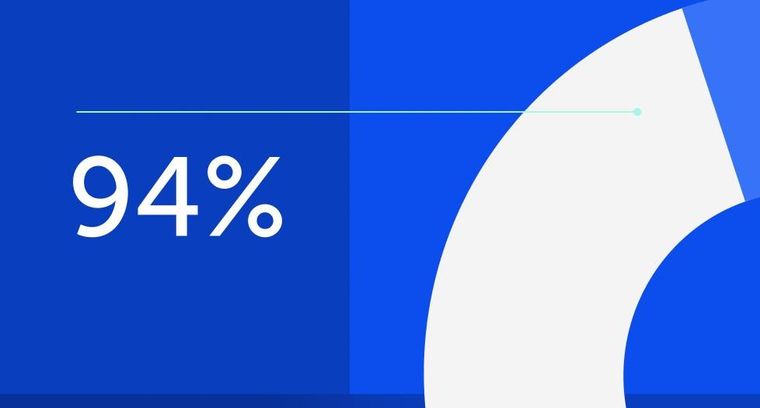
94% of researchers rate our articles as excellent or good
Learn more about the work of our research integrity team to safeguard the quality of each article we publish.
Find out more
REVIEW article
Front. Immunol., 26 January 2024
Sec. Microbial Immunology
Volume 15 - 2024 | https://doi.org/10.3389/fimmu.2024.1321395
This article is part of the Research TopicOvercoming Challenges in Microbial Immunology: 2022View all 10 articles
The gut microbiome is a heterogeneous population of microbes comprising viruses, bacteria, fungi, and protozoa. Such a microbiome is essential for sustaining host equilibrium, and its impact on human health can be altered by a variety of factors such as external variables, social behavior, age, nutrition, and genetics. Gut microbes’ imbalances are related to a variety of chronic diseases including cancer, obesity, and digestive disorders. Globally, recent findings show that intestinal microbes have a significant role in the formation of cardiovascular disease (CVD), which is still the primary cause of fatalities. Atherosclerosis, hypertension, diabetes, inflammation, and some inherited variables are all cardiovascular risk variables. However, studies found correlations between metabolism, intestinal flora, and dietary intake. Variations in the diversity of gut microbes and changes in their activity are thought to influence CVD etiology. Furthermore, the gut microbiota acts as an endocrine organ, producing bioactive metabolites such as TMA (trimethylamine)/TMAO (trimethylamine N-oxide), SCFA (short-chain fatty acids), and bile acids, which have a substantial impact on host wellness and disease by multiple mechanisms. The purpose of this overview is to compile current evidence highlighting the intricate links between gut microbiota, metabolites, and the development of CVD. It focuses on how intestinal dysbiosis promotes CVD risk factors such as heart failure, hypertension, and atherosclerosis. This review explores the normal physiology of intestinal microbes and potential techniques for targeting gut bacteria for CVD treatment using various microbial metabolites. It also examines the significance of gut bacteria in disease treatment, including supplements, prebiotics, probiotics, antibiotic therapies, and fecal transplantation, which is an innovative approach to the management of CVD. As a result, gut bacteria and metabolic pathways become increasingly attractive as potential targets for CVD intervention.
Graphical Abstract The significance of microbial-mediated metabolites in the emergence of CVD. CVD, cardiovascular disease; SCFAs, short-chain fatty acids; TMA, trimethylamine; TMAO, trimethylamine N-oxide; LPS, lipopolysaccharides.
Understanding the evolution of the gut microbiota and its internal and external impacts on the intestine, as well as the risk factors for cardiovascular diseases (CVDs), such as metabolic syndrome, has attracted a great deal of attention (1, 2). Globally, CVDs are the primary causes of death, encompassing conditions including coronary artery disease (CAD), atherosclerosis, thrombosis, aneurysms, arterial hypertension, and cardiomyopathies that reinforce heart failure and cerebrovascular diseases (3–5). The current studies predict 17.5 million deaths per year by CVD, accounting for around 31% of all overall mortality (6). Among them, heart attack and stroke are directly linked to 85% of the cases (7, 8). Inflammation, dyslipidemia (i.e., elevated serum cholesterol, triglycerides, and low-density lipoproteins), and diabetes mellitus are prevalent pathological mechanisms and risk factors that can impact the progression and emergence of CVD (9, 10). In addition to hereditary factors, environmental factors such as nutrition and gut microbiota composition are going to play a significant influence in the development of CVDs. Furthermore, the rise of obesity and diabetes has been related to intestinal dysbiosis (11, 12), insulin resistance, and sedentary behaviors such as smoking, insufficient exercise, and poor nutrition are all identified risk variables for CVD (13, 14). The research into how the human gut microbiome affects CVD and metabolic diseases has expanded dramatically (15). Gut dysbiosis is a condition defined by changes in intestinal bacteria in adults, can be induced by a range of events such as dietary choices, environmental effects, intestinal infections, or external variables, and it can result in inflammation and metabolic disorders (16). The human gut microbiome comprises an array of over 10 trillion diverse microbes, encompassing bacteria, viruses, protozoa, methanogen archaea, and fungi. This collective term, microbiota, is synonymous with the entirety of these microbial inhabitants residing within the human body (17), while Actinobacteria, Firmicutes, Proteobacteria, and Bacteroides are the four major bacterial genera that comprise a healthy microbiota (18, 19). Before birth, an infant’s gut has very few germs (20), but after birth, the body begins to receive a steady stream of stimulation from the environment. It promotes a gradual rise in the number of bacteria in the colon, eventually leading to the formation of a dynamic and balanced balance in the gut microbiota (21).
The intestinal mucosal surface serves as the interface between the gut microbiota and performs a number of tasks that keep the intestinal epithelial barrier functioning (22). Endotoxins, microbes, and their byproducts can move more easily through the gut wall and into the bloodstream, where they can cause autoimmune disorders. Immune dysregulation and inflammation are at the basis of many CVDs, including atherosclerosis, myocardial infarction (MI), rhythm disorders, pericardial disease, cardiomyopathies, and heart failure (23). Moreover, it is important to highlight that the intestinal tract can be seen as an extensive and diverse ecosystem that produces a significant number of microbial metabolites (24). The host food is broken down by the gut flora into a variety of metabolically active products, including trimethylamine N-Oxide (TMAO), short-chain fatty acids (SCFAs), primary and secondary bile acids, tryptophan and indole derivatives, phenylacetylglutamine (PAGln), and branched-chain amino acids (BCAA), these may contribute to the CVD progression (25). Moreover, N-oxide TMA/TMAO and bile acids are fascinating biomarkers for CVD progression (26), however other gut microbiota components or similar chemicals should be investigated for use as early CVD markers (11). The loss of SCFA-producing intestinal microbes would disrupt the equilibrium of glucose metabolism, raising the risk of CVD (27). Trimethylamine stimulates macrophage stimulation, producing vascular damage; excessive TMAO levels owing to intestinal dysbiosis promote atherosclerosis, raise the risk of CAD, and hasten arterial plaque development that leads to cardiovascular disease (6). As shown in Figure 1, reducing dietary TMAO precursor intake is a promising strategy for lowering the risk of CVD due to the high amounts of trimethylamine (TMA) and TMAO generation by choline-induced gut flora (28, 29). Microbial sequencing analysis has emerged as a valuable tool for uncovering distinct gut microbiota patterns linked to cardiovascular disease CVD (30–32).
Figure 1 A diagram depicting the effect of gut bacteria and metabolites on CVD risk factors. SCFAs, short-chain fatty acids; TMAO, trimethylamine N-oxide.
The gut microbiota plays a crucial role in influencing overall health, either through direct mechanisms or indirect pathways. The intricate interactions involving variations in microbiome composition, metabolites, and CVD susceptibility underscore the significance of intestinal microbes as a novel modulator of CVD. The identified association between gut microbes and CVD suggests that modifying the intestinal microbiota could be beneficial in preventing and managing the development of CVD. Nutritional therapy, the use of pre/probiotics and antibiotics, fecal microbiota transplantation, TMAO reduction, and regular exercise are all current ways to manage gut bacteria to improve cardiovascular function (11). The latest study highlights the possible importance of microbial imbalance in CVD disorders. The advent of genomic and metabolomic technologies has allowed for more thorough characterization and molecular research of these microbiota and their metabolites. However, most evidence continues to indicate associations and the particular chemical processes driving a majority of visible events remain unidentified (33). Future studies focusing on microbe-microbe and microbe-host interactions could reveal how specific metabolites influence the disease process. It is also critical to have a better understanding of the bacterial mechanisms involved in the production of CVD-related metabolites, and also their functional roles. These results could provide a solid theoretical basis for the invention of therapeutic methods for CVD individuals. The present paper covers the usual composition and functional significance of intestinal bacteria and also provides new insights into the gut microbiota and its linked metabolites, which are implicated in CVDs. Scientific studies, putative biological explanations, and therapeutic outcomes are of significant interest to researchers. In addition, we discuss studies relating the gut microbiota to inflammatory processes, lipid metabolic disorders, and diabetes, all of which are linked to an elevated risk of cardiovascular disease. As a result, this overview focuses primarily on studying the role of gut microbiota-related metabolites and their therapeutic potential in CVDs, which may eventually provide more insight into the development of CVD prevention.
The intestinal Bacteroidetes are one of the most significant bacterial colonies in the gastrointestinal microbiota. Despite their wide species composition, these cultures display stability in many gut regions, and some exhibit location-specific differentiation, mainly in the ascending colon (34). A total of around 1,000 different species of intestinal microbes, comprising about 1014, and bacterial-to-human cell ratio varied between 10:1 and 1:1 (35). In cardiovascular patients, more than 90% of these bacteria had an impact on the growth of Bacteroidetes and Firmicutes, keeping a stable Firmicutes/Bacteroidetes (F/B) ratio (36). The emergence of CVD is dependent on a compromised mucosal barrier and decreased intestinal mucosal barrier function, and is mostly caused by gram-negative microbes, such as lipopolysaccharide (LPS), which plays a significant role in the emergence of cardiometabolic diseases (37, 38). A high-fat diet has been shown to reduce gram-positive Bifidobacteria levels in the digestive system while increasing the amount of intestinal microbes that hold LPS, both of which contribute to obesity, the primary risk factor for CVD (39). The F/B ratio become a crucial role in the context of obesity, particularly in children (40). This ratio is linked to low-grade inflammation, which increases the probability of diabetes, a known risk factor for CVDs (41).
Because the gut acts as a bridge between them, the interaction between the host and the gut microbiota is crucial for preserving intestinal integrity. Several microbial metabolites have been linked to CVD (25) including bile acids, SCFAs, branched-chain amino acids, TMAO, tryptophan, and indole derivatives (42). The TMAO is formed when foods rich in choline, lecithin, and L-carnitine, primarily found in animal products, with limited plant-based sources, are ingested. In the gut, lecithin (comprising phosphatidylcholine, a choline source) and dietary choline are metabolized into TMA by the gut microbiota having specialized enzymes TMA lyses transcribed by cutC/cutD genes found in various bacterial strains. Recent data suggests that elevated circulating levels of TMAO are associated with an increased risk of CVD and mortality (43–46). Increased TMAO levels in the bloodstream encourage lipid accumulation in the arteries, which contributes to atherosclerosis. Figure 2 depicts how the inflammatory response influences the development of glucose intolerance, diabetes, and CVD (47–50). A dysbiotic microbe was found to decrease the amount of cholesterol eliminated by feces while increasing absorption and plasma levels of low-density lipoproteins, signaling that dysbiosis may increase the risk of atherosclerosis and CVD (39).
Figure 2 The gut microbiota of the target body’s functioning mechanisms. A low-fiber diet corresponds with decreased short-chain fatty acid butyrate formation, exacerbating dysbiosis and sustaining local and systemic inflammation via bacterial toxin leaks, most notably LPS. A modern Western diet strong in red meat promotes the synthesis of TMA by bacteria, which is then oxidized in the liver to the pro-atherosclerotic metabolite. CVD, cardiovascular disease; TMA, trimethylamine; TMAO, trimethylamine N-oxide.
The bacterial composition, diversity, and abundance are highly influenced by genetic changes in the host’s genome, and by external variables such as the host’s lifestyle, diet, sanitation, health, and the use of antibiotics and probiotics (51). The gut microbiota has small genetic differences in various parts of the intestine. Eckburg et al., (24) used metagenomic analysis to discover the gut bacterial community is made up of six phyla: Firmicutes, Bacteroidetes, Proteobacteria, Actinobacteria, Fusobacteria, and Verrucomicrobia, with the majority of the organisms in a healthy bacterial community being anaerobic population, as shown in Figure 3 (52). They inhabit unique biological niches on mucosal surfaces and in the gut lumen, where they form sophisticated biochemical interaction networks with both their hosts and with others (53). The synergistic interaction between the host species and the gut microbiome fosters the proliferation of beneficial microbes while inhibiting the growth of harmful bacteria (54). The gut flora regulates many bodily functions, such as providing metabolic fuel to the host, supporting growth and immune system regulation, removing harmful microbes, keeping intestinal wall integrity, and maintaining overall homeostasis (55). Microbial life has a significant impact on immune function and metabolism, with well-balanced gut microbiota playing an important role in the health of the host. (56). Inadequate dietary intake, excessive stress, significant life events, and antibiotic administration can all impact the diversity of the gut microbiota, leading to a disorder called dysbiosis (20). Elie Metchnikoff, a Russian immunologist and microbiologist renowned for his contributions to the understanding of the immune system, particularly the concept of phagocytosis, is not credited with coining the term “dysbiosis” (57, 58). An imbalance in the typical microbial composition (microbiota) of the colon or other bodily regions is described by this term, which is used in the current discipline of microbiology (59). In particular, Metchnikoff’s study has little work with the current concept of dysbiosis, which emerged as knowledge of the function of the human microbiome in health has increased (60). As seen in Figure 3, several risk factors were put out as potential causes of intestinal dysbiosis. There is a lot of literature known about the use of antibiotics, which has been seen to alter the composition of the gut’s microbiome and have both short-term and long-term effects (61–64). Obesity and high-fat and sugar meals are all related to persistent variations in the gut microbiota (65–68).
It is believed that external factors at various stages of life influence the formation of gut dysbiosis. The style of delivery, type of feeding, and hospital milieu are all related to the diversity of the bacteria during childhood (69, 70). Further, social stresses and exposure to xenobiotics including pesticides and heavy metals have been related to gut dysbiosis (71, 72). The emergence of a gut microbiome has a genetic basis as opposed to social factors, based on twin studies. Given that identical twins have nearly identical DNA, any changes in their gut microbes must be the result of non-genetic variables like food, medical history, or use of antibiotics (73, 74). The study of bacterial genomes has transformed the field of microbial research. Metagenomic sequencing and 16S rDNA sequencing are two types of sequencing that are frequently utilized to assess the abundance of microbial components. By focusing on the conserved sections that surround the hypervariable regions, the 16S sequencing approach can detect variations in bacterial genomes (75, 76).
A broad spectrum of diseases is considered in CVD, including atherosclerosis, aortic valve disease, peripheral artery disease, hypertension, and stroke. Heart failure, hypertension, and atherosclerosis are associated with gut dysbiosis as shown in Table 1. In recent years, significant progress has been made in understanding how the gut microbiome impacts cardiovascular function and the development of these diseases. In this specific section, we intend to highlight several well-supported studies that deliver compelling evidence regarding the role of gut microbiota in the development of cardiovascular disease as depicted in Figure 4 (92).
Table 1 Modifications in the diversity of the intestinal microbes attributed to CVD. CVD-related changes in the diversity of the gut’s microbes.
Figure 4 The contribution of the gastrointestinal microbiome to CVD. Choline, phosphatidylcholine, and carnitine are all available in high-cholesterol, high-fat diets. Intestinal microbes convert phosphatidylcholine in the diet to choline, which is then turned into trimethylamine. Hepatic flavin monooxygenases convert TMA to TMAO in the liver. By increasing atherosclerosis and generating agonist-induced platelet activation, TMAO promotes thrombosis. High levels of TMAO in the blood are linked to an increased risk of CVD. Furthermore, a high-fat diet raises the levels of microbe-associated molecular patterns like LPS. Increased intestinal uptake of microbe-associated molecular patterns results in metabolic endotoxemia and low-grade inflammation, both of which worsen atherosclerosis. TLR2 induces arterial thrombosis by increasing the interaction between von Willebrand factor and platelet integrin. Furthermore, intestinal bacteria convert carbs to SCFA and produced by gut microbial fermentation regulate blood pressure, a risk factor for CVD progression. FMO: flavin monooxygenases, SCFAs, short-chain fatty acids; TMAO, trimethylamine N-oxide; TLR2, toll-like receptor-2; LPS, lipopolysaccharide.
