- VIB-Center for Cancer Biology, KU Leuven, Leuven, Belgium
Microglia are the brain’s resident macrophages that play pivotal roles in immune surveillance and maintaining homeostasis of the Central Nervous System (CNS). Microglia are functionally implicated in various cerebrovascular diseases, including stroke, aneurysm, and tumorigenesis as they regulate neuroinflammatory responses and tissue repair processes. Here, we review the manifold functions of microglia in the brain under physiological and pathological conditions, primarily focusing on the implication of microglia in glioma propagation and progression. We further review the current status of therapies targeting microglial cells, including their re-education, depletion, and re-population approaches as therapeutic options to improve patient outcomes for various neurological and neuroinflammatory disorders, including cancer.
1 Introduction
The brain is a highly specialized and complex organ that controls vital functions ranging from cognitive processing, motor skills, vision, generation and regulation of emotions, and body homeostasis. Most regions of the brain are shielded in the form of a blood-brain barrier (BBB) against potentially harmful substances to maintain a stable and tightly regulated microenvironment essential for optimal brain function, (1, 2).
The brain harbors a complex network of cell types, including neurons, glial cells, innate immune cells, and mesenchymal cells, each contributing to specialized functions (3). While neurons facilitate signal transduction and communication within the brain and throughout the body by enabling sensory, motor, and cognitive functions (4), glial cell types play a crucial role in supporting and protecting the brain’s structure and functionality. Astrocytes are the most abundant glial cells, which regulate cerebral blood flow, the blood-brain barrier, and tissue repair following infection and traumatic injuries (5). Oligodendrocytes produce myelin sheaths for the protective insulation of nerve cells, facilitating rapid signal transmission (6). Brain-resident macrophages entail perivascular, meningeal, and choroid plexus macrophages that are localized at the interface between the parenchyma and the circulation (7), and microglial cells which are distributed throughout the entire brain and serve as immune sentinels, actively surveilling the brain microenvironment, participating in both innate and adaptive immune responses, and protecting the brain from potential threats such as injury and infection (8–10). Mesenchymal cells include endothelial cells, pericytes, and smooth muscle cells that form a widespread and extensive vascular network to provide nutrients and oxygen, remove waste products, and support the maintenance of neighboring cells. The intricate interplay between blood vessels, astrocytes, and microglial cells ensures the proper function of the blood-brain-barrier, contributing to overall neurological health (8, 9, 11).
In this review, we will focus on microglial cells and discuss their responsibilities under physiological conditions and their impact on cerebrovascular diseases (i.e., stroke, aneurysm, and glioma), taking a recent perspective of microglial experts into consideration that proposes a revised nomenclature to display the dynamic and multilayered states of microglial cells. We explore 1) microglia functions in health and disease, 2) the differences between brain-resident macrophages and monocyte-derived infiltrating macrophages, 3) the mechanisms of microglia re-education, depletion, and repopulation, and 4) highlight new insights into myeloid adoptive cell transfer as a new therapeutic approach for cerebrovascular diseases. This will provide a better understanding of how microglial cells modify brain homeostasis upon injury and pave the way to improve the efficacy of current therapies for stroke, aneurysm, and glioma by targeting the brain resident macrophages.
2 Microglial cells
2.1 Microglia origin and function
Microglial cells derive from primitive macrophages that originated in the yolk sac during early embryonic development, setting them apart transcriptionally from other tissue-resident macrophages and bone marrow-derived macrophages (Figure 1) (10, 12). In mice, primitive hematopoiesis occurs in the yolk sac around day seven (E.7) of embryonic development and contributes to producing the first erythrocytes and macrophages. Primitive macrophages appear in the blood islands of the yolk sac around day nine of embryonic development (E.9). These yolk sac-derived primitive macrophages migrate to different tissues, including the brain, through the blood after the establishment of the circulatory system (from E8.5 to E10) where they differentiate into fetal macrophage populations (13). They enter the brain at E9.5, before the BBB formation, and colonize the brain parenchyma via migration and proliferation mechanisms (14, 15). Microglia proliferate in response to colony-stimulating factor 1 (CSF-1), granulocyte-macrophage colony-stimulating factor, interleukin 4 (IL-4), and interleukin 5 (IL-5) (16). They reach their highest population two weeks after birth and are maintained until adulthood (17, 18). While microglia can self-renew to sustain their population, other fetal macrophage populations are replaced by fetal liver-derived monocytes that colonize the tissue later and differentiate into macrophages (19). The latter is conceivable with the observation that the generation of the BBB in rodents coincides with the release of fetal liver monocytes into the blood circulation at about E13.5. Yolk sac-derived primitive macrophages, however, start to invade the brain tissues earlier at E9.5 (20) (Figure 1).
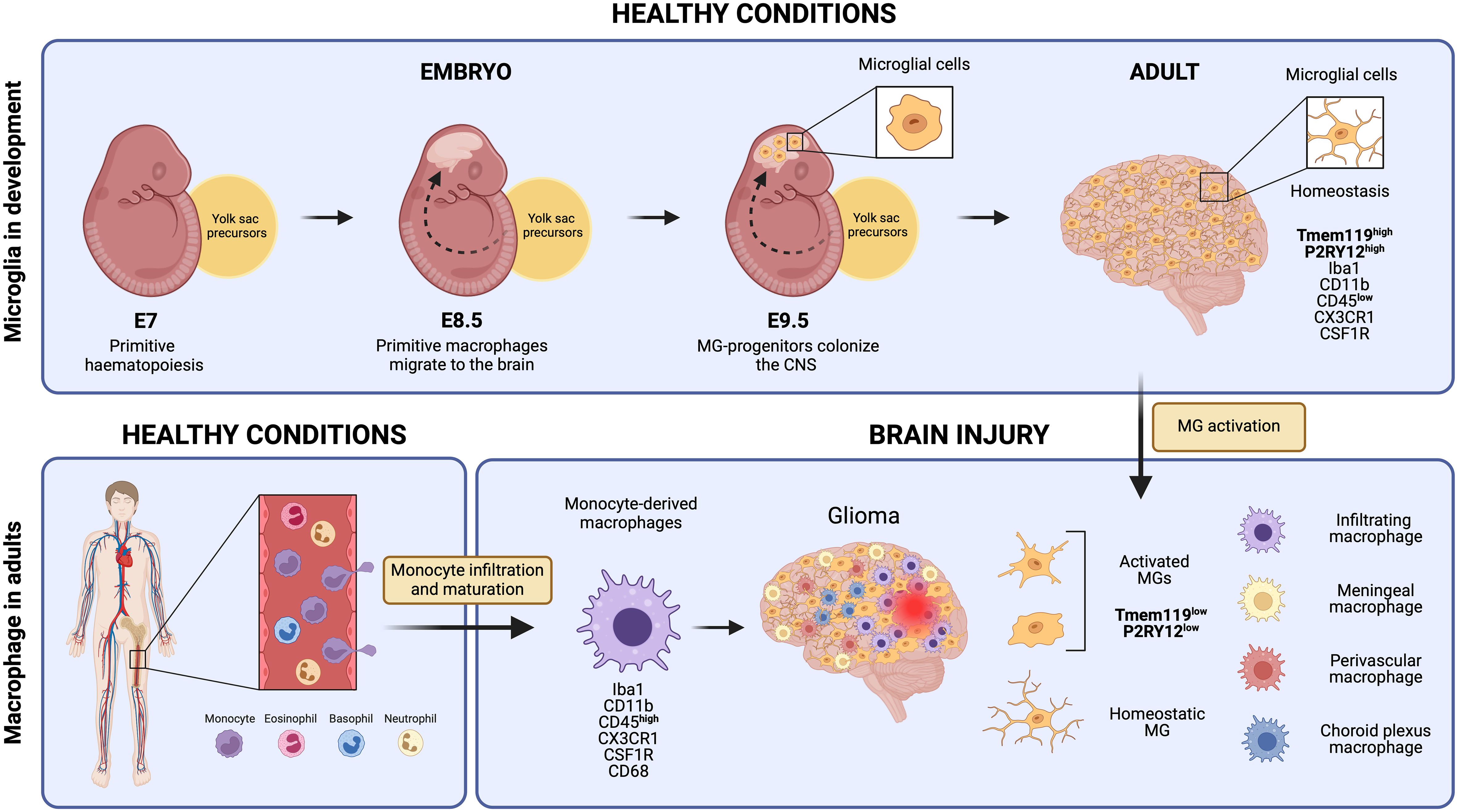
Figure 1 Microglial cells originate from yolk sac precursors around day 9.5 of embryonic development and populate the entire Central Nervous System. Under normal, healthy conditions, microglia maintain a homeostatic state expressing unique markers that set them apart from monocyte-derived macrophages, such as Tmem119 and P2RY12. However, in response to brain injury, microglial cells undergo activation and subsequently down-regulate some of these specific markers. This downregulation makes it extremely challenging to distinguish them from monocyte-derived macrophages that reach the injury site through monocyte infiltration and maturation, as well as other brain-resident macrophages like meningeal macrophages, perivascular macrophages, and choroid plexus macrophages. Created by Biorender.
Different mechanisms have been proposed by which microglial distribution may occur in the developing brain parenchyma. Microglial cells could migrate along developing blood vessels because migration happens at the same time as vascularization and blood circulation (E10), and vascular sprouts can modulate microglial migration during mouse embryonic development (14, 21). In addition, microglial migration could also be influenced by the modulation of the extracellular matrix by matrix metalloproteinases (MMPs) as inhibition of MMP-8 and MMP-9 impaired microglial spreading (22). Other studies demonstrated that microglia in the postnatal Central Nervous System (CNS) can migrate along the vasculature via the C-X3-C motif ligand 1 (CX3CL1)/C-X3-C receptor 1 (CX3CR1) axis (23). In support, microglial CX3CR1 knockout delayed the microglial recruitment in the early postnatal hippocampus and somatosensory cortex by several days (24).
Once microglia have colonized the brain parenchyma, they will undergo differentiation, which relies on environmental cues. Thereby, microglia require signaling through the colony-stimulating factor 1 receptor (CSF1R) by macrophage colony-stimulating factor 1 (CSF-1) produced primarily by oligodendrocytes and astrocytes for their survival, differentiation, and maintenance as underscored by the observation that CSF1R knockout mice are severely diminished in the number of microglial cells (17, 25). In addition, interleukin 34 (IL-34), another CSF1R ligand, secreted by neurons, and the hematopoietic transcription factor PU.1 can also contribute to microglial homeostasis and development, respectively (22, 26). Interestingly, transforming growth factor β (TGF-β) and interleukin-33 (IL-33) secreted by mature neurons and astrocytes, respectively, were shown to trigger post-natal microglial differentiation (27, 28). In addition, a recent study indicated that brain-resident CD4+ T-cells (CD69+) play a key role in the post-natal transcriptional and morphological maturation of microglia, both in mice and humans. The absence of murine CD4+ T-cells impaired microglial maturation to the final adult state, resulting in immature neuronal synapsis, defective synaptic pruning, and behavioral abnormalities (29).
Throughout adulthood, microglia undergo turnover approximately every four years. This means that over a lifetime, they will be renewed several times, primarily through self-renewal mechanisms among existing microglia (30, 31). Interestingly, studies have demonstrated that peripherally derived macrophages can replace depleted microglia in certain situations. However, it is essential to note that these replacement cells retain their unique identity and differ from microglial cells (32).
2.2 Microglia communication with other cell types in the brain environment
Under steady-state conditions, MGs actively interact with various brain cell types, including neurons, astrocytes, oligodendrocytes, pericytes, and endothelial cells, to maintain a functional and healthy brain (33–35). For instance, MGs support neuronal activity in different ways. Firstly, MGs can prune defective synapses and regulate neuronal excitability (36, 37). They can clear debris produced by dying neurons and modify the synapsis by partial phagocytosis (trogocytosis) and by interposing themselves between pre- and post-synaptic membranes (synaptic stripping) (38–40). Secondly, they can also influence neuron proliferation and migration and promote their survival by secreting growth factors such as insulin-like growth factor-1 (IGF-1), essential for protection against cytokine-induced neuronal cell death (41) (Figure 2).
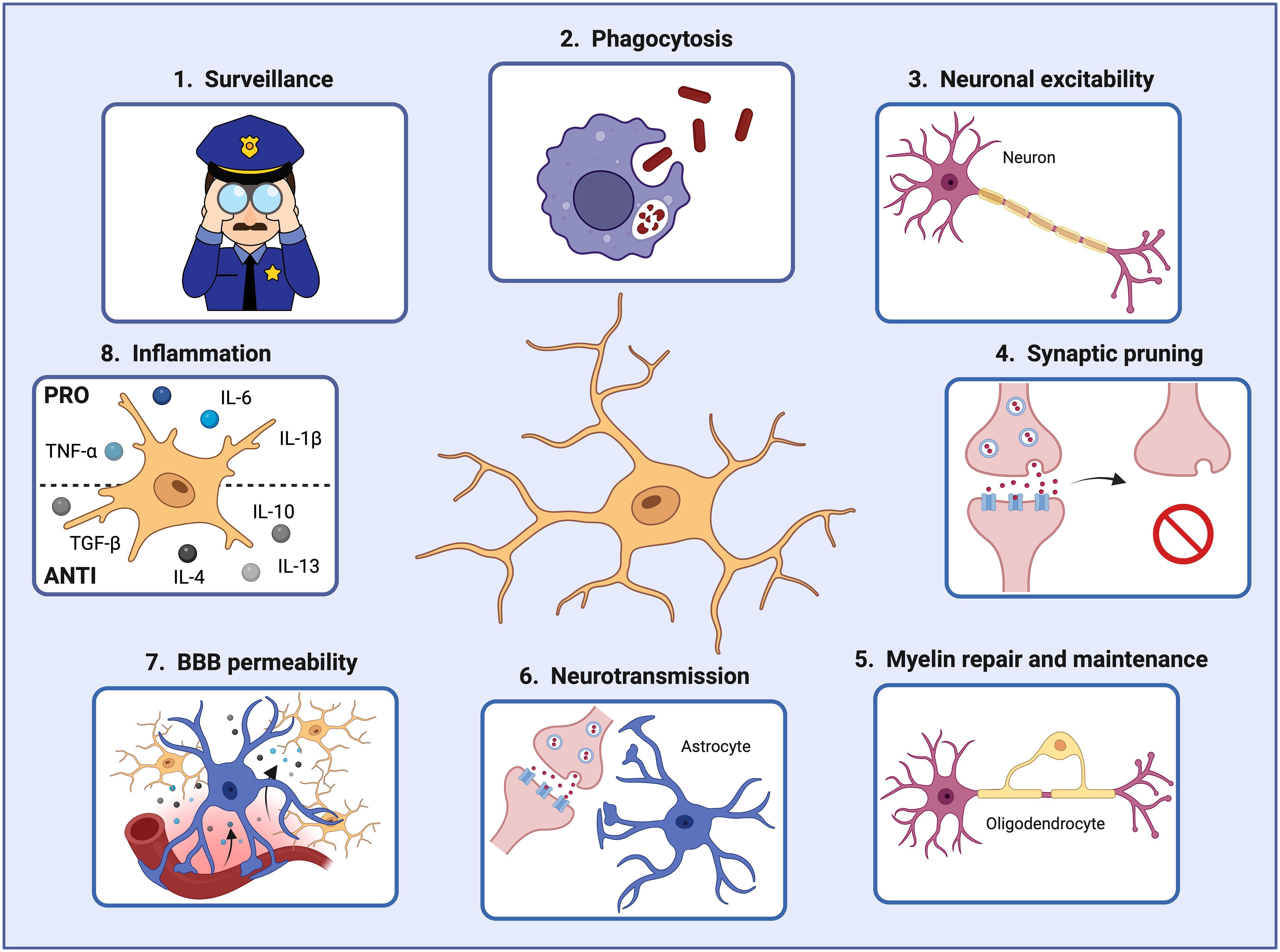
Figure 2 Microglial cells play crucial roles in the brain during development and adulthood. In order to maintain brain homeostasis, microglial cells 1) act as sentinels constantly surveilling the brain environment and adapting their functions to specific triggers, 2) phagocytose pathogens and damaged cells that may threaten the brain homeostasis and function, 3) regulate neuronal excitability, 4) prune defective synapses, 5) interact with oligodendrocytes for myelin repair and maintenance, 6) assist astrocytes in controlling neurotransmitter levels, 7) modify the blood-brain barrier permeability by secreting factors that increase the expression of cell adhesion molecules in endothelial cells, and 8) secrete pro- and anti-inflammatory factors controlling the inflammatory response. Created by Biorender.
