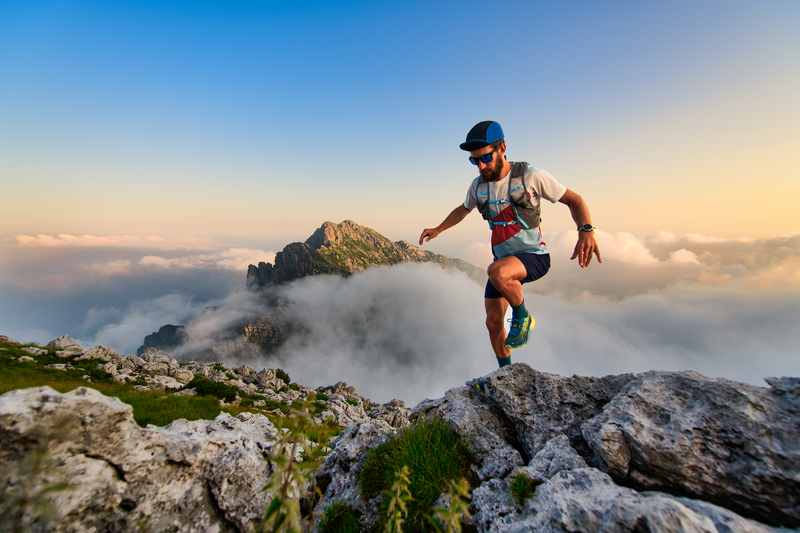
95% of researchers rate our articles as excellent or good
Learn more about the work of our research integrity team to safeguard the quality of each article we publish.
Find out more
REVIEW article
Front. Immunol. , 21 February 2024
Sec. Microbial Immunology
Volume 15 - 2024 | https://doi.org/10.3389/fimmu.2024.1324018
The bidirectional communication between the gut and brain or gut-brain axis is regulated by several gut microbes and microbial derived metabolites, such as short-chain fatty acids, trimethylamine N-oxide, and lipopolysaccharides. The Gut microbiota (GM) produce neuroactives, specifically neurotransmitters that modulates local and central neuronal brain functions. An imbalance between intestinal commensals and pathobionts leads to a disruption in the gut microbiota or dysbiosis, which affects intestinal barrier integrity and gut-immune and neuroimmune systems. Currently, fecal microbiota transplantation (FMT) is recommended for the treatment of recurrent Clostridioides difficile infection. FMT elicits its action by ameliorating inflammatory responses through the restoration of microbial composition and functionality. Thus, FMT may be a potential therapeutic option in suppressing neuroinflammation in post-stroke conditions and other neurological disorders involving the neuroimmune axis. Specifically, FMT protects against ischemic injury by decreasing IL-17, IFN-γ, Bax, and increasing Bcl-2 expression. Interestingly, FMT improves cognitive function by lowering amyloid-β accumulation and upregulating synaptic marker (PSD-95, synapsin-1) expression in Alzheimer’s disease. In Parkinson’s disease, FMT was shown to inhibit the expression of TLR4 and NF-κB. In this review article, we have summarized the potential sources and methods of administration of FMT and its impact on neuroimmune and cognitive functions. We also provide a comprehensive update on the beneficial effects of FMT in various neurological disorders by undertaking a detailed interrogation of the preclinical and clinical published literature.
The Gut-brain axis (GBA), also known as the microbiota-gut-brain axis, refers to the complex bidirectional connections between gut and brain occurring through the central, autonomic, and enteric nerves systems, endocrine system, and innate and acquired immune systems (1, 2). The gut microbiota (GM) refers to the collection of microorganisms such as bacteria, viruses, protozoa, archaea, and fungi, which are present in the highest density in the colon. The collective genome of the GM in the gastrointestinal tract (GIT) is called the gut microbiome (3). An imbalance between intestinal commensal and pathobionts leads to a disruption in the gut microbiota or gut dysbiosis (GD), which in turn affects gut-immune and neuroimmune systems. The crosstalk between the gut and brain suggests that the GM plays an important role in regulating the metabolism, immune system, and vascular system of the host (4).
The human GM comprises 10–100 trillion microbes constituting a diverse population belonging to both commensal and opportunistic microbes (5). There are more than 100 bacterial phyla; the major two phyla are Firmicutes and Bacteroidetes, and minor phyla are Actinobacteria, Proteobacteria, and Verrucomicrobia (6). A compositional study on the gut microbiome by Hollister et al. revealed that sex, race/ethnicity, environmental factors, and age contribute to variations in the human GM composition (7). The GM and its metabolites mediate the wellness of the body by regulating immunological, metabolic, and neuroendocrinological functions. The functions of the GM and metabolites are diverse, ranging from supporting the absorption of nutrients and minerals, the synthesis of enzymes, vitamins, and amino acids, and the production of bacterial-derived metabolites such as short-chain fatty acids (SCFAs) (acetate, propionate, valeric acid, and butyrate), phenylacetylglutamine, and trimethylamine N-oxide (TMAO); all of which maintain host homeostasis (8). The GM therefore acts as a defense barrier by mediating colonization resistance (preventing pathogenic colonization) through competing for attachment sites and nutrients, and by secretion and production of antimicrobials (9). Bacterial-derived metabolites, particularly SCFAs, help to modulate the maturation and functions of microglia in the brain, implicating its regulatory potential in modifying neuro-inflammatory responses and thus play a role in neurodegenerative diseases (NDDs) (10). The GM also plays an important role in immunomodulation by altering B-cell activation to produce IgA and Group 3 innate lymphoid cells (immune cells found in barrier sites), gut-associated lymphoid tissues, macrophages, dendritic cells in the lamina propria, and effector and regulatory T cells (11). For example, GD is associated with numerous diseases, including Inflammatory bowel disease (IBD) (12), diabetes (13), Parkinson’s disease (PD) (14), Alzheimer’s disease (AD) (15), autism (16), multiple sclerosis (17), and stroke (18). These reports indicate a strong involvement of GD in various pathological conditions, particularly altering the inflammatory milieu. GD impacts neurological disorders mainly through altering immune responses. Similarly, use of FMT is shown to improve immune dysregulation in Clostridioides difficile infection (CDI) and other inflammatory diseases (19, 20). Herein, we comprehensively demonstrate the mechanistic impact of FMT in various neurological disorder with a central focus on inflammation.
Dysregulation of the gut-brain axis has been implicated in various neurological disorders including Alzheimer’s disease (AD), autism spectrum disorder (ASD), Parkinson’s disease (PD), and stroke. These neurological conditions have been shown to cause changes in the bidirectional link, resulting in the emergence of brain-gut disorders including irritable bowel syndrome (IBS) (21). AD etiology has also been strongly linked to GD and microbial-derived metabolites that are directly linked with phosphorylated tau/Aβ42 accumulation in AD (22). Interestingly, Helicobacter pylori infection has been linked to AD pathology. It causes abnormal hyperphosphorylation of tau protein as well as the release of inflammatory mediators and β-amyloids (23). GD has increased recognition in PD wherein the initiation of α-synucleinopathy is produced in the enteric nervous system (ENS) during the early stages of the disease (24). PD patients experience a wide range of clinical symptoms including difficulties in swallowing, drooling, delayed gastric emptying, small intestinal bacterial overgrowth, and constipation (25). Furthermore, gut microbes belonging to Prevotellaceae, Christensenella, Akkermansia, and Lactobacillus were previously demonstrated to be as associated with PD (26, 27). On the other hand, several studies have reported the GM composition differences between ASD and healthy children, where studies have reported a reduced abundance of beneficial bacterium Bifidobacterium, and an increased abundance of pathobionts such as taxa belonging to Clostridium cluster XVIII and Escherichia/Shigella (28, 29). These reports indicate that the GM regulates GBA functions and the pathophysiology of neurological disorders.
The GM exerts diverse beneficial effects on brain health, the neuroimmune system, and defense against pathogenic infections (30). A well-coordinated inflammatory response within the brain is known as a neuroinflammatory reaction, and it is mediated by a variety of cell populations, including astrocytes and microglia. Astrocytes, microglia, and peripherally derived immune cells are the primary producers of inflammatory mediators such as reactive oxygen species, cytokines, and chemokines (31). Strong experimental evidence suggests that the GM and immune-mediated inflammatory responses interact in a complex, dynamic, and bidirectional manner. Germ-free animals serve as an excellent tool for demonstrating the interaction between the GM on the innate and adaptive immune systems in health and disease states (32).
The innate immune response of the gut mucosa is directed against one or more pathogens and antigens (33). The GM in association with antigen-presenting cells (APCs) protects the body against infection. Also, to maintain immune tolerance to the normal gut flora, the dendritic cells (DCs) of Peyer’s patches (lymphoid nodules embedded in the gut wall) produce anti-inflammatory cytokines e.g., interleukin (IL)-10 and TGF-β (34). In the adaptive immune system, CD4+ T cells play an important role. The lamina propria of the intestine contains the majority of the intestinal CD4+ T lymphocytes. Naive CD4+ T cells can develop into one of four main subtypes after being stimulated: T helper 1 (Th1), Th2, Th17, or regulatory T cell (Treg) (35). However, in GD, the adaptive immune system activates CD4+ T cells which further interact with intestinal immune cells such as Th1, Th2, Th17, and Tregs, which lead to neuroinflammation and neuronal death (36).
Pathogenic microbes/microbial components trigger inflammatory reactions via pattern recognition receptor (PRP) families that include pathogen-associated molecular patterns/microbe-associated molecular patterns (PAMPs/MAMPs), or by specific molecular structures released by host cells called danger-associated molecular patterns (DAMPs) (dead or dying cells, cancer cells, toxic, or allergenic structures (37). These stereotyped molecular patterns are recognized by tissue-resident immune cells (mast cells and macrophages) and as a result, they elicit an innate immune response resulting in the increased production of cytokines and chemokines and also induce complement activation (38). Localized dendritic cells and macrophages serve as antigen-presenting cells (APCs). To initiate an adaptive immune response, APCs translocate to tissue-draining lymph nodes where they expose local immune cells to foreign antigens via molecules of the major histocompatibility complex (MHC). A persistent inflammatory response results in the recruitment of circulating leukocytes, particularly T-lymphocytes, which enter the tissue and act as effectors of cellular adaptive immunity (Figure 1).
Figure 1 Immune response to the pathobionts. The pathobionts trigger inflammatory reactions via pathogen-associated molecular patterns/microbe-associated molecular patterns (PAMPs/MAMPs), or danger-associated molecular patterns (DAMPs). These stereotyped molecular patterns activate the innate immune response (mast cells and macrophages) and adaptive immune response resulting in the increased production inflammatory markers. Subsequently, antigen-presenting cells (APCs) translocate to tissue-draining lymph nodes and form major histocompatibility complex (MHC) and T-lymphocytes will fight against the pathobionts.
Neurological disorders are closely associated with GD. The factors affecting GD are reduced abundance of beneficial bacteria, excessive proliferation of pathobionts, and a reduction in the overall microbial diversity in the gut (39). Numerous observational and animal studies suggest that the GM plays a vital role in the neuropathogenesis of CNS diseases via alteration in the GBA (40). GD leads to the production of metabolites and cytokines, initiating inflammation, influencing the BBB and brain volume, and potentially acting as pseudo neurotransmitters. These effects contribute to the disruption of brain physiology and neuronal function in various neurological disorders, including those characterized by demyelination or neuropsychiatric manifestations (41–43).
The American Heart Association/American Stroke Association defines stroke as a “disease that affects the arteries leading to and within the brain”. Stroke remains the second leading cause of death globally, with high morbidity, disability, and recurrence rates (44). The World Stroke Organization reported over 12.2 million new stroke cases each year and more than 101 million people that are currently alive have experienced a stroke (45). Globally, 9.6 million cases of ischemic stroke and 4.1 million cases of hemorrhagic stroke have been documented, with 90% of these cases being triggered by modifiable risk factors. In India, stroke is the fourth and fifth leading causes of death and disability, respectively. The occurrence of stroke ranges from 105 to 152/100,000 persons annually in India (46).
