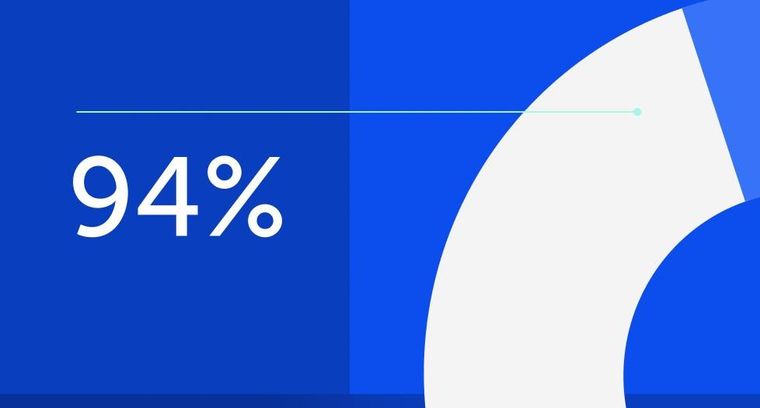
94% of researchers rate our articles as excellent or good
Learn more about the work of our research integrity team to safeguard the quality of each article we publish.
Find out more
REVIEW article
Front. Immunol., 20 March 2024
Sec. Autoimmune and Autoinflammatory Disorders : Autoimmune Disorders
Volume 15 - 2024 | https://doi.org/10.3389/fimmu.2024.1339714
This article is part of the Research TopicThymus Dysfunction As A Neglected Issue In Autoimmunity DisordersView all 5 articles
The intricate balance of immune reactions towards invading pathogens and immune tolerance towards self is pivotal in preventing autoimmune diseases, with the thymus playing a central role in establishing and maintaining this equilibrium. The induction of central immune tolerance in the thymus involves the elimination of self-reactive T cells, a mechanism essential for averting autoimmunity. Disruption of the thymic T cell selection mechanisms can lead to the development of autoimmune diseases. In the dynamic microenvironment of the thymus, T cell migration and interactions with thymic stromal cells are critical for the selection processes that ensure self-tolerance. Thymic epithelial cells are particularly significant in this context, presenting self-antigens and inducing the negative selection of autoreactive T cells. Further, the synergistic roles of thymic fibroblasts, B cells, and dendritic cells in antigen presentation, selection and the development of regulatory T cells are pivotal in maintaining immune responses tightly regulated. This review article collates these insights, offering a comprehensive examination of the multifaceted role of thymic tissue homeostasis in the establishment of immune tolerance and its implications in the prevention of autoimmune diseases. Additionally, the developmental pathways of the thymus are explored, highlighting how genetic aberrations can disrupt thymic architecture and function, leading to autoimmune conditions. The impact of infections on immune tolerance is another critical area, with pathogens potentially triggering autoimmunity by altering thymic homeostasis. Overall, this review underscores the integral role of thymic tissue homeostasis in the prevention of autoimmune diseases, discussing insights into potential therapeutic strategies and examining putative avenues for future research on developing thymic-based therapies in treating and preventing autoimmune conditions.
The adaptive immune system relies on the capability to recognize foreign peptides and fight intruding pathogens, thereby providing protection against the infection by viruses, bacteria or fungi. The broad range of foreign peptide recognition depends on the high receptor diversity of the patrolling immune cells. This receptor diversity is key in order to prevent infections, but this mechanism is also increasing the risk of producing immune cell receptors that react towards the host's own peptides and thereby leading to autoimmune diseases. The selection mechanisms that developing T-lymphocytes undergo in the thymus are indispensable checkpoint mechanisms during T cell maturation, leading to the removal of autoreactive T cells by deletion or fate-diversion into regulatory T cells.
Lymphocyte pools are under a steady turnover and get constantly replenished by newly developing cells. The progenitors of T-lymphocytes originate from haematopoietic progenitor cells (HPCs) in the bone marrow (BM) and traffic to the thymus where they differentiate and undergo maturation and selection (i.e. positive and negative selection). This requires the directed migration of the precursor cells via the blood towards the thymus as well as intravascular adhesion and egress from venules into the thymic stroma. Proper thymus function essentially depends on the continuous thymic homing of lymphoid progenitor cells. Even though thymus-resident T cell progenitors have been reported to be capable of maintaining T cell development for several months when the bone marrow is deprived of T cell progenitors (1, 2), the absence of intrathymic competition may lead to T-lineage acute lymphoblastic leukemia (3). The seeding of the thymus during embryonic development (E11.5 in mice and at the 8th week of gestation in humans) is mediated by a vasculature-independent pathway and later in embryonic development and postnatally via a vasculature-dependent pathway (4).
Thymic tolerance induction is mediated by thymic antigen-presenting cells (APCs) including thymic epithelial cells (i.e., cortical thymic epithelial cells (cTECs) and medullary thymic epithelial cells (mTECs)), dendritic cells (DCs) and thymic B cells (5–9). These thymic APCs present endogenously transcribed and imported peripheral peptides by major histocompatibility complex (MHC) class I and II molecules on their surfaces to thymocytes, selecting non-self-reactive thymocytes. Autoimmune diseases develop under circumstances where tolerance towards self-antigens in impaired, either due to a loss of central tolerance selection or impaired peripheral suppressive immune response regulation (10–12). Peripheral tolerance is mediated by CD4+CD25+FOXP3+ Treg (regulatory T cells) which can either develop intrathymically, these Tregs are referred to as natural Tregs (nTregs) or in the periphery after antigen contact which are referred to as induced Tregs (iTregs) (13–15). The pathologies of several autoimmune conditions, such as type 1 diabetes, rheumatoid arthritis, multiple sclerosis, systemic lupus erythematosus and myasthenia gravis (MG) are based on dysfunctional Tregs (16–18). The vast variety of cell types involved in the regulation of thymocyte development and selection, central tolerance induction and Treg development, ranging from B cells, natural killer (NK) cells, Tuft cell, macrophages over endothelial cells and fibroblasts to epithelial cells and dendritic cells keeps increasing as we unveil the mechanisms of central tolerance. In this review we will focus on the importance of thymic tissue homeostasis and the involved cell-cell interactions during thymic immune tolerance induction providing an integrated overview over recent findings and how thymocytes, epithelial cells, dendritic cells, B cells, endothelial cells and fibroblasts interact with each other to maintain self-tolerance.
The progenitors of T-lymphocytes originate from haematopoietic progenitor cells (HPCs) in the bone marrow and traffic to the thymus where they differentiate and undergo maturation and selection (i.e. positive and negative selection). T cell progenitors are reported to preferentially immigrate at the cortex-medulla-junction (CMJ) of the thymus at venules and then migrate through the thymic cortex and medulla regions and encounter the self-repertoire presented by epithelial and dendritic cells (19–21).
Intercellular communication among APCs, endothelial cells (ECs) and developing T cells is key to proper thymic tissue homeostasis and central immune tolerance induction. The lympho-stromal crosstalk between stromal cells and thymocytes is known to be essential for T cell development and thymic epithelial cell maintenance (22, 23) (Figure 1). The bilateral regulation of migrating thymocytes and stromal cells is an intertwined dependency of high clinical relevance in which the immune-metabolic interactions in the thymus are changing in an age-dependent manner (24). A better understanding of the involved interactions and signaling pathways is a prerequisite to be able to develop potential future treatments. Of particular interest are the involved recruiting and guiding signals for thymocyte migration. Homing of T cell progenitors to the thymus has been demonstrated to rely on a multistep adhesion cascade involving selectin ligands, chemokine receptors and integrins, which is similar to, but molecularly distinct from the adhesion cascades that guide leukocytes to lymph nodes or the bone marrow (25). Among the chemokines, CCL21 and CCL25 and their respective receptors, CCR7 and CCR9, were shown to be involved in fetal thymus colonization (26–30). During adult thymus seeding several lymphocyte-endothelial cell interactions such as P-selectin glycoprotein ligand (PSGL-1)/P-selectin, α4β1/VCAM-1, LFA1/ICAM-1 as well as lymphocyte-epithelial cell CCR9/CCL25 interactions were shown to be involved (25, 31). For instance, the CCR9 ligand CCL25 (TECK), which plays a key role in the multi-step adhesion cascades that guides leukocytes to the thymus, is expressed by medullary and cortical epithelial cells (32, 33). Thymic immigration and egress of T cell progenitors and mature T cells was proposed to occur mainly in post-capillary venules at the cortex-medulla-junction based on electron microscopy studies and an ex vivo study using CFSE-labeling of cells (19). These ex vivo studies could demonstrate the localization of T cells in close proximity to venules, however, it was not possible to determine whether the cells were entering or exiting the thymus. In vivo intravascular staining of CD4 SP T cells showed a preference for a transmigration at the CMJ, labeling presumably egressing cells (34).
Figure 1 T cell maturation and selection in the thymus. T cell progenitor cells originate from the bone marrow from HSCs and migrate into the thymus through blood vessels at the cortex-medulla-junction (immigration). Depicted is one lobule of the thymus. The maturation from DN1-DN2-DN3-DN4 into DP thymocytes takes place preferentially in the cortex region of the thymus, where cTECs mediate positive selection of DP thymocytes. SP thymocytes migrate into the medulla where they encounter self-peptides presented by mTECs and DCs and undergo negative selection. High reactivity towards self-antigens leads to apoptosis, intermediate reactivity towards self-antigens leads to the development into Tregs and low reactivity towards self-antigens leads to the maturation of CD4+ and CD8+ mature naïve T cells which egress from the thymus through blood vessels. T cell migration towards the blood vessels and egress is facilitated by S1P gradients. HSC, haematopoietic stem cell; TCP, T cell progenitor; DN, double negative; DP, double positive; SP, single positive; cTEC, cortical thymic epithelial cell; mTEC, medullary thymic epithelial cell; TRA, tissue restricted antigen; MHC, major histocompatibility complex; TCR, T cell receptor; Tregs, regulatory T cells; S1P, spingosine-1-phosphate.
Thymocyte migration in the thymus is dependent on sphingosine-1-phosphate (S1P) signaling which is essential for the egress of mature thymocytes (34–36). The substrate for S1P synthesis is sphingosine, which is phosphorylated by sphingosine kinase 1 (Sphk1) or sphingosine kinase 2 (Sphk2) to generate S1P. Degradation on the opposite is mediated by S1P phosphatase (SPP), S1P lyase 1 (Sgpl1), S1P lyase 2 (Sgpl2) or phospholipid phosphatase 1, 2, or 3 (Plpp1, Plpp2, Plpp3). After negative selection in the thymus medulla, mature T cells upregulate the sphingosine-1-phosphate receptor 1 (S1PR1) making them responsive to S1P gradients within the tissue guiding them to egress from the thymic tissue. Premature S1PR1 expression in thymocytes is associated with the development of autoimmunity, probably due to incomplete negative selection and the egress of self-reactive T cells (34). Red blood cells are a major source of S1P in the blood (37). Another source of S1P within the thymic tissue are pericytes (34). Further, endothelial cells are implicated in the regulation of S1P gradients, as lymphatic endothelial expression of the S1P transporter Spns2 was shown to be crucial for peripheral T cell migration and affects thymic T cell egress (38–41). Local S1P secretion by endothelial cells is thought to be a crucial guiding cue for T cell egress from the thymus (42).
Beyond playing a role in thymocyte immigration and egress, EC-derived factors were shown to be involved in the developmental progression during thymocyte maturation. The EC-derived factor Kit ligand (KitL, also called stem cell factor (SCF)) is essential for early thymocyte proliferation (43). Membrane-bound KitL (mKitL) is expressed by cortical thymic epithelial cells and endothelial cells in the cortex (44). Interestingly, thymocyte development at the DN1 stage was shown to depend on EC-derived mKitL, whereas the consecutive stage of DN2 was shown to depend on cTEC-derived mKitL. Additionally, EC-derived factors were demonstrated to play a crucial role in thymic tissue regeneration in adult mice. The ability of the thymus to undergo tissue regeneration after damage caused by infection, chemotherapy or radiation is critical in order to prevent T cell deficiency associated health conditions. Following radiation-induced damage, thymic endothelial cells secrete bone morphogenetic protein 4 (BMP4) which leads to the upregulation of Foxn1 expression in thymic epithelial cells and increased expression of Foxn1 target genes such as delta-like ligand 4 (DLL4) (45). Foxn1 target genes are involved in the regulation of TEC development, maintenance and regeneration. Moreover, multi-organ dataset analysis shown that neighboring cells can induce tissue-specific behavior in endothelial cells, the other way around endothelial cells can affect the gene expression programs of adjacent cells. Examples for such cross-influence are described for cardiac endothelial cells that were reported to express cardiac muscle-specific genes. Another example are sinusoidal endothelial cells that were shown to express genes usually expressed by hepatocytes (46, 47).
The thymus consists of two lobes which are structured into two different compartments, the outer cortex region and the inner medulla region (Figure 2). Thymic epithelial cells are morphologically distinct to epithelial cells of other tissues in the body, where cuboidal, squamous or columnar morphologies that are organized in polarized mono- or multilayers are the predominant features (48). In contrast, thymic epithelial cells build a 3D meshwork of epithelial cells permeated by endothelial cells and populated by T cells, dendritic cells and thymic B cells (49–52). Cortical thymic epithelial cells show a characteristic structure of flattened ovoid cells forming long looping structures, engulfing developing thymocytes. Whereas cTECs are described to show a radially oriented alignment towards the capsule, mTECs are positioned in the medulla seemingly without a clear orientation or polarization. Moreover, the mTEC morphologies are highly diverse and no definitive pattern of the mTEC structure could be detected in an elegant study using Confetti (Brainbow2.1) mice labeling of individual cells and analysis by confocal microscopy (53).
Figure 2 Contribution of DCs, TECs, B cells and fibroblasts to central tolerance induction. Hematoxylin and eosin staining of a mouse thymus. Depicted are the two lobes of the thymus and the two compartments of outer cortex (red) and inner medulla regions (green). The respective expression of chemokines, surface and intracellular markers that are characteristic for cDC1, cDC2, mTECs, cTECs, thymic B cells, capFbs and mFbs are shown. The contribution to antigen presentation and the extracellular matrix in the thymus is indicated. The location of cDC1, cDC2, mTECs, cTECs, thymic B cells, capFbs and mFbs in the cortex (red circle) and/or medulla (green circle) are shown. cDC, conventional dendritic cell; cTEC, cortical thymic epithelial cell; mTEC, medullary thymic epithelial cell; capFbs, capsular fibroblasts; mFbs, medullary fibroblasts.
