- 1School of Basic Medical Sciences, Southwest Medical University, Luzhou, Sichuan, China
- 2Department of Pathology, Affiliated Hospital of Southwest Medical University, Luzhou, Sichuan, China
- 3The School of Clinical Medical Sciences, Southwest Medical University, Sichuan, Luzhou, China
- 4Department of Pathology, Yaan People’s Hospital (Yaan Hospital of West China Hospital of Sichuan University), Yaan, Sichuan, China
Non-small cell lung cancer (NSCLC) is the most common and lethal type of lung cancer, with limited treatment options and poor prognosis. Immunotherapy offers hope for improving the survival and quality of life of NSCLC patients, but its efficacy depends on the tumor immune microenvironment (TME). Tissue-resident immune cells are a subset of immune cells that reside in various tissues and organs, and play an important role in fighting tumors. In NSCLC, tissue-resident immune cells are heterogeneous in their distribution, phenotype, and function, and can either promote or inhibit tumor progression and response to immunotherapy. In this review, we summarize the current understanding on the characteristics, interactions, and roles of tissue-resident immune cells in NSCLC. We also discuss the potential applications of tissue-resident immune cells in NSCLC immunotherapy, including immune checkpoint inhibitors (ICIs), other immunomodulatory agents, and personalized cell-based therapies. We highlight the challenges and opportunities for developing targeted therapies for tissue-resident immune cells and optimizing existing immunotherapeutic approaches for NSCLC patients. We propose that tissue-resident immune cells are a key determinant of NSCLC outcome and immunotherapy response, and warrant further investigation in future research.
1 Introduction
Non-small cell lung cancer (NSCLC) represents the majority of lung cancer cases, accounting for approximately 80% to 85% of diagnoses and contributing to a significant number of cancer-related deaths worldwide (1, 2). Despite advancements in surgical techniques, chemotherapy, radiotherapy, and targeted therapy, the prognosis for NSCLC patients remains discouraging with a 5-year survival rate of only 19% (1). Consequently, there is an urgent and imperative need to develop more innovative and effective therapeutic strategies to combat NSCLC.
In recent years, immunotherapy has emerged as a promising approach in the treatment of various cancers, including NSCLC (3, 4). This novel therapeutic technique aims to harness and enhance the body’s natural immune system to recognize and eliminate tumor cells. The efficacy and mechanism of immunotherapy largely depend on the intricate interplay of immune cells, which serve as the main drivers of the immune response. Within this vast array of immune cells, tissue-resident immune cells play a pivotal role in tumor immunotherapy. Distinguished by their long-lasting presence, these immune cells possess memory and effector functions (5). Notable examples of tissue-resident immune cells include tissue-resident memory T (TRM) cells, B cells, macrophages, natural killer (NK) cells, etc. (6). Tissue-resident immune cells can establish immune memories within tissues, enabling them to initiate robust immune responses upon encountering similar or identical antigens. Additionally, tissue-resident immune cells can serve as targets or biomarkers for immunotherapy, imparting both synergistic and antagonistic effects when used in combination with different immunotherapies.
In this review, we address the following question: How do tissue-resident immune cells regulate NSCLC immunity and immunotherapy? We hypothesize that tissue-resident immune cells have a dual role in NSCLC, exerting both anti-tumor and pro-tumor effects depending on their subsets and activation states. We argue that tissue-resident immune cells represent a promising target for improving the efficacy and overcoming the resistance of immunotherapy for NSCLC.
We will first introduce the distribution, phenotype, and function of tissue-resident immune cells in NSCLC tissues. Then, we will discuss the role of tissue-resident immune cells in NSCLC control, including their anti-tumor or pro-tumor effects, and their interactions with other immune or non-immune cells. Next, we will examine the application of tissue-resident immune cells in NSCLC immunotherapy, covering the currently employed immune checkpoint inhibitors (ICIs), along with other state-of-the-art immunotherapeutic approaches and ongoing research advancements. Finally, we will conclude by emphasizing the importance and uniqueness of tissue-resident immune cells in NSCLC immunity and immunotherapy, and by proposing future research directions in this field.
With this comprehensive introduction, it is our intent to emphasize the crucial role and unique status of tissue-resident immune cells in the context of NSCLC. Further studies in this direction will contribute significantly to the development of novel therapeutic strategies, including the targeted modulation of tissue-resident immune cells, exploration of synergistic effects with other therapeutic modalities, and optimization of the application of immune cells in individualized therapy. We firmly believe that by fully harnessing the potential of immunotherapy, we will drastically improve the prognosis of NSCLC patients and pave the way for the future advancements in this field.
2 Tissue-resident immune cells in NSCLC
2.1 Phenotype and distribution of tissue-resident immune cells in NSCLC tissues
In NSCLC, the phenotypes of tissue-resident immune cells are diverse, including T lymphocytes, macrophages, B lymphocytes, NK cells, dendritic cells (DC), and neutrophils (6). Tumor-associated macrophages (TAM) and tissue-resident memory T cells (TRM) are two important subsets of immune cells that play crucial roles in the tumor immunology in NSCLC.
TAM are derived from circulating monocytes that are recruited to the tumor site by various chemokines and cytokines, such as CCL2, CSF-1, and VEGF (7, 8). Macrophages differentiate differentially in tissues with different micro-environments and can be divided into two distinct polarization states: M1 macrophages (M1) and M2 macrophages (M2). But TAM are generally characterized by M2-like macrophages (9), which exhibit immunosuppressive and protumorigenic functions, such as promoting angiogenesis, lymphangiogenesis, invasion, metastasis, and resistance to therapy (10). TAM are distributed unevenly within the tumor tissue, and their spatial localization can affect their interaction with other immune cells and tumor cells (9). Tissue-resident macrophages gather near tumor cells at an early stage of tumor initiation, enhancing epithelial-mesenchymal transition and the ability of tumor cells to invade (11). Additionally, tissue-resident macrophages trigger a strong response from regulatory T cells, which shields tumor cells from adaptive immunity (11). TAM are potential targets for immunotherapy in NSCLC, and several strategies have been developed to modulate TAM, such as limiting monocytes recruitment, targeting TAM activation, reprogramming TAM into anti-tumor activity, and targeting TAM specific markers (12). However, there are still many challenges and limitations in TAM-targeted therapy, such as the lack of specific and reliable biomarkers, the complexity and diversity of TAM subsets, the dynamic and context-dependent nature of TAM, and the potential adverse effects of TAM manipulation.
TRM is a subset of memory T cells that reside in non-lymphoid tissues, such as the lung, skin, and gut, and provide rapid and robust protection against local infections and tumors (13). TRM differentiation is guided by transcription programs common to both effector and memory T cells (14). CD103 promotes the localization and retention of CD8+ T cells in tumor tissues (15) through binding to E-calmodulin (16) and also modulates the CD8+ T cell response to anti-tumor immunotherapy (17). CD8+ T cells accumulate in non-small cell lung cancer tumors and accumulate mainly in the tumor center (18). TRM can exert immune pressure on tumor cells at the early stage of tumor development, affecting tumor evolution and escape (19). The number and function of TRM are closely related to tumor prognosis and treatment response (20). TRM is a promising target for NSCLC immunotherapy, for example as a target for improved cancer vaccines, as a target for immune checkpoint blocking, and as a target for adoptive cell therapy (21). However, there are also many challenges and limitations in TRM-targeted therapy, such as the lack of specific and reliable biomarkers, the complexity and diversity of TRM subsets, the dynamic nature of TRM, and the potential adverse effects of TRM manipulation.
In addition to TAM and TRM, other immune cells also play important roles in NSCLC. For example, in early NSCLC tissues, there are large numbers of tissue-resident macrophages and tumor-infiltrating lymphocytes (TILs) (11, 22). The distribution of NK cells in lung tumors is not homogeneous and is concentrated in the central regions of the tumors (18). NK cells are the first line of defense against environmental destruction, pathogens and cancers, enriched in the tumor microenvironment of NSCLC (23), lung trNK cells are prone to produce CCL5, MIP-1β, and GM-CSF, which may always alter their microenvironment by attracting other immune cells (e. g., monocytes, T cells, and eosinophils) (24). A study has shown that tissue-resident CD69+ CXCR6+ NK cells with depletion phenotype accumulate in human non-small cell lung cancer and are a promising target for immune checkpoint inhibitors in the treatment of NSCLC (25). For DC, a study has shown that DC derived from NSCLC has immunosuppressive effects, and the co-suppressor molecule B7-H3 plays a crucial role in mediating the T cell inhibition of DC under tumor conditions (26). Tissue-resident B cells are a subset of B cells that reside in the tissues and form ectopic lymphoid organs called tertiary lymphoid structures (TLS) in the margins of solid tumors. TLS are associated with improved patient survival and response to immune checkpoint blockade in NSCLC (27). Another study suggests that neoadjuvant chemotherapy may improve the efficacy of ICBs by increasing the density and diversity of tumor-infiltrating lymphocytes, including B cells, as well as inducing immunogenic cell death and antigen release (28). TIL-B is only present in patients with specific tumors, but its increase in number can positively affect clinical outcomes (29). According to in vitro assays, TIL-B can be used as local APCs for secondary stimulation of CD4+ TILs in NSCLC, ultimately changing its phenotype and function (29). Among the TILs, T cells dominate, while B cells and NK cells also hold importance (22). Tissue resident neutrophils (TRNs) of NSCLC include TANs and NANs (30),NSCLC has immune features dominated by neutrophils (31). TANs are closely involved in tumor proliferation, invasion, and metastasis through the synthesis and secretion of related proteases, such as neutrophil enzymes (NE) and matrix metalloproteinases (MMPs) (32). Salcher et al. demonstrated that tissue-resident neutrophils play a role in NSCLC by participating in TLS formation, acquiring new functional properties in the tumor microenvironment, and expressing ERV antigens. Their presence and function may be modulated by different therapeutic interventions, such as ICB and targeted therapy (30).
In single-cell sequencing, new immune cell subpopulations, such as CD45 AMS, TAN-1, TAN-2, TAN-3, TAN-4, CD45 AMs Can support the proliferation of lung cancer cells in vivo (33). TAN-1 shows a high expression of interleukin 1 receptor antagonist (IL 1 RN), which negatively regulates IL-8 secretion to control excessive neutrophil inflammatory activity (34), and the potent NF- κ B activators RIPK271 and CD44, regulating cell recruitment and adhesion (35, 36). The TAN-2 subcluster is characterized by the expression of the major histocompatibility complex (MHC) class II genes HLA-DRA, CD74, HLA-DMB, and HLA-DRB 1, indicating that the phenotype is characterized by immunogenic antigen presentation. The TAN-3 subcluster is characterized by high expression of proinflammatory cytokines (C15orf48, CCL 3, CCL 4, CSTB) and galectin 3 (LGALS3), which is associated with neutrophil activation and migration. TAN-4 shows high expression of ribosomal genes (e. g., RPS 12, RPL 3, RPN 2, RPL 23) and eventually changes to other cellular phenotypes, such as tumor endothelial cells (30).
These results suggest that tissue-resident immune cells have a complex and diverse phenotype and distribution in NSCLC, and they play an important role in the regulation of tumor immune response and immunotherapy.
2.2 Interaction of tissue-resident immune cells with the tumor microenvironment
The tumor microenvironment (TME) of NSCLC is a complex and dynamic system comprising various elements, such as cancer cells, immune cells, stroma cells, blood vessels and extracellular matrix (ECM) (37). The TME modulates the behavior and fate of tissue-resident immune cells through multiple mechanisms, influencing the efficacy of the antitumor immunity. In this section, we explore how tissue-resident immune cells interact with the TME and how this affects NSCLC progression and therapy.