In mice following stroke, T lymphocytes migrate from the small intestinal lamina propria or intestinal Peyer’s patches to the brain and/or the leptomeninges. Immune cells such as Th1 cells, Th17 cells, monocytes, and toxic chemicals from the gut, and bacteria migrate to the infarct region, which inhibits the migration of anti-inflammatory Treg cells (47). Pathobionts stimulate dendritic cell migration to mesenteric lymph nodes, which promotes T cells to differentiate into Treg cells (48). Impaired Treg cell migration to the lamina propria increases γδT-cell differentiation. The migration of these pro-inflammatory T cells from the lamina propria to the meninges increases the inflammatory milieu and infarct size (49). Additionally, the cytokines released by activated microglia and the DAMPs produced as a result of brain damage stimulate the vagus nerve, which ultimately results in GD (50). The increased translocation of bacterial toxins, pathogenic bacteria, and toxic metabolites produced by these alterations in the GIT subsequently leak additional gut inflammatory and immune cells into the bloodstream, leading to stroke-damaged areas (51). As a result, the outcome of post-stroke treatment is negatively impacted by the inflammatory loop which is triggered by GD. Cheng et al. demonstrated raised plasma levels of LPS and pro-inflammatory cytokines in cynomolgus monkeys post-stroke state, which corresponded with the relative abundance of the phylum Bacteroidetes (52). LPS is an immunogenic endotoxin produced by the Gram-negative bacteria (Yersinia pestis, Klebsiella pneumonia, Pseudomonas aeruginosa, Porphyromonas gingivalis, bifidobacterial species, Chlamydia trachomatis and Francisella tularensis) that can either directly cause neuroinflammation or peripheral immune cells to migrate into the brain (50).
Ischemic stroke is caused by atherosclerotic plaque or emboli occluding blood vessels, which reduces blood flow and oxygen availability, causing apoptosis and neuronal death (53, 54). Hemorrhagic stroke occurs as a result of deep perforating vasculopathy associated with high blood pressure, amyloid angiopathy of cerebral arteries with microbleeds, and clinical hemorrhages typically affecting the basal ganglia, cerebellum, pons, or thalamus (55). Clinical risk factors for stroke are hypertension, physical inactivity, unhealthy diet, tobacco use, use of alcohol, atrial fibrillation, raised blood lipid levels, genetic disposition, obesity, stress, diabetes, and depression. Post-stroke GI complications such as dysphagia, constipation, fecal incontinence, and GI bleeding are more frequently reported and are characterized by severe inflammation, bowel obstruction, and gut dysbiosis. These complications fuel disease progression and slow down the neuronal recovery of the degenerating brain (56).
Some studies have reported that GM composition influences stroke prognosis (57, 58). The GBA regulates immunological functions in the post-stroke condition while altered GM composition and abundance affect post-stroke outcomes mainly via gut leakiness, endotoxemia, and the neuroimmune axis. These mechanisms allow the dissemination and translocation of resident microflora, cellular, and humoral factors, including metabolites, immune cells, and cytokines/chemokines from the gut to the brain (59).
In ischemic stroke (IS), local and systemic inflammatory responses are augmented (60). Within 24 hours, monocytes (innate response) penetrate the brain, reaching peak numbers 3-5 days after acute ischemic stroke (AIS) (61). Neutrophils play a controversial role in AIS. Neutrophils have been linked to several mechanisms, including the generation of reactive oxygen species and the release of metalloproteinases, but it has also been shown that N2 neutrophils are neuroprotective (62). Additionally, neurotoxic mechanisms (oxidative stress, neuroinflammation, apoptosis, genotoxic activity, and impaired signaling pathways) activate the release of pro-inflammatory cytokines including interleukin-21 from a cluster of differentiating CD4+ T cells within 24 h post-AIS. Th1 and Th17 subpopulations promote neuroinflammation, whereas Tregs act as neuroprotectors by reducing inflammation (63). Moreover, T cells release cytokines and chemokines into the bloodstream which impact the microbiota composition and lead to GD (64). Stroke is frequently reported to be associated with gut dysmotility, increased intestinal barrier permeability, and translocation of microorganisms and a spike in the concentration of metabolites, such as TMAO and lipopolysaccharide (LPS), to enter the bloodstream (65, 66). These alterations further promote the systemic inflammatory response and disease progression and result in a poor prognosis.
In a Japanese cohort study in ischemic stroke patients, the relative abundance of Atopobium cluster and Lactobacillus ruminis were elevated and the relative abundance of Lactobacillus sakeiakei subgroup decreased and these changes triggered IL-6 detection in the circulation. Furthermore, the decreased concentration of acetic acid was negatively correlated with the levels of glycated hemoglobin and low-density lipoprotein cholesterol. In contrast, elevated fecal valeric acid concentration was positively correlated with the level of high-sensitive C-reactive protein and white blood cell counts. The changes in metabolites associated with GD in patients with IS are correlated with host metabolism and inflammation (67). These observations suggest a strong pathological involvement of GD in the IS condition.
As in AD, GD causes altered release of neurotransmitters, which affects the GBA, resulting in behavioral changes, increased anxiety, mood swings, sleep deprivation, and depression. GD is primarily characterized by an elevation in the Firmicutes/Bacteroidetes ratio, which has been linked to the accumulation of gut APP from the early stages of AD (68). Alterations in the composition of the GM in APP/PS1 mice were linked to elevated Aβ levels in the CNS as well as impaired memory performances (69). Similarly, in Tg2576 animals, GD, intestinal epithelial barrier dysfunction, and vascular Aβ accumulation in the intestine occur before the development of cerebral Aβ depositions. The occurrence of Aβ deposition is also noted in intestinal autopsies of AD patients (22). Nevertheless, the relationship between GD, intestinal Aβ deposition, and AD onset still needs to be elucidated.
GD has been postulated to act as a potential cause of PD. Clinical studies have revealed the incidence of GD in PD patients compared with healthy individuals, and both fecal and mucosal GM compositions have been described to change in PD patients (27, 70, 71). Likewise, in the mouse model of PD (Thy1-αSyn transgenic animals), GM exacerbates motor impairments, microglial activation, and α-Syn accumulation. However, in the absence of GM, the severity of motor impairments decreased but did not eliminate (72). In the rat PD model (wild-type Groningen rats), the treatment with dopamine agonists showed improvement in the GD with decreased abundance of pathobionts (Lachnospiraceae and Prevotellaceae) and increased abundance of beneficial gut flora (Lactobacillus and Bifidobacterium) (73, 74). Hence, the evidence of both clinical and preclinical studies has confirmed that GD plays a crucial role in PD pathology. However, more research is required to study the GM changes which occur in the post-PD phase.
Researchers have reported many reasons for the association of GD with ASD symptoms development. For example, colonization of germ-free mice (GF) with fecal microbiota from ASD children exhibits ASD-like behavior. Mice colonized with microbiota from ASD subjects exhibited increased autistic behavior compared to normal mice (75). ASD patients were shown to have altered GM composition when compared to normal children (76). However, no specific GM species are causally linked to ASD (28, 77). However, in ASD, the data consistently reports alterations in the phyla Bacteroidetes/Firmicutes ratios (28, 78). In addition, a few studies have demonstrated an increased abundance of Candida albicans, which produce ammonia and other toxins that are likely to cause autism-related behaviors, in the intestines of ASD children compared to non-ASD children (79, 80). Similarly, S. cerevisiae stimulates the production of TNF-α and IL-6 by altering immune functions via activation of TLR ligands, and it is thought these immunological mechanisms may play a role in the onset of ASD (81).
Currently, several potential approaches, such as the use of antibiotics as prophylactics, fecal microbiota transplantation (FMT), prebiotics, probiotics, and dietary interventions, have been shown to reverse post-stroke gut GD and improve quality of life (56). FMT is a microbiota-based therapeutic intervention and involves the transfer of fecal matter from a carefully selected donor into the GIT of a recipient to directly modify the recipient’s microbial composition and provide a health benefit (82). FMT is shown to be an effective therapy for GI and non-GI diseases, including neurological disorders such as stroke (83). In the United States, FMT is regulated as a biological agent by the Food and Drug Administration (FDA) with the source of FMT being largely supported by “stool banks” operated by clinical investigators or by OpenBiome. Since the FDA classifies FMT as an investigational new drug (IND), physicians and scientists are required to submit an IND application (84). However, at the European Union level, FMT still occupies a regulatory grey area where it falls to member states to decide how to regulate FMT. For instance, the UK regulates FMT as a medicinal product, where it is recommended for the treatment of recurrent Clostridioides difficile infection (CDI) under national guidelines (85). In Italy and Belgium, FMT is regulated as a tissue or cells under their respective national tissue and cell legislation (86). In Australia, all FMT products are regulated as biologicals with the level of regulation varying with the level of external governance and clinical oversight (87, 88). China has formed an FMT consensus expert group under the Committee of Gut Microbiota in Chinese Society of Gastroenterology since 2016 (89). Elsewhere, the regulations regarding FMT are still taking shape (90).
The safety of FMT is an important factor in its implementation. Generally, FMT has been used to treat both GI and non-GI diseases and typically is associated with few or minimal side effects with strict donor screening (91–93) (Table 1). Most short-term risks are mild and known to be related to the delivery method. However, longer-term side effects have not been established (116). In addition, FMT has demonstrated variable degrees of efficacy in treating different GI problems and is the most effective therapy for the treatment of CDI. In the FMT process, stringent donor screening is necessary to avoid the transmission of infectious diseases. However, there is also a theoretical risk of FMT modulating susceptibility for developing conditions or diseases associated with the intestinal microbiota (117, 118). Donors must not have a family history of metabolic, autoimmune, and malignant conditions. Donors are also extensively screened for potential pathogens (85). The preparation of fecal matter involves the mixing of feces with water or normal saline and is followed by the removal of particulate matter through filtration, as shown in (Figure 2).
Figure 2 FMT preparation. A fecal sample is collected from donors, weighed, and dissolved in a fixed volume of sterile saline and homogenized. The mixture is filtered to remove large particulate matter, which may obstruct the endoscope channel. The filtrate is centrifuged and the supernatant is usually stored at -80°C until required for use.
FMT aims to treat a disease by restoring phylogenetic diversity and microbiota composition and function. Donor feces can be administered via the upper or lower GIT through different delivery routes (Figure 3) (119). In the upper GIT, FMT administration via capsule is relatively quick, convenient, inexpensive, and technically simple (120). The FMT procedure via the lower GIT necessitates bowel cleansing, followed by recolonizing the entire colon with favorable bacteria. This delivery route avoids the likelihood of aspiration and vomiting but requires a colonoscopy which is an invasive procedure (121). FMT enemas are easy to administer and are inexpensive. However, some patients have reported an aversion to handling stool, which obviously might interfere with the acceptability of fecal enemas (122). Although in the US, the FDA has reported six incidents of serious infections following FMT therapy (123), FMT is considered a safe, effective, and generally well-tolerated therapy with virtually no interim adverse effects if performed correctly. However, the evidence available on long-term safety is limited. Thus, it is crucial to establish clinical protocols that allow clinicians to operate with the highest degree of quality and safety assurance with the fewest possible risks associated with the process.