The endoderm-derived epithelial cells in the thymus are differentiated into functionally distinct cortical thymic epithelial cells (cTECs) in the cortex and medullary thymic epithelial cells (mTECs) in the medulla, each having specific function during the sequential T cell developmental steps. The different stages of T cell development are well studied (54, 55) and are reviewed elsewhere in detail (56–60). In brief, double-negative (CD4-CD8-) thymocytes migrate through the cortex and undergo T cell receptor gene recombination. The newly generated TCRs are subsequently subjected to a selection process, called positive selection, in which the newly generated pool of thymocytes is tested for TCRs that are capable to bind and recognize MHC on antigen-presenting cells such as cTECs, providing a survival signal to the developing thymocyte (8, 61, 62). Positively selected thymocytes further develop into single-positive CD4+- and CD8+-T cells which are undergoing a second selection step, called negative selection, in which T cells carrying TCRs with high reactivity towards self-antigens are depleted from the T cell population. Intermediate binding intensities at this selection step will lead to the development into Foxp3-expressing CD4+ regulatory T cells (Tregs) (Figure 1) (63). The presentation of self-peptides and mediation of negative selection is accomplished mainly by mTECs as well as by DCs and thymic B cells in the medulla region of the thymus (5–9). The endogenous self-antigen expression by mTECs is referred to as promiscuous gene expression (pGE) or ectopic gene expression.
One of the main characteristics of promiscuous gene expression is the so-called mosaic expression pattern of tissue restricted antigens (TRAs). Each TRA is expressed in about 1-5% of the mTECs at a certain point in time. This characteristic is conserved between mouse, rat and human (64–69). Despite the high degree of variance at the individual cell level, the mTEC population as a whole manages to reflect the entire self-repertoire (70–74). Due to the high heterogeneity of thymic self-peptide expression the cellular and molecular regulations involved remain challenging to identify (75–80). The promiscuously expressed genes in mTECs comprise a diverse range of biological functions and tissue specificities and vary greatly in their regulatory elements and promoter regions. Notably, in the context of thymic self-antigen expression by mTECs, several peripheral tissue-specific transcription factors were shown to be dispensable for the respective thymic gene expression (69, 81, 82). In the past, several studies focused on investigating the regulatory mechanisms of the self-antigen mosaic expression patterns evaluating to which degree this phenomenon arises based on stochastic or regulated processes. Originally, studies performed on bulk mTEC populations were unable to detect recurrent gene expression patterns (66, 69). Likely, due to the high heterogeneity in gene expression from cell-to-cell, the patterns of subpopulations were not detectable on the population level at the time. However, the development of single cell sequencing technology and methods for selective enrichment of TRA-expressing mTEC subsets enabled the detection of recurring gene expression patterns in mTECs in mouse and human, thus arguing for yet unidentified regulatory mechanisms rather than a solely random process (67, 68, 70, 71, 73). Early during embryonal development, the thymus is developing through clonal expansion (83). Different developmental models of how the co-expression patterns of promiscuous gene expression evolve postnatally, such as a lineage diversification model and a linear developmental program model have been proposed (67, 68). Distinct conserved groups of self- antigens that are co-expressed in mTECs might share regulatory features that establish sub-lineages of mTECs. Alternatively, the co-expressed groups of self-antigens might be the result of a developmental program over the lifespan of a mTEC. This would lead to different sets of self-antigens being expressed and presented over time and an agile representation of the self-repertoire in the thymus. Interestingly, based on ex-vivo culturing experiments the linear developmental model is more likely to be accurate for the mature mTEC compartment (67). In difference to the early and mature mTEC populations, late developmental stages are more likely to develop based on lineage diversification into sub-lineages. The late mTEC stages comprise of post-Aire cornified (Krt10+) mTECs (84, 85) as well as Tuft-mTECs (Dclk1+) (72, 77, 86, 87), microfold mTECs (Gp2+), enterocyte/hepatocyte mTECs (Hnf4+), keratinocyte-like mTECs (Grhl+), neuroendocrine mTECs (Foxa+), cilitated mTECs (Foxj1+) and other mimetic mTECs (88, 89). Previous reports on thymic cell populations that represent characteristics of peripheral differentiated cell types and expression of mimetic cell genes in the thymus were initially difficult to distinguish from features of promiscuous gene expression of peripheral antigens, however single-cell technologies and high throughput assays enabled a revised view on those findings, leading to the identification of several distinct mimetic cell populations in the thymus with characteristic gene expression profiles, lineage-specific transcription factors, phenotypical characteristics and probably specific functions in the thymus reviewed in other review articles in more detail (90, 91). The mature mTEC population is subject to high turnover with a half-life of 12-14 days (92, 93), thus leading to a constant change in self-antigen co-expression groups over the course of mTEC development and lifetime. The mTEC population is not synchronized in their development, thus at any given age of the thymus different developmental stages of mTECs can be found. Thereby the two variables of I.) different self-antigens being expressed at different developmental timepoints and II.) the spatial differences within the thymic tissue at which developmental step the mTECs currently are leads to a highly versatile and heterogeneous presentation of the self-repertoire to developing T cells. The migration speed and distance of thymocytes as well as the number of contacts with APCs are defining factors in the efficiency of self-tolerance induction. Several studies assessed the migration and selection characteristics of developing T cells in the thymus using ex vivo culturing of tissue sections, reaggregation of thymic tissue or thymic explants (94, 95). In vivo microscopy of murine thymi is difficult first due to the opaqueness of the tissue which limits the penetration and visibility in two-photon microscopy and second due to its positioning on top of the moving heart, which makes in vivo analysis difficult. The interaction or contact time during positive selection between thymocytes and stromal cells was analyzed in a three-dimensional thymic organ culture and identified to be of either “dynamic” short interactions of 13-36 minutes or “stable” longer interactions of 6-12 hours (96). The migration speed of thymocyte was analyzed using ex vivo two-photon microscopy analysis of thick thymus sections before and after antigen encounter (97). The interval speed before antigen contact was about 11 µm/min and 9 µm/min after antigen contact. SP thymocytes are estimated to reside in the medulla region of the thymus for about 4-5 days as measured using BrdU-labelling, intrathymic injection of a cellular tag or RAG2p-GFP reporter mice (98). Elimination of self-reactive T cells upon self-antigen encounter and strong affinity towards self is executed swiftly, resulting in a rapid calcium influx and migratory arrest within a few minutes followed by caspase 3 activation and thymocyte death after a few hours (97). Imaging of ex vivo agarose-embedded sections of thymic explants was used in another study of transgenic reporter mice labelling thymocytes and DC interactions during negative selection in the medulla region (99). Accelerated migration rates of 10-20 µm/min were detected for thymocytes migrating towards the medulla after positive selection and within the medulla during negative selection in this setting. Further, short dynamic contacts between DCs and thymocytes of 1-2 minutes with multiple contacts per hour were described in this setup. The migration range that thymocytes travel was shown to be limited to 30-50 µm in total which was surprising due to the previously expected scanning of T cells with multiple APC contacts before egressing the thymus (100). It is important to note, that different experimental setups with different temperatures will result in differences in the observed migration speed and distance of cells and hence has to be kept in mind when analyzing ex vivo imaging analysis or imaging setups where the thymus is exposed from the chest of mice.
The transcriptional and epigenetic regulation of self-antigen expression in mTECs might in difference to the respective gene regulation in peripheral tissues be dependent on different thymus-specific regulatory mechanisms (69, 81, 82). Two factors regulating mTEC development and affecting the expression of self-peptides in mTECs are the transcriptional regulator Autoimmune regulator (Aire) and the transcription factor Forebrain Embryonic Zinc Finger-like protein 2 (Fezf2) (76, 78). Aire is a transcriptional regulator known to be essential for mTEC maturation and T cell selection (85, 101–103). Aire-deficiency leads to impaired thymocyte selection due to missing tissue restricted antigen (TRA) expression by mTECs and results in the development of autoimmune symptoms in Aire-deficient mice and patients with mutations in the AIRE gene (76, 104, 105). The loss of AIRE is one of the prominent examples for monogenic autoimmune disorders in which the loss of central tolerance is causative for the development of the autoimmune disorder autoimmune polyendocrinopathy candidiasis ectodermal dystrophy (APECED) (or Autoimmune polyendocrine syndrome type 1 (APS1)) leading to multiorgan autoimmunity (106). The mode of action of the transcriptional regulator Aire is at the epigenetic level, by acting on transcriptional elongation and by acting on superenhancers (107, 108). Aire is interacting with a multitude of different factors identified so far, including proteins involved in nuclear transport (HDAC1, HDAC2) (109), chromatin-modifiers (H3K4me0) (110, 111), transcriptional regulators (RNA PolII, DNA-PK, P-TEFb, HNRNPL, BRD4) (102, 112–116), and proteins involved in mRNA processing (JMJD6) (117) which is reviewed in other articles in more detail (91, 101, 118). The mode of action of the transcription factor Fezf2 on the other hand, is through consensus binding site recognition. The consensus sequence for the Fezf2 TFBM in mice was recently identified based on Fezf2 ChIP-seq experiments and a Stamp-based comparison to the Fezf2 TFBM in zebrafish (119). Fezf2-deficiency in mice was shown to lead to disturbed TRA expression and negative selection leading to the development of autoimmune symptoms (78). Recently, Fezf2 was identified to regulate late mTEC development and thymic Tuft cell development (119). Aire and Fezf2 are regulating distinct sets of self-antigens in mTECs and might likely reflect regulatory mechanisms at different timepoints during mature mTEC development or of sublineages of mature mTECs. Noteworthy, these two regulatory factors act relatively broadly on mTEC development and maturation and regulate the gene expression of several thousands of genes. Possibly, Aire and Fezf2 might be involved in the regulation of a downstream regulatory network of transcription factors that are regulating self-antigens leading to the particular expression patterns of TRAs in a mosaic expression pattern (119). Further factors involved in the regulation of those recurring gene expression patterns in mTECs remain to be identified. The question arises as to which degree Aire and Fezf2 are specific transcriptional regulators of self-antigen gene expression or whether their impact on mTEC development and maturation indirectly affects self-antigen expression. To this end, a recent study analyzed promiscuous gene expression and Aire-dependent gene expression over the course of aging using single-cell RNA-sequencing analysis and lineage-tracing showing, that AIRE-controlled genes were downregulated during mTEC development despite the continued expression of Aire and Fezf2, hence different mechanisms of transcription reliant on other regulators might be involved (120). The regulation of Aire itself and the mechanisms through which it regulates gene expression in mTECs has been intensively studied. One of the interaction partners and regulators of Aire is Hipk2, which is involved in regulating the activity of Aire. Hipk2-dependent phosphorylation was shown to inhibit the co-transcriptional activator function of Aire (121). Further, the protein deacetylase Sirtuin-1 (Sirt1) was shown to be involved in Aire-dependent gene regulation and induction of immunological self-tolerance by deacetylation of Aire (122). Additionally, the acetyltransferase KAT7 was identified to be important for Aire-dependent gene expression and tolerance induction by increasing chromatin accessibility at Aire-induced gene loci (123). Other posttranslational modifiers of Aire such as CBP, FBXO3, PIAS1 (124–126) and factors which are involved in the regulation of the Aire gene such as IRF4, IRF8, TBX21, TCF7 and CTCF were identified (127).
Besides the regulatory role of Aire in the promotion of mTEC maturation and promiscuous gene expression, Aire was also shown to be involved in chemokine production, the positioning of DCs within the thymus and extrathymic Aire functions early during embryogenesis are described (128). Aire deficiency leads to the reduced expression of the CCR4 and CCR7 ligands which are expressed by mTECs and are involved in thymocyte migration within the tissue, being CCL5, CCL17, and CCL22 for CCR4 and CCL19 and CCL21 for CCR7 (129). The mTEC-DC interaction of XCR1 on DCs and XCL1 on mTECs is important for the positioning of thymic DCs in the medulla and Aire-deficiency leads to a lack of XCL1 expression in mTECs, a lack of DCs in the medulla region, defective generation of Tregs and the development of autoimmune dacryoadenitis (130). Aire has also been described to be expressed in other tissues such as lymph node and spleen, in cells, termed extrathymic Aire-expressing cells (eTACs), which are of hematopoietic origin, exhibit DC-like features and express Aire-regulated genes that are distinct to thymic TRAs (131–133).
Besides Aire and Fezf2 other transcription factors are important for the regulation of mTEC gene expression and mTEC development. Insulinoma-associated 1 (Insm1), a zinc finger protein was shown to be expressed in Aire-expressing mTECs and neuroendocrine mimetic cells (FoxA+ mimetic cells) and is involved in the regulation of Aire expression, TRA expression and tolerance induction (134). The zinc finger transcription factor Ikaros (Ikzf1) regulates Aire+mTEC development, thymic mimetic cell development, TRA expression and immune tolerance induction (135). The absence of Ikzf1 led to an expansion of Dclk1+ tuft cells and a reduction in Gp2+ microfold cells, hence altering the composition of the mimetic cell populations in the thymus. Additionally, the transcription factors Ehf, Klf4 and Elf3 were shown to be involved in the regulation of gene expression in mTECs (119). The complexity of the medullary thymic epithelial cell population and the heterogeneity in gene expression in mTECs have historically made it challenging to identify the regulatory mechanisms. Notably, advancements such as single-cell sequencing have enabled us to gain an increasingly better understanding of these regulatory mechanisms.
Beyond the transcriptional regulation of promiscuous (or ectopic) gene expression other factors are influencing the efficiency of central tolerance induction. Among these, the MHCII-TCR interaction between thymic APCs and thymocytes, referred to as thymic crosstalk, influences mature mTEC development, TRA expression and immune tolerance induction, leading to autoimmune phenotypes if abrogated (22, 23, 136). Moreover, the butyrophilin surface receptor Btn2a2 expressed on thymic epithelial cells is important for central T cell tolerance induction (137, 138). Btn2a2-deficient mice showed enhanced effector CD4+ and CD8+ T cell responses, impaired CD4+ regulatory T cell induction, potentiated antitumor responses, and exacerbated experimental autoimmune encephalomyelitis (137). While another study reported increased TCR signaling and CD5 levels for developing thymocytes in Btn2a2-deficient mice with otherwise unchanged TEC compartments and thymic T cell populations (138).