The disease termed heart failure is defined by the heart’s decreased ability to efficiently pump enough blood and oxygen to satisfy the demands of the body (93, 94). It serves as the final stage of multiple CVDs, which are highly prevalent, have significant mortality rates, and pose a significant threat to human well-being (95). While chronic exposure is defined by an altered inflammatory state related to pro-inflammatory aspects that are critical to the beginning of HF, immediate exposure is associated with a variety of inflammation-related symptoms (96). Our understanding of the pathophysiological processes behind HF has greatly increased. The recognition of the vital role of managing neurohumoral processes rather than focusing solely on changes in blood flow is a key shift in this understanding (97, 98). More evidence indicates the stomach is implicated in decreased heart rate and higher systemic congestion, both of which can contribute to intestinal mucosal ischemia and edema. As a result, bacterial translocation may be enhanced, allowing endotoxins into the bloodstream and contributing to the inflammation seen in HF patients (99). Niebauer et al. (100) conducted a study that revealed a significant association between peripheral edema in HF patients and higher plasma levels of endotoxins and inflammatory cytokines. Specifically, patients experiencing peripheral edema demonstrated elevated concentrations of these markers compared to those without edema. However, the study found a decrease in serum amounts of endotoxins but not cytokines following short-term diuretic treatment. This finding raises the prospect that edema and gut-associated inflammation in cardiac failure may be linked while diuretic medication might be effective (100). Pasini et al. (88) showed a recent comparison of the bacterial and fungal profiles in the feces of heart failure patients and the findings revealed people with chronic heart failure (CHF) had a greater risk of perilous bacterial growth compared with control group.
The presence of Candida, Campylobacter, and Shigella species was linked to 78.3% of CHF disease severity that had significantly greater intestinal permeability. Between TMAO and the risk of atherosclerosis, there was a strong positive correlation between gut permeability and right atrial pressure. An increased TMAO levels have been linked to poor outcomes in people with heart failure (101, 102). Scientists evaluated the levels of TMAO in 2,490 patients with chronic heart disease in the study with a 9.7-year follow-up. The data showed increasing TMAO levels coincided with increased rates of morbidity and mortality, particularly in HFrEF patients. This study suggests that TMAO could be utilized as a biomarker to predict poor outcomes in HfrEF patients (103). A recent meta-analysis offered that some reliable insights into the prognostic importance of TMAO in HF (102). The higher TMAO precursor trimethyl lysine (TML)-derived N, N, N-trimethyl-5-aminovaleric acid (TMAVA) synthesis by the gut microbiota was linked to a progressive reduction in fatty acid oxidation (104). Several studies have consistently reported that patients with HF exhibited a decrease in butyrate-producing bacteria, particularly within the Lachnospiraceaand Ruminococcaceae families (105). However, the absence of butyrate-producing microbes such Eubacterium Halli and Lachnospiracea is associated with higher mortality, increased inflammation, and severity of disease. This association implies that the abundance of these beneficial gut bacteria may have a major impact on the progress and results of cardiac failure (106). Dysbiosis has been consistently linked to reduced butyrate production in various heart failure cohorts (105). Additionally, bile acids, particularly secondary bile acids produced through the transformation by gut microbiota, play a crucial role in heart failure. Research has indicated a rise in secondary bile acids among individuals with CHF(97). Indoxyl sulfate produced by gut microbial metabolism, has also been linked to cardiac fibrosis and ventricular remodeling. These findings underscore the importance of the gut microbiota and its metabolites in heart health and offer possible therapeutic targets for heart failure management (107).
Atherosclerotic cardiovascular disease is a persistent inflammatory state primarily impacting sizeable and intermediate arteries. Numerous firmly established factors are correlated with atherosclerosis, including hypertension, dyslipidemia, advanced age, and smoking (108). This is characterized by the accumulation of low-density lipoprotein within the artery walls, leading to the formation of atheroma and distinct plaques consisting of proliferative fibrous tissue and calcifications (109, 110). Recent decades, there has been a growing interest in finding out how the gut microbiota plays a significant role in the emergence of atherosclerotic lesions (111). Koren et al. did an analysis using shotgun DNA sequencing focused on the gut metagenome that indicated significant changes in the diversity of gut microbial populations between patients with symptomatic atherosclerosis and those assumed to be healthy controls. These findings strongly suggest that the gut microbiota may play a significant role in the atherosclerosis (30, 31). Further, extensive metagenome-wide association research done on a cohort of 218 atherosclerosis patients and 187 healthy controls confirmed a link between the diseases with changed gut microbiome composition. In particular, the study found that people with atherosclerosis had significantly higher concentrations of Enterobacteriaceae, Ruminococcusgnavus, and Eggerthellalenta (112, 113). Introducing prebiotics and probiotic strains which enhance the production of SCFA and boost the diversity of beneficial microbes might be a valuable strategy in atherosclerosis prevention strategies (114). Various animal studies, including the work by Chan et al., (115) have explored the impact of probiotics and telmisartan on mitigating atherosclerosis induced by a high-fat diet, resulted in an increase in the Firmicutes to Bacteroidetes ratio. A diet rich in fats was found to decrease the prevalence of Eubacterium, Anaeroplasma, Oscillospira, Roseburia, and Dehalobacterium, while simultaneously elevating the quantities of Allobaculum, Clostridium, Lactobacillus, and Bifidobacteria (116). The findings show B. fragilis can produce extracellular vesicles (EVs), which are lipid bilayer particles. Human research has revealed a relationship between infectious and non-infectious disorders, as well as changes in the systemic levels of EVs derived from gut bacteria (117). It was identified as an essential cell-cell communicator with the potential to increase the knowledge of atherosclerotic disease, ranging from biomarkers to disease pathogenesis (118). Proteomic study has revealed unique protein compositions for EV subtypes, with some indicators assisting in the differentiation of EVs via biogenesis processes. Endosomal sorting complexes required for transport (ESCRT) proteins, Alix, and tetraspanins, for example, are exosome markers, whereas ectosome markers include Annexin A2/A5, ARF6, and Enolase 1 (119). EVs are involved in the immunological response, vascular remodeling, endothelial dysfunction, and apoptosis, which all contribute to atherosclerosis, and EVs in plasma may be useful as atherosclerosis indicators (120).
Scientists used the terminal restriction fragment length polymorphism technique for insight into the gut bacteria contents with coronary artery patients. Their research showed that the microbial diversity in the individuals studied experienced unique alterations. Notably, the levels of Bacteroides were decreased while the abundance of Lactobacillales and Clostridium subcluster XIVa increased in the fecal samples of people. These findings suggest the gut microbiota may contribute to the progression of coronary artery disease. Novel strategies for treating or preventing cardiovascular diseases may be developed as a result of a better understanding of such microbial modification (79). A significant negative association found between the decrease in (1) Eubacterium and the rise of inflammatory cytokines like matrix metalloproteinase-9 (MMP-9) and E-selectin; (2) Dehalobacterium and adipocyte fatty acid binding protein (A-FABP); and (3) Roseburia and MMP-9. This confirms a connection between imbalanced gut microbiota and the development of atherosclerosis (115). Similarly, Stepankova showed experiments signifying the beneficial impact of gut microbiota in inhibiting the atherosclerotic lesions progression (121).The aortas of the germ-free mice fed a low-cholesterol diet showed atherosclerotic plaques. The results of the study offer strong evidence that microorganisms suppress the progress of atherosclerosis (122).
On the contrary, systems depending on metabolism can be affected by gut dysbiosis, which alters a wide variety of metabolites and may have an effect on the onset and development of atherosclerosis (6). One of the several metabolites produced by the gut bacteria, TMAO, plays a crucial role in the formation of atherosclerosis (123). The accumulation of TMAO in the body has been related to an increase in the risk of atherosclerosis and cardiovascular diseases (124). The blood plasma levels of TMAO in mice with normal gut microbiota grew as they were fed a diet high in choline. In contrast, animals given the same choline-rich diet and antibiotic treatment, which changed their gut microbiota, had minimal TMAO amount (43). Compared to the control TMAO levels, the mice with greater TMAO levels displayed a higher amount of foam cell formation and the development of atherosclerotic plaques. The risk of sudden cardiovascular events is increased by TMAO linked to plaque vulnerability to its involvement in developing atherosclerosis (28, 47). High levels of TMAO in the bloodstream have been associated with conditions such as obesity, Type 2 diabetes mellitus, chronic kidney disease (CKD), and CVD (125, 126). Moreover, numerous other studies have shown that SCFAs may have a positive impact on atherosclerosis by suppressing inflammation (127, 128). As a result, SCFAs may reduce cholesterol levels and stop the host from developing lipid deposits. Dyslipidemia can result from decreased SCFA production, whereas probiotics (Lactobicili) are effective in reducing cholesterol. According to Dieck et al., (129) probiotic anti-cholesterolemic effect can be induced via bile salt hydrolysis (BSH), interference with hepatic de novo lipid synthesis by regulation of SCFA, or satiety hormones. It indicates that SCFAs may have a preventive effect by reducing the risk factors for cardiac disease (130).
Hypertension is a significant worldwide public health concern and stands as the foremost risk factor for cardiovascular diseases, leading to a substantial economic burden on society. Its epidemiology is defined by a high prevalence, notable levels of disability and mortality, and often insufficient awareness (131, 132). As of 2021, it was estimated that around 330 million people in China were affected by cardiovascular diseases, with approximately 245 million individuals having been diagnosed with hypertension (133). The causes of hypertension involve a combination of factors, including genetic predisposition, lifestyle choices, environmental influences, hormonal imbalances, inflammatory processes, and changes in hemodynamic mechanisms (134). The American College of Cardiology, the American Heart Association, and the European Society of Hypertension have collaboratively formulated behavioral guidelines aimed at maintaining optimal blood pressure levels, with a particular emphasis on non-pharmacological strategies (135). These include using the dietary methods to stop hypertension (DASH) diet, which highlights a high intake of fruits and vegetables while minimizing fat consumption, increasing physical activity through specific aerobic exercises, slicing off salt and alcohol consumption, losing weight, and increasing salt and alcohol consumption (136–138).
A small number of studies mostly in animal models have shown an explicit link between gut microbiota and the control of blood pressure (139–142). For instance, Yang et al. (140) conducted a study where they investigated changes in the fecal microbiota of animal models with hypertension, specifically comparing alterations in the spontaneously hypertensive rat and chronic angiotensin II infusion rat models. They observed a notable gut dysbiosis in hypertensive animals characterized by a decrease in microbial richness, diversity, and consistency (140). Kim (143) also found that among hypertension patients, the presence of butyrate-producing bacteria, such as Butyricimonas and anaerobic Corynebacterium, had significantly decreased. Studies have revealed a positive correlation between blood pressure and the levels of Ruminococcaceae, Streptococcus, and Turicibacter (140, 144, 145). The metabolite production derived from microbes may also be impacted by changes in gut microbiota. Since they are created by bacterial digestion of dietary fiber and are closely related to good health, SCFAs are of particular significance among these metabolites that are formed from microbes (146), play a vital role in the HTN development. A larger amount of research indicates the potential of SCFAs may effectively decrease the host’s blood pressure by interacting with G protein-coupled receptor 41 (147–149). Although TMAO is required for disease start, an animal model was first used to demonstrate the relationship between TMAO and CVD in 2011 (28). Recently, Wang et al. (150) have provided compelling evidence of a causal link between TMAO and its precursors with blood pressure by employing a Mendelian Randomization approach. Moreover, multiple studies have validated the strong association between elevated TMAO levels and an increased prevalence of hypertension (151–153). Ge et al. (153) proved that a rise of 5 and 10 mol/L in TMAO levels corresponded to a 9% and 20% escalation in the risk of hypertension, respectively. Apart from TMAO, other gut microbiota-derived metabolites, including corticosterone, H2S, choline, BAs, indole sulfate, and LPS, are also produced. The SCFAs, TMAO, BAs, H2S, and LPS metabolites have been closely linked to the development of hypertension. So, the intestinal microbiota may have an interconnected role in regulating blood pressure, and any disruptions in their function could be linked to hypertension. Studies have proposed that Lactobacillus probiotics might play a beneficial role in blood pressure regulation (154) Additionally, a meta-analysis has shown that probiotics treatment can lead to a significant reduction in blood pressure in patients (155).
We will briefly discuss the association between trimethylamine N-Oxide and CVD, and focus on other microbial metabolites in this review as illustrated in Figure 5 (156).
Figure 5 Representation of microbial-derived metabolites to CVD. Variations in the composition of the gut microbiome can change the metabolism, allowing bacteria or its fragments and metabolites to enter the circulation more easily. This can aggravate the pro-inflammatory milieu and produce metabolic disturbances, which can lead to CVD. BA, Bile acid; SCFA, short-chain fatty acids; TMA, trimethylamine; TMAO, trimethylamine-N-oxide.
The gut microbial digestion of phosphatidylcholine, the main dietary source of choline, was found to produce a proatherogenic metabolite called trimethylamine-N-oxide (157). Among the numerous physiologically active metabolites of microbial metabolism, TMAO is a biologically active molecule that has been linked to an increased risk of adverse cardiovascular events, including acute coronary syndrome (ACS), stroke, and mortality (47, 158, 159). TMAO production occurs secondary to the ingestion of nutrients containing the trimethylamine moiety, such as choline, phosphatidylcholine, and L-carnitine, all of which are found in high concentrations in animal products, including red meat, fish, milk, and eggs. The metabolism of these nutrients by microbial TMA lyases produces TMA, which enters the portal circulation, is oxidized to TMAO by hepatic flavin monooxygenases, primarily FMO3 (160), and subsequently enters the general circulation (28, 161). It is believed that TMAO may contribute to the development of atherosclerosis, following a proatherogenic pathway. Elevated levels of TMAO in the bloodstream have been positively associated with early atherosclerosis in humans. Moreover, monitoring TMAO levels can be useful in predicting the risk of mortality in patients with stable coronary artery disease and acute coronary syndrome (151, 162). Research has indicated that higher TMAO levels in the bloodstream are linked to the severity of peripheral artery disease and a greater risk of cardiovascular mortality among individuals affected by this condition (163).
In-depth analyses, including meta-analysis and dose-response studies, have further revealed that elevated plasma TMAO levels are associated with a higher occurrence of major adverse cardiovascular events in patients with coronary heart disease (164). Additionally, proinflammatory monocytes and elevated TMAO levels were substantially associated in stroke patients. According to Haghikia et al. (165) higher cardiovascular events such as myocardial infarction, recurrent stroke, and cardiovascular death were also linked to a raised TMAO plasma level. Numerous human investigations have also supported the involvement of TMAO in CVD. Compared to controls, patients with chronic heart failure had higher plasma levels of TMAO, choline, and betaine in a prospective observational analysis of stable CAD and healthy people (166). Similarly, in patients who experienced a myocardial infarction, TMAO was identified as an independent predictor of mortality at the two-year follow-up. The ratio stood at 1.21 (with a 95% confidence interval of 1.03-1.43, P = 0.023), as observed in a study involving 292 events (167). Another study conducted by Tang et al. (48), observed a correlation between elevated TMAO levels and a higher risk of major adverse cardiac events. However, the precise mechanisms by which TMAO affects cardiovascular disease have not been fully investigated.
The human digestive system cannot break down complex carbohydrates, such as dietary fiber, to support cell activity. Nevertheless, the gut microbiota can utilize fibers by fermenting them, resulting in the production of SCFAs (168). SCFAs are saturated fatty acids composed of carbon chains ranging from one to six carbons. Acetate, propionate, and butyrate are the main types of SCFAs found in the human body (169). The primary bacteria responsible for producing SCFAs are found in the clostridial clusters IV and XIVa within the Firmicutes phylum and include various species of bacteria such as Eubacterium, Roseburia, Faecalibacterium, and Coprococcus (170). It plays crucial roles in regulating anti-inflammatory responses, lipid metabolism, and gluconeogenesis. Notably, butyrate, one of the SCFAs, is considered a significant energy source for intestinal epithelial cells (171). A significant amount of research shows that SCFAs protect against heart failure and are essential for preserving the integrity of the intestinal barrier by encouraging mucus formation and reducing inflammation (172). The presence of high SCFA levels in fecal samples is linked to markers of hypertension, central obesity, and subclinical indicators of cardiometabolic disorders (173) and to the development of atherosclerosis (174).
Butyric acid in the diet effectively slowed the progression of atherosclerotic plaques in the arterial walls of mice missing apolipoprotein E (Apo-E) in a trial utilizing rodent as a model. The favorable benefits were obtained by slowing macrophage migration, boosting collagen deposition, and improving plaque stability (175). Multiple studies show that the SCFAs contribute to manage blood pressure. For example, when fecal material from hypertension human donors was introduced into germ-free mice vs normotensive donors, researchers saw an increase in blood pressure (176). SCFAs are linked to blood pressure regulation through G-protein coupled receptor (GPCR) pathways, specifically in renin secretion and blood control. SCFA activation of the olfactory receptor (Olfr) 78 and the free fatty acid receptor GPR41 causes an increase in blood pressure and a decrease in blood pressure, respectively. The acetate and propionate have antihypertensive properties due to their ability to reduce systemic inflammation and atherosclerotic lesions, both of which are independent predictors of hypertension (177). However, SCFAs have been implicated in causing damage to the organs affected by hypertension in mice infused with angiotensin II, indicating their role in hypertensive organ damage (178). As a result, a lot of evidence points to the gut microbial community’s influence on blood pressure regulation in the host, with SCFAs functioning as one of the microbial components that contribute to vasomotor tone and blood pressure regulation. Recent research has revealed more evidence that SCFAs play a role in a variety of CVD processes, including ischemia-reperfusion injury, heart repair after myocardial infarction, and arterial compliance impairment (179, 180).
