- Departamento de Genética, Facultad de Biología, Universidad de Sevilla, Sevilla, Spain
Salmonella enterica serovar Typhimurium expresses two type III secretion systems, T3SS1 and T3SS2, which are encoded in Salmonella pathogenicity island 1 (SPI1) and SPI2, respectively. These are essential virulent factors that secrete more than 40 effectors that are translocated into host animal cells. This study focuses on three of these effectors, SlrP, SspH1, and SspH2, which are members of the NEL family of E3 ubiquitin ligases. We compared their expression, regulation, and translocation patterns, their role in cell invasion and intracellular proliferation, their ability to interact and ubiquitinate specific host partners, and their effect on cytokine secretion. We found that transcription of the three genes encoding these effectors depends on the virulence regulator PhoP. Although the three effectors have the potential to be secreted through T3SS1 and T3SS2, the secretion of SspH1 and SspH2 is largely restricted to T3SS2 due to their expression pattern. We detected a role for these effectors in proliferation inside fibroblasts that is masked by redundancy. The generation of chimeric proteins allowed us to demonstrate that the N-terminal part of these proteins, containing the leucine-rich repeat motifs, confers specificity towards ubiquitination targets. Furthermore, the polyubiquitination patterns generated were different for each effector, with Lys48 linkages being predominant for SspH1 and SspH2. Finally, our experiments support an anti-inflammatory role for SspH1 and SspH2.
1 Introduction
Salmonella enterica serovar Typhimurium is an important bacterial pathogen that is responsible for millions of gastrointestinal infections every year in humans (1). As many other Gram negative pathogens and symbionts, Salmonella relies on type III secretion systems (T3SS) for its interactions with mammalian host cells (2). These systems, also known as injectisomes, are complex molecular devices (3) that allow some bacteria to inject specific proteins, known as effectors (4), directly into host cells. S. enterica possesses two distinct T3SS, T3SS1 and T3SS2, which are encoded by Salmonella pathogenicity island 1 (SPI1) and Salmonella pathogenicity island 2 (SPI2), respectively. T3SS1 is involved in host cell invasion and in initial colonization of the intestine (5). Some of the effectors secreted through this system are responsible for cytoskeletal rearrangements and the formation of membrane ruffles, which facilitate the entry of bacteria into host cells. T3SS2 is activated when Salmonella is inside the host cell and plays a crucial role in intracellular survival and replication of bacteria. The effectors of this system are involved in the generation of a specialized intracellular compartment called the Salmonella-containing vacuole (SCV), which is important for the evasion of host immune defenses (6).
Ubiquitination is a post-translational modification of proteins that involves the covalent attachment of one or more ubiquitins, small proteins consisting of 76 amino acids. It plays a pivotal role in the regulation of cellular processes such as protein degradation, signal transduction, and immune responses (7). Ubiquitin is bound to a protein in a sequential manner involving a ubiquitin-activating enzyme (E1), a ubiquitin-conjugating enzyme (E2), and a ubiquitin ligase (E3), which is essential for the selection of the specific target (8). Ubiquitin can be attached to target proteins as single ubiquitin molecules or as polyubiquitin chains. These chains can have different linkage types, with different roles (9). For example, Lys48 (K48)-linked ubiquitin chains are generally associated with protein degradation by the proteasome (10), and K63-linked chains are often involved in signaling processes (11). Although ubiquitination is commonly associated with eukaryotic cells, bacterial pathogens have evolved a variety of virulence factors, including T3SS effectors, to manipulate the host ubiquitination system (12, 13). Among them there are effectors with E3 ubiquitin ligase activity that are structurally similar to RING-type eukaryotic E3 ligases, such as NleG from enterohemorrhagic Escherichia coli (14), or HECT-type E3 ligases, such as SopA in S. enterica serovar Typhimurium (15). Interestingly, some effectors belong to a new family of E3 ligases that is not found in eukaryotic cells and is known as the NEL (for novel E3 ligase) family. Members of this family are the IpaH proteins from Shigella flexneri, as well as SlrP, SspH1, and SspH2 from S. enterica serovar Typhimurium (16).
In spite of their functional relationship with T3SS1 and T3SS2, the genes encoding these three effectors are located outside of SPI1 and SPI2, in other horizontally acquired regions of the chromosome (16). SlrP is secreted through T3SS1 and T3SS2 (17, 18), interacts with mammalian thioredoxin and catalyzes its ubiquitination, reducing its activity and leading to host cell death (19). This effector also binds to ERdj3, a chaperone located in the endoplasmic reticulum, and interferes with its folding activity, which can also contribute to cell death (20). A recently described ubiquitination target for SlrP is SNRPD2, a core component of the spliceosome (21). SspH1 is also secreted through both systems but localizes in the nucleus of host cells where it ubiquitinates PKN1 (22), leading to its proteasome-dependent degradation. SspH2 is specifically secreted through T3SS2 and interacts with the actin binding proteins filamin and profilin (23), the cell cycle regulator and NLR cochaperone SGT1 (24), and several other proteins (25). SspH2 and SGT1 form a trimeric complex with Nod1 leading to monoubiquitination and activation of the latter (24). Nod1 is a canonical NLR involved in the expression of pro-inflammatory chemokines such as IL-8 (26).
The presence of several effectors of one family in the same bacteria, displaying the same biochemical activity, as is the case for SlrP, SspH1, and SspH2 in S. enterica serovar Typhimurium, raises the interesting question of the degree of redundancy and specificity of these effectors. Here, we address this issue through a direct comparison of the three proteins in terms of patterns of expression, regulation, and translocation into eukaryotic cells. We also analyze their role in invasion and intracellular proliferation, and their ability to interact and ubiquitinate specific targets. Finally, we compare the ability of these effectors to interfere with the immune response of host cells.
2 Materials and methods
2.1 Bacterial strains and plasmids
The bacterial strains and plasmids used in this study are described in Table 1. S. enterica serovar Typhimurium strains derived from the wild-type strain 14028. Transductional crosses using the P22 HT105/1 int-201 phage (37) were used for the construction of Salmonella strains.
2.2 Bacterial culture
The standard culture medium for bacteria was LB broth. For SPI1-inducing conditions, S. enterica strains were grown overnight at 37°C in LB-0.3 M NaCl medium without shaking. For SPI2-inducing conditions, the bacteria were inoculated in low magnesium minimal medium (LPM) at pH 5.8, and incubated at 37°C with shaking. LPM contained 80 mM 2-(N-morpholino) ethanesulfonic acid (pH 5.8), 5 mM KCl, 7.5 mM (NH4)2SO4, 0.5mM K2SO4, 0.1% casamino acids, 38 mM glycerol, 337.5 μM K2HPO4-KH2PO4 (pH 7.4), and 8 μM MgCl2. The solid media contained 1.5% agar. Antibiotics were used at the following concentrations in LB: kanamycin (Km), 50 μg/ml; chloramphenicol (Cm), 20 μg/ml; ampicillin (Ap), 100 μg/ml. In minimal medium antibiotics were used at these concentrations: Km, 125 μg/ml; Cm, 5 μg/ml; Ap, 50 μg/ml.
2.3 Yeast strains, culture, and two-hybrid methods
The Saccharomyces cerevisiae strain used was L40 (38). The culture media for yeasts were YPD: 1% yeast extract, 2% peptone, 2% glucose; and synthetic drop-out (SD) medium: 0.15% yeast nitrogen base without amino acids and ammonium sulfate, 0.5% ammonium sulfate, 2% glucose; and yeast synthetic drop-out supplements (Formedium) lacking the appropriate components: tryptophan to select for the presence of derivatives of pLEX10 (34), leucine for derivatives of pGAD1318 (32), and histidine to check interactions. Solid medium contained 1.5% agar. For two-hybrid assays, strain L40 was transformed with the appropriate combinations of pLEX10 and pGAD1318 derivatives using the lithium acetate procedure (39). The transformants were selected in SD without tryptophan and leucine. Interactions were analyzed by checking the growth in SD lacking tryptophan, leucine, and histidine.
2.4 Mammalian cell culture, lysis, transfection, and analysis of cytokine secretion
HeLa (human epithelial; ECACC no. 93021013), RAW264.7 (murine macrophages; ECACC no. 91062702), and NRK-49F (normal rat kidney fibroblasts; ATCC CRL-1570) cells were cultured in DMEM supplemented with 10% fetal calf serum, 2 mM L-glutamine, 100 U/ml penicillin, and 100 μg/ml streptomycin. The cells were kept in a humidified atmosphere with 5% CO2 at 37°C. For cell lysis, 2 × 107 to 108 cells per ml were incubated at 4°C in NP40 buffer (10 mM Tris-HCl pH 7.4, 150 mM NaCl, 10% glycerol, 1% NP40, 1 mM PMSF, 1% protease inhibitor cocktail P8849 from Sigma-Aldrich) for 20 min. The extract was centrifuged at 20,000× g for 20 min and the supernatant was stored at -80°C. For transient transfection assays, 2–5 × 106 HeLa cells/assay were resuspended in 200 μL of 15 mM HEPES-buffered serum-containing medium, mixed with 50 μL of 210 mM NaCl containing 5–10 μg of plasmid DNA and electroporated using a BTX Electrocell Manipulator 600 set at 240 V, 950 μF, resistance = None. Cells were processed 24 h after electroporation. The supernatants of transfected cells were tested for CCL5 secretion using the human CCL5/RANTES DuoSet ELISA kit (R&D Systems) and for the secretion of IFNγ, IL-1β, IL-6 and IL-8 using the appropriate uncoated ELISA kits (Thermo Fisher Scientific).
2.5 Bacterial infection of cultured cells, invasion, proliferation, and protein translocation assays
Mammalian cells were plated in 24-well plates at 1.5 x 105 cells per well and incubated 24 h at 37°C with 5% CO2 in media without antibiotics. For infections under SPI1-inducing (invasive) conditions, bacteria grown overnight in LB with 0.3 M NaCl in a tightly closed tube without shaking were added at a multiplicity of infection of 150. For infections of RAW264.7 cells under non-invasive conditions, the bacteria were grown in LB for 24 h at 37°C with shaking. Cell culture was washed twice with phosphate-buffered saline (PBS) at 1 h post-infection (p.i.), overlaid with DMEM containing 100 μg/ml gentamicin, and incubated for another hour. The culture was then washed twice with PBS, covered with DMEM with gentamicin 16 μg/ml, and incubated for 6 h. Following the infections described above, the translocation of CyaA’ fusions into eukaryotic cells was monitored by measuring the levels of cyclic AMP (cAMP) 2 h or 8 h p.i. Infected cells were lysed and the level of cAMP in the lysates was determined using a colorimetric direct cAMP enzyme immunoassay kit (Arbor Assays) according to the manufacturer’s instructions. For invasion and proliferation assays, infections were carried out using a 10:1 mix of a mutant strain and a trg::MudJ mutant (wild type for invasion and intracellular proliferation, but Lac+ due to the MudJ insertion). Competitive indices for invasion and proliferation were calculated as previously described (29) after plating appropriate dilutions and enumerating white colonies and blue colonies (trg::MudJ) in LB plates supplemented with 40 μg/ml 5-bromo-4-chloro-galactopyranoside (X-Gal). For invasion, the input was the initial mix of bacteria used in the infection and the output, bacteria recovered 2 h p.i. For intracellular proliferation, bacteria were recovered 2 h p.i. (input) and 24 h p.i. (output).
2.6 Luminescence measurements
Salmonella strains were grown in triplicate in the appropriate media and samples of 150 μl of each culture were used to measure luminescence and OD600, in 96-well white clear bottom plates using a FLUOstar Omega plate reader (BMG LABTECH). To measure the luminescence of intracellular bacteria, RAW264.7 macrophages were plated in 96-well white clear bottom plates at 3 x 104 cells per well, and were infected 24 h later with non-invasive bacteria (grown in LB for 24 h at 37°C with shaking) at a multiplicity of infection of 500. The cell culture was washed twice with PBS 30 min p.i., overlaid with DMEM containing 100 μg/ml gentamicin, and incubated for 1.5 h. The culture was then washed twice with PBS, covered with DMEM with 16 μg/ml gentamicin and incubated for 6 additional h. Luminescence was measured at 2, 4 and 8 h p.i. and the colony forming units per well were calculated after incubation with 1% Triton X-100 in PBS for 10 min at 37°C to release bacteria, plating appropriate dilutions in LB with Ap, and counting colonies after 24 h incubation at 37°C.