First, the migration and settlement of tissue-resident immune cells in the TME are intricately regulated by chemokines and adhesion molecules. Chemokines, such as small molecules like CCL2, CCL7, CXCL248, play a pivotal role in inducing immune cells to move in a specific direction (38). On the other hand, adhesion molecules, such as ICAM-1, VCAM-1, CD103, facilitate immune cell attachment to other cells or ECM (39). In the case of NSCLC, tumor cells and mesenchymal stromal cells exhibit a remarkable ability to secrete diverse chemokines and express various adhesion molecules. These results in the attraction or repulsion of different types of tissue-resident immune cells into or out of tumor tissues. For instance, CCL2 has the capability to recruit M2-type macrophages into tumor tissues (8), thereby inhibiting the effector function and antitumor effects of CD8+ T cells (40), which is detrimental to the survival of non-small cell lung cancer patients (41); CD103 promotes the localization and retention of CD8+ T cells in tumor tissues (15) through binding to E-calmodulin (16) and also modulates the CD8+ T cell response to anti-tumor immunotherapy (17). Hence, it is evident that chemokines and adhesion molecules play crucial regulatory roles in the migration and settlement of tissue-resident immune cells in NSCLC.
Second, the activation and proliferation of tissue-resident immune cells in the tumor microenvironment are tightly regulated by antigen presentation and co-stimulatory signals. Antigen presentation involves immune cells recognizing and binding specific antigens, such as tumor-specific or mutant antigens, through special receptors. This recognition then triggers an immune response, which plays a crucial role in fighting against tumors (42). On the other hand, co-stimulatory signaling refers to the process through which immune cells receive and transmit positive or negative signals via non-specific receptors, thus either enhancing or inhibiting the immune response (43). In NSCLC, several types of antigen-presenting cells (APCs) are present in the tumor microenvironment, such as dendritic cells, macrophages, and B cells (44). These APCs can present antigens to CD8+ T cells or CD4+ T cells via major histocompatibility complex (MHC) class I or class II molecules, which activate the effector functions of tissue-resident immune cells (42),and these APCs also play a crucial role in regulating the proliferation and survival of tissue-resident immune cells. They achieve this by expressing a wide range of co-stimulatory molecules, such as CD80, CD86, PD-L1, and PD-L2. For example, CD80 and CD86 can bind to CD28, thereby providing positive co-stimulatory signals to promote the proliferation and survival of tissue-resident immune cells (45); PD-L1 and PD-L2 can bind to PD-1(programmed death protein 1), thereby providing negative co-stimulatory signals to inhibit the proliferation and survival of tissue-resident immune cells, promoting NSCLC (46, 47). Thus, antigen presentation and co-stimulatory signaling have important regulatory roles in NSCLC for the activation and proliferation of tissue-resident immune cells.
Third, the differentiation and polarization of tissue-resident immune cells are regulated by cytokines in the tumor microenvironment. Cytokines are proteins that act as messengers between immune cells, which including IL-2, IL-4, IL-10, IFN-γ, and TGF-β (48). The tumor microenvironment of NSCLC contains different types of cytokines that can bind to specific receptors on tissue-resident immune cells and change their phenotype and function. For instance, IL-4 can make CD4+ T cells become Th2 cells and help B cells produce antibodies (49); IFN-γ can make CD4+ T cells become Th1 cells and boost the effector function of CD8+ T cells (50); and TGF-β can make CD4+ T cells become Treg cells and suppress the effector function of CD8+ T cells (51). Therefore, cytokines are important regulators of tissue-resident immune cells differentiation and polarization in NSCLC (Figure 1).
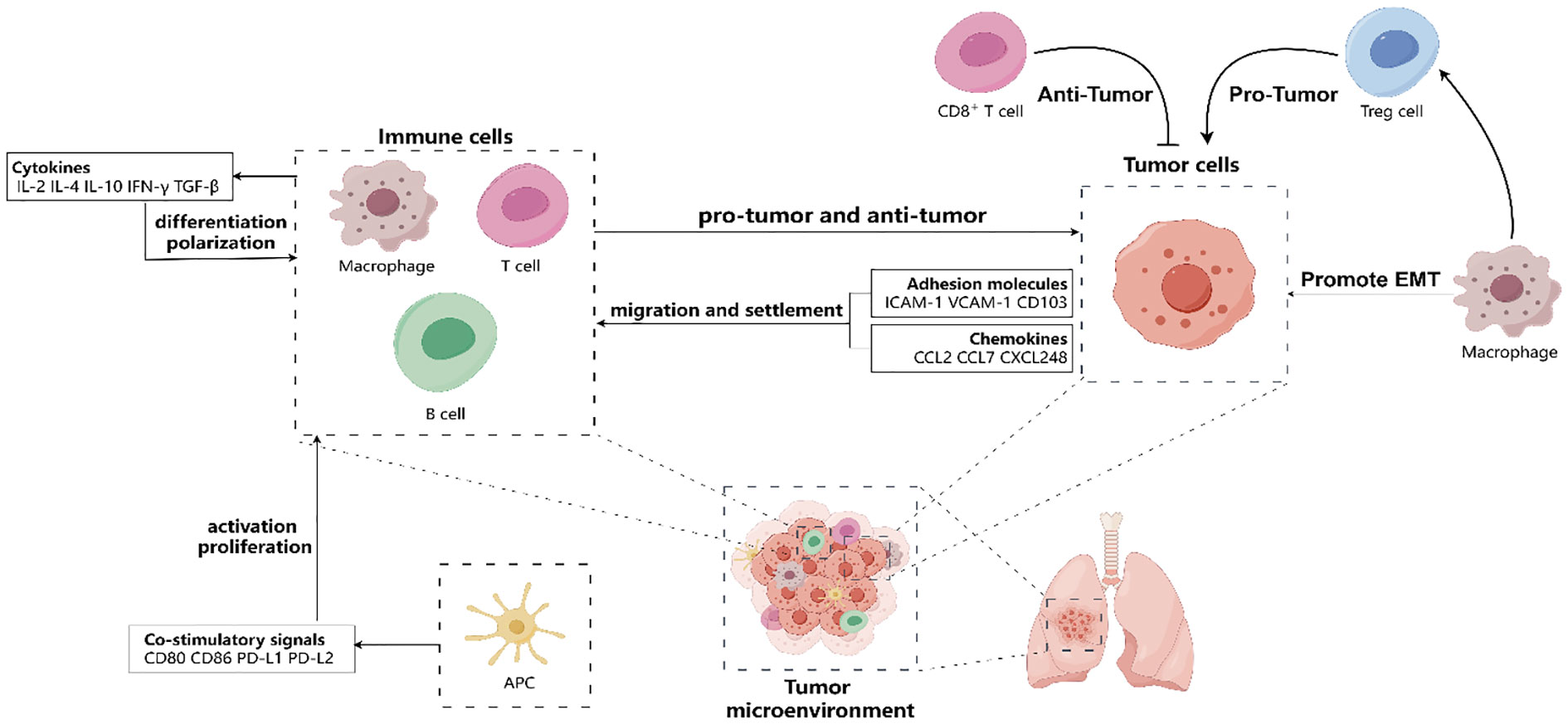
Figure 1 Tumor microenvironment (TME) affect the migration and settlement of tissue-resident immune cells (such as T cells, B cells, macrophages, antigen-presenting cells) by releasing various chemokines (such as CCL2, CCL7, CXCL248, etc.) and expressing various adhesion molecules (such as ICAM-1, VCAM-1, etc.). Besides, tissue-resident immune cells regulate their activation and proliferation by receiving and transmitting co-stimulatory signals (such as CD28/CD80/CD86 or PD-1/PD-L1/PD-L2, etc.) in the TME. Moreover, tissue-resident immune cells regulate their differentiation and polarization by cytokines (such as IL-2, IL-4, IL-10, IFN-γ, TGF-β, etc.) in the TME. In TME, CD8+ cells play an anti-tumor role, but macrophages can promote tumor progression directly through epithelial-mesenchymal transformation (EMT) and indirectly through regulating the function of Treg cells. Hence, the TME has a significant impact on the behavior and fate of tissue-resident immune cells, which in turn affects NSCLC progression and therapy.
2.3 Identification and characterization of tissue-resident immune cells in NSCLC
Understanding the role and potential of tissue-resident immune cells in NSCLC requires their identification and characterization. However, this task is challenging due to the heterogeneity, diversity, and plasticity of tissue-resident immune cells. Multiple methods and criteria are necessary to identify and characterize tissue-resident immune cells in NSCLC tissues accurately.
One such method is flow cytometry, a technique that can assess the expression of various surface or intracellular markers on individual cells. This allows for quantifying and examining different immune cell subsets and their phenotypes (52, 53). Flow cytometry can also sort the immune cells based on their markers for further analysis (54). Nevertheless, flow cytometry requires predefined antibodies and panels for specific markers, which may limit the discovery of novel or rare immune cell subsets. Furthermore, flow cytometry does not provide information about the spatial distribution and localization of immune cells within NSCLC tissues.
Another method is immunohistochemistry (IHC). IHC utilizes specific antibodies to detect the expression of a small number of markers on tissue sections. Staining or using fluorescence allows for the visualization of immune cells (55). IHC can provide valuable information about immune cells’ spatial distribution and localization and their interactions with tumor cells in the TME. For example, Righi et al. (56) used IHC to classify NSCLC into different subtypes based on the expression of cytokeratin (CK), thyroid transcription factor-1 (TTF-1), and p63. They found that the immunophenotype of NSCLC-not otherwise specified (NOS) was associated with treatment outcome and survival. Specifically, they found that NOS with adenocarcinoma-like (ADC-like) features had a similar survival as ADC. In contrast, NOS with squamous cell carcinoma-like (SCC-like) features had a similar survival as SCC. Moreover, NOS with no differentiation features (“null” group) had a significantly worse survival than the other groups (56). These results suggest that IHC can improve the accuracy of NSCLC typing and guide the optimal treatment strategy. Nevertheless, IHC has low sensitivity and specificity and cannot quantify immune cell subsets’ expression level or frequency. Moreover, it does not offer functional information regarding immune cells in NSCLC tissues. Multiplex immunohistochemistry (mIHC) has been developed to simultaneously detect multiple antigens in a single tissue section using chromogens or fluorophores to overcome these challenges. mIHC can increase the number of parameters that can be measured in a single experiment, reduce tissue consumption, and enable the automated quantification and analysis of the data. For example, Sun et al. (57) used an automated chromogenic mIHC assay to simultaneously detect six biomarkers (CD3, CD8, CD163, PD-L1, FoxP3, and CK) and DAPI in NSCLC. They found that this assay could accurately reveal the spatial distribution and relationship of tumor cells and immune cells in the TME without compromising the antigenicity, cross-reactivity, or staining intensity. They also found that this assay could be performed on standard antigen retrieval and automated staining protocols, reducing the need for validation strategies. Furthermore, mIHC can be enhanced by optimizing the staining protocol, the antibody panel, and the image analysis software. For example, Sun et al. (58) developed an enhanced 7-color mIHC protocol. They used this protocol to analyze the specimens of NSCLC, and found the differences in the composition and distribution of immune cells among different tumor types. These examples demonstrate the advances of mIHC in immunophenotype profiling of NSCLC.
A third method is single-cell sequencing, which can analyze the transcriptome or proteome of individual cells and reveal the gene expression or protein abundance of thousands of genes or proteins. Single-cell sequencing can identify and characterize novel or rare immune cell subsets based on their gene expression or protein abundance profiles, as well as their functional states and pathways (59). Single-cell sequencing can also reveal the heterogeneity and diversity of immune cells in NSCLC tissues (60). Nevertheless, single-cell sequencing is expensive and complex, and requires high-quality samples and bioinformatics tools for data processing and analysis.
Therefore, identifying and characterizing immune cells in NSCLC tissues requires a combination of multiple methods and criteria that can complement each other’s strengths and weaknesses. Using these methods and criteria, researchers can better identify and characterize the tissue-resident immune cells in NSCLC tissues and understand their role in tumor immunity. (All mechanisms in this chapter are summarized in Table 1).