In addition, microglia can interact with astrocytes and help to control the brain’s neurotransmitter levels. By releasing small amounts of ATP, astrocytes are recruited, and glutamate is released. Astrocyte-secreted glutamate increases the excitatory postsynaptic currents via neuronal metabotropic glutamate receptor 5 (mGluR5), improving the synaptic transmission and keeping the brain homeostasis (42). Moreover, microglia also interact with oligodendrocytes, the cells responsible for myelin production. A recent study demonstrated that microglia depletion in adult mice resulted in enlarged inner tongues and ticker myelin (43). Another study discovered that microglia help repair demyelinated lesions via post-squalene sterol synthesis (44). Thus, microglia are essential in helping oligodendrocytes maintain and repair the myelin for optimal neuronal communication and overall brain function (33) (Figure 2).
Furthermore, microglia, being macrophages, communicate with endothelial cells. Microglia can secrete factors such as TNF-α and IL-1β that increase the expression of leukocyte adhesion molecules (i.e., ICAM-1 and VCAM- 1) on vascular endothelial cells, promoting the infiltration of peripheral immune cells into the brain parenchyma (11, 34). Thereby, microglia can also interact with the infiltrating immune cells via MHC-II-like molecules, activating T-cells to become pro-inflammatory and consequently releasing inflammatory factors that will initiate and regulate the immune response within the brain during pathological conditions (45, 46) (Figure 2).
In conclusion, microglial cells interact with other cell constituents in the brain, like neurons, astrocytes, oligodendrocytes, endothelial cells, and immune cells, via different mechanisms aiming at protecting the immune-privileged brain and maintaining homeostasis.
2.3 Microglial cell states
Recently, a consortium of experts in the field has put forward a revised view of the dynamic and complex microglia states in relationship to their specific functions in development, homeostasis, aging, and disease and provided recommendations on the use of a revised microglial terminology that is more appropriately reflective of their complex and dynamic states (47). In line with their proposition, microglia should be considered highly dynamic and plastic cells that display co-existing and interchangeable phenotypes in response to environmental cues like “wearing several hats which they can continuously exchange”. There is a common census that the historical and still current view of dividing microglia function into dichotomic categories (e.g., M1 versus M2, resting versus activated) is prone to misconceptions, while a multidimensional integration of data on their transcriptomics, metabolomics, morphology, location, or epigenetics will help to reveal a more complete picture of their nature and function.
For example, microglia have been commonly described to be in a “resting state” during homeostatic conditions as characterized by their ramified morphology and modest local proliferation. However, homeostatic microglial cells are anything but resting as they continuously patrol the brain environment and promptly react when they detect the first signs of infection or tissue damage. While microglia respond locally to minor tissue insults detected, they react much more rigorously upon severe injury or formation of neuroinflammatory and neurodegenerative disturbances. This is commonly accompanied by morphological changes, sometimes adopting an amoeboid morphology with rapid clonal expansion throughout the brain, making it challenging to distinguish microglia from bone marrow-derived macrophages (Figure 1) or other monocytes that are recruited to the injury site and elicit immune responses (48).
Upon activation by injury or inflammation, microglia, like macrophages, set out to eliminate any threats first but then subsequently support tissue repair and wound healing. Due to their phagocytic capability, they are instrumental in removing cell debris and foreign microorganisms. They are also involved in synaptic pruning, transmission, and regulation of neuronal excitability, as well as astrocyte activation (12, 49) (Figure 2).
In the context of injury, microglia exposed to microbial products or damaged tissue, release pro-inflammatory molecules such as interleukin-1 β (IL-1β), interleukin-6 (IL-6), tumor necrosis factor-alpha (TNF-α), and reactive oxygen species (ROS) that help initiate and amplify inflammatory responses, recruiting and activating immune cells (i.e., T and B cells) and promoting phagocytosis of pathogens or damaged cells. In contrast, during tissue repair and regeneration, microglia secrete anti-inflammatory cytokines, such as interleukin 10 (IL-10), transforming growth factor β (TGF-β), interleukin 4 (IL-4), and interleukin 13 (IL-13) to abrogate inflammation and promote wound healing (50, 51) (Figure 2). In addition, a recent study demonstrated the pivotal role of microglia in neocortex inflammation since they produce and secrete CCL2, a chemokine involved in immune cell recruitment and BBB-microvessel leakage. Notably, decreased MG-CCL2 expression was associated with a more restored BBB and diminished neuroinflammation (52). It is important to note that the immune status, referred to as polarization of microglia or macrophages, will be determined by the net effect of a variety of pro-inflammatory and anti-inflammatory stimuli, being the outcome of the response a balance between the strength of the detected signals. This fine-tuned process is influenced by complex interactions with neighboring cells, including neurons, astrocytes, and endothelial cells, as well as by specific signaling molecules (50, 53).
Changes in the activation state of microglial cells, and macrophages in general, also require metabolic rewiring. Microglia display high glycolytic activity and glutaminolysis to meet their energy demands for surveillance, motility, and immune responses (54, 55). Pro-inflammatory microglia obtain their ATP energy via aerobic glycolysis by increasing their glucose uptake and lactate production (56). In addition, they activate their pentose phosphate pathway (PPP) to support DNA replication and RNA transcription (56, 57). In contrast, immunosuppressive/anti-inflammatory microglia/macrophages use mostly mitochondrial oxidative phosphorylation for ATP production (56, 58) and increase fatty acid oxidation to fulfill their energy demands (56, 59). The ability of microglia to use different energy sources enables them to promptly adapt to variable metabolic conditions. Metabolic rewiring eases their response by adapting quickly to a changing microenvironment in the healthy brain and in disease.
Transcriptional profiling has helped to identify specific gene signatures that are distinct between microglia and monocyte/macrophage populations. The genes P2ry13, P2ry12, Slc2a5, Sall1, Olfml3, and Tmem119 are highly expressed in microglial cells, while monocytes and macrophages exhibit higher levels of F10, Emilin2, F5, Gda, Mki67, Sell, and Hp (60). Both macrophage cell types share IBA-1, CX3CR1, F4/80, CD11b, CD45, and CD68 expression. So far, transmembrane protein 119 (TMEM119) and purinergic receptor P2Y12R are the most specific and broadly used markers for (homeostatic) microglia but activated microglia tend to downregulate their expression (61–63) (Figure 1). Using flow cytometry, microglial cells can be distinguished from bone marrow-derived macrophages by differential expression of the CD11b, CD45, Lys6C, and Lys6G markers because microglia are CD11b+ CD45low Lys6C- Lys6G- while infiltrating monocytes/macrophages are CD11b+ CD45high Lys6Clow Lys6G- (64). This distinction, however, becomes challenging during inflammation and aging in which microglia can upregulate the expression of these markers (i.e., CD45) (60, 65). In summary, microglia quickly adapt to their environment and modify their functions with a spectrum of activation states which is reflected in their distinct expression profiles.
2.4 Understanding microglia plasticity by inducing microglia re-population
Microglial cells play a crucial role in maintaining the delicate balance of the brain’s microenvironment. Their essential functions and distinct origin from other infiltrating macrophages have drawn attention to understanding the consequences of depleting microglia in the brain. Microglia depletion has provided insight into the ability of the brain to repopulate microglia from different cell resources, underscoring their essential functions. These studies also exposed the extent of the repopulating cell constituents in immune surveillance and neural-supporting functions during brain homeostasis.
2.5 Microglia depletion in the brain
To date, several methods, using pharmacological and genetic approaches have been used to deplete microglial cells by abrogating crucial signaling cues. For instance, colony-stimulating factor 1 receptor (CSF1R) inhibitors (i.e., PLX5622, PLX3397 (Plexxikon), BLZ945 (Novartis)) that pass the BBB, have been extensively used to deplete microglia in several animal models (66–68). These inhibitors block the CSF1R activation, which is essential for microglial differentiation and survival. However, it is important to note that blocking CSF1R signaling will affect both infiltrating monocyte-derived macrophages and resident microglial cells (69) (Figure 3).
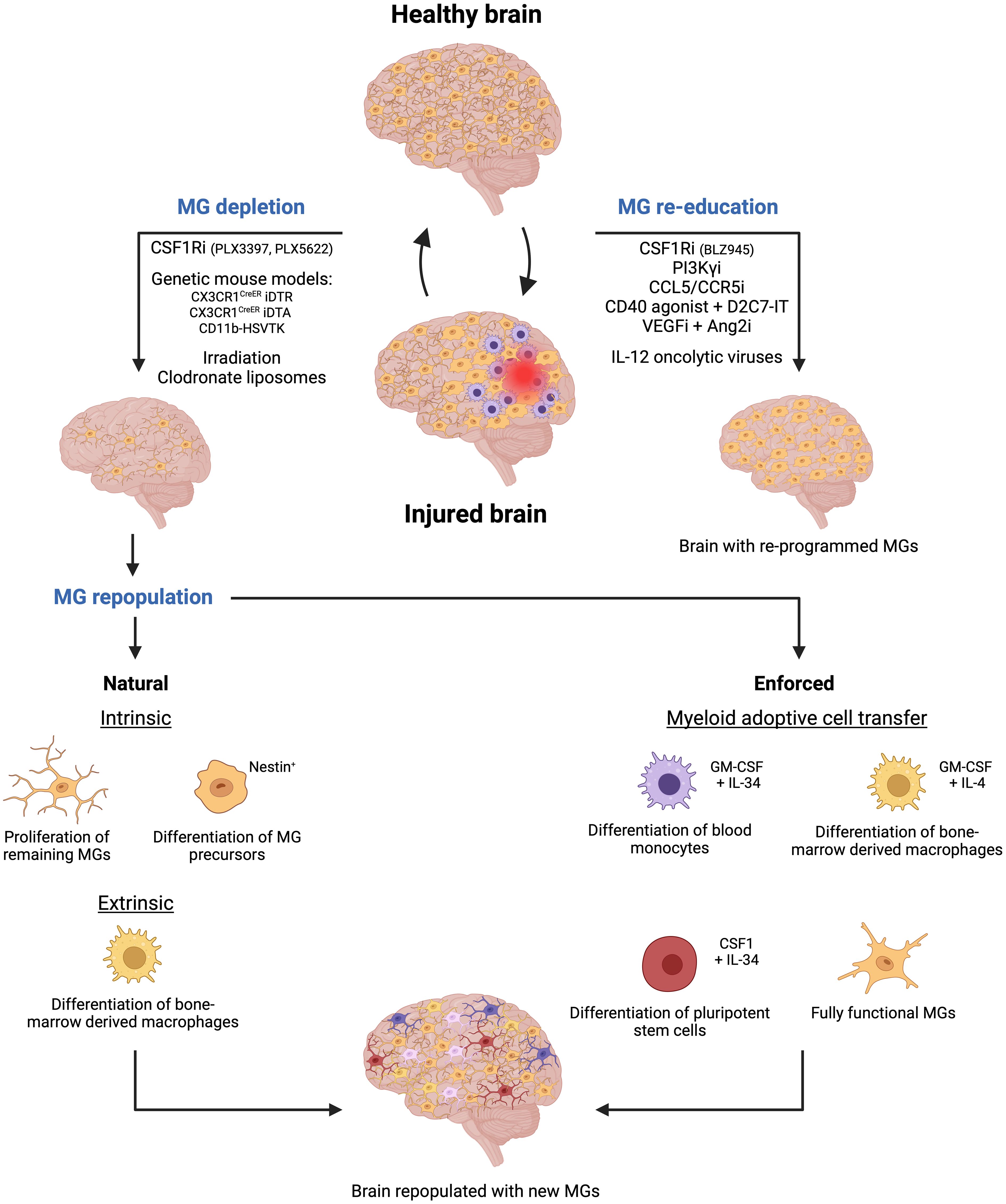
Figure 3 Microglia-targeted therapies are emerging as a novel therapeutic approach for cerebrovascular diseases. Two primary strategies are being explored to target microglial cells following brain injury. The first strategy involves depleting microglial cells using methods such as pharmaceutical inhibitors or genetic approaches. Subsequently, new microglial cells will repopulate the empty niche through natural processes like proliferation of remaining microglial cells, differentiation of nestin+ microglia precursors, and differentiation of bone marrow-derived macrophages. However, enforced repopulation can occur through myeloid adoptive cell transfer leading to the emergence of new microglia from the differentiation of blood monocytes, bone marrow-derived macrophages, and pluripotent stem cells, or upon direct transfer of fully functional and activated microglial cells. The second strategy focuses on re-educating existing microglial cells using specific inhibitors or oncolytic viruses, thereby activating them to contribute to maintaining brain homeostasis following injury. Created by Biorender.
In addition, cell-specific genetic strategies have been used to either genetically delete the gene of interest whose products are crucial for microglial survival or use a cell-type-specific approach to kill microglial cells directly. For example, the Cre-loxP technology has been utilized to drive the expression of the diphtheria toxin receptor (DTR) in microglia cells in CX3CR1CreER iDTR transgenic mice. Thus, when the diphtheria toxin is administered upon tamoxifen administration in mice, it selectively targets and kills the DTR-expressing microglial cells (70, 71). Similarly, microglia were depleted through the administration of tamoxifen, which induced the expression of the toxic DTA (Diphtheria Toxin A subunit) protein in CX3CR1CreER DTA transgenic mice (69, 72) (Figure 3).
Another approach is the depletion of microglial cells in the CD11b-HSVTK transgenic model. In this mouse model, the herpes simplex virus thymidine kinase (HSVTK) gene is under the control of the CD11b gene promoter, a gene for integrin alpha that is expressed in macrophages and microglial cells. HSVTK is an enzyme that converts the antiviral drug ganciclovir (GCV) into a toxic form. Thus, upon ganciclovir administration to the mice, HSVTK converts GCV into a toxic compound in CD11b+ cells. As a result, microglia and infiltrating macrophages are depleted in the brain (69, 73) (Figure 3).
Additionally, other alternative methods have been used to deplete microglial cells. In certain experimental settings, irradiation has been shown to deplete microglia, since it affects rapidly dividing cells, like activated microglia, leading to their depletion. This method is often combined with bone marrow transplantation to replace the depleted microglia with microglia, derived from donor-derived bone marrow cells (74, 75). Moreover, liposome encapsulating clodronate, a drug that induces cell death in phagocytes, has also been used to deplete MGs. When these liposomes are administered to mice, microglial cells, but also other myeloid cell types including neutrophils, internalize clodronate and undergo apoptosis (76, 77) (Figure 3).
Importantly, circulating monocytes and peripheral tissue macrophages can also be affected upon microglial depletion as genetic and pharmacological approaches cannot distinguish between these populations. Only when pharmacological inhibitors were directly delivered to the brain, were circulating monocytes and peripheral tissue macrophages not reduced. Thus, specific delivery strategies should be taken into consideration for microglia depletion and replacement therapies (78).
Nevertheless, recent research has identified promising potential markers, such as Tmem119 and P2RY12, which are uniquely expressed in resident microglia (61–63). Notably, Tmem119-CreER and P2ry12-CreER mice have already been generated to manipulate gene expression in brain-resident microglial cells without altering monocyte-derived macrophages (79, 80).
2.6 Microglia repopulation in the brain
The depletion of microglia can be very efficient, but microglial repopulation is quickly stimulated in the brain, restoring the CNS niche in about 1-2 weeks, specifically when the depleting agent is removed (81).
In detail, microglial cells can repopulate the brain in three different ways: 1) the residual microglia can self-renew by increasing their proliferative capabilities and repopulating the empty niche (81, 82), 2) nestin+ microglial progenitors can differentiate into mature microglia replenishing the brain (69, 83), and 3) bone marrow-derived macrophages can infiltrate the brain and acquire a “microglia-like” identity with a distinct transcriptomic profile compared to the original resident microglia (32, 69, 73) (Figure 3). The latter restoration process is also very powerful in other organs. For example, circulating monocytes can fully replace Kupffer cells in the liver and alveolar macrophages in the lung with nearly identical cellular phenotypes (84, 85).
Importantly, depending on the method of depletion, the repopulation sources may be different. For instance, it has been shown that microglia depletion with the CSF1R-inhibitor PLX5622 leads to repopulation by which remaining resident microglia increase proliferation (86). Similarly, MG-depletion using the CX3CR1CreER iDTR mouse model induced repopulation by self-renewal of residual microglia (87). However, genetic ablation using the CX3CR1CreER DTA mouse model induced MG-repopulation via both differentiation of monocyte-derived macrophages and proliferation of local microglia (88). Interestingly, the depletion of microglial cells using the CD11b-HSVTK transgenic model promoted the infiltration and differentiation of monocyte-derived macrophages to replenish microglia in the brain (73).
In addition, repopulation of microglia upon depletion may happen at different time points depending on the depletion methods, previously used. For instance, administration of liposome-encapsulating clodronate leads to microglia depletion on day 1, lasting the effects for 3 days, and promoting MG-repopulation 5 days after treatment (76). However, in the CX3CR1CreER iDTR mouse model, most of the MGs are depleted after 3 days upon diphtheria toxin administration, and their repopulation occurred later on day 14 (87). Similarly, in the CD11b-HSVTK mouse model, MG-repopulation happens after 2 weeks (73). Furthermore, CSF1R inhibition using PLX5622 depleted microglial cells after 1-2 weeks of administration and promoted their repopulation 2 weeks after the treatment stopped (89).