2.7 DNA amplification with the polymerase chain reaction, cloning, mutagenesis, and sequencing
Amplification reactions were carried out on a T100 Thermal Cycler (Bio-Rad) using Q5 High-Fidelity DNA polymerase (New England Biolabs) or MyTaq Red DNA polymerase (Bioline) according to the supplier’s instructions. Oligonucleotides are described in Table 2. Plasmid construction was carried out either using a classical cloning strategy based on enzymatic digestion or by Gibson assembly (40). The plasmids were sequenced with an automated DNA sequencer (Stab Vida). The disruption of sspH1 or sspH2 and replacement in strain 14028 with a gene conferring resistance to Km was carried out as previously described (41) using primers listed in Table 2 and plasmid pKD4. The antibiotic resistance cassette introduced by the gene targeting procedure was eliminated by recombination using the FLP helper plasmid pCP20. The addition of a DNA fragment encoding the 3xFLAG epitope tag at the 3’ end of sspH1 or sspH2 was carried out as described (42) using primers listed in Table 2 and the plasmid pSUB11. The protocol to generate chromosomal cyaA’ translational fusions was also previously described (43). Overlapping PCR was used to generate point mutations in the gene encoding human ubiquitin, UBC, using pIZ3683 as template and primers indicated in Table 2, and the PCR products were cloned in pCS2 with EcoRI/XbaI restriction endonucleases. The constructs for the purification of HA ubiquitin were made by Gibson assembly using primers P2pTYB21-Fw and P1pTYB21-Rv to amplify the plasmid pTYB21, and primers P1pTYB21-HA-UB-Fw and P2pTYB21-HA-UB-Rv to amplify the different ubiquitins of plasmids pIZ3657, pIZ3682, and pIZ3683. Double mutant ubiquitin was constructed by overlapping PCR using pIZ3683 as a template with the following primers: PCR1: P1-pTYB21-HA-UB-Fw and UB-K48R-Rv; PCR2: UB-K48R-Fw and pCS2-3; PCR3: P1-pTYB21-HA-UB-Fw and P1-pTYB21-HA-UB-Rv and cloning into pTYB21 by Gibson assembly. The presence of the inserts was confirmed with primers pTYB21-ext-Fw and pTYB21-ext-Rv.
2.8 Expression and purification of HA-ubiquitin
The plasmids pIZ3698, pIZ3695, pIZ3696, and pIZ3716 were transformed into E. coli ER2566. Overnight cultures of these strains were diluted 50 times in 500 ml of ampicillin-supplemented LB and incubated at 37°C until an OD600 of 0.5. The expression of target proteins was induced by adding 0.4 mM isopropyl-β-D-thiogalactoside and incubating overnight at 20°C with shaking. Subsequently, the cultures were centrifuged at 5000 g, 15 min, 4°C, the pellets were washed with PBS and centrifuged again to remove PBS. The pellets were lysed by sonication (output 2, duty cycle 30%, 1 min, 5 times) in column buffer (10 mM Tris-HCl pH 7.5, 150 mM NaCl, 10% glycerol, 0.5% NP40) containing 1 mM PMSF and protease inhibitor cocktail (1:200) (Sigma-Aldrich). The supernatants were separated from the pellets by centrifugation at 15000 g, 20 min, 4°C and loaded into the affinity chromatography column according to the IMPACT E6901 Manual (New England Biolabs), with the following modifications: crystal columns of 1.5 x 20 cm and 5 ml of chitin beads were used. To release target proteins from the column, beads were incubated with cleavage buffer with DTT 100 mM, 72 h at 4°C. The eluted fractions were dialyzed in 10 mM HEPES buffer pH 7.5 with 5% glycerol using the Slide-A-Lyzer® Dialysis cassette 3,500 MWCO, 3-12 ml capacity and incubated overnight at 4°C with slight shaking. To concentrate the proteins centrifugal filter units (Amicon® Ultra-15) were used. The aliquots were stored at -80°C and the protein concentration was quantified using the Coomassie (Bradford) protein assay kit from Thermo Scientific.
2.9 Purification of fusion proteins, electrophoresis, and immunoblotting
The expression of GST fusion proteins was induced by the addition of 1 mM isopropyl-β-D-thiogalactoside to E. coli BL21 (DE3) containing pGEX-4T-1, pGEX-4T-2 or their derivatives. Bacteria were sonicated in NP40 buffer and fusion proteins were isolated from bacterial lysates by affinity chromatography with glutathione-agarose beads (Sigma-Aldrich). Proteins tagged with 6His were produced after the addition of 1 mM isopropyl-β-D-thiogalactoside to E. coli XL1-Blue containing derivatives of pQE30 or pQE80L, purified with Ni-NTA agarose beads (Sigma-Aldrich) and eluted with 300 mM imidazole in binding buffer (50 mM NaH2PO4, 300 mM NaCl). For electrophoresis, bacterial and mammalian lysates were mixed with an equal volume of 4x Laemmli sample buffer and boiled for 5 min. Proteins were separated by SDS-PAGE using mini-protean TGX precast gels, 4-15% gradient (BioRad), and electrophoretically transferred to nitrocellulose filters for western blot analysis. Primary antibodies were: anti-FLAG M2 (mouse, monoclonal, 1:5,000, Sigma-Aldrich), anti-HA-peroxidase 3F10 (rat, 1:2000, Roche), anti-GroEL (rabbit, polyclonal, 1:20,000, Sigma-Aldrich), anti-DnaK 8E2/2 (mouse, monoclonal, 1:5,000, Assay Designs). Secondary antibodies were goat anti-mouse IRDye 800CW-conjugated or goat anti-rabbit IRDye 680RD-conjugated antibodies (LI-COR). The bands were detected using the Odyssey Fc imaging system (LI-COR).
2.10 In vitro ubiquitination assays
Ubiquitination reactions were carried out in a 20-μl mixture containing buffer A (25 mM Tris-HCl, pH 7.5, 50 mM NaCl, 5 mM ATP, 10 mM MgCl2, 0.1 mM DTT), 2 μg of HA-tagged ubiquitin, 0.25 μg of E1 (Boston Biochem, Cambridge, MA, USA) and 1 μg of E2 (human recombinant UbcH5b from Boston Biochem) in the presence or absence of ubiquitin ligases and substrates. Reactions were incubated at 37°C for 1 h with shaking and stopped by adding an equal volume of Laemmli sample buffer 4X containing 100 mM DTT and boiling. Some reactions were carried out with GST fusion proteins bound to glutathione-agarose beads, and the beads were washed five times with NP40 buffer before boiling in Laemmli sample buffer with 100 mM DTT.
2.11 Statistical analysis
Each competitive index and each cytokine concentration value are the mean of at least three independent infections or transfections. Means and standard deviations were calculated and a Student’s t-test was used to evaluate if each competitive index was significantly different from 1 or if every cytokine concentration was significantly different from the concentration in the control assay. P values of 0.05 or less are considered significant.
3 Results
3.1 In vitro expression and regulation of slrP, sspH1, and sspH2
S. enterica serovar Typhimurium has three orthologous genes, slrP, sspH1, and sspH2, which encode E3 ubiquitin ligases belonging to the NEL family of type III secretion effectors. To compare the expression of these genes, we cloned their promoter regions into the plasmid pSB377 to generate luxCDABE transcriptional fusions. Plasmids were introduced into S. enterica serovar Typhimurium strain 14028 and luminescence was measured after growth under conditions inducing SPI1 expression (LB with 0.3 M NaCl without aeration) and conditions inducing SPI2 expression (LPM at pH 5.8 with high aeration). Although the three genes were preferentially expressed under SPI2-inducing conditions (Figure 1A), there were dramatic differences in the ratios between SPI2- and SPI1-inducing conditions. While slrP was significantly expressed under SPI1-inducing conditions and the SPI2/SPI1 ratio was only 3.2, expression of sspH1 and sspH2 was more restricted and the SPI2/SPI1 ratios were 58.8 and 367.3, respectively. Luminescence was also measured using Salmonella strains lacking virulence regulators PhoP or SsrB. This analysis revealed that the two-component system PhoQ/PhoP was strictly necessary for the expression of slrP, sspH1, and sspH2 under SPI2-inducing conditions. Furthermore, SsrB, the positive regulator of SPI2, was essential for the expression of sspH2, but has only a partial influence on the expression of slrP and sspH1 (Figure 1B). These results were confirmed at the protein level by immunoblot using 3xFLAG chromosomal fusions (Figure 1C).
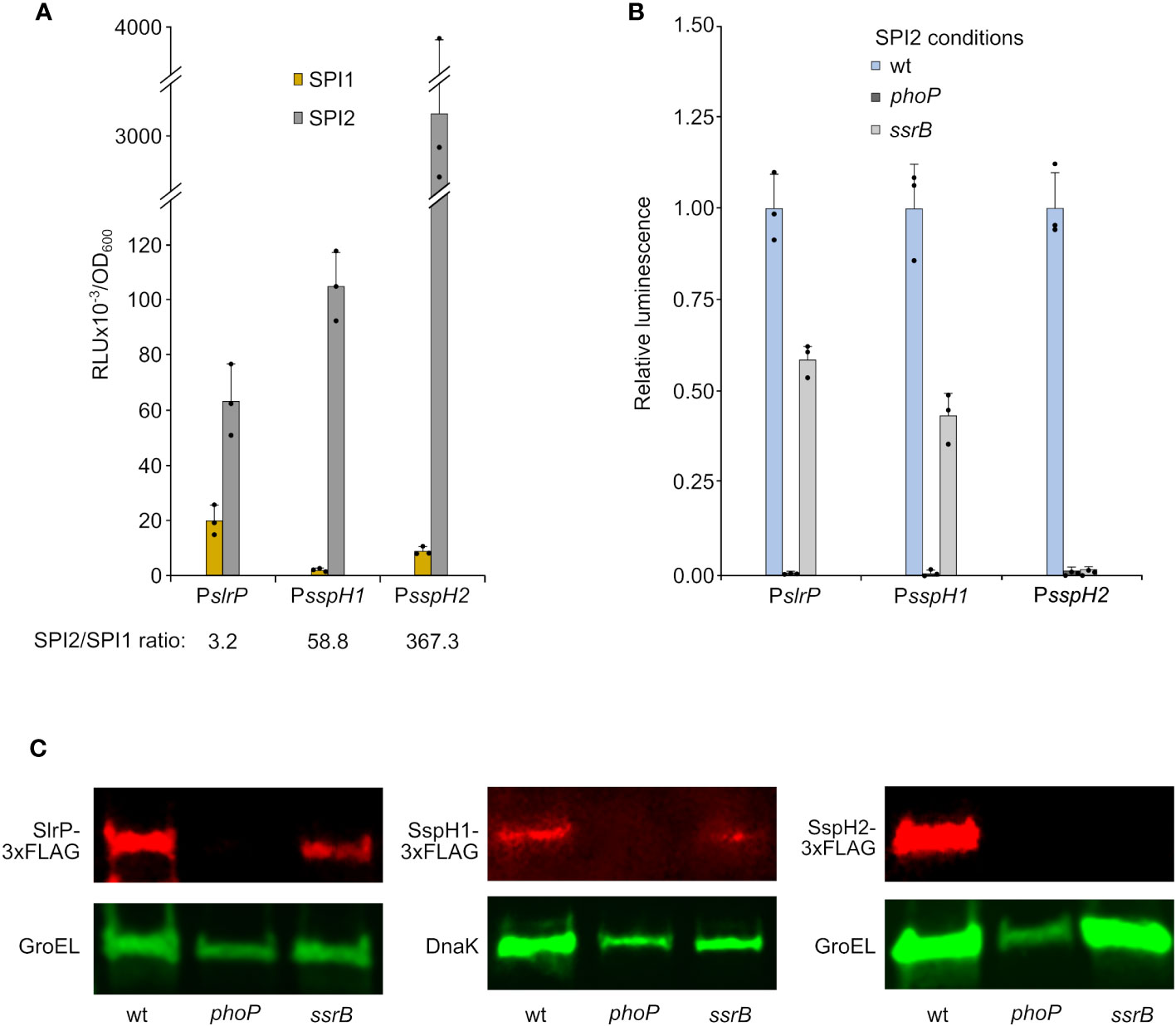
Figure 1 Expression and regulation of slrP, sspH1, and sspH2. DNA fragments containing the promoters of slrP, sspH1, or sspH2 were cloned into plasmid pSB377 to generate luxCDABE transcriptional fusions. (A) Luminescence was measured in cultures of S. enterica serovar Typhimurium strain 14028 carrying these plasmids grown to stationary phase in LB 0.3 M NaCl (SPI1-inducing conditions) and LPM (SPI2-inducing conditions). (B) Luminescence was measured under SPI2-inducing conditions in different genetic backgrounds: wild-type (wt), phoP null mutant or ssrB null mutant. RLU, relative light units. Means and standard deviations of three independent measurements are represented. Dots represent individual values. (C) Protein extracts of derivatives of S. enterica serovar Typhimurium strains producing 3xFLAG-tagged SlrP, SspH1, or SspH2 grown under SPI2-inducing conditions were resolved by SDS-PAGE. Immunoblotting was performed with monoclonal anti-FLAG antibodies. Anti-GroEL or anti-DNAK antibodies were used as loading control. Replicate experiments for this panel are shown in Supplementary Material.