Figure 3 Routes of administration for FMT. Through the upper GI route, FMT can be administered via nasogastric tube which is placed through the nose and reaches the stomach or the jejunum (nasojunal tube) to deliver the fecal transplant. The most currently preferred delivery technique involves oral administration of encapsulated FMT, which is both safe and efficient, and is well-tolerated by patients. To deliver the processed fecal matter, the supply tube is inserted into the mouth and passed through the pharynx, oesophagus, stomach, and duodenum (EGD). In the lower GIT, FMT is administered with a colonoscopy, in which the physician inserts a colonoscope into the colon to administer the liquefied donor’s stool. Similar to a colonoscopy, a sigmoidoscopy primarily examines and delivers fecal materials to the lower third of the colon. A percutaneous endoscopic cecostomy (PEC) refers to when the C-tube is inserted under endoscopic or imaging guidance to the cecum. FMT can be delivered into the small intestine with esophagogastroduodenoscopy and using a longer conventional endoscope, a double-balloon endoscope (double-balloon enteroscopy) which may be passed through mouth or anus. During endoscopy, the colonic transendoscopic enteral (TET) tube is introduced into the ileocecal junction and connected to the cecum using titanium clips. The first technique to be used was the traditional enema, also referred to as a clyster, which involves injecting the stool preparation into the lower colon via the rectum.
FMT increases intestinal microbial diversity and restores a healthy immune system (124). The potential therapeutic effects of FMT are correlated with decreased GD-induced neuroinflammation (Figure 4). For example, FMT therapy downregulates NLRP3 and IL-1 expression levels, which inhibit the NLRP3 inflammasome’s activation and prevents the appearance of pathogenic Th17 cells in a rat model of chronic cerebral hypoperfusion. In the intestinal mucosa, activation (phosphorylation) of Stat3 serves as a master regulator of Th17 cell development by upregulating the production of IL-6, IL-17A, IL-22, and RAR-related orphan receptor gamma (RORγt) (125). FMT treatment reduces the activation of microglial cells and inflammatory gene expression in a mouse model of traumatic brain injury (TBI) (126). Another study found that FMT reduced the proliferative capacity of colonic mucosal T cells in colitic mice compared with animals who did not receive FMT. The frequencies of CD8+ T and CD4+ T cells, which express the cytotoxicity-related molecule CD107a, were also decreased in FMT-treated animals. Indeed, colonic T cells from FMT animals treated showed a decreased pro-inflammatory phenotype (127).
Figure 4 FMT modulates the neuroimmune axis. Effect of FMT on the immune system and metabolites in the context of neurological disease and gut dysbiosis (GD) state via the GBA. Left side: Pathobionts induce an inflammatory state during the GD condition. In GD, the adaptive immune system activates CD4+ T cells which further interact with intestinal immune cells [T helper 1 (Th1), Th2, Th17, or regulatory T cell (Treg)] and produce inflammatory cytokines such as IL-6, IL17A, IL22, IL-1Ra, LPS and TMAO, which leads to neuroinflammation and neural cell death. In addition, GD reduces the levels of fecal SCFAs, causing BBB disruption, activating astrocytes and microglia, and inducing neuroinflammation followed by neuronal cell death. Right side: The recolonized GM (beneficial GM) by FMT promotes the production of neurotransmitters, SCFAs, and regulatory T cells through interactions with intestinal immune cells (dendritic cells). Furthermore, FMT restores gut barrier integrity, decreases the production and translocation of TMAO and LPS to the periphery, reduces BBB disruption and activation of brain immune cells, and improves brain functions.
In a clinical study, Jacob et al. performed a single FMT delivery by colonoscopy for active UC patients using a 2-donor fecal microbiota preparation. Post-FMT, mucosal CD4+ T-cell analysis showed a decrease in both Th1 and Treg cells (128). Crothers et al. reported that in a single-center prospective study, post-oral FMT treatment reduced the frequencies of Treg and mucosal-associated invariant T (MAIT) cell populations in the peripheral circulation of ulcerative colitis (UC) subjects (106). Wang et al. carried out a prospective study in UC patients, in which FMT administration significantly reduced the serum levels of IL-1Ra, IL-6, interferon-inducible protein (IP)-10, and epithelial neutrophil-activating peptide (ENA)-78. In addition, granulocyte-colony stimulating factor (G-CSF), vascular cell adhesion molecule (VCAM)-1, and mucosae-associated epithelial chemokine (MEC) levels were significantly decreased in sera from UC patients (129).
In recent years, many animal experiments have been performed to define the relationship between GD associated with metabolic, neuropsychiatric, and sleep disorders, as well as intestinal and cardiovascular diseases (130, 131). Emerging evidence from animal studies has shown that manipulating the GM via FMT as a potential therapeutic approach for the treatment of stroke and post-stroke complications. A recent systematic review has summarized the preclinical animal evidence for the beneficial effects of FMT on stroke management (83). Inflammatory processes and altered metabolites have a detrimental impact on the GM in post-stroke conditions (132). In one study, post-stroke recolonization in germ-free mice resulted in increased levels of pro-inflammatory cytokines such as IFN-γ and IL-17, T-cell migration from the intestine to the post-stroke brain, and an aggravation of the lesion volume and functional impairments. In a separate study, FMT was associated with the reversal of GD and stroke outcomes with neuroprotective action (64). FMT in middle cerebral artery occlusion (MCAO) animal models fed a Western-style high-fat diet was found to inhibit apoptosis by lowering the level of Bax (Bcl-2-associated X protein) and cleaved caspase-3 and increasing Bcl-2, thereby attenuating the cerebral ischemic injury possibly via reduction in oxidative stress and brain apoptosis (133). In MCAO aged mice, FMT by gavaging stool from young mice was shown to improve post-stroke recovery and survival, while the converse was observed when FMT was administered from aged mice. In this latter group, FMT worsened post-stroke mortality indicating the role of age in affecting the gut microenvironment (134). Similarly, a gender-derived GM transplantation study revealed the dominance of microbial communities from female mice in increasing the survival rate, protection from brain damage and decreasing the systemic level of inflammatory cytokines in the post-stroke condition, suggesting sex as another critical factor influencing the gut microenvironment (135) (Figure 5, Table 2).
Figure 5 Gut dysbiosis and impact of FMT in stroke. Left panel – Stroke-associated gut dysbiosis leads to increased pathobionts and decreased beneficial bacteria in the gut. This leads to decreased levels of short-chain fatty acids (SCFAs), and elevated levels of LPS and TMAO. This triggers immune cells and the secretion of proinflammatory cytokines/chemokines. Increased cytokines and inflammation disrupt the tight junction integrity that induces gut leakiness. Consequently, excessive LPS, TMAO, cytokines, and immune cells pass through loose junctions into the systemic circulation to cause endotoxemia and systemic inflammatory reactions. Right panel - FMT reduces pathobionts and elevates abundance of beneficial bacteria i.e., restores gut microbial homeostasis and gut barrier integrity. Consequently, neuroinflammation and cognitive functions in stroke models would be improved.
Lactobacillus species have been shown to decrease oxidative stress, neuronal apoptosis, and cerebral infarction volume via inhibition of TLR-4/NF-κB signaling, and prevented barrier dysfunction by repairing epithelial cells in the gut following cerebral ischemia reperfusion-injury (140). In antibiotic-treated C57BL/6 mice, transplantation of gut microbiota from db/db mice treated with sodium butyrate showed a reduction in cerebral infarct volume with better gut barrier and blood-brain barrier (BBB) function. These mice also exhibited an increased abundance of butyrate-producing bacteria such as Ruminococcaceae, Ruminococcu, Lachnospiraceae, and Oscillospira and showed reduced levels of serum LPS and inflammatory cytokines (IL-6, TNF-α, and IL-1β) in ischemic mice. Furthermore, attenuation of IS injury in the butyrate-treated mice correlated with reduced levels of systemic inflammatory markers such as intercellular adhesion molecule-1 (ICAM-1), vascular cell adhesion molecule-1 (VCAM-1), and matrix metalloproteinase-9 (MMP-9) in the brain. The latter may also be due to the protection of the BBB, marked by the prevention of cerebral capillary endothelial glycocalyx degradation, reflected by the lower levels of syndecan-1 and heparan sulfate in serum (136). FMT therapy improved GM-derived metabolites including SCFAs in the recipient group as compared to the saline group (137). SCFAs support the growth of beneficial bacterial populations such as Lactobacillus, Butyricicoccus, and Meganonas. Additionally, FMT also alleviated the level of serum total cholesterol levels, cerebral infarct volume, and intestinal permeability in an MCAO mouse model (137).
Based on these preclinical findings, GD exerts chronic inflammatory responses both peripherally and centrally that accelerate stroke prognosis. Certain bacterial phyla such as Bacteroidetes, Firmicutes, and Actinobacteria are altered in the post-stroke condition as depicted by increased F/B ratio (141), Therefore, targeting these bacterial phyla via FMT represents a promising adjuvant therapeutic and prophylactic strategy to protect the brain and gut against stroke-related complications.
The pathophysiology of stroke is strongly linked with gut inflammation and immune responses. This could be a key therapeutic target in post-stroke management. Studies reported that patients with stroke showed altered GM composition compared to healthy subjects, particularly in specific bacterial phyla as mentioned in (Table 3) (145). The GM-derived SCFAs alter brain functions directly or indirectly via immune, vagal, endocrine, and humoral pathways (146). Na Li et al. reported that in ischemic stroke patients, the fecal microbiota composition of SCFA-producing taxa Odoribacter and Akkermansia was decreased. Additionally, the genus Christensenellaceae_R-7_group, norank_f_Ruminococcaceae, and Enterobacter levels were correlated to stroke severity, whilst Christensenellaceae_R-7_group levels were positively correlated with IS outcomes (147).
In acute ischemic stroke patients, there is an abundance of Enterobacteriaceae and decreased levels of Fecalibacterium, Parabacteroides Clostridiaceae, and Lachnospira. Animals receiving FMT from subjects with a high stroke dysbiosis index (SDI) showed a higher abundance of Enterobacteriaceae and lower levels of Lachnospiraceae which are associated with severe brain injury and poor stroke outcomes, in part displayed by a downward trend of T cells which act as neuroprotectants (143).
In stroke, thrombin causes direct vascular disruption, cellular dysfunction, oxidative stress, and inflammation. In addition, there is a direct correlation between thrombin activity in the affected brain hemisphere and the infarction volume (148). Yassene Mohammed et al. reported that the FMT from healthy donors suppressed the onset of thrombin generation in metabolic disorder patients (149). However, the effect of FMT on delaying the onset of thrombin generation in stroke patients is yet to be investigated.
Despite the different aetiologies of neurological disorders, neuronal damage is commonly associated with chronic activation of an innate immune response in the central nervous system (CNS). The GM composition differs in patients compared to healthy controls, indicating a pathophysiological role of the gut microbiome in CNS functions. However, GM restoration by FMT could be an effective treatment approach for several neurological disorders despite the available limited evidence (113).
Alzheimer’s Disease (AD) is characterized by the accumulation of specific proteins inside or outside cells such as misfolded amyloid-β (Aβ) and tau hyperphosphorylation which forms neurofibrillary tangles. The GM plays a vital role in brain functions including myelinization, neurogenesis, and microglial activation which are closely related to behavioral, mood, and cognitive modulations (130). Mounting evidence indicates that AD is associated with abnormal GM (150). The abundance of Desulfovibrionaceae and Helicobacteraceae at the family level and Helicobacter and Odoribacter at the genus level were significantly increased in APPswe/PS1dE9 transgenic (Tg) mice compared with wild-type (WT) mice (69). In an APPswe/PS1dE9 Tg mouse model, FMT improved cognition as evidenced by increased synaptic markers (PSD-95, synapsin-1) and decreased Aβ accumulation and neuroinflammatory markers (COX-2, CD11b). FMT treatment enriched bacterial like Proteobacteria, Verrucomicrobia, at phylum levels and Desulfovibrio and Akkermansia at genus levels and decreased the abundance of Bacteroidetes at phylum levels and Alloprevotella at genus levels in the Tg animals (100). Furthermore, FMT restored the levels of Acidobacteria and Bifidobacterium which significantly delayed AD progression in 3xTg-AD mice (151). Dodiya et al. reported that FMT between age- and sex-matched AD male mice without antibiotics and antibiotic-treated AD male mice partially restored microglial morphology and recolonized intestinal bacteria. Post-FMT in antibiotic-treated male mice, anti-inflammatory cytokines such as IL-10 were expressed more frequently, while pro-inflammatory cytokines like IL-1β, IL-2, IL-3, IL-17A, LIX (CXC5), RANTES (CCL5) and a cluster of differentiation 30 (CD30) and CD40 were reduced (152). Elangovan et al. demonstrated the 5XFAD mouse model showed significantly improved novel object recognition and spatial memory and reduction in amyloid pathology after 7 days of treatment of FMT from wild-type donor mice (101). These data demonstrate that FMT is an effective treatment for AD-like disorders, but further studies are required.