Bile acids are produced in the liver through the breakdown of cholesterol, are crucial in controlling the absorption of lipids. Primary and secondary BAs can be identified based on their structural features. BAs can also be classed as bound or free based on whether they are conjugated with glycine or taurine (181). In a healthy adult, the liver produces primary bile acids at a daily rate of 500 mg that constitute approximately 72.5% of the total bile acid pool; chenodeoxycholic acid comprises 35%, while cholic acid constitutes 37.5% (182, 183). The synthesis of bile acids occurs through two distinct pathways: the classic (or neutral) pathway and the alternative (or acidic) pathway, each regulated by a specific enzyme. Cholesterol 7-hydroxylase (CYP7A1) enzyme responsible for the classic pathway, whereas oxysterol 7-hydroxylase (CYP7B1) is involved in the alternative pathway (184). Bile acids are stored in the gallbladder and released during digestion into the small intestine. The primary role of bile acids is to emulsify dietary fats and fat-soluble vitamins, facilitating their absorption and transport in the digestive system. Primary bile acids released into the duodenum have a critical function in emulsifying food components and vitamins that are lipid-soluble, enabling their digestion and absorption (185). Secondary bile acids are created when bacterial enzymes change the primary bile acids, which make up roughly 27.5% of bile acid (186).
However, Deoxycholic acid accounts for 25% of the overall bile acid pool, while lithocholic acid and ursodeoxycholic acid collectively make up 2.5% of these bile acids. In a healthy individual, almost 95% of BAs are efficiently reabsorbed in the distal ileum, primarily due to the process of enterohepatic circulation (187). The bile acids that are reabsorbed in the distal ileum are then transported back to the liver to build an effective recycling process. Surprisingly, bile acids, which make up to 2-4 grams of the body’s total weight and play a crucial function, are controlled by a relatively tiny pool (188). This process happens multiple times a day, typically ranging from 5 to 10 cycles daily (189, 190). In order to prevent bile acids from building up to hepatotoxic levels and to limit their impact on cholesterol metabolism, the size of the bile pool is carefully managed through feedback regulation of bile acid synthesis (191). Bile acids also possess strong microbial activity and serve as signaling molecules, acting as ligands for nuclear receptors, thereby impacting various metabolic processes (192). For instance, Farnesoid X-receptor (FXR) activation leads to the suppression of the cholesterol 7a-hydroxylase enzyme. By regulating this enzyme, FXR helps maintain the balance of bile acid synthesis and contributes to the overall control of cholesterol metabolism (193). The gut microbiota plays a significant role in modifying primary bile acids through bacterial salt hydrolase activity. This enzymatic process involves removing the 2OH groups from primary bile acids, transforming them into secondary bile acids (194). Bacteria can lessen BA toxicity by increasing their solubility, giving the gut microbiota a way to defend itself. Additionally, the gut microbiota might change bile acids further before they return to the liver for reconjugation and rejoin the circulation (195). Bile acids serve as a crucial pathway for cholesterol elimination through excretion in feces helping to decrease circulating cholesterol levels and reduce the risk of plaque accumulation (193). However, alterations in the gut microbiome can influence the bile acid synthesis rate, potentially leading to increased plasma levels of LDL cholesterol and an elevated risk of atherosclerosis (196). Thus, maintaining a healthy gut microbiome is essential for regulating bile acid metabolism and its impact on cholesterol levels and cardiovascular health. Additionally, microbial metabolites such as tryptophan and indole have been identified to have significant roles in the development of cardiovascular diseases.
The novel research implies that the gut microbiota plays a critical role in the progression of cardiovascular illnesses. Therapeutic techniques for influencing the composition and metabolic activity of the gut microbiota have been developed. As shown in Figure 6, these options include dietary changes, the use of probiotics and prebiotics, antibiotic treatments, and even fecal transplantation. Notably, these therapies have shown the potential to improve blood pressure control, restore lipid profiles to normal levels, and reduce body weight in people with cardiovascular disease (33). In a vicious cycle, the intricate interaction between dietary components and other variables affects the gut microbiota and pathogenesis of many cardiovascular diseases as shown in Figure 7 (168).
Figure 6 Potential treatments related to improved cardiovascular disease results and improved gut microbiota. The figure illustrates six strategies, i.e., dietary modifications, probiotics, antibiotics, FMT, bioengineering, and herbal treatment.
Figure 7 The correlation between the intestinal microbiome, metabolites, and cardiovascular disease. The intricate connection between dietary components absorbed and other factors influencing the gut microbiota, whose composition then influences their functionality and metabolite production and release, a disruption which leads to dysbiosis, thereby affecting host health and the onset and cause of different cardiovascular disorders. ATP, adenosine triphosphate; BA, bile acid; CO2, carbon dioxide; CH4, methane; CVD, cardiovascular disease; H2S, hydrogen sulfide; PBA, primary bile acid; ROS, reactive oxygen species; SBA, secondary bile acid; SCFA, short-chain fatty acids; TMAO, trimethylamine-N-oxide.
Numerous scientific studies have provided persuasive evidence supporting the idea that dietary interventions can significantly decrease the risk of cardiovascular problems (197, 198). Diets that frequently occur in Western industrialized societies that feature high consumption of red meat or animal proteins, saturated fats, and simple carbohydrates have been associated with an increased risk of CVD (199, 200). An increasing mass of evidence refers to the intestinal microbiota as a possible avenue for CVD treatment. Current clinical trials on microbe targeting for CVD therapy are summarized in Table 2 (39). Conversely, the composition of our diet can influence the structure and functioning of the gut microbiome (201). The gut microbiota is greatly influenced by essential food elements such as macronutrients, fiber, polyphenols, prebiotics, and probiotics, which also have an impact on the production and release of major gut microbiome metabolites including SCFAs (202). In a prior study, it was found that diets rich in fiber promote the growth of beneficial symbiotic microbes while preventing the spread of known infectious diseases (203). Moreover, the consumption of a high-fiber diet increased acetate-producing microbiota, which was associated with lower blood pressure and a reduction in cardiac hypertrophy and fibrosis (204). The mediterranean diet, which consists of a high intake of vegetables, fruits, grains, and legumes combined with a low intake of red meat and processed carbohydrates, has been shown to be effective in the prevention of CVD (205). This is mostly due to the high levels of antioxidants, nitrates, and fiber in this diet, and to the low levels of saturated/Trans fatty acids, salt, and phosphate. These elements are expected to reduce inflammation and oxidative stress, promote antioxidant activity, increase nitric oxide bioavailability, and microbiota modulation to improve vascular and cardiac function (206). The Western diet, compared to the Mediterranean diet, is known to raise CVD risk by lowering gut microbiota diversity and beneficial bacteria such as Bifidobacterium (207). A study involving mice fed a Western diet, the findings revealed higher plasma concentrations of TMAO and the development of cardiac dysfunction and heart fibrosis (208). The expression of pro-inflammatory cytokines (IL-10) and tumor necrosis factor (TNF-α) as well as interleukin-1 (IL-1), both of which are indicative of increased inflammation, was shown to be altered (208). In a study involving 153 volunteers from four cities in Italy, researchers found that the consumption of fruits, vegetables, and legumes is consistent with the Mediterranean diet led to an increase in fecal SCFA levels (209). This effect is due to fermentation by a greater abundance of bacteria from the Firmicutes and Bacteroidetes groups (209).
Table 2 Clinical trials targeting the gut bacteria in the treatment of cardiovascular diseases (39).
ATP, adenosine triphosphate; BA, bile acid; CO2, carbon dioxide; CH4, methane; CVD, cardiovascular disease; H2S, hydrogen sulfide; PBA, primary bile acid; ROS, reactive oxygen species; SBA, secondary bile acid; SCFA, short-chain fatty acids; TMAO, trimethylamine-N-oxide.
Following a mediterranean diet leads to reduced TMAO levels, thereby helping to prevent cardiovascular issues and heart failure (210, 211). A particular study revealed that incorporating ginger supplements into the diet influenced the gut microbiota composition, resulting in a notable rise in fatty acid metabolism (212). Moreover, the presence of miRNAs within ginger-derived exosome-like nanoparticles has the potential to modify bacterial gene expression, influencing the host genome (213). Currently, there is an obvious association between nutrition and intestinal microbes, resulting in varied gut bacterial communities across various diets and geographic locations. However, there is still a huge gap in our understanding of how our food influences the gut microbiome and the impact of the gut microbiota on general host health. More study is needed since nutrition is a low-cost, easy to manage strategy for the possible prevention, control, and management of cardiovascular disease.
The human colon is filled with probiotics, which are primarily made up of bifidobacteria and lactobacilli, and are essential for maintaining healthy immune systems, colon microflora, and the production of healthy compounds and also have ability to stop the spread of cancer, lower cholesterol, increase the synthesis of vital cytokines and vitamins, and prevent infections (214, 215). According to Gibson and Roberfroid, prebiotics are defined as non-digestible poly or oligosaccharides that have a positive impact on the host by selectively promoting the growth or activity of specific beneficial bacteria in the colon (216). The Lactobacillus Plantarum led to an improvement in the diversity of gut microbial flora, this consumption was linked to a reduction in the incidence of CVD incidents (217). Another study showed by Naruszewicz et al. (218), involving 36 healthy volunteers who were active smokers, revealed that there was a negative correlation between the intake of Lactobacillus Plantarum and various health markers, including blood pressure levels, fibrinogen levels, monocyte adhesion, and proinflammatory cytokine levels. These findings suggest that Lactobacillus Plantarum may have potential in the primary prevention of atherosclerosis. The normal or moderately elevated cholesterol levels in females experienced low LDL after consuming fermented milk containing Lactobacillus acidophilus and Bifidobacterium longum (219).
A recent study conducted by Catry et al.(220) revealed a 15-day supplementation of inulin-type fructans (ITFs) had a positive impact on endothelial function in the arteries of n-3 PUFA-depleted ApoE-/- mice. The improvement in endothelial function might be attributed to an increase in bacteria capable of producing nitric oxide, as it helps dilate blood vessels and improve blood flow, ultimately benefiting cardiovascular health. The findings highlight the ITFs potential in promoting cardiovascular well-being, particularly in the context of n-3 PUFA-depleted conditions (221). Similarly, a comprehensive analysis revealed that intake of isolated triterpene fraction yields favorable results on LDL cholesterol levels in the human (222). In addition to ITFs, beta-glucan supplements have also shown the capacity to lower total and LDL cholesterol levels while boosting endothelial vascular reactivity in people in the great health (223). It is crucial to recognize that prebiotics are formed up of a diverse range of chemicals that are controlled by different gut flora (224–227). A fiber-rich diet has been demonstrated to alter the gut microbiota by increasing acetate-producing bacteria, resulting in reduced gut dysbiosis and cardiovascular protection, most notably the transcription factor Egr1, are related to acetate regulation and govern CVD through inflammation, heart fibrosis, and hypertrophy (204).
Another prebiotic, beta-glucan was demonstrated to influence cholesterol levels and glucose homeostasis. A 2-month study that included a beta-glucan dietary plan indicated a significant reduction in LDL and total cholesterol levels. The endothelial function improved in healthy people, showing cardioprotective effects. These effects are mostly due to the production of beneficial SCFA by the gut flora (228). In animal tests, arabinoxylans showed potential as a possible prebiotic. It was discovered that their role in encouraging the growth of bifidobacteria and the production of propionate reduces cholesterol and fat deposition (206). Dietary arabinoxylan oligosaccharides raised bacterial populations and butyrate levels in stools in individuals (229). Probiotics help to improve human metabolism by boosting digestive enzyme output, suppressing bacterial enzyme activity, and lowering ammonia generation. Lactobacillus and Bifidobacterium have beneficial effects on intestinal barrier function and play a protective role in inflammatory diseases by modulating inflammatory and proinflammatory cytokines, which may potentially delay or improve CVD (230, 231). Akkermansia muciniphila is also renowned for its probiotic features, and it is related to glucose, insulin, and leptin, all of which have roles in the metabolism of lipids and glucose (232). However, Lactobacillus plantarum efficiently lowered LDL-C and total cholesterol levels while also inhibiting the formation of atherosclerotic plaques in hypercholesterolaemic individuals (233). The preceding research concentrated on the effects of prebiotics and probiotics on cardiovascular risk factors such as inflammation and hypertension, as well as impacts on glucose and lipid metabolism, rather than the direct benefit of atherosclerosis. However, considering their beneficial effect on several CVD risk variables, more research into how these medications affect the onset and progression of CVD is essential.
In the regulation of host health, the gut microbiome has a huge impact on the host as a result of antibiotic usage. The use of antibiotics may damage the host’s health in a variety of methods, both directly and indirectly. This impact can alter a variety of bodily processes, including immunological control, metabolism, and ultimately general health (48, 234–237). A variety of antibiotics have shown evidence of affecting blood pressure and intestinal flora. A prime example is the drug minocycline, which has been studied for its capacity to alter the nature of the gut microbiota and control blood pressure (BP) in hypertensive rats (140). Using erythromycin, tetracycline, or doxycycline within the previous five years did not reduce the chance of developing a first MI, according to another population-based trial, and its authors disputed their efficacy in avoiding primary coronary heart disease (CHD) (238). Macrolides antibiotics, such as azithromycin, erythromycin, and clarithromycin, comprise a significant class of orally active antibiotics that function as bacteriostatic agents. In 2013, 51.5 million drugs for azithromycin were prescribed in the United States (239). The use of macrolide antibiotics is believed to increase the risk of cardiovascular diseases such as myocardial infarction (MI), ventricular tachyarrhythmias, and sudden cardiac death (SCD) (240, 241). Further, quadruple antibiotic therapy was shown to significantly lower high systolic and diastolic blood pressures in salt-induced hypertensive rats (242). It helps to realize the research on the effect of antibiotics on blood pressure regulation produced varying outcomes (243).
For instance, minocycline and vancomycin medication in rats led to lower Firmicutes levels in the gut, resulting in lower blood pressure in hypertensive rats. It’s noteworthy to note that identical antibiotic therapy actually raised blood pressure in salt-sensitive rats. This difference underlines the intricacy of the gut microbiota-blood pressure interaction and the significance of taking into account a variety of variables that may affect the results of such mediations (244). In a study by Rune et al., they found that ampicillin had the ability to lower mice’s levels of LDL and VLDL cholesterol. Atherosclerosis risk is associated with these forms of cholesterol. As a result, the mice’s aortic atherosclerotic lesions were reduced in size (245). Although a few studies provide promising results, indicating possible benefits in this regard, the efficiency of antibiotics for offering preventive benefits against CVD in trials involving patients is yet unknown (246). However, certain analyses have failed to demonstrate a distinct and obvious link between the use of antibiotics and protection against CVD, yielding unclear outcomes. As a result, more research and analysis are needed to determine whether antibiotics can significantly reduce the risk of cardiovascular disease (240). Furthermore, universal antibiotics can have a variety of impacts on the body, making methods of treating CVD with antibiotics contentious. While certain studies have suggested that taking antibiotics to treat CVD may have some benefits, their broad action can have a number of adverse reactions. Thus, any possible benefits of using antibiotics in treating CVD must be carefully balanced against any dangers and adverse effects that could result from their use. Before making antibiotics a common therapy choice, more study is required to better understand their precise mechanisms of action and potential advantages in the management of CVD.
Fecal microbiota transplantation (FMT) is a therapeutic method intended to restore a healthy balance of gut microbiota in a recipient (247), by transferring fecal matter from a donor who is in a healthy condition (248). It gained a lot of attention for its safety and efficacy in therapeutic applications after being extensively studied in an array of mammalian species (249).The more complicated nature of propagating gut bacteria compared to those that inhabit the mouth cavity is one of the difficulties that FMT still challenges (250). The FMT involves transferring fecal matter from an adult donor to a recipient with an unbalanced intestinal microbiota and the fecal matter is rich in various microbial populations such as Clostridioides difficile (251, 252).This transplantation has shown promising results in treating several intestinal and other chronic diseases and has been researched as a viable therapeutic alternative in clinical applications (253). Notably, FMT has confirmed effectiveness in treating various conditions, including recurrent Clostridium difficile infection (254), inflammatory bowel disease (255), and irritable bowel syndrome (256). New research has explored the potential of FMT as a promising approach for addressing cardiometabolic disorders (257).
In 2013, the US Food and Drug Administration (FDA) granted its initial approval for FMT, specifically for managing recurrent Clostridium difficile infection. Since then, FMT has gained recognition as a therapy for a wide range of gastrointestinal as well as non-gastrointestinal conditions. However, there remains limited understanding of its mechanism of action and potential long-term side effects (258). The probable therapeutic effects of FMT have also been demonstrated in a number of animal models involving people with severe multiple sclerosis, autism, multidrug-resistant (MDR) infections, and multiple organ failure in seriously confined people (259–261). Recent findings have indicated a lower abundance of Clostridia strains that produce butyrate in the intestines with type 2 diabetes mellitus. Conversely, studies have shown a higher prevalence of non-butyrate-producing Clostridiales in these patients by demonstrated that both insulin sensitivity and levels of butyrate-producing intestinal microbiota significantly improved following microbiota transplantation (262). Experiments on mice raised the possibility of a brain-gut-microbiota axis that goes in each direction. Various neurological conditions like anxiety, depression, dementia such as Alzheimer’s, and Parkinson’s disorder are caused by an imbalance in this axis (263, 264).