Notably, defects in the expression of specific self-antigens by mTECs can predispose to the onset of organ-specific autoimmune diseases. One example is the development of autoimmune diabetes in cases where the thymic insulin (Ins2) expression is abrogated (139–141). The development of type 1 diabetes is associated with a causal polymorphism in the insulin gene that leads to reduced thymic expression and the occurrence of autoreactivity (141). Other examples are the development of uveitis when the expression of interphotoreceptor retinoid-binding protein (IRBP) in thymic epithelial cells was faulty (142) and the development of autoimmune myocarditis in mice and human upon loss of α-isoform of myosin heavy chain (α-MyHC) gene expression by mTECs (143). Moreover, variants in the gene encoding the α-subunit of the muscle acetylcholine receptor (Chrna1), which is the main target of pathogenic auto-antibodies in autoimmune myasthenia gravis, caused reduced thymic expression and is associated with the development of the disease (144).
Mesenchyme-derived thymic fibroblasts can be differentiated into medullary (mFbs) and capsular (capFbs) fibroblasts based on their location in the thymus and the expression of Dpp4, which is expressed by capFbs but not by mFbs (145, 146). Due to the expression of the matrix metalloprotease (Mmp9), different regulators involved in cell migration (Ccl19, Enpp2, Cd300lg) and cytokine receptors (Csf2rb, Csf2rb2) by mFbs a role in the regulation of cell migration in the medulla was postulated. Interestingly, the development of mFbs is dependent on SP thymocyte population of the thymus, but independent of mTEC development. Conditional knock-out of LtßR in fibroblasts led to autoimmune phenotypes in lung, pancreas, salivary gland and liver, identifying thymic fibroblasts as one of the receptor-bearing target cells for thymic lymphotoxin-dependent signaling and their contribution to central tolerance induction (145). Fibroblasts play an important role in the production of the extracellular matrix (ECM) in diverse tissues and are implicated in tissue repair and healing processes (147). Tissue damage induced secretion of IL-25 by thymic tuft cells and IL-33 by fibroblasts leads to the activation of ILC2 (innate lymphoid cell type 2) production of amphiregulin and Il-13 and tissue regeneration in a dexamethasone-induced acute thymic involution mouse model (148).
Thymic B cells are one of the antigen-presenting cells in the thymus that are involved in the mediation of negative selection (5). Two types of thymic B cells are described, resident B cells, which develop intrathymically from early fetal liver derived progenitor cells (149–152), and circulatory B cells which immigrate from the periphery and acquire a specific thymus-resident B cell phenotype, including the expression of Aire (5, 153). Different localizations of thymic B cells have been described with B cell progenitors being preferentially localized in the thymic cortex, whereas mature B cells are primarily found in the corticomedullar and medullary regions (151) and B cells that are found in perivascular spaces (154, 155). The age-associated increase in the frequency of thymic B cells was attributed to an increase in peripheral-derived B cells in the thymus with a preferred localization in perivascular spaces. Further, B cells which are localized to the perivascular spaces express CD21, CD72, CD37 and the chemokine receptor CXCR3 (154, 155). Despite the increase in thymic B cell numbers upon aging, thymic B cells were found to exhibit lower expression of MHCII, CD80, CD83, CD86, Aire and Aire-dependent genes in adult and aged mice compared to young mice (154, 156). These age-dependent changes during the course of aging offer the possibility, that also the reduction of thymic B cells as one type of thymic APCs, similarly to the age-dependent alterations in mTEC physiology, can contribute to the phenomena of thymic involution and impaired thymopoiesis in aged thymi. Thymic hyperplasia is often observed in patients with myasthenia gravis. The thymic architecture in patients with myasthenia gravis and thymic hyperplasia is disturbed and B cell follicles containing active germinal centers (GCs) are formed, which give rise to plasma cells and are a source of autoantibodies. Increased chemotactic signal through CXCL13 and a higher release of the B cell activating factor (BAFF) by thymic epithelial cells are thought to promote B cell recruitment to the thymus in myasthenia gravis (153, 157, 158). In difference to myasthenia gravis associated hyperplasia of the thymus, patients with systemic lupus erythematosus show thymus atrophy with disorganized medulla regions, diminished cortex regions and enlarged perivascular spaces with lymphocytic follicles and plasma cells (153, 159).
The significance of dendritic cells in initiating immunological tolerance was demonstrated through mutant mouse models. In these models, the lack of DCs led to compromised negative selection of thymocytes (160, 161). Thymic dendritic cells consist of subsets that are distinct both phenotypically and functionally, being conventional DCs (cDC1 and cDC2), plasmacytoid DCs (pDCs), and monocyte-derived DCs (moDCs) or activated DCs (sDCs) that are either derived from intrathymic progenitors or are migrating towards the thymus from peripheral tissues (162). Murine conventional DCs are distinguished into cDC1 and cDC2 populations based on surface marker expression and their distinct developmental regulators. Thus, cDC1s are identified by their surface expression of XCR1, CD8α, CLEC9A, and DEC205, and they are developmentally dependent on IRF8, Id2, and BATF3 (163). CD8α +CD11b– cDC1s comprises the majority of cDCs in the murine thymus. Most cells in this DC subset are thought to originate intrathymically from a common lymphoid progenitor (CLP) (164–166). Similar to CD8α+ cDCs in the periphery, thymic CD8α+CD11b– cDC1s are very efficient at cross-presenting mTEC-derived antigens on MHC class I, which was shown using in vitro experiments (167–169). In vivo, thymic cross-presentation of self-antigens by DCs contributes to the induction of CD8+ T cell tolerance, however, the identity of the cross-presenting DC subset or the contribution of different subsets is currently not sufficiently addressed and necessitates further analysis (169, 170). Instead, cDC2s are defined by their surface expression of CD11b and CD172a (SIRPα), are developmentally dependent on IRF4, IRF2 and RelB, and preferentially activate CD4+ helper T cell responses (171, 172). Thymic cDC2s and pDCs are migrating towards the thymus from the periphery, importing peripheral antigens for central tolerance induction (173, 174). Moreover, thymic epithelial cells and thymic dendritic cells contribute non-redundant antigens to facilitate the process of negative selection (175) by using different antigen processing machineries, leading to the generation of distinct self-peptides. The self-antigen diversity presented by mTECs is further enhanced due to mechanisms of mis-initiation, alternative splicing and the expression of endogenous retroelements which increase the self-peptidome presented to developing T-lymphocytes (176). Thereby DCs and mTECs represent the self-repertoire presented in the context of thymic negative selection in an integrated manner.
Additionally, a specific group of dendritic cells in both murine and human thymi, termed transendothelial dendritic cells (TE-DCs), has been identified for their unique location within the vascular walls of thymic microvessels (9). From these positions, TE-DCs extend their cellular processes into the bloodstream to gather circulating antigenic materials. The unique transendothelial placement of these antigen-sampling TE-DCs has been found to rely on the interaction between the chemokine receptor CX3CR1, expressed on the DCs, and its ligand CX3CL1, which is abundantly present in thymic capillaries. Furthermore, TE-DCs are capable of capturing and cross-presenting blood-derived proteins, leading to the specific deletion of developing antigen-specific T cells. Together, the different thymic APC populations, achieve to present the self-repertoire in an astonishingly comprehensive manner, ensuring the successful selection of newly generated T cells to continuously generate non-self-reactive naïve mature T cells to replenish the peripheral T cell pool.
During the, 1960s, the functional role of the thymus was revealed. Until then the thymus itself and the presence of lymphocytes in the thymus was described, however, the thymus was thought of as a terminal storage or graveyard for lymphocytes and its actual role was unknown at that point (177). The immunological role and the importance of the thymus for survival and its tolerogenic role were described by J. F. A. P. Miller (178, 179). The removal of the thymus (i.e. thymectomy) in mice at the age of 3 weeks was shown to lead to the development of autoimmunity (180). Positive selection was first described by M. J. Bevan (181) and the process of intrathymic clonal elimination for tolerance induction was described 10 years later (182, 183). The importance of the thymic epithelium in tolerance induction was identified using mouse chimeras (184) and finally, the identification of promiscuous gene expression of self-peptides in mTECs was described in, 2001 by J. Derbinski, A. Schulte, B. Kyewski and L. Klein (185) after several individual reports on genes that were found to be expressed by the thymic epithelium (141, 186–189). Since then, research on the thymus and the immunological processes taking place in the thymus has increased immensely. Several factors were identified to be essential for thymus development and the prevention of autoimmune reactions, most importantly the transcription factor Foxn1 (forkhead box protein 1) which is essential for thymic epithelial cell development and consecutive T cell progenitor recruitment (190). Nude mice with a mutation in the Foxn1 gene have congenital athymia and show severe immunodeficiency besides their name giving deficiency in hair growth (191). Athymic nude mice are used as a model system to study thymus development and the development of autoimmune reactions in thymic transplantation experiments. The Foxn1 gene promoter is also widely used in conditional knock-out systems to delete and study gene functions in thymic epithelial cells by crossing Foxn1-cre lines to the “gene of interest”-flox mouse line. Other transcription factors and signaling pathways involved in thymus morphogenesis are reviewed elsewhere (192).
Several monogenic autoimmune diseases have been described to date, in which proper thymic development of the thymic stromal compartment or thymocytes is abrogated. The autoimmune polyendocrinopathy syndrome type 1 (APS1), also known as autoimmune polyendocrinopathy candidiasis ectodermal dystrophy (APECED), is a monogenetic autoimmune disease affecting multiple organs. It is characterized by a mutation in the autoimmune regulator (AIRE) gene, leading to a disruption in central immune tolerance (193). Similarly, autoimmune lymphoproliferative syndrome (ALS) is another monogenic autoimmune condition, distinguished by an accumulation of polyclonal double-negative (DN) T cells. This accumulation results from mutations in either Fas or Fas ligand, or in caspases that are part of the Fas signaling pathway (194). Additionally, the monogenic autoimmune disorder IPEX (immunodysregulation polyendocrinopathy enteropathy X-linked syndrome) arises due to a defect in the Foxp3 gene, leading to a loss of Tregs (CD4+CD25+) and consequently, a breakdown in peripheral tolerance (195). The identification and characterization of such monogenic autoimmune diseases in mouse and human have been crucial to identify the mechanisms involved in central tolerance induction.
In the course of aging the thymus tissue is undergoing gradual atrophy, a process called involution. Thymic involution occurs in mice and humans and starts approximately at young adulthood or during puberty at the age of about 8-10 weeks in mice and 15-20 years in humans (93, 196). In the context of thymic involution different models are discussed as to whether the process is initiated by a reduction of thymocyte-derived signals or reduced TEC-derived signaling (120, 197–200). Initially a reduced production of progenitor cells originating from the haematopoietic stem cells (HSCs) in the bone marrow and reduced numbers of early T cell progenitors (ETPs) entering the thymus were proposed as initial changes at the onset of involution (201). However, recruitment of progenitor cells into the thymus does not appear to be the sole initiating factor for age-dependent thymic involution, as the ability of aged thymi to recruit intravenously injected lymphoid progenitor cells was shown to be similar to that of young thymi (200). Furthermore, fetal thymic transplants under the kidney capsule of aged mice led to reestablished normal thymic lymphopoiesis and thymocyte subpopulations, including ETPs, DN subsets, DP, and CD4+ and CD8+ SP T cells. Thus, demonstrating that haematopoietic progenitor cells of aged mice were sufficiently capable to home and proliferate in the young thymic tissue. Conversely, intrathymic injection of ETPs isolated from young mice into old mice did not restore normal thymic lymphopoiesis (200). Instead of a reduction in progenitor cells, changes in the thymic microenvironment and tissue homeostasis came into focus as causative factors for the age-dependent thymic involution. Aged thymi show reduced numbers of cTECs and mTECs and an increase in adipocytes and perivascular spaces (196, 199, 200). Further, a shift in the composition of the TEC population from primarily perinatal cTECs (1-week-old mice) over mature mTECs (4-week-old mice) to mature cTECs and intertypical TECs (showing mTEC and cTEC expression features) (from 16 weeks onwards) can be observed in mice of different ages (120). Furthermore, changes in the gene expression profiles of aged mTECs can be observed, with genes involved in inflammatory signaling, apoptosis and KRAS signaling being upregulated, whereas genes involved in cholesterol homeostasis and oxidative phosphorylation get downregulated (120). The expression of the transcription factor Foxn1, which is essential for TEC development, is reduced in aged thymi, alongside with a downregulation of Foxn1-target genes (202, 203).
Crucially, in aged mice with fully involuted thymi, the upregulation of FOXN1 in thymic epithelial cells (TECs) triggered thymic regeneration. This regeneration was marked by the activation of genes essential for TEC development or for T cell differentiation and repertoire selection. Key genes involved include Delta-like 4 (Dll4), chemokine (C-C motif) ligand 25 (Ccl25), kit ligand (Kitl), and chemokine (C-X-C motif) ligand 12 (Cxcl12). This activation led to enhanced thymopoiesis and an increased production of naïve T cells (202). Furthermore, in young mice, the overexpression of Foxn1 resulted in a delay in thymic involution (204). Conversely, reducing Foxn1 expression levels in young mice induced premature thymic involution (205, 206). Further, it was shown that Fgf7 (also known as keratinocyte growth factor (KGF)) signaling increases the rate of thymic epithelial cell proliferation both at steady state and after thymic insult, such as infections or chemotherapy in rhesus macaques and mice (207). Furthermore, fetal thymic mesenchyme produces FGF7 and FGF10 that promote TEC differentiation and proliferation (208, 209). Additionally, TEC-derived FGF21 and the age-dependent downregulation thereof was implicated in mediating thymic involution (210). Age-related reduction of thymic tissue results in a decrease in the number of peripheral T cells and a reduction in T cell diversity. Differentiated αβCD8+-T cells, CD4+CD28–T cells and memory T cells subsequently accumulate (211, 212), which is a common characteristic of patients with chronic inflammatory diseases such as SLE, rheumatoid arthritis, multiple sclerosis or Graves’ disease (213, 214).
