59). In the acute setting, radiation has previously been shown to increase endothelial Notch signaling, especially Notch1 and Notch2 (60–62), which were supported by the upregulation of the Notch pathway components Jagged1 and Hey1 (59, 61). Kondelaji observed that 8 Gy of irradiation in pulmonary endothelial cells increased transcription of Notch2 target genes Hes1 and Hey2 at 6, 24, and 72 h following irradiation (62). These results further validate the important role of the Notch pathway in the regulation of radioresistance, suggesting that Notch activation may be required for radioresistance.
In the aforementioned studies, we found that B cells and their subsets were damaged to varying degrees after radiation exposure, such as cell apoptosis. Therefore, the extensive generation of ROS and the impact of DNA damage on B cells during radiotherapy still require our attention. More studies indicated that the Notch signaling pathway is activated under conditions of oxidative stress induced by radiation (57). It is worth further investigating whether the Notch signaling pathway, a crucial regulator in B cells lineage development, plays a regulatory role in B cells after radiation injury.
3 Notch signaling pathway
The Notch signaling pathway is a G protein-coupled receptor (GPCR) and enzyme-linked receptors-mediated meristem signaling pathway controlling diverse aspects of the differentiation and maturation of lymphocytes and HSC (63, 64). As shown in Figure 2, the Notch signaling pathway consists of four components: the receptors, ligands, the CSL DNA-binding proteins, and downstream target genes (65). Currently, four Notch receptors are known, namely Notch1, Notch2, Notch3, and Notch4. Five ligands namely Jagged1, 2, and Delta-like ligands 1, 3 and 4 (63). Structurally, the Notch receptor is a single transmembrane heterodimer consisting of an extracellular ligand-binding domain and intracellular structural domain (66), which constitutes a transmembrane region and the intracellular part that mediates the receptor ligation signal (67, 68). The extracellular region of the Notch receptor is an elongated structure (69), the N-terminal end of the protein located outside the cell contains multiple epidermal growth factor-like receptor (EGF-like receptor) repeats (63). The numbers of EGF-like repeats vary among Notch family members (68). The EGF-like receptors are followed by the negative regulatory region (NRR) (63), which prevents premature signaling of the Notch receptor by blocking protein hydrolysis cleavage sites (70). Near the transmembrane structural region of the NRR are Furin protease cleavage site 1 (S1), a disintegrin and metalloproteinase domain (ADAM) cleavage site 2 (S2), and a γ disintegrin and metalloproteinase cleavage site 3 (S3) (71). The RBP-Jκ association module (RAM), ankyrin repeat sequence (ankyrin, ANK), transcriptional activation domain (TAD) (72), and proline/serine/threonine-rich motifs (PEST) are composed of the intracellular domain of Notch receptor (ICN) (73–75). The PEST structural domain located at the C-terminal end contributes to Notch degradation (73–75). The TAD is capable of autonomous transcriptional activity and directly binds to the coactivators PCAF and GNC5 (64, 76). Upon binding of the Notch receptor and ligand, the Notch receptor is cleaved by ADAM family proteins at site 2 (S2), followed by the cleavage of site 3 (S3) by γ-secretase (77), which ultimately releases the Notch intracellular domain (NICD), making the NICD readily localized to the nucleus, where it binds to the coactivator (Mastermind-like-1, MAML1) and the transcriptional repressor, RBP-Jκ, to promote the activation of target gene expression such as Hes, Hey and Dtx gene families (78–80).
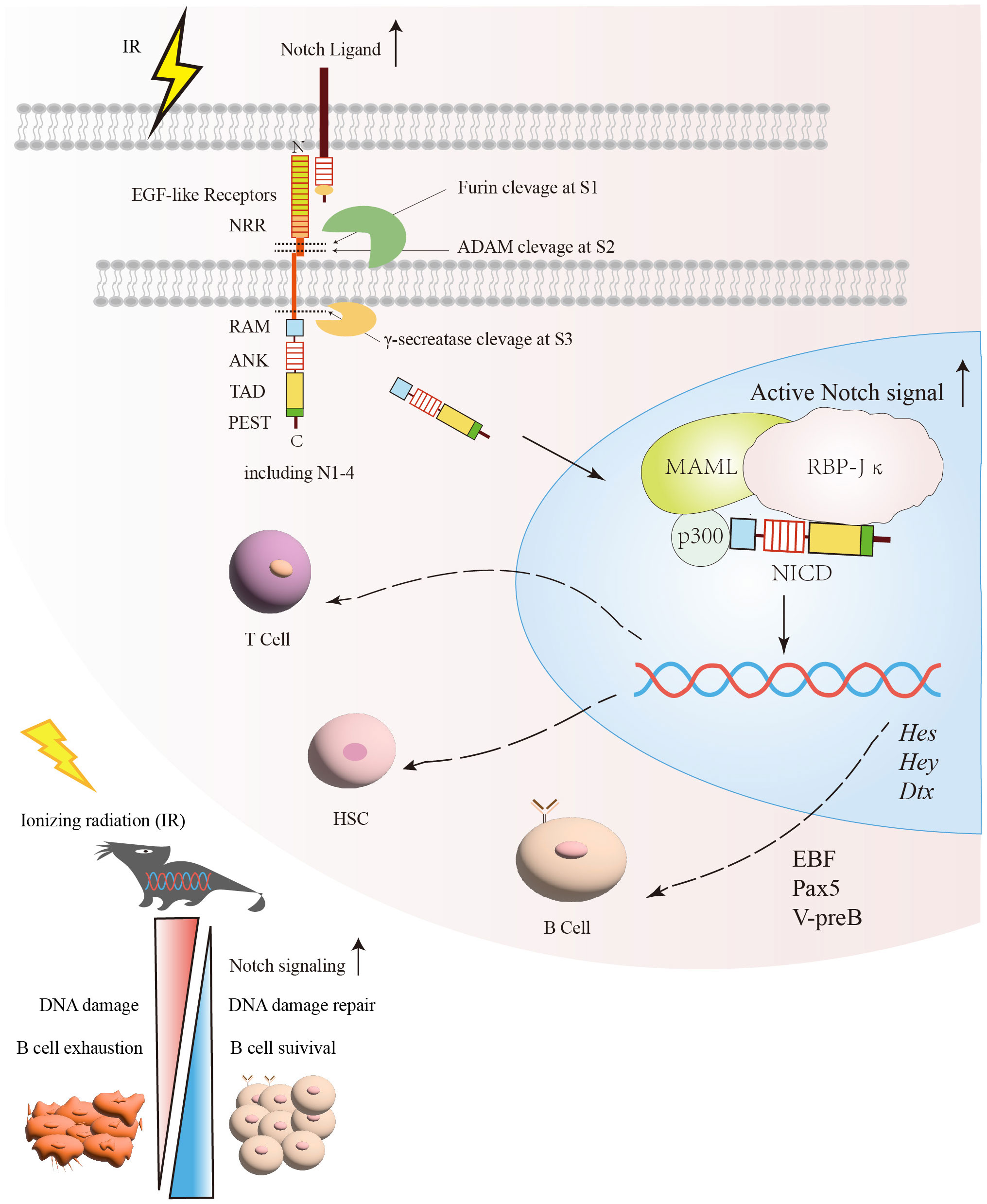
Figure 2 The protective effect of the Notch signaling pathway on B cells under irradiation exposure. After exposure to radiation, DNA damage and other associated harmful effects can lead to B cells exhaustion. Following radiation injury, the activation of Notch and its related signaling pathways facilitates DNA damage repair and promotes B cells survival. Subsequently, the relative enzymes are released and target specific sites (S1, S2, S3) within the Notch signaling pathway for cleavage in order to generate NICD. Once NICD enters the nucleus, it can recruit MAML and RBP-Jκ, releasing corepressors and recruiting coactivators. This process promotes the transcription of Notch target genes, such as Hes, Hey, and Dtx. Through the regulation of Notch signaling, it promotes the recovery of B cells after irradiation. NICD, Notch intracellular domain; RBP-Jκ, Recombination signal-binding protein for immunoglobulin kappa J region; MAMLs, Mastermind-like proteins.
Notch signaling regulates B cells maturation depending on RBP-Jκ (also called REPJ), the sequence-specific transcription factor, which is formed in B cells to promote B cells development under certain special conditions (81–83). Interestingly, the ADAM family is particularly important in regulating Notch signaling activation involved in lymphocyte development and maturation. It has been shown that ADAM10 is required for initiating Notch2 signaling in B cells and plays an important role in the development of the entire marginal zone B cells lineage (84–86). In ADAM10 deficient gene mice, the expression of Dtx1 and Hes1, Hes10, and other downstream target genes of the Notch signaling pathway were significantly suppressed in T1 B and MZB (84, 85). Taken together, Notch signaling, especially Notch2, is critical for proper B cells development.