3.2 Expression and regulation of slrP, sspH1, and sspH2 in host cells
Salmonella carrying transcriptional lux fusions were also used to infect cultures of epithelial HeLa cells, NRK fibroblasts, and RAW264.7 macrophages. Luminescence measurements at different times p.i. suggested that in most cases maximal expression was reached after 8 h (Figure 2), when Salmonella inside the SCV is also expressing SPI2. In vivo expression inside macrophages required activation by PhoP, which is consistent with the in vitro expression and regulation data shown in the previous section. However, expression of sspH2 inside HeLa and NRK cells was at least partially independent of PhoP.
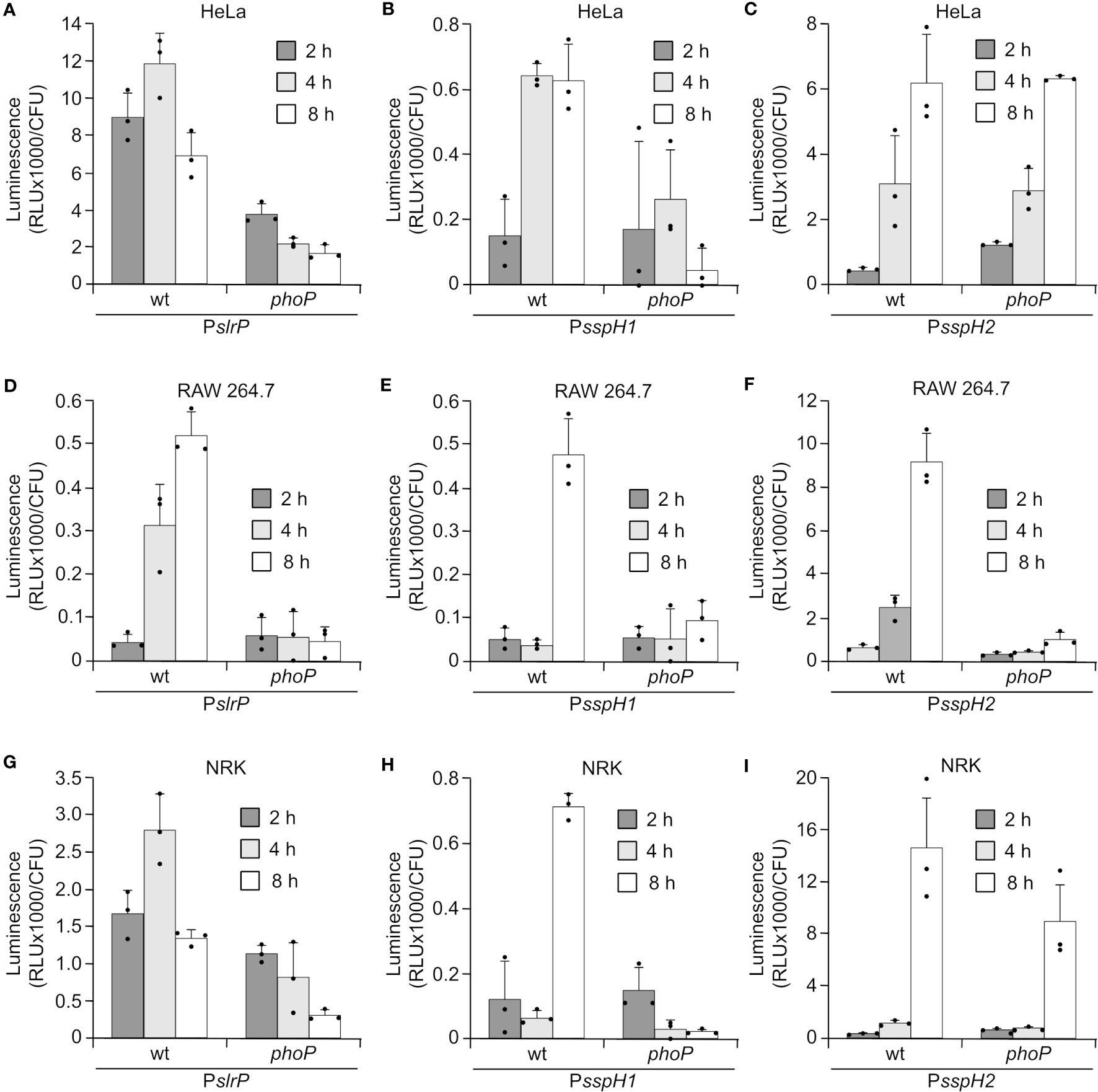
Figure 2 Expression of slrP, sspH1, and sspH2 during host cells infection. S. enterica serovar Typhimurium carrying plasmids expressing PslrP::luxCDABE (A, D, G), PsspH1:luxCDABE (B, E, H) or PsspH2::luxCDABE (C, F, I) were grown under invasive conditions (16 h in LB with 0.3 M NaCl without aeration) to infect HeLa cells (A–C) and NRK cells (G–I), or non-invasive conditions (24 h in LB at 37°C with aeration) to infect RAW264.7 macrophages (D–F). Luminescence produced by intracellular bacteria was measured 2, 4, and 8 h p.i.. Dots represent individual values.
3.3 Patterns of translocation of SlrP, SspH1, and SspH2 into host cells
The translocation of the three effectors into host cells was studied using two types of CyaA’ translational fusions: plasmid fusions under the control of a constitutive promoter, and chromosomal fusions controlled by native promoters. Fusions were introduced into wild-type Salmonella, as well as a prgH mutant (lacking T3SS1) and a ssaV mutant (lacking T3SS2), and these bacteria were used to infect human epithelial HeLa cells, murine RAW264.7 macrophages, and rat NRK fibroblasts. Translocation was detected by an increase in cAMP concentration in cell cultures that was measured 2 h and 8 h p.i. The bacteria were grown under SPI1-inducing conditions (invasive conditions) except for RAW264.7 8 h infections to avoid triggering early pyroptosis. As seen in Figure 3, the observed translocation patterns were very complex. Some general conclusions are: (i) translocation is more promiscuous when effectors are constitutively produced (plasmid fusions); (ii) under more physiological conditions (chromosomal fusions) SlrP can be detected at short and long times p.i., while SspH1 and SspH2 tend to be secreted only at long times; (iii) translocation is T3SS2-dependent for RAW264.7 at 8 h.
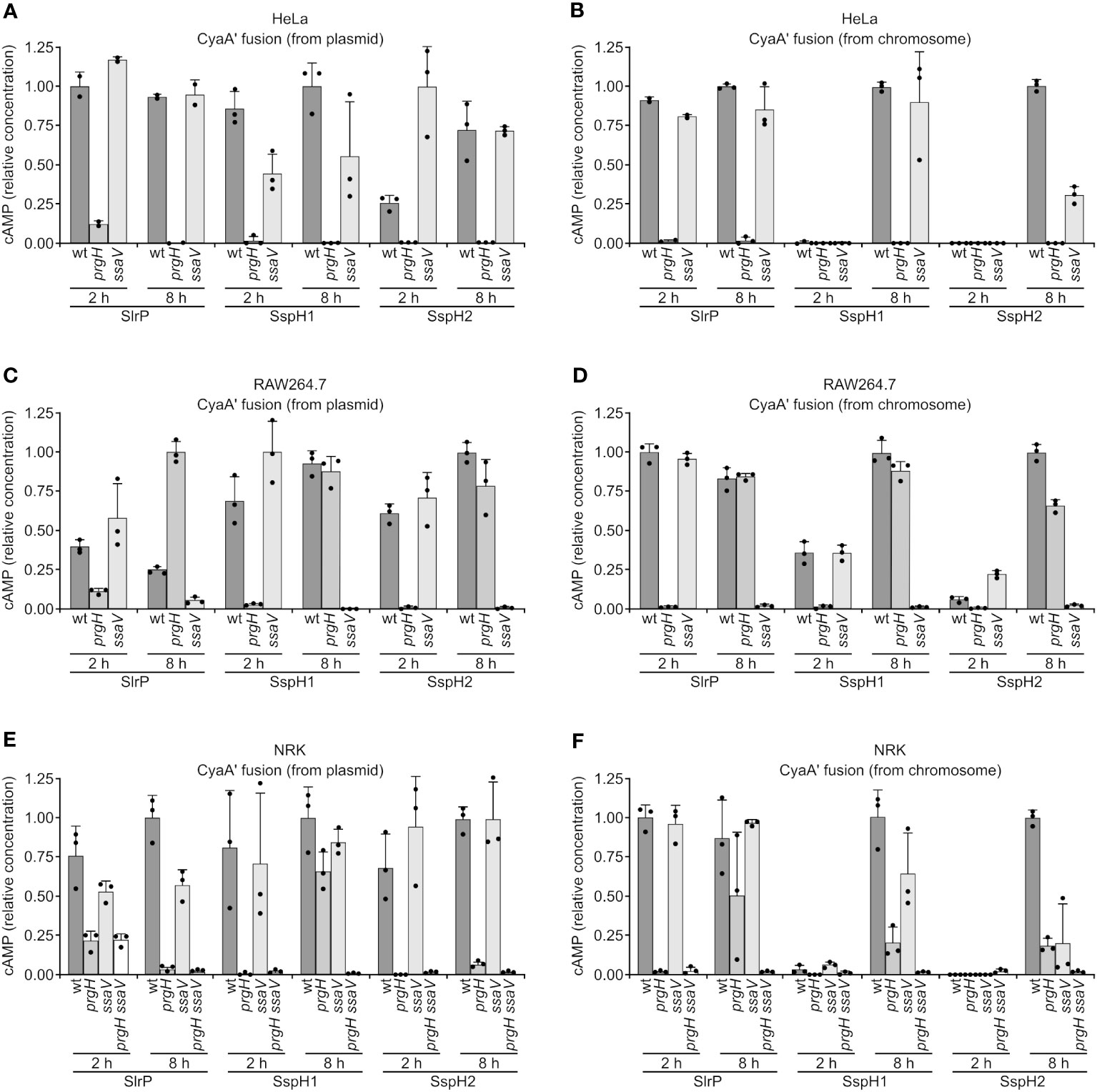
Figure 3 Translocation of SlrP, SspH1 and SspH2 into mammalian cells. Human epithelial HeLa cells (A, B), RAW264.7 murine macrophages (C, D), and NRK rat kidney fibroblasts (E, F) were infected with derivatives of S. enterica serovar Typhimurium 14028 (wild-type, wt, prgH, ssaV, and prgH ssaV strains) carrying a plasmid expressing SlrP-CyaA’, SspH1-CyaA’, or SspH2-CyaA’ fusions from a constitutive promoter (A, C, E) or chromosomal fusions expressed under native promoters (B, D, F). Bacteria were grown under SPI1-inducing conditions (invasive conditions) except for infections of RAW264.7 cells for 8 h (non-invasive conditions to prevent early cell death). Levels of cAMP were measured as and indication of translocation. For each effector and cell type, these levels were relativized to the level obtained for the wild-type infection at 2 h or 8 h, that was set to 1. Means and standard deviations from triplicate experiments are represented. Dots represent individual values.