Abnormal thymus atrophy can be caused by infections, stress or medical interventions and lead to reduced thymopoiesis and a reduction in the generation of naïve mature T cells (215). Pharmacological application of synthetic glucocorticoids can also lead to thymic atrophy by engaging with intracellular glucocorticoid receptors (GRs), which trigger the activation of the mitochondrial apoptotic pathway in double-positive (DP) thymocytes (216, 217). Thymus hyperplasia can be caused by two forms of thymic enlargement, either due to thymic hyperplasia of normally organized thymic tissue caused by chemotherapy, corticosteroid use, irradiation or thermal burns or due to thymic lymphocytic hyperplasia which is characterized by increased number of lymphoid follicles with germinal centers in the thymus. Thymic lymphocytic hyperplasia has been associated with the development of autoimmune diseases such as myasthenia gravis and Graves’ disease (218). Another form of thymus enlargement can occur due to thymoma, which are often benign epithelioid neoplasm, which are also associated with the development of autoimmune conditions such as myasthenia gravis and pure red cell aplasia (219–222). Thymomas are classified based on morphological features and the content of lymphocytes into type A, type B (B1-B3) and micronodular thymoma with lymphoid stroma (MNT) which are distinguished from thymic carcinoma (TC) (223). Epithelial cells of thymomas show reduced expression levels of MHCII and Aire (224), thereby leading to inefficient T cell selection and the development of autoreactive lymphocytes (225). In a recent study, 116 thymomas from patients of which 34 developed myasthenia gravis were analyzed for myasthenia gravis specific characteristics using bulk- and single cell RNA-sequencing (226). A development of atypical immune microenvironments in the thymus with germinal center formation, B cell maturation, and ectopic neuromuscular expression of GABRA5, MAP2, NEFL, NEFM, SOX15 by neuromuscular mTECs (KRT6+ GABRA5+ nmTECs) was described for MG-associated thymomas (226, 227). In this case the authors suggest that the presentation of neuronal autoantigens by mTECs in this scenario might lead to the development of autoimmunity with GC formations and affecting thymic B cell maturation in line with previous reports (227, 228), but in difference to the general dogma of self-antigen expression in mTECs for negative selection (i.e. deletion via apoptosis) of autoreactive T cells. Further analysis of the implications of thymic antigen expression in the formation of thymic GCs will be needed to analyze possible connections.
Among the environmental influences believed to potentially initiate the onset of autoimmune diseases, infections with pathogens in particular bacterial and viral infections are major suspects. The connection between viral infections and the initiation of autoimmune reactions through mechanisms such as molecular mimicry, bystander activation and epitope spreading have been proposed and recently reviewed elsewhere (229). Further, peripheral infections with pathogens such as gastrointestinal microbiota can cause thymus atrophy. Similarly, thymic atrophy is also known to occur after lipopolysaccharide injection which is used as a model system to study the mechanisms in mice (230). However, also thymic infections with viruses or bacteria are reported to cause thymic atrophy due to impaired thymocyte development and increased apoptosis of thymocytes (215). Even though the event of a direct infection of the thymic tissue is infrequent and a blood-thymus barrier similar to a blood-brain barrier has been proposed in the past and the selectivity and restrictiveness is controversially discussed (231–233), thymic infections with viruses and bacteria such as Mycobacteria have been described (234). Especially with regards to the recently identified thymic Tuft cells, which in mucosal tissues, are involved in pathogen clearance, a role of thymic tuft cells in the immune response towards thymic infections requires further investigations.
Thymic viral infections have been reported to disrupt T cell maturation and selection, suggesting them as potential causes for autoimmunity. Infection of the thymus with human and mouse roseoloviruses, belonging to the herpesvirus family, results in a temporary reduction of CD4+ SP and CD4+CD8+ DP thymocytes (235, 236). Furthermore, neonatal infections with roseoloviruses in mice have shown a connection to the development of autoimmune gastritis. Twelve weeks post-infection, these mice exhibited autoimmune characteristics, including stomach inflammation characterized by the production of autoantibodies and autoreactive CD4+ T cells (237). Notably, after such neonatal roseolovirus infections in mice, there is a transient decrease in numbers of medullary thymic epithelial cells (mTECs) and dendritic cells (DCs), along with diminished expression of Aire and tissue-restricted antigens (TRAs). The Coxsackievirus B (CVB), particularly the enterovirus strain CVB4 E2 is one of the viral environmental risk factors for the development of diabetes mellitus type 1 (DMT1) by influencing the inflammatory milieu, inducing bystander activation of CD8+ T cells and possibly through mechanisms of molecular mimicry (229). Thymic infections with CVB were investigated as a possible cause for the development of DMT1 using in vitro TEC systems and in vivo orally-inoculated mice (238–240).
Therapeutic interventions targeting thymic tissue homeostasis and restoring thymopoiesis is often discussed as one of the aims to circumvent inflammaging and age-associated appearances of diseases (241). Perturbations of thymopoiesis due to infections, stress or interventions such as chemotherapy or irradiation are of high clinical relevance and much effort has gone into research to develop strategies which allow for a renormalization of thymic tissue homeostasis and thymopoiesis. Then again, an interference with the physiological age-related involution has to be carefully considered weighing the risk for the development of leukemic transformed T cells or autoimmunity (197). In this context, the relation of haematopoietic progenitor cell availability and the proportion of thymic epithelial compartments providing the niches for thymopoiesis needs to be considered. Different pre-clinical and clinical trial studies assessed thymic epithelial cell regeneration as one possible angle for thymus rejuvenation. One of the factors known to influence TEC maintenance is KGF. Recombinant human KGF was shown to enhance the regenerative capacity of the thymic epithelial compartment and showed cytoprotective effects (242). In a clinical trial study (NCT00071240), the regenerative effect of growth hormones was analyzed. The human recombinant growth hormone somatropin was administered in HIV-infected patients and was shown to increase thymic cellularity, naïve CD4+ and CD8+ T cells and peripheral immune responses (243). In a different clinical trial, known as the TRIIM (Thymus Regeneration, Immunorestoration and Insulin Mitigation) Study, healthy male participants were administered a combination of recombinant human growth hormone (rhGH), dehydroepiandrosterone (DHEA), and metformin. This regimen led to enhanced thymic function, beneficial immunological alterations, and improved risk profiles for several age-related diseases (244). Another ongoing trial (NCT04375657, TRIIM X trial) is currently underway and will analyze the potential therapeutic benefit of recombinant growth hormones in men and women. Other clinical trials analyzed the therapeutic potential of recombinant human IL-7 administration or sex steroid ablation to increase thymopoiesis (215). Further, ex vivo approaches and in vitro methods to enhance thymus function and generate transplantable thymic organoids are explored for their putative therapeutic potential (245, 246). During T cell development many thymocytes die in the course of positive and negative selection. At steady-state the presence of apoptotic thymocytes was shown to suppress the production of the regenerative factors IL-23 and BMP4 through TAM receptor signaling and activation of Rho-GTPase Rac1 and NOD2-mediated suppression (247). Upon thymocyte depletion the suppression is lacking and the production of IL-23 and BMP4 is increased. Interestingly pharmacological inhibition of Rac1 led to an enhancement in thymic function after acute damage offering possible treatment strategies for patients with compromised thymic function (247).
FS: Visualization, Writing –review & editing. LH: Data curation, Visualization, Writing – review & editing. KR: Conceptualization, Supervision, Visualization, Writing – original draft, Writing – review & editing.
The author(s) declare financial support was received for the research, authorship, and/or publication of this article. This work was funded by the Deutsche Forschungsgemeinschaft (DFG, German Research Foundation) grant, 535139916 to Kristin Rattay and funding by the University of Marburg to Kristin Rattay. Open access funding was provided by the Open Access Publishing Fund of the Philipps-Universität Marburg with support of the Deutsche Forschungsgemeinschaft (DFG, German Research Foundation).
The figures in this review were created with BioRender.com.
The authors declare that the research was conducted in the absence of any commercial or financial relationships that could be construed as a potential conflict of interest.
All claims expressed in this article are solely those of the authors and do not necessarily represent those of their affiliated organizations, or those of the publisher, the editors and the reviewers. Any product that may be evaluated in this article, or claim that may be made by its manufacturer, is not guaranteed or endorsed by the publisher.
1. Peaudecerf L, Lemos S, Galgano A, Krenn G, Vasseur F, Di Santo JP, et al. Thymocytes may persist and differentiate without any input from bone marrow progenitors. J Exp Med. (2012) 209:1401–8. doi: 10.1084/jem.20120845
2. Martins VC, Ruggiero E, Schlenner SM, Madan V, Schmidt M, Fink PJ, et al. Thymus-autonomous T cell development in the absence of progenitor import. J Exp Med. (2012) 209:1409–17. doi: 10.1084/jem.20120846
3. Martins VC, Busch K, Juraeva D, Blum C, Ludwig C, Rasche V, et al. Cell competition is a tumour suppressor mechanism in the thymus. Nature. (2014) 509:465–70. doi: 10.1038/nature13317
4. Luis TC, Luc S, Mizukami T, Boukarabila H, Thongjuea S, Woll PS, et al. Initial seeding of the embryonic thymus by immune-restricted lympho-myeloid progenitors. Nat Immunol. (2016) 17:1424–35. doi: 10.1038/ni.3576
5. Yamano T, Nedjic J, Hinterberger M, Steinert M, Koser S, Pinto S, et al. Thymic B cells are licensed to present self antigens for central T cell tolerance induction. Immunity. (2015) 42:1048–61. doi: 10.1016/j.immuni.2015.05.013
6. Starr TK, Jameson SC, Hogquist KA. Positive and negative selection of T cells. Annu Rev Immunol. (2003) 21:139–76. doi: 10.1146/annurev.immunol.21.120601.141107
7. Takaba H, Takayanagi H. The mechanisms of T cell selection in the thymus. Trends Immunol. (2017) 38:805–16. doi: 10.1016/j.it.2017.07.010
8. Klein L, Kyewski B, Allen PM, Hogquist KA. Positive and negative selection of the T cell repertoire: What thymocytes see (and don't see). Nat Rev Immunol. (2014) 14:377–91. doi: 10.1038/nri3667
9. Vollmann EH, Rattay K, Barreiro O, Thiriot A, Fuhlbrigge RA, Vrbanac V, et al. Specialized transendothelial dendritic cells mediate thymic T-cell selection against blood-borne macromolecules. Nat Commun. (2021) 12:6230. doi: 10.1038/s41467-021-26446-x
10. Sogkas G, Atschekzei F, Adriawan IR, Dubrowinskaja N, Witte T, Schmidt RE. Cellular and molecular mechanisms breaking immune tolerance in inborn errors of immunity. Cell Mol Immunol. (2021) 18:1122–40. doi: 10.1038/s41423-020-00626-z
11. Mercadante ER, Lorenz UM. Breaking free of control: How conventional T cells overcome regulatory T cell suppression. Front Immunol. (2016) 7:193. doi: 10.3389/fimmu.2016.00193
12. Cho JH, Gregersen PK. Genomics and the multifactorial nature of human autoimmune disease. N Engl J Med. (2011) 365:1612–23. doi: 10.1056/NEJMra1100030
13. Josefowicz SZ, Lu LF, Rudensky AY. Regulatory T cells: Mechanisms of differentiation and function. Annu Rev Immunol. (2012) 30:531–64. doi: 10.1146/annurev.immunol.25.022106.141623
14. Kanamori M, Nakatsukasa H, Okada M, Lu Q, Yoshimura A. Induced regulatory T cells: Their development, stability, and applications. Trends Immunol. (2016) 37:803–11. doi: 10.1016/j.it.2016.08.012