PD is a chronic, progressive, neurodegenerative condition characterized by accumulation of the presynaptic neuronal protein α-synuclein (αSyn) and dopaminergic neuron loss (110). It is not only associated with motor and non-motor deficits but also affects GI function causing altered bowel movement patterns and abdominal bloating (153). Several studies have reported a relationship between Toll-like receptors (TRLs), particularly TRL4, GM, and αSyn pathology. TLR4 depletion inhibited upward regulation of AP-1 in dopaminergic neurons in the substantia nigra of PD mice, suggesting that TLR4 may play a potential role in PD (154). TLRs have also been demonstrated to activate several signaling pathways, including PI3K/AKT/GSK3 and NF-κB. Additionally, TLR4 activation stimulates microglial cells and α-syn, which in turn enhances the synthesis of TNF-α and nuclear translocation of NF-κB (155).
Studies have reported that patients with PD have an increased abundance of genera including Akkermansia, Lactobacillus, Bifidobacterium, Enterobacteriaceae, and decreased levels of Blautia, Faecalibacterium, and Prevotella compared to healthy controls (14, 156). Very recent reports also reveal that FMT treatment improved the motor and non-motor functions in PD patients and reduced the symptoms of constipation (110, 157). FMT treatment inhibited the expression of p-PI3K, p-AKT, TLR4, and NF-κB, as well as TNF-α, confirming a close link between the TLR4/PI3K/AKT/NF-κB signaling and gut microbial dysbiosis in PD (158).
Autism spectrum disorder (ASD) are severe brain or neurodevelopmental disorder that is characterized by deficits in social communication with restricted, repetitive, and stereotyped behaviors that can vary in individuals along a continuum of severity. The pathophysiology of ASD is significantly influenced by the neuroimmune system’s involvement and dysregulation, which stimulate microglia, astrocytes, and the release of pro-inflammatory cytokines (159). Inga J´acome et al. reported that in patients (3 to 9-year-old children) with ASD, concentrations of pro-inflammatory cytokines such as IL-1β, IL-6, IL-17, IL-12p40, and TNF-α in plasma were high and correlated with disease severity and progression in comparison with healthy subjects (160). Additionally, children with autism have also shown signs of immune system activation, such as an aberrant CD4:CD8 T cell ratio, a high proportion of DR+ (activated) T cells, elevated neopterin levels in the urine, and increased cytokine production (161). The systemic and neuroinflammatory effects of GM are anticipated to have a direct impact on lymphoid cells and the adaptive immune response. In general, microbial components are known to directly interact with antigen-presenting cells, TLRs, differentiated B cells, T cells, and CD4+ T cells (162). The GM induces activation of Th17 and Th17 lymphocytes which increases systemic inflammation and promotes BBB disruption and CNS inflammation (163).
In recent years, emerging research reported the association between ASD and GM, which was also identified as a contributing component to the prognosis of ASD through the GBA (28, 164). Patients with ASD had higher abundance of Bacteroides, Parabacteroides, Faecalibacterium, Clostridium, and Phascolarctobacterium, according to a metanalysis report. On the other hand, control subjects have higher levels of Coprococcus and Bifidobacterium (164). In a clinical cohort study of ASD patients, Ning Li et al. found that the levels of the phyla Verrucomicrobia, and genera Eubacterium Coprostanoligenes, Akkermansia, Ruminococcus, Corprococcus, and Christensenellaceae were significantly altered and that of 5-HT transporter (SERT or 5-HTT) or 5-HT levels were elevated. FMT treatment through oral and rectal delivery led to significant improvement (gastrointestinal symptoms and autism-like behaviors) in ASD patients by reducing Eubacterium and Coprostanoligenes. In addition, FMT decreased the serum levels of neurotransmitters 5-HT and GABA (114). In a single case report, FMT by colposcopy and oral capsule was shown to improve gastrointestinal and ASD-related symptoms and decrease the Childhood Autism Rating Scale (CARS scores) in a patient with ASD (165).
FMT from healthy human GM significantly reduced anxiety-like repetitive behaviors and raised serum levels of chemokines, including GRO-1 (CXCL1), MRIP-1 (CCL3), MCP-3 (CCL7), Eotaxin (CCL11) and RANTES (CCL5) which are crucial for neurogenesis and synaptic transmission in the central nervous system, in ASD mice (166). In another study, FMT from naive wild-type mice significantly reduced levels of TNFα and Iba1 in the mouse brain, normalized A. muciniphila abundance to wild-type levels, and restored memory impairments and social disengagement in Fmr1 knockout mice (167).
The GM affects numerous essential host functions, including the immune response and the nervous system. Both human and animal data do seem to indicate that FMT may improve ASD symptoms. However, as data on the safety profile of FMT and the long-term effects of this treatment in ASD is still limited, further research is needed.
In the current review, we have summarized the relationship between GD and the development and prognosis of various neurological diseases including AD, PD, ASD, and stroke. The GM may be considered a potential contributory factor in the pathogenesis of neurological disorders. Restoration of the GM by FMT may attenuate symptoms or progression of neurological disorders mainly via GM-mediated immunological and neural pathways. Neurodegenerative diseases have yet to attract effective disease-altering/modifying therapies, despite decades of intensive research. Current therapies typically try to relieve symptoms but impose severe adverse reactions that restrict usage. Thus, there is an essential need for microbiome-inspired therapeutic alternatives that enhance the quality of life or significantly alter the course of the disease. The information compiled in this review indicates that FMT may be a viable and promising therapeutic option for treating several GI and neurological diseases. Nevertheless, large double-blinded randomized clinical tests (RCTs) are desirable to further elucidate the effect of FMT in numerous disorders of the microbiota GBA.
There is a lack of molecular understanding, despite clinical and preclinical data supporting the use of FMT in neurological diseases. Most studies did not investigate the possible detrimental effects of FMT, and long-term adverse effects were seldom identified. The efficacy of FMT is influenced by several factors, including donors, antibiotic types, treatment approaches, and microbial composition. To further validate the safety and rule out any potential adverse effects, long-term follow-up and appropriate controls are required. In addition, there has been no uniformity in dose, FMT delivery route, stool filtering technique, frequency of administration, and diet in the studies. Also, there is currently no approved standard for choosing donors for specific disorders, thus more research in this line is necessary. Several studies have shown that FMT improves neurological disorders by improving GI symptoms, and host immune function. However, in future, robust taxonomic resolution methods may be employed for identifying the biomarkers for specific neurological disorders. Finally, further mechanistic studies are warranted to understand the direct neuroprotective effects of specific species or bacterial-derived metabolite(s) on the gut-brain axis using molecular techniques such as human organotypic cultures, synthetic communities and microbiome depleted germ free mouse models to determine causality.
TH: Formal analysis, Methodology, Software, Writing – original draft, Investigation, Project administration. CV: Writing – original draft. NA: Writing – original draft. MB: Writing – original draft. SE: Investigation, Writing – original draft. PK: Writing – original draft. MW: Writing – original draft. MA: Writing – original draft. RK-G: Writing – original draft. AM: Writing – original draft. JY: Writing – original draft. BS: Data curation, Investigation, Supervision, Writing – review & editing. TM: Conceptualization, Data curation, Supervision, Writing – review & editing. MS: Conceptualization, Data curation, Supervision, Writing – review & editing. SC: Conceptualization, Data curation, Supervision, Writing – review & editing.
The author(s) declare financial support was received for the research, authorship, and/or publication of this article. The work was supported by the “Public Health and Nutrition Division”, Department of Biotechnology, Ministry of Science and Technology, Govt of India, (BT/PR38038/PFN/20/1528/2020), and JSS AHER grant (order no. REG/DIR(R)/URG/54/2011-12/5662). Also partly funded by Agricultural Development Fund (ADF) grant from the Provincial Government of Saskatchewan, Canada (422905).
The authors thank their respective institutions for providing the required infrastructure to produce this manuscript.
The authors declare that the research was conducted in the absence of any commercial or financial relationships that could be construed as a potential conflict of interest.
All claims expressed in this article are solely those of the authors and do not necessarily represent those of their affiliated organizations, or those of the publisher, the editors and the reviewers. Any product that may be evaluated in this article, or claim that may be made by its manufacturer, is not guaranteed or endorsed by the publisher.
αSyn- α-synuclein; AIS- Acute ischemic stroke; AD- Alzheimer’s Disease; ASD-Autism spectrum disorder; Aβ- Amyloid Beta; BBB- Blood brain barrier; CNS- Central nervous system; CDI- Clostridioides difficile infection; DAMP- Damage-associated molecular patterns; ENS- Enteric nervous system; DSS- Dextrean sodium sufate; FMT- Fecal microbiota transplantation; GI- Gastrointestinal; GD- Gut dysbiosis; GM- Gut Microbiota; GBA- Gut-brain axis; IBD- Inflammatory bowel disease; IS- Ischemic stroke; MCAO- Middle cerebral artery occlusion; PD- Parkinson’s disease; TMAO-trimethylamine N-oxide; Tg-Transgenic.