4 Expression of Notch receptors in B cells and its subsets
The four Notch receptors have different functions in different cells due to the specificity of their receptor structures (87). Notch1 and Notch3 are highly expressed in thymus T-cells (88). Notch4 is less expressed in mouse B cells (89–91). In particular, it was recently reported that Notch2 is mainly expressed in B-cells (92–94). In BM, Notch1 signaling inhibits the developmental differentiation of HSPCs to B cells, thereby promoting early T cells development (72, 95). The inclination of Notch1 to promote T cells development is more pronounced in the BM (96), thus prompting the inquiry into how B cells respond to Notch1 signaling to sustain their own developmental processes (97). The B-cell lineage factor Pax5 has been identified as capable of inhibiting the expression of Notch1 and attenuating the tendency of T cell differentiation, thereby ensuring the development of the B-cell lineage (98–100).
To further investigate the function of Notch on B cells development, recent studies have suggested that Notch2 is expressed throughout B cells development which is particularly highly expressed on a subpopulation of spleen-matured B cells (101, 102). Notch2-mediated RBP-Jκ signaling is essential for MZB development (83, 103, 104). The data supporting a role for Notch2 signaling in MZB were obtained using CD19-Cre knockdown of the RBJ-Jκ allele in B cells, which exhibited a significant reduction in the number of MZB and a modest increase in the number of FoB (83, 105).
The Msx2 interacting nuclear target protein (MINT) promotes FoB development by interacting with RBP-Jκ, thereby inhibiting Notch-RBP-Jκ-mediated signaling (90). MINT was found to be a negative regulator of Notch/RBP-J-dependent signaling (90), and more highly expressed in FoB than that in MZB (90, 106). MINT deficiency resulted in more efficient differentiation of splenic B cells into MZB with a concomitant decrease in FoB (92). Notch2 influences B cells lineage differentiation toward MZB and FoB by regulating the expression profile of RBP-Jκ (92). A related report found that Notch2 expression was low in B-1 cells in the spleen, but higher in B-2 cells including FoB and MZB (107–109). This is further evidence that Notch2 plays a crucial role in late developmental differentiation of B cells (103, 110, 111). Notch1 is preferentially expressed in immature T cells (112–114) while Notch2 is expressed in mature B cells (93), indicating that Notch1 and Notch2 have functionally distinct roles in the lymphocyte development (93, 115–117). This is due to their different expression patterns or specific regulation in lymphogenesis (93). The function of Notch3, due to its low expression levels in mouse B cells, is not clear during B cells development (93). The distinguishing feature of Notch4 is its reduced number of EGF repeats, absence of a transcriptional activation domain, and lack of cytokine response proteins while exhibiting robust expression in endothelial cells (118). The activation of the Notch4 signaling pathway enhances the activity of HSPCs and promotes the proliferation of immature T cells lineage, resulting in impaired B cells development. These findings indicate that Notch4 may impede the differentiation of HSPCs into B cells (119, 120).
5 Regulation of irradiated-B cells by Notch signaling
Since the body requires a sufficient number of lymphocytes for immune monitoring (21), it is essential to maintain a pool of primary lymphocytes at different stages (121). Radiation-induced immunosuppression leads to the emergence of opportunistic infections (121, 122). The damage caused by these infections can be fatal depending on the radiation dose, dose rate, and duration of exposure (122). Therefore, protection of immunoreactive cells from radiation-induced damage is important for immune hemostasis. Among these immune cells, B cells play a major role in the humoral immune response. Extensive studies of B cells development have helped to determine the severity of radiation damage to B cells at various stages (21). Epidemiological data showed that exposure of infants or young adult mice to IR increases the risk of precursor B-cell tumors (123).
The impact of irradiation on B cells development was assessed, revealing an augmentation in the populations of Pro-B and Pre-B cells within the BM of irradiated mice, while a substantial reduction was observed in the numbers of Pre-pro-B cells (124–126). Pre-B, as the next cell subset in the developmental stage of Pro-B, is the earliest type of cell to produce Pre-B cells signaling receptors (Pre-BCRs), which stimulates the proliferation of developing B cells (33). Interestingly, the numbers of immature B cells in BM significantly decreased on 3 days after irradiation and reversed to a significant increase after 14 days (30). The resistance to radiation exhibited by Pro-B and later developing Pre-B may be related to the differentiation stages of the cells. Differentiated cells are usually more resistant to radiation than undifferentiated cells (127). According to the aforementioned analysis, relevant studies have revealed significant alterations in the total count of B lymphocytes at various stages subsequent to total body irradiation (30, 128, 129). Further analysis on these points showed that a significant increase in immunoglobulin heavy chain rearrangements and a decrease in immunoglobulin light chains in B cells 1-2 weeks after irradiation (21, 128, 130, 131). Importantly, heavy and light chain immunoglobulin genes were recombined by V(D)J and rearranged at the Pro-B and Pre-B stages, respectively, depending on RAG-1 and RAG-2 DNA nucleic acid endonucleases (30, 132, 133). The body achieves DNA repair through NHEJ. Radiation activates the DNA damage repair response pathway, which includes the NHEJ pathway required for B-cell development (30, 133). This suggests that after irradiation 1-2 weeks, the period of transition from pro-B to pre-B cells, is a critical period for early B cell subsets to process DNA damage repair (21, 134).
Although precursor B cells are highly sensitive to radiation-induced DNA damage within 1-2 weeks (21). To attenuate radiation-induced damage to the lymphatic system (135), a subpopulation of B cells achieves rapid regeneration and differentiation under the regulation of Notch signaling (27, 136). Notch signaling is involved in the stages of early B-cell development probably through the regulation of early B-cell factor (EBF) (121, 137). Pax5 functions to activate pre-B cell-restricted target initiation factors (e.g. Cd79a, λ5, V-preB, and B29) (137). A regulatory network consisting of the transcription factors EBF1, Pax5, E2A, and Foxo1 is closely associated with B-cell gene activation and lineage formation (121). In this network, both EBF1 and Pax5 are involved in B cell development by repressing genes (98, 121, 137–139), which are associated with T cell lineage development (99, 121). Pax5 represses genes encoding cell surface receptors (99), such as Notch1, while EBF1 represses genes encoding T cell lineage-promoting transcription factors, such as TCF1 and GATA3 (82, 98–100, 121, 137). EBF may be a key regulator of Notch signaling in pre-B cells generation, mainly through genes encoding key components of the pre-B cells receptor (99, 121, 140, 141). In addition, 72% of the genomic binding sites in pre-B cells were found to overlap with EBF1 binding sites (82, 121, 137). MZB expressing EFB1 also requires Notch2 signaling for maintenance, suggesting that Notch signaling activates these transcription factors involved in B cells development (93, 121). To investigate whether different Notch ligands influence early B-cell differentiation, Delta-1 and Jagged-1 were found to have different effects on early B-cell differentiation (128). Delta-1-4 signaling prevented Pro-B cells differentiation while promoting the development of cell populations with T/Nk progenitor cell phenotypes (128). In contrast, Jagged-1 did not interfere with the development of HSPCs to B lymphocytes (128, 134). To investigate the effects of radiation on peripheral splenic B cells subsets, relevant data showed that B-regs cells, memory B cells, transitional (T1, T2) B cells, and mature B cells showed different degrees of reduction in numbers within 24 hours after irradiation, whereas plasma cells differentiated from MZB showed a high degree of resistance to radiation (8, 30, 142). BAFF signaling and NF-κB signaling are required for the development of T2 B cells into FoB, which recirculates back to secondary lymphoid organs through the bloodstream and lymphatics (30, 93). Thus, FoB tends to be more genetically diverse than MZB in terms of IgV(D) genes (93). FoB interacts with T helper (Th) cells to form germinal centers, undergo class-switch recombination (CSR) and somatic hypermutation (SHM), and ultimately produce high-affinity antibodies or memory B cells (62, 77). Moreover, Notch2 is important for the development of T2 B cells into MZB (93). MZB is located in the marginal sinus at the outer edge of the splenic follicle, which is the junction of the red and white pulp (80). MZB participates in the thymic-independent antigenic immune response, allowing for the production of large numbers of IgM-producing, short-lived plasma cells (81). In addition, ADAM10 has been shown to play a key role in Notch2-mediated MZB development. Bone marrow transplantation of irradiated mice with recombinant ADAM10 revealed that the lymph nodes of the transplanted mice had normal lymphoid structure and the MZB in the cortical area were restored to normal (143). The study demonstrated that Notch-mediated ADAM10 expression restored secondary lymphoid structures and promoted the neogenesis of splenic germinal centers in irradiated mice (84, 144). In the spleen, the Notch2 ligand (delta-like 1, DL1) is present at high concentrations in the small splenic veins which is considered a key activator of MZB development (82, 84).