3.4 Role in host cell invasion and intracellular proliferation
To test the relevance of effectors of the NEL family in cell invasion and intracellular proliferation, competitive indices were calculated after mixed infections of host cells with the triple mutant slrP sspH1 sspH2 and a strain carrying a trg::lacZ fusion. The trg mutant has no invasion or proliferation defect (29) and forms blue colonies in X-Gal supplemented plates, providing a way to distinguish between colonies of both strains. A ratio 10:1, slrP sspH1 sspH2: trg::lacZ, was used for the infection input in order to prevent the possibility of complementation of the triple mutant by the control strain. Invasion and proliferation were tested in epithelial HeLa cells and NRK fibroblasts. Proliferation was also studied in RAW264.7 macrophages. As seen in Figure 4A, a significant defect was only detected for proliferation inside NRK cells. Then we measured intracellular proliferation in NRK of single and double mutants. Although single mutants were not defective, double mutants slrP sspH1 and slrP sspH2 exhibited a slightly but significantly reduced ability to proliferate inside these cells (Figure 4B). These results suggest the existence of a certain redundancy between the three effectors of this family and an additive effect of the mutations studied for this particular phenotype.
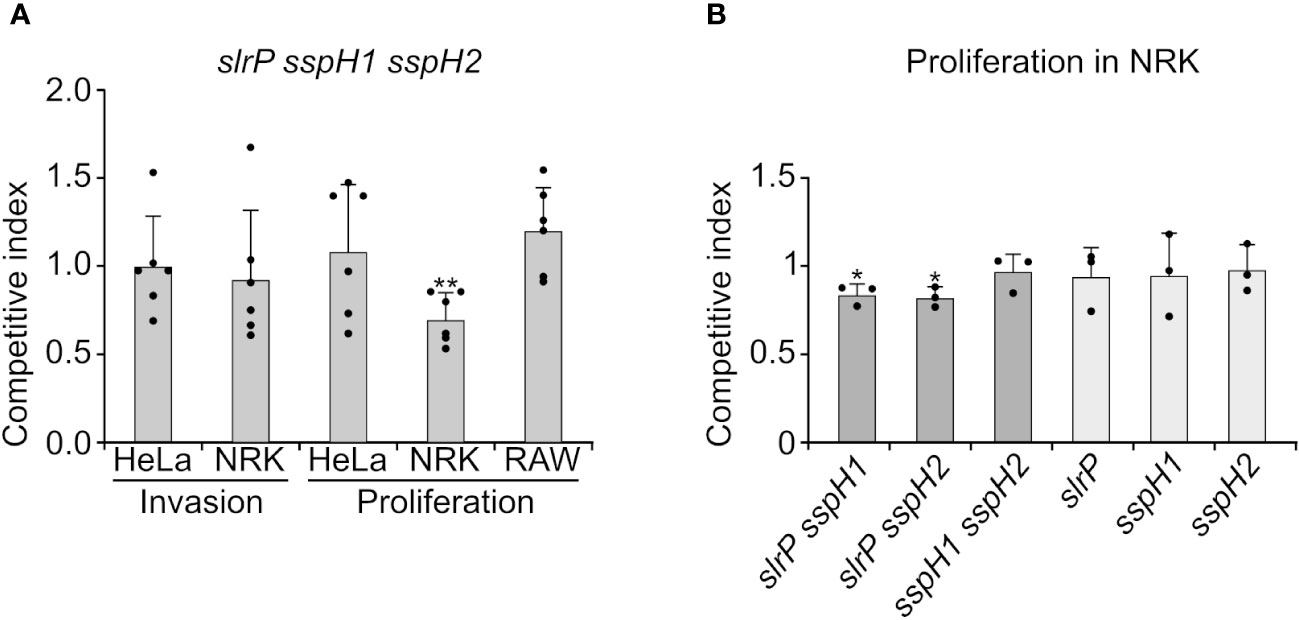
Figure 4 Effect of SlrP, SspH1, and SspH2 on host cell invasion and proliferation. (A) Analysis of invasion and intracellular proliferation of the triple mutant slrP sspH1 sspH2 in mixed infections with a trg::MudJ mutant used as the wild-type strain. (B) Analysis of intracellular proliferation in NRK cells of the indicated mutants in mixed infections with a trg::MudJ mutant used as the control strain. Competitive indices are the means of three infections. The error bars represent the standard deviations. Dots represent individual values. Asterisks denote that the indices are significantly different from 1 for a t-test: *P-value < 0.05, **P-value < 0.01.
3.5 Specificity of interactions and ubiquitination of known substrates
Effectors of the NEL family are typically composed of a N-terminal secretion motif, a leucine-rich repeat (LRR) domain, and a C-terminal novel E3 ubiquitin ligase (NEL) domain (16). While the NEL domain is necessary for the ubiquitination of host proteins, the LRR domain is supposed to be involved in the interaction with substrates. We wondered whether the selection of substrates relied entirely on this domain. To test this hypothesis, we cloned DNA fragments encoding the N- or C-part of SlrP, SspH1 and SspH2, and combinations of these fragments to generate all possible chimeric proteins (Figure 5). We used the vector pLEX10 to test interactions using the yeast two-hybrid system and the vector pQE80L to produce and purify proteins with the 6-His tag and test them in ubiquitination assays.
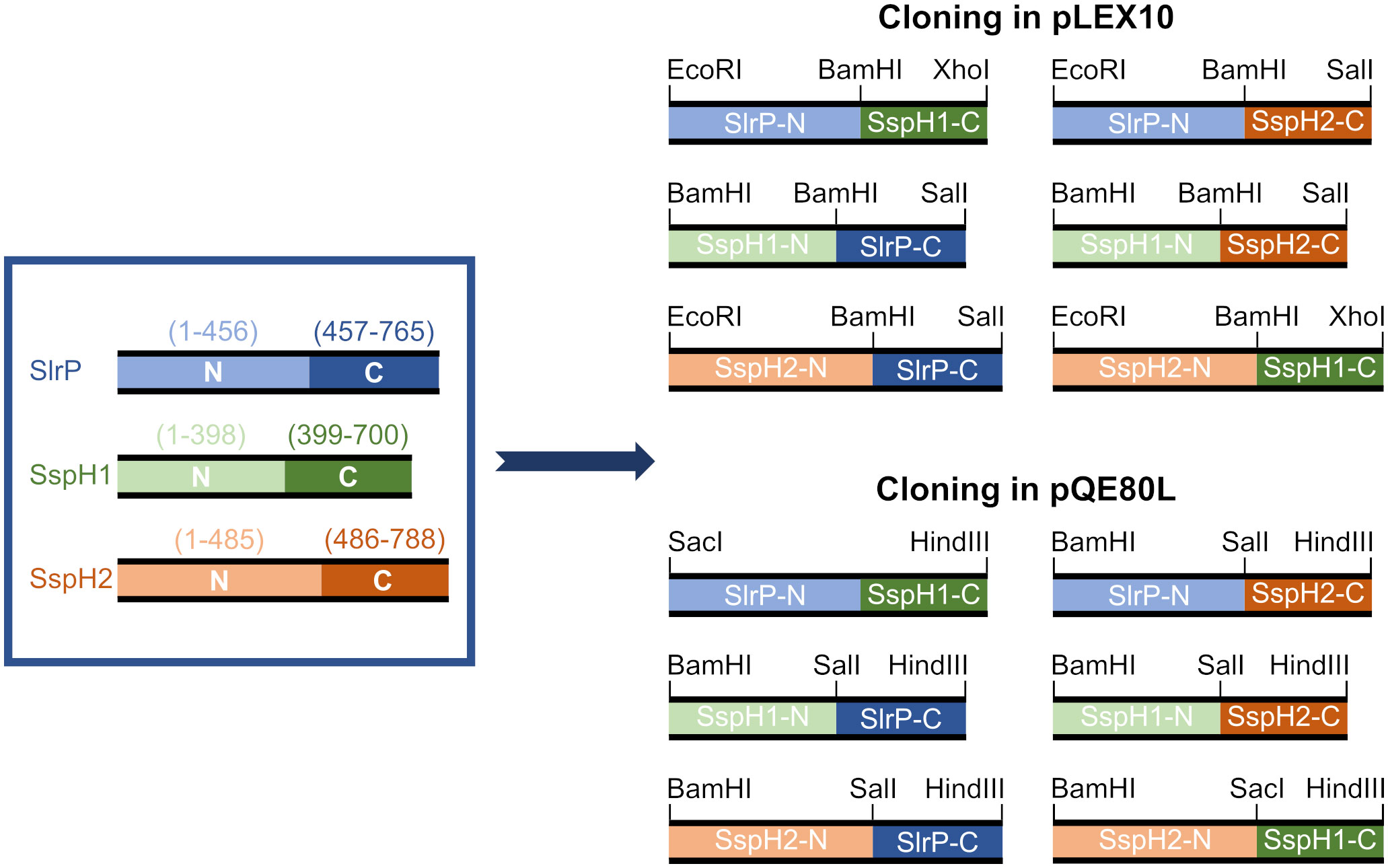
Figure 5 Design of clones for the expression of chimeric NEL effectors. The represented N- and C-terminal fragments of SlrP, SspH1 and SspH2 were cloned into the vectors pLEX10 (for yeast two-hybrid experiments) and pQE80L (for 6His fusions generation) using the indicated restriction enzymes.
Physical interactions were studied for cognate substrates of these effectors: SNRPD2 (21) for SlrP, PKN1HR1b subdomain (44, 45) for SspH1 and NOD1 (24) for SspH2. The C-terminal fragment of SlrP (SlrP-C) and the N-terminal fragment of SspH1 (SspH1-N) exhibited autoactivation problems that precluded the analysis of interactions in the yeast two-hybrid system (Figure 6). However, some interesting conclusions can be drawn from this analysis. (i) The N-terminal fragments (that included the LRR domains) of SlrP and SspH1 direct the interaction with SNRPD2 and PKN1, respectively, alone or in fusion with the C-terminal domain of SspH2. (ii) No interaction was detected for NOD1, which may be due to the necessity of the formation of a ternary complex between SspH2, NOD1 and SGT1 (24).
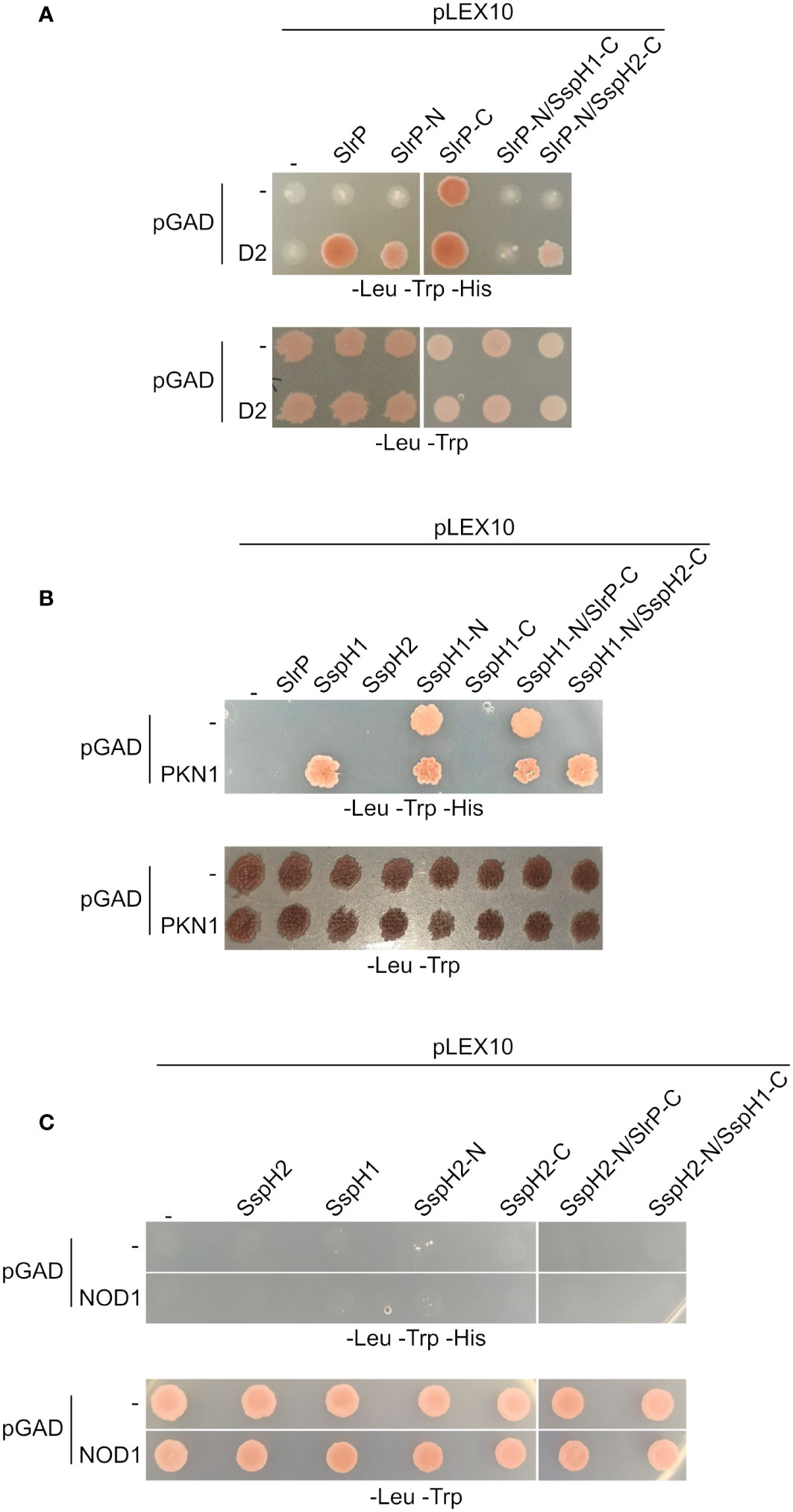
Figure 6 Interaction of SlrP, SspH1 and SspH2 with host proteins in the yeast two-hybrid system. Derivatives of pLEX10 and pGAD1318 (pGAD) were introduced into yeast strain L40 by transformation. Transformants were selected in media lacking tryptophan and leucine. (A) Study of the interaction of SlrP or derivatives in which the C-terminal part was replaced by that of SspH1 or SspH2 with SNRPD2 (D2). (B) Study of the interaction of SspH1 or derivatives in which the C-terminal part was replaced by that of SlrP or SspH2 with PKN1. (C) Study of the interaction of SspH2 or derivatives in which the C-terminal part was replaced by that of SlrP or SspH1 with NOD1.