These observations highlight the different mechanisms of microglia repopulation upon their depletion in the brain. Understanding the timing and modes of repopulation is crucial for anticipating the consequences of microglia targeting, not only in the context of brain homeostasis but also in the context of brain diseases as detailed in the following chapters. It is pivotal to characterize the repopulated microglial cell population in response to the depletion method. This knowledge is essential for identifying the origin and function of the newly replenished microglia, which may likely display some differences in their transcriptomic profiles and functions compared to the original microglial population.
3 Microglia in cerebrovascular diseases
3.1 Stroke, aneurysm, glioma
Given microglia’s functions in tissue repair, it is not surprising that they are involved in shaping the outcome of cerebrovascular diseases such as stroke, aneurysms, and gliomas. (90–92). However, their responses to these CNS insults can exacerbate or reduce disease progression depending on their conversion into specific states, as recognized by specific molecular signatures. For example, disease-associated microglia (DAM), which are found at sites of neurodegeneration, have protective effects. They are endowed with a sensory mechanism that involves the Trem2 signaling pathway, to detect damage within the CNS in the form of neurodegeneration-associated molecular patterns (NAMPs). NAMPs are danger signals present on apoptotic bodies of dying neural cells, myelin debris, lipid degradation products, and extracellular protein aggregates typical for neurodegenerative diseases. NAMP binding to microglia triggers their transition into DAMs, whose primary function is to contain and remove the damage (93). Notwithstanding, there is also increasing evidence that microglia actively contribute to the initiation and progression of CNS disorders, including Alzheimer’s disease, amyotrophic lateral sclerosis, and brain tumors (94–99).
3.2 Microglial cell behavior in stroke
Stroke is a common cerebrovascular disease characterized by interrupting the blood flow to the brain, leading to oxygen and nutrient deprivation. As a result, events like inflammation, oxidative stress, and cell death occur in the affected areas. There are two main types of strokes: 1) ischemic stroke, the most common stroke caused by a blockage of an artery or a vein interrupting the blood flow to the brain, and 2) hemorrhagic stroke, caused by bleeding into or around the brain. Ischemic stroke is the most common type of stroke since it accounts for around 70-80% of all stroke cases, while hemorrhagic stroke accounts for around 10-20% (2). Both stroke types will promote BBB disruption, neuronal death, and neuroinflammation, which will lead to severe neurological problems (1, 3). So far, treatments for both kinds of strokes are minimal, urging the need to identify therapies that decrease the chances of brain injury and improve recovery upon stroke (4).
After stroke injury, microglial cells can be rapidly activated and migrate into the damaged areas to regulate brain recovery (8). Depending on the activation signals they receive, they can exert dual roles, either promoting injury or facilitating repair (90). In the acute phase of ischemic stroke, microglia/macrophages produce anti-inflammatory cytokines like IL-10, protecting neurons against oxygen and glucose deprivation while promoting tissue repair and regeneration. However, after the acute phase, microglia/macrophages contribute to the exacerbation of inflammation and promote cell death expressing cytokines such as IL-6 and TNF-α (100, 101). In addition, the depletion of microglia 24 hours after an ischemic stroke worsened the ischemic lesion, most probably due to an increased inflammatory response of the remaining macrophages.
Interestingly, in a hemorrhagic stroke, microglia/macrophages experience a distinct phenotypic switch from an immune-activating phenotype during early/acute hemorrhagic stroke towards an immuno-suppressive phenotype in later stages (within 3-7 days after injury) (102, 103).
3.3 Microglial cell behavior in brain aneurysm
Cerebral aneurysms are abnormal swellings or bulges in the blood vessels of the brain (91, 92). There are four main types of intracranial aneurysms: saccular, fusiform, mycotic, and dissecting. Saccular aneurysms are the most common type, accounting for around 90% of all aneurysm cases. They are formed by a blood-filled sac that protrudes from the main artery or one of its branches (104).
A vessel leak or rupture by an aneurysm leads to bleeding into the surrounding tissue in the brain, causing a hemorrhagic stroke, also called a subarachnoid hemorrhage. Studies in animals with subarachnoid hemorrhage have shown that microglial cells undergo dynamic changes transitioning from proinflammatory to anti-inflammatory states (105, 106). Congruently, several clinical studies have also provided evidence that microglial cells accumulate and respond to subarachnoid hemorrhage (107, 108). Thereby, microglial cells release pro-inflammatory mediators like IL-6 and TNF-α that may contribute to secondary brain injuries, neuronal cell death, increased blood-brain barrier permeability and vascular damage, and recruitment of perivascular macrophages, perpetuating the cycle of injury and inflammation (91, 109). However, erythropoietin, a hormone, that regulates red blood cell production, can induce a shift in microglial polarization toward an immunosuppressive phenotype (91, 110). Thereby, microglial cells secrete the anti-inflammatory cytokines and chemokines IL-4 and IL-17 (111), upregulate glutamate receptor 5 (GLUT5) and reduce the production of pro-inflammatory cytokines (i.e. IL-1β, IL-6, TNF-α) (112). This decreases neuronal cell death and clears cell debris, altogether facilitating the resolution of cerebral injury and hemorrhage resulting in a neuroprotective effect (91, 110).
3.4 Microglial cell behavior in glioma
Gliomas are the most common malignant brain tumors in the CNS. Among the different types of gliomas, glioblastoma multiforme (GBM) is the most prevalent and deadly primary brain tumor in human adults. GBMs entail several features of aggressive growth, including a high proliferative rate, resistance to apoptosis, diffuse infiltration and invasion, propensity for necrosis and hypoxia, and vigorous and abnormal angiogenesis (113, 114). In addition, the capacity of GBMs and lower-grade gliomas to invade and infiltrate normal brain tissues makes their complete surgical removal impossible. The residual tumor remnants and their paucity of standard and targeted therapies provide GBM patients with a poor prognosis and a mean survival time of 15 months only (115–117). Thus, the most important survival predictor is still the extent of surgical resection urging the need to identify treatments that block the invasive growth of glioma in order to completely resect the tumor and improve overall survival (118).
The GBM microenvironment consists of a wide variety of immune, stromal, and glial cells with a highly abnormal tumor vasculature that together can form distinct vascular tumor niches (113). In these niches, tumor and brain resident cells interact via cell contact and paracrine signals to promote tumor cell maintenance, growth, and protection against cancer therapies. From the rim of the tumor, tumor cells commonly infiltrate the normal brain parenchyma directly or by moving along nerves and basement membranes including those of vascular structures where they become closely associated with microglial cells (113, 117). It is believed that microglial cells are recruited to and accumulate around tumors as part of a wounding response. Under the influence of glioma cells, microglial cells acquire pro-tumorigenic properties (96). It has been shown that microglial cells can favor tumor progression by releasing pro-tumorigenic growth factors (119), boosting angiogenesis and invasion (120), and helping the tumor to escape the immune response by suppressing cytotoxic T-cell functions and inducing a Treg immunosuppressive response (121).
In addition, peripheral macrophages accumulate within GBMs due to chemoattractive signals emanating from the tumor, which enable macrophage infiltration through the abnormal and leaky tumor vasculature (113). Tumor-associated macrophages (TAMs), including microglial cells and monocyte-derived macrophages, can represent up to 50% of the tumor mass in GBMs (122). While GBM-infiltrating macrophages are predominant in the tumor (Tumor-associated macrophages (TAMs)), resident microglia reside preferentially in the tumor periphery (64, 123).
Several studies have shown that although microglial cells in tumor-bearing mice change their homeostatic microglia signature, one can, to a certain extent, still distinguish glioma-activated microglia from infiltrating TAMs, in part by determining their transcription profile by scRNAseq (Figures 1, 4) (124).
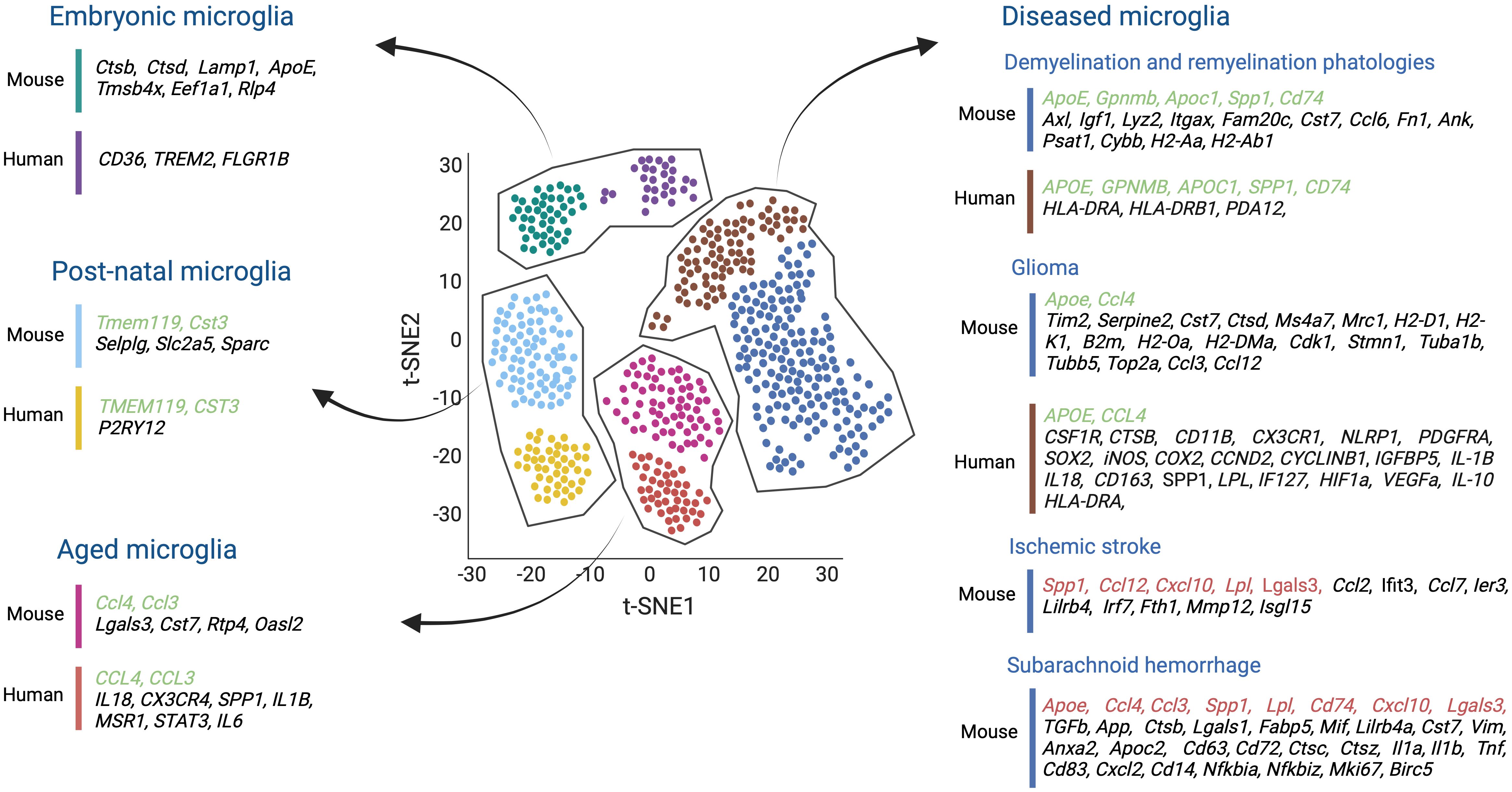
Figure 4 Single-cell transcriptomics studies both in mouse and human microglia, conducted across various stages of development, aging, and in the context of several brain diseases such as demyelination and remyelination pathologies, glioma, ischemic stroke, and subarachnoid hemorrhage, have shed light on the molecular diversity of microglial cells. These studies have helped us to understand the transcriptomic similarities (highlighted in green) and differences (highlighted in black) between mice and humans in healthy and diseased brains. Moreover, comparisons were made between mouse microglial gene expression during ischemic stroke and subarachnoid hemorrhage, and the transcriptional profile of other disease-associated microglia, proving insights into shared molecular responses across different contexts (highlighted in red). Created by Biorender.
TAMs, like microglia, comprise a diverse population capable of both anti-inflammatory/pro-tumorigenic and pro-inflammatory/anti-tumorigenic phenotypes. Pro-inflammatory TAMs can be cytotoxic and have the ability to present antigens to recruit cytotoxic T cells to attack the tumor cells (51). Conversely, anti-inflammatory TAMs can participate in tissue remodeling and angiogenesis, while secreting anti-inflammatory cytokines such as IL-10 and TFG-β to promote tumor growth (125). It is important to note that recent studies revealed that glioma-associated microglia/macrophages display a broader profile of states reflected in their distinct expression profiles that go beyond the immune-related phenotypes. For instance, murine and human TAMs display a higher expression of Gpnmb and Spp1 compared to naïve microglia or peripheral macrophages, which has been correlated with poor patient survival (126). Additionally, RNAseq analysis showed that microglia cells in close conjunction with glioma cells presented a different expression profile than those that did not interact with tumor cells. For example, glioma-associated microglial cells downregulated transcription of factors that promoted tumor sensing and killing like Siglech, Cd33, Gpr34 (microglia sensors for GBM cell ligands), P2ry12 and P2ry13 (microglial ATP receptors responsible for trigger an acute inflammatory response) respectively. Moreover, they upregulated the expression of genes, elevating molecules that are involved in the degradation of the extracellular matrix (ECM), promoting tumor migration and growth (i.e. Mmp12, Mmp13, Mmp14), and immune suppression such as Pd-l1 and Pd-l2 (127). All these observations infer that TAMs are diverse cell populations that most likely play distinct roles in tumor progression and immune evasion.
Given the convincing results of microglia/macrophage implication in glioma growth and progression, targeting approaches similar to those described for microglial cells in the healthy brain, have been assessed. The colony-stimulating factor-1 (CSF1)-CSF1R signaling axis in macrophages/microglia not only regulates microglia/macrophage differentiation and survival, but it also enhances an immune-tolerant state (95, 128). Congruently, pharmacological inhibition of CSF1R (BLZ945) in a PDGFB-driven GBM mouse model skewed macrophages to an immune-stimulating state blocking glioma progression and enhancing survival without depleting microglial cells (95). However, several other preclinical and clinical studies did not observe any significant beneficial effects of CSF1Ri therapy. While recent studies in mice have confirmed that CSF1R inhibition decreased tumor growth in PDGFB-driven GBM, they also reported that it failed to show beneficial effects in mice bearing mesenchymal RAS-driven GBMs or other proneural and mesenchymal GBMs (129). Further, TAM depletion with CSF1Ri (PLX5622) did not confer any survival benefit in oligodendrocyte progenitor cell lineage-associated GBMs or subventricular zone neural stem cell-associated GBMs (130). In line with the preclinical studies, the CSF1Ri PLX3397 did not produce beneficial effects in a GBM Phase I/II clinical trial (131).
Another signaling pathway implicated in promoting protumorigenic features in macrophages has been described for PI3Kγ, a myeloid cell-specific PI3K subunit. PI3Kγ promotes immune suppression by activating mTOR-S6Kα-C/EBPβ and inhibiting NFkβ in macrophages. While PI3Kγ-mTOR-mediated immunosuppression promoted tumor growth, PI3Kγ inhibition reverses these effects by shifting macrophages towards NFkβ -dependent pro-inflammatory polarization (132–134). Moreover, a recent study has shown that PI3Kγ inhibition decreased TAM accumulation in the GBM microenvironment, enhancing temozolomide (TMZ) treatment response in orthotopic murine GBM models (119).
Microglia can also support GBM growth by becoming oxidatively stressed and impairing antigen presentation to CD8+ T-cells. Under these conditions, microglia upregulate the transcription factor Nr4a2, which alters cholesterol homeostasis. Myeloid-specific Nr4a2 knockout reversed immunosuppression by upregulating the MHC-I complex and enhancing antigen presentation for CD8 T cells, subsequently reducing GBM growth (135).
Additionally, inhibiting HMOX1 activity in microglia reduced IL-10-produced T cell exhaustion whereas the JAK1/2 inhibitor ruxolitinib boosted T cell activation by reducing immunosuppressive macrophages (136). Moreover, targeting P-selectin (SELP), a leukocyte adhesion molecule, delayed GBM growth, prolonged survival, and improved immune cell infiltration in vivo. scRNAseq analysis of GBM-shSELP-derived microglia/macrophages showed an increase in pro-inflammation and T-cell activity (137). Thus, P-selectin may play a crucial role in modulating the microglia/macrophage immune response in GBMs.