Recently, Park et al. (265) from Inho University Hospital in Incheon, South Korea, used FMT to treat a 90-year-old woman who had severe CDI and Alzheimer’s dementia. Her fecal microbiota diversity drastically altered after the transplant, and her cognitive abilities significantly improved, according to a comparison of the results from before and after the procedure. The study also demonstrated a strong correlation between gut flora and cognitive function. Segal et al. (266) conducted distinct clinical research at Soroka University Medical Centre in Israel with six individuals suffering from both Parkinson’s disease and constipation. These patients were given treatment that included Fecal Microbiota Transplantation (FMT). Moreover, Doll et al.(267) used transplantation of fecal microbiota as add on therapy in two patients with major depression. After 4 weeks, both patient signs of depression improved, and study suggested that FMT be tested extensively for MDD treatment. It was found that transferring feces microbiota from healthy rats with normal heart rates to rats with naturally elevated levels produced positive results. The results included lower systolic blood pressure, enhanced blood vessel functionality, lower levels of oxidative stress and inflammation within blood vessels, and a more favorable balance between two unique types of immune cells, Th17 and Tregs (126, 268). However, the curative benefits of FMT can be linked to a broader variety of bacteria, viruses, fungi, and archaea that can engraft into the recipient host and increase the functional variety of a microbiota FMT is also being examined in almost 300 clinical trials for a variety of disease indications, including autoimmune diseases, neurological difficulties, cancer, host disease, and metabolic and gastrointestinal disorders. There is currently insufficient data to support the relevance of fecal microbiome transplantation about gut microbiota in human patients with CVD, necessitating more research in this field. Different approaches and processing variations, such as donor selection and testing, fecal microbiome transplantation via the upper gastrointestinal tract, enema, or colonoscopy, as well as short- and long-term patient monitoring for adverse effects and treatment efficacy, introduce new challenges to be investigated.
Physical inactivity holds substantial significance as a risk factor for a range of metabolic disorders, and roughly 1/3 of world’s population contributes in inadequate levels of physical activity, which has implications for health (269). Statistics indicate that roughly 3.2million deaths annually can be attributed to inadequate levels of physical activity (270), with healthcare expenses amounting to $117 billion yearly, attributed to conditions resulting from a lack of exercise (271). People who adopt a sedentary lifestyle and fail to engage in regular physical activity are more prone to the development of cardiovascular disease (272), and who are less active face a 30-50% higher risk of developing high blood pressure (273). The researchers predicted that lack of exercise was responsible for 12% of myocardial infarctions (MI), a risk proportion that fell within high blood pressure (18%), CVD cases (6%) and diabetic mellitus (10%) recognized risk factors for heart disease whose incidence is also inversely related to physical activity levels (274, 275). The study confirmed that exercise can enrich the microflora diversity; improve the F/M ratio, which may contribute to weight loss, obesity-related pathologies, and gastrointestinal disorders; and stimulate the proliferation of bacteria, which can modulate mucosal immunity and maintain homeostasis (276–278). Research has demonstrated that exercise has the capacity to increase the levels of the bacterial metabolite known as butyrate (279).While human research in this area is limited, data from several laboratories, including our own, indicate that exercise training can exert a noteworthy influence on the gut microbiota in animal models (280–282). Moreover, the modifications in the gut microbiota brought about by exercise are linked to changes in the host’s physiology, such changes include metabolic rate modifications (283), immunity (280), and even behavior (281). Certainly, exercise training has been demonstrated to increase the concentrations of short-chain fatty acids derived from the gut microbiota in mouse models (284), comprising of two to six carbon atoms, play a crucial role as an energy source for various tissues and are associated with beneficial effects such as reducing inflammation (285), improving insulin sensitivity (286), and inducing the morphology of the central nervous system (287). Notably, levels of LPS are elevated in cardiovascular disease and specific cardio metabolic disorders (288). However, high-endurance training has been shown to have the potential to decrease plasma LPS levels. This suggests that exercise may have a positive impact on reducing inflammation associated with CVD and related metabolic conditions (289). A crucial observation to highlight is that the advantages provided by the gut microbiota due to exercise training were not enduring. This emphasizes the necessity for consistent and regular exercise to sustain a constructive impact on the gut microbiota and the associated health benefits (279). The findings highlighted the broad-ranging benefits of influencing the gut microbiota via physical exercise, stretching beyond the realm of cardiovascular health. Nonetheless, it’s important to recognize that substantial and enduring advantages necessitate prolonged periods and higher-intensity aerobic training. Participating in more extended and intense exercise sessions seems to be pivotal in achieving lasting enhancements in gut microbiota composition and the correlated health advantages for the individual. Consistently adhering to such exercise routines is pivotal for maximizing the influence on the gut microbiota and overall well-being (290). To protect the heart and arteries, physical activity can increase insulin sensitivity, reduce plasma dyslipidemia, proper raise blood pressure, decrease blood viscosity, promote endothelial nitric oxide generation, and improve leptin sensitivity. Furthermore, the preventive impact of exercise on the body involves not only laboratory animal models but also clinical studies, as proven by WHO recommendations (291). Numerous studies have illustrated a clear dose-response correlation between physical activity levels and a decreased incidence of CVD and characterized by reductions in factors such as blood pressure, body weight, oxidized low-density lipoprotein (ox-LDL), and improved glucose tolerance as physical activity increases (292, 293). Although it’s known that exercise protects against CVD by reducing sympathetic impulses, arterial pressure, and heart rate, increasing blood flow and endothelial NO production, causing vessel dilation, and decreasing inflammatory cytokines and oxygen radical formation, the precise processes that lead to transcriptional factor modifications are unknown. Future research could focus on the mechanisms of exercise’s protective effects on the heart and arteries.
The human intestine is the habitat of the most enormous and varied population of microbes. The main purpose of the gut microbes is to prevent the expansion of potentially lethal germs. However, there is a rising acknowledgment of the intestinal microbiota as a risk variable for developing cardiovascular disease (CVD). Metabolites derived from the gut microbiota, such as short-chain fatty acids, trimethylamine-N-oxide, bile acids, and polyphenols, are critical in maintaining normal cardiovascular function. When these metabolites are out of balance, it has the potential to contribute to an outbreak of CVD. Variations in the composition and diversity of the gut microbiota, known as dysbiosis, have been associated with disorders such as heart failure, atherosclerosis, hypertension, myocardial fibrosis, myocardial infarction, and coronary artery disease. However, the specific mechanisms behind these relationships are still unknown. As a result, the microbiota and its metabolites have emerged as a novel therapeutic target for both CVD prevention and treatment. Ongoing attempts are being made to widen the application of microbiota therapies not only for CVD but also for a variety of other human disorders. Innovations in genomic and metabolomic technology have enabled improved characterization and molecular research of bacteria and their metabolites. Individual microbiome may be profiled in the future utilizing metabolomic/biomarker analysis to measure individual health, potentially delivering specific guidance on food and lifestyle changes. Dietary treatments, the use of pre/probiotics and antibiotics, FMT, TMAO reduction, and regular exercise are current strategies for regulating gut bacteria to improve cardiac function. Further research on microbe-microbe and microbe-host associations may explain how specific metabolites affect the disease process. Improving our understanding of the complex interplay between gut microbiota, host characteristics, and therapeutic response is critical for developing breakthrough precision therapies for cardiovascular disease.
AL: Writing – original draft. AH: Writing – review & editing. MU: Writing – review & editing. SN: Writing – review & editing. MehrajU: Writing – review & editing. LZ: Writing – review & editing. AU: Writing – review & editing. KU: Writing – review & editing. WA: Writing – review & editing. GW: Writing – review & editing, Funding acquisition, Supervision.
The author(s) declare financial support was received for the research, authorship, and/or publication of this article. This work was supported by grants from the National Natural Science Foundation of China (12032007, 31971242); the Science and Technology Innovation Project of JinFeng Laboratory, Chongqing, China (jfkyjf202203001); Chongqing University of Science and Technology, natural science fund surface project, (CSTB2023NSCQ-MSX1060)
We are thankful for the First Batch of Key Disciplines on Public Health in Chongqing and the Public Experiment Centre of State Bioindustrial Base (Chongqing), China.
The authors declare that the research was conducted in the absence of any commercial or financial relationships that could be construed as a potential conflict of interest.
All claims expressed in this article are solely those of the authors and do not necessarily represent those of their affiliated organizations, or those of the publisher, the editors and the reviewers. Any product that may be evaluated in this article, or claim that may be made by its manufacturer, is not guaranteed or endorsed by the publisher.
1. Hou K, Wu Z-X, Chen X-Y, Wang J-Q, Zhang D, Xiao C, et al. Microbiota in health and diseases. Signal transduction targeted Ther (2022) 7:135. doi: 10.1038/s41392-022-00974-4
2. Rahman MM, Islam F, Or-Rashid MH, Mamun AA, Rahaman MS, Islam MM, et al. The gut microbiota (microbiome) in cardiovascular disease and its therapeutic regulation. Front Cell Infection Microbiol (2022) 12:903570. doi: 10.3389/fcimb.2022.903570
3. Ding Y-N, Tang X, Chen H-Z, Liu D-P. Epigenetic regulation of vascular aging and age-related vascular diseases. Aging Aging-Related Diseases: Mech Interventions (2018), 55–75. doi: 10.1007/978-981-13-1117-8_4
4. Velasquez MT, Centron P, Barrows I, Dwivedi R, Raj DS. Gut microbiota and cardiovascular uremic toxicities. Toxins (2018) 10:287. doi: 10.3390/toxins10070287
5. Ren S-C, Chen X, Gong H, Wang H, Wu C, Li P-H, et al. SIRT6 in vascular diseases, from bench to bedside. Aging Dis (2022) 13:1015. doi: 10.14336/AD.2021.1204
6. Hemmati M, Kashanipoor S, Mazaheri P, Alibabaei F, Babaeizad A, Asli S, et al. Importance of gut microbiota metabolites in the development of cardiovascular diseases (CVD). Life Sci (2023) 121947. doi: 10.1016/j.lfs.2023.121947
7. Marzullo P, Di Renzo L, Pugliese G, De Siena M, Barrea L, Muscogiuri G, et al. From obesity through gut microbiota to cardiovascular diseases: a dangerous journey. Int J Obes Suppl (2020) 10:35–49. doi: 10.1038/s41367-020-0017-1
8. Rehman S, Rehman E, Ikram M, Jianglin Z. Cardiovascular disease (CVD): assessment, prediction and policy implications. BMC Public Health (2021) 21:1–14. doi: 10.1186/s12889-021-11334-2
9. Zoungas S, Curtis AJ, Mcneil JJ, Tonkin AM. Treatment of dyslipidemia and cardiovascular outcomes: the journey so far—Is this the end for statins? Clin Pharmacol Ther (2014) 96:192–205. doi: 10.1038/clpt.2014.86
10. Haybar H, Shokuhian M, Bagheri M, Davari N, Saki N. Involvement of circulating inflammatory factors in prognosis and risk of cardiovascular disease. J Mol Cell Cardiol (2019) 132:110–9. doi: 10.1016/j.yjmcc.2019.05.010
11. Xu H, Wang X, Feng W, Liu Q, Zhou S, Liu Q, et al. The gut microbiota and its interactions with cardiovascular disease. Microbial Biotechnol (2020) 13:637–56. doi: 10.1111/1751-7915.13524
12. Almeida C, Barata P, Fernandes R. The influence of gut microbiota in cardiovascular diseases—a brief review. Porto Biomed J (2021) 6. doi: 10.1097/j.pbj.0000000000000106
13. Naghavi M, Wang H, Lozano R, Davis A, Liang X, Zhou M. GBD 2013 Mortality and Causes of Death Collaborators. Global, regional, and national age-sex specific all-cause and cause-specific mortality for 240 causes of death 1990-2013: a systematic analysis for the Global Burden of Disease Study 2013. Lancet (2015) 385:117–71. doi: 10.1016/S0140-6736(14)61682-2
14. Mozaffarian D, Benjamin EJ, Go AS, Arnett DK, Blaha MJ, Cushman M, et al. Heart disease and stroke statistics—2016 update: a report from the American Heart Association. circulation (2016) 133:e38–e360.
15. Novakovic M, Rout A, Kingsley T, Kirchoff R, Singh A, Verma V, et al. Role of gut microbiota in cardiovascular diseases. World J Cardiol (2020) 12:110. doi: 10.4330/wjc.v12.i4.110
16. Degruttola AK, Low D, Mizoguchi A, Mizoguchi E. Current understanding of dysbiosis in disease in human and animal models. Inflammation Bowel Dis (2016) 22:1137–50. doi: 10.1097/MIB.0000000000000750
17. Rinninella E, Raoul P, Cintoni M, Franceschi F, Miggiano G, Gasbarrini A, et al. What is the healthy gut microbiota composition? A changing ecosystem across age, environment, diet, and diseases. Microorganisms (2019) 7:14. doi: 10.3390/microorganisms7010014
18. Canfora EE, Blaak EE. Acetate: a diet-derived key metabolite in energy metabolism: good or bad in context of obesity and glucose homeostasis? Curr Opin Clin Nutr Metab Care (2017) 20:477–83. doi: 10.1097/MCO.0000000000000408
19. Dekaboruah E, Suryavanshi MV, Chettri D, Verma AK. Human microbiome: an academic update on human body site specific surveillance and its possible role. Arch Microbiol (2020) 202:2147–67. doi: 10.1007/s00203-020-01931-x
20. Jandhyala SM, Talukdar R, Subramanyam C, Vuyyuru H, Sasikala M, Reddy DN. Role of the normal gut microbiota. World J gastroenterology: WJG (2015) 21:8787. doi: 10.3748/wjg.v21.i29.8787
21. Yatsunenko T, Rey FE, Manary MJ, Trehan I, Dominguez-Bello MG, Contreras M, et al. Human gut microbiome viewed across age and geography. nature (2012) 486:222–7. doi: 10.1038/nature11053
22. Ramezani A, Raj DS. The gut microbiome, kidney disease, and targeted interventions. J Am Soc Nephrology: JASN (2014) 25:657. doi: 10.1681/ASN.2013080905
23. Kumari S, Taliyan R, Dubey SK. Comprehensive review on potential signaling pathways involving the transfer of α-synuclein from the gut to the brain that leads to Parkinson’s disease. ACS Chem Neurosci (2023) 14:590–602. doi: 10.1021/acschemneuro.2c00730
24. Eckburg PB, Bik EM, Bernstein CN, Purdom E, Dethlefsen L, Sargent M, et al. Diversity of the human intestinal microbial flora. science (2005) 308:1635–8. doi: 10.1126/science.1110591
25. Wen Y, Sun Z, Xie S, Hu Z, Lan Q, Sun Y, et al. Intestinal flora derived metabolites affect the occurrence and development of cardiovascular disease. J Multidiscip Healthcare (2022), 2591–603. doi: 10.2147/JMDH.S367591
26. Li X, Fan Z, Cui J, Li D, Lu J, Cui X, et al. Trimethylamine N-oxide in heart failure: a meta-analysis of prognostic value. Front Cardiovasc Med (2022) 9:817396. doi: 10.3389/fcvm.2022.817396
27. He J, Zhang P, Shen L, Niu L, Tan Y, Chen L, et al. Short-chain fatty acids and their association with signalling pathways in inflammation, glucose and lipid metabolism. Int J Mol Sci (2020) 21:6356. doi: 10.3390/ijms21176356
28. Wang Z, Klipfell E, Bennett BJ, Koeth R, Levison BS, Dugar B, et al. Gut flora metabolism of phosphatidylcholine promotes cardiovascular disease. Nature (2011) 472:57–63. doi: 10.1038/nature09922
29. Ahmadmehrabi S, Tang WW. Gut microbiome and its role in cardiovascular diseases. Curr Opin Cardiol (2017) 32:761. doi: 10.1097/HCO.0000000000000445
30. Koren O, Spor A, Felin J, Fåk F, Stombaugh J, Tremaroli V, et al. Human oral, gut, and plaque microbiota in patients with atherosclerosis. Proc Natl Acad Sci (2011) 108:4592–8. doi: 10.1073/pnas.1011383107
31. Karlsson FH, Fåk F, Nookaew I, Tremaroli V, Fagerberg B, Petranovic D, et al. Symptomatic atherosclerosis is associated with an altered gut metagenome. Nat Commun (2012) 3:1245. doi: 10.1038/ncomms2266
32. Yamashiro K, Tanaka R, Urabe T, Ueno Y, Yamashiro Y, Nomoto K, et al. Gut dysbiosis is associated with metabolism and systemic inflammation in patients with ischemic stroke. PloS One (2017) 12:e0171521.
33. Jin L, Shi X, Yang J, Zhao Y, Xue L, Xu L, et al. Gut microbes in cardiovascular diseases and their potential therapeutic applications. Protein Cell (2021) 12:346–59. doi: 10.1007/s13238-020-00785-9
34. Hemarajata P, Versalovic J. Effects of probiotics on gut microbiota: mechanisms of intestinal immunomodulation and neuromodulation. Ther Adv Gastroenterol (2013) 6:39–51. doi: 10.1177/1756283X12459294
35. Sender R, Fuchs S, Milo R. Revised estimates for the number of human and bacteria cells in the body. PloS Biol (2016) 14:e1002533. doi: 10.1371/journal.pbio.1002533
36. Vaiserman A, Romanenko M, Piven L, Moseiko V, Lushchak O, Kryzhanovska N, et al. Differences in the gut Firmicutes to Bacteroidetes ratio across age groups in healthy Ukrainian population. BMC Microbiol (2020) 20:1–8. doi: 10.1186/s12866-020-01903-7
37. Zhi C, Huang J, Wang J, Cao H, Bai Y, Guo J, et al. Connection between gut microbiome and the development of obesity. Eur J Clin Microbiol Infect Dis (2019) 38:1987–98. doi: 10.1007/s10096-019-03623-x
38. Anhê FF, Barra NG, Cavallari JF, Henriksbo BD, Schertzer JD. Metabolic endotoxemia is dictated by the type of lipopolysaccharide. Cell Rep (2021) 36.
