- National Clinical Research Center for Infectious Disease, Shenzhen Third People's Hospital, Shenzhen, Guangdong, China
The central nervous system (CNS) harbors its own special immune system composed of microglia in the parenchyma, CNS-associated macrophages (CAMs), dendritic cells, monocytes, and the barrier systems within the brain. Recently, advances in the immune cells in the CNS provided new insights to understand the development of tuberculous meningitis (TBM), which is the predominant form of Mycobacterium tuberculosis (M.tb) infection in the CNS and accompanied with high mortality and disability. The development of the CNS requires the protection of immune cells, including macrophages and microglia, during embryogenesis to ensure the accurate development of the CNS and immune response following pathogenic invasion. In this review, we summarize the current understanding on the CNS immune cells during the initiation and development of the TBM. We also explore the interactions of immune cells with the CNS in TBM. In the future, the combination of modern techniques should be applied to explore the role of immune cells of CNS in TBM.
1 Introduction
Tuberculosis (TB) is one of the communicable diseases and the leading causes of health problems, which causes more deaths from a single agent of infection [ranking above human immunodeficiency virus/acquired immunodeficiency syndrome (HIV/AIDS)] (1). TB patients suffered from bacillus Mycobacterium tuberculosis (M.tb) infection when exposed to air containing M.tb after coughing by TB patients. Although approximately a quarter of the world’s population is supposed to be infected with M.tb, most people will not develop TB (2). TB is the first cause of death by a single infectious agent and ranks as the 13th leading cause of death worldwide. However, the Mycobacterium bovis bacille Calmette–Guérin (BCG), which is the only licensed vaccine in clinical practice, is applied to prevent tuberculosis. Notably, in global TB deaths among HIV-negative or HIV-positive people, 54% or 51% were men, 32% or 38% were women, and 14% or 11% were children, respectively. In summary, approximately 90% of the TB patients are adults, with the number of men bigger than that of women in adult TB patients (3). In TB patients, M.tb mainly affects the lungs (pulmonary TB), and extrapulmonary sites including the central nervous system (CNS), lymph nodes, and pleura could also be affected (4–6). Diffusion of M.tb to the brain may induce the most severe form of extrapulmonary TB, tuberculous meningitis (TBM), which is the predominant form of M.tb infection in the CNS and accompanied with high mortality and disability. Moreover, TBM is the most serious extrapulmonary form of TB with high mortality up to 50% in the HIV co-infected patients (7, 8). Although the morbidity of TBM in TB patients is approximately 1%, TBM causes a higher rate of mortality and morbidity in young children compared to that in adults (9).
A retrospective, descriptive study in Beijing Children’s Hospital revealed that approximately 50% of the TB patients had extrapulmonary TB and approximately 39% had TBM in extrapulmonary TB (10). An investigation of national surveillance data of Germany from 2002 to 2009 showed that of the pediatric TB patients, approximately 3.9% of those younger than 5 years, 2.2% of those aged 5–9 years, and 1.3% of those aged 10–14 years had TBM (11). In the referral center for infectious diseases in Thessaloniki, 43 children aged 7 months to 13 years, of whom 14 children (33%) were identified as having an extrameningeal site of infection, 14 children (33%) were identified as having pulmonary tuberculosis, and 1 child was diagnosed with spondylitis, had been diagnosed with TBM from 1984 to 2008 with a gradually decreasing trend over the years (12). Despite the development and application of many methods for detecting M.tb, the diagnosis of TBM and some other extrapulmonary TB continues to face a huge challenge. Nicholas et al. found that a caseating focus existed in brain parenchyma or meninges in TBM patients (13). Moreover, modified Ziehl–Neelsen (ZN) staining in the cerebrospinal fluid (CSF), GeneXpert, and culture of cerebrospinal fluid were applied to improve the microbiological diagnosis of TBM (14). High levels of CSF volume and lactate and lower blood glucose ratio were independently related with microbiological confirmation of TBM. M.tb infects the CNS from the lungs through hematogenous transmission. Briefly, the deposition of M.tb in the brain is known as “Rich foci” during bacteremia in the early stage of TB. In the CNS, the “Rich foci” existed in the subpial or subependymal areas of the brain, bacilli, and meninges kept in a dormant state for a long time. The initiation of TBM was made by the growing and rupturing of those “Rich foci” into the ventricular system or subarachnoid space (15). Generally, primary infection in the brain may lead to the occurrence of TBM from 6 to 12 months (16). The M.tb has the potential to invade and migrate into the CNS by crossing the blood–brain barrier (BBB) and blood–CSF barrier (BCSFB) with the existence of virulence and immune factors, exocytosis, and longer intracellular survival (17, 18). Although the role of immune system in the diffusion of M.tb to the peripheral system has been well studied, the role of immune cells in the CNS has not been well reviewed. Here, we summarize the role of immune cells in the CNS during the progress of TBM with an aim of helping to understand the interaction between TBM and immune system in the CNS.
The brain had been regarded as “immune privileged” by wrapping with some barriers in the early twentieth century. This hypothesis had been supported by two experiments that injection of a dye in peripheral blood did not enter the brain (19) and the survival of tissue grafts was prolonged by transplantation of the brain tissue compared to transplantation of peripheral tissues (20). These may lead to a conclusion that the brain is an independent immune system isolated from the peripheral immune system. Otherwise, microglia, the major brain-resident immune cell in the brain, is considered as an argument to support the brain as an independent immune system. However, the fact that the lymphatic system had been found in the brain as early as the early twentieth century has been ignored and did not apply to oppose the “immune privilege” of the brain (21). Moreover, some adaptive immune cells have been identified in CNS in some studies that have been carried out in mammal models of neuroinflammatory diseases (22–25). The interaction between the brain and peripheral immune system has been suggested to be achieved in some mammal models, in which bone-marrow-produced T cells (26) and macrophages (27) exert a protection and repair role in the CNS. In addition, T cells play a critical role in maintaining brain function by recognizing brain self-antigens (26). Thus, these studies demonstrate that the protective autoimmunity in the CNS can enhance brain repair and maintain brain homeostasis by self-recognizing immune cells. The adaptive immune cells are thought to support the formation of new neurons in the CNS (28, 29). Thus, adaptive immune cells in the CNS are involved in social behavior (30–33), emotional stress (34, 35), and cognition (36, 37). In this review, we aim to summarize the role of immune cells in the CNS during the initiation and development of TBM and then explore the interaction of immune cells with the CNS in TBM.
2 Biological roles of CNS macrophages in TBM
The development of CNS requires the protection of immune cells, including macrophages and microglia, during embryogenesis to ensure the accurate development of CNS and immune response following pathogenic invasion (38, 39). Therefore, macrophages exert their immune functions in the brain very early during embryogenesis and development. The differentiation in CNS needs a highly integrated process during the embryogenesis and development by genetic and extrinsic factors. Macrophages act as the main reservoir for M.tb, and its survival depends on its ability to evade the killing mechanisms of macrophages (40, 41). Some macrophages infected with M.tb act as a “Trojan horse”, which carry M.tb across the BBB (42). It is difficult for the anti-tuberculosis drugs to cross the BBB and target the CNS macrophages, which cannot effectively eliminate M.tb, bringing a great challenge to the treatment of TBM (43).
Neuroinflammation in the CNS caused by pathogenic infections recruits macrophages and releases chemokines and cytokines in the parenchyma, which then opens the BBB (44). TBM patients who received a 7-day course of standard first-line anti-tuberculosis therapy showed excellent improvement, with increased numbers of macrophages and lymphocytes in the CSF capable of engulfing M.tb (45, 46). Then, the macrophages induced the host innate immunity after M.tb infection through inducing inflammatory response and pathogen recognition (47). Shao et al. demonstrated that detection of mycobacterial antigens in the CSF macrophages by using immunocytochemical staining had a sensitivity or specificity of 73.5% or 90.7%, respectively, and could be applied in diagnosing TBM in clinical practice (48).
The CNS macrophages exerted its protection by equipping nucleotide-binding oligomerization domain-like receptors (NLRs), PRRs, and TLRs in the CNS (49–54). Clearance of invading pathogens through phagocytosis is accompanied by the release of proinflammatory cytokines and chemokines (55), which then activate neighboring microglia and recruit other immune cells to treat this infection (56–62). The cell apoptosis or programmed cell death occurs after M.tb infection and then reduce the viability of mycobacteria. The level of apoptosis in alveolar macrophages after infection with attenuated M. tuberculosis H37Ra is higher than those with the virulent H37Rv strain infection (63). Therefore, the cell apoptosis in macrophage may exert a protective role in M.tb infection.
3 Biological roles of microglia in TBM
M.tb, the infectious agent of TB, replicates and propagates from the respiratory epithelium and then enters the CNS by breaking through the BBB and causes primary infection in the meninges or the brain. Microglia are resident macrophages and are major components of the brain’s immune system, working with other immune cells and neurons to maintain the brain’s homeostasis and prevent the invasion of harmful pathogens. Microglia fulfill their physiological roles throughout their lives, which is independent from the blood circle, and maintain apoptosis and proliferation under physiological conditions (64–66). Recently, studies have illustrated that microglia only originate in the erythromyeloid progenitors (EMPs) in the embryonic yolk sac (67, 68) and that microglia have a long lifespan with a low-rate clonal expansion (69, 70). Microglia are the first immune cells in the CNS when infected with M.tb, and the activation of microglia results in the progression of infection. However, Tucker et al. have demonstrated that microglia have been activated in a pediatric rabbit model of TBM (71). Zhou et al. showed that microRNA-124 modulates the proliferation of Mycobacterium by inhibiting the signal transducer and activator of transcription 3 signaling pathway (72). Although microglia play an immune supervisor role in the CNS, the long-lasting activation of microglia induces hyper-neuroinflammation, which may induce neurotoxicity and impair the cognitive ability in TB patients (73). As the main component of myeloid cells, microglia infiltration reflects the brain inflammatory activation status in TBM (74). The proinflammatory cytokines and chemokines secreted by activated microglia after the M.tb infection trigger neurotoxicity and tissue injuries in the CNS. Microglia have both “resting” and “activated” status, and various factors including viral infection in the peripheral nervous system and brain trauma may alter the balance between “resting” and “activated” state (75). Microglia are primarily involved in the maintenance of a “resting” state and perform kinds of functions including pathogen protection, neuron nourishment, and debris removal (76–78).