15. Bacchetta R, Weinberg K. Thymic origins of autoimmunity-lessons from inborn errors of immunity. Semin Immunopathol. (2021) 43:65–83. doi: 10.1007/s00281-020-00835-8
16. Dominguez-Villar M, Hafler DA. Regulatory T cells in autoimmune disease. Nat Immunol. (2018) 19:665–73. doi: 10.1038/s41590-018-0120-4
17. Balandina A, Lecart S, Dartevelle P, Saoudi A, Berrih-Aknin S. Functional defect of regulatory cd4(+)Cd25+ T cells in the thymus of patients with autoimmune myasthenia gravis. Blood. (2005) 105:735–41. doi: 10.1182/blood-2003-11-3900
18. Itoh M, Takahashi T, Sakaguchi N, Kuniyasu Y, Shimizu J, Otsuka F, et al. Thymus and autoimmunity: Production of cd25+Cd4+ Naturally anergic and suppressive T cells as a key function of the thymus in maintaining immunologic self-tolerance. J Immunol. (1999) 162:5317–26. doi: 10.4049/jimmunol.162.9.5317
19. Lind EF, Prockop SE, Porritt HE, Petrie HT. Mapping precursor movement through the postnatal thymus reveals specific microenvironments supporting defined stages of early lymphoid development. J Exp Med. (2001) 194:127–34. doi: 10.1084/jem.194.2.127
20. Takahama Y. Journey through the thymus: Stromal guides for T-cell development and selection. Nat Rev Immunol. (2006) 6:127–35. doi: 10.1038/nri1781
21. Love PE, Bhandoola A. Signal integration and crosstalk during thymocyte migration and emigration. Nat Rev Immunol. (2011) 11:469–77. doi: 10.1038/nri2989
22. van Ewijk W, Shores EW, Singer A. Crosstalk in the mouse thymus. Immunol Today. (1994) 15:214–7. doi: 10.1016/0167-5699(94)90246-1
23. Lopes N, Serge A, Ferrier P, Irla M. Thymic crosstalk coordinates medulla organization and T-cell tolerance induction. Front Immunol. (2015) 6:365. doi: 10.3389/fimmu.2015.00365
24. Dixit VD. Impact of immune-metabolic interactions on age-related thymic demise and T cell senescence. Semin Immunol. (2012) 24:321–30. doi: 10.1016/j.smim.2012.04.002
25. Scimone ML, Aifantis I, Apostolou I, von Boehmer H, von Andrian UH. A multistep adhesion cascade for lymphoid progenitor cell homing to the thymus. Proc Natl Acad Sci U.S.A. (2006) 103:7006–11. doi: 10.1073/pnas.0602024103
26. Bleul CC, Boehm T. Chemokines define distinct microenvironments in the developing thymus. Eur J Immunol. (2000) 30:3371–9. doi: 10.1002/(ISSN)1521-4141
27. Liu C, Ueno T, Kuse S, Saito F, Nitta T, Piali L, et al. The role of ccl21 in recruitment of T-precursor cells to fetal thymi. Blood. (2005) 105:31–9. doi: 10.1182/blood-2004-04-1369
28. Wurbel MA, Malissen M, Guy-Grand D, Meffre E, Nussenzweig MC, Richelme M, et al. Mice lacking the ccr9 cc-chemokine receptor show a mild impairment of early T- and B-cell development and a reduction in T-cell receptor gammadelta(+) gut intraepithelial lymphocytes. Blood. (2001) 98:2626–32. doi: 10.1182/blood.V98.9.2626
29. Calderon L, Boehm T. Synergistic, context-dependent, and hierarchical functions of epithelial components in thymic microenvironments. Cell. (2012) 149:159–72. doi: 10.1016/j.cell.2012.01.049
30. Liu C, Saito F, Liu Z, Lei Y, Uehara S, Love P, et al. Coordination between ccr7- and ccr9-mediated chemokine signals in prevascular fetal thymus colonization. Blood. (2006) 108:2531–9. doi: 10.1182/blood-2006-05-024190
31. Rossi FMV, Corbel SY, Merzaban JS, Carlow DA, Gossens K, Duenas J, et al. Recruitment of adult thymic progenitors is regulated by P-selectin and its ligand psgl-1. Nat Immunol. (2005) 6:626–34. doi: 10.1038/ni1203
32. Wurbel MA, Philippe JM, Nguyen C, Victorero G, Freeman T, Wooding P, et al. The chemokine teck is expressed by thymic and intestinal epithelial cells and attracts double- and single-positive thymocytes expressing the teck receptor ccr9. Eur J Immunol. (2000) 30:262–71. doi: 10.1002/1521-4141(200001)30:1262::AID-IMMU2623.3.CO;2-S
33. Gossens K, Naus S, Corbel SY, Lin S, Rossi FM, Kast J, et al. Thymic progenitor homing and lymphocyte homeostasis are linked via S1p-controlled expression of thymic P-selectin/ccl25. J Exp Med. (2009) 206:761–78. doi: 10.1084/jem.20082502
34. Zachariah MA, Cyster JG. Neural crest-derived pericytes promote egress of mature thymocytes at the corticomedullary junction. Science. (2010) 328:1129–35. doi: 10.1126/science.1188222
35. Matloubian M, Lo CG, Cinamon G, Lesneski MJ, Xu Y, Brinkmann V, et al. Lymphocyte egress from thymus and peripheral lymphoid organs is dependent on S1p receptor 1. Nature. (2004) 427:355–60. doi: 10.1038/nature02284
36. Yagi H, Kamba R, Chiba K, Soga H, Yaguchi K, Nakamura M, et al. Immunosuppressant fty720 inhibits thymocyte emigration. Eur J Immunol. (2000) 30:1435–44. doi: 10.1002/(ISSN)1521-4141
37. Pappu R, Schwab SR, Cornelissen I, Pereira JP, Regard JB, Xu Y, et al. Promotion of lymphocyte egress into blood and lymph by distinct sources of sphingosine-1-phosphate. Science. (2007) 316:295–8. doi: 10.1126/science.1139221
38. Mendoza A, Breart B, Ramos-Perez WD, Pitt LA, Gobert M, Sunkara M, et al. The transporter spns2 is required for secretion of lymph but not plasma sphingosine-1-phosphate. Cell Rep. (2012) 2:1104–10. doi: 10.1016/j.celrep.2012.09.021
39. Hisano Y, Kobayashi N, Yamaguchi A, Nishi T. Mouse spns2 functions as a sphingosine-1-phosphate transporter in vascular endothelial cells. PLoS One. (2012) 7:e38941. doi: 10.1371/journal.pone.0038941
40. Nijnik A, Clare S, Hale C, Chen J, Raisen C, Mottram L, et al. The role of sphingosine-1-phosphate transporter spns2 in immune system function. J Immunol. (2012) 189:102–11. doi: 10.4049/jimmunol.1200282
41. Fukuhara S, Simmons S, Kawamura S, Inoue A, Orba Y, Tokudome T, et al. The sphingosine-1-phosphate transporter spns2 expressed on endothelial cells regulates lymphocyte trafficking in mice. J Clin Invest. (2012) 122:1416–26. doi: 10.1172/JCI60746
42. Dixit D, Okuniewska M, Schwab SR. Secrets and lyase: Control of sphingosine 1-phosphate distribution. Immunol Rev. (2019) 289:173–85. doi: 10.1111/imr.12760
43. Rodewald HR, Kretzschmar K, Swat W, Takeda S. Intrathymically expressed C-kit ligand (Stem cell factor) is a major factor driving expansion of very immature thymocytes in vivo. Immunity. (1995) 3:313–9. doi: 10.1016/1074-7613(95)90116-7
44. Buono M, Facchini R, Matsuoka S, Thongjuea S, Waithe D, Luis TC, et al. A dynamic niche provides kit ligand in a stage-specific manner to the earliest thymocyte progenitors. Nat Cell Biol. (2016) 18:157–67. doi: 10.1038/ncb3299
45. Wertheimer T, Velardi E, Tsai J, Cooper K, Xiao S, Kloss CC, et al. Production of bmp4 by endothelial cells is crucial for endogenous thymic regeneration. Sci Immunol. (2018) 3:1–11. doi: 10.1126/sciimmunol.aal2736
46. Halpern KB, Shenhav R, Massalha H, Toth B, Egozi A, Massasa EE, et al. Paired-cell sequencing enables spatial gene expression mapping of liver endothelial cells. Nat Biotechnol. (2018) 36:962–70. doi: 10.1038/nbt.4231
47. Paik DT, Tian L, Williams IM, Rhee S, Zhang H, Liu C, et al. Single-cell rna sequencing unveils unique transcriptomic signatures of organ-specific endothelial cells. Circulation. (2020) 142:1848–62. doi: 10.1161/CIRCULATIONAHA.119.041433
48. Lemke SB, Nelson CM. Dynamic changes in epithelial cell packing during tissue morphogenesis. Curr Biol. (2021) 31:R1098–R110. doi: 10.1016/j.cub.2021.07.078
49. van Ewijk W, Wang B, Hollander G, Kawamoto H, Spanopoulou E, Itoi M, et al. Thymic microenvironments, 3-D versus 2-D? Semin Immunol. (1999) 11:57–64. doi: 10.1006/smim.1998.0158
50. van Ewijk W, Holländer G, Terhorst C, Wang B. Stepwise development of thymic microenvironments in vivo is regulated by thymocyte subsets. Development. (2000) 127:1583–91. doi: 10.1242/dev.127.8.1583
51. Guo J, Rahman M, Cheng L, Zhang S, Tvinnereim A, Su DM. Morphogenesis and maintenance of the 3d thymic medulla and prevention of nude skin phenotype require foxn1 in pre- and post-natal K14 epithelium. J Mol Med (Berl). (2011) 89:263–77. doi: 10.1007/s00109-010-0700-8
52. Clevers H. Modeling development and disease with organoids. Cell. (2016) 165:1586–97. doi: 10.1016/j.cell.2016.05.082
53. Venables T, Griffith AV, DeAraujo A, Petrie HT. Dynamic changes in epithelial cell morphology control thymic organ size during atrophy and regeneration. Nat Commun. (2019) 10:4402. doi: 10.1038/s41467-019-11879-2
54. Kernfeld EM, Genga RMJ, Neherin K, Magaletta ME, Xu P, Maehr R. A single-cell transcriptomic atlas of thymus organogenesis resolves cell types and developmental maturation. Immunity. (2018) 48:1258–70 e6. doi: 10.1016/j.immuni.2018.04.015
55. Park JE, Botting RA, Dominguez Conde C, Popescu DM, Lavaert M, Kunz DJ, et al. A cell atlas of human thymic development defines T cell repertoire formation. Science. (2020) 367:1–11. doi: 10.1126/science.aay3224
56. Koch U, Radtke F. Mechanisms of T cell development and transformation. Annu Rev Cell Dev Biol. (2011) 27:539–62. doi: 10.1146/annurev-cellbio-092910-154008
57. Kumar BV, Connors TJ, Farber DL. Human T cell development, localization, and function throughout life. Immunity. (2018) 48:202–13. doi: 10.1016/j.immuni.2018.01.007
58. Irla M. Instructive cues of thymic T cell selection. Annu Rev Immunol. (2022) 40:95–119. doi: 10.1146/annurev-immunol-101320-022432
59. Chopp L, Redmond C, O'Shea JJ, Schwartz DM. From thymus to tissues and tumors: A review of T-cell biology. J Allergy Clin Immunol. (2023) 151:81–97. doi: 10.1016/j.jaci.2022.10.011
60. Ashby KM, Hogquist KA. A guide to thymic selection of T cells. Nat Rev Immunol. (2024) 24:103–17. doi: 10.1038/s41577-023-00911-8
61. Huesmann M, Scott B, Kisielow P, von Boehmer H. Kinetics and efficacy of positive selection in the thymus of normal and T cell receptor transgenic mice. Cell. (1991) 66:533–40. doi: 10.1016/0092-8674(81)90016-7
62. Shortman K, Vremec D, Egerton M. The kinetics of T cell antigen receptor expression by subgroups of cd4+8+ Thymocytes: Delineation of cd4+8+3(2+) thymocytes as post-selection intermediates leading to mature T cells. J Exp Med. (1991) 173:323–32. doi: 10.1084/jem.173.2.323
63. Aschenbrenner K, D'Cruz LM, Vollmann EH, Hinterberger M, Emmerich J, Swee LK, et al. Selection of foxp3+ Regulatory T cells specific for self antigen expressed and presented by aire+ Medullary thymic epithelial cells. Nat Immunol. (2007) 8:351–8. doi: 10.1038/ni1444
64. Kyewski B, Klein L. A central role for central tolerance. Annu Rev Immunol. (2006) 24:571–606. doi: 10.1146/annurev.immunol.23.021704.115601
65. Cloosen S, Arnold J, Thio M, Bos GMJ, Kyewski B, Germeraad WTV. Expression of tumor-associated differentiation antigens, muc1 glycoforms and cea, in human thymic epithelial cells: implications for self-tolerance and tumor therapy. Cancer Res. (2007) 67:3919–26. doi: 10.1158/0008-5472.CAN-06-2112
66. Derbinski J, Pinto S, Rösch S, Hexel K, Kyewski B. Promiscuous gene expression patterns in single medullary thymic epithelial cells argue for a stochastic mechanism. Proc Natl Acad Sci. (2008) 105:657–62. doi: 10.1073/pnas.0707486105
67. Pinto S, Michel C, Schmidt-Glenewinkel H, Harder N, Rohr K, Wild S, et al. Overlapping gene coexpression patterns in human medullary thymic epithelial cells generate self-antigen diversity. Proc Natl Acad Sci. (2013) 110:E3497–E505. doi: 10.1073/pnas.1308311110
68. Rattay K, Meyer HV, Herrmann C, Brors B, Kyewski B. Evolutionary conserved gene co-expression drives generation of self-antigen diversity in medullary thymic epithelial cells. J Autoimmun. (2016) 67:65–75. doi: 10.1016/j.jaut.2015.10.001
69. Villaseñor J, Besse W, Benoist C, Mathis D. Ectopic expression of peripheral-tissue antigens in the thymic epithelium: Probabilistic, monoallelic, misinitiated. Proc Natl Acad Sci. (2008) 105:15854–9. doi: 10.1073/pnas.0808069105
70. Brennecke P, Reyes A, Pinto S, Rattay K, Nguyen M, Kuchler R, et al. Single-cell transcriptome analysis reveals coordinated ectopic gene-expression patterns in medullary thymic epithelial cells. Nat Immunol. (2015) 16:933–41. doi: 10.1038/ni.3246
71. Dhalla F, Baran-Gale J, Maio S, Chappell L, Hollander GA, Ponting CP. Biologically indeterminate yet ordered promiscuous gene expression in single medullary thymic epithelial cells. EMBO J. (2020) 39:e101828. doi: 10.15252/embj.2019101828
72. Bornstein C, Nevo S, Giladi A, Kadouri N, Pouzolles M, Gerbe F, et al. Single-cell mapping of the thymic stroma identifies il-25-producing tuft epithelial cells. Nature. (2018) 559:622–6. doi: 10.1038/s41586-018-0346-1
73. Meredith M, Zemmour D, Mathis D, Benoist C. Aire controls gene expression in the thymic epithelium with ordered stochasticity. Nat Immunol. (2015) 16:942–9. doi: 10.1038/ni.3247