6 Notch signaling pathway is involved in recovery of B cells and HSPCs under irradiation exposure
HSC is a type of cells with self-renewal and differentiation potential in the hematopoietic system (145). It has been shown that exposure to IR doses (>1 Gy) within a short period of time can cause acute radiation sickness, with myeloid acute radiation sickness being the most serious (146). IR inhibits the self-renewal of HSCs and induces the senescence of HSCs mediated by an abnormal increase ROS production, which leads to premature senescence and dysfunction of HSCs (147). In addition, DNA damage induced by IR results in abnormal proliferation and differentiation of HSCs (147, 148), leading to hematopoietic-related diseases such as acute myeloid leukemia (149). Accidental or intentional exposure to moderate to high doses of IR leads to not only acute myelosuppression, but also long-term residual hematopoietic damage manifested as defective HSC self-renewal (150). Correspondingly, it has been recently reported that mice exposed to different doses of IR (2, 4, 6 Gy) within 1 month after exposure had a decrease in the total number of HSPCs and a decrease in the ability of colony formation in vitro (55, 150). Recent findings proved that both endothelial cells and osteoblasts express Notch ligands and promote ex-vivo HSPCs maintenance, suggesting that direct ligand or receptor interactions are a key component of the HSPCs ecological niche. In addition, conditional Notch1 deletion in BM endothelial cells results in reduced HSPCs after irradiation. These data proved for the importance of Notch signaling in maintaining HSPCs in the BM (81, 151).
HSPCs are found in the BM which is the ultimate source of all blood cell lineages (152). Although most hematopoietic lineages develop in the BM, B cell is unique in that it must complete its maturation in peripheral immune organs (153). Furthermore, recent studies have unveiled that Notch signaling can regulate HSC embryonic development, maintenance of “sternness”, and in vitro expansion (152, 154). Notch signaling is not only involved in the maintenance of hematopoietic homeostasis (155), but also regulates the development of HSC and B lymphocytes. Endothelial cells express various Notch receptors and ligands to regulate hematopoietic reconstruction in the absence of homeostasis (156, 157). Relevant studies have found that high purity novel Notch ligand heavy histone delta-like receptor 1 (D1R) has the biological effect of targeting anchored endothelial cells and activating the Notch signaling pathway (158). When the BM is acutely or chronically damaged by ionizing radiation, its long-term hematopoietic reconstruction ability is impaired (57). Recombinant protein D1R has the ability to exogenously activate the Notch signaling pathway, a classical pathway of hematopoietic cells within the hematopoietic niche (158, 159). D1R promotes the reconstitution of HSPCs in radiation-damaged mice, endogenously expands hematopoietic cell populations, and contributes to the spectral remodeling of the lymphocyte cells, thus improving the immunity of the body (158, 159).
The BM is the main tissue that produces HSPCs and carries some of the transition from stem cells to differentiated cells, including precursor cells for the different stages of B cells development (160). The stem cells or precursor B cells in the BM are highly susceptible to IR resulting in a dramatic decrease in peripheral B lymphocytes. The hematopoietic system has a strong repair and regenerative capacity. The feature compensates for the decrease in stem cells and lymphoid precursor cells through the activation of the Notch signaling pathway to reestablish the hematopoiesis and maintain homeostatic balance of hematopoiesis in vivo (21, 55, 161). It has been demonstrated that the potential role of Notch in regulating the self-renewal of HSPCs and in determining B cells fates (162). Indeed, a commitment of HSPCs into the B lineage needs to inhibit the Notch1 signal (163). For instance, when Pro-B cells undergo maturation in the BM, bone marrow stromal cells secrete the cytokine CXCL12, which effectively suppresses the expression of Notch ligands (144). With the Pro-B cells continuing to develop, Notch signaling plays an increasingly important role in subsequent developmental processes (134). The differentiation of HSPCs into the B-cell lineage is influenced by distinct Notch ligands and receptors, each playing specific roles (162, 164, 165). For example, Delta-like ligands-1 (Delta 1), as the important Notch2 ligand, induces immature B cells homing to the spleen, where Notch2 activation DLL1-mediated induces immature B2 cells to differentiate into MZB (144). However, the lower densities of Delta 1 in BM is inhibited B lineage development because the induced Notch signaling was not sufficient (162). Relevant researchers found that early B lineage was strongly inhibited in the Delta 1 transgenic NOG mice (NOG-D1-Tg) which have been irradiated 2.5 Gy and transplanted HSC (166). Interestingly, the researcher also showed decreased numbers of B cells in NOG-D1-Tg mice, a similar differentiation rate in B-cell subsets was observed for both NOG-D1-Tg and non-Tg mice (166). This implies that irradiation, in the presence of the Notch signaling ligand Delta, reduced the number of early B cells in the BM, but did not affect the differentiation capacity of B cells. Based on the above studies, we speculate that the depletion effect of radiation on early B cells in BM may be related to the insufficient number of Delta 1 ligands and the silencing of Notch signaling in early B cells. With the continuous differentiation of B cell lineages (134) and the activation of Notch signaling (144, 162), subsequent developing B cells become increasingly resistant to radiation, such as plasma cells (30).
7 Prospect and conclusion
Most of the studies on the effects of radiation on the immune system have focused on HSPCs and T cells, but little is known about the influences of radiation on the development and differentiation of B cells. B cells are a specialized class of antigen-presenting cells that produces antibodies to mediate humoral immune responses and activate a large number of cytokines involved in immune regulation, inflammatory responses, and hematopoiesis. B cells are one of the most radiosensitive cells in mammalian cells (4, 23–25)while the mechanisms involved in irradiation-induced B cells damage are still unknown. Notch is an evolutionarily conserved intercellular signaling pathway that regulates cellular differentiation and function at different developmental stages in the spleen, BM, thymus, etc. Interestingly, The Notch pathway has an important role in inducing the development of Pro-B cells to mature B cells during hemopoietic and immune system.
Our present review provides insight into B cells injury from IR and how Notch signaling activates progenitors and precursor B cells to initiate proliferation and differentiation by regulating transcription factors, such as EBF and Pax5, to replenish damaged B cells in a timely manner. Given that previous research, it is conceivable that Notch regulates B cells to perform non-homologous end-joining for repairing damaged DNA. It is worthwhile to further study that effector B cells (plasma cells) are highly resistant to radiation, which may provide a new idea for radiation therapy of B cells malignancy.
Author contributions
XS: Writing – original draft. JW: Writing – review & editing. HZ: Writing – review & editing. LS: Funding acquisition, Validation, Writing – review & editing.
Funding
The author(s) declare financial support was received for the research, authorship, and/or publication of this article. This work was supported by the National Natural Science Foundation of China (Grant No.82073484,82260117,81960104,81860026), Key R & D plan of Jiangxi Provincial Science and Technology Department (Grant No. 20202ACB206009) and Long-term project of “double thousand plan” in Jiangxi Province (Grant No. jsp2018101037), Project of Traditional Chinese Medicine in Jiangxi Province (Grant No. 2019A176), Graduate Innovation Special Fund of Jiangxi Province (Grant No.YC2022-s083).
Conflict of interest
The authors declare that the research was conducted in the absence of any commercial or financial relationships that could be construed as a potential conflict of interest.
Publisher’s note
All claims expressed in this article are solely those of the authors and do not necessarily represent those of their affiliated organizations, or those of the publisher, the editors and the reviewers. Any product that may be evaluated in this article, or claim that may be made by its manufacturer, is not guaranteed or endorsed by the publisher.