In view of these results, we decided to focus on the study of the ubiquitination of SNRPD2 and PKN1. In vitro ubiquitination assays shown in Figure 7 indicated that SNRPD2 can be ubiquitinated by SlrP, but not by SspH1 or SspH2, as previously described (21). Interestingly, this host protein is also ubiquitinated in the presence of the chimeric proteins SlrP-N/SspH1-C and SlrP-N/SspH2-C. In addition, PKN1 can be ubiquitinated by SspH1, but not SlrP or SspH2, and by the chimeric protein SspH1-N/SspH2-C. These results are fully consistent with the conclusions obtained from the physical interaction analysis and support our hypothesis that substrate specificity for these effectors resides in the LRR domain.
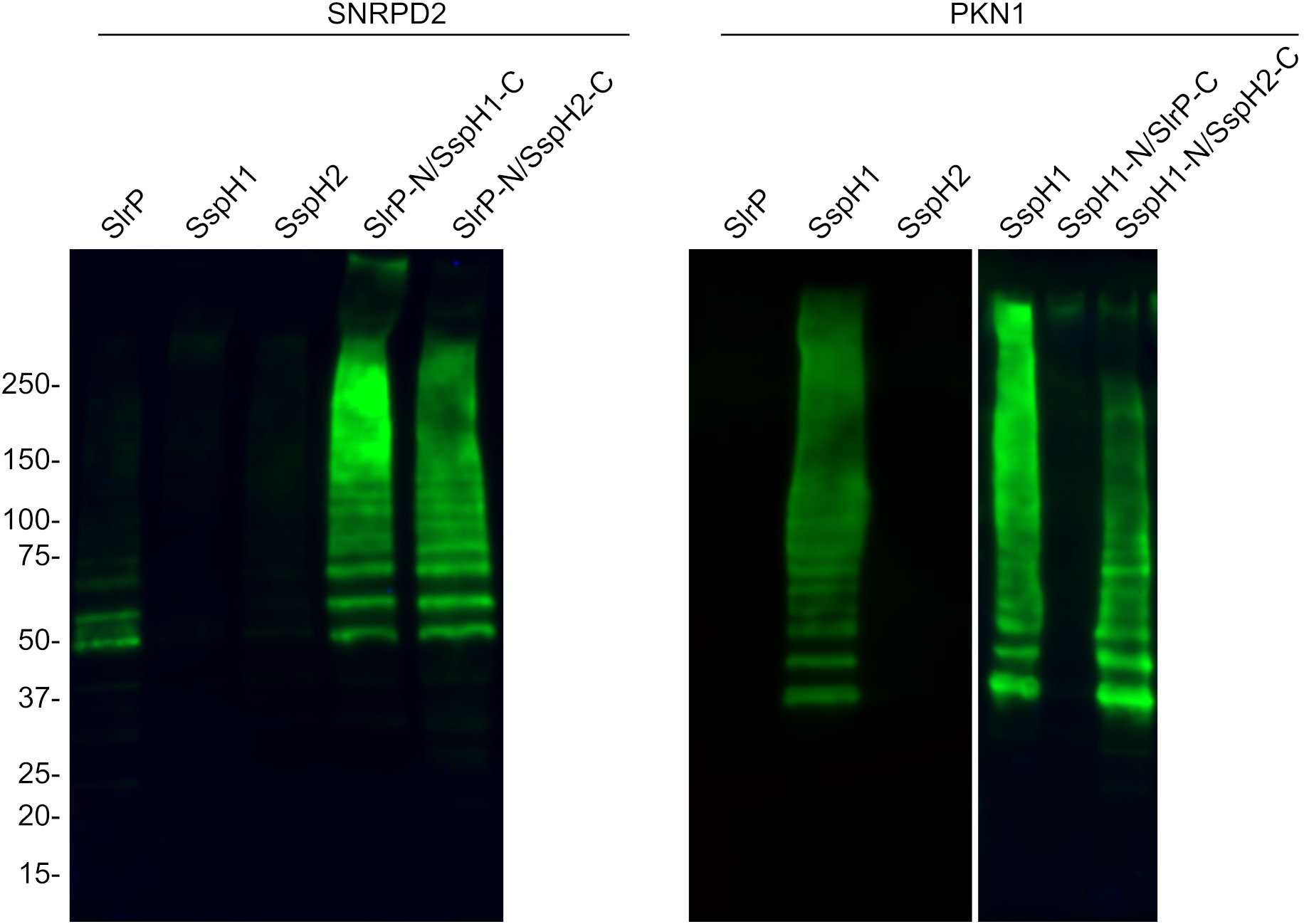
Figure 7 Ubiquitination of SNRPD2 and PKN1 by SlrP, SspH1, SspH2 and chimeric effectors. The ubiquitination of GST-SNRPD2 or GST-PKN1 bound to glutathione-agarose beads was tested in the presence of HA-ubiquitin, E1, E2, and a Salmonella effector fused to 6His. The beads were washed prior to immunoblot analysis. The sizes in kDa of the molecular weight markers are shown on the left. Replicate experiments for this figure are shown in Supplementary Material.
Lysines 48 and 63 are the main ubiquitin residues involved in the formation of polyubiquitin chains and the use of a particular Lys results in different outcomes in terms of stability or activity of the ubiquitinated protein. Therefore, we next produced different forms of HA-tagged ubiquitin: wild-type ubiquitin and mutants with specific Lys changed into Arg (K48R, K63R and K48R/K63R). Then we used these ubiquitin forms in ubiquitination assays with the three effectors (Figure 8). The results of these experiments suggest that, while the polyubiquitination catalyzed by SspH1 and SspH2 is at least partially dependent on Lys 48, other lysines may be used by SlrP.
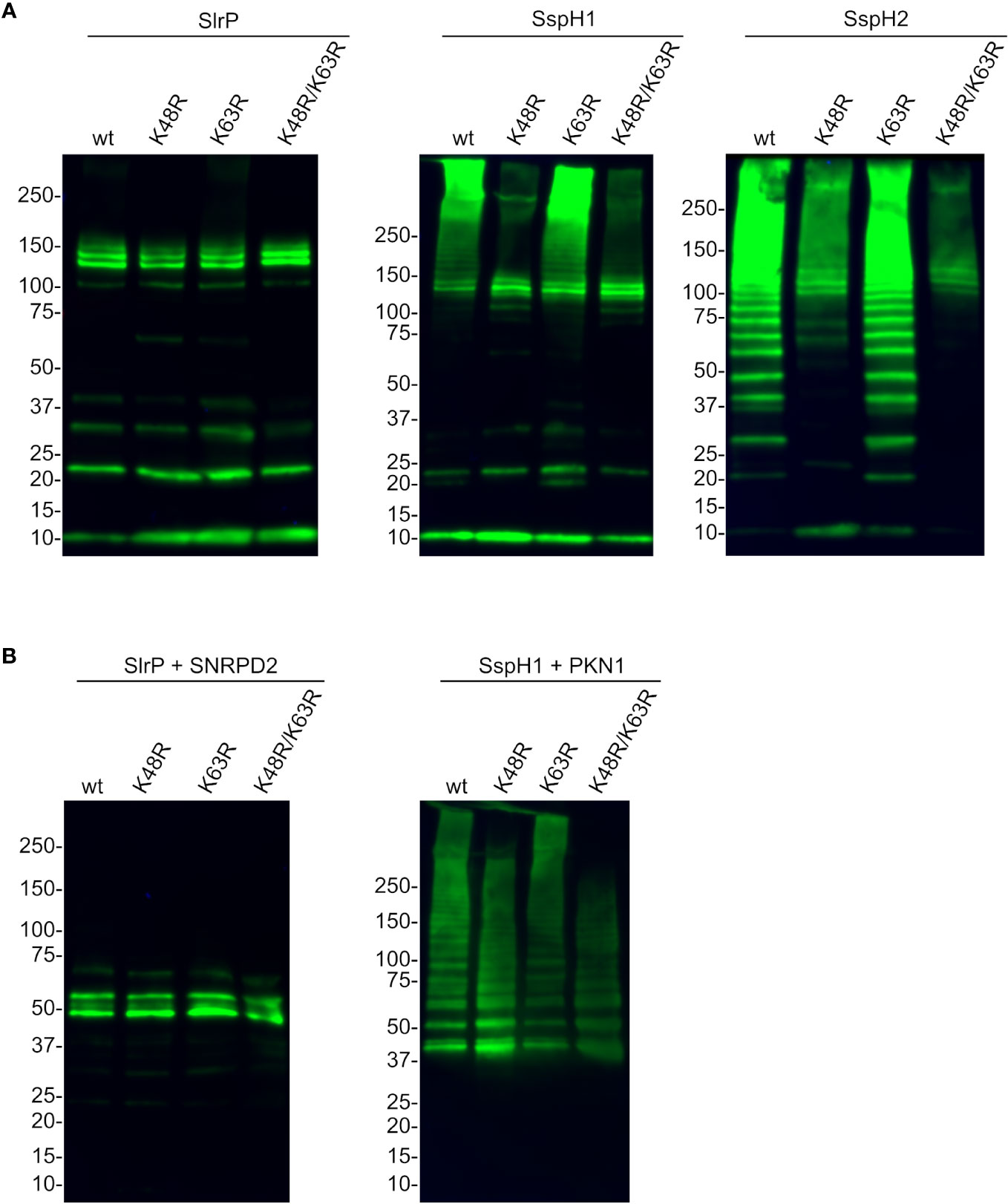
Figure 8 Analysis of the specific type of polyubiquitination catalyzed by SlrP, SspH1, and SspH2. HA-ubiquitin and its derivatives with the indicated mutations replacing lysines by arginines (K48R, K63R, K48R/K63R) were used in ubiquitination assays with 6His fusions of SlrP, SspH1, and SspH2. (A) No additional substrate was added and the whole reaction was analyzed to detect polyubiquitination of ubiquitin. (B) GST-SNRPD2 or GST-PKN1 bound to glutathione-agarose beads were added as substrates and beads were washed prior to immunoblot analysis with anti-HA antibodies. The sizes in kDa of the molecular weight markers are shown on the left. Replicate experiments for this figure are shown in Supplementary Material.
3.6 Effects in cytokine secretion
Previous studies have shown that SspH1 inhibits IL-8 production in host cells (46). In contrast, SspH2 enhances IL-8 secretion (24) and may decrease expression of genes that encode several cytokines (47). These experiments were carried out using different methodologies and different host cell models. Therefore, we decided to measure the effect of SlrP, SspH1, and SspH2 on the secretion of several cytokines using a comparable approach. Human HeLa cells were transfected with plasmids expressing slrP, sspH1, sspH2, or the empty vector as a control. Then, CCL5, IFN-γ, IL-1β, IL-6 and IL-8 concentrations were measured in the supernatants of cell cultures 24 h after transfection. Secretion of IFN-γ and IL-1β was not detected in this model. As seen in Figure 9, while SlrP had no effect on the secretion of the assayed cytokines, SspH1 and SspH2 caused a decrease in the secretion of CCL5 and IL-6, suggesting an anti-inflammatory role for these effectors.