74. Miragaia RJ, Zhang X, Gomes T, Svensson V, Ilicic T, Henriksson J, et al. Single-cell rna-sequencing resolves self-antigen expression during mtec development. Sci Rep. (2018) 8:685. doi: 10.1038/s41598-017-19100-4
75. Ucar O, Rattay K. Promiscuous gene expression in the thymus: A matter of epigenetics, mirna, and more? Front Immunol. (2015) 6:93. doi: 10.3389/fimmu.2015.00093
76. Anderson MS, Venanzi ES, Klein L, Chen Z, Berzins SP, Turley SJ, et al. Projection of an immunological self shadow within the thymus by the aire protein. Science. (2002) 298:1395–401. doi: 10.1126/science.1075958
77. Miller CN, Proekt I, von Moltke J, Wells KL, Rajpurkar AR, Wang H, et al. Thymic tuft cells promote an il-4-enriched medulla and shape thymocyte development. Nature. (2018) 559:627–31. doi: 10.1038/s41586-018-0345-2
78. Takaba H, Morishita Y, Tomofuji Y, Danks L, Nitta T, Komatsu N, et al. Fezf2 orchestrates a thymic program of self-antigen expression for immune tolerance. Cell. (2015) 163:975–87. doi: 10.1016/j.cell.2015.10.013
79. Tomofuji Y, Takaba H, Suzuki HI, Benlaribi R, Martinez CDP, Abe Y, et al. Chd4 choreographs self-antigen expression for central immune tolerance. Nat Immunol. (2020) 21:892–901. doi: 10.1038/s41590-020-0717-2
80. Goldfarb Y, Kadouri N, Levi B, Sela A, Herzig Y, Cohen RN, et al. Hdac3 is a master regulator of mtec development. Cell Rep. (2016) 15:651–65. doi: 10.1016/j.celrep.2016.03.048
81. Tykocinski L-O, Sinemus A, Rezavandy E, Weiland Y, Baddeley D, Cremer C, et al. Epigenetic regulation of promiscuous gene expression in thymic medullary epithelial cells. Proc Natl Acad Sci United States America. (2010) 107:19426–31. doi: 10.1073/pnas.1009265107
82. Danso-Abeam D, Staats KA, Franckaert D, Van Den Bosch L, Liston A, Gray DHD, et al. Aire mediates thymic expression and tolerance of pancreatic antigens via an unconventional transcriptional mechanism. Eur J Immunol. (2013) 43:75–84. doi: 10.1002/eji.201242761
83. Rodewald HR, Paul S, Haller C, Bluethmann H, Blum C. Thymus medulla consisting of epithelial islets each derived from a single progenitor. Nature. (2001) 414:763–8. doi: 10.1038/414763a
84. Kadouri N, Nevo S, Goldfarb Y, Abramson J. Thymic epithelial cell heterogeneity: Tec by tec. Nat Rev Immunol. (2020) 20:239–53. doi: 10.1038/s41577-019-0238-0
85. Yano M, Kuroda N, Han H, Meguro-Horike M, Nishikawa Y, Kiyonari H, et al. Aire controls the differentiation program of thymic epithelial cells in the medulla for the establishment of self-tolerance. J Exp Med. (2008) 205:2827–38. doi: 10.1084/jem.20080046
86. Panneck AR, Rafiq A, Schutz B, Soultanova A, Deckmann K, Chubanov V, et al. Cholinergic epithelial cell with chemosensory traits in murine thymic medulla. Cell Tissue Res. (2014) 358:737–48. doi: 10.1007/s00441-014-2002-x
87. Soultanova A, Voigt A, Chubanov V, Gudermann T, Meyerhof W, Boehm U, et al. Cholinergic chemosensory cells of the thymic medulla express the bitter receptor tas2r131. Int Immunopharmacol. (2015) 29:143–7. doi: 10.1016/j.intimp.2015.06.005
88. Michelson DA, Hase K, Kaisho T, Benoist C, Mathis D. Thymic epithelial cells co-opt lineage-defining transcription factors to eliminate autoreactive T cells. Cell. (2022) 185:1–17. doi: 10.1016/j.cell.2022.05.018
89. Givony T, Leshkowitz D, Del Castillo D, Nevo S, Kadouri N, Dassa B, et al. Thymic mimetic cells function beyond self-tolerance. Nature. (2023) 622:164–72. doi: 10.1038/s41586-023-06512-8
90. Michelson DA, Mathis D. Thymic mimetic cells: Tolerogenic masqueraders. Trends Immunol. (2022) 43:782–91. doi: 10.1016/j.it.2022.07.010
91. Matsumoto M, Yoshida H, Tsuneyama K, Oya T, Matsumoto M. Revisiting aire and tissue-restricted antigens at single-cell resolution. Front Immunol. (2023) 14:1176450. doi: 10.3389/fimmu.2023.1176450
92. Gäbler J, Arnold J, Kyewski B. Promiscuous gene expression and the developmental dynamics of medullary thymic epithelial cells. Eur J Immunol. (2007) 37:3363–72. doi: 10.1002/eji.200737131
93. Gray D, Abramson J, Benoist C, Mathis D. Proliferative arrest and rapid turnover of thymic epithelial cells expressing aire. J Exp Med. (2007) 204:2521–8. doi: 10.1084/jem.20070795
94. Ehrlich LI, Oh DY, Weissman IL, Lewis RS. Differential contribution of chemotaxis and substrate restriction to segregation of immature and mature thymocytes. Immunity. (2009) 31:986–98. doi: 10.1016/j.immuni.2009.09.020
95. Lancaster JN, Thyagarajan HM, Srinivasan J, Li Y, Hu Z, Ehrlich LIR. Live-cell imaging reveals the relative contributions of antigen-presenting cell subsets to thymic central tolerance. Nat Commun. (2019) 10:2220. doi: 10.1038/s41467-019-09727-4
96. Bousso P, Bhakta NR, Lewis RS, Robey E. Dynamics of thymocyte-stromal cell interactions visualized by two-photon microscopy. Science. (2002) 296:1876–80. doi: 10.1126/science.1070945
97. Dzhagalov IL, Chen KG, Herzmark P, Robey EA. Elimination of self-reactive T cells in the thymus: A timeline for negative selection. PLoS Biol. (2013) 11:e1001566. doi: 10.1371/journal.pbio.1001566
98. McCaughtry TM, Wilken MS, Hogquist KA. Thymic emigration revisited. J Exp Med. (2007) 204:2513–20. doi: 10.1084/jem.20070601
99. Le Borgne M, Ladi E, Dzhagalov I, Herzmark P, Liao YF, Chakraborty AK, et al. The impact of negative selection on thymocyte migration in the medulla. Nat Immunol. (2009) 10:823–30. doi: 10.1038/ni.1761
100. Klein L. Dead man walking: How thymocytes scan the medulla. Nat Immunol. (2009) 10:809–11. doi: 10.1038/ni0809-809
101. Abramson J, Anderson G. Thymic epithelial cells. Annu Rev Immunol. (2017) 35:85–118. doi: 10.1146/annurev-immunol-051116-052320
102. Oven I, Brdickova N, Kohoutek J, Vaupotic T, Narat M, Peterlin BM. Aire recruits P-tefb for transcriptional elongation of target genes in medullary thymic epithelial cells. Mol Cell Biol. (2007) 27:8815–23. doi: 10.1128/MCB.01085-07
103. Anderson MS, Venanzi ES, Chen Z, Berzins SP, Benoist C, Mathis D. The cellular mechanism of aire control of T cell tolerance. Immunity. (2005) 23:227–39. doi: 10.1016/j.immuni.2005.07.005
104. Liston A, Lesage S, Wilson J, Peltonen L, Goodnow CC. Aire regulates negative selection of organ-specific T cells. Nat Immunol. (2003) 4:350–4. doi: 10.1038/ni906
105. Mathis D, Benoist C. Aire. Annu Rev Immunol. (2009) 27:287–312. doi: 10.1146/annurev.immunol.25.022106.141532
106. Akirav EM, Ruddle NH, Herold KC. The role of aire in human autoimmune disease. Nat Rev Endocrinol. (2011) 7:25–33. doi: 10.1038/nrendo.2010.200
107. Bansal K, Yoshida H, Benoist C, Mathis D. The transcriptional regulator aire binds to and activates super-enhancers. Nat Immunol. (2017) 18:263–73. doi: 10.1038/ni.3675
108. Abramson J, Giraud M, Benoist C, Mathis D. Aire's partners in the molecular control of immunological tolerance. Cell. (2010) 140:123–35. doi: 10.1016/j.cell.2009.12.030
109. Incani F, Serra ML, Meloni A, Cossu C, Saba L, Cabras T, et al. Aire acetylation and deacetylation: effect on protein stability and transactivation activity. J Biomed Sci. (2014) 21:1–11. doi: 10.1186/s12929-014-0085-z
110. Org T, Chignola F, Hetényi C, Gaetani M, Rebane A, Liiv I, et al. The autoimmune regulator phd finger binds to non-methylated histone H3k4 to activate gene expression. EMBO Rep. (2008) 9:370–6. doi: 10.1038/sj.embor.2008.11
111. Koh AS, Kuo AJ, Park SY, Cheung P, Abramson J, Bua D, et al. Aire employs a histone-binding module to mediate immunological tolerance, linking chromatin regulation with organ-specific autoimmunity. Proc Natl Acad Sci U.S.A. (2008) 105:15878–83. doi: 10.1073/pnas.0808470105
112. Giraud M, Yoshida H, Abramson J, Rahl PB, Young RA, Mathis D, et al. Aire unleashes stalled rna polymerase to induce ectopic gene expression in thymic epithelial cells. Proc Natl Acad Sci United States America. (2012) 109:535–40. doi: 10.1073/pnas.1119351109
113. Liiv I, Rebane A, Org T, Saare M, Maslovskaja J, Kisand K, et al. DNA-pk contributes to the phosphorylation of aire: importance in transcriptional activity. Biochim Biophys Acta. (2008) 1783:74–83. doi: 10.1016/j.bbamcr.2007.09.003
114. Yoshida H, Bansal K, Schaefer U, Chapman T, Rioja I, Proekt I, et al. Brd4 bridges the transcriptional regulators, aire and P-tefb, to promote elongation of peripheral-tissue antigen transcripts in thymic stromal cells. Proc Natl Acad Sci U.S.A. (2015) 112:E4448–57. doi: 10.1073/pnas.1512081112
115. Giraud M, Jmari N, Du L, Carallis F, Nieland TJF, Perez-Campo FM, et al. An rnai screen for aire cofactors reveals a role for hnrnpl in polymerase release and aire-activated ectopic transcription. Proc Natl Acad Sci United States America. (2014) 111(4):1491–6. doi: 10.1073/pnas.1323535111
116. Waterfield M, Khan IS, Cortez JT, Fan U, Metzger T, Greer A, et al. The transcriptional regulator aire coopts the repressive atf7ip-mbd1 complex for the induction of immunotolerance. Nat Immunol. (2014) 15:258–65. doi: 10.1038/ni.2820
117. Yanagihara T, Sanematsu F, Sato T, Uruno T, Duan X, Tomino T, et al. Intronic regulation of aire expression by jmjd6 for self-tolerance induction in the thymus. Nat Commun. (2015) 6:8820. doi: 10.1038/ncomms9820
118. Passos GA, Speck-Hernandez CA, Assis AF, Mendes-da-Cruz DA. Update on aire and thymic negative selection. Immunology. (2018) 153:10–20. doi: 10.1111/imm.12831
119. Lammers S, Barrera V, Brennecke P, Miller C, Yoon J, Balolong J, et al. Ehf and fezf2 regulate late medullary thymic epithelial cell and thymic tuft cell development. Front Immunol. (2024) 14:1277365. doi: 10.3389/fimmu.2023.1277365
120. Baran-Gale J, Morgan MD, Maio S, Dhalla F, Calvo-Asensio I, Deadman ME, et al. Ageing compromises mouse thymus function and remodels epithelial cell differentiation. Elife. (2020) 9:1–27. doi: 10.7554/eLife.56221
121. Rattay K, Claude J, Rezavandy E, Matt S, Hofmann TG, Kyewski B, et al. Homeodomain-interacting protein kinase 2, a novel autoimmune regulator interaction partner, modulates promiscuous gene expression in medullary thymic epithelial cells. J Immunol. (2015) 194:921–8. doi: 10.4049/jimmunol.1402694
122. Chuprin A, Avin A, Goldfarb Y, Herzig Y, Levi B, Jacob A, et al. The deacetylase sirt1 is an essential regulator of aire-mediated induction of central immunological tolerance. Nat Immunol. (2015) 16:737–45. doi: 10.1038/ni.3194
123. Heinlein M, Gandolfo LC, Zhao K, Teh CE, Nguyen N, Baell JB, et al. The acetyltransferase kat7 is required for thymic epithelial cell expansion, expression of aire target genes, and thymic tolerance. Sci Immunol. (2022) 7:eabb6032. doi: 10.1126/sciimmunol.abb6032
124. Pitkänen J, Doucas V, Sternsdorf T, Nakajima T, Aratani S, Jensen K, et al. The autoimmune regulator protein has transcriptional transactivating properties and interacts with the common coactivator creb-binding protein. J Biol Chem. (2000) 275:16802–9. doi: 10.1074/jbc.M908944199
125. Shao W, Zumer K, Fujinaga K, Peterlin BM. Fbxo3 protein promotes ubiquitylation and transcriptional activity of aire (Autoimmune regulator). J Biol Chem. (2016) 291:17953–63. doi: 10.1074/jbc.M116.724401
126. Ilmarinen T, Kangas H, Kytömaa T, Eskelin P, Saharinen J, Seeler J-S, et al. Functional interaction of aire with pias1 in transcriptional regulation. Mol Immunol. (2008) 45:1847–62. doi: 10.1016/j.molimm.2007.10.045
127. Herzig Y, Nevo S, Bornstein C, Brezis MR, Ben-Hur S, Shkedy A, et al. Transcriptional programs that control expression of the autoimmune regulator gene aire. Nat Immunol. (2017) 18:161–72. doi: 10.1038/ni.3638
128. Nishikawa Y, Hirota F, Yano M, Kitajima H, J-i M, Kawamoto H, et al. Biphasic aire expression in early embryos and in medullary thymic epithelial cells before end-stage terminal differentiation. J Exp Med. (2010) 207:963–71. doi: 10.1084/jem.20092144
129. Laan M, Kisand K, Kont V, Moll K, Tserel L, Scott HS, et al. Autoimmune regulator deficiency results in decreased expression of ccr4 and ccr7 ligands and in delayed migration of cd4+ Thymocytes. J Immunol. (2009) 183:7682–91. doi: 10.4049/jimmunol.0804133
130. Lei Y, Ripen AM, Ishimaru N, Ohigashi I, Nagasawa T, Jeker LT, et al. Aire-dependent production of xcl1 mediates medullary accumulation of thymic dendritic cells and contributes to regulatory T cell development. J Exp Med. (2011) 208:383–94. doi: 10.1084/jem.20102327
131. Gardner JM, Devoss JJ, Friedman RS, Wong DJ, Tan YX, Zhou X, et al. Deletional tolerance mediated by extrathymic aire-expressing cells. Science. (2008) 321:843–7. doi: 10.1126/science.1159407