These examples underscore the impact of blocking immunosuppressive key signaling nodes in macrophages, which albeit not microglia-specific, limit the overall immunosuppressive milieu in GBM. As targeting macrophages with CSF1Ri or blocking negative immune checkpoint regulators like PD1, PD-L1 or CTLA4 are not sufficient as stand-alone therapies to improve the outcome in GBM patients, combining these strategies, also in conjunction with standard chemo- and radiation therapies or other immunotherapies are highly anticipated as they may hold great potential for improving the treatment of gliomas (138).
Overall, the studies exemplified above, provide ample evidence of microglia and macrophage implications in cerebrovascular diseases, including stroke, aneurysm, and glioma. Their responses are plastic and influenced by the complex and dynamic microenvironment of the CNS in the respective conditions. Transcriptomics have greatly helped to gain more insight into the different microglia and macrophage states and their involvement in CNS disease conditions. Understanding the underlying mechanisms of microglial activation and participation in these diseases may offer opportunities for developing targeted therapies to modulate microglial and macrophage activity and improve patient outcomes.
4 Lessons learned from microglia transcriptomics
4.1 Microglial during development and in homeostasis
Advances in single-cell transcriptomic studies have provided invaluable insights into the molecular diversity of microglial cells in humans and mice at different stages of life and in several disease contexts. For instance, the transcriptional profile of different microglial populations was analyzed across several developmental stages in healthy and diseased mouse brains and compared to human MG populations. Single microglial cells from multiple brain regions of healthy embryonic E16.5, juvenile (3 weeks), and adult (16 weeks) mice were analyzed and compared to microglia isolated from mice with neurodegenerative and demyelinating conditions. Unsupervised clustering inferred thirteen different clusters corresponding to developmental microglia (C1-C10), microglia during neurodegeneration (C11), and microglia under demyelination and remyelination conditions (C12-C13). Interestingly, microglia clustered separately depending on the pathological state,’ (139).
Among the microglial developmental clusters (C1-C10), two central states were identified reminiscent of embryonic microglia (C1-C6) and postnatal microglia (C7-C10), which include juvenile and adult mouse samples. All embryonic-related microglia clusters (C1 to C6) generally exhibited the highest heterogeneity. They all expressed lysosome-related genes such as Ctsb, Ctsd, and Lamp1. The C1, C2, and C5 clusters displayed Apoe, and high levels of Tmsb4x, Eef1a1, while Rlp4 gene expression characterized the C6 microglia cluster. Interestingly, factors associated with homeostatic microglia like Tmem119, Selplg, and Slc2a5 inferred the highest expression in the postnatal clusters (C7-C10). In addition, clusters C9 and C10 highly expressed Cst3, a cysteine proteinase inhibitor involved in neurodegenerative diseases of the CNS, and Sparc (Figure 4).
Microglia in both demyelination and remyelination conditions (C12-C13) increased the expression of Apoe, Axl, Igf1, Lyz2, Itgax, Gpnmb, and Apoc1. The C12 cluster corresponding to microglia in demyelination was enriched in Fam20c, Cst7, Ccl6, Fn1, Ank, Psat1, and Spp1 genes. Still, the C13 remyelination cluster was characterized by high Cybb and MHC II gene expression (i.e., Cd74, H2-Aa, H2-Ab1) being reflective of an immune-activated state (Figure 4).
Finally, to compare the similarities and discrepancies between murine and human microglia cells, microglial cells were obtained from healthy human brain tissues. Unbiased clustering showed four main clusters (HHu-C1 to HHu-C4) that partially overlapped with the adult murine microglia clusters. For example, CST3, enriched in the mouse C9 and C10 clusters, was also upregulated in HHu-C1 and HHu-C2 clusters. Moreover, CD45+ cells isolated from the brains of patients with early active multiple sclerosis (MS) were analyzed and compared to the human microglia of healthy patients. Unbiased clustering revealed ten different clusters (Hu-C1 to Hu-C10), but only Hu-C2 to Hu-C8 expressed microglial core genes (i.e. TMEM112 and P2RY12). Hu-C5, Hu-C6, and Hu-C7 clusters corresponded to microglia from healthy brains and expressed high levels of the MG-core genes, resembling an expression profile of healthy mouse postnatal C7 to C10 clusters. In addition, the microglial Hu-C2, Hu-C3, and Hu-C8 clusters from the diseased MS brains resembled the C12 and C13 clusters of disease-related mice. All clusters were enriched in the expression of APOE and downregulated the typical MG-core genes. Specifically, Hu-C2 had high APOC1 and GPNMB levels, and Hu-C3, like the murine C13 cluster, exhibited increased transcription of MHC II- related genes (i.e., CD74, HLA-DRA, and HLA-DRB1). Hu-C8, similar to the mouse C12 cluster, showed high levels of SPP1 and PADI2 expression (139) (Figure 4).
Taken together, during homeostasis different microglia subpopulations were found from embryonic to adulthood, but they did not cluster separately and showed a somewhat similar expression profile with the human homologs. However, microglia responses in distinct disease settings (i.e., neurodegeneration, demyelination, and remyelination) were transcriptionally quite different from each other as their responses were tailored to the specific pathology.
4.2 Microglia during aging
Other scRNAseq studies have investigated transcriptional changes of aging microglial cells during murine and human lifespans. In a first study, microglial cells were isolated from mouse embryos at E14.5, from postnatal brains at P4/5, from brains of late juvenile mice at P30, P100, and aged mice at P540. Aged microglia were found to express inflammatory-related genes such as Lgals3, Cst7, Ccl4, Ccl3, Rtp4, and Oasl2, and revealed a limited transcriptomics heterogeneity compared to microglia from younger mice (140) (Figure 4). These results were supported by another study which demonstrated that early postnatal microglia were more heterogeneous than adult microglia. They also discovered an early postnatal phagocytic state of proliferative-region-associated microglia (PAM) with a similar gene signature to that of degenerative disease-associated microglia (DAM) (141). Interestingly, scRNAseq on human microglia isolated from pre-natal (2nd trimester), pediatric (18 months – 24 months), adolescent (10-14 years old), and adult (40-62 years old) brain samples revealed pre-natal microglia with a higher phagocytic activity (i.e., upregulation of CD36, TREM2, and FCGR1B) compared to those at an adult stage. Adult microglia, however, were more immune responsive (i.e., upregulation of the pro-inflammatory cytokines IL18 and CX3CR4, the interferon-response genes CCL3 and CCL4 as well as SPP1, IL1B, and MSR1) (142) (Figure 4). Likewise, microglia from patients aged 50 years and older had higher SPP1 expression levels compared to microglia from younger adults (143) (Figure 4). Further support for an increased microglial inflammation state during aging stems from Lopes et al., exposing upregulated inflammatory pathway activities in microglia of humans with increasing age, including STAT3 and IL-6 signaling, the (LXR)/retinoid X receptor (RXR) activation and liver X receptor (144) (Figure 4).
Taken together, scRNA-seq analyses revealed reduced transcript heterogeneity but increased inflammatory signatures in aging microglial cells, both in mice and humans.
It is noteworthy that these studies would further gain from revealing potential gender differences in microglia expression profiles and subsequent function as several studies already described differences in microglia among genders from several developmental states (i.e. embryonic, early postnatal, adult, and aged) and neurodegenerative diseases. For example, in a model of ischemic stroke, transplanting female microglia into male mice reduced the progression of ischemic damage compared to transplanting male microglia, suggesting a protective role of female microglia in ischemia (145, 146).
4.3 Microglial transcriptomics in CNS disease
4.3.1 Glioma
A recent study performed scRNA-seq analysis of murine GL261 tumors and discovered two new microglia states compared with microglia from naive brains. Both states showed an upregulation of Timp2, Serpine2, Cst7, and Ctsd, genes related to the reorganization of the extracellular matrix, and Apoe, Ms4a7, and Mrc1. However, while one of these GBM-related microglia states was enriched in transcripts for MHC I (i.e., H2-D1, H2-K1, and B2m) and MHC II (i.e., H2-Oa and H2-DMa), the other one mainly expressed genes related to cell proliferation, including Cdk1, Stmn1, Tuba1b, Tubb5, and Top2a. Transcriptional network analysis further demonstrated that MHC IIhigh-activated microglia express more chemokine-encoding genes (i.e., Ccl3, Ccl4, and Ccl12), suggesting that this subset of microglial cells may help to recruit other immune cells (124) (Figure 4).
Similarly, scRNA-seq analysis of human microglia from five glioma patients also identified a subset of high-grade glioma-associated microglial cells (HGG-AM) in IDH1-WT/SETD2-mutant GBM. This specific microglia subset expressed core microglia markers, including CSF1R, CTSB, and CD11B. It also upregulated CX3CR1, NLRP1, PDGFRA, and SOX2, while decreasing the expression of several homeostatic genes, including P2RY12 and TMEM119. Interestingly, this cluster exhibited pro-inflammatory and proliferative signatures (i.e., NLRP1, iNOS, COX2, CCND2, CYCLINB1, IGFBP5) and shaped the cytokine microenvironment (i.e., IL-1B and IL18) as previously reported for the murine glioma-associated microglial cells (147) (Figure 4). In line, Sankowski et al., identified two human glioma-associated microglia (GAMs) clusters with low expression of the microglia core genes, including CX3CR1 and SELPLG, and high expression of metabolic, inflammatory, and interferon-associated genes such as CD163, SPP1, APOE, LPL, and IF127. In addition, these clusters presented a MHC II gene signature similar to glioma-associated microglia cells in mice. Some cells also expressed hypoxia-associated genes such as HIF1a and VEGF-A (143) (Figure 4). Moreover, another single-cell study revealed immunosuppressive CD163+HMOX1+ microglia in human GBM samples that expressed tumor-associated macrophage genes such as CD163, CCL4, APOE, and HLA-DRA and showed a significant enrichment of pathways involved in antigen-processing and cytokine signaling similar to the mouse glioma-associated microglial cells. Additionally, this population induced T-cell exhaustion via the release of IL-10 (136) (Figure 4).
Taken together, murine and human glioma-associated microglia cells exhibit a similar pro-inflammatory and proliferative phenotype with a characteristic upregulation of several metabolic pathways and reduced expression of microglia core genes.
4.3.2 Stroke
scRNAseq analysis of brain areas with an ischemic stroke helped to identify unique microglia subpopulations with specific transcriptional profiles. For example, Zheng et al., performed scRNAseq on the MCAO (middle cerebral artery occlusion) mouse model of ischemic stroke and identified five unique microglia states (MG 0-4) (148). All microglia clusters generally expressed the typical microglial markers (i.e., Gpr34, Olfml3, P2ry12, TMEM119, Selplg, and Siglech) (Figure 4). However, most ischemic-associated microglia clusters (MG 2-4) had a relatively low expression of these markers and contained, to some extent, inflammatory signatures. For instance, these microglia upregulated several monocyte chemotactic protein family genes such as CCL12, CCL2, and CCL7, as well as IER3 (a gene associated with hypoxia). In addition, typical genes of neurodegeneration-associated microglial subgroups (i.e., Lgals3, Lilrb4, Lpl, Spp1, and Fth1) (8) and MMP12 (matrix metalloproteinases 12) known to cause damage to the BBB upon ischemic stroke (149) were also upregulated (Figure 4). Moreover, the expression levels of genes involved in IFN signaling, such as Isgl15, Ifit3, Irf7, and Cxcl10, were also increased. Additionally, transcription of genes implemented in cell proliferation like Top2a, Stmn1, Birc5, and Ube2c were also upregulated (148) (Figure 4).
4.3.3 Subarachnoid hemorrhage
A scRNAseq study of microglia post subarachnoid hemorrhage (SAH) compared to healthy mouse brain microglia identified three clusters in the SAH-affected brains (SMG-C5, SMG-C6, and SMG-C7), with all exhibiting inflammatory signatures accompanied by high expression of CCL, GALECTIN, TGFb, APP, and SPP1. The SMG-C5 cluster revealed high expression of Spp1, Lpl, Apoe, Ctsb, Lgals1, Lgals3, Fabp5, Mif, Lilrb4a, Cst7, and Vim (Figure 4). Noticeably, some of these genes were upregulated in disease-associated microglia (DAM) and injury-responsive microglia (IRM). In addition, a gene expression profile entailing Anxa2, Apoc2, CD63, CD72, Ctsc, and Ctsz was also upregulated and similar to that of microglia in developing brain and in mouse models of Alzheimer’s disease and lysolecithin (LCP) injury (140, 150). The SMG-C6 cluster expressed several cytokines (i.e. Il1a, Il1b, Tnf, Ccl4, and Ccl3), chemokines (i.e. Cxcl10 and Cxcl2), and other immune signal-regulating genes (i.e. CD83, CD74, CD14, Nfkbia, and Nfkbiz). Some of these markers were previously identified in IRM subpopulations in Alzheimer’s disease and multiple sclerosis (MS) (140, 150, 151) (Figure 4). The SMG-C7 cluster increased the transcription of genes promoting proliferation, such as Birc5, Mki67, and Fabp,5 like in developing microglia (152) (Figure 4).
In summary, single-cell studies have provided an excellent overview of the distinct microglial transcriptomic profiles in neurovascular diseases such as glioma, ischemic stroke, and subarachnoid hemorrhage. Independently of the number of microglia clusters found, some presented similarities. For instance, the disease-associated microglia (GAMs in glioma, ischemic-associated MG in MCAO, and DAM and IRM in SAH exhibit a decrease in several microglial core genes such as CX3CR1 and TMEM119, a high presence of inflammatory signatures, and an upregulation of distinct proliferation markers. These findings underscore the dynamic nature of microglial responses in diverse neurological conditions, providing valuable insights for potential therapeutic interventions targeting these intricate immune cells.
5 Microglia targeting for treating cerebrovascular diseases
5.1 Microglia depletion in brain disease
In the context of a stroke, researchers have explored microglial depletion through various approaches to better understand its impact on disease outcomes. For instance, pharmacological agents such as PLX5622 and PLX3397 have been used to deplete microglia before middle cerebral artery occlusion. Surprisingly, results indicated that microglial depletion worsened disease outcomes, leading to increased brain injury and altered neuronal activity. Thus, it is conceivable that, at an early stage, microglia may still be able to elicit protective functions against injury (153, 154). Similarly, experiments involving microglia depletion using PLX3397 and CX3CR1CreER iDTR transgenic mice, both before and after middle cerebral artery occlusion, demonstrated aggravated neurological functions, brain inflammation, cell death, and leukocyte infiltration within the initial days following stroke (66, 155, 156). These findings confirm that microglial cells provide beneficial effects during the first days after stroke, reducing cell death, neurological deficits, leukocyte infiltration, and neuroinflammatory markers (66, 155, 156). However, long-term microglia depletion using PLX5622 post-ischemic stroke decreased neuroinflammation, affecting the delicate homeostatic balance (157). Currently, the optimal window for microglia depletion to enhance recovery remains unknown. Emerging data suggest that microglial activity and function are essential both before and within the initial days of stroke to reduce peripheral cell infiltration and cytotoxicity. Nevertheless, prolonged depletion may become detrimental (158), highlighting the need to target microglia within an optimal therapeutic window.
In summary, MGs exert both beneficial and detrimental effects in the context of a stroke. Their presence during the early onset of a stroke appears to have a neuroprotective role, while long-term depletion may disrupt the delicate balance of neuroinflammatory responses. Understanding the precise timing and duration of microglial interventions may pave the way for targeted therapeutic strategies to enhance stroke recovery and improve patient outcomes.
In the setting of aneurysms, microglia play a crucial role in the inflammatory response within the surrounding area, which may contribute to secondary brain injuries, perpetuating the cycle of inflammation after an initial injury (91, 109). Therefore, several studies have focused on the depletion of MGs via genetic and pharmacological approaches to study its impact on the disease’s development and its effect on the recovery post-aneurysm. For instance, studies have reported that microglia depletion in vivo using the CD11b-HSVTK mouse model, in which all CD11b+ cells are depleted upon ganciclovir administration in the brain, resulted in reduced neuronal cell death and improved vasospasm following aneurysmal subarachnoid hemorrhage (SAH) (108). Similar results were observed using liposome-encapsulating clodronate. Upon intracerebroventricular injection of clodronate in early-phase SAH, a decrease in neuronal cell death was observed, suggesting that neuronal apoptosis was microglial-dependent. However, in late-phase SAH, neuronal cell death was associated with TLR4- toll receptor-associated activator of interferon (TRIF) and was independent of the microglia presence. In both early and late-phase SAH, microglia were shown to be necessary and sufficient to cause vasospasm since microglia depletion improved the vasospasm outcome (159). In addition, MG depletion with the CSF1R inhibitor PLX3397 following SAH reduced neuronal cell death due to a decrease in microglia accumulation and activation (160).