1. Madhogaria B, Bhowmik P, Kundu A. Correlation between human gut microbiome and diseases. Infect Med. (2022) 1:180–91. doi: 10.1016/j.imj.2022.08.004
2. Ortega MA, Álvarez-Mon MA, García-Montero C, Fraile-Martínez Ó, Monserrat J, Martinez-Rozas L, et al. Microbiota-gut-brain axis mechanisms in the complex network of bipolar disorders: potential clinical implications and translational opportunities. Mol Psychiatry. (2023) 28:2645–73. doi: 10.1038/s41380-023-01964-w
3. Gupta S, Allen-Vercoe E, Petrof EO. Fecal microbiota transplantation: in perspective. Therap Adv Gastroenterol. (2016) 9:229–39. doi: 10.1177/1756283X15607414
4. Cryan JF, O’Riordan KJ, Cowan CSM, Sandhu KV, Bastiaanssen TFS, Boehme M, et al. The microbiota-gut-brain axis. Physiol Rev. (2019) 99:1877–2013. doi: 10.1152/physrev.00018.2018
5. Qin J, Li R, Raes J, Arumugam M, Burgdorf KS, Manichanh C, et al. A human gut microbial gene catalogue established by metagenomic sequencing. Nature. (2010) 464:59–65. doi: 10.1038/nature08821
6. Lloyd-Price J, Mahurkar A, Rahnavard G, Crabtree J, Orvis J, Hall AB, et al. Strains, functions and dynamics in the expanded Human Microbiome Project. Nature. (2017) 550:61–6. doi: 10.1038/nature23889
7. Hollister EB, Gao C, Versalovic J. Compositional and functional features of the gastrointestinal microbiome and their effects on human health. Gastroenterology. (2014) 146:1449–58. doi: 10.1053/j.gastro.2014.01.052
8. Dash S, Syed YA, Khan MR. Understanding the role of the gut microbiome in brain development and its association with neurodevelopmental psychiatric disorders. Front Cell Dev Biol. (2022) 10:880544. doi: 10.3389/fcell.2022.880544
9. Chung H, Pamp SJ, Hill JA, Surana NK, Edelman SM, Troy EB, et al. Gut immune maturation depends on colonization with a host-specific microbiota. Cell. (2012) 149:1578–93. doi: 10.1016/j.cell.2012.04.037
10. Ho L, Ono K, Tsuji M, Mazzola P, Singh R, Pasinetti GM. Protective roles of intestinal microbiota derived short chain fatty acids in Alzheimer’s disease-type beta-amyloid neuropathological mechanisms. Expert Rev Neurother. (2018) 18:83–90. doi: 10.1080/14737175.2018.1400909
11. Jandhyala SM, Talukdar R, Subramanyam C, Vuyyuru H, Sasikala M, Reddy DN. Role of the normal gut microbiota. World J Gastroenterol. (2015) 21:8787–803. doi: 10.3748/wjg.v21.i29.8787
12. Buttó LF, Haller D. Dysbiosis in intestinal inflammation: Cause or consequence. Int J Med Microbiol. (2016) 306:302–9. doi: 10.1016/j.ijmm.2016.02.010
13. Karlsson FH, Tremaroli V, Nookaew I, Bergström G, Behre CJ, Fagerberg B, et al. Gut metagenome in European women with normal, impaired and diabetic glucose control. Nature. (2013) 498:99–103. doi: 10.1038/nature12198
14. Scheperjans F, Aho V, Pereira PAB, Koskinen K, Paulin L, Pekkonen E, et al. Gut microbiota are related to Parkinson’s disease and clinical phenotype. Mov Disord. (2015) 30:350–8. doi: 10.1002/mds.26069
15. Liu S, Gao J, Zhu M, Liu K, Zhang H-L. Gut microbiota and dysbiosis in alzheimer’s disease: implications for pathogenesis and treatment. Mol Neurobiol. (2020) 57:5026–43. doi: 10.1007/s12035-020-02073-3
16. Taniya MA, Chung H-J, Al Mamun A, Alam S, Aziz MA, Emon NU, et al. Role of gut microbiome in autism spectrum disorder and its therapeutic regulation. Front Cell Infection Microbiol. (2022) 12:915701. doi: 10.3389/fcimb.2022.915701
17. Schepici G, Silvestro S, Bramanti P, Mazzon E. The gut microbiota in multiple sclerosis: an overview of clinical trials. Cell Transplant. (2019) 28:1507–27. doi: 10.1177/0963689719873890
18. Yamashiro K, Kurita N, Urabe T, Hattori N. Role of the gut microbiota in stroke pathogenesis and potential therapeutic implications. ANM. (2021) 77:36–44. doi: 10.1159/000516398
19. Yadegar A, Pakpoor S, Ibrahim FF, Nabavi-Rad A, Cook L, Walter J, et al. Beneficial effects of fecal microbiota transplantation in recurrent Clostridioides difficile infection. Cell Host Microbe. (2023) 31:695–711. doi: 10.1016/j.chom.2023.03.019
20. Quraishi MN, Shaheen W, Oo YH, Iqbal TH. Immunological mechanisms underpinning faecal microbiota transplantation for the treatment of inflammatory bowel disease. Clin Exp Immunol. (2020) 199:24–38. doi: 10.1111/cei.13397
21. Mayer EA, Ryu HJ, Bhatt RR. The neurobiology of irritable bowel syndrome. Mol Psychiatry. (2023) 28:1451–65. doi: 10.1038/s41380-023-01972-w
22. Honarpisheh P, Reynolds CR, Blasco Conesa MP, Moruno Manchon JF, Putluri N, Bhattacharjee MB, et al. Dysregulated gut homeostasis observed prior to the accumulation of the brain amyloid-β in tg2576 mice. Int J Mol Sci. (2020) 21:1711. doi: 10.3390/ijms21051711
23. Wang X-L, Zeng J, Yang Y, Xiong Y, Zhang Z-H, Qiu M, et al. Helicobacter pylori filtrate induces alzheimer-like tau hyperphosphorylation by activating glycogen synthase kinase-3β. J Alzheimer’s Dis. (2015) 43:153–65. doi: 10.3233/JAD-140198
24. Chen M, Mor DE. Gut-to-brain α-synuclein transmission in parkinson’s disease: evidence for prion-like mechanisms. Int J Mol Sci. (2023) 24:7205. doi: 10.3390/ijms24087205
25. Fasano A, Visanji NP, Liu LWC, Lang AE, Pfeiffer RF. Gastrointestinal dysfunction in Parkinson’s disease. Lancet Neurol. (2015) 14:625–39. doi: 10.1016/S1474-4422(15)00007-1
26. Petrov VA, Saltykova IV, Zhukova IA, Alifirova VM, Zhukova NG, Dorofeeva YB, et al. Analysis of gut microbiota in patients with parkinson’s disease. Bull Exp Biol Med. (2017) 162:734–7. doi: 10.1007/s10517-017-3700-7
27. Bedarf JR, Hildebrand F, Coelho LP, Sunagawa S, Bahram M, Goeser F, et al. Functional implications of microbial and viral gut metagenome changes in early stage L-DOPA-naïve Parkinson’s disease patients. Genome Med. (2017) 9:39. doi: 10.1186/s13073-017-0428-y
28. Strati F, Cavalieri D, Albanese D, De Felice C, Donati C, Hayek J, et al. New evidences on the altered gut microbiota in autism spectrum disorders. Microbiome. (2017) 5:24. doi: 10.1186/s40168-017-0242-1
29. West KA, Yin X, Rutherford EM, Wee B, Choi J, Chrisman BS, et al. Multi-angle meta-analysis of the gut microbiome in Autism Spectrum Disorder: a step toward understanding patient subgroups. Sci Rep. (2022) 12:17034. doi: 10.1038/s41598-022-21327-9
30. Heiman ML, Greenway FL. A healthy gastrointestinal microbiome is dependent on dietary diversity. Mol Metab. (2016) 5:317–20. doi: 10.1016/j.molmet.2016.02.005
31. DiSabato DJ, Quan N, Godbout JP. Neuroinflammation: the devil is in the details. J Neurochem. (2016) 139:136–53. doi: 10.1111/jnc.13607
32. Smith K, McCoy KD, Macpherson AJ. Use of axenic animals in studying the adaptation of mammals to their commensal intestinal microbiota. Semin Immunol. (2007) 19:59–69. doi: 10.1016/j.smim.2006.10.002
33. Thaiss CA, Elinav E. The remedy within: will the microbiome fulfill its therapeutic promise? J Mol Med. (2017) 95:1021–7. doi: 10.1007/s00109-017-1563-z
34. Suárez LJ, Arboleda S, Angelov N, Arce RM. Oral versus gastrointestinal mucosal immune niches in homeostasis and allostasis. Front Immunol. (2021) 12:705206. doi: 10.3389/fimmu.2021.705206
35. Wu H-J, Wu E. The role of gut microbiota in immune homeostasis and autoimmunity. Gut Microbes. (2012) 3:4–14. doi: 10.4161/gmic.19320
36. Zheng D, Liwinski T, Elinav E. Interaction between microbiota and immunity in health and disease. Cell Res. (2020) 30:492–506. doi: 10.1038/s41422-020-0332-7
37. Schaefer L. Complexity of danger: the diverse nature of damage-associated molecular patterns *. J Biol Chem. (2014) 289:35237–45. doi: 10.1074/jbc.R114.619304
38. Jin HS, Suh H-W, Kim S-J, Jo E-K. Mitochondrial control of innate immunity and inflammation. Immune Netw. (2017) 17:77–88. doi: 10.4110/in.2017.17.2.77
39. DeGruttola AK, Low D, Mizoguchi A, Mizoguchi E. Current understanding of dysbiosis in disease in human and animal models. Inflammatory Bowel Dis. (2016) 22:1137–50. doi: 10.1097/MIB.0000000000000750
40. Yang D, Zhao D, Ali Shah SZ, Wu W, Lai M, Zhang X, et al. The role of the gut microbiota in the pathogenesis of parkinson’s disease. Front Neurol. (2019) 10:1155. doi: 10.3389/fneur.2019.01155
41. Bermúdez-Humarán LG, Salinas E, Ortiz GG, Ramirez-Jirano LJ, Morales JA, Bitzer-Quintero OK. From probiotics to psychobiotics: live beneficial bacteria which act on the brain-gut axis. Nutrients. (2019) 11:890. doi: 10.3390/nu11040890
42. Guo R, Chen L-H, Xing C, Liu T. Pain regulation by gut microbiota: molecular mechanisms and therapeutic potential. Br J Anaesthesia. (2019) 123:637–54. doi: 10.1016/j.bja.2019.07.026
43. Karakan T, Ozkul C, Küpeli Akkol E, Bilici S, Sobarzo-Sánchez E, Capasso R. Gut-brain-microbiota axis: antibiotics and functional gastrointestinal disorders. Nutrients. (2021) 13:389. doi: 10.3390/nu13020389
44. Regenhardt RW, Das AS, Ohtomo R, Lo EH, Ayata C, Gurol ME. Pathophysiology of lacunar stroke: history’s mysteries and modern interpretations. J Stroke Cerebrovascular Dis. (2019) 28:2079–97. doi: 10.1016/j.jstrokecerebrovasdis.2019.05.006
45. Feigin VL, Brainin M, Norrving B, Martins S, Sacco RL, Hacke W, et al. World stroke organization (WSO): global stroke fact sheet 2022. Int J Stroke. (2022) 17:18–29. doi: 10.1177/17474930211065917
46. Jones SP, Baqai K, Clegg A, Georgiou R, Harris C, Holland E-J, et al. Stroke in India: A systematic review of the incidence, prevalence, and case fatality. Int J Stroke. (2022) 17:132–40. doi: 10.1177/17474930211027834
47. Gelderblom M, Weymar A, Bernreuther C, Velden J, Arunachalam P, Steinbach K, et al. Neutralization of the IL-17 axis diminishes neutrophil invasion and protects from ischemic stroke. Blood. (2012) 120:3793–802. doi: 10.1182/blood-2012-02-412726