132. Gardner JM, Fletcher AL, Anderson MS, Turley SJ. Aire in the thymus and beyond. Curr Opin Immunol. (2009) 21:582–9. doi: 10.1016/j.coi.2009.08.007
133. Gardner JM, Metzger TC, McMahon EJ, Au-Yeung BB, Krawisz AK, Lu W, et al. Extrathymic aire-expressing cells are a distinct bone marrow-derived population that induce functional inactivation of cd4(+) T cells. Immunity. (2013) 39:560–72. doi: 10.1016/j.immuni.2013.08.005
134. Tao W, Ye Z, Wei Y, Wang J, Yang W, Yu G, et al. Insm1 regulates mtec development and immune tolerance. Cell Mol Immunol. (2023) 20:1472–86. doi: 10.1038/s41423-023-01102-0
135. Sin JH, Sucharov J, Kashyap S, Wang Y, Proekt I, Liu X, et al. Ikaros is a principal regulator of aire(+) mtec homeostasis, thymic mimetic cell diversity, and central tolerance. Sci Immunol. (2023) 8:eabq3109. doi: 10.1126/sciimmunol.abq3109
136. Lopes N, Boucherit N, Santamaria JC, Provin N, Charaix J, Ferrier P, et al. Thymocytes trigger self-antigen-controlling pathways in immature medullary thymic epithelial stages. Elife. (2022) 11:1–31. doi: 10.7554/eLife.69982
137. Sarter K, Leimgruber E, Gobet F, Agrawal V, Dunand-Sauthier I, Barras E, et al. Btn2a2, a T cell immunomodulatory molecule coregulated with mhc class ii genes. J Exp Med. (2016) 213:177–87. doi: 10.1084/jem.20150435
138. Frech M, Danzer H, Uchil P, Azizov V, Schmid E, Schalter F, et al. Butyrophilin 2a2 (Btn2a2) expression on thymic epithelial cells promotes central T cell tolerance and prevents autoimmune disease. J Autoimmun. (2023) 139:103071. doi: 10.1016/j.jaut.2023.103071
139. Chentoufi AA, Polychronakos C. Insulin expression levels in the thymus modulate insulin-specific autoreactive T-cell tolerance: the mechanism by which the iddm2 locus may predispose to diabetes. Diabetes. (2002) 51:1383–90. doi: 10.2337/diabetes.51.5.1383
140. Fan Y, Rudert WA, Grupillo M, He J, Sisino G, Trucco M. Thymus-specific deletion of insulin induces autoimmune diabetes. EMBO J. (2009) 28:2812–24. doi: 10.1038/emboj.2009.212
141. Pugliese A, Zeller M, Fernandez A Jr., Zalcberg LJ, Bartlett RJ, Ricordi C, et al. The insulin gene is transcribed in the human thymus and transcription levels correlated with allelic variation at the ins vntr-iddm2 susceptibility locus for type 1 diabetes. Nat Genet. (1997) 15:293–7. doi: 10.1038/ng0397-293
142. DeVoss J, Hou Y, Johannes K, Lu W, Liou GI, Rinn J, et al. Spontaneous autoimmunity prevented by thymic expression of a single self-antigen. J Exp Med. (2006) 203:2727–35. doi: 10.1084/jem.20061864
143. Lv H, Havari E, Pinto S, Gottumukkala RV, Cornivelli L, Raddassi K, et al. Impaired thymic tolerance to alpha-myosin directs autoimmunity to the heart in mice and humans. J Clin Invest. (2011) 121:1561–73. doi: 10.1172/JCI44583
144. Giraud M, Taubert R, Vandiedonck C, Ke X, Levi-Strauss M, Pagani F, et al. An irf8-binding promoter variant and aire control chrna1 promiscuous expression in thymus. Nature. (2007) 448:934–7. doi: 10.1038/nature06066
145. Nitta T, Tsutsumi M, Nitta S, Muro R, Suzuki EC, Nakano K, et al. Fibroblasts as a source of self-antigens for central immune tolerance. Nat Immunol. (2020) 21:1172–80. doi: 10.1038/s41590-020-0756-8
146. Nitta T. Mesenchymal stromal cells in the thymus. Inflammation Regener. (2022) 42:33. doi: 10.1186/s41232-022-00219-5
147. Plikus MV, Wang X, Sinha S, Forte E, Thompson SM, Herzog EL, et al. Fibroblasts: origins, definitions, and functions in health and disease. Cell. (2021) 184:3852–72. doi: 10.1016/j.cell.2021.06.024
148. Nevo S, Frenkel N, Kadouri N, Gome T, Rosenthal N, Givony T, et al. Tuft cells and fibroblasts promote thymus regeneration through ilc2-mediated type 2 immune response. Sci Immunol. (2024) 9:eabq6930. doi: 10.1126/sciimmunol.abq6930
149. Isaacson PG, Norton AJ, Addis BJ. The human thymus contains a novel population of B lymphocytes. Lancet. (1987) 2:1488–91. doi: 10.1016/s0140-6736(87)92622-5
150. Nango K, Inaba M, Inaba K, Adachi Y, Than S, Ishida T, et al. Ontogeny of thymic B cells in normal mice. Cell Immunol. (1991) 133:109–15. doi: 10.1016/0008-8749(91)90183-C
151. Akashi K, Richie LI, Miyamoto T, Carr WH, Weissman IL. B lymphopoiesis in the thymus. J Immunol. (2000) 164:5221–6. doi: 10.4049/jimmunol.164.10.5221
152. Mori S, Inaba M, Sugihara A, Taketani S, Doi H, Fukuba Y, et al. Presence of B cell progenitors in the thymus. J Immunol. (1997) 158:4193–9. doi: 10.4049/jimmunol.158.9.4193
153. Castaneda J, Hidalgo Y, Sauma D, Rosemblatt M, Bono MR, Nunez S. The multifaceted roles of B cells in the thymus: From immune tolerance to autoimmunity. Front Immunol. (2021) 12:766698. doi: 10.3389/fimmu.2021.766698
154. Nunez S, Moore C, Gao B, Rogers K, Hidalgo Y, Del Nido PJ, et al. The human thymus perivascular space is a functional niche for viral-specific plasma cells. Sci Immunol. (2016) 1:1–10. doi: 10.1126/sciimmunol.aah4447
155. Flores KG, Li J, Hale LP. B cells in epithelial and perivascular compartments of human adult thymus. Hum Pathol. (2001) 32:926–34. doi: 10.1053/hupa.2001.27106
156. Cepeda S, Cantu C, Orozco S, Xiao Y, Brown Z, Semwal MK, et al. Age-associated decline in thymic B cell expression of aire and aire-dependent self-antigens. Cell Rep. (2018) 22:1276–87. doi: 10.1016/j.celrep.2018.01.015
157. Cufi P, Dragin N, Ruhlmann N, Weiss JM, Fadel E, Serraf A, et al. Central role of interferon-beta in thymic events leading to myasthenia gravis. J Autoimmun. (2014) 52:44–52. doi: 10.1016/j.jaut.2013.12.016
158. Meraouna A, Cizeron-Clairac G, Panse RL, Bismuth J, Truffault F, Tallaksen C, et al. The chemokine cxcl13 is a key molecule in autoimmune myasthenia gravis. Blood. (2006) 108:432–40. doi: 10.1182/blood-2005-06-2383
159. Goldstein G, Mackay IR. The thymus in systemic lupus erythematosus: A quantitative histopathological analysis and comparison with stress involution. Br Med J. (1967) 2:475–8. doi: 10.1136/bmj.2.5550.475
160. Loschko J, Schreiber HA, Rieke GJ, Esterhazy D, Meredith MM, Pedicord VA, et al. Absence of mhc class ii on cdcs results in microbial-dependent intestinal inflammation. J Exp Med. (2016) 213:517–34. doi: 10.1084/jem.20160062
161. Ohnmacht C, Pullner A, King SB, Drexler I, Meier S, Brocker T, et al. Constitutive ablation of dendritic cells breaks self-tolerance of cd4 T cells and results in spontaneous fatal autoimmunity. J Exp Med. (2009) 206:549–59. doi: 10.1084/jem.20082394
162. Brezina J, Voboril M, Filipp D. Mechanisms of direct and indirect presentation of self-antigens in the thymus. Front Immunol. (2022) 13:926625. doi: 10.3389/fimmu.2022.926625
163. Eisenbarth SC. Dendritic cell subsets in T cell programming: location dictates function. Nat Rev Immunol. (2019) 19:89–103. doi: 10.1038/s41577-018-0088-1
164. Corcoran L, Ferrero I, Vremec D, Lucas K, Waithman J, O'Keeffe M, et al. The lymphoid past of mouse plasmacytoid cells and thymic dendritic cells. J Immunol. (2003) 170:4926–32. doi: 10.4049/jimmunol.170.10.4926
165. Feyerabend TB, Terszowski G, Tietz A, Blum C, Luche H, Gossler A, et al. Deletion of notch1 converts pro-T cells to dendritic cells and promotes thymic B cells by cell-extrinsic and cell-intrinsic mechanisms. Immunity. (2009) 30:67–79. doi: 10.1016/j.immuni.2008.10.016
166. Ardavin C, Wu L, Li CL, Shortman K. Thymic dendritic cells and T cells develop simultaneously in the thymus from a common precursor population. Nature. (1993) 362:761–3. doi: 10.1038/362761a0
167. Joffre OP, Segura E, Savina A, Amigorena S. Cross-presentation by dendritic cells. Nat Rev Immunol. (2012) 12:557–69. doi: 10.1038/nri3254
168. Proietto AI, Lahoud MH, Wu L. Distinct functional capacities of mouse thymic and splenic dendritic cell populations. Immunol Cell Biol. (2008) 86:700–8. doi: 10.1038/icb.2008.63
169. Gallegos AM, Bevan MJ. Central tolerance to tissue-specific antigens mediated by direct and indirect antigen presentation. J Exp Med. (2004) 200:1039–49. doi: 10.1084/jem.20041457
170. Koble C, Kyewski B. The thymic medulla: A unique microenvironment for intercellular self-antigen transfer. J Exp Med. (2009) 206:1505–13. doi: 10.1084/jem.20082449
171. Collin M, Bigley V, Haniffa M, Hambleton S. Human dendritic cell deficiency: the missing id? Nat Rev Immunol. (2011) 11:575–83. doi: 10.1038/nri3046
172. Collin M, Bigley V. Human dendritic cell subsets: an update. Immunology. (2018) 154:3–20. doi: 10.1111/imm.12888
173. Bonasio R, Scimone ML, Schaerli P, Grabie N, Lichtman AH, von Andrian UH. Clonal deletion of thymocytes by circulating dendritic cells homing to the thymus. Nat Immunol. (2006) 7:1092–100. doi: 10.1038/ni1385
174. Hadeiba H, Lahl K, Edalati A, Oderup C, Habtezion A, Pachynski R, et al. Plasmacytoid dendritic cells transport peripheral antigens to the thymus to promote central tolerance. Immunity. (2012) 36:438–50. doi: 10.1016/j.immuni.2012.01.017
175. Hinterberger M, Aichinger M, Prazeres da Costa O, Voehringer D, Hoffmann R, Klein L. Autonomous role of medullary thymic epithelial cells in central cd4(+) T cell tolerance. Nat Immunol. (2010) 11:512–9. doi: 10.1038/ni.1874
176. Carter JA, Stromich L, Peacey M, Chapin SR, Velten L, Steinmetz LM, et al. Transcriptomic diversity in human medullary thymic epithelial cells. Nat Commun. (2022) 13:4296. doi: 10.1038/s41467-022-31750-1
177. Miller JFAP. The golden anniversary of the thymus. Nat Rev Immunol. (2011) 11:489–95. doi: 10.1038/nri2993
178. Miller JF. Analysis of the thymus influence in leukaemogenesis. Nature. (1961) 191:248–9. doi: 10.1038/191248a0
179. Miller JF. Immunological function of the thymus. Lancet. (1961) 2:748–9. doi: 10.1016/S0140-6736(61)90693-6
180. Nishizuka Y, Sakakura T. Thymus and reproduction: sex-linked dysgenesia of the gonad after neonatal thymectomy in mice. Science. (1969) 166:753–5. doi: 10.1126/science.166.3906.753
181. Bevan MJ. In a radiation chimaera, host H-2 antigens determine immune responsiveness of donor cytotoxic cells. Nature. (1977) 269:417–8. doi: 10.1038/269417a0
182. Kappler JW, Roehm N, Marrack P. T cell tolerance by clonal elimination in the thymus. Cell. (1987) 49:273–80. doi: 10.1016/0092-8674(87)90568-X
183. Kisielow P, Bluthmann H, Staerz UD, Steinmetz M, von Boehmer H. Tolerance in T-cell-receptor transgenic mice involves deletion of nonmature cd4+8+ Thymocytes. Nature. (1988) 333:742–6. doi: 10.1038/333742a0
184. Salaun J, Bandeira A, Khazaal I, Calman F, Coltey M, Coutinho A, et al. Thymic epithelium tolerizes for histocompatibility antigens. Science. (1990) 247:1471–4. doi: 10.1126/science.247.4949.1471
185. Derbinski J, Schulte A, Kyewski B, Klein L. Promiscuous gene expression in medullary thymic epithelial cells mirrors the peripheral self. Nat Immunol. (2001) 2:1032–9. doi: 10.1038/ni723
186. Klein L, Klein T, Rüther U, Kyewski B. Cd4 T cell tolerance to human C-reactive protein, an inducible serum protein, is mediated by medullary thymic epithelium. J Exp Med. (1998) 188:5–16. doi: 10.1084/jem.188.1.5
187. Egwuagu CE, Charukamnoetkanok P, Gery I. Thymic expression of autoantigens correlates with resistance to autoimmune disease. J Immunol. (1997) 159:3109–12. doi: 10.4049/jimmunol.159.7.3109
188. Heath VL, Moore NC, Parnell SM, Mason DW. Intrathymic expression of genes involved in organ specific autoimmune disease. J Autoimmun. (1998) 11:309–18. doi: 10.1006/jaut.1998.0210
189. Jolicoeur C, Hanahan D, Smith KM. T-cell tolerance toward a transgenic beta-cell antigen and transcription of endogenous pancreatic genes in thymus. Proc Natl Acad Sci United States America. (1994) 91:6707–11. doi: 10.1073/pnas.91.14.6707
190. Nehls M, Kyewski B, Messerle M, Waldschutz R, Schuddekopf K, Smith AJ, et al. Two genetically separable steps in the differentiation of thymic epithelium. Science. (1996) 272:886–9. doi: 10.1126/science.272.5263.886
191. Nehls M, Pfeifer D, Schorpp M, Hedrich H, Boehm T. New member of the winged-helix protein family disrupted in mouse and rat nude mutations. Nature. (1994) 372:103–7. doi: 10.1038/372103a0
192. Figueiredo M, Zilhao R, Neves H. Thymus inception: molecular network in the early stages of thymus organogenesis. Int J Mol Sci. (2020) 21:1–20. doi: 10.3390/ijms21165765
193. Aaltonen J, Björses P, Perheentupa J, Horelli–Kuitunen N, Palotie A, Peltonen L, et al. An autoimmune disease, apeced, caused by mutations in a novel gene featuring two phd-type zinc-finger domains. Nat Genet. (1997) 17:399–403. doi: 10.1038/ng1297-399
194. Fisher GH, Rosenberg FJ, Straus SE, Dale JK, Middleton LA, Lin AY, et al. Dominant interfering fas gene mutations impair apoptosis in a human autoimmune lymphoproliferative syndrome. Cell. (1995) 81:935–46. doi: 10.1016/0092-8674(95)90013-6