References
1. Gauci ML, Quero L, Ram-Wolff C, Guillerm S, M'Barek B, Lebbé C, et al. Outcomes of radiation therapy of indolent cutaneous B-cell lymphomas and literature review. J Eur Acad Dermatol Venereol. (2018) 32:1668–73. doi: 10.1111/jdv.14972
2. Takahashi J, Nagasawa S. Immunostimulatory effects of radiotherapy for local and systemic control of melanoma: A review. Int J Mol Sci. (2020) 21(23):9324. doi: 10.3390/ijms21239324
3. Rodriguez-Ruiz ME, Rodriguez I, Garasa S, Barbes B, Solorzano JL, Perez-Gracia JL, et al. Abscopal effects of radiotherapy are enhanced by combined immunostimulatory mAbs and are dependent on CD8 T cells and crosspriming. Cancer Res. (2016) 76:5994–6005. doi: 10.1158/0008-5472.CAN-16-0549
4. Paganetti H. A review on lymphocyte radiosensitivity and its impact on radiotherapy. Front Oncol. (2023) 13:1201500. doi: 10.3389/fonc.2023.1201500
5. Wang H, Morse HC 3rd, Bolland S. Transcriptional control of mature B cell fates. Trends Immunol. (2020) 41:601–13. doi: 10.1016/j.it.2020.04.011
6. Bala S, Chugh NA, Bansal SC, Koul A. Aloe vera modulates X-ray induced hematological and splenic tissue damage in mice. Hum Exp Toxicol. (2019) 38:1195–211. doi: 10.1177/0960327119860174
7. Malik N, Sansom OJ, Michie AM. The role of mTOR-mediated signals during haemopoiesis and lineage commitment. Biochem Soc Trans. (2018) 46:1313–24. doi: 10.1042/BST20180141
8. Zhou Y, Zhang Y, Han J, Yang M, Zhu J, Jin T. Transitional B cells involved in autoimmunity and their impact on neuroimmunological diseases. J Transl Med. (2020) 18:131. doi: 10.1186/s12967-020-02289-w
9. Wang Y, Liu J, Burrows PD, Wang JY. B cell development and maturation. Adv Exp Med Biol. (2020) 1254:1–22. doi: 10.1007/978-981-15-3532-1
10. Loder F, Mutschler B, Ray RJ, Paige CJ, Sideras P, Torres R, et al. B cell development in the spleen takes place in discrete steps and is determined by the quality of B cell receptor-derived signals. J Exp Med. (1999) 190:75–89. doi: 10.1084/jem.190.1.75
11. Kabashima K, Honda T, Ginhoux F, Egawa G. The immunological anatomy of the skin. Nat Rev Immunol. (2019) 19:19–30. doi: 10.1038/s41577-018-0084-5
12. Sims GP, Ettinger R, Shirota Y, Yarboro CH, Illei GG, Lipsky PE. Identification and characterization of circulating human transitional B cells. Blood. (2005) 105:4390–8. doi: 10.1182/blood-2004-11-4284
13. Fujimoto M. Regulatory B cells in skin and connective tissue diseases. J Dermatol Sci. (2010) 60:1–7. doi: 10.1016/j.jdermsci.2010.08.010
14. Su TT, Rawlings DJ. Transitional B lymphocyte subsets operate as distinct checkpoints in murine splenic B cell development. J Immunol. (2002) 168:2101–10. doi: 10.4049/jimmunol.168.5.2101
15. Hardy RR, Hayakawa K. B cell development pathways. Annu Rev Immunol. (2001) 19:595–621. doi: 10.1146/annurev.immunol.19.1.595
16. Luo Y, Wang J, Li K, Li M, Xu S, Liu X, et al. Single-cell genomics identifies distinct B1 cell developmental pathways and reveals aging-related changes in the B-cell receptor repertoire. Cell Biosci. (2022) 12:57. doi: 10.1186/s13578-022-00795-6
17. Mabbott NA, Gray D. Identification of co-expressed gene signatures in mouse B1, marginal zone and B2 B-cell populations. Immunology. (2014) 141:79–95. doi: 10.1111/imm.12171
18. Allman D, Srivastava B, Lindsley RC. Alternative routes to maturity: branch points and pathways for generating follicular and marginal zone B cells. Immunol Rev. (2004) 197:147–60. doi: 10.1111/j.0105-2896.2004.0108.x
19. Gorelik L, Gilbride K, Dobles M, Kalled SL, Zandman D, Scott ML. Normal B cell homeostasis requires B cell activation factor production by radiation-resistant cells. J Exp Med. (2003) 198:937–45. doi: 10.1084/jem.20030789
20. Trowell OA. The sensitivity of lymphocytes to ionising radiation. J Pathol Bacteriol. (1952) 64:687–704. doi: 10.1002/path.1700640403
21. Han D, Zhang M, Ma J, Hong J, Chen C, Zhang B, et al. Transition pattern and mechanism of B-lymphocyte precursors in regenerated mouse bone marrow after subtotal body irradiation. PloS One. (2012) 7:e46560. doi: 10.1371/journal.pone.0046560
22. Vral A, Thierens H, Bryant P, De Ridder L. A higher micronucleus yield in B-versus T-cells after low-dose gamma-irradiation is not linked with defective Ku86 protein. Int J Radiat Biol. (2001) 77(3):329–39. doi: 10.1080/0955300001004237
23. Louagie H, Van Eijkeren M, Philippe J, Thierens H, de Ridder L. Changes in peripheral blood lymphocyte subsets in patients undergoing radiotherapy. Int J Radiat Biol. (1999) 75(6):767–71. doi: 10.1080/095530099140113
24. Anderson RE, Sprent J, Miller JF. Radiosensitivity of T and B lymphocytes. I. Effect of irradiation on cell migration. Eur J Immunol. (1974) 4:199–203. doi: 10.1002/eji.1830040309
25. Heylmann D, Ponath V, Kindler T, Kaina B. Comparison of DNA repair and radiosensitivity of different blood cell populations. Sci Rep. (2021) 11:2478. doi: 10.1038/s41598-021-81058-1
26. Heylmann D, Rödel F, Kindler T, Kaina B. Radiation sensitivity of human and murine peripheral blood lymphocytes, stem and progenitor cells. Biochim Biophys Acta. (2014) 1846:121–9. doi: 10.1016/j.bbcan.2014.04.009
27. Nishii K, Gibbons DL, Titley I, Papworth D, Goodhead DT, Greaves M. Regulation of the apoptotic response to radiation damage in B cell development. Cell Death Differ. (1998) 5:77–86. doi: 10.1038/sj.cdd.4400317
28. Griffiths SD, Goodhead DT, Marsden SJ, Wright EG, Krajewski S, Reed JC, et al. Interleukin 7-dependent B lymphocyte precursor cells are ultrasensitive to apoptosis. J Exp Med. (1994) 179:1789–97. doi: 10.1084/jem.179.6.1789
29. Griffiths SD, Marsden SJ, Wright EG, Greaves MF, Goodhead DT. Lethality and mutagenesis of B lymphocyte progenitor cells following exposure to alpha-particles and X-rays. Int J Radiat Biol. (1994) 66(2):197–205. doi: 10.1080/09553009414551101
30. Franiak-Pietryga I, Miyauchi S, Kim SS, Sanders PD, Sumner W, Zhang L, et al. Activated B cells and plasma cells are resistant to radiation therapy. Int J Radiat Oncol Biol Phys. (2022) 112:514–28. doi: 10.1016/j.ijrobp.2021.08.037
31. Otero DC, Poli V, David M, Rickert RC. Cutting edge: inherent and acquired resistance to radiation-induced apoptosis in B cells: a pivotal role for STAT3. J Immunol. (2006) 177:6593–7. doi: 10.4049/jimmunol.177.10.6593
32. Tanaka S, Baba Y. B cell receptor signaling. Adv Exp Med Biol. (2020) 1254:23–36. doi: 10.1007/978-981-15-3532-1_2
33. Monroe JG. ITAM-mediated tonic signalling through pre-BCR and BCR complexes. Nat Rev Immunol. (2006) 6:283–94. doi: 10.1038/nri1808
34. Kim SS, Shen S, Miyauchi S, Sanders PD, Franiak-Pietryga I, Mell L, et al. B cells improve overall survival in HPV-associated squamous cell carcinomas and are activated by radiation and PD-1 blockade. Clin Cancer Res. (2020) 26:3345–59. doi: 10.1158/1078-0432.CCR-19-3211
35. Singh VK, Seed TM. A review of radiation countermeasures focusing on injury-specific medicinals and regulatory approval status: part I. Radiation sub-syndromes, animal models and FDA-approved countermeasures. Int J Radiat Biol. (2017) 93:851–69. doi: 10.1080/09553002.2017.1332438
36. Wang A, Shi Z, Wang L, Wang Y, Chen X, He C, et al. The injuries of spleen and intestinal immune system induced by 2-Gy (60)Co γ-ray whole-body irradiation. Int J Radiat Biol. (2023) 99:406–18. doi: 10.1080/09553002.2022.2094017