While neurons and astrocytes may be potential targets for M.tb infection, microglia have been considered to be the first target in the CNS due to their properties related to macrophages (79, 80). Studies have shown that human microglia and astrocytes have been intracellularly infected with M.tb H37Rv strain after 24 h in vitro treatment. Notably, most of the microglia infected with M.tb were infected with 4.2 bacilli per cell. However, only 15% astrocytes were infected with 1.3 bacilli per cell (80). The difference in the infection rate of M.tb between microglia and astrocytes may be due to the expression of CD14 receptors in microglia (81, 82).
The M.tb could be recognized by microglia via the innate immune and neuro-specific receptors. After CNS infection, activated microglia not only produce tumor necrosis factor-α (TNF-α) and interleukin-8 but also express an amount of immune-recognition molecules in the innate immunity (83, 84). The particular pattern recognition receptors (PRRs) in microglia are essential for initiating a fast response during the invasion of M.tb in microglia (85, 86). In the antigen recognition process, M.tb could seriously damage microglia (87). The Toll-like receptors (TLRs), intracellular PRRs, C-type lectin receptors, complement receptor 3, triggering receptors expressed on myeloid cells (TREM), and myeloid DAP-12-associated lectin (MDL-1) are a member of intracellular PRRs in microglia (88). TLR co-receptors CD14, TLR1-TLR4, and TLR5-TLR9 are mostly expressed in microglia. Furthermore, previous studies have shown that TLR1, TLR2, and TLR4 are mainly presented on the surface of microglia, whereas TLR3, TLR7, and TLR8 are predominantly located intracellularly (49, 52, 88). Although studies have found that the TLR mechanisms in neurodegenerative disorders [Alzheimer’s disease (AD), spinal cord injury, amyotrophic lateral sclerosis, and Parkinson’s disease (PD)], pathogenic infections (HIV, Japanese encephalitis virus (JEV), and Neisseria meningitidis), and ischemic brain injury have been well studied (89–95), the TLR mechanisms in TBM remain unclear. Previous findings revealed that the TLR4 binds to CD14 on the surface of microglia through lipopolysaccharides (LPS) in the internalization of M.tb (82). On the other hand, TLR2, TLR4, and TLR9 pathways activated their downstream phosphorylation and cytokine production during the internalization of M.tb in macrophages (96–98). Understanding the mechanism of the role of PRRs on macrophages in M.tb pathogenic infection and damage recognition will help to explore the mechanism of PRRs in microglia during the progress of TBM. Another report has shown that both murine microglial TLR2 and dectin-1 were not participated in inflammatory responses induced by M.tb (99). Consequently, the mechanism by which microglia recognize M.tb after infection remains unclear.
Mycobacterium marinum has been applied to explore the role of microglial autophagy in TBM. Mycobacterium marinum infection induces the formation of granulomas in the zebrafish brain. It also induces the microglial autophagy in response to its replication (100). However, the production of interleukin-6 (IL-6) from macrophages inhibits interferon-gamma (IFN-γ)-induced autophagy and then combats innate immunity after M. marinum infection and increases the intracellular persistence (101).
Recently, a macrophage-inducible C-type lectin (Mincle), which belongs to the C-type lectin receptor family, may be become a potential target for Mincle agonist (102). Trehalose-6,6-dibehenate (TDB), a novel adjuvant for TB vaccines and a synthetic analog of trehalose6,6-dimycolate (TDM), was applied in human clinical studies. Both TDM and TDB induce inflammatory gene expression in macrophages and dendritic cells by binding to Mincle (103, 104). Although the expression of Mincle is limited in the primary microglia, TDB reduces the TLR4-mediated neuroinflammation in Mincle-knockout microglia and mice. Notably, these results in Mincle-knockout microglia and mice differed from their performance in macrophages. However, the mechanism of TDB in modulating C-gamma 1 (PLC-γ1) signaling pathway remains unclear. The M.tb may persist and multiply intracellularly after being internalized by microglia (81, 105). In the development of TBM, M.tb leads to microglial accumulation and activation and then triggers the granulomatous formation (106, 107). Three distinct granuloma types including non-necrotizing, necrotizing gummatous, and necrotizing abscess were distinguished. All types of granulomatous were observed in each patient and mainly located in the perivascular areas of the leptomeninges. The size of non-necrotizing granuloma (0.1–0.5 mm) was smaller than the necrotizing gummatous (≥ 5 mm) and necrotizing abscess (10 mm). In TBM patients, granulomas were wrapped with a blood vessel and then damaged by vasculitis in a later stage.
Yang et al. found that inhibiting secretory phospholipase A2 (sPLA2) could attenuate the ROS and various inflammatory mediators production after M.tb infection in murine microglial BV-2 cells. Inhibition of the Ras/Raf-1/MEK1/ERK1/2 signaling pathway diminished sPLA2 activity after M.tb infection in BV-2 cells (108). Mitochondrial ROS, potassium efflux, and lysosomal proteases cathepsin B promote activation of nucleotide binding and oligomerization of the domain-like receptor family pyrin domain containing 3 protein (NLRP3) inflammasome activation in response to M.tb infection (109).
4 Biological roles of B and T lymphocytes in TBM
Activated T and B lymphocytes have been found in the CSF of TBM patients from the clinical onset of disease. In addition, T and B lymphocytes were involved in the adaptive cellular immune response in TBM patients (106). Meningeal B lymphocytes were present in secondary progressive multiple sclerosis (SPMS) and were responsible for inducing pathology by producing inflammatory mediators and participating in antigen presentation (110–112). Anti-CD20 may be a potential therapeutic target for meningeal B-lymphocytes aggregation (113). During the development of TBM, the humoral response was sustained for approximately 2 months and the cellular immune response was maintained for another 3 months (114). Zhang et al. illustrated that the distribution of lymphocyte subpopulations and the ratio of CD4:CD8 in CSF were different in patients with anti-N-methyl-D-aspartate receptor AE (NMDAR-AE), herpes simplex virus encephalitis (HSVE), and TBM (115). After clinical therapy of TBM, the number of CD4- and CD45RO-positive T cells increased significantly in CSF (116). Therefore, the levels of CD4 and CD45RO positive T cells were useful biomarkers for diagnosing TBM. The T lymphocytes and CD4-positive helper T (Th) cells play a key role in granuloma formation in TBM patient, and the interactions between CD4+ Th cells and T lymphocytes induce the IFN-γ production and then activate the macrophages to engulf and digest M.tb (117–120). Another report indicated that IL-2-positive T lymphocytes in the CSF of TBM patients can produce specific and reactive antigen when stimulated with IL-2, antigen-presenting cells (APC), or Muromonab CD3 (OKT3) antibody (121). Xu et al. found that the expression levels of Th1, Th2, Th 17, TNF-α, and TNF-β were elevated in TBM patients compared to those without the CNS infections in HIV-infected persons (122). Moreover, the expression levels of IFN-γ, regulated upon activation normal T cells expressed and presumably secreted (RANTES) and interferon-inducible protein (IP-10) in TBM patients were higher than in non-TBM with HIV infection. The cytokines/chemokines of Th1, Th2, and Th17 exerted a critical role in the pathogenesis of TBM. The upregulation of IL-17a, TNF-β, IL-5, IL-12p40, and IL-1Rα in CSF has been demonstrated to be related with meningitis. Thus, Kösters et al. have developed a valuable diagnostic tool for the diagnosis of neurotuberculosis including TBM by using a T-cell IFN-γ release assay in clinical practice (123). More diagnostic tools for measuring cytokines/chemokines in TBM should be developed in clinical practice.
5 Biological roles of neutrophil in TBM
Neutrophils do not exist in the border zone encephalitis areas of parenchyma under physiological conditions. The absence of neutrophils in the brain is due to the protection of CNS against the aggressive cells, including neutrophils, across the BBB. Neutrophils have a large number of enzymes in their membrane-bound granules that produce reactive oxygen species and thereby affect the cellular environment. In the early stage of TBM, the BBB prevents neutrophils from entering the CNS and avoids the neural damage (106). In TBM patients, the number of CSF neutrophils and M.tb-positive presentation were considered for the characterization of the immune reconstitution inflammatory syndrome (IRIS) (124). Additionally, CSF neutrophil mediators, including S100A8/9, were significantly increased in patients with TBM-IRIS compared to those without (125, 126). Marais et al. illustrated that neutrophil-abundant transcripts increased in the progression of developing IRIS until the onset of TBM-IRIS by performing longitudinal microarray analysis of the blood (127, 128). After the diagnosis of TBM was confirmed, TBM patients received first-line anti-tuberculosis treatment. After 7 days treatments of anti-TB, all patients showed excellent improvement, with 82% lymphocytes and macrophages in CSF participated in engulfing M.tb. Then, the number of neutrophils and the protein level were increased in CSF after reducing the dose of dexamethasone during the first-line therapy of anti-TB; those findings indicated a relapse of TBM. Moreover, the strong relationship between neutrophils and matrix metalloproteinase-9 (MMP-9) in CSF has been found in the TBM after dexamethasone treatment (129–131). However, a high level of neutrophils in CSF has a positive impact on the chance of survival in TBI patients and was associated with the occurrence of cerebral infarction by using head MRI in adult TBM patients (132–135). In addition, the neutrophil proportion in the CSF is independently associated with TBM, and doctors can use five indexes including neutrophil proportion, disease course, white blood cell count, serum sodium, and total white cell count in the CSF to distinguish TBM from bacterial meningitis (136–142).