3 The role of tissue-resident immune cells in the control of non-small cell lung cancer
3.1 Antitumor effects of tissue-resident immune cells
Tissue-resident immune cells can exert anti-tumor effects by directly killing tumor cells, producing cytokines and chemokines, activating and recruiting other immune cells, and influencing the TME. Several studies have shown that tissue-resident immune cells are associated with better clinical outcomes and response to immunotherapy in NSCLC patients. For example, tissue-resident memory T (TRM) cells are characterized by the expression of CD69 and CD103 (61). These cells have been found to be enriched in non-small cell lung cancer tumors and are associated with improved survival and reduced recurrence rates (20, 62, 63). TRM cells can recognize tumor antigens and mediate cytotoxicity and cytokine secretion (62, 64). Moreover, TRM cells can enhance the function of other immune cells, such as dendritic cells and NK cells, through direct contact or soluble factors (65). TRM cells can also respond to immunotherapy by upregulating PD-1 expression and producing IFN-γ (20, 66).
Another example of tissue-resident immune cells with anti-tumor activity is tissue-resident natural killer (trNK) cells, which are a subset of innate lymphoid cells that express CD69 and CD49a (24). trNK cells are abundant in the lung and can recognize and kill tumor cells through various mechanisms, such as antibody-dependent cellular cytotoxicity (ADCC), natural cytotoxicity receptors (NCRs) and NKG2D (67–69). trNK cells can also produce cytokines, such as IFN-γ, TNF-α, and IL-15, that modulate the TME and promote the activation of other immune cells (24, 25).
Subcentral clusters in the TRN of NSCLC include TANs and NANs (30), and TAN abundance in the TME was considered as the primary predictor of adverse outcome (70). A high neutrophil to CD8 T-cell ratio within the tumor is a poor prognostic indicator of relapse-free survival and OS (71). The antitumor activity of TANs includes activation of the expression of immune cytokines, low levels of arginase, high H2O2 production, and increased ability to kill tumor cells in vitro (72). At the same time, it can also directly produce cytotoxic mediators or release TNF-related apoptosis-inducing ligand to inhibit tumor growth (73). In resected lung cancer tissues, TANs crosstalk with activated T cells, resulting in a significant upregulation of co-stimulatory molecules on the surface of neutrophils, thereby promoting the proliferation of T cells in the positive feedback cycle (74).
Other types of tissue-resident immune cells, such as tissue-resident macrophages, tissue-resident B cells, and non-classical T cells (such as γδ T cells) (75), may also contribute to anti-tumor immunity in NSCLC by various mechanisms. However, the exact anti-tumor role and function of these tissue-resident immune cells in NSCLC remain to be elucidated.
3.2 Synergistic or antagonistic effects of tissue-resident immune cells with other immune or non-immune cells
Tissue-resident immune cells in non-small cell lung cancer do not act in isolation, but rather interact with other immune cells in the tumor microenvironment. These interactions can have either synergistic or antagonistic effects on the anti-tumor immunity and the tumor progression. For example, in human, CD103+ TRM cells can produce granzyme B (GZMB) and IFN-γ, which can enhance recruitment of monocytes, NK cells, and XCR1+ cDC1 to the tumor site, resulting in anti-tumor (76, 77). Nevertheless, several dysfunctional subtypes of TRM cells exist in human NSCLC tissues, including NKG2A+CD8+ T cells, NKG2A is an inhibitor of both T cells and NK cells. Persistent activation thereof may cause T cells and NK cells to express NKG2A and potentially lead to chronic infection or cancer (78). In NSCLC, MDSC, expressing Arg-1 and iNOS, participates in inhibiting CD8+ T cell proliferation and reducing CD3 ζ expression (79, 80). MDSCs also release various immunosuppressive molecules and upregulate repression checkpoints to promote differentiation or development of Tregs and interfere with homing and trafficking of tumor-specific T cells (81). Myeloid DC plays a central role in antitumor immunity because of their ability to initiate T cells in lymph nodes. DC can also produce TGF- β, which can induce the differentiation of CD4+ T lymphocytes to Treg, thereby inhibiting T cell proliferation (82). CD103 DC controls CD8+ T cell activation in a variety of tumor types (83).
On the other hand, tissue-resident macrophages accumulate around tumor cells early during tumorigenesis to promote epithelial-mesenchymal transition and tumor cell invasiveness, as well as to elicit a potent regulatory T-cell response protecting tumor cells from adaptive immunity. Depletion of tissue-resident macrophages reduced the number and altered the phenotype of Treg cells. This led to the accumulation of CD8+ T cells and reduced tumor invasiveness and growth (11).
3.3 Tumor-promoting and injury-promoting effects of tissue-resident immune cells
Tissue-resident immune cells play a complex role in cancer, as they can exert both anti-tumor and pro-tumor effects. Tissue-resident immune cells can promote tumor initiation, progression, and metastasis by various mechanisms, such as inducing inflammation and epithelial-mesenchymal transition (EMT). Moreover, tissue-resident immune cells can also compromise the anti-tumor immunity or cause tissue damage, for instance through immune exhaustion.
Inflammation is a key factor that links chronic infection, tissue injury, and cancer. Tissue-resident immune cells can induce inflammation by secreting cytokines, chemokines, growth factors, which can recruit and activate other immune cells or non-immune cells in the TME. Whereas activated immune cells and inflammatory cells produce reactive oxygen species (ROS) and reactive nitrogen species (RNS), which exert chemical effects in inflammation-driven carcinogenesis (84). And ROS-induced DNA damage includes DNA strand breaks, DNA base modifications, and DNA cross-links, leading to replication errors and genomic instability, which in turn lead to tumorigenesis (85). Moreover, in the tumor microenvironment, multiple pro-inflammatory mediators are involved in complex inflammatory signaling, which promotes tumor progression by promoting tumor cell extravasation through the stroma (86). Inflammation can also promote tumor metastasis by enhancing tumor cell intravasation, extravasation, and colonization at distant sites (87).
EMT is the process of acquiring mesenchymal features and losing epithelial features by epithelial cells (88). Using lineage tracing, it has been shown that the development of NSCLC lesions in mice initially accumulates TRMs in the vicinity of the tumor cells to promote epithelial-mesenchymal transition and invasiveness in the tumor cells, and that these TRMs also induced potent Treg cell responses that protected the tumor cells from adaptive immune responses (11, 89). In summary, EMT can promote tumor progression by enhancing tumor cell invasion, migration and resistance to apoptosis or therapy. EMT can also promote tumor metastasis by facilitating tumor cell detachment from the primary site and attachment to the secondary site (90, 91).
Immune exhaustion is the process of losing effector functions and becoming unresponsive to stimulation. Some tissue-resident immune cells, especially CD8+ T cells, can become exhausted after chronic exposure to tumor antigens or immunosuppressive signals. This exhaustion can also be identified by means of CD38 labeling (92). And, it has been found that a tissue-resident CD69 CXCR6 NK cell with an exhaustive phenotype accumulates in NSCLC. Its dysfunction can be partially ameliorated by PD-1 and CTLA-4 blockade (25). Thus, these findings suggest a close interrelationship between tissue-resident immune cells exhaustion and tumor. Understanding and intervening in this interrelationship through research could help develop more effective tumor treatments.
4 The application of tissue-resident immune cells in non-small cell lung cancer immunotherapy
4.1 The role, limitations, and clinical studies of immune checkpoint inhibitors
Immune checkpoint inhibitors (ICIs) are a type of immunotherapy that block the negative signals between tumor cells and immune cells, thereby enhancing the anti-tumor immune response (93). ICIs targeting PD-1/PD-L1 or CTLA-4 have shown remarkable efficacy and survival benefit in patients with advanced NSCLC, especially those with high PD-L1 expression or tumor mutational burden (94, 95). However, ICIs are not effective for a subset of patients, and some patients may develop primary or secondary resistance, or experience immune-related adverse events (96, 97). Therefore, it is important to understand the mechanisms of resistance and toxicity, and to identify biomarkers that can predict the response and outcome of ICIs.
One of the factors that may influence the efficacy of ICIs is the presence and function of tissue-resident immune cells in the TME. Tissue-resident immune cells can provide local immune surveillance and protection against pathogens and tumors, but they can also be modulated by the TME to acquire pro-tumoral or immunosuppressive phenotypes (5). Therefore, tissue-resident immune cells may play a dual role in NSCLC immunotherapy, either enhancing or inhibiting the anti-tumor effect of ICIs.
Several studies have investigated the impact of tissue-resident immune cells on the response and resistance to ICIs in NSCLC. For example, a recent study by Zhang et al. (19) showed that tissue-resident memory T (TRM) cells exerted early immune pressure on NSCLC cells and shaped their clonal evolution and neoantigen landscape. The authors found that TRM cells were enriched in tumors with high PD-L1 expression and low tumor mutational burden, and that they recognized shared neoantigens that were present in most tumor cells (19). However, TRM also induced the emergence of subclones that escaped TRM recognition by losing or mutating the neoantigens (19). These subclones were resistant to PD-1 blockade and had poor prognosis. The authors suggested that targeting TRM-induced neoantigens or combining PD-1 blockade with other therapies that prevent tumor evolution may improve the outcome of NSCLC immunotherapy (19).
Another study by Lee et al. (98) investigates the presence and expansion of TRM in the peripheral blood of patients with lung cancer who are undergoing immune checkpoint inhibitor therapy. This study reveals that treatment with ICIs leads to the clonal expansion of TRM in the peripheral blood, suggesting that these cells play a crucial role in the anti-tumor immune response induced by ICIs.
These studies reveal a pivotal role of tissue-resident immune cells in modulating the TME and the response to ICIs in NSCLC, highlighting the potential of these cells as therapeutic targets. However, more studies are needed to elucidate the diversity, plasticity and function of tissue-resident immune cells in different types and stages of NSCLC, and to explore how to manipulate them to enhance the efficacy and safety of ICIs.
4.2 Other immunotherapeutic approaches and research progress
Besides ICIs, other immunotherapeutic approaches have been explored for NSCLC, such as vaccines, adoptive cell therapy, and oncolytic viruses. These strategies aim to enhance the anti-tumor activity of tissue-resident immune cells or recruit more tissue-resident immune cells to the tumor site.
Vaccines are designed to elicit specific immune responses against tumor-associated antigens (TAAs) or neoantigens (99, 100). Several types of vaccines have been tested in NSCLC, including peptide-based, dendritic cell-based, tumor cell-based, and viral vector-based vaccines (101–104). Some of these vaccines have shown promising results in combination with ICIs or chemotherapy (100, 105). However, the efficacy of vaccines is limited by the heterogeneity of TAAs, the low immunogenicity of neoantigens, and the immunosuppressive TME. Therefore, more efforts are needed to identify optimal antigens, delivery systems, and combination regimens for NSCLC vaccines.
Adoptive cell therapy (ACT) involves the infusion of ex vivo expanded or genetically modified immune cells into patients. The most widely used ACT for NSCLC is chimeric antigen receptor (CAR) T cell therapy, which targets specific antigens expressed by tumor cells or stroma (106, 107). CAR T cells have shown remarkable efficacy in hematological malignancies (108, 109), but their application in solid tumors is challenging due to the lack of tumor-specific antigens, the poor infiltration and persistence of CAR T cells in the TME, and the hostile TME that inhibits CAR T cell function (110). Several strategies have been proposed to overcome these obstacles, such as engineering CAR T cells with enhanced migratory and survival abilities, co-expressing cytokines or checkpoint modulators, and combining CAR T cells with other immunotherapies (111, 112).