37. Wang A, Wang L, Fu Q, Shi Z, Chen X, Zhang X, et al. Yiqi Jiedu herbal decoction attenuates the 2 Gy (60)Co γ ray induced spleen injury by inhibiting apoptosis and modulating the immune balance. J Ethnopharmacol. (2022) 286:114925. doi: 10.1016/j.jep.2021.114925
38. Kaspler P, Pintilie M, Hill RP. Dynamics of micronuclei in rat skin fibroblasts after X irradiation. Radiat Res. (2009) 172:106–13. doi: 10.1667/RR1649.1
39. Noda A. Radiation-induced unrepairable DSBs: their role in the late effects of radiation and possible applications to biodosimetry. J Radiat Res. (2018) 59:ii114–20. doi: 10.1093/jrr/rrx074
40. O’Brien TJ, Ceryak S, Patierno SR. Complexities of chromium carcinogenesis: role of cellular response, repair and recovery mechanisms. Mutat Res. (2003) 533:3–36. doi: 10.1016/j.mrfmmm.2003.09.006
41. Ceccaldi R, Rondinelli B, D’Andrea AD. Repair pathway choices and consequences at the double-strand break. Trends Cell Biol. (2016) 26:52–64. doi: 10.1016/j.tcb.2015.07.009
42. Innes CL, Hesse JE, Morales AJ, Helmink BA, Schurman SH, Sleckman BP, et al. DNA damage responses in murine Pre-B cells with genetic deficiencies in damage response genes. Cell Cycle. (2020) 19:67–83. doi: 10.1080/15384101.2019.1693118
43. Bredemeyer AL, Helmink BA, Innes CL, Calderon B, McGinnis LM, Mahowald GK, et al. DNA double-strand breaks activate a multi-functional genetic program in developing lymphocytes. Nature. (2008) 456:819–23. doi: 10.1038/nature07392
44. Innes CL, Hesse JE, Palii SS, Helmink BA, Holub AJ, Sleckman BP, et al. DNA damage activates a complex transcriptional response in murine lymphocytes that includes both physiological and cancer-predisposition programs. BMC Genomics. (2013) 14:163. doi: 10.1186/1471-2164-14-163
45. Delia D, Mizutani S. The DNA damage response pathway in normal hematopoiesis and Malignancies. Int J Hematol. (2017) 106:328–34. doi: 10.1007/s12185-017-2300-7
46. Gueiderikh A, Maczkowiak-Chartois F, Rosselli F. A new frontier in Fanconi anemia: From DNA repair to ribosome biogenesis. Blood Rev. (2022) 52:100904. doi: 10.1016/j.blre.2021.100904
47. Li N, Chen H, Wang J. DNA damage and repair in the hematopoietic system. Acta Biochim Biophys Sin (Shanghai). (2022) 54:847–57. doi: 10.3724/abbs.2022053
48. Barzilai A, Yamamoto K. DNA damage responses to oxidative stress. DNA Repair (Amst). (2004) 3:1109–15. doi: 10.1016/j.dnarep.2004.03.002
49. Su YJ, Wang PW, Weng SW. The role of mitochondria in immune-cell-mediated tissue regeneration and ageing. Int J Mol Sci. (2021) 22(5):2668. doi: 10.3390/ijms22052668
50. Yahata T, Takanashi T, Muguruma Y, Ibrahim AA, Matsuzawa H, Uno T, et al. Accumulation of oxidative DNA damage restricts the self-renewal capacity of human hematopoietic stem cells. Blood. (2011) 118:2941–50. doi: 10.1182/blood-2011-01-330050
51. Li D, Tian Z, Tang W, Zhang J, Lu L, Sun Z, et al. The protective effects of 5-methoxytryptamine-α-lipoic acid on ionizing radiation-induced hematopoietic injury. Int J Mol Sci. (2016) 17(6):935. doi: 10.3390/ijms17060935
52. Muri J, Kopf M. Redox regulation of immunometabolism. Nat Rev Immunol. (2021) 21:363–81. doi: 10.1038/s41577-020-00478-8
53. Lorimore SA, Coates PJ, Scobie GE, Milne G, Wright EG. Inflammatory-type responses after exposure to ionizing radiation in vivo: a mechanism for radiation-induced bystander effects? Oncogene. (2001) 20:7085–95. doi: 10.1038/sj.onc.1204903
54. Jung H, Kim MJ, Kim DO, Kim WS, Yoon SJ, Park YJ, et al. TXNIP maintains the hematopoietic cell pool by switching the function of p53 under oxidative stress. Cell Metab. (2013) 18:75–85. doi: 10.1016/j.cmet.2013.06.002
55. Wang Y, Liu L, Pazhanisamy SK, Li H, Meng A, Zhou D. Total body irradiation causes residual bone marrow injury by induction of persistent oxidative stress in murine hematopoietic stem cells. Free Radic Biol Med. (2010) 48:348–56. doi: 10.1016/j.freeradbiomed.2009.11.005
56. Paul MK, Bisht B, Darmawan DO, Chiou R, Ha VL, Wallace WD, et al. Dynamic changes in intracellular ROS levels regulate airway basal stem cell homeostasis through Nrf2-dependent Notch signaling. Cell Stem Cell. (2014) 15:199–214. doi: 10.1016/j.stem.2014.05.009
57. Kim JH, Thimmulappa RK, Kumar V, Cui W, Kumar S, Kombairaju P, et al. NRF2-mediated Notch pathway activation enhances hematopoietic reconstitution following myelosuppressive radiation. J Clin Invest. (2014) 124:730–41. doi: 10.1172/JCI70812
58. Ooi A, Dykema K, Ansari A, Petillo D, Snider J, Kahnoski R, et al. CUL3 and NRF2 mutations confer an NRF2 activation phenotype in a sporadic form of papillary renal cell carcinoma. Cancer Res. (2013) 73:2044–51. doi: 10.1158/0008-5472.CAN-12-3227
59. Wang J, Wakeman TP, Lathia JD, Hjelmeland AB, Wang XF, White RR, et al. Notch promotes radioresistance of glioma stem cells. Stem Cells. (2010) 28:17–28. doi: 10.1002/stem.261
60. Banerjee D, Barton SM, Grabham PW, Rumeld AL, Okochi S, Street C, et al. High-dose radiation increases Notch1 in tumor vasculature. Int J Radiat Oncol Biol Phys. (2020) 106:857–66. doi: 10.1016/j.ijrobp.2019.11.010
61. Scharpfenecker M, Kruse JJ, Sprong D, Russell NS, Ten Dijke P, Stewart FA. Ionizing radiation shifts the PAI-1/ID-1 balance and activates notch signaling in endothelial cells. Int J Radiat Oncol Biol Phys. (2009) 73:506–13. doi: 10.1016/j.ijrobp.2008.09.052
62. Kondelaji MHR, Sharma GP, Jagtap J, Shafiee S, Hansen C, Gasperetti T, et al. 2(nd) window NIR imaging of radiation injury mitigation provided by reduced notch-Dll4 expression on vasculature. Mol Imaging Biol. (2024) 26(1):124–37. doi: 10.1007/s11307-023-01840-7
63. Zhou B, Lin W, Long Y, Yang Y, Zhang H, Wu K, et al. Notch signaling pathway: architecture, disease, and therapeutics. Signal Transduct Target Ther. (2022) 7:95. doi: 10.1038/s41392-022-00934-y
64. Shao L, Sottoriva K, Palasiewicz K, Zhang J, Hyun J, Soni SS, et al. A Tie2-Notch1 signaling axis regulates regeneration of the endothelial bone marrow niche. Haematologica. (2019) 104:2164–77. doi: 10.3324/haematol.2018.208660
65. Meurette O, Mehlen P. Notch signaling in the tumor microenvironment. Cancer Cell. (2018) 34:536–48. doi: 10.1016/j.ccell.2018.07.009
66. Andersson ER, Sandberg R, Lendahl U. Notch signaling: simplicity in design, versatility in function. Development. (2011) 138:3593–612. doi: 10.1242/dev.063610
67. Gordon WR, Vardar-Ulu D, Histen G, Sanchez-Irizarry C, Aster JC, Blacklow SC. Structural basis for autoinhibition of Notch. Nat Struct Mol Biol. (2007) 14:295–300. doi: 10.1038/nsmb1227
68. Cordle J, Johnson S, Tay JZ, Roversi P, Wilkin MB, de Madrid BH, et al. A conserved face of the Jagged/Serrate DSL domain is involved in Notch trans-activation and cis-inhibition. Nat Struct Mol Biol. (2008) 15:849–57. doi: 10.1038/nsmb.1457
69. Cai J, Qiao Y, Chen L, Lu Y, Zheng D. Regulation of the Notch signaling pathway by natural products for cancer therapy. J Nutr Biochem. (2024) 123:109483. doi: 10.1016/j.jnutbio.2023.109483
70. Weisshuhn PC, Sheppard D, Taylor P, Whiteman P, Lea SM, Handford PA, et al. Non-linear and flexible regions of the human Notch1 extracellular domain revealed by high-resolution structural studies. Structure. (2016) 24:555–66. doi: 10.1016/j.str.2016.02.010