48. Benakis C, Brea D, Caballero S, Faraco G, Moore J, Murphy M, et al. Commensal microbiota affects ischemic stroke outcome by regulating intestinal γδ T cells. Nat Med. (2016) 22:516–23. doi: 10.1038/nm.4068
49. Crapser J, Ritzel R, Verma R, Venna VR, Liu F, Chauhan A, et al. Ischemic stroke induces gut permeability and enhances bacterial translocation leading to sepsis in aged mice. Aging (Albany NY). (2016) 8:1049–63. doi: 10.18632/aging.100952
50. Kalyan M, Tousif AH, Sonali S, Vichitra C, Sunanda T, Praveenraj SS, et al. Role of endogenous lipopolysaccharides in neurological disorders. Cells. (2022) 11:4038. doi: 10.3390/cells11244038
51. Tang W, Zhu H, Feng Y, Guo R, Wan D. The impact of gut microbiota disorders on the blood–brain barrier. Infect Drug Resist. (2020) 13:3351–63. doi: 10.2147/IDR.S254403
52. Cheng Y-W, Phelps E, Nemes S, Rogers N, Sagi S, Bohm M, et al. Fecal microbiota transplant decreases mortality in patients with refractory severe or fulminant clostridioides difficile infection. Clin Gastroenterol Hepatol. (2020) 18:2234–2243.e1. doi: 10.1016/j.cgh.2019.12.029
53. Woodruff TM, Thundyil J, Tang S-C, Sobey CG, Taylor SM, Arumugam TV. Pathophysiology, treatment, and animal and cellular models of human ischemic stroke. Mol Neurodegeneration. (2011) 6:11. doi: 10.1186/1750-1326-6-11
54. Ortega MA, Fraile-Martinez O, García-Montero C, Haro S, Álvarez-Mon MÁ, De Leon-Oliva D, et al. A comprehensive look at the psychoneuroimmunoendocrinology of spinal cord injury and its progression: mechanisms and clinical opportunities. Military Med Res. (2023) 10:26. doi: 10.1186/s40779-023-00461-z
55. Cordonnier C, Demchuk A, Ziai W, Anderson CS. Intracerebral haemorrhage: current approaches to acute management. Lancet. (2018) 392:1257–68. doi: 10.1016/S0140-6736(18)31878-6
56. Tuz AA, Hasenberg A, Hermann DM, Gunzer M, Singh V. Ischemic stroke and concomitant gastrointestinal complications- a fatal combination for patient recovery. Front Immunol. (2022) 13:1037330. doi: 10.3389/fimmu.2022.1037330
57. Dinan TG, Cryan JF. The microbiome-gut-brain axis in health and disease. Gastroenterol Clin North Am. (2017) 46:77–89. doi: 10.1016/j.gtc.2016.09.007
58. Chen J, Chi B, Ma J, Zhang J, Gu Q, Xie H, et al. Gut microbiota signature as predictors of adverse outcomes after acute ischemic stroke in patients with hyperlipidemia. Front Cell Infect Microbiol. (2022) 12:1073113. doi: 10.3389/fcimb.2022.1073113
59. Koszewicz M, Jaroch J, Brzecka A, Ejma M, Budrewicz S, Mikhaleva LM, et al. Dysbiosis is one of the risk factor for stroke and cognitive impairment and potential target for treatment. Pharmacol Res. (2021) 164:105277. doi: 10.1016/j.phrs.2020.105277
60. Chu HX, Kim HA, Lee S, Moore JP, Chan CT, Vinh A, et al. Immune cell infiltration in Malignant middle cerebral artery infarction: comparison with transient cerebral ischemia. J Cereb Blood Flow Metab. (2014) 34:450–9. doi: 10.1038/jcbfm.2013.217
61. Kronenberg G, Uhlemann R, Richter N, Klempin F, Wegner S, Staerck L, et al. Distinguishing features of microglia- and monocyte-derived macrophages after stroke. Acta Neuropathol. (2018) 135:551–68. doi: 10.1007/s00401-017-1795-6
62. Cuartero MI, Ballesteros I, Moraga A, Nombela F, Vivancos J, Hamilton JA, et al. N2 neutrophils, novel players in brain inflammation after stroke: modulation by the PPARγ agonist rosiglitazone. Stroke. (2013) 44:3498–508. doi: 10.1161/STROKEAHA.113.002470
63. Clarkson BDS, Ling C, Shi Y, Harris MG, Rayasam A, Sun D, et al. T cell–derived interleukin (IL)-21 promotes brain injury following stroke in mice. J Exp Med. (2014) 211:595–604. doi: 10.1084/jem.20131377
64. Singh V, Roth S, Llovera G, Sadler R, Garzetti D, Stecher B, et al. Microbiota dysbiosis controls the neuroinflammatory response after stroke. J Neurosci. (2016) 36:7428–40. doi: 10.1523/JNEUROSCI.1114-16.2016
65. Praveenraj SS, Sonali S, Anand N, Tousif HA, Vichitra C, Kalyan M, et al. The role of a gut microbial-derived metabolite, trimethylamine N-oxide (TMAO), in neurological disorders. Mol Neurobiol. (2022) 59:6684–700. doi: 10.1007/s12035-022-02990-5
66. Zhu W, Romano KA, Li L, Buffa JA, Sangwan N, Prakash P, et al. Gut microbes impact stroke severity via the trimethylamine N-oxide pathway. Cell Host Microbe. (2021) 29:1199–1208.e5. doi: 10.1016/j.chom.2021.05.002
67. Chen Y, Liang J, Ouyang F, Chen X, Lu T, Jiang Z, et al. Persistence of gut microbiota dysbiosis and chronic systemic inflammation after cerebral infarction in cynomolgus monkeys. Front Neurol. (2019) 10:661. doi: 10.3389/fneur.2019.00661
68. Brandscheid C, Schuck F, Reinhardt S, Schäfer K-H, Pietrzik CU, Grimm M, et al. Altered gut microbiome composition and tryptic activity of the 5xFAD alzheimer’s mouse model. J Alzheimer’s Dis. (2017) 56:775–88. doi: 10.3233/JAD-160926
69. Shen L, Liu L, Ji H-F. Alzheimer’s Disease Histological and Behavioral Manifestations in Transgenic Mice Correlate with Specific Gut Microbiome State. J Alzheimer’s Dis. (2017) 56:385–90. doi: 10.3233/JAD-160884
70. Unger MM, Spiegel J, Dillmann K-U, Grundmann D, Philippeit H, Bürmann J, et al. Short chain fatty acids and gut microbiota differ between patients with Parkinson’s disease and age-matched controls. Parkinsonism Related Disord. (2016) 32:66–72. doi: 10.1016/j.parkreldis.2016.08.019
71. Pietrucci D, Cerroni R, Unida V, Farcomeni A, Pierantozzi M, Mercuri NB, et al. Dysbiosis of gut microbiota in a selected population of Parkinson’s patients. Parkinsonism Related Disord. (2019) 65:124–30. doi: 10.1016/j.parkreldis.2019.06.003
72. Sampson TR, Debelius JW, Thron T, Janssen S, Shastri GG, Ilhan ZE, et al. Gut microbiota regulate motor deficits and neuroinflammation in a model of parkinson’s disease. Cell. (2016) 167:1469–1480.e12. doi: 10.1016/j.cell.2016.11.018
73. van Kessel SP, Bullock A, van Dijk G, El Aidy S. Parkinson’s disease medication alters small intestinal motility and microbiota composition in healthy rats. mSystems. (2022) 7:e01191–21. doi: 10.1128/msystems.01191-21
74. García-Montero C, Fraile-Martinez O, Rodriguez-Martín S, Saz JV, Rodriguez RA, Moreno JMP, et al. The use of prebiotics from pregnancy and its complications: health for mother and offspring—A narrative review. Foods. (2023) 12:1148. doi: 10.3390/foods12061148
75. Sharon G, Cruz NJ, Kang D-W, Gandal MJ, Wang B, Kim Y-M, et al. Human gut microbiota from autism spectrum disorder promote behavioral symptoms in mice. Cell. (2019) 177:1600–1618.e17. doi: 10.1016/j.cell.2019.05.004
76. Sivamaruthi BS, Suganthy N, Kesika P, Chaiyasut C. The role of microbiome, dietary supplements, and probiotics in autism spectrum disorder. Int J Environ Res Public Health. (2020) 17:2647. doi: 10.3390/ijerph17082647
77. Pulikkan J, Maji A, Dhakan DB, Saxena R, Mohan B, Anto MM, et al. Gut microbial dysbiosis in Indian children with autism spectrum disorders. Microb Ecol. (2018) 76:1102–14. doi: 10.1007/s00248-018-1176-2
78. Settanni CR, Bibbò S, Ianiro G, Rinninella E, Cintoni M, Mele MC, et al. Gastrointestinal involvement of autism spectrum disorder: focus on gut microbiota. Expert Rev Gastroenterol Hepatol. (2021) 15:599–622. doi: 10.1080/17474124.2021.1869938
79. Iovene MR, Bombace F, Maresca R, Sapone A, Iardino P, Picardi A, et al. Intestinal dysbiosis and yeast isolation in stool of subjects with autism spectrum disorders. Mycopathologia. (2017) 182:349–63. doi: 10.1007/s11046-016-0068-6
80. Kantarcioglu AS, Kiraz N, Aydin A. Microbiota–gut–brain axis: yeast species isolated from stool samples of children with suspected or diagnosed autism spectrum disorders and in vitro susceptibility against nystatin and fluconazole. Mycopathologia. (2016) 181:1–7. doi: 10.1007/s11046-015-9949-3
81. Zou R, Wang Y, Duan M, Guo M, Zhang Q, Zheng H. Dysbiosis of gut fungal microbiota in children with autism spectrum disorders. J Autism Dev Disord. (2021) 51:267–75. doi: 10.1007/s10803-020-04543-y
82. Smits LP, Bouter KEC, de Vos WM, Borody TJ, Nieuwdorp M. Therapeutic potential of fecal microbiota transplantation. Gastroenterology. (2013) 145:946–53. doi: 10.1053/j.gastro.2013.08.058
83. Pasokh A, Farzipour M, Mahmoudi J, Sadigh-Eteghad S. The effect of fecal microbiota transplantation on stroke outcomes: A systematic review. J Stroke Cerebrovascular Dis. (2022) 31:106727. doi: 10.1016/j.jstrokecerebrovasdis.2022.106727
84. Thanush D, Venkatesh MP. Fecal microbiota transplantation: History, procedure and regulatory considerations. La Presse Médicale. (2023) 52:104204. doi: 10.1016/j.lpm.2023.104204
85. Mullish BH, Quraishi MN, Segal JP, McCune VL, Baxter M, Marsden GL, et al. The use of faecal microbiota transplant as treatment for recurrent or refractory Clostridium difficile infection and other potential indications: joint British Society of Gastroenterology (BSG) and Healthcare Infection Society (HIS) guidelines. Gut. (2018) 67:1920–41. doi: 10.1136/gutjnl-2018-316818
86. Faecal Microbiota Transplantation - EU-IN Horizon Scanning Report. (2021) 9(2):e0004221. Available at: https://www.ema.europa.eu/en/documents/report/faecal-microbiota-transplantation-eu-horizon-scanning-report_en.pdf.
87. Haifer C, Kelly CR, Paramsothy S, Andresen D, Papanicolas LE, McKew GL, et al. Australian consensus statements for the regulation, production and use of faecal microbiota transplantation in clinical practice. Gut. (2020) 69:801–10. doi: 10.1136/gutjnl-2019-320260
88. Administration (TGA) TG. Faecal Microbiota Transplant (FMT) product regulation | Therapeutic Goods Administration (TGA). (2023). Available at: https://www.tga.gov.au/resources/resource/guidance/faecal-microbiota-transplant-fmt-product-regulation (Accessed January 29, 2024).