Oncolytic viruses (OVs) are genetically modified or naturally occurring viruses that selectively infect and lyse tumor cells, while stimulating innate and adaptive immune responses. OVs can also be used as vectors to deliver therapeutic genes, such as cytokines, co-stimulatory molecules, or checkpoint inhibitors (113, 114). Several OVs have been evaluated in NSCLC preclinical and clinical studies, such as Coxsackievirus A21 (V937), Coxsackievirus A11, influenza viruses and Vesicular stomatitis virus (VSV) (115–117). A study that tested the safety and effectiveness of a modified version of CVA11, called V937, in patients with advanced solid tumors, including NSCLC. V937 was given alone or with another drug called pembrolizumab, which blocks a molecule called PD-1 that can weaken the immune system. The combination of V937 and pembrolizumab showed some signs of shrinking the tumors (117).
Bispecific antibodies are a promising strategy that targets both PD-L1 and TGF-β, two key immunosuppressive factors in the tumor microenvironment. TGF-β is a pleiotropic cytokine that can inhibit the activation, proliferation, and cytotoxicity of T cells (118, 119), as well as induce the differentiation of Treg cells and myeloid-derived suppressor cells (119). Moreover, TGF-β can promote the epithelial-mesenchymal transition of tumor cells, which increases their invasiveness and resistance to ICIs (120). By blocking both PD-L1 and TGF-β, bispecific antibodies can synergistically enhance the antitumor immune response and overcome the resistance to PD-L1 blockade (121). Several anti-TGF-β/PD-L1 bispecific antibodies have been developed and tested in preclinical and clinical studies, such as YM101, BiTP, and M7824. YM101 is a bispecific antibody that targets murine PD-L1 and TGF-β, and has shown potent antitumor activity in mouse models of triple-negative breast cancer (122). BiTP is a humanized version of YM101 that targets human PD-L1 and TGF-β, and has demonstrated superior antitumor efficacy to anti-PD-L1 and anti-TGF-β monotherapy in humanized mouse models of TNBC (122). M7824 is a bifunctional fusion protein that consists of a PD-L1 antibody and the extracellular domain of TGF-β receptor II, and has entered phase I/II trials for various solid tumors, including NSCLC (123). These bispecific antibodies have the potential to improve the clinical outcomes of NSCLC patients who are refractory or resistant to PD-L1 blockade.
Other immunotherapeutic approaches that are under investigation for NSCLC include cytokine therapy, innate immune stimulators, and microbiome modulation (124–126). Some examples of other immunotherapeutic approaches related to NSCLC are LunX-CART, CD147-CART, and OSE2101 vaccine. LunX-CART is a CAR T cell therapy that targets LunX, a molecular marker of overexpression in NSCLC (106). LunX-CART has been shown to effectively eradicate LUNX-positive NSCLC cells by secreting cytokines (106). Cd147-cart is another CAR-T cell therapy targeting CD147. CD147, a glycoprotein overexpressed in non-small cell lung cancer, plays an important role in NSCLC progression and is a promising target for CART therapy in NSCLC (127). CD147-CART has demonstrated substantial antitumor activity and safety in preclinical models of non-small cell lung cancer (127). OSE2101 is a peptide-based vaccine that contains modified epitopes from five tumor-associated antigens restricted to HLA-A2+ patients (128). OSE2101 has been evaluated in a randomized controlled trial of NSCLC patients who failed immune checkpoint inhibitors. The results showed that OSE2101 improved overall survival compared to standard care in patients with secondary resistance to immune checkpoint inhibitors (128). These examples illustrate how the immune system can be reignited to fight against tumors by targeting specific antigens, enhancing CAR T cell function, or inducing neoantigen-specific responses. These approaches aim to enhance the activation and recruitment of tissue-resident immune cells or modulate the TME to favor anti-tumor immunity. However, the optimal combination and sequencing of these therapies with ICIs or conventional treatments remain to be determined.
4.3 Challenges and issues of immunotherapy for NSCLC
Although immunotherapy for NSCLC has made remarkable achievements, there are still many challenges and issues that need to be addressed. These include the identification of reliable biomarkers, the management of immune-related adverse events (irAEs), the overcoming of primary and acquired resistance, and the optimization of combination and sequential therapies.
Biomarkers are essential for selecting suitable patients, predicting treatment response, monitoring disease progression, and evaluating treatment efficacy. The most widely used biomarker for NSCLC immunotherapy is PD-L1 expression, which is measured by immunohistochemistry (IHC) on tumor biopsy samples (129). However, PD-L1 expression is not a perfect predictor of response. First, only patients with both high PD-L1 expression and high immune infiltration could benefit from chemotherapy plus immunotherapy in first-line treatment of advanced NSCLC (130). Moreover, PD-L1 expression is dynamic and heterogeneous, and may vary depending on the tumor site, stage, histology, and prior treatments. Therefore, there is an urgent need to identify more robust and comprehensive biomarkers for NSCLC immunotherapy. Some potential candidates include tumor mutational burden (TMB), microsatellite instability (MSI), (131), gene expression profiles (GEPs) and microbiome (131–135). TMB is defined as the total number of somatic/acquired mutations per coding area of a tumor genome (Mut/Mb). It reflects the neogenic antigen load of the tumor and correlates with the response to immunotherapy (136). MSI refers to microsatellite instability caused by the accumulation of DNA replication errors due to the loss or mutation of mismatch repair genes, leading to abnormal expression of proteins in tumor cells and activation of the immune system (137). GEPs refer to gene expression profiles, which can reflect the molecular characteristics and status of tumor cells and immune cells, as well as the immune phenomena in the TME (138).
The performance of these biomarkers and their limitations still require further validation and optimization. For example, the measurement methods, standards, and thresholds of TMB have not been unified, and different detection platforms and algorithms may lead to different results (139). There are also some issues with the detection of MSI, such as the selection of microsatellite loci, the sensitivity and specificity of detection techniques, and the dynamic changes of MSI. More clinical studies on the relationship between MSI-related sites and tumor resistance are needed to improve the therapeutic effect of chemotherapy (140). The application of GEPs is also limited by factors such as sample quality and quantity, data analysis and interpretation, and cost considerations.
In order to improve the predictive ability of biomarkers, it is necessary to integrate these different biomarkers and construct more precise and personalized models. Some studies have already attempted this approach, such as combining TMB and PD-L1, which has been found to be more predictive of the response to immunotherapy in NSCLC patients than individual biomarkers (141). In addition, some studies have used machine learning and artificial intelligence methods to extract the most valuable features from a large amount of biomarker data and establish more complex and refined prediction models (142).
The application of these biomarkers also needs to consider their clinical feasibility and practicality. The detection of some biomarkers requires sufficient tumor tissue samples, which is a barrier for patients who cannot undergo or have difficulty with biopsies. Therefore, it is necessary to develop non-invasive or minimally invasive detection methods, such as using circulating tumor DNA (ctDNA) or circulating tumor cells (CTCs) in blood or other fluids, to predict the clinical response of anti-PD-1/PD-L1 therapy in cancer (143). On the other hand, the detection of some biomarkers requires the use of high-throughput sequencing or chip technologies, which are costly and require specialized equipment and personnel, limiting their use in resource-limited areas. Therefore, it is necessary to develop more convenient and economical detection methods, such as using multiplex PCR or multiplex IHC to detect specific genes or proteins (57, 144).
The research on these biomarkers also needs to explore their future directions and prospects. Some biomarker studies are still in their early stages and require more clinical trials and cohort studies to validate their effectiveness and reliability. Some biomarker studies also need to consider their interaction and impact with other treatment modalities, such as radiotherapy, chemotherapy, targeted therapy, etc., as well as the influence of different treatment regimens and sequences on biomarkers. Some biomarker studies also need to focus on the differences and applicability of biomarkers in different tumor subtypes and molecular subtypes, as well as in different populations and regions. Some biomarker studies also need to seek more new and potential biomarkers, as well as new and potential detection methods and technologies.
In conclusion, biomarkers are an important area in tumor immunotherapy, which requires more research and innovation to discover and develop more effective biomarkers. Future research should focus on improving the detection and predictive capabilities of biomarkers, integrating different biomarkers to construct more precise and personalized models, considering the clinical feasibility and practicality of biomarkers, and exploring new directions and prospects for biomarkers.
irAEs are a unique spectrum of side effects caused by immunotherapy, which result from the activation of immune cells against normal tissues (145). irAEs can affect various organs and systems, such as skin, gastrointestinal tract, liver, endocrine glands, lungs, kidneys, nervous system, and cardiovascular system (146, 147). The incidence and severity of irAEs vary depending on the type and combination of ICIs, the tumor type and stage, and the patient characteristics (148). The management of irAEs usually involves the use of corticosteroids or other immunosuppressive agents, which may compromise the anti-tumor efficacy of ICIs (149, 150). Therefore, it is important to monitor and diagnose irAEs early, and to develop strategies to prevent or treat them without affecting the anti-tumor response.
Resistance to immunotherapy is a major obstacle for achieving durable clinical benefits in NSCLC patients. Resistance can be classified into primary resistance, which occurs when tumors do not respond to ICIs at all, and acquired resistance, which occurs when tumors initially respond to ICIs but then relapse or progress (151). The mechanisms of resistance are complex and multifactorial, involving both intrinsic factors of tumor cells and extrinsic factors of TME. Some of the intrinsic factors include genetic alterations in tumor antigens (152), antigen presentation machinery (153), interferon signaling pathway (154), chromatin remodeling (155), and other inhibitory immune checkpoints (156). Some of the extrinsic factors include immunosuppressive cells (such as Treg cells, myeloid-derived suppressor cells, tumor-associated macrophages) (157, 158), soluble mediators (such as cytokines, chemokines, metabolites) (159) and vascular factors (such as hypoxia) (160). To overcome resistance to immunotherapy, several approaches have been proposed or tested in preclinical or clinical studies. These include identifying novel immune checkpoints or targets, modulating TME to enhance immune infiltration or activation, combining ICIs with other immunotherapies or conventional therapies (such as chemotherapy, radiotherapy, targeted therapy), and developing personalized or neoantigen-based vaccines.
Combination and sequential therapies are promising strategies to improve the efficacy and overcome the resistance of ICIs for NSCLC. However, there are many challenges and issues that need to be addressed before these strategies can be widely applied in clinical practice. These include the selection of optimal agents or modalities to combine with ICIs, the determination of optimal doses and schedules of each component, the evaluation of potential synergistic or antagonistic effects between different therapies, the identification of predictive biomarkers for each combination or sequence, the assessment of cost-effectiveness and quality of life for each combination or sequence, and the management of increased toxicity or irAEs caused by each combination or sequence.
5 Conclusion
In this review, we have discussed the role of tissue-resident immune cells in non-small cell lung cancer (NSCLC) immunity and immunotherapy. Tissue-resident immune cells are a heterogeneous population of immune cells that reside in various tissues, including the lung. Tissue-resident immune cells have distinct phenotypes, functions, and interactions with the tumor microenvironment (TME) compared to their circulating counterparts. Tissue-resident immune cells can exert anti-tumor or pro-tumor effects depending on their subsets and activation states. Tissue-resident immune cells are also involved in the response and resistance to immunotherapy, especially immune checkpoint inhibitors (ICIs), which are the mainstay of treatment for advanced NSCLC.
Tissue-resident immune cells represent a promising target for improving the efficacy and overcoming the resistance of ICIs for NSCLC. Several strategies have been proposed or tested to modulate tissue-resident immune cells in NSCLC, such as enhancing their recruitment, activation, or persistence in the TME, or inhibiting their immunosuppressive or tumor-promoting functions. Moreover, tissue-resident immune cells can be combined with other immunotherapeutic approaches, such as vaccines, adoptive cell therapy, oncolytic viruses, or bispecific antibodies, to achieve synergistic anti-tumor effects. However, there are still many challenges and issues that need to be addressed before tissue-resident immune cells can be widely applied in clinical practice. These include the identification of reliable biomarkers for tissue-resident immune cells, the optimization of delivery systems and dosing regimens for tissue-resident immune cells-based therapies, the evaluation of potential toxicities or adverse events caused by tissue-resident immune cells manipulation, and the development of personalized or precision medicine based on tissue-resident immune cells.