These findings highlight the importance of microglial cells in the pathophysiology of aneurysmal SAH and suggest that strategies to modulate microglial activation could mitigate secondary brain injuries and vasospasms in individuals with aneurysms. Further research may provide valuable insights into novel treatment strategies for this serious medical condition.
Microglia/TAM-targeting therapies have also been conducted in gliomas, given their role in promoting glioma progression, angiogenesis, and invasion (120). Several studies have indicated that targeting MGs via CSF1R inhibition can delay recurrence and extend overall survival in several glioma models by inducing a more pro-inflammatory phenotype in immune cells (95, 161). Despite these promising results, CSF1R inhibition has not demonstrated efficacy in pre-clinical and clinical glioma studies (131, 162, 163). Several reasons have been identified for this lack of success. CSF1R inhibition monotherapy may not be sufficient to switch the balance of TAMs/microglia to an immunostimulating state and it can promote the development of acquired resistance mechanisms such as 1) increased insulin-like growth factor-1 receptor (IGF-1R) expression and aberrant phosphoinositide 3-kinase (PI3K) activity on cancer cells (162), 2) enhanced influx of Foxp3+ regulatory T cells (Tregs) (164), 3), high recruitment of pro-tumorigenic cell populations such as myeloid-derived suppressor cells (165), 4) elevated levels of glioma-secreted factors such as granulocyte-macrophage CSF (GM-CSF) and interferon-y (IFN-y) (95), and 5) up-regulation of T-cell immune checkpoint molecules such as programmed cell death 1 ligand (PD-L1) and cytotoxic T-lymphocyte-associated protein 4 (CTLA-4) (166, 167), increased recruitment of tumor-infiltrating lymphocytes (TILs) (168).
Due to the failure of monotherapy using CSF1R inhibitors, researchers are currently exploring combinatorial therapies as a promising approach to target the tumor from different angles. For instance, in the context of gliomas, the combination of CSF1R inhibitors and radiotherapy has shown synergistic results by effectively suppressing tumor growth (169). Likewise, when CSF1R inhibitors were combined with anti-PD-1 therapy, there was a notable increase in overall survival in mice (161, 168). In addition, CSF1R inhibition was also combined with anti-angiogenic therapy using cediranib (a VEGFR2 inhibitor), reducing cell proliferation and altering the tumor morphology (129). Most interesting, a new phase I study using SYHA1813, a VEGFR and CSF1R dual inhibitor, has been reported to show anti-tumor activity in patients with recurrent High-Grade Gliomas and Advanced Solid Tumors (170).
Further research is needed to explore combination therapies and strategies that can potentiate the effects of CSF1R inhibition while addressing the mechanisms of acquired resistance. Understanding the complex interplay between microglial cells and the tumor microenvironment will be critical in developing effective treatments for glioma.
5.2 MG repopulation in brain disease
To date, there are no reported studies on the outcomes of microglial repopulation upon depletion in stroke, aneurysm, or glioma. Further research in this area is essential to understand the dynamics of microglial repopulation and its potential implications on the recovery of these diseases upon previous microglia depletion. However, one can speculate about the potential positive effects of repopulating microglia. For instance, in the context of stroke, repopulated microglia that elicit anti-inflammatory properties could help decrease the inflammation in the injured area, facilitating a faster recovery. This, however, is challenging as it would require stable reprogramming of repopulating microglia to hinder their polarization to a pro-inflammatory state in the brain, which would exacerbate the stroke-induced injury (45, 90).
Similarly, in the context of aneurysms, the phenotypic state of the newly re-populated microglia will be essential for the outcomes of the inflammatory responses that will affect the stability of the aneurysm wall and the resolution of the injury upon aneurysm disruption. Since anti-inflammatory macrophages have been shown to secrete several cytokines and chemokines, the best possible outcome would be that repopulating microglia adopt a wound-healing state to facilitate the resolution of the cerebral injury and hemorrhage upon aneurysm disruption (91). However, the same concern as in stroke arises if re-populating microglia become pro-inflammatory and perpetuate inflammation (91).
In the situation of gliomas, repopulating microglia should be immune-stimulating to enable restoration of immune surveillance and anti-tumor immune responses. This would also facilitate the recruitment of other immune cells, such as T cells, natural killer (NK) cells, and dendritic cells, thereby contributing to a more effective anti-tumor immune response (171). However, it is essential to consider the potential negative effects as well. Re-populating microglia may promote immunosuppression, angiogenesis, and tissue remodeling, creating a tumor-supportive environment (96, 113, 172). Additionally, in some cases, re-populated microglia may contribute to the suppression of anti-tumor immunity by inducing the recruitment of regulatory T cells (Tregs) or myeloid-derived suppressor cells (MDSCs), which can dampen the immune response (121, 171).
Expanding our knowledge in this field will help to decipher whether microglia-targeted therapies could be a good treatment option for these devastating diseases. Further research is needed to ensure that MG-targeting or depletion strategies do not back-fire and improve the patient’s prognosis and quality of life.
5.3 Microglia re-education or re-programming in brain disease
An alternative strategy that circumvents the need for microglia depletion and repopulation is an endogenous re-education of microglia/macrophages.
Microglia re-programming has long been studied in the context of gliomas given the key role that microglia/macrophages play in this disease progression. Some studies have shown that CSF1R inhibition with BLZ945 and PLX3397 depleted macrophages in healthy tissues. However, glioma-TAMs were not depleted with BLZ945 but reprogrammed toward a less pro-tumoral phenotype (95, 173). Why certain CSF1R inhibitors deplete microglia while others reeducate microglia and TAMs, is unknown. As it may be, CSF1R inhibitors have only shown modest improvement in the short-term survival of mice bearing PDGF-B driven gliomas (162). Thus, other strategies have been used to re-educate TAMs, including the inhibition of the myeloid PI3K pathway, thereby shifting macrophage polarization toward a pro-inflammatory phenotype in preclinical studies of GBM (119) (Figure 3).
Another method to re-program TAMs is via blocking the CCL5/CCR5 signaling either by targeting CCR5 (a G-protein coupled receptor) or by using a neutralizing antibody against CCL5. CCL5 and CCR5 expression correlates with poor prognosis and shorter patient survival in GBMs. It has been shown that glioma invasion depends on the CCL5/CCR5 signaling axis and it is inhibited by the CCR5 antagonist maraviroc (174). Moreover, CCR5 is also expressed in microglia/macrophages and has been linked to inducing their activation and polarization mediating immunosuppression in glioma (174, 175). Maraviroc treatment was able to shift TAMs to become more anti-tumoral by decreasing the gene expression of Arg1 and IL-10, and increasing the levels of NO and IL-1β upon microglia/macrophages LPS-IFNγ-conditioned medium stimulation in vitro (175) (Figure 3).
CD40 agonist treatment in combination with D2C7-IT, a dual immunotoxin that targets specifically the human epidermal growth factor receptor (EGFR) and mutant EGFR variant III (vIIII) proteins, has also been reported to repolarize macrophages and boost their antitumoral immune response in GBM models increasing patient survival outcomes (176) (Figure 3). Interestingly, a phase I trial with D2C7-IT in combination with 2141-V11 (an anti-CD40 monoclonal antibody) has recently started in patients with recurrent malignant glioma (NCT04547777).
Moreover, engineered oncolytic viruses specifically designed to kill cancer cells have shown a significant impact on GBM progression since these viral particles expressing IL-12 increased tumor cell death and shifted the TAMs towards a pro-inflammatory phenotype, improving anti-PD1 and anti-CTLA-4 immunotherapies outcomes (177) (Figure 3). Additionally, certain studies also showed that dual-inhibition of VEGF and Ang2 in glioma models prolonged survival compared with single VEGF inhibition by reprograming Tie2+ TAMs toward a pro-inflammatory/anti-tumoral phenotype (178) (Figure 3).
In summary, microglia/TAM re-education offers a promising alternative strategy to overcome any obstacles that may appear upon their depletion and consequent re-population. Several approaches of re-educating microglia/TAMs have provided promising effects on reducing tumor growth in pre-clinical studies, but the effects in GBM remain modest. Thus, TAM/microglia repolarization toward a more pro-inflammatory/anti-tumoral phenotype will be more beneficial in combination with other immune-related therapies such as anti-PD1, anti-CTLA-4, or CAR-T, or even just standard chemo-or radiation therapy improving tumor growth reduction and overall patient survival.
5.4 Myeloid adoptive cell transfer
Another option is to re-populate microglial cells via adoptive cell transfer of pre-defined myeloid cells. Functional blood-derived monocytes, bone marrow-derived macrophages, or stem cell-derived myeloid cells could be used to re-populate the microglia-depleted brain in different stages, including pre-activated or genetically modified forms (Figure 3). It has been demonstrated that blood monocytes can be differentiated into blood monocyte-derived microglial cells via treatment with GM-CSF and IL-34. These cells share striking similarities with the brain-resident microglia, exhibiting a ramified morphology and expressing microglial genes such as Tmem119 and P2ry12. Moreover, they display efficient phagocytic capabilities, contributing to debris clearance. Interestingly, these microglia-like cells display distinct characteristics from monocytes, macrophages, and dendritic cells (179, 180).
A different approach for myeloid adoptive cell transfer would be the generation of microglia-like cells from bone marrow-derived macrophages. For instance, it has been reported that bone marrow-derived mononuclear cells treated with GM-CSF and IL-4 became microglia-like cells, demonstrating therapeutic effects in a mouse model of amyotrophic lateral sclerosis (181) (Figure 3).
Another more recent approach is the generation of microglia-like cells from human inducible pluripotent stem cells (hiPSCs). For example, it has been shown that CSF1 and IL-34 can differentiate hiPSCs into Tmem119+/P2RY12+ microglia-like cells (182). Furthermore, complementation with specific factors and co-culture with astrocytes have also been successful in converting hiPSCs into microglia-like cells (183) (Figure 3).
All the above-mentioned options rely on the differentiation of “precursor cells” into functional microglial-like cells. The newly derived MGs could be transplanted in cases where the existing MGs/macrophages are depleted, effectively restoring functional macrophages aiming at the resolution of cerebrovascular diseases. Furthermore, these MG-like cells could be stimulated in vitro prior to cell transfer, enhancing their ability to resolve injuries or fight against the tumor cells in the context of GBMs.
Another unexplored alternative involves the transplantation of fully functional microglial cells into patients (Figure 3). This cell-based therapy has already been studied in the context of stroke, aiming at attenuating brain injury and improving neurological outcomes. For instance, transplantation of HMO6 cells (a human microglia cell line) into rats that had chronic cerebral ischemia resulted in a reduction in white matter damage caused by ischemia, attributed to the downregulation of matrix metalloproteinase (MMP)-2 levels in microglia (184). Moreover, other studies explored the transplantation of M2-like activated murine microglia cell line BV2 into recipient mice post-middle cerebral artery occlusion (MCAO). This intervention led to a decrease in brain damage resulting from ischemia and the promotion of angiogenesis (185).
As such, microglial cell transplantation has emerged as a novel therapeutic strategy to reduce the impact of stroke injuries and to improve neurological recovery upon such injuries. However, it is essential to note that further research is also required in this area which is still in its infancy. This is particularly important because some studies revealed that microglial transplantation in rats did not confer any beneficial effects in cases of permanent cerebral ischemia (186).
Overall, the potential of reprogramming and repopulating microglia through adoptive myeloid cell therapy holds significant promise for enhancing outcomes in both cerebrovascular diseases and neurological disorders. Exploiting the plasticity of these immune cells and directing them toward beneficial phenotypes could open up novel therapeutic pathways.
6 Conclusions and future perspectives
In recent years, significant progress has been made in our understanding of microglial functions in maintaining homeostasis in the healthy brain, and their implication in neuroinflammatory diseases. Thereby, a major advancement has been the development of single-cell and multi-omics technologies and the integration of gene and protein expression profiles, which have identified diverse and context-dependent microglial states across species and models. These analyses have provided new insight into the molecular mechanisms of microglia functions, revealing multiple cell states reflective of their plasticity to display their context-dependent multifunctionality during development, homeostasis, and CNS disease. These studies also underline the recent request for a new microglial terminology to more faithfully describe microglia’s dynamic states and depict their complex nature and function in a context-dependent manner (47).
Notwithstanding, while researchers have made substantial contributions regarding the origin, morphology, and states of microglial cells in healthy conditions, it is still challenging to distinguish microglia from infiltrating macrophages in several disease settings, and, importantly, to specifically target microglia without affecting other macrophage populations. Such a distinction is crucial to infer to which extent and by which means the regulation of the neuroinflammatory response and tissue repair is commonly or distinctly orchestrated by microglial cells or other macrophage types. So far, current therapeutic strategies aimed at harnessing the neuroprotective potential or decreasing harmful responses of microglia cells affecting also other macrophages. To specifically modulate microglial behavior, there is an urgent need to gain more insight into the complex function of and interplay between microglia, other brain-resident macrophages, and infiltrating macrophages, and their communication with other cell types in the brain.
So far, targeting both microglia and macrophages has already shown some promising results in several pre-clinical settings of stroke, aneurysm, and glioma. However, as most of these approaches are not sufficient yet to mount a clinically relevant response, further research in this field is urgently needed to improve patient outcome. This may be achieved by current approaches that include combinatorial strategies with other standard or targeted treatment modalities and the right timing and duration to target microglial cells. An important asset for these strategies is also the application of myeloid adoptive cell transfers, which introduces new treatment opportunities by exploiting the surveillance and sentinel functions of microglial cells. Although still in its infancy, such an approach may hold grand promise in harnessing the patrolling capabilities inherent in microglial cells and directing them toward injury sites to facilitate prompt and targeted therapeutic interventions.
Finally, there are also new exciting developments on the horizon of which one is the potential involvement of microglial cells in the gut-brain axis. There is increasing evidence of the bidirectional communication between the gut and brain in the maintenance of physiologic homeostasis, as well as in the onset and development of several pathologic conditions, including neurodegenerative disorders, such as Parkinson’s disease and Alzheimer’s disease. Patients with neurodegenerative diseases often exhibit gastrointestinal disorders and those with inflammatory bowel diseases can experience neurological disorders. In these patients, for example, microglial cells appear to activate astrocytes (reactive astrocytes) that are implicated in perpetuating neuroinflammatory processes that contribute to apoptosis of oligodendrocytes and synaptic nerve degeneration (187, 188).
Further research in these exciting research arenas is needed to deepen our understanding not only of the microenvironmental but also of the macroenvironmental influences on microglial behavior and biology and pave the way for new and innovative treatments that will enhance brain health and combat neurological disorders.
Author contributions
CP-M: Writing – review & editing, Writing – original draft. GB: Writing – review & editing, Writing – original draft.
Funding
The author(s) declare that financial support was received for the research, authorship, and/or publication of this article. This work was supported by grants from the Flemish government FWO (11F1421N to CP-M), Stichting tegen Kanker (STK 2018-086 FAF-F/2018/1303 to GB), and has received funding from the European Union’s Horizon Europe research and innovation programme under the Marie Sklodowska-Curie Dorctoral Networks grant agreement No. 101073386 (Glioresolve to GB).
Conflict of interest
The authors declare that the research was conducted in the absence of any commercial or financial relationships that could be construed as a potential conflict of interest.
Publisher’s note
All claims expressed in this article are solely those of the authors and do not necessarily represent those of their affiliated organizations, or those of the publisher, the editors and the reviewers. Any product that may be evaluated in this article, or claim that may be made by its manufacturer, is not guaranteed or endorsed by the publisher.
References
1. Obermeier B, Daneman R, Ransohoff RM. Development, maintenance and disruption of the blood-brain barrier. Nat Med. (2013) 19:1584–96. doi: 10.1038/nm.3407
2. Kadry H, Noorani B, Cucullo L. A blood–brain barrier overview on structure, function, impairment, and biomarkers of integrity. Fluids Barriers CNS. (2020) 17:69. doi: 10.1186/s12987-020-00230-3
3. Herculano-Houzel S. The human brain in numbers: a linearly scaled-up primate brain. Front Hum Neurosci. (2009) 3. doi: 10.3389/neuro.09.031.2009
4. Lovinger DM. Communication networks in the brain: neurons, receptors, neurotransmitters, and alcohol. Alcohol Res Health. (2008) 31(3):196–214.