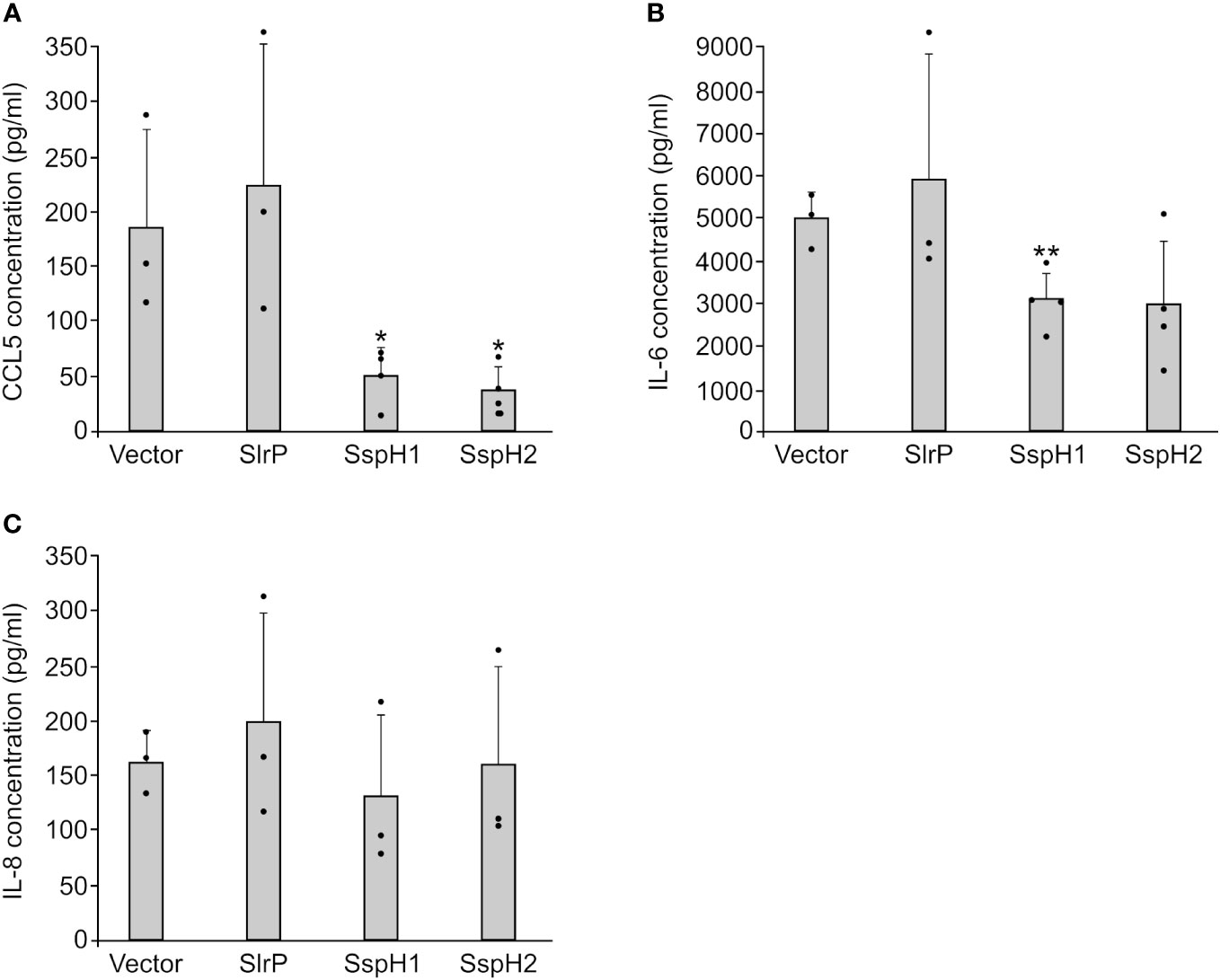
Figure 9 Effect of SlrP, SspH1 and SspH2 on the secretion of cytokines by mammalian cells. HeLa cells were transfected with a plasmid expressing SlrP, SspH1 or SspH2 or with the empty vector and the concentration of CCL5 (A), IL-6 (B), and IL-8 (C) was measured in the supernatants of cell cultures 24 h after transfection. Data are presented as mean values + standard deviations of at least three biological replicates. Dots represent individual values. * p < 0.05, ** p < 0.01, for a two-tailed Student’s t-test comparing cells expressing each effector with cells transfected with the empty vector.
4 Discussion
Salmonella injects more than 40 effectors into host cells to manipulate their behavior and facilitate the survival and replication of the pathogen. Some of these effectors act as adaptor proteins or contribute to modifying the lipid content of host membranes, but most of these effectors exhibit some biochemical activity that mediates post-translational modifications of host proteins (48). These modifications include ubiquitination, deubiquitination, phosphorylation, dephosphorylation, hydrolysis, deamidation, glycosylation, and acetylation. Interestingly, there are effectors that share biochemical activity and have similarities in sequence and structure, so that they are part of the same family. Examples of effector families found in S. enterica serovar Typhimurium are the family of effectors with arginine N-glycosyltransferase activity, with SseK1, SseK2 and SseK3 (49), and the NEL family of E3 ubiquitin ligases, with SlrP, SspH1 and SspH2 (16). Redundancies can provide an additional level of robustness to the Salmonella virulence strategy: if one effector is inactivated by host defenses, another one can carry out the function. However, there are several ways in which members of the same family can accomplish different missions: (i) they can differ in the promoter region of the genes encoding them, and so their regulation may be different; (ii) the N-terminal portion of the protein, which directs translocation, may be adapted to a particular T3SS apparatus, or the timing of expression of the protein can restrict translocation to that particular apparatus; (iii) specific domains can direct selection of interaction and biochemical targets; (iv) subcellular localization can be different and may lead to functional specialization.
This study was initiated to analyze all these aspects in the case of the NEL family of E3 ubiquitin ligases. Regarding regulation, our experiments show that synthesis of the three effectors is totally dependent on PhoP in vitro under SPI2-inducing conditions (Figure 1). PhoP is the cytosolic response regulator of the two-component regulatory system PhoQ/PhoP. The membrane-associated sensor PhoQ is activated by a variety of signals, including low periplasmic Mg2+ concentration, mildly acidic pH in the cytosol, increased osmolarity, and sublethal concentrations of cationic antimicrobial peptides (50). Once activated, PhoP is a master regulator of Salmonella virulence. It represses the transcription of hilA, which encodes the major transcriptional activator of SPI1 genes, and activates the transcription of ssrB, whose product is the response regulator of the SsrA/SsrB two-component regulatory system, which is necessary for the expression of SPI2 genes. Interestingly, SsrB is essential for the transcription of sspH2, whereas the regulation of slrP and sspH1 by SsrB is only partial (Figure 1B). The results obtained with transcriptional lux fusions were confirmed at the protein level by immunoblotting (Figure 1C). The expression of the genes encoding the three effectors is also dependent on PhoP in vivo during macrophage infection (Figure 2). Interestingly, transcription of sspH2 is PhoP independent inside HeLa and NRK cells. These results suggest that there may be other signals inside these cells that are able to activate SPI2 expression. This is consistent with previous data showing, for instance, that the PhoQ/PhoP pathway is not required for SPI2 expression in the presence of phosphorylated OmpR (51).
The expression and regulation pattern of sspH2 is consistent with its translocation into host cells at 8 h p.i., when SPI2 is expressed, but not 2 h p.i., when only SPI1 has been expressed during invasion (Figure 3B, D, F). In contrast, SlrP translocation was detected at 2 h and 8 h p.i. since, as previously described (18), it is expected to be translocated both by T3SS1 and T3SS2. The timing of translocation of SspH1 is more similar to that observed for SspH2 and appears to occur preferentially through T3SS2. In these experiments, we expressed the genes encoding the effectors from their native promoters (CyaA’ fusion in the chromosome) or from a constitutive promoter (CyaA’ fusions in a plasmid). This analysis provides an additional conclusion: the three effectors have the ability to be translocated by T3SS1 and T3SS2, only the lack of expression under native conditions prevents SspH1 and SspH2 from being translocated through T3SS1 (compare, for instance, Figures 3A, B at 2 h p.i.). Translocation was studied in three different cell lines: HeLa (human epithelial), RAW264.7 (murine macrophages), and NRK (rat fibroblasts). The diverse patterns observed can be attributed, at least in part, to the different ways of entry used by Salmonella. Invasion of HeLa cells occurs only by the trigger system induced by T3SS1. Therefore, a prgH mutant, lacking T3SS1, is unable to invade and so translocation of effectors cannot be detected at any time in these cells (Figure 3B). In contrast, the prgH mutant is capable of entering macrophages by phagocytosis and can translocate SlrP, SspH1, and SspH2 into these cells at 8 h p.i., but translocation is lost in the ssaV mutant, indicating that T3SS2 is the relevant system under these conditions (Figure 3D). The situation is more complex in fibroblasts, with significant secretion of SlrP through both systems (Figure 3F, the double mutation is needed to prevent translocation). This can be explained because Salmonella invades fibroblasts through multiple routes (52). It should be taken into account that the method used to detect translocation of effectors into host cells has some limitations. In particular, in HeLa and NRK cells the interpretation is more difficult than in RAW264.7 cells because we cannot clearly separate T3SS1-dependent from T3SS2-dependent secretion since T3SS1 expression (invasive conditions) are needed in order to infect these cells. Previous data indicated that half-lives of effectors of this family are longer than several hours (53, 54). As a consequence, the high levels of translocation observed for SlrP in HeLa cells (Figure 3B) at 8 h with the ssaV mutant (lacking T3SS2) could be due to the translocation that occurred previously through T3SS1.
The multifactorial nature of bacterial virulence makes it prone to be affected by redundancy phenomena. In fact, the lack of only one of the more than 40 T3SS effectors, which can be obtained with a single null mutant, usually does not lead to a significant decrease in Salmonella pathogenicity. This is what we observe for SlrP, SspH1, and SspH2 in our in vitro cellular infection models (Figure 4). However, a mutant lacking the three effectors is significantly attenuated for intracellular proliferation in NRK cells compared to wild-type bacteria. These results suggest that these three effectors work redundantly to allow Salmonella survival inside fibroblasts. They also show that there are different requirements and mechanisms for the adaptation of this pathogen to different host cell lines since no defects were detected in epithelial cells or macrophages.
SlrP, SspH1, and SspH2 share a similar structure and a similar autoinhibition mechanism in which the activity of the NEL domain is repressed by the LRR domain in the absence of substrate (55). There are several well defined host substrates for these effectors that include SNRPD2, PKN1, and NOD1, respectively. We have studied the level of specificity in the interaction and ubiquitination of these substrates and the determinants of this specificity. To do that, we have generated all possible combinations of the LRR and NEL domains of the three effectors (Figure 5) and tested their interaction with host proteins using the yeast two-hybrid system. Our previous results showed that SNRPD2 interacts specifically with SlrP but not with SspH1 or SspH2 (21). Here, we show that the N-terminal region of SlrP, including the LRR domain, directs this interaction (Figure 6A). Analogous results were obtained for PKN1, whose Hr1b domain interacts specifically with SspH1. In this case, the interaction with the isolated N-terminal region, containing the LRR domain, could not be tested due to transactivation of the reporter gene in the yeast two-hybrid system. However, the fact that this interaction occurs through this part of the protein was confirmed by the chimeric protein SspH1-N/SspH2-C, which did not show transactivation (Figure 6B). These results confirm the notion that host proteins interact with these effectors through their LRR domains. More interestingly, the chimeric proteins SlrP-N/SspH1-C and SlrP-N/SspH2-C were able to ubiquitinate SNRPD2 (Figure 7), indicating that the NEL domains of the three effectors can catalyze the ubiquitination of this host target if fused to the interaction determinant provided by the LRR domain of SlrP. In fact, the NEL domains of SspH1 and SspH2 demonstrated greater ubiquitination activity on SNRPD2 than the NEL domain of SlrP under these conditions. Similar results were obtained for PKN1 and SspH1, although in this case ubiquitination was not detected with the chimeric protein SspH1-N/SlrP-C. This may be due to lower activity of the catalytic domain of SlrP or to an improper folding of the chimera.