In conclusion, tissue-resident immune cells play an important role and have a special status in NSCLC immunity and immunotherapy. Future research directions should focus on developing novel tissue-resident immune cells-targeted drugs, exploring the synergistic effects of tissue-resident immune cells with other treatment modalities, and optimizing the application of tissue-resident immune cells in individualized therapy.
Author contributions
RT: Writing – original draft. HW: Writing – original draft. MT: Funding acquisition, Writing – review & editing.
Funding
The author(s) declare financial support was received for the research, authorship, and/or publication of this article. The authors thank the financial support provided by the Scientific Research Starting Foundation for Doctor of Southwest Medical University of China (2011204 to MT); the Joint Research Fund for Sichuan Province Science and Technology Agency and Southwest Medical University of China (14JC0161 to MT).
Acknowledgments
Figure drawing was supported by Figdraw.
Conflict of interest
The authors declare that the research was conducted in the absence of any commercial or financial relationships that could be construed as a potential conflict of interest.
Publisher’s note
All claims expressed in this article are solely those of the authors and do not necessarily represent those of their affiliated organizations, or those of the publisher, the editors and the reviewers. Any product that may be evaluated in this article, or claim that may be made by its manufacturer, is not guaranteed or endorsed by the publisher.
References
1. Ettinger DS, Wood DE, Aisner DL, Akerley W, Bauman JR, Bharat A, et al. Non-small cell lung cancer, version 3.2022, nccn clinical practice guidelines in oncology. J Natl Compr Canc Netw (2022) 20:497–530. doi: 10.6004/jnccn.2022.0025
2. Punekar SR, Shum E, Grello CM, Lau SC, Velcheti V. Immunotherapy in non-small cell lung cancer: past, present, and future directions. Front Oncol (2022) 12:877594. doi: 10.3389/fonc.2022.877594
3. Wang M, Herbst RS, Boshoff C. Toward personalized treatment approaches for non-small-cell lung cancer. Nat Med (2021) 27:1345–56. doi: 10.1038/s41591-021-01450-2
4. Reck M, Remon J, Hellmann MD. First-line immunotherapy for non-small-cell lung cancer. J Clin Oncol (2022) 40:586–97. doi: 10.1200/JCO.21.01497
5. Del Prete A, Wu Q. Editorial: tissue-resident immune cells in tumor immunity and immunotherapy. Front Cell Dev Biol (2022) 10:1068720. doi: 10.3389/fcell.2022.1068720
6. Gray JI, Farber DL. Tissue-resident immune cells in humans. Annu Rev Immunol (2022) 40:195–220. doi: 10.1146/annurev-immunol-093019-112809
7. Mantovani A, Allavena P, Sica A, Balkwill F. Cancer-related inflammation. Nature (2008) 454:436–44. doi: 10.1038/nature07205
8. Xu M, Wang Y, Xia R, Wei Y, Wei X. Role of the CCL2-CCR2 signalling axis in cancer: Mechanisms and therapeutic targeting. Cell Prolif (2021) 54:E13115. doi: 10.1111/cpr.13115
9. Zheng X, Weigert A, Reu S, Guenther S, Mansouri S, Bassaly B, et al. Spatial density and distribution of tumor-associated macrophages predict survival in non-small cell lung carcinoma. Cancer Res (2020) 80:4414–25. doi: 10.1158/0008-5472.CAN-20-0069
10. Yin M, Li X, Tan S, Zhou HJ, Ji W, Bellone S, et al. Tumor-associated macrophages drive spheroid formation during early transcoelomic metastasis of ovarian cancer. J Clin Invest (2016) 126:4157–73. doi: 10.1172/JCI87252
11. Casanova-Acebes M, Dalla E, Leader AM, Leberichel J, Nikolic J, Morales BM, et al. Tissue-resident macrophages provide A pro-tumorigenic niche to early nsclc cells. Nature (2021) 595:578–84. doi: 10.1038/s41586-021-03651-8
12. Pan Y, Yu Y, Wang X, Zhang T. Tumor-associated macrophages in tumor immunity. Front Immunol (2020) 11:583084. doi: 10.3389/fimmu.2020.583084
13. Okła K, Farber DL, Zou W. Tissue-resident memory T cells in tumor immunity and immunotherapy. J Exp Med (2021) 218:2. doi: 10.1084/jem.20201605
14. Milner JJ, Goldrath AW. Transcriptional programming of tissue-resident memory cd8(+) T cells. Curr Opin Immunol (2018) 51:162–9. doi: 10.1016/j.coi.2018.03.017
15. Boutet M, Gauthier L, Leclerc M, Gros G, De Montpreville V, Théret N, et al. Tgfβ Signaling intersects with cd103 integrin signaling to promote T-lymphocyte accumulation and antitumor activity in the lung tumor microenvironment. Cancer Res (2016) 76:1757–69. doi: 10.1158/0008-5472.CAN-15-1545
16. Franciszkiewicz K, Le Floc’h A, Boutet M, Vergnon I, Schmitt A, Mami-Chouaib F. Cd103 or lfa-1 engagement at the immune synapse between cytotoxic T cells and tumor cells promotes maturation and regulates T-cell effector functions. Cancer Res (2013) 73:617–28. doi: 10.1158/0008-5472.CAN-12-2569
17. Le Floc’h A, Jalil A, Franciszkiewicz K, Validire P, Vergnon I, Mami-Chouaib F. Minimal engagement of cd103 on cytotoxic T lymphocytes with an E-cadherin-fc molecule triggers lytic granule polarization via A phospholipase cgamma-dependent pathway. Cancer Res (2011) 71:328–38. doi: 10.1158/0008-5472.CAN-10-2457
18. Brownlie D, Von Kries A, Valenzano G, Wild N, Yilmaz E, Säfholm J, et al. Accumulation of tissue-resident natural killer cells, innate lymphoid cells, and cd8(+) T cells towards the center of human lung tumors. Oncoimmunology (2023) 12:2233402. doi: 10.1080/2162402X.2023.2233402
19. Weeden CE, Gayevskiy V, Marceaux C, Batey D, Tan T, Yokote K, et al. Early immune pressure initiated by tissue-resident memory T cells sculpts tumor evolution in non-small cell lung cancer. Cancer Cell (2023) 41:837–852.E6. doi: 10.1016/j.ccell.2023.03.019
20. Djenidi F, Adam J, Goubar A, Durgeau A, Meurice G, De Montpréville V, et al. Cd8+Cd103+ Tumor-infiltrating lymphocytes are tumor-specific tissue-resident memory T cells and A prognostic factor for survival in lung cancer patients. J Immunol (2015) 194:3475–86. doi: 10.4049/jimmunol.1402711
21. Dhodapkar MV, Dhodapkar KM. Tissue-resident memory-like T cells in tumor immunity: clinical implications. Semin Immunol (2020) 49:101415. doi: 10.1016/j.smim.2020.101415
22. O’brien SM, Klampatsa A, Thompson JC, Martinez MC, Hwang WT, Rao AS, et al. Function of human tumor-infiltrating lymphocytes in early-stage non-small cell lung cancer. Cancer Immunol Res (2019) 7:896–909. doi: 10.1158/2326-6066.CIR-18-0713
23. Russick J, Joubert PE, Gillard-Bocquet M, Torset C, Meylan M, Petitprez F, et al. Natural killer cells in the human lung tumor microenvironment display immune inhibitory functions. J Immunother Cancer (2020) 8:3. doi: 10.1136/jitc-2020-001054
24. Marquardt N, Kekäläinen E, Chen P, Lourda M, Wilson JN, Scharenberg M, et al. Unique transcriptional and protein-expression signature in human lung tissue-resident nk cells. Nat Commun (2019) 10:3–7. doi: 10.1038/s41467-019-11632-9
25. Chen X, Chen Y, Xin Z, Lin M, Hao Z, Chen D, et al. Tissue-resident cd69(+) cxcr6(+) natural killer cells with exhausted phenotype accumulate in human non-small cell lung cancer. Eur J Immunol (2022) 52:1993–2005. doi: 10.1002/eji.202149608
26. Schneider T, Hoffmann H, Dienemann H, Schnabel PA, Enk AH, Ring S, et al. Non-small cell lung cancer induces an immunosuppressive phenotype of dendritic cells in tumor microenvironment by upregulating B7-H3. J Thorac Oncol (2011) 6:1162–8. doi: 10.1097/JTO.0b013e31821c421d
27. Ng KW, Boumelha J, Enfield KSS, Almagro J, Cha H, Pich O, et al. Antibodies against endogenous retroviruses promote lung cancer immunotherapy. Nature (2023) 616:563–73. doi: 10.1038/s41586-023-05771-9
28. Gaudreau PO, Negrao MV, Mitchell KG, Reuben A, Corsini EM, Li J, et al. Neoadjuvant chemotherapy increases cytotoxic T cell, tissue resident memory T cell, and B cell infiltration in resectable nsclc. J Thorac Oncol (2021) 16:127–39. doi: 10.1016/j.jtho.2020.09.027
29. Tang J, Ramis-Cabrer D, Curull V, Wang X, Mateu-Jiménez M, Pijuan L, et al. B cells and tertiary lymphoid structures influence survival in lung cancer patients with resectable tumors. Cancers (Basel) (2020) 12:3. doi: 10.3390/cancers12092644
30. Salcher S, Sturm G, Horvath L, Untergasser G, Kuempers C, Fotakis G, et al. High-resolution single-cell atlas reveals diversity and plasticity of tissue-resident neutrophils in non-small cell lung cancer. Cancer Cell (2022) 40:1503–1520.E8. doi: 10.1016/j.ccell.2022.10.008
31. Kargl J, Busch SE, Yang GH, Kim KH, Hanke ML, Metz HE, et al. Neutrophils dominate the immune cell composition in non-small cell lung cancer. Nat Commun (2017) 8:14381. doi: 10.1038/ncomms14381
32. Yang M, Lin C, Wang Y, Chen K, Zhang H, Li W. Identification of a cytokine-dominated immunosuppressive class in squamous cell lung carcinoma with implications for immunotherapy resistance. Genome Med (2022) 14:72. doi: 10.1186/s13073-022-01079-x
33. Taniguchi S, Matsui T, Kimura K, Funaki S, Miyamoto Y, Uchida Y, et al. Inivonductionfctivin A-roducinglveolaracrophagesupportsherogressionfungellarcinoma. Nat Commun (2023) 14:143. doi: 10.1038/s41467-022-35701-8
34. Hattar K, Fink L, Fietzner K, Himmel B, Grimminger F, Seeger W, et al. Cell density regulates neutrophil il-8 synthesis: role of il-1 receptor antagonist and soluble tnf receptors. J Immunol (2001) 166:6287–93. doi: 10.4049/jimmunol.166.10.6287
35. Katayama Y, Hidalgo A, Chang J, Peired A, Frenette PS. Cd44 is A physiological E-selectin ligand on neutrophils. J Exp Med (2005) 201:1183–9. doi: 10.1084/jem.20042014
36. Yang Q, Tian S, Liu Z, Dong W. Knockdown of RIPK2 inhibits proliferation and migration, and induces apoptosis via the NF-κB signaling pathway in gastric cancer. Front Genet (2021) 12:627464. doi: 10.3389/fgene.2021.627464