71. Sanchez-Irizarry C, Carpenter AC, Weng AP, Pear WS, Aster JC, Blacklow SC. Notch subunit heterodimerization and prevention of ligand-independent proteolytic activation depend, respectively, on a novel domain and the LNR repeats. Mol Cell Biol. (2004) 24:9265–73. doi: 10.1128/MCB.24.21.9265-9273.2004
72. Katoh M, Katoh M. Precision medicine for human cancers with Notch signaling dysregulation (Review). Int J Mol Med. (2020) 45:279–97. doi: 10.3892/ijmm
73. Gordon WR, Zimmerman B, He L, Miles LJ, Huang J, Tiyanont K, et al. Mechanical allostery: evidence for a force requirement in the proteolytic activation of notch. Dev Cell. (2015) 33:729–36. doi: 10.1016/j.devcel.2015.05.004
74. Seo D, Southard KM, Kim JW, Lee HJ, Farlow J, Lee JU, et al. A mechanogenetic toolkit for interrogating cell signaling in space and time. Cell. (2016) 165:1507–18. doi: 10.1016/j.cell.2016.04.045
75. Yu X, Alder JK, Chun JH, Friedman AD, Heimfeld S, Cheng L, et al. HES1 inhibits cycling of hematopoietic progenitor cells via DNA binding. Stem Cells. (2006) 24:876–88. doi: 10.1634/stemcells.2005-0598
76. Kim HE, Bae E, Jeong DY, Kim MJ, Jin WJ, Park SW, et al. Lipin1 regulates PPARγ transcriptional activity. Biochem J. (2013) 453:49–60. doi: 10.1042/BJ20121598
77. Martinez Lyons A, Boulter L. The developmental origins of Notch-driven intrahepatic bile duct disorders. Dis Model Mech. (2021) 14(9):dmm048413. doi: 10.1242/dmm.048413
78. Brou C, Logeat F, Gupta N, Bessia C, LeBail O, Doedens JR, et al. A novel proteolytic cleavage involved in Notch signaling: the role of the disintegrin-metalloprotease TACE. Mol Cell. (2000) 5:207–16. doi: 10.1016/S1097-2765(00)80417-7
79. Tanwar A, Stanley P. Synergistic regulation of Notch signaling by different O-glycans promotes hematopoiesis. Front Immunol. (2023) 14:1097332. doi: 10.3389/fimmu.2023.1097332
80. D’Souza B, Miyamoto A, Weinmaster G. The many facets of Notch ligands. Oncogene. (2008) 27:5148–67. doi: 10.1038/onc.2008.229
81. Garis M, Garrett-Sinha LA. Notch signaling in B cell immune responses. Front Immunol. (2020) 11:609324. doi: 10.3389/fimmu.2020.609324
82. Lu F, Chen HS, Kossenkov AV, DeWispeleare K, Won KJ, Lieberman PM. EBNA2 drives formation of new chromosome binding sites and target genes for B-cell master regulatory transcription factors RBP-jκ and EBF1. PloS Pathog. (2016) 12:e1005339. doi: 10.1371/journal.ppat.1005339
83. Tanigaki K, Han H, Yamamoto N, Tashiro K, Ikegawa M, Kuroda K, et al. Notch-RBP-J signaling is involved in cell fate determination of marginal zone B cells. Nat Immunol. (2002) 3:443–50. doi: 10.1038/ni793
84. Gibb DR, El Shikh M, Kang DJ, Rowe WJ, El Sayed R, Cichy J, et al. ADAM10 is essential for Notch2-dependent marginal zone B cell development and CD23 cleavage in vivo. J Exp Med. (2010) 207:623–35. doi: 10.1084/jem.20091990
85. Mathews JA, Gibb DR, Chen BH, Scherle P, Conrad DH. CD23 Sheddase A disintegrin and metalloproteinase 10 (ADAM10) is also required for CD23 sorting into B cell-derived exosomes. J Biol Chem. (2010) 285:37531–41. doi: 10.1074/jbc.M110.141556
86. Sakamoto K, Jin SP, Goel S, Jo JH, Voisin B, Kim D, et al. Disruption of the endopeptidase ADAM10-Notch signaling axis leads to skin dysbiosis and innate lymphoid cell-mediated hair follicle destruction. Immunity. (2021) 54(10):2321–2337.e2310. doi: 10.1016/j.immuni.2021.09.001
87. Handford PA, Korona B, Suckling R, Redfield C, Lea SM. Structural insights into notch receptor-ligand interactions. Adv Exp Med Biol. (2018) 1066:33–46. doi: 10.1007/978-3-319-89512-3_2
88. Herranz D, Ambesi-Impiombato A, Palomero T, Schnell SA, Belver L, Wendorff AA, et al. A NOTCH1-driven MYC enhancer promotes T cell development, transformation and acute lymphoblastic leukemia. Nat Med. (2014) 20:1130–7. doi: 10.1038/nm.3665
89. James AC, Szot JO, Iyer K, Major JA, Pursglove SE, Chapman G, et al. Notch4 reveals a novel mechanism regulating Notch signal transduction. Biochim Biophys Acta. (2014) 1843:1272–84. doi: 10.1016/j.bbamcr.2014.03.015
90. Radtke F, Wilson A, MacDonald HR. Notch signaling in T- and B-cell development. Curr Opin Immunol. (2004) 16:174–9. doi: 10.1016/j.coi.2004.01.002
91. Harris KM, Clements MA, Kwilasz AJ, Watkins LR. T cell transgressions: Tales of T cell form and function in diverse disease states. Int Rev Immunol. (2022) 41:475–516. doi: 10.1080/08830185.2021.1921764
92. Kuroda K, Han H, Tani S, Tanigaki K, Tun T, Furukawa T, et al. Regulation of marginal zone B cell development by MINT, a suppressor of Notch/RBP-J signaling pathway. Immunity. (2003) 18:301–12. doi: 10.1016/S1074-7613(03)00029-3
93. Saito T, Chiba S, Ichikawa M, Kunisato A, Asai T, Shimizu K, et al. Notch2 is preferentially expressed in mature B cells and indispensable for marginal zone B lineage development. Immunity. (2003) 18:675–85. doi: 10.1016/S1074-7613(03)00111-0
94. Zhu X, Schweitzer BL, Romer EJ, Sulentic CE, DeKoter RP. Transgenic expression of Spi-C impairs B-cell development and function by affecting genes associated with BCR signaling. Eur J Immunol. (2008) 38:2587–99. doi: 10.1002/eji.200838323
95. Edelmann J, Dokal AD, Vilventhraraja E, Holzmann K, Britton D, Klymenko T, et al. Rituximab and obinutuzumab differentially hijack the B cell receptor and NOTCH1 signaling pathways. iScience. (2021) 24:102089. doi: 10.1016/j.isci.2021.102089
96. González-García S, García-Peydró M, Alcain J, Toribio ML. Notch1 and IL-7 receptor signalling in early T-cell development and leukaemia. Curr Top Microbiol Immunol. (2012) 360:47–73. doi: 10.1007/82_2012_231
97. García-Peydró M, de Yébenes VG, Toribio ML. Notch1 and IL-7 receptor interplay maintains proliferation of human thymic progenitors while suppressing non-T cell fates. J Immunol. (2006) 177:3711–20. doi: 10.4049/jimmunol.177.6.3711
98. Calderón L, Schindler K, Malin SG, Schebesta A, Sun Q, Schwickert T, et al. Pax5 regulates B cell immunity by promoting PI3K signaling via PTEN down-regulation. Sci Immunol. (2021) 6(61):eabg5003. doi: 10.1126/sciimmunol.abg5003
99. Souabni A, Cobaleda C, Schebesta M, Busslinger M. Pax5 promotes B lymphopoiesis and blocks T cell development by repressing Notch1. Immunity. (2002) 17:781–93. doi: 10.1016/S1074-7613(02)00472-7
100. Gruenbacher S, Jaritz M, Hill L, Schäfer M, Busslinger M. Essential role of the Pax5 C-terminal domain in controlling B cell commitment and development. J Exp Med. (2023) 220(12):e20230260. doi: 10.1084/jem.20230260
101. Lechner M, Engleitner T, Babushku T, Schmidt-Supprian M, Rad R, Strobl LJ, et al. Notch2-mediated plasticity between marginal zone and follicular B cells. Nat Commun. (2021) 12:1111. doi: 10.1038/s41467-021-21359-1
102. Mangolini M, Götte F, Moore A, Ammon T, Oelsner M, Lutzny-Geier G, et al. Notch2 controls non-autonomous Wnt-signalling in chronic lymphocytic leukaemia. Nat Commun. (2018) 9:3839. doi: 10.1038/s41467-018-06069-5
103. Zhang Y, Zhu G, Xiao H, Liu X, Han G, Chen G, et al. CD19 regulates ADAM28-mediated Notch2 cleavage to control the differentiation of marginal zone precursors to MZ B cells. J Cell Mol Med. (2017) 21:3658–69. doi: 10.1111/jcmm.13276