39. Wang L, Wang S, Zhang Q, He C, Fu C, Wei Q. The role of the gut microbiota in health and cardiovascular diseases. Mol biomedicine (2022) 3:30. doi: 10.1186/s43556-022-00091-2
40. Indiani C.M.D.S.P., Rizzardi KF, Castelo PM, Ferraz LFC, Darrieux M, Parisotto TM. Childhood obesity and Firmicutes/Bacteroidetes ratio in the gut microbiota: a systematic review. Childhood Obes (2018) 14:501–9. doi: 10.1089/chi.2018.0040
41. Pascale A, Marchesi N, Govoni S, Coppola A, Gazzaruso C. The role of gut microbiota in obesity, diabetes mellitus, and effect of metformin: new insights into old diseases. Curr Opin Pharmacol (2019) 49:1–5. doi: 10.1016/j.coph.2019.03.011
42. Sanchez-Gimenez R, Ahmed-Khodja W, Molina Y, Peiró OM, Bonet G, Carrasquer A, et al. Gut microbiota-derived metabolites and cardiovascular disease risk: a systematic review of prospective cohort studies. Nutrients (2022) 14:2654. doi: 10.3390/nu14132654
43. Hoyles L, Jiménez-Pranteda ML, Chilloux J, Brial F, Myridakis A, Aranias T, et al. Metabolic retroconversion of trimethylamine N-oxide and the gut microbiota. Microbiome (2018) 6:1–14. doi: 10.1186/s40168-018-0461-0
44. Mutengo KH, Masenga SK, Mweemba A, Mutale W, Kirabo A. Gut microbiota dependant trimethylamine N-oxide and hypertension. Front Physiol (2023) 14:1075641. doi: 10.3389/fphys.2023.1075641
45. Shanmugham M, Bellanger S, Leo CH. Gut-derived metabolite, trimethylamine-N-oxide (TMAO) in cardio-metabolic diseases: detection, mechanism, and potential therapeutics. Pharmaceuticals (2023) 16:504. doi: 10.3390/ph16040504
46. Tacconi E, Palma G, De Biase D, Luciano A, Barbieri M, De Nigris F, et al. Microbiota effect on trimethylamine N-oxide production: from cancer to fitness—A practical preventing recommendation and therapies. Nutrients (2023) 15:563. doi: 10.3390/nu15030563
47. Koeth RA, Wang Z, Levison BS, Buffa JA, Org E, Sheehy BT, et al. Intestinal microbiota metabolism of L-carnitine, a nutrient in red meat, promotes atherosclerosis. Nat Med (2013) 19:576–85. doi: 10.1038/nm.3145
48. Tang WW, Wang Z, Levison BS, Koeth RA, Britt EB, Fu X, et al. Intestinal microbial metabolism of phosphatidylcholine and cardiovascular risk. New Engl J Med (2013) 368:1575–84. doi: 10.1056/NEJMoa1109400
49. Lemos BS, Medina-Vera I, Malysheva OV, Caudill MA, Fernandez ML. Effects of egg consumption and choline supplementation on plasma choline and trimethylamine-N-oxide in a young population. J Am Coll Nutr (2018) 37:716–23. doi: 10.1080/07315724.2018.1466213
50. Jing L, Zhang H, Xiang Q, Shen L, Guo X, Zhai C, et al. Targeting trimethylamine N-oxide: A new therapeutic strategy for alleviating atherosclerosis. Front Cardiovasc Med (2022) 9:864600. doi: 10.3389/fcvm.2022.864600
51. Bu J, Wang Z. Cross-talk between gut microbiota and heart via the routes of metabolite and immunity. Gastroenterol Res Pract (2018) 2018. doi: 10.1155/2018/6458094
52. Jiang C, Hou X, Gao X, Liu P, Guo X, Hu G, et al. The 16S rDNA high-throughput sequencing correlation analysis of milk and gut microbial communities in mastitis Holstein cows. BMC Microbiol (2023) 23:1–12. doi: 10.1186/s12866-023-02925-7
53. Shkoporov AN, Hill C. Bacteriophages of the human gut: the “known unknown” of the microbiome. Cell Host Microbe (2019) 25:195–209. doi: 10.1016/j.chom.2019.01.017
54. Mukherjee S, Joardar N, Sengupta S, Babu SPS. Gut microbes as future therapeutics in treating inflammatory and infectious diseases: lessons from recent findings. J Nutr Biochem (2018) 61:111–28. doi: 10.1016/j.jnutbio.2018.07.010
55. Jones RM. Focus: Microbiome: The influence of the gut microbiota on host physiology: In pursuit of mechanisms. Yale J Biol Med (2016) 89:285.
56. Neish A. Reviews in basic and clinical gastroenterology. Gastroenterology (2009) 136:65–80. doi: 10.1053/j.gastro.2008.10.080
57. Gordon S. Phagocytosis: the legacy of metchnikoff. Cell (2016) 166:1065–8. doi: 10.1016/j.cell.2016.08.017
58. Di Stefano M, Santonocito S, Polizzi A, Mauceri R, Troiano G, Lo Giudice A, et al. A reciprocal link between oral, gut microbiota during periodontitis: the potential role of probiotics in reducing dysbiosis-induced inflammation. Int J Mol Sci (2023) 24:1084. doi: 10.3390/ijms24021084
59. Mendoza-León MJ, Mangalam AK, Regaldiz A, González-Madrid E, Rangel-Ramírez MA, Álvarez-Mardonez O, et al. Gut microbiota short-chain fatty acids and their impact on the host thyroid function and diseases. Front Endocrinol (2023) 14.
60. Doskaliuk B. ÉLIE METCHNIKOFF’S LEGACY IN THE FIELD OF ORTHOBIOSIS. Anti-Aging Eastern Europe (2023) 2:54–8. doi: 10.56543/aaeeu.2023.2.1.10
61. Adamsson I, Nord CE, Lundquist P, Sjöstedt S, Edlund C. Comparative effects of omeprazole, amoxycillin plus metronidazole versus omeprazole, clarithromycin plus metronidazole on the oral, gastric and intestinal microflora in Helicobacter pylori-infected patients. J Antimicrobial Chemotherapy (1999) 44:629–40. doi: 10.1093/jac/44.5.629
62. Jernberg C, Löfmark S, Edlund C, Jansson JK. Long-term ecological impacts of antibiotic administration on the human intestinal microbiota. ISME J (2007) 1:56–66. doi: 10.1038/ismej.2007.3
63. Dethlefsen L, Huse S, Sogin ML, Relman DA. The pervasive effects of an antibiotic on the human gut microbiota, as revealed by deep 16S rRNA sequencing. PloS Biol (2008) 6:e280. doi: 10.1371/journal.pbio.0060280
64. Jakobsson HE, Jernberg C, Andersson AF, Sjölund-Karlsson M, Jansson JK, Engstrand L. Short-term antibiotic treatment has differing long-term impacts on the human throat and gut microbiome. PloS One (2010) 5:e9836. doi: 10.1371/journal.pone.0009836
65. Ley RE, Bäckhed F, Turnbaugh P, Lozupone CA, Knight RD, Gordon JI. Obesity alters gut microbial ecology. Proc Natl Acad Sci (2005) 102:11070–5. doi: 10.1073/pnas.0504978102
66. Turnbaugh PJ, Ridaura VK, Faith JJ, Rey FE, Knight R, Gordon JI. The effect of diet on the human gut microbiome: a metagenomic analysis in humanized gnotobiotic mice. Sci Trans Med (2009) 1:6ra14–16ra14. doi: 10.1126/scitranslmed.3000322
67. Bisanz JE, Upadhyay V, Turnbaugh JA, Ly K, Turnbaugh PJ. Meta-analysis reveals reproducible gut microbiome alterations in response to a high-fat diet. Cell Host Microbe (2019) 26:265–272. e264. doi: 10.1016/j.chom.2019.06.013
68. Ju M, Liu Y, Li M, Cheng M, Zhang Y, Deng G, et al. Baicalin improves intestinal microecology and abnormal metabolism induced by high-fat diet. Eur J Pharmacol (2019) 857:172457. doi: 10.1016/j.ejphar.2019.172457
69. Rodríguez JM, Murphy K, Stanton C, Ross RP, Kober OI, Juge N, et al. The composition of the gut microbiota throughout life, with an emphasis on early life. Microbial Ecol Health Dis (2015) 26:26050. doi: 10.3402/mehd.v26.26050
70. Cong X, Xu W, Romisher R, Poveda S, Forte S, Starkweather A, et al. Focus: Microbiome: Gut microbiome and infant health: Brain-gut-microbiota axis and host genetic factors. Yale J Biol Med (2016) 89:299.
71. Tsiaoussis J, Antoniou MN, Koliarakis I, Mesnage R, Vardavas CI, Izotov BN, et al. Effects of single and combined toxic exposures on the gut microbiome: Current knowledge and future directions. Toxicol Lett (2019) 312:72–97. doi: 10.1016/j.toxlet.2019.04.014
72. Werbner M, Barsheshet Y, Werbner N, Zigdon M, Averbuch I, Ziv O, et al. Social-stress-responsive microbiota induces stimulation of self-reactive effector T helper cells. MSystems (2019) 4:e00292–00218. doi: 10.1128/mSystems.00292-18
73. Chang C-S, Kao C-Y. Current understanding of the gut microbiota shaping mechanisms. J Biomed Sci (2019) 26:1–11. doi: 10.1186/s12929-019-0554-5
74. Cahana I, Iraqi FA. Impact of host genetics on gut microbiome: Take-home lessons from human and mouse studies. Anim Models Exp Med (2020) 3:229–36. doi: 10.1002/ame2.12134
75. Carding S, Verbeke K, Vipond DT, Corfe BM, Owen LJ. Dysbiosis of the gut microbiota in disease. Microbial Ecol Health Dis (2015) 26:26191. doi: 10.3402/mehd.v26.26191
76. Peterson D, Bonham KS, Rowland S, Pattanayak CW, Consortium R, Klepac-Ceraj V. Comparative analysis of 16S rRNA gene and metagenome sequencing in pediatric gut microbiomes. Front Microbiol (2021) 12:670336. doi: 10.3389/fmicb.2021.670336
77. Jie Z, Xia H, Zhong S-L, Feng Q, Li S, Liang S, et al. The gut microbiome in atherosclerotic cardiovascular disease. Nat Commun (2017) 8:845. doi: 10.1038/s41467-017-00900-1
78. The HC, Le S-NH. Dynamic of the human gut microbiome under infectious diarrhea. Curr Opin Microbiol (2022) 66:79–85. doi: 10.1016/j.mib.2022.01.006
79. Emoto T, Yamashita T, Kobayashi T, Sasaki N, Hirota Y, Hayashi T, et al. Characterization of gut microbiota profiles in coronary artery disease patients using data mining analysis of terminal restriction fragment length polymorphism: gut microbiota could be a diagnostic marker of coronary artery disease. Heart vessels (2017) 32:39–46. doi: 10.1007/s00380-016-0841-y
80. Aly AM, Adel A, El-Gendy AO, Essam TM, Aziz RK. Gut microbiome alterations in patients with stage 4 hepatitis C. Gut Pathog (2016) 8:1–12. doi: 10.1186/s13099-016-0124-2
81. Ziganshina EE, Sharifullina DM, Lozhkin AP, Khayrullin RN, Ignatyev IM, Ziganshin AM. Bacterial communities associated with atherosclerotic plaques from Russian individuals with atherosclerosis. PloS One (2016) 11:e0164836. doi: 10.1371/journal.pone.0164836
82. Li Z, Liu K, Zhao J, Yang L, Chen G, Liu A, et al. Antibiotics in elderly Chinese population and their relations with hypertension and pulse pressure. Environ Sci pollut Res (2022) 29:67026–45. doi: 10.1007/s11356-022-20613-3
83. Yan Q, Gu Y, Li X, Yang W, Jia L, Chen C, et al. Alterations of the gut microbiome in hypertension. Front Cell infection Microbiol (2017) 7:381. doi: 10.3389/fcimb.2017.00381
84. Altemani F, Barrett HL, Gomez-Arango L, Josh P, Mcintyre HD, Callaway LK, et al. Pregnant women who develop preeclampsia have lower abundance of the butyrate-producer Coprococcus in their gut microbiota. Pregnancy hypertension (2021) 23:211–9. doi: 10.1016/j.preghy.2021.01.002
85. Cui X, Wang X, Chang X, Bao L, Wu J, Tan Z, et al. A new capacity of gut microbiota: Fermentation of engineered inorganic carbon nanomaterials into endogenous organic metabolites. Proc Natl Acad Sci (2023) 120:e2218739120. doi: 10.1073/pnas.2218739120
86. Luedde M, Winkler T, Heinsen FA, Rühlemann MC, Spehlmann ME, Bajrovic A, et al. Heart failure is associated with depletion of core intestinal microbiota. ESC Heart failure (2017) 4:282–90. doi: 10.1002/ehf2.12155
87. Lupu VV, Adam Raileanu A, Mihai CM, Morariu ID, Lupu A, Starcea IM, et al. The implication of the gut microbiome in heart failure. Cells (2023) 12:1158. doi: 10.3390/cells12081158
88. Pasini E, Aquilani R, Testa C, Baiardi P, Angioletti S, Boschi F, et al. Pathogenic gut flora in patients with chronic heart failure. JACC: Heart Failure (2016) 4:220–7. doi: 10.1016/j.jchf.2015.10.009
89. Kamo T, Akazawa H, Suda W, Saga-Kamo A, Shimizu Y, Yagi H, et al. Dysbiosis and compositional alterations with aging in the gut microbiota of patients with heart failure. PloS One (2017) 12:e0174099. doi: 10.1371/journal.pone.0174099
90. Cui X, Ye L, Li J, Jin L, Wang W, Li S, et al. Metagenomic and metabolomic analyses unveil dysbiosis of gut microbiota in chronic heart failure patients. Sci Rep (2018) 8:635. doi: 10.1038/s41598-017-18756-2
91. Zuo K, Li J, Li K, Hu C, Gao Y, Chen M, et al. Disordered gut microbiota and alterations in metabolic patterns are associated with atrial fibrillation. Gigascience (2019) 8:giz058. doi: 10.1093/gigascience/giz058
92. Ascher S, Reinhardt C. The gut microbiota: an emerging risk factor for cardiovascular and cerebrovascular disease. Eur J Immunol (2018) 48:564–75. doi: 10.1002/eji.201646879
93. Ponikowski P, Anker SD, Alhabib KF, Cowie MR, Force TL, Hu S, et al. Heart failure: preventing disease and death worldwide. ESC Heart failure (2014) 1:4–25. doi: 10.1002/ehf2.12005
94. Rogers C, Bush N. Heart failure: pathophysiology, diagnosis, medical treatment guidelines, and nursing management. Nurs Clinics (2015) 50:787–99.
96. Shirazi LF, Bissett J, Romeo F, Mehta JL. Role of inflammation in heart failure. Curr Atheroscl Rep (2017) 19:1–9. doi: 10.1007/s11883-017-0660-3
97. Dantzer R, Cohen S, Russo SJ, Dinan TG. Resilience and immunity. Brain behavior Immun (2018) 74:28–42. doi: 10.1016/j.bbi.2018.08.010
98. Moshkelgosha S, Masetti G, Berchner-Pfannschmidt U, Verhasselt HL, Horstmann M, Diaz-Cano S, et al. Gut microbiome in BALB/c and C57BL/6J mice undergoing experimental thyroid autoimmunity associate with differences in immunological responses and thyroid function. Hormone Metab Res (2018) 50:932–41. doi: 10.1055/a-0653-3766
99. Sandek A, Bauditz J, Swidsinski A, Buhner S, Weber-Eibel J, Von Haehling S, et al. Altered intestinal function in patients with chronic heart failure. J Am Coll Cardiol (2007) 50:1561–9. doi: 10.1016/j.jacc.2007.07.016
100. Niebauer J, Yolk ID, Kemp M, Dominguez M, Schumann RR, Rauchhaus M, et al. Endotoxin and inunune activation in chronic heart failure: a prospective cohort study. Lancet (1999) 353:1838–42. doi: 10.1016/S0140-6736(98)09286-1
101. Tang WW, Kitai T, Hazen SL. Gut microbiota in cardiovascular health and disease. Circ Res (2017) 120:1183–96. doi: 10.1161/CIRCRESAHA.117.309715
102. Li H, Liu W, Lei Y, Zhou H, Wang P, Li J. Professor jun li treating vascular dementia from mutual conclusion of phlegm and blood stasis. J Clin Nurs Res (2022) 6:67–75. doi: 10.26689/jcnr.v6i1.2904
103. Schuett K, Kleber ME, Scharnagl H, Lorkowski S, März W, Niessner A, et al. Trimethylamine-N-oxide and heart failure with reduced versus preserved ejection fraction. J Am Coll Cardiol (2017) 70:3202–4. doi: 10.1016/j.jacc.2017.10.064
104. Zhao M, Wei H, Li C, Zhan R, Liu C, Gao J, et al. Gut microbiota production of trimethyl-5-aminovaleric acid reduces fatty acid oxidation and accelerates cardiac hypertrophy. Nat Commun (2022) 13:1757. doi: 10.1038/s41467-022-29060-7
105. Trøseid M, Andersen G.Ø, Broch K, Hov JR. The gut microbiome in coronary artery disease and heart failure: Current knowledge and future directions. EBioMedicine (2020) 52.