37. Remark R, Becker C, Gomez JE, Damotte D, Dieu-Nosjean MC, Sautès-Fridman C, et al. The non-small cell lung cancer immune contexture. A major determinant of tumor characteristics and patient outcome. Am J Respir Crit Care Med (2015) 191:377–90. doi: 10.1164/rccm.201409-1671PP
38. Zlotnik A, Yoshie O. The chemokine superfamily revisited. Immunity (2012) 36:705–16. doi: 10.1016/j.immuni.2012.05.008
39. Harjunpää H, Llort Asens M, Guenther C, Fagerholm SC. Cell adhesion molecules and their roles and regulation in the immune and tumor microenvironment. Front Immunol (2019) 10. doi: 10.3389/fimmu.2019.01078
40. Chow A, SChad S, Green MD, Hellmann MD, Allaj V, Ceglia N, et al. Tim-4(+) cavity-resident macrophages impair anti-tumor cd8(+) T cell immunity. Cancer Cell (2021) 39:973–988.E9. doi: 10.1016/j.ccell.2021.05.006
41. Li L, Liu YD, Zhan YT, Zhu YH, Li Y, Xie D, et al. High levels of ccl2 or ccl4 in the tumor microenvironment predict unfavorable survival in lung adenocarcinoma. Thorac Cancer (2018) 9:775–84. doi: 10.1111/1759-7714.12643
42. Kawasaki T, Ikegawa M, Kawai T. Antigen presentation in the lung. Front Immunol (2022) 13:860915. doi: 10.3389/fimmu.2022.860915
43. Kraehenbuehl L, Weng CH, Eghbali S, Wolchok JD, Merghoub T. Enhancing immunotherapy in cancer by targeting emerging immunomodulatory pathways. Nat Rev Clin Oncol (2022) 19:37–50. doi: 10.1038/s41571-021-00552-7
44. Cohen M, Giladi A, Barboy O, Hamon P, Li B, Zada M, et al. The interaction of cd4(+) helper T cells with dendritic cells shapes the tumor microenvironment and immune checkpoint blockade response. Nat Cancer (2022) 3:303–17. doi: 10.1038/s43018-022-00338-5
45. Zhang YQ, Joost Van Neerven RJ, Van Gool SW, Coorevits L, De Boer M, Ceuppens JL. B7-d28nteractions Aatectingo-timulatoryignaloruman Tellesponses. Int Immunol (1997) 9:1095–102. doi: 10.1093/intimm/9.8.1095
46. Chen X, Gao A, Zhang F, Yang Z, Wang S, Fang Y, et al. Ilt4 inhibition prevents tam- and dysfunctional T cell-mediated immunosuppression and enhances the efficacy of anti-pd-L1 therapy in nsclc with egfr activation. Theranostics (2021) 11:3392–416. doi: 10.7150/thno.52435
47. Tu E, Mcglinchey K, Wang J, Martin P, Ching SL, Floc’h N, et al. Anti-PD-L1 and anti-CD73 combination therapy promotes T cell response to EGFR-mutated NSCLC. JCI Insight (2022) 7:4. doi: 10.1172/jci.insight.142843
48. Propper DJ, Balkwill FR. Harnessing cytokines and chemokines for cancer therapy. Nat Rev Clin Oncol (2022) 19:237–53. doi: 10.1038/s41571-021-00588-9
49. Kalies K, Blessenohl M, Nietsch J, Westermann J. T cell zones of lymphoid organs constitutively express th1 cytokine mrna: specific Changes during the early phase of an immune response. J Immunol (2006) 176:741–9. doi: 10.4049/jimmunol.176.2.741
50. Whitmire JK, Eam B, Benning N, Whitton JL. Directnterferon-ammaignalingramaticallynhancesd4+ Andd8+ Tellemory. J Immunol (2007) 179:1190–7. doi: 10.4049/jimmunol.179.2.1190
51. Van Der Veeken J, Gonzalez AJ, Cho H, Arvey A, Hemmers S, Leslie CS, et al. Memory of inflammation in regulatory T cells. Cell (2016) 166:977–90. doi: 10.1016/j.cell.2016.07.006
52. Stankovic B, Bjørhovde HAK, Skarshaug R, Aamodt H, Frafjord A, Müller E, et al. Immune cell composition in human non-small cell lung cancer. Front Immunol (2018) 9. doi: 10.3389/fimmu.2018.03101
53. Yan Y, Wang X, Liu C, Jia J. Association of lymphocyte subsets with efficacy and prognosis of immune checkpoint inhibitor therapy in advanced non-small cell lung carcinoma: a retrospective study. BMC Pulm Med (2022) 22:166. doi: 10.1186/s12890-022-01951-x
54. Gustafson MP, Lin Y, Laplant B, Liwski CJ, Maas ML, League SC, et al. Immune monitoring using the predictive power of immune profiles. J Immunother Cancer (2013) 1:7. doi: 10.1186/2051-1426-1-7
55. Hung YP, Sholl LM. Diagnostic and predictive immunohistochemistry for non-small cell lung carcinomas. Adv Anat Pathol (2018) 25:374–86. doi: 10.1097/PAP.0000000000000206
56. Righi L, Vavalà T, Rapa I, Vatrano S, Giorcelli J, Rossi G, et al. Impact of non-small-cell lung cancer-not otherwise specified immunophenotyping on treatment outcome. J Thorac Oncol (2014) 9:1540–6. doi: 10.1097/JTO.0000000000000271
57. Ilie M, Beaulande M, Hamila M, Erb G, Hofman V, Hofman P. Automated chromogenic multiplexed immunohistochemistry assay for diagnosis and predictive biomarker testing in non-small cell lung cancer. Lung Cancer (2018) 124:90–4. doi: 10.1016/j.lungcan.2018.07.037
58. Sun Z, Nyberg R, Wu Y, Bernard B, Redmond WL. Developing an enhanced 7-color multiplex IHC protocol to dissect immune infiltration in human cancers. PloS One (2021) 16:E0247238. doi: 10.1371/journal.pone.0247238
59. Pang J, Yu Q, Chen Y, Yuan H, Sheng M, Tang W. Integrating single-cell rna-seq to construct A neutrophil prognostic model for predicting immune responses in non-small cell lung cancer. J Transl Med (2022) 20:531. doi: 10.1186/s12967-022-03723-x
60. Wlosik J, Fattori S, Rochigneux P, Goncalves A, Olive D, Chretien AS. Immune biology of NSCLC revealed by single-cell technologies: implications for the development of biomarkers in patients treated with immunotherapy. Semin Immunopathol (2023) 45:29–41. doi: 10.1007/s00281-022-00973-1
61. Thome JJ, Yudanin N, Ohmura Y, Kubota M, Grinshpun B, Sathaliyawala T, et al. Spatial map of human T cell compartmentalization and maintenance over decades of life. Cell (2014) 159:814–28. doi: 10.1016/j.cell.2014.10.026
62. Ganesan AP, Clarke J, Wood O, Garrido-Martin EM, Chee SJ, Mellows T, et al. Tissue-resident memory features are linked to the magnitude of cytotoxic T cell responses in human lung cancer. Nat Immunol (2017) 18:940–50. doi: 10.1038/ni.3775
63. Duhen T, Duhen R, Montler R, Moses J, Moudgil T, De Miranda NF, et al. Co-expression of cd39 and cd103 identifies tumor-reactive cd8 T cells in human solid tumors. Nat Commun (2018) 9:7. doi: 10.1038/s41467-018-05072-0
64. Corgnac S, Malenica I, Mezquita L, Auclin E, Voilin E, Kacher J, et al. Cd103(+)Cd8(+) T(Rm) cells accumulate in tumors of anti-pd-1-responder lung cancer patients and are tumor-reactive lymphocytes enriched with tc17. Cell Rep Med (2020) 1:100127. doi: 10.1016/j.xcrm.2020.100127
65. Reina-Campos M, Heeg M, Kennewick K, Mathews IT, Galletti G, Luna V, et al. Metabolic programs of T cell tissue residency empower tumour immunity. Nature (2023) 621:179–87. doi: 10.1038/s41586-023-06483-w
66. Milner JJ, Toma C, Yu B, Zhang K, Omilusik K, Phan AT, et al. Runx3 programs cd8(+) T cell residency in non-lymphoid tissues and tumours. Nature (2017) 552:253–7. doi: 10.1038/nature24993
67. Gunther S, Ostheimer C, Stangl S, Specht HM, Mozes P, Jesinghaus M, et al. Correlation of hsp70 serum levels with gross tumor volume and composition of lymphocyte subpopulations in patients with squamous cell and adeno non-small cell lung cancer. Front Immunol (2015) 6:556. doi: 10.3389/fimmu.2015.00556
68. Wang D, Gu X, Liu X, Liu X, Wang B, Lao F, et al. “Acquired”. Nkg2d Ligand Stimulates Nk Cell-Mediated Tumor Immunosurveillance. J Immunother (2019) 42:189–96. doi: 10.1097/CJI.0000000000000276
69. Hamilton G, Plangger A. The impact of nk cell-based therapeutics for the treatment of lung cancer for biologics: targets and therapy. Biologics (2021) 15:265–77. doi: 10.3389/fimmu.2015.00556
70. Gentles AJ, Newman AM, Liu CL, Bratman SV, Feng W, Kim D, et al. The prognostic landscape of genes and infiltrating immune cells across human cancers. Nat Med (2015) 21:938–45. doi: 10.1038/nm.3909
71. Ilie M, Hofman V, Ortholan C, Bonnetaud C, Coëlle C, Mouroux J, et al. Predictive clinical outcome of the intratumoral cd66b-positive neutrophil-to-cd8-positive T-cell ratio in patients with resectable nonsmall cell lung cancer. Cancer (2012) 118:1726–37. doi: 10.1002/cncr.26456
72. Fridlender ZG, Sun J, Kim S, Kapoor V, Cheng G, Ling L, et al. Polarization of tumor-associated neutrophil phenotype by tgf-beta: “N1”. Versus “N2” Tan (2009) 16:183–94. doi: 10.1016/j.ccr.2009.06.017
73. Amarante-Mendes GP, Griffith TS. Therapeutic applications of trail receptor agonists in cancer and beyond. Pharmacol Ther (2015) 155:117–31. doi: 10.1016/j.pharmthera.2015.09.001
74. Eruslanov EB, Bhojnagarwala PS, Quatromoni JG, Stephen TL, Ranganathan A, Deshpande C, et al. Tumor-associated neutrophils stimulate T cell responses in early-stage human lung cancer. J Clin Invest (2014) 124:5466–80. doi: 10.1172/JCI77053
75. Wu Y, Biswas D, Usaite I, Angelova M, Boeing S, Karasaki T, et al. A local human Vδ1 T cell population is associated with survival in nonsmall-cell lung cancer. Nat Cancer (2022) 3:696–709. doi: 10.1038/s43018-022-00376-z
76. Spranger S, Dai D, Horton B, Gajewski TF. Tumor-residing Batf3 dendritic cells are required for effector T Cell trafficking and adoptive T Cell therapy. Cancer Cell (2017) 31:711–723.E4. doi: 10.1016/j.ccell.2017.04.003
77. Amsen D, Van Gisbergen K, Hombrink P, Van Lier RAW. Tissue-resident memory T cells at the center of immunity to solid tumors. Nat Immunol (2018) 19:538–46. doi: 10.1038/s41590-018-0114-2
78. Chen Y, Xin Z, Huang L, Zhao L, Wang S, Cheng J, et al. Cd8(+) T cells form the predominant subset of nkg2a(+) cells in human lung cancer. Front Immunol (2019) 10. doi: 10.3389/fimmu.2019.03002
79. Liu CY, Wang YM, Wang CL, Feng PH, Ko HW, Liu YH, et al. Population alterations of L-arginase- and inducible nitric oxide synthase-expressed cd11b+/cd14⁻/cd15+/cd33+ Myeloid-derived suppressor cells and cd8+ T lymphocytes in patients with advanced-stage non-small cell lung cancer. J Cancer Res Clin Oncol (2010) 136:35–45. doi: 10.1007/s00432-009-0634-0
80. Feng PH, Lee KY, Chang YL, Chan YF, Kuo LW, Lin TY, et al. Cd14(+)S100a9(+) monocytic myeloid-derived suppressor cells and their clinical relevance in non-small cell lung cancer. Am J Respir Crit Care Med (2012) 186:1025–36. doi: 10.1164/rccm.201204-0636OC