5. Clarke LE, Barres BA. Emerging roles of astrocytes in neural circuit development. Nat Rev Neurosci. (2013) 14:311–21. doi: 10.1038/nrn3484
6. Simons M, Nave K-A. Oligodendrocytes: myelination and axonal support. Cold Spring Harbor Perspect Biol. (2016) 8:a020479. doi: 10.1101/cshperspect.a020479
7. Li Q, Barres BA. Microglia and macrophages in brain homeostasis and disease. Nat Rev Immunol. (2018) 18:225–42. doi: 10.1038/nri.2017.125
8. Butovsky O, Weiner HL. Microglial signatures and their role in health and disease. Nat Rev Neurosci. (2018) 19:622–35. doi: 10.1038/s41583-018-0057-5
9. Prinz M, Jung S, Priller J. Microglia biology: one century of evolving concepts. Cell. (2019) 179:292–311. doi: 10.1016/j.cell.2019.08.053
10. Zhang L, Cao Y, Zhang X, Gu X, Mao Y, Peng B, et al. The origin and repopulation of microglia. Dev Neurobiol. (2022) 82:112–24. doi: 10.1002/dneu.22862
11. Ronaldson PT, Davis TP. Regulation of blood–brain barrier integrity by microglia in health and disease: A therapeutic opportunity. J Cereb Blood Flow Metab. (2020) 40:S6–S24. doi: 10.1177/0271678X20951995
12. Borst K, Schwabenlanda M, Prinz M. Microglia metabolism in health and disease. Neurochemistry Int. (2019) 130:104331. doi: 10.1016/j.neuint.2018.11.006
13. McGrath KE, Koniski AD, Malik J, Palis J. Circulation is established in a stepwise pattern in the mammalian embryo. Blood. (2003) 101:1669–75. doi: 10.1182/blood-2002-08-2531
14. Rigato C, Buckinx R, Le-Corronc H, Rigo JM, Legendre P. Pattern of invasion of the embryonic mouse spinal cord by microglial cells at the time of the onset of functional neuronal networks. Glia. (2011) 59:675–95. doi: 10.1002/glia.21140
15. Swinnen N, Smolders S, Avila A, Notelaers K, Paesen R, Ameloot M. Complex invasion pattern of the cerebral cortex bymicroglial cells during development of the mouse embryo. Glia. (2013) 61:150–63. doi: 10.1002/glia.22421
16. Navascués J, Calvente R, Marín-Teva JL, Cuadros MA. Entry, dispersion and differentiation of microglia in the developing central nervous system. Anais da Academia Bras Ciências. (2000) 72:91–102. doi: 10.1590/S0001-37652000000100013
17. Ginhoux F, Greter M, Leboeuf M, Nandi S, See P, Gokhan S, et al. Fate mapping analysis reveals that adult microglia derive from primitive macrophages. Science. (2010) 330:841–5. doi: 10.1126/science.1194637
18. Nikodemova M, Kimyon RS, De I, Small AL, Collier LS, Watters JJ, et al. Microglial numbers attain adult levels after undergoing a rapid decrease in cell number in the third postnatal week. J Neuroimmunology. (2015) 278:280–8. doi: 10.1016/j.jneuroim.2014.11.018
19. Hoeffel G, Wang Y, Greter M, See P, Teo P, Malleret B, et al. Adult Langerhans cells derive predominantly from embryonic fetal liver monocytes with a minor contribution of yolk sac–derived macrophages. J Exp Med. (2012) 209:1167–81. doi: 10.1084/jem.20120340
20. Ginhoux F, Merad M. Ontogeny and homeostasis of Langerhans cells. Immunol Cell Biol. (2010) 88:387–92. doi: 10.1038/icb.2010.38
21. Arnold T, Betsholtz C. The importance of microglia in the development of the vasculature in the central nervous system. Vasc Cell. (2013) 5:4. doi: 10.1186/2045-824X-5-4
22. Kierdorf K, Erny D, Goldmann T, Sander V, Schulz C, Perdiguero EG, et al. Microglia emerge from erythromyeloid precursors via Pu.1- and Irf8-dependent pathways. Nat Neurosci. (2013) 16:273–80. doi: 10.1038/nn.3318
23. Mondo E, Becker SC, Kautzman AG, Schifferer M, Baer CE, Chen J. A developmental analysis of juxtavascular microglia dynamics and interactions with the vasculature. J Neurosci. (2020) 40:6503–21. doi: 10.1523/JNEUROSCI.3006-19.2020
24. Hoshiko M, Arnoux I, Avignone E, Yamamoto N, Audinat E. Deficiency of the microglial receptor CX3CR1 impairs postnatal functional development of thalamocortical synapses in the barrel cortex. J Neurosci. (2012) 32:15106–11. doi: 10.1523/JNEUROSCI.1167-12.2012
25. Erblich B, Zhu L, Etgen AM, Dobrenis K, Pollard JW. Absence of colony stimulation factor-1 receptor results in loss of microglia, disrupted brain development and olfactory deficits. PloS One. (2011) 6:e26317. doi: 10.1371/journal.pone.0026317
26. Garceau V, Smith J, Paton IR, Davey M, Fares MA, Sester DP, et al. Pivotal Advance: Avian colony-stimulating factor 1 ( CSF-1 ), interleukin-34 ( IL-34 ), and CSF-1 receptor genes and gene products. J Leukocyte Biol. (2010) 87:753–64. doi: 10.1189/jlb.0909624
27. Attaai A, Neidert N, von Ehr A, Potru PS, Zoller T, Spittau B, et al. Postnatal maturation of microglia is associated with alternative activation and activated TGFβ signaling. Glia. (2018) 66:1695–708. doi: 10.1002/glia.23332
28. Vainchtein ID, Chin G, Cho FS, Kelley KW, Miller JG, Chien EC. Astrocyte-derived interleukin-33 promotes microglial synapse engulfment and neural circuit development. Science. (2018) 359:1269–73. doi: 10.1126/science.aal3589
29. Pasciuto E, Burton OT, Roca CP, Lagou V, Rajan WD, Theys T. Microglia require CD4 T cells to complete the fetal-to-adult transition. Cell. (2020) 182:625–640.e24. doi: 10.1016/j.cell.2020.06.026
30. Réu P, Khosravi A, Bernard S, Mold JE, Salehpour M, Alkass K, et al. The lifespan and turnover of microglia in the human brain. Cell Rep. (2017) 20:779–84. doi: 10.1016/j.celrep.2017.07.004
31. Huang Y, Xu Z, Xiong S, Sun F, Qin G, Hu G, et al. Repopulated microglia are solely derived from the proliferation of residual microglia after acute depletion. Nat Neurosci. (2018) 21:530–40. doi: 10.1038/s41593-018-0090-8
32. Cronk JC, Filiano AJ, Louveau A, Marin I, Marsh R, Ji E, et al. Peripherally derived macrophages can engraft the brain independent of irradiation and maintain an identity distinct from microglia. J Exp Med. (2018) 215:1627–47. doi: 10.1084/jem.20180247
33. Domingues HS, Portugal CC, Socodato R, Relvas JB. Oligodendrocyte, astrocyte, and microglia crosstalk in myelin development, damage, and repair. Front Cell Dev Biol. (2016) 4. doi: 10.3389/fcell.2016.00071
34. Kang R, Gamdzyk M, Lenahan C, Tang J, Tan S, Zhang JH, et al. The dual role of microglia in blood-brain barrier dysfunction after stroke. Curr Neuropharmacology. (2020) 18:1237–49. doi: 10.2174/1570159X18666200529150907
35. Wang Y, Huang Z. Microglia interact with neurons by forming somatic junctions. Neurosci Bull. (2020) 36:1085–8. doi: 10.1007/s12264-020-00517-3
36. Paolicelli RC, Bolasco G, Pagani F, Maggi L, Scianni M, Panzanelli P, et al. Synaptic pruning by microglia is necessary for normal brain development. Science. (2011) 333:1456–8. doi: 10.1126/science.1202529
37. Peng J, Liu Y, Umpierre AD, Xie M, Tian D-S, Richardson JR, et al. Microglial P2Y12 receptor regulates ventral hippocampal CA1 neuronal excitability and innate fear in mice. Mol Brain. (2019) 12:71. doi: 10.1186/s13041-019-0492-x
38. Bishop DL, Misgeld T, Walsh MK, Gan W-B, Lichtman JW, et al. Axon branch removal at developing synapses by axosome shedding. Neuron. (2004) 44:651–61. doi: 10.1016/j.neuron.2004.10.026
39. Weinhard L, di Bartolomei G, Bolasco G, Machado P, Schieber NL, Neniskyte U, et al. Microglia remodel synapses by presynaptic trogocytosis and spine head filopodia induction. Nat Commun. (2018) 9:1228. doi: 10.1038/s41467-018-03566-5
40. Lim TK, Ruthazer ES. Microglial trogocytosis and the complement system regulate axonal pruning in vivo. eLife. (2021) 10:e62167. doi: 10.7554/eLife.62167
41. Suh H-S, Zhao M-L, Derico L, Choi N, Lee SC. Insulin-like growth factor 1 and 2 (IGF1, IGF2) expression in human microglia: differential regulation by inflammatory mediators. J Neuroinflamm. (2013) 10:805. doi: 10.1186/1742-2094-10-37
42. Pascual O, Achour SB, Rostaing P, Triller A, Bessis A. Microglia activation triggers astrocyte-mediated modulation of excitatory neurotransmission. Proc Natl Acad Sci. (2012) 109(4):E197–205. doi: 10.1073/pnas.1111098109
43. McNamara NB, Munro DAD, Bestard-Cuche N, Uyeda A, Bogie JFJ, Hoffmann A, et al. Microglia regulate central nervous system myelin growth and integrity. Nature. (2023) 613:120–9. doi: 10.1038/s41586-022-05534-y
44. Berghoff SA, Spieth L, Sun T, Hosang L, Schlaphoff L, Depp C, et al. Microglia facilitate repair of demyelinated lesions via post-squalene sterol synthesis. Nat Neurosci. (2021) 24:47–60. doi: 10.1038/s41593-020-00757-6
45. Berchtold D, Priller J, Meisel C, Meisel A. Interaction of microglia with infiltrating immune cells in the different phases of stroke. Brain Pathol. (2020) 30:1208–18. doi: 10.1111/bpa.12911
46. Xu Y, Li Y, Wang C, Han T, Liu H, Sun L, et al. The reciprocal interactions between microglia and T cells in Parkinson’s disease: a double-edged sword. J Neuroinflamm. (2023) 20:33. doi: 10.1186/s12974-023-02723-y
47. Paolicelli R, Sierra A, Stevens B, Tremblay M-E, Aguzzi A, Ajami B, et al. Microglia states and nomenclature: A field at its crossroads. Neuron. (2022) 110:3458–83. doi: 10.1016/j.neuron.2022.10.020
48. Jurga AM, Paleczna M, Kuter KZ. Overview of general and discriminating markers of differential microglia phenotypes. Front Cell Neurosci. (2020) 14:198. doi: 10.3389/fncel.2020.00198
49. Garaschuk O, Verkhratsky A. Microglia: methods and protocols. New York, NY: Springer New York (Methods in Molecular Biology (2019).
50. Hanisch U-K, Kettenmann H. Microglia: active sensor and versatile effector cells in the normal and pathologic brain. Nat Neurosci. (2007) 10:1387–94. doi: 10.1038/nn1997
51. Colonna M, Butovsky O. Microglia function in the central nervous system during health and neurodegeneration. Annu Rev Immunol. (2017) 35:441–68. doi: 10.1146/annurev-immunol-051116-052358
52. Errede M, Annese T, Petrosino V, Longo G, Girolamo F, de Trizio I, et al. Microglia-derived CCL2 has a prime role in neocortex neuroinflammation. Fluids Barriers CNS. (2022) 19:68. doi: 10.1186/s12987-022-00365-5
53. Xue J, Schmidt SV, Sander J, Draffehn A, Krebs W, Quester I, et al. Transcriptome-based network analysis reveals a spectrum model of human macrophage activation. Immunity. (2014) 40:274–88. doi: 10.1016/j.immuni.2014.01.006
54. Kalsbeek MJT, Mulder L, Yi C-X. Microglia energy metabolism in metabolic disorder. Mol Cell Endocrinol. (2016) 438:27–35. doi: 10.1016/j.mce.2016.09.028
55. Wang L, Pavlou S, Du X, Bhuckory M, Xu H, Chen M, et al. Glucose transporter 1 critically controls microglial activation through facilitating glycolysis. Mol Neurodegeneration. (2019) 14:2. doi: 10.1186/s13024-019-0305-9
56. Orihuela R, McPherson CA, Harry GJ. Microglial M1/M2 polarization and metabolic states: Microglia bioenergetics with acute polarization. Br J Pharmacol. (2016) 173:649–65. doi: 10.1111/bph.13139
57. Haschemi A, Kosma P, Gille L, Evans CR, Burant CF, Starkl P, et al. The sedoheptulose kinase CARKL directs macrophage polarization through control of glucose metabolism. Cell Metab. (2012) 15:813–26. doi: 10.1016/j.cmet.2012.04.023
58. Cordes T, Wallace M, Michelucci A, Divakaruni AS, Sapcariu SC, Sousa C, et al. Immunoresponsive gene 1 and itaconate inhibit succinate dehydrogenase to modulate intracellular succinate levels. J Biol Chem. (2016) 291:14274–84. doi: 10.1074/jbc.M115.685792
59. Amici SA, Dong J, Guerau-de-Arellano M. Molecular mechanisms modulating the phenotype of macrophages and microglia. Front Immunol. (2017) 8:1520. doi: 10.3389/fimmu.2017.01520
60. Haage V, Semtner M, Vidal RO, Hernandez DP, Pong WW, Chen Z, et al. Comprehensive gene expression meta-analysis identifies signature genes that distinguish microglia from peripheral monocytes/macrophages in health and glioma. Acta Neuropathologica Commun. (2019) 7:20. doi: 10.1186/s40478-019-0665-y
61. Gautier EL, Shay T, Miller J, Greter M, Jakubzick C, Ivanov S, et al. Gene-expression profiles and transcriptional regulatory pathways that underlie the identity and diversity of mouse tissue macrophages. Nat Immunol. (2012) 13:1118–28. doi: 10.1038/ni.2419
62. Butovsky O, Jedrychowski MP, Moore CS, Cialic R, Lanser AJ, Gabriely G, et al. Identification of a unique TGF-β–dependent molecular and functional signature in microglia. Nat Neurosci. (2014) 17:131–43. doi: 10.1038/nn.3599
63. Bennett ML, Bennett CF, Liddelow SA, Ajami B, Zamanian JL, Fernhoff NB, et al. New tools for studying microglia in the mouse and human CNS. Proc Natl Acad Sci. (2016) 113(12):E1738–1746. doi: 10.1073/pnas.1525528113
64. Chen Z, Feng X, Herting CJ, Garcia VA, Nie K, Pong WW, et al. Cellular and molecular identity of tumor-associated macrophages in glioblastoma. Cancer Res. (2017) 77:2266–78. doi: 10.1158/0008-5472.CAN-16-2310
65. Benmamar-Badel A, Owens T, Wlodarczyk A. Protective microglial subset in development, aging, and disease: lessons from transcriptomic studies. Front Immunol. (2020) 11:430. doi: 10.3389/fimmu.2020.00430
66. Jin W-N, Shi SX-Y, Li Z, Li M, Wood K, Gonzales RJ, et al. Depletion of microglia exacerbates postischemic inflammation and brain injury. J Cereb Blood Flow Metab. (2017) 37:2224–36. doi: 10.1177/0271678X17694185
67. Spangenberg E, Severson PL, Hohsfield LA, Crapser J, Zhang J, Burton EA, et al. Sustained microglial depletion with CSF1R inhibitor impairs parenchymal plaque development in an Alzheimer’s disease model. Nat Commun. (2019) 10:3758. doi: 10.1038/s41467-019-11674-z
68. Spiteri A, King, Nicholas JC. Putting PLX5622 into perspective: microglia in central nervous system viral infection. Neural Regeneration Res. (2023) 18:1269. doi: 10.4103/1673-5374.360170
69. Han J, Zhu K, Zhang X-M, Harris RA. Enforced microglial depletion and repopulation as a promising strategy for the treatment of neurological disorders. Glia. (2019) 67:217–31. doi: 10.1002/glia.23529
70. Parkhurst CN, Yang G, Ninan I, Savas JN, Yates JR III, Lafaille JJ, et al. Microglia promote learning-dependent synapse formation through brain-derived neurotrophic factor. Cell. (2013) 155:1596–609. doi: 10.1016/j.cell.2013.11.030
71. Eme-Scolan E, Dando SJ. Tools and approaches for studying microglia in vivo. Front Immunol. (2020) 11:583647. doi: 10.3389/fimmu.2020.583647