6 Biological roles of natural killer cells in TBM
Natural killer (NK) cells are upregulated in the CSF of TBM patients, and the predominance of NK cells is associated with a better outcome and survival (143). However, the function of NK cells in TBM patients has not been well addressed. Limited literature has reported the role of NK cells in the inflammatory process related with TBM. NK cells have been demonstrated to be implicated in the pathogenesis of viral and bacterial infections in the CNS. Notably, the activity of NK cells may inhibit herpes virus’s infection (144, 145). The total number of NK cells and CD56bright NK cells in the blood of TBM patients was less than latent TB infection (LTBI) and pulmonary TB (PTB) patients. Cytokines produced by NK cells may contribute to the pathogenic alterations of some CNS bacterial infections (146). Accordingly, the reduction in the total number of NK cells and CD56bright NK cells in the blood of TBM patients may be responsible for the migration of NK cells to the CNS. NK cells in the brain may release proinflammatory cytokines, which in turn induce brain injury in TBM patients. On the other hand, the TBM patients have a higher level of CD56dimCD16+ NK cells in peripheral circulation. The increased number of CD56dimCD16+ NK cells in the blood of TBM patients may induce these NK cells to enter the CNS for the purpose of controlling CNS infection. The cytotoxicity induced by NK cells may exert a protective effect for some neurological complications after bacterial infection (147, 148). Meanwhile, van Laarhoven et al. illustrated that the decrease in blood NK cells leads to the enrichment of NK cells in the CNS (143). In addition, they also found that CD69 NK cells reflect the active mobilization of NK cells to the CNS in TBM patients (143). Nevertheless, more information of phenotypical characteristics of NK cells in the CNS during TBM should be studied.
7 Interaction of the microglia with CNS macrophages in TBM
Microglia and macrophages are thought to orchestrate the CNS after M.tb infection. The intrinsic transcriptional programs, differentiation, and maturation of CNS-associated macrophages (CAMs) and microglia are triggered by environmental factors during the embryonic and postnatal development (149). The CNS is formed earlier than any other organs during embryogenesis with the involvement of immune system to protect the new-born CNS from pathogens (150), and the macrophages exist in the brain during the embryogenesis. Microglia and CAMs are present in the brain parenchyma and perivascular space, respectively (65, 68, 151). Interestingly, foamy macrophages are surrounded by microglia, lymphocytes, epithelioid histiocytes, and new blood vessels and are involved in the formation of CNS granuloma (106, 107). M.tb has established a strategy for survival within macrophages by influencing phagosome–lysosome fusion and intervening normal host trafficking events (152, 153). The interaction network between microglia and macrophage mediates the production of cytokines and chemokines in response to M.tb invasion (80, 81). Previous studies have shown expression of TNF-α, six different interleukins (IL-1α, IL-1β, IL-6, IL-10, IL-12p40, and IL-18), C-X-C motif chemokine ligands (CXCL8 and CXCL10), matrix metallopeptidases (MMP-1, MMP-3, and MMP-9), granulocyte-macrophage colony-stimulating factor (GM-CSF), macrophage inflammatory protein-1β (MIP-1β), and granulocyte colony-stimulating factor (G-CSF) (67, 80). Lee et al. have revealed that the activation of primary murine microglia in conditioned media from M.tb-infected macrophages was associated with the maturation of caspase-1 and IL-1β in microglia during the M.tb infection (109). Generally, M.tb could be maintained in a silencing status by regulating the expression of interleukins in the homeostatic immune response (154, 155). Moreover, signal transducer and activator of transcription 1 (Stat1) and interferon regulatory factor1 (IRF1) have been verified to be involved in the inflammation response of macrophages and microglia after TBM induced by attenuated M.tb (74).
8 Interaction of the microglia with astrocytes in TBM
M.tb induces both microgliosis and astrogliosis in the CNS, and neurobiological mechanisms are involved in the pathogenesis, which plays a key role in modulating neuronal–glial interactions and synaptic function after M.tb infection. The activation of microglia and astrocytes induces neuroimmune interactions in the CNS, producing both pro- and anti-inflammatory cytokines. In addition, the activated microglia and astrocytes have been found in the meningeal exudate (156). Rock et al. have found that dexamethasone, an adjunctive therapy for TBM, could modulate the content of proinflammatory cytokines and chemokines secreted by the CNS macrophages while inhibiting the release of TNF and IL-6 in microglia (80). The astrocyte–microglia lactate shuttle (AMLS) hypothesis states that lactic acid produced by astrocytes through glycolysis in response to the initiation of immune response plays a key role in microglia in the development of TBM (157). Lactic acid is a crucial energy substrate in energy metabolism, and its upregulation contributes to the glucose metabolism in microglia of TBM, thereby performing neuroprotective effects (158, 159).
9 Interaction of the macrophages with other cells in the CNS of TBM
Previous studies have found that T lymphocytes and CD4+ Th cells play a critical role in granuloma formation. IFN-γ induced by the communication of CD4+ Th cells and T lymphocytes activates the macrophages to eliminate the M.tb (160–162). Results showed that CD4+ and CD8+ T cells were gathered around all granuloma types in biopsy specimens, but limited CD4+ and CD8+ T cells were found in the post-mortem tissues. Moreover, NK cells and monocytes (myeloid mononuclear cells) can kill extracellular M.tb and activate a series of signaling pathways including reactive oxygen species (ROS) and glutathione (GSH)-related signaling pathways in macrophages (163). The GSH in NK cells has been reported to be involved in inhibiting the growth of M.tb. Thus, exploring the interaction between macrophages and NK cells or lymphocytes may contribute to understanding the infection of the M.tb during TBM.
10 Conclusions
The development of CNS requires the protection of immune cells, including macrophages and microglia, during embryogenesis to ensure the accurate development of CNS and immune response following pathogenic invasion. Microglia are resident macrophages and the major component of the brain’s immune system, working with other immune cells and neurons to maintain the brain’s homeostasis and prevent the invasion of M.tb. Moreover, activated T and B lymphocytes have been found in the CSF of TBM patients from the clinical onset of disease, and more diagnostic tools to measure the cytokines/chemokines secreted by lymphocytes in TBM have been developed in clinical practice. Moreover, the interaction of microglia between CNS macrophages or astrocytes has been involved in TBM (Figure 1).
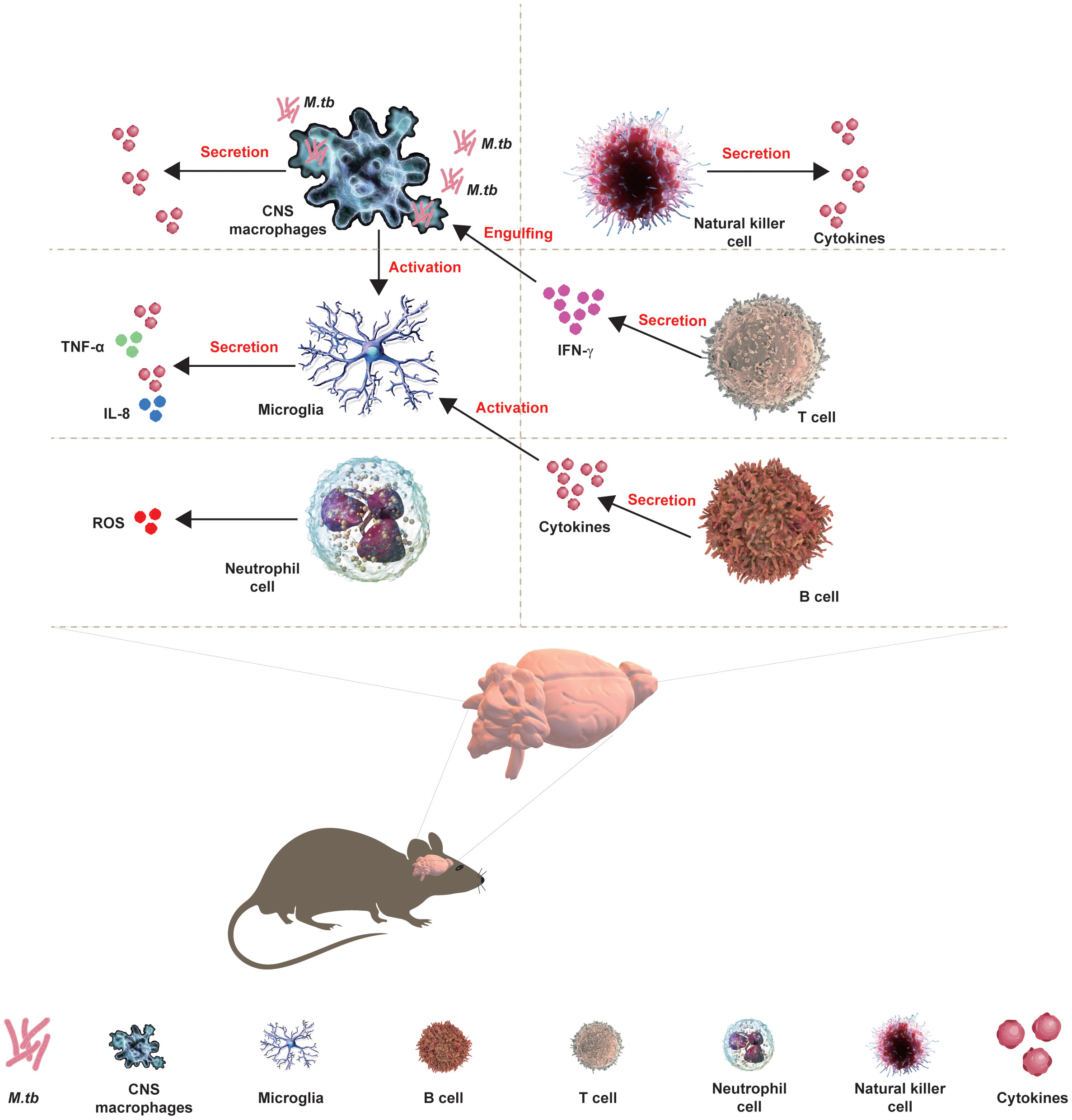
Figure 1 Interaction of CNS with immune cells in TBM. The development of CNS requires the protection of immune cells, including macrophages and microglia, during embryogenesis to ensure the accurate development of CNS and immune response following pathogenic invasion. Microglia are resident macrophages and the major component of the brain’s immune system, working with other immune cells and neurons to maintain the brain’s homeostasis and prevent the invasion of M.tb. Moreover, activated T and B lymphocytes have been found in the CSF of TBM patients from the clinical onset of the disease, and more diagnostic tools for TBM measuring the cytokines/chemokines that are secreted by lymphocytes have been developed in clinical practice. Moreover, the interaction of microglia between CNS macrophages or astrocytes has been involved in the TBM.