104. Case JB, Bonami RH, Nyhoff LE, Steinberg HE, Sullivan AM, Kendall PL. Bruton’s tyrosine kinase synergizes with Notch2 to govern marginal zone B cells in nonobese diabetic mice. J Immunol. (2015) 195:61–70. doi: 10.4049/jimmunol.1400803
105. Hampel F, Ehrenberg S, Hojer C, Draeseke A, Marschall-Schröter G, Kühn R, et al. CD19-independent instruction of murine marginal zone B-cell development by constitutive Notch2 signaling. Blood. (2011) 118:6321–31. doi: 10.1182/blood-2010-12-325944
106. Tanigaki K, Kuroda K, Han H, Honjo T. Regulation of B cell development by Notch/RBP-J signaling. Semin Immunol. (2003) 15:113–9. doi: 10.1016/S1044-5323(03)00008-3
107. Tan JB, Xu K, Cretegny K, Visan I, Yuan JS, Egan SE, et al. Lunatic and manic fringe cooperatively enhance marginal zone B cell precursor competition for delta-like 1 in splenic endothelial niches. Immunity. (2009) 30:254–63. doi: 10.1016/j.immuni.2008.12.016
108. Montecino-Rodriguez E, Dorshkind K. New perspectives in B-1 B cell development and function. Trends Immunol. (2006) 27:428–33. doi: 10.1016/j.it.2006.07.005
109. Song H, Cerny J. Functional heterogeneity of marginal zone B cells revealed by their ability to generate both early antibody-forming cells and germinal centers with hypermutation and memory in response to a T-dependent antigen. J Exp Med. (2003) 198:1923–35. doi: 10.1084/jem.20031498
110. Pillai S, Cariappa A. The follicular versus marginal zone B lymphocyte cell fate decision. Nat Rev Immunol. (2009) 9:767–77. doi: 10.1038/nri2656
111. Iwahashi S, Maekawa Y, Nishida J, Ishifune C, Kitamura A, Arimochi H, et al. Notch2 regulates the development of marginal zone B cells through Fos. Biochem Biophys Res Commun. (2012) 418:701–7. doi: 10.1016/j.bbrc.2012.01.082
112. Zhu G, Wang X, Xiao H, Liu X, Fang Y, Zhai B, et al. Both Notch1 and its ligands in B cells promote antibody production. Mol Immunol. (2017) 91:17–23. doi: 10.1016/j.molimm.2017.08.021
113. Tan JB, Visan I, Yuan JS, Guidos CJ. Requirement for Notch1 signals at sequential early stages of intrathymic T cell development. Nat Immunol. (2005) 6:671–9. doi: 10.1038/ni1217
114. Harman BC, Jenkinson WE, Parnell SM, Rossi SW, Jenkinson EJ, Anderson G. T/B lineage choice occurs prior to intrathymic Notch signaling. Blood. (2005) 106:886–92. doi: 10.1182/blood-2004-12-4881
115. Radtke F, Wilson A, Mancini SJ, MacDonald HR. Notch regulation of lymphocyte development and function. Nat Immunol. (2004) 5:247–53. doi: 10.1038/ni1045
116. Hubmann R, Schwarzmeier JD, Shehata M, Hilgarth M, Duechler M, Dettke M, et al. Notch2 is involved in the overexpression of CD23 in B-cell chronic lymphocytic leukemia. Blood. (2002) 99:3742–7. doi: 10.1182/blood.V99.10.3742
117. Chiang MY, Radojcic V, Maillard I. Oncogenic Notch signaling in T-cell and B-cell lymphoproliferative disorders. Curr Opin Hematol. (2016) 23:362–70. doi: 10.1097/MOH.0000000000000254
118. Vercauteren SM, Sutherland HJ. Constitutively active Notch4 promotes early human hematopoietic progenitor cell maintenance while inhibiting differentiation and causes lymphoid abnormalities in vivo. Blood. (2004) 104:2315–22. doi: 10.1182/blood-2004-01-0204
119. Ng HL, Quail E, Cruickshank MN, Ulgiati D. To be, or notch to be: mediating cell fate from embryogenesis to lymphopoiesis. Biomolecules. (2021) 11(6):849. doi: 10.3390/biom11060849
120. Harb H, Benamar M, Lai PS, Contini P, Griffith JW, Crestani E, et al. Notch4 signaling limits regulatory T-cell-mediated tissue repair and promotes severe lung inflammation in viral infections. Immunity. (2021) 54:1186–1199.e1187. doi: 10.1016/j.immuni.2021.04.002
121. Bayer M, Boller S, Ramamoothy S, Zolotarev N, Cauchy P, Iwanami N, et al. Tnpo3 enables EBF1 function in conditions of antagonistic Notch signaling. Genes Dev. (2022) 36:901–15. doi: 10.1101/gad.349696.122
122. Maisin JR, Wambersie A, Gerber GB, Mattelin G, Lambiet-Collier M, De Coster B, et al. Life-shortening and disease incidence in mice after exposure to gamma rays or high-energy neutrons. Radiat Res. (1991) 128:S117–123. doi: 10.2307/3578013
123. Tachibana H, Morioka T, Daino K, Shang Y, Ogawa M, Fujita M, et al. Early induction and increased risk of precursor B-cell neoplasms after exposure of infant or young-adult mice to ionizing radiation. J Radiat Res. (2020) 61:648–56. doi: 10.1093/jrr/rraa055
124. Zhang QF, Li J, Jiang K, Wang R, Ge JL, Yang H, et al. CDK4/6 inhibition promotes immune infiltration in ovarian cancer and synergizes with PD-1 blockade in a B cell-dependent manner. Theranostics. (2020) 10:10619–33. doi: 10.7150/thno.44871
125. Monroe JG, Dorshkind K. Fate decisions regulating bone marrow and peripheral B lymphocyte development. Adv Immunol. (2007) 95:1–50. doi: 10.1016/S0065-2776(07)95001-4
126. Nagasawa T. Microenvironmental niches in the bone marrow required for B-cell development. Nat Rev Immunol. (2006) 6:107–16. doi: 10.1038/nri1780
127. Uckun FM, Mitchell JB, Obuz V, Park CH, Waddick K, Friedman N, et al. Radiation sensitivity of human B-lineage lymphoid precursor cells. Int J Radiat Oncol Biol Phys. (1991) 21:1553–60. doi: 10.1016/0360-3016(91)90332-X
128. Jaleco AC, Neves H, Hooijberg E, Gameiro P, Clode N, Haury M, et al. Differential effects of Notch ligands Delta-1 and Jagged-1 in human lymphoid differentiation. J Exp Med. (2001) 194:991–1002. doi: 10.1084/jem.194.7.991
129. Yuan T, Han X, Liu H, Zhang J, Fan S. Mouse parabiosis model promotes recovery of lymphocytes in irradiated mice. Int J Radiat Biol. (2021) 97:1589–96. doi: 10.1080/09553002.2021.1969464
130. Gallenstein N, Tichy L, Weigand MA, Schenz J. Notch signaling in acute inflammation and sepsis. Int J Mol Sci. (2023) 24(4):3458. doi: 10.3390/ijms24043458
131. Šućur A, Filipović M, Flegar D, Kelava T, Šisl D, Lukač N, et al. Notch receptors and ligands in inflammatory arthritis - a systematic review. Immunol Lett. (2020) 223:106–14. doi: 10.1016/j.imlet.2020.04.010
132. Hardy RR, Carmack CE, Shinton SA, Kemp JD, Hayakawa K. Resolution and characterization of pro-B and pre-pro-B cell stages in normal mouse bone marrow. J Exp Med. (1991) 173:1213–25. doi: 10.1084/jem.173.5.1213
133. Edry E, Melamed D. Receptor editing in positive and negative selection of B lymphopoiesis. J Immunol. (2004) 173:4265–71. doi: 10.4049/jimmunol.173.7.4265
134. Benne C, Lelievre JD, Balbo M, Henry A, Sakano S, Levy Y. Notch increases T/NK potential of human hematopoietic progenitors and inhibits B cell differentiation at a pro-B stage. Stem Cells. (2009) 27:1676–85. doi: 10.1002/stem.94
135. Baker KP. BLyS–an essential survival factor for B cells: basic biology, links to pathology and therapeutic target. Autoimmun Rev. (2004) 3:368–75. doi: 10.1016/j.autrev.2004.02.001
136. Cheng P, Zhou J, Gabrilovich D. Regulation of dendritic cell differentiation and function by Notch and Wnt pathways. Immunol Rev. (2010) 234:105–19. doi: 10.1111/j.0105-2896.2009.00871.x
137. Smith EM, Akerblad P, Kadesch T, Axelson H, Sigvardsson M. Inhibition of EBF function by active Notch signaling reveals a novel regulatory pathway in early B-cell development. Blood. (2005) 106:1995–2001. doi: 10.1182/blood-2004-12-4744