106. Kummen M, Mayerhofer CC, Vestad B, Broch K, Awoyemi A, Storm-Larsen C, et al. Gut microbiota signature in heart failure defined from profiling of 2 independent cohorts. J Am Coll Cardiol (2018) 71:1184–6. doi: 10.1016/j.jacc.2017.12.057
107. Wu C-C, Hsieh M-Y, Hung S-C, Kuo K-L, Tsai T-H, Lai C-L, et al. Serum indoxyl sulfate associates with postangioplasty thrombosis of dialysis grafts. J Am Soc Nephrology: JASN (2016) 27:1254. doi: 10.1681/ASN.2015010068
108. Mendelsohn AR, Larrick JW. Dietary modification of the microbiome affects risk for cardiovascular disease. Rejuvenation Res (2013) 16:241–4. doi: 10.1089/rej.2013.1447
109. Bentzon JF, Otsuka F, Virmani R, Falk E. Mechanisms of plaque formation and rupture. Circ Res (2014) 114:1852–66. doi: 10.1161/CIRCRESAHA.114.302721
110. Soehnlein O, Libby P. Targeting inflammation in atherosclerosis—from experimental insights to the clinic. Nat Rev Drug Discovery (2021) 20:589–610. doi: 10.1038/s41573-021-00198-1
111. Forkosh E, Ilan Y. The heart-gut axis: new target for atherosclerosis and congestive heart failure therapy. Open Heart (2019) 6:e000993. doi: 10.1136/openhrt-2018-000993
112. Guiducci L, Nicolini G, Forini F. Dietary patterns, gut microbiota remodeling, and cardiometabolic disease. Metabolites (2023) 13:760. doi: 10.3390/metabo13060760
113. Jie Z, Zhu Q, Zou Y, Wu Q, Qin M, He D, et al. A consortium of three-bacteria isolated from human feces inhibits formation of atherosclerotic deposits and lowers lipid levels in a mouse model. iScience (2023) 26. doi: 10.1016/j.isci.2023.106960
114. Markowiak-Kopeć P, Śliżewska K. The effect of probiotics on the production of short-chain fatty acids by human intestinal microbiome. Nutrients (2020) 12:1107. doi: 10.3390/nu12041107
115. Chan YK, Brar MS, Kirjavainen PV, Chen Y, Peng J, Li D, et al. High fat diet induced atherosclerosis is accompanied with low colonic bacterial diversity and altered abundances that correlates with plaque size, plasma A-FABP and cholesterol: a pilot study of high fat diet and its intervention with Lactobacillus rhamnosus GG (LGG) or telmisartan in ApoE–/– mice. BMC Microbiol (2016) 16:1–13. doi: 10.1186/s12866-016-0883-4
116. Zhang H, Jiang F, Zhang J, Wang W, Li L, Yan J. Modulatory effects of polysaccharides from plants, marine algae and edible mushrooms on gut microbiota and related health benefits: A review. Int J Biol Macromolecules (2022) 204:169–92. doi: 10.1016/j.ijbiomac.2022.01.166
117. Tulkens J, Vergauwen G, Van Deun J, Geeurickx E, Dhondt B, Lippens L, et al. Increased levels of systemic LPS-positive bacterial extracellular vesicles in patients with intestinal barrier dysfunction. Gut (2020) 69:191–3. doi: 10.1136/gutjnl-2018-317726
118. Dickhout A, Koenen RR. Extracellular vesicles as biomarkers in cardiovascular disease; chances and risks. Front Cardiovasc Med (2018) 5:113. doi: 10.3389/fcvm.2018.00113
119. Brás IC, Khani MH, Riedel D, Parfentev I, Gerhardt E, Van Riesen C, et al. Ectosomes and exosomes are distinct proteomic entities that modulate spontaneous activity in neuronal cells. bioRxiv (2021). 2021.2006. 2024.449731.
120. Patel S, Guo MK, Abdul Samad M, Howe KL. Extracellular vesicles as biomarkers and modulators of atherosclerosis pathogenesis. Front Cardiovasc Med (2023) 10:1202187. doi: 10.3389/fcvm.2023.1202187
121. Stepankova R, Tonar Z, Bartova J, Nedorost L, Rossman P, Poledne R, et al. Absence of microbiota (germ-free conditions) accelerates the atherosclerosis in ApoE-deficient mice fed standard low cholesterol diet. J Atheroscl Thromb (2010) 17:796–804. doi: 10.5551/jat.3285
122. Kiouptsi K, Pontarollo G, Todorov H, Braun J, Jäckel S, Koeck T, et al. Germ-free housing conditions do not affect aortic root and aortic arch lesion size of late atherosclerotic low-density lipoprotein receptor-deficient mice. Gut Microbes (2020) 11:1809–23. doi: 10.1080/19490976.2020.1767463
123. Gatarek P, Kaluzna-Czaplinska J. Trimethylamine N-oxide (TMAO) in human health. EXCLI J (2021) 20:301.
124. Janeiro MH, Ramírez MJ, Milagro FI, Martínez JA, Solas M. Implication of trimethylamine N-oxide (TMAO) in disease: potential biomarker or new therapeutic target. Nutrients (2018) 10:1398. doi: 10.3390/nu10101398
125. Holmes E, Li JV, Marchesi JR, Nicholson JK. Gut microbiota composition and activity in relation to host metabolic phenotype and disease risk. Cell Metab (2012) 16:559–64. doi: 10.1016/j.cmet.2012.10.007
126. Tang WW, Wang Z, Kennedy DJ, Wu Y, Buffa JA, Agatisa-Boyle B, et al. Gut microbiota-dependent trimethylamine N-oxide (TMAO) pathway contributes to both development of renal insufficiency and mortality risk in chronic kidney disease. Circ Res (2015) 116:448–55. doi: 10.1161/CIRCRESAHA.116.305360
127. Li M, Van Esch BC, Henricks PA, Folkerts G, Garssen J. The anti-inflammatory effects of short chain fatty acids on lipopolysaccharide-or tumor necrosis factor α-stimulated endothelial cells via activation of GPR41/43 and inhibition of HDACs. Front Pharmacol (2018) 9:533. doi: 10.3389/fphar.2018.00533
128. Tayyeb JZ, Popeijus HE, Mensink RP, Konings MC, Mokhtar FB, Plat J. Short-chain fatty acids (except hexanoic acid) lower NF-kB transactivation, which rescues inflammation-induced decreased apolipoprotein AI transcription in HepG2 cells. Int J Mol Sci (2020) 21:5088. doi: 10.3390/ijms21145088
129. Tom Dieck H, Schön C, Wagner T, Pankoke HC, Fluegel M, Speckmann B. A synbiotic formulation comprising Bacillus subtilis DSM 32315 and L-alanyl-L-glutamine improves intestinal butyrate levels and lipid metabolism in healthy humans. Nutrients (2021) 14:143. doi: 10.3390/nu14010143
130. Larkin TA, Astheimer LB, Price WE. Dietary combination of soy with a probiotic or prebiotic food significantly reduces total and LDL cholesterol in mildly hypercholesterolaemic subjects. Eur J Clin Nutr (2009) 63:238–45. doi: 10.1038/sj.ejcn.1602910
131. Wu Y, Jin A, Xie G, Wang L, Liu K, Jia G, et al. The 20 most important and most preventable health problems of China: a Delphi consultation of Chinese experts. Am J Public Health (2018) 108:1592–8. doi: 10.2105/AJPH.2018.304684
132. Burström B, Tao W. Social determinants of health and inequalities in COVID-19. European Public Health Association, Oxford, United Kingdom: Oxford University Press (2020).
134. Brantsæter AL, Myhre R, Haugen M, Myking S, Sengpiel V, Magnus P, et al. Intake of probiotic food and risk of preeclampsia in primiparous women: the Norwegian Mother and Child Cohort Study. Am J Epidemiol (2011) 174:807–15. doi: 10.1093/aje/kwr168
135. Thomson A. Health professionals' knowledge and attitudes toward vitamin D in the general population, pregnancy, and infancy: a thesis presented in partial fulfilment of the requirements for the degree of Master of Science in Nutrition and Dietetics. Massey University, Albany, New Zealand: Massey University (2020).
137. Williams B, Mancia G, Spiering W, Agabiti Rosei E, Azizi M, Burnier M, et al. 2018 Practice Guidelines for the management of arterial hypertension of the European Society of Cardiology and the European Society of Hypertension. Blood Pressure (2018) 27:314–40. doi: 10.1080/08037051.2018.1527177
138. Cao L, Li X, Yan P, Wang X, Li M, Li R, et al. The effectiveness of aerobic exercise for hypertensive population: a systematic review and meta-analysis. J Clin Hypertension (2019) 21:868–76. doi: 10.1111/jch.13583
139. Mell B, Jala VR, Mathew AV, Byun J, Waghulde H, Zhang Y, et al. Evidence for a link between gut microbiota and hypertension in the Dahl rat. Physiol Genomics (2015) 47:187–97. doi: 10.1152/physiolgenomics.00136.2014
140. Yang T, Santisteban MM, Rodriguez V, Li E, Ahmari N, Carvajal JM, et al. Gut dysbiosis is linked to hypertension. hypertension (2015) 65:1331–40. doi: 10.1161/HYPERTENSIONAHA.115.05315
141. Adnan S, Nelson JW, Ajami NJ, Venna VR, Petrosino JF, Bryan RM Jr., et al. Alterations in the gut microbiota can elicit hypertension in rats. Physiol Genomics (2017) 49:96–104. doi: 10.1152/physiolgenomics.00081.2016
142. Vickers NJ. Animal communication: when i’m calling you, will you answer too? Curr Biol (2017) 27:R713–5. doi: 10.1016/j.cub.2017.05.064
143. Kim S, Goel R, Kumar A, Qi Y, Lobaton G, Hosaka K, et al. Imbalance of gut microbiome and intestinal epithelial barrier dysfunction in patients with high blood pressure. Clin Sci (2018) 132:701–18. doi: 10.1042/CS20180087
144. Zhang Z, Zhao J, Tian C, Chen X, Li H, Wei X, et al. Targeting the gut microbiota to investigate the mechanism of lactulose in negating the effects of a high-salt diet on hypertension. Mol Nutr Food Res (2019) 63:1800941. doi: 10.1002/mnfr.201800941
145. Guo H, Hao Y, Fan X, Richel A, Everaert N, Yang X, et al. Administration with quinoa protein reduces the blood pressure in spontaneously hypertensive rats and modifies the fecal microbiota. Nutrients (2021) 13:2446. doi: 10.3390/nu13072446
146. Huart J, Leenders J, Taminiau B, Descy J, Saint-Remy A, Daube G, et al. Gut microbiota and fecal levels of short-chain fatty acids differ upon 24-hour blood pressure levels in men. Hypertension (2019) 74:1005–13. doi: 10.1161/HYPERTENSIONAHA.118.12588
147. Le Poul E, Loison C, Struyf S, Springael J-Y, Lannoy V, Decobecq M-E, et al. Functional characterization of human receptors for short chain fatty acids and their role in polymorphonuclear cell activation. J Biol Chem (2003) 278:25481–9. doi: 10.1074/jbc.M301403200
148. Pluznick JL, Protzko RJ, Gevorgyan H, Peterlin Z, Sipos A, Han J, et al. Olfactory receptor responding to gut microbiota-derived signals plays a role in renin secretion and blood pressure regulation. Proc Natl Acad Sci (2013) 110:4410–5. doi: 10.1073/pnas.1215927110
149. Natarajan N, Pluznick JL. From microbe to man: the role of microbial short chain fatty acid metabolites in host cell biology. Am J Physiology-Cell Physiol (2014) 307:C979–85. doi: 10.1152/ajpcell.00228.2014
150. Wang H, Luo Q, Ding X, Chen L, Zhang Z. Trimethylamine N-oxide and its precursors in relation to blood pressure: A mendelian randomization study. Front Cardiovasc Med (2022) 9:922441. doi: 10.3389/fcvm.2022.922441
151. Senthong V, Wang Z, Li XS, Fan Y, Wu Y, Wilson Tang W, et al. Intestinal microbiota-generated metabolite trimethylamine-N-oxide and 5-year mortality risk in stable coronary artery disease: the contributory role of intestinal microbiota in a COURAGE-like patient cohort. J Am Heart Assoc (2016) 5:e002816. doi: 10.1161/JAHA.115.002816
152. Suzuki T, Heaney LM, Bhandari SS, Jones DJ, Ng LL. Trimethylamine N-oxide and prognosis in acute heart failure. Heart (2016) 102:841–8. doi: 10.1136/heartjnl-2015-308826
153. Ge X, Zheng L, Zhuang R, Yu P, Xu Z, Liu G, et al. The gut microbial metabolite trimethylamine N-oxide and hypertension risk: a systematic review and dose–response meta-analysis. Adv Nutr (2020) 11:66–76. doi: 10.1093/advances/nmz064
154. Lewis-Mikhael A-M, Davoodvandi A, Jafarnejad S. Effect of Lactobacillusplantarum containing probiotics on blood pressure: A systematic review and meta-analysis. Pharmacol Res (2020) 153:104663. doi: 10.1016/j.phrs.2020.104663
155. Khalesi S, Sun J, Buys N, Jayasinghe R. Effect of probiotics on blood pressure: a systematic review and meta-analysis of randomized, controlled trials. Hypertension (2014) 64:897–903. doi: 10.1161/HYPERTENSIONAHA.114.03469
156. Murphy K, O’donovan AN, Caplice NM, Ross RP, Stanton C. Exploring the gut microbiota and cardiovascular disease. Metabolites (2021) 11:493. doi: 10.3390/metabo11080493
157. Centner AM, Khalili L, Ukhanov V, Kadyan S, Nagpal R, Salazar G. The role of phytochemicals and gut microbiome in atherosclerosis in preclinical mouse models. Nutrients (2023) 15:1212. doi: 10.3390/nu15051212
158. Zeisel SH, Warrier M. Trimethylamine N-oxide, the microbiome, and heart and kidney disease. Annu Rev Nutr (2017) 37:157–81. doi: 10.1146/annurev-nutr-071816-064732
159. Li XS, Obeid S, Klingenberg R, Gencer B, Mach F, Räber L, et al. Gut microbiota-dependent trimethylamine N-oxide in acute coronary syndromes: a prognostic marker for incident cardiovascular events beyond traditional risk factors. Eur Heart J (2017) 38:814–24. doi: 10.1093/eurheartj/ehw582
160. Bennett BJ, De Aguiar Vallim TQ, Wang Z, Shih DM, Meng Y, Gregory J, et al. Trimethylamine-N-oxide, a metabolite associated with atherosclerosis, exhibits complex genetic and dietary regulation. Cell Metab (2013) 17:49–60. doi: 10.1016/j.cmet.2012.12.011
161. Crisci G, Israr MZ, Cittadini A, Bossone E, Suzuki T, Salzano A. Heart failure and trimethylamine N-oxide: time to transform a ‘gut feeling’in a fact? ESC Heart Failure (2023) 10:1. doi: 10.1002/ehf2.14205
162. Randrianarisoa E, Lehn-Stefan A, Wang X, Hoene M, Peter A, Heinzmann SS, et al. Relationship of serum trimethylamine N-oxide (TMAO) levels with early atherosclerosis in humans. Sci Rep (2016) 6:26745. doi: 10.1038/srep26745
163. Roncal C, Martínez-Aguilar E, Orbe J, Ravassa S, Fernandez-Montero A, Saenz-Pipaon G, et al. Trimethylamine-N-oxide (TMAO) predicts cardiovascular mortality in peripheral artery disease. Sci Rep (2019) 9:15580. doi: 10.1038/s41598-019-52082-z
164. Hoseini-Tavassol Z, Ejtahed H-S, Larijani B, Hasani-Ranjbar S. Trimethylamine N-Oxide as a potential risk factor for non-communicable diseases: A systematic review. Endocrine Metab Immune Disorders-Drug Targets (Formerly Curr Drug Targets-Immune Endocrine Metab Disorders) (2023) 23:617–32. doi: 10.2174/1871530323666221103120410
165. Querio G, Antoniotti S, Geddo F, Levi R, Gallo MP. Modulation of endothelial function by TMAO, a gut microbiota-derived metabolite. Int J Mol Sci (2023) 24:5806. doi: 10.3390/ijms24065806
166. Trøseid M, Ueland T, Hov J, Svardal A, Gregersen I, Dahl C, et al. Microbiota-dependent metabolite trimethylamine-N-oxide is associated with disease severity and survival of patients with chronic heart failure. J Internal Med (2015) 277:717–26. doi: 10.1111/joim.12328
167. Suzuki T, Heaney LM, Jones DJ, Ng LL. Trimethylamine N-oxide and risk stratification after acute myocardial infarction. Clin Chem (2017) 63:420–8. doi: 10.1373/clinchem.2016.264853
168. Ahmad AF, Dwivedi G, O’gara F, Caparros-Martin J, Ward NC. The gut microbiome and cardiovascular disease: current knowledge and clinical potential. Am J Physiology-Heart Circulatory Physiol (2019) 317:H923–38. doi: 10.1152/ajpheart.00376.2019
169. Blacher E, Levy M, Tatirovsky E, Elinav E. Microbiome-modulated metabolites at the interface of host immunity. J Immunol (2017) 198:572–80. doi: 10.4049/jimmunol.1601247
170. Fiorucci S, Distrutti E. Bile acid-activated receptors, intestinal microbiota, and the treatment of metabolic disorders. Trends Mol Med (2015) 21:702–14. doi: 10.1016/j.molmed.2015.09.001
171. Donohoe DR, Garge N, Zhang X, Sun W, O'connell TM, Bunger MK, et al. The microbiome and butyrate regulate energy metabolism and autophagy in the mammalian colon. Cell Metab (2011) 13:517–26. doi: 10.1016/j.cmet.2011.02.018