In sum, in the recent work, there remains some doubt about the role of CNS immune cells for TBM. The combination of modern techniques, including single-cell sequencing, multiomics technologies, and deep transcriptomics, will contribute to understanding the role of immune cells of CNS and explore the interaction of immune cells in the development of TBM.
Author contributions
QM: Conceptualization, Writing – original draft. JC: Writing – original draft. XK: Writing – review & editing. YZ: Writing – review & editing. ZC: Writing – review & editing. HL: Writing – review & editing. LL: Writing – review & editing. SL: Conceptualization, Supervision, Writing – review & editing. XW: Conceptualization, Supervision, Writing – review & editing.
Funding
The author(s) declare financial support was received for the research, authorship, and/or publication of this article. This work was supported by the Shenzhen Fund for Guangdong Provincial High-level Clinical Key Specialties (No. SZGSP010), Shenzhen High-level Hospital Construction Fund (No. G2022061)and the National Nature Science Foundation of China (Grant Numbers 32260883 and 31700130).
Conflict of interest
The authors declare that the research was conducted in the absence of any commercial or financial relationships that could be construed as a potential conflict of interest.
Publisher’s note
All claims expressed in this article are solely those of the authors and do not necessarily represent those of their affiliated organizations, or those of the publisher, the editors and the reviewers. Any product that may be evaluated in this article, or claim that may be made by its manufacturer, is not guaranteed or endorsed by the publisher.
References
1. Makam P, Matsa R. “Big three” Infectious diseases: tuberculosis, malaria and Hiv/Aids. Curr Top Med Chem (2021) 21(31):2779–99. doi: 10.2174/1568026621666210916170417
2. Bagcchi S. Who’s global tuberculosis report 2022. Lancet Microbe (2023) 4(1):e20. doi: 10.1016/S2666-5247(22)00359-7
3. Akpobolokemi T, Martinez-Nunez RT, Raimi-Abraham BT. Tackling the global impact of substandard and falsified and unregistered/unlicensed anti-tuberculosis medicines. J Med Access (2022) 6. doi: 10.1177/23992026211070406
4. Rohlwink UK, Walker NF, Ordonez AA, Li YJ, Tucker EW, Elkington PT, et al. Matrix metalloproteinases in pulmonary and central nervous system tuberculosis-a review. Int J Mol Sci (2019) 20(6):1350. doi: 10.3390/ijms20061350
5. Sahoo H, Garg RK, Rizvi I, Malhotra HS, Kumar N, Jain A, et al. Extra-central nervous system tuberculosis in hiv-uninfected patients of tuberculous meningitis: A prospective evaluation. J Infect Public Health (2020) 13(8):1101–6. doi: 10.1016/j.jiph.2020.04.011
6. Thwaites G, Fisher M, Hemingway C, Scott G, Solomon T, Innes J, et al. British infection society guidelines for the diagnosis and treatment of tuberculosis of the central nervous system in adults and children. J Infect (2009) 59(3):167–87. doi: 10.1016/j.jinf.2009.06.011
7. Garg RK, Sinha MK. Tuberculous meningitis in patients infected with human immunodeficiency virus. J Neurol (2011) 258(1):3–13. doi: 10.1007/s00415-010-5744-8
8. Khan MK, Islam MN, Ferdous J, Alam MM. An overview on epidemiology of tuberculosis. Mymensingh Med J (2019) 28(1):259–66.
9. Thwaites GE, van Toorn R, Schoeman J. Tuberculous meningitis: more questions, still too few answers. Lancet Neurol (2013) 12(10):999–1010. doi: 10.1016/S1474-4422(13)70168-6
10. Wu XR, Yin QQ, Jiao AX, Xu BP, Sun L, Jiao WW, et al. Pediatric tuberculosis at beijing children’s hospital: 2002-2010. Pediatrics (2012) 130(6):e1433–40. doi: 10.1542/peds.2011-3742
11. Ducomble T, Tolksdorf K, Karagiannis I, Hauer B, Brodhun B, Haas W, et al. The burden of extrapulmonary and meningitis tuberculosis: an investigation of national surveillance data, Germany, 2002 to 2009. Euro Surveill (2013) 18(12):20436. doi: 10.2807/ese.18.12.20436-en
12. Mihailidou E, Goutaki M, Nanou A, Tsiatsiou O, Kavaliotis J. Tuberculous meningitis in greek children. Scand J Infect Dis (2012) 44(5):337–43. doi: 10.3109/00365548.2011.639030
13. Be NA, Kim KS, Bishai WR, Jain SK. Pathogenesis of central nervous system tuberculosis. Curr Mol Med (2009) 9(2):94–9. doi: 10.2174/156652409787581655
14. Heemskerk AD, Donovan J, Thu DDA, Marais S, Chaidir L, Dung VTM, et al. Improving the microbiological diagnosis of tuberculous meningitis: A prospective, international, multicentre comparison of conventional and modified ziehl-neelsen stain, genexpert, and culture of cerebrospinal fluid. J Infect (2018) 77(6):509–15. doi: 10.1016/j.jinf.2018.09.003
15. Isabel BE, Rogelio HP. Pathogenesis and immune response in tuberculous meningitis. Malays J Med Sci (2014) 21(1):4–10.
16. Kalita J, Misra UK. Outcome of tuberculous meningitis at 6 and 12 months: A multiple regression analysis. Int J Tuberc Lung Dis (1999) 3(3):261–5.
17. Jain SK, Paul-Satyaseela M, Lamichhane G, Kim KS, Bishai WR. Mycobacterium tuberculosis invasion and traversal across an in vitro human blood-brain barrier as a pathogenic mechanism for central nervous system tuberculosis. J Infect Dis (2006) 193(9):1287–95. doi: 10.1086/502631
18. Castellani G, Croese T, Peralta Ramos JM, Schwartz M. Transforming the understanding of brain immunity. Science (2023) 380(6640):eabo7649. doi: 10.1126/science.abo7649
19. Hahn PF, Ross JF, Bale WF, Balfour WM, Whipple GH. Red cell and plasma volumes (Circulating and total) as determined by radio iron and by dye. J Exp Med (1942) 75(2):221–32. doi: 10.1084/jem.75.2.221