138. Banerjee A, Northrup D, Boukarabila H, Jacobsen SE, Allman D. Transcriptional repression of Gata3 is essential for early B cell commitment. Immunity. (2013) 38:930–42. doi: 10.1016/j.immuni.2013.01.014
139. Nechanitzky R, Akbas D, Scherer S, Györy I, Hoyler T, Ramamoorthy S, et al. Transcription factor EBF1 is essential for the maintenance of B cell identity and prevention of alternative fates in committed cells. Nat Immunol. (2013) 14:867–75. doi: 10.1038/ni.2641
140. Sigvardsson M, Clark DR, Fitzsimmons D, Doyle M, Akerblad P, Breslin T, et al. Early B-cell factor, E2A, and Pax-5 cooperate to activate the early B cell-specific mb-1 promoter. Mol Cell Biol. (2002) 22:8539–51. doi: 10.1128/MCB.22.24.8539-8551.2002
141. Sigvardsson M. Overlapping expression of early B-cell factor and basic helix-loop-helix proteins as a mechanism to dictate B-lineage-specific activity of the lambda5 promoter. Mol Cell Biol. (2000) 20:3640–54. doi: 10.1128/.20.10.3640-3654.2000
142. Vossenkämper A, Spencer J. Transitional B cells: how well are the checkpoints for specificity understood? Arch Immunol Ther Exp (Warsz). (2011) 59:379–84. doi: 10.1007/s00005-011-0135-0
143. Folgosa L, Zellner HB, El Shikh ME, Conrad DH. Disturbed follicular architecture in B cell A disintegrin and metalloproteinase (ADAM)10 knockouts is mediated by compensatory increases in ADAM17 and TNF-α shedding. J Immunol. (2013) 191:5951–8. doi: 10.4049/jimmunol.1302042
144. Mesini N, Fiorcari S, Atene CG, Maffei R, Potenza L, Luppi M, et al. Role of Notch2 pathway in mature B cell Malignancies. Front Oncol. (2022) 12:1073672. doi: 10.3389/fonc.2022.1073672
145. Shao L, Paik NY, Sanborn MA, Bandara T, Vijaykumar A, Sottoriva K, et al. Hematopoietic Jagged1 is a fetal liver niche factor required for functional maturation and engraftment of fetal hematopoietic stem cells. Proc Natl Acad Sci U S A. (2023) 120:e2210058120. doi: 10.1073/pnas.2210058120
146. Hu L, Yin X, Zhang Y, Pang A, Xie X, Yang S, et al. Radiation-induced bystander effects impair transplanted human hematopoietic stem cells via oxidative DNA damage. Blood. (2021) 137:3339–50. doi: 10.1182/blood.2020007362
147. Shao L, Li H, Pazhanisamy SK, Meng A, Wang Y, Zhou D. Reactive oxygen species and hematopoietic stem cell senescence. Int J Hematol. (2011) 94:24–32. doi: 10.1007/s12185-011-0872-1
148. Ito K, Hirao A, Arai F, Takubo K, Matsuoka S, Miyamoto K, et al. Reactive oxygen species act through p38 MAPK to limit the lifespan of hematopoietic stem cells. Nat Med. (2006) 12:446–51. doi: 10.1038/nm1388
149. Engel M, Sidwell T, Vasanthakumar A, Grigoriadis G, Banerjee A. Thymic regulatory T cell development: role of signalling pathways and transcription factors. Clin Dev Immunol. (2013) 2013:617595. doi: 10.1155/2013/617595
150. Wang Y, Schulte BA, LaRue AC, Ogawa M, Zhou D. Total body irradiation selectively induces murine hematopoietic stem cell senescence. Blood. (2006) 107:358–66. doi: 10.1182/blood-2005-04-1418
151. Vanderbeck A, Maillard I. Notch signaling at the crossroads of innate and adaptive immunity. J Leukoc Biol. (2021) 109:535–48. doi: 10.1002/JLB.1RI0520-138R
152. Nolan DJ, Ginsberg M, Israely E, Palikuqi B, Poulos MG, James D, et al. Molecular signatures of tissue-specific microvascular endothelial cell heterogeneity in organ maintenance and regeneration. Dev Cell. (2013) 26:204–19. doi: 10.1016/j.devcel.2013.06.017
153. Awong G, Singh J, Mohtashami M, Malm M, La Motte-Mohs RN, Benveniste PM, et al. Human proT-cells generated in vitro facilitate hematopoietic stem cell-derived T-lymphopoiesis in vivo and restore thymic architecture. Blood. (2013) 122:4210–9. doi: 10.1182/blood-2012-12-472803
154. Bigas A, Espinosa L. Hematopoietic stem cells: to be or Notch to be. Blood. (2012) 119:3226–35. doi: 10.1182/blood-2011-10-355826
155. Bjornson CR, Cheung TH, Liu L, Tripathi PV, Steeper KM, Rando TA. Notch signaling is necessary to maintain quiescence in adult muscle stem cells. Stem Cells. (2012) 30:232–42. doi: 10.1002/stem.773
156. Dzierzak E, Bigas A. Blood development: hematopoietic stem cell dependence and independence. Cell Stem Cell. (2018) 22:639–51. doi: 10.1016/j.stem.2018.04.015
157. Konantz M, Alghisi E, Müller JS, Lenard A, Esain V, Carroll KJ, et al. Evi1 regulates Notch activation to induce zebrafish hematopoietic stem cell emergence. EMBO J. (2016) 35:2315–31. doi: 10.15252/embj.201593454
158. Tian DM, Liang L, Zhao XC, Zheng MH, Cao XL, Qin HY, et al. Endothelium-targeted Delta-like 1 promotes hematopoietic stem cell expansion ex vivo and engraftment in hematopoietic tissues in vivo. Stem Cell Res. (2013) 11:693–706. doi: 10.1016/j.scr.2013.04.008
159. Ebens CL, Maillard I. Notch signaling in hematopoietic cell transplantation and T cell alloimmunity. Blood Rev. (2013) 27:269–77. doi: 10.1016/j.blre.2013.08.001
160. Mauch P, Constine L, Greenberger J, Knospe W, Sullivan J, Liesveld JL, et al. Hematopoietic stem cell compartment: acute and late effects of radiation therapy and chemotherapy. Int J Radiat Oncol Biol Phys. (1995) 31:1319–39. doi: 10.1016/0360-3016(94)00430-S
161. Greenberger JS, Epperly M. Bone marrow-derived stem cells and radiation response. Semin Radiat Oncol. (2009) 19:133–9. doi: 10.1016/j.semradonc.2008.11.006
162. Dallas MH, Varnum-Finney B, Delaney C, Kato K, Bernstein ID. Density of the Notch ligand Delta1 determines generation of B and T cell precursors from hematopoietic stem cells. J Exp Med. (2005) 201:1361–6. doi: 10.1084/jem.20042450
163. Carotta S, Brady J, Wu L, Nutt SL. Transient Notch signaling induces NK cell potential in Pax5-deficient pro-B cells. Eur J Immunol. (2006) 36:3294–304. doi: 10.1002/eji.200636325
164. Varnum-Finney B, Purton LE, Yu M, Brashem-Stein C, Flowers D, Staats S, et al. The Notch ligand, Jagged-1, influences the development of primitive hematopoietic precursor cells. Blood. (1998) 91:4084–91. doi: 10.1182/blood.V91.11.4084.411k05_4084_4091
165. Varnum-Finney B, Brashem-Stein C, Bernstein ID. Combined effects of Notch signaling and cytokines induce a multiple log increase in precursors with lymphoid and myeloid reconstituting ability. Blood. (2003) 101:1784–9. doi: 10.1182/blood-2002-06-1862
Keywords: radiation, B cells, Notch signaling, spleen, HSC
Citation: Shu X, Wang J, Zeng H and Shao L (2024) Progression of Notch signaling regulation of B cells under radiation exposure. Front. Immunol. 15:1339977. doi: 10.3389/fimmu.2024.1339977
Received: 17 November 2023; Accepted: 14 February 2024;
Published: 08 March 2024.
Edited by:
Maria Pia Felli, Sapienza University of Rome, ItalyReviewed by:
Qing Min, Fudan University, ChinaAmit Kumar Singh, National Institute on Aging, United States
Copyright © 2024 Shu, Wang, Zeng and Shao. This is an open-access article distributed under the terms of the Creative Commons Attribution License (CC BY). The use, distribution or reproduction in other forums is permitted, provided the original author(s) and the copyright owner(s) are credited and that the original publication in this journal is cited, in accordance with accepted academic practice. No use, distribution or reproduction is permitted which does not comply with these terms.
*Correspondence: Huihong Zeng, zenghuihong@ncu.edu.cn; Lijian Shao, lshao@ncu.edu.cn