81. Genova C, Dellepiane C, Carrega P, Sommariva S, Ferlazzo G, Pronzato P, et al. Therapeutic implications of tumor microenvironment in lung cancer: focus on immune checkpoint blockade. Front Immunol (2021) 12:799455. doi: 10.3389/fimmu.2021.799455
82. Li T, Fu J, Zeng Z, Cohen D, Li J, Chen Q, et al. Timer2.0 for analysis of tumor-infiltrating immune cells. Nucleic Acids Res (2020) 48:W509–14. doi: 10.1093/nar/gkaa407
83. Li T, Fan J, Wang B, Traugh N, Chen Q, Liu JS, et al. Timer: A web server for comprehensive analysis of tumor-infiltrating immune cells. Cancer Res (2017) 77:E108–10. doi: 10.1158/1538-7445.AM2017-108
84. Hu K, Li Y, Rotenberg SA, Amatore C, Mirkin MV. Electrochemical measurements of reactive oxygen and nitrogen species inside single phagolysosomes of living macrophages. J Am Chem Soc (2019) 141:4564–8. doi: 10.1021/jacs.9b01217
85. Srinivas US, Tan BWQ, Vellayappan BA, Jeyasekharan AD. ROS and the DNA damage response in cancer. Redox Biol (2019) 25:101084. doi: 10.1016/j.redox.2018.101084
86. Ariztia EV, Lee CJ, Gogoi R, Fishman DA. The tumor microenvironment: key to early detection. Crit Rev Clin Lab Sci (2006) 43:393–425. doi: 10.1080/10408360600778836
87. Singh R, Mishra MK, Aggarwal H. Inflammation, immunity, and cancer. Mediators Inflammation (2017) 2017:6027305. doi: 10.1155/2017/6027305
88. Dongre A, Weinberg RA. New insights into the mechanisms of epithelial-mesenchymal transition and implications for cancer. Nat Rev Mol Cell Biol (2019) 20:69–84. doi: 10.1038/s41580-018-0080-4
89. Loyher PL, Hamon P, Laviron M, Meghraoui-Kheddar A, Goncalves E, Deng Z, et al. Macrophages of distinct origins contribute to tumor development in the lung. J Exp Med (2018) 215:2536–53. doi: 10.1084/jem.20180534
90. Taki M, Abiko K, Ukita M, Murakami R, Yamanoi K, Yamaguchi K, et al. Tumor immune microenvironment during epithelial-mesenchymal transition. Clin Cancer Res (2021) 27:4669–79. doi: 10.1158/1078-0432.CCR-20-4459
91. Huang Y, Hong W, Wei X. The molecular mechanisms and therapeutic strategies of emt in tumor progression and metastasis J Hematol Oncol (2022) 15:129. doi: 10.1186/s13045-022-01347-8
92. Reolo MJY, Otsuka M, Seow JJW, Lee J, Lee YH, Nguyen PHD, et al. Cd38 marks the exhausted cd8(+) tissue-resident memory T cells in hepatocellular carcinoma. Front Immunol (2023) 14:1182016. doi: 10.3389/fimmu.2023.1182016
93. Qu J, Jiang M, Wang L, Zhao D, Qin K, Wang Y, et al. Mechanism and potential predictive biomarkers of immune checkpoint inhibitors in nsclc. BioMed Pharmacother (2020) 127:109996. doi: 10.1016/j.biopha.2020.109996
94. Brahmer JR, Tykodi SS, Chow LQ, Hwu WJ, Topalian SL, Hwu P, et al. Safety and activity of anti-pd-L1 antibody in patients with advanced cancer. N Engl J Med (2012) 366:2455–65. doi: 10.1056/NEJMoa1200694
95. Borghaei H, Paz-Ares L, Horn L, Spigel DR, Steins M, Ready NE, et al. Nivolumab versus docetaxel in advanced nonsquamous non-small-cell lung cancer. N Engl J Med (2015) 373:1627–39. doi: 10.1056/NEJMoa1507643
96. Postow MA, Sidlow R, Hellmann MD. Immune-related adverse events associated with immune checkpoint blockade. N Engl J Med (2018) 378:158–68. doi: 10.1056/NEJMra1703481
97. Shankar B, Zhang J, Naqash AR, Forde PM, Feliciano JL, Marrone KA, et al. Multisystem immune-related adverse events associated with immune checkpoint inhibitors forreatmentfon-mallellungancer. JAMA Oncol (2020) 6:1952–6. doi: 10.1001/jamaoncol.2020.5012
98. Kim H, Park S, Han KY, Lee N, Kim H, Jung HA, et al. Clonal expansion of resident memory T cells in peripheral blood of patients with non-small cell lung cancer during immune checkpoint inhibitor treatment. J Immunother Cancer (2023) 11:8. doi: 10.1136/jitc-2022-005509
99. De Pas T, Giovannini M, Rescigno M, Catania C, Toffalorio F, Spitaleri G, et al. Vaccines in non-small cell lung cancer: rationale, combination strategies and update on clinical trials. Crit Rev Oncol Hematol (2012) 83:432–43. doi: 10.1016/j.critrevonc.2011.12.005
100. Awad MM, Govindan R, Balogh KN, Spigel DR, Garon EB, Bushway ME, et al. Personalized neoantigen vaccine neo-pv-01 with chemotherapy and anti-pd-1 as first-line treatment for non-squamous non-small cell lung cancer. Cancer Cell (2022) 40:1010–1026.E11. doi: 10.1016/j.ccell.2022.08.003
101. Sharma S, Srivastava MK, Harris-White M, Lee JM, Dubinett S. MUC1 peptide vaccine mediated antitumor activity in non-small cell lung cancer. Expert Opin Biol Ther (2011) 11:987–90. doi: 10.1517/14712598.2011.598146
102. Limacher JM, Quoix E. Tg4010: A therapeutic vaccine against muc1 expressing tumors. Oncoimmunology (2012) 1:791–2. doi: 10.4161/onci.19863
103. Wang QT, Nie Y, Sun SN, Lin T, Han RJ, Jiang J, et al. Tumor-associated antigen-based personalized dendritic cell vaccine in solid tumor patients. Cancer Immunol Immunother (2020) 69:1375–87. doi: 10.1007/s00262-020-02496-w
104. Adotévi O, Vernerey D, Jacoulet P, Meurisse A, Laheurte C, Almotlak H, et al. Safety, immunogenicity, and 1-year efficacy of universal cancer peptide-based vaccine in patients with refractory advanced non-small-cell lung cancer: A phase ib/phase iia de-escalation study. J Clin Oncol (2023) 41:373–84. doi: 10.1200/JCO.22.00096
105. Ott PA, Hu-Lieskovan S, Chmielowski B, Govindan R, Naing A, Bhardwaj N, et al. A phase ib trial of personalized neoantigen therapy plus anti-pd-1 in patients with advanced melanoma, non-small cell lung cancer, or bladder cancer. Cell (2020) 183:347–362.E24. doi: 10.1016/j.cell.2020.08.053
106. Hu Z, Zheng X, Jiao D, Zhou Y, Sun R, Wang B, et al. Lunx-car T cells as A targeted therapy for non-small cell lung cancer. Mol Ther Oncolytics (2020) 17:361–70. doi: 10.1016/j.omto.2020.04.008
107. Reppel L, Tsahouridis O, Akulian J, Davis IJ, Lee H, Fucà G, et al. Targeting disialoganglioside gd2 with chimeric antigen receptor-redirected T cells in lung cancer. J Immunother Cancer (2022) 10:9. doi: 10.1136/jitc-2021-003897
108. Ying Z, Huang XF, Xiang X, Liu Y, Kang X, Song Y, et al. Aafendotentnti-d19ar Tellherapy. Nat Med (2019) 25:947–53. doi: 10.1038/s41591-019-0421-7
109. Patel AJ, Richter A, Drayson MT, Middleton GW. The role of B lymphocytes in the immuno-biology of non-small-cell lung cancer. Cancer Immunol Immunother (2020) 69:325–42. doi: 10.1007/s00262-019-02461-2
110. Fucà G, Reppel L, Landoni E, Savoldo B, Dotti G. Enhancing chimeric antigen receptor T-cell efficacy in solid tumors. Clin Cancer Res (2020) 26:2444–51. doi: 10.1158/1078-0432.CCR-19-1835
111. Li J, Li W, Huang K, Zhang Y, Kupfer G, Zhao Q. Chimeric antigen receptor T cell (Car-T) immunotherapy for solid tumors: lessons learned and strategies for moving forward. J Hematol Oncol (2018) 11:22. doi: 10.1186/s13045-018-0568-6
112. Tian Y, Li Y, Shao Y, Zhang Y. Gene modification strategies for next-generation CAR T cells against solid cancers. J Hematol Oncol (2020) 13:54. doi: 10.1186/s13045-020-00890-6
113. Kaufman HL, Kohlhapp FJ, Zloza A. Oncolytic viruses: A new class of immunotherapy drugs. Nat Rev Drug Discovery (2015) 14:642–62. doi: 10.1038/nrd4663
114. Bommareddy PK, Shettigar M, Kaufman HL. Integrating oncolytic viruses in combination cancer immunotherapy. Nat Rev Immunol (2018) 18:498–513. doi: 10.1038/s41577-018-0014-6
115. Patel MR, Dash A, Jacobson BA, Ji Y, Baumann D, Ismail K, et al. Jak/stat inhibition with ruxolitinib enhances oncolytic virotherapy in non-small cell lung cancer models. Cancer Gene Ther (2019) 26:411–8. doi: 10.1038/s41417-018-0074-6
116. Masemann D, Meissner R, Schied T, Lichty BD, Rapp UR, Wixler V, et al. Synergistic anti-tumor efficacy of oncolytic influenza viruses and B7-H3 immune- checkpoint inhibitors against ic-resistant lung cancers. Oncoimmunology (2021) 10:1885778. doi: 10.1080/2162402X.2021.1885778
117. Rudin CM, Pandha HS, Zibelman M, Akerley WL, Harrington KJ, Day D, et al. Phase 1, open-label, dose-escalation study on the safety, pharmacokinetics, and preliminary efficacy of intravenous coxsackievirus A21 (V937), with or without pembrolizumab, in patients with advanced solid tumors. J Immunother Cancer (2023) 11:9. doi: 10.1136/jitc-2022-005007
118. Brabletz T, Pfeuffer I, Schorr E, Siebelt F, Wirth T, Serfling E. Transforming growth factor beta and cyclosporin A inhibit the inducible activity of the interleukin-2 gene in T cells through A noncanonical octamer-binding site. Mol Cell Biol (1993) 13:1155–62. doi: 10.1128/MCB.13.2.1155
119. Batlle E, Massagué J. Transforming growth factor-β Signaling in immunity and cancer. Immunity (2019) 50:924–40. doi: 10.1016/j.immuni.2019.03.024
120. Li L, Liu J, Xue H, Li C, Liu Q, Zhou Y, et al. A tgf-β-mta1-sox4-ezh2 signaling axis drives epithelial-mesenchymal transition in tumor metastasis. Oncogene (2020) 39:2125–39. doi: 10.1038/s41388-019-1132-8
121. Strauss J, Heery CR, Schlom J, Madan RA, Cao L, Kang Z, et al. Phase I trial of M7824 (MSB0011359C), a bifunctional fusion protein targeting PD-L1 and TGFβ, in advanced solid tumors. Clin Cancer Res (2018) 24:1287–95. doi: 10.1158/1078-0432.CCR-17-2653
122. Yi M, Wu Y, Niu M, Zhu S, Zhang J, Yan Y, et al. Anti-TGF-β/PD-L1 bispecific antibody promotes T cell infiltration and exhibits enhanced antitumor activity in triple-negative breast cancer. J Immunother Cancer (2022) 10:9. doi: 10.1136/jitc-2022-005543
123. Paz-Ares L, Kim TM, Vicente D, Felip E, Lee DH, Lee KH, et al. Bintrafusp alfa, A bifunctional fusion protein targeting tgf-β And pd-L1, in second-line treatment of patients with nsclc: results from an expansion cohort of A phase 1 trial. J Thorac Oncol (2020) 15:1210–22. doi: 10.1016/j.jtho.2020.03.003