172. Mayerhofer CC, Kummen M, Holm K, Broch K, Awoyemi A, Vestad B, et al. Low fibre intake is associated with gut microbiota alterations in chronic heart failure. ESC Heart Failure (2020) 7:456–66. doi: 10.1002/ehf2.12596
173. Chakaroun RM, Olsson LM, Bäckhed F. The potential of tailoring the gut microbiome to prevent and treat cardiometabolic disease. Nat Rev Cardiol (2023) 20:217–35. doi: 10.1038/s41569-022-00771-0
174. Aguilar EC, Dos Santos LC, Leonel AJ, De Oliveira JS, Santos EA, Navia-Pelaez JM, et al. Oral butyrate reduces oxidative stress in atherosclerotic lesion sites by a mechanism involving NADPH oxidase down-regulation in endothelial cells. J Nutr Biochem (2016) 34:99–105. doi: 10.1016/j.jnutbio.2016.05.002
175. Aguilar E, Leonel A, Teixeira L, Silva A, Silva J, Pelaez J, et al. Butyrate impairs atherogenesis by reducing plaque inflammation and vulnerability and decreasing NFκB activation. Nutrition Metab Cardiovasc Dis (2014) 24:606–13. doi: 10.1016/j.numecd.2014.01.002
176. Li J, Zhao F, Wang Y, Chen J, Tao J, Tian G, et al. Gut microbiota dysbiosis contributes to the development of hypertension. Microbiome (2017) 5:1–19. doi: 10.1186/s40168-016-0222-x
177. Masenga SK, Hamooya B, Hangoma J, Hayumbu V, Ertuglu LA, Ishimwe J, et al. Recent advances in modulation of cardiovascular diseases by the gut microbiota. J Hum Hypertension (2022) 36:952–9. doi: 10.1038/s41371-022-00698-6
178. Bartolomaeus H, Balogh A, Yakoub M, Homann S, Markó L, Höges S, et al. Short-chain fatty acid propionate protects from hypertensive cardiovascular damage. Circulation (2019) 139:1407–21. doi: 10.1161/CIRCULATIONAHA.118.036652
179. Battson ML, Lee DM, Li Puma LC, Ecton KE, Thomas KN, Febvre HP, et al. Gut microbiota regulates cardiac ischemic tolerance and aortic stiffness in obesity. Am J Physiology-Heart Circulatory Physiol (2019) 317:H1210–20. doi: 10.1152/ajpheart.00346.2019
180. Tang TW, Chen H-C, Chen C-Y, Yen CY, Lin C-J, Prajnamitra RP, et al. Loss of gut microbiota alters immune system composition and cripples postinfarction cardiac repair. Circulation (2019) 139:647–59. doi: 10.1161/CIRCULATIONAHA.118.035235
181. Hanafi NI, Mohamed AS, Sheikh Abdul Kadir SH, Othman MHD. Overview of bile acids signaling and perspective on the signal of ursodeoxycholic acid, the most hydrophilic bile acid, in the heart. Biomolecules (2018) 8:159. doi: 10.3390/biom8040159
182. Insull W Jr. Clinical utility of bile acid sequestrants in the treatment of dyslipidemia: a scientific review. South Med J (2006) 99:257–74. doi: 10.1097/01.smj.0000208120.73327.db
183. Yamaoka-Tojo M, Tojo T, Izumi T. Beyond cholesterol lowering: pleiotropic effects of bile acid binding resins against cardiovascular disease risk factors in patients with metabolic syndrome. Curr Vasc Pharmacol (2008) 6:271–81. doi: 10.2174/157016108785909698
184. Sun J, Fan J, Li T, Yan X, Jiang Y. Nuciferine protects against high-fat diet-induced hepatic steatosis via modulation of gut microbiota and bile acid metabolism in rats. J Agric Food Chem (2022) 70:12014–28. doi: 10.1021/acs.jafc.2c04817
185. Ahmed M. Functional, diagnostic and therapeutic aspects of bile. Clin Exp Gastroenterol (2022), 105–20. doi: 10.2147/CEG.S360563
186. Charach G, Argov O, Geiger K, Charach L, Rogowski O, Grosskopf I. Diminished bile acids excretion is a risk factor for coronary artery disease: 20-year follow up and long-term outcome. Ther Adv Gastroenterol (2018) 11:1756283X17743420. doi: 10.1177/1756283X17743420
187. Choudhuri S, Klaassen CD. Molecular regulation of bile acid homeostasis. Drug Metab Disposition (2022) 50:425–55. doi: 10.1124/dmd.121.000643
188. Raghunatha Reddy R. Biochemical studies on the antilithogenic effect of dietary fenugreek seeds (Trigonella foenum-graecum). India: University of Mysore (2010).
189. Packard C, Shepherd J. The hepatobiliary axis and lipoprotein metabolism: effects of bile acid sequestrants and ileal bypass surgery. J Lipid Res (1982) 23:1081–98. doi: 10.1016/S0022-2275(20)38045-7
190. Einarsson K, Ericsson S, Ewerth S, Reihner E, Rudling M, Ståhlberg D, et al. Bile acid sequestrants: mechanisms of action on bile acid and cholesterol metabolism. Eur J Clin Pharmacol (1991) 40:S53–8. doi: 10.1007/BF03216291
191. Di Ciaula A, Bonfrate L, Baj J, Khalil M, Garruti G, Stellaard F, et al. Recent advances in the digestive, metabolic and therapeutic effects of farnesoid X receptor and fibroblast growth factor 19: from cholesterol to bile acid signaling. Nutrients (2022) 14:4950. doi: 10.3390/nu14234950
192. Yokota A, Fukiya S, Islam KS, Ooka T, Ogura Y, Hayashi T, et al. Is bile acid a determinant of the gut microbiota on a high-fat diet? Gut Microbes (2012) 3:455–9. doi: 610.4161/gmic.21216
193. Lau K, Srivatsav V, Rizwan A, Nashed A, Liu R, Shen R, et al. Bridging the gap between gut microbial dysbiosis and cardiovascular diseases. Nutrients (2017) 9:859. doi: 10.3390/nu9080859
194. Wang Z, Zhao Y. Gut microbiota derived metabolites in cardiovascular health and disease. Protein Cell (2018) 9:416–31. doi: 10.1007/s13238-018-0549-0
195. Rowland I, Gibson G, Heinken A, Scott K, Swann J, Thiele I, et al. Gut microbiota functions: metabolism of nutrients and other food components. Eur J Nutr (2018) 57:1–24. doi: 10.1007/s00394-017-1445-8
196. Jia B, Zou Y, Han X, Bae J-W, Jeon CO. Gut microbiome-mediated mechanisms for reducing cholesterol levels: Implications for ameliorating cardiovascular disease. Trends Microbiol (2023). doi: 10.1016/j.tim.2022.08.003
197. Cavalu S, Banica F, Gruian C, Vanea E, Goller G, Simon V. Microscopic and spectroscopic investigation of bioactive glasses for antibiotic controlled release. J Mol Structure (2013) 1040:47–52. doi: 10.1016/j.molstruc.2013.02.016
198. Sindhu RK, Goyal A, Algın Yapar E, Cavalu S. Bioactive compounds and nanodelivery perspectives for treatment of cardiovascular diseases. Appl Sci (2021) 11:11031. doi: 10.3390/app112211031
199. Myles IA. Fast food fever: reviewing the impacts of the Western diet on immunity. Nutr J (2014) 13:1–17. doi: 10.1186/1475-2891-13-61
200. Perler BK, Friedman ES, Wu GD. The role of the gut microbiota in the relationship between diet and human health. Annu Rev Physiol (2023) 85:449–68. doi: 10.1146/annurev-physiol-031522-092054
201. Rothschild D, Weissbrod O, Barkan E, Kurilshikov A, Korem T, Zeevi D, et al. Environment dominates over host genetics in shaping human gut microbiota. Nature (2018) 555:210–5. doi: 10.1038/nature25973
202. Danneskiold-Samsøe NB, Barros HDDFQ, Santos R, Bicas JL, Cazarin CBB, Madsen L, et al. Interplay between food and gut microbiota in health and disease. Food Res Int (2019) 115:23–31. doi: 10.1016/j.foodres.2018.07.043
203. Foye OT, Huang I-F, Chiou CC, Walker WA, Shi HN. Early administration of probiotic Lactobacillus acidophilus and/or prebiotic inulin attenuates pathogen-mediated intestinal inflammation and Smad 7 cell signaling. FEMS Immunol Med Microbiol (2012) 65:467–80. doi: 10.1111/j.1574-695X.2012.00978.x
204. Marques FZ, Nelson E, Chu P-Y, Horlock D, Fiedler A, Ziemann M, et al. High-fiber diet and acetate supplementation change the gut microbiota and prevent the development of hypertension and heart failure in hypertensive mice. Circulation (2017) 135:964–77. doi: 10.1161/CIRCULATIONAHA.116.024545
205. Eckel R, Jakicic J, Ard J. 2013 AHA/ACC Guideline on Lifestyle Management to Reduce Cardiovascular Risk: A Report of the American College of Cardiology/American Heart Association Task Force on Practice Guidelines. J Am Coll Cardiol 2013 Nov 12 [E-pub ahead of print. J Am Coll Cardiol (2014) 63:3027–8. doi: 10.1016/j.jacc.2013.11.003
206. Kerley CP. Dietary patterns and components to prevent and treat heart failure: a comprehensive review of human studies. Nutr Res Rev (2019) 32:1–27. doi: 10.1017/S0954422418000148
207. Battson ML, Lee DM, Jarrell DK, Hou S, Ecton KE, Weir TL, et al. Suppression of gut dysbiosis reverses Western diet-induced vascular dysfunction. Am J Physiology-Endocrinology Metab (2018) 314:E468–77. doi: 10.1152/ajpendo.00187.2017
208. Chen K, Zheng X, Feng M, Li D, Zhang H. Gut microbiota-dependent metabolite trimethylamine N-oxide contributes to cardiac dysfunction in western diet-induced obese mice. Front Physiol (2017) 8:139. doi: 10.3389/fphys.2017.00139
209. De Filippis F, Pellegrini N, Vannini L, Jeffery IB, La Storia A, Laghi L, et al. High-level adherence to a Mediterranean diet beneficially impacts the gut microbiota and associated metabolome. Gut (2016) 65:1812–21. doi: 10.1136/gutjnl-2015-309957
210. Papadaki A, Martínez-González MÁ., Alonso-Gómez A, Rekondo J, Salas-Salvadó J, Corella D, et al. Mediterranean diet and risk of heart failure: results from the PREDIMED randomized controlled trial. Eur J Heart Failure (2017) 19:1179–85. doi: 10.1002/ejhf.750
211. Estruch R, Ros E, Salas-Salvadó J, Covas M-I, Corella D, Arós F, et al. Primary prevention of cardiovascular disease with a Mediterranean diet supplemented with extra-virgin olive oil or nuts. New Engl J Med (2018) 378:e34. doi: 10.1056/NEJMoa1800389
212. Wang J, Wang P, Li D, Hu X, Chen F. Beneficial effects of ginger on prevention of obesity through modulation of gut microbiota in mice. Eur J Nutr (2020) 59:699–718. doi: 10.1007/s00394-019-01938-1
213. Teng Y, Ren Y, Sayed M, Hu X, Lei C, Kumar A, et al. Plant-derived exosomal microRNAs shape the gut microbiota. Cell Host Microbe (2018) 24:637–652. e638. doi: 10.1016/j.chom.2018.10.001
214. Yeşilyurt N, Yılmaz B, Ağagündüz D, Capasso R. Involvement of probiotics and postbiotics in the immune system modulation. Biologics (2021) 1:89–110. doi: 10.3390/biologics1020006
215. Singh D, Singh A, Kumar S. Probiotics: friend or foe to the human immune system. Bull Natl Res Centre (2023) 47:1–9. doi: 10.1186/s42269-023-01098-7
216. Gibson GR, Roberfroid MB. Dietary modulation of the human colonic microbiota: introducing the concept of prebiotics. J Nutr (1995) 125:1401–12. doi: 10.1093/jn/125.6.1401
217. Karlsson C, Ahrné S, Molin G, Berggren A, Palmquist I, Fredrikson GN, et al. Probiotic therapy to men with incipient arteriosclerosis initiates increased bacterial diversity in colon: a randomized controlled trial. Atherosclerosis (2010) 208:228–33. doi: 10.1016/j.atherosclerosis.2009.06.019
218. Naruszewicz M, Johansson M-L, Zapolska-Downar D, Bukowska H. Effect of Lactobacillus plantarum 299v on cardiovascular disease risk factors in smokers. Am J Clin Nutr (2002) 76:1249–55. doi: 10.1093/ajcn/76.6.1249
219. Andrade S, Borges N. Effect of fermented milk containing Lactobacillus acidophilus and Bifidobacterium longum on plasma lipids of women with normal or moderately elevated cholesterol. J dairy Res (2009) 76:469–74. doi: 10.1017/S0022029909990173
220. Catry E, Bindels LB, Tailleux A, Lestavel S, Neyrinck AM, Goossens J-F, et al. Targeting the gut microbiota with inulin-type fructans: preclinical demonstration of a novel approach in the management of endothelial dysfunction. Gut (2018) 67:271–83. doi: 10.1136/gutjnl-2016-313316
221. Neyrinck AM, Goossens J-F, Lobysheva I, Plovier H, Essaghir A, Demoulin J-B, et al. Targeting the gut microbiota with inulin-type fructans: preclinical demonstration of a novel approach in the management of endothelial dysfunction. (2017).
222. Liu F, Prabhakar M, Ju J, Long H, Zhou H. Effect of inulin-type fructans on blood lipid profile and glucose level: a systematic review and meta-analysis of randomized controlled trials. Eur J Clin Nutr (2017) 71:9–20. doi: 10.1038/ejcn.2016.156
223. Cosola C, De Angelis M, Rocchetti MT, Montemurno E, Maranzano V, Dalfino G, et al. Beta-glucans supplementation associates with reduction in p-cresyl sulfate levels and improved endothelial vascular reactivity in healthy individuals. PloS One (2017) 12:e0169635. doi: 10.1371/journal.pone.0169635
224. Sonnenburg ED, Smits SA, Tikhonov M, Higginbottom SK, Wingreen NS, Sonnenburg JL. Diet-induced extinctions in the gut microbiota compound over generations. Nature (2016) 529:212–5. doi: 10.1038/nature16504
225. Griffin NW, Ahern PP, Cheng J, Heath AC, Ilkayeva O, Newgard CB, et al. Prior dietary practices and connections to a human gut microbial metacommunity alter responses to diet interventions. Cell Host Microbe (2017) 21:84–96. doi: 10.1016/j.chom.2016.12.006
226. Holscher HD. Dietary fiber and prebiotics and the gastrointestinal microbiota. Gut Microbes (2017) 8:172–84. doi: 10.1080/19490976.2017.1290756
227. Kootte RS, Levin E, Salojärvi J, Smits LP, Hartstra AV, Udayappan SD, et al. Improvement of insulin sensitivity after lean donor feces in metabolic syndrome is driven by baseline intestinal microbiota composition. Cell Metab (2017) 26:611–619. e616. doi: 10.1016/j.cmet.2017.09.008
228. Jayachandran M, Chen J, Chung SSM, Xu B. A critical review on the impacts of β-glucans on gut microbiota and human health. J Nutr Biochem (2018) 61:101–10. doi: 10.1016/j.jnutbio.2018.06.010
229. Walton GE, Lu C, Trogh I, Arnaut F, Gibson GR. A randomised, double-blind, placebo controlled cross-over study to determine the gastrointestinal effects of consumption of arabinoxylan-oligosaccharides enriched bread in healthy volunteers. Nutr J (2012) 11:1–11. doi: 10.1186/1475-2891-11-36
230. Wang H, Zhang W, Zuo L, Zhu W, Wang B, Li Q, et al. Bifidobacteria may be beneficial to intestinal microbiota and reduction of bacterial translocation in mice following ischaemia and reperfusion injury. Br J Nutr (2013) 109:1990–8. doi: 10.1017/S0007114512004308
231. Jacouton E, Chain F, Sokol H, Langella P, Bermudez-Humaran LG. Probiotic strain Lactobacillus casei BL23 prevents colitis-associated colorectal cancer. Front Immunol (2017) 8:1553. doi: 10.3389/fimmu.2017.01553
232. Schneeberger M, Everard A, Gómez-Valadés AG, Matamoros S, Ramírez S, Delzenne NM, et al. Akkermansia muciniphila inversely correlates with the onset of inflammation, altered adipose tissue metabolism and metabolic disorders during obesity in mice. Sci Rep (2015) 5:16643. doi: 10.1038/srep16643
233. Fuentes MC, Lajo T, Carrión JM, Cuné J. Cholesterol-lowering efficacy of Lactobacillus plantarum CECT 7527, 7528 and 7529 in hypercholesterolaemic adults. Br J Nutr (2013) 109:1866–72. doi: 10.1017/S000711451200373X
234. Cani PD, Bibiloni R, Knauf C, Waget A, Neyrinck AM, Delzenne NM, et al. Changes in gut microbiota control metabolic endotoxemia-induced inflammation in high-fat diet–induced obesity and diabetes in mice. Diabetes (2008) 57:1470–81. doi: 10.2337/db07-1403
235. Membrez M, Blancher F, Jaquet M, Bibiloni R, Cani PD, Burcelin RG, et al. Gut microbiota modulation with norfloxacin and ampicillin enhances glucose tolerance in mice. FASEB J (2008) 22:2416–26. doi: 10.1096/fj.07-102723
236. Kuczynski J, Lauber CL, Walters WA, Parfrey LW, Clemente JC, Gevers D, et al. Experimental and analytical tools for studying the human microbiome. Nat Rev Genet (2012) 13:47–58. doi: 10.1038/nrg3129
237. Fujisaka S, Ussar S, Clish C, Devkota S, Dreyfuss JM, Sakaguchi M, et al. Antibiotic effects on gut microbiota and metabolism are host dependent. J Clin Invest (2016) 126:4430–43. doi: 10.1172/JCI86674
