- 1Department of Clinical Laboratory, The Second Hospital of Jilin University, Jilin University, Changchun, China
- 2Institute of Theoretical Chemistry, Jilin University, Changchun, China
- 3Department of Pathogenobiology, College of Basic Medical Sciences, Jilin University, Changchun, China
Protozoan diseases cause great harm in animal husbandry and require human-provided medical treatment. Protozoan infection can induce changes in cyclooxygenase-2 (COX-2) expression. The role played by COX-2 in the response to protozoan infection is complex. COX-2 induces and regulates inflammation by promoting the synthesis of different prostaglandins (PGs), which exhibit a variety of biological activities and participate in pathophysiological processes in the body in a variety of ways. This review explains the roles played by COX-2 in protozoan infection and analyzes the effects of COX-2-related drugs in protozoan diseases.
1. Introduction
Protozoan infection is one of the most common parasitic infections in humans and plays a very important role in worldwide morbidity and mortality (1). Protozoan infections are found around the world but are mainly concentrated in developing countries. The most important diseases in humans include malaria (2), leishmaniasis (3), Chagas disease (4), and giardiasis (5). Malaria is a life-threatening disease caused by Plasmodium, with parasites transmitted to humans through the bite of infected female Anopheles mosquitoes (3). World Health Organization (WHO) statistics show that five parasites are the main causes of malaria in humans, with Plasmodium falciparum (P. falciparum) and Plasmodium vivax (P. vivax) posing the greatest malaria threats (https://www.who.int/). Combined, these species caused 241 million malaria cases in 2020, with 627,000 malarial deaths (6). Leishmaniasis is a neglected tropical disease and the second leading cause of parasite-associated death, with 700,000 to 1 million new cases diagnosed each year (7). There are three main forms of leishmaniasis: visceral leishmaniasis (VL; also known as black fever and the most serious leishmaniasis), cutaneous leishmaniasis (the most common form), and mucocutaneous leishmaniasis. Left untreated, VL results in death in more than 95% of cases (8). Chagas disease is one of the most important diseases. It is a neglected tropical disease that has a high public health impact in the area. People can become infected through vector-borne transmission, congenital transmission, blood transfusions, organ transplantation and other transmission routes. Even in developed countries such as Germany, less than 1% of affected people receive adequate treatment (9). Giardia lamblia (G. lamblia) is the most common intestinal parasite worldwide (10). Immune-compromised individuals and undernourished children in developing countries are the most prone to severe manifestations of untreated giardiasis (11). The prevalence of Giardia in developing countries is estimated to range from 20% to 30% due to unsafe water supplies, ineffective environmental sanitation, and poor personal hygiene (12).
Despite several initiatives to reduce the incidence of protozoan disease, including treatment of giardiasis with the antibiotic metronidazole (13); treatment of malaria with artemisinin (14); and improvements in water, sanitation and hygiene conditions, protozoan infection remains a serious global health concern. Furthermore, drug resistance is a growing problem in veterinary medicine and may develop in humans; for example, metronidazole resistance has been reported in Endamoeba histolytica (E. histolytica) (15), Trichomonas vaginalis (T. vaginalis) (16) and G. lamblia (17). Clearly, there is a pressing need to develop new methods to control protozoan infection. One possible way to meet this need is to strengthen our understanding of the interactions between protozoa and hosts. Moreover, here, in addition to the four most relevant diseases, we also discuss other diseases, such as toxoplasmosis and babesiosis.
2. The role played by cyclooxygenase-2 in different diseases
After the protozoa invade the host organism, the protozoa and the host immune system are destined to fight. The result of this not only determines the fate of the parasite itself but also determines whether the host can survive and recover. The result of the fight depends on the location of the parasite within the host. Some intracellular parasites (for example, Plasmodium, Leishmania, Trypanosome and Toxoplasma) can enter and even reproduce in the host cells, but the host can destroy the infected cells. Extracellular parasites (e. g., Giardia), which are parasitic in the intestinal lumen, can be excreted due to the failure to adhere to epithelial cells or the destruction of cells after adhesion. Prostaglandin (PG) products play an important role for pathogens, and they are involved in many processes, such as inflammation, platelet aggregation, etc.
COX is the rate-limiting enzyme in prostaglandin-endoperoxide synthesis (18). It can metabolize arachidonic acid (AA) to form various PG products (Figure 1) (19–34). PGs play an important role in the regulation of human physiology and are involved in many processes, such as inflammation (35), platelet aggregation (36) and tumor development (37). COX is expressed in the following three isoforms: epoxygenase-1 (COX-1), COX-2 and COX-3 (38). The COX-1 enzyme was first identified in 1976 (39). The mouse gene (Ptgs1) encoding COX-1 was first isolated in 1993 (40). COX-1 is mainly expressed in blood vessels, interstitial cells, smooth muscle cells, and platelets (24), and after stimulation by growth factors or hormones, its expression level is increased 2-4-fold. Studies have shown that overexpression of COX-1 is associated with the development of various carcinomas (41, 42). COX-2 was identified in 1991 (43), and its discovery led to an understanding of the differences in PGs in normal function and disease. The gene (Ptgs2) encoding COX-2 is located on chromosome 1 (44). COX-2 is predominantly expressed in parenchymal cells in many tissues except the heart (24). Upon stimulation with IL-1, TNF, lipopolysaccharide (LPS), cAMP or other inflammatory factors, the COX-2 expression level can increase by approximately 80-fold, promoting a high rate of PG-endoperoxide and triggering an inflammatory response. PGs produced by COX-2 have multiple biological activities and can participate in pathophysiological processes in the body through various pathways (45, 46). The expression of COX-3 in human body is tissue specific, and COX-3 is common in the cerebral cortex and the heart. The typical remission period of chronic inflammatory diseases such as rheumatoid arthritis may be related to the expression of COX-3 (25).AA can be metabolized by COX into PGH2, which is then metabolized into four different PGs and tromboxanes (Txs), such as TxA2. For example, prostacyclin synthase catalyzes the isomerization of PGH2 to form PGI2. TxA2 is formed from PGH2 by Tx synthase. In addition, AA can also produce inflammatory substances through the 5-lipoxygenase (5-LOX) pathway, such as leukotrienes (LTs).
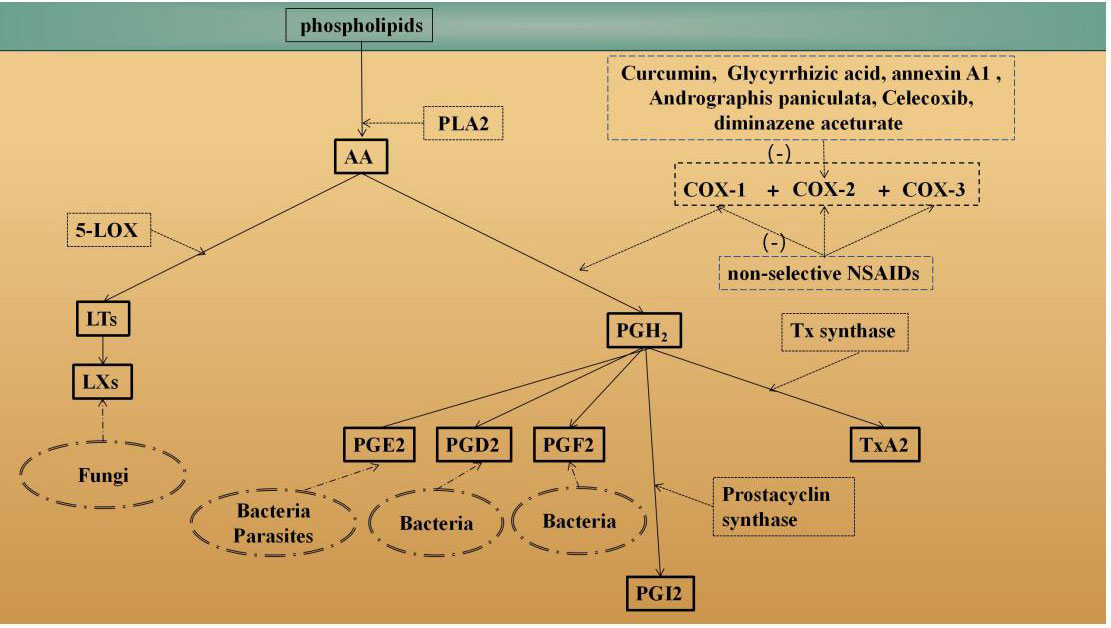
Figure 1 Overview of the AA metabolism pathways. Phospholipase enzymes (such as PLA2) can release AA from membrane-bound phospholipids (19) COX is the rate-limiting enzyme in prostaglandin-endoperoxide synthesis. AA can be metabolized by COX to PGH2, which is metabolized into four different PGs and tromboxane (Tx) (20–23). For example, prostacyclin synthase catalyzes the isomerization of PGH2 to form PG12 (20). TxA2 is formed via PGH2 by Tx synthase. In addition, AA can also produce inflammatory substances, such as leukotrienes (ET), through the 5-lipoxygenase (5-LOX) pathway (23). Different parts of the AA degradation pathway that occur in different pathogens (such as fungi, bacteria parasities) are also shown (24–27). Moreover, how anti-protozoal drugs are though to operate controlling COX-2 are also shown (28–34).
In tumor-related studies, PGE2 has been shown to be a proinflammatory cytokine and is overexpressed in a variety of human malignancies (47, 48). In various pathways, PGE2 binds to prostaglandin receptor (EP) in cancer cells, inducing tumorigenesis or promoting tumor progression (49). In intestinal tumors, overexpression of COX-2 contributed to the production of aberrant levels of PGE2 in epithelial cells. PGE2 plays various roles in cancer progression, including proliferation, migration, and immune escape. Tumor cells can acquire migratory capacity, which promotes metastatic colonization (50). Notably, ω-6 polyunsaturated fatty acids (PUFAs) were found to enhance the metastatic potential of gastric cancer cells via COX-2/PGE2 (51, 52). Thus, PGE2 has been shown to be a conventional target with pleiotropic effects in cancer onset and progression and can be used to develop novel potential treatments with clinical implications.
In addition, COX-2 plays an important role in vascular remodeling (53). COX-2 is an induced enzyme in the synthesis of PG intermediates, and its expression is closely related to vascular remodeling. Different induction factors upregulate the expression level of COX-2 by activating different signaling pathways, which subsequently activate different PGs, such as PGE2 or PGI2, and their respective receptors EP or IP to regulate vascular remodeling with the same or opposite effects. Moreover, regulator of calcineurin 1 (Rcan1) may be an endogenous negative regulator of COX-2 expression and activity, maintaining normal contractility and vascular stiffness in the aorta and mesenteric arterioles by inhibiting calcineurin and NF-κB pathway activation, respectively (54).
Alternatively, COX-2 plays an important role in endometrial-related lesions (55). Studies have shown that treatment with PGE2 and the PTGER2 agonist butaprost can induce the proliferation of bovine endometrial epithelial cells (bEECs). However, bEEC proliferation was attenuated by the PTGER2 antagonist AH6809 and CDK inhibitors (56).
Therefore, COX-2 plays an important role in many pathological processes, such as inflammation, coagulation, cell growth and tumor development. Because COX-2-related reports in protozoan infections are rare, in this review, we present an analysis of the function of COX-2 in different protozoan infections as reported in recent years and evaluate the correlations and differences.
3. Intracellular parasites
3.1. COX-2 in Chagas disease
3.1.1. The azards of Chagas disease
Trypanosoma cruzi (T. cruzi), the main pathogen that causes Chagas disease, is estimated to infect approximately 6 to 7 million people worldwide (57). In Latin America, transmission to humans is mainly established through triatomine vectors (58). However, oral transmission, blood transfusion, maternal-neonatal transmission and organ transplantation are also known transmission routes (59). Hotez et al. (60) found that the prevalence of Chagas disease increased by 16% from 2000 to 2017. The WHO established World Chagas Disease Day in 2020 (April 14th) to call attention to Chagas disease (https://www.who.int/).