72. Lund H, Pieber M, Parsa R, Grommisch D, Ewing E, Kular. Fatal demyelinating disease is induced by monocyte-derived macrophages in the absence of TGF-β signaling. Nat Immunol. (2018) 19:1–7. doi: 10.1038/s41590-018-0091-5
73. Varvel NH, Grathwohla SA, Baumanna F, Liebiga C, Boscha A, Brawek B, et al. Microglial repopulation model reveals a robust homeostatic process for replacing CNS myeloid cells. Proc Natl Acad Sci. (2012) 109:18150–5. doi: 10.1073/pnas.1210150109
74. Han W, Umekawa T, Zhou K, Zhang X-M, Ohshima M, Dominguez CA, et al. Cranial irradiation induces transient microglia accumulation, followed by long-lasting inflammation and loss of microglia. Oncotarget. (2016) 7:82305–23. doi: 10.18632/oncotarget.12929
75. Xu Z, Zhou X, Peng B, Rao Y. Microglia replacement by bone marrow transplantation (Mr BMT) in the central nervous system of adult mice. STAR Protoc. (2021) 2:100666. doi: 10.1016/j.xpro.2021.100666
76. Han X, Li Q, Lan X, EL-Mufti L, Ren H, Wang J, et al. Microglial depletion with clodronate liposomes increases proinflammatory cytokine levels, induces astrocyte activation, and damages blood vessel integrity. Mol Neurobiol. (2019) 56:6184–96. doi: 10.1007/s12035-019-1502-9
77. Ravichandran S, Manickam N, Kandasamy M. Liposome encapsulated clodronate mediated elimination of pathogenic macrophages and microglia: A promising pharmacological regime to defuse cytokine storm in COVID-19. Med Drug Discovery. (2022) 15:100136. doi: 10.1016/j.medidd.2022.100136
78. Han J, Fan Y, Zhou K, Zhu K, Blomgren K, Lund H, et al. Underestimated peripheral effects following pharmacological and conditional genetic microglial depletion. Int J Mol Sci. (2020) 21(22). doi: 10.3390/ijms21228603
79. Kaiser T, Feng G. Tmem119-EGFP and tmem119-creERT2 transgenic mice for labeling and manipulating microglia. eneuro. (2019) 6:ENEURO.0448–18.2019. doi: 10.1523/ENEURO.0448-18.2019
80. McKinsey GL, Lizama CO, Keown-Lang AE, Niu A, Santander N, Larpthaveesarp A, et al. A new genetic strategy for targeting microglia in development and disease. eLife. (2020) 9:e54590. doi: 10.7554/eLife.54590
81. Elmore MRP, Lee RJ, West BL, Green KN. Characterizing newly repopulated microglia in the adult mouse: impacts on animal behavior, cell morphology, and neuroinflammation. PloS One. (2015) 10:e0122912. doi: 10.1371/journal.pone.0122912
82. Han J, Harris RA, Zhang X-M. An updated assessment of microglia depletion: current concepts and future directions. Mol Brain. (2017) 10:25. doi: 10.1186/s13041-017-0307-x
83. Elmore MRP, Najafi AR, Koike MA, Dagher NN, Spangenberg EA, Rice RA, et al. Colony-stimulating factor 1 receptor signaling is necessary for microglia viability, unmasking a microglia progenitor cell in the adult brain. Neuron. (2014) 82:380–97. doi: 10.1016/j.neuron.2014.02.040
84. Scott CL, Zheng F, De Baetselier P, Martens L, Saeys Y, De Prijck S, et al. Bone marrow-derived monocytes give rise to self-renewing and fully differentiated Kupffer cells. Nat Commun. (2016) 7:10321. doi: 10.1038/ncomms10321
85. van de Laar L, Saelens W, De Prijck S, Martens L, Scott CL, Van Isterdael G, et al. Yolk sac macrophages, fetal liver, and adult monocytes can colonize an empty niche and develop into functional tissue-resident macrophages. Immunity. (2016) 44:755–68. doi: 10.1016/j.immuni.2016.02.017
86. Weber MD, McKim DB, Niraula A, Witcher KG, Yin W, Sobol CG, et al. The influence of microglial elimination and repopulation on stress sensitization induced by repeated social defeat. Biol Psychiatry. (2019) 85:667–78. doi: 10.1016/j.biopsych.2018.10.009
87. Bruttger J, Karram K, Wortge S, Regen T, Marini F, Hoppmann N, et al. Genetic cell ablation reveals clusters of local self-renewing microglia in the mammalian central nervous system. Immunity. (2015) 43:92–106. doi: 10.1016/j.immuni.2015.06.012
88. Lund H, Pieber M, Parsa R, Han J, Pieber M, Parsa R, Han J, Grommisch D, Ewing E, et al. Competitive repopulation of an empty microglial niche yields functionally distinct subsets of microglia-like cells. Nat Commun. (2018) 9:4845. doi: 10.1038/s41467-018-07295-7
89. Huang Y, Xu Z, Xiong S, Qin G, Sun F, Yan J, et al. Dual extra-retinal origins of microglia in the model of retinal microglia repopulation. Cell Discovery. (2018) 4:9. doi: 10.1038/s41421-018-0011-8
90. Qin C, Zhou L-Q, Ma X-T, Hu Z-W, Yang S, Chen M, et al. Dual functions of microglia in ischemic stroke. Neurosci Bull. (2019) 35:921–33. doi: 10.1007/s12264-019-00388-3
91. Timis TL, Florian IA, Susman S, Florian IS. Involvement of microglia in the pathophysiology of intracranial aneurysms and vascular malformations—A short overview. Int J Mol Sci. (2021) 22:6141. doi: 10.3390/ijms22116141
92. Magid-Bernstein J, Girard R, Polster S, Srinath A, Romanos S, Awad IA, et al. Cerebral hemorrhage: pathophysiology, treatment, and future directions. Circ Res. (2022) 130:1204–29. doi: 10.1161/CIRCRESAHA.121.319949
93. Deczkowska A, Keren-Shaul H, Weiner A, Colonna M, Schwartz M, Amit I, et al. Disease-associated microglia: A universal immune sensor of neurodegeneration. Cell. (2018) 173:1073–81. doi: 10.1016/j.cell.2018.05.003
94. Liu S, Liu Y, Hao W, Wolf L, Kiliaan AJ, Penke B, et al. TLR2 is a primary receptor for alzheimer’s amyloid β Peptide to trigger neuroinflammatory activation. J Immunol. (2012) 188:1098–107. doi: 10.4049/jimmunol.1101121
95. Pyonteck SM, Akkari L, Schuhmacher AJ, Bowman RL, Sevenich L, Quail DF, et al. CSF-1R inhibition alters macrophage polarization and blocks glioma progression. Nat Med. (2013) 19:1264–72. doi: 10.1038/nm.3337
96. Hambardzumyan D, Gutmann DH, Kettenmann H. The role of microglia and macrophages in glioma maintenance and progression. Nat Neurosci. (2016) 19:20–7. doi: 10.1038/nn.4185
97. Geloso MC, Corvino V, Marchese E, Serrano A, Michetti F, D’Ambrosi N, et al. The dual role of microglia in ALS: mechanisms and therapeutic approaches. Front Aging Neurosci. (2017) 9:242. doi: 10.3389/fnagi.2017.00242
98. Mathys H, Adaikkan C, Gao F, Young JZ, Manet E, Hemberg M, et al. Temporal tracking of microglia activation in neurodegeneration at single-cell resolution. Cell Rep. (2017) 21:366–80. doi: 10.1016/j.celrep.2017.09.039
99. Volonté C, Amadio S, Fabbrizio P, Apolloni S. Functional microglia neurotransmitters in amyotrophic lateral sclerosis. Semin Cell Dev Biol. (2019) 94:121–8. doi: 10.1016/j.semcdb.2019.04.014
100. Hu X, Li P, Guo Y, Wang H, Leak RK, Chen S, et al. Microglia/macrophage polarization dynamics reveal novel mechanism of injury expansion after focal cerebral ischemia. Stroke. (2012) 43:3063–70. doi: 10.1161/STROKEAHA.112.659656
101. Perego C, Fumagalli S, Zanier ER, Carlino E, Paninic N, Erba E, et al. Macrophages are essential for maintaining a M2 protective response early after ischemic brain injury. Neurobiol Dis. (2016) 96:284–93. doi: 10.1016/j.nbd.2016.09.017
102. Taylor RA, Chang C-F, Goods BA, Hammond MD, Mac Grory B, Ai Y, et al. TGF-β1 modulates microglial phenotype and promotes recovery after intracerebral hemorrhage. J Clin Invest. (2016) 127:280–92. doi: 10.1172/JCI88647
103. Lan X, Han X, Li Q, Li Q, Gao Y, Cheng T, et al. Pinocembrin protects hemorrhagic brain primarily by inhibiting toll-like receptor 4 and reducing M1 phenotype microglia. Brain Behavior Immun. (2017) 61:326–39. doi: 10.1016/j.bbi.2016.12.012
104. Keedy A. An overview of intracranial aneurysms. McGill J Med. (2020) 9(2):141–6. doi: 10.26443/mjm.v9i2.672
105. Peng J, Pang J, Huang L, Enkhjargal B, Zhang T, Mo J, et al. LRP1 activation attenuates white matter injury by modulating microglial polarization through Shc1/PI3K/Akt pathway after subarachnoid hemorrhage in rats. Redox Biol. (2019) 21:101121. doi: 10.1016/j.redox.2019.101121
106. Zheng ZV, Lyu H, Lam SYE, Lam PK, Poon WS, Wong GKC, et al. The dynamics of microglial polarization reveal the resident neuroinflammatory responses after subarachnoid hemorrhage. Trans Stroke Res. (2020) 11:433–49. doi: 10.1007/s12975-019-00728-5
107. Roa JA, Sarkar D, Zanaty M, ishii D, Lu Y, Karandikar NJ, et al. Preliminary results in the analysis of the immune response after aneurysmal subarachnoid hemorrhage. Sci Rep. (2020) 10:11809. doi: 10.1038/s41598-020-68861-y
108. Schneider UC, Davids A-M, Brandenburg S, Müller A, Elke A, Magrini S, et al. Microglia inflict delayed brain injury after subarachnoid hemorrhage. Acta Neuropathologica. (2015) 130:215–31. doi: 10.1007/s00401-015-1440-1
109. Coulibaly AP, Provencio JJ. Aneurysmal subarachnoid hemorrhage: an overview of inflammation-induced cellular changes. Neurotherapeutics. (2020) 17:436–45. doi: 10.1007/s13311-019-00829-x
110. Wei S, Luob C, Yu S, Gao J, Liu C, Wei Z, et al. Erythropoietin ameliorates early brain injury after subarachnoid haemorrhage by modulating microglia polarization via the EPOR/JAK2-STAT3 pathway. Exp Cell Res. (2017) 361:342–52. doi: 10.1016/j.yexcr.2017.11.002
111. Galea J, Ogungbenro K, Hulme S, Patel H, Scarth S, Hoadley M, et al. Reduction of inflammation after administration of interleukin-1 receptor antagonist following aneurysmal subarachnoid hemorrhage: results of the Subcutaneous Interleukin-1Ra in SAH (SCIL-SAH) study. J Neurosurg. (2018) 128:515–23. doi: 10.3171/2016.9.JNS16615
112. Zhang Z, Sun B-L, Liu J-K, Yang M-F, Li D-W, Fang J, et al. Activation of mGluR5 attenuates microglial activation and neuronal apoptosis in early brain injury after experimental subarachnoid hemorrhage in rats. Neurochemical Res. (2015) 40:1121–32. doi: 10.1007/s11064-015-1572-7
113. Hambardzumyan D, Bergers G. Glioblastoma: defining tumor niches. Trends Cancer. (2015) 1:252–65. doi: 10.1016/j.trecan.2015.10.009
114. Louis DN, Perry A, Reifenberger G, von Deimling A, Figarella-Branger D, Cavenee WK, et al. The 2016 world health organization classification of tumors of the central nervous system: a summary. Acta Neuropathologica. (2016) 131:803–20. doi: 10.1007/s00401-016-1545-1
115. Robertson FL, Marqués-Torrejó M-A, Morrison GM, Pollard SM. Experimental models and tools to tackle glioblastoma. Dis Models Mech. (2019) 12:dmm040386. doi: 10.1242/dmm.040386
116. Lu KV, Bergers G. Mechanisms of evasive resistance to anti-VEGF therapy in glioblastoma. CNS Oncol. (2013) 2:49–65. doi: 10.2217/cns.12.36
117. Cuddapah VA, Robel S, Watkins S, Sontheimer H. A neurocentric perspective on glioma invasion. Nat Rev Neurosci. (2014) 15:455–65. doi: 10.1038/nrn3765
119. Li J, Kanedab MM, Maa J, Lia M, Shepardb RM, Patel K, et al. PI3Kγ inhibition suppresses microglia/TAM accumulation in glioblastoma microenvironment to promote exceptional temozolomide response. Proc Natl Acad Sci. (2021) 118:e2009290118. doi: 10.1073/pnas.2009290118
120. Ugel S, De Sanctis F, Mandruzzato S, Bronte V. Tumor-induced myeloid deviation: when myeloid-derived suppressor cells meet tumor-associated macrophages. J Clin Invest. (2015) 125:3365–76. doi: 10.1172/JCI80006
121. Dubinski D, Wolfer J, Hasselblatt M, Schneider-Hohendorf T, Bogdahn U, Stummer W, et al. CD4 + T effector memory cell dysfunction is associated with the accumulation of granulocytic myeloid-derived suppressor cells in glioblastoma patients. Neuro-Oncology. (2016) 18:807–18. doi: 10.1093/neuonc/nov280
122. Charles NA, Holland EC, Gilbertson R, Glass R, Kettenmann H. The brain tumor microenvironment. Glia. (2011) 59:1169–80. doi: 10.1002/glia.21136
123. Darmanis S, Sloan SA, Croote D, Mignardi M, Chernikova S, Samghababi P, et al. Single-cell RNA-seq analysis of infiltrating neoplastic cells at the migrating front of human glioblastoma. Cell Rep. (2017) 21:1399–410. doi: 10.1016/j.celrep.2017.10.030
124. Ochocka N, Segit P, Walentynowicz KA, Wojnicki K, Cyranowski S, Swatler J, et al. Single-cell RNA sequencing reveals functional heterogeneity of glioma-associated brain macrophages. Nat Commun. (2021) 12:1151. doi: 10.1038/s41467-021-21407-w
125. Murray PJ, Allen JE, Biswas SK, Fisher EA, Gilroy DW, Goerdt S, et al. Macrophage activation and polarization: nomenclature and experimental guidelines. Immunity. (2014) 41:14–20. doi: 10.1016/j.immuni.2014.06.008
126. Szulzewsky F, Pelz A, Feng X, Synowitz M, Markovic D, Langmann T, et al. Glioma-associated microglia/macrophages display an expression profile different from M1 and M2 polarization and highly express gpnmb and spp1. PloS One. (2015) 10:e0116644. doi: 10.1371/journal.pone.0116644
127. Maas SLN, Abels ER, Van De Haar LL, Zhang X, Morsett L, Sil S, et al. Glioblastoma hijacks microglial gene expression to support tumor growth. J Neuroinflamm. (2020) 17:120. doi: 10.1186/s12974-020-01797-2
128. Sica A, Allavena P, Mantovani A. Cancer related inflammation: The macrophage connection. Cancer Lett. (2008) 267:204–15. doi: 10.1016/j.canlet.2008.03.028
129. Rao R, Han R, Ogurek S, Xue C, Man Wu L, Zhang L, et al. Glioblastoma genetic drivers dictate the function of tumor-associated macrophages/microglia and responses to CSF1R inhibition. Neuro-Oncology. (2022) 24:584–97. doi: 10.1093/neuonc/noab228
130. Chipman ME, Wang Z, Sun D, Pedrazaa AM, Bale TA, Parada LF, et al. Tumor progression is independent of tumor-associated macrophages in cell lineage-based mouse models of glioblastoma. Proc Natl Acad Sci USA. (2023) 120(16):e2222084120. doi: 10.1073/pnas.2222084120
131. Butowski N, Colman H, De Groot JF, Omuro AM, Nayak L, Wen PY, et al. Orally administered colony stimulating factor 1 receptor inhibitor PLX3397 in recurrent glioblastoma: an Ivy Foundation Early Phase Clinical Trials Consortium phase II study. Neuro-Oncology. (2016) 18:557–64. doi: 10.1093/neuonc/nov245
132. Rivera LB, Meyronet D, Hervieu V, Frederick MJ, Bergsland E, Bergers G, et al. ' Intratumoral myeloid cells regulate response and resistance to antiangiogenic therapy'. Cell Rep. (2015) 11:577–91. doi: 10.1016/j.celrep.2015.03.055
133. Kaneda MM, Messer KS, Ralainirina N, Li H, Leem CJ, Gorjestani S, et al. PI3Kγ is a molecular switch that controls immune suppression. Nature. (2016) 539:437–42. doi: 10.1038/nature19834