89. Shi Y, Yang Y. Fecal microbiota transplantation: Current status and challenges in China. JGH Open. (2018) 2:114–6. doi: 10.1002/jgh3.12071
90. Zhang F, Luo W, Shi Y, Fan Z, Ji G. Should we standardize the 1,700-year-old fecal microbiota transplantation? Off J Am Coll Gastroenterol ACG. (2012) 107:1755. doi: 10.1038/ajg.2012.251
91. Kumar V, Fischer M. Expert opinion on fecal microbiota transplantation for the treatment of Clostridioides difficile infection and beyond. Expert Opin Biol Ther. (2020) 20:73–81. doi: 10.1080/14712598.2020.1689952
92. Mazzawi T, Lied GA, Sangnes DA, El-Salhy M, Hov JR, Gilja OH, et al. The kinetics of gut microbial community composition in patients with irritable bowel syndrome following fecal microbiota transplantation. PloS One. (2018) 13:e0194904. doi: 10.1371/journal.pone.0194904
93. Choi HH, Cho Y-S. Fecal microbiota transplantation: current applications, effectiveness, and future perspectives. Clin Endosc. (2016) 49:257–65. doi: 10.5946/ce.2015.117
94. Zhou J, Zhou Z, Ji P, Ma M, Guo J, Jiang S. Effect of fecal microbiota transplantation on experimental colitis in mice. Exp Ther Med. (2019) 17:2581. doi: 10.3892/etm.2019.7263
95. Yang Y, Zheng X, Wang Y, Tan X, Zou H, Feng S, et al. Human fecal microbiota transplantation reduces the susceptibility to dextran sulfate sodium-induced germ-free mouse colitis. Front Immunol. (2022) 13:836542. doi: 10.3389/fimmu.2022.836542
96. Seekatz AM, Theriot CM, Molloy CT, Wozniak KL, Bergin IL, Young VB. Fecal microbiota transplantation eliminates clostridium difficile in a murine model of relapsing disease. Infect Immun. (2015) 83:3838–46. doi: 10.1128/IAI.00459-15
97. Jiang Z-D, Alexander A, Ke S, Valilis EM, Hu S, Li B, et al. Stability and efficacy of frozen and lyophilized fecal microbiota transplant (FMT) product in a mouse model of Clostridium difficile infection (CDI). Anaerobe. (2017) 48:110–4. doi: 10.1016/j.anaerobe.2017.08.003
98. Sun M-F, Zhu Y-L, Zhou Z-L, Jia X-B, Xu Y-D, Yang Q, et al. Neuroprotective effects of fecal microbiota transplantation on MPTP-induced Parkinson’s disease mice: Gut microbiota, glial reaction and TLR4/TNF-α signaling pathway. Brain Behavior Immun. (2018) 70:48–60. doi: 10.1016/j.bbi.2018.02.005
99. Zhang T, Wang T, Chen X, Zhao Z, Chen Z. Gut microbiota relieves inflammation in the substantia nigra of chronic Parkinson’s disease by protecting the function of dopamine neurons. Exp Ther Med. (2022) 23:52. doi: 10.3892/etm.2021.10974
100. Sun J, Xu J, Ling Y, Wang F, Gong T, Yang C, et al. Fecal microbiota transplantation alleviated Alzheimer’s disease-like pathogenesis in APP/PS1 transgenic mice. Transl Psychiatry. (2019) 9:189. doi: 10.1038/s41398-019-0525-3
101. Elangovan S, Borody TJ, Holsinger RMD. Fecal microbiota transplantation reduces pathology and improves cognition in a mouse model of alzheimer’s disease. Cells. (2022) 12:119. doi: 10.3390/cells12010119
102. Abuaish S, Al-Otaibi NM, Abujamel TS, Alzahrani SA, Alotaibi SM, AlShawakir YA, et al. Fecal transplant and bifidobacterium treatments modulate gut clostridium bacteria and rescue social impairment and hippocampal BDNF expression in a rodent model of autism. Brain Sci. (2021) 11:1038. doi: 10.3390/brainsci11081038
103. Bermudez-Martin P, Becker JAJ, Caramello N, Fernandez SP, Costa-Campos R, Canaguier J, et al. The microbial metabolite p-Cresol induces autistic-like behaviors in mice by remodeling the gut microbiota. Microbiome. (2021) 9:157. doi: 10.1186/s40168-021-01103-z
104. Costello SP, Hughes PA, Waters O, Bryant RV, Vincent AD, Blatchford P, et al. Effect of fecal microbiota transplantation on 8-week remission in patients with ulcerative colitis: A randomized clinical trial. JAMA. (2019) 321:156–64. doi: 10.1001/jama.2018.20046
105. Moayyedi P, Surette MG, Kim PT, Libertucci J, Wolfe M, Onischi C, et al. Fecal microbiota transplantation induces remission in patients with active ulcerative colitis in a randomized controlled trial. Gastroenterology. (2015) 149:102–109.e6. doi: 10.1053/j.gastro.2015.04.001
106. Crothers JW, Chu ND, Nguyen LTT, Phillips M, Collins C, Fortner K, et al. Daily, oral FMT for long-term maintenance therapy in ulcerative colitis: results of a single-center, prospective, randomized pilot study. BMC Gastroenterol. (2021) 21:281. doi: 10.1186/s12876-021-01856-9
107. Cold F, Browne PD, Günther S, Halkjaer SI, Petersen AM, Al-Gibouri Z, et al. Multidonor FMT capsules improve symptoms and decrease fecal calprotectin in ulcerative colitis patients while treated – an open-label pilot study. Scandinavian J Gastroenterol. (2019) 54:289–96. doi: 10.1080/00365521.2019.1585939
108. Kelly CR, Khoruts A, Staley C, Sadowsky MJ, Abd M, Alani M, et al. Effect of fecal microbiota transplantation on recurrence in multiply recurrent clostridium difficile infection. Ann Intern Med. (2016) 165:609–16. doi: 10.7326/M16-0271
109. Camacho-Ortiz A, Gutiérrez-Delgado EM, Garcia-Mazcorro JF, Mendoza-Olazarán S, Martínez-Meléndez A, Palau-Davila L, et al. Randomized clinical trial to evaluate the effect of fecal microbiota transplant for initial Clostridium difficile infection in intestinal microbiome. PloS One. (2017) 12:e0189768. doi: 10.1371/journal.pone.0189768
110. Segal A, Zlotnik Y, Moyal-Atias K, Abuhasira R, Ifergane G. Fecal microbiota transplant as a potential treatment for Parkinson’s disease – A case series. Clin Neurol Neurosurg. (2021) 207:106791. doi: 10.1016/j.clineuro.2021.106791
111. Kuai X, Yao X, Xu L, Zhou Y, Zhang L, Liu Y, et al. Evaluation of fecal microbiota transplantation in Parkinson’s disease patients with constipation. Microbial Cell Factories. (2021) 20:98. doi: 10.1186/s12934-021-01589-0
112. Xue L-J, Yang X-Z, Tong Q, Shen P, Ma S-J, Wu S-N, et al. Fecal microbiota transplantation therapy for Parkinson’s disease: A preliminary study. Medicine. (2020) 99:e22035. doi: 10.1097/MD.0000000000022035
113. Hazan S. Rapid improvement in Alzheimer’s disease symptoms following fecal microbiota transplantation: a case report. J Int Med Res. (2020) 48:300060520925930. doi: 10.1177/0300060520925930
114. Li N, Chen H, Cheng Y, Xu F, Ruan G, Ying S, et al. Fecal microbiota transplantation relieves gastrointestinal and autism symptoms by improving the gut microbiota in an open-label study. Front Cell Infection Microbiol. (2021) 11:759435. doi: 10.3389/fcimb.2021.759435
115. Kang D-W, Adams JB, Coleman DM, Pollard EL, Maldonado J, McDonough-Means S, et al. Long-term benefit of Microbiota Transfer Therapy on autism symptoms and gut microbiota. Sci Rep. (2019) 9:5821. doi: 10.1038/s41598-019-42183-0
116. Park S-Y, Seo GS. Fecal microbiota transplantation: is it safe? Clin Endosc. (2021) 54:157–60. doi: 10.5946/ce.2021.072
117. Aràjol C, Aira Gómez A, González-Suárez B, Casals-Pascual C, Martí Martí S, Domínguez Luzón MÁ, et al. Donor selection for faecal microbiota transplantation. Consensus document of the Catalan Society of Gastroenterology and the Catalan Society of Infectious Diseases and Clinical Microbiology. Gastroenterología Y Hepatología (English Edition). (2021) 44:175–80. doi: 10.1016/j.gastre.2020.07.005
118. Duvallet C, Zellmer C, Panchal P, Budree S, Osman M, Alm EJ. Framework for rational donor selection in fecal microbiota transplant clinical trials. PloS One. (2019) 14:e0222881. doi: 10.1371/journal.pone.0222881
119. Hirsch BE, Saraiya N, Poeth K, Schwartz RM, Epstein ME, Honig G. Effectiveness of fecal-derived microbiota transfer using orally administered capsules for recurrent Clostridium difficile infection. BMC Infect Dis. (2015) 15:191. doi: 10.1186/s12879-015-0930-z
120. Youngster I, Russell GH, Pindar C, Ziv-Baran T, Sauk J, Hohmann EL. Oral, capsulized, frozen fecal microbiota transplantation for relapsing Clostridium difficile infection. JAMA. (2014) 312:1772–8. doi: 10.1001/jama.2014.13875
121. Gough E, Shaikh H, Manges AR. Systematic review of intestinal microbiota transplantation (Fecal bacteriotherapy) for recurrent clostridium difficile infection. Clin Infect Dis. (2011) 53:994–1002. doi: 10.1093/cid/cir632
122. Zipursky JS, Sidorsky TI, Freedman CA, Sidorsky MN, Kirkland KB. Patient attitudes toward the use of fecal microbiota transplantation in the treatment of recurrent Clostridium difficile infection. Clin Infect Dis. (2012) 55:1652–8. doi: 10.1093/cid/cis809
123. FDA. Commissioner O of the. Fecal Microbiota for Transplantation: Safety Alert - Risk of Serious Adverse Events Likely Due to Transmission of Pathogenic Organisms. (2020). Available at: https://www.fda.gov/safety/medical-product-safety-information/fecal-microbiota-transplantation-safety-alert-risk-serious-adverse-events-likely-due-transmission (Accessed March 7, 2023).