Our ubiquitination experiments showed in all cases the classical ladders that are indicative of polyubiquitination. The type of linkage between ubiquitins within the ubiquitin polymer determines the fate of the marked protein. As mentioned above, K48-linked chains are typically related to proteasomal degradation, while K63-linked chains are generally involved in signaling processes. In order to find out the type of polyubiquitination that was catalyzed by each effector, we generated and used mutant forms of ubiquitin. Assays carried out without a substrate, where polyubiquitination of ubiquitin is observed, revealed a strong dependence on Lys48 for the activity of SspH2 and also SspH1, although in the latter the effect is observed more clearly in very high molecular weight polyubiquitin bands (Figure 8A). The pattern of SlrP-induced polyubiquitination did not change dramatically using K48R or K63R mutant ubiquitins, suggesting that other residues may be involved. In fact, ubiquitin has five additional lysine residues (K6, K11, K27, K29, and K33) that, together with methionine 1 (M1), can be ubiquitinated. Ubiquitination assays using SNRPD2 or PKN1HR1b as substrates confirm these conclusions (Figure 8B).
S. enterica serovar Typhimurium manipulates host inflammatory responses in complex ways (56, 57). Stimulation of inflammatory signaling depends on the T3SS effectors SopB, SopE, and SopE2, which act at the beginning of infection to help Salmonella compete with the normal intestinal microbiota. However, to prevent an excessive inflammatory response that would be detrimental to the bacteria, Salmonella limits this response by expressing other effectors, including SptP, PipA, GogA, GtgA and AvrA (58–61). SspH1 from S. enterica serovar Typhimurium also down-regulates the production of the pro-inflammatory factor IL-8 (46). In contrast, SspH2 was shown to enhance IL-8 secretion (24), and the effect of SlrP was not previously reported. Here, we studied the effect of the three effectors on several pro-inflammatory cytokines. Although we did not detect significant effects on IL-8 secretion, there was a reduction in CCL5 and IL-6 secretion in the presence of SspH1 or SspH2, but not SlrP (Figure 9). Previous experiments in a mouse model of anorexia suggested that SlrP from S. enterica serovar Typhimurium inhibited inflammasome activation and IL-1β maturation in the small intestine (62). Furthermore, SspH2 from S. enterica serovar Enteritidis was shown to reduce the expression of pro-inflammatory factors, including IL-1β, IFN-γ, and iNOS, in Caco-2 BBE cells (47). Together, these results and the results presented here support a predominant anti-inflammatory role for Salmonella NEL effectors that can be expressed in different ways depending on the biological model and context. Interestingly, SlrP and SspH2 are involved in the inhibition of antigen presentation in dendritic cells (63) and in preventing the migration of these cells toward the chemokine CCL19 (64). Furthermore, Salmonella has been shown to interfere with MHC class II antigen presentation, inducing polyubiquitination and reduction of cell surface HLA-DR expression (65). This effect depends on T3SS2, so it is tempting to speculate with the involvement of Salmonella NEL effectors. However, previous experiments failed to identify them as potential candidates and showed that the T3SS2 effector SteD decreased surface levels of MHC class II in dendritic cells by recruiting the host E3 ubiquitin ligase Wwp2 (66, 67). Further experiments will be required to clarify the role of these effectors in this function.
In conclusion, despite their similar structure and biochemical activity, the three members of the NEL family of T3SS effectors of S. enterica serovar Typhimurium have different expression and translocation patterns and recognize specific host targets that are modified with different polyubiquitination patterns. Unfortunately, few targets of these effectors have been described so far. Therefore, additional research is needed to obtain a complete list of these targets and a clearer picture of all the effects of these Salmonella proteins on the host.
Data availability statement
The original contributions presented in the study are included in the article/Supplementary Material. Further inquiries can be directed to the corresponding authors.
Author contributions
AB-B: Formal analysis, Investigation, Methodology, Visualization, Writing – review & editing. PM-M: Formal analysis, Investigation, Methodology, Visualization, Writing – review & editing. CV-G: Formal analysis, Investigation, Methodology, Visualization, Writing – review & editing. JB-B: Formal analysis, Funding acquisition, Project administration, Resources, Supervision, Writing – review & editing. FR-M: Formal analysis, Funding acquisition, Resources, Supervision, Writing – original draft, Writing – review & editing.
Funding
The author(s) declare financial support was received for the research, authorship, and/or publication of this article. This research was supported by “Proyecto PID2022-136863NB-I00 financiado por MCIN/AEI /10.13039/501100011033/ y por FEDER Una manera de hacer Europa”.
Acknowledgments
The authors are grateful to Laura Tomás Gallardo (Servicio de Proteómica y Bioquímica, Universidad Pablo de Olavide, Sevilla) for help in the intein-mediated purification of HA-ubiquitin. We also thank the contribution of grant PID2019-106132RB-I00 funded by MCIN/AEI/10.13039/501100011033, grant P20_00576 funded by Fondo Europeo de Desarrollo Regional (FEDER) and Consejería de Transformación Económica, Industria, Conocimiento y Universidades de la Junta de Andalucía, and grant US-1380805 funded by Universidad de Sevilla, FEDER and Consejería de Transformación Económica, Industria, Conocimiento y Universidades de la Junta de Andalucía.
Conflict of interest
The authors declare that the research was conducted in the absence of any commercial or financial relationships that could be construed as a potential conflict of interest.
Publisher’s note
All claims expressed in this article are solely those of the authors and do not necessarily represent those of their affiliated organizations, or those of the publisher, the editors and the reviewers. Any product that may be evaluated in this article, or claim that may be made by its manufacturer, is not guaranteed or endorsed by the publisher.
Supplementary material
The Supplementary Material for this article can be found online at: https://www.frontiersin.org/articles/10.3389/fimmu.2024.1328707/full#supplementary-material
References
1. Teklemariam AD, Al-Hindi RR, Albiheyri RS, Alharbi MG, Alghamdi MA, Filimban AAR, et al. Human salmonellosis: A continuous global threat in the farm-to-fork food safety continuum. Foods Basel Switz (2023) 12:1756. doi: 10.3390/foods12091756
2. Pais SV, Kim E, Wagner S. Virulence-associated type III secretion systems in Gram-negative bacteria. Microbiol Read Engl (2023) 169:1328. doi: 10.1099/mic.0.001328
3. Chen P, Goldberg MB. Recent insights into type-3 secretion system injectisome structure and mechanism of human enteric pathogens. Curr Opin Microbiol (2023) 71:102232. doi: 10.1016/j.mib.2022.102232
4. De Ryck J, Van Damme P, Goormachtig S. From prediction to function: Current practices and challenges towards the functional characterization of type III effectors. Front Microbiol (2023) 14:1113442. doi: 10.3389/fmicb.2023.1113442
5. Fattinger SA, Sellin ME, Hardt W-D. Salmonella effector driven invasion of the gut epithelium: breaking in and setting the house on fire. Curr Opin Microbiol (2021) 64:9–18. doi: 10.1016/j.mib.2021.08.007
6. Vaughn B, Abu Kwaik Y. Idiosyncratic biogenesis of intracellular pathogens-containing vacuoles. Front Cell Infect Microbiol (2021) 11:722433. doi: 10.3389/fcimb.2021.722433
7. Damgaard RB. The ubiquitin system: from cell signalling to disease biology and new therapeutic opportunities. Cell Death Differ (2021) 28:423–6. doi: 10.1038/s41418-020-00703-w
8. Yang Q, Zhao J, Chen D, Wang Y. E3 ubiquitin ligases: styles, structures and functions. Mol BioMed (2021) 2:23. doi: 10.1186/s43556-021-00043-2
9. Tracz M, Bialek W. Beyond K48 and K63: non-canonical protein ubiquitination. Cell Mol Biol Lett (2021) 26:1. doi: 10.1186/s11658-020-00245-6
10. Grice GL, Nathan JA. The recognition of ubiquitinated proteins by the proteasome. Cell Mol Life Sci CMLS (2016) 73:3497–506. doi: 10.1007/s00018-016-2255-5
11. Madiraju C, Novack JP, Reed JC, Matsuzawa S-I. K63 ubiquitination in immune signaling. Trends Immunol (2022) 43:148–62. doi: 10.1016/j.it.2021.12.005
12. Roberts CG, Franklin TG, Pruneda JN. Ubiquitin-targeted bacterial effectors: rule breakers of the ubiquitin system. EMBO J (2023) 42:e114318. doi: 10.15252/embj.2023114318
13. Vozandychova V, Stojkova P, Hercik K, Rehulka P, Stulik J. The ubiquitination system within bacterial host-pathogen interactions. Microorganisms (2021) 9:638. doi: 10.3390/microorganisms9030638
14. Valleau D, Little DJ, Borek D, Skarina T, Quaile AT, Di Leo R, et al. Functional diversification of the NleG effector family in enterohemorrhagic. Escherichia coli. Proc Natl Acad Sci U.S.A. (2018) 115:10004–9. doi: 10.1073/pnas.1718350115
15. Zhang Y, Higashide WM, McCormick BA, Chen J, Zhou D. The inflammation-associated Salmonella SopA is a HECT-like E3 ubiquitin ligase. Mol Microbiol (2006) 62:786–93. doi: 10.1111/j.1365-2958.2006.05407.x
16. Bullones-Bolaños A, Bernal-Bayard J, Ramos-Morales F. The NEL family of bacterial E3 ubiquitin ligases. Int J Mol Sci (2022) 23:7725. doi: 10.3390/ijms23147725
17. Miao EA, Scherer CA, Tsolis RM, Kingsley RA, Adams LG, Baumler AJ, et al. Salmonella typhimurium leucine-rich repeat proteins are targeted to the SPI1 and SPI2 type III secretion systems. Mol Microbiol (1999) 34:850–64. doi: 10.1046/j.1365-2958.1999.01651.x
18. Cordero-Alba M, Ramos-Morales F. Patterns of expression and translocation of the ubiquitin ligase SlrP in Salmonella enterica serovar Typhimurium. J Bacteriol (2014) 196:3912–22. doi: 10.1128/JB.02158-14
19. Bernal-Bayard J, Ramos-Morales F. Salmonella type III secretion effector SlrP is an E3 ubiquitin ligase for mammalian thioredoxin. J Biol Chem (2009) 284:27587–95. doi: 10.1074/jbc.M109.010363
20. Bernal-Bayard J, Cardenal-Muñoz E, Ramos-Morales F. The Salmonella type III secretion effector, Salmonella leucine-rich repeat protein (SlrP), targets the human chaperone ERdj3. J Biol Chem (2010) 285:16360–8. doi: 10.1074/jbc.M110.100669
21. Bullones-Bolaños A, Araujo-Garrido JL, Fernández-García J, Romero F, Bernal-Bayard J, Ramos-Morales F. SNRPD2 is a novel substrate for the ubiquitin ligase activity of the Salmonella type III secretion effector SlrP. Biology (2022) 11:1517. doi: 10.3390/biology11101517
22. Rohde JR, Breitkreutz A, Chenal A, Sansonetti PJ, Parsot C. Type III secretion effectors of the IpaH family are E3 ubiquitin ligases. Cell Host Microbe (2007) 1:77–83. doi: 10.1016/j.chom.2007.02.002
23. Miao EA, Brittnacher M, Haraga A, Jeng RL, Welch MD, Miller SI. Salmonella effectors translocated across the vacuolar membrane interact with the actin cytoskeleton. Mol Microbiol (2003) 48:401–15. doi: 10.1046/j.1365-2958.2003.t01-1-03456.x
24. Bhavsar AP, Brown NF, Stoepel J, Wiermer M, Martin DDO, Hsu KJ, et al. The Salmonella type III effector SspH2 specifically exploits the NLR co-chaperone activity of SGT1 to subvert immunity. PloS Pathog (2013) 9:e1003518. doi: 10.1371/journal.ppat.1003518
25. Auweter SD, Bhavsar AP, De Hoog CL, Li Y, Chan YA, van der Heijden J, et al. Quantitative mass spectrometry catalogues Salmonella pathogenicity island-2 effectors and identifies their cognate host binding partners. J Biol Chem (2011) 286:24023–35. doi: 10.1074/jbc.M111.224600
26. da Silva Correia J, Miranda Y, Leonard N, Hsu J, Ulevitch RJ. Regulation of Nod1-mediated signaling pathways. Cell Death Differ (2007) 14:830–9. doi: 10.1038/sj.cdd.4402070
27. Hanahan D. Studies on transformation of Escherichia coli with plasmids. J Mol Biol (1983) 166:557–80. doi: 10.1016/S0022-2836(83)80284-8
28. Bullock WO, Fernandez JM, Short JM. XL1-Blue : A high efficiency plasmid transforming recA Escherichia coli strain with betagalactosidase selection. Bio Tech (1987) 5:376–9.