195. Bennett CL, Christie J, Ramsdell F, Brunkow ME, Ferguson PJ, Whitesell L, et al. The immune dysregulation, polyendocrinopathy, enteropathy, X-linked syndrome (Ipex) is caused by mutations of foxp3. Nat Genet. (2001) 27:20–1. doi: 10.1038/83713
196. Manley NR, Richie ER, Blackburn CC, Condie BG, Sage J. Structure and function of the thymic microenvironment. Front Biosci (Landmark Ed). (2011) 16:2461–77. doi: 10.2741/3866
197. Boehm T, Swann JB. Thymus involution and regeneration: two sides of the same coin? Nat Rev Immunol. (2013) 13:831–8. doi: 10.1038/nri3534
198. Chinn IK, Blackburn CC, Manley NR, Sempowski GD. Changes in primary lymphoid organs with aging. Semin Immunol. (2012) 24:309–20. doi: 10.1016/j.smim.2012.04.005
199. Gray DH, Seach N, Ueno T, Milton MK, Liston A, Lew AM, et al. Developmental kinetics, turnover, and stimulatory capacity of thymic epithelial cells. Blood. (2006) 108:3777–85. doi: 10.1182/blood-2006-02-004531
200. Gui J, Zhu X, Dohkan J, Cheng L, Barnes PF, Su DM. The aged thymus shows normal recruitment of lymphohematopoietic progenitors but has defects in thymic epithelial cells. Int Immunol. (2007) 19:1201–11. doi: 10.1093/intimm/dxm095
201. Min H, Montecino-Rodriguez E, Dorshkind K. Reduction in the developmental potential of intrathymic T cell progenitors with age. J Immunol. (2004) 173:245–50. doi: 10.4049/jimmunol.173.1.245
202. Bredenkamp N, Nowell CS, Blackburn CC. Regeneration of the aged thymus by a single transcription factor. Development. (2014) 141:1627–37. doi: 10.1242/dev.103614
203. Rode I, Martins VC, Kublbeck G, Maltry N, Tessmer C, Rodewald HR. Foxn1 protein expression in the developing, aging, and regenerating thymus. J Immunol. (2015) 195:5678–87. doi: 10.4049/jimmunol.1502010
204. Zook EC, Krishack PA, Zhang S, Zeleznik-Le NJ, Firulli AB, Witte PL, et al. Overexpression of foxn1 attenuates age-associated thymic involution and prevents the expansion of peripheral cd4 memory T cells. Blood. (2011) 118:5723–31. doi: 10.1182/blood-2011-03-342097
205. Chen L, Xiao S, Manley NR. Foxn1 is required to maintain the postnatal thymic microenvironment in a dosage-sensitive manner. Blood. (2009) 113:567–74. doi: 10.1182/blood-2008-05-156265
206. Cheng L, Guo J, Sun L, Fu J, Barnes PF, Metzger D, et al. Postnatal tissue-specific disruption of transcription factor foxn1 triggers acute thymic atrophy. J Biol Chem. (2010) 285:5836–47. doi: 10.1074/jbc.M109.072124
207. Seggewiss R, Lore K, Guenaga FJ, Pittaluga S, Mattapallil J, Chow CK, et al. Keratinocyte growth factor augments immune reconstitution after autologous hematopoietic progenitor cell transplantation in rhesus macaques. Blood. (2007) 110:441–9. doi: 10.1182/blood-2006-12-065623
208. Jenkinson WE, Jenkinson EJ, Anderson G. Differential requirement for mesenchyme in the proliferation and maturation of thymic epithelial progenitors. J Exp Med. (2003) 198:325–32. doi: 10.1084/jem.20022135
209. Erickson M, Morkowski S, Lehar S, Gillard G, Beers C, Dooley J, et al. Regulation of thymic epithelium by keratinocyte growth factor. Blood. (2002) 100:3269–78. doi: 10.1182/blood-2002-04-1036
210. Youm YH, Horvath TL, Mangelsdorf DJ, Kliewer SA, Dixit VD. Prolongevity hormone fgf21 protects against immune senescence by delaying age-related thymic involution. Proc Natl Acad Sci U.S.A. (2016) 113:1026–31. doi: 10.1073/pnas.1514511113
211. Elyahu Y, Hekselman I, Eizenberg-Magar I, Berner O, Strominger I, Schiller M, et al. Aging promotes reorganization of the cd4 T cell landscape toward extreme regulatory and effector phenotypes. Sci Adv. (2019) 5:eaaw8330. doi: 10.1126/sciadv.aaw8330
212. Thome JJ, Yudanin N, Ohmura Y, Kubota M, Grinshpun B, Sathaliyawala T, et al. Spatial map of human T cell compartmentalization and maintenance over decades of life. Cell. (2014) 159:814–28. doi: 10.1016/j.cell.2014.10.026
213. Watad A, Bragazzi NL, Adawi M, Amital H, Toubi E, Porat BS, et al. Autoimmunity in the elderly: insights from basic science and clinics - a mini-review. Gerontology. (2017) 63:515–23. doi: 10.1159/000478012
214. Prelog M. Aging of the immune system: A risk factor for autoimmunity? Autoimmun Rev. (2006) 5:136–9. doi: 10.1016/j.autrev.2005.09.008
215. Duah M, Li L, Shen J, Lan Q, Pan B, Xu K. Thymus degeneration and regeneration. Front Immunol. (2021) 12:706244. doi: 10.3389/fimmu.2021.706244
216. Li T, Yan F, Meng X, Wang J, Ting Kam RK, Zeng X, et al. Improvement of glucocorticoid-impaired thymus function by dihydromyricetin via up-regulation of ppargamma-associated fatty acid metabolism. Pharmacol Res. (2018) 137:76–88. doi: 10.1016/j.phrs.2018.09.011
217. Billard MJ, Gruver AL, Sempowski GD. Acute endotoxin-induced thymic atrophy is characterized by intrathymic inflammatory and wound healing responses. PLoS One. (2011) 6:e17940. doi: 10.1371/journal.pone.0017940
218. Zhang K, Wu W, Wu Y, Bai M, Li X, Zhang J, et al. Thymic lymphoid hyperplasia with graves' Disease in a 28-year-old female: A case report. Gland Surg. (2020) 9:437–41. doi: 10.21037/gs.2019.12.18
219. Singhal S, Hellyer J, Ouseph MM, Wakelee HA, Padda SK. Autoimmune disease in patients with advanced thymic epithelial tumors. JTO Clin Res Rep. (2022) 3:100323. doi: 10.1016/j.jtocrr.2022.100323
220. Bernard C, Frih H, Pasquet F, Kerever S, Jamilloux Y, Tronc F, et al. Thymoma associated with autoimmune diseases: 85 cases and literature review. Autoimmun Rev. (2016) 15:82–92. doi: 10.1016/j.autrev.2015.09.005
221. Blum TG, Misch D, Kollmeier J, Thiel S, Bauer TT. Autoimmune disorders and paraneoplastic syndromes in thymoma. J Thorac Dis. (2020) 12:7571–90. doi: 10.21037/jtd-2019-thym-10
222. Mollaeian A, Haas C. A tale of autoimmunity: thymoma, thymectomy, and systemic lupus erythematosus. Clin Rheumatol. (2020) 39:2227–34. doi: 10.1007/s10067-020-05061-z
223. Marx A, Chan JKC, Chalabreysse L, Dacic S, Detterbeck F, French CA, et al. The 2021 who classification of tumors of the thymus and mediastinum: what is new in thymic epithelial, germ cell, and mesenchymal tumors? J Thorac Oncol. (2022) 17:200–13. doi: 10.1016/j.jtho.2021.10.010
224. Strobel P, Murumagi A, Klein R, Luster M, Lahti M, Krohn K, et al. Deficiency of the autoimmune regulator aire in thymomas is insufficient to elicit autoimmune polyendocrinopathy syndrome type 1 (Aps-1). J Pathol. (2007) 211:563–71. doi: 10.1002/path.2141
225. Shelly S, Agmon-Levin N, Altman A, Shoenfeld Y. Thymoma and autoimmunity. Cell Mol Immunol. (2011) 8:199–202. doi: 10.1038/cmi.2010.74
226. Yasumizu Y, Ohkura N, Murata H, Kinoshita M, Funaki S, Nojima S, et al. Myasthenia gravis-specific aberrant neuromuscular gene expression by medullary thymic epithelial cells in thymoma. Nat Commun. (2022) 13:4230. doi: 10.1038/s41467-022-31951-8
227. Lefeuvre CM, Payet CA, Fayet OM, Maillard S, Truffault F, Bondet V, et al. Risk factors associated with myasthenia gravis in thymoma patients: The potential role of thymic germinal centers. J Autoimmun. (2020) 106:102337. doi: 10.1016/j.jaut.2019.102337
228. Shiono H, Wong YL, Matthews I, Liu JL, Zhang W, Sims G, et al. Spontaneous production of anti-ifn-alpha and anti-il-12 autoantibodies by thymoma cells from myasthenia gravis patients suggests autoimmunization in the tumor. Int Immunol. (2003) 15:903–13. doi: 10.1093/intimm/dxg088
229. Sundaresan B, Shirafkan F, Ripperger K, Rattay K. The role of viral infections in the onset of autoimmune diseases. Viruses. (2023) 15:1–32. doi: 10.3390/v15030782
230. Luo M, Xu L, Qian Z, Sun X. Infection-associated thymic atrophy. Front Immunol. (2021) 12:652538. doi: 10.3389/fimmu.2021.652538
231. Raviola E, Karnovsky MJ. Evidence for a blood-thymus barrier using electron-opaque tracers. J Exp Med. (1972) 136:466–98. doi: 10.1084/jem.136.3.466
232. Ribatti D. The discovery of the blood-thymus barrier. Immunol Lett. (2015) 168:325–8. doi: 10.1016/j.imlet.2015.10.014
233. Nagatake T, Zhao YC, Ito T, Itoh M, Kometani K, Furuse M, et al. Selective expression of claudin-5 in thymic endothelial cells regulates the blood-thymus barrier and T-cell export. Int Immunol. (2021) 33:171–82. doi: 10.1093/intimm/dxaa069
234. Nobrega C, Cardona PJ, Roque S, Pinto do OP, Appelberg R, Correia-Neves M. The thymus as a target for mycobacterial infections. Microbes Infect. (2007) 9:1521–9. doi: 10.1016/j.micinf.2007.08.006
235. Morse SS, Valinsky JE, Mouse Thymic Virus (Mtlv). A mammalian herpesvirus cytolytic for cd4+ (L3t4+) T lymphocytes. J Exp Med. (1989) 169:591–6. doi: 10.1084/jem.169.2.591
236. Patel SJ, Zhao G, Penna VR, Park E, Lauron EJ, Harvey IB, et al. A murine herpesvirus closely related to ubiquitous human herpesviruses causes T-cell depletion. J Virol. (2017) 91:1–15. doi: 10.1128/JVI.02463-16
237. Bigley TM, Yang L, Kang LI, Saenz JB, Victorino F, Yokoyama WM. Disruption of thymic central tolerance by infection with murine roseolovirus induces autoimmune gastritis. J Exp Med. (2022) 219:1–20. doi: 10.1084/jem.20211403
238. Jaidane H, Gharbi J, Lobert PE, Lucas B, Hiar R, M'Hadheb MB, et al. Prolonged viral rna detection in blood and lymphoid tissues from coxsackievirus B4 E2 orally-inoculated swiss mice. Microbiol Immunol. (2006) 50:971–4. doi: 10.1111/j.1348-0421.2006.tb03874.x
239. Jaidane H, Caloone D, Lobert PE, Sane F, Dardenne O, Naquet P, et al. Persistent infection of thymic epithelial cells with coxsackievirus B4 results in decreased expression of type 2 insulin-like growth factor. J Virol. (2012) 86:11151–62. doi: 10.1128/JVI.00726-12
240. Michaux H, Martens H, Jaidane H, Halouani A, Hober D, Geenen V. How does thymus infection by coxsackievirus contribute to the pathogenesis of type 1 diabetes? Front Immunol. (2015) 6:338. doi: 10.3389/fimmu.2015.00338
241. Elyahu Y, Monsonego A. Thymus involution sets the clock of the aging T-cell landscape: implications for declined immunity and tissue repair. Ageing Res Rev. (2021) 65:101231. doi: 10.1016/j.arr.2020.101231
242. Alpdogan O, Hubbard VM, Smith OM, Patel N, Lu S, Goldberg GL, et al. Keratinocyte growth factor (Kgf) is required for postnatal thymic regeneration. Blood. (2006) 107:2453–60. doi: 10.1182/blood-2005-07-2831
243. Tesselaar K, Miedema F. Growth hormone resurrects adult human thymus during hiv-1 infection. J Clin Invest. (2008) 118:844–7. doi: 10.1172/JCI35112
244. Fahy GM, Brooke RT, Watson JP, Good Z, Vasanawala SS, Maecker H, et al. Reversal of epigenetic aging and immunosenescent trends in humans. Aging Cell. (2019) 18:e13028. doi: 10.1111/acel.13028
245. Sharma H, Moroni L. Recent advancements in regenerative approaches for thymus rejuvenation. Adv Sci (Weinh). (2021) 8:2100543. doi: 10.1002/advs.202100543
246. Ramos SA, Armitage LH, Morton JJ, Alzofon N, Handler D, Kelly G, et al. Generation of functional thymic organoids from human pluripotent stem cells. Stem Cell Rep. (2023) 18:829–40. doi: 10.1016/j.stemcr.2023.02.013
Keywords: thymus, autoimmune disease, tolerance, tissue homeostasis, mTEC, thymocytes, Aire, Fezf2
Citation: Shirafkan F, Hensel L and Rattay K (2024) Immune tolerance and the prevention of autoimmune diseases essentially depend on thymic tissue homeostasis. Front. Immunol. 15:1339714. doi: 10.3389/fimmu.2024.1339714
Received: 16 November 2023; Accepted: 11 March 2024;
Published: 20 March 2024.
Edited by:
Vincent Geenen, University of Liège, BelgiumReviewed by:
Bin Zhao, Central South University, ChinaCopyright © 2024 Shirafkan, Hensel and Rattay. This is an open-access article distributed under the terms of the Creative Commons Attribution License (CC BY). The use, distribution or reproduction in other forums is permitted, provided the original author(s) and the copyright owner(s) are credited and that the original publication in this journal is cited, in accordance with accepted academic practice. No use, distribution or reproduction is permitted which does not comply with these terms.
*Correspondence: Kristin Rattay, a3Jpc3Rpbi5yYXR0YXlAdW5pLW1hcmJ1cmcuZGU=
†ORCID: Fatemeh Shirafkan, orcid.org/0000-0002-0931-1468
Kristin Rattay, orcid.org/0000-0003-3503-2136
Disclaimer: All claims expressed in this article are solely those of the authors and do not necessarily represent those of their affiliated organizations, or those of the publisher, the editors and the reviewers. Any product that may be evaluated in this article or claim that may be made by its manufacturer is not guaranteed or endorsed by the publisher.
Research integrity at Frontiers
Learn more about the work of our research integrity team to safeguard the quality of each article we publish.