124. Zeng W, Shen J, Bo T, Peng L, Xu H, Nasser MI, et al. Cutting edge: probiotics and fecal microbiota transplantation in immunomodulation. J Immunol Res. (2019) 2019. doi: 10.1155/2019/1603758
125. Su S-H, Wu Y-F, Lin Q, Zhang L, Wang D-P, Hai J. Fecal microbiota transplantation and replenishment of short-chain fatty acids protect against chronic cerebral hypoperfusion-induced colonic dysfunction by regulating gut microbiota, differentiation of Th17 cells, and mitochondrial energy metabolism. J Neuroinflamm. (2022) 19:313. doi: 10.1186/s12974-022-02675-9
126. Davis BTI, Chen Z, Islam MBAR, Timken ME, Procissi D, Schwulst SJ. Postinjury fecal microbiome transplant decreases lesion size and neuroinflammation in traumatic brain injury. Shock. (2022) 58:287. doi: 10.1097/SHK.0000000000001979
127. Burrello C, Giuffrè MR, Macandog AD, Diaz-Basabe A, Cribiù FM, Lopez G, et al. Fecal microbiota transplantation controls murine chronic intestinal inflammation by modulating immune cell functions and gut microbiota composition. Cells. (2019) 8:E517. doi: 10.3390/cells8060517
128. Jacob V, Crawford C, Cohen-Mekelburg S, Viladomiu M, Putzel GG, Schneider Y, et al. Single delivery of high-diversity fecal microbiota preparation by colonoscopy is safe and effective in increasing microbial diversity in active ulcerative colitis. Inflammatory Bowel Dis. (2017) 23:903–11. doi: 10.1097/MIB.0000000000001132
129. Wang Y, Ren R, Sun G, Peng L, Tian Y, Yang Y. Pilot study of cytokine changes evaluation after fecal microbiota transplantation in patients with ulcerative colitis. Int Immunopharmacol. (2020) 85:106661. doi: 10.1016/j.intimp.2020.106661
130. Cenit MC, Sanz Y, Codoñer-Franch P. Influence of gut microbiota on neuropsychiatric disorders. World J Gastroenterol. (2017) 23:5486. doi: 10.3748/wjg.v23.i30.5486
131. Wang J-W, Kuo C-H, Kuo F-C, Wang Y-K, Hsu W-H, Yu F-J, et al. Fecal microbiota transplantation: Review and update. J Formosan Med Assoc. (2019) 118:S23–31. doi: 10.1016/j.jfma.2018.08.011
132. Chidambaram SB, Rathipriya AG, Mahalakshmi AM, Sharma S, Hediyal TA, Ray B, et al. The influence of gut dysbiosis in the pathogenesis and management of ischemic stroke. Cells. (2022) 11:1239. doi: 10.3390/cells11071239
133. Xie T, Yang R, Zhang X, Shen X, Yu L, Liao J, et al. Fecal microbiota transplantation alleviated cerebral ischemia reperfusion injury in obese rats. Tohoku J Exp Med. (2023) 259:49–55. doi: 10.1620/tjem.2022.J094
134. Spychala MS, Venna VR, Jandzinski M, Doran SJ, Durgan DJ, Ganesh BP, et al. Age-related changes in the gut microbiota influence systemic inflammation and stroke outcome. Ann Neurol. (2018) 84:23–36. doi: 10.1002/ana.25250
135. Wang J, Zhong Y, Zhu H, Mahgoub OK, Jian Z, Gu L, et al. Different gender-derived gut microbiota influence stroke outcomes by mitigating inflammation. J Neuroinflamm. (2022) 19:245. doi: 10.1186/s12974-022-02606-8
136. Wang H, Song W, Wu Q, Gao X, Li J, Tan C, et al. Fecal transplantation from db/db mice treated with sodium butyrate attenuates ischemic stroke injury. Microbiol Spectr (2021) 9:e00042–21. doi: 10.1128/Spectrum.00042-21
137. Chen R, Xu Y, Wu P, Zhou H, Lasanajak Y, Fang Y, et al. Transplantation of fecal microbiota rich in short chain fatty acids and butyric acid treat cerebral ischemic stroke by regulating gut microbiota. Pharmacol Res. (2019) 148:104403. doi: 10.1016/j.phrs.2019.104403
138. Lee J, d’Aigle J, Atadja L, Quaicoe V, Honarpisheh P, Ganesh BP, et al. Gut microbiota-derived short-chain fatty acids promote poststroke recovery in aged mice. Circ Res. (2020) 127:453–65. doi: 10.1161/CIRCRESAHA.119.316448
139. Du D, Tang W, Zhou C, Sun X, Wei Z, Zhong J, et al. Fecal microbiota transplantation is a promising method to restore gut microbiota dysbiosis and relieve neurological deficits after traumatic brain injury. Oxid Med Cell Longev. (2021) 2021:5816837. doi: 10.1155/2021/5816837
140. Wanchao S, Chen M, Zhiguo S, Futang X, Mengmeng S. Protective effect and mechanism of Lactobacillus on cerebral ischemia reperfusion injury in rats. Braz J Med Biol Res. (2018) 51:e7172. doi: 10.1590/1414-431x20187172
141. Wang Y, Tang J, Lv Q, Tan Y, Dong X, Liu H, et al. Establishment and resilience of transplanted gut microbiota in aged mice. iScience. (2022) 25:103654. doi: 10.1016/j.isci.2021.103654
142. Tan C, Wu Q, Wang H, Gao X, Xu R, Cui Z, et al. Dysbiosis of gut microbiota and short-chain fatty acids in acute ischemic stroke and the subsequent risk for poor functional outcomes. J Parenteral Enteral Nutr. (2021) 45:518–29. doi: 10.1002/jpen.1861
143. Xia G-H, You C, Gao X-X, Zeng X-L, Zhu J-J, Xu K-Y, et al. Stroke dysbiosis index (SDI) in gut microbiome are associated with brain injury and prognosis of stroke. Front Neurol. (2019) 10:397. doi: 10.3389/fneur.2019.00397
144. Stanley D, Moore RJ, Wong CHY. An insight into intestinal mucosal microbiota disruption after stroke. Sci Rep. (2018) 8:568. doi: 10.1038/s41598-017-18904-8
145. Yin J, Liao S-X, He Y, Wang S, Xia G-H, Liu F-T, et al. Dysbiosis of gut microbiota with reduced trimethylamine-N-oxide level in patients with large-artery atherosclerotic stroke or transient ischemic attack. J Am Heart Assoc. (2015) 4:e002699. doi: 10.1161/JAHA.115.002699
146. Chidambaram SB, Essa MM, Rathipriya AG, Bishir M, Ray B, Mahalakshmi AM, et al. Gut dysbiosis, defective autophagy and altered immune responses in neurodegenerative diseases: Tales of a vicious cycle. Pharmacol Ther. (2022) 231:107988. doi: 10.1016/j.pharmthera.2021.107988
147. Li N, Wang X, Sun C, Wu X, Lu M, Si Y, et al. Change of intestinal microbiota in cerebral ischemic stroke patients. BMC Microbiol. (2019) 19:191. doi: 10.1186/s12866-019-1552-1
148. Pleşeru A-M, Mihailă RG. The role of thrombin in central nervous system activity and stroke. Clujul Med. (2018) 91:368–71. doi: 10.15386/cjmed-973
149. Mohammed Y, Kootte RS, Kopatz WF, Borchers CH, Büller HR, Versteeg HH, et al. The intestinal microbiome potentially affects thrombin generation in human subjects. J Thromb Haemostasis. (2020) 18:642–50. doi: 10.1111/jth.14699
150. Zhuang Z-Q, Shen L-L, Li W-W, Fu X, Zeng F, Gui L, et al. Gut microbiota is altered in patients with alzheimer’s disease. J Alzheimers Dis. (2018) 63:1337–46. doi: 10.3233/JAD-180176
151. Bonfili L, Cecarini V, Cuccioloni M, Angeletti M, Berardi S, Scarpona S, et al. SLAB51 probiotic formulation activates SIRT1 pathway promoting antioxidant and neuroprotective effects in an AD mouse model. Mol Neurobiol. (2018) 55:7987–8000. doi: 10.1007/s12035-018-0973-4
152. Dodiya HB, Kuntz T, Shaik SM, Baufeld C, Leibowitz J, Zhang X, et al. Sex-specific effects of microbiome perturbations on cerebral Aβ amyloidosis and microglia phenotypes. J Exp Med. (2019) 216:1542–60. doi: 10.1084/jem.20182386
153. Zhao Z, Ning J, Bao X, Shang M, Ma J, Li G, et al. Fecal microbiota transplantation protects rotenone-induced Parkinson’s disease mice via suppressing inflammation mediated by the lipopolysaccharide-TLR4 signaling pathway through the microbiota-gut-brain axis. Microbiome. (2021) 9:226. doi: 10.1186/s40168-021-01107-9
154. Gorecki AM, Anyaegbu CC, Anderton RS. TLR2 and TLR4 in Parkinson’s disease pathogenesis: the environment takes a toll on the gut. Transl Neurodegener. (2021) 10:47. doi: 10.1186/s40035-021-00271-0
155. Li Y, Xia Y, Yin S, Wan F, Hu J, Kou L, et al. Targeting microglial α-synuclein/TLRs/NF-kappaB/NLRP3 inflammasome axis in parkinson’s disease. Front Immunol. (2021) 12:719807. doi: 10.3389/fimmu.2021.719807
156. Scheperjans F, Derkinderen P, Borghammer P. The gut and parkinson’s disease: hype or hope? J Parkinsons Dis. (2018) 8:S31–9. doi: 10.3233/JPD-181477
157. Huang H, Xu H, Luo Q, He J, Li M, Chen H, et al. Fecal microbiota transplantation to treat Parkinson’s disease with constipation: A case report. Med (Baltimore). (2019) 98:e16163. doi: 10.1097/MD.0000000000016163
158. Zhong Z, Chen W, Gao H, Che N, Xu M, Yang L, et al. Fecal microbiota transplantation exerts a protective role in MPTP-induced parkinson’s disease via the TLR4/PI3K/AKT/NF-κB pathway stimulated by α-synuclein. Neurochem Res. (2021) 46:3050–8. doi: 10.1007/s11064-021-03411-0
159. Matta SM, Hill-Yardin EL, Crack PJ. The influence of neuroinflammation in Autism Spectrum Disorder. Brain Behav Immun. (2019) 79:75–90. doi: 10.1016/j.bbi.2019.04.037
160. Inga Jácome MC, Morales Chacòn LM, Vera Cuesta H, Maragoto Rizo C, Whilby Santiesteban M, Ramos Hernandez L, et al. Peripheral inflammatory markers contributing to comorbidities in autism. Behav Sci. (2016) 6:29. doi: 10.3390/bs6040029
161. Hughes HK, Rose D, Ashwood P. The gut microbiota and dysbiosis in autism spectrum disorders. Curr Neurol Neurosci Rep. (2018) 18:81. doi: 10.1007/s11910-018-0887-6
162. Singh RK, Chang H-W, Yan D, Lee KM, Ucmak D, Wong K, et al. Influence of diet on the gut microbiome and implications for human health. J Trans Med. (2017) 15:73. doi: 10.1186/s12967-017-1175-y
163. Eshraghi RS, Davies C, Iyengar R, Perez L, Mittal R, Eshraghi AA. Gut-induced inflammation during development may compromise the blood-brain barrier and predispose to autism spectrum disorder. J Clin Med. (2020) 10:27. doi: 10.3390/jcm10010027
164. Fattorusso A, Di Genova L, Dell’Isola GB, Mencaroni E, Esposito S. Autism spectrum disorders and the gut microbiota. Nutrients. (2019) 11:E521. doi: 10.3390/nu11030521
165. Jain M, Swaminathan S, Teresa S, Srividhya G, Govindarao B, Gopal L, et al. Fecal microbiota transplantation for autism. Trop Gastroenterol. (2021) 41:147–8. doi: 10.7869/tg.599
166. Chen K, Fu Y, Wang Y, Liao L, Xu H, Zhang A, et al. Therapeutic effects of the in vitro cultured human gut microbiota as transplants on altering gut microbiota and improving symptoms associated with autism spectrum disorder. Microb Ecol. (2020) 80:475–86. doi: 10.1007/s00248-020-01494-w
Keywords: gut microbiota, gut-brain axis, immune cells, neuroimmune axis, neuroinflammation, fecal microbiota transplantation, ischemic stroke, neurological disorders
Citation: Hediyal TA, Vichitra C, Anand N, Bhaskaran M, Essa SM, Kumar P, Qoronfleh MW, Akbar M, Kaul-Ghanekar R, Mahalakshmi AM, Yang J, Song B-J, Monaghan TM, Sakharkar MK and Chidambaram SB (2024) Protective effects of fecal microbiota transplantation against ischemic stroke and other neurological disorders: an update. Front. Immunol. 15:1324018. doi: 10.3389/fimmu.2024.1324018
Received: 08 November 2023; Accepted: 01 February 2024;
Published: 21 February 2024.
Edited by:
Daniel Erny, University of Freiburg Medical Center, GermanyReviewed by:
Miguel A. Ortega, University of Alcalá, SpainCopyright © 2024 Hediyal, Vichitra, Anand, Bhaskaran, Essa, Kumar, Qoronfleh, Akbar, Kaul-Ghanekar, Mahalakshmi, Yang, Song, Monaghan, Sakharkar and Chidambaram. This is an open-access article distributed under the terms of the Creative Commons Attribution License (CC BY). The use, distribution or reproduction in other forums is permitted, provided the original author(s) and the copyright owner(s) are credited and that the original publication in this journal is cited, in accordance with accepted academic practice. No use, distribution or reproduction is permitted which does not comply with these terms.
*Correspondence: Saravana Babu Chidambaram, YmFidXB1YmxpY2F0aW9uc0BnbWFpbC5jb20=; c2FyYXZhbmFiYWJ1LmNAanNzdW5pLmVkdS5pbg==; Meena Kishore Sakharkar, bWVlbmEuc2FraGFya2FyQHVzYXNrLmNh
†ORCID: Tousif Ahmed Hediyal, orcid.org/0000-0001-5605-4989
M. Walid Qoronfleh, orcid.org/0000-0001-6757-1922
Mohammed Akbar, orcid.org/0000-0002-8820-7406
Byoung-Joon Song, orcid.org/0000-0002-3954-3497
Meena Kishore Sakharkar, orcid.org/0000-0002-9289-3314
Saravana Babu Chidambaram, orcid.org/0000-0003-2357-056X
Disclaimer: All claims expressed in this article are solely those of the authors and do not necessarily represent those of their affiliated organizations, or those of the publisher, the editors and the reviewers. Any product that may be evaluated in this article or claim that may be made by its manufacturer is not guaranteed or endorsed by the publisher.
Research integrity at Frontiers
Learn more about the work of our research integrity team to safeguard the quality of each article we publish.