29. Segura I, Casadesus J, Ramos-Morales F. Use of mixed infections to study cell invasion and intracellular proliferation of Salmonella enterica in eukaryotic cell cultures. J Microbiol Methods (2004) 56:83–91. doi: 10.1016/j.mimet.2004.03.013
30. García-Calderón CB, Casadesús J, Ramos-Morales F. Rcs and PhoPQ regulatory overlap in the control of Salmonella enterica virulence. J Bacteriol (2007) 189:6635–44. doi: 10.1128/JB.00640-07
31. Baisón-Olmo F, Cardenal-Muñoz E, Ramos-Morales F. PipB2 is a substrate of the Salmonella pathogenicity island 1-encoded type III secretion system. Biochem Biophys Res Commun (2012) 423:240–6. doi: 10.1016/j.bbrc.2012.05.095
32. Van Aelst L. Two-hybrid analysis of Ras-Raf interactions. Methods Mol Biol Clifton NJ (1998) 84:201–22. doi: 10.1385/0-89603-488-7:201
33. Cardenal-Muñoz E, Ramos-Morales F. Analysis of the expression, secretion and translocation of the Salmonella enterica type III secretion system effector SteA. PloS One (2011) 6:e26930. doi: 10.1371/journal.pone.0026930
34. Selig L, Benichou S, Rogel ME, Wu LI, Vodicka MA, Sire J, et al. Uracil DNA glycosylase specifically interacts with Vpr of both human immunodeficiency virus type 1 and simian immunodeficiency virus of sooty mangabeys, but binding does not correlate with cell cycle arrest. J Virol (1997) 71:4842–6. doi: 10.1128/jvi.71.6.4842-4846.1997
35. Winson MK, Swift S, Hill PJ, Sims CM, Griesmayr G, Bycroft BW, et al. Engineering the luxCDABE genes from Photorhabdus luminescens to provide a bioluminescent reporter for constitutive and promoter probe plasmids and mini-Tn5 constructs. FEMS Microbiol Lett (1998) 163:193–202. doi: 10.1016/S0378-1097(98)00173-6
36. Valinsky L, Nisan I, Tu X, Nisan G, Rosenshine I, Hanski E, et al. A host-specific virulence protein of Erwinia herbicola pv. gypsophilae is translocated into human epithelial cells by the Type III secretion system of enteropathogenic Escherichia coli. Mol Plant Pathol (2002) 3:97–101. doi: 10.1046/j.1464-6722.2002.00099.x
37. Schmieger H. Phage P22-mutants with increased or decreased transduction abilities. Mol Gen Genet MGG (1972) 119:75–88. doi: 10.1007/BF00270447
38. Vojtek AB, Hollenberg SM, Cooper JA. Mammalian Ras interacts directly with the serine/threonine kinase Raf. Cell (1993) 74:205–14. doi: 10.1016/0092-8674(93)90307-C
39. Sherman F, Fink GR, Hicks JB. Methods in yeast genetics: a laboratory course manual. Cold Spring Harbor Lab (1987).
40. Gibson DG, Young L, Chuang RY, Venter JC, Hutchison CA, Smith HO. Enzymatic assembly of DNA molecules up to several hundred kilobases. Nat Methods (2009) 6:343–5. doi: 10.1038/NMETH.1318
41. Datsenko KA, Wanner BL. One-step inactivation of chromosomal genes in Escherichia coli K-12 using PCR products. Proc Natl Acad Sci U.S.A. (2000) 97:6640–5. doi: 10.1073/pnas.120163297
42. Uzzau S, Figueroa-Bossi N, Rubino S, Bossi L. Epitope tagging of chromosomal genes in Salmonella. Proc Natl Acad Sci U.S.A. (2001) 98:15264–9. doi: 10.1073/pnas.261348198
43. Ramos-Morales F, Cardenal-Muñoz E, Cordero-Alba M, Baisón-Olmo F. Generation and Use of Site-Directed Chromosomal cyaA′ Translational Fusions in Salmonella enterica. Methods Mol Biol (2015) 1225:93–104. doi: 10.1007/978-1-4939-1625-2_6
44. Haraga A, Miller SI. Salmonella type III secretion effector interacts with the mammalian serine/threonine protein kinase PKN1. Cell Microbiol (2006) 8:837–46. doi: 10.1111/j.1462-5822.2005.00670.x
45. Keszei A, Tang X, McCormick C, Zeqiraj E, Rohde JR, Tyers M, et al. Structure of an SspH1-PKN1 complex reveals the basis for host substrate recognition and mechanism of activation for a bacterial E3 ubiquitin ligase. Mol Cell Biol (2014) 34:362–73. doi: 10.1128/MCB.01360-13
46. Haraga A, Miller SI. Salmonella enterica serovar Typhimurium translocated leucine-rich repeat effector protein inhibits NF-kappa B-dependent gene expression. Infect Immun (2003) 71:4052–8. doi: 10.1128/IAI.71.7.4052
47. Shappo MOE, Li Q, Lin Z, Hu M, Ren J, Xu Z, et al. SspH2 as anti-inflammatory candidate effector and its contribution in Salmonella Enteritidis virulence. Microb Pathog (2020) 142:104041. doi: 10.1016/j.micpath.2020.104041
48. Pillay TD, Hettiarachchi SU, Gan J, Diaz-Del-Olmo I, Yu X-J, Muench JH, et al. Speaking the host language: how Salmonella effector proteins manipulate the host. Microbiol Read Engl (2023) 169:1342. doi: 10.1099/mic.0.001342
49. Araujo-Garrido JL, Bernal-Bayard J, Ramos-Morales F. Type III secretion effectors with arginine N-glycosyltransferase activity. Microorganisms (2020) 8:357. doi: 10.3390/microorganisms8030357
50. Groisman EA, Duprey A, Choi J. How the PhoP/PhoQ system controls virulence and Mg2+ Homeostasis: lessons in signal transduction, pathogenesis, physiology, and evolution. Microbiol Mol Biol Rev MMBR (2021) 85:e0017620. doi: 10.1128/MMBR.00176-20
51. Kim CC, Falkow S. Delineation of upstream signaling events in the Salmonella pathogenicity island 2 transcriptional activation pathway. J Bacteriol (2004) 186:4694–704. doi: 10.1128/JB.186.14.4694-4704.2004
52. Aiastui A, Pucciarelli MG, García-del Portillo F. Salmonella enterica serovar Typhimurium invades fibroblasts by multiple routes differing from the entry into epithelial cells. Infect Immun (2010) 78:2700–13. doi: 10.1128/IAI.01389-09
53. Chou Y-C, Keszei AFA, Rohde JR, Tyers M, Sicheri F. Conserved structural mechanisms for autoinhibition in IpaH ubiquitin ligases. J Biol Chem (2012) 287:268–75. doi: 10.1074/jbc.M111.316265
54. Suzuki S, Mimuro H, Kim M, Ogawa M, Ashida H, Toyotome T, et al. Shigella IpaH7.8 E3 ubiquitin ligase targets glomulin and activates inflammasomes to demolish macrophages. Proc Natl Acad Sci U.S.A. (2014) 111:E4254–4263. doi: 10.1073/pnas.1324021111
55. Keszei AFA, Sicheri F. Mechanism of catalysis, E2 recognition, and autoinhibition for the IpaH family of bacterial E3 ubiquitin ligases. Proc Natl Acad Sci U.S.A. (2017) 114:1311–6. doi: 10.1073/pnas.1611595114
56. Bernal-Bayard J, Ramos-Morales F. Molecular mechanisms used by Salmonella to evade the immune system. Curr Issues Mol Biol (2018) 25:133–68. doi: 10.21775/cimb.025.133
57. Zhou G, Zhao Y, Ma Q, Li Q, Wang S, Shi H. Manipulation of host immune defenses by effector proteins delivered from multiple secretion systems of Salmonella and its application in vaccine research. Front Immunol (2023) 14:1152017. doi: 10.3389/fimmu.2023.1152017
58. Lin SL, Le TX, Cowen DS. SptP, a Salmonella typhimurium type III-secreted protein, inhibits the mitogen-activated protein kinase pathway by inhibiting Raf activation. Cell Microbiol (2003) 5:267–75. doi: 10.1046/j.1462-5822.2003.t01-1-00274.x
59. Sun H, Kamanova J, Lara-Tejero M, Galán JE. A family of Salmonella type III secretion effector proteins selectively targets the NF-κB signaling pathway to preserve host homeostasis. PloS Pathog (2016) 12:e1005484. doi: 10.1371/journal.ppat.1005484
60. Yin C, Liu Z, Xian H, Jiao Y, Yuan Y, Li Y, et al. AvrA Exerts Inhibition of NF-κB Pathway in Its Naïve Salmonella Serotype through Suppression of p-JNK and Beclin-1 Molecules. Int J Mol Sci (2020) 21:6063. doi: 10.3390/ijms21176063
61. Liao AP, Petrof EO, Kuppireddi S, Zhao Y, Xia Y, Claud EC, et al. Salmonella type III effector AvrA stabilizes cell tight junctions to inhibit inflammation in intestinal epithelial cells. PloS One (2008) 3:e2369. doi: 10.1371/journal.pone.0002369
62. Rao S, Schieber AMP, O’Connor CP, Leblanc M, Michel D, Ayres JS. Pathogen-mediated inhibition of anorexia promotes host survival and transmission. Cell (2017) 168:503–516.e12. doi: 10.1016/j.cell.2017.01.006
63. Halici S, Zenk SF, Jantsch J, Hensel M. Functional analysis of the Salmonella pathogenicity island 2-mediated inhibition of antigen presentation in dendritic cells. Infect Immun (2008) 76:4924–33. doi: 10.1128/IAI.00531-08
64. McLaughlin LM, Xu H, Carden SE, Fisher S, Reyes M, Heilshorn SC, et al. A microfluidic-based genetic screen to identify microbial virulence factors that inhibit dendritic cell migration. Integr Biol Quant Biosci Nano Macro (2014) 6:438–49. doi: 10.1039/c3ib40177d
65. Lapaque N, Hutchinson JL, Jones DC, Méresse S, Holden DW, Trowsdale J, et al. Salmonella regulates polyubiquitination and surface expression of MHC class II antigens. Proc Natl Acad Sci U.S.A. (2009) 106:14052–7. doi: 10.1073/pnas.0906735106
66. Bayer-Santos E, Durkin CH, Rigano LA, Kupz A, Alix E, Cerny O, et al. The Salmonella effector SteD mediates MARCH8-dependent ubiquitination of MHC II molecules and inhibits T cell activation. Cell Host Microbe (2016) 20:584–95. doi: 10.1016/j.chom.2016.10.007
Keywords: Salmonella enterica, type III secretion systems, E3 ubiquitin ligase, SlrP, SspH1, SspH2
Citation: Bullones-Bolaños A, Martín-Muñoz P, Vallejo-Grijalba C, Bernal-Bayard J and Ramos-Morales F (2024) Specificities and redundancies in the NEL family of bacterial E3 ubiquitin ligases of Salmonella enterica serovar Typhimurium. Front. Immunol. 15:1328707. doi: 10.3389/fimmu.2024.1328707
Received: 27 October 2023; Accepted: 17 January 2024;
Published: 01 February 2024.
Edited by:
Jiri Stulik, University of Defence, CzechiaReviewed by:
Nishanth Gopala Krishna, Hannover Medical School, GermanySusan M Bueno, Pontificia Universidad Católica de Chile, Chile
Copyright © 2024 Bullones-Bolaños, Martín-Muñoz, Vallejo-Grijalba, Bernal-Bayard and Ramos-Morales. This is an open-access article distributed under the terms of the Creative Commons Attribution License (CC BY). The use, distribution or reproduction in other forums is permitted, provided the original author(s) and the copyright owner(s) are credited and that the original publication in this journal is cited, in accordance with accepted academic practice. No use, distribution or reproduction is permitted which does not comply with these terms.
*Correspondence: Joaquín Bernal-Bayard, amJiYXlhcmRAdXMuZXM=; Francisco Ramos-Morales, ZnJhbW9zQHVzLmVz
†These authors have contributed equally to this work and share first authorship