3.1.2. Role played by COX-2 in Chagas disease
Chronic Chagas disease cardiomyopathy (CCC) is one of the most common types of chronic myocarditis in the world, with symptoms ranging from mild to severe cardiac remodeling with associated inflammation, fibrosis, arrhythmias and thromboembolisms, which may cause congestive heart failure and sudden death (61). Because of its important role in regulating inflammation and fibrosis in the heart, COX-2 has attracted great interest in the context of Chagas disease (62).
Studies have shown that macrophages and other innate immune cells form the first line of defense to inhibit parasite reproduction after parasite infection, as indicated by the immediate increase observed in the expression of proinflammatory cytokines and the production of the highly cytotoxic oxidant peroxynitrite (ONOO-) (63). Inhibition of COX activity may increase NO levels and thus restore antiparasitic activity in macrophages. Recently, obtained evidence suggests that COX is involved in the T. cruzi invasion process during infection (64). In the early stages of T. cruzi interaction with host cells, the parasites regulate cellular metabolism to enhance their own survival (65). Furthermore, although the effects of ROS on parasites are complex, some nonimmune cells, such as cardiomyocytes, respond to T. cruzi infection by producing ROS. In fact, several studies have identified mitochondria as the primary sources of ROS in T. cruzi-infected cardiomyocytes (66, 67). Typically, disruption of the mitochondrial membrane potential or loss of mitochondrial membrane structural integrity adversely affects the electron transport chain and causes increased mitochondrial ROS (mtROS) production (66). ROS production can then increase the nuclear localization of NF-κB and increase the NF-κB-mediated transcription of inflammation-related genes to promote an inflammatory response (68). Notably, when cardiomyocytes were injured, NF-κB activation induced the upregulation of COX-2 expression, and inhibition of COX-2 activity reduced the parasite-induced transcription of proinflammatory factors and enhanced ROS activity, conferring protection on cardiomyocytes (69).
3.1.3. Drugs used to treat Chagas disease regulate COX-2 expression
Multiple drugs are currently available for the treatment of Chagas disease, including aspirin, benznidazole, and nifurtimox (4, 70). Among these drugs, aspirin triggers resolvin D1 production during the early chronic phase of T. cruzi infection, which modulates systemic infection levels and inflammatory responses in cardiac tissue and reduces immune cell infiltration, cardiomyocyte hypertrophy, fibrosis and the parasite load in heart tissue (70). Moreover, curcumin treatment in mice with acute Chagas disease improved mouse survival and prevented the activation of associated inflammatory processes in the heart (28). The curcumin mechanism of action may include inhibition of the Ca2+/NFAT-dependent pathological COX-2/mPGES-1/PGE2 pathway in trypanosome-infected cardiomyocytes, potentially conferring cardioprotection in infected mice (62). Moreover, inhibition of PGE2 synthesis with aspirin synergistically enhanced the activity of nitrofurantoin and benznidazole in infected RAW 264.7 cells (70, 71).
In conclusion, the role played by COX-2 in Chagas disease may be related to the attenuation of increased NO levels, negatively affecting the antiparasitic activity of macrophages. It may also be related to a reduction in the ROS content in cardiomyocytes. From the perspective of protecting cardiomyocytes, the use of anti-inflammatory drugs can exert a protective effect on cardiomyocytes by regulating inflammation and fibrosis in the heart.
3.2. COX-2 in leishmaniasis
3.2.1. The hazards of leishmaniasis
Since the WHO first recognized leishmaniasis as a neglected tropical disease and the second leading cause of parasite-related death, more than 1 billion people in 98 countries still face a risk of contracting this disease (https://www.who.int/). Depending on the type of leishmaniasis, symptoms may include fever, weight loss, and partial or total destruction of the mucous membranes of the nose, mouth, and throat (72).
In 2020, most VL cases were diagnosed in Brazil, Ethiopia, India, Kenya, Somalia, and Sudan (https://www.who.int/). According to one estimate, 500,000 patients are first infected with VL each year, and approximately 12 million people are infected with Leishmania parasites (73). Dogs are considered the main urban sources of L. infantum, which strongly parasitizes canine skin, and people in contact with infected dogs can be infected.
3.2.2. Role played by COX-2 in leishmaniasis
Leishmania can upregulate COX-2 expression and PGE2 synthesis (20), and PGE2 can regulate the microbicidal properties of macrophages in a manner mediated by NO2 (74). A COX-2 inhibitor (NS-398) has been reported to reduce the parasite load in peritoneal macrophages of mice infected with Leishmania donovani (L. donovani) (75). Moreover, antibodies against serine protease (SP) inhibited COX-2-mediated PGE2 synthesis and reduced ROS production while enhancing the expression of T helper 1 cell (Th1) cytokines, such as IL-12 (76). Since defense against Leishmania relies on Th1 inflammatory responses (77, 78), SP is suggested to be an important novel target for treatment of VL.
De novo linoleic acid synthesis in Leishmania is required for parasite survival in the extracellular promastigote and the intracellular amastigote stages (79). Due to the anti-inflammatory properties of linoleic acid, it may regulate COX-2. Western blotting showed that infection with a linoleic acid-deficient Leishmania (KO) mutant led to increased phosphorylation of NF-κB p65, IkB and IKKb in RAW264.7 cells. Similarly, RAW264.7 cells infected with KO Leishmania showed a significant increase in COX-2 expression and TNF-α secretion compared with other cell lines. Therefore, we speculate that COX-2 and TNF-α expression in macrophages may be increased by the activation of the NF-κB signaling pathway. In addition, after incubation for 2 h to 6 h, the adhesion rate of promastigotes was the same in all groups. However, the internalization rate of promastigotes in the KO group at 24 h was lower than that in the other groups. Most infected macrophages in the control group still contained parasites after 72 h of incubation; however, after 72 h of incubation, the number of parasites in the KO group was significantly smaller than that in the control group. When RAW264.7 cells were pretreated with BSA-bound linoleic acid, the KO group was found to have a higher infectious capacity than the control group. Thus, KO parasites are more likely to be killed within macrophages (79).
3.3.3. Drugs used to treat leishmaniasis regulate COX-2 expression
Resveratrol is found in various medicinal plants (33). In addition to its cytotoxic, antifungal, antimicrobial and cardioprotective effects, resveratrol also inhibits COX-1 and COX-2 activity (80). Recent in vitro and in vivo assays demonstrated that resveratrol at all concentrations and resveratrol nanoemulsions exert important inhibitory effects against Leishmania (81). This effective antiparasitic activity may be related to potential mitochondrial membrane depolarization, increased plasma membrane permeability, and interference with cell cycle progression (82).
Glycyrrhizic acid (GA) is the main active ingredient of licorice and has played an important role in traditional Chinese medicine and research since ancient times (29). Recently, GA has been found to have antiparasitic activity (83). Experimental GA treatment in vitro resulted in enhanced expression of inducible NO synthase (iNOS) and DUSP4 and inhibition of COX-2 expression. GA treatment of infected macrophages enhanced the expression of IL-12 and TNF-α, which was accompanied by the downregulation of IL-10 and TGF-β expression (83, 84). GA increased the macrophage effector response by inhibiting COX-2-mediated PGE2 synthesis in L. donovani-infected macrophages (85). GA also reduced the parasite load in the liver and spleen and increased T-cell proliferation in BALB/c mice infected with Leishmania (79, 86).
In conclusion, although COX-2-related signaling pathways involved in Leishmania infection have not been extensively studied, genetic mutations have indicated that COX-2 expression may be increased by NF-κB signaling pathway activation. Therefore, drugs that can inhibit NF-κB signaling expression, COX-2 expression and PGE2 synthesis might be a future direction in drug research.
3.3. COX-2 in toxoplasmosis
3.3.1. The hazards of toxoplasmosis
Toxoplasmosis is a common disease caused by Toxoplasma gondii (T. gondii), a parasite with a high prevalence in the tropics (87). The T gondii life cycle largely progresses in cats (88). Most T. gondii infections lead to mild symptoms, but immunocompromised patients tend to have a poor prognosis. Therefore, toxoplasmosis can be a significant disease in patients infected with human immunodeficiency virus and with acquired immunodeficiency syndrome (HIV/AIDS) or other immunosuppressive diseases (89).
3.3.2. Role of COX-2 in toxoplasmosis
Many studies have demonstrated a role of COX-2 and PGE2 in T. gondii infection (32, 90–92). Notably, COX-2 and PGE2 promote the survival of different pathogens in host cells, induce the replication and dissemination of pathogens, and downregulate the immune response (93, 94). COX-2 expression has been shown to be significantly increased in T. gondii infection, confirming that this parasite is a potent inducer of COX-2 (95). T. gondii-infected macrophages may exhibit modulated AA content (a COX-2 substrate) mediated through the calcium signal transduction pathway, and the PKC-dependent COX-2 metabolic pathway has been shown to regulate PGE2 synthesis (91). Moreover, the induction of lipid droplet (LD) formation by T. gondii in host cells was found to be closely associated with COX-2 expression. LD formation primarily maintains T. gondii survival but is also important for the production of inflammatory mediators (96). An LD core consists of phospholipids and neutral lipids, and when cells detect parasites, these LDs release AA, which can be converted to PGE2 via COX-2 activity (97).
Moreover, since T. gondii tachyzoites pass through the placenta and can reach fetal tissue during pregnancy, which can cause serious birth defects that exert impacts into adulthood, the study of T. gondii replication in trophoblast cells and chorionic cells is important (98). Studies have shown that the COX-2 expression levels and PGE2 production levels are significantly increased, proinflammatory cytokine (IL-6 and MIF) expression is induced, and the level of anti-inflammatory cytokines (IL-4 and IL-10) and the number of LDs are increased in toxoplasmosis (32). Thus, COX-2 facilitates intracellular T. gondii proliferation in human trophoblast cells and human chorionic villi, downregulates proinflammatory mediator expression and increases LD production in cells, thereby inhibiting immune responses in the environment where the parasite lives.
3.3.3. Drugs or proteins used to treat toxoplasmosis regulate COX-2 expression
Many anti-Toxoplasma drugs have been developed, and the combination of sulfadiazine and pyrimethamine is currently the gold standard for the treatment of toxoplasmosis (99). In addition, spiramycin and some Chinese medicine ingredients, such as curcumin and artemether, can significantly inhibit the proliferation of Toxoplasma (100–102). Among these medicines, the effect of curcumin on T. gondii may be similar to its effect on T. cruzi. Recent studies have shown that annexin A1 (ANXA1) expression in cytotrophoblasts is decreased after T. gondii infection. ANXA1 is a calcium-dependent phospholipid-binding protein that mediates glucocorticoid action, inhibits PG synthesis, and limits the abundance of COX-2. Treatment with ANXA1 reduces the parasitism rate of placental explants in the third trimester of pregnancy (32). In vitro cultured trophoblast cells provide a cellular model for studying the mechanism by which various substances cross the placental barrier; furthermore, these cells provide the cytological basis for studying the pathogenesis of mother-to-child transmitted diseases. Since T. gondii tachyzoites reach the fetal tissue through the placenta during pregnancy, it is important to investigate T. gondii replication in trophoblast and chorionic cells (27). It has been reported that COX-2 inhibitors reduced T. gondii replication in trophoblast and chorionic tumor cells and altered the expression of inflammation-associated cytokines (32). For example, in BeWo cells (human chorionic tumor cells), COX-2 inhibitors induced an increase in proinflammatory cytokines (IL-6 and MIF) and reduced the expression of anti-inflammatory cytokines (IL-4 and IL-10). In HTR-8/SVneo (human chorionic trophoblast) cells, COX-2 inhibitors (meloxicam or celecoxib) induced an increase in IL-6 and nitrite and a decrease in IL-4 and TGF-1 levels (32). In addition, COX-2 inhibitors reduced the number of LDs in the two cell types.
In conclusion, the increase in COX-2 expression and PGE2 production induced by T. gondii infection in macrophages and other cells is clear. However, the mechanism directing this increase is not clear, with multiple pathways possibly regulating the increase in COX-2 expression and PGE2 production. Studies targeting LDs may be a direction for future research.