124. Vernocchi P, Gili T, Conte F, Del Chierico F, Conta G, Miccheli A, et al. Network analysis of gut microbiome and metabolome to giscover microbiota-linked biomarkers in patients affected by non-small vell lung cancer. Int J Mol Sci (2020) 21:9. doi: 10.1158/2159-8290.CD-20-0116
125. Yun J, Lee SH, Kim SY, Jeong SY, Kim JH, Pyo KH, et al. Antitumor Activity of Amivantamab (JNJ-61186372), an EGFR-MET Bispecific Antibody, in Diverse Models of EGFR Exon 20 Insertion-Driven NSCLC. Cancer Discov (2020) 10:1194–209. doi: 10.1158/2159-8290.CD-20-0116
126. Srivastava S, Mohanty A, Nam A, Singhal S, Salgia R. Chemokines and NSCLC: Emerging role in prognosis, heterogeneity, and therapeutics. Semin Cancer Biol (2022) 86:233–46. doi: 10.1016/j.semcancer.2022.06.010
127. Chen XH, Chen R, Shi MY, Tian RF, Zhang H, Xin ZQ, et al. Chimeric antigen receptor T cells targeting cd147 for non-small cell lung cancer therapy. Transl Oncol (2022) 16:101309. doi: 10.1016/j.tranon.2021.101309
128. Besse B, Felip E, Garcia Campelo R, Cobo M, Mascaux C, Madroszyk A, et al. Randomized open-label controlled study of cancer vaccine ose2101 versus chemotherapy in hla-A2-positive patients with advanced non-small-cell lung cancer with resistance to immunotherapy: atalante-1. Ann Oncol (2023) 34:920–33. doi: 10.1016/j.annonc.2023.07.006
129. Doroshow DB, Bhalla S, Beasley MB, Sholl LM, Kerr KM, Gnjatic S, et al. Pd-L1 as A biomarker of response to immune-checkpoint inhibitors. Nat Rev Clin Oncol (2021) 18:345–62. doi: 10.1038/s41571-021-00473-5
130. Sun D, Liu J, Zhou H, Shi M, Sun J, Zhao S, et al. Classification of tumor immune microenvironment according to programmed death-ligand 1 expression and immune infiltration predicts response to immunotherapy plus chemotherapy in advanced patients with NSCLC. J Thorac Oncol (2023) 18:869–81. doi: 10.1016/j.jtho.2023.03.012
131. Park S, Ock CY, Kim H, Pereira S, Park S, Ma M, et al. Artificial intelligence-powered spatial analysis of tumor-infiltrating lymphocytes as complementary biomarker for immune checkpoint inhibition in non-small-cell lung cancer. J Clin Oncol (2022) 40:1916–28. doi: 10.1200/JCO.21.02010
132. Zhao P, Li L, Jiang X, Li Q. Mismatch repair deficiency/microsatellite instability-high as a predictor for anti-PD-1/PD-L1 immunotherapy efficacy. J Hematol Oncol (2019) 12:54. doi: 10.1186/s13045-019-0738-1
133. Lurienne L, Cervesi J, Duhalde L, De Gunzburg J, Andremont A, Zalcman G, et al. Nsclc immunotherapy efficacy and antibiotic use: A systematic review and meta-analysis. J Thorac Oncol (2020) 15:1147–59. doi: 10.1016/j.jtho.2020.03.002
134. Foy JP, Karabajakian A, Ortiz-Cuaran S, Boussageon M, Michon L, Bouaoud J, et al. Immunologically active phenotype by gene expression profiling is associated with clinical benefit from pd-1/pd-L1 inhibitors in real-world head and neck and lung cancer patients. Eur J Cancer (2022) 174:287–98. doi: 10.1016/j.ejca.2022.06.034
135. Nassar AH, Adib E, Abou Alaiwi S, El Zarif T, Groha S, Akl EW, et al. Ancestry-driven recalibration of tumor mutational burden and disparate clinical outcomes in response to immune checkpoint inhibitors. Cancer Cell (2022) 40:1161–1172.E5. doi: 10.1016/j.ccell.2022.08.022
136. Bravaccini S, Bronte G, Ulivi P. Tmb in nsclc: A broken dream? Int J Mol Sci (2021) 22:10. doi: 10.3390/ijms22126536
137. Chang L, Chang M, Chang HM, Chang F. Microsatellite instability: A predictive biomarker for cancer immunotherapy. Appl Immunohistochem Mol Morphol (2018) 26:E15–21. doi: 10.1097/PAI.0000000000000575
138. De Marchi P, Leal LF, Da Silva LS, Cavagna RO, Da Silva FAF, Da Silva VD, et al. Gene expression profiles (Geps) of immuno-oncologic pathways as predictors of response to checkpoint inhibitors in advanced nsclc. Transl Oncol (2023) 39:101818. doi: 10.1016/j.tranon.2023.101818
139. Addeo A, Friedlaender A, Banna GL, Weiss GJ. Tmb or not tmb as A biomarker: that is the question. Crit Rev Oncol Hematol (2021) 163:103374. doi: 10.1016/j.critrevonc.2021.103374
140. Li K, Luo H, Huang L, Luo H, Zhu X. Microsatellite instability: A review of what the oncologist should know. Cancer Cell Int (2020) 20:16. doi: 10.1186/s12935-019-1091-8
141. Shi Y, Lei Y, Liu L, Zhang S, Wang W, Zhao J, et al. Integration of comprehensive genomic profiling, tumor mutational burden, and PD-L1 expression to identify novel biomarkers of immunotherapy in non-small cell lung cancer. Cancer Med (2021) 10:2216–31. doi: 10.1002/cam4.3649
142. Wang F, Su Q, Li C. dentidication of novel biomarkers in non-small cell lung cancer using machine learning. Sci Rep (2022) 12:16693. doi: 10.1038/s41598-022-21050-5
143. Yi M, Jiao D, Xu H, Liu Q, Zhao W, Han X, et al. Biomarkers for predicting efficacy of PD-1/PD-L1 inhibitors. Mol Cancer (2018) 17:129. doi: 10.1186/s12943-018-0864-3
144. Leatham B, Mcnall K, Subramanian HKK, Jacky L, Alvarado J, Yurk D, et al. A rapid, multiplex digital pcr assay to detect gene variants and fusions in non-small cell lung cancer. Mol Oncol (2023) 17:2221–34. doi: 10.1002/1878-0261.13523
145. Okiyama N, Tanaka R. Immune-related adverse events in various organs caused by immune checkpoint inhibitors. Allergol Int (2022) 71:169–78. doi: 10.1016/j.alit.2022.01.001
146. Geisler AN, Phillips GS, Barrios DM, Wu J, Leung DYM, Moy AP, et al. Immune checkpoint inhibitor-related dermatologic adverse events. J Am Acad Dermatol (2020) 83:1255–68. doi: 10.1016/j.jaad.2020.03.132
147. Alruwaii ZI, Montgomery EA. Gastrointestinal and hepatobiliary immune-related adverse events: A histopathologic review. Adv Anat Pathol (2022) 29:183–93. doi: 10.1097/PAP.0000000000000346
148. Liu X, Shi Y, Zhang D, Zhou Q, Liu J, Chen M, et al. Risk factors for immune-related adverse events: what have we learned and what lies ahead? biomark Res (2021) 9:79. doi: 10.1186/s40364-021-00314-8
149. Luo J, Beattie JA, Fuentes P, Rizvi H, Egger JV, Kern JA, et al. Beyond steroids: immunosuppressants in steroid-refractory or resistant immune-related adverse events. J Thorac Oncol (2021) 16:1759–64. doi: 10.1016/j.jtho.2021.06.024
150. Nadelmann ER, Yeh JE, Chen ST. Management of cutaneous immune-related adverse events in patients with cancer treated with immune checkpoint inhibitors: A systematic review. JAMA Oncol (2022) 8:130–8. doi: 10.1001/jamaoncol.2021.4318
151. Schoenfeld AJ, Antonia SJ, Awad MM, Felip E, Gainor J, Gettinger SN, et al. Clinical definition of acquired resistance to immunotherapy in patients with metastatic non-small-cell lung cancer. Ann Oncol (2021) 32:1597–607. doi: 10.1016/j.annonc.2021.08.2151
152. Sun C, Nagaoka K, Kobayashi Y, Maejima K, Nakagawa H, Nakajima J, et al. Immunotherapies targeting neoantigens are effective in PD-1 blockade-resistant tumors. Int J Cancer (2023) 152:1463–75. doi: 10.1002/ijc.34382
153. Maier B, Leader AM, Chen ST, Tung N, Chang C, Leberichel J, et al. A conserved dendritic-cell regulatory program limits antitumour immunity. Nature (2020) 580:257–62. doi: 10.1038/s41586-020-2134-y
154. Garcia-Diaz A, Shin DS, Moreno BH, Saco J, Escuin-Ordinas H, Rodriguez GA, et al. Interferon receptor signaling pathways regulating pd-L1 and pd-L2 expression. Cell Rep (2017) 19:1189–201. doi: 10.1016/j.celrep.2017.04.031
155. Pan D, Kobayashi A, Jiang P, Ferrari De Andrade L, Tay RE, Luoma AM, et al. A major chromatin regulator determines resistance of tumor cells to T cell-mediated killing. Science (2018) 359:770–5. doi: 10.1126/science.aao1710
156. Beavis PA, Henderson MA, Giuffrida L, Davenport AJ, Petley EV, House IG, et al. Dual pd-1 and ctla-4 checkpoint blockade promotes antitumor immune responses through cd4+Foxp3– cell–mediated modulation of cd103+ Dendritic cells. Cancer Immunol Res (2018) 6:1069–81. doi: 10.1158/2326-6066.CIR-18-0291
157. Highfill SL, Cui Y, Giles AJ, Smith JP, Zhang H, Morse E, et al. Disruption of cxcr2-mediated mdsc tumor trafficking enhances anti-pd1 efficacy. Sci Transl Med (2014) 6:237ra67. doi: 10.1126/scitranslmed.3007974
158. Arce Vargas F, Furness AJS, Solomon I, Joshi K, Mekkaoui L, Lesko MH, et al. Fc-optimized anti-cd25 depletes tumor-infiltrating regulatory T cells and synergizes with pd-1 blockade to eradicate established tumors. Immunity (2017) 46:577–86. doi: 10.1016/j.immuni.2017.03.013
159. Do HTT, Lee CH, Cho J. Chemokines and their receptors: multifaceted roles in cancer progression and potential value as cancer prognostic markers. Cancers (Basel) (2020) 12:11. doi: 10.3390/cancers12020287
Keywords: non-small cell lung cancer, tissue-resident immune cells, immunotherapy, tumor immune microenvironment, immune checkpoint inhibitors
Citation: Tang R, Wang H and Tang M (2023) Roles of tissue-resident immune cells in immunotherapy of non-small cell lung cancer. Front. Immunol. 14:1332814. doi: 10.3389/fimmu.2023.1332814
Received: 03 November 2023; Accepted: 23 November 2023;
Published: 07 December 2023.
Edited by:
Sam Hanash, University of Texas MD Anderson Cancer Center, United StatesReviewed by:
Yihui Chen, University of Texas MD Anderson Cancer Center, United StatesMing Yi, Zhejiang University, China
Copyright © 2023 Tang, Wang and Tang. This is an open-access article distributed under the terms of the Creative Commons Attribution License (CC BY). The use, distribution or reproduction in other forums is permitted, provided the original author(s) and the copyright owner(s) are credited and that the original publication in this journal is cited, in accordance with accepted academic practice. No use, distribution or reproduction is permitted which does not comply with these terms.
*Correspondence: Mingxi Tang, bXh0YW5nNjlAMTYzLmNvbQ==
†These authors have contributed equally to this work and share first authorship