20. Whitmore WF 3rd, Gittes RF. The rate of skin allografts within the prostate. Invest Urol (1976) 14(2):153–5.
21. Clark PF, Fraser FR, Amoss HL. The relation to the blood of the virus of epidemic poliomyelitis. J Exp Med (1914) 19(3):223–33. doi: 10.1084/jem.19.3.223
22. Cuzner ML, Hayes GM, Newcombe J, Woodroofe MN. The nature of inflammatory components during demyelination in multiple sclerosis. J Neuroimmunol (1988) 20(2-3):203–9. doi: 10.1016/0165-5728(88)90161-0
23. Hickey WF, Hsu BL, Kimura H. T-lymphocyte entry into the central nervous system. J Neurosci Res (1991) 28(2):254–60. doi: 10.1002/jnr.490280213
24. Hickey WF, Vass K, Lassmann H. Bone marrow-derived elements in the central nervous system: an immunohistochemical and ultrastructural survey of rat chimeras. J Neuropathol Exp Neurol (1992) 51(3):246–56. doi: 10.1097/00005072-199205000-00002
25. Naqvi SH, Dunkle LM, Naseer S, Barth C. Significance of neutrophils in cerebrospinal fluid samples processed by cytocentrifugation. Clin Pediatr (Phila) (1983) 22(9):608–11. doi: 10.1177/000992288302200904
26. Moalem G, Leibowitz-Amit R, Yoles E, Mor F, Cohen IR, Schwartz M. Autoimmune T cells protect neurons from secondary degeneration after central nervous system axotomy. Nat Med (1999) 5(1):49–55. doi: 10.1038/4734
27. Rapalino O, Lazarov-Spiegler O, Agranov E, Velan GJ, Yoles E, Fraidakis M, et al. Implantation of stimulated homologous macrophages results in partial recovery of paraplegic rats. Nat Med (1998) 4(7):814–21. doi: 10.1038/nm0798-814
28. Wolf SA, Steiner B, Akpinarli A, Kammertoens T, Nassenstein C, Braun A, et al. Cd4-positive T lymphocytes provide a neuroimmunological link in the control of adult hippocampal neurogenesis. J Immunol (2009) 182(7):3979–84. doi: 10.4049/jimmunol.0801218
29. Ziv Y, Ron N, Butovsky O, Landa G, Sudai E, Greenberg N, et al. Immune cells contribute to the maintenance of neurogenesis and spatial learning abilities in adulthood. Nat Neurosci (2006) 9(2):268–75. doi: 10.1038/nn1629
30. Alves de Lima K, Rustenhoven J, Da Mesquita S, Wall M, Salvador AF, Smirnov I, et al. Meningeal gammadelta T cells regulate anxiety-like behavior via il-17a signaling in neurons. Nat Immunol (2020) 21(11):1421–9. doi: 10.1038/s41590-020-0776-4
31. Filiano AJ, Xu Y, Tustison NJ, Marsh RL, Baker W, Smirnov I, et al. Unexpected role of interferon-gamma in regulating neuronal connectivity and social behaviour. Nature (2016) 535(7612):425–9. doi: 10.1038/nature18626
32. Reed MD, Yim YS, Wimmer RD, Kim H, Ryu C, Welch GM, et al. Il-17a promotes sociability in mouse models of neurodevelopmental disorders. Nature (2020) 577(7789):249–53. doi: 10.1038/s41586-019-1843-6
33. Ribeiro M, Brigas HC, Temido-Ferreira M, Pousinha PA, Regen T, Santa C, et al. Meningeal gammadelta T cell-derived il-17 controls synaptic plasticity and short-term memory. Sci Immunol (2019) 4(40):eaay5199. doi: 10.1126/sciimmunol.aay5199
34. Hlaca N, Zagar T, Kastelan M, Brajac I, Prpic-Massari L. Current concepts of vitiligo immunopathogenesis. Biomedicines (2022) 10(7):1639. doi: 10.3390/biomedicines10071639
35. Hong H, Ji M, Lai D. Chronic stress effects on tumor: pathway and mechanism. Front Oncol (2021) 11:738252. doi: 10.3389/fonc.2021.738252
36. Pape K, Tamouza R, Leboyer M, Zipp F. Immunoneuropsychiatry - novel perspectives on brain disorders. Nat Rev Neurol (2019) 15(6):317–28. doi: 10.1038/s41582-019-0174-4
37. Xu H, Jia J. Single-cell rna sequencing of peripheral blood reveals immune cell signatures in alzheimer’s disease. Front Immunol (2021) 12:645666. doi: 10.3389/fimmu.2021.645666
38. Carrithers MD. Innate immune viral recognition: relevance to cns infections. Handb Clin Neurol (2014) 123:215–23. doi: 10.1016/B978-0-444-53488-0.00009-2
39. Tan Z, Lei Z, Zhang Z, Zhang H, Shu K, Hu F, et al. Identification and characterization of microglia/macrophages in the granuloma microenvironment of encephalic schistosomiasis japonicum. BMC Infect Dis (2019) 19(1):1088. doi: 10.1186/s12879-019-4725-5
40. Ernst JD. The immunological life cycle of tuberculosis. Nat Rev Immunol (2012) 12(8):581–91. doi: 10.1038/nri3259
41. Weiss G, Schaible UE. Macrophage defense mechanisms against intracellular bacteria. Immunol Rev (2015) 264(1):182–203. doi: 10.1111/imr.12266
42. Nguyen L, Pieters J. The trojan horse: survival tactics of pathogenic mycobacteria in macrophages. Trends Cell Biol (2005) 15(5):269–76. doi: 10.1016/j.tcb.2005.03.009
43. Ma W, Peng H, Liu K, Wang Y, Wang W, Qu S, et al. Efficacy of dual-targeting combined anti-tuberculosis drug delivery system in the treatment of tuberculous meningitis. J BioMed Nanotechnol (2021) 17(10):2034–42. doi: 10.1166/jbn.2021.3169
44. Cain MD, Salimi H, Diamond MS, Klein RS. Mechanisms of pathogen invasion into the central nervous system. Neuron (2019) 103(5):771–83. doi: 10.1016/j.neuron.2019.07.015
45. Chen Y, Liu X, Zhang X, Zhang Z, Zhou X, Wang Y, et al. Longitudinal cerebrospinal fluid assessment in a patient with tuberculous meningitis-a case report. J Clin Lab Anal (2020) 34(7):e23286. doi: 10.1002/jcla.23286
46. Ransohoff RM, Engelhardt B. The anatomical and cellular basis of immune surveillance in the central nervous system. Nat Rev Immunol (2012) 12(9):623–35. doi: 10.1038/nri3265
47. Thuong NT, Dunstan SJ, Chau TT, Thorsson V, Simmons CP, Quyen NT, et al. Identification of tuberculosis susceptibility genes with human macrophage gene expression profiles. PloS Pathog (2008) 4(12):e1000229. doi: 10.1371/journal.ppat.1000229
48. Shao Y, Xia P, Zhu T, Zhou J, Yuan Y, Zhang H, et al. Sensitivity and specificity of immunocytochemical staining of mycobacterial antigens in the cytoplasm of cerebrospinal fluid macrophages for diagnosing tuberculous meningitis. J Clin Microbiol (2011) 49(9):3388–91. doi: 10.1128/JCM.01323-11
49. Bsibsi M, Ravid R, Gveric D, van Noort JM. Broad expression of toll-like receptors in the human central nervous system. J Neuropathol Exp Neurol (2002) 61(11):1013–21. doi: 10.1093/jnen/61.11.1013
50. Dalpke AH, Schafer MK, Frey M, Zimmermann S, Tebbe J, Weihe E, et al. Immunostimulatory cpg-DNA activates murine microglia. J Immunol (2002) 168(10):4854–63. doi: 10.4049/jimmunol.168.10.4854
51. Laflamme N, Rivest S. Toll-like receptor 4: the missing link of the cerebral innate immune response triggered by circulating gram-negative bacterial cell wall components. FASEB J (2001) 15(1):155–63. doi: 10.1096/fj.00-0339com
52. Olson JK, Miller SD. Microglia initiate central nervous system innate and adaptive immune responses through multiple tlrs. J Immunol (2004) 173(6):3916–24. doi: 10.4049/jimmunol.173.6.3916
53. Shiau CE, Monk KR, Joo W, Talbot WS. An anti-inflammatory nod-like receptor is required for microglia development. Cell Rep (2013) 5(5):1342–52. doi: 10.1016/j.celrep.2013.11.004
54. Zhang YK, Liu JT, Peng ZW, Fan H, Yao AH, Cheng P, et al. Different tlr4 expression and microglia/macrophage activation induced by hemorrhage in the rat spinal cord after compressive injury. J Neuroinflamm (2013) 10:112. doi: 10.1186/1742-2094-10-112
55. Ribes S, Ebert S, Czesnik D, Regen T, Zeug A, Bukowski S, et al. Toll-like receptor prestimulation increases phagocytosis of escherichia coli dh5alpha and escherichia coli K1 strains by murine microglial cells. Infect Immun (2009) 77(1):557–64. doi: 10.1128/IAI.00903-08
56. Boddeke EW, Meigel I, Frentzel S, Biber K, Renn LQ, Gebicke-Harter P. Functional expression of the fractalkine (Cx3c) receptor and its regulation by lipopolysaccharide in rat microglia. Eur J Pharmacol (1999) 374(2):309–13. doi: 10.1016/s0014-2999(99)00307-6
57. Cheeran MC, Hu S, Yager SL, Gekker G, Peterson PK, Lokensgard JR. Cytomegalovirus induces cytokine and chemokine production differentially in microglia and astrocytes: antiviral implications. J Neurovirol (2001) 7(2):135–47. doi: 10.1080/13550280152058799
58. Hanisch UK. Microglia as a source and target of cytokines. Glia (2002) 40(2):140–55. doi: 10.1002/glia.10161
59. McManus CM, Brosnan CF, Berman JW. Cytokine induction of mip-1 alpha and mip-1 beta in human fetal microglia. J Immunol (1998) 160(3):1449–55. doi: 10.4049/jimmunol.160.3.1449
60. Peterson PK, Hu S, Salak-Johnson J, Molitor TW, Chao CC. Differential production of and migratory response to beta chemokines by human microglia and astrocytes. J Infect Dis (1997) 175(2):478–81. doi: 10.1093/infdis/175.2.478