238. Anderson JL, Muhlestein JB. Antibiotic trials for coronary heart disease. Texas Heart Institute J (2004) 31:33.
239. Albert RK, Schuller JL, Network CCR. Macrolide antibiotics and the risk of cardiac arrhythmias. Am J Respir Crit Care Med (2014) 189:1173–80. doi: 10.1164/rccm.201402-0385CI
240. Cheng Y-J, Nie X-Y, Chen X-M, Lin X-X, Tang K, Zeng W-T, et al. The role of macrolide antibiotics in increasing cardiovascular risk. J Am Coll Cardiol (2015) 66:2173–84. doi: 10.1016/j.jacc.2015.09.029
241. Wong AY, Root A, Douglas IJ, Chui CS, Chan EW, Ghebremichael-Weldeselassie Y, et al. Cardiovascular outcomes associated with use of clarithromycin: population based study. bmj (2016) 352. doi: 10.1136/bmj.h6926
242. Yan X, Jin J, Su X, Yin X, Gao J, Wang X, et al. Intestinal flora modulates blood pressure by regulating the synthesis of intestinal-derived corticosterone in high salt-induced hypertension. Circ Res (2020) 126:839–53. doi: 10.1161/CIRCRESAHA.119.316394
243. Jose PA, Raj D. Gut microbiota in hypertension. Curr Opin Nephrol hypertension (2015) 24:403. doi: 10.1097/MNH.0000000000000149
244. Galla S, Chakraborty S, Cheng X, Yeo J, Mell B, Zhang H, et al. Disparate effects of antibiotics on hypertension. Physiol Genomics (2018) 50:837–45. doi: 10.1152/physiolgenomics.00073.2018
245. Rune I, Rolin B, Larsen C, Nielsen DS, Kanter JE, Bornfeldt KE, et al. Modulating the gut microbiota improves glucose tolerance, lipoprotein profile and atherosclerotic plaque development in ApoE-deficient mice. PloS One (2016) 11:e0146439. doi: 10.1371/journal.pone.0146439
246. Li Z, Wu Z, Yan J, Liu H, Liu Q, Deng Y, et al. Gut microbe-derived metabolite trimethylamine N-oxide induces cardiac hypertrophy and fibrosis. Lab Invest (2019) 99:346–57. doi: 10.1038/s41374-018-0091-y
247. Yang R, Chen Z, Cai J. Fecal microbiota transplantation: Emerging applications in autoimmune diseases. J Autoimmun (2023) 103038. doi: 10.1016/j.jaut.2023.103038
248. Tuniyazi M, Hu X, Fu Y, Zhang N. Canine fecal microbiota transplantation: Current application and possible mechanisms. Veterinary Sci (2022) 9:396. doi: 10.3390/vetsci9080396
249. Valles-Colomer M, Blanco-Míguez A, Manghi P, Asnicar F, Dubois L, Golzato D, et al. The person-to-person transmission landscape of the gut and oral microbiomes. Nature (2023) 614:125–35. doi: 10.1038/s41586-022-05620-1
250. Yu D, Meng X, De Vos WM, Wu H, Fang X, Maiti AK. Implications of gut microbiota in complex human diseases. Int J Mol Sci (2021) 22:12661. doi: 10.3390/ijms222312661
251. Settanni CR, Ianiro G, Bibbò S, Cammarota G, Gasbarrini A. Gut microbiota alteration and modulation in psychiatric disorders: Current evidence on fecal microbiota transplantation. Prog Neuropsychopharmacol Biol Psychiatry (2021) 109:110258. doi: 10.1016/j.pnpbp.2021.110258
252. Beyi AF, Wannemuehler M, Plummer PJ. Impacts of gut microbiota on the immune system and fecal microbiota transplantation as a re-emerging therapy for autoimmune diseases. Antibiotics (2022) 11:1093. doi: 10.3390/antibiotics11081093
253. Ooijevaar RE, Terveer EM, Verspaget HW, Kuijper EJ, Keller JJ. Clinical application and potential of fecal microbiota transplantation. Annu Rev Med (2019) 70:335–51. doi: 10.1146/annurev-med-111717-122956
254. Khan MY, Dirweesh A, Khurshid T, Siddiqui WJ. Comparing fecal microbiota transplantation to standard-of-care treatment for recurrent Clostridium difficile infection: a systematic review and meta-analysis. Eur J Gastroenterol Hepatol (2018) 30:1309–17. doi: 10.1097/MEG.0000000000001243
255. D'odorico I, Di Bella S, Monticelli J, Giacobbe DR, Boldock E, Luzzati R. Role of fecal microbiota transplantation in inflammatory bowel disease. J digestive Dis (2018) 19:322–34. doi: 10.1111/1751-2980.12603
256. El-Salhy M, Mazzawi T. Fecal microbiota transplantation for managing irritable bowel syndrome. Expert Rev Gastroenterol Hepatol (2018) 12:439–45. doi: 10.1080/17474124.2018.1447380
257. Vrieze A, Van Nood E, Holleman F, Salojärvi J, Kootte RS, Bartelsman JF, et al. Transfer of intestinal microbiota from lean donors increases insulin sensitivity in individuals with metabolic syndrome. Gastroenterology (2012) 143:913–916. e917. doi: 10.1053/j.gastro.2012.06.031
258. Mahmoudi H, Hossainpour H. Application and development of fecal microbiota transplantation in the treatment of gastrointestinal and metabolic diseases: A review. Saudi J gastroenterology: Off J Saudi Gastroenterol Assoc (2023) 29:3. doi: 10.4103/sjg.sjg_131_22
259. Innes AJ, Mullish BH, Ghani R, Szydlo RM, Apperley JF, Olavarria E, et al. Fecal microbiota transplant mitigates adverse outcomes seen in patients colonized with multidrug-resistant organisms undergoing allogeneic hematopoietic cell transplantation. Front Cell Infection Microbiol (2021) 11:684659. doi: 10.3389/fcimb.2021.684659
260. Li N, Chen H, Cheng Y, Xu F, Ruan G, Ying S, et al. Fecal microbiota transplantation relieves gastrointestinal and autism symptoms by improving the gut microbiota in an open-label study. Front Cell infection Microbiol (2021) 11:948.
261. Boussamet L, Rajoka MSR, Berthelot L. Microbiota, IgA and multiple sclerosis. Microorganisms (2022) 10:617. doi: 10.3390/microorganisms10030617
262. Arora T, Tremaroli V. Therapeutic potential of butyrate for treatment of type 2 diabetes. Front Endocrinol (2021) 12:761834. doi: 10.3389/fendo.2021.761834
263. Knox EG, Aburto MR, Tessier C, Nagpal J, Clarke G, O’driscoll CM, et al. Microbial-derived metabolites induce actin cytoskeletal rearrangement and protect blood-brain barrier function. iscience (2022) 25. doi: 10.1016/j.isci.2022.105648
264. Su L, Hong Z, Zhou T, Jian Y, Xu M, Zhang X, et al. Health improvements of type 2 diabetic patients through diet and diet plus fecal microbiota transplantation. Sci Rep (2022) 12:1152. doi: 10.1038/s41598-022-05127-9
265. Park S-H, Lee JH, Shin J, Kim J-S, Cha B, Lee S, et al. Cognitive function improvement after fecal microbiota transplantation in Alzheimer’s dementia patient: A case report. Curr Med Res Opin (2021) 37:1739–44. doi: 10.1080/03007995.2021.1957807
266. Segal A, Zlotnik Y, Moyal-Atias K, Abuhasira R, Ifergane G. Fecal microbiota transplant as a potential treatment for Parkinson's disease–A case series. Clin Neurol Neurosurg (2021) 207:106791. doi: 10.1016/j.clineuro.2021.106791
267. Doll JP, Vázquez-Castellanos JF, Schaub A-C, Schweinfurth N, Kettelhack C, Schneider E, et al. Fecal microbiota transplantation (FMT) as an adjunctive therapy for depression—case report. Front Psychiatry (2022) 13:815422. doi: 10.3389/fpsyt.2022.815422
268. Luo X, Han Z, Kong Q, Wang Y, Mou H, Duan X. Clostridium butyricum prevents dysbiosis and the rise in blood pressure in spontaneously hypertensive rats. Int J Mol Sci (2023) 24:4955. doi: 10.3390/ijms24054955
269. Park JH, Moon JH, Kim HJ, Kong MH, Oh YH. Sedentary lifestyle: overview of updated evidence of potential health risks. Korean J Family Med (2020) 41:365. doi: 10.4082/kjfm.20.0165
270. Wang L, Lei J, Wang R, Li K. Non-traditional risk factors as contributors to cardiovascular disease. Rev Cardiovasc Med (2023) 24:134. doi: 10.31083/j.rcm2405134
271. Piercy KL, Troiano RP, Ballard RM, Carlson SA, Fulton JE, Galuska DA, et al. The physical activity guidelines for Americans. Jama (2018) 320:2020–8. doi: 10.1001/jama.2018.14854
272. Al-Saber A, May A-N. Effect of mindful meditation, physical activity, and diet to reduce the risk to develop or reduce severity of cardiovascular diseases in Saudi Arabia: a systematic review. World J Cardiovasc Dis (2023) 13:46–72. doi: 10.4236/wjcd.2023.131005
273. Lippi G, Henry BM, Sanchis-Gomar F. Physical inactivity and cardiovascular disease at the time of coronavirus disease 2019 (COVID-19). Eur J Prev Cardiol (2020) 27:906–8. doi: 10.1177/2047487320916823
274. Lee I-M, Shiroma EJ, Lobelo F, Puska P, Blair SN, Katzmarzyk PT. Effect of physical inactivity on major non-communicable diseases worldwide: an analysis of burden of disease and life expectancy. Lancet (2012) 380:219–29. doi: 10.1016/S0140-6736(12)61031-9
275. Albalak G, Stijntjes M, Van Bodegom D, Jukema JW, Atsma DE, Van Heemst D, et al. Setting your clock: associations between timing of objective physical activity and cardiovascular disease risk in the general population. Eur J Prev Cardiol (2023) 30:232–40. doi: 10.1093/eurjpc/zwac239
276. Petriz BA, Castro AP, Almeida JA, Gomes CP, Fernandes GR, Kruger RH, et al. Exercise induction of gut microbiota modifications in obese, non-obese and hypertensive rats. BMC Genomics (2014) 15:1–13. doi: 10.1186/1471-2164-15-511
277. Lambert JE, Myslicki JP, Bomhof MR, Belke DD, Shearer J, Reimer RA. Exercise training modifies gut microbiota in normal and diabetic mice. Appl Physiology Nutrition Metab (2015) 40:749–52. doi: 10.1139/apnm-2014-0452
278. Jurdana M, Maganja DB. Regular physical activity influences gut microbiota with positive health effects. (2023). doi: 10.5772/intechopen.110725
279. Allen JM, Mailing LJ, Niemiro GM, Moore R, Cook MD, White BA, et al. Exercise alters gut microbiota composition and function in lean and obese humans. Med Sci Sports Exerc (2018) 50:747–57. doi: 10.1249/MSS.0000000000001495
280. Choi JJ, Eum SY, Rampersaud E, Daunert S, Abreu MT, Toborek M. Exercise attenuates PCB-induced changes in the mouse gut microbiome. Environ Health Perspect (2013) 121:725–30. doi: 10.1289/ehp.1306534
281. Kang SS, Jeraldo PR, Kurti A, Miller MEB, Cook MD, Whitlock K, et al. Diet and exercise orthogonally alter the gut microbiome and reveal independent associations with anxiety and cognition. Mol neurodegeneration (2014) 9:1–12. doi: 10.1186/1750-1326-9-36
282. Allen JM, Miller MEB, Pence BD, Whitlock K, Nehra V, Gaskins HR, et al. Voluntary and forced exercise differentially alters the gut microbiome in C57BL/6J mice. J Appl Physiol (2015). doi: 10.1152/japplphysiol.01077.2014
283. Evans CC, Lepard KJ, Kwak JW, Stancukas MC, Laskowski S, Dougherty J, et al. Exercise prevents weight gain and alters the gut microbiota in a mouse model of high fat diet-induced obesity. PloS One (2014) 9:e92193. doi: 10.1371/journal.pone.0092193
284. Matsumoto M, Inoue R, Tsukahara T, Ushida K, Chiji H, Matsubara N, et al. Voluntary running exercise alters microbiota composition and increases n-butyrate concentration in the rat cecum. Bioscience biotechnology Biochem (2008) 72:572–6. doi: 10.1271/bbb.70474
285. Kaczmarczyk MM, Miller MJ, Freund GG. The health benefits of dietary fiber: beyond the usual suspects of type 2 diabetes mellitus, cardiovascular disease and colon cancer. Metabolism (2012) 61:1058–66. doi: 10.1016/j.metabol.2012.01.017
286. Watterson KR, Hudson BD, Ulven T, Milligan G. Treatment of type 2 diabetes by free fatty acid receptor agonists. Front Endocrinol (2014) 5:137. doi: 10.3389/fendo.2014.00137
287. De Vadder F, Kovatcheva-Datchary P, Goncalves D, Vinera J, Zitoun C, Duchampt A, et al. Microbiota-generated metabolites promote metabolic benefits via gut-brain neural circuits. Cell (2014) 156:84–96. doi: 10.1016/j.cell.2013.12.016
288. Kallio KE, Hätönen KA, Lehto M, Salomaa V, Männistö S, Pussinen PJ. Endotoxemia, nutrition, and cardiometabolic disorders. Acta diabetologica (2015) 52:395–404. doi: 10.1007/s00592-014-0662-3
289. Lira FS, Rosa JC, Pimentel GD, Souza HA, Caperuto EC, Carnevali LC, et al. Endotoxin levels correlate positively with a sedentary lifestyle and negatively with highly trained subjects. Lipids Health Dis (2010) 9:1–5. doi: 10.1186/1476-511X-9-82
290. Simmons A. Treated like animals: improving the lives of the creatures we own, eat and use. Wenlock Road, London, UK: Pelagic Publishing Ltd (2023).
291. Tian D, Meng J. Exercise for prevention and relief of cardiovascular disease: prognoses, mechanisms, and approaches. Oxid Med Cell Longevity (2019) 2019. doi: 10.1155/2019/3756750
292. Li J, Siegrist J. Physical activity and risk of cardiovascular disease—a meta-analysis of prospective cohort studies. Int J Environ Res Public Health (2012) 9:391–407. doi: 10.3390/ijerph9020391
Keywords: CVD, HF, HTN, TMAO, SCFAs, FMT
Citation: Luqman A, Hassan A, Ullah M, Naseem S, Ullah M, Zhang L, Din AU, Ullah K, Ahmad W and Wang G (2024) Role of the intestinal microbiome and its therapeutic intervention in cardiovascular disorder. Front. Immunol. 15:1321395. doi: 10.3389/fimmu.2024.1321395
Received: 14 October 2023; Accepted: 08 January 2024;
Published: 26 January 2024.
Edited by:
Nathella Pavan Kumar, National Institute of Research in Tuberculosis (ICMR), IndiaReviewed by:
Abdullah Saeed, City of Hope National Medical Center, United StatesCopyright © 2024 Luqman, Hassan, Ullah, Naseem, Ullah, Zhang, Din, Ullah, Ahmad and Wang. This is an open-access article distributed under the terms of the Creative Commons Attribution License (CC BY). The use, distribution or reproduction in other forums is permitted, provided the original author(s) and the copyright owner(s) are credited and that the original publication in this journal is cited, in accordance with accepted academic practice. No use, distribution or reproduction is permitted which does not comply with these terms.
*Correspondence: Guixue Wang, d2FuZ2d4QGNxdS5lZHUuY24=
†These authors have contributed equally to this work
Disclaimer: All claims expressed in this article are solely those of the authors and do not necessarily represent those of their affiliated organizations, or those of the publisher, the editors and the reviewers. Any product that may be evaluated in this article or claim that may be made by its manufacturer is not guaranteed or endorsed by the publisher.
Research integrity at Frontiers
Learn more about the work of our research integrity team to safeguard the quality of each article we publish.