3.4. COX-2 in malaria
3.4.1. The hazards of malaria
A 2021 study reported a total of 241 million malaria cases worldwide in 2020, and the total number of malaria-related deaths worldwide was 627,000. Of these, 95% of malaria cases and 96% of malaria deaths occurred in Africa.
Plasmodium mainly infects two types of hosts, mosquitoes and humans; it undergoes sexual reproduction in mosquitoes and asexual proliferation in humans (103). Plasmodium-carrying Anopheles mosquitoes transmit the parasite by biting humans (104). Then, the parasite migrates through the dermis and enters the blood, where it is carried to the liver (105). The parasite then enters hepatocytes and develops schizonts. The schizonts are released from hepatocytes into the bloodstream, where they invade red blood cells (106). They begin the next phase of their life cycle in these red blood cells, progressing through ring stages to the trophozoite site and then the schizont stage (107). Malaria clinical outcomes range from the complete absence of symptoms to severe disease and death. Children under the age of 5 years and pregnant women are the most vulnerable groups affected by malaria (108).
3.4.2. Role of COX-2 in malaria
Pregnant women are particularly susceptible to malaria due to immunological changes during pregnancy (109). Placental malaria caused by Plasmodium berghei (P. berghei) and P. falciparum can upregulate PG synthesis by increasing COX-2 enzyme activity (110). COX-2 and PGs cause uterine contractions and can therefore induce miscarriage or preterm birth (31). Thus, regulating COX-2 and PG levels is very important to fetal health.
Moreover, COX-2 regulates severe malarial anemia (SMA). SNP variations and their genetic combination in the COX-2 promoter have been reported to be related to the longitudinal risk of malaria, SMA and all-cause mortality in children living in areas with high Plasmodium falciparum transmission (111). Notably, in contrast to the high expression of COX-2 in pregnant mice infected with P. berghei, PGE2 levels in the monocytes of mice which infected with P. falciparum were significantly associated with the increase in plasma IL-10 levels (112). In P. falciparum infection, the induction of high PGE2 levels by COX-2 is an important host defense mechanism (113). In vitro studies have shown that reduced PGE2 production is caused by the downregulation of COX-2 expression due to the associated production of parasite-produced hemozoin (114). Hence, a central feature of SMA pathogenesis was found to be the systemic inhibition of PGE2 production, and reduced systemic PGE2 levels during infection are at least partially mediated by leukocyte phagocytosis of hemozoin (115). In vitro and in vivo experimental results suggest that inhibition of COX-2-mediated PGE2 production is associated with TNF-α overproduction in children with malaria (115).
Furthermore, in P. falciparum-transmitted areas, children with malaria often present with an increased incidence of other infections, such as bacterial infections and HIV-1 (116, 117). Therefore, the COX-2 expression and PGE2 production in children with malaria and children coinfected with bacteria or HIV-1 were also analyzed. The results showed that the COX-2 expression levels in peripheral blood and bicyclo-PGE2/creatinine levels in plasma were significantly reduced in coinfected children compared to levels in malaria monoinfected children. Furthermore, inhibition of circulating bicyclo-PGE2 was significantly associated with reduced hemoglobin levels in children with either malaria mono- or coinfection (112), suggesting that bicyclo-PGE may be a marker and mediator of malaria pathogenesis.
COX-2 also plays a very important role in cerebral malaria (CM). COX-2-derived PGE2 is negatively correlated with the disease severity of CM caused by P. falciparum (118). Altered blood brain barrier integrity and cytokine expression patterns are key determinants of central nervous system lesion formation in patients with CM caused by P. falciparum (119). Moreover, in clinical trials, the peripheral blood of CM patients showed altered PG concentrations (112). PG synthesis is controlled by many types of cyclooxygenases, and COX expression has been found to play a key role in immune regulation, hemostasis, and inflammatory reactions in multiple pathologically altered brain tissues. COX expression in CM patient brains was determined through immunohistochemistry. The accumulation of COX-2-expressing endothelial cells and astrocytes was detected in CM brain samples (120).
Similar to elevated COX-2 expression in infected pregnant mice, COX-2 expression was shown to be elevated in C57BL/6 mouse models of CM established with P. berghei (121). In a comparison study with CM-susceptible CBA mice, C57BL/6 mice and malaria-resistant BALB/c mice, all three mouse types responded to Plasmodium. The mice exhibited increases in the number of LDs in macrophages and a significant inflammatory response. Furthermore, the expression of COX-2 and 5-lipoxygenase (5-LOX) was enhanced in the brain tissue cells and blood vessels of C57BL/6 mice and CBA mice. However, neither COX-2 nor 5-LOX was expressed in the brain tissue cells or blood vessels of BALB/c mice. In the macrophages of infected BALB/c mice, PPAR expression levels were increased in the nucleus and decreased in the cytoplasm, suggesting PPAR-γ nuclear translocation (122, 123). PPAR-γ translocation to the nucleus may result in downregulation of proinflammatory cytokine production (124), delaying disease severity in BALB/c mice; this possibility may explain the increased infection resistance in BALB/c mice.
3.4.3. Drugs used to treat malaria regulate COX-2 expression
P. berghei caused elevated COX-2 expression in pregnant mice, but Andrographis paniculata (AS201-01) tablets significantly reduced placental COX-2 expression and PG production in pregnant mice infected with P. berghei (31). The anti-inflammatory activity of AS201-01 may inhibit iNOS and COX-2 expression by inhibiting p38/MAPK signaling pathway activation (125). AS201-01 can increase TGF-β and decrease TLR-4 expression and the apoptotic index, ultimately inhibiting P. berghei growth (31).
Studies have shown that although aspirin and celecoxib are effective in T. cruzi-induced diseases, caution must be taken when they are used to treat malaria. The reason for this may be related to platelets, because human platelets are important for a variety of Plasmodium parasites. Thus, the inhibition of platelet function by platelet inhibitors such as aspirin may also eliminate the positive effects (126). Erythrocytic parasite analysis indicates that platelet-associated parasite killing is characterized by intracellular erythrocytic accumulation of platelet factor 4 (PF4) (127). This function of PF4 is critically dependent on the Duffy antigen (Fy) receptor binding to PF4. Previous studies have shown that evolutionary selection of African Fy -negative alleles provides protection against P. vivax infection (128). Meanwhile, recent studies showed that Fy binds the platelet effector molecule PF4 and is required for platelet-mediated killing of P. falciparum (129). Platelet killing of P. falciparum requires platelet-iRBC contact, the release of PF4, and the binding of PF4 to Fy receptors. The mechanism may be that the release of PF4 upon direct platelet-iRBC contact allows access to P. falciparum via the Fy (129).
In recent years, artesunate has been used as a first-line treatment for severe malaria in adults and children. The use of artesunate has been shown to reduce mortality in patients with severe malaria (130). Artemisinin binds to a very wide range of parasitic proteins and can affect diverse organelles and cellular processes, including hemoglobin endocytosis, glycolysis, protein synthesis and degradation, and cell cycle regulation (131). Artemisinin-induced decreases in COX-2 expression have mainly been observed in cancer (132). Artesunate significantly inhibited gastric cancer cell proliferation in a time- and dose-dependent manner and induced the apoptosis of gastric cancer cells in a dose-dependent manner, and this apoptotic effect was found to be associated with decreased COX-2 expression (133). Furthermore, artesunate prevents neuroinflammation in BV2 microglia by interfering with the NF-κB and p38/MAPK signaling pathways (134). However, whether COX-2 regulates neuroinflammation in malaria has not been extensively investigated.
In conclusion, hemozoin is an insoluble crystalline pigment produced by Plasmodium after digestion of host hemoglobin within erythrocytes. After rupture of infected erythrocytes, hemozoin is released into the bloodstream and is phagocytosed by circulating monocytes and tissue macrophages (135). Experiments with cultured blood monocytes have shown that hemozoin increases the release of cytokines, such as the proinflammatory cytokine TNF-α and the anti-inflammatory cytokine IL-10. Then, TNF-α induces high levels of persistent COX-2 gene expression (136), whereas reduced peripheral PGE2 biosynthesis induced by Plasmodium occurs through hemozoin-induced suppression of blood mononuclear cell COX-2 gene expression via increased IL-10 expression (113); hence, the regulatory effects of hemozoin on COX-2 expression may be complex.
3.5. COX-2 in babesiosis
3.5.1. The hazards of babesiosis
Babesiosis, also known as redwater, is a blood protozoonotic disease transmitted by hard ticks (137). The clinical symptoms of acute bovine babesiosis are fever, limb weakness, anemia, and sometimes animal death (138). Babesiosis is endemic in many countries in Europe, Asia and Africa (139). Babesiosis is mainly transmitted between domestic animals and wild animals, but certain strains are zoonotic (140).
3.5.2. Role of COX-2 in babesiosis
LDs are key regulators of not only inflammation and toxoplasmosis but also babesiosis. Increases in the number of liposomes during bovine Babesia infection have been reported to be associated with Babesia strains and the expression of COX-2 and other enzymes (141, 142). The attenuated strain R1A (LA) and toxic strain S2P (LV) induced TNF-α and IL-6 secretion by mouse peritoneal macrophages after infection with both parasites. Moreover, the LA strain elevates liposome content and COX-2 expression, which is associated with TLR2 and TLR6 expression, respectively (141, 142).
3.5.3. Drugs used to treat babesiosis regulate COX-2 expression
Different Babesia species differ in their drug susceptibility. Large canine Babesia species, such as Babesia canis and Babesia rossi, are sensitive to imidocarb dipropionate and diminazene aceturate, but small species, such as Babesia gibsoni and Babesia conradae, are relatively resistant to these drugs. Therefore, combination treatment with hydroxynaphthoquinone atovaquone and the antibiotic azithromycin is usually used (143). Azithromycin and other antibiotics with antiprotozoal properties mainly target the apicoplast, a residual plastid found in protozoa, and eventually cause parasite death (144). Moreover, tafenoquine may be a highly useful drug to treat B. microti infection (145). The regulatory effect of these drugs on COX-2 has been less frequently reported than the effects of other drugs.
Studies have shown that diminazene aceturate exerts a regulatory effect on COX-2. For example, the local use of diminazene aceturate in the treatment of endotoxic uveitis downregulated the mRNA expression levels of inflammatory factors and mediators, such as COX-2 and iNOS, in the iris and ciliary body. Azithromycin inhibited PGE2 synthesis in human leukocytes by inhibiting the expression of cPLA2, COX-1, and COX-2 mRNA, suggesting an anti-inflammatory mechanism of action (146).
In conclusion, the drug sensitivity of different Babesia species may be related to the apicoplast or the proteins encoded by the mitochondrial genome. For example, certain apicoplasts or proteins encoded by the mitochondrial genome can be targeted by antibiotics such as ciprofloxacin and rifampicin. However, only anti-Babesia drugs, such as diminazene aceturate, have shown a regulatory effect on COX-2; however, whether other drugs have a regulatory effect on COX-2 expression remains to be determined.
4. Extracellular parasites
4.1. COX-2 in giardiasis
4.1.1. Hazards for giardiasis
Giardiasis is caused by the protozoan parasite Giardia (147); these parasites are transmitted through the fecal-oral route, usually after ingestion of contaminated water or food or contact between individuals (148). Approximately half of Giardia-infected children are asymptomatic. Other children develop either acute or chronic diarrhea (149). Metronidazole and tinidazole are the preferred drugs for treating giardiasis (13), but resistance to common anti-Giardia drugs has increased in recent years (150). Therefore, the search for new molecular targets against Giardia has become urgent.
4.1.2. The role of COX-2 in giardiasis
COX-2 plays a very important role in inflammation during Giardia infection (149). Studies have shown that the levels of COX-2 and proinflammatory cytokines (TNF-α, IL-6 and IL-1) are increased in the intestinal tissue of infected mice. Moreover, in a coculture with Giardia, the expression levels of COX-2 and proinflammatory cytokines were significantly increased in J774A.1 macrophages (151). These results indicate that Giardia can induce the upregulation of COX-2 expression levels in both macrophage infection and mouse infection models, suggesting a possible role played by COX-2 in Giardia infection. Furthermore, pretreatment with the COX-2 inhibitor NS398 attenuated the upregulated expression of these proinflammatory cytokines, suggesting that Giardia-induced upregulation of inflammatory cytokine expression may be mediated through COX-2. Administration of SB203580 (an inhibitor of p38), SCH772984 (an inhibitor of ERK1/2) or JSH-23 (an inhibitor of NF-κB) after infection with Giardia led to COX-2-mediated inflammatory factor expression activation via the NF-κB and p38/ERK1/2/MAPK signaling pathways (151).