61. Smith JA, Das A, Ray SK, Banik NL. Role of pro-inflammatory cytokines released from microglia in neurodegenerative diseases. Brain Res Bull (2012) 87(1):10–20. doi: 10.1016/j.brainresbull.2011.10.004
62. Sorensen TL, Tani M, Jensen J, Pierce V, Lucchinetti C, Folcik VA, et al. Expression of specific chemokines and chemokine receptors in the central nervous system of multiple sclerosis patients. J Clin Invest (1999) 103(6):807–15. doi: 10.1172/JCI5150
63. Keane J, Balcewicz-Sablinska MK, Remold HG, Chupp GL, Meek BB, Fenton MJ, et al. Infection by mycobacterium tuberculosis promotes human alveolar macrophage apoptosis. Infect Immun (1997) 65(1):298–304. doi: 10.1128/iai.65.1.298-304.1997
64. Askew K, Li K, Olmos-Alonso A, Garcia-Moreno F, Liang Y, Richardson P, et al. Coupled proliferation and apoptosis maintain the rapid turnover of microglia in the adult brain. Cell Rep (2017) 18(2):391–405. doi: 10.1016/j.celrep.2016.12.041
65. Ginhoux F, Greter M, Leboeuf M, Nandi S, See P, Gokhan S, et al. Fate mapping analysis reveals that adult microglia derive from primitive macrophages. Science (2010) 330(6005):841–5. doi: 10.1126/science.1194637
66. Prinz M, Jung S, Priller J. Microglia biology: one century of evolving concepts. Cell (2019) 179(2):292–311. doi: 10.1016/j.cell.2019.08.053
67. McMenamin PG, Wealthall RJ, Deverall M, Cooper SJ, Griffin B. Macrophages and dendritic cells in the rat meninges and choroid plexus: three-dimensional localisation by environmental scanning electron microscopy and confocal microscopy. Cell Tissue Res (2003) 313(3):259–69. doi: 10.1007/s00441-003-0779-0
68. Schulz C, Gomez Perdiguero E, Chorro L, Szabo-Rogers H, Cagnard N, Kierdorf K, et al. A lineage of myeloid cells independent of myb and hematopoietic stem cells. Science (2012) 336(6077):86–90. doi: 10.1126/science.1219179
69. Fuger P, Hefendehl JK, Veeraraghavalu K, Wendeln AC, Schlosser C, Obermuller U, et al. Microglia turnover with aging and in an alzheimer’s model via long-term in vivo single-cell imaging. Nat Neurosci (2017) 20(10):1371–6. doi: 10.1038/nn.4631
70. Tay TL, Mai D, Dautzenberg J, Fernandez-Klett F, Lin G, Sagar, et al. A new fate mapping system reveals context-dependent random or clonal expansion of microglia. Nat Neurosci (2017) 20(6):793–803. doi: 10.1038/nn.4547
71. Tucker EW, Pokkali S, Zhang Z, DeMarco VP, Klunk M, Smith ES, et al. Microglia activation in a pediatric rabbit model of tuberculous meningitis. Dis Model Mech (2016) 9(12):1497–506. doi: 10.1242/dmm.027326
72. Alkondon M, Ray A, Sen P. Nonstereoselective aspects of propranolol pharmacodynamics. Can J Physiol Pharmacol (1986) 64(12):1455–62. doi: 10.1139/y86-247
73. Davis AG, Dreyer AJ, Albertyn C, Maxebengula M, Stek C, Wasserman S, et al. Cognitive impairment in tuberculous meningitis. Clin Infect Dis (2023) 76(5):842–9. doi: 10.1093/cid/ciac831
74. Zhang X, Zhao Z, Wu Q, Wang L, Li L, Wang M, et al. Single-cell analysis reveals changes in bcg vaccine-injected mice modeling tuberculous meningitis brain infection. Cell Rep (2023) 42(3):112177. doi: 10.1016/j.celrep.2023.112177
75. Streit WJ, Graeber MB, Kreutzberg GW. Functional plasticity of microglia: A review. Glia (1988) 1(5):301–7. doi: 10.1002/glia.440010502
76. Sierra A, Tremblay ME, Wake H. Never-resting microglia: physiological roles in the healthy brain and pathological implications. Front Cell Neurosci (2014) 8:240. doi: 10.3389/fncel.2014.00240
77. Wes PD, Sayed FA, Bard F, Gan L. Targeting microglia for the treatment of alzheimer’s disease. Glia (2016) 64(10):1710–32. doi: 10.1002/glia.22988
78. Xue Y, Nie D, Wang LJ, Qiu HC, Ma L, Dong MX, et al. Microglial polarization: novel therapeutic strategy against ischemic stroke. Aging Dis (2021) 12(2):466–79. doi: 10.14336/AD.2020.0701
79. Randall PJ, Hsu NJ, Lang D, Cooper S, Sebesho B, Allie N, et al. Neurons are host cells for mycobacterium tuberculosis. Infect Immun (2014) 82(5):1880–90. doi: 10.1128/IAI.00474-13
80. Rock RB, Hu S, Gekker G, Sheng WS, May B, Kapur V, et al. Mycobacterium tuberculosis-induced cytokine and chemokine expression by human microglia and astrocytes: effects of dexamethasone. J Infect Dis (2005) 192(12):2054–8. doi: 10.1086/498165
81. Curto M, Reali C, Palmieri G, Scintu F, Schivo ML, Sogos V, et al. Inhibition of cytokines expression in human microglia infected by virulent and non-virulent mycobacteria. Neurochem Int (2004) 44(6):381–92. doi: 10.1016/j.neuint.2003.08.012
82. Peterson PK, Gekker G, Hu S, Sheng WS, Anderson WR, Ulevitch RJ, et al. Cd14 receptor-mediated uptake of nonopsonized mycobacterium tuberculosis by human microglia. Infect Immun (1995) 63(4):1598–602. doi: 10.1128/iai.63.4.1598-1602.1995
83. Rock RB, Gekker G, Hu S, Sheng WS, Cheeran M, Lokensgard JR, et al. Role of microglia in central nervous system infections. Clin Microbiol Rev (2004) 17(4):942–64. doi: 10.1128/CMR.17.4.942-964.2004
84. Rock RB, Olin M, Baker CA, Molitor TW, Peterson PK. Central nervous system tuberculosis: pathogenesis and clinical aspects. Clin Microbiol Rev (2008) 21(2):243–61. doi: 10.1128/CMR.00042-07
85. Carson MJ, Doose JM, Melchior B, Schmid CD, Ploix CC. Cns immune privilege: hiding in plain sight. Immunol Rev (2006) 213:48–65. doi: 10.1111/j.1600-065X.2006.00441.x
86. Krishnaswamy JK, Chu T, Eisenbarth SC. Beyond pattern recognition: nod-like receptors in dendritic cells. Trends Immunol (2013) 34(5):224–33. doi: 10.1016/j.it.2012.12.003
87. Huynh J, Donovan J, Phu NH, Nghia HDT, Thuong NTT, Thwaites GE. Tuberculous meningitis: progress and remaining questions. Lancet Neurol (2022) 21(5):450–64. doi: 10.1016/S1474-4422(21)00435-X
88. Kumar V. Toll-like receptors in the pathogenesis of neuroinflammation. J Neuroimmunol (2019) 332:16–30. doi: 10.1016/j.jneuroim.2019.03.012
89. Barichello T, Generoso JS, Goularte JA, Collodel A, Pitcher MR, Simoes LR, et al. Does infection-induced immune activation contribute to dementia? Aging Dis (2015) 6(5):342–8. doi: 10.14336/AD.2015.0521
90. Chakrabarty P, Li A, Ladd TB, Strickland MR, Koller EJ, Burgess JD, et al. Tlr5 decoy receptor as a novel anti-amyloid therapeutic for alzheimer’s disease. J Exp Med (2018) 215(9):2247–64. doi: 10.1084/jem.20180484
91. Chhatbar C, Prinz M. The roles of microglia in viral encephalitis: from sensome to therapeutic targeting. Cell Mol Immunol (2021) 18(2):250–8. doi: 10.1038/s41423-020-00620-5
92. Kouli A, Horne CB, Williams-Gray CH. Toll-like receptors and their therapeutic potential in parkinson’s disease and alpha-synucleinopathies. Brain Behav Immun (2019) 81:41–51. doi: 10.1016/j.bbi.2019.06.042
93. Perez-Bercoff D, Laude H, Lemaire M, Hunewald O, Thiers V, Vignuzzi M, et al. Sustained high expression of multiple apobec3 cytidine deaminases in systemic lupus erythematosus. Sci Rep (2021) 11(1):7893. doi: 10.1038/s41598-021-87024-1
94. Tajalli-Nezhad S, Karimian M, Beyer C, Atlasi MA, Azami Tameh A. The regulatory role of toll-like receptors after ischemic stroke: neurosteroids as tlr modulators with the focus on tlr2/4. Cell Mol Life Sci (2019) 76(3):523–37. doi: 10.1007/s00018-018-2953-2
95. Zhang P, Zhang N, Liu L, Zheng K, Zhu L, Zhu J, et al. Polymorphisms of toll-like receptors 2 and 9 and severity and prognosis of bacterial meningitis in chinese children. Sci Rep (2017) 7:42796. doi: 10.1038/srep42796
96. Kiemer AK, Senaratne RH, Hoppstadter J, Diesel B, Riley LW, Tabeta K, et al. Attenuated activation of macrophage tlr9 by DNA from virulent mycobacteria. J Innate Immun (2009) 1(1):29–45. doi: 10.1159/000142731
97. Liu L, Liu J, Niu G, Xu Q, Chen Q. Mycobacterium tuberculosis 19-kda lipoprotein induces toll-like receptor 2-dependent peroxisome proliferator-activated receptor gamma expression and promotes inflammatory responses in human macrophages. Mol Med Rep (2015) 11(4):2921–6. doi: 10.3892/mmr.2014.3070
98. Sanchez D, Rojas M, Hernandez I, Radzioch D, Garcia LF, Barrera LF. Role of tlr2- and tlr4-mediated signaling in mycobacterium tuberculosis-induced macrophage death. Cell Immunol (2010) 260(2):128–36. doi: 10.1016/j.cellimm.2009.10.007