134. Dumas AA, Pomella N, Rosser G, Guglielmi L, Vinel C, Millner TO, et al. Microglia promote glioblastoma via mTOR-mediated immunosuppression of the tumour microenvironment. EMBO J. (2020) 39:e103790. doi: 10.15252/embj.2019103790
135. Ye Z, Ai X, Yang K, Yang Z, Fei F, Liao X, et al. Targeting microglial metabolic rewiring synergizes with immune-checkpoint blockade therapy for glioblastoma. Cancer Discov. (2023) 13:974–1001. doi: 10.1158/2159-8290.CD-22-0455
136. Ravi V, Neidert N, Will P, Joseph K, Maier JP, Kückelhaus J, et al. T-cell dysfunction in the glioblastoma microenvironment is mediated by myeloid cells releasing interleukin-10. Nat Commun. (2022) 13:925. doi: 10.1038/s41467-022-28523-1
137. Yeini E, Ofek P, Pozzi S, Albeck N, Ben-Shushan D, Tiram G, et al. P-selectin axis plays a key role in microglia immunophenotype and glioblastoma progression. Nat Commun. (2021) 12:1912. doi: 10.1038/s41467-021-22186-0
138. Khan F, Pang L, Dunterman M, Lesniak MS, Heimberger AB, Chen P. Macrophages and microglia in glioblastoma: heterogeneity, plasticity, and therapy. J Clin Invest. (2023) 133(1). doi: 10.1172/JCI163446
139. Masuda T, Staszewski \Sankowski R, Böttcher O, Amann C L, Sagar. Spatial and temporal heterogeneity of mouse and human microglia at single-cell resolution. Nature. (2019) 566:388–92. doi: 10.1038/s41586-019-0924-x
140. Hammond TR, Dufort C, Dissing-Olesen L, Giera S, Young A, Wysoker A. Single-cell RNA sequencing of microglia throughout the mouse lifespan and in the injured brain reveals complex cell-state changes. Immunity. (2019) 50:253–271.e256. doi: 10.1016/j.immuni.2018.11.004
141. Li Q, Cheng Z, Zhou L, Darmanis S, Neff NF, Okamoto J, et al. Developmental heterogeneity of microglia and brain myeloid cells revealed by deep single-cell RNA sequencing. Neuron. (2019) 101:207–223.e210. doi: 10.1016/j.neuron.2018.12.006
142. Yaqubi M, Groh AMR, Dorion M-F, Afanasiev E, Xiao J, Luo X, et al. Analysis of the microglia transcriptome across the human lifespan using single cell RNA sequencing. J Neuroinflamm. (2023) 20:132. doi: 10.1186/s12974-023-02809-7
143. Sankowski R, Böttcher C, Masuda T, Geirsdottir L, Sagar, Sindram E, et al. Mapping microglia states in the human brain through the integration of high-dimensional techniques. Nat Neurosci. (2019) 22:2098–110. doi: 10.1038/s41593-019-0532-y
144. Lopes KP, Snijders GJL, Humphrey J, Allan A, Sneeboer MAM, Navarro E, et al. Genetic analysis of the human microglial transcriptome across brain regions, aging and disease pathologies. Nat Genet. (2022) 54:4–17. doi: 10.1038/s41588-021-00976-y
145. Villa A, Gelosa P, Castiglioni L, Cimino M, Rizzi N, Pepe G, et al. Sex-specific features of microglia from adult mice. Cell Rep. (2018) 23:3501–11. doi: 10.1016/j.celrep.2018.05.048
146. Lynch MA. Exploring sex-related differences in microglia may be a game-changer in precision medicine. Front Aging Neurosci. (2022) 14:868448. doi: 10.3389/fnagi.2022.868448
147. Liu H, Sun Y, Zhang Q, Jin W, Gordon RE, Zhang Y, et al. Pro-inflammatory and proliferative microglia drive progression of glioblastoma. Cell Rep. (2021) 36:109718. doi: 10.1016/j.celrep.2021.109718
148. Zheng K, Lin L, Jiang W, Chen L, Zhang X, Zhang Q, et al. Single-cell RNA-seq reveals the transcriptional landscape in ischemic stroke. J Cereb Blood Flow Metab. (2022) 42:56–73. doi: 10.1177/0271678X211026770
149. Chelluboina B, Klopfenstein JD, Pinson DM, Wang DZ, Vemuganti R, Veeravalli KK, et al. Matrix metalloproteinase-12 induces blood-brain barrier damage after focal cerebral ischemia. Stroke. (2015) 46:3523–31. doi: 10.1161/STROKEAHA.115.011031
150. Keren-Shaul H, Spinrad A, Weiner A, Matcovitch-Natan O, Dvir-Szternfeld R, Ulland TK, et al. A unique microglia type associated with restricting development of alzheimer's disease. Cell. (2017) 169:1276–1290.e1217. doi: 10.1016/j.cell.2017.05.018
151. Masuda T, Sankowski R, Staszewski O, Prinz M. Microglia heterogeneity in the single-cell era. Cell Rep. (2020) 30:1271–81. doi: 10.1016/j.celrep.2020.01.010
152. Chen J, Sun L, Lyu H, Zheng Z, Lai H, Wang Y, et al. Single-cell analysis of microglial transcriptomic diversity in subarachnoid haemorrhage. Clin Transl Med. (2022) 12:e783. doi: 10.1002/ctm2.783
153. Szalay G, Martinecz B, Lénárt N, Kornyei Z, Orsolits B, Judák L, et al. Microglia protect against brain injury and their selective elimination dysregulates neuronal network activity after stroke. Nat Commun. (2016) 7:11499. doi: 10.1038/ncomms11499
154. Barca C, Foray C, Hermann S, Herrlinger U, Remory I, Laoui D, et al. The colony stimulating factor-1 receptor (CSF-1R)-mediated regulation of microglia/macrophages as a target for neurological disorders (Glioma, stroke). Front Immunol. (2021) 12:787307. doi: 10.3389/fimmu.2021.787307
155. Otxoa-de-Amezaga A, Miró‑Mur F, Pedragosa J, Gallizioli M, Justicia C, Gaja‑Capdevila N, et al. Microglial cell loss after ischemic stroke favors brain neutrophil accumulation. Acta Neuropathologica. (2019) 137:321–41. doi: 10.1007/s00401-018-1954-4
156. Li T, Zhao J, Xie W, Yuan W, Guo J, Pang S, et al. Specific depletion of resident microglia in the early stage of stroke reduces cerebral ischemic damage. J Neuroinflamm. (2021) 18:81. doi: 10.1186/s12974-021-02127-w
157. Barca C, Kiliaan AJ, Foray C, Wachsmuth L, Hermann S, Faber C, et al. A longitudinal PET/MRI study of colony-stimulating factor 1 receptor–mediated microglia depletion in experimental stroke. J Nucl Med. (2022) 63:446–52. doi: 10.2967/jnumed.121.262279
158. Jiang C, Wu W-F, Deng Y-H, Ge J-W. Modulators of microglia activation and polarization in ischemic stroke. Mol Med Rep. (2020) 21(5):2006–18. doi: 10.3892/mmr.2020.11003
159. Hanafy KA. The role of microglia and the TLR4 pathway in neuronal apoptosis and vasospasm after subarachnoid hemorrhage. J Neuroinflamm. (2013) 10:868. doi: 10.1186/1742-2094-10-83
160. Heinz R, Brandenburg S, Nieminen-Kelhä M, Kremenetskaia I, Boehm-Sturm P, Vajkoczy P, et al. Microglia as target for anti-inflammatory approaches to prevent secondary brain injury after subarachnoid hemorrhage (SAH). J Neuroinflamm. (2021) 18:36. doi: 10.1186/s12974-021-02085-3
161. Przystal JM, Becker H, Canjuga D, Tsiami F, Anderle N, Keller A-L, et al. Targeting CSF1R alone or in combination with PD1 in experimental glioma. Cancers. (2021) 13:2400. doi: 10.3390/cancers13102400
162. Quail DF, Bowman RL, Akkari L, Quick ML, Schuhmacher AJ, Huse JT, et al. The tumor microenvironment underlies acquired resistance to CSF-1R inhibition in gliomas. Science. (2016) 352:aad3018. doi: 10.1126/science.aad3018
163. Lamb YN. Pexidartinib: first approval. Drugs. (2019) 79:1805–12. doi: 10.1007/s40265-019-01210-0
164. Gyori D, Lim EL, Grant FM, Spensberger D, Roychoudhuri R, Shuttleworth SJ, et al. Compensation between CSF1R+ macrophages and Foxp3+ Treg cells drives resistance to tumor immunotherapy. JCI Insight. (2018) 3:e120631. doi: 10.1172/jci.insight.120631
165. Kumar V, Donthireddy L, Marvel D, Condamine T, Wang F, Lavilla-Alonso S, et al. Cancer-associated fibroblasts neutralize the anti-tumor effect of CSF1 receptor blockade by inducing PMN-MDSC infiltration of tumors. Cancer Cell. (2017) 32:654–668.e5. doi: 10.1016/j.ccell.2017.10.005
166. Strachan DC, Ruffell B, Oei Y, Bissell MJ, Coussens LM, Pryer N, et al. CSF1R inhibition delays cervical and mammary tumor growth in murine models by attenuating the turnover of tumor-associated macrophages and enhancing infiltration by CD8 + T cells. OncoImmunology. (2013) 2:e26968. doi: 10.4161/onci.26968
167. Zhu Y, Knolhoff BL, Meyer MA, Nywening TM, West BL, Luo J, et al. CSF1/CSF1R blockade reprograms tumor-infiltrating macrophages and improves response to T-cell checkpoint immunotherapy in pancreatic cancer models. Cancer Res. (2014) 74:5057–69. doi: 10.1158/0008-5472.CAN-13-3723
168. Antonios JP, Soto H, Everson RG, Moughon D, Orpilla JR, Shin NP, et al. Immunosuppressive tumor-infiltrating myeloid cells mediate adaptive immune resistance via a PD-1/PD-L1 mechanism in glioblastoma. Neuro-Oncology. (2017) 19(6):796–807. doi: 10.1093/neuonc/now287
169. Stafford JH, Hirai T, Deng L, Chernikova SB, Urata K, West BL, et al. Colony stimulating factor 1 receptor inhibition delays recurrence of glioblastoma after radiation by altering myeloid cell recruitment and polarization. Neuro-Oncology. (2016) 18:797–806. doi: 10.1093/neuonc/nov272
170. Kang Z, Li S, Lin Y, Li Y, Mao Y, Zhang J, et al. ‘A phase I dose-escalation study of SYHA1813, a VEGFR and CSF1R inhibitor, in patients with recurrent High-Grade Gliomas or Advanced Solid Tumors. Investigational New Drugs. (2023) 41:296–305. doi: 10.1007/s10637-022-01325-4
171. Lanza M, Casili G, Campolo M, Paterniti I, Colarossi C, Mare M, et al. Immunomodulatory effect of microglia-released cytokines in gliomas. Brain Sci. (2021) 11:466. doi: 10.3390/brainsci11040466
172. Lin C, Wang N, Xu C. Glioma-associated microglia/macrophages (GAMs) in glioblastoma: Immune function in the tumor microenvironment and implications for immunotherapy. Front Immunol. (2023) 14:1123853. doi: 10.3389/fimmu.2023.1123853
173. Yan D, Kowal J, Akkari L, Schuhmacher AJ, Huse JT, West BL, et al. Inhibition of colony stimulating factor-1 receptor abrogates microenvironment-mediated therapeutic resistance in gliomas. Oncogene. (2017) 36:6049–58. doi: 10.1038/onc.2017.261
174. Novak M, Krajnc MK, Hrastar B, Breznik B, Majc B, Mlinar M, et al. CCR5-mediated signaling is involved in invasion of glioblastoma cells in its microenvironment. Int J Mol Sci. (2020) 21:4199. doi: 10.3390/ijms21124199
175. Laudati E, Currò D, Navarra P, Lisi L. Blockade of CCR5 receptor prevents M2 microglia phenotype in a microglia-glioma paradigm. Neurochemistry Int. (2017) 108:100–8. doi: 10.1016/j.neuint.2017.03.002
176. Parker S, McDowall C, Sanchez-Perez L, Osorio C, Duncker PC, Briley A, et al. Immunotoxin-αCD40 therapy activates innate and adaptive immunity and generates a durable antitumor response in glioblastoma models. Sci Trans Med. (2023) 15:eabn5649. doi: 10.1126/scitranslmed.abn5649
177. Saha D, Martuza RL, Rabkin SD. Macrophage polarization contributes to glioblastoma eradication by combination immunovirotherapy and immune checkpoint blockade. Cancer Cell. (2017) 32:253–267.e5. doi: 10.1016/j.ccell.2017.07.006
178. Kloepper J, Riedemanna L, Amoozgara Z, Seanoa G, Suseka K, Yu V, et al. Ang-2/VEGF bispecific antibody reprograms macrophages and resident microglia to anti-tumor phenotype and prolongs glioblastoma survival. Proc Natl Acad Sci. (2016) 113:4476–81. doi: 10.1073/pnas.1525360113
179. Quek H, Cuní‑López C, Stewart R, Colletti T, Notaro A, Nguyen TH, et al. ‘ALS monocyte-derived microglia-like cells reveal cytoplasmic TDP-43 accumulation, DNA damage, and cell-specific impairment of phagocytosis associated with disease progression. J Neuroinflamm. (2022) 19:58. doi: 10.1186/s12974-022-02421-1
180. Banerjee A, Lu Y, Do K, Mize T, Wu X, Chen X, et al. Validation of induced microglia-like cells (iMG cells) for future studies of brain diseases. Front Cell Neurosci. (2021) 15:629279. doi: 10.3389/fncel.2021.629279
181. Kobashi S, Terashima T, Katagi M, Urushitani M, Kojima H, et al. Bone marrow-derived inducible microglia-like cells ameliorate motor function and survival in a mouse model of amyotrophic lateral sclerosis. Cytotherapy. (2022) 24:789–801. doi: 10.1016/j.jcyt.2022.02.001
182. Muffat J, Li Y, Yuan B, Mitalipova M, Omer A, Corcoran S, et al. Efficient derivation of microglia-like cells from human pluripotent stem cells. Nat Med. (2016) 22:1358–67. doi: 10.1038/nm.4189
183. Pandya H, Shen MJ, Ichikawa DM, Sedlock AB, Choi Y, Johnson KR, et al. Differentiation of human and murine induced pluripotent stem cells to microglia-like cells. Nat Neurosci. (2017) 20:753–9. doi: 10.1038/nn.4534
184. Narantuya D, Nagaib A, Sheikhb A, Wakabayashia K, Shiotaa Y, Watanabe T, et al. Microglia transplantation attenuates white matter injury in rat chronic ischemia model via matrix metalloproteinase-2 inhibition. Brain Res. (2010) 1316:145–52. doi: 10.1016/j.brainres.2009.12.043
185. Tian Y, Zhu P, Liu S, Jin Z, Li D, Zhao H, et al. IL-4-polarized BV2 microglia cells promote angiogenesis by secreting exosomes. Adv Clin Exp Med. (2019) 28:421–30. doi: 10.17219/acem/91826
186. Jiang C, Wang J, Yu L, Ou C, Liu X, Zhao X, et al. Comparison of the therapeutic effects of bone marrow mononuclear cells and microglia for permanent cerebral ischemia. Behav Brain Res. (2013) 250:222–9. doi: 10.1016/j.bbr.2013.05.011
187. Morais LH, Schreiber HL 4th, Mazmanian SK. The gut microbiota-brain axis in behaviour and brain disorders. Nat Rev Microbiol. (2021) 19:241–55. doi: 10.1038/s41579-020-00460-0
Keywords: microglia, neuroinflammation, depletion, repopulation, re-education, stroke, aneurysm, glioblastoma
Citation: Pallarés-Moratalla C and Bergers G (2024) The ins and outs of microglial cells in brain health and disease. Front. Immunol. 15:1305087. doi: 10.3389/fimmu.2024.1305087
Received: 30 September 2023; Accepted: 19 March 2024;
Published: 11 April 2024.
Edited by:
Zhiqiang Dong, Huazhong Agricultural University, ChinaReviewed by:
Antonio d’Amati, University of Bari Aldo Moro, ItalyRobert Adam Harris, Karolinska Institutet (KI), Sweden
Copyright © 2024 Pallarés-Moratalla and Bergers. This is an open-access article distributed under the terms of the Creative Commons Attribution License (CC BY). The use, distribution or reproduction in other forums is permitted, provided the original author(s) and the copyright owner(s) are credited and that the original publication in this journal is cited, in accordance with accepted academic practice. No use, distribution or reproduction is permitted which does not comply with these terms.
*Correspondence: Gabriele Bergers, Z2FicmllbGUuYmVyZ2Vyc0BrdWxldXZlbi5iZQ==; Carla Pallarés-Moratalla, Y2FybGEucGFsbGFyZXNAa3VsZXV2ZW4uYmU=