The effect of COX-2 on the apoptosis of intestinal epithelial cells (IECs) may be another key pathogenic factor in giardiasis. After treatment for Giardia infection, the amount of nitric oxide (NO) released from IECs gradually decreased. Moreover, a COX-2 inhibitor diminished cell viability, exacerbated the reduction in NO release, and increased the cell apoptosis rate (152). In contrast, COX-2 overexpression induced by the agonist rebamipide inhibited the apoptosis of IECs induced by Giardia infection. Cell apoptosis is closely related to the MAPK/AKT/NF-κB signaling pathway. For example, the MAPK/AKT/NF-κB signaling pathway has been reported to modulate COX-2 expression (149). First, the p38 inhibitor SB202190, ERK1/2 inhibitor SCH772984, and AKT inhibitor MK-22062 were found to block the upregulated COX-2 expression induced by Giardia infection, revealing an association between p38/ERK/AKT signaling and COX-2. Then, the TLR4 inhibitor TAK-242 was found to significantly block p38 phosphorylation and COX-2 expression in IECs infected with Giardia, suggesting that TLR4-dependent p38 signaling plays a role in regulating COX-2 expression. Moreover, in the p38-NF-κB signaling pathway, COX-2 expression was inhibited by the NF-κB p65 inhibitor JSH-23.
4.1.3. Drugs used to treat giardiasis regulate COX-2 expression
The antiprotozoan activity of indazole derivatives has been recently reported (153). The indazole core is a very important basic structure in pharmacochemistry, and it is widely used to regulate the inflammatory response (154, 155). Considering that protozoan infections are associated with the inflammatory response, researchers designed a group of 2H indazole derivatives to treat parasite infections. Among these derivatives, compound No. 18 showed 12.8-fold greater activity than metronidazole against Giardia. Interestingly, compound No. 18 also showed inhibitory activity against COX-2 in vitro (153). The design of novel antiparasitic compounds with additional COX-2 inhibitory properties may be an interesting direction for future drug production. However, other studies have shown that Giardia-triggered apoptosis may increase IEC permeability and aid in the development of giardiasis through the action of the protease Giardipain-1 (156); thus, antiapoptotic therapy is thought to be an effective strategy to mitigate giardiasis without harming uninfected tissues.
Overall, inhibition of COX-2 activity can promote a reduction in the IEC activity induced by Giardia. Moreover, increasing the production of reactive oxygen species (ROS) and reducing the release of NO aggravates the apoptosis of IEC cells. Therefore, inhibition of COX-2 activity does not exert a protective effect on IECs but plays a role in promoting apoptosis. In contrast, COX-2 overexpression can reduce IEC apoptosis induced by Giardia. Increased ROS levels and decreased NO levels have been identified as stimuli leading to IEC apoptosis (157, 158). The levels of ROS/NO may be influenced by COX-2 expression, which is regulated by MAPK/AKT/NF-κB signaling (159, 160).
However, it is worth noting that IECs are renewed every 3-5 days (161, 162); therefore, Giardia trophozoites must always adhere to new IECs to avoid being eliminated by intestinal movement (163). In this case, apoptosis of some IEC cells may play a positive role in giardiasis by preventing Giardia from colonizing the intestinal epithelia.
In conclusion, we found that the effects of COX-2 on giardiasis may be complex. Although inhibition of COX-2 activity does not protect IECs, it promotes apoptosis in IECs. However, the results of promoting IEC apoptosis may be diverse. On the one hand, IEC apoptosis can prevent parasite infection by preventing Giardia colonization. On the other hand, it may also cause epithelial damage and aggravate inflammatory reactions. Therefore, the current research seems to be focused on exploring which method (COX-2 overexpression or COX-2 inhibition) is more favorable for controlling giardiasis. However, the results of current studies are not consistent.
4.2. COX-2 in Acanthamoeba Keratitis (Acanthamoebiasis)
4.2.1. The hazards of Acanthamoeba Keratitis
Acanthamoeba spp. are pathogenic and opportunistic free-living parasites that cause AK and granulomatous amoebic encephalitis (GAE) in immunocompromised individuals (164). The biological and pathogenic features underlying these opportunistic protozoa are not fully understood. Therefore, a focus on the relationship between the parasites and hosts is needed. The symptoms of AK are mostly redness, photophobia, tears, conjunctival congestion and eye pain, and AK is usually misdiagnosed as herpetic, bacterial or fungal keratitis (165).
4.2.2. Role of COX-2 in AK
In the eyes, PGE2 formation may be attributed to both COX-1 and COX-2 expression. PGE2 is one of the most extensively studied PGs. PGE2 is critical for producing fever and pain and in neurotransmitter modulation. The role played by PGE2 in the eyes has been studied, especially in the context of low intraocular pressure (IOP) (166, 167). Increased PG production may exacerbate inflammation and downregulate the immune response and cytokine production (IL-1, IL-2, IFN-γ and TNF-α), key determinants controlling disease severity and outcome. Moreover, the synthesis of PGs, especially PGE2, is increased under parasite stimulation (168). PGE2 signals binding to the G protein-coupled receptor EP4 can stimulate adenylate cyclase (AC) and then promote cAMP production. Elevated cAMP expression can stimulate cyst formation (169). Thus, increased generation of PGs affects cyst formation (169).
4.2.3. Drugs used to treat AK regulate COX-2 expression
Agahan et al. (170) reported three cases of AK that were successfully treated with nonsteroidal anti-inflammatory drug (NSAID) eye drops. NSAIDs inhibit COX activity. Studies have shown that diclofenac sodium and indomethacin significantly inhibit Acanthamoeba castellanii (A. castellanii) growth (171). Moreover, both diclofenac sodium and indomethacin inhibited cyst formation. In 2015, Aqeel et al. (172) observed that the inhibitory effect of G protein-coupled receptors affected the growth of A. castellanii in vitro. These findings, together with our observations of the inhibitory effect of NSAIDs on A. castellanii growth, suggest that inhibition of COX expression reduces PG synthesis and thus downregulates G protein-coupled receptor activity, leading to downstream cascade blockade and ultimately causing cell cycle arrest. Acetaminophen is a weak inhibitor of PG synthesis, which may explain its ineffectiveness against A. castellanii. NSAIDs are frequently used in clinical practice, and they may be informative for the design and improvement of therapeutics and may lead to better prevention strategies when combined with other antiamoebic drugs.
5. Conclusion
In this review, we discussed the effects of COX-2 expression in host cells in several different parasitic diseases, including Chagas disease, leishmaniasis, giardiasis, trichomoniasis, amebiasis, malaria and babesiosis (Table 1). For intracellular parasitic parasites, the role of COX-2 may be related to the regulation of inflammatory factor expression in the immunological cells of infected animals. For example, aspirin can trigger resolvin D1 production in the early chronic stage of T. cruzi infection, and resolvin D1 regulates systemic infection levels and inflammatory responses in heart tissue by reducing immune cell infiltration, cardiomyocyte hypertrophy, fibrosis, and parasite load in heart tissue. Notably, the effects of different Plasmodium species on COX-2 may be complex. For example, P. berghei can induce increased COX-2 expression, but P. falciparum can reduce COX-2 expression. Extracellular parasites such as Giardia can cause increased COX-2 expression in macrophages. However, the inhibition of COX-2 activity can change its adhesion ability; for example, inhibiting the COX-2 pathway can change IEC cell activity, promote the apoptosis of IEC, and affect the subsequent pathogenic ability of the parasite. It is important to note that changes in COX-2 activity may also affect the cell cycle. Therefore, in addition to its role in cancer, COX-2 may also inhibit different stages of cell cycle progression, such as that of Acanthamoeba. Many inflammatory factor-related signaling pathways are also involved in these diseases, such as the NF-κB and p38/ERK1/2/MAPK pathways. Therefore, clarifying the effect of COX-2 on parasites may lead to the development of better antiparasitic drugs. It should be noted that the most widely available COX-2 inhibitors, NSAIDs, have limited application in the treatment of certain diseases, such as cancer, due to certain side effects. Therefore, further studies are needed to identify drugs that can effectively regulate COX-2 or PGE2 and are better for use against parasites.
Author contributions
JZ performed the majority of the research and data analyses and helped draft the manuscript. XW and JC analyzed and interpreted the raw data. All authors contributed to the article and approved the submitted version.
Funding
This work was supported by the Bethune Research Plan of Jilin University (grant number: 2020-31), the Training Plan for Lixin Excellent Young Teachers of Jilin University (grant number: 2021) and the Foundation of Jilin Provincial Department of Education (grant number: JJKH20201026KJ). Jilin Provincial Science and Technology Development Plan, 20200202046YY (Which belongs to JZ).
Conflict of interest
The authors declare that this research was conducted in the absence of any commercial or financial relationships that could be construed as potential conflicts of interest.
Publisher’s note
All claims expressed in this article are solely those of the authors and do not necessarily represent those of their affiliated organizations, or those of the publisher, the editors and the reviewers. Any product that may be evaluated in this article, or claim that may be made by its manufacturer, is not guaranteed or endorsed by the publisher.
References
1. Cunha-Neto E, Chevillard C, Rodrigues MM, Bozza MT. Immunology and infection by protozoan parasites. Mediators Inflammation (2015) 2015:504951. doi: 10.1155/2015/504951