99. Yang CS, Lee HM, Lee JY, Kim JA, Lee SJ, Shin DM, et al. Reactive oxygen species and P47phox activation are essential for the mycobacterium tuberculosis-induced pro-inflammatory response in murine microglia. J Neuroinflamm (2007) 4:27. doi: 10.1186/1742-2094-4-27
100. Chen Z, Shao XY, Wang C, Hua MH, Wang CN, Wang X, et al. Mycobacterium marinum infection in zebrafish and microglia imitates the early stage of tuberculous meningitis. J Mol Neurosci (2018) 64(2):321–30. doi: 10.1007/s12031-018-1026-1
101. Dutta RK, Kathania M, Raje M, Majumdar S. Il-6 inhibits ifn-gamma induced autophagy in mycobacterium tuberculosis H37rv infected macrophages. Int J Biochem Cell Biol (2012) 44(6):942–54. doi: 10.1016/j.biocel.2012.02.021
102. Mohanraj M, Sekar P, Liou HH, Chang SF, Lin WW. The mycobacterial adjuvant analogue tdb attenuates neuroinflammation via mincle-independent plc-gamma1/pkc/erk signaling and microglial polarization. Mol Neurobiol (2019) 56(2):1167–87. doi: 10.1007/s12035-018-1135-4
103. Ishikawa E, Ishikawa T, Morita YS, Toyonaga K, Yamada H, Takeuchi O, et al. Direct recognition of the mycobacterial glycolipid, trehalose dimycolate, by C-type lectin mincle. J Exp Med (2009) 206(13):2879–88. doi: 10.1084/jem.20091750
104. Strasser D, Neumann K, Bergmann H, Marakalala MJ, Guler R, Rojowska A, et al. Syk kinase-coupled C-type lectin receptors engage protein kinase C-delta to elicit card9 adaptor-mediated innate immunity. Immunity (2012) 36(1):32–42. doi: 10.1016/j.immuni.2011.11.015
105. Cannas S, Molicotti P, Bua A, Usai D, Sechi LA, Scanu AM, et al. Interaction between mycobacterium tuberculosis, mycobacterium bovis, mycobacterium avium subspecies paratuberculosis with the enteric glia and microglial cells. Gut Pathog (2011) 3:19. doi: 10.1186/1757-4749-3-19
106. Zaharie SD, Franken DJ, van der Kuip M, van Elsland S, de Bakker BS, Hagoort J, et al. The immunological architecture of granulomatous inflammation in central nervous system tuberculosis. Tuberculosis (Edinb) (2020) 125:102016. doi: 10.1016/j.tube.2020.102016
107. Zucchi FC, Pelegrini-da-Silva A, Neder L, Silva CL, Tsanaclis AM, Takayanagui OM. The contribution of a murine cns-tb model for the understanding of the host-pathogen interactions in the formation of granulomas. J Neurosci Methods (2012) 206(1):88–93. doi: 10.1016/j.jneumeth.2012.02.015
108. Yang CS, Yuk JM, Shin DM, Kang J, Lee SJ, Jo EK. Secretory phospholipase A2 plays an essential role in microglial inflammatory responses to mycobacterium tuberculosis. Glia (2009) 57(10):1091–103. doi: 10.1002/glia.20832
109. Lee HM, Kang J, Lee SJ, Jo EK. Microglial activation of the nlrp3 inflammasome by the priming signals derived from macrophages infected with mycobacteria. Glia (2013) 61(3):441–52. doi: 10.1002/glia.22448
110. Duddy M, Niino M, Adatia F, Hebert S, Freedman M, Atkins H, et al. Distinct effector cytokine profiles of memory and naive human B cell subsets and implication in multiple sclerosis. J Immunol (2007) 178(10):6092–9. doi: 10.4049/jimmunol.178.10.6092
111. Weber MS, Prod’homme T, Patarroyo JC, Molnarfi N, Karnezis T, Lehmann-Horn K, et al. B-cell activation influences T-cell polarization and outcome of anti-cd20 B-cell depletion in central nervous system autoimmunity. Ann Neurol (2010) 68(3):369–83. doi: 10.1002/ana.22081
112. Molnarfi N, Schulze-Topphoff U, Weber MS, Patarroyo JC, Prod’homme T, Varrin-Doyer M, et al. Mhc class ii-dependent B cell apc function is required for induction of cns autoimmunity independent of myelin-specific antibodies. J Exp Med (2013) 210(13):2921–37. doi: 10.1084/jem.20130699
113. Roodselaar J, Zhou Y, Leppert D, Hauser AE, Urich E, Anthony DC. Anti-cd20 disrupts meningeal B-cell aggregates in a model of secondary progressive multiple sclerosis. Neurol Neuroimmunol Neuroinflamm (2021) 8(3):e975. doi: 10.1212/NXI.0000000000000975
114. Pelc S, De Maertelaere E. Csf cells in tuberculous meningitis. Humoral and cellular immune response. J Neurol Sci (1981) 49(2):223–8. doi: 10.1016/0022-510x(81)90080-0
115. Zhang QQ, Zhang YF, Yu N, Lin XJ, Di Q. Differential diagnosis of autoimmune encephalitis from infectious lymphocytic encephalitis by analysing the lymphocyte subsets of cerebrospinal fluid. Anal Cell Pathol (Amst) (2019) 2019:9684175. doi: 10.1155/2019/9684175
116. Yu N, Zhang QQ, Zhang K, Xie Y, Zhu HQ, Lin XJ, et al. Comparative study of cd4 and cd45ro T cells and cd20 B cells in cerebrospinal fluid of syphilitic meningitis and tuberculous meningitis patients. APMIS (2016) 124(9):764–9. doi: 10.1111/apm.12572
117. Green AM, Difazio R, Flynn JL. Ifn-gamma from cd4 T cells is essential for host survival and enhances cd8 T cell function during mycobacterium tuberculosis infection. J Immunol (2013) 190(1):270–7. doi: 10.4049/jimmunol.1200061
118. Kumar P. Ifngamma-producing cd4(+) T lymphocytes: the double-edged swords in tuberculosis. Clin Transl Med (2017) 6(1):21. doi: 10.1186/s40169-017-0151-8
119. Rothchild AC, Stowell B, Goyal G, Nunes-Alves C, Yang Q, Papavinasasundaram K, et al. Role of granulocyte-macrophage colony-stimulating factor production by T cells during mycobacterium tuberculosis infection. mBio (2017) 8(5):e01514-17. doi: 10.1128/mBio.01514-17
120. Kamboj D, Gupta P, Basil MV, Mohan A, Guleria R, Bhatnagar A, et al. Improved mycobacterium tuberculosis clearance after the restoration of ifn-gamma(+) tnf-alpha(+) cd4(+) T cells: impact of pd-1 inhibition in active tuberculosis patients. Eur J Immunol (2020) 50(5):736–47. doi: 10.1002/eji.201948283
121. Clark RB, Lingenheld EG, Donaldson JO, Pollard MK. Compartmentalized immune responses: antigen-specificity of cerebrospinal fluid T-cell lines maintained in the absence of antigen. Clin Immunol Immunopathol (1985) 36(2):176–86. doi: 10.1016/0090-1229(85)90119-9
122. Xu L, Xu Y, Zheng Y, Peng X, Yang Z, Cao Q, et al. Differences in cytokine and chemokine profiles in cerebrospinal fluid caused by the etiology of cryptococcal meningitis and tuberculous meningitis in hiv patients. Clin Exp Immunol (2021) 206(1):82–90. doi: 10.1111/cei.13644
123. Kosters K, Nau R, Bossink A, Greiffendorf I, Jentsch M, Ernst M, et al. Rapid diagnosis of cns tuberculosis by a T-cell interferon-gamma release assay on cerebrospinal fluid mononuclear cells. Infection (2008) 36(6):597–600. doi: 10.1007/s15010-007-7316-0
124. Marais S, Meintjes G, Pepper DJ, Dodd LE, Schutz C, Ismail Z, et al. Frequency, severity, and prediction of tuberculous meningitis immune reconstitution inflammatory syndrome. Clin Infect Dis (2013) 56(3):450–60. doi: 10.1093/cid/cis899
125. Marais S, Wilkinson KA, Lesosky M, Coussens AK, Deffur A, Pepper DJ, et al. Neutrophil-associated central nervous system inflammation in tuberculous meningitis immune reconstitution inflammatory syndrome. Clin Infect Dis (2014) 59(11):1638–47. doi: 10.1093/cid/ciu641
126. Muefong CN, Owolabi O, Donkor S, Charalambous S, Mendy J, Sey ICM, et al. Major neutrophil-derived soluble mediators associate with baseline lung pathology and post-treatment recovery in tuberculosis patients. Front Immunol (2021) 12:740933. doi: 10.3389/fimmu.2021.740933
127. Donovan J, Tram TTB, Phu NH, Hiep NTT, Van VTT, Mui DTH, et al. Influence of strongyloides stercoralis coinfection on the presentation, pathogenesis, and outcome of tuberculous meningitis. J Infect Dis (2022) 225(9):1653–62. doi: 10.1093/infdis/jiaa672
128. Marais S, Lai RPJ, Wilkinson KA, Meintjes G, O’Garra A, Wilkinson RJ. Inflammasome activation underlying central nervous system deterioration in hiv-associated tuberculosis. J Infect Dis (2017) 215(5):677–86. doi: 10.1093/infdis/jiw561
129. Green JA, Tran CT, Farrar JJ, Nguyen MT, Nguyen PH, Dinh SX, et al. Dexamethasone, cerebrospinal fluid matrix metalloproteinase concentrations and clinical outcomes in tuberculous meningitis. PloS One (2009) 4(9):e7277. doi: 10.1371/journal.pone.0007277
130. Li K, Tang H, Yang Y, Li Q, Zhou Y, Ren M, et al. Clinical features, long-term clinical outcomes, and prognostic factors of tuberculous meningitis in west China: A multivariate analysis of 154 adults. Expert Rev Anti Infect Ther (2017) 15(6):629–35. doi: 10.1080/14787210.2017.1309974
131. Grobbelaar M, van Toorn R, Solomons R. Lumbar cerebrospinal fluid evolution in childhood tuberculous meningitis. J Child Neurol (2018) 33(11):700–7. doi: 10.1177/0883073818785553