2. World Health Organisation. World malaria report 2019. Geneva, Switzerland: World Health Organization (2019).
3. Ghorbani M, Farhoudi R. Leishmaniasis in humans: drug or vaccine therapy? Drug Des Devel Ther (2018) 12:25–40. doi: 10.2147/DDDT.S146521
4. Kratz JM. Drug discovery for chagas disease: A viewpoint. Acta Trop (2019) 198:105107. doi: 10.1016/j.actatropica.2019.105107
5. Fehlberg HF, Ribeiro CM, Junior PAB, Oliveira BCM, Santos CAD, Alvarez MRDV, et al. Detection of Cryptosporidium spp. and Giardia duodenalis in small wild mammals in northeastern Brazil. PloS One (2021) 16:e0256199. doi: 10.1371/journal.pone.0256199
6. Bekci T, Cakir IM, Aslan S. Cerebral malaria: A life-threatening complication. Rev Soc Bras Med Trop (2021) 54:e01862021. doi: 10.1590/0037-8682-0186-2021
7. Karunaweera ND, Ferreira MU. Leishmaniasis: Current challenges and prospects for elimination with special focus on the south Asian region. Parasitology (2018) 145:425–9. doi: 10.1017/S0031182018000471
8. da Silva GA, Sugui D, Nunes RF, de Azevedo K, de Azevedo M, Marques A, et al. Mucocutaneous Leishmaniasis/HIV coinfection presented as a diffuse desquamative rash. Case Rep Infect Dis (2014) 2014:293761. doi: 10.1155/2014/293761
9. Noller JMG, Froeschl G, Eisermann P, Jochum J, Theuring S, Reiter-Owona I, et al. Describing nearly two decades of chagas disease in Germany and the lessons learned: a retrospective study on screening, detection, diagnosis, and treatment of trypanosoma cruzi infection from 2000-2018. BMC Infect Dis (2020) 20:919. doi: 10.1186/s12879-020-05600-8
10. van den Bijllaardt W, Overdevest IT, Buiting AG, Verweij JJ. Rapid clearance of Giardia lamblia DNA from the gut after successful treatment. Clin Microbiol Infect (2014) 20:O972–4. doi: 10.1111/1469-0691.12626
11. Solaymani-Mohammadi S. Mucosal defense against giardia at the intestinal epithelial cell interface. Front Immunol (2022) 13:817468. doi: 10.3389/fimmu.2022.817468
12. Hajare ST, Chekol Y, Chauhan NM. Assessment of prevalence of Giardia lamblia infection and its associated factors among government elementary school children from sidama zone, SNNPR, Ethiopia. PloS One (2022) 17:e0264812. doi: 10.1371/journal.pone.0264812
13. Beard DK, Bristol S, Cosby K, Davis A, Manning C, Perry L, et al. Crystal structure of a hypothetical protein from giardia lamblia. Acta Crystallogr F Struct Biol Commun (2022) 78:59–65. doi: 10.1107/S2053230X21013595
14. Lopes EA, Mestre R, Fontinha D, Legac J, Pei JV, Sanches-Vaz M, et al. Discovery of spirooxadiazoline oxindoles with dual-stage antimalarial activity. Eur J Med Chem (2022) 236:114324. doi: 10.1016/j.ejmech.2022.114324
15. Juarez-Saldivar A, Barbosa-Cabrera E, Lara-Ramirez EE, Paz-Gonzalez AD, Martinez-Vazquez AV, Bocanegra-Garcia V, et al. Virtual screening of FDA-approved drugs against triose phosphate isomerase from entamoeba histolytica and Giardia lamblia identifies inhibitors of their trophozoite growth phase. Int J Mol Sci (2021) 22:5943. doi: 10.3390/ijms22115943
16. Huang PJ, Huang CY, Li YX, Liu YC, Chu LJ, Yeh YM, et al. Dissecting the transcriptomes of multiple metronidazole-resistant and sensitive Trichomonas vaginalis strains identified distinct genes and pathways associated with drug resistance and cell death. Biomedicines (2021) 9:1817. doi: 10.3390/biomedicines9121817
17. Padia J, Kulakova L, Galkin A, Herzberg O. Discovery and preclinical development of antigiardiasis fumagillol derivatives. Antimicrob Agents Chemother (2020) 64:e00582–20. doi: 10.1128/AAC.00582-20
18. Koeberle SC, Gollowitzer A, Laoukili J, Kranenburg O, Werz O, Koeberle A, et al. Distinct and overlapping functions of glutathione peroxidases 1 and 2 in limiting NF-kappaB-driven inflammation through redox-active mechanisms. Redox Biol (2020) 28:101388. doi: 10.1016/j.redox.2019.101388
19. Markworth JF, Cameron-Smith D. Arachidonic acid supplementation enhances in vitro skeletal muscle cell growth via a COX-2-dependent pathway. Am J Physiol Cell Physiol (2013) 304:C56–67. doi: 10.1152/ajpcell.00038.2012
20. Giroux M, Descoteaux A. Cyclooxygenase-2 expression in macrophages: modulation by protein kinase c-alpha. J Immunol (2000) 165:3985–91. doi: 10.4049/jimmunol.165.7.3985
21. Zidar N, Odar K, Glavac D, Jerse M, Zupanc T, Stajer D. Cyclooxygenase in normal human tissues–is COX-1 really a constitutive isoform, and COX-2 an inducible isoform? J Cell Mol Med (2009) 13:3753–63. doi: 10.1111/j.1582-4934.2008.00430.x
22. Aleem SAA, Simmons DL, Donaldson LF. Distribution of cyclooxygenase-3 (COX-3) in rat nervous system. Med J Cairo Univ (2009) 77:245–50.
23. Maphasa RE, Meyer M, Dube A. The macrophage response to Mycobacterium tuberculosis and opportunities for autophagy inducing nanomedicines for tuberculosis therapy. Front Cell Infect Microbiol (2021) 10:618414. doi: 10.3389/fcimb.2020.618414
24. Deng Y, Liu B, Mao W, Shen Y, Fu C, Gao L, et al. Regulatory roles of PGE2 in LPS-induced tissue damage in bovine endometrial explants. Eur J Pharmacol (2019) 852:207–17. doi: 10.1016/j.ejphar.2019.03.044
25. Wu J, Bai F, Mao W, Liu B, Yang X, Zhang J, et al. Anti-inflammatory effects of the prostaglandin D(2)/prostaglandin DP1 receptor and lipocalin-type prostaglandin D(2) synthase/prostaglandin D(2) pathways in bacteria-induced bovine endometrial tissue. Vet Res (2022) 53:98. doi: 10.1186/s13567-022-01100-6
26. Xiong W, Wen Q, Du X, Wang J, He W, Wang R, et al. Novel function of cyclooxygenase-2: Suppressing mycobacteria by promoting autophagy via the protein kinase B/Mammalian target of rapamycin pathway. J Infect Dis (2018) 217:1267–79. doi: 10.1093/infdis/jiy033
27. de Souza G, Silva RJ, Milian ICB, Rosini AM, de Araujo TE, Teixeira SC, et al. Cyclooxygenase (COX)-2 modulates Toxoplasma gondii infection, immune response and lipid droplets formation in human trophoblast cells and villous explants. Sci Rep (2021) 11:12709. doi: 10.1038/s41598-021-92120-3
28. Zhu X, Peng X, Lin J, Zhang Y, He H, Zhao G. Lipoxin A4 activates ALX/FPR2 to attenuate inflammation in Aspergillus fumigatus keratitis. Int Immunopharmacol (2021) 96:107785. doi: 10.1016/j.intimp.2021.107785
29. Biteman B, Hassan IR, Walker E, Leedom AJ, Dunn M, Seta F, et al. Interdependence of lipoxin A4 and heme-oxygenase in counter-regulating inflammation during corneal wound healing. FASEB J (2007) 21:2257–66. doi: 10.1096/fj.06-7918com
30. Novaes RD, Sartini MV, Rodrigues JP, Goncalves RV, Santos EC, Souza RL, et al. Curcumin enhances the anti-Trypanosoma cruzi activity of benznidazole-based chemotherapy in acute experimental chagas disease. Antimicrob Agents Chemother (2016) 60:3355–64. doi: 10.1128/AAC.00343-16
31. Dinesh N, Neelagiri S, Kumar V, Singh S. Glycyrrhizic acid attenuates growth of Leishmania donovani by depleting ergosterol levels. Exp Parasitol (2017) 176:21–9. doi: 10.1016/j.exppara.2017.02.015
32. Cardoso MFDO, Moreli JB, Gomes AO, de Freitas Zanon C, Silva AE, Paulesu LR, et al. Annexin A1 peptide is able to induce an anti-parasitic effect in human placental explants infected by toxoplasma gondii. Microb Pathog (2018) 123:153–61. doi: 10.1016/j.micpath.2018.07.005
33. Prasetyo B, Indriani ED, Viandika N, Ilmi H, Tumewu L, Widyawaruyanti A. Activities of Andrographis paniculata (AS201-01) tablet on cox-2 and prostaglandin expression of placental of Plasmodium berghei infected mice. Iran J Parasitol (2021) 16:43–51. doi: 10.18502/ijpa.v16i1.5510
34. Malaguarnera L. Influence of resveratrol on the immune response. Nutrients (2019) 11:946. doi: 10.3390/nu11050946
35. Toth AD, Schell R, Levay M, Vettel C, Theis P, Haslinger C, et al. Inflammation leads through PGE/EP(3) signaling to HDAC5/MEF2-dependent transcription in cardiac myocytes. EMBO Mol Med (2018) 10:e8536. doi: 10.15252/emmm.201708536
36. Philipose S, Konya V, Sreckovic I, Marsche G, Lippe IT, Peskar BA, et al. The prostaglandin E2 receptor EP4 is expressed by human platelets and potently inhibits platelet aggregation and thrombus formation. Arterioscler Thromb Vasc Biol (2010) 30:2416–23. doi: 10.1161/ATVBAHA.110.216374
37. Kalinski P. Regulation of immune responses by prostaglandin E2. J Immunol (2012) 188:21–8. doi: 10.4049/jimmunol.1101029
38. Chan PC, Liao MT, Hsieh PS. The dualistic effect of COX-2-mediated signaling in obesity and insulin resistance. Int J Mol Sci (2019) 20:3115. doi: 10.3390/ijms20133115
39. Moncada S, Gryglewski R, Bunting S, Vane JR. An enzyme isolated from arteries transforms prostaglandin endoperoxides to an unstable substance that inhibits platelet aggregation. Nature (1976) 263:663–5. doi: 10.1038/263663a0
40. Wen PZ, Warden C, Fletcher BS, Kujubu DA, Herschman HR, Lusis AJ. Chromosomal organization of the inducible and constitutive prostaglandin synthase/cyclooxygenase genes in mouse. Genomics (1993) 15:458–60. doi: 10.1006/geno.1993.1091
41. Malerba P, Crews BC, Ghebreselasie K, Daniel CK, Jashim E, Aleem AM, et al. Targeted detection of cyclooxygenase-1 in ovarian cancer. ACS Med Chem Lett (2020) 11:1837–42. doi: 10.1021/acsmedchemlett.9b00280
42. Uddin MJ, Wilson AJ, Crews BC, Malerba P, Uddin MI, Kingsley PJ, et al. Discovery of furanone-based radiopharmaceuticals for diagnostic targeting of COX-1 in ovarian cancer. ACS Omega (2019) 4:9251–61. doi: 10.1021/acsomega.9b01093
43. Hla T, Neilson K. Human cyclooxygenase-2 cDNA. Proc Natl Acad Sci U.S.A. (1992) 89:7384–8. doi: 10.1073/pnas.89.16.7384
44. Mongini PK, Kramer JM, Ishikawa TO, Herschman H, Esposito D. Candidate chromosome 1 disease susceptibility genes for sjogren’s syndrome xerostomia are narrowed by novel NOD.B10 congenic mice. Clin Immunol (2014) 153:79–90. doi: 10.1016/j.clim.2014.03.012
45. Abramson SB. The role of COX-2 produced by cartilage in arthritis. Osteoarthr Cartil (1999) 7:380–1. doi: 10.1053/joca.1998.0217
46. Tanioka T, Nakatani Y, Semmyo N, Murakami M, Kudo I. Molecular identification of cytosolic prostaglandin E2 synthase that is functionally coupled with cyclooxygenase-1 in immediate prostaglandin E2 biosynthesis. J Biol Chem (2000) 275:32775–82. doi: 10.1074/jbc.M003504200
47. Baryawno N, Sveinbjornsson B, Eksborg S, Orrego A, Segerstrom L, Oqvist CO, et al. Tumor-growth-promoting cyclooxygenase-2 prostaglandin E2 pathway provides medulloblastoma therapeutic targets. Neuro Oncol (2008) 10:661–74. doi: 10.1215/15228517-2008-035
48. Salvado MD, Alfranca A, Escolano A, Haeggstrom JZ, Redondo JM. COX-2 limits prostanoid production in activated HUVECs and is a source of PGH2 for transcellular metabolism to PGE2 by tumor cells. Arterioscler Thromb Vasc Biol (2009) 29:1131–7. doi: 10.1161/ATVBAHA.109.188540
49. Chen C, Guan J, Gu X, Chu Q, Zhu H. Prostaglandin E2 and receptors: Insight into tumorigenesis, tumor progression, and treatment of hepatocellular carcinoma. Front Cell Dev Biol (2022) 10:834859. doi: 10.3389/fcell.2022.834859
50. Ballerini P, Contursi A, Bruno A, Mucci M, Tacconelli S, Patrignani P. Inflammation and cancer: From the development of personalized indicators to novel therapeutic strategies. Front Pharmacol (2022) 13:838079. doi: 10.3389/fphar.2022.838079
51. Luo JF, Yao YD, Cheng CS, Lio CK, Liu JX, Huang YF, et al. Sinomenine increases the methylation level at specific GCG site in mPGES-1 promoter to facilitate its specific inhibitory effect on mPGES-1. Biochim Biophys Acta Gene Regul Mech (2022) 1865:194813. doi: 10.1016/j.bbagrm.2022.194813
52. Ma J, Zhang C, Liang W, Li L, Du J, Pan C, et al. Omega-3 and omega-6 polyunsaturated fatty acids regulate the proliferation, invasion and angiogenesis of gastric cancer through COX/PGE signaling pathway. Front Oncol (2022) 12:802009. doi: 10.3389/fonc.2022.802009
53. Rudic RD, Brinster D, Cheng Y, Fries S, Song WL, Austin S, et al. COX-2-derived prostacyclin modulates vascular remodeling. Circ Res (2005) 96:1240–7. doi: 10.1161/01.RES.0000170888.11669.28
54. Garcia-Redondo AB, Esteban V, Briones AM, Campo LSDD, Gonzalez-Amor M, Mendez-Barbero N, et al. Regulator of calcineurin 1 modulates vascular contractility and stiffness through the upregulation of COX-2-derived prostanoids. Pharmacol Res (2018) 133:236–49. doi: 10.1016/j.phrs.2018.01.001
55. Pinheiro A, Antunes A Jr., Andrade L, De Brot L, Pinto-Neto AM, Costa-Paiva L. Expression of hormone receptors, Bcl−2, Cox−2 and Ki67 in benign endometrial polyps and their association with obesity. Mol Med Rep (2014) 9:2335–41. doi: 10.3892/mmr.2014.2125
56. Peng-Fei H, Na AR, Hui C, Hong-Yu W, Jin-Shan C. Activation of alpha7 nicotinic acetylcholine receptor protects bovine endometrial tissue against LPS-induced inflammatory injury via JAK2/STAT3 pathway and COX-2 derived prostaglandin E(2). Eur J Pharmacol (2021) 900:174067. doi: 10.1016/j.ejphar.2021.174067
57. Zuma AA, de Souza W. Chagas disease chemotherapy: What do we know so far? Curr Pharm Des (2021) 27:3963–95. doi: 10.2174/1381612827666210216152654
58. Bern C, Messenger LA, Whitman JD, Maguire JH. Chagas disease in the united states: A public health approach. Clin Microbiol Rev (2019) 33:e00023–19. doi: 10.1128/CMR.00023-19
59. Coura JR. The main sceneries of chagas disease transmission. the vectors, blood and oral transmissions–a comprehensive review. Mem Inst Oswaldo Cruz (2015) 110:277–82. doi: 10.1590/0074-0276140362
60. Hotez PJ, Damania A, Bottazzi ME. Central Latin America: Two decades of challenges in neglected tropical disease control. PloS Negl Trop Dis (2020) 14:e0007962. doi: 10.1371/journal.pntd.0007962
61. Cunha-Neto E, Chevillard C. Chagas disease cardiomyopathy: Immunopathology and genetics. Mediators Inflammation (2014) 2014:683230. doi: 10.1155/2014/683230
62. Hernandez M, Wicz S, Corral RS. Cardioprotective actions of curcumin on the pathogenic NFAT/COX-2/prostaglandin E(2) pathway induced during Trypanosoma cruzi infection. Phytomedicine (2016) 23:1392–400. doi: 10.1016/j.phymed.2016.06.017
63. Alvarez MN, Peluffo G, Piacenza L, Radi R. Intraphagosomal peroxynitrite as a macrophage-derived cytotoxin against internalized Trypanosoma cruzi: Consequences for oxidative killing and role of microbial peroxiredoxins in infectivity. J Biol Chem (2011) 286:6627–40. doi: 10.1074/jbc.M110.167247
64. Alcaide P, Fresno M. AgC10, a mucin from Trypanosoma cruzi, destabilizes TNF and cyclooxygenase-2 mRNA by inhibiting mitogen-activated protein kinase p38. Eur J Immunol (2004) 34:1695–704. doi: 10.1002/eji.200324660
65. Moraes KC, Diniz LF, Bahia MT. Role of cyclooxygenase-2 in Trypanosoma cruzi survival in the early stages of parasite host-cell interaction. Mem Inst Oswaldo Cruz (2015) 110:181–91. doi: 10.1590/0074-02760140311
66. Gupta S, Bhatia V, Wen JJ, Wu Y, Huang MH, Garg NJ. Trypanosoma cruzi infection disturbs mitochondrial membrane potential and ROS production rate in cardiomyocytes. Free Radic Biol Med (2009) 47:1414–21. doi: 10.1016/j.freeradbiomed.2009.08.008
67. Corral RS, Guerrero NA, Cuervo H, Girones N, Fresno M. Trypanosoma cruzi infection and endothelin-1 cooperatively activate pathogenic inflammatory pathways in cardiomyocytes. PloS Negl Trop Dis (2013) 7:e2034. doi: 10.1371/journal.pntd.0002034
68. Ba X, Gupta S, Davidson M, Garg NJ. Trypanosoma cruzi induces the reactive oxygen species-PARP-1-RelA pathway for up-regulation of cytokine expression in cardiomyocytes. J Biol Chem (2010) 285:11596–606. doi: 10.1074/jbc.M109.076984
69. Maldonado E, Rojas DA, Urbina F, Solari A. The oxidative stress and chronic inflammatory process in chagas disease: Role of exosomes and contributing genetic factors. Oxid Med Cell Longev (2021) 2021:4993452. doi: 10.1155/2021/4993452
70. Carrillo I, Rabelo RAN, Barbosa C, Rates M, Fuentes-Retamal S, Gonzalez-Herrera F, et al. Aspirin-triggered resolvin D1 reduces parasitic cardiac load by decreasing inflammation in a murine model of early chronic chagas disease. PloS Negl Trop Dis (2021) 15:e0009978. doi: 10.1371/journal.pntd.0009978
71. Pereira RS, Malvezi AD, Lovo-Martins MI, Lucchetti BFC, Santos JP, Tavares ER, et al. Combination therapy using benznidazole and aspirin during the acute phase of experimental chagas disease prevents cardiovascular dysfunction and decreases typical cardiac lesions in the chronic phase. Antimicrob Agents Chemother (2020) 64:e00069–20. doi: 10.1128/AAC.00069-20
72. Rama M, Kumar NV, Balaji S. A comprehensive review of patented antileishmanial agents. Pharm Pat Anal (2015) 4:37–56. doi: 10.4155/ppa.14.55
73. Fallahi P, Elia G, Bonatti A. Leishmaniasis and IFN-gamma dependent chemokines. Clin Ter (2016) 167:e117–22. doi: 10.7417/CT.2016.1954
74. Venturin GL, Bragato JP, Melo LM, Rebech GT, Costa SF, de Siqueira CE, et al. Regulatory effect of PGE(2) on microbicidal activity and inflammatory cytokines in canine leishmaniasis. Parasite Immunol (2020) 42:e12713. doi: 10.1111/pim.12713
75. Das P, De T, Chakraborti T. Leishmania donovani secretory serine protease alters macrophage inflammatory response via COX-2 mediated PGE-2 production. Indian J Biochem Biophys (2014) 51:542–51.
76. Das P, Paik D, Naskar K, Chakraborti T. Leishmania donovani serine protease encapsulated in liposome elicits protective immunity in experimental visceral leishmaniasis. Microbes Infect (2018) 20:37–47. doi: 10.1016/j.micinf.2017.09.011
77. Diaz-Gandarilla JA, Osorio-Trujillo C, Hernandez-Ramirez VI, Talamas-Rohana P. PPAR activation induces M1 macrophage polarization via cPLA(2)-COX-2 inhibition, activating ROS production against leishmania mexicana. BioMed Res Int (2013) 2013:215283. doi: 10.1155/2013/215283
78. Ratnapriya S, Keerti, Yadav NK, Dube A, Sahasrabuddhe AA. A chimera of Th1 stimulatory proteins of Leishmania donovani offers moderate immunotherapeutic efficacy with a Th1-inclined immune response against visceral leishmaniasis. BioMed Res Int (2021) 2021:8845826. doi: 10.1155/2021/8845826
79. Mukherjee A, Adhikari A, Das P, Biswas S, Mukherjee S, Adak S. Loss of virulence in NAD(P)H cytochrome b5 oxidoreductase deficient leishmania major. Biochem Biophys Res Commun (2018) 503:371–7. doi: 10.1016/j.bbrc.2018.06.037
80. Kang SS, Cuendet M, Endringer DC, Croy VL, Pezzuto JM, Lipton MA. Synthesis and biological evaluation of a library of resveratrol analogues as inhibitors of COX-1, COX-2 and NF-kappaB. Bioorg Med Chem (2009) 17:1044–54. doi: 10.1016/j.bmc.2008.04.031
81. Mousavi P, Rahimi Esboei B, Pourhajibagher M, Fakhar M, Shahmoradi Z, Hejazi SH, et al. Anti-leishmanial effects of resveratrol and resveratrol nanoemulsion on leishmania major. BMC Microbiol (2022) 22:56. doi: 10.1186/s12866-022-02455-8
82. Antinarelli LMR, Meinel RS, Coelho EAF, da Silva AD, Coimbra ES. Resveratrol analogues present effective antileishmanial activity against promastigotes and amastigotes from distinct leishmania species by multitarget action in the parasites. J Pharm Pharmacol (2019) 71:1854–63. doi: 10.1111/jphp.13177
83. Bhattacharjee S, Bhattacharjee A, Majumder S, Majumdar SB, Majumdar S. Glycyrrhizic acid suppresses cox-2-mediated anti-inflammatory responses during Leishmania donovani infection. J Antimicrob Chemother (2012) 67:1905–14. doi: 10.1093/jac/dks159
84. Parveen S, Chowdhury AR, Jawed JJ, Majumdar SB, Saha B, Majumdar S. Immunomodulation of dual specificity phosphatase 4 during visceral leishmaniasis. Microbes Infect (2018) 20:111–21. doi: 10.1016/j.micinf.2017.10.009
85. Richard SA. Exploring the pivotal immunomodulatory and anti-inflammatory potentials of glycyrrhizic and glycyrrhetinic acids. Mediators Inflammation (2021) 2021:6699560. doi: 10.1155/2021/6699560
86. Bandyopadhyay S, Bhattacharjee A, Banerjee S, Halder K, Das S, Chowdhury BP, et al. Glycyrrhizic acid-mediated subdual of myeloid-derived suppressor cells induces antileishmanial immune responses in a susceptible host. Infect Immun (2015) 83:4476–86. doi: 10.1128/IAI.00729-15
87. Elreheim AA, Farid A, Mahana N, Bauiomy I, Elameer A. Sandwich-Elisa development for the diagnosis of toxoplasma gondii. J Egypt Soc Parasitol (2016) 46:429–40. doi: 10.21608/jesp.2016.88726
88. Dubey JP, Cerqueira-Cezar CK, Murata FHA, Kwok OCH, Yang YR, Su C. All about toxoplasmosis in cats: The last decade. Vet Parasitol (2020) 283:109145. doi: 10.1016/j.vetpar.2020.109145
89. Robert-Gangneux F, Darde ML. Epidemiology of and diagnostic strategies for toxoplasmosis. Clin Microbiol Rev (2012) 25:264–96. doi: 10.1128/CMR.05013-11
90. Kim JY, Ahn MH, Song HO, Choi JH, Ryu JS, Min DY, et al. Involvement of MAPK activation in chemokine or COX-2 productions by toxoplasma gondii. Korean J Parasitol (2006) 44:197–207. doi: 10.3347/kjp.2006.44.3.197
91. Peng BW, Lin JY, Zhang T. Toxoplasma gondii induces prostaglandin E2 synthesis in macrophages via signal pathways for calcium-dependent arachidonic acid production and PKC-dependent induction of cyclooxygenase-2. Parasitol Res (2008) 102:1043–50. doi: 10.1007/s00436-007-0873-4
92. Pereira ACA, Silva RJ, Franco PS, Gomes ADO, Souza G, Milian ICB, et al. Cyclooxygenase (COX)-2 inhibitors reduce Toxoplasma gondii infection and upregulate the pro-inflammatory immune response in Calomys callosus rodents and human monocyte cell line. Front Microbiol (2019) 10:225. doi: 10.3389/fmicb.2019.00225
93. Alfajaro MM, Choi JS, Kim DS, Seo JY, Kim JY, Park JG, et al. Activation of COX-2/PGE2 promotes sapovirus replication via the inhibition of nitric oxide production. J Virol (2017) 91:e01656–16. doi: 10.1128/JVI.01656-16
94. Wang Y, Liu S, Li B, Jiang Y, Zhou X, Chen J, et al. Staphylococcus aureus induces COX-2-dependent proliferation and malignant transformation in oral keratinocytes. J Oral Microbiol (2019) 11:1643205. doi: 10.1080/20002297.2019.1643205
95. Milian ICB, Silva RJ, Manzan-Martins C, Barbosa BF, Guirelli PM, Ribeiro M, et al. Increased Toxoplasma gondii intracellular proliferation in human extravillous trophoblast cells (HTR8/SVneo line) is sequentially triggered by MIF, ERK1/2, and COX-2. Front Microbiol (2019) 10:852. doi: 10.3389/fmicb.2019.00852
96. Dias SSG, Soares VC, Ferreira AC, Sacramento CQ, Fintelman-Rodrigues N, Temerozo JR, et al. Lipid droplets fuel SARS-CoV-2 replication and production of inflammatory mediators. PloS Pathog (2020) 16:e1009127. doi: 10.1371/journal.ppat.1009127
97. Gomes AF, Magalhaes KG, Rodrigues RM, de Carvalho L, Molinaro R, Bozza PT, et al. Toxoplasma gondii-skeletal muscle cells interaction increases lipid droplet biogenesis and positively modulates the production of IL-12, IFN-g and PGE2. Parasit Vectors (2014) 7:47. doi: 10.1186/1756-3305-7-47
98. Rudzki EN, Ander SE, Coombs RS, Alrubaye HS, Cabo LF, Blank ML, et al. Toxoplasma gondii GRA28 is required for placenta-specific induction of the regulatory chemokine CCL22 in human and mouse. mBio (2021) 12:e0159121. doi: 10.1128/mBio.01591-21
99. Dunay IR, Gajurel K, Dhakal R, Liesenfeld O, Montoya JG. Treatment of toxoplasmosis: historical perspective, animal models, and current clinical practice. Clin Microbiol Rev (2018) 31:e00057–17. doi: 10.1128/CMR.00057-17
100. Goo YK, Yamagishi J, Ueno A, Terkawi MA, Aboge GO, Kwak D, et al. Characterization of Toxoplasma gondii glyoxalase 1 and evaluation of inhibitory effects of curcumin on the enzyme and parasite cultures. Parasit Vectors (2015) 8:654. doi: 10.1186/s13071-015-1268-5
101. Mikaeiloo H, Ghaffarifar F, Dalimi A, Sharifi Z, Hassan ZM. Apoptotic activity and anti-toxoplasma effects of artemether on the tachyzoites and experimental infected vero and J774 cell lines by toxoplasma gondii. Indian J Pharmacol (2016) 48:179–85. doi: 10.4103/0253-7613.178838
102. Montazeri M, Mehrzadi S, Sharif M, Sarvi S, Tanzifi A, Aghayan SA, et al. Drug resistance in toxoplasma gondii. Front Microbiol (2018) 9:2587. doi: 10.3389/fmicb.2018.02587
103. Gu W, Killeen GF, Mbogo CM, Regens JL, Githure JI, Beier JC. An individual-based model of Plasmodium falciparum malaria transmission on the coast of Kenya. Trans R Soc Trop Med Hyg (2003) 97:43–50. doi: 10.1016/s0035-9203(03)90018-6
104. Rossati A, Bargiacchi O, Kroumova V, Zaramella M, Caputo A, Garavelli PL. Climate, environment and transmission of malaria. Infez Med (2016) 24:93–104.
105. Loubens M, Vincensini L, Fernandes P, Briquet S, Marinach C, Silvie O. Plasmodium sporozoites on the move: Switching from cell traversal to productive invasion of hepatocytes. Mol Microbiol (2021) 115:870–81. doi: 10.1111/mmi.14645
106. Yang ASP, van Waardenburg YM, van de Vegte-Bolmer M, van Gemert GA, Graumans W, de Wilt JHW, et al. Zonal human hepatocytes are differentially permissive to Plasmodium falciparum malaria parasites. EMBO J (2021) 40:e106583. doi: 10.15252/embj.2020106583
107. Zhang J, Feng GH, Zou CY, Su PC, Liu HE, Yang ZQ. Overview of the improvement of the ring-stage survival assay-a novel phenotypic assay for the detection of artemisinin-resistant plasmodium falciparum. Zool Res (2017) 38:317–20. doi: 10.24272/j.issn.2095-8137.2017.075
108. Gimenez AM, Marques RF, Regiart M, Bargieri DY. Diagnostic methods for non-falciparum malaria. Front Cell Infect Microbiol (2021) 11:681063. doi: 10.3389/fcimb.2021.681063
109. McLean AR, Ataide R, Simpson JA, Beeson JG, Fowkes FJ. Malaria and immunity during pregnancy and postpartum: A tale of two species. Parasitology (2015) 142:999–1015. doi: 10.1017/S0031182015000074
110. Sarr D, Aldebert D, Marrama L, Frealle E, Gaye A, Brahim HO, et al. Chronic infection during placental malaria is associated with up-regulation of cycloxygenase-2. Malar J (2010) 9:45. doi: 10.1186/1475-2875-9-45
111. Anyona SB, Hengartner NW, Raballah E, Ong’echa JM, Lauve N, Cheng Q, et al. Cyclooxygenase-2 haplotypes influence the longitudinal risk of malaria and severe malarial anemia in Kenyan children from a holoendemic transmission region. J Hum Genet (2020) 65:99–113. doi: 10.1038/s10038-019-0692-3
112. Anyona SB, Kempaiah P, Davenport GC, Vulule JM, Hittner JB, Ong’echa JM, et al. Suppressed circulating bicyclo-PGE2 levels and leukocyte COX-2 transcripts in children co-infected with p. falciparum malaria and HIV-1 or bacteremia. Biochem Biophys Res Commun (2013) 436:585–90. doi: 10.1016/j.bbrc.2013.05.089
113. Keller CC, Hittner JB, Nti BK, Weinberg JB, Kremsner PG, Perkins DJ. Reduced peripheral PGE2 biosynthesis in Plasmodium falciparum malaria occurs through hemozoin-induced suppression of blood mononuclear cell cyclooxygenase-2 gene expression via an interleukin-10-independent mechanism. Mol Med (2004) 10:45–54. doi: 10.2119/2004-00035.perkins
114. Keller CC, Davenport GC, Dickman KR, Hittner JB, Kaplan SS, Weinberg JB, et al. Suppression of prostaglandin E2 by malaria parasite products and antipyretics promotes overproduction of tumor necrosis factor-alpha: Association with the pathogenesis of childhood malarial anemia. J Infect Dis (2006) 193:1384–93. doi: 10.1086/503047
115. Anyona SB, Kempaiah P, Raballah E, Davenport GC, Were T, Konah SN, et al. Reduced systemic bicyclo-prostaglandin-E2 and cyclooxygenase-2 gene expression are associated with inefficient erythropoiesis and enhanced uptake of monocytic hemozoin in children with severe malarial anemia. Am J Hematol (2012) 87:782–9. doi: 10.1002/ajh.23253
116. Otieno RO, Ouma C, Ong’echa JM, Keller CC, Were T, Waindi EN, et al. Increased severe anemia in HIV-1-exposed and HIV-1-positive infants and children during acute malaria. AIDS (2006) 20:275–80. doi: 10.1097/01.aids.0000200533.56490.b7
117. Abuga KM, Muriuki JM, Uyoga SM, Mwai K, Makale J, Mogire RM, et al. Hepcidin regulation in Kenyan children with severe malaria and non-typhoidal Salmonella bacteremia. Haematologica (2022) 107:1589–98. doi: 10.3324/haematol.2021.279316
118. Deininger MH, Kremsner PG, Meyermann R, Schluesener HJ. Focal accumulation of cyclooxygenase-1 (COX-1) and COX-2 expressing cells in cerebral malaria. J Neuroimmunol (2000) 106:198–205. doi: 10.1016/s0165-5728(00)00187-9
119. Ball HJ, MacDougall HG, McGregor IS, Hunt NH. Cyclooxygenase-2 in the pathogenesis of murine cerebral malaria. J Infect Dis (2004) 189:751–8. doi: 10.1086/381503
120. Perkins DJ, Hittner JB, Mwaikambo ED, Granger DL, Weinberg JB, Anstey NM. Impaired systemic production of prostaglandin E2 in children with cerebral malaria. J Infect Dis (2005) 191:1548–57. doi: 10.1086/429332
121. Anand SS, Maruthi M, Babu PP. The specific, reversible JNK inhibitor SP600125 improves survivability and attenuates neuronal cell death in experimental cerebral malaria (ECM). Parasitol Res (2013) 112:1959–66. doi: 10.1007/s00436-013-3352-0
122. Delahaye NF, Coltel N, Puthier D, Barbier M, Benech P, Joly F, et al. Gene expression analysis reveals early changes in several molecular pathways in cerebral malaria-susceptible mice versus cerebral malaria-resistant mice. BMC Genomics (2007) 8:452. doi: 10.1186/1471-2164-8-452
123. Borges TK, Alves EA, Vasconcelos HA, Carneiro FP, Nicola AM, Magalhaes KG, et al. Differences in the modulation of reactive species, lipid bodies, cyclooxygenase-2, 5-lipoxygenase and PPAR-gamma in cerebral malaria-susceptible and resistant mice. Immunobiology (2017) 222:604–19. doi: 10.1016/j.imbio.2016.11.010
124. Neri T, Armani C, Pegoli A, Cordazzo C, Carmazzi Y, Brunelleschi S, et al. Role of NF-kappaB and PPAR-gamma in lung inflammation induced by monocyte-derived microparticles. Eur Respir J (2011) 37:1494–502. doi: 10.1183/09031936.00023310
125. Liu J, Wang ZT, Ge BX. Andrograpanin, isolated from andrographis paniculata, exhibits anti-inflammatory property in lipopolysaccharide-induced macrophage cells through down-regulating the p38 MAPKs signaling pathways. Int Immunopharmacol (2008) 8:951–8. doi: 10.1016/j.intimp.2007.12.014
126. McMorran BJ, Marshall VM, de Graaf C, Drysdale KE, Shabbar M, Smyth GK, et al. Platelets kill intraerythrocytic malarial parasites and mediate survival to infection. Science (2009) 323:797–800. doi: 10.1126/science.1166296
127. Kho S, Barber BE, Johar E, Andries B, Poespoprodjo JR, Kenangalem E, et al. Platelets kill circulating parasites of all major Plasmodium species in human malaria. Blood (2018) 132:1332–44. doi: 10.1182/blood-2018-05-849307
128. Miller LH, Mason SJ, Clyde DF, McGinniss MH. The resistance factor to Plasmodium vivax in blacks: The Duffy-blood-group genotype. N Engl J Med (1976) 295:302–4. doi: 10.1056/nejm197608052950602
129. McMorran BJ, Wieczorski L, Drysdale KE, Chan JA, Huang HM, Smith C, et al. Platelet factor 4 and Duffy antigen required for platelet killing of plasmodium falciparum. Science (2012) 338:1348–51. doi: 10.1126/science.1228892
130. Lefèvre A, Léonard P. Artesunate and severe malaria in paediatrics. Rev Med Liege (2019) 74:503–7.
131. Wicht KJ, Mok S, Fidock DA. Molecular mechanisms of drug resistance in Plasmodium falciparum malaria. Annu Rev Microbiol (2020) 74:431–54. doi: 10.1146/annurev-micro-020518-115546
132. Su T, Li F, Guan J, Liu L, Huang P, Wang Y, et al. Artemisinin and its derivatives prevent helicobacter pylori-induced gastric carcinogenesis via inhibition of NF-kappaB signaling. Phytomedicine (2019) 63:152968. doi: 10.1016/j.phymed.2019.152968
133. Zhang P, Luo HS, Li M, Tan SY. Artesunate inhibits the growth and induces apoptosis of human gastric cancer cells by downregulating COX-2. Onco Targets Ther (2015) 8:845–54. doi: 10.2147/OTT.S81041
134. Okorji UP, Olajide OA. A semi-synthetic derivative of artemisinin, artesunate inhibits prostaglandin E2 production in LPS/IFNgamma-activated BV2 microglia. Bioorg Med Chem (2014) 22:4726–34. doi: 10.1016/j.bmc.2014.07.007
135. Lautenschlager SOS, Kim T, Bidoia DL, Nakamura CV, Anders HJ, Steiger S. Plasma proteins and platelets modulate neutrophil clearance of malaria-related hemozoin crystals. Cells (2019) 9:93. doi: 10.3390/cells9010093
136. Prato M, Giribaldi G, Polimeni M, Gallo V, Arese P. Phagocytosis of hemozoin enhances matrix metalloproteinase-9 activity and TNF-alpha production in human monocytes: role of matrix metalloproteinases in the pathogenesis of falciparum malaria. J Immunol (2005) 175:6436–42. doi: 10.4049/jimmunol.175.10.6436
137. Johnson N, Phipps LP, McFadzean H, Barlow AM. An outbreak of bovine babesiosis in February, 2019, triggered by above average winter temperatures in southern England and co-infection with babesia divergens and anaplasma phagocytophilum. Parasit Vectors (2020) 13:305. doi: 10.1186/s13071-020-04174-3
138. He L, Bastos RG, Sun Y, Hua G, Guan G, Zhao J, et al. Babesiosis as a potential threat for bovine production in China. Parasit Vectors (2021) 14:460. doi: 10.1186/s13071-021-04948-3
139. Xia Z, Hui W, Jing-Bo X, Shang X, Xiao-Nong Z. Epidemic and research progress of babesiosis. Zhongguo Xue Xi Chong Bing Fang Zhi Za Zhi (2019) 31:63–70. doi: 10.16250/j.32.1374.2018293
140. Hussain S, Hussain A, Aziz MU, Song B, Zeb J, George D, et al. A review of zoonotic babesiosis as an emerging public health threat in Asia. Pathogens (2021) 11:23. doi: 10.3390/pathogens11010023
141. Gimenez G, Magalhaes KG, Belaunzaran ML, Poncini CV, Lammel EM, Cappa SMG, et al. Lipids from attenuated and virulent Babesia bovis strains induce differential TLR2-mediated macrophage activation. Mol Immunol (2010) 47:747–55. doi: 10.1016/j.molimm.2009.10.014
142. Gimenez G, Belaunzaran ML, Magalhaes KG, Poncini CV, Lammel EM, Cappa SMG, et al. Involvement of TLR6 in the induction of COX-2, PGE2 and IL-10 in macrophages by lipids from virulent S2P and attenuated R1A Babesia bovis strains. Vet Parasitol (2016) 223:127–32. doi: 10.1016/j.vetpar.2016.04.033
143. Baneth G. Antiprotozoal treatment of canine babesiosis. Vet Parasitol (2018) 254:58–63. doi: 10.1016/j.vetpar.2018.03.001
144. Burns AL, Sleebs BE, Siddiqui G, De Paoli AE, Anderson D, Liffner B, et al. Retargeting azithromycin analogues to have dual-modality antimalarial activity. BMC Biol (2020) 18:133. doi: 10.1186/s12915-020-00859-4
145. Mordue DG, Wormser GP. Could the drug tafenoquine revolutionize treatment of babesia microti infection? J Infect Dis (2019) 220:442–7. doi: 10.1093/infdis/jiz119
146. Zheng C, Lei C, Chen Z, Zheng S, Yang H, Qiu Y, et al. Topical administration of diminazene aceturate decreases inflammation in endotoxin-induced uveitis. Mol Vis (2015) 21:403–11.
147. Leung AKC, Leung AAM, Wong AHC, Sergi CM, Kam JKM. Giardiasis: an overview. Recent Pat Inflammation Allergy Drug Discovery (2019) 13:134–43. doi: 10.2174/1872213X13666190618124901
148. Cibot M, McLennan MR, Kvac M, Sak B, Asiimwe C, Petrzelkova K. Sparse evidence for Giardia intestinalis, Cryptosporidium spp. and microsporidia infections in humans, domesticated animals and wild nonhuman primates sharing a farm-forest mosaic landscape in Western Uganda. Pathogens (2021) 10:933. doi: 10.3390/pathogens10080933
149. Yang Y, Zhao Y, Liu L, Zhu W, Jia S, Li X, et al. The anti-apoptotic role of COX-2 during in vitro infection of human intestinal cell line by Giardia duodenalis and the potential regulators. Infect Immun (2022) 90:e0067221. doi: 10.1128/iai.00672-21
150. Carter ER, Nabarro LE, Hedley L, Chiodini PL. Nitroimidazole-refractory giardiasis: a growing problem requiring rational solutions. Clin Microbiol Infect (2018) 24:37–42. doi: 10.1016/j.cmi.2017.05.028
151. Zhao YD. COX-2 mediates giardia duodenalis induced inflammatory response in J774A.1 macrophages [MS thesis]. China: Northeast Agricultural University (2021).
152. Al-Ashy R, Chakroun I, El-Sabban ME, Homaidan FR. The role of NF-kappaB in mediating the anti-inflammatory effects of IL-10 in intestinal epithelial cells. Cytokine (2006) 36:1–8. doi: 10.1016/j.cyto.2006.10.003
153. Perez-Villanueva J, Yepez-Mulia L, Gonzalez-Sanchez I, Palacios-Espinosa JF, Soria-Arteche O, Sainz-Espunes TDR, et al. Synthesis and biological evaluation of 2H-indazole derivatives: Towards antimicrobial and anti-inflammatory dual agents. Molecules (2017) 22:1864. doi: 10.3390/molecules22111864
154. Garcia-Valdivia AA, Garcia-Garcia A, Jannus F, Zabala-Lekuona A, Mendez-Arriaga JM, Fernandez B, et al. Antiparasitic, anti-inflammatory and cytotoxic activities of 2D coordination polymers based on 1H-indazole-5-carboxylic acid. J Inorg Biochem (2020) 208:111098. doi: 10.1016/j.jinorgbio.2020.111098
155. Ibanez-Escribano A, Reviriego F, Vela N, Fonseca-Berzal C, Nogal-Ruiz JJ, Aran VJ, et al. Promising hit compounds against resistant trichomoniasis: Synthesis and antiparasitic activity of 3-(omega-aminoalkoxy)-1-benzyl-5-nitroindazoles. Bioorg Med Chem Lett (2021) 37:127843. doi: 10.1016/j.bmcl.2021.127843
156. Ortega-Pierres G, Arguello-Garcia R, Laredo-Cisneros MS, Fonseca-Linan R, Gomez-Mondragon M, Inzunza-Arroyo R, et al. Giardipain-1, a protease secreted by Giardia duodenalis trophozoites, causes junctional, barrier and apoptotic damage in epithelial cell monolayers. Int J Parasitol (2018) 48:621–39. doi: 10.1016/j.ijpara.2018.01.006
157. Ito J, Uchida H, Yokote T, Ohtake K, Kobayashi J. Fasting-induced intestinal apoptosis is mediated by inducible nitric oxide synthase and interferon-gamma in rat. Am J Physiol Gastrointest Liver Physiol (2010) 298:G916–26. doi: 10.1152/ajpgi.00429.2009
158. Feng W, Wang J, Li B, Liu Y, Xu D, Cheng K, et al. Graphene oxide leads to mitochondrial-dependent apoptosis by activating ROS-p53-mPTP pathway in intestinal cells. Int J Biochem Cell Biol (2022) 146:106206. doi: 10.1016/j.biocel.2022.106206
159. Chun J, Choi RJ, Khan S, Lee DS, Kim YC, Nam YJ, et al. Alantolactone suppresses inducible nitric oxide synthase and cyclooxygenase-2 expression by down-regulating NF-kappaB, MAPK and AP-1 via the MyD88 signaling pathway in LPS-activated RAW 264.7 cells. Int Immunopharmacol (2012) 14:375–83. doi: 10.1016/j.intimp.2012.08.011
160. Sun K, Luo J, Jing X, Xiang W, Guo J, Yao X, et al. Hyperoside ameliorates the progression of osteoarthritis: An in vitro and in vivo study. Phytomedicine (2021) 80:153387. doi: 10.1016/j.phymed.2020.153387
161. Lopez-Romero G, Quintero J, Astiazarán-García H, Velazquez C. Host defences against giardia lamblia. Parasite Immunol (2015) 37:394–406. doi: 10.1111/pim.12210
162. Rath E, Moschetta A, Haller D. Mitochondrial function - gatekeeper of intestinal epithelial cell homeostasis. Nat Rev Gastroenterol Hepatol (2018) 15:497–516. doi: 10.1038/s41575-018-0021-x
163. Hagen KD, McInally SG, Hilton ND, Dawson SC. Microtubule organelles in giardia. Adv Parasitol (2020) 107:25–96. doi: 10.1016/bs.apar.2019.11.001
164. Rodriguez-Exposito RL, Sifaoui I, Reyes-Batlle M, Maciver SK, Pinero JE, Lorenzo-Morales J. Statins induce actin cytoskeleton disassembly and an apoptosis-like process in Acanthamoeba spp. Antibiotics (Basel) (2022) 11:280. doi: 10.3390/antibiotics11020280
165. Kot K, Kolodziej D, Kupnicka P, Kosik-Bogacka DI, Lanocha-Arendarczyk N. Concentrations of PGE(2) and TXB(2) in the eyes of mice with disseminated acanthamoebiasis. Pathogens (2022) 11:438. doi: 10.3390/pathogens11040438
166. Fuwa M, Toris CB, Fan S, Taniguchi T, Ichikawa M, Odani-Kawabata N, et al. Effects of a novel selective EP2 receptor agonist, omidenepag isopropyl, on aqueous humor dynamics in laser-induced ocular hypertensive monkeys. J Ocul Pharmacol Ther (2018) 34:531–7. doi: 10.1089/jop.2017.0146
167. Ida Y, Furuhashi M, Watanabe M, Umetsu A, Hikage F, Ohguro H. Prostaglandin F2 and EP2 agonists exert different effects on 3D 3T3-L1 spheroids during their culture phase. biomedicines (2021) 9:1821. doi: 10.3390/biomedicines9121821
168. Hadas E, Mazur T. Biosynthesis of prostaglandins in pathogenic and nonpathogenic strains of acanthamoeba spp. Parasitol Res (1997) 83:296–9. doi: 10.1007/s004360050250
169. Sebastian-Perez V, Sifaoui I, Reyes-Batlle M, Dominguez-De Barros A, Lopez-Arencibia A, Campillo NE, et al. Discovery of amoebicidal compounds by combining computational and experimental approaches. Antimicrob Agents Chemother (2021) 65:e01749–20. doi: 10.1128/AAC.01749-20
170. Agahan AL, Lim RB, Valenton MJ. Successful treatment of Acanthamoeba keratitis without anti-amoebic agents. Ann Acad Med Singap (2009) 38:175–6. doi: 10.47102/annals-acadmedsg.V38N2p175
171. Siddiqui R, Lakhundi S, Iqbal J, Khan NA. Effect of non-steroidal anti-inflammatory drugs on biological properties of Acanthamoeba castellanii belonging to the T4 genotype. Exp Parasitol (2016) 168:45–50. doi: 10.1016/j.exppara.2016.06.011
Keywords: COX-2, protozoan infection, protozoa, AA, PGs
Citation: Wang X, Chen J and Zheng J (2023) The roles of COX-2 in protozoan infection. Front. Immunol. 14:955616. doi: 10.3389/fimmu.2023.955616
Received: 29 May 2022; Accepted: 06 February 2023;
Published: 16 February 2023.
Edited by:
Dario S. Zamboni, University of São Paulo, BrazilReviewed by:
Fátima Ribeiro-Dias, Universidade Federal de Goiás, BrazilWanderley De Souza, Federal University of Rio de Janeiro, Brazil
Milad Badri, Qazvin University of Medical Sciences, Iran
Tracey Lamb, The University of Utah, United States
Copyright © 2023 Wang, Chen and Zheng. This is an open-access article distributed under the terms of the Creative Commons Attribution License (CC BY). The use, distribution or reproduction in other forums is permitted, provided the original author(s) and the copyright owner(s) are credited and that the original publication in this journal is cited, in accordance with accepted academic practice. No use, distribution or reproduction is permitted which does not comply with these terms.
*Correspondence: Jingtong Zheng, emhlbmdqaW5ndG9uZ0BqbHUuZWR1LmNu