132. Thwaites GE, Simmons CP, Than Ha Quyen N, Thi Hong Chau T, Phuong Mai P, Thi Dung N, et al. Pathophysiology and prognosis in Vietnamese adults with tuberculous meningitis. J Infect Dis (2003) 188(8):1105–15. doi: 10.1086/378642
133. van Laarhoven A, Dian S, Ruesen C, Hayati E, Damen M, Annisa J, et al. Clinical parameters, routine inflammatory markers, and lta4h genotype as predictors of mortality among 608 patients with tuberculous meningitis in Indonesia. J Infect Dis (2017) 215(7):1029–39. doi: 10.1093/infdis/jix051
134. Cresswell FV, Davis AG, Sharma K, Basu Roy R, Ganiem AR, Kagimu E, et al. Recent developments in tuberculous meningitis pathogenesis and diagnostics. Wellcome Open Res (2019) 4:164. doi: 10.12688/wellcomeopenres.15506.3
135. Harahap MIR, Soetikno RD, Anwary F. Relationship between neutrophil count in cerebrospinal fluid and cerebral infarct appearance in head mri on tuberculous meningitis patients. Eur J Radiol Open (2023) 10:100469. doi: 10.1016/j.ejro.2022.100469
136. Karandanis D, Shulman JA. Recent survey of infectious meningitis in adults: review of laboratory findings in bacterial, tuberculous, and aseptic meningitis. South Med J (1976) 69(4):449–57. doi: 10.1097/00007611-197604000-00021
137. Jakka S, Veena S, Rao AR, Eisenhut M. Cerebrospinal fluid adenosine deaminase levels and adverse neurological outcome in pediatric tuberculous meningitis. Infection (2005) 33(4):264–6. doi: 10.1007/s15010-005-5005-4
138. Pinto VL Jr., Lima MA, Rolla VC, Rebelo MC, Boia MN. Atypical cerebrospinal fluid profile in tuberculous meningitis. Trop Doct (2009) 39(2):76–8. doi: 10.1258/td.2008.080242
139. Jongeling AC, Pisapia D. Pearls and oy-sters: tuberculous meningitis: not a diagnosis of exclusion. Neurology (2013) 80(4):e36–9. doi: 10.1212/WNL.0b013e31827f0832
140. He H, Zou Y, He J, Bu H, Liu Y. A diagnostic scoring system for distinguishing between tuberculous and bacterial meningitis based on clinical and laboratory findings. BioMed Res Int (2021) 2021:1220650. doi: 10.1155/2021/1220650
141. Lu Y, Ma C, Chen R, Hu Z, Yao H, Zhang Q, et al. Development and validation of a new scoring system for the early diagnosis of tuberculous meningitis in adults. Diagn Microbiol Infect Dis (2021) 101(2):115393. doi: 10.1016/j.diagmicrobio.2021.115393
142. Luo Y, Xue Y, Lin Q, Mao L, Tang G, Song H, et al. Diagnostic model for discrimination between tuberculous meningitis and bacterial meningitis. Front Immunol (2021) 12:731876. doi: 10.3389/fimmu.2021.731876
143. van Laarhoven A, Dian S, van Dorp S, Purnama F, Koeken V, Diandini E, et al. Immune cell characteristics and cytokine responses in adult hiv-negative tuberculous meningitis: an observational cohort study. Sci Rep (2019) 9(1):884. doi: 10.1038/s41598-018-36696-3
144. Adler H, Beland JL, Del-Pan NC, Kobzik L, Sobel RA, Rimm IJ. In the absence of T cells, natural killer cells protect from mortality due to hsv-1 encephalitis. J Neuroimmunol (1999) 93(1-2):208–13. doi: 10.1016/s0165-5728(98)00236-7
145. Almerigogna F, Fassio F, Giudizi MG, Biagiotti R, Manuelli C, Chiappini E, et al. Natural killer cell deficiencies in a consecutive series of children with herpetic encephalitis. Int J Immunopathol Pharmacol (2011) 24(1):231–8. doi: 10.1177/039463201102400128
146. Mitchell AJ, Yau B, McQuillan JA, Ball HJ, Too LK, Abtin A, et al. Inflammasome-dependent ifn-gamma drives pathogenesis in streptococcus pneumoniae meningitis. J Immunol (2012) 189(10):4970–80. doi: 10.4049/jimmunol.1201687
147. Stach JL, Dufrenoy E, Roffi J, Bach MA. T-cell subsets and natural killer activity in plasmodium falciparum-infected children. Clin Immunol Immunopathol (1986) 38(1):129–34. doi: 10.1016/0090-1229(86)90130-3
148. Shieh TM, Carter DL, Blosser RL, Mankowski JL, Zink MC, Clements JE. Functional analyses of natural killer cells in macaques infected with neurovirulent simian immunodeficiency virus. J Neurovirol (2001) 7(1):11–24. doi: 10.1080/135502801300069593
149. Oschwald A, Petry P, Kierdorf K, Erny D. Cns macrophages and infant infections. Front Immunol (2020) 11:2123. doi: 10.3389/fimmu.2020.02123
150. Ransohoff RM, Schafer D, Vincent A, Blachere NE, Bar-Or A. Neuroinflammation: ways in which the immune system affects the brain. Neurotherapeutics (2015) 12(4):896–909. doi: 10.1007/s13311-015-0385-3
151. Goldmann T, Wieghofer P, Jordao MJ, Prutek F, Hagemeyer N, Frenzel K, et al. Origin, fate and dynamics of macrophages at central nervous system interfaces. Nat Immunol (2016) 17(7):797–805. doi: 10.1038/ni.3423
152. Pieters J. Mycobacterium tuberculosis and the macrophage: maintaining a balance. Cell Host Microbe (2008) 3(6):399–407. doi: 10.1016/j.chom.2008.05.006
153. Jayachandran R, BoseDasgupta S, Pieters J. Surviving the macrophage: tools and tricks employed by mycobacterium tuberculosis. Curr Top Microbiol Immunol (2013) 374:189–209. doi: 10.1007/82_2012_273
154. Carow B, Ye X, Gavier-Widen D, Bhuju S, Oehlmann W, Singh M, et al. Silencing suppressor of cytokine signaling-1 (Socs1) in macrophages improves mycobacterium tuberculosis control in an interferon-gamma (Ifn-gamma)-dependent manner. J Biol Chem (2011) 286(30):26873–87. doi: 10.1074/jbc.M111.238287
155. Jung BG, Vankayalapati R, Samten B. Mycobacterium tuberculosis stimulates il-1beta production by macrophages in an esat-6 dependent manner with the involvement of serum amyloid A3. Mol Immunol (2021) 135:285–93. doi: 10.1016/j.molimm.2021.04.022
156. Tripathi S, Patro I, Mahadevan A, Patro N, Phillip M, Shankar SK. Glial alterations in tuberculous and cryptococcal meningitis and their relation to hiv co-infection–a study on human brains. J Infect Dev Ctries (2014) 8(11):1421–43. doi: 10.3855/jidc.3894
157. Mason S. Lactate shuttles in neuroenergetics-homeostasis, allostasis and beyond. Front Neurosci (2017) 11:43. doi: 10.3389/fnins.2017.00043
158. Mason S, van Furth AM, Mienie LJ, Engelke UF, Wevers RA, Solomons R, et al. A hypothetical astrocyte-microglia lactate shuttle derived from a (1)H nmr metabolomics analysis of cerebrospinal fluid from a cohort of South African children with tuberculous meningitis. Metabolomics (2015) 11(4):822–37. doi: 10.1007/s11306-014-0741-z
159. Mason S, Reinecke CJ, Kulik W, van Cruchten A, Solomons R, van Furth AM. Cerebrospinal fluid in tuberculous meningitis exhibits only the L-enantiomer of lactic acid. BMC Infect Dis (2016) 16:251. doi: 10.1186/s12879-016-1597-9
160. Russell MS, Dudani R, Krishnan L, Sad S. Ifn-gamma expressed by T cells regulates the persistence of antigen presentation by limiting the survival of dendritic cells. J Immunol (2009) 183(12):7710–8. doi: 10.4049/jimmunol.0901274
161. Abebe F. Is interferon-gamma the right marker for bacille calmette-guerin-induced immune protection? The missing link in our understanding of tuberculosis immunology. Clin Exp Immunol (2012) 169(3):213–9. doi: 10.1111/j.1365-2249.2012.04614.x
162. Carow B, Reuschl AK, Gavier-Widen D, Jenkins BJ, Ernst M, Yoshimura A, et al. Critical and independent role for socs3 in either myeloid or T cells in resistance to mycobacterium tuberculosis. PloS Pathog (2013) 9(7):e1003442. doi: 10.1371/journal.ppat.1003442
Keywords: central nervous system, immune cells, tuberculous meningitis, microglia, macrophages
Citation: Ma Q, Chen J, Kong X, Zeng Y, Chen Z, Liu H, Liu L, Lu S and Wang X (2024) Interactions between CNS and immune cells in tuberculous meningitis. Front. Immunol. 15:1326859. doi: 10.3389/fimmu.2024.1326859
Received: 24 October 2023; Accepted: 10 January 2024;
Published: 01 February 2024.
Edited by:
Sofiati Dian, Radboud University Medical Centre, NetherlandsReviewed by:
Kathirvel Maruthai, Johns Hopkins University, United StatesCopyright © 2024 Ma, Chen, Kong, Zeng, Chen, Liu, Liu, Lu and Wang. This is an open-access article distributed under the terms of the Creative Commons Attribution License (CC BY). The use, distribution or reproduction in other forums is permitted, provided the original author(s) and the copyright owner(s) are credited and that the original publication in this journal is cited, in accordance with accepted academic practice. No use, distribution or reproduction is permitted which does not comply with these terms.
*Correspondence: Xiaomin Wang, V1hNX1pNVUAxNjMuY29t; Shuihua Lu, bHVzaHVpaHVhNjZAMTI2